- 1Department of Psychiatry, Loma Linda University, Loma Linda, CA, United States
- 2Patton State Hospital, San Bernardino, CA, United States
- 3Department of Psychiatry, Loma Linda University, Loma Linda, CA, United States
- 4Instituto Nacional de Cardiologia Ignacio Chavez, Mexico City, Mexico
- 5Department of Medicine, The University of Texas Rio Grande Valley, Edinburg, TX, United States
- 6International Agency For Research On Cancer (IARC), Lyon, France
SARS-CoV-2 virus, the etiologic agent of COVID-19, has affected almost every aspect of human life, precipitating stress-related pathology in vulnerable individuals. As the prevalence rate of posttraumatic stress disorder in pandemic survivors exceeds that of the general and special populations, the virus may predispose to this disorder by directly interfering with the stress-processing pathways. The SARS-CoV-2 interactome has identified several antigens that may disrupt the blood-brain-barrier by inducing premature senescence in many cell types, including the cerebral endothelial cells. This enables the stress molecules, including angiotensin II, endothelin-1 and plasminogen activator inhibitor 1, to aberrantly activate the amygdala, hippocampus, and medial prefrontal cortex, increasing the vulnerability to stress related disorders. This is supported by observing the beneficial effects of angiotensin receptor blockers and angiotensin converting enzyme inhibitors in both posttraumatic stress disorder and SARS-CoV-2 critical illness. In this narrative review, we take a closer look at the virus-host dialog and its impact on the renin-angiotensin system, mitochondrial fitness, and brain-derived neurotrophic factor. We discuss the role of furin cleaving site, the fibrinolytic system, and Sigma-1 receptor in the pathogenesis of psychological trauma. In other words, learning from the virus, clarify the molecular underpinnings of stress related disorders, and design better therapies for these conditions. In this context, we emphasize new potential treatments, including furin and bromodomains inhibitors.
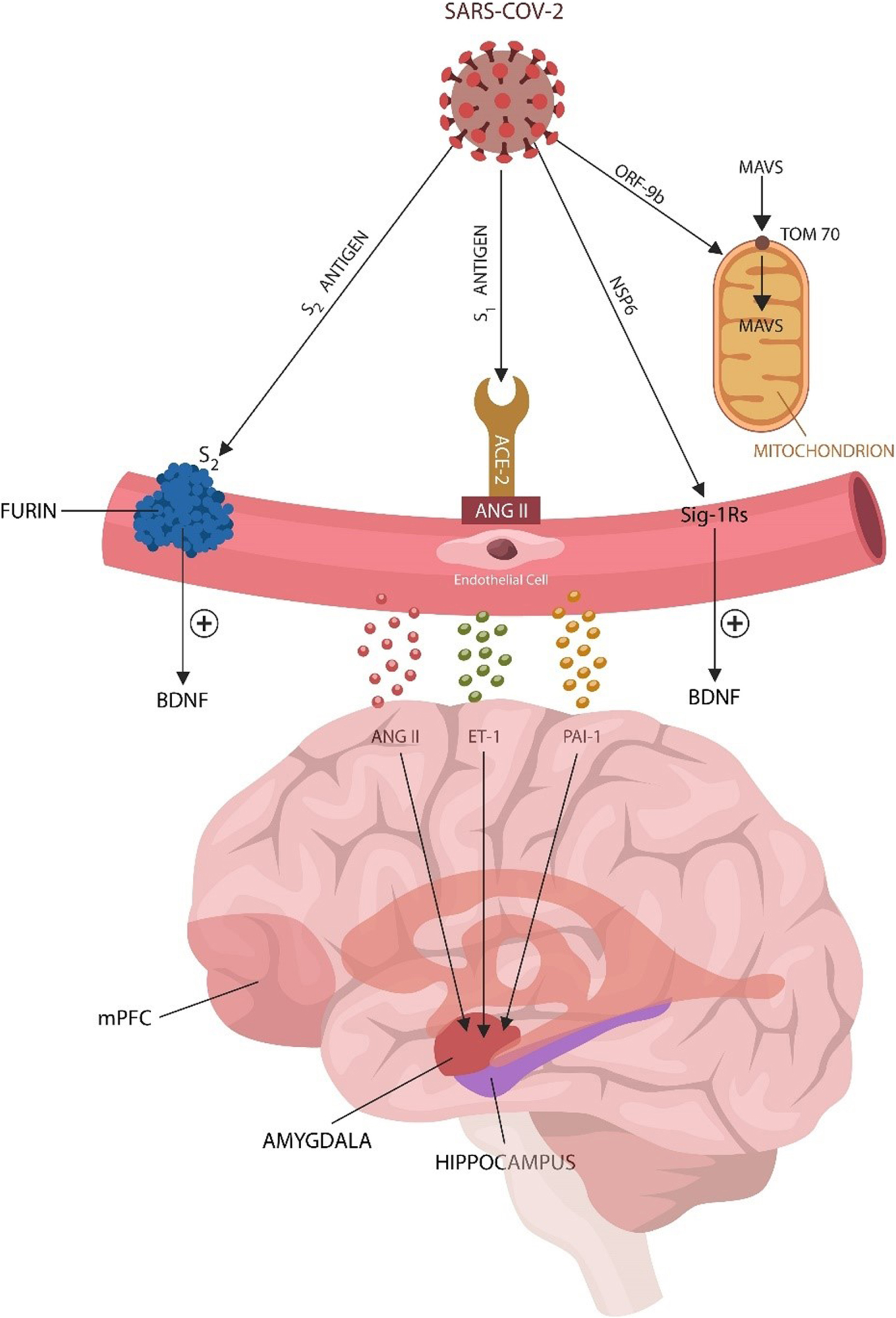
Graphical Abstract 1. Covid-19 triggers endothelial cell (EC) senescence and dysfunction, likely predisposing to PTSD by increasing microvascular permeability that enables the extravasation of stress molecules into the brain trauma-processing networks in amygdala, hippocampus and the medial prefrontal cortex. The virus upregulates host angiotensin II (ANG II) (via S1 antigen), usurps furin/plasmin (via S2 antigen), mitochondria (via ORF9b), and Sigma-1 receptors (Sig-1Rs) via NSP6. These structures, previously associated with PTSD, link the SARS-CoV-2 virus to increased susceptibility for stress related disorders. As ECs are major producers of brain derived neurotrophic factor (BDNF), a neurotrophin altered in PTSD, senescent ECs lower this molecule further, predisposing to stress related disorders.
Highlights
- The SARS-CoV-2 virus triggers endothelial senescence by usurping host serine proteases, the renin angiotensin system, and mitochondria.
- Senescent endothelial cells disrupt the BBB tight junctions, allowing stress molecules, including ANGc II, ET-1 and PAI-1, access to the amygdala, hippocampus, and mPFC, increasing the susceptibility for PTSD and other stress related disorders.
- Virus-damaged mitochondria predispose to PTSD by shifting cellular metabolism from OXPHOS to glycolysis in a Warburg effect.
- Virus-usurped furin and plasmin alter the pro-BDNF/BDNF ratio, predisposing to PTSD.
Introduction
Severe acute respiratory syndrome coronavirus-2 (SARS-CoV-2), the etiological agent of COVID-19, spread rapidly throughout the world and was declared a pandemic in March 2020 (Wu and McGoogan, 2020). Restrictive measures, including mandatory isolation, social distancing, and absence of family support affected fragile populations, including the psychiatric patients, more than the society at large. In this group, many individuals have experienced recurrence of depression, anxiety, and use of substances, often culminating in posttraumatic stress disorder (PTSD) (Chamberlain et al., 2021; Janiri et al., 2021). Moreover, the experience of being hospitalized with COVID-19, facing intubation, tracheostomy, and the possibility of death, amplified the perception of life-threat, facilitating the development of stress related disorders (SRDs) as well as depression and anxiety (Dutheil et al., 2020; Tarsitani et al., 2021).
PTSD is a chronic debilitating syndrome that can originate in vulnerable individuals after exposure to real or threatened death, sexual assault, or severe illness. Impaired fear extinction and excessive anxiety are believed to drive the symptoms of this disorder (Myers and Davis, 2007). Clinical manifestations include re-experiencing the traumatic event(s), hypervigilance, exaggerated startle reflex, intrusive memories, nightmares, and dissociative phenomena (Lancaster et al., 2016).
Although primarily treated by psychiatrists, PTSD may be conceptualized as a systemic disease, considering its frequent association with medical conditions, including metabolic, autoimmune, and vascular disorders (Edmondson et al., 2013; Yapici-Eser et al., 2021). In this regard, the comorbidity of PTSD with cardiovascular and cerebrovascular disease, suggests that dysfunctional endothelia may play a key role in the pathophysiology of this disorder (Vaccarino et al., 2013; Chen et al., 2015; Grenon et al., 2016).
In the general population, about 8% of trauma-exposed individuals develop PTSD, while in military personnel and war veterans, the PTSD prevalence can reach up to 16% (Gates et al., 2012). Interestingly, some viral infections, including SARS-CoV-2, human immunodeficiency virus (HIV) and Ebola were associated with PTSD rates upward of 30%, indicating that these pathogens may interact directly with microvessels and/or the stress-processing centers of the brain (Lam et al., 2009; Siyahhan Julnes et al., 2016; de Solis et al., 2017; Spottswood et al., 2017; Kumar et al., 2021). Indeed, recent epidemiological data reported a PTSD prevalence of up to 30.2% in COVID-19 survivors, 39.5% in Ebola and 34% in HIV, suggesting that these viruses may directly target the cells of brain stress-processing pathways (Israelski et al., 2007; Bah et al., 2020; Janiri et al., 2021). In addition, as these pathogens contain a furin cleaving site (FCS), PTSD may be precipitated by the viral exploitation of furin and plasmin. These serine proteases were previously implicated in SRDs as they convert a precursor protein, pro-BDNF, into brain derived neurotrophic factor (BDNF) (Levin et al., 1997; Aksu et al., 2018; Miranda et al., 2019).
Numerous studies have associated PTSD with the stress mediators of the hypothalamic-pituitary-adrenal (HPA) axis and autonomic nervous system, the two main drivers of stress related phenotypes. However, here we only discuss the SARS-CoV-2-usurped renin-angiotensin system (RAS) that can also trigger this pathology (Chrousos and Zapanti, 2014; Griffin et al., 2014; Marinzalda Mde et al., 2014; Terock et al., 2019; Yu et al., 2019; Shkreli et al., 2020; Seligowski et al., 2021). Indeed, angiotensin receptor blockers (ARBs) and angiotensin converting enzyme inhibitors (ACEi) showed beneficial effects in both PTSD and severe COVID-19, suggesting that dysfunctional RAS may play an essential role in both conditions (Khoury et al., 2012; Marvar et al., 2014; Vian et al., 2017). For instance, the SARS-CoV-2 attachment to angiotensin converting enzyme 2 (ACE-2), disrupts the blood-brain barrier (BBB) by inducing senescence in cerebral endothelial cells ECs (Buzhdygan et al., 2020; Mehta et al., 2021; Rhea et al., 2021; Sanchez-Vazquez et al., 2021). This may enable ANG II to aberrantly activate its receptors in the amygdala, hippocampus, and the medial prefrontal cortex (mPFC), lowering the PTSD resilience (Marinzalda Mde et al., 2014; Wang L. et al., 2016; Terock et al., 2019; Yu et al., 2019; Shkreli et al., 2020). Increased BBB permeability facilitates endothelin-1 (ET-1) and plasminogen activator inhibitor 1 (PAI-1) extravasation and interaction with their respective receptors in the stress-processing pathways, predisposing to PTSD (Kurihara et al., 2000; Jiang et al., 2016; Chen et al., 2017a; Bouarab et al., 2021). Moreover, as mitochondria express ANG II type 1 receptors (AT-1Rs), the virus may disrupt these organelles, shifting metabolism, from oxidative phosphorylation (OXPHOS) to glycolysis and lactate accumulation (Barron et al., 2004; Doughan et al., 2008; Mellon et al., 2019; Sabbatinelli et al., 2019; Gordon et al., 2020; Sher et al., 2020; Icard et al., 2021). It has been established that lactate triggers flashbacks and panic attacks in PTSD patients, suggesting that unchecked glycolysis may account for the increased prevalence of SRDs in COVID-19 (Jensen et al., 1997; Johnson et al., 2013).
In summary, the SARS-CoV-2 viral antigens may upregulate ANG II, ET-1 and PAI-1, promoting mitochondrial dysfunction, EC senescence and increased BBB permeability. Extravasation of these stress-molecules into the brain parenchyma may reprogram the amygdala, hippocampus and mPFC, predisposing to PTSD.
In our previous work, we discussed ANG II-mediated mitochondrial dysfunction and premature EC senescence (Sfera et al., 2020, 2021a,b). Here, based on the SARS-CoV-2 interactome, we go a step further, linking PTSD vulnerability to virus-exploited furin, Sigma-1 receptors (Sig-1Rs), plasmin, bromodomains and mitochondria, moving from specific pathways to organelle structure. In other words, attempting to learn from the virus, may clarify the SRDs molecular underpinnings, and develop better therapies for patients with PTSD. We also discuss potential new treatments, including the inhibitors of furin and bromodomains.
Stress, Aging Vessels, and the SARS-CoV-2 Virus
SARS-CoV-2 is an enveloped, positive-sense single-stranded RNA virus that ingresses human cells through several proteins, including ACE-2 (Bourgonje et al., 2020; Hoffmann et al., 2020). The virus consists a genome of about 29.9 kb, encoding for 29 structural, non-structural (NSP) and open-reading frame (ORF) antigens that interact with numerous human proteins, triggering pathological changes (Gordon et al., 2020). For example, virus-upregulated ANG II interacts with AT-1Rs on cerebral ECs, inducing premature senescence and increased BBB permeability (Fleegal-DeMotta et al., 2009; Marinzalda Mde et al., 2014; Marvar et al., 2014; Yu et al., 2019). A similar pathology is triggered by viral interaction with mitochondria, bromodomain 4 (BRD4), furin, plasmin, and Sig-1Rs (Table 1; Kunieda et al., 2006; Doughan et al., 2008; Gordon et al., 2020; Chamberlain et al., 2021). The connection between FCS and PTSD, as well as Sig-1Rs and PTSD are presented in detail below:
Furin Cleaving Site and PTSD
Novel studies reported premature endothelial senescence in veterans and non-veterans with PTSD, indicating that BBB disruption likely plays a pivotal role in this disorder (Boscarino, 2008; Grenon et al., 2016; Thurston et al., 2018). Others have found that war veterans with PTSD, like older individuals, present with defective mitochondria, telomeres, furin/plasmin, and BDNF, connecting cerebral EC senescence with dysfunctional subcellular structures (Levin et al., 1997; Doughan et al., 2008; Gray and Ellis, 2008; Fleegal-DeMotta et al., 2009; Khoury et al., 2012; Grenon et al., 2016; Aksu et al., 2018; Connolly et al., 2018; Stein et al., 2018; Miranda et al., 2019; Seligowski et al., 2021; Cleveland et al., 2021).
The SARS-CoV-2 antigen S1 usurps ACE-2, upregulating ANG II, while S2 protein exploits furin and plasmin (Table 1). Furin and plasmin are cell membrane serine proteases that under normal circumstances promote the maturation of BDNF, a neurotrophin involved in both PTSD and COVID-19 (Angelucci et al., 2014; Miller et al., 2017; Azoulay et al., 2020). Viral S2 antigen activates the FCS, hijacking host furin and depleting BDNF that in turn increases the risk of COVID-19 and SRDs (Gray and Ellis, 2008; Johnson et al., 2020; Table 1). Indeed, other viruses expressing FCS, including HIV and Ebola, were associated with a high PTSD prevalence, linking this motif to SRDs (Hallenberger et al., 1992; Volchkov et al., 1998; Figure 1). There are also PTSD comorbidities observed with further viral infections, however the scientific basis of those correlations remains an active field of research (Papić et al., 2011; Coughlin, 2012; Morais-de-Jesus et al., 2014). Moreover, as furin and plasmin regulate synaptic plasticity and the fibrinolytic system, an impaired tissue-type plasminogen activator (tPA)/PAI-1 ratio, may lower the resilience for both PTSD and COVID-19. Indeed, severe COVID-19 and impaired fear extinction were associated with tPA/PAI-1 dyshomeostasis (Bouarab et al., 2021; Zuo et al., 2021).
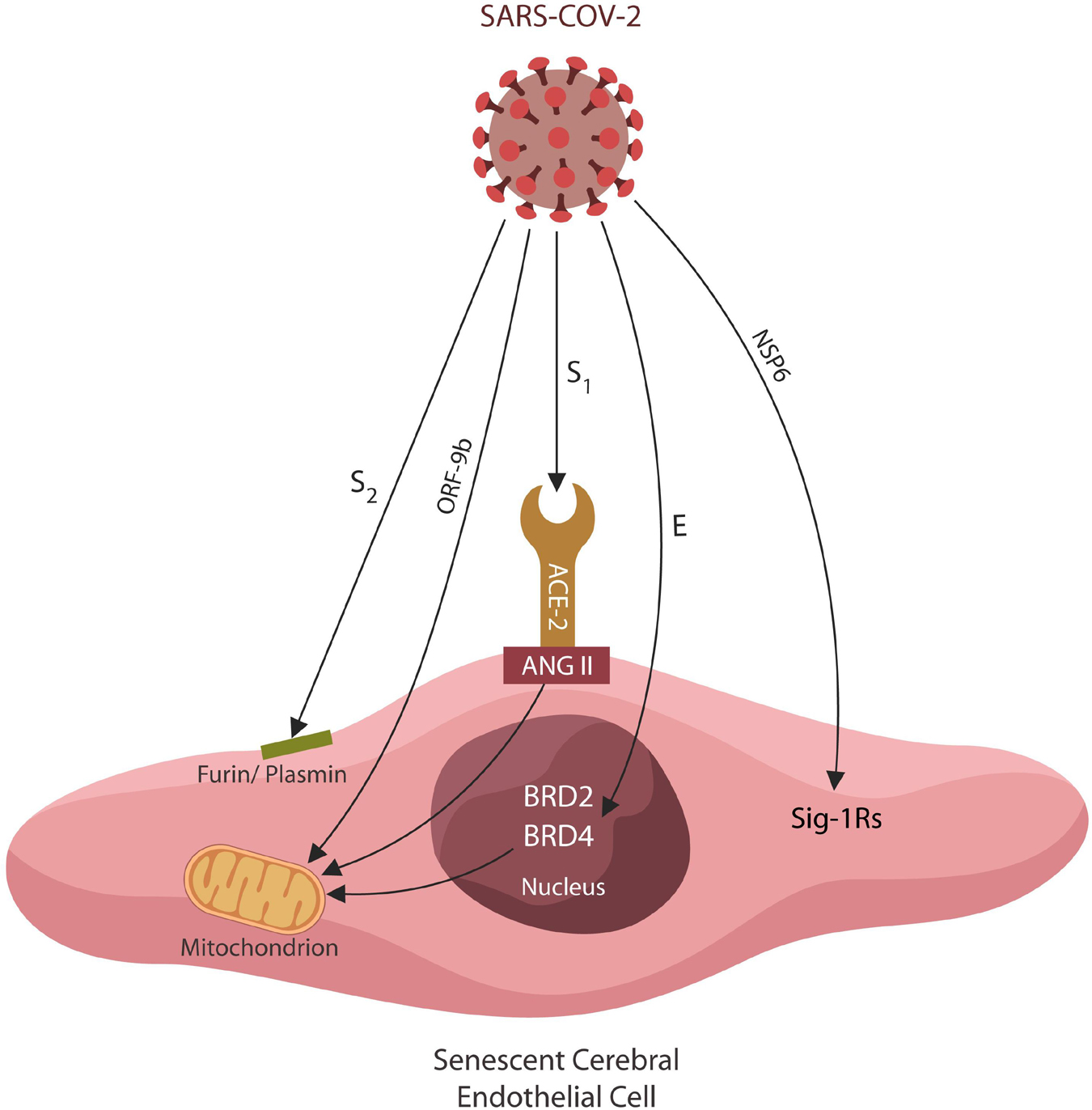
Figure 1. Several SARS-CoV-2 antigens interact directly with host EC proteins, inducing cellular senescence and altering the BBB permeability. Viral hijacking of furin/plasmin by antigen S2 impairs BDNF maturation, increasing PTSD susceptibility. The S1/ACE-2 attachment upregulates ANG II, inducing mitochondrial damage and cellular senescence. Viral ORF9b disrupts mitochondria directly, altering antiviral defenses and cellular metabolism. The SARS-CoV-2 antigen E exploits host epigenetic readers BRD-2 and BRD-4, altering the expression of mitochondrial proteins encoded in the nuclear DNA. Viral antigen NSP6 interacts with Sigma-1 receptors, inducing cellular senescence by an alternative pathway.
Another peripheral molecule that alters BDNF maturation is ET-1, a potent vasoconstrictor upregulated by ANG II (Lin Y.J. et al., 2014; Chen et al., 2017b; Ward et al., 2018; Notaras and van den Buuse, 2020; Diwakar et al., 2021). Since ET-1 was directly corelated with PTSD, its exploitation by the SARS-CoV-2 virus (via ANG II) may also contribute to the high comorbidity of PTSD and COVID-19 (Yammine et al., 2014; Fox et al., 2018). Interestingly, ET-1 is synthesized as a biologically inactive protein, proET-1, that requires processing by furin to convert to bigET-1 and subsequently to ET-1 (Denault et al., 1995). Along these lines, HIV, a FCS-containing virus, was associated with upregulated ET-1, likely accounting for the high PTSD comorbidity (Kanmogne et al., 2005; Neigh et al., 2016; Jain and Mehrotra, 2020).
Taken together, dysfunctional subcellular components, including furin/plasmin and BDNF, trigger cerebral EC senescence and BBB disruption. The FCS motif of the SARS-CoV-2 virus usurps human furin and plasmin, altering BDNF homeostasis that in turn predispose to SRDs (Kanmogne et al., 2005; Figure 1).
Sigma-1receptors and PTSD
The SARS-CoV-2 antigen NSP6 exploits host Sig-1R, a positive BDNF regulator, likely disrupting synaptic plasticity and predisposing to SRDs (Fujimoto et al., 2012; Hashimoto, 2015, 2021; Xu et al., 2015; Gordon et al., 2020; Figure 1). In addition, as ECs are major sources of BDNF and senescent ECs are depleted of this neurotrophin, the susceptibility for both COVID-19 and PTSD is likely increased (Nakahashi et al., 2000; Descamps et al., 2018; Marie et al., 2018; Table 1).
Under physiological circumstances, Sig-1Rs agonists upregulate BDNF, protecting against EC damage triggered by either psychological stress and possibly the SARS-CoV-2 virus (Kimura et al., 2013). In addition, activated Sig-1Rs increase mitochondrial pregnenolone, protecting the organelle from psychological stress and virus-mediated dysfunction (Marriott et al., 2012) (see the section on mitochondria and neurosteroids). Indeed, the Sig-1Rs agonist, fluvoxamine, was shown to lower the intensity of stressful memories in SRD patients and to ameliorate COVID-19 outcomes (De Boer et al., 1992; Escalona et al., 2002; Pal et al., 2012; Hashimoto, 2013, 2021; Ji et al., 2017; Inserra, 2018; Sukhatme et al., 2021). Moreover, Sig-1R activating drugs were reported to protect against ANG II-induced EC damage, suggesting that combining ARBs/ACEi with Sig-1R agonists could have a superior therapeutic value in both COVID-19 and PTSD (Meunier and Hayashi, 2010; Hirano et al., 2014; Natsvlishvili et al., 2015; Liu et al., 2018; Lewis et al., 2019). Furthermore, virus-upregulated ANG II and hijacked Sig-1Rs may explain the early development of neurodegeneration observed in many PTSD patients (Yaffe et al., 2010; Mohlenhoff et al., 2017; Sobczak et al., 2021).
In response to stressors, cells undergo senescence, a state of proliferation arrest and active metabolism fueled primarily by glycolysis-derived lactate (Wiley and Campisi, 2016). Interestingly, under normal circumstances, ECs obtain over 80% of adenosine triphosphate (ATP) from lactate despite oxygen availability (Warburg effect), probably explaining the SARS-CoV-2 predilection for these cells (Sabbatinelli et al., 2019; Leung and Shi, 2021). Indeed, as senescent ECs upregulate lactate even more than their younger counterparts, they engender an optimal environment for viral replication (Schmid et al., 2007; Wong et al., 2017). Upon crossing into the brain parenchyma, lactate generates a local acidic microenvironment that may activate the acid-sensing ion channel-1a (ASIC1a), a protein implicated in SRDs (Coryell et al., 2009; Ziemann et al., 2009; Smoller et al., 2014; Quagliato et al., 2018). For example, low brain pH activates amiloride-sensitive cation channel, an member of the ASIC1a family, triggering PTSD symptoms (Taugher et al., 2017; Quagliato et al., 2018). Indeed, lactate was demonstrated to precipitate flashbacks and anxiety in PTSD and panic disorder patients, connecting unchecked glycolysis to the high prevalence of SRDs in COVID-19 survivors (Jensen et al., 1997; Filipović et al., 2020). Indeed, ASIC1a receptor antagonist, amiloride, was reported beneficial in anxiety and SRDs, further implicating glycolysis in these conditions (Pidoplichko et al., 2014; Battaglia et al., 2019). For this reason, amiloride nasal spray is considered therapeutic for PTSD as it readily crosses the BBB, inhibiting ASIC1a (Dwyer et al., 2009; Yellepeddi et al., 2020). Interestingly, under physiological circumstances, Sig-1R agonists also inhibit ASIC1a, indicating that drugs like fluvoxamine may counteract the detrimental effects of excessive brain lactate (Herrera et al., 2008). In consequence, a combination of fluvoxamine and amiloride may be a superior PTSD therapy compared to each drug individually.
Hijacking Sig-1Rs, the SARS-CoV-2 virus likely increases the detrimental effects of lactate on brain stress-processing pathways, explaining the high prevalence of SRDs in this infection (Vela, 2020; Figure 1). Moreover, ASIC1a receptors are abundantly expressed in cerebral arteries, suggesting that, like ANG II, upregulated lactate may increase the BBB permeability (Lin L.H. et al., 2014). This is in line with preclinical studies showing that lactate operates hand in hand with ANG II to engender anxiety (Shekhar et al., 2006; Yu et al., 2019).
Taken together, Sig-1R agonists, such as fluvoxamine, may reverse the negative effects of virus-upregulated ANG II on endothelia. As Sig-1R are downregulated by psychological and cellular stress, agonists at these receptors in combination with ARBs, ACEi or amiloride may offer superior therapeutic efficacy to patients with PTSD and severe SARS-CoV-2 (Spottswood et al., 2017; Hashimoto, 2021; Janiri et al., 2021).
The SARS-CoV-2 Virus, Psychological Stress, BDNF and the Fibrinolytic System
Beyond the direct interaction of SARS-CoV-2 proteins with specific pathways in the interactome, the following paragraphs take a wider, more systematic perspective, focusing on psychological stress and BDNF as well as the fibrinolytic system.
The Virus and BDNF
Brain derived neurotrophic factor is a growth factor belonging to the neurotrophin family that promotes angiogenesis, neurogenesis, and synaptic plasticity, enhancing the CNS recovery after insults (Alhusban et al., 2016). BDNF is synthesized as a biologically inactive precursor protein, pro-BDNF, that is activated upon furin or plasmin processing (Luchkina and Bolshakov, 2019).
Brain derived neurotrophic factor signals with tropomyosin receptor kinase B (TrkB), promoting hippocampal long-term potentiation (LTP), synaptic plasticity and memory formation (Jia et al., 2010; Leal et al., 2015). In contrast, pro-BDNF activates p75 neurotrophin receptor (p75NTR), inducing hippocampal long-term depression (LTD), dysfunctional synaptic plasticity and likely impaired fear learning (Rösch et al., 2005; Martinowich et al., 2012). Indeed, PTSD was associated with LTD, defective fear extinction, and impaired memory, likely accounting for the hypermnesia and dissociative amnesia documented in these patients (Graves et al., 2016; He et al., 2018).
In the adult brain, p75NTR is expressed almost exclusively in the cholinergic neurons of the basal forebrain and is altered by psychological stress, connecting neurotrophins to acetylcholine signaling (Woo et al., 2005; Martinowich et al., 2012; Green et al., 2013; Aksu et al., 2018; Boskovic et al., 2018; Ilchibaeva et al., 2018). Along these lines, neuroimaging studies in PTSD patients found abnormal activation of basal forebrain cholinergic neurons, suggesting that p75NTR may be upregulated (Hughes and Shin, 2011; Sherin and Nemeroff, 2011; Turchi et al., 2018). In addition, the adverse effects of acetylcholinesterase inhibitors often resemble PTSD symptoms, indicating that hyperactive cholinergic signaling may play a role in SRDs (Kaufer et al., 1998; McLay and Ho, 2007). Indeed, preclinical studies associated PTSD with dysfunctional p75NTR in basal forebrain (Martinowich et al., 2012). This is in line with the clinical studies in combat veterans with PTSD and non-veterans with anxiety and aggression that documented abnormal pro-BDNF/BDNF ratio, likely due to p75NTR upregulation (Matsuoka et al., 2015; Ilchibaeva et al., 2018; Kowiański et al., 2018).
Despite the established connection between BDNF and PTSD, the neurotrophin serum levels have been inconclusive, indicating that more studies are needed to clarify this issue (Angelucci et al., 2014; Martinotti et al., 2015; Mojtabavi et al., 2020). For example, low BDNF was found in individuals that developed PTSD shortly after stress exposure, while others found increased BDNF levels in patients with established PTSD (Angelucci et al., 2014; Mojtabavi et al., 2020). This discrepancy might be reconciled if BDNF is considered together with nitric oxide (NO) and peroxinitrate levels (Biojone et al., 2015; Banoujaafar et al., 2016). While NO itself lowers BDNF, peroxynitrate was shown to upregulate astrocytic growth factors, probably including the BDNF (Canossa et al., 2002; Vargas et al., 2004). Psychological stress was associated with the overexpression of nitric oxide synthase and peroxynitrate upregulation, linking oxidative stress with psychosocial trauma (Kumar and Chanana, 2017). On the other hand, NO has antioxidant properties and was reported to decrease anxiety and depressive-like behaviors in animal models (Kumar and Chanana, 2017). Interestingly, peroxynitrate is a TrkB receptor agonist that may compete with BDNF for the receptor site, accounting for the elevated BDNF levels documented in patients with chronic PTSD (Yuen et al., 2000; Amoureux et al., 2008; Krolow et al., 2014). Moreover, ebselen, a peroxynitrate scavenger, was found therapeutic in COVID-19, probably by restoring the physiologic BDNF/TrkB signaling, indicating a potential therapeutic value in PTSD (Chen et al., 2007; Amporndanai et al., 2021). Along these lines, several studies found that NO inhibitors, including methylene blue, can lower anxiety and depression, ameliorating many SRD symptoms (Chen et al., 2007; Zoellner et al., 2017). Furthermore, a polymorphism of NO gene, nitric oxide synthase 1 adaptor protein (NOS1AP) and a BDNF variant, Val66Met, were associated with severe PTSD, emphasizing the importance of NO/BDNF dialog in this disorder (Chen et al., 2006; Bruenig et al., 2017; Pitts et al., 2019). Indeed, a dysfunctional NO/BDNF interaction with resultant EC senescence, was found in both PTSD and COVID-19 critical illness (Azoulay et al., 2020; Nehme et al., 2020). Interestingly, NO is a furin inhibitor that may lower SARS-CoV-2 infectivity by denying this serine protease to the virus (Wolf and Morrison, 2017). For this reason, NO is currently in clinical trials for COVID-19 (NCT04388683).
Other furin inhibitors, including diminazene, are currently being evaluated for efficacy against COVID-19, suggesting that withholding furin from the SARS-CoV-2 virus may comprise a valuable therapeutic strategy (AbdelMassih et al., 2020; Wu et al., 2020). As diminazene also blocks ASIC1a channels, attenuating the detrimental effect of brain lactate, this agent may also be therapeutic in PTSD (Qaradakhi et al., 2020). Furthermore, spironolactone, another furin inhibitor, was found to protect against COVID-19 critical illness, suggesting a potential role in PTSD (Schmidt et al., 2017).
Aside from neurons, BDNF also protects ECs and type II pneumocytes (both cell types targeted by SARS-CoV-2), emphasizing that this neurotrophin likely attenuates viral replication (Takeda et al., 2013; Wilcox and Pitt, 2020). Indeed, COVID-19 critical illness was associated with low BDNF, while recovery was directly corelated with the levels of this growth factor (Azoulay et al., 2020). Moreover, as ACE-2 is essential for BDNF release, viral exploitation of this protein, likely impairs synaptic plasticity, predisposing to SRDs (Paris et al., 2020). On the other hand, ARBs and ACEi were shown to upregulate BDNF, improving synaptic plasticity, further linking dysfunctional RAS to PTSD (Ishrat et al., 2015; Alhusban et al., 2016; Andhavarapu et al., 2021; Table 2).
The Virus and Fibrinolytic System
The fibrinolytic system regulates thrombolysis (via plasmin activation) and neuroplasticity (via BDNF) (Wang X.L. et al., 2016). Both severe COVID-19 and PTSD were associated with upregulated PAI-1, a negative tPA regulator, indicating that targeting this protein may be therapeutic for both diseases (Cesari et al., 2010; Idell et al., 2017; Bouarab et al., 2021). Indeed, PAI-1 has been identified as a potential target in COVID-19 and its antagonists, such as statins, are currently in clinical trials (NCT04634799) (NCT04472611) (Bouarab et al., 2021; Kellici et al., 2021; Figure 2).
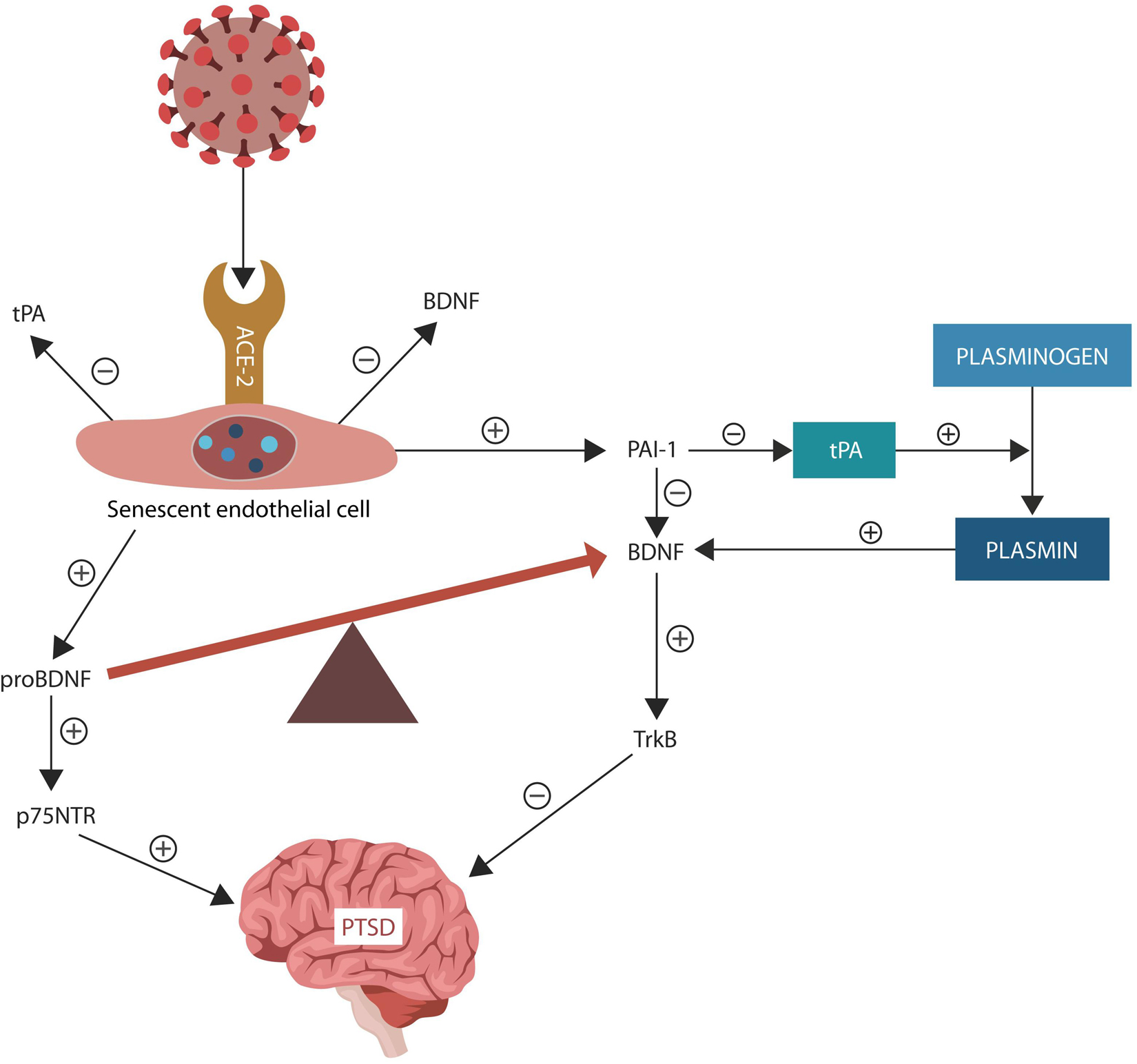
Figure 2. Under normal circumstances, tPA facilitates conversion of plasminogen to plasmin. PAI-1 is a tPA inhibitor that downregulates plasmin, a protein necessary for BDNF maturation. The SARS-CoV-2 interaction with ACE-2, increases ANG II inducing EC senescence. Senescent ECs upregulate PAI-1, lowering tPA and plasmin levels. SARS-CoV-2 antigen S2 usurps furin and plasmin (not shown), disrupting the conversion of pro-BDNF into BDNF, that in return impairs synaptic plasticity, predisposing to PTSD. Upregulated pro-BDNF increases PTSD vulnerability further.
COVID-19-induced endothelial senescence and altered BBB may facilitate PAI-1 extravasation and interference with the stress-processing centers in amygdala, hippocampus, and mPFC. Several studies connected tPA/PAI-1 imbalance to PTSD, fear, anxiety, and depression, suggesting that restoring the fibrinolytic homeostasis may ameliorate the symptoms of SRDs (Pawlak et al., 2003; Bouarab et al., 2021). In addition, senescent ECs upregulate PAI-1, disrupting both plasmin activation and BDNF maturation, predisposing to PTSD (Pawlak et al., 2003; Tsai, 2017; Vaughan et al., 2017; Figure 2). On the other hand, ARBs and ACEi upregulate plasmin and downregulate PAI-1, promoting BDNF maturation and synaptic plasticity (Fogari and Zoppi, 2006; Baluta and Vintila, 2015; Chen et al., 2017c).
Attempts to increase BDNF levels with exogenous neurotrophin have been frustrating because of the unfavorable pharmacodynamic properties of this molecule, including poor BBB crossing. For this reason, a new modality, BDNF delivery via minimally invasive nasal depot (MIND), appears to be a promising PTSD treatment (Szekeres et al., 2010). In addition, several TrkB receptor agonists have been tested in veterans with PTSD, including the small molecule, 7,8-dihydroxyflavone, that showed beneficial effects in many patients (Padmakumar et al., 2021). In addition, as lithium displays TrkB agonism, it was found helpful, especially for the PTSD patients with impulsivity and affective instability (Sanz-García et al., 2016). Another TrkB agonist, LM22A-4, has been suggested for use in PTSD as it showed some positive results in animal models (Forster et al., 1995). Interestingly, candesartan was demonstrated to upregulate BDNF and promote angiogenesis, suggesting a potential treatment for dysfunctional endothelia and PTSD (Andero and Ressler, 2012; Table 2).
Taken together, psychological stress causes overexpression of nitric oxide synthase and peroxynitrate formation, disrupting the pro-BDNF/BDNF balance. Peroxynitrate scavengers and TrkB agonists, including intranasal BDNF, may have therapeutic value in SRDs.
Of Stress and Water
Cerebral ECs form the BBB with astrocytic end-feet, structures rich in aquaporin-4 (AQP-4) channels that also engender the glymphatic system, a waste disposal apparatus, located between astrocytic processes and ECs (Daneman and Prat, 2015; Krikov et al., 2008). Interstitial fluid (ISF) circulation through this space facilitates the clearance of molecular debris and contributes to the brain-wide diffusion of peptides, neurotransmitters, BDNF and viral particles (Jessen et al., 2015; Plog and Nedergaard, 2018; Figure 3). A growing body of evidence connected that AQP-4 to BDNF and neuroplasticity in the hippocampus, amygdala and mPFC (Szu and Binder, 2016; Huber et al., 2018). On the other hand, dysfunctional AQP-4 channels were associated with depression, insomnia, dysfunctional synaptic plasticity, and impaired fear extinction (Scharfman and Binder, 2013; Berntsen and Rubin, 2014). For example, AQP-4 knockout mice displayed both corticosterone-induced depression and defective memory, symptoms reversed by mifepristone and fluoxetine, linking the brain water circulation to SRDs (Kong et al., 2014; Di Benedetto et al., 2016). Moreover, astrocytic end-feet were demonstrated to contain abundant angiotensinogen, a molecule regulated by AQP-4, connecting this protein to RAS (Wei et al., 2019). Interestingly, ARBs and ACEi were demonstrated to upregulate peritoneal AQP-4, suggesting that these agents may have a similar effect in astrocytic end-feet (Matsushita et al., 2010). In addition, as COVID-19 was reported to target hippocampal astrocytes, the virus likely alters AQP-4 (via ANG II), disrupting synaptic plasticity and fear extinction (Imai et al., 2001; Tavčar et al., 2021).
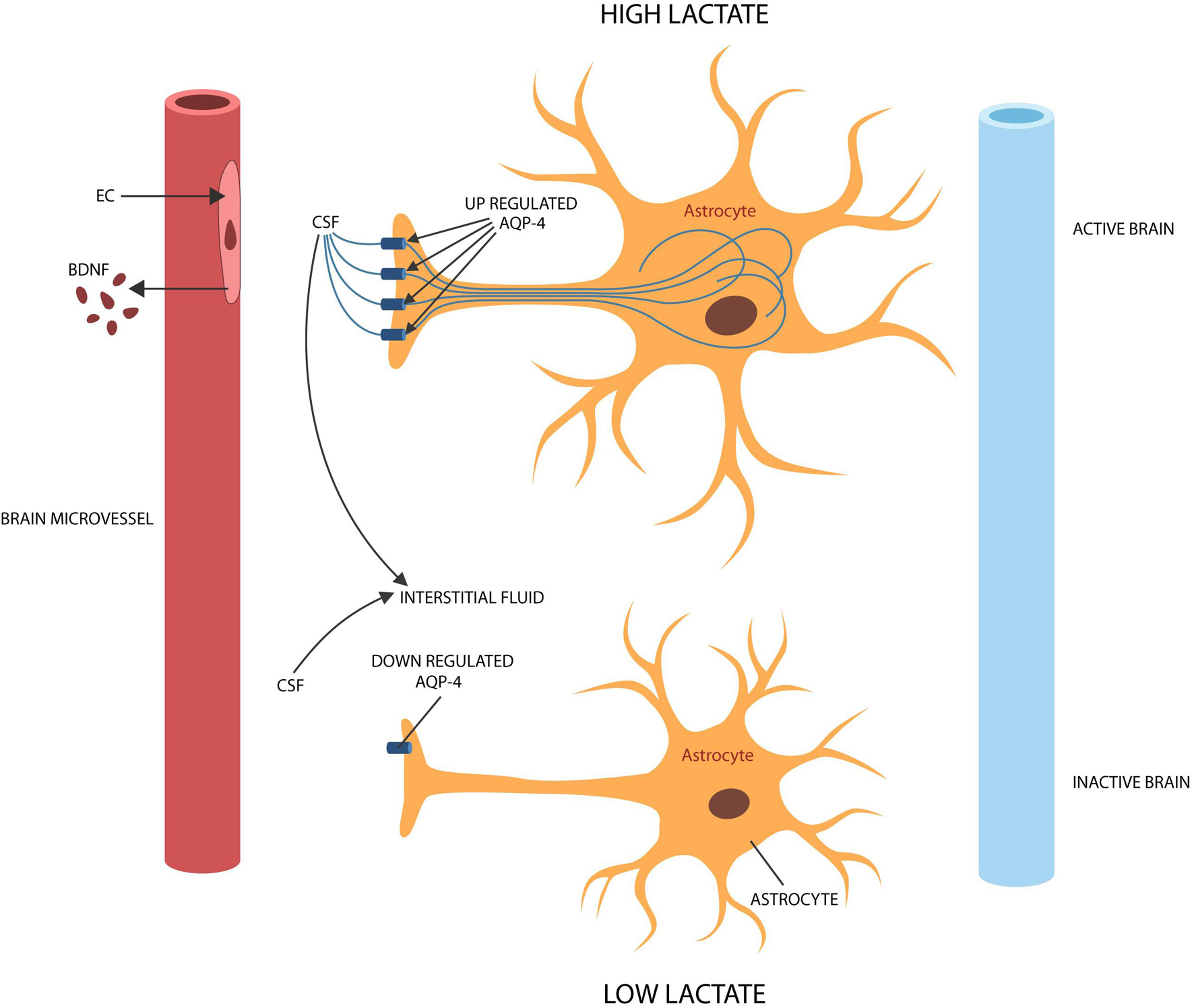
Figure 3. During brain activation and information processing, ISF enters active astrocytes via AQP-4 channels, leaving less fluid in the extracellular space and upregulating lactate (shuttled to neurons). During idle time or slow wave sleep, ISF exits the astrocyte, widening the extracellular space and lowering lactate to facilitate waste clearance. To accomplish their physiological functions, astrocytes require activation by BDNF. Senescent cerebral ECs produce less BDNF, probably leading to astrocyte deactivation and dysfunctional glymphatic clearance.
In our previous work on delirium, we hypothesized that dysfunctional AQP-4, led to impaired information processing, connecting these proteins to neuroplasticity and memory formation (Sfera et al., 2015; Ritchie et al., 2020). This is now supported by data, demonstrating that AQP-4 receptors are essential for brain activation during mental work and deactivation during downtime or sleep, revealing an inverse relationship between memory formation and ISF circulation (Sfera and Osorio, 2014; DiNuzzo and Nedergaard, 2017). Indeed, astrocytic end feet, express the most AQP-4 channels in the entire CNS, playing a key role in brain activation and deactivation (Mader and Brimberg, 2019).
Astrocytes have previously been implicated in PTSD as they regulate synaptic transmission, plasticity, and the formation of aversive memories (Nagelhus and Ottersen, 2013; Saur et al., 2016). To participate in neuroplasticity as well as in the glymphatic circulation, astrocytes require activation by BDNF (Brigadski and Leßmann, 2020). In this regard, PTSD-associated hypermnesia and dissociative amnesia may be traced to astrocytic deactivation (Kol et al., 2020). For example, preclinical studies have associated fear with dysfunctional glymphatic circulation, further connecting the astrocyte to stressful experiences (Li et al., 2020). As astrocytic activation restores the glymphatic circulation, lowering anxiety and fear in animal models, stimulation of these cells may emerge as a therapeutic strategy for SRDs (Martin-Fernandez et al., 2017; Verkhratsky and Nedergaard, 2018; Figure 3). Other preclinical studies have reported that ANG II inhibits GABAergic transmission in the CNS, triggering anxiety and fear, further connecting dysfunctional RAS to SRDs (Li and Pan, 2005; Wang et al., 2018; Zhou et al., 2019). This is significant as it may explain the high prevalence of PTSD in COVID-19 survivors.
Astrocytes have been reported to drive LTP as they shuttle lactate to active neurons, increasing plasticity and memory formation (Hagiwara and Kubo, 2007). Indeed, preclinical studies have associated amnesia with impaired astrocytic lactate transporters, while studies in humans linked psychological stress to the upregulated plasma lactate (Suzuki et al., 2011; Descalzi et al., 2019). Moreover, patients with neuromyelitis optica, a rare autoimmune disease marked by autoantibodies against AQP-4, demonstrated elevated lactate concentrations, suggesting an inverse relationship between glycolysis and the expression of water channels in astrocytic end-feet (Kubera et al., 2012). Along these lines, novel studies have shown that lactate circulates through AQP-4 and that glycolysis is inversely corelated with the expression of water channels (Jarius et al., 2014). Indeed, lactate is upregulated during brain activation, likely to facilitate water entry into astrocytes, while during idle time or slow wave sleep, AQP-4 channels are downregulated to facilitate the glymphatic clearance (Jarius et al., 2014).
Several viruses, including HIV and SARS-CoV-2, were associated with AQP-4 autoantibodies, suggesting that disabling the water channels to upregulate lactate may be a strategy adopted by select pathogens (Lundgaard et al., 2017; Rahimy et al., 2017; Mariajoseph-Antony et al., 2020; Tice et al., 2020; Erickson et al., 2021). Indeed, AQP-4 autoantibodies were found in a subset of COVID-19 patients with neurological symptoms, linking defective water channels to COVID-19 critical illness (Corrêa et al., 2021). In contrast, normal aging was associated with upregulated AQP-4 and loss of glycolysis, further emphasizing the inverse relationship between water channels and brain lactate (Owasil et al., 2020; Corrêa et al., 2021).
Taken together, dysfunctional AQP-4 interferes with RAS and the brain metabolism. As viruses thrive in lactate-rich environments, they may have developed the capability to disable AQP-4 channels, promoting glycolysis and excessive lactate that increases SRD vulnerability.
Mitochondria and PTSD
As the final part of the manuscript, we are looking into the higher cellular structures, i.e., organelles and in particular mitochondria, their relation to COVID-19, and potential insights into PTSD.
Mitochondria are dynamic intracellular organelles that participate in multiple physiological functions, including metabolism, ROS generation, cellular senescence, and innate antiviral immunity (Goyal et al., 2017). The latter is initiated by mitochondrial import of antiviral signaling protein (MAVS) through the pore TOM70 (translocase of the outer mitochondrial membrane 70) (Seth et al., 2005; Tang et al., 2014; Gordon et al., 2020). Indeed, most of the mitochondrial proteome is encoded by the nuclear genome and imported into the organelle as precursor proteins. Recently, TOM70 was associated with mitochondrial bioenergetics, indicating that viral exploitation of this protein could also alter cellular metabolism (Liu et al., 2010; Figure 4).
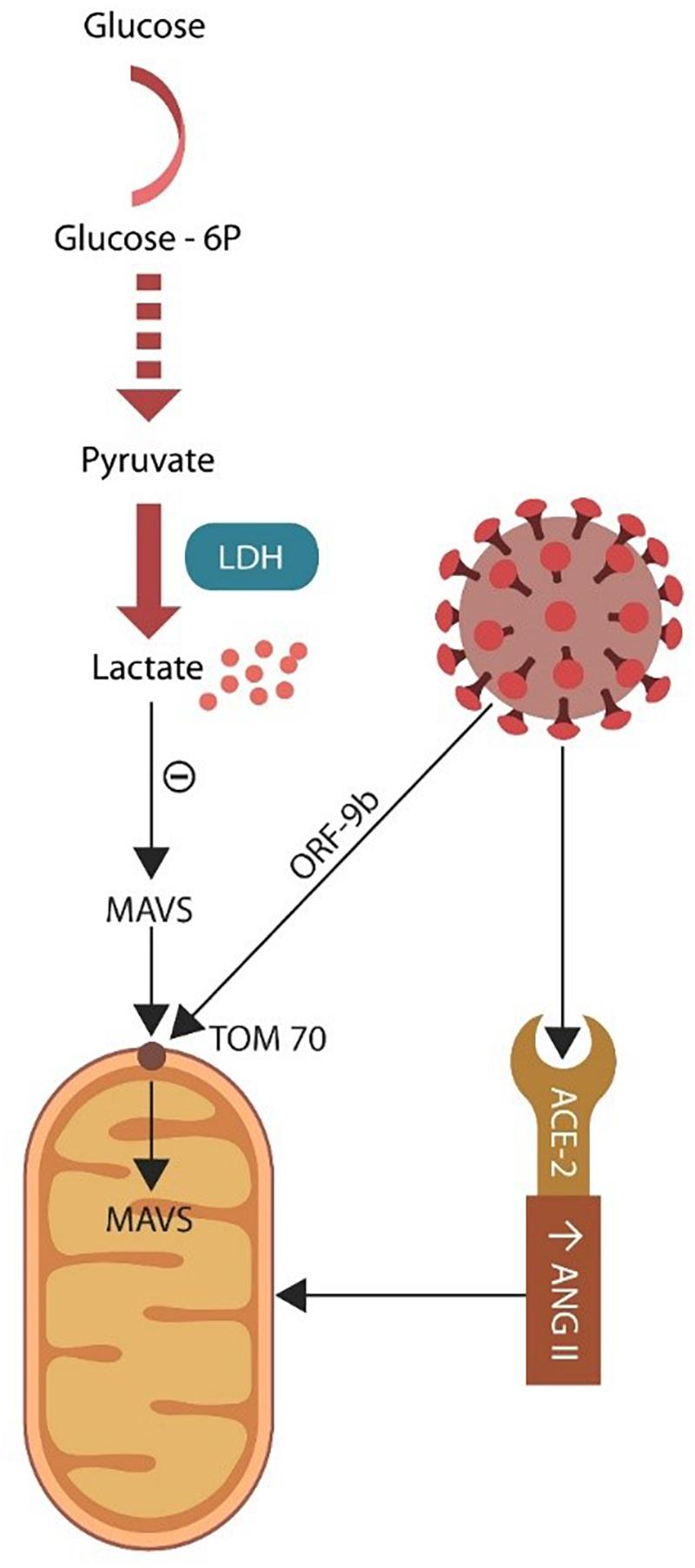
Figure 4. Glycolysis takes place in the cytoplasm where glucose is converted to pyruvate. Under normal circumstances pyruvate enters the mitochondrion and generates ATP via OXPHOS. Damaged mitochondria may be incapable of sustaining OXPHOS, forcing the cell to rely on lactate (generated via LDH). Excess lactate blocks the antiviral MAVS entry into the mitochondrion through the TOM70 pore. SARS-CoV-2 virus targets TOM70 (via ORF-9b), emphasizing the importance of this channel for both antiviral defenses and cellular metabolism.
Mitochondria are rich in iron as they house the iron-sulfur clusters (ISCs) and heme prosthetic groups. Since infectious agents, including the SARS-CoV-2 virus may require iron for replication, they target the mitochondrion. However, the host immune system is also dependent on iron for clonal expansion and sequestrates this biometal, initiating the “battle for iron” (Filadi et al., 2018). To extract iron, the SARS-CoV-2 virus may damage the mitochondrion directly via ORF9b and indirectly via bromodomains and ANG II (Gordon et al., 2020; Figure 1). As mitochondria express AT-1R and ET-1Rs, the virus may also disrupt the organelle via ANG II accumulation (Carver, 2018; Ganji and Reddy, 2021). For example, ANG II-upregulated ET-1 damages the organelle by interfering with mitochondrial genome and the cellular metabolism (Dikalov and Nazarewicz, 2013). Indeed, several studies found that ROS upregulate mitochondrial AT-1Rs, while ARBs or ACEi lower ROS, restoring mitochondrial function (de Cavanagh et al., 2003; Chaphalkar et al., 2020).
Psychological stress was shown to upregulate ROS, damaging the mitochondrion that in turn triggers cellular senescence in many cell types, including the cerebral ECs (Dai et al., 2011; Toda and Nakanishi-Toda, 2011; Salim, 2014; Kershaw et al., 2017). On the other hand, preclinical studies have reported that mitochondrial antioxidants, such as elamipretide or SS-31, restore organelles’ homeostasis, restoring endothelial function (Tarantini et al., 2018; Chiao et al., 2020). Along these lines, several studies on combat veterans with PTSD found a direct relationship between symptom severity and the degree of mitochondrial damage, emphasizing the role of these organelles in SRDs (Flaquer et al., 2015; Bersani et al., 2016; Chen et al., 2017d). Moreover, psychological stress-upregulated ROS trigger telomere attrition, a phenomenon encountered in both PTSD and severe COVID-19, linking these conditions to premature aging (Atanackovic et al., 2002; Yegorov et al., 2020). Interestingly, it was reported that aside from AT-1Rs and ET-1Rs, mitochondria also expressed gamma-aminobutyric acid (GABA), glucocorticoid, and monoamine oxidase A and B receptors, further connecting the organelle to SRDs (Krueger, 1995; Mongelli et al., 2021).
Mitochondria and Lactate
Most cells throughout the body obtain ATP from mitochondria-associated OXPHOS but under hypoxic conditions switch to glycolysis, deriving energy from lactate (Naoi et al., 2006; Owasil et al., 2020). The SARS-CoV-2 virus may target mitochondria to acquire iron and block the import of MAVS, while at the same time it rewires the cellular metabolism to aerobic glycolysis in a Warburg-like effect encountered in malignant cells (Wilson, 2017; Figure 4). Damaged organelles release mitochondrial DNA (mDNA), including copy number (mtDNAcn) and cell-free mitochondrial DNA (cf-mtDNA), emphasizing potential biomarkers for both PTSD and COVID-19 critical illness (Bersani et al., 2016; Chauhan et al., 2019; Scozzi et al., 2021; Trumpff et al., 2021).
As the SARS-CoV-2 virus thrives on lactate, it preferentially targets ECs, that under normal circumstances, derive most of their energy from glycolysis. In addition, as the virus induces EC senescence, it may upregulates lactate further, generating a friendly microenvironment for its replication. Excessive brain lactate, as discussed above, likely triggers PTSD symptoms by activating ASIC1a and downregulating AQP-4 (Smoller et al., 2014; Quagliato et al., 2018; Li et al., 2020).
Propranolol, an established glycolysis inhibitor, has been utilized in PTSD for decades as it facilitates the extinction of fear learning, linking this cognitive defect to excessive lactate (Vaiva et al., 2003; Brohée et al., 2018; Ganji and Reddy, 2021). In addition, propranolol protects ECs by restoring mitochondrial integrity, increasing PTSD resilience (Giustino et al., 2016). Propranolol also blocks the Warburg effect, disrupting the energy supply of both malignant and virus-infected cells, emphasizing the anticancer and antiviral properties of this drug (Iwai et al., 2002; Lucido et al., 2018; Barbieri et al., 2020; Vasanthakumar, 2020).
Aside from its role as a metabolite, lactate is also a signaling molecule that interacts with G-protein-coupled receptor 81 (GPR81), increasing angiogenesis and opposing ECs senescence (Morland et al., 2015, 2017). Interestingly, preclinical studies reported that GPR81 agonists trigger anxiety via cAMP, suggesting that blocking these receptors could be therapeutic in SRDs (Morland et al., 2015; Shan et al., 2020). Interestingly, a cAMP transcription factor, cAMP response element-binding (CREB) protein, was implicated in PTSD, and can be inhibited by dopamine blockers (Tsang and Lal, 1977; Martini et al., 2013; Keil et al., 2016).
Another mitochondria-protective agent with therapeutic potential in PTSD is dichloroacetate (DCA), a lactate inhibitor with established anticancer and antiviral properties (Kho et al., 2019). Despite these benefits, DCA use in PTSD is likely limited by its serious adverse effects such as peripheral neuropathy and delirium (Table 2).
Mitochondrial transplant, used with some success in pediatric patients with Pearson’s syndrome, may also benefit PTSD patients by replacing damaged organelles (Emani and McCully, 2018). However, as of this time, it is unclear whether transplanted mitochondria can survive in the extracellular environment or selectively enter in the defective cells, indicating that this intervention is not ready for clinical practice at this time. However, another procedure based on introducing functional mitochondria directly into dysfunctional cells may be more promising for PTSD (Gomzikova et al., 2021).
Mitochondria and Neurosteroids
Neurosteroids, synthesized in the CNS, adrenals, and gonads, are agonists at GABA-A receptors, that play a major role in regulating multiple brain signaling pathways (Lightowlers et al., 2020). Mitochondria initiate steroidogenesis by importing cholesterol to synthesize pregnenolone, a precursor molecule, that exits the organelle and is converted to progesterone, pregnanolone, and allopregnanolone in the cytoplasm (Zorumski et al., 2013).
Women with PTSD demonstrated low CSF levels of allopregnanolone and pregnanolone, further linking this disorder to mitochondrial dysfunction (Rasmusson et al., 2006; Almeida et al., 2021). Interestingly, allopregnanolone, also known as brexanolone, was approved by the Food and Drug Administration (FDA) for the treatment of postpartum depression (Pineles et al., 2018; Morrison et al., 2019). Earlier studies found that allopregnanolone improved the BBB permeability, indicating potential benefits for senescent endothelia and PTSD (brexanolone is currently in clinical trials for this disorder) (NCT04468360). Moreover, allopregnanolone was found therapeutic in PTSD and severe COVID-19, as it might reverse some aspects of endothelial senescence (Lüscher and Möhler, 2019). In addition, progesterone was reported beneficial for both COVID-19 and PTSD, possibly by increasing NO in cerebral ECs (Balan et al., 2019; Ney et al., 2019; Seligowski et al., 2020). Indeed, as mentioned above, NO pathology contributes to PTSD and is currently in clinical trials for COVID-19 (Ghandehari et al., 2021) (NCT04601077) (Table 2).
Taken together, neurosteroids may be therapeutic for PTSD and COVID-19 as they improve mitochondrial function and restore endothelial integrity.
Mitochondria and Bromodomains
The E (envelope) antigen of SARS-CoV-2 virus hijacks BRD2 and BRD4, possibly damaging mitochondria that in return trigger cellular senescence, glycolytic states, and impaired antiviral immunity (Gordon et al., 2020). In animal models, defective BRD4 was associated with impaired synaptic plasticity and memory formation, likely linking this protein to PTSD-related amnesia, depression, and anxiety, while at the same time indicating that BRD4 inhibitors may be therapeutic for SRD (Bruenig et al., 2017; Wang et al., 2021). The SARS-CoV-2 virus may target BDR4 as it regulates antiviral immunity, including the interferon-gamma (IFN-gamma), a molecule previously associated with both PTSD and affective disorders (Bruenig et al., 2018; Gibbons et al., 2019; Huang et al., 2021). Interestingly, in humans, IFN-gamma was shown to drive EC senescence, linking COVID-19 further to premature cellular aging (Bam et al., 2016).
Bromodomains are epigenetic readers that regulate the chromatin landscape in cell nucleus, influencing multiple genes. For example, BRD4 regulates the expression of mitochondrial proteins encoded in nuclear DNA, including the ones in charge of metabolism, suggesting that viral exploitation of this protein may inhibit OXPHOS and activate glycolysis (Kim et al., 2009, 2020). Indeed, BRD4 engenders the glycolytic states associated with senescent cells and their secretome, the senescence-associated secretory phenotype (SASP) (Barrow et al., 2016). On the other hand, BRD4 inhibitors, including JQ1 or SF2523, act as senolytics by promoting the clearance of senescent and virus-infected cells (Tasdemir et al., 2016; Go et al., 2021; Table 2). Moreover, as senolytics were found therapeutic against idiopathic pulmonary fibrosis, a BRD4-associated condition, these agents may be helpful to SRD patients (Tang et al., 2013; Acharya et al., 2021). As senolytic agents facilitate the clearance of all damaged cells, including the malignant and virus-infected ones, they are currently being evaluated for COVID-19 (Hu et al., 2020; Go et al., 2021).
Taken together, this data links SRDs to dysfunctional mitochondria and EC senescence, while, on the other hand, BRD4 inhibitors protect the mitochondrion, preempting premature endothelial aging.
Molecular Mimicry and Inflammation
Recent bioinformatic studies established that some SARS-CoV-2 antigens mimic human proteins expressed by neurons, astrocytes, and CECs, predisposing to PTSD. For example, viral N protein shares amino acid residues with the human zonula occludens-1 (ZO-1) and solute carrier family 12 member 6 (SLC12A6), molecules linked to BBB permeability (Hu et al., 2020). In addition, tryptophan 5-hydroxylase 2 (TPH2), an enzyme involved in serotonin synthesis, was found to have a strong mimicry with the S protein of SARS-CoV-2, potentially contributing to anxiety and depression (Yapici-Eser et al., 2021). This is significant as TPH2 plays a major role in PTSD, as well as in premature ECs senescence (Goçi Uka et al., 2019; Wu, 2021). Moreover, the molecular mimicry between the SARS-CoV-2 protein S and human anti-inflammatory proteins was associated with inflammation, a pathology demonstrated in PTSD (Kanduc, 2020). As mitochondrial damage can directly activate inflammasomes, leading to inflammation, the virus may utilize several mechanisms to ignite this pathology (Zhou et al., 2011).
Limitations/Gaps/Challenges
Epidemiologic studies have associated some viruses with anxiety, depression, and PTSD however, only a handful of researchers have systematically studied this connection. More studies are needed to elucidate the interaction between communicable diseases and psychiatric disorders, including SRDs. To fill the knowledge gap between endothelia and PTSD, a combination of different expertise domains is required, an endeavor often difficult to accomplish in clinical context.
Despite these drawbacks, available studies appear to support the concept that, like psychosocial trauma, some viruses promote cellular senescence in many cell types, including ECs and T cells, emphasizing the role of premature aging in these conditions (Justice et al., 2019; Saleh et al., 2020). Indeed, trauma-focused psychotherapy, was shown to restore the physiological T cell phenotypes and reduce PTSD symptoms, suggesting that psychotherapy may have a place in infectious diseases, including the long COVID-19 (Prather et al., 2018). When this is considered together with the fact that IFN-gamma, a regulator of T cell aging, is depleted in both PTSD and COVID-19, a bromodomain-mediated epigenetic mechanism is emerging (Morath et al., 2014; De Biasi et al., 2020). Indeed, several studies have pointed to the fact that in old brains’ T cells express abundant IFN-gamma, a molecule that promotes BBB leakage (Tewari et al., 2007; Michopoulos et al., 2017; Bonney et al., 2019; Dulken et al., 2019). Although some studies failed to demonstrate endothelial dysfunction in PTSD, they connected this disorder to impaired coagulation, a pathology validated by several other studies (von Känel et al., 2008; Robicsek et al., 2011). Systematic studies in this area would be beneficial, especially in the age that has seen increased incidence of viral infections and SRDs.
Conclusion
COVID-19 has been associated with a high PTSD prevalence, suggesting that aside from the psychological burden of the disease itself, the virus may directly interfere with the stress-processing brain areas. The virus-host interactome reveals several pathogen-induced states, such as cellular senescence and associated glycolysis, that facilitate viral replication, while at the same time disrupt the BBB. The virus likely increases PTSD susceptibility as, even in the absence of viral infection, this disorder has been associated with EC senescence and glycolytic states. This two-strike model not only may explain the high comorbidity of COVID-19 and PTSD, but also opens vistas for novel interventions, including ARBs, ACEi, ASIC1a blockers, senolytics, furin inhibitors, BRD4 inhibitors and anti-glycolytic interventions.
Author Contributions
All authors listed have made a substantial, direct and intellectual contribution to the work, and approved it for publication.
Author Disclaimer
Where authors are identified as personnel of the International Agency for Research on Cancer/WHO, the authors alone are responsible for the views expressed in this article and they do not necessarily represent the decisions, policy or views of the International Agency for Research on Cancer/WHO.
Conflict of Interest
The authors declare that the research was conducted in the absence of any commercial or financial relationships that could be construed as a potential conflict of interest.
Publisher’s Note
All claims expressed in this article are solely those of the authors and do not necessarily represent those of their affiliated organizations, or those of the publisher, the editors and the reviewers. Any product that may be evaluated in this article, or claim that may be made by its manufacturer, is not guaranteed or endorsed by the publisher.
Abbreviations
ANGII, angiotensin II; ET-1, endothelin-1; PAI-1, plasminogen activator inhibitor 1; RAS, renin-angiotensin system; Sig-1R, Sigma-1 receptor; FCS, furin cleaving site; CECs, cerebral endothelial cells; ECs, endothelial cells; BDNF, brain derived neurotrophic factor; MAVS, mitochondrial antiviral signaling; PTSD, posttraumatic stress disorder; SRDs, stress related disorders; HPA, hypothalamic-pituitary axis; ARBs, angiotensin receptor blockers; ACEi, angiotensin converting enzyme inhibitors; ACE-2, angiotensin converting enzyme 2; OXPHOS, oxidative phosphorylation; BRDs, bromodomains; LTP, long term potentiation; LTD, long term depression; ASC1a, acid-sensitive ion channel-1a; TrkB, tropomyosin receptor kinase B; AQP-4, aquaporin-4.
References
AbdelMassih, A. F., Ye, J., Kamel, A., Mishriky, F., Ismail, H. A., Ragab, H. A., et al. (2020). A multicenter consensus: a role of furin in the endothelial tropism in obese patients with COVID-19 infection. Obes. Med. 19:100281. doi: 10.1016/j.obmed.2020.100281
Acharya, A., Pandey, K., Thurman, M., Challagundala, K. B., Vann, K. R., Kutateladze, T. G., et al. (2021). Blockade of SARS-CoV-2 infection in vitro by highly potent PI3K-α/mTOR/BRD4 inhibitor. bioRxiv [Preprint]. doi: 10.1101/2021.03.02.433604
Aksu, S., Unlu, G., Kardesler, A. C., Cakaloz, B., and Aybek, H. (2018). Altered levels of brain-derived neurotrophic factor, proBDNF and tissue plasminogen activator in children with posttraumatic stress disorder. Psychiatry Res. 268, 478–483. doi: 10.1016/j.psychres.2018.07.013
Alhusban, A., Fouda, A. Y., and Fagan, S. C. (2016). ARBs improve stroke outcome through an AT2-dependent, BDNF-induced proangiogenic and prorecovery response. Neural Regen. Res. 11, 912–913. doi: 10.4103/1673-5374.184484
Almeida, F. B., Barros, H. M. T., and Pinna, G. (2021). Neurosteroids and neurotrophic factors: what is their promise as biomarkers for major depression and PTSD? Int. J. Mol. Sci. 22:1758. doi: 10.3390/ijms22041758
Amoureux, S., Sicard, P., Korandji, C., Borey, A., Benkhadra, S., Sequeira-Le Grand, A., et al. (2008). Increase in levels of BDNF is associated with inflammation and oxidative stress during cardiopulmonary bypass. Int. J. Biomed. Sci. 4, 204–211.
Amporndanai, K., Meng, X., Shang, W., Jin, Z., Rogers, M., Zhao, Y., et al. (2021). Inhibition mechanism of SARS-CoV-2 main protease by ebselen and its derivatives. Nat. Commun. 12:3061. doi: 10.1038/s41467-021-23313-7
Andero, R., and Ressler, K. J. (2012). Fear extinction and BDNF: translating animal models of PTSD to the clinic. Genes Brain Behav. 11, 503–512. doi: 10.1111/j.1601-183X.2012.00801.x
Andhavarapu, S., Bryant, J., Gerzanich, V., Simard, M. J., and Makar, T. K. (2021). The Potential Therapeutic Value of BDNF-TrkB Pathway in COVID-19 Associated Stroke. J. Neurol. Neurosci. 12:366.
Angelucci, F., Ricci, V., Gelfo, F., Martinotti, G., Brunetti, M., Sepede, G., et al. (2014). BDNF serum levels in subjects developing or not post-traumatic stress disorder after trauma exposure. Brain Cogn. 84, 118–122. doi: 10.1016/j.bandc.2013.11.012
Atanackovic, D., Brunner-Weinzierl, M. C., Kröger, H., Serke, S., and Deter, H. C. (2002). Acute psychological stress simultaneously alters hormone levels, recruitment of lymphocyte subsets, and production of reactive oxygen species. Immunol. Invest. 31, 73–91. doi: 10.1081/imm-120004800
Azoulay, D., Shehadeh, M., Chepa, S., Shaoul, E., Baroum, M., Horowitz, N. A., et al. (2020). Recovery from SARS-CoV-2 infection is associated with serum BDNF restoration. J. Infect. 81, e79–e81. doi: 10.1016/j.jinf.2020.06.038
Bah, A. J., James, P. B., Bah, N., Sesay, A. B., Sevalie, S., and Kanu, J. S. (2020). Prevalence of anxiety, depression and post-traumatic stress disorder among Ebola survivors in northern Sierra Leone: a cross-sectional study. BMC Public Health 20:1391. doi: 10.1186/s12889-020-09507-6
Balan, I., Beattie, M. C., O’Buckley, T. K., Aurelian, L., and Morrow, A. L. (2019). Endogenous neurosteroid (3α,5α)3-hydroxypregnan-20-one inhibits toll-like-4 receptor activation and pro-inflammatory signaling in macrophages and brain. Sci. Rep. 9:1220. doi: 10.1038/s41598-018-37409-6
Baluta, M. M., and Vintila, M. M. (2015). PAI-1 inhibition - another therapeutic option for cardiovascular protection. Maedica 10, 147–152.
Bam, M., Yang, X., Zhou, J., Ginsberg, J. P., Leyden, Q., Nagarkatti, P. S., et al. (2016). Evidence for epigenetic regulation of pro-inflammatory cytokines, interleukin-12 and interferon gamma, in peripheral blood mononuclear cells from PTSD Patients. J. Neuroimmune Pharmacol. 11, 168–181. doi: 10.1007/s11481-015-9643-8
Banoujaafar, H., Monnier, A., Pernet, N., Quirié, A., Garnier, P., Prigent-Tessier, A., et al. (2016). Brain BDNF levels are dependent on cerebrovascular endothelium-derived nitric oxide. Eur. J. Neurosci. 44, 2226–2235. doi: 10.1111/ejn.13301
Barbieri, A., Robinson, N., Palma, G., Maurea, N., Desiderio, V., and Botti, G. (2020). Can beta-2-adrenergic pathway be a new target to combat SARS-CoV-2 hyperinflammatory syndrome?-lessons learned from cancer. Front. Immunol. 11:588724. doi: 10.3389/fimmu.2020.588724
Barron, J. T., Sasse, M. F., and Nair, A. (2004). Effect of angiotensin II on energetics, glucose metabolism and cytosolic NADH/NAD and NADPH/NADP redox in vascular smooth muscle. Mol. Cell Biochem. 262, 91–99. doi: 10.1023/b:mcbi.0000038221.44904.a1
Barrow, J. J., Balsa, E., Verdeguer, F., Tavares, C. D., Soustek, M. S., Hollingsworth, L. R., et al. (2016). Bromodomain inhibitors correct bioenergetic deficiency caused by mitochondrial disease complex I mutations. Mol. Cell. 64, 163–175. doi: 10.1016/j.molcel.2016.08.023
Battaglia, M., Rossignol, O., Bachand, K., D’Amato, F. R., and De Koninck, Y. (2019). Amiloride modulation of carbon dioxide hypersensitivity and thermal nociceptive hypersensitivity induced by interference with early maternal environment. J. Psychopharmacol. 33, 101–108. doi: 10.1177/0269881118784872
Berntsen, D., and Rubin, D. C. (2014). Involuntary memories and dissociative amnesia: assessing key assumptions in PTSD research. Clin. Psychol. Sci. 2, 174–186. doi: 10.1177/2167702613496241
Bersani, F. S., Morley, C., Lindqvist, D., Epel, E. S., Picard, M., Yehuda, R., et al. (2016). Mitochondrial DNA copy number is reduced in male combat veterans with PTSD. Prog. Neuropsychopharmacol. Biol. Psychiatry 64, 10–17. doi: 10.1016/j.pnpbp.2015.06.012
Biojone, C., Casarotto, P. C., Joca, S. R., and Castrén, E. (2015). Interplay between nitric oxide and brain-derived neurotrophic factor in neuronal plasticity. CNS Neurol. Disord. Drug Targets 14, 979–987. doi: 10.2174/1871527314666150909113727
Bonney, S., Seitz, S., Ryan, C. A., Jones, K. L., Clarke, P., Tyler, K. L., et al. (2019). Gamma interferon alters junctional integrity via rho kinase, resulting in blood-brain barrier leakage in experimental viral encephalitis. mBio 10:e016750-19. doi: 10.1128/mBio.01675-19
Boscarino, J. A. (2008). A prospective study of PTSD and early-age heart disease mortality among Vietnam veterans: implications for surveillance and prevention. Psychosom. Med. 70, 668–676. doi: 10.1097/PSY.0b013e31817bccaf
Boskovic, Z., Milne, M. R., Qian, L., Clifton, H. D., McGovern, A. E., Turnbull, M. T., et al. (2018). Cholinergic basal forebrain neurons regulate fear extinction consolidation through p75 neurotrophin receptor signaling. Transl. Psychiatry 8:199. doi: 10.1038/s41398-018-0248-x
Bouarab, C., Roullot-Lacarrière, V., Vallée, M., Le, Roux A, Guette, C., Mennesson, M., et al. (2021). PAI-1 protein is a key molecular effector in the transition from normal to PTSD-like fear memory. Mol. Psychiatry [Epub ahead of print]. doi: 10.1038/s41380-021-01024-1
Bourgonje, A. R., Abdulle, A. E., Timens, W., Hillebrands, J. L., Navis, G. J., Gordijn, S. J., et al. (2020). Angiotensin-converting enzyme 2 (ACE2), SARS-CoV-2 and the pathophysiology of coronavirus disease 2019 (COVID-19). J. Pathol. 251, 228–248. doi: 10.1002/path.5471
Brigadski, T., and Leßmann, V. (2020). The physiology of regulated BDNF release. Cell Tissue Res. 382, 15–45. doi: 10.1007/s00441-020-03253-2
Brohée, L., Peulen, O., Nusgens, B., Castronovo, V., Thiry, M., Colige, A. C., et al. (2018). Propranolol sensitizes prostate cancer cells to glucose metabolism inhibition and prevents cancer progression. Sci. Rep. 8:7050. doi: 10.1038/s41598-018-25340-9
Bruenig, D., Mehta, D., Morris, C. P., Lawford, B., Harvey, W., McD Young, R., et al. (2018). Correlation between interferon γ and interleukin 6 with PTSD and resilience. Psychiatry Res. 260, 193–198. doi: 10.1016/j.psychres.2017.11.069
Bruenig, D., Morris, C. P., Mehta, D., Harvey, W., Lawford, B., Young, R. M., et al. (2017). Nitric oxide pathway genes (NOS1AP and NOS1) are involved in PTSD severity, depression, anxiety, stress and resilience. Gene 625, 42–48. doi: 10.1016/j.gene.2017.04.048
Buzhdygan, T. P., DeOre, B. J., Baldwin-Leclair, A., Bullock, T. A., McGary, H. M., Khan, J. A., et al. (2020). The SARS-CoV-2 spike protein alters barrier function in 2D static and 3D microfluidic in-vitro models of the human blood-brain barrier. Neurobiol. Dis. 146:105131. doi: 10.1016/j.nbd.2020.105131
Canossa, M., Giordano, E., Cappello, S., Guarnieri, C., and Ferri, S. (2002). Nitric oxide down-regulates brain-derived neurotrophic factor secretion in cultured hippocampal neurons. Proc. Natl. Acad. Sci. U.S.A. 99, 3282–3287. doi: 10.1073/pnas.042504299
Carver, P. L. (2018). The battle for iron between humans and microbes. Curr. Med. Chem. 25, 85–96. doi: 10.2174/0929867324666170720110049
Cesari, M., Pahor, M., and Incalzi, R. A. (2010). Plasminogen activator inhibitor-1 (PAI-1): a key factor linking fibrinolysis and age-related subclinical and clinical conditions. Cardiovasc. Ther. 28, e72–e91. doi: 10.1111/j.1755-5922.2010.00171.x
Chamberlain, S. R., Grant, J. E., Trender, W., Hellyer, P., and Hampshire, A. (2021). Post-traumatic stress disorder symptoms in COVID-19 survivors: online population survey. BJPsych. Open 7:e47. doi: 10.1192/bjo.2021.3
Chaphalkar, R. M., Stankowska, D. L., He, S., Kodati, B., Phillips, N., Prah, J., et al. (2020). Endothelin-1 mediated decrease in mitochondrial gene expression and bioenergetics contribute to neurodegeneration of retinal ganglion cells. Sci. Rep. 10:3571. doi: 10.1038/s41598-020-60558-6
Chauhan, A. S., Zhuang, L., and Gan, B. (2019). Antagonism between antiviral signaling and glycolysis. Trends Endocrinol. Metab. 30, 571–573. doi: 10.1016/j.tem.2019.07.010
Chen, J., Park, H. C., Patschan, S., Brodsky, S. V., Gealikman, O., Kuo, M. C., et al. (2007). Premature vascular senescence in metabolic syndrome: could it be prevented and reversed by a selenorganic antioxidant and peroxynitrite scavenger ebselen? Drug Discov. Today Ther. Strateg. 4, 93–99. doi: 10.1016/j.ddstr.2007.10.006
Chen, M., Shu, S., Yan, H. H., Pei, L., Wang, Z. F., Wan, Q., et al. (2017a). Hippocampal Endothelin-1 decreases excitability of pyramidal neurons and produces anxiolytic effects. Neuropharmacology 118, 242–250. doi: 10.1016/j.neuropharm.2017.03.014
Chen, M., Yan, H. H., Shu, S., Pei, L., Zang, L. K., Fu, Y., et al. (2017b). Amygdalar endothelin-1 regulates pyramidal neuron excitability and affects anxiety. Sci. Rep. 7:2316. doi: 10.1038/s41598-017-02583-6
Chen, M. H., Pan, T. L., Li, C. T., Lin, W. C., Chen, Y. S., Lee, Y. C., et al. (2015). Risk of stroke among patients with post-traumatic stress disorder: nationwide longitudinal study. Br. J. Psychiatry 206, 302–307. doi: 10.1192/bjp.bp.113.143610
Chen, S., Jiang, H., Liu, Y., Hou, Z., Yue, Y., Zhang, Y., et al. (2017c). Combined serum levels of multiple proteins in tPA-BDNF pathway may aid the diagnosis of five mental disorders. Sci. Rep. 7:6871. doi: 10.1038/s41598-017-06832-6
Chen, Y., Meyer, J. N., Hill, H. Z., Lange, G., Condon, M. R., Klein, J. C., et al. (2017d). Role of mitochondrial DNA damage and dysfunction in veterans with gulf war illness. PLoS One 12:e0184832. doi: 10.1371/journal.pone.0184832
Chen, Z. Y., Jing, D., Bath, K. G., Ieraci, A., Khan, T., Siao, C. J., et al. (2006). Genetic variant BDNF (Val66Met) polymorphism alters anxiety-related behavior. Science 314, 140–143. doi: 10.1126/science.1129663
Chiao, Y. A., Zhang, H., Sweetwyne, M., Whitson, J., Ting, Y. S., Basisty, N., et al. (2020). Late-life restoration of mitochondrial function reverses cardiac dysfunction in old mice. eLife 9:e55513. doi: 10.7554/eLife.55513
Chrousos, G. P., and Zapanti, E. D. (2014). Hypothalamic-pituitary-adrenal axis in HIV infection and disease. Endocrinol. Metab. Clin. North Am. 43, 791–806. doi: 10.1016/j.ecl.2014.06.002
Cleveland, S., Reed, K., Thomas, J. L., Ajijola, O. A., Ebrahimi, R., Hsiai, T., et al. (2021). Key dimensions of post-traumatic stress disorder and endothelial dysfunction: a protocol for a mechanism-focused cohort study. BMJ Open 11:e043060. doi: 10.1136/bmjopen-2020-043060
Connolly, S. L., Stoop, T. B., Logue, M. W., Orr, E. H., De Vivo, I., Miller, M. W., et al. (2018). Posttraumatic stress disorder symptoms, temperament, and the pathway to cellular senescence. J. Trauma Stress 31, 676–686. doi: 10.1002/jts.22325
Corrêa, D. G., de Souza Lima, F. C., da Cruz Bezerra, D., Coutinho, A. C. Jr., and Hygino da Cruz, L. C. Jr. (2021). COVID-19 associated with encephalomyeloradiculitis and positive anti-aquaporin-4 antibodies: cause or coincidence? Mult. Scler. 27, 973–976. doi: 10.1177/1352458520949988
Coryell, M. W., Wunsch, A. M., Haenfler, J. M., Allen, J. E., Schnizler, M., Ziemann, A. E., et al. (2009). Acid-sensing ion channel-1a in the amygdala, a novel therapeutic target in depression-related behavior. J. Neurosci. 29, 5381–5388. doi: 10.1523/JNEUROSCI.0360-09.2009
Coughlin, S. S. (2012). Anxiety and depression: linkages with viral diseases. Public Health Rev. 34:7. doi: 10.1007/BF03391675
Dai, D. F., Johnson, S. C., Villarin, J. J., Chin, M. T., Nieves-Cintrón, M., Chen, T., et al. (2011). Mitochondrial oxidative stress mediates angiotensin II-induced cardiac hypertrophy and Galphaq overexpression-induced heart failure. Circ. Res. 108, 837–846. doi: 10.1161/CIRCRESAHA.110.232306
Daneman, R., and Prat, A. (2015). The blood-brain barrier. Cold Spring Harb. Perspect. Biol. 7:a020412. doi: 10.1101/cshperspect.a020412
De Biasi, S., Meschiari, M., Gibellini, L., Bellinazzi, C., Borella, R., Fidanza, L., et al. (2020). Marked T cell activation, senescence, exhaustion and skewing towards TH17 in patients with COVID-19 pneumonia. Nat. Commun. 11:3434. doi: 10.1038/s41467-020-17292-4
De Boer, M., Op den Velde, W., Falger, P. J., Hovens, J. E., De, Groen JH, and Van Duijn, H. (1992). Fluvoxamine treatment for chronic PTSD: a pilot study. Psychother. Psychosom. 57, 158–163. doi: 10.1159/000288593
de Cavanagh, E. M., Piotrkowski, B., Basso, N., Stella, I., Inserra, F., Ferder, L., et al. (2003). Enalapril and losartan attenuate mitochondrial dysfunction in aged rats. FASEB J. 17, 1096–1098. doi: 10.1096/fj.02-0063fje
de Solis, C. A., Hosek, M. P., Holehonnur, R., Ho, A., Banerjee, A., Luong, J. A., et al. (2017). Adeno-associated viral serotypes differentially transduce inhibitory neurons within the rat amygdala. Brain Res. 1672, 148–162. doi: 10.1016/j.brainres.2017.07.023
Denault, J. B., Claing, A., D’Orléans-Juste, P., Sawamura, T., Kido, T., Masaki, T., et al. (1995). Processing of proendothelin-1 by human furin convertase. FEBS Lett. 362, 276–280. doi: 10.1016/0014-5793(95)00249-9
Descalzi, G., Gao, V., Steinman, M. Q., Suzuki, A., and Alberini, C. M. (2019). Lactate from astrocytes fuels learning-induced mRNA translation in excitatory and inhibitory neurons. Commun. Biol. 2:247. doi: 10.1038/s42003-019-0495-2
Descamps, B., Saif, J., Benest, A. V., Biglino, G., Bates, D. O., Chamorro-Jorganes, A., et al. (2018). BDNF (brain-derived neurotrophic factor) promotes embryonic stem cells differentiation to endothelial cells via a molecular pathway, including MicroRNA-214, EZH2 (Enhancer of Zeste Homolog 2), and eNOS (endothelial nitric oxide synthase). Arterioscler. Thromb. Vasc. Biol. 38, 2117–2125. doi: 10.1161/ATVBAHA.118.311400
Di Benedetto, B., Malik, V. A., Begum, S., Jablonowski, L., Gómez-González, G. B., Neumann, I. D., et al. (2016). Fluoxetine requires the endfeet protein aquaporin-4 to enhance plasticity of astrocyte processes. Front. Cell Neurosci. 10:8. doi: 10.3389/fncel.2016.00008
Dikalov, S. I., and Nazarewicz, R. R. (2013). Angiotensin II-induced production of mitochondrial reactive oxygen species: potential mechanisms and relevance for cardiovascular disease. Antioxid. Redox Signal. 19, 1085–1094. doi: 10.1089/ars.2012.4604
DiNuzzo, M., and Nedergaard, M. (2017). Brain energetics during the sleep-wake cycle. Curr. Opin. Neurobiol. 47, 65–72. doi: 10.1016/j.conb.2017.09.010
Diwakar, L., Gowaikar, R., Chithanathan, K., Gnanabharathi, B., Tomar, D. S., and Ravindranath, V. (2021). Endothelin-1 mediated vasoconstriction leads to memory impairment and synaptic dysfunction. Sci. Rep. 11:4868. doi: 10.1038/s41598-021-84258-x
Doughan, A. K., Harrison, D. G., and Dikalov, S. I. (2008). Molecular mechanisms of angiotensin II-mediated mitochondrial dysfunction: linking mitochondrial oxidative damage and vascular endothelial dysfunction. Circ. Res. 102, 488–496. doi: 10.1161/CIRCRESAHA.107.162800
Dulken, B. W., Buckley, M. T., Navarro Negredo, P., Saligrama, N., Cayrol, R., Leeman, D. S., et al. (2019). Single-cell analysis reveals T cell infiltration in old neurogenic niches. Nature 571, 205–210. doi: 10.1038/s41586-019-1362-5
Dutheil, F., Mondillon, L., and Navel, V. (2020). PTSD as the second tsunami of the SARS-Cov-2 pandemic [published online ahead of print, 2020 Apr 24]. Psychol. Med. [Epub ahead of print]. doi: 10.1017/S0033291720001336
Dwyer, J. M., Rizzo, S. J., Neal, S. J., Lin, Q., Jow, F., Arias, R. L., et al. (2009). Acid sensing ion channel (ASIC) inhibitors exhibit anxiolytic-like activity in preclinical pharmacological models. Psychopharmacology 203, 41–52. doi: 10.1007/s00213-008-1373-7
Edmondson, D., Kronish, I. M., Shaffer, J. A., Falzon, L., and Burg, M. M. (2013). Posttraumatic stress disorder and risk for coronary heart disease: a meta-analytic review. Am. Heart J. 166, 806–814. doi: 10.1016/j.ahj.2013.07.031
Emani, S. M., and McCully, J. D. (2018). Mitochondrial transplantation: applications for pediatric patients with congenital heart disease. Transl. Pediatr. 7, 169–175. doi: 10.21037/tp.2018.02.02
Erickson, M. A., Rhea, E. M., Knopp, R. C., and Banks, W. A. (2021). Interactions of SARS-CoV-2 with the blood-brain barrier. Int. J. Mol. Sci. 22:2681. doi: 10.3390/ijms22052681
Escalona, R., Canive, J. M., Calais, L. A., and Davidson, J. R. (2002). Fluvoxamine treatment in veterans with combat-related post-traumatic stress disorder. Depress Anxiety 15, 29–33. doi: 10.1002/da.1082
Filadi, R., Leal, N. S., Schreiner, B., Rossi, A., Dentoni, G., Pinho, C. M., et al. (2018). TOM70 sustains cell bioenergetics by promoting IP3R3-mediated ER to mitochondria Ca2+ transfer. Curr. Biol. 28, 369.e6–382.e6. doi: 10.1016/j.cub.2017.12.047
Filipović, D., Perić, I., Costina, V., Stanisavljević, A., Gass, P., and Findeisen, P. (2020). Social isolation stress-resilient rats reveal energy shift from glycolysis to oxidative phosphorylation in hippocampal nonsynaptic mitochondria. Life Sci. 254:117790. doi: 10.1016/j.lfs.2020.117790
Flaquer, A., Baumbach, C., Ladwig, K. H., Kriebel, J., Waldenberger, M., Grallert, H., et al. (2015). Mitochondrial genetic variants identified to be associated with posttraumatic stress disorder. Transl. Psychiatry 5:e524. doi: 10.1038/tp.2015.18
Fleegal-DeMotta, M. A., Doghu, S., and Banks, W. A. (2009). Angiotensin II modulates BBB permeability via activation of the AT(1) receptor in brain endothelial cells. J. Cereb. Blood Flow Metab. 29, 640–647. doi: 10.1038/jcbfm.2008.158
Fogari, R., and Zoppi, A. (2006). Antihypertensive drugs and fibrinolytic function. Am. J. Hypertens. 19, 1293–1299. doi: 10.1016/j.amjhyper.2006.04.013
Forster, P. L., Schoenfeld, F. B., Marmar, C. R., and Lang, A. J. (1995). Lithium for irritability in post-traumatic stress disorder. J. Trauma Stress 8, 143–149. doi: 10.1007/BF02105412
Fox, B. M., Becker, B. K., Loria, A. S., Hyndman, K. A., Jin, C., Clark, H., et al. (2018). Acute pressor response to psychosocial stress is dependent on endothelium-derived endothelin-1. J. Am. Heart Assoc. 7:e007863. doi: 10.1161/JAHA.117.007863
Fujimoto, M., Hayashi, T., Urfer, R., Mita, S., and Su, T. P. (2012). Sigma-1 receptor chaperones regulate the secretion of brain-derived neurotrophic factor. Synapse 66, 630–639. doi: 10.1002/syn.21549
Ganji, R., and Reddy, P. H. (2021). Impact of COVID-19 on mitochondrial-based immunity in aging and age-related diseases. Front. Aging Neurosci. 12:614650. doi: 10.3389/fnagi.2020.614650
Gates, M. A., Holowka, D. W., Vasterling, J. J., Keane, T. M., Marx, B. P., and Rosen, R. C. (2012). Posttraumatic stress disorder in veterans and military personnel: epidemiology, screening, and case recognition. Psychol. Serv. 9, 361–382. doi: 10.1037/a0027649
Ghandehari, S., Matusov, Y., Pepkowitz, S., Stein, D., Kaderi, T., Narayanan, D., et al. (2021). Progesterone in addition to standard of care vs standard of care alone in the treatment of men hospitalized with moderate to severe COVID-19: a randomized. Control. Pilot Trial. Chest. 160, 74–84. doi: 10.1016/j.chest.2021.02.024
Gibbons, H. R., Mi, D. J., Farley, V. M., Esmond, T., Kaood, M. B., and Aune, T. M. (2019). Bromodomain inhibitor JQ1 reversibly blocks IFN-γ production. Sci. Rep. 9:10280. doi: 10.1038/s41598-019-46516-x
Giustino, T. F., Fitzgerald, P. J., and Maren, S. (2016). Revisiting propranolol and PTSD: memory erasure or extinction enhancement? Neurobiol. Learn. Mem. 130, 26–33. doi: 10.1016/j.nlm.2016.01.009
Go, S., Kang, M., Kwon, S. P., Jung, M., Jeon, O. H., and Kim, B. S. (2021). The senolytic drug JQ1 removes senescent cells via ferroptosis. Tissue Eng. Regen. Med. 18, 841–850. doi: 10.1007/s13770-021-00346-z
Goçi Uka, A., Agani, F., Blyta, A., Hoxha, B., Haxhibeqiri, S., Haxhibeqiri, V., et al. (2019). Role of the allelic variation in the 5-hydroxytryptamine receptor 1A (HTR1A) and the tryptophan hydroxylase 2 (TPH2) genes in the development of PTSD. Psychiatr. Danub. 31, 256–262. doi: 10.24869/psyd.2019.256
Gomzikova, M. O., James, V., and Rizvanov, A. A. (2021). Mitochondria donation by mesenchymal stem cells: current understanding and mitochondria transplantation strategies. Front. Cell Dev. Biol. 9:653322. doi: 10.3389/fcell.2021.653322
Gordon, D. E., Jang, G. M., Bouhaddou, M., Xu, J., Obernier, K., White, K. M., et al. (2020). A SARS-CoV-2 protein interaction map reveals targets for drug repurposing. Nature 583, 459–468. doi: 10.1038/s41586-020-2286-9
Goyal, M. S., Vlassenko, A. G., Blazey, T. M., Su, Y., Couture, L. E., Durbin, T. J., et al. (2017). Loss of brain aerobic glycolysis in normal human aging. Cell Metab. 26, 353.e3–360.e3. doi: 10.1016/j.cmet.2017.07.010
Graves, A. R., Moore, S. J., Spruston, N., Tryba, A. K., and Kaczorowski, C. C. (2016). Brain-derived neurotrophic factor differentially modulates excitability of two classes of hippocampal output neurons. J. Neurophysiol. 116, 466–471. doi: 10.1152/jn.00186.2016
Gray, K., and Ellis, V. (2008). Activation of pro-BDNF by the pericellular serine protease plasmin. FEBS Lett. 582, 907–910. doi: 10.1016/j.febslet.2008.02.026
Green, C. R., Corsi-Travali, S., and Neumeister, A. (2013). The role of BDNF-TrkB signaling in the pathogenesis of PTSD. J. Depress Anxiety 2013:006. doi: 10.4172/2167-1044.S4-006
Grenon, S. M., Owens, C. D., Alley, H., Perez, S., Whooley, M. A., Neylan, T. C., et al. (2016). Posttraumatic stress disorder is associated with worse endothelial function among veterans. J. Am. Heart Assoc. 5:e003010. doi: 10.1161/JAHA.115.003010
Griffin, G. D., Charron, D., and Al-Daccak, R. (2014). Post-traumatic stress disorder: revisiting adrenergics, glucocorticoids, immune system effects and homeostasis. Clin. Transl. Immunology 3:e27. doi: 10.1038/cti.2014.26
Hagiwara, Y., and Kubo, T. (2007). Intracerebroventricular injection of losartan inhibits angiotensin II-sensitive neurons via GABA inputs in the anterior hypothalamic area of rats. Neurosci. Lett. 416, 150–154. doi: 10.1016/j.neulet.2007.01.059
Hallenberger, S., Bosch, V., Angliker, H., Shaw, E., Klenk, H. D., and Garten, W. (1992). Inhibition of furin-mediated cleavage activation of HIV-1 glycoprotein gp160. Nature 360, 358–361. doi: 10.1038/360358a0
Hashimoto, K. (2013). Sigma-1 receptor chaperone and brain-derived neurotrophic factor: emerging links between cardiovascular disease and depression. Prog. Neurobiol. 100, 15–29. doi: 10.1016/j.pneurobio.2012.09.001
Hashimoto, K. (2015). Activation of sigma-1 receptor chaperone in the treatment of neuropsychiatric diseases and its clinical implication. J. Pharmacol. Sci. 127, 6–9. doi: 10.1016/j.jphs.2014.11.010
Hashimoto, K. (2021). Repurposing of CNS drugs to treat COVID-19 infection: targeting the sigma-1 receptor. Eur. Arch. Psychiatry Clin. Neurosci. 271, 249–258. doi: 10.1007/s00406-020-01231-x
He, M., Wei, J. X., Mao, M., Zhao, G. Y., Tang, J. J., Feng, S., et al. (2018). Synaptic plasticity in PTSD and associated comorbidities: the function and mechanism for diagnostics and therapy. Curr. Pharm. Des. 24, 4051–4059. doi: 10.2174/1381612824666181120094749
Herrera, Y., Katnik, C., Rodriguez, J. D., Hall, A. A., Willing, A., Pennypacker, K. R., et al. (2008). sigma-1 receptor modulation of acid-sensing ion channel a (ASIC1a) and ASIC1a-induced Ca2+ influx in rat cortical neurons. J. Pharmacol. Exp. Ther. 327, 491–502. doi: 10.1124/jpet.108.143974
Hirano, K., Tagashira, H., and Fukunaga, K. (2014). [Cardioprotective effect of the selective sigma-1 receptor agonist, SA4503]. Yakugaku Zasshi. 134, 707–713. doi: 10.1248/yakushi.13-00255-3
Hoffmann, M., Kleine-Weber, H., Schroeder, S., Krüger, N., Herrler, T., Erichsen, S., et al. (2020). SARS-CoV-2 cell entry depends on ACE2 and TMPRSS2 and is blocked by a clinically proven protease inhibitor. Cell 181, 271.e8–280.e8. doi: 10.1016/j.cell.2020.02.052
Hu, C., Tao, L., Cao, X., and Chen, L. (2020). The solute carrier transporters and the brain: physiological and pharmacological implications. Asian J. Pharm. Sci. 15, 131–144. doi: 10.1016/j.ajps.2019.09.002
Huang, F. L., Li, F., Zhang, W. J., Li, S. J., Yang, Z. H., Yang, T. L., et al. (2021). Brd4 participates in epigenetic regulation of the extinction of remote auditory fear memory. Neurobiol. Learn. Mem. 179:107383. doi: 10.1016/j.nlm.2021.107383
Huber, V. J., Igarashi, H., Ueki, S., Kwee, I. L., and Nakada, T. (2018). Aquaporin-4 facilitator TGN-073 promotes interstitial fluid circulation within the blood-brain barrier: [17O]H2O JJVCPE MRI study. Neuroreport 29, 697–703. doi: 10.1097/WNR.0000000000000990
Hughes, K. C., and Shin, L. M. (2011). Functional neuroimaging studies of post-traumatic stress disorder. Expert Rev. Neurother. 11, 275–285. doi: 10.1586/ern.10.198
Icard, P., Lincet, H., Wu, Z., Coquerel, A., Forgez, P., Alifano, M., et al. (2021). The key role of Warburg effect in SARS-CoV-2 replication and associated inflammatory response. Biochimie 180, 169–177. doi: 10.1016/j.biochi.2020.11.010
Idell, R. D., Florova, G., Komissarov, A. A., Shetty, S., Girard, R. B., and Idell, S. (2017). The fibrinolytic system: a new target for treatment of depression with psychedelics. Med. Hypotheses. 100, 46–53. doi: 10.1016/j.mehy.2017.01.013
Ilchibaeva, T. V., Tsybko, A. S., Kozhemyakina, R. V., Kondaurova, E. M., Popova, N. K., and Naumenko, V. S. (2018). Genetically defined fear-induced aggression: focus on BDNF and its receptors. Behav. Brain Res. 343, 102–110. doi: 10.1016/j.bbr.2018.01.034
Imai, H., Nakamoto, H., Ishida, Y., Yamanouchi, Y., Inoue, T., Okada, H., et al. (2001). Renin-angiotensin system plays an important role in the regulation of water transport in the peritoneum. Adv. Perit. Dial. 17, 20–24.
Inserra, A. (2018). Hypothesis: the psychedelic ayahuasca heals traumatic memories via a sigma 1 receptor-mediated epigenetic-mnemonic process. Front. Pharmacol. 9:330. doi: 10.3389/fphar.2018.00330
Ishrat, T., Pillai, B., Soliman, S., Fouda, A. Y., Kozak, A., Johnson, M. H., et al. (2015). Low-dose candesartan enhances molecular mediators of neuroplasticity and subsequent functional recovery after ischemic stroke in rats. Mol. Neurobiol. 51, 1542–1553. doi: 10.1007/s12035-014-8830-6
Israelski, D. M., Prentiss, D. E., Lubega, S., Balmas, G., Garcia, P., Muhammad, M., et al. (2007). Psychiatric co-morbidity in vulnerable populations receiving primary care for HIV/AIDS. AIDS Care 19, 220–225.
Iwai, T., Tanonaka, K., Kasahara, S., Inoue, R., and Takeo, S. (2002). Protective effect of propranolol on mitochondrial function in the ischaemic heart. Br. J. Pharmacol. 136, 472–480. doi: 10.1038/sj.bjp.0704724
Jain, A., and Mehrotra, V. (2020). ET-traps as a potential treatment for COVID-19. Med. Drug Discov. 7:100054. doi: 10.1016/j.medidd.2020.100054
Janiri, D., Carfì, A., Kotzalidis, G. D., Bernabei, R., Landi, F., and Sani, G. (2021). Gemelli against COVID-19 post-acute care study group. posttraumatic stress disorder in patients after severe COVID-19 infection. JAMA Psychiatry 78, 567–569. doi: 10.1001/jamapsychiatry.2021.0109
Jarius, S., Wildemann, B., and Paul, F. (2014). Neuromyelitis optica: clinical features, immunopathogenesis and treatment. Clin. Exp. Immunol. 176, 149–164. doi: 10.1111/cei.12271
Jensen, C. F., Keller, T. W., Peskind, E. R., McFall, M. E., Veith, R. C., Martin, D., et al. (1997). Behavioral and neuroendocrine responses to sodium lactate infusion in subjects with posttraumatic stress disorder. Am. J. Psychiatry 154, 266–268. doi: 10.1176/ajp.154.2.266
Jessen, N. A., Munk, A. S., Lundgaard, I., and Nedergaard, M. (2015). The glymphatic system: a beginner’s guide. Neurochem. Res. 40, 2583–2599. doi: 10.1007/s11064-015-1581-6
Ji, L. L., Peng, J. B., Fu, C. H., Tong, L., and Wang, Z. Y. (2017). Sigma-1 receptor activation ameliorates anxiety-like behavior through NR2A-CREB-BDNF signaling pathway in a rat model submitted to single-prolonged stress. Mol. Med. Rep. 16, 4987–4993. doi: 10.3892/mmr.2017.7185
Jia, Y., Gall, C. M., and Lynch, G. (2010). Presynaptic BDNF promotes postsynaptic long-term potentiation in the dorsal striatum. J. Neurosci. 30, 14440–14445. doi: 10.1523/JNEUROSCI.3310-10.2010
Jiang, H., Li, X., Chen, S., Lu, N., Yue, Y., Liang, J., et al. (2016). Plasminogen activator inhibitor-1 in depression: results from animal and clinical studies. Sci. Rep. 6:30464. doi: 10.1038/srep30464
Johnson, B. A., Xie, X., Kalveram, B., Lokugamage, K. G., Muruato, A., Zou, J., et al. (2020). Furin Cleavage Site Is Key to SARS-CoV-2 Pathogenesis. bioRxiv [Preprint]. doi: 10.1101/2020.08.26.268854
Johnson, P. L., Sajdyk, T. J., Fitz, S. D., Hale, M. W., Lowry, C. A., Hay-Schmidt, A., et al. (2013). Angiotensin II’s role in sodium lactate-induced panic-like responses in rats with repeated urocortin 1 injections into the basolateral amygdala: amygdalar angiotensin receptors and panic. Prog. Neuropsychopharmacol. Biol. Psychiatry 44, 248–256. doi: 10.1016/j.pnpbp.2013.02.014
Justice, J. N., Nambiar, A. M., Tchkonia, T., LeBrasseur, N. K., Pascual, R., Hashmi, S. K., et al. (2019). Senolytics in idiopathic pulmonary fibrosis: results from a first-in-human, open-label, pilot study. EBioMedicine 40, 554–563. doi: 10.1016/j.ebiom.2018.12.052
Kanduc, D. (2020). From Anti-SARS-CoV-2 immune responses to COVID-19 via molecular mimicry. Antibodies 9:33. doi: 10.3390/antib9030033
Kanmogne, G. D., Primeaux, C., and Grammas, P. (2005). Induction of apoptosis and endothelin-1 secretion in primary human lung endothelial cells by HIV-1 gp120 proteins. Biochem. Biophys. Res. Commun. 333, 1107–1115. doi: 10.1016/j.bbrc.2005.05.198
Kaufer, D., Friedman, A., Seidman, S., and Soreq, H. (1998). Acute stress facilitates long-lasting changes in cholinergic gene expression. Nature 393, 373–377. doi: 10.1038/30741
Keil, M. F., Briassoulis, G., Stratakis, C. A., and Wu, T. J. (2016). Protein kinase A and anxiety-related behaviors: a mini-review. Front. Endocrinol. 7:83. doi: 10.3389/fendo.2016.00083
Kellici, T. F., Pilka, E. S., and Bodkin, M. J. (2021). Therapeutic potential of targeting plasminogen activator inhibitor-1 in COVID-19. Trends Pharmacol. Sci. 42, 431–433. doi: 10.1016/j.tips.2021.03.006
Kershaw, K. N., Lane-Cordova, A. D., Carnethon, M. R., Tindle, H. A., and Liu, K. (2017). Chronic stress and endothelial dysfunction: the multi-ethnic study of atherosclerosis (MESA). Am. J. Hypertens. 30, 75–80. doi: 10.1093/ajh/hpw103
Kho, A. R., Choi, B. Y., Lee, S. H., Hong, D. K., Jeong, J. H., Kang, B. S., et al. (2019). The effects of sodium dichloroacetate on mitochondrial dysfunction and neuronal death following hypoglycemia-induced injury. Cells 8:405. doi: 10.3390/cells8050405
Khoury, N. M., Marvar, P. J., Gillespie, C. F., Wingo, A., Schwartz, A., Bradley, B., et al. (2012). The renin-angiotensin pathway in posttraumatic stress disorder: angiotensin-converting enzyme inhibitors and angiotensin receptor blockers are associated with fewer traumatic stress symptoms. J. Clin. Psychiatry 73, 849–855. doi: 10.4088/JCP.11m07316
Kim, K. S., Kang, K. W., Seu, Y. B., Baek, S. H., and Kim, J. R. (2009). Interferon-gamma induces cellular senescence through p53-dependent DNA damage signaling in human endothelial cells. Mech. Ageing Dev. 130, 179–188. doi: 10.1016/j.mad.2008.11.004
Kim, S. Y., Zhang, X., Schiattarella, G. G., Altamirano, F., Ramos, T. A. R., French, K. M., et al. (2020). Epigenetic reader BRD4 (bromodomain-containing protein 4) governs nucleus-encoded mitochondrial transcriptome to regulate cardiac function. Circulation 142, 2356–2370. doi: 10.1161/CIRCULATIONAHA.120.047239
Kimura, Y., Fujita, Y., Shibata, K., Mori, M., and Yamashita, T. (2013). Sigma-1 receptor enhances neurite elongation of cerebellar granule neurons via TrkB signaling. PLoS One 8:e75760. doi: 10.1371/journal.pone.0075760
Kol, A., Adamsky, A., Groysman, M., Kreisel, T., London, M., and Goshen, I. (2020). Astrocytes contribute to remote memory formation by modulating hippocampal-cortical communication during learning. Nat. Neurosci. 23, 1229–1239. doi: 10.1038/s41593-020-0679-6
Kong, H., Zeng, X. N., Fan, Y., Yuan, S. T., Ge, S., Xie, W. P., et al. (2014). Aquaporin-4 knockout exacerbates corticosterone-induced depression by inhibiting astrocyte function and hippocampal neurogenesis. CNS Neurosci. Ther. 20, 391–402. doi: 10.1111/cns.12222
Kowiański, P., Lietzau, G., Czuba, E., Waśkow, M., Steliga, A., and Moryś, J. B. D. N. F. (2018). A key factor with multipotent impact on brain signaling and synaptic plasticity. Cell Mol. Neurobiol. 38, 579–593. doi: 10.1007/s10571-017-0510-4
Krikov, M., Thone-Reineke, C., Müller, S., Villringer, A., and Unger, T. (2008). Candesartan but not ramipril pretreatment improves outcome after stroke and stimulates neurotrophin BNDF/TrkB system in rats. J. Hypertens. 26, 544–552. doi: 10.1097/HJH.0b013e3282f2dac9
Krolow, R., Arcego, D. M., Noschang, C., Weis, S. N., and Dalmaz, C. (2014). Oxidative imbalance and anxiety disorders. Curr. Neuropharmacol. 12, 193–204. doi: 10.2174/1570159X11666131120223530
Krueger, K. E. (1995). Molecular and functional properties of mitochondrial benzodiazepine receptors. Biochim. Biophys. Acta 1241, 453–470. doi: 10.1016/0304-4157(95)00016-x
Kubera, B., Hubold, C., Otte, S., Lindenberg, A. S., Zeiss, I., Krause, R., et al. (2012). Rise in plasma lactate concentrations with psychosocial stress: a possible sign of cerebral energy demand. Obes. Facts 5, 384–392. doi: 10.1159/000339958
Kumar, A., and Chanana, P. (2017). Role of nitric oxide in stress-induced anxiety: from pathophysiology to therapeutic target. Vitam. Horm. 103, 147–167. doi: 10.1016/bs.vh.2016.09.004
Kumar, S., Veldhuis, A., and Malhotra, T. (2021). Neuropsychiatric and cognitive sequelae of COVID-19. Front. Psychol. 12:577529. doi: 10.3389/fpsyg.2021.577529
Kunieda, T., Minamino, T., Nishi, J., Tateno, K., Oyama, T., Katsuno, T., et al. (2006). Angiotensin II induces premature senescence of vascular smooth muscle cells and accelerates the development of atherosclerosis via a p21-dependent pathway. Circulation 114, 953–960. doi: 10.1161/CIRCULATIONAHA.106.626606
Kurihara, Y., Kurihara, H., Morita, H., Cao, W. H., Ling, G. Y., Kumada, M., et al. (2000). Role of endothelin-1 in stress response in the central nervous system. Am. J. Physiol. Regul. Integr. Comp. Physiol. 279, R515–R521. doi: 10.1152/ajpregu.2000.279.2.R515
Lam, M. H. B., Wing, Y. K., Yu, M. W. M., Leung, C. M., Ma, R. C. W., Kong, A. P. S., et al. (2009). Mental morbidities and chronic fatigue in severe acute respiratory syndrome survivors long-term follow-up. Arch. Intern. Med. 169, 2142–2147.
Lancaster, C. L., Teeters, J. B., Gros, D. F., and Back, S. E. (2016). Posttraumatic stress disorder: overview of evidence-based assessment and treatment. J. Clin. Med. 5:105. doi: 10.3390/jcm5110105
Leal, G., Afonso, P. M., Salazar, I. L., and Duarte, C. B. (2015). Regulation of hippocampal synaptic plasticity by BDNF. Brain Res. 1621, 82–101. doi: 10.1016/j.brainres.2014.10.019
Leung, S. W. S., and Shi, Y. (2021). The glycolytic process in endothelial cells and its implications. Acta Pharmacol. Sin. [Epub ahead of print]. doi: 10.1038/s41401-021-00647-y
Levin, E. G., Santell, L., and Osborn, K. G. (1997). The expression of endothelial tissue plasminogen activator in vivo: a function defined by vessel size and anatomic location. J. Cell Sci. 110(Pt 2), 139–148.
Lewis, R., Li, J., McCormick, P. J., L-H Huang, C., and Jeevaratnam, K. (2019). Is the sigma-1 receptor a potential pharmacological target for cardiac pathologies? A systematic review [published correction appears in Int J Cardiol Heart Vasc. 2020; 32:100700]. Int. J. Cardiol. Heart Vasc. 26:100449. doi: 10.1016/j.ijcha.2019.100449
Li, D. P., and Pan, H. L. (2005). Angiotensin II attenuates synaptic GABA release and excites paraventricular-rostral ventrolateral medulla output neurons. J. Pharmacol. Exp. Ther. 313, 1035–1045. doi: 10.1124/jpet.104.082495
Li, Y., Li, L., Wu, J., Zhu, Z., Feng, X., Qin, L., et al. (2020). Activation of astrocytes in hippocampus decreases fear memory through adenosine A1 receptors. eLife 9:e57155. doi: 10.7554/eLife.57155
Lightowlers, R. N., Chrzanowska-Lightowlers, Z. M., and Russell, O. M. (2020). Mitochondrial transplantation-a possible therapeutic for mitochondrial dysfunction?: mitochondrial transfer is a potential cure for many diseases but proof of efficacy and safety is still lacking. EMBO Rep. 21:e50964. doi: 10.15252/embr.202050964
Lin, L. H., Jin, J., Nashelsky, M. B., and Talman, W. T. (2014). Acid-sensing ion channel 1 and nitric oxide synthase are in adjacent layers in the wall of rat and human cerebral arteries. J. Chem. Neuroanat. 61-62, 161–168. doi: 10.1016/j.jchemneu.2014.10.002
Lin, Y. J., Kwok, C. F., Juan, C. C., Hsu, Y. P., Shih, K. C., Chen, C. C., et al. (2014). Angiotensin II enhances endothelin-1-induced vasoconstriction through upregulating endothelin type A receptor. Biochem. Biophys. Res. Commun. 451, 263–269. doi: 10.1016/j.bbrc.2014.07.119
Liu, D. Y., Chi, T. Y., Ji, X. F., Liu, P., Qi, X. X., Zhu, L., et al. (2018). Sigma-1 receptor activation alleviates blood-brain barrier dysfunction in vascular dementia mice. Exp. Neurol. 308, 90–99. doi: 10.1016/j.expneurol.2018.07.002
Liu, X. Y., Wei, B., Shi, H. X., Shan, Y. F., and Wang, C. (2010). Tom70 mediates activation of interferon regulatory factor 3 on mitochondria. Cell Res. 20, 994–1011. doi: 10.1038/cr.2010.103
Luchkina, N. V., and Bolshakov, V. Y. (2019). Mechanisms of fear learning and extinction: synaptic plasticity-fear memory connection. Psychopharmacology 236, 163–182. doi: 10.1007/s00213-018-5104-4
Lucido, C. T., Miskimins, W. K., and Vermeer, P. D. (2018). Propranolol promotes glucose dependence and synergizes with dichloroacetate for anti-cancer activity in HNSCC. Cancers 10:476. doi: 10.3390/cancers10120476
Lundgaard, I., Lu, M. L., Yang, E., Peng, W., Mestre, H., Hitomi, E., et al. (2017). Glymphatic clearance controls state-dependent changes in brain lactate concentration. J. Cereb. Blood Flow Metab. 37, 2112–2124. doi: 10.1177/0271678X16661202
Lüscher, B., and Möhler, H. (2019). Brexanolone, a neurosteroid antidepressant, vindicates the GABAergic deficit hypothesis of depression and may foster resilience. F1000Research 8:F1000 Faculty Rev-751. doi: 10.12688/f1000research.18758.1
Mader, S., and Brimberg, L. (2019). Aquaporin-4 water channel in the brain and its implication for health and disease. Cells 8:90. doi: 10.3390/cells8020090
Mariajoseph-Antony, L. F., Kannan, A., Panneerselvam, A., Loganathan, C., Anbarasu, K., and Prahalathan, C. (2020). Could aquaporin modulators be employed as prospective drugs for COVID-19 related pulmonary comorbidity? Med. Hypotheses. 143:110201. doi: 10.1016/j.mehy.2020.110201
Marie, C., Pedard, M., Quirié, A., Tessier, A., Garnier, P., Totoson, P., et al. (2018). Brain-derived neurotrophic factor secreted by the cerebral endothelium: a new actor of brain function? J. Cereb. Blood Flow Metab. 38, 935–949. doi: 10.1177/0271678X18766772
Marinzalda Mde, L., Pérez, P. A., Gargiulo, P. A., Casarsa, B. S., Bregonzio, C., and Baiardi, G. (2014). Fear-potentiated behaviour is modulated by central amygdala angiotensin II AT1 receptors stimulation. Biomed. Res. Int. 2014:183248. doi: 10.1155/2014/183248
Marriott, K. S., Prasad, M., Thapliyal, V., and Bose, H. S. (2012). σ-1 receptor at the mitochondrial-associated endoplasmic reticulum membrane is responsible for mitochondrial metabolic regulation. J. Pharmacol. Exp. Ther. 343, 578–586. doi: 10.1124/jpet.112.198168
Martin-Fernandez, M., Jamison, S., Robin, L. M., Zhao, Z., Martin, E. D., Aguilar, J., et al. (2017). Synapse-specific astrocyte gating of amygdala-related behavior. Nat. Neurosci. 20, 1540–1548. doi: 10.1038/nn.4649
Martini, C., Da Pozzo, E., Carmassi, C., Cuboni, S., Trincavelli, M. L., Massimetti, G., et al. (2013). Cyclic adenosine monophosphate responsive element binding protein in post-traumatic stress disorder. World J. Biol. Psychiatry 14, 396–402. doi: 10.3109/15622975.2011.577189
Martinotti, G., Sepede, G., Brunetti, M., Ricci, V., Gambi, F., Chillemi, E., et al. (2015). BDNF concentration and impulsiveness level in post-traumatic stress disorder. Psychiatry Res. 229, 814–818. doi: 10.1016/j.psychres.2015.07.085
Martinowich, K., Schloesser, R. J., Lu, Y., Jimenez, D. V., Paredes, D., Greene, J. S., et al. (2012). Roles of p75(NTR), long-term depression, and cholinergic transmission in anxiety and acute stress coping. Biol. Psychiatry 71, 75–83. doi: 10.1016/j.biopsych.2011.08.014
Marvar, P. J., Goodman, J., Fuchs, S., Choi, D. C., Banerjee, S., and Ressler, K. J. (2014). Angiotensin type 1 receptor inhibition enhances the extinction of fear memory. Biol. Psychiatry 75, 864–872. doi: 10.1016/j.biopsych.2013.08.024
Matsuoka, Y., Nishi, D., Tanima, Y., Itakura, M., Kojima, M., Hamazaki, K., et al. (2015). Serum pro-BDNF/BDNF as a treatment biomarker for response to docosahexaenoic acid in traumatized people vulnerable to developing psychological distress: a randomized controlled trial. Transl. Psychiatry 5:e596.
Matsushita, T., Isobe, N., Kawajiri, M., Mogi, M., Tsukuda, K., Horiuchi, M., et al. (2010). CSF angiotensin II and angiotensin-converting enzyme levels in anti-aquaporin-4 autoimmunity. J. Neurol Sci. 295, 41–45. doi: 10.1016/j.jns.2010.05.014
McLay, R. N., and Ho, J. (2007). Posttraumatic stress disorder-like symptoms after treatment with acetylcholinesterase inhibitors. J. Neuropsychiatry Clin. Neurosci. 19, 92–93. doi: 10.1176/jnp.2007.19.1.92
Mehta, S. R., Iudicello, J. E., Lin, J., Ellis, R. J., Morgan, E., Okwuegbuna, O., et al. (2021). Telomere length is associated with HIV infection, methamphetamine use, inflammation, and comorbid disease risk. Drug Alcohol Depend. 221:108639. doi: 10.1016/j.drugalcdep.2021.108639
Mellon, S. H., Bersani, F. S., Lindqvist, D., Hammamieh, R., Donohue, D., Dean, K., et al. (2019). Metabolomic analysis of male combat veterans with post traumatic stress disorder. PLoS One 14:e0213839. doi: 10.1371/journal.pone.0213839
Meunier, J., and Hayashi, T. (2010). Sigma-1 receptors regulate Bcl-2 expression by reactive oxygen species-dependent transcriptional regulation of nuclear factor kappaB. J. Pharmacol. Exp. Ther. 332, 388–397. doi: 10.1124/jpet.109.160960
Michopoulos, V., Powers, A., Gillespie, C. F., Ressler, K. J., and Jovanovic, T. (2017). Inflammation in fear- and anxiety-based disorders: PTSD, GAD, and beyond. Neuropsychopharmacology 42, 254–270. doi: 10.1038/npp.2016.146
Miller, J. K., McDougall, S., Thomas, S., and Wiener, J. (2017). The impact of the brain-derived neurotrophic factor gene on trauma and spatial processing. J. Clin. Med. 6:108. doi: 10.3390/jcm6120108
Miranda, M., Morici, J. F., Zanoni, M. B., and Bekinschtein, P. (2019). Brain-derived neurotrophic factor: a key molecule for memory in the healthy and the pathological brain. Front. Cell Neurosci. 13:363. doi: 10.3389/fncel.2019.00363
Mohlenhoff, B. S., O’Donovan, A., Weiner, M. W., and Neylan, T. C. (2017). Dementia risk in posttraumatic stress disorder: the relevance of sleep-related abnormalities in brain structure, amyloid, and inflammation. Curr. Psychiatry Rep. 19:89. doi: 10.1007/s11920-017-0835-1
Mojtabavi, H., Saghazadeh, A., van den Heuvel, L., Bucker, J., and Rezaei, N. (2020). Peripheral blood levels of brain-derived neurotrophic factor in patients with post-traumatic stress disorder (PTSD): a systematic review and meta-analysis. PLoS One 15:e0241928. doi: 10.1371/journal.pone.0241928
Mongelli, A., Barbi, V., Gottardi Zamperla, M., Atlante, S., Forleo, L., Nesta, M., et al. (2021). Evidence for biological age acceleration and telomere shortening in COVID-19 survivors. Int. J. Mol. Sci. 22:6151. doi: 10.3390/ijms22116151
Morais-de-Jesus, M., Daltro-Oliveira, R., Pettersen, K. M., Dantas-Duarte, A., Amaral, L. D., Cavalcanti-Ribeiro, P., et al. (2014). Hepatitis C virus infection as a traumatic experience. PLoS One 9:e110529. doi: 10.1371/journal.pone.0110529
Morath, J., Gola, H., Sommershof, A., Hamuni, G., Kolassa, S., Catani, C., et al. (2014). The effect of trauma-focused therapy on the altered T cell distribution in individuals with PTSD: evidence from a randomized controlled trial. J. Psychiatr. Res. 54, 1–10. doi: 10.1016/j.jpsychires.2014.03.016
Morland, C., Andersson, K. A., Haugen, ØP., Hadzic, A., Kleppa, L., Gille, A., et al. (2017). Exercise induces cerebral VEGF and angiogenesis via the lactate receptor HCAR1. Nat. Commun. 8:15557. doi: 10.1038/ncomms15557
Morland, C., Lauritzen, K. H., Puchades, M., Holm-Hansen, S., Andersson, K., Gjedde, A., et al. (2015). The lactate receptor, G-protein-coupled receptor 81/hydroxycarboxylic acid receptor 1: expression and action in brain. J. Neurosci. Res. 93, 1045–1055. doi: 10.1002/jnr.23593
Morrison, K. E., Cole, A. B., Thompson, S. M., and Bale, T. L. (2019). Brexanolone for the treatment of patients with postpartum depression. Drugs Today 55, 537–544. doi: 10.1358/dot.2019.55.9.3040864
Myers, K., and Davis, M. (2007). Mechanisms of fear extinction. Mol. Psychiatry 12, 120–150. doi: 10.1038/sj.mp.4001939
Nagelhus, E. A., and Ottersen, O. P. (2013). Physiological roles of aquaporin-4 in brain. Physiol. Rev. 93, 1543–1562. doi: 10.1152/physrev.00011.2013
Nakahashi, T., Fujimura, H., Altar, C. A., Li, J., Kambayashi, J., Tandon, N. N., et al. (2000). Vascular endothelial cells synthesize and secrete brain-derived neurotrophic factor. FEBS Lett. 470, 113–117. doi: 10.1016/s0014-5793(00)01302-8
Naoi, M., Maruyama, W., Akao, Y., Yi, H., and Yamaoka, Y. (2006). Involvement of type A monoamine oxidase in neurodegeneration: regulation of mitochondrial signaling leading to cell death or neuroprotection. J. Neural Transm. Suppl. 71, 67–77. doi: 10.1007/978-3-211-33328-0_8
Natsvlishvili, N., Goguadze, N., Zhuravliova, E., and Mikeladze, D. (2015). Sigma-1 receptor directly interacts with Rac1-GTPase in the brain mitochondria. BMC Biochem. 16:11. doi: 10.1186/s12858-015-0040-y
Nehme, J., Borghesan, M., Mackedenski, S., Bird, T. G., and Demaria, M. (2020). Cellular senescence as a potential mediator of COVID-19 severity in the elderly. Aging Cell 19:e13237. doi: 10.1111/acel.13237
Neigh, G. N., Rhodes, S. T., Valdez, A., and Jovanovic, T. (2016). PTSD co-morbid with HIV: separate but equal, or two parts of a whole? Neurobiol. Dis. 92(Pt B), 116–123. doi: 10.1016/j.nbd.2015.11.012
Ney, L. J., Gogos, A., Ken Hsu, C. M., and Felmingham, K. L. (2019). An alternative theory for hormone effects on sex differences in PTSD: the role of heightened sex hormones during trauma. Psychoneuroendocrinology 109:104416. doi: 10.1016/j.psyneuen.2019.104416
Notaras, M., and van den Buuse, M. (2020). Neurobiology of BDNF in fear memory, sensitivity to stress, and stress-related disorders. Mol. Psychiatry 25, 2251–2274. doi: 10.1038/s41380-019-0639-2
Owasil, R., O’Neill, R., Keable, A., Nimmo, J., MacGregor Sharp, M., Kelly, L., et al. (2020). The pattern of AQP4 expression in the ageing human brain and in cerebral amyloid angiopathy. Int. J. Mol. Sci. 21:1225. doi: 10.3390/ijms21041225
Padmakumar, S., Jones, G., Pawar, G., Khorkova, O., Hsiao, J., Kim, J., et al. (2021). Minimally Invasive Nasal Depot (MIND) technique for direct BDNF AntagoNAT delivery to the brain. J. Control Release 331, 176–186. doi: 10.1016/j.jconrel.2021.01.027
Pal, A., Fontanilla, D., Gopalakrishnan, A., Chae, Y. K., Markley, J. L., and Ruoho, A. E. (2012). The sigma-1 receptor protects against cellular oxidative stress and activates antioxidant response elements. Eur. J. Pharmacol. 682, 12–20. doi: 10.1016/j.ejphar.2012.01.030
Papić, N., Židovec Lepej, S., Kurelac, I., Čajić, V., Budimir, J., Dušek, D., et al. (2011). Treatment of chronic hepatitis C in Croatian war veterans: experiences from Croatian reference center for viral hepatitis. Croat Med. J. 52, 35–40. doi: 10.3325/cmj.2011.52.35
Paris, A. J., Hayer, K. E., Oved, J. H., Avgousti, D. C., Toulmin, S. A., Zepp, J. A., et al. (2020). STAT3-BDNF-TrkB signalling promotes alveolar epithelial regeneration after lung injury. Nat. Cell Biol. 22, 1197–1210. doi: 10.1038/s41556-020-0569-x
Pawlak, R., Magarinos, A. M., Melchor, J., McEwen, B., and Strickland, S. (2003). Tissue plasminogen activator in the amygdala is critical for stress-induced anxiety-like behavior. Nat. Neurosci. 6, 168–174. doi: 10.1038/nn998
Pidoplichko, V. I., Aroniadou-Anderjaska, V., Prager, E. M., Figueiredo, T. H., Almeida-Suhett, C. P., Miller, S. L., et al. (2014). ASIC1a activation enhances inhibition in the basolateral amygdala and reduces anxiety. J. Neurosci. 34, 3130–3141. doi: 10.1523/JNEUROSCI.4009-13.2014
Pineles, S. L., Nillni, Y. I., Pinna, G., Irvine, J., Webb, A., Arditte, Hall KA, et al. (2018). PTSD in women is associated with a block in conversion of progesterone to the GABAergic neurosteroids allopregnanolone and pregnanolone measured in plasma. Psychoneuroendocrinology 93, 133–141. doi: 10.1016/j.psyneuen.2018.04.024
Pitts, B. L., Whealin, J. M., Harpaz-Rotem, I., Duman, R. S., Krystal, J. H., Southwick, S. M., et al. (2019). BDNF Val66Met polymorphism and posttraumatic stress symptoms in U.S. military veterans: protective effect of physical exercise. Psychoneuroendocrinology 100, 198–202. doi: 10.1016/j.psyneuen.2018.10.011
Plog, B. A., and Nedergaard, M. (2018). The glymphatic system in central nervous system health and disease: past, present, and future. Annu. Rev. Pathol. 13, 379–394. doi: 10.1146/annurev-pathol-051217-111018
Prather, A. A., Epel, E. S., Portela Parra, E., Coccia, M., Puterman, E., Aiello, A. E., et al. (2018). Associations between chronic caregiving stress and T cell markers implicated in immunosenescence. Brain Behav. Immun. 73, 546–549. doi: 10.1016/j.bbi.2018.06.019
Qaradakhi, T., Gadanec, L. K., McSweeney, K. R., Tacey, A., Apostolopoulos, V., Levinger, I., et al. (2020). The potential actions of angiotensin-converting enzyme II (ACE2) activator diminazene aceturate (DIZE) in various diseases. Clin. Exp. Pharmacol. Physiol. 47, 751–758. doi: 10.1111/1440-1681.13251
Quagliato, L. A., Freire, R. C., and Nardi, A. E. (2018). The role of acid-sensitive ion channels in panic disorder: a systematic review of animal studies and meta-analysis of human studies. Transl. Psychiatry 8:185. doi: 10.1038/s41398-018-0238-z
Rahimy, E., Li, F. Y., Hagberg, L., Fuchs, D., Robertson, K., Meyerhoff, D. J., et al. (2017). Blood-brain barrier disruption is initiated during primary HIV infection and not rapidly altered by antiretroviral therapy. J. Infect Dis. 215, 1132–1140. doi: 10.1093/infdis/jix013
Rasmusson, A. M., Pinna, G., Paliwal, P., Weisman, D., Gottschalk, C., Charney, D., et al. (2006). Decreased cerebrospinal fluid allopregnanolone levels in women with posttraumatic stress disorder. Biol. Psychiatry 60, 704–713. doi: 10.1016/j.biopsych.2006.03.026
Rhea, E. M., Logsdon, A. F., Hansen, K. M., Williams, L. M., Reed, M. J., Baumann, K. K., et al. (2021). The S1 protein of SARS-CoV-2 crosses the blood–brain barrier in mice. Nat. Neurosci. 24, 368–378. doi: 10.1038/s41593-020-00771-8
Ritchie, K., Chan, D., and Watermeyer, T. (2020). The cognitive consequences of the COVID-19 epidemic: collateral damage? Brain Commun. 2:fcaa069. doi: 10.1093/braincomms/fcaa069
Robicsek, O., Makhoul, B., Klein, E., Brenner, B., and Sarig, G. (2011). Hypercoagulation in chronic post-traumatic stress disorder. ISR Med. Assoc. J. 13, 548–552.
Rösch, H., Schweigreiter, R., Bonhoeffer, T., Barde, Y. A., and Korte, M. (2005). The neurotrophin receptor p75NTR modulates long-term depression and regulates the expression of AMPA receptor subunits in the hippocampus. Proc. Natl. Acad. Sci. U.S.A. 102, 7362–7367. doi: 10.1073/pnas.0502460102
Sabbatinelli, J., Prattichizzo, F., Olivieri, F., Procopio, A. D., Rippo, M. R., and Giuliani, A. (2019). Where metabolism meets senescence: focus on endothelial cells. Front. Physiol. 10:1523. doi: 10.3389/fphys.2019.01523
Saleh, T., Carpenter, V. J., Tyutyunyk-Massey, L., Murray, G., Leverson, J. D., Souers, A. J., et al. (2020). Clearance of therapy-induced senescent tumor cells by the senolytic ABT-263 via interference with BCL-XL -BAX interaction. Mol. Oncol. 14, 2504–2519. doi: 10.1002/1878-0261.12761
Salim, S. (2014). Oxidative stress and psychological disorders. Curr. Neuropharmacol. 12, 140–147. doi: 10.2174/1570159X11666131120230309
Sanchez-Vazquez, R., Guío-Carrión, A., Zapatero-Gaviria, A., Martínez, P., and Blasco, M. A. (2021). Shorter telomere lengths in patients with severe COVID-19 disease. Aging 13, 1–15. doi: 10.18632/aging.202463
Sanz-García, A., Knafo, S., Pereda-Pérez, I., Esteban, J. A., Venero, C., and Armario, A. (2016). Administration of the TrkB receptor agonist 7,8-dihydroxyflavone prevents traumatic stress-induced spatial memory deficits and changes in synaptic plasticity. Hippocampus 26, 1179–1188. doi: 10.1002/hipo.22599
Saur, L., Baptista, P. P., Bagatini, P. B., Neves, L. T., de Oliveira, R. M., Vaz, S. P., et al. (2016). Experimental Post-traumatic Stress Disorder Decreases Astrocyte Density and Changes Astrocytic Polarity in the CA1 Hippocampus of Male Rats. Neurochem. Res. 41, 892–904. doi: 10.1007/s11064-015-1770-3
Scharfman, H. E., and Binder, D. K. (2013). Aquaporin-4 water channels and synaptic plasticity in the hippocampus. Neurochem. Int. 63, 702–711. doi: 10.1016/j.neuint.2013.05.003
Schmid, S. A., Gaumann, A., Wondrak, M., Eckermann, C., Schulte, S., Mueller-Klieser, W., et al. (2007). Lactate adversely affects the in vitro formation of endothelial cell tubular structures through the action of TGF-beta1. Exp. Cell Res. 313, 2531–2549. doi: 10.1016/j.yexcr.2007.05.016
Schmidt, A., Rossetti, G., Joussen, S., and Gründer, S. (2017). Diminazene is a slow pore blocker of acid-sensing ion channel 1a (ASIC1a). Mol. Pharmacol. 92, 665–675. doi: 10.1124/mol.117.110064
Scozzi, D., Cano, M., Ma, L., Zhou, D., Zhu, J. H., O’Halloran, J. A., et al. (2021). Circulating mitochondrial DNA is an early indicator of severe illness and mortality from COVID-19. JCI Insight 6:e143299. doi: 10.1172/jci.insight.143299
Seligowski, A. V., Duffy, L. A., Merker, J. B., Michopoulos, V., Gillespie, C. F., Marvar, P. J., et al. (2021). The renin-angiotensin system in PTSD: a replication and extension. Neuropsychopharmacology 46, 750–755. doi: 10.1038/s41386-020-00923-1
Seligowski, A. V., Hurly, J., Mellen, E., Ressler, K. J., and Ramikie, T. S. (2020). Translational studies of estradiol and progesterone in fear and PTSD. Eur. J. Psychotraumatol. 11:1723857. doi: 10.1080/20008198.2020.1723857
Seth, R. B., Sun, L., Ea, C. K., and Chen, Z. J. (2005). Identification and characterization of MAVS, a mitochondrial antiviral signaling protein that activates NF-kappaB and IRF 3. Cell 122, 669–682. doi: 10.1016/j.cell.2005.08.012
Sfera, A., and Osorio, C. (2014). Water for thought: is there a role for aquaporin channels in delirium? Front. Psychiatry 5:57. doi: 10.3389/fpsyt.2014.00057
Sfera, A., Osorio, C., Jafri, N., Diaz, E. L., and Campo Maldonado, J. E. (2020). Intoxication with endogenous angiotensin II: a COVID-19 hypothesis. Front. Immunol. 11:1472. doi: 10.3389/fimmu.2020.01472
Sfera, A., Osorio, C., Maguire, G., Rahman, L., Afzaal, J., Cummings, M., et al. (2021a). COVID-19, ferrosenescence and neurodegeneration, a mini-review. Prog. Neuropsychopharmacol. Biol. Psychiatry 109:110230. doi: 10.1016/j.pnpbp.2020.110230
Sfera, A., Osorio, C., Price, A. I., Gradini, R., and Cummings, M. (2015). Delirium from the gliocentric perspective. Front. Cell Neurosci. 9:171. doi: 10.3389/fncel.2015.00171
Sfera, A., Osorio, C., Zapata Martín del Campo, C. M., Pereida, S., Maurer, S., Maldonado, J. C., et al. (2021b). Endothelial senescence and chronic fatigue syndrome, a COVID-19 based hypothesis. Front. Cell. Neurosci. 15:673217. doi: 10.3389/fncel.2021.673217
Shan, B., Ai, Z., Zeng, S., Song, Y., Song, J., Zeng, Q., et al. (2020). Gut microbiome-derived lactate promotes to anxiety-like behaviors through GPR81 receptor-mediated lipid metabolism pathway. Psychoneuroendocrinology 117:104699. doi: 10.1016/j.psyneuen.2020.104699
Shekhar, A., Johnson, P. L., Sajdyk, T. J., Fitz, S. D., Keim, S. R., Kelley, P. E., et al. (2006). Angiotensin-II is a putative neurotransmitter in lactate-induced panic-like responses in rats with disruption of GABAergic inhibition in the dorsomedial hypothalamus. J Neurosci. 26, 9205–9215. doi: 10.1523/JNEUROSCI.2491-06.2006
Sher, L. D., Geddie, H., Olivier, L., Cairns, M., Truter, N., Beselaar, L., et al. (2020). Chronic stress and endothelial dysfunction: mechanisms, experimental challenges, and the way ahead. Am. J. Physiol. Heart Circ. Physiol. 319, H488–H506. doi: 10.1152/ajpheart.00244.2020
Sherin, J. E., and Nemeroff, C. B. (2011). Post-traumatic stress disorder: the neurobiological impact of psychological trauma. Dialog. Clin. Neurosci. 13, 263–278. doi: 10.31887/DCNS.2011.13.2/jsherin
Shkreli, L., Woud, M. L., Ramsbottom, R., Rupietta, A. E., Waldhauser, G. T., Kumsta, R., et al. (2020). Angiotensin involvement in trauma processing-exploring candidate neurocognitive mechanisms of preventing post-traumatic stress symptoms. Neuropsychopharmacology 45, 507–514. doi: 10.1038/s41386-019-0553-y
Siyahhan Julnes, P., Auh, S., Krakora, R., Withers, K., Nora, D., Matthews, L., et al. (2016). The association between post-traumatic stress disorder and markers of inflammation and immune activation in hiv-infected individuals with controlled viremia. Psychosomatics 57, 423–430. doi: 10.1016/j.psym.2016.02.015
Smoller, J. W., Gallagher, P. J., Duncan, L. E., McGrath, L. M., Haddad, S. A., Holmes, A. J., et al. (2014). The human ortholog of acid-sensing ion channel gene ASIC1a is associated with panic disorder and amygdala structure and function. Biol. Psychiatry 76, 902–910. doi: 10.1016/j.biopsych.2013.12.018
Sobczak, S., Olff, M., Rutten, B., Verhey, F., and Deckers, K. (2021). Comorbidity rates of Posttraumatic Stress Disorder in dementia: a systematic literature review. Eur. J. Psychotraumatol. 12:1883923. doi: 10.1080/20008198.2021.1883923
Spottswood, M., Davydow, D. S., and Huang, H. (2017). The prevalence of posttraumatic stress disorder in primary care: a systematic review. Harv. Rev. Psychiatry 25, 159–169. doi: 10.1097/HRP.0000000000000136
Stein, J. Y., Levin, Y., Uziel, O., Abumock, H., and Solomon, Z. (2018). Traumatic stress and cellular senescence: the role of war-captivity and homecoming stressors in later life telomere length. J. Affect. Disord. 238, 129–135. doi: 10.1016/j.jad.2018.05.037
Sukhatme, V. P., Reiersen, A. M., Vayttaden, S. J., and Sukhatme, V. V. (2021). Fluvoxamine: a review of its mechanism of action and its role in COVID-19. Front. Pharmacol. 12:652688. doi: 10.3389/fphar.2021.652688
Suzuki, A., Stern, S. A., Bozdagi, O., Huntley, G. W., Walker, R. H., Magistretti, P. J., et al. (2011). Astrocyte-neuron lactate transport is required for long-term memory formation. Cell 144, 810–823. doi: 10.1016/j.cell.2011.02.018
Szekeres, M., Nádasy, G. L., Turu, G., Süpeki, K., Szidonya, L., Buday, L., et al. (2010). Angiotensin II-induced expression of brain-derived neurotrophic factor in human and rat adrenocortical cells. Endocrinology 151, 1695–1703. doi: 10.1210/en.2009-1060
Szu, J. I., and Binder, D. K. (2016). The role of astrocytic aquaporin-4 in synaptic plasticity and learning and memory. Front. Integr. Neurosci. 10:8. doi: 10.3389/fnint.2016.00008
Takeda, K., Kermani, P., Anastasia, A., Obinata, Y., Hempstead, B. L., and Kurihara, H. (2013). BDNF protects human vascular endothelial cells from TNFα-induced apoptosis. Biochem. Cell Biol. 91, 341–349. doi: 10.1139/bcb-2013-0005
Tang, X., Luo, Y. X., Chen, H. Z., and Liu, D. P. (2014). Mitochondria, endothelial cell function, and vascular diseases. Front. Physiol. 5:175. doi: 10.3389/fphys.2014.00175
Tang, X., Peng, R., Phillips, J. E., Deguzman, J., Ren, Y., Apparsundaram, S., et al. (2013). Assessment of Brd4 inhibition in idiopathic pulmonary fibrosis lung fibroblasts and in vivo models of lung fibrosis. Am. J. Pathol. 183, 470–479. doi: 10.1016/j.ajpath.2013.04.020
Tarantini, S., Valcarcel-Ares, N. M., Yabluchanskiy, A., Fulop, G. A., Hertelendy, P., Gautam, T., et al. (2018). Treatment with the mitochondrial-targeted antioxidant peptide SS-31 rescues neurovascular coupling responses and cerebrovascular endothelial function and improves cognition in aged mice. Aging Cell. 17:e12731. doi: 10.1111/acel.12731
Tarsitani, L., Vassalini, P., Koukopoulos, A., Borrazzo, C., Alessi, F., Di Nicolantonio, C., et al. (2021). Post-traumatic stress disorder among COVID-19 survivors at 3-month follow-up after hospital discharge. J. Gen. Intern. Med. 9, 1–6. doi: 10.1007/s11606-021-06731-7
Tasdemir, N., Banito, A., Roe, J. S., Alonso-Curbelo, D., Camiolo, M., Tschaharganeh, D. F., et al. (2016). BRD4 connects enhancer remodeling to senescence immune surveillance. Cancer Discov. 6, 612–629. doi: 10.1158/2159-8290.CD-16-0217
Taugher, R. J., Lu, Y., Fan, R., Ghobbeh, A., Kreple, C. J., Faraci, F. M., et al. (2017). ASIC1A in neurons is critical for fear-related behaviors. Genes Brain Behav. 16, 745–755. doi: 10.1111/gbb.12398
Tavčar, P., Potokar, M., Kolenc, M., Korva, M., Avšič-Županc, T., Zorec, R., et al. (2021). Neurotropic viruses, astrocytes, and COVID-19. Front. Cell Neurosci. 15:662578. doi: 10.3389/fncel.2021.662578
Terock, J., Hannemann, A., Janowitz, D., Freyberger, H. J., Felix, S. B., Dörr, M., et al. (2019). Associations of trauma exposure and post-traumatic stress disorder with the activity of the renin-angiotensin-aldosterone-system in the general population. Psychol. Med. 49, 843–851. doi: 10.1017/S0033291718001496
Tewari, K., Nakayama, Y., and Suresh, M. (2007). Role of direct effects of IFN-gamma on T cells in the regulation of CD8 T cell homeostasis. J. Immunol. 179, 2115–2125. doi: 10.4049/jimmunol.179.4.2115
Thurston, R. C., Barinas-Mitchell, E., von Känel, R., Chang, Y., Koenen, K. C., and Matthews, K. A. (2018). Trauma exposure and endothelial function among midlife women. Menopause 25, 368–374. doi: 10.1097/GME.0000000000001036
Tice, C., McDevitt, J., and Langford, D. (2020). Astrocytes, HIV and the glymphatic system: a disease of disrupted waste management? Front. Cell Infect. Microbiol. 10:523379. doi: 10.3389/fcimb.2020.523379
Toda, N., and Nakanishi-Toda, M. (2011). How mental stress affects endothelial function. Pflugers Arch. 462, 779–794. doi: 10.1007/s00424-011-1022-6
Trumpff, C., Michelson, J., Lagranha, C. J., Taleon, V., Karan, K. R., Sturm, G., et al. (2021). Stress and circulating cell-free mitochondrial DNA: a systematic review of human studies, physiological considerations, and technical recommendations. Mitochondrion 59, 225–245. doi: 10.1016/j.mito.2021.04.002
Tsai, S. J. (2017). Role of tissue-type plasminogen activator and plasminogen activator inhibitor-1 in psychological stress and depression. Oncotarget 8, 113258–113268.
Tsang, D., and Lal, S. (1977). Effect of monoamine receptor agonists and antagonists on cyclic AMP accumulation in human cerebral cortex slices. Can. J. Physiol. Pharmacol. 55, 1263–1269. doi: 10.1139/y77-172
Turchi, J., Chang, C., Ye, F. Q., Russ, B. E., Yu, D. K., Cortes, C. R., et al. (2018). The basal forebrain regulates global resting-state fMRI fluctuations. Neuron 97, 940.e4–952.e4. doi: 10.1016/j.neuron.2018.01.032
Vaccarino, V., Goldberg, J., Rooks, C., Shah, A. J., Veledar, E., Faber, T. L., et al. (2013). Post-traumatic stress disorder and incidence of coronary heart disease: a twin study. J. Am. Coll. Cardiol. 62, 970–978. doi: 10.1016/j.jacc.2013.04.085
Vaiva, G., Ducrocq, F., Jezequel, K., Averland, B., Lestavel, P., Brunet, A., et al. (2003). Immediate treatment with propranolol decreases posttraumatic stress disorder two months after trauma. Biol. Psychiatry 54, 947–949. doi: 10.1016/s0006-3223(03)00412-8
Vargas, M. R., Pehar, M., Cassina, P., Estévez, A. G., Beckman, J. S., and Barbeito, L. (2004). Stimulation of nerve growth factor expression in astrocytes by peroxynitrite. In Vivo 18, 269–274.
Vasanthakumar, N. (2020). Can beta-adrenergic blockers be used in the treatment of COVID-19? Med. Hypotheses 142:109809. doi: 10.1016/j.mehy.2020.109809
Vaughan, D. E., Rai, R., Khan, S. S., Eren, M., and Ghosh, A. K. (2017). Plasminogen activator inhibitor-1 is a marker and a mediator of senescence. Arterioscler. Thromb. Vasc. Biol. 37, 1446–1452. doi: 10.1161/ATVBAHA.117.309451
Vela, J. M. (2020). Repurposing sigma-1 receptor ligands for COVID-19 therapy? Front. Pharmacol. 11:582310. doi: 10.3389/fphar.2020.582310
Verkhratsky, A., and Nedergaard, M. (2018). Physiology of astroglia. Physiol. Rev. 98, 239–389. doi: 10.1152/physrev.00042.2016
Vian, J., Pereira, C., Chavarria, V., Köhler, C., Stubbs, B., Quevedo, J., et al. (2017). The renin-angiotensin system: a possible new target for depression. BMC Med. 15:144. doi: 10.1186/s12916-017-0916-3
Volchkov, V. E., Feldmann, H., Volchkova, V. A., and Klenk, H. D. (1998). Processing of the Ebola virus glycoprotein by the proprotein convertase furin. Proc. Natl. Acad. Sci. U.S.A. 95, 5762–5767. doi: 10.1073/pnas.95.10.5762
von Känel, R., Hepp, U., Traber, R., Kraemer, B., Mica, L., Keel, M., et al. (2008). Measures of endothelial dysfunction in plasma of patients with posttraumatic stress disorder. Psychiatry Res. 158, 363–373. doi: 10.1016/j.psychres.2006.12.003
Wang, L., de Kloet, A. D., Pati, D., Hiller, H., Smith, J. A., Pioquinto, D. J., et al. (2016). Increasing brain angiotensin converting enzyme 2 activity decreases anxiety-like behavior in male mice by activating central Mas receptors. Neuropharmacology 105, 114–123. doi: 10.1016/j.neuropharm.2015.12.026
Wang, W., Wang, R., Jiang, Z., Li, H., Zhu, Z., Khalid, A., et al. (2021). Inhibiting Brd4 alleviated PTSD-like behaviors and fear memory through regulating immediate early genes expression and neuroinflammation in rats. J. Neurochem. 158, 912–927. doi: 10.1111/jnc.15439
Wang, X. L., Iwanami, J., Min, L. J., Tsukuda, K., Nakaoka, H., Bai, H. Y., et al. (2016). Deficiency of angiotensin-converting enzyme 2 causes deterioration of cognitive function. NPJ Aging Mech. Dis. 2:16024. doi: 10.1038/npjamd.2016.24
Wang, Y., Xie, L., Gao, C., Zhai, L., Zhang, N., and Guo, L. (2018). Astrocytes activation contributes to the antidepressant-like effect of ketamine but not scopolamine. Pharmacol. Biochem. Behav. 170, 1–8. doi: 10.1016/j.pbb.2018.05.001
Ward, R., Abdul, Y., and Ergul, A. (2018). Endothelin-1 inhibition improves the mBDNF/proBDNF ratio in endothelial cells and HT22 neurons under high glucose/palmitate growth conditions. Physiol. Res. 67(Suppl. 1), S237–S246. doi: 10.33549/physiolres.933837
Wei, F., Song, J., Zhang, C., Lin, J., Xue, R., Shan, L. D., et al. (2019). Chronic stress impairs the aquaporin-4-mediated glymphatic transport through glucocorticoid signaling. Psychopharmacology 236, 1367–1384. doi: 10.1007/s00213-018-5147-6
Wilcox, C. S., and Pitt, B. (2020). Is spironolactone the preferred renin-angiotensin-aldosterone inhibitor for protection against COVID-19? J. Cardiovasc. Pharmacol. 77, 323–331. doi: 10.1097/FJC.0000000000000960
Wiley, C. D., and Campisi, J. (2016). From ancient pathways to aging cells-connecting metabolism and cellular senescence. Cell Metab. 23, 1013–1021. doi: 10.1016/j.cmet.2016.05.010
Wilson, D. F. (2017). Oxidative phosphorylation: regulation and role in cellular and tissue metabolism. J. Physiol. 595, 7023–7038. doi: 10.1113/JP273839
Wolf, E. J., and Morrison, F. G. (2017). Traumatic stress and accelerated cellular aging: from epigenetics to cardiometabolic disease. Curr. Psychiatry Rep. 19:75. doi: 10.1007/s11920-017-0823-5
Wong, B. W., Marsch, E., Treps, L., Baes, M., and Carmeliet, P. (2017). Endothelial cell metabolism in health and disease: impact of hypoxia. EMBO J. 36, 2187–2203. doi: 10.15252/embj.201696150
Woo, N. H., Teng, H. K., Siao, C. J., Chiaruttini, C., Pang, P. T., Milner, T. A., et al. (2005). Activation of p75NTR by proBDNF facilitates hippocampal long-term depression. Nat. Neurosci. 8, 1069–1077. doi: 10.1038/nn1510
Wu, C., Zheng, M., Yang, Y., Gu, X., Yang, K., Li, M., et al. (2020). Furin: a potential therapeutic target for COVID-19. iScience 23, 101642. doi: 10.1016/j.isci.2020.101642
Wu, K. K. (2021). Control of mesenchymal stromal cell senescence by tryptophan metabolites. Int. J. Mol. Sci. 22:697. doi: 10.3390/ijms22020697
Wu, Z., and McGoogan, J. M. (2020). Characteristics of and important lessons from the coronavirus disease 2019 (covid-19) outbreak in china: summary of a report of 72 314 cases from the chinese center for disease control and prevention. JAMA 323, 1239–1242. doi: 10.1001/jama.2020.2648
Xu, Q., Ji, X. F., Chi, T. Y., Liu, P., Jin, G., Gu, S. L., et al. (2015). Sigma 1 receptor activation regulates brain-derived neurotrophic factor through NR2A-CaMKIV-TORC1 pathway to rescue the impairment of learning and memory induced by brain ischaemia/reperfusion. Psychopharmacology 232, 1779–1791. doi: 10.1007/s00213-014-3809-6
Yaffe, K., Vittinghoff, E., Lindquist, K., Barnes, D., Covinsky, K. E., Neylan, T., et al. (2010). Posttraumatic stress disorder and risk of dementia among US veterans. Arch. Gen. Psychiatry 67, 608–613. doi: 10.1001/archgenpsychiatry.2010.61
Yammine, L., Kang, D. H., Baun, M. M., and Meininger, J. C. (2014). Endothelin-1 and psychosocial risk factors for cardiovascular disease: a systematic review. Psychosom. Med. 76, 109–121.
Yapici-Eser, H., Koroglu, Y. E., Oztop-Cakmak, O., Keskin, O., Gursoy, A., and Gursoy-Ozdemir, Y. (2021). Neuropsychiatric symptoms of COVID-19 explained by SARS-CoV-2 proteins’ mimicry of human protein interactions. Front. Hum. Neurosci. 15:656313. doi: 10.3389/fnhum.2021.656313
Yegorov, Y. E., Poznyak, A. V., Nikiforov, N. G., Sobenin, I. A., and Orekhov, A. N. (2020). The link between chronic stress and accelerated aging. Biomedicines 8:1982020.
Yellepeddi, V., Sayre, C., Burrows, A., Watt, K., Davies, S., Strauss, J., et al. (2020). Stability of extemporaneously compounded amiloride nasal spray. PLoS One 15:e0232435. doi: 10.1371/journal.pone.0232435
Yu, Z., Swiercz, A. P., Moshfegh, C. M., Hopkins, L., Wiaderkiewicz, J., Speth, R. C., et al. (2019). Angiotensin II type 2 receptor-expressing neurons in the central amygdala influence fear-related behavior. Biol. Psychiatry 86, 899–909. doi: 10.1016/j.biopsych.2019.05.027
Yuen, E. C., Gunther, E. C., and Bothwell, M. (2000). Nitric oxide activation of TrkB through peroxynitrite. Neuroreport 11, 3593–3597. doi: 10.1097/00001756-200011090-00038
Zhou, R., Yazdi, A. S., Menu, P., and Tschopp, J. (2011). A role for mitochondria in NLRP3 inflammasome activation. Nature 469, 221–225. doi: 10.1038/nature09663
Zhou, X., Xiao, Q., Xie, L., Yang, F., Wang, L., and Tu, J. (2019). Astrocyte, a promising target for mood disorder interventions. Front. Mol. Neurosci. 12:136. doi: 10.3389/fnmol.2019.00136
Ziemann, A. E., Allen, J. E., Dahdaleh, N. S., Drebot, I. I., Coryell, M. W., Wunsch, A. M., et al. (2009). The amygdala is a chemosensor that detects carbon dioxide and acidosis to elicit fear behavior. Cell 139, 1012–1021. doi: 10.1016/j.cell.2009.10.029
Zoellner, L. A., Telch, M., Foa, E. B., Farach, F. J., McLean, C. P., Gallop, R., et al. (2017). Enhancing extinction learning in posttraumatic stress disorder with brief daily imaginal exposure and methylene blue: a randomized controlled trial. J. Clin. Psychiatry 78, e782–e789. doi: 10.4088/JCP.16m10936
Zorumski, C. F., Paul, S. M., Izumi, Y., Covey, D. F., and Mennerick, S. (2013). Neurosteroids, stress and depression: potential therapeutic opportunities. Neurosci. Biobehav. Rev. 37, 109–122. doi: 10.1016/j.neubiorev.2012.10.005
Keywords: PTSD, endothelia, SARS-CoV-2, COVID-19, mitochondria, lactate
Citation: Sfera A, Osorio C, Rahman L, Zapata-Martín del Campo CM, Maldonado JC, Jafri N, Cummings MA, Maurer S and Kozlakidis Z (2021) PTSD as an Endothelial Disease: Insights From COVID-19. Front. Cell. Neurosci. 15:770387. doi: 10.3389/fncel.2021.770387
Received: 03 September 2021; Accepted: 11 October 2021;
Published: 29 October 2021.
Edited by:
Abel Viejo-Borbolla, Hannover Medical School, GermanyReviewed by:
Hale Yapici Eser, Koç University, TurkeyIsabella Zanella, University of Brescia, Italy
Copyright © 2021 Sfera, Osorio, Rahman, Zapata-Martín del Campo, Maldonado, Jafri, Cummings, Maurer and Kozlakidis. This is an open-access article distributed under the terms of the Creative Commons Attribution License (CC BY). The use, distribution or reproduction in other forums is permitted, provided the original author(s) and the copyright owner(s) are credited and that the original publication in this journal is cited, in accordance with accepted academic practice. No use, distribution or reproduction is permitted which does not comply with these terms.
*Correspondence: Adonis Sfera, ZHIuc2ZlcmFAZ21haWwuY29t