- Almazov Federal Medical Research Centre, Saint Petersburg, Russia
Neurons in the somatic, sympathetic, and parasympathetic ganglia are surrounded by envelopes consisting of satellite glial cells (SGCs). Recently, it has become clear that SGCs are highly altered after nerve injury, which influences neuronal excitability and, consequently, the development and maintenance of pain in different animal models of chronic pain. However, the exact mechanism underlying chronic pain is not fully understood yet because it is assumed that SGCs in different ganglia share many common peculiarities, making the process complex. Here, we review recent data on morphological and functional heterogeneity and changes in SGCs in various pain conditions and their role in response to injury. More research is required to decipher the role of SGCs in diseases, such as chronic pain, neuropathology, and neurodegenerative diseases.
Introduction
The nervous system consists of two main cell types: neurons and glial cells. Glial cells have diverse functions in various physiological processes, including central nervous system (CNS) and peripheral nervous system (PNS) development (Pfrieger and Barres, 1997; Ullian et al., 2001; Christopherson et al., 2005; Pascual et al., 2005; Zuchero and Barres, 2015; Lago-Baldaia et al., 2020), pathogen recognition (Kofler and Wiley, 2011; Kigerl et al., 2014), cytotoxicity (Banati et al., 1993; Benn et al., 2001), extracellular matrix regulation (De Luca et al., 2020), lipid transport (Barber and Raben, 2019), cell-to-cell communication (Koizumi et al., 2005; Paolicelli et al., 2019; Schiera et al., 2019), and modulation of inflammation (Vallejo et al., 2010). Considering the great variety of peripheral glial functions, various mechanisms might play a role in different pain conditions, including the release of proinflammatory substances and neurotrophins (Suzumura et al., 2006; Vallejo et al., 2010; Mitterreiter et al., 2017), sensitizing neurons (Hossain et al., 2017).
The main types of glial cells in the CNS are astrocytes, microglia, oligodendrocytes, and ependymal cells, glial cells in the PNS include myelinating and non-myelinating Schwann cells, satellite glial cells (SGCs) and enteric glial cells. SGCs were functionally compared with astrocytes since astrocytes and SGCs, the main homeostatic glial cells, seem to share similar functions (Hanani and Verkhratsky, 2021). For many years, astrocytes have been a topic of great research interest compared to SGCs. Thus, comparing SGCs to astrocytes might be inaccurate and lead to imprecise impressions of SGCs functions and communication with neurons. Over the past few years, knowledge has been gained regarding the morphology, molecular heterogeneity, and involvement of SGCs in different pain conditions.
However, there is a lack of studies providing a comprehensive view of the recent advancements in our understanding of the SGCs. Therefore, this review aims to summarize the latest information about SGCs biology to advance our understanding of these glial cells.
Morphological characteristics of satellite glial cells
The PNS is part of the nervous system that extends beyond the brain and spinal cord. It consists of cranial and spinal nerves, and plexuses of the autonomic nervous system. Cranial and spinal nerve bodies are located within the brainstem nuclei or in the dorsal root ganglia (DRG), while autonomic neurons are organized in the sympathetic and parasympathetic ganglia. As mentioned above, SGCs are one of the main types of glial cells in the PNS, including Schwann and enteric glial cells (Peripheral Glial Cells, 2013). SGCs are located in the sensory and autonomic ganglia of the PNS and form a tight sheath around the neuronal soma (Pannese, 1981). In some areas, SGCs simply contact each other, whereas, in other areas, the lamellar extensions of each SGC may intertwine and overlap (Pannese, 2010).
The sensory ganglia, being DRG, trigeminal ganglia and other ganglia associated with cranial nerves, contain sensory neurons, SGCs, Schwann cells and other non-neuronal cells such as endothelial cells and immune cells (Haberberger et al., 2019; Vermeiren et al., 2020). SGCs in the sensory ganglia are laminar cells, usually a sheath of several SGCs that surround each neuron (Figure 1). The number of SGCs that make up the sheath increases in proportion to the surrounding neurons' volume (Hanani, 2005). Additionally, the envelope volume increases in proportion to the volume and surface area of the neuron. The distance of the extracellular space between the sheath and plasma membrane of the neuron is 20 nm, which allows DRG neurons and their SGC sheets to form a single anatomical and functional unit (Pannese, 1981). The patches of connective tissue separated these individual units. However, some sensory neurons occupy the same place in the connective tissue and are therefore divided by two or three neurons, which are primarily in newborn or young animals (Pannese et al., 1991).
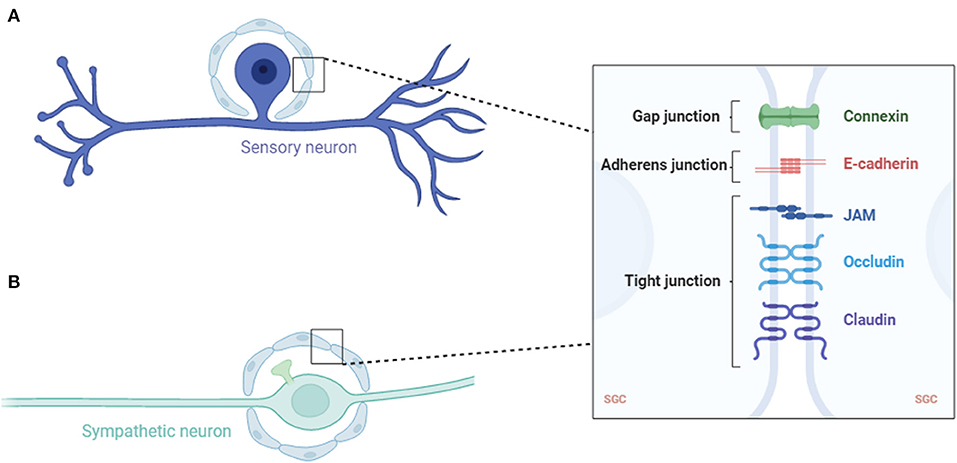
Figure 1. Schematic of a sensory (A) and sympathetic (B) neuron covered with an SGC envelope. SGC, satellite glial cell; JAM, junctional adhesion molecule.
In the sympathetic ganglia, SGCs are one of the three main cell types, with the other two being sympathetic ganglion neurons and small highly fluorescent (SIF) cells (Hanani, 2010). The SIF cells of the sympathetic ganglia are divided into several groups, each surrounded by an SGC sheath. The SGCs of the sympathetic ganglia had the same basic structure as the sensory ganglia, except that the sympathetic ganglia also received synapses (Figure 2). Therefore, the SGC envelope of the sympathetic neurons must extend further to cover the axonal ganglia near the soma. Similar to the sheath area near the glial nucleus, axonal hillocks are thicker than those in the rest of the surrounding neurons (Hanani, 2010).
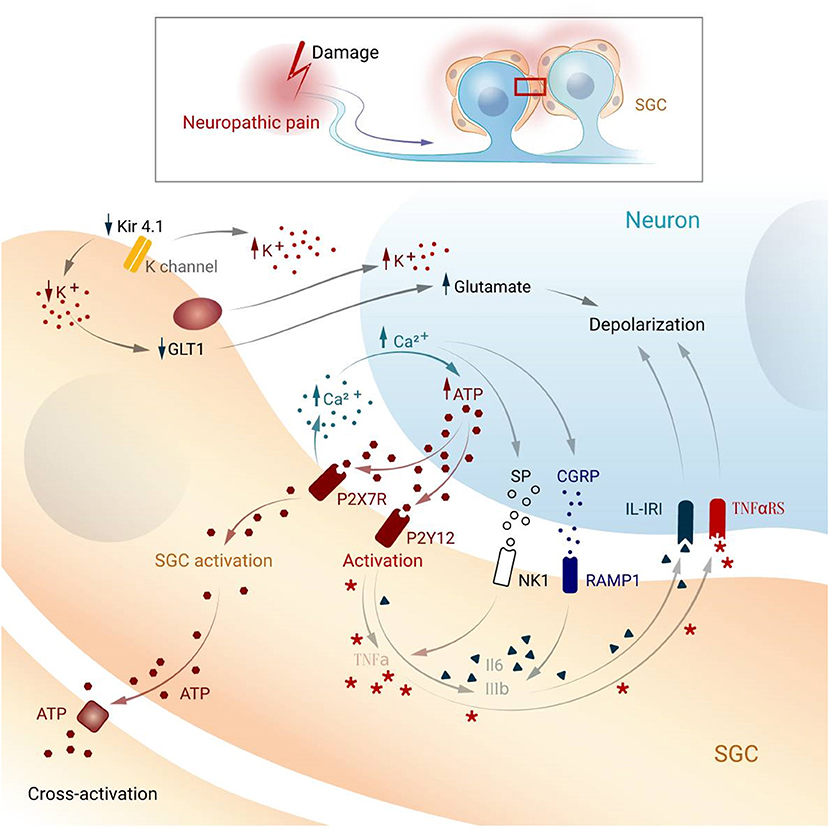
Figure 2. Possible mechanism underlying activation and cross-activation in SGCs and neurons while pain. SGC, satellite glial cell; Kir4.1, potassium channel; GLT1, glutamate transporter; P2X7R, P2X purinoceptor 7; P2Y12, purinergic receptor; NK1, Neurokinin 1; RAMP1, Receptor activity modifying protein 1; IL1-β, interleukin-1 beta; IK-IR, Interleukin-1 receptor; TNF-α, tumor necrosis factor-alpha; TNFaRS, tumor necrosis factor receptor; CGRP, Calcitonin gene-related peptide; SP, Substance P.
Much less knowledge has been gained about SGCs in the parasympathetic ganglia due to their location, which makes it difficult to access the ganglion. However, the general organization of the SGCs envelope is very similar to that in the sympathetic ganglia in guinea pig urinary bladder (Gabella, 1990), guinea pig trachea (Bałuk et al., 1985), guinea pig pancreas (Liu et al., 1997), mouse pulmonary vein ganglia (Bałuk and Gabella, 1987), mouse salivary duct ganglia (Pomeroy et al., 1996), cat pancreatic ganglia (Sha et al., 1996), human choroidal ganglia (May et al., 2004), and human cardiac ganglia (Pauziene and Pauza, 2003).
In all types of ganglia, SGCs have adhesive, tight, and gap junctions (Pannese et al., 1977, 1994; Sakuma et al., 2001; Liu et al., 2014). Molecules with a mass of up to 1 kDa can be transported through these gap junctions (Hanani et al., 2002; Pannese et al., 2003; Huang et al., 2006). This suggests that SGCs function in a synaptic context, thereby influencing the synaptic transmission.
Despite more than 50 years of studying SGCs morphology, our knowledge in this area is still limited. It is assumed that SGCs in different ganglia share many common peculiarities, therefore, more research dedicated to its complexity is needed.
Heterogeneity of satellite glial cells
Sensory neurons in the DRG are extremely diverse depending on their size, expression, and signaling activity (Körner and Lampert, 2022). Because each neuron has a sheath consisting of SGCs, the diversity of neurons is accompanied by a variety of SGCs consisting of this sheath.
Studies using electron microscopy have demonstrated at least two morphological types of SGC (Siemionow et al., 2006; Nascimento et al., 2008): the first type (~50–60% of the population) is the most typical SGC, forming a sheath around the neuron with a very thin layer of cytoplasm and invaginating a lot inside the neuron. The second type consists of SGCs that are associated with an axon.
Modern molecular biology methods enable the isolation of cell subtypes based on analysis of their expression. Thus, RNA-seq analysis shows that SGCs exhibit a great variety in their molecular nature (Avraham et al., 2020; Tasdemir-Yilmaz et al., 2020; van Weperen et al., 2021; Mapps et al., 2022). The expression analysis of SGCs isolated from different locations showed specifically expressed various proteins. For example, SGCs of the cochlear ganglion share common markers (GATA 2, NPY, and Epha3) with other glial cells (Tasdemir-Yilmaz et al., 2020). Another study demonstrated a difference in protein expression of SGCs in the sensory and sympathetic ganglia (Mapps et al., 2022). The sensory subpopulation of SGCs is enriched in steroid biosynthesis and exclusively expresses Brevican core protein (Bcan), a member of the lectican family (Mapps et al., 2022). In addition, in both the DRG and sympathetic ganglia, there are three common types of SGCs: subpopulations enriched (1) for cytokine and interleukin signaling, (2) for ECM and cell adhesion pathways, and (3) for classical immediate early genes (Avraham et al., 2020; Tasdemir-Yilmaz et al., 2020; van Weperen et al., 2021; Mapps et al., 2022) also identified a specific SGCs subpopulation after injury enriched with (Prada et al., 2011) transcriptional factor, which regulates gliosecretion in astrocytes (Prada et al., 2011).
Thus, it is possible to distinguish several SGCs populations both within and outside the same ganglion: three populations that occur in each ganglion: SGCs expressing (1) proinflammatory molecules, (2) ECM and cell adhesion molecules, and (3) early genes, as well as a population that differs from ganglion to ganglion: (4) sympathetic SGCs and (5) sensory SGCs. Further study of the parasympathetic and other sympathetic and sensory ganglia SGCs will improve our understanding of the variety in morphological and molecular properties of SGCs, which is sufficient to understand their function in normal and pathological conditions, such as chronic pain, neuropathology, and neurodegenerative diseases.
Participation of satellite glial cells in pain conditions
Pain is an unpleasant sensory experience associated with injury and/or damage. Pain is mainly mediated by nociceptors, the body of which lies in the DRG or trigeminal ganglia (Treede et al., 2019). Nociceptors are a unique neuronal population characterized by a high threshold of activation and unencapsulated nerve endings (Mertens et al., 2015). Nociceptive receptors are multimodal, non-myelinated, or lightly myelinated primary afferent nerve fibers. Their transmission is mainly mediated by glutamate, which modulates postsynaptic ionotropic receptors, which in turn can be modulated by the co-expression of substance P and calcitonin gene-related peptide (CGRP) (Zieglgänsberger, 2019). Pain plays a key role in the healing process, and consequently, in survival. People unable to experience pain rarely survive into adulthood, inevitably hurt themselves, and thus, decrease their life expectancy (Verpoorten et al., 2006).
Historically, pain has been divided into two main categories, representing the clinical aspects of pain syndrome (Bennett, 2006). Neuropathic pain is mainly caused by peripheral nerve injury, which leads to hyperalgesia due to enhanced sensory neuron excitability and reduced neuronal excitation threshold (Campbell and Meyer, 2006; Colloca et al., 2017). Inflammatory pain is usually considered to be an acute condition linked to nociceptor excitation and strong neuroimmune interactions that occur in response to tissue damage (Kidd and Urban, 2001).
Recent studies have identified the effects of neuropathic pain on SGCs (Ohara et al., 2008; Siemionow et al., 2009; Zhang et al., 2009; Ji et al., 2013; Yoon et al., 2013; Lee and Kim, 2020; Yuan et al., 2020). After nerve injury, SGCs may play an important role in the transmission of spinal cord injury signals (Ji et al., 2013). Studies have shown that gap junctions between glial cells may play an important role in neuropathic pain (Yoon et al., 2013). After nerve injury, the number of gap junctions between SGCs increases significantly (Ohara et al., 2008) but gradually returns to normal levels after a period of time. In models of neuropathic conditions, the number of activated SGCs connected with SGCs of other neurons is increased (Hanani et al., 2002; Lee and Kim, 2020; Yuan et al., 2020), while under physiological conditions, only a few neurons share a common sheath.
SGC activation is traditionally associated with increasing levels of glial fibrillary acidic protein (GFAP) (Siemionow et al., 2009; Zhang et al., 2009) and many other molecular properties. SGCs activation caused by increased GFAP expression leads to enhanced glial cohesion, whereas blocking gap junctions induces analgesia (Warwick and Hanani, 2013).
In the DRG, a large number of ions exist between cells to maintain the stability of cell potential, and satellite glia in the ganglion play an essential role in potassium ion buffering (Tang et al., 2010). Intracellular potassium homeostasis maintains neuronal excitability, which increases when the extracellular potassium concentration increases (Bellot-Saez et al., 2017). SGCs express the inwardly rectifying potassium channel Kir4.1, which buffers potassium concentrations in the ganglia (Tang et al., 2010). Studies have shown that Kir4.1 expression is downregulated upon nerve injury, and siRNA silencing of Kir4.1 induces spontaneous and evoked facial pain-like behaviors in freely moving rats (Ohara et al., 2008).
Glutamate is an important excitatory neurotransmitter in the CNS and PNS. Excessive glutamate can lead to increased neuronal excitation, which can lead to pain (Pereira and Goudet, 2019). The PNS does not contain glutamate-degrading enzymes; therefore, glutamate removal relies on high-affinity glutamate transporters on SGCs. SGCs take up extracellular glutamate and maintain homeostasis of extracellular glutamate (Fonseca et al., 2005; Chiang et al., 2007, 2008). The glutamate transporter, GLT-1, is involved in the transport of glutamate into glial cells (Maeda et al., 2008; Zhao et al., 2018). Following nerve injury, decreasing of Kir4.1 channel and sequential an increase of extracellular K+ can downregulate GLT-1 (Vit et al., 2008), accumulating glutamate, which increases the excitability of the postsynaptic neurons (Sung et al., 2003). Studies have shown that, in the pathological pain model, the glutamate content in the cell bodies of sensory neurons is increased (Kung et al., 2013; Cho et al., 2021). Additionally, SGCs also express glutamate-aspartate receptors and glutamine synthetase, so glutamate can be taken up outside the cell and converted into glutamine inside the cell. Glutamate uptake by SGCs can maintain normal extracellular glutamate levels, thereby maintaining neuronal excitability (Ohara et al., 2009).
After nerve injury, a large number of inflammatory cells at the injury site aggregate and release inflammatory transmitters, which induce chemical signals to generate electrical signals and transmit them to the DRG or trigeminal ganglion. Studies have shown that Adenosine triphosphate (ATP) is one of the main signal transmitters involved in communication between neurons and satellite glial cells (Hanani, 2012). While ATP cannot pass through the membrane, it is released by vesicles or channels such as P2X7R or P2Y12. When nerve impulses reach the DRG, SGCs and neurons release a large quantity of ATP, thereby increasing intracellular calcium concentration (Weick et al., 2003; Zhang et al., 2007; Suadicani et al., 2010; Villa et al., 2010). Activation of the purinergic receptor P2Y12R increases calcium influx into SGCs, which in turn increases cell excitability (Ceruti et al., 2008; Takeda et al., 2009; Katagiri et al., 2012). On the other hand, P2X7R is selectively expressed in SGCs and is involved in the modulation of nociceptive signals in the DRGs (North, 2002; Liu and Salter, 2005; Nakatsuka and Jianguo, 2006; Chen et al., 2008). For instance, P2X7R in SGCs promotes the release of proinflammatory cytokines including tumor necrosis factor-alpha (TNF-α), interleukin-1 beta, and interleukin-6 (IL-6) (Arulkumaran et al., 2011). In HIV treatment-induced neuropathic models, SGCs demonstrate increased GFAP expression and P2Y212 receptor activation (Zhou et al., 2019).
Transient receptor potential (TRP) channels are a group of ion channels that mediates sensory transduction. TRP type A1 (TRPA1) modulates calcium homeostasis in astrocytes (Shigetomi et al., 2011, 2013). A recent study revealed that inflammation and nerve injury enhance the expression of TRPA1 in neurons and SGCs, disrupting intracellular calcium signaling and leading to pain generation (Shin et al., 2020).
After nerve injury, electrical signals are transmitted into the ganglia, resulting in the massive release of neurotransmitters, as well as neural and immune factors such as glutamate, ATP, substance P, CGRP, brain-derived neurotrophic factor, IL-6, and CCL2 (Scholz and Woolf, 2007; Ren and Dubner, 2008; Milligan and Watkins, 2009). These mediators increase the sensitivity of postsynaptic neurons and activate satellite glia around the neurons. Peripherally released immune factors such as proinflammatory cytokines (e.g., IL-6) may also activate central glial cells (Schöbitz et al., 1992; Vallières and Rivest, 1997). Peripheral IL-6 can be transported to the CNS through the circulation, increasing COX-2 activity and PGE2 release in cerebral vascular endothelial cells, resulting in a central immune response (Schöbitz et al., 1992; Vallières and Rivest, 1997).
Glial cell activation and neuron-glia interactions play key roles in chronic pain. Accumulated data associate pain syndromes with various states of glial activation, occurring in SGCs as well as: glial response through upregulation of glial markers (i.e., GFAP), activation of ATP and glutamate transporters, and expression of glial mediators (e.g., cytokines, chemokines, growth factors). In this review, we report on recent developments in the involvement of SGCs in pathological conditions; however, our understanding is far from complete.
Acute and chronic pain conditions
Acute and chronic pain are another category for pain. An acute pain condition has a brief onset and lasts <3 months, whereas chronic pain lasts longer than normal healing process. Acute pain characterizes a variety of inflammatory mediators expression (ATP, bradykinin, sodium, potassium, histamine and serotonin and others (Feizerfan and Sheh, 2015). These substances interact with cells surrounding injured cells, leading to depolarization and systemic inflammation, inducing up-regulation of P substances, activation TRP vanilloid receptors (TRPV) and, thus, to hyperalgesia (Pe et al., 2010). Repetitive stimulation may result a prolonged inflammation and release of different cytokines (such as IL-6, TNF-α and others), up-regulation of voltage-gated sodium channels, phosphorilation of protein kinases A and C. As described above SGCs involve in both acute and chronic conditions through different cellular mechanisms.
Conclusion
Here, we describe the latest evidence on SGCs morphology, heterogeneity, and its role in various pain conditions. Despite the apparent importance of SGCs in normal and pathological conditions, our knowledge of their cell biology is still incomplete. Understanding SGCs biology might be indispensable to improving our understanding of chronic pain and other neurodegenerative diseases.
Author contributions
DA and VD planned the study. AD, BD, LM, and NB wrote the manuscript. All authors read and approved the final version of the manuscript.
Funding
This work was financially supported by the Ministry of Science and Higher Education of the Russian Federation (Agreement No. 075-15-2022-301). Open access funding was provided by the Almazov Federal Medical Research Center (Saint Petersburg, Russia).
Acknowledgments
We thank Olga Kharchenko for the drawings presented in Figures.
Conflict of interest
The authors declare that the research was conducted in the absence of any commercial or financial relationships that could be construed as a potential conflict of interest.
Publisher's note
All claims expressed in this article are solely those of the authors and do not necessarily represent those of their affiliated organizations, or those of the publisher, the editors and the reviewers. Any product that may be evaluated in this article, or claim that may be made by its manufacturer, is not guaranteed or endorsed by the publisher.
References
Arulkumaran, N., Unwin, R. J., and Tam, F. W. K. (2011). “A Potential therapeutic role for P2X7 receptor (P2X7R) antagonists in the treatment of inflammatory diseases. Expert Opinion Invest. Drugs. 20, 897–915. doi: 10.1517/13543784.2011.578068
Avraham, O., Deng, P. Y., Jones, S., Kuruvilla, R., Semenkovich, C. F., Klyachko, V. A., et al. (2020). Satellite glial cells promote regenerative growth in sensory neurons. Nat. Commun. 11, 1–17. doi: 10.1038/s41467-020-18642-y
Bałuk, P., Fujiwara, T., and Matsuda, S. (1985). The fine structure of the ganglia of the guinea-pig trachea. Cell Tissue Res. 239, 51–60. doi: 10.1007/BF00214902
Bałuk, P., and Gabella, G. (1987). Fine structure of the autonomic ganglia of the mouse pulmonary vein. J. Neurocytol. 16, 169–184. doi: 10.1007/BF01795301
Banati, R. B., Gehrmann, J., Schubert, P., and Kreutzberg, G. W. (1993). Cytotoxicity of microglia. Glia 7, 111–118. doi: 10.1002/glia.440070117
Barber, C. N., and Raben, D. M. (2019). Lipid metabolism crosstalk in the brain: glia and neurons. Front. Cell. Neurosci. 13, 212. doi: 10.3389/fncel.2019.00212
Bellot-Saez, A., Kékesi, O., Morley, J. W., and Buskila, Y. (2017). Astrocytic modulation of neuronal excitability through K+ spatial buffering. Neurosci. Biobehav. Rev. 77, 87–97. doi: 10.1016/j.neubiorev.2017.03.002
Benn, T., Halfpenny, C., and Scolding, N. (2001). Glial cells as targets for cytotoxic immune mediators. Glia 36, 200–211. doi: 10.1002/glia.1109
Bennett, G. J. (2006). Can we distinguish between inflammatory and neuropathic pain? Pain Res. Manag. 11, 11A−15A. doi: 10.1155/2006/237251
Campbell, J. N., and Meyer, R. A. (2006). Mechanisms of neuropathic pain. Neuron 52, 77–92. doi: 10.1016/j.neuron.2006.09.021
Ceruti, S., Fumagalli, M., Villa, G., Verderio, C., and Abbracchio, M. P. (2008). Purinoceptor-mediated calcium signaling in primary neuron-glia trigeminal cultures. Cell Calcium 43, 576–590. doi: 10.1016/j.ceca.2007.10.003
Chen, Y., Zhang, X., Wang, C., Li, G., Gu, Y., and Huang, L. -Y. M. (2008). Activation of P2X7 receptors in glial satellite cells reduces pain through downregulation of P2X3 receptors in nociceptive neurons. Proc. Natl. Acad. Sci. U. S. A. 105, 16773–16778. doi: 10.1073/pnas.0801793105
Chiang, C. Y., Li, Z., Dostrovsky, J. O., Hu, J. W., and Sessle, B. J. (2008). Glutamine uptake contributes to central sensitization in the medullary dorsal horn. Neuroreport 19, 1151–1154. doi: 10.1097/WNR.0b013e3283086781
Chiang, C. Y., Wang, J., Xie, Y. F., Zhang, S., Hu, J. W., Dostrovsky, J. O., et al. (2007). Astroglial glutamate-glutamine shuttle is involved in central sensitization of nociceptive neurons in rat medullary dorsal horn. J. Neurosci. 27, 9068–9076. doi: 10.1523/JNEUROSCI.2260-07.2007
Cho, Y. S., Ko, H. G., Mah, W., Kim, Y. S., Bae, J. Y., Youn, D. H., et al. (2021). Glutamate Signaling, Associated With Satellite Glial Cells that Envelop Small Trigeminal Ganglion Neurons is Highly Involved in the Neuropathic Pain. In Review. doi: 10.21203/rs.3.rs-445113/v1
Christopherson, K. S., Ullian, E. M., Stokes, C. C. A., Mullowney, C. E., Hell, J. W., Agah, A., et al. (2005). Thrombospondins are astrocyte-secreted proteins that promote CNS synaptogenesis. Cell 120, 421–433. doi: 10.1016/j.cell.2004.12.020
Colloca, L., Ludman, T., Bouhassira, D., Baron, R., Dickenson, A. H., Yarnitsky, D., et al. (2017). Neuropathic pain. Nat. Rev. Dis. Primer 3, 1–19. doi: 10.1038/nrdp.2017.2
De Luca, C., Colangelo, A. M., Virtuoso, A., Alberghina, L., and Papa, M. (2020). Neurons, glia, extracellular matrix and neurovascular unit: a systems biology approach to the complexity of synaptic plasticity in health and disease. Int. J. Mol. Sci. 21, 1539. doi: 10.3390/ijms21041539
Feizerfan, A., and Sheh, G. (2015). “Transition from acute to chronic pain. Continuing Education in Anaesthesia Critical Care & Pain. 15, 98–102. doi: 10.1093/bjaceaccp/mku044
Fonseca, L. L., Monteiro, M. A. R., Alves, P. M., Carrondo, M. J. T., and Santos, H. (2005). Cultures of rat astrocytes challenged with a steady supply of glutamate: new model to study flux distribution in the glutamate-glutamine cycle. Glia 51, 286–296. doi: 10.1002/glia.20209
Gabella, G. (1990). Intramural neurons in the urinary bladder of the guinea-pig. Cell Tissue Res. 261, 231–237. doi: 10.1007/BF00318664
Haberberger, R. V., Barry, C., Dominguez, N., and Matusica, D. (2019). Human dorsal root ganglia. Front. Cell. Neurosci. 13, 271. doi: 10.3389/fncel.2019.00271
Hanani, M. (2005). Satellite glial cells in sensory ganglia: from form to function. Brain Res. Rev. 48, 457–476. doi: 10.1016/j.brainresrev.2004.09.001
Hanani, M. (2010). Satellite glial cells in sympathetic and parasympathetic ganglia: in search of function. Brain Res. Rev. 64, 304–327. doi: 10.1016/j.brainresrev.2010.04.009
Hanani, M. (2012). Intercellular communication in sensory ganglia by purinergic receptors and gap junctions: implications for chronic pain. Brain Res. 1487, 183–191. doi: 10.1016/j.brainres.2012.03.070
Hanani, M., Huang, T. Y., Cherkas, P. S., Ledda, M., and Pannese, E. (2002). Glial cell plasticity in sensory ganglia induced by nerve damage. Neuroscience 114, 279–283. doi: 10.1016/S0306-4522(02)00279-8
Hanani, M., and Verkhratsky, A. (2021). Satellite glial cells and astrocytes, a comparative review. Neurochem. Res. 46, 2525–2537. doi: 10.1007/s11064-021-03255-8
Hossain, M. Z., Unno, S., Ando, H., Masuda, Y., and Kitagawa, J. (2017). Neuron–glia crosstalk and neuropathic pain: involvement in the modulation of motor activity in the orofacial region. Int. J. Mol. Sci. 18, 2051. doi: 10.3390/ijms18102051
Huang, T. Y., Hanani, M., Ledda, M., De Palo, S., and Pannese, E. (2006). Aging is associated with an increase in dye coupling and in gap junction number in satellite glial cells of murine dorsal root ganglia. Neuroscience 137, 1185–1192. doi: 10.1016/j.neuroscience.2005.10.020
Ji, R.-R., Berta, T., and Nedergaard, M. (2013). Glia and pain: is chronic pain a gliopathy? Pain 154 (Suppl 1), S10–S28. doi: 10.1016/j.pain.2013.06.022
Katagiri, A., Shinoda, M., Honda, K., Toyofuku, A., Sessle, B. J., and Iwata, K. (2012). Satellite glial cell P2Y12 receptor in the trigeminal ganglion is involved in lingual neuropathic pain mechanisms in rats. Mol. Pain 8, 23. doi: 10.1186/1744-8069-8-23
Kidd, B. L., and Urban, L. A. (2001). Mechanisms of inflammatory pain. Br. J. Anaesth. 87, 3–11. doi: 10.1093/bja/87.1.3
Kigerl, K. A., de Rivero Vaccari, J. P., Dietrich, W. D., Popovich, P. G., and Keane, R. W. (2014). Pattern recognition receptors and central nervous system repair. Exp. Neurol. 258, 5–16. doi: 10.1016/j.expneurol.2014.01.001
Kofler, J., and Wiley, C. A. (2011). Microglia: key innate immune cells of the brain. Toxicol. Pathol. 39, 103–114. doi: 10.1177/0192623310387619
Koizumi, S., Fujishita, K., and Inoue, K. (2005). Regulation of cell-to-cell communication mediated by astrocytic ATP in the CNS. Purinergic Signal. 1, 211–217. doi: 10.1007/s11302-005-6321-y
Körner, J., and Lampert, A. (2022). Functional subgroups of rat and human sensory neurons: a systematic review of electrophysiological properties. Pflugers Arch. 474, 367–385. doi: 10.1007/s00424-021-02656-6
Kung, L.-H., Gong, K., Adedoyin, M., Ng, J., Bhargava, A., Ohara, P. T., et al. (2013). Evidence for glutamate as a neuroglial transmitter within sensory ganglia. PLoS ONE 8, e68312. doi: 10.1371/journal.pone.0068312
Lago-Baldaia, I., Fernandes, V. M., and Ackerman, S. D. (2020). More than mortar: glia as architects of nervous system development and disease. Front. Cell Dev. Biol. 8, 611269. doi: 10.3389/fcell.2020.611269
Lee, J. H., and Kim, W. (2020). The role of satellite glial cells, astrocytes, and microglia in oxaliplatin-induced neuropathic pain. Biomedicines 8, 324. doi: 10.3390/biomedicines8090324
Liu, H. P., Tay, S. S., and Leong, S. K. (1997). An ultrastructural study of the innervation of the guinea pig pancreas. J. Hirnforsch. 38, 107–117.
Liu, W., Glueckert, R., Linthicum, F. H., Rieger, G., Blumer, M., Bitsche, M., et al. (2014). Possible role of gap junction intercellular channels and connexin 43 in satellite glial cells (SGCs) for preservation of human spiral ganglion neurons : a comparative study with clinical implications. Cell Tissue Res. 355, 267–278. doi: 10.1007/s00441-013-1735-2
Liu, X. J., and Salter, M. W. (2005). “Purines and Pain Mechanisms: Recent Developments.” Current Opinion in Investigational Drugs. (London, England: 2000) 6, 65–75.
Maeda, S., Kawamoto, A., Yatani, Y., Shirakawa, H., Nakagawa, T., and Kaneko, S. (2008). Gene transfer of GLT-1, a glial glutamate transporter, into the spinal cord by recombinant adenovirus attenuates inflammatory and neuropathic pain in rats. Mol. Pain 4, 65. doi: 10.1186/1744-8069-4-65
Mapps, A. A., Thomsen, M. B., Boehm, E., Zhao, H., Hattar, S., and Kuruvilla, R. (2022). Diversity of satellite glia in sympathetic and sensory ganglia. Cell Rep. 38, 110328. doi: 10.1016/j.celrep.2022.110328
May, C. A., Neuhuber, W., and Lütjen-Drecoll, E. (2004). Immunohistochemical classification and functional morphology of human choroidal ganglion cells. Invest. Ophthalmol. Vis. Sci. 45, 361–367. doi: 10.1167/iovs.03-0624
Mertens, P., Blond, S., David, R., and Rigoard, P. (2015). Anatomy, physiology and neurobiology of the nociception: A focus on low back pain (part A). Neurochirurgie. 61, S22–S34. doi: 10.1016/j.neuchi.2014.09.001
Milligan, E. D., and Watkins, L. R. (2009). Pathological and protective roles of glia in chronic pain. Nat. Rev. Neurosci. 10, 23–36. doi: 10.1038/nrn2533
Mitterreiter, J. G., Ouwendijk, W. J. D., van Velzen, M., van Nierop, G. P., Osterhaus, A. D. M. E., and Verjans, G. M. G. M. (2017). Satellite glial cells in human trigeminal ganglia have a broad expression of functional Toll-like receptors. Eur. J. Immunol. 47, 1181–1187. doi: 10.1002/eji.201746989
Nakatsuka, T., and Jianguo, G. G. (2006). “P2X purinoceptors and sensory transmission.” Pflugers Archiv: European J. Physiol. 452, 598–607. doi: 10.1007/s00424-006-0057-6
Nascimento, R. S. M. F., Santiago, Santiago, M. F., Marques, S. A., Allodi, S., Martinez, A. M. B., et al. (2008). Diversity among satellite glial cells in dorsal root ganglia of the rat. Braz. J. Med. Biol. Res. 41, 1011–1017. doi: 10.1590/S0100-879X2008005000051
North, R. A. (2002). “Molecular Physiology of P2X Receptors.” Physiol. Rev. 82, 1013–67. doi: 10.1152/physrev.00015.2002
Ohara, P. T., Vit, J. P., Bhargava, A., and Jasmin, L. (2008). Evidence for a role of connexin 43 in trigeminal pain using RNA interference in vivo. J. Neurophysiol. 100, 3064–3073. doi: 10.1152/jn.90722.2008
Ohara, P. T., Vit, J. P., Bhargava, A., Romero, M., Sundberg, C., Charles, A. C., et al. (2009). Gliopathic pain: when satellite glial cells go bad. Neurosci. Rev. J. Bringing Neurobiol. Neurol. Psychiatry 15, 450–463. doi: 10.1177/1073858409336094
Pannese, E. (1981). “Introduction,” in The Satellite Cells of the Sensory Ganglia Advances in Anatomy Embryology and Cell Biology, eds Pannese, E., (Berlin, Heidelberg: Springer), 1–2.
Pannese, E. (2010). The structure of the perineuronal sheath of satellite glial cells (SGCs) in sensory ganglia*. Neuron Glia Biol. 6, 3–10. doi: 10.1017/S1740925X10000037
Pannese, E., Ledda, M., Arcidiacono, G., and Rigamonti, L. (1991). Clusters of nerve cell bodies enclosed within a common connective tissue envelope in the spinal ganglia of the lizard and rat. Cell Tissue Res. 264, 209–214. doi: 10.1007/BF00313957
Pannese, E., Ledda, M., Cherkas, P. S., Huang, T. Y., and Hanani, M. (2003). Satellite cell reactions to axon injury of sensory ganglion neurons: increase in number of gap junctions and formation of bridges connecting previously separate perineuronal sheaths. Anat. Embryol. (Berl.) 206, 337–347. doi: 10.1007/s00429-002-0301-6
Pannese, E., Luciano, L., Iurato, S., and Reale, E. (1977). Intercellular junctions and other membrane specializations in developing spinal ganglia: a freeze-fracture study. J. Ultrastruct. Res. 60, 169–180. doi: 10.1016/S0022-5320(77)80063-4
Pannese, E., Rigamonti, L., Ledda, M., and Arcidiacono, G. (1994). Perikaryal projections of spinal ganglion neurons: quantitative differences between membrane domains in contact with different microenvironments. J. Anat. 185 (Pt 3), 497–502.
Paolicelli, R. C., Bergamini, G., and Rajendran, L. (2019). Cell-to-cell communication by extracellular vesicles: focus on microglia. Neuroscience 405, 148–157. doi: 10.1016/j.neuroscience.2018.04.003
Pascual, O., Casper, K. B., Kubera, C., Zhang, J., Revilla-Sanchez, R., Sul, J.-Y., et al. (2005). Astrocytic purinergic signaling coordinates synaptic networks. Science 310, 113–116. doi: 10.1126/science.1116916
Pauziene, N., and Pauza, D. H. (2003). Electron microscopic study of intrinsic cardiac ganglia in the adult human. Ann. Anat. Anat. Anz. 185, 135–148. doi: 10.1016/S0940-9602(03)80077-8
Pe, M., Schug, Sa, Scott, Da, Visser, Ej, and Walker, Sm APM:SE Working Group of the Australian New Zealand College of Anaesthetists Faculty of Pain Medicine. (2010). Acute Pain Management: Scientific Evidence. 3rd Edition. Australian and New Zealand College of Anaesthetists.
Pereira, V., and Goudet, C. (2019). Emerging trends in pain modulation by metabotropic glutamate receptors. Front. Mol. Neurosci. 11, 464. doi: 10.3389/fnmol.2018.00464
Peripheral Glial Cells (2013). Glial Physiology and Pathophysiology. West Sussex: John Wiley and Sons, Ltd.
Pfrieger, F. W., and Barres, B. A. (1997). Synaptic efficacy enhanced by glial cells in vitro. Science 277, 1684–1687. doi: 10.1126/science.277.5332.1684
Pomeroy, S. L., Zurakowski, D., Khoxayo, S., Endara, M., and Dikkes, P. (1996). Postnatal addition of satellite cells to parasympathetic neurons. J. Comp. Neurol. 375, 518–525. doi: 10.1002/(SICI)1096-9861(19961118)375:3<518::AID-CNE12>3.0.CO;2-H
Prada, I., Marchaland, J., Podini, P., Magrassi, L., D'Alessandro, R., Bezzi, P., et al. (2011). REST/NRSF governs the expression of dense-core vesicle gliosecretion in astrocytes. J. Cell Biol. 193, 537–549. doi: 10.1083/jcb.201010126
Ren, K., and Dubner, R. (2008). Neuron-glia crosstalk gets serious: role in pain hypersensitivity. Curr. Opin. Anaesthesiol. 21, 570–579. doi: 10.1097/ACO.0b013e32830edbdf
Sakuma, E., Wang, H. J., Asai, Y., Tamaki, D., Amano, K., Mabuchi, Y., et al. (2001). Gap junctional communication between the satellite cells of rat dorsal root ganglia. Kaibogaku Zasshi 76, 297–302.
Schiera, G., Di Liegro, C. M., and Di Liegro, I. (2019). Cell-to-cell communication in learning and memory: from neuro- and glio-transmission to information exchange mediated by extracellular vesicles. Int. J. Mol. Sci. 21, 266. doi: 10.3390/ijms21010266
Schöbitz, B., Voorhuis, D. A., and De Kloet, E. R. (1992). Localization of interleukin 6 mRNA and interleukin 6 receptor mRNA in rat brain. Neurosci. Lett. 136, 189–192. doi: 10.1016/0304-3940(92)90046-A
Scholz, J., and Woolf, C. J. (2007). The neuropathic pain triad: neurons, immune cells and glia. Nat. Neurosci. 10, 1361–1368. doi: 10.1038/nn1992
Sha, L., Ou, L. L., Miller, S. M., Ma, R., and Szurszewski, J. H. (1996). Cat pancreatic neurons: morphology, electrophysiological properties, and responses to 5-HT. Pancreas 13, 111–124. doi: 10.1097/00006676-199608000-00001
Shigetomi, E., Jackson-Weaver, O., Huckstepp, R. T., O'Dell, T. J., and Khakh, B. S. (2013). TRPA1 channels are regulators of astrocyte basal calcium levels and long-term potentiation via constitutive d-serine release. J. Neurosci. 33, 10143–10153. doi: 10.1523/JNEUROSCI.5779-12.2013
Shigetomi, E., Tong, X., Kwan, K. Y., Corey, D. P., and Khakh, B. S. (2011). TRPA1 channels regulate astrocyte resting calcium and inhibitory synapse efficacy through GAT-3. Nat. Neurosci. 15, 70–80. doi: 10.1038/nn.3000
Shin, S. M., Itson-Zoske, B., Cai, Y., Qiu, C., Pan, B., Stucky, C. L., et al. (2020). Satellite glial cells in sensory ganglia express functional transient receptor potential ankyrin 1 that is sensitized in neuropathic and inflammatory pain. Mol. Pain 16, 1744806920925425. doi: 10.1177/1744806920925425
Siemionow, K., Klimczak, A., Brzezicki, G., Siemionow, M., and McLain, R. F. (2009). The Effects of inflammation on glial fibrillary acidic protein expression in satellite cells of the dorsal root ganglion. Spine 34, 1631–1637. doi: 10.1097/BRS.0b013e3181ab1f68
Siemionow, K., Weinstein, J. N., and McLain, R. F. (2006). Support and satellite cells within the rabbit dorsal root ganglion: ultrastructure of a perineuronal support cell. Spine 31, 1882–1887. doi: 10.1097/01.brs.0000228721.18242.42
Suadicani, S. O., Cherkas, P. S., Zuckerman, J., Smith, D. N., Spray, D. C., and Hanani, M. (2010). Bidirectional calcium signaling between satellite glial cells and neurons in cultured mouse trigeminal ganglia. Neuron Glia Biol. 6, 43–51. doi: 10.1017/S1740925X09990408
Sung, B., Lim, G., and Mao, J. (2003). Altered expression and uptake activity of spinal glutamate transporters after nerve injury contribute to the pathogenesis of neuropathic pain in rats. J. Neurosci. 23, 2899–2910. doi: 10.1523/JNEUROSCI.23-07-02899.2003
Suzumura, A., Takeuchi, H., Zhang, G., Kuno, R., and Mizuno, T. (2006). Roles of glia-derived cytokines on neuronal degeneration and regeneration. Ann. N. Y. Acad. Sci. 1088, 219–229. doi: 10.1196/annals.1366.012
Takeda, M., Takahashi, M., and Matsumoto, S. (2009). Contribution of the activation of satellite glia in sensory ganglia to pathological pain. Neurosci. Biobehav. Rev. 33, 784–792. doi: 10.1016/j.neubiorev.2008.12.005
Tang, X., Schmidt, T. M., Perez-Leighton, C. E., and Kofuji, P. (2010). Inwardly rectifying potassium channel Kir4.1 is responsible for the native inward potassium conductance of satellite glial cells in sensory ganglia. Neuroscience 166, 397–407. doi: 10.1016/j.neuroscience.2010.01.005
Tasdemir-Yilmaz, O. E., Druckenbrod, N. R., Olukoya, O. O., Yung, A. R., Bastille, I., Pazyra-Murphy, M. F., et al. (2020). Diversity of developing peripheral glia revealed by single cell RNA sequencing. Dev. Cell 56, 2516–2535.e8. doi: 10.1101/2020.12.04.411975
Treede, R. D., Rief, W., Barke, A., Aziz, Q., Bennett, M. I., Benoliel, R., et al. (2019). Chronic pain as a symptom or a disease: the IASP Classification of Chronic Pain for the International Classification of Diseases (ICD-11). Pain 160, 19–27. doi: 10.1097/j.pain.0000000000001384
Ullian, E. M., Sapperstein, S. K., Christopherson, K. S., and Barres, B. A. (2001). Control of synapse number by glia. Science 291, 657–661. doi: 10.1126/science.291.5504.657
Vallejo, R., Tilley, D. M., Vogel, L., and Benyamin, R. (2010). The role of glia and the immune system in the development and maintenance of neuropathic pain. Pain Pract. 10, 167–184. doi: 10.1111/j.1533-2500.2010.00367.x
Vallières, L., and Rivest, S. (1997). Regulation of the genes encoding interleukin-6, its receptor, and gp130 in the rat brain in response to the immune activator lipopolysaccharide and the proinflammatory cytokine interleukin-1beta. J. Neurochem. 69, 1668–1683. doi: 10.1046/j.1471-4159.1997.69041668.x
van Weperen, V. Y. H., Littman, R. J., Arneson, D. V., Contreras, J., Yang, X., and Ajijola, O. A. (2021). Single-cell transcriptomic profiling of satellite glial cells in stellate ganglia reveals developmental and functional axial dynamics. Glia 69, 1281–1291. doi: 10.1002/glia.23965
Vermeiren, S., Bellefroid, E. J., and Desiderio, S. (2020). Vertebrate sensory ganglia: common and divergent features of the transcriptional programs generating their functional specialization. Front. Cell Dev. Biol. 8, 587699. doi: 10.3389/fcell.2020.587699
Verpoorten, N., Claeys, K. G., Deprez, L., Jacobs, A., Van Gerwen, V., Lagae, L., et al. (2006). Novel frameshift and splice site mutations in the neurotrophic tyrosine kinase receptor type 1 gene (NTRK1) associated with hereditary sensory neuropathy type IV. Neuromuscul. Disord. 16, 19–25. doi: 10.1016/j.nmd.2005.10.007
Villa, G., Fumagalli, M., Verderio, C., Abbracchio, M. P., and Ceruti, S. (2010). Expression and contribution of satellite glial cells purinoceptors to pain transmission in sensory ganglia: an update. Neuron Glia Biol. 6, 31–42. doi: 10.1017/S1740925X10000086
Vit, J.-P., Ohara, P. T., Bhargava, A., Kelley, K., and Jasmin, L. (2008). Silencing the Kir4.1 potassium channel subunit in satellite glial cells of the rat trigeminal ganglion results in pain-like behavior in the absence of nerve injury. J. Neurosci. 28, 4161–4171. doi: 10.1523/JNEUROSCI.5053-07.2008
Warwick, R. A., and Hanani, M. (2013). The contribution of satellite glial cells to chemotherapy-induced neuropathic pain. Eur. J. Pain 17, 571–580. doi: 10.1002/j.1532-2149.2012.00219.x
Weick, M., Cherkas, P. S., Härtig, W., Pannicke, T., Uckermann, O., Bringmann, A., et al. (2003). P2 receptors in satellite glial cells in trigeminal ganglia of mice. Neuroscience 120, 969–977. doi: 10.1016/S0306-4522(03)00388-9
Yoon, S. Y., Robinson, C. R., Zhang, H., and Dougherty, P. M. (2013). Spinal astrocyte gap junctions contribute to oxaliplatin-induced mechanical hypersensitivity. J. Pain 14, 205–214. doi: 10.1016/j.jpain.2012.11.002
Yuan, Q., Liu, X., Xian, Y., Yao, M., Zhang, X., Huang, P., et al. (2020). Satellite glia activation in dorsal root ganglion contributes to mechanical allodynia after selective motor fiber injury in adult rats. Biomed. Pharmacother. 127, 110187. doi: 10.1016/j.biopha.2020.110187
Zhang, H., Mei, X., Zhang, P., Ma, C., White, F. A., Donnelly, D. F., et al. (2009). Altered functional properties of satellite glial cells in compressed spinal ganglia. Glia 57, 1588–1599. doi: 10.1002/glia.20872
Zhang, X., Chen, Y., Wang, C., and Huang, L. Y. (2007). Neuronal somatic ATP release triggers neuron-satellite glial cell communication in dorsal root ganglia. Proc. Natl. Acad. Sci. U S A. 104, 9864–9869. doi: 10.1073/pnas.0611048104
Zhao, Z., Hiraoka, Y., Ogawa, H., and Tanaka, K. (2018). Region-specific deletions of the glutamate transporter GLT1 differentially affect nerve injury-induced neuropathic pain in mice. Glia 66, 1988–1998. doi: 10.1002/glia.23452
Zhou, J., Feng, D., Zhang, X., Xia, C., Zhang, Z., Kang, J., et al. (2019). Involvement of P2X7 receptors in satellite glial cells of dorsal root ganglia in the BmK I -induced pain model of rats. Gen. Physiol. Biophys. 38, 407–416. doi: 10.4149/gpb_2019026
Zieglgänsberger, W. (2019). Substance P and pain chronicity. Cell Tissue Res. 375, 227–241. doi: 10.1007/s00441-018-2922-y
Keywords: satellite glial cells, chronic pain, pain conditions, nerve injury, heterogeneity
Citation: Andreeva D, Murashova L, Burzak N and Dyachuk V (2022) Satellite Glial Cells: Morphology, functional heterogeneity, and role in pain. Front. Cell. Neurosci. 16:1019449. doi: 10.3389/fncel.2022.1019449
Received: 15 August 2022; Accepted: 20 September 2022;
Published: 06 October 2022.
Edited by:
Jean-Pierre Hornung, Université de Lausanne, SwitzerlandReviewed by:
Alfredo Ribeiro-da-Silva, McGill University, CanadaCopyright © 2022 Andreeva, Murashova, Burzak and Dyachuk. This is an open-access article distributed under the terms of the Creative Commons Attribution License (CC BY). The use, distribution or reproduction in other forums is permitted, provided the original author(s) and the copyright owner(s) are credited and that the original publication in this journal is cited, in accordance with accepted academic practice. No use, distribution or reproduction is permitted which does not comply with these terms.
*Correspondence: Vyacheslav Dyachuk, ZHlhY2h1a192YUBhbG1hem92Y2VudHJlLnJ1