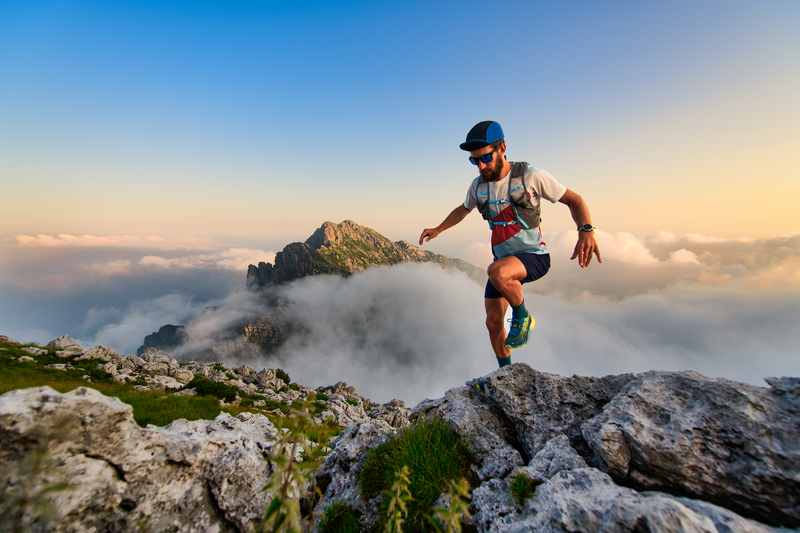
95% of researchers rate our articles as excellent or good
Learn more about the work of our research integrity team to safeguard the quality of each article we publish.
Find out more
REVIEW article
Front. Cell. Neurosci. , 22 June 2022
Sec. Cellular Neuropathology
Volume 16 - 2022 | https://doi.org/10.3389/fncel.2022.915587
This article is part of the Research Topic The Role of Inflammation in Neurodegenerative Diseases View all 8 articles
Neuroinflammatory disorder is a general term that is associated with the progressive loss of neuronal structure or function. At present, the widely studied diseases with neuroinflammatory components are mainly divided into neurodegenerative and neuropsychiatric diseases, namely, Alzheimer’s disease, Parkinson’s disease, depression, stroke, and so on. An appropriate neuroinflammatory response can promote brain homeostasis, while excessive neuroinflammation can inhibit neuronal regeneration and damage the central nervous system. Apart from the symptomatic treatment with cholinesterase inhibitors, antidepressants/anxiolytics, and neuroprotective drugs, the treatment of neuroinflammation is a promising therapeutic method. Sirtuins are a host of class III histone deacetylases, that require nicotinamide adenine dinucleotide for their lysine residue deacetylase activity. The role of sirtuin 2 (SIRT2), one of the sirtuins, in modulating senescence, myelin formation, autophagy, and inflammation has been widely studied. SIRT2 is associated with many neuroinflammatory disorders considering it has deacetylation properties, that regulate the entire immune homeostasis. The aim of this review was to summarize the latest progress in regulating the effects of SIRT2 on immune homeostasis in neuroinflammatory disorders. The overall structure and catalytic properties of SIRT2, the selective inhibitors of SIRT2, the relationship between immune homeostasis and SIRT2, and the multitasking role of SIRT2 in several diseases with neuroinflammatory components were discussed.
With aging populations worldwide, the central nervous system (CNS) diseases associated with aging have been on the rise (Hou et al., 2019). Neurological diseases such as depression and stroke have also been increasing in young and middle-aged people (Pascoe and Parker, 2019; Putaala, 2020). Recent studies have found that the occurrence and development of these diseases have a common feature related to neuroinflammation, which generally refers to the noxious effects caused by immunological activation of microglia and astrocytes in various diseases of the CNS (Kwon and Koh, 2020; Griciuc and Tanzi, 2021). It is a physiological response of the immune system to harmful stimuli (Akbari et al., 2020; Jia et al., 2021). In response to harmful stimuli, neutrophils, monocytes/macrophages, different subtypes of T cells, and other inflammatory cells are commonly activated to maintain the immune system’s balance (Maida et al., 2020). An appropriate neuroinflammatory response can promote brain homeostasis, while excessive neuroinflammation can inhibit neuronal regeneration (Yang B. M. et al., 2019). An immune imbalance has been considered to be the mechanism of the deterioration of a variety of CNS diseases, including Alzheimer’s disease (AD) (Armstrong, 2019), Parkinson’s disease (PD) (Schonhoff et al., 2020), depression (Hayley et al., 2021), stroke (Iadecola et al., 2020), and so on. Thus, restoring the balance of the immune homeostasis has been suggested as a new treatment approach for diseases with neuroinflammatory components. These biologic molecules of immune regulation have moved toward the focus of research. Based on the specific effects on inflammatory factors, sirtuin 2 (SIRT2) has attracted much academic attention. We further reviewed the specific and detailed role of SIRT2 in neuroinflammatory disorders in the CNS.
Sirtuin 2 is predominantly found in the cytoplasm of cells in the (CNS), where it balances the whole immune homeostasis via the nicotinamide adenine dinucleotide (NAD)-dependent histone deacetylases (HDACs) (Gu et al., 2020; Hong et al., 2020; Zhang et al., 2021a; Figure 1). SIRT2 has a generic catalytic core domain like other Sirtuin family members and unique N-terminal and C-terminal sequences (Yang et al., 2020). Substrate and the catalytic core domain are vital during SIRT2 deacetylation (Minten et al., 2021). Therefore, SIRT2 participates in many biological processes by catalyzing the deacetylation of various substrates, such as senescence, myelin formation, autophagy, and inflammation (Puigoriol-Illamola et al., 2020; Lu et al., 2021). Yet, the exact role of SIRT2 in the immune regulation of neuroinflammatory disorders is still not fully understood. This review summarized the latest advances in SIRT2 in neuroinflammatory disorders, and the role of SIRT2 in balancing the immune homeostasis and inflammation in the CNS.
Figure 1. Subcellular location of 7 mammalian SIRTs (SIRT1–7) and SIRT2 related to neuroinflammatory disorders. SIRT1, SIRT6, and SIRT7 were found in the nucleus of the cell. Under certain conditions, SIRT1 can be transported from the nucleus to the cytoplasm. SIRT6 was also found in heterochromatin and the endoplasmic reticulum. SIRT7 was located at the nucleolus. SIRT3, SIRT4, and SIRT5 were found in the mitochondria and contribute to oxidative stress alleviation by regulating the activity of specific metabolic enzymes. SIRT3 is moved between the nucleus and mitochondria under cellular stress. The main site of SIRT2 was the cytoplasm, but in some phases of the cell cycle it was also found in the nucleus. SIRT2 was involved in the pathogenesis and progression of neuroinflammatory disorders.
Sirtuin 2, an NAD+ dependent protein deacetylase, is the only sirtuin protein that is predominantly found in the cytoplasm. Yet, SIRT2 is also found in the mitochondria and nucleus (Lin et al., 2021).
The human SIRT2 gene, mapped at chromosome 19q13.2, is composed of 16 exons and spans a region of 20,960 bp (Yang W. T. et al., 2017; Yang Y. et al., 2017). SIRT2 has a 323 amino acid catalytic core, which is flanked by a 19-residue N-terminal and a C-terminal helical extension (Li et al., 2015; Figure 2B). Two isoforms of the SIRT2 protein are encoded: a 389-aa protein with a predictive molecular weight of 43.2 kDa and an isoelectric point of 5.22 is encoded by isoform 1, a 352-aa protein with a predictive molecular weight of 39.5 kDa, and an isoelectric point of 6.05 is encoded by isoform 2, which lacks the first three exons (Alcaín and Villalba, 2009).
Figure 2. The overall structure of SIRT2. (A) Synthesis of SIRT2 inhibitors. (B) Schematic representation of human SIRT2. The conserved large catalytic domain was shown in gray. Numbers referred to amino acid residues in the proteins. (C) SIRT2 consisted of two domains that were connected by several conserved loops. (D) The SIRT2 enzymatic reaction was composed of three sequential steps, two of which were reversible and the final one irreversible.
The catalytic core has an elongated shape containing two domains, namely, a larger domain (residues 53–89, 146–186, and 241–356) and a smaller domain (residues 90–145 and 187–240) (Finnin et al., 2001). The former is a variant of the Rossmann fold, which is present in many diverse NAD(H)/NADP(H) binding enzymes (Hou et al., 2014). And the latter is composed of a helical module and a zinc-binding module (Carafa et al., 2012; Karaman et al., 2018). The two domains are connected by a hinge region that consists of four crossovers of the polypeptide chain (Finnin et al., 2001). At the interface of the two domains, the four crossovers and three loops of the large domain form a large groove (Liu Y. M. et al., 2019; Figure 2C).
The larger domain consists of six β strands and eight α helices (North and Verdin, 2004). The β strands form a parallel β sheet, and the α helices pack against the β sheet (Finnin et al., 2001). The Rossmann fold contains various features of a typical NAD+ binding site, such as a conserved Gly-X-Gly sequence important for NAD+ phosphate binding, a charged amino acid residue responsible for ribose group binding, and a small pocket to accommodate an NAD+ molecule (Satoh et al., 2011; Zhang X. et al., 2021; Figure 2C).
The smaller domain contains two structural modules that stem from two insertions in the Rossmann fold of the large domain (North and Verdin, 2004). The first insertion comprises four α helices that fold to form the helical module (Finnin et al., 2001). The second insertion consists of a β sheet, an α helix, and a zinc atom coordinated by four Cys residues (Finnin et al., 2001). A pocket in the helical module lines with hydrophobic residues and could intersect with the large groove between the larger and smaller domains, which is a class-specific binding site for proteins. The zinc-binding module has a topology, that mediates the interactions between protein and protein in diverse proteins (Figure 2C).
The SIRT2 enzymatic reaction consists of three sequential steps. Two of them are reversible, and one, the final step, is irreversible. The enzyme catalyzes the deacetylation of an acetylated substrate with the NAD+. The Acetyl Lys protein is an acetylated substrate. These three steps are involved in the binding of NAD+ and an acetylated lysine substrate; the formation of an enzyme-ADP-Ribose (ADPR)-acetylated lysine (AcLys-protein) intermediate (enzyme-ADPR-AcLys-protein) with the release of nicotinamide (Nic); and at last, 2′-O-acetyl-ADP-ribose (AADPR) and the deacetylated protein release. The reversibility of the second step is the basis of the NER assay, as only in this way the excess of labeled nicotinamide can reverse the reaction, and the labeled NAD+ can be produced de novo (Figure 2D).
The sirtuin family is an NAD+ dependent HDACs family, which has been highly conserved during its evolution (Dai et al., 2018). SIRT2 is a member of the Sirtuin family that mediates transcriptional silencing at telomeres, mating-type loci, and ribosomal gene clusters (Finnin et al., 2001). Its major characteristic is that it can realize deacetylation of acetyllysine substrate (Wang et al., 2019). NAD+ is the basis of this catalytic reaction. Moreover, it influences the direct response of SIRT2 to the changes in intracellular metabolism (Jêśko et al., 2017). As a redox-active metabolite, NAD+ can be converted from NADH or into NADH. Thus, the ratio of NAD+/NADH affects the activity of SIRT2 (Ren et al., 2020). In addition, the activity of SIRT2 is also influenced by nicotinamide, which is the product of the deacetylation process (Ma et al., 2014; Li et al., 2018).
During the deacetylation reaction mediated by SIRT2, NAD+, SIRT2, and acetyllysine substrate interact to produce three products, i.e., the nicotinamide, the deacetylated substrate, and AADPR (Sedlackova and Korolchuk, 2020). The detailed steps of the NAD+ dependent deacetylation are roughly divided into three parts (Tatone et al., 2015; Grabowska et al., 2017; Carafa et al., 2019; Lewis et al., 2019): (1) the first part is mediated by NAD+, where SIRT2 recognizes and binds to the substrate; during this process, the NAD+, SIRT2, and substrate can form a ternary complex. Also, the combination between NAD+ and SIRT2 is related to the NAD+ binding site on the Rossmann fold of SIRT2; (2) the second part mainly includes NAD+ releasing the free nicotinamide and the activated ADP-ribose, which is realized by SIRT2 cleaving the glycosidic bond between nicotinamide and ADP-ribose of NAD+; and (3) the third part, the acetyl group of the substrate is transferred to the ADP-ribose via SIRT2 catalysis, after which two products (the deacetylated substrate and the AADPR) are obtained.
The benefits of SIRT2 modulation by small molecules have been demonstrated in cancer (Chen et al., 2020) and various metabolic (Yang P. H. et al., 2019) and neurodegenerative disorders (Zhang et al., 2020). Gradually, the potential of the unexplored regulator scaffolds in developing SIRT2 ligands has been rationalized. The regulator scaffolds of SIRT2 include two main modes, namely, the activators and inhibitors (Villalba and Alcaín, 2012). Almost all sirtuin activators are described for SIRT1 (Villalba and Alcaín, 2012), while SIRT2 is rarely involved (Dai et al., 2018). However, the inhibitors of SIRT2 have been widely studied.
The core scaffolds of SIRT2 inhibitors are synthesized by morpholine-catalyzed Knoevenagel condensation/lactonization of hydroxy naphthaldehydes with β-keto esters (Figure 2A).
In view of the variety of SIRT2 functions in cells, they are a druggable class of enzymes that have beneficial effects on various human diseases when selectively activated or inhibited by different molecules. This review focuses on those compounds that act as sirtuin inhibitors, which are potentially useful as therapeutic agents. Several specific inhibitors have been described, including sirtinol (Zhang M. M. et al., 2021), AGK2 (Yu et al., 2018), cambinol (Mahajan et al., 2014), salermide (Mu et al., 2019), suramin (Trapp et al., 2007), CSC8, and CSC13 (Schlicker et al., 2011; Figure 3).
Sirtinol is a widely used sirtuin inhibitor that affects the activation of both yeast Sir2p and human SIRT2 in vitro (Grozinger et al., 2001; Alcaín and Villalba, 2009). Sirtinol has an IC50 of 38 μM against SIRT2 in an in vitro assay, which shows only approximately threefold weaker potency against SIRT1 (Schlicker et al., 2011). Two further analogs, m- and p-sirtinol, have been demonstrated to be more potent than sirtinol against human SIRT2 (Villalba and Alcaín, 2012).
Sirtuin 2 has an important role in deacetylating phosphoenolpyruvate carboxykinase 1 (PEPCK1), a critical enzyme for gluconeogenesis (Jiang et al., 2011; Knyphausen et al., 2016). Also, sirtinol has been previously used to treat type II diabetes (Zhang M. M. et al., 2021). Zhang M. M. et al. (2021) have demonstrated that sirtinol has anti-diabetic effects on cell gluconeogenesis in vivo and in vitro. In SIRT2-knockdown cells, sirtinol exerts little influence on endogenous PEPCK1 levels (Zhang M. M. et al., 2021). Thus, SIRT2 may be a key target for cetirizine in the treatment of diabetes. In addition, sirtinol administration has also been reported to effectively kill cancer cells when combined with dichloroacetic acid (DCA) and another SIRT2 inhibitor, AGK2 (Ma et al., 2018). AGK2 and sirtinol increase the lysine-acetylation and decrease the serine-phosphorylation of pyruvate dehydrogenase α 1 (PDHA1). Also, the two inhibitors synergize with DCA to further activate PDHA1, which further decreases glucose consumption and lactate production, and increases oxygen consumption rate (OCR) and reactive oxygen species (ROS) generation, which is the function of oxidative phosphorylation (OXPHOS) (Ma et al., 2018). Furthermore, a recent study has demonstrated that lipopolysaccharide (LPS)-stimulated production of tumor necrosis factor-α (TNF-α) and prostaglandin E2 (PGE2) in HAPI rat microglial cells can be inhibited by sirtinol, which protects SH-SY5Y cells from excessive inflammation (Zhang Y. Q. et al., 2021). This research provides theoretical support for further investigation of the therapeutic effect of SIRT2 regulators on LPS-induced neuroinflammation and neurodegeneration.
AGK2, a selective SIRT2 inhibitor, has a calculated IC50 for SIRT2 of 3.5 μM (Alcaín and Villalba, 2009). AGK2 can enter cells easily and act on endogenous SIRT2 in its natural environment. Even at the higher compound concentrations, AGK2 shows only minimal toxicity (Alcaín and Villalba, 2009).
AGK2 reduces the α-synuclein (α-syn) toxicity of dorsomedial dopamine neurons (Outeiro et al., 2007; Suresh and Manjithaya, 2019). Treatment with AGK2 leads to C-6 glioma cell apoptosis via the caspase-3-dependent pathway (He et al., 2012; Qin et al., 2014). AGK2 also downregulates the forkhead box O3 (FOXO3a) and mitogen-activated protein kinase (MAPK) signaling pathways, which confer neuroprotection in ischemic stroke (She et al., 2018). In addition, AGK2 treatment decreases the RNAs of hepatitis B virus (HBV), the replication of intermediates, and the secretion of HBeAg and HBsAg, which inhibits HBV replication (Yu et al., 2018). Furthermore, AGK2 reduces the expression of Evans blue pigmentation by inhibiting mast cell activation and lung barrier dysfunction by inhibiting inflammatory responses (Kim Y. Y. et al., 2020).
Cambinol, a chemically stable compound, shares a β-naphthol pharmacophore with sirtinol (Heltweg et al., 2006; Medda et al., 2009). It is a dual SIRT1/SIRT2 inhibitor, which inhibits the activity of human SIRT2 deacetylase in vitro with an IC50 value of 59 μM (Alcaín and Villalba, 2009).
Cambinol has cytotoxic activity against cancer cells in vitro. Other studies have shown that cambinol induces apoptosis through the hyperacetylation of BCL6 and p53, which inhibits the growth of Burkitt lymphoma xenografts (Chen, 2011; Chowdhury et al., 2020). Besides, cambinol affects the survival and migration of cellular through modulating the acetylation of p53 and FoxO1 in HepG2 and Huh7 cells. Furthermore, it has been confirmed that SIRT2 activity blockage can be beneficial during hepatocellular carcinoma therapy (Ceballos et al., 2018).
Salermide, a reverse amide compound histone deacetylase inhibitor (HDACI), has a strong inhibitory effect on SIRT2. For example, salermide inhibits 80% of SIRT2 activity in an in vitro assay at 25 μM (Trapp et al., 2007). Also, in vivo studies suggest that salermide is well-tolerated by mice at concentrations up to 100 μM (Alcaín and Villalba, 2009).
Moreover, studies have found that inhibition of SIRT2 can induce apoptosis in cancer cells, having a multifaceted role in regulating autophagy (Kim et al., 2011). Salermide upregulates heat shock protein family A (Hsp70) member 5 (HSPA5) acetylation and induces pro-survival autophagy via the ATF4-DDIT4-mTORC1 axis in human lung cancer cells (Mu et al., 2019). In addition, salermide induces cell death and p53 acetylation by targeting SIRT2 (Peck et al., 2010).
Suramin is a potent inhibitor of SIRT2 NAD+ dependent deacetylase activity, with an IC50 value for SIRT2 of 1.15 μM (Trapp et al., 2007). It is also a polyanionic naphthylurea with antiproliferative and antiviral activity (Alcaín and Villalba, 2009). Nevertheless, the severe neurotoxicity and other systemic side effects of suramin seriously hinder its application in therapeutic treatment (Peltier and Russell, 2002).
CSC8 and CSC13 are SIRT2 inhibitors. The virtual docking of the compound library into the peptide-binding pockets of SIRT2 crystal structures is exploited, which yields compounds discriminating between different isoforms. In activity assays, two compounds show the largest effects in the screen for further characterization. The compounds CSC8 and CSC13 inhibit SIRT2 to ∼80% in the screen (Schlicker et al., 2011). So, CSC8 and CSC13 with micromolar potency and high specificity for SIRT2 are the novel sirtuin inhibitors that were developed based on structure (Botta et al., 2012).
Neuroinflammation is considered a critical factor in the progression of multiple neurological diseases (Bai et al., 2021). The inflammatory factors can rapidly activate inflammatory cells and promote the infiltration of inflammatory cells in the injured area, which aggravates brain damage and advances disease progression (Jayaraj et al., 2019).
Sirtuin 2 protects the CNS by reducing excessive inflammation. A previous study found that SIRT2 regulators effectively inhibited LPS (5 ng/mL)-stimulated TNF- and PGE2 production in HAPI rat microglial cells and protected SH-SY5Y human neuroblastoma cells from excessive inflammation (Zhang Y. Q. et al., 2021). In non-neuronal cells, SIRT2 has been shown to function as a tubulin deacetylase and a key regulator of cell division and differentiation. Moreover, the distribution and function of the SIRT2 microtubule (MT) deacetylase are related to the differentiation of cells (Maxwell et al., 2011). Studies have also demonstrated that isoform 3 of SIRT2 is an age-dependent accumulation protein in the CNS (Sola-Sevilla et al., 2021). Also, a preclinical study found a significant increase in the pro-inflammatory cytokine interleukin-1β (IL-1β) in the model mice overexpressing SIRT2.3 (Sola-Sevilla et al., 2021). The most recent study discovered that reduced levels of the intermediate filament protein glial fibrillary acidic protein (GFAP), IL-1β, IL-6, and TNF-α indicated that early SIRT2 inhibition prevents neuroinflammation, which explains the improvement in cognitive deficits shown by 33i-treated senescence accelerated mouse prone 8 (SAMP8) mice (Diaz-Perdigon et al., 2020). Moreover, SIRT2 in microglia can prevent N-methyl-D-aspartic (NMDA)-mediated excitotoxicity in hippocampal slices by resisting the inflammatory signal from LPS (Sade Almeida et al., 2020). In addition, a study found that AGK2 suppresses the expression of inflammatory cytokines like inducible nitric oxide synthase (iNOS), TNF-α, and IL-1β in BV2 mouse microglial cells induced with LPS (Jiao et al., 2020). Also, the same study indicated that AGK2 could reduce the increase of phosphorylation p38, c-Jun N-terminal kinase (JNK), and extracellular signal-regulated kinase (ERK) (Jiao et al., 2020). Besides, AK7, an inhibitor of SIRT2, can inhibit sevoflurane (3%)-induced neuroinflammation and microglial activation by switching microglia from the M1 to M2 phenotype (Wu et al., 2020). The expression of forkhead box P3 (Foxp3), immunosuppression-associated molecules in Treg cells, can be promoted by inhibiting the activity of SIRT2 (Shu et al., 2019). Also, the anti-inflammatory effect of Treg cells on pro-inflammatory macrophages is weakened by SIRT2 using a lentiviral system (Shu et al., 2019). The nuclear factor-kappa B (NF-κB) drives the transcription of pro-inflammatory mediators involved in the terminal effector pathways (Liu X. X. et al., 2019). SIRT2 inhibition increases acetylation and nuclear translocation of NF-κB p65 protein, resulting in the up-regulation of NF-κB targets such as aquaporin 4 (AQP4), matrix metalloproteinases 9 (MMP-9), pro-inflammatory cytokines and chemokines, which exacerbates neuroinflammation (Yuan et al., 2016; Zhang and Chi, 2018). Therefore, the overexpression of SIRT2 alleviates neuroinflammation through the deacetylation of NF-κB.
Sirtuin 2 protects against traumatic brain injury (TBI) by regulating chemokines. Studies have found that secondary injury occurs after TBI, resulting in chronic and progressive neurodegenerative changes (Thapa et al., 2021). These mechanisms are strongly related to microglial-mediated neuroinflammation (Aires et al., 2021). In the patients with severe TBI under neurointensive care, high levels of endothelial chemokine (C-X-C motif) ligand 1 (CXCL1), CXCL10, metaphase chromosome protein 1 (MCP1), MCP2, and IL-8, as well as low levels of the chemokine CC-chemokine ligand 28 (CCL28) and MCP4 have been found (Dyhrfort et al., 2019). Besides, cross-correlation analysis revealed that leukemia inhibitory factor (LIF) and CXCL5 have a central role in the inflammatory cascade (Dyhrfort et al., 2019). Whether SIRT2 can regulate these chemokines and participate in the secondary injury of neuroinflammation after TBI should be addressed by future studies (Dyhrfort et al., 2019).
Sirtuin 2 protects spinal muscular atrophy and Charcot-Marie-Tooth disease by regulating the deacetylation of microrchidia 2 (MORC2), an effector of epigenetic silencing. Missense mutations in MORC2 cause neuropathies, including spinal muscular atrophy and Charcot-Marie-Tooth disease (Douse et al., 2018). In addition, SIRT2 is also the main deacetylase of MORC2 deacetylation (Liu et al., 2020). Knock-down of SIRT2 using siRNAs resulted in an increase in k767AC of MORC2, which further suggested that ectopic expression of SIRT2 reduces the k767AC of MORC2 (Liu et al., 2020). Also, the deacetylase activity of SIRT2 is necessary for the deacetylation of MORC2 (Liu et al., 2020). Therefore, SIRT2 has a potential role in the deacetylation of MORC2 to protect against the excessive neuroinflammation-related diseases.
Microglial cells, which are the immunocompetent cells and specific to the CNS, have emerged as crucial players in neuroinflammatory conditions (Rodrigues et al., 2018; Ahmad et al., 2022). In addition, studies have shown that the deacetylases SIRT2 can exert its neuroprotective effects through the reduction in neuroinflammation in the CNS, attenuating the microglial response, and releasing pro-inflammatory cytokines (Zhang Y. Q. et al., 2021).
Sirtuin 2 has an important role in the LPS-induced activation of BV2 microglia (Chen et al., 2015; Belarbi et al., 2020). The treatment of BV2 microglia with LPS increases nitric oxide (NO), iNOS, TNF-α, and IL-6 expression, indicating that microglia are activated. SIRT2 silencing significantly reversed this process in microglia cells (Chen et al., 2015). Besides, it was found that SIRT2 mediates the significant increase of the intracellular ATP level and the Akt phosphorylation in BV2 microglia induced by NAD and NADH, while SIRT2 siRNA and the SIRT2 inhibitor AGK2 can reverse this process (Zhang et al., 2018).
Similar research found that the administration of SIRT2 deacetylase inhibitors (AGK2, AK-1, or AK-7) significantly promotes the dectin-induced expression of proinflammatory genes in mouse microglia (Sun et al., 2021). SIRT2 modulators (sirtinol, AGK2) reduce LPS-induced excessive inflammation in HAPI microglial cells and protect SH-SY5Y neuronal cells in vitro (Zhang Y. Q. et al., 2021). In addition, the SIRT2 inhibitor AGK2 alleviates LPS-induced neuroinflammation by regulating MAPK phosphatase-1 (MKP-1) in BV2 microglial cells (Jiao et al., 2020). AK7, an inhibitor of SIRT2, attenuates sevoflurane-induced learning and memory deficits in developing rats via modulating neuroinflammation and microglial activation (Wu et al., 2020).
It was also observed that SIRT2 protein was mainly expressed in the cytoplasm of neurons but not in astrocytes and microglia (Xie et al., 2017). In this regard, future studies are needed to further explore the role of SIRT2 in microglia physiopathology.
The nervous system is known as one of the most complex systems in the human body (Aslani et al., 2021). Lesions of the nervous system seriously affect the quality of life in humans (Fang et al., 2020). With the development of neurobiology, the role of neuroinflammation in nervous system disorders has been widely investigated. Studies have shown that neuroinflammation has a critical role in the pathogenesis of neurological disorders (Jayaraj et al., 2019; Mukhara et al., 2020; de Brito Toscano et al., 2021). SIRT2, a type of NAD+ dependent deacetylases, is predominantly expressed in the cytoplasm of cells in the mammalian CNS (Ma et al., 2020; Chen et al., 2021), which suggests that SIRT2 has a significant role in diseases with neuroinflammatory components (Wang et al., 2016, 2020; Figure 4 and Table 1).
Alzheimer’s disease is the most common cause of dementia globally (Kim S. et al., 2020). Its incidence has been increasing over the years, especially due to the aging of the population, bringing a heavy burden to individuals and society (Soria Lopez et al., 2019). Typical characteristics of AD progress are two hallmark pathologies: β-amyloid (Aβ) plaque deposition and neurofibrillary tangles of hyperphosphorylated tau (Weller and Budson, 2018). Besides the two pathologies, sustained neuroinflammation is also included in the process of AD, which involves the activation of microglia and astrocytes (Laurent et al., 2018).
A previous study found that microglial SIRT2 has a protective role in amnesic deficits associated with neuroinflammation (Sade Almeida et al., 2020). In vivo study, LPS significantly reduced the long-term potentiation (LTP) of hippocampal slices in microglia-specific SIRT2 deficient mice (Sade Almeida et al., 2020), while NMDA receptor antagonists restored the LTP values. The results suggest that microglial SIRT2 can prevent NMDA-mediated excitotoxicity in hippocampal slices in response to an inflammatory signal (Sade Almeida et al., 2020).
Another study found that early inhibition of SIRT2 prevents excessive neuroinflammation by decreasing the levels of IL-1β, IL-6, GFAP, and TNF-α, which suggests that SIRT2 is an emerging target for improving excessive neuroinflammation or AD progression (Diaz-Perdigon et al., 2020). A recent study showed that the pharmacological inactivation of SIRT2 exhibits a protective role in AD. For example, in the amyloid precursor protein/presenilin 1 transgenic mouse, the inhibitor of SIRT2 improved cognitive impairment and decreased the production of Aβ in the hippocampus (Wang et al., 2020). A similar protective role of SIRT2 for AD was also shown in another study (Zhang et al., 2020). AK1, an inhibitor of SIRT2, increases the acetylation level of tubulin, the completion of autophagy vesicular, and the aggregation of Aβ in AD cell models. It has been clarified that the inhibition of SIRT2 plays a beneficial role in inducing tubulin acetylation and strengthening autophagic trafficking (Zhang et al., 2020). Other evidence also suggests SIRT2 as a novel target for controlling AD (Jêśko et al., 2017; Silva et al., 2017; Shen et al., 2020).
Parkinson’s disease, a progressive neurodegenerative disease characterized by tremors and bradykinesia, causes heavy losses in productivity and medical resources (Hayes, 2019). The loss of dopaminergic neurons in the substantia nigra striatal pathway and the formation of neuronal inclusions are the main causes of PD (Xie et al., 2021). Yet, recent data have suggested chronic neuroinflammation as another feature of PD (Belarbi et al., 2020).
Some evidence suggests that SIRT2 is an emerging target in controlling PD by participating in the processes of PD pathogenesis, including the aggregation of α-syn, inflammation, oxidative stress, autophagy, and microtubule function (Singh et al., 2021). For example, a recent study found that SIRT2 inhibition exhibited the effects of neuroprotection and anti-inflammation in vitro (Liu Y. M. et al., 2019). Meanwhile, the expression level of SIRT2 in degenerating SNpc in the brain of PD was also mentioned (Liu Y. M. et al., 2019), suggesting that SIRT2 might be a potential target in treating PD.
To specifically address the role of SIRT2 in sporadic PD, a SIRT2 knock-out mouse was used to verify the pathogenic role of SIRT2. The SIRT2 knock-out mouse, which is cultured by the neurotoxin 1-methyl-4-phenylpyridinium (MPP+), induced a decrease in mitochondrial membrane potential in mesencephalic neuronal cells (Esteves et al., 2018). The knock-out of SIRT2 deacetylase enhanced α-tubulin acetylation and facilitated the trafficking and clearance of misfolded proteins (Esteves et al., 2018). These results indicated that mitochondrial, microtubule, and autophagy dysfunction are involved in neurodegeneration observed in sporadic PD.
Furthermore, studies have shown that inhibiting SIRT2 activity via miR-212-5p transfection can prevent dopaminergic neuron loss and reduce dopamine transporter (DAT), implying that miR-212-5p can control PD by targeting SIRT2 (Sun et al., 2018). These studies all indicate that SIRT2 has an important role in PD.
Depression is a common mental disorder characterized by low mood, poor cognition, and anhedonia as its core symptoms (Zheng et al., 2019). Currently, depression is one of the essential contributors to disability globally and one of the leading causes of the global burden of disease (Yao et al., 2019; Sarno et al., 2021). It has been estimated that depression will become the primary factor of disability by 2030 (Subermaniam et al., 2020).
Depression, as a neuropsychiatric disease, also belongs to the category of neuroinflammatory disorders. Among the mainstream hypotheses of the cause of depression, the hypothesis of immune and inflammation has been a hot spot in studying its pathological mechanism (Sharma, 2016; Leonard, 2018; Beurel et al., 2020). Polymorphism studies have shown that SIRT2-related genes are associated with postpartum depression (Luo et al., 2020) and AD depression (Porcelli et al., 2013).
A previous study found that 33i, a selective SIRT2 inhibitor, led to the increase of serotonin levels and glutamate receptor subunits, inducing an antidepressant-like action in the chronic mild stress (CMS) model of depression (Erburu et al., 2017). Another study found that the SIRT2 inhibitors sirtinol and AGK2 significantly decrease the secretion of TNF-α and reduce the production of PGE2 in LPS-stimulated HAPI rat microglial cells (Zhang Y. Q. et al., 2021). In addition, the pretreatment of Sirtinol and AGK2 protects the cells from LPS-stimulated HAPI supernatant in differentiated SH-SY5Y cells (Zhang Y. Q. et al., 2021). SIRT2 inhibition can induce an antidepressant-like action. Moreover, as new antidepressant agents, 33i, sirtinol, and AGK2 have the therapeutic potential to treat depression.
Stroke with a high lifelong disability and fatality rate is one of the most common diseases in modern society (Campbell and Khatri, 2020). It is an acute cerebrovascular disease, resulting in brain tissue damage due to the sudden rupture of blood vessels in the brain (hemorrhagic strokes) (Saand et al., 2019) or vascular occlusion preventing blood from flowing into the brain (ischemic strokes) (Patel et al., 2020). Ischemic stroke is the most common type of stroke (Wei et al., 2020).
Recent studies suggest that secondary neuroinflammation promotes further brain damage, leading to cell death in patients with acute stroke (Jayaraj et al., 2019; Huang et al., 2020). Conversely, the secretion of inflammatory factors is also beneficial as it promotes immune recovery (Jayaraj et al., 2019). SIRT2 has a vital role in stroke as well. It was found that the downregulation of SIRT2 protects the mouse brain against ischemic stroke (Xie et al., 2017). In addition, SIRT2 serves as a risk and prognosis marker for acute ischemic stroke in clinical practice (Zhang et al., 2021b).
Sirtuin 2 induces neuronal cell death in ischemic stroke. Previous studies reported that the SIRT2 inhibitors AK1 and AGK2 could reduce the cleaved caspase-3, Bim, and Bad, by attenuating apoptotic cell death caused by oxygen-glucose deprivation (She et al., 2018). Moreover, the neuroprotective effect of SIRT2 inhibition on ischemic stroke is modulated via the downregulation of the protein kinase B (AKT)/FOXO3a axis and the MAPK pathways (She et al., 2018). Another study showed that the anti-inflammatory effect of Treg cells was weakened by SIRT2 on pro-inflammatory macrophages, which indirectly impacted the process of stroke in the model of middle cerebral artery occlusion (Shu et al., 2019).
In this review, the latest reports on the role of SIRT2 in regulating neuroinflammatory disorders were summarized and highlighted. As one of the most special sirtuins in the CNS, SIRT2 regulates multiple biological functions by NAD+ dependent deacetylation effects, including senescence, myelin formation, autophagy, and inflammation. We focused on the immunomodulatory effects of SIRT2 in the immunology response through molecules of immune regulation of neuroinflammatory disorders.
The immunomodulatory properties and the therapeutic potential of SIRT2 have been demonstrated in vivo or in vitro models of neuroinflammatory disorders. Moreover, previous studies have indicated that SIRT2 modulates immune balance in nervous system diseases via key molecules in different cellular signaling pathways. Herein, we summarized terminal effector molecules of the inflammatory response and reviewed the specific inhibitors of SIRT2, while the specific mechanisms of SIRT2 were inversely verified by relevant molecular biology experiments. Also, the regulatory details of different diseases with neuroinflammatory components, including neurodegenerative and neuropsychiatric diseases, were summed up to a certain extent.
Although the regulatory mechanism of SIRT2 in neuroinflammatory disorders has been studied, some questions still remain. First, the molecular regulating mechanism of SIRT2 has not yet been elaborated. Second, due to the lack of effective activators, it is difficult to assess the potency of SIRT2 inhibitors. Moreover, clinical evidence of immunomodulatory and immune-balancing effects of SIRT2 is lacking. Therefore, further studies should focus on investigating precise targets of SIRT2, which will contribute to developing treatment strategies for neuroinflammatory disorders.
With the state-of-art methods for studying SIRT2 that are expected to be available in the future, studies with an interdisciplinary perspective will ultimately provide a more comprehensive understanding of SIRT2 during the development of neuroinflammatory disorders. We hope this review will draw more attention to preventing and treating neuroinflammatory disorders by targeting SIRT2.
ZF conceived and designed the framework of the review, performed literature research and sorting, analyzed most of the data, prepared the figures, and wrote the manuscript. LB conceived and designed the framework of the review, supervised the execution of this project, and contributed to data interpretation and manuscript preparation. Both authors read and approved the final manuscript.
This work was supported by grants from the National Natural Science Foundation of China (grant number 82074547) and the National Key Research and Development Plan (grant number 2019YFC1709703).
The authors declare that the research was conducted in the absence of any commercial or financial relationships that could be construed as a potential conflict of interest.
All claims expressed in this article are solely those of the authors and do not necessarily represent those of their affiliated organizations, or those of the publisher, the editors and the reviewers. Any product that may be evaluated in this article, or claim that may be made by its manufacturer, is not guaranteed or endorsed by the publisher.
This work would not have been possible without the enormous efforts of our collaborators and scientific friends over the years. We thank the Beijing Institute of Chinese Medicine for support in the process.
CNS, central nervous system; SIRT2, sirtuin 2; NAD, nicotinamide adenine dinucleotide; HDACs, histone deacetylases; AADPR, the 2 ′ -O-acetyl-ADP-ribose; PEPCK1, phosphoenolpyruvate carboxykinase 1; DCA, dichloroacetic acid; PDHA1, pyruvate dehydrogenase α 1; OCR, oxygen consumption rate; ROS, reactive oxygen species; OXPHOS, oxidative phosphorylation; LPS, lipopolysaccharide; TNF- α, tumor necrosis factor- α; PGE2, prostaglandin E2; α -syn, α -synuclein; FOXO3a, forkhead box O3; MAPK, mitogen-activated protein kinase; HBV, hepatitis B virus; HSPA5, heat shock protein family A (Hsp70) member 5; IL-1 β, interleukin-1 β; GFAP, glial fibrillary acidic protein; SAMP8, senescence accelerated mouse prone 8; NMDA, N-methyl -D-aspartic; iNOS, inducible nitric oxide synthase; JNK, c-JunN-terminal kinase; ERK, extracellular signal-regulated kinase; Foxp3, forkhead box P3; NF- κ B, nuclear factor-kappa B; AQP4, aquaporin 4; MMP-9, matrix metalloproteinases 9; TBI, traumatic brain injury; CXCL1, chemokine (C-X-C motif) ligand 1; MCP1, metaphase chromosome protein 1; CCL28, CC-chemokine ligand 28; LIF, leukemia inhibitory factor; MORC2, microrchidia 2; NO, nitric oxide; MKP-1, MAPK phosphatase-1; AD, Alzheimer’s disease; A β, β -amyloid; LTP, long-term potentiation; PD, Parkinson’s disease; DAT, dopamine transporter; MPP+, neurotoxin 1-methyl-4-phenylpyridinium; CMS, chronic mild stress; AKT, protein kinase B.
Ahmad, M. A., Kareem, O., Khushtar, M., Akbar, M., Haque, M. R., Iqubal, A., et al. (2022). Neuroinflammation: A Potential Risk for Dementia. Int. J. Mol. Sci. 23:616. doi: 10.3390/ijms23020616
Aires, I. D., Ribeiro-Rodrigues, T., Boia, R., Ferreira-Rodrigues, M., Girão, H., Ambrósio, A. F., et al. (2021). Microglial Extracellular Vesicles as Vehicles for Neurodegeneration Spreading. Biomolecules 11:770. doi: 10.3390/biom11060770
Akbari, Z., Reisi, P., Torkaman-Boutorabi, A., and Farahmandfar, M. (2020). Effect of Pentoxifylline on Apoptotic-Related Gene Expression Profile, Learning and Memory Impairment Induced by Systemic Lipopolysaccharide Administration in the Rat Hippocampus. Int. J. Prev. Med. 11, 151. doi: 10.4103/ijpvm.IJPVM_170_19
Alcaín, F. J., and Villalba, J. M. (2009). Sirtuin inhibitors. Expert Opin. Ther. Pat. 19, 283–294. doi: 10.1517/13543770902755111
Armstrong, R. A. (2019). Risk factors for Alzheimer’s disease. Folia Neuropathol. 57, 87–105. doi: 10.5114/fn.2019.85929
Aslani, M., Mortazavi-Jahromi, S. S., and Mirshafiey, A. (2021). Efficient roles of miR-146a in cellular and molecular mechanisms of neuroinflammatory disorders: An effectual review in neuroimmunology. Immunol. Lett. 238, 1–20. doi: 10.1016/j.imlet.2021.07.004
Bai, Y. M., Feng, Y. L., Jiang, B., Yang, Y., Pei, Z. W., Yang, Q., et al. (2021). The Role of Exercise in Reducing Hyperlipidemia-Induced Neuronal Damage in Apolipoprotein E-Deficient Mice. Biomed. Res. Int. 2021:5512518. doi: 10.1155/2021/5512518
Belarbi, K., Cuvelier, E., Bonte, M. A., Desplanque, M., Gressier, B., Devos, D., et al. (2020). Glycosphingolipids and neuroinflammation in Parkinson’s disease. Mol. Neurodegener. 15:59. doi: 10.1186/s13024-020-00408-1
Beurel, E., Toups, M., and Nemeroff, C. B. (2020). The Bidirectional Relationship of Depression and Inflammation: Double Trouble. Neuron 107, 234–256. doi: 10.1016/j.neuron.2020.06.002
Botta, G., De Santis, L. P., and Saladino, R. (2012). Current advances in the synthesis and antitumoral activity of SIRT1-2 inhibitors by modulation of p53 and pro-apoptotic proteins. Curr. Med. Chem. 19, 5871–5884. doi: 10.2174/092986712804143303
Campbell, B. C. V., and Khatri, P. (2020). Stroke. Lancet 396, 129–142. doi: 10.1016/s0140-6736(20)31179-x
Carafa, V., Altucci, L., and Nebbioso, A. (2019). Dual Tumor Suppressor and Tumor Promoter Action of Sirtuins in Determining Malignant Phenotype. Front. Pharmacol. 10:38. doi: 10.3389/fphar.2019.00038
Carafa, V., Nebbioso, A., and Altucci, L. (2012). Sirtuins and disease: the road ahead. Front. Pharmacol. 3:4. doi: 10.3389/fphar.2012.00004
Ceballos, M. P., Decándido, G., Quiroga, A. D., Comanzo, C. G., Livore, V. I., Lorenzetti, F., et al. (2018). Inhibition of sirtuins 1 and 2 impairs cell survival and migration and modulates the expression of P-glycoprotein and MRP3 in hepatocellular carcinoma cell lines. Toxicol. Lett. 289, 63–74. doi: 10.1016/j.toxlet.2018.03.011
Chen, G. Y., Huang, P., and Hu, C. (2020). The role of SIRT2 in cancer: A novel therapeutic target. Int. J. Cancer 147, 3297–3304. doi: 10.1002/ijc.33118
Chen, H. Y., Wu, D. H., Ding, X. T., and Ying, W. H. (2015). SIRT2 is required for lipopolysaccharide-induced activation of BV2 microglia. Neuroreport 26, 88–93. doi: 10.1097/WNR.0000000000000305
Chen, L. (2011). Medicinal chemistry of sirtuin inhibitors. Curr. Med. Chem. 18, 1936–1946. doi: 10.2174/09298671179559005
Chen, X. Q., Lu, W. M., and Wu, D. H. (2021). Sirtuin 2 (SIRT2): Confusing Roles in the Pathophysiology of Neurological Disorders. Front. Neurosci. 15:614107. doi: 10.3389/fnins.2021.614107
Chowdhury, S., Sripathy, S., Webster, A., Park, A., Lao, U., Hsu, J. H., et al. (2020). Discovery of Selective SIRT2 Inhibitors as Therapeutic Agents in B-Cell Lymphoma and Other Malignancies. Molecules 25:455. doi: 10.3390/molecules25030455
Dai, H., Sinclair, D. A., Ellis, J. L., and Steegborn, C. (2018). Sirtuin activators and inhibitors: Promises, achievements, and challenges. Pharmacol. Ther. 188, 140–154. doi: 10.1016/j.pharmthera.2018.03.004
de Brito Toscano, E. C., Rocha, N. P., Lopes, B. N. A., Suemoto, C. K., and Teixeira, A. L. (2021). Neuroinflammation in Alzheimer’s disease: focus on NLRP1 and NLRP3 inflammasomes. Curr. Protein Pept. Sci. 22, 584–598. doi: 10.2174/1389203722666210916141436
Diaz-Perdigon, T., Belloch, F. B., Ricobaraza, A., Elboray, E. E., Suzuki, T., Tordera, R. M., et al. (2020). Early sirtuin 2 inhibition prevents age-related cognitive decline in a senescence-accelerated mouse model. Neuropsychopharmacology 45, 347–357. doi: 10.1038/s41386-019-0503-8
Douse, C. H., Bloor, S., Liu, Y. C., Shamin, M., Tchasovnikarova, I. A., Timms, R. T., et al. (2018). Neuropathic MORC2 mutations perturb GHKL ATPase dimerization dynamics and epigenetic silencing by multiple structural mechanisms. Nat. Commun. 9:651. doi: 10.1038/s41467-018-03045-x
Dyhrfort, P., Shen, Q. J., Clausen, F., Thulin, M., Enblad, P., Kamali-Moghaddam, M., et al. (2019). Monitoring of Protein Biomarkers of Inflammation in Human Traumatic Brain Injury Using Microdialysis and Proximity Extension Assay Technology in Neurointensive Care. J. Neurotrauma 36, 2872–2885. doi: 10.1089/neu.2018.6320
Erburu, M., Muñoz-Cobo, I., Diaz-Perdigon, T., Mellini, P., Suzuki, T., Puerta, E., et al. (2017). SIRT2 inhibition modulate glutamate and serotonin systems in the prefrontal cortex and induces antidepressant-like action. Neuropharmacology 117, 195–208. doi: 10.1016/j.neuropharm.2017.01.033
Esteves, A. R., Arduíno, D. M., Silva, D. F., Viana, S. D., Pereira, F. C., and Cardoso, S. M. (2018). Mitochondrial Metabolism Regulates Microtubule Acetylome and Autophagy Trough Sirtuin-2: Impact for Parkinson’s Disease. Mol. Neurobiol. 55, 1440–1462. doi: 10.1007/s12035-017-0420-y
Fang, X., Zhou, X. T., Miao, Y. Q., Han, Y. W., Wei, J., and Chen, T. T. (2020). Therapeutic effect of GLP-1 engineered strain on mice model of Alzheimer’s disease and Parkinson’s disease. AMB Express 10:80. doi: 10.1186/s13568-020-01014-6
Finnin, M. S., Donigian, J. R., and Pavletich, N. P. (2001). Structure of the histone deacetylase SIRT2. Nat. Struct. Biol. 8, 621–625. doi: 10.1038/89668
Grabowska, W., Sikora, E., Bielak-Zmijewska, A., and Sirtuins. (2017). a promising target in slowing down the ageing process. Biogerontology 18, 447–476. doi: 10.1007/s10522-017-9685-9
Griciuc, A., and Tanzi, R. E. (2021). The role of innate immune genes in Alzheimer’s disease. Curr. Opin. Neurol. 34, 228–236. doi: 10.1097/wco.0000000000000911
Grozinger, C. M., Chao, Blackwell, H. E., Moazed, D., and Schreiber, S. L. (2001). Identification of a class of small molecule inhibitors of the sirtuin family of NAD-dependent deacetylases by phenotypic screening. J Biol. Chem. 276, 38837–38843. doi: 10.1074/jbc.M106779200
Gu, J. Y., Chen, C., Wang, J., Chen, T. T., Yao, W. J., Yan, T. D., et al. (2020). Withaferin A Exerts Preventive Effect on Liver Fibrosis through Oxidative Stress Inhibition in a Sirtuin 3-Dependent Manner. Oxid Med Cell Longev. 2020:2452848. doi: 10.1155/2020/2452848
Hayes, M. T. (2019). Parkinson’s Disease and Parkinsonism. Am. J. Med. 132, 802–807. doi: 10.1016/j.amjmed.2019.03.001
Hayley, S., Hakim, A. M., and Albert, P. R. (2021). Depression, dementia and immune dysregulation. Brain 144, 746–760. doi: 10.1093/brain/awaa405
He, X., Nie, H., Hong, Y. Y., Sheng, C. B., Xia, W. L., and Ying, W. H. (2012). SIRT2 activity is required for the survival of C6 glioma cells. Biochem. Biophys. Res. Commun. 417, 468–472. doi: 10.1016/j.bbrc.2011.11.141
Heltweg, B., Gatbonton, T., Schuler, A. D., Posakony, J., Li, H., Goehle, S., et al. (2006). Antitumor activity of a small-molecule inhibitor of human silent information regulator 2 enzymes. Cancer Res. 66, 4368–4377. doi: 10.1158/0008-5472.Can-05-3617
Hong, Y. A., Kim, J. E., Jo, M., and Ko, G. J. (2020). The Role of Sirtuins in Kidney Diseases. Int. J. Mol. Sci. 21:6686. doi: 10.3390/ijms21186686
Hou, B. B., Liu, X. M., Zheng, F. L., Xu, X. Z., and Zhang, Z. Y. (2014). Molecular cloning, modeling and differential expression of a gene encoding a silent information regulator-like protein from Sporothrix schenckii. Int. J. Mol. Med. 33, 1415–1422. doi: 10.3892/ijmm.2014.1719
Hou, Y. J., Dan, X. L., Babbar, M., Wei, Y., Hasselbalch, S. G., Croteau, D. L., et al. (2019). Ageing as a risk factor for neurodegenerative disease. Nat. Rev. Neurol. 15, 565–581. doi: 10.1038/s41582-019-0244-7
Huang, J. B., Chen, N. C., Chen, C. L., Fu, M. H., Pan, H. Y., Hsu, C. Y., et al. (2020). Serum Levels of Soluble Triggering Receptor Expressed on Myeloid Cells-1 Associated with the Severity and Outcome of Acute Ischemic Stroke. J. Clin. Med. 10:61. doi: 10.3390/jcm10010061
Iadecola, C., Buckwalter, M. S., and Anrather, J. (2020). Immune responses to stroke: mechanisms, modulation, and therapeutic potential. J. Clin. Invest. 130, 2777–2788. doi: 10.1172/jci135530
Jayaraj, R. L., Azimullah, S., Beiram, R., Jalal, F. Y., and Rosenberg, G. A. (2019). Neuroinflammation: friend and foe for ischemic stroke. J. Neuroinflamm. 16:142. doi: 10.1186/s12974-019-1516-2
Jêśko, H., Wencel, P., Strosznajder, R. P., and Strosznajder, J. B. (2017). Sirtuins and Their Roles in Brain Aging and Neurodegenerative Disorders. Neurochem. Res. 42, 876–890. doi: 10.1007/s11064-016-2110-y
Jia, Y., Zhang, D. D., Yin, H., Li, H. R., Du, J., and Bao, H. K. (2021). Ganoderic Acid A Attenuates LPS-Induced Neuroinflammation in BV2 Microglia by Activating Farnesoid X Receptor. Neurochem. Res. 46, 1725–1736. doi: 10.1007/s11064-021-03303-3
Jiang, W. Q., Wang, S. W., Xiao, M. T., Lin, Y., Zhou, L. S., Lei, Q. Y., et al. (2011). Acetylation regulates gluconeogenesis by promoting PEPCK1 degradation via recruiting the UBR5 ubiquitin ligase. Mol. Cell 43, 33–44. doi: 10.1016/j.molcel.2011.04.028
Jiao, F. Z., Wang, Y., Zhang, W. B., Zhang, H. Y., Chen, Q., Wang, L. W., et al. (2020). AGK2 Alleviates Lipopolysaccharide Induced Neuroinflammation through Regulation of Mitogen-Activated Protein Kinase Phosphatase-1. J. Neuroimmune Pharmacol. 15, 196–208. doi: 10.1007/s11481-019-09890-x
Karaman, B., Alhalabi, Z., Swyter, S., Mihigo, S. O., Andrae-Marobela, K., Jung, M., et al. (2018). Identification of Bichalcones as Sirtuin Inhibitors by Virtual Screening and In Vitro Testing. Molecules 23:416. doi: 10.3390/molecules23020416
Kim, H. S., Vassilopoulos, A., Wang, R. H., Lahusen, T., Xiao, Z., Xu, X., et al. (2011). SIRT2 maintains genome integrity and suppresses tumorigenesis through regulating APC/C activity. Cancer Cell 20, 487–499. doi: 10.1016/j.ccr.2011.09.004
Kim, S., Nam, Y., Kim, C., Lee, H., Hong, S., Kim, H. S., et al. (2020). Neuroprotective and Anti-Inflammatory Effects of Low-Moderate Dose Ionizing Radiation in Models of Alzheimer’s Disease. Int. J. Mol. Sci. 21:3678. doi: 10.3390/ijms21103678
Kim, Y. Y., Hur, G., Lee, S. W., Lee, S. J., Lee, S., Kim, S. H., et al. (2020). AGK2 ameliorates mast cell-mediated allergic airway inflammation and fibrosis by inhibiting FcεRI/TGF-β signaling pathway. Pharmacol. Res. 159:105027. doi: 10.1016/j.phrs.2020.105027
Knyphausen, P., de Boor, S., Kuhlmann, N., Scislowski, L., Extra, A., Baldus, L., et al. (2016). Insights into Lysine Deacetylation of Natively Folded Substrate Proteins by Sirtuins. J. Biol. Chem. 291, 14677–14694. doi: 10.1074/jbc.M116.726307
Kwon, H. S., and Koh, S. H. (2020). Neuroinflammation in neurodegenerative disorders: the roles of microglia and astrocytes. Transl. Neurodegener. 9:42. doi: 10.1186/s40035-020-00221-2
Laurent, C., Buée, L., and Blum, D. (2018). Tau and neuroinflammation: What impact for Alzheimer’s Disease and Tauopathies? Biomed. J. 41, 21–33. doi: 10.1016/j.bj.2018.01.003
Leonard, B. E. (2018). Inflammation and depression: a causal or coincidental link to the pathophysiology? Acta Neuropsychiatr. 30, 1–16. doi: 10.1017/neu.2016.69
Lewis, J. E., Singh, N., Holmila, R. J., Sumer, B. D., Williams, N. S., Furdui, C. M., et al. (2019). Targeting NAD(+) Metabolism to Enhance Radiation Therapy Responses. Semin. Radiat. Oncol. 29, 6–15. doi: 10.1016/j.semradonc.2018.10.009
Li, J. Y., Flick, F., Verheugd, P., Carloni, P., Lüscher, B., and Rossetti, G. (2015). Insight into the Mechanism of Intramolecular Inhibition of the Catalytic Activity of Sirtuin 2 (SIRT2). PLoS One 10:e0139095. doi: 10.1371/journal.pone.0139095
Li, X. J., Egervari, G., Wang, Y. G., Berger, S. L., and Lu, Z. M. (2018). Regulation of chromatin and gene expression by metabolic enzymes and metabolites. Nat. Rev. Mol. Cell Biol. 19, 563–578. doi: 10.1038/s41580-018-0029-7
Lin, J. L., Xiong, Z. C., Gu, J. H., Sun, Z. R., Jiang, S., Fan, D. W., et al. (2021). Sirtuins: Potential Therapeutic Targets for Defense against Oxidative Stress in Spinal Cord Injury. Oxid. Med. Cell Longev. 2021:7207692. doi: 10.1155/2021/7207692
Liu, H. Y., Liu, Y. Y., Yang, F., Zhang, L., Zhang, F. L., Hu, X., et al. (2020). Acetylation of MORC2 by NAT10 regulates cell-cycle checkpoint control and resistance to DNA-damaging chemotherapy and radiotherapy in breast cancer. Nucleic Acids Res. 48, 3638–3656. doi: 10.1093/nar/gkaa130
Liu, X. X., Liu, F. H., Ma, Y. F., Li, H. R., Ju, X. H., and Xu, J. Q. (2019). Effect of Puerarin, Baicalin and Berberine Hydrochloride on the Regulation of IPEC-J2 Cells Infected with Enterotoxigenic Escherichia coli. Evid. Based Complement Alternat. Med. 2019:7438593. doi: 10.1155/2019/7438593
Liu, Y. M., Zhang, Y. Y., Zhu, K. H., Chi, S., Wang, C., and Xie, A. M. (2019). Emerging Role of Sirtuin 2 in Parkinson’s Disease. Front. Aging Neurosci. 11:372. doi: 10.3389/fnagi.2019.00372
Lu, W. M., Wang, Q., Xu, C., Yuan, H. H., Fan, Q., Chen, B. Y., et al. (2021). SUMOylation is essential for Sirt2 tumor-suppressor function in neuroblastoma. Neoplasia 23, 129–139. doi: 10.1016/j.neo.2020.11.013
Luo, S. C., Duan, K. M., Fang, C., Li, D. Y., Zheng, S. S., Yang, S. Q., et al. (2020). Correlations Between SIRT Genetic Polymorphisms and Postpartum Depressive Symptoms in Chinese Parturients Who Had Undergone Cesarean Section. Neuropsychiatr. Dis. Treat. 16, 3225–3238. doi: 10.2147/ndt.S278248
Ma, L., Maruwge, W., Strambi, A., D’Arcy, P., Pellegrini, P., Kis, L., et al. (2014). SIRT1 and SIRT2 inhibition impairs pediatric soft tissue sarcoma growth. Cell Death Dis. 5:e1483. doi: 10.1038/cddis.2014.385
Ma, W. J., Zhao, X. P., Wang, K. Y., Liu, J. J., and Huang, G. (2018). Dichloroacetic acid (DCA) synergizes with the SIRT2 inhibitor Sirtinol and AGK2 to enhance anti-tumor efficacy in non-small cell lung cancer. Cancer Biol. Ther. 19, 835–846. doi: 10.1080/15384047.2018.1480281
Ma, Y. G., Deng, M., Zhao, X. Q., and Liu, M. (2020). Alternatively Polarized Macrophages Regulate the Growth and Differentiation of Ependymal Stem Cells through the SIRT2 Pathway. Exp. Neurobiol. 29, 150–163. doi: 10.5607/en19078
Mahajan, S. S., Scian, M., Sripathy, S., Posakony, J., Lao, U., Loe, T. K., et al. (2014). Development of pyrazolone and isoxazol-5-one cambinol analogues as sirtuin inhibitors. J. Med. Chem. 57, 3283–3294. doi: 10.1021/jm4018064
Maida, C. D., Norrito, R. L., Daidone, M., Tuttolomondo, A., and Pinto, A. (2020). Neuroinflammatory Mechanisms in Ischemic Stroke: Focus on Cardioembolic Stroke, Background, and Therapeutic Approaches. Int. J. Mol. Sci. 21:6454. doi: 10.3390/ijms21186454
Maxwell, M. M., Tomkinson, E. M., Nobles, J., Wizeman, J. W., Amore, A. M., Quinti, L., et al. (2011). The Sirtuin 2 microtubule deacetylase is an abundant neuronal protein that accumulates in the aging CNS. Hum. Mol. Genet. 20, 3986–3996. doi: 10.1093/hmg/ddr326
Medda, F., Russell, R. J., Higgins, M., McCarthy, A. R., Campbell, J., Slawin, A. M., et al. (2009). Novel cambinol analogs as sirtuin inhibitors: synthesis, biological evaluation, and rationalization of activity. J. Med. Chem. 52, 2673–2682. doi: 10.1021/jm8014298
Minten, E. V., Kapoor-Vazirani, P., Li, C., Zhang, H., Balakrishnan, K., and Yu, D. S. (2021). SIRT2 promotes BRCA1-BARD1 heterodimerization through deacetylation. Cell Rep. 34:108921. doi: 10.1016/j.celrep.2021.108921
Mu, N., Lei, Y. J., Wang, Y., Wang, Y. Y., Duan, Q. H., Ma, G. L., et al. (2019). Inhibition of SIRT1/2 upregulates HSPA5 acetylation and induces pro-survival autophagy via ATF4-DDIT4-mTORC1 axis in human lung cancer cells. Apoptosis 24, 798–811. doi: 10.1007/s10495-019-01559-3
Mukhara, D., Oh, U., and Neigh, G. N. (2020). Neuroinflammation. Handb. Clin. Neurol. 175, 235–259. doi: 10.1016/b978-0-444-64123-6.00017-5
North, B. J., and Verdin, E. (2004). Sirtuins: Sir2-related NAD-dependent protein deacetylases. Genom. Biol. 5:224. doi: 10.1186/gb-2004-5-5-224
Outeiro, T. F., Kontopoulos, E., Altmann, S. M., Kufareva, I., Strathearn, K. E., Amore, A. M., et al. (2007). Sirtuin 2 inhibitors rescue alpha-synuclein-mediated toxicity in models of Parkinson’s disease. Science 317, 516–519. doi: 10.1126/science.1143780
Pascoe, M. C., and Parker, A. G. (2019). Physical activity and exercise as a universal depression prevention in young people: A narrative review. Early Interv. Psychiatr. 13, 733–739. doi: 10.1111/eip.12737
Patel, P., Yavagal, D., and Khandelwal, P. (2020). Hyperacute Management of Ischemic Strokes: JACC Focus Seminar. J. Am. Coll. Cardiol. 75, 1844–1856. doi: 10.1016/j.jacc.2020.03.006
Peck, B., Chen, C. Y., Ho, K. K., Di Fruscia, P., Myatt, S. S., Coombes, R. C., et al. (2010). SIRT inhibitors induce cell death and p53 acetylation through targeting both SIRT1 and SIRT2. Mol. Cancer Ther. 9, 844–855. doi: 10.1158/1535-7163.Mct-09-0971
Peltier, A. C., and Russell, J. W. (2002). Recent advances in drug-induced neuropathies. Curr. Opin. Neurol. 15, 633–638. doi: 10.1097/00019052-200210000-00015
Porcelli, S., Salfi, R., Politis, A., Atti, A. R., Albani, D., Chierchia, A., et al. (2013). Association between Sirtuin 2 gene rs10410544 polymorphism and depression in Alzheimer’s disease in two independent European samples. J. Neural Transm. 120, 1709–1715. doi: 10.1007/s00702-013-1045-6
Puigoriol-Illamola, D., Martínez-Damas, M., Griñán-Ferré, C., and Pallàs, M. (2020). Chronic Mild Stress Modified Epigenetic Mechanisms Leading to Accelerated Senescence and Impaired Cognitive Performance in Mice. Int. J. Mol. Sci. 21:1154. doi: 10.3390/ijms21031154
Putaala, J. (2020). Ischemic Stroke in Young Adults. Continuum 26, 386–414. doi: 10.1212/con.0000000000000833
Qin, B., Panickar, K. S., and Anderson, R. A. (2014). Cinnamon polyphenols regulate S100β, sirtuins, and neuroactive proteins in rat C6 glioma cells. Nutrition 30, 210–217. doi: 10.1016/j.nut.2013.07.001
Ren, Y. R., Ye, Y. L., Feng, Y., Xu, T. F., Shen, Y., Liu, J., et al. (2020). SL010110, a lead compound, inhibits gluconeogenesis via SIRT2-p300-mediated PEPCK1 degradation and improves glucose homeostasis in diabetic mice. Acta Pharmacol. Sin. 42, 1834–1846. doi: 10.1038/s41401-020-00609-w
Rodrigues, A. C., Aires, I. D., Vindeirinho, J., Boia, R., Madeira, M. H., Gonçalves, F. Q., et al. (2018). Elevated Pressure Changes the Purinergic System of Microglial Cells. Front. Pharmacol. 9:16. doi: 10.3389/fphar.2018.00016
Saand, A. R., Yu, F., Chen, J., and Chou, S. H. (2019). Systemic inflammation in hemorrhagic strokes - A novel neurological sign and therapeutic target? J. Cereb. Blood Flow Metab. 39, 959–988. doi: 10.1177/0271678x19841443
Sade Almeida, J., Vargas, M., Fonseca-Gomes, J., Tanqueiro, S. R., Belo, R. F., et al. (2020). Microglial Sirtuin 2 Shapes Long-Term Potentiation in Hippocampal Slices. Front. Neurosci. 14:614. doi: 10.3389/fnins.2020.00614
Sarno, E., Moeser, A. J., and Robison, A. J. (2021). Neuroimmunology of depression. Adv. Pharmacol. 91, 259–292. doi: 10.1016/bs.apha.2021.03.004
Satoh, A., Stein, L., and Imai, S. (2011). The role of mammalian sirtuins in the regulation of metabolism, aging, and longevity. Handb. Exp. Pharmacol. 206, 125–162. doi: 10.1007/978-3-642-21631-2_7
Schlicker, C., Boanca, G., Lakshminarasimhan, M., and Steegborn, C. (2011). Structure-based development of novel sirtuin inhibitors. Aging 3, 852–872. doi: 10.18632/aging.100388
Schonhoff, A. M., Williams, G. P., Wallen, Z. D., Standaert, D. G., and Harms, A. S. (2020). Innate and adaptive immune responses in Parkinson’s disease. Prog. Brain Res. 252, 169–216. doi: 10.1016/bs.pbr.2019.10.006
Sedlackova, L., and Korolchuk, V. I. (2020). The crosstalk of NAD. ROS and autophagy in cellular health and ageing. Biogerontology 21, 381–397. doi: 10.1007/s10522-020-09864-0
Sharma, A. (2016). Systems Genomics Support for Immune and Inflammation Hypothesis of Depression. Curr. Neuropharmacol. 14, 749–758. doi: 10.2174/1570159x14666160106155331
She, D. T., Wong, L. J., Baik, S. H., and Arumugam, T. V. (2018). SIRT2 Inhibition Confers Neuroprotection by Downregulation of FOXO3a and MAPK Signaling Pathways in Ischemic Stroke. Mol. Neurobiol. 55, 9188–9203. doi: 10.1007/s12035-018-1058-0
Shen, Y. Y., Chen, L. Y., Zhang, S. J., and Xie, L. Q. (2020). Correlation Between SIRT2 3’UTR Gene Polymorphism and the Susceptibility to Alzheimer’s Disease. J. Mol. Neurosci. 70, 878–886. doi: 10.1007/s12031-020-01513-y
Shu, L., Xu, C. Q., Yan, Z. Y., Yan, Y., Jiang, S. Z., and Wang, Y. R. (2019). Post-Stroke Microglia Induce Sirtuin2 Expression to Suppress the Anti-inflammatory Function of Infiltrating Regulatory T Cells. Inflammation 42, 1968–1979. doi: 10.1007/s10753-019-01057-3
Silva, D. F., Esteves, A. R., Oliveira, C. R., and Cardoso, S. M. (2017). Mitochondrial Metabolism Power SIRT2-Dependent Deficient Traffic Causing Alzheimer’s-Disease Related Pathology. Mol. Neurobiol. 54, 4021–4040. doi: 10.1007/s12035-016-9951-x
Singh, A. P., Nigam, L., Yadav, Y., Shekhar, S., Subbarao, N., and Dey, S. (2021). Design and in vitro analysis of SIRT2 inhibitor targeting Parkinson’s disease. Mol. Divers. 25, 2261–2270. doi: 10.1007/s11030-020-10116-z
Sola-Sevilla, N., Ricobaraza, A., Hernandez-Alcoceba, R., Aymerich, M. S., Tordera, R. M., and Puerta, E. (2021). Understanding the Potential Role of Sirtuin 2 on Aging: Consequences of SIRT2.3 Overexpression in Senescence. Int. J. Mol. Sci. 22:3017. doi: 10.3390/ijms22063107
Soria Lopez, J. A., González, H. M., and Léger, G. C. (2019). Alzheimer’s disease. Handb. Clin. Neurol. 167, 231–255. doi: 10.1016/b978-0-12-804766-8.00013-3
Subermaniam, K., Yow, Y. Y., Lim, S. H., Koh, O. H., and Wong, K. H. (2020). Malaysian macroalga Padina australis Hauck attenuates high dose corticosterone-mediated oxidative damage in PC12 cells mimicking the effects of depression. Saud. J. Biol. Sci. 27, 1435–1445. doi: 10.1016/j.sjbs.2020.04.042
Sun, S. F., Han, X. J., Li, X. T., Song, Q. Q., Lu, M., Jia, M. M., et al. (2018). MicroRNA-212-5p Prevents Dopaminergic Neuron Death by Inhibiting SIRT2 in MPTP-Induced Mouse Model of Parkinson’s Disease. Front. Mol. Neurosci. 11:381. doi: 10.3389/fnmol.2018.00381
Sun, W. W., Ma, X. J., Wang, H. P., Du, Y. Y., Chen, J. W., Hu, H. J., et al. (2021). MYO1F regulates antifungal immunity by regulating acetylation of microtubules. Proc. Natl. Acad. Sci. USA 118:e2100230118. doi: 10.1073/pnas.2100230118
Suresh, S. N., and Manjithaya, R. (2019). A small molecule autophagy inducer exerts cytoprotection against α-synuclein toxicity. Eur. J. Pharmacol. 862:172635. doi: 10.1016/j.ejphar.2019.172635
Tatone, C., Di Emidio, G., Vitti, M., Di Carlo, M., Santini, S. Jr., D’Alessandro, A. M., et al. (2015). Sirtuin Functions in Female Fertility: Possible Role in Oxidative Stress and Aging. Oxid Med Cell Longev 2015, 659687. doi: 10.1155/2015/659687
Thapa, K., Khan, H., Singh, T. G., and Kaur, A. (2021). Traumatic Brain Injury: Mechanistic Insight on Pathophysiology and Potential Therapeutic Targets. J. Mol. Neurosci. 71, 1725–1742. doi: 10.1007/s12031-021-01841-7
Trapp, J., Meier, R., Hongwiset, D., Kassack, M. U., Sippl, W., and Jung, M. (2007). Structure-activity studies on suramin analogues as inhibitors of NAD+-dependent histone deacetylases (sirtuins). Chem. Med. Chem. 2, 1419–1431. doi: 10.1002/cmdc.200700003
Villalba, J. M., and Alcaín, F. J. (2012). Sirtuin activators and inhibitors. Biofactors 38, 349–359. doi: 10.1002/biof.1032
Wang, B., Zhang, Y. J., Cao, W., Wei, X. B., Chen, J., and Ying, W. H. (2016). SIRT2 Plays Significant Roles in Lipopolysaccharides-Induced Neuroinflammation and Brain Injury in Mice. Neurochem. Res. 41, 2490–2500. doi: 10.1007/s11064-016-1981-2
Wang, Y., Yang, J. Q., Hong, T. T., Chen, X. J., and Cui, L. L. (2019). SIRT2: Controversy and multiple roles in disease and physiology. Ageing Res Rev 55, 100961. doi: 10.1016/j.arr.2019.100961
Wang, Y., Yang, J. Q., Hong, T. T., Sun, Y. H., Huang, H. L., Chen, F., et al. (2020). RTN4B-mediated suppression of Sirtuin 2 activity ameliorates β-amyloid pathology and cognitive impairment in Alzheimer’s disease mouse model. Aging Cell 19:e13194. doi: 10.1111/acel.13194
Wei, Z. Q., Qi, X. D., Chen, Y., Xia, X. S., Zheng, B. Y., Sun, X. G., et al. (2020). Bioinformatics method combined with logistic regression analysis reveal potentially important miRNAs in ischemic stroke. Biosci. Rep. 40:BSR20201154. doi: 10.1042/bsr20201154
Weller, J., and Budson, A. (2018). Current understanding of Alzheimer’s disease diagnosis and treatment. F1000Res 7:F1000FacultyRev–1161. doi: 10.12688/f1000research.14506.1
Wu, Z. Y., Zhang, Y., Zhang, Y. N., and Zhao, P. (2020). Sirtuin 2 Inhibition Attenuates Sevoflurane-Induced Learning and Memory Deficits in Developing Rats via Modulating Microglial Activation. Cell Mol. Neurobiol. 40, 437–446. doi: 10.1007/s10571-019-00746-9
Xie, J., Chen, S. T., Bopassa, J. C., and Banerjee, S. (2021). Drosophila tubulin polymerization promoting protein mutants reveal pathological correlates relevant to human Parkinson’s disease. Sci. Rep. 11:13614. doi: 10.1038/s41598-021-92738-3
Xie, X. Q., Zhang, P., Tian, B., and Chen, X. Q. (2017). Downregulation of NAD-Dependent Deacetylase SIRT2 Protects Mouse Brain Against Ischemic Stroke. Mol. Neurobiol. 54, 7251–7261. doi: 10.1007/s12035-016-0173-z
Yang, B. M., Figueroa, D. M., Hou, Y. J., Babbar, M., Baringer, S. L., Croteau, D. L., et al. (2019). NEIL1 stimulates neurogenesis and suppresses neuroinflammation after stress. Free Radic. Biol. Med. 141, 47–58. doi: 10.1016/j.freeradbiomed.2019.05.037
Yang, P. H., Xu, C., Reece, E. A., Chen, X., Zhong, J. X., Zhan, M., et al. (2019). Tip60- and sirtuin 2-regulated MARCKS acetylation and phosphorylation are required for diabetic embryopathy. Nat. Commun. 10:282. doi: 10.1038/s41467-018-08268-6
Yang, Q. J., Zhou, Y. X., Sun, Y. T., Luo, Y., Shen, Y., and Shao, A. W. (2020). Will Sirtuins Be Promising Therapeutic Targets for TBI and Associated Neurodegenerative Diseases? Front. Neurosci. 14:791. doi: 10.3389/fnins.2020.00791
Yang, W. T., Gao, F., Zhang, P., Pang, S. C., Cui, Y. H., Liu, L. X., et al. (2017). Functional genetic variants within the SIRT2 gene promoter in acute myocardial infarction. PLoS One 12:e0176245. doi: 10.1371/journal.pone.0176245
Yang, Y., Ding, J., Gao, Z. G., and Wang, Z. J. (2017). A variant in SIRT2 gene 3’-UTR is associated with susceptibility to colorectal cancer. Oncotarget 8, 41021–41025. doi: 10.18632/oncotarget.17460
Yao, Y. C., Ding, G. Q., Wang, L. L., Jin, Y., Lin, J. W., Zhai, Y. J., et al. (2019). Risk Factors for Depression in Empty Nesters: A Cross-Sectional Study in a Coastal City of Zhejiang Province and China. Int. J. Environ. Res. Public Health 16:4106. doi: 10.3390/ijerph16214106
Yu, H. B., Jiang, H., Cheng, S. T., Hu, Z. W., Ren, J. H., and Chen, J. (2018). AGK2, A SIRT2 Inhibitor, Inhibits Hepatitis B Virus Replication In Vitro And In Vivo. Int. J. Med. Sci. 15, 1356–1364. doi: 10.7150/ijms.26125
Yuan, F., Xu, Z. M., Lu, L. Y., Nie, H., Ding, J., Ying, W. H., et al. (2016). SIRT2 inhibition exacerbates neuroinflammation and blood-brain barrier disruption in experimental traumatic brain injury by enhancing NF-κB p65 acetylation and activation. J. Neurochem. 136, 581–593. doi: 10.1111/jnc.13423
Zhang, J., Wang, C. X., and Ying, W. H. (2018). SIRT2 and Akt mediate NAD+-induced and NADH-induced increases in the intracellular ATP levels of BV2 microglia under basal conditions. Neuroreport 29, 65–70. doi: 10.1097/WNR.0000000000000876
Zhang, X., Ameer, F. S., Azhar, G., and Wei, J. Y. (2021). Alternative Splicing Increases Sirtuin Gene Family Diversity and Modulates Their Subcellular Localization and Function. Int. J. Mol. Sci. 22:473. doi: 10.3390/ijms22020473
Zhang, Y., Anoopkumar-Dukie, S., and Davey, A. K. (2021a). SIRT1 and SIRT2 Modulators: Potential Anti-Inflammatory Treatment for Depression? Biomolecules 11:353. doi: 10.3390/biom11030353
Zhang, Y., and Chi, D. C. (2018). Overexpression of SIRT2 Alleviates Neuropathic Pain and neuroinflammation Through Deacetylation of Transcription Factor Nuclear Factor-Kappa B. Inflammation 41, 569–578. doi: 10.1007/s10753-017-0713-3
Zhang, Y., Yan, Q. F., and Zhang, Y. (2021b). Overexpression of sirtuin 2 and its association with prognosis in acute ischemic stroke patients. J. Clin Lab. Anal. 35:e23707. doi: 10.1002/jcla.23707
Zhang, Y. Q., Anoopkumar-Dukie, S., Arora, D., and Davey, A. K. (2020). Review of the anti-inflammatory effect of SIRT1 and SIRT2 modulators on neurodegenerative diseases. Eur J Pharmacol 867, 172847. doi: 10.1016/j.ejphar.2019.172847
Zhang, Y. Q., Anoopkumar-Dukie, S., Mallik, S. B., and Davey, A. K. (2021). SIRT1 and SIRT2 modulators reduce LPS-induced inflammation in HAPI microglial cells and protect SH-SY5Y neuronal cells in vitro. J. Neural Transm. 128, 631–644. doi: 10.1007/s00702-021-02331-1
Zhang, M. M., Pan, Y. D., Dorfman, R. G., Yin, Y. Y., Zhou, Q., Huang, S., et al. (2021). Sirtinol promotes PEPCK1 degradation and inhibits gluconeogenesis by inhibiting deacetylase SIRT2. Sci. Rep. 7:7. doi: 10.1038/s41598-017-00035-9
Keywords: sirtuin 2 (SIRT2), deacetylation, neuroinflammatory disorders, inflammatory, neuroprotection, promising target
Citation: Fan Z and Bin L (2022) Will Sirtuin 2 Be a Promising Target for Neuroinflammatory Disorders? Front. Cell. Neurosci. 16:915587. doi: 10.3389/fncel.2022.915587
Received: 08 April 2022; Accepted: 30 May 2022;
Published: 22 June 2022.
Edited by:
Maryam Sardari, University of Tehran, IranReviewed by:
Yu Song, Xinxiang Medical University, ChinaCopyright © 2022 Fan and Bin. This is an open-access article distributed under the terms of the Creative Commons Attribution License (CC BY). The use, distribution or reproduction in other forums is permitted, provided the original author(s) and the copyright owner(s) are credited and that the original publication in this journal is cited, in accordance with accepted academic practice. No use, distribution or reproduction is permitted which does not comply with these terms.
*Correspondence: Li Bin, bGliaW5ianp5QDE2My5jb20=
Disclaimer: All claims expressed in this article are solely those of the authors and do not necessarily represent those of their affiliated organizations, or those of the publisher, the editors and the reviewers. Any product that may be evaluated in this article or claim that may be made by its manufacturer is not guaranteed or endorsed by the publisher.
Research integrity at Frontiers
Learn more about the work of our research integrity team to safeguard the quality of each article we publish.