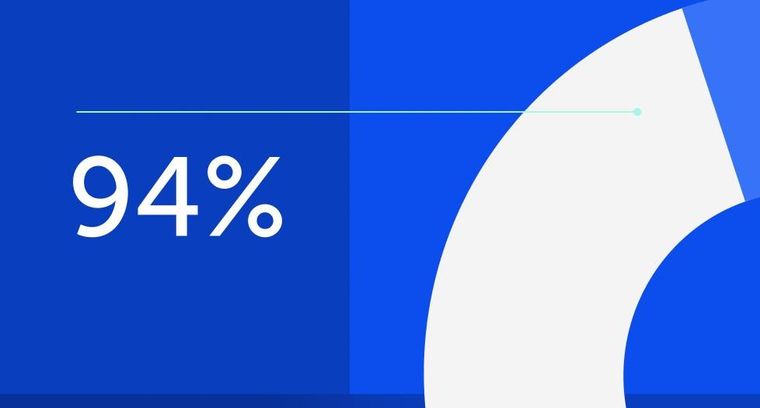
94% of researchers rate our articles as excellent or good
Learn more about the work of our research integrity team to safeguard the quality of each article we publish.
Find out more
REVIEW article
Front. Cell. Neurosci., 20 September 2022
Sec. Cellular Neuropathology
Volume 16 - 2022 | https://doi.org/10.3389/fncel.2022.979856
This article is part of the Research TopicNew Insights Into Schizophrenia-Related Neural and Behavioral PhenotypesView all 12 articles
Neuropathy is a growing public health problem in the aging, adolescent, and sport-playing populations, and the number of individuals at risk of neuropathy is growing; its risks include aging, violence, and conflicts between players. The signal pathways underlying neuronal aging and damage remain incompletely understood and evidence-based treatment for patients with neuropathy is insufficiently delivered; these are two of the reasons that explain why neuropathy is still not completely curable and why the progression of the disease cannot be inhibited. Extracellular vesicles (EVs) shuttling is an important pathway in disease progression. Previous studies have focused on the EVs of cells that support and protect neurons, such as astrocytes and microglia. This review aims to address the role of neuronal EVs by delineating updated mechanisms of neuronal damage and summarizing recent findings on the function of neuronal EVs. Challenges and obstacles in isolating and analyzing neuronal EVs are discussed, with an emphasis on neuron as research object and modification of EVs on translational medicine.
Neurons are the cells that make up the brain and the nervous system. They are the fundamental units that send and receive signals, allowing us to move our muscles, feel the external world, think, form memories, and much more. A great number of studies have shown that neurons are more vulnerable to various stresses, including oxidative stress, hypoxia, ischemia, inflammation, or neurotoxin than other neural cells and are considered less capable of regeneration and self-repair (Gonzalez et al., 2016). The disease burden resulting from neuronal damage induced by pollution, violence, and other factors is a growing health problem. Examples include Alzheimer’s disease (AD), Parkinson’s disease (PD), multiple sclerosis, amyotrophic lateral sclerosis (ALS), cerebral ischemia, cerebral hemorrhage, and traumatic brain injury, among others. The etiology and mechanism of neuron impairment are complex and remain to be elucidated. Numerous studies have shown that the dysregulation of cellular function underlying the process of neurodegeneration results from both abnormal cellular communication and misfolded proteins that cannot be effectively degraded or cleaned up. The exact mechanisms leading to these defects remain to be investigated (Peng et al., 2020), but studies have shown that major contributors are the classical pathway, including diffusion, phagocytosis, and exocytosis (Asai et al., 2015), and the unconventional secretion pathway, such as pore-mediated translocation, ATP-binding cassette transporter-based secretion, membrane-bound organelle-based secretion, the Golgi bypass pathway, and extracellular vesicles (EVs) secretion.
EVs have recently caught more attention due to their physicochemical characteristics and biological function. EVs are one type of small lipid bilayer vesicles, including large vesicles (in the size range of ∼50 nm to 1 μm) and exosomes [in the size range of ∼40–160 nm (average ∼100 nm in diameter)] and have an important role in the therapy and development of neurodegenerative diseases. Although many research approaches on EVs have been established and applied, it is challenging to study alone the large vesicles or exosomes due to the resemblance of their biogenesis pathway and regulatory mechanisms. Therefore, in this manuscript we favor the term EVs.
EVs were first observed and termed “platelet dust” 50 years ago (Wolf, 1967). EVs contain many cell components, depending on the original cell, including nucleic acid, lipids, metabolites, cytosolic and cell-surface proteins, and can be found in all biological fluids and tissues, including the central nervous system (CNS). However, the physiological purpose of generating EVs remains largely unknown and needs to be investigated. One speculated role is that EVs remove excess and/or unnecessary constituents from cells to maintain cellular homeostasis, a process that would be similar to autophagy, which was discovered earlier and is also considered to eliminate the cellular garbage (Mizushima and Komatsu, 2011; Babuta et al., 2019). Meanwhile, studies in these years found that both processes play a vital role in cellular homeostasis and intercellular communication (Pan and Johnstone, 1983; Harding et al., 1984) and affect each other through common elements (Babuta et al., 2019; Zhang X. W. et al., 2020). Notably, studies indicated a negative correlation between the ratio of the release of EVs with the ratio of formation of autophagosome—the intermediate material of the autophagy process (Zhang X. W. et al., 2020), and the EVs biogenesis and release are closely related to the formation of the multivesicular body (MVB), which is also involved in autophagy (Baixauli et al., 2014). These studies supported the conclusion that EVs may play a role in the process of cellular homeostasis.
Recent studies reviewed here also indicate a functional, targeted, and active accumulation of specific cellular components in EVs, suggesting that they may play an important role in regulating intercellular communication and trigger phenotypic changes in the recipient cells (Pegtel and Gould, 2019). For instance, EVs from nicotine-stimulated macrophages promote the migration and proliferation of vascular smooth muscle cells (VSMC), whereas neuron-derived EVs promote the polarization of M1 microglia in culture (Zhu et al., 2019; Yin et al., 2020). In addition, several lines of evidence showed that EVs from CNS might directly alter the function of the nearby cell via the paracrine pathway or leave the brain and betray the state of CNS as potential biomarkers released into the biological fluids, including cerebrospinal fluid (CSF), plasma, serum, saliva, urine, semen, breast milk, amniotic fluid, and ascites fluid (Wang and Zhang, 2020; Yu et al., 2020). In the meantime, many studies revealed that EVs derived from neurons and/or glial cells might be involved in the initial development of neurodegeneration. However, the culprit of neurodegenerative diseases remains to be investigated (Singh and Muqit, 2020; Yin et al., 2020; You et al., 2020). This review focuses on the regulation mechanism of biogenesis, the release of the neuronal EVs, and its effects on the receptor cells in the pathogenesis, diagnosis, treatment, and prognosis of neurodegeneration. Glia-derived EVs and their effects in neurons have been the focus of several studies and will not be addressed in this review (Lemaire et al., 2019; Upadhya et al., 2020).
The biogenesis and release of neuronal EVs are partially similar to other cells (Figure 1). For example, the biogenesis of neuronal EVs also begins with the endosome system and is released into the extracellular space through fusion with the plasma membrane (Hessvik and Llorente, 2018). However, this mode is not the only pathway due to multiple lines of evidence showing that EVs also directly bud from the plasma membrane (Nabhan et al., 2012; Bianchi et al., 2014; Nager et al., 2017). The general process of EVs biogenesis and release mainly include three steps: the first step, the formation of early endosome mediated by endocytosis; the second step, the production of intracellular MVBs regulated by endosomal sorting complexes required for transport (ESCRT) machinery, including ESCRT-0, ESCRT-I, ESCRT-II, and ESCRT-III (Hessvik and Llorente, 2018) or ESCRT-I, ESCRT-II, and ESCRT-III (Vietri et al., 2020), with their accessory proteins and then the intraluminal vesicles (ILVs) formed by inward budding from the limiting membrane of MVBs; and the last step, in which ILVs are secreted through the MVBs containing ILVs fused with the plasma membrane and exocytosis.
Figure 1. A common process for EVs synthesis and release. Cells internalized the cellular components/extracellular ligands and then formed the early endosome. Subsequently, the early endosome matured into MVB, and the inward budding of early endosomes formed ILVs. In the last step, MVB either digests and degrades after fusion with lysosomes or releases exosomes through fusion with cell membranes.
In general, the ESCRT machinery is considered to play a vital role during the assembled MVBs and the budding of ILV (Colombo et al., 2013; Vietri et al., 2020). However, the pathway of independent-ESCRT is also uncovered, such as the interaction of RAB31 with the epidermal growth factor receptor (EGFR) (Wei et al., 2021) or the tetraspanin pathway (Kenific et al., 2021). Indeed, loss of VPS4 function, an essential factor for the ESCRT function (Vietri et al., 2020), did not affect the secretion of EVs marker proteins such as CD63 (Fang et al., 2007; Trajkovic et al., 2008; Colombo et al., 2013). These studies demonstrated that other molecules might regulate EVs biogenesis beyond the ESCRT machinery. In addition, evidence collected during the last 20 years also indicated that the dynamics of protein trafficking are also involved in the process of EVs biogenesis and release. However, the regulatory mechanisms of these processes remain to be elucidated due to its high precision and complexity (Pegtel and Gould, 2019). For instance, a vast number of studies found that the Ras-related protein GTPase Rab (Ostrowski et al., 2010), Sytenin-1, tumor susceptibility gene 101 (TSG101), apoptosis-linked gene 2-interacting protein X (ALIX), syndecan-1 (Baietti et al., 2012), ESCRT proteins (Colombo et al., 2013), tetraspanins (Escola et al., 1998), ceramides, sphingomyelinases, and soluble N-ethylmaleimide–sensitive factor attachment protein receptor (SNARE) complex proteins (Vilcaes et al., 2021) are involved in the origin and biogenesis process of EVs (D’Souza-Schorey and Schorey, 2018; Pegtel and Gould, 2019). However, their precise rate-limiting actions and functions in EVs biogenesis require further in-depth exploration, especially in vivo.
The Rab protein family has received more attention due to their roles in regulating vesicle trafficking between different compartments along the endo-lysosomal and secretory pathways (Pfeffer, 2017). For example, studies found that Rab27, Rab35, or Rab11 cooperation with their effectors Slp4, Slac2b and Munc13-4, and regulator TBC1D10B play important roles in MVB biogenesis and positioning. The loss of Rab27 or Rab35 function results in a ∼50–75% drop or little effect on EVs biogenesis upon differentiation of a cell line (Hsu et al., 2010; Ostrowski et al., 2010), overexpressing Rab11 within RAW macrophages elevated the amounts of EVs by about 50% through promoting homotypic fusion/maturation of MVBs (Savina et al., 2002). It is worth noting that there is controversy about how Rab proteins regulate EVs biogenesis. For instance, studies have shown that Rab27/Rab11 proteins promote MVB maturation and deliver MVB to the plasma membrane (Savina et al., 2002; Ostrowski et al., 2010). Others revealed that Rab27a is also required for assembling plasma membrane microdomains (Booth et al., 2006). Thus, it appears that Rab27 proteins control exosome biogenesis at both endosome and plasma membranes. In addition, Ostrowski et al. (2010) found that inhibition of Rab11a did not significantly modify EVs secretion in the HeLa B6H4 cell line. These results indicated that EVs biogenesis and secretion, which do not have to comply with the canonical regulations, were regulated by multiple pathways.
Although most of our knowledge is about the mechanism of EVs biogenesis and secretion in cancer (non-neuronal) cells, many of these mechanisms also function in neurons. However, it is known that the regulatory mechanism of EVs biogenesis and secretion depends on the cell type and state. Therefore, both processes of neuronal EVs have a different pathway compared to cancer cells due to the differential expression of genes and their extreme morphologies. For example, previous research found that endocannabinoid 2-arachadonoylglycerol (2-AG) containing EVs derived from midbrain dopaminergic (DA) neurons can be released in the Arf6-myosin light chain kinase (MLCK) pathway (Nakamura et al., 2019). However, the Arf6-MLCK pathway is specifically attributed to microvesicle (MV) release in tumor cells (Muralidharan-Chari et al., 2009). Moreover, neuronal EVs are released in response to stimulation or depolarization. Furthermore, a positive correlation was observed between the concentration of neuronal EVs and the degree of neuronal depolarization (Faure et al., 2006; Blanchette and Rodal, 2020). Meanwhile, previous studies suggest that neuronal somatodendritic compartments or axons may be the release site of neuronal EVs (Coulter et al., 2018; Polanco et al., 2018). However, the above-mentioned correlation or similar bioconstructors remain to be explored in cancer cells. The same genes may even play different roles in regulating the biogenesis or release of EVs depending in different types of cells. For instance, knockdown of the autophagy-related gene 5 (ATG5) in the MDA-MB-231 cells (a human breast cancer cell line) not only inhibited the autophagy but also induced a severely decreased EVs production (Guo et al., 2017). In contrast, knockout of ATG5 in neuronal cells greatly increases the release of EVs and EV-associated prions from neuronal cells (Abdulrahman et al., 2018). This result showed that the regulatory mechanism of EVs biogenesis and release is not common.
Due to above reasons, studies about EVs derived from neurons need to have neurons as their research object rather than other cell lines such as HEK293 (Zheng et al., 2017, 2018). The exciting news is that some experiments using neurons as research objects have been implemented and acquired some achievements in healthy or disease models. For example, a study in hippocampus neurons found that brain fibroblast growth factors-2 (bFGF2) can greatly improve the fusion of MVB with the plasma membrane and the release of neuronal exosome by promoting the v-SNARE vesicle-associated membrane protein 3 (VAMP3) translocation to the exosome rather than VAMP2 (Kumar et al., 2020). Moreover, the bFGF2 system regulates neuronal calcium homeostasis and plasticity and promotes neuroprotection and repair in response to neural tissue damage (Guillemot and Zimmer, 2011). Blocked Drp1 in N27 or SH-SY5Y cells treated with PFF (Human α-synuclein preformed fibrils) can enhance the autophagy flux, inhibit the release of EVs, and prevent the transmission of aggregative or misfolded α-synuclein (α-syn) protein between neurons via EVs (Fan et al., 2019). KIBRA regulates the secretion of EVs derived from the mouse hippocampal and cortex neuron rather than the liver or muscle tissue via inhibiting the proteasomal degradation of Rab27a (Song et al., 2019c). Although the function of these proteins or genes may also be involved with other disorders and needs to be investigated, it has been confirmed that the disorder of these proteins or their gene expression is involved in the progress or development of neurodegeneration such as major depressive disorder, bipolar disorder (BPD), PD, or AD (Evans et al., 2004; Gaughran et al., 2006; Song et al., 2019b; Zhang W. et al., 2019). Therefore, based on the above results, we suggested that disorders in the neuronal EVs biogenesis or release contribute to the neurological disorder. More interestingly, Pastuzyn et al. (2018) found that neuronal Arc protein self-assembles into virus-like capsids that encapsulated Arc mRNA and mediated the transmission of Arc protein and Arc mRNA between neurons in EVs, where it can undergo activity-dependent translation to regulate the synaptic plasticity of recipient neurons. This finding provides new insight into EVs biogenesis or release mechanism. Moreover, the secretion of neuron-derived EVs was also modulated by the activities of sphingolipid metabolizing enzymes, including neutral sphingomyelinase 2 (nSMase2) and sphingomyelin synthase 2 (SMS2) (Yuyama et al., 2012); however, nSMase2 or SMS2 expression can be detected in other cells (Hitomi et al., 2020), the regulated biogenesis or secrete mechanism of EVs by nSMase2 or SMS2 in other cells are few.
EVs derived from neurons are continuously secreted into the extracellular space and are up taken by the glia cells and nearby neurons or other cells through neuronal junctions (Faure et al., 2006). The cargo of EVs, like non-coding RNAs (ncRNAs) or proteins and other molecules, influences the function of recipient cells by binding their target molecules. Although how and why neuronal EVs are delivered into other cells are being investigated, some studies have shown that the neuronal EVs containing ncRNA or proteins may modulate the neuronal survival or activity, neural circuits, and the function of other organs by regulating the specific gene expression of receptor cells. For example, Morel et al. (2013) found that exosome-containing miRNA-124a derived from a cortical neuron can be engulfed by astrocytes and indirectly regulate glutamate transporter-1(GLT-1). Furthermore, they confirmed that the translational regulation of GLT-1 protein in astrocytes is dependent on neuronal exosome-containing miRNA-124a (Morel et al., 2013). However, they did not identify the target genes of miRNA-124a that regulate the translation of the GLT-1 protein in astrocytes. Men et al. also confirmed that secreted neuronal exosome-containing miRNAs could be shuttled into astrocytes and influence the function of astrocytes (Men et al., 2019). Furthermore, overexpressed miRNA-124-3p in exosomes can strongly upregulate the expression of GLT-1 via the abolition of the inhibitory effects of miR-132 or miR-128 on GLT-1 in astrocytes (Men et al., 2019). Our previous studies indicated that downregulation of GLT-1 expression in astrocytes results in the drastic increase of glutamate concentration in the dopaminergic (DA) neuronal synaptic cleft and then induces DA degeneration via glutamate excitatory toxicity (Zhang Y. et al., 2020). There is also evidence indicating that miRNA-124-3p is involved in the perioperative neurocognitive disorders in patients after cardiac surgery (Xiao Q. X. et al., 2020). Furthermore, neuron axonal exosome-containing miRNA-124-3p can suppress the activation of M1 microglia and A1 astrocyte via inhibiting MYH9 by directly targeting its 3′-UTR and thereby modulating the PI3K/Akt/NF-κB pathway improving over the motor function of spinal cord injury (SCI) mice (Jiang D. et al., 2020). However, the exact role of miRNA-124-3p in neurodegenerative diseases remains to be investigated.
The effects of EVs on recipient cells depend on the state of neuron. EVs derived from the neurons with physiological status or drug treatment may protect other cells. For instance, healthy cortical neurons released the miRNA-181-3p-containing exosome, which suppressed the neuroinflammation by targeting the CXCL1 gene in astrocytes. However, the expression of neuronal and exosomal miRNA-181-3p is significantly elevated in the ischemic brain injury (IBI) (Song et al., 2019a). CD2019 improved the deficiency of locomotor and sensory via promoting the regeneration of neuronal axonal and decreasing the glial scar formation in SCI rats. The underlying mechanism of the protective effect may be the induction of the translocation of PTEN and promotion of the secretion of enriched PTEN-containing exosome via activating the retinoic acid receptor β (RARβ). These PTEN-containing exosomes are then delivered to astrocytes, inhibiting the proliferation and activation of the cells (Goncalves et al., 2015). In contrast, the gene expression in damaged neurons causes the dysregulation of the cargo that is packaged into EVs. Subsequently, these cargo molecules are delivered to other cells and then disrupt the function of recipient cells. For example, Yin and his colleagues found that the expression of miRNA-21-5p is significantly elevated in PC12 cells and exosomes from PC12 cells after scratch injury. These EV-containing miRNA-21-5p shuttle into the BV2 cells, inducing the polarization of cells, and then the polarized BV2 cells cause chronic neuro-inflammation via secreting pro-inflammation factors like IL-1β, IL-6, and TNF-α (Yin et al., 2020). Irreversible neuro-inflammation is considered to play an important role in the onset and development of neurodegenerative disease (Calsolaro and Edison, 2016; Han X. et al., 2019; Jiao et al., 2019; Passamonti et al., 2019). SH-SY5Y cells transfected with wild-type human SNCA gene secreted exosomes with a higher expression of miRNA-19a-3p. The miRNA-19a-3p-containing exosomes elevated the expression of miRNA-19a-3p in microglia via delivering miRNA-19a-3p into the microglia, and then the miRNA inhibited the autophagy of microglia by targeting the PTEN/AKT/mTOR signal pathway (Zhou T. et al., 2019). However, a healthy autophagy-lysosome pathway of microglia plays a crucial role in the process that cleans up toxic molecules such as misfolded α-syn (Nash et al., 2017; Choi et al., 2020), abnormal tau, amyloid-β (Aβ) protein (Cho et al., 2014; Heckmann et al., 2019; Houtman et al., 2019), and cellular debris (Pluvinage et al., 2019). On the contrary, the dysregulated autophagy of microglia impacted the degradation of phagocytized cargo and induced an accumulation of misfolded proteins or cellular debris in the microglia or extracellular space, which ultimately contributes to the pathophysiology of neurodegeneration (Qin et al., 2021).
Neuronal EVs in CNS may be absorbed by glial cells; however, neuronal EVs of the peripheral nervous system (PNS) regulated the function of other cells by shuttling to other organ cells and neurites (Figure 2). For instance, the impaired sensory neuron in dorsal root ganglia secreted exosomes with a high miRNA-21 expression that macrophages may engulf by phagocytosis, inducing their activation and release of pro-inflammation cytokines (Simeoli et al., 2017). These cytokines recruit more macrophages, which infiltrate into the site of injury, amplifying and deteriorating the immunity response, ultimately causing neuro-inflammation and neurodegeneration (Harland et al., 2020; Zhang Y. et al., 2020). Xu et al. (2017) also found that neurons transfer miR-132 via secreting exosomes to endothelial cells (ECs) and then regulate the expression of vascular endothelial cadherin (VE-cadherin), an important adherens junction protein, by directly targeting the eukaryotic elongation factor 2 kinase (eef2k) to maintain brain vascular integrity. However, an adequate supply of blood and structural and functional integrity of blood vessels are key to normal brain functioning. In contrast, cerebral blood flow shortfalls and blood-brain barrier dysfunction can be earlier discovered in neurodegenerative disorders in humans and animal models (Iturria-Medina et al., 2016; Sweeney et al., 2018; Zhang C. et al., 2020). The abnormal structure and function of blood vessels result in the destruction of blood-brain barrier integrity, and peripheral immune cells like T cells infiltrated into CNS and evoked neuro-inflammation. For instance, evidence from postmortem or animal models of neurodegenerative disorder showed that T cells are abundantly present in the impaired region of the brain (Machado-Santos et al., 2018; Liu et al., 2019; Lodygin et al., 2019; Haque et al., 2020). Meanwhile, we reported that the expression of miRNA-132 is significantly reduced in primary hippocampus neurons treated by soluble Aβ oligomers, a classical cell model of AD (Wei et al., 2020). Furthermore, oxidative stress after SCI injured neurons and altered the release of the neuronal exosome; secreted exosomes promoted the apoptosis of transplanted bone marrow-derived stem cells with oxidative stress (Luo et al., 2019).
Figure 2. Schematic representation of the neuronal EVs that regulated the function of receptor cells. EVs derived from neurons undergoing various stress deliver their cargoes, such as ncRNA, mRNA, or misfold proteins, into other cells in CNS or PNS via paracrine or active transport. These cargoes are abnormal expressions and are packaged into EVs, which disturb the gene expression or signal pathway of the recipient cells, ultimately leading to cell dysfunction. Meanwhile, dysfunctional recipient cells secrete “detrimental molecules” and then damage the neurons or nearby cells, and vice versa.
In addition to ncRNA, proteins such as Tau and α-syn within EVs also regulate the function of recipient cells and even disturb the cellular homeostasis (Meng et al., 2020). For example, NSC-34 cell, a kind of motor neuron-like cell, mutated superoxide dismutase 1 (mSOD1, G93A) as donor cells released exosome-containing mSOD1, which are phagocytosed by N9-microglial cells. Internalized exosome-containing mSOD1 upregulated the expression of IL-1β, TNF-α, MHC-II, and iNOS gene via activating the NF-κB pathway in N9 cells and reducing the phagocytic ability of N9 cells (Pinto et al., 2017). Meanwhile, neuronal PTEN can be transferred to astrocytes by EVs and inhibit the information of glia scar (Goncalves et al., 2015). The formatted glial scar is considered an obstacle to the regeneration of neuronal axons (Anderson et al., 2016). Tau pathology or misfolded α-syn protein spreading is also achieved by direct transmission of exosomes between neurons and then induced Tau protein aggregation or misfolded endogenous α-syn in the receptor neurons (Wang et al., 2017; Sardar Sinha et al., 2018); ultimately, these abnormal proteins induced neurodegenerative diseases.
Based on the above results, we proposed that the expressed dysregulation encapsulated in neuronal EVs containing ncRNAs or pathological proteins induced by diverse factors, such as neurotoxic or mild traumatic brain injury, may result in neurodegeneration. It is noteworthy that almost all studies supposed that the level of cargo in EVs depends on the parental cells. However, Men et al. (2019) found that secreted neuronal exosomes contain a subset of microRNAs that is drastically different from the miRNA profile of neurons. This result is significantly distinct from previous reports (Morel et al., 2013; Xu et al., 2017; Ying et al., 2017; Zhu et al., 2019). Therefore, the sorting mechanism of exosomal cargo remains to be investigated.
The postmortem studies identified that the stereotypical and temporal distribution of pathology is limited due to a lack of longitudinal data and therefore do not provide direct evidence of the sequential evolvement of different brain regions during disease progression. Nevertheless, numerous studies from cell and animal models have shown that pathological proteins such as α-syn and Tau, or the abnormal regulator such as miRNA, lncRNA, and rRNA undergo neuron-to-neuron transmission and partially contribute to the progress of neurodegenerative diseases (Emmanouilidou et al., 2010; Zhou W. et al., 2019; Ruan et al., 2021; Vilcaes et al., 2021). Although the modes of interplay with neurons are various, EV-based secretion and uptake, one of the unconventional secretion pathways, is the most extensively studied pathway for the transmission of excessive regulators of pathological proteins. EVs containing an excessive level of regulators or misfolded proteins are engulfed into the nearby or distal neurons. The former induced a dysfunction in neurons by disturbing the expression of a specific gene in the recipient neurons. The latter work as “pathological seeds” that damage the function of neurons via inducing their normal endogenous counterpart protein to misfold, leading to the amplification of the pathological protein conformation in the receptor cell (Wang et al., 2017; Figure 3). Previous research has revealed that EVs derived from dysfunctional neurons may contain the “misfolded proteins” such as α-syn oligomer in PD (Niu et al., 2020), Aβ and tau aggregates in AD (Guix et al., 2018; Sardar Sinha et al., 2018), TAR DNA-binding protein 43(TDP43) and mSOD1 pathology in ALS (Neumann et al., 2006; Iguchi et al., 2016; Silverman et al., 2019), and mutated huntingtin (HTT) (DiFiglia et al., 1997; Jeon et al., 2016). These “unusual EVs” are taken up by other neurons and disturb cellular homeostasis by perturbing the gene expression or protein folding. For example, Han et al. found that the intravenous or intrastriatal treatment of mice with exosomes derived from patients with PD containing α-syn, IL-1β, and TNF-α could trigger dopamine neuron degeneration via inducing α-syn, ubiquitin, and p62 aggregation in recipient cells (Han C. et al., 2019). Sinha et al. also found that tau-containing exosomes derived from SH-SY5Y cells when treated with Aβ shuttled to the normal SH-SY5Y cells and increased the LDH level of normal SH-SY5Y cells; dynasore, which is an inhibitor of endocytosis, can inhibit this cytotoxicity effect (Sardar Sinha et al., 2018). Furthermore, Park et al. also confirmed that the Fas-associated factor 1 (FAF1) of SY5Y cells was secreted and transmitted to neighboring cells via exocytosis as well as an exosomal pathway, where it induced cell death through apoptotic and necrotic pathways (Park et al., 2020).
Figure 3. Schematic representation of the interplay between neurons by EVs. EVs is an important tool for maintaining the completion of neuronal circuit and the delivery of neurotransmitters. EVs derived from impaired neurons containing abnormal regulators or neurotransmitters are absorbed into healthy neurons, perturbing the neuronal function. The completion of neuronal circuit depends on the neuronal function. Especially, those EVs containing misfold proteins, such as Tau, α-syn, and TDP43, spread to other neurons; these misfold proteins acting as “pathological seeds” induce misfolding of endogenous protein, cause a dissemination from local brain region to others, and accelerate disease progression.
The dysfunction of a neuron may cause the disorder of package and release of the exosome. For example, studies have shown that the release of cortex neuronal exosome is regulated upon the calcium homeostasis in the cytoplasm and glutamatergic synaptic activity (Faure et al., 2006; Lachenal et al., 2011); however, both contribute to neuronal impairment (Cheng et al., 2016; Malik and Willnow, 2019; Alvarez et al., 2020). In contrast, the cargo of normal neuronal EVs contribute to the homeostasis of neuronal function and circuit. For example, Sharma et al. found that exosomes derived from hiPSC-derived neurons increased neurogenesis by promoting cultured primary neuronal proliferation and differentiation and rescued the aberrant neural circuit development mediated by X-linked Methyl-CpG-binding protein 2 (MECP2) gene mutation, which is involved with Rett syndrome (Sharma et al., 2019). Although their study did not reveal the effector within the exosome, these results confirmed the protected role of exosomal cargo in neurons. Subsequently, studies indicate that miRNA-containing exosomes derived from normal cells play a protective role in maintaining the function of other cells (de Rivero Vaccari et al., 2016; Mead and Tomarev, 2017). For instance, HT-22 secreted miRNA-21-5p-containing exosomes exert a protection effect by targeting the Rab11a to inhibit the autophagy flux of injured HT-22 (Li et al., 2019). Furthermore, miRNA-126-3p-enriched EVs from hypoxia-preconditioned VSC 4.1 neurons attenuate ischemia-reperfusion-induced pain hypersensitivity by regulating the PIK3R2-mediated pathway (Wang et al., 2021). Together, these papers uncovered the partial underlying mechanism of the gradual progress of neurodegeneration and confirmed the role of neuronal EVs in the disease process. Meanwhile, they also verified that the interplay between neurons is vital for maintaining neuronal homeostasis except for the glial cells which deliver nerve trophic factor into neurons (Upadhya et al., 2020) or maintain the synaptic plasticity of neurons using EVs (Wilton et al., 2019).
EVs are released into various body fluids or across the various biological membranes into the cells due to their physicochemical characteristics, such as the small size and similar composition with the cellular membrane. Meanwhile, EV contents are cellular-specific due to the existence of different donor cells. Furthermore, EVs secretion is a constitutive phenomenon involved in both physiological and pathological processes. Hence, many studies focused on the EVs and their carried cargo as biomarkers, vaccines, and drug carriers, and have modified them rationally for therapeutic interventions (Figure 4). In particular, the development of EVs for cancer is exploding (Rayamajhi et al., 2019; Zhang W. et al., 2019; Guo et al., 2020; Nie et al., 2020; Xiao Y. et al., 2020; Liu et al., 2021; Yang et al., 2021; Zhao et al., 2021). It is worth mentioning that the development of exosome-containing GPC-1(GPC-1+ crExos) for pancreatic ductal adenocarcinoma (PDAC) is inspiring. For example, Melo et al. (2015) found that GPC-1+ crExos derived from PDAC and breast cancer patients’ serum is significantly elevated. They found that GPC-1+ crExos strongly correlates with the PDAC rather than breast cancer. The most impressive finding was that the GPC-1+ crExos distinguished patients with PDAC and pancreatic cancer precursor lesions (PCPL) from healthy donors and BPD patients; the sensitivity and specificity of GPC-1+ crExos prediction are 100% compared with CA19-9 (the clinical standard tumor biomarker for patients with PDAC). These findings are outstanding because no biomarker performs with 100% sensitivity and specificity in the history of cancer biomarker identification and validation, especially for an early stage of the disease. These findings remain to be validated before being applied in clinical settings because the GPC-1+ cross is also higher in others cancer such as breast cancer (Su et al., 2006). Studies about EVs have made great progress. However, there are still huge challenges concerning nerve diseases, especially in CNS diseases, due to the insufficiency of samples and contradictory conclusions. For instance, Winston et al. (2018) found that plasma exosomes containing neurogranin were highly diagnostic (sensitivity = 86.1 ± 0.037, confidence interval = 63.4–100) in distinguishing mild cognitive impairment patients from cognitively normal controls. However, a great number of published studies indicated that L1 cell adhesion molecule (L1CAM, CD147) positive exosome-containing Aβ42, T-tau, P-T181-tau, α-syn, and other proteins or ncRNA had higher diagnostic power for neurodegenerative diseases (Shi et al., 2014; Fiandaca et al., 2015; Yang et al., 2018; Jia et al., 2019; Jiang C. et al., 2020; Wang and Zhang, 2020). That said, the conclusions remain to be confirmed in longitudinal studies due to the small sample size. In addition, the enriched method of neuronal exosomes in immunoprecipitation with anti-L1CAM antibody further needs to be validated because the L1CAM protein is not only expressed in the nervous system but also expressed in other tissues or cells, such as soft tissue, fallopian tube, kidney cells, and epithelial cells (according to the Human Protein Atlas)1 (Pace et al., 2019; Asano et al., 2020), which may mean that the EVs pulled down with anti-L1CAM antibodies are not solely derived from neural tissues. Meanwhile, EVs derived from CSF are valuable for diagnosing CNS diseases (Jia et al., 2019; Muraoka et al., 2020). However, CSF samples are commonly obtained by lumbar puncture, which is an invasive test that can cause headaches and other side effects (Doherty and Forbes, 2014). Thus, it is challenging to recruit patients and healthy volunteers who agree to undergo lumbar puncture. Moreover, most research on the neuronal EVs as a biomarker has relied on the patient’s serum or plasma. Although serum or plasma is easily collected compared to CSF, the EVs in the serum or plasma is pool of EVs that come from various cells and organs. This factor may compromise the repeatability and accuracy of the results.
Figure 4. Schematic representation of the clinical application of neuronal EVs. The cargoes in EVs are derived from the parental cells, which partly reflect the physiological or pathological state of the donor cells. Moreover, EVs can effectively protect their contents from being degraded by various enzymes in body fluids, prolonging the half-life of various biomarkers. Therefore, isolating and purifying EVs from body fluids using various methods, including ultracentrifugation, and then analyzing and studying them are of great significance for predicting the state of cells. However, clinical application of EVs is still quite difficult because there is no effective method to purify EVs from a defined cell. Additionally, based on the physical and chemical characteristics of EVs, such as the ability to pass the blood-brain barrier, EVs can package some targeted drugs via genetic manipulation to deal with neuropathy.
During the past 5 years, EVs research has confirmed the transmission of EVs cargo between cells. Given the important role and therapeutic significance of EVs in neurological diseases, this topic is likely to continue under the spotlight.
One challenge for the field is that most studies were performed using a patient’s serum plasma or animal model. The limited availability of brain tissue currently constrains these studies. However, accumulating evidence demonstrates that isolating and purifying unmixed neuronal EVs from these samples is extremely difficult. Moreover, results from cultured primary neurons or cell lines as research objects in vitro are inconsistent due to the difference between protocols, operators, and devices. More importantly, in vitro studies cannot replicate exactly the state of physiology or pathology in vivo, which precludes a direct clinical translation. In the future, developing improved methods to isolate and purify EVs from neuropathological patients’ humor will be extremely beneficial for this field.
Another major challenge is understanding the underlying molecular mechanisms of packaging and transmission of neuronal EVs cargo. Analyzing the interaction of different trafficking proteins involved with both processes is extremely challenging. Although several studies found that many trafficking proteins and complexes contribute to the packaging and transmission of EVs cargo, both processes are unclear. Many proteins are associated with various cellular functions and participate in multiple pathways; the regulated mechanism remains to be investigated.
Despite the progress made, there are several key gaps in our knowledge. First, we do not yet know the physiological purpose of the generated EVs. Second, whether EVs maintain an essential cellular function is not known. Finally, although the different sizes of EVs have now been identified, how these different EVs are generated is unclear. The development of new technologies to track, isolate, and analyze EVs will be essential in addressing these important questions.
ZJ and TZ designed the structure of the manuscript and finalized the manuscript. ZJ drafted the manuscript. ZH, NL, and YL provided critical revisions and improvements to the manuscript. All authors approved the final version of the manuscript.
This work was supported by the Key Laboratory of Prevention and Treatment of Cardiovascular and Cerebrovascular Diseases, Ministry of Education (XN202014).
We thank everyone who contributed to this manuscript.
The authors declare that the research was conducted in the absence of any commercial or financial relationships that could be construed as a potential conflict of interest.
All claims expressed in this article are solely those of the authors and do not necessarily represent those of their affiliated organizations, or those of the publisher, the editors and the reviewers. Any product that may be evaluated in this article, or claim that may be made by its manufacturer, is not guaranteed or endorsed by the publisher.
Abdulrahman, B. A., Abdelaziz, D. H., and Schatzl, H. M. (2018). Autophagy regulates exosomal release of prions in neuronal cells. J. Biol. Chem. 293, 8956–8968.
Alvarez, J., Alvarez-Illera, P., Garcia-Casas, P., Fonteriz, R. I., and Montero, M. (2020). The Role of Ca(2+) Signaling in Aging and Neurodegeneration: Insights from Caenorhabditis elegans Models. Cells 9:204. doi: 10.3390/cells9010204
Anderson, M. A., Burda, J. E., Ren, Y., Ao, Y., O’Shea, T. M., Kawaguchi, R., et al. (2016). Astrocyte scar formation aids central nervous system axon regeneration. Nature 532, 195–200. doi: 10.1038/nature17623
Asai, H., Ikezu, S., Tsunoda, S., Medalla, M., Luebke, J., Haydar, T., et al. (2015). Depletion of microglia and inhibition of exosome synthesis halt tau propagation. Nat. Neurosci. 18, 1584–1593. doi: 10.1038/nn.4132
Asano, H., Hatanaka, K. C., Matsuoka, R., Dong, P., Mitamura, T., Konno, Y., et al. (2020). L1CAM Predicts Adverse Outcomes in Patients with Endometrial Cancer Undergoing Full Lymphadenectomy and Adjuvant Chemotherapy. Ann. Surg. Oncol. 27, 2159–2168. doi: 10.1245/s10434-019-08103-2
Babuta, M., Furi, I., Bala, S., Bukong, T. N., Lowe, P., Catalano, D., et al. (2019). Dysregulated autophagy and lysosome function are linked to exosome production by micro-rna 155 in alcoholic liver disease. Hepatology 70, 2123–2141. doi: 10.1002/hep.30766
Baietti, M. F., Zhang, Z., Mortier, E., Melchior, A., Degeest, G., Geeraerts, A., et al. (2012). Syndecan-syntenin-ALIX regulates the biogenesis of exosomes. Nat. Cell Biol. 14, 677–685.
Baixauli, F., Lopez-Otin, C., and Mittelbrunn, M. (2014). Exosomes and autophagy: Coordinated mechanisms for the maintenance of cellular fitness. Front. Immunol. 5:403. doi: 10.3389/fimmu.2014.00403
Bianchi, E., Doe, B., Goulding, D., and Wright, G. J. (2014). Juno is the egg Izumo receptor and is essential for mammalian fertilization. Nature 508, 483–487.
Blanchette, C. R., and Rodal, A. A. (2020). Mechanisms for biogenesis and release of neuronal extracellular vesicles. Curr. Opin. Neurobiol. 63, 104–110.
Booth, A. M., Fang, Y., Fallon, J. K., Yang, J. M., Hildreth, J. E., and Gould, S. J. (2006). Exosomes and HIV Gag bud from endosome-like domains of the T cell plasma membrane. J. Cell Biol. 172, 923–935. doi: 10.1083/jcb.200508014
Calsolaro, V., and Edison, P. (2016). Neuroinflammation in Alzheimer’s disease: Current evidence and future directions. Alzheimers Dement 12, 719–732.
Cheng, A., Yang, Y., Zhou, Y., Maharana, C., Lu, D., Peng, W., et al. (2016). Mitochondrial SIRT3 Mediates Adaptive Responses of Neurons to Exercise and Metabolic and Excitatory Challenges. Cell Metab. 23, 128–142. doi: 10.1016/j.cmet.2015.10.013
Cho, M. H., Cho, K., Kang, H. J., Jeon, E. Y., Kim, H. S., Kwon, H. J., et al. (2014). Autophagy in microglia degrades extracellular beta-amyloid fibrils and regulates the NLRP3 inflammasome. Autophagy 10, 1761–1775. doi: 10.4161/auto.29647
Choi, I., Zhang, Y., Seegobin, S. P., Pruvost, M., Wang, Q., Purtell, K., et al. (2020). Microglia clear neuron-released alpha-synuclein via selective autophagy and prevent neurodegeneration. Nat. Commun. 11:1386. doi: 10.1038/s41467-020-15119-w
Colombo, M., Moita, C., van Niel, G., Kowal, J., Vigneron, J., Benaroch, P., et al. (2013). Analysis of ESCRT functions in exosome biogenesis, composition and secretion highlights the heterogeneity of extracellular vesicles. J. Cell Sci. 126, 5553–5565. doi: 10.1242/jcs.128868
Coulter, M. E., Dorobantu, C. M., Lodewijk, G. A., Delalande, F., Cianferani, S., Ganesh, V. S., et al. (2018). The ESCRT-III Protein CHMP1A Mediates Secretion of Sonic Hedgehog on a Distinctive Subtype of Extracellular Vesicles. Cell Rep. 24, 973–986e8. doi: 10.1016/j.celrep.2018.06.100
de Rivero Vaccari, J. P., Brand, F., Adamczak, S., Lee, S. W., Perez-Barcena, J., and Wang, M. Y. (2016). Exosome-mediated inflammasome signaling after central nervous system injury. J. Neurochem. 136, 39–48. doi: 10.1111/jnc.13036
DiFiglia, M., Sapp, E., Chase, K. O., Davies, S. W., Bates, G. P., Vonsattel, J. P., et al. (1997). Aggregation of huntingtin in neuronal intranuclear inclusions and dystrophic neurites in brain. Science 277, 1990–1993. doi: 10.1126/science.277.5334.1990
D’Souza-Schorey, C., and Schorey, J. S. (2018). Regulation and mechanisms of extracellular vesicle biogenesis and secretion. Essays Biochem. 62, 125–133.
Emmanouilidou, E., Melachroinou, K., Roumeliotis, T., Garbis, S. D., Ntzouni, M., and Margaritis, L. H. (2010). Cell-produced alpha-synuclein is secreted in a calcium-dependent manner by exosomes and impacts neuronal survival. J. Neurosci. 30, 6838–6851. doi: 10.1523/JNEUROSCI.5699-09.2010
Escola, J. M., Kleijmeer, M. J., Stoorvogel, W., Griffith, J. M., Yoshie, O., and Geuze, H. J. (1998). Selective enrichment of tetraspan proteins on the internal vesicles of multivesicular endosomes and on exosomes secreted by human B-lymphocytes. J. Biol. Chem. 273, 20121–20127. doi: 10.1074/jbc.273.32.20121
Evans, S. J., Choudary, P. V., Neal, C. R., Li, J. Z., Vawter, M. P., Tomita, H., et al. (2004). Dysregulation of the fibroblast growth factor system in major depression. Proc. Natl. Acad. Sci. U.S.A. 101, 15506–15511.
Fan, R. Z., Guo, M., Luo, S., Cui, M., and Tieu, K. (2019). Exosome release and neuropathology induced by alpha-synuclein: New insights into protective mechanisms of Drp1 inhibition. Acta Neuropathol. Commun. 7:184. doi: 10.1186/s40478-019-0821-4
Fang, Y., Wu, N., Gan, X., Yan, W., Morrell, J. C., and Gould, S. J. (2007). Higher-order oligomerization targets plasma membrane proteins and HIV gag to exosomes. PLoS Biol. 5:e158. doi: 10.1371/journal.pbio.0050158
Faure, J., Lachenal, G., Court, M., Hirrlinger, J., Chatellard-Causse, C., Blot, B., et al. (2006). Exosomes are released by cultured cortical neurones. Mol. Cell Neurosci. 31, 642–648.
Fiandaca, M. S., Kapogiannis, D., Mapstone, M., Boxer, A., Eitan, E., Schwartz, J. B., et al. (2015). Identification. Alzheimers Dement 11, 600–7e1.
Gaughran, F., Payne, J., Sedgwick, P. M., Cotter, D., and Berry, M. (2006). Hippocampal FGF-2 and FGFR1 mRNA expression in major depression, schizophrenia and bipolar disorder. Brain Res. Bull. 70, 221–227. doi: 10.1016/j.brainresbull.2006.04.008
Goncalves, M. B., Malmqvist, T., Clarke, E., Hubens, C. J., Grist, J., Hobbs, C., et al. (2015). Neuronal RARbeta Signaling Modulates PTEN Activity Directly in Neurons and via Exosome Transfer in Astrocytes to Prevent Glial Scar Formation and Induce Spinal Cord Regeneration. J. Neurosci. 35, 15731–15745. doi: 10.1523/JNEUROSCI.1339-15.2015
Gonzalez, R., Hamblin, M. H., and Lee, J. P. (2016). Neural Stem Cell Transplantation and CNS Diseases. CNS Neurol. Disord. Drug Targets 15, 881–886.
Guillemot, F., and Zimmer, C. (2011). From cradle to grave: The multiple roles of fibroblast growth factors in neural development. Neuron 71, 574–588. doi: 10.1016/j.neuron.2011.08.002
Guix, F. X., Corbett, G. T., Cha, D. J., Mustapic, M., Liu, W., Mengel, D., et al. (2018). Detection of Aggregation-Competent Tau in Neuron-Derived Extracellular Vesicles. Int. J. Mol. Sci. 19:663. doi: 10.3390/ijms19030663
Guo, C., Liu, J., Zhou, Q., Song, J., Zhang, Z., Li, Z., et al. (2020). Exosomal Noncoding RNAs and Tumor Drug Resistance. Cancer Res. 80, 4307–4313.
Guo, H., Chitiprolu, M., Roncevic, L., Javalet, C., Hemming, F. J., Trung, M. T., et al. (2017). Atg5 disassociates the v1v0-atpase to promote exosome production and tumor metastasis independent of canonical macroautophagy. Dev. Cell 43, 716–730.e7. doi: 10.1016/j.devcel.2017.11.018
Han, C., Xiong, N., Guo, X., Huang, J., Ma, K., Liu, L., et al. (2019). Exosomes from patients with Parkinson’s disease are pathological in mice. J. Mol. Med. 97, 1329–1344.
Han, X., Sun, S., Sun, Y., Song, Q., Zhu, J., Song, N., et al. (2019). Small molecule-driven NLRP3 inflammation inhibition via interplay between ubiquitination and autophagy: Implications for Parkinson disease. Autophagy 15, 1860–1881. doi: 10.1080/15548627.2019.1596481
Haque, A., Samantaray, S., Knaryan, V. H., Capone, M., Hossain, A., Matzelle, D., et al. (2020). Calpain mediated expansion of CD4+ cytotoxic T cells in rodent models of Parkinson’s disease. Exp. Neurol. 330:113315. doi: 10.1016/j.expneurol.2020.113315
Harding, C., Heuser, J., and Stahl, P. (1984). Endocytosis and intracellular processing of transferrin and colloidal gold-transferrin in rat reticulocytes: Demonstration of a pathway for receptor shedding. Eur. J. Cell Biol. 35, 256–263.
Harland, M., Torres, S., Liu, J., and Wang, X. (2020). Neuronal Mitochondria Modulation of LPS-Induced Neuroinflammation. J. Neurosci. 40, 1756–1765.
Heckmann, B. L., Teubner, B. J. W., Tummers, B., Boada-Romero, E., Harris, L., Yang, M., et al. (2019). LC3-Associated Endocytosis Facilitates beta-Amyloid Clearance and Mitigates Neurodegeneration in Murine Alzheimer’s Disease. Cell 178, 536–551.e14.
Hessvik, N. P., and Llorente, A. (2018). Current knowledge on exosome biogenesis and release. Cell. Mol. Life Sci. 75, 193–208.
Hitomi, K., Okada, R., Loo, T. M., Miyata, K., Nakamura, A. J., and Takahashi, A. (2020). DNA Damage Regulates Senescence-Associated Extracellular Vesicle Release via the Ceramide Pathway to Prevent Excessive Inflammatory Responses. Int. J. Mol. Sci. 21:3720. doi: 10.3390/ijms21103720
Houtman, J., Freitag, K., Gimber, N., Schmoranzer, J., Heppner, F. L., and Jendrach, M. (2019). Beclin1-driven autophagy modulates the inflammatory response of microglia via NLRP3. EMBO J. 38:e99430. doi: 10.15252/embj.201899430
Hsu, C., Morohashi, Y., Yoshimura, S., Manrique-Hoyos, N., Jung, S., Lauterbach, M. A., et al. (2010). Regulation of exosome secretion by Rab35 and its GTPase-activating proteins TBC1D10A-C. J. Cell Biol. 189, 223–232. doi: 10.1083/jcb.200911018
Iguchi, Y., Eid, L., Parent, M., Soucy, G., Bareil, C., Riku, Y., et al. (2016). Exosome secretion is a key pathway for clearance of pathological TDP-43. Brain 139, 3187–3201. doi: 10.1093/brain/aww237
Iturria-Medina, Y., Sotero, R. C., Toussaint, P. J., Mateos-Perez, J. M., Evans, A. C., and Alzheimer’s Disease Neuroimaging, I. (2016). Early role of vascular dysregulation on late-onset Alzheimer’s disease based on multifactorial data-driven analysis. Nat. Commun. 7:11934. doi: 10.1038/ncomms11934
Jeon, I., Cicchetti, F., Cisbani, G., Lee, S., Li, E., Bae, J., et al. (2016). Human-to-mouse prion-like propagation of mutant huntingtin protein. Acta Neuropathol. 132, 577–592. doi: 10.1007/s00401-016-1582-9
Jia, L., Qiu, Q., Zhang, H., Chu, L., Du, Y., Zhang, J., et al. (2019). Concordance between the assessment of Abeta42, T-tau, and P-T181-tau in peripheral blood neuronal-derived exosomes and cerebrospinal fluid. Alzheimers Dement 15, 1071–1080. doi: 10.1016/j.jalz.2019.05.002
Jiang, C., Hopfner, F., Katsikoudi, A., Hein, R., Catli, C., Evetts, S., et al. (2020). Serum neuronal exosomes predict and differentiate Parkinson’s disease from atypical parkinsonism. J. Neurol. Neurosurg. Psychiatry 91, 720–729. doi: 10.1136/jnnp-2019-322588
Jiang, D., Gong, F., Ge, X., Lv, C., Huang, C., Feng, S., et al. (2020). Neuron-derived exosomes-transmitted miR-124-3p protect traumatically injured spinal cord by suppressing the activation of neurotoxic microglia and astrocytes. J. Nanobiotechnol. 18:105. doi: 10.1186/s12951-020-00665-8
Jiao, Z., Zhang, W., Chen, C., Zhu, X., Chen, X., Zhou, M., et al. (2019). Gene Dysfunction Mediates Immune Response to Dopaminergic Degeneration in Parkinson’s Disease. ACS Chem. Neurosci. 10, 803–811.
Kenific, C. M., Zhang, H., and Lyden, D. (2021). An exosome pathway without an ESCRT. Cell Res. 31, 105–106.
Kumar, R., Tang, Q., Muller, S. A., Gao, P., Mahlstedt, D., Zampagni, S., et al. (2020). Fibroblast Growth Factor 2-Mediated Regulation of Neuronal Exosome Release Depends on VAMP3/Cellubrevin in Hippocampal Neurons. Adv. Sci. 7:1902372. doi: 10.1002/advs.201902372
Lachenal, G., Pernet-Gallay, K., Chivet, M., Hemming, F. J., Belly, A., Bodon, G., et al. (2011). Release of exosomes from differentiated neurons and its regulation by synaptic glutamatergic activity. Mol. Cell Neurosci. 46, 409–418.
Lemaire, Q., Raffo-Romero, A., Arab, T., Van Camp, C., Drago, F., Forte, S., et al. (2019). Isolation of microglia-derived extracellular vesicles: Towards miRNA signatures and neuroprotection. J. Nanobiotechnol. 17:119. doi: 10.1186/s12951-019-0551-6
Li, D., Huang, S., Zhu, J., Hu, T., Han, Z., Zhang, S., et al. (2019). Exosomes from MiR-21-5p-increased neurons play a role in neuroprotection by suppressing RAB11A-mediated neuronal autophagy in vitro after traumatic brain injury. Med. Sci. Monit. 25, 1871–1885. doi: 10.12659/MSM.915727
Liu, X. N., Zhang, C. B., Lin, H., Tang, X. Y., Zhou, R., Wen, H. L., et al. (2021). microRNA-204 shuttled by mesenchymal stem cell-derived exosomes inhibits the migration and invasion of non-small-cell lung cancer cells via the KLF7/AKT/HIF-1alpha axis. Neoplasma 68, 719–731. doi: 10.4149/neo_2021_201208N1328
Liu, Z., Qiu, A. W., Huang, Y., Yang, Y., Chen, J. N., Gu, T. T., et al. (2019). IL-17A exacerbates neuroinflammation and neurodegeneration by activating microglia in rodent models of Parkinson’s disease. Brain Behav. Immun. 81, 630–645. doi: 10.1016/j.bbi.2019.07.026
Lodygin, D., Hermann, M., Schweingruber, N., Flugel-Koch, C., Watanabe, T., Schlosser, C., et al. (2019). beta-Synuclein-reactive T cells induce autoimmune CNS grey matter degeneration. Nature 566, 503–508.
Luo, Z., Wu, F., Xue, E., Huang, L., Yan, P., Pan, X., et al. (2019). Hypoxia preconditioning promotes bone marrow mesenchymal stem cells survival by inducing HIF-1alpha in injured neuronal cells derived exosomes culture system. Cell Death Dis. 10:134. doi: 10.1038/s41419-019-1410-y
Machado-Santos, J., Saji, E., Troscher, A. R., Paunovic, M., Liblau, R., Gabriely, G., et al. (2018). The compartmentalized inflammatory response in the multiple sclerosis brain is composed of tissue-resident CD8+ T lymphocytes and B cells. Brain 141, 2066–2082. doi: 10.1093/brain/awy151
Malik, A. R., and Willnow, T. E. (2019). Excitatory Amino Acid Transporters in Physiology and Disorders of the Central Nervous System. Int. J. Mol. Sci. 20:5671.
Mead, B., and Tomarev, S. (2017). Bone Marrow-Derived Mesenchymal Stem Cells-Derived Exosomes Promote Survival of Retinal Ganglion Cells Through miRNA-Dependent Mechanisms. Stem Cells Transl. Med. 6, 1273–1285. doi: 10.1002/sctm.16-0428
Melo, S. A., Luecke, L. B., Kahlert, C., Fernandez, A. F., Gammon, S. T., Kaye, J., et al. (2015). Glypican-1 identifies cancer exosomes and detects early pancreatic cancer. Nature 523, 177–182.
Men, Y., Yelick, J., Jin, S., Tian, Y., Chiang, M. S. R., Higashimori, H., et al. (2019). Exosome reporter mice reveal the involvement of exosomes in mediating neuron to astroglia communication in the CNS. Nat. Commun. 10:4136. doi: 10.1038/s41467-019-11534-w
Meng, Y., Ding, J., Li, C., Fan, H., He, Y., and Qiu, P. (2020). Transfer of pathological alpha-synuclein from neurons to astrocytes via exosomes causes inflammatory responses after METH exposure. Toxicol. Lett. 331, 188–199. doi: 10.1016/j.toxlet.2020.06.016
Mizushima, N., and Komatsu, M. (2011). Autophagy: Renovation of cells and tissues. Cell 147, 728–741.
Morel, L., Regan, M., Higashimori, H., Ng, S. K., Esau, C., Vidensky, S., et al. (2013). Neuronal exosomal miRNA-dependent translational regulation of astroglial glutamate transporter GLT1. J. Biol. Chem. 288, 7105–7116. doi: 10.1074/jbc.M112.410944
Muralidharan-Chari, V., Clancy, J., Plou, C., Romao, M., Chavrier, P., Raposo, G., et al. (2009). ARF6-regulated shedding of tumor cell-derived plasma membrane microvesicles. Curr. Biol. 19, 1875–1885. doi: 10.1016/j.cub.2009.09.059
Muraoka, S., Jedrychowski, M. P., Yanamandra, K., Ikezu, S., Gygi, S. P., and Ikezu, T. (2020). Proteomic Profiling of Extracellular Vesicles Derived from Cerebrospinal Fluid of Alzheimer’s Disease Patients: A Pilot Study. Cells 9:1959. doi: 10.3390/cells9091959
Nabhan, J. F., Hu, R., Oh, R. S., Cohen, S. N., and Lu, Q. (2012). Formation and release of arrestin domain-containing protein 1-mediated microvesicles (ARMMs) at plasma membrane by recruitment of TSG101 protein. Proc. Natl. Acad. Sci. U.S.A. 109, 4146–4151. doi: 10.1073/pnas.1200448109
Nager, A. R., Goldstein, J. S., Herranz-Perez, V., Portran, D., Ye, F., Garcia-Verdugo, J. M., et al. (2017). An Actin Network Dispatches Ciliary GPCRs into Extracellular Vesicles to Modulate Signaling. Cell 168, 252–263.e14. doi: 10.1016/j.cell.2016.11.036
Nakamura, Y., Dryanovski, D. I., Kimura, Y., Jackson, S. N., Woods, A. S., Yasui, Y., et al. (2019). Cocaine-induced endocannabinoid signaling mediated by sigma-1 receptors and extracellular vesicle secretion. elife 8:e47209. doi: 10.7554/eLife.47209
Nash, Y., Schmukler, E., Trudler, D., Pinkas-Kramarski, R., and Frenkel, D. (2017). DJ-1 deficiency impairs autophagy and reduces alpha-synuclein phagocytosis by microglia. J. Neurochem. 143, 584–594. doi: 10.1111/jnc.14222
Neumann, M., Sampathu, D. M., Kwong, L. K., Truax, A. C., Micsenyi, M. C., Chou, T. T., et al. (2006). Ubiquitinated TDP-43 in frontotemporal lobar degeneration and amyotrophic lateral sclerosis. Science 314, 130–133.
Nie, W., Wu, G., Zhang, J., Huang, L. L., Ding, J., Jiang, A., et al. (2020). Responsive Exosome Nano-bioconjugates for Synergistic Cancer Therapy. Angew. Chem. Int. Ed. Engl. 59, 2018–2022. doi: 10.1002/anie.201912524
Niu, M., Li, Y., Li, G., Zhou, L., Luo, N., Yao, M., et al. (2020). A longitudinal study on alpha-synuclein in plasma neuronal exosomes as a biomarker for Parkinson’s disease development and progression. Eur. J. Neurol. 27, 967–974. doi: 10.1111/ene.14208
Ostrowski, M., Carmo, N. B., Krumeich, S., Fanget, I., Raposo, G., Savina, A., et al. (2010). Rab27a and Rab27b control different steps of the exosome secretion pathway. Nat. Cell Biol. 12, 19–30.
Pace, K. R., Dutt, R., and Galileo, D. S. (2019). Exosomal L1CAM Stimulates Glioblastoma Cell Motility, Proliferation, and Invasiveness. Int. J. Mol. Sci. 20:3982.
Pan, B. T., and Johnstone, R. M. (1983). Fate of the transferrin receptor during maturation of sheep reticulocytes in vitro: Selective externalization of the receptor. Cell 33, 967–978.
Park, G., Kim, B. S., and Kim, E. (2020). A novel function of FAF1, which induces dopaminergic neuronal death through cell-to-cell transmission. Cell Commun. Signal. 18:133. doi: 10.1186/s12964-020-00632-8
Passamonti, L., Tsvetanov, K. A., Jones, P. S., Bevan-Jones, W. R., Arnold, R., Borchert, R. J., et al. (2019). Neuroinflammation and functional connectivity in alzheimer’s disease: Interactive influences on cognitive performance. J. Neurosci. 39, 7218–7226.
Pastuzyn, E. D., Day, C. E., Kearns, R. B., Kyrke-Smith, M., Taibi, A. V., McCormick, J., et al. (2018). The Neuronal Gene Arc Encodes a Repurposed Retrotransposon Gag Protein that Mediates Intercellular RNA Transfer. Cell 172, 275–288.e18.
Peng, C., Trojanowski, J. Q., and Lee, V. M. (2020). Protein transmission in neurodegenerative disease. Nat. Rev. Neurol. 16, 199–212.
Pfeffer, S. R. (2017). Rab GTPases: Master regulators that establish the secretory and endocytic pathways. Mol. Biol. Cell 28, 712–715.
Pinto, S., Cunha, C., Barbosa, M., Vaz, A. R., and Brites, D. (2017). Exosomes from nsc-34 cells transfected with hsod1-g93a are enriched in mir-124 and drive alterations in microglia phenotype. Front. Neurosci. 11:273. doi: 10.3389/fnins.2017.00273
Pluvinage, J. V., Haney, M. S., Smith, B. A. H., Sun, J., Iram, T., Bonanno, L., et al. (2019). CD22 blockade restores homeostatic microglial phagocytosis in ageing brains. Nature 568, 187–192.
Polanco, J. C., Li, C., Durisic, N., Sullivan, R., and Gotz, J. (2018). Exosomes taken up by neurons hijack the endosomal pathway to spread to interconnected neurons. Acta Neuropathol. Commun. 6:10. doi: 10.1186/s40478-018-0514-4
Qin, Y., Qiu, J., Wang, P., Liu, J., Zhao, Y., Jiang, F., et al. (2021). Impaired autophagy in microglia aggravates dopaminergic neurodegeneration by regulating NLRP3 inflammasome activation in experimental models of Parkinson’s disease. Brain Behav. Immun. 91, 324–338. doi: 10.1016/j.bbi.2020.10.010
Rayamajhi, S., Nguyen, T. D. T., Marasini, R., and Aryal, S. (2019). Macrophage-derived exosome-mimetic hybrid vesicles for tumor targeted drug delivery. Acta Biomater. 94, 482–494. doi: 10.1016/j.actbio.2019.05.054
Ruan, Z., Pathak, D., Venkatesan Kalavai, S., Yoshii-Kitahara, A., Muraoka, S., and Bhatt, N. (2021). Alzheimer’s disease brain-derived extracellular vesicles spread tau pathology in interneurons. Brain 144, 288–309.
Sardar Sinha, M., Ansell-Schultz, A., Civitelli, L., Hildesjo, C., Larsson, M., Lannfelt, L., et al. (2018). Alzheimer’s disease pathology propagation by exosomes containing toxic amyloid-beta oligomers. Acta Neuropathol. 136, 41–56. doi: 10.1007/s00401-018-1868-1
Savina, A., Vidal, M., and Colombo, M. I. (2002). The exosome pathway in K562 cells is regulated by Rab11. J. Cell Sci. 115, 2505–2515. doi: 10.1242/jcs.115.12.2505
Sharma, P., Mesci, P., Carromeu, C., McClatchy, D. R., Schiapparelli, L., and Yates, J. R. III, et al. (2019). Exosomes regulate neurogenesis and circuit assembly. Proc. Natl. Acad. Sci. U.S.A. 116, 16086–16094. doi: 10.1073/pnas.1902513116
Shi, M., Liu, C., Cook, T. J., Bullock, K. M., Zhao, Y., Ginghina, C., et al. (2014). Plasma exosomal alpha-synuclein is likely CNS-derived and increased in Parkinson’s disease. Acta Neuropathol. 128, 639–650.
Silverman, J. M., Christy, D., Shyu, C. C., Moon, K. M., Fernando, S., Gidden, Z., et al. (2019). CNS-derived extracellular vesicles from superoxide dismutase 1 (SOD1)(G93A) ALS mice originate from astrocytes and neurons and carry misfolded SOD1. J. Biol. Chem. 294, 3744–3759. doi: 10.1074/jbc.RA118.004825
Simeoli, R., Montague, K., Jones, H. R., Castaldi, L., Chambers, D., Kelleher, J. H., et al. (2017). Exosomal cargo including microRNA regulates sensory neuron to macrophage communication after nerve trauma. Nat. Commun. 8:1778. doi: 10.1038/s41467-017-01841-5
Singh, P. K., and Muqit, M. M. K. (2020). Parkinson’s: A disease of aberrant vesicle trafficking. Annu. Rev. Cell Dev. Biol. 36, 237–264. doi: 10.1146/annurev-cellbio-100818-125512
Song, L., Tang, S., Han, X., Jiang, Z., Dong, L., Liu, C., et al. (2019c). KIBRA controls exosome secretion via inhibiting the proteasomal degradation of Rab27a. Nat. Commun. 10:1639. doi: 10.1038/s41467-019-09720-x
Song, L., Tang, S., Dong, L., Han, X., Cong, L., Dong, J., et al. (2019b). The Neuroprotection of KIBRA in Promoting Neuron Survival and Against Amyloid beta-Induced Apoptosis. Front. Cell Neurosci. 13:137. doi: 10.3389/fncel.2019.00137
Song, H., Zhang, X., Chen, R., Miao, J., Wang, L., Cui, L., et al. (2019a). Cortical neuron-derived exosomal microrna-181c-3p inhibits neuroinflammation by downregulating cxcl1 in astrocytes of a rat model with ischemic brain injury. Neuroimmunomodulation 26, 217–233. doi: 10.1159/000502694
Su, G., Meyer, K., Nandini, C. D., Qiao, D., Salamat, S., and Friedl, A. (2006). Glypican-1 is frequently overexpressed in human gliomas and enhances FGF-2 signaling in glioma cells. Am. J. Pathol. 168, 2014–2026. doi: 10.2353/ajpath.2006.050800
Sweeney, M. D., Sagare, A. P., and Zlokovic, B. V. (2018). Blood-brain barrier breakdown in Alzheimer disease and other neurodegenerative disorders. Nat. Rev. Neurol. 14, 133–150.
Trajkovic, K., Hsu, C., Chiantia, S., Rajendran, L., Wenzel, D., Wieland, F., et al. (2008). Ceramide triggers budding of exosome vesicles into multivesicular endosomes. Science 319, 1244–1247. doi: 10.1126/science.1153124
Upadhya, R., Zingg, W., Shetty, S., and Shetty, A. K. (2020). Astrocyte-derived extracellular vesicles: Neuroreparative properties and role in the pathogenesis of neurodegenerative disorders. J. Control Release 323, 225–239. doi: 10.1016/j.jconrel.2020.04.017
Vietri, M., Radulovic, M., and Stenmark, H. (2020). The many functions of ESCRTs. Nat. Rev. Mol. Cell Biol. 21, 25–42.
Vilcaes, A. A., Chanaday, N. L., and Kavalali, E. T. (2021). Interneuronal exchange and functional integration of synaptobrevin via extracellular vesicles. Neuron 109, 971–983.e5. doi: 10.1016/j.neuron.2021.01.007
Wang, H., Chen, F. S., Zhang, Z. L., Zhou, H. X., Ma, H., and Li, X. Q. (2021). MiR-126-3p-enriched extracellular vesicles from hypoxia-preconditioned VSC 4.1 neurons attenuate ischaemia-reperfusion-induced pain hypersensitivity by regulating the PIK3R2-mediated pathway. Mol. Neurobiol. 58, 821–834. doi: 10.1007/s12035-020-02159-y
Wang, L., and Zhang, L. (2020). Circulating Exosomal miRNA as Diagnostic Biomarkers of Neurodegenerative Diseases. Front. Mol. Neurosci. 13:53. doi: 10.3389/fnmol.2020.00053
Wang, Y., Balaji, V., Kaniyappan, S., Kruger, L., Irsen, S., Tepper, K., et al. (2017). The release and trans-synaptic transmission of Tau via exosomes. Mol. Neurodegener. 12:5. doi: 10.1186/s13024-016-0143-y
Wei, D., Zhan, W., Gao, Y., Huang, L., Gong, R., Wang, W., et al. (2021). RAB31 marks and controls an ESCRT-independent exosome pathway. Cell Res. 31, 157–177. doi: 10.1038/s41422-020-00409-1
Wei, Z., Meng, X., El Fatimy, R., Sun, B., Mai, D., Zhang, J., et al. (2020). Environmental enrichment prevents Abeta oligomer-induced synaptic dysfunction through mirna-132 and hdac3 signaling pathways. Neurobiol. Dis. 134:104617. doi: 10.1016/j.nbd.2019.104617
Wilton, D. K., Dissing-Olesen, L., and Stevens, B. (2019). Neuron-Glia Signaling in Synapse Elimination. Annu. Rev. Neurosci. 42, 107–127.
Winston, C. N., Goetzl, E. J., Baker, L. D., Vitiello, M. V., and Rissman, R. A. (2018). Growth Hormone-Releasing Hormone Modulation of Neuronal Exosome Biomarkers in Mild Cognitive Impairment. J. Alzheimers Dis. 66, 971–981. doi: 10.3233/JAD-180302
Wolf, P. (1967). The nature and significance of platelet products in human plasma. Br. J. Haematol. 13, 269–288.
Xiao, Q. X., Cheng, C. X., Deng, R., Liu, Q., Ren, Y. B., He, L., et al. (2020). LncRNA-MYL2-2 and miR-124-3p Are Associated with Perioperative Neurocognitive Disorders in Patients after Cardiac Surgery. J. Invest. Surg. 34, 1297–1303. doi: 10.1080/08941939.2020.1797949
Xiao, Y., Zhong, J., Zhong, B., Huang, J., Jiang, L., Jiang, Y., et al. (2020). Exosomes as potential sources of biomarkers in colorectal cancer. Cancer Lett. 476, 13–22.
Xu, B., Zhang, Y., Du, X. F., Li, J., Zi, H. X., Bu, J. W., et al. (2017). Neurons secrete miR-132-containing exosomes to regulate brain vascular integrity. Cell Res. 27, 882–897. doi: 10.1038/cr.2017.62
Yang, K. S., Ciprani, D., O’Shea, A., Liss, A. S., Yang, R., Fletcher-Mercaldo, S., et al. (2021). Extracellular Vesicle Analysis Allows for Identification of Invasive IPMN. Gastroenterology 160, 1345–1358.e11. doi: 10.1053/j.gastro.2020.11.046
Yang, T. T., Liu, C. G., Gao, S. C., Zhang, Y., and Wang, P. C. (2018). The Serum Exosome Derived MicroRNA-135a, -193b, and -384 Were Potential Alzheimer’s Disease Biomarkers. Biomed. Environ. Sci. 31, 87–96. doi: 10.3967/bes2018.011
Yin, Z., Han, Z., Hu, T., Zhang, S., Ge, X., Huang, S., et al. (2020). Neuron-derived exosomes with high miR-21-5p expression promoted polarization of M1 microglia in culture. Brain Behav. Immun. 83, 270–282.
Ying, W., Riopel, M., Bandyopadhyay, G., Dong, Y., Birmingham, A., Seo, J. B., et al. (2017). Adipose Tissue Macrophage-Derived Exosomal miRNAs Can Modulate In Vivo and In Vitro Insulin Sensitivity. Cell 171, 372–384.e12. doi: 10.1016/j.cell.2017.08.035
You, Y., Borgmann, K., Edara, V. V., Stacy, S., Ghorpade, A., and Ikezu, T. (2020). Activated human astrocyte-derived extracellular vesicles modulate neuronal uptake, differentiation and firing. J. Extracell. Vesicles 9:1706801. doi: 10.1080/20013078.2019.1706801
Yu, H., Sun, T., An, J., Wen, L., Liu, F., Bu, Z., et al. (2020). Potential roles of exosomes in parkinson’s disease: From pathogenesis, diagnosis, and treatment to prognosis. Front. Cell Dev. Biol. 8:86. doi: 10.3389/fcell.2020.00086
Yuyama, K., Sun, H., Mitsutake, S., and Igarashi, Y. (2012). Sphingolipid-modulated exosome secretion promotes clearance of amyloid-beta by microglia. J. Biol. Chem. 287, 10977–10989. doi: 10.1074/jbc.M111.324616
Zhang, X. W., Zhou, J. C., Peng, D., Hua, F., Li, K., Yu, J. J., et al. (2020). Disrupting the TRIB3-SQSTM1 interaction reduces liver fibrosis by restoring autophagy and suppressing exosome-mediated HSC activation. Autophagy 16, 782–796. doi: 10.1080/15548627.2019.1635383
Zhang, C., Wu, B., Wang, X., Chen, C., Zhao, R., Lu, H., et al. (2020). Vascular, flow and perfusion abnormalities in Parkinson’s disease. Parkinsonism Relat. Disord. 73, 8–13.
Zhang, Y., Meng, X., Jiao, Z., Liu, Y., Zhang, X., and Qu, S. (2020). Generation of a Novel Mouse Model of Parkinson’s Disease via Targeted Knockdown of Glutamate Transporter GLT-1 in the Substantia Nigra. ACS Chem. Neurosci. 11, 406–417. doi: 10.1021/acschemneuro.9b00609
Zhang, Q., Hu, C., Huang, J., Liu, W., Lai, W., Leng, F., et al. (2019). ROCK1 induces dopaminergic nerve cell apoptosis via the activation of Drp1-mediated aberrant mitochondrial fission in Parkinson’s disease. Exp. Mol. Med. 51, 1–13. doi: 10.1038/s12276-019-0318-z
Zhang, W., Zhu, J., He, X., Liu, X., Li, J., Li, W., et al. (2019). Exosome complex genes mediate RNA degradation and predict survival in mantle cell lymphoma. Oncol. Lett. 18, 5119–5128. doi: 10.3892/ol.2019.10850
Zhao, S., Mi, Y., Guan, B., Zheng, B., Wei, P., Gu, Y., et al. (2021). Correction to: Tumor-derived exosomal miR-934 induces macrophage M2 polarization to promote liver metastasis of colorectal cancer. J. Hematol. Oncol. 14:33. doi: 10.1186/s13045-021-01042-0
Zheng, T., Pu, J., Chen, Y., Guo, Z., Pan, H., Zhang, L., et al. (2017). Exosomes Secreted from HEK293-APP Swe/Ind Cells Impair the Hippocampal Neurogenesis. Neurotox. Res. 32, 82–93. doi: 10.1007/s12640-017-9713-1
Zheng, T., Wu, X., Wei, X., Wang, M., and Zhang, B. (2018). The release and transmission of amyloid precursor protein via exosomes. Neurochem. Int. 114, 18–25. doi: 10.1016/j.neuint.2017.12.009
Zhou, T., Lin, D., Chen, Y., Peng, S., Jing, X., Lei, M., et al. (2019). alpha-synuclein accumulation in SH-SY5Y cell impairs autophagy in microglia by exosomes overloading miR-19a-3p. Epigenomics 11, 1661–1677. doi: 10.2217/epi-2019-0222
Zhou, W., Woodson, M., Sherman, M. B., Neelakanta, G., and Sultana, H. (2019). Exosomes mediate Zika virus transmission through SMPD3 neutral Sphingomyelinase in cortical neurons. Emerg. Microbes Infect. 8, 307–326. doi: 10.1080/22221751.2019.1578188
Keywords: neuron, extracellular vesicles, neuropathy, biomarker, neurodegeneration
Citation: Jiao Z, He Z, Liu N, Lai Y and Zhong T (2022) Multiple roles of neuronal extracellular vesicles in neurological disorders. Front. Cell. Neurosci. 16:979856. doi: 10.3389/fncel.2022.979856
Received: 28 June 2022; Accepted: 10 August 2022;
Published: 20 September 2022.
Edited by:
Ulises Gomez-Pinedo, Health Research Institute of Hospital Clínico San Carlos, SpainReviewed by:
Edwin Estefan Reza, Instituto de Tecnología y Educación Superior de Monterrey (ITESM), MexicoCopyright © 2022 Jiao, He, Liu, Lai and Zhong. This is an open-access article distributed under the terms of the Creative Commons Attribution License (CC BY). The use, distribution or reproduction in other forums is permitted, provided the original author(s) and the copyright owner(s) are credited and that the original publication in this journal is cited, in accordance with accepted academic practice. No use, distribution or reproduction is permitted which does not comply with these terms.
*Correspondence: Tianyu Zhong, emhvbmd0aWFueXVAZ21haWwuY29t; Zhigang Jiao, amlhb3poaWdhbmc4MDQzQDE2My5jb20=
Disclaimer: All claims expressed in this article are solely those of the authors and do not necessarily represent those of their affiliated organizations, or those of the publisher, the editors and the reviewers. Any product that may be evaluated in this article or claim that may be made by its manufacturer is not guaranteed or endorsed by the publisher.
Research integrity at Frontiers
Learn more about the work of our research integrity team to safeguard the quality of each article we publish.