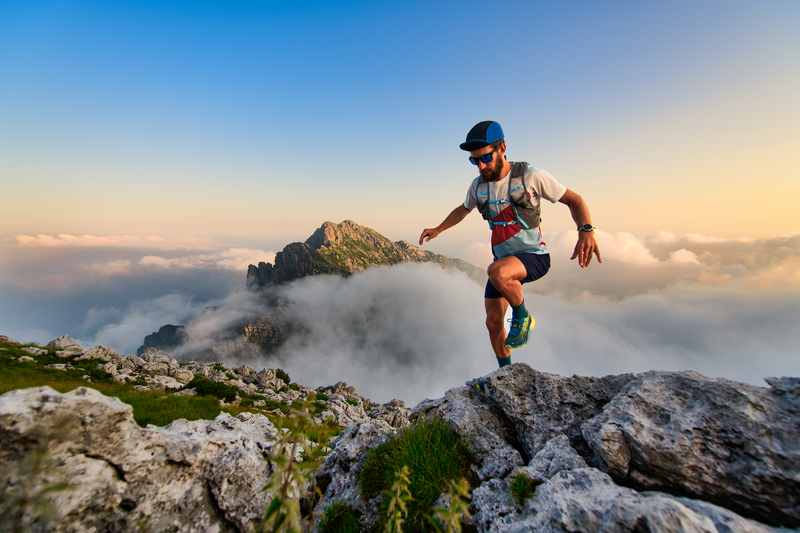
94% of researchers rate our articles as excellent or good
Learn more about the work of our research integrity team to safeguard the quality of each article we publish.
Find out more
BRIEF RESEARCH REPORT article
Front. Cell. Neurosci. , 08 December 2022
Sec. Cellular Neuropathology
Volume 16 - 2022 | https://doi.org/10.3389/fncel.2022.999303
Background: The cell cycle is a critical mechanism for proper cellular growth, development and viability. The p16INK4a and p21Waf1/Cip1 are important regulators of the cell cycle progression in response to internal and external stimuli (e.g., stress). Accumulating evidence indicates that the prefrontal cortex (PFC) is particularly vulnerable to stress, where stress induces, among others, molecular and morphological alterations, reflecting behavioral changes. Here, we investigated if the p16INK4a and p21Waf1/Cip1 expression are associated with behavioral outcomes.
Methods: Prefrontal cortex mRNA and protein levels of p16INK4A and p21Waf1/Cip1 of mice (six independent groups of C57BL/6J, eight mice/group, 50% female) exposed from 0 to 35 days of chronic restraint stress (CRS) were quantified by qPCR and Western Blot, respectively. Correlation analyses were used to investigate the associations between cyclin-dependent kinase inhibitors (CKIs) expression and anxiety- and depression-like behaviors.
Results: Our results showed that the PFC activated the cell cycle regulation pathways mediated by both CKIs p16INK4A and p21Waf1/Cip1 in mice exposed to CRS, with overall decreased mRNA expression and increased protein expression. Moreover, correlation analysis revealed that mRNA and protein levels are statistically significant correlated with anxiety and depressive-like behavior showing a greater effect in males than females.
Conclusion: Our present study extends the existing literature providing evidence that PFC cells respond to chronic stress exposure by overexpressing CKIs. Furthermore, our findings indicated that abnormal expression of p16INK4A and p21Waf1/Cip1 may significantly contribute to non-adaptive behavioral responses.
The cell cycle is a succession of coordinated events which lead to cell division, critical for both the development and viability of multicellular organisms (Kumari and Jat, 2021). The transition from one cell cycle stage to another is regulated by several molecules, including cyclins, cyclin-dependent kinases (CDKs), and cyclin-dependent kinases inhibitors (CKIs). CDKs drive the cell cycle through the accumulation of the dephosphorylated form of the retinoblastoma tumor suppressor protein (pRb) (Mitra et al., 1999; Li et al., 2009). The pRb protein holds an important role in the cell cycle, its inactivation by cyclin-dependent kinases 4 and 6 (CDK4 and CDK6, respectively) releases E2F transcription factors and allows the expression of genes that mediate entry of the transition from G1 to S phase (Giacinti and Giordano, 2006). Of the four phases of the cell cycle, G1 is a dynamic stage marked by high rates of biosynthesis and responsiveness to extracellular regulatory signals required for the cell to progress toward division (Bertoli et al., 2013). Control of the G1 phase is an essential gatekeeper in the rate of cell cycle progression and is negatively regulated by two main families of CKIs: the Ink4 (p16INK4a, p15INK4b, p18INK4c, and p19INK4d) and Cip/Kip (p21Waf1/Cip1, p27Kip1, and p57Kip2). The CKI p16INK4A binds directly to CDK4 and CDK6, blocking phosphorylation of the pRb and subsequent traversal of the G1/S cell cycle checkpoint. In addition, the CKI p21Waf1/Cip1 maintains cell quiescence, blocking progression into S-phase, by inhibiting the cyclin-CDK2, cyclin-CDK1, and cyclin-CDK4, 6 complexes (Georgakilas et al., 2017). If upregulated, p21Waf1/Cip1 causes cell growth arrest at the G2 phase and is required for sustained G2 arrest following DNA damage (Bunz et al., 1998). The cell cycle machinery is known predominantly for regulating cellular proliferation, although accumulating evidence indicates that cell cycle proteins may also control cellular senescence and apoptosis, especially in postmitotic cells (Sapieha and Mallette, 2018). Postmitotic cells, also known as terminally differentiated cells, have long been considered to be permanently in G0. However, increasing evidence indicates that postmitotic cells such as neurons can re-enter the cell cycle in response to internal and external stimuli such as stress and this can be critical for their functions (Pajalunga et al., 2007; Sah et al., 2021).
Stress is characterized as a state in which intrinsic or extrinsic stimuli evoke a dynamic and complex repertoire of biological, physiological, and behavioral adaptive responses of the organism (Chrousos and Gold, 1992; Chrousos, 2009; Yaribeygi et al., 2017). Adaptive responses are required to reestablish homeostasis and range from transient molecular changes to cell death based on the stimulus’s type, timing, and severity (Lupien et al., 2009). Several studies have shown that stress can be either a triggering or aggravating factor for many pathological conditions, including cancer (Moreno-Smith et al., 2010), depression (Yang et al., 2015), post-traumatic stress disorder (Sherin and Nemeroff, 2011), and neurodegenerative disorders (Hemmerle et al., 2014; Justice, 2018; Song et al., 2020). Stress response involves a range of well-conserved signaling pathways that depend upon highly interconnected neuroendocrine, cellular, and molecular pathways (Harding et al., 2003; Poon, 2016; Nava et al., 2020). The hypothalamic-pituitary-adrenal axis and the autonomic nervous system are key components of the stress system, and they interact with different brain regions and tissues/organs in the periphery to elicit the cellular and molecular adaptive responses against the stimuli (Miller and O’Callaghan, 2002). To date, it is mechanistically not clarified how senescence status in brain cells is activated by stress and how it is connected to maladaptive behavioral responses. The literature consistently shows that suppression of adult hippocampal neurogenesis caused by stress (Besnard and Sahay, 2016; Drew and Huckleberry, 2017; Huckleberry et al., 2018) might contribute to a maladaptive emotional and stress-coping strategy. Several lines of evidence have indicated that the prefrontal cortex (PFC) is particularly vulnerable to stress, where stress induces volumetric (de Lange et al., 2008; McEwen et al., 2016; Misquitta et al., 2021), cytoarchitectural (Misquitta et al., 2021), and morphological changes, including the reduction of dendrite length, branching, and spine density (McEwen, 1997; McEwen and Magarinos, 1997; Radley et al., 2006; Arnsten, 2009; Kang et al., 2012; Arnsten et al., 2015; Qiao et al., 2016; Moreno et al., 2017; Banasr et al., 2021; Bernardo et al., 2022). In addition, altered PFC neuronal activity is associated with social defeat-induced depression- and anxiety-like behavior in mice, and learning and memory impairments (Sullivan and Gratton, 2002; Lupien et al., 2009; Felix-Ortiz et al., 2016; Burgos-Robles et al., 2017; Planchez et al., 2019).
Previous studies have shown that p16INK4a and p21Waf1/Cip1 play critical roles in cell cycle arrest in neurons in the hippocampus by preserving cellular integrity and avoiding cell death (Sapieha and Mallette, 2018; Sah et al., 2021). Building on observations that p16INK4a and p21Waf1/Cip1 expression in the brain is sustained postnatally, Le et al. (2018) have shown that p16INK4a expression mediates the loss of neurogenesis in the hippocampus of mice exposed to ionizing radiation. In a recent report, p16INK4a appears to prevent a neural stem cell release from quiescence when neurogenic stimuli are present, suggesting that p16INK4a is crucial to the self-renewal capacity during aging (Micheli et al., 2019). Moreover, Pechnick et al. (2011) showed that proliferation of adult neurons in the hippocampus is restrained by CKIs and loss of p21Waf1/Cip1 function results in the release of progenitors from cell cycle block. Although the cellular bases of senescence underlying the stress response have been extensively investigated in the hippocampus, the effects of cell cycle arrest in PFC remain unknown.
In order to investigate whether the cell cycle regulators are involved in behavioral changes, we first measure the mRNA and protein levels of CKIs p16INK4a and p21Waf1/Cip1 in the PFC of mice exposed to chronic restraint stress (CRS). Then we assessed whether changes in p16INK4a and p21Waf1/mRNA and protein levels Cip1 were associated with anxiety- and depressive-like behaviors. Our findings open new avenues for cell cycle-related research and provide evidence that postmitotic cells have a complex stress response involving CKIs, extending our understanding of cellular senescence underlying depressive symptoms.
Mouse brain samples were obtained from the chronic stress mouse brain bank of Drs. Prevot and Banasr. Behavioral outcomes induced by CRS are described in Prevot et al. (2021). All procedures followed guidelines set by the Canadian Council on Animal Care (CCAC) and were approved by CAMH Animal Care Committee. Briefly, 8-week-old C57BL/6J mice (Jackson Laboratory, Bar Harbor, ME, USA; n = 48, eight animals/group, 50% females) were housed under standard conditions with a 12 h light/dark cycle and provided with ad libitum access to food and water. Animals were subjected to either 0, or 3, 14, 21, 28, or 35 days of CRS. Each restraint stress session consists of placing mice in a 50 ml Falcon® tube (Thermo Fisher Scientific, Waltham, MA, USA) perforated on each end to allow air circulation. Restraint sessions occurred twice daily for 1 h during the light cycle (7:00 a.m.–7:00 p.m.), separated by a minimum of 2 h. CRS-exposed animals were single-housed throughout the stress exposure, and control animals were group-housed until behavioral assessment.
Behavioral outcomes were assessed at each time point, and the methods and results were presented in Prevot et al. (2021). Animals were tested in the sucrose consumption test and the Phenotyper™ test. Each animal coat degradation and weight were also measured.
Anhedonia was assessed on each time point of the CRS protocol using a sucrose consumption test. The test consists of habituation to sucrose (1%) for 48 h and presentation of pre-weighted drinking bottles for 1 h after 16 h of fluid deprivation. Water consumption was measured on a consecutive day following the same procedure. Sucrose preference was calculated according to the formula: sucrose preference = (sucrose intake)/(sucrose intake + water intake) × 100.
The PhenoTyper™ test was performed according to a previous publication (Prevot et al., 2019) on each time point. Residual avoidance (RA) was calculated for each mouse based on the time spent in food (RA FZ) and shelter (RA SZ) zones after the light challenge, as previous described (2019).
Animals’ coat state quality was scored across seven anatomical areas 0, 0.5, or 1, from maintained to unkempt, and a sum was calculated. Weight was tracked weekly and expressed as percent change (%) to measure deviation from normal development.
After completing each time point of CRS protocol, all animals were euthanized, the brain was removed, and the PFC was dissected, collected, and separated into 1.5 ml Eppendorf tubes (Eppendorf, UK) and then rapidly shock-frozen on dry ice and stored at –80°C until used. Protein and RNA were extracted from the samples using the AllPrep RNA/Protein Kit (Qiagen, Hilden, Germany) and stored at –80°C.
Total protein concentration was determined using a BCA protein assay. Protein homogenates (30 μg) were loaded and separated by sodium dodecyl sulfate-polyacrylamide gel electrophoresis (SDS-PAGE). The membranes were incubated overnight with primary antibodies against p16INK4a (rabbit polyclonal antibody ab189034, Abcam, Cambridge, MA; 1:1,000), p21Waf1/Cip1 (mouse monoclonal antibody sc-6246, Santa Cruz Biotechnology, Santa Cruz, CA; 1:1,000), GAPDH (rabbit polyclonal antibody E-AB-20072, Elabscience Biotechnology, Wuhan, China; 1:2000), and then incubated with the related secondary antibodies at a 1:10,000 dilution. The GAPDH levels were used to normalize the levels of the targeted proteins quantified by Western blot.
Extracted RNA was converted into cDNA by reverse transcription (Kadakkuzha et al., 2013). Briefly, 1 μg of RNA was used with SuperScript VILO cDNA Synthesis Kit (Thermo Fisher, Waltham, Massachusetts, EUAUSA) according to the manufacturer’s instructions. Quantitative real-time reverse transcription-polymerase chain reaction (RT-PCR) was performed using gene-specific primers for p16INK4a (Forward 5′-CCGAACTCTTTCGGTCGTAC-3′; Reverse 5′-AGTTCGAATCTGCACCGTAGT-3′; amplicon size 94 bp), p21Waf1/Cip1 (Forward 5′-TGTCGCTGTCTT GCACTCTG-3′; Reverse 5′-GACCAATCTGCGCTTGGAGT-3′; amplicon size 128 bp) and GAPDH (Forward 5′-AACTCCCACTCTTCCACCT-3′; Reverse 5′-CACCACC CTGTTGCTGTA-3′; amplicon size 111 bp) and SYBR Green PCR master mix (Applied Biosystems Carlsbad, CA, USA) for detection in Bio-rad CFX96 Touch PCR Detection System (Bio-Rad Laboratories, Hercules, CA, USA). All the qPCR amplifications were performed in triplicate in a total volume of 20 μl containing 4 μl of H2O, 3 μl of cDNA, 10 μl of 2X Master Mix, 1.5 μl of 10 μm (each) forward and reverse primers. Data were analyzed by standard ΔCq method (2–Δ Δ Cq) where ΔCq is the difference between the genes of interest and GAPDH control Cq values for each sample (Livak and Schmittgen, 2001).
Data analysis was performed using SPSS software (IBSM SPSS statistic 24). A priori, we evaluated the normal distribution of the data. Data following normal distribution were analyzed using one-way ANOVA followed by Dunnett’s post hoc test to compare the impact of CRS exposure (control vs. each CRS group) on p16INK4a and p21Waf1/Cip1 expression. Moreover, data with a non-normal distribution were analyzed using a non-parametric Kruskal–Wallis test, followed by Bonferroni/Dunn post hoc tests to compare the impact of CRS exposure (control vs. each CRS group) on p16INK4a and p21Waf1/Cip1 expression. Analysis of covariance (ANCOVA) was applied to investigate the influence of sex on p16INK4a and p21Waf1/Cip1 expression and anxiety- and depression-like behaviors. Z-scores were calculated to assess consistency of behavioral phenotypes across tests, referred to as z-emotionality using averaged z-scores of behavioral tests as described previously (Guilloux et al., 2011). We carried out Pearson’s or Spearman’s correlation analysis when adequate to investigate the association between an overall expression of mRNA and protein of p16INK4a and p21Waf1/Cip1, and anxiety- and depression-like behaviors. Significance was set at a p-value ≤ 0.05.
Full report of the behavioral outcomes was presented in Prevot et al. (2021). In summary, Prevot et al. (2021) showed that shorter durations of chronic stress exposure induced anxiety-like behaviors (within 7 days), while the longer duration induced anxiety- and anhedonia-like behaviors (35 days). The anxiety- and anhedonia-like behaviors were reflected by increased shelter zone time and decreased food zone time, lower sucrose consumption, lower weight gain, and worse coat state.
Real-time qPCR was used to measure p16INK4a and p21Waf1/Cip1 mRNA expression in PFC samples obtained from the whole cohort and the size and specificity of each amplicon was confirmed by agarose gel electrophoresis (Figure 1C) and melting curve (Supplementary Figure 4). Analysis of variance showed that C57BL/6J mice PFC presented a significant main effect of stress on the p16INK4a mRNA expression across groups (F(5,39) = 3.907, p = 0.006). Repeated-measures ANCOVA of p16INK4a mRNA levels revealed that the significant main effect of CRS (F(11,33) = 2.509, p = 0.02) remained after controlling for sex. The ANCOVA model also showed that there were no main effects of both sex (F(1,33) = 1.895, p = 0.178) and stress*sex interaction (F(5,33) = 1.147, p = 0.355). Pairwise comparisons of CRS groups versus control showed that p16INK4a mRNA expression reduced significantly on days 3 (p = 0.031), 21 (p = 0.007), and 35 (p = 0.029; Figure 1A). In addition, there were no significant differences in p16INK4a mRNA expression on days 14 (p = 0.877) and 28 (p = 0.466) when compared to the control group (Figure 1A).
Figure 1. Chronic restraint stress (CRS) induces abnormal mRNA and protein expression of p16INK4a and p21Waf1/Cip1 in mice. (A) The p16INK4a mRNA expression levels were lower in mice submitted to CRS than the control group after 3, 21, and 35 days of stress exposure. (B) The p21Waf1/Cip1 mRNA expression levels were lower in mice submitted to CRS compared to the control group after 28 days of stress exposure. (C) Full length electrophoresis gel image showing single amplicon bands after RT-qPCR for p21 and p16INK4a genes expressed in PFC of mice submitted to CRS (0–35 days of exposure). (D) The p16INK4a protein expression levels were higher in mice submitted to CRS compared to the control group after 14 days of stress exposure. (E) The p21Waf1/Cip1 protein expression levels were higher in mice submitted to CRS compared to the control group after 3, 14, 21, 28, and 35 days of stress exposure. (F) Western blot analysis showing the protein expression levels of p16INK4a, p21, and GAPDH in PFC of mice submitted to CRS (0–35 days of exposure). Data showed were obtained in both male and female, and were presented as mean ± SEM. *p < 0.05, **p < 0.01.
Results of ANOVA analysis showed that p21Waf1/Cip1 mRNA expression was different among groups (F(5,40) = 2.924, p = 0.024). When sex was added as a co-factor, the difference in the p21Waf1/Cip1 mRNA expression across groups did not remain statistically significant (F(11,34) = 1.475, p = 0.186). In addition, we found no main effect of both sex (F(1,34) = 0.044, p = 0.836) and stress*sex interaction (F(5,34) = 0.545, p = 0.741) on p21Waf1/Cip1 mRNA expression. Pairwise analysis of CRS groups versus control showed that p21Waf1/Cip1 mRNA expression reduced significantly on day 28 (p = 0.037; Figure 1B). There were no significant differences in p21Waf1/Cip1 mRNA expression on days 3 (p = 0.330), 14 (p = 0.194), 21 (p = 0.180), and 35 (p = 0.387) when compared to the control group (Figure 1B).
Western blot was performed on PFC samples obtained from the whole cohort (Figure 1F). Kruskal-Wallis test revealed that p16INK4a protein expression was different among groups [X2(5,42) = 19.003, p = 0.002]. After including sex as a co-factor, the difference in the protein expression of p16INK4a across groups remained statistically significant [F(11,30) = 5.043, p = 0.0001]. The ANCOVA model revealed a significant main effect of stress*sex interaction [F(5,30) = 3.665, p = 0.010], and no main effect of sex [F(1,30) = 2.164, p = 0.152]. Pairwise analysis showed that mice submitted to 14 days of CRS increased significantly p16INK4a protein levels (p = 0.025) compared to control group (Figure 1D). There were no significant differences in p16INK4a protein expression on days 3 (p = 0.749), 21 (p = 0.355), 28 (p = 0.338), and 35 (p = 0.083) when compared to the control group (Figure 1D).
We found a marginally significant difference on p21Waf1/Cip1 protein expression among groups [X2(5,39) = 10.790, p = 0.056]. When sex was considered as a covariate, there was no significant difference in the p21Waf1/Cip1 protein expression across groups [F(11,33) = 2.066, p = 0.061], but a main effect of sex [F(1,33) = 4.518, p = 0.043] was found. In addition, there was no main effect of stress × sex interaction [F(5,33) = 1.294, p = 0.296]. Pairwise contrast analysis were performed and results showed that p21Waf1/Cip1 protein expression increased significantly on days 3 (p = 0.016), 14 (p = 0.005), 21 (p = 0.005), 28 (p = 0.045), and 35 (p = 0.028) when compared to the control group (Figure 1E).
Correlation analyses were performed to assess potential relationships between PFC mRNA and protein expression of p16INK4a and p21Waf1/Cip1 and behavioral outcomes. The p16INK4a mRNA expression was correlated negatively with RA in shelter zone (r = −0.318, p = 0.034; Figure 2A), correlated positively with weight gain (r = 0.337, p = 0.024; Figure 2B), and sucrose consumption (r = 0.355, p = 0.018; Figure 2C). There were no significant correlations with RA in food zone (r = −0.251, p = 0.097) and coat state (r = −0.164, p = 0.280; Supplementary Figures 2A,B, respectively). The p21Waf1/Cip1 mRNA expression was correlated negatively with coat state (r = −0.400; p = 0.006; Figure 2D), but not showed a significant correlation with RA in shelter (r = −0.009; p = 0.952) and food zones (r = −0.068; p = 0.651), weight gain (r = −0.223; p = 0.136), or sucrose consumption (r = 0.094; p = 0.539; Supplementary Figures 2C–F, respectively).
Figure 2. Chronic restraint stress (CRS)-induced alterations in PFC p16INK4a and p21Waf1/Cip1 mRNA and protein expression levels correlates with behavioral performances. The p16INK4a mRNA expression levels were significantly correlated with residual avoidance in shelter zone (RA SZ) (A), weight gain (B), and sucrose consumption (C). The p21Waf1/Cip1 mRNA levels showed a significant negative correlation with coat state (D). The p16INK4a protein expression levels were negatively correlated with coat state (E) and the p21Waf1/Cip1 protein expression levels were negatively correlated with sucrose consumption (F). *p < 0.05, **p < 0.01.
The p16INK4a protein levels were correlated negatively with coat state (r = −0.408, p = 0.007; Figure 2E), but showed no significant correlation with RA in food (r = −0.066, p = 0.679) and shelter zones (r = −0.003, p = 0.985), weight gain (r = 0.209, p = 0.185), or sucrose consumption (r = 0.217, p = 0.173; Supplementary Figures 2G–J, respectively). The p21Waf1/Cip1 protein expression was correlated negatively with sucrose consumption (r = −0.342, p = 0.036; Figure 2F), but showed no significant correlation with RA in food (r = 0.128, p = 0.436) and shelter zones (r = −0.063, p = 0.705), weight gain (r = −0.160, p = 0.332), or coat state (r = 0.250, p = 0.124; Supplementary Figures 2K–N, respectively).
Based on the observed mRNA and protein expression associations with behavioral outcomes, we decided to split the dataset by sex to investigate potential sexual dimorphism effects. In females, correlations analysis revealed a significant association of p16INK4a mRNA expression with weight gain (r = 0.418, p = 0.042; Figure 3A), and p21Waf1/Cip1 with worsened coat state (r = −0.438, p = 0.032; Figure 3B). In males, correlation analysis showed a significant association of p21Waf1/Cip1 mRNA expression and weight gain (r = −0.455, p = 0.033; Figure 3C). Moreover, p16INK4a and p21Waf1/Cip1 protein expression were correlated with behavior exclusively in males. The p16INK4a protein levels were correlated positively with sucrose consumption (r = 0.458, p = 0.049; Figure 3D) and negatively with coat state (r = −0.495, p = 0.031; Figure 3E). In addition, p21Waf1/Cip1 protein levels were correlated positively with RA in shelter zone (r = 0.547, p = 0.012; Figure 3F) and negatively with sucrose consumption (r = −0.609, p = 0.004; Figure 3G). No other correlations reached statistical significance in females or males; results are shown in Supplementary Figure 3.
Figure 3. Chronic restraint stress (CRS) leads to sexually dimorphic p16INK4a and p21Waf1/Cip1 mRNA and protein expression changes in the PFC with associated behavioral responses. In females, correlations analysis revealed a significant association of p16INK4a mRNA expression with weight gain (A), and p21Waf1/Cip1 with worsened coat state (B). In males, we found a significant correlation of p21Waf1/Cip1 mRNA expression and weight gain (C). Higher protein levels of p16INK4a showed a positive association with sucrose consumption (D) and worsened coat state (E), and higher protein levels of p21Waf1/Cip1 were associated with greater (RA SZ) (F) and reduced sucrose consumption (G). Data showed were obtained in both male and female. *p < 0.05, **gp < 0.01.
Finally, we did not find a significant correlation between mRNA and protein expression levels of p16INK4a (r = 0.208, p = 0.192) and p21Waf1/Cip1 (r = −0.113, p = 0.494).
In this exploratory study, we used CRS as a tool to investigate the influence of cell cycle regulators (p16INK4a and p21Waf1/Cip1) expression on mice behavior. Our results demonstrated that mice exposed to CRS showed a decreased mRNA expression of p16INK4a (within 3, 21, and 35 days) and p21Waf1/Cip1 (within 21 days), but increased protein levels of p16INK4a (within 14 days) and p21Waf1/Cip1 (within 3, 14, 21, 28, and 35 days) in the PFC. Further, the PFC mRNA and protein expression of p16INK4a and p21Waf1/Cip1 were correlated with anxiety- and depressive-like behaviors showing a greater effect in males than females. To best of our knowledge, this is the first study to revealed that p16INK4a and p21Waf1/Cip1 are involved in the PFC response to chronic stress and their expression are significantly associated with behavioral performances.
Accumulating evidence indicates that cellular responses to exposure to different stimuli are largely adaptive by means of a complex protein network responsible for the recovery and maintenance of genetic integrity (Bartek and Lukas, 2007; Jackson and Bartek, 2009; Poon, 2016). However, if the damage caused by the stimuli such as stress cannot be repaired, the cell will commit to two primary fates: senescence, or apoptosis (Poon, 2016; Nava et al., 2020). Our results suggested a differential changes in PFC mRNA and protein expression of p16INK4a and p21Waf1/Cip1 during stress exposure. The mRNA expression seems to be inhibited by stress while is observed overexpression of protein with longer exposure to CRS. Assuming that mRNA and protein abundance is regulated through a dynamic balance between their synthesis, degradation rates, and mean lifetime, a direct correlation between the amounts of an mRNA and its corresponding protein might not be observed (Maier et al., 2009; Hoernes et al., 2016). Taking together these results may suggest a cooperative stress response eliciting two distinct damage-response pathways responsible for (1) inhibit transcription, avoiding increase the DNA damage, and (2) cell cycle arrest by overexpression of p16INK4a and p21CIP1/WAF1 proteins. Evidence suggests that inhibition of DNA synthesis may prevent the accumulation of DNA damage and worse consequences such as apoptosis (Kim and Jinks-Robertson, 2012). Moreover, increased levels of p16INK4a and p21Waf1/Cip1 proteins may suggest a secondary activation of the Rb protein phosphorylation which also inhibits the DNA synthesis and triggers the cell to arrest in G1 (Kim and Jinks-Robertson, 2012). Kfoury et al. (2018) showed that mRNA and protein of p16INK4a and p21Waf1/Cip1 dynamically changed and presented a cooperative phenomenon that is necessary to protect astrocytes against an aberrant proliferation and DNA mutations.
The transition from acute senescence state to a permanent one typically involves prolonged CDK–cyclin activity inhibition by increased CKIs expression. In line with results published by Purvis et al. (2012), we observed an increased protein levels of p21Waf1/Cip1 and transient increased p16Ink4a protein levels over CRS exposure. Several findings of a senescent-like phenotype postmitotic neurons and glia in mice and human indicate that this mechanism might not be exclusive to proliferating cells (Sedelnikova et al., 2004; Jurk et al., 2012; Piechota et al., 2016; Fielder et al., 2017). A recent study showed that both p21WAF1/CIP1 and p16INK4a were expressed by astrocyte and induced senescence state in early stages of the and amyotrophic lateral sclerosis, whereas p21WAF1/CIP1 was exclusively expressed by neurons and might reflect a more general mechanism of age-related cell-cycle dysregulation (Vazquez-Villaseñor et al., 2020). Le et al. (2018) found that the absence of p16INK4a mRNA expression could to some extent restore neurogenesis in the dentate gyrus following exposure to ionizing radiation. In astrocytes and microglia, senescence seems to contribute to the disruption of the glia–neuron interaction, glial loss and neuronal atrophy and may underlie, in part, the development of age-related brain pathologies such as Alzheimer’s disease and depression (Cotter et al., 2001; Drevets, 2001; Rajkowska and Miguel-Hidalgo, 2007; Banasr et al., 2010; Mombach et al., 2015; Crowe et al., 2016; Safaiyan et al., 2016; Christman et al., 2020). Cellular senescence might be a key mechanism in which cells temporarily lose their replicative phenotype as an attempt to respond to stress and reverse or compensate for decreased cell number and loss of neuroplasticity (Sikora et al., 2021). However, given that the primary consequence of senescence activation in postmitotic cells are still not completely understood.
Combining our findings, the correlation analysis demonstrated that lower p16INK4a/p21CIP1/WAF1 mRNA levels are associated with greater anxiety, and anhedonia deficits, whereas higher p16INK4a/p21CIP1/WAF1 protein levels are associated with greater coat deterioration and reduced sucrose consumption. The mechanisms underlying how stress affects body weight and food intake are not well understood. Weight gain or lack thereof in CRS animals was associated with chronic elevation of corticosterone level and HPA axis dysregulation without change in food intake (Jeong et al., 2013). It is possible that the significant association between p16INK4a/p21CIP1/WAF1 expression and weight gain is linked to this mechanism. Different studies will need to be designed to specifically address this question. Although the main focus of this study was investigating the effects of PFC CKI’s expression in behavior we included an equal number of male and female mice to account for sex. In CRS female mice, p16INK4a mRNA levels correlated positively with weight gain and p21Waf1/Cip1 mRNA expression levels correlated with worsened coat state. In CRS male mice, p21CIP1/WAF1 mRNA expression levels correlated negatively with weight gain, p16INK4a protein expression levels correlated positively with greater sucrose consumption and correlated negatively with coat state, and p21Waf1/Cip1 protein expression levels correlated positively with RA SZ and correlated negatively with sucrose consumption. The literature suggests that females are more affected by stress and exhibit more symptoms of stress-related disorders (Giovanniello et al., 2021; Woodburn et al., 2021), although we were unable to find significant correlations between CKI’s protein levels and behavioral outcomes in females. This could be due to variability in the estrus cycle which we did not monitor in this study or to potential sexual dimorphism. Indeed, in prior studies, sexual dimorphism in cell cycle regulation and DNA repair by p16INK4a and p21CIP1/WAF1 was shown to have a crucial role in cancer incidence such as glioblastoma (Ostrom et al., 2016; Kfoury et al., 2018). Future studies specifically designed for studying sexual dimorphism and defining the cellular effects of an abnormal CKI’s expression in PFC would be needed to answer this question.
This study has several limitations. First, the relatively small number of animals per group and per sex, the cross-sectional design also limit the interpretation of our findings regarding the progression of the response to chronic stress. Additionally, the study design did not allow for drawing any causal link between cell cycle regulators’ expression and depression-like behavior. We will need to investigate further to understand the underlying mechanism of time-dependent effects of stress on cortical p16INK4a and p21CIP1/WAF1 expression, as well as its potential influence on a difference in cell cycle response between males and females. Although cognitive deficits are part of depressive symptoms and could have brought additional information related to PFC changes, we chose not to perform any cognitive tasks driven by feasibility concerns with a high volume of animals. Further studies will be necessary to investigate the effects of p16INK4a and p21CIP1/WAF1 expression changes on cognitive functions and memory. Finally, we also did not measure p16INK4a and p21CIP1/WAF1 levels in specific brain cells or other brain regions. Future studies should investigate the induction of senescence through p16INK4a and p21Waf1/Cip1 in human brain tissue from patients with stress-related disorders to confirm the translation of our findings.
Despite these limitations, the present exploratory study extends the existing literature providing evidence that postmitotic cells have a complex stress response involving senescence markers, and. suggests a link between p16INK4a and p21Waf1/Cip1 expression and anxiety- and depressive-like behaviors in mice submitted to CRS.
The original contributions presented in this study are included in the article/Supplementary material, further inquiries can be directed to the corresponding author.
This animal study was reviewed and approved by the CAMH Animal Care Committee.
AM-S conceptualized the study, conducted all the wet lab and statistical analyses, interpretation of results, and wrote the original manuscript draft. TP conceptualized the study, performed the behavioral tests, and contributed to the original manuscript draft. MB and ES contributed to the manuscript reviewing, editing, and providing critical intellectual input about the analysis and results. BD developed the study concept, oversaw all analyses, and all stages of the manuscript preparation. All authors reviewed and approved the manuscript before submission.
This work was supported by the CAMH’s intramural funds mechanisms.
The authors declare that the research was conducted in the absence of any commercial or financial relationships that could be construed as a potential conflict of interest.
All claims expressed in this article are solely those of the authors and do not necessarily represent those of their affiliated organizations, or those of the publisher, the editors and the reviewers. Any product that may be evaluated in this article, or claim that may be made by its manufacturer, is not guaranteed or endorsed by the publisher.
The Supplementary Material for this article can be found online at: https://www.frontiersin.org/articles/10.3389/fncel.2022.999303/full#supplementary-material
Arnsten, A. F. (2009). Stress signalling pathways that impair prefrontal cortex structure and function. Nat. Rev. Neurosci. 10, 410–422. doi: 10.1038/nrn2648
Arnsten, A. F., Raskind, M. A., Taylor, F. B., and Connor, D. F. (2015). The effects of stress exposure on prefrontal cortex: translating basic research into successful treatments for post-traumatic stress disorder. Neurobiol. Stress 1, 89–99. doi: 10.1016/j.ynstr.2014.10.002
Banasr, M., Chowdhury, G. M., Terwilliger, R., Newton, S. S., Duman, R. S., Behar, K. L., et al. (2010). Glial pathology in an animal model of depression: reversal of stress-induced cellular, metabolic and behavioral deficits by the glutamate-modulating drug riluzole. Mol. Psychiatry 15, 501–511. doi: 10.1038/mp.2008.106
Banasr, M., Sanacora, G., and Esterlis, I. (2021). Macro- and microscale stress-associated alterations in brain structure: translational link with depression. Biol. Psychiatry 90, 118–127. doi: 10.1016/j.biopsych.2021.04.004
Bartek, J., and Lukas, J. (2007). DNA damage checkpoints: from initiation to recovery or adaptation. Curr. Opin. Cell Biol. 19, 238–245. doi: 10.1016/j.ceb.2007.02.009
Bernardo, A., Lee, P., Marcotte, M., Mian, M. Y., Rezvanian, S., Sharmin, D., et al. (2022). Symptomatic and neurotrophic effects of GABAA receptor positive allosteric modulation in a mouse model of chronic stress. Neuropsychopharmacology 47, 1608–1619. doi: 10.1038/s41386-022-01360-y
Bertoli, C., Skotheim, J. M., and de Bruin, R. A. (2013). Control of cell cycle transcription during G1 and S phases. Nat. Rev. Mol. Cell Biol. 14, 518–528. doi: 10.1038/nrm3629
Besnard, A., and Sahay, A. (2016). Adult hippocampal neurogenesis, fear generalization, and stress. Neuropsychopharmacology 41, 24–44. doi: 10.1038/npp.2015.167
Bunz, F., Dutriaux, A., Lengauer, C., Waldman, T., Zhou, S., Brown, J. P., et al. (1998). Requirement for p53 and p21 to sustain G2 arrest after DNA damage. Science 282, 1497–1501. doi: 10.1126/science.282.5393.1497
Burgos-Robles, A., Kimchi, E. Y., Izadmehr, E. M., Porzenheim, M. J., Ramos-Guasp, W. A., Nieh, E. H., et al. (2017). Amygdala inputs to prefrontal cortex guide behavior amid conflicting cues of reward and punishment. Nat. Neurosci. 20, 824–835. doi: 10.1038/nn.4553
Christman, S., Bermudez, C., Hao, L., Landman, B. A., Boyd, B., Albert, K., et al. (2020). Accelerated brain aging predicts impaired cognitive performance and greater disability in geriatric but not midlife adult depression. Transl. Psychiatry 10:317. doi: 10.1038/s41398-020-01004-z
Chrousos, G. P. (2009). Stress and disorders of the stress system. Nat. Rev. Endocrinol. 5, 374–381. doi: 10.1038/nrendo.2009.106
Chrousos, G. P., and Gold, P. W. (1992). The concepts of stress and stress system disorders. Overview of physical and behavioral homeostasis. JAMA 267, 1244–1252. doi: 10.1001/jama.1992.03480090092034
Cotter, D., Mackay, D., Landau, S., Kerwin, R., and Everall, I. (2001). Reduced glial cell density and neuronal size in the anterior cingulate cortex in major depressive disorder. Arch. Gen. Psychiatry 58, 545–553. doi: 10.1001/archpsyc.58.6.545
Crowe, E. P., Tuzer, F., Gregory, B. D., Donahue, G., Gosai, S. J., Cohen, J., et al. (2016). Changes in the transcriptome of human astrocytes accompanying oxidative stress-induced senescence. Front. Aging Neurosci. 8:208. doi: 10.3389/fnagi.2016.00208
de Lange, F. P., Koers, A., Kalkman, J. S., Bleijenberg, G., Hagoort, P., van der Meer, J. W., et al. (2008). Increase in prefrontal cortical volume following cognitive behavioural therapy in patients with chronic fatigue syndrome. Brain 131(Pt 8), 2172–2180. doi: 10.1093/brain/awn140
Drevets, W. C. (2001). Neuroimaging and neuropathological studies of depression: implications for the cognitive-emotional features of mood disorders. Curr. Opin. Neurobiol. 11, 240–249. doi: 10.1016/S0959-4388(00)00203-8
Drew, M. R., and Huckleberry, K. A. (2017). Modulation of Aversive Memory by Adult Hippocampal Neurogenesis. Neurotherapeutics 14, 646–661. doi: 10.1007/s13311-017-0528-9
Felix-Ortiz, A. C., Burgos-Robles, A., Bhagat, N. D., Leppla, C. A., and Tye, K. M. (2016). Bidirectional modulation of anxiety-related and social behaviors by amygdala projections to the medial prefrontal cortex. Neuroscience 321, 197–209. doi: 10.1016/j.neuroscience.2015.07.041
Fielder, E., von Zglinicki, T., and Jurk, D. (2017). The DNA damage response in neurons: die by apoptosis or survive in a senescence-like state? J. Alzheimers Dis. 60, S107–S131. doi: 10.3233/JAD-161221
Georgakilas, A. G., Martin, O. A., and Bonner, W. M. (2017). p21: A two-faced genome guardian. Trends Mol. Med. 23, 310–319. doi: 10.1016/j.molmed.2017.02.001
Giacinti, C., and Giordano, A. (2006). RB and cell cycle progression. Oncogene 25, 5220–5227. doi: 10.1038/sj.onc.1209615
Giovanniello, J., Ahrens, S., Yu, K., and Li, B. (2021). Sex-specific stress-related behavioral phenotypes and central amygdala dysfunction in a mouse model of 16p11.2 microdeletion. Biol. Psychiatry Glob. Open Sci. 1, 59–69. doi: 10.1016/j.bpsgos.2021.01.001
Guilloux, J. P., Seney, M., Edgar, N., and Sibille, E. (2011). Integrated behavioral z-scoring increases the sensitivity and reliability of behavioral phenotyping in mice: relevance to emotionality and sex. J. Neurosci. Methods 197, 21–31. doi: 10.1016/j.jneumeth.2011.01.019
Harding, H. P., Zhang, Y., Zeng, H., Novoa, I., Lu, P. D., Calfon, M., et al. (2003). An integrated stress response regulates amino acid metabolism and resistance to oxidative stress. Mol. Cell 11, 619–633. doi: 10.1016/S1097-2765(03)00105-9
Hemmerle, A. M., Dickerson, J. W., Herman, J. P., and Seroogy, K. B. (2014). Stress exacerbates experimental Parkinson’s disease. Mol. Psychiatry 19, 638–640. doi: 10.1038/mp.2013.108
Hoernes, T. P., Hüttenhofer, A., and Erlacher, M. D. (2016). mRNA modifications: Dynamic regulators of gene expression? RNA Biol. 13, 760–765. doi: 10.1080/15476286.2016.1203504
Huckleberry, K. A., Shue, F., Copeland, T., Chitwood, R. A., Yin, W., and Drew, M. R. (2018). Dorsal and ventral hippocampal adult-born neurons contribute to context fear memory. Neuropsychopharmacology 43, 2487–2496. doi: 10.1038/s41386-018-0109-6
Jackson, S. P., and Bartek, J. (2009). The DNA-damage response in human biology and disease. Nature 461, 1071–1078. doi: 10.1038/nature08467
Jeong, J. Y., Lee, D. H., and Kang, S. S. (2013). Effects of chronic restraint stress on body weight, food intake, and hypothalamic gene expressions in mice. Endocrinol. Metab. 28, 288–296. doi: 10.3803/EnM.2013.28.4.288
Jurk, D., Wang, C., Miwa, S., Maddick, M., Korolchuk, V., Tsolou, A., et al. (2012). Postmitotic neurons develop a p21-dependent senescence-like phenotype driven by a DNA damage response. Aging Cell 11, 996–1004. doi: 10.1111/j.1474-9726.2012.00870.x
Justice, N. J. (2018). The relationship between stress and Alzheimer’s disease. Neurobiol. Stress 8, 127–133. doi: 10.1016/j.ynstr.2018.04.002
Kadakkuzha, B. M., Akhmedov, K., Capo, T. R., Carvalloza, A. C., Fallahi, M., and Puthanveettil, S. V. (2013). Age-associated bidirectional modulation of gene expression in single identified R15 neuron of Aplysia. BMC Genomics 14:880. doi: 10.1186/1471-2164-14-880
Kang, H. J., Voleti, B., Hajszan, T., Rajkowska, G., Stockmeier, C. A., Licznerski, P., et al. (2012). Decreased expression of synapse-related genes and loss of synapses in major depressive disorder. Nat. Med. 18, 1413–1417. doi: 10.1038/nm.2886
Kfoury, N., Sun, T., Yu, K., Rockwell, N., Tinkum, K. L., Qi, Z., et al. (2018). Cooperative p16 and p21 action protects female astrocytes from transformation. Acta Neuropathol. Commun. 6:12. doi: 10.1186/s40478-018-0513-5
Kim, N., and Jinks-Robertson, S. (2012). Transcription as a source of genome instability. Nat. Rev. Genet. 13, 204–214. doi: 10.1038/nrg3152
Kumari, R., and Jat, P. (2021). Mechanisms of cellular senescence: cell cycle arrest and senescence associated secretory phenotype. Front. Cell Dev. Biol. 9:645593. doi: 10.3389/fcell.2021.645593
Le, O., Palacio, L., Bernier, G., Batinic-Haberle, I., Hickson, G., and Beauséjour, C. (2018). INK4a/ARF Expression impairs neurogenesis in the brain of irradiated mice. Stem Cell Rep. 10, 1721–1733. doi: 10.1016/j.stemcr.2018.03.025
Li, W., Kotoshiba, S., Berthet, C., Hilton, M. B., and Kaldis, P. (2009). Rb/Cdk2/Cdk4 triple mutant mice elicit an alternative mechanism for regulation of the G1/S transition. Proc. Natl. Acad. Sci. U.S.A. 106, 486–491. doi: 10.1073/pnas.0804177106
Livak, K. J., and Schmittgen, T. D. (2001). Analysis of relative gene expression data using real-time quantitative PCR and the 2(-Delta Delta C(T)) Method. Methods 25, 402–408. doi: 10.1006/meth.2001.1262
Lupien, S. J., McEwen, B. S., Gunnar, M. R., and Heim, C. (2009). Effects of stress throughout the lifespan on the brain, behaviour and cognition. Nat. Rev. Neurosci. 10, 434–445. doi: 10.1038/nrn2639
Maier, T., Güell, M., and Serrano, L. (2009). Correlation of mRNA and protein in complex biological samples. FEBS Lett. 583, 3966–3973. doi: 10.1016/j.febslet.2009.10.036
McEwen, B. S. (1997). Possible mechanisms for atrophy of the human hippocampus. Mol. Psychiatry 2, 255–262. doi: 10.1038/sj.mp.4000254
McEwen, B. S., and Magarinos, A. M. (1997). Stress effects on morphology and function of the hippocampus. Ann. N. Y. Acad. Sci. 821, 271–284. doi: 10.1111/j.1749-6632.1997.tb48286.x
McEwen, B. S., Nasca, C., and Gray, J. D. (2016). Stress effects on neuronal structure: hippocampus, amygdala, and prefrontal cortex. Neuropsychopharmacology 41, 3–23. doi: 10.1038/npp.2015.171
Micheli, L., D’Andrea, G., Ceccarelli, M., Ferri, A., Scardigli, R., and Tirone, F. (2019). p16Ink4a prevents the activation of aged quiescent dentate Gyrus stem cells by physical exercise. Front. Cell Neurosci. 13:10. doi: 10.3389/fncel.2019.00010
Miller, D. B., and O’Callaghan, J. P. (2002). Neuroendocrine aspects of the response to stress. Metabolism 51(6 Suppl. 1), 5–10. doi: 10.1053/meta.2002.33184
Misquitta, K. A., Miles, A., Prevot, T. D., Knoch, J. K., Fee, C., Newton, D. F., et al. (2021). Reduced anterior cingulate cortex volume induced by chronic stress correlates with increased behavioral emotionality and decreased synaptic puncta density. Neuropharmacology 190:108562. doi: 10.1016/j.neuropharm.2021.108562
Mitra, J., Dai, C. Y., Somasundaram, K., El-Deiry, W. S., Satyamoorthy, K., Herlyn, M., et al. (1999). Induction of p21(WAF1/CIP1) and inhibition of Cdk2 mediated by the tumor suppressor p16(INK4a). Mol. Cell Biol. 19, 3916–3928. doi: 10.1128/MCB.19.5.3916
Mombach, J. C., Vendrusculo, B., and Bugs, C. A. A. (2015). Model for p38MAPK-induced astrocyte senescence. PLoS One 10:e0125217. doi: 10.1371/journal.pone.0125217
Moreno, G. L., Bruss, J., and Denburg, N. L. (2017). Increased perceived stress is related to decreased prefrontal cortex volumes among older adults. J. Clin. Exp. Neuropsychol. 39, 313–325. doi: 10.1080/13803395.2016.1225006
Moreno-Smith, M., Lutgendorf, S. K., and Sood, A. K. (2010). Impact of stress on cancer metastasis. Future Oncol. 6, 1863–1881. doi: 10.2217/fon.10.142
Nava, M. M., Miroshnikova, Y. A., Biggs, L. C., Whitefield, D. B., Metge, F., Boucas, J., et al. (2020). Heterochromatin-driven nuclear softening protects the genome against mechanical stress-induced damage. Cell 181, 800–17.e22. doi: 10.1016/j.cell.2020.03.052
Ostrom, Q. T., Gittleman, H., Xu, J., Kromer, C., Wolinsky, Y., Kruchko, C., et al. (2016). CBTRUS statistical report: primary brain and other central nervous system tumors diagnosed in the United States in 2009-2013. Neuro Oncol. 18(Suppl._5), v1–v75. doi: 10.1093/neuonc/now207
Pajalunga, D., Mazzola, A., Salzano, A. M., Biferi, M. G., De Luca, G., and Crescenzi, M. (2007). Critical requirement for cell cycle inhibitors in sustaining nonproliferative states. J. Cell Biol. 176, 807–818. doi: 10.1083/jcb.200608109
Pechnick, R. N., Zonis, S., Wawrowsky, K., Cosgayon, R., Farrokhi, C., Lacayo, L., et al. (2011). Antidepressants stimulate hippocampal neurogenesis by inhibiting p21 expression in the subgranular zone of the hipppocampus. PLoS One 6:e27290. doi: 10.1371/journal.pone.0027290
Piechota, M., Sunderland, P., Wysocka, A., Nalberczak, M., Sliwinska, M. A., Radwanska, K., et al. (2016). Is senescence-associated β-galactosidase a marker of neuronal senescence? Oncotarget 7, 81099–81109. doi: 10.18632/oncotarget.12752
Planchez, B., Surget, A., and Belzung, C. (2019). Animal models of major depression: drawbacks and challenges. J. Neural. Transm. 126, 1383–1408. doi: 10.1007/s00702-019-02084-y
Poon, R. Y. (2016). Cell cycle control: a system of interlinking oscillators. Methods Mol. Biol. 1342, 3–19. doi: 10.1007/978-1-4939-2957-3_1
Prevot, T. D., Chatterjee, D., Knoch, J., Codeluppi, S., Misquitta, K. A., Fee, C. J. E., et al. (2021). Dynamic behavioral and molecular changes induced by chronic stress exposure in mice. bioRxiv [Preprint] doi: 10.1101/2021.05.07.443011v1.full.pdf
Prevot, T. D., Misquitta, K. A., Fee, C., Newton, D. F., Chatterjee, D., Nikolova, Y. S., et al. (2019). Residual avoidance: A new, consistent and repeatable readout of chronic stress-induced conflict anxiety reversible by antidepressant treatment. Neuropharmacology 153, 98–110. doi: 10.1016/j.neuropharm.2019.05.005
Purvis, J. E., Karhohs, K. W., Mock, C., Batchelor, E., Loewer, A., and Lahav, G. (2012). p53 dynamics control cell fate. Science 336, 1440–1444. doi: 10.1126/science.1218351
Qiao, H., Li, M. X., Xu, C., Chen, H. B., An, S. C., and Ma, X. M. (2016). Dendritic spines in depression: what we learned from animal models. Neural Plast. 2016:8056370. doi: 10.1155/2016/8056370
Radley, J. J., Rocher, A. B., Miller, M., Janssen, W. G., Liston, C., Hof, P. R., et al. (2006). Repeated stress induces dendritic spine loss in the rat medial prefrontal cortex. Cereb. Cortex. 16, 313–320. doi: 10.1093/cercor/bhi104
Rajkowska, G., and Miguel-Hidalgo, J. J. (2007). Gliogenesis and glial pathology in depression. CNS Neurol. Disord. Drug Targets 6, 219–233. doi: 10.2174/187152707780619326
Safaiyan, S., Kannaiyan, N., Snaidero, N., Brioschi, S., Biber, K., Yona, S., et al. (2016). Age-related myelin degradation burdens the clearance function of microglia during aging. Nat. Neurosci. 19, 995–998. doi: 10.1038/nn.4325
Sah, E., Krishnamurthy, S., Ahmidouch, M. Y., Gillispie, G. J., Milligan, C., and Orr, M. E. (2021). The cellular senescence stress response in post-mitotic brain cells: cell survival at the expense of tissue degeneration. Life 11:229. doi: 10.3390/life11030229
Sapieha, P., and Mallette, F. A. (2018). Cellular senescence in postmitotic cells: beyond growth arrest. Trends Cell Biol. 28, 595–607. doi: 10.1016/j.tcb.2018.03.003
Sedelnikova, O. A., Horikawa, I., Zimonjic, D. B., Popescu, N. C., Bonner, W. M., and Barrett, J. C. (2004). Senescing human cells and ageing mice accumulate DNA lesions with unrepairable double-strand breaks. Nat. Cell Biol. 6, 168–170. doi: 10.1038/ncb1095
Sherin, J. E., and Nemeroff, C. B. (2011). Post-traumatic stress disorder: the neurobiological impact of psychological trauma. Dialogues Clin. Neurosci. 13, 263–278. doi: 10.31887/DCNS.2011.13.2/jsherin
Sikora, E., Bielak-Zmijewska, A., Dudkowska, M., Krzystyniak, A., Mosieniak, G., Wesierska, M., et al. (2021). Cellular senescence in brain aging. Front. Aging Neurosci. 13:646924. doi: 10.3389/fnagi.2021.646924
Song, H., Sieurin, J., Wirdefeldt, K., Pedersen, N. L., Almqvist, C., Larsson, H., et al. (2020). Association of stress-related disorders with subsequent neurodegenerative diseases. JAMA Neurol. 77, 700–709. doi: 10.1001/jamaneurol.2020.0117
Sullivan, R. M., and Gratton, A. (2002). Behavioral effects of excitotoxic lesions of ventral medial prefrontal cortex in the rat are hemisphere-dependent. Brain Res. 927, 69–79. doi: 10.1016/S0006-8993(01)03328-5
Vazquez-Villaseñor, I., Garwood, C. J., Heath, P. R., Simpson, J. E., Ince, P. G., and Wharton, S. B. (2020). Expression of p16 and p21 in the frontal association cortex of ALS/MND brains suggests neuronal cell cycle dysregulation and astrocyte senescence in early stages of the disease. Neuropathol. Appl. Neurobiol. 46, 171–185. doi: 10.1111/nan.12559
Woodburn, S. C., Bollinger, J. L., and Wohleb, E. S. (2021). Synaptic and behavioral effects of chronic stress are linked to dynamic and sex-specific changes in microglia function and astrocyte dystrophy. Neurobiol. Stress 14:100312. doi: 10.1016/j.ynstr.2021.100312
Yang, L., Zhao, Y., Wang, Y., Liu, L., Zhang, X., Li, B., et al. (2015). The effects of psychological stress on depression. Curr. Neuropharmacol. 13, 494–504. doi: 10.2174/1570159X1304150831150507
Keywords: cell cycle, cyclin-dependent kinase inhibitors, senescence, prefrontal cortex, anxiety- and depression-like behavior, stress-signaling, chronic restraint stress
Citation: Mendes-Silva AP, Prevot TD, Banasr M, Sibille E and Diniz BS (2022) Abnormal expression of cortical cell cycle regulators underlying anxiety and depressive-like behavior in mice exposed to chronic stress. Front. Cell. Neurosci. 16:999303. doi: 10.3389/fncel.2022.999303
Received: 20 July 2022; Accepted: 22 November 2022;
Published: 08 December 2022.
Edited by:
Susana Cardoso, University of Coimbra, PortugalReviewed by:
Bogdan Catalin, University of Medicine and Pharmacy of Craiova, RomaniaCopyright © 2022 Mendes-Silva, Prevot, Banasr, Sibille and Diniz. This is an open-access article distributed under the terms of the Creative Commons Attribution License (CC BY). The use, distribution or reproduction in other forums is permitted, provided the original author(s) and the copyright owner(s) are credited and that the original publication in this journal is cited, in accordance with accepted academic practice. No use, distribution or reproduction is permitted which does not comply with these terms.
*Correspondence: Ana Paula Mendes-Silva, YW5hLXBhdWxhLnNpbHZhQGNhbWguY2E=
Disclaimer: All claims expressed in this article are solely those of the authors and do not necessarily represent those of their affiliated organizations, or those of the publisher, the editors and the reviewers. Any product that may be evaluated in this article or claim that may be made by its manufacturer is not guaranteed or endorsed by the publisher.
Research integrity at Frontiers
Learn more about the work of our research integrity team to safeguard the quality of each article we publish.