- 1Department of Neuroscience, Università Cattolica del Sacro Cuore, Rome, Italy
- 2Fondazione Policlinico Universitario A. Gemelli IRCCS, Rome, Italy
Transcranial direct current stimulation (tDCS) is a non-invasive brain stimulation technique (NIBS) that has been proven to promote beneficial effects in a range of neurological and psychiatric disorders. Unfortunately, although has been widely investigated, the mechanism comprehension around tDCS effects presents still some gaps. Therefore, scientists are still trying to uncover the cellular and molecular mechanisms behind its positive effects to permit a more suitable application. Experimental models have provided converging evidence that tDCS elicits improvements in learning and memory by modulating both excitability and synaptic plasticity in neurons. Recently, among tDCS neurobiological effects, neural synchronization and dendritic structural changes have been reported in physiological and pathological conditions, suggesting possible effects at the neuronal circuit level. In this review, we bring in to focus the emerging effects of tDCS on the structural plasticity changes and neuronal rewiring, with the intent to match these two aspects with the underpinning molecular mechanisms identified so far, providing a new perspective to work on to unveil novel tDCS therapeutic use to treat brain dysfunctions.
Introduction
Transcranial direct current stimulation (tDCS) is a low-intensity constant electric current given through the scalp by using two electrodes. During stimulation, the current delivered flows through the brain layers and, depending upon the polarity of the applied stimulation, tDCS can have a depolarizing (anodal) or hyperpolarizing (cathodal) effect (Antal et al., 2017). Different electrode configurations are commonly used: (i) unilateral configuration (i.e., one electrode positioned over the target cortical area and the other one over the contralateral supraorbital region or, in some cases, extracephalically); (ii) bilateral (or bihemispheric) configuration (i.e., one electrode positioned over the target cortical area and the other one over the contralateral side).
Early tDCS studies demonstrated how tDCS was able to induce long-lasting and polarity-specific excitability changes in the human motor cortex and changes in synaptic efficacy including long-term potentiation (LTP)-like and long-term depression (LTD)-like effects (Nitsche and Paulus, 2000; unilateral tDCS, 0.286 A/m2, 5 min; Lang et al., 2004; unilateral tDCS, 0.286 A/m2, 10 min). At the functional level, tDCS application over the motor cortex of healthy subjects enhanced motor learning and motor task performance (Nitsche et al., 2003; unilateral tDCS, 0.286 A/m2, 15 min). Later, the application of tDCS over different human brain areas showed multiple beneficial effects on both cognitive and motor function domains primarily modulating short- and long-lasting synaptic plasticity (Di Pino et al., 2014).
Many works and clinical trials provided solid evidence for its use in the neurological disorders, namely, stroke and epilepsy, movement disorders, Parkinson’s disease (PD), and Alzheimer’s disease (AD) (Flöel, 2014). It has also been shown that in aged subjects, anodal tDCS improved memory to a level equal to younger controls (Meinzer et al., 2013; unilateral anodal tDCS 0.286 A/m2, 20 min). At last, in the patients with stroke, tDCS was able to ameliorate fine motor control as well as the recovery of upper limb function (Tedesco Triccas et al., 2016). Despite the numerous existing studies, there is still great variability in the protocols adopted as well as in the outcome obtained which makes optimal clinical translation for tDCS difficult. Nevertheless, consistency seems to emerge in cellular/molecular mechanisms engaged by tDCS, especially anodal tDCS.
Indeed, cellular and molecular studies mainly performed in animal models, provided evidence of plasticity mechanisms based on tDCS after-effects thus justifying and supporting its therapeutic potential for brain disorders based on impaired synaptic plasticity (Korai et al., 2021).
Within this frame, in this review, we will summarize recent findings reporting plastic and metaplastic effects of tDCS and the beneath molecular mechanisms, focusing on those involved in the synaptic and dendritic spine changes, which are at the basis of neuronal network and connectivity rearrangements. In particular, we narrowed our review on preclinical and clinical works reporting positive functional outcomes specifically linked to tDCS-induced changes in plasticity and/or connectivity. Unless otherwise stated, the efficacy of treatment has been reported by the cited studies compared with the control groups receiving sham stimulation.
Experimental and clinical results will be discussed and paralleled to pin down a clearer picture of the mechanisms underlying tDCS modulatory effects providing elements to perfection the therapeutic application of this non-invasive brain stimulation technique (NIBS) and to use its properties to shape the plastic elements of the brain.
Plastic and metaplastic effects of transcranial direct current stimulation
Many works have documented plasticity-related effects of tDCS in animal models. The ability of tDCS to modulate Hebbian plasticity might well explain its positive effects on motor learning and cognitive enhancement observed in animal and human models both in physiological and pathological conditions.
Podda et al. (2016) showed increased LTP in the hippocampus of mice subjected to unilateral anodal tDCS (current density, 56 A/m2 for 20 min) and shed light on the molecular mechanisms underlying tDCS plastic effects. Basically, tDCS transiently increases intracellular Ca2+ initiating a molecular cascade that leads to an increased level of pCREBSer133 and its binding on BDNF promoter I. This event facilitates CREB binding protein (CBP) recruitment on the promoter region which, in turn, increases H3K9 promoter acetylation thus enhancing BDNF exon I expression (Figure 1). Histone acetylation on BDNF promoter I was previously shown to affect LTP and long-term memories (Alarcón et al., 2004) and, in keeping with this, Podda and collaborators demonstrated in this study that tDCS improved hippocampus-dependent spatial and recognition memory performance as revealed by the Morris Water Maze and Novel Object Recognition tasks. Consistent with epigenetic regulation, both LTP and memory enhancement induced by anodal tDCS persisted 1 week after the end of the stimulation protocol (Podda et al., 2016).
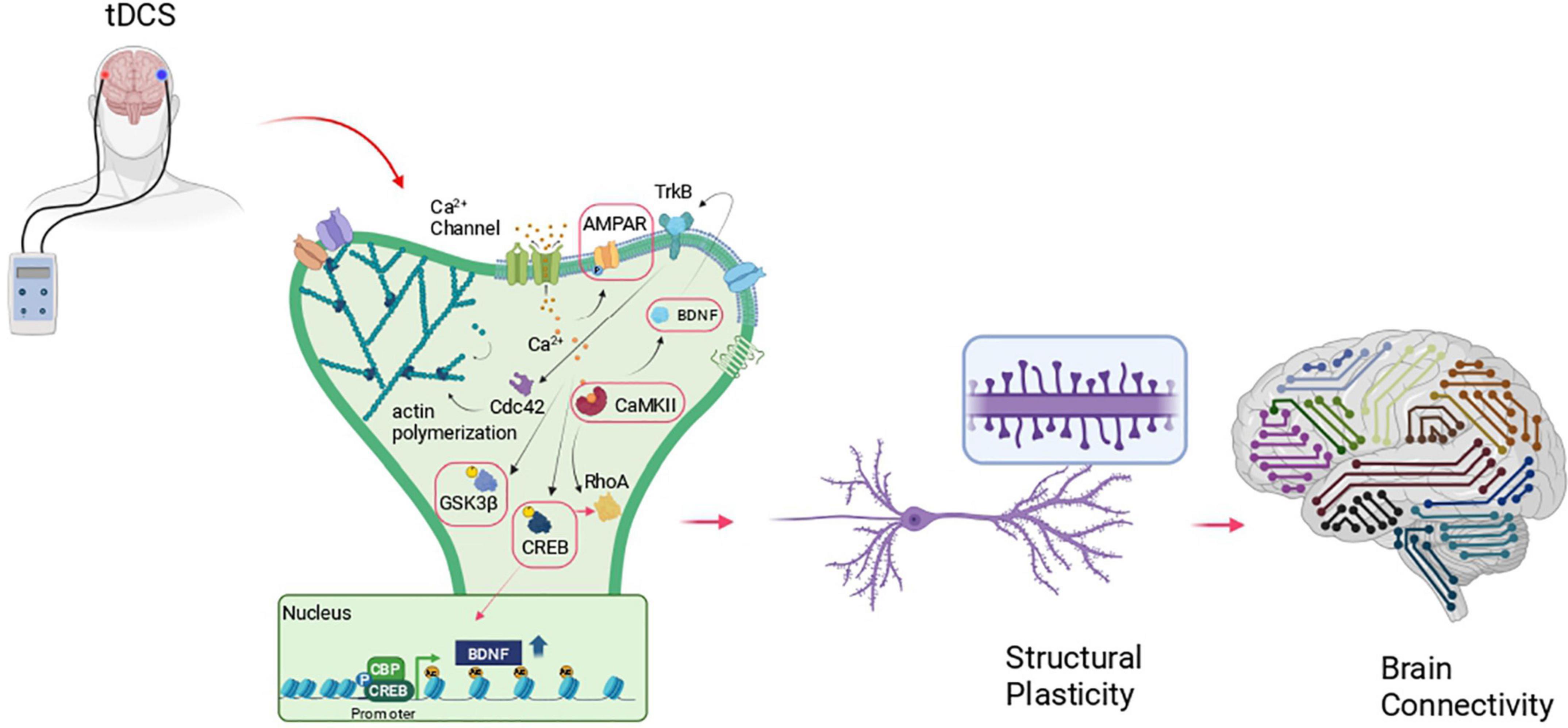
Figure 1. Schematic representation of the molecular cascade at the basis of structural plasticity and its possible recruitment by tDCS. The red boxes indicate those molecules engaged by transcranial direct current stimulation (tDCS)- in different brain areas, in animal models that, so far, have been correlated to changes at synapses (i.e., enhanced LTP, synaptic transmission, dendritic spine density), increased learning and memory and enhanced connectivity. Created with BioRender.com.
Hence, while the immediate effects of tDCS can be explained by membrane potential changes, long-term after-effects are the result of intracellular calcium dynamics and secondary synaptic plasticity key elements modulation (Korai et al., 2021). This activity-dependent modulation called “metaplasticity” is fundamental for the modulation and the maintenance of synaptic strength that is at the basis of the learning process (Cooper and Bear, 2012). Interestingly, unilateral anodal tDCS of the hippocampus has been shown to induce BDNF-mediated priming after-effects on synaptic plasticity and memory, making synapses susceptible to LTP induction in the rodent hippocampus (Podda et al., 2016; Yu et al., 2019; 0.1 A/m2, 30 min).
tDCS metaplastic outcomes have been observed in several clinical studies. Indeed, unilateral anodal tDCS (0.43 A/m2, 15 min) over the primary motor cortex (M1) followed by a repetitive transcranial magnetic stimulation (TMS) protocol elicits polarity-dependent facilitation on motor-evoked potentials (MEPs) in the healthy human subjects (Cosentino et al., 2012). Similarly, Bocci et al. (2014) showed that unilateral anodal tDCS (0.43 A/m2, 15 min) preconditioning of the primary visual cortex followed by the application of TMS repetitive stimulation protocol can induce and modulate synaptic strength in healthy subjects, proving the occurrence of tDCS metaplastic mechanisms (Hurley and Machado, 2017). At last, Monte-Silva et al. (2013) showed the presence of metaplastic interactions in response to two consecutive unilateral sessions of anodal tDCS of M1 (0.57 A/m2, 13 min) when these were separated by a time interval resulting in an increase in MEPs as a metaplastic outcome in healthy subjects.
In line with this, Barbati et al. (2020) showed that unilateral anodal tDCS of the mouse M1 (35.4 A/m2 for 20 min, single daily sessions for 3 consecutive days) induced metaplastic-like effects resulting in motor skill performance improvements similarly to what observed in humans (Reis et al., 2008; Roji et al., 2015; Angius et al., 2018). Specifically, the authors demonstrated that tDCS-induced increase in LTP at layer II-III synapses and such effect was accompanied by increased phosphorylation at Ser831 on the subunit 1 of AMPAR (pGluA1Ser831), a residue particularly important for the channel permeabilization and LTP expression providing the evidence of tDCS effect on LTP through a metaplastic modification.
Transcranial direct current stimulation modulation of structural plasticity
Physiologically dendritic spines respond to synaptic plasticity stimulation by modifying their structural and functional features, contributing to learning and memory formation (Rogerson et al., 2014). On the contrary, synaptic dysfunction has been widely recognized as a prodromal sign of both neurodegenerative (Taoufik et al., 2018) and neuropsychiatric disorders (Wang et al., 2018). Indeed, the perturbation of synaptic structure and function is thought to be the basis of the clinical symptoms and the progressive appearance of cognitive deficits (Spires-Jones and Knafo, 2012).
Spines undergo activity-dependent changes playing a fundamental role in LTP and LTD through spine enlargement and shrinkage, respectively (Harris et al., 2003). In addition to this, synaptic activity not only regulates the number of dendritic spines but also their shape and volume by modifying their internal sub-structures (Bosch and Hayashi, 2012; Alimohamadi et al., 2021). Indeed, the modulation local actin polymerization together with its interaction with scaffolding molecules, modifies spine architecture by sturdly positioning channels, cell adhesion proteins, and sub-spine structure, e.g., endosomes—at postsynaptic densities (Spence and Soderling, 2015; Bertling and Hotulainen, 2017; Dutta et al., 2021).
Structural changes occur within minutes from spine or dendritic shaft stimulation, including ex novo spine formation or spine enlargement. The first event is represented by calcium entrance inside the cell where it binds to Ca2+-binding protein calmodulin (CaM) which in turn activates the holoenzyme CaMKII (Lisman et al., 2012). Activated CaMKII forms complexes with postsynaptic density (PSD) molecules stabilizing NMDARs and enhancing AMPAR activity (Henley and Wilkinson, 2013) and expression (Opazo et al., 2010). At the same time, CaMKII interacts with key regulators of spine morphogenesis and LTP such as the small GTPase protein including Ras, RhoA, Rac1, and Cdc42 (Nishiyama and Yasuda, 2015). These GTPases activate several kinases including p21-activated kinase (PAK), Rho kinase (ROCK), and LIM kinase (LIMK), which in turn regulate actin remodeling and structural LTP via interaction and regulation of actin-binding partners such as Profilin, Cofilin, and Arp2/3 (Figure 1).
Long-lasting plasticity effects including spine enlargement and structural maintenance require de novo protein synthesis either via local protein synthesis (Holt et al., 2019; Runge et al., 2020) or via gene transcription in the nucleus through the activation of activity-dependent transcription factors such as CREB or MEF2C. Among neurotrophins, BDNF has a central role in numerous processes of functional and structural plasticity (Renna et al., 2022). BDNF via TrkB receptor binding and signaling regulates post-synaptic function by modifying NMDAR and AMPAR properties, hence favoring the induction and maintenance of LTP (Rauti et al., 2020) via actin cytoskeletal changes (Huang and Kandel, 2005; Rex et al., 2007). Indeed, BDNF–TrkB signaling has been shown to promote local protein synthesis of several proteins including Arc, Homer, LIMK1, which in end regulate the turnover of the dendritic actin cytoskeleton proteins (Ying et al., 2002; Messaoudi et al., 2007; Bramham, 2008).
Considering the aforementioned reviewed synaptic plasticity modifications induced by tDCS and also the activated molecular cascade underlying these effects, some recent works pointed the attention not only at the functional but also the structural changes (Paciello et al., 2018; Barbati et al., 2020; Gellner et al., 2020; Longo et al., 2022).
In this view, Paciello et al. (2018) have studied the effect of unilateral anodal tDCS of the auditory cortex (56 A/m2, 20 min, single daily sessions for 2 consecutive days) on healthy rats (normal-hearing, NH) and on rats with altered auditory cortex structural plasticity (i.e., animals exposed to acoustic trauma, NIHL). In this work comes up very clearly that tDCS exerts a global trophic action by increasing the number of dendritic spines in the auditory cortex, targeting apical dendrites of pyramidal neurons of layer II-III and V-VI in NH rats, while in NIHL rats, tDCS specifically targeted the most noise-affected layer II-III. In addition, the authors demonstrated that tDCS can modulate dendritic spine shape, increasing the number of both thin- and mushroom-shaped spines in NIHL, while in NH rats it increased only the number of thin spines. These results suggest that in the lesioned auditory cortex tDCS induces the formation of new spines but at the same time it stabilizes those already existing to preserve plasticity. TDCS-related structural changes were accompanied by increased synaptophysin levels and causally linked to increased level of BDNF in both NH and NIHL rats. TDCS effects on spinogenesis were, indeed, completely abolished in presence of ANA-12, the BDNF/TrkB receptor blocker.
Similarly, Barbati et al. (2020) showed increased spine density at both apical and basal dendrites of M1 layer II-III pyramidal neurons following repeated tDCS stimulation protocol (unilateral anodal tDCS 35.4 A/m2 for 20 min, single daily sessions for 3 consecutive days). This result was matched by enhanced forelimb strength and motor skilled performance and by increased synaptic transmission and plasticity at M1 layer II-III horizontal connections in tDCS-mice compared with the relative sham-controls. At the cellular level, tDCS increased neurotransmitter release and AMPA/NMDA ratio, events that are consistent with the increased spine density observed in tDCS mice. At the molecular level, the dendritic spine structural changes were accompanied by an increased level of BDNF and by an increased level of phosphorylation of key synaptic and structural plasticity players including CaMKII, CREB, and GluA1. Moreover, tDCS activated nitric oxide pathway leading to GluA1 S-nitrosylation and consecutively GluA1 phosphorylation at Ser831 (pGluA1Ser831) thus increasing single-channel conductance (Selvakumar et al., 2013; Barbati et al., 2020). As a proof-of-concept, the authors showed that mouse treatment with the NOS inhibitor, L-NAME, abolished tDCS-induced increases of pGluA1Ser831. This effect likely cooperated with other tDCS-induced epigenetic mechanisms including the pCREB-dependent recruitment of the histone acetyltransferase, CBP, at the promoter region of the BDNF gene (Figure 1).
These recent works provided the first evidence on how tDCS promotes not only functional but also structural plasticity changes at dendritic spines encouraging further investigation to clarify the molecular mechanisms at the basis of the actin remodeling events induced by tDCS and to focus on tDCS effects on brain connectivity.
Transcranial direct current stimulation and rewiring
Recently, there has been growing interest in “brain connectome” to understand how brain and therefore neuronal structures gives rise to brain function, and ultimately, to behavior. The outline connection between neurons, determines how the stream of information flows through neural circuits, and therefore, how these circuits function (Sporns et al., 2005; Bota et al., 2015).
Recent works indicate that in the mammalian brain neuronal connections may undergo rewiring during learning and experience-dependent plasticity (Bennett et al., 2018). This network reorganization reinforces some neuron-to-neuron connections and weakened some others by synapse elimination.
Rewiring is a phenomenon that consists of two different mechanisms. The first one includes formation and elimination of individual synapses at existing connections determining a local event where the number of synapses at the connection change. The second type involves a radical reconfiguration of neural connection by incorporating or removing neurons from the existing circuit (Barnes and Finnerty, 2010). The functional and structural changes on the basis of this phenomenon are the consequence of electrical activity and hence, rewiring provides the strategy through which the brain responds to experience in a long-lasting way.
Given tDCS ability to modulate cortical excitability and to promote plasticity mechanisms by changing synaptic efficacy and structure some works have pointed attention to its ability to modulate brain connectivity.
In line with this, Polanía et al. (2011) showed that, on healthy subjects, unilateral anodal tDCS (0.625 A/m2, 10 min) has an effect on functional network synchronization not only within the target motor area but also coupling the former with neighbored premotor and sensorimotor areas.
Neuroplastic events and the reorganization of motor cortical connections have also been described as crucial processes in stroke recovery (Rossini et al., 2003; Murphy and Corbett, 2009; Xing and Bai, 2020). In patients with stroke, cortical reorganization, with an increased excitability of the contralesional hemisphere has been observed recurrently (Butefisch et al., 2003, 2008). Therefore, good functional recovery has frequently been associated with a rebalancing of interhemispheric inhibition (Nowak et al., 2009; Calabrò et al., 2019). As such, the most common tDCS configuration used in human and rodent stroke studies is the bilateral stimulation with the anode over the lesioned cortex and cathode over the contralateral side, providing the simultaneous stimulation of the two cortices with facilitating and inhibiting currents on affected and unaffected hemispheres, respectively.
In keeping with this, bilateral tDCS effects in stroke recovery have been tested in clinical studies showing encouraging results (Chew et al., 2020; Orrù et al., 2020). Furthermore, works investigating tDCS capacity to induce changes in cortical electroencephalogram oscillations, suggested that motor recovery might be enhanced by early stimulation that seeks to increase functional connectivity (FC) of motor relays and pathways (Bolognini et al., 2020; bilateral tDCS delivered at 0.57 A/m2, 15 min; Vecchio et al., 2018; bilateral tDCS delivered at 0.40 A/m2, 12 min). Lefebvre et al. (2017) showed that one single session of bilateral tDCS (0.28 A/m2, 30 min) applied over M1-modulated FC in patients with stroke. In particular, seed-based analysis of FC established that tDCS-enhanced FC within the motor and premotor regions in the lesioned hemispheres 1 week after the end of the stimulation protocol.
Moreover, in a recent preclinical study, Longo et al. (2022) applied bilateral tDCS over M1 (35.4 A/m2, 20 min, single daily sessions for 3 consecutive days) in a mouse model of ischemic stroke and showed that tDCS accelerated motor recovery by enhancing forelimb strength and ameliorating performances in both skilled and non-skilled motor tasks. The authors looked at possible effects on FC by recording local field potentials through epidurally implanted electrodes over M1 and somatosensory cortices and analyzing total coherence (TotCoh)—an index expressing global functional coupling of the LFP rhythms (Vecchio et al., 2019). Interestingly, they found that functional coupling between M1 and somatosensory cortices of both the hemispheres was decreased at all frequency bands and time points in stroke mice and, more importantly, tDCS significantly increased connectivity. Particularly, tDCS in stroke mice restored TotCoh values back to those observed in sham-healthy mice and, in addition, increased this parameter in healthy mice, suggesting that a structural network reorganization occurs following tDCS. In support of this, the analysis of Golgi-Cox staining of the peri-infarct cortex showed increased spine density at both apical and basal dendrites at layer II-III pyramidal neurons following tDCS, a result that corroborates the mounting evidence of tDCS- induced spinogenesis (Barbati et al., 2020; Gellner et al., 2020). Notably, the authors provided a causal link between BDNF and tDCS-dependent effects by demonstrating that blockade of BDNF/TrkB receptor hindered: (i) improvements of functional outcomes; (ii) increases in spine density and TotCoh, and also (iii) the activation of plasticity related proteins, such as ERK, CaMKII, and MEF2C.
These results provide the first evidence linking tDCS effects on structural plasticity to changes in connectivity, substantiating a novel role for tDCS in shaping neuronal connections. Given the translational relevance of such a tool, further studies are warranted to look more in-depth at tDCS effects on the FC by advanced analysis (e.g., graph theory) on EEG and functional MRI signals and at mechanism of action on dendritic spine structure and dynamics to optimize its use to modulate, preserve, and restructure neural circuits.
Conclusion
In the last two decades, tDCS’ positive impact on the cognitive and motor functions has been proven in the subjects with diverse psychiatric and neurological disorders. This NIBS has also been used on animal models to clarify the mechanisms behind its effects on brain plasticity and to understand, more broadly, the relationship between structural and functional changes at synapses.
In literature, tDCS has been shown to modulate synaptic plasticity and, at the same time, to regulate the expression, activation, and localization of plasticity-related proteins such as BDNF, CaMKII, and GluA1. Intriguingly, these molecules are part of well-known signaling pathways at the basis of spine remodeling and actin polymerization.
Recent works have indeed shown that tDCS can modify the number and structure of dendritic spine, indicating that this NIBS not only changes the efficacy of a synapse, but it also shapes it. These structural changes at synapses might favor rewiring processes improving the neuronal networks and resulting in enhanced learning and memory as well as amelioration of cognitive and motor functions clinically and experimentally observed following tDCS.
In conclusion, in this review, we recapitulated the most recent evidence around tDCS’ plastic effects bringing into focus its capability to trigger dendritic spine rearrangement in neurons, thus, changing neuronal wiring patterns. Currently, treatments based on boosting rewiring are explored by the scientific community to treat acute brain damage as well as permanent and progressive brain dysfunctions. A deeper comprehension of tDCS effects on rewiring and structural plasticity at the basis of neuronal network rearrangement will allow tDCS to be exploited therapeutically for treating brain pathologies characterized by the widespread brain wiring abnormalities.
Author contributions
SAB conceived the work and drafted the manuscript. MVP conceived the work and contributed making a critical revision of the manuscript. CG contributed by making a critical revision of the manuscript. All authors read and approved the final version of the review.
Funding
This work was supported by Ministero della Salute—Ricerca Corrente 2022.
Conflict of interest
The authors declare that the research was conducted in the absence of any commercial or financial relationships that could be construed as a potential conflict of interest.
Publisher’s note
All claims expressed in this article are solely those of the authors and do not necessarily represent those of their affiliated organizations, or those of the publisher, the editors and the reviewers. Any product that may be evaluated in this article, or claim that may be made by its manufacturer, is not guaranteed or endorsed by the publisher.
References
Alarcón, J. M., Malleret, G., Touzani, K., Vronskaya, S., Ishii, S., Kandel, E. R., et al. (2004). Chromatin acetylation, memory, and LTP are impaired in CBP+/- mice: a model for the cognitive deficit in Rubinstein-Taybi syndrome and its amelioration. Neuron 42, 947–959. doi: 10.1016/j.neuron.2004.05.021
Alimohamadi, H., Bell, M. K., Halpain, S., and Rangamani, P. (2021). Mechanical Principles Governing the Shapes of Dendritic Spines. Front. Physiol. 12:657074. doi: 10.3389/fphys.2021.657074
Angius, L., Mauger, A. R., Hopker, J., Pascual-Leone, A., Santarnecchi, E., and Marcora, S. M. (2018). Bilateral extracephalic transcranial direct current stimulation improves endurance performance in healthy individuals. Brain Stimul. 11, 108–117. doi: 10.1016/j.brs.2017.09.017
Antal, A., Alekseichuk, I., Bikson, M., Brockmöller, J., Brunoni, A. R., Chen, R., et al. (2017). Low intensity transcranial electric stimulation: safety, ethical, legal regulatory and application guidelines. Clin. Neurophysiol. 128, 1774–1809. doi: 10.1016/j.clinph.2017.06.001
Barbati, S. A., Cocco, S., Longo, V., Spinelli, M., Gironi, K., Mattera, A., et al. (2020). Enhancing plasticity mechanisms in the mouse motor cortex by anodal transcranial direct-current stimulation: the contribution of nitric oxide signaling. Cereb. Cortex 30, 2972–2985. doi: 10.1093/cercor/bhz288
Barnes, S. J., and Finnerty, G. T. (2010). Sensory experience and cortical rewiring. Neuroscientist 16, 186–198. doi: 10.1177/1073858409343961
Bennett, S. H., Kirby, A. J., and Finnerty, G. T. (2018). Rewiring the connectome: evidence and effects. Neurosci. Biobehav. Rev. 88, 51–62. doi: 10.1016/j.neubiorev.2018.03.001
Bertling, E., and Hotulainen, P. (2017). New waves in dendritic spine actin cytoskeleton: from branches and bundles to rings, from actin binding proteins to post-translational modifications. Mol. Cell Neurosci. 84, 77–84. doi: 10.1016/j.mcn.2017.05.002
Bocci, T., Caleo, M., Tognazzi, S., Francini, N., Briscese, L., Maffei, L., et al. (2014). Evidence for metaplasticity in the human visual cortex. J. Neural Trans. 121, 221–231. doi: 10.1007/s00702-013-1104-z
Bolognini, N., Russo, C., Souza Carneiro, M. I., Nicotra, A., Olgiati, E., Spandri, V., et al. (2020). Bi-hemispheric transcranial direct current stimulation for upper-limb hemiparesis in acute stroke: a randomized, double-blind, sham-controlled trial. Eur. J. Neurol. 27, 2473–2482. doi: 10.1111/ene.14451
Bosch, M., and Hayashi, Y. (2012). Structural plasticity of dendritic spines. Curr. Opin. Neurobiol. 22, 383–388. doi: 10.1016/j.conb.2011.09.002
Bota, M., Sporns, O., and Swanson, L. W. (2015). Architecture of the cerebral cortical association connectome underlying cognition. Proc. Natl. Acad. Sci. U. S. A. 112, E2093–E2101. doi: 10.1073/pnas.1504394112
Bramham, C. R. (2008). Local protein synthesis, actin dynamics, and LTP consolidation. Curr. Opin. Neurobiol. 18, 524–531. doi: 10.1016/j.conb.2008.09.013
Butefisch, C. M., Netz, J., Wessling, M., Seitz, R. J., and Homberg, V. (2003). Remote changes in cortical excitability after stroke. Brain 126, 470–481. doi: 10.1093/brain/awg044
Butefisch, C. M., Wessling, M., Netz, J., Seitz, R. J., and Homberg, V. (2008). Relationship between interhemispheric inhibition and motor cortex excitability in subacute stroke patients. Neurorehabil. Neural. Rep. 22, 4–21. doi: 10.1177/1545968307301769
Calabrò, R. S., Accorinti, M., Porcari, B., Carioti, L., Ciatto, L., Billeri, L., et al. (2019). Does hand robotic rehabilitation improve motor function by rebalancing interhemispheric connectivity after chronic stroke? Encouraging data from a randomised-clinical-trial. Clin. Neurophysiol. 130, 767–780. doi: 10.1016/j.clinph.2019.02.013
Chew, E., Teo, W. P., Tang, N., Ang, K. K., Ng, Y. S., Zhou, J. H., et al. (2020). Using Transcranial Direct Current Stimulation to Augment the Effect of Motor Imagery-Assisted Brain-Computer Interface Training in Chronic Stroke Patients-Cortical Reorganization Considerations. Front. Neurol. 11:948. doi: 10.3389/fneur.2020.00948
Cooper, L. N., and Bear, M. F. (2012). The BCM theory of synapse modification at 30: interaction of theory with experiment. Nat. Rev. Neurosci. 13, 798–810. doi: 10.1038/nrn3353
Cosentino, G., Fierro, B., Paladino, P., Talamanca, S., Vigneri, S., Palermo, A., et al. (2012). Transcranial direct current stimulation preconditioning modulates the effect of high-frequency repetitive transcranial magnetic stimulation in the human motor cortex. Eur. J. Neurosci. 35, 119–124. doi: 10.1111/j.1460-9568.2011.07939.x
Di Pino, G., Pellegrino, G., Assenza, G., Capone, F., Ferreri, F., Formica, D., et al. (2014). Modulation of brain plasticity in stroke: a novel model for neurorehabilitation. Nat. Rev. Neurol. 10, 597–608. doi: 10.1038/nrneurol.2014.162
Dutta, P., Bharti, P., Kumar, J., and Maiti, S. (2021). Role of actin cytoskeleton in the organization and function of ionotropic glutamate receptors. Curr. Res. Struct. Biol. 3, 277–289. doi: 10.1016/j.crstbi.2021.10.001
Flöel, A. (2014). tDCS-enhanced motor and cognitive function in neurological diseases. Neuroimage 85, 934–947. doi: 10.1016/j.neuroimage.2013.05.098
Gellner, A. K., Reis, J., Holtick, C., Schubert, C., and Fritsch, B. (2020). Direct current stimulation-induced synaptic plasticity in the sensorimotor cortex: structure follows function. Brain Stimul. 13, 80–88. doi: 10.1016/j.brs.2019.07.026
Harris, K. M., Fiala, J. C., and Ostroff, L. (2003). Structural changes at dendritic spine synapses during long-term potentiation. Philos. Trans. R. Soc. Lond. B Biol. Sci. 358, 745–748. doi: 10.1098/rstb.2002.1254
Henley, J. M., and Wilkinson, K. A. (2013). AMPA receptor trafficking and the mechanisms underlying synaptic plasticity and cognitive aging. Dial. Clin. Neurosci. 15, 11–27. doi: 10.31887/DCNS.2013.15.1/jhenley
Holt, C. E., Martin, K. C., and Schuman, E. M. (2019). Local translation in neurons: visualization and function. Nat. Struct. Mol. Biol. 26, 557–566. doi: 10.1038/s41594-019-0263-5
Huang, Y. Y., and Kandel, E. R. (2005). Theta frequency stimulation induces a local form of late phase LTP in the CA1 region of the hippocampus. Learn Mem. 12, 587–593.
Hurley, R., and Machado, L. (2017). Using tDCS priming to improve brain function: can metaplasticity provide the key to boosting outcomes? Neurosci. Biobehav. Rev. 83, 155–159. doi: 10.1016/j.neubiorev.2017.09.029
Korai, S. A., Ranieri, F., Di Lazzaro, V., Papa, M., and Cirillo, G. (2021). Neurobiological after-effects of low intensity transcranial electric stimulation of the human nervous system: from basic mechanisms to metaplasticity. Front. Neurol. 12:587771. doi: 10.3389/fneur.2021.587771
Lang, N., Siebner, H. R., Ernst, D., Nitsche, M. A., Paulus, W., Lemon, R. N., et al. (2004). Preconditioning with transcranial direct current stimulation sensitizes the motor cortex to rapid-rate transcranial magnetic stimulation and controls the direction of after-effects. Biol. Psychiat. 56, 634–639. doi: 10.1016/j.biopsych.2004.07.017
Lefebvre, S., Dricot, L., Laloux, P., Desfontaines, P., Evrard, F., Peeters, A., et al. (2017). Increased functional connectivity one week after motor learning and tDCS in stroke patients. Neuroscience 340, 424–435. doi: 10.1016/j.neuroscience.2016.10.066
Lisman, J., Yasuda, R., and Raghavachari, S. (2012). Mechanisms of CaMKII action in long-term potentiation. Nat. Rev. Neurosci. 13, 169–182. doi: 10.1038/nrn3192
Longo, V., Barbati, S. A., Re, A., Paciello, F., Bolla, M., Rinaudo, M., et al. (2022). Transcranial Direct Current Stimulation Enhances Neuroplasticity and Accelerates Motor Recovery in a Stroke Mouse Model. Stroke 53, 1746–1758. doi: 10.1161/STROKEAHA.121.034200
Meinzer, M., Lindenberg, R., Antonenko, D., Flaisch, T., and Flöel, A. (2013). Anodal transcranial direct current stimulation temporarily reverses age-associated cognitive decline and functional brain activity changes. J. Neurosci. 33, 12470–12478. doi: 10.1523/JNEUROSCI.5743-12.2013
Messaoudi, E., Kanhema, T., Soulé, J., Tiron, A., Dagyte, G., da Silva, B., et al. (2007). Sustained Arc/Arg3.1 synthesis controls long-term potentiation consolidation through regulation of local actin polymerization in the dentate gyrus in vivo. J. Neurosci. 27, 10445–10455. doi: 10.1523/JNEUROSCI.2883-07.2007
Monte-Silva, K., Kuo, M. F., Hessenthaler, S., Fresnoza, S., Liebetanz, D., Paulus, W., et al. (2013). Induction of late LTP-like plasticity in the human motor cortex by repeated non-invasive brain stimulation. Brain Stimul. 6, 424–432. doi: 10.1016/j.brs.2012.04.011
Murphy, T. H., and Corbett, D. (2009). Plasticity during stroke recovery: from synapse to behaviour. Nat. Rev. Neurosci. 10, 861–872. doi: 10.1038/nrn2735
Nishiyama, J., and Yasuda, R. (2015). Biochemical computation for spine structural plasticity. Neuron 87, 63–75. doi: 10.1016/j.neuron.2015.05.043
Nitsche, M. A., and Paulus, W. (2000). Excitability changes induced in the human motor cortex by weak transcranial direct current stimulation. J. Physiol. 527, 633–639. doi: 10.1111/j.1469-7793.2000.t01-1-00633.x
Nitsche, M. A., Schauenburg, A., Lang, N., Liebetanz, D., Exner, C., Paulus, W., et al. (2003). Facilitation of implicit motor learning by weak transcranial direct current stimulation of the primary motor cortex in the human. J. Cogn. Neurosci. 15, 619–626. doi: 10.1162/089892903321662994
Nowak, D. A., Grefkes, C., Ameli, M., and Fink, G. R. (2009). Interhemispheric competition after stroke: brain stimulation to enhance recovery of function of the affected hand. Neurorehabil. Neural. Rep. 23, 641–656. doi: 10.1177/1545968309336661
Opazo, P., Labrecque, S., Tigaret, C. M., Frouin, A., Wiseman, P. W., De Koninck, P., et al. (2010). CaMKII triggers the diffusional trapping of surface AMPARs through phosphorylation of stargazin. Neuron 67, 239–252. doi: 10.1016/j.neuron.2010.06.007
Orrù, G., Conversano, C., Hitchcott, P. K., and Gemignani, A. (2020). Motor stroke recovery after tDCS: a systematic review. Rev. Neurosci. 31, 201–218. doi: 10.1515/revneuro-2019-0047
Paciello, F., Podda, M. V., Rolesi, R., Cocco, S., Petrosini, L., Troiani, D., et al. (2018). Anodal transcranial direct current stimulation affects auditory cortex plasticity in normal-hearing and noise-exposed rats. Brain Stimul. 11, 1008–1023. doi: 10.1016/j.brs.2018.05.017
Podda, M. V., Cocco, S., Mastrodonato, A., Fusco, S., Leone, L., Barbati, S. A., et al. (2016). Anodal transcranial direct current stimulation boosts synaptic plasticity and memory in mice via epigenetic regulation of Bdnf expression. Sci. Rep. 6:22180. doi: 10.1038/srep22180
Polanía, R., Nitsche, M. A., and Paulus, W. (2011). Modulating functional connectivity patterns and topological functional organization of the human brain with transcranial direct current stimulation. Hum. Brain Mapp. 32, 1236–1249. doi: 10.1002/hbm.21104
Rauti, R., Cellot, G., D’Andrea, P., Colliva, A., Scaini, D., Tongiorgi, E., et al. (2020). BDNF impact on synaptic dynamics: extra or intracellular long-term release differently regulates cultured hippocampal synapses. Mol. Brain 13:43. doi: 10.1186/s13041-020-00582-9
Reis, J., Robertson, E., Krakauer, J. W., Rothwell, J., Marshall, L., Gerloff, C., et al. (2008). Consensus: “Can tDCS and TMS enhance motor learning and memory formation?” Brain Stimul. 1, 363–369. doi: 10.1016/j.brs.2008.08.001
Renna, P., Ripoli, C., Dagliyan, O., Pastore, F., Rinaudo, M., Re, A., et al. (2022). Engineering a switchable single-chain TEV protease to control protein maturation in living neurons. Bioeng. Transl. Med. 7:e10292. doi: 10.1002/btm2.10292
Rex, C. S., Lin, C. Y., Kramár, E. A., Chen, L. Y., Gall, C. M., and Lynch, G. (2007). Brain-derived neurotrophic factor promotes long-term potentiation-related cytoskeletal changes in adult hippocampus. Learn Mem. 12, 587–593. doi: 10.1101/lm.98905
Rogerson, T., Cai, D. J., Frank, A., Sano, Y., Shobe, J., Lopez-Aranda, M. F., et al. (2014). Synaptic tagging during memory allocation. Nat. Rev. Neurosci. 15, 157–169. doi: 10.1038/nrn3667
Roji, O., van Kuyck, K., Nuttin, B., and Wenderoth, N. (2015). Anodal tDCS over the primary motor cortex facilitates long-term memory formation reflecting use-dependent plasticity. PLoS One 10:e0127270. doi: 10.1371/journal.pone.0127270
Rossini, P. M., Calautti, C., Pauri, F., and Baron, J. C. (2003). Post-stroke plastic reorganisation in the adult brain. Lancet Neurol. 2, 493–502. doi: 10.1016/s1474-4422(03)00485-x
Runge, K., Cardoso, C., and de Chevigny, A. (2020). Dendritic spine plasticity: function and mechanisms. Front. Synaptic. Neurosci. 12:36. doi: 10.3389/fnsyn.2020.00036
Selvakumar, B., Jenkins, M. A., Hussain, N. K., Huganir, R. L., Traynelis, S. F., and Snyder, S. H. (2013). S-nitrosylation of AMPA receptor GluA1 regulates phosphorylation, single-channel conductance, and endocytosis. Proc. Natl. Acad. Sci. U. S. A. 110, 1077–1082. doi: 10.1073/pnas.1221295110
Spence, E. F., and Soderling, S. H. (2015). Actin out: regulation of the synaptic cytoskeleton. J. Biol. Chem. 290, 28613–28622. doi: 10.1074/jbc.R115.655118
Spires-Jones, T., and Knafo, S. (2012). Spines, plasticity, and cognition in Alzheimer’s model mice. Neural. Plast. 2012:319836. doi: 10.1155/2012/319836
Sporns, O., Tononi, G., and Kötter, R. (2005). The human connectome: a structural description of the human brain. PLoS Comput. Biol. 1:e42. doi: 10.1371/journal.pcbi.0010042
Taoufik, E., Kouroupi, G., Zygogianni, O., and Matsas, R. (2018). Synaptic dysfunction in neurodegenerative and neurodevelopmental diseases: an overview of induced pluripotent stem-cell-based disease models. Open Biol. 8:180138. doi: 10.1098/rsob.180138
Tedesco Triccas, L., Burridge, J. H., Hughes, A. M., Pickering, R. M., Desikan, M., Rothwell, J. C., et al. (2016). Multiple sessions of transcranial direct current stimulation and upper extremity rehabilitation in stroke: a review and meta-analysis. Clin. Neurophysiol. 127, 946–955. doi: 10.1016/j.clinph.2015.04.067
Vecchio, F., Di Iorio, R., Miraglia, F., Granata, G., Romanello, R., Bramanti, P., et al. (2018). Transcranial direct current stimulation generates a transient increase of small-world in brain connectivity: an EEG graph theoretical analysis. Exp. Brain Res. 236, 1117–1127. doi: 10.1007/s00221-018-5200-z
Vecchio, F., Miraglia, F., and Rossini, P. M. (2019). Tracking neuronal connectivity from electric brain signals to predict performance. Neuroscientist 25, 86–93. doi: 10.1177/1073858418776891
Wang, X., Christian, K. M., Song, H., and Ming, G. L. (2018). Synaptic dysfunction in complex psychiatric disorders: from genetics to mechanisms. Genome Med. 10:9. doi: 10.1186/s13073-018-0518-5
Xing, Y., and Bai, Y. (2020). A review of exercise-induced neuroplasticity in ischemic stroke: pathology and mechanisms. Mol. Neurobiol. 57, 4218–4231. doi: 10.1007/s12035-020-02021-1
Ying, S. W., Futter, M., Rosenblum, K., Webber, M. J., Hunt, S. P., Bliss, T. V., et al. (2002). Brain-derived neurotrophic factor induces long-term potentiation in intact adult hippocampus: requirement for ERK activation coupled to CREB and upregulation of Arc synthesis. J. Neurosci. 22, 1532–1540. doi: 10.1523/JNEUROSCI.22-05-01532.2002
Keywords: BDNF, brain connectivity, memory, metaplasticity, neurological disorder, stroke, structural plasticity, tDCS
Citation: Barbati SA, Podda MV and Grassi C (2022) Tuning brain networks: The emerging role of transcranial direct current stimulation on structural plasticity. Front. Cell. Neurosci. 16:945777. doi: 10.3389/fncel.2022.945777
Received: 16 May 2022; Accepted: 29 June 2022;
Published: 21 July 2022.
Edited by:
Natalia Arias, King’s College London, United KingdomReviewed by:
Sorinel A. Oprisan, College of Charleston, United StatesThomas Freret, Université de Caen Normandie, France
Copyright © 2022 Barbati, Podda and Grassi. This is an open-access article distributed under the terms of the Creative Commons Attribution License (CC BY). The use, distribution or reproduction in other forums is permitted, provided the original author(s) and the copyright owner(s) are credited and that the original publication in this journal is cited, in accordance with accepted academic practice. No use, distribution or reproduction is permitted which does not comply with these terms.
*Correspondence: Maria Vittoria Podda, bWFyaWF2aXR0b3JpYS5wb2RkYUB1bmljYXR0Lml0