- 1State Key Laboratory of Agricultural Microbiology, College of Veterinary Medicine, Huazhong Agricultural University, Wuhan, China
- 2Key Laboratory of Preventive Veterinary Medicine in Hubei Province, The Cooperative Innovation Center for Sustainable Pig Production, Wuhan, China
- 3Key Laboratory of Development of Veterinary Diagnostic Products, Ministry of Agriculture of the People’s Republic of China, Wuhan, China
- 4International Research Center for Animal Disease, Ministry of Science and Technology of the People’s Republic of China, Wuhan, China
Bacterial meningitis is a life-threatening infectious disease with high morbidity and mortality worldwide, among which meningitic Escherichia coli is a common Gram-negative pathogenic bacterium causing meningitis. It can penetrate the blood–brain barrier (BBB), invoke local inflammatory responses and consequently disrupt the integrity of the BBB. Interleukin-17A (IL-17A) is recognized as a pro-inflammatory cytokine that is released during meningitic E. coli infection. It has been reported that IL-17A is involved in several pathological tissue injuries. However, the function of IL-17A in BBB breakdown remains rarely discussed. Here, our study found that E. coli-induced IL-17A led to the degradation of tight junction proteins (TJs) and adherens junction proteins (AJs) in human brain microvascular endothelial cells (hBMECs) through inhibiting protease proteinase 3 (PRTN3)/protease-activated receptor 2 (PAR-2) axis, thus increasing the permeability of BBB. In summary, this study uncovered the involvement of IL-17A in regulating BBB integrity and proposed a novel regulatory mechanism, which could be potential therapeutic targets of E. coli meningitis.
Introduction
Bacterial meningitis is a severe life-threatening infectious disease of the central nervous system (CNS) and a major cause of death or disability worldwide, especially in newborns (Leib and Tauber, 1999). There are a variety of pathogens that are classically associated with bacterial meningitis including Group B Streptococcus, Streptococcus pneumoniae, Escherichia coli, Neisseria meningitidis, and Haemophilus influenzae type B (Snyder and Brunjes, 1965; Booy and Kroll, 1998; Orihuela et al., 2009; Mook-Kanamori et al., 2011). Among these, meningitic E. coli is the most common Gram-negative bacillary organism (Janowski and Newland, 2017). To cause meningitis, E. coli must persist in the blood long enough, interact with and cross the blood–brain barrier (BBB), and invoke inflammatory responses (Kim, 2003).
Blood–brain barrier is a microvasculature that coordinates the movement of molecules and cells between the CNS and bloodstream (Zlokovic, 2008). It comprises brain microvascular endothelial cells (BMECs), pericytes, and astrocyte endfeet and maintains CNS homeostasis (Hawkins and Davis, 2005). Among these component cells, BMECs are the most direct and functional structural component of BBB and are characterized by the presence of tight junction proteins (TJs) and adherens junction proteins (AJs) (Dejana et al., 2009; Edwards et al., 2013). TJs are mainly composed of zonula occludens (ZOs), occludin, claudins, and AJs are primarily composed of vascular endothelial cadherin (VE-cadherin) (Bayir and Sendemir, 2021). The distribution or decrease of these TJs and AJs leads to an increased BBB permeability. Increased BBB permeability has been reported in numerous diseases, such as neoplasia, hypertension, experimental allergic encephalomyelitis, trauma, and neurotropic viral infections (Scholz et al., 2007; Candelario-Jalil et al., 2009). Inflammatory factors such as tumor necrosis factor alpha (TNF-α), interleukin-6 (IL-6), C-C motif ligand 2 (CCL2, also known as MCP-1) are reported to mediate BBB breakdown, which ultimately leads to the infiltration of peripheral leukocytes and brain injury (Sarami Foroshani et al., 2018; Siqueira et al., 2021; Xu D. et al., 2021). However, the underlying mechanisms by which these factors regulate BBB permeability in response to infection remain largely unclear.
Interleukin-17A (IL-17A) is the first identified member of the IL-17 family. Both αβT-cells and γδT-cells are associated with its production in infectious diseases (Papotto et al., 2017; Stockinger and Omenetti, 2017). Increased understanding of the biology of IL-17A has revealed that this inflammatory cytokine is involved in the modulation of acute or chronic bacterial infections, as well as other inflammation-associated diseases (Veldhoen, 2017). It has been shown that overproduced IL-17A promotes hyperinflammation and tissue damage in various diseases. For example, IL-17A mediates the production of vascular endothelial growth factor (VEGF), a major manager for vasculopathy, and aggravates neovascular retinopathy (Talia et al., 2016). Moreover, IL-17A also increases the permeability of alveolar epithelia (Bai et al., 2021). Notably, increasing studies support that IL-17A is involved in the breakdown of BBB integrity and subsequent neuroinflammation, for example, in multiple sclerosis (MS) or Group A Streptococcus infection (Tzartos et al., 2008; Dileepan et al., 2016). Despite these, whether IL-17A mediates BBB disruption in meningitic E. coli and the detailed mechanism by which IL-17A disrupts the BBB remain poorly understood.
In the present study, we characterized IL-17A as significantly up-regulated and an essential inflammatory cytokine in mouse brains in response to E. coli. Our in vivo and in vitro results demonstrated that IL-17A contributed to the disruption of BBB integrity by decreasing TJs and AJs. Further investigation suggested IL-17A down-regulated TJs and AJs of BMECs at the post-transcriptional level by inhibiting serine protease proteinase 3 (PRTN3)/protease-activated receptor 2 (PAR-2) axis. These observations indicated a novel strategy regarding the mechanism of meningitic E. coli-induced IL-17A in disrupting BBB and aggravating CNS dysfunction, which might be a potential therapeutic target for E. coli meningitis.
Materials and Methods
Bacterial Strains and Cell Culture
Escherichia coli strain PCN033 used herein was initially isolated from swine in cerebrospinal fluid from a diseased farm in China, 2006 (Liu et al., 2015). Bacterial cells were routinely grown in Luria-Bertani medium at 37 °C.
The human brain microvascular endothelial cells (hBMECs) were routinely cultured in RPMI1640 supplemented with 10% fetal bovine serum (FBS), 2 mM L-glutamine, 1 mM sodium pyruvate, essential amino acids, non-essential amino acids, vitamins, and penicillin and streptomycin (100 U/mL) in 37°C incubator under 5% CO2 until monolayer confluence (Stins et al., 2001). Confluent cells were washed with Hanks’ balanced salt solution and starved in serum-free medium (1:1 mixture of Ham’s F-12 and M-199) for 16–18 h before the experiment.
Animal Infection Assay
The C57BL/6 wild-type mice and IL-17A knockout mice (kindly provided by Prof. Anding Zhang in Huazhong Agricultural University) were challenged with E. coli through the tail vein at 1 × 107 CFUs suspended and diluted in phosphate-buffered saline (PBS; pH 7.4). The mice were anesthetized and the brains were harvested after cardiac perfusion for further assays.
For survival assay, anesthetized mice were pre-treated with IL-17A (10 ng/mouse, i.p.) or PBS 12 h before injection E. coli, followed by the injection (i.v.) of E. coli. The survival of each group of mice (n = 14) was recorded during the observation period of 24 h after E. coli infection.
Evan’s Blue Assay
The BBB permeability of C57BL/6 wild-type mice and IL-17A knockout mice was evaluated using Evan’s blue dye (St. Louis, MO, United States), 500 μL Evan’s blue (5 mg/mL) was injected via the tail vein to allow circulation for 10 min before the mice were sacrificed and perfused. Brains were then taken and photographed for extravascular staining of the dye.
Reverse Transcription and Real-Time Polymerase Chain Reaction
TRIzol reagent (Aidlab Biotech, Beijing, China) was utilized to isolate total RNA of brains or hBMECs. Aliquots (500 ng) of the total RNA in each sample were subjected to cDNA synthesis using the HiScript II Q RT SuperMix (Vazyme, Nanjing, China). Real-time PCR was performed with the real-time PCR thermal cycler qTOWER3 (Analytikjena, Jena, Germany) using MonAmp™ SYBR® Green qPCR Mix (Monad Biotech, China) according to the manufacturers’ recommendations. Primers for real-time PCR were listed in Supplementary Table 1. Transcriptional levels of the target mRNA were normalized to GAPDH.
Cytokine mRNA Assay
QuantiGene Plex 2.0 Assay (Panomics, Santa Clara, CA, United States) was used for cytokine mRNA quantification in the mouse brains as described elsewhere (Kottilil et al., 2009).
Cytokine Protein Assay
Procartaplex Multiplex Immunoassays (eBioscience, San Diego, CA, United States) was used for measuring cytokines of brain lysates according to the manufacturer’s instructions (Lu et al., 2015).
Western Blotting
Mouse brains or hBMECs were lysed using radioimmunoprecipitation assay buffer (EpiZyme, Shanghai, China) with protease inhibitor cocktail (MedChemExpress, Monmouth, NJ, United States), followed by centrifugation at 12,000 rpm for 15 min at 4°C to remove the insoluble cell debris. The protein concentrations were measured by using the bicinchoninic acid protein assay kit (CWBiotech, Beijing, China). Equal amounts of protein were further separated by 12% SDS-PAGE and electrophoretically transferred to polyvinylidene difluoride (PVDF) membranes. The blots were blocked with Tris-buffered saline-Tween (TBST) containing 5% bovine serum albumin for 2 h, and subsequently incubated with primary antibodies against ZO-1 (1:1,000; 220 kDa; Abcam, Cambridge, MA, United States), Occludin (1:1,000; 59 kDa; Abcam, Cambridge, MA, United States), Claudin-5 (1:1,000; 23 kDa; Affinity Biosciences, Changzhou, China), VE-Cadherin (1:1,000; 120 kDa; Affinity Biosciences, Changzhou, China), IL-17A (1:1,000; 18 kDa; Proteintech, Chicago, IL, United States), PRTN3 (1:1,000; 28 kDa; Proteintech, Chicago, IL, United States), β-actin (1:5,000; 42 kDa; Proteintech, Chicago, IL, United States). Membranes were subsequently washed and incubated with horseradish peroxidase-conjugated anti-rabbit or anti-mouse secondary antibodies (1:5,000; Biodragon, Beijing, China). The blots were visualized with the Super electrochemiluminescence Prime kit (US Everbright, Suzhou, China) and densitometrically analyzed using Image Lab software (Bio-Rad, Hercules, CA, United States).
Histopathological Examination
For hematoxylin and eosin (H&E) assay, mice brain tissues were immersed in 4% paraformaldehyde for 4h, and transferred to 70% ethanol. Individual lobes of tissues biopsy material were placed in processing cassettes, dehydrated through a serial alcohol gradient, and embedded in paraffin wax blocks. Before staining, 5 μm-thick tissue sections were dewaxed in xylene, rehydrated through decreasing ethanol concentrations, and washed in PBS, and then stained with hematoxylin and eosin. After staining, sections were dehydrated through increasing concentrations of ethanol and xylene.
Immunofluorescence Analysis
For immunofluorescence (IF) assay, sections were incubated with the primary antibody against ZO-1, Occludin (Abcam, Cambridge, MA, United States), Claudin-5, VE-Cadherin (Affinity Biosciences, Changzhou, China), followed by incubation with Cy3 conjugated secondary antibody. The same sections were then incubated with CD31 (Proteintech, Chicago, IL, United States) primary antibody, followed by incubation with FITC conjugated secondary antibody prior to the final nucleus staining with DAPI. Sections were photographed and analyzed using BX41 Microscopy (Olympus, Tokyo, Japan).
Electrical Cell-Substrate Impedance Sensing
To evaluate the real-time alteration of the monolayer cell resistance, electrical cell-substrate impedance sensing (ECIS) system (Applied BioPhysics, Troy, MI, United States) was applied to compare the transendothelial electrical resistance (TEER) values in hBMECs with or without recombinant IL-17A protein seeded on the collagen-coated, gold-plate electrodes in 96-well chamber slides (96W1E+) as previously described. Two ECIS parameters, R (Ω), representing the electrical cell-cell contacts, and Rb (Ωcm2), representing paracellular barrier, were extracted from the continuously recorded impedance spectra to reflect the real-time changes of the monolayer barrier function.
Statistics
All results are displayed as mean ± SD, and the significance of differences between groups was evaluated by one-way ANOVA. The survival curve analysis, log-rank (Mantel–Cox) was performed to test the significance of the difference between the evaluation groups. All statistical analyses were performed using GraphPad Prism software.
Results
Interleukin-17A Was Significantly Up-Regulated in Escherichia coli-Challenged Mice
To evaluate the intensity of inflammatory responses elicited by meningitic E. coli, the mRNA transcription, as well as protein expression of cytokines and chemokines, were assessed in the brain by using QuantiGene Plex 2.0 Multiplex assay and Procartaplex Multiplex Immunoassays. Among these data, IL-17A was significantly up-regulated within hours of infection at the transcriptional level (Figure 1A) and expression level (Figure 1B). By applying qPCR and Western blotting assay, we further verified that the expression of IL-17A in the brain was increased in a time-dependent manner (Figures 1C,D). These findings suggested that meningitic E. coli infection in mice can cause a significant increase of IL-17A in the brain.
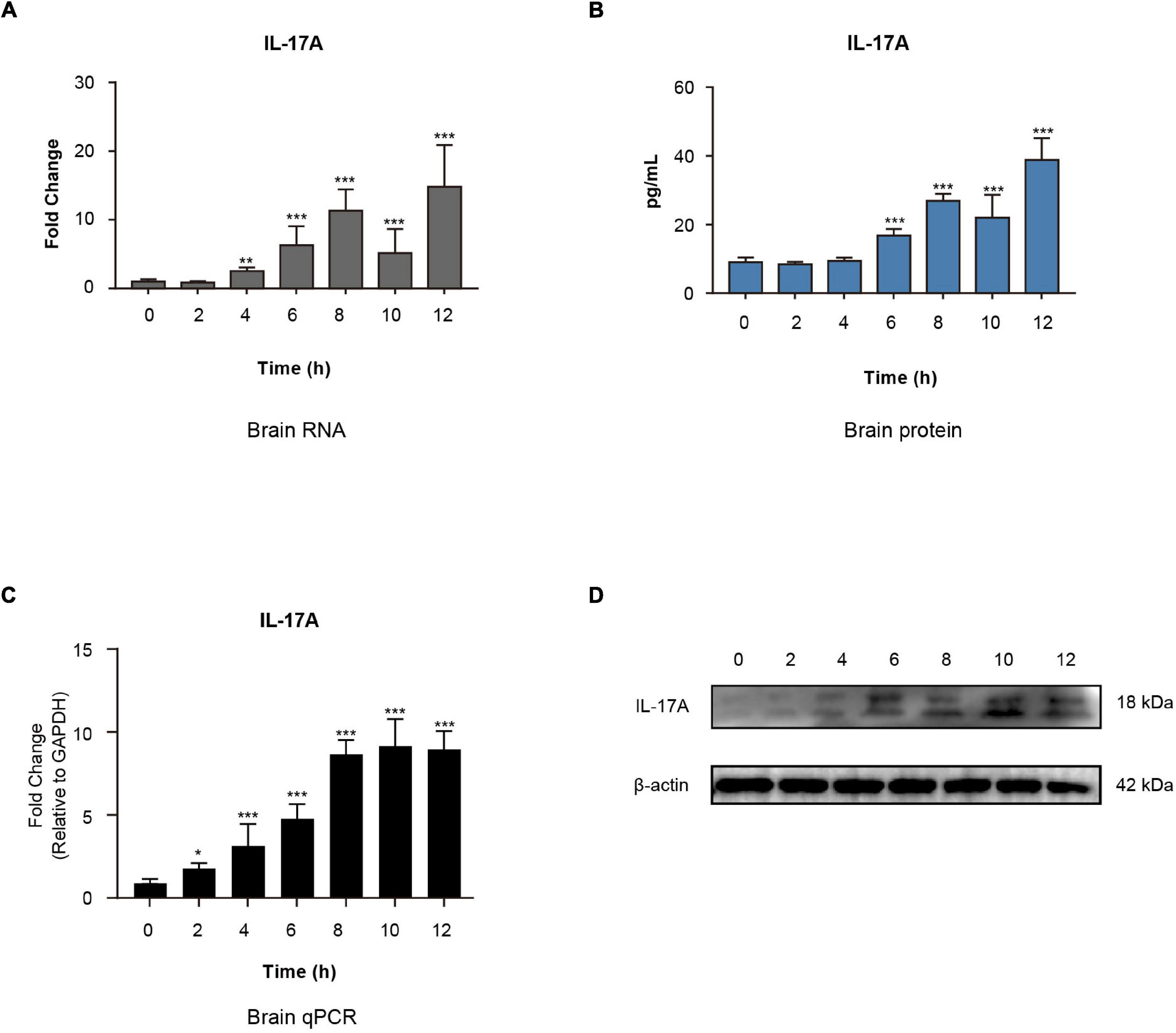
Figure 1. Interleukin-17A expression in mouse brains triggered by E. coli infection. Quantification of IL-17A expression in the brains of E. coli challenged mice at both the transcriptional and protein levels by applying QuantiGene Plex 2.0 Assay (A) or Procartaplex Multiplex Immunoassays (B). **p < 0.01 and ***p < 0.001 by one-way ANOVA analysis. Data were collected and presented as mean ± SD from three replicates at each time point. (C) Real-time PCR verification of the IL-17A mRNA transcription in the brains of E. coli challenged mice. Data were collected and presented as mean ± SD from three replicates at each time point. *p < 0.05 and ***p < 0.001 by one-way ANOVA analysis. (D) Western blot verification of the IL-17A protein expression in the brains of E. coli challenged mice. β-Actin was used as the loading control.
Interleukin-17A Promoted the Disruption of Blood–Brain Barrier Integrity
Since a high level of IL-17A was detected in mouse brains after E. coli infection, we next investigated the function of IL-17A in regulating BBB permeability. As Figure 2A shown, intravenous (tail vein) injection of recombinant IL-17A (1, 5, 10, and 20 ng/mouse) for 12 h could dose-dependently increase the amount of Evans blue dye leaking out of the blood vessels compared with the control, which meant the increase of BBB permeability. In addition, IL-17A could also time-dependently increase the permeability of BBB (Supplementary Figure 2A). Further observation indicated that E. coli-caused increase of BBB permeability in WT mice could be restored by knocking out IL-17A (Figure 2B).
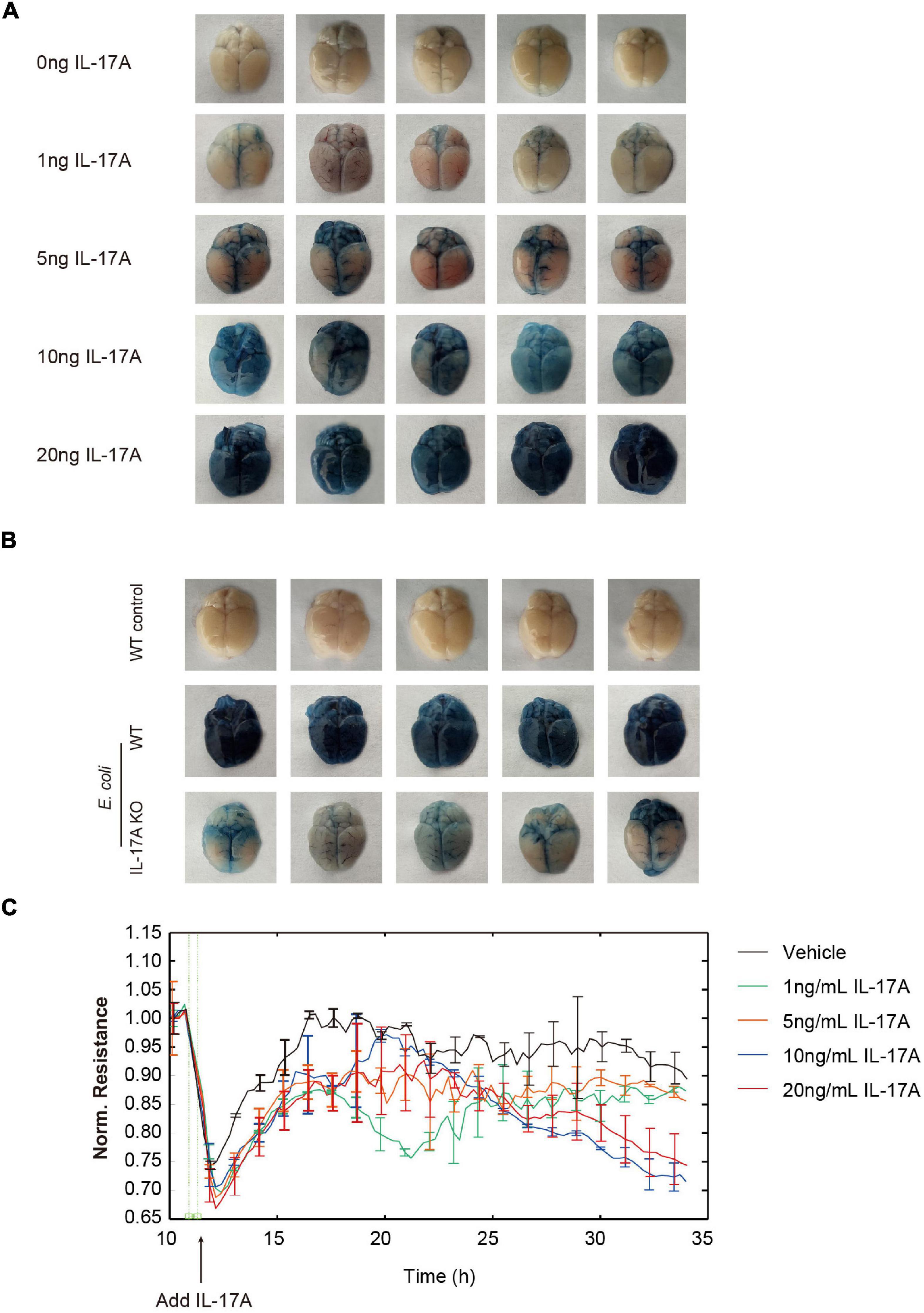
Figure 2. Interleukin-17A contributes to the increase of BBB permeability. (A) Effects of the recombinant mouse IL-17A (0, 1, 5, 10, and 20 ng/mouse) on the permeability of the mice brain evaluated by Evan’s blue approach. (B) The permeability of control mice, E. coli challenged WT or IL-17A KO mice evaluated by Evan’s blue approach. (C) TEER changes of hBMECs in the treatment of multiple dosage of IL-17A monitored by the ECIS system. Data were collected and presented as mean ± SD from three replicated wells at each time point.
The hBMECs were subsequently used as in vitro model. We additionally tested the effects of IL-17A on TEER value, an important indicator of the monolayer permeability (Fu et al., 2021), by application of ECIS system. The results showed that IL-17A could dose-dependently down-regulate the TEER value of hBMECs monolayer (Figure 2C). Taken together, these observations indicated that E. coli-induced IL-17A could negatively affect the integrity of BBB.
Interleukin-17A Disrupted Blood–Brain Barrier Integrity by Downregulating Tight Junction Proteins and Adherens Junction Proteins
As mentioned above, TJs and AJs determine the integrity of BBB, and we investigated whether TJs or AJs were involved in the IL-17A mediated disruption of BBB. In vivo, IF was performed to examine the distribution and expression of ZO-1, Occludin, Claudin-5, and VE-Cadherin in mouse brain tissues. The results showed that ZO-1, Occludin, Claudin-5, and VE-Cadherin were well-organized and distributed around the vascular in the control mice brain. In contrast, when treated with E. coli, the TJs and AJs around the vascular became inconsecutively distributed, irregular gapped, or down-regulated in WT mice. In contrast, these adverse effects of infection on TJs and AJs were significantly reversed by knocking-out IL-17A (Figures 3A–D).
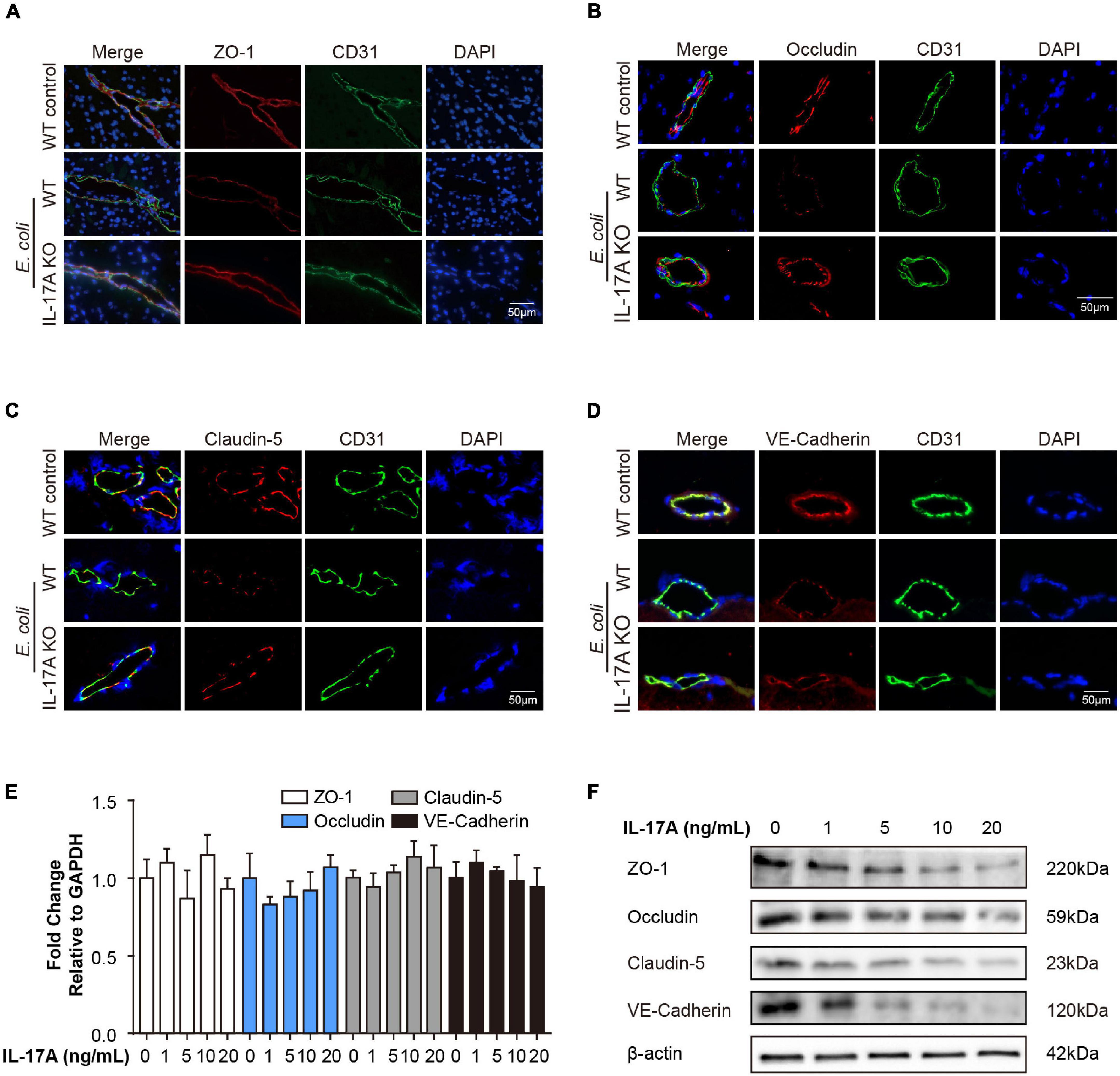
Figure 3. Interleukin-17A treatment downregulates the expression of TJs and AJs. IF analysis of vascular endothelium integrity in infected WT or IL-17A KO mice. ZO-1 (A), Occludin (B), Claudin-5 (C), and VE-Cadherin (D) were selected as the markers reflecting the integrity of the vascular endothelium. CD31 was applied explicitly for labeling the microvessels. Scale bar indicates 50 μm. (E) The qPCR analysis of ZO-1, Occludin, Claudin-5, and VE-Cadherin transcription in hBMECs treated by multiple dosages of IL-17A (0, 1, 5, 10, and 20 ng/mL). GAPDH was used as the internal reference. Data were presented as mean ± SD from three independent experiments. (F) Western blot analysis of ZO-1, Occludin, Claudin-5, and VE-Cadherin in hBMECs in response to multiple dosages of IL-17A. β-Actin was used as the loading control.
In vitro, by treating hBMECs with different dosages of recombinant IL-17A, the expression of ZO-1, Occludin, Claudin-5, and VE-Cadherin at the protein level was significantly decreased. However, there was no significant difference at the transcriptional level (Figures 3E,F). These findings suggested that in meningitic E. coli infection, IL-17A was able to affect the integrity of BBB by down-regulating TJs and AJs at the post-transcriptional level.
The Post-transcriptional Regulation of Interleukin-17A to Tight Junction Proteins and Adherens Junction Proteins Through Inhibiting Proteinase 3/Protease-Activated Receptor 2 Axis
As we have demonstrated that IL-17A could post-transcriptionally regulate the expression of TJs and AJs, further investigations were taken to determine a more detailed regulatory mechanism of this phenomenon. It was reported that the ubiquitin-proteasome system and autophagy were the primary mechanisms for degrading protein; however, neither the proteasome inhibitor MG-132 nor the autophagy inhibitor chloroquine showed reverse decrease of TJs and AJs in hBMECs induced by IL-17A (Figures 4A,B).
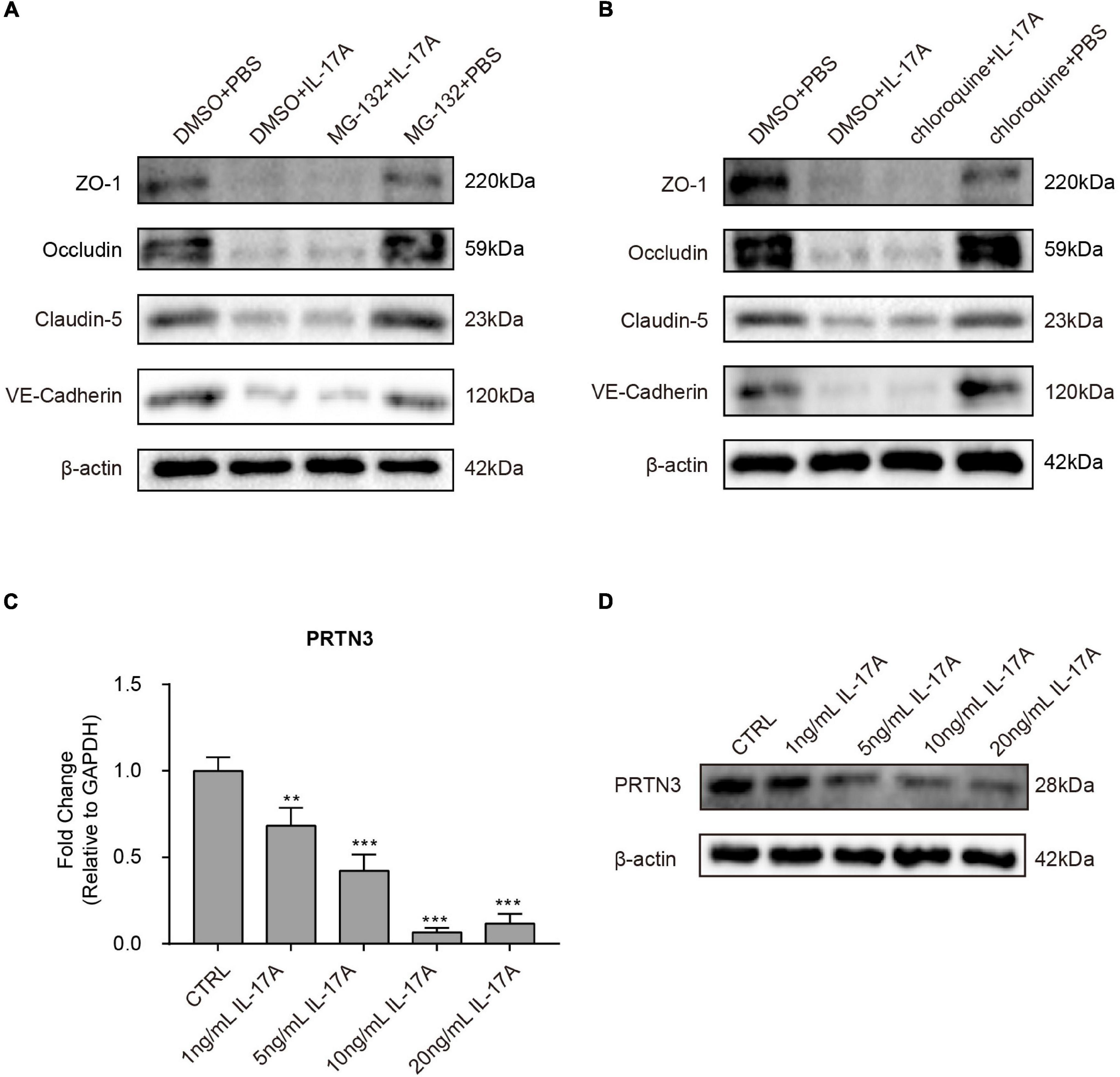
Figure 4. Interleukin-17A treatment decreases PRTN3 expression hBMECs. Western blot analysis of ZO-1, Occludin, Claudin-5, and VE-Cadherin extracted from IL-17A untreated hBMECs and IL-17A treated hBMECs with or without the presence of Proteasome inhibitor MG-132 (A) or autophagy inhibitor chloroquine (B). β-Actin was used as the loading control. (C) The qPCR analysis of PRTN3 transcription in hBMECs treated by multiple dosages of IL-17A (0, 1, 5, 10, and 20 ng/mL). GAPDH was used as the internal reference. Data were presented as mean ± SD from three independent experiments. **p < 0.01 and ***p < 0.001 by one-way ANOVA analysis. (D) Western blot analysis of PRTN3 in hBMECs in response to multiple dosages of IL-17A. β-Actin was used as the loading control.
Previous studies have demonstrated that a serine protease PRTN3 was able to enhance endothelial cell barrier and thus vascular integrity through cleaving and activating PAR-2 (Kuckleburg and Newman, 2013). Therefore, we next focused on the expression of PRTN3, and the observation showed that the mRNA transcription (Figure 4C) and protein expression (Figure 4D) of PRTN3 were significantly down-regulated in hBMECs in response to IL-17A treatment in a dose-dependent manner.
To examine whether the decrease of PRTN3 reduces the barrier function of hBMECs, PRTN3 overexpression constructs were used to validate its regulative effects on TJs. As demonstrated, PRTN3 overexpression did not influence the mRNA transcription of the ZO-1, Occludin, Claudin-5, and VE-Cadherin (Figure 5A). Meanwhile, at the protein level, PRTN3 overexpression could significantly restore the decrease of ZO-1, Occludin, Claudin-5, and VE-Cadherin in hBMECs caused by IL-17A (Figure 5B). As mentioned above, PRTN3 enhances the endothelial cell barrier through activating PAR-2; we subsequently determined the function of PAR-2 in TJs and AJs disruption. We pre-treated hBMECs with AC-55541, a novel small molecule agonist of PAR-2. The results revealed that PAR-2 agonist significantly inhibited the decrease of TJs and AJs protein expression induced by IL-17A (Figure 5D), and it also had no effect on the transcription of TJs and AJs (Figure 5C). Correspondingly, the ECIS assay showed that PAR-2 agonist could ameliorate the loss of TEER value of hBMECs monolayer induced by IL-17A (Figure 5E). These results indicated that IL-17A could decrease the expression of TJs and AJs of hBMECs at the post-transcriptional level via inhibiting PRTN3/PAR-2 axis, thus augmented the vascular permeability of BBB.
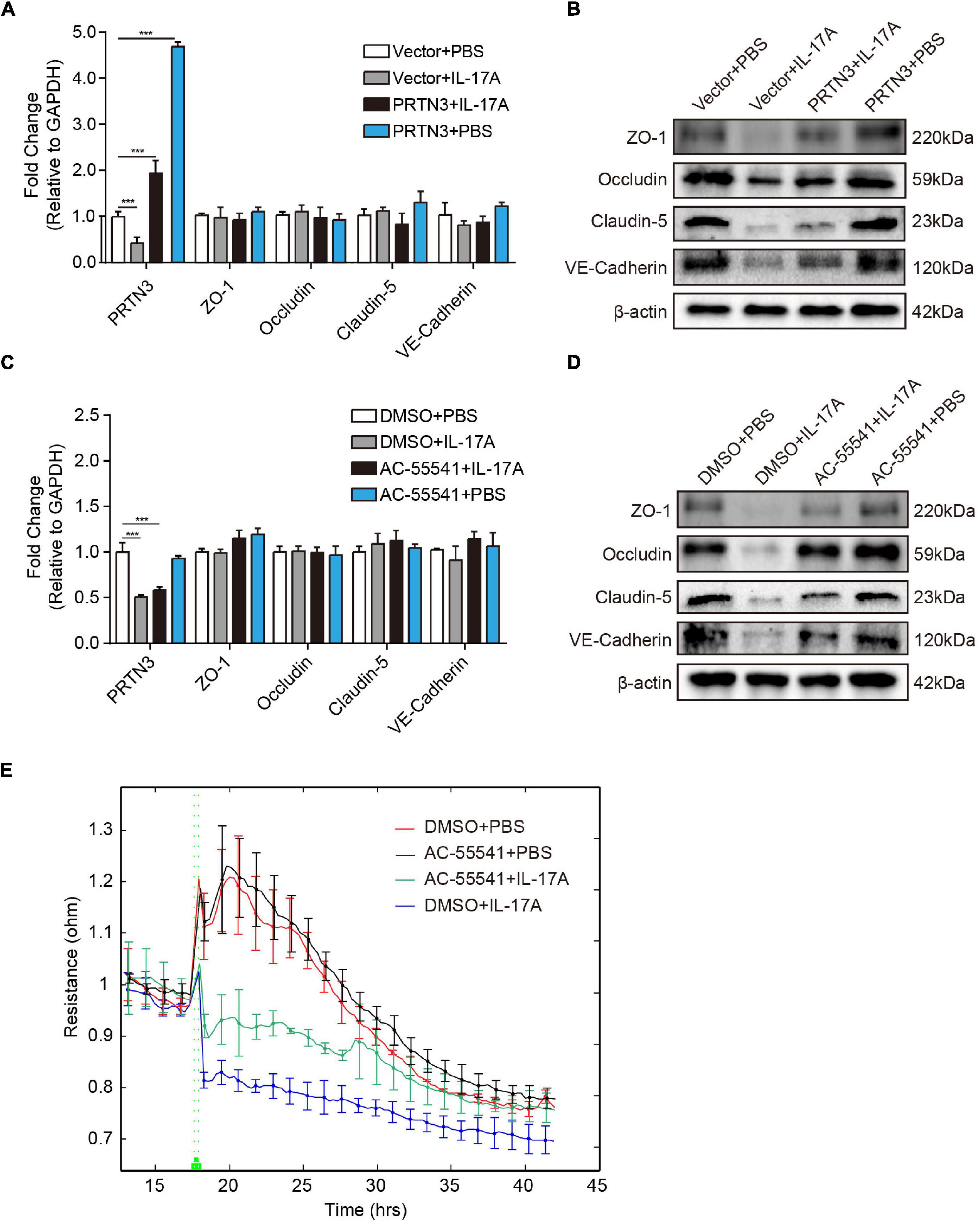
Figure 5. Interleukin-17A disrupts TJs and AJs by inhibiting PRTN3/PAR-2 axis. (A) The qPCR analysis of ZO-1, Occludin, Claudin-5, and VE-Cadherin extracted from IL-17A untreated hBMECs and IL-17A treated hBMECs with or without the overexpression of PRTN3. GAPDH was used as the internal reference. Data were presented as mean ± SD from three independent experiments. ***p < 0.001 by one-way ANOVA analysis. (B) Western blot of ZO-1, Occludin, Claudin-5 and VE-Cadherin extracted from IL-17A untreated hBMECs and IL-17A treated hBMECs with or without overexpression of PRTN3. β-Actin was used as the loading control. (C) The qPCR analysis of ZO-1, Occludin, Claudin-5, and VE-Cadherin extracted from IL-17A untreated hBMECs and IL-17A treated hBMECs with or without PAR-2 agonist AC-55541. GAPDH was used as the internal reference. Data were presented as mean ± SD from three independent experiments. ***p < 0.001 by one-way ANOVA analysis. (D) Western blot of ZO-1, Occludin, Claudin-5, and VE-Cadherin extracted from IL-17A untreated hBMECs and IL-17A treated hBMECs with or without PAR-2 agonist AC-55541. β-Actin was used as the loading control. (E) TEER changes of the IL-17A untreated hBMECs and IL-17A treated hBMECs with or without PAR-2 agonist AC-55541 monitored by the ECIS system. Data were collected and presented as mean ± SD from three replicated wells at each time point.
Interleukin-17A Aggravated Brain Tissue Damage and Reduced Survival Rate in Escherichia coli-Challenged Mice
We further determined the effects of IL-17A in mice throughout E. coli meningitis pathogenesis. Histologic sections of the brain showed that E. coli infection in WT mice could induce tissue damage such as meningeal thickening and hemorrhage. This pathological phenomenon was severer than that in IL-17A KO mice. Notably, we observed that this phenomenon could be well restored by the addition of recombinant IL-17A (Figure 6A).
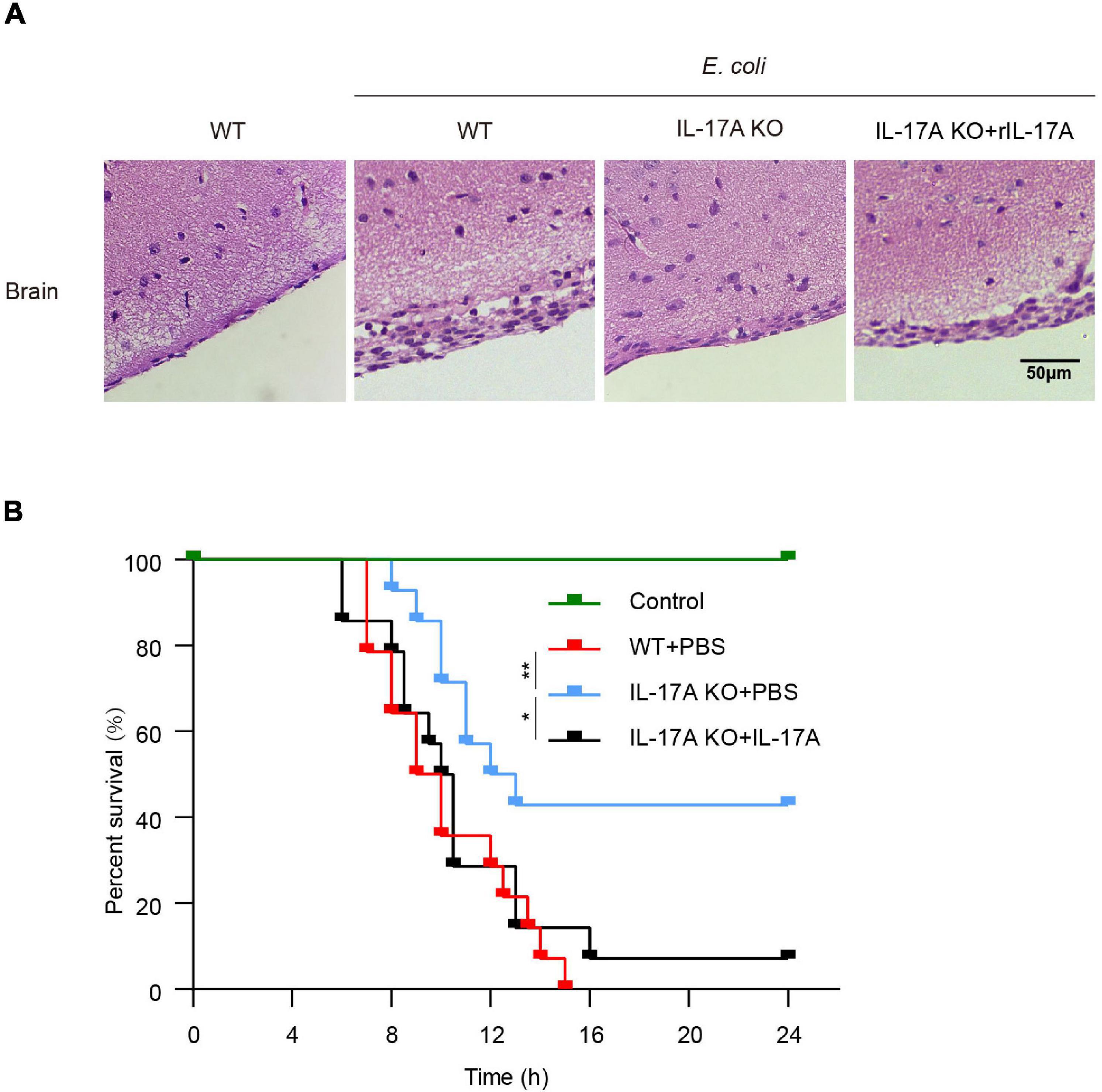
Figure 6. Interleukin-17A aggravated brain tissue damage and reduced survival rate in E. coli-infected mice. (A) WT mice, IL-17A KO mice with or without adding recombinant IL-17A, were infected with E. coli, the histopathological changes in the brain were investigated by H&E staining. Scale bar indicates 50 μm. (B) Survival analysis curves for WT mice, IL-17A KO mice with or without adding recombinant IL-17A infected with E. coli. Data were collected and shown as Kaplan–Meier survival curves from 14 individual mice. *p < 0.05 and **p < 0.01.
Based on these observations, we evaluated the effects of IL-17A on the lethality of mice. As shown in Figure 6B, when challenged with E. coli, mice with IL-17A knocked out had a higher survival rate than WT mice. In contrast, IL-17A KO mice pre-injected with recombinant IL-17A could not improve the survival rate of the infection. These data suggested IL-17A aggravated the damage to the brain and thus led to increased mortality.
Discussion
The BBB is a highly specialized structure that maintains CNS homeostasis (Tajes et al., 2014). In bacterial meningitis, pathogens such as E. coli, Neisseria, and Group B Streptococcus always penetrate BBB, induce local CNS inflammatory responses, and lead to the disruption of BBB (Booy and Kroll, 1998; Kim, 2000; Herbert et al., 2004). BBB disruption is a typical pathological phenomenon in many diseases related to CNS disorders, including bacterial meningitis, Japanese encephalitis, ischemic stroke, etc. (Wang et al., 2018; Liu C. et al., 2020; Xu B. et al., 2021). Accumulating evidence has suggested that host factors and cytokines are involved in the regulation of TJs expression and contribute to BBB dysfunction. For example, cytokines such as TNF-α and IL-6 (Sarami Foroshani et al., 2018; Siqueira et al., 2021), chemokines such as CCL2 and IP-10 (Wang et al., 2018; Xu D. et al., 2021), and growth factors such as VEGF-A and PDGF-BB (Yang et al., 2016, 2019) are all reported to induce BBB breakdown. However, there are limited reports on whether IL-17A directly impacts BBB permeability. In the current study, we reported that, as an essential pro-inflammatory cytokine, IL-17A was significantly increased in mouse brains after meningitic E. coli PCN033 infection. With further in vivo and in vitro verification in response to infection, we revealed that meningitic E. coli-induced IL-17A significantly down-regulated the expression of TJs and AJs of hBMECs at the post-transcriptional level through inhibiting PRTN3/PAR-2 axis, thus augmenting endothelial permeability and disrupting BBB integrity. It ultimately facilitates brain damage and promotes the death of mice.
Interleukin-17A is a key inflammatory factor that contributes to the occurrence and development of several pathogenic injuries such as severe intestinal injury, pancreatic injury, and acute kidney injury (Chan et al., 2014; Kim et al., 2015). In Bacteroides infection, IL-17A is significantly increased and helps disrupt the intestinal barrier (Liu W. et al., 2020). In acute necrotizing pancreatitis, the large number of induced IL-17A is correlated significantly with TJs reduction and pancreatic injury (Guo et al., 2019). It has also been reported that IL-17A harms proximal tubule epithelium integrity and mediates renal injury (Dudas et al., 2011). As for the brain, our in vivo and in vitro data confirmed that IL-17A increases the permeability of BBB by down-regulating the expression of TJs at the protein level rather than the transcription level. To clarify its mechanism, many genes reported to be involved in BBB breakdown were validated by us. It was widely agreed that matrix metalloproteinases-2 (MMP-2), MMP-3, and MMP-9 are involved in the degradation of TJs (Rosenberg and Yang, 2007; Oppenheim et al., 2013; Weekman and Wilcock, 2016), however, in our study, IL-17A did not up-regulate the expression of these MMPs (Supplementary Figure 1). Furthermore, the ubiquitin-proteasome system and autophagy are considered the important pathways for protein degradation (Varshavsky, 2017). However, the results showed that these pathways were not involved in the IL-17A-induced degradation of TJs. It reminds us that IL-17A affects BBB permeability through a novel pathway.
Interestingly, it was reported that a serine protease called PRTN3 could enhance endothelial barrier function and thus vascular integrity by cleaving and activating PAR-2, a cathepsin S cleaves protease-activated receptor (Kuckleburg and Newman, 2013). Our in vitro studies also found that IL-17A caused a reduction of PRTN3 in both time-dependent and dose-dependent manners (Supplementary Figures 2B,C), while the application of PRTN3 overexpression construct or PAR-2 agonist can well restore the reduction of TJs and AJs caused by IL-17A, suggesting that IL-17A negatively regulates the BBB function at the post-transcriptional level through inhibiting PRTN3/PAR2.
Dysfunction of BBB has been reported to cause severe neurological complications, such as electrolyte disturbance, intracerebral hemorrhage, and increased intracranial pressure, all of which ultimately result in death (Duan et al., 2020; Yang et al., 2021). When challenged with meningitic E. coli, due to the BBB disruption induced by IL-17A, WT mice or IL-17A pre-treated KO mice suffered more severe brain injuries, such as hemorrhage and meningeal thickening compared to IL-17A KO mice. This pathological change accelerated the death process of mice and increased the mortality of mice. Nonetheless, it is increasingly recognized that inflammation is a double-edged sword during infection, which can be both a destroyer of tissue damage and a helper of eliminating infection (Chaudhry et al., 2013). For example, TNF-α was reported to trigger a sustained inflammation response to destroy the invading of Mycobacterium tuberculosis (MTB). However, excessive TNF-α can cause tissue damage and necrosis, thus giving rise to organ dysfunction (Mootoo et al., 2009). Our other study found that IL-17A might mediate E. coli clearance via increasing antimicrobial peptides, which require further investigation to comprehensively interpret the function of IL-17A throughout E. coli meningitis pathogenesis.
Conclusion
In conclusion, the evidence highlights the importance of IL-17A in meningitic E. coli-induced BBB disruption. We demonstrate that IL-17A mediates TJs and AJs breakdown, thereby augmenting BBB permeability via inhibiting PRTN3/PAR2 axis during meningitic E. coli infection, leading to severe neuroinflammation and neuronal injury. Elucidating mechanisms of the IL-17A-induced BBB disruption may provide an accurate and effective target for preventing BBB breakdown in meningitis.
Data Availability Statement
The original contributions presented in the study are included in the article/Supplementary Material, further inquiries can be directed to the corresponding author.
Ethics Statement
The animal study was reviewed and approved by the Institutional Animal Care and Use Committee of Huazhong Agricultural University.
Author Contributions
XW, BX, and JC designed the study, performed the experiments, and drafted the manuscript. JF, RY, and BY helped acquire data and process samples. BX and DH analyzed and interpreted the data. CT and HC provided technical support. XW and RY revised the manuscript. All authors have read and approved the final version of this article.
Funding
This work was funded by grants from the National Key Research and Development Program of China (2021YFD1800800), the National Natural Science Foundation of China, China (32122086 and 31772736), the China Agriculture Research System of MOF and MARA, Hubei Postdoctoral Preferential Foundation, China (590320102), and China Postdoctoral Science Foundation, China (2020M672379).
Conflict of Interest
The authors declare that the research was conducted in the absence of any commercial or financial relationships that could be construed as a potential conflict of interest.
Publisher’s Note
All claims expressed in this article are solely those of the authors and do not necessarily represent those of their affiliated organizations, or those of the publisher, the editors and the reviewers. Any product that may be evaluated in this article, or claim that may be made by its manufacturer, is not guaranteed or endorsed by the publisher.
Acknowledgments
We want to thank Anding Zhang at Huazhong Agricultural University for providing the IL-17A knockout mice. We also want to thank Kwang Sik Kim at Johns Hopkins University School of Medicine for providing the hBMECs cells.
Supplementary Material
The Supplementary Material for this article can be found online at: https://www.frontiersin.org/articles/10.3389/fncel.2022.814867/full#supplementary-material
Supplementary Figure 1 | The qPCR analysis of MMP2 (A), MMP3 (B), MMP9 (C), and MMP12 (D) transcription in hBMECs treated by multiple dosages of IL17A (0, 1, 5, 10, and 20 ng/mL). GAPDH was used as the internal reference. Data were presented as mean ± SD from three independent experiments.
Supplementary Figure 2 | The time course of the effects of IL-17A on BBB permeability in vivo and in vitro. (A) IL-17A time-dependently increased the permeability of BBB evaluated by Evan’s blue approach. (B) The transcription of PRTN3 in hBMECs treated with IL-17A for 3, 6, 9, and 12 h. GAPDH was used as the internal reference. Data were presented as mean ± SD from three independent experiments. **p < 0.01 and ***p < 0.001 by one-way ANOVA analysis. (C) Western blot analysis of PRTN3 in hBMECs treated with IL-17A for 3, 6, 9, and 12 h. β-Actin was used as the loading control.
Supplementary Table 1 | Overview of used RT-qPCR primer sequences.
References
Bai, S., Wang, W., Ye, L., Fang, L., Dong, T., Zhang, R., et al. (2021). IL-17 stimulates neutrophils to release S100A8/A9 to promote lung epithelial cell apoptosis in Mycoplasma pneumoniae-induced pneumonia in children. Biomed. Pharmacother. 143:112184. doi: 10.1016/j.biopha.2021.112184
Bayir, E., and Sendemir, A. (2021). Role of intermediate filaments in blood-brain barrier in health and disease. Cells 10:1400. doi: 10.3390/cells10061400
Booy, R., and Kroll, J. S. (1998). Bacterial meningitis and meningococcal infection. Curr. Opin. Pediatr. 10, 13–18. doi: 10.1097/00008480-199802000-00004
Candelario-Jalil, E., Yang, Y., and Rosenberg, G. A. (2009). Diverse roles of matrix metalloproteinases and tissue inhibitors of metalloproteinases in neuroinflammation and cerebral ischemia. Neuroscience 158, 983–994. doi: 10.1016/j.neuroscience.2008.06.025
Chan, A. J., Alikhan, M. A., Odobasic, D., Gan, P. Y., Khouri, M. B., Steinmetz, O. M., et al. (2014). Innate IL-17A-producing leukocytes promote acute kidney injury via inflammasome and Toll-like receptor activation. Am. J. Pathol. 184, 1411–1418. doi: 10.1016/j.ajpath.2014.01.023
Chaudhry, H., Zhou, J., Zhong, Y., Ali, M. M., McGuire, F., Nagarkatti, P. S., et al. (2013). Role of cytokines as a double-edged sword in sepsis. In Vivo 27, 669–684.
Dejana, E., Tournier-Lasserve, E., and Weinstein, B. M. (2009). The control of vascular integrity by endothelial cell junctions: molecular basis and pathological implications. Dev. Cell 16, 209–221. doi: 10.1016/j.devcel.2009.01.004
Dileepan, T., Smith, E. D., Knowland, D., Hsu, M., Platt, M., Bittner-Eddy, P., et al. (2016). Group A Streptococcus intranasal infection promotes CNS infiltration by streptococcal-specific Th17 cells. J. Clin. Invest. 126, 303–317. doi: 10.1172/JCI80792
Duan, H., Zhao, S., Xiang, J., Ju, C., Chen, X., Gramaglia, I., et al. (2020). Targeting the CD146/Galectin-9 axis protects the integrity of the blood-brain barrier in experimental cerebral malaria. Cell Mol. Immunol. 18, 2443–2454. doi: 10.1038/s41423-020-00582-8
Dudas, P. L., Sague, S. L., Elloso, M. M., and Farrell, F. X. (2011). Proinflammatory/profibrotic effects of interleukin-17A on human proximal tubule epithelium. Nephron Exp. Nephrol. 117, e114–e123. doi: 10.1159/000320177
Edwards, V. L., Wang, L. C., Dawson, V., Stein, D. C., and Song, W. (2013). Neisseria gonorrhoeae breaches the apical junction of polarized epithelial cells for transmigration by activating EGFR. Cell Microbiol. 15, 1042–1057. doi: 10.1111/cmi.12099
Fu, J., Li, L., Huo, D., Yang, R., Yang, B., Xu, B., et al. (2021). Meningitic Escherichia coli alpha-hemolysin aggravates blood-brain barrier disruption via targeting TGFbeta1-triggered hedgehog signaling. Mol. Brain 14:116. doi: 10.1186/s13041-021-00826-2
Guo, Y., Huang, C., Liu, L., Fu, X., Lu, Y., Zheng, J., et al. (2019). Paneth cell ablation aggravates pancreatic and intestinal injuries in a rat model of acute necrotizing pancreatitis after normal and high-fat diet. Mediators Inflamm. 2019:8474523. doi: 10.1155/2019/8474523
Hawkins, B. T., and Davis, T. P. (2005). The blood-brain barrier/neurovascular unit in health and disease. Pharmacol. Rev. 57, 173–185. doi: 10.1124/pr.57.2.4
Herbert, M. A., Beveridge, C. J., and Saunders, N. J. (2004). Bacterial virulence factors in neonatal sepsis: group B streptococcus. Curr. Opin. Infect. Dis. 17, 225–229. doi: 10.1097/00001432-200406000-00009
Janowski, A., and Newland, J. (2017). Of the Phrensy: an update on the epidemiology and pathogenesis of bacterial meningitis in the pediatric population. F1000Res 6:F1000 Faculty Rev-86. doi: 10.12688/f1000research.8533.1
Kim, K. S. (2000). E. coli invasion of brain microvascular endothelial cells as a pathogenetic basis of meningitis. Subcell Biochem. 33, 47–59. doi: 10.1007/978-1-4757-4580-1_3
Kim, K. S. (2003). Pathogenesis of bacterial meningitis: from bacteraemia to neuronal injury. Nat. Rev. Neurosci. 4, 376–385. doi: 10.1038/nrn1103
Kim, S. R., Kim, H. J., Kim, D. I., Lee, K. B., Park, H. J., Jeong, J. S., et al. (2015). Blockade of Interplay between IL-17A and endoplasmic reticulum stress attenuates LPS-induced lung injury. Theranostics 5, 1343–1362. doi: 10.7150/thno.11685
Kottilil, S., Yan, M. Y., Reitano, K. N., Zhang, X., Lempicki, R., Roby, G., et al. (2009). Human immunodeficiency virus and hepatitis C infections induce distinct immunologic imprints in peripheral mononuclear cells. Hepatology 50, 34–45. doi: 10.1002/hep.23055
Kuckleburg, C. J., and Newman, P. J. (2013). Neutrophil proteinase 3 acts on protease-activated receptor-2 to enhance vascular endothelial cell barrier function. Arterioscler. Thromb. Vasc. Biol. 33, 275–284. doi: 10.1161/ATVBAHA.112.300474
Leib, S. L., and Tauber, M. G. (1999). Pathogenesis of bacterial meningitis. Infect. Dis. Clin. North Am. 13, 527–548, v–vi. doi: 10.1016/s0891-5520(05)70093-3
Liu, C., Xie, J., Sun, S., Li, H., Li, T., Jiang, C., et al. (2020). Hemorrhagic transformation after tissue plasminogen activator treatment in acute ischemic stroke. Cell Mol. Neurobiol. doi: 10.1007/s10571-020-00985-1 [Epub ahead of print].
Liu, C., Zheng, H., Yang, M., Xu, Z., Wang, X., Wei, L., et al. (2015). Genome analysis and in vivo virulence of porcine extraintestinal pathogenic Escherichia coli strain PCN033. BMC Genomics 16:717. doi: 10.1186/s12864-015-1890-9
Liu, W., Zhou, Y., Qin, Y., Yu, L., Li, R., Chen, Y., et al. (2020). Quercetin intervention alleviates offspring’s oxidative stress, inflammation, and tight junction damage in the colon induced by maternal fine particulate matter (PM2.5) exposure through the reduction of Bacteroides. Nutrients 12:3095. doi: 10.3390/nu12103095
Lu, X., Ding, Z. C., Cao, Y., Liu, C., Habtetsion, T., Yu, M., et al. (2015). Alkylating agent melphalan augments the efficacy of adoptive immunotherapy using tumor-specific CD4+ T cells. J. Immunol. 194, 2011–2021. doi: 10.4049/jimmunol.1401894
Mook-Kanamori, B. B., Geldhoff, M., van der Poll, T., and van de Beek, D. (2011). Pathogenesis and pathophysiology of pneumococcal meningitis. Clin. Microbiol. Rev. 24, 557–591. doi: 10.1128/CMR.00008-11
Mootoo, A., Stylianou, E., Arias, M. A., and Reljic, R. (2009). TNF-alpha in tuberculosis: a cytokine with a split personality. Inflamm. Allergy Drug Targets 8, 53–62. doi: 10.2174/187152809787582543
Oppenheim, H. A., Lucero, J., Guyot, A. C., Herbert, L. M., McDonald, J. D., Mabondzo, A., et al. (2013). Exposure to vehicle emissions results in altered blood brain barrier permeability and expression of matrix metalloproteinases and tight junction proteins in mice. Part Fibre Toxicol. 10:62. doi: 10.1186/1743-8977-10-62
Orihuela, C. J., Mahdavi, J., Thornton, J., Mann, B., Wooldridge, K. G., Abouseada, N., et al. (2009). Laminin receptor initiates bacterial contact with the blood brain barrier in experimental meningitis models. J. Clin. Invest. 119, 1638–1646. doi: 10.1172/JCI36759
Papotto, P. H., Ribot, J. C., and Silva-Santos, B. (2017). IL-17(+) gammadelta T cells as kick-starters of inflammation. Nat. Immunol. 18, 604–611. doi: 10.1038/ni.3726
Rosenberg, G. A., and Yang, Y. (2007). Vasogenic edema due to tight junction disruption by matrix metalloproteinases in cerebral ischemia. Neurosurg. Focus 22:E4. doi: 10.3171/foc.2007.22.5.5
Sarami Foroshani, M., Sobhani, Z. S., Mohammadi, M. T., and Aryafar, M. (2018). Fullerenol nanoparticles decrease blood-brain barrier interruption and brain edema during cerebral ischemia-reperfusion injury probably by reduction of interleukin-6 and matrix metalloproteinase-9 transcription. J. Stroke Cerebrovasc. Dis. 27, 3053–3065. doi: 10.1016/j.jstrokecerebrovasdis.2018.06.042
Scholz, M., Cinatl, J., Schadel-Hopfner, M., and Windolf, J. (2007). Neutrophils and the blood-brain barrier dysfunction after trauma. Med. Res. Rev. 27, 401–416. doi: 10.1002/med.20064
Siqueira, M., Araujo, A. P. B., Gomes, F. C. A., and Stipursky, J. (2021). Ethanol gestational exposure impairs vascular development and endothelial potential to control BBB-associated astrocyte function in the developing cerebral cortex. Mol. Neurobiol. 58, 1755–1768. doi: 10.1007/s12035-020-02214-8
Snyder, S. N., and Brunjes, S. (1965). Hemophilus influenzae meningitis in adults. Review of the literature and report of 18 cases. Am. J. Med. Sci. 250, 658–667.
Stins, M. F., Badger, J., and Sik Kim, K. (2001). Bacterial invasion and transcytosis in transfected human brain microvascular endothelial cells. Microb. Pathog. 30, 19–28. doi: 10.1006/mpat.2000.0406
Stockinger, B., and Omenetti, S. (2017). The dichotomous nature of T helper 17 cells. Nat. Rev. Immunol. 17, 535–544. doi: 10.1038/nri.2017.50
Tajes, M., Ramos-Fernandez, E., Weng-Jiang, X., Bosch-Morato, M., Guivernau, B., Eraso-Pichot, A., et al. (2014). The blood-brain barrier: structure, function and therapeutic approaches to cross it. Mol. Membr. Biol. 31, 152–167. doi: 10.3109/09687688.2014.937468
Talia, D. M., Deliyanti, D., Agrotis, A., and Wilkinson-Berka, J. L. (2016). Inhibition of the nuclear receptor RORgamma and interleukin-17A suppresses neovascular retinopathy: involvement of immunocompetent microglia. Arterioscler. Thromb. Vasc. Biol. 36, 1186–1196. doi: 10.1161/ATVBAHA.115.307080
Tzartos, J. S., Friese, M. A., Craner, M. J., Palace, J., Newcombe, J., Esiri, M. M., et al. (2008). Interleukin-17 production in central nervous system-infiltrating T cells and glial cells is associated with active disease in multiple sclerosis. Am. J. Pathol. 172, 146–155. doi: 10.2353/ajpath.2008.070690
Varshavsky, A. (2017). The ubiquitin system, autophagy, and regulated protein degradation. Annu. Rev. Biochem. 86, 123–128. doi: 10.1146/annurev-biochem-061516-044859
Veldhoen, M. (2017). Interleukin 17 is a chief orchestrator of immunity. Nat. Immunol. 18, 612–621. doi: 10.1038/ni.3742
Wang, K., Wang, H., Lou, W., Ma, L., Li, Y., Zhang, N., et al. (2018). IP-10 promotes blood-brain barrier damage by inducing tumor necrosis factor alpha production in japanese encephalitis. Front. Immunol. 9:1148. doi: 10.3389/fimmu.2018.01148
Weekman, E. M., and Wilcock, D. M. (2016). Matrix metalloproteinase in blood-brain barrier breakdown in dementia. J. Alzheimers Dis. 49, 893–903. doi: 10.3233/JAD-150759
Xu, B., Yang, R., Fu, J., Yang, B., Chen, J., Tan, C., et al. (2021). LncRSPH9-4 facilitates meningitic Escherichia coli-caused blood-brain barrier disruption via miR-17-5p/MMP3 axis. Int. J. Mol. Sci. 22:6343. doi: 10.3390/ijms22126343
Xu, D., Gao, Q., Wang, F., Peng, Q., Wang, G., Wei, Q., et al. (2021). Sphingosine-1-phosphate receptor 3 is implicated in BBB injury via the CCL2-CCR2 axis following acute intracerebral hemorrhage. CNS Neurosci. Ther. 27, 674–686. doi: 10.1111/cns.13626
Yang, B., Yang, R., Xu, B., Fu, J., Qu, X., Li, L., et al. (2021). miR-155 and miR-146a collectively regulate meningitic Escherichia coli infection-mediated neuroinflammatory responses. J. Neuroinflammation 18:114. doi: 10.1186/s12974-021-02165-4
Yang, R. C., Qu, X. Y., Xiao, S. Y., Li, L., Xu, B. J., Fu, J. Y., et al. (2019). Meningitic Escherichia coli-induced upregulation of PDGF-B and ICAM-1 aggravates blood-brain barrier disruption and neuroinflammatory response. J. Neuroinflammation 16:101. doi: 10.1186/s12974-019-1497-1
Yang, R., Liu, W., Miao, L., Yang, X., Fu, J., Dou, B., et al. (2016). Induction of VEGFA and Snail-1 by meningitic Escherichia coli mediates disruption of the blood-brain barrier. Oncotarget 7, 63839–63855. doi: 10.18632/oncotarget.11696
Keywords: IL-17A, Escherichia coli meningitis, blood-brain barrier, permeability, PRTN3, PAR-2
Citation: Xu B, Chen J, Fu J, Yang R, Yang B, Huo D, Tan C, Chen H and Wang X (2022) Meningitic Escherichia coli-Induced Interleukin-17A Facilitates Blood–Brain Barrier Disruption via Inhibiting Proteinase 3/Protease-Activated Receptor 2 Axis. Front. Cell. Neurosci. 16:814867. doi: 10.3389/fncel.2022.814867
Received: 14 November 2021; Accepted: 24 January 2022;
Published: 11 February 2022.
Edited by:
Vasudharani Devanathan, Indian Institute of Science Education and Research, Tirupati, IndiaReviewed by:
Ritu Mishra, National Institute of Immunology (NII), IndiaYukari Shigemoto-mogami, National Institute of Health Sciences (NIHS), Japan
Copyright © 2022 Xu, Chen, Fu, Yang, Yang, Huo, Tan, Chen and Wang. This is an open-access article distributed under the terms of the Creative Commons Attribution License (CC BY). The use, distribution or reproduction in other forums is permitted, provided the original author(s) and the copyright owner(s) are credited and that the original publication in this journal is cited, in accordance with accepted academic practice. No use, distribution or reproduction is permitted which does not comply with these terms.
*Correspondence: Xiangru Wang, d2FuZ3hyMjI4QG1haWwuaHphdS5lZHUuY24=