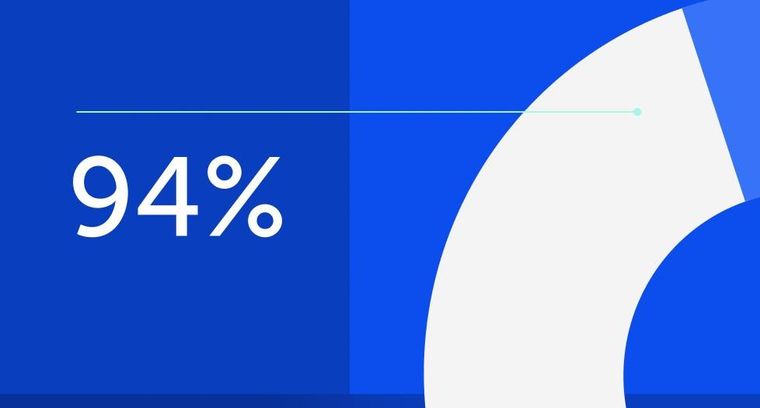
94% of researchers rate our articles as excellent or good
Learn more about the work of our research integrity team to safeguard the quality of each article we publish.
Find out more
REVIEW article
Front. Cell. Neurosci., 04 October 2021
Sec. Non-Neuronal Cells
Volume 15 - 2021 | https://doi.org/10.3389/fncel.2021.759571
Alpha-synuclein accumulation in dopaminergic neurons is one of the primary features of Parkinson’s disease (PD). Despite its toxic properties during PD, alpha-synuclein has some important physiological functions. Although the activity of the protein has been extensively studied in neurons, the protein is also expressed in other cell types including immune cells and glia. Genetic studies show that mutations in synuclein alpha (SNCA), the gene that encodes alpha-synuclein, and alterations in its expression levels are a significant risk factor for PD, which likely impact the functions of a broad range of cell types. The consequences of altered SNCA expression in other cell types is beginning to be explored. Microglia, the primary macrophage population in the Central Nervous System (CNS), for example, are affected by variations in alpha-synuclein levels and functions. Studies suggest that deviations of alpha-synuclein’s normal activity influence hematopoiesis, the process that gives rise to microglia, and microglia’s immune functions. Alpha-synuclein levels also dictate the efficiency of SNARE-mediated vesicle formation, which could influence autophagy and cytokine release in microglia. Starting from the time of conception, these effects could impact one’s risk for developing PD. Further studies are needed to determine the physiological role of alpha-synuclein and how the protein is affected during PD in non-neuronal cells such as microglia. In this review we will discuss the known roles of alpha-synuclein in differentiation, immune responses, and vesicle formation, with insights into how abnormal alpha-synuclein expression and activity are linked to altered functions of microglia during PD.
Parkinson’s disease (PD) is characterized by the loss of dopaminergic neurons in the substantia nigra, leading to impaired motor function. Another pathological feature of the disease is the presence of intraneuronal aggregates of misfolded alpha-synuclein. Due to the complex ways in which disease risk is influenced by the interaction of genetics and environment, most PD is idiopathic and there are still no treatments to cure PD or slow its progression. Therapies targeting the protein alpha-synuclein, in efforts to reduce its aggregation within dopaminergic neurons, have, however, shown promising therapeutic potential (Brys et al., 2019; Cole et al., 2021). Despite the use of alpha-synuclein as a disease modifying target, there is still little known about its functions in a broad range of cell types and how the activity of the protein influences PD risk via such cell types.
A substantial body of research has focused on the role of alpha-synuclein in neurons, as its aggregation primarily occurs in, and is most highly expressed by this cell type (Bendor et al., 2013; Del Tredici and Braak, 2016). A disruption of alpha-synuclein’s normal function in other cell types, however, may contribute to PD initiation and/or progression. Genetic studies show that highly penetrant mutations, in addition to more common PD risk variants, affect the expression of synuclein alpha (SNCA) in PD (Chiba-Falek and Nussbaum, 2001; Farrer et al., 2004; Campelo and Silva, 2017). Cell types such as astrocytes, oligodendrocytes, microglia, and other immune cells do indeed express alpha-synuclein and are, thus, likely impacted by these genetic risk factors (Zhang et al., 2016; Reynolds et al., 2019). Microglia in particular have gained significant attention due to their involvement with prolonged CNS inflammation in correlation with alpha-synuclein aggregation and neurodegeneration in PD patients (Qian and Flood, 2008). Microglia release proinflammatory cytokines in the presence of exogenous alpha-synuclein when they become activated, and are one of the main cell types contributing to transmission of the misfolded protein between neurons (Sanchez-Guajardo et al., 2013). The extent to which microglia in PD patients show morphological and functional changes in correlation with alpha-synuclein aggregation has been extensively reviewed elsewhere (Ferreira and Romero-Ramos, 2018; Lecours et al., 2018). Most studies of microglia show that PD-associated genetic mutations give rise to abnormal microglia morphology (Lee et al., 2002; Su et al., 2009). However, these changes have been attributed to the interaction that microglia have with alpha-synuclein originating from other cell-types or outside sources. Importantly, such changes could be independent from those that are induced by exogenous alpha-synuclein mutant forms or aggregates, but this is still an emerging topic. Indeed there are studies showing functional changes in microglia as a result of endogenous SNCA expression changes (Grozdanov and Danzer, 2020). Investigating the function of endogenous alpha-synuclein in immune cell types, such as microglia, may reveal novel disease mechanisms involved in disease initiation and progression and provide additional strategies for therapeutic intervention. In this review we will discuss the known roles for alpha-synuclein with a focus on the relevance of its function in microglia during PD.
SNCA was the first reported PD-associated gene to have a missense point mutation, resulting in an amino acid change from alanine to threonine at position 53 (A53T) in the alpha-synuclein protein (Polymeropoulos et al., 1997). Since then, four other mutations (A30P, H50Q, G51D, and E46K) have been found in patients with familial PD (Zarranz et al., 2004; Appel-Cresswell et al., 2013; Kiely et al., 2013; Rutherford et al., 2014). A triplication at the SNCA locus, leading to increased levels of alpha-synuclein, was also reported for autosomal dominant PD (Singleton et al., 2003; Miller et al., 2004). More recently, unbiased genome-wide association studies (GWAS) have identified common risk variants/single nucleotide polymorphisms (SNPs) in non-coding and coding regions in and around SNCA, that are associated with sporadic PD (Billingsley et al., 2018; Nalls et al., 2019). For example, the most highly significant PD-associated SNP, rs356182 (meta p = 1.85 × 10–82), determined in the latest metanalysis of PD, is located within an enhancer at the SNCA locus (Nalls et al., 2019). Interestingly, the protective allele (A) is associated with increased expression of SNCA, whereas the allele that carries the risk (G) is linked to lower expression levels of SNCA in cerebellar and substantia nigra tissue (Cooper et al., 2017). This SNP is situated in an enhancer that has pleiotropic consequences in neurons (Prahl et al., 2020). The data suggests that higher and lower levels of alpha-synuclein are associated with PD. In line with this complexity, eQTL (expression quantitative trait loci) data from GTeX (Genotype Tissue Expression Project) also shows that rs356182 has allele-dependent expression of SNCA, which is increased in some brain tissues and decreased in others. These results are derived from bulk tissue, making it difficult to interpret which cell types are truly affected by allelic differences. Taken together, the genetic data suggest a strong correlation between increased and decreased expression of SNCA leading to elevated risk for PD. However, the mechanisms and the cell types influenced by these genetic associations remain to be explored.
Genetic risk may in part dictate alterations in pathways that influence microglia’s functions or abundance in the brain (Figure 1). For example, GWAS support a role for inflammatory pathways, like those involved in cytokine-mediated signaling, in PD susceptibility (Durrenberger et al., 2012; Holmans et al., 2013). Genes highly expressed in microglia, including LRRK2 and lysosomal genes, also show enrichment of PD genetic risk variants (in or around the genes) that affect their expression levels (Reynolds et al., 2019). Genetic risk is, however, complex and although PD has been tied to genes, like LRRK2, the complete set of genes in microglia and their functions that lead to PD susceptibility are only beginning to be explored. In a previous study (Booms et al., 2020), we searched for PD risk SNPs that reside in regulatory DNA in microglia and found one such SNP (rs2737004—highlighted in yellow in Supplementary Table 1) located in an intergenic enhancer at SNCA. The full list of SNPs at the SNCA locus can also be found in Supplementary Table 1. We believe that rs2737004 is exerting its effects through the regulation of SNCA expression. Although in general, SNCA is known to be lowly expressed in microglia, our study indicates that a portion of PD risk may be acting through this genetic variant, which could influence SNCA expression in microglia. Indeed, studies (discussed below) that modulate SNCA expression in microglia show an effect on their functions (Grozdanov and Danzer, 2020). Furthermore, few studies have evaluated SNCA expression in microglia depending on maturation or activation status (also discussed below). We therefore argue that more emphasis should be placed on examining the mechanisms in microglia involving the regulation of SNCA dictated by genetic risk variants or more highly penetrant mutations. Figure 1 outlines a general depiction of genetic risk at the SNCA locus. To further dissect the link between genetic risk for PD and alterations in SNCA expression, it will be important to continue to uncover the full scope of alpha-synuclein functions in cell types such as microglia in addition to neurons.
Figure 1. PD-associated genetic variation at the SNCA locus could affect microglia function at the precursor and mature stages. PD risk SNPs in regulatory regions of DNA, such as enhancers and promoters at the SNCA locus, along with more highly penetrant mutations or multiplications in SNCA coding regions, could impact the process of differentiation of hematopoietic precursors into mature microglia. Once differentiated, microglia’s functions, such as cytokine release and phagocytosis, could also be influenced by these genetic risk factors, contributing to initiation or progression of PD starting from the time of conception. This figure was created with BioRender.com.
The molecular functions of alpha-synuclein are still incompletely understood, but studies investigating its activity have shown that under normal conditions it facilitates the release and transport of dopamine, promotes fibrilization of microtubule associated protein tau (MAPT), and prevents caspase-3 activation through p53 inhibition to protect against cell death (da Costa et al., 2000; Alves Da Costa et al., 2002; Siddiqui et al., 2016). In PD, SNCA adopts more neurotoxic forms, especially due to mutations, such as A53T, that promote its aggregation (de Oliveira and Silva, 2019). These findings have been primarily demonstrated in neuronal cells. However, alpha-synuclein is also involved in processes regulating differentiation, induction of inflammatory responses, and SNARE-mediated vesicle formation, which may impact the behavior of other non-neuronal cell types. These studies are discussed below.
Multiple studies provide evidence that alpha-synuclein plays a key role in differentiation. For example, erythrocytes express a substantial amount of alpha-synuclein, with expression increasing during terminal differentiation to promote protein enucleation of erythroblasts and stabilize erythroid membranes (Satoh and Kuroda, 2001; Kim et al., 2018). In the MG63 osteosarcoma cell line, overexpression of alpha-synuclein caused these cells to adopt a more differentiated phenotype (Huang et al., 2017). Furthermore, NTera2 teratocarcinoma cells showed an increase in alpha-synuclein expression levels during neuronal differentiation (Kuure et al., 2007). Barbour et al. (2008) also demonstrated that alpha-synuclein affects neuronal differentiation. Overexpression of wild-type or mutant A53T alpha-synuclein interfered with retinoic acid-induced differentiation in SH-SY5Y cells, indicating that PD-associated mutations may also influence the normal differentiation process. These cells had lower levels of tyrosine hydroxylase and dopamine transporter, that correlated with a reduction in neurite outgrowth compared to control cells. Results from this study were also confirmed in murine primary cultured mesencephalic dopaminergic neurons expressing A53T mutant alpha-synuclein. Interestingly, the mechanism leading to the loss of the differentiation phenotype was through interference with GSK-3β/β-catenin signaling, a key pathway in neurogenesis and differentiation of other cell types and fetal tissues, such as the mesoderm where microglia originate (Fujita et al., 2007; Araki et al., 2018). In line with these observations, iPSC-derived neurons from a PD patient with an SNCA triplication had a reduced capacity to differentiate into dopaminergic and GABAergic neurons (Oliveira et al., 2015). Multiple studies, thus indicate that differentiation is affected by changes in alpha-synuclein that could potentially influence PD risk.
Microglia originate from yolk sac-primitive macrophages and colonize the brain at a very early stage in embryonic development as a part of “primitive hematopoiesis.” Hematopoietic stem cells generated during “definitive hematopoiesis” migrate from the yolk-sac to the fetal liver and bone marrow, where they then differentiate into monocytes, macrophages, and lymphocytes (Alliot et al., 1999; Ginhoux et al., 2010; Kierdorf et al., 2013; Ginhoux and Prinz, 2015). Although microglia and other immune cell lineages arise during two distinct hematopoietic processes, cells like macrophages, monocytes, and dendritic cells are classified as mononuclear phagocytes, along with microglia, based on common cell surface markers, functions, and the possibility that they are derived from a common early hematopoietic precursor (Ransohoff and Cardona, 2010). There may, therefore, be similarities between microglia and peripheral monocyte hematopoietic precursor functions.
In addition to the cell types discussed above, some evidence suggests that alpha-synuclein plays a prominent role in differentiation of cells derived from the hematopoietic lineage (Pei and Maitta, 2019). SNCA expression is relatively strong in peripheral hematopoietic precursor cells and mature erythrocytes, with knockout of SNCA in mice leading to dysfunctional hematopoiesis (Tashkandi et al., 2018; Grozdanov and Danzer, 2020). Furthermore, Xiao et al. (2014) showed that alpha-synuclein is important during late hematopoiesis and B cell lymphopoiesis, suggesting a role for alpha-synuclein in differentiation of immune cells. Moreover, abnormalities in hematopoiesis have been observed in PD (Savica et al., 2009; Pei and Maitta, 2019). For example, PD patients who experienced anemia early in life were more likely to develop PD later in life (Kasten et al., 2010). In addition, a greater percentage of monocyte precursors were observed in the blood of PD patients, suggesting that these cells may not properly differentiate (Funk et al., 2013). The involvement of SNCA in hematopoiesis is still elusive. However, low levels of SNCA transcripts were found in PD patient’s blood samples and correlated with cognitive decline (Locascio et al., 2015). Animal studies demonstrate the involvement of SNCA in differentiation of cells derived from the hematopoietic lineage and human studies show that disruption in hematopoiesis is linked to PD. Therefore, more emphasis should be placed on the investigation of hematopoietic changes linked to SNCA expression abnormalities as this may be an early event that drives disease initiation.
Microglia are derived through the hematopoietic lineage and display many of the same immune properties as peripheral monocytes and macrophages, but the role of alpha-synuclein in the differentiation of these cells has not yet been explored. Microglia can self-renew and undergo mitosis to increase their numbers in the affected area during insult (Ajami et al., 2007). Therefore, the capacity of these cells to differentiate is critical to maintain the health of the CNS. Differentiation of microglia during primitive hematopoiesis at the time of embryogenesis could also affect the number of mature microglia residing in the CNS at the time of birth. The evidence showing that SNCA is expressed at high levels in hematopoietic precursors and influences the function of the hematopoietic system, which gives rise to immune cells, provokes the question of whether alpha-synuclein influences differentiation of microglia. RNA-seq data from mice indicates that Snca is expressed at higher levels in embryonic microglia compared to early post-natal and more mature microglia (Li et al., 2019). It is unknown, however, if SNCA is expressed in microglia precursors in humans and how that expression changes with differentiation. We thus evaluated existing data sets to determine if SNCA is expressed at higher levels in microglia precursors compared to cells that are further along the differentiation process into mature microglia (Figure 2).
Figure 2. SNCA, P2RY12, and TMEM119 expression in microglia precursors across differentiation in vivo and in vitro. This data is a re-analysis of publicly available data from Svoboda et al. (2019). CPM normalized expression levels of SNCA, P2RY12, and TMEM119 are shown for microglial iMPs (induced microglia precursors) that have been transplanted into mice and then differentiation in vivo (left) or differentiated in vitro (right) for 10 and 60 days. Significance between timepoints was determined using a Kruskal-Wallis test with Dunn’s Multiple Comparison (∗p ≤ 0.05, ∗∗p ≤ 0.01, ∗∗∗p ≤ 0.001, ****p ≤ 0.0001).
To evaluate expression of SNCA in microglia precursors and throughout differentiation, we analyzed previously published data from Svoboda et al. (2019), who conducted a study using immature hiPSC-derived microglia that were either cultured in vitro or transplanted into mice at the precursor stage (iMP). They performed bulk RNA-seq of in vivo cells at 10- and 60-days post implantation and in in vitro cultured cells at 10- and 60- days of differentiation. After examining this data set for SNCA expression in microglia precursors compared to more mature in vivo and in vitro microglia, the data showed changes in SNCA across maturation time points. TMM normalized RNA-seq counts were derived using edgeR, and the significance between timepoints was determined using a Kruskal-Wallis test with Dunn’s Multiple Comparison to account for some of the within group variation (Figure 2). In line with what Svoboda et al. (2019) showed, the data demonstrates that SNCA expression significantly increased after 60 days cultured in vitro, whereas SNCA expression remained relatively similar across time points in vivo.
The data confirm that SNCA is expressed in microglia precursors, although the pattern across differentiation is different between in vivo and in vitro conditions. We observed a general pattern in SNCA expression for in vivo microglia, where SNCA expression is highest prior to or at the start of differentiation (day 10), with levels decreasing as the cells mature (Figure 2). However, the difference was not significant. This pattern is like what is seen in other cell types. For example, in vitro, dividing oligodendrocyte precursors show high SNCA expression and alpha-synuclein levels, which are lost during maturation (Djelloul et al., 2015). This is contradictory to what is seen in neurons where alpha-synuclein levels increase upon maturation (Pierce et al., 2018). These results highlight a possible role for alpha-synuclein in differentiation in non-neuronal cell types, whereas in neurons, in addition to differentiation, alpha-synuclein facilitates neuronal specific processes like synaptic transmission following maturation. Although the in vivo data in microglia for SNCA expression across timepoints is inconclusive, a more extensive evaluation in human microglia, by conducting a trajectory analysis using single-cell data from the human brain, could give better insights into the pattern of SNCA expression during the transition from hematopoietic stem cells to mature microglia.
An intriguing observation from this data is that SNCA expression increases throughout differentiation in vitro. Although this could be a factor of differentiation conditions, it is unknown whether the differences between in vivo and in vitro SNCA expression across time is related to activation status. Using single-cell data, the authors showed that iMG differentiated in vitro, more closely represent cells in a disease state than microglia differentiated in vivo, which could indicate that they are more activated. Indeed, it has been shown that microglia in culture adopt a more activated state and tend to lose expression of microglia specific markers, such as P2RY12 and TMEM119 (Butovsky et al., 2014; Bohlen et al., 2017; Gosselin et al., 2017). Svoboda et al. (2019)’s data, indicates this to be partially true, as P2RY12 expression decrease over the course of differentiation in vitro, but increased over time in vivo. Whereas TMEM119 expression increased by day 60 in both conditions (Figure 2). However, the data points for expression of TMEM119 at day 60 in the in vitro group appear to have a large bimodal distribution. With having no prior knowledge on the necessary experimental design for each bulk RNA-seq sample, we attempted to do a batch correction using RUVseq, which helps to remove unknown sources of unwanted variation (Risso et al., 2014). Still, the bimodal distribution remained. We thus interpret these results with caution. Based on this data, the relationship between SNCA expression and microglia activation state is still unclear. Further studies are needed to explore the role of SNCA in microglia precursors and activated microglia to determine if alpha-synuclein influences differentiation or changes with activation state under physiological or disease conditions.
Infections from bacterial and viral pathogens are associated with high risk for PD. Patients with PD have been observed to have altered gut microbiota that consists of increases in certain types of bacteria such as Lactobacillus and decreases in others such as Prevotella (Scheperjans, 2018; Johnson et al., 2019). There is also a causative relationship between viruses like influenza, Herpes Simplex Virus 1, Epstein Barr Virus, and others with the development of Parkinsonism symptoms (Limphaibool et al., 2019). These infections are thought to act as one of the initiating factors that, in combination with genetic and other environmental risk factors, leads to elevated alpha-synuclein levels.
Although alpha-synuclein is present in enteric nerves of both PD and healthy patients, it is speculated that inflammation associated with infection leads to increased alpha-synuclein aggregation (Johnson et al., 2019). Exposures to infection are indeed linked to changes in alpha-synuclein expression, which also correlate with immune cell recruitment (Labrie and Brundin, 2017). For example, alpha-synuclein expression increased in primary neurons following infection with West Nile virus (Beatman et al., 2015). In addition, alpha-synuclein knock-out mice had an increase of infectious viral particles that correlated with lower survival, indicating a role for alpha-synuclein in restricting infection (Beatman et al., 2015). Another study by Stolzenberg et al. (2017) showed elevated levels of alpha-synuclein in enteric neurites of the upper GI tract of pediatric patients with gastric and duodenal inflammation. Expression of alpha-synuclein in enteric neurons was also induced in an additional cohort of patients with confirmed cases of norovirus (Stolzenberg et al., 2017). In the same study, oligomeric forms of alpha-synuclein acted as chemoattractant for neutrophils and monocytes. Thus, convincing evidence indicates that alpha-synuclein has a role in early immune responses.
The early immune response involving alpha-synuclein is not restricted to neurons. Human macrophages in culture had increased levels of alpha-synuclein following exposure to LPS and IL-β, indicating that infection affects the levels of endogenous alpha-synuclein, which consequently influences macrophage function (Tanji et al., 2002). Microglia, the resident macrophages in the brain, are highly implicated in PD pathogenies through their immune response to exogenous alpha-synuclein, but there is evidence to suggest that alpha-synuclein expression within microglia influences their immune responses as well (Grozdanov and Danzer, 2020). One of the first studies demonstrating the effects of endogenous alpha-synuclein on microglia function was done in 2006 by Austin et al. (2011). They found that microglia isolated from Snca-/- mice had an increased reactive phenotype at baseline and following stimulation with LPS as compared to microglia from Snca wild-type mice. After stimulation, Snca-/- microglia also had elevated levels of cytokine release accompanying an impairment in phagocytosis. In drosophila, overexpression of wild-type alpha-synuclein under the control of a pan-glial promoter (repo-GAL4) led to alpha-synuclein aggregation, death of dopamine neurons, and locomotion and autonomic dysfunction that was worsened in combination with co-expression of alpha-synuclein in both glia and neurons (Olsen and Feany, 2019).
Although these experiments highlight the potential for alterations in SNCA expression or alpha-synuclein levels to lead to functional changes in microglia, most of these studies relied on episomal expression constructs or whole-body knock-out animal models, which may not mimic physiological conditions related to genetic alterations in microglia SNCA expression in humans. Haenseler et al. (2017), however, generated iPSC-derived macrophages from PD patients with SNCA A53T mutations or SNCA triplications. The A53T mutant cell lines showed no effects, whereas iPSC-derived macrophages from patients with an SNCA triplication showed elevated levels of alpha-synuclein and a decrease in phagocytosis and cytokine release. Although macrophages share similar functions to microglia, they may behave differently under normal conditions or during disease (Yamasaki et al., 2014; Grozdanov and Danzer, 2020). More work is needed to understand the influence of endogenous SNCA expression on microglia’s immune functions during PD.
One of the prominent functions for monomeric alpha-synuclein, primarily demonstrated in neurons, is its facilitation of synaptic vesicle formation. The protein associates with fatty acids and lipids within vesicle membranes to promote the assembly of the SNARE complex, which drives the fusion of membranes (Huang et al., 2019). In mice hippocampal neuron cultures lacking alpha-synuclein showed a reduction in the number of pre-synaptic vesicles, which correlated with lower dopamine levels in the striatum (Abeliovich et al., 2000; Cabin et al., 2002). In line with this observation, transgenic expression of alpha-synuclein rescued neurodegeneration in mice lacking CSPα, a presynaptic chaperone protein important in SNARE complex formation (Chandra et al., 2005; Burre et al., 2010). Conversely, deletion of alpha-synuclein accelerated neurodegeneration in mice (Chandra et al., 2005; Burre et al., 2010). These experiments show that monomeric alpha-synuclein is necessary for SNARE complex assembly and deficits in the protein can impact vesicle formation. Alternatively, aggregated alpha-synuclein inhibits SNARE-mediated membrane fusion (Hawk et al., 2019). The data explains a possible mechanism for impairments in SNARE-mediated neuronal dopamine release leading to neurodegeneration resulting from a lack of monomeric alpha-synuclein and/or increased aggregation of insoluble alpha-synuclein. However, as SNARE proteins are essential for a wide variety of processes involving membrane fusion, alterations in alpha-synuclein could impact vesicle formation in a broad range of cell types, especially during fetal development at the time when alpha-synuclein is most ubiquitously expressed (Ltic et al., 2004).
The effects of alpha-synuclein deficits or aggregation on SNARE-mediated vesicle formation in microglia have not yet been demonstrated. However, because vesicle formation during autophagy is critical for microglia to phagocytose and deliver extracellular cargo to the lysosome for degradation, the lack of endogenous alpha-synuclein could impact vesicle formation like what is observed in neurons. SNARE proteins are indeed required for the formation of precursor vesicles that give rise to the phagophore during the initiation phase of autophagy (Wang et al., 2016). This mechanism in microglia aids in the removal of toxic proteins and is linked to protection against the accumulation of neuron-derived alpha-synuclein (Choi et al., 2020). Any impairments in vesicle formation during autophagy could influence microglia’s ability to clear misfolded alpha-synuclein and thus contribute to its aggregation. Although, defective autophagy is seen in PD patients and in vivo studies show that impairments in microglial autophagy specifically, leads to neurodegeneration in mice, there is still a loose connection between impaired autophagy in microglia and PD in humans, with no studies investigating alpha-synuclein’s role in this paradigm (Karabiyik et al., 2017; Qin et al., 2021).
Vesicle exocytosis during cytokine release from microglia is also mediated by the SNARE complex (Murray et al., 2005). Evidence suggests that this process involves alpha-synuclein because cytokine release in microglia is impaired by both over-expression and deficient levels of alpha-synuclein in mice (Austin et al., 2006, 2011; Gardai et al., 2013). Gardai et al. (2013) data suggests that a possible mechanism leading to impaired cytokine release could be via alpha-synuclein’s interaction with SNAP23, a component of the SNARE complex. However, this was tested in the H4 neuroglioma cell line. This and other studies show there are multiple functions involving vesicle formation that are potentially facilitated by alpha-synuclein. Vesicle formation is critical for many processes in microglia that provide protection against toxic protein accumulation and to facilitate inflammatory responses in the brain. Further research is needed to determine if alpha-synuclein interacts with SNARE complexes in microglia and what the impacts of alterations in endogenous alpha-synuclein are on autophagic clearance of toxic proteins and cytokine release in microglia.
Gene-environment interactions play a significant role in PD risk (Cannon and Greenamyre, 2013). For example, as discussed above, viral infections may trigger an inflammatory event that lead to the seeding of pathological alpha-synuclein aggregates, which could be aggravated in the presence of PD-associated mutations. Most of the studies mentioned in previous sections have been done in in vivo and in vitro models that rely on transgenic or viral over-expression, which represents genetic related changes in endogenous microglial alpha-synuclein. The PFF model, however, represents an environmental trigger or extracellular source of fibrillar alpha-synuclein. In the PFF model, injection of alpha-synuclein fibrils leads to the conversion of endogenous alpha-synuclein into pathological aggregates (Volpicelli-Daley et al., 2011, 2014; Chung et al., 2020). The levels of alpha-synuclein in these models are more physiologically relevant and the spatial and temporal spread of pathology better mimics what is observed in the PD brain (Duffy et al., 2018a). Furthermore, dopamine neuronal dysfunction is observed prior to the onset of motor symptoms as is seen in PD patients (Kordower et al., 2013; Chung et al., 2020). For these reasons PFF models are considered one of the best tools for studying neurodegeneration in synucleinopathies and should be used in combination with transgenic models to understand the compounding effects of genetic and environmental risk. Indeed, it has been demonstrated that viral-mediated overexpression of alpha-synuclein in dopamine neurons exacerbates alpha-synuclein pathology and neuroinflammation in combination with PFF injection (Thakur et al., 2017). The question remains as to whether genetic variants and mutations that impact the endogenous expression of SNCA or alpha-synuclein function in microglia specifically, has a synergistic effect on neurodegeneration induced by exogenous alpha-synuclein in a PFF model.
Findings from PFF models alone indicate that microglia display phenotypes that influence neurodegeneration. Duffy et al. (2018b) showed a positive correlation with alpha-synuclein inclusion load and the number of MHC-II positive microglia prior to neurodegeneration in a rat PFF model, suggesting that microglia activation, in response to exogenous phosphorylated alpha-synuclein, is an early event that may contribute to neuronal vulnerability. This same phenotype has been reported in PD patients (Croisier et al., 2005). Other alpha-synuclein PFF models further demonstrate persistent microglial reactivity and cytokine release that is associated with neurodegeneration (Zhang et al., 2005; Yun et al., 2018). In marmosets injected with PFFs of alpha-synuclein, microglia also seem to play a protective role through phagocytic clearance of alpha-synuclein (Shimozawa et al., 2017). These studies demonstrate that alpha-synuclein PFF models displays robust immune responses in microglia. However, the mechanisms in which alpha-synuclein fibrils exert their effects are not well characterized. Proteomics data from Sarkar et al. (2020) showed that alpha-synuclein PFFs induce expression changes of microglial genes involved in RNA binding, mitochondrial stress, and lysosomal and autophagic function. They confirmed that some of these genes, like Grn which codes for progranulin, have been implicated in PD risk through GWAS. Models utilizing PFFs have thus provided novel insights into microglia behavior when exposed to toxic alpha-synuclein fibrils. The molecular pathways affected by fibrillar alpha-synuclein may converge on pathways influenced by genetic risk variants and mutations and thus dictate the severity of functional changes that increase risk for PD.
Limitations of the PFF model have posed difficulties in defining their immune responses. Processes triggered by alpha-synuclein fibrils seem to be highly dependent on the site of PFF injection, the phosphorylation status of the seed, the method of PFF preparation, the compatibility of fibrils with the host species, and the timing of injection/pathological examination (Karampetsou et al., 2017; Polinski et al., 2018; Chung et al., 2020; Chmielarz and Domanskyi, 2021), all of which may affect immune phenotypes. Furthermore, the PFF model shows a slower stepwise progression of alpha-synuclein pathology that may not lead to as exaggerated inflammatory responses comparable to what is displayed in over-expression models. These factors likely contribute to variations in the degree of pathology seen in PFF models (Karampetsou et al., 2017). Some work has focused on overcoming this issue by standardizing and publishing best methods for model generation (Polinski et al., 2018). Despite the limitations of the PFF model, it is well suited for investigating the effects of neuroinflammation on PD as it closely recapitulates the events in idiopathic PD. Moving forward alpha-synuclein PFF models will be an important tool for evaluating how PD risk genes, like SNCA, in microglia, function in an already established disease state or in the context of compounding risk through exposure to environmental triggers for PD.
Alpha-synuclein dysfunction or alterations in expression levels are one of the most well-known contributing factors to PD. These changes affect the function of neurons, especially at later stages in the disease. Genetic studies, however, indicate that alterations in SNCA expression occur in multiple cell types. In some cases, SNCA is expressed at highest levels in precursor cell populations like hematopoietic stem cells, with fluctuations in the levels of alpha-synuclein leading to observable effects on differentiation. This suggests that abnormalities in SNCA expression beginning from conception could dictate the composition of mature cell populations residing in the brain and their function later in life.
More recent studies have shown that alpha-synuclein influences the function of immune cells, with important implications for PD risk. For example, macrophages from PD patients appear to be affected by mutant forms, and loss or gain of alpha-synuclein levels (Haenseler et al., 2017). Microglia are the resident macrophages in the brain, and functional changes in this cell type leading to excess inflammation are associated with PD. Microglia have been extensively studied for their contribution to neurodegeneration, yet there is little known about the function of endogenous alpha-synuclein in these cells, especially during their development. As discussed above, SNCA is expressed at high levels in hematopoietic stem cells, with varying levels of alpha-synuclein affecting hematopoiesis of peripheral immune and blood cells. Microglia also originate from hematopoietic precursors, and as the in vivo data from Svoboda et al. (2019) suggest, SNCA expression is higher in microglia precursors compared to more mature cells. Alpha-synuclein may therefore be most active during the differentiation process in microglia. Consequently, hematopoietic dysfunction could influence microglia inflammatory responses in the brain following differentiation. The studies investigating the activity of endogenous alpha-synuclein in mature microglia do show that altered SNCA expression is linked to impaired cytokine release and phagocytosis (Austin et al., 2011; Grozdanov and Danzer, 2020). The evidence demonstrating that alpha-synuclein influences hematopoiesis and inflammatory responses provides precedence to explore the functions of alpha-synuclein in microglia as they develop and mature, to determine if dysfunctional differentiation and immune responses in microglia contribute to increased risk for PD.
We briefly discussed the involvement of alpha-synuclein in vesicle formation, which appears to be a mechanism that is linked to altered dopamine signaling in neurons during PD. Since this mechanism requires the involvement of alpha-synuclein in SNARE complex formation, one could speculate that alpha-synuclein also plays a role in SNARE complex formation in microglia, which is a critical step for the formation of vesicles involved in autophagic clearance of toxic proteins such as aggregated alpha-synuclein, and cytokine release. Although there is little evidence of genetic linked alterations in SNCA expression and alpha-synuclein function in microglia leading to changes in vesicle-mediated processes such as autophagy, it is worth exploring as a possible mechanism leading to alpha-synuclein aggregation and neurodegeneration in PD.
It is difficult to dissect the influence of certain cell populations early on in disease. However, models using PFFs of alpha-synuclein and iPSCs derived from PD patients are beginning to give us clues to the changes that occur in PD patients prior to the onset of neurodegeneration. Understanding the early biological processes involving imbalances in alpha-synuclein expression in cell types like hematopoietic stem cells and microglia, will contribute to the understanding of the influence of genetics on PD risk and may provide avenues for therapeutic intervention before PD manifests into motor impairment.
AB designed and drafted all sections of the manuscript and performed all data analysis and prepared the figures. GC contributed to manuscript design and review. Both authors contributed to the article and approved the submitted version.
This work was supported by the internal funding at the Van Andel Institute and the Van Andel Institute Graduate School.
The authors declare that the research was conducted in the absence of any commercial or financial relationships that could be construed as a potential conflict of interest.
All claims expressed in this article are solely those of the authors and do not necessarily represent those of their affiliated organizations, or those of the publisher, the editors and the reviewers. Any product that may be evaluated in this article, or claim that may be made by its manufacturer, is not guaranteed or endorsed by the publisher.
We acknowledge contributions from the GC lab members, especially Elizabeth Ensink, Steven Pierce and Trevor Tyson. We would also like to acknowledge the bioinformatics and biostatistics core, especially Zach Madaj, for advice on statistical methods. Lastly, we acknowledge Lee Marshall for guidance on bioinformatics methods.
The Supplementary Material for this article can be found online at: https://www.frontiersin.org/articles/10.3389/fncel.2021.759571/full#supplementary-material
Supplementary Table 1 | PD risk SNPs at the SNCA locus. The SNPs listed in this table were determined to be associated with PD risk by Nalls et al. (2019). The one that we believe to be relevant to microglia function, based on its location in open regulatory DNA (marked using ATAC- and H3K27ac-seq; Booms et al., 2020), is highlighted in yellow. IUPAC codes are listed at the bottom.
Abeliovich, A., Schmitz, Y., Farinas, I., Choi-Lundberg, D., Ho, W. H., Castillo, P. E., et al. (2000). Mice lacking alpha-synuclein display functional deficits in the nigrostriatal dopamine system. Neuron 25, 239–252. doi: 10.1016/S0896-6273(00)80886-7
Ajami, B., Bennett, J. L., Krieger, C., Tetzlaff, W., and Rossi, F. M. (2007). Local self-renewal can sustain CNS microglia maintenance and function throughout adult life. Nat. Neurosci. 10, 1538–1543. doi: 10.1038/nn2014
Alliot, F., Godin, I., and Pessac, B. (1999). Microglia derive from progenitors, originating from the yolk sac, and which proliferate in the brain. Brain Res. Dev. Brain Res. 117, 145–152. doi: 10.1016/S0165-3806(99)00113-3
Alves Da Costa, C., Paitel, E., Vincent, B., and Checler, F. (2002). Alpha-synuclein lowers p53-dependent apoptotic response of neuronal cells. Abolishment by 6-hydroxydopamine and implication for Parkinson’s disease. J. Biol. Chem. 277, 50980–50984. doi: 10.1074/jbc.M207825200
Appel-Cresswell, S., Vilarino-Guell, C., Encarnacion, M., Sherman, H., Yu, I., Shah, B., et al. (2013). Alpha-synuclein p.H50Q, a novel pathogenic mutation for Parkinson’s disease. Mov. Disord. 28, 811–813. doi: 10.1002/mds.25421
Araki, K., Sugawara, K., Hayakawa, E. H., Ubukawa, K., Kobayashi, I., Wakui, H., et al. (2018). The localization of alpha-synuclein in the process of differentiation of human erythroid cells. Int. J. Hematol. 108, 130–138. doi: 10.1007/s12185-018-2457-8
Austin, S. A., Floden, A. M., Murphy, E. J., and Combs, C. K. (2006). Alpha-synuclein expression modulates microglial activation phenotype. J. Neurosci. 26, 10558–10563. doi: 10.1523/JNEUROSCI.1799-06.2006
Austin, S. A., Rojanathammanee, L., Golovko, M. Y., Murphy, E. J., and Combs, C. K. (2011). Lack of alpha-synuclein modulates microglial phenotype in vitro. Neurochem. Res. 36, 994–1004. doi: 10.1007/s11064-011-0439-9
Barbour, R., Kling, K., Anderson, J. P., Banducci, K., Cole, T., Diep, L., et al. (2008). Red blood cells are the major source of alpha-synuclein in blood. Neurodegener. Dis. 5, 55–59. doi: 10.1159/000112832
Beatman, E. L., Massey, A., Shives, K. D., Burrack, K. S., Chamanian, M., Morrison, T. E., et al. (2015). Alpha-Synuclein Expression Restricts RNA Viral Infections in the Brain. J. Virol. 90, 2767–2782. doi: 10.1128/JVI.02949-15
Bendor, J. T., Logan, T. P., and Edwards, R. H. (2013). The function of alpha-synuclein. Neuron 79, 1044–1066. doi: 10.1016/j.neuron.2013.09.004
Billingsley, K. J., Bandres-Ciga, S., Saez-Atienzar, S., and Singleton, A. B. (2018). Genetic risk factors in Parkinson’s disease. Cell Tissue Res. 373, 9–20. doi: 10.1007/s00441-018-2817-y
Bohlen, C. J., Bennett, F. C., Tucker, A. F., Collins, H. Y., Mulinyawe, S. B., and Barres, B. A. (2017). Diverse requirements for microglial survival, specification, and function revealed by defined-medium cultures. Neuron 94, 759–773e8. doi: 10.1016/j.neuron.2017.04.043
Booms, A., Pierce, S. E., and Coetzee, G. A. (2020). Parkinson’s disease genetic risk evaluation in microglia highlights autophagy and lysosomal genes. bioRxiv [Preprint]. doi: 10.1101/2020.08.17.254276
Brys, M., Fanning, L., Hung, S., Ellenbogen, A., Penner, N., Yang, M., et al. (2019). Randomized phase I clinical trial of anti-alpha-synuclein antibody BIIB054. Mov. Disord. 34, 1154–1163. doi: 10.1002/mds.27738
Burre, J., Sharma, M., Tsetsenis, T., Buchman, V., Etherton, M. R., and Sudhof, T. C. (2010). Alpha-synuclein promotes SNARE-complex assembly in vivo and in vitro. Science 329, 1663–1667. doi: 10.1126/science.1195227
Butovsky, O., Jedrychowski, M. P., Moore, C. S., Cialic, R., Lanser, A. J., Gabriely, G., et al. (2014). Identification of a unique TGF-beta-dependent molecular and functional signature in microglia. Nat. Neurosci. 17, 131–143. doi: 10.1038/nn.3599
Cabin, D. E., Shimazu, K., Murphy, D., Cole, N. B., Gottschalk, W., McIlwain, K. L., et al. (2002). Synaptic vesicle depletion correlates with attenuated synaptic responses to prolonged repetitive stimulation in mice lacking alpha-synuclein. J. Neurosci. 22, 8797–8807. doi: 10.1523/JNEUROSCI.22-20-08797.2002
Campelo, C., and Silva, R. H. (2017). Genetic variants in SNCA and the risk of Sporadic Parkinson’s disease and clinical outcomes: a review. Parkinsons Dis. 2017:4318416. doi: 10.1155/2017/4318416
Cannon, J. R., and Greenamyre, J. T. (2013). Gene-environment interactions in Parkinson’s disease: specific evidence in humans and mammalian models. Neurobiol. Dis. 57, 38–46. doi: 10.1016/j.nbd.2012.06.025
Chandra, S., Gallardo, G., Fernandez-Chacon, R., Schluter, O. M., and Sudhof, T. C. (2005). Alpha-synuclein cooperates with CSPalpha in preventing neurodegeneration. Cell 123, 383–396. doi: 10.1016/j.cell.2005.09.028
Chiba-Falek, O., and Nussbaum, R. L. (2001). Effect of allelic variation at the NACP-Rep1 repeat upstream of the alpha-synuclein gene (SNCA) on transcription in a cell culture luciferase reporter system. Hum. Mol. Genet. 10, 3101–3109. doi: 10.1093/hmg/10.26.3101
Chmielarz, P., and Domanskyi, A. (2021). Alpha-synuclein preformed fibrils: a tool to understand Parkinson’s disease and develop disease modifying therapy. Neural. Regen. Res. 16, 2219–2221. doi: 10.4103/1673-5374.310686
Choi, I., Zhang, Y., Seegobin, S. P., Pruvost, M., Wang, Q., Purtell, K., et al. (2020). Microglia clear neuron-released alpha-synuclein via selective autophagy and prevent neurodegeneration. Nat. Commun. 11:1386. doi: 10.1038/s41467-020-15119-w
Chung, H. K., Ho, H. A., Perez-Acuna, D., and Lee, S. J. (2020). Modeling alpha-Synuclein propagation with preformed fibril injections. J. Mov. Disord. 13, 77–79. doi: 10.14802/jmd.19046.e
Cole, T. A., Zhao, H., Collier, T. J., Sandoval, I., Sortwell, C. E., Steece-Collier, K., et al. (2021). α-Synuclein antisense oligonucleotides as a disease-modifying therapy for Parkinson’s disease. JCI Insight 6:e135633. doi: 10.1172/jci.insight.135633
Cooper, C. A., Jain, N., Gallagher, M. D., Weintraub, D., Xie, S. X., Berlyand, Y., et al. (2017). Common variant rs356182 near SNCA defines a Parkinson’s disease endophenotype. Ann. Clin. Transl. Neurol. 4, 15–25. doi: 10.1002/acn3.371
Croisier, E., Moran, L. B., Dexter, D. T., Pearce, R. K., and Graeber, M. B. (2005). Microglial inflammation in the parkinsonian substantia nigra: relationship to alpha-synuclein deposition. J. Neuroinflammation 2:14. doi: 10.1186/1742-2094-2-14
da Costa, C. A., Ancolio, K., and Checler, F. (2000). Wild-type but not Parkinson’s disease-related ala-53 –> Thr mutant alpha -synuclein protects neuronal cells from apoptotic stimuli. J. Biol. Chem. 275, 24065–24069. doi: 10.1074/jbc.M002413200
de Oliveira, G. A. P., and Silva, J. L. (2019). Alpha-synuclein stepwise aggregation reveals features of an early onset mutation in Parkinson’s disease. Commun. Biol. 2:374. doi: 10.1038/s42003-019-0598-9
Del Tredici, K., and Braak, H. (2016). Review: sporadic Parkinson’s disease: development and distribution of alpha-synuclein pathology. Neuropathol. Appl. Neurobiol. 42, 33–50. doi: 10.1111/nan.12298
Djelloul, M., Holmqvist, S., Boza-Serrano, A., Azevedo, C., Yeung, M. S., Goldwurm, S., et al. (2015). Alpha-Synuclein expression in the oligodendrocyte lineage: an In vitro and in vivo study using rodent and human models. Stem Cell Rep. 5, 174–184. doi: 10.1016/j.stemcr.2015.07.002
Duffy, M. F., Collier, T. J., Patterson, J. R., Kemp, C. J., Fischer, D. L., Stoll, A. C., et al. (2018a). Quality over quantity: advantages of using alpha-synuclein preformed fibril triggered synucleinopathy to model idiopathic Parkinson’s disease. Front. Neurosci. 12:621. doi: 10.3389/fnins.2018.00621
Duffy, M. F., Collier, T. J., Patterson, J. R., Kemp, C. J., Luk, K. C., Tansey, M. G., et al. (2018b). Lewy body-like alpha-synuclein inclusions trigger reactive microgliosis prior to nigral degeneration. J. Neuroinflammation 15:129. doi: 10.1186/s12974-018-1202-9
Durrenberger, P. F., Grunblatt, E., Fernando, F. S., Monoranu, C. M., Evans, J., Riederer, P., et al. (2012). Inflammatory Pathways in Parkinson’s Disease; A BNE Microarray Study. Parkinsons Dis. 2012:214714. doi: 10.1155/2012/214714
Farrer, M., Kachergus, J., Forno, L., Lincoln, S., Wang, D. S., Hulihan, M., et al. (2004). Comparison of kindreds with parkinsonism and alpha-synuclein genomic multiplications. Ann. Neurol. 55, 174–179. doi: 10.1002/ana.10846
Ferreira, S. A., and Romero-Ramos, M. (2018). Microglia response during Parkinson’s Disease: alpha-Synuclein Intervention. Front. Cell Neurosci. 12:247. doi: 10.3389/fncel.2018.00247
Fujita, M., Sugama, S., Nakai, M., Takenouchi, T., Wei, J., Urano, T., et al. (2007). alpha-Synuclein stimulates differentiation of osteosarcoma cells: relevance to down-regulation of proteasome activity. J. Biol. Chem. 282, 5736–5748. doi: 10.1074/jbc.M606175200
Funk, N., Wieghofer, P., Grimm, S., Schaefer, R., Buhring, H. J., Gasser, T., et al. (2013). Characterization of peripheral hematopoietic stem cells and monocytes in Parkinson’s disease. Mov. Disord. 28, 392–395. doi: 10.1002/mds.25300
Gardai, S. J., Mao, W., Schule, B., Babcock, M., Schoebel, S., Lorenzana, C., et al. (2013). Elevated alpha-synuclein impairs innate immune cell function and provides a potential peripheral biomarker for Parkinson’s disease. PLoS One 8:e71634. doi: 10.1371/journal.pone.0071634
Ginhoux, F., Greter, M., Leboeuf, M., Nandi, S., See, P., Gokhan, S., et al. (2010). Fate mapping analysis reveals that adult microglia derive from primitive macrophages. Science 330, 841–845. doi: 10.1126/science.1194637
Ginhoux, F., and Prinz, M. (2015). Origin of microglia: current concepts and past controversies. Cold Spring Harb. Perspect. Biol. 7:a020537. doi: 10.1101/cshperspect.a020537
Gosselin, D., Skola, D., Coufal, N. G., Holtman, I. R., Schlachetzki, J. C. M., Sajti, E., et al. (2017). An environment-dependent transcriptional network specifies human microglia identity. Science 356:eaal3222. doi: 10.1126/science.aal3222
Grozdanov, V., and Danzer, K. M. (2020). Intracellular Alpha-Synuclein and immune cell function. Front. Cell Dev. Biol. 8:562692. doi: 10.3389/fcell.2020.562692
Haenseler, W., Zambon, F., Lee, H., Vowles, J., Rinaldi, F., Duggal, G., et al. (2017). Excess alpha-synuclein compromises phagocytosis in iPSC-derived macrophages. Sci. Rep. 7:9003. doi: 10.1038/s41598-017-09362-3
Hawk, B. J. D., Khounlo, R., and Shin, Y. K. (2019). Alpha-Synuclein continues to enhance SNARE-dependent vesicle docking at exorbitant concentrations. Front. Neurosci. 13:216. doi: 10.3389/fnins.2019.00216
Holmans, P., Moskvina, V., Jones, L., Sharma, M., International Parkinson’s Disease Genomics Consortium [IPDGC] Vedernikov, A., et al. (2013). A pathway-based analysis provides additional support for an immune-related genetic susceptibility to Parkinson’s disease. Hum. Mol. Genet. 22, 1039–1049. doi: 10.1093/hmg/dds492
Huang, J., Guo, X., Li, W., and Zhang, H. (2017). Activation of Wnt/beta-catenin signalling via GSK3 inhibitors direct differentiation of human adipose stem cells into functional hepatocytes. Sci. Rep. 7:40716. doi: 10.1038/srep40716
Huang, M., Wang, B., Li, X., Fu, C., Wang, C., and Kang, X. (2019). α-Synuclein: a multifunctional player in exocytosis, endocytosis, and vesicle recycling. Front. Neurosci. 13:28. doi: 10.3389/fnins.2019.00028
Johnson, M. E., Stecher, B., Labrie, V., Brundin, L., and Brundin, P. (2019). Triggers, facilitators, and aggravators: redefining Parkinson’s Disease pathogenesis. Trends Neurosci. 42, 4–13. doi: 10.1016/j.tins.2018.09.007
Karabiyik, C., Lee, M. J., and Rubinsztein, D. C. (2017). Autophagy impairment in Parkinson’s disease. Essays Biochem. 61, 711–720. doi: 10.1042/EBC20170023
Karampetsou, M., Ardah, M. T., Semitekolou, M., Polissidis, A., Samiotaki, M., Kalomoiri, M., et al. (2017). Phosphorylated exogenous alpha-synuclein fibrils exacerbate pathology and induce neuronal dysfunction in mice. Sci. Rep. 7:16533. doi: 10.1038/s41598-017-15813-8
Kasten, M., Tadic, V., Klein, C., Rocca, W. A., Savica, R., Eric Ahlskog, J., et al. (2010). Anemia or low hemoglobin levels preceding Parkinson disease: a case-control study. Neurology 74:1655. doi: 10.1212/WNL.0b013e3181d8a3d6
Kiely, A. P., Asi, Y. T., Kara, E., Limousin, P., Ling, H., Lewis, P., et al. (2013). α-Synucleinopathy associated with G51D SNCA mutation: a link between Parkinson’s disease and multiple system atrophy? Acta Neuropathol. 125, 753–769. doi: 10.1007/s00401-013-1096-7
Kierdorf, K., Erny, D., Goldmann, T., Sander, V., Schulz, C., Perdiguero, E. G., et al. (2013). Microglia emerge from erythromyeloid precursors via Pu.1- and Irf8-dependent pathways. Nat. Neurosci. 16, 273–280. doi: 10.1038/nn.3318
Kim, S., Lim, J., Bang, Y., Moon, J., Kwon, M. S., Hong, J. T., et al. (2018). Alpha-Synuclein suppresses retinoic acid-induced neuronal differentiation by targeting the glycogen synthase Kinase-3beta/beta-Catenin signaling pathway. Mol. Neurobiol. 55, 1607–1619. doi: 10.1007/s12035-016-0370-9
Kordower, J. H., Olanow, C. W., Dodiya, H. B., Chu, Y., Beach, T. G., Adler, C. H., et al. (2013). Disease duration and the integrity of the nigrostriatal system in Parkinson’s disease. Brain 136, 2419–2431. doi: 10.1093/brain/awt192
Kuure, S., Popsueva, A., Jakobson, M., Sainio, K., and Sariola, H. (2007). Glycogen synthase kinase-3 inactivation and stabilization of beta-catenin induce nephron differentiation in isolated mouse and rat kidney mesenchymes. J. Am. Soc. Nephrol. 18, 1130–1139. doi: 10.1681/ASN.2006111206
Labrie, V., and Brundin, P. (2017). Alpha-Synuclein to the rescue: immune cell recruitment by alpha-synuclein during gastrointestinal infection. J. Innate Immun. 9, 437–440. doi: 10.1159/000479653
Lecours, C., Bordeleau, M., Cantin, L., Parent, M., Paolo, T. D., and Tremblay, M. E. (2018). Microglial implication in Parkinson’s Disease: loss of beneficial physiological roles or gain of inflammatory functions? Front. Cell Neurosci. 12:282. doi: 10.3389/fncel.2018.00282
Lee, M. K., Stirling, W., Xu, Y., Xu, X., Qui, D., Mandir, A. S., et al. (2002). Human alpha-synuclein-harboring familial Parkinson’s disease-linked Ala-53 –> Thr mutation causes neurodegenerative disease with alpha-synuclein aggregation in transgenic mice. Proc. Natl. Acad. Sci. U. S. A. 99, 8968–8973. doi: 10.1073/pnas.132197599
Li, Q., Cheng, Z., Zhou, L., Darmanis, S., Neff, N. F., Okamoto, J., et al. (2019). Developmental Heterogeneity of Microglia and Brain Myeloid Cells Revealed by Deep Single-Cell RNA Sequencing. Neuron 101, 207–223.e10. doi: 10.1016/j.neuron.2018.12.006
Limphaibool, N., Iwanowski, P., Holstad, M. J. V., Kobylarek, D., and Kozubski, W. (2019). Infectious etiologies of Parkinsonism: pathomechanisms and clinical implications. Front. Neurol. 10:652. doi: 10.3389/fneur.2019.00652
Locascio, J. J., Eberly, S., Liao, Z., Liu, G., Hoesing, A. N., Duong, K., et al. (2015). Association between alpha-synuclein blood transcripts and early, neuroimaging-supported Parkinson’s disease. Brain 138, 2659–2671. doi: 10.1093/brain/awv202
Ltic, S., Perovic, M., Mladenovic, A., Raicevic, N., Ruzdijic, S., Rakic, L., et al. (2004). Alpha-synuclein is expressed in different tissues during human fetal development. J. Mol. Neurosci. 22, 199–204. doi: 10.1385/JMN:22:3:199
Miller, D. W., Hague, S. M., Clarimon, J., Baptista, M., Gwinn-Hardy, K., Cookson, M. R., et al. (2004). Alpha-synuclein in blood and brain from familial Parkinson disease with SNCA locus triplication. Neurology 62, 1835–1838. doi: 10.1212/01.WNL.0000127517.33208.F4
Murray, R. Z., Kay, J. G., Sangermani, D. G., and Stow, J. L. (2005). A role for the phagosome in cytokine secretion. Science 310, 1492–1495. doi: 10.1126/science.1120225
Nalls, M. A., Blauwendraat, C., Vallerga, C. L., Heilbron, K., Bandres-Ciga, S., Chang, D., et al. (2019). Identification of novel risk loci, causal insights, and heritable risk for Parkinson’s disease: a meta-analysis of genome-wide association studies. Lancet Neurol. 18, 1091–1102. doi: 10.1016/S1474-4422(19)30320-5
Oliveira, L. M., Falomir-Lockhart, L. J., Botelho, M. G., Lin, K. H., Wales, P., Koch, J. C., et al. (2015). Elevated alpha-synuclein caused by SNCA gene triplication impairs neuronal differentiation and maturation in Parkinson’s patient-derived induced pluripotent stem cells. Cell Death Dis. 6:e1994. doi: 10.1038/cddis.2015.318
Olsen, A. L., and Feany, M. B. (2019). Glial alpha-synuclein promotes neurodegeneration characterized by a distinct transcriptional program in vivo. Glia 67, 1933–1957. doi: 10.1002/glia.23671
Pei, Y., and Maitta, R. W. (2019). Alpha synuclein in hematopoiesis and immunity. Heliyon 5:e02590. doi: 10.1016/j.heliyon.2019.e02590
Pierce, S. E., Tyson, T., Booms, A., Prahl, J., and Coetzee, G. A. (2018). Parkinson’s disease genetic risk in a midbrain neuronal cell line. Neurobiol. Dis. 114, 53–64. doi: 10.1016/j.nbd.2018.02.007
Polinski, N. K., Volpicelli-Daley, L. A., Sortwell, C. E., Luk, K. C., Cremades, N., Gottler, L. M., et al. (2018). Best practices for generating and using alpha-synuclein pre-formed fibrils to model parkinson’s disease in rodents. J. Parkinsons Dis. 8, 303–322. doi: 10.3233/JPD-171248
Polymeropoulos, M. H., Lavedan, C., Leroy, E., Ide, S. E., Dehejia, A., Dutra, A., et al. (1997). Mutation in the alpha-synuclein gene identified in families with Parkinson’s disease. Science 276, 2045–2047. doi: 10.1126/science.276.5321.2045
Prahl, J., Pierce, S. E., van der Schans, E. J. C., Coetzee, G. A., and Tyson, T. (2020). Global effects of a PD Risk-SNP at the Alpha-Synuclein locus. bioRxiv [Preprint]. doi: 10.1101/2021.07.06.451330
Qian, L., and Flood, P. M. (2008). Microglial cells and Parkinson’s disease. Immunol. Res. 41, 155–164. doi: 10.1007/s12026-008-8018-0
Qin, Y., Qiu, J., Wang, P., Liu, J., Zhao, Y., Jiang, F., et al. (2021). Impaired autophagy in microglia aggravates dopaminergic neurodegeneration by regulating NLRP3 inflammasome activation in experimental models of Parkinson’s disease. Brain Behav. Immun. 91, 324–338. doi: 10.1016/j.bbi.2020.10.010
Ransohoff, R. M., and Cardona, A. E. (2010). The myeloid cells of the central nervous system parenchyma. Nature 468, 253–262. doi: 10.1038/nature09615
Reynolds, R. H., Botia, J., Nalls, M. A., International Parkinson’s Disease Genomics Consortium [IPDGC] System Genomics of Parkinson’s Disease [SGPD] Hardy, J., et al. (2019). Moving beyond neurons: the role of cell type-specific gene regulation in Parkinson’s disease heritability. NPJ Parkinsons Dis. 5:6. doi: 10.1038/s41531-019-0076-6
Risso, D., Ngai, J., Speed, T. P., and Dudoit, S. (2014). Normalization of RNA-seq data using factor analysis of control genes or samples. Nat. Biotechnol. 32, 896–902. doi: 10.1038/nbt.2931
Rutherford, N. J., Moore, B. D., Golde, T. E., and Giasson, B. I. (2014). Divergent effects of the H50Q and G51D SNCA mutations on the aggregation of alpha-synuclein. J. Neurochem. 131, 859–867. doi: 10.1111/jnc.12806
Sanchez-Guajardo, V., Barnum, C. J., Tansey, M. G., and Romero-Ramos, M. (2013). Neuroimmunological processes in Parkinson’s disease and their relation to alpha-synuclein: microglia as the referee between neuronal processes and peripheral immunity. ASN Neuro 5, 113–139. doi: 10.1042/AN20120066
Sarkar, S., Dammer, E. B., Malovic, E., Olsen, A. L., Raza, S. A., Gao, T., et al. (2020). Molecular signatures of neuroinflammation induced by alphaSynuclein aggregates in microglial cells. Front. Immunol. 11:33. doi: 10.3389/fimmu.2020.00033
Satoh, J. I., and Kuroda, Y. (2001). Alpha-synuclein expression is up-regulated in NTera2 cells during neuronal differentiation but unaffected by exposure to cytokines and neurotrophic factors. Parkinsonism Relat. Disord. 8, 7–17. doi: 10.1016/S1353-8020(00)00075-4
Savica, R., Grossardt, B. R., Carlin, J. M., Icen, M., Bower, J. H., Ahlskog, J. E., et al. (2009). Anemia or low hemoglobin levels preceding Parkinson disease: a case-control study. Neurology 73, 1381–1387. doi: 10.1212/WNL.0b013e3181bd80c1
Shimozawa, A., Ono, M., Takahara, D., Tarutani, A., Imura, S., Masuda-Suzukake, M., et al. (2017). Propagation of pathological alpha-synuclein in marmoset brain. Acta Neuropathol. Commun. 5:12. doi: 10.1186/s40478-017-0413-0
Siddiqui, I. J., Pervaiz, N., and Abbasi, A. A. (2016). The Parkinson Disease gene SNCA: evolutionary and structural insights with pathological implication. Sci. Rep. 6:24475. doi: 10.1038/srep24475
Singleton, A. B., Farrer, M., Johnson, J., Singleton, A., Hague, S., Kachergus, J., et al. (2003). alpha-Synuclein locus triplication causes Parkinson’s disease. Science 302:841. doi: 10.1126/science.1090278
Stolzenberg, E., Berry, D., Yang, D., Lee, E. Y., Kroemer, A., Kaufman, S., et al. (2017). A role for neuronal alpha-synuclein in gastrointestinal immunity. J. Innate Immun. 9, 456–463. doi: 10.1159/000477990
Su, X., Federoff, H. J., and Maguire-Zeiss, K. A. (2009). Mutant alpha-synuclein overexpression mediates early proinflammatory activity. Neurotox. Res. 16, 238–254. doi: 10.1007/s12640-009-9053-x
Svoboda, D. S., Barrasa, M. I., Shu, J., Rietjens, R., Zhang, S., Mitalipova, M., et al. (2019). Human iPSC-derived microglia assume a primary microglia-like state after transplantation into the neonatal mouse brain. Proc. Natl. Acad. Sci. U. S. A. 116, 25293–25303. doi: 10.1073/pnas.1913541116
Tanji, K., Mori, F., Imaizumi, T., Yoshida, H., Matsumiya, T., Tamo, W., et al. (2002). Upregulation of alpha-synuclein by lipopolysaccharide and interleukin-1 in human macrophages. Pathol. Int. 52, 572–577. doi: 10.1046/j.1440-1827.2002.01385.x
Tashkandi, H., Shameli, A., Harding, C. V., and Maitta, R. W. (2018). Ultrastructural changes in peripheral blood leukocytes in alpha-synuclein knockout mice. Blood Cells Mol. Dis. 73, 33–37. doi: 10.1016/j.bcmd.2018.09.001
Thakur, P., Breger, L. S., Lundblad, M., Wan, O. W., Mattsson, B., Luk, K. C., et al. (2017). Modeling Parkinson’s disease pathology by combination of fibril seeds and alpha-synuclein overexpression in the rat brain. Proc. Natl. Acad. Sci. U. S. A. 114, E8284–E8293. doi: 10.1073/pnas.1710442114
Volpicelli-Daley, L. A., Luk, K. C., and Lee, V. M. (2014). Addition of exogenous α-synuclein preformed fibrils to primary neuronal cultures to seed recruitment of endogenous α-synuclein to Lewy body and Lewy neurite-like aggregates. Nat. Protoc. 9, 2135–2146. doi: 10.1038/nprot.2014.143
Volpicelli-Daley, L. A., Luk, K. C., Patel, T. P., Tanik, S. A., Riddle, D. M., Stieber, A., et al. (2011). Exogenous alpha-synuclein fibrils induce Lewy body pathology leading to synaptic dysfunction and neuron death. Neuron 72, 57–71. doi: 10.1016/j.neuron.2011.08.033
Wang, Y., Li, L., Hou, C., Lai, Y., Long, J., Liu, J., et al. (2016). SNARE-mediated membrane fusion in autophagy. Semin. Cell Dev. Biol. 60, 97–104. doi: 10.1016/j.semcdb.2016.07.009
Xiao, W., Shameli, A., Harding, C. V., Meyerson, H. J., and Maitta, R. W. (2014). Late stages of hematopoiesis and B cell lymphopoiesis are regulated by alpha-synuclein, a key player in Parkinson’s disease. Immunobiology 219, 836–844. doi: 10.1016/j.imbio.2014.07.014
Yamasaki, R., Lu, H., Butovsky, O., Ohno, N., Rietsch, A. M., Cialic, R., et al. (2014). Differential roles of microglia and monocytes in the inflamed central nervous system. J. Exp. Med. 211, 1533–1549. doi: 10.1084/jem.20132477
Yun, S. P., Kam, T. I., Panicker, N., Kim, S., Oh, Y., Park, J. S., et al. (2018). Block of A1 astrocyte conversion by microglia is neuroprotective in models of Parkinson’s disease. Nat. Med. 24, 931–938. doi: 10.1038/s41591-018-0051-5
Zarranz, J. J., Alegre, J., Gomez-Esteban, J. C., Lezcano, E., Ros, R., Ampuero, I., et al. (2004). The new mutation, E46K, of alpha-synuclein causes Parkinson and Lewy body dementia. Ann. Neurol. 55, 164–173. doi: 10.1002/ana.10795
Zhang, W., Wang, T., Pei, Z., Miller, D. S., Wu, X., Block, M. L., et al. (2005). Aggregated alpha-synuclein activates microglia: a process leading to disease progression in Parkinson’s disease. FASEB J. 19, 533–542. doi: 10.1096/fj.04-2751com
Keywords: SNCA, alpha-synuclein, microglia, hematopoietic progenitors, differentiation, immune response
Citation: Booms A and Coetzee GA (2021) Functions of Intracellular Alpha-Synuclein in Microglia: Implications for Parkinson’s Disease Risk. Front. Cell. Neurosci. 15:759571. doi: 10.3389/fncel.2021.759571
Received: 16 August 2021; Accepted: 06 September 2021;
Published: 04 October 2021.
Edited by:
Hiroaki Wake, Nagoya University, JapanReviewed by:
Daisuke Kato, Nagoya University, JapanCopyright © 2021 Booms and Coetzee. This is an open-access article distributed under the terms of the Creative Commons Attribution License (CC BY). The use, distribution or reproduction in other forums is permitted, provided the original author(s) and the copyright owner(s) are credited and that the original publication in this journal is cited, in accordance with accepted academic practice. No use, distribution or reproduction is permitted which does not comply with these terms.
*Correspondence: Alix Booms, YWxpeC5ib29tc0B2YWkub3Jn
Disclaimer: All claims expressed in this article are solely those of the authors and do not necessarily represent those of their affiliated organizations, or those of the publisher, the editors and the reviewers. Any product that may be evaluated in this article or claim that may be made by its manufacturer is not guaranteed or endorsed by the publisher.
Research integrity at Frontiers
Learn more about the work of our research integrity team to safeguard the quality of each article we publish.