- 1Instituto de Fisiología Celular, División de Neurociencias, Universidad Nacional Autónoma de México, Mexico City, Mexico
- 2Laboratory of Neurobiology of Appetitive, Department of Pharmacology, Center for Research and Advanced Studies of the National Polytechnic Institute, CINVESTAV, Mexico City, Mexico
Taste memory involves storing information through plasticity changes in the neural network of taste, including the insular cortex (IC) and ventral tegmental area (VTA), a critical provider of dopamine. Although a VTA-IC dopaminergic pathway has been demonstrated, its role to consolidate taste recognition memory remains poorly understood. We found that photostimulation of dopaminergic neurons in the VTA or VTA-IC dopaminergic terminals of TH-Cre mice improves the salience to consolidate a subthreshold novel taste stimulus regardless of its hedonic value, without altering their taste palatability. Importantly, the inhibition of the D1-like receptor into the IC impairs the salience to facilitate consolidation of an aversive taste recognition memory. Finally, our results showed that VTA photostimulation improves the salience to consolidate a conditioned taste aversion memory through the D1-like receptor into the IC. It is concluded that the dopamine activity from the VTA into IC is required to increase the salience enabling the consolidation of a taste recognition memory. Notably, the D1-like receptor activity into the IC is required to consolidate both innate and learned aversive taste memories but not appetitive taste memory.
Introduction
Taste memory evolves as a critical system for animal survival through the detection of taste attributes related to the hedonic value, degree of familiarity, and to remember their nutritive or toxic consequences of food to form a memory for future acceptance or avoidance responses (Bermúdez-Rattoni, 2004; Scott, 2005). Taste memory involves encoding, storing, and retrieving taste information due to neural plastic changes taking place in a complex network comprising many different brain areas, encompassing the insular cortex (IC), medial prefrontal cortex, basolateral amygdala, nucleus accumbens, and ventral tegmental area (VTA), among others (Yamamoto, 2006, 2008; Ramírez-Lugo et al., 2007; Fontanini et al., 2009; Jezzini et al., 2013).
Several studies have demonstrated that taste learning requires dopaminergic neurotransmission to consolidate the memory representation of tastants (Guzmán-Ramos and Bermúdez-Rattoni, 2011; Yiannakas and Rosenblum, 2017). In this regard, the VTA is a vital dopamine supplier that serves a central role in motivating behavior and reward processing. Some evidence suggests that VTA dopaminergic neurons increase their firing rate to signal reward (Berridge and Robinson, 1998; Nomoto et al., 2010; Fiorillo, 2013). However, evidence shows VTA dopaminergic neurons also increase their activity after the presentation of an aversive stimuli (Brischoux et al., 2009; Bromberg-Martin et al., 2010; Núñez-Jaramillo et al., 2010). In this sense, it has been reported that the modulation of the dopaminergic neurons by rewarding or aversive stimuli depends on the brain area(s) to which these dopaminergic neurons project (Lammel et al., 2011; de Jong et al., 2019).
We recently described the functional characterization of the dopaminergic VTA-IC pathway. However, its role in consolidating taste recognition memory remains poorly understood. The photoactivation of ChR2 + neurons in TH-Cre mice (20 Hz, 15 mW laser power, 473 nm and 5 ms pulse width) for 20 min induces electrophysiological responses in VTA neurons, dopamine release (measured by in vivo microdialysis), and neuronal modulation in the IC (Gil-Lievana et al., 2020). Importantly, the IC contains the primary gustatory cortex, which serves as a critical structure to consolidate taste recognition memory (Bermudez-Rattoni and McGaugh, 1991; Rosenblum et al., 1993; Bermúdez-Rattoni, 2004; Chen et al., 2011; Bermudez-Rattoni, 2014). Accordingly, in vivo microdialysis studies show dopamine release triggered in the IC when a novel appetitive or aversive taste is presented (Guzmán-Ramos et al., 2010; Osorio-Gómez et al., 2017). Interestingly, dopamine released during the presentation of novelty enables memory consolidation through the D1-like receptor since post-trial cortical microinjection of D1-like receptor antagonist impedes consolidation of taste recognition memory (Osorio-Gómez et al., 2021). It has been suggested that phasic dopamine activity plays a major role in salience (Bromberg-Martin et al., 2010; Cho et al., 2017) mainly via D1-like receptor (Brenhouse et al., 2008). Salience can be signaled by multiple factors, including intrinsic physical and chemical properties of the stimuli, the association with valenced stimuli, and the physiological state of the organisms, among others (Hyman, 2005; Cowan et al., 2021). Salient stimuli prioritize the consolidation of the relevant over neutral information to drive goal-relevant behaviors (Payne et al., 2008; Moessnang et al., 2012; Alger et al., 2019). We define stimulus salience as allowing subthreshold taste stimuli to be consolidated in long-term memory.
In this work, we aimed to increase the salience of subthreshold aversive and appetitive taste stimuli through the photostimulation of the VTA-IC dopaminergic pathway to facilitate the consolidation of taste recognition memories. Here we found that the photostimulation of VTA increases the salience to facilitate consolidation of taste stimuli regardless of the natural hedonic value and without altering its taste palatability. Consequently, photostimulation of the VTA dopaminergic terminals into IC also increases the salience to facilitate the consolidation of appetitive and aversive taste stimuli. However, the D1-like receptor activity into the IC is only required to consolidate both innate and learned aversive taste recognition memory.
Materials and Methods
Animals
TH-Cre mice (Tyrosine Hydroxylase, FI12 line) express Cre-recombinase protein under the control of the endogenous tyrosine hydroxylase (TH) promoter. Breeder mice were kindly donated by Rui M. Costa from the Champalimaud (Center for the Unknown) and crossed onto C57BL/6J mice for at least six generations. Two-month-old (25–30 g bodyweight) female and male TH-Cre mice were used for all experiments. No differences were found between male and female mice in all experiments. Mice were housed individually at 20 ± 2°C, 50 ± 5% humidity, under a 12:12 h light/dark cycle with free access to food and water all time except during behavioral testing. All experiments were conducted during the light phase of the room illumination cycle. All experiments were approved by Instituto de Fisiología Celular (FBR125-18) and complied per the Official Mexican Standard (NOM-062-ZOO-1999).
Genotyping
The genotyping procedure was previously reported (Gil-Lievana et al., 2020). Once the mice were 1 month old, a tail snipping procedure was performed, and 1 mm of the tail was removed with sanitized sharp scissors. We used the HotSHOT method for DNA extraction. Briefly, the tail snip was lysed in an alkaline reagent (25 mM NaOH, 0.2 mM disodium EDTA) under heat conditions (95°C, 1 h) and further neutralization with a suitable buffer (1 M Tris-HCl, pH 7.4). After centrifugation (2,500 rpm, 2 min, Hermle Z 233 MK-2), the DNA’s supernatant was recovered. The DNA was used for PCR amplification (201443, QIAGEN). Primers sequences were as follows: Cre forward primer 5′-AGC CTG TTT TGC ACG TTC ACC-3″; Cre reverse primer 5′-GGT TTC CCG CAG AAC CTG AA-3′ (both primers were purchased from Sigma-Aldrich).
Viral Vector
The Cre-inducible adeno-associated virus (AAV) was obtained from the University of North Carolina (UNC) Gene Therapy Center Vector Core. The viral concentrations were as follows: 5.2 × 1012 viral units/ml for rAAV5/EfIα-DIO-hChR2(H134R)-eYFP (ChR2); 6.0 × 1012 viral units/ml for rAAV5/EfIα-DIO-eYFP (eYFP). All viruses were aliquoted and stored at −80°C until use.
Stereotaxic Surgery
Mice were induced to anesthesia with 3% Isoflurane and maintained with 1–1.5% isoflurane (VETone FlurisoTM; Matrix VIP 3000, Midmark) until the end of surgery. Once anesthetized, mice were placed in a stereotaxic apparatus (51603, Stoelting) with an incisive adapter (923-B, KOPF instruments). A small incision in the scalp was made, and the head was adjusted to the horizontal plane. The microinjection needles (29-G) were connected to a 10 μl Hamilton syringe and filled with AAV. For all experiments, the mice were bilaterally injected with AAV (0.5 μl) at a rate of 0.1 μl/min with an additional 5 min for diffusion. Mice were implanted with core optic fibers (200 μm) through zirconia ferrules (1.25-mm-wide) in each hemisphere. The AAV was injected into the VTA [from Bregma (mm) AP: −3.08; ML: ± 0.60 ML; DV −4.80]. The optic fibers were implanted above the VTA [from Bregma (mm) AP: −3.08; ML: ± 1.20; DV: −4.30 at 10° angle] or above the IC [from Bregma (mm) AP: + 1.40; ML: ± 3.30, DV: −3.5]. For pharmacological experiments, mice were implanted with bilateral 23-gauge stainless steel cannulas (8 mm long, Small Parts, Logansport, Indiana, United States) into IC [from Bregma (mm) AP: + 1.40; ML: ± 3.30; DV: −3.0]. Coordinates were taken from Allens reference atlas of the mouse brain. The cannulas and ferrules were anchored with dental adhesive and dental acrylic cement. Stylets were inserted into guide cannulas to prevent clogging. Mice were allowed to recover for 3 weeks before behavioral procedures.
Optogenetic Stimulation
The conditions for photostimulation were previously reported (Gil-Lievana et al., 2020), briefly: optogenetic stimulation consisted of a diode-pumped-solid-state blue laser (473 nm, 15 mW, 20 Hz, 5 ms width; OEM Laser Systems) coupled to 62.5 μm core, 0.22 NA standard multimode hard-cladding optical fiber (ThorLabs, New Jersey, United States) that passed through a single-channel optical rotary joint (Doric Lenses) before being split 50:50 with a fused optical coupler. The light intensity output was 12–15 mW per split fiber for all experiments. These photostimulation parameters have previously been shown to increase dopamine release by in vivo microdialysis experiments, producing electrophysiological responses in VTA neurons and modulation of IC neurons (Gil-Lievana et al., 2020).
Behavioral Procedures
Mice were water-deprived only during experimental days. Every afternoon, mice were supplied with water for 10 min to avoid dehydration. All experiments were conducted during the light phase of the illumination cycle in an acrylic bowl (height 36 cm, diameter 40 cm, CMA 120 bowl, Harvard apparatus). During five consecutive days, two randomized bottles of water were presented for 20 min (baseline). The inclusion criteria were that mice must consume from both bottles. The mice with a bottle preference (consumption index >0.6) during the acquisition session were discarded. A new cohort of mice was used for each behavioral experiment.
Taste Intensity Detection Test
The next day after the last baseline session, two bottles of quinine (low: 126 μM or high: 504 μM) or saccharin (low: 5 mM or high: 15 mM) were presented for 20 min. Twenty-four hours later, during the memory test, two bottles with water/quinine (low or high) or water/saccharin (low or high) were presented to mice for 20 min. The consumption index during the novel tastant exposure session was calculated dividing the volume of the tastant consumed (ml) by the sum of the volume of tastant (ml) and the mean of the baseline consumed (ml): tastant/(tastant + mean baseline).
Concomitant Optogenetic Stimulation and Tastant Exposure
Next day after baseline, two bottles of 126 μM quinine or 5 Mm saccharin were presented for 20 min (novel tastant exposure); at the same time, photoactivation of VTA, or VTA projections in the IC was performed during the 20 min session.
Brief Access Taste Task
Mice were water-deprived for 23 h and placed in an operant chamber equipped with a central sipper (Med Associates Inc., Fairfax, VT, United States), where one of three tastants could be delivered (water, quinine 126 μM, or saccharin 5 mM) controlled by a solenoid valve (Parker, Mayfield Heights, OH, United States). In each trial, mice randomly received one tastant for 5 s (2 μl drop in each lick), mice decided whether they lick during the entire reward period (Garcia et al., 2021). To start a new trial, mice needed to refrain from licking for a 1–3 s inter-trial interval (ITI) and lick once again after the ITI was finished. Mice were trained during five sessions, and three additional sessions were performed with laser stimulation, in which mice were opto-stimulated during the entire task at 20 Hz.
Conditioned Taste Aversion and Pharmacological Manipulations
The next day after the last baseline session, mice were injected into IC with vehicle (0.9% saline solution), or dopamine D1-like receptor antagonist SCH23390 (2 μg/μl, dissolved in 0.9% saline solution, D054, Sigma-Aldrich). Ten minutes after the drug injection, two bottles of 5 mM saccharin were presented for 20 min; simultaneously, photoactivation of VTA was performed during the 20 min session (the photostimulation conditions were like those previously described). Ten minutes after the tastant exposure, mice received an intraperitoneal injection of 0.15 M LiCl at 48 mg/kg body weight or 0.15 M NaCl at a dose of 66 mg/kg body weight and returned to their households. The consumption index during the training session were calculated by dividing the volume of the tastant (ml) by the sum of the volume of the tastant (ml) and mean of the baseline (ml): tastant/(tastant + mean baseline).
Twenty-four hours after the training session, a memory test was performed in all cases; one bottle that contained the taste (used during the training session) and one bottle of water were presented to mice for 20 min. Consumption indexes for the test were calculated by dividing the volume of the tastant by the total volume consumed: tastant/(tastant + water).
Immunofluorescence
After the test, mice were sacrificed with an overdose of intraperitoneal pentobarbital monosodium (200 mg/kg). Intracardiac perfusion was performed with 0.9% saline solution and pre-fixed with 4% paraformaldehyde in 0.1 M phosphate buffer solution. Brains were removed and fixed in 4% paraformaldehyde and stored for 1 week. Brains were treated with 30% sucrose at least 2 days before slicing. Brains were sliced in 40 μm sections using a cryostat (Leica, CM1520). Free-floating sections were incubated with anti-TH (1:1,000, rabbit, P40101, Pel-Freez, Rogers, AR) overnight, at 4°C. Sections were washed with trizma buffer solution added with a triton (TBST; 150 mM NaCl, 100 mM trizma base, 0.1% triton X-100; all purchased from Sigma-Aldrich) incubated with CY3-conjugated goat anti-rabbit (1:250, AP132C, Millipore, Darm-Stadt, Germany) for 2 h. Antibodies were incubated in 5% bovine serum albumin in TBST. Sections were washed with TBST and incubated with 300 nM 4’,6-diamidino-2-fenilindol (DAPI, D9542, Sigma-Aldrich) for 1 min. After a final wash with TBST, sections were mounted in Dako fluorescence mounting medium. Immunofluorescence was observed using a ZEISS LSM 800 confocal microscope.
Statistical Analysis
For experiments, we used the minimum sample necessary to obtain a mean and standard deviation required for a Cohen’s d parameter equals or greater than 0.8 (Lakens, 2013). Data were analyzed using GraphPad Prism software version 8.0 and Matlab R2021a. The Kolmogorov-Smirnoff test was performed for normal distribution. Data were plotted as mean ± SEM. One sample Student’s t-test against 0.5 was performed for experiments showing consumption index during the memory tests and two-way ANOVA were used for raw data during the novel tastant exposure and the brief access taste task (BATT). For all statistical analyses p < 0.05 threshold was considered statistically significant.
Results
The Activity of the Ventral Tegmental Area Dopaminergic Neurons Increases the Intensity of Appetitive and Aversive Taste Stimuli
To determine the role of VTA dopaminergic neurons to increase the intensity of innate appetitive and aversive taste stimuli, we tested the performance of mice to consolidate taste recognition memory (TRM) when two different concentrations of saccharin or quinine were presented. We did not find differences in consumption index for low and high saccharin (Figure 1A) nor quinine in intact mice during the novel tastant exposure session (Figure 1B). Interestingly, we found that only high, but not low, concentration of saccharin (15 mM, Figure 1A) or quinine (504 μM, Figure 1B) solution produced a reliable preference for saccharin and avoidance for quinine in mice during the memory test. However, mice did not recognize a very low concentration of either saccharin (5 mM, Figure 1A) or quinine solution (126 μM, Figure 1B) in comparison to water when presented during the memory test. Therefore, the low concentration of saccharin (5 mM) or quinine solution (126 μM) was insufficient to produce a TRM, and they were used as less-salient taste concentrations for our further experiments.
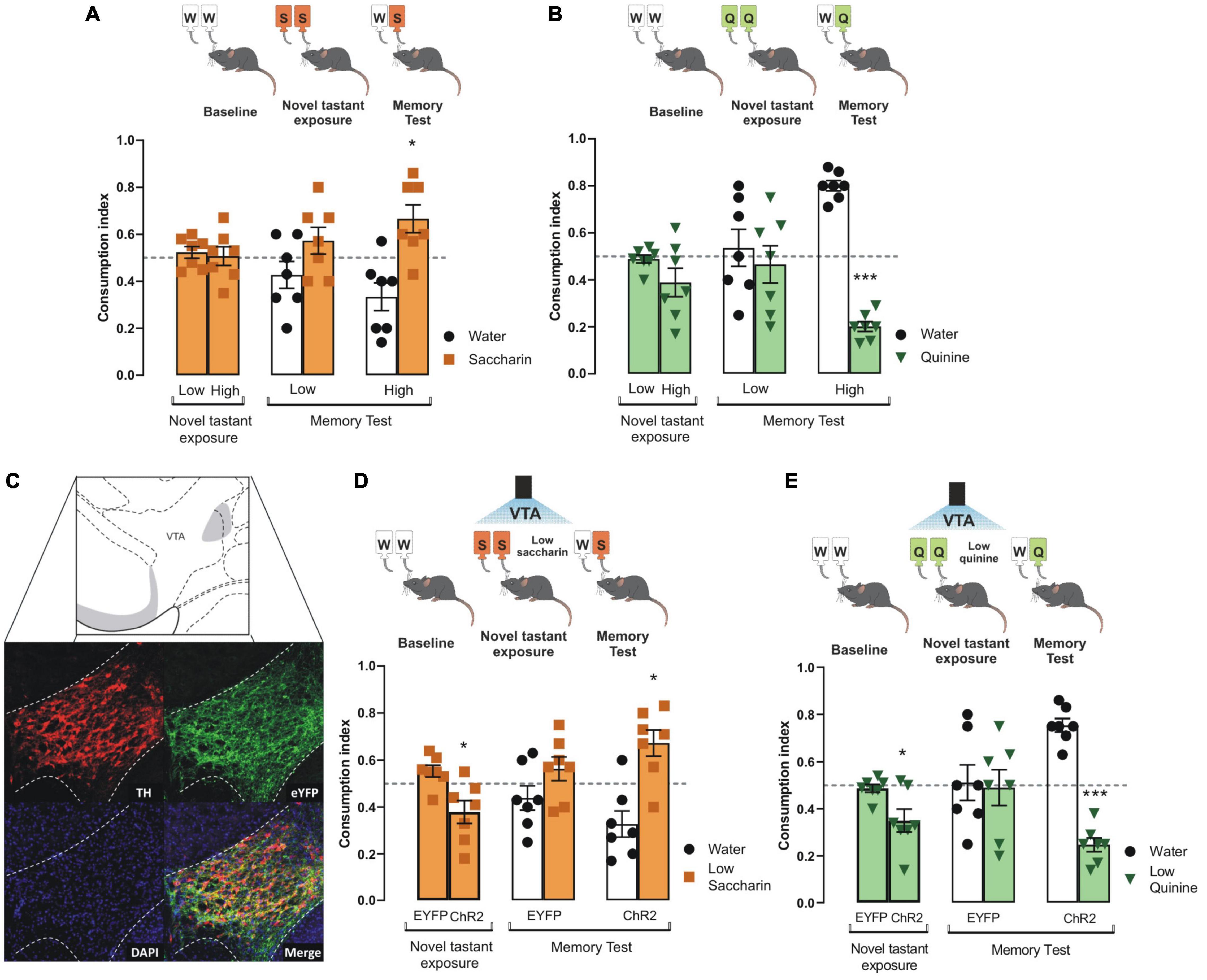
Figure 1. Photoactivation of VTA dopaminergic neurons increases the salience of tastant stimuli regardless of the hedonic value. (A) During the novel tastant exposure session, no significant differences were reported between low and high saccharin (n = 7) in intact mice vs. randomness 0.5 [Low: one sample t-test, t(6) = 0.9305, p = 0.3880; High: one sample t-test, t(6) = 0.1805, p = 0.8627]. During the memory test, mice were not capable of discriminating low saccharin (5 mM)/water (n = 7) vs. randomness 0.5 [one sample t-test, t(6) = 1.283, p = 0.2470]. In comparison, high saccharin (15 mM)/water (n = 7) was discriminated vs. randomness 0.5 [one sample t-test, t(6) = 2.799, p = 0.0312]. (B) Mice do not show statistical significance in consumption index vs. randomness 0.5 for neither low or high quinine during the novel tastant exposure session vs. randomness 0.5 [Low: one sample t-test, t(6) = 0.6737, p = 0.5256; High: one sample t-test, t(6) = 1.834, p = 0.1164]. Accordingly, quinine memory test shows that low quinine (126 μM)/water (n = 7) was not discriminated vs. randomness 0.5 [one sample t-test, t(6) = 0.4336, p = 0.6797], while higher quinine (504 μM)/water (n = 7) was distinguished vs. randomness 0.5 [one sample t-test, t(6) = 14.01, p < 0.0001]. (C) Micrographs of the adenoviral infection in ventral tegmental area (VTA). Green shows the reporter protein enhanced yellow fluorescent protein (eYFP), red shows the expression of tyrosine hydroxylase (TH) protein, and the last micrograph shows the colocalization between eYFP and TH expression. VTA diagram adapted from Dong (2008). (D) During novel taste presentation, the optogenetic stimulation of VTA decreases the consumption index of low saccharin vs. randomness 0.5 [EYFP: one sample t-test, t(6) = 2.103, p = 0.0802; ChR2: one sample t-test, t(6) = 2.485, p = 0.0475]. The ability to discriminate saccharin solution was improved vs. randomness 0.5 during the memory test in ChR2 mice [ChR2 group n = 7, one sample t-test, t(6) = 3.101, p = 0.0211; EYFP group n = 7, one sample t-test, t(6) = 1.240, p = 0.2613]. (E) During novel taste presentation, the optogenetic of VTA decreases the consumption index of low quinine vs. randomness 0.5 [EYFP: one sample t-test, t(6) = 0.7756, p = 0.4675; ChR2: one sample t-test, t(6) = 3.086, p = 0.0215]. Accordingly, the performance of ChR2 mice to discriminate quinine vs. randomness 0.5 was improved during the memory test [EYFP group n = 7, one sample t-test, t(6) = 0.1320, p = 0.8993; ChR2 group n = 7, one sample t-test, t(6) = 8.526, p = 0.0001]. All data are shown as mean ± SEM. *p < 0.05, ***p < 0.001.
To study whether the VTA dopaminergic neurons increase the salience of appetitive and aversive taste stimuli, we injected TH-Cre mice with an adeno-associated virus encoding Cre-dependent channelrhodopsin-2 protein (ChR2). Enhanced yellow fluorescent protein (eYFP) was used as a reporter protein. Expression of eYFP reporter and TH immunoreactive neurons in coronal slices of VTA neurons is shown in Figure 1C. Colocalization analysis showed high expression of eYFP in VTA dopaminergic neurons with endogenous TH immunoreactivity (Figure 1C, merge). We found that the photostimulation of the VTA coupled to the presentation of low concentration saccharin (Figure 1D) or quinine (Figure 1E) solutions during the novel tastant exposure session decreased the consumption index in ChR2 mice, but not in eYFP mice. We found that the ChR2 mice showed a reliable TRM, measured by the strong preference for low saccharin solution (Figure 1D) or strong avoidance for low quinine solution (Figure 1E). However, during the memory test, eYFP mice did not consolidate a TRM to low concentrated saccharin or quinine solutions. These results suggest that VTA dopaminergic neurons increase the salience of low concentration appetitive and aversive taste stimuli to consolidate into TRM.
Photostimulation of Ventral Tegmental Area Dopaminergic Neurons Does Not Alter the Palatability of Appetitive or Aversive Tastes
To determine whether the behavioral effects induced by VTA dopaminergic neurons were due to a change in taste palatability, mice were placed in a Brief Access Taste Task (BATT). A BATT measures the oromotor responses (palatability) using the lick rate evoked by tastants during the 5 s reward period (Villavicencio et al., 2018). If dopaminergic neurons are related to taste palatability, we hypothesize that the stimulation would change the lick rate of familiar tastants (Garcia et al., 2021). During this task, mice received either low concentrated saccharin 5 mM, quinine 126 μM, or water for 5 s per trial (Figure 2A). As expected, eYFP mice exhibited a higher lick rate elicited by saccharin and a lower licking rate after quinine delivery (Figure 2B). Similar results were also observed when mice were tested at high tastants concentrations (Figures 2E,F). Importantly, taste palatability was not altered by the photostimulation of VTA dopaminergic neurons while mice licked for familiar tastes (Figure 2C). Specifically, the lick bout duration, a measure of palatability, in the ChR2 mice was not significantly different from eYFP mice (Figure 2D; two-way ANOVA, Factor mice, F(1,21) = 1.188, p = 0.28). Collectively, our data demonstrate that the behavioral effects induced by photostimulation of VTA dopaminergic neurons were not related to taste palatability. Thus, it is more likely that stimulation of DA neurons affected saliency rather than taste palatability.
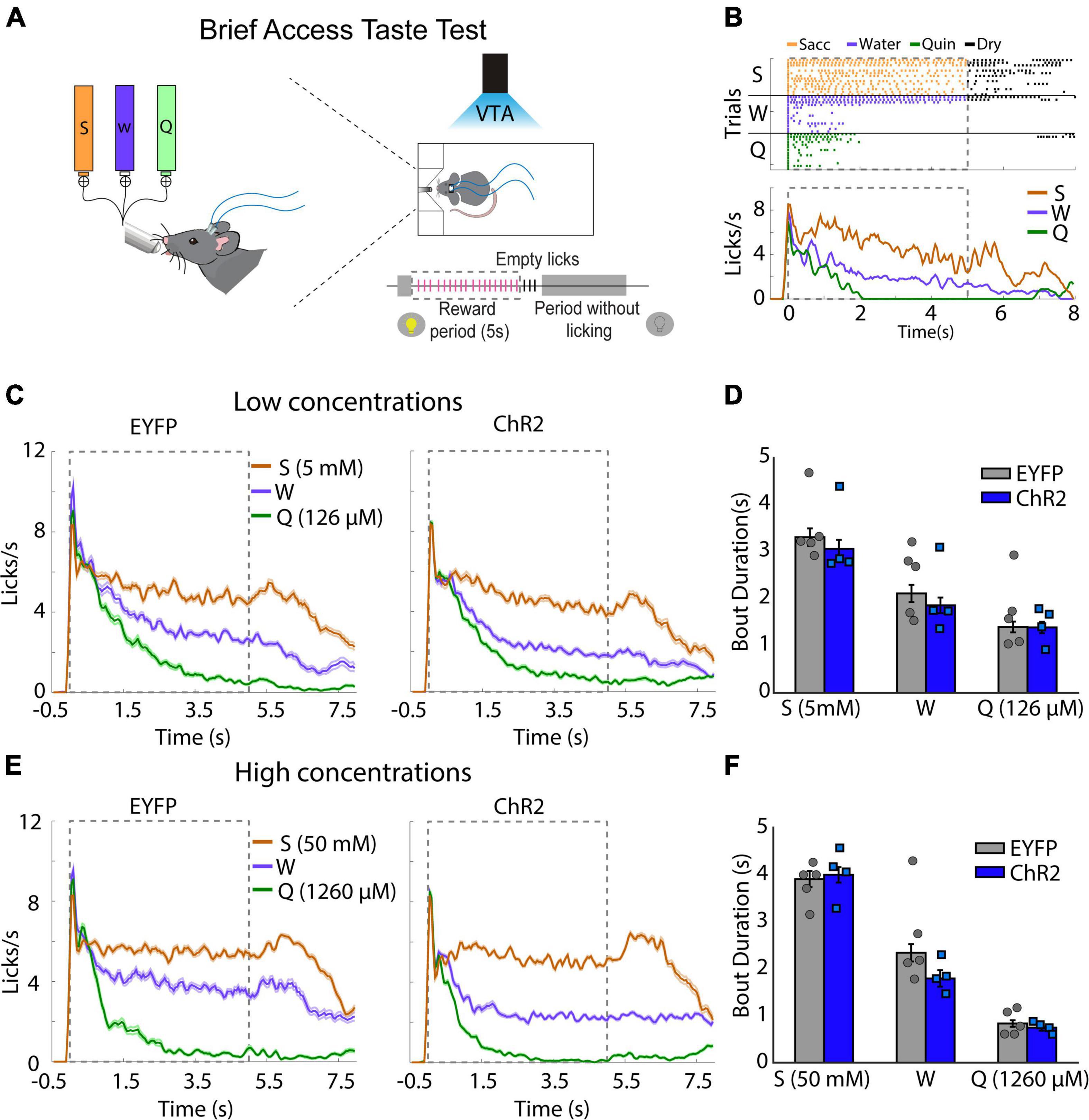
Figure 2. Photoactivation of ventral tegmental area (VTA) dopaminergic neurons does not impact taste palatability. (A) Schematic representation of each trial, in which mice had access to different tastants for a brief time (5 s reward period) to either saccharin, water, or quinine, randomly. Mice received photostimulation during the complete session. (B) A representative raster plot for licking of one mouse and session. Each tick indicates a single lick and the color the tastant delivered (orange saccharin, blue water, green quinine); black ticks indicate empty (dry) licks. Below is the Peristimulus time histogram (PSTH) from a representative mouse. The dash and gray square indicate the reward period. (C) Population PSTH for all subjects and sessions for low concentrations. (D) Mean of lick bout duration (a measure of palatability, i.e., the longer, the more palatable) for each taste during the rewarded period. (E,F) like (C,D), but for high concentrations. S, saccharin, W, water, or Q, quinine hydrochloride. Enhanced yellow fluorescent protein (eYFP) group (n = 5) and ChR2 group (n = 4). Differences were not observed in the bout duration between subjects for low concentrations [two-way ANOVA, Factor mice, F(1,21) = 1.188, p = 0.28] or high concentrations [two-way ANOVA, Factor mice, F(1,21) = 1.386, p = 0.25].
Specific Photostimulation of the Ventral Tegmental Area-Insular Cortex Dopaminergic Terminals Increases the Salience to Consolidate Taste Stimuli
To determine whether the VTA dopaminergic projections in the IC would solely consolidate taste information (Bermudez-Rattoni, 2014), we photostimulated the VTA dopaminergic terminals in the IC during the novel tastant exposure session. Congruently with prior studies, we found a greater level of off-target EYFP expression in the IC due to the tyrosine hydroxylase promoter used to control the expression of the transgenes in the TH-Cre mice (Lammel et al., 2015). The photostimulation of VTA dopaminergic projections into the IC (Figure 3A) did not alter the consumption indexes in ChR2 mice of novel saccharin (Figure 3B) or quinine (Figure 3C) at low concentrations during the novel tastant exposure session. In contrast, ChR2 mice exhibited a reliable TRM during the memory test, as seen by the strong preference for saccharin relative to water (Figure 3B) and a strong avoidance for the quinine solution vs. water (Figure 3C). Our results showed that the photostimulation of the VTA-IC inputs is sufficient to consolidate a TRM in ChR2 mice but not in eYFP control mice.
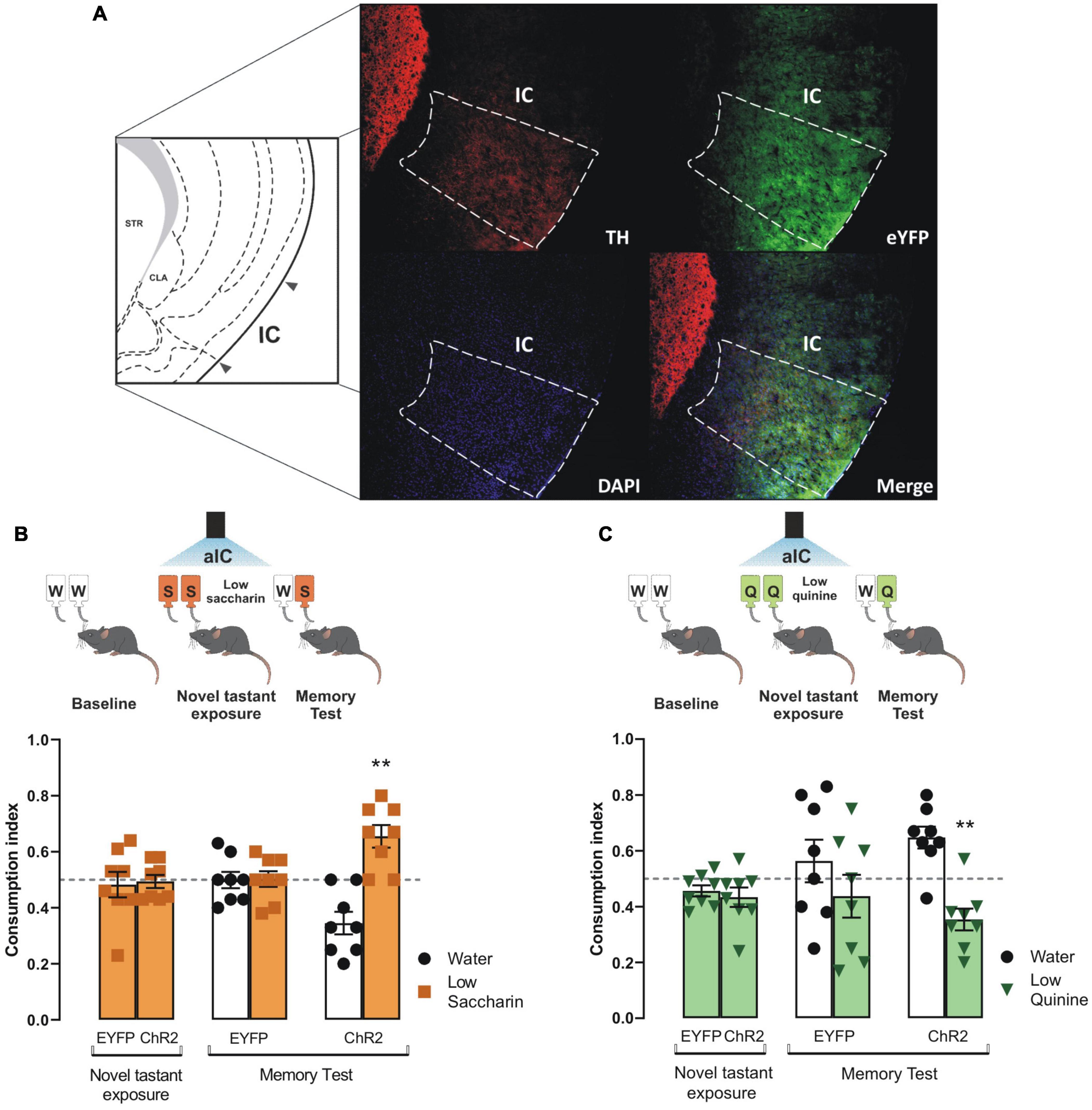
Figure 3. Photostimulation of the ventral tegmental area (VTA)-insular cortex (IC) dopaminergic pathway increases the salience of taste stimuli. (A) Representative micrographs of triple immunofluorescence for tyrosine hydroxylase (TH) immunoreactive fibers in the IC (top, left), enhanced yellow fluorescent protein (eYFP) immunoreactive projections from the VTA into the IC (top, right), DAPI (bottom, left), and merge (bottom, right). IC diagram adapted from Dong (2008). (B) Optogenetic stimulation of VTA-IC dopaminergic projections during the novel tastant exposure session does not alter consumption index of low saccharin in ChR2 mice vs. randomness 0.5 [ChR2: one sample t-test, t(7) = 0.2666, p = 0.7975; EYFP: one sample t-test, t(7) = 0.3856, p = 0.7113]. However, in the memory test, the optogenetic stimulation of the VTA-IC dopaminergic projections in ChR2 mice (n = 8) enabled the preference for low saccharin solution vs. randomness 0.5 [one sample t-test, t(7) = 3.854, p = 0.0063], but not in EYFP mice (n = 8) [one sample t-test, t(7) = 0.08904, p = 0.9315]. (C) Optogenetic stimulation of VTA-IC dopaminergic projections in ChR2 mice during the novel tastant exposure session does not modify the consumption index of low quinine vs. randomness 0.5 [EYFP: one sample t-test, t(7) = 2.178, p = 0.0658; ChR2: one sample t-test, t(7) = 1.910, p = 0.0978]. Accordingly, during the memory test, the optogenetic stimulation of the VTA dopaminergic projections into the IC led ChR2 mice (n = 8) to avoid low quinine solution [one sample t-test, t(7) = 3.745, p = 0.0072]. However, EYFP mice (n = 8) did not show a significant difference in consumption between low quinine vs. randomness 0.5 [one sample t-test, t(7) = 0.8147, p = 0.4421]. All data are shown as mean ± SEM. **p < 0.01.
The Salience to Consolidate Aversive, but Not Appetitive Taste Recognition Memory, Requires D1-Like Receptor Activity Into Insular Cortex
Having demonstrated the role of dopamine from VTA into the IC to process appetitive and aversive taste stimuli, we administered an antagonist of D1-like receptors (SCH23390) into the IC before the photostimulation of the VTA dopaminergic neurons in ChR2 and eYFP mice during the presentation of saccharin and quinine solutions. We found that the blockage of the D1-like receptors into the IC did not alter the consumption index during the novel tastant exposure session nor impair the salience to consolidate an appetitive TRM (Figure 4A), as measured by a preference for saccharin vs. water during the memory test. Nevertheless, the blockage of the D1-like receptors did not affect the consumption index during the novel tastant exposure session but impaired the salience to consolidate an aversive TRM (Figure 4B). These results suggest that the VTA-IC dopaminergic pathway increases the salience of taste stimuli regardless of the hedonic value to consolidate a TRM. Notably, the aversive but not appetitive taste stimuli require D1-like receptors into the IC to process the salience of TRM.
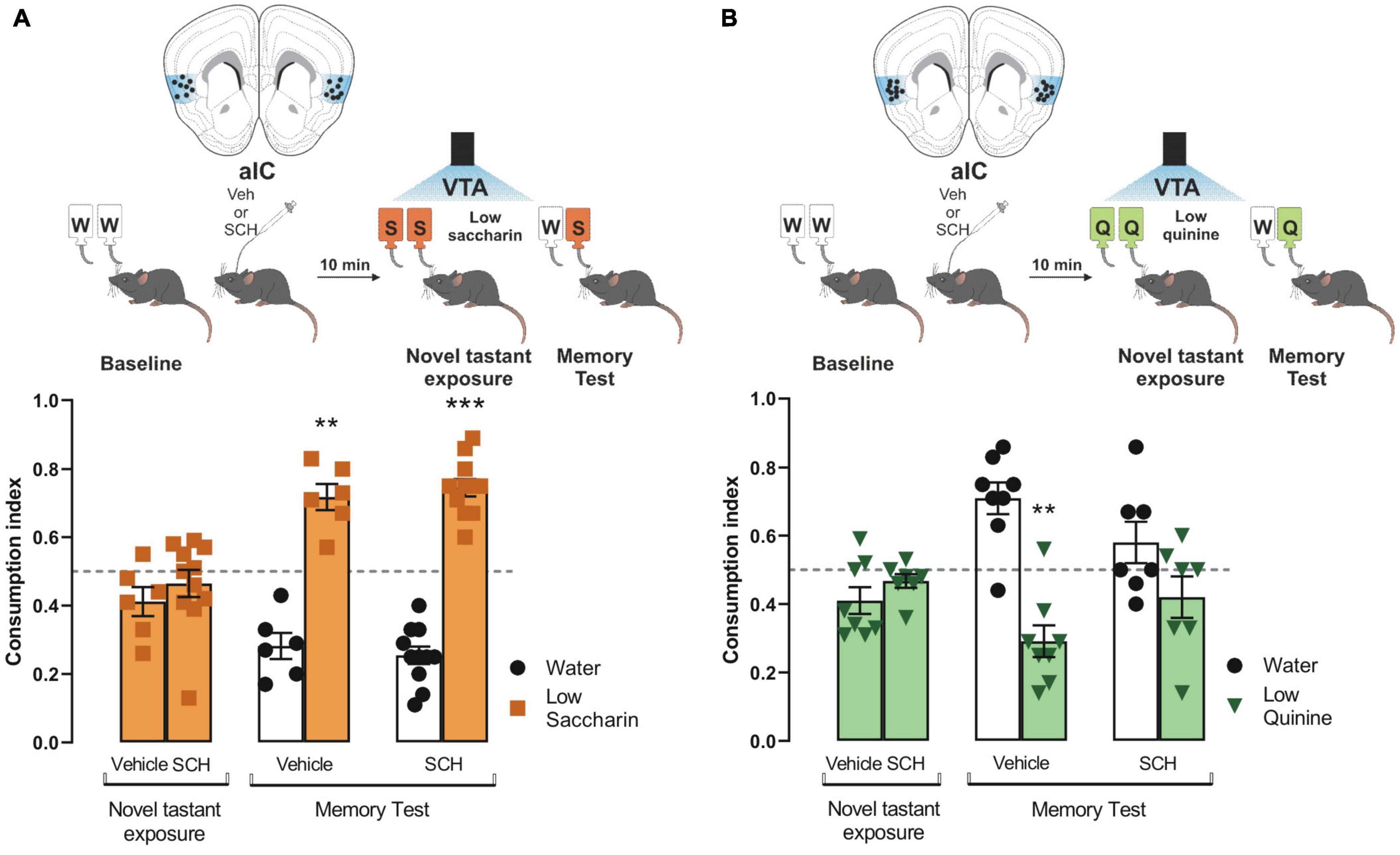
Figure 4. Processing of aversive but not appetitive taste requires D1-like receptor activity into insular cortex (IC). Dopaminergic activity blockade in the IC impedes consolidation of aversive but not appetitive taste recognition memory (TRM). (A) ChR2 mice were administered with SCH23390 (n = 11) or vehicle (n = 6) before the photostimulation of the ventral tegmental area (VTA) dopaminergic neurons. No statistical significance was shown for consumption index of low saccharin in ChR2 mice/vehicle nor ChR2/SCH23390 mice vs. randomness 0.5 [ChR2/Vehicle mice: one sample t-test, t(5) = 2.077, p = 0.0925; ChR2/SCH23390 mice: one sample t-test, t(10) = 0.8933, p = 0.3927]. During the memory test, both mice groups exhibited a strong preference for the low saccharin solution vs. randomness [ChR2/Vehicle: one sample t-test, t(5) = 5.722, p = 0.0023; ChR2/SCH23390: one sample t-test, t(10) = 9.670, p < 0.0001]. IC coronal plane adapted from Dong (2008). (B) ChR2 mice were administered with SCH23390 (n = 7) or vehicle (n = 8) before the photostimulation of the VTA dopaminergic neurons. No statistical significance was shown for consumption index of low quinine in ChR2/Vehicle nor ChR2/SCH23390 vs. randomness 0.5 [ChR2/Vehicle: one sample t-test, t(7) = 2.313, p = 0.0540; ChR2/SCH23390: one sample t-test, t(6) = 1.636, p = 0.1530]. During the memory test, the group administered with SCH23390 before the optogenetic stimulation of the VTA-IC dopaminergic pathway impaired the avoidance behavior for the low quinine solution in the ChR2 mice vs. randomness 0.5 (n = 7) [one sample t-test, t(7) = 4.489, p = 0.0028] but not in the ChR2 mice administered with vehicle vs. randomness 0.5 (n = 8) [one sample t-test, t(6) = 1.320, p = 0.2349]. IC coronal plane adapted from Dong (2008). All data are shown as mean ± SEM. **p < 0.01, ***p < 0.001.
Salience to Consolidate Conditioned Taste Aversion Requires D1-Like Receptor Activity Into Insular Cortex
Since the results showed that the VTA-IC dopaminergic pathways potentiated the salience of an innate aversive taste stimulus to consolidate a TRM through the D1-like receptors; we extended our research to study whether the same pathway is required to process the salience of associative aversive taste memories, such as a conditioned taste aversion memory. First, ChR2 and eYFP mice received VTA dopaminergic neurons photostimulation concomitantly with the presentation of the low saccharin solution (training session). ChR2 mice showed reduced consumption of low saccharin solution compared with eYFP mice (Figure 5A). Ten minutes later, mice were randomly injected with a neutral stimulus (NaCl, 66 mg/kg) or a subthreshold of an unconditioned aversive stimulus (LiCl, 48 mg/kg) intraperitoneally. We found that neutral stimulation (NaCl) did not induce conditioned taste aversion. Moreover, eYFP mice could not discriminate between the low saccharin solution against water, but ChR2 mice preferred the low saccharin solution from water (Figure 5A). Interestingly, eYFP mice injected with the subthreshold LiCl (48 mg/kg) did not discriminate the low saccharin solution against water. Instead, ChR2 mice showed a robust conditioned taste aversion response due to the aversive association between the gastric malaise produced by the LiCl and the low saccharin solution (Figure 5A). Finally, using the protocol described above, ChR2 mice were injected with SCH23390 or vehicle into the IC 10 min before the conditioned taste aversion. Our results showed that the ChR2 mice injected with the vehicle had a strong conditioned aversive response, with a reliable avoidance behavior for the low saccharin solution associated with the gastric malaise produced by the LiCl. On the other hand, the ChR2 mice administered with SCH23390 were incapable of associating the unconditioned aversive stimulus with the low saccharin solution, showing a preference for the low saccharin solution rather than the water (Figure 5B). In sum, these results suggest that dopamine terminals activity from VTA to the IC also increases the salience of subthreshold stimuli, enhancing the association of an unconditioned aversive stimulus with an appetitive taste via D1-like receptors.
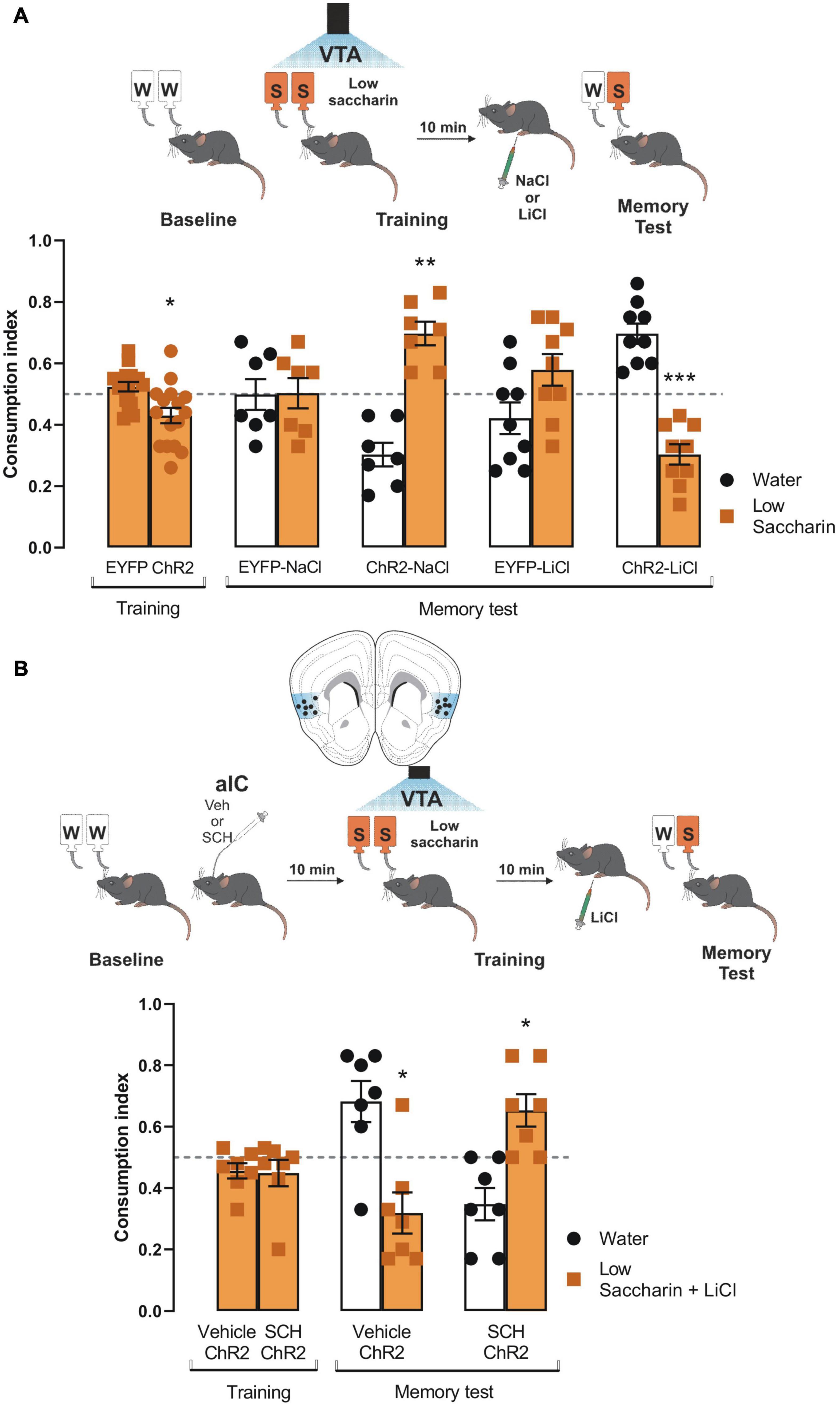
Figure 5. Ventral tegmental area (VTA) photostimulation increases the salience of conditioned taste aversion for an appetitive taste. (A) ChR2 (n = 16) or EYFP (n = 16) mice were photostimulated into VTA when the low saccharin was presented during the training session. No statistical significance was shown for consumption index of low saccharin in EYFP nor ChR2 mice vs. randomness 0.5 [EYFP: one sample t-test, t(15) = 1.550, p = 0.1421; ChR2: one sample t-test, t(15) = 2.793, p = 0.0137]. After the training session, mice were intraperitoneally injected with an unconditioned stimulus NaCl (66 mg/kg) or LiCl (48 mg/kg). The administration of NaCl in ChR2 mice (n = 7) did not affect the preference for the low saccharin vs. randomness 0.5 due to the photostimulation of the VTA dopaminergic neurons [ChR2-NaCl: one sample t-test, t(6) = 5.109, p = 0.0022] but the consumption in EYFP was not affected [EYFP-NaCl: one sample t-test, t(6) = 0.05799, p = 0.9556]. The administration of LiCl in ChR2 mice decreased the consumption of the low saccharin vs. randomness 0.5 [ChR2-LiCl: one sample t-test, t(8) = 5.930, p = 0.0003], but the consumption of EYFP mice was not affected [EYFP-LiCl: one sample t-test, t(8) = 1.532, p = 0.1640]. (B) Diagram of coronal slice for insular cortex (IC) cannulation in ChR2 mice. SCH23390 (n = 7) or vehicle (n = 7) in ChR2 mice was administered before the photostimulation of the VTA dopaminergic neurons. No statistical significance was shown for consumption index of low saccharin in Vehicle/ChR2 mice nor SHC23390/ChR2 mice vs. randomness 0.5 [Vehicle/ChR2: one sample t-test, t(6) = 1.767, p = 0.1276; SCH23390/ChR2: one sample t-test, t(6) = 1.190, p = 0.2791]. Ten minutes later mice were intraperitoneally injected with a low dose of LiCl. The blockage of the D1-like receptors into the IC impairs the consolidation of the conditioned taste aversion in the SCH23390/ChR2 mice [one sample t-test, t(6) = 2.897, p = 0.0274]. In contrast, the vehicle/ChR2 mice showed a reliable conditioned taste aversion [one sample t-test, t(6) = 2.703, p = 0.0355]. IC coronal plane adapted from Dong (2008). All data are shown as mean ± SEM. *p < 0.05; **p < 0.01.
Discussion
Our results demonstrate that the VTA photostimulation increases the salience of subthreshold taste stimuli, which are not likely to form long-term memory. The VTA photostimulation facilitates the consolidation of a taste recognition memory generated through naturally appetitive or aversive stimuli without an evident alteration on its taste palatability. In this sense, midbrain dopaminergic neurons modulate brain networks through phasic responses to encode not only the novelty, prominence, or surprise of rewarding stimuli but also aversive experiences (Schultz, 1998; Redgrave et al., 1999; Horvitz, 2000; Di Chiara, 2002; Joseph et al., 2003; Pezze and Feldon, 2004; Ungless et al., 2004; Lisman and Grace, 2005; Redgrave and Gurney, 2006; Schultz, 2007a; Moriya et al., 2018). The salience of stimuli, including taste, is adaptive because salient information tends to be preferentially consolidated into long-term memory (Shohamy and Adcock, 2010; Cowan et al., 2021). Importantly, we further extended our previous proposal by demonstrating that the increased VTA dopaminergic neuronal activity did not alter taste palatability. Our results agree with previous results, showing that dopaminergic neurotransmission affects novel taste intake without affecting taste evoked orofacial responses, another parameter of taste palatability (Treit and Berridge, 1990). Therefore, our findings suggest that the dopaminergic activity of the VTA pathway is involved in salience to consolidate the taste memory trace without affecting palatability. We observed a reduced consumption of the novel low concentration of tastants with the optogenetic stimulation of the VTA dopaminergic neurons, but not during the photostimulation of the VTA-IC dopaminergic pathway. However, the photostimulation of either VTA or VTA-IC terminals with low taste concentrations induced taste memory consolidation in both cases. These results suggest that in the first case, the photostimulation of VTA might trigger dopamine release into different brain structures potentially implicated in motivated behavior (Bromberg-Martin et al., 2010), motor control (Bourdy and Barrot, 2012), or even reward processing (Lammel et al., 2011). However, this unspecific effect does not interfere with taste memory consolidation.
After novel food consumption, c-fos activity is increased in the VTA and its projection areas within the mesolimbic and mesocortical dopaminergic systems, necessary to form memory (Kest et al., 2012; Dela Cruz et al., 2016). Additionally, we recently reported that photoactivation of the VTA dopaminergic neurons at 20 Hz for 20 min induces dopamine release in the IC, which modulates the neuronal activity in this cortical structure (Gil-Lievana et al., 2020). Here we found that VTA-IC dopaminergic activity increases the salience needed to preferentially facilitate consolidation of a taste recognition memory regardless of the hedonic value of taste stimuli. Similar outcomes were obtained by Kutlu et al. (2021), who show that dopamine contributes to fit valence-independent perceived salience across different learning paradigms. We hypothesize that the increased salience to facilitate consolidation into IC results from the synaptic potentiation induced by photostimulation of the VTA dopaminergic neurons. It is widely known that tonic and phasic dopaminergic release differentially modifies and modulates the synaptic strength and neuronal activity in many different structures, including the hippocampus, prefrontal, and insular cortices, to serve as a cellular mechanism to establish memory (Matsuda et al., 2006; Sheynikhovich et al., 2013; Rodríguez-Durán and Escobar, 2014; Otani et al., 2015; Navakkode et al., 2017; Papaleonidopoulos et al., 2018). In fact, findings support that brief exposure to a novel environment reduces the threshold to induce long-term potentiation. This facilitatory effect occurs for a short period of time following novelty exposure and depends on the activation of D1-like receptors but is absent in animals that explore a familiar environment (Li et al., 2003). Moreover, it has been demonstrated that aversive stimuli selectively modify synapses on dopaminergic neurons that project to cortical areas (Lammel et al., 2011). Furthermore, the dysfunctional dopaminergic activity alters the synaptic plasticity in the BLA-IC pathway to transform the long-term potentiation into long-term depression associated with memory impairment (Moreno-Castilla et al., 2016).
Although our results show that the VTA-IC dopaminergic activity is sufficient to increase the salience of aversive and appetitive stimuli, we found that D1-like receptor activity is necessary to integrate aversive taste information to facilitate the consolidation of an aversive taste recognition memory. Interestingly, the consumption index during the novel tastant exposure (acquisition) was not altered, regardless of the inhibition of D1-like receptors, but impairs the aversive long-term memory. Accordingly, the presentation of a novel taste stimulus, regardless of its valence, induces an increment of basal concentration of dopamine in the IC (Osorio-Gómez et al., 2021). However, previous reports have shown that the D1-like receptor is critical for aversive recognition memories formation, increasing neural activity within cortical networks (Heath et al., 2015; Saito et al., 2020). It is parsimonious to hypothesize that the VTA-IC dopaminergic pathway is one of many circuits encoding salience of taste stimuli regardless of the hedonic value due to the processing of salience to consolidate taste information involves multiple brain areas (Bromberg-Martin et al., 2010; Shiner et al., 2015; Cai et al., 2020; Kutlu et al., 2021). However, a D1-like receptor within IC is required for the consolidation and storage of mainly aversive taste recognition memory. Moreover, we found that D1-like receptor activity is also required to establish a conditioned taste aversion. Importantly, the blockage of the D1-like receptor into the IC before a conditioned taste aversion impairs the consolidation, but not the short-term memory, suggesting that the D1-like receptor is involved in the cognitive processing to consolidate the conditioned taste aversion (Osorio-Gómez et al., 2021). This study’s results agree with reports suggesting that D1-like, but not D2/D3 dopaminergic receptor activity is associated with conditioned taste aversion (Fenu et al., 2001).
Dopamine plays a critical role in mediating reward and aversive signals of various stimuli, including visual, auditory, and mechanosensory stimuli (Wise, 2004; Schultz, 2007b; Zweifel et al., 2011; McCutcheon et al., 2012; Stelly et al., 2019). We show that although dopamine signaling in the IC is required to consolidate aversive and appetitive taste memories, the downstream molecular events may differ. Indeed, the PKC activity, a kinase involved in neural plasticity processes, is needed in the IC to establish aversive taste memory, but not for appetitive taste memory (Núñez-Jaramillo et al., 2007). We propose that dopamine from VTA encodes the salience of appetitive and aversive taste, but the interaction of different brain structures (e.g., basolateral amygdala and nucleus accumbens) and other neurotransmitters (i.e., glutamate) within the IC leads to the activation of different signaling pathways to facilitate consolidation of the aversive appetitive nature of the taste memory.
All in all, we found that the activity of the VTA-IC dopaminergic pathway increases the salience to facilitate the consolidation of aversive and appetitive taste stimuli. However, the IC only consolidates the aversive taste information through the D1-like dopaminergic receptors. Given the complexity of VTA connectivity, it is reasonable to hypothesize that the encoding of tastant’s salience requires the processing of multiple parallel brain regions. Here we unveiled the VTA-IC dopaminergic pathway as an essential component of this complex circuitry involved in salience to facilitate the consolidation of taste recognition memories. Importantly, it is very likely our photostimulation parameters do not replicate any endogenous process in the VTA-IC pathway. Therefore, future studies should identify a more defined temporal window in which the VTA-IC dopaminergic pathway is required to increase the salience of novel taste stimuli to consolidate taste memory.
Data Availability Statement
The raw data supporting the conclusions of this article will be made available by the authors, without undue reservation.
Ethics Statement
The animal study was reviewed and approved by Ethics Committee of Instituto de Fisiología Celular (FBR125-18).
Author Contributions
EG-L, GR-M, OU-M, RG, and FB-R designed the research and wrote the manuscript. EG-L, GR-M, and OU-M performed behavioral and optogenetic experiments. JL-I performed experiments for brief access taste test. EG-L and GR-M performed imaging analysis. All authors contributed to the article and approved the submitted version.
Funding
This work was supported by Productos Medix 3247, Cátedra Marcos Moshinsky (to RG), and the CONACyT grants 250870, FOINS 474, and DGAPA-PAPIIT-UNAM IN212919 to FB-R.
Conflict of Interest
The authors declare that the research was conducted in the absence of any commercial or financial relationships that could be construed as a potential conflict of interest.
Publisher’s Note
All claims expressed in this article are solely those of the authors and do not necessarily represent those of their affiliated organizations, or those of the publisher, the editors and the reviewers. Any product that may be evaluated in this article, or claim that may be made by its manufacturer, is not guaranteed or endorsed by the publisher.
Acknowledgments
We thank Rui M. Costa and Fatuel Tecuapetla for the TH-Cre mice. We thank Daniel Osorio Gómez for their comments on an earlier version of the manuscript. Luis Rodríguez Durán, Josué O. Ramírez Jarquín, and Cecilia Acevedo Huerta for technical assistance. Ruth Rincon Heredia and Abraham Rosas Arellano for imaging support.
References
Alger, S. E., Chen, S., and Payne, J. D. (2019). Do different salience cues compete for dominance in memory over a daytime nap? Neurobiol. Learn. Mem. 160, 48–57. doi: 10.1016/j.nlm.2018.06.005
Bermúdez-Rattoni, F. (2004). Molecular mechanisms of taste-recognition memory. Nat. Rev. Neurosci. 5, 209–217. doi: 10.1038/nrn1344
Bermudez-Rattoni, F. (2014). The forgotten insular cortex: its role on recognition memory formation. Neurobiol. Learn. Mem. 109, 207–216. doi: 10.1016/j.nlm.2014.01.001
Bermudez-Rattoni, F., and McGaugh, J. L. (1991). Insular cortex and amygdala lesions differentially affect acquisition on inhibitory avoidance and conditioned taste aversion. Brain Res. 549, 165–170. doi: 10.1016/0006-8993(91)90616-4
Berridge, K. C., and Robinson, T. E. (1998). What is the role of dopamine in reward: hedonic impact, reward learning, or incentive salience? Brain Res. Brain Res. Rev. 28, 309–369. doi: 10.1016/s0165-0173(98)00019-8
Bourdy, R., and Barrot, M. (2012). A new control center for dopaminergic systems: pulling the VTA by the tail. Trends Neurosci. 35, 681–690. doi: 10.1016/j.tins.2012.06.007
Brenhouse, H. C., Sonntag, K. C., and Andersen, S. L. (2008). Transient D1 dopamine receptor expression on prefrontal cortex projection neurons: relationship to enhanced motivational salience of drug cues in adolescence. J. Neurosci. 28, 2375–2382. doi: 10.1523/JNEUROSCI.5064-07.2008
Brischoux, F., Chakraborty, S., Brierley, D. I., and Ungless, M. A. (2009). Phasic excitation of dopamine neurons in ventral VTA by noxious stimuli. Proc. Natl. Acad. Sci. U.S.A. 106, 4894–4899. doi: 10.1073/pnas.0811507106
Bromberg-Martin, E. S., Matsumoto, M., and Hikosaka, O. (2010). Dopamine in motivational control: rewarding, aversive, and alerting. Neuron 68, 815–834. doi: 10.1016/j.neuron.2010.11.022
Cai, L. X., Pizano, K., Gundersen, G. W., Hayes, C. L., Fleming, W. T., Holt, S., et al. (2020). Distinct signals in medial and lateral VTA dopamine neurons modulate fear extinction at different times. eLife 9:e54936. doi: 10.7554/eLife.54936
Chen, X., Gabitto, M., Peng, Y., Ryba, N. J., and Zuker, C. S. (2011). A gustotopic map of taste qualities in the mammalian brain. Science 333, 1262–1266. doi: 10.1126/science.1204076
Cho, J. R., Treweek, J. B., Robinson, J. E., Xiao, C., Bremner, L. R., Greenbaum, A., et al. (2017). Dorsal raphe dopamine neurons modulate arousal and promote wakefulness by salient stimuli. Neuron 94, 1205–1219.e8. doi: 10.1016/j.neuron.2017.05.020
Cowan, E. T., Schapiro, A. C., Dunsmoor, J. E., and Murty, V. P. (2021). Memory consolidation as an adaptive process. Psychon. Bull. Rev. 28, 1796–1810. doi: 10.3758/s13423-021-01978-x
de Jong, J. W., Afjei, S. A., Pollak Dorocic, I., Peck, J. R., Liu, C., Kim, C. K., et al. (2019). A neural circuit mechanism for encoding aversive stimuli in the mesolimbic dopamine system. Neuron 101, 133–151.e7. doi: 10.1016/j.neuron.2018.11.005
Dela Cruz, J. A., Coke, T., and Bodnar, R. J. (2016). Simultaneous detection of c-fos activation from mesolimbic and mesocortical dopamine reward sites following naive sugar and fat ingestion in rats. J. Vis. Exp. 114:53897. doi: 10.3791/53897
Di Chiara, G. (2002). Nucleus accumbens shell and core dopamine: differential role in behavior and addiction. Behav. Brain Res. 137, 75–114. doi: 10.1016/s0166-4328(02)00286-3
Dong, H. W. (2008). Allen Reference Atlas. A Digital Color Brain Atlas of the C57BL/6J Male Mouse. John Wiley and Sons Ltd.
Fenu, S., Bassareo, V., and Di Chiara, G. (2001). A role for dopamine D1 receptors of the nucleus accumbens shell in conditioned taste aversion learning. J. Neurosci. 21, 6897–6904. doi: 10.1523/JNEUROSCI.21-17-06897.2001
Fiorillo, C. D. (2013). Two dimensions of value: dopamine neurons represent reward but not aversiveness. Science 341, 546–549. doi: 10.1126/science.1238699
Fontanini, A., Grossman, S. E., Figueroa, J. A., and Katz, D. B. (2009). Distinct subtypes of basolateral amygdala taste neurons reflect palatability and reward. J. Neurosci. 29, 2486–2495. doi: 10.1523/JNEUROSCI.3898-08.2009
Garcia, A., Coss, A., Luis-Islas, J., Puron-Sierra, L., Luna, M., Villavicencio, M., et al. (2021). Lateral hypothalamic GABAergic neurons encode and potentiate sucrose’s palatability. Front. Neurosci. 14:608047. doi: 10.3389/fnins.2020.608047
Gil-Lievana, E., Balderas, I., Moreno-Castilla, P., Luis-Islas, J., McDevitt, R. A., Tecuapetla, F., et al. (2020). Glutamatergic basolateral amygdala to anterior insular cortex circuitry maintains rewarding contextual memory. Commun. Biol. 3:139. doi: 10.1038/s42003-020-0862-z
Guzmán-Ramos, K., and Bermúdez-Rattoni, F. (2011). Post-learning molecular reactivation underlies taste memory consolidation. Front. Syst. Neurosci. 5:79. doi: 10.3389/fnsys.2011.00079
Guzmán-Ramos, K., Osorio-Gómez, D., Moreno-Castilla, P., and Bermúdez-Rattoni, F. (2010). Off-line concomitant release of dopamine and glutamate involvement in taste memory consolidation. J. Neurochem. 114, 226–236. doi: 10.1111/j.1471-4159.2010.06758.x
Heath, F. C., Jurkus, R., Bast, T., Pezze, M. A., Lee, J. L., Voigt, J. P., et al. (2015). Dopamine D1-like receptor signalling in the hippocampus and amygdala modulates the acquisition of contextual fear conditioning. Psychopharmacology 232, 2619–2629. doi: 10.1007/s00213-015-3897-y
Horvitz, J. C. (2000). Mesolimbocortical and nigrostriatal dopamine responses to salient non-reward events. Neuroscience 96, 651–656. doi: 10.1016/s0306-4522(00)00019-1
Hyman, S. E. (2005). Addiction: a disease of learning and memory. Am. J. Psychiatry 162, 1414–1422. doi: 10.1176/appi.ajp.162.8.1414
Jezzini, A., Mazzucato, L., La Camera, G., and Fontanini, A. (2013). Processing of hedonic and chemosensory features of taste in medial prefrontal and insular networks. J. Neurosci. 33, 18966–18978. doi: 10.1523/JNEUROSCI.2974-13.2013
Joseph, M. H., Datla, K., and Young, A. M. (2003). The interpretation of the measurement of nucleus accumbens dopamine by in vivo dialysis: The kick, the craving or the cognition? Neurosci. Biobehav. Rev. 27, 527–541. doi: 10.1016/j.neubiorev.2003.09.001
Kest, K., Cruz, I., Chen, D. H., Galaj, E., and Ranaldi, R. (2012). A food-associated CS activates c-Fos in VTA DA neurons and elicits conditioned approach. Behav. Brain Res. 235, 150–157. doi: 10.1016/j.bbr.2012.07.044
Kutlu, M. G., Zachry, J. E., Melugin, P. R., Cajigas, S. A., Chevee, M. F., Kelly, S. J., et al. (2021). Dopamine release in the nucleus accumbens core signals perceived saliency. Curr. Biol. 31, 4748–4761.e8. doi: 10.1016/j.cub.2021.08.052
Lakens, D. (2013). Calculating and reporting effect sizes to facilitate cumulative science: a practical primer for t-tests and ANOVAs. Front. Psychol. 4:863. doi: 10.3389/fpsyg.2013.00863
Lammel, S., Ion, D. I., Roeper, J., and Malenka, R. C. (2011). Projection-specific modulation of dopamine neuron synapses by aversive and rewarding stimuli. Neuron 70, 855–862. doi: 10.1016/j.neuron.2011.03.025
Lammel, S., Steinberg, E. E., Földy, C., Wall, N. R., Beier, K., Luo, L., et al. (2015). Diversity of transgenic mouse models for selective targeting of midbrain dopamine neurons. Neuron 85, 429–438. doi: 10.1016/j.neuron.2014.12.036
Li, S., Cullen, W. K., Anwyl, R., and Rowan, M. J. (2003). Dopamine-dependent facilitation of LTP induction in hippocampal CA1 by exposure to spatial novelty. Nat. Neurosci. 6, 526–531. doi: 10.1038/nn1049
Lisman, J. E., and Grace, A. A. (2005). The hippocampal-VTA loop: controlling the entry of information into long-term memory. Neuron 46, 703–713. doi: 10.1016/j.neuron.2005.05.002
Matsuda, Y., Marzo, A., and Otani, S. (2006). The presence of background dopamine signal converts long-term synaptic depression to potentiation in rat prefrontal cortex. J. Neurosci. 26, 4803–4810. doi: 10.1523/JNEUROSCI.5312-05.2006
McCutcheon, J. E., Ebner, S. R., Loriaux, A. L., and Roitman, M. F. (2012). Encoding of aversion by dopamine and the nucleus accumbens. Front. Neurosci. 6:137. doi: 10.3389/fnins.2012.00137
Moessnang, C., Habel, U., Schneider, F., and Siegel, S. J. (2012). The electrophysiological signature of motivational salience in mice and implications for schizophrenia. Neuropsychopharmacology 37, 2846–2854. doi: 10.1038/npp.2012.156
Moreno-Castilla, P., Rodriguez-Duran, L. F., Guzman-Ramos, K., Barcenas-Femat, A., Escobar, M. L., and Bermudez-Rattoni, F. (2016). Dopaminergic neurotransmission dysfunction induced by amyloid-β transforms cortical long-term potentiation into long-term depression and produces memory impairment. Neurobiol. Aging 41, 187–199. doi: 10.1016/j.neurobiolaging.2016.02.021
Moriya, S., Yamashita, A., Kawashima, S., Nishi, R., Yamanaka, A., and Kuwaki, T. (2018). Acute aversive stimuli rapidly increase the activity of ventral tegmental area dopamine neurons in awake mice. Neuroscience 386, 16–23. doi: 10.1016/j.neuroscience.2018.06.027
Navakkode, S., Chew, K. C. M., Tay, S. J. N., Lin, Q., Behnisch, T., and Soong, T. W. (2017). Bidirectional modulation of hippocampal synaptic plasticity by Dopaminergic D4-receptors in the CA1 area of hippocampus. Sci. Rep. 7:15571. doi: 10.1038/s41598-017-15917-1
Nomoto, K., Schultz, W., Watanabe, T., and Sakagami, M. (2010). Temporally extended dopamine responses to perceptually demanding reward-predictive stimuli. J. Neurosci. 30, 10692–10702. doi: 10.1523/JNEUROSCI.4828-09.2010
Núñez-Jaramillo, L., Delint-Ramirez, I., and Bermúdez-Rattoni, F. (2007). PKC blockade differentially affects aversive but not appetitive gustatory memories. Brain Res. 1148, 177–182. doi: 10.1016/j.brainres.2007.02.032
Núñez-Jaramillo, L., Ramírez-Lugo, L., Herrera-Morales, W., and Miranda, M. I. (2010). Taste memory formation: latest advances and challenges. Behav. Brain Res. 207, 232–248. doi: 10.1016/j.bbr.2009.10.040
Osorio-Gómez, D., Bermúdez-Rattoni, F., and Guzmán-Ramos, K. R. (2021). Cortical neurochemical signaling of gustatory stimuli and their visceral consequences during the acquisition and consolidation of taste aversion memory. Neurobiol. Learn. Mem. 181:107437. doi: 10.1016/j.nlm.2021.107437
Osorio-Gómez, D., Guzmán-Ramos, K., and Bermúdez-Rattoni, F. (2017). Memory trace reactivation and behavioral response during retrieval are differentially modulated by amygdalar glutamate receptors activity: interaction between amygdala and insular cortex. Learn. Mem. 24, 14–23. doi: 10.1101/lm.042895.116
Otani, S., Bai, J., and Blot, K. (2015). Dopaminergic modulation of synaptic plasticity in rat prefrontal neurons. Neurosci. Bull. 31, 183–190. doi: 10.1007/s12264-014-1507-3
Papaleonidopoulos, V., Kouvaros, S., and Papatheodoropoulos, C. (2018). Effects of endogenous and exogenous D1/D5 dopamine receptor activation on LTP in ventral and dorsal CA1 hippocampal synapses. Synapse 72:e22033. doi: 10.1002/syn.22033
Payne, J. D., Stickgold, R., Swanberg, K., and Kensinger, E. A. (2008). Sleep preferentially enhances memory for emotional components of scenes. Psychol. Sci. 19, 781–788. doi: 10.1111/j.1467-9280.2008.02157.x
Pezze, M. A., and Feldon, J. (2004). Mesolimbic dopaminergic pathways in fear conditioning. Prog. Neurobiol. 74, 301–320. doi: 10.1016/j.pneurobio.2004.09.004
Ramírez-Lugo, L., Núñez-Jaramillo, L., and Bermúdez-Rattoni, F. (2007). Taste memory formation: role of nucleus accumbens. Chem. Senses 32, 93–97. doi: 10.1093/chemse/bjl023
Redgrave, P., and Gurney, K. (2006). The short-latency dopamine signal: a role in discovering novel actions? Nat. Rev. Neurosci. 7, 967–975. doi: 10.1038/nrn2022
Redgrave, P., Prescott, T. J., and Gurney, K. (1999). Is the short-latency dopamine response too short to signal reward error? Trends Neurosci. 22, 146–151. doi: 10.1016/s0166-2236(98)01373-3
Rodríguez-Durán, L. F., and Escobar, M. L. (2014). NMDA receptor activation and PKC but not PKA lead to the modification of the long-term potentiation in the insular cortex induced by conditioned taste aversion: differential role of kinases in metaplasticity. Behav. Brain Res. 266, 58–62. doi: 10.1016/j.bbr.2014.02.049
Rosenblum, K., Meiri, N., and Dudai, Y. (1993). Taste memory: the role of protein synthesis in gustatory cortex. Behav. Neural Biol. 59, 49–56. doi: 10.1016/0163-1047(93)91145-d
Saito, N., Tainaka, K., Macpherson, T., Hikida, T., Yamaguchi, S., and Sasaoka, T. (2020). Neurotransmission through dopamine D1 receptors is required for aversive memory formation and arc activation in the cerebral cortex. Neurosci. Res. 156, 58–65. doi: 10.1016/j.neures.2020.04.006
Schultz, W. (1998). Predictive reward signal of dopamine neurons. J. Neurophysiol. 80, 1–27. doi: 10.1152/jn.1998.80.1.1
Schultz, W. (2007a). Behavioral dopamine signals. Trends Neurosci. 30, 203–210. doi: 10.1016/j.tins.2007.03.007
Schultz, W. (2007b). Multiple dopamine functions at different time courses. Annu. Rev. Neurosci. 30, 259–288. doi: 10.1146/annurev.neuro.28.061604.135722
Scott, K. (2005). Taste recognition: food for thought. Neuron 48, 455–464. doi: 10.1016/j.neuron.2005.10.015
Sheynikhovich, D., Otani, S., and Arleo, A. (2013). Dopaminergic control of long-term depression/long-term potentiation threshold in prefrontal cortex. J. Neurosci. 33, 13914–13926. doi: 10.1523/JNEUROSCI.0466-13.2013
Shiner, T., Symmonds, M., Guitart-Masip, M., Fleming, S. M., Friston, K. J., and Dolan, R. J. (2015). Dopamine, salience, and response set shifting in prefrontal cortex. Cereb. Cortex 25, 3629–3639. doi: 10.1093/cercor/bhu210
Shohamy, D., and Adcock, R. A. (2010). Dopamine and adaptive memory. Trends Cogn. Sci. 14, 464–472. doi: 10.1016/j.tics.2010.08.002
Stelly, C. E., Haug, G. C., Fonzi, K. M., Garcia, M. A., Tritley, S. C., Magnon, A. P., et al. (2019). Pattern of dopamine signaling during aversive events predicts active avoidance learning. Proc. Natl. Acad. Sci. U.S.A. 116, 13641–13650. doi: 10.1073/pnas.1904249116
Treit, D., and Berridge, K. C. (1990). A comparison of benzodiazepine, serotonin, and dopamine agents in the taste-reactivity paradigm. Pharmacol. Biochem. Behav. 37, 451–456. doi: 10.1016/0091-3057(90)90011-6
Ungless, M. A., Magill, P. J., and Bolam, J. P. (2004). Uniform inhibition of dopamine neurons in the ventral tegmental area by aversive stimuli. Science 303, 2040–2042. doi: 10.1126/science.1093360
Villavicencio, M., Moreno, M. G., Simon, S. A., and Gutierrez, R. (2018). Encoding of sucrose’s palatability in the nucleus accumbens shell and its modulation by exteroceptive auditory cues. Front. Neurosci. 12:265. doi: 10.3389/fnins.2018.00265
Wise, R. A. (2004). Dopamine, learning and motivation. Nat. Rev. Neurosci. 5, 483–494. doi: 10.1038/nrn1406
Yamamoto, T. (2006). Neural substrates for the processing of cognitive and affective aspects of taste in the brain. Arch. Histol. Cytol. 69, 243–255. doi: 10.1679/aohc.69.243
Yamamoto, T. (2008). Central mechanisms of roles of taste in reward and eating. Acta Physiol. Hung. 95, 165–186. doi: 10.1556/APhysiol.95.2008.2.2
Yiannakas, A., and Rosenblum, K. (2017). The insula and taste learning. Front. Mol. Neurosci. 10:335. doi: 10.3389/fnmol.2017.00335
Keywords: insular cortex, ventral tegmental area, salience, consolidation, aversive taste, D1-like receptor
Citation: Gil-Lievana E, Ramírez-Mejía G, Urrego-Morales O, Luis-Islas J, Gutierrez R and Bermúdez-Rattoni F (2022) Photostimulation of Ventral Tegmental Area-Insular Cortex Dopaminergic Inputs Enhances the Salience to Consolidate Aversive Taste Recognition Memory via D1-Like Receptors. Front. Cell. Neurosci. 16:823220. doi: 10.3389/fncel.2022.823220
Received: 26 November 2021; Accepted: 08 February 2022;
Published: 11 March 2022.
Edited by:
Sheri Mizumori, University of Washington, United StatesReviewed by:
Matthew Wanat, University of Texas at San Antonio, United StatesAnna K. Radke, Miami University, United States
Copyright © 2022 Gil-Lievana, Ramírez-Mejía, Urrego-Morales, Luis-Islas, Gutierrez and Bermúdez-Rattoni. This is an open-access article distributed under the terms of the Creative Commons Attribution License (CC BY). The use, distribution or reproduction in other forums is permitted, provided the original author(s) and the copyright owner(s) are credited and that the original publication in this journal is cited, in accordance with accepted academic practice. No use, distribution or reproduction is permitted which does not comply with these terms.
*Correspondence: Federico Bermúdez-Rattoni, ZmJlcm11ZGVAaWZjLnVuYW0ubXg=