- Department of Neurosurgery, Fujian Medical University Union Hospital, Fuzhou, China
Spinal cord injury (SCI) not only affects the quality of life of patients but also poses a heavy burden on their families. Therefore, it is essential to prevent the occurrence of SCI; for unpreventable SCI, it is critical to develop effective treatments. In recent years, various major breakthroughs have been made in cell therapy to protect and regenerate the damaged spinal cord via various mechanisms such as immune regulation, paracrine signaling, extracellular matrix (ECM) modification, and lost cell replacement. Nevertheless, many recent studies have shown that the cell therapy has many disadvantages, such as tumorigenicity, low survival rate, and immune rejection. Because of these disadvantages, the clinical application of cell therapy is limited. In recent years, the role of exosomes in various diseases and their therapeutic potential have attracted much attention. The same is true for exosomal noncoding RNAs (ncRNAs), which do not encode proteins but affect transcriptional and translational processes by targeting specific mRNAs. This review focuses on the mechanism of action of exosomes obtained from different cell sources in the treatment of SCI and the regulatory role and therapeutic potential of exosomal ncRNAs. This review also discusses the future opportunities and challenges, proposing that exosomes and exosomal ncRNAs might be promising tools for the treatment of SCI.
Introduction
According to the etiology, spinal cord injury (SCI) can be divided into traumatic and nontraumatic. Traumatic SCI is often caused by severe damage to the spinal cord due to external physical impacts (for example, car accidents, falls, sports-related injuries, or violence). Nontraumatic SCI often occurs during acute or chronic diseases (for example, tumors, infections, disc herniation, or vertebral fracture-dislocations), causing spinal cord compression to produce primary and secondary injuries. SCI not only affects the quality of life of patients but also poses a heavy burden on their families. Therefore, it is essential to prevent the occurrence of SCI; for unpreventable SCI, it is critical to develop effective treatments (Ahuja et al., 2017).
A central concept in managing any SCI patient has been “time is the spine” (Ahuja et al., 2017). SCI is characterized by the progressive loss of neurologic function within a few hours. Therefore, it is essential to rapidly diagnose patients and provide neuroprotective interventions in the acute injury stage. Because of these treatments including hemodynamics (Ryken et al., 2013), hormonal therapy (Hurlbert et al., 2015), and surgical decompression (Ramakonar and Fehlings, 2021), long-term functional recovery is improved in patients. Management of patients with SCI is complex and involves multiple stages of care, often lasting several years after the initial injury. Thus, later rehabilitation is also an integral part of the treatment process (Gómara-Toldrà et al., 2014; van der Scheer et al., 2021).
However, these treatments are not adequate for long-term functional recovery in SCI patients. In recent years, various major breakthroughs have been made in cell therapy to protect and regenerate the damaged spinal cord via various mechanisms such as immune regulation, paracrine signaling, extracellular matrix (ECM) modification, and lost cell replacement. Among them, the most commonly studied and promising cell types include induced pluripotent stem cells (iPSC), mesenchymal stem cells (MSCs), neural stem cells (NSCs), oligodendrocyte progenitor cells (OPCs), Schwann cells (SCs), and olfactory ensheathing cells (OECs; Harrop et al., 2012; Shao et al., 2019; Ahuja et al., 2020). Nevertheless, many recent studies have shown that the cell therapy has many disadvantages, such as tumorigenicity, low survival rate, and immune rejection. Because of these disadvantages, the clinical application of cell therapy is limited (Feng et al., 2021; Liu J. et al., 2021).
The remarkable effect of cell therapy can be attributed to its dominant paracrine effect. Exosomes, an intercellular communication tool, affect normal and pathological conditions. In recent years, the role of exosomes in various diseases and their therapeutic potential have attracted much attention. The same is true for exosomal noncoding RNAs (ncRNAs), which do not encode proteins but affect transcriptional and translational processes by targeting specific mRNAs. Their diverse functions have attracted much interest (Colombo et al., 2014; Quinzaños-Fresnedo and Sahagún-Olmos, 2015; Shi et al., 2018; Dutta et al., 2021). The types of source cells can influence the heterogeneity of exosomes, which have different contents and specific markers from varied cell sources. For example, ERBB2 is specifically expressed in breast cancer cell-derived exosomes, and TSPAN8 is a specific marker of epithelial cell-derived exosomes. The inherent biology and the microenvironment of the cells can also give exosomes distinct functions such as uptake by specific cells and tropism to certain organs (Kalluri and LeBleu, 2020). Especially, exosomal miRNAs have attracted substantial attention because of their various functions in the context of SCI treatment. They vary widely with different cell sources (Cho et al., 2019). For example, neuron-derived exosomes were enriched for miRNA-383, whereas glial cells-derived exosomes were not (Pomper et al., 2020). It is noteworthy that a study directly compared the efficacy of human pluripotent stem cells (hPSCs)-derived and MSCs-derived exosomes in an animal model of ischemic stroke. Results showed that hPSCs-derived exosomes were more effective than MSCs-derived (Webb et al., 2018).
MSCs-derived exosomes are the most widely studied to date in the treatment of SCI. However, no studies have directly compared the efficacy of exosomes from different cell sources in the treatment of SCI (Dutta et al., 2021). Therefore, it is essential to directly compare the therapeutic potential of exosomes from varied cell sources in the treatment of SCI. This review focuses on the mechanism of action and therapeutic potential of exosomes and exosomal ncRNAs from different cell sources in the treatment of SCI. This review also discusses the future opportunities and challenges, proposing that exosomes and exosomal ncRNAs from different cell sources might be promising tools for the treatment of SCI.
Pathophysiological Process of SCI
According to its pathological process, traumatic SCI has a complex pathophysiological process, and it can be broadly classified into primary and secondary injuries. The primary damage can immediately cause mechanical destruction and dislocation of the spine, causing spinal cord compression or transection. Subsequently, the primary injury leads to a continuous cascade of secondary injuries, causing further damage to the spinal cord and neurological dysfunction. Then, this injury causes damage to myelin, axons, and neurons. Moreover, it also disrupts the blood-spinal cord barrier. The primary damage is often irreversible, while the secondary injury usually causes more severe damage than the primary injury (Tator, 1995; McDonald and Sadowsky, 2002; Ahuja et al., 2017).
SCI is divided into five stages depending on the pathophysiological process and the time of injury: hyperacute phase (0–2 h), acute phase (2–48 h), subacute phase (2–14 days), intermediate phase (2 weeks to 6 months), and chronic phase (>6 months; Rowland et al., 2008). In the hyperacute phase (0–2 h), it is characterized by traumatic axons, hemorrhagic necrosis of gray matter, and microglial activation releasing proinflammatory cytokines. The proinflammatory cytokines TNFα and IL-1β can be immediately released by the microglial after injury (Donnelly and Popovich, 2008). In the acute phase (2–48 h), it is characterized by vasogenic and cytotoxic edema, persistent hemorrhage and necrosis of glutamate-mediated excitotoxicity, disruption of blood-spinal cord barrier (BSCB) permeability, early demyelination, axonal swelling, and neuronal death. The BSCB can remain disrupted even at 28 days after SCI and spread along the entire length of the cord (Whetstone et al., 2003). The complex network of tight junction (TJ) proteins, which are the major protein component of the BSCB, can be modulated by inflammatory cytokines (Lee et al., 2012; Kumar et al., 2017). In the subacute phase (2–14 days), it is characterized by persistent edema, thrombosis, and vasospasm aggravate ischemia. A continual inflammatory cell infiltration causes further cell death, forming cavities. In addition, reactive astrocytes act as a barrier to prevent damage from aggravating but secrete some inhibitory ECM molecules around the lesion. Chondroitin sulfate proteoglycans (CSPGs), which are a key component of the ECM, can inhibit axon regeneration through binding to their major cognate receptor, Protein Tyrosine Phosphatase Sigma (PTPσ; Sakamoto et al., 2019). Therefore, blocking the combination of them can effectively promote axonal regeneration. In the intermediate phase (2 weeks to 6 months), the axons continue to degenerate, and reactive astrocyte scars mature, becoming an effective regenerative inhibitor, and the cysts merge to limit axonal regeneration and cell migration. The scars have two distinct components after SCI: the lesion core, which primarily includes macrophages and fibroblasts, is generally considered as the fibrotic scar, and the lesion border, which is predominantly composed of microglia, reactive astrocytes, and NG2+ oligodendrocyte progenitor cells, is commonly regarded as a glial scar (Bradbury and Burnside, 2019; Tran et al., 2021). In the traditional concept, the glial scar is considered to exert a detrimental function for neurological recovery, which not only secretes some inhibitory extracellular matrix molecules, cytokines, and oxidative stress products but also inhibits axonal regeneration as a chemical barrier (Silver and Miller, 2004). However, many studies have confirmed that glial scar plays a vital role in neuroprotection. It can limit the spread of inflammation at the injury site to the surrounding injury as a barrier. And it can also secrete neurotrophic factors (nerve growth factor and fibroblast growth factor) and extracellular matrix proteins (laminin and fibronectin; Lukovic et al., 2015). Therefore, an increasing number of studies are focusing on the beneficial function of the glial scar for neurological recovery. In the chronic phase (>6 months), the Wallerian degeneration process of severed axons continues, and the severed axons and their cell bodies may take years to be completely removed (Ehlers, 2004). Unfortunately, neurological dysfunction and neuropathic pain will be caused by the formation of syringomyelia (Todor et al., 2000). Therefore, therapeutic strategies aim to improve axonal degeneration and demyelination by drug or cell transplantation.
The Role of Exosomes and Exosomal ncRNAs from Different Cell Sources in The Treatment of SCI
Exosomes are small extracellular vesicles (EVs) of 40–150 nm, endosome-derived, secreted by most cells. They have been isolated from many biological fluids, including blood, urine, semen, and cerebrospinal fluid (Kalra et al., 2016). During the formation of exosomes, the first phase is the early invagination of the endosome membrane allows intracellular components to be engulfed in early sorting endosomes (ESE), with the participation of mitochondria, Golgi apparatus, and the endoplasmic reticulum (Hessvik and Llorente, 2018). Then, the ESEs can mature into the late sorting endosomes (LSEs) with the participation of the endosomal-sorting complex necessary for transport (ESCRT) proteins (Vietri et al., 2020). Eventually, the LSEs generate multivesicular bodies (MVBs) after the selective integration of substances. The MVBs contain several vesicular intraluminal vesicles (ILVs; van Niel et al., 2018). Then, MVBs fuse with the plasma membrane and then release ILVs as exosomes into the extracellular space. Also, they can fuse with lysosomes or autophagosomes to be degraded (Thery et al., 2002; Colombo et al., 2014; van Niel et al., 2018; Jeppesen et al., 2019; Figure 1) Moreover, exosomes can be taken up by recipient cells through phagocytosis, direct fusion, endocytosis, and ligand-receptor interactions. Then, the contents of the exosomes can be deposited into the cytoplasm (Kalluri and LeBleu, 2020).
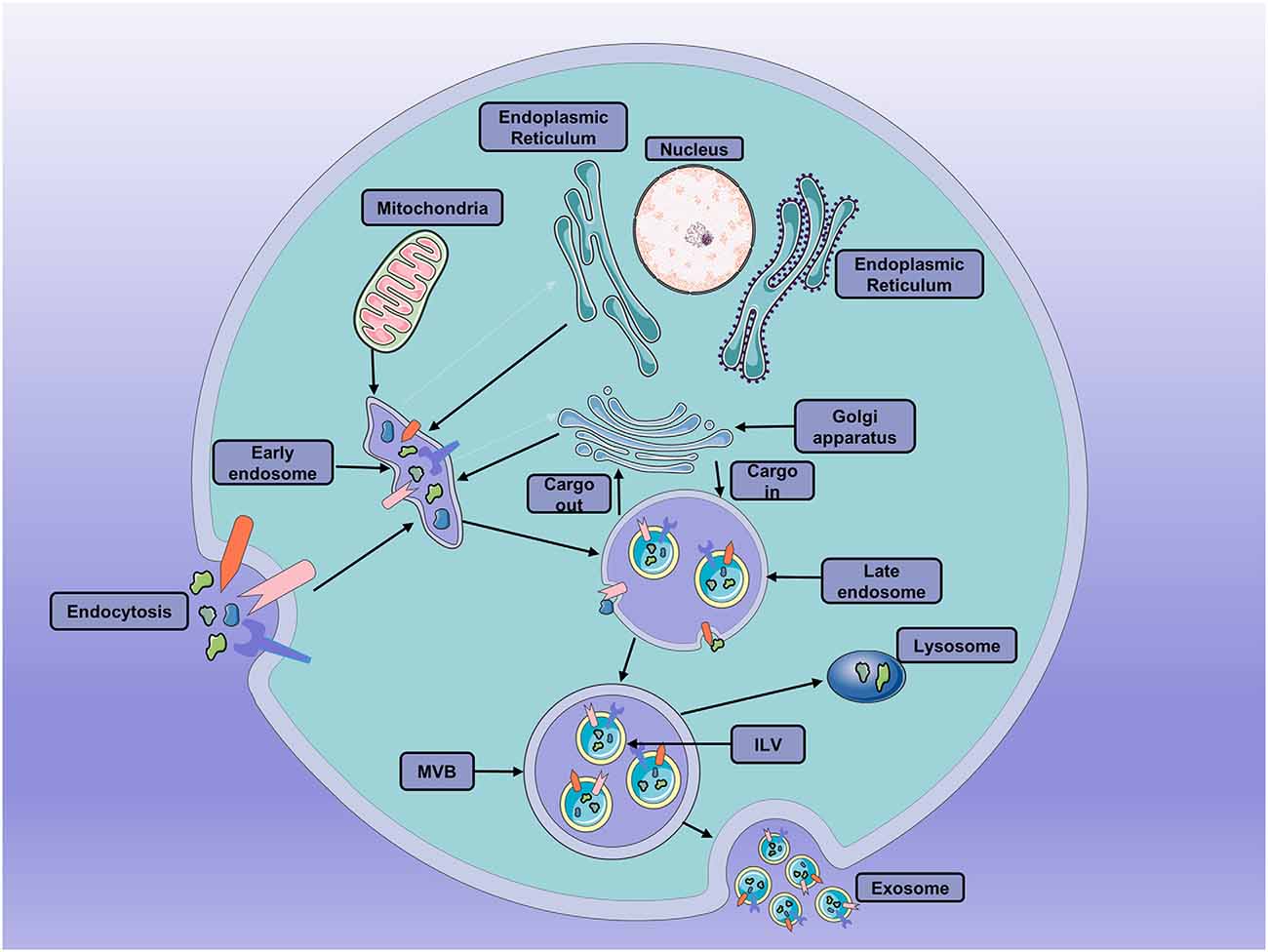
Figure 1. The processes of exosomes formation, secretion and fusion. Early invagination of the endosome membrane allows intracellular components to be engulfed in vesicular intraluminal vesicles (ILVs). Then, the late endosomes become multivesicular bodies (MVBs) after the selective integration of substances by early endosomes. MVBs can either fuse with the plasma membrane and then release ILVs as exosomes into the extracellular space or fuse with lysosomes or autophagosomes to be degraded.
Because exosomes are derived from endosomes, these substances, including the proteins involved in MVB formation (Alix and TSG101), membrane transport and fusion (annexins, GTPases), adhesion (integrins), tetraspanins (CD9, CD63, CD81), antigen presentation [major histocompatibility complex (MHC) class molecules], heat shock proteins (HSP70, HSP90), and other related proteins, are commonly used to identify exosomes regardless of the cell type of origin. In addition to proteins, exosomes are rich in specific lipids, mainly containing ceramide, cholesterol, and sphingolipids. And the lipids contribute to the formation and structural stability of exosomes (Mashouri et al., 2019). Exosomes also contain surface polysaccharides and glycans, mainly containing mannose, α-2,6-sialic acid, and polyglactin. They present at the plasma membrane of exosomes, contributing to the docking and attachment of these exosomes to recipient cells, especially the Glypican 1 (Melo et al., 2015). Exosomes have been reported to carry DNA and RNAs, including mRNAs and some ncRNAs (Lotvall et al., 2014; Kalra et al., 2016; Thery et al., 2018; Figure 2) Although the ability of exosomes to contain DNA remains controversial, there have been many pieces of research showing that they are used for identification (Thakur et al., 2014; Hagey et al., 2021). Exosomal RNAs are secreted to regulate intercellular communication; and miRNAs, in particular, play a vital role in various biological mechanisms (Treiber et al., 2019). However, the composition and function of exosomes remain to be fully elucidated.
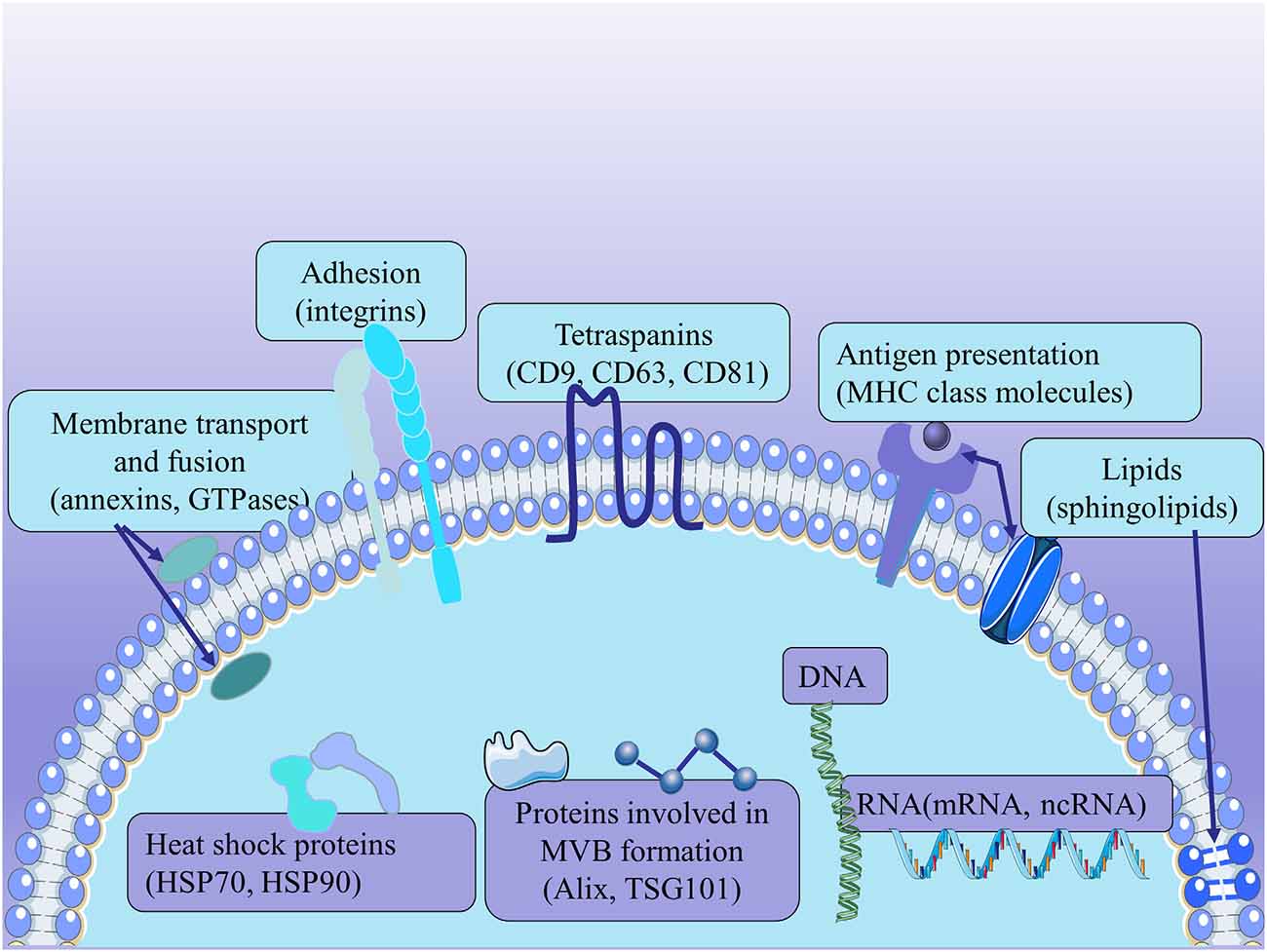
Figure 2. The structure of exosomes. Exosomes express the proteins involved in MVB formation (Alix and TSG101), membrane transport and fusion (annexins, GTPases), adhesion (integrins), tetraspanins (CD9, CD63, CD81), antigen presentation [major histocompatibility complex (MHC) class molecules], and heat shock proteins (HSP70, HSP90). Exosomes derived from MSCs carry a complex cargo, including nucleic acids, proteins, lipids, and enzymes.
Around 98% of all the genomic output is ncRNAs in the data from genome-wide transcriptional analysis in humans (Mattick, 2001). According to the size of ncRNAs, ncRNAs are mainly divided into two groups: long ncRNAs with more than 200 nucleotides and small ncRNAs with no more than 200 nucleotides (Mercer et al., 2009). Interestingly, although ncRNAs do not code for proteins, they have diverse functions in physiology and development of the organisms (Amaral and Mattick, 2008). For this reason, an increasing number of studies have shown that many ncRNAs, particularly noncoding small RNAs (microRNAs), long ncRNAs (lncRNAs), and circular RNAs (circRNAs) become differentially expressed after SCI. In the treatment of SCI, these ncRNAs regulate the translation and transcription mainly by targeting specific mRNAs to affect neuronal survival, axonal regeneration, and glial cell phenotype (Zhou et al., 2016; Bie et al., 2021). MicroRNAs (miRNAs) are 20–24 nucleotide RNA molecules with the function of regulating the protein expression levels by affecting mRNA. Their pivotal role in SCI can be attributed to individual miRNAs that can target the translation of many mRNAs (Bhalala et al., 2013). There are two modes of action for miRNAs binding to the 3’-untranslated region (3’-UTR) of target mRNA. One is that the perfect binding of miRNAs to targets induces mRNA degradation, and the other is that imperfect binding of miRNAs to targets represses translation (Shahzad et al., 2021). The two modes of action will prevent protein accumulation by an unknown mechanism. Furthermore, lncRNAs will act as endogenous RNA to compete for miRNAs binding to regulate gene expression. lncRNAs are defined as thousands of RNA transcripts of more than 200 nucleotides in length without protein-coding potential, which has attracted much attention in various fields (Shi et al., 2018). A study showed that the effect of miR-203 and miR-101, which can down-regulate the expression of BARD1 protein, can be counteracted by a novel lncRNA (BARD1 9’L). These findings subvert our perception of the biological function of ncRNAs, from thinking that they are nonfunctional transcriptional junk to gaining insight into their involvement in the pathogenesis of various diseases (Lee, 2012). It is reported that the exosomes play a role in intercellular communication. The attachment of RNA-induced silencing complexes (RISCs) to the ESCRT components makes the ncRNAs recruit to the exosomes (Sato-Kuwabara et al., 2015) and the ncRNAs, which are one of the enriched cargo in exosomes, can be exported outside cells to target specific mRNAs. Ceramide-dependent machinery controls the release of exosomal ncRNAs (Kosaka et al., 2010). For this reason, an increasing number of studies are focusing on the role of the exosomal ncRNAs as potential therapeutic strategies in SCI (Pant et al., 2021). Exosomes derived from different cells exhibit their functions, both in vivo or in vitro, and in healthy or disease states (Lotvall et al., 2014; Thery et al., 2018). Their differential expression in diverse states can be used as a particular biomarker, provide new therapeutic targets for diseases, and even used as a therapeutic approach to replace or assist cell therapy (Chen et al., 2017). Therefore, we summarize the respective roles of exosomes and exosomal ncRNAs obtained from different cell sources in SCI treatment with great confidence for their promotion of functional recovery after SCI (Figure 3).
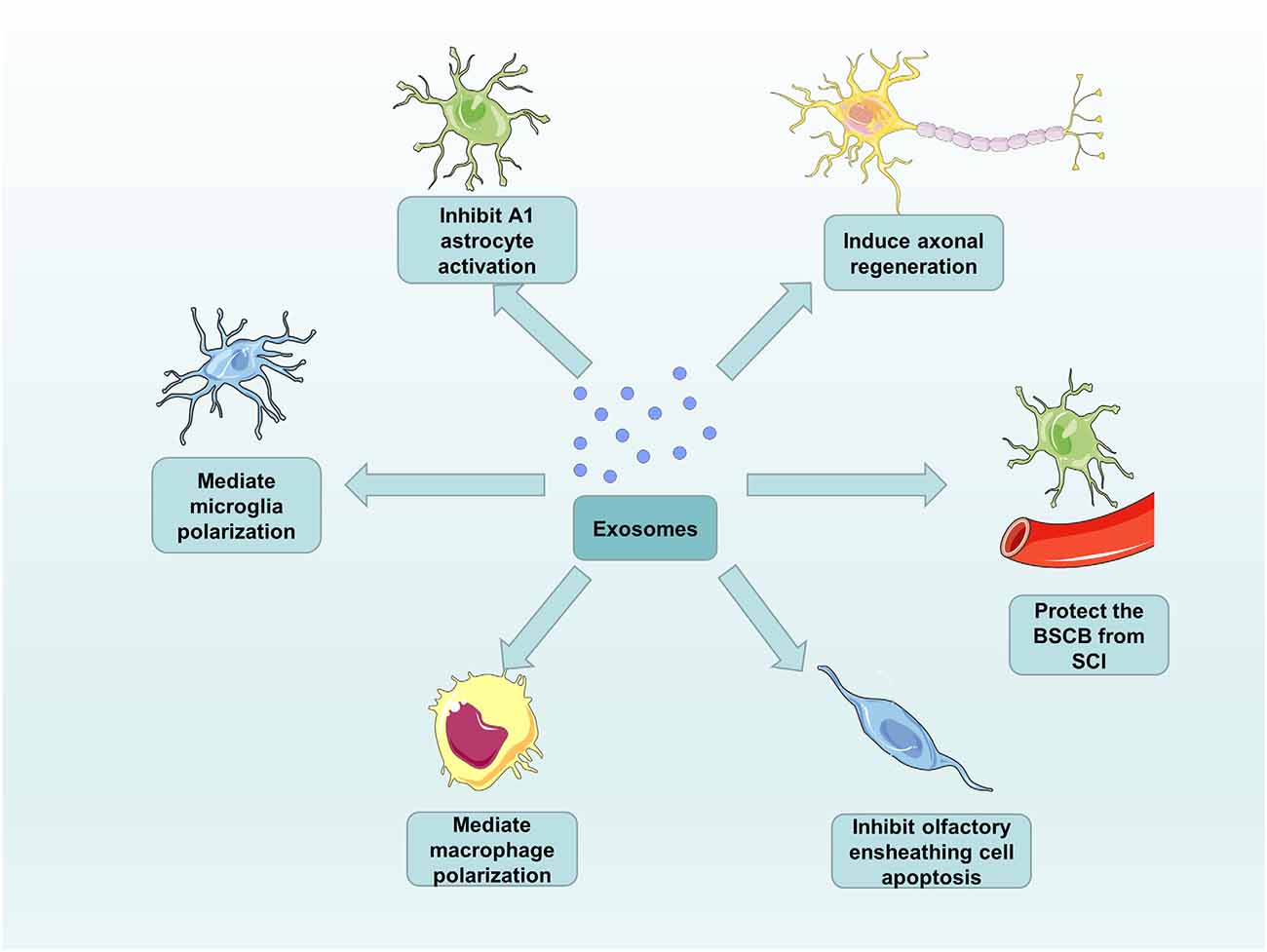
Figure 3. The functions of exosomes in SCI repair. Exosomes from different cell sources can inhibit A1 astrocyte activation and olfactory ensheathing cell apoptosis, as well as induce axonal regeneration, mediate microglia and macrophage polarization, and protect the BSCB from SCI.
MSCs Sources
In the treatment of SCI, the beneficial effects of MSC transplants have been demonstrated in different experimental studies. There are many sources of MSCs, such as bone marrow-derived MSCs, adipose-derived MSCs, and umbilical cord-derived MSCs. Among them, bone marrow-derived MSCs are widely studied as well as MSC-derived exosomes (Liau et al., 2020; Ren et al., 2020; Andrzejewska et al., 2021). It has been reported that BMSCs-Exos effectively promotes the formation of capillaries and improves the migration of human umbilical vein endothelial cells (HUVECs) in vitro. In the GLU-induced excitotoxicity model, the number of TUNEL-positive neuronal cells was significantly reduced after the treatment of BMSCs-Exos, indicating that BMSCs-Exos have neuroprotective function. In an SCI rat model intravenously injected with BMSCs-Exos, the lesion area became smaller, and CSPG deposition was significantly reduced, indicating that it inhibited glial scar formation. The expression levels of inflammatory markers including TNF-α, IL-1β, and IL-6 significantly decreased, indicating the alleviation of the inflammatory response. The degree of NF200 staining reduction at the injury site was significantly lower than that in the untreated group, indicating that it promoted axonal regeneration and neuronal survival. The number of C3-positive reactive astrocytes was significantly reduced, indicating that BMSCs-Exos inhibited the activation of A1 neurotoxic reactive astrocytes (Liu et al., 2019). Moreover, some studies have shown that BMSCs-Exos effectively inhibited pericyte apoptosis and maintained BSCB integrity by regulating NOD1-related signaling pathways in vitro. Therefore, the pericyte level is increased, thereby enhancing the functional recovery after SCI (Zhou et al., 2022). After the treatment of BMSCs-Exos, the expression levels of autophagy-related proteins LC3B and Beclin-1 were increased. Also, the formation of autophagosomes was promoted. Besides, the expression level of caspase-3 cleaved by proapoptotic proteins was significantly decreased, while the expression of antiapoptotic protein Bcl-2 was upregulated. These results show that BMSCs-Exos can reduce neuronal apoptosis and promote the recovery of functional behavior in SCI rats by promoting autophagy, thus providing a new target for the treatment of SCI (Gu et al., 2020). Studies have shown that the complementary levels such as C6, C4 binding protein α, and complement factor H increase after SCI. Otherwise, BMSCs-Exos treatment can effectively attenuate the increasing trend of complementary levels. BMSCs-Exos can also bind to microglia at the injury site and inhibit the nuclear factor kappa-B (NF-κB) activated by SCI, thus exerting a protective effect (Zhao et al., 2019).
Studies have shown that the intravenous injection of human placental stem cell-derived exosomes (hpMSCs-Exos) significantly increased the expression of neural stem/progenitor cell markers in the spinal cord. The proliferation ability of nerve progenitor cells (NPCs) also increases, indicating that hpMSCs-Exos can promote endogenous NPC and neurogenesis activation and promote the recovery of motor and autonomic function after SCI (Zhou et al., 2021). Essentially, hpMSCs-Exos promoted vessel formation and migration of HUVECs in vitro, but also significantly increased the vessel number, vessel volume fraction, and vascular connectivity in a rat model of SCI (Zhang et al., 2020). The experimental results of other studies have shown that human Wharton’s jelly stem cell-derived extracellular vesicles (WJMSCs-EVs) can inhibit neuroinflammation after SCI, reduce cell death, and thus restore the motor function. WJMSCs-EVs can also decrease the GFAP expression, prevent glial scar formation, and promote regeneration by stimulating NPC (Noori et al., 2021). In in vivo and in vitro experiments, human umbilical cord stem cell-derived exosomes (hucMSCs-Exos) inhibited the secretion of their proinflammatory factors and promoted the production of anti-inflammatory factors by promoting the polarization of M1 macrophages to M2 macrophages. Ultimately, hucMSCs-Exos plays a role in controlling the inflammatory response (Sun et al., 2018). Recently, it has been shown that epidural adipose-derived exosomes (EF-MSCs-Exos) injected into the tail vein of SCI rats can improve their neurological recovery and reduce the lesion volume in SCI rats. Besides, EF-MSCs-Exos can inhibit the activation of NLRP3 inflammasome and reduce the expression of proinflammatory factors. In addition, EF-MSCs-Exos treatment can also reduce the expression level of proapoptotic protein Bax and upregulate the expression level of antiapoptotic protein Bcl-2 (Huang et al., 2020a).
Recently, it has been demonstrated that miRNAs discovery in exosomes can be exported outside cells and affect gene expression in distant cells (Colombo et al., 2014). Therefore, exosomes derived from miRNA mimics or antisense miRNAs-modified cells can overexpress or inhibit miRNAs in exosomes, thereby being used to treat SCI (Bhalala et al., 2013). Because exosomes and exosomal miRNAs from MSCs are the most widely studied to date, we summarize the existing studies on the use of MSC-derived exosomes and exosomal miRNAs for the treatment of SCI as follows (Table 1). Recent studies have also shown that the expression of lncRNAs changes after SCI, and lncRNAs might play a crucial role in the pathological process of SCI, unlike miRNAs, and lncRNAs have their specific characteristics (Yu et al., 2015). Recently, a study showed that lncRNA-Gm37494 expression was upregulated in exosomes (Exos) produced by adipose-derived stem cells (ADSCs) under hypoxia. After overexpressing in ADSCs-Exos by transfection with lncRNA-Gm37494, lncRNA-Gm37494 inhibited BV2 microglia polarization to M1 type and promote their polarization to M2 type by inhibiting miR-130b-3p and promoting PPARγ expression, ultimately achieving the purpose of repairing SCI (Shao et al., 2020).
NSCs/NPCs Sources
NSCs/NPCs exhibit nerve regeneration and neuroprotective effects; the transplantation of such cells into damaged tissue sites is a promising SCI therapy. Similarly, a large number of preclinical studies and some clinical studies have shown its unique advantages (Csobonyeiova et al., 2019). Nonetheless, NSCs/NPCs also suffer from the same problems as others, such as tumorigenicity and immune rejection. Fortunately, the neural stem/progenitor cell-derived exosomes (NSCs/NPCs-Exos) discovered in recent years can overcome these disadvantages of stem cell transplantation and might play the same role to a certain extent. At present, NSCs/NPCs-Exos have been gradually studied in nerve-related diseases such as stroke and brain injury, but they still need to be developed for SCI treatment (Vogel et al., 2018). Only some studies have evaluated NSCs/NPCs-Exos for the treatment of SCI. It has been demonstrated that NSCs-Exos can significantly reduce the extent of SCI and promote functional recovery and microglial activation in rats. More importantly, NSCs-Exos treatments increased the expression levels of autophagy-related proteins LC3B and Beclin-1. Also, they could promote the formation of autophagosomes. Furthermore, the expression level of caspase-3 cleaved by proapoptotic proteins clearly decreased, while the expression of antiapoptotic protein Bcl-2 was upregulated. These results indicate that NSCs-Exos reduced neuronal apoptosis and benefited the recovery of functional behavior in SCI rats by improving autophagy (Rong et al., 2019). It has also been shown that NSCs-Exos can promote the migration, proliferation, and angiogenesis of spinal cord microvascular endothelial cells (SCMECs) after trauma in vitro. SCMECs can also increase the microvessel density, spinal canal shrinkage, and motor function recovery in the rat models of SCI. Moreover, this study further showed that NSCs-Exos exhibited proangiogenic effects on SCMECs by transferring vascular endothelial growth factor A (VEGF-A) and enhancing microvascular regeneration and tissue healing (Zhong et al., 2020). Recently, a study showed that miR-29b expression was upregulated in exosomes (Exos) produced by human neuroepithelial stem cells (HNESCs). After overexpressing in HNESCs-Exos by transfection with miR-29b, miR-29b subsequently suppressed the apoptosis of neuron cells by down-regulating the expression of PTEN/caspase-3, ultimately achieving the purpose of repairing SCI (Kang et al., 2020). Furthermore, another study showed that miR-219a-2–3p expression was upregulated in exosomes (Exos) produced by neural stem cells (NSCs). After overexpressing in NSCs-Exos by transfection with miR-219a-2–3p, miR-219a-2–3p attenuated apoptosis and neuroinflammation by down-regulating the expression of YY1/NF-κB, ultimately achieving the purpose of repairing SCI (Ma et al., 2019).
Other Cell Sources
Nerve regeneration is related to various cells in the tissue microenvironment, and different types of cells in diverse states produce the corresponding effects on the target recipient cells. For example, SCs cannot only migrate to the damaged tissue area to become a key component of nerve regeneration but also secrete signaling molecules to attract macrophages, activate local MSC, and interact with other cell types (Min et al., 2021). SC-derived exosomes obtained from the skin (SKP-SCs-Exos) have also been studied; they can regulate cell growth and death signaling pathways mediated by Akt/mTOR/p70S6K. SKP-SCs-Exos can enhance the recovery of neuronal viability and axonal regeneration in the in vivo and in vitro models (Wu et al., 2020). It has also been shown that SC-derived exosomes (SCDEs) obtained from the sciatic nerve can reduce the deposition of chondroitin sulfate proteoglycans (CSPGs) deposition by increasing Toll-like receptor 2 (TLR2) expression on astrocytes through the NF-κB/PI3K signaling pathway, thereby promoting the functional recovery in mice after SCI (Pan et al., 2021). Recently, it has been demonstrated that peripheral macrophages (PMs) can effectively improve the microenvironment of the lesion site, and they are a pivotal factor in promoting the repair after SCI (Tsarouchas et al., 2018). Then, the mechanism of peripheral macrophage-derived exosomes (PMs-Exos) in the treatment of SCI has also been elucidated. PMs-Exos can activate microglial autophagy and enhance the polarization of anti-inflammatory microglia (M2) by inhibiting the PI3K/AKT/mTOR signaling pathway, thus playing a meaningful role in the anti-inflammation process of SCI repair (Zhang B. et al., 2021). Transplantation of OECs also has its unique advantages in SCI treatment, and it has a strong growth force. Particularly, it provides a suitable microenvironment and strong migration characteristics for axonal growth (Kato et al., 2000). HOECs-Exos can stimulate NPC proliferation to promote nerve regeneration in the in vitro models and improve NPC cytotoxicity during oxidative stress. Otherwise, the in vivo therapeutic effect in SCI rat model remains to be studied (Tu and Hsueh, 2020). Pericytes, a vital part of the neurovascular unit, have the same characteristics as stem cells. Moreover, pericytes interact with endothelial cells and maintain the stability of endothelial barrier. Treatment with pericyte-derived exosomes can promote blood flow and endothelial function to protect BSCB. Moreover, pericyte-derived exosomes can improve the functional and behavioral recovery after SCI by reducing the apoptotic response, and they can be cocultured with endothelial cells under hypoxic conditions in vitro can also reduce their permeability and play a protective role (Yuan et al., 2019). Recently, a study showed that miR-421-3p expression was upregulated in exosomes (Exos) produced by M2 bone marrow-derived macrophages (BMDMs). After overexpressing in BMDMs-Exos by transfection with miR-421-3p, miR-421-3p enhanced protective autophagy in neuronal cells by inhibiting the expression of mTOR protein, ultimately achieving the purpose of repairing SCI (Wang et al., 2020).
The Separation and Concentration Methods of Each Cell-Derived Exosomes in The Treatment of SCI
MSCs-Exos were separated and concentrated by ultracentrifugation and differential centrifugation in many studies (Huang et al., 2017, 2020a; Lankford et al., 2018; Wang et al., 2018; Guo et al., 2019; Ji et al., 2019; Kang et al., 2019; Li et al., 2019; Li C. et al., 2020; Li et al., 2021; Chen et al., 2021; Cheng et al., 2021; Han et al., 2021; Jia et al., 2021; Jiang and Zhang, 2021; Liu W. Z. et al., 2021; Liu et al., 2022; Nakazaki et al., 2021; Nie and Jiang, 2021; Noori et al., 2021; Sheng et al., 2021; Xiao et al., 2021; Xin et al., 2021; Zhang A. et al., 2021; Liang et al., 2022; Zhou et al., 2022) and by precipitation kits/polymer (PEG or others) in some studies (Li et al., 2018; Ren et al., 2019; Xu et al., 2019; Yu et al., 2019; Zhao et al., 2019; Fan et al., 2021; Jia et al., 2021a, b; Kang and Guo, 2022). To achieve better specificity of MSCs-Exos separation, many researchers isolated MSCs-Exos using ultrafiltration-centrifugation combined with density-gradient ultracentrifugation (Liu et al., 2020; Shao et al., 2020; Chang et al., 2021; Luo et al., 2021; Huang et al., 2022). Furthermore, some researchers used one or more techniques following the ultracentrifugation, such as density-gradient ultracentrifugation (Liu et al., 2019), size-exclusion chromatography (Li L. et al., 2020), and magnetic sorting (Kim et al., 2018). NSCs-Exos were separated and concentrated by ultracentrifugation (Ma et al., 2019), ultrafiltration-centrifugation (Zhong et al., 2020), and by kits methods (Kang et al., 2020). To achieve better specificity of NSCs-Exos separation, some researchers isolated NSCs-Exos using ultrafiltration-centrifugation combined with density-gradient ultracentrifugation (Rong et al., 2019). SKP-SCs-Exos were separated and concentrated by kits methods (Wu et al., 2020). SC-Exos (Pan et al., 2021), HOECs-Exos (Tu and Hsueh, 2020), and PMs-Exos (Zhang B. et al., 2021) were separated and concentrated by ultracentrifugation. BMDMs-Exos were separated and concentrated by ultracentrifugation and kits methods (Wang et al., 2020). There are no specific separation and concentration methods for exosomes from different cell sources. However, as reviewed in the 2018 guidelines, different isolation methods have their advantages and disadvantages (Thery et al., 2018). For example, the ultracentrifugation method has intermediate recovery and intermediate specificity. The kit method has high recovery but low specificity. Moreover, ultrafiltration-centrifugation combined with density-gradient ultracentrifugation has low recovery but high specificity. Although there are no high recovery and high specificity exosome isolation methods, dendritic cells (DCs)-derived exosomes have been applied in clinical trials to treat patients with malignant melanoma and non-small cell lung carcinoma and achieved some efficacy (Nikfarjam et al., 2020).
Prospects
Traditional drugs have numerous disadvantages: poor water solubility, rapid in vivo clearance, poor biocompatibility, unsatisfactory in vivo distribution, and low permeability. New drug carriers are being continuously developed to optimize and improve the bioavailability to solve these problems. In recent years, exosomes have also been developed for drug loading, which can improve the stability of drugs and exhibit natural targeting ability based on donor cells. Because it is a nanomolecule with cell surface substances, it can readily and selectively penetrate biological barriers (Batrakova and Kim, 2015; Antimisiaris et al., 2018). At present, there are two main methods of exosomes transplantation in the treatment of SCI (Table 1), including intrathecal injection and tail vein injection. Moreover, a meta-analysis showed that intrathecal therapy seems to be more effective than tail vein injection therapy (Yi and Wang, 2021). Interestingly, a study showed that exosomes could also be used to treat SCI by intranasal injection. More specifically, intranasal exosomes led to significant locomotor recovery as compared to the intrathecal exosomes group (Shahzad et al., 2021). However, so far, there is no uniform standard for the isolation methods of exosomes. Exosomes are obtained using different isolation methods with different purities and specificities. At present, the most used isolation method for exosomes is ultracentrifugation, but the purity of obtained exosomes is low. Therefore, the isolation methods of exosomes still should be developed to obtain a higher purity and specificity. For questions regarding exosome preservation and transport, refer to the 2018 International Association for Extracellular Vesicles statement (Gardiner et al., 2016; Thery et al., 2018). When exosomes are used as drugs or carriers for treatments, the dosage, timing, and administration route are still not known. Therefore, it is essential to assess their half-life and in vivo distribution characteristics in advance (Smyth et al., 2015; Yi and Wang, 2021). Regarding the content and function of exosomes, as mentioned earlier, the results obtained from different cell sources and culture conditions are different, and the diversity in their therapeutic effects remains to be studied. Specific exosomes can be selected according to their studies. Moreover, more exosomes obtained from cell sources and culture conditions can be sought.
Currently, in the field of treatment of SCI, most studies on exosomal ncRNAs focused on miRNAs, lncRNAs, and circRNAs. In the future, more RNAs should be developed, such as rRNA, tRNA, and piRNA (Chandran et al., 2017; Jogia and Ruitenberg, 2020). For the targeted therapy of ncRNAs, the biological regulatory pathway is very complex, and a single targeted axis might play only a limited role. Therefore, to clarify the regulatory network, multicellular, multitarget, and multipathway validation is one of the directions to elucidate the specific regulatory mechanism for future research (Li X. et al., 2020; Wang W. Z. et al., 2021). Nevertheless, ncRNA-targeted therapy has many disadvantages. For example, it is easy to miss the target. Even it has a low transfection efficiency and a short half-life. What’s worse, it is difficult to overcome the limitations of blood-spinal cord barrier (Shahzad et al., 2021). Fortunately, targeting and half-life can be improved by the carrier delivery of drugs such as viruses, siRNAs, lipids, polyethylene glycol, and exosomes (Guo et al., 2019; Xu et al., 2019; Li L. et al., 2020; Segel et al., 2021). What’s more, as mentioned earlier, many studies have achieved some results using ncRNAs in combination with exosomes for the treatment of SCI. Because exosomes have the ability to penetrate the BSCB, they can deliver ncRNAs to the lesioned area of SCI to enhance efficacy (Ding et al., 2019). Moreover, exosomes have a lower risk of tumorigenicity, toxic effects, and autoimmune responses (Lai et al., 2013). However, the clinical application of exosomes remains problematic. It has been shown that the amount of exosomes produced by cellular secretion is small (Katsuda et al., 2013), and it is hard to meet clinical needs. Therefore, regulating exosomes release is particularly important. It is reported that the pH of the microenvironment has a role in the secretion of exosomes (Parolini et al., 2009). Regulating the pH of the microenvironment may result in increased exosomes production. Furthermore, most studies have focused on the mechanism of miRNAs in combination with exosomes for the treatment of SCI, and only a few studies have used other RNAs.
Conclusion
In summary, the treatment of SCI is still a huge concern, and no effective methods are available to promote neurological recovery. SCI is the result of multiple factors, hindering the development of rehabilitation because of its complexity. Therefore, understanding the pathomechanism will facilitate better treatment of SCI. As a mediator of intercellular communication, exosomes are advantageous in treating SCI. Exosomal ncRNAs have also been shown to contribute to nerve regeneration. Exosomes derived from different cells play very much the same role, and exosomal ncRNAs such as miRNAs, lncRNAs, and circRNAs have tremendous therapeutic potential in SCI. We must optimize and enrich exosomes and exosomal ncRNAs of various cellular sources and combine both of them effectively to improve their therapeutic efficacy in SCI. Then, more studies are needed to elucidate the specific mechanism of action of exosomes and exosomal ncRNAs from different cell sources in SCI. These searches provide a comprehensive theoretical basis for the clinical translation of exosomes and exosomal ncRNAs from different cell sources in SCI treatment and provide great hope for the clinical treatment of SCI.
Author Contributions
All the listed authors directly, substantially, and intellectually contributed to the preparation of this manuscript and approved the publication. All authors contributed to the article and approved the submitted version.
Conflict of Interest
The authors declare that the research was conducted in the absence of any commercial or financial relationships that could be construed as a potential conflict of interest.
Publisher’s Note
All claims expressed in this article are solely those of the authors and do not necessarily represent those of their affiliated organizations, or those of the publisher, the editors and the reviewers. Any product that may be evaluated in this article, or claim that may be made by its manufacturer, is not guaranteed or endorsed by the publisher.
Abbreviations
SCI, spinal cord injury; ECM, extracellular matrix; ncRNAs, noncoding RNAs; iPSC, induced pluripotent stem cells; MSCs, mesenchymal stem cells; NSCs, neural stem cells; OPCs, oligodendrocyte progenitor cells; SCs, schwann cells; OECs, olfactory ensheathing cells; BSCB, blood-spinal cord barrier; CSPGs, chondroitin sulfate proteoglycans; PTPσ, Protein Tyrosine Phosphatase Sigma; EVs, extracellular vesicles; ESE, early sorting endosomes; LSEs, late sorting endosomes; ESCRT, endosomal-sorting complex necessary for transport; MVBs, multivesicular bodies; ILVs, intraluminal vesicles; MHC, major histocompatibility complex; HSP, heat shock proteins; HUVECs, human umbilical vein endothelial cells; BMSCs-Exos, bone marrow mesenchymal stem cells-derived exosomes; NF-κB, nuclear factor kappa-B; hpMSCs-Exos, human placental stem cell-derived exosomes; NPCs, nerve progenitor cells; WJMSCs-EVs, Wharton’s jelly stem cell-derived extracellular vesicles; hucMSCs-Exos, human umbilical cord stem cell-derived exosomes; EF-MSCs-Exos, epidural adipose-derived exosomes; NSCs/NPCs-Exos, neural stem/progenitor cell-derived exosomes; SCMECs, spinal cord microvascular endothelial cells; VEGF-A, vascular endothelial growth factor A; SKP-SCs-Exos, skin schwann cells-derived exosomes; SCDEs, SC-derived exosomes; TLR2, toll-like receptor 2; PMs, peripheral macrophages; PMs-Exos, peripheral macrophage-derived exosomes; noncoding small RNAs, microRNAs; lncRNAs, long ncRNAs; circRNAs, circular RNAs; miRNAs, microRNAs; PTENP1, phosphatase and tension protein homologous pseudogene 1; PTEN, phosphatase and tension protein homolog; ADSCs, adipose-derived stem cells; Exos, exosomes; BMDMs, bone marrow-derived macrophages; HNESCs, human neuroepithelial stem cells; DCs, dendritic cells.
References
Ahuja, C. S., Mothe, A., Khazaei, M., Badhiwala, J. H., Gilbert, E. A., van der Kooy, D., et al. (2020). The leading edge: emerging neuroprotective and neuroregenerative cell-based therapies for spinal cord injury. Stem Cells Transl. Med. 9, 1509–1530. doi: 10.1002/sctm.19-0135
Ahuja, C. S., Wilson, J. R., Nori, S., Kotter, M. R. N., Druschel, C., Curt, A., et al. (2017). Traumatic spinal cord injury. Nat. Rev. Dis. Primers 3:17018. doi: 10.1038/nrdp.2017.18
Amaral, P. P., and Mattick, J. S. (2008). Noncoding RNA in development. Mamm. Genome 19, 454–492. doi: 10.1007/s00335-008-9136-7
Andrzejewska, A., Dabrowska, S., Lukomska, B., and Janowski, M. (2021). Mesenchymal stem cells for neurological disorders. Adv. Sci. (Weinh) 8:2002944. doi: 10.1002/advs.202002944
Antimisiaris, S. G., Mourtas, S., and Marazioti, A. (2018). Exosomes and exosome-inspired vesicles for targeted drug delivery. Pharmaceutics 10:218. doi: 10.3390/pharmaceutics10040218
Batrakova, E. V., and Kim, M. S. (2015). Using exosomes, naturally-equipped nanocarriers, for drug delivery. J. Control. Release 219, 396–405. doi: 10.1016/j.jconrel.2015.07.030
Bhalala, O. G., Srikanth, M., and Kessler, J. A. (2013). The emerging roles of microRNAs in CNS injuries. Nat. Rev. Neurol. 9, 328–339. doi: 10.1038/nrneurol.2013.67
Bie, F., Wang, K., Xu, T., Yuan, J., Ding, H., Lv, B., et al. (2021). The potential roles of circular RNAs as modulators in traumatic spinal cord injury. Biomed. Pharmacother. 141:111826. doi: 10.1016/j.biopha.2021.111826
Bradbury, E. J., and Burnside, E. R. (2019). Moving beyond the glial scar for spinal cord repair. Nat. Commun. 10:3879. doi: 10.1038/s41467-019-11707-7
Chandran, R., Mehta, S. L., and Vemuganti, R. (2017). Non-coding RNAs and neuroprotection after acute CNS injuries. Neurochem. Int. 111, 12–22. doi: 10.1016/j.neuint.2017.01.015
Chang, Q., Hao, Y., Wang, Y., Zhou, Y., Zhuo, H., and Zhao, G. (2021). Bone marrow mesenchymal stem cell-derived exosomal microRNA-125a promotes M2 macrophage polarization in spinal cord injury by downregulating IRF5. Brain Res. Bull. 170, 199–210. doi: 10.1016/j.brainresbull.2021.02.015
Chen, B., Li, Q., Zhao, B., and Wang, Y. (2017). Stem cell-derived extracellular vesicles as a novel potential therapeutic tool for tissue repair. Stem Cells Transl. Med. 6, 1753–1758. doi: 10.1002/sctm.16-0477
Chen, Y., Tian, Z., He, L., Liu, C., Wang, N., Rong, L., et al. (2021). Exosomes derived from miR-26a-modified MSCs promote axonal regeneration via the PTEN/AKT/mTOR pathway following spinal cord injury. Stem Cell Res. Ther. 12:224. doi: 10.1186/s13287-021-02282-0
Cheng, J., Chen, Z., Liu, C., Zhong, M., Wang, S., Sun, Y., et al. (2021). Bone mesenchymal stem cell-derived exosome-loaded injectable hydrogel for minimally invasive treatment of spinal cord injury. Nanomedicine (Lond) 16, 1567–1579. doi: 10.2217/nnm-2021-0025
Cho, K. H. T., Xu, B., Blenkiron, C., and Fraser, M. (2019). Emerging roles of miRNAs in brain development and perinatal brain injury. Front. Physiol. 10:227. doi: 10.3389/fphys.2019.00227
Colombo, M., Raposo, G., and Théry, C. (2014). Biogenesis, secretion and intercellular interactions of exosomes and other extracellular vesicles. Annu. Rev. Cell Dev. Biol. 30, 255–289. doi: 10.1146/annurev-cellbio-101512-122326
Csobonyeiova, M., Polak, S., Zamborsky, R., and Danisovic, L. (2019). Recent progress in the regeneration of spinal cord injuries by induced pluripotent stem cells. Int. J. Mol. Sci. 20:3838. doi: 10.3390/ijms20153838
Ding, S. Q., Chen, J., Wang, S. N., Duan, F. X., Chen, Y. Q., Shi, Y. J., et al. (2019). Identification of serum exosomal microRNAs in acute spinal cord injured rats. Exp. Biol. Med. (Maywood) 244, 1149–1161. doi: 10.1177/1535370219872759
Donnelly, D. J., and Popovich, P. G. (2008). Inflammation and its role in neuroprotection, axonal regeneration and functional recovery after spinal cord injury. Exp. Neurol. 209, 378–388. doi: 10.1016/j.expneurol.2007.06.009
Dutta, D., Khan, N., Wu, J., and Jay, S. M. (2021). Extracellular vesicles as an emerging frontier in spinal cord injury pathobiology and therapy. Trends Neurosci. 44, 492–506. doi: 10.1016/j.tins.2021.01.003
Ehlers, M. D. (2004). Deconstructing the axon: wallerian degeneration and the ubiquitin-proteasome system. Trends Neurosci. 27, 3–6. doi: 10.1016/j.tins.2003.10.015
Fan, L., Dong, J., He, X., Zhang, C., and Zhang, T. (2021). Bone marrow mesenchymal stem cells-derived exosomes reduce apoptosis and inflammatory response during spinal cord injury by inhibiting the TLR4/MyD88/NF-κB signaling pathway. Hum. Exp. Toxicol. 40, 1612–1623. doi: 10.1177/09603271211003311
Feng, J., Zhang, Y., Zhu, Z., Gu, C., Waqas, A., and Chen, L. (2021). Emerging exosomes and exosomal MiRNAs in spinal cord injury. Front. Cell. Dev. Biol. 9:703989. doi: 10.3389/fcell.2021.703989
Gardiner, C., Di Vizio, D., Sahoo, S., Thery, C., Witwer, K. W., Wauben, M., et al. (2016). Techniques used for the isolation and characterization of extracellular vesicles: results of a worldwide survey. J. Extracell. Vesicles 5:32945. doi: 10.3402/jev.v5.32945
Gómara-Toldrà, N., Sliwinski, M., and Dijkers, M. P. (2014). Physical therapy after spinal cord injury: a systematic review of treatments focused on participation. J. Spinal Cord Med. 37, 371–379. doi: 10.1179/2045772314Y.0000000194
Gu, J., Jin, Z. S., Wang, C. M., Yan, X. F., Mao, Y. Q., and Chen, S. (2020). Bone marrow mesenchymal stem cell-derived exosomes improves spinal cord function after injury in rats by activating autophagy. Drug Des. Devel. Ther. 14, 1621–1631. doi: 10.2147/DDDT.S237502
Guo, S., Perets, N., Betzer, O., Ben-Shaul, S., Sheinin, A., Michaelevski, I., et al. (2019). Intranasal delivery of mesenchymal stem cell derived exosomes loaded with phosphatase and tensin homolog siRNA repairs complete spinal cord injury. ACS Nano 13, 10015–10028. doi: 10.1021/acsnano.9b01892
Hagey, D. W., Kordes, M., Gorgens, A., Mowoe, M. O., Nordin, J. Z., Moro, C. F., et al. (2021). Extracellular vesicles are the primary source of blood-borne tumour-derived mutant KRAS DNA early in pancreatic cancer. J. Extracell. Vesicles 10:e12142. doi: 10.1002/jev2.12142
Han, T., Song, P., Wu, Z., Xiang, X., Liu, Y., Wang, Y., et al. (2021). MSC secreted extracellular vesicles carrying TGF-beta upregulate Smad 6 expression and promote the regrowth of neurons in spinal cord injured rats. Stem Cell Rev. Rep. . [Online ahead of print]. doi: 10.1007/s12015-021-10219-6
Harrop, J. S., Hashimoto, R., Norvell, D., Raich, A., Aarabi, B., Grossman, R. G., et al. (2012). Evaluation of clinical experience using cell-based therapies in patients with spinal cord injury: a systematic review. J. Neurosurg. Spine 17, 230–246. doi: 10.3171/2012.5.AOSPINE12115
Hessvik, N. P., and Llorente, A. (2018). Current knowledge on exosome biogenesis and release. Cell. Mol. Life Sci. 75, 193–208. doi: 10.1007/s00018-017-2595-9
Huang, J. H., Fu, C. H., Xu, Y., Yin, X. M., Cao, Y., and Lin, F. Y. (2020a). Extracellular vesicles derived from epidural fat-mesenchymal stem cells attenuate NLRP3 inflammasome activation and improve functional recovery after spinal cord injury. Neurochem. Res. 45, 760–771. doi: 10.1007/s11064-019-02950-x
Huang, J. H., Xu, Y., Yin, X. M., and Lin, F. Y. (2020b). Exosomes derived from miR-126-modified MSCs promote angiogenesis and neurogenesis and attenuate apoptosis after spinal cord injury in rats. Neuroscience 424, 133–145. doi: 10.1016/j.neuroscience.2019.10.043
Huang, T., Jia, Z., Fang, L., Cheng, Z., Qian, J., Xiong, F., et al. (2022). Extracellular vesicle-derived miR-511-3p from hypoxia preconditioned adipose mesenchymal stem cells ameliorates spinal cord injury through the TRAF6/S1P axis. Brain Res. Bull. 180, 73–85. doi: 10.1016/j.brainresbull.2021.12.015
Huang, J. H., Yin, X. M., Xu, Y., Xu, C. C., Lin, X., Ye, F. B., et al. (2017). Systemic administration of exosomes released from mesenchymal stromal cells attenuates apoptosis, inflammation and promotes angiogenesis after spinal cord injury in rats. J. Neurotrauma 34, 3388–3396. doi: 10.1089/neu.2017.5063
Hurlbert, R. J., Hadley, M. N., Walters, B. C., Aarabi, B., Dhall, S. S., Gelb, D. E., et al. (2015). Pharmacological therapy for acute spinal cord injury. Neurosurgery 76, S71–S83. doi: 10.1227/01.neu.0000462080.04196.f7
Jeppesen, D. K., Fenix, A. M., Franklin, J. L., Higginbotham, J. N., Zhang, Q., Zimmerman, L. J., et al. (2019). Reassessment of exosome composition. Cell 177, 428–445.e18. doi: 10.1016/j.cell.2019.02.029
Ji, W., Jiang, W., Li, M., Li, J., and Li, Z. (2019). miR-21 deficiency contributes to the impaired protective effects of obese rat mesenchymal stem cell-derived exosomes against spinal cord injury. Biochimie 167, 171–178. doi: 10.1016/j.biochi.2019.10.002
Jia, X., Huang, G., Wang, S., Long, M., Tang, X., Feng, D., et al. (2021). Extracellular vesicles derived from mesenchymal stem cells containing microRNA-381 protect against spinal cord injury in a rat model via the BRD4/WNT5A axis. Bone Joint Res. 10, 328–339. doi: 10.1302/2046-3758.105.BJR-2020-0020.R1
Jia, Y., Lu, T., Chen, Q., Pu, X., Ji, L., Yang, J., et al. (2021a). Exosomes secreted from sonic hedgehog-modified bone mesenchymal stem cells facilitate the repair of rat spinal cord injuries. Acta Neurochir. (Wien) 163, 2297–2306. doi: 10.1007/s00701-021-04829-9
Jia, Y., Yang, J., Lu, T., Pu, X., Chen, Q., Ji, L., et al. (2021b). Repair of spinal cord injury in rats via exosomes from bone mesenchymal stem cells requires sonic hedgehog. Regen. Ther. 18, 309–315. doi: 10.1016/j.reth.2021.08.007
Jiang, Z., and Zhang, J. (2021). Mesenchymal stem cell-derived exosomes containing miR-145-5p reduce inflammation in spinal cord injury by regulating the TLR4/NF-κB signaling pathway. Cell Cycle 20, 993–1009. doi: 10.1080/15384101.2021.1919825
Jogia, T., and Ruitenberg, M. J. (2020). Traumatic spinal cord injury and the gut microbiota: current insights and future challenges. Front. Immunol. 11:704. doi: 10.3389/fimmu.2020.00704
Kalluri, R., and LeBleu, V. S. (2020). The biology, function and biomedical applications of exosomes. Science 367:eaau6977. doi: 10.1126/science.aau6977
Kalra, H., Drummen, G. P., and Mathivanan, S. (2016). Focus on extracellular vesicles: introducing the next small big thing. Int. J. Mol. Sci. 17:170. doi: 10.3390/ijms17020170
Kang, J., and Guo, Y. (2022). Human umbilical cord mesenchymal stem cells derived exosomes promote neurological function recovery in a rat spinal cord injury model. Neurochem. Res. . [Online ahead of print]. doi: 10.1007/s11064-022-03545-9
Kang, J., Li, Z., Zhi, Z., Wang, S., and Xu, G. (2019). MiR-21 derived from the exosomes of MSCs regulates the death and differentiation of neurons in patients with spinal cord injury. Gene Ther. 26, 491–503. doi: 10.1038/s41434-019-0101-8
Kang, J., Zhang, C., Zhi, Z., Wang, Y., Liu, J., Wu, F., et al. (2020). Stem-like cells of various origins showed therapeutic effect to improve the recovery of spinal cord injury. Artif. Cells Nanomed. Biotechnol. 48, 627–638. doi: 10.1080/21691401.2020.1725031
Kato, T., Honmou, O., Uede, T., Hashi, K., and Kocsis, J. D. (2000). Transplantation of human olfactory ensheathing cells elicits remyelination of demyelinated rat spinal cord. Glia 30, 209–218. doi: 10.1002/(sici)1098-1136(200005)30:3<209::aid-glia1>3.0.co;2-8
Katsuda, T., Tsuchiya, R., Kosaka, N., Yoshioka, Y., Takagaki, K., Oki, K., et al. (2013). Human adipose tissue-derived mesenchymal stem cells secrete functional neprilysin-bound exosomes. Sci. Rep. 3:1197. doi: 10.1038/srep01197
Kim, H. Y., Kumar, H., Jo, M. J., Kim, J., Yoon, J. K., Lee, J. R., et al. (2018). Therapeutic efficacy-potentiated and diseased organ-targeting nanovesicles derived from mesenchymal stem cells for spinal cord injury treatment. Nano Lett. 18, 4965–4975. doi: 10.1021/acs.nanolett.8b01816
Kosaka, N., Iguchi, H., and Ochiya, T. (2010). Circulating microRNA in body fluid: a new potential biomarker for cancer diagnosis and prognosis. Cancer Sci. 101, 2087–2092. doi: 10.1111/j.1349-7006.2010.01650.x
Kumar, H., Ropper, A. E., Lee, S. H., and Han, I. (2017). Propitious therapeutic modulators to prevent blood-spinal cord barrier disruption in spinal cord injury. Mol. Neurobiol. 54, 3578–3590. doi: 10.1007/s12035-016-9910-6
Lai, R. C., Yeo, R. W., Tan, K. H., and Lim, S. K. (2013). Exosomes for drug delivery - a novel application for the mesenchymal stem cell. Biotechnol. Adv. 31, 543–551. doi: 10.1016/j.biotechadv.2012.08.008
Lankford, K. L., Arroyo, E. J., Nazimek, K., Bryniarski, K., Askenase, P. W., and Kocsis, J. D. (2018). Intravenously delivered mesenchymal stem cell-derived exosomes target M2-type macrophages in the injured spinal cord. PLoS One 13:e0190358. doi: 10.1371/journal.pone.0190358
Lee, J. T. (2012). Epigenetic regulation by long noncoding RNAs. Science 338, 1435–1439. doi: 10.1126/science.1231776
Lee, J. Y., Kim, H. S., Choi, H. Y., Oh, T. H., and Yune, T. Y. (2012). Fluoxetine inhibits matrix metalloprotease activation and prevents disruption of blood-spinal cord barrier after spinal cord injury. Brain 135, 2375–2389. doi: 10.1093/brain/aws171
Li, C., Jiao, G., Wu, W., Wang, H., Ren, S., Zhang, L., et al. (2019). Exosomes from bone marrow mesenchymal stem cells inhibit neuronal apoptosis and promote motor function recovery via the Wnt/β-catenin signaling pathway. Cell Transplant. 28, 1373–1383. doi: 10.1177/0963689719870999
Li, C., Li, X., Zhao, B., and Wang, C. (2020). Exosomes derived from miR-544-modified mesenchymal stem cells promote recovery after spinal cord injury. Arch. Physiol. Biochem. 126, 369–375. doi: 10.1080/13813455.2019.1691601
Li, X., Lou, X., Xu, S., Du, J., and Wu, J. (2020). Hypoxia inducible factor-1 (HIF-1α) reduced inflammation in spinal cord injury via miR-380-3p/ NLRP3 by Circ 0001723. Biol. Res. 53:35. doi: 10.1186/s40659-020-00302-6
Li, C., Qin, T., Zhao, J., He, R., Wen, H., Duan, C., et al. (2021). Bone marrow mesenchymal stem cell-derived exosome-educated macrophages promote functional healing after spinal cord injury. Front. Cell. Neurosci. 15:725573. doi: 10.3389/fncel.2021.725573
Li, L., Zhang, Y., Mu, J., Chen, J., Zhang, C., Cao, H., et al. (2020). Transplantation of human mesenchymal stem-cell-derived exosomes immobilized in an adhesive hydrogel for effective treatment of spinal cord injury. Nano Lett. 20, 4298–4305. doi: 10.1021/acs.nanolett.0c00929
Li, D., Zhang, P., Yao, X., Li, H., Shen, H., Li, X., et al. (2018). Exosomes derived from miR-133b-modified mesenchymal stem cells promote recovery after spinal cord injury. Front. Neurosci. 12:845. doi: 10.3389/fnins.2018.00845
Li, R., Zhao, K., Ruan, Q., Meng, C., and Yin, F. (2020). Bone marrow mesenchymal stem cell-derived exosomal microRNA-124-3p attenuates neurological damage in spinal cord ischemia-reperfusion injury by downregulating Ern1 and promoting M2 macrophage polarization. Arthritis Res. Ther. 22:75. doi: 10.1186/s13075-020-2146-x
Liang, Y., Wu, J. H., Zhu, J. H., and Yang, H. (2022). Exosomes secreted by hypoxia-pre-conditioned adipose-derived mesenchymal stem cells reduce neuronal apoptosis in rats with spinal cord injury. J. Neurotrauma . [Online ahead of print]. doi: 10.1089/neu.2021.0290
Liau, L. L., Looi, Q. H., Chia, W. C., Subramaniam, T., Ng, M. H., and Law, J. X. (2020). Treatment of spinal cord injury with mesenchymal stem cells. Cell Biosci. 10:112. doi: 10.1186/s13578-020-00475-3
Liu, C., Hu, F., Jiao, G., Guo, Y., Zhou, P., Zhang, Y., et al. (2022). Dental pulp stem cell-derived exosomes suppress M1 macrophage polarization through the ROS-MAPK-NFκB P65 signaling pathway after spinal cord injury. J. Nanobiotechnology 20:65. doi: 10.1186/s12951-022-01273-4
Liu, J., Lin, M., Qiao, F., and Zhang, C. (2021). Exosomes derived from lncRNA TCTN2-modified mesenchymal stem cells improve spinal cord injury by miR-329-3p/IGF1R Axis. J. Mol. Neurosci. 72, 482–495. doi: 10.1007/s12031-021-01914-7
Liu, W. Z., Ma, Z. J., Li, J. R., and Kang, X. W. (2021). Mesenchymal stem cell-derived exosomes: therapeutic opportunities and challenges for spinal cord injury. Stem Cell Res. Ther. 12:102. doi: 10.1186/s13287-021-02153-8
Liu, W., Rong, Y., Wang, J., Zhou, Z., Ge, X., Ji, C., et al. (2020). Exosome-shuttled miR-216a-5p from hypoxic preconditioned mesenchymal stem cells repair traumatic spinal cord injury by shifting microglial M1/M2 polarization. J. Neuroinflammation 17:47. doi: 10.1186/s12974-020-1726-7
Liu, W., Wang, Y., Gong, F., Rong, Y., Luo, Y., Tang, P., et al. (2019). Exosomes derived from bone mesenchymal stem cells repair traumatic spinal cord injury by suppressing the activation of A1 neurotoxic reactive astrocytes. J. Neurotrauma 36, 469–484. doi: 10.1089/neu.2018.5835
Lotvall, J., Hill, A. F., Hochberg, F., Buzas, E. I., Di Vizio, D., Gardiner, C., et al. (2014). Minimal experimental requirements for definition of extracellular vesicles and their functions: a position statement from the International Society for Extracellular Vesicles. J. Extracell. Vesicles 3:26913. doi: 10.3402/jev.v3.26913
Lukovic, D., Stojkovic, M., Moreno-Manzano, V., Jendelova, P., Sykova, E., Bhattacharya, S. S., et al. (2015). Concise review: reactive astrocytes and stem cells in spinal cord injury: good guys or bad guys? Stem Cells 33, 1036–1041. doi: 10.1002/stem.1959
Luo, Y., Xu, T., Liu, W., Rong, Y., Wang, J., Fan, J., et al. (2021). Exosomes derived from GIT1-overexpressing bone marrow mesenchymal stem cells promote traumatic spinal cord injury recovery in a rat model. Int. J. Neurosci. 131, 170–182. doi: 10.1080/00207454.2020.1734598
Ma, K., Xu, H., Zhang, J., Zhao, F., Liang, H., Sun, H., et al. (2019). Insulin-like growth factor-1 enhances neuroprotective effects of neural stem cell exosomes after spinal cord injury via an miR-219a-2-3p/YY1 mechanism. Aging (Albany NY) 11, 12278–12294. doi: 10.18632/aging.102568
Mashouri, L., Yousefi, H., Aref, A. R., Ahadi, A. M., Molaei, F., and Alahari, S. K. (2019). Exosomes: composition, biogenesis and mechanisms in cancer metastasis and drug resistance. Mol. Cancer 18:75. doi: 10.1186/s12943-019-0991-5
Mattick, J. S. (2001). Non-coding RNAs: the architects of eukaryotic complexity. EMBO Rep. 2, 986–991. doi: 10.1093/embo-reports/kve230
McDonald, J. W., and Sadowsky, C. (2002). Spinal-cord injury. Lancet 359, 417–425. doi: 10.1016/S0140-6736(02)07603-1
Melo, S. A., Luecke, L. B., Kahlert, C., Fernandez, A. F., Gammon, S. T., Kaye, J., et al. (2015). Glypican-1 identifies cancer exosomes and detects early pancreatic cancer. Nature 523, 177–182. doi: 10.1038/nature14581
Mercer, T. R., Dinger, M. E., and Mattick, J. S. (2009). Long non-coding RNAs: insights into functions. Nat. Rev. Genet. 10, 155–159. doi: 10.1038/nrg2521
Min, Q., Parkinson, D. B., and Dun, X. P. (2021). Migrating Schwann cells direct axon regeneration within the peripheral nerve bridge. Glia 69, 235–254. doi: 10.1002/glia.23892
Nakazaki, M., Morita, T., Lankford, K. L., Askenase, P. W., and Kocsis, J. D. (2021). Small extracellular vesicles released by infused mesenchymal stromal cells target M2 macrophages and promote TGF-β upregulation, microvascular stabilization and functional recovery in a rodent model of severe spinal cord injury. J. Extracell. Vesicles 10:e12137. doi: 10.1002/jev2.12137
Nie, H., and Jiang, Z. (2021). Bone mesenchymal stem cell-derived extracellular vesicles deliver microRNA-23b to alleviate spinal cord injury by targeting toll-like receptor TLR4 and inhibiting NF-κB pathway activation. Bioengineered 12, 8157–8172. doi: 10.1080/21655979.2021.1977562
Nikfarjam, S., Rezaie, J., Kashanchi, F., and Jafari, R. (2020). Dexosomes as a cell-free vaccine for cancer immunotherapy. J. Exp. Clin. Cancer Res. 39:258. doi: 10.1186/s13046-020-01781-x
Noori, L., Arabzadeh, S., Mohamadi, Y., Mojaverrostami, S., Mokhtari, T., Akbari, M., et al. (2021). Intrathecal administration of the extracellular vesicles derived from human Wharton’s jelly stem cells inhibit inflammation and attenuate the activity of inflammasome complexes after spinal cord injury in rats. Neurosci. Res. 170, 87–98. doi: 10.1016/j.neures.2020.07.011
Pan, D., Li, Y., Yang, F., Lv, Z., Zhu, S., Shao, Y., et al. (2021). Increasing toll-like receptor 2 on astrocytes induced by Schwann cell-derived exosomes promotes recovery by inhibiting CSPGs deposition after spinal cord injury. J. Neuroinflammation 18:172. doi: 10.1186/s12974-021-02215-x
Pant, T., Juric, M., Bosnjak, Z. J., and Dhanasekaran, A. (2021). Recent insight on the non-coding RNAs in mesenchymal stem cell-derived exosomes: regulatory and therapeutic role in regenerative medicine and tissue engineering. Front. Cardiovasc. Med. 8:737512. doi: 10.3389/fcvm.2021.737512
Parolini, I., Federici, C., Raggi, C., Lugini, L., Palleschi, S., De Milito, A., et al. (2009). Microenvironmental pH is a key factor for exosome traffic in tumor cells. J. Biol. Chem. 284, 34211–34222. doi: 10.1074/jbc.M109.041152
Pomper, N., Liu, Y., Hoye, M. L., Dougherty, J. D., and Miller, T. M. (2020). CNS microRNA profiles: a database for cell type enriched microRNA expression across the mouse central nervous system. Sci. Rep. 10:4921. doi: 10.1038/s41598-020-61307-5
Quinzaños-Fresnedo, J., and Sahagún-Olmos, R. C. (2015). [Micro RNA and its role in the pathophysiology of spinal cord injury - a further step towards neuroregenerative medicine]. Cir. Cir. 83, 442–447. doi: 10.1016/j.circir.2015.05.045
Ramakonar, H., and Fehlings, M. G. (2021). ’Time is Spine’: new evidence supports decompression within 24 h for acute spinal cord injury. Spinal Cord 59, 933–934. doi: 10.1038/s41393-021-00654-0
Ren, Z., Qi, Y., Sun, S., Tao, Y., and Shi, R. (2020). Mesenchymal stem cell-derived exosomes: hope for spinal cord injury repair. Stem Cells Dev. 29, 1467–1478. doi: 10.1089/scd.2020.0133
Ren, Z. W., Zhou, J. G., Xiong, Z. K., Zhu, F. Z., and Guo, X. D. (2019). Effect of exosomes derived from MiR-133b-modified ADSCs on the recovery of neurological function after SCI. Eur. Rev. Med. Pharmacol. Sci. 23, 52–60. doi: 10.26355/eurrev_201901_16747
Rong, Y., Liu, W., Wang, J., Fan, J., Luo, Y., Li, L., et al. (2019). Neural stem cell-derived small extracellular vesicles attenuate apoptosis and neuroinflammation after traumatic spinal cord injury by activating autophagy. Cell Death Dis. 10:340. doi: 10.1038/s41419-019-1571-8
Rowland, J. W., Hawryluk, G. W., Kwon, B., and Fehlings, M. G. (2008). Current status of acute spinal cord injury pathophysiology and emerging therapies: promise on the horizon. Neurosurg. Focus 25:E2. doi: 10.3171/FOC.2008.25.11.E2
Ryken, T. C., Hurlbert, R. J., Hadley, M. N., Aarabi, B., Dhall, S. S., Gelb, D. E., et al. (2013). The acute cardiopulmonary management of patients with cervical spinal cord injuries. Neurosurgery 72, 84–92. doi: 10.1227/NEU.0b013e318276ee16
Sakamoto, K., Ozaki, T., Ko, Y. C., Tsai, C. F., Gong, Y., Morozumi, M., et al. (2019). Glycan sulfation patterns define autophagy flux at axon tip via PTPRσ-cortactin axis. Nat. Chem. Biol. 15, 699–709. doi: 10.1038/s41589-019-0274-x
Sato-Kuwabara, Y., Melo, S. A., Soares, F. A., and Calin, G. A. (2015). The fusion of two worlds: non-coding RNAs and extracellular vesicles–diagnostic and therapeutic implications (Review). Int. J. Oncol. 46, 17–27. doi: 10.3892/ijo.2014.2712
Segel, M., Lash, B., Song, J., Ladha, A., Liu, C. C., Jin, X., et al. (2021). Mammalian retrovirus-like protein PEG10 packages its own mRNA and can be pseudotyped for mRNA delivery. Science 373, 882–889. doi: 10.1126/science.abg6155
Shahzad, U., Krumholtz, S., Rutka, J. T., and Das, S. (2021). Noncoding RNAs in glioblastoma: emerging biological concepts and potential therapeutic implications. Cancers (Basel) 13:1555. doi: 10.3390/cancers13071555
Shao, M., Jin, M., Xu, S., Zheng, C., Zhu, W., Ma, X., et al. (2020). Exosomes from long noncoding RNA-Gm37494-ADSCs repair spinal cord injury via shifting microglial M1/M2 polarization. Inflammation 43, 1536–1547. doi: 10.1007/s10753-020-01230-z
Shao, A., Tu, S., Lu, J., and Zhang, J. (2019). Crosstalk between stem cell and spinal cord injury: pathophysiology and treatment strategies. Stem Cell Res. Ther. 10:238. doi: 10.1186/s13287-019-1357-z
Sheng, X., Zhao, J., Li, M., Xu, Y., Zhou, Y., Xu, J., et al. (2021). Bone marrow mesenchymal stem cell-derived exosomes accelerate functional recovery after spinal cord injury by promoting the phagocytosis of macrophages to clean myelin debris. Front. Cell. Dev. Biol. 9:772205. doi: 10.3389/fcell.2021.772205
Shi, Z., Pan, B., and Feng, S. (2018). The emerging role of long non-coding RNA in spinal cord injury. J. Cell. Mol. Med. 22, 2055–2061. doi: 10.1111/jcmm.13515
Silver, J., and Miller, J. H. (2004). Regeneration beyond the glial scar. Nat. Rev. Neurosci. 5, 146–156. doi: 10.1038/nrn1326
Smyth, T., Kullberg, M., Malik, N., Smith-Jones, P., Graner, M. W., and Anchordoquy, T. J. (2015). Biodistribution and delivery efficiency of unmodified tumor-derived exosomes. J. Control. Release 199, 145–155. doi: 10.1016/j.jconrel.2014.12.013
Sun, G., Li, G., Li, D., Huang, W., Zhang, R., Zhang, H., et al. (2018). hucMSC derived exosomes promote functional recovery in spinal cord injury mice via attenuating inflammation. Mater. Sci. Eng. C Mater. Biol. Appl. 89, 194–204. doi: 10.1016/j.msec.2018.04.006
Tator, C. H. (1995). Update on the pathophysiology and pathology of acute spinal cord injury. Brain Pathol. 5, 407–413. doi: 10.1111/j.1750-3639.1995.tb00619.x
Thakur, B. K., Zhang, H., Becker, A., Matei, I., Huang, Y., Costa-Silva, B., et al. (2014). Double-stranded DNA in exosomes: a novel biomarker in cancer detection. Cell Res. 24, 766–769. doi: 10.1038/cr.2014.44
Thery, C., Witwer, K. W., Aikawa, E., Alcaraz, M. J., Anderson, J. D., Andriantsitohaina, R., et al. (2018). Minimal information for studies of extracellular vesicles 2018 (MISEV2018): a position statement of the International Society for Extracellular Vesicles and update of the MISEV2014 guidelines. J. Extracell. Vesicles 7:1535750. doi: 10.1080/20013078.2018.1535750
Thery, C., Zitvogel, L., and Amigorena, S. (2002). Exosomes: composition, biogenesis and function. Nat. Rev. Immunol. 2, 569–579. doi: 10.1038/nri855
Todor, D. R., Mu, H. T., and Milhorat, T. H. (2000). Pain and syringomyelia: a review. Neurosurg. Focus 8:E11. doi: 10.3171/foc.2000.8.3.11
Tran, A. P., Warren, P. M., and Silver, J. (2021). New insights into glial scar formation after spinal cord injury. Cell Tissue Res. . [Online ahead of print]. doi: 10.1007/s00441-021-03477-w
Treiber, T., Treiber, N., and Meister, G. (2019). Regulation of microRNA biogenesis and its crosstalk with other cellular pathways. Nat. Rev. Mol. Cell Biol. 20, 5–20. doi: 10.1038/s41580-018-0059-1
Tsarouchas, T. M., Wehner, D., Cavone, L., Munir, T., Keatinge, M., Lambertus, M., et al. (2018). Dynamic control of proinflammatory cytokines Il-1beta and Tnf-alpha by macrophages in zebrafish spinal cord regeneration. Nat. Commun. 9:4670. doi: 10.1038/s41467-018-07036-w
Tu, Y. K., and Hsueh, Y. H. (2020). Extracellular vesicles isolated from human olfactory ensheathing cells enhance the viability of neural progenitor cells. Neurol. Res. 42, 959–967. doi: 10.1080/01616412.2020.1794371
van der Scheer, J. W., Goosey-Tolfrey, V. L., Valentino, S. E., Davis, G. M., and Ho, C. H. (2021). Functional electrical stimulation cycling exercise after spinal cord injury: a systematic review of health and fitness-related outcomes. J. Neuroeng. Rehabil. 18:99. doi: 10.1186/s12984-021-00882-8
van Niel, G., D’Angelo, G., and Raposo, G. (2018). Shedding light on the cell biology of extracellular vesicles. Nat. Rev. Mol. Cell Biol. 19, 213–228. doi: 10.1038/nrm.2017.125
Vietri, M., Radulovic, M., and Stenmark, H. (2020). The many functions of ESCRTs. Nat. Rev. Mol. Cell Biol. 21, 25–42. doi: 10.1038/s41580-019-0177-4
Vogel, A., Upadhya, R., and Shetty, A. K. (2018). Neural stem cell derived extracellular vesicles: attributes and prospects for treating neurodegenerative disorders. EBioMedicine 38, 273–282. doi: 10.1016/j.ebiom.2018.11.026
Wang, Y., Lai, X., Wu, D., Liu, B., Wang, N., and Rong, L. (2021). Umbilical mesenchymal stem cell-derived exosomes facilitate spinal cord functional recovery through the miR-199a-3p/145-5p-mediated NGF/TrkA signaling pathway in rats. Stem Cell Res. Ther. 12:117. doi: 10.1186/s13287-021-02148-5
Wang, W. Z., Li, J., Liu, L., Zhang, Z. D., Li, M. X., Li, Q., et al. (2021). Role of circular RNA expression in the pathological progression after spinal cord injury. Neural Regen. Res. 16, 2048–2055. doi: 10.4103/1673-5374.308100
Wang, L., Pei, S., Han, L., Guo, B., Li, Y., Duan, R., et al. (2018). Mesenchymal stem cell-derived exosomes reduce A1 astrocytes via downregulation of phosphorylated NFκB P65 subunit in spinal cord injury. Cell. Physiol. Biochem. 50, 1535–1559. doi: 10.1159/000494652
Wang, J., Rong, Y., Ji, C., Lv, C., Jiang, D., Ge, X., et al. (2020). MicroRNA-421-3p-abundant small extracellular vesicles derived from M2 bone marrow-derived macrophages attenuate apoptosis and promote motor function recovery via inhibition of mTOR in spinal cord injury. J. Nanobiotechnol. 18:72. doi: 10.1186/s12951-020-00630-5
Webb, R. L., Kaiser, E. E., Scoville, S. L., Thompson, T. A., Fatima, S., Pandya, C., et al. (2018). Human neural stem cell extracellular vesicles improve tissue and functional recovery in the murine thromboembolic stroke model. Transl. Stroke Res. 9, 530–539. doi: 10.1007/s12975-017-0599-2
Whetstone, W. D., Hsu, J. Y., Eisenberg, M., Werb, Z., and Noble-Haeusslein, L. J. (2003). Blood-spinal cord barrier after spinal cord injury: relation to revascularization and wound healing. J. Neurosci. Res. 74, 227–239. doi: 10.1002/jnr.10759
Wu, X., Wang, L., Cong, M., Shen, M., He, Q., Ding, F., et al. (2020). Extracellular vesicles from skin precursor-derived Schwann cells promote axonal outgrowth and regeneration of motoneurons via Akt/mTOR/p70S6K pathway. Ann. Transl. Med. 8:1640. doi: 10.21037/atm-20-5965
Xiao, X., Li, W., Rong, D., Xu, Z., Zhang, Z., Ye, H., et al. (2021). Human umbilical cord mesenchymal stem cells-derived extracellular vesicles facilitate the repair of spinal cord injury via the miR-29b-3p/PTEN/Akt/mTOR axis. Cell Death Discov. 7:212. doi: 10.1038/s41420-021-00572-3
Xin, W., Qiang, S., Jianing, D., Jiaming, L., Fangqi, L., Bin, C., et al. (2021). Human bone marrow mesenchymal stem cell-derived exosomes attenuate blood-spinal cord barrier disruption via the TIMP2/MMP pathway after acute spinal cord injury. Mol. Neurobiol. 58, 6490–6504. doi: 10.1007/s12035-021-02565-w
Xu, G., Ao, R., Zhi, Z., Jia, J., and Yu, B. (2019). miR-21 and miR-19b delivered by hMSC-derived EVs regulate the apoptosis and differentiation of neurons in patients with spinal cord injury. J. Cell. Physiol. 234, 10205–10217. doi: 10.1002/jcp.27690
Yi, H., and Wang, Y. (2021). A meta-analysis of exosome in the treatment of spinal cord injury. Open Med. (Wars) 16, 1043–1060. doi: 10.1515/med-2021-0304
Yu, T., Zhao, C., Hou, S., Zhou, W., Wang, B., and Chen, Y. (2019). Exosomes secreted from miRNA-29b-modified mesenchymal stem cells repaired spinal cord injury in rats. Braz. J. Med. Biol. Res. 52:e8735. doi: 10.1590/1414-431X20198735
Yu, B., Zhou, S., Yi, S., and Gu, X. (2015). The regulatory roles of non-coding RNAs in nerve injury and regeneration. Prog. Neurobiol. 134, 122–139. doi: 10.1016/j.pneurobio.2015.09.006
Yuan, X., Wu, Q., Wang, P., Jing, Y., Yao, H., Tang, Y., et al. (2019). Exosomes derived from pericytes improve microcirculation and protect blood-spinal cord barrier after spinal cord injury in mice. Front. Neurosci. 13:319. doi: 10.3389/fnins.2019.00319
Zhang, A., Bai, Z., Yi, W., Hu, Z., and Hao, J. (2021). Overexpression of miR-338-5p in exosomes derived from mesenchymal stromal cells provides neuroprotective effects by the Cnr1/Rap1/Akt pathway after spinal cord injury in rats. Neurosci. Lett. 761:136124. doi: 10.1016/j.neulet.2021.136124
Zhang, B., Lin, F., Dong, J., Liu, J., Ding, Z., and Xu, J. (2021). Peripheral macrophage-derived exosomes promote repair after spinal cord injury by inducing local anti-inflammatory type microglial polarization via increasing autophagy. Int. J. Biol. Sci. 17, 1339–1352. doi: 10.7150/ijbs.54302
Zhang, M., Wang, L., Huang, S., and He, X. (2021). Exosomes with high level of miR-181c from bone marrow-derived mesenchymal stem cells inhibit inflammation and apoptosis to alleviate spinal cord injury. J. Mol. Histol. 52, 301–311. doi: 10.1007/s10735-020-09950-0
Zhang, C., Zhang, C., Xu, Y., Li, C., Cao, Y., and Li, P. (2020). Exosomes derived from human placenta-derived mesenchymal stem cells improve neurologic function by promoting angiogenesis after spinal cord injury. Neurosci. Lett. 739:135399. doi: 10.1016/j.neulet.2020.135399
Zhao, C., Zhou, X., Qiu, J., Xin, D., Li, T., Chu, X., et al. (2019). Exosomes derived from bone marrow mesenchymal stem cells inhibit complement activation in rats with spinal cord injury. Drug Des. Devel. Ther. 13, 3693–3704. doi: 10.2147/DDDT.S209636
Zhong, D., Cao, Y., Li, C. J., Li, M., Rong, Z. J., Jiang, L., et al. (2020). Neural stem cell-derived exosomes facilitate spinal cord functional recovery after injury by promoting angiogenesis. Exp. Biol. Med. (Maywood) 245, 54–65. doi: 10.1177/1535370219895491
Zhou, X., Chu, X., Yuan, H., Qiu, J., Zhao, C., Xin, D., et al. (2019). Mesenchymal stem cell derived EVs mediate neuroprotection after spinal cord injury in rats via the microRNA-215p/FasL gene axis. Biomed. Pharmacother. 115:108818. doi: 10.1016/j.biopha.2019.108818
Zhou, S., Ding, F., and Gu, X. (2016). Non-coding RNAs as emerging regulators of neural injury responses and regeneration. Neurosci. Bull. 32, 253–264. doi: 10.1007/s12264-016-0028-7
Zhou, W., Silva, M., Feng, C., Zhao, S., Liu, L., Li, S., et al. (2021). Exosomes derived from human placental mesenchymal stem cells enhanced the recovery of spinal cord injury by activating endogenous neurogenesis. Stem Cell Res. Ther. 12:174. doi: 10.1186/s13287-021-02248-2
Keywords: spinal cord injury, exosome, mesenchymal stem cell, neural stem cell, noncoding RNAs
Citation: Yang Z-L, Rao J, Lin F-B, Liang Z-Y, Xu X-J, Lin Y-K, Chen X-Y, Wang C-H and Chen C-M (2022) The Role of Exosomes and Exosomal Noncoding RNAs From Different Cell Sources in Spinal Cord Injury. Front. Cell. Neurosci. 16:882306. doi: 10.3389/fncel.2022.882306
Received: 23 February 2022; Accepted: 21 March 2022;
Published: 18 April 2022.
Edited by:
Xiaohuan Xia, Tongji University, ChinaReviewed by:
He-Zuo Lü, Bengbu Medical College, ChinaLitao Tao, Creighton University, United States
Copyright © 2022 Yang, Rao, Lin, Liang, Xu, Lin, Chen, Wang and Chen. This is an open-access article distributed under the terms of the Creative Commons Attribution License (CC BY). The use, distribution or reproduction in other forums is permitted, provided the original author(s) and the copyright owner(s) are credited and that the original publication in this journal is cited, in accordance with accepted academic practice. No use, distribution or reproduction is permitted which does not comply with these terms.
*Correspondence: Chun-Mei Chen, MTczMTAxMjk0OEBxcS5jb20=; Chun-Hua Wang, d2NobWFpbEAxMjYuY29t
† These authors have contributed equally to this work and share first authorship