- Department of Psychiatry, BIDMC, Harvard Medical School, Boston, MA, United States
The primary aim of this research was to study the developmental trajectory of oscillatory synchronization in neural networks of normal healthy rats during adolescence, corresponding to the vulnerable age of schizophrenia prodrome in human. To monitor the development of oscillatory networks through adolescence we used a “pseudo-longitudinal” design. Recordings were performed in terminal experiments under urethane anesthesia, every day from PN32 to PN52 using rats-siblings from the same mother, to reduce individual innate differences between subjects. We found that hippocampal theta power decreased and delta power in prefrontal cortex increased through adolescence, indicating that the oscillations in the two different frequency bands follow distinct developmental trajectories to reach the characteristic oscillatory activity found in adults. Perhaps even more importantly, theta rhythm showed age-dependent stabilization toward late adolescence. Furthermore, sex differences was found in both networks, more prominent in the prefrontal cortex compared with hippocampus. Delta increase was stronger in females and theta stabilization was completed earlier in females, in postnatal days PN41-47, while in males it was only completed in late adolescence. Our finding of a protracted maturation of theta-generating networks in late adolescence is overall consistent with the findings of longitudinal studies in human adolescents, in which oscillatory networks demonstrated a similar pattern of maturation.
1. Introduction
Adolescence and early adulthood is commonly recognized as a critical period of psychiatric diseases, including schizophrenia (SZ). During this period, the brain undergoes dramatic developmental changes characterized, in general, by pruning of excitatory synapses, increased myelination, and proliferation of inhibitory circuits (Feinberg, 1982; Weinberger, 1987; Insel, 2010; Uhlhaas and Singer, 2011). All these components may affect the development of oscillations and long-range interregional coupling. There are also striking alterations in different neurotransmitter systems including those involved in generating network oscillations, such as GABA and glutamate, and NMDA receptor mechanisms (Monyer et al., 1994; Wenzel et al., 1997; Dumas, 2005a; Tseng and O’Donnell, 2007; Benoit-Marand and O’Donnell, 2008; Lewis and Gonzalez-Burgos, 2008; Hoftman and Lewis, 2011; Kilb, 2011; O’Donnell, 2011, 2012; Sturman and Moghaddam, 2011b; Huppe-Gourgues and O’Donnell, 2012). These changes occur at different time points and at a different pace during periadolescent stages of development. There are also discrepancies between neuronal developments of different structures resulting in a period between childhood and adulthood characterized by interregional imbalance (Casey et al., 2008; Somerville and Casey, 2010; Sturman and Moghaddam, 2011b). In particular, structural and functional development of the prefrontal cortex (PFC) and limbic circuits are more protracted into early adulthood compared with sensory cortex (Cressman et al., 2010). These data were mostly collected, however, using cross-sectional designs which have limited potential to decipher timing and trajectories of complex pathological processes.
The importance of longitudinal observations has been recognized in human studies using EEG and fMRI as indicators of adolescent brain maturation (Almli et al., 2007; Whitford et al., 2007a; Possel et al., 2008; Campbell and Feinberg, 2009; Uhlhaas et al., 2009; Feinberg and Campbell, 2010; Cragg et al., 2011; Feinberg et al., 2011; Gmehlin et al., 2011; Maurer et al., 2011; Uhlhaas and Singer, 2011; Mikanmaa et al., 2019). The common finding of human studies was a late maturation of oscillatory networks, indicated e.g., by increases in evoked and induced gamma oscillations in the auditory and visual cortex (Rojas et al., 2006; Poulsen et al., 2009; Uhlhaas et al., 2009; Werkle-Bergner et al., 2009). Developmental trends, also peaking in late adolescence and early adulthood, were also reported in low-frequency oscillations (Whitford et al., 2007b; Muller et al., 2009) and in long-range coupling between frontal and parietal regions (Srinivasan, 1999; Uhlhaas et al., 2009). A similarly delayed maturation of PFC-dependent executive control is well-established (Cressman et al., 2010; Miller et al., 2012; Naneix et al., 2012; O’Donnell, 2012).
Yet, the common approach to cellular and network-level investigation of development in animal models has until now been limited to comparing data from pre-adolescents (Spear, 2000; Kilb, 2011) with adults (Pen et al., 2006; Hogsden and Dringenberg, 2009; Gvilia et al., 2011; Sturman and Moghaddam, 2011a). Strong and rigorous research was primarily aimed at the role and mechanisms of oscillations, both local and transmitted between regions, in neonates (Leinekugel et al., 1997, 2002; Garaschuk et al., 1998; Khazipov et al., 2004; Buhl and Buzsaki, 2005; Ben-Ari et al., 2007; Minlebaev et al., 2007; Mohns et al., 2007; Brockmann et al., 2011; Del Rio-Bermudez et al., 2017; Griguoli and Cherubini, 2017; Ahlbeck et al., 2018) while periadolescent stages remain much less explored (Sato et al., 1979; Konopacki et al., 1988; Slawecki, 2002; Slawecki and Ehlers, 2003; Lychakov et al., 2007; Burton et al., 2008; Pian et al., 2008; Ehlers et al., 2013, 2018; Caban et al., 2018; Buzzell et al., 2019; Medlej et al., 2019; Muessig et al., 2019; Zhang et al., 2019).
In normal healthy brain of adults, slow and fast oscillations functionally interact to create an oscillator hierarchy which normally operates across multiple spatial and temporal scales (Bragin et al., 1995; Lakatos et al., 2004, 2005; Schroeder and Lakatos, 2009a,b; Belluscio et al., 2012). Neuronal ensembles dynamically synchronize their activities at fast (40–100 Hz gamma and >120 Hz high frequency oscillations) and characteristic slow oscillations (delta and theta). These latter also serve for long-range oscillatory coupling between distant networks. For example, hippocampal theta rhythm can modulate spike activity and locally generated gamma rhythm in target structures, including the PFC and vice versa, PFC slow oscillations in the delta range may in turn modulate spike and network activity in the hippocampus (Fujisawa and Buzsaki, 2011; Roy et al., 2017). Dynamic coupling between these structures is particularly important for specific cognitive functions in adults and plays an important role in early neurodevelopment (Leinekugel et al., 2002; Khazipov et al., 2004; Karlsson et al., 2006; Mohns et al., 2007; Mohns and Blumberg, 2008; Brockmann et al., 2011; Janiesch et al., 2011). Abnormal oscillations in the adult brain are well-established in SZ (Kwon et al., 1999; Spencer et al., 2008; Spencer, 2011; Gandal et al., 2012; Gonzalez-Burgos and Lewis, 2012; Gonzalez-Burgos et al., 2015; Hirano et al., 2015; Pittman-Polletta et al., 2015; Ferrarelli and Tononi, 2017; Hunt et al., 2017; Pratt et al., 2017; Uhlhaas et al., 2017; Dienel and Lewis, 2019); having been formulated as the concept of “gamma oscillatory endophenotype” (Gandal et al., 2012) that may underlie downstream phenotypic deficits characteristic for SZ. Slow oscillations are also altered but these are less clear and less studied (Boutros et al., 2008, 2014; Bates et al., 2009; Ducharme et al., 2012; Basar, 2013; Narayanan et al., 2014; Cousijn et al., 2015; Michaels et al., 2018; Kaefer et al., 2019).
Neural oscillations are directly related to parvalbumin-expressing (PV+) GABA interneurons which were shown impaired in SZ—representing one of the few signs consistently found in post-mortem examinations in human SZ (Blum and Mann, 2002; Heckers and Konradi, 2015; Kaar et al., 2019) and common in rodent models (Steullet et al., 2017). NMDA receptor (NMDA-R) input to PV+ neurons is essential for setting the level of their overall activity affecting the excitation/inhibition (E/I) balance, crucial for precise network activity. Dysregulation of E/I balance was implicated in SZ and NMDA-R antagonists were shown to mimic some of the positive and negative symptoms of SZ (Javitt and Zukin, 1991; Olney and Farber, 1995; Homayoun and Moghaddam, 2007), along with disrupting oscillator hierarchy (Pinault, 2008; Kittelberger et al., 2012; Kocsis, 2012b; Pittman-Polletta et al., 2018). The major input controlling PV+ cell activity, specifically targeting NMDA receptors expressing the NR2A (GluN2A) subunit, develops weeks after birth, well after the switch in GABA transmission from excitatory to inhibitory.
In this study we designed longitudinal investigations through the peri-adolescent period of normal rats, males and females to define how the oscillatory hierarchy develops in hippocampus and PFC. We hypothesized that maturation of oscillatory cortical networks is a protracted process occurring over the length of adolescence and into early adulthood during which the different components of the oscillatory hierarchy may follow different trajectories to arrive to the pattern of well-coordinated neural synchronization at different frequencies in adults. The study was limited to healthy rats to create a basis for future investigations aimed at development in psychiatric diseases.
2. Materials and methods
2.1. Animals
A total of 27 Spraque-Dawley rats were used to perform this experiment. All experiments were performed in accordance to the Beth Israel Deaconess Medical Center’s Institutional Animal Care and Use Committee (IACUC). A total of 22 Sprague-Dawley rats [both genders (10 males and 12 females) pups-siblings from two mothers (Charles River Laboratories, Cambridge, MA, USA) were used for recordings under urethane anesthesia in adolescence. Weaning occurred at postnatal day 21 (PN21) and the animals were recorded one a day, every day through adolescence, from PN32 to PN52 (body weight: 115–310 g). Three male rats from a third mother was delivered early after weaning and recorded under urethane anesthesia inPN25. Longitudinal recordings in unanesthetized, freely moving conditions were recorded in two rats, for several days through adolescence (PN41–PN52) and early adulthood (PN66–PN73).
2.2. Surgery
Surgery was performed under urethane anesthesia, which unlike other anesthetics, allows theta generation under controlled circumstances, in adult rats. The rat was injected intraperitoneally with two doses of urethane based on a concentration of 1 g/kg, with a 1 h interval. A total of six electrodes were implanted in the rat for recording in accordance to the stereotaxic coordinates, using the rat brain atlas of Paxinos & Watson (Paxinos and Watson, 1986). Specifically, two single-wire electrodes were implanted in the right and left prefrontal cortex (AP: +3.2, DV: -4.8, ML: +0.5 and -0.5, respectively) and a twisted double-wire electrodes implanted in the dorsal hippocampus (AP: -3.7, DV: -3.5, ML: +2.2)—the coordinates reflect the location of the longer electrode of the twisted pair whereas the shorter was located 0.8–1.2 mm dorsally. The electrodes were cemented to the bone using methyl methacrylate to secure them in place and then connected to the recording apparatus (A.M. Systems) for data collection.
2.3. Brain cryosectioning and histology
After the surgery and the data recording, the rats were euthanized with a 1 ml injection of ketamine and decapitated for the removal of the brain. The brain was stored at a low temperature in a 10% formalin solution (Fisher Scientific) in a glass vial. The formalin solution in the glass vial was exchanged for a 20% sucrose solution (Fisher Scientific), prior to sectioning. After removing the brain from the sucrose solution and freezing it with dry ice, a freezing microtome (Microm HM 450, Thermo Scientific) was used for slicing sections 50 μm thick. The brain slices were separated according to the rat brain atlas in a petri dish containing PBS, and then mounted on Superfrost plus microscope slides using gelatin buffer [Gelatin type A (Acros), chromium (III) potassium sulfate (Fisher Science Education), Sodium Azide (Fisher Scientific)]. The slices were left to dry before being stained in Cresyl Violet (Fisher Scientific) staining. After drying from the staining, the slides were cover-slipped with Permaslip (Alban Scientific Inc.) for preservation and a light microscope was used for the analyses of the electrode placements.
2.4. Electrophysiological recording and data analysis
Electrodes were connected to an amplifier (A-M systems) and local field potentials (LFPs) were recorded via the implanted electrodes and saved in DASYLab 7.0 Acquistion System Laboratory for the data collection. Each recording session lasted approximately two hours and was followed by euthanasia of the rat using an 1 ml injection of Ketamine. The signals as ∼.DDF-files in DASYLab 7.0 were extracted into Spike2 Cambridge Electronic Devices) for further filtering (low-pass filter set to pass signals under 10 Hz) and signal analysis in Spike2’s waveform and “sonogram” (spike-frequency plots) mode. Low-pass filter was set to pass signals under 10 Hz. Signals were subjected to Fast Fourier Transform and power density spectra were calculated. Frequencies with the greatest power (peak frequencies) were identified in the hippocampus and prefrontal cortex to define theta and delta oscillations, respectively. Peak power values were then calculated and Microsoft Excel formulas and functions (version 16.40) were used for the statistical computations and data analysis. Pearson’s correlation coefficient (r) was calculated between peak power and age for both theta and delta oscillations. It’s significance was tested using the excel procedure p = TDIST(x) where x = r*SQRT[(N-2)/(1–r*r)] approximately follows a t-distribution. Group averages of theta and delta power were compared using t-test for the entire population and two-way ANOVA for age-sex groups.
3. Results
3.1. Development of hippocampal theta rhythm through adolescence–urethane anesthetized rats
The presence of adult-type lasting theta oscillations under urethane anesthesia was verified at an early age after weaning, in PN25, in three rats-siblings (all male). Theta and wide-band delta activity alternated in all these experiments. Theta rhythm appeared at ∼4 Hz in the hippocampus as well as in the PFC (Figure 1). Narrow-band delta rhythm, present in the PFC in adults during theta states, was less obvious, it was either missing or spread in a wider range below 3–4 Hz (Figure 1).
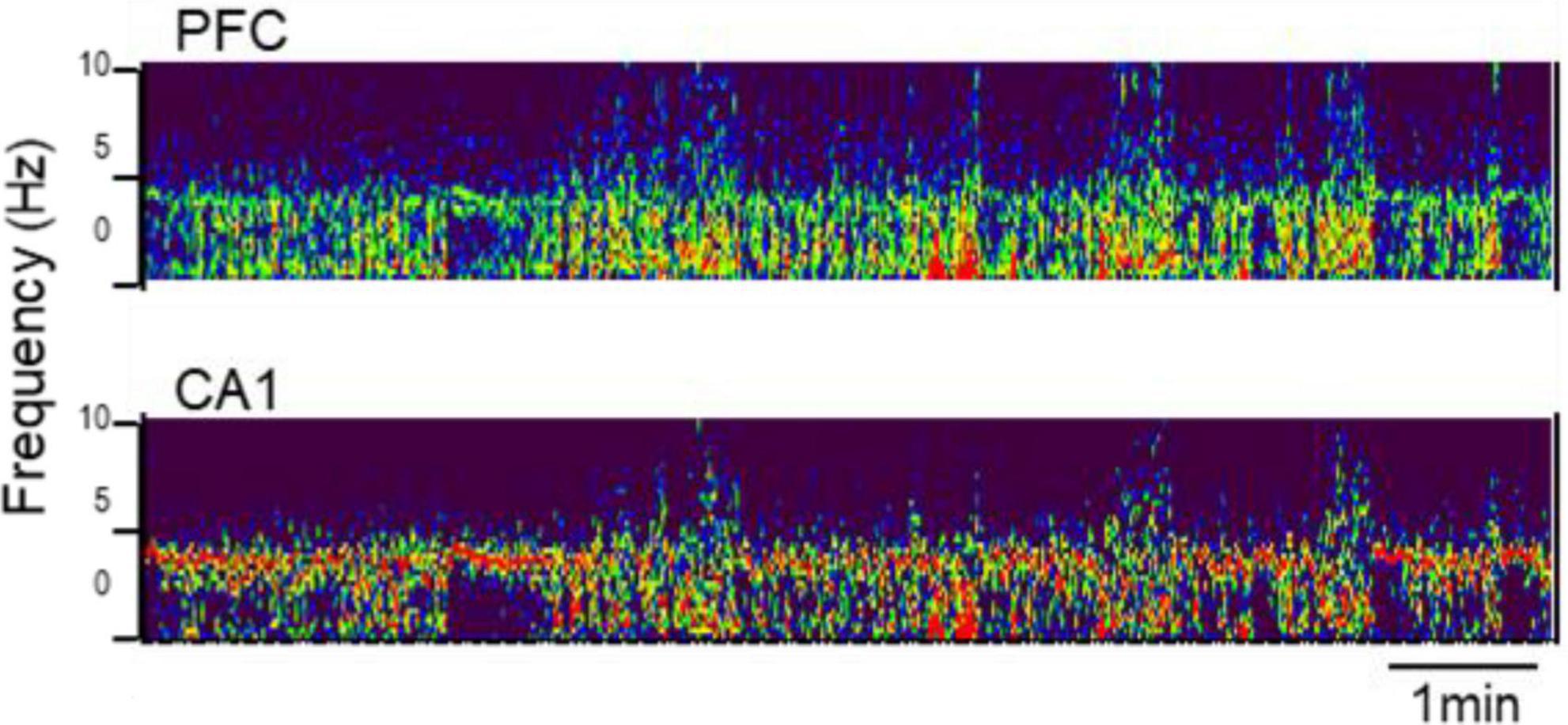
Figure 1. Rhythmic synchronization in prefrontal cortex (PFC) (top) and hippocampus [CA1, (bottom)] at PN25. Time-frequency plot (850 s × 0–10 Hz) under urethane anesthesia.
The development of low frequency oscillations was then analyzed in this model through adolescence, in detail. Urethane has an important advantage compared with other common anesthetics: intrinsic oscillations in forebrain networks, including hippocampal theta rhythm are preserved in adult rats under urethane. Therefore, this model provided major help in studying network mechanisms of oscillatory synchrony in adults, over decades (Kocsis and Vertes, 1994, 1996, 1997; Li et al., 2007; Sorman et al., 2011; Thorn et al., 2022). On the other hand, recordings under Urethane represent terminal procedures; the rats are sacrificed at the end of experiments. Thus, to monitor the development of oscillatory networks through adolescence we used a “pseudo-longitudinal” design. Recordings were performed every day from PN32 to PN52 using rats-siblings from the same mother, to reduce individual innate differences between subjects. A total of 22 rats (10 males and 12 females) from two mothers were included in this study. As shown in Figure 2, we recorded one or two rats a day with progressively increasing body weights from 115 to 190 grams in females and 120 to 310 grams in males.
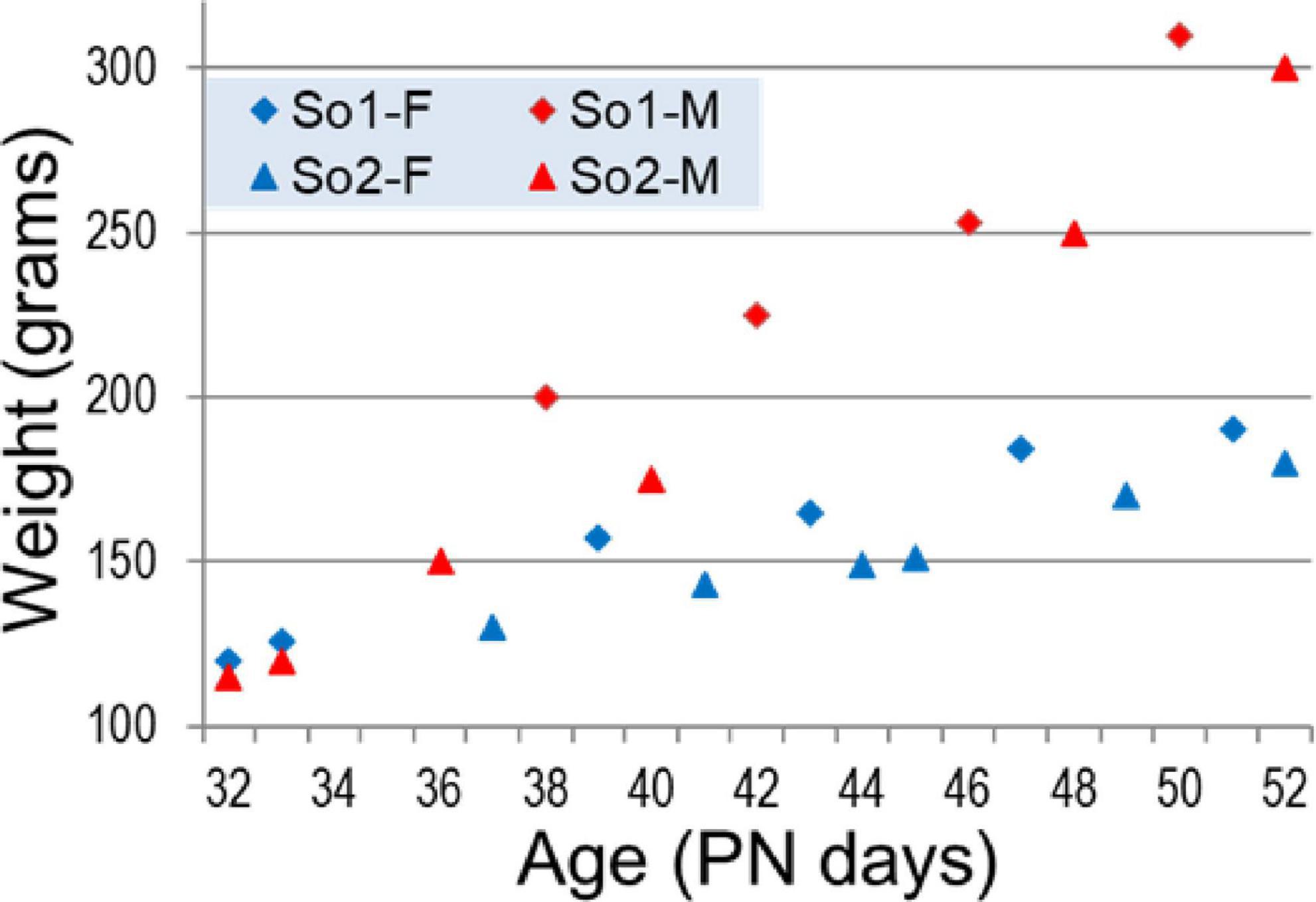
Figure 2. Weight of female (blue) and male (red) rat siblings born to two mothers (So1 and So2) in adolescence (age PN32–PN52).
Electroencephalograms (EEG) over frontal and parietal cortices and local field potentials (LFP) in deep electrodes placed in the prefrontal cortex (PFC) and hippocampus CA1 and DG (dentate gyrus) showed the pattern of activity typically recorded in urethane-anesthetized adult rats. Segments of wide band activity mostly in the delta range (1–4 Hz) alternated with segments showing strong oscillations. These episodes occurred simultaneously in all EEG and LFP channels; their occurrence and length was unpredictable, and not elicited by experimental interventions of any kind (Figure 3). Theta rhythm was dominant in parietal cortex and hippocampus in all rats, both male and female, from PN32 and PN52. It was also present in frontal cortex and PFC in lesser extent, simultaneously with a dominant rhythm in the delta range (Figure 4). Theta frequency varied between 3.66 and 5.37 Hz in individual rats. There was no significant difference between individuals in the two families (labeled as So1 and So2 in all figures) or between males and females (M and F in figures). Theta frequency did not change over adolescent development from PN32 to PN52 (Figure 5). Regular (not wide band) delta oscillations did not change either, remained in the narrow range of 1.22–2.65 Hz, similar in So1 and So2, and in the two sexes (Figure 5).
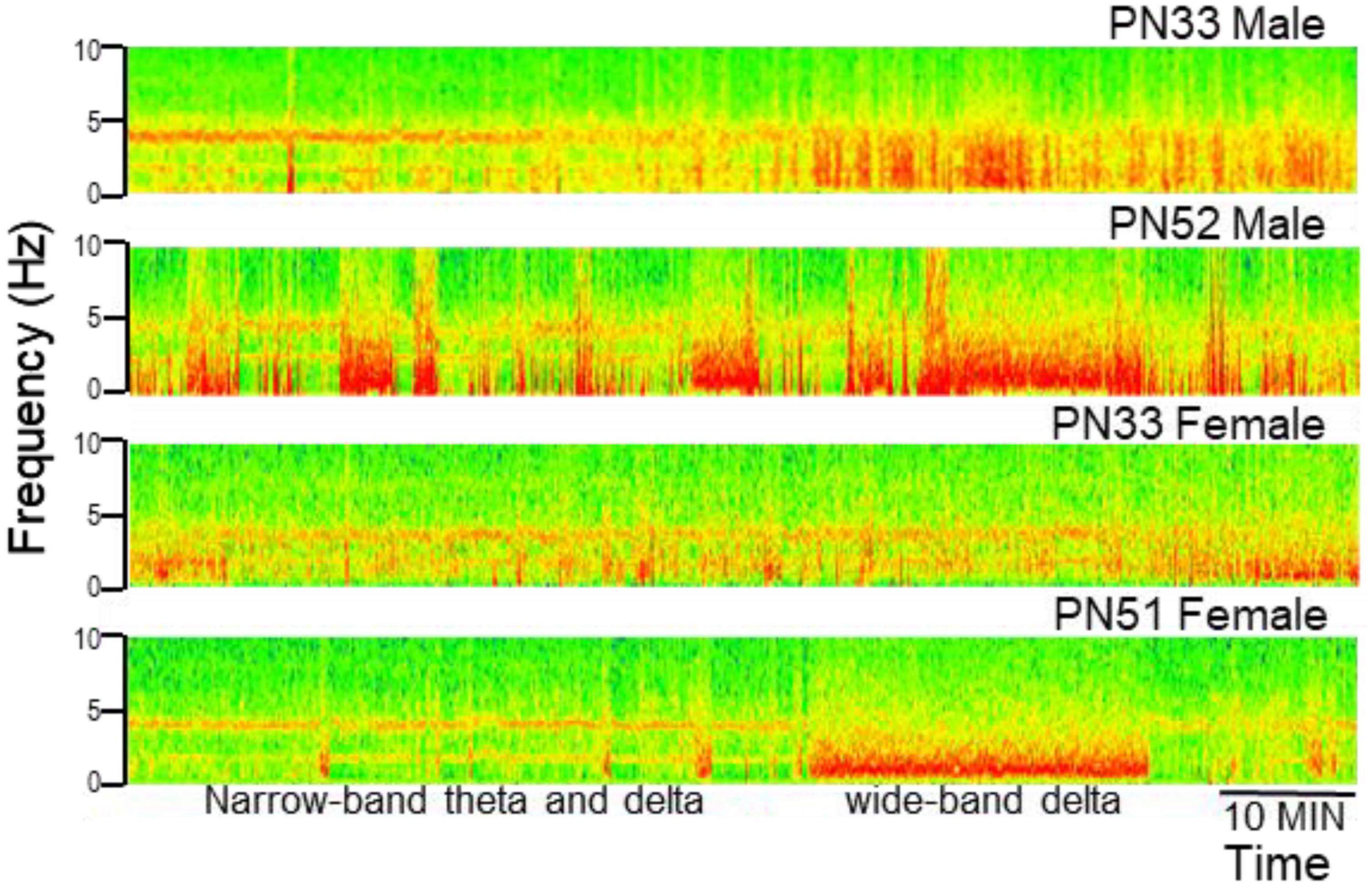
Figure 3. Examples of electrophysiological recordings (time frequency plots, power shown by color) in the hippocampus (CA1) in male and female rats at early (PN33) and late (PN51–52) adolescence. Note alternating states with narrow-band oscillations in theta and delta ranges and states characterized by wide-band delta activity (labeled in the last example).
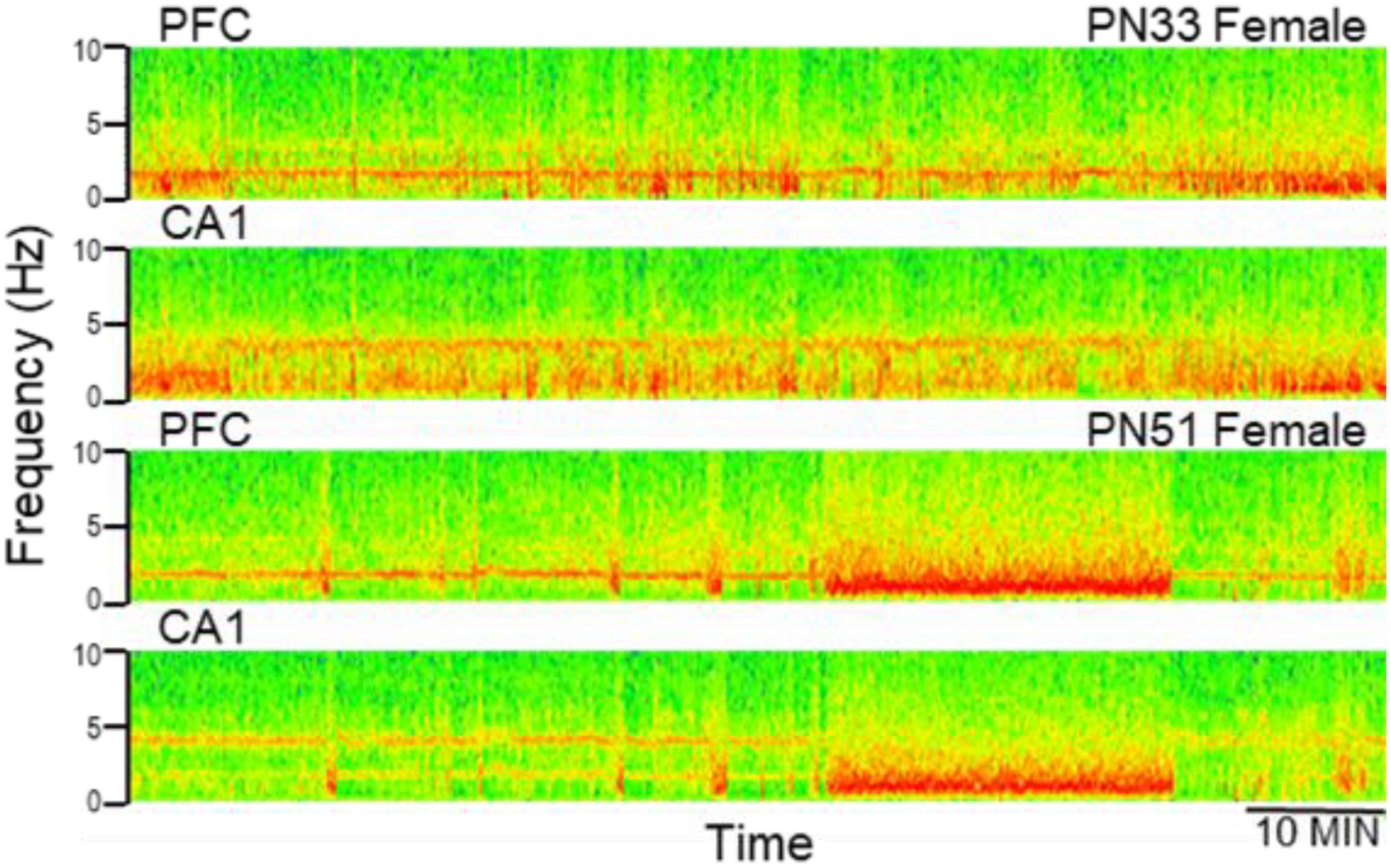
Figure 4. Examples of electrophysiological recordings (time frequency plots, power shown by color) in the prefrontal cortex (PFC) and hippocampus (CA1) at early (PN33) and late (PN51) adolescence. Note more dominant delta oscillations in PFC and theta in CA1, both at PN33 and PN51.
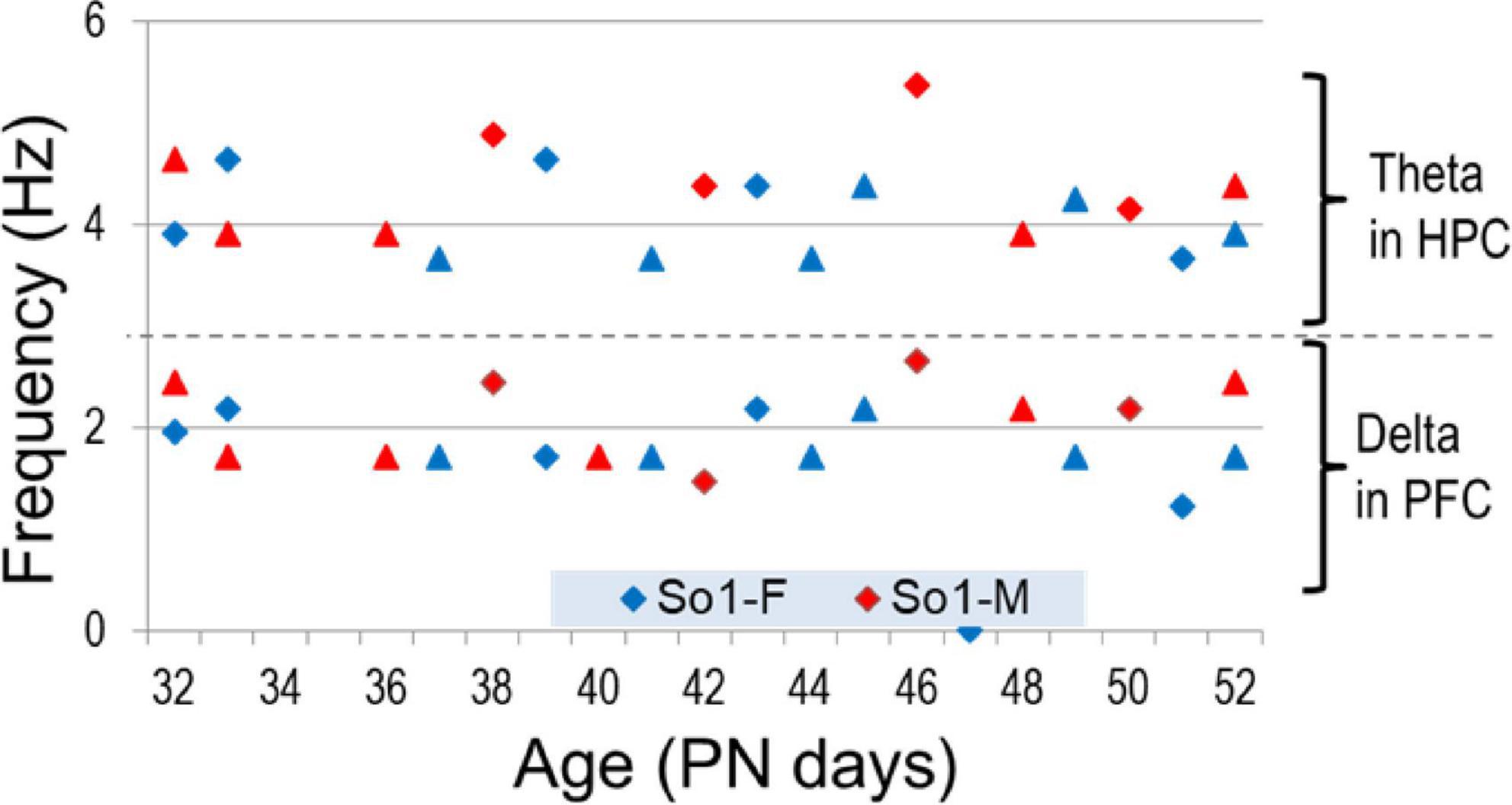
Figure 5. Frequency of theta and delta rhythms measured in hippocampal (HPC) and PFC recordings, respectively, through adolescence.
Theta was dominant in hippocampal recordings, the peak amplitude was 3.75 + 0.7 times higher than delta power (Figure 6A). In the PFC, peak delta power was larger (14.74 times) than local theta and varied in a wide range (1.39–28.04) (Figure 6B). Importantly however, the two rhythms in the two areas showed different developmental trends. Whereas theta dominance in the hippocampus showed no changes over time (p > 0.3), delta dominance in PFC became stronger to late adolescence (PN48–PN52) compared with the first (PN32–PN39; p = 0.02) and second weeks (PN41–PN47; p = 0.003), i.e., indicating either an increase in delta power in the PFC or a relative decrease in theta power transmitted from the hippocampus.
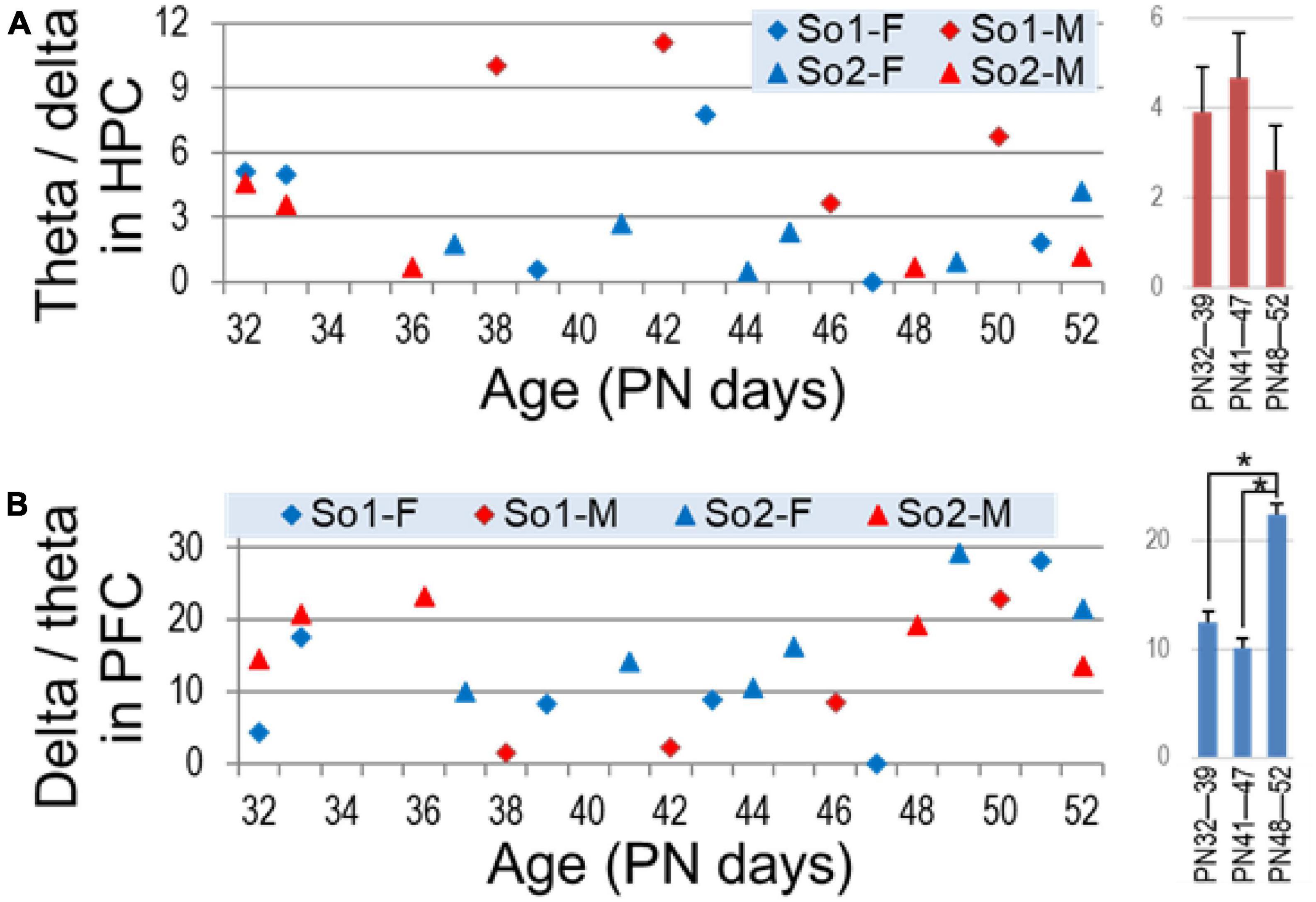
Figure 6. Dominance of theta (theta/delta ratio) in the hippocampus (A) and delta (delta/theta ratio) in the prefrontal cortex (PFC) (B) through adolescence. Right: average ± S.E.M of these parameters in age groups (early PN32—PN39, middle PN41—PN47, and late adolescence PN48—PN52). Asterisks show significant differences (p < 0.05).
Next we analyzed the temporal evolution of theta power in the hippocampus and delta in the PFC. Hippocampal theta power decreased through adolescence, shown both by significant differences between age groups (Figure 7A) and significant declines within the youngest (PN32—PN39, n = 7, r[age, theta] correlation, p = 0.014) and middle-age groups (PN41—PN47, n = 7, p = 0.003) to reach stable level in the oldest group (PN48—PN52, n = 6, p = 41) (Figure 7B). Perhaps even more importantly, theta power stabilized with age, as indicated by drastic reduction of standard deviation (Figure 7A) from the youngest group to middle-aged and then to older adolescents. The correlation between age and theta power was significant in both males and females for the entire period of adolescence (p < 0.001). Theta stabilization, i.e., drastic reduction of standard deviation, was also apparent in both sex groups, but whereas in females it was complete by the middle-age group, in males this process appeared slower or delayed (Figure 7C), although sex differences were not statistically different (two-way ANOVA, factors: age and sex with 3 and 2 levels, respectively).
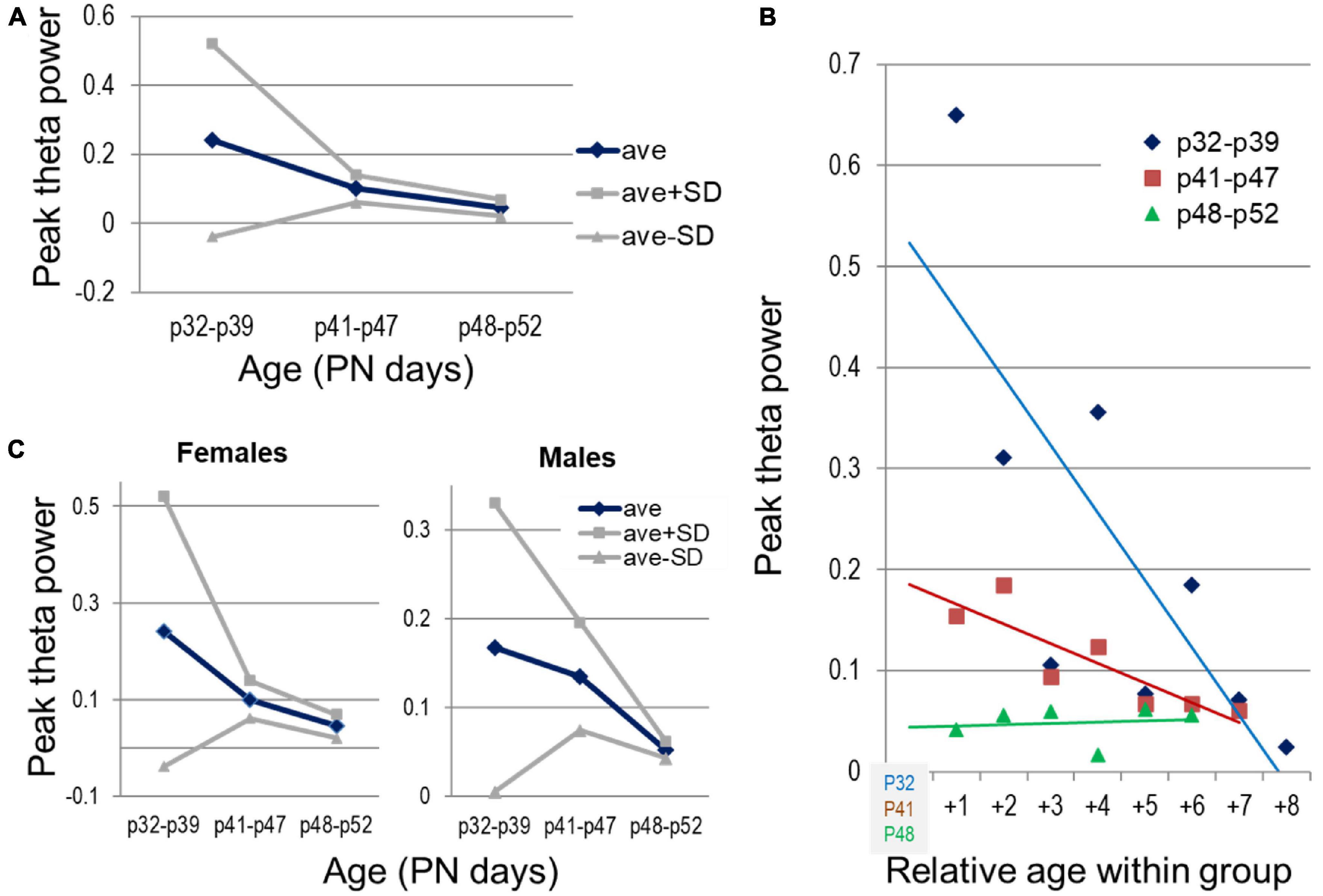
Figure 7. Development of theta oscillations in the hippocampus through adolescence. (A) Changes in peak theta power (average and standard deviations) with age, between three age groups, early (PN32—PN39, n = 7), middle (PN41—PN47, n = 7), and late adolescence (PN48—PN52, n = 6). (B) Peak theta power in individual experiments grouped by age (blue, brown, green). Age shown relative to the youngest age in each group. (C) Sex differences of the average theta power and its spreading within different age groups (n = 4, 4, 3 females and n = 3, 3, 3 males in the three age groups).
Development of narrow-band delta oscillations in PFC followed a different route. PFC delta was not seen at PN25 (Figure 1), but delta peak power significantly increased through adolescence (p = 0.038), with strong sex differences (p = 0.0007 for females and not significant in males: p = 0.413; p = 0.07 for sex-age interaction in two-way ANOVA) (Figure 8).
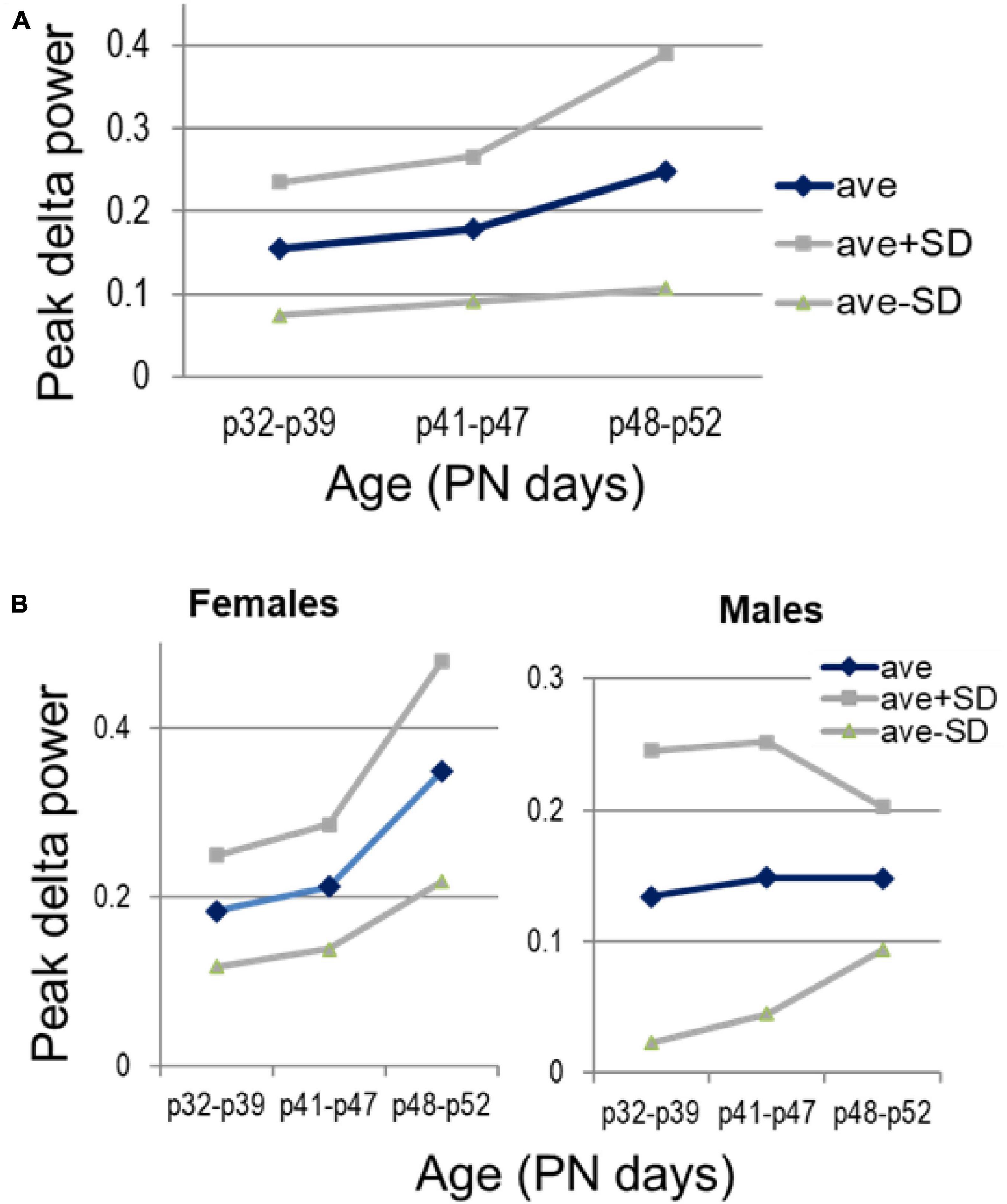
Figure 8. Development of delta oscillations in the prefrontal cortex (PFC) through adolescence. (A) Changes in peak delta power with age, between three age groups, early (PN32—PN39, n = 7), middle (PN41—PN47, n = 7), and late adolescence (PN48—PN52, n = 6). (B) Sex differences of the average delta power and its spreading within different age groups (n = 4, 4, 3 females and n = 3, 3, 3 males, in the three age groups).
3.2. Timing of theta development relative to gamma rhythm—in a small sample of freely moving rats
Oscillatory network activity is undoubtedly affected by anesthesia, both by the lack of normal behavior and sleep-wake sates and by side effects of urethane. Importantly, while slow oscillations (delta, theta) are present under urethane, gamma rhythm is generally suppressed. Gamma is a key component of oscillatory synchrony, involved in cognitive processing and expressed in all cortical structures in a behavior-dependent manner. Although investigations in freely behaving animals were beyond the scope of this study, we tested in a small sample (n = 2) how trends of development might compare for theta and gamma rhythms which normally co-occur in adult animals in wake exploration and REM sleep. In this pilot experiment, 24 h EEG recordings were made in a longitudinal study through adolescence and early adulthood (PN41–PN72), every other day over the frontal and parietal cortices. We found a tendency of theta development resembling that under urethane in adolescence i.e., a decrease from PN43–PN46 to PN48–PN52, which however, was followed by a massive increase in theta power after the rats reached adulthood (PN65–PN72) (Figure 9A). In contrast, gamma activity showed increase with age which appeared faster than theta, i.e., gamma power already reached the adult level in late adolescence (PN49–PN51; green in Figure 9B), around the time of theta stabilization under urethane. This finding suggests that slow and fast oscillations might be controlled by divergent rules; their relative trajectories of maturation remain to be investigated.
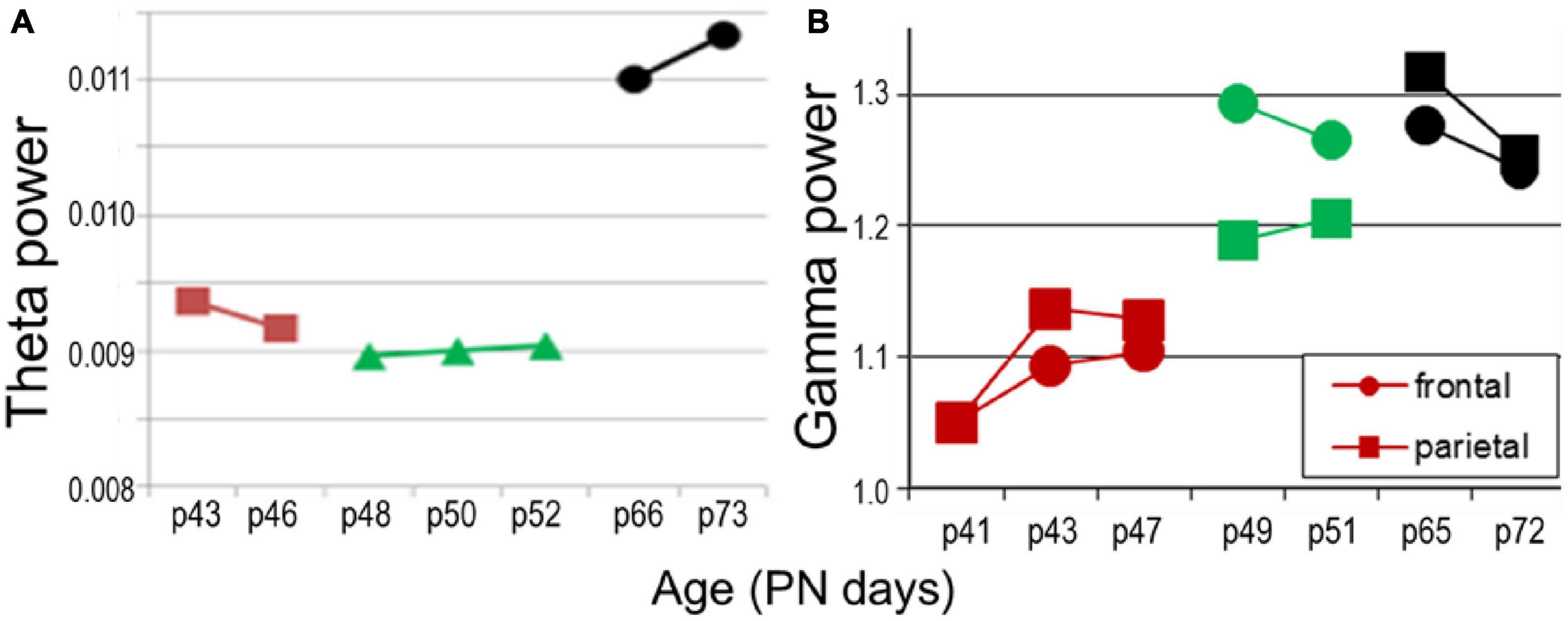
Figure 9. Network oscillations in longitudinal recordings in freely moving rats (n = 2) in age PN41–PN52 (colors matching those in Figure 7C) compared with adult (PN66–PN73, black). (A) Theta power (average in 5–10 Hz band). (B) Gamma power.
4. Discussion
The primary findings of this study was that both theta and delta oscillations were already present at age PN32 and then hippocampal theta peak power decreased and PFC delta peak power increased through adolescence, indicating that the oscillations in the two different frequency bands follow distinct developmental trajectories to reach the characteristic oscillatory activity found in adults. Perhaps even more importantly, theta rhythm showed age-dependent stabilization toward late adolescence. Standard deviation of peak theta power over the group drastically dropped, potentially symbolizing maturation of oscillatory networks in the hippocampus during adolescence. Furthermore, prominent sex difference was found in both networks. In the hippocampus, theta stabilization was completed earlier in females, in mid-adolescence, while in males it was completed in late adolescence. In the PFC, large interindividual variations in early adolescence appeared only in males and stabilized by late adolescence. These dissimilarities in time of stabilization of both rhythms highlights a potential gender difference in maturation where the development of oscillatory networks in males appears a more extended process. Our finding of a protracted maturation of theta-generating networks in late adolescence is overall consistent with the findings of longitudinal studies in human adolescents, in which oscillatory networks demonstrated a similar pattern of maturation (Rojas et al., 2006; Uhlhaas et al., 2009).
The findings are, in general, also consistent with the principles of development of oscillatory networks and the mechanisms involved, at another critical time of development, in neonates (Brockmann et al., 2011; Janiesch et al., 2011). Adult-like oscillations were confirmed in urethane-anesthetized rats as early as PN25, i.e., a few days after weaning. This is consistent with understanding of the development of oscillatory networks in neonates dependent on the critical switch between the function of GABAergic synapses from excitatory in neonates to inhibitory at later stages (Khirug et al., 2008; Kaila et al., 2014; Rinetti-Vargas et al., 2017). Thus, synchronized activity in neonates, appear as giant depolarizing potentials (Ben-Ari et al., 1989; Griguoli and Cherubini, 2017), or intermittent short oscillations (Leinekugel et al., 2002; Ben-Ari et al., 2007; Brockmann et al., 2011; Ahlbeck et al., 2018) different from network oscillations which are based on inhibitory GABA signaling which appear later.
Critical changes in synaptic function continue at later stages of development, as well. This concerns for example the reorganization of NMDA receptors (NMDA-Rs) commonly found in different species, including rodents, cat, rabbit, birds, frog, and human (Laurie et al., 1997; Chen and Steinmetz, 2000; Law et al., 2003), in which NMDA-Rs expressing the NR2B subunit, dominant from birth, are progressively replaced by NR2A subunit expressing receptors. The two receptors have divergent roles in synaptic transmission, plasticity, and neurodevelopment [see rev. Hensch, 2004; Paoletti et al., 2013; Shipton and Paulsen, 2014; Baez et al., 2018; Franchini et al., 2020; Vieira et al., 2020]. In rats, NR2A expression peaks in PN week 2–3 (Monyer et al., 1994; Riva et al., 1994; Zhong et al., 1995; Wenzel et al., 1997), i.e., after the change of GABA receptors from excitatory to inhibitory is completed, and reaches adult levels 3–5 weeks after birth. The timeline depends on activity and varies in different brain regions [rev. Dumas, 2005a,b]. The changes involve developmental shift (Sheng et al., 1994) in which NR2A expression sharply increases in the synapse and NR2Bs move to extrasynaptic receptors, resulting in preferential synaptic NR2A enrichment (Steigerwald et al., 2000) and extrasynaptic NR2B abundance (Groc et al., 2006). Functional differences were identified between synaptic vs. extrasynaptic NMDA-Rs and were traced back to differences in intracellular signaling to pro-survival or pro-apoptotic pathways, respectively (Hardingham and Bading, 2010).
This shift may also have direct, complex consequences on the development of neural oscillations. PV + GABA neurons, which are essential for rhythmogenesis (Buzsaki, 2006; Sohal et al., 2009), are specifically vulnerable to NR2A-selective NMDA-R blockade. NR2A subunit-containing NMDA-Rs play a specific role in the maintenance of the phenotype of PV+ interneurons. Exposure of cultured PV+ neurons to ketamine or NR2A-R antagonist induced time and dose-dependent decrease in PV and GAD67 whereas NR2B-R antagonist had no effect on PV and only partially reduced GAD67 (Kinney et al., 2006). In adult rats, NMDA-R blockade was also shown to act on oscillations in a subunit-specific manner, In agreement with the disproportional distribution of NR2A-containing NMDA-Rs on PV+ GABA neurons in rodents (Kinney et al., 2006; Xi et al., 2009), abnormal gamma enhancement for example, a well-known effect of non-specific NMDA-R antagonists, was only elicited by NR2A subunit-preferring but not by NR2B-selective antagonist (Kocsis, 2012a). Furthermore, slow rhythms modulating the amplitude of fast oscillations are also differentially affected by NR2A-active compounds. While enhanced theta modulation was prominent or potentiated by non-specific of NR2B-selective blockade, NR2A-preferring antagonist switched the balance in the opposite direction, from theta to delta modulation (Pittman-Polletta et al., 2018).
In this study we used a “pseudo-longitudinal” design in anesthetized rats, pursuing the obvious technical advantages of this model. The findings will however, require further validation in freely moving animals. Studies under urethane have traditionally been tested in parallel with freely moving experiments, from the very beginning (Kramis et al., 1975; Buzsaki et al., 1983, 1986; Brankack et al., 1993). It is important to note that even though different types of brain oscillations better survive urethane anesthesia than other anesthetics (Kramis et al., 1975; Kocsis and Gyimesi-Pelczer, 1998; Granata and Cohen, 2004; Szkudlarek et al., 2012; Totah et al., 2018), the comparison with natural states has important limitations. Since theta oscillations are preserved under urethane, this preparation has been widely used for mechanistic investigations of generating this rhythm (Buzsaki and Eidelberg, 1983; Vertes et al., 1993; Leung et al., 1994; Kocsis and Vertes, 1997; Vertes and Kocsis, 1997; Kocsis et al., 2001; Jackson and Bland, 2006; Shinohara et al., 2013; Vandecasteele et al., 2014; Bland et al., 2016) and related neuropharmacology (Li et al., 2007; Sorman et al., 2011; Ly et al., 2013; Stoiljkovic et al., 2016; Skovgard et al., 2018; Ahnaou et al., 2020). The urethane model provides important information regarding neuronal organization but theta “state” under urethane is not an analog of theta states in freely moving animals, i.e., active waking (where theta is always associated with locomotion) or REM sleep (always short, never lasting longer than a few minutes). As for delta rhythm, the states with wide-band delta activity and states with narrow-band delta oscillations mostly accompanying theta (see Figures 3, 4) are spontaneously alternating under urethane. In freely moving rats, the first has been commonly associated with delta activity during slow wave sleep for a long time while the second was shown more recently in waking in a behavior-dependent manner. Side effects of urethane at low frequencies were reported in previous studies across widespread areas of the neocortex. Urethane may trigger slow (typically, <1.0 Hz) oscillations and a prominent large-amplitude and low frequency (1 Hz) rhythm, which is similar to the oscillatory patterns in deactivated states of slow wave sleep (Steriade et al., 1993; Amzica and Steriade, 1995) and suppresses hippocampal subthreshold activity and neuronal synchronization (Yagishita et al., 2020).
Specifically important for this study is the suppression of gamma activity by urethane. Although excluding of the effect of external inputs such as sensory input and inputs related to motor activity which generate higher frequency oscillations that interfere with slower rhythms may have advantages studying delta-theta rhythms, suppression of gamma activity represent a major distortion of the oscillatory hierarchy in normal neural networks. Its assessment will require longitudinal recordings in freely moving animals. Our pilot study on a small sample of drug-free animals is promising in this direction. It showed that an increase in gamma activity in late adolescence may be matching the trajectory of stabilization of theta rhythm (compare Figures 7, 9). These changes, if replicated in an adequate sample, might advocate for a controlled developmental process with different timing of theta and gamma synchronizations., i.e., indicating an orderly process in which hippocampal theta activity assumes a progressively stable standard drive modulating gamma oscillations locally as well as in the PFC. Such mechanism, involving hippocampal theta, would adhere to the same principle as that found in early development [PN6-14 (Brockmann et al., 2011; Janiesch et al., 2011)], when theta rhythm originating first in the hippocampus was shown to shape the development of different forms of ensemble activity in structures showing slower development, e.g., PFC. We hypothesize that a similar mechanism may also function during the similarly protracted process of maturation in adolescence which can only be understood by the analysis of PFC-hippocampal interactions in a longitudinal design of simultaneous multisite recordings.
Data availability statement
The original contributions presented in this study are included in the article/supplementary material, further inquiries can be directed to the corresponding author.
Ethics statement
This animal study was reviewed and approved by BIDMC IACUC.
Author contributions
SS, RM, and BK contributed to the experiments, analysis, interpreting the results, and writing the report. All authors contributed to the article and approved the submitted version.
Funding
This work was supported by the National Institute of Mental Health grants R01 MH100820 and MH127483 to BK.
Conflict of interest
The authors declare that the research was conducted in the absence of any commercial or financial relationships that could be construed as a potential conflict of interest.
Publisher’s note
All claims expressed in this article are solely those of the authors and do not necessarily represent those of their affiliated organizations, or those of the publisher, the editors and the reviewers. Any product that may be evaluated in this article, or claim that may be made by its manufacturer, is not guaranteed or endorsed by the publisher.
References
Ahlbeck, J., Song, L., Chini, M., Bitzenhofer, S. H., and Hanganu-Opatz, I. L. (2018). Glutamatergic drive along the septo-temporal axis of hippocampus boosts prelimbic oscillations in the neonatal mouse. eLife 7:e33158. doi: 10.7554/eLife.33158
Ahnaou, A., Broadbelt, T., Biermans, R., Huysmans, H., Manyakov, N. V., and Drinkenburg, W. H. (2020). The phosphodiesterase-4 and glycine transporter-1 inhibitors enhance in vivo hippocampal theta network connectivity and synaptic plasticity, whereas D-serine does not. Transl. Psychiatry 10:197.
Almli, C. R., Rivkin, M. J., and McKinstry, R. C. (2007). The NIH MRI study of normal brain development (Objective-2): Newborns, infants, toddlers, and preschoolers. Neuroimage 35, 308–325. doi: 10.1016/j.neuroimage.2006.08.058
Amzica, F., and Steriade, M. (1995). Short- and long-range neuronal synchronization of the slow (< 1 Hz) cortical oscillation. J. Neurophysiol. 73, 20–38. doi: 10.1152/jn.1995.73.1.20
Baez, M. V., Cercato, M. C., and Jerusalinsky, D. A. (2018). NMDA receptor subunits change after synaptic plasticity induction and learning and memory acquisition. Neural Plast. 2018:5093048.
Basar, E. (2013). Brain oscillations in neuropsychiatric disease. Dialog. Clin. Neurosci. 15, 291–300.
Bates, A. T., Kiehl, K. A., Laurens, K. R., and Liddle, P. F. (2009). Low-frequency EEG oscillations associated with information processing in schizophrenia. Schizophr. Res. 115, 222–230.
Belluscio, M. A., Mizuseki, K., Schmidt, R., Kempter, R., and Buzsaki, G. (2012). Cross-frequency phase-phase coupling between theta and gamma oscillations in the hippocampus. J. Neurosci. 32, 423–435.
Ben-Ari, Y., Cherubini, E., Corradetti, R., and Gaiarsa, J. L. (1989). Giant synaptic potentials in immature rat CA3 hippocampal neurones. J. Physiol. 416, 303–325.
Ben-Ari, Y., Gaiarsa, J. L., Tyzio, R., and Khazipov, R. (2007). GABA: A pioneer transmitter that excites immature neurons and generates primitive oscillations. Physiol. Rev. 87, 1215–1284. doi: 10.1152/physrev.00017.2006
Benoit-Marand, M., and O’Donnell, P. (2008). D2 dopamine modulation of corticoaccumbens synaptic responses changes during adolescence. Eur. J. Neurosci. 27, 1364–1372. doi: 10.1111/j.1460-9568.2008.06107.x
Bland, B. H., Bland, C. E., and MacIver, M. B. (2016). Median raphe stimulation-induced motor inhibition concurrent with suppression of type 1 and type 2 hippocampal theta. Hippocampus 26, 289–300. doi: 10.1002/hipo.22521
Blum, B. P., and Mann, J. J. (2002). The GABAergic system in schizophrenia. Int. J. Neuropsychopharmacol. 5, 159–179.
Boutros, N. N., Arfken, C., Galderisi, S., Warrick, J., Pratt, G., and Iacono, W. (2008). The status of spectral EEG abnormality as a diagnostic test for schizophrenia. Schizophr. Res. 99, 225–237. doi: 10.1016/j.schres.2007.11.020
Boutros, N. N., Mucci, A., Vignapiano, A., and Galderisi, S. (2014). Electrophysiological aberrations associated with negative symptoms in schizophrenia. Curr. Top. Behav. Neurosci. 21, 129–156.
Bragin, A., Jand, G., Nádasdy, Z., Hetke, J., Wise, K., and Buzsáki, G. (1995). Gamma (40-100 Hz) oscillation in the hippocampus of the behaving rat. J. Neurosci. 15, 47–60.
Brankack, J., Stewart, M., and Fox, S. E. (1993). Current source density analysis of the hippocampal theta rhythm: Associated sustained potentials and candidate synaptic generators. Brain Res. 615, 310–327. doi: 10.1016/0006-8993(93)90043-m
Brockmann, M. D., Poschel, B., Cichon, N., and Hanganu-Opatz, I. L. (2011). Coupled oscillations mediate directed interactions between prefrontal cortex and hippocampus of the neonatal rat. Neuron 71, 332–347. doi: 10.1016/j.neuron.2011.05.041
Buhl, D. L., and Buzsaki, G. (2005). Developmental emergence of hippocampal fast-field “ripple” oscillations in the behaving rat pups. Neuroscience 134, 1423–1430. doi: 10.1016/j.neuroscience.2005.05.030
Burton, B. G., Economo, M. N., Lee, G. J., and White, J. A. (2008). Development of theta rhythmicity in entorhinal stellate cells of the juvenile rat. J. Neurophysiol. 100, 3144–3157. doi: 10.1152/jn.90424.2008
Buzsaki, G., and Eidelberg, E. (1983). Phase relations of hippocampal projection cells and interneurons to theta activity in the anesthetized rat. Brain Res. 266, 334–339.
Buzsaki, G., Czopf, J., Kondakor, I., and Kellenyi, L. (1986). Laminar distribution of hippocampal rhythmic slow activity (RSA) in the behaving rat: Current-source density analysis, effects of urethane and atropine. Brain Res. 365, 125–137. doi: 10.1016/0006-8993(86)90729-8
Buzsaki, G., Leung, L. W., and Vanderwolf, C. H. (1983). Cellular bases of hippocampal EEG in the behaving rat. Brain Res. 287, 139–171. doi: 10.1016/0165-0173(83)90037-1
Buzzell, G. A., Barker, T. V., Troller-Renfree, S. V., Bernat, E. M., Bowers, M. E., Morales, S., et al. (2019). Adolescent cognitive control, theta oscillations, and social observation. Neuroimage 198, 13–30.
Caban, B., Staszelis, A., Kazmierska, P., Kowalczyk, T., and Konopacki, J. (2018). Postnatal development of the posterior hypothalamic theta rhythm and local cell discharges in rat brain slices. Dev. Neurobiol. 78, 1049–1063. doi: 10.1002/dneu.22628
Campbell, I. G., and Feinberg, I. (2009). Longitudinal trajectories of non-rapid eye movement delta and theta EEG as indicators of adolescent brain maturation. Proc. Natl. Acad. Sci. U.S.A. 106, 5177–5180.
Chen, G., and Steinmetz, J. E. (2000). Intra-cerebellar infusion of NMDA receptor antagonist AP5 disrupts classical eyeblink conditioning in rabbits. Brain Res. 887, 144–156. doi: 10.1016/s0006-8993(00)03005-5
Cousijn, H., Tunbridge, E. M., Rolinski, M., Wallis, G., Colclough, G. L., Woolrich, M. W., et al. (2015). Modulation of hippocampal theta and hippocampal-prefrontal cortex function by a schizophrenia risk gene. Hum. Brain Mapp. 36, 2387–2395. doi: 10.1002/hbm.22778
Cragg, L., Kovacevic, N., McIntosh, A. R., Poulsen, C., Martinu, K., Leonard, G., et al. (2011). Maturation of EEG power spectra in early adolescence: A longitudinal study. Dev. Sci. 14, 935–943.
Cressman, V. L., Balaban, J., Steinfeld, S., Shemyakin, A., Graham, P., Parisot, N., et al. (2010). Prefrontal cortical inputs to the basal amygdala undergo pruning during late adolescence in the rat. J. Comp. Neurol. 518, 2693–2709. doi: 10.1002/cne.22359
Del Rio-Bermudez, C., Kim, J., Sokoloff, G., and Blumberg, M. S. (2017). Theta oscillations during active sleep synchronize the developing rubro-hippocampal sensorimotor network. Curr. Biol. 27, 1413–1424.e4. doi: 10.1016/j.cub.2017.03.077
Dienel, S. J., and Lewis, D. A. (2019). Alterations in cortical interneurons and cognitive function in schizophrenia. Neurobiol. Dis. 131:104208.
Ducharme, G., Lowe, G. C., Goutagny, R., and Williams, S. (2012). Early alterations in hippocampal circuitry and theta rhythm generation in a mouse model of prenatal infection: Implications for schizophrenia. PLoS One 7:e29754. doi: 10.1371/journal.pone.0029754
Dumas, T. C. (2005a). Developmental regulation of cognitive abilities: Modified composition of a molecular switch turns on associative learning. Prog. Neurobiol. 76, 189–211. doi: 10.1016/j.pneurobio.2005.08.002
Dumas, T. C. (2005b). Late postnatal maturation of excitatory synaptic transmission permits adult-like expression of hippocampal-dependent behaviors. Hippocampus 15, 562–578. doi: 10.1002/hipo.20077
Ehlers, C. L., Desikan, A., and Wills, D. N. (2013). Developmental differences in EEG and sleep responses to acute ethanol administration and its withdrawal (hangover) in adolescent and adult Wistar rats. Alcohol 47, 601–610. doi: 10.1016/j.alcohol.2013.09.040
Ehlers, C. L., Sanchez-Alavez, M., and Wills, D. (2018). Effect of gabapentin on sleep and delta and theta EEG power in adult rats exposed to chronic intermittent ethanol vapor and protracted withdrawal during adolescence. Psychopharmacology 235, 1783–1791. doi: 10.1007/s00213-018-4888-6
Feinberg, I. (1982). Schizophrenia: Caused by a fault in programmed synaptic elimination during adolescence? J. Psychiatr. Res. 17, 319–334. doi: 10.1016/0022-3956(82)90038-3
Feinberg, I., and Campbell, I. G. (2010). Sleep EEG changes during adolescence: An index of a fundamental brain reorganization. Brain Cogn. 72, 56–65.
Feinberg, I., de Bie, E., Davis, N. M., and Campbell, I. G. (2011). Topographic differences in the adolescent maturation of the slow wave EEG during NREM sleep. Sleep 34, 325–333.
Ferrarelli, F., and Tononi, G. (2017). Reduced sleep spindle activity point to a TRN-MD thalamus-PFC circuit dysfunction in schizophrenia. Schizophr. Res. 180, 36–43. doi: 10.1016/j.schres.2016.05.023
Franchini, L., Carrano, N., Di Luca, M., and Gardoni, F. (2020). Synaptic GluN2A-Containing NMDA receptors: From physiology to pathological synaptic plasticity. Int. J. Mol. Sci. 21:1538.
Fujisawa, S., and Buzsaki, G. (2011). A 4 Hz oscillation adaptively synchronizes prefrontal, VTA, and hippocampal activities. Neuron 72, 153–165. doi: 10.1016/j.neuron.2011.08.018
Gandal, M. J., Edgar, J. C., Klook, K., and Siegel, S. J. (2012). Gamma synchrony: Towards a translational biomarker for the treatment-resistant symptoms of schizophrenia. Neuropharmacology 62, 1504–1518. doi: 10.1016/j.neuropharm.2011.02.007
Garaschuk, O., Hanse, E., and Konnerth, A. (1998). Developmental profile and synaptic origin of early network oscillations in the CA1 region of rat neonatal hippocampus. J. Physiol. 507, 219–236. doi: 10.1111/j.1469-7793.1998.219bu.x
Gmehlin, D., Thomas, C., Weisbrod, M., Walther, S., Resch, F., and Oelkers-Ax, R. (2011). Development of brain synchronisation within school-age–individual analysis of resting (alpha) coherence in a longitudinal data set. Clin. Neurophysiol. 122, 1973–1983. doi: 10.1016/j.clinph.2011.03.016
Gonzalez-Burgos, G., and Lewis, D. A. (2012). NMDA receptor hypofunction, parvalbumin-positive neurons, and cortical gamma oscillations in schizophrenia. Schizophr. Bull. 38, 950–957.
Gonzalez-Burgos, G., Cho, R. Y., and Lewis, D. A. (2015). Alterations in cortical network oscillations and parvalbumin neurons in schizophrenia. Biol. Psychiatry 77, 1031–1040.
Granata, A. R., and Cohen, M. I. (2004). Different types of barosensory synaptic inputs to rostral ventrolateral medulla neurons of the rat. Brain Res. 998, 36–47. doi: 10.1016/j.brainres.2003.10.055
Griguoli, M., and Cherubini, E. (2017). Early correlated network activity in the hippocampus: Its putative role in shaping neuronal circuits. Front. Cell. Neurosci. 11:255. doi: 10.3389/fncel.2017.00255
Groc, L., Heine, M., Cousins, S. L., Stephenson, F. A., Lounis, B., Cognet, L., et al. (2006). NMDA receptor surface mobility depends on NR2A-2B subunits. Proc. Natl. Acad. Sci. U.S.A. 103, 18769–18774. doi: 10.1073/pnas.0605238103
Gvilia, I., Suntsova, N., Angara, B., McGinty, D., and Szymusiak, R. (2011). Maturation of sleep homeostasis in developing rats: A role for preoptic area neurons. Am. J. Physiol. Regul. Integr. Comp. Physiol. 300, R885–R894.
Hardingham, G. E., and Bading, H. (2010). Synaptic versus extrasynaptic NMDA receptor signalling: Implications for neurodegenerative disorders. Nat. Rev. Neurosci. 11, 682–696. doi: 10.1038/nrn2911
Heckers, S., and Konradi, C. (2015). GABAergic mechanisms of hippocampal hyperactivity in schizophrenia. Schizophr. Res. 167, 4–11.
Hirano, Y., Oribe, N., Kanba, S., Onitsuka, T., Nestor, P. G., Spencer, K. M., et al. (2015). Spontaneous Gamma Activity in Schizophrenia. JAMA Psychiatry 72, 813–821.
Hoftman, G. D., and Lewis, D. A. (2011). Postnatal developmental trajectories of neural circuits in the primate prefrontal cortex: Identifying sensitive periods for vulnerability to schizophrenia. Schizophr. Bull. 37, 493–503. doi: 10.1093/schbul/sbr029
Hogsden, J. L., and Dringenberg, H. C. (2009). Decline of long-term potentiation (LTP) in the rat auditory cortex in vivo during postnatal life: Involvement of NR2B subunits. Brain Res. 1283, 25–33. doi: 10.1016/j.brainres.2009.06.001
Homayoun, H., and Moghaddam, B. (2007). NMDA receptor hypofunction produces opposite effects on prefrontal cortex interneurons and pyramidal neurons. J. Neurosci. 27, 11496–11500. doi: 10.1523/JNEUROSCI.2213-07.2007
Hunt, M. J., Kopell, N. J., Traub, R. D., and Whittington, M. A. (2017). Aberrant network activity in schizophrenia. Trends Neurosci. 40, 371–382.
Huppe-Gourgues, F., and O’Donnell, P. D. (2012). (1)-NMDA receptor interactions in the rat nucleus accumbens change during adolescence. Synapse 66, 584–591. doi: 10.1002/syn.21544
Jackson, J., and Bland, B. H. (2006). Medial septal modulation of the ascending brainstem hippocampal synchronizing pathways in the anesthetized rat. Hippocampus 16, 1–10.
Janiesch, P. C., Kruger, H. S., Poschel, B., and Hanganu-Opatz, I. L. (2011). Cholinergic control in developing prefrontal-hippocampal networks. J. Neurosci. 31, 17955–17970. doi: 10.1523/JNEUROSCI.2644-11.2011
Javitt, D. C., and Zukin, S. R. (1991). Recent advances in the phencyclidine model of schizophrenia. Am. J. Psychiatry 148, 1301–1308.
Kaar, S. J., Angelescu, I., Marques, T. R., and Howes, O. D. (2019). Pre-frontal parvalbumin interneurons in schizophrenia: A meta-analysis of post-mortem studies. J. Neural. Transm. 126, 1637–1651. doi: 10.1007/s00702-019-02080-2
Kaefer, K., Malagon-Vina, H., Dickerson, D., O’Neill, J., Trossbach, S., Korth, C., et al. (2019). Disrupted-in-schizophrenia 1 overexpression disrupts hippocampal coding and oscillatory synchronization. Hippocampus 29, 802–816. doi: 10.1002/hipo.23076
Kaila, K., Price, T. J., Payne, J. A., Puskarjov, M., and Voipio, J. (2014). Cation-chloride cotransporters in neuronal development, plasticity and disease. Nat. Rev. Neurosci. 15, 637–654.
Karlsson, K. A., Mohns, E. J., di Prisco, G. V., and Blumberg, M. S. (2006). On the co-occurrence of startles and hippocampal sharp waves in newborn rats. Hippocampus 16, 959–965. doi: 10.1002/hipo.20224
Khazipov, R., Sirota, A., Leinekugel, X., Holmes, G. L., Ben-Ari, Y., and Buzsáki, G. (2004). Early motor activity drives spindle bursts in the developing somatosensory cortex. Nature 432, 758–761.
Khirug, S., Yamada, J., Afzalov, R., Voipio, J., Khiroug, L., and Kaila, K. (2008). GABAergic depolarization of the axon initial segment in cortical principal neurons is caused by the Na-K-2Cl cotransporter NKCC1. J. Neurosci. 28, 4635–4639. doi: 10.1523/JNEUROSCI.0908-08.2008
Kilb, W. (2011). Development of the GABAergic System from Birth to Adolescence. Neuroscientist 18, 613–630.
Kinney, J. W., Davis, C. N., Tabarean, I., Conti, B., Bartfai, T., and Behrens, M. M. (2006). A specific role for NR2A-containing NMDA receptors in the maintenance of parvalbumin and GAD67 immunoreactivity in cultured interneurons. J. Neurosci. 26, 1604–1615. doi: 10.1523/JNEUROSCI.4722-05.2006
Kittelberger, K., Hur, E. E., Sazegar, S., Keshavan, V., and Kocsis, B. (2012). Comparison of the effects of acute and chronic administration of ketamine on hippocampal oscillations: Relevance for the NMDA receptor hypofunction model of schizophrenia. Brain Struct. Funct. 217, 395–409. doi: 10.1007/s00429-011-0351-8
Kocsis, B. (2012b). State-dependent increase of cortical gamma activity during REM sleep after selective blockade of NR2B subunit containing NMDA Receptors. Sleep 35, 1011–1016. doi: 10.5665/sleep.1972
Kocsis, B. (2012a). Differential role of NR2A and NR2B subunits in N-methyl-D-aspartate receptor antagonist-induced aberrant cortical gamma oscillations. Biol. Psychiatry 71, 987–995. doi: 10.1016/j.biopsych.2011.10.002
Kocsis, B., and Gyimesi-Pelczer, K. (1998). Spinal segments communicating resting sympathetic activity to postganglionic nerves of the stellate ganglion. Am. J. Physiol. 275, R400–R409. doi: 10.1152/ajpregu.1998.275.2.R400
Kocsis, B., and Vertes, R. P. (1994). Characterization of neurons of the supramammillary nucleus and mammillary body that discharge rhythmically with the hippocampal theta rhythm in the rat. J. Neurosci. 14, 7040–7052.
Kocsis, B., and Vertes, R. P. (1996). Midbrain raphe cell firing and hippocampal theta rhythm in urethane-anaesthetized rats. Neuroreport 7, 2867–2872. doi: 10.1097/00001756-199611250-00012
Kocsis, B., and Vertes, R. P. (1997). Phase relations of rhythmic neuronal firing in the supramammillary nucleus and mammillary body to the hippocampal theta activity in urethane anesthetized rats. Hippocampus 7, 204–214. doi: 10.1002/(SICI)1098-1063(1997)7:2<204::AID-HIPO7>3.0.CO;2-M
Kocsis, B., Di Prisco, G. V., and Vertes, R. P. (2001). Theta synchronization in the limbic system: The role of Gudden’s tegmental nuclei. Eur. J. Neurosci. 13, 381–388.
Konopacki, J., Bland, B. H., and Roth, S. H. (1988). The development of carbachol-induced EEG ‘theta’ examined in hippocampal formation slices. Brain Res. 466, 229–232. doi: 10.1016/0165-3806(88)90048-x
Kramis, R., Vanderwolf, C. H., and Bland, B. H. (1975). Two types of hippocampal rhythmical slow activity in both the rabbit and the rat: Relations to behavior and effects of atropine, diethyl ether, urethane, and pentobarbital. Exp. Neurol. 49, 58–85. doi: 10.1016/0014-4886(75)90195-8
Kwon, J. S., O’Donnell, B. F., Wallenstein, G. V., Greene, R. W., Hirayasu, Y., Nestor, P. G., et al. (1999). Gamma frequency-range abnormalities to auditory stimulation in schizophrenia. Arch. Gen. Psychiatry 56, 1001–1005.
Lakatos, P., Shah, A. S., Knuth, K. H., Ulbert, I., Karmos, G., and Schroeder, C. E. (2005). An oscillatory hierarchy controlling neuronal excitability and stimulus processing in the auditory cortex. J. Neurophysiol. 94, 1904–1911. doi: 10.1152/jn.00263.2005
Lakatos, P., Szilágyi, N., Pincze, Z., Rajkai, C., Ulbert, I., and Karmos, G. (2004). Attention and arousal related modulation of spontaneous gamma-activity in the auditory cortex of the cat. Brain Res. Cogn. Brain Res. 19, 1–9. doi: 10.1016/j.cogbrainres.2003.10.023
Laurie, D. J., Bartke, I., Schoepfer, R., Naujoks, K., and Seeburg, P. H. (1997). Regional, developmental and interspecies expression of the four NMDAR2 subunits, examined using monoclonal antibodies. Brain Res. Mol. Brain Res. 51, 23–32. doi: 10.1016/s0169-328x(97)00206-4
Law, A. J., Weickert, C. S., Webster, M. J., Herman, M. M., Kleinman, J. E., Harrison, P. J., et al. (2003). Changes in NMDA receptor subunit mRNAs and cyclophilin mRNA during development of the human hippocampus. Ann. N. Y. Acad. Sci. 1003, 426–430. doi: 10.1196/annals.1300.043
Leinekugel, X., Khazipov, R., Cannon, R., Hirase, H., Ben-Ari, Y., and Buzsáki, G. (2002). Correlated bursts of activity in the neonatal hippocampus in vivo. Science 296, 2049–2052.
Leinekugel, X., Medina, I., Khalilov, I., Ben-Ari, Y., and Khazipov, R. (1997). Ca2+ oscillations mediated by the synergistic excitatory actions of GABA(A) and NMDA receptors in the neonatal hippocampus. Neuron 18, 243–255.
Leung, L. S., Martin, L. A., and Stewart, D. J. (1994). Hippocampal theta rhythm in behaving rats following ibotenic acid lesion of the septum. Hippocampus 4, 136–147. doi: 10.1002/hipo.450040204
Lewis, D. A., and Gonzalez-Burgos, G. (2008). Neuroplasticity of neocortical circuits in schizophrenia. Neuropsychopharmacology 33, 141–165.
Li, S., Topchiy, I., and Kocsis, B. (2007). The effect of atropine administered in the medial septum or hippocampus on high- and low-frequency theta rhythms in the hippocampus of urethane anesthetized rats. Synapse 61, 412–419.
Ly, S., Pishdari, B., Lok, L. L., Hajos, M., and Kocsis, B. (2013). Activation of 5-HT6 receptors modulates sleep-wake activity and hippocampal theta oscillation. ACS Chem. Neurosci. 4, 191–199. doi: 10.1021/cn300184t
Lychakov, D. V., Aristakesian, E. A., and Oganesian, G. A. (2007). [Effect of swinging on EEG of rats of juvenile age in the wakefulness state]. Zh Evol. Biokhim. Fiziol. 43, 427–434.
Maurer, U., Schulz, E., Brem, S., Mark, S v, Bucher, K., Martin, E., et al. (2011). The development of print tuning in children with dyslexia: Evidence from longitudinal ERP data supported by fMRI. Neuroimage 57, 714–722. doi: 10.1016/j.neuroimage.2010.10.055
Medlej, Y., Asdikian, R., Wadi, L., Salah, H., Dosh, L., Hashash, R., et al. (2019). Enhanced setup for wired continuous long-term EEG monitoring in juvenile and adult rats: Application for epilepsy and other disorders. BMC Neurosci. 20:8. doi: 10.1186/s12868-019-0490-z
Michaels, T. I., Long, L. L., Stevenson, I. H., Chrobak, J. J., and Chen, C. A. (2018). Effects of chronic ketamine on hippocampal cross-frequency coupling: Implications for schizophrenia pathophysiology. Eur. J. Neurosci. 48, 2903–2914. doi: 10.1111/ejn.13822
Mikanmaa, E., Grent-’t-Jong, T., Hua, L., Recasens, M., Thune, H., and Uhlhaas, P. J. (2019). Towards a neurodynamical understanding of the prodrome in schizophrenia. Neuroimage 190, 144–153. doi: 10.1016/j.neuroimage.2017.11.026
Miller, D. J., Duka, T., Stimpson, C. D., Schapiro, S. J., Baze, W. B., McArthur, M. J., et al. (2012). Prolonged myelination in human neocortical evolution. Proc. Natl. Acad. Sci. U.S.A. 109, 16480–16485.
Minlebaev, M., Ben-Ari, Y., and Khazipov, R. (2007). Network mechanisms of spindle-burst oscillations in the neonatal rat barrel cortex in vivo. J. Neurophysiol. 97, 692–700. doi: 10.1152/jn.00759.2006
Mohns, E. J., and Blumberg, M. S. (2008). Synchronous bursts of neuronal activity in the developing hippocampus: Modulation by active sleep and association with emerging gamma and theta rhythms. J. Neurosci. 28, 10134–10144.
Mohns, E. J., Karlsson, K. A., and Blumberg, M. S. (2007). Developmental emergence of transient and persistent hippocampal events and oscillations and their association with infant seizure susceptibility. Eur. J. Neurosci. 26, 2719–2730. doi: 10.1111/j.1460-9568.2007.05928.x
Monyer, H., Burnashev, N., Laurie, D. J., Sakmann, B., and Seeburg, P. H. (1994). Developmental and regional expression in the rat brain and functional properties of four NMDA receptors. Neuron 12, 529–540.
Muessig, L., Lasek, M., Varsavsky, I., Cacucci, F., and Wills, T. J. (2019). Coordinated emergence of hippocampal replay and theta sequences during post-natal development. Curr. Biol. 29, 834–840.e4. doi: 10.1016/j.cub.2019.01.005
Muller, V., Gruber, W., Klimesch, W., and Lindenberger, U. (2009). Lifespan differences in cortical dynamics of auditory perception. Dev. Sci. 12, 839–853.
Naneix, F., Marchand, A. R., Di Scala, G., Pape, J. R., and Coutureau, E. (2012). Parallel maturation of goal-directed behavior and dopaminergic systems during adolescence. J. Neurosci. 32, 16223–16232.
Narayanan, B., O’Neil, K., Berwise, C., Stevens, M. C., Calhoun, V. D., Clementz, B. A., et al. (2014). Resting state electroencephalogram oscillatory abnormalities in schizophrenia and psychotic bipolar patients and their relatives from the bipolar and schizophrenia network on intermediate phenotypes study. Biol. Psychiatry 76, 456–465. doi: 10.1016/j.biopsych.2013.12.008
O’Donnell, P. (2011). Adolescent onset of cortical disinhibition in schizophrenia: Insights from animal models. Schizophr. Bull. 37, 484–492. doi: 10.1093/schbul/sbr028
O’Donnell, P. (2012). Cortical disinhibition in the neonatal ventral hippocampal lesion model of schizophrenia: New vistas on possible therapeutic approaches. Pharmacol. Ther. 133, 19–25. doi: 10.1016/j.pharmthera.2011.07.005
Olney, J. W., and Farber, N. B. (1995). Glutamate receptor dysfunction and schizophrenia. Arch. Gen. Psychiatry 52, 998–1007.
Paoletti, P., Bellone, C., and Zhou, Q. (2013). NMDA receptor subunit diversity: Impact on receptor properties, synaptic plasticity and disease. Nat. Rev. Neurosci. 14, 383–400.
Paxinos, G., and Watson, C. (1986). The Rat Brain in Stereotaxic Coordinates. Sydney: Academic Press.
Pen, G. L., Gourevitch, R., Hazane, F., Hoareau, C., Jay, T. M., and Krebs, M. (2006). Peri-pubertal maturation after developmental disturbance: A model for psychosis onset in the rat. Neuroscience 143, 395–405. doi: 10.1016/j.neuroscience.2006.08.004
Pian, J. P., Criado, J. R., Walker, B. M., and Ehlers, C. L. (2008). Differential effects of acute alcohol on EEG and sedative responses in adolescent and adult Wistar rats. Brain Res. 1194, 28–36. doi: 10.1016/j.brainres.2007.11.057
Pinault, D. (2008). N-methyl d-aspartate receptor antagonists ketamine and MK-801 induce wake-related aberrant gamma oscillations in the rat neocortex. Biol. Psychiatry 63, 730–735. doi: 10.1016/j.biopsych.2007.10.006
Pittman-Polletta, B. R., Kocsis, B., Vijayan, S., Whittington, M. A., and Kopell, N. J. (2015). Brain rhythms connect impaired inhibition to altered cognition in schizophrenia. Biol. Psychiatry 77, 1020–1030. doi: 10.1016/j.biopsych.2015.02.005
Pittman-Polletta, B., Hu, K., and Kocsis, B. (2018). Subunit-specific NMDAR antagonism dissociates schizophrenia subtype-relevant oscillopathies associated with frontal hypofunction and hippocampal hyperfunction. Sci. Rep. 8:11588. doi: 10.1038/s41598-018-29331-8
Possel, P., Lo, H., Fritz, A., and Seemann, S. (2008). A longitudinal study of cortical EEG activity in adolescents. Biol. Psychol. 78, 173–178.
Poulsen, C., Picton, T. W., and Paus, T. (2009). Age-related changes in transient and oscillatory brain responses to auditory stimulation during early adolescence. Dev. Sci. 12, 220–235. doi: 10.1111/j.1467-7687.2008.00760.x
Pratt, J., Dawson, N., Morris, B. J., Grent-’t-Jong, T., Roux, F., and Uhlhaas, P. J. (2017). Thalamo-cortical communication, glutamatergic neurotransmission and neural oscillations: A unique window into the origins of ScZ? Schizophr. Res. 180, 4–12. doi: 10.1016/j.schres.2016.05.013
Rinetti-Vargas, G., Phamluong, K., Ron, D., and Bender, K. J. (2017). Periadolescent Maturation of GABAergic Hyperpolarization at the Axon Initial Segment. Cell Rep. 20, 21–29. doi: 10.1016/j.celrep.2017.06.030
Riva, M. A., Tascedda, F., Molteni, R., and Racagni, G. (1994). Regulation of NMDA receptor subunit mRNA expression in the rat brain during postnatal development. Brain Res. Mol. Brain Res. 25, 209–216.
Rojas, D. C., Maharajh, K., Teale, P. D., Kleman, M. R., Benkers, T. L., Carlson, J. P., et al. (2006). Development of the 40Hz steady state auditory evoked magnetic field from ages 5 to 52. Clin. Neurophysiol. 117, 110–117. doi: 10.1016/j.clinph.2005.08.032
Roy, A., Svensson, F. P., Mazeh, A., and Kocsis, B. (2017). Prefrontal-hippocampal coupling by theta rhythm and by 2-5 Hz oscillation in the delta band: The role of the nucleus reuniens of the thalamus. Brain Struct. Funct. 222, 2819–2830. doi: 10.1007/s00429-017-1374-6
Sato, H., Sakamoto, Y., Kamei, C., and Shimizu, M. (1979). EEG activity changes in juvenile rats chronically treated with phenobarbital. Fol. Psychiatr. Neurol. Jpn. 33, 323–327. doi: 10.1111/j.1440-1819.1979.tb00764.x
Schroeder, C. E., and Lakatos, P. (2009a). Low-frequency neuronal oscillations as instruments of sensory selection. Trends Neurosci. 32, 9–18. doi: 10.1016/j.tins.2008.09.012
Schroeder, C. E., and Lakatos, P. (2009b). The gamma oscillation: Master or slave? Brain Topogr. 22, 24–26.
Sheng, M., Cummings, J., Roldan, L. A., Jan, Y. N., and Jan, L. Y. (1994). Changing subunit composition of heteromeric NMDA receptors during development of rat cortex. Nature 368, 144–147. doi: 10.1038/368144a0
Shinohara, Y., Hosoya, A., and Hirase, H. (2013). Experience enhances gamma oscillations and interhemispheric asymmetry in the hippocampus. Nat. Commun. 4:1652. doi: 10.1038/ncomms2658
Shipton, O. A., and Paulsen, O. (2014). GluN2A and GluN2B subunit-containing NMDA receptors in hippocampal plasticity. Philos. Trans. R. Soc. Lond. B Biol. Sci. 369:20130163.
Skovgard, K., Agerskov, C., Kohlmeier, K. A., and Herrik, K. F. (2018). The 5-HT3 receptor antagonist ondansetron potentiates the effects of the acetylcholinesterase inhibitor donepezil on neuronal network oscillations in the rat dorsal hippocampus. Neuropharmacology 143, 130–142. doi: 10.1016/j.neuropharm.2018.09.017
Slawecki, C. J. (2002). Altered EEG responses to ethanol in adult rats exposed to ethanol during adolescence. Alcohol Clin. Exp. Res. 26, 246–254.
Slawecki, C. J., and Ehlers, C. L. (2003). The effects of corticotropin-releasing factor on the cortical EEG are reduced following adolescent nicotine exposure. Neuropeptides 37, 66–73. doi: 10.1016/s0143-4179(03)00006-4
Sohal, V. S., Zhang, F., Yizhar, O., and Deisseroth, K. (2009). Parvalbumin neurons and gamma rhythms enhance cortical circuit performance. Nature 459, 698–702. doi: 10.1038/nature07991
Somerville, L. H., and Casey, B. J. (2010). Developmental neurobiology of cognitive control and motivational systems. Curr. Opin. Neurobiol. 20, 236–241.
Sorman, E., Wang, D., Hajos, M., and Kocsis, B. (2011). Control of hippocampal theta rhythm by serotonin: Role of 5-HT2c receptors. Neuropharmacology 61, 489–494.
Spear, L. P. (2000). The adolescent brain and age-related behavioral manifestations. Neurosci. Biobehav. Rev. 24, 417–463.
Spencer, K. M. (2011). Baseline gamma power during auditory steady-state stimulation in schizophrenia. Front. Hum. Neurosci. 5:190. doi: 10.3389/fnhum.2011.00190
Spencer, K. M., Niznikiewicz, M. A., Shenton, M. E., and McCarley, R. W. (2008). Sensory-evoked gamma oscillations in chronic schizophrenia. Biol. Psychiatry 63, 744–747.
Srinivasan, R. (1999). Spatial structure of the human alpha rhythm: Global correlation in adults and local correlation in children. Clin. Neurophysiol. 110, 1351–1362. doi: 10.1016/s1388-2457(99)00080-2
Steigerwald, F., Schulz, T. W., Schenker, L. T., Kennedy, M. B., Seeburg, P. H., Köhr, G., et al. (2000). C-Terminal truncation of NR2A subunits impairs synaptic but not extrasynaptic localization of NMDA receptors. J. Neurosci. 20, 4573–4581.
Steriade, M., McCormick, D. A., and Sejnowski, T. J. (1993). Thalamocortical oscillations in the sleeping and aroused brain. Science 262, 679–685.
Steullet, P., Cabungcal, J., Coyle, J., Didriksen, M., Gill, K., Grace, A. A., et al. (2017). Oxidative stress-driven parvalbumin interneuron impairment as a common mechanism in models of schizophrenia. Mol. Psychiatry 22, 936–943. doi: 10.1038/mp.2017.47
Stoiljkovic, M., Kelley, C., Nagy, D., Leventhal, L., and Hajos, M. (2016). Selective activation of alpha7 nicotinic acetylcholine receptors augments hippocampal oscillations. Neuropharmacology 110, 102–108.
Sturman, D. A., and Moghaddam, B. (2011b). The neurobiology of adolescence: Changes in brain architecture, functional dynamics, and behavioral tendencies. Neurosci. Biobehav. Rev. 35, 1704–1712. doi: 10.1016/j.neubiorev.2011.04.003
Sturman, D. A., and Moghaddam, B. (2011a). Reduced neuronal inhibition and coordination of adolescent prefrontal cortex during motivated behavior. J. Neurosci. 31, 1471–1478. doi: 10.1523/JNEUROSCI.4210-10.2011
Szkudlarek, H. J., Orlowska, P., and Lewandowski, M. H. (2012). Light-induced responses of slow oscillatory neurons of the rat olivary pretectal nucleus. PLoS One 7:e33083. doi: 10.1371/journal.pone.0033083
Thorn, C. W., Kafetzopoulos, V., and Kocsis, B. (2022). Differential Effect of Dopamine D4 Receptor Activation on Low-Frequency Oscillations in the Prefrontal Cortex and Hippocampus May Bias the Bidirectional Prefrontal-Hippocampal Coupling. Int. J. Mol. Sci. 23:11705. doi: 10.3390/ijms231911705
Totah, N. K., Neves, R. M., Panzeri, S., Logothetis, N. K., and Eschenko, O. (2018). The Locus Coeruleus Is a Complex and Differentiated Neuromodulatory System. Neuron 99, 1055–1068.e6.
Tseng, K. Y., and O’Donnell, P. (2007). Dopamine modulation of prefrontal cortical interneurons changes during adolescence. Cereb. Cortex 17, 1235–1240.
Uhlhaas, P. J., and Singer, W. (2011). The development of neural synchrony and large-scale cortical networks during adolescence: Relevance for the pathophysiology of schizophrenia and neurodevelopmental hypothesis. Schizophr. Bull. 37, 514–523. doi: 10.1093/schbul/sbr034
Uhlhaas, P. J., Liddle, P., Linden, D. E., Nobre, A. C., Singh, K. D., and Gross, J. (2017). Magnetoencephalography as a Tool in Psychiatric Research: Current Status and Perspective. Biol. Psychiatry Cogn. Neurosci. Neuroimaging 2, 235–244. doi: 10.1016/j.bpsc.2017.01.005
Uhlhaas, P. J., Roux, F., Singer, W., Haenschel, C., Sireteanu, R., and Rodriguez, E. (2009). The development of neural synchrony reflects late maturation and restructuring of functional networks in humans. Proc. Natl. Acad. Sci. U.S.A. 106, 9866–9871. doi: 10.1073/pnas.0900390106
Vandecasteele, M., Varga, V., Berényi, A., Papp, E., Barth, P., Venance, L., et al. (2014). Optogenetic activation of septal cholinergic neurons suppresses sharp wave ripples and enhances theta oscillations in the hippocampus. Proc. Natl. Acad. Sci. U.S.A. 111, 13535–13540.
Vertes, R. P., and Kocsis, B. (1997). Brainstem-diencephalo-septohippocampal systems controlling the theta rhythm of the hippocampus. Neuroscience 81, 893–926. doi: 10.1016/s0306-4522(97)00239-x
Vertes, R. P., Colom, L. V., Fortin, W. J., and Bland, B. H. (1993). Brainstem sites for the carbachol elicitation of the hippocampal theta rhythm in the rat. Exp. Brain Res. 96, 419–429. doi: 10.1007/BF00234110
Vieira, M., Yong, X. L. H., Roche, K. W., and Anggono, V. (2020). Regulation of NMDA glutamate receptor functions by the GluN2 subunits. J. Neurochem. 154, 121–143.
Weinberger, D. R. (1987). Implications of normal brain development for the pathogenesis of schizophrenia. Arch. Gen. Psychiatry 44, 660–669.
Wenzel, A., Fritschy, J. M., Mohler, H., and Benke, D. (1997). NMDA receptor heterogeneity during postnatal development of the rat brain: Differential expression of the NR2A, NR2B, and NR2C subunit proteins. J. Neurochem. 68, 469–478. doi: 10.1046/j.1471-4159.1997.68020469.x
Werkle-Bergner, M., Shing, Y. L., Muller, V., Li, S. C., and Lindenberger, U. (2009). EEG gamma-band synchronization in visual coding from childhood to old age: Evidence from evoked power and inter-trial phase locking. Clin. Neurophysiol. 120, 1291–1302. doi: 10.1016/j.clinph.2009.04.012
Whitford, T. J., Farrow, T. F., Rennie, C. J., Grieve, S. M., Gomes, L., Brennan, J., et al. (2007a). Longitudinal changes in neuroanatomy and neural activity in early schizophrenia. Neuroreport 18, 435–439. doi: 10.1097/WNR.0b013e3280119d31
Whitford, T. J., Rennie, C. J., Grieve, S. M., Clark, C. R., Gordon, E., and Williams, L. M. (2007b). Brain maturation in adolescence: Concurrent changes in neuroanatomy and neurophysiology. Hum. Brain Mapp. 28, 228–237. doi: 10.1002/hbm.20273
Xi, D., Keeler, B., Zhang, W., Houle, J. D., and Gao, W. J. (2009). NMDA receptor subunit expression in GABAergic interneurons in the prefrontal cortex: Application of laser microdissection technique. J. Neurosci. Methods 176, 172–181. doi: 10.1016/j.jneumeth.2008.09.013
Yagishita, H., Nishimura, Y., Noguchi, A., Shikano, Y., Ikegaya, Y., and Sasaki, T. (2020). Urethane anesthesia suppresses hippocampal subthreshold activity and neuronal synchronization. Brain Res. 1749:147137. doi: 10.1016/j.brainres.2020.147137
Zhang, H., Li, Q., Shang, Y., Xiao, X., Xu, X., Zhang, J., et al. (2019). Effect of prenatal stress on neural oscillations in developing hippocampal formation. Prog. Neuropsychopharmacol. Biol. Psychiatry 89, 456–464. doi: 10.1016/j.pnpbp.2018.10.019
Keywords: theta rhythm, narrow-band delta oscillations, animal models, prefrontal cortex, hippocampus, oscillatory coupling, schizophrenia, urethane anesthesia
Citation: Sibilska S, Mofleh R and Kocsis B (2023) Development of network oscillations through adolescence in male and female rats. Front. Cell. Neurosci. 17:1135154. doi: 10.3389/fncel.2023.1135154
Received: 31 December 2022; Accepted: 19 April 2023;
Published: 05 May 2023.
Edited by:
George C. McConnell, Stevens Institute of Technology, United StatesReviewed by:
Sorinel A. Oprisan, College of Charleston, United StatesWen-Jun Gao, Drexel University, United States
Copyright © 2023 Sibilska, Mofleh and Kocsis. This is an open-access article distributed under the terms of the Creative Commons Attribution License (CC BY). The use, distribution or reproduction in other forums is permitted, provided the original author(s) and the copyright owner(s) are credited and that the original publication in this journal is cited, in accordance with accepted academic practice. No use, distribution or reproduction is permitted which does not comply with these terms.
*Correspondence: Bernat Kocsis, YmtvY3Npc0BobXMuaGFydmFyZC5lZHU=