- 1Department of Anesthesiology, Tongji Hospital affiliated to Tongji University School of Medicine, Shanghai, China
- 2Translational Research Center, Shanghai Yangzhi Rehabilitation Hospital affiliated to Tongji University School of Medicine, Shanghai, China
- 3Center for Translational Neurodegeneration and Regenerative Therapy, Tongji Hospital affiliated to Tongji University School of Medicine, Shanghai, China
- 4Shanghai Frontiers Science Center of Nanocatalytic Medicine, Shanghai, China
- 5Translational Research Institute of Brain and Brain-Like Intelligence, Shanghai Fourth People’s Hospital affiliated to Tongji University School of Medicine, Shanghai, China
- 6Collaborative Innovation Center for Brain Science, Tongji University, Shanghai, China
Astrocytes, the most numerous glial cells in the brain, play an important role in preserving normal neural functions and mediating the pathogenesis of neurological disorders. Recent studies have shown that astrocytes are GABAceptive and GABAergic astrocytes express GABAA receptors, GABAB receptors, and GABA transporter proteins to capture and internalize GABA. GABAceptive astrocytes thus influence both inhibitory and excitatory neurotransmission by controlling the levels of extracellular GABA. Furthermore, astrocytes synthesize and release GABA to directly regulate brain functions. In this review, we highlight recent research progresses that support astrocytes as GABAceptive and GABAergic cells. We also summarize the roles of GABAceptive and GABAergic astrocytes that serve as an inhibitory node in the intercellular communication in the brain. Besides, we discuss future directions for further expanding our knowledge on the GABAceptive and GABAergic astrocyte signaling.
Introduction
Astrocytes, the most abundant glial cells in the central nervous system (CNS), account for about 20% of the glial cells in the neocortex of the human brain (Pelvig et al., 2008). They form complex connections with neurons, blood vessels, and other glial cells, and play an important role in preserving normal brain functions via providing energy and nutritional support for neurons, maintaining metabolic homeostasis of the CNS, and regulating cerebral blood flow (Anderson and Nedergaard, 2003; Bélanger et al., 2011; Scheiber and Dringen, 2013).
A single astrocyte can touch more than 100,000 synapses in the mouse cortex via a tripartite synapse, a structure that the astrocyte associates with the pre- and post-synapse areas of neurons (Bushong et al., 2002). Astrocytes induce synaptic formation, regulate the release and uptake of synaptic neurotransmitters, and maintain synaptic cleft transmitter homeostasis (Allen, 2014). More importantly, astrocytes directly regulate synaptic plasticity and synaptic transmission by releasing gliotransmitters such as glutamate, adenosine triphosphate (ATP), taurine, glycine, and D-serine (Barakat and Bordey, 2002; Hussy, 2002; Henneberger et al., 2010; Bernardinelli et al., 2014; Mederos and Perea, 2019). In the recent decade, gamma-aminobutyric acid (GABA) has emerged as a novel gliotransmitter (Yoon and Lee, 2014). Astrocytes express GABA receptors to interact with an extracellular GABA, suggesting astrocytes as GABAceptive cells (Le Meur et al., 2012; Yoon et al., 2012). Astrocytes also contain a considerable amount of GABA that can be released to modulate the activities of GABA receptors-expressing cells, indicating a GABAergic role of astrocytes (Le Meur et al., 2012; Yoon et al., 2012).
Astrocytes Are GABAceptive Cells
Astrocytes Internalize GABA via GABA Receptors and Transporters
GABA is the main inhibitory transmitter in adults, which binds to GABA receptors (ionotropic GABAA receptors, GABAARs and metabotropic GABAB receptors, GABABRs) on neurons and inhibits neuronal activities via reducing exocytosis, hyperpolarizing membranes, and shunting depolarization. Astrocytes are also with GABA uptake capacity which requires at least two sodium ions per transportable GABA molecule (Figure 1; Larsson et al., 1980). Astrocytes express both GABAARs and GABABRs in the soma, the synapse-surrounding processes, and the brain vessel-contacting endfeet (Nilsson et al., 1993; Charles et al., 2003; Meier et al., 2008). The GABAARs consist of five protein subunits arranged around a central pore that constitutes the ion channel. Each subunit has a large extracellular N-terminal domain, three membrane spanning domains (M1-3), an intracellular loop of variable length, and a fourth membrane spanning domain (M4) with extracellular C-terminal end. The GABAAR family comprises 19 discovered subunits: α1–6, β1–3, γ1–3, ρ1–3, δ, ε, π, and θ, and the subunit combinations lead to a great diversity of GABAARs (Olsen and Sieghart, 2008, 2009; Sequeira et al., 2019). Indeed, about 20 widely occurring native GABAARs have been identified, with the major combinations being α1β2/3γ2, α2β3γ2, α3β3γ2 (Barnard et al., 1998; Mohler, 2006). The structure diversity confers GABAARs with distinct topology, channel kinetics, affinity for GABA, rate of desensitization, and ability for transient chemical modification such as phosphorylation (Mohler, 2006). Although the exact types of astroglial GABAARs in the brain have not been clearly distinguished, functional GABAARs have been found on astrocytes (Fraser et al., 1995). The mRNAs of many GABAARs subunits including α1–5, β1–3, γ1–3, and δ have been detected in cultured primary astrocytes isolated from rodent cerebella (Bovolin et al., 1992; Zheng et al., 1993). A recent study on human brains reported expressions of genes encoding α2, β1, and γ1 subunits in astrocytes, indicating the existence of functional astroglial α2β1γ1 receptors in humans (Sequeira et al., 2019). Extracellular GABA can activate astroglial GABAARs to open Cl− channels in astrocytes in primary cell culture and rodent hippocampal slices (Kettenmann et al., 1987; MacVicar et al., 1989). The Cl−-mediated depolarization results in an influx of Ca2+ from the extracellular space through L- and T-type voltage-gated calcium channels (VGCC; Young et al., 2010). GABA also activates astroglial GABABRs. Unlike GABAARs, GABABRs, belonging to class C of G-protein coupled receptors (GPCRs), mediate slow and prolonged inhibitory signaling in the brain via the activation of Gi/o type G-proteins, thus lead to inhibition of adenylyl cyclase (AC; Munk et al., 2016). GABABRs are obligate heterodimers composed of GABAB1 and GABAB2 subunits (Evenseth et al., 2020). Each subunit consists of an extracellular Venus flytrap (VFT) domain and a heptahelical transmembrane (7TM) domain (Chun et al., 2012). The VFT is connected to the 7TM by a linker without the cysteine rich domain (CRD; Chun et al., 2012). There are multiple isoforms of the GABAB1 subunit, but the most abundant are GABAB1a and GABAB1b, encoded by the same gene, GABBR1 (Kaupmann et al., 1997). GABAB1a, GABAB1b, and GABAB2 receptor subunits are all expressed on astrocytes (Charles et al., 2003). The activation of astroglial GABABRs increases intracellular Ca2+, which triggers the release of Ca2+ from intracellular IP3-sensitive Ca2+ pools (Lee et al., 2011; Vélez-Fort et al., 2012; Mariotti et al., 2016). Gamma-hydroxybutyric acid, a metabolite of GABA, also activates GABABRs, which in turn stimulates astrocytes (Gould et al., 2014). GABA is depolarizing in astrocytes as their chloride equilibrium potential is more depolarized than their resting membrane potential due to the lack of chloride-extruding transporter, potassium chloride cotransporter 2 (KCC2; Kolta, 2018). The expression of GABA receptors in astrocytes is affected by many factors. For instance, GABAARs in astrocytes decrease with in vitro aging and cerebral ischemia, possibly due to the overproduction of S100B in activated astrocytes (Tateishi et al., 2006).
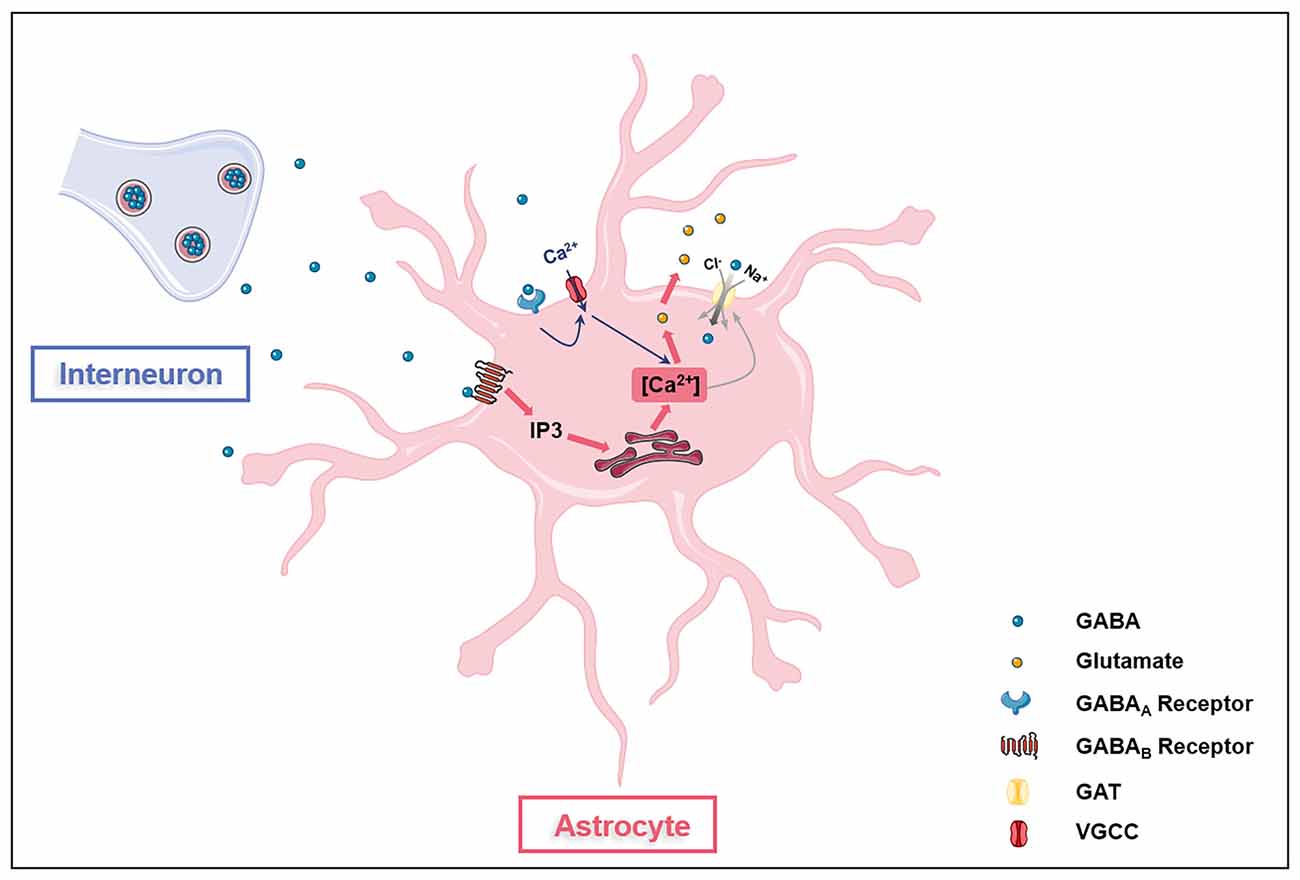
Figure 1. Astrocytes are GABAceptive cells. Astrocytes express GABAA receptors (GABAARs), GABAB receptors (GABABRs), and GABA transporter proteins (GATs). Activation of GAGAARs on astrocytes opens voltage-gated calcium channels (VGCC), leading to the influx of extracellular Ca2+ into the cell (blue arrow). GAGABRs activation induces the release of Ca2+ from the intracellular IP3-sensitive Ca2+ pool (red arrow). Ca2+ oscillations in astrocytes affect glutamate release and GATs expression. GATs directly transport extracellular GABA into astrocytes.
Besides, astrocytes express multiple GABA transporter proteins (GATs), including GAT-1, GAT-3, and betaine-GABA transporter (BGT-1; Schousboe et al., 2017). Although GAT-1 is predominantly expressed in GABAergic neurons for the recycling of GABA in presynapse (Conti et al., 2004), the GAT-1 subtype is found in astrocytes to be responsible for glial GABA transport (Radian et al., 1990; Schousboe et al., 2017). Unlike GAT-1, GAT-3 is expressed exclusively by astrocytes and is mainly located at the astroglial processes to modulate tonic inhibitory currents in postsynaptic cells (Durkin et al., 1995; Minelli et al., 1996; Kersante et al., 2013; Melone et al., 2015). GAT-3 activities influence various astroglial functions including astrocyte synaptic proximity, inhibitory synapse efficacy regulation, excitatory neurotransmission modulation, and heroin seeking, indicating GAT-3 as a key glial GABA transport (Shigetomi et al., 2011; Boddum et al., 2016; Kruyer et al., 2021). Studies have reported conflicting results regarding the expression of BGT-1 in astrocytes (Bitoun and Tappaz, 2000; Olsen et al., 2005; Zhou et al., 2012; Schousboe et al., 2017). BGT-1 expression can be detected in cultured astrocytes (Olsen et al., 2005), however, the expression of BGT-1 in astrocytes in vivo may be very low (Bitoun and Tappaz, 2000; Zhou et al., 2012; Schousboe et al., 2017). Thus, GAT-1 and GAT-3 appear to be the two GABA transporters that are mainly responsible for GABA uptake (Kersante et al., 2013) and intracellular Ca2+ signaling of astrocytes (Doengi et al., 2009; Matos et al., 2018), even though BGT-1 may also play a role (Schousboe et al., 2017). Interestingly, the levels of Ca2+ signaling influence GATs expression as the enhanced extrusion of cytosolic Ca2+ via plasma membrane Ca2+ pump PMCA2 upregulates GATs expression in astrocytes (Yu et al., 2018). Hence, aforementioned literatures indicate that astroglial GATs act in concert with GABA receptors to regulate extracellular GABA levels in the brain.
GABA Regulates the Differentiation, Metabolism, and Functions of Astrocytes
GABA plays an important role in the differentiation, maturation, and morphology of astrocytes. GABA treatment significantly promotes the morphological differentiation of neonatal and adult astrocytes in vitro and in vivo (Matsutani and Yamamoto, 1997; Mong et al., 2002; Runquist and Alonso, 2003). The effects can be blocked by GABAA antagonist, suggesting the involvement of GABAAR in GABA-induced neonatal astrocyte differentiation (Matsutani and Yamamoto, 1997).
GABA also influences the metabolism of astrocytes. GABA released by starvation- or ghrelin treatment-activated agouti-related protein (AgRP) neurons can replace glutamate as an energy source and affect the metabolic fate of glutamate and glucose in astrocytes, thus inducing the depolarization of astrocytes, expression of the glial fibrillary acidic protein (GFAP), and mitochondrial fission (McKenna and Sonnewald, 2005; Varela et al., 2021). GABA metabolism in astrocytes is perturbed in neurological disorders. GABA metabolism has been found to be downregulated in astrocytes with APP or PSEN-1 mutations, which was associated with the decline of GAT-3 expression and GABA uptake (Salcedo et al., 2021). In cortical astrocytes, both the nitrogen and carbon skeleton of GABA can be used for glutamine synthesis (Andersen et al., 2020). Although exogenous GABA may not directly stimulate glycolysis or oxidative metabolism in astrocytes, it is used as an additional substrate for uncoupled respiration to enhance this reaction.
The functions of astrocytes are under the regulation of GABA as well. Astrocytes are an important unit of blood-brain-barrier (BBB) via the interaction of endothelial cells (Abbott et al., 2006). GABA released by interneurons in the basal forebrain (BF) activates GABAARs in astrocytes that attach to microvessels or vascular walls, thus inducing astrocyte-mediated vascular dilation (Kaupmann et al., 1997). Similarly, astrocyte GABA uptake trigger vasoconstriction in developing olfactory bulb (Vélez-Fort et al., 2012).
Except for BBB regulation, GABA also influences the molecule release capacity of astrocytes. GABA inhibited cultured rat astrocytes from releasing endozepine (Patte et al., 1999). Endozepines are a family of astroglia-secreted proteins, namely diazepam-binding inhibitor/acyl-CoA-binding protein (DBI/ACBP) and its processing fragments, triakontatetraneuropeptide (TTN), and octadecaneuropeptide (ODN; Guidotti et al., 1983; Knudsen et al., 1989; Rothstein et al., 1992; Farzampour et al., 2015; Masmoudi-Kouki et al., 2018; Lebrun et al., 2021). Although the term endozepines has been around 40 years, endozepines remain a controversial theme due to unclarity of the exact roles of proposed endozepines in the brain (Tonon et al., 2020). Endozepines have been originally isolated and characterized as natural ligands of central-type benzodiazepine receptor (BZR), located on the GABAA receptor complex (Guidotti et al., 1983). Following studies have reported that endozepines interact with another BZR, the mitochondrial translocator protein (TSPO; Slobodyansky et al., 1989), and GPCR coupled to the PLC/PKC and/or AC/PKA pathways (Patte et al., 1995; Marino et al., 2003). Afterwards, growing evidence has strongly suggested that endozepines act as endogenous regulators of anxiety-related behaviors (Guidotti et al., 1983; De Mateos-Verchere et al., 1998), energy balance (Guillebaud et al., 2017; Lebrun et al., 2021), neuroprotection (Ghouili et al., 2018; Masmoudi-Kouki et al., 2018), neurogenesis, tumorigenesis (Dumitru et al., 2017; Duman et al., 2019), and hormonal secretions (Yoshida et al., 1999; Tonon et al., 2020). It is also worth-noting that the identification of endozepines is still an ongoing work. There are other endozepines such as endozepine-2 and endozepine-4 that may be associated with the pathogenesis of stupor (Rothstein et al., 1992). Hence, GABA modulates astroglial function under physiological and pathological conditions via inducing endozepines secretion.
Besides, GABA also regulates the release of ATP and adenosine from astrocytes, which, modulates neuronal function (Orellana and Stehberg, 2014; Matos et al., 2018). Astroglial ATP acts on presynaptic P2X receptors to trigger a prolonged increase of GABA release, therefore switching the plasticity of GABA synapses in the dorsomedial hypothalamus (Crosby et al., 2018). The GABA-driven release of astroglial adenosine acts on presynaptic A1 receptors to mediate heterosynaptic depression and propagation of glial activation (Newman, 2003; Serrano et al., 2006), therefore regulating mnemonic processes (Vogt and Nicoll, 1999; Guetg et al., 2009) and the pathogenesis of various neurological disorders including epilepsy (Maitre et al., 1974; Heja, 2014).
GABAceptive Astrocytes Fine-Tune Astrocyte-Neuron Crosstalk
The GABA uptake capacity confers astrocytes an essential role in regulating inhibitory networks in the brain (Figure 2). Interneuron-derived GABA increases GAT-1 and GAT-3 activity in astrocytes, which modulates synaptic activities of thalamocortical neurons and striatal output neurons, thus maintaining the tonic inhibition in the thalamus and striatum, respectively (Pirttimaki et al., 2013; Wójtowicz et al., 2013; Boddum et al., 2016). The activities of GAT-1 and GAT-3 also influence GABAAR-mediated inhibitory transmission (Moldavan et al., 2017). The inhibition or knockout of GATs induces the accumulation of extracellular GABA in the brain, leading to extrasynaptic GABAARs activation and GABAAR-mediated tonic current induction (Chiu et al., 2005; Song et al., 2013). Besides, astrocytes from the somatosensory cortex and the hippocampus can sense GABA released from parvalbumin (PV)-expressing interneurons via GABABR, which influences inhibitory post-synaptic current potentiation at the medial prefrontal cortex (mPFC) circuits (Perea et al., 2016; Covelo and Araque, 2018; Mariotti et al., 2018; Mederos et al., 2021). The GABABR-mediated coordination of excitation-inhibitory balance and gamma oscillations plays an important role in goal-directed behaviors (Mederos et al., 2021). The response of astrocytes to GABA signals depends on the type of interneurons involved. The synaptic activities of somatostatin-expressing interneurons (SOM-INs) can be detected by hippocampal astrocytes via GABABR and GAT-3-dependent Ca2+ signaling mechanisms, leading to the release of ATP and the production of adenosine to activate SOM-IN synaptic inhibition of pyramidal cells (Mariotti et al., 2016; Matos et al., 2018; Losi et al., 2021). GABA-stimulated astrocytes also release prostaglandin E2 (PGE2) to activate AgRP-expressing neurons via EP2 receptor (Varela et al., 2021). Astrocytes also regulate dopaminergic neurotransmission by controlling extracellular uptake of GABA (Roberts et al., 2020). Under normal circumstances, GABA released by GABAergic striatum neurons acts on GABA receptors located in dopamine (DA) axons, thereby inhibiting the co-release of DA and GABA in DA axons. This process is controlled by the activity of astrocyte GATs which internalize GABA from extracellular space.
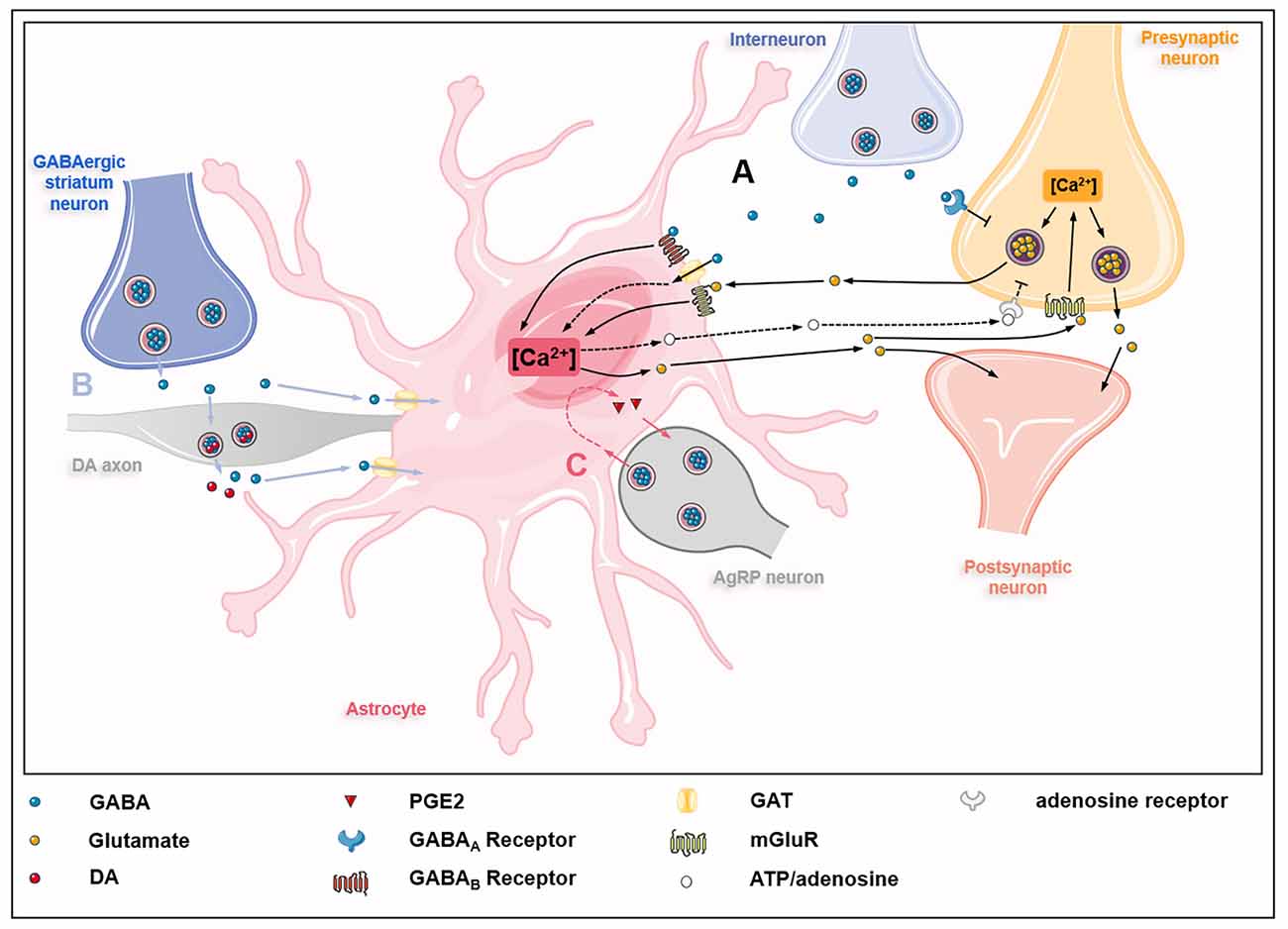
Figure 2. GABAceptive astrocytes fine-tune astrocyte-neuron crosstalk. (A) GABA released by interneurons on one hand inhibits glutamate release from presynaptic neurons by acting on GABAA receptors (GABAARs) on presynaptic neurons; on the other hand, GABA acts on GABAB receptors (GABABRs), metabotropic glutamate receptors (mGluRs), and GABA transporter proteins (GATs) on astrocytes, causing Ca2+ oscillations in astrocytes. Ca2+ oscillations regulate the release of glutamate and ATP/adenosine. Glutamate derived from astrocytes acts on mGluRs on presynaptic neurons, thus promoting the release of glutamate from presynaptic neurons into the synaptic cleft. In addition, astrocyte-derived glutamate also acts directly on postsynaptic neurons to partially counteract the inhibitory effect of GABA. Besides, astrocyte-derived ATP/adenosine inhibits glutamate release from presynaptic neurons by activating presynaptic adenosine receptors (dashed lines). (B) GABA released by GABAergic striatum neurons acts on dopamine (DA) axons and inhibits the co-release of GABA and dopamine, which is modulated by GATs on astrocytes (blue lines). (C) GABA released by agouti-related protein (AgRP) neurons acts on astrocytes, causing astrocytes to release prostaglandin E2 (PGE2) to activate AgRP neurons (red lines).
Interestingly, the activation of GABAceptive astrocytes has effects on excitatory neurotransmission as well. Astrocyte GAT3-mediated regulation of extracellular GABA in the hippocampus plays an important role in controlling the excitability of hippocampal cells in response to increased network activity (Kersante et al., 2013). The GABA-induced activation of GABABRs results in glutamate release from astrocytes and activation of presynaptic group I metabotropic glutamate receptors (mGluRs), which persists in the bursts of interneuron action potential seven during the interneuron down-state, leading to enhancement of excitatory neurotransmission (Perea et al., 2016). Furthermore, computational modeling has recently been utilized to investigate the effects of exposure of astrocytes to different concentrations of exogenous GABA on excitatory presynaptic and postsynaptic endings (Li et al., 2020). The results show that increased GABA concentration not only reduces neuronal spikes but also facilitates astrocyte glutamate release by inducing Ca2+ oscillations, leading to astrocyte-mediated presynaptic release and enhanced postsynaptic slow inward current (i.e., depolarizing currents). Thus GABA-activated astrocytes induce neuronal excitation that partially counteracts GABA inhibition.
In addition, GABA-stimulated astrocytes can affect the differentiation and maturation of neurons. During early development, astrocytes internalize neuronal precursor-derived GABA to create a microenvironment that strictly regulates the level of GABA and the activation of GABAAR, which is conducive to controlling the migration rate of neuronal precursors during development (Bolteus and Bordey, 2004).
GABA-Stimulated Astrocytes Contribute to Neurological Disorders
GABA neurotransmission disorders have been reported in various neurological diseases. For instance, the decline of GABA uptake and metabolism in AD astrocytes leads to GATs expression reduction, hereby contributing to neurotransmitter disturbances and cognitive impairment (Salcedo et al., 2021). In mouse models of early Parkinson’s disease, the downregulated GATs expression in the dorsal striatum also reduces the co-release of DA and GABA in DA axons, enhancing tonic inhibition of DA release and accelerating disease progression (Roberts et al., 2020). Furthermore, the activation of astrocyte GABABR by striatal medium spiny neurons results in acute behavioral hyperactivity and disrupted attention, revealing the activation of GABAceptive astrocytes as a causal factor for hyperactivity, attention deficit, and related psychiatric disorders (Nagai et al., 2019). In hyperammonemia, astrocyte activation and neuroinflammation have been reported to participate in GABA neurotransmission alteration, which causes cognitive dysfunction in hepatic encephalopathy (Malaguarnera et al., 2019, 2021). Treatment by GABAAR antagonist bicuculline can restore GABA neurotransmission, leading to the recovery of spatial learning and reduction of anxiety (Malaguarnera et al., 2019, 2021). Moreover, enhanced GABAAR responses of astrocytes are required for endozepines actions in the thalamic reticular nucleus (nRT), which mediates antiepileptic and sleep-promoting effects (Christian and Huguenard, 2013). Besides, dysfunction of GABAceptive astrocytes alters GABAergic transmission, thus contributing to epilepsy.
Taken together, astrocytes express GABA receptors and transporters. The uptake of GABA regulates astrocyte differentiation and function, therefore influencing GABA neurotransmission and contributing to neurological disorders.
Astrocytes Are GABAergic Cells
Astrocytes Produce and Release GABA
GABA was previously thought to be produced in and released from neurons only. However, emerging evidence has demonstrated that similar to neurons, astrocytes are capable of producing and releasing GABA, suggesting astrocytes as GABAergic cells (Figure 3; Le Meur et al., 2012). Astrocytes synthesize GABA using diverse enzymes like monoamine oxidase B (MAO-B) and glutamic acid decarboxylase (GAD) in different brain regions (Wu et al., 2014; Yoon et al., 2014). In the thalamus, diamine oxidase (DAO) and aldehyde dehydrogenase 1 family A1 (Aldh1a1) in astrocytes convert putrescine to GABA through two metabolic steps and release by bestrophin (Kwak et al., 2020). In the cerebellum and hippocampus, MAO-B converts putrescine into GABA in astrocytes (Yoon et al., 2014; Park et al., 2019). Besides, hippocampal astrocytes utilize two glutamate decarboxylases (GAD65 and GAD67, 65- and 67-kD isoforms, respectively) to convert glutamate into GABA (Kwak et al., 2020). Putrescine in astrocytes can be catabolized to GABA under the catalysis of copper amine oxidases (CAOs; Szabó et al., 2021).
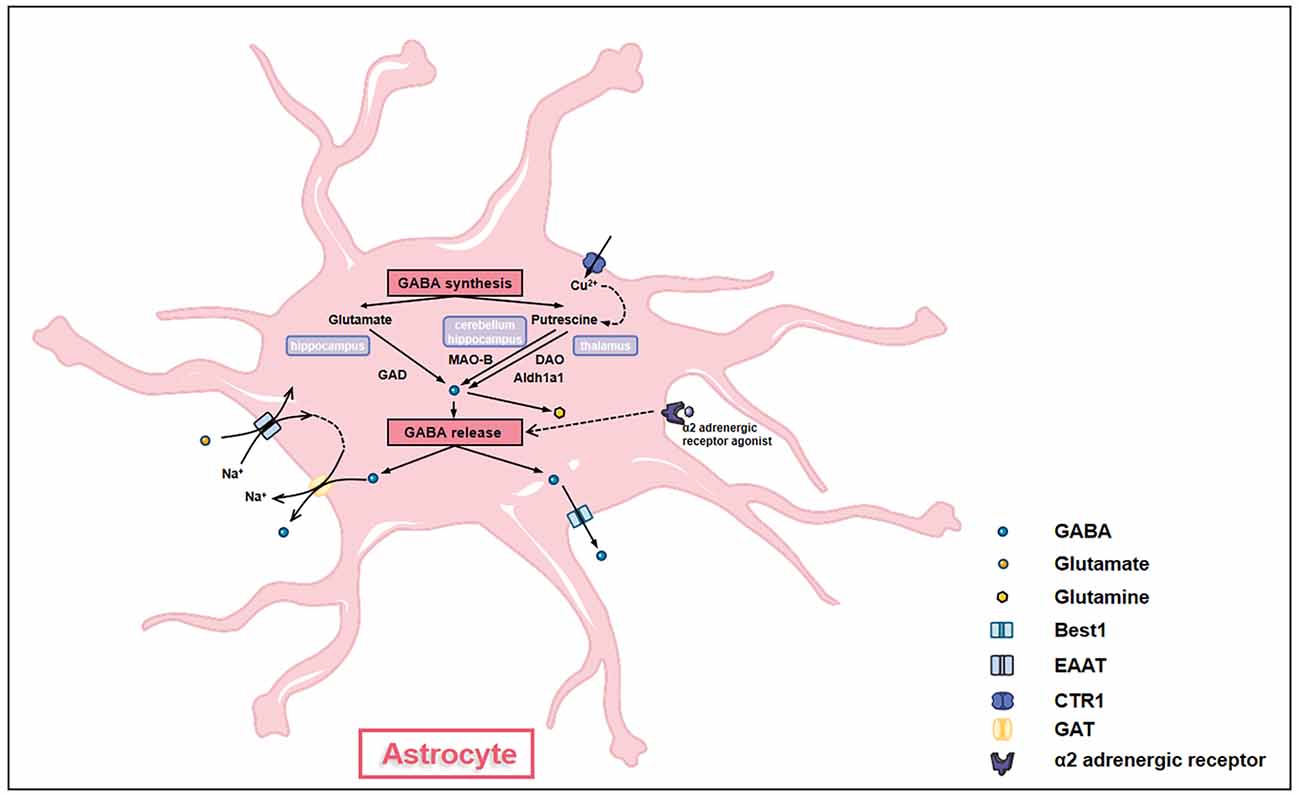
Figure 3. Astrocytes synthesize and release GABA. GABA synthesis in astrocytes has different pathways in different brain regions. In the hippocampus, glutamate in astrocytes is converted into GABA under the action of glutamate acid decarboxylase (GAD). In the cerebellum and the hippocampus, putrescine in astrocytes is converted into GABA under the action of monoamine oxidase B (MAO-B). In the thalamus, putrescine in astrocytes generates GABA through a two-step interaction of diamine oxidase (DAO) and aldehyde dehydrogenase 1 family A1 (Aldh1a1). The transformation of putrescine to GABA is affected by the levels of intracellular copper ion which is regulated by copper transporter (CTR). GABA synthesized by astrocytes can be further converted into glutamine to enter glutamine-glutamate cycle. GABA is released out of cells by either GABA transporter proteins (GATs) under the action of glutamate transporter (EAAT) or through bestrophin (Best1). α-2 adrenergic receptors also modulate the release of GABA through Giβγ subunit-associated signaling pathways.
Astrocytes release GABA post various stimulations. For example, intracellular copper levels affect the amount of GABA accumulated in astrocytes. After being taken up by copper transporter (CTR1), copper enhances CAOs’ activities and GABA production, causing GABA release and tonic inhibition (Szabó et al., 2021). Astrocyte GABA can also be released into the extrasynaptic space by Na+ influx mediated by glutamate transporter (EAAT; Szabó et al., 2021). Besides, activation of α-2 adrenergic receptors also stimulates the release of GABA by astrocytes through Giβγ subunit-associated signaling pathways (Gaidin et al., 2020a). Thus, the GABAceptive and GABAergic characteristics confer essential roles of GABA homeostasis maintenance to astrocytes, making GABAergic astrocytes key nodes of inhibitory networks in the brain. Moreover, astrocyte GABA is co-released with glutamate, acetylcholine, dopamine, or histamine in the presynaptic terminal, providing temporal-spatial precise signals and regulating synaptic plasticity (Tritsch et al., 2016).
GABAergic Astrocytes Contribute to the Pathogenesis of Neurological Disorders
GABAergic astrocytes play a part in the pathogenesis of many diseases including Alzheimer’s disease (AD), stroke, epilepsy, and other neurological diseases.
In middle age AD mice, the size of astrocytes increases significantly. Astrocytic GABA in the cortex and dentate gyrus showed an approximately normal distribution with animals’ age. In normal mice, only a brief increase in GABA levels occurred in middle age. Excessive GABA is found accumulated in and released from astrocytes even in absence of amyloidosis in AD mice (Brawek et al., 2018). In the dentate gyrus of AD mice, astrocyte-derived GABA inhibits the number of ridges of granulosa cells, therefore impairing cognitive functions (Jo et al., 2014). The blockage of GABA production and release in reactive astrocytes restores the memory of AD mice (Jo et al., 2014). An abnormal amount of GABA released by astrocytes was also observed in AD patient samples (Le Meur et al., 2012). In addition, human hippocampal astrocytes release GABA to induce slow outward currents (SOCs) of neurons, leading to neurotransmission inhibition.
Besides neurodegenerative diseases, GABAergic astrocytes dysfunction is involved in other neurological disorders. In stroke, the internal capsular infarct induces reactive astrocyte proliferation and GABA release in the motor cortex (Nam et al., 2020). Reactive astrocyte-derived GABA inhibits neuronal glucose metabolism, which can be erased by inhibiting GABA synthase MAO-B. Therefore, MAO-B inhibitor combined with rehabilitation therapy may be a new strategy to promote functional recovery after stroke. In epilepsy, GABA has been found to be progressively accumulated in reactive astrocytes (Müller et al., 2020). The overproduction of GABA in reactive astrocytes is mediated by both decarboxylation of glutamate and putrescine degradation, and the excessive release of GABA preserves tonic inhibitory currents in the epileptic brain (Eid et al., 2013; Müller et al., 2020). In depression, the blockage of GABA synthesis in astrocytes restores prominent plasticity in the prefrontal cortex in depressed rats (Srivastava et al., 2020). Under acute hyperammonemia, the release of astrocyte GABA induced by α-2 adrenergic receptor agonists plays a neuroprotective role (Gaidin et al., 2020b). One possible mechanism is that astrocyte GABA act on GABAceptive microglia with GABAARs and GABABRs, which may inhibit microglial activation and alleviate neuroinflammation (Malaguarnera et al., 2021).
Future Directions to Combine with Current Research Hotspots
Although mounting evidence has indicated important roles of astrocytes as GABAceptive and GABAergic cells in the regulation of neural functions, there are still many questions to be addressed. Here, we summarize current research hotspots and provide our thoughts that may inspire future studies.
Are Exosomes Able to Mediate GABAceptive and GABAergic Astrocyte-Dependent Regulation of Neuronal Cells?
Exosomes, a subtype of small bilipid layer extracellular vesicles (EVs), serve as an essential regulator of neural functions (Chivet et al., 2012, 2014). We recently proposed a model that exosomes might serve as novel neurotransmitters (Xia et al., 2022). Given that, whether exosomes mediate GABAceptive and GABAergic astrocyte-dependent regulation of neuronal cells has emerged as an interesting question. It has been reported that exosomes derived from GABA-treated intestinal cells or from the serum of GABA-treated mice are able to activate neuronal cells in vitro by affecting the expression of genes related to memory in the hippocampus (Inotsuka et al., 2021). Besides, although in the absence of direct causal evidence, hyperactivation of GABA receptors and the abnormal release of exosomes have been closely linked to neurological disorders including epilepsy (Khalyfa and Sanz-Rubio, 2019). These studies implied a positive answer to this question, which needs to be exhaustively examined in future works.
Is GABA Able to Regulate Inflammatory Responses of Astrocytes?
Since the first publication that proposed an A1/A2 model for reactive astrocytes in 2017, this field has explosively expanded (Liddelow et al., 2017). Currently, multiple neurotoxic or inflammatory stimuli have been identified to trigger A1 or A2-like reactive phenotypes of astrocytes (Li et al., 2019; Peng et al., 2020). However, the roles that GABA plays in astrocyte inflammatory responses remain controversial. It has been reported that GABA receptors participate in the activation of astrocytes post lipopolysaccharide (LPS) and interferon-gamma (IFNγ) stimulation (Lee et al., 2011). This study implicated GABA as an anti-inflammatory molecule that decreases astroglial activation and inhibits pro-inflammatory pathways. In contrast, another study has demonstrated that GABA treatment and the subsequent activation of GABAARs induce activation of astrocytes, ascertained by enhanced expression of GFAP (Runquist and Alonso, 2003). These conflicting observations indicate that astrocytes are a highly heterogeneous population and the effects of GABA on astrocyte inflammatory responses are highly dynamic. Therefore, more comprehensive investigations are urgently needed to expand our understanding in this field.
Is GABA Able to Mediate Metabolic Reprogramming of Astrocytes?
Metabolic reprogramming is the alteration of energy metabolism modes that were firstly reported in cancer cells. Cancer cells can switch their metabolism mode to a glycolytic one even under aerobic conditions for rapid energy generation. This switch meets cancer cells’ bioenergetic and biosynthetic demands to support their rapid proliferation (Ward and Thompson, 2012). Hence, cancer cells get energy via high consumption of glucose and its conversion into lactic acid by glycolysis mostly, whereas normal cells mainly utilize mitochondrial oxidative phosphorylation (Biswas, 2015). Recent studies reveal that activated normal cells also undergo a distinct metabolic shift that significantly impacts their biological functions. Under resting conditions, astrocytes in adult brains almost exclusively utilize the complete oxidative metabolism of glucose for energy supply (Hertz, 2011). Under other conditions, however, astrocytes have the capacity to switch to a mode with a high glycolytic rate and lower oxidative metabolism as evidenced by the high expression of 6-phosphofructo-2-kinase/fructose-2, 6-biphosphatase 3 (Pfkfb3), a key positive modulator of glycolysis, and low activities of pyruvate dehydrogenase (PDH), the enzymatic complex that generates TCA cycle substrate acetyl-CoA (Herrero-Mendez et al., 2009; Halim et al., 2010). These observations reveal that the metabolic states of astrocytes are altered with environmental changes. Interestingly, 2-deoxy-D-glucose (2-DG), a glucose analog that inhibits glycolytic enzymes, has been reported to potentiate GABAergic tonic inhibition via neurosteroid-mediated activation of extrasynaptic GABAARs in the brain granule cells (Forte et al., 2016). A similar phenomenon may exist in astrocytes as well. In addition, the integration between glycolysis and the glutamate-glutamine cycle has been reported to participate in the regulation of astroglial activation (Hertz and Chen, 2017). Since GABA is the substrate and product of glutamate-glutamine cycle, intracellular GABA is likely to modulate glycolytic rates of astrocytes via modulating glutamate production (Cabrera-Pastor et al., 2019). Therefore, the potential reciprocal regulation between GABA and metabolic reprogramming may significantly manipulate astrocyte functions, especially under inflammatory conditions.
In summary, there are knowledge gaps in current understandings of the functions and regulations of GABAceptive and GABAergic astrocytes. More in-depth and systematic researches are needed to unmask the unknowns of GABAceptive and GABAergic astrocytes in the future.
Conclusions
Recent studies identified GABA as a novel gliotransmitter in the CNS. The activities of GABAceptive astrocytes driven by inhibitory cells regulate both inhibitory and excitatory neurotransmission. More importantly, astrocytes themselves produce and release GABA to influence the brain function directly. The GABAceptive and GABAergic features make astrocytes a key regulator in both the maintenance of the proper function of the CNS and the pathogenesis of various neurological disorders. More comprehensive investigations will unveil the physiological and pathological roles of GABAceptive and GABAergic astrocytes yet to be discovered, and will greatly promote the progress of neuroscience to shed light on the development of novel astrocyte-dependent therapeutic strategies in treating neurological disorders.
Author Contributions
JL, XX, and JZ conceived the manuscript. JL, XF, and XX collected references. JL, XF, XX, and YW wrote the manuscript. All authors contributed to the article and approved the submitted version.
Funding
This work was supported in part by research grants from the National Natural Science Foundation of China (No. 91949204 and No. 81830037 to JZ, No. 82171194 and No. 81974155 to JL, No. 81971145 and No. 81901333 to XX).
Acknowledgments
We thank Jie Zhu, Yanyan Zhang, Drs. Ling Ye and Xinrui Qi for proofreading the manuscript.
Abbreviations
2-DG, 2-deoxy-D-glucose; 7TM, Heptahelical transmembrane; AC, Adenylyl cyclase; ACBP, acyl-CoA-binding protein; AgRP, Agouti-related protein; Aldh1a1, Aldehyde dehydrogenase 1 family A1; ATP, Adenosine triphosphate; BBB, Blood-brain-barrier; BF, Basal forebrain; BGT-1, Betaine-GABA transporter; BZR, Benzodiazepine receptor; CAOs, Copper amine oxidases; CNS, Central nervous system; CRD, Cysteine rich domain; CTR1, Copper transporter; Cx43, Connexin 43; DA, Dopamine; DAO, Diamine oxidase; DBI, Diazepam-binding inhibitor; EAAT, Glutamate transporter; EVs, Extracellular vesicles; GABA, Gamma-aminobutyric acid; GABAARs, GABAA receptors; GABABRs, GABAB receptors; GAD, Glutamic acid decarboxylase; GAT, GABA transporter protein; GFAP, Glial fibrillary acidic protein; GPCR, G-protein coupled receptors; IFNγ, Interferon-gamma; KCC2, Potassium chloride cotransporter 2; LPS, Lipopolysaccharide; MAO-B, Monoamine oxidase B; mGluR, Metabotropic glutamate receptor; mPFC, Medial prefrontal cortex; nRT, Thalamic reticular nucleus; ODN, Octadecaneuropeptide; PDH, Pyruvate dehydrogenase; Pfkfb3, 6-phosphofructo-2-kinase/fructose-2, 6-biphosphatase 3; PGE2, Prostaglandin E2; PV, Parvalbumin; SOCs, Slow outward currents; SOM-INs, Somatostatin-expressing interneurons; TSPO, Translocator protein; TTN, Triakontatetraneuropeptide; VFT, Venus flytrap; VGCC, Voltage-gated calcium channels.
Conflict of Interest
The authors declare that the research was conducted in the absence of any commercial or financial relationships that could be construed as a potential conflict of interest.
Publisher’s Note
All claims expressed in this article are solely those of the authors and do not necessarily represent those of their affiliated organizations, or those of the publisher, the editors and the reviewers. Any product that may be evaluated in this article, or claim that may be made by its manufacturer, is not guaranteed or endorsed by the publisher.
References
Abbott, N. J., Rönnbäck, L., and Hansson, E. (2006). Astrocyte-endothelial interactions at the blood-brain barrier. Nat. Rev. Neurosci. 7, 41–53. doi: 10.1038/nrn1824
Allen, N. J. (2014). Astrocyte regulation of synaptic behavior. Annu. Rev. Cell Dev. Biol. 30, 439–463. doi: 10.1146/annurev-cellbio-100913-013053
Andersen, J. V., Jakobsen, E., Westi, E. W., Lie, M. E. K., Voss, C. M., Aldana, B. I., et al. (2020). Extensive astrocyte metabolism of γ-aminobutyric acid (GABA) sustains glutamine synthesis in the mammalian cerebral cortex. Glia 68, 2601–2612. doi: 10.1002/glia.23872
Anderson, C. M., and Nedergaard, M. (2003). Astrocyte-mediated control of cerebral microcirculation. Trends Neurosci. 26, 340–344. doi: 10.1016/S0166-2236(03)00141-3
Bélanger, M., Allaman, I., and Magistretti, P. J. (2011). Brain energy metabolism: focus on astrocyte-neuron metabolic cooperation. Cell Metab. 14, 724–738. doi: 10.1016/j.cmet.2011.08.016
Barakat, L., and Bordey, A. (2002). GAT-1 and reversible GABA transport in Bergmann glia in slices. J. Neurophysiol. 88, 1407–1419. doi: 10.1152/jn.2002.88.3.1407
Barnard, E. A., Skolnick, P., Olsen, R. W., Mohler, H., Sieghart, W., Biggio, G., et al. (1998). International Union of Pharmacology. XV. Subtypes of gamma-aminobutyric acidA receptors: classification on the basis of subunit structure and receptor function. Pharmacol. Rev. 50, 291–313.
Bernardinelli, Y., Muller, D., and Nikonenko, I. (2014). Astrocyte-synapse structural plasticity. Neural. Plast. 2014:232105. doi: 10.1155/2014/232105
Biswas, S. K. (2015). Metabolic reprogramming of immune cells in cancer progression. Immunity 43, 435–449. doi: 10.1016/j.immuni.2015.09.001
Bitoun, M., and Tappaz, M. (2000). Gene expression of taurine transporter and taurine biosynthetic enzymes in brain of rats with acute or chronic hyperosmotic plasma. A comparative study with gene expression of myo-inositol transporter, betaine transporter and sorbitol biosynthetic enzyme. Brain Res. Mol. Brain Res. 77, 10–18. doi: 10.1016/s0169-328x(00)00034-6
Boddum, K., Jensen, T. P., Magloire, V., Kristiansen, U., Rusakov, D. A., Pavlov, I., et al. (2016). Astrocytic GABA transporter activity modulates excitatory neurotransmission. Nat. Commun. 7:13572. doi: 10.1038/ncomms13572
Bolteus, A. J., and Bordey, A. (2004). GABA release and uptake regulate neuronal precursor migration in the postnatal subventricular zone. J. Neurosci. 24, 7623–7631. doi: 10.1523/JNEUROSCI.1999-04.2004
Bovolin, P., Santi, M. R., Puia, G., Costa, E., and Grayson, D. (1992). Expression patterns of gamma-aminobutyric acid type a receptor subunit mRNAs in primary cultures of granule neurons and astrocytes from neonatal rat cerebella. Proc. Natl. Acad. Sci. U S A 89, 9344–9348. doi: 10.1073/pnas.89.19.9344
Brawek, B., Chesters, R., Klement, D., Müller, J., Lerdkrai, C., Hermes, M., et al. (2018). A bell-shaped dependence between amyloidosis and GABA accumulation in astrocytes in a mouse model of Alzheimer’s disease. Neurobiol. Aging 61, 187–197. doi: 10.1016/j.neurobiolaging.2017.09.028
Bushong, E. A., Martone, M. E., Jones, Y. Z., and Ellisman, M. H. (2002). Protoplasmic astrocytes in CA1 stratum radiatum occupy separate anatomical domains. J. Neurosci. 22, 183–192. doi: 10.1523/JNEUROSCI.22-01-00183.2002
Cabrera-Pastor, A., Arenas, Y. M., Taoro-Gonzalez, L., Montoliu, C., and Felipo, V. (2019). Chronic hyperammonemia alters extracellular glutamate, glutamine and GABA and membrane expression of their transporters in rat cerebellum. Modulation by extracellular cGMP. Neuropharmacology 161:107496. doi: 10.1016/j.neuropharm.2019.01.011
Charles, K. J., Deuchars, J., Davies, C. H., and Pangalos, M. N. (2003). GABA B receptor subunit expression in glia. Mol. Cell. Neurosci. 24, 214–223. doi: 10.1016/s1044-7431(03)00162-3
Chiu, C. S., Brickley, S., Jensen, K., Southwell, A., McKinney, S., Cull-Candy, S., et al. (2005). GABA transporter deficiency causes tremor, ataxia, nervousness and increased GABA-induced tonic conductance in cerebellum. J. Neurosci. 25, 3234–3245. doi: 10.1523/JNEUROSCI.3364-04.2005
Chivet, M., Hemming, F., Pernet-Gallay, K., Fraboulet, S., and Sadoul, R. (2012). Emerging role of neuronal exosomes in the central nervous system. Front. Physiol. 3:145. doi: 10.3389/fphys.2012.00145
Chivet, M., Javalet, C., Laulagnier, K., Blot, B., Hemming, F. J., Sadoul, R., et al. (2014). Exosomes secreted by cortical neurons upon glutamatergic synapse activation specifically interact with neurons. J. Extracell. Vesicles 3:24722. doi: 10.3402/jev.v3.24722
Christian, C. A., and Huguenard, J. R. (2013). Astrocytes potentiate GABAergic transmission in the thalamic reticular nucleus via endozepine signaling. Proc. Natl. Acad. Sci. U S A 110, 20278–20283. doi: 10.1073/pnas.1318031110
Chun, L., Zhang, W. H., and Liu, J. F. (2012). Structure and ligand recognition of class C GPCRs. Acta Pharmacol. Sin. 33, 312–323. doi: 10.1038/aps.2011.186
Conti, F., Minelli, A., and Melone, M. (2004). GABA transporters in the mammalian cerebral cortex: localization, development and pathological implications. Brain Res. Brain Res. Rev. 45, 196–212. doi: 10.1016/j.brainresrev.2004.03.003
Covelo, A., and Araque, A. (2018). Neuronal activity determines distinct gliotransmitter release from a single astrocyte. eLife 7:e32237. doi: 10.7554/eLife.32237
Crosby, K. M., Murphy-Royal, C., Wilson, S. A., Gordon, G. R., Bains, J. S., Pittman, Q. J., et al. (2018). Cholecystokinin switches the plasticity of GABA synapses in the dorsomedial hypothalamus via astrocytic ATP release. J. Neurosci. 38, 8515–8525. doi: 10.1523/JNEUROSCI.0569-18.2018
De Mateos-Verchere, J. G., Leprince, J., Tonon, M. C., Vaudry, H., and Costentin, J. (1998). The octadecaneuropeptide ODN induces anxiety in rodents: possible involvement of a shorter biologically active fragment. Peptides 19, 841–848. doi: 10.1016/s0196-9781(98)00037-0
Doengi, M., Hirnet, D., Coulon, P., Pape, H. C., Deitmer, J. W., and Lohr, C. (2009). GABA uptake-dependent Ca2+ signaling in developing olfactory bulb astrocytes. Proc. Natl. Acad. Sci. U S A 106, 17570–17575. doi: 10.1073/pnas.0809513106
Duman, C., Yaqubi, K., Hoffmann, A., Acikgoz, A. A., Korshunov, A., Bendszus, M., et al. (2019). Acyl-CoA-binding protein drives glioblastoma tumorigenesis by sustaining fatty acid oxidation. Cell Metab. 30, e5274–e5289. doi: 10.1016/j.cmet.2019.04.004
Dumitru, I., Neitz, A., Alfonso, J., and Monyer, H. (2017). Diazepam binding inhibitor promotes stem cell expansion controlling environment-dependent neurogenesis. Neuron 94, e5125–e5137. doi: 10.1016/j.neuron.2017.03.003
Durkin, M. M., Smith, K. E., Borden, L. A., Weinshank, R. L., Branchek, T. A., and Gustafson, E. L. (1995). Localization of messenger RNAs encoding three GABA transporters in rat brain: an in situ hybridization study. Brain Res. Mol. Brain Res. 33, 7–21. doi: 10.1016/0169-328x(95)00101-w
Eid, T., Tu, N., Lee, T. S., and Lai, J. C. (2013). Regulation of astrocyte glutamine synthetase in epilepsy. Neurochem. Int. 63, 670–681. doi: 10.1016/j.neuint.2013.06.008
Evenseth, L. S. M., Gabrielsen, M., and Sylte, I. (2020). The GABAB receptor-structure, ligand binding and drug development. Molecules 25:3093. doi: 10.3390/molecules25133093
Farzampour, Z., Reimer, R. J., and Huguenard, J. (2015). Endozepines. Adv. Pharmacol. 72, 147–164. doi: 10.1016/bs.apha.2014.10.005
Forte, N., Medrihan, L., Cappetti, B., Baldelli, P., and Benfenati, F. (2016). 2-Deoxy-d-glucose enhances tonic inhibition through the neurosteroid-mediated activation of extrasynaptic GABAA receptors. Epilepsia 57, 1987–2000. doi: 10.1111/epi.13578
Fraser, D. D., Duffy, S., Angelides, K. J., Perez-Velazquez, J. L., Kettenmann, H., and MacVicar, B. A. (1995). GABAA/benzodiazepine receptors in acutely isolated hippocampal astrocytes. J. Neurosci. 15, 2720–2732. doi: 10.1523/JNEUROSCI.15-04-02720.1995
Gaidin, S. G., Zinchenko, V. P., and Kosenkov, A. M. (2020a). Mechanisms of ammonium-induced neurotoxicity. Neuroprotective effect of alpha-2 adrenergic agonists. Arch. Biochem. Biophys. 693:108593. doi: 10.1016/j.abb.2020.108593
Gaidin, S. G., Zinchenko, V. P., Sergeev, A. I., Teplov, I. Y., Mal’tseva, V. N., Kosenkov, A. M., et al. (2020b). Activation of alpha-2 adrenergic receptors stimulates GABA release by astrocytes. Glia 68, 1114–1130. doi: 10.1002/glia.23763
Ghouili, I., Bahdoudi, S., Morin, F., Amri, F., Hamdi, Y., Coly, P. M., et al. (2018). Endogenous expression of ODN-related peptides in astrocytes contributes to cell protection against oxidative stress: astrocyte-neuron crosstalk relevance for neuronal survival. Mol. Neurobiol. 55, 4596–4611. doi: 10.1007/s12035-017-0630-3
Gould, T., Chen, L., Emri, Z., Pirttimaki, T., Errington, A. C., Crunelli, V., et al. (2014). GABAB receptor-mediated activation of astrocytes by gamma-hydroxybutyric acid. Philos. Trans. R. Soc. Lond. B. Biol. Sci. 369:20130607. doi: 10.1098/rstb.2013.0607
Guetg, N., Seddik, R., Vigot, R., Turecek, R., Gassmann, M., Vogt, K. E., et al. (2009). The GABAB1a isoform mediates heterosynaptic depression at hippocampal mossy fiber synapses. J. Neurosci. 29, 1414–1423. doi: 10.1523/JNEUROSCI.3697-08.2009
Guidotti, A., Forchetti, C. M., Corda, M. G., Konkel, D., Bennett, C. D., Costa, E., et al. (1983). Isolation, characterization and purification to homogeneity of an endogenous polypeptide with agonistic action on benzodiazepine receptors. Proc. Natl. Acad. Sci. U S A 80, 3531–3535. doi: 10.1073/pnas.80.11.3531
Guillebaud, F., Girardet, C., Abysique, A., Gaige, S., Barbouche, R., Verneuil, J., et al. (2017). Glial endozepines inhibit feeding-related autonomic functions by acting at the brainstem level. Front. Neurosci. 11:308. doi: 10.3389/fnins.2017.00308
Halim, N. D., McFate, T., Mohyeldin, A., Okagaki, P., Korotchkina, L. G., Patel, M. S., et al. (2010). Phosphorylation status of pyruvate dehydrogenase distinguishes metabolic phenotypes of cultured rat brain astrocytes and neurons. Glia 58, 1168–1176. doi: 10.1002/glia.20996
Heja, L. (2014). Astrocytic target mechanisms in epilepsy. Curr. Med. Chem. 21, 755–763. doi: 10.2174/0929867320666131119160445
Henneberger, C., Papouin, T., Oliet, S. H., and Rusakov, D. A. (2010). Long-term potentiation depends on release of D-serine from astrocytes. Nature 463, 232–236. doi: 10.1038/nature08673
Herrero-Mendez, A., Almeida, A., Fernández, E., Maestre, C., Moncada, S., Bolaños, J. P., et al. (2009). The bioenergetic and antioxidant status of neurons is controlled by continuous degradation of a key glycolytic enzyme by APC/C-Cdh1. Nat. Cell Biol. 11, 747–752. doi: 10.1038/ncb1881
Hertz, L. (2011). Astrocytic energy metabolism and glutamate formation–relevance for 13C-NMR spectroscopy and importance of cytosolic/mitochondrial trafficking. Magn. Reson. Imaging 29, 1319–1329. doi: 10.1016/j.mri.2011.04.013
Hertz, L., and Chen, Y. (2017). Integration between glycolysis and glutamate-glutamine cycle flux may explain preferential glycolytic increase during brain activation, requiring glutamate. Front. Integr. Neurosci. 11:18. doi: 10.3389/fnint.2017.00018
Hussy, N. (2002). Glial cells in the hypothalamo-neurohypophysial system: key elements of the regulation of neuronal electrical and secretory activity. Prog. Brain Res. 139, 95–112. doi: 10.1016/s0079-6123(02)39010-1
Inotsuka, R., Udono, M., Yamatsu, A., Kim, M., and Katakura, Y. (2021). Exosome-mediated activation of neuronal cells triggered by γ-aminobutyric acid (GABA). Nutrients 13:2544. doi: 10.3390/nu13082544
Jo, S., Yarishkin, O., Hwang, Y. J., Chun, Y. E., Park, M., Woo, D. H., et al. (2014). GABA from reactive astrocytes impairs memory in mouse models of Alzheimer’s disease. Nat. Med. 20, 886–896. doi: 10.1038/nm.3639
Kaupmann, K., Huggel, K., Heid, J., Flor, P. J., Bischoff, S., Mickel, S. J., et al. (1997). Expression cloning of GABAB receptors uncovers similarity to metabotropic glutamate receptors. Nature 386, 239–246. doi: 10.1038/386239a0
Kersante, F., Rowley, S. C., Pavlov, I., Gutierrez-Mecinas, M., Semyanov, A., Reul, J. M., et al. (2013). A functional role for both -aminobutyric acid (GABA) transporter-1 and GABA transporter-3 in the modulation of extracellular GABA and GABAergic tonic conductances in the rat hippocampus. J. Physiol. 591, 2429–2441. doi: 10.1113/jphysiol.2012.246298
Kettenmann, H., Backus, K. H., and Schachner, M. (1987). γ-Aminobutyric acid opens Cl-channels in cultured astrocytes. Brain Res. 404, 1–9. doi: 10.1016/0006-8993(87)91349-7
Khalyfa, A., and Sanz-Rubio, D. (2019). Genetics and extracellular vesicles of pediatrics sleep disordered breathing and epilepsy. Int. J. Mol. Sci. 20:5483. doi: 10.3390/ijms20215483
Knudsen, J., Hojrup, P., Hansen, H. O., Hansen, H. F., and Roepstorff, P. (1989). Acyl-CoA-binding protein in the rat. Purification, binding characteristics, tissue concentrations and amino acid sequence. Biochem. J. 262, 513–519. doi: 10.1042/bj2620513
Kolta, A. (2018). Astrocyte-mediated primary afferent depolarization: a new twist to a complicated tale? J. Physiol. 596, 4809–4810. doi: 10.1113/JP276949
Kruyer, A., Dixon, D., Angelis, A., Amato, D., and Kalivas, P. W. (2021). Astrocytes in the ventral pallidum extinguish heroin seeking through GAT-3 upregulation and morphological plasticity at D1-MSN terminals. Mol. Psychiatry 27, 855–864. doi: 10.1038/s41380-021-01333-5
Kwak, H., Koh, W., Kim, S., Song, K., Shin, J. I., Lee, J. M., et al. (2020). Astrocytes control sensory acuity via tonic inhibition in the thalamus. Neuron 108, e10691–e10706. doi: 10.1016/j.neuron.2020.08.013
Larsson, O. M., Hertz, L., and Schousboe, A. (1980). GABA uptake in astrocytes in primary cultures: coupling with two sodium ions. J. Neurosci. Res. 5, 469–477. doi: 10.1002/jnr.490050602
Le Meur, K., Mendizabal-Zubiaga, J., Grandes, P., and Audinat, E. (2012). GABA release by hippocampal astrocytes. Front. Comput. Neurosci. 6:59. doi: 10.3389/fncom.2012.00059
Lebrun, B., Barbot, M., Tonon, M. C., Prevot, V., Leprince, J., Troadec, J. D., et al. (2021). Glial endozepines and energy balance: old peptides with new tricks. Glia 69, 1079–1093. doi: 10.1002/glia.23927
Lee, M., Schwab, C., and McGeer, P. L. (2011). Astrocytes are GABAergic cells that modulate microglial activity. Glia 59, 152–165. doi: 10.1002/glia.21087
Li, T., Chen, X., Zhang, C., Zhang, Y., and Yao, W. (2019). An update on reactive astrocytes in chronic pain. J. Neuroinflammation 16:140. doi: 10.1186/s12974-019-1524-2
Li, L., Zhou, J., Sun, H., Liu, J., Wang, H., Liu, X., et al. (2020). A computational model to investigate GABA-activated astrocyte modulation of neuronal excitation. Comput. Math. Methods Med. 2020:8750167. doi: 10.1155/2020/8750167
Liddelow, S. A., Guttenplan, K. A., Clarke, L. E., Bennett, F. C., Bohlen, C. J., Schirmer, L., et al. (2017). Neurotoxic reactive astrocytes are induced by activated microglia. Nature 541, 481–487. doi: 10.1038/nature21029
Losi, G., Gomez-Gonzalo, M., Zonta, M., Chiavegato, A., and Carmignoto, G. (2021). Cellular and molecular mechanisms of new onset seizure generation. Aging Clin. Exp. Res. 33, 1713–1716. doi: 10.1007/s40520-019-01396-z
Müller, J., Timmermann, A., Henning, L., Müller, H., Steinhäuser, C., Bedner, P., et al. (2020). Astrocytic GABA accumulation in experimental temporal lobe epilepsy. Front. Neurol. 11:614923. doi: 10.3389/fneur.2020.614923
MacVicar, B. A., Tse, F. W., Crichton, S. A., and Kettenmann, H. (1989). GABA-activated Cl- channels in astrocytes of hippocampal slices. J. Neurosci. 9, 3577–3583. doi: 10.1523/JNEUROSCI.09-10-03577.1989
Maitre, M., Chesielski, L., Lehmann, A., Kempf, E., and Mandel, P. (1974). Protective effect of adenosine and nicotinamide against audiogenic seizure. Biochem. Pharmacol. 23, 2807–2816. doi: 10.1016/0006-2952(74)90054-9
Malaguarnera, M., Balzano, T., Castro, M. C., Llansola, M., and Felipo, V. (2021). The dual role of the GABAA receptor in peripheral inflammation and neuroinflammation: a study in hyperammonemic rats. Int. J. Mol. Sci. 22:6772. doi: 10.3390/ijms22136772
Malaguarnera, M., Llansola, M., Balzano, T., Gómez-Giménez, B., Antúnez-Muñoz, C., Martínez-Alarcón, N., et al. (2019). Bicuculline reduces neuroinflammation in hippocampus and improves spatial learning and anxiety in hyperammonemic rats role of glutamate receptors. Front. Pharmacol. 10:132. doi: 10.3389/fphar.2019.00132
Marino, F., Cosentino, M., Fietta, A. M., Ferrari, M., Cattaneo, S., Frigo, G., et al. (2003). Interleukin-8 production induced by the endozepine triakontatetraneuropeptide in human neutrophils: role of calcium and pharmacological investigation of signal transduction pathways. Cell Signal. 15, 511–517. doi: 10.1016/s0898-6568(02)00134-1
Mariotti, L., Losi, G., Lia, A., Melone, M., Chiavegato, A., Gómez-Gonzalo, M., et al. (2018). Interneuron-specific signaling evokes distinctive somatostatin-mediated responses in adult cortical astrocytes. Nat. Commun. 9:82. doi: 10.1038/s41467-017-02642-6
Mariotti, L., Losi, G., Sessolo, M., Marcon, I., and Carmignoto, G. (2016). The inhibitory neurotransmitter GABA evokes long-lasting Ca2+ oscillations in cortical astrocytes. Glia 64, 363–373. doi: 10.1002/glia.22933
Masmoudi-Kouki, O., Hamdi, Y., Ghouili, I., Bahdoudi, S., Kaddour, H., Leprince, J., et al. (2018). Neuroprotection with the endozepine octadecaneuropeptide, ODN. Curr. Pharm. Des. 24, 3918–3925. doi: 10.2174/1381612824666181112111746
Matos, M., Bosson, A., Riebe, I., Reynell, C., Vallée, J., Laplante, I., et al. (2018). Astrocytes detect and upregulate transmission at inhibitory synapses of somatostatin interneurons onto pyramidal cells. Nat. Commun. 9:4254. doi: 10.1038/s41467-018-06731-y
Matsutani, S., and Yamamoto, N. (1997). Neuronal regulation of astrocyte morphology in vitro is mediated by GABAergic signaling. Glia 20, 1–9. doi: 10.1002/(sici)1098-1136(199705)20:1<1::aid-glia1>3.0.co;2-e
McKenna, M. C., and Sonnewald, U. (2005). GABA alters the metabolic fate of [U-13C]glutamate in cultured cortical astrocytes. J. Neurosci. Res. 79, 81–87. doi: 10.1002/jnr.20309
Mederos, S., and Perea, G. (2019). GABAergic-astrocyte signaling: a refinement of inhibitory brain networks. Glia 67, 1842–1851. doi: 10.1002/glia.23644
Mederos, S., Sánchez-Puelles, C., Esparza, J., Valero, M., Ponomarenko, A., Perea, G., et al. (2021). GABAergic signaling to astrocytes in the prefrontal cortex sustains goal-directed behaviors. Nat. Neurosci. 24, 82–92. doi: 10.1038/s41593-020-00752-x
Meier, S. D., Kafitz, K. W., and Rose, C. R. (2008). Developmental profile and mechanisms of GABA-induced calcium signaling in hippocampal astrocytes. Glia 56, 1127–1137. doi: 10.1002/glia.20684
Melone, M., Ciappelloni, S., and Conti, F. (2015). A quantitative analysis of cellular and synaptic localization of GAT-1 and GAT-3 in rat neocortex. Brain Struct. Funct. 220, 885–897. doi: 10.1007/s00429-013-0690-8
Minelli, A., DeBiasi, S., Brecha, N. C., Zuccarello, L. V., and Conti, F. (1996). GAT-3, a high-affinity GABA plasma membrane transporter, is localized to astrocytic processes and it is not confined to the vicinity of GABAergic synapses in the cerebral cortex. J. Neurosci. 16, 6255–6264. doi: 10.1523/JNEUROSCI.16-19-06255.1996
Mohler, H. (2006). GABAA receptor diversity and pharmacology. Cell Tissue Res. 326, 505–516. doi: 10.1007/s00441-006-0284-3
Moldavan, M., Cravetchi, O., and Allen, C. N. (2017). GABA transporters regulate tonic and synaptic GABAA receptor-mediated currents in the suprachiasmatic nucleus neurons. J. Neurophysiol. 118, 3092–3106. doi: 10.1152/jn.00194.2017
Mong, J. A., Nuñez, J. L., and McCarthy, M. M. (2002). GABA mediates steroid-induced astrocyte differentiation in the neonatal rat hypothalamus. J. Neuroendocrinol. 14, 45–55. doi: 10.1046/j.1365-2826.2002.00737.x
Munk, C., Isberg, V., Mordalski, S., Harpsoe, K., Rataj, K., Hauser, A. S., et al. (2016). GPCRdb: the G protein-coupled receptor database - an introduction. Br. J. Pharmacol. 173, 2195–2207. doi: 10.1111/bph.13509
Nagai, J., Rajbhandari, A. K., Gangwani, M. R., Hachisuka, A., Coppola, G., Masmanidis, S. C., et al. (2019). Hyperactivity with disrupted attention by activation of an astrocyte synaptogenic cue. Cell 177, e201280–e201292. doi: 10.1016/j.cell.2019.03.019
Nam, M. H., Cho, J., Kwon, D. H., Park, J. Y., Woo, J., Lee, J. M., et al. (2020). Excessive astrocytic GABA causes cortical hypometabolism and impedes functional recovery after subcortical stroke. Cell Rep. 32:107975. doi: 10.1016/j.celrep.2020.107861
Newman, E. A. (2003). Glial cell inhibition of neurons by release of ATP. J. Neurosci. 23, 1659–1666. doi: 10.1523/JNEUROSCI.23-05-01659.2003
Nilsson, M., Eriksson, P. S., Rönnbäck, L., and Hansson, E. (1993). GABA induces Ca2+ transients in astrocytes. Neuroscience 54, 605–614. doi: 10.1016/0306-4522(93)90232-5
Olsen, M., Sarup, A., Larsson, O. M., and Schousboe, A. (2005). Effect of hyperosmotic conditions on the expression of the betaine-GABA-transporter (BGT-1) in cultured mouse astrocytes. Neurochem. Res. 30, 855–865. doi: 10.1007/s11064-005-6879-3
Olsen, R. W., and Sieghart, W. (2008). International union of pharmacology. LXX. Subtypes of gamma-aminobutyric acidA receptors: classification on the basis of subunit composition, pharmacology and function. Update. Pharmacol. Rev. 60, 243–260. doi: 10.1124/pr.108.00505
Olsen, R. W., and Sieghart, W. (2009). GABAA receptors: subtypes provide diversity of function and pharmacology. Neuropharmacology 56, 141–148. doi: 10.1016/j.neuropharm.2008.07.045
Orellana, J. A., and Stehberg, J. (2014). Hemichannels: new roles in astroglial function. Front. Physiol. 5:193. doi: 10.3389/fphys.2014.00193
Park, J. H., Ju, Y. H., Choi, J. W., Song, H. J., Jang, B. K., Woo, J., et al. (2019). Newly developed reversible MAO-B inhibitor circumvents the shortcomings of irreversible inhibitors in Alzheimer’s disease. Sci. Adv. 5:eaav0316. doi: 10.1126/sciadv.aav0316
Patte, C., Gandolfo, P., Leprince, J., Thoumas, J. L., Fontaine, M., Vaudry, H., et al. (1999). GABA inhibits endozepine release from cultured rat astrocytes. Glia 25, 404–411.
Patte, C., Vaudry, H., Desrues, L., Gandolfo, P., Strijdveen, I., Lamacz, M., et al. (1995). The endozepine ODN stimulates polyphosphoinositide metabolism in rat astrocytes. FEBS Lett. 362, 106–110. doi: 10.1016/0014-5793(95)00209-r
Pelvig, D. P., Pakkenberg, H., Stark, A. K., and Pakkenberg, B. (2008). Neocortical glial cell numbers in human brains. Neurobiol. Aging 29, 1754–1762. doi: 10.1016/j.neurobiolaging.2007.04.013
Peng, A. Y. T., Agrawal, I., Ho, W. Y., Yen, Y. C., Pinter, A. J., Liu, J., et al. (2020). Loss of TDP-43 in astrocytes leads to motor deficits by triggering A1-like reactive phenotype and triglial dysfunction. Proc. Natl. Acad. Sci. U S A 117, 29101–29112. doi: 10.1073/pnas.2007806117
Perea, G., Gómez, R., Mederos, S., Covelo, A., Ballesteros, J. J., Schlosser, L., et al. (2016). Activity-dependent switch of GABAergic inhibition into glutamatergic excitation in astrocyte-neuron networks. eLife 5:e20362. doi: 10.7554/eLife.20362
Pirttimaki, T., Parri, H. R., and Crunelli, V. (2013). Astrocytic GABA transporter GAT-1 dysfunction in experimental absence seizures. J. Physiol. 591, 823–833. doi: 10.1113/jphysiol.2012.242016
Radian, R., Ottersen, O. P., Storm-Mathisen, J., Castel, M., and Kanner, B. I. (1990). Immunocytochemical localization of the GABA transporter in rat brain. J. Neurosci. 10, 1319–1330. doi: 10.1523/JNEUROSCI.10-04-01319.1990
Roberts, B. M., Doig, N. M., Brimblecombe, K. R., Lopes, E. F., Siddorn, R. E., Threlfell, S., et al. (2020). GABA uptake transporters support dopamine release in dorsal striatum with maladaptive downregulation in a parkinsonism model. Nat. Commun. 11:4958. doi: 10.1038/s41467-020-18247-5
Rothstein, J. D., Garland, W., Puia, G., Guidotti, A., Weber, R. J., Costa, E., et al. (1992). Purification and characterization of naturally occurring benzodiazepine receptor ligands in rat and human brain. J. Neurochem. 58, 2102–2115. doi: 10.1111/j.1471-4159.1992.tb10952.x
Runquist, M., and Alonso, G. (2003). Gabaergic signaling mediates the morphological organization of astrocytes in the adult rat forebrain. Glia 41, 137–151. doi: 10.1002/glia.10166
Salcedo, C., Wagner, A., Andersen, J. V., Vinten, K. T., Waagepetersen, H. S., Schousboe, A., et al. (2021). Downregulation of GABA transporter 3 (GAT3) is associated with deficient oxidative GABA metabolism in human induced pluripotent stem cell-derived astrocytes in Alzheimer’s disease. Neurochem. Res. 46, 2676–2686. doi: 10.1007/s11064-021-03276-3
Scheiber, I. F., and Dringen, R. (2013). Astrocyte functions in the copper homeostasis of the brain. Neurochem. Int. 62, 556–565. doi: 10.1016/j.neuint.2012.08.017
Schousboe, A., Wellendorph, P., Frølund, B., Clausen, R. P., and Krogsgaard-Larsen, P. (2017). Astrocytic GABA transporters: pharmacological properties and targets for antiepileptic drugs. Adv. Neurobiol. 16, 283–296. doi: 10.1007/978-3-319-55769-4_14
Sequeira, A., Shen, K., Gottlieb, A., and Limon, A. (2019). Human brain transcriptome analysis finds region- and subject-specific expression signatures of GABAAR subunits. Commun. Biol. 2:153. doi: 10.1038/s42003-019-0413-7
Serrano, A., Haddjeri, N., Lacaille, J. C., and Robitaille, R. (2006). GABAergic network activation of glial cells underlies hippocampal heterosynaptic depression. J. Neurosci. 26, 5370–5382. doi: 10.1523/JNEUROSCI.5255-05.2006
Shigetomi, E., Tong, X., Kwan, K. Y., Corey, D. P., and Khakh, B. S. (2011). TRPA1 channels regulate astrocyte resting calcium and inhibitory synapse efficacy through GAT-3. Nat. Neurosci. 15, 70–80. doi: 10.1038/nn.3000
Slobodyansky, E., Guidotti, A., Wambebe, C., Berkovich, A., and Costa, E. (1989). Isolation and characterization of a rat brain triakontatetraneuropeptide, a posttranslational product of diazepam binding inhibitor: specific action at the Ro 5–4864 recognition site. J. Neurochem. 53, 1276–1284. doi: 10.1111/j.1471-4159.1989.tb07425.x
Song, I., Volynski, K., Brenner, T., Ushkaryov, Y., Walker, M., Semyanov, A., et al. (2013). Different transporter systems regulate extracellular GABA from vesicular and non-vesicular sources. Front. Cell. Neurosci. 7:23. doi: 10.3389/fncel.2013.00023
Srivastava, I., Vazquez-Juarez, E., Henning, L., Gómez-Galán, M., and Lindskog, M. (2020). Blocking astrocytic GABA restores synaptic plasticity in prefrontal cortex of rat model of depression. Cells 9:1705. doi: 10.3390/cells9071705
Szabó, Z., Péter, M., Héja, L., and Kardos, J. (2021). Dual role for astroglial copper-assisted polyamine metabolism during intense network activity. Biomolecules 11:604. doi: 10.3390/biom11040604
Tateishi, N., Shimoda, T., Manako, J., Katsumata, S., Shinagawa, R., and Ohno, H. (2006). Relevance of astrocytic activation to reductions of astrocytic GABAA receptors. Brain Res. 1089, 79–91. doi: 10.1016/j.brainres.2006.02.139
Tonon, M. C., Vaudry, H., Chuquet, J., Guillebaud, F., Fan, J., Masmoudi-Kouki, O., et al. (2020). Endozepines and their receptors: structure, functions and pathophysiological significance. Pharmacol. Ther. 208:107386. doi: 10.1016/j.pharmthera.2019.06.008
Tritsch, N. X., Granger, A. J., and Sabatini, B. L. (2016). Mechanisms and functions of GABA co-release. Nat. Rev. Neurosci. 17, 139–145. doi: 10.1038/nrn.2015.21
Vélez-Fort, M., Audinat, E., and Angulo, M. C. (2012). Central role of GABA in neuron-glia interactions. Neuroscientist 18, 237–250. doi: 10.1177/1073858411403317
Varela, L., Stutz, B., Song, J. E., Kim, J. G., Liu, Z. W., Gao, X. B., et al. (2021). Hunger-promoting AgRP neurons trigger an astrocyte-mediated feed-forward autoactivation loop in mice. J. Clin. Invest. 131:e144239. doi: 10.1172/JCI144239
Vogt, K. E., and Nicoll, R. A. (1999). Glutamate and gamma-aminobutyric acid mediate a heterosynaptic depression at mossy fiber synapses in the hippocampus. Proc. Natl. Acad. Sci. U S A 96, 1118–1122. doi: 10.1073/pnas.96.3.1118
Wójtowicz, A. M., Dvorzhak, A., Semtner, M., and Grantyn, R. (2013). Reduced tonic inhibition in striatal output neurons from Huntington mice due to loss of astrocytic GABA release through GAT-3. Front. Neural Circuits 7:188. doi: 10.3389/fncir.2013.00188
Ward, P. S., and Thompson, C. B. (2012). Metabolic reprogramming: a cancer hallmark even warburg did not anticipate. Cancer Cell 21, 297–308. doi: 10.1016/j.ccr.2012.02.014
Wu, Z., Guo, Z., Gearing, M., and Chen, G. (2014). Tonic inhibition in dentate gyrus impairs long-term potentiation and memory in an Alzheimer’s (corrected) disease model. Nat. Commun. 5:4159. doi: 10.1038/ncomms5159
Xia, X., Wang, Y., Qin, Y., Zhao, S., and Zheng, J. C. (2022). Exosome: a novel neurotransmission modulator or non-canonical neurotransmitter? Ageing Res. Rev. 74:101558. doi: 10.1016/j.arr.2021.101558
Yoon, B. E., and Lee, C. J. (2014). GABA as a rising gliotransmitter. Front. Neural Circuits 8:141. doi: 10.3389/fncir.2014.00141
Yoon, B. E., Woo, J., Chun, Y. E., Chun, H., Jo, S., Bae, J. Y., et al. (2014). Glial GABA, synthesized by monoamine oxidase B, mediates tonic inhibition. J. Physiol. 592, 4951–4968. doi: 10.1113/jphysiol.2014.278754
Yoon, B. E., Woo, J., and Lee, C. J. (2012). Astrocytes as GABA-ergic and GABA-ceptive cells. Neurochem. Res. 37, 2474–2479. doi: 10.1007/s11064-012-0808-z
Yoshida, H., Tsunoda, Y., and Owyang, C. (1999). Diazepam-binding inhibito r33–50 elicits Ca2+ oscillation and CCK secretion in STC-1 cells via L-type Ca2+ channels. Am. J. Physiol. 276, G694–G702. doi: 10.1152/ajpgi.1999.276.3.G694
Young, S. Z., Platel, J. C., Nielsen, J. V., Jensen, N. A., and Bordey, A. (2010). GABAA Increases calcium in subventricular zone astrocyte-like cells through L- and T-Type voltage-gated calcium channels. Front. Cell. Neurosci. 4:8. doi: 10.3389/fncel.2010.00008
Yu, X. A., Taylor, M. W., Nagai, J., Golshani, P., Evans, C. J., Coppola, G., et al. (2018). Reducing astrocyte calcium signaling in vivo alters striatal microcircuits and causes repetitive behavior. Neuron 99, 1170–1187. doi: 10.1016/j.neuron.2018.08.015
Zheng, T., Santi, M. R., Bovolin, P., Marlier, L. N., and Grayson, D. R. (1993). Developmental expression of the alpha 6 GABAA receptor subunit mRNA occurs only after cerebellar granule cell migration. Brain Res. Dev. Brain Res. 75, 91–103. doi: 10.1016/0165-3806(93)90068-l
Keywords: astrocyte, GABA, brain, neuron, microglia, gliotransmitter
Citation: Liu J, Feng X, Wang Y, Xia X, and Zheng JC (2022) Astrocytes: GABAceptive and GABAergic Cells in the Brain. Front. Cell. Neurosci. 16:892497. doi: 10.3389/fncel.2022.892497
Received: 09 March 2022; Accepted: 17 May 2022;
Published: 10 June 2022.
Edited by:
Eleonora Palma, Sapienza University of Rome, ItalyReviewed by:
Agenor Limon, University of Texas Medical Branch at Galveston, United StatesAtsuo Fukuda, Hamamatsu University School of Medicine, Japan
Copyright © 2022 Liu, Feng, Wang, Xia and Zheng. This is an open-access article distributed under the terms of the Creative Commons Attribution License (CC BY). The use, distribution or reproduction in other forums is permitted, provided the original author(s) and the copyright owner(s) are credited and that the original publication in this journal is cited, in accordance with accepted academic practice. No use, distribution or reproduction is permitted which does not comply with these terms.
*Correspondence: Jialin C. Zheng, amlhbGluemhlbmdAdG9uZ2ppLmVkdS5jbg==; Xiaohuan Xia, eGlhb2h1YW5feGlhMUAxNjMuY29t
† These authors have contributed equally to this work