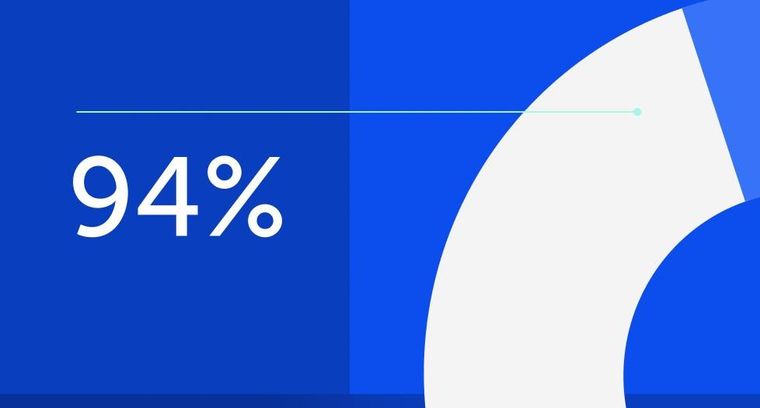
94% of researchers rate our articles as excellent or good
Learn more about the work of our research integrity team to safeguard the quality of each article we publish.
Find out more
REVIEW article
Front. Cell. Neurosci., 02 March 2022
Sec. Cellular Neuropathology
Volume 16 - 2022 | https://doi.org/10.3389/fncel.2022.786926
This article is part of the Research TopicNeurodegenerative Eye Diseases: Molecular Mechanisms of Neurogenesis and Therapeutic PerspectivesView all 6 articles
Thymoquinone is a naturally occurring compound and is the major component of Nigella sativa, also known as black seed or black cumin. For centuries thymoquinone has been used especially in the Middle East traditionally to treat wounds, asthma, allergies, fever, headache, cough, hypertension, and diabetes. Studies have suggested beneficial effects of thymoquinone to be attributed to its antioxidant, antibacterial, anti-oxidative stress, anti-inflammatory, and neuroprotective properties. Recently, there has been a surge of interest in thymoquinone as a treatment for neurodegeneration in the brain, such as that seen in Alzheimer’s (AD) and Parkinson’s diseases (PD). In vitro and in vivo studies on animal models of AD and PD suggest the main neuroprotective mechanisms are based on the anti-inflammatory and anti-oxidative properties of thymoquinone. Neurodegenerative conditions of the eye, such as Age-related Macular Degeneration (AMD) and glaucoma share at least in part similar mechanisms of neuronal cell death with those occurring in AD and PD. This review aims to summarize and critically analyze the evidence to date of the effects and potential neuroprotective actions of thymoquinone in the eye and ocular neurodegenerations.
Thymoquinone is the main extract present in Nigella sativa, a traditional remedy widely used in Middle East countries. Recent growing evidence has shown antioxidant, anti-inflammatory, anti-bacterial, anti-fungal, and anti-neurodegenerative properties of thymoquinone in various models (Sedaghat et al., 2014; Parlar and Arslan, 2020; Pop et al., 2020).
Neurodegeneration is associated with progressive deterioration of neuronal structures and functions. In the eye the process of neurodegeneration is implicated in several degenerative diseases of the retina. These include glaucoma, age-related macular degeneration (AMD), diabetic retinopathy (DR) and inherited retinal disorders which cause loss of vision (Jadeja and Martin, 2021). Over the years, increasing cases of vision loss has been identified along with increasing age and life span. According to statistical data from World Health Organization (WHO) from 2010, it is estimated that out of 285 million visually impaired people worldwide, 39 million of them were blind, of which 82% were over 50 years old, and 246 million presented low vision. (Pascolini and Mariotti, 2012). Currently, 43.3 million individuals are estimated to be blind, with this number being predicted to increase to 61 million by 2050 (Bourne et al., 2020, 2021). This data highlights the global problem of blindness faced around the world, especially in countries with longer life span and improved healthcare.
Oxidative stress and inflammation are among the main factors associated with neurodegeneration of the retina (Domènech and Marfany, 2020; Jadeja and Martin, 2021). With aging, the metabolic function in the retina is impaired leading to increase in free radicals such as reactive oxygen species (ROS) and reactive nitrogen species (Domènech and Marfany, 2020). Accumulation of ROS leads to various downstream effects including increase in oxidative stress, accumulation of advanced glycation end products, impaired mitochondrial function and changes in signaling pathways which lead to inflammation, cell death, and apoptosis (Giacco and Brownlee, 2010).
Overall, current treatment options of ocular neurodegeneration are focused only at treating the symptoms and delaying the progression of the disease. To date, there is no cure for these diseases. By altering the signaling pathways related to oxidative stress and inflammatory processes, neuronal cell death processes may be delayed. Alternative and newer treatment options, including from plant origins are being explored to increase the available armamentarium of treatment modalities to delay and prevent loss of vision. Thymoquinone is one of potential therapeutic agent to be used in treating eye diseases.
Pubmed database searches on thymoquinone resulted in 1190 research reports from 1960 to October 2020. The first few thymoquinone studies were designed to investigate its effects in relation to allergic reaction (Coenraads et al., 1975; Chakravarty, 1993). In subsequent studies, thymoquinone was shown to exert protective effects against histamine release by decreasing intracellular calcium uptake, inhibiting protein kinase C and inhibition of oxidative energy metabolism, contributing to some inhibition of histamine release. Interestingly, there were 651 study reports made available from the last 5 years, showing a strongly increasing interest in this compound. Recent reports were further focused on the effect of thymoquinone with anti-inflammatory, antioxidant, and anti-cancer properties. Given the growing evidence of its effect on inflammatory and oxidative stress pathways, thymoquinone emerges as a compound with therapeutic potential for managing ocular neurodegenerative diseases.
Despite the advancement of medical management, herbal-based medicine is still being practiced in certain parts of the world. The wide utilization of herbal drugs as traditional remedies has encouraged scientists to investigate their active ingredients and their effects on health. Black seed or Nigella sativa is widely used as traditional medicine in the Middle East and North America (Badary et al., 2003). Thymoquinone (chemical name 2-isopropyl-5methyl-1,4-benzoquinone; Figure 1; Silachev et al., 2015) is the active compound with the most pharmacological properties isolated from Nigella sativa. Its molecular formula is C10H12O2 and has a molar mass 164.20 g/mol. Thymoquinone is a hydrophobic molecule with solubility in 0.1N hydrochloric acid and PBS at different pH levels ranging from 5 to 9. Thymoquinone is shown to be more stable at a lower pH and its stability will decrease with increasing pH. In addition, thymoquinone is found to be light and heat sensitive, which contributes to its short half-life (Goyal et al., 2017).
The protective effect and therapeutic values of thymoquinone have been documented for various conditions such as asthma (Ammar el et al., 2011; Kalemci et al., 2013; Keyhanmanesh et al., 2014), wound healing (Selçuk et al., 2013; Siriwattanasatorn et al., 2020), liver damage (Zeinvand-Lorestani et al., 2018; Atteya et al., 2019), diabetes (El-Shemi et al., 2018; Rani et al., 2018), degenerative diseases (Angeles et al., 2016; Ebrahimi et al., 2017; Elibol et al., 2020), and inflammation-related diseases (Pop et al., 2020). Thymoquinone has been recognized to exert anti-inflammatory and antioxidant properties (Parlar and Arslan, 2020; Savran et al., 2020). However, the main concern in using thymoquinone is its delivery system. Thymoquinone is an oil-based compound with poor oral bioavailability and low solubility. Currently, two lipid-based drug delivery system has been used to improve the solubility and bioavailability of thymoquinone namely thymoquinone-solid-lipid nanostructure (TQ-SLN) and thymoquinone- nanostructured lipid carrier (TQ-NLC). Between these two, TQ-NLC was shown to provide a better and sustained drug release pattern which is a better option to maintain drug concentration for therapeutic approach. TQ-NLC is designed to be 50nm in size with improved encapsulation efficiency and can be stable for up to 24 months. Comparison on the efficacy of oral and intravenous administration of TQ-NLC in terms of thymoquinone delivery and distribution in rat organs indicate that absorption is higher when administered intravenously compared to orally. However, in terms of relative bioavailability, the oral route is superior (Zakarial Ansar et al., 2020).
The increasing number of studies on thymoquinone’s effect on various conditions and diseases demonstrate the compound’s significance as a therapeutic target, which is primarily mediated through changes in signaling pathways related to ROS scavenging and antioxidant production in inflammatory and degenerative diseases (Parlar and Arslan, 2020; Tabeshpour et al., 2020; Tian et al., 2020; TÜrkÖz et al., 2020). Recently, there has been a surge of interest in thymoquinone as a treatment for neurodegeneration in the brain, such as that seen in Alzheimer’s disease (AD) and Parkinson’s disease (PD) (Ebrahimi et al., 2017; Elibol et al., 2020). Both AD and PD share disease mechanisms, owing to an increase in oxidative stress and inflammatory activation which occur with aging and these two factors also play an important role in AMD.
Alzheimer’s disease is a neurodegenerative disorder characterized by accumulation of amyloid beta in the hippocampus. PD is a disease caused by degeneration of dopaminergic neurons in a specific area of the brain called substantia nigra. Several in vitro and in vivo neuroprotective studies have been conducted on various cell lines and animal models of AD and PD (Table 1). Thymoquinone dose ranging from 100 nm to 12.5 μM were used in in vitro neurodegenerative study models (Radad et al., 2009; Khan et al., 2012; Alhebshi et al., 2013, 2014; Ismail et al., 2013; Angeles et al., 2016; Cobourne-Duval et al., 2016, 2018; Alhibshi et al., 2019; Dong et al., 2020). In animal studies, different doses of thymoquinone were administered either by intraperitonial, intravenous or intragastric routes. The experimental studies’ dosing of thymoquinone were between 10 mg/kg and 500 mg/kg, however 10 mg/kg and 20 mg/kg were the most common concentrations used (Ibrahim AbdEl Fattah et al., 2016; Ebrahimi et al., 2017; Ismail et al., 2017a,b; Abulfadl et al., 2018b,c; Dalli et al., 2018; Poorgholam et al., 2018; Ardah et al., 2019; Elibol et al., 2020). The results of these studies in AD and PD clearly showed that thymoquinone could ameliorate the factors that lead to neurodegeneration suggesting that thymoquinone exhibits neuroprotective activities.
Table 1. In vitro and In vivo studies on the neuroprotective effects of thymoquinone in AD and PD models.
Taken together, these studies showed that thymoquinone has a neuroprotective effect mediated via modulation of oxidative stress, reduced formation of AB plaques, control of mitochondrial integrity and functions, as well as regulation of inflammation-related genes, thus preventing neuronal cell death and apoptosis. These findings suggested that thymoquinone could be an effective neuroprotective agent in treating AD and PD. Due to similar neurodegenerative mechanisms, it was also suggested that thymoquinone could be exploited to study its possible protective effect for ocular neurodegeneration diseases (Kaarniranta et al., 2011).
There are limited studies on the positive effects of thymoquinone in other eye conditions. Studies of the effect of thymoquinone on the eye focused on dry eyes (Kocatürk et al., 2018), conjunctivitis (Hayat et al., 2011), cataract (Fouad and Alwadani, 2015; Taysi et al., 2015), glaucoma (Fahmy et al., 2018), and oxidative stress in the RPE (Hu et al., 2019). Table 2 summarizes the findings of these studies on various eye conditions.
With regards to allergic conjunctivitis, Hayat et al. (2011) studied the effect of thymoquinone on a mice model of ovalbumin (OVA)-induced allergic conjunctivitis by looking into eosinophil recruitment, IgE levels, histamine and cytokines releases (Hayat et al., 2011). OVA administration caused increase in IgE and OVA-specific immunoglobulin E (IgE) levels, eosinophils recruitment, histamine level, mRNA expressions and protein level of pro-inflammatory cytokines as compared to control groups and thymoquinone was shown to ameliorate this effect. Thymoquinone concentration as low as 0.05% w/v was able to attenuate eosinophil recruitment to the site of allergy. However, at a concentration of 0.5%, thymoquinone showed convincing suppression of ocular symptoms, inflammatory cell infiltration in the conjunctiva, blood and OLF, increased levels of serum and OVA-specific IgE, and histamine levels in OVA-exposed mice. In addition, administration of thymoquinone markedly reduced mRNA expression and serum level of interleukins including 1L-4, IL-5, IL-13 in mice exposed to OVA. In another study, Fouad and Alwadani (2015) investigated the protective effects of thymoquinone against lens changes in streptozotocin-induced diabetic rats (Fouad and Alwadani, 2015). Thymoquinone (20, 40, and 80 mg/kg/day) was administered for 12 weeks. At 40 and 80 mg/kg concentrations, there was significant decrease of many pro-inflammatory factors such as malondialdehyde, and tumor necrosis factor-α, glutathione peroxidase, superoxide dismutase (SOD), and catalase activities. Decreased levels of glycated proteins, aldose reductase activity, sorbitol level, caspase-3 activity, and total and soluble protein contents in the lens were also noted among diabetic rats. These data suggest that thymoquinone protects lens tissue against changes induced by diabetes through its antioxidant, anti-inflammatory, and antidiabetic effects. Kocatürk et al. (2018) studied the effects of thymoquinone on a dry eye model. They showed that thymoquinone was able to cause inflammation observed through pathological examination, but no significant changes were observed in the level of cytokines.
Next, Taysi et al. (2015) studied the antioxidant and radioprotective effects of thymoquinone against ionizing radiation-induced cataracts in the lens of rats exposed to total cranial irradiation (IR) with a single dose of 5 gray (Gy) (Taysi et al., 2015). Thymoquinone (50 mg/kg/day) reduced cataract formation rate from 80 to 50% compared to control. Nitric oxide synthase activity, nitric oxide and peroxynitrite levels were also reduced with thymoquinone administration. The findings of this study indicated that thymoquinone may be a potential compound for preventing cataract formation in IR-exposed lenses by inhibiting the production of oxidative stress.
In the most recent study, Xu et al. (2018) examined the effect of thymoquinone against hydrogen peroxide (H2O2) -induced oxidative stress in human RPE cells (Xu et al., 2018). Their results showed that thymoquinone (5, 10, 20, and 40 μM) improved cell viability and reduced apoptosis in H2O2- induced ARPE cells. They also found that the levels of ROS, malondialdehyde, glutathione and SOD activity induced by H2O2 decreased following thymoquinone pretreatment. The changes caused by thymoquinone were thought to be through Nrf2/Ho-1 signaling pathway as thymoquinone was shown to enhance the activation of Nrf2/heme oxygenase 1 (HO-1) pathway in H2O2-induced ARPE cells. To support their hypothesis, they showed that this thymoquinone effect was absent in Nrf2-knockout mice model.
These data mentioned above can be a preliminary data that shows the possible protective ability of thymoquinone in eye-related problems. Although, these studies are more focus toward the protective effect of thymoquinone on the disease focusing on the frontal area of the eye, based on evidence of thymoquinone on neurodegenerative disease discussed earlier, it is possible that thymoquinone can be exploited to be a new treatment for neurodegeneration in the eye.
The effect of thymoquinone on AD- and PD-related neurodegeneration warrants further studies investigating the direct protective effects of thymoquinone in ocular neurodegenerative diseases. The neuroprotective effect of thymoquinone might be cell-specific, thus the effect seen in AD and PD might differ from ocular degeneration models. However, it is important to note that the neuroprotective mechanisms in AD and PD are shown to occur via the anti-inflammatory and anti-oxidative properties of thymoquinone (Sedaghat et al., 2014; Elibol et al., 2019). These include modulation of oxidative stress, anti-inflammatory activity, apoptosis and involve regulation of mitochondria function, NF-kB and Nrf2 signaling pathways and restoration of redox homeostasis (Alhebshi et al., 2013; Ardah et al., 2019).
Intake of antioxidants such as Vitamin C, E, beta-carotene, lutein, and zeaxanthin have been shown to prevent neurodegeneration, thus preserving vision (Payne et al., 2014). Thymoquinone is a naturally occuring plant-based antioxidant and its consumption may potentially provide similar neuroprotective effects like Vitamin C. However further studies into improving delivery methods are required to optimize thymoquinone absorption. For instance, comparison between two delivery routes (intranasal and intravenous route) of 5 mg/kg/day thymoquinone loaded with PLGA-chitosan nanoparticles showed that the intranasal route was better at facilitating the delivery of thymoquinone to the brain (Xiao et al., 2016). Data showed a concentration of 996.43 ± 119.36 ng/mL was delivered to the brain intranasally compared to intravenous at 390.61 ± 54.44 ng/mL. Apart from a better concentration, intranasal route also provides a longer half-life (118.23 ± 3.97 h) and lower elimination rate of thymoquinone. Hence, this study may be a steppingstone in investigating the delivery system of thymoquinone in the case of ocular diseases.
Redox homeostasis is defined as the endogenous ability of the cells in dealing with challenges from various stressors generated by metabolic activities or any exogenous sources. Persistent exposure to the stressor and inadequate ability of the cells to restore the balance leads to oxidative stress formation. Hence, restoration of the redox homeostasis is essential to prevent oxidative stress conditions. For this purpose, the cells increase production of oxidants that acts as signaling species resulting in balance equilibrium in production of pro-inflammatory reaction and pro-oxidant enzymes. The whole process is only aimed to diminish the challenge and restore the homeostasis (Ursini et al., 2016).
In the eye, ROS are not only generated endogenously due to robust metabolic activities. Intense exposure to light, and high atmospheric oxygen exposure also contributed to generation of ROS in the eye (Beatty et al., 2000; Tokarz et al., 2013). Neutralization of this abundantly generated ROS in the eye is crucial for cellular homeostasis. At a low and moderate level, the ROS acts a signaling molecule that sustain cellular proliferation and differentiation as well as activate stress-responsive survival pathways by increasing the expression of pro-oxidant related genes. Persistent exposure to ROS would implicated to various eye diseases such as cataract (Ho et al., 2010), glaucoma (Izzotti et al., 2006), and AMD (Beatty et al., 2000). GSH, NADH, and NADPH are examples of the cellular redox presented in the eye mainly generated from mitochondria-related pathways and their level are reduced with aging (Bradshaw, 2019; Pan et al., 2021).
Thymoquinone has antioxidant capacity that has been found to be able to maintain redox homeostasis in the cells. Thymoquinone has been shown to decrease the level of GSH and increase the level of NADH and NAPDH (Sankaranarayanan and Pari, 2011). These molecules serve important roles in biological oxidation-reduction processes, as a cofactor for many enzymes, and a detoxifying and free radical scavenging agent, hence retain the cell homeostasis. GSH is responsible in maintaining the sulfhydryl (–SH) group of enzymes in its reduced state. The sulfhydryl residues of GSH molecules are easily oxidized to form GSH disulfide (GSSG), which is then reduced back to GSH by the reaction with GSH reductase (Aoyama and Nakaki, 2015). Since RNS and ROS may interact with cysteine thiol group to form electrophile containing –SH, GSH is responsible to attack these molecules and retain it at less reactive state (Armutcu et al., 2018). Decrease in GSH level is accompanied with increase in GSSH is observed in oxidative stress related diseases which include AD and PD (Calabrese et al., 2006; Mischley et al., 2016). The redox capacity of cells is determined by the ratio of GSH/GSSG. In an in vivo study, decreased level of GSH was observed in the brain tissue treated with sodium nitrite and it was restored with thymoquinone treatment. In addition, thymoquinone treatment also shown to decrease the level of TNF-α and IL1β and increase level of IL-10 in a concentration-dependent manner (Hamdan et al., 2019). Similar reaction was observed in the model of brain ischemia (Al-Majed et al., 2006). Mass spectrometry analysis suggest that thymoquinone react with GSH by rapid binding of the thymoquinone to the cysteine residues of the GSH molecules in the 3-position of benzoquinone ring, hence prevent GSH oxidation to GSSG (Khalife and Lupidi, 2007).
NADH is the reduced form of NAD+ plays important role in mitochondrial metabolism. The NAD+/NADH ratio is a known regulator of various biological processes in the cells including glycolysis, energy metabolism, mitochondrial function, and gene expression (Xiao et al., 2018). The NADH is essential for ATP production and oxidative phosphorylation in the electron transport chain system. Decrease in the NAD+/NADH ratio, reduced cellular NAD + level, and increased NADH have been observed during aging (Braidy et al., 2011). NADPH is responsible for maintaining cells’ redox state and biosynthesis of fatty acids, cholesterol, and deoxynucleotides. NADPH decreased in aging as a result of decreased of its immediate precursor, NADP+. Therapies that increase NAD + levels and NADP + could greatly benefit subjects with aging-related disorders. Study in BV2 microglial cells showed that thymoquinone was able to reduce LPS-induce ROS by increasing the level of NAD+/NADH ratio. This reaction is followed by the attenuation of cellular ROS level (Velagapudi et al., 2017a). In addition, thymoquinone was also showed to play a major role in hyperglycemia model which is important in DR pathogenesis. A significant drop of 45 and 30% of NADH and NADPH was observed with 5 μm of thymoquinone incubation (Gray et al., 2016). The mechanism on how thymoquinone regulate the NAD+, NADH, NADP+, and NADPH is still not clear. Some research suggest it happens through inhibition of p47PHOX, a cytosolic subunit of the NADPH oxidase, phosphorylation on Ser-304 and Ser-328 (Boudiaf et al., 2016).
Oxidative stress has long been linked to aging and neurodegenerative disorders, including those affecting the eyes (Jadeja and Martin, 2021). An imbalance in the accumulation of harmful substances in cells and the cell’s normal ability to avoid oxidative damage by using its anti-oxidative properties is referred to as oxidative stress (Beatty et al., 2000; Burton and Jauniaux, 2011; Zafrilla et al., 2013). Under normal homeostasis, the antioxidant would neutralize the oxidative stress level to ensure cell survival. Persistent stress, on the other hand, will disrupt this protective mechanism, causing cells to undergo apoptosis, necrosis, or autophagic cell death. Oxidative stress is accompanied by the increase in ROS in the cells. The major types of ROS are superoxide anion, hydrogen peroxide, hydroxyl radical, peroxy radical, and the second messenger nitric oxide (Burton and Jauniaux, 2011). A major source of ROS production in cells is the mitochondrial respiratory chain. Oxidative stress accumulation in cells is detrimental because it causes cellular damages to proteins, lipids and nucleic acids (Cui et al., 2012). Excessive accumulation of ROS can trigger downstream signaling pathways such as NF-κB, ERK1/2, p38 MAPK, and autophagy-related signaling (Zhang et al., 2016).
Due to a variety of factors, including high oxygen intake, excessive light exposure, and the presence of polyunsaturated fatty acids, the retina is extremely susceptible to oxidative stress harm. Furthermore, since the outer segments of photoreceptors are constantly shed, the RPE’s phagocytic role adds to the oxidative burden (Beatty et al., 2000; Tokarz et al., 2013). The accumulation of oxidative stress disrupts cell homeostasis and triggers a defensive cell response in photoreceptors and retinal ganglion cells (Nita and Grzybowski, 2016). These two cell types are high-metabolizing neurons that are constantly exposed to oxidative stress insults (Beatty et al., 2000).
Thymoquinone is known to reduce in vitro and in vivo oxidative stress levels. Various studies showed the ability of thymoquinone to reduce oxidative stressors such as TNFα, lipopolysaccharides, IL-1β and Il-1α levels (Wang D. et al., 2015; Cobourne-Duval et al., 2018). In the case of neurodegenerations, Sedaghat et al. (2014) has shown that thymoquinone was able to ameliorate the oxidative stress marker [malondialdehyde (MDA) and nitrile] levels in unilateral intrastriatal 6-hydroxydopamine (6-OHDA)-lesioned rats as a model of PD (Sedaghat et al., 2014). In another study, thymoquinone significantly decreased MDA, nitrile oxide and TNF-α level in an AD model (Abulfadl et al., 2018b). A similar observation was made in LPS-induced oxidative stress in BV2 microglialcell, where 10um of thymoquinone was able to reduce the inflammatory cytokines expressions through qPCR and ELISA (Cobourne-Duval et al., 2018). In addition, thymoquinone treatment (2.5, 5, and 10 mM) was shown to reduce the levels of TNF-a, IL-6, and IL-1b in BV2 cells challenged by LPS at both mRNA and protein levels (Velagapudi et al., 2017a). Thymoquinone (3, 6, 12 μM) was found to exert its effect by reducing the level of nitrite oxide and PGE2 production indicated in BV2 cells (Wang Y. et al., 2015). On the contrary however, Elibol et al. (2019) showed that in the case of AD thymoquinone did not change the level of TNF-α, IL-1α, and IL-1β groups with pretreatment of thymoquinone (Elibol et al., 2019).
In addition to reducing oxidative stressors, thymoquinone has been shown to increase inherent intracellular antioxidants such as SOD, catalase and glutathione. In an in vivo study of arsenic-induced neurotoxicity, 10 mg/kg of thymoquinone suppressed levels of oxidative stressors (NO and TNF-α) and increasing antioxidants (glutathione, glutathione peroxidase, SOD, and catalase) in the rat’s nervous system (Kassab and El-Hennamy, 2017). The same effect was seen in lead (Mabrouk, 2017) and paraquat toxicity (Zeinvand-Lorestani et al., 2018) in rat’s liver. Co-treatment with 5 mg/kg/day of thymoquinone for 5 weeks resulted in increased in reduced glutathione levels, but also SOD, glutathione peroxidase, catalase and glutathione reductase activities (Mabrouk, 2017). Meanwhile, pretreatment with 10 mg/kg thymoquinone was shown to restore SOD activity in paraquat-induced toxicity (Zeinvand-Lorestani et al., 2018). These studies show the ability of thymoquinone in ameliorating the level of antioxidant in hepatotoxicity injury.
The effect of thymoquinone on different cell types may vary and possibly are cell specific. However, it is possible that thymoquinone provide protective effects on the retina by reducing oxidative stress and increasing antioxidant properties. Therefore, it is worthwhile to examine the effects of thymoquinone on ocular neurodegenerative models.
Inflammatory response occurs as a result of modulation of oxidative stress involving a series of cellular events that allow the body to defense itself from intruders or further damage (Hussain et al., 2016). Accumulation and prolong exposure to oxidative stress triggers exaggerated inflammatory response of cells termed as chronic inflammation. Studies showed that chronic inflammation does play an important role in age-related disease (Sharif et al., 2019). Inflammation is considered as the primary immune system reaction to eliminate pathogens or other stimuli in order to restore the cells to normal state (Chen et al., 2017).
In the eye, there five neuronal cells present namely photoreceptors, bipolar cells, ganglion cells, horizontal cells, and amacrine cells. These cells are responsible in providing vision. With aging, these neuronal cells undergo various physiological and morphological changes due to various endogenous and exogenous factors including oxidative stress and inflammation, which leads to impaired vision. Recently, inflammation has become a concern for AMD, DR and macular edema as a secondary to DR (Das, 2016; Tan et al., 2020). The importance of inflammation in these diseases has been discussed (Ascaso et al., 2014; Kauppinen et al., 2016; Forrester et al., 2020). Apart from anti-VEGF treatment, anti-inflammatory agents such as corticosteroids has been used to delay the progression of these disease (Wang et al., 2011). Macrophages and giant cells have been reported to localize near drusen, at the breakdown of Bruch’s membrane, and in the CNV membrane of AMD. Furthermore, increase in inflammatory cytokines such as tumor necrosis factor-α (TNF-α) and IL-1, was also found in the RPE, thereby inducing additional inflammatory cellular infiltration and accelerates angiogenesis and CNV formation in AMD (Wang et al., 2011). VEGF is a growth factor responsible in promoting angiogenesis. However in DR and AMD, the presence of VEGF cause uncontrolled growth of blood vessels and promote disease progression. Increase of VEGF and its involvement in ocular disease is well studied (Homayouni, 2009; Ahuja et al., 2019).
Microglia activation is considered first line of defense against inflammation followed by release of anti-inflammatory mediators and resolution of the inflammatory response. It is well known that microglia activation is an important in pathogenesis of AD and PD (Qian and Flood, 2008; Mandrekar-Colucci and Landreth, 2010). Analysis on microglia cells exposed to thymoquinone demonstrated a significant anti-inflammatory property of thymoquinone observed through attenuation of IL-6, CCL12/MCP-5, and CCL2/MCP-1 protein and mRNA expression (Taka et al., 2015). In another study, thymoquinone showed anti-inflammatory properties by inhibition of nitric oxide (NO), PGE2, TNF-α, and IL-1β production in BV2 microglial cells (Wang Y. et al., 2015). Besides, thymoquinone anti-inflammatory activity in AD has been observed through alleviation of TLR signaling pathway, a signaling pathway responsible for innate response. Different thymoquinone dosage ranging from 10 mg/kg/day to 40 mg/kg/day was able to reduce TLR-2, TLR-4, MyD88, TRIF and IRF-3, which are related to TLR signaling (Abulfadl et al., 2018a). In conclusion, these findings suggest a possible anti-inflammatory effect of thymoquinone through attenuation of various inflammatory related cytokines, which could provide therapeutic value in neurodegenerative diseases and ocular disease.
Accumulation of oxidative stress are reported to cause peroxidation of polyunsaturated fatty acids in the cell membrane of the brain resulting in the formation of toxic metabolites such as MDA and 4HNE, as well as advanced glycation end products (AGEs). Long-lived proteins and protein deposits in human and animal tissues, including the retina, have been shown to contain these byproduct metabolites. The AGEs is a byproduct of Maillard reaction through interaction of aldehyde or carbonyl sugar with amino group of proteins, lipids or nucleic acids (Goldin et al., 2006; Luevano-Contreras and Chapman-Novakofski, 2010). In the eye specifically, AGEs are associated with age-related macular degeneration (AMD), cataract formation, diabetic retinopathy and glaucoma (Ishibashi et al., 1998; Howes et al., 2004).
Advanced glycation end products accumulation can affect the eye through two mechanisms, either by damage to the extracellular matrix layer or interactions with receptor proteins. AGEs accumulate with age (Araki et al., 1992; Schleicher et al., 1997). Accumulation of AGEs in the eye would leads to abnormal crosslinking of extracellular matrix proteins, increase vascular stiffness, leading to changes in vascular structure and function. As a result, damage and leakage in the blood vessels and hyperproliferation can occur in diabetic retinopathy and AMD (Vlassara et al., 1994). AGEs formation is enhanced in hyperglycemic conditions in diabetic patients and reflects the severity of diabetic complications (Ono et al., 1998). Localization of AGEs in retinal blood vessels has been observed in patients with type 2 diabetes (Stitt et al., 1997). AGEs-induce oxidative stress cause consequent apoptosis of retinal pericytes (Bergers and Song, 2005). Pericytes play an important role in the maintenance of microvascular homeostasis. Loss of pericytes leads to angiogenesis, thrombogenesis, and endothelial cell injury, thus leading to diabetic retinopathy development (Eilken et al., 2017). In addition, AGEs interact with RAGE, resulting in increased intracellular production of free radicals and oxidative stress, which is then phosphorylates by MAP kinase and activates and increases expression of NF-κβ controlled genes causing vasoconstriction, angiogenesis, increased adhesion molecule expression, and inducing a procoagulant state associated with AMD and diabetic retinopathy (Tong and Yao, 2006; Sun et al., 2017).
There has been growing evidence suggesting that thymoquinone may be attributed to reducing the levels of AGEs (MDA, 4HNE) in cells. Administration of thymoquinone (10 mg/kg) in AD models has shown to lower the MDA level (Zaher et al., 2019). Besides, the protective effect of thymoquinone through reduction of 4HNE was observed in the model of renal (Dera et al., 2020a) and pulmonary diseases (Dera et al., 2020b). Reduction of MDA was also accompanied by increased levels of SOD, catalase and reduced glutathione (GSH). The findings were supported by an in vitro study Alhebshi et al. (2013), which showed that thymoquinone administration restored the redox balance in cultured rat hippocampal and cortical neurons displaying Aβ aggregation, as seen in AD (Alhebshi et al., 2013). Several studies also showed the same results in various condition such as in the liver and kidney of the rat model (Hosseinian et al., 2017), spinal cord injury model (Chen et al., 2018), diabetic model (Desai et al., 2015), renal induced toxicity (Sener et al., 2016), and ischemic brain model (Xiao et al., 2016).
Blocking of the Maillard reaction has been shown to be beneficial in preventing AGEs formation. Thymoquinone acts on AGEs by blocking the attachment of sugar to proteins, thereby attenuating glycosylation and oxidative stress while preventing protein crosslinking in a dose dependent manner (Losso et al., 2011; Anwar et al., 2014). Several studies have been shown to reduce glycation in sugar-induced AGEs model following administration of thymoquinone (Losso et al., 2011; Mahmood et al., 2013; Zafar et al., 2013; Benvidi et al., 2018; Pandey et al., 2018). Mahmood et al. (2013) and Zafar et al. (2013) observed the effect of glucose in inducing AGEs in model of diabetic. Both studies showed thymoquinone’s ability in reducing AGEs formation measured through thiobarbituric assay and AGEs fluorescence read at 480 nm (Mahmood et al., 2013; Zafar et al., 2013). In cases of fructose and BSA glycation, Pandey et al. (2018) shows thymoquinone reduced browning effect, indication of glycation process during AGEs formation (Pandey et al., 2018). The group also looked into carbonyl content and glycation aggregation index, which is a part of late stage of AGEs formation process. Data showed thymoquinone can reduce glycation aggregation index.
In support of those study, Losso et al. (2011) assessed the potential inhibitory effect of thymoquinone against AGEs from serum samples of diabetic patients through the use of hemoglobin-δ-gluconolactone, human serum albumin-glucose and the N-acetyl-glycyl-lysine methyl ester-ribose assay. Their results suggested thymoquinone at a dose as low as 10mM was able to suppress 78% of AGEs in blood plasma (Losso et al., 2011). The effect of thymoquinone on AGEs was also linked to the binding of thymoquinone to arginine-409 of thymoquinone as observed in BSA-induced AGEs (Benvidi et al., 2018). In addition, reduction of AGEs formation was also postulated to be due to the ability of thymoquinone in preventing the inactivation of SOD, an enzyme responsible as a defense mechanism in prevention of glycation (Anwar et al., 2014; Benvidi et al., 2018). Incubation of SOD with glycating agent, glucose, methyglyoxyl or both, has been shown to increase structural changes by SOD, thus alter it defense mechanism. However, thymoquinone was shown to reverse the effect by reducing the amount of SOD structural changes, attenuate AGEs formation as well as reduced protein crosslinking (Anwar et al., 2014). These data show the important of thymoquinone as an anti-glycating agent that may be useful to tackle AGEs formation in the ocular disorder.
Apoptosis is defined as a programmed cell death intend to remove unwanted or damaged beyond repairs cells in the body. Shrinkage of the cell, fragmentation into membrane-bound apoptotic bodies and rapid phagocytosis by neighboring cells are the common characteristic of apoptosis (Nickells and Zack, 1996). Under normal circumstances, apoptosis is important to prevent accumulation of unwanted cells and making a way for new young cells to grow. Dysregulation of apoptosis can cause health problems. In cancer for instance, apoptosis process is absent followed with uncontrolled growth of new cells. In addition, excessive apoptosis also can create degeneration conditions in the body as seen in AD and PD, where the brain lose some part that is not supposed to die.
In the eye, apoptosis is considered a mechanism of cell death in ocular diseases including glaucoma, retinitis pigmentosa, cataract, AMD and diabetic retinopathy. Apoptosis has been found precedes these disease progression (Charakidas et al., 2005; Feenstra et al., 2013). DNA fragmentation analysis using terminal digoxigenin-labeled dUTP nick end labeling (TUNEL) in cataract patient revealed that apoptosis in cataract patient is higher compared to non-cataract patient. 92.6% of the samples tested shown average of 21.7 apoptotic cells per mm2, or 0.0047% of overall cell population and only three apoptotic epithelial cells per mm2 was detected from the non-cataract samples (Charakidas et al., 2005). Analysis of apoptosis modulator, Bcl-2 and Bax would allow determination of the susceptibility of a cell to apoptosis. Increase in the expression of both these proteins during disease progression was observed in glaucoma (Zalewska et al., 2004), retinitis pigmentosa (Comitato et al., 2014), cataract (Osnes-Ringen et al., 2016), AMD (Dunaief et al., 2002), and diabetic retinopathy (Khalfaoui et al., 2010). These findings suggest the important of apoptosis in the ocular disease development and study on possible modulation of these important process may leads to a crucial opportunity to deliver treatment sooner and hence preserve vision.
Apart from the earlier mentioned protective capability of thymoquinone, it also serves a dual function in apoptosis. In cancer cells, thymoquinone has been shown to promote apoptosis. In bladder cancer, thymoquinone was showed to induce apoptosis by enhancing the expression of the anti-apoptotic protein Bcl-2, blocking the release of cytochrome c and enhance the translocation of Bax from the cytoplasm to mitochondria (Zhang et al., 2018). Similar reaction was observed in breast cancer. Proapoptotic capacity of thymoquinone was observed through inhibition of anti-apoptotic genes, such as XIAP, survivin, Bcl-xL and Bcl-2, and increase in DNA fragmentation (Woo et al., 2013). Further study showed that pro-apoptotic activity of thymoquinone is followed with reduction in telomerase activity, an enzyme responsible for cellular resistance to apoptosis (Gurung et al., 2010). Thus, inhibition of telomerase by thymoquinone may induce apoptosis which is essential to prevent cancer.
On contrary, thymoquinone has been found to regulate apoptosis by reducing DNA fragmentation, downregulating of Bax and upregulating the Bcl-2 expression as well as decreasing downstream caspases 8, 9, and 3 in model of hepatic ischemia reperfusion injury, hence blocking apoptosis (Abd El-Ghany et al., 2009). In an in vitro model of AD, thymoquinone was shown to prevent apoptosis by blocking αβ-induced neurotoxicity (Alhibshi et al., 2019). Treatment with αβ1–42 for 48 h showed a significant decreased in cell viability to 63.5% as compared to the control group and cotreatment with 100 nM of thymoquinone restored the cell viability to 90%. The restoration of cell viability by thymoquinone was accompanied with significant reduction in ROS level to 2.6-fold as compared to sixfold in αβ1–42 only treatment. Ismail et al. (2013), also observed similar results with additional preserved intact cell bodies, extensive neurite networks, a loss of condensed chromatin and less free radical generation in primary cultured cerebellar granule neurons than those exposed to Aβ1–40 alone (Ismail et al., 2013). From these data, it is shown the selective capability of thymoquinone in inducing or inhibiting apoptosis in cells. This raises another interesting topic in studying the mechanism of thymoquinone in cells and applied it to ocular research.
Apoptosis is closely related to mitochondrial dysfunction. Mitochondrial dysfunction is described as a decrease in electron transport chain efficiency and a decrease in the synthesis of high-energy molecules like adenosine-5′-triphosphate (ATP). Mitochondrial dysfunction is a common feature in aging, and it also occurs in chronic diseases such as AD, muscular dystrophy, diabetes and cancer. It is important to note that ROS is primarily produced in the mitochondria, where they inhibit the mitochondrial electron transport chain’s complex I and reduce membrane integrity, resulting in higher ROS production (He et al., 2008). Mitochondrial-related mutation in the eye was first observed in Leber hereditary optic neuropathy (LHON). Mitochondrial DNA mutation in LHON affects the complex I of the electron transport chain, altering ATP synthesis. The resulting retinal ganglion cell apoptosis and optic nerve injury subsequently leads to blindness (Ghelli et al., 2003).
The mitochondria in retinal ganglion cells generate a large amount of energy required for nerve function in the unmyelinated ganglion cell axons. Glaucoma, like LHON, is an optic nerve disease characterized by accelerated apoptosis of retinal ganglion cells and their axons. It has been hypothesized that during the onset of glaucoma, an imbalance in the retinal ganglion cell axon energy requirement is caused by a change in blood flow dynamics in the optic nerve head, rendering the ganglion cells vulnerable to additional insults such as light entering the eye, which can further affect ganglion cell axon mitochondrial activity, and molecules released by astrocytes. If the mitochondria are unable to maintain normal function in these conditions, ganglion cell death can occur. Loss of retinal ganglion cells in glaucoma mouse models has been documented and this loss is due to the reduction in quantity of mitochondria and impairment of the mitochondrial function (Kim et al., 2015; Takihara et al., 2015).
More recently, the role of mitochondrial damage was investigated in diabetic retinopathy and AMD. An important cause of these conditions is an excess of pro-oxidants induced by the overproduction of ROS by the mitochondria throughout the retina. Increased retinal mitochondrial damage and disrupted mitochondrial membrane were observed in diabetic mouse models, together with elevated levels of ROS (Kanwar et al., 2007). In addition, in a diabetic rat model, the retinal mitochondria were shown to be leaky with diabetes, hence cytochrome c began to accumulate in the cytosol of retinal cells, observed in vitro rat models (Kowluru and Abbas, 2003). The RPE contains a high density of mitochondria to produce sufficient ATP to perform all its physiological processes. As a result, age-related mitochondrial dysfunction will lead to an increase in oxidative stress in the RPE, resulting in AMD. Wang et al. (2008) showed that mitochondrial damage was increased with age in the RPE of mice and rats (Wang et al., 2008). They concluded that the increase in mitochondrial damage in aging was due to a decrease in the cell’s DNA repair capability. In the case of AMD, Ferrington et al. (2017) and Golestaneh et al. (2017) investigated the mitochondrial function in RPE of AMD and healthy donor RPE. Their results showed reduction in mitochondrial function in RPE of AMD patients as compared to healthy donors (Ferrington et al., 2017; Golestaneh et al., 2017). It is also important to note, mitochondrial dysfunction can result in further increase in oxidative stress and thus leads to RPE functional decline and apoptosis. Taking these data together, maintaining the mitochondrial function and integrity is important to reduce the damage to the retinal ganglion cell and RPE cells in order to prevent blindness.
Like in the retina, the energy requirement in the brain is high to maintain its physiological function, thus mitochondrial dysfunction may pose a threat and lead to neurodegeneration as seen in AD and PD (Picone et al., 2014). The effect of thymoquinone on arsenic induced hippocampal toxicity and mitochondrial dysfunction in Wistar rats was studied (Firdaus et al., 2018). Arsenic is a compound found in pollutants and was found to induce oxidative stress as well as interruption in mitochondrial functions. Exposure to arsenic caused significant increase in intracellular ROS generation, mitochondrial dysfunction and apoptotic events. Administration of thymoquinone (2.5 and 5 mg/kg) was shown to inhibit the mitochondrial dysfunction by reducing the mitochondrial membrane potential in the rats hippocampal. Administration of thymoquinone concentration of 30 mg/kg for 10 consecutive days was shown to prevent mitochondrial dysfunction in liver of warm ischemia-reperfusion (I/R) injury rat model (Bouhlel et al., 2017, 2018). Thymoquinone was shown to also lessen the release of cytochrome C, an activator for caspase 9 and caspase 3 apoptotic pathways and thus preserve the mitochondrial function and prevent cell apoptosis. The prevention of liver mitochondrial damage could also be explained by the antioxidant and free radical scavenging activities of thymoquinone which in turn stabilized mitochondrial membrane permeability. Khan et al. (2012) showed the protective effect of thymoquinone against mitochondrial dysfunction in an in vitro model of AD using differentiated PC 12 cell line (Khan et al., 2012). In their study, thymoquinone pretreatment (1, 2, 4 μm) significantly counteracted the decreased in mitochondrial membrane potential. This showed an important mechanism of thymoquinone in combating mitochondrial dysfunction in cells. In addition, administration of 10 mg/kg of thymoquinone was shown to increase ATP synthesis in the renal cortex of kidney disease models (Dera et al., 2020a). These data together show a promising use of thymoquinone in maintaining retinal mitochondrial integrity and functions to prevent ocular neurodegeneration.
The cells undergo cell-to-cell communication through signaling pathways. Various signaling pathways have been identified and associated with different diseases in the eye. Among all, NF-κβB is widely studied due to its important role in regulating key cellular processes such as inflammation, apoptosis, stress response, wound healing, and angiogenesis (Morgan and Liu, 2011). NF-κβ is a transcription factor present ubiquitously in the cell cytoplasm as a dimer bound to an inhibitory complex, Iκβ. NF- κβ molecule activation is triggered by a variety of stimuli including growth factors, cytokines, bacterial lipopolysaccharides, UV, and oxidative stress. Activation and translocation of NF-κβ molecules involved a series of phosphorylation and ubiquitination processes leading to gene transcription through binding of the NF-κβ dimer to the κβ sites of DNA sequences. Binding of NF-κβ dimer to the κβ sites of DNA sequences NF-κβ will induce gene transcription of specific proteins including various cytokines (Lingappan, 2018). NF-κβ is thought to be cell specific and it can even exert a dual function, for cell survival and induce cell death.
In the eye, the NF-κβ signaling is activated by retinal degeneration (Wu et al., 2002; Zeng et al., 2008). Zeng et al. (2008) studied the alteration of NF-κβ activity during retinal degeneration in rd mice (Zeng et al., 2008). Increase of phosphorylated NF-κB P65 proteins and augmented DNA binding activity were observed in rd mice with retinal degeneration. Wu et al. (2002) also demonstrated intense light exposure activates NF-κB in the photoreceptor cells of rd mouse model followed with increase in NF-κβ DNA–binding activity, and increased in expression of mRNA of Iκβα, a target gene of NF-κβ (Wu et al., 2002). Since NF-κβ activation resulted in the production of proinflammatory cytokines such as TNF-a and IL-1, which mediate inflammatory and immune responses, these findings may indicate that altering NF-κβ activity promotes photoreceptor apoptosis in the rd retina by initiating and perpetuating chronic inflammation. This hypothesis is supported by an in vitro study, where 60–70% of the 661W photoreceptor cells were found to undergo apoptosis with light exposure and level of P65 proteins were downregulated (Yang et al., 2007). Pretreatment with minocycline or sulforaphane countered the downregulation of P65 protein and as a result, photoreceptor apoptosis was inhibited. These data highlight NF-κβ as an extremely attractive target for therapeutic intervention in retinal degeneration.
Thymoquinone was shown to reduce activation of NF-κβ in cells. In one of the studies, BV2 microglial cells were stimulated with LPS after thymoquinone incubation. LPS-induced NF-κβ activation was studied by measuring the secretion of TNFα and other NF- κβ related inflammatory cytokines such as IL-6 and IL-1β (Nagi et al., 1999; Wang Y. et al., 2015; Velagapudi et al., 2017a). The levels of these NF- κβ related cytokines were significantly decreased. In addition, exposure of LPS on rat basophil cell lines showed a marked increase in TNFα production, and thymoquinone was able to reverse this effect at a concentration as low as 10 μM concentration (El Gazzar et al., 2007). Similar findings were noted on studies of thymoquinone on NF-κβ signaling and TNFα cytokines release in the rat’s liver (Sayed and Morcos, 2007).
Thymoquinone (10, 15, 20, 25 μmol/L) suppressed NF-κβ activation in dose dependent manner, specifically by suppression direct binding of nuclear p65 and recombinant p65 to the DNA in 0.1 nmol/L TNFα and LPS stimulated cells. To confirm the finding, cells were transfected with p65 plasmid containing cysteine residue 38 mutated to serine and the binding inhibition by thymoquinone were flattened. Their data showed that thymoquinone suppressed NF-κβ activation correlated with sequential inhibition of the activation of IKK, IKβα phosphorylation, IKβα degradation, p65 phosphorylation, p65 nuclear translocation, and the NF-κβ-dependent reporter gene expression. Expression of NF-κβ regulated antiapoptotic genes (IAP1, IAP2, XIAP Bcl-2, Bcl-xL, and survivin), proliferative (cyclin D1, cyclooxygenase-2, and c-Myc), and angiogenic (matrix metalloproteinase-9 and vascular endothelial growth factor) gene products were also downregulated with thymoquinone treatment (Sethi et al., 2008). Similar findings were reported by Velagapudi et al. (2017b).
Wang Y. et al. (2015) and Velagapudi et al. (2017b) suggested that alteration of NF- κβ by thymoquinone may also be mediated through inhibition of Iκβα phosphorylation or P65 phosphorylation (Wang Y. et al., 2015; Velagapudi et al., 2017b). Thymoquinone has also been reported to exert inhibitory effects on AGE-induced NF-κB-activation in human proximal tubular epithelial cells (pTECs) stimulated with advanced glycation end products (AGEs) (Sayed and Morcos, 2007). All these findings showed the mechanism of thymoquinone in reducing the pro-inflammatory NF-κβ signaling pathways, which can be a reflection of its potential neuroprotective effects in ocular degeneration.
Apart from NF-κβ signaling, thymoquinone also affects – Nrf2 signaling, a master regulator of oxidative stress. Both NF-kB and Nrf2 signaling pathways are inter-related. Increase in NF-κB activation is followed by a decline in Nrf2 signaling pathway, thus further increasing inflammation response. Nrf2 is a transcription factor that is involved in the cellular defense against oxidative stress by controlling the expression of antioxidant-related gene expression resulting in anti-inflammatory response in the cells (Vomund et al., 2017). Nrf2 is thought to play a pivotal role in inflammation because its protein products are responsible for detoxification and elimination of oxidative stress in the cells (Nguyen et al., 2009). Mounting evidence suggests that aging induces a decline in the antioxidant capacity via a reduction in Nrf2 signaling.
The importance of Nrf2 signaling in the pathogenesis of AMD was highlighted when Nrf2-deficient mice developed features similar to human AMD. These mice exhibit pathological characteristics of human AMD including progressive RPE and Bruch’s membrane degeneration, drusen deposits and lipofuscin accumulation, and decreased electroretinography responses (Zhao et al., 2011).
In the RPE, under oxidative stress conditions, the Nrf2 signaling is impaired. Nrf2 transcription factors are lowered, and hence are not able to translocate to the nucleus to induce an antioxidant response. This renders the RPE and photoreceptors vulnerable to damage. Catanzaro et al. (2020) studied the role of Nrf2 on wild type (WT) and Nrf2-silenced (siNrf2)- ARPE-19 cells exposed to various AMD-related noxae (H2O2, 4-HNE, MG132 + Bafilomycin) (Catanzaro et al., 2020). The study showed that in Nrf2 knockout group, cells present a higher susceptibility to oxidative insults as compared to WT groups, suggesting that the Nrf2 induction represents an efficient defensive strategy to prevent the stress-induced damage. Increasing Nrf2-regulated glutathione levels and NAD(P)H attenuated ultraviolet light-induced RPE damage by Gao and Talalay (2004). Carotenoids zeaxanthin and lutein were shown to preserve photoreceptors against light damage by mitigating oxidative stress through Nrf2 signaling (Frede et al., 2017; Yu et al., 2018).
Thymoquinone has been shown to increase activation of Nrf2 signaling. Anti-inflammatory effects of thymoquinone in BV2 microglial cells occurred via the Nrf2 signaling pathway. Activation of Nrf2 signaling by thymoquinone was found to be accompanied by increased nuclear localization, DNA binding and transcriptional activity of Nrf2, as well as increasing protein levels of HO-1 and NQO1. Suppression of Nrf2 activity through siRNA or with the use of trigonelline resulted in the loss of this effect (Velagapudi et al., 2017b). The same results were also observed in models of lung fibrosis (Ahmad et al., 2020), kidney injury (Al Fayi et al., 2020), lung injury (Mao et al., 2020), and allergy response (Dera et al., 2020c). Taken together, these data demonstrated the anti-inflammatory effect of thymoquinone through Nrf2 signaling.
Proteostasis is regulated by a complex network of cellular mechanisms that monitors the concentration, folding, cellular localization, and interactions of proteins from their synthesis through their cellular degradation. Decline in proteostasis is one of the hallmarks of aging and accumulation of damaged protein leads to age related diseases such AD, PD, AMD, glaucoma and cataract. Proteosomes complex is a non-lysosomal ATP-dependent degradation system responsible in removal of damaged, oxidized and misfolded proteins in cells through a process called autophagy (Saez and Vilchez, 2014).
In the eye, autophagy occurs in the lens, cornea, photoreceptors, and RPE cells. Autophagy plays a role in lens fiber cells and corneal maturation (Costello et al., 2013). And maintains the inner segment turnover and survival of the photoreceptor. Within RPE cells, autophagy is involved in aspects of development, cell survival in response to stress, melanin degradation, as well as the degradation of toxic cellular components or damaged organelles. The importance of autophagy in these tissue has been discussed (Frost et al., 2014).
Damaged protein degradation is crucial in cells before the accumulated proteins aggregated. The 20S proteasome is the central core of this system and acts as a part of the intracellular antioxidant defense system (Bonfili et al., 2008). It is estimated that more than 20% of proteins in mammalian cells are degraded by the 20S proteasomes (Baugh et al., 2009). The 20S proteasomes recognized damage proteins through specific degradation signals such as multi-ubiquitin chain tag (Sahu et al., 2021). For a protein to be tagged for degradation, a series of reaction was carried out by E1 (ubiquitin-activating), E2 (ubiquitin-conjugating), and E3 (ubiquitin-ligating) enzymes to create a ubiquitin tag that consist of at least 4 ubiquitin molecules. The chain of ubiquitin directs the damaged proteins to the 20S proteasomes where proteolytic degradation occurs (Ravid and Hochstrasser, 2008; Saez and Vilchez, 2014).
Thymoquinone has been demonstrated to induces selective and time-dependent proteasome complex modulation. However, the mechanism of thymoquinone has only been observed in cancer cells (Cecarini et al., 2010). In glioblastoma cells, thymoquinone has been shown to induce proteosome complex inhibition. This inhibition is due to compromised 20S proteosomes complex functionality and not to downregulation of its synthesis as observed through western blotting and 20S proteosomes activity analysis. In addition, E3 ubiquitin ligase was also downregulated with thymoquinone treatment in acute leukemia model (Alghamdi et al., 2020). Based on this evidence in cancer subject and with the importance of autophagy in the eye, study on thymoquinone in proteosome might provide a novel mechanism of thymoquinone in ocular disease application.
Thymoquinone has been traditionally and still widely used to treat various conditions due to its medicinal properties. Modern scientific studies showed the beneficial neuroprotective effects of thymoquinone on AD and PD experimental models. Further studies demonstrated the mechanistic pathways involved in the anti-inflammatory and antioxidant properties of thymoquinone in various in vitro and in vivo models. Thymoquinone has been shown to exert its protective effects through the NF-κβ and Nrf2 signaling pathways, and the anti-oxidative activity. These findings provide the basis of the potential therapeutic effects of thymoquinone in ocular degenerative diseases as similar mechanisms of action occur and signaling pathways are involved in degenerative retinal conditions such as AMD. More studies of thymoquinone effects on the retina are needed to provide a deeper understanding of the mechanism of thymoquinone on a cellular level, before clinical trials can be performed.
NM drafted the manuscript. TK, NM, and LP reviewed and revised the initial manuscript. NK reviewed the manuscript draft. All authors read and approved the final manuscript.
This research was supported by University of Malaya Research Grant (UMRG Program) – HTM (Wellness) (Grant Number: RP033-14HTM), Alcon Research Institute Grant (IF011-2020), High Impact Research Grant, Ministry of Higher Education, Malaysia (Grant Number: H-20001-00-E000059), and UMSC CareFund 2021.
The authors declare that the research was conducted in the absence of any commercial or financial relationships that could be construed as a potential conflict of interest.
All claims expressed in this article are solely those of the authors and do not necessarily represent those of their affiliated organizations, or those of the publisher, the editors and the reviewers. Any product that may be evaluated in this article, or claim that may be made by its manufacturer, is not guaranteed or endorsed by the publisher.
Abd El-Ghany, R. M., Sharaf, N. M., Kassem, L. A., Mahran, L. G., and Heikal, O. A. (2009). Thymoquinone triggers anti-apoptotic signaling targeting death ligand and apoptotic regulators in a model of hepatic ischemia reperfusion injury. Drug Discov. Ther. 3, 296–306.
Abulfadl, Y. S., El-Maraghy, N. N., Ahmed, A. A. E., Nofal, S., and Badary, O. A. (2018b). Protective effects of thymoquinone on D-galactose and aluminum chloride induced neurotoxicity in rats: biochemical, histological and behavioral changes. Neurol. Res. 40, 324–333. doi: 10.1080/01616412.2018.1441776
Abulfadl, Y. S., El-Maraghy, N. N., Ahmed, A. E., Nofal, S., Abdel-Mottaleb, Y., and Badary, O. A. (2018c). Thymoquinone alleviates the experimentally induced Alzheimer’s disease inflammation by modulation of TLRs signaling. Hum. Exp. Toxicol. 37, 1092–1104. doi: 10.1177/0960327118755256
Abulfadl, Y. S., El-Maraghy, N., Ahmed, A. E., Nofal, S., Abdel-Mottaleb, Y., and Badary, O. A. (2018a). Thymoquinone alleviates the experimentally induced Alzheimer’s disease inflammation by modulation of TLRs signaling. Hum. Exp. Toxicol. 37, 1092–1104.
Ahmad, A., Alkharfy, K. M., Jan, B. L., Ahad, A., Ansari, M. A., Al-Jenoobi, F. I., et al. (2020). Thymoquinone treatment modulates the Nrf2/HO-1 signaling pathway and abrogates the inflammatory response in an animal model of lung fibrosis. Exp. Lung Res. 46, 53–63. doi: 10.1080/01902148.2020.1726529
Ahuja, S., Saxena, S., Akduman, L., Meyer, C. H., Kruzliak, P., and Khanna, V. K. (2019). Serum vascular endothelial growth factor is a biomolecular biomarker of severity of diabetic retinopathy. Int. J. Retina Vitreous 5:29. doi: 10.1186/s40942-019-0179-6
Al Fayi, M., Otifi, H., Alshyarba, M., Dera, A. A., and Rajagopalan, P. (2020). Thymoquinone and curcumin combination protects cisplatin-induced kidney injury, nephrotoxicity by attenuating NFκB, KIM-1 and ameliorating Nrf2/HO-1 signalling. J. Drug Target 28, 913–922. doi: 10.1080/1061186x.2020.1722136
Alghamdi, R., Hassan, M., Kaleem, M., Kayali, A., Halwani, M., Zamzami, M., et al. (2020). Targeting Itch/p73 pathway by thymoquinone as a novel therapeutic strategy for cancers with p53 mutation. Eur. J. Cell Sci. 2, 20–26.
Alhebshi, A. H., Gotoh, M., and Suzuki, I. (2013). Thymoquinone protects cultured rat primary neurons against amyloid β-induced neurotoxicity. Biochem. Biophys. Res. Commun. 433, 362–367. doi: 10.1016/j.bbrc.2012.11.139
Alhebshi, A. H., Odawara, A., Gotoh, M., and Suzuki, I. (2014). Thymoquinone protects cultured hippocampal and human induced pluripotent stem cells-derived neurons against α-synuclein-induced synapse damage. Neurosci. Lett. 570, 126–131. doi: 10.1016/j.neulet.2013.09.049
Alhibshi, A. H., Odawara, A., and Suzuki, I. (2019). Neuroprotective efficacy of thymoquinone against amyloid beta-induced neurotoxicity in human induced pluripotent stem cell-derived cholinergic neurons. Biochem. Biophys. Rep. 17, 122–126. doi: 10.1016/j.bbrep.2018.12.005
Al-Majed, A. A., Al-Omar, F. A., and Nagi, M. N. (2006). Neuroprotective effects of thymoquinone against transient forebrain ischemia in the rat hippocampus. Eur. J. Pharmacol. 543, 40–47. doi: 10.1016/j.ejphar.2006.05.046
Ammar el, S. M., Gameil, N. M., Shawky, N. M., and Nader, M. A. (2011). Comparative evaluation of anti-inflammatory properties of thymoquinone and curcumin using an asthmatic murine model. Int. Immunopharmacol. 11, 2232–2236. doi: 10.1016/j.intimp.2011.10.013
Angeles, D. C., Ho, P., Dymock, B. W., Lim, K. L., Zhou, Z. D., and Tan, E. K. (2016). Antioxidants inhibit neuronal toxicity in Parkinson’s disease-linked LRRK2. Ann. Clin. Transl. Neurol. 3, 288–294. doi: 10.1002/acn3.282
Anwar, S., Khan, M. A., Sadaf, A., and Younus, H. (2014). A structural study on the protection of glycation of superoxide dismutase by thymoquinone. Int. J. Biol. Macromol. 69, 476–481. doi: 10.1016/j.ijbiomac.2014.06.003
Aoyama, K., and Nakaki, T. (2015). Glutathione in Cellular Redox Homeostasis: association with the Excitatory Amino Acid Carrier 1 (EAAC1). Molecules 20, 8742–8758. doi: 10.3390/molecules20058742
Araki, N., Ueno, N., Chakrabarti, B., Morino, Y., and Horiuchi, S. (1992). Immunochemical evidence for the presence of advanced glycation end products in human lens proteins and its positive correlation with aging. J. Biol. Chem. 267, 10211–10214.
Ardah, M. T., Merghani, M. M., and Haque, M. E. (2019). Thymoquinone prevents neurodegeneration against MPTP in vivo and modulates α-synuclein aggregation in vitro. Neurochem. Int. 128, 115–126. doi: 10.1016/j.neuint.2019.04.014
Armutcu, F., Akyol, S., and Akyol, O. (2018). The interaction of glutathione and thymoquinone and their antioxidant properties. Electr. J. Gener. Med. 15, 1–8.
Ascaso, F. J., Huerva, V., and Grzybowski, A. (2014). The Role of Inflammation in THE Pathogenesis of Macular Edema Secondary to Retinal Vascular Diseases. Mediat. inflammat. 2014:432685. doi: 10.1155/2014/432685
Atteya, M., Ahmed, A. M., AlRabiah, A., Al-Matrafi, T. A., Arafah, M. M., Al-Saggaf, S., et al. (2019). Thymoquinone and curcumin modify inducible nitric oxide synthase, caspase 3, and thioredoxin immunohistochemical expression in acetaminophen hepatotoxicity. Folia Morphol. 78, 773–788. doi: 10.5603/FM.a2019.0027
Badary, O. A., Taha, R. A., Gamal El-Din, A. M., and Abdel-Wahab, M. H. (2003). Thymoquinone Is a Potent Superoxide Anion Scavenger. Drug Chem. Toxicol. 26, 87–98. doi: 10.1081/DCT-120020404
Baugh, J. M., Viktorova, E. G., and Pilipenko, E. V. (2009). Proteasomes Can Degrade a Significant Proportion of Cellular Proteins Independent of Ubiquitination. J. Mol. Biol. 386, 814–827. doi: 10.1016/j.jmb.2008.12.081
Beatty, S., Koh, H.-H., Phil, M., Henson, D., and Boulton, M. (2000). The role of oxidative stress in the pathogenesis of age-related macular degeneration. Survey Ophthalmol. 45, 115–134.
Benvidi, A., Rezaeinasab, M., Gharaghani, S., and Abbasi, S. (2018). Monitoring the protective ability of thymoquinone mixture with p-cymene against bovine serum albumin (BSA) glycation: mCR-ALS analysis based on combined spectroscopic and electrochemical methods. Int. J. Biol. Macromol. 107, 2465–2474. doi: 10.1016/j.ijbiomac.2017.10.135
Bergers, G., and Song, S. (2005). The role of pericytes in blood-vessel formation and maintenance. Neuro Oncol. 7, 452–464. doi: 10.1215/S1152851705000232
Bonfili, L., Cecarini, V., Amici, M., Cuccioloni, M., Angeletti, M., Keller, J. N., et al. (2008). Natural polyphenols as proteasome modulators and their role as anti-cancer compounds. Febs J. 275, 5512–5526. doi: 10.1111/j.1742-4658.2008.06696.x
Boudiaf, K., Hurtado-Nedelec, M., Belambri, S. A., Marie, J.-C., Derradji, Y., Benboubetra, M., et al. (2016). Thymoquinone strongly inhibits fMLF-induced neutrophil functions and exhibits anti-inflammatory properties in vivo. Biochem. Pharmacol. 104, 62–73. doi: 10.1016/j.bcp.2016.01.006
Bouhlel, A., Bejaoui, M., Ben Mosbah, I., Hadj Abdallah, N., Ribault, C., Viel, R., et al. (2018). Thymoquinone protects rat liver after partial hepatectomy under ischaemia/reperfusion through oxidative stress and endoplasmic reticulum stress prevention. Clin. Exp. Pharmacol. Physiol. 45, 943–951. doi: 10.1111/1440-1681.12961
Bouhlel, A., Ben Mosbah, I., Hadj Abdallah, N., Ribault, C., Viel, R., Mannaï, S., et al. (2017). Thymoquinone prevents endoplasmic reticulum stress and mitochondria-induced apoptosis in a rat model of partial hepatic warm ischemia reperfusion. Biomed. Pharmacother. 94, 964–973. doi: 10.1016/j.biopha.2017.08.018
Bourne, R. R., Adelson, J., Flaxman, S., Briant, P., Bottone, M., Vos, T., et al. (2020). Global Prevalence of Blindness and Distance and Near Vision Impairment in 2020: progress towards the Vision 2020 targets and what the future holds. Investig. Ophthalmol. Visual Sci. 61:2317.
Bourne, R., Steinmetz, J. D., Flaxman, S., Briant, P. S., Taylor, H. R., Resnikoff, S., et al. (2021). Trends in prevalence of blindness and distance and near vision impairment over 30 years: an analysis for the Global Burden of Disease Study. Lancet Global Health 9, e130–e143. doi: 10.1016/S2214-109X(20)30425-3
Bradshaw, P. C. (2019). Cytoplasmic and Mitochondrial NADPH-Coupled Redox Systems in the Regulation of Aging. Nutrients 11:504.
Braidy, N., Guillemin, G. J., Mansour, H., Chan-Ling, T., Poljak, A., and Grant, R. (2011). Age related changes in NAD+ metabolism oxidative stress and Sirt1 activity in wistar rats. PLoS One 6:e19194. doi: 10.1371/journal.pone.0019194
Burton, G. J., and Jauniaux, E. (2011). Oxidative stress. Best Pract. Res. Clin. Obstetr. Gynaecol. 25, 287–299.
Calabrese, V., Sultana, R., Scapagnini, G., Guagliano, E., Sapienza, M., Bella, R., et al. (2006). Nitrosative stress, cellular stress response, and thiol homeostasis in patients with Alzheimer’s disease. Antioxid. Redox Signal. 8, 1975–1986.
Catanzaro, M., Lanni, C., Basagni, F., Rosini, M., Govoni, S., and Amadio, M. (2020). Eye-light on age-related macular degeneration: targeting nrf2-pathway as a novel therapeutic strategy for retinal pigment epithelium. Front. Pharmacol. 11:844.
Cecarini, V., Quassinti, L., Di Blasio, A., Bonfili, L., Bramucci, M., Lupidi, G., et al. (2010). Effects of thymoquinone on isolated and cellular proteasomes. Febs J. 277, 2128–2141. doi: 10.1111/j.1742-4658.2010.07629.x
Chakravarty, N. (1993). Inhibition of histamine release from mast cells by nigellone. Ann. Allergy 70, 237–242.
Charakidas, A., Kalogeraki, A., Tsilimbaris, M., Koukoulomatis, P., Brouzas, D., and Delides, G. (2005). Lens Epithelial Apoptosis and Cell Proliferation in Human Age-Related Cortical Cataract. Eur. J. Ophthalmol. 15, 213–220. doi: 10.1177/112067210501500206
Chen, L., Deng, H., Cui, H., Fang, J., Zuo, Z., Deng, J., et al. (2017). Inflammatory responses and inflammation-associated diseases in organs. Oncotarget 9, 7204–7218. doi: 10.18632/oncotarget.23208
Chen, Y., Wang, B., and Zhao, H. (2018). Thymoquinone reduces spinal cord injury by inhibiting inflammatory response, oxidative stress and apoptosis via PPAR-γ and PI3K/Akt pathways. Exp. Ther. Med. 15, 4987–4994. doi: 10.3892/etm.2018.6072
Cobourne-Duval, M. K., Taka, E., Mendonca, P., and Soliman, K. F. (2018). Thymoquinone increases the expression of neuroprotective proteins while decreasing the expression of pro-inflammatory cytokines and the gene expression NFκB pathway signaling targets in LPS/IFNγ-activated BV-2 microglia cells. J. Neuroimmunol. 320, 87–97.
Cobourne-Duval, M. K., Taka, E., Mendonca, P., Bauer, D., and Soliman, K. F. (2016). The Antioxidant Effects of Thymoquinone in Activated BV-2 Murine Microglial Cells. Neurochem. Res. 41, 3227–3238. doi: 10.1007/s11064-016-2047-1
Coenraads, P. J., Bleumink, E., and Nater, J. P. (1975). Susceptibility to primary irritants: age dependence and relation to contact allergic reactions. Contact Dermat. 1, 377–381. doi: 10.1111/j.1600-0536.1975.tb05478.x
Comitato, A., Sanges, D., Rossi, A., Humphries, M. M., and Marigo, V. (2014). Activation of Bax in Three Models of Retinitis Pigmentosa. Investig. Ophthalmol. Vis. Sci. 55, 3555–3561. doi: 10.1167/iovs.14-13917
Costello, M. J., Brennan, L. A., Basu, S., Chauss, D., Mohamed, A., Gilliland, K. O., et al. (2013). Autophagy and mitophagy participate in ocular lens organelle degradation. Exp. Eye Res. 116, 141–150. doi: 10.1016/j.exer.2013.08.017
Cui, H., Kong, Y., and Zhang, H. (2012). Oxidative stress, mitochondrial dysfunction, and aging. J. Signal Transd. 2012:646354. doi: 10.1155/2012/646354
Dalli, T., Beker, M., Terzioglu-Usak, S., Akbas, F., and Elibol, B. (2018). Thymoquinone activates MAPK pathway in hippocampus of streptozotocin-treated rat model. Biomed. Pharmacother. 99, 391–401. doi: 10.1016/j.biopha.2018.01.047
Das, U. N. (2016). Diabetic macular edema, retinopathy and age-related macular degeneration as inflammatory conditions. Arch. Med. Sci. 12, 1142–1157. doi: 10.5114/aoms.2016.61918
Dera, A. A., Al Fayi, M., Otifi, H., Alshyarba, M., Alfhili, M., and Rajagopalan, P. (2020a). Thymoquinone (Tq) protects necroptosis induced by autophagy/mitophagy-dependent oxidative stress in human bronchial epithelial cells exposed to cigarette smoke extract (CSE). J. Food Biochem. 44:e13366. doi: 10.1111/jfbc.13366
Dera, A. A., Rajagopalan, P., Alfhili, M. A., Ahmed, I., and Chandramoorthy, H. C. (2020b). Thymoquinone attenuates oxidative stress of kidney mitochondria and exerts nephroprotective effects in oxonic acid-induced hyperuricemia rats. Biofactors 46, 292–300. doi: 10.1002/biof.1590
Dera, A., Rajagopalan, P., Ahmed, I., Alfhili, M., Alsughayyir, J., and Chandramoorthy, H. C. (2020c). Thymoquinone attenuates IgE-mediated allergic response via pi3k-Akt-NFκB pathway and upregulation of the Nrf2-HO1 axis. J. Food Biochem. 44:e13216. doi: 10.1111/jfbc.13216
Desai, S., Saheb, S. H., Das, K. K., and Haseena, S. (2015). Effect of thymoquinone on MDA and SOD levels in sterptozotocine induced diabetic albino rats. J. Pharmaceut. Sci. Res. 7:523.
Domènech, E. B., and Marfany, G. (2020). The Relevance of Oxidative Stress in the Pathogenesis and Therapy of Retinal Dystrophies. Antioxidants 9:347. doi: 10.3390/antiox9040347
Dong, J., Zhang, X., Wang, S., Xu, C., Gao, M., Liu, S., et al. (2020). Thymoquinone Prevents Dopaminergic Neurodegeneration by Attenuating Oxidative Stress Via the Nrf2/ARE Pathway. Front. Pharmacol. 11:615598. doi: 10.3389/fphar.2020.615598
Dunaief, J. L., Dentchev, T., Ying, G. S., and Milam, A. H. (2002). The role of apoptosis in age-related macular degeneration. Arch. Ophthalmol. 120, 1435–1442. doi: 10.1001/archopht.120.11.1435
Ebrahimi, S. S., Oryan, S., Izadpanah, E., and Hassanzadeh, K. (2017). Thymoquinone exerts neuroprotective effect in animal model of Parkinson’s disease. Toxicol. Lett. 276, 108–114. doi: 10.1016/j.toxlet.2017.05.018
Eilken, H. M., Diéguez-Hurtado, R., Schmidt, I., Nakayama, M., Jeong, H.-W., Arf, H., et al. (2017). Pericytes regulate VEGF-induced endothelial sprouting through VEGFR1. Nat. Commun. 8:1574. doi: 10.1038/s41467-017-01738-3
El Gazzar, M. A., El Mezayen, R., Nicolls, M. R., and Dreskin, S. C. (2007). Thymoquinone attenuates proinflammatory responses in lipopolysaccharide-activated mast cells by modulating NF-kappaB nuclear transactivation. Biochim. Biophys. Acta 1770, 556–564. doi: 10.1016/j.bbagen.2007.01.002
Elibol, B., Beker, M., Terzioglu-Usak, S., Dalli, T., and Kilic, U. (2020). Thymoquinone administration ameliorates Alzheimer’s disease-like phenotype by promoting cell survival in the hippocampus of amyloid beta(1-42) infused rat model. Phytomedicine 79:153324. doi: 10.1016/j.phymed.2020.153324
Elibol, B., Terzioglu-Usak, S., Beker, M., and Sahbaz, C. (2019). Thymoquinone (TQ) demonstrates its neuroprotective effect via an anti-inflammatory action on the Aβ(1–42)-infused rat model of Alzheimer’s disease. Psychiatry Clin. Psychopharmacol. 29, 379–386. doi: 10.1080/24750573.2019.1673945
El-Shemi, A. G., Kensara, O. A., Alsaegh, A., and Mukhtar, M. H. (2018). Pharmacotherapy with Thymoquinone Improved Pancreatic β-Cell Integrity and Functional Activity, Enhanced Islets Revascularization, and Alleviated Metabolic and Hepato-Renal Disturbances in Streptozotocin-Induced Diabetes in Rats. Pharmacology 101, 9–21. doi: 10.1159/000480018
Fahmy, H. M., Saad, E., Sabra, N. M., El-Gohary, A. A., Mohamed, F. F., and Gaber, M. H. (2018). Treatment merits of Latanoprost/Thymoquinone - Encapsulated liposome for glaucomatus rabbits. Int. J. Pharm. 548, 597–608. doi: 10.1016/j.ijpharm.2018.07.012
Feenstra, D. J., Yego, E. C., and Mohr, S. (2013). Modes of Retinal Cell Death in Diabetic Retinopathy. J. Clin. Exp. Ophthalmol. 4, 298–298. doi: 10.4172/2155-9570.1000298
Ferrington, D. A., Ebeling, M. C., Kapphahn, R. J., Terluk, M. R., Fisher, C. R., Polanco, J. R., et al. (2017). Altered bioenergetics and enhanced resistance to oxidative stress in human retinal pigment epithelial cells from donors with age-related macular degeneration. Redox Biol. 13, 255–265. doi: 10.1016/j.redox.2017.05.015
Firdaus, F., Zafeer, M. F., Waseem, M., Ullah, R., Ahmad, M., and Afzal, M. (2018). Thymoquinone alleviates arsenic induced hippocampal toxicity and mitochondrial dysfunction by modulating mPTP in Wistar rats. Biomed. Pharmacother. 102, 1152–1160. doi: 10.1016/j.biopha.2018.03.159
Forrester, J. V., Kuffova, L., and Delibegovic, M. (2020). The Role of Inflammation in Diabetic Retinopathy. Front. Immunol. 11:583687. doi: 10.3389/fimmu.2020.583687
Fouad, A. A., and Alwadani, F. (2015). Ameliorative effects of thymoquinone against eye lens changes in streptozotocin diabetic rats. Environ. Toxicol. Pharmacol. 40, 960–965. doi: 10.1016/j.etap.2015.09.010
Frede, K., Ebert, F., Kipp, A. P., Schwerdtle, T., and Baldermann, S. (2017). Lutein activates the transcription factor Nrf2 in human retinal pigment epithelial cells. J. Agric. Food Chem. 65, 5944–5952.
Frost, L. S., Mitchell, C. H., and Boesze-Battaglia, K. (2014). Autophagy in the eye: implications for ocular cell health. Exp. Eye Res. 124, 56–66. doi: 10.1016/j.exer.2014.04.010
Gao, X., and Talalay, P. (2004). Induction of phase 2 genes by sulforaphane protects retinal pigment epithelial cells against photooxidative damage. Proc. Natl. Acad. Sci. U. S. A. 101, 10446–10451. doi: 10.1073/pnas.0403886101
Ghelli, A., Zanna, C., Porcelli, A. M., Schapira, A. H. V., Martinuzzi, A., Carelli, V., et al. (2003). Leber’s Hereditary Optic Neuropathy (LHON) Pathogenic Mutations Induce Mitochondrial-dependent Apoptotic Death in Transmitochondrial Cells Incubated with Galactose Medium*. J. Biol. Chem. 278, 4145–4150. doi: 10.1074/jbc.M210285200
Giacco, F., and Brownlee, M. (2010). Oxidative stress and diabetic complications. Circ. Res. 107, 1058–1070. doi: 10.1161/CIRCRESAHA.110.223545
Goldin, A., Beckman, J. A., Schmidt, A. M., and Creager, M. A. (2006). Advanced Glycation End Products: Sparking the Development of Diabetic Vascular Injury. Circulation 114, 597–605. doi: 10.1161/circulationaha.106.621854
Golestaneh, N., Chu, Y., Xiao, Y. Y., Stoleru, G. L., and Theos, A. C. (2017). Dysfunctional autophagy in RPE, a contributing factor in age-related macular degeneration. Cell Death Dis. 8:e2537. doi: 10.1038/cddis.2016.453
Goyal, S. N., Prajapati, C. P., Gore, P. R., Patil, C. R., Mahajan, U. B., Sharma, C., et al. (2017). Therapeutic potential and pharmaceutical development of thymoquinone: a multitargeted molecule of natural origin. Front. Pharmacol. 8:656.
Gray, J. P., Burgos, D. Z., Yuan, T., Seeram, N., Rebar, R., Follmer, R., et al. (2016). Thymoquinone, a bioactive component of Nigella sativa, normalizes insulin secretion from pancreatic β-cells under glucose overload via regulation of malonyl-CoA. Am. J. Physiol. Endocrinol. Metabol. 310, E394–E404. doi: 10.1152/ajpendo.00250.2015
Gurung, R. L., Lim, S. N., Khaw, A. K., Soon, J. F. F., Shenoy, K., Mohamed Ali, S., et al. (2010). Thymoquinone induces telomere shortening. DNA damage and apoptosis in human glioblastoma cells. PLoS One 5:e12124. doi: 10.1371/journal.pone.0012124
Hamdan, A. M., Al-Gayyar, M. M., Shams, M. E. E., Alshaman, U. S., Prabahar, K., Bagalagel, A., et al. (2019). Thymoquinone therapy remediates elevated brain tissue inflammatory mediators induced by chronic administration of food preservatives. Sci. Rep. 9:7026. doi: 10.1038/s41598-019-43568-x
Hayat, K., Asim, M. B., Nawaz, M., Li, M., Zhang, L., and Sun, N. (2011). Ameliorative effect of thymoquinone on ovalbumin-induced allergic conjunctivitis in Balb/c mice. Curr. Eye Res. 36, 591–598. doi: 10.3109/02713683.2011.573898
He, Y., Leung, K. W., Zhang, Y.-H., Duan, S., Zhong, X.-F., Jiang, R.-Z., et al. (2008). Mitochondrial Complex I Defect Induces ROS Release and Degeneration in Trabecular Meshwork Cells of POAG Patients: protection by Antioxidants. Investig. Ophthalmol. Vis. Sci. 49, 1447–1458. doi: 10.1167/iovs.07-1361
Ho, M.-C., Peng, Y.-J., Chen, S.-J., and Chiou, S.-H. (2010). Senile cataracts and oxidative stress. J. Clin. Gerontol. Geriatr. 1, 17–21. doi: 10.1016/j.jcgg.2010.10.006
Homayouni, M. (2009). Vascular endothelial growth factors and their inhibitors in ocular neovascular disorders. J. Ophthalm. Vision Res. 4, 105–114.
Hosseinian, S., Rad, A. K., Bideskan, A. E., Soukhtanloo, M., Sadeghnia, H., Shafei, M. N., et al. (2017). Thymoquinone ameliorates renal damage in unilateral ureteral obstruction in rats. Pharmacol. Rep. 69, 648–657. doi: 10.1016/j.pharep.2017.03.002
Howes, K. A., Liu, Y., Dunaief, J. L., Milam, A., Frederick, J. M., Marks, A., et al. (2004). Receptor for advanced glycation end products and age-related macular degeneration. Investig. Ophthalmol. Vis. Sci. 45, 3713–3720.
Hu, X., Liang, Y., Zhao, B., and Wang, Y. (2019). Thymoquinone protects human retinal pigment epithelial cells against hydrogen peroxide induced oxidative stress and apoptosis. J. Cell. Biochem. 120, 4514–4522. doi: 10.1002/jcb.27739
Hussain, T., Tan, B., Yin, Y., Blachier, F., Tossou, M. C. B., and Rahu, N. (2016). Oxidative Stress and Inflammation: what Polyphenols Can Do for Us? Oxidat. Med. Cell. Longev. 2016:7432797. doi: 10.1155/2016/7432797
Ibrahim AbdEl Fattah, L., Zickri, M. B., Aal, L. A., Heikal, O., and Osama, E. (2016). The Effect of Thymoquinone, α7 Receptor Agonist and α7 Receptor Allosteric Modulator on the Cerebral Cortex in Experimentally Induced Alzheimer’s Disease in Relation to MSCs Activation. Int. J. Stem Cells 9, 230–238. doi: 10.15283/ijsc16021
Ishibashi, T., Murata, T., Hangai, M., Nagai, R., Horiuchi, S., Lopez, P. F., et al. (1998). Advanced glycation end products in age-related macular degeneration. Arch. Ophthalmol. 116, 1629–1632.
Ismail, N., Ismail, M., Azmi, N. H., Bakar, M. F. A., Yida, Z., Abdullah, M. A., et al. (2017a). Thymoquinone-rich fraction nanoemulsion (TQRFNE) decreases Aβ40 and Aβ42 levels by modulating APP processing, up-regulating IDE and LRP1, and down-regulating BACE1 and RAGE in response to high fat/cholesterol diet-induced rats. Biomed. Pharmacother. 95, 780–788. doi: 10.1016/j.biopha.2017.08.074
Ismail, N., Ismail, M., Azmi, N. H., Bakar, M. F. A., Yida, Z., Stanslas, J., et al. (2017b). Beneficial effects of TQRF and TQ nano- and conventional emulsions on memory deficit, lipid peroxidation, total antioxidant status, antioxidants genes expression and soluble Aβ levels in high fat-cholesterol diet-induced rats. Chem. Biol. Interact. 275, 61–73. doi: 10.1016/j.cbi.2017.07.014
Ismail, N., Ismail, M., Mazlan, M., Latiff, L. A., Imam, M. U., Iqbal, S., et al. (2013). Thymoquinone prevents β-amyloid neurotoxicity in primary cultured cerebellar granule neurons. Cell Mol. Neurobiol. 33, 1159–1169. doi: 10.1007/s10571-013-9982-z
Izzotti, A., Bagnis, A., and Saccà, S. C. (2006). The role of oxidative stress in glaucoma. Mutat. Res. 612, 105–114. doi: 10.1016/j.mrrev.2005.11.001
Jadeja, R. N., and Martin, P. M. (2021). Oxidative Stress and Inflammation in Retinal Degeneration. Switzerland: Multidisciplinary Digital Publishing Institute.
Kaarniranta, K., Salminen, A., Haapasalo, A., Soininen, H., and Hiltunen, M. (2011). Age-related macular degeneration (AMD): Alzheimer’s disease in the eye? J. Alzheimers Dis. 24, 615–631.
Kalemci, S., Cilaker Micili, S., Acar, T., Senol, T., Dirican, N., Omeroglu, G., et al. (2013). Effectiveness of thymoquinone in the treatment of experimental asthma. Clin. Ter. 164, e155–e158. doi: 10.7417/ct.2013.1559
Kanwar, M., Chan, P. S., Kern, T. S., and Kowluru, R. A. (2007). Oxidative damage in the retinal mitochondria of diabetic mice: possible protection by superoxide dismutase. Invest. Ophthalmol. Vis. Sci. 48, 3805–3811. doi: 10.1167/iovs.06-1280
Kassab, R. B., and El-Hennamy, R. E. (2017). The role of thymoquinone as a potent antioxidant in ameliorating the neurotoxic effect of sodium arsenate in female rat. Egypt. J. Basic Appl. Sci. 4, 160–167. doi: 10.1016/j.ejbas.2017.07.002
Kauppinen, A., Paterno, J. J., Blasiak, J., Salminen, A., and Kaarniranta, K. (2016). Inflammation and its role in age-related macular degeneration. Cell. Mol. Life Sci. 73, 1765–1786. doi: 10.1007/s00018-016-2147-8
Keyhanmanesh, R., Pejman, L., Omrani, H., Mirzamohammadi, Z., and Shahbazfar, A. A. (2014). The effect of single dose of thymoquinone, the main constituents of Nigella sativa, in guinea pig model of asthma. Bioimpacts 4, 75–81. doi: 10.5681/bi.2014.006
Khalfaoui, T., Basora, N., and Ouertani-Meddeb, A. (2010). Apoptotic factors (Bcl-2 and Bax) and diabetic retinopathy in type 2 diabetes. J. Mol. Histol. 41, 143–152. doi: 10.1007/s10735-010-9271-9
Khalife, K. H., and Lupidi, G. (2007). Nonenzymatic reduction of thymoquinone in physiological conditions. Free Radic. Res. 41, 153–161. doi: 10.1080/10715760600978815
Khan, A., Vaibhav, K., Javed, H., Khan, M. M., Tabassum, R., Ahmed, M. E., et al. (2012). Attenuation of Aβ-induced neurotoxicity by thymoquinone via inhibition of mitochondrial dysfunction and oxidative stress. Mol. Cell Biochem. 369, 55–65. doi: 10.1007/s11010-012-1368-x
Kim, K. Y., Perkins, G. A., Shim, M. S., Bushong, E., Alcasid, N., Ju, S., et al. (2015). DRP1 inhibition rescues retinal ganglion cells and their axons by preserving mitochondrial integrity in a mouse model of glaucoma. Cell Death Dis. 6:e1839. doi: 10.1038/cddis.2015.180
Kocatürk, T., Erkan, E., Meteoğlu, l̇, Ekici, M., Karul Büyüköztürk, A., and Yavaşoğlu, l̇, et al. (2018). Effects of Topical Thymoquinone in an Experimental Dry Eye Model. Turk. J. Ophthalmol. 48, 281–287. doi: 10.4274/tjo.50146
Kowluru, R. A., and Abbas, S. N. (2003). Diabetes-induced mitochondrial dysfunction in the retina. Invest. Ophthalmol. Vis. Sci. 44, 5327–5334. doi: 10.1167/iovs.03-0353
Lingappan, K. (2018). NF-κB in Oxidative Stress. Curr. Opin. Toxicol. 7, 81–86. doi: 10.1016/j.cotox.2017.11.002
Losso, J. N., Bawadi, H. A., and Chintalapati, M. (2011). Inhibition of the formation of advanced glycation end products by thymoquinone. Food Chem. 128, 55–61. doi: 10.1016/j.foodchem.2011.02.076
Luevano-Contreras, C., and Chapman-Novakofski, K. (2010). Dietary advanced glycation end products and aging. Nutrients 2, 1247–1265.
Mabrouk, A. (2017). Protective effect of thymoquinone against lead-induced antioxidant defense system alteration in rat liver. Acta Biol. Hung 68, 248–254. doi: 10.1556/018.68.2017.3.2
Mahmood, T., Moin, S., Faizy, A. F., Naseem, S., and Aman, S. (2013). Nigella sativa as an antiglycating agent for human serum albumin. Med. Sci. 2, 25–27.
Mandrekar-Colucci, S., and Landreth, G. E. (2010). Microglia and inflammation in Alzheimer’s disease. CNS Neurol. Disord. Drug Targets 9, 156–167. doi: 10.2174/187152710791012071
Mao, M., Li, J., Bi, A., Jia, H., Li, Q., Liu, Y., et al. (2020). Thymoquinone ameliorates the PM2.5-induced lung injury in rats. Exp. Lung Res. 46, 297–307. doi: 10.1080/01902148.2020.1801895
Mischley, L. K., Standish, L. J., Weiss, N. S., Padowski, J. M., Kavanagh, T. J., White, C. C., et al. (2016). Glutathione as a Biomarker in Parkinson’s Disease: associations with Aging and Disease Severity. Oxid. Med. Cell. Longev. 2016:9409363. doi: 10.1155/2016/9409363
Morgan, M. J., and Liu, Z.-G. (2011). Crosstalk of reactive oxygen species and NF-κB signaling. Cell Res. 21, 103–115. doi: 10.1038/cr.2010.178
Nagi, M. N., Alam, K., Badary, O. A., Al-Shabanah, O. A., Al-Sawaf, H. A., and Al-Bekairi, A. M. (1999). Thymoquinone protects against carbon tetrachloride hetatotoxicity in mice via an antioxidant mechanism. IUBMB Life 47, 153–159. doi: 10.1080/15216549900201153
Nguyen, T., Nioi, P., and Pickett, C. B. (2009). The Nrf2-antioxidant response element signaling pathway and its activation by oxidative stress. J. Biol. Chem. 284, 13291–13295. doi: 10.1074/jbc.R900010200
Nickells, R. W., and Zack, D. J. (1996). Apoptosis in ocular disease: a molecular overview. Ophthalm. Genet. 17, 145–165. doi: 10.3109/13816819609057889
Nita, M., and Grzybowski, A. (2016). The Role of the Reactive Oxygen Species and Oxidative Stress in the Pathomechanism of the Age-Related Ocular Diseases and Other Pathologies of the Anterior and Posterior Eye Segments in Adults. Oxid. Med. Cell. Longev. 2016:3164734. doi: 10.1155/2016/3164734
Ono, Y., Aoki, S., Ohnishi, K., Yasuda, T., Kawano, K., and Tsukada, Y. (1998). Increased serum levels of advanced glycation end-products and diabetic complications. Diabetes Res. Clin. Pract. 41, 131–137. doi: 10.1016/s0168-8227(98)00074-6
Osnes-Ringen, Ø, Berg, K. H., Moe, M. C., Zetterström, C., Røger, M., and Nicolaissen, B. (2016). Cell death pattern in lens epithelium of cataract patients. Acta Ophthalmol. 94, 514–520. doi: 10.1111/aos.13009
Pan, W. W., Wubben, T. J., and Besirli, C. G. (2021). Photoreceptor metabolic reprogramming: current understanding and therapeutic implications. Commun. Biol. 4:245. doi: 10.1038/s42003-021-01765-3
Pandey, R., Kumar, D., and Ahmad, A. (2018). Nigella sativa seed extracts prevent the glycation of protein and DNA. Curr. Perspect. Med. Aromat. Plants 1, 1–7.
Parlar, A., and Arslan, S. O. (2020). Thymoquinone reduces ischemia and reperfusion-induced intestinal injury in rats, through anti-oxidative and anti-inflammatory effects. Turk. J. Surg. 36, 96–104. doi: 10.5578/turkjsurg.4583
Pascolini, D., and Mariotti, S. P. (2012). Global estimates of visual impairment: 2010. Br. J. Ophthalmol. 96, 614–618. doi: 10.1136/bjophthalmol-2011-300539
Payne, A. J., Kaja, S., Naumchuk, Y., Kunjukunju, N., and Koulen, P. (2014). Antioxidant drug therapy approaches for neuroprotection in chronic diseases of the retina. Int. J. Mol. Sci. 15, 1865–1886. doi: 10.3390/ijms15021865
Picone, P., Nuzzo, D., Caruana, L., Scafidi, V., and Di Carlo, M. (2014). Mitochondrial dysfunction: different routes to Alzheimer’s disease therapy. Oxid. Med. Cell. Longev. 2014:780179. doi: 10.1155/2014/780179
Poorgholam, P., Yaghmaei, P., and Hajebrahimi, Z. (2018). Thymoquinone recovers learning function in a rat model of Alzheimer’s disease. Avicenna J. Phytomed. 8, 188–197.
Pop, R. M., Sabin, O., Suciu, Ş, Vesa, S. C., Socaci, S. A., Chedea, V. S., et al. (2020). Nigella sativa’s Anti-Inflammatory and Antioxidative Effects in Experimental Inflammation. Antioxidants 9:921. doi: 10.3390/antiox9100921
Qian, L., and Flood, P. M. (2008). Microglial cells and Parkinson’s disease. Immunol. Res. 41, 155–164. doi: 10.1007/s12026-008-8018-0
Radad, K., Moldzio, R., Taha, M., and Rausch, W. D. (2009). Thymoquinone protects dopaminergic neurons against MPP+ and rotenone. Phytother. Res. 23, 696–700. doi: 10.1002/ptr.2708
Rani, R., Dahiya, S., Dhingra, D., Dilbaghi, N., Kim, K. H., and Kumar, S. (2018). Improvement of antihyperglycemic activity of nano-thymoquinone in rat model of type-2 diabetes. Chem. Biol. Interact. 295, 119–132. doi: 10.1016/j.cbi.2018.02.006
Ravid, T., and Hochstrasser, M. (2008). Diversity of degradation signals in the ubiquitin-proteasome system. Nat. Rev. Mol. Cell Biol. 9, 679–690. doi: 10.1038/nrm2468
Saez, I., and Vilchez, D. (2014). The Mechanistic Links Between Proteasome Activity. Aging and Age-related Diseases. Curr. Genomics 15, 38–51. doi: 10.2174/138920291501140306113344
Sahu, I., Mali, S. M., Sulkshane, P., Xu, C., Rozenberg, A., Morag, R., et al. (2021). The 20S as a stand-alone proteasome in cells can degrade the ubiquitin tag. Nat. Commun. 12:6173. doi: 10.1038/s41467-021-26427-0
Sankaranarayanan, C., and Pari, L. (2011). Thymoquinone ameliorates chemical induced oxidative stress and β-cell damage in experimental hyperglycemic rats. Chem. Biol. Interact. 190, 148–154. doi: 10.1016/j.cbi.2011.02.029
Savran, M., Ascı, H., Armagan, l̇, Erzurumlu, Y., Azırak, S., Kaya, Ozer, M., et al. (2020). Thymoquinone could be protective against valproic acid-induced testicular toxicity by antioxidant and anti-inflammatory mechanisms. Andrologia 52:e13623. doi: 10.1111/and.13623
Sayed, A. A., and Morcos, M. (2007). Thymoquinone decreases AGE-induced NF-kappaB activation in proximal tubular epithelial cells. Phytother. Res. 21, 898–899. doi: 10.1002/ptr.2177
Schleicher, E. D., Wagner, E., and Nerlich, A. G. (1997). Increased accumulation of the glycoxidation product N(epsilon)-(carboxymethyl)lysine in human tissues in diabetes and aging. J. Clin. Invest. 99, 457–468. doi: 10.1172/jci119180
Sedaghat, R., Roghani, M., and Khalili, M. (2014). Neuroprotective effect of thymoquinone, the Nigella sativa bioactive compound, in 6-hydroxydopamine-induced hemi-parkinsonian rat model. Iran J. Pharm. Res. 13, 227–234.
Selçuk, C. T., Durgun, M., Tekin, R., Yolbas, L., Bozkurt, M., Akçay, C., et al. (2013). Evaluation of the effect of thymoquinone treatment on wound healing in a rat burn model. J. Burn Care Res. 34, e274–e281. doi: 10.1097/BCR.0b013e31827a2be1
Sener, U., Uygur, R., Aktas, C., Uygur, E., Erboga, M., Balkas, G., et al. (2016). Protective effects of thymoquinone against apoptosis and oxidative stress by arsenic in rat kidney. Ren. Fail 38, 117–123. doi: 10.3109/0886022x.2015.1103601
Sethi, G., Ahn, K. S., and Aggarwal, B. B. (2008). Targeting nuclear factor-kappa B activation pathway by thymoquinone: role in suppression of antiapoptotic gene products and enhancement of apoptosis. Mol. Cancer Res. 6, 1059–1070. doi: 10.1158/1541-7786.mcr-07-2088
Sharif, U., Mahmud, N. M., Kay, P., Yang, Y. C., Harding, S. P., Grierson, I., et al. (2019). Advanced glycation end products-related modulation of cathepsin L and NF-κB signalling effectors in retinal pigment epithelium lead to augmented response to TNFα. J. Cell. Mol. Med. 23, 405–416. doi: 10.1111/jcmm.13944
Silachev, D. N., Plotnikov, E. Y., Zorova, L. D., Pevzner, I. B., Sumbatyan, N. V., Korshunova, G. A., et al. (2015). Neuroprotective effects of mitochondria-targeted plastoquinone and thymoquinone in a rat model of brain ischemia/reperfusion injury. Molecules 20, 14487–14503.
Siriwattanasatorn, M., Itharat, A., Thongdeeying, P., and Ooraikul, B. (2020). In Vitro Wound Healing Activities of Three Most Commonly Used Thai Medicinal Plants and Their Three Markers. Evid Based Complement. Alternat. Med. 2020:6795383. doi: 10.1155/2020/6795383
Stitt, A. W., Li, Y. M., Gardiner, T. A., Bucala, R., Archer, D. B., and Vlassara, H. (1997). Advanced glycation end products (AGEs) co-localize with AGE receptors in the retinal vasculature of diabetic and of AGE-infused rats. Am. J. Pathol. 150, 523–531.
Sun, L., Huang, T., Xu, W., Sun, J., Lv, Y., and Wang, Y. (2017). Advanced glycation end products promote VEGF expression and thus choroidal neovascularization via Cyr61-PI3K/AKT signaling pathway. Sci. Rep. 7:14925. doi: 10.1038/s41598-017-14015-6
Tabeshpour, J., Mehri, S., Abnous, K., and Hosseinzadeh, H. (2020). Role of Oxidative Stress, MAPKinase and Apoptosis Pathways in the Protective Effects of Thymoquinone Against Acrylamide-Induced Central Nervous System Toxicity in Rat. Neurochem. Res. 45, 254–267. doi: 10.1007/s11064-019-02908-z
Taka, E., Mazzio, E. A., Goodman, C. B., Redmon, N., Flores-Rozas, H., Reams, R., et al. (2015). Anti-inflammatory effects of thymoquinone in activated BV-2 microglial cells. J. Neuroimmunol. 286, 5–12. doi: 10.1016/j.jneuroim.2015.06.011
Takihara, Y., Inatani, M., Eto, K., Inoue, T., Kreymerman, A., Miyake, S., et al. (2015). In vivo imaging of axonal transport of mitochondria in the diseased and aged mammalian CNS. Proc. Natl. Acad. Sci. 112, 10515–10520. doi: 10.1073/pnas.1509879112
Tan, W., Zou, J., Yoshida, S., Jiang, B., and Zhou, Y. (2020). The Role of Inflammation in Age-Related Macular Degeneration. Int. J. Biol. Sci. 16, 2989–3001. doi: 10.7150/ijbs.49890
Taysi, S., Abdulrahman, Z. K., Okumus, S., Demir, E., Demir, T., Akan, M., et al. (2015). The radioprotective effect of Nigella sativa on nitrosative stress in lens tissue in radiation-induced cataract in rat. Cutan Ocul. Toxicol. 34, 101–106. doi: 10.3109/15569527.2014.910802
Tian, F., Liu, R., Fan, C., Sun, Y., Huang, X., Nie, Z., et al. (2020). Effects of Thymoquinone on Small-Molecule Metabolites in a Rat Model of Cerebral Ischemia Reperfusion Injury Assessed using MALDI-MSI. Metabolites 10:27. doi: 10.3390/metabo10010027
Tokarz, P., Kaarniranta, K., and Blasiak, J. (2013). Role of antioxidant enzymes and small molecular weight antioxidants in the pathogenesis of age-related macular degeneration (AMD). Biogerontology 14, 461–482. doi: 10.1007/s10522-013-9463-2
Tong, J. P., and Yao, Y. F. (2006). Contribution of VEGF and PEDF to choroidal angiogenesis: a need for balanced expressions. Clin. Biochem. 39, 267–276. doi: 10.1016/j.clinbiochem.2005.11.013
TÜrkÖz, D., TÜrkÖz, A., Önger, M. E., and KuruoĞlu, E. (2020). The stereological and histopathological evaluation of the effect of Thymoquinone on peridural fibrosis following laminectomy in rats. Turk. J. Med. Sci. 51, 375–381. doi: 10.3906/sag-2006-147
Ursini, F., Maiorino, M., and Forman, H. J. (2016). Redox homeostasis: the Golden Mean of healthy living. Redox Biol. 8, 205–215. doi: 10.1016/j.redox.2016.01.010
Velagapudi, R., El-Bakoush, A., Lepiarz, I., Ogunrinade, F., and Olajide, O. A. (2017a). AMPK and SIRT1 activation contribute to inhibition of neuroinflammation by thymoquinone in BV2 microglia. Mol. Cell. Biochem. 435, 149–162. doi: 10.1007/s11010-017-3064-3
Velagapudi, R., Kumar, A., Bhatia, H. S., El-Bakoush, A., Lepiarz, I., Fiebich, B. L., et al. (2017b). Inhibition of neuroinflammation by thymoquinone requires activation of Nrf2/ARE signalling. Int. Immunopharmacol. 48, 17–29. doi: 10.1016/j.intimp.2017.04.018
Vlassara, H., Bucala, R., and Striker, L. (1994). Pathogenic effects of advanced glycosylation: biochemical, biologic, and clinical implications for diabetes and aging. Lab. Invest. 70, 138–151.
Vomund, S., Schäfer, A., Parnham, M. J., Brüne, B., and von Knethen, A. (2017). Nrf2, the Master Regulator of Anti-Oxidative Responses. Int. J. Mol. Sci. 18:2772. doi: 10.3390/ijms18122772
Wang, A. L., Lukas, T. J., Yuan, M., and Neufeld, A. H. (2008). Increased mitochondrial DNA damage and down-regulation of DNA repair enzymes in aged rodent retinal pigment epithelium and choroid. Mol. Vis. 14, 644–651.
Wang, D., Qiao, J., Zhao, X., Chen, T., and Guan, D. (2015). Thymoquinone Inhibits IL-1β-Induced Inflammation in Human Osteoarthritis Chondrocytes by Suppressing NF-κB and MAPKs Signaling Pathway. Inflammation 38, 2235–2241. doi: 10.1007/s10753-015-0206-1
Wang, Y., Gao, H., Zhang, W., Zhang, W., and Fang, L. (2015). Thymoquinone inhibits lipopolysaccharide-induced inflammatory mediators in BV2 microglial cells. Int. Immunopharmacol. 26, 169–173. doi: 10.1016/j.intimp.2015.03.013
Wang, Y., Wang, V., and Chan, C. (2011). The role of anti-inflammatory agents in age-related macular degeneration (AMD) treatment. Eye 25, 127–139.
Woo, C. C., Hsu, A., Kumar, A. P., Sethi, G., and Tan, K. H. B. (2013). Thymoquinone Inhibits Tumor Growth and Induces Apoptosis in a Breast Cancer Xenograft Mouse Model: the Role of p38 MAPK and ROS. PLoS One 8:e75356. doi: 10.1371/journal.pone.0075356
Wu, T., Chen, Y., Chiang, S. K. S., and Tso, M. O. M. (2002). NF-κB Activation in Light-Induced Retinal Degeneration in a Mouse Model. Investig. Ophthalmol. Vis. Sci. 43, 2834–2840.
Xiao, W., Wang, R.-S., Handy, D. E., and Loscalzo, J. (2018). NAD (H) and NADP (H) redox couples and cellular energy metabolism. Antioxid Redox Signal 28, 251–272.
Xiao, X. Y., Zhu, Y. X., Bu, J. Y., Li, G. W., Zhou, J. H., and Zhou, S. P. (2016). Evaluation of Neuroprotective Effect of Thymoquinone Nanoformulation in the Rodent Cerebral Ischemia-Reperfusion Model. Biomed. Res. Int. 2016:2571060. doi: 10.1155/2016/2571060
Xu, J., Zhu, L., Liu, H., Li, M., Liu, Y., Yang, F., et al. (2018). Thymoquinone reduces cardiac damage caused by hypercholesterolemia in apolipoprotein E-deficient mice. Lipids Health Dis. 17:173. doi: 10.1186/s12944-018-0829-y
Yang, L. P., Zhu, X. A., and Tso, M. O. (2007). Role of NF-kappaB and MAPKs in light-induced photoreceptor apoptosis. Invest. Ophthalmol. Vis. Sci. 48, 4766–4776. doi: 10.1167/iovs.06-0871
Yu, M., Yan, W., and Beight, C. (2018). Lutein and Zeaxanthin Isomers Protect against Light-Induced Retinopathy via Decreasing Oxidative and Endoplasmic Reticulum Stress in BALB/cJ Mice. Nutrients 10:842. doi: 10.3390/nu10070842
Zafar, H., Hussain, F., Zafar, S., and Yasmin, R. (2013). Glycation inhibition by Nigella sativa (Linn)–an in vitro model. Asian J. Agric. Biol. 1, 187–189.
Zafrilla, P., Losada, M., Perez, A., Caravaca, G., and Mulero, J. (2013). Biomarkers of oxidative stress in patients with wet age related macular degeneration. J. Nutr. Health Aging 17, 219–222.
Zaher, M. F., Bendary, M. A., Abd El-Aziz, G. S., and Ali, A. S. (2019). Potential Protective Role of Thymoquinone on Experimentally-induced Alzheimer Rats. J. Pharmaceut. Res. Int. 31, 1–18.
Zakarial Ansar, F. H., Latifah, S. Y., Wan Kamal, W. H. B., Khong, K. C., Ng, Y., Foong, J. N., et al. (2020). Pharmacokinetics and Biodistribution of Thymoquinone-loaded Nanostructured Lipid Carrier After Oral and Intravenous Administration into Rats. Int. J. Nanomed. 15, 7703–7717. doi: 10.2147/ijn.s262395
Zalewska, R., Reszeć, J., Mariak, Z., and Sulkowski, S. (2004). [The expression of Bcl-2, Bcl-xl, Bak and Bax proteins in axons of the optic nerve in closed-angle glaucoma]. Klin Oczna 106, 155–157.
Zeinvand-Lorestani, H., Nili-Ahmadabadi, A., Balak, F., Hasanzadeh, G., and Sabzevari, O. (2018). Protective role of thymoquinone against paraquat-induced hepatotoxicity in mice. Pestic. Biochem. Physiol. 148, 16–21. doi: 10.1016/j.pestbp.2018.03.006
Zeng, H.-Y., Tso, M. O. M., Lai, S., and Lai, H. (2008). Activation of nuclear factor-kappaB during retinal degeneration in rd mice. Mol. Vis. 14, 1075–1080.
Zhang, J., Wang, X., Vikash, V., Ye, Q., Wu, D., Liu, Y., et al. (2016). ROS and ROS-Mediated Cellular Signaling. Oxid. Med. Cell. Longev. 2016:4350965. doi: 10.1155/2016/4350965
Zhang, M., Du, H., Huang, Z., Zhang, P., Yue, Y., Wang, W., et al. (2018). Thymoquinone induces apoptosis in bladder cancer cell via endoplasmic reticulum stress-dependent mitochondrial pathway. Chem. Biol. Interact. 292, 65–75. doi: 10.1016/j.cbi.2018.06.013
Keywords: thymoquinone, neuroprotection, retinal pigment epithelium, oxidative stress, age-related macular degeneration
Citation: Mahmud NM, Paraoan L, Khaliddin N and Kamalden TA (2022) Thymoquinone in Ocular Neurodegeneration: Modulation of Pathological Mechanisms via Multiple Pathways. Front. Cell. Neurosci. 16:786926. doi: 10.3389/fncel.2022.786926
Received: 30 September 2021; Accepted: 09 February 2022;
Published: 02 March 2022.
Edited by:
Pooi Ling Mok, Universiti Putra Malaysia, MalaysiaReviewed by:
Birsen Elibol, Bezmiâlem Vakıf Üniversitesi, TurkeyCopyright © 2022 Mahmud, Paraoan, Khaliddin and Kamalden. This is an open-access article distributed under the terms of the Creative Commons Attribution License (CC BY). The use, distribution or reproduction in other forums is permitted, provided the original author(s) and the copyright owner(s) are credited and that the original publication in this journal is cited, in accordance with accepted academic practice. No use, distribution or reproduction is permitted which does not comply with these terms.
*Correspondence: Tengku Ain Kamalden, dGFmdGthbWFsZGVuQHVtLmVkdS5teQ==
Disclaimer: All claims expressed in this article are solely those of the authors and do not necessarily represent those of their affiliated organizations, or those of the publisher, the editors and the reviewers. Any product that may be evaluated in this article or claim that may be made by its manufacturer is not guaranteed or endorsed by the publisher.
Research integrity at Frontiers
Learn more about the work of our research integrity team to safeguard the quality of each article we publish.