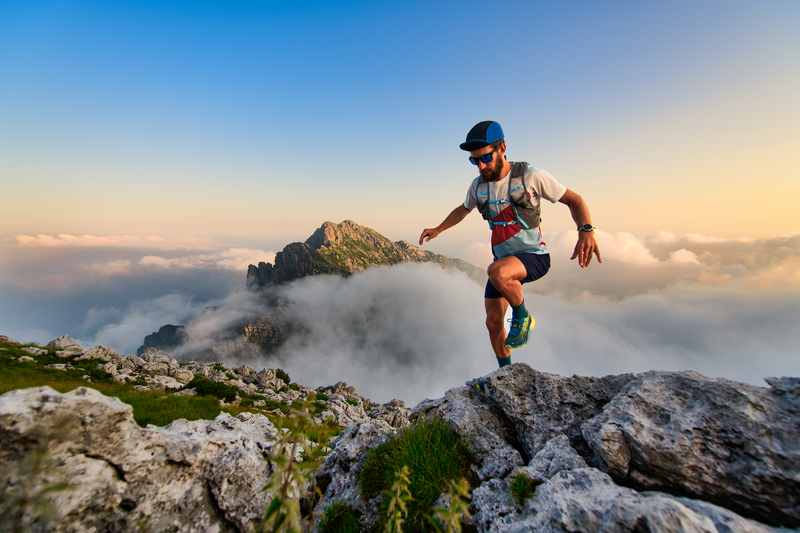
94% of researchers rate our articles as excellent or good
Learn more about the work of our research integrity team to safeguard the quality of each article we publish.
Find out more
REVIEW article
Front. Cell. Neurosci. , 03 June 2022
Sec. Cellular Neuropathology
Volume 16 - 2022 | https://doi.org/10.3389/fncel.2022.862673
This article is part of the Research Topic Innovative Therapies Using Neural Stem/Progenitor Cell Transplants for Spinal Cord Injury and Stroke View all 4 articles
Spinal cord injury (SCI) often leads to severe motor, sensory, and autonomic dysfunction in patients and imposes a huge economic cost to individuals and society. Due to its complicated pathophysiological mechanism, there is not yet an optimal treatment available for SCI. Mesenchymal stromal cells (MSCs) are promising candidate transplant cells for use in SCI treatment. The multipotency of MSCs, as well as their rich trophic and immunomodulatory abilities through paracrine signaling, are expected to play an important role in neural repair. At the same time, the simplicity of MSCs isolation and culture and the bypassing of ethical barriers to stem cell transplantation make them more attractive. However, the MSCs concept has evolved in a specific research context to encompass different populations of cells with a variety of biological characteristics, and failure to understand this can undermine the quality of research in the field. Here, we review the development of the concept of MSCs in order to clarify misconceptions and discuss the controversy in MSCs neural differentiation. We also summarize a potential role of MSCs in SCI treatment, including their migration and trophic and immunomodulatory effects, and their ability to relieve neuropathic pain, and we also highlight directions for future research.
Approximately 27 million people worldwide live with disability due to spinal cord injury (SCI), and approximately 0.92 million new patients are reported each year (James et al., 2019). SCI often results in partial or complete loss of sensory and motor functions in patients, resulting in huge physical and social consequences and a heavy medical burden for both patients’ families and society. The difficulty of treating SCI derives from its complex pathophysiology. First, adult neurons are terminally differentiated cells that cannot divide, and the number and distribution of endogenous neural stem cells in the spinal cord are very limited. Second, primary injury in the spinal cord often triggers a cascade of secondary damage, including the release of excitatory amino acids, loss of ionic homeostasis, cellular calcium overload, mitochondrial dysfunction, and multiple immune and inflammatory responses, which further aggravate tissue ischemia and inflammation, and lead to a cycle of neuronal and glial apoptosis. Thus, the secondary damage often exceeds the primary injury. Finally, glial scars and cystic cavities develop during the later stages of SCI and act as a physical barrier to axon regeneration (Ahuja et al., 2017a; Badhiwala et al., 2018). Thus, SCI often results in permanent neurological functional deficits. Regarding the low survival of patients and the huge consumption of medical resources, the interest in developing new treatments for SCI continues. However, achievements have been limited. In fact, according to the clinical guidelines, the only drug currently available for SCI treatment is methylprednisolone, along with strict timing requirements, unclear efficacy, and high risk of complications (Fehlings et al., 2017a,b). Another drug, ganglioside GM1, was once thought to be effective, but has been withdrawn from clinical practice amid great controversy (Hurlbert et al., 2013; Walters et al., 2013).
Stem cell therapy has broadened the field of SCI research because of its potential to protect, rescue, or replace damaged nerve cells. Many types of stem cells, including embryonic stem cells (ESCs), neural precursor/stem cells (NPCs/NSCs), olfactory ensheathing cells (OECs), Schwann cells (SCs), mesenchymal stromal cells (MSCs), and induced pluripotent stem cells (iPSCs), have been investigated for the treatment of SCI (Vismara et al., 2017). Therapies using these cells have achieved promising results on the bench. However, when it comes to clinical applications, especially for ESCs and NSCs, the cell source problem, ethical dilemmas, and capricious changes in policy are unavoidable problems (Murugan, 2009; Trawczynski et al., 2019). MSCs have been considered somatic stem cells. They are easily accessible and have strong self-renewal ability and multidirectional differentiation potential, which make them attractive candidates for cell therapy for SCI treatment. MSCs can be obtained from a variety of sources for autologous transplantation, avoiding the immune and ethical issues associated with ESCs. In addition, MSCs are safer than ESCs or iPSCs in teratoma formation and gene manipulation (Sacchetti et al., 2007; Bianco et al., 2008).
Over the past two decades, studies have shown that MSCs may have potential of cell replacement due to their multilineage differentiation abilities. In addition, MSCs may play a neuroprotective role and promote neuronal repair after transplantation into the injured spinal cord via multiple biological mechanisms. For example, their paracrine activity may produce neurotrophic, immunomodulatory, and anti-inflammatory effects. However, there are still some ambiguities in this field of research, including the controversy of neural differentiation and premature clinical trials. The emergence of novel cellular and biological techniques has deepened our understanding of MSCs at the genomic, transcription, and proteome levels, which also provides new interpretations and directions in the development of MSCs treatments for SCI. As the diverse functions of MSCs are revealed, the narrative of its potential for treating SCI has been constantly updated. In this review, we discuss the development of MSCs treatments for SCI and the underlying biological mechanisms, and summarize progress and further development in this field.
In 1968, Friedenstein discovered that a small number of bone marrow cells could adhere to Petri dishes and form fibroblast-like cells with osteogenic potential (Friedenstein et al., 1968, 1970). He also found that these cells could form colony-forming unit fibroblasts (CFU-Fs) from a single cell in vitro (Friedenstein et al., 1970). Subsequent studies found that these cells could differentiate into a variety of bone tissues both in vivo and in vitro, including bone, cartilage, and fat (Caplan, 1994; Horwitz et al., 2005). Thus, these multipotential cells were originally named osteogenic stem cells or bone marrow stromal stem cells (Friedenstein et al., 1987; Owen, 1988). For a long time, it was widely believed that hematopoietic stem cells were the only stem cells residing in the adult bone marrow. The discovery of these multipotential cells has brought attention to non-hematopoietic stem cells in bone marrow. Caplan et al. used the term “mesenchymal stem cell” to refer to these cells based on their multipotency to attract the attention of orthopedic research (Caplan, 1991; Pittenger et al., 1999). During this period, research in ESCs progressed rapidly, and the multipotential differentiation ability of MSCs seemed to make up the vacancy in the research of adult stem cells. In this context, many scientists considered that mesenchymal stem cells had a broader differentiation potential than expected. This hypothesis has aroused many studies investigating the trans-germ differentiation of MSCs, along with confusion and controversy (Grove et al., 2004; Lakshmipathy and Verfaillie, 2005). However, little experimental data has confirmed the stem cell properties of MSCs, and the term mesenchymal stem cells convey an assumption that deviates from the original concept of non-hematopoietic stem cells. The International Society for Cell & Gene Therapy (ISCT) suggested that these cells be named “mesenchymal stromal cells” (Horwitz et al., 2005). “Mesenchymal” indicates that the cells arise from embryonic loose connective tissue derived from mesoderm cells. “Stromal” indicates that the cells reside in the stromal/connective tissues of the supportive structures. Because the acronym “MSCs” has been widely used, retaining it avoids unnecessary confusion and maintains historical coherence. The removal of “stem” is hoped to evoke caution about its stemness and avoid exaggerating its potential. ISCT has drawn up criteria for categorizing MSCs that are widely used: MSCs (1) are plastic adherent, (2) express CD73, CD90, and CD105, but do not express hematopoietic and endothelial markers CD11b, CD14, CD19, CD34, CD45, CD79a, and HLA-DR, and (3) are capable of differentiating into adipocyte, chondrocyte, and osteoblast lineages in vitro (Dominici et al., 2006). Although this minimum standard has promoted research on MSCs, it has also led to the misconception that standard-compliant MSCs are identical in features and functions. In fact, later studies have demonstrated that the concept of MSCs under this framework includes different populations of cells with different biological functions.
As research has progressed, the concept of MSCs has expanded from bone marrow to other tissues. MSCs have been found in almost all blood vessel-containing adult tissues, including fat (Halvorsen et al., 2000), the umbilical cord (Romanov et al., 2003), skin (Richardson et al., 2005), radices dentis (Miura et al., 2003), and menstrual blood (Bozorgmehr et al., 2020; Figure 1). The widespread distribution of MSCs may be explained by the association between its origin and blood vessels. Accumulating evidence has suggested a close association between MSCs and pericytes around vessels (Sacchetti et al., 2016). Crisan et al. (2008) demonstrated that MSCs and pericytes share a high degree of overlap in cell surface labeling, in vivo location, and multilineage differentiation abilities. Therefore, MSCs are hypothesized to originate from pericytes and migrate to the capillary walls of fibrous tissue after embryonic development or injury (Corselli et al., 2012; Murray et al., 2014). However, despite the similarities between pericytes and MSCs, many differences between the two cells remain, and some studies have questioned their identities (Blocki et al., 2013; Guimaraes-Camboa et al., 2017). In addition, the consistency of MSCs populations cultured in vivo and in vitro is questionable. The multipotency of MSCs may be the product of in vitro culture, as they retain their identity in vivo and do not differentiate into other lineages (Guimaraes-Camboa et al., 2017).
Figure 1. The isolation, culture, and neuronal differentiation research procedure of MSCs. MSCs can be isolated from a variety of tissues including bone marrow, fat, placenta, and so on. These cells can be cultured adherently in Petri dish, and spread out in a flat spindle shape [as shown in microscope picture (A): red, Phalloidin; blue, DAPI]. The neural induction protocols of MSCs usually share some common features, including pharmacological drugs, neural trophic factors, and neuronal conditioned medium. The morphology of the differentiated MSCs will change to form neuron-like cells [as shown in microscope picture (B): red, Phalloidin; blue, DAPI. Unpublished data from the author]. The trans-differentiated cells are usually verified by cell morphology, neuronal markers, and electrophysiology examination to assess the effect of treatment. MSCs, mesenchymal stromal cells; RA, retinoic acid; SHH, sonic hedgehog; IBMX, 3-isobutyl-1-methylxanthine; BDNF, brain-derived neurotrophic factor; GDNF, glial cell-derived neurotrophic factor; NGF, nerve growth factor; bFGF, basic fibroblast growth factor.
As the research grows, our knowledge of the biological function of MSCs in SCI treatment has undergone a paradigm shift from cell replacement to paracrine actions. Some cytokines secreted by MSCs play a major role in tissue repair and microenvironment regulation through their trophic, angiogenic, immunomodulatory, and anti-inflammatory activities (Kordelas et al., 2014). Despite the multilineage differentiation potential of MSCs, their therapeutic effects in vivo differ from that of conventional stem cells. In addition, MSCs do not form teratomas due to their limited differentiation capacity in vivo, contributing to the safety of MSCs transplantation. Based on their secretory function, Caplan (2010) suggested abandoning the notion that MSCs are stem cells and changing their name to medical signaling cells. The ISCT has also recently reiterated its position on the concept of MSCs, supporting the use of the term “mesenchymal stromal cells.” This term now describes a large unisolated heterogeneous cell population that may include fibroblasts, myoblasts, and even a small number of stem/progenitor cells, but not hematopoietic or endothelial cells (Viswanathan et al., 2019). The term “mesenchymal stem cells” should only be applied for the cell with rigorous evidence of stem cell properties both in vitro and in vivo (Viswanathan et al., 2019). The specific functions of MSCs may vary according to their source, culture method, and stimulant factors. The specific characteristics of MSCs trophic secretion, immune regulation, and vasogenic effects can be characterized using functional matrix assays, including quantitative RNA analysis of specific genes, cell marker flow cytometry, and secretory proteome analysis (Galipeau et al., 2016; Chinnadurai et al., 2018).
The evolution of the concept of MSCs shows the dynamic development in this field, and clarifying its context is helpful to deepen our understanding of MSCs. Outdated and vague understandings of concepts can and have led to obstacles to scientific research. At the same time, as a widely carried out experimental therapy in clinic, MSCs must be well-understood for both clinicians and patients to avoid misplaced expectations of its efficacy.
Due to the multipotential differentiation ability of MSCs into mesodermal cells, researchers speculated about whether MSCs could differentiate into neuronal lineages (Figure 1). MSCs are thought to develop mainly from the mesoderm (Dennis and Charbord, 2002; Wislet-Gendebien et al., 2012). The neuroectoderm produces transient populations of cells characterized as MSCs, which can persist in some adult tissues (Dupin and Coelho-Aguiar, 2013). Takashima et al. (2007) showed that MSCs in embryos are derived from SOX1+ neuroepithelial cells. MSCs express nestin, βIII tubulin, or neuronal characteristics in soft gels which mimic bone marrow or nerve tissue environments (Mendez-Ferrer et al., 2010; Lee et al., 2016). Transcriptome analysis of bone marrow MSCs have shown that they also express mRNA of neural tissues (Tremain et al., 2001). Genomic analyses of MSCs have shown significant overlap with master transcriptional regulators (e.g., RUNX2, C/EBPβ) that are epigenetically reduced in size after differentiation, and these promoter regions are highly plastic, leading to the trans-differentiation ability of MSCs (Wu et al., 2017). These studies serve as theoretical support for the neural differentiation potential of MSCs. Another theoretical support comes from embryological studies, which have shown that cells from one tissue can be implanted into another tissue and be locally controlled by the new environment and serve different functions.
In the last 20 years, many pioneering studies have explored the possibility neuronal differentiation of MSCs in the treatment for SCI. By transplanting MSCs into the lateral ventricles of newborn mice, Kopen et al. (1999) observed the expression of GFAP and neurofilament proteins in MSCs, suggesting that they may differentiate into nerve cells. Subsequently, MSCs cultured in vitro were stimulated with chemical reagents and were morphologically transformed into neuron-like cells with several different nerve cell surface markers (Sanchez-Ramos et al., 2000; Woodbury et al., 2000). Brazelton et al. (2000) observed that MSCs injected intravenously migrate to the brain and differentiate into neuron-like cells. A large number of experiments have demonstrated that there do have many culture conditions, differentiation protocols, and gene regulations that can induce MSCs to differentiate into neuron-like cells in vitro, express proteins that are typically expressed in nerve cells such as nerve filaments and excitatory amino acid receptors, or exhibit some electrophysiological activity (Kopen et al., 1999; Jiang et al., 2003; Dezawa et al., 2004; Hermann et al., 2004; Kondo et al., 2005; Bi et al., 2010; Ma et al., 2011; Aguilera-Castrejon et al., 2017; Kang et al., 2019). Although the culture conditions for these studies differed, they shared some common features in general, including the use of neural stem cell conditioned media, the addition of neurotrophic factors or pharmacological drugs to stimulate specific signaling pathways, and the artificial manipulation of neural cell-specific gene expressions. The pathways involved primarily included retinoic acid, Hedgehog, cAMP, Wnt, neurotrophin-activated pathways, and MAPK (Neirinckx et al., 2013; Choudhary et al., 2021). Some studies have also shown that physical methods, such as surface morphology, elasticity, and even acoustic waves, can trigger neural differentiation of MSCs (Choi et al., 2016; Mung et al., 2016; Yang et al., 2020). In addition, some adjuvant components such as butylated hydroxyanisole (BHA), β-mercaptoethanol (BME), and dimethyl sulfoxide (DMSO) are added to the medium to induce neural differentiation of MSCs, but lacking detailed explanation of their mechanisms (Woodbury et al., 2000; Kondo et al., 2005; Naghdi et al., 2009).
Although numerous studies demonstrating MSCs differentiation into neurons have been published, most are not as rigorous as the studies of the mesodermal differentiation of MSCs, and detailed experimental conditions and criteria require further identification. The reprograming of stem cell phenotypes depends on the appropriate cellular environment and sustained application of instructive agents. The developmental biology of MSCs in the embryonic stage is far different from that of adult tissue repair and induction culture environments. Most existing studies assessed the neuronal induction of MSCs via cell morphology and the expression of classical neural markers, and some have examined ion channel properties (Tropel et al., 2006; Neirinckx et al., 2013). However, the criteria of cell differentiation requires further clarification: differentiation into neurons does not simply mean that the cell has a similar morphology or immune-phenotype to that of a nerve cell, but more importantly, that the differentiated cell has the ability to receive and transmit neural signals, including releasing neurotransmitters or activating action potentials. Morphological changes and neural marker expression of trans-differentiated MSCs may be artifacts, and electrophysiological activity cannot be equated with action potential triggering and conduction (Montzka et al., 2009). In fact, it has been suggested that although trans-differentiated MSCs have a neuronal phenotype, they lack functional action potentials (Barnabe et al., 2009). Lu et al. (2004) summarized several studies of the drug-induced differentiation of MSCs into nerve cells. Although cytoplasmic wrinkling and increased expression of neural markers were observed in different cell types treated with these drugs, these phenomena were also observed when MSCs were treated with cytotoxic agents. Therefore, Lu et al. (2004) suggested that neural differentiation of MSCs may be morphological changes and neural marker expression artifacts caused by cytoplasmic shrinkage, which was itself triggered by cellular stress responses. Neuhuber et al. (2004) reported similar observations. Neural markers are a necessary but not sufficient condition for neural differentiation of MSCs. The neural ectodermal origin of some MSCs may explain the early results showing that MSCs share some surface markers with neural lineage cells (Phinney and Prockop, 2007). For example, nestin, a classic marker of neural stem cells, is also expressed in subtypes of MSCs that are capable of osteogenesis and angiogenesis (Mendez-Ferrer et al., 2010; Baryawno et al., 2019). In addition, transcriptome analysis of MSCs after induction has shown that the increased expression of nestin may be due to the upregulation of cytoskeleton-related proteins; that is, it may be based on morphological changes rather than changes in cell function (Khan et al., 2020).
In conclusion, current research indicates that the evidence for neural differentiation of MSCs is not strong enough, and it remains difficult to differentiate MSCs into functional mature nerve cells in vitro using current culture regimens. Therefore, a more cautious attitude is needed to review studies of neural differentiation of MSCs in multiple dimensions.
Although the neural differentiation and cell replacement functions of MSCs are controversial, the beneficial effects of MSCs on SCI have indeed been observed by researchers. These benefits may not result from the cellular replacement of MSCs, but rather from the paracrine and immune regulatory roles of MSCs. Contrary to the belief that cells behave the same way in tissue culture as they do in vivo, MSCs did not differentiate into cartilage, bone, or fat in vivo (Caplan, 2017). Zwolanek et al. (2017) found that MSCs did not differentiate directly into cartilage during cartilage repair as expected, but rather acted through non-progenitor cell pathways. A similar situation occurred during nerve damage repair. One possible explanation is that due to the role of MSCs themselves in supporting and maintaining the hematopoietic microenvironment and the blood sinus network in bone marrow, the transplantation of MSCs may lead to the transfer of their inherent biological functions to target organs, namely, non-hematopoietic cells may obtain the nursing effect from direct or paracrine interaction with MSCs (Caplan and Dennis, 2006; Bianco et al., 2008). Gene expression analysis of MSCs has shown that MSCs are not only involved in hematopoietic support but are also involved in angiogenic, anti-inflammatory, and immunomodulatory activities (Tremain et al., 2001; Pittenger et al., 2019). Many studies have found that growth factors, cytokines, and other bioactive substances produced by MSCs are contained in exosomes and microvesicles, which play a paracrine role in stimulating tissue repair, regulating inflammation, modulating immunity, promoting angiogenesis, and repairing nerve injury (Table 1; Baglio et al., 2015; Phinney and Pittenger, 2017). These secretory effects change dynamically with the microenvironment in vivo or at the site of injury, and in turn, affect the local microenvironment. This “plasticity” and “crosstalk” are the key to the therapeutic effects of MSCs (Phinney and Sensebe, 2013). At the same time, this also makes exploration of the therapeutic effects of MSCs more complicated, and it is difficult to identify the specific role of certain cell factors in neuroprotection. The comprehensive role of these pathways is not fully understood because of the synergies and overlap of these mechanisms in function, and how this process dynamically responds to SCI damage environmental cues, cell cloning, and culture environment has not been fully revealed. Therefore, reviewing the secretory characteristics of MSCs in SCI is the key to understanding their biological functions (Figure 2).
Table 1. Mesenchymal stromal cells (MSCs) secrete a variety of growth factors, cytokines, and bioactive substances to take effect in spinal cord injury.
Figure 2. Typical mechanisms of MSCs transplantation in the treatment of SCI. MSCs can be administrated by intravenous, intraspinal, and subarachnoid injection. Regardless of the administration route, MSCs can migrate and accumulate to the injury site. (A) The migration of MSCs is conducted through the SDF-1/CXCR4 axis. (B) MSCs secrete a variety of neurotrophic and immunoregulatory factors through a bystander effect to regulate the microenvironment of injury site, rescue cell death and promote axon growth. (C) Most MSCs reside around the vessels and have some functionalities in common with those of pericytes. Besides, MSCs secrete VEGF constitutively without being regulated by inflammatory factors, which has a positive effect on improving vascular injury, ischemia, hypoxia, and the accumulation of inflammatory substances after SCI. (D) MSCs can migrate to the DRG through SDF-1/CXCR4, and secrete anti-inflammatory factors such as TGF-β1, IL-10, LIF-10 to inhibit hyperexcitability of DRG cells, alleviate opioid tolerance, and relieve hyperalgesia. CXCR4, CXC chemokine receptor type 4; SDF-1, stromal cell-derived factor-1; VEGF, vascular endothelial growth factor; TGF-β1, transforming growth factor beta 1; IL-10, interleukin-10; LIF-10, leukemia inhibitory factor.
The migration, or homing, of MSCs is their ability to migrate to injured tissues/organs. This migration concentrates the biological function of MSCs at the site of SCI and is the basis for the feasibility of intravenous or intrathecal injection of MSCs. MSCs migration is regulated by a variety of factors, and the key chemokine is stromal cell-derived factor-1 (SDF-1, also known as CXCL12) (Liu et al., 2011). SDF-1 levels can be locally elevated due to pathological conditions such as inflammation, ischemia, and hypoxia (Li and Ransohoff, 2008). SDF-1 binds to CXC chemokine receptor 4 (CXCR4) on the surfaces of MSCs to activate signaling molecules, including PI3K, ERK, and Akt, which attract MSCs to the site of injury (Bang et al., 2017). In addition, substance P released after SCI impairs transforming growth factor β (TGF-β) mediated MSCs mobilization but does not affect the function of SDF-1 (Nam et al., 2020). A variety of growth factors, including basic fibroblast growth factor (bFGF), hepatocyte growth factor (HGF), leukemia inhibitory factor (LIF), vascular endothelial growth factor (VEGF), IGF-1, and VE-cadherin, also participate in the migration of MSCs (Karp and Leng Teo, 2009; Nitzsche et al., 2017; Fu et al., 2019).
A widely proposed mechanism by which MSCs promote SCI recovery is the trophic factors or cytokines secreted by MSCs, which play an important role in regulating endogenous repair after SCI in many aspects. Chopp and Li (2002) demonstrated the presence of important neuroregulatory molecules in the secretome of MSCs, including vascular endothelial growth factor (VEGF), nerve growth factor (NGF), brain-derived neurotrophic factor (BDNF), basic fibroblast growth factor (bFGF), insulin-like growth factor-1 (IGF-1), and glial cell derived neurotrophic factor (GDNF), which play an important role in neurogenesis and angiogenesis. Furthermore, Teixeira et al. (2017) analyzed untreated bone marrow MSCs and observed secretion of other factors, such as interleukin-6 (IL-6), cystatin C, glia-derived nexin (GDN), galectin-1, and pigment epithelium-derived factor (PEDF), which plays an important role in cell migration, differentiation, and neuroprotection. BDNF is believed to have the ability to induce neuronal differentiation and axon growth (Fujii et al., 2015; Ritfeld et al., 2015), and its protective effect is mediated partly by activation of the AKT pathway (Wilkins et al., 2009). Direct transplantation of BDNF-overexpressing bone marrow MSCs to treat nerve injury increases nerve tissue volume and vascular density (Ritfeld et al., 2015). Gunther et al. (2015) used an alginate scaffold to carry BDNF-modified MSCs and observed a large number of axons regenerated and penetrated across the injury site. However, Brock et al. (2016) transplanted MSCs genetically modified to secrete BDNF into spinal cord contusion injuries in rats and observed no reduction in the size of the damaged area or improvement in motor function. GDNF plays an important role in the improvement of motor function and has antioxidant effects, enhancing the activity of enzymes involved in detoxification of reactive oxygen species (e.g., superoxide dismutase, catalase, and glutathione) (Hoban et al., 2015). Up-regulation of PEDF stimulates the cascade activation of the NF-kB signal, induces the expression of BDNF and GDNF, and triggers improvement in behavior and survival of neurons (Falk et al., 2010). In addition, Sanchez et al. (2012) found that PEDF can reduce the oxidant-induced neuronal death through reducing phosphorylation of extracellular signal-regulated kinase 1/2. Samper Agrelo et al. (2020) analyzed the secretome of bone marrow MSCs transplanted into the nervous system and found increased survival of neural stem cells stimulated by transplanted MSCs. Moreover, the tissue inhibitor metalloproteinase type 1 (TIMP-1) secreted by MSCs promoted the differentiation of NSCs into oligodendrocytes, which promoted the formation of regenerative myelin sheaths (Samper Agrelo et al., 2020). MSCs can also secrete VEGF constitutively without being regulated by inflammatory factors, which has a positive effect on improving vascular injury, ischemia, hypoxia, and the accumulation of inflammatory substances after SCI, thereby promoting the repair of nerve tissues (Okon et al., 2013; Teixeira et al., 2017). In addition, it was found that hypoxia (O2 < 5%) can induce MSCs to enhance nerve growth and angiogenesis capacity (Lennon et al., 2001; Rosova et al., 2008; Tsai et al., 2011; Liu et al., 2013). The up-regulated expression of growth factors such as BDNF, GDNF, IGF-1, and bFGF after MSCs transplantation can also promote the formation of immature blood vessels (Li et al., 2021).
Multiple factors secreted by MSCs are involved in local inflammatory responses following SCI. Chinnadurai et al. (2018) analyzed the immunomodulatory function of the secretome of MSCs using a combinatorial assay matrix method and found that it involves altering the active regulatory pathway in immune response, which plays a major role in the immune regulation of host tissues. MSCs can also exert anti-inflammatory or immune regulation effects by responding to inflammatory stimuli. For example, exposure to interferon-gamma (IFN-γ) causes MSCs to bring forth immunosuppressive activity by expressing indoleamine 2, 3-dioxygenase (IDO1), an enzyme that consumes tryptophan to reduce inflammation (Krampera et al., 2006). In addition, Galleu et al. (2017) reported that the apoptosis of MSCs induced by recipient cytotoxic cells after transplantation is essential to initiate immunosuppression. Apoptotic MSCs are engulfed by phagocytes and induce the latter to produce IDO1. The MSCs also secrete the TNF-α-stimulated gene 6 protein (TSG-6) when activated by inflammatory signals and suppress the inflammation cascade induced by NF-κB signaling pathway in resident macrophages, and shift macrophages from pro-inflammatory to anti-inflammatory phenotype (Choi et al., 2011; Mittal et al., 2016). In addition, IFN-γ and tumor necrosis factor (TNF-α) promoted the polarization of MSCs toward a secretory phenotype characterized by the expression of IL-4, IL-10, CD274, PD-L1, and IDO (Jin et al., 2016). Besides, the transplanted MSCs may also be phagocytosed by monocytes and induce the latter shift to immunomodulatory intermediate phenotype with anti-inflammatory abilities (Bernardo and Fibbe, 2013; de Witte et al., 2018). However, exposure to inflammatory stimuli also has adverse effects on MSCs. For example, IFN-γ can induce programed cell death of MSCs (Bernardo and Fibbe, 2013). In addition, procedures in cell transplantation, such as freezing and thawing, result in a defective secretome of MSCs and reduce their survival rate in inflammatory environments (Pollock et al., 2015; Chinnadurai et al., 2016).
Neuropathic pain is a common complication following SCI and often seriously affects the physical and mental state of patients. Opioids are a common drug to treat neuropathic pain but often cause opioid tolerance and induce hyperalgesia. MSCs transplantation has shown favorable analgesic effects in these patients. Chen et al. (2015) found that bone marrow MSCs injected into the subarachnoid space of the spinal cord recruited to the dorsal root ganglion (DRG) through SDF-1/CXCR4, and secreted TGF-β1 to inhibit hyperexcitability of DRG cells and relieve hyperalgesia. Both intrathecal and intravenous injection of MSCs can alleviate opioid tolerance and opioid-induced hyperalgesia, the mechanisms of which may be related to TGF, IL-10, and LIF-10 secreted by MSCs (Li et al., 2018). Hua et al. (2016) reported similar results. Vaquero et al. (2018a) reported that MSCs transplantation significantly improved neuropathic pain in patients in a clinical trial. In conclusion, the relief of neuropathic pain conducted by MSCs transplantation brings additional benefits for SCI treatment.
Nestin, a type VI intermediate filament protein, is regarded as a neural stem cell marker, as well as expressed in fibroblasts, endothelial progenitor cells, and some bone marrow MSCs (Lendahl et al., 1990; Mokry et al., 2004; Xie et al., 2015). Nestin+ MSCs co-localize with hematopoietic stem cells (HSCs) and constitute an important component of the HSCs niche (Mendez-Ferrer et al., 2010). The Nestin+ group of MSCs has some potential superior characteristics that may make it a candidate for the treatment of SCI. Nestin+ MSCs have stronger self-renewal and multilineage differentiation potential than Nestin– MSCs and express higher levels of chemokine SDF-1, stem cell factor, angiopoietin-1, and vascular cell adhesion molecule 1 (VCAM1) (Lindsay and Barnett, 2017; Lu et al., 2019). The secretion of SDF-1 by Nestin+ MSCs is regulated by the sympathetic nerve, and the SDF-1 not only promotes the recruitment of NSCs to the injury site but also provides guiding cues for axons and neurites, thus promoting axon growth and neurogenesis (Li et al., 2012; Guyon, 2014). Lindsay et al. (2013) found that Nestin+ MSCs isolated from olfactory mucosa could better promote the myelination of the central nervous system in vitro. In addition, Nestin+ MSCs show strong potential for angiogenesis (Pacini and Petrini, 2014). Due to these unique biological characteristics of Nestin+ MSCs, they may be a good candidate for repairing SCI, or one of the entry points for us to understand the biological function of MSCs fully.
The efficacy of MSCs transplantation is affected by the time and route of administration, as well as the number of cells. Administration routes include intravenous, intraspinal, and subarachnoid injection. Many preclinical and clinical studies have reported on the safety of MSCs transplantation by these routes, but there is no sufficient evidence to show that one route is superior to others (Table 2; Zhao et al., 2019; Zholudeva and Lane, 2019). The intravenous injection is less invasive, but there is a risk of cells sticking together that forms microemboli and block the vascular system. In addition, a large proportion of cells are trapped in peripheral organs and circulation due to the blood-spinal barrier. Direct intraspinal injection of MSCs into the injured area of the spinal cord can achieve a high number of cells in the site of transplantation. However, the harsh microenvironment of the injured spinal cord contains inflammatory factors and ion disturbances, which affect the survival and proliferation of transplanted cells. In addition, the volume effect of injecting a large number of cells may cause secondary damage. Since MSCs function through bystander effect rather than cell replacement, transplanting them around the injury site instead right in the epicenter can make them take effect while avoiding the adverse effects of the harsh microenvironment and improving the survival rate of cells. Current clinical studies have shown no significant difference in the efficacy of intravenous or intrathecal injection of MSCs, which may be due to the migration effect of MSCs (Muthu et al., 2021).
Table 2. Main clinical studies on mesenchymal stromal cells (MSCs) therapy for spinal cord injury (SCI) in recent 10 years.
The timing of cell transplantation is also a key issue to consider because the changes in the local environment over time after injury will affect the survival and proliferation of the transplanted cells, as well as the mechanism by which they take effect. SCI can be temporally divided into acute (<48 h), subacute (48 h to 14 days), intermediate (14 days to 6 months), and chronic phases (>6 months) (Ahuja et al., 2017b). The major problem of cell transplantation during the acute phase is that the pro-inflammatory environment at the lesion site adversely affects the cell survival, but in turn, the surviving cells can secrete anti-inflammatory or neuroprotective factors to take effect in the early stage. The subacute phase is characterized by continuous anatomical and biochemical changes in the spinal cord, during which cell transplantation may take effect in trophic support and angiogenesis, with a relatively better survival rate (Lin et al., 2017). Four weeks after the SCI, the anatomy and physiology condition of the injured spinal cord become stable, and glial scars are formed as a barrier to nerve regeneration. It is unclear whether the paracrine ability of MSCs can affect the chronic glial scars, and whether the benefits of transplantation during this stage are comparable to those of earlier treatments. However, the research on cell transplantation in the chronic phase is of great significance, as most SCI patients are living in this period, and more research should focus on this area.
The dose of cell transplantation varies widely, ranging from 1 × 105 to 1 × 109 (Andrzejewska et al., 2021). A meta-analysis of clinical studies on MSCs transplantation for SCI showed that therapeutic effects occurred with a low dose of MSCs (<5 × 107 cells) injected intravenously or intrathecally (Muthu et al., 2021). Another meta-analysis showed that transplant dose 1–5 × 107 between 10–20 × 107 may provide more benefits for patients with SCIs (Zhao et al., 2019). However, Krupa et al. (2018) showed a dose-dependent effect of intrathecal injection of MSCs in the treatment of SCI, and multiple and high-dose cell transplants (1.5 million × 3 times) have better efficacy. Similar results have been reported in studies by Vaquero et al. (2016). It is difficult to identify the ideal dose of transplanted cells, but a large dose does not seem to affect the safety of MSCs transplantation.
The ethical rationality of MSCs transplantation is based more on the observed safety than the in-depth understanding of its mechanisms. The ISCT minimal standards for MSCs have promoted research on this field, but relying on these standards alone has led to the misconception that standards-compliant MSCs are identical in characteristics and functions. In fact, MSCs are not merely a collection of surface markers but have huge heterogeneity due to differences in cell sources, culture methods, and stimulation methods, and the biological features of MSCs in vivo and in vitro are not exactly the same (Le Blanc and Davies, 2018; Pittenger et al., 2019). In addition, while MSCs play a complex role in vivo and affect the local environment, they can also be influenced by the host tissues, which increases the complexity of their function. These scientific uncertainties bring about ethical flaws, since in the absence of thoroughly clarifying conditions and mechanisms, the clinical application of MSCs in the treatment of SCI lacks a steady scientific foundation. However, from a practical point of view, the research history of cell transplantation and an abundance of clinical trial data do show that there are no significant adverse reactions to MSCs in the treatment of SCI, no matter what kind of administration route. Given the plight of SCI patients, such a treatment may be worth taking a try, but it must be performed with strict supervision and full informed consent.
Given the heterogeneity of MSCs and their susceptibility in culture conditions in vitro, researchers must recognize the fact that different culture conditions will produce different MSCs products, even if they meet the minimum standards of MSCs. There are dozens of clinical trials of MSCs for the treatment of SCI on the registry; however, the heterogeneity of the MSCs in these studies reduces the comparability of data (Pittenger et al., 2019). To address this issue, bioequivalence standards have been derived from the iPSC library to create a stable, differentiable benchmark against which MSCs products can be compared (Viswanathan et al., 2014; Prockop, 2017). The study of MSCs therapy requires the incorporation of technical systems for the isolating, culturing, and purifying of MSCs from different sources into research, and carrying out standardized testing of in vitro genetic stability and efficacy testing of disease-specific mechanisms (Yin et al., 2019). Standardizing the quality and characteristics of MSCs products using these methods would improve the comparability of studies and reduce ethical issues.
We reviewed the conceptual evolution of MSCs and summarized the mechanisms of MSCs in treating SCI. The ease of acquisition and expansion of MSCs, as well as their abundant trophic functions, anti-inflammatory, and immunomodulatory activities, make them an attractive cellular tool for the treatment of SCI. However, the complexity of the identity and function of MSCs is the other side of the coin, and we should admit that our understanding of MSCs is incomplete. Our understanding of MSCs and its role in the treatment of SCI updates with the development of biological methods. As a next step, efforts to trans-differentiate MSCs into neural lineages should be incorporated into a more rigorous framework. Another goal is to identify the specific mechanisms of MSCs with different characteristics in SCI and how they respond to the changing environments of injury. Clarifying these issues is essential for the final clinical application of MSCs therapy.
J-LX illustrated the figures. All authors provided input, co-wrote the manuscript, and approved the submitted version.
This work was supported by the National Key Research and Development Program (No. 2020YFC1107402), the Innovation and Entrepreneurship Program of Jiangsu Province, and a Priority Academic Program Development of Jiangsu Higher Education Institutions.
The authors declare that the research was conducted in the absence of any commercial or financial relationships that could be construed as a potential conflict of interest.
All claims expressed in this article are solely those of the authors and do not necessarily represent those of their affiliated organizations, or those of the publisher, the editors and the reviewers. Any product that may be evaluated in this article, or claim that may be made by its manufacturer, is not guaranteed or endorsed by the publisher.
Aguilera-Castrejon, A., Pasantes-Morales, H., Montesinos, J. J., Cortes-Medina, L. V., Castro-Manrreza, M. E., Mayani, H., et al. (2017). Improved proliferative capacity of NP-like cells derived from human mesenchymal stromal cells and neuronal transdifferentiation by small molecules. Neurochem. Res. 42, 415–427. doi: 10.1007/s11064-016-2086-7
Ahuja, C. S., Nori, S., Tetreault, L., Wilson, J., Kwon, B., Harrop, J., et al. (2017a). Traumatic spinal cord injury-repair and regeneration. Neurosurgery 80, S9–S22. doi: 10.1093/neuros/nyw080
Ahuja, C. S., Wilson, J. R., Nori, S., Kotter, M. R. N., Druschel, C., Curt, A., et al. (2017b). Traumatic spinal cord injury. Nat. Rev. Dis. Primers 3:17018. doi: 10.1038/nrdp.2017.18
Andrzejewska, A., Dabrowska, S., Lukomska, B., and Janowski, M. (2021). Mesenchymal stem cells for neurological disorders. Adv. Sci. 8:2002944. doi: 10.1002/advs.202002944
Badhiwala, J. H., Ahuja, C. S., and Fehlings, M. G. (2018). Time is spine: a review of translational advances in spinal cord injury. J. Neurosurg. Spine 30, 1–18. doi: 10.3171/2018.9.SPINE18682
Baglio, S. R., Rooijers, K., Koppers-Lalic, D., Verweij, F. J., Perez Lanzon, M., Zini, N., et al. (2015). Human bone marrow- and adipose-mesenchymal stem cells secrete exosomes enriched in distinctive miRNA and tRNA species. Stem Cell Res. Ther. 6:127. doi: 10.1186/s13287-015-0116-z
Bang, O. Y., Moon, G. J., Kim, D. H., Lee, J. H., Kim, S., Son, J. P., et al. (2017). Stroke induces mesenchymal stem cell migration to infarcted brain areas via CXCR4 and C-Met signaling. Transl. Stroke Res. doi: 10.1007/s12975-017-0538-2 Online ahead of print,
Barnabe, G. F., Schwindt, T. T., Calcagnotto, M. E., Motta, F. L., Martinez, G. Jr., de Oliveira, A. C., et al. (2009). Chemically-induced RAT mesenchymal stem cells adopt molecular properties of neuronal-like cells but do not have basic neuronal functional properties. PLoS One 4:e5222. doi: 10.1371/journal.pone.0005222
Baryawno, N., Przybylski, D., Kowalczyk, M. S., Kfoury, Y., Severe, N., Gustafsson, K., et al. (2019). A cellular taxonomy of the bone marrow stroma in homeostasis and leukemia. Cell 177, 1915–1932.e16. doi: 10.1016/j.cell.2019.04.040
Bernardo, M. E., and Fibbe, W. E. (2013). Mesenchymal stromal cells: sensors and switchers of inflammation. Cell Stem Cell 13, 392–402. doi: 10.1016/j.stem.2013.09.006
Bi, Y., Gong, M., Zhang, X., Zhang, X., Jiang, W., Zhang, Y., et al. (2010). Pre-activation of retinoid signaling facilitates neuronal differentiation of mesenchymal stem cells. Dev. Growth Differ. 52, 419–431. doi: 10.1111/j.1440-169X.2010.01182.x
Bianco, P., Robey, P. G., and Simmons, P. J. (2008). Mesenchymal stem cells: revisiting history, concepts, and assays. Cell Stem Cell 2, 313–319. doi: 10.1016/j.stem.2008.03.002
Blocki, A., Wang, Y., Koch, M., Peh, P., Beyer, S., Law, P., et al. (2013). Not all MSCs can act as pericytes: functional in vitro assays to distinguish pericytes from other mesenchymal stem cells in angiogenesis. Stem Cells Dev. 22, 2347–2355. doi: 10.1089/scd.2012.0415
Bozorgmehr, M., Gurung, S., Darzi, S., Nikoo, S., Kazemnejad, S., Zarnani, A. H., et al. (2020). Endometrial and menstrual blood mesenchymal stem/stromal cells: biological properties and clinical application. Front. Cell Dev. Biol. 8:497. doi: 10.3389/fcell.2020.00497
Brazelton, T. R., Rossi, F. M., Keshet, G. I., and Blau, H. M. (2000). From marrow to brain: expression of neuronal phenotypes in adult mice. Science 290, 1775–1779. doi: 10.1126/science.290.5497.1775
Brock, J. H., Graham, L., Staufenberg, E., Collyer, E., Koffler, J., and Tuszynski, M. H. (2016). Bone marrow stromal cell intraspinal transplants fail to improve motor outcomes in a severe model of spinal cord injury. J. Neurotrauma 33, 1103–1114. doi: 10.1089/neu.2015.4009
Caplan, A. I. (1991). Mesenchymal stem cells. J. Orthop. Res. 9, 641–650. doi: 10.1002/jor.1100090504
Caplan, A. I. (2010). What’s in a name? Tissue Eng. Part A 16, 2415–2417. doi: 10.1089/ten.TEA.2010.0216
Caplan, A. I. (2017). Mesenchymal stem cells: time to change the name! Stem Cells Transl. Med. 6, 1445–1451. doi: 10.1002/sctm.17-0051
Caplan, A. I., and Dennis, J. E. (2006). Mesenchymal stem cells as trophic mediators. J. Cell Biochem. 98, 1076–1084. doi: 10.1002/jcb.20886
Chen, G., Park, C. K., Xie, R. G., and Ji, R. R. (2015). Intrathecal bone marrow stromal cells inhibit neuropathic pain via TGF-beta secretion. J. Clin. Invest. 125, 3226–3240. doi: 10.1172/JCI80883
Cheng, H., Liu, X., Hua, R., Dai, G., Wang, X., Gao, J., et al. (2014). Clinical observation of umbilical cord mesenchymal stem cell transplantation in treatment for sequelae of thoracolumbar spinal cord injury. J. Transl. Med. 12:253. doi: 10.1186/s12967-014-0253-7
Chinnadurai, R., Copland, I. B., Garcia, M. A., Petersen, C. T., Lewis, C. N., Waller, E. K., et al. (2016). Cryopreserved mesenchymal stromal cells are susceptible to T-cell mediated apoptosis which is partly rescued by IFNgamma licensing. Stem Cells 34, 2429–2442. doi: 10.1002/stem.2415
Chinnadurai, R., Rajan, D., Qayed, M., Arafat, D., Garcia, M., Liu, Y., et al. (2018). Potency analysis of mesenchymal stromal cells using a combinatorial assay matrix approach. Cell Rep. 22, 2504–2517. doi: 10.1016/j.celrep.2018.02.013
Choi, H., Lee, R. H., Bazhanov, N., Oh, J. Y., and Prockop, D. J. (2011). Anti-inflammatory protein TSG-6 secreted by activated MSCs attenuates zymosan-induced mouse peritonitis by decreasing TLR2/NF-kappaB signaling in resident macrophages. Blood 118, 330–338. doi: 10.1182/blood-2010-12-327353
Choi, Y., Park, J. E., Jeong, J. S., Park, J. K., Kim, J., and Jeon, S. (2016). Sound waves induce neural differentiation of human bone marrow-derived mesenchymal stem cells via ryanodine receptor-induced calcium release and Pyk2 activation. Appl. Biochem. Biotechnol. 180, 682–694. doi: 10.1007/s12010-016-2124-6
Chopp, M., and Li, Y. (2002). Treatment of neural injury with marrow stromal cells. Lancet Neurol. 1, 92–100. doi: 10.1016/s1474-4422(02)00040-6
Choudhary, P., Gupta, A., and Singh, S. (2021). Therapeutic advancement in neuronal transdifferentiation of mesenchymal stromal cells for neurological disorders. J. Mol. Neurosci. 71, 889–901. doi: 10.1007/s12031-020-01714-5
Corselli, M., Chen, C. W., Sun, B., Yap, S., Rubin, J. P., and Peault, B. (2012). The tunica adventitia of human arteries and veins as a source of mesenchymal stem cells. Stem Cells Dev. 21, 1299–1308. doi: 10.1089/scd.2011.0200
Crisan, M., Yap, S., Casteilla, L., Chen, C. W., Corselli, M., Park, T. S., et al. (2008). A perivascular origin for mesenchymal stem cells in multiple human organs. Cell Stem Cell 3, 301–313. doi: 10.1016/j.stem.2008.07.003
Dai, G., Liu, X., Zhang, Z., Yang, Z., Dai, Y., and Xu, R. (2013). Transplantation of autologous bone marrow mesenchymal stem cells in the treatment of complete and chronic cervical spinal cord injury. Brain Res. 1533, 73–79. doi: 10.1016/j.brainres.2013.08.016
de Witte, S. F. H., Luk, F., Sierra Parraga, J. M., Gargesha, M., Merino, A., Korevaar, S. S., et al. (2018). Immunomodulation by therapeutic Mesenchymal Stromal Cells (MSC) is triggered through phagocytosis of MSC by monocytic cells. Stem Cells 36, 602–615. doi: 10.1002/stem.2779
Dennis, J. E., and Charbord, P. (2002). Origin and differentiation of human and murine stroma. Stem Cells 20, 205–214. doi: 10.1634/stemcells.20-3-205
Dezawa, M., Kanno, H., Hoshino, M., Cho, H., Matsumoto, N., Itokazu, Y., et al. (2004). Specific induction of neuronal cells from bone marrow stromal cells and application for autologous transplantation. J. Clin. Investig. 113, 1701–1710. doi: 10.1172/jci200420935
Dominici, M., Le Blanc, K., Mueller, I., Slaper-Cortenbach, I., Marini, F., Krause, D., et al. (2006). Minimal criteria for defining multipotent mesenchymal stromal cells. The international society for cellular therapy position statement. Cytotherapy 8, 315–317. doi: 10.1080/14653240600855905
Dupin, E., and Coelho-Aguiar, J. M. (2013). Isolation and differentiation properties of neural crest stem cells. Cytometry A 83, 38–47. doi: 10.1002/cyto.a.22098
El-Kheir, W. A., Gabr, H., Awad, M. R., Ghannam, O., Barakat, Y., Farghali, H. A., et al. (2014). Autologous bone marrow-derived cell therapy combined with physical therapy induces functional improvement in chronic spinal cord injury patients. Cell Transplant. 23, 729–745. doi: 10.3727/096368913X664540
Falk, T., Gonzalez, R. T., and Sherman, S. J. (2010). The yin and yang of VEGF and PEDF: multifaceted neurotrophic factors and their potential in the treatment of Parkinson’s Disease. Int. J. Mol. Sci. 11, 2875–2900. doi: 10.3390/ijms11082875
Fehlings, M. G., Tetreault, L. A., Wilson, J. R., Kwon, B. K., Burns, A. S., Martin, A. R., et al. (2017a). A clinical practice guideline for the management of acute spinal cord injury: introduction, rationale, and scope. Glob. Spine J. 7(3 Suppl.), 84S–94S. doi: 10.1177/2192568217703387
Fehlings, M. G., Wilson, J. R., Harrop, J. S., Kwon, B. K., Tetreault, L. A., Arnold, P. M., et al. (2017b). Efficacy and safety of methylprednisolone sodium succinate in acute spinal cord injury: a systematic review. Glob. Spine J. 7(3 Suppl.), 116S–137S. doi: 10.1177/2192568217706366
Friedenstein, A. J., Chailakhjan, R. K., and Lalykina, K. S. (1970). The development of fibroblast colonies in monolayer cultures of guinea-pig bone marrow and spleen cells. Cell Tissue Kinet. 3, 393–403. doi: 10.1111/j.1365-2184.1970.tb00347.x
Friedenstein, A. J., Chailakhyan, R. K., and Gerasimov, U. V. (1987). Bone marrow osteogenic stem cells: in vitro cultivation and transplantation in diffusion chambers. Cell Tissue Kinet. 20, 263–272. doi: 10.1111/j.1365-2184.1987.tb01309.x
Friedenstein, A. J., Petrakova, K. V., Kurolesova, A. I., and Frolova, G. P. (1968). Heterotopic of bone marrow. Analysis of precursor cells for osteogenic and hematopoietic tissues. Transplantation 6, 230–247.
Fu, X., Liu, G., Halim, A., Ju, Y., Luo, Q., and Song, A. G. (2019). Mesenchymal stem cell migration and tissue repair. Cells 8:784. doi: 10.3390/cells8080784
Fujii, H., Matsubara, K., Sakai, K., Ito, M., Ohno, K., Ueda, M., et al. (2015). Dopaminergic differentiation of stem cells from human deciduous teeth and their therapeutic benefits for Parkinsonian rats. Brain Res. 1613, 59–72. doi: 10.1016/j.brainres.2015.04.001
Galipeau, J., Krampera, M., Barrett, J., Dazzi, F., Deans, R. J., DeBruijn, J., et al. (2016). International society for cellular therapy perspective on immune functional assays for mesenchymal stromal cells as potency release criterion for advanced phase clinical trials. Cytotherapy 18, 151–159. doi: 10.1016/j.jcyt.2015.11.008
Galleu, A., Riffo-Vasquez, Y., Trento, C., Lomas, C., Dolcetti, L., Cheung, T. S., et al. (2017). Apoptosis in mesenchymal stromal cells induces in vivo recipient-mediated immunomodulation. Sci. Transl. Med. 9:eaam7828. doi: 10.1126/scitranslmed.aam7828
Grove, J. E., Bruscia, E., and Krause, D. S. (2004). Plasticity of bone marrow-derived stem cells. Stem Cells 22, 487–500. doi: 10.1634/stemcells.22-4-487
Guimaraes-Camboa, N., Cattaneo, P., Sun, Y., Moore-Morris, T., Gu, Y., Dalton, N. D., et al. (2017). Pericytes of multiple organs do not behave as mesenchymal stem cells in vivo. Cell Stem Cell 20, 345–359.e5. doi: 10.1016/j.stem.2016.12.006
Gunther, M. I., Weidner, N., Muller, R., and Blesch, A. (2015). Cell-seeded alginate hydrogel scaffolds promote directed linear axonal regeneration in the injured rat spinal cord. Acta Biomater. 27, 140–150. doi: 10.1016/j.actbio.2015.09.001
Guyon, A. (2014). CXCL12 chemokine and its receptors as major players in the interactions between immune and nervous systems. Front. Cell Neurosci. 8:65. doi: 10.3389/fncel.2014.00065
Halvorsen, Y. C., Wilkison, W. O., and Gimble, J. M. (2000). Adipose-derived stromal cells–their utility and potential in bone formation. Int. J. Obes. Relat. Metab. Disord. 24, (Suppl. 4), S41–S44. doi: 10.1038/sj.ijo.0801503
Hermann, A., Gastl, R., Liebau, S., Popa, M. O., Fiedler, J., Boehm, B. O., et al. (2004). Efficient generation of neural stem cell-like cells from adult human bone marrow stromal cells. J. Cell Sci. 117(Pt. 19), 4411–4422. doi: 10.1242/jcs.01307
Hoban, D. B., Howard, L., and Dowd, E. (2015). GDNF-secreting mesenchymal stem cells provide localized neuroprotection in an inflammation-driven rat model of Parkinson’s disease. Neuroscience 303, 402–411. doi: 10.1016/j.neuroscience.2015.07.014
Hoffmann, M. C., Nitsch, C., Scotti, A. L., Reinhard, E., and Monard, D. (1992). The prolonged presence of glia-derived nexin, an endogenous protease inhibitor, in the hippocampus after ischemia-induced delayed neuronal death. Neuroscience 49, 397–408. doi: 10.1016/0306-4522(92)90105-b
Horwitz, E. M., Le Blanc, K., Dominici, M., Mueller, I., Slaper-Cortenbach, I., Marini, F. C., et al. (2005). Clarification of the nomenclature for MSC: the international society for cellular therapy position statement. Cytotherapy 7, 393–395. doi: 10.1080/14653240500319234
Hua, Z., Liu, L., Shen, J., Cheng, K., Liu, A., Yang, J., et al. (2016). Mesenchymal stem cells reversed morphine tolerance and opioid-induced hyperalgesia. Sci. Rep. 6:32096. doi: 10.1038/srep32096
Hur, J. W., Cho, T. H., Park, D. H., Lee, J. B., Park, J. Y., and Chung, Y. G. (2016). Intrathecal transplantation of autologous adipose-derived mesenchymal stem cells for treating spinal cord injury: a human trial. J. Spinal Cord Med. 39, 655–664. doi: 10.1179/2045772315Y.0000000048
Hurlbert, R. J., Hadley, M. N., Walters, B. C., Aarabi, B., Dhall, S. S., Gelb, D. E., et al. (2013). Pharmacological therapy for acute spinal cord injury. Neurosurgery 72, (Suppl. 2), 93–105. doi: 10.1227/NEU.0b013e31827765c6
James, S. L., Theadom, A., Ellenbogen, R. G., Bannick, M. S., Montjoy-Venning, W., and Lucchesi, L. R. (2019). Global, regional, and national burden of traumatic brain injury and spinal cord injury, 1990–2016: a systematic analysis for the Global Burden of Disease Study 2016. Lancet Neurol. 18, 56–87. doi: 10.1016/s1474-4422(18)30415-0
Jiang, P. C., Xiong, W. P., Wang, G., Ma, C., Yao, W. Q., Kendell, S. F., et al. (2013). A clinical trial report of autologous bone marrow-derived mesenchymal stem cell transplantation in patients with spinal cord injury. Exp. Ther. Med. 6, 140–146. doi: 10.3892/etm.2013.1083
Jiang, Y., Henderson, D., Blackstad, M., Chen, A., Miller, R. F., and Verfaillie, C. M. (2003). Neuroectodermal differentiation from mouse multipotent adult progenitor cells. Proc. Natl. Acad. Sci. U.S.A. 100, (Suppl. 1), 11854–11860. doi: 10.1073/pnas.1834196100
Jin, P., Zhao, Y., Liu, H., Chen, J., Ren, J., Jin, J., et al. (2016). Interferon-gamma and tumor necrosis factor-alpha polarize bone marrow stromal cells uniformly to a Th1 phenotype. Sci. Rep. 6:26345. doi: 10.1038/srep26345
Kang, Y. H., Shivakumar, S. B., Son, Y. B., Bharti, D., Jang, S. J., Heo, K. S., et al. (2019). Comparative analysis of three different protocols for cholinergic neuron differentiation in vitro using mesenchymal stem cells from human dental pulp. Anim. Cells Syst. 23, 275–287. doi: 10.1080/19768354.2019.1626280
Karp, J. M., and Leng Teo, G. S. (2009). Mesenchymal stem cell homing: the devil is in the details. Cell Stem Cell 4, 206–216. doi: 10.1016/j.stem.2009.02.001
Khan, A. A., Huat, T. J., Al Mutery, A., El-Serafi, A. T., Kacem, H. H., Abdallah, S. H., et al. (2020). Significant transcriptomic changes are associated with differentiation of bone marrow-derived mesenchymal stem cells into neural progenitor-like cells in the presence of bFGF and EGF. Cell Biosci. 10:126. doi: 10.1186/s13578-020-00487-z
Kilroy, G. E., Foster, S. J., Wu, X., Ruiz, J., Sherwood, S., Heifetz, A., et al. (2007). Cytokine profile of human adipose-derived stem cells: expression of angiogenic, hematopoietic, and pro-inflammatory factors. J. Cell Physiol. 212, 702–709. doi: 10.1002/jcp.21068
Kolar, M. K., Itte, V. N., Kingham, P. J., Novikov, L. N., Wiberg, M., and Kelk, P. (2017). The neurotrophic effects of different human dental mesenchymal stem cells. Sci. Rep. 7:12605. doi: 10.1038/s41598-017-12969-1
Kondo, T., Johnson, S. A., Yoder, M. C., Romand, R., and Hashino, E. (2005). Sonic hedgehog and retinoic acid synergistically promote sensory fate specification from bone marrow-derived pluripotent stem cells. Proc. Natl. Acad. Sci. U.S.A. 102, 4789–4794. doi: 10.1073/pnas.0408239102
Kopen, G. C., Prockop, D. J., and Phinney, D. G. (1999). Marrow stromal cells migrate throughout forebrain and cerebellum, and they differentiate into astrocytes after injection into neonatal mouse brains. Proc. Natl. Acad. Sci. U.S.A. 96, 10711–10716. doi: 10.1073/pnas.96.19.10711
Kordelas, L., Rebmann, V., Ludwig, A. K., Radtke, S., Ruesing, J., Doeppner, T. R., et al. (2014). MSC-derived exosomes: a novel tool to treat therapy-refractory graft-versus-host disease. Leukemia 28, 970–973. doi: 10.1038/leu.2014.41
Krampera, M., Cosmi, L., Angeli, R., Pasini, A., Liotta, F., Andreini, A., et al. (2006). Role for interferon-gamma in the immunomodulatory activity of human bone marrow mesenchymal stem cells. Stem Cells 24, 386–398. doi: 10.1634/stemcells.2005-0008
Krupa, P., Vackova, I., Ruzicka, J., Zaviskova, K., Dubisova, J., Koci, Z., et al. (2018). The effect of human mesenchymal stem cells derived from wharton’s jelly in spinal cord injury treatment is dose-dependent and can be facilitated by repeated application. Int. J. Mol. Sci. 19:1503. doi: 10.3390/ijms19051503
Lakshmipathy, U., and Verfaillie, C. (2005). Stem cell plasticity. Blood Rev. 9, 29–38. doi: 10.1016/j.blre.2004.03.001
Larocca, T. F., Macedo, C. T., Souza, B. S. F., Andrade-Souza, Y. M., Villarreal, C. F., Matos, A. C., et al. (2017). Image-guided percutaneous intralesional administration of mesenchymal stromal cells in subjects with chronic complete spinal cord injury: a pilot study. Cytotherapy 19, 1189–1196. doi: 10.1016/j.jcyt.2017.06.006
Le Blanc, K., and Davies, L. C. (2018). MSCs-cells with many sides. Cytotherapy 20, 273–278. doi: 10.1016/j.jcyt.2018.01.009
Lee, J., Abdeen, A. A., Tang, X., Saif, T. A., and Kilian, K. A. (2016). Matrix directed adipogenesis and neurogenesis of mesenchymal stem cells derived from adipose tissue and bone marrow. Acta Biomater. 42, 46–55. doi: 10.1016/j.actbio.2016.06.037
Lendahl, U., Zimmerman, L. B., and McKay, R. D. (1990). CNS stem cells express a new class of intermediate filament protein. Cell 60, 585–595. doi: 10.1016/0092-8674(90)90662-x
Lennon, D. P., Edmison, J. M., and Caplan, A. I. (2001). Cultivation of rat marrow-derived mesenchymal stem cells in reduced oxygen tension: effects on in vitro and in vivo osteochondrogenesis. J. Cell Physiol. 187, 345–355. doi: 10.1002/jcp.1081
Li, F., Liu, L., Cheng, K., Chen, Z., and Cheng, J. (2018). The use of stem cell therapy to reverse opioid tolerance. Clin. Pharmacol. Ther. 103, 971–974. doi: 10.1002/cpt.959
Li, J., Zhang, Q., Wang, W., Lin, F., Wang, S., and Zhao, J. (2021). Mesenchymal stem cell therapy for ischemic stroke: a look into treatment mechanism and therapeutic potential. J. Neurol. 268, 4095–4107. doi: 10.1007/s00415-020-10138-5
Li, M., and Ransohoff, R. M. (2008). Multiple roles of chemokine CXCL12 in the central nervous system: a migration from immunology to neurobiology. Prog. Neurobiol. 84, 116–131. doi: 10.1016/j.pneurobio.2007.11.003
Li, M., Hale, J. S., Rich, J. N., Ransohoff, R. M., and Lathia, J. D. (2012). Chemokine CXCL12 in neurodegenerative diseases: an SOS signal for stem cell-based repair. Trends Neurosci. 35, 619–628. doi: 10.1016/j.tins.2012.06.003
Lin, C. C., Lai, S. R., Shao, Y. H., Chen, C. L., and Lee, K. Z. (2017). The therapeutic effectiveness of delayed fetal spinal cord tissue transplantation on respiratory function following mid-cervical spinal cord injury. Neurotherapeutics 14, 792–809. doi: 10.1007/s13311-016-0509-4
Lindsay, S. L., and Barnett, S. C. (2017). Are nestin-positive mesenchymal stromal cells a better source of cells for CNS repair? Neurochem. Int. 106, 101–107. doi: 10.1016/j.neuint.2016.08.001
Lindsay, S. L., Johnstone, S. A., McGrath, M. A., Mallinson, D., and Barnett, S. C. (2016). Comparative miRNA-based fingerprinting reveals biological differences in human olfactory mucosa- and bone-marrow-derived mesenchymal stromal cells. Stem Cell Rep. 6, 729–742. doi: 10.1016/j.stemcr.2016.03.009
Lindsay, S. L., Johnstone, S. A., Mountford, J. C., Sheikh, S., Allan, D. B., Clark, L., et al. (2013). Human mesenchymal stem cells isolated from olfactory biopsies but not bone enhance CNS myelination in vitro. Glia 61, 368–382. doi: 10.1002/glia.22440
Liu, L., Gao, J., Yuan, Y., Chang, Q., Liao, Y., and Lu, F. (2013). Hypoxia preconditioned human adipose derived mesenchymal stem cells enhance angiogenic potential via secretion of increased VEGF and bFGF. Cell Biol. Int. 37, 551–560. doi: 10.1002/cbin.10097
Liu, X., Duan, B., Cheng, Z., Jia, X., Mao, L., Fu, H., et al. (2011). SDF-1/CXCR4 axis modulates bone marrow mesenchymal stem cell apoptosis, migration and cytokine secretion. Protein Cell 2, 845–854. doi: 10.1007/s13238-011-1097-z
Lu, D., Liao, Y., Zhu, S. H., Chen, Q. C., Xie, D. M., Liao, J. J., et al. (2019). Bone-derived Nestin-positive mesenchymal stem cells improve cardiac function via recruiting cardiac endothelial cells after myocardial infarction. Stem Cell Res Ther. 10:127. doi: 10.1186/s13287-019-1217-x
Lu, P., Blesch, A., and Tuszynski, M. H. (2004). Induction of bone marrow stromal cells to neurons: differentiation, transdifferentiation, or artifact? J. Neurosci. Res. 77, 174–191. doi: 10.1002/jnr.20148
Ma, K., Fox, L., Shi, G., Shen, J., Liu, Q., Pappas, J. D., et al. (2011). Generation of neural stem cell-like cells from bone marrow-derived human mesenchymal stem cells. Neurol. Res. 33, 1083–1093. doi: 10.1179/1743132811Y.0000000053
Mendez-Ferrer, S., Michurina, T. V., Ferraro, F., Mazloom, A. R., Macarthur, B. D., Lira, S. A., et al. (2010). Mesenchymal and haematopoietic stem cells form a unique bone marrow niche. Nature 466, 829–834. doi: 10.1038/nature09262
Mendonca, M. V., Larocca, T. F., de Freitas Souza, B. S., Villarreal, C. F., Silva, L. F., Matos, A. C., et al. (2014). Safety and neurological assessments after autologous transplantation of bone marrow mesenchymal stem cells in subjects with chronic spinal cord injury. Stem Cell Res. Ther. 5:126. doi: 10.1186/scrt516
Mittal, M., Tiruppathi, C., Nepal, S., Zhao, Y. Y., Grzych, D., Soni, D., et al. (2016). TNFalpha-stimulated gene-6 (TSG6) activates macrophage phenotype transition to prevent inflammatory lung injury. Proc. Natl. Acad. Sci. U.S.A. 113, E8151–E8158. doi: 10.1073/pnas.1614935113
Miura, M., Gronthos, S., Zhao, M., Lu, B., Fisher, L. W., Robey, P. G., et al. (2003). SHED: stem cells from human exfoliated deciduous teeth. Proc. Natl. Acad. Sci. U.S.A. 100, 5807–5812. doi: 10.1073/pnas.0937635100
Mokry, J., Cizkova, D., Filip, S., Ehrmann, J., Osterreicher, J., Kolar, Z., et al. (2004). Nestin expression by newly formed human blood vessels. Stem Cells Dev. 13, 658–664. doi: 10.1089/scd.2004.13.658
Montzka, K., Lassonczyk, N., Tschoke, B., Neuss, S., Fuhrmann, T., Franzen, R., et al. (2009). Neural differentiation potential of human bone marrow-derived mesenchymal stromal cells: misleading marker gene expression. BMC Neurosci. 10:16. doi: 10.1186/1471-2202-10-16
Mung, K. L., Tsui, Y. P., Tai, E. W., Chan, Y. S., Shum, D. K., and Shea, G. K. (2016). Rapid and efficient generation of neural progenitors from adult bone marrow stromal cells by hypoxic preconditioning. Stem Cell Res. Ther. 7:146. doi: 10.1186/s13287-016-0409-x
Murray, I. R., West, C. C., Hardy, W. R., James, A. W., Park, T. S., Nguyen, A., et al. (2014). Natural history of mesenchymal stem cells, from vessel walls to culture vessels. Cell Mol. Life Sci. 71, 1353–1374. doi: 10.1007/s00018-013-1462-6
Murugan, V. (2009). Embryonic stem cell research: a decade of debate from Bush to Obama. Yale J. Biol. Med. 82, 101–103.
Muthu, S., Jeyaraman, M., Gulati, A., and Arora, A. (2021). Current evidence on mesenchymal stem cell therapy for traumatic spinal cord injury: systematic review and meta-analysis. Cytotherapy 23, 186–197. doi: 10.1016/j.jcyt.2020.09.007
Naghdi, M., Tiraihi, T., Namin, S. A., and Arabkheradmand, J. (2009). Transdifferentiation of bone marrow stromal cells into cholinergic neuronal phenotype: a potential source for cell therapy in spinal cord injury. Cytotherapy 11, 137–152. doi: 10.1080/14653240802716582
Nam, D., Park, A., Dubon, M. J., Yu, J., Kim, W., Son, Y., et al. (2020). Coordinated regulation of mesenchymal stem cell migration by various chemotactic stimuli. Int. J. Mol. Sci. 21:8561. doi: 10.3390/ijms21228561
Neirinckx, V., Coste, C., Rogister, B., and Wislet-Gendebien, S. (2013). Concise review: adult mesenchymal stem cells, adult neural crest stem cells, and therapy of neurological pathologies: a state of play. Stem Cells Transl. Med. 2, 284–296. doi: 10.5966/sctm.2012-0147
Neuhuber, B., Gallo, G., Howard, L., Kostura, L., Mackay, A., and Fischer, I. (2004). Reevaluation of in vitro differentiation protocols for bone marrow stromal cells: disruption of actin cytoskeleton induces rapid morphological changes and mimics neuronal phenotype. J. Neurosci. Res. 77, 192–204. doi: 10.1002/jnr.20147
Nitzsche, F., Muller, C., Lukomska, B., Jolkkonen, J., Deten, A., and Boltze, J. (2017). Concise review: MSC adhesion cascade-insights into homing and transendothelial migration. Stem Cells 35, 1446–1460. doi: 10.1002/stem.2614
Oh, S. K., Choi, K. H., Yoo, J. Y., Kim, D. Y., Kim, S. J., and Jeon, S. R. (2016). A phase III clinical trial showing limited efficacy of autologous mesenchymal stem cell therapy for spinal cord injury. Neurosurgery 78, 436–447;discussion447. doi: 10.1227/NEU.0000000000001056
Okon, E. B., Streijger, F., Lee, J. H., Anderson, L. M., Russell, A. K., and Kwon, B. K. (2013). Intraparenchymal microdialysis after acute spinal cord injury reveals differential metabolic responses to contusive versus compressive mechanisms of injury. J. Neurotrauma 30, 1564–1576. doi: 10.1089/neu.2013.2956
Oraee-Yazdani, S., Akhlaghpasand, M., Golmohammadi, M., Hafizi, M., Zomorrod, M. S., Kabir, N. M., et al. (2021). Combining cell therapy with human autologous Schwann cell and bone marrow-derived mesenchymal stem cell in patients with subacute complete spinal cord injury: safety considerations and possible outcomes. Stem Cell Res. Ther. 12:445. doi: 10.1186/s13287-021-02515-2
Owen, M. (1988). Marrow stromal stem cells. J. Cell Sci. Suppl. 10, 63–76. doi: 10.1242/jcs.1988.supplement_10.5
Pacini, S., and Petrini, I. (2014). Are MSCs angiogenic cells? New insights on human nestin-positive bone marrow-derived multipotent cells. Front. Cell Dev. Biol. 2:20. doi: 10.3389/fcell.2014.00020
Phinney, D. G., and Pittenger, M. F. (2017). Concise review: MSC-derived exosomes for cell-free therapy. Stem Cells 35, 851–858. doi: 10.1002/stem.2575
Phinney, D. G., and Prockop, D. J. (2007). Concise review: mesenchymal stem/multipotent stromal cells: the state of transdifferentiation and modes of tissue repair–current views. Stem Cells 25, 2896–2902. doi: 10.1634/stemcells.2007-0637
Phinney, D. G., and Sensebe, L. (2013). Mesenchymal stromal cells: misconceptions and evolving concepts. Cytotherapy 15, 140–145. doi: 10.1016/j.jcyt.2012.11.005
Pittenger, M. F., Discher, D. E., Peault, B. M., Phinney, D. G., Hare, J. M., and Caplan, A. I. (2019). Mesenchymal stem cell perspective: cell biology to clinical progress. NPJ Regen. Med. 4:22. doi: 10.1038/s41536-019-0083-6
Pittenger, M. F., Mackay, A. M., Beck, S. C., Jaiswal, R. K., Douglas, R., Mosca, J. D., et al. (1999). Multilineage potential of adult human mesenchymal stem cells. Science 284, 143–147. doi: 10.1126/science.284.5411.143
Pollock, K., Sumstad, D., Kadidlo, D., McKenna, D. H., and Hubel, A. (2015). Clinical mesenchymal stromal cell products undergo functional changes in response to freezing. Cytotherapy 17, 38–45. doi: 10.1016/j.jcyt.2014.06.008
Prockop, D. J. (2017). The exciting prospects of new therapies with mesenchymal stromal cells. Cytotherapy 19, 1–8. doi: 10.1016/j.jcyt.2016.09.008
Ribeiro, C. A., Fraga, J. S., Graos, M., Neves, N. M., Reis, R. L., Gimble, J. M., et al. (2012). The secretome of stem cells isolated from the adipose tissue and Wharton jelly acts differently on central nervous system derived cell populations. Stem Cell Res. Ther. 3:18. doi: 10.1186/scrt109
Richardson, G. D., Arnott, E. C., Whitehouse, C. J., Lawrence, C. M., Hole, N., and Jahoda, C. A. (2005). Cultured cells from the adult human hair follicle dermis can be directed toward adipogenic and osteogenic differentiation. J. Invest. Dermatol. 124, 1090–1091. doi: 10.1111/j.0022-202X.2005.23734.x
Ritfeld, G. J., Patel, A., Chou, A., Novosat, T. L., Castillo, D. G., Roos, R. A., et al. (2015). The role of brain-derived neurotrophic factor in bone marrow stromal cell-mediated spinal cord repair. Cell Transplant 24, 2209–2220. doi: 10.3727/096368915X686201
Romanov, Y. A., Svintsitskaya, V. A., and Smirnov, V. N. (2003). Searching for alternative sources of postnatal human mesenchymal stem cells: candidate MSC-like cells from umbilical cord. Stem Cells 21, 105–110. doi: 10.1634/stemcells.21-1-105
Rosova, I., Dao, M., Capoccia, B., Link, D., and Nolta, J. A. (2008). Hypoxic preconditioning results in increased motility and improved therapeutic potential of human mesenchymal stem cells. Stem Cells 26, 2173–2182. doi: 10.1634/stemcells.2007-1104
Sacchetti, B., Funari, A., Michienzi, S., Di Cesare, S., Piersanti, S., Saggio, I., et al. (2007). Self-renewing osteoprogenitors in bone marrow sinusoids can organize a hematopoietic microenvironment. Cell 131, 324–336. doi: 10.1016/j.cell.2007.08.025
Sacchetti, B., Funari, A., Remoli, C., Giannicola, G., Kogler, G., Liedtke, S., et al. (2016). No identical “Mesenchymal Stem Cells” at different times and sites: human committed progenitors of distinct origin and differentiation potential are incorporated as adventitial cells in microvessels. Stem Cell Rep. 6, 897–913. doi: 10.1016/j.stemcr.2016.05.011
Samper Agrelo, I., Schira-Heinen, J., Beyer, F., Groh, J., Butermann, C., Estrada, V., et al. (2020). Secretome analysis of mesenchymal stem cell factors fostering oligodendroglial differentiation of neural stem cells in vivo. Int. J. Mol. Sci. 21:4350. doi: 10.3390/ijms21124350
Sanchez, A., Tripathy, D., Yin, X., Luo, J., Martinez, J., and Grammas, P. (2012). Pigment epithelium-derived factor (PEDF) protects cortical neurons in vitro from oxidant injury by activation of extracellular signal-regulated kinase (ERK) 1/2 and induction of Bcl-2. Neurosci. Res. 72, 1–8. doi: 10.1016/j.neures.2011.09.003
Sanchez-Ramos, J., Song, S., Cardozo-Pelaez, F., Hazzi, C., Stedeford, T., Willing, A., et al. (2000). Adult bone marrow stromal cells differentiate into neural cells in vitro. Exp. Neurol. 164, 247–256. doi: 10.1006/exnr.2000.7389
Satti, H. S., Waheed, A., Ahmed, P., Ahmed, K., Akram, Z., Aziz, T., et al. (2016). Autologous mesenchymal stromal cell transplantation for spinal cord injury: a phase I pilot study. Cytotherapy 18, 518–522. doi: 10.1016/j.jcyt.2016.01.004
Takashima, Y., Era, T., Nakao, K., Kondo, S., Kasuga, M., Smith, A. G., et al. (2007). Neuroepithelial cells supply an initial transient wave of MSC differentiation. Cell 129, 1377–1388. doi: 10.1016/j.cell.2007.04.028
Teixeira, F. G., Carvalho, M. M., Panchalingam, K. M., Rodrigues, A. J., Mendes-Pinheiro, B., Anjo, S., et al. (2017). Impact of the secretome of human mesenchymal stem cells on brain structure and animal behavior in a rat model of parkinson’s disease. Stem Cells Transl. Med. 6, 634–646. doi: 10.5966/sctm.2016-0071
Trawczynski, M., Liu, G., David, B. T., and Fessler, R. G. (2019). Restoring motor neurons in spinal cord injury with induced pluripotent stem cells. Front. Cell. Neurosci. 13:369. doi: 10.3389/fncel.2019.00369
Tremain, N., Korkko, J., Ibberson, D., Kopen, G. C., DiGirolamo, C., and Phinney, D. G. (2001). MicroSAGE analysis of 2,353 expressed genes in a single cell-derived colony of undifferentiated human mesenchymal stem cells reveals mRNAs of multiple cell lineages. Stem Cells 19, 408–418. doi: 10.1634/stemcells.19-5-408
Tropel, P., Platet, N., Platel, J. C., Noel, D., Albrieux, M., Benabid, A. L., et al. (2006). Functional neuronal differentiation of bone marrow-derived mesenchymal stem cells. Stem Cells 24, 2868–2876. doi: 10.1634/stemcells.2005-0636
Tsai, C. C., Chen, Y. J., Yew, T. L., Chen, L. L., Wang, J. Y., Chiu, C. H., et al. (2011). Hypoxia inhibits senescence and maintains mesenchymal stem cell properties through down-regulation of E2A-p21 by HIF-TWIST. Blood 117, 459–469. doi: 10.1182/blood-2010-05-287508
Unsicker, K., Meier, C., Krieglstein, K., Sartor, B. M., and Flanders, K. C. (1996). Expression, localization, and function of transforming growth factor-beta s in embryonic chick spinal cord, hindbrain, and dorsal root ganglia. J. Neurobiol. 29, 262–276. doi: 10.1002/(SICI)1097-4695(199602)29:2<262::AID-NEU10<3.0.CO;2-D
Vaquero, J., Zurita, M., Rico, M. A., Aguayo, C., Fernandez, C., Gutierrez, R., et al. (2018a). Intrathecal administration of autologous bone marrow stromal cells improves neuropathic pain in patients with spinal cord injury. Neurosci. Lett. 670, 14–18. doi: 10.1016/j.neulet.2018.01.035
Vaquero, J., Zurita, M., Rico, M. A., Aguayo, C., Fernandez, C., Rodriguez-Boto, G., et al. (2018b). Cell therapy with autologous mesenchymal stromal cells in post-traumatic syringomyelia. Cytotherapy 20, 796–805. doi: 10.1016/j.jcyt.2018.04.006
Vaquero, J., Zurita, M., Rico, M. A., Bonilla, C., Aguayo, C., Fernandez, C., et al. (2017). Repeated subarachnoid administrations of autologous mesenchymal stromal cells supported in autologous plasma improve quality of life in patients suffering incomplete spinal cord injury. Cytotherapy 19, 349–359. doi: 10.1016/j.jcyt.2016.12.002
Vaquero, J., Zurita, M., Rico, M. A., Bonilla, C., Aguayo, C., Montilla, J., et al. (2016). An approach to personalized cell therapy in chronic complete paraplegia: the Puerta de Hierro phase I/II clinical trial. Cytotherapy 18, 1025–1036. doi: 10.1016/j.jcyt.2016.05.003
Vismara, I., Papa, S., Rossi, F., Forloni, G., and Veglianese, P. (2017). Current options for cell therapy in spinal cord injury. Trends Mol. Med. 23, 831–849. doi: 10.1016/j.molmed.2017.07.005
Viswanathan, S., Keating, A., Deans, R., Hematti, P., Prockop, D., Stroncek, D. F., et al. (2014). Soliciting strategies for developing cell-based reference materials to advance mesenchymal stromal cell research and clinical translation. Stem Cells Dev. 23, 1157–1167. doi: 10.1089/scd.2013.0591
Viswanathan, S., Shi, Y., Galipeau, J., Krampera, M., Leblanc, K., Martin, I., et al. (2019). Mesenchymal stem versus stromal cells: international society for cell & gene therapy (ISCT(R)) mesenchymal stromal cell committee position statement on nomenclature. Cytotherapy 21, 1019–1024. doi: 10.1016/j.jcyt.2019.08.002
Walters, B. C., Hadley, M. N., Hurlbert, R. J., Aarabi, B., Dhall, S. S., Gelb, D. E., et al. (2013). Guidelines for the management of acute cervical spine and spinal cord injuries: 2013 update. Neurosurgery 60, 82–91. doi: 10.1227/01.neu.0000430319.32247.7f
Wang, J., Wang, B., Jiang, L., Zhou, K., Yang, G. Y., and Jin, K. (2020). The effect of IDO on neural progenitor cell survival under oxygen glucose deprivation. Front. Cell Neurosci. 14:581861. doi: 10.3389/fncel.2020.581861
Wilkins, A., Kemp, K., Ginty, M., Hares, K., Mallam, E., and Scolding, N. (2009). Human bone marrow-derived mesenchymal stem cells secrete brain-derived neurotrophic factor which promotes neuronal survival in vitro. Stem Cell Res. 3, 63–70. doi: 10.1016/j.scr.2009.02.006
Wislet-Gendebien, S., Laudet, E., Neirinckx, V., Alix, P., Leprince, P., Glejzer, A., et al. (2012). Mesenchymal stem cells and neural crest stem cells from adult bone marrow: characterization of their surprising similarities and differences. Cell Mol. Life Sci. 69, 2593–2608. doi: 10.1007/s00018-012-0937-1
Woodbury, D., Schwarz, E. J., Prockop, D. J., and Black, I. B. (2000). Adult rat and human bone marrow stromal cells differentiate into neurons. J. Neurosci. Res. 61, 364–370. doi: 10.1002/1097-4547(20000815)61:4<364::Aid-jnr2>3.0.Co;2-c
Woodbury, M. E., and Ikezu, T. (2014). Fibroblast growth factor-2 signaling in neurogenesis and neurodegeneration. J. Neuroimmune Pharmacol. 9, 92–101. doi: 10.1007/s11481-013-9501-5
Wu, H., Gordon, J. A., Whitfield, T. W., Tai, P. W., van Wijnen, A. J., Stein, J. L., et al. (2017). Chromatin dynamics regulate mesenchymal stem cell lineage specification and differentiation to osteogenesis. Biochim. Biophys. Acta Gene Regul. Mech. 1860, 438–449. doi: 10.1016/j.bbagrm.2017.01.003
Xie, L., Zeng, X., Hu, J., and Chen, Q. (2015). Characterization of Nestin, a selective marker for bone marrow derived mesenchymal stem cells. Stem Cells Int. 2015:762098. doi: 10.1155/2015/762098
Yang, L., Jurczak, K. M., Ge, L., and van Rijn, P. (2020). High-throughput screening and hierarchical topography-mediated neural differentiation of mesenchymal stem cells. Adv. Healthc. Mater. 9:e2000117. doi: 10.1002/adhm.202000117
Yang, Y., Pang, M., Du, C., Liu, Z. Y., Chen, Z. H., Wang, N. X., et al. (2021). Repeated subarachnoid administrations of allogeneic human umbilical cord mesenchymal stem cells for spinal cord injury: a phase 1/2 pilot study. Cytotherapy 23, 57–64. doi: 10.1016/j.jcyt.2020.09.012
Yi, J. J., Barnes, A. P., Hand, R., Polleux, F., and Ehlers, M. D. (2010). TGF-beta signaling specifies axons during brain development. Cell 142, 144–157. doi: 10.1016/j.cell.2010.06.010
Yin, J. Q., Zhu, J., and Ankrum, J. A. (2019). Manufacturing of primed mesenchymal stromal cells for therapy. Nat. Biomed. Eng. 3, 90–104. doi: 10.1038/s41551-018-0325-8
Zhao, H., Sun, Q. L., Duan, L. J., Yang, Y. D., Gao, Y. S., Zhao, D. Y., et al. (2019). Is cell transplantation a reliable therapeutic strategy for spinal cord injury in clinical practice? A systematic review and meta-analysis from 22 clinical controlled trials. Eur. Spine J. 28, 1092–1112. doi: 10.1007/s00586-019-05882-w
Zholudeva, L. V., and Lane, M. A. (2019). Transplanting cells for spinal cord repair: who, what, when, where and why? Cell Transplant 28, 388–399. doi: 10.1177/0963689718824097
Keywords: mesenchymal stromal cell, cell transplanting, cell differentiation, neuroregeneration, spinal cord injury
Citation: Xie J-L, Wang X-R, Li M-M, Tao Z-H, Teng W-W and Saijilafu (2022) Mesenchymal Stromal Cell Therapy in Spinal Cord Injury: Mechanisms and Prospects. Front. Cell. Neurosci. 16:862673. doi: 10.3389/fncel.2022.862673
Received: 26 January 2022; Accepted: 09 May 2022;
Published: 03 June 2022.
Edited by:
Paul Lu, University of California, San Diego, United StatesReviewed by:
Arianna Merlini, University Medical Center Göttingen, GermanyCopyright © 2022 Xie, Wang, Li, Tao, Teng and Saijilafu. This is an open-access article distributed under the terms of the Creative Commons Attribution License (CC BY). The use, distribution or reproduction in other forums is permitted, provided the original author(s) and the copyright owner(s) are credited and that the original publication in this journal is cited, in accordance with accepted academic practice. No use, distribution or reproduction is permitted which does not comply with these terms.
*Correspondence: Saijilafu, c2FpamlsYWZ1QHN1ZGEuZWR1LmNu
Disclaimer: All claims expressed in this article are solely those of the authors and do not necessarily represent those of their affiliated organizations, or those of the publisher, the editors and the reviewers. Any product that may be evaluated in this article or claim that may be made by its manufacturer is not guaranteed or endorsed by the publisher.
Research integrity at Frontiers
Learn more about the work of our research integrity team to safeguard the quality of each article we publish.