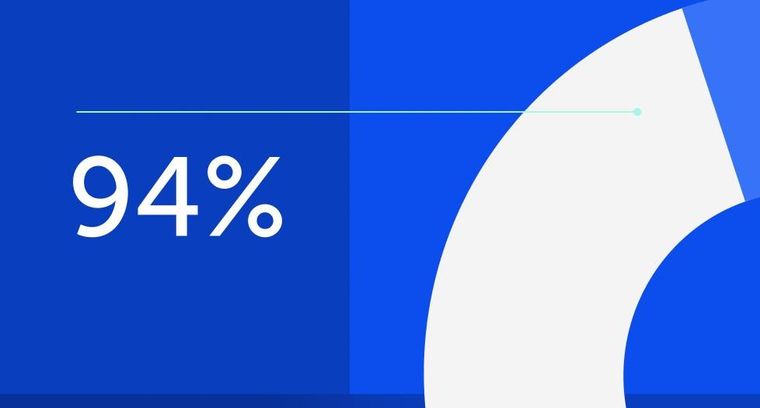
94% of researchers rate our articles as excellent or good
Learn more about the work of our research integrity team to safeguard the quality of each article we publish.
Find out more
REVIEW article
Front. Cell. Neurosci., 15 July 2022
Sec. Non-Neuronal Cells
Volume 16 - 2022 | https://doi.org/10.3389/fncel.2022.922181
This article is part of the Research TopicThe Brain Barriers in Diseases of the Nervous SystemView all 7 articles
Differential diagnosis of brain lesion pathologies is complex, but it is nevertheless crucial for appropriate clinical management. Advanced imaging methods, including diffusion-weighted imaging and apparent diffusion coefficient, can help discriminate between brain mass lesions such as glioblastoma, brain metastasis, brain abscesses as well as brain lymphomas. These pathologies are characterized by blood-brain barrier alterations and have been extensively studied. However, the changes in the blood-brain barrier that are observed around brain pathologies and that contribute to the development of vasogenic brain edema are not well described. Some infiltrative brain pathologies such as glioblastoma are characterized by glioma cell infiltration in the brain tissue around the tumor mass and thus affect the nature of the vasogenic edema. Interestingly, a common feature of primary and secondary brain tumors or tumor-like brain lesions characterized by vasogenic brain edema is the formation of various molecules that lead to alterations of tight junctions and result in blood-brain barrier damage. The resulting vasogenic edema, especially blood-brain barrier disruption, can be visualized using advanced magnetic resonance imaging techniques, such as diffusion-weighted imaging and apparent diffusion coefficient. This review presents a comprehensive overview of blood-brain barrier changes contributing to the development of vasogenic brain edema around glioblastoma, brain metastases, lymphomas, and abscesses.
Brain edema, a pathologic state defined by excessive fluid accumulation within the brain parenchyma, can be divided into cytotoxic, ionic, and vasogenic types. Cytotoxic brain edema is characterized by swelling of the cells caused by the influx of osmolytes such as Na+ and Cl– and fluids intracellularly. This type of brain edema is dominant following brain ischemia and paucity of ATP. Subsequently, ionic or osmotic edema develops as a result of fluids and osmolytes redistribution between the extravascular and intravascular spaces. These differences lead to the water transfer into the brain parenchyma across the blood-brain barrier (BBB). Vasogenic edema results from the breakdown of BBB and typically occurs in the brain parenchyma surrounding nearly all malignant central nervous system (CNS) tumors, as well as brain abscesses (Leinonen et al., 2018; White et al., 2019).
The BBB comprises interacting cells such as endothelial cells, pericytes, and astrocytes. These cells, along with neurons, microglia, vascular smooth muscle cells, the basement membrane, and the extracellular matrix, form part of the so-called neurovascular unit (Muoio et al., 2014; Gorelick et al., 2017). An intact BBB is essential for maintaining homeostasis of the CNS by separating the intravascular from the interstitial compartment of the brain (Abbott et al., 2006; Coureuil et al., 2017). Therefore, any alteration in the BBB results in vasogenic brain edema (Wahl et al., 1988), which can be detected by magnetic resonance imaging (MRI; Dalby et al., 2021). Studies so far have focused mainly on changes in the BBB in the enhancing brain lesions (Arvanitis et al., 2020; Rosińska and Gavard, 2021). These sites are characterized by a leaky BBB that allows passage of large molecules such as contrast agents used for MRI showing up as enhancement on T1 weighted images (Cramer and Larsson, 2014). However, little is known about the differences in the BBB in the perilesional edema beyond the margins of the T1 contrast-enhancement ring. The presence of water within brain tissue manifests as long T2 relaxation times that lead to increased T2 values and thus determine the presence or absence of brain edema (Obenaus and Ashwal, 2008). However, T2 values do not specify the compartment in which water accumulates. Therefore, advanced MRI parameters such as quantitative diffusion-weighted imaging (DWI) and apparent diffusion coefficient (ADC) are used to visualize water movement between brain compartments. Low ADC values reflect restricted water diffusion caused by cellular swelling and reduced extracellular space (Badaut et al., 2011). On the other hand, high ADC values are a consequence of increased water mobility through a disrupted BBB (Yang et al., 2017). The movement of water in the extracellular space is also affected by increased cellularity, which is seen as a decline in ADC values (Guzman et al., 2008; Klimas et al., 2013). In some cases, however, even advanced MRI techniques, such as DWI and ADC, fail to differentiate pathologies characterized by perilesional edema that require different therapeutic approaches (Suh et al., 2018). Thus, it is more appropriate to focus on finding other possible methods of ADC map analysis to differentiate these brain pathologies. For this purpose, it is necessary to understand how perilesional edema differs at the cellular and molecular level. Our review focuses on pathophysiological mechanisms responsible for BBB changes in common brain lesions, which are characteristically accompanied by perifocal edema such as GBM, brain metastases, lymphomas, and abscesses.
The BBB is composed of non-fenestrated capillaries and strictly regulates the movement of molecules from the intravascular to the interstitial compartment of the brain (Kang et al., 2013). The main cellular components of BBB are endothelial cells (ECs), astrocytes, and pericytes (Ballabh et al., 2004). Endothelial cells line the vascular lumen and are connected at the molecular level by different junctional protein complexes such as tight junctions (TJ), adherent junctions (AJ), and gap junctions (Begley and Brightman, 2003; Solár et al., 2022). TJs are located between endothelial cells and consist of transmembrane proteins, including occludin, claudin, junctional adhesion molecule (JAM) as well as cytoplasmatic proteins that anchor transmembrane proteins to the cytoskeleton of endothelial cells (Stamatovic et al., 2016). The transmembrane protein occludin is considered important to maintaining BBB integrity. Moreover, occludin stretches the TJ and limits the transfer of small molecules through the BBB and thus participates in controlling endothelial paracellular permeability (Hashimoto and Campbell, 2020). Claudins, as other transmembrane proteins, belong to a group of more than 20 proteins that affect BBB permeability to molecules of a certain size and play a role in barrier formation (Hashimoto and Campbell, 2020). Claudins also contribute to high electrical resistance across the barrier by selectively limiting paracellular ion movement (Van Itallie and Anderson, 2004). The role of JAMs (mainly JAM-A but also JAM-B and JAM-C) is to maintain TJ permeability (Aurrand-Lions et al., 2001). Moreover, these TJ proteins regulate the level of integrin expression during pathological conditions and can thus affect leukocyte trafficking into the brain (Kummer and Ebnet, 2018). The transmembrane TJ proteins bind to the cell cytoskeleton through cytoplasmic proteins called zonulins [(ZO), ZO-1, ZO-2, and ZO-3] and cingulin, members of the membrane-associated guanylate kinase (MAGUK) family of proteins (Mitic et al., 2000; Hawkins and Davis, 2005).
Adherent junctions (AJ) are formed by transmembrane glycoproteins, cadherins, cytoplasmic proteins, p120, and catenins α, β, and γ. These proteins are located on the basolateral cell membrane. Cadherins interact with the cytoskeleton of endothelial cells through catenins and p120, members of the Armadillo protein family (Li et al., 2018). Vascular endothelial cadherin (VE-cadherin), a cadherin family protein, plays an important role during the morphogenesis of blood vessels as well as in maintaining cell-cell junction stability and BBB integrity (Vestweber, 2008; Li et al., 2018).
Gap junctions (GJ) are specialized membrane protein structures between adjacent endothelial cells that contribute to maintaining TJ integrity and allow direct intercellular communication (Sweeney et al., 2019). GJ comprise intercellular channels made up of transmembrane proteins called connexins, through which ions and small molecules can pass (Meşe et al., 2007; Stamatovic et al., 2016).
The basement membrane is a layer formed by a complex of extracellular matrix proteins and provides structural support for endothelial cells. The vascular basement membrane consists of a three-dimensional network and contains four major protein complexes made of laminins, collagen IV isoforms, heparan sulfate proteoglycans (perlecan), and nidogens. Collagen IV is the most abundant component of the basement membrane and interacts with endothelial cells and other basement membrane components (Xu et al., 2018). Laminins, a group of extracellular matrix glycoproteins, comprise three α, β, and γ chains and play a role in the organization of the basement membrane (Mak and Mei, 2017). Adhesion receptors play a vital role in maintaining BBB properties. These receptors, including integrins α1β1, α3β1, α6β1, αvβ1/αvβ3, and dystroglycan anchor cells to the basement membrane (Engelhardt and Sorokin, 2009). Integrins, as well as dystroglycan, connect the cytoskeleton of endothelial cells, pericytes, and astrocyte endfeet with extracellular matrix proteins (Del Zoppo et al., 2006; Engelhardt and Sorokin, 2009).
Pericytes are multifunctional cells with cytoplasmic processes attached to the basement membrane and surround the endothelial cells. Pericytes and endothelial cells are connected by different types of proteinaceous junctions such as TJ, GJ, and AJ (Allt and Lawrenson, 2001; Armulik et al., 2011). Moreover, synapse-like peg-socket contacts appear in places where endothelial cells and pericytes are in close contact. These specialized junctions are probably involved in the regulation of brain microcirculation. Other functions of pericytes include participating in the development of the BBB, influencing vascular permeability and inflammatory processes, as well as maintaining vessel stability through growth factors and angiogenic molecules (Dore-Duffy and LaManna, 2007; Nakagawa et al., 2007; Armulik et al., 2011).
Astrocytes are also an important component of the BBB. They are glial cells that interact with endothelial cells through their endfeet projections surrounding the abluminal side of capillaries. Astrocytes maintain homeostasis in the brain microenvironment, control neurotransmitter and ion concentrations, modulate synaptic transmission, and regulate immune reactions (Abbott et al., 2006; Keaney and Campbell, 2015).
GBM IDH-wild-type is the most common primary tumor of the CNS in adults and is characterized by rapid diffuse infiltrative growth of neoplastic glial cells, predominantly with astrocytic differentiation (Ostrom et al., 2021). Tumor cells extend within the CNS mainly along white matter tracts, perivascular space, and subpial surface, which are detectable as secondary structures of diffusely growing GBM in histology samples (Zagzag et al., 2008). Generally, GBM is incurable with a dismal prognosis. Median overall survival is 16 months, notwithstanding standard treatment involving surgical resection followed by adjuvant concomitant chemo-radiotherapy with the alkylating agent temozolomide (Stupp et al., 2005; Lakomy et al., 2020; Tan et al., 2020). Even though GBM is one of the most vascularized neoplasms, antiangiogenic treatment-bevacizumab has exhibited limited effect in primary GBM and its use is currently limited for recurrent GBM (Tan et al., 2020).
Precipitative GBM growth necessitates a robust vascular system to supply the increasing nutrient and metabolic demands of the tumor mass. In contrast to the healthy brain vasculature with a highly organized physiological BBB, tumor vessels in GBM are remarkably irregular, structurally disorganized, and highly permeable, which results in irregular blood flow, vascular leakage, and brain edema. Morphologically GBM microvasculature might be divided into microvascular sprouting, which represents classic angiogenesis forming thin-walled capillaries, vascular mimicries, and bizarre vascular formations (Figure 1; Birner et al., 2003; Preusser et al., 2006; Chen et al., 2015).
Figure 1. GBM microvasculature. Different microvasculature types detected in GBM, including vascular clusters, glomeruloid vascular proliferations, vascular garlands, and vascular mimicry. Vascular mimicry, unlike other microvascular types, shows a lumen lined by tumor cells.
Vascular mimicry, also called vasculogenic mimicry, is the uncanny ability of tumor cells to assume endothelial-like properties, including expression of junction proteins such as VE-cadherin, and form a network of channels independent of endothelial cells reminiscent of a capillary system (Angara et al., 2017). The tumor cells are also surrounded by a rich extracellular matrix forming a basal membrane, which also contains laminins, proteoglycans, such as heparan sulfate as well as collagen IV and VI (Angara et al., 2017; Fernández-Cortés et al., 2019). Vascular mimicry is commonly found in aggressive malignant neoplasms and is linked with poorer prognosis, likely functionally contributing to tumor progression (Liu et al., 2011; Wang et al., 2013; Mei et al., 2020). Bizarre vascular formations, so-called microvascular proliferations (MPVs), are a histological hallmark of GBM. Nonetheless, their presence in the brain is not limited to GBM, as they have also been described in other primary or metastatic brain tumors. MVPs include vascular clusters, vascular garlands as well as glomeruloid vascular proliferations. Vascular clusters are local aggregations of more than three vessels lacking connective tissue ensheathing them (Figure 2). Vascular garlands are multiple vessels structured into complex spacious formations with garland-like architecture, and unlike glomeruloid vascular proliferations, they consist of tight vascular clusters encompassed by connective tissue. The presence of either vascular garlands or glomeruloid vascular proliferations is associated with worse disease outcomes in terms of progression-free survival (Chen et al., 2015). MPV density tends to increase from the tumor infiltrative zone toward the central perinecrotic zone in GBM (Takeuchi et al., 2010). MPVs are multilayered vessels formed of two distinct cell types. The inner luminar site is lined with flat endothelial cells enveloped by the smooth muscle cells/pericytes creating the outer abluminal surface, which is separated from the surrounding brain tissue infiltrated with GBM tumor cells by a network of reticulin/type III collagen fibers (Wesseling et al., 1995; Takeuchi et al., 2010). The disproportional cellular composition of MVPs with hypertrophic and hyperplastic pericytes is linked to their higher proliferation activity in relation to flattened endothelial cells (Díaz-Flores et al., 2021).
Figure 2. Vascular morphology. Microvascular proliferations at the invasive edge of GBM—(A) glomeruloid vascular proliferation (white arrow) and vascular garlands (black arrows). (B) CD31 expression in a vascular garland seen by immunohistochemistry. (C) Metastasis of clear cell renal cell carcinoma to the brain seen on the right side surrounded by microvascular proliferation (arrow). (D) Pulmonary non-mucinous adenocarcinoma metastatic to the brain (bottom left corner) surrounded by multiple glomeruloid microvascular proliferations (arrows). (E) Perivascular infiltration of the PCNSL intermingled with reactive lymphocytes forming vascular cuffs. (F) Reticulin-specific stain highlights the complex reticulin web encompassing the tumor cell caused by neoplastic lymphoid cells penetrating through the vascular wall. (G) Vessels in acute abscess in the brain with leukostasis and neutrophil transmigration across the BBB. H, vessels in chronic abscess display reactive pericytes prominently (white arrows), and no microvascular proliferations are seen. Panels (A–D) magnification 100x, panels (E–H) magnification 400x.
The neurovascular unit is a central component of the BBB that is dynamically modulated through tumor-neurovascular signaling. Glioma-induced BBB injury sequentially progresses to endothelial dysfunction followed by vasogenic edema. Subsequently, paracellular channels in the endothelium allow diffusion of plasmatic water molecules and proteins into the brain parenchyma, leading to characteristic peritumoral brain edema.
The formation of vasogenic edema follows the structural disintegration of the endothelial BBB (Figure 3). Alteration in BBB has been shown to correspond to peritumoral edema in gliomas, suggesting a role for them in the formation of glioma-related edema. Downregulation of claudin-1, claudin-5, and occludin has been repeatedly reported in gliomas (Liebner et al., 2000; Karnati et al., 2014; Zhao et al., 2019). Moreover, the expression levels of claudin-1 and claudin-5 decrease with increasing severity of the glioma, according to World Health Organization (WHO) grading (Karnati et al., 2014). As for TJs expression, metalloproteinase-9 (MMP9) expression, which significantly contributes to GBM invasivity, leads to claudin-5 and occludin degradation (Xue et al., 2017; Zhao et al., 2019).
Figure 3. Disruption of the blood-brain barrier in glioblastoma. GBM cells infiltrate the perivascular space with subsequent astrocytic end-feet displacement. Tumor, stromal, and immune cells occupy a specific tumor niche with a diverse proteomic profile but most importantly show upregulated VEGF and TGF-ß. VEGF binds to its receptor on endothelial cells leading to increased transendothelial permeability and downregulation of specific TJs (e.g., claudin-5, occludin, and ZO-1) and subsequent increased paracellular influx. Matrix metalloproteinases, most importantly MMP-9, that are secreted by tumor cells contribute to the disruption of the basal membrane by cleaving the ECM. Inset shows a more detailed view of the transcellular and paracellular movements across the BBB. The endothelium forms the inner layer of the BBB. GBM promotes a “leaky” phenotype in endothelial cells with increased transcellular transport and endothelial fenestrations. Additionally, down-regulation of TJs leads to increased paracellular transport with subsequent edema formation.
Similarly, down-regulation of claudin-1 and ZO-1 occurred through TGF-ß secretion, which is usually overexpressed in GBM (Ishihara et al., 2008; Han et al., 2015). However, the administration of anti-TGF-ß antibodies restored the barrier properties of the BBB (Ishihara et al., 2008). Up-regulation of claudin-5 and occludin is also seen upon glucocorticoid administration, a commonly used therapy to alleviate peritumoral edema in patients with GBM (Na et al., 2017). Interestingly, downregulation of plasma occludin levels has been shown to correlate with peritumoral brain edema volume and severity, suggesting that it may be used as a potential peritumoral edema biomarker (Park et al., 2006; Shi et al., 2020).
Several other mechanisms, including dysregulation in the expression of VEGF and aquaporins as well as pro-inflammatory changes, contribute to disruption in BBB and result in the development of vasogenic brain edema around GBM (Table 1).
Table 1. Table summarizing molecular and cellular changes in edematous brain tissue around glioblastoma, brain metastasis, lymphoma, and abscess.
Vascular endothelial growth factors (VEGF) and their receptors (VEGFR) represent the main proangiogenic growth factors commonly involved in tumor neovascularization (Apte et al., 2019). Despite the proangiogenic properties of VEGF, it seems that overexpression of VEGF induces down-regulation of claudin-5 and occludin and thus potentiates the paracellular permeability of the BBB (Argaw et al., 2009).
Six VEGF isoforms have so far been identified, but it is the VEGF-A isoform that is most commonly implicated in the glioma-VEGF mediated signaling pathway (Greenberg and Jin, 2005). Needless to say, one of the intrinsic characteristics of glioma is VEGF overexpression (Shweiki et al., 1992; Tamura et al., 2018). VEGF overexpression has been shown to be driven by specific genetic mutations, by HIF-1α under hypoxic conditions, or through autocrine secretion in the tumor microenvironment (Lin et al., 2004; Steiner et al., 2004; Wang et al., 2014; Wen et al., 2017; Almiron Bonnin et al., 2018). Interestingly, VEGF mRNA expression corresponds to the WHO glioma grade, and VEGF-A distribution is specific, with the highest levels of VEGF in the hypoxic tumor core and lower values in the peritumoral zone (Tamura et al., 2018; Ahir et al., 2020). By binding to its respective receptors in the adjacent endothelium and glioma stem cells, VEGF promotes the invasive and proliferative properties of the glioma, thus leading to a hyperpermeable endothelium (Xu et al., 2013; Atzori et al., 2017). This VEGF-induced hyperpermeable endothelium with plasmatic extravasation into the brain parenchyma is the most common reason for the formation of inter-endothelial gaps, endothelial fenestrations, or fragmentations, and alterations in the basement membrane (Dobrogowska et al., 1998). Indeed, VEGF mRNA expression has been shown to correlate with the degree of capillary permeability (Machein et al., 1999). The administration of axitinib, a VEGF/VEGFR inhibitor, leads to increased integrity of the BBB (Wen et al., 2017). Further, VEGF also induces endothelium secreted matrix metalloproteinases with the subsequent disintegration of the extracellular matrix (ECM) and the basal membrane, as was described above (Ahir et al., 2020).
Alternatively, VEGF can induce hyperpermeability by forming vesiculo-vacuolar organelles (VVO) and thus increasing endothelial transcellular transport (Lin et al., 2008). One of the ways GBM harnesses VEGF overexpression is aberrant phosphorylation of STAT3 commonly found in GBM (Brantley et al., 2008; Lin et al., 2014; Ou et al., 2021). Phosphorylated STAT3 (pSTAT3) then upregulates transcription from the VEGF promoter and thus VEGF overexpression (Wang et al., 2014). Phosphorylated STAT3 (pSTAT3) then regulates the VEGF from the VEGF promoter leading to VEGF overexpression (Wang et al., 2014).
Aquaporin is an integral membranous protein that acts as a water channel across cellular membranes (Takata et al., 2004). Numerous isoforms have been recognized, but aquaporin 1 (AQP1) and aquaporin 4 (AQP4) are the most prominent isoforms in the CNS (Verkman et al., 2011). In particular, numerous studies addressed the possible role of AQP4 in glioma-related edema, as well as in the regulation of glioma cell proliferation, invasivity, cell migration, and the brain glymphatic system (Yang et al., 2011; Lan et al., 2017; Hubbard et al., 2018; Simone et al., 2019; Toh and Siow, 2021). AQP4 is usually expressed at the astrocytic end-feet of the gliovascular interface of the BBB (Warth et al., 2004; Noell et al., 2012). These specific microdomains of the neurovascular unit facilitate dynamic changes in cellular volume and contribute to the regulation of the brain glymphatic system. However, gliomas are associated with aberrant spatial expression of astrocytic AQP4 and AQP4 redistribution across the glioma cell surface (Warth et al., 2007). Additionally, AQP4 k/o mice formed aberrant TJs and displayed astrocytic end-feet swelling (Zhou et al., 2008). In parallel, inactivation of the AQP4-associated Na+ channel SUR1-TRPM4 protein complex reverted astrocytic end-feet swelling (Stokum et al., 2018). Taken together, AQP4 expression is essential for the maintenance of the gliovascular interface (Zhou et al., 2008). However, upregulation of AQP4 was also linked to brain edema formation and BBB breakdown (Saadoun et al., 2002a; Yang et al., 2012). Interestingly, this upregulation was specific to certain WHO glioma grades, with the highest expression levels in grade I and IV gliomas (Warth et al., 2007). This correlates with the increased extent of peritumoral edema and increasing peritumoral AQP4 expression (Mou et al., 2010). Correspondingly, overexpression of VEGF in gliomas induces upregulation of AQP4 mRNA expression (Rite et al., 2008). Similarly, alterations in dystroglycan and argin complexes by MMP-2, MMP-3, and MMP-9 lead to edema formation through redistribution and abnormally directed AQP4 mediated water flow (Rauch et al., 2011; Noell et al., 2012).
In-depth molecular studies of AQP4 in GBM suggest that the state of AQP4 aggregation into AQP4 orthogonal arrays of particles (AQP4-OAPs) and disintegration of AQP4 into tetramers may be involved in determining the fate of glioma cells (Noell et al., 2012; Wolburg et al., 2012; Simone et al., 2019). Generally, AQP4-OAPs favor apoptotic glioma cell fate, while AQP4 tetramers increase glioma cell invasiveness. Interestingly, even though glioma tissue shows upregulated AQP4 expression, the expression levels of AQP4-OAPs remain unchanged (Lan et al., 2017).
The role of the specific immune microenvironment in cancer pathogenesis has been extensively investigated in the past few years. These dynamic interactions between glioma tumor cells and immune cells in the tumor microenvironment were shown to influence glioma invasion, infiltration, angiogenesis, or peritumoral edema (Hambardzumyan et al., 2016; Herting et al., 2019; Buonfiglioli and Hambardzumyan, 2021). Apart from glioma tumor cells, tumor-associated macrophages (TAMs) is the dominant non-neoplastic cell type in GBM (Buonfiglioli and Hambardzumyan, 2021). They represent a supportive stromal cell type providing advantageous conditions for GBM potentiation. Historically, an anti-inflammatory M2-like phenotype of TAMs was considered more implicated in GBM pathogenesis. However, recent transcriptomic studies have demonstrated that TAMs in GBM are rather heterogeneous comprising M0-, M1- and M2-like phenotypes leading to the production of both inflammatory and anti-inflammatory signaling molecules (Chen et al., 2020). Interestingly, TAMs express VEGF, IL-1, MMPs, or AQP4, which are all implicated in the pathogenesis of glioma-related edema (Saadoun et al., 2002a; Carlson et al., 2007; Gabrusiewicz et al., 2011; Herting et al., 2019). The upregulation of interleukin-1ß by TAMs has been associated with the suppression of astrocytic sonic-hedgehog signaling. This suppression leads to the downregulation of TJs and increased BBB permeability (Hambardzumyan et al., 2016). Herting et al. (2019) demonstrated that coculture of bone marrow-derived macrophages and organotypic tumor slices stimulated the secretion of both IL-1α and IL-1ß, while coculture with microglia yielded the opposite outcome. Additionally, inhibition of the IL-1 signaling pathway by ablation of the IL-1 receptor reduced BBB permeability and decreased edema formation in a mouse model. Furthermore, dexamethasone administration showed a similar IL-1α and IL-1ß down-regulation (Herting et al., 2019). Correspondingly, upon administration of sulfasalazine, an inhibitor of IL-1ß, cerebral edema decreased in size in the rat glioma model (Sehm et al., 2016). Apart from this, amplified expression of IL-6 has been associated with upregulation of VEGF secretion by GBM cells (Loeffler et al., 2005). Finally, glioma-related edema decreased upon administration of recombinant IL-1 receptor antagonists (Shevtsov et al., 2015).
Brain metastases are tumors primarily originating outside the CNS and spreading into the brain mainly through the hematogenous route or by direct infiltration from adjacent anatomic structures. Among those with the highest propensity to metastasize into the CNS are lung, breast, and renal cell carcinomas, along with melanomas. Brain metastases form solitary or multiple well-circumscribed masses with collateral brain edema (Ostrom et al., 2021).
Disruption of BBB is considered one of the main causes of developing perilesional edema around brain metastases (Tran et al., 2019). Similar to GBM, decreased expression of the TJ protein occludin in brain metastasis contributes to the higher permeability of the BBB and the formation of peritumoral brain edema (Figure 4 and Table 1). Decreased expression of occludin was found not only in the tumor cells themselves but also in non-neoplastic endothelial cells adjacent to brain metastasis. As with GBM, VEGF plays an important role in these changes. Alterations in occludin expression may be caused by both upregulated VEGF and the secreted cytokine, Scatter Factor/Hepatocyte Growth Factor (SF/HGF). These secreted proteins are overexpressed by metastatic cells and may diffuse into peritumoral brain tissue (Papadopoulos et al., 2001). VEGF as well as SF/HGF induces the phosphorylation of occludin leading to its downregulation and subsequent opening of the BBB (Davies, 2002). Moreover, VEGF may induce fenestration in the endothelium and thus increase capillary permeability partially by inhibiting the TJ proteins claudin-1 and claudin-5 (Roberts and Palade, 1995; Song et al., 2018). Along with metastatic cells, tumor-infiltrating lymphocytes in the peritumoral edema also contribute to VEGF expression. This suggests that peritumoral edema is controlled not only by angiogenic cytokines from metastatic cells but also by infiltrating immune cells (Trembath et al., 2020).
Figure 4. Disruption of the peritumoral blood-brain barrier in brain metastasis. Expression of VEGF and SH/VEGF by metastatic cells leads to alteration in TJ proteins such as occludin, claudin-1, and claudin-5 resulting in increased paracellular water influx. In addition to metastatic cells, tumor-infiltrating lymphocytes contribute to the production of VEGF as well as some cytokines including IL-2. Degradation of TJ proteins and the basal membrane is also potentiated by increased expression of matrix metalloproteinases such as MMP-2 and MMP-9. Activation of metalloproteinases is induced by overexpression of ET-1, which leads to up-regulation of ROS and eNOS. Increased expression of AQP4, a part of the glymphatic system on astrocytic endfeet around metastatic lesions, contributes to the development of vasogenic edema by the accumulation of CSF into the brain interstitium.
VEGF action involves the synthesis and release of nitric oxide, activation of soluble guanylate cyclase and the consequent conversion of GTP to cGMP. This pathway of VEGF action contributes to BBB alteration and the development of peritumoral brain edema (Chi et al., 1999; Mayhan, 1999). The effect of NO on the development of edema around metastatic brain tumors can also be enhanced by increased expression of endothelial nitric oxide synthase (eNOS), not only in the tumor itself but also in the peritumoral brain area (Broholm et al., 2003).
VEGF has been found to cooperate with integrin αvβ5 and FAK and activate the Ras-ERK cascade. αvβ5 integrin expression was found on peritumoral vessels as well as on blood vessels in the surrounding brain parenchyma. On the other hand, increased expression of αvβ3 was found only on angiogenic, sprouting peritumoral vessels containing multilayered endothelial cells. Similarly, αvβ3, in collaboration with Basic Fibroblast Growth Factor (bFGF) receptor, activates downstream RAS and c-Raf, which leads to sustained Ras-ERK activation. These pathways regulate the expression of genes involved in cell survival, proliferation, differentiation, and migration (Hood et al., 2003; Berghoff et al., 2013). Moreover, it was shown experimentally that an increase in integrin αvβ3 leads to increased expression of MMP-2 and MMP-9, internalization of occludin and ZO-1, and the disruption of VE-cadherin localization (Edwards and Bix, 2019).
Activation of VEGF receptor 2 regulates the functions of vascular endothelium, leading to the generation of inositol trisphosphate (IP3) and diacylglycerol (DAG). These molecules increase the intracellular concentration of Ca2+, which activates eNOS and, in turn, generates NO. The elevated level of intracellular calcium also stimulates the cytosolic phospholipase A (cPLA) and the production of prostaglandins. Vasoactive molecules, including NO and prostaglandins, lead to increased vascular cell permeability (Song et al., 2018). Other factors such as AQP4 and metalloproteinases are implicated in the development of brain peritumoral edema (Tran et al., 2019). This is supported by increased expression of AQP4 that was observed within peritumoral astrocytes adjacent to the brain metastasis (Saadoun et al., 2002b). Unlike peritumoral edema, AQP4 expression is decreased in the blood-tumor barrier (Zhao et al., 2015). In addition to AQP4, upregulation of Kir4.1 and α- syntrophin expression was found in astrocytes surrounding carcinoma metastasis (Saadoun et al., 2003). As part of the brain glymphatic system, increased expression of AQP4 channels in the peritumoral edema may be involved in elevated periarterial influx of CSF into the brain interstitium (Toh et al., 2021). Sulfonylurea receptor 1 (SUR1) may play a role in the development of cerebral metastatic-related vasogenic edema. Expression of SUR1 was found in glial and endothelial cells in the pericellular areas of the metastatic cells. Inhibition of SUR1 leads to decreased ZO-1 gap formation, suggesting that it acts on TJ proteins and thus on the BBB (Thompson et al., 2013). Moreover, SUR1 regulates the activity of the non-selective cation channel NCCa-ATP. Activation of these channels leads to Na+ influx followed by water influx to maintain osmotic neutrality resulting in cytotoxic edema. Elevated intracellular Na+ leads to alteration in the actin cytoskeleton and thus in the integrity of tight junctions. These changes increase paracellular plasma transfer and result in the development of vasogenic, plasma-rich edema (Simard et al., 2006, 2009).
Reactive glial cells participate in NO-independent pathways that may be involved in the development of peritumoral brain edema around brain metastases. Cells such as microglia and astrocytes were found to contribute to an inflammatory response in these areas (Utsuki et al., 2007; Fitzgerald et al., 2008). Reactive astrocytes produce endothelins that are released into the fluid microenvironment of the peritumoral brain (Zhang and Olsson, 1995). Endothelin-1 (ET-1), for instance, is known as a potent vasoconstrictor. In addition to the vasoconstrictive effect of ET-1, its overexpression in astrocytes could contribute to the development of cytotoxic edema (Yeung et al., 2009). ET-1 activates the ETB receptor, which subsequently induces the expression of eNOS, which in turn causes the activation of MMP-9 resulting in ZO-1 degradation and vasogenic edema formation (Kim et al., 2015). Moreover, activation of ETB receptors in astrocyte endfeet may generate intracellular reactive oxygen species (ROS) through the action of NADPH oxidase and cause dystrophin, the anchor protein for AQP4 to malfunction (Kim et al., 2013).
The microvascular density of brain metastases may influence the size of brain edema. High neovascularization and microvascular density were associated with large peritumoral edema (Figure 2). On the other hand, low microvascular density was found in brain metastases with small peritumoral edema. A similar pattern was found for hypoxia-induced factor 1 alpha (HIF1α) expression (Spanberger et al., 2013). The size of the peritumoral edema may reflect the extent of immune activation against brain metastases. In support of this, a correlation has been observed between the amount of peritumoral brain edema and the density of tumor-infiltrating CD8 + lymphocytes in brain metastases. The formation of edema is probably related to the release of cytokines such as IL-2 that stimulate T-lymphocytes (Berghoff et al., 2016).
Glymphatic pathways may also play a role in the development of peritumoral edema. Recently, it was suggested that brain metastases may disrupt movement in the glymphatic pathways. Altered glymphatic pathways complicate interstitial fluid clearance and contribute to its accumulation in the tumor interstitium and peritumoral edema formation (Toh et al., 2021).
The CNS might be affected by a wide range of hematological neoplasms, either primarily originating within the CNS or by secondary spread from lymphomas that produce systemic disease. Primary CNS lymphomas (PCNSL) are a type of extranodal non-Hodgkin’s lymphoma, accounting for less than 1% of non-Hodgkin’s lymphomas. Of these PCNSLs, diffuse large cell B-cell lymphoma of the CNS is the most common histologically defined tumor (Wang et al., 2021). PCNSL typically forms either single or multiple hypercellular, diffusely growing lesions (Krebs et al., 2021). Predominant perivascular aggregation, in the form of tight vascular cuffs of neoplastic cells intermingled with reactive non-neoplastic infiltrating inflammatory lymphocytes and macrophages, is typically detected at the periphery (Marcelis et al., 2020). Nevertheless, PCNSLs are still poorly understood compared to GBM due to their significantly lower incidence.
A higher vascular permeability in PCNSL was detected by MRI imaging compared to GBM, which implies a greater degree of BBB disruption within the bulk tumor (Kickingereder et al., 2014). This BBB disruption within the tumor mass is seen as increased vessel permeability and corresponds to structural changes in microscopic observations. Vascular involvement is uneven, where neoplastic cells predominantly affect large vessels and not capillaries. Initially, the basal membrane and glial end-feet maintain an impermeable barrier, holding tumor cells within the vascular wall (Aho et al., 1993). Neoplastic PCNSL cells readily widen the co-opted vascular wall by penetrating through its rich reticulin fiber network, consisting of collagen type III and IV, laminin and fibronectin, thus forming a delicate web encompassing angiocentric tumor cells (Aho et al., 1993; Kickingereder et al., 2014). Later, the outer vascular reticulin layer in the PCNSL as well as in the secondary CNS lymphoma opens, which allows neoplastic lymphoid cells to infiltrate surrounding brain tissue (Figure 5 and Table 1). This brain tissue infiltration is rarely seen in reactive lymphocytic infiltration (Figure 2; Aho et al., 1993).
Figure 5. Development of primary CNS lymphoma. Progression of PCNSL (clockwise from top). Initially, neoplastic lymphoid cells accumulate within the vascular wall of arteries and venules fragmenting the reticular fiber network. The outer vascular layer with the glial end-feet maintains an impermeable barrier preventing tumor cells from infiltrating the brain tissue. Later, tumor cells fragment the outer layer and infiltrate brain tissue, where multiple reactive astrocytes can be detected. Endothelial cells undergo regressive as well as reparative changes throughout, leading to disruption of the endothelial lining.
The endothelial cell lining, originating from vascular progenitor cells or presumably even from tumor cells, is frequently flattened with fenestrations, or is discontinuous, exposing a duplicated or frayed basal membrane (Ruggieri et al., 2017). Interestingly, a similar frequency of apoptotic endothelial cells was observed regardless of corticosteroid drug use. Intact endothelial cells display various degrees of regressive as well as regenerative changes. Endothelial cells frequently lack direct contact with normal astrocytic processes. Intercellular junctions between endothelial cells vary considerably in length, width, and density but appear to be functional. Pericytes are preserved in many cases and are intertwined in irregular and discontinued basal membranes (Molnár et al., 1999). These vascular changes are accompanied by reactive gliosis in the surrounding brain tissue (Soussain et al., 2007). Furthermore, significant variation has been detected in the preservation of BBB function in tumor-affected brain vessels in PCNSL showing varied VEGF expression. Tumors expressing VEGF presented with high microvascular density contain newly formed vessels and lack a well-formed BBB. Endothelial cells in immature PCNSL capillaries were fenestrated, similar to VEGF-induced hyperpermeable endothelium. Furthermore, the expression of occludin and ZO-1 was also significantly reduced. VEGF expressing PCNSL patients survived significantly longer when compared with the VEGF negative group, which exhibited preserved BBB function (Takeuchi et al., 2007). On the other hand, VEGF overexpression in non-Hodgkin’s lymphoma outside of the CNS is associated with a worse prognosis (Yang et al., 2015). Preserving BBB function in some PCNSL limits therapeutic agents from acting and thus might be directly linked with worse clinical outcomes. Furthermore, alterations in BBB function might occur to different degrees in various tumor lesions, of which some might remain completely unaltered and thus be virtually undetectable by current imaging while also being less available for therapeutic agents. This suggestion is supported clinically by frequent tumor relapse at a site distant from that of the initial tumor (Calimeri et al., 2021). Moreover, leaky BBB reconstitutes after therapy-induced tumor shrinkage and subsequent treatment is thus rendered less effective (Maza et al., 2009; Iorio-Morin et al., 2020). Autopsy studies have demonstrated that where imaging failed to detect the full extent of the tumor burden, PCNSL recurrence infiltrates the CNS diffusely (Lai et al., 2002).
The most common causes of developing brain abscess are hematogenous spread, infected emboli, the extension of extracranial infections, penetrating head trauma, or complications of neurosurgical procedures. Nonetheless, in some cases, there is no obvious source. The most common organisms causing bacterial abscesses are Staphylococcus aureus and Streptococcus species (Mathisen and Johnson, 1997).
Disruption of the BBB following brain abscess leads to the development of vasogenic brain edema (Figure 6 and Table 1; Papadopoulos and Verkman, 2007). Changes in edematous brain tissue include shrunken cells with nuclear condensation resulting in pyknotic nuclei caused by neurotoxins such as α-hemolysin in Staphylococcus infection (Dahlberg et al., 2015b).
Figure 6. Molecular and cellular changes in the blood-brain barrier around brain abscess. Bacteria and other microorganisms in brain abscess release PAMPs that are recognized by TLR2 on astrocytes and microglia. Activation of TLR2 receptors leads to increased expression of various cytokines and chemokines, including TNF-α, IL-1β, IL-12, and MIP-2. These pro-inflammatory molecules increase the expression of adhesion molecules such as ICAM-1 and VCAM-1 that interact with integrins (LFA-1 and VLA-4) on immune cells and thus potentiate the transfer of leukocytes across the BBB. The pro-inflammatory molecules, as well as ROS, lead to increased expression and activation of matrix metalloproteinases resulting in disruption of TJ proteins. The formation of ROS is potentiated by metal ions, mainly potassium, zinc, and copper, that are released from the abscess. Moreover, decreased expression of TJ proteins such as claudin-5 and occludin is also potentiated by increased expression of VEGF from reactive astrocytes.
Cellular changes in the BBB include gaps in the endothelial cell layer (Wallenfang et al., 1980). Activated astrocytes form a diffusible barrier from fibrous tissue which surrounds the abscess. Some metal ions, mainly potassium and zinc, and others, including iron and copper, may penetrate from the abscess cavity into the brain tissue around the abscess. The elevated level of these ions in brain tissue leads to neuronal depolarization, inhibition of glutamate and GABA receptors, and formation of ROS that can contribute to BBB disruption (Pun et al., 2009; Hassel et al., 2014; Dahlberg et al., 2015a; Vazana et al., 2016). Moreover, higher concentrations of ammonia were found in brain edema. Elevated ammonia level in brain tissue around the abscess leads to swelling of astrocytes (Dahlberg et al., 2016). On the other hand, increased expression of the glial fibrillary acidic protein (GFAP) in activated astrocytes surrounding the brain abscess probably contributes to reducing the spread of these molecules and pathogens within the brain (Stenzel et al., 2004).
Reactive astrocytes and inflammatory cells are responsible for the production of VEGF, which affects BBB integrity in brain tissue surrounding the abscess (Vaquero et al., 2001). Upregulation of VEGF in reactive astrocytes leads to downregulation of claudin-5 and occludin, resulting in BBB disruption and the development of brain edema (Archie et al., 2021).
Accumulation of extracellular fluid following BBB disruption is reduced by upregulation of AQP4 expression in reactive astrocytes. Increased AQP4 expression was found in the capsule surrounding the abscess. AQP4 protection probably takes the form of fluid reabsorption from the brain tissue around the abscess and the consequent attenuation of vasogenic edema (Bloch et al., 2005). However, this effect may be attenuated by AQP4 downregulation in edematous brain vessels in the neighboring area of the brain (Ampawong and Luplertlop, 2019).
TNF-α plays an important role in the development of brain edema and the regulation of the immune response to brain abscesses (Stenzel et al., 2005a,b). Astrocytes and microglia are involved in the production of pro-inflammatory cytokines and chemokines that lead to BBB disruption and cause albumin and IgG accumulation in brain parenchyma resulting in the development of vasogenic edema. Pathogen-associated molecular patterns (PAMPs) released from bacteria are recognized by toll-like receptor 2 (TLR2) on astrocytes and microglia. Increased expression of TLR2 mediates glial activation in response to gram-positive bacteria such as Staphylococcus aureus (Esen et al., 2004; Kielian et al., 2005a). MyD88, the adapter protein for the majority of TLRs, is involved in downstream pathways and plays a role in the protective host CNS response during brain abscess development, thus also affecting the magnitude of brain edema (Kielian et al., 2007). Activation of TLR2 leads to increased expression of various cytokines such as TNF-α, IL-1β, IL-12, and chemokines including MIP-2, MIP-1α, β, MCP-1, and RANTES. It appears that TLR2 induces overexpression of pro-inflammatory molecules mainly during the acute stage of brain abscess (Kielian et al., 2005b). The released pro-inflammatory cytokines increase the expression of adhesion molecules, ICAM and VCAM that facilitate the movement of macrophages, neutrophils, and T cells across the BBB into the abscess (Figure 2; Kielian, 2004). Based on the observation that the fibrous capsule does not form a diffusion barrier (Dahlberg et al., 2015a), it is likely that these pro-inflammatory molecules can diffuse into the surrounding brain tissue and alter the BBB. CD3+, CD4+, Th1 and Th17 cells also seem to play an important role in the regulation of immune cell infiltration in the later stages of brain abscess. Th1 and Th17 regulate the release of inflammatory mediators from infiltrating macrophages and neutrophils (Holley and Kielian, 2012). Immune cells (mainly macrophages and neutrophils) release degradative enzymes such as metalloproteinases which lead to proteolysis of the basement membrane and TJ proteins and thus potentiate extravasation of peripheral immune cells through the BBB into the brain parenchyma (Baldwin and Kielian, 2004; Rempe et al., 2016). Rapid and sustained expression of CXC chemokines such as keratinocytes-derived chemokine (KC) and MIP-2 by resident glial cells play an important role in the recruitment of neutrophils (Kielian et al., 2001). BBB disruption and increased permeability in the early phase of brain abscess are important events in the inflammatory response and the development of abscess-associated brain edema (Lo et al., 1994).
As described in this review, primary and secondary brain tumors or tumor-like brain lesions showed characteristic features of BBB damage resulting in the development of vasogenic brain edema. In general, BBB damage is mainly due to varying degrees of perilesional tumor cell infiltration, formation of new vessels, destruction of ECM, alteration of TJ proteins between endothelial cells, impaired cellular expression of growth factors, cellular channels, or inflammatory reactions. These changes are part of the complex pathophysiology of brain edema and present themselves to different extents in diagnoses like GBM, brain metastases, lymphomas, or abscesses. Future research should therefore focus on finding specific pattern(s) in vasogenic edema in different brain lesions that can be detected and quantified by advanced MRI techniques such as ADC maps. These patterns can then contribute to improved diagnosis so that appropriate treatment may be applied for these brain pathologies.
PS, MiH, and MB designed the study and wrote the manuscript. HV prepared the figures and participated in the preparation of the manuscript. MaH and RJ participated in the supervision and editing of the manuscript. All authors contributed to the article and approved the submitted version.
This work was supported by an internal grant from the St. Anne’s University Hospital Brno (grant no. FNDN_IP21IPV15AXA_PN), the Internal Grant Agency of Masaryk University (MUNI/A/1408/2021), and the Ministry of Education, Youth and Sports, Czechia—INTER-EXCELLENCE, project n. LTC20027 (program INTER-COST).
The authors declare that the research was conducted in the absence of any commercial or financial relationships that could be construed as a potential conflict of interest.
All claims expressed in this article are solely those of the authors and do not necessarily represent those of their affiliated organizations, or those of the publisher, the editors and the reviewers. Any product that may be evaluated in this article, or claim that may be made by its manufacturer, is not guaranteed or endorsed by the publisher.
ADC, apparent diffusion coefficient; CNS, central nervous system; GBM, glioblastoma; DWI, diffusion-weighted imaging; ADC, apparent diffusion coefficient; ECs, endothelial cells; TJ, tight junctions; AJ, adherent junctions; GJ, gap junctions; JAM, junctional adhesion molecule; ZO, zonula occludens; VE-cadherin, vascular endothelial cadherin; MPVs, microvascular proliferations; MMP, matrix metalloproteinase; VEGF, vascular endothelial growth factors; ECM, extracellular matrix; VVO, vesiculo-vacuolar organelles; pSTAT3, phosphorylated STAT3; AQP, aquaporin; AQP4-OAPs, orthogonal arrays of AQP4 particles; TAMs, tumor-associated macrophages; SF/HGF, scatter factor/hepatocyte growth factor; eNOS, endothelial nitric oxide synthase; bFGF, basic fibroblast growth factor; VEGFR2, vascular endothelial growth factor 2; IP3, inositol trisphosphate; DAG, diacylglycerol; cPLA, cytosolic phospholipase A; SUR1, sulfonylurea receptor 1; NCCa-ATP, ATP/Ca2 + non-selective cation channel; ET-1, endothelin-1; ROS, reactive oxygen species; HIF1 α, hypoxia-induced factor 1 alpha; PCNSL, primary CNS lymphomas; GFAP, glial fibrillary acidic protein; PAMPs, pathogen-associated molecular patterns; TLR2, toll-like receptor 2; KC, keratinocytes-derived chemokine.
Abbott, N. J., Rönnbäck, L., and Hansson, E. (2006). Astrocyte-endothelial interactions at the blood-brain barrier. Nat. Rev. Neurosci. 7, 41–53. doi: 10.1038/nrn1824
Ahir, B. K., Engelhard, H. H., and Lakka, S. S. (2020). Tumor Development and Angiogenesis in Adult Brain Tumor: glioblastoma. Mol. Neurobiol. 57, 2461–2478. doi: 10.1007/s12035-020-01892-8
Aho, R., Ekfors, T., Haltia, M., and Kalimo, H. (1993). Pathogenesis of primary central nervous system lymphoma: invasion of malignant lymphoid cells into and within the brain parenchyme. Acta Neuropathol. 86, 71–76. doi: 10.1007/BF00454901
Allt, G., and Lawrenson, J. G. (2001). Pericytes: cell biology and pathology. Cells Tissues Organs 169, 1–11. doi: 10.1159/000047855
Almiron Bonnin, D. A., Havrda, M. C., Lee, M. C., Liu, H., Zhang, Z., Nguyen, L. N., et al. (2018). Secretion-mediated STAT3 activation promotes self-renewal of glioma stem-like cells during hypoxia. Oncogene 37, 1107–1118. doi: 10.1038/onc.2017.404
Ampawong, S., and Luplertlop, N. (2019). Experimental Scedosporiosis Induces Cerebral Oedema Associated with Abscess regarding Aquaporin-4 and Nrf-2 Depletions. Biomed. Res. Int. 2019:6076571. doi: 10.1155/2019/6076571
Angara, K., Borin, T. F., and Arbab, A. S. (2017). Vascular Mimicry: a Novel Neovascularization Mechanism Driving Anti-Angiogenic Therapy (AAT) Resistance in Glioblastoma. Transl. Oncol. 10, 650–660. doi: 10.1016/j.tranon.2017.04.007
Apte, R. S., Chen, D. S., and Ferrara, N. (2019). VEGF in Signaling and Disease: beyond Discovery and Development. Cell 176, 1248–1264. doi: 10.1016/j.cell.2019.01.021
Archie, S. R., Al Shoyaib, A., and Cucullo, L. (2021). Blood-Brain Barrier Dysfunction in CNS Disorders and Putative Therapeutic Targets: an Overview. Pharmaceutics 13:1779. doi: 10.3390/pharmaceutics13111779
Argaw, A. T., Gurfein, B. T., Zhang, Y., Zameer, A., and John, G. R. (2009). VEGF-mediated disruption of endothelial CLN-5 promotes blood-brain barrier breakdown. Proc. Natl. Acad. Sci. U S A 106, 1977–1982. doi: 10.1073/pnas.0808698106
Armulik, A., Genové, G., and Betsholtz, C. (2011). Pericytes: developmental, physiological, and pathological perspectives, problems, and promises. Dev. Cell 21, 193–215. doi: 10.1016/j.devcel.2011.07.001
Arvanitis, C. D., Ferraro, G. B., and Jain, R. K. (2020). The blood-brain barrier and blood-tumour barrier in brain tumours and metastases. Nat. Rev. Cancer 20, 26–41. doi: 10.1038/s41568-019-0205-x
Atzori, M. G., Tentori, L., Ruffini, F., Ceci, C., Lisi, L., Bonanno, E., et al. (2017). The anti-vascular endothelial growth factor receptor-1 monoclonal antibody D16F7 inhibits invasiveness of human glioblastoma and glioblastoma stem cells. J. Exp. Clin. Cancer Res. 36:106. doi: 10.1186/s13046-017-0577-2
Aurrand-Lions, M., Johnson-Leger, C., Wong, C., Du Pasquier, L., and Imhof, B. A. (2001). Heterogeneity of endothelial junctions is reflected by differential expression and specific subcellular localization of the three JAM family members. Blood 98, 3699–3707. doi: 10.1182/blood.v98.13.3699
Badaut, J., Ashwal, S., and Obenaus, A. (2011). Aquaporins in cerebrovascular disease: a target for treatment of brain edema? Cerebrovasc. Dis. 31, 521–531. doi: 10.1159/000324328
Baldwin, A. C., and Kielian, T. (2004). Persistent immune activation associated with a mouse model of Staphylococcus aureus-induced experimental brain abscess. J. Neuroimmunol. 151, 24–32. doi: 10.1016/j.jneuroim.2004.02.002
Ballabh, P., Braun, A., and Nedergaard, M. (2004). The blood-brain barrier: an overview: structure, regulation, and clinical implications. Neurobiol. Dis. 16, 1–13. doi: 10.1016/j.nbd.2003.12.016
Begley, D. J., and Brightman, M. W. (2003). ““Structural and functional aspects of the blood-brain barrier,”,” in Peptide Transport and Delivery into the Central Nervous System Progress in Drug Research, eds L. Prokai and K. Prokai-Tatrai (Basel: Birkhäuser), 39–78. doi: 10.1007/978-3-0348-8049-7_2
Berghoff, A. S., Fuchs, E., Ricken, G., Mlecnik, B., Bindea, G., Spanberger, T., et al. (2016). Density of tumor-infiltrating lymphocytes correlates with extent of brain edema and overall survival time in patients with brain metastases. Oncoimmunology 5:e1057388. doi: 10.1080/2162402X.2015.1057388
Berghoff, A. S., Rajky, O., Winkler, F., Bartsch, R., Furtner, J., Hainfellner, J. A., et al. (2013). Invasion patterns in brain metastases of solid cancers. Neuro. Oncol. 15, 1664–1672. doi: 10.1093/neuonc/not112
Birner, P., Piribauer, M., Fischer, I., Gatterbauer, B., Marosi, C., Ambros, P. F., et al. (2003). Vascular patterns in glioblastoma influence clinical outcome and associate with variable expression of angiogenic proteins: evidence for distinct angiogenic subtypes. Brain Pathol. 13, 133–143. doi: 10.1111/j.1750-3639.2003.tb00013.x
Bloch, O., Papadopoulos, M. C., Manley, G. T., and Verkman, A. S. (2005). Aquaporin-4 gene deletion in mice increases focal edema associated with staphylococcal brain abscess. J. Neurochem. 95, 254–262. doi: 10.1111/j.1471-4159.2005.03362.x
Brantley, E. C., Nabors, L. B., Gillespie, G. Y., Choi, Y.-H., Palmer, C. A., Harrison, K., et al. (2008). Loss of PIAS3 Expression in Glioblastoma Multiforme Tumors: implications for STAT-3 Activation and Gene Expression. Clin. Cancer Res. 14, 4694–4704. doi: 10.1158/1078-0432.CCR-08-0618
Broholm, H., Rubin, I., Kruse, A., Braendstrup, O., Schmidt, K., Skriver, E. B., et al. (2003). Nitric oxide synthase expression and enzymatic activity in human brain tumors. Clin. Neuropathol. 22, 273–281.
Buonfiglioli, A., and Hambardzumyan, D. (2021). Macrophages and microglia: the cerberus of glioblastoma. Acta Neuropathol. Commun. 9:54. doi: 10.1186/s40478-021-01156-z
Calimeri, T., Marcucci, F., and Corti, A. (2021). Overcoming the blood-brain barrier in primary central nervous system lymphoma: a review on new strategies to solve an old problem. Ann. Lymphoma 5, doi: 10.21037/aol-20-54
Carlson, M. R. J., Pope, W. B., Horvath, S., Braunstein, J. G., Nghiemphu, P., Tso, C.-L., et al. (2007). Relationship between survival and edema in malignant gliomas: role of vascular endothelial growth factor and neuronal pentraxin 2. Clin. Cancer Res. 13, 2592–2598. doi: 10.1158/1078-0432.CCR-06-2772
Chen, L., Lin, Z.-X., Lin, G.-S., Zhou, C.-F., Chen, Y.-P., Wang, X.-F., et al. (2015). Classification of microvascular patterns via cluster analysis reveals their prognostic significance in glioblastoma. Hum. Pathol. 46, 120–128. doi: 10.1016/j.humpath.2014.10.002
Chen, Z., Herting, C. J., Ross, J. L., Gabanic, B., Puigdelloses Vallcorba, M., Szulzewsky, F., et al. (2020). Genetic driver mutations introduced in identical cell-of-origin in murine glioblastoma reveal distinct immune landscapes but similar response to checkpoint blockade. Glia 68, 2148–2166. doi: 10.1002/glia.23883
Chi, O. Z., Liu, X., and Weiss, H. R. (1999). Effects of cyclic GMP on microvascular permeability of the cerebral cortex. Microvasc. Res. 58, 35–40. doi: 10.1006/mvre.1999.2152
Coureuil, M., Lécuyer, H., Bourdoulous, S., and Nassif, X. (2017). A journey into the brain: insight into how bacterial pathogens cross blood-brain barriers. Nat. Rev. Microbiol. 15, 149–159. doi: 10.1038/nrmicro.2016.178
Cramer, S. P., and Larsson, H. B. (2014). Accurate Determination of Blood–Brain Barrier Permeability Using Dynamic Contrast-Enhanced T1-Weighted MRI: a Simulation and in vivo Study on Healthy Subjects and Multiple Sclerosis Patients. J. Cereb. Blood Flow Metab. 34, 1655–1665. doi: 10.1038/jcbfm.2014.126
Dahlberg, D., Ivanovic, J., and Hassel, B. (2016). Toxic levels of ammonia in human brain abscess. J. Neurosurg. 124, 854–860. doi: 10.3171/2015.1.JNS142582
Dahlberg, D., Mariussen, E., Goverud, I. L., Tønjum, T., Mæhlen, J., Antal, E.-A., et al. (2015b). Staphylococcal α-hemolysin is neurotoxic and causes lysis of brain cells in vivo and in vitro. Neurotoxicology 48, 61–67. doi: 10.1016/j.neuro.2015.03.001
Dahlberg, D., Ivanovic, J., Mariussen, E., and Hassel, B. (2015a). High extracellular levels of potassium and trace metals in human brain abscess. Neurochem. Int. 82, 28–32. doi: 10.1016/j.neuint.2015.02.003
Dalby, T., Wohl, E., Dinsmore, M., Unger, Z., Chowdhury, T., and Venkatraghavan, L. (2021). Pathophysiology of Cerebral Edema—A Comprehensive Review. J. Neuroanaesth. Crit. Care 08, 163–172. doi: 10.1055/s-0040-1721165
Davies, D. C. (2002). Blood-brain barrier breakdown in septic encephalopathy and brain tumours. J. Anat. 200, 639–646. doi: 10.1046/j.1469-7580.2002.00065.x
Del Zoppo, G. J., Milner, R., Mabuchi, T., Hung, S., Wang, X., and Koziol, J. A. (2006). Vascular matrix adhesion and the blood-brain barrier. Biochem. Soc. Trans. 34, 1261–1266. doi: 10.1042/BST0341261
Díaz-Flores, L., Gutiérrez, R., González-Gómez, M., García, M.-D.-P., Díaz-Flores, L., González-Marrero, I., et al. (2021). Disproportion in Pericyte/Endothelial Cell Proliferation and Mechanisms of Intussusceptive Angiogenesis Participate in Bizarre Vessel Formation in Glioblastoma. Cells 10:2625. doi: 10.3390/cells10102625
Dobrogowska, D. H., Lossinsky, A. S., Tarnawski, M., and Vorbrodt, A. W. (1998). Increased blood-brain barrier permeability and endothelial abnormalities induced by vascular endothelial growth factor. J. Neurocytol. 27, 163–173. doi: 10.1023/a:1006907608230
Dore-Duffy, P., and LaManna, J. C. (2007). Physiologic angiodynamics in the brain. Antioxid. Redox Signal 9, 1363–1371. doi: 10.1089/ars.2007.1713
Edwards, D. N., and Bix, G. J. (2019). Roles of blood-brain barrier integrins and extracellular matrix in stroke. Am. J. Physiol.-Cell Physiol. 316, C252–C263. doi: 10.1152/ajpcell.00151.2018
Engelhardt, B., and Sorokin, L. (2009). The blood-brain and the blood-cerebrospinal fluid barriers: function and dysfunction. Semin. Immunopathol. 31, 497–511. doi: 10.1007/s00281-009-0177-0
Esen, N., Tanga, F. Y., DeLeo, J. A., and Kielian, T. (2004). Toll-like receptor 2 (TLR2) mediates astrocyte activation in response to the Gram-positive bacterium Staphylococcus aureus. J. Neurochem. 88, 746–758. doi: 10.1046/j.1471-4159.2003.02202.x
Fernández-Cortés, M., Delgado-Bellido, D., and Oliver, F. J. (2019). Vasculogenic Mimicry: become an Endothelial Cell “But Not So Much.”. Front. Oncol. 9:803. doi: 10.3389/fonc.2019.00803
Fitzgerald, D. P., Palmieri, D., Hua, E., Hargrave, E., Herring, J. M., Qian, Y., et al. (2008). Reactive glia are recruited by highly proliferative brain metastases of breast cancer and promote tumor cell colonization. Clin. Exp. Metastasis 25, 799–810. doi: 10.1007/s10585-008-9193-z
Gabrusiewicz, K., Ellert-Miklaszewska, A., Lipko, M., Sielska, M., Frankowska, M., and Kaminska, B. (2011). Characteristics of the alternative phenotype of microglia/macrophages and its modulation in experimental gliomas. PLoS One 6:e23902. doi: 10.1371/journal.pone.0023902
Gorelick, P. B., Furie, K. L., Iadecola, C., Smith, E. E., Waddy, S. P., Lloyd-Jones, D. M., et al. (2017). Defining Optimal Brain Health in Adults: a Presidential Advisory From the American Heart Association/American Stroke Association. Stroke 48:e284–e303. doi: 10.1161/STR.0000000000000148
Greenberg, D. A., and Jin, K. (2005). From angiogenesis to neuropathology. Nature 438, 954–959. doi: 10.1038/nature04481
Guzman, R., Altrichter, S., El-Koussy, M., Gralla, J., Weis, J., Barth, A., et al. (2008). Contribution of the apparent diffusion coefficient in perilesional edema for the assessment of brain tumors. J. Neuroradiol. 35, 224–229. doi: 10.1016/j.neurad.2008.02.003
Hambardzumyan, D., Gutmann, D. H., and Kettenmann, H. (2016). The role of microglia and macrophages in glioma maintenance and progression. Nat. Neurosci. 19, 20–27. doi: 10.1038/nn.4185
Han, J., Alvarez-Breckenridge, C. A., Wang, Q.-E., and Yu, J. (2015). TGF-β signaling and its targeting for glioma treatment. Am. J. Cancer Res. 5, 945–955.
Hashimoto, Y., and Campbell, M. (2020). Tight junction modulation at the blood-brain barrier: current and future perspectives. Biochim. Biophys. Acta Biomembr. 1862:183298. doi: 10.1016/j.bbamem.2020.183298
Hassel, B., Dahlberg, D., Mariussen, E., Goverud, I. L., Antal, E.-A., Tønjum, T., et al. (2014). Brain infection with Staphylococcus aureus leads to high extracellular levels of glutamate, aspartate, γ-aminobutyric acid, and zinc. J. Neurosci. Res. 92, 1792–1800. doi: 10.1002/jnr.23444
Hawkins, B. T., and Davis, T. P. (2005). The blood-brain barrier/neurovascular unit in health and disease. Pharmacol. Rev. 57, 173–185. doi: 10.1124/pr.57.2.4
Herting, C. J., Chen, Z., Maximov, V., Duffy, A., Szulzewsky, F., Shayakhmetov, D. M., et al. (2019). Tumour-associated macrophage-derived interleukin-1 mediates glioblastoma-associated cerebral oedema. Brain 142, 3834–3851. doi: 10.1093/brain/awz331
Holley, M. M., and Kielian, T. (2012). Th1 and Th17 cells regulate innate immune responses and bacterial clearance during central nervous system infection. J. Immunol. 188, 1360–1370. doi: 10.4049/jimmunol.1101660
Hood, J. D., Frausto, R., Kiosses, W. B., Schwartz, M. A., and Cheresh, D. A. (2003). Differential αv integrin–mediated Ras-ERK signaling during two pathways of angiogenesis. J. Cell Biol. 162, 933–943. doi: 10.1083/jcb.200304105
Hubbard, J. A., Szu, J. I., and Binder, D. K. (2018). The role of aquaporin-4 in synaptic plasticity, memory and disease. Brain Res. Bull. 136, 118–129. doi: 10.1016/j.brainresbull.2017.02.011
Iorio-Morin, C., Gahide, G., Morin, C., Vanderweyen, D., Roy, M.-A., St-Pierre, I., et al. (2020). Management of Primary Central Nervous System Lymphoma Using Intra-Arterial Chemotherapy With Osmotic Blood-Brain Barrier Disruption: retrospective Analysis of the Sherbrooke Cohort. Front. Oncol. 10:543648. doi: 10.3389/fonc.2020.543648
Ishihara, H., Kubota, H., Lindberg, R. L. P., Leppert, D., Gloor, S. M., Errede, M., et al. (2008). Endothelial Cell Barrier Impairment Induced by Glioblastomas and Transforming Growth Factor β2 Involves Matrix Metalloproteinases and Tight Junction Proteins. J. Neuropathol. Exp. Neurol. 67, 435–448. doi: 10.1097/NEN.0b013e31816fd622
Kang, E. J., Major, S., Jorks, D., Reiffurth, C., Offenhauser, N., Friedman, A., et al. (2013). Blood-brain barrier opening to large molecules does not imply blood-brain barrier opening to small ions. Neurobiol. Dis. 52, 204–218. doi: 10.1016/j.nbd.2012.12.007
Karnati, H. K., Panigrahi, M., Shaik, N. A., Greig, N. H., Bagadi, S. A. R., Kamal, M., et al. (2014). Down Regulated Expression of Claudin-1 and Claudin-5 and Up Regulation of B-Catenin: association with Human Glioma Progression. CNS Neurol. Disord. Drug Targets 13, 1413–1426. doi: 10.2174/1871527313666141023121550
Keaney, J., and Campbell, M. (2015). The dynamic blood-brain barrier. FEBS J. 282, 4067–4079. doi: 10.1111/febs.13412
Kickingereder, P., Sahm, F., Wiestler, B., Roethke, M., Heiland, S., Schlemmer, H.-P., et al. (2014). Evaluation of microvascular permeability with dynamic contrast-enhanced MRI for the differentiation of primary CNS lymphoma and glioblastoma: radiologic-pathologic correlation. AJNR Am. J. Neuroradiol. 35, 1503–1508. doi: 10.3174/ajnr.A3915
Kielian, T. (2004). Immunopathogenesis of brain abscess. J. Neuroinflamm. 1:16. doi: 10.1186/1742-2094-1-16
Kielian, T., Barry, B., and Hickey, W. F. (2001). CXC chemokine receptor-2 ligands are required for neutrophil-mediated host defense in experimental brain abscesses. J. Immunol. 166, 4634–4643. doi: 10.4049/jimmunol.166.7.4634
Kielian, T., Esen, N., and Bearden, E. D. (2005a). Toll-like receptor 2 (TLR2) is pivotal for recognition of S. aureus peptidoglycan but not intact bacteria by microglia. Glia 49, 567–576. doi: 10.1002/glia.20144
Kielian, T., Haney, A., Mayes, P. M., Garg, S., and Esen, N. (2005b). Toll-like receptor 2 modulates the pro-inflammatory milieu in Staphylococcus aureus-induced brain abscess. Infect. Immun. 73, 7428–7435. doi: 10.1128/IAI.73.11.7428-7435.2005
Kielian, T., Phulwani, N. K., Esen, N., Syed, M. Md, Haney, A. C., McCastlain, K., et al. (2007). MyD88-Dependent Signals Are Essential for the Host Immune Response in Experimental Brain Abscess. J. Immunol. 178, 4528–4537. doi: 10.4049/jimmunol.178.7.4528
Kim, J.-E., Ryu, H. J., and Kang, T.-C. (2013). Status Epilepticus Induces Vasogenic Edema via Tumor Necrosis Factor-α/ Endothelin-1-Mediated Two Different Pathways. PLoS One 8:e74458. doi: 10.1371/journal.pone.0074458
Kim, J. Y., Ko, A.-R., Hyun, H.-W., and Kang, T.-C. (2015). ETB receptor-mediated MMP-9 activation induces vasogenic edema via ZO-1 protein degradation following status epilepticus. Neuroscience 304, 355–367. doi: 10.1016/j.neuroscience.2015.07.065
Klimas, A., Drzazga, Z., Kluczewska, E., and Hartel, M. (2013). Regional ADC measurements during normal brain aging in the clinical range of b values: a DWI study. Clin. Imaging 37, 637–644. doi: 10.1016/j.clinimag.2013.01.013
Krebs, S., Barasch, J. G., Young, R. J., Grommes, C., and Schöder, H. (2021). Positron emission tomography and magnetic resonance imaging in primary central nervous system lymphoma-a narrative review. Ann. Lymph. 5:15. doi: 10.21037/aol-20-52
Kummer, D., and Ebnet, K. (2018). Junctional Adhesion Molecules (JAMs): the JAM-Integrin Connection. Cells 7:E25. doi: 10.3390/cells7040025
Lai, R., Rosenblum, M. K., and DeAngelis, L. M. (2002). Primary CNS lymphoma: a whole-brain disease? Neurology 59, 1557–1562. doi: 10.1212/01.wnl.0000034256.20173.ea
Lakomy, R., Kazda, T., Selingerova, I., Poprach, A., Pospisil, P., Belanova, R., et al. (2020). Real-World Evidence in Glioblastoma: stupp’s Regimen After a Decade. Front. Oncol. 10:840. doi: 10.3389/fonc.2020.00840
Lan, Y.-L., Wang, X., Lou, J.-C., Ma, X.-C., and Zhang, B. (2017). The potential roles of aquaporin 4 in malignant gliomas. Oncotarget 8, 32345–32355. doi: 10.18632/oncotarget.16017
Leinonen, V., Vanninen, R., and Rauramaa, T. (2018). ““Chapter 4 - Raised intracranial pressure and brain edema,”,” in Handbook of Clinical Neurology Neuropathology, eds G. G. Kovacs and I. Alafuzoff (Amsterdam: Elsevier), 25–37. doi: 10.1016/B978-0-12-802395-2.00004-3
Li, W., Chen, Z., Chin, I., Chen, Z., and Dai, H. (2018). The Role of VE-cadherin in Blood-brain Barrier Integrity Under Central Nervous System Pathological Conditions. Curr. Neuropharmacol. 16, 1375–1384. doi: 10.2174/1570159X16666180222164809
Liebner, S., Fischmann, A., Rascher, G., Duffner, F., Grote, E. H., Kalbacher, H., et al. (2000). Claudin-1 and claudin-5 expression and tight junction morphology are altered in blood vessels of human glioblastoma multiforme. Acta Neuropathol. 100, 323–331. doi: 10.1007/s004010000180
Lin, C., McGough, R., Aswad, B., Block, J. A., and Terek, R. (2004). Hypoxia induces HIF-1alpha and VEGF expression in chondrosarcoma cells and chondrocytes. J. Orthop. Res. 22, 1175–1181. doi: 10.1016/j.orthres.2004.03.002
Lin, G.-S., Chen, Y.-P., Lin, Z.-X., Wang, X.-F., Zheng, Z.-Q., and Chen, L. (2014). STAT3 serine 727 phosphorylation influences clinical outcome in glioblastoma. Int. J. Clin. Exp. Pathol. 7, 3141–3149.
Lin, Z. X., Yang, L. J., Huang, Q., Lin, J. H., Ren, J., Chen, Z. B., et al. (2008). Inhibition of tumor-induced edema by antisense VEGF is mediated by suppressive vesiculo-vacuolar organelles (VVO) formation. Cancer Sci. 99, 2540–2546. doi: 10.1111/j.1349-7006.2008.00974.x
Liu, X., Zhang, Q., Mu, Y., Zhang, X., Sai, K., Pang, J. C.-S., et al. (2011). Clinical significance of vasculogenic mimicry in human gliomas. J. Neurooncol. 105, 173–179. doi: 10.1007/s11060-011-0578-5
Lo, W. D., Wolny, A., and Boesel, C. (1994). Blood-brain barrier permeability in staphylococcal cerebritis and early brain abscess. J. Neurosurg. 80, 897–905. doi: 10.3171/jns.1994.80.5.0897
Loeffler, S., Fayard, B., Weis, J., and Weissenberger, J. (2005). Interleukin-6 induces transcriptional activation of vascular endothelial growth factor (VEGF) in astrocytes in vivo and regulates VEGF promoter activity in glioblastoma cells via direct interaction between STAT3 and Sp1. Int. J. Cancer 115, 202–213. doi: 10.1002/ijc.20871
Machein, M. R., Kullmer, J., Fiebich, B. L., Plate, K. H., and Warnke, P. C. (1999). Vascular endothelial growth factor expression, vascular volume, and, capillary permeability in human brain tumors. Neurosurgery 44, 732–740. doi: 10.1097/00006123-199904000-00022
Mak, K. M., and Mei, R. (2017). Basement Membrane Type IV Collagen and Laminin: an Overview of Their Biology and Value as Fibrosis Biomarkers of Liver Disease. Anat. Rec. 300, 1371–1390. doi: 10.1002/ar.23567
Marcelis, L., Antoranz, A., Delsupehe, A.-M., Biesemans, P., Ferreiro, J. F., Debackere, K., et al. (2020). In-depth characterization of the tumor microenvironment in central nervous system lymphoma reveals implications for immune-checkpoint therapy. Cancer Immunol. Immunother. 69, 1751–1766. doi: 10.1007/s00262-020-02575-y
Mathisen, G. E., and Johnson, J. P. (1997). Brain abscess. Clin. Infect. Dis. 25, 763–779. doi: 10.1086/515541
Mayhan, W. G. (1999). VEGF increases permeability of the blood-brain barrier via a nitric oxide synthase/cGMP-dependent pathway. Am. J. Physiol. 276, C1148–C1153. doi: 10.1152/ajpcell.1999.276.5.C1148
Maza, S., Kiewe, P., Munz, D. L., Korfel, A., Hamm, B., Jahnke, K., et al. (2009). First report on a prospective trial with yttrium-90-labeled ibritumomab tiuxetan (Zevalin) in primary CNS lymphoma. Neuro. Oncol. 11, 423–429. doi: 10.1215/15228517-2008-108
Mei, X., Chen, Y.-S., Zhang, Q.-P., Chen, F.-R., Xi, S.-Y., Long, Y.-K., et al. (2020). Association between glioblastoma cell-derived vessels and poor prognosis of the patients. Cancer Commun. 40, 211–221. doi: 10.1002/cac2.12026
Meşe, G., Richard, G., and White, T. W. (2007). Gap junctions: basic structure and function. J. Invest. Dermatol. 127, 2516–2524. doi: 10.1038/sj.jid.5700770
Mitic, L. L., Van Itallie, C. M., and Anderson, J. M. (2000). Molecular physiology and pathophysiology of tight junctions I. Tight junction structure and function: lessons from mutant animals and proteins. Am. J. Physiol. Gastrointest. Liver Physiol. 279, G250–G254. doi: 10.1152/ajpgi.2000.279.2.G250
Molnár, P. P., O’Neill, B. P., Scheithauer, B. W., and Groothuis, D. R. (1999). The blood-brain barrier in primary CNS lymphomas: ultrastructural evidence of endothelial cell death. Neuro. Oncol. 1, 89–100. doi: 10.1093/neuonc/1.2.89
Mou, K., Chen, M., Mao, Q., Wang, P., Ni, R., Xia, X., et al. (2010). AQP-4 in peritumoral edematous tissue is correlated with the degree of glioma and with expression of VEGF and HIF-alpha. J. Neurooncol. 100, 375–383. doi: 10.1007/s11060-010-0205-x
Muoio, V., Persson, P. B., and Sendeski, M. M. (2014). The neurovascular unit - concept review. Acta Physiol. 210, 790–798. doi: 10.1111/apha.12250
Na, W., Shin, J. Y., Lee, J. Y., Jeong, S., Kim, W.-S., Yune, T. Y., et al. (2017). Dexamethasone suppresses JMJD3 gene activation via a putative negative glucocorticoid response element and maintains integrity of tight junctions in brain microvascular endothelial cells. J. Cereb. Blood Flow Metab. 37, 3695–3708. doi: 10.1177/0271678X17701156
Nakagawa, S., Deli, M. A., Nakao, S., Honda, M., Hayashi, K., Nakaoke, R., et al. (2007). Pericytes from brain microvessels strengthen the barrier integrity in primary cultures of rat brain endothelial cells. Cell Mol. Neurobiol. 27, 687–694. doi: 10.1007/s10571-007-9195-4
Noell, S., Wolburg-Buchholz, K., Mack, A. F., Ritz, R., Tatagiba, M., Beschorner, R., et al. (2012). Dynamics of expression patterns of AQP4, dystroglycan, agrin and matrix metalloproteinases in human glioblastoma. Cell Tissue Res. 347, 429–441. doi: 10.1007/s00441-011-1321-4
Obenaus, A., and Ashwal, S. (2008). Magnetic resonance imaging in cerebral ischemia: focus on neonates. Neuropharmacology 55, 271–280. doi: 10.1016/j.neuropharm.2008.06.010
Ostrom, Q. T., Cioffi, G., Waite, K., Kruchko, C., and Barnholtz-Sloan, J. S. (2021). CBTRUS Statistical Report: primary Brain and Other Central Nervous System Tumors Diagnosed in the United States in 2014-2018. Neuro. Oncol. 23, iii1–iii105. doi: 10.1093/neuonc/noab200
Ou, A., Ott, M., Fang, D., and Heimberger, A. B. (2021). The Role and Therapeutic Targeting of JAK/STAT Signaling in Glioblastoma. Cancers 13:437. doi: 10.3390/cancers13030437
Papadopoulos, M. C., Saadoun, S., Woodrow, C. J., Davies, D. C., Costa-Martins, P., Moss, R. F., et al. (2001). Occludin expression in microvessels of neoplastic and non-neoplastic human brain. Neuropathol. Appl. Neurobiol. 27, 384–395. doi: 10.1046/j.0305-1846.2001.00341.x
Papadopoulos, M. C., and Verkman, A. S. (2007). Aquaporin-4 and brain edema. Pediatr. Nephrol. 22, 778–784. doi: 10.1007/s00467-006-0411-0
Park, M.-W., Kim, C.-H., Cheong, J.-H., Bak, K.-H., Kim, J.-M., and Oh, S.-J. (2006). Occludin Expression in Brain Tumors and its Relevance to Peritumoral Edema and Survival. Cancer Res. Treat. 38, 139–143. doi: 10.4143/crt.2006.38.3.139
Preusser, M., Heinzl, H., Gelpi, E., Schonegger, K., Haberler, C., Birner, P., et al. (2006). Histopathologic assessment of hot-spot microvessel density and vascular patterns in glioblastoma: poor observer agreement limits clinical utility as prognostic factors: a translational research project of the European Organization for Research and Treatment of Cancer Brain Tumor Group. Cancer 107, 162–170. doi: 10.1002/cncr.21973
Pun, P. B. L., Lu, J., and Moochhala, S. (2009). Involvement of ROS in BBB dysfunction. Free Radic. Res. 43, 348–364. doi: 10.1080/10715760902751902
Rauch, S. M., Huen, K., Miller, M. C., Chaudry, H., Lau, M., Sanes, J. R., et al. (2011). Changes in Brain β-Amyloid Deposition and Aquaporin 4 Levels in Response to Altered Agrin Expression in Mice. J. Neuropathol. Exp. Neurol. 70, 1124–1137. doi: 10.1097/NEN.0b013e31823b0b12
Rempe, R. G., Hartz, A. M., and Bauer, B. (2016). Matrix metalloproteinases in the brain and blood–brain barrier: versatile breakers and makers. J. Cereb. Blood Flow Metab. 36, 1481–1507. doi: 10.1177/0271678X16655551
Rite, I., Machado, A., Cano, J., and Venero, J. L. (2008). Intracerebral VEGF injection highly upregulates AQP4 mRNA and protein in the perivascular space and glia limitans externa. Neurochem. Int. 52, 897–903. doi: 10.1016/j.neuint.2007.10.004
Roberts, W. G., and Palade, G. E. (1995). Increased microvascular permeability and endothelial fenestration induced by vascular endothelial growth factor. J. Cell Sci. 108, 2369–2379. doi: 10.1242/jcs.108.6.2369
Rosińska, S., and Gavard, J. (2021). Tumor Vessels Fuel the Fire in Glioblastoma. Int. J. Mol. Sci. 22:6514. doi: 10.3390/ijms22126514
Ruggieri, S., Tamma, R., Resta, N., Albano, F., Coccaro, N., Loconte, D., et al. (2017). Stat3-positive tumor cells contribute to vessels neoformation in primary central nervous system lymphoma. Oncotarget 8, 31254–31269. doi: 10.18632/oncotarget.16115
Saadoun, S., Papadopoulos, M. C., Bell, B. A., Krishna, S., and Davies, D. C. (2002a). The aquaporin-4 water channel and brain tumour oedema. J. Anat. 200, 523–534. doi: 10.1046/j.1469-7580.2002.00047_16.x
Saadoun, S., Papadopoulos, M. C., Davies, D. C., Krishna, S., and Bell, B. A. (2002b). Aquaporin-4 expression is increased in oedematous human brain tumours. J. Neurol. Neurosurg. Psychiatr. 72, 262–265. doi: 10.1136/jnnp.72.2.262
Saadoun, S., Papadopoulos, M. C., and Krishna, S. (2003). Water transport becomes uncoupled from K+ siphoning in brain contusion, bacterial meningitis, and brain tumours: immunohistochemical case review. J. Clin. Pathol. 56, 972–975. doi: 10.1136/jcp.56.12.972
Sehm, T., Fan, Z., Ghoochani, A., Rauh, M., Engelhorn, T., Minakaki, G., et al. (2016). Sulfasalazine impacts on ferroptotic cell death and alleviates the tumor microenvironment and glioma-induced brain edema. Oncotarget 7, 36021–36033. doi: 10.18632/oncotarget.8651
Shevtsov, M. A., Nikolaev, B. P., Yakovleva, L. Y., Dobrodumov, A. V., Zhakhov, A. V., Mikhrina, A. L., et al. (2015). Recombinant interleukin-1 receptor antagonist conjugated to superparamagnetic iron oxide nanoparticles for theranostic targeting of experimental glioblastoma. Neoplasia 17, 32–42. doi: 10.1016/j.neo.2014.11.001
Shi, S., Cheng, J., Zhang, C., Liang, T., Zhang, Y., Sun, Y., et al. (2020). Peripheral Blood Occludin Level as a Biomarker for Perioperative Cerebral Edema in Patients with Brain Tumors. Dis. Markers 2020, 8813535. doi: 10.1155/2020/8813535
Shweiki, D., Itin, A., Soffer, D., and Keshet, E. (1992). Vascular endothelial growth factor induced by hypoxia may mediate hypoxia-initiated angiogenesis. Nature 359, 843–845. doi: 10.1038/359843a0
Simard, J. M., Chen, M., Tarasov, K. V., Bhatta, S., Ivanova, S., Melnitchenko, L., et al. (2006). Newly expressed SUR1-regulated NC(Ca-ATP) channel mediates cerebral edema after ischemic stroke. Nat. Med. 12, 433–440. doi: 10.1038/nm1390
Simard, J. M., Geng, Z., Woo, S. K., Ivanova, S., Tosun, C., Melnichenko, L., et al. (2009). Glibenclamide reduces inflammation, vasogenic edema, and caspase-3 activation after subarachnoid hemorrhage. J. Cereb. Blood Flow Metab. 29, 317–330. doi: 10.1038/jcbfm.2008.120
Simone, L., Pisani, F., Mola, M. G., De Bellis, M., Merla, G., Micale, L., et al. (2019). AQP4 Aggregation State Is a Determinant for Glioma Cell Fate. Cancer Res. 79, 2182–2194. doi: 10.1158/0008-5472.CAN-18-2015
Solár, P., Zamani, A., Lakatosová, K., and Joukal, M. (2022). The blood–brain barrier and the neurovascular unit in subarachnoid hemorrhage: molecular events and potential treatments. Fluids Barriers CNS 19, 1–79. doi: 10.1186/s12987-022-00312-4
Song, Y., Liu, B., Guan, M., and Liu, M. (2018). Successful treatment using apatinib in intractable brain edema: a case report and literatures review. Cancer Biol. Ther. 19, 1093–1096. doi: 10.1080/15384047.2018.1491502
Soussain, C., Muldoon, L. L., Varallyay, C., Jahnke, K., DePaula, L., and Neuwelt, E. A. (2007). Characterization and magnetic resonance imaging of a rat model of human B-cell central nervous system lymphoma. Clin. Cancer Res. 13, 2504–2511. doi: 10.1158/1078-0432.CCR-06-2379
Spanberger, T., Berghoff, A. S., Dinhof, C., Ilhan-Mutlu, A., Magerle, M., Hutterer, M., et al. (2013). Extent of peritumoral brain edema correlates with prognosis, tumoral growth pattern. HIF1a expression and angiogenic activity in patients with single brain metastases. Clin. Exp. Metastas. 30, 357–368. doi: 10.1007/s10585-012-9542-9
Stamatovic, S. M., Johnson, A. M., Keep, R. F., and Andjelkovic, A. V. (2016). Junctional proteins of the blood-brain barrier: new insights into function and dysfunction. Tissue Barriers 4, e1154641. doi: 10.1080/21688370.2016.1154641
Steiner, H.-H., Karcher, S., Mueller, M. M., Nalbantis, E., Kunze, S., and Herold-Mende, C. (2004). Autocrine pathways of the vascular endothelial growth factor (VEGF) in glioblastoma multiforme: clinical relevance of radiation-induced increase of VEGF levels. J. Neurooncol. 66, 129–138. doi: 10.1023/b:neon.0000013495.08168.8f
Stenzel, W., Dahm, J., Sanchez-Ruiz, M., Miletic, H., Hermann, M., Courts, C., et al. (2005a). Regulation of the inflammatory response to Staphylococcus aureus-induced brain abscess by interleukin-10. J. Neuropathol. Exp. Neurol. 64, 1046–1057. doi: 10.1097/01.jnen.0000189836.48704.ca
Stenzel, W., Soltek, S., Miletic, H., Hermann, M. M., Körner, H., Sedgwick, J. D., et al. (2005b). An essential role for tumor necrosis factor in the formation of experimental murine Staphylococcus aureus-induced brain abscess and clearance. J. Neuropathol. Exp. Neurol. 64, 27–36. doi: 10.1093/jnen/64.1.27
Stenzel, W., Soltek, S., Schlüter, D., and Deckert, M. (2004). The intermediate filament GFAP is important for the control of experimental murine Staphylococcus aureus-induced brain abscess and Toxoplasma encephalitis. J. Neuropathol. Exp. Neurol. 63, 631–640. doi: 10.1093/jnen/63.6.631
Stokum, J. A., Kwon, M. S., Woo, S. K., Tsymbalyuk, O., Vennekens, R., Gerzanich, V., et al. (2018). SUR1-TRPM4 and AQP4 form a heteromultimeric complex that amplifies ion/water osmotic coupling and drives astrocyte swelling. Glia 66, 108–125. doi: 10.1002/glia.23231
Stupp, R., Mason, W. P., van den Bent, M. J., Weller, M., Fisher, B., Taphoorn, M. J. B., et al. (2005). Radiotherapy plus Concomitant and Adjuvant Temozolomide for Glioblastoma. N. Engl. J. Med. 352, 987–996. doi: 10.1056/NEJMoa043330
Suh, C. H., Kim, H. S., Jung, S. C., and Kim, S. J. (2018). Diffusion-Weighted Imaging and Diffusion Tensor Imaging for Differentiating High-Grade Glioma from Solitary Brain Metastasis: a Systematic Review and Meta-Analysis. Am. J. Neuroradiol. 39, 1208–1214. doi: 10.3174/ajnr.A5650
Sweeney, M. D., Zhao, Z., Montagne, A., Nelson, A. R., and Zlokovic, B. V. (2019). Blood-Brain Barrier: from Physiology to Disease and Back. Physiol. Rev. 99, 21–78. doi: 10.1152/physrev.00050.2017
Takata, K., Matsuzaki, T., and Tajika, Y. (2004). Aquaporins: water channel proteins of the cell membrane. Prog. Histochem. Cytochem. 39, 1–83. doi: 10.1016/j.proghi.2004.03.001
Takeuchi, H., Hashimoto, N., Kitai, R., Kubota, T., and Kikuta, K. (2010). Proliferation of vascular smooth muscle cells in glioblastoma multiforme. J. Neurosurg. 113, 218–224. doi: 10.3171/2009.10.JNS08631
Takeuchi, H., Matsuda, K., Kitai, R., Sato, K., and Kubota, T. (2007). Angiogenesis in primary central nervous system lymphoma (PCNSL). J. Neurooncol. 84, 141–145. doi: 10.1007/s11060-007-9363-x
Tamura, R., Ohara, K., Sasaki, H., Morimoto, Y., Yoshida, K., and Toda, M. (2018). Histopathological vascular investigation of the peritumoral brain zone of glioblastomas. J. Neurooncol. 136, 233–241. doi: 10.1007/s11060-017-2648-9
Tan, A. C., Ashley, D. M., López, G. Y., Malinzak, M., Friedman, H. S., and Khasraw, M. (2020). Management of glioblastoma: state of the art and future directions. CA Cancer J. Clin. 70, 299–312. doi: 10.3322/caac.21613
Thompson, E. M., Pishko, G. L., Muldoon, L. L., and Neuwelt, E. A. (2013). Inhibition of SUR1 Decreases the Vascular Permeability of Cerebral Metastases. Neoplasia 15, 535–543. doi: 10.1593/neo.13164
Toh, C. H., and Siow, T. Y. (2021). Factors Associated With Dysfunction of Glymphatic System in Patients With Glioma. Front. Oncol. 11:744318. doi: 10.3389/fonc.2021.744318
Toh, C. H., Siow, T. Y., and Castillo, M. (2021). Peritumoral Brain Edema in Metastases May Be Related to Glymphatic Dysfunction. Front. Oncol. 11:4144. doi: 10.3389/fonc.2021.725354
Tran, T. T., Mahajan, A., Chiang, V. L., Goldberg, S. B., Nguyen, D. X., Jilaveanu, L. B., et al. (2019). Perilesional edema in brain metastases: potential causes and implications for treatment with immune therapy. J. Immunother. Cancer 7:200. doi: 10.1186/s40425-019-0684-z
Trembath, D. G., Davis, E. S., Rao, S., Bradler, E., Saada, A. F., Midkiff, B. R., et al. (2020). Brain Tumor Microenvironment and Angiogenesis in Melanoma Brain Metastases. Front. Oncol. 10:604213. doi: 10.3389/fonc.2020.604213
Utsuki, S., Oka, H., Sato, S., Shimizu, S., Suzuki, S., Tanizaki, Y., et al. (2007). Histological examination of false positive tissue resection using 5-aminolevulinic acid-induced fluorescence guidance. Neurol. Med. Chir. 47, 210–213. doi: 10.2176/nmc.47.210
Van Itallie, C. M., and Anderson, J. M. (2004). The role of claudins in determining paracellular charge selectivity. Proc. Am. Thorac. Soc. 1, 38–41. doi: 10.1513/pats.2306013
Vaquero, J., Zurita, M., and Morales, C. (2001). Possible Role for Vascular Permeability Factor in the Pathophysiology of Vasogenic Oedema Associated to Brain Abscess. Acta Neurochir. 143, 1039–1040. doi: 10.1007/s007010170009
Vazana, U., Veksler, R., Pell, G. S., Prager, O., Fassler, M., Chassidim, Y., et al. (2016). Glutamate-Mediated Blood-Brain Barrier Opening: implications for Neuroprotection and Drug Delivery. J. Neurosci. 36, 7727–7739. doi: 10.1523/JNEUROSCI.0587-16.2016
Verkman, A. S., Ratelade, J., Rossi, A., Zhang, H., and Tradtrantip, L. (2011). Aquaporin-4: orthogonal array assembly, CNS functions, and role in neuromyelitis optica. Acta Pharmacol. Sin. 32, 702–710. doi: 10.1038/aps.2011.27
Vestweber, D. (2008). VE-cadherin: the major endothelial adhesion molecule controlling cellular junctions and blood vessel formation. Arterioscler. Thromb. Vasc. Biol. 28, 223–232. doi: 10.1161/ATVBAHA.107.158014
Wahl, M., Unterberg, A., Baethmann, A., and Schilling, L. (1988). Mediators of blood-brain barrier dysfunction and formation of vasogenic brain edema. J. Cereb. Blood Flow Metab. 8, 621–634. doi: 10.1038/jcbfm.1988.109
Wallenfang, T., Bohl, J., and Kretzschmar, K. (1980). Evolution of brain abscess in cats formation of capsule and resolution of brain edema. Neurosurg. Rev. 3, 101–111. doi: 10.1007/BF01644062
Wang, H.-C., Hsiao, H.-H., Du, J.-S., Cho, S.-F., Yeh, T.-J., Gau, Y.-C., et al. (2021). Effect of Tumor Microenvironment and Angiogenesis on Clinical Outcomes of Primary Central Nervous System Lymphoma. BioMed. Res. Int. 2021:e3291762. doi: 10.1155/2021/3291762
Wang, S.-Y., Ke, Y.-Q., Lu, G.-H., Song, Z.-H., Yu, L., Xiao, S., et al. (2013). Vasculogenic mimicry is a prognostic factor for postoperative survival in patients with glioblastoma. J. Neurooncol. 112, 339–345. doi: 10.1007/s11060-013-1077-7
Wang, X.-F., Lin, G.-S., Lin, Z.-X., Chen, Y.-P., Chen, Y., Zhang, J.-D., et al. (2014). Association of pSTAT3-VEGF signaling pathway with peritumoral edema in newly diagnosed glioblastoma: an immunohistochemical study. Int. J. Clin. Exp. Pathol. 7, 6133–6140.
Warth, A., Kröger, S., and Wolburg, H. (2004). Redistribution of aquaporin-4 in human glioblastoma correlates with loss of agrin immunoreactivity from brain capillary basal laminae. Acta Neuropathol. 107, 311–318. doi: 10.1007/s00401-003-0812-0
Warth, A., Simon, P., Capper, D., Goeppert, B., Tabatabai, G., Herzog, H., et al. (2007). Expression pattern of the water channel aquaporin-4 in human gliomas is associated with blood-brain barrier disturbance but not with patient survival. J. Neurosci. Res. 85, 1336–1346. doi: 10.1002/jnr.21224
Wen, L., Tan, Y., Dai, S., Zhu, Y., Meng, T., Yang, X., et al. (2017). VEGF-mediated tight junctions pathological fenestration enhances doxorubicin-loaded glycolipid-like nanoparticles traversing BBB for glioblastoma-targeting therapy. Drug Deliv. 24, 1843–1855. doi: 10.1080/10717544.2017.1386731
Wesseling, P., Schlingemann, R. O., Rietveld, F. J., Link, M., Burger, P. C., and Ruiter, D. J. (1995). Early and extensive contribution of pericytes/vascular smooth muscle cells to microvascular proliferation in glioblastoma multiforme: an immuno-light and immuno-electron microscopic study. J. Neuropathol. Exp. Neurol. 54, 304–310. doi: 10.1097/00005072-199505000-00003
White, M. L., Moore, D. W., Zhang, Y., Mark, K. D., Greiner, T. C., and Bierman, P. J. (2019). Primary central nervous system post-transplant lymphoproliferative disorders: the spectrum of imaging appearances and differential. Insights Imaging 10:46. doi: 10.1186/s13244-019-0726-6
Wolburg, H., Noell, S., Fallier-Becker, P., Mack, A. F., and Wolburg-Buchholz, K. (2012). The disturbed blood-brain barrier in human glioblastoma. Mol. Aspects Med. 33, 579–589. doi: 10.1016/j.mam.2012.02.003
Xu, C., Wu, X., and Zhu, J. (2013). VEGF promotes proliferation of human glioblastoma multiforme stem-like cells through VEGF receptor 2. Sci. WorldJ. 2013:417413. doi: 10.1155/2013/417413
Xu, L., Nirwane, A., and Yao, Y. (2018). Basement membrane and blood–brain barrier. Stroke Vasc. Neurol. 4, 78–82. doi: 10.1136/svn-2018-000198
Xue, Q., Cao, L., Chen, X.-Y., Zhao, J., Gao, L., Li, S.-Z., et al. (2017). High expression of MMP9 in glioma affects cell proliferation and is associated with patient survival rates. Oncol. Lett. 13, 1325–1330. doi: 10.3892/ol.2017.5567
Yang, J., Li, Q., Wang, Z., Qi, C., Han, X., Lan, X., et al. (2017). Multimodality MRI assessment of grey and white matter injury and blood-brain barrier disruption after intracerebral haemorrhage in mice. Sci. Rep. 7:40358. doi: 10.1038/srep40358
Yang, J., Li, W., He, X., Zhang, G., Yue, L., and Chai, Y. (2015). VEGF Overexpression Is a Valuable Prognostic Factor for Non-Hodgkin’s Lymphoma Evidence from a Systemic Meta-Analysis. Dis. Mark 2015:786790. doi: 10.1155/2015/786790
Yang, L., Wang, X., Zhen, S., Zhang, S., Kang, D., and Lin, Z. (2012). Aquaporin-4 upregulated expression in glioma tissue is a reaction to glioma-associated edema induced by vascular endothelial growth factor. Oncol. Rep. 28, 1633–1638. doi: 10.3892/or.2012.1973
Yang, N., Ng, Y. H., Pang, Z. P., Südhof, T. C., and Wernig, M. (2011). Induced neuronal cells: how to make and define a neuron. Cell Stem Cell 9, 517–525. doi: 10.1016/j.stem.2011.11.015
Yeung, P. K. K., Lo, A. C. Y., Leung, J. W. C., Chung, S. S. M., and Chung, S. K. (2009). Targeted Overexpression of Endothelin-1 in Astrocytes Leads to More Severe Cytotoxic Brain Edema and Higher Mortality. J. Cereb. Blood Flow Metab. 29, 1891–1902. doi: 10.1038/jcbfm.2009.175
Zagzag, D., Esencay, M., Mendez, O., Yee, H., Smirnova, I., Huang, Y., et al. (2008). Hypoxia- and vascular endothelial growth factor-induced stromal cell-derived factor-1alpha/CXCR4 expression in glioblastomas: one plausible explanation of Scherer’s structures. Am. J. Pathol. 173, 545–560. doi: 10.2353/ajpath.2008.071197
Zhang, M., and Olsson, Y. (1995). Reactions of astrocytes and microglial cells around hematogenous metastases of the human brain. Expression of endothelin-like immunoreactivity in reactive astrocytes and activation of microglial cells. J. Neurol. Sci. 134, 26–32. doi: 10.1016/0022-510x(95)00227-9
Zhao, B., Wang, H., Wang, X., Zhao, H., and Liu, J. (2015). Multiple intracranial metastatic tumor case report and aquaporin water channel-related research. Cell Biochem. Biophys. 71, 1015–1021. doi: 10.1007/s12013-014-0303-z
Zhao, X., Sun, Q., Dou, C., Chen, Q., and Liu, B. (2019). BMP4 inhibits glioblastoma invasion by promoting E-cadherin and claudin expression. Front. Biosci. 24:1060–1070. doi: 10.2741/4768
Keywords: blood-brain barrier, glioblastoma multiforme, brain edema, brain metastasis, brain lymphoma, brain abscess
Citation: Solar P, Hendrych M, Barak M, Valekova H, Hermanova M and Jancalek R (2022) Blood-Brain Barrier Alterations and Edema Formation in Different Brain Mass Lesions. Front. Cell. Neurosci. 16:922181. doi: 10.3389/fncel.2022.922181
Received: 17 April 2022; Accepted: 20 June 2022;
Published: 15 July 2022.
Edited by:
Lucy Vulchanova, University of Minnesota Twin Cities, United StatesReviewed by:
Paolo Rosa, Sapienza University of Rome, ItalyCopyright © 2022 Solar, Hendrych, Barak, Valekova, Hermanova and Jancalek. This is an open-access article distributed under the terms of the Creative Commons Attribution License (CC BY). The use, distribution or reproduction in other forums is permitted, provided the original author(s) and the copyright owner(s) are credited and that the original publication in this journal is cited, in accordance with accepted academic practice. No use, distribution or reproduction is permitted which does not comply with these terms.
*Correspondence: Radim Jancalek, cmFkaW0uamFuY2FsZWtAZm51c2EuY3o=
†These authors have contributed equally to this work
Disclaimer: All claims expressed in this article are solely those of the authors and do not necessarily represent those of their affiliated organizations, or those of the publisher, the editors and the reviewers. Any product that may be evaluated in this article or claim that may be made by its manufacturer is not guaranteed or endorsed by the publisher.
Research integrity at Frontiers
Learn more about the work of our research integrity team to safeguard the quality of each article we publish.