- Department of Biological Sciences, Lehigh University, Bethlehem, PA, United States
Electrical synapses are the neurophysiological product of gap junctional pores between neurons that allow bidirectional flow of current between neurons. They are expressed throughout the mammalian nervous system, including cortex, hippocampus, thalamus, retina, cerebellum, and inferior olive. Classically, the function of electrical synapses has been associated with synchrony, logically following that continuous conductance provided by gap junctions facilitates the reduction of voltage differences between coupled neurons. Indeed, electrical synapses promote synchrony at many anatomical and frequency ranges across the brain. However, a growing body of literature shows there is greater complexity to the computational function of electrical synapses. The paired membranes that embed electrical synapses act as low-pass filters, and as such, electrical synapses can preferentially transfer spike after hyperpolarizations, effectively providing spike-dependent inhibition. Other functions include driving asynchronous firing, improving signal to noise ratio, aiding in discrimination of dissimilar inputs, or dampening signals by shunting current. The diverse ways by which electrical synapses contribute to neuronal integration merits furthers study. Here we review how functions of electrical synapses vary across circuits and brain regions and depend critically on the context of the neurons and brain circuits involved. Computational modeling of electrical synapses embedded in multi-cellular models and experiments utilizing optical control and measurement of cellular activity will be essential in determining the specific roles performed by electrical synapses in varying contexts.
Introduction
Across the nervous system, neurons couple to other neurons at gap junctions formed by plaques of paired and docked hemichannel pores composed of connexin or innexin proteins (Phelan et al., 1996, 1998; Starich et al., 1996; Condorelli et al., 1998; Landesman et al., 1999; Rash et al., 2000, 2001a,b; Stebbings et al., 2000; Söhl and Willecke, 2003) that allow ions to pass between neurons. Gap junctions are the biophysical substrate for the neurophysiological component of electrical synapses (Furshpan and Potter, 1957, 1959; Watanabe, 1958; Bennett, 1966), which couple neurons in the mature mammalian brain. These unique structures enable current to flow directly between neurons without relying on energetically costly neurotransmitters or a presynaptic spike in order to initiate inter-neuronal communication. Similar to their more abundant counterpart, chemical synapses, the function of electrical synapses is of great interest in determining how neurons integrate inputs and information.
Much early work focused on the potential for electrical coupling to synchronize firing between neurons. Electrical synapses pass ions proportionally to the difference in membrane voltage between coupled neurons, and their most basic effect is to reduce that difference, resulting in minimization of differences in voltage or activity. This is thought to be the simplest, though not sole, mechanism that underlies synchrony between coupled neurons. Synchrony, generally considered, can be appreciated at the level of ongoing repetitive activity, or for individual spikes. Synchrony of spiking activity in broader heterogenous networks, while more complex in mechanisms, often relies on contributions from the inhibitory neurons that gap junctions frequently couple. Thus, electrical synapses contribute to synchrony both directly and indirectly. Because neuronal electrical synapses take diverse values of strength amongst brain areas, their effects only rarely approach the perfect synchronization that would occur between coupled neurons with infinitely strong electrical synapses. This opens the door to a variety of diverse effects mediated by electrical synapses within neural circuitry. Previous reviews have covered aspects of electrical synapses (Bennett and Zukin, 2004; Connors and Long, 2004; Haas et al., 2016; Connors, 2017; Nagy et al., 2018; Alcami and Pereda, 2019; Trenholm and Awatramani, 2019; Curti et al., 2022). Here, we detail the progress in understanding the functions of electrical synapses within, and as a result of, coupled circuits and networks. We organize our review by the different functions of electrical synapses, followed by comments on future directions for studying electrical synapses. Because so much work involves interrogating the role of electrical synapses in synchrony of rhythmic activity, that section is divided into subsections based on the source of the evidence.
Synchrony of Rhythmic Activity
Reports of synchrony are a hallmark of electrical synapse work across neural tissue (Figure 1A). Watanabe (1958) made the earliest inference, noting that the synchronous subthreshold fluctuations of lobster heart ganglion cells were a function of common presynaptic inputs and electrical coupling. This was followed by pioneering demonstrations of electrical coupling by Bennett (1966), who inferred that electrical synapses were likely to be associated with synchronization of activity, based on observations of electrical coupling in fish electromotor neurons and toad swim bladder motor neurons. Later, an early report of synchrony in feline inferior olive was associated with the presence of electrical synapses (Llinas et al., 1974). This was eventually followed by paired-patch recordings that identified electrical coupling between inhibitory interneurons in murine cortex and also found that coupled neurons were inclined to spike together when depolarized (Galarreta and Hestrin, 1999; Gibson et al., 1999; Beierlein et al., 2000). Electrical synapses have long been known to contribute to synchrony in crustacean neural networks (Eisen and Marder, 1982; Hooper and Marder, 1987; Gutierrez et al., 2013).
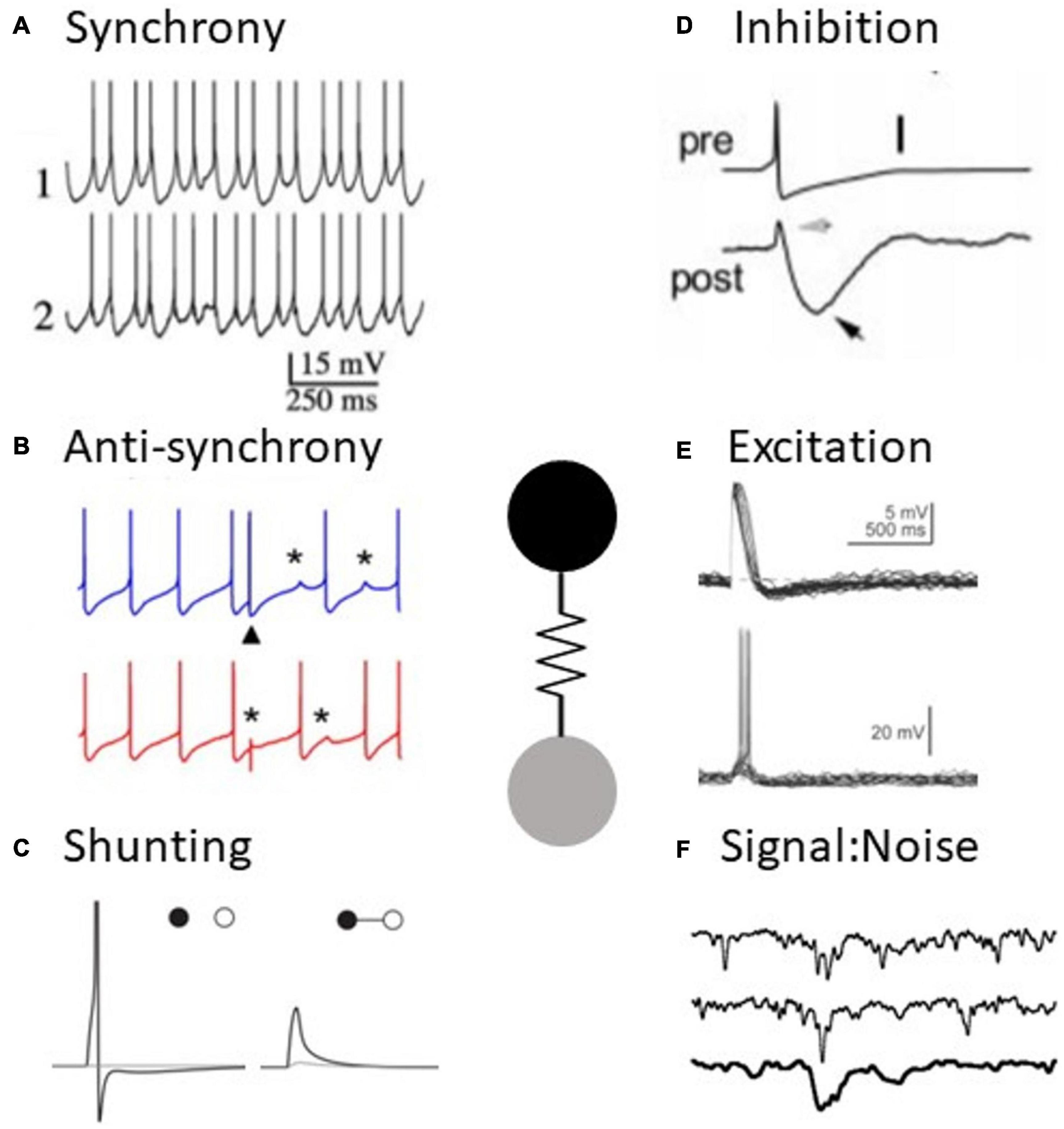
Figure 1. Electrical synapses have diverse functions in neural processing. (A) Example of synchronous activity in coupled neurons (adapted from Long et al., 2004). (B) Example of anti-synchronous firing in coupled neurons (adapted from Vervaeke et al., 2010). (C) An electrical synapse (right) shunts an excitatory signal to one cell to subthreshold levels, compared to the uncoupled case (left) (adapted from Hjorth et al., 2009). (D) Slow spike afterhyperpolarizations appear as inhibition in a coupled neuron (adapted from Galarreta and Hestrin, 2001). (E) Spikes result in excitation in a coupled neuron (adapted from Apostolides and Trussell, 2014). (F) Example of a signal amidst noise in retinal neurons, impacted by the presence of electrical synapses (adapted from Dunn et al., 2006).
Neocortex
Cortical rhythms are diverse and include gamma-range oscillations, which are linked to higher-order function (Benchenane et al., 2011); beta oscillations, which are involved in sensorimotor processes (Kilavik et al., 2013); theta oscillations associated with learning, memory, spatial navigation, and speech (Benchenane et al., 2011; Giraud and Poeppel, 2012); delta oscillations that are related to speech processing and decision making (Giraud and Poeppel, 2012; Nácher et al., 2013); and alpha oscillations that are tied to attention (Buzsáki and Draguhn, 2004; Hanslmayr et al., 2011). In electrically coupled cortical interneurons, simultaneous depolarization drives coordinated spiking (Gibson et al., 1999, 2005; Beierlein et al., 2000; Blatow et al., 2003; Mancilla et al., 2007; Hu and Agmon, 2015). Carbachol-induced spiking is synchronous in coupled inhibitory neurons as well, and that synchrony is correlated with electrical synapse conductance, while uncoupled neurons displayed no correlation in spiking (Caputi et al., 2009). Similarly, correlated spiking induced by ACPD or carbachol is desynchronized by connexin36 knockout or pharmacological blockade of gap junctions (Deans et al., 2001; Blatow et al., 2003). For layers 2 and 3 basket cells, gamma frequency stimulation in one cell entrains gamma frequency spiking in coupled basket cells, albeit with a phase lag of about 10 ms; electrical coupling in addition to GABAergic connections shortened gap junction mediated postsynaptic potentials and promoted synchronous gamma activity (Tamás et al., 2000). Synchronization of cortical interneurons can entrain synchrony of rhythmic spiking in cortical pyramidal neurons (Whittington et al., 1995, 2000; Traub et al., 1996; Whittington and Traub, 2003; Cardin, 2016); synchronized network rhythms may be important for sensory processing, working memory, and attention (Buzsáki and Draguhn, 2004; Wang, 2010; Benchenane et al., 2011; Hanslmayr et al., 2011).
Thalamus
The thalamic reticular nucleus (TRN) receives excitatory input from ascending thalamic relay nuclei (Jones, 1975; Ohara and Lieberman, 1985; Fosse et al., 1986; Fitzpatrick et al., 1994; Liu and Jones, 1999), feedback excitation from layers 5 and 6 of cortex (Jones, 1975; Bromberg et al., 1981; Fonnum et al., 1981; Feig and Harting, 1998; Zhang and Jones, 2004), and a myriad of modulatory input (Wilson, 1985; Cornwall et al., 1990; Gandia et al., 1993; Reardon and Mitrofanis, 2000; Freeman et al., 2001; Prensa and Parent, 2001; Anaya-Martinez et al., 2006; Zikopoulos and Barbas, 2012; Leon-Dominguez et al., 2013); the exclusively GABAergic neurons of the TRN provide the main source of inhibitory drive onto thalamic nuclei (Scheibel and Scheibel, 1966; Jones, 1975; Houser et al., 1980), and thus control thalamocortical relay of sensory information. The TRN is integral in thalamocortical spindle oscillations during sleep and memory formation during sleep (Pinault, 2004; Steriade, 2005; Halassa et al., 2011; Latchoumane et al., 2017). Paired depolarization of coupled TRN neurons drives correlated spiking (Landisman et al., 2002; Haas and Landisman, 2011), and depolarization resulting from ACPD application also causes correlated spiking (Long et al., 2004). The cross-correlation of mGluR-induced subthreshold rhythms is positively correlated with the conductance of the electrical synapse within the pair.
Hippocampus
The nested gamma and theta rhythms of the hippocampal formation are essential to its spatial and memory functions (Colgin and Moser, 2010), and hippocampal GABAergic neurons are connected via dendrodendritic gap junctions (Fukuda and Kosaka, 2000). Hippocampus basket cells initiate gamma oscillations when depolarized with potassium, glutamate, kainite, or carbachol; the measured power of those gamma oscillations through field potentials are reduced with gap junction blockers or connexin knockout (Hormuzdi et al., 2001; Traub et al., 2001). Furthermore, IPSCs onto pyramidal cells become more variable in connexin knock outs (Hormuzdi et al., 2001). The effect of gap junctions synchronizing gamma oscillations was reinforced by a following study recording field potentials of CA1 pyramidal neurons in vivo, where connexin36 knockout reduced the power of gamma oscillations (Buhl et al., 2003).
Synchronous high frequency (<150 hz) bursts of activity occur in hippocampal pyramidal neurons, and gap junctions are necessary for the high frequency bursts to occur in vitro (Draguhn et al., 1998), although this was not confirmed in vivo (Buhl et al., 2003). Seizing activity in hippocampal slices induced by Ca2+ free ACSF is reduced and desynchronized by weakening electrical coupling through acidification (Perez-Velazquez et al., 1994). Similarly, another hippocampal seizure model with no Mg2+ and 4-aminopyridine in the ACSF had its population bursts greatly reduced by gap junction blockers, suggesting that electrical synapses were partially responsible for simultaneous activity seen in the seizure model (Ross et al., 2000).
Cerebellum
The cerebellum is responsible for timing of motor control, and precise temporal precision of spiking is key to that function. Both low frequency (7–30 hz) oscillations and high frequency (>40 hz) oscillations have been observed in the cerebellum and may contribute to motor execution and learning (D’Angelo et al., 2009). In vitro, spontaneous spiking in coupled Golgi inhibitory interneurons of the cerebellum is correlated. Further, coupled Golgi neurons oscillate synchronously or spike synchronously when depolarized with kainate (Dugué et al., 2009). Computational modeling of Golgi neurons suggests that transfer of the after hyperpolarization through the gap junction is critical for synchronized spiking. The afterhyperpolarization (AHP) is inhibitory to the coupled cell and determines the delay period after which a coupled neuron can next spike (Dugué et al., 2009). In vivo cell-attached recordings demonstrated that coupled Golgi interneuron spiking is correlated within a few ms (van Welie et al., 2016); knock-out of connexin36 results in uncorrelated spiking. Paired recordings showed that spiking in one neuron resulted in a depolarizing spikelet in the other, and in some instances, the spikelet was sufficient to drive a correlated spike within milliseconds. Altogether, these heroic in vivo experiments suggest that coupled Golgi interneurons spike together due to electrical synapses correlating subthreshold potentials and by driving a coupled neighbor past threshold through an excitatory spikelet.
Inferior Olive
The coupled neurons of the inferior olive provide error-signal input to cerebellar Purkinje cell dendrites and exhibit strong subthreshold and spiking oscillations in the 1–10 Hz range of frequency (Armstrong et al., 1968; De Zeeuw et al., 1998). Paired recordings of inferior olive (IO) neurons show that spontaneous spiking and subthreshold oscillations are highly correlated amongst coupled neurons. Knockout of connexin36 causes an increase in uncorrelated spiking and desynchronization of subthreshold oscillations, which still persist (Long et al., 2002; Leznik and Llinas, 2005). Long et al. (2002) suggest that coupled IO neurons spike synchronously in part due to coupled neurons faithfully spiking at the peak of membrane fluctuations, where decoupled neurons sometimes spike in the trough of their oscillation. Electrical synapses reduce excitability by shunting current, and therefore, they make IO neurons less likely to spike during the trough of a subthreshold membrane oscillation.
Synchronization of IO neurons via gap junctions affects the downstream synchrony of cerebellar neurons. In vivo injections of gap junction blockers into the IO or knockout of connexin36 reduces synchrony of complex spikes in postsynaptic Purkinje cells (Blenkinsop and Lang, 2006; Marshall et al., 2007) and interferes with motor learning (Van Der Giessen et al., 2008). This effect is presumably the result of desynchronized activity in the uncoupled IO.
Suprachiasmatic Nucleus
The suprachiasmatic nucleus (SCN) is a central regulator of circadian rhythms. Dye-coupling, which images the spread of gap junction-permeable dyes through coupled networks, is greatest during the sleep cycle, when there is more synchronous activity, than during the active cycle (Colwell, 2000). Coupling is positively correlated with synchronous spiking, while spiking was uncorrelated in uncoupled neurons (Long et al., 2005). The circadian rhythms of connexin36 knockout mice are irregular, so the synchronous firing during the wake cycle may be a component of circadian rhythm maintenance (Long et al., 2005). Long et al. (2005) also showed evidence that coupling between cells changes in strength over 24 h and that knockout rats lose rhythmicity of circadian activity in 24 h dark conditions.
Brainstem
Evidence for the synchronizing effects of gap junctions in brainstem is mixed. One study using adult rats found that gap junction blockers decreased synchrony of phrenic bursts (Solomon et al., 2003). In contrast, an earlier study found that gap junction blockers in neonatal brain stem slices showed that gap junctions reduce synchronized activity of the phrenic nerve in the short term (Bou-Flores and Berger, 2001). In the mesencephalic trigeminal nucleus, strong electrical synapses can drive a quiescent neighbor to spike within 2 ms of the spikes of coupled neurons. Further, subthreshold depolarizations of coupled neurons resulted in membrane potential oscillations that were correlated between coupled pairs (Curti et al., 2012). Synchronization of the mesencephalic trigeminal nucleus could be important for coordinated inputs to relax the jaw.
Spinal Cord
Electrical coupling is present between juvenile motor neurons in rats, but it does not persist with maturation (Walton and Navarrete, 1991; Chang et al., 1999). Juvenile motor neuron spiking is correlated, even the presence of TTX, but spiking becomes uncorrelated with pharmacological blockade of gap junctions (Tresch and Kiehn, 2000; Personius et al., 2001). Electrical synapses may have an important role in guiding development of motor neurons by promoting synchronous activity. Inhibition of acetylcholine release by motor neurons during development causes electrical synapses to persist into adolescence, suggesting that activity at the neuromuscular junction communicates the need to remove electrical synapses (Pastor et al., 2003).
Olfactory Bulb
Mitral cells in the olfactory bulb synchronously oscillate at 30–80 hz when stimulated with one or more odorants (Kashiwadani et al., 1999). Oscillations in the olfactory bulb have a role in odorant discrimination, and it is speculated that synchronous activity facilities the summation of EPSPs in piriform cortex (Schoppa and Urban, 2003; Rojas-Líbano and Kay, 2008). Electrical synapses contribute to synchrony in the olfactory bulb; depolarizing coupled mitral cells in the olfactory bulb drives correlated spiking that is absent when connexin36 is knocked out (Christie et al., 2005).
Retina
Retina is a major hub where electrical synapses exert influence on processing light signals. All layers of the retina express gap junctions, and their electrical synapse strength is regulated by brightness via dopamine, nitric oxide, and adenosine (Hampson et al., 1992; McMahon and Brown, 1994; Mills and Massey, 1995; Lee et al., 2002; Mills et al., 2007; Ribelayga et al., 2008; Bloomfield and Volgyi, 2009; Kothmann et al., 2009; Li et al., 2013; Jacoby et al., 2018; Trenholm and Awatramani, 2019). Electrical synapse regulation in retina is critical for adaptation to different light intensities.
The developing retina undergoes slow waves of activity that spread across ganglion cells with an interval on the order of minutes (Firth et al., 2005). Retinal waves require electrical synapses and L-type Ca2+ channels. It has been inferred that electrical synapses propagate the wave by transferring excitation driven by L-type Ca2+ channels (Singer et al., 2001; Firth et al., 2005). Further, electrical synapses contribute to the responsiveness of developing retina to light, and those electrical synapses are depressed by retinal waves and dopamine release (Arroyo and Feller, 2016).
Computational modeling of electrical synapses robustly supports the role of electrical synapses in synchronization (Mulloney et al., 1981; Sherman and Rinzel, 1992; Traub, 1995; Chow and Kopell, 2000; Moortgat et al., 2000; Velazquez and Carlen, 2000; Nomura et al., 2003; Pfeuty et al., 2003; Bem and Rinzel, 2004; Kopell and Ermentrout, 2004; Mancilla et al., 2007; Ostojic et al., 2009). Modeling suggests that electrical synapses can switch from promoting synchrony to anti-synchrony in different contexts (Mulloney et al., 1981; Sherman and Rinzel, 1992; Chow and Kopell, 2000; Lewis and Rinzel, 2003; Nomura et al., 2003; Pfeuty et al., 2003; Bem and Rinzel, 2004; Gibson et al., 2005; Mancilla et al., 2007). Some models point to voltage equalization as a key part of electrical synapse promotion of synchrony (Sherman and Rinzel, 1992; Montbrió and Pazó, 2020). In addition, models have shown that synchrony is promoted by strong electrical synapses, while asynchrony is promoted by weak electrical synapses (Chow and Kopell, 2000; Nomura et al., 2003; Bem and Rinzel, 2004).
Models also suggest that electrical synapses can promote anti-synchrony by preferentially passing the slower afterhyperpolarization that follows a spike. This causes electrical synapses to function as reciprocal inhibitory synapses, which optimizes firing when the neurons are 180° out of phase (Sherman and Rinzel, 1992; Bem and Rinzel, 2004; Ostojic et al., 2009; Vervaeke et al., 2010). Similarly, modeling by Ostojic et al. (2009) suggests that electrical synapses facilitate in-phase synchrony when they are primarily excitatory but facilitate bistable synchrony and asynchrony when they are primarily inhibitory; these models also suggest that electrical synapses are best able to promote synchrony when neurons are active at their resonant frequency. In addition, models by Pfeuty et al. (2003) demonstrate that electrical synapses promote anti-synchrony when there is a strong persistent sodium current and weak potassium current, but promote synchrony when there is a strong persistent potassium current. Other models suggest that generation of either synchronous or anti-synchronous activity require reciprocal GABAergic connections along with electrical synapses (Pfeuty et al., 2003; Bem et al., 2005; Gibson et al., 2005; Lau et al., 2010).
Although anti-synchronous firing between coupled neurons is a robust phenomenon across a wide spectrum of parameter space, experimental evidence for this phenomenon is notably lacking. One example of simulated electrical synapses and reciprocal GABAergic synapses, implemented using dynamic clamp in snail neurons, was able to produce anti-synchrony. Further, stimulation of sparse mossy fiber inputs to Golgi cells transiently switches coupled neurons from spiking synchronously to spiking asynchronously in vitro (Vervaeke et al., 2010; Figure 1B). However, anti-synchronous firing in coupled Golgi neurons was not observed in vivo (van Welie et al., 2016).
Contrary to the persistent thread of evidence connecting electrical synapses to synchronization, a few studies have suggested that electrical synapses fail to play a central role in synchronizing spiking in certain systems. In cortical inhibitory neurons, knockout of connexin36 did not impair synchrony of gamma activity, and synchrony of gamma activity was not correlated with electrical synapse strength (Salkoff et al., 2015; Neske and Connors, 2016).
Beyond Synchrony
Excitation
As mentioned above, by passing depolarizations between coupled neurons gap junctions can act as excitatory synapses (Figure 1E). Several studies have identified electrical synapses as actors in lateral excitation, resulting in enhanced sensitivity to stimuli or neural input. Retinal ganglion cells exhibit increased responses to low-contrast and moving stimuli due to lateral excitation from electrical synapses between bipolar, amacrine, or ganglion cells (Trenholm et al., 2013; Kuo et al., 2016). Moreover, excitatory electrical relay from AII amacrine cells to rod bipolar cells is critical for rod-mediated responses in ganglion cells (Güldenagel et al., 2001; Deans et al., 2002). For guinea pigs, the receptive fields of ON center medium retinal ganglion cells (RGCs) are effectively expanded by lateral excitation from electrically coupled ON center α-RGCs (Puller et al., 2020). Under scotopic conditions, electrically coupled directionally sensitive ganglion cells (DSCGs) broaden their tuning for their preferred direction, while uncoupled DSCGs tuning remain stable. Consequently, coupled DSCGs enhance their detection of movement at the cost of discrimination of movement direction through lateral excitation (Yao et al., 2018). It is suspected that coupled DSCGs could potentiate coupling under scotopic conditions to induce that effect, but it remains to be directly measured. In the olfactory bulb, mitral cells enhance reactions to odorants through electrical lateral excitation (Christie and Westbrook, 2006). In addition, depolarizing a Golgi cell in cerebellum increases firing rate in its neighbors. Glutamate uncaging experiments in conjunction with gap junction blockers revealed that gap junctions helped compensate for the decay of the many chemical synaptic inputs at distal dendrites (Vervaeke et al., 2012).
Direct excitatory drive by electrical synapses is a physiological certainty in several cases. Strong electrical synapses between TRN neurons enable bursts in one cell to drive a spike in its coupled neighbor (Parker et al., 2009; Haas et al., 2011). Similarly, Curti et al. (2012) demonstrated the excitatory power of strong electrical synapses in the mesencephalic trigeminal nucleus. In a pair with a coupling coefficient of 0.51, spiking in the presynaptic cell was sufficient to drive spiking in the postsynaptic cell. Interneurons in the stratum lacunosum moleculare of the hippocampus can generate large depolarizations, up to 10 mV, in their coupled neighbors when they burst, due to slower depolarizations passing the gap junction (Zsiros et al., 2007). Goldfish club endings excite Mauthner cells through a mixed chemical and electrical synapse to trigger an escape reflex. The electrical synapses at the club ending experience a diverse array of plasticity mechanism, which may finely tune how the Mauthner cell responds to different auditory inputs (Pereda et al., 1992, 1994, 1998, 2004). M5 Intrinsically photosensitive retinal ganglion cells (ipRGCs) directly stimulate GABAergic amacrine cells through gap junctions (Pottackal et al., 2021). The amacrine cells then inhibit M5 and M4 ipRGCs; in this circuit, electrical synapses function as the excitatory component of feedback and feedforward inhibition. Striatal medium spiny neurons depolarize cholinergic interneurons through gap junctions, and electrical synapses contribute to the tonic activity observed in cholinergic interneurons (Ren et al., 2021). Electrical synapses also function to excite cortical parvalbumin neurons and cooperate with NMDAR inputs to drive bursts (Lee et al., 2021). In the TRN, spiking depolarizes coupled neighbors, and potentiation of an electrical synapse can transform a synapse from previously subthreshold to spike-driving (Fricker et al., 2021). Modeling of thalamocortical inhibitory feedback circuits revealed that excitation through electrical synapses in the TRN can affect the temporal separation of inputs relayed to cortex by thalamus (Pham and Haas, 2018). In this scenario, one TRN cell receiving earlier input from thalamus drives a coupled TRN cell to spike, which then delays spiking in its other thalamic cells. The degree of separation is directly tied to the strength of the electrical synapse, which can increase separation between spikes in thalamic relay cells by tens of ms. In a feed forward model circuit, powerful electrical synapses can drive coupled interneurons to spike and shorten the integration window for downstream cortical neurons (Pham and Haas, 2019).
Further, electrical synapses are capable of passing subthreshold chemical synaptic events. Subthreshold EPSPs in dorsal cochlear nucleus fusiform cells excite coupled stellate cells, and that excitation can be sufficient to drive spikes in the stellate cells (Apostolides and Trussell, 2014). Interneurons in the stratum lacunosum moleculare are excited by GABA due to HCO3– leaving the cell (Perkins and Wong, 1996); excitatory GABAergic postsynaptic potential are passed through the gap junction. Inhibitory Renshaw cells in the spinal cord also excite each other by passing cholinergic EPSPs through gap junctions (d’Incamps et al., 2012). Thus, subthreshold chemical synaptic inputs in one cell are computational relevant in its electrical coupled neighbor and may be an understudied feature of electrical synapses.
Inhibition
By passing hyperpolarizations such as AHPs or IPSPs between coupled neurons, gap junctions act as inhibitory synapses (Figure 1D). Due to the low-pass filtering that results from current flowing across two cell membranes, slower signals are preferentially transferred through electrical synapses compared to faster events such as action potentials. In the case of spikes, slow AHPs are passed more effectively than the spike itself, making the event as a whole net inhibitory to the coupled cell. This effect was noted in fast spiking cortical interneurons (Galarreta and Hestrin, 2001). Inhibition from electrical synapses can be compounded with an accompanying inhibitory chemical synapse between coupled neurons, enhancing the inhibitory effect after spiking (Galarreta and Hestrin, 2001). The spike waveform in the presynaptic cell is critical in determining whether electrical synapses will excite, inhibit, or both after a spike. Spikes with larger half widths or broad depolarizations underlying a burst are better at exciting the postsynaptic cells, while fast spikes and large AHPs are net inhibitory. Gibson et al. (2005) demonstrated that fast-spiking inhibitory neurons send effective inhibition after spiking, while low-threshold spiking neurons primarily depolarize the postsynaptic neurons after spiking. Membrane potential also affects the net valence of post synaptic potentials from electrical synapses via differences in postsynaptic membrane conductances. Fast-spiking neurons almost exclusively depolarize postsynaptic cells after spiking when the postsynaptic neuron is hyperpolarized, but they primarily inhibit depolarized postsynaptic cells (Otsuka and Kawaguchi, 2013).
Inhibition carried by gap junction-mediated AHPs was also described in Golgi interneurons (Vervaeke et al., 2010; Yaeger and Trussell, 2016; Hoehne et al., 2020). Interestingly, in this case electrical synapse inhibition was shown to function similar to feedforward inhibition. Stimulation of excitatory parallel fiber inputs to electrically coupled basket cells results in an EPSP followed by electrically mediated inhibitory postsynaptic potential. The inhibition delivered by electrical synapses in this case sharpens the EPSPs that precede them and reduces temporal summation, hallmarks of feedforward inhibition (Hoehne et al., 2020). In striatum, burst firing between 25 to 60 Hz of fast-spiking interneurons inhibits spiking in coupled neighbors by transferring AHPs (Russo et al., 2013). In the dorsal cochlear nucleus, fusiform cells are able to transfer subthreshold synaptic voltage fluctuations to stellate cells via electrical synapses (Apostolides and Trussell, 2014). EPSPs in fusiform cells drive depolarization followed by a hyperpolarization caused by closing HCN channels (Apostolides and Trussell, 2014). Both the depolarizing and hyperpolarizing aspects of the chemical postsynaptic potential in the fusiform cell is transferred to stellate cells through the gap junction. The ability for electrical synapses to hyperpolarize neurons and inhibit spiking is an important aspect of their function that should not be overlooked.
Shunting
Unlike chemical synapses, electrical synapses passively contribute to the excitability of neurons without requiring activation of the synapse for that effect. When one neuron in an electrically coupled pair is stimulated, the gap junction to the neighboring cell shunts current away and renders the first neurons less likely to spike (Llinas et al., 1974; Van Der Giessen et al., 2008; Hjorth et al., 2009; Chatzigeorgiou and Schafer, 2011; Kawano et al., 2011; Rabinowitch et al., 2013; Figure 1C). Consequently, each hyperpolarized neuron in a coupled network inhibits spike generation, and depolarization of those neurons lifts that opposition. Shunting by electrical synapses can be especially impactful when one neuron is coupled to many other neurons. In such a network, a central neuron is strongly inhibited from spiking until a threshold of neighbors are also depolarized (Chatzigeorgiou and Schafer, 2011; Rabinowitch et al., 2013), effectively offering a mass-coincidence detection or insurance against erroneous responses.
Moreover, regulation of electrical synapse strength affects the overall excitability of a network. Coupled nNOS-1 amacrine cells in retina release nitric oxide in response to light, which consequently decouple the amacrine cells. Decoupling the amacrine cells increases their individual excitability by reducing the shunting of current to its neighbors (Jacoby et al., 2018).
Coincidence Detection
The same principles that enable electrical synapses to facilitate synchrony also allow them to act as coincident detectors. Typically, coincidence detection is conceptualized as the summation of near-simultaneous excitatory chemical inputs to one cell, driving spiking only when they are sufficiently coincident. Inputs arriving through electrical synapses can work in the same additive fashion. In addition, as mentioned earlier and in contrast to excitatory synapses, inactive electrically coupled neurons shunt current away from active neurons until they become depolarized themselves. As a result, electrical synapses can act as detectors of coincident depolarization between coupled neurons by effectively checking if both neurons in a pair are simultaneously depolarized before allowing spike generation. Furthermore, the spikelet generated by one neuron can drive spiking in a peri threshold neighbor, ensuring that coincident inputs are likely to result in both neurons spiking.
Galarreta and Hestrin (2001) injected current simulating subthreshold EPSPs into coupled cortical fast spiking interneurons, which drove spikes when they occurred within 1 ms, but failed to drive spikes when EPSPs were separated by 5 ms. Subthreshold current injections to each neuron of a pair that are mismatched in time fail to produce spiking in coupled amacrine cells, but generate spikes in both coupled cells when applied to both cells in a pair simultaneously (Veruki and Hartveit, 2002b). Similarly, Trenholm et al. (2013) stimulated one retinal ganglion cell with light and a coupled neighbor with current injection. Weaker light stimuli failed to drive spikes unless there was coincident stimulation of the coupled neighbor (Trenholm et al., 2013). In Golgi basket cells, simultaneous stimulation of both cells in a coupled pair has greater action potential probability compared to stimulation of one cell alone (Alcami, 2018). Computational modeling of striatal inhibitory neurons showed that electrical synapses reduce the overall firing of neurons in the circuit, but that effect was minimized when coupled neurons received coincident inputs (Hjorth et al., 2009). Experiments in C. elegans have found that RIH neurons act as a hub with electrical synaptic connections to multiple neural pathways; coincident stimulation from electrically connected partners were necessary to sufficiently stimulate RIH neurons and facilitate C. elegans escape response after a nose touch (Chatzigeorgiou and Schafer, 2011). Future modeling of the nose-touch-response circuit supports that electrical synapses from multiple pathways shunt current and make RIH neurons less responsive, while multiple simultaneous active neurons promote activation of RIH neurons and consequently the escape response (Rabinowitch et al., 2013). Taken together, the combined ability of electrical synapses to shunt current when a neuron is inactive, and promote activity when it is active, helps to cause synchronous activity and acts as detector of coincident activity across multiple neurons.
Signal to Noise Ratio
Neurons are subject to a variety of stochastic factors, including the random opening and closing of ionic channels and spontaneous synaptic activity. A fundamental task for the nervous system is to distinguish between signal and noise. Electrical synapses that shunt current and decrease excitability implement the functional effect of noise dampening (Figure 1F). Computational modeling supports the possibility of this mechanism for noise reduction via electrical synapses (Lamb and Simon, 1976; Vardi and Smith, 1996; Usher et al., 1999; DeVries et al., 2002; Medvedev, 2009).
Improvement of signal to noise ratio by electrical synapses has been investigated in multiple systems. Noise was measured in retinal preparations by recording membrane fluctuations of AII amacrine cells in complete darkness; noise was greater in amacrine cells with genetically knocked out connexin36 (Dunn et al., 2006). In the fish olfactory bulb, the variance of mitral cell response to an odorant increases with pharmacological blockade of gap junctions (Zhu et al., 2013). Electrical synapses suppress membrane oscillations entirely in drosophila lobula plate tangential cells, likely via shunting noisy intrinsic currents of in the cell (Ammer et al., 2022). It has also been inferred that electrical synapses improve signal to noise ratio in the fly olfactory bulb (Kazama and Wilson, 2009; Yaksi and Wilson, 2010) and monkey locus coeruleus (Usher et al., 1999). However, experiments comparing noise in the presence and absence of electrical synapses in those systems are still needed.
Asymmetry
Bidirectional flow of current is a notable distinguishing property of electrical synapses. Yet the magnitudes of signals relayed in each direction quite often show some degree of direction dependence. In the mammalian nervous system, asymmetry of synapses has been noted in neocortex (Galarreta and Hestrin, 2002), TRN (Haas et al., 2011; Sevetson and Haas, 2015; Zolnik and Connors, 2016), inferior olive (Devor and Yarom, 2002), cerebellum (Mann-Metzer and Yarom, 1999; Alcami and Marty, 2013; Szoboszlay et al., 2016), dorsal cochlear nucleus (Apostolides and Trussell, 2013), and mesencephalic trigeminal nucleus (Curti et al., 2012). Asymmetry could result from heterotypy of gap junction channels and plaques, where oligomerization or docking of different connexin or innexin proteins can asymmetrically pass current (Bukauskas et al., 1995; Phelan et al., 2008; Rash et al., 2013), and differences in hemichannel scaffolding (Marsh et al., 2017) could also contribute to the rectifying ability of electrical synapses. Even for homotypic gap junctions based on connexin36, which are largely voltage-independent (Srinivas et al., 1999), differences between cell properties, such as input resistance or cable properties, create functional asymmetry for signals sent across the gap junction (Mann-Metzer and Yarom, 1999; Veruki and Hartveit, 2002a; Nadim and Golowasch, 2006; Alcami and Marty, 2013; Amsalem et al., 2016; Mendoza and Haas, 2022). Furthermore, those different sources of asymmetry can also compensate for or exacerbate genuine junctional asymmetry between electrical synapses (Mendoza and Haas, 2022). For example, at the Mauthner mixed synapse, heterotypic asymmetrical gap junctions composed of connexin34.7 and connexin35 preferentially pass current from the Mauthner cell to the club endings. At that synapse, rectification compensates for the large dendrite of the Mauthner cell, making the electrical synapse more functionally bidirectional (Rash et al., 2013).
Asymmetry of electrical synapses directly impacts their function. Full rectification can create nearly unidirectional communication, such as in the giant motor neuron of crayfish or pyloric circuit of the spiny lobster (Furshpan and Potter, 1957, 1959; Graubard and Hartline, 1987; Johnson et al., 1993). Voltage-dependent gating of gap junction channels allows crayfish presynaptic giant interneurons to unidirectionally excite the giant motor neuron and engage an escape reflex (Jaslove and Brink, 1986). For the pyloric circuit of the spiny lobster, the rectified synapse allows the lateral pyloric neuron to drive the pyloric neuron to burst, and then send a delayed chemical inhibitory signal to terminate the burst (Graubard and Hartline, 1987; Mamiya et al., 2003). In the dorsal cochlear nucleus (Apostolides and Trussell, 2014), near-complete asymmetry is a result of input resistance mismatch, and results in fusiform cells driving spikes in more-compact stellate cells. Asymmetry in this case ensures recruitment of local inhibition within the circuit even during subthreshold excitation of the fusiform cells.
For more moderate asymmetry, modeling results reveal an effect of asymmetry on detailed spike timing, even to the extent of reversing spike order in a coupled cells (Sevetson and Haas, 2015), and controls the phase of synchronous rhythmic activity (Mendoza and Haas, 2022). Modeling of larger networks shows that rectifying electrical synapses can improve the robustness of rhythmic activity (Gutierrez et al., 2013). Because electrical synapse plasticity has been shown to systematically alter the degree of asymmetry (Haas et al., 2011; Fricker et al., 2021), asymmetry may be actively regulated in order to tune spike timing and network activity. Collectively, our understanding of the function of asymmetry is still limited, but its potential impacts are worthy of additional investigation.
Future of Electrical Synapse Study
The study of electrical synapse function has a dense focus on the contribution of electrical synapses to synchronization of activity between coupled pairs. This attention is merited, and there is ample evidence to support this function, especially when neurons in a coupled network receive prolonged, simultaneous excitation. The next decade of research would greatly benefit from studies focusing on how electrical synapses in different systems affect transient inputs, and from studies that look at how temporal variation in the activity of multiple electrically coupled neurons in a network affect activity. In C. elegans, a hub and spoke model has been proposed, where a central neuron integrates the information from multiple electrical synapses to determine whether to fire. Such a computational process has not yet been explored in mammalian electrical networks.
One limitation to understanding the functions of electrical synapses within and beyond a network is that the gold standard for identification and measurement of coupling between neurons is dual whole-cell recordings, which is difficult and limited to two cells at a time. In this manner, the network is neglected. Extracellular methods, such as multi-electrode arrays, field recordings, and wide-scale imaging of fluorophores overcome this limitation, but do not reveal which neurons are coupled in a network or the strengths or spatial distributions of their coupling. One could hope that cell-specific optogenetics could mitigate the barrier (Dakin and Li, 2006; Qiao and Sanes, 2016), and some all-imaging approaches have identified GJ-mediated signals (Tian et al., 2021). In computational studies of neural networks, electrical synapses are most often neglected entirely, which we regard as a vast missed opportunity.
Improved imaging could bring new insight into the function of electrical synapses. Currently, we lack a dye that can pass through connexin36-based gap junctions and be imaged in live tissue, which limits our ability to identify and image specific gap junctions. A new GCaMP connexin36 hybrid gene has been created and used in HeLa cells (Moore et al., 2020). It is possible that replacement of the connexin36 gene with the GCaMP connexin36 hybrid would allow researchers to look at localized activity in the dendrites and near the gap junction. However, this is early speculation, and the viability of such a method needs to be vetted. Another major complication is differentiating connexin36 in the cytoplasm from connexin36 that forms gap junction pores.
A recent innovation has created novel connexin genes through an iterative mutation approach. Mutated perch connexin34.7 and connexin35 proteins form exclusively heterotypic gap junctions (Ransey et al., 2021a) resulting in asymmetrical electrical synapses (Ransey et al., 2021b), and expression of each protein can be directed toward specific cell types. This technique could be useful for interrogating how the addition or substitution of asymmetric electrical synapses modifies a circuit and behavior.
Electrical synapses have profound and complicated impacts on the processing of neural signals. The functional consequence of electrical synapses is collectively impacted by the membrane voltages of pre- and post-synaptic neurons, the waveform of spikes, the frequency of spiking, and their location on dendrites. Wherever electrical synapses are present, their function should be thoroughly interrogated. Moreover, it is important for connectome projects (Van Essen et al., 2013; Oh et al., 2014; Zhang et al., 2019) to include electrical connections in addition to chemical connections to achieve a whole picture of neural communication pathways.
Author Contributions
JH and MV contributed equally to conceptualization and writing. Both authors contributed to the article and approved the submitted version.
Funding
This research was funded by the Whitehall Grant in Aid to JH.
Conflict of Interest
The authors declare that the research was conducted in the absence of any commercial or financial relationships that could be construed as a potential conflict of interest.
Publisher’s Note
All claims expressed in this article are solely those of the authors and do not necessarily represent those of their affiliated organizations, or those of the publisher, the editors and the reviewers. Any product that may be evaluated in this article, or claim that may be made by its manufacturer, is not guaranteed or endorsed by the publisher.
References
Alcami, P. (2018). Electrical synapses enhance and accelerate interneuron recruitment in response to coincident and sequential excitation. Front. Cell. Neurosci. 12:156. doi: 10.3389/fncel.2018.00156
Alcami, P., and Marty, A. (2013). Estimating functional connectivity in an electrically coupled interneuron network. Proc. Natl. Acad. Sci. 110, E4798–E4807. doi: 10.1073/pnas.1310983110
Alcami, P., and Pereda, A. E. (2019). Beyond plasticity: the dynamic impact of electrical synapses on neural circuits. Nat. Rev. Neurosci. 20, 253–271. doi: 10.1038/s41583-019-0133-5
Ammer, G., Vieira, R. M., Fendl, S., and Borst, A. (2022). Anatomical distribution and functional roles of electrical synapses in Drosophila. Curr. Biol. 32, 2022–2036.e4. doi: 10.1016/j.cub.2022.03.040
Amsalem, O., Van Geit, W., Muller, E., Markram, H., and Segev, I. (2016). From neuron biophysics to orientation selectivity in electrically coupled networks of neocortical L2/3 large basket cells. Cereb. Cortex 26, 3655–3668. doi: 10.1093/cercor/bhw166
Anaya-Martinez, V., Martinez-Marcos, A., Martinez-Fong, D., Aceves, J., and Erlij, D. (2006). Substantia nigra compacta neurons that innervate the reticular thalamic nucleus in the rat also project to striatum or globus pallidus: implications for abnormal motor behavior. Neuroscience 143, 477–486. doi: 10.1016/j.neuroscience.2006.08.033
Apostolides, P. F., and Trussell, L. O. (2013). Regulation of interneuron excitability by gap junction coupling with principal cells. Nat. Neurosci. 16, 1764–1772. doi: 10.1038/nn.3569
Apostolides, P. F., and Trussell, L. O. (2014). Control of interneuron firing by subthreshold synaptic potentials in principal cells of the dorsal cochlear nucleus. Neuron 83, 324–330. doi: 10.1016/j.neuron.2014.06.008
Armstrong, D., Eccles, J., Harvey, R., and Matthews, P. (1968). Responses in the dorsal accessory olive of the cat to stimulation of hind limb afferents. J. Physiol. 194, 125–145. doi: 10.1113/jphysiol.1968.sp008398
Arroyo, D. A., and Feller, M. B. (2016). Spatiotemporal features of retinal waves instruct the wiring of the visual circuitry. Front. Neural Circuits 10:54. doi: 10.3389/fncir.2016.00054
Beierlein, M., Gibson, J. R., and Connors, B. W. (2000). A network of electrically coupled interneurons drives synchronized inhibition in neocortex. Nat. Neurosci. 3, 904–910. doi: 10.1038/78809
Bem, T., Le Feuvre, Y., Rinzel, J., and Meyrand, P. (2005). Electrical coupling induces bistability of rhythms in networks of inhibitory spiking neurons. Eur. J. Neurosci. 22, 2661–2668. doi: 10.1111/j.1460-9568.2005.04405.x
Bem, T., and Rinzel, J. (2004). Short duty cycle destabilizes a half-center oscillator, but gap junctions can restabilize the anti-phase pattern. Journal of neurophysiology 91, 693–703. doi: 10.1152/jn.00783.2003
Benchenane, K., Tiesinga, P. H., and Battaglia, F. P. (2011). Oscillations in the prefrontal cortex: a gateway to memory and attention. Curr. Opin. Neurobiol. 21, 475–485. doi: 10.1016/j.conb.2011.01.004
Bennett, M. V. (1966). Physiology of electrotonic junctions. Ann. N. Y. Acad. Sci. 137, 509–539. doi: 10.1111/j.1749-6632.1966.tb50178.x
Bennett, M. V., and Zukin, R. S. (2004). Electrical coupling and neuronal synchronization in the Mammalian brain. Neuron 41, 495–511. doi: 10.1016/s0896-6273(04)00043-1
Blatow, M., Rozov, A., Katona, I., Hormuzdi, S. G., Meyer, A. H., Whittington, M. A., et al. (2003). A novel network of multipolar bursting interneurons generates theta frequency oscillations in neocortex. Neuron 38, 805–817. doi: 10.1016/s0896-6273(03)00300-3
Blenkinsop, T. A., and Lang, E. J. (2006). Block of inferior olive gap junctional coupling decreases Purkinje cell complex spike synchrony and rhythmicity. J. Neurosci. 26, 1739–1748. doi: 10.1523/JNEUROSCI.3677-05.2006
Bloomfield, S. A., and Volgyi, B. (2009). The diverse functional roles and regulation of neuronal gap junctions in the retina. Nat. Rev. Neurosci. 10, 495–506. doi: 10.1038/nrn2636
Bou-Flores, C., and Berger, A. J. (2001). Gap junctions and inhibitory synapses modulate inspiratory motoneuron synchronization. J. Neurophysiol. 85, 1543–1551. doi: 10.1152/jn.2001.85.4.1543
Bromberg, M. B., Penne, J. B. Jr., Stephenson, B. S., and Young, A. B. (1981). Evidence for glutamate as the neurotransmitter of corticothalamic and corticorubral pathways. Brain Res. 215, 369–374. doi: 10.1016/0006-8993(81)90519-9
Buhl, D. L., Harris, K. D., Hormuzdi, S. G., Monyer, H., and Buzsáki, G. (2003). Selective impairment of hippocampal gamma oscillations in connexin-36 knock-out mouse in vivo. J. Neurosci. 23, 1013–1018. doi: 10.1523/JNEUROSCI.23-03-01013.2003
Bukauskas, F. F., Elfgang, C., Willecke, K., and Weingart, R. (1995). Heterotypic gap junction channels (connexin26–connexin32) violate the paradigm of unitary conductance. Pflügers Arch. 429, 870–872. doi: 10.1007/BF00374812
Buzsáki, G., and Draguhn, A. (2004). Neuronal oscillations in cortical networks. Science 304, 1926–1929.
Caputi, A., Rozov, A., Blatow, M., and Monyer, H. (2009). Two calretinin-positive GABAergic cell types in layer 2/3 of the mouse neocortex provide different forms of inhibition. Cereb. Cortex 19, 1345–1359. doi: 10.1093/cercor/bhn175
Cardin, J. A. (2016). Snapshots of the brain in action: local circuit operations through the lens of γ oscillations. J. Neurosci. 36, 10496–10504. doi: 10.1523/JNEUROSCI.1021-16.2016
Chang, Q., Gonzalez, M., Pinter, M. J., and Balice-Gordon, R. J. (1999). Gap junctional coupling and patterns of connexin expression among neonatal rat lumbar spinal motor neurons. J. Neurosci. 19, 10813–10828. doi: 10.1523/JNEUROSCI.19-24-10813.1999
Chatzigeorgiou, M., and Schafer, W. R. (2011). Lateral facilitation between primary mechanosensory neurons controls nose touch perception in C. elegans. Neuron 70, 299–309. doi: 10.1016/j.neuron.2011.02.046
Chow, C. C., and Kopell, N. (2000). Dynamics of spiking neurons with electrical coupling. Neural Comput. 12, 1643–1678.
Christie, J. M., Bark, C., Hormuzdi, S. G., Helbig, I., Monyer, H., and Westbrook, G. L. (2005). Connexin36 mediates spike synchrony in olfactory bulb glomeruli. Neuron 46, 761–772. doi: 10.1016/j.neuron.2005.04.030
Christie, J. M., and Westbrook, G. L. (2006). Lateral excitation within the olfactory bulb. J. Neurosci. 26, 2269–2277.
Colgin, L. L., and Moser, E. I. (2010). Gamma oscillations in the hippocampus. Physiology 25, 319–329.
Colwell, C. S. (2000). Rhythmic coupling among cells in the suprachiasmatic nucleus. J. Neurobiol. 43, 379–388. doi: 10.1002/1097-4695(20000615)43:4<379::aid-neu6>3.0.co;2-0
Condorelli, D. F., Parenti, R., Spinella, F., Salinaro, A. T., Belluardo, N., Cardile, V., et al. (1998). Cloning of a new gap junction gene (Cx36) highly expressed in mammalian brain neurons. Eur. J. Neurosci. 10, 1202–1208. doi: 10.1046/j.1460-9568.1998.00163.x
Connors, B. W. (2017). Synchrony and so much more: diverse roles for electrical synapses in neural circuits. Dev. Neurobiol. 77, 610–624. doi: 10.1002/dneu.22493
Connors, B. W., and Long, M. A. (2004). Electrical synapses in the mammalian brain. Annu. Rev. Neurosci. 27, 393–418.
Cornwall, J., Cooper, J. D., and Phillipson, O. T. (1990). Projections to the rostral reticular thalamic nucleus in the rat. Exp. Brain Res. 80, 157–171. doi: 10.1007/BF00228857
Curti, S., Davoine, F., and Dapino, A. (2022). Function and plasticity of electrical synapses in the mammalian brain: role of non-junctional mechanisms. Biology 11:81. doi: 10.3390/biology11010081
Curti, S., Hoge, G., Nagy, J. I., and Pereda, A. E. (2012). Synergy between electrical coupling and membrane properties promotes strong synchronization of neurons of the mesencephalic trigeminal nucleus. J. Neurosci. 32, 4341–4359. doi: 10.1523/JNEUROSCI.6216-11.2012
Dakin, K., and Li, W.-H. (2006). Infrared-LAMP: two-photon uncaging and imaging of gap junctional communication in three dimensions. Nat. Methods 3, 959–959. doi: 10.1038/nmeth1206-959
D’Angelo, E., Koekkoek, S., Lombardo, P., Solinas, S., Ros, E., Garrido, J., et al. (2009). Timing in the cerebellum: oscillations and resonance in the granular layer. Neuroscience 162, 805–815. doi: 10.1016/j.neuroscience.2009.01.048
De Zeeuw, C. I., Hoogenraad, C. C., Koekkoek, S., Ruigrok, T. J., Galjart, N., and Simpson, J. I. (1998). Microcircuitry and function of the inferior olive. Trends Neurosci. 21, 391–400. doi: 10.1016/s0166-2236(98)01310-1
Deans, M. R., Gibson, J. R., Sellitto, C., Connors, B. W., and Paul, D. L. (2001). Synchronous activity of inhibitory networks in neocortex requires electrical synapses containing connexin36. Neuron 31, 477–485. doi: 10.1016/s0896-6273(01)00373-7
Deans, M. R., Volgyi, B., Goodenough, D. A., Bloomfield, S. A., and Paul, D. L. (2002). Connexin36 is essential for transmission of rod-mediated visual signals in the mammalian retina. Neuron 36, 703–712. doi: 10.1016/s0896-6273(02)01046-2
Devor, A., and Yarom, Y. (2002). Electrotonic coupling in the inferior olivary nucleus revealed by simultaneous double patch recordings. J. Neurophysiol. 87, 3048–3058. doi: 10.1152/jn.2002.87.6.3048
DeVries, S. H., Qi, X., Smith, R., Makous, W., and Sterling, P. (2002). Electrical coupling between mammalian cones. Curr. Biol. 12, 1900–1907. doi: 10.1016/s0960-9822(02)01261-7
d’Incamps, B. L., Krejci, E., and Ascher, P. (2012). Mechanisms shaping the slow nicotinic synaptic current at the motoneuron–Renshaw cell synapse. J. Neurosci. 32, 8413–8423. doi: 10.1523/JNEUROSCI.0181-12.2012
Draguhn, A., Traub, R., Schmitz, D., and Jefferys, J. (1998). Electrical coupling underlies high-frequency oscillations in the hippocampus in vitro. Nature 394, 189–192. doi: 10.1038/28184
Dugué, G. P., Brunel, N., Hakim, V., Schwartz, E., Chat, M., Lévesque, M., et al. (2009). Electrical coupling mediates tunable low-frequency oscillations and resonance in the cerebellar Golgi cell network. Neuron 61, 126–139. doi: 10.1016/j.neuron.2008.11.028
Dunn, F. A., Doan, T., Sampath, A. P., and Rieke, F. (2006). Controlling the gain of rod-mediated signals in the mammalian retina. J. Neurosci. 26, 3959–3970. doi: 10.1523/JNEUROSCI.5148-05.2006
Eisen, J. S., and Marder, E. (1982). Mechanisms underlying pattern generation in lobster stomatogastric ganglion as determined by selective inactivation of identified neurons. III. Synaptic connections of electrically coupled pyloric neurons. J. Neurophysiol. 48, 1392–1415. doi: 10.1152/jn.1982.48.6.1392
Feig, S., and Harting, J. K. (1998). Corticocortical communication via the thalamus: ultrastructural studies of corticothalamic projections from area 17 to the lateral posterior nucleus of the cat and inferior pulvinar nucleus of the owl monkey. J. Comp. Neurol. 395, 281–295. doi: 10.1002/(sici)1096-9861(19980808)395:3<281::aid-cne2>3.0.co;2-z
Firth, S. I., Wang, C.-T., and Feller, M. B. (2005). Retinal waves: mechanisms and function in visual system development. Cell Calcium 37, 425–432. doi: 10.1016/j.ceca.2005.01.010
Fitzpatrick, D., Usrey, W. M., Schofield, B. R., and Einstein, G. (1994). The sublaminar organization of corticogeniculate neurons in layer 6 of macaque striate cortex. Vis. Neurosci. 11, 307–315. doi: 10.1017/s0952523800001656
Fonnum, F., Storm-Mathisen, J., and Divac, I. (1981). Biochemical evidence for glutamate as neurotransmitter in corticostriatal and corticothalamic fibres in rat brain. Neuroscience 6, 863–873. doi: 10.1016/0306-4522(81)90168-8
Fosse, V. M., Kolstad, J., and Fonnum, F. (1986). A bioluminescence method for the measurement of l-glutamate: applications to the study of changes in the release of l-glutamate from lateral geniculate nucleus and superior colliculus after visual cortex ablation in rats. J. Neurochem. 47, 340–349. doi: 10.1111/j.1471-4159.1986.tb04507.x
Freeman, A., Ciliax, B., Bakay, R., Daley, J., Miller, R. D., Keating, G., et al. (2001). Nigrostriatal collaterals to thalamus degenerate in parkinsonian animal models. Ann. Neurol. 50, 321–329. doi: 10.1002/ana.1119
Fricker, B., Heckman, E., Cunningham, P. C., Wang, H., and Haas, J. S. (2021). Activity-dependent long-term potentiation of electrical synapses in the mammalian thalamus. J. Neurophysiol. 125, 476–488. doi: 10.1152/jn.00471.2020
Fukuda, T., and Kosaka, T. (2000). Gap junctions linking the dendritic network of GABAergic interneurons in the hippocampus. J. Neurosci. 20, 1519–1528. doi: 10.1523/JNEUROSCI.20-04-01519.2000
Furshpan, E., and Potter, D. (1957). Mechanism of nerve-impulse transmission at a crayfish synapse. Nature 180, 342–343. doi: 10.1038/180342a0
Furshpan, E., and Potter, D. (1959). Transmission at the giant motor synapses of the crayfish. J. Physiol. 145:289. doi: 10.1113/jphysiol.1959.sp006143
Galarreta, M., and Hestrin, S. (1999). A network of fast-spiking cells in the neocortex connected by electrical synapses. Nature 402, 72–75. doi: 10.1038/47029
Galarreta, M., and Hestrin, S. (2001). Spike transmission and synchrony detection in networks of GABAergic interneurons. Science 292, 2295–2299. doi: 10.1126/science.1061395
Galarreta, M., and Hestrin, S. (2002). Electrical and chemical synapses among parvalbumin fast-spiking GABAergic interneurons in adult mouse neocortex. Proc. Natl. Acad. Sci. 99, 12438–12443. doi: 10.1073/pnas.192159599
Gandia, J. A., De Las Heras, S., Garcia, M., and Gimenez-Amaya, J. M. (1993). Afferent projections to the reticular thalamic nucleus from the globus pallidus and the substantia nigra in the rat. Brain Res. Bull. 32, 351–358. doi: 10.1016/0361-9230(93)90199-l
Gibson, J. R., Beierlein, M., and Connors, B. W. (1999). Two networks of electrically coupled inhibitory neurons in neocortex. Nature 402, 75–79. doi: 10.1038/47035
Gibson, J. R., Beierlein, M., and Connors, B. W. (2005). Functional properties of electrical synapses between inhibitory interneurons of neocortical layer 4. J. Neurophysiol. 93, 467–480. doi: 10.1152/jn.00520.2004
Giraud, A.-L., and Poeppel, D. (2012). Cortical oscillations and speech processing: emerging computational principles and operations. Nat. Neurosci. 15, 511–517. doi: 10.1038/nn.3063
Graubard, K., and Hartline, D. K. (1987). Full-wave rectification from a mixed electrical-chemical synapse. Science 237, 535–537. doi: 10.1126/science.2885921
Güldenagel, M., Ammermüller, J., Feigenspan, A., Teubner, B., Degen, J., Söhl, G., et al. (2001). Visual transmission deficits in mice with targeted disruption of the gap junction gene connexin36. J. Neurosci. 21, 6036–6044. doi: 10.1523/JNEUROSCI.21-16-06036.2001
Gutierrez, G. J., O’Leary, T., and Marder, E. (2013). Multiple mechanisms switch an electrically coupled, synaptically inhibited neuron between competing rhythmic oscillators. Neuron 77, 845–858. doi: 10.1016/j.neuron.2013.01.016
Haas, J. S., Greenwald, C. M., and Pereda, A. E. (2016). Activity-dependent plasticity of electrical synapses: increasing evidence for its presence and functional roles in the mammalian brain. BMC Cell Biol. 17 (Suppl. 1):14. doi: 10.1186/s12860-016-0090-z
Haas, J. S., and Landisman, C. E. (2011). State-dependent modulation of gap junction signaling by the persistent sodium current. Front. Cell Neurosci. 5:31. doi: 10.3389/fncel.2011.00031
Haas, J. S., Zavala, B., and Landisman, C. E. (2011). Activity-dependent long-term depression of electrical synapses. Science 334, 389–393. doi: 10.1126/science.1207502
Halassa, M. M., Siegle, J. H., Ritt, J. T., Ting, J. T., Feng, G., and Moore, C. I. (2011). Selective optical drive of thalamic reticular nucleus generates thalamic bursts and cortical spindles. Nat. Neurosci. 14, 1118–1120. doi: 10.1038/nn.2880
Hampson, E., Vaney, D. I., and Weiler, R. (1992). Dopaminergic modulation of gap junction permeability between amacrine cells in mammalian retina. J. Neurosci. 12, 4911–4922. doi: 10.1523/JNEUROSCI.12-12-04911.1992
Hanslmayr, S., Gross, J., Klimesch, W., and Shapiro, K. L. (2011). The role of alpha oscillations in temporal attention. Brain Res. Rev. 67, 331–343. doi: 10.1016/j.brainresrev.2011.04.002
Hjorth, J., Blackwell, K. T., and Kotaleski, J. H. (2009). Gap junctions between striatal fast-spiking interneurons regulate spiking activity and synchronization as a function of cortical activity. J. Neurosci. 29, 5276–5286. doi: 10.1523/JNEUROSCI.6031-08.2009
Hoehne, A., McFadden, M. H., and DiGregorio, D. A. (2020). Feed-forward recruitment of electrical synapses enhances synchronous spiking in the mouse cerebellar cortex. Elife 9:e57344. doi: 10.7554/eLife.57344
Hooper, S. L., and Marder, E. (1987). Modulation of the lobster pyloric rhythm by the peptide proctolin. J. Neurosci. 7, 2097–2112. doi: 10.1523/JNEUROSCI.07-07-02097.1987
Hormuzdi, S. G., Pais, I., LeBeau, F. E., Towers, S. K., Rozov, A., Buhl, E. H., et al. (2001). Impaired electrical signaling disrupts gamma frequency oscillations in connexin 36-deficient mice. Neuron 31, 487–495. doi: 10.1016/s0896-6273(01)00387-7
Houser, C. R., Vaughn, J. E., Barber, R. P., and Roberts, E. (1980). GABA neurons are the major cell type of the nucleus reticularis thalami. Brain Res. 200, 341–354. doi: 10.1016/0006-8993(80)90925-7
Hu, H., and Agmon, A. (2015). Properties of precise firing synchrony between synaptically coupled cortical interneurons depend on their mode of coupling. J. Neurophysiol. 114, 624–637. doi: 10.1152/jn.00304.2015
Jacoby, J., Nath, A., Jessen, Z. F., and Schwartz, G. W. (2018). A self-regulating gap junction network of amacrine cells controls nitric oxide release in the retina. Neuron 100, 1149–1162.e5. doi: 10.1016/j.neuron.2018.09.047
Jaslove, S. W., and Brink, P. R. (1986). The mechanism of rectification at the electrotonic motor giant synapse of the crayfish. Nature 323, 63–65. doi: 10.1038/323063a0
Johnson, B. R., Peck, J. H., and Harris-Warrick, R. M. (1993). Dopamine induces sign reversal at mixed chemical-electrical synapses. Brain Res. 625, 159–164. doi: 10.1016/0006-8993(93)90149-h
Jones, E. (1975). Some aspects of the organization of the thalamic reticular complex. J. Comp. Neurol. 162, 285–308. doi: 10.1002/cne.901620302
Kashiwadani, H., Sasaki, Y. F., Uchida, N., and Mori, K. (1999). Synchronized oscillatory discharges of mitral/tufted cells with different molecular receptive ranges in the rabbit olfactory bulb. J. Neurophysiol. 82, 1786–1792. doi: 10.1152/jn.1999.82.4.1786
Kawano, T., Po, M. D., Gao, S., Leung, G., Ryu, W. S., and Zhen, M. (2011). An imbalancing act: gap junctions reduce the backward motor circuit activity to bias C. elegans for forward locomotion. Neuron 72, 572–586. doi: 10.1016/j.neuron.2011.09.005
Kazama, H., and Wilson, R. I. (2009). Origins of correlated activity in an olfactory circuit. Nat. Neurosci. 12, 1136–1144. doi: 10.1038/nn.2376
Kilavik, B. E., Zaepffel, M., Brovelli, A., MacKay, W. A., and Riehle, A. (2013). The ups and downs of beta oscillations in sensorimotor cortex. Exp. Neurol. 245, 15–26. doi: 10.1016/j.expneurol.2012.09.014
Kopell, N., and Ermentrout, B. (2004). Chemical and electrical synapses perform complementary roles in the synchronization of interneuronal networks. Proc. Natl. Acad. Sci. 101, 15482–15487. doi: 10.1073/pnas.0406343101
Kothmann, W. W., Massey, S. C., and O’Brien, J. (2009). Dopamine-stimulated dephosphorylation of connexin 36 mediates AII amacrine cell uncoupling. J. Neurosci. 29, 14903–14911. doi: 10.1523/JNEUROSCI.3436-09.2009
Kuo, S. P., Schwartz, G. W., and Rieke, F. (2016). Nonlinear spatiotemporal integration by electrical and chemical synapses in the retina. Neuron 90, 320–332. doi: 10.1016/j.neuron.2016.03.012
Lamb, T., and Simon, E. (1976). The relation between intercellular coupling and electrical noise in turtle photoreceptors. J. Physiol. 263, 257–286. doi: 10.1113/jphysiol.1976.sp011631
Landesman, Y., White, T. W., Starich, T. A., Shaw, J. E., Goodenough, D. A., and Paul, D. L. (1999). Innexin-3 forms connexin-like intercellular channels. J. Cell Sci. 112, 2391–2396. doi: 10.1242/jcs.112.14.2391
Landisman, C. E., Long, M. A., Beierlein, M., Deans, M. R., Paul, D. L., and Connors, B. W. (2002). Electrical synapses in the thalamic reticular nucleus. J. Neurosci. 22, 1002–1009.
Latchoumane, C.-F. V., Ngo, H.-V. V., Born, J., and Shin, H.-S. (2017). Thalamic spindles promote memory formation during sleep through triple phase-locking of cortical, thalamic, and hippocampal rhythms. Neuron 95, 424–435.e6. doi: 10.1016/j.neuron.2017.06.025
Lau, T., Gage, G. J., Berke, J. D., and Zochowski, M. (2010). Local dynamics of gap-junction-coupled interneuron networks. Phys. Biol. 7:016015. doi: 10.1088/1478-3975/7/1/016015
Lee, E., Lee, S., Shin, J. J., Choi, W., Chung, C., Lee, S., et al. (2021). Excitatory synapses and gap junctions cooperate to improve Pv neuronal burst firing and cortical social cognition in Shank2-mutant mice. Nat. Commun. 12, 1–20. doi: 10.1038/s41467-021-25356-2
Lee, K. W., Hong, J. H., Choi, I. Y., Che, Y., Lee, J. K., Yang, S. D., et al. (2002). Impaired D2 dopamine receptor function in mice lacking type 5 adenylyl cyclase. J. Neurosci. 22, 7931–7940. doi: 10.1523/JNEUROSCI.22-18-07931.2002
Leon-Dominguez, U., Vela-Bueno, A., Froufe-Torres, M., and Leon-Carrion, J. (2013). A chronometric functional sub-network in the thalamo-cortical system regulates the flow of neural information necessary for conscious cognitive processes. Neuropsychologia 51, 1336–1349. doi: 10.1016/j.neuropsychologia.2013.03.012
Lewis, T. J., and Rinzel, J. (2003). Dynamics of spiking neurons connected by both inhibitory and electrical coupling. J. Comput. Neurosci. 14, 283–309. doi: 10.1023/a:1023265027714
Leznik, E., and Llinas, R. (2005). Role of gap junctions in synchronized neuronal oscillations in the inferior olive. J. Neurophysiol. 94, 2447–2456. doi: 10.1152/jn.00353.2005
Li, H., Zhang, Z., Blackburn, M. R., Wang, S. W., Ribelayga, C. P., and O’Brien, J. (2013). Adenosine and dopamine receptors coregulate photoreceptor coupling via gap junction phosphorylation in mouse retina. J. Neurosci. 33, 3135–3150. doi: 10.1523/JNEUROSCI.2807-12.2013
Liu, X. B., and Jones, E. G. (1999). Predominance of corticothalamic synaptic inputs to thalamic reticular nucleus neurons in the rat. J. Comp. Neurol. 414, 67–79.
Llinas, R., Baker, R., and Sotelo, C. (1974). Electrotonic coupling between neurons in cat inferior olive. J. Neurophysiol. 37, 560–571. doi: 10.1152/jn.1974.37.3.560
Long, M. A., Deans, M. R., Paul, D. L., and Connors, B. W. (2002). Rhythmicity without synchrony in the electrically uncoupled inferior olive. J. Neurosci. 22, 10898–10905. doi: 10.1523/JNEUROSCI.22-24-10898.2002
Long, M. A., Jutras, M. J., Connors, B. W., and Burwell, R. D. (2005). Electrical synapses coordinate activity in the suprachiasmatic nucleus. Nat. Neurosci. 8, 61–66. doi: 10.1038/nn1361
Long, M. A., Landisman, C. E., and Connors, B. W. (2004). Small clusters of electrically coupled neurons generate synchronous rhythms in the thalamic reticular nucleus. J. Neurosci. 24, 341–349. doi: 10.1523/JNEUROSCI.3358-03.2004
Mamiya, A., Manor, Y., and Nadim, F. (2003). Short-term dynamics of a mixed chemical and electrical synapse in a rhythmic network. J. Neurosci. 23, 9557–9564. doi: 10.1523/JNEUROSCI.23-29-09557.2003
Mancilla, J. G., Lewis, T. J., Pinto, D. J., Rinzel, J., and Connors, B. W. (2007). Synchronization of electrically coupled pairs of inhibitory interneurons in neocortex. J. Neurosci. 27, 2058–2073. doi: 10.1523/JNEUROSCI.2715-06.2007
Mann-Metzer, P., and Yarom, Y. (1999). Electrotonic coupling interacts with intrinsic properties to generate synchronized activity in cerebellar networks of inhibitory interneurons. J. Neurosci. 19, 3298–3306. doi: 10.1523/JNEUROSCI.19-09-03298.1999
Marsh, A. J., Michel, J. C., Adke, A. P., Heckman, E. L., and Miller, A. C. (2017). Asymmetry of an intracellular scaffold at vertebrate electrical synapses. Curr. Biol. 27, 3561–3567.e4. doi: 10.1016/j.cub.2017.10.011
Marshall, S. P., Van Der Giessen, R. S., De Zeeuw, C. I., and Lang, E. J. (2007). Altered olivocerebellar activity patterns in the connexin36 knockout mouse. Cerebellum 6, 287–299. doi: 10.1080/14734220601100801
McMahon, D. G., and Brown, D. R. (1994). Modulation of gap-junction channel gating at zebrafish retinal electrical synapses. J. Neurophysiol. 72, 2257–2268. doi: 10.1152/jn.1994.72.5.2257
Medvedev, G. S. (2009). Electrical coupling promotes fidelity of responses in the networks of model neurons. Neural Comput. 21, 3057–3078. doi: 10.1162/neco.2009.07-08-813
Mendoza, A. J., and Haas, J. S. (2022). Intrinsic sources and functional impacts of asymmetry at electrical synapses. eNeuro 9:ENEURO.0469-21.2022. doi: 10.1523/ENEURO.0469-21.2022
Mills, S. L., and Massey, S. C. (1995). Differential properties of two gap junctional pathways made by AII amacrine cells. Nature 377, 734–737. doi: 10.1038/377734a0
Mills, S. L., Xia, X. B., Hoshi, H., Firth, S. I., Rice, M. E., Frishman, L. J., et al. (2007). Dopaminergic modulation of tracer coupling in a ganglion-amacrine cell network. Vis. Neurosci. 24, 593–608. doi: 10.1017/S0952523807070575
Montbrió, E., and Pazó, D. (2020). Exact mean-field theory explains the dual role of electrical synapses in collective synchronization. Phys. Rev. Lett. 125:248101. doi: 10.1103/PhysRevLett.125.248101
Moore, K. B., Mitchell, C. K., Lin, Y.-P., Lee, Y.-H., Shihabeddin, E., and O’brien, J. (2020). Localized calcium signaling and the control of coupling at Cx36 gap junctions. Eneuro 7:ENEURO.0445-19.2020. doi: 10.1523/ENEURO.0445-19.2020
Moortgat, K. T., Bullock, T. H., and Sejnowski, T. J. (2000). Gap junction effects on precision and frequency of a model pacemaker network. J. Neurophysiol. 83, 984–997. doi: 10.1152/jn.2000.83.2.984
Mulloney, B., Perkel, D. H., and Budelli, R. W. (1981). Motor-pattern production: interaction of chemical and electrical synapses. Brain Res. 229, 25–33. doi: 10.1016/0006-8993(81)90742-3
Nácher, V., Ledberg, A., Deco, G., and Romo, R. (2013). Coherent delta-band oscillations between cortical areas correlate with decision making. Proc. Natl. Acad. Sci. 110, 15085–15090. doi: 10.1073/pnas.1314681110
Nadim, F., and Golowasch, J. (2006). Signal transmission between gap-junctionally coupled passive cables is most effective at an optimal diameter. J. Neurophysiol. 95, 3831–3843. doi: 10.1152/jn.00033.2006
Nagy, J. I., Pereda, A. E., and Rash, J. E. (2018). Electrical synapses in mammalian CNS: past eras, present focus and future directions. Biochim. Biophys. Acta Biomembr. 1860, 102–123. doi: 10.1016/j.bbamem.2017.05.019
Neske, G. T., and Connors, B. W. (2016). Synchronized gamma-frequency inhibition in neocortex depends on excitatory-inhibitory interactions but not electrical synapses. J. Neurophysiol. 116, 351–368. doi: 10.1152/jn.00071.2016
Nomura, M., Fukai, T., and Aoyagi, T. (2003). Synchrony of fast-spiking interneurons interconnected by GABAergic and electrical synapses. Neural Comput. 15, 2179–2198. doi: 10.1162/089976603322297340
Oh, S. W., Harris, J. A., Ng, L., Winslow, B., Cain, N., Mihalas, S., et al. (2014). A mesoscale connectome of the mouse brain. Nature 508, 207–214.
Ohara, P. T., and Lieberman, A. (1985). The thalamic reticular nucleus of the adult rat: experimental anatomical studies. J. Neurocytol. 14, 365–411. doi: 10.1007/BF01217752
Ostojic, S., Brunel, N., and Hakim, V. (2009). Synchronization properties of networks of electrically coupled neurons in the presence of noise and heterogeneities. J. Comput. Neurosci. 26, 369–392. doi: 10.1007/s10827-008-0117-3
Otsuka, T., and Kawaguchi, Y. (2013). Common excitatory synaptic inputs to electrically connected cortical fast-spiking cell networks. J. Neurophysiol. 110, 795–806. doi: 10.1152/jn.00071.2013
Parker, P. R., Cruikshank, S. J., and Connors, B. W. (2009). Stability of electrical coupling despite massive developmental changes of intrinsic neuronal physiology. J. Neurosci. 29, 9761–9770. doi: 10.1523/JNEUROSCI.4568-08.2009
Pastor, A. M., Mentis, G. Z., De la Cruz, R. R., Díaz, E., and Navarrete, R. (2003). Increased electrotonic coupling in spinal motoneurons after transient botulinum neurotoxin paralysis in the neonatal rat. J. Neurophysiol. 89, 793–805. doi: 10.1152/jn.00498.2002
Pereda, A., Triller, A., Korn, H., and Faber, D. S. (1992). Dopamine enhances both electrotonic coupling and chemical excitatory postsynaptic potentials at mixed synapses. Proc. Natl. Acad. Sci. 89, 12088–12092. doi: 10.1073/pnas.89.24.12088
Pereda, A. E., Bell, T. D., Chang, B. H., Czernik, A. J., Nairn, A. C., Soderling, T. R., et al. (1998). Ca2+/calmodulin-dependent kinase II mediates simultaneous enhancement of gap-junctional conductance and glutamatergic transmission. Proc. Natl. Acad. Sci. U. S. A. 95, 13272–13277. doi: 10.1073/pnas.95.22.13272
Pereda, A. E., Nairn, A. C., Wolszon, L. R., and Faber, D. S. (1994). Postsynaptic modulation of synaptic efficacy at mixed synapses on the Mauthner cell. J. Neurosci. 14, 3704–3712. doi: 10.1523/JNEUROSCI.14-06-03704.1994
Pereda, A. E., Rash, J. E., Nagy, J. I., and Bennett, M. V. (2004). Dynamics of electrical transmission at club endings on the Mauthner cells. Brain Res. Rev. 47, 227–244. doi: 10.1016/j.brainresrev.2004.06.010
Perez-Velazquez, J., Valiante, T. A., and Carlen, P. L. (1994). Modulation of gap junctional mechanisms during calcium-free induced field burst activity: a possible role for electrotonic coupling in epileptogenesis. J. Neurosci. 14, 4308–4317. doi: 10.1523/JNEUROSCI.14-07-04308.1994
Perkins, K. L., and Wong, R. (1996). Ionic basis of the postsynaptic depolarizing GABA response in hippocampal pyramidal cells. J. Neurophysiol. 76, 3886–3894. doi: 10.1152/jn.1996.76.6.3886
Personius, K., Chang, Q., Bittman, K., Panzer, J., and Balice-Gordon, R. (2001). Gap junctional communication among motor and other neurons shapes patterns of neural activity and synaptic connectivity during development. Cell Commun. Adhes. 8, 329–333. doi: 10.3109/15419060109080748
Pfeuty, B., Mato, G., Golomb, D., and Hansel, D. (2003). Electrical synapses and synchrony: the role of intrinsic currents. J. Neurosci. 23, 6280–6294. doi: 10.1523/JNEUROSCI.23-15-06280.2003
Pham, T., and Haas, J. S. (2018). Electrical synapses between inhibitory neurons shape the responses of principal neurons to transient inputs in the thalamus: a modeling study. Sci. Rep. 8:7763. doi: 10.1038/s41598-018-25956-x
Pham, T., and Haas, J. S. (2019). Electrical synapses regulate both subthreshold integration and population activity of principal cells in response to transient inputs within canonical feedforward circuits. PLoS Comput. Biol. 15:e1006440. doi: 10.1371/journal.pcbi.1006440
Phelan, P., Goulding, L. A., Tam, J. L., Allen, M. J., Dawber, R. J., Davies, J. A., et al. (2008). Molecular mechanism of rectification at identified electrical synapses in the Drosophila giant fiber system. Curr. Biol. 18, 1955–1960. doi: 10.1016/j.cub.2008.10.067
Phelan, P., Nakagawa, M., Wilkin, M. B., Moffat, K. G., O’Kane, C. J., Davies, J. A., et al. (1996). Mutations in shaking-B prevent electrical synapse formation in the Drosophila giant fiber system. J. Neurosci. 16, 1101–1113. doi: 10.1523/JNEUROSCI.16-03-01101.1996
Phelan, P., Stebbings, L. A., Baines, R. A., Bacon, J. P., Davies, J. A., and Ford, C. (1998). Drosophila Shaking-B protein forms gap junctions in paired Xenopus oocytes. Nature 391, 181–184. doi: 10.1038/34426
Pinault, D. (2004). The thalamic reticular nucleus: structure, function and concept. Brain Res. Brain Res. Rev. 46, 1–31. doi: 10.1016/j.brainresrev.2004.04.008
Pottackal, J., Walsh, H. L., Rahmani, P., Zhang, K., Justice, N. J., and Demb, J. B. (2021). Photoreceptive ganglion cells drive circuits for local inhibition in the mouse retina. J. Neurosci. 41, 1489–1504. doi: 10.1523/JNEUROSCI.0674-20.2020
Prensa, L., and Parent, A. (2001). The nigrostriatal pathway in the rat: a single-axon study of the relationship between dorsal and ventral tier nigral neurons and the striosome/matrix striatal compartments. J. Neurosci. 21, 7247–7260. doi: 10.1523/JNEUROSCI.21-18-07247.2001
Puller, C., Duda, S., Lotfi, E., Arzhangnia, Y., Block, C. T., Ahlers, M. T., et al. (2020). Electrical coupling of heterotypic ganglion cells in the mammalian retina. J. Neurosci. 40, 1302–1310. doi: 10.1523/JNEUROSCI.1374-19.2019
Qiao, M., and Sanes, J. R. (2016). Genetic method for labeling electrically coupled cells: application to retina. Front. Mol. Neurosci. 8:81. doi: 10.3389/fnmol.2015.00081
Rabinowitch, I., Chatzigeorgiou, M., and Schafer, W. R. (2013). A gap junction circuit enhances processing of coincident mechanosensory inputs. Current Biology 23, 963–967. doi: 10.1016/j.cub.2013.04.030
Ransey, E., Chesnov, K., Bursac, N., and Dzirasa, K. (2021a). FETCH: a platform for high-throughput quantification of gap junction hemichannel docking. bioRxiv[Preprint] doi: 10.1101/2021.06.07.447352
Ransey, E., Chesnov, K., Wisdom, E., Bowman, R., Rodriguez, T., Adamson, E., et al. (2021b). Long-term precision editing of neural circuits using engineered gap junction hemichannels. bioRxiv [Preprint] doi: 10.1101/2021.08.24.457429
Rash, J., Staines, W., Yasumura, T., Patel, D., Furman, C., Stelmack, G., et al. (2000). Immunogold evidence that neuronal gap junctions in adult rat brain and spinal cord contain connexin-36 but not connexin-32 or connexin-43. Proc. Natl. Acad. Sci. 97, 7573–7578. doi: 10.1073/pnas.97.13.7573
Rash, J. E., Yasumura, T., Davidson, K., Furman, C., Dudek, F., and Nagy, J. (2001a). Identification of cells expressing Cx43, Cx30, Cx26, Cx32 and Cx36 in gap junctions of rat brain and spinal cord. Cell Commun. Adhes. 8, 315–320. doi: 10.3109/15419060109080745
Rash, J. E., Curti, S., Vanderpool, K. G., Kamasawa, N., Nannapaneni, S., Palacios-Prado, N., et al. (2013). Molecular and functional asymmetry at a vertebrate electrical synapse. Neuron 79, 957–969. doi: 10.1016/j.neuron.2013.06.037
Rash, J. E., Yasumura, T., Dudek, F. E., and Nagy, J. I. (2001b). Cell-specific expression of connexins and evidence of restricted gap junctional coupling between glial cells and between neurons. J. Neurosci. 21, 1983–2000. doi: 10.1523/JNEUROSCI.21-06-01983.2001
Reardon, F., and Mitrofanis, J. (2000). Organisation of the amygdalo-thalamic pathways in rats. Anat. Embryol. 201, 75–84. doi: 10.1007/pl00008229
Ren, Y., Liu, Y., and Luo, M. (2021). Gap junctions between striatal d1 neurons and cholinergic interneurons. Front. Cell. Neurosci. 15:674399. doi: 10.3389/fncel.2021.674399
Ribelayga, C., Cao, Y., and Mangel, S. C. (2008). The circadian clock in the retina controls rod-cone coupling. Neuron 59, 790–801. doi: 10.1016/j.neuron.2008.07.017
Rojas-Líbano, D., and Kay, L. M. (2008). Olfactory system gamma oscillations: the physiological dissection of a cognitive neural system. Cogn. Neurodyn. 2, 179–194. doi: 10.1007/s11571-008-9053-1
Ross, F., Gwyn, P., Spanswick, D., and Davies, S. (2000). Carbenoxolone depresses spontaneous epileptiform activity in the CA1 region of rat hippocampal slices. Neuroscience 100, 789–796. doi: 10.1016/s0306-4522(00)00346-8
Russo, G., Nieus, T. R., Maggi, S., and Taverna, S. (2013). Dynamics of action potential firing in electrically connected striatal fast-spiking interneurons. Front. Cell. Neurosci. 7:209. doi: 10.3389/fncel.2013.00209
Salkoff, D. B., Zagha, E., Yüzgeç, Ö, and McCormick, D. A. (2015). Synaptic mechanisms of tight spike synchrony at gamma frequency in cerebral cortex. J. Neurosci. 35, 10236–10251. doi: 10.1523/JNEUROSCI.0828-15.2015
Scheibel, M. E., and Scheibel, A. B. (1966). The organization of the nucleus reticularis thalami: a Golgi study. Brain Res. 1, 43–62. doi: 10.1016/0006-8993(66)90104-1
Schoppa, N. E., and Urban, N. N. (2003). Dendritic processing within olfactory bulb circuits. Trends Neurosci. 26, 501–506. doi: 10.1016/S0166-2236(03)00228-5
Sevetson, J., and Haas, J. S. (2015). Asymmetry and modulation of spike timing in electrically coupled neurons. J. Neurophysiol. 113, 1743–1751. doi: 10.1152/jn.00843.2014
Sherman, A., and Rinzel, J. (1992). Rhythmogenic effects of weak electrotonic coupling in neuronal models. Proc. Natl. Acad. Sci. 89, 2471–2474. doi: 10.1073/pnas.89.6.2471
Singer, J. H., Mirotznik, R., and Feller, M. (2001). Potentiation of L-type calcium channels reveals nonsynaptic mechanisms that correlate spontaneous activity in the developing mammalian retina. J. Neurosci. 21, 8514–8522. doi: 10.1523/JNEUROSCI.21-21-08514.2001
Söhl, G., and Willecke, K. (2003). An update on connexin genes and their nomenclature in mouse and man. Cell Commun. Adhes. 10, 173–180. doi: 10.1080/cac.10.4-6.173.180
Solomon, I. C., Chon, K. H., and Rodriguez, M. N. (2003). Blockade of brain stem gap junctions increases phrenic burst frequency and reduces phrenic burst synchronization in adult rat. J. Neurophysiol. 89, 135–149. doi: 10.1152/jn.00697.2002
Srinivas, M., Rozental, R., Kojima, T., Dermietzel, R., Mehler, M., Condorelli, D. F., et al. (1999). Functional properties of channels formed by the neuronal gap junction protein connexin36. J. Neurosci. 19, 9848–9855. doi: 10.1523/JNEUROSCI.19-22-09848.1999
Starich, T. A., Lee, R., Panzarella, C., Avery, L., and Shaw, J. E. (1996). eat-5 and unc-7 represent a multigene family in Caenorhabditis elegans involved in cell-cell coupling. J. Cell Biol. 134, 537–548. doi: 10.1083/jcb.134.2.537
Stebbings, L. A., Todman, M. G., Phelan, P., Bacon, J. P., and Davies, J. A. (2000). Two Drosophila innexins are expressed in overlapping domains and cooperate to form gap-junction channels. Mol. Biol. Cell 11, 2459–2470. doi: 10.1091/mbc.11.7.2459
Steriade, M. (2005). Sleep, epilepsy and thalamic reticular inhibitory neurons. Trends Neurosci. 28, 317–324. doi: 10.1016/j.tins.2005.03.007
Szoboszlay, M., Lõrincz, A., Lanore, F., Vervaeke, K., Silver, R. A., and Nusser, Z. (2016). Functional properties of dendritic gap junctions in cerebellar Golgi cells. Neuron 90, 1043–1056. doi: 10.1016/j.neuron.2016.03.029
Tamás, G., Buhl, E. H., Lörincz, A., and Somogyi, P. (2000). Proximally targeted GABAergic synapses and gap junctions synchronize cortical interneurons. Nat. Neurosci. 3, 366–371. doi: 10.1038/73936
Tian, H., Davis, H. C., Wong-Campos, J. D., Fan, L. Z., Gmeiner, B., Begum, S., et al. (2021). All-optical electrophysiology with improved genetically encoded voltage indicators reveals interneuron network dynamics in vivo. bioRxiv[Preprint] doi: 10.1101/2021.11.22.469481
Traub, R. D. (1995). Model of synchronized population bursts in electrically coupled interneurons containing active dendritic conductances. J. Comput. Neurosci. 2, 283–289. doi: 10.1007/BF00961440
Traub, R. D., Kopell, N., Bibbig, A., Buhl, E. H., LeBeau, F. E., and Whittington, M. A. (2001). Gap junctions between interneuron dendrites can enhance synchrony of gamma oscillations in distributed networks. J. Neurosci. 21, 9478–9486. doi: 10.1523/JNEUROSCI.21-23-09478.2001
Traub, R. D., Whittington, M. A., Stanford, I. M., and Jefferys, J. G. (1996). A mechanism for generation of long-range synchronous fast oscillations in the cortex. Nature 383, 621–624. doi: 10.1038/383621a0
Trenholm, S., and Awatramani, G. B. (2019). “Myriad roles for gap junctions in retinal circuits,” in Webvision: The Organization of the Retina and Visual System, eds H. Kolb., E. Fernandez and R. Nelson (Salt Lake City (UT): University of Utah Health Sciences Center)
Trenholm, S., McLaughlin, A. J., Schwab, D. J., and Awatramani, G. B. (2013). Dynamic tuning of electrical and chemical synaptic transmission in a network of motion coding retinal neurons. J. Neurosci. 33, 14927–14938. doi: 10.1523/JNEUROSCI.0808-13.2013
Tresch, M. C., and Kiehn, O. (2000). Motor coordination without action potentials in the mammalian spinal cord. Nat. Neurosci. 3, 593–599. doi: 10.1038/75768
Usher, M., Cohen, J. D., Servan-Schreiber, D., Rajkowski, J., and Aston-Jones, G. (1999). The role of locus coeruleus in the regulation of cognitive performance. Science 283, 549–554. doi: 10.1126/science.283.5401.549
Van Der Giessen, R. S., Koekkoek, S. K., van Dorp, S., De Gruijl, J. R., Cupido, A., Khosrovani, S., et al. (2008). Role of olivary electrical coupling in cerebellar motor learning. Neuron 58, 599–612. doi: 10.1016/j.neuron.2008.03.016
Van Essen, D. C., Smith, S. M., Barch, D. M., Behrens, T. E., Yacoub, E., Ugurbil, K., et al. (2013). The WU-Minn human connectome project: an overview. Neuroimage 80, 62–79. doi: 10.1016/j.neuroimage.2013.05.041
van Welie, I., Roth, A., Ho, S. S., Komai, S., and Häusser, M. (2016). Conditional spike transmission mediated by electrical coupling ensures millisecond precision-correlated activity among interneurons in vivo. Neuron 90, 810–823. doi: 10.1016/j.neuron.2016.04.013
Vardi, N., and Smith, R. G. (1996). The AII amacrine network: coupling can increase correlated activity. Vis. Res. 36, 3743–3757. doi: 10.1016/0042-6989(96)00098-3
Velazquez, J. L. P., and Carlen, P. L. (2000). Gap junctions, synchrony and seizures. Trends Neurosci. 23, 68–74. doi: 10.1016/s0166-2236(99)01497-6
Veruki, M. L., and Hartveit, E. (2002b). Electrical synapses mediate signal transmission in the rod pathway of the mammalian retina. J. Neurosci. 22, 10558–10566. doi: 10.1523/JNEUROSCI.22-24-10558.2002
Veruki, M. L., and Hartveit, E. (2002a). AII (Rod) amacrine cells form a network of electrically coupled interneurons in the mammalian retina. Neuron 33, 935–946. doi: 10.1016/s0896-6273(02)00609-8
Vervaeke, K., Lõrincz, A., Gleeson, P., Farinella, M., Nusser, Z., and Silver, R. A. (2010). Rapid desynchronization of an electrically coupled interneuron network with sparse excitatory synaptic input. Neuron 67, 435–451. doi: 10.1016/j.neuron.2010.06.028
Vervaeke, K., Lõrincz, A., Nusser, Z., and Silver, R. A. (2012). Gap junctions compensate for sublinear dendritic integration in an inhibitory network. Science 335, 1624–1628. doi: 10.1126/science.1215101
Walton, K. D., and Navarrete, R. (1991). Postnatal changes in motoneurone electrotonic coupling studied in the in vitro rat lumbar spinal cord. J. Physiol. 433, 283–305. doi: 10.1113/jphysiol.1991.sp018426
Wang, X.-J. (2010). Neurophysiological and computational principles of cortical rhythms in cognition. Physiol. Rev. 90, 1195–1268. doi: 10.1152/physrev.00035.2008
Watanabe, A. (1958). The interaction of electrical activity among neurons of lobster cardiac ganglion. Jpn. J. Physiol. 8, 305–318. doi: 10.2170/jjphysiol.8.305
Whittington, M. A., and Traub, R. D. (2003). Interneuron diversity series: inhibitory interneurons and network oscillations in vitro. Trends Neurosci. 26, 676–682. doi: 10.1016/j.tins.2003.09.016
Whittington, M. A., Traub, R. D., and Jefferys, J. G. (1995). Synchronized oscillations in interneuron networks driven by metabotropic glutamate receptor activation. Nature 373, 612–615. doi: 10.1038/373612a0
Whittington, M. A., Traub, R. D., Kopell, N., Ermentrout, B., and Buhl, E. H. (2000). Inhibition-based rhythms: experimental and mathematical observations on network dynamics. Int. J. Psychophysiol. 38, 315–336. doi: 10.1016/s0167-8760(00)00173-2
Wilson, P. M. (1985). A photographic perspective on the origins, form, course and relations of the acetylcholinesterase-containing fibres of the dorsal tegmental pathway in the rat brain. Brain Res. 357, 85–118. doi: 10.1016/0165-0173(85)90001-3
Yaeger, D. B., and Trussell, L. O. (2016). Auditory Golgi cells are interconnected predominantly by electrical synapses. J. Neurophysiol. 116, 540–551. doi: 10.1152/jn.01108.2015
Yaksi, E., and Wilson, R. I. (2010). Electrical coupling between olfactory glomeruli. Neuron 67, 1034–1047. doi: 10.1016/j.neuron.2010.08.041
Yao, X., Cafaro, J., McLaughlin, A. J., Postma, F. R., Paul, D. L., Awatramani, G., et al. (2018). Gap junctions contribute to differential light adaptation across direction-selective retinal ganglion cells. Neuron 100, 216–228.e6. doi: 10.1016/j.neuron.2018.08.021
Zhang, L., and Jones, E. G. (2004). Corticothalamic inhibition in the thalamic reticular nucleus. J. Neurophysiol. 91, 759–766. doi: 10.1152/jn.00624.2003
Zhang, Z., Allen, G. I., Zhu, H., and Dunson, D. (2019). Tensor network factorizations: Relationships between brain structural connectomes and traits. Neuroimage 197, 330–343. doi: 10.1016/j.neuroimage.2019.04.027
Zhu, P., Frank, T., and Friedrich, R. W. (2013). Equalization of odor representations by a network of electrically coupled inhibitory interneurons. Nat. Neurosci. 16, 1678–1686. doi: 10.1038/nn.3528
Zikopoulos, B., and Barbas, H. (2012). Pathways for emotions and attention converge on the thalamic reticular nucleus in primates. J. Neurosci. 32, 5338–5350. doi: 10.1523/JNEUROSCI.4793-11.2012
Zolnik, T. A., and Connors, B. W. (2016). Electrical synapses and the development of inhibitory circuits in the thalamus. J. Physiol. 594, 2579–2592. doi: 10.1113/JP271880
Keywords: electrical synapse, gap junction, connexin, synchrony, integration
Citation: Vaughn MJ and Haas JS (2022) On the Diverse Functions of Electrical Synapses. Front. Cell. Neurosci. 16:910015. doi: 10.3389/fncel.2022.910015
Received: 31 March 2022; Accepted: 25 May 2022;
Published: 09 June 2022.
Edited by:
Arianna Maffei, Stony Brook University, United StatesReviewed by:
Ernest Montbrio, Pompeu Fabra University, SpainBarry Connors, Brown University, United States
Copyright © 2022 Vaughn and Haas. This is an open-access article distributed under the terms of the Creative Commons Attribution License (CC BY). The use, distribution or reproduction in other forums is permitted, provided the original author(s) and the copyright owner(s) are credited and that the original publication in this journal is cited, in accordance with accepted academic practice. No use, distribution or reproduction is permitted which does not comply with these terms.
*Correspondence: Julie S. Haas, anVsaWUuaGFhc0BsZWhpZ2guZWR1