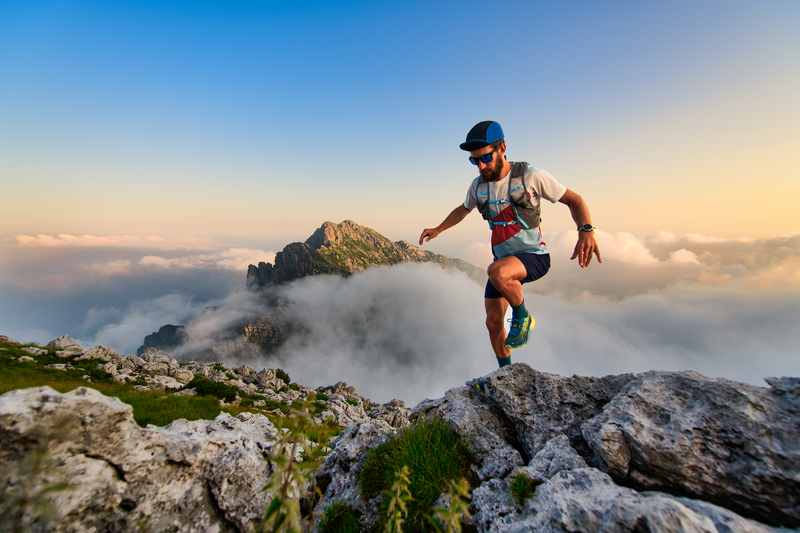
95% of researchers rate our articles as excellent or good
Learn more about the work of our research integrity team to safeguard the quality of each article we publish.
Find out more
REVIEW article
Front. Cell. Neurosci. , 21 March 2023
Sec. Non-Neuronal Cells
Volume 17 - 2023 | https://doi.org/10.3389/fncel.2023.1117218
This article is part of the Research Topic 15 Years of Frontiers in Cellular Neuroscience: Blood Brain Barrier Modulation and Dysfunction in Brain Diseases View all 6 articles
Stroke, a serious systemic inflammatory disease, features neurological deficits and cardiovascular dysfunction. Neuroinflammation is characterized by the activation of microglia after stroke, which disrupts the cardiovascular-related neural network and the blood–brain barrier. Neural networks activate the autonomic nervous system to regulate the cardiac and blood vessels. Increased permeability of the blood–brain barrier and the lymphatic pathways promote the transfer of the central immune components to the peripheral immune organs and the recruitment of specific immune cells or cytokines, produced by the peripheral immune system, and thus modulate microglia in the brain. In addition, the spleen will also be stimulated by central inflammation to further mobilize the peripheral immune system. Both NK cells and Treg cells will be generated to enter the central nervous system to suppress further inflammation, while activated monocytes infiltrate the myocardium and cause cardiovascular dysfunction. In this review, we will focus on microglia-mediated inflammation in neural networks that result in cardiovascular dysfunction. Furthermore, we will discuss neuroimmune regulation in the central–peripheral crosstalk, in which the spleen is a vital part. Hopefully, this will benefit in anchoring another therapeutic target for neuro-cardiovascular dysfunction.
- The cardiovascular-related neural network mediated by microglial inflammation contributes to cardiovascular dysfunction.
- The central and peripheral organs are connected via brain-derived antigen delivery and the spleen's immune response.
- Spleen-targeted neuroimmunomodulation may improve cardiovascular dysfunction after stroke.
Stroke is focal neurological dysfunction caused by a hemorrhage or infarction in the brain, retina, spinal cord, or subarachnoid space. Severe neurological dysfunctions, including unilateral weakness, numbness, loss of vision, diplopia, ataxia, and non-upright vertigo, are the common causes of death. Even worse, an injury caused by a secondary stroke is more serious with higher risks (Hankey, 2014). Approximately 25% of patients died within 1 year and only 50% survived for 5 years (Hankey, 2017).
Growing evidence suggests that stroke would not only lead to neurological dysfunction, but also cause systemic inflammatory response, immune dysregulations, and further damage to peripheral organs (Saand et al., 2019). In other words, damage may spread to the heart, lungs, gastrointestinal tract, liver, kidneys, spleen, and bone after stroke (McDonald et al., 2020). Although most patients with stroke die of neurological damage, cardiovascular complications appear to be the second major reason for death after a stroke (Chen et al., 2017). However, we still do not know whether cardiovascular diseases are unrelated or secondary to stroke. Diffusion tensor imaging (DTI) can be used to view the fibrous connection between the cardiovascular-related nucleus, which forms a neural network. The release of inflammatory molecules after stroke will destroy the neural network and cause serious neurological complications (Ghosh and Basu, 2012). Most importantly, activated microglia would engage in short-term (acute stage) and long-term (chronic stage) neuroinflammation after stroke (Wang et al., 2021). Our review will focus on how microglia contribute to the cardiovascular-related neural network.
In addition to the heart, the spleen is also regulated by neural networks and leads to the peripheral immune response of stroke. The spleen will release inflammatory cells to infiltrate cardiomyocytes and cause dysfunction. The removal of the spleen can alleviate neurodegeneration and other cellular degeneration of the body during an ischemic stroke (Pennypacker and Offner, 2015). Current therapeutics and interventions for stroke rehabilitation can be further improved to live up to patients' needs (Winstein et al., 2016). We need to elucidate central and peripheral interactions to investigate the possible pathway targets and explore effective strategies for stroke treatment.
A literature search was performed on PubMed and Web of Science databases without restrictions to publication types and languages between the years 2000 and 2021. The following terms are not limited to an individual or multiple combined queries: stroke, microglia, neuroinflammation, cytokine, neural network, cardiovascular, spleen, and crosstalk. We also studied the references listed in the literature found in accordance with the above search principles and selected the ones we thought were useful and relevant. We tried to demonstrate the possible mediators and complex interaction mechanisms between microglia polarization and heart injury, but our research has limitations as there were few relevant experimental or clinical studies.
Neuro-cardiovascular dysfunction refers to cardiovascular disease caused by the disruption of the neural network after stroke, which generally includes blood pressure variability, arrhythmias, unstable angina, myocardial infarction, or heart failure. Although neurological defects are the leading cause of death after stroke, the number of patients who die of heart damage cannot be ignored. Recent studies have even proposed “stroke-heart syndrome” (Scheitz et al., 2021). More and more studies believe that stroke will cause not only neuroinflammation, but also systemic damage. Some specific detection biomarkers of heart injury will change significantly, and can even be used as a stratification of post-stroke risk (Chen et al., 2017). Scheitz et al. (2021) provide prognostic implications of elevated cardiovascular troponin levels in patients with acute ischemic stroke. Approximately 30–60% of patients have elevated CTn levels at admission, which is higher than expected in older adults without acute stroke. The CTn level of 15% of patients was increased even beyond the threshold used in the emergency department to classify patients with chest pain as “suspected myocardial infarction.” Suleiman et al. (2017) also reported that the mean values of serum cTnT and CK-MB (creatine kinase) were higher in patients with acute ischemic stroke compared to controls. Although there are no clear guidelines to clarify myocardial markers as post-stroke risks, cardiac functions in patients with stroke are supposed to be comprehensively evaluated.
Many brain regions that control emotion, stress, and homeostasis, including the anterior insula, anterior cingulate cortex, amygdala, hypothalamus, periurethral gray matter, parabrachial nucleus, and medulla, would influence the heart rate and cardiovascular contractility through the sympathetic and parasympathetic nervous systems (Palma and Benarroch, 2014). The adverse consequences of various neurological diseases on the heart emphasize the need for a better understanding of the functional, anatomical, and neurochemical mechanisms of cardiovascular nerve control, especially severe arrhythmias and cardiovascular injuries following neurotraumatic events (Palma and Benarroch, 2014). The sympathetic and parasympathetic nervous systems, characterized as powerful regulators of the cardiovascular system, remain largely unknown at the molecular and functional diversity levels of their constituent neurons and circuits (Palma and Benarroch, 2014).
Cardiovascular parasympathetic effluents originate from the brain stem preganglionic neurons, mainly located in the ambiguus (Amb), and a few cardiovascular parasympathetic nerves located in the motor nucleus of the dorsal vagus nerve. Avin Veerakumar's recent dissection of cardiovascular parasympathetic control circuits in mice revealed cardiovascular innervation neurons in the brainstem. Amb is composed of two molecules that are anatomically and functionally distinct subtypes called ambiguus cardiopulmonary (ACP) and ambiguus cardiovascular (ACV). ACV will sense changes in the peripheral blood pressure and selectively activate the cholinergic cardiovascular ganglion neurons, which regulate the cardiovascular function, whereas ACP is recently found and activates the dive reflex which can also induce bronchoconstriction simultaneously (Veerakumar et al., 2022). Ching-Yi Tsai used DTI to revise the baroreflex neural circuits that consist of nucleus tractus solitarii (NTS) and Amb or the rostral ventrolateral medulla (RVLM) and the caudal ventrolateral medulla (CVLM). The NTS will project an excitatory projection onto the Amb to activate the vagus nerve and inhibit cardiovascular activity. In addition, NTS also regulates blood pressure by activating the CVLM and RVLM. Simultaneously, the CVLM also projects onto the inhibitory projective fibers to RVLM (Tsai et al., 2019) to restrain vasoconstriction and cardiovascular excitation. Stressful conditions in the brain can reduce activity in the medial prefrontal cortex, leading to the overactivation of the amygdala. This leads to the continued activation of the noradrenaline-producing locus coeruleus and chronic activation of the sympathetic nervous system both of which promote aseptic inflammation (Yang et al., 2021). Glutamic acid circuits in the hypothalamus link the contralateral supraoptic nucleus (SON) and the ipsilateral paraventricular nucleus of the hypothalamus (PVN) large cell neurons together and activate their synchronization. The PVN and RVLM are key components of the neural network that generate and regulate sympathetic nerve activity (Boudaba and Tasker, 2006) (Figure 1).
Figure 1. Cardiovascular-related neural network. Amb includes ACV and ACP. ACV will sense changes in peripheral blood pressure and regulate cardiovascular function, whereas ACP induces bronchoconstriction simultaneously. NTS will project an excitatory projection onto the Amb to activate the vagus nerve and inhibit cardiovascular activity. NTS also activates CVLM and RVLM. In the meantime, CVLM would inhibit RVLM to inhibit vasoconstriction and cardiovascular excitation. Overactivation of the amygdala leads to the continued activation of the noradrenaline-producing locus coeruleus and chronic activation of the sympathetic nervous system. PVN regulates the sympathetic nerve and SON synchronizes with PVN through the glutamic acid circuits.
Previous studies have suggested that microglia affect the peripheral organs by regulating the autonomic nerve, especially through sympathetic and parasympathetic regulation (Li Y. et al., 2020), i.e., the hypothalamic-pituitary-adrenal (HPA) axis and catecholamine surge (Chen et al., 2017). Furthermore, modulation of microglial activity has been shown to regulate the incidence and progression of cardiovascular diseases. Microglia have been indicated as a potential regulator of hypertension, myocardial infarction, and ischemia/reperfusion injury (Wang et al., 2022). The dysregulation of cytokine secretion and neurotoxic metabolites released from microglia seriously damage neural-network activity, leading to pathological outcomes (Li Y. et al., 2020). M1 microglia are detected immediately within hours of stroke, stimulating the pro-inflammatory mechanisms to mediate the neurotoxic effects, while M2 microglia occur a few days after stroke, mediating the anti-inflammatory mechanisms to exert neuroprotective effects (Wang et al., 2021). Therefore, future exploration of the molecular linkage of microglia may provide various therapeutic targets.
Toll-like receptors belong to the innate immune receptor family, which play a significant part in neuroinflammation (Fang et al., 2013). The activation of TLR4 in microglia could initiate the myeloid differentiation primary response protein 88 (MyD88) pathway. The Toll-receptor-associated activator of the interferon (TRIF) pathway is triggered to express the nuclear factor-κB (NF-κB) and release inflammatory cytokines such as TNF-α and IL-6 (Wang et al., 2021). AngII Type 1 receptor (AT1R), widely expressed by various central nervous system cell types, directly affects neuronal activity by binding neuron AT1R or affecting the endothelial cells and perivascular macrophages. However, endogenous AngII will promote the interactions between AT1R and TLR4, thereby activating microglia and disrupting the BBB. Tac-242 (resatorvid), a specific TLR4 inhibitor, will improve neurogenic hypertension (Mowry et al., 2021). Masson et al. (2015a) also found the expression of TLR4 in PVN and that acute LPS treatment would influence heart rate. Therefore, therapy targeting TLR4 may be a potential strategy for alleviating neurogenic cardiovascular disease.
The high-mobility group protein box 1 (HMGB1) and the advanced glycation end-product receptor (RAGE) are involved in microglia polarization and hypertension (Li et al., 2022). The ubiquitous protein in nucleoprotein is known as the high-mobility group protein box 1 (HMGB1). This nuclear protein is released into the peripheral environment to promote inflammation (Andersson et al., 2018) after cell activation, stress, injury, or death. Upon neuroinflammation, the microglia secrete HMGB1 that can activate TLR4 expression and then promote the secretion of inflammatory cytokines through the NF-κB pathway, thus regulating the polarization of microglia (Li et al., 2022). In addition, studies have shown that HMGB1 can increase TNF-α and IL-1β to aggravate neuroinflammation (Yang et al., 2017). In spontaneously hypertensive rats, HMGB1, IL-6, and TNF-α levels were higher in the PVN (Masson et al., 2015b), which suggest the significant role of microglia involved in the cardiovascular network.
P2X receptor, a subtype of P2 receptor, located on the cell membrane of microglia is an ion-gated channel that is activated by the ATP in microglia. Some studies have shown that mice with knocked-out P2X receptor genes express less TNF-α and IL-1β and reduce neuroinflammation (Subauste, 2019). The P2X7 receptor is the key to microglia-mediated cardiovascular injury. In an animal experiment, the P2X7 receptor was co-located with the microglia in the PVN of mice and the increased ATP content in the PVN leads to an increase in P2X7R expression. Activated microglia release TNF-α and IL-1β that stimulate the release of oxytocin and vasopressin through the P2X7 receptor. Oxytocin and vasopressin participate in the regulation of the cardiovascular system by activating the sympathetic nerve (Du et al., 2015).
TGF-β1 stimulates the M2 phenotype microglial transition that contributes to play an anti-inflammatory function. Tissue regeneration and neurological recovery are promoted by the TGF-β/Smad2/3 pathway in mice that have gone through stroke (Zhang et al., 2021). Furthermore, TGF-β can suppress hypertension by modulating microglia (Li Y. et al., 2020). In normotensive rats, TGF-β would inhibit neuroinflammation, the kidney norepinephrine level, and blood pressure, which are mediated through microglia (Li et al., 2017). Tmem119, Olfml3, and Klf10 can be direct Tgfβ1-Smad2 target genes, which are associated with microglia maturation and maintenance (Spittau et al., 2013; Attaai et al., 2018; Neidert et al., 2018). Therefore, TGF-β is a unique microglial signature that provides the possibility for targeting microglia for treatment (Butovsky et al., 2014) (Figure 2).
Figure 2. Inflammatory pathways related to cardiovascular diseases expressed in microglia: P2X7, TLR4-AT1R, and HMGB1-RAGE would activate TNF-α, IL-6, and IL-1β, while TGF-β would activate Tmem119/Olfml3/Klf10 induce microglia maturation.
Endothelial cells, astrocyte end-feet, and pericytes compose the BBB (Sweeney et al., 2019) that could prevent neurotoxic plasma components, blood cells, and pathogens from entering the brain and modulate the molecules into and out of the central nervous system (CNS) (Sweeney et al., 2019; Zhang et al., 2020). Increased BBB permeability is a major pathophysiological change after stroke that promotes the entry of inflammatory molecules. Interestingly, the BBB permeability is increased not only during inflammatory responses. Hendy and Hall (2019) reported in their study that up to 50% of patients with cardiovascular disease showed a temporary increase in BBB permeability after surgery and other studies also show that peripheral leukocytes (Greenwood et al., 2011), cytokines, chemokines, proteases, and adhesive proteins (Rahman and Dandekar, 2021) may change when the BBB gets disrupted which suggests that the brain–heart interaction is likely to play a role in immune molecules passing through the BBB. In addition, cardiovascular diseases are likely to be one of the systemic inflammatory reactions of stroke.
Injured astrocytes, neurons, and oligodendrocytes (Zhang et al., 2020) release brain-derived antigens such as glial fibrillary acidic protein, S100, and myelin basic protein (MBP) (Chen et al., 2017). Hochmeister et al. (2008) injected antigens into both the CNS and cerebrospinal fluid and found them in the deep cervical lymph nodes, mesenteric lymph nodes, and spleen. Furthermore, antigen-presenting cells (APCs) and soluble anti-inflammatory proteins can also be detected in the peripheral lymph nodes during inflammation (Planas et al., 2012). Although the brain has no lymphatic vessels, the brain antigens and lymphocytes break through the BBB and flow out along the arterial wall to the cervical lymph nodes (Clapham et al., 2010). However, there is also communication between the cerebrospinal fluid and lymphatic vessels, and other antigenic substances can also enter the peripheral lymph nodes in this way (Johnston et al., 2004). Latest research shows that the high-resolution real-time imaging technology makes the landmark discovery of the peridural lymphatic system of the brain possible, which provides a deeper understanding dimension for neuroimmunology, and the central nervous system will no longer be an immune-privileged area (Negi and Das, 2018) (Figure 3).
Figure 3. Possible mechanism of brain–spleen–heart crosstalk: after stroke, the permeability of the blood–brain barrier increases, and the immune cells and inflammatory molecules in the brain reach the peripheral lymph nodes and spleen through the BBB to further regulate the occurrence of immune inflammation. The inflammatory molecules released by spleen activation mediate myocardial damage. Simultaneously, the spleen also releases NK cells and Treg cells into the brain to inhibit neuroinflammation while the monocytes would promote neuroinflammation. In addition, the inflammatory reaction near the hypothalamus can also promote the hypothalamic adrenaline axis to regulate the immune response of the spleen and heart damage.
A specific brain network exists that connects to the spleen through the splenic nerve and produces a rhythmic discharge; even severing the link between the spleen and the central nervous system may treat refractory hypertension (Carnevale et al., 2016), which indicates the emerging role of neural networks in systematic inflammation. A neural network modulates the production of T cells that in turn produces the neurotransmitters to inhibit innate immunity (Rosas-Ballina et al., 2011). Although it has been proposed that the brain–spleen connection may be an important mechanism of neuroimmunity in cardiovascular injury, more experiments need to be conducted to prove it.
Splenic activation is associated with neuroimmune activation to respond to systemic inflammation and regulate the immune system and impaired neurological recovery (Saand et al., 2019). The spleen has been proposed to play an important role in the brain–heart crosstalk (Chen et al., 2017). Most studies have preliminarily shown that the spleen is involved in brain injury after ischemic stroke and hemorrhagic stroke (Ajmo et al., 2008). A reduction in splenic volume from 48 to 96 h after a stroke has been observed (Saand et al., 2019) in an MCAO model. Interestingly, the splenic volume will return to its previous level around the 10th day after stroke. Experiments in animals have shown that administering simvastatin immediately after the stroke can reduce spleen atrophy and splenocyte apoptosis in a mouse MCAO model, thereby inhibiting the expression of brain IFN-γ and reducing the risk of pulmonary bacterial infection (Lee et al., 2008) and proving that there is a close relationship between spleen and stroke. As the main peripheral immune organ of the human body, the spleen may be connected with the brain through the immune system, such as various immune cells and inflammatory molecules (Saand et al., 2019). Central immune cells have been shown to escape from the central to cervical lymph nodes through the cerebrospinal fluid lymphatic pathway and it is worth noting that many central immune cells have also been found in the spleen (Hochmeister et al., 2008).
Some studies conducted in rats have shown that the cells of the spleen have undergone great changes after stroke while the number of neutrophils and macrophages in the spleen did not change significantly after stroke. The T cells and B cell levels of the spleen decrease significantly after stroke, but the false spleen did not undergo changes in the control group. Liu, Q found that spleen macrophages, T cells, and B cells were negatively correlated with the neurological dysfunction score (NDS), suggesting that these spleen immune cells may contribute to stroke recovery. Some studies believe that spleen-derived monocytes are consistent with the increase in monocytes in the brain after stroke and play an anti-inflammatory role in ischemic stroke (Kim et al., 2014; Han et al., 2020).
NK cells were used as a bridge in an animal experiment using MCAO mice that can be shown that the activation of catecholamine and HPA axis may be the reason for the spatiotemporal fluctuation of the number of NK cells in the central nervous system and peripheral system. After a stroke, the activation of catecholamine and the HPA axis can reduce the number of peripheral NK cells through the JAK-STAT signal pathway and induce the NK cells of the peripheral system to enter the brain through the blood–brain barrier (Liu et al., 2017). In another period, through the regulation of the brain immune axis, spleen atrophy and the spleen-mediated anti-inflammatory mechanism began to protect nerve cells by increasing the Treg cells (Offner et al., 2006a,b; Vendrame et al., 2006). Treg cells are released from the spleen, which can regulate the immune system and inhibit the inflammatory response and autoimmunity. Simultaneously, the presence of Tregs can induce the polarization of microglia to the M2 type, which is more conducive to reducing the damage caused by the inflammatory response (Zhou et al., 2017). The expansion of the number of Tregs in the brain after ischemic stroke is considered to be one of the endogenous anti-inflammatory mechanisms (Yang et al., 2014) and its function has been confirmed (Offner et al., 2006b; Urra et al., 2009). However, the effect and mechanism of Tregs in ischemic stroke still need more experimental research. Interestingly, in splenectomized animals, the neuroprotective effects seem to disappear which suggests that the spleen is essential for the mechanisms of these neuroprotective strategies. Studies have shown that spleen-derived Tregs cells can also prevent the activation of the TLR4 and NF-κB pathways in the brain to reduce the inflammatory response of the central system (Kim et al., 2015; Wang et al., 2016). In general, regulation of the brain immune axis would activate the spleen-mediated peripheral immune response, which can be fed back to the brain to achieve the effect of inflammation or anti-inflammation (Venkat et al., 2018).
The spleen correlated with the inflammation closely, and hemodynamic congestion, and sympathetic stimulation would affect the size of the spleen (Maeda et al., 2021). However, the inflammation mechanisms require more investigation. Dunford et al. (2017) used fluoro-18-fluorodeoxyglucose (18F-FDG) PET/CT to investigate the spleen–heart axis. Monocyte activation is the key to heart damage, and the processing of antigens by the spleen enhances their activation and promotes the immune-mediated injury response (Ismahil et al., 2014). Some studies have shown that IL-17A, which may be derived from pathogenic Th17 cells and effector/memory T cells, in the spleen and blood would prominently affect the heart (Zamora et al., 2021). Similarly, the release of inflammatory factors into the blood after heart injury will also lead to the remodeling of spleen tissue (Jahng et al., 2016). A mice model study concluded that the release of proinflammatory cytokines and inflammatory cells infiltrate the myocardium after ICH leading to cardiovascular pathological remodeling and cardiovascular dysfunction (Zhao et al., 2019; Yan et al., 2020). As the regulator of peripheral immunity, the spleen will continue to release inflammatory cells with the activation of the peripheral immune response which will infiltrate the myocardium and result in acute or chronic cardiovascular dysfunction (Zhao et al., 2019). After splenectomy, the number of cardiovascular-infiltrated macrophages and leukocytes decreased significantly and the ICH-induced cardiovascular dysfunction was improved (Mebius and Kraal, 2005; Li W. et al., 2020). In animal models, IL-6 inhibition can significantly reduce cardiovascular insufficiency, inflammation, and fibrosis (González et al., 2015). The ICH and splenectomy groups significantly reduce MCP-1 expression and macrophage and monocyte infiltration compared with the ICH group without treatment. Therefore, spleen-mediated immune responses may be the main cause of cardiovascular dysfunction after ICH, which may be a bridge of the brain–heart interaction after stroke (Li W. et al., 2020).
Neuroimmune regulation has long been neglected by cardiologists. A pioneering work in David Harrison's lab (Harrison, 2013) has brilliantly demonstrated that lymphocytes are needed to raise blood pressure and also Tracey (2009) found that stimulating the nerve reflex in the spleen protected the mice from septic shock. Specific regions of the perception of peripheral circulation can integrate the immune and vascular lesion reactions and discover the key role of the spleen. This secondary lymphoid organ produces prime immune cells that are subsequently recruited to target organs and are crucial for blood pressure regulation (Carnevale and Lembo, 2018). However, this discovery still needs further investigations and it is necessary to map the cerebral splenic nerve and the cerebral cardiosympathetic nerve with microneurography.
ANS activation, brain-derived antigen, and activation of chemokines have been proven to play crucial roles in brain–spleen after stroke (Wang et al., 2019). Since the spleen is the neuroimmunomodulatory center of the systemic inflammatory response after stroke, its treatment strategy has received increasing attention. While splenectomy can help to reduce the infarct size after stroke (Saand et al., 2019), Carvedilol, a beta blocker, can significantly reduce infarct size due to the massive release of catecholamines on the splenic nerve after stroke that activates the immune function of the spleen (Ajmo et al., 2009). Simvastatin can also ameliorate peripheral immunodepression after stroke by attenuating spleen atrophy (Jin et al., 2013). However, immunomodulatory regulation is an important means of activating the spleen. Stem cell therapy targeting the spleen is a promising strategy for treating systemic inflammatory responses after stroke. Intravenously injected stem cells modulate the inflammatory response by targeting the brain and spleen after stroke, and microglia also transform from the M1 proinflammatory phenotype to the M2 anti-inflammatory phenotype, providing a favorable environment for nerve regeneration and angiogenesis (Wang et al., 2019). In addition, we found that selective thermal ablation of splenic nerves prevents T-cell expulsion (Carnevale et al., 2016). Interleukin (IL)-33 has been found to increase IL-4, IL-10, and TGF-beta levels in the spleen which would exert the M2-phenotype microglia and promote nerve regeneration (Xiao et al., 2019) (Table 1).
This is a review of the mechanisms of severe neuroinflammation and cardiovascular dysfunction after stroke (Figure 4). Degeneration products generated after stroke will stimulate specific immune receptors of microglia and also lead to a disruption of the neural network and cause systemic inflammation. Cardiovascular dysfunction is the most serious injury in peripheral organs, and the microglia-mediated inflammatory response can regulate ANS activation to impact the peripheral organs. More importantly, brain-derived antigens and other cytokines can reach the spleen through the BBB and the lymphatic pathway, thus influencing the immune response of the spleen. Monocyte cells can infiltrate the myocardia to mediate heart damage. Treg cells and NK cells can also enter the central nervous system to inhibit the occurrence of central nervous inflammation, while the monocytes have the opposite effect. Therefore, the crosstalk between the brain, spleen, and heart may be an important mechanism of cardiovascular dysfunction after stroke, and the spleen plays an important role in it. The treatment of neuroimmunomodulation targeting the spleen has been investigated but effective strategies for regulating cardiovascular-related neural networks still need development.
Figure 4. Possible mechanism of microglia-mediated inflammatory destruction of neuro-cardiovascular dysfunction.
JW and HH initiated this project. JD, CC, and SX performed the literature research and contributed to the original draft. DS and WP participated in the revision of the manuscript. All authors contributed to the article and approved the submitted version.
This study was supported by grants from the Natural Science Fund of Guangdong Province (2017A030313597), the Natural Science Fund of Guangzhou in Basic and Applied Research (202201010875), and the Southern Medical University (S202012121088S, X202012121354, 202212121027, and S202212121113S).
JW really appreciates the great help of the Neural Networks Surgery team of the Southern Medical University, Guangzhou, China toward this study.
The authors declare that the research was conducted in the absence of any commercial or financial relationships that could be construed as a potential conflict of interest.
All claims expressed in this article are solely those of the authors and do not necessarily represent those of their affiliated organizations, or those of the publisher, the editors and the reviewers. Any product that may be evaluated in this article, or claim that may be made by its manufacturer, is not guaranteed or endorsed by the publisher.
Amb, Ambiguss; ANXA1, Annexin A1; AT1R, AngII Type 1 Receptor; APC, Antigen-presenting cell; BBB, Blood–Brain Barrier; CK-MB, Creatine kinase; CTn, Cardiovascular troponin; DAMPs, Damage-associated molecular patterns; GR, Glucocorticoid receptor; HMGB1, High-mobility group box 1; HPA, Hypothalamic-pituitary-adrenal; ICH, Intracerebral hemorrhage; MCAO, Middle cerebral artery occlusion; MPO, Myeloperoxidase; MyD88, Myeloid differentiation primary response protein 88; NDS, Neurological dysfunction score; NF-κB, Nuclear factor-κB; NTS, Nucleus tractus solitarii; NSC, Neurogenic stress cardiomyopathy; NVU, Neurovascular unit; PAMP, Pathogen-associated pattern molecules; PMN, Polymorphonuclear neutrophils; PVN, Paraventricular nucleus; RAGE, Receptors for advanced glycation end products; TLR4, Toll-like receptor 4; TRIF, Toll receptor-associated activator of interferon; USP18, Ubiquitin-specific protease 18.
Ajmo, C. T., Collier, L. A., Leonardo, C. C., Hall, A. A., Green, S. M., Womble, T. A., et al. (2009). Blockade of adrenoreceptors inhibits the splenic response to stroke. Exp. Neurol. 218, 47–55. doi: 10.1016/j.expneurol.2009.03.044
Ajmo, C. T. Jr., Vernon, D. O., Collier, L., Hall, A. A., Garbuzova-Davis, S., Willing, A., et al. (2008). The spleen contributes to stroke-induced neurodegeneration. J. Neurosci. Res. 86, 2227–2234. doi: 10.1002/jnr.21661
Andersson, U., Yang, H., and Harris, H. (2018). Extracellular HMGB1 as a therapeutic target in inflammatory diseases. Expert Opin. Ther. Targets 22, 263–277. doi: 10.1080/14728222.2018.1439924
Attaai, A., Neidert, N., von Ehr, A., Potru, P. S., Zöller, T., and Spittau, B. (2018). Postnatal maturation of microglia is associated with alternative activation and activated TGFβ signaling. Glia 66, 1695–1708. doi: 10.1002/glia.23332
Boudaba, C., and Tasker, J. G. (2006). Internuclear coupling of hypothalamic magnocellular nuclei by glutamate synaptic circuits. Am. J. Physiol. Regulat. Integr. Compar. Physiol. 291, R102–R111. doi: 10.1152/ajpregu.00795.2005
Butovsky, O., Jedrychowski, M. P., Moore, C. S., Cialic, R., Lanser, A. J., Gabriely, G., et al. (2014). Identification of a unique TGF-β-dependent molecular and functional signature in microglia. Nat. Neurosci. 17, 131–143. doi: 10.1038/nn.3599
Carnevale, D., and Lembo, G. (2018). Heart, spleen, brain: neuroimmune axis of cardiovascular control. Circulation 138, 1917–1919. doi: 10.1161/CIRCULATIONAHA.118.035628
Carnevale, D., Perrotta, M., Pallante, F., Fardella, V., Iacobucci, R., Fardella, S., et al. (2016). A cholinergic-sympathetic pathway primes immunity in hypertension and mediates brain-to-spleen communication. Nat. Commun. 7, 13035. doi: 10.1038/ncomms13035
Chen, Z., Venkat, P., Seyfried, D., Chopp, M., Yan, T., and Chen, J. (2017). Brain-heart interaction: cardiac complications after stroke. Circ. Res. 121, 451–468. doi: 10.1161/CIRCRESAHA.117.311170
Clapham, R., O'Sullivan, E., Weller, R. O., and Carare, R. O. (2010). Cervical lymph nodes are found in direct relationship with the internal carotid artery: significance for the lymphatic drainage of the brain. Clin. Anat. 23, 43–47. doi: 10.1002/ca.20887
Du, D., Jiang, M., Liu, M., Wang, J., Xia, C., Guan, R., et al. (2015). Microglial P2X7 receptor in the hypothalamic paraventricular nuclei contributes to sympathoexcitatory responses in acute myocardial infarction rat. Neurosci. Lett. 587, 22–28. doi: 10.1016/j.neulet.2014.12.026
Dunford, A., Keramida, G., Anagnostopoulos, C. D., and Peters, A. M. (2017). The cardiosplenic axis: another obscure pathophysiological function of the spleen and its investigation using molecular imagingd. Nucl. Med. Commun. 38, 205–208. doi: 10.1097/MNM.0000000000000635
Fang, H., Wang, P. F., Zhou, Y., Wang, Y. C., and Yang, Q. W. (2013). Toll-like receptor 4 signaling in intracerebral hemorrhage-induced inflammation and injury. J. Neuroinflammation 10, 27. doi: 10.1186/1742-2094-10-27
Ghosh, S., and Basu, A. (2012). Network medicine in drug design: implications for neuroinflammation. Drug Discov. Today 17, 600–607. doi: 10.1016/j.drudis.2012.01.018
González, G. E., Rhaleb, N. E., D'Ambrosio, M. A., Nakagawa, P., Liu, Y., Leung, P., et al. (2015). Deletion of interleukin-6 prevents cardiac inflammation, fibrosis and dysfunction without affecting blood pressure in angiotensin II-high salt-induced hypertension. J. Hypertens. 33, 144–152. doi: 10.1097/HJH.0000000000000358
Greenwood, J., Heasman, S. J., Alvarez, J. I., Prat, A., Lyck, R., and Engelhardt, B. (2011). Leucocyte-endothelial cell crosstalk at the blood-brain barrier: A prerequisite for successful immune cell entry to the brain. Neuropathol. Appl. Neurobiol. 37, 24–39. doi: 10.1111/j.1365-2990.2010.01140.x
Han, D., Liu, H., and Gao, Y. (2020). The role of peripheral monocytes and macrophages in ischemic stroke. Neurol. Sci. 41, 3589–3607. doi: 10.1007/s10072-020-04777-9
Hankey, G. J. (2014). Secondary stroke prevention. Lancet Neurol. 13, 178–194. doi: 10.1016/S1474-4422(13)70255-2
Harrison, D. G. (2013). The mosaic theory revisited: common molecular mechanisms coordinating diverse organ and cellular events in hypertension. J. Am. Soc. Hypertens. 7, 68–74. doi: 10.1016/j.jash.2012.11.007
Hendy, A., and Hall, R. (2019). Cardiac surgery and the blood-brain barrier. Anesthesiol. Clin. 37, 787–800. doi: 10.1016/j.anclin.2019.08.009
Hochmeister, S., Zeitelhofer, M., Bauer, J., Nicolussi, E. M., Fischer, M. T., Heinke, B., et al. (2008). After injection into the striatum, in vitro-differentiated microglia- and bone marrow-derived dendritic cells can leave the central nervous system via the blood stream. Am. J. Pathol. 173, 1669–1681. doi: 10.2353/ajpath.2008.080234
Ismahil, M. A., Hamid, T., Bansal, S. S., Patel, B., Kingery, J. R., and Prabhu, S. D. (2014). Remodeling of the mononuclear phagocyte network underlies chronic inflammation and disease progression in heart failure. Circ. Res. 114, 266–282. doi: 10.1161/CIRCRESAHA.113.301720
Jahng, J. W. S., Song, E., and Sweeney, G. (2016). Crosstalk between the heart and peripheral organs in heart failure. Experimental Mol. Med. 48, e217. doi: 10.1038/emm.2016.20
Jin, R., Zhu, X., Liu, L., Nanda, A., Granger, D. N., and Li, G. (2013). Simvastatin attenuates stroke-induced splenic atrophy and lung susceptibility to spontaneous bacterial infection in mice. Stroke 44, 1135–1143. doi: 10.1161/STROKEAHA.111.000633
Johnston, M., Zakharov, A., Papaiconomou, C., Salmasi, G., and Armstrong, D. (2004). Evidence of connections between cerebrospinal fluid and nasal lymphatic vessels in humans, non-human primates and other mammalian species. Cerebrospinal Fluid Res. 1, 2. doi: 10.1186/1743-8454-1-2
Kim, B. S., Nishikii, H., Baker, J., Pierini, A., Schneidawind, D., Pan, Y., et al. (2015). Treatment with agonistic DR3 antibody results in expansion of donor Tregs and reduced graft-versus-host disease. Blood 126, 546–557. doi: 10.1182/blood-2015-04-637587
Kim, E., Yang, J., Beltran, C. D., and Cho, S. (2014). Role of spleen-derived monocytes/macrophages in acute ischemic brain injury. J. Cerebral Blood Flow Metab. 34, 1411–1419. doi: 10.1038/jcbfm.2014.101
Lee, S. T., Chu, K., Jung, K. H., Kim, S. J., Kim, D. H., Kang, K. M., et al. (2008). Anti-inflammatory mechanism of intravascular neural stem cell transplantation in haemorrhagic stroke. Brain131, 616–629. doi: 10.1093/brain/awm306
Li, R., Zhou, Y., Zhang, S., Li, J., Zheng, Y., and Fan, X. (2022). The natural (poly)phenols as modulators of microglia polarization via TLR4/NF-κB pathway exert anti-inflammatory activity in ischemic stroke. Eur. J. Pharmacol. 914, 174660. doi: 10.1016/j.ejphar.2021.174660
Li, W., Li, L., Li, W., Chopp, M., Venkat, P., Zacharek, A., et al. (2020). Spleen associated immune-response mediates brain-heart interaction after intracerebral hemorrhage. Exp. Neurol. 327, 113209. doi: 10.1016/j.expneurol.2020.113209
Li, Y., Shen, X. Z., Li, L., Zhao, T. V., Bernstein, K. E., Johnson, A. K., et al. (2017). Brain transforming growth factor-β resists hypertension via regulating microglial activation. Stroke 48, 2557–2564. doi: 10.1161/STROKEAHA.117.017370
Li, Y., Wei, B., Liu, X., Shen, X. Z., and Shi, P. (2020). Microglia, autonomic nervous system, immunity and hypertension: is there a link? Pharmacol. Res. 155, 104451. doi: 10.1016/j.phrs.2019.104451
Liu, Q., Jin, W. N., Liu, Y., Shi, K., Sun, H., Zhang, F., et al. (2017). Brain ischemia suppresses immunity in the periphery and brain via different neurogenic innervations. Immunity 46, 474–487. doi: 10.1016/j.immuni.2017.02.015
Maeda, D., Sakane, K., Kanzaki, Y., Horai, R., Akamatsu, K., Tsuda, K., et al. (2021). Splenic volume index determined using computed tomography upon admission is associated with readmission for heart failure among patients with acute decompensated heart failure. Int. Heart J. 62, 584–591. doi: 10.1536/ihj.20-564
Masson, G. S., Nair, A. R., Dange, R. B., Silva-Soares, P. P., Michelini, L. C., and Francis, J. (2015a). Toll-like receptor 4 promotes autonomic dysfunction, inflammation and microglia activation in the hypothalamic paraventricular nucleus: role of endoplasmic reticulum stress. PLoS ONE 10, e0122850. doi: 10.1371/journal.pone.0122850
Masson, G. S., Nair, A. R., Silva Soares, P. P., Michelini, L. C., and Francis, J. (2015b). Aerobic training normalizes autonomic dysfunction, HMGB1 content, microglia activation and inflammation in hypothalamic paraventricular nucleus of SHR. Am. J. Physiol. Heart Circ. Physiol. 309, H1115–1122. doi: 10.1152/ajpheart.00349.2015
McDonald, S. J., Sharkey, J. M., Sun, M., Kaukas, L. M., Shultz, S. R., Turner, R. J., et al. (2020). Beyond the brain: peripheral interactions after traumatic brain injury. J. Neurotrauma 37, 770–781. doi: 10.1089/neu.2019.6885
Mebius, R. E., and Kraal, G. (2005). Structure and function of the spleen. Nat. Rev. Immunol. 5, 606–616. doi: 10.1038/nri1669
Mowry, F. E., Peaden, S. C., Stern, J. E., and Biancardi, V. C. (2021). TLR4 and AT1R mediate blood-brain barrier disruption, neuroinflammation, and autonomic dysfunction in spontaneously hypertensive rats. Pharmacol. Res. 174, 105877. doi: 10.1016/j.phrs.2021.105877
Negi, N., and Das, B. K. (2018). CNS: Not an immunoprivilaged site anymore but a virtual secondary lymphoid organ. Int. Rev. Immunol. 37, 57–68. doi: 10.1080/08830185.2017.1357719
Neidert, N., von Ehr, A., Zöller, T., and Spittau, B. (2018). Microglia-specific expression of Olfml3 is directly regulated by transforming growth factor β1-induced Smad2 signaling. Front. Immunol. 9, 1728. doi: 10.3389/fimmu.2018.01728
Offner, H., Subramanian, S., Parker, S. M., Afentoulis, M. E., Vandenbark, A. A., and Hurn, P. D. (2006a). Experimental stroke induces massive, rapid activation of the peripheral immune system. J. Cerebral Blood Flow Metab. 26, 654–665. doi: 10.1038/sj.jcbfm.9600217
Offner, H., Subramanian, S., Parker, S. M., Wang, C., Afentoulis, M. E., Lewis, A., et al. (2006b). Splenic atrophy in experimental stroke is accompanied by increased regulatory T cells and circulating macrophages. J. Immunol. 176, 6523–6531. doi: 10.4049/jimmunol.176.11.6523
Palma, J. A., and Benarroch, E. E. (2014). Neural control of the heart: recent concepts and clinical correlations. Neurology 83, 261–271. doi: 10.1212/WNL.0000000000000605
Pennypacker, K. R., and Offner, H. (2015). The role of the spleen in ischemic stroke. J. Cerebral Blood Flow Metab. 35, 186–187. doi: 10.1038/jcbfm.2014.212
Planas, A. M., Gómez-Choco, M., Urra, X., Gorina, R., Caballero, M., and Chamorro, Á. (2012). Brain-derived antigens in lymphoid tissue of patients with acute stroke. J. Immunol. 188, 2156–2163. doi: 10.4049/jimmunol.1102289
Rahman, Z., and Dandekar, M. P. (2021). Crosstalk between gut microbiome and immunology in the management of ischemic brain injury. J. Neuroimmunol. 353, 577498. doi: 10.1016/j.jneuroim.2021.577498
Rosas-Ballina, M., Olofsson, P. S., Ochani, M., Valdes-Ferrer, S. I., Levine, Y. A., Reardon, C., et al. (2011). Acetylcholine-synthesizing T cells relay neural signals in a vagus nerve circuit. Science 334, 98–101. doi: 10.1126/science.1209985
Saand, A. R., Yu, F., Chen, J., and Chou, S. H. (2019). Systemic inflammation in hemorrhagic strokes - a novel neurological sign and therapeutic target? J. Cerebral Blood Flow Metab. 39, 959–988. doi: 10.1177/0271678X19841443
Scheitz, J. F., Stengl, H., Nolte, C. H., Landmesser, U., and Endres, M. (2021). Neurological update: use of cardiac troponin in patients with stroke. J. Neurol. 268, 2284–2292. doi: 10.1007/s00415-020-10349-w
Spittau, B., Wullkopf, L., Zhou, X., Rilka, J., Pfeifer, D., and Krieglstein, K. (2013). Endogenous transforming growth factor-beta promotes quiescence of primary microglia in vitro. Glia 61, 287–300. doi: 10.1002/glia.22435
Subauste, C. S. (2019). The CD40-ATP-P2X (7) Receptor pathway: cell to cell cross-talk to promote inflammation and programmed cell death of endothelial cells. Front. Immunol. 10, 2958. doi: 10.3389/fimmu.2019.02958
Suleiman, H. M., Aliyu, I. S., Abubakar, S. A., Isa, M. S., El-Bashir, J. M., Adamu, R., et al. (2017). Cardiac troponin T and creatine kinase MB fraction levels among patients with acute ischemic stroke in Nigeria. Niger. J. Clin. Pract. 20, 1618–1621. doi: 10.4103/njcp.njcp_78_17
Sweeney, M. D., Zhao, Z., Montagne, A., Nelson, A. R., and Zlokovic, B. V. (2019). Blood-brain barrier: from physiology to disease and back. Physiol. Rev. 99, 21–78. doi: 10.1152/physrev.00050.2017
Tracey, K. J. (2009). Reflex control of immunity. Nat. Rev. Immunol. 9, 418–428. doi: 10.1038/nri2566
Tsai, C. Y., Poon, Y. Y., Chan, J. Y. H., and Chan, S. H. H. (2019). Baroreflex functionality in the eye of diffusion tensor imaging. J. Physiol. 597, 41–55. doi: 10.1113/JP277008
Urra, X., Cervera, A., Villamor, N., Planas, A. M., and Chamorro, A. (2009). Harms and benefits of lymphocyte subpopulations in patients with acute stroke. Neuroscience 158, 1174–1183. doi: 10.1016/j.neuroscience.2008.06.014
Veerakumar, A., Yung, A. R., Liu, Y., and Krasnow, M. A. (2022). Molecularly defined circuits for cardiovascular and cardiopulmonary control. Nature 606, 739–746. doi: 10.1038/s41586-022-04760-8
Vendrame, M., Gemma, C., Pennypacker, K. R., Bickford, P. C., Davis Sanberg, C., Sanberg, P. R., et al. (2006). Cord blood rescues stroke-induced changes in splenocyte phenotype and function. Exp. Neurol. 199, 191–200. doi: 10.1016/j.expneurol.2006.03.017
Venkat, P., Chen, J., and Chopp, M. (2018). Exosome-mediated amplification of endogenous brain repair mechanisms and brain and systemic organ interaction in modulating neurological outcome after stroke. J. Cerebral Blood Flow Metab. 38, 2165–2178. doi: 10.1177/0271678X18782789
Wang, J., Liang, J., Deng, J., Liang, X., Wang, K., Wang, H., et al. (2021). Emerging role of microglia-mediated neuroinflammation in epilepsy after subarachnoid hemorrhage. Mol. Neurobiol. 58, 2780–2791. doi: 10.1007/s12035-021-02288-y
Wang, M., Pan, W., Xu, Y., Zhang, J., Wan, J., and Jiang, H. (2022). Microglia-mediated neuroinflammation: a potential target for the treatment of cardiovascular diseases. J. Inflamm. Res. 15, 3083–3094. doi: 10.2147/JIR.S350109
Wang, Y., Mao, L., Zhang, L., Zhang, L., Yang, M., Zhang, Z., et al. (2016). Adoptive regulatory T-cell therapy attenuates subarachnoid hemor-rhage-induced cerebral inflammation by suppressing TLR4/NF-B signaling pathway. Curr. Neurovasc. Res. 13, 121–126. doi: 10.2174/1567202613666160314151536
Wang, Z., He, D., Zeng, Y. Y., Zhu, L., Yang, C., Lu, Y. J., et al. (2019). The spleen may be an important target of stem cell therapy for stroke. J. Neuroinflammation 16, 1–24. doi: 10.1186/s12974-019-1400-0
Winstein, C. J., Stein, J., Arena, R., Bates, B., Cherney, L. R., Cramer, S. C., et al. (2016). Guidelines for adult stroke rehabilitation and recovery: a guideline for healthcare professionals From the American Heart Association/American Stroke Association. Stroke 47, e98–e169. doi: 10.1161/STR.0000000000000098
Xiao, W., Guo, S., Chen, L., and Luo, Y. (2019). The role of interleukin-33 in the modulation of splenic T-cell immune responses after experimental ischemic stroke. J. Neuroimmunol. 333, 576970. doi: 10.1016/j.jneuroim.2019.576970
Yan, T., Chen, Z., Chopp, M., Venkat, P., Zacharek, A., Li, W., et al. (2020). Inflammatory responses mediate brain-heart interaction after ischemic stroke in adult mice. J. Cerebral Blood Flow Metab. 40, 1213–1229. doi: 10.1177/0271678X18813317
Yang, H. J., Koh, E., and Kang, Y. (2021). Susceptibility of women to cardiovascular disease and the prevention potential of mind-body intervention by changes in neural circuits and cardiovascular physiology. Biomolecules 11, 708. doi: 10.3390/biom11050708
Yang, W., Li, J., Shang, Y., Zhao, L., Wang, M., Shi, J., et al. (2017). HMGB1-TLR4 axis plays a regulatory role in the pathogenesis of mesial temporal lobe epilepsy in immature rat model and children via the p38MAPK signaling pathway. Neurochem. Res. 42, 1179–1190. doi: 10.1007/s11064-016-2153-0
Yang, Z., Yu, A., Liu, Y., Shen, H., Lin, C., Lin, L., et al. (2014). Regulatory T cells inhibit microglia activation and protect against inflammatory injury in intracerebral hemorrhage. Int. Immunopharmacol. 22, 522–525. doi: 10.1016/j.intimp.2014.06.037
Zamora, R., Chavan, S., Zanos, T., Simmons, R. L., Billiar, T. R., and Vodovotz, Y. (2021). Spatiotemporally specific roles of TLR4, TNF, and IL-17A in murine endotoxin-induced inflammation inferred from analysis of dynamic networks. Mol. Med. 27, 1. doi: 10.1186/s10020-021-00333-z
Zhang, L., Wei, W., Ai, X., Kilic, E., Hermann, D. M., Venkataramani, V., et al. (2021). Extracellular vesicles from hypoxia-preconditioned microglia promote angiogenesis and repress apoptosis in stroke mice via the TGF-β/Smad2/3 pathway. Cell Death Dis. 12, 1068. doi: 10.1038/s41419-021-04363-7
Zhang, S., Kim, B., Zhu, X., Gui, X., Wang, Y., Lan, Z., et al. (2020). Glial type specific regulation of CNS angiogenesis by HIFα-activated different signaling pathways. Nat. Commun. 11, 2027. doi: 10.1038/s41467-020-15656-4
Zhao, Q., Yan, T., Li, L., Chopp, M., Venkat, P., Qian, Y., et al. (2019). Immune response mediates cardiac dysfunction after traumatic brain injury. J. Neurotrauma 36, 619–629. doi: 10.1089/neu.2018.5766
Zhou, K., Zhong, Q., Wang, Y. C., Xiong, X. Y., Meng, Z. Y., Zhao, T., et al. (2017). Regulatory T cells ameliorate intracerebral hemorrhage-induced inflammatory injury by modulating microglia/macrophage polarization through the IL-10/GSK3β/PTEN axis. J. Cerebral Blood Flow Metab. 37, 967–979. doi: 10.1177/0271678X16648712
Keywords: microglia, stroke, spleen, cardiac, inflammation, neural-network
Citation: Deng J, Chen C, Xue S, Su D, Poon WS, Hou H and Wang J (2023) Microglia-mediated inflammatory destruction of neuro-cardiovascular dysfunction after stroke. Front. Cell. Neurosci. 17:1117218. doi: 10.3389/fncel.2023.1117218
Received: 06 December 2022; Accepted: 23 February 2023;
Published: 21 March 2023.
Edited by:
Liang Tan, Army Medical University, ChinaReviewed by:
Sheng Zhang, Mayo Clinic, United StatesCopyright © 2023 Deng, Chen, Xue, Su, Poon, Hou and Wang. This is an open-access article distributed under the terms of the Creative Commons Attribution License (CC BY). The use, distribution or reproduction in other forums is permitted, provided the original author(s) and the copyright owner(s) are credited and that the original publication in this journal is cited, in accordance with accepted academic practice. No use, distribution or reproduction is permitted which does not comply with these terms.
*Correspondence: Jun Wang, c211d2FuZ2p1bkAxNjMuY29t; Honghao Hou, c3MuaGhoODlAaG90bWFpbC5jb20=; Wai Sang Poon, d2FpcG9vbkBoa3Utc3poLm9yZw==
†These authors have contributed equally to this work
‡ORCID: Wai Sang Poon orcid.org/0000-0001-5368-3480
Honghao Hou orcid.org/0000-0002-7133-9760
Jun Wang orcid.org/0000-0003-3509-0790
Disclaimer: All claims expressed in this article are solely those of the authors and do not necessarily represent those of their affiliated organizations, or those of the publisher, the editors and the reviewers. Any product that may be evaluated in this article or claim that may be made by its manufacturer is not guaranteed or endorsed by the publisher.
Research integrity at Frontiers
Learn more about the work of our research integrity team to safeguard the quality of each article we publish.