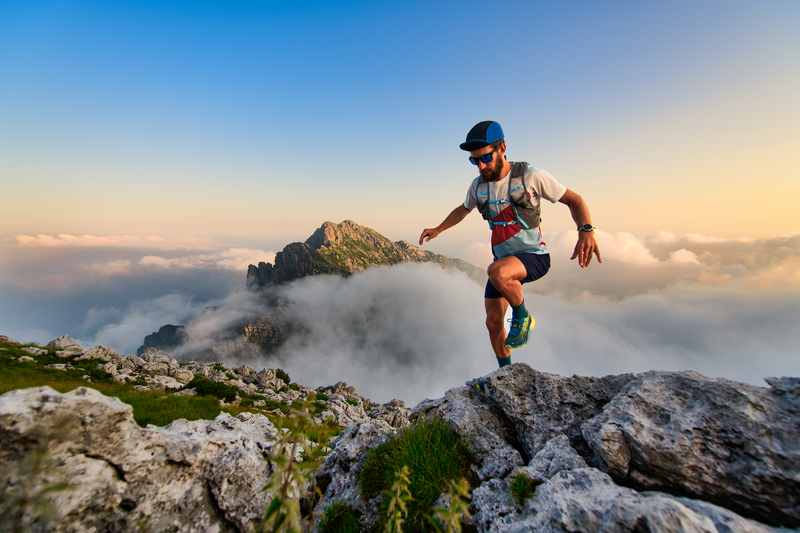
95% of researchers rate our articles as excellent or good
Learn more about the work of our research integrity team to safeguard the quality of each article we publish.
Find out more
REVIEW article
Front. Cell. Neurosci. , 17 November 2022
Sec. Cellular Neuropathology
Volume 16 - 2022 | https://doi.org/10.3389/fncel.2022.949939
This article is part of the Research Topic Neuropathophysiological Mechanisms of Spinal Cord Injury and Therapeutic Options View all 6 articles
Spinal cord injury (SCI) often brings devastating consequences to patients and their families. Pathophysiologically, the primary insult causes irreversible damage to neurons and glial cells and initiates the secondary damage cascade, further leading to inflammation, ischemia, and cells death. In SCI, the release of various inflammatory mediators aggravates nerve injury. Pyroptosis is a new pro-inflammatory pattern of regulated cell death (RCD), mainly mediated by caspase-1 or caspase-11/4/5. Gasdermins family are pore-forming proteins known as the executor of pyroptosis and the gasdermin D (GSDMD) is best characterized. Pyroptosis occurs in multiple central nervous system (CNS) cell types, especially plays a vital role in the development of SCI. We review here the evidence for pyroptosis in SCI, and focus on the pyroptosis of different cells and the crosstalk between them. In addition, we discuss the interaction between pyroptosis and other forms of RCD in SCI. We also summarize the therapeutic strategies for pyroptosis inhibition, so as to provide novel ideas for improving outcomes following SCI.
Spinal cord injury (SCI) is a devastating injury, which results in serious motor and neurological dysfunctions and places tremendous financial burdens on patients. Statistics show that the costs for patients with SCI are staggering at $14.5 billion in American (Habtemariam, 2019) and $2.67 billion in Canada per year (Katoh et al., 2019). Despite clinical urgent need, no effective treatment to date is uniquely specific for SCI. Thus, further understanding of the potential mechanisms of SCI, especially the pattern of cell death, is needed to explore underlying therapeutic targets.
The pathophysiology of SCI involves two stages: (i) the primary injury, due to mechanical injury to spinal cord and leading to destruction of neural parenchyma, including cell membrane rupture and tissue necrosis (Mortezaee et al., 2018), and (ii) the secondary injury, triggering by the primary injury and producing deleterious contents, involving glutamate, potassium, K+ flux, reactive oxygen species (ROS), and cathepsin B (Dixon, 2017). These contents are highly pro-inflammatory, and initiate a persistent secondary injury cascade. This cascade leads to inflammatory reaction of neurons and glial cells, ER, mitochondrial dysfunction and excitotoxicity, and ultimately changes in organization and structural architecture of spinal cord and permanent neurological deficits as a result of axonal destruction, demyelination and neuronal death (Alizadeh et al., 2019). Accordingly, inflammation is the key regulator in SCI.
Cell death plays a vital role in the pathophysiology of SCI. Three different patterns of cell death in SCI, including apoptosis, autophagy death, and necrosis have been extensively studied in the past (Tang et al., 2014; Bao et al., 2019). Recently, researchers have defined a new type of regulated cell death (RCD): pyroptosis, a pro-inflammatory (infectious and sterile) form of lytic cell death, which promotes cell swelling, plasma membrane dissolution, chromatin fragmentation, and release of inflammatory cytokines, such as interleukin (IL) –18 and IL-1β, through gasdermins pores. The pathophysiology of SCI involves two stages: (i) gasdermins plasma membrane pore formation and releasing of IL-1β/18 and ionic fluxes, and (ii) subsequent plasma membrane rupture (PMR), cell swelling and lysis, and release of LDH (Kayagaki et al., 2021). Neuroinflammation, a double-edged sword in SCI, involves numerous cell types such as microglia, astrocytes, neurons, and pericytes (Miron and Franklin, 2014). After SCI, these cells and the multicellular interactions are interrupted and disorganized, resulting in impaired neurological recovery. Substantial evidence indicates inflammation is an important determinant in the pathogenesis of SCI, particularly, pyroptosis has emerged as a critical factor. Thus, understanding the mechanism of pyroptosis on SCI provides new therapeutic approaches for clinical treatments. This review focuses on recent findings on the mechanisms and function of pyroptosis in SCI, especially the interactions between different cell lines as well as the crosstalk between different RCD patterns. This article also highlights the potential drugs that can alleviate pyroptosis, so as to provide new ideas for the potential treatment of SCI.
Friedlander (1986) found that anthrax lethal toxin could cause the death of macrophages and the rapid release of intracellular contents, which may be the earliest description of pyroptosis. In Zychlinsky et al. (1992) found cell death in macrophages infected with Shigella flexneri, which was different from apoptosis. The term pyroptosis was initially coined by Cookson and Brennan (2001) in to describe a form of macrophage death suffering Salmonella infection. Pyroptosis is a lytic, pro−inflammatory RCD program and characterized by its reliance on gasdermin proteins as the executioners of cell death. Activation of pyroptosis has been classified into a canonical pathway and non-canonical pathway. Generally, in a canonical pyroptotic pathway, activation of inflammasome occurs through a multitude of pathogen-associated molecular patterns (PAMPs) or damage-associated molecular patterns (DAMPs), including nucleic acids, flagellin, bacterial toxins, microbial cell wall components, alum, ATP, and uric acid. Inflammasome assembly is triggered when sensor proteins recognize their ligands. This nucleates the inflammasome and initiates polymerization of the scaffolding protein apoptosis-associated speck-like protein containing a CARD (ASC), a non-enzymatic scaffolding protein couples the sensor protein to the effector protease. Cross-linked ASC fibers recruit pro-inflammatory caspases that undergo proximal-induced autoactivation for proteolytic activity (Franklin et al., 2018). Upon activation, inflammasomes recruit caspase-1 via CARD-CARD interactions. The caspase-1 is localized on inflammasomes and clustering of caspase-1 generates a transient caspase-1 p33/p10 species, obligated to most substrate cleavage events. Cleavage of the CARD domain from the p33 fragment generates a caspase-1 p20/p10 species that speedily detaches from the inflammasome and becomes inactive (Boucher et al., 2018).
Inflammasomes, large cytosolic multiprotein complexes that detect a broad range of PAMPs and DAMPs and serve as platforms upon which activated proinflammatory caspases cleave and activate their protein substrates (Broz and Dixit, 2016). Depending on the type of caspases involved, the inflammasome are also divided into typical and atypical inflammasomes (Zhou C. et al., 2022). Canonical inflammasome complex is composed of three components, including a sensor protein, a caspase-1 family protease and an ASC. Danger signals such as extracellular ATP and mitochondrial dysfunction are potent inflammasome activators during SCI (Rathinam and Fitzgerald, 2016). Assembly of the inflammasome complex leads to caspase-1 activation by an autoproteolysis process. In addition to the cleavage of precursor cytokines pro-IL-1β/18 into IL-1β/18, the activated caspase-1 substrates also involve in the cleavage of Gasdermin D (GSDMD) into N-terminal fragment of GSDMD (GSDMD-NT), which inserts pores in the cell membrane and compromises membrane integrity, accompanied by the secretion of inflammatory signaling molecule, including IL-1β/18 (Liu et al., 2016). Additionally, non-canonical inflammasome directly senses cytosolic LPS from Gram-negative bacteria and their outer membrane vesicles through caspase-4/5 in humans or caspase-11 in mice. Like caspase-1, activated caspase-4/5/11 cleave GSDMD to induce pyroptosis (Shi et al., 2015). Notably, while caspase4/5/11 cannot process pro-IL-1β/18 directly, they can facilitate the maturation and secretion of the IL-1β/18 via the activation of the NLRP3 in an indirect way (Humphries et al., 2018; Yang et al., 2022). The receptors in the inflammasome complex belong in most cases to the Nod-like receptor (NLR) and absent in melanoma (AIM) 2-like receptor (ALR) families. Currently, known inflammasomes include the NLRP1, NLRP2, NLRP3, NAIP/NLRC4, NLRC5, NLRP6, NLRP12, AIM2, and pyrin (Linder et al., 2020). The best-studied inflammasomes in central nervous system (CNS) are NLRP1, NLRP3, and AIM2, although NLRC4 is also identified (Zendedel et al., 2018; Han et al., 2019). NLRP1, the first inflammasome identified, remains somewhat mysterious owing to its unique activation mechanism (Lamkanfi and Dixit, 2014). NLRP1 can be activated by intracellular ATP depletion, ion flux downstream of the P2 × 4/7 receptors and extracellular Aβ (Chavarría-Smith et al., 2016). Whereas NLRP1 in neurons is different from macrophages because it contains inhibitors of caspase 11 and apoptosis protein X-linked inhibitor of apoptosis protein (XIAP) (Mortezaee et al., 2018). The activation mechanism of caspase-11 in CNS injury remains unclear. Whether activation of the NLRP1 inflammasome induces cleavage of caspase-11 or caspase-11 forms independent of caspase-1 are still under investigation. Among SCI studies, NLRP3 inflammasome is the most extensively studied, which is expressed in microglia, neuron and astrocytes and detects perturbations to cellular homeostasis, although the unifying mechanism for NLRP3 activation remains controversial (Lamkanfi and Dixit, 2014). NLRP3 can be selectively blocked by concentrated extracellular K+, MCC950, ketogenic diet, glyburide, and minocycline (Muñoz-Planillo et al., 2013; Wallisch et al., 2017). AIM2 is a non-NLR inflammasome, which recognizes double-stranded DNA (dsDNA), either pathogen or host-derived, through its C terminal HIN200 domain (Lamkanfi and Dixit, 2014). Widespread necrotic cell death after SCI releases cell-free DNA into the cerebrospinal fluid, which may act as a ligand for AIM2. Binding of dsDNA to the HIN domain of AIM2 recruits ASC and procaspase-1, leading to the maturation of caspase-1, and pro-inflammatory cytokines (de Zoete et al., 2014).
Gasdermins (GSDMs) are a family of porogens containing six gene clusters [GSDMA-E and pejvakin (PJVK)] (Qin et al., 2022), and activated by proteolytic removal of autoinhibitory carboxy-terminal domains, typically by caspase modulators (LaRock et al., 2022). To date, little was known about GSDMs other than GSDMD. GSDMA is present in mammals, reptiles and birds. Notably, GSDMB, GSDMC, and GSDMD are only expressed in mammals (Broz et al., 2020), intriguingly, unlike other GSDMs, GSDMB lacks a mouse ortholog, which hinders the study of its in vivo function (Ivanov et al., 2022). Another unique feature of GSDMB is that it binds to phosphatidylinositol phosphate and sulfide (Chao et al., 2017). In epithelial cells, GSDMB can be cleaved by GzmA to induce pyroptosis (Zhou et al., 2020). GSDMD, one member of GSDMs family, is the major driver of pyroptosis, which contains dual domain with autoinhibitory mechanism, whereby the C terminal domain possessing repressor activity constitutively interacts with and represses the N terminal domain possessing pore-forming activity (Liu et al., 2018). The dual domain structure of GSDMD in humans is linked by a domain containing a caspase-1/4 (or caspase-1/11 in mice) cleavage site (Shi et al., 2015; Kovacs and Miao, 2017). Upon cleavage by caspase-1/4 (or caspase-1/11 in mice), the autoinhibition of the C terminus is eliminated, releasing the p30 N terminal fragment with pore-forming activity (GSDMD-N). Lipid binding triggers the oligomerization of GSDMD-N to form transmembrane pores in the plasma membrane, leading to disruption flocal osmotic potential, swelling, and terminal membrane rupture (Liu et al., 2016). GSDMD pore (10–15 nm in diameter) provides a logical conduit through which IL-1β (4.5 nm in diameter) and IL-18 (5.0 nm in diameter) escape the cell (Kovacs and Miao, 2017). Except for GSDMD, recent study implicates that chemotherapy drugs could induce pyroptosis through caspase-3 cleavage of GSDME, another member of the gasdermins family (Wang et al., 2017). The content described below, unless otherwise specified, refers to GSDMD-mediated pyroptosis (Figure 1).
Ninjurin (NINJ) is a cell adhesion molecule and membrane protein with homophilic binding properties. Studies have shown that its expression is increased in neurons and Schwann cells of distal nerve segments after nerve transection or crush injury and promotes axonal sprouting and nerve repair in rat sciatic nerves (Araki and Milbrandt, 1996; Araki et al., 1997). In mammals, NINJ family is divided into NINJ1 and NINJ2. It has been reported that NINJ1 is involved in inflammatory processes. After acute cerebral ischemia in rats, NINJ1 is dynamically expressed in a variety of immune cells and involved in cell trafficking and axonal growth following neuroinflammation (Lee et al., 2016, 2018). Similarly, expression level of NINJ1 was up-regulated in the injured spinal cord in a rat model of spinal cord crush injury. As touched upon earlier, PMR is a catastrophic event in the second stage of pyroptosis, a process distinct from GSDMs-dependent pore formation, which is closely related to the regulation of NINJ1 (Kayagaki et al., 2015; Rühl and Broz, 2022). NINJ1 releases LDH and intracellular DAMPs, such as HMGB1, by modulating protrusive membrane dynamics. NINJ1 silencing (NINJ1–/–) can attenuate PMR and its associated inflammatory dissemination response. Of note, NINJ1 is dispensable for the formation of GSDMs-dependent pore (Ahn et al., 2014; Kayagaki et al., 2021). Intriguingly, NINJ2, a homolog of NINJ1, is identified as a key pro-survival factor in neurons and glial cells, which can be targeted for silencing by microRNA-764 (miR-764) (Jing et al., 2018). Although NINJ has a central role in both the nervous system and pyroptosis, the mechanism of NINJ in SCI deserves further investigation.
IL-1β/18 are fully studied substrates of proinflammatory caspases. Release of IL-1β/18 from cells is a widely used assay for inflammasome induction. Despite closely related to pyroptosis, cleavage and release of IL-1β/18 is neither necessary nor sufficient for the occurrence of pyroptosis (McKenzie et al., 2018). In non-canonical pyroptotic pathway, mouse caspase-11 and human caspase-4/5 are sensitized by intracellular lipopolysaccharide (LPS) generated by bacteria, resulting in pyroptosis through the cleavage of GSDMD. In the process of pyroptosis, IL-1β and IL-18 are released into the extracellular environment, which elicit intensive inflammatory reactions via activating the IL-1R/IL-18R-MyD88-NFκB pathway. The other inflammatory stimulators, such as IL-1α and HMGB1, released during pyroptosis also act to magnify the inflammatory reactions. During SCI, inflammasome activation is initiated by acute perturbations in homeostasis (e.g., ion flux, mitochondrial dysfunction) together with danger signals in the microenvironment (e.g., extracellular ATP, double-stranded DNA, microbial molecules) (de Rivero Vaccari et al., 2016). Microglia, neuron and oligodendrocytes, etc. may undergo pyroptosis in response to stimuli. Here we specifically focus upon evidence for pyroptosis in each cell populations of spinal cord (Figure 2 and Table 1).
Activated microglia are major innate immune cells in the spinal cord and play an important role in neuroinflammation (Walsh et al., 2014; David et al., 2015). Microglia are quickly activated after SCI, resulting in increased expression of pro-inflammatory cytokines mediated by multiple signaling cascades, while hyperactivation of microglia causes overproduction of pro-inflammatory cytokines and ROS, which in turn aggravates the inflammatory response and secondary injury (Gaudet and Fonken, 2018).
Activation of the inflammasome is a key step in neuroinflammation induced by secondary injury in SCI. CD73 is an immunosuppressive molecule that regulates the conversion of extracellular ATP to adenosine, maintaining immune homeostasis by regulating the balance between pro-inflammatory ATP and immunosuppressive adenosine to prevent excessive immune responses (Antonioli et al., 2013; Yu et al., 2018). Xu et al. (2021) observed that NLRP3 and GSDMD expression were significantly up-regulated in blood samples isolated from SCI patients. In a mouse spinal cord squeezed injury model, it was found that CD73 inhibits microglial pyroptosis by suppressing the expression of GSDMD at the transcriptional level in Foxo1 via the PI3K/AKT signaling axis. CD73 knockout exacerbated SCI, while CD73 overexpression can alleviate microglial pyroptosis in vivo and in vitro dependent on activation of PI3K/AKT and NLRP3 inflammasome. Furthermore, inhibiting the activity of hypoxia-inducible factor (HIF)-1α can reduce the expression of CD73 after SCI. Interestingly, the expression of HIF-1α in microglia was CD73-dependent, meaning that a positive feedback regulatory loop may be formed between CD73 and HIF-1α, which involved in regulating microglial pyroptosis and inhibiting neuroinflammatory response after SCI (Xu et al., 2021). Another study by the same researching group unmasked that toll-like receptor (TLR4) was activated after SCI, promoting the expression of long non-coding RNA (lncRNA)-F630028O10Rik, and also enhanced microglial pyroptosis by activating the PI3K/AKT axis and NLRP3 inflammasome (Xu et al., 2020). Subsequently, they found in TLR 4 knockout mice that TLR4 upregulated the expression of dead-box helicase 3 X-linked (DDX3X) by activating the JAK2/STAT1 pathway, which further aggravated NLPR3-induced pyroptosis in microglia (Wang et al., 2022). Intriguingly, this academic team believes that either the promotion or inhibition of microglia pyroptosis is through the activation of the PI3K/AKT axis in a similar way. Whether it was due to regulation of different PI3K isoforms remains unknown.
Oxidative stress, as an important pathogenic inducement of secondary SCI, refers to the imbalance between the oxidative damage derived from the ROS and the antioxidant defense system. As novel biomarkers of oxidative stress, advanced oxidation protein products (AOPPs) are formed by the biological reaction of chlorinated oxidants (HOCl/OCl–) and proteins, including dityrosine- and cross-linking protein products (Skvarilová et al., 2005; Cristani et al., 2016), and involved in different neuroinflammatory diseases, such as Parkinson’s disease, and amyotrophic disease (Pasquali et al., 2015). AOPPs can enhance ROS generation by activating nicotinamide adenine dinucleotide phosphate (NADPH) oxidase. Liu et al. (2020) discovered that the expression levels of AOPPs in plasma, cerebrospinal fluid and spinal cord were significantly elevated. APPOS induced microglia activation in vivo after SCI and regulated BV2 cells to generate intracellular ROS by activating NADPH in vitro. Subsequent experiments suggested that AOPPs induced activation of the NLRP3 inflammasome, caspase-1, IL-18, and IL-1β and cleavage of GSDMD in BV2 cells through Nox4/ROS/Mitogen-activated protein kinase (MAPK)/NF-κB signaling pathway, ultimately leading to pyroptosis. Afterward, the investigators applied apocynin, the NADPH oxidase inhibitor, in rat hemi-contusion injury model. Results showed that apocynin significantly reduced the level of AOPPs after SCI, attenuated microglia pyroptosis by inhibiting MAPK/NF-κB axis and meanwhile ameliorated forelimb motor function. Another research conducted by this academic group demonstrated that similar to apocynin, kaempferol pretreatment reduced the ROS production by impeding NADPH oxidase 4 and inhibiting the phosphorylation of p38 MAPK and c-Jun N-terminal kinase (JNK), thereby suppressed the nuclear translocation of NF-κB p65 to induce pro-inflammatory cytokines, which subsequently alleviated NLRP3-mediated microglial pyroptosis (Liu et al., 2021). Upregulation of Nrf2 expression facilitates functional recovery after SCI (Xia et al., 2018). Zhang D. et al. (2022) discovered that Nrf2 overexpression reduced pyroptosis after SCI by facilitating miR–146a transcription. Interestingly, GSDMD was a downstream target of miR–146a. MiR–146a knockdown or GSDMD overexpression counteracted the inhibitory effect of Nrf2 on microglial pyroptosis (Zhang D. et al., 2022).
Celastrol and Polygotin are isolated from plants and possesses various biological properties, including anti-inflammatory and neuroprotection. In SCI model, these natural products inhibited the activation of NLRP3 via NF-κB pathway in microglia and alleviated the inflammatory response by reducing the release of inflammatory factors such as tumor necrosis factor (TNF) -α and IL-1β, thus promoted the restoration of limb motor functionality in rats (Dai et al., 2019; Lv et al., 2019). Another plant extract, paeonol, prevents recovery after SCI by inhibiting the pyroptosis of microglia via TLR4/MyD88/NF-κB (p65) pathway (Zhao et al., 2022b).
The above statements collectively indicate that microglia are important mediators of innate immune responses following SCI and are critical for subsequent inflammatory responses. Although the current researches have not fully documented the mechanism of microglial pyroptosis after SCI, it is undeniable that inhibiting microglial pyroptosis has broad application prospects for the treatment of SCI.
Neuron are the basic unit of structure and function of the CNS and the main carrier of neural activity. Pyroptosis performs a key role in neuronal death after SCI, in which GSDMD overexpression can lead to massive neuronal loss (Xu et al., 2021). The most extensively studied inflammasomes in spinal neurons are NLRP3, NLRP1, and AIM2. Accumulating evidence now points to significant anti-inflammatory biological effect of carbon monoxide (CO) (Onyiah et al., 2013; Nikolic et al., 2014). CO releasing molecule-3 (CORM-3), a classical donor of CO, inhibited inositol-requiring enzyme 1(IRE1) phosphorylation in neurons after SCI, reduced the expression levels of NLRP1 and NLRP3, and restrained the activation of caspase-1 and caspase-11, thereby preventing neuronal death and promoting motor function after SCI by alleviating pyroptosis (Zheng et al., 2019). MCC950, an NLRP3-specific inflammasome inhibitor, is a recently developed selective small molecule, and been reported to inhibit the activation of NLRP3 inflammasome in spinal cord tissue and the expression levels of pro-inflammatory factors such as caspase-1, TNF-a, IL-1β, and IL-18 after SCI, as a result, improved the hindlimb motor strength of mice and histopathological score. In vitro, MCC950 can also inhibit LPS or oxygen–glucose deprivation (OGD)-induced NLRP3 inflammasome activation and alleviated neuronal damage (Perera et al., 2018; Jiao et al., 2020). Metformin is a commonly used hypoglycemic drug for the treatment of type 2 diabetes with anti-inflammatory, anti-apoptotic and antioxidant properties, therefore it is also a candidate drug for the treatment of neurological diseases. A recent study confirmed that metformin improved motor function recovery after SCI through phosphorylating AMPK and reducing the release of pro-inflammatory cytokines [IL-1β, IL-6, and tumor necrosis factor alpha (TNF-α)] and NLRP3 activation (Yuan et al., 2022).
Zinc is essential for cell physiological processes and involved in the activities of enzymes and transcription factors (Wang et al., 2014). It is known that zinc may inhibit the catalytic activity of NO synthase, thereby reducing oxidative damage and neuroinflammation (Jurowski et al., 2014). Lithium is used to treat bipolar disorder and exerts a neuroprotective role in neurological diseases. In SCI model, both zinc and lithium chloride (LiCl) treatment resulted in down-regulation of NLRP3 inflammasome, ROS and malondialdehyde in spinal cord, which protected against neuroinflammation and motor deficits (Li D. et al., 2020; Zhao et al., 2022d). Interestingly, the anti-pyroptosis effects of these two ions were both via the Nrf2/heme oxygenase-1(Nrf2/HO-1) pathway. Thus, exogenous CORM-3, inhalable CO, MCC950, Metformin, zinc, and LiCl are potential therapeutic targets for SCI.
AIM2 recognizes cytosolic or foreign dsDNA in neurons (Adamczak et al., 2014). Spinal cord ischemia-reperfusion injury (SCIRI) is a common complication after temporary thoracoabdominal aortic occlusion, resulting in motor function and sensory impairment (Miranda et al., 2018). Recent study suggested that motor dysfunction after reperfusion was closely related to elevation of dsDNA levels in serum and cerebrospinal fluid in SCIRI model (Li et al., 2019). Application of AIM2-targeting siRNA blocked the activation of AIM2, which decreased the expression levels of AIM2, ASC, caspase-1, and IL-1β and inhibited neuronal pyroptosis. However, poly(dA)-oligo(dT), nucleotides composed of a 2:1 mix of poly(dA) and 18-mer oligo(dT) (Masuda et al., 2018), can reverse the above effect and accelerate neuronal pyroptosis.
Guo et al. (2022) proposed that PC12 suffering OGD/R or SCIRI could increase the expression level of lncRNA H19 and aggravate neuronal pyroptosis. MiR-181a-5p, a negative regulator of HMGB1, was inhibited by upstream lncRNA H19. LncRNA H19 silence or knockdown alleviated SCIRI induced neuronal pyroptosis by downregulating HMGB1 (Guo et al., 2022). Exosomes (EXOs) are important messengers for intercellular communication in various biological processes. Proteins, DNA fragments, RNAs, lipids and other substances rich in exosomes play a role in intercellular communication (Xin et al., 2021). A recent study indicated that circ_003564, a non-coding circular RNA contained in bone marrow mesenchymal stem cell (BMSC) -derived EXOs, attenuated neuronal NLPR3-related pyroptosis when BMSC-EMO and neuron were co-cultured (Zhao et al., 2022c). Due to the wide variety of circRNAs, they may regulate pyroptosis through various pathways and multiple targets.
Studies carried out by Zhu et al. (2020) implied that the expression levels of caspase-1 and IL-1β in mice were significantly reduced when treated with Smad3 inhibitor, which alleviated inflammatory neuronal death after SCI. Interestingly, the researchers denied that AIM2 or NLRP1 were involved in the regulation of Samd3, but did not confirm which inflammasomes or gasdermins were crucial. Growing evidence has documented that NLRP1 inflammasome can activated by extracellular K+ ions via pannexin-1 channels (de Rivero Vaccari et al., 2009) or other intracellular or extracellular substances, such as intracellular ATP depletion, intracellular ion flux downstream of the purinergic P2 × 4/7 receptors and extracellular Ab (Liao and Mogridge, 2013; Chavarría-Smith and Vance, 2015). During the process of CNS injury, both K+ efflux and ATP release are engaged in the formation of inflammasomes. Purinergic P2 × 4 receptors are abundantly expressed in neurons. SCI causes excess ATP release and triggers these purinergic receptors, ultimately promoting the formation of the NLRP1 inflammasome (Wang et al., 2004; Zhou et al., 2016). These statements collectively demonstrate that regulation of K+ and ATP emerged as a potential way for the treatment of pyroptosis after SCI.
Under physiological conditions, microglia play the roles of immune surveillance, nourishing neurons and maintaining the homeostasis of the CNS. Nevertheless, the hyperactivation of microglia leads to the production of a large number of pro-inflammatory factors, including nitric oxide (NO), free radicals and ROS, which cause neuronal death (Block et al., 2007). That is, neuron and microglia reciprocally influence each other.
Recent studies identified that mitochondrial ROS (mtROS) and mitochondrial DNA (mtDNA) released from damaged mitochondria in microglia after SCI acted as assembly activators to promote and amplify the activation of NLRP3 (Elliott and Sutterwala, 2015). Quercetin, a natural bioflavonoid, promoted impaired mitochondrial clearance by enhancing microglial mitophagy to reduce mtROS accumulation, NF-kB activation and subsequent NLRP3 inflammasome assembly, thereby reducing neuronal degeneration caused by excessive inflammatory toxicity and exerting neuroprotective effect (Han et al., 2021). The microglial voltage-gated proton channel (Hv1) maintained the intracellular physiological pH and was involved in ROS-induced neuronal damage in ischemic stroke (Tian et al., 2016). Knockdown of Hv1 in microglia reduced ROS production following SCI, then attenuated neuronal apoptosis and NLRP3-inflammasome-mediated pyroptosis, thereby promoting axonal regeneration and improving motor function. In the PC12 cell oxygen-glucose deprivation/reoxygenation (OGD/R) model, NLRP3, ASC, and caspase-1 p20 were significantly up-regulated, contrarily, ROS scavengers could reverse neuronal NLRP3 inflammasome activation and pyroptosis (Li X. et al. 2020). LPS induces BV2 cells to produce IL-6, TNF-α, NO, and ROS and leads to the death of HT22 hippocampal neurons. Ginsenoside Re (Ginsenoside Re) protects hippocampal neurons by inhibiting the production and release of pro-inflammatory mediators in microglia. In addition, LPS induced BV2 cells to produce IL-6, TNF-α, NO, and ROS and led to the death of HT22 hippocampal neurons. Ginsenoside Re prevented hippocampal neurons death by causing reduced microglial activation and pro-inflammatory mediators release in microglia (Madhi et al., 2021). The mechanism of interaction between microglia and neurons following SCI warrants deeper examination, which can provide a more comprehensive theoretical basis for the treatment of SCI.
Oligodendrocytes (ODCs) play an important role in the process of myelination and regeneration in the spinal cord to maintain neuronal electrophysiological signal conduction. ODCs are easily affected by the local environment after SCI and undergo necrosis and apoptosis, leading to demyelination and impaired axonal function. Recent studies have revealed that ODCs can undergo caspase-1-dependent pyroptosis. In the multiple sclerosis model, ODCs pyroptosis caused inflammatory demyelinating lesions, however, the caspase-1 small molecule inhibitor VX-765 reversed ODCs pyroptosis and alleviated demyelinating lesions (McKenzie et al., 2018). ODCs and microglial pyroptosis was observed in vitro following SCI and was alleviated by estradiol (Zendedel et al., 2018). Differentiation of oligodendrocyte precursor cells (OPCs) into mature oligodendrocytes is a critical step in remyelination. Thioredoxin interacting protein (TXNIP), an endoplasmic reticulum (ER)-induced protein, is increased in SCI. TXNIP is an important linking factor between ER stress and inflammasome, which can trigger the activation of NLRP3 and caspase-1, ultimately activate pyroptosis (Oslowski et al., 2012). ER activates caspase-2 via NLRP3, resulting in mitochondrial dysfunction. Damaged mitochondria release mitochondria-derived DAMPs that activate inflammasomes, leading to caspase-1 activation, and pyroptosis (Zhou et al., 2011; Bronner et al., 2015). Yanagisawa et al. (2019) reported that the expressions of TXNIP, NLRP3, ASC, and caspase-2 were significantly increased in OPCs, while the expression levels were lower in astrocytes. OPCs are less tolerant to ER stress than other cell types. Inhibition of TXNIP and NLRP3 inflammasome provides a crucial means to alleviate the pyroptosis and demyelinating injury of OPCs. Although OPCs pyroptosis can be induced by ER, more studies are needed to confirm whether there are other pathways causing pyroptosis.
Astrocytes, the most numerous and widely distributed glial cells in the CNS (Han et al., 2020), play a more active role in maintaining the BBB/BSCB, antioxidant and neural circuit formation (Lin et al., 2020). Current evidence has noted the important role of pyroptosis in astrocytes. Unconjugated bilirubin induced brain astrocyte pyroptosis through a caspase-1-dependent pathway (Feng et al., 2018). Dynamin-related protein 1 (Drp1) mediated mitochondrial fission and triggers NLRP3 inflammasome activation in astrocytes and microglia. FK866 inhibited Drp1-mediated activation of NLRP3 and pyroptosis and protected against cerebral ischemia-reperfusion injury (Zou et al., 2020).
Ethanol induced mitochondrial ROS production and NLRP3/caspase-1-dependent pyroptosis in astrocytes, and this biological effect can be reversed by the ROS scavenger mito-TEMPO, NLRP3 blocking peptide, or the caspase-1 inhibitor Z-YVAD-FMK (Alfonso-Loeches et al., 2014). In the primary neuron-astrocyte co-culture OGD/R model, Zhang et al. (2020) demonstrated that NLRP6 was mainly expressed in astrocytes, with little expression in neurons and microglia. NLRP6 induced astrocytes pyroptosis by activating caspase-1, which aggravated neuronal damage, which NLRP6 silence reversed this effect. One group demonstrated that inflammasome levels in astrocytes were significantly elevated on day 3 after SCI (Yanagisawa et al., 2019). However, astrocytes were resistant to inflammasome-mediated pyroptosis, which may be one of the reasons for the survival of astrocytes after SCI and resulted in the formation of glial scarring (Leal-Filho, 2011).
Pericytes participate in the formation of blood vessels and maintain the function of endothelial cells. Pericytes are also a kind of pluripotent stem cells, which are engaged in the formation of the blood-brain barrier (BBB) and the blood-spinal cord barrier (BSCB) in the central system. In human brain pericytes, there is no typical inflammasome activation of NLRP1, NLRP2, NLRP3, or NLRC4, but only atypical activation of the nucleotide-binding oligomerization domain containing (Nod) 1, Nod2, and TLR2 (Nyúl-Tóth et al., 2017). SCI inevitably leads to pericyte damage, disruption of microvascular stability, and increased BSCB leakage (Figley et al., 2014). The therapeutic potential of stem cell-derived exosomes has attracted increasing attention as they cannot only exert stem cell-like paracrine functions but also overcome the limitations of stem cell transplantation (Wu D. et al., 2021). BMSC-derived EXOs significantly reduced the expression of caspase-1 and IL-1β in pericytes after SCI, and increased the pericyte/endothelial cell coverage of the vessel wall and BSCB integrity, ultimately, prevented myelin loss and spinal neuron death. BMSC-EXOs were confirmed to improve BSCB integrity by inhibiting the activation of Nod1 inflammasome and pericyte pyroptosis (Zhou Y. et al., 2022). Inhibition of pericyte pyroptosis to alleviate BSCB leakage after SCI warrants deeper examination.
RCD is an important way of CNS development, maintaining homeostasis and removing damaged cells. Dozens of types of RCD exist, ranging from non-inflammatory (e.g., autophagy, apoptosis) to proinflammatory (e.g., pyroptosis, ferroptosis, necroptosis), which is fundamentally different from necrosis (Niu et al., 2022). It bears consideration that each RCD is defined by a distinct molecular signature and may interact with each other. Single stimulus factor may trigger multiple forms of cell death programs in a given cell population.
Autophagy is an evolutionarily conserved catabolic process that degrades damaged proteins and organelles through lysosomes. The role of autophagy in CNS injury is considered a double-edged sword. On the one hand, Autophagy maintains cellular homeostasis by recycling energy and substances, on the other hand, excessive or inappropriate autophagy may induce autophagic cell death (Chen et al., 2012). There are various crosstalks between pyroptosis and autophagy, especially with the mitochondria as the core organelle. Several groups have visualized that autophagy can negatively regulate NLRP3 inflammasome activation by removing endogenous activators of the inflammasome, including ROS from damaged mitochondria, inflammatory factors or cytokines. Meanwhile, the NLRP3 inflammasome can restrain autophagy induced by TLR4-Toll/IL-1R domain-containing adaptor-inducing IFN-β (TRIF) signaling pathway by activating caspase-1 to cleave the TIR domain adaptor protein (Jabir et al., 2014; Lai et al., 2018). Studies have shown that cathepsin B (CTSB), a cathepsin released from lysosomal rupture, not only directly activates NLRP3 (Chevriaux et al., 2020), but also inhibits autophagy by regulating the activity of the kinase ULK1, which negatively regulates the activation of the NLRP3 (Qi et al., 2016; Zhao et al., 2021). Recent studies have implicated that inhibition of the NLRP3 inflammasome by enhancing autophagy prevented neuronal deficits during cerebral ischemia-reperfusion (He et al., 2017; Wang et al., 2019). In addition, inflammasomes can also inhibit mitophagy in macrophages in a caspase-1-dependent manner, leading to mitochondrial dysfunction (Zhao et al., 2021). While most investigators believed that autophagy reduced inflammatory injury by inhibiting the inflammasome, some scholars have found that autophagy can promote the activation of the NLRP3 in yeast cells (Dupont et al., 2011). Accordingly, a complex interaction mechanism may exist between pyroptosis and autophagy.
Accumulating evidence suggests that secondary injury after SCI is closely related to mitochondrial damage and excessive ROS production (Chen X. et al., 2018). In a SCI model of mice, treatment with piperine, a natural compound present in spices, has been found to prevent neurons from SCI by inhibiting oxidative stress, and pyroptosis, mediated by the promotion of autophagy (Zhang H. et al., 2022). Damaged mitochondria lead to massive accumulation of ROS, which induces NLRP3 inflammasome formation and subsequent activation of caspase-1-dependent pyroptosis (Lin et al., 2019). In addition, mtDNA released into the cytoplasm is also an important activator of the inflammasomes. ROS oxidation leads to the opening of the mitochondrial permeability transition (MPT) pore, through which mtDNA is released into the cytoplasm (Nakahira et al., 2011; Yu et al., 2019a). Although one research has demonstrated that the oxidized form of mtDNA mainly activates NLRP3, and the non-oxidized form of mtDNA mainly tends to activate the AIM2 inflammasome, these have not been further confirmed in subsequent studies (Shimada et al., 2012). Mitophagy, a selective autophagic degradation of damaged mitochondria, can reduce the accumulation of ROS and the release of mitochondria-related damage factors (Li et al., 2018; Chu, 2019), just like the protective mechanism of quercetin mentioned above. In support of this hypothesis, it was recently elucidated that betulinic acid restored autophagy flux in neuron following SCI, which accelerated mitophagy to eliminate ROS accumulation and inhibit pyroptosis. As an important member of the MiT family, the transcription factor EB (TFEB) is involved in the regulation of the lysosome-autophagosome pathway. Betulinic acid induced phosphorylation of 5′ adenosine-monophosphate-activated protein kinase (AMPK) and inhibited mammalian target of rapamycin (mTOR) phosphorylation. Briefly, betulinic acid enhanced neuronal mitophagy and inhibited pyroptosis through the AMPK-mTOR-TFEB signaling pathway to promote functional recovery following SCI (Wu C. et al., 2021). Another research by the same study group confirmed that baicalein, namely 5,6,7-trihydroxyflavone, a component derived from the root of the herb scutellaria baicalensis, inhibited NLRP3 inflammasome activation and ER stress-mediated apoptosis via promoting neuronal autophagy to attenuate spinal cord defect-reperfusion injury. This neuroprotective effect could be counteracted by the autophagy-specific inhibitor 3MA (Wu et al., 2020). Although autophagy plays a vital role in neuroinflammation by regulating the inflammasome, some questions remain to be elucidated, such as whether autophagy can affect pyroptosis through other pathways or inhibiting neuroinflammation by promoting autophagy brings additional damage? With the research continued, it may provide new methods for the treatment of SCI by regulating pyroptosis and autophagy.
Apoptosis is one of the most common forms of RCD in SCI, in which cell membrane integrity is maintained, whereas pyroptosis involves rapid rupture of membrane integrity. Molecular interactions between pyroptotic and apoptosis signaling pathways are becoming increasingly apparent. Numerous markers of apoptosis are shared with pyroptosis owing to the overlaps, including TUNEL (Jorgensen and Miao, 2015), Annexin-V positivity (de Vasconcelos et al., 2019), and PARP cleavage (Taabazuing et al., 2017). Annexin-V-positive and PI-negative dual-labeling paradigm are considered to characterize early apoptotic cells, but similar labels can also be identified in pyroptotic cells after either NLRP1 or NLRC4 activation (de Vasconcelos et al., 2019).
Early studies have shown that activation of executioner caspases, principally caspase-3, have long been considered important markers of apoptosis (Mattson, 2000). Apoptotic stimuli can trigger caspase-3-mediated inactivation of GSDMD by generating a non-pore-forming truncated N-terminus, thereby preventing pyroptosis. Intriguingly, the cleavage of GSDMD by caspase-3 is an inactivating event (Taabazuing et al., 2017). However, It has also been shown that activated caspase-3 can trigger the cleavage of GSDME and induce pyroptosis (Rogers et al., 2017). Caspase-8 is also an important participator in the cell death cascade. Caspase-8 can cleave and activate both GSDMD and GSDME to cause pyroptosis (Sarhan et al., 2018; Chen et al., 2019). Activated caspase-8 can drive classical caspase-dependent apoptosis and inhibit recombinant receptor-interacting serine-threonine kinase 3 (RIPK3)-mediated necroptosis. In addition, caspase-8 can promote NLRP3 activation by cleaving GSDMD to induce pyroptosis, a process that does not require RIPK3 kinase activity (Bertheloot et al., 2021). Furthermore, caspase-8 also plays a key role in pro-IL-1β synthesis and maturation (Moriwaki et al., 2016).
By contrast, caspase-1 is the important checkpoint in the regulation of apoptosis by pyroptosis. One academic team has elucidated caspase-1-dependent mitochondrial depolarization and cytochrome C release downstream of NLRP3 and AIM2 inflammasomes (Yu et al., 2014). There is some evidence that apoptotic executioner caspase-3 and caspase-7, the caspase-1 substrates, are proteolytically active during pyroptosis (Taabazuing et al., 2017). Caspase-1 can sensitize caspases-3/7, either directly through proteolysis or indirectly through activation of the upstream activator caspases-8/9 (McKenzie et al., 2020). Nevertheless, in the absence of the proteins required for pyroptosis can prompt cells toward apoptosis rather than pyroptosis. Pyroptotic stimuli can induct caspase-1-dependent apoptosis in the absence of GSDMD (DiPeso et al., 2017; Taabazuing et al., 2017; Tsuchiya et al., 2019) or caspase-1-independent apoptosis in the absence of caspase-1 (Sagulenko et al., 2013). Nonetheless, evidence for activation of caspase-3/7 downstream of caspase-1 in GSDMD-mediated pyroptosis is abundant. The unifying mechanism underlying the interactions between pyroptotic and apoptotic signaling pathways warrant further investigation in the context of SCI.
SCI is one of the most serious traumas that not only reduces the quality of life and the activity level of patients, but also imposes a heavy financial burden on families and society. Although years of research have yielded some fruitful approaches, great challenges remain in the treatment of this complex and multifaceted disease. Neuroinflammation is the central process of secondary phase of SCI after initial cell death. Pyroptosis induced by intense inflammatory response accelerates the process of cell death and inhibits the recovery of nerve function after injury (Okada, 2016; Chen L. et al., 2018). Researchers have explored a variety of methods and drugs to inhibit pyroptosis after SCI to alleviate neurological dysfunction, opening a new channel for clinical treatment of SCI, but the molecular mechanism of pyroptosis remains to be further investigated.
Nevertheless, less research has been done on other inflammasome receptors during SCI, such as Pyrin (detects changes in cellular homeostasis mediated by bacterial toxins) (de Torre-Minguela et al., 2017), NLRP2 (responds to extracellular ATP) (Minkiewicz et al., 2013), NLRP6 (Has a negative regulatory effect on CNS) (Anand et al., 2012), NLRC5 (responds to bacterial ligands and DAMPs) (Davis et al., 2011). In addition, NLRX1, a member of NLR-family proteins, have immunomodulatory effects independent of inflammasome formation (Guo et al., 2016; Gharagozloo et al., 2019). Pyroptosis typically involves the cleavage and activation of GSDMD by caspase-1, with notable exceptions including caspase-8/3 (Wang et al., 2017; Orning et al., 2018; Sarhan et al., 2018). Although there is evidence that caspase-8 activation can induce NLRP3 inflammasome-triggered pyroptosis, and the caspase-8/GSDMD signaling pathway may be another initiating factor of pyroptosis (Vince and Silke, 2016), the mechanism of action of casapase-8/3-dependent pyroptosis in SCI remains unclear. At present, the research on pyroptosis after SCI mainly focuses on the caspase-1-dependent pathway, and the caspase-4/5/11-dependent pathway needs additional research. Similar to the regulation of pyroptosis by GSDMD, secondary necrosis mediated by GSDME (Aizawa et al., 2020) or GSDMA (Zhao et al., 2022a), as well as GSDMD mediated by GzmA can also induce pyroptosis, but there are few related experimental studies concerning SCI. Whether these pyroptosis signaling pathway are active in SCI remains to be determined.
Multiple cell death mechanisms can affect concurrently on the progression of SCI. A systematic comparison of different cell death modalities in SCI is also something to be expanded in future work. Recent evidence has implicated that NLRP3 inflammasome activation not only contributes to pyroptosis, but also contributes to other types of cell death, such as apoptosis, necroptosis, and ferroptosis (Huang et al., 2021). Notably, not all investigations point to similar conclusions regarding inflammasome activation and pyroptosis in different cell types. Most studies focus on only one type of cell exposure to inflammation-relevant stimuli, the mechanism of the crosstalk between neuron and glial cells within SCI is still unclear. Species, strains, ages, animal model, ex vivo isolation methods as well as culture conditions likely impact the experimental results. A brief overview of strategies for the inhibition of pyroptosis, such as ROS scavenger (e.g., prussian blue nanozyme) (Ma et al., 2022), caspase-1 inhibitors (e.g., VX-765, VX-740, and Z-YVAD-FMK) (Linton, 2005; Cornelis et al., 2007; Zhang et al., 2019), NLRP3 inhibitors (e.g., MCC950, CY09, OLT1177, Bay 11-7082, Tranilast, Rubesin, and microRNAs) (Juliana et al., 2010; Coll et al., 2015; Huang et al., 2018; Marchetti et al., 2018; Yang et al., 2019; Yu et al., 2019b; Cheng et al., 2021) and GSDMD inhibitors (e.g., disulfiram, Ac-FLTD-CMK) (Yang et al., 2018; Tan C. et al., 2022) are summarized. Additionally, NIMA-related kinase 7 (NEK7), a mitotic serine/threonine kinase and an upstream regulator of NLRP3 inflammasome activation, tend to be a point of intervention (Zhou C. et al., 2022). These compounds tend to have acceptable safety profiles in vivo and targeting pyroptosis has already established beneficial outcomes in preclinical models. As touched upon earlier, as a biomarker of SCI neuropathogenesis, the role of NINJ in CNS inflammation is still controversial. Given the important role of NINJ1 in PMR and pyroptosis, reducing neuroinflammation by modulating NINJ may also become a potentially effective approach. Strikingly, knockout or silencing of GSDMs is an important method to attenuate pyroptosis, which has been widely used in the treatment of tumors and pulmonary inflammation (Song et al., 2022; Tan G. et al., 2022), nevertheless, it is rarely used in SCI research and deserves further exploration. In addition, lncRNAs and EXOs are promising regulators of inflammation and pyroptosis during SCI. This work highlights key developments in pyroptosis as a driver of neuroinflammation during SCI and its potential therapeutic approaches targeting pyroptosis. A comprehensive understanding of pyroptosis within SCI may help develop viable neuroprotective strategies.
JY and GG: writing the manuscript. WW: modification of the manuscript. XL: guidance and revision of the manuscript. All authors contributed to the article and approved the submitted version.
This study was supported by the Natural Science Foundation of Jiangsu Province (BK20191120), Hospital Project of Jinling Hospital of Nanjing University (YYQN2021097), and Nanjing Health Science and Technology Development Special Fund Project (YKK22222).
The authors declare that the research was conducted in the absence of any commercial or financial relationships that could be construed as a potential conflict of interest.
All claims expressed in this article are solely those of the authors and do not necessarily represent those of their affiliated organizations, or those of the publisher, the editors and the reviewers. Any product that may be evaluated in this article, or claim that may be made by its manufacturer, is not guaranteed or endorsed by the publisher.
Adamczak, S., Vaccari, J. P., Dale, G., Brand, F. J. III, Nonner, D., Bullock, M. R., et al. (2014). Pyroptotic neuronal cell death mediated by the AIM2 inflammasome. J. Cereb. Blood Flow Metab. 34, 621–629. doi: 10.1038/jcbfm.2013.236
Ahn, B., Le, H., Shin, M. W., Bae, S. J., Lee, E. J., Lee, S. Y., et al. (2014). Ninjurin1 enhances the basal motility and transendothelial migration of immune cells by inducing protrusive membrane dynamics. J. Biol. Chem. 289, 21926–21936.
Aizawa, E., Karasawa, T., Watanabe, S., Komada, T., Kimura, H., Kamata, R., et al. (2020). GSDME-dependent incomplete pyroptosis permits selective IL-1α release under caspase-1 inhibition. iScience 23:101070. doi: 10.1016/j.isci.2020.101070
Alfonso-Loeches, S., Ureña-Peralta, J., Morillo-Bargues, M., Oliver-De La Cruz, J., and Guerri, C. (2014). Role of mitochondria ROS generation in ethanol-induced NLRP3 inflammasome activation and cell death in astroglial cells. Front. Cell. Neurosci. 8:216. doi: 10.3389/fncel.2014.00216
Alizadeh, A., Dyck, S., and Karimi-Abdolrezaee, S. (2019). Traumatic spinal cord injury: An overview of pathophysiology, models and acute injury mechanisms. Front. Neurol. 10:282. doi: 10.3389/fneur.2019.00282
Anand, P., Malireddi, R. K., Lukens, J. R., Vogel, P., Bertin, J., Lamkanfi, M., et al. (2012). NLRP6 negatively regulates innate immunity and host defence against bacterial pathogens. Nature 488, 389–393. doi: 10.1038/nature11250
Antonioli, L., Pacher, P., Vizi, E., and Haskó, G. (2013). CD39 and CD73 in immunity and inflammation. Trends Mol. Med. 19, 355–367. doi: 10.1016/j.molmed.2013.03.005
Araki, T., and Milbrandt, J. (1996). Ninjurin, a novel adhesion molecule, is induced by nerve injury and promotes axonal growth. Neuron 17, 353–361. doi: 10.1016/s0896-6273(00)80166-x
Araki, T., Zimonjic, D., Popescu, N., and Milbrandt, J. (1997). Mechanism of homophilic binding mediated by ninjurin, a novel widely expressed adhesion molecule. J. Biol. Chem. 272, 21373–21380. doi: 10.1074/jbc.272.34.21373
Bao, Z., Fan, L., Zhao, L., Xu, X., Liu, Y., Chao, H., et al. (2019). Silencing of A20 aggravates neuronal death and inflammation after traumatic brain injury: A potential trigger of necroptosis. Front. Mol. Neurosci. 12:222. doi: 10.3389/fnmol.2019.00222
Bertheloot, D., Latz, E., and Franklin, B. (2021). Necroptosis, pyroptosis and apoptosis: An intricate game of cell death. Cell. Mol. Immunol. 18, 1106–1121. doi: 10.1038/s41423-020-00630-3
Block, M., Zecca, L., and Hong, J. (2007). Microglia-mediated neurotoxicity: Uncovering the molecular mechanisms. Nat. Rev. Neurosci. 8, 57–69. doi: 10.1038/nrn2038
Boucher, D., Monteleone, M., Coll, R. C., Chen, K. W., Ross, C. M., Teo, J. L., et al. (2018). Caspase-1 self-cleavage is an intrinsic mechanism to terminate inflammasome activity. J. Exp. Med. 215, 827–840. doi: 10.1084/jem.20172222
Bronner, D., Abuaita, B. H., Chen, X., Fitzgerald, K. A., Nuñez, G., He, Y., et al. (2015). Endoplasmic reticulum stress activates the inflammasome via NLRP3- and Caspase-2-driven mitochondrial damage. Immunity 43, 451–462. doi: 10.1016/j.immuni.2015.08.008
Broz, P., and Dixit, V. (2016). Inflammasomes: Mechanism of assembly, regulation and signalling. Nat. Rev. Immunol. 16, 407–420. doi: 10.1038/nri.2016.58
Broz, P., Pelegrín, P., and Shao, F. (2020). The gasdermins, a protein family executing cell death and inflammation. Nat. Rev. Immunol. 20, 143–157. doi: 10.1038/s41577-019-0228-2
Chao, K., Kulakova, L., and Herzberg, O. (2017). Gene polymorphism linked to increased asthma and IBD risk alters gasdermin-B structure, a sulfatide and phosphoinositide binding protein. Proc. Natl. Acad. Sci. U.S.A. 114, E1128–E1137. doi: 10.1073/pnas.1616783114
Chavarría-Smith, J., and Vance, R. (2015). The NLRP1 inflammasomes. Immunol. Rev. 265, 22–34. doi: 10.1111/imr.12283
Chavarría-Smith, J., Mitchell, P., Ho, A., Daugherty, M., and Vance, R. (2016). Functional and evolutionary analyses identify proteolysis as a general mechanism for NLRP1 inflammasome activation. PLoS Pathog. 12:e1006052. doi: 10.1371/journal.ppat.1006052
Chen, K, Demarco, B, Heilig, R, Shkarina, K, Boettcher, A, Farady, CJ., et al. (2019). Extrinsic and intrinsic apoptosis activate pannexin-1 to drive NLRP3 inflammasome assembly. EMBO J. 38:e101638 doi: 10.15252/embj.2019101638
Chen, X, Cui, J, Zhai, X, Zhang, J, Gu, Z, Zhi, X, et al. (2018). Inhalation of hydrogen of different concentrations ameliorates spinal cord injury in mice by protecting spinal cord neurons from apoptosis, oxidative injury and mitochondrial structure damages. Cell. Physiol. Biochem. 47:176–190 doi: 10.1159/000489764
Chen, L, Deng, H, Cui, H, Fang, J, Zuo, Z, Deng, J, et al. (2018). Inflammatory responses and inflammation-associated diseases in organs. Oncotarget 9:7204–7218 doi: 10.18632/oncotarget.23208
Chen, G., Ke, Z., Xu, M., Liao, M., Wang, X., Qi, Y., et al. (2012). Autophagy is a protective response to ethanol neurotoxicity. Autophagy 8, 1577–1589. doi: 10.4161/auto.21376
Cheng, J., Hao, J., Jiang, X., Ji, J., Wu, T., Chen, X., et al. (2021). Ameliorative effects of miR-423-5p against polarization of microglial cells of the M1 phenotype by targeting a NLRP3 inflammasome signaling pathway. Int. Immunopharmacol. 99:108006. doi: 10.1016/j.intimp.2021.108006
Chevriaux, A., Pilot, T., Derangère, V., Simonin, H., Martine, P., Chalmin, F., et al. (2020). Cathepsin B is required for NLRP3 inflammasome activation in macrophages, through NLRP3 interaction. Front. Cell Dev. Biol. 8:167. doi: 10.3389/fcell.2020.00167
Chu, C. (2019). Mechanisms of selective autophagy and mitophagy: Implications for neurodegenerative diseases. Neurobiol. Dis. 122, 23–34. doi: 10.1016/j.nbd.2018.07.015
Coll, R., Robertson, A. A., Chae, J. J., Higgins, S. C., Muñoz-Planillo, R., Inserra, M. C., et al. (2015). A small-molecule inhibitor of the NLRP3 inflammasome for the treatment of inflammatory diseases. Nat. Med. 21, 248–255. doi: 10.1038/nm.3806
Cookson, B., and Brennan, M. (2001). Pro-inflammatory programmed cell death. Trends Microbiol. 9, 113–114. doi: 10.1016/s0966-842x(00)01936-3
Cornelis, S., Kersse, K., Festjens, N., Lamkanfi, M., and Vandenabeele, P. (2007). Inflammatory caspases: Targets for novel therapies. Curr. Pharm. Des. 13, 367–385. doi: 10.2174/138161207780163006
Cristani, M., Speciale, A., Saija, A., Gangemi, S., Minciullo, P., and Cimino, F. (2016). Circulating advanced oxidation protein products as oxidative stress biomarkers and progression mediators in pathological conditions related to inflammation and immune dysregulation. Curr. Med. Chem. 23, 3862–3882. doi: 10.2174/0929867323666160902154748
Dai, W., Wang, X., Teng, H., Li, C., Wang, B., and Wang, J. (2019). Celastrol inhibits microglial pyroptosis and attenuates inflammatory reaction in acute spinal cord injury rats. Int. Immunopharmacol. 66, 215–223. doi: 10.1016/j.intimp.2018.11.029
David, S., Greenhalgh, A., and Kroner, A. (2015). Macrophage and microglial plasticity in the injured spinal cord. Neuroscience 307, 311–318. doi: 10.1016/j.neuroscience.2015.08.064
Davis, B., Roberts, R. A., Huang, M. T., Willingham, S. B., Conti, B. J., Brickey, W. J., et al. (2011). Cutting edge: NLRC5-dependent activation of the inflammasome. J. Immunol. 186, 1333–1337. doi: 10.4049/jimmunol.1003111
de Rivero Vaccari, J., Dietrich, W., and Keane, R. (2016). Therapeutics targeting the inflammasome after central nervous system injury. Transl. Res. 167, 35–45. doi: 10.1016/j.trsl.2015.05.003
de Rivero Vaccari, J., Lotocki, G., Alonso, O., Bramlett, H., Dietrich, W., and Keane, R. (2009). Therapeutic neutralization of the NLRP1 inflammasome reduces the innate immune response and improves histopathology after traumatic brain injury. J. Cereb. Blood Flow Metab. 29, 1251–1261. doi: 10.1038/jcbfm.2009.46
de Torre-Minguela, C., Mesa Del Castillo, P., and Pelegrín, P. (2017). The NLRP3 and pyrin inflammasomes: Implications in the pathophysiology of autoinflammatory diseases. Front. Immunol. 8:43. doi: 10.3389/fimmu.2017.00043
de Vasconcelos, N., Van Opdenbosch, N., Van Gorp, H., Parthoens, E., and Lamkanfi, M. (2019). Single-cell analysis of pyroptosis dynamics reveals conserved GSDMD-mediated subcellular events that precede plasma membrane rupture. Cell Death Differ. 26, 146–161. doi: 10.1038/s41418-018-0106-7
de Zoete, M., Palm, N., Zhu, S., and Flavell, R. (2014). Inflammasomes. Cold Spring Harb. Perspect. Biol. 6:a016287. doi: 10.1101/cshperspect.a016287
DiPeso, L., Ji, D., Vance, R., and Price, J. (2017). Cell death and cell lysis are separable events during pyroptosis. Cell Death Discov. 3:17070. doi: 10.1038/cddiscovery.2017.70
Dixon, K. (2017). Pathophysiology of traumatic brain injury. Phys. Med. Rehabil. Clin. N. Am. 28, 215–225. doi: 10.1016/j.pmr.2016.12.001
Dupont, N., Jiang, S., Pilli, M., Ornatowski, W., Bhattacharya, D., and Deretic, V. (2011). Autophagy-based unconventional secretory pathway for extracellular delivery of IL-1β. EMBO J. 30, 4701–4711. doi: 10.1038/emboj.2011.398
Elliott, E., and Sutterwala, F. (2015). Initiation and perpetuation of NLRP3 inflammasome activation and assembly. Immunol. Rev. 265, 35–52. doi: 10.1111/imr.12286
Feng, J., Li, M., Wei, Q., Li, S., Song, S., and Hua, Z. (2018). Unconjugated bilirubin induces pyroptosis in cultured rat cortical astrocytes. J. Neuroinflamm. 15:23. doi: 10.1186/s12974-018-1064-1
Figley, S., Khosravi, R., Legasto, J., Tseng, Y., and Fehlings, M. (2014). Characterization of vascular disruption and blood-spinal cord barrier permeability following traumatic spinal cord injury. J. Neurotrauma 31, 541–552. doi: 10.1089/neu.2013.3034
Franklin, B., Latz, E., and Schmidt, F. (2018). The intra- and extracellular functions of ASC specks. Immunol. Rev. 281, 74–87. doi: 10.1111/imr.12611
Friedlander, A. (1986). Macrophages are sensitive to anthrax lethal toxin through an acid-dependent process. J. Biol. Chem. 261, 7123–7126.
Gaudet, A., and Fonken, L. (2018). Glial cells shape pathology and repair after spinal cord injury. Neurotherapeutics 15, 554–577. doi: 10.1007/s13311-018-0630-7
Gharagozloo, M., Mahmoud, S., Simard, C., Yamamoto, K., Bobbala, D., Ilangumaran, S., et al. (2019). NLRX1 inhibits the early stages of CNS inflammation and prevents the onset of spontaneous autoimmunity. PLoS Biol. 17:e3000451. doi: 10.1371/journal.pbio.3000451
Guo, H., önig, R., Deng, M., Riess, M., Mo, J., Zhang, L., et al. (2016). NLRX1 sequesters STING to negatively regulate the interferon response, thereby facilitating the replication of HIV-1 and DNA viruses. Cell Host Microbe 19, 515–528. doi: 10.1016/j.chom.2016.03.001
Guo, L., Wang, D., Alexander, H., Ren, X., and Ma, H. (2022). Long non-coding RNA H19 contributes to spinal cord ischemia/reperfusion injury through increasing neuronal pyroptosis by miR-181a-5p/HMGB1 axis. Aging 14, 5449–5463. doi: 10.18632/aging.204160
Habtemariam, S. (2019). Antioxidant and anti-inflammatory mechanisms of neuroprotection by ursolic acid: Addressing brain injury. Cerebral ischemia, cognition deficit, anxiety, and depression. Oxid. Med.Cell. Longev. 2019:8512048. doi: 10.1155/2019/8512048
Han, X., Sun, S., Sun, Y., Song, Q., Zhu, J., Song, N., et al. (2019). Small molecule-driven NLRP3 inflammation inhibition via interplay between ubiquitination and autophagy: Implications for Parkinson disease. Autophagy 15, 1860–1881. doi: 10.1080/15548627.2019.1596481
Han, X., Xu, T., Fang, Q., Zhang, H., Yue, L., Hu, G., et al. (2021). Quercetin hinders microglial activation to alleviate neurotoxicity via the interplay between NLRP3 inflammasome and mitophagy. Redox Biol. 44:102010. doi: 10.1016/j.redox.2021.102010
Han, X., Zhang, T., Liu, H., Mi, Y., and Gou, X. (2020). Astrocyte senescence and Alzheimer’s disease: A review. Front. Aging Neurosci. 12:148. doi: 10.3389/fnagi.2020.00148
He, Q., Li, Z., Wang, Y., Hou, Y., Li, L., and Zhao, J. (2017). Resveratrol alleviates cerebral ischemia/reperfusion injury in rats by inhibiting NLRP3 inflammasome activation through Sirt1-dependent autophagy induction. Int. Immunopharmacol. 50, 208–215. doi: 10.1016/j.intimp.2017.06.029
Huang, Y., Jiang, H., Chen, Y., Wang, X., Yang, Y., Tao, J., et al. (2018). Tranilast directly targets NLRP3 to treat inflammasome-driven diseases. EMBO Mol. Med. 10:e8689. doi: 10.15252/emmm.201708689
Huang, Y., Xu, W., and Zhou, R. (2021). NLRP3 inflammasome activation and cell death. Cell.Mol. Immunol. 18, 2114–2127. doi: 10.1038/s41423-021-00740-6
Humphries, F., Bergin, R., Jackson, R., Delagic, N., Wang, B., Yang, S., et al. (2018). The E3 ubiquitin ligase Pellino2 mediates priming of the NLRP3 inflammasome. Nat. Commun. 9:1560. doi: 10.1038/s41467-018-03669-z
Ivanov, A., Rana, N., Privitera, G., and Pizarro, T. (2022). The enigmatic roles of epithelial gasdermin B : Recent discoveries and controversies. Trends Cell Biol. **VP. doi: 10.1016/j.tcb.2022.06.006
Jabir, M., Ritchie, N. D., Li, D., Bayes, H. K., Tourlomousis, P., Puleston, D., et al. (2014). Caspase-1 cleavage of the TLR adaptor TRIF inhibits autophagy and β-interferon production during Pseudomonas aeruginosa infection. Cell Host Microbe 15, 214–227. doi: 10.1016/j.chom.2014.01.010
Jiao, J., Zhao, G., Wang, Y., Ren, P., and Wu, M. (2020). MCC950, a selective inhibitor of NLRP3 inflammasome. reduces the inflammatory response and improves neurological outcomes in mice model of spinal cord injury. Front. Mol. Biosci. 7:37. doi: 10.3389/fmolb.2020.00037
Jing, D., Yinzhu, L., Jinjing, P., Lishuang, L., and Guozhuan, Z. (2018). Targeting ninjurin 2 by miR-764 regulates hydrogen peroxide (HO)-induced neuronal cell death. Biochem.Biophys. Res. Commun. 505, 1180–1188. doi: 10.1016/j.bbrc.2018.09.184
Jorgensen, I., and Miao, E. (2015). Pyroptotic cell death defends against intracellular pathogens. Immunol. Rev. 265, 130–142. doi: 10.1111/imr.12287
Juliana, C., Fernandes-Alnemri, T., Wu, J., Datta, P., Solorzano, L., Yu, J. W., et al. (2010). Anti-inflammatory compounds parthenolide and Bay 11-7082 are direct inhibitors of the inflammasome. J. Biol. Chem. 285, 9792–9802. doi: 10.1074/jbc.M109.082305
Jurowski, K., Szewczyk, B., Nowak, G., and Piekoszewski, W. (2014). Biological consequences of zinc deficiency in the pathomechanisms of selected diseases. J. Biol. Inorg. Chem. 19, 1069–1079. doi: 10.1007/s00775-014-1139-0
Katoh, H., Yokota, K., and Fehlings, M. (2019). Regeneration of spinal cord connectivity through stem cell transplantation and biomaterial scaffolds. Front. Cell. Neurosci. 13:248. doi: 10.3389/fncel.2019.00248
Kayagaki, N., Kornfeld, O. S., Lee, B. L., Stowe, I. B., O’Rourke, K., Li, Q., et al. (2021). NINJ1 mediates plasma membrane rupture during lytic cell death. Nature 591, 131–136. doi: 10.1038/s41586-021-03218-7
Kayagaki, N., Stowe, I. B., Lee, B. L., O’Rourke, K., Anderson, K., Warming, S., et al. (2015). Caspase-11 cleaves gasdermin D for non-canonical inflammasome signalling. Nature 526, 666–671. doi: 10.1038/nature15541
Kovacs, S., and Miao, E. (2017). Gasdermins: Effectors of pyroptosis. Trends Cell Biol. 27, 673–684. doi: 10.1016/j.tcb.2017.05.005
Lai, M., Yao, H., Shah, S. Z. A., Wu, W., Wang, D., Zhao, Y., et al. (2018). The NLRP3-caspase 1 inflammasome negatively regulates autophagy via TLR4-TRIF in prion peptide-infected microglia. Front. Aging Neurosci. 10:116. doi: 10.3389/fnagi.2018.00116
Lamkanfi, M., and Dixit, V. (2014). Mechanisms and functions of inflammasomes. Cell 157, 1013–1022. doi: 10.1016/j.cell.2014.04.007
LaRock, D., Johnson, A., Wilde, S., Sands, J., Monteiro, M., and LaRock, C. (2022). Group a Streptococcus induces GSDMA-dependent pyroptosis in keratinocytes. Nature 605, 527–531. doi: 10.1038/s41586-022-04717-x
Leal-Filho, M. (2011). Spinal cord injury: From inflammation to glial scar. Surg. Neurol. Int. 2:112. doi: 10.4103/2152-7806.83732
Lee, H., Kim, I., Lee, H., Luo, L., Kim, S., and Lee, J. (2018). Neuroprotective and Anti-inflammatory effects of a dodecamer peptide harboring ninjurin 1 cell adhesion motif in the postischemic brain. Mol. Neurobiol. 55, 6094–6111. doi: 10.1007/s12035-017-0810-1
Lee, H., Lee, H., Luo, L., and Lee, J. (2016). Induction of nerve injury-induced protein 1 (Ninjurin 1) in myeloid cells in rat brain after transient focal cerebral ischemia. Exp. Neurobiol. 25, 64–74. doi: 10.5607/en.2016.25.2.64
Li, D., Tian, H., Li, X., Mao, L., Zhao, X., Lin, J., et al. (2020). Zinc promotes functional recovery after spinal cord injury by activating Nrf2/HO-1 defense pathway and inhibiting inflammation of NLRP3 in nerve cells. Life Sci. 245:117351. doi: 10.1016/j.lfs.2020.117351
Li, X., Yu, Z., Zong, W., Chen, P., Li, J., Wang, M., et al. (2020). Deficiency of the microglial Hv1 proton channel attenuates neuronal pyroptosis and inhibits inflammatory reaction after spinal cord injury. J. Neuroinflamm. 17:263. doi: 10.1186/s12974-020-01942-x
Li, Q., Gao, S., Kang, Z., Zhang, M., Zhao, X., Zhai, Y., et al. (2018). Rapamycin enhances mitophagy and attenuates apoptosis after spinal ischemia-reperfusion injury. Front. Neurosci. 12:865. doi: 10.3389/fnins.2018.00865
Li, X., Yu, Q., Fang, B., Zhang, Z., and Ma, H. (2019). Knockdown of the AIM2 molecule attenuates ischemia-reperfusion-induced spinal neuronal pyroptosis by inhibiting AIM2 inflammasome activation and subsequent release of cleaved caspase-1 and IL-1β. Neuropharmacology 160:107661. doi: 10.1016/j.neuropharm.2019.05.038
Liao, K., and Mogridge, J. (2013). Activation of the Nlrp1b inflammasome by reduction of cytosolic ATP. Infect. Immun. 81, 570–579. doi: 10.1128/iai.01003-12
Lin, A., Liu, J., Gong, P., Chen, Y., Zhang, H., Zhang, Y., et al. (2020). Serum amyloid A inhibits astrocyte migration via activating p38 MAPK. J. Neuroinflamm. 17:254. doi: 10.1186/s12974-020-01924-z
Lin, D., Huang, Y. W., Ho, C. S., Hung, P. L., Hsu, M. H., Wang, T. J., et al. (2019). Oxidative insults and mitochondrial DNA mutation promote enhanced autophagy and mitophagy compromising cell viability in pluripotent cell model of mitochondrial disease. Cells 8:65. doi: 10.3390/cells8010065
Linder, A., Bauernfried, S., Cheng, Y., Albanese, M., Jung, C., Keppler, O. T., et al. (2020). CARD8 inflammasome activation triggers pyroptosis in human T cells. EMBO J. 39:e105071. doi: 10.15252/embj.2020105071
Linton, S. (2005). Caspase inhibitors: A pharmaceutical industry perspective. Curr. Top. Med. Chem. 5, 1697–1717. doi: 10.2174/156802605775009720
Liu, X., Zhang, Z., Ruan, J., Pan, Y., Magupalli, V. G., Wu, H., et al. (2016). Inflammasome-activated gasdermin D causes pyroptosis by forming membrane pores. Nature 535, 153–158. doi: 10.1038/nature18629
Liu, Z., Wang, C., Rathkey, J. K., Yang, J., Dubyak, G. R., Abbott, D. W., et al. (2018). Structures of the gasdermin D C-terminal domains reveal mechanisms of autoinhibition. Structure 26, 778.e–784.e. doi: 10.1016/j.str.2018.03.002
Liu, Z., Yao, X., Jiang, W., Li, W., Zhu, S., Liao, C., et al. (2020). Advanced oxidation protein products induce microglia-mediated neuroinflammation via MAPKs-NF-κB signaling pathway and pyroptosis after secondary spinal cord injury. J. neuroinflamm. 17:90. doi: 10.1186/s12974-020-01751-2
Liu, Z., Yao, X., Sun, B., Jiang, W., Liao, C., Dai, X., et al. (2021). Pretreatment with kaempferol attenuates microglia-mediate neuroinflammation by inhibiting MAPKs-NF-κB signaling pathway and pyroptosis after secondary spinal cord injury. Free Radic. Biol. Med. 168, 142–154. doi: 10.1016/j.freeradbiomed.2021.03.037
Lv, R., Du, L., Liu, X., Zhou, F., Zhang, Z., and Zhang, L. (2019). Polydatin alleviates traumatic spinal cord injury by reducing microglial inflammation via regulation of iNOS and NLRP3 inflammasome pathway. Int. Immunopharmacol. 70, 28–36. doi: 10.1016/j.intimp.2019.02.006
Ma, X., Hao, J., Wu, J., Li, Y., Cai, X., and Zheng, Y. (2022). Prussian blue nanozyme as a pyroptosis inhibitor alleviates neurodegeneration. Adv. Mater. 34:e2106723. doi: 10.1002/adma.202106723
Madhi, I., Kim, J., Shin, J., and Kim, Y. (2021). Ginsenoside Re exhibits neuroprotective effects by inhibiting neuroinflammation via CAMK/MAPK/NF-κB signaling in microglia. Mol. Med. Rep. 24:698. doi: 10.3892/mmr.2021.12337
Marchetti, C., Swartzwelter, B., Gamboni, F., Neff, C. P., Richter, K., Azam, T., et al. (2018). OLT1177, a β-sulfonyl nitrile compound, safe in humans, inhibits the NLRP3 inflammasome and reverses the metabolic cost of inflammation. Proc.Natl. Acad. Sci.U. S.A. 115, E1530–E1539. doi: 10.1073/pnas.1716095115
Masuda, Y., Mitsuyuki, S., Kanao, R., Hishiki, A., Hashimoto, H., and Masutani, C. (2018). Regulation of HLTF-mediated PCNA polyubiquitination by RFC and PCNA monoubiquitination levels determines choice of damage tolerance pathway. Nucleic Acids Res. 46, 11340–11356. doi: 10.1093/nar/gky943
Mattson, M. (2000). Apoptosis in neurodegenerative disorders. Nat. Rev. Mol. Cell Biol. 1, 120–129. doi: 10.1038/35040009
McKenzie, B., Dixit, V., and Power, C. (2020). Fiery cell death: Pyroptosis in the central nervous system. Trends Neurosci. 43, 55–73. doi: 10.1016/j.tins.2019.11.005
McKenzie, B., Mamik, M. K., Saito, L. B., Boghozian, R., Monaco, M. C., Major, E. O., et al. (2018). Caspase-1 inhibition prevents glial inflammasome activation and pyroptosis in models of multiple sclerosis. Proc. Natl. Acad. Sci. U.S.A. 115, E6065–E6074. doi: 10.1073/pnas.1722041115
Minkiewicz, J., de Rivero Vaccari, J., and Keane, R. (2013). Human astrocytes express a novel NLRP2 inflammasome. Glia 61, 1113–1121. doi: 10.1002/glia.22499
Miranda, V., Sousa, J., and Mansilha, A. (2018). Spinal cord injury in endovascular thoracoabdominal aortic aneurysm repair: Prevalence, risk factors and preventive strategies. Int. Angiol. 37, 112–126. doi: 10.23736/s0392-9590.18.03960-3
Miron, V., and Franklin, R. (2014). Macrophages and CNS remyelination. J. Neurochem. 130, 165–171. doi: 10.1111/jnc.12705
Moriwaki, K., Balaji, S., and Chan, F. (2016). Border security: The role of RIPK3 in epithelium homeostasis. Front. Cell Dev. Biol. 4:70. doi: 10.3389/fcell.2016.00070
Mortezaee, K., Khanlarkhani, N., Beyer, C., and Zendedel, A. (2018). Inflammasome: Its role in traumatic brain and spinal cord injury. J. Cell. Physiol. 233, 5160–5169. doi: 10.1002/jcp.26287
Muñoz-Planillo, R., Kuffa, P., Martínez-Colón, G., Smith, B., Rajendiran, T., and Núñez, G. (2013). K+ efflux is the common trigger of NLRP3 inflammasome activation by bacterial toxins and particulate matter. Immunity 38, 1142–1153. doi: 10.1016/j.immuni.2013.05.016
Nakahira, K., Haspel, J. A., Rathinam, V. A., Lee, S. J., Dolinay, T., Lam, H. C., et al. (2011). Autophagy proteins regulate innate immune responses by inhibiting the release of mitochondrial DNA mediated by the NALP3 inflammasome. Nat. Immunol 12, 222–230. doi: 10.1038/ni.1980
Nikolic, I., Saksida, T., Mangano, K., Vujicic, M., Stojanovic, I., Nicoletti, F., et al. (2014). Pharmacological application of carbon monoxide ameliorates islet-directed autoimmunity in mice via anti-inflammatory and anti-apoptotic effects. Diabetologia 57, 980–990. doi: 10.1007/s00125-014-3170-7
Niu, X., Chen, L., Li, Y., Hu, Z., and He, F. (2022). Ferroptosis, necroptosis, and pyroptosis in the tumor microenvironment: Perspectives for immunotherapy of SCLC. Semin. Cancer Biol. 86, 273–285. doi: 10.1016/j.semcancer.2022.03.009
Nyúl-Tóth, Á, Kozma, M., Nagyõszi, P., Nagy, K., Fazakas, C., Haskó, J., et al. (2017). Expression of pattern recognition receptors and activation of the non-canonical inflammasome pathway in brain pericytes. Brain Behav. Immun. 64, 220–231. doi: 10.1016/j.bbi.2017.04.010
Okada, S. (2016). The pathophysiological role of acute inflammation after spinal cord injury. Inflamm. Regen. 36:20. doi: 10.1186/s41232-016-0026-1
Onyiah, J., Sheikh, S. Z., Maharshak, N., Steinbach, E. C., Russo, S. M., Kobayashi, T., et al. (2013). Carbon monoxide and heme oxygenase-1 prevent intestinal inflammation in mice by promoting bacterial clearance. Gastroenterology 144, 789–798. doi: 10.1053/j.gastro.2012.12.025
Orning, P., Weng, D., Starheim, K., Ratner, D., Best, Z., Lee, B., et al. (2018). Pathogen blockade of TAK1 triggers caspase-8-dependent cleavage of gasdermin D and cell death. Science 362, 1064–1069. doi: 10.1126/science.aau2818
Oslowski, C., Hara, T., O’Sullivan-Murphy, B., Kanekura, K., Lu, S., Hara, M., et al. (2012). Thioredoxin-interacting protein mediates ER stress-induced β cell death through initiation of the inflammasome. Cell Metab. 16, 265–273. doi: 10.1016/j.cmet.2012.07.005
Pasquali, L., Pecori, C., Lucchesi, C., LoGerfo, A., Iudice, A., Siciliano, G., et al. (2015). Plasmatic oxidative stress biomarkers in multiple sclerosis: Relation with clinical and demographic characteristics. Clin. Biochem. 48, 19–23. doi: 10.1016/j.clinbiochem.2014.09.024
Perera, A., Fernando, R., Shinde, T., Gundamaraju, R., Southam, B., Sohal, S. S., et al. (2018). MCC950, a specific small molecule inhibitor of NLRP3 inflammasome attenuates colonic inflammation in spontaneous colitis mice. Sci. Rep. 8:8618. doi: 10.1038/s41598-018-26775-w
Qi, X., Man, S. M., Malireddi, R. K., Karki, R., Lupfer, C., Gurung, P., et al. (2016). Cathepsin B modulates lysosomal biogenesis and host defense against Francisella novicida infection. J. Exp. Med. 213, 2081–2097. doi: 10.1084/jem.20151938
Qin, Z., Zhou, F., and Zhang, L. (2022). Novel pyroptosis-independent functions of gasdermins. Signal Transduc. Target. Ther. 7:127. doi: 10.1038/s41392-022-00991-3
Rathinam, V., and Fitzgerald, K. (2016). Inflammasome complexes: Emerging mechanisms and effector functions. Cell 165, 792–800. doi: 10.1016/j.cell.2016.03.046
Rogers, C., Fernandes-Alnemri, T., Mayes, L., Alnemri, D., Cingolani, G., and Alnemri, E. (2017). Cleavage of DFNA5 by caspase-3 during apoptosis mediates progression to secondary necrotic/pyroptotic cell death. Nat. Commun. 8:14128. doi: 10.1038/ncomms14128
Rühl, S., and Broz, P. (2022). Regulation of lytic and non-lytic functions of gasdermin pores. J. Mol. Biol. 434:167246. doi: 10.1016/j.jmb.2021.167246
Sagulenko, V., Thygesen, S. J., Sester, D. P., Idris, A., Cridland, J. A., Vajjhala, P. R., et al. (2013). AIM2 and NLRP3 inflammasomes activate both apoptotic and pyroptotic death pathways via ASC. Cell Death Differ. 20, 1149–1160. doi: 10.1038/cdd.2013.37
Sarhan, J., Liu, B. C., Muendlein, H. I., Li, P., Nilson, R., Tang, A. Y., et al. (2018). Yersinia Caspase-8 induces cleavage of gasdermin D to elicit pyroptosis during infection. Proc.Natl. Acad. Sci. U.S.A. 115, E10888–E10897. doi: 10.1073/pnas.1809548115
Shi, J., Zhao, Y., Wang, K., Shi, X., Wang, Y., Huang, H., et al. (2015). Cleavage of GSDMD by inflammatory caspases determines pyroptotic cell death. Nature 526, 660–665. doi: 10.1038/nature15514
Shimada, K., Crother, T. R., Karlin, J., Dagvadorj, J., Chiba, N., Chen, S., et al. (2012). Oxidized mitochondrial DNA activates the NLRP3 inflammasome during apoptosis. Immunity 36, 401–414. doi: 10.1016/j.immuni.2012.01.009
Skvarilová, M., Bulava, A., Stejskal, D., Adamovská, S., and Bartek, J. (2005). Increased level of advanced oxidation products (AOPP) as a marker of oxidative stress in patients with acute coronary syndrome. Biomed. Pap. Med. Fac. Univ. Palacky Olomouc Czech. Repub. 149, 83–87. doi: 10.5507/bp.2005.009
Song, M., Wang, J., Sun, Y., Pang, J., Li, X., Zhou, Y., et al. (2022). Inhibition of gasdermin D-dependent pyroptosis attenuates the progression of silica-induced pulmonary inflammation and fibrosis. Acta Pharm. Sin. B 12, 1213–1224. doi: 10.1016/j.apsb.2021.10.006
Taabazuing, C., Okondo, M., and Bachovchin, D. (2017). Pyroptosis and Apoptosis Pathways Engage in Bidirectional Crosstalk in Monocytes and Macrophages. Cell Chem. Biol. 24, 507.e–514.e. 507-514.e4. doi: 10.1016/j.chembiol.2017.03.009
Tan, C., Reilly, B., Jha, A., Murao, A., Lee, Y., Brenner, M., et al. (2022). Active Release of eCIRP via gasdermin D channels to induce inflammation in Sepsis. J. Immunol. 208, 2184–2195. doi: 10.4049/jimmunol.2101004
Tan, G., Lin, C., Huang, C., Chen, B., Chen, J., Shi, Y., et al. (2022). Radiosensitivity of colorectal cancer and radiation-induced gut damages are regulated by gasdermin E. Cancer Lett. 529, 1–10. doi: 10.1016/j.canlet.2021.12.034
Tang, P., Hou, H., Zhang, L., Lan, X., Mao, Z., Liu, D., et al. (2014). Autophagy reduces neuronal damage and promotes locomotor recovery via inhibition of apoptosis after spinal cord injury in rats. Mol. Neurobiol. 49, 276–287. doi: 10.1007/s12035-013-8518-3
Tian, D., Li, C., Qin, C., Murugan, M., Wu, L., and Liu, J. (2016). Deficiency in the voltage-gated proton channel Hv1 increases M2 polarization of microglia and attenuates brain damage from photothrombotic ischemic stroke. J. Neurochem. 139, 96–105. doi: 10.1111/jnc.13751
Tsuchiya, K., Nakajima, S., Hosojima, S., Thi, Nguyen D, Hattori, T., Manh, Le, et al. (2019). Caspase-1 initiates apoptosis in the absence of gasdermin D. Nat. Commun. 10:2091. doi: 10.1038/s41467-019-09753-2
Vince, J., and Silke, J. (2016). The intersection of cell death and inflammasome activation. Cell. Mol. Life Sci. 73, 2349–2367. doi: 10.1007/s00018-016-2205-2
Wallisch, J., Simon, D., Bayır, H., Bell, M., Kochanek, P., and Clark, R. (2017). cerebrospinal fluid NLRP3 is increased after severe traumatic brain injury in infants and children. Neurocrit. Care 27, 44–50. doi: 10.1007/s12028-017-0378-7
Walsh, J., Muruve, D., and Power, C. (2014). Inflammasomes in the CNS. Nat. Rev. Neurosci. 15, 84–97. doi: 10.1038/nrn3638
Wang, J., Zhang, F., Xu, H., Yang, H., Shao, M., Xu, S., et al. (2022). TLR4 aggravates microglial pyroptosis by promoting DDX3X-mediated NLRP3 inflammasome activation via JAK2/STAT1 pathway after spinal cord injury. Clin. Transl. Med. 12:e894. doi: 10.1002/ctm2.894
Wang, X., Arcuino, G., Takano, T., Lin, J., Peng, W. G., Wan, P., et al. (2004). P2X7 receptor inhibition improves recovery after spinal cord injury. Nat. Med. 10, 821–827. doi: 10.1038/nm1082
Wang, Y., Gao, W., Shi, X., Ding, J., Liu, W., He, H., et al. (2017). Chemotherapy drugs induce pyroptosis through caspase-3 cleavage of a gasdermin. Nature 547, 99–103. doi: 10.1038/nature22393
Wang, Y., Meng, C., Zhang, J., Wu, J., and Zhao, J. (2019). Inhibition of GSK-3β alleviates cerebral ischemia/reperfusion injury in rats by suppressing NLRP3 inflammasome activation through autophagy. Int. Immunopharmacol. 68, 234–241. doi: 10.1016/j.intimp.2018.12.042
Wang, Y., Su, R., Lv, G., Cao, Y., Fan, Z., Wang, Y., et al. (2014). Supplement zinc as an effective treatment for spinal cord ischemia/reperfusion injury in rats. Brain Res. 1545, 45–53. doi: 10.1016/j.brainres.2013.12.015
Wu, D., Chang, X., Tian, J., Kang, L., Wu, Y., Liu, J., et al. (2021). Bone mesenchymal stem cells stimulation by magnetic nanoparticles and a static magnetic field: Release of exosomal miR-1260a improves osteogenesis and angiogenesis. J. Nanobiotechnol. 19:209. doi: 10.1186/s12951-021-00958-6
Wu, C., Chen, H., Zhuang, R., Zhang, H., Wang, Y., Hu, X., et al. (2021). Betulinic acid inhibits pyroptosis in spinal cord injury by augmenting autophagy via the AMPK-mTOR-TFEB signaling pathway. Int. J. Biol. Sci. 17, 1138–1152. doi: 10.7150/ijbs.57825
Wu, C., Xu, H., Li, J., Hu, X., Wang, X., Huang, Y., et al. (2020). viaBaicalein attenuates pyroptosis and endoplasmic reticulum stress following spinal cord ischemia-reperfusion injury autophagy enhancement. Front. Pharmacol. 11:1076. doi: 10.3389/fphar.2020.01076
Xia, P., Gao, X., Duan, L., Zhang, W., and Sun, Y. (2018). Mulberrin (Mul) reduces spinal cord injury (SCI)-induced apoptosis, inflammation and oxidative stress in rats via miroRNA-337 by targeting Nrf-2. Biomed. Pharmacother. 107, 1480–1487. doi: 10.1016/j.biopha.2018.07.082
Xin, W., Qiang, S., Jianing, D., Jiaming, L., Fangqi, L., Bin, C., et al. (2021). Human bone marrow mesenchymal stem cell-derived exosomes attenuate blood-spinal cord barrier disruption via the TIMP2/MMP pathway after acute spinal cord injury. Mol. Neurobiol. 58, 6490–6504. doi: 10.1007/s12035-021-02565-w
Xu, S., Wang, J., Jiang, J., Song, J., Zhu, W., Zhang, F., et al. (2020). TLR4 promotes microglial pyroptosis via lncRNA-F630028O10Rik by activating PI3K/AKT pathway after spinal cord injury. Cell Death Dis. 11:693. doi: 10.1038/s41419-020-02824-z
Xu, S., Wang, J., Zhong, J., Shao, M., Jiang, J., Song, J., et al. (2021). CD73 alleviates GSDMD-mediated microglia pyroptosis in spinal cord injury through PI3K/AKT/Foxo1 signaling. Clin. Transl. Med. 11:e269. doi: 10.1002/ctm2.269
Yanagisawa, S., Katoh, H., Imai, T., Nomura, S., and Watanabe, M. (2019). The relationship between inflammasomes and the endoplasmic reticulum stress response in the injured spinal cord. Neurosci. Lett. 705, 54–59. doi: 10.1016/j.neulet.2019.04.033
Yang, B., Zhong, W., Gu, Y., and Li, Y. (2022). Emerging mechanisms and targeted therapy of pyroptosis in central nervous system trauma. Front. Cell Dev. Biol. 10:832114. doi: 10.3389/fcell.2022.832114
Yang, J., Liu, Z., Wang, C., Yang, R., Rathkey, J. K., Pinkard, O. W., et al. (2018). Mechanism of gasdermin D recognition by inflammatory caspases and their inhibition by a gasdermin D-derived peptide inhibitor. Proc. Natl. Acad. Sci. U.S.A. 115, 6792–6797. doi: 10.1073/pnas.1800562115
Yang, Y., Wang, H., Kouadir, M., Song, H., and Shi, F. (2019). Recent advances in the mechanisms of NLRP3 inflammasome activation and its inhibitors. Cell Death Dis. 10:128. doi: 10.1038/s41419-019-1413-8
Yu, J., Nagasu, H., Murakami, T., Hoang, H., Broderick, L., Hoffman, H. M., et al. (2014). Inflammasome activation leads to Caspase-1-dependent mitochondrial damage and block of mitophagy. Proc. Natl. Acad. Sci. U.S.A. 111, 15514–15519. doi: 10.1073/pnas.1414859111
Yu, J., Wang, X., Lu, Q., Wang, J., Li, L., Liao, X., et al. (2018). Extracellular 5’-nucleotidase (CD73) promotes human breast cancer cells growth through AKT/GSK-3β/β-catenin/cyclinD1 signaling pathway. Int. J. Cancer 142, 959–967. doi: 10.1002/ijc.31112
Yu, T., Dohl, J., Elenberg, F., Chen, Y., and Deuster, P. (2019a). Curcumin induces concentration-dependent alterations in mitochondrial function through ROS in C2C12 mouse myoblasts. J. Cell. Physiol. 234, 6371–6381. doi: 10.1002/jcp.27370
Yu, T., Xie, W., and Sun, Y. (2019b). Oridonin inhibits LPS-induced inflammation in human gingival fibroblasts by activating PPARγ. Int. Immunopharmacol. 72, 301–307. doi: 10.1016/j.intimp.2019.04.006
Yuan, Y., Fan, X., Guo, Z., Zhou, Z., and Gao, W. (2022). Metformin protects against spinal cord injury and cell pyroptosis via AMPK/NLRP3 inflammasome pathway. Anal. Cell. Pathol. (Amst) 2022:3634908. doi: 10.1155/2022/3634908
Zendedel, A., Qian, J., Zhang, P., Li, H., Shen, H., Li, X., et al. (2018). Estrogen attenuates local inflammasome expression and activation after spinal cord injury. Mol. Neurobiol. 55, 1364–1375. doi: 10.1007/s12035-017-0400-2
Zhang, D., Mao, F., Wang, S., Wu, H., Wang, S., and Liao, Y. (2022). Role of transcription factor Nrf2 in pyroptosis in spinal cord injury by regulating GSDMD. Neurochem. Res. doi: 10.1007/s11064-022-03719-5
Zhang, H., Wu, C., Yu, D., Su, H., Chen, Y., and Ni, W. (2022). Piperine attenuates the inflammation, oxidative stress, and pyroptosis to facilitate recovery from spinal cord injury via autophagy enhancement. Phytother. Res. 1–14. doi: 10.1002/ptr.7625
Zhang, D., Qian, J., Zhang, P., Li, H., Shen, H., Li, X., et al. (2019). Gasdermin D serves as a key executioner of pyroptosis in experimental cerebral ischemia and reperfusion model both in vivo and in vitro. J. Neurosci. Res. 97, 645–660. doi: 10.1002/jnr.24385
Zhang, J., Jiang, N., Zhang, L., Meng, C., Zhao, J., and Wu, J. (2020). NLRP6 expressed in astrocytes aggravates neurons injury after OGD/R through activating the inflammasome and inducing pyroptosis. Int. Immunopharmacol. 80:106183. doi: 10.1016/j.intimp.2019.106183
Zhao, H., Wang, X., Liu, S., and Zhang, Q. (2022b). Paeonol regulates NLRP3 inflammasomes and pyroptosis to alleviate spinal cord injury of rat. BMC Neurosci. 23:16. doi: 10.1186/s12868-022-00698-9
Zhao, Y., Qiao, H., Liu, D. F., Li, J., Li, J. X., Chang, S. E., et al. (2022d). Lithium promotes recovery after spinal cord injury. Neural Regen. Res. 17, 1324–1333. doi: 10.4103/1673-5374.327348
Zhao, Y., Chen, Y., Wang, Z., Xu, C., Qiao, S., Liu, T., et al. (2022c). Bone marrow mesenchymal stem cell exosome attenuates inflammasome-related pyroptosis via delivering circ_003564 to improve the recovery of spinal cord injury. Mol. Neurobiol. 59, 6771–6789. doi: 10.1007/s12035-022-03006-y
Zhao, A., Kirkby, M., and Man, S. (2022a). Streptococcus makes the cut: Gasdermin A-induced pyroptosis. Cell Host Microbe 30, 410–412. doi: 10.1016/j.chom.2022.03.003
Zhao, S., Li, X., Wang, J., and Wang, H. (2021). The role of the effects of autophagy on NLRP3 inflammasome in inflammatory nervous system diseases. Front. Cell Dev. Biol. 9:657478. doi: 10.3389/fcell.2021.657478
Zheng, G., Zhan, Y., Wang, H., Luo, Z., Zheng, F., Zhou, Y., et al. (2019). Carbon monoxide releasing molecule-3 alleviates neuron death after spinal cord injury via inflammasome regulation. EBioMedicine 40, 643–654. doi: 10.1016/j.ebiom.2018.12.059
Zhou, C., Zheng, J., Fan, Y., and Wu, J. (2022). TI: NLRP3 Inflammasome-dependent pyroptosis in CNS Trauma: A potential therapeutic target. Front. Cell Dev. Biol. 10:821225. doi: 10.3389/fcell.2022.821225
Zhou, Y., Wen, L. L., Li, Y. F., Wu, K. M., Duan, R. R., Yao, Y. B., et al. (2022). Exosomes derived from bone marrow mesenchymal stem cells protect the injured spinal cord by inhibiting pericyte pyroptosis. Neural Regen. Res. 17, 194–202. doi: 10.4103/1673-5374.314323
Zhou, K., Shi, L., Wang, Y., Chen, S., and Zhang, J. (2016). Recent advances of the NLRP3 inflammasome in central nervous system disorders. J. Immunol. Res. 2016:9238290. doi: 10.1155/2016/9238290
Zhou, R., Yazdi, A., Menu, P., and Tschopp, J. (2011). A role for mitochondria in NLRP3 inflammasome activation. Nature 469, 221–225. doi: 10.1038/nature09663
Zhou, Z., He, H., Wang, K., Shi, X., Wang, Y., Su, Y., et al. (2020). Granzyme A from cytotoxic lymphocytes cleaves GSDMB to trigger pyroptosis in target cells. Science 368:eaaz7548. doi: 10.1126/science.aaz7548
Zhu, J., Fu, Y., and Tu, G. (2020). Role of Smad3 inhibitor and the pyroptosis pathway in spinal cord injury. Exp. Ther. Med. 20, 1675–1681. doi: 10.3892/etm.2020.8832
Zou, X., Xie, L., Wang, W., Zhao, G., Tian, X., and Chen, M. (2020). FK866 alleviates cerebral pyroptosis and inflammation mediated by Drp1 in a rat cardiopulmonary resuscitation model. Int. Immunopharmacol. 89:107032. doi: 10.1016/j.intimp.2020.107032
Keywords: spinal cord injury, pyroptosis, inflammasome, gasdermins, regulated cell death
Citation: Yin J, Gong G, Wan W and Liu X (2022) Pyroptosis in spinal cord injury. Front. Cell. Neurosci. 16:949939. doi: 10.3389/fncel.2022.949939
Received: 27 May 2022; Accepted: 03 November 2022;
Published: 17 November 2022.
Edited by:
Zhaoyang Yin, Nanjing Medical University, ChinaReviewed by:
Marcin Poreba, Wrocław University of Science and Technology, PolandCopyright © 2022 Yin, Gong, Wan and Liu. This is an open-access article distributed under the terms of the Creative Commons Attribution License (CC BY). The use, distribution or reproduction in other forums is permitted, provided the original author(s) and the copyright owner(s) are credited and that the original publication in this journal is cited, in accordance with accepted academic practice. No use, distribution or reproduction is permitted which does not comply with these terms.
*Correspondence: Xinhui Liu, bGl1eGluaHVpam55eUAxNjMuY29t
†These authors have contributed equally to this work
Disclaimer: All claims expressed in this article are solely those of the authors and do not necessarily represent those of their affiliated organizations, or those of the publisher, the editors and the reviewers. Any product that may be evaluated in this article or claim that may be made by its manufacturer is not guaranteed or endorsed by the publisher.
Research integrity at Frontiers
Learn more about the work of our research integrity team to safeguard the quality of each article we publish.