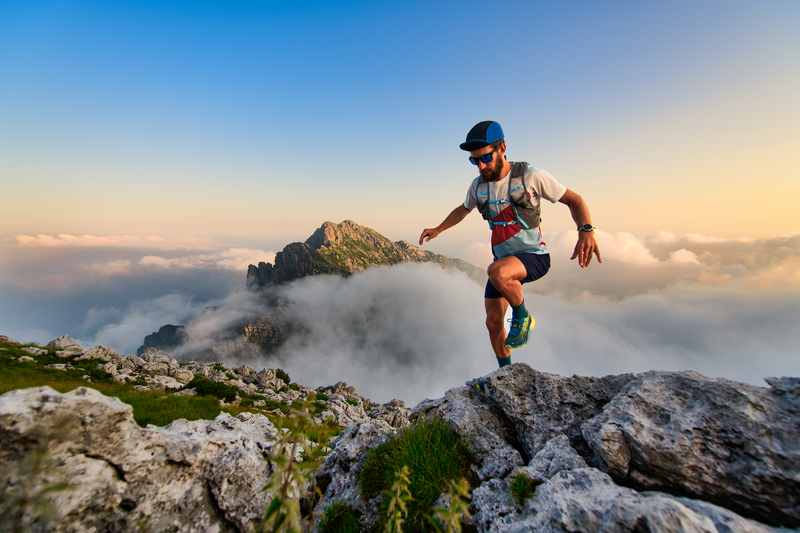
95% of researchers rate our articles as excellent or good
Learn more about the work of our research integrity team to safeguard the quality of each article we publish.
Find out more
REVIEW article
Front. Cell. Neurosci. , 13 June 2022
Sec. Cellular Neuropathology
Volume 16 - 2022 | https://doi.org/10.3389/fncel.2022.898497
This article is part of the Research Topic Blood-Brain Barrier Dysregulation and Recovery Following Brain Ischemia: Cellular Constituents, Molecular Mechanisms, and Therapeutic Strategies Enabling Successful Brain Remodeling View all 6 articles
Intracerebral hemorrhage (ICH), a common lethal subtype of stroke accounting for nearly 10–15% of the total stroke disease and affecting two million people worldwide, has a high mortality and disability rate and, thus, a major socioeconomic burden. However, there is no effective treatment available currently. The role of mesenchymal stem cells (MSCs) in regenerative medicine is well known owing to the simplicity of acquisition from various sources, low immunogenicity, adaptation to the autogenic and allogeneic systems, immunomodulation, self-recovery by secreting extracellular vesicles (EVs), regenerative repair, and antioxidative stress. MSC therapy provides an increasingly attractive therapeutic approach for ICH. Recently, the functions of MSCs such as neuroprotection, anti-inflammation, and improvement in synaptic plasticity have been widely researched in human and rodent models of ICH. MSC transplantation has been proven to improve ICH-induced injury, including the damage of nerve cells and oligodendrocytes, the activation of microglia and astrocytes, and the destruction of blood vessels. The improvement and recovery of neurological functions in rodent ICH models were demonstrated via the mechanisms such as neurogenesis, angiogenesis, anti-inflammation, anti-apoptosis, and synaptic plasticity. Here, we discuss the pathological mechanisms following ICH and the therapeutic mechanisms of MSC-based therapy to unravel new cues for future therapeutic strategies. Furthermore, some potential strategies for enhancing the therapeutic function of MSC transplantation have also been suggested.
Intracerebral hemorrhage (ICH) comprises 10–15% of all strokes; more than two million patients worldwide per year suffer from this hemorrhagic type of stroke with a complex pathophysiology and 1-month mortality of about 70% (Keep et al., 2012; Biffi et al., 2015; Bosche et al., 2020; Schrag and Kirshner, 2020; Jain et al., 2021; Rajashekar and Liang, 2021; Witsch et al., 2021). With the development of medical knowledge, the ICH incidence showed an increasing trend worldwide (van Asch et al., 2010; Wu and Anderson, 2020), resulting from the spiraling increment of older adults; the application of anticoagulants, antiplatelets, and thrombolytics; and other issues (Carpenter et al., 2016; Wu et al., 2019). Although significant progress in potential treatment after ICH models has been developed in preclinical research (Bentz et al., 2010; Xiong et al., 2014; Alharbi et al., 2016; Chen-Roetling et al., 2021), the lack of available evidence-based therapeutic strategies still limits the improvement of ICH prognosis in the clinical setting, where only active first-stage rehabilitation and general rehabilitation may lead to a modification of the outcomes (Saulle and Schambra, 2016).
Stem cell therapy is a promising method that has been actively applied in preclinical research of neurological diseases in recent years. Mesenchymal stem cells (MSCs) possess unique properties, including extensive proliferation and differentiation potential, simplicity of acquisition from various sources, low immunogenicity, secretome for extracellular vesicles (EVs), immunomodulation, and anti-inflammatory properties (Schipani and Kronenberg, 2008; Zheng et al., 2018; Dornen and Dittmar, 2021). Until now, MSC therapy has been recognized as a promising therapy in regenerative medicine research and tissue engineering (Bentz et al., 2010; Zheng et al., 2018).
Previously, accumulated evidence from preclinical studies has confirmed the protective effects of MSC therapy after ICH. However, the exact mechanisms of MSC transplantation in clinical translation are still undefined. Therefore, here, we have summarized the mechanisms of MSC application that facilitate neurological restoration after ICH. Simultaneously, some current challenges such as ICH-induced mass effect, iron overload, inflammation, oxidative stress, and limitations of clinical translation for MSC therapies are also emphasized when MSC treatment is applied to the ICH.
The brain injury after ICH, which always manifests a high risk of ischemia and recurrent bleeding (Baang and Sheth, 2021), is traditionally described as a cascade of disease courses including two different successive pathological processes: the primary brain injury stage and the secondary brain injury stage (Huang et al., 2020). In the first few hours of the primary brain injury stage after ICH occurs, the blood released from the ruptured blood vessels develops to form a consistent mass effect. Hematoma formation induces mechanical dissection and compression of the brain tissue, leading to high intracranial pressure forming herniation (Huang et al., 2020), which is always addressed by advanced surgical techniques in clinical practice (Tschoe et al., 2020). The secondary brain injury (SBI) stage is composed of damage caused by hemolytic products of erythrolysis, excitotoxicity, oxidative stress, and neuroinflammation-induced neurological deficits (Aronowski and Zhao, 2011; Babu et al., 2012; Duan et al., 2016; Lan et al., 2017), accompanied by successive pathological changes, including hemodynamic change-induced ischemia, enhancement of cerebral edema, destruction of the blood–brain barrier (BBB), and direct cellular toxicity (Aronowski and Zhao, 2011; Keep et al., 2012; Xiong et al., 2014; Chen S. et al., 2015; Mohammed Thangameeran et al., 2020; Zheng et al., 2022). During this process, the hemoglobin, heme, and free iron, released from erythrolysis and other blood derivatives, infiltrate into the perihematoma, activating microglia/macrophages to accelerate hematoma clearance displaying neuroprotection (Bosche et al., 2020; Lua et al., 2021; Wei et al., 2022; Zheng et al., 2022). Thrombin activation after ICH implies the vasculogenic edema formation associated with the destruction of endothelial cells and the BBB (Wilkinson et al., 2018). Perihematomal edema (PHE), associated with a worse prognosis, has been recognized as an evident marker of SBI after ICH and a likely therapeutic pathophysiological target for attenuating SBI (Brouwers and Greenberg, 2013; Bautista et al., 2021; Chen et al., 2021). Furthermore, it was displayed that intracranial hematoma expands to further and adjacent brain tissues through perivascular spaces, white matter tracts, and their perineurium (Yin et al., 2013; Fu et al., 2021), particularly if ICH is combined with an intraventricular hemorrhage (Bosche et al., 2020). All these pathological changes in the hematoma site make the nerve fibers distended and distorted and finally disrupted to a point at which they cannot be rescued. Therefore, the pathological changes of SBI after ICH induced permanent impairment of brain tissues, and severe neurological deficits should continuously be paid close attention to Qureshi et al. (2009) and Wilkinson et al. (2018).
The pathophysiological changes after ICH are mainly characterized by demyelination and axonal injury. Oligodendrocytes are the only source of myelin formation, insulating myelin sheaths for neurons to enhance the propagation of action potentials and protect the integrity of neurons and axons (Bacmeister et al., 2020). After ICH, there is a sharp increase in intracellular Fe2+ released from the hemoglobin of dead erythrocytes, which destroy oligodendrocytes. Demyelination and axonal damage are observed at the edge of the hematoma 6 h after ICH that peaks at the highest level of impairment at 3 days, and the axonal damage gradually extends to the adjacent parenchyma over time (Wasserman and Schlichter, 2008; Fu et al., 2021). Zille et al. (2017) have verified that the hemin and hemoglobin-induced toxic mediators from lysed blood after ICH participate in the death of primary cortical neurons through ferroptosis and necroptosis, rather than caspase-dependent apoptosis or autophagy in vitro and in vivo. Moreover, Palumbo et al. (2021) observed a time-dependent morphological degeneration of axons in hemin-induced primary cortical neurons, resulting in a declination of the axon area and enhancement of axonal swelling and fragment areas depending on a novel microfluidic device and a deep learning tool on microscopy. Hemorrhages in the internal capsule block the axonal transport to induce axonal dysfunction closely associated with an early decline of motor performance in mice with ICH (Hijioka et al., 2016). The capacity of proliferation and differentiation to oligodendrocyte precursors [NG2(+) Olig2(+)] presented a dramatically increasing trend in the perihematomal region over the first week, which provided the valid re-myelination chance on axon tracts in the rat striatum after ICH (Joseph et al., 2016). Ultrastructural features in mice with injured striatum after ICH examined by transmission electron microscopy at 3, 6, and 28 days have demonstrated remarkable axonal demyelination and degeneration, degenerated neurons, abnormal synapses, and infiltrating macrophages engulfing debris of different degenerated cells (Li et al., 2018). In short, severe destruction of myelin and axons can be seen clearly in rodents with ICH, contributing to the loss of motor function. Therefore, viewing the aforementioned accumulating solid evidence on the ICH, it is critical to point toward the primary brain injury and the second brain injury to benefit from controlling the outcomes following brain injury (Figure 1).
Figure 1. Proposed schematic diagram linking outcomes after ICH. After ischemic cerebral hemorrhage onset, primary and secondary brain injury is going. The prior brain injury mechanism starts from an occulted blood vessel. And is followed by blood vessel ruptured, extravasated red blood cells causing dynamic hematoma expansion, resulting in the adjacent brain tissue immediately compressed, followed by brain tissue damaged finally. This process disrupts the surrounding brain structures, resulting in early neurological dysfunction. The multiple hemolytic products, including ferrous ions, hemoglobin, heme, and other lytic molecules, cause secondary brain injury within the period from hours to days after the primary brain injury. During this process, nerve and glial cells suffer from oxidative stress, inflammation, excitotoxicity, and death signals.
Some studies have verified that MSCs can be isolated from various adult tissues such as the bone marrow, adipose tissue, synovium, and neonatal tissues, including the umbilical cord (blood), placenta, amniotic fluid, and amniotic membrane, and possess the potential of differentiating into diverse cell lineages and tissues like bone, adipose tissue, cartilage, nerves, and liver, both in vivo and in vitro (Gao et al., 2018; Sumer et al., 2018; Mathot et al., 2019; Gong et al., 2021; Li J. et al., 2021; Li Q. Y. et al., 2021; Ramesh et al., 2021; Souza et al., 2021; Zhu et al., 2021; Bian et al., 2022). Others have verified that MSC treatment holding promise exerts indirect therapeutic mechanisms such as anti-inflammation (Huang et al., 2019), secretion of growth factors (Tang W. et al., 2021; Ulpiano et al., 2021), and EVs (Bang and Kim, 2022), which are associated with the recovery of damaged tissues. Moreover, caution is generally needed while using these or similar MSC-related therapeutic approaches (Molcanyi et al., 2013, 2014; Levy et al., 2020; Kim D. et al., 2021). The implantation of stem cells was accompanied by a massive invasion of macrophages into transplantation sites, reactivation of astrocytes, and activated microglia following brain injury inflammatory response, in which the survival rate and integration of implanted stem cells are always impeded (Molcanyi et al., 2007).
Bone-derived MSCs (BMSCs) are frequently used for treating brain injuries not only because of the ease of acquisition from the host but also because of infiltrating capability via the BBB without disrupting the structure (Kopen et al., 1999; Gao et al., 2018) to differentiate into neurons or neuron-like cells for tissue reparation (Wislet-Gendebien et al., 2005; Nagai et al., 2007; Bae et al., 2011). Various studies have demonstrated that BMSCs could limit neurological deficits and BBB dysfunction in ICH rats (Chen M. et al., 2015; Wang et al., 2015).
Neonatal tissue-derived MSCs, including human umbilical cord MSCs (HUC-MSCs), umbilical cord blood (HUCB-MSCs), placenta MSCs (HP-MSCs), amniotic fluid (HAF-MSCs), and amniotic membrane (HAM-MSCs), are another kind of widely used MSCs, which have been used to treat neurological deficits in animal models and patients with ICH and displayed apparent therapeutic effects (Nan et al., 2005; Chang et al., 2016; Xie et al., 2016).
Adipose-derived MSCs (ADMSCs) are also used for regenerative medicine isolated from adipose tissues, which are easily accessible and abundant (Gimble et al., 2010). Chen et al. (2012) found that the transplantation of rat ADMSCs for treating ICH rats demonstrated the differentiation of neuron-like and astrocyte-like cells around the injured site and improved the expression level of vascular endothelial growth factor (VEGF) for the recovery of neurological function. Yang et al. (2012) employed the injection of ADMSCs generated from a 65-year-old male donor’s fat tissue into the right femoral vein of ICH-induced stroke rats, which suggested that ADMSC transplantation could facilitate functional recovery of the experimental animals.
Dental pulp stem cells (DPSCs) originate from the neural crest and exhibit neuro-ectodermal features having multilineage differentiation potentials, which were first discovered in the pulp tissue (Gronthos et al., 2000; Pierdomenico et al., 2005). They are a subpopulation of dental pulp cells (DPCs) owing to MSC properties, including the similarity of morphology like fibroblast, adherence, surface marker expression, proliferation, and colony-forming behavior (Lan et al., 2019). Given the attractive characteristics of DPSCs such as ease of acquisition, powerful proliferation ability, and long-time cryopreservation with no loss of multi-directional differentiation capacity, DPSC therapy displays an increasing interest in stroke disease in preclinical and clinical research (Song et al., 2017; Sowa et al., 2018; Lan et al., 2019; Nito et al., 2022). In a preclinical study, Nito et al. (2018) have reported that transplantation of human DPSCs via the femoral vein ameliorates infarct volume and motor functional deficits in rats following acute cerebral ischemia. Moreover, the clinical evidence shows that the intracranial transplantation of autologous DPSCs is safe and feasible in patients with chronic stroke, and the maximum tolerable dose is also verified in human subjects, which provides guidance for the design of future clinical trials (Nagpal et al., 2016).
In rodents with ICH, three different ways, including intracerebral, intravenous, and intra-arterial injection, for transplantation of MSCs have often been used (Li J. et al., 2021). Stereotactic intracerebral injection of HUC-MSCs transduced with hepatocyte growth factor (HGF) into the left ventricle improved neurological deficits in rats after ICH, which was due to the improvement of demyelination and axonal regeneration (Liu et al., 2010). Allogeneic and syngeneic BMSCs injected intravenously 24 h after stroke in rats ameliorated the neurological deficits without immunologic sensitization by enhancing the reactive oligodendrocytes and astrocytes or axon–glia units to remodel the injured axons and promote effective reparation of white matter (Li et al., 2006; Rosenzweig and Carmichael, 2015). However, the experiments of Fischer et al. (2009) revealed that after intravenous injection of MSCs, the majority of them were trapped inside the lungs via flow cytometry to detect labeled cells reaching the arterial circulation and harvested the lungs, heart, spleen, kidney, and liver. Additionally, many clinical trials have also verified the safety and efficient neuroprotective effects of allogeneic and syngeneic HUC-MSCs, BMSCs, and ADMSCs through intra-arterial injection for different brain diseases such as ICH, ischemic stroke, and traumatic brain injury (Li J. et al., 2021). The adverse events or complications, including microembolisms and decreased cerebral blood flow due to cell dose and infusion velocity, were recently reported after intra-arterial cell delivery in rodent models (Cui et al., 2015). These parameters should be considered before preclinical studies in rats and clinical research in patients with stroke. Cui L. L. et al. (2017) found that MSCs restrained in microvessels occasionally formed conspicuous cell aggregations, giving rise to local blood flow interruptions in vivo. Overexpressing integrin α4 (ITGA4) via lentiviral transfection on MSCs improved transendothelial migration in vitro and furthered safety by alleviating cell aggregations and ameliorating the induced cerebral embolism after intracarotid transplantation of MSCs into rats with stroke (Cui L. L. et al., 2017). Moreover, although the number of clinical trials using MSCs for regeneration and immunomodulation for stroke therapy increases, the fact that the proliferative and immunomodulatory functions of MSCs decrease with aging due to the complex ICH environment cannot be ignored (Li et al., 2017; Fafian-Labora et al., 2019). Furthermore, it has been verified that the condition of the transplanted cells impacts the subsequent therapeutic effects. Weise et al. (2014) have demonstrated that the intravenous administration of cryopreserved human umbilical cord blood mononuclear cells (HUCB-MNCs) did not display neurorestorative properties in spontaneously hypertensive rats with stroke, which suggests that translating cord blood therapy into clinical stroke trials requires further knowledge about its precise functions. Moreover, the immunomodulatory activity of cryopreserved MSCs can be reduced after thawing when compared to freshly prepared MSCs (Moll et al., 2016).
Ongoing research efforts focus on the potential plasticity and therapeutic applications of MSCs in regenerative medicine. Accompanied by the properties of trans-differentiation into lineages derived from the neuro-ectoderm and migration to the injured sites, MSCs have been suggested to be a promising candidate in regenerative medicine (Chen et al., 2008). It is a fact that MSC therapy has demonstrated its function in improving ICH-induced neuronal defects, neural network reconstruction, and neurological functions via anti-inflammation, neurogenesis, angiogenesis, and anti-apoptosis (Zhu et al., 2021).
Many secreted trophic molecules as components of their secretome from MSCs transplanted by orthotopic and caudal vein injection facilitated the endogenous reparatory mechanism, which ultimately improved the recovery of neurological function after stroke (Smith and Gavins, 2012; Shichinohe et al., 2015; Karagyaur et al., 2021). MSC transplantation is promising for promoting angiogenesis. It has been found that HA-MSCs upregulated the human placental endothelial cells (hPECs) viability due to its crucial angiogenic potential (Pfeiffer et al., 2019). Furthermore, HP-MSCs significantly increased the viability, migration, and network formation of endothelial cells, exerting angiogenic potential depending on released angiogenic factors in vitro, such as VEGF, angiogenin, IL-6, IL-8, and matrix metalloproteinase (MMP)-1 and 2 (Konig et al., 2015). Moreover, HPMSCs have also been found to promote neovascularization in vivo (Kinzer et al., 2014; Tuca et al., 2016; Ertl et al., 2018).
Following administration via different routes, they migrate to the damaged tissues or organs, where they might face severe surroundings coupled with death signals because of the disordered formation between the cells and matrix. Preconditioning with various physical, chemical, and biological factors; genetic modification; and optimization of MSC culture conditions are pivotal strategies to facilitate their functions in vitro and in vivo, which will contribute to improving the efficacy of MSC administration in regenerative medicine (Hu and Li, 2018; Zhao et al., 2019).
Preconditioning HUCB-MSCs with hypoxia remarkably enhances their proliferative capacity and the expression of the neural gene GFAP in vitro (Kheirandish et al., 2017). Although hypoxia reduces the cell viability and proliferation of MSCs initially, the following reoxygenation process improves their rehabilitation, and the approach of hypoxia and reoxygenation (H/R) promotes the expression of pro-survival genes and the release of various trophic factors in MSCs (Kim et al., 2015). On account of the advantages of H/R, current studies have focused on optimizing oxygen concentrations to promote the cell activities and therapeutic effects of MSCs. Recently, the potential of electroacupuncture (EA) in promoting neurofunctional recovery through the NT4/5-TrkB-CREB signaling pathway has been identified (Ahn et al., 2016). The combined function of EA and transfected MSCs with modified TrkB gene (TrkB-MSCs) has been investigated in mice with stroke (Ahn et al., 2019). Ahn et al. (2019) found that EA can remarkably enhance the survival and differentiation of grafted TrkB-MSCs to neuronal cells via the BDNF/NT4-TrkB-CREB signaling pathway. EA directly upregulated the gene expression of plasticity-related gene 5 (PRG5), which is a critical neurogenesis factor, and also upregulated the protein expression of postsynaptic density 95 (PSD95) and synaptophysin (SYP). Moreover, EA downregulated the expression of neurogenesis inhibitory molecules, including NogoA, lysophosphatidic acid, and RhoA, to improve the proliferation and differentiation of endogenous neural stem cells and synaptic plasticity in stroke rats (Tan et al., 2018; Yang et al., 2022). Deng et al. (2021) have reported that EA promoted differentiation of transplanted MSCs into neuron-like cells and expressions of BDNF and NGF proteins displaying therapeutic efficacy in ICH rats. Furthermore, Zhang et al. (2015) suggested that the combination of direct stereotactic intracerebral injection of HUC-MSCs and minimally invasive hematoma aspiration was better than either therapy alone in reducing neuronal damage and improving neuronal functions. Although some benefits of pre-conditioning MSCs have been reported in animal experiments, the experiments of Mello et al. (2020) presented a warning conclusion that intravenous administration of HUC-MSCs decreased the hematoma volume in moderate collagenase-induced brain hemorrhage in rats but failed to reduce the hematoma volume, and continuous neurological impairments can be observed in animals with a severe ICH.
Brain-derived neurotrophic factor (BDNF), recognized as a nerve growth factor and released from MSCs, has been widely researched in different brain diseases, including stroke, neurodegenerative diseases, and others (Ko et al., 2018; Kim H. J. et al., 2021; Sharma et al., 2021). BMSCs overexpressing glial cell-derived neurotrophic factor (GDNF) and placental growth factor (PlGF) display better neuroprotective effects in the rodent model of ICH and cerebral ischemia (Liu et al., 2006; Yang et al., 2011). It has been proved that overexpressing BDNF of HUCB-MSCs induced their neural differentiation via the TrkB-mediated phosphorylated ERKs and β-catenin in the developing brain (Lim et al., 2011). Moreover, others also found that HUCB-MSCs and their secreted BDNF exert therapeutic effects in intraventricular hemorrhage and ameliorate neuronal loss and neurocognitive deficits via the BDNF-TrkB-CREB signaling pathway (Ko et al., 2018). Zhang et al. (2018) have demonstrated a pronounced downregulation of microRNA-21 (miR-21) in ICH patients’ blood and brain tissue. Therefore, they treated ICH rats with the modified MSCs overexpressing miR-21 and observed improved MSC survival that could be conveyed to neurons depending on influent exosomes derived from MSCs, alleviating the neuronal injury by targeting transient receptor potential melastatin 7 (TRPM7) (Zhang et al., 2018). MiR-126-modified MSCs also alleviated the neuronal apoptosis in collagenase-induced ICH rats’ injured brain tissues (Wang et al., 2020). The expression level of growth-associated protein 43 (GAP-43), which is not only known as a growth cone-specific protein to developing neurons but also recognized as a novel axonal phosphoprotein, which has a synchronous effect on BDNF, was highly upregulated on immature growing axonal terminals along with enhancement of synaptic plasticity, but its downregulation suggested the formation of matured synaptogenesis (Gupta et al., 2009; Morita and Miyata, 2013). Cui J. et al. (2017) have reported that MSCs can enhance the expression level of GAP-43 to ameliorate neurological deficits and improve axonal regeneration via the ERK1/2 and PI3K/Akt signaling pathways in rats with ICH. Taken together, it is suggested that MSC therapy can enhance improvement of the axonal damage and synaptic plasticity to some extent, whereas GAP-43 and BDNF may be taken into account as the potential therapeutic target after ICH for MSC treatment.
The corticospinal tract (CST) is the sole descending fiber bundle responsible for conducting the electrical activity, where the axons from nerve cells immediately contact spinal motor neurons through the synaptic connections, associating with the experienced voluntary movement in people and motor function in animals. Critically, early motor dysfunction after stroke attributes to the destruction of the framework and function of axons due to the hemorrhage of the caudate nucleus disrupting the CST in the internal capsule (Hijioka et al., 2016; Jang et al., 2018; Chen W. et al., 2019), where the injury lasted for at least 5 weeks, suggesting that the structural integrity of the CST has sustained damage in ICH (Ng et al., 2020). Meanwhile, the longitudinal pathological alterations in the cervical portion of the spinal cord of the CST in mice with ICH were also confirmed by confocal microscopy and transmission electron microscopy (Ng et al., 2020). In short, nerve regeneration after ICH mainly depends on axonal sprouting of existing surviving nerve cells, new forming synapses, synaptic plasticity, and nerve growth factors such as BDNF and GAP-43. Many studies have verified that MSCs can facilitate neurogenesis based on their characteristics of differentiation, secretion, and axonal plasticity, which ameliorate worse outcomes after brain injury. MSCs may also hinder hematoma expansion and decrease acute mortality during the hyperacute course in rats with ICH by improving the endothelial integrity to cerebral vasculature and enhancing the tight junction protein levels, including zona occludens-1 (ZO-1) and occludin (Choi et al., 2018). Pretreatment of HP-MSCs with apocynin, an NADPH oxidase inhibitor, enhanced the endovascular integrity of cerebral vasculature, demonstrating therapeutic efficacy in the ICH acute stage (Min et al., 2018). Meanwhile, the experiments conducted by Wang et al. (2020) also reported that miR-126-modified MSCs diminished the mRNA expression of protease-activated receptor-1 and MMP-9 while enhancing the ZO-1 and claudin-5 expression levels for the repairment of BBB in the ICH rats.
Except for its role in regenerative medicine, MSC therapy has also been experimentally investigated in other indications, including its immunomodulatory capabilities (Diehl et al., 2017). It has been known that MSCs prevent T-cell response indirectly through the modulation of dendritic cells and directly by suppressing the natural killer cell function of CD8+ and CD4+ T cells. Specifically, they can well restrain or regulate immune responses in complex interactions of T and B lymphocytes, dendritic, and NK cells (Wang and Zhao, 2009). Their immunosuppressive effects of T lymphocyte proliferation, rather than induction of apoptosis, are not only based on soluble factors including transforming growth factor beta 1 (TGFβ1), hepatocyte growth factor (HGF), and other mediators but also depend on direct interactions of cells (Di Nicola et al., 2002; Krampera et al., 2003; Le Blanc et al., 2003). A preclinical investigation was performed by Azevedo et al. (2020) to observe the potential functions of MSCs on CD4 T cells, and the data suggested that MSCs induced CD4 T cells into Treg-like cells via TGF-β and/or programmed death-1 (PD-1)/programmed death ligand 1 (PD-L1) pathways. It has been verified that PD-L1 decreased the infiltration of CD4+ T cells to the brain and resulted in upregulation of Th2 and Treg cells but downregulation of Th1 and Th17 cells through the mTOR pathway by in vitro and in vivo experiments (Han et al., 2017). Moreover, other studies have also proved similar results in the ICH rodent model, a well-defined B10.D2 [H-2(d)) donor to BALB/c (H-2(d)] recipient mice model and experimental autoimmune neuritis (EAN) rat model (Fujiwara et al., 2014; Ding et al., 2016; Han et al., 2017).
There is a well-acknowledged fact that neuroinflammation aggravated the progress of ICH-induced brain damage. Thus, the strategy for regulating the immunoreaction could attenuate ICH-induced brain injury. The remarkable properties of anti-inflammation and immunomodulation make MSC transplantation an appropriate therapeutic candidate for responding against inflammatory diseases like ICH through regulating microglia and neutrophils while enhancing the protective function of anti-inflammatory cytokines and inhibiting the disadvantages of pro-inflammatory cytokines. Kim J. M. et al. (2007) found that ADMSC transplantation for the rats’ ICH model could alleviate the acute inflammation and chronic brain degradation to improve long-term functional recovery.
Recent studies have confirmed that MSCs are effective modifiers maintaining a resting microglial phenotype, preventing microglial activation by downregulating pro-inflammatory cytokines/chemokines and upregulating hypoxia-inducible factor 1-alpha (HIF-1α) and growth factors including VEGF, BDNF, GDNF, stromal-derived factor-1 (SDF-1), and erythropoietin (EPO) following stroke (Wei et al., 2012; Yan et al., 2013). Other studies have shown that hypoxic preconditioning augmented MSC survival and tissue-protective capability, displaying better therapeutic efficacy than the single MSC transplantation by improving the miR-326/PTBP1/PI3K-mediated autophagy and alleviated microglial activation to downregulate IL-1β and TNF-α expression levels and microglial pyroptosis after ICH (Liu et al., 2021a,b). Human ADMSCs improved the neurological deficits in the ICH mice model by suppressing the acute inflammation mediated by the CD11+CD45+ subpopulation of cells (Kuramoto et al., 2019). In the rat middle cerebral artery occlusion (MCAO) model, microglial activation and their pro-inflammatory phenotype were significantly downregulated by interferon-γ (INF-γ)-activated MSCs, along with the improvement in oligodendrogenesis by the upregulation of neuron-glial antigen 2, a hallmark protein of oligodendrocyte progenitor cells, for promyelination and minimizing the infarct and penumbra (Tobin et al., 2020). The microglial activation is mainly regulated by CX3CR1, and some studies have verified that MSCs are known to polarize M1 macrophages to M2 phenotypes via CX3CR1 (Min et al., 2016; Li et al., 2019). Du et al. (2020) found that in the global cerebral ischemia mice, downregulated CX3CR1 remarkably alleviated microglial activation and peripheral inflammatory responses, including the downregulation of IL-6, IL-1β, and TNF-α in the serum, which promoted the differentiation and maturation of mature oligodendrocytes from oligodendrocyte progenitor cells in the striatum, cortex, and hippocampus and thus, attenuated further dysfunction of myelin from ischemia-induced brain injury. Similarly, Hamzei Taj et al. (2018) found that after transplantation of genetically modified IL-13-engineered MSCs (IL-13-MSCs) for knock-in fluorescent protein reporter mice (CX3CR1eGFP/+ CCR2RFP/+), the brain-resident microglia can be recognized from freshly infiltrated macrophages after stroke. The results suggested that the graft of IL-13-MSCs switched the microglia/macrophages into an alternative activation state (an anti-inflammatory and neuroprotective phenotype) by significantly increasing Arg-1 and decreasing MHC-II expression after 14 days of ischemia (Hamzei Taj et al., 2018). Engineered MSCs have also been applied in malignant glioma tumor models (Sun et al., 2011). The exosomes derived from the miR-146a-5p-rich BMSCs could reduce neuronal apoptosis and inflammation via inhibiting microglial M1 polarization by downregulating the expression of IL-1 receptor-associated kinase 1 (IRAK1) and nuclear factor of activated T cells 5 (NFAT5) (Duan et al., 2020). Furthermore, miR-183-5p is also implicated in brain injury and found to be decreased in db/db rat brain tissues after ICH (Ding et al., 2021). It has been verified that EVs derived from BMSCs repressed the inflammatory response through the microRNA-183-5p/PDCD4/NLRP3 pathway (Ding et al., 2021; Nakano and Fujimiya, 2021).
Astrocytes, one of the primary components of glial cells, have also been confirmed to have a reparative function in the injured brain tissue after ICH (Zhang et al., 2006; Chen et al., 2020b), although adequate research is not recorded. After transplantation of MSCs in ICH mice, astrocytes underwent astroglial–mesenchymal phenotype switching and became capable of proliferating and were protected from apoptosis via downregulation of p-MST1 and p-YAP (Chen et al., 2020b). Together, the Hippo pathway-mediated favorable impacts of MSCs can be recognized as a unique therapeutic target in ICH injury (Chen et al., 2020b). Experiments performed by Chen et al. (2020a) also found that transplanting BMSCs led to an elevation of glial fibrillary acidic protein (GFAP), a biomarker of astrocytes, and the level of expression and improved astroglial–mesenchymal phenotype switching and anti-apoptotic abilities through the Cx43/Nrf2/HO-1 axis. Interestingly, Donega et al. (2014) reported that MSCs transplanted via intranasal administration for the hypoxic–ischemic (HI) neonatal mice model migrating specifically toward the lesion site successfully downregulated the expression level of GFAP while decreasing the formation of glial scars, which is a crucial step for promoting neurogenesis. Chen et al. (2020a) maintained that astrocytes serve as the primary defense system, just like a double-edged sword for responding to ICH injury. Fortunately, the graft of MSCs for ICH-injured mice exerting anti-inflammatory and angiogenic properties significantly alleviated the dysfunction of cognition, movement, and hematoma volume, which corresponded with previous studies (Bedini et al., 2018). Comprehending how to enlarge the advantages and minimize the disadvantages of reactive astrocytes is the critical challenge in improving ICH-induced brain injury. Another factor is IL-33, a member of the IL-1 family having pro-inflammatory and anti-inflammatory properties displaying a double-edged sword function that improves wound healing by enhancing M2 macrophage polarization, collagen accumulation, and angiogenesis in the wound sites (Schmitz et al., 2005; He et al., 2017). It is mainly expressed in microglia, astrocytes, and oligodendrocytes in the central nervous system (CNS), leading to severe pathological changes in mucosal organs (Schmitz et al., 2005). Chen Z. et al. (2019) found that IL-33 attenuated neurological deficits, neuronal degeneration, and secondary brain injury by a favorable regulation of ICH-induced microglial responses, which led to microglial polarization from M1 to M2; thus, IL-33 maybe another novel therapeutic target for ICH intervention.
MSCs display immunomodulatory functions by secreting multifunctional paracrine signaling factors, including cytokines, growth factors, and chemokines, the combined effect of which regulates the cellular immune function (Zhang et al., 2013; Zhao et al., 2013; Liu et al., 2014; Zhou et al., 2019). Transplanted MSCs by intravenous infusion can infiltrate through the BBB and express a protein marker phenotype for neuronal cells (Chen et al., 2001). It has been widely accepted that the functional benefits derived from MSC grafts arise from their improvement of the trophic support and anti-inflammatory effect, thereby controlling the potentially toxic environment (Caplan and Dennis, 2006; Hess and Borlongan, 2008). Some investigations suggested that the bystander mechanism of MSC protective functions depends on some soluble factors, including IL-10, indoleamine 2,3-dioxygenase (IDO), PGE2, TGF-β1, TNF-α, and TNF-α-stimulated gene/protein 6 (TSG-6) (Nemeth et al., 2009). Cell-secreted EVs commonly encapsulate these different kinds of molecules, and according to the dimension and origination, they are called exosomes, microvesicles (MVs), and apoptotic bodies. TSG-6, an anti-inflammatory factor, can restrain neutrophils infiltrating into the inflammatory region, display functions on resident macrophages through interaction with the CD44 receptor, and block the NF-κB signaling pathway (Chen M. et al., 2015). Tang B. et al. (2021) reported that TSG−6 derived from BMSCs moderated reactive astrocytes by downregulating the NF−κB signaling pathway to attenuate BBB dysfunction after ICH. The BMSC transplantation from the jugular vein alleviated the inflammatory response via decreasing the pro-inflammatory cytokines and alleviated BBB dysfunction in ICH-bearing rats by releasing TSG-6, downregulating the expression levels of iNOS, MMP-9, and peroxynitrite [ONOO (-)] (Chen M. et al., 2015). Recently, researchers have confirmed in the monkey that the treatment with intravenous infusions of MSC-derived EVs (MSC-EVs) alleviated injury-induced hyperexcitability and regulated the relationship between excitation and inhibition around the injured ventral premotor cortex for reducing pathological changes (Medalla et al., 2020). In addition, MSC-EVs increase the conversion from M1-like pro-inflammatory phenotypes to anti-inflammatory M2 phenotypes to trigger the macrophage polarization by downregulating IL-23 and IL-22 (Li J. et al., 2021).
The antioxidative characteristics of MSCs have been verified in an Escherichia coli-induced acute lung injury (ALI) in the mouse model (Shalaby et al., 2014). In the pathophysiological condition of ischemia diseases, including ischemic stroke and heart disease, the PI3k/Akt pathway participating in angiogenesis, oxidative stress, and survival of the MSC graft could be further enhanced by preconditioning of MSCs with pharmacological factors, such as statins (Samakova et al., 2019), which can further their survival capacity and properties of secretome, paracrine, and autocrine secretion (Hu and Li, 2018; Samakova et al., 2019).
Despite various pieces of preclinical research evidence concerning the mechanisms of MSCs against ICH, there is still a need for further investigation about its therapeutic and preventive role to promote its use as a clinical therapy (Figure 2) since not all the promising MSC techniques have been proved clinically beneficial in a randomized controlled trial (RCT) (Fernandez Vallone et al., 2013; Azad et al., 2016; Squillaro et al., 2016; Levy et al., 2020).
Figure 2. Mechanisms of MSCs application in ICH. The interactions between MSCs and tissue environments have occurred via two effective mechanisms cell-to-cell communication and cell-to-extracellular vesicles communication. MSCs interact with the adjacent cells, including the immune cells, nerve cells, glial cells, and endothelial cells, promoting regenerative repair and structural remodeling of damaged tissue. MSCs generate the extracellular vesicles (EVs) that contain lipids, proteins, microRNAs (miR), and cytokines representing an efficient way to transfer functional cargoes between each other. Biological processes are positively modulated, including autophagy, pyroptosis, apoptosis, angiogenesis, inflammation, cell plasticity, cell migration, and oxidative stress. These communications differentiate MSCs into replacement cell types and modulate immune cell responses.
Multiple or ongoing trials have been performed that use MSCs. To date, clinical trials of MSC-based therapies for stroke first have focused on assessing safety and efficacy. A significant concern is the risk of tumor formation, and understanding the underlying biology is critical to avoid such adverse effects (Jandial and Snyder, 2009). An open-label, observer-blinded RCT of a long-term follow-up study had verified significant functional recovery after intravenous autologous MSC transplantation based on the modified Rankin Scale (mRS) score along with no difference in adverse events (Lee et al., 2010), and other clinical trials have also suggested the safety and feasibility of MSC administration in ischemic stroke (Bhasin et al., 2011, 2013). After searching the clinical trials on MSC therapy for ICH on the official website, the results display nearly six related projects (clinicaltrials.gov) (Table 1). The table shows that the clinical trials of the MSCs on ICH are rudimentary, and some of them with unknown causes have passed their completion date.
Compared with the effective positive results in preclinical research, including reducing the extent of damage, inflammation response, and free radicals, as well as reversing markers of neurodegeneration, therapeutic effects of MSC-based administration in clinical studies have shown to be unsatisfactory (Fernandez Vallone et al., 2013; Azad et al., 2016; Squillaro et al., 2016; Gutierrez-Vargas and Cardona-Gomez, 2020). Previously, the inadequate quality of preclinical tests has been recognized as a major reason for explaining the failure of translation from the preclinic research into the clinical setting (Cui L. L. et al., 2019).
Although an increasing number of investigations for early phase clinical studies of cell therapy have been conducted in stroke and other brain diseases, the convincing evidence of effectiveness is still far deficient (Kode et al., 2009; Moniche et al., 2012; Hess et al., 2017; Li J. et al., 2021). It is thought-provoking that current clinical studies of cell therapy for stroke are in the early stage of clinical trials, which fail to elucidate the critical therapeutic effects. Nevertheless, remarkable design differences between preclinical and clinical studies were detected, including cell immunogenicity, cryopreservation, cell type, recipient comorbidities, recipient sex and age, cerebral vessel occlusion modalities, the time window of cell transplantation, delivery route, and methodological limitations, which may affect clinical translation (Cui L. L. et al., 2019; Dabrowski et al., 2019). Future research should focus on applying proper biomarkers so that research investigators can estimate or discover biological targets to optimize efficacy during clinical trials. For the progress of preclinical to clinical translation, an iterative process between the clinic and the laboratory is necessary to improve the ways for MSC-based treatment and ultimately accomplish the expected results.
Remarkable development has been made in understanding the underlying mechanisms of ICH-induced brain damage during the past two decades (Balami and Buchan, 2012; Fang et al., 2013; Chen S. et al., 2015; Duan et al., 2016; Bobinger and Burkardt, 2018). Most significantly, various studies have proved that MSCs can secrete various neurotrophic, angiogenic, and immunomodulatory factors displaying potential functions in the injured brain (Chen et al., 2008; Bedini et al., 2018; Galipeau and Sensebe, 2018). The neuroprotection of MSCs has been wholly verified in many studies. Improving the therapeutic effects and immunomodulatory properties of MSC application could give investigators the potential targets in the following research work.
It has been confirmed that numerous signaling molecular pathways are related to secondary brain damage with inflammatory responses (Zhou et al., 2014; Zhu et al., 2019). Although some promising results of modulation of immunologic response after ICH have been revealed in some studies, it is also critical to conduct extended trials for further verification. There are still several issues that should be addressed. Although animal models have been commonly used for inquiring into the ICH mechanisms associated with etiology and pathophysiology, advanced animal models are still lacking. Since rodents are endowed with extraordinary spontaneous rehabilitation of sensorimotor deficits and limited evidence for cognitive disorders, recent commonly used animal models did not well recapitulate the synthetic etiology of spontaneous ICH in humans (Zille et al., 2022). Therefore, some researchers suggested that the multiple common risk factors within models (including hypertension and anticoagulants) are included to perfectly imitate the clinical scenario. Due to the varied therapeutic response of different species, it is critical that potential therapies be investigated in at least two species, rather than two rodents, which ameliorates the limitations of a single species and widely enhances the applicable reliability of valid mechanisms (Hemorrhagic Stroke Academia Industry Roundtable Participants, 2018). Second, the pathological mechanisms in human ICH disease cannot be completely simulated and displayed in experimental models. Due to the paucity of translational animal models in preclinical research, large animal models were used to investigate pivotal pathophysiological parameters. Using 1.5T MRI, including structural as well as perfusion and diffusion, weighted neuroimaging reflected the critical aspects of human ICH disease and can be comparatively researched in different aspects of human ICH, including hematoma expansion, white matter injury, and hematoma evacuation (Boltze et al., 2019). Third, many studies on ICH-induced brain injury only focus on single-factor intervention. Therefore, to potentially exploit agents with multiple targets or improve the multidirectional development of drug therapy, strategies need to be well researched in further work. Last but not least, the choice of sources, quantity, and quality of MSCs are critical challenges for MSC therapy and a topic of concern for future research. Several questions related to the safety, efficacy, and critical mechanisms of MSC infusion therapy because of different aspects of cell dosage, cell source or the approaches of cell transplantation, and timing prior to clinical trials still exist and need to be further verified and elucidated (Kode et al., 2009; Singh et al., 2016; Yong et al., 2018).
ND and LW: conceptualization. GY and XF: writing—original draft preparation. MM: writing—review and editing. GY: visualization. HX, SY, and LW: project administration, science and technology project of Sichuan province, National Natural Science Foundation of China, and funding acquisition. All authors have read and agreed to the published version of the manuscript.
This research was funded by the National Traditional Chinese Medicine Inheritance and Innovation Team (No. ZYYCXTD-C-202207), the Science and Technology Project of Sichuan Province (No. 2019YFS0543), the Luzhou-Southwest Medical University Science and Technology Strategic Cooperation Project (No. 2021LZXNYD-P04), the National Natural Science Foundation of China (No. 2021XJYJS02), the Brain Disease Innovation Team of the Affiliated Traditional Chinese Medicine Hospital of Southwest Medical University (No. 2022-CXTD-05), the Luzhou Science and Technology Project (No. 2020, 124), the Sichuan Traditional Chinese Medicine Project (2021) No. 13, the Southwestern Medical University Hospital (2020) No. 33, and the Project of Southwest Medical University (No. 2021ZKQN125).
The authors declare that the research was conducted in the absence of any commercial or financial relationships that could be construed as a potential conflict of interest.
All claims expressed in this article are solely those of the authors and do not necessarily represent those of their affiliated organizations, or those of the publisher, the editors and the reviewers. Any product that may be evaluated in this article, or claim that may be made by its manufacturer, is not guaranteed or endorsed by the publisher.
We thank the Chinese Scholarship Council (CSC) for the financial support for XF. We also thank Lubin Tang from the Physical Culture Institute of Southwest Medical University for drawing support for Figure 1.
Ahn, S. M., Kim, Y. R., Kim, H. N., Shin, Y. I., Shin, H. K., and Choi, B. T. (2016). Electroacupuncture ameliorates memory impairments by enhancing oligodendrocyte regeneration in a mouse model of prolonged cerebral hypoperfusion. Sci. Rep. 6:28646. doi: 10.1038/srep28646
Ahn, S. M., Kim, Y. R., Shin, Y. I., Ha, K. T., Lee, S. Y., Shin, H. K., et al. (2019). Therapeutic potential of a combination of electroacupuncture and TrkB-Expressing mesenchymal stem cells for ischemic stroke. Mol. Neurobiol. 56, 157–173. doi: 10.1007/s12035-018-1067-z
Alharbi, B. M., Tso, M. K., and Macdonald, R. L. (2016). Animal models of spontaneous intracerebral hemorrhage. Neurol. Res. 38, 448–455.
Aronowski, J., and Zhao, X. (2011). Molecular pathophysiology of cerebral hemorrhage: secondary brain injury. Stroke 42, 1781–1786. doi: 10.1161/STROKEAHA.110.596718
Azad, T. D., Veeravagu, A., and Steinberg, G. K. (2016). Neurorestoration after stroke. Neurosurg. Focus. 40:E2.
Azevedo, R. I., Minskaia, E., Fernandes-Platzgummer, A., Vieira, A. I. S., da Silva, C. L., Cabral, J. M. S., et al. (2020). Mesenchymal stromal cells induce regulatory T cells via epigenetic conversion of human conventional CD4 T cells in vitro. Stem Cells 38, 1007–1019. doi: 10.1002/stem.3185
Baang, H. Y., and Sheth, K. N. (2021). Stroke prevention after intracerebral hemorrhage: where are we now? Curr. Cardiol. Rep. 23:162. doi: 10.1007/s11886-021-01594-0
Babu, R., Bagley, J. H., Di, C., Friedman, A. H., and Adamson, C. (2012). Thrombin and hemin as central factors in the mechanisms of intracerebral hemorrhage-induced secondary brain injury and as potential targets for intervention. Neurosurg. Focus. 32:E8. doi: 10.3171/2012.1.FOCUS11366
Bacmeister, C. M., Barr, H. J., McClain, C. R., Thornton, M. A., Nettles, D., Welle, C. G., et al. (2020). Motor learning promotes remyelination via new and surviving oligodendrocytes. Nat. Neurosci. 23, 819–831. doi: 10.1038/s41593-020-0637-3
Bae, K. S., Park, J. B., Kim, H. S., Kim, D. S., Park, D. J., and Kang, S. J. (2011). Neuron-like differentiation of bone marrow-derived mesenchymal stem cells. Yonsei Med. J. 52, 401–412.
Balami, J. S., and Buchan, A. M. (2012). Complications of intracerebral haemorrhage. Lancet. Neurol. 11, 101–118.
Bang, O. Y., and Kim, J. E. (2022). Stem cell-derived extracellular vesicle therapy for acute brain insults and neurodegenerative diseases. BMB Rep. 55, 20–29.
Bautista, W., Adelson, P. D., Bicher, N., Themistocleous, M., Tsivgoulis, G., and Chang, J. J. (2021). Secondary mechanisms of injury and viable pathophysiological targets in intracerebral hemorrhage. Ther. Adv. Neurol. Disord. 14:49208. doi: 10.1177/17562864211049208
Bedini, G., Bersano, A., Zanier, E. R., Pischiutta, F., and Parati, E. A. (2018). Mesenchymal stem cell therapy in intracerebral haemorrhagic stroke. Curr. Med. Chem. 25, 2176–2197. doi: 10.2174/0929867325666180111101410
Bentz, K., Molcanyi, M., Schneider, A., Riess, P., Maegele, M., Bosche, B., et al. (2010). Extract derived from rat brains in the acute phase following traumatic brain injury impairs survival of undifferentiated stem cells and induces rapid differentiation of surviving cells. Cell Physiol. Biochem. 26, 821–830. doi: 10.1159/000323991
Bhasin, A., Srivastava, M. V., Kumaran, S. S., Mohanty, S., Bhatia, R., Bose, S., et al. (2011). Autologous mesenchymal stem cells in chronic stroke. Cerebrovasc. Dis. Extra. 1, 93–104.
Bhasin, A., Srivastava, M. V., Mohanty, S., Bhatia, R., Kumaran, S. S., and Bose, S. (2013). Stem cell therapy: a clinical trial of stroke. Clin. Neurol. Neurosurg. 115, 1003–1008. doi: 10.1016/j.clineuro.2012.10.015
Bian, D., Wu, Y., Song, G., Azizi, R., and Zamani, A. (2022). The application of mesenchymal stromal cells (MSCs) and their derivative exosome in skin wound healing: a comprehensive review. Stem Cell Res. Ther. 13:24. doi: 10.1186/s13287-021-02697-9
Biffi, A., Anderson, C. D., Battey, T. W., Ayres, A. M., Greenberg, S. M., Viswanathan, A., et al. (2015). Association Between Blood Pressure Control and Risk of Recurrent Intracerebral Hemorrhage. JAMA 314, 904–912. doi: 10.1001/jama.2015.10082
Bobinger, T., and Burkardt, P. (2018). H BH, Manaenko A. Programmed Cell Death after Intracerebral Hemorrhage. Curr. Neuropharmacol. 16, 1267–1281. doi: 10.2174/1570159X15666170602112851
Boltze, J., Ferrara, F., Hainsworth, A. H., Bridges, L. R., Zille, M., Lobsien, D., et al. (2019). Lesional and perilesional tissue characterization by automated image processing in a novel gyrencephalic animal model of peracute intracerebral hemorrhage. J. Cereb. Blood Flow Metab. 39, 2521–2535. doi: 10.1177/0271678X18802119
Bosche, B., Mergenthaler, P., Doeppner, T. R., Hescheler, J., and Molcanyi, M. (2020). Complex clearance mechanisms after intraventricular hemorrhage and rt-PA Treatment-a Review on Clinical Trials. Transl. Stroke Res. 11, 337–344. doi: 10.1007/s12975-019-00735-6
Brouwers, H. B., and Greenberg, S. M. (2013). Hematoma expansion following acute intracerebral hemorrhage. Cerebrovasc. Dis. 35, 195–201.
Caplan, A. I., and Dennis, J. E. (2006). Mesenchymal stem cells as trophic mediators. J. Cell Biochem. 98, 1076–1084.
Carpenter, A. M., Singh, I. P., Gandhi, C. D., and Prestigiacomo, C. J. (2016). Genetic risk factors for spontaneous intracerebral haemorrhage. Nat. Rev. Neurol. 12, 40–49. doi: 10.1038/nrneurol.2015.226
Chang, Z., Mao, G., Sun, L., Ao, Q., Gu, Y., and Liu, Y. (2016). Cell therapy for cerebral hemorrhage: five year follow-up report. Exp. Ther. Med. 12, 3535–3540. doi: 10.3892/etm.2016.3811
Chen, J., Li, Y., Wang, L., Zhang, Z., Lu, D., Lu, M., et al. (2001). Therapeutic benefit of intravenous administration of bone marrow stromal cells after cerebral ischemia in rats. Stroke 32, 1005–1011. doi: 10.1161/01.str.32.4.1005
Chen, J., Tang, Y. X., Liu, Y. M., Chen, J., Hu, X. Q., Liu, N., et al. (2012). Transplantation of adipose-derived stem cells is associated with neural differentiation and functional improvement in a rat model of intracerebral hemorrhage. CNS Neurosci. Ther. 18, 847–854. doi: 10.1111/j.1755-5949.2012.00382.x
Chen, M., Li, X., Zhang, X., He, X., Lai, L., Liu, Y., et al. (2015). The inhibitory effect of mesenchymal stem cell on blood-brain barrier disruption following intracerebral hemorrhage in rats: contribution of TSG-6. J. Neuroinflam. 12:61. doi: 10.1186/s12974-015-0284-x
Chen, S., Yang, Q., Chen, G., and Zhang, J. H. (2015). An update on inflammation in the acute phase of intracerebral hemorrhage. Transl. Stroke Res. 6, 4–8. doi: 10.1007/s12975-014-0384-4
Chen, W., Xia, M., Guo, C., Jia, Z., Wang, J., Li, C., et al. (2019). Modified behavioural tests to detect white matter injury- induced motor deficits after intracerebral haemorrhage in mice. Sci. Rep. 9:16958. doi: 10.1038/s41598-019-53263-6
Chen, X., Liang, H., Xi, Z., Yang, Y., Shan, H., Wang, B., et al. (2020a). BM-MSC Transplantation alleviates intracerebral hemorrhage-induced brain injury, promotes astrocytes vimentin expression, and enhances astrocytes antioxidation via the Cx43/Nrf2/HO-1 Axis. Front. Cell Dev. Biol. 8:302. doi: 10.3389/fcell.2020.00302
Chen, X., Xu, C. X., Liang, H., Xi, Z., Pan, J., Yang, Y., et al. (2020b). Bone marrow mesenchymal stem cells transplantation alleviates brain injury after intracerebral hemorrhage in mice through the Hippo signaling pathway. Aging 12, 6306–6323. doi: 10.18632/aging.103025
Chen, Y., Chen, S., Chang, J., Wei, J., Feng, M., and Wang, R. (2021). Perihematomal edema after intracerebral hemorrhage: an update on pathogenesis, risk factors, and therapeutic advances. Front. Immunol. 12:740632. doi: 10.3389/fimmu.2021.740632
Chen, Y., Shao, J. Z., Xiang, L. X., Dong, X. J., and Zhang, G. R. (2008). Mesenchymal stem cells: a promising candidate in regenerative medicine. Int. J. Biochem. Cell Biol. 40, 815–820. doi: 10.1016/j.biocel.2008.01.007
Chen, Z., Xu, N., Dai, X., Zhao, C., Wu, X., Shankar, S., et al. (2019). Interleukin-33 reduces neuronal damage and white matter injury via selective microglia M2 polarization after intracerebral hemorrhage in rats. Brain Res. Bull. 150, 127–135. doi: 10.1016/j.brainresbull.2019.05.016
Chen-Roetling, J., Li, Y., Cao, Y., Yan, Z., Lu, X., and Regan, R. F. (2021). Effect of hemopexin treatment on outcome after intracerebral hemorrhage in mice. Brain Res. 1765:147507. doi: 10.1016/j.brainres.2021.147507
Choi, B. Y., Kim, O. J., Min, S. H., Jeong, J. H., Suh, S. W., and Chung, T. N. (2018). Human placenta-derived mesenchymal stem cells reduce mortality and hematoma size in a rat intracerebral hemorrhage model in an acute phase. Stem Cells Int. 2018:1658195. doi: 10.1155/2018/1658195
Cui, J., Cui, C., Cui, Y., Li, R., Sheng, H., Jiang, X., et al. (2017). Bone Marrow mesenchymal stem cell transplantation increases GAP-43 Expression via ERK1/2 and PI3K/Akt Pathways in Intracerebral Hemorrhage. Cell Physiol. Biochem. 42, 137–144. doi: 10.1159/000477122
Cui, L. L., Golubczyk, D., Tolppanen, A. M., Boltze, J., and Jolkkonen, J. (2019). Cell therapy for ischemic stroke: Are differences in preclinical and clinical study design responsible for the translational loss of efficacy? Ann. Neurol. 86, 5–16. doi: 10.1002/ana.25493
Cui, L. L., Kerkela, E., Bakreen, A., Nitzsche, F., Andrzejewska, A., Nowakowski, A., et al. (2015). The cerebral embolism evoked by intra-arterial delivery of allogeneic bone marrow mesenchymal stem cells in rats is related to cell dose and infusion velocity. Stem Cell Res. Ther. 6:11. doi: 10.1186/scrt544
Cui, L. L., Nitzsche, F., Pryazhnikov, E., Tibeykina, M., Tolppanen, L., Rytkonen, J., et al. (2017). Integrin alpha4 overexpression on rat mesenchymal stem cells enhances transmigration and reduces cerebral embolism after intracarotid injection. Stroke 48, 2895–2900. doi: 10.1161/STROKEAHA.117.017809
Dabrowski, A., Robinson, T. J., and Felling, R. J. (2019). Promoting Brain Repair and regeneration after stroke: a plea for cell-based therapies. Curr. Neurol. Neurosci. Rep. 19:5. doi: 10.1007/s11910-019-0920-4
Deng, L., Zhou, L., Zhu, Y., Fan, G., Tang, H., Zheng, Y., et al. (2021). Electroacupuncture enhance therapeutic efficacy of mesenchymal stem cells transplantation in rats with intracerebral hemorrhage. Stem Cell Rev. Rep. 18, 570–584. doi: 10.1007/s12015-021-10144-8
Di Nicola, M., Carlo-Stella, C., Magni, M., Milanesi, M., Longoni, P. D., Matteucci, P., et al. (2002). Human bone marrow stromal cells suppress T-lymphocyte proliferation induced by cellular or nonspecific mitogenic stimuli. Blood 99, 3838–3843.
Diehl, R., Ferrara, F., Muller, C., Dreyer, A. Y., McLeod, D. D., Fricke, S., et al. (2017). Immunosuppression for in vivo research: state-of-the-art protocols and experimental approaches. Cell Mol. Immunol. 14, 146–179. doi: 10.1038/cmi.2016.39
Ding, H., Jia, Y., Lv, H., Chang, W., Liu, F., and Wang, D. (2021). Extracellular vesicles derived from bone marrow mesenchymal stem cells alleviate neuroinflammation after diabetic intracerebral hemorrhage via the miR-183-5p/PDCD4/NLRP3 pathway. J. Endocrinol. Invest. 44, 2685–2698. doi: 10.1007/s40618-021-01583-8
Ding, Y., Han, R., Jiang, W., Xiao, J., Liu, H., Chen, X., et al. (2016). Programmed Death Ligand 1 plays a neuroprotective role in experimental autoimmune neuritis by controlling peripheral nervous system inflammation of rats. J. Immunol. 197, 3831–3840. doi: 10.4049/jimmunol.1601083
Donega, V., Nijboer, C. H., Braccioli, L., Slaper-Cortenbach, I., Kavelaars, A., van Bel, F., et al. (2014). Intranasal administration of human MSC for ischemic brain injury in the mouse: in vitro and in vivo neuroregenerative functions. PLoS One 9:e112339. doi: 10.1371/journal.pone.0112339
Dornen, J., and Dittmar, T. (2021). The Role of MSCs and cell fusion in tissue regeneration. Int. J. Mol. Sci. 22:20. doi: 10.3390/ijms222010980
Du, B., Liang, M., Zheng, H., Fan, C., Zhang, H., Lu, X., et al. (2020). Anti-mouse CX3CR1 antibody alleviates cognitive impairment, neuronal loss and myelin deficits in an animal model of brain ischemia. Neuroscience 438, 169–181. doi: 10.1016/j.neuroscience.2020.05.011
Duan, S., Wang, F., Cao, J., and Wang, C. (2020). Exosomes Derived from MicroRNA-146a-5p-enriched bone marrow mesenchymal stem cells alleviate intracerebral hemorrhage by inhibiting neuronal apoptosis and microglial M1 Polarization. Drug Des. Devel. Ther. 14, 3143–3158. doi: 10.2147/DDDT.S255828
Duan, X., Wen, Z., Shen, H., Shen, M., and Chen, G. (2016). Intracerebral Hemorrhage, oxidative stress, and antioxidant therapy. Oxid. Med. Cell Longev. 2016:1203285. doi: 10.1155/2016/1203285
Ertl, J., Pichlsberger, M., Tuca, A. C., Wurzer, P., Fuchs, J., Geyer, S. H., et al. (2018). Comparative study of regenerative effects of mesenchymal stem cells derived from placental amnion, chorion and umbilical cord on dermal wounds. Placenta 65, 37–46. doi: 10.1016/j.placenta.2018.04.004
Fafian-Labora, J. A., Morente-Lopez, M., and Arufe, M. C. (2019). Effect of aging on behaviour of mesenchymal stem cells. World J. Stem Cells 11, 337–346. doi: 10.4252/wjsc.v11.i6.337
Fang, H., Wang, P. F., Zhou, Y., Wang, Y. C., and Yang, Q. W. (2013). Toll-like receptor 4 signaling in intracerebral hemorrhage-induced inflammation and injury. J. Neuroinflam. 10:27. doi: 10.1186/1742-2094-10-27
Fernandez Vallone, V. B., Romaniuk, M. A., Choi, H., Labovsky, V., Otaegui, J., and Chasseing, N. A. (2013). Mesenchymal stem cells and their use in therapy: what has been achieved? Differentiation 85, 1–10. doi: 10.1016/j.diff.2012.08.004
Fischer, U. M., Harting, M. T., Jimenez, F., Monzon-Posadas, W. O., Xue, H., Savitz, S. I., et al. (2009). Pulmonary passage is a major obstacle for intravenous stem cell delivery: the pulmonary first-pass effect. Stem Cells Dev. 18, 683–692. doi: 10.1089/scd.2008.0253
Fu, X., Zhou, G., Zhuang, J., Xu, C., Zhou, H., Peng, Y., et al. (2021). White matter injury after intracerebral hemorrhage. Front. Neurol. 12:562090.
Fujiwara, H., Maeda, Y., Kobayashi, K., Nishimori, H., Matsuoka, K., Fujii, N., et al. (2014). Programmed death-1 pathway in host tissues ameliorates Th17/Th1-mediated experimental chronic graft-versus-host disease. J. Immunol. 193, 2565–2573. doi: 10.4049/jimmunol.1400954
Galipeau, J., and Sensebe, L. (2018). Mesenchymal stromal cells: clinical challenges and therapeutic opportunities. Cell Stem Cell. 22, 824–833. doi: 10.1016/j.stem.2018.05.004
Gao, L., Xu, W., Li, T., Chen, J., Shao, A., Yan, F., et al. (2018). Stem Cell Therapy: a promising therapeutic method for intracerebral hemorrhage. Cell Transpl. 27, 1809–1824. doi: 10.1177/0963689718773363
Gimble, J. M., Guilak, F., and Bunnell, B. A. (2010). Clinical and preclinical translation of cell-based therapies using adipose tissue-derived cells. Stem Cell Res. Ther. 1:19. doi: 10.1186/scrt19
Gong, Y. H., Hao, S. L., and Wang, B. C. (2021). Mesenchymal stem cells transplantation in intracerebral hemorrhage: application and challenges. Front. Cell Neurosci. 15:653367. doi: 10.3389/fncel.2021.653367
Gronthos, S., Mankani, M., Brahim, J., Robey, P. G., and Shi, S. (2000). Postnatal human dental pulp stem cells (DPSCs) in vitro and in vivo. Proc. Natl. Acad. Sci. USA 97, 13625–13630. doi: 10.1073/pnas.240309797
Gupta, S. K., Mishra, R., Kusum, S., Spedding, M., Meiri, K. F., Gressens, P., et al. (2009). GAP-43 is essential for the neurotrophic effects of BDNF and positive AMPA receptor modulator S18986. Cell Death Differ. 16, 624–637. doi: 10.1038/cdd.2008.188
Gutierrez-Vargas, J. A., and Cardona-Gomez, G. P. (2020). Considering risk factors for the effectiveness of translational therapies in brain stroke. J. Neurol. Sci. 408:116547. doi: 10.1016/j.jns.2019.116547
Hamzei Taj, S., Le Blon, D., Hoornaert, C., Daans, J., Quarta, A., Praet, J., et al. (2018). Targeted intracerebral delivery of the anti-inflammatory cytokine IL13 promotes alternative activation of both microglia and macrophages after stroke. J. Neuroinflam. 15:174. doi: 10.1186/s12974-018-1212-7
Han, R., Luo, J., Shi, Y., Yao, Y., and Hao, J. P. D. (2017). L1 (Programmed Death Ligand 1) protects against experimental intracerebral hemorrhage-induced brain injury. Stroke 48, 2255–2262. doi: 10.1161/STROKEAHA.117.016705
He, R., Yin, H., Yuan, B., Liu, T., Luo, L., Huang, P., et al. (2017). IL-33 improves wound healing through enhanced M2 macrophage polarization in diabetic mice. Mol. Immunol. 90, 42–49. doi: 10.1016/j.molimm.2017.06.249
Hemorrhagic Stroke Academia Industry Roundtable Participants (2018). Basic and translational research in intracerebral hemorrhage: limitations, priorities, and recommendations. Stroke 49, 1308–1314. doi: 10.1161/STROKEAHA.117.019539
Hess, D. C., and Borlongan, C. V. (2008). Stem cells and neurological diseases. Cell Prolif. 41, 94–114.
Hess, D. C., Wechsler, L. R., Clark, W. M., Savitz, S. I., Ford, G. A., Chiu, D., et al. (2017). Safety and efficacy of multipotent adult progenitor cells in acute ischaemic stroke (MASTERS): a randomised, double-blind, placebo-controlled, phase 2 trial. Lancet Neurol. 16, 360–368. doi: 10.1016/S1474-4422(17)30046-7
Hijioka, M., Anan, J., Matsushita, H., Ishibashi, H., Kurauchi, Y., Hisatsune, A., et al. (2016). Axonal dysfunction in internal capsule is closely associated with early motor deficits after intracerebral hemorrhage in mice. Neurosci. Res. 106, 38–46. doi: 10.1016/j.neures.2015.10.006
Hu, C., and Li, L. (2018). Preconditioning influences mesenchymal stem cell properties in vitro and in vivo. J. Cell Mol. Med. 22, 1428–1442. doi: 10.1111/jcmm.13492
Huang, A. P., Hsu, Y. H., Wu, M. S., Tsai, H. H., Su, C. Y., Ling, T. Y., et al. (2020). Potential of stem cell therapy in intracerebral hemorrhage. Mol. Biol. Rep. 47, 4671–4680. doi: 10.1007/s11033-020-05457-9
Huang, P., Freeman, W. D., Edenfield, B. H., Brott, T. G., Meschia, J. F., and Zubair, A. C. (2019). Safety and efficacy of intraventricular delivery of bone marrow-derived mesenchymal stem cells in hemorrhagic stroke model. Sci. Rep. 9:5674. doi: 10.1038/s41598-019-42182-1
Jain, A., Malhotra, A., and Payabvash, S. (2021). Imaging of Spontaneous Intracerebral Hemorrhage. Neuroimag. Clin. N. Am. 31, 193–203.
Jandial, R., and Snyder, E. Y. A. (2009). safer stem cell: on guard against cancer. Nat. Med. 15, 999–1001. doi: 10.1038/nm0909-999
Jang, S., Kwon, Y., and Kwak, S. (2018). Change of an injured corticospinal tract during 3 Weeks’ rehabilitation after putaminal hemorrhage. Am. J. Phys. Med. Rehabil. 97, e29–e30. doi: 10.1097/PHM.0000000000000787
Joseph, M. J., Caliaperumal, J., and Schlichter, L. C. (2016). After Intracerebral hemorrhage, oligodendrocyte precursors proliferate and differentiate inside white-matter tracts in the rat striatum. Transl. Stroke Res. 7, 192–208. doi: 10.1007/s12975-015-0445-3
Karagyaur, M., Dzhauari, S., Basalova, N., Aleksandrushkina, N., Sagaradze, G., Danilova, N., et al. (2021). MSC Secretome as a promising tool for neuroprotection and neuroregeneration in a model of intracerebral hemorrhage. Pharmaceutics 13:12. doi: 10.3390/pharmaceutics13122031
Keep, R. F., Hua, Y., and Xi, G. (2012). Intracerebral haemorrhage: mechanisms of injury and therapeutic targets. Lancet Neurol. 11, 720–731. doi: 10.1016/S1474-4422(12)70104-7
Kheirandish, M., Gavgani, S. P., and Samiee, S. (2017). The effect of hypoxia preconditioning on the neural and stemness genes expression profiling in human umbilical cord blood mesenchymal stem cells. Transfus. Apher. Sci. 56, 392–399. doi: 10.1016/j.transci.2017.03.015
Kim, D., Lee, A. E., Xu, Q., Zhang, Q., and Le, A. D. (2021). Gingiva-derived mesenchymal stem cells: potential application in tissue engineering and regenerative medicine - a comprehensive review. Front. Immunol. 12:667221. doi: 10.3389/fimmu.2021.667221
Kim, H. J., Bayarsaikhan, D., Lee, J., Bayarsaikhan, G., and Lee, B. (2021). Brain-derived neurotrophic factor secreting human mesenchymal stem cells improve outcomes in rett syndrome mouse models. Front. Neurosci. 15:725398. doi: 10.3389/fnins.2021.725398
Kim, J. M., Lee, S. T., Chu, K., Jung, K. H., Song, E. C., Kim, S. J., et al. (2007). Systemic transplantation of human adipose stem cells attenuated cerebral inflammation and degeneration in a hemorrhagic stroke model. Brain Res. 1183, 43–50. doi: 10.1016/j.brainres.2007.09.005
Kim, Y. S., Noh, M. Y., Cho, K. A., Kim, H., Kwon, M. S., Kim, K. S., et al. (2015). Hypoxia/Reoxygenation-preconditioned human bone marrow-derived mesenchymal stromal cells rescue ischemic rat cortical neurons by enhancing trophic factor release. Mol. Neurobiol. 52, 792–803. doi: 10.1007/s12035-014-8912-5
Kinzer, M., Hingerl, K., Konig, J., Reinisch, A., Strunk, D., Huppertz, B., et al. (2014). Mesenchymal stromal cells from the human placenta promote neovascularization in a mouse model in vivo. Placenta 35, 517–519. doi: 10.1016/j.placenta.2014.04.004
Ko, H. R., Ahn, S. Y., Chang, Y. S., Hwang, I., Yun, T., Sung, D. K., et al. (2018). Human UCB-MSCs treatment upon intraventricular hemorrhage contributes to attenuate hippocampal neuron loss and circuit damage through BDNF-CREB signaling. Stem Cell Res. Ther. 9:326. doi: 10.1186/s13287-018-1052-5
Kode, J. A., Mukherjee, S., Joglekar, M. V., and Hardikar, A. A. (2009). Mesenchymal stem cells: immunobiology and role in immunomodulation and tissue regeneration. Cytotherapy 11, 377–391. doi: 10.1080/14653240903080367
Konig, J., Weiss, G., Rossi, D., Wankhammer, K., Reinisch, A., Kinzer, M., et al. (2015). Placental mesenchymal stromal cells derived from blood vessels or avascular tissues: what is the better choice to support endothelial cell function? Stem Cells Dev. 24, 115–131. doi: 10.1089/scd.2014.0115
Kopen, G. C., Prockop, D. J., and Phinney, D. G. (1999). Marrow stromal cells migrate throughout forebrain and cerebellum, and they differentiate into astrocytes after injection into neonatal mouse brains. Proc. Natl. Acad. Sci. USA 96, 10711–10716. doi: 10.1073/pnas.96.19.10711
Krampera, M., Glennie, S., Dyson, J., Scott, D., Laylor, R., Simpson, E., et al. (2003). Bone marrow mesenchymal stem cells inhibit the response of naive and memory antigen-specific T cells to their cognate peptide. Blood 101, 3722–3729. doi: 10.1182/blood-2002-07-2104
Kuramoto, Y., Takagi, T., Tatebayashi, K., Beppu, M., Doe, N., Fujita, M., et al. (2019). Intravenous administration of human adipose-derived stem cells ameliorates motor and cognitive function for intracerebral hemorrhage mouse model. Brain Res. 1711, 58–67. doi: 10.1016/j.brainres.2018.12.042
Lan, X., Han, X., Li, Q., Yang, Q. W., and Wang, J. (2017). Modulators of microglial activation and polarization after intracerebral haemorrhage. Nat. Rev. Neurol. 13, 420–433. doi: 10.1038/nrneurol.2017.69
Lan, X., Sun, Z., Chu, C., Boltze, J., and Li, S. (2019). Dental pulp stem cells: an attractive alternative for cell therapy in ischemic stroke. Front. Neurol. 10:824. doi: 10.3389/fneur.2019.00824
Le Blanc, K., Tammik, L., Sundberg, B., Haynesworth, S. E., and Ringden, O. (2003). Mesenchymal stem cells inhibit and stimulate mixed lymphocyte cultures and mitogenic responses independently of the major histocompatibility complex. Scand. J. Immunol. 57, 11–20. doi: 10.1046/j.1365-3083.2003.01176.x
Lee, J. S., Hong, J. M., Moon, G. J., Lee, P. H., Ahn, Y. H., Bang, O. Y., et al. (2010). A long-term follow-up study of intravenous autologous mesenchymal stem cell transplantation in patients with ischemic stroke. Stem Cells. 28, 1099–1106. doi: 10.1002/stem.430
Levy, O., Kuai, R., Siren, E. M. J., Bhere, D., Milton, Y., Nissar, N., et al. (2020). Shattering barriers toward clinically meaningful MSC therapies. Sci. Adv. 6:eaba6884. doi: 10.1126/sciadv.aba6884
Li, G., Yu, H., Liu, N., Zhang, P., Tang, Y., Hu, Y., et al. (2019). Overexpression of CX3CR1 in adipose-derived stem cells promotes cell migration and functional recovery after experimental intracerebral hemorrhage. Front. Neurosci. 13:462. doi: 10.3389/fnins.2019.00462
Li, J., Xiao, L., He, D., Luo, Y., and Sun, H. (2021). Mechanism of white matter injury and promising therapeutic strategies of MSCs After Intracerebral Hemorrhage. Front. Aging Neurosci. 13:632054. doi: 10.3389/fnagi.2021.632054
Li, Q. Y., Chen, J., Luo, Y. H., Zhang, W., and Xiao, E. H. (2021). Sodium Butyrate pre-treatment enhance differentiation of bone marrow mesenchymal stem cells (BM-MSCs) into hepatocytes and improve liver injury. Curr. Mol. Med. 2021:1716. doi: 10.2174/1566524021666211014161716
Li, Q., Weiland, A., Chen, X., Lan, X., Han, X., Durham, F., et al. (2018). Ultrastructural characteristics of neuronal death and white matter injury in mouse brain tissues after intracerebral hemorrhage: coexistence of ferroptosis, autophagy, and necrosis. Front. Neurol. 9:581. doi: 10.3389/fneur.2018.00581
Li, Y., McIntosh, K., Chen, J., Zhang, C., Gao, Q., Borneman, J., et al. (2006). Allogeneic bone marrow stromal cells promote glial-axonal remodeling without immunologic sensitization after stroke in rats. Exp. Neurol. 198, 313–325. doi: 10.1016/j.expneurol.2005.11.029
Li, Y., Wu, Q., Wang, Y., Li, L., Bu, H., and Bao, J. (2017). Senescence of mesenchymal stem cells (Review). Int. J. Mol. Med. 39, 775–782.
Lim, J. Y., Park, S. I., Kim, S. M., Jun, J. A., Oh, J. H., Ryu, C. H., et al. (2011). Neural differentiation of brain-derived neurotrophic factor-expressing human umbilical cord blood-derived mesenchymal stem cells in culture via TrkB-mediated ERK and beta-catenin phosphorylation and following transplantation into the developing brain. Cell Transpl. 20, 1855–1866. doi: 10.3727/096368910X557236
Liu, A. M., Lu, G., Tsang, K. S., Li, G., Wu, Y., Huang, Z. S., et al. (2010). Umbilical cord-derived mesenchymal stem cells with forced expression of hepatocyte growth factor enhance remyelination and functional recovery in a rat intracerebral hemorrhage model. Neurosurgery 67, 357–365. doi: 10.1227/01.NEU.0000371983.06278.B3
Liu, H., Honmou, O., Harada, K., Nakamura, K., Houkin, K., Hamada, H., et al. (2006). Neuroprotection by PlGF gene-modified human mesenchymal stem cells after cerebral ischaemia. Brain 129, 2734–2745. doi: 10.1093/brain/awl207
Liu, J., He, J., Ge, L., Xiao, H., Huang, Y., Zeng, L., et al. (2021a). Hypoxic preconditioning rejuvenates mesenchymal stem cells and enhances neuroprotection following intracerebral hemorrhage via the miR-326-mediated autophagy. Stem Cell Res. Ther. 12:413. doi: 10.1186/s13287-021-02480-w
Liu, J., He, J., Huang, Y., Ge, L., Xiao, H., Zeng, L., et al. (2021b). Hypoxia-preconditioned mesenchymal stem cells attenuate microglial pyroptosis after intracerebral hemorrhage. Ann. Transl. Med. 9:1362. doi: 10.21037/atm-21-2590
Liu, L., Yu, Y., Hou, Y., Chai, J., Duan, H., Chu, W., et al. (2014). Human umbilical cord mesenchymal stem cells transplantation promotes cutaneous wound healing of severe burned rats. PLoS One 9:e88348. doi: 10.1371/journal.pone.0088348
Lua, J., Ekanayake, K., Fangman, M., and Dore, S. (2021). Potential role of soluble toll-like receptors 2 and 4 as therapeutic agents in stroke and brain hemorrhage. Int. J. Mol. Sci. 22:18. doi: 10.3390/ijms22189977
Mathot, F., Shin, A. Y., and Van Wijnen, A. J. (2019). Targeted stimulation of MSCs in peripheral nerve repair. Gene 710, 17–23. doi: 10.1016/j.gene.2019.02.078
Medalla, M., Chang, W., Calderazzo, S. M., Go, V., Tsolias, A., Goodliffe, J. W., et al. (2020). Treatment with mesenchymal-derived extracellular vesicles reduces injury-related pathology in pyramidal neurons of monkey perilesional ventral premotor cortex. J. Neurosci. 40, 3385–3407. doi: 10.1523/JNEUROSCI.2226-19.2020
Mello, T. G., Rosado-de-Castro, P. H., Campos, R. M. P., Vasques, J. F., Rangel-Junior, W. S., Mattos, R., et al. (2020). Intravenous human umbilical cord-derived mesenchymal stromal cell administration in models of moderate and severe intracerebral hemorrhage. Stem Cells Dev. 29, 586–598. doi: 10.1089/scd.2019.0176
Min, H., Jang, Y. H., Cho, I. H., Yu, S. W., and Lee, S. J. (2016). Alternatively activated brain-infiltrating macrophages facilitate recovery from collagenase-induced intracerebral hemorrhage. Mol. Brain 9:42. doi: 10.1186/s13041-016-0225-3
Min, S., Kim, O. J., Bae, J., and Chung, T. N. (2018). Effect of Pretreatment with the NADPH oxidase inhibitor apocynin on the therapeutic efficacy of human placenta-derived mesenchymal stem cells in intracerebral hemorrhage. Int. J. Mol. Sci. 19:11. doi: 10.3390/ijms19113679
Mohammed Thangameeran, S. I., Tsai, S. T., Hung, H. Y., Hu, W. F., Pang, C. Y., Chen, S. Y., et al. (2020). A Role for endoplasmic reticulum stress in intracerebral hemorrhage. Cells 9:3. doi: 10.3390/cells9030750
Molcanyi, M., Bosche, B., Kraitsy, K., Patz, S., Zivcak, J., Riess, P., et al. (2013). Pitfalls and fallacies interfering with correct identification of embryonic stem cells implanted into the brain after experimental traumatic injury. J. Neurosci. Methods 215, 60–70. doi: 10.1016/j.jneumeth.2013.02.012
Molcanyi, M., Mehrjardi, N. Z., Schafer, U., Haj-Yasein, N. N., Brockmann, M., Penner, M., et al. (2014). Impurity of stem cell graft by murine embryonic fibroblasts - implications for cell-based therapy of the central nervous system. Front. Cell Neurosci. 8:257. doi: 10.3389/fncel.2014.00257
Molcanyi, M., Riess, P., Bentz, K., Maegele, M., Hescheler, J., Schafke, B., et al. (2007). Trauma-associated inflammatory response impairs embryonic stem cell survival and integration after implantation into injured rat brain. J. Neurotrauma. 24, 625–637. doi: 10.1089/neu.2006.0180
Moll, G., Geissler, S., Catar, R., Ignatowicz, L., Hoogduijn, M. J., Strunk, D., et al. (2016). Cryopreserved or fresh mesenchymal stromal cells: only a matter of taste or key to unleash the full clinical potential of MSC Therapy? Adv. Exp. Med. Biol. 951, 77–98. doi: 10.1007/978-3-319-45457-3_7
Moniche, F., Gonzalez, A., Gonzalez-Marcos, J. R., Carmona, M., Pinero, P., Espigado, I., et al. (2012). Intra-arterial bone marrow mononuclear cells in ischemic stroke: a pilot clinical trial. Stroke 43, 2242–2244. doi: 10.1161/STROKEAHA.112.659409
Morita, S., and Miyata, S. (2013). Synaptic localization of growth-associated protein 43 in cultured hippocampal neurons during synaptogenesis. Cell Biochem. Funct. 31, 400–411. doi: 10.1002/cbf.2914
Nagai, A., Kim, W. K., Lee, H. J., Jeong, H. S., Kim, K. S., Hong, S. H., et al. (2007). Multilineage potential of stable human mesenchymal stem cell line derived from fetal marrow. PLoS One 2:e1272. doi: 10.1371/journal.pone.0001272
Nagpal, A., Kremer, K. L., Hamilton-Bruce, M. A., Kaidonis, X., Milton, A. G., Levi, C., et al. (2016). TOOTH (The Open study Of dental pulp stem cell Therapy in Humans): Study protocol for evaluating safety and feasibility of autologous human adult dental pulp stem cell therapy in patients with chronic disability after stroke. Int. J. Stroke 11, 575–585. doi: 10.1177/1747493016641111
Nakano, M., and Fujimiya, M. (2021). Potential effects of mesenchymal stem cell derived extracellular vesicles and exosomal miRNAs in neurological disorders. Neural. Regen. Res. 16, 2359–2366. doi: 10.4103/1673-5374.313026
Nan, Z., Grande, A., Sanberg, C. D., Sanberg, P. R., and Low, W. C. (2005). Infusion of human umbilical cord blood ameliorates neurologic deficits in rats with hemorrhagic brain injury. Ann. N Y Acad. Sci. 1049, 84–96. doi: 10.1196/annals.1334.009
Nemeth, K., Leelahavanichkul, A., Yuen, P. S., Mayer, B., Parmelee, A., Doi, K., et al. (2009). Bone marrow stromal cells attenuate sepsis via prostaglandin E(2)-dependent reprogramming of host macrophages to increase their interleukin-10 production. Nat. Med. 15, 42–49. doi: 10.1038/nm.1905
Ng, A. C. K., Yao, M., Cheng, S. Y., Li, J., Huang, J. D., Wu, W., et al. (2020). Protracted morphological changes in the corticospinal tract within the cervical spinal cord after intracerebral hemorrhage in the right striatum of mice. Front. Neurosci. 14:506. doi: 10.3389/fnins.2020.00506
Nito, C., Sowa, K., Nakajima, M., Sakamoto, Y., Suda, S., Nishiyama, Y., et al. (2018). Transplantation of human dental pulp stem cells ameliorates brain damage following acute cerebral ischemia. Biomed. Pharmacother. 108, 1005–1014. doi: 10.1016/j.biopha.2018.09.084
Nito, C., Suda, S., Nitahara-Kasahara, Y., Okada, T., and Kimura, K. (2022). Dental-Pulp stem cells as a therapeutic strategy for ischemic stroke. Biomedicines 10:4. doi: 10.3390/biomedicines10040737
Palumbo, A., Gruning, P., Landt, S. K., Heckmann, L. E., Bartram, L., Pabst, A., et al. (2021). Deep learning to decipher the progression and morphology of axonal degeneration. Cells 10:2539. doi: 10.3390/cells10102539
Pfeiffer, D., Wankhammer, K., Stefanitsch, C., Hingerl, K., Huppertz, B., Dohr, G., et al. (2019). Amnion-derived mesenchymal stem cells improve viability of endothelial cells exposed to shear stress in ePTFE grafts. Int. J. Artif. Organs. 42, 80–87. doi: 10.1177/0391398818815470
Pierdomenico, L., Bonsi, L., Calvitti, M., Rondelli, D., Arpinati, M., Chirumbolo, G., et al. (2005). Multipotent mesenchymal stem cells with immunosuppressive activity can be easily isolated from dental pulp. Transplantation 80, 836–842. doi: 10.1097/01.tp.0000173794.72151.88
Qureshi, A. I., Mendelow, A. D., and Hanley, D. F. (2009). Intracerebral haemorrhage. Lancet. 373, 1632–1644.
Ramesh, S., Govarthanan, K., Ostrovidov, S., Zhang, H., Hu, Q., Camci-Unal, G., et al. (2021). Cardiac differentiation of mesenchymal stem cells: impact of biological and chemical inducers. Stem Cell Rev. Rep. 17, 1343–1361.
Rosenzweig, S., and Carmichael, S. T. (2015). The axon-glia unit in white matter stroke: mechanisms of damage and recovery. Brain Res. 1623, 123–134. doi: 10.1016/j.brainres.2015.02.019
Samakova, A., Gazova, A., Sabova, N., Valaskova, S., Jurikova, M., and Kyselovic, J. (2019). The PI3k/Akt pathway is associated with angiogenesis, oxidative stress and survival of mesenchymal stem cells in pathophysiologic condition in ischemia. Physiol. Res. 68, S131–S138. doi: 10.33549/physiolres.934345
Saulle, M. F., and Schambra, H. M. (2016). Recovery and rehabilitation after intracerebral hemorrhage. Semin. Neurol. 36, 306–312.
Schmitz, J., Owyang, A., Oldham, E., Song, Y., Murphy, E., McClanahan, T. K., et al. (2005). IL-33, an interleukin-1-like cytokine that signals via the IL-1 receptor-related protein ST2 and induces T helper type 2-associated cytokines. Immunity 23, 479–490. doi: 10.1016/j.immuni.2005.09.015
Schrag, M., and Kirshner, H. (2020). Management of intracerebral hemorrhage: JACC Focus Seminar. J. Am. Coll. Cardiol. 75, 1819–1831. doi: 10.1016/j.jacc.2019.10.066
Shalaby, S. M., El-Shal, A. S., Abd-Allah, S. H., Selim, A. O., Selim, S. A., Gouda, Z. A., et al. (2014). Mesenchymal stromal cell injection protects against oxidative stress in Escherichia coli-induced acute lung injury in mice. Cytotherapy 16, 764–775. doi: 10.1016/j.jcyt.2013.12.006
Sharma, G. P., Frei, A. C., Narayanan, J., Gasperetti, T., Veley, D., Amjad, A., et al. (2021). Brain-derived neurotrophic factor promotes immune reconstitution following radiation injury via activation of bone marrow mesenchymal stem cells. PLoS One 16:e0259042. doi: 10.1371/journal.pone.0259042
Shichinohe, H., Ishihara, T., Takahashi, K., Tanaka, Y., Miyamoto, M., Yamauchi, T., et al. (2015). Bone marrow stromal cells rescue ischemic brain by trophic effects and phenotypic change toward neural cells. Neurorehabil. Neural. Repair. 29, 80–89. doi: 10.1177/1545968314525856
Singh, A., Singh, A., and Sen, D. (2016). Mesenchymal stem cells in cardiac regeneration: a detailed progress report of the last 6 years (2010-2015). Stem Cell Res. Ther. 7:82. doi: 10.1186/s13287-016-0341-0
Smith, H. K., and Gavins, F. N. (2012). The potential of stem cell therapy for stroke: is PISCES the sign? FASEB J. 26, 2239–2252. doi: 10.1096/fj.11-195719
Song, M., Lee, J. H., Bae, J., Bu, Y., and Kim, E. C. (2017). Human dental pulp stem cells are more effective than human bone marrow-derived mesenchymal stem cells in cerebral ischemic injury. Cell Transpl. 26, 1001–1016. doi: 10.3727/096368916X694391
Souza, A. T. P., Freitas, G. P., Lopes, H. B., Totoli, G. G. C., Tarone, A. G., Marostica-Junior, M. R., et al. (2021). Jabuticaba peel extract modulates adipocyte and osteoblast differentiation of MSCs from healthy and osteoporotic rats. J. Bone Miner Metab. 39, 163–173. doi: 10.1007/s00774-020-01152-8
Sowa, K., Nito, C., Nakajima, M., Suda, S., Nishiyama, Y., Sakamoto, Y., et al. (2018). Impact of dental pulp stem cells overexpressing hepatocyte growth factor after cerebral ischemia/reperfusion in rats. Mol. Ther. Methods Clin. Dev. 10, 281–290. doi: 10.1016/j.omtm.2018.07.009
Squillaro, T., Peluso, G., and Galderisi, U. (2016). Clinical trials with mesenchymal stem cells: an update. Cell Transpl. 25, 829–848.
Sumer, H., Liu, J., and Roh, S. (2018). Mesenchymal stem cells and regenerative medicine. Stem Cells Int. 2018, 9810972.
Sun, X. L., Xu, Z. M., Ke, Y. Q., Hu, C. C., Wang, S. Y., Ling, G. Q., et al. (2011). Molecular targeting of malignant glioma cells with an EphA2-specific immunotoxin delivered by human bone marrow-derived mesenchymal stem cells. Cancer Lett. 312, 168–177. doi: 10.1016/j.canlet.2011.07.035
Tan, F., Wang, J., Liu, J. X., Wang, C., Li, M., and Gu, Y. (2018). Electroacupuncture stimulates the proliferation and differentiation of endogenous neural stem cells in a rat model of ischemic stroke. Exp. Ther. Med. 16, 4943–4950. doi: 10.3892/etm.2018.6848
Tang, B., Song, M., Xie, X., Le, D., Tu, Q., Wu, X., et al. (2021). Tumor Necrosis Factor-stimulated Gene-6 (TSG-6) Secreted by BMSCs Regulates Activated Astrocytes by Inhibiting NF-kappaB signaling pathway to ameliorate blood brain barrier damage after intracerebral hemorrhage. Neurochem. Res. 46, 2387–2402. doi: 10.1007/s11064-021-03375-1
Tang, W., Lv, X., Huang, J., Wang, B., Lin, L., Shen, Y., et al. (2021). Neuroprotective effect of stroke pretreated MSCs against cerebral ischemia/reperfusion injury in rats. World Neurosurg. 2021:114. doi: 10.1016/j.wneu.2021.04.114
Tobin, M. K., Stephen, T. K. L., Lopez, K. L., Pergande, M. R., Bartholomew, A. M., Cologna, S. M., et al. (2020). Activated mesenchymal stem cells induce recovery following stroke via regulation of inflammation and oligodendrogenesis. J. Am. Heart Assoc. 9:e013583. doi: 10.1161/JAHA.119.013583
Tschoe, C., Bushnell, C. D., Duncan, P. W., Alexander-Miller, M. A., and Wolfe, S. Q. (2020). Neuroinflammation after intracerebral hemorrhage and potential therapeutic targets. J. Stroke 22, 29–46.
Tuca, A. C., Ertl, J., Hingerl, K., Pichlsberger, M., Fuchs, J., Wurzer, P., et al. (2016). Comparison of Matrigel and Matriderm as a carrier for human amnion-derived mesenchymal stem cells in wound healing. Placenta 48, 99–103. doi: 10.1016/j.placenta.2016.10.015
Ulpiano, C., da Silva, C. L., and Monteiro, G. A. (2021). Mesenchymal Stromal Cells (MSCs): a promising tool for cell-based angiogenic therapy. Curr. Gene. Ther. 21, 382–405. doi: 10.2174/1566523221666210917114353
van Asch, C. J., Luitse, M. J., Rinkel, G. J., van der Tweel, I., Algra, A., and Klijn, C. J. (2010). Incidence, case fatality, and functional outcome of intracerebral haemorrhage over time, according to age, sex, and ethnic origin: a systematic review and meta-analysis. Lancet. Neurol. 9, 167–176. doi: 10.1016/S1474-4422(09)70340-0
Wang, C., Cao, J., Duan, S., Xu, R., Yu, H., Huo, X., et al. (2020). Effect of MicroRNA-126a-3p on bone marrow mesenchymal stem cells repairing blood-brain barrier and nerve injury after intracerebral hemorrhage. J. Stroke Cerebrovasc. Dis. 29:104748. doi: 10.1016/j.jstrokecerebrovasdis.2020.104748
Wang, C., Fei, Y., Xu, C., Zhao, Y., and Pan, Y. (2015). Bone marrow mesenchymal stem cells ameliorate neurological deficits and blood-brain barrier dysfunction after intracerebral hemorrhage in spontaneously hypertensive rats. Int. J. Clin. Exp. Pathol. 8, 4715–4724.
Wang, L., and Zhao, R. C. (2009). Mesenchymal stem cells targeting the GVHD. Sci. China C Life Sci. 52, 603–609. doi: 10.1007/s11427-009-0091-7
Wasserman, J. K., and Schlichter, L. C. (2008). White matter injury in young and aged rats after intracerebral hemorrhage. Exp. Neurol. 214, 266–275.
Wei, L., Fraser, J. L., Lu, Z. Y., Hu, X., and Yu, S. P. (2012). Transplantation of hypoxia preconditioned bone marrow mesenchymal stem cells enhances angiogenesis and neurogenesis after cerebral ischemia in rats. Neurobiol. Dis. 46, 635–645. doi: 10.1016/j.nbd.2012.03.002
Wei, Y., Song, X., Gao, Y., Gao, Y., Li, Y., and Gu, L. (2022). Iron toxicity in intracerebral hemorrhage: Physiopathological and therapeutic implications. Brain Res. Bull. 178, 144–154. doi: 10.1016/j.brainresbull.2021.11.014
Weise, G., Lorenz, M., Posel, C., Maria Riegelsberger, U., Storbeck, V., Kamprad, M., et al. (2014). Transplantation of cryopreserved human umbilical cord blood mononuclear cells does not induce sustained recovery after experimental stroke in spontaneously hypertensive rats. J. Cereb. Blood Flow Metab. 34, e1–e9. doi: 10.1038/jcbfm.2013.185
Wilkinson, D. A., Pandey, A. S., Thompson, B. G., Keep, R. F., Hua, Y., and Xi, G. (2018). Injury mechanisms in acute intracerebral hemorrhage. Neuropharmacology 134, 240–248. doi: 10.1016/j.neuropharm.2017.09.033
Wislet-Gendebien, S., Hans, G., Leprince, P., Rigo, J. M., Moonen, G., and Rogister, B. (2005). Plasticity of cultured mesenchymal stem cells: switch from nestin-positive to excitable neuron-like phenotype. Stem Cells 23, 392–402. doi: 10.1634/stemcells.2004-0149
Witsch, J., Siegerink, B., Nolte, C. H., Sprugel, M., Steiner, T., Endres, M., et al. (2021). Prognostication after intracerebral hemorrhage: a review. Neurol. Res. Pract. 3:22. doi: 10.1186/s42466-021-00120-5
Wu, S., and Anderson, C. S. A. (2020). need to re-focus efforts to improve long-term prognosis after stroke in China. Lancet Glob. Health 8, e468–e469. doi: 10.1016/S2214-109X(20)30086-3
Wu, S., Wu, B., Liu, M., Chen, Z., Wang, W., Anderson, C. S., et al. (2019). Stroke in China: advances and challenges in epidemiology, prevention, and management. Lancet Neurol. 18, 394–405. doi: 10.1016/S1474-4422(18)30500-3
Xie, J., Wang, B., Wang, L., Dong, F., Bai, G., and Liu, Y. (2016). Intracerebral and intravenous transplantation represents a favorable approach for application of human umbilical cord mesenchymal stromal cells in intracerebral hemorrhage rats. Med. Sci. Monit. 22, 3552–3561. doi: 10.12659/msm.900512
Xiong, X. Y., Wang, J., Qian, Z. M., and Yang, Q. W. (2014). Iron and intracerebral hemorrhage: from mechanism to translation. Transl. Stroke Res. 5, 429–441. doi: 10.1007/s12975-013-0317-7
Yan, K., Zhang, R., Sun, C., Chen, L., Li, P., Liu, Y., et al. (2013). Bone marrow-derived mesenchymal stem cells maintain the resting phenotype of microglia and inhibit microglial activation. PLoS One 8:e84116. doi: 10.1371/journal.pone.0084116
Yang, C., Zhou, L., Gao, X., Chen, B., Tu, J., Sun, H., et al. (2011). Neuroprotective effects of bone marrow stem cells overexpressing glial cell line-derived neurotrophic factor on rats with intracerebral hemorrhage and neurons exposed to hypoxia/reoxygenation. Neurosurgery 68, 691–704. doi: 10.1227/NEU.0b013e3182098a8a
Yang, G., Zhu, J., Zhan, G., Fan, G., Deng, L., Tang, H., et al. (2022). Mesenchymal stem cell-derived neuron-like cell transplantation combined with electroacupuncture improves synaptic plasticity in rats with intracerebral hemorrhage via mTOR/p70S6K Signaling. Stem Cells Int. 2022:527. doi: 10.1155/2022/6450527
Yang, K. L., Lee, J. T., Pang, C. Y., Lee, T. Y., Chen, S. P., Liew, H. K., et al. (2012). Human adipose-derived stem cells for the treatment of intracerebral hemorrhage in rats via femoral intravenous injection. Cell Mol. Biol. Lett. 17, 376–392. doi: 10.2478/s11658-012-0016-5
Yin, J., Lu, T. M., Qiu, G., Huang, R. Y., Fang, M., Wang, Y. Y., et al. (2013). Intracerebral hematoma extends via perivascular spaces and perineurium. Tohoku J. Exp. Med. 230, 133–139. doi: 10.1620/tjem.230.133
Yong, K. W., Choi, J. R., Mohammadi, M., Mitha, A. P., Sanati-Nezhad, A., and Sen, A. (2018). Mesenchymal stem cell therapy for ischemic tissues. Stem Cells Int. 2018:8179075. doi: 10.1155/2018/8179075
Zhang, H., Huang, Z., Xu, Y., and Zhang, S. (2006). Differentiation and neurological benefit of the mesenchymal stem cells transplanted into the rat brain following intracerebral hemorrhage. Neurol. Res. 28, 104–112. doi: 10.1179/016164106X91960
Zhang, H., Wang, Y., Lv, Q., Gao, J., Hu, L., and He, Z. (2018). MicroRNA-21 overexpression promotes the neuroprotective efficacy of mesenchymal stem cells for treatment of intracerebral hemorrhage. Front. Neurol. 9:931. doi: 10.3389/fneur.2018.00931
Zhang, Q., Shang, X., Hao, M., Zheng, M., Li, Y., Liang, Z., et al. (2015). Effects of human umbilical cord mesenchymal stem cell transplantation combined with minimally invasive hematoma aspiration on intracerebral hemorrhage in rats. Am. J. Transl. Res. 7, 2176–2186.
Zhang, R., Liu, Y., Yan, K., Chen, L., Chen, X. R., Li, P., et al. (2013). Anti-inflammatory and immunomodulatory mechanisms of mesenchymal stem cell transplantation in experimental traumatic brain injury. J. Neuroinflam. 10:106. doi: 10.1186/1742-2094-10-106
Zhao, L., Hu, C., Zhang, P., Jiang, H., and Chen, J. (2019). Preconditioning strategies for improving the survival rate and paracrine ability of mesenchymal stem cells in acute kidney injury. J. Cell Mol. Med. 23, 720–730. doi: 10.1111/jcmm.14035
Zhao, Y., Gao, J., and Lu, F. (2013). Human adipose-derived stem cell adipogenesis induces paracrine regulation of the invasive ability of MCF-7 human breast cancer cells in vitro. Exp. Ther. Med. 6, 937–942. doi: 10.3892/etm.2013.1237
Zheng, H., Zhang, B., Chhatbar, P. Y., Dong, Y., Alawieh, A., Lowe, F., et al. (2018). Mesenchymal stem cell therapy in stroke: a systematic review of literature in pre-clinical and clinical research. Cell Transpl. 27, 1723–1730. doi: 10.1177/0963689718806846
Zheng, Y., Tan, X., and Cao, S. (2022). The critical role of erythrolysis and microglia/macrophages in clot resolution after intracerebral hemorrhage: a review of the mechanisms and potential therapeutic targets. Cell Mol. Neurobiol. 2022:1175. doi: 10.1007/s10571-021-01175-3
Zhou, Y., Wang, Y., Wang, J., Anne Stetler, R., and Yang, Q. W. (2014). Inflammation in intracerebral hemorrhage: from mechanisms to clinical translation. Prog. Neurobiol. 115, 25–44. doi: 10.1016/j.pneurobio.2013.11.003
Zhou, Y., Yamamoto, Y., Xiao, Z., and Ochiya, T. (2019). The Immunomodulatory functions of mesenchymal stromal/stem cells mediated via paracrine activity. J. Clin. Med. 8:7. doi: 10.3390/jcm8071025
Zhu, H., Wang, Z., Yu, J., Yang, X., He, F., Liu, Z., et al. (2019). Role and mechanisms of cytokines in the secondary brain injury after intracerebral hemorrhage. Prog. Neurobiol. 178:101610.
Zhu, X., Yan, T., Cheng, C., Ma, J., Xiang, J., Lv, Y., et al. (2021). Mesenchymal Stem Cells (MSCs) in targeted drug delivery: literature review and exploratory data on migrating and differentiation capacities of bone MSCs into hepatic progenitor cells. Curr. Top Med. Chem. 21, 1251–1267.
Zille, M., Farr, T. D., Keep, R. F., Romer, C., Xi, G., and Boltze, J. (2022). Novel targets, treatments, and advanced models for intracerebral haemorrhage. EBioMedicine 76:103880. doi: 10.1016/j.ebiom.2022.103880
Keywords: intracerebral hemorrhage, mesenchymal stem cells, brain injury, neuroprotection, immunomodulators
Citation: Yang G, Fan X, Mazhar M, Yang S, Xu H, Dechsupa N and Wang L (2022) Mesenchymal Stem Cell Application and Its Therapeutic Mechanisms in Intracerebral Hemorrhage. Front. Cell. Neurosci. 16:898497. doi: 10.3389/fncel.2022.898497
Received: 17 March 2022; Accepted: 18 May 2022;
Published: 13 June 2022.
Edited by:
Yong Gu, Hainan Provincial Hospital of Traditional Chinese Medicine, ChinaReviewed by:
Johannes Boltze, University of Warwick, United KingdomCopyright © 2022 Yang, Fan, Mazhar, Yang, Xu, Dechsupa and Wang. This is an open-access article distributed under the terms of the Creative Commons Attribution License (CC BY). The use, distribution or reproduction in other forums is permitted, provided the original author(s) and the copyright owner(s) are credited and that the original publication in this journal is cited, in accordance with accepted academic practice. No use, distribution or reproduction is permitted which does not comply with these terms.
*Correspondence: Nathupakorn Dechsupa, bmF0aHVwYWtvcm4uZEBjbXUuYWMudGg=; Li Wang, d2FuZ2xpMTIwQHN3bXUuZWR1LmNu
†These authors have contributed equally to this work and share first authorship
Disclaimer: All claims expressed in this article are solely those of the authors and do not necessarily represent those of their affiliated organizations, or those of the publisher, the editors and the reviewers. Any product that may be evaluated in this article or claim that may be made by its manufacturer is not guaranteed or endorsed by the publisher.
Research integrity at Frontiers
Learn more about the work of our research integrity team to safeguard the quality of each article we publish.