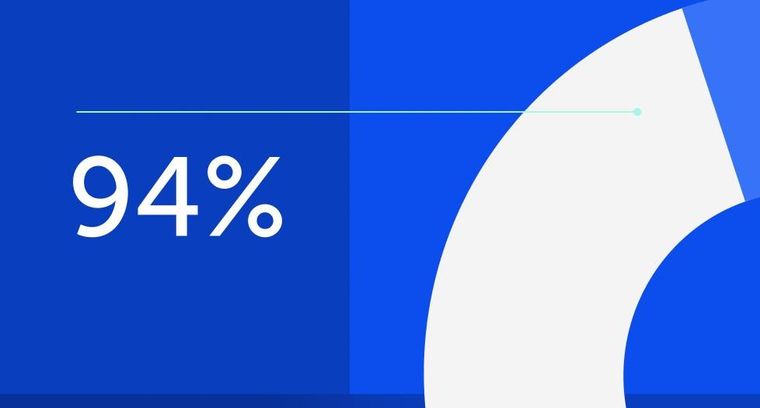
94% of researchers rate our articles as excellent or good
Learn more about the work of our research integrity team to safeguard the quality of each article we publish.
Find out more
ORIGINAL RESEARCH article
Front. Cell. Neurosci., 24 November 2021
Sec. Non-Neuronal Cells
Volume 15 - 2021 | https://doi.org/10.3389/fncel.2021.750373
This article is part of the Research TopicRole of Neuroinflammation in the Neuropsychiatric and Neurological Aspects of COVID-19View all 7 articles
The coronavirus disease 2019 (COVID-19) pandemic has generated a lot of stress and anxiety among not only infected patients but also the general population across the globe, which disturbs cerebral immune homeostasis and potentially exacerbates the SARS-CoV-2 virus-induced neuroinflammation, especially among people susceptible to neuropsychiatric disorders. Here, we used a chronic unpredictable mild stress (CUMS) mouse model to study its effects on glia-mediated neuroinflammation and expression of SARS-CoV2 viral receptors. We observed that female mice showed depressive-like behavior after CUMS, whereas male mice showed enhanced anxiety and social withdrawal. Interestingly, CUMS led to increased amounts of total and MHCII+ microglia in the hippocampi of female mice but not male mice. mRNA levels of SARS-CoV-2 viral receptors angiotensin-converting enzyme 2 (Ace2) and basigin (Bsg) were also upregulated in the prefrontal cortices of stressed female mice but not male mice. Similarly, sex-specific changes in SARS-CoV-2 viral receptors FURIN and neuropilin-1 (NRP1) were also observed in monocytes of human caregivers enduring chronic stress. Our findings provided evidence on detrimental effects of chronic stress on the brain and behavior and implied potential sex-dependent susceptibility to SARS-CoV-2 infection after chronic stress.
The coronavirus disease 2019 (COVID-19) pandemic caused by the severe acute respiratory syndrome coronavirus 2 (SARS-CoV-2) has been giving a significant psychosocial impact on human beings leading to our prolonged state of mental stress (Saladino et al., 2020). Recent surveys have suggested that not only children and young adults (Orgilés et al., 2020) but also health caregivers and aging people (Iodice et al., 2021) are at high risk of developing anxious symptoms and COVID-19 comorbidities, which include neurological diseases and psychiatric disorders (Kempuraj et al., 2020; Nuzzo and Picone, 2020). Furthermore, psychiatric patients were also reported to have higher COVID-19 mortality than common patients (Lega et al., 2021; Vai et al., 2021). Notably, women are suggested to be mentally and behaviorally more aware of the pandemic as a serious health problem (Bwire, 2020; Galasso et al., 2020) and more susceptible to disease-related anxiety and depression (Hou et al., 2020; Thibaut and van Wijngaarden-Cremers, 2020) but in contrary male COVID-19 patients have more severe symptoms and outcomes (Huang et al., 2020; Jin et al., 2020; Peckham et al., 2020; Vahidy et al., 2021). Considering the huge population being directly or indirectly affected by waves of the pandemic globally, the detrimental effect of psychosocial stress on mental health during and after the ongoing pandemic should not be neglected in the society.
The main host cell receptor for the SARS-CoV-2 virus is angiotensin-converting enzyme 2 (ACE2) (Hoffmann et al., 2020; Yang et al., 2020). Another associated viral receptor is basic immunoglobulin (Basigin, BSG) (Wang et al., 2020), which is coregulated by viral infection together with ACE2 and modulates ACE2 abundance. In addition, neuropilin-1 (NRP1) and Furin also assist ACE2 in viral attachment (Xia et al., 2020). These viral receptors are expressed by different cell types including brain cells (Fodoulian et al., 2020; Vargas et al., 2020; Chen et al., 2021) and can be regulated by neurological pathologies (Davies et al., 2020; Fodoulian et al., 2020; Qiao et al., 2020). The cerebral entry routes of the virus are suggested to possibly include the vasculature, the olfactory and trigeminal nerves, the cerebrospinal fluid, and the lymphatic system (Desforges et al., 2019; Boldrini et al., 2021).
Glia-induced neuroinflammation is a common pathological observation in neurological cases of COVID-19 (Tremblay et al., 2020; Vargas et al., 2020), as microglia and astrocytes are key pathogen-combating players in the brain during SARS-CoV-2 infection (McMahon et al., 2021; Yang et al., 2021). However, when primed by stress, they can cause serious neurological and psychiatric complications (Tay et al., 2017; Madore et al., 2020). So far, the stress effect on COVID-19-associated neuropathology and the underlying glia-mediated mechanisms have not been reported. To address this question, we hereby used a chronic unpredictable mild stress (CUMS) mouse model aligned with a human monocyte transcriptomic dataset published by a previous study on human subjects who endured stress caused by caregiving of their family members (Miller et al., 2014). We found that the abundancy of microglia, especially MHCII+ microglia in the hippocampus and mRNA levels of Ace2 and Bsg in the prefrontal cortex (PFC), was upregulated only in stressed female mice but not in males. Similarly, viral receptor FURIN was also increased in monocytes of human female caregivers enduring chronic stress, whereas NRP1 was decreased in stressed male caregivers.
Wild-type C57BL/6NTac female and male mice (3 months old) were housed and bred in the laboratory animal facility at the Institute of Biomedicine and Translational Medicine, University of Tartu. Mice of the same sex from different litters were housed in the same 1264C Euro standard type II cages (Tecniplast, West Chester, PA, United States) measuring 268 mm × 215 mm × 141 mm. Cages contained aspen chips for bedding and aspen wool for nesting material, which were replaced once a week. Mice were kept under standard conditions with unlimited access to food and water on a 12/12 h light/dark cycle (light on during 07:00–19:00). All animal procedures in this study were performed in accordance with the European Communities Directive with license No. 171 (July 1, 2020) issued from the Estonian National Board of Animal Experiments.
Mice from each litter were randomly assigned into two groups: Control and CUMS group, n = 5 per group. One female from the CUMS group died during the experiment. We used the resource equation to calculate the sample size used for the present study (Mead, 1988). The scheme of experimental design is shown in Figure 1A. The CUMS procedure was adapted from Liu et al. (2019). Briefly, after a week of transfer adaptation, mice were daily subjected to one of seven CUMS stressors for five consecutive weeks including: food and water deprivation overnight, rat odor and isolation overnight, restraint in 50-ml tube for 2 h, wet bedding and tilted cage, stroboscopic illumination overnight, flipped light/dark exposure, and swimming at 18°C for 10 min. All stressors were applied individually and were randomly scheduled at different time points per day, and no single stressor was applied for two consecutive days to sustain an unpredictable procedure. Following the CUMS procedure, the same mice were used for behavioral tasks, flow cytometry, and real-time quantitative PCR (RT-qPCR).
Figure 1. Chronic unpredictable mild stress (CUMS) induced depressive-like behavior in female mice and anxious and social withdrawal in male mice. (A) A schema representing experimental design. (B) In the open field test, control male mice traveled less in the corners than control female mice, whereas CUMS males traveled more than control male mice. (C) Control female mice consumed more sucrose than control male mice, whereas CUMS female mice consumed less than control female mice. (D,E). In the three chamber sociability test, control male mice were more social than control female mice, in terms of ratios of the time spent in a chamber with a stranger mouse vs. that with an empty container (D) and the number of interactions with the stranger mouse vs. the empty container (E), respectively, whereas CUMS male mice showed less sociability than control male mice. The symbols * and # represent CUMS and sex differences, respectively (two-way ANOVA). *p < 0.05, **p < 0.01, and #p < 0.05.
Before experimentation, mice were habituated to room light for 1 h. Locomotor and exploratory activities of individual mouse were measured for 30 min in a box (44.8 cm × 44.8 cm × 45 cm) connected to a computer, which registered the distance traveled, number of rearing, corner visits, distance in corner, and time spent in the central part of the box. Data were recorded by a behavioral analytic system (Technical and Scientific Equipment GmbH, Bad Homburg Germany). The floor of the box was cleaned with 70% ethanol and dried thoroughly after each mouse.
Mice were single caged, and two bottles of fresh water were kept for adaptation for 3 days. On the 4th day, one bottle was replaced with 1% sucrose-containing water. The two bottles were weighed before and 12 h afterward. Sucrose preference (SP) was calculated according to the following formula, SP = sucrose intake/(sucrose intake + water intake) × 100%.
A rectangular three-chamber box made from clear Plexiglas was divided into an open middle section and two other identical side sections, which each accommodated a lid-covered and wire-structured cup-like container large enough to enclose a single mouse, allowing free exchange of air but not direct physical contacts between the mice on either side of it. A test mouse was first habituated in the central chamber for 5 min, then introduced to a stranger-1 mouse located in a container for the sociability test. Mice were left to freely explore the three chambers for the next 10 min. All stranger mice of both sexes were at the same age as test mice and habituated to the apparatus during the previous day (30 min habituation for three times). The box and wire containers were cleaned with 70% ethanol and dried thoroughly after each test. Social exploration was defined as time spent in each chamber and social interaction as number of contacts when the head and front paws of a test mouse were within 3 cm vicinity of the container wall as recorded by a camera (Noldus, Wageningen, Netherlands). The ratios of time spent with a stranger mouse or in an empty chamber and number of contacts with a stranger mouse or in an empty container were calculated as sociability indices, respectively.
Mice were euthanized with CO2. Dissected hippocampi were gently homogenized through 70 μm cell strainers (#352350, BD Bioscience, San Jose, CA, United States) in ice-cold phosphate-buffered saline (PBS) with 1% fetal calf serum. Homogenates were washed and centrifuged at 500 × g for 5 min. Isolated cells were blocked with 10% rat serum in ice-cold PBS for 1 h. Brain cells were stained with 0.5 μl anti-mouse MHCII-Brilliant Violet (BV) 711 (#107643, BioLegend, San Diego, CA, United States), CD11b-BV 421 (#101251, BioLegend, San Diego, CA, United States), CD45-BV 650 (#103151, BioLegend, San Diego, CA, United States), Glast-APC (#130-123-555, Miltenyi Biotech, Bergisch Gladbach, Germany), and O4-PE (#130-117-357, Miltenyi Biotech, Bergisch Gladbach, Germany) with the corresponding isotype control antibodies (all from BioLegend, San Diego, CA, United States): rat IgG2b-BV711 (#400653), IgG2b-BV421 (#400639), IgG2b-BV650 (#400651), IgG2b-APC (#400219), and IgM-PE (#401611) in PBS with 1% fetal calf serum on ice for 1 h. Cells were washed, resuspended in 500 ml PBS and acquired with a Fortessa flow cytometer (BD Bioscience, San Jose, CA, United States). Data were analyzed by Kaluza v2.1 software (Beckman Coulter, Indianapolis, IN, United States). Astrocytes were defined as Glast+ cells, oligodendrocyte precursor cells (OPCs) as O4+ cells, microglia as CD45lowCD11bhi and MHCII+ cells. Cell populations were calculated as the percentages among total brain cells or among microglia.
Total RNAs were extracted from the PFC tissue by TRIzol (Molecular Research Center, Cincinnati, OH, United States) and reversely transcribed with a RevertAid First Strand cDNA Synthesis Kit (Thermo Fisher Scientific, Waitham, MA, United States). RT-QPCR was performed by using corresponding primers and 5 × HOT FIREPol® EvaGreen® qPCR Supermix (Solis BioDyne, Tartu, Estonia) on a PCR instrument equipped with QuantStudio 12KFlex Software v.1.2.2 (Thermo Fisher Scientific, Waitham, MA, United States) according to the instructions of the respective manufacturers. The primers (Ma et al., 2020) listed in Supplementary Table 1 were purchased from TAG Copenhagen A/S (Frederiksberg, Denmark). Quantification was performed by normalizing Ct values of target genes to that of the reference gene (β-actin) and expressed as exponential fold changes against the average ΔCt value of the control group (2–ΔΔCt).
Microarray dataset of a human stress study on monocytes (GSE52319) was retrieved from Gene Expression Omnibus (GEO) and analyzed by GEO2R therein for log values of fold changes (Log2FC) and adjusted p-values of differential expression. Identified target genes were counterchecked in various brain RNAseq databases (Supplementary Figures 2–5). To analyze spatial distributions of Ace, Ace2, Nrp1, Nrp2, Bsg, and Furin, images of Nissl and in situ hybridization (ISH) staining in the mouse hippocampus and prefrontal cortical areas were downloaded from Allen Mouse Brain Atlas database1 with their permission (Lein et al., 2007; Bicks et al., 2015), and the Brain explorer® 2 software (Sunkin et al., 2013) was used to closely visualize and quantify gene expression levels based on staining intensity in ISH images.
GraphPad 8.0.1 (San Diego, CA, United States) was used for statistical analyses and graphical presentations. Two-way ANOVA was used to evaluate the interaction effect between CUMS and sex and their main effects, with Tukey’s test used for post hoc multiple comparisons only if significant interaction effects were found. Statistical significance was set at p < 0.05, and data were reported as mean ± SEM.
To assess the impact of CUMS procedure (experimental design shown in Figure 1A), female and male C57Bl/6N mice were evaluated behaviorally. Bodyweights did not show any significant CUMS and sex interaction or CUMS main effect on both female and male mice during the 5 weeks of CUMS (Supplementary Figure 1A). In the open field test, there was a significant interaction between CUMS and sex in distance moved in corners [F (1, 15) = 6.35, p < 0.05], showing that only male mice traveled more distances in corners after CUMS, whereas a lower level in control males compared to control females was also observed (Figure 1B). Meanwhile, no significant changes in total locomotor activities were observed in either sex or treatment groups (Supplementary Figure 1B). In addition, although no interaction effect was seen, there was a significant main effect of stress in the ratio of time spent in corners vs. center [F (1, 15) = 7.29, p < 0.05], and male mice had more such a trend (Supplementary Figure 1C). These results indicated increased anxiety in male mice after CUMS. To further assess anhedonic behavior, we performed the sucrose preference test. A significant interaction between CUMS and sex in sucrose preference was found [F (1, 15) = 8.09, p < 0.05], showing that female mice had significant reduction in sucrose preference, hence showing anhedonia, after CUMS as compared to their control counterparts, whereas male mice did not respond to CUMS but only control male mice showed less sucrose preference compared to control female mice (Figure 1C). Interaction between CUMS and sex was also noticed in the three-chamber sociability test, showing significances in the ratio of time spent in the social chamber vs. the empty container chamber [F (1, 15) = 6.43, p < 0.05] (Figure 1D) and the ratio of a number of interactions with a stranger mouse vs. an empty container [F (1, 15) = 6.65, p < 0.05] (Figure 1E), respectively. Specifically, control male mice showed more social time and interactions compared to control female mice, both of which were dampened by CUMS in male mice, whereas no CUMS effect was found in female mice (Figures 1D,E).
To understand CUMS-induced neuroinflammation, we first used flow cytometry to quantitate astrocytes, OPCs, and microglia. Gating strategy was depicted in representative dotplots in Figure 2A and Supplementary Figure 2B, and isotype antibody stainings used as negative controls in the experiment were shown in Supplementary Figure 2A. Significant interactions between CUMS and sex were found in the percentages of total microglia [F (1, 15) = 8.22, p < 0.05] and MHCII+ subtype of microglia [F (1, 15) = 27.27, p < 0.001]. In female mice, the percentages of total microglia (Figure 2B) and MHCII+ microglia (Figure 2C) in CUMS group were both increased compared to controls, indicating stress-induced neuroinflammation in female mice. By contrast, there was a decrease in MHCII+ microglia in CUMS male mice compared to controls (Figure 2C), implying immunosuppressive effect of CUMS in male mice. We noticed no significant interaction effects between CUMS and sex on astrocytes and OPCs. However, main sex and stress effects were observed on astrocytes [F (1, 15) = 8.5, p < 0.01] and OPCs [F (1, 15) = 9.44, p < 0.01], respectively. Astrocytes were trendily higher in control male mice compared to control female mice (Supplementary Figure 2C), and OPCs were trendily decreased in CUMS male mice compared to control male mice (Supplementary Figure 2D).
Figure 2. Hippocampal microglia were activated in CUMS female mice. (A) Representative graphs showing gating strategy for astrocytes, OPCs, microglia, and MHCII+ microglia; (B) percentage of microglia among total brain cells present in the hippocampus; (C) percentage of MHCII+ microglia among total microglia. The symbols * and # represent CUMS and sex differences, respectively (two-way ANOVA). *p < 0.05, **p < 0.01, #p < 0.05, and ####p < 0.0001.
Furthermore, we analyzed spatial distributions of the SARS-CoV-2 receptors in the normal mouse brain using the ISH data in the Allen Mouse Brain Atlas covering the hippocampus (Figure 3A) and the forebrain (Figure 3B, the PFC marked in red). Expressions of most of these receptors were observed in both regions, especially Bsg, Nrp2, and Furin (Figures 3A,B), whereas Ace and Ace2 levels were low in the parenchyma (except for high level of Ace in the choroid plexus in Figure 3B) and Nrp1 was expressed intermediately in the hippocampus (Figure 3A). Using the Brain explorer® 2 software, we further scrutinized the spatial distributions of these genes within the PFC areas, namely the anterior cingulate cortex, prelimbic area, infralimbic area, and orbital area, and we found their overall enrichment in the orbital area except Ace2, as summarized in Supplementary Table 2. Gene expressions of these receptors were also checked in the human PFC using a human protein atlas dataset, also showing that ACE2 had very low expression, whereas the other genes were expressed more abundantly but with low regional specificity (Supplementary Figure 3).
Figure 3. Spatial distributions of the SARS-CoV-2 receptors in the sagittal sections of mouse hippocampus and PFC region. Spatial distributions of the SARS-CoV-2 receptors Ace, Ace2, Nrp1, Nrp2, Bsg, and Furin in the mouse hippocampus (A) and cerebral cortex region (B) were studied. In representative Nissl images, the cornu ammonis (CA)1, CA2, CA3, dentate gyrus (DG), and subiculum (SUB), and PFC are labeled in red. The middle and right columns represent ISH and pseudo-colored expression images, respectively. Blue, green, and red pseudo-colors encode staining intensities (low, medium, and high) in expression images, respectively. All images were downloaded from the Allen Mouse Brain Atlas (©2004 Allen Institute for Brain Science) (Lein et al., 2007).
As we were interested of glial cell expression of these genes, we further explored the Stanford brain RNAseq database (Zhang et al., 2014), showing they were enriched in different brain cell types in the human and mouse brains (Supplementary Figure 4). Specifically, Ace was expressed most abundantly in OPCs in mice but not detectable in human glial cells. Ace2 was enriched in endothelia but not present in any glial cells in humans and mice. Bsg was present not only in human and mouse endothelia but also in human astrocytes. Furin was highly expressed in microglia in mice and astrocytes in humans. Nrp1 was present mainly in endothelia in mice but in astrocytes and microglia in humans, whereas Nrp2 was enriched in both human and mouse microglia. Furthermore, among these genes, Ace and Nrp2 were expressed in mouse neurons. We also explored the mouse vascular single-cell transcriptomic database from Belsholtz lab (He et al., 2018; Supplementary Figure 5). Ace, Ace2, and Bsg were not enriched in any glial types compared to the other cell types studied there, with Ace and Bsg well enriched in endothelial cells and Ace2 in smooth muscle cells. Furin was expressed broadly including astrocytes, whereas Nrp1 and Nrp2 were enriched not only in endothelial cells and fibroblasts but also present in microglia and astrocytes. Exploring yet another single-cell transcriptomic database on the mouse PFC (Bhattacherjee et al., 2019), we observed that all genes were enriched in endothelia. Meanwhile, Nrp1, Bsg, and Furin were all enriched in astrocytes, Nrp1, Nrp2, Bsg, and Furin in microglia, Bsg in oligodendrocytes and OPCs, whereas Ace, Nrp1, Nrp2, and Bsg in neurons as well (Supplementary Figure 6). In summary, a consensus result of these public datasets suggests that Ace, Ace2, and Bsg were enriched in vascular endothelia and associated smooth muscle cells, besides neurons, whereas Nrp1, Nrp2, Bsg, and Furin were expressed by glial cell types.
We next quantified gene expressions of the viral receptors and some pro-inflammation cytokines in the PFC of CUMS and control mice. Significant interaction effects between CUMS and sex on the expressions of Ace2a [F (1, 15) = 5.17, p < 0.05] and Bsg [F (1, 15) = 7.15, p < 0.05] were seen, implying a possible sex-specific effect of CUMS on potentiating viral entry and/or spreading in the brain. Specifically, Ace2a (Figure 4A) and Bsg (Figure 4B) were upregulated only in female mice but not in male mice after CUMS, whereas enhanced level of Ace2a (Figure 4A) in control male mice and suppressed level of Bsg (Figure 4B) in CUMS male mice, respectively, were seen compared to female mice. No interaction effects between CUMS and sex on other viral receptors and cytokines were found. However, main stress and sex effects were observed on Ace2b [F (1, 15) = 9.37, p < 0.01] and Nrp1 [F (1, 15) = 8.0, p < 0.05], respectively. A trend of enhancing effect of CUMS on Ace2b was seen in female mice (Supplementary Figure 7B), and Nrp1 had trendily higher basal level in control male mice compared to control female mice (Supplementary Figure 7C). Furthermore, cytokine Il7 showed CUMS main effect too [F (1, 15) = 11.77, p < 0.01] and was trendily dampened in CUMS male mice compared to control male mice (Supplementary Figure 7G), whereas cytokines TNF and Il1b showed no significant changes in both female and male mice (Supplementary Figures 7F,H).
Figure 4. SARS-CoV-2 receptors were upregulated in the PFC of female mice. Sex-dependent upregulations of Ace2a (A) and Bsg (B) were detected using RT-qPCR. The symbols * and # represent CUMS and sex differences, respectively (two-way ANOVA). *p < 0.05, **p < 0.01, and #p < 0.05.
To further characterize stress effect on viral receptors and immune responses in human context, we searched GEO and discovered a dataset (GSE52319) that studied the influence of chronic stress, caused by caregiving to patients, on signaling pathways that regulate inflammation in human monocytes. This study found increased expression of genes bearing response elements for nuclear-factor kappa B, a key pro-inflammatory transcription factor among chronically stressed (CS) caregivers compared to non-stressed (NS) subjects. Simultaneously, CS caregivers showed reduced expression of genes with response elements for the glucocorticoid receptor, a transcription factor that conveys cortisol’s anti-inflammatory signals to monocytes (Miller et al., 2014). Their transcriptomic result revealed that NRP1 was downregulated in stressed male caregivers, whereas a significant upregulation of FURIN was observed in stressed female caregivers (Table 1).
Table 1. Expressions of ACE, ACE2, NRP1, NRP2, BSG, and FURIN in monocytes of human subjects enduring chronic stress (CS) as compared to non-stressed (NS) controls.
Since there is still a knowledge gap on the relationship between psychosocial stress and COVID-19 disease development, the present study aims to investigate whether there is an exacerbating effect of chronic stress on glial activation that may contribute to SARS-CoV-2-induced brain infection and pathology. Our most interesting finding was sex-dependent linkage of chronic stress to anxiety- and depressive-related behaviors, microglial activation, and expression of SARS-CoV-2 receptors.
We first used a CUMS mouse model showing its behavioral effects in both female and male mice. Here, we found that female CUMS mice showed anhedonia, whereas male CUMS mice showed more anxiogenic phenotype and social withdrawal, giving us a first glimpse on sex differences in response to chronic stress and facial validation of our CUMS protocol.
Later, we examined how different glial cell types, namely microglia, MHCII+ microglia, OPCs, and astrocytes, responded to stress in the hippocampi of CUMS mice. The expression of MHCII is low in microglia in homeostatic conditions but can be rapidly upregulated and is therefore often used as a marker of microglial activation and neuroinflammation in pathological conditions (Wyss-Coray and Mucke, 2002). In macrophages, MHCII has not only been shown to be enriched in M1 type but also expressed by M2b type that more closely resembles M1 macrophages than other M2 subtypes (Edwards et al., 2006). However, whether this expression pattern of MHCII can be directly extrapolated into microglial subtypes is uncertain as the definition of M1/M2 microglia in vivo has been objected by many researchers (Ransohoff, 2016). It should also be noted that increase of MHCII+ microglia may reflect an adaptive and beneficial response of microglia to stress. In fact, MHCII-expressing microglia were suggested to have neuroprotective role in some inflammatory and neurological conditions (Butovsky et al., 2005; Thored et al., 2009), which is worthy to be examined in psychiatric disorders more carefully.
Stress has a significant effect on neuroplasticity and behavior, staging especially the hippocampal region of the brain (Kim and Diamond, 2002). Again, we observed sex effect, as microglia, particularly the MHCII+ subtype of microglia, were increased only in female mice after CUMS, suggesting their increased neuroinflammatory response to stress. By contrast, decreases in percentages of MHCII+ microglia and OPCs in CUMS male mice compared to controls suggest immunosuppression by stress possibly via glucocorticoids. Sex difference in microglial activation involving the hippocampus has been documented (Nelson et al., 2019). For instance, a previous study observed more elevated corticosterone level and enhanced pro-inflammatory response in the hippocampus of female mice compared to male mice after CUMS (Liu et al., 2019). Increased microglia in female mice could be mediated by their glucocorticoid receptors, which are known to cause neuroinflammation that exacerbates anxiety and depression (Frank et al., 2012; Johnson et al., 2019). Corroboratively, glucocorticoid receptors and associated signaling genes were reported to be differentially expressed in female human subjects and rodents after stress (Miller et al., 2014; Bekhbat et al., 2018). Furthermore, female sex hormones, such as estrogens and progesterone, brought about sex differences in behavior due to stress (ter Horst et al., 2012). Our findings are also in line with a previous work showing that CUMS animals exhibited reduced proliferation of oligodendrocytes and endothelial cells in male rodents (Banasr et al., 2007).
Microglia are known to regulate neurogenesis in neurogenic niches including the hippocampal dentate gyrus (Tay et al., 2017). An earlier study showed that neurogenesis in the adult hippocampal dentate gyrus conferred an important mechanism for stress resilience (Anacker et al., 2018). Stimulation of hippocampal microglial proliferation was previously found to partially or completely reverse the depressive-like behavior and increase hippocampal neurogenesis after CUMS (Kreisel et al., 2014). Moreover, a recent study showed that decreasing Arg1+ microglia in the hippocampus by knocking down the microglial IL4R suppressed hippocampal neurogenesis and enhanced vulnerability to CUMS, whereas increasing Arg1+ microglia in the hippocampus by enhancing IL4 signaling restored hippocampal neurogenesis and the resilience to stress-induced depression via brain-derived neurotropic factor (BDNF) (Zhang et al., 2021). Activated microglia along with impaired adult hippocampal neurogenesis during chronic stress may hence jointly contribute to the pathophysiology underlying stress susceptibility in psychiatric disorders (Madore et al., 2020; Gomes-Leal, 2021). Whether microglial regulation of adult hippocampal neurogenesis in stress susceptibility or resilience is sex-dependent warrants future research.
Sex-specific stress response was also found at gene levels, as SARS-CoV-2 receptors Ace2 and Bsg showed significant increases only in CUMS female mice, but not in male mice. Bsg is expressed by glial cells according to several public transcriptomic databases. Corroboratively, gender-dependent responses of SARS-CoV-2 receptors expressed in monocytes of human subjects enduring chronic stress were found in a GEO dataset as well, showing a significant increase of FURIN in stressed women but a decrease of NRP1 in stressed men. Monocytes and monocyte-derived macrophages share myeloid features with activated microglia, and furthermore are postulated to contribute to various psychiatric disorders (Prinz and Priller, 2014; McKim et al., 2018), which was why we chose this human GEO dataset to compare to our findings in the rodent model. Our findings altogether suggest that immune cells may be more vulnerable to SARS-CoV-2 infection after being primed by stress and support recent clinical observations that women were more susceptible to the pandemic-induced anxiety and depression (Hou et al., 2020; Thibaut and van Wijngaarden-Cremers, 2020).
However, although we observed increase of MHCII+ microglia in stressed female mice, indicating enhanced microglia-mediated neuroinflammation, we did not see significant sex-dependent changes in cytokines Il1b and TNF after CUMS, which may require a big sample size for reliable detection and hence was a limitation in our experiment. Nevertheless, we found Il7 was reduced in male CUMS mice. IL-7 is known to be vital for T-cell homeostasis and autoimmune inflammatory condition (Lawson et al., 2015) and was reported to be upregulated in patients with COVID-19 with neurological conditions (Huang et al., 2020; Pacheco-Herrero et al., 2021), but its importance for neuroinflammation and glial activation needs to be confirmed still.
The spatial distribution and glial expression of SARS-CoV-2 viral receptors in the human and mouse brains were noteworthy too. Ace2 was shown to be expressed in the olfactory area of the human brain and in pericytes and endothelial cells (Fodoulian et al., 2020; Chen et al., 2021). Here, multiple viral receptors were seen enriched in the hippocampus and the orbital, anterior cingulate and prelimbic areas of the PFC in the mouse brain. Both the hippocampus and the PFC are known key regulators for executive functions associated with social cognition, mood regulation, decision-making, etc. (Bicks et al., 2015) and are the most sensitive brain regions to stress-induced epigenetic and transcriptomic changes (Hervé et al., 2017; Musaelyan et al., 2020). This suggests the potential sensitivities of these regions for viral entry or residence in the brain. However, it should be cautioned that supportive evidence on brain invasion of the SARS-CoV-2 virus is currently sparse. Furthermore, both its entry route and cellular host in the brain are still a mystery, with many postulations that lack solid experimental evidence to support (Desforges et al., 2019; Boldrini et al., 2021). In this regard, knowledge on differential expressions of these viral receptors among brain cell types is valuable for hypothesis-driven research, and the possible roles of glial cells in reception and propagation of the virus in the brain due to stress should be investigated more carefully in the future.
It should be noted that our preliminary study has many limitations. First of all, we did not provide any direct evidence demonstrating that stress and microglial activation would contribute to actual viral infection in the brain. Our correlational observations, therefore, need to be proved or disproved in a viral infection model. Second, we had small animal numbers in our experiments, which may cause biases in our data analysis and interpretation. Third, due to the high demand of tissue size in our flow cytometric experiment, we needed to use both hemispheric hippocampal tissues of each mouse and hence were unable to analyze gene expressions by qPCR in this region as well, which limited our understanding of region-dependent changes in viral receptors and cytokines in the mouse brain after stress. Therefore, more vigorous future studies are needed to examine whether there is a truly sex-dependent susceptibility of COVID-19 and associated neuroinflammatory responses post chronic stress.
Our findings provided evidence showing detrimental effects of chronic stress on brain and behavior and implied potential sex-dependent susceptibility to SARS-CoV-2 infection after chronic stress.
The original contributions presented in the study are included in the article/Supplementary Material, further inquiries can be directed to the corresponding author/s.
The animal study was reviewed and approved by Estonian National Board of Animal Experiments.
LY designed and performed all the CUMS animal experiments, made the data analysis, and prepared the figures. MJ helped with the behavioral tests in CUMS, analyzed the transcriptomic data, prepared the figures, and wrote the manuscript. KC did the flow cytometry experiment in CUMS. AZ critically analyzed and improved the manuscript. LT supervised the project and CUMS experiments, analyzed the human transcriptomic data, and wrote the manuscript. All authors contributed to the article and approved the submitted version.
LT is supported by the Estonian Research Council-European Union Regional Developmental Fund Mobilitas Pluss Program No. MOBTT77. AZ is supported by the Estonian Research Council personal research funding team grant project No. PRG878.
The authors declare that the research was conducted in the absence of any commercial or financial relationships that could be construed as a potential conflict of interest.
All claims expressed in this article are solely those of the authors and do not necessarily represent those of their affiliated organizations, or those of the publisher, the editors and the reviewers. Any product that may be evaluated in this article, or claim that may be made by its manufacturer, is not guaranteed or endorsed by the publisher.
We would like to thank Kersti Lilleväli for helping with animal ordering and bookkeeping in this study. We would also like to thank the Allen Institute for Brain Science (https://alleninstitute.org/), the Human Protein Atlas (https://www.proteinatlas.org/), the PFC explorer (http://djeknad.pythonanywhere.com/), the Brain RNA-Seq Database (https://www.brainrnaseq.org/), the Brain Vascular Single-Cell Database (http://betsholtzlab.org/VascularSingleCells/database.html), and the GTExportal Database (https://www.gtexportal.org) for their publicly shared datasets.
The Supplementary Material for this article can be found online at: https://www.frontiersin.org/articles/10.3389/fncel.2021.750373/full#supplementary-material
Anacker, C., Luna, V. M., Stevens, G. S., Millette, A., Shores, R., Jimenez, J. C., et al. (2018). Hippocampal neurogenesis confers stress resilience by inhibiting the ventral dentate gyrus. Nature 559, 98–102. doi: 10.1038/s41586-018-0262-4
Banasr, M., Valentine, G. W., Li, X. Y., Gourley, S. L., Taylor, J. R., and Duman, R. S. (2007). Chronic unpredictable stress decreases cell proliferation in the cerebral cortex of the adult rat. Biol. Psychiatry 62, 496–504. doi: 10.1016/j.biopsych.2007.02.006
Bekhbat, M., Glasper, E. R., Rowson, S. A., Kelly, S. D., and Neigh, G. N. (2018). Measuring corticosterone concentrations over a physiological dynamic range in female rats. Physiol. Behav. 194, 73–76. doi: 10.1016/j.physbeh.2018.04.033
Bhattacherjee, A., Djekidel, M. N., Chen, R., Chen, W., Tuesta, L. M., and Zhang, Y. (2019). Cell type-specific transcriptional programs in mouse prefrontal cortex during adolescence and addiction. Nat. Commun. 10:4169. doi: 10.1038/s41467-019-12054-3
Bicks, L. K., Koike, H., Akbarian, S., and Morishita, H. (2015). Prefrontal cortex and social cognition in mouse and man. Front. Psychol. 6:1805. doi: 10.3389/fpsyg.2015.01805
Boldrini, M., Canoll, P. D., and Klein, R. S. (2021). How COVID-19 affects the brain. JAMA Psychiatry 78, 682–683. doi: 10.1001/jamapsychiatry.2021.0500
Butovsky, O., Talpalar, A. E., Ben-Yaakov, K., and Schwartz, M. (2005). Activation of microglia by aggregated beta-amyloid or lipopolysaccharide impairs MHC-II expression and renders them cytotoxic whereas IFN-gamma and IL-4 render them protective. Mol. Cell. Neurosci. 29, 381–393. doi: 10.1016/j.mcn.2005.03.005
Bwire, G. M. (2020). Coronavirus: why men are more vulnerable to Covid-19 than women? SN Compr. Clin. Med. 2, 874–876. doi: 10.1007/s42399-020-00341-w
Chen, R. E., Zhang, X., Case, J. B., Winkler, E. S., Liu, Y., VanBlargan, L. A., et al. (2021). Resistance of SARS-CoV-2 variants to neutralization by monoclonal and serum-derived polyclonal antibodies. Nat. Med. 27, 717–726. doi: 10.1038/s41591-021-01294-w
Davies, J., Randeva, H. S., Chatha, K., Hall, M., Spandidos, D. A., Karteris, E., et al. (2020). Neuropilin-1 as a new potential SARS-CoV-2 infection mediator implicated in the neurologic features and central nervous system involvement of COVID-19. Mol. Med. Rep. 22, 4221–4226. doi: 10.3892/mmr.2020.11510
Desforges, M., Le Coupanec, A., Dubeau, P., Bourgouin, A., Lajoie, L., Dubé, M., et al. (2019). Human coronaviruses and other respiratory viruses: underestimated opportunistic pathogens of the central nervous system? Viruses 12:14. doi: 10.3390/v12010014
Edwards, J. P., Zhang, X., Frauwirth, K. A., and Mosser, D. M. (2006). Biochemical and functional characterization of three activated macrophage populations. J. Leukoc. Biol. 80, 1298–1307. doi: 10.1189/jlb.0406249
Fodoulian, L., Tuberosa, J., Rossier, D., Boillat, M., Kan, C., Pauli, V., et al. (2020). SARS-CoV-2 receptors and entry genes are expressed in the human olfactory neuroepithelium and brain. iScience 23:101839. doi: 10.1016/j.isci.2020.101839
Frank, M. G., Thompson, B. M., Watkins, L. R., and Maier, S. F. (2012). Glucocorticoids mediate stress-induced priming of microglial pro-inflammatory responses. Brain Behav. Immun. 26, 337–345. doi: 10.1016/j.bbi.2011.10.005
Galasso, V., Pons, V., Profeta, P., Becher, M., Brouard, S., and Foucault, M. (2020). Gender differences in COVID-19 attitudes and behavior: panel evidence from eight countries. Proc. Natl. Acad. Sci. U.S.A. 117, 27285–27291. doi: 10.1073/pnas.2012520117
Gomes-Leal, W. (2021). Adult hippocampal neurogenesis and affective disorders: new neurons for psychic well-being. Front. Neurosci. 15:594448. doi: 10.3389/fnins.2021.594448
He, L., Vanlandewijck, M., Mäe, M. A., Andrae, J., Ando, K., Del Gaudio, F., et al. (2018). Single-cell RNA sequencing of mouse brain and lung vascular and vessel-associated cell types. Sci. data 5:180160. doi: 10.1038/sdata.2018.160
Hervé, M., Bergon, A., Le Guisquet, A. M., Leman, S., Consoloni, J. L., Fernandez-Nunez, N., et al. (2017). Translational identification of transcriptional signatures of major depression and antidepressant response. Front. Mol. Neurosci. 10:248. doi: 10.3389/fnmol.2017.00248
Hoffmann, M., Kleine-Weber, H., Schroeder, S., Krüger, N., Herrler, T., Erichsen, S., et al. (2020). SARS-CoV-2 cell entry depends on ACE2 and TMPRSS2 and is blocked by a clinically proven protease inhibitor. Cell 181, 271–280.e8. doi: 10.1016/j.cell.2020.02.052
Hou, F., Bi, F., Jiao, R., Luo, D., and Song, K. (2020). Gender differences of depression and anxiety among social media users during the COVID-19 outbreak in China: a cross-sectional study. BMC Public Health 20:1648. doi: 10.1186/s12889-020-09738-7
Huang, C., Wang, Y., Li, X., Ren, L., Zhao, J., Hu, Y., et al. (2020). Clinical features of patients infected with 2019 novel coronavirus in Wuhan, China. Lancet 395, 497–506. doi: 10.1016/S0140-6736(20)30183-5
Iodice, F., Cassano, V., and Rossini, P. M. (2021). Direct and indirect neurological, cognitive, and behavioral effects of COVID-19 on the healthy elderly, mild-cognitive-impairment, and Alzheimer’s disease populations. Neurol. Sci. 42, 455–465. doi: 10.1007/s10072-020-04902-8
Jin, J. M., Bai, P., He, W., Wu, F., Liu, X. F., Han, D. M., et al. (2020). Gender differences in patients with COVID-19: focus on severity and mortality. Front. Public Health 8:152. doi: 10.3389/fpubh.2020.00152
Johnson, J. D., Barnard, D. F., Kulp, A. C., and Mehta, D. M. (2019). Neuroendocrine regulation of brain cytokines after psychological stress. J. Endocr. Soc. 3, 1302–1320. doi: 10.1210/js.2019-00053
Kempuraj, D., Selvakumar, G. P., Ahmed, M. E., Raikwar, S. P., Thangavel, R., Khan, A., et al. (2020). COVID-19, mast cells, cytokine storm, psychological stress, and neuroinflammation. Neuroscientist 26, 402–414. doi: 10.1177/1073858420941476
Kim, J. J., and Diamond, D. M. (2002). The stressed hippocampus, synaptic plasticity and lost memories. Nat. Rev. Neurosci. 3, 453–462. doi: 10.1038/nrn849
Kreisel, T., Frank, M. G., Licht, T., Reshef, R., Ben-Menachem-Zidon, O., Baratta, M. V., et al. (2014). Dynamic microglial alterations underlie stress-induced depressive-like behavior and suppressed neurogenesis. Mol. Psychiatry 19, 699–709. doi: 10.1038/mp.2013.155
Lawson, B. R., Gonzalez-Quintial, R., Eleftheriadis, T., Farrar, M. A., Miller, S. D., Sauer, K., et al. (2015). Interleukin-7 is required for CD4(+) T cell activation and autoimmune neuroinflammation. Clin. Immunol. 161, 260–269. doi: 10.1016/j.clim.2015.08.007
Lega, I., Nisticò, L., Palmieri, L., Caroppo, E., Lo Noce, C., Donfrancesco, C., et al. (2021). Psychiatric disorders among hospitalized patients deceased with COVID-19 in Italy. EClinicalMedicine 35:100854. doi: 10.1016/j.eclinm.2021.100854
Lein, E. S., Hawrylycz, M. J., Ao, N., Ayres, M., Bensinger, A., Bernard, A., et al. (2007). Genome-wide atlas of gene expression in the adult mouse brain. Nature 445, 168–176. doi: 10.1038/nature05453
Liu, L. L., Li, J. M., Su, W. J., Wang, B., and Jiang, C. L. (2019). Sex differences in depressive-like behaviour may relate to imbalance of microglia activation in the hippocampus. Brain Behav. Immun. 81, 188–197. doi: 10.1016/j.bbi.2019.06.012
Ma, D., Chen, C. B., Jhanji, V., Xu, C., Yuan, X. L., Liang, J. J., et al. (2020). Expression of SARS-CoV-2 receptor ACE2 and TMPRSS2 in human primary conjunctival and pterygium cell lines and in mouse cornea. Eye 34, 1212–1219. doi: 10.1038/s41433-020-0939-4
Madore, C., Yin, Z., Leibowitz, J., and Butovsky, O. (2020). Microglia, lifestyle stress, and neurodegeneration. Immunity 52, 222–240. doi: 10.1016/j.immuni.2019.12.003
McKim, D. B., Weber, M. D., Niraula, A., Sawicki, C. M., Liu, X., Jarrett, B. L., et al. (2018). Microglial recruitment of IL-1β-producing monocytes to brain endothelium causes stress-induced anxiety. Mol. Psychiatry 23, 1421–1431. doi: 10.1038/mp.2017.64
McMahon, C. L., Staples, H., Gazi, M., Carrion, R., and Hsieh, J. (2021). SARS-CoV-2 targets glial cells in human cortical organoids. Stem Cell Rep. 16, 1156–1164. doi: 10.1016/j.stemcr.2021.01.016
Miller, G. E., Murphy, M. L., Cashman, R., Ma, R., Ma, J., Arevalo, J. M., et al. (2014). Greater inflammatory activity and blunted glucocorticoid signaling in monocytes of chronically stressed caregivers. Brain Behav. Immun. 41, 191–199. doi: 10.1016/j.bbi.2014.05.016
Musaelyan, K., Yildizoglu, S., Bozeman, J., Du Preez, A., Egeland, M., Zunszain, P. A., et al. (2020). Chronic stress induces significant gene expression changes in the prefrontal cortex alongside alterations in adult hippocampal neurogenesis. Brain Commun. 2:fcaa153. doi: 10.1093/braincomms/fcaa153
Nelson, L. H., Saulsbery, A. I., and Lenz, K. M. (2019). Small cells with big implications: microglia and sex differences in brain development, plasticity and behavioral health. Prog. Neurobiol. 176, 103–119. doi: 10.1016/j.pneurobio.2018.09.002
Nuzzo, D., and Picone, P. (2020). Potential neurological effects of severe COVID-19 infection. Neurosci. Res. 158, 1–5. doi: 10.1016/j.neures.2020.06.009
Orgilés, M., Morales, A., Delvecchio, E., Mazzeschi, C., and Espada, J. P. (2020). Immediate psychological effects of the COVID-19 quarantine in youth from Italy and Spain. Front. Psychol. 11:579038. doi: 10.3389/fpsyg.2020.579038
Pacheco-Herrero, M., Soto-Rojas, L. O., Harrington, C. R., Flores-Martinez, Y. M., Villegas-Rojas, M. M., León-Aguilar, A. M., et al. (2021). Elucidating the neuropathologic mechanisms of SARS-CoV-2 infection. Front. Neurol. 12:660087. doi: 10.3389/fneur.2021.660087
Peckham, H., de Gruijter, N. M., Raine, C., Radziszewska, A., Ciurtin, C., Wedderburn, L. R., et al. (2020). Male sex identified by global COVID-19 meta-analysis as a risk factor for death and ITU admission. Nat. Commun. 11:6317. doi: 10.1038/s41467-020-19741-6
Prinz, M., and Priller, J. (2014). Microglia and brain macrophages in the molecular age: from origin to neuropsychiatric disease. Nat. Rev. Neurosci. 15, 300–312. doi: 10.1038/nrn3722
Qiao, J., Li, W., Bao, J., Peng, Q., Wen, D., Wang, J., et al. (2020). The expression of SARS-CoV-2 receptor ACE2 and CD147, and protease TMPRSS2 in human and mouse brain cells and mouse brain tissues. Biochem. Biophys. Res. Commun. 533, 867–871. doi: 10.1016/j.bbrc.2020.09.042
Ransohoff, R. M. (2016). A polarizing question: do M1 and M2 microglia exist? Nat. Neurosci. 19, 987–991. doi: 10.1038/nn.4338
Saladino, V., Algeri, D., and Auriemma, V. (2020). The psychological and social impact of Covid-19: new perspectives of well-being. Front. Psychol. 11:577684. doi: 10.3389/fpsyg.2020.577684
Sunkin, S. M., Ng, L., Lau, C., Dolbeare, T., Gilbert, T. L., Thompson, C. L., et al. (2013). Allen Brain Atlas: an integrated spatio-temporal portal for exploring the central nervous system. Nucleic Acids Res. 41, D996–D1008. doi: 10.1093/nar/gks1042
Tay, T. L., Savage, J. C., Hui, C. W., Bisht, K., and Tremblay, M. È (2017). Microglia across the lifespan: from origin to function in brain development, plasticity and cognition. J. Physiol. 595, 1929–1945. doi: 10.1113/JP272134
ter Horst, J. P., de Kloet, E. R., Schächinger, H., and Oitzl, M. S. (2012). Relevance of stress and female sex hormones for emotion and cognition. Cell. Mol. Neurobiol. 32, 725–735. doi: 10.1007/s10571-011-9774-2
Thibaut, F., and van Wijngaarden-Cremers, P. J. M. (2020). Women’s mental health in the time of Covid-19 pandemic. Front. Glob. Women’s Health 17:588372. doi: 10.3389/fgwh.2020.588372
Thored, P., Heldmann, U., Gomes-Leal, W., Gisler, R., Darsalia, V., Taneera, J., et al. (2009). Long-term accumulation of microglia with proneurogenic phenotype concomitant with persistent neurogenesis in adult subventricular zone after stroke. Glia 57, 835–849. doi: 10.1002/glia.20810
Tremblay, M. E., Madore, C., Bordeleau, M., Tian, L., and Verkhratsky, A. (2020). Neuropathobiology of COVID-19: the role for glia. Front. Cell. Neurosci. 14:592214. doi: 10.3389/fncel.2020.592214
Vahidy, F. S., Pan, A. P., Ahnstedt, H., Munshi, Y., Choi, H. A., Tiruneh, Y., et al. (2021). Sex differences in susceptibility, severity, and outcomes of coronavirus disease 2019: cross-sectional analysis from a diverse US metropolitan area. PLoS One 16:e0245556. doi: 10.1371/journal.pone.0245556
Vai, B., Mazza, M. G., Delli Colli, C., Foiselle, M., Allen, B., Benedetti, F., et al. (2021). Mental disorders and risk of COVID-19-related mortality, hospitalisation, and intensive care unit admission: a systematic review and meta-analysis. Lancet Psychiatry 8, 797–812. doi: 10.1016/S2215-0366(21)00232-7
Vargas, G., Medeiros Geraldo, L. H., Gedeão Salomão, N., Viana Paes, M., Regina Souza Lima, F., and Carvalho Alcantara Gomes, F. (2020). Severe acute respiratory syndrome coronavirus 2 (SARS-CoV-2) and glial cells: insights and perspectives. Brain Behav. Immun. 7:100127. doi: 10.1016/j.bbih.2020.100127
Wang, K., Chen, W., Zhang, Z., Deng, Y., Lian, J. Q., Du, P., et al. (2020). CD147-spike protein is a novel route for SARS-CoV-2 infection to host cells. Signal Transduct. Target. Ther. 5:283. doi: 10.1038/s41392-020-00426-x
Wyss-Coray, T., and Mucke, L. (2002). Inflammation in neurodegenerative disease–a double-edged sword. Neuron 35, 419–432. doi: 10.1016/s0896-6273(02)00794-8
Xia, S., Lan, Q., Su, S., Wang, X., Xu, W., Liu, Z., et al. (2020). The role of furin cleavage site in SARS-CoV-2 spike protein-mediated membrane fusion in the presence or absence of trypsin. Signal Transduct. Target. Ther. 5:92. doi: 10.1038/s41392-020-0184-0
Yang, A. C., Kern, F., Losada, P. M., Agam, M. R., Maat, C. A., Schmartz, G. P., et al. (2021). Dysregulation of brain and choroid plexus cell types in severe COVID-19. Nature 595, 565–571. doi: 10.1038/s41586-021-03710-0
Yang, J., Petitjean, S., Koehler, M., Zhang, Q., Dumitru, A. C., Chen, W., et al. (2020). Molecular interaction and inhibition of SARS-CoV-2 binding to the ACE2 receptor. Nat. Commun. 11:4541. doi: 10.1038/s41467-020-18319-6
Zhang, J., Rong, P., Zhang, L., He, H., Zhou, T., Fan, Y., et al. (2021). IL4-driven microglia modulate stress resilience through BDNF-dependent neurogenesis. Sci. Adv. 7:eabb9888. doi: 10.1126/sciadv.abb9888
Keywords: chronic unpredictable mild stress, neuroinflammation, glial cells, COVID-19, SARS-CoV-2, neuropsychiatric disorders
Citation: Yan L, Jayaram M, Chithanathan K, Zharkovsky A and Tian L (2021) Sex-Specific Microglial Activation and SARS-CoV-2 Receptor Expression Induced by Chronic Unpredictable Stress. Front. Cell. Neurosci. 15:750373. doi: 10.3389/fncel.2021.750373
Received: 30 July 2021; Accepted: 25 October 2021;
Published: 24 November 2021.
Edited by:
Kathryn M. Lenz, The Ohio State University, United StatesReviewed by:
Jessica Lynn Bolton, University of California, Irvine, United StatesCopyright © 2021 Yan, Jayaram, Chithanathan, Zharkovsky and Tian. This is an open-access article distributed under the terms of the Creative Commons Attribution License (CC BY). The use, distribution or reproduction in other forums is permitted, provided the original author(s) and the copyright owner(s) are credited and that the original publication in this journal is cited, in accordance with accepted academic practice. No use, distribution or reproduction is permitted which does not comply with these terms.
*Correspondence: Li Tian, bGkudGlhbkB1dC5lZQ==; Mohan Jayaram, bW9oYW4uamF5YXJhbUB1dC5lZQ==
Disclaimer: All claims expressed in this article are solely those of the authors and do not necessarily represent those of their affiliated organizations, or those of the publisher, the editors and the reviewers. Any product that may be evaluated in this article or claim that may be made by its manufacturer is not guaranteed or endorsed by the publisher.
Research integrity at Frontiers
Learn more about the work of our research integrity team to safeguard the quality of each article we publish.