- 1Department of Microbiology and Immunology, Medical School, Southeast University, Nanjing, China
- 2Jiangsu Provincial Key Laboratory of Critical Care Medicine, Department of Critical Care Medicine, School of Medicine, Zhongda Hospital, Southeast University, Nanjing, China
- 3Key Laboratory of Developmental Genes and Human Disease, Ministry of Education, Southeast University, Nanjing, China
- 4Jiangsu Key Laboratory of Molecular and Functional Imaging, Medical School, Zhongda Hospital, Southeast University, Nanjing, China
The expression and function of immune molecules, such as major histocompatibility complex (MHC), within the developing and adult brain have been discovered over the past few years. Studies utilizing classical class I MHC knockout animals suggest that these molecules, in fact, play essential roles in the establishment, function, and modification of synapses in the CNS. Altered neuronal expression of class I MHC, as has been reported in pathological conditions, leads to aberrations in neuronal development and repair. In the hippocampus, cellular and molecular mechanisms that regulate synaptic plasticity have heretofore been extensively studied. It is for this reason that multiple studies directed at better understanding the expression, regulation, and function of class I MHC within the hippocampus have been undertaken. Since several previous reviews have addressed the roles of class I MHC in the formation and function of hippocampal connections, the present review will focus on describing the spatial and temporal expression of class I MHC in developing, healthy adult, and aging hippocampus. Herein, we also review current literatures exploring mechanisms that regulate class I MHC expression in murine hippocampus. With this review, we aim to facilitate a deeper mechanistic understanding into the complex tight regulation of MHC I expression in hippocampus, which are needed as we explore the potential for targeting MHC I for therapeutic intervention in normal aging and in neurodegenerative diseases in the future.
Introduction
Major histocompatibility complex (MHC) is considered to play central roles in the immune system for presenting peptides and activating lymphocytes. The MHC complex can be divided into three regions: class I, II, and III, and class I MHC genes can be subdivided into classical and non-classical groups (Milner and Campbell, 2001). Over the past decades, the expression of classical class I MHC genes (referred to as MHC I in the following text) within the healthy central nervous system (CNS) has become increasingly recognized and studied. HLA (human leucocyte antigen) is the MHC antigen specific to humans, and HLA-A, HLA-B and HLA-C are the three types of classical human MHC class I proteins. Mouse MHC antigen is called H-2 antigen, and there are three classical mouse class I proteins: H2-K, H2-D, and H2-L. C57BL/6 mice only express two of them: H2-Kb and H2-Db (MHC haplotype b) (Tetruashvily et al., 2016). As of today, the roles of classical MHC I proteins in the establishment, function, and modification of synapses have been extensively reviewed (Boulanger and Shatz, 2004; Boulanger, 2009; Shatz, 2009; Fourgeaud and Boulanger, 2010; Elmer and McAllister, 2012; McAllister, 2014). A well-characterized model system for studying neural connection in the CNS is the hippocampus, which is known for its function in regulating learning, memory, and emotion (Martin et al., 2000). Abnormal expression of classical MHC I in the hippocampus resulted in altered synapse number and morphology with irregular synaptic transmission in animal models (Huh et al., 2000; Goddard et al., 2007; Fourgeaud et al., 2010; Wu et al., 2011; Dixon-Salazar et al., 2014; Lee et al., 2014). Since inappropriate classical MHC I expression in hippocampal neurons is deleterious, the expression of MHC I molecules should be maintained under tight regulation. In this review, we focus on describing the spatial and temporal expression of MHC I, especially classical MHC I genes, in the hippocampus of different species, and we also introduce several reports investigating the molecular mechanisms used by hippocampal neurons to regulate MHC I expression.
Spatial and Temporal Expression of MHC I Molecules in Hippocampus, From Rodent to Primate
MHC I mRNA and Protein Expression in the Developing and Adult Hippocampus
MHC I expression was generally considered to be limited to non-neuronal cells in healthy uninjured brain (Neumann et al., 1995). In 1998, MHC I mRNA was discovered when researchers screened for genes that were regulated by neural activity in the developing retino-geniculate pathway of cats. These authors also detected MHC I mRNA in hippocampal pyramidal neurons by in situ hybridization. During feline development, the expression of MHC I mRNA in hippocampus was found to begin at postnatal day 10 (P10) and was maintained at a relatively high level until P91. The expression of MHC I mRNA and proteins in the dentate gyrus (DG) and cornu ammonis (CA) of normal P22 rat hippocampus was also confirmed by the same group (Corriveau et al., 1998). This was the first report of MHC I molecules that are present normally in subsets of neurons and are required for synapse formation in the LGN.
Since MHC I knockout models were only available in mouse, MHC I expression in the murine hippocampus has been extensively studied. MHC I mRNA in hippocampus was detected in granule and pyramidal cells where P40 mice showed much weaker expression than P6 mice (Huh et al., 2000). Different MHC I alleles showed distinct levels of expression, with H2-Db showing stronger expression in pyramidal cell layers of hippocampus than that of H2-Kb (Huh et al., 2000). By checking with in situ hybridization using specific probes, our group also observed that H2-Db mRNA was expressed postnatally (P4) in CA, DG, and also in the mossy fiber of the hilus in mouse hippocampus. H2-Kb signals ran parallel with H2-Db, and their expression levels were almost identical. Evidence of expression continued at P8 and P15, while at P30 and in adult mice, the signal decreased and became limited to DG and CA1 regions of hippocampus (Liu et al., 2013). Since the expression level of MHC I alleles we reported during hippocampal development differs from other reports, we later measured H2-Kb and H2-Db expression using qRT-PCR, a more quantitative method. The result demonstrated that MHC I mRNA increased steadily from P0 to P60 in mouse hippocampal tissues with higher expression of H2-Kb than H2-Db, suggesting an uneven expression of different MHC I alleles as other reports (Li et al., 2020). Meanwhile, we also detected MHC I proteins by immunofluorescence in hippocampal neurons, but not in GFAP-positive astrocytes (Lv et al., 2015; Li et al., 2020). While the mRNA levels of MHC I increased steadily, the change of MHC I proteins only mirrored MHC I mRNAs levels from P0 to P15. After that, expression of MHC I proteins declined sharply at P30 and P60, suggesting a posttranscriptional regulation (Lv et al., 2015; Patel et al., 2020). Detailed cell-type-specific difference in mouse MHC I gene expression within the hippocampus were also recently identified. Multiple MHC I genes were found to be transcribed in hippocampal tissues collected from P13 to P16. A single-cell mRNA-based approach was used to examine neurons collected from hippocampal tissue. These studies revealed that H2-Kb and H2-Db mRNA were largely present in CA1 pyramidal neurons. Expression profiles of MHC I mRNA in CA1 were significantly different from those of CA3 and DG, which helped to clarify that certain phenotypes, such as increased synapse number, were only observed in restricted regions of the hippocampus of the systemic MHC I knockout mice (Tetruashvily et al., 2016). In general, MHC I gene expression is confirmed in the developing murine hippocampus. However, individual hippocampal neurons can express more than one MHC I gene and their gene expression profiles differ significantly from each other.
The organization, structure, and function of many genes can be quite different from rodents to primates. Therefore, the expression of MHC I proteins in higher vertebrates was investigated. We collected fixed human brain tissues from aborted human fetus, infants, and adults who died without major pathological findings in the CNS (Zhang et al., 2013). Since these specimens were already fixed with paraformaldehyde, immunohistochemistry was used to detect MHC I protein expression by two fully validated allele-specific anti-MHC antibodies: HC-10 and HLA-A2, which recognized HLA-B/C and HLA-A proteins, respectively, in fixed human tumor tissue (Patel et al., 2020). In human hippocampus, MHC I expression was very low at 20 gestational weeks (GW), slowly increased at 27–33 GW, and was maintained to postnatal 55 days. We cannot detect any MHC I protein expression in neurons of the adult brain. Just as reported in the rodents, MHC I proteins were mainly expressed in neurons but not in astrocytes, with most MHC I-expressing neurons being glutamatergic (Zhang et al., 2013). Because human fetal brain tissue is often unavailable, there is only one study about MHC I expressing in the development of human brain. Further studies on the human brain are highly required before we can draw any conclusions. In common marmosets, a non-human primate, expression of classical MHC I proteins in hippocampus was also detected by immunostaining with HC-10 and HLA-A2 antibodies (Rolleke et al., 2006; Ribic et al., 2010). MHC I expression was within mossy fibers and co-localized with vGlut1 immuno-positive signals, suggesting a role of MHC I proteins in excitatory transmission at mossy fiber-CA3 synapses. The expression of MHC I proteins was found to be identical from 1 day postnatal to 6 years old in common marmoset monkeys. In addition, the expression of MHC I mRNAs was examined by in situ hybridization. However, the authors concluded that the signal detected belongs to non-classical MHC I molecules.
MHC I mRNA and Protein Expression in the Aging Hippocampus
An age-related increase in MHC I mRNA was observed in the synaptosomal parts of hippocampus neuron in aged rats (26–28 months old) compared with the adult rats (12 months old). The expression level of MHC I protein was also increased in aged rats as compared to adult rats (VanGuilder Starkey et al., 2012). To date, most researchers believe that the increased expression of MHC I mRNA and proteins in aged animals is important in regulating synaptic plasticity and cognitive function. Complete absence of MHC I (constitutive knockout of H-2K/D, β2M, or TAP) resulted in decreased dendritic complexity, altered dendritic spine type and increased GluN2B NMDA receptor in CA1 pyramidal neurons in aged mice (Lazarczyk et al., 2016). β2M, light chain of MHC I complex, is elevated in the blood of aging humans and mice and promotes age-related cognitive dysfunction and impairs neurogenesis, raising the possible therapies targeting β2M for treatment of age-related cognitive dysfunction (Smith et al., 2015). However, whether MHC I proteins are increased in neurons of aging human and mouse hippocampus and how MHC I is involved in age-related cognitive loss remain unknown.
Regulation of MHC I Expression in Mouse Hippocampal Neurons
Since MHC I has been reported to play an essential role in the establishment and modification of neuronal connections in the hippocampus, it must be under tight regulation. MHC I was discovered among a set of genes whose expression was decreased when endogenous action potential activity was blocked by intracranial infusion of TTX; thus, its expression was determined to be regulated by neural activity (Corriveau et al., 1998). Several reports have additionally indicated that MHC I expression is significantly regulated by seizure-induced overexcitation in the hippocampus (Corriveau et al., 1998; Lee et al., 2021). However, the signaling pathways that relay neuronal activity to MHC I expression have remained poorly defined.
Transcriptional Regulation of MHC I Expression by Neural Activity
The regulation of MHC I expression has been extensively studied in immune cells and in other cell types. It has been determined that tissue-specific expression of MHC I is regulated by distal and proximal upstream regulatory elements, which are located at −800 to −700 bp and −220 to −95 bp regions of the promoters, respectively (Howcroft et al., 2005). In cell types with low endogenous MHC I expression, a silencer element in the promoter distal regulatory elements has been found showing increased activity (Weissman and Singer, 1991). For example, in one such cell line, human neuroblastoma CHP-126, the absence expression of MHC I was a direct result of repressor activity in upstream silencer elements (Murphy et al., 1996).
Although neurons were considered to be deficient in MHC I expression before 1998, they have been found to react to cytokine treatment, such as IFN-γ and TNF-β, by increasing MHC I transcription, suggesting their ability to quickly induce MHC I expression under pathogenic conditions and to activate the immune system (Neumann et al., 1997). Besides differences in silencer activity within the promoter distal regulatory elements, basal and activated transcription of MHC I genes is dependent on different transcriptional factors binding to the core promoter domain and is initiated at different sites within the MHC I promoter (Lee et al., 2010). It is unknown whether neural activity could induce MHC I transcription via a similar mechanism used by cytokines. In neurons, changes in activity always result in a remodeling of the strength of synapses (also called activity dependent plasticity), which involves a series of gene transcriptional changes (Gainey et al., 2015; Joseph and Turrigiano, 2017). The transcription factor CREB has been reported to be necessary for activity-dependent plasticity in hippocampal neurons (Josselyn and Nguyen, 2005) and has also been reported to be one of the key regulators of MHC I expression in immune cells (Meissner et al., 2012). Thus, CREB may be a central player in neural-activity-induced MHC I transcription. We reported on the induction of MHC I expression in cultured hippocampus neurons by enhanced neural activity with KA treatment. We also have shown that transient intracellular Calcium [Ca2+] flux induced by KA activated protein kinase A and C, which served as upstream regulators to increase the amount of NF-κB, IRF-1, and CREB translocation into the nucleus and finally upregulated MHC I transcription. Thus, membrane activity can be connected to neuronal MHC I expression through the modulation of intracellular Ca2+ concentration and Ca2+ regulated signal transduction cascades that share the same transcription factors as those induced by cytokines (Lv et al., 2015). In short, transcription of MHC I can be regulated by tissue-specific (basal) and cytokine/membrane activity (activated) mechanisms in hippocampal neurons. However, our study was performed using cultured neurons with KA treatment that can induce action potentials. Whether changes in physiological level of neural activity in hippocampal neurons in vivo during specific development stages activate the same pathways to regulate MHC I transcription requires further investigation.
Transcriptional Regulation of MHC I Expression by NLRC5, a New Transcriptional Regulator in Hippocampal Neurons
The expression profile of MHC I in neurons behaves in much the same way as classical class II MHC molecules (MHC II) in the peripheral immune system, with cell-type-specific expression and inducible expression by cytokines (Muhlethaler-Mottet et al., 1997). Class II major histocompatibility complex transactivator (CIITA) is the key factor that determines the expression profile of MHC II (Accolla et al., 2019). However, along with other groups, we have also reported that CIITA is not found expressed in normal neurons, so it does not determine the spatial and temporal expression of MHC I in neurons (Castillo et al., 2008; Li et al., 2020). NLRC5, a recently defined transactivator of MHC I genes, attracted our attention for its functional similarity to CIITA that coordinates other transcription factors to form an enhanceosome on the MHC I promoter (Meissner et al., 2010). Abolishing NLRC5 expression resulted in reduced MHC I expression in several immune cells, although to different extents (Biswas et al., 2012; Staehli et al., 2012). Just as has been shown in the immune system, we identified dramatically decreased MHC I mRNA expression in the hippocampus from P0 to P60 in NLRC5 knockout mice, suggesting that NLRC5 is a key regulator of constitutive neural MHC I expression. In the murine neuroblastoma cell line, Neuro2a, NLRC5-induced MHC I activation requires the recruitment of NLRC5 to other transcriptional factors that bind to W/S-X-Y promoter domains (Li et al., 2020). Besides the activation of MHC I transcription, other molecules involved in surface MHC I expression such as β2-microgloubulin, TAP, and LMP are also regulated by NLRC5 in Nero2a cells, implying the potential value of NLRC5 as a target for manipulating neuron MHC I expression (Li et al., 2020). In summary, the working model of transcriptional regulation of MHC I expression in hippocampal neuron is shown in Figure 1.
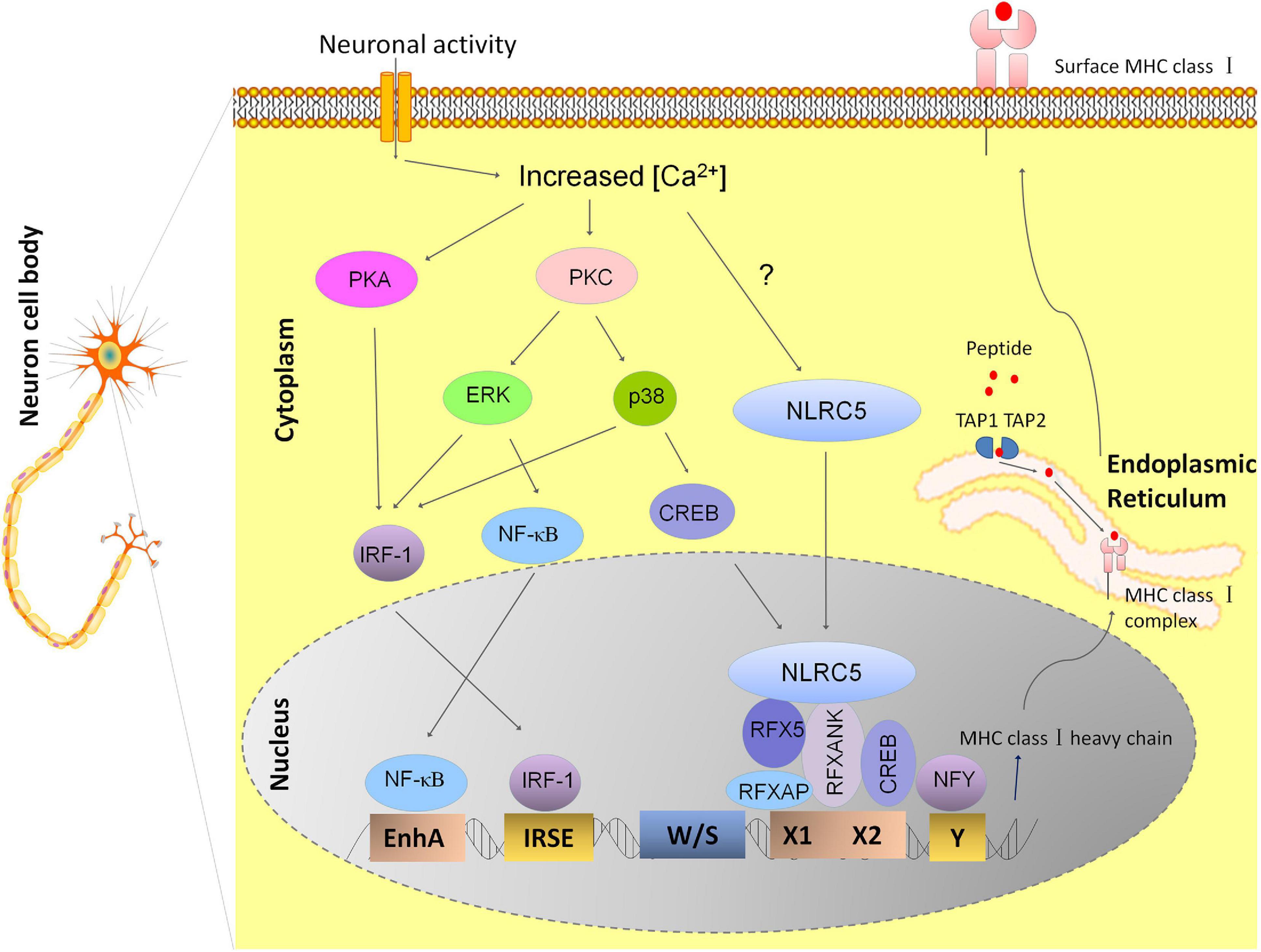
Figure 1. Model depicting the transcriptional regulation of MHC I expression in hippocampal neurons. The transcriptional regulation of MHC I genes is dependent on different transcriptional factors binding to the conserved DNA sequence motifs within the MHC I promoter. Neuronal activity may leads to activation of calcium-dependent Protein Kinase A and C (PKA and PKC), thus results in activation of ERK and P38 of the MAPK pathways and increases the nuclear translocation of transcription factors NF-κB, CREB, and IRF-1. These molecules finally leads to enhanced expression of MHC I by binding to its promoter elements: enhancer A, ISRE, and X box, respectively (Lv et al., 2015). RFX complex also binds to X box besides CREB and the nuclear transcription factor Y (NFY) binds to the Y box. NLRC5 is recruited to the enhanceosome by RFX complex. However, how neuronal activity affects NLRC5 is still not known (Li et al., 2020). Newly synthesized MHC class I heavy chains and beta-2 microglobulin (β2m) proteins form MHC I complex with antigenic peptides in endoplasmic reticulum (ER) and MHC I complex loaded with antigens are transported to the cell surface.
Posttranscriptional Regulation of MHC I Expression in Neurons
Although the expression of MHC I molecules is in large part transcriptionally regulated, the dissociated expression of MHC I mRNA and proteins from P15 to P60 in mouse hippocampus indicates the existence of posttranscriptional regulatory mechanisms. In the immune system, MHC I expression is regulated by microRNAs, RNA binding proteins, and ubiquitin E3 ligase, which affects mRNA stability or indirectly changes the translation efficiency (Kulkarni et al., 2011, 2013; Reches et al., 2016). The ubiquitination system also targeted MHC I proteins to regulate protein transport or proteasomal degradation in the cytosol (Cano et al., 2012). The expression of miR-34a increases rapidly at P30 and P60 in mouse hippocampal tissue, making it an ideal candidate for posttranscriptional regulation of MHC I expression in hippocampal neurons. As we expected, miR-34a showed the ability to regulate MHC I expression in cultured hippocampus neurons. Since MHC I mRNA and protein expression were both decreased upon enhanced expression of miR-34a, we cannot tell whether miR-34a exerts its function through reducing the stability of MHC I mRNA or inhibiting the protein translation (Hu et al., 2020). However, additional in vivo data exploring the regulation of MHC I by miR-34a are still needed.
Discussion
In summary, the expression of MHC I mRNA and protein in postnatal, adult, and aging hippocampal neurons of several species has been detailed by multiple independent studies (Table 1). However, reports of dynamic expression of MHC I molecules in hippocampus throughout the entire life span (prenatal to aged adult) of one species are scarce since most research focuses only on specific developmental stages. Another shortcoming is that the expression of MHC I proteins in mouse neurons remains to be further validated since antibodies that can specifically recognize mouse MHC I are lacking. Most anti-mouse MHC I antibodies available today are only suitable for detecting the fully folded MHC I proteins in situ at the cell surface, and they are not useful for detecting denatured MHC I proteins by Western blots or by immunofluorescence (Wu et al., 2011). Although there have been reports describing a new way for surface labeling of MHC I proteins by immunofluorescence in cortical neurons (Glynn et al., 2011), the staining steps still involved the fixation of coverslips, and using these antibodies for immunofluorescence was not widely accepted. OX18 is the common antibody used for studying neuronal MHC I in rodents, and it can recognize native and denatured rat and mouse MHC I molecules. However, it recognizes both classical and non-classical MHC I molecules (Needleman et al., 2010; Wu et al., 2011). Generating and validating new anti-MHC I antibodies that can be used for detecting denatured MHC I proteins in mice is necessary for precisely determining how protein expression corresponds to the abundance of MHC I mRNAs in neurons. Furthermore, high-specificity antibodies that can accurately discriminate between individual members of the MHC I family will be indispensable for identifying which MHC I genes participate in the establishment and refinement of hippocampal circuits. Finally, it remains important to accurately define the expression of MHC I proteins across different regions of the hippocampus since abnormal synapse structure and function have been observed in specific parts of the hippocampus in global MHC I knockout mice and in H2-Db overexpression mice (Wu et al., 2011; Dixon-Salazar et al., 2014). In order to clearly elucidate this, studies favoring regional or single-cell hippocampal neurons should be prioritized over whole hippocampus homogenates.
Transactivation of MHC I is normally suppressed in neurons, but it was readily inducible in response to stimuli such as cytokines or neural activity (Neumann et al., 1995). After the critical developmental time frame, reduction of neural activity can decrease MHC I expression, suggesting that this is a very dynamic system. In the development of murine hippocampus, MHC I protein level was dramatically decreased after P15 while MHC I mRNA was kept at high level from P0 to P60 (Li et al., 2020). We confirmed that NLRC5 was responsible for promoting constitutive transcription of MHC I gene, and Ca2+-regulated signal transduction cascades were important for relaying membrane activity to upregulated MHC I expression in hippocampal neurons (Lv et al., 2015; Li et al., 2020). We also found that miR34a, which increased dramatically after P15, can decrease MHC I expression, suggesting that it contributed to divergent expression of MHC I mRNA and protein expression after P15 (Hu et al., 2020). Although some mechanisms have been discovered, the information about the pathways that are responsible for temporal neuronal MHC I expression is still very limited. First, most of these results were performed in in vitro cultured system. Bear in mind that results may be in opposition when studied using in vitro vs. in vivo systems. Furthermore, cell-type-specific difference in MHC I gene expression within the hippocampus indicates that regulatory mechanisms may differ between neurons located within distinct regions of the hippocampus. In addition, posttranscriptional regulation could not be excluded since there is disparity between MHC I mRNA and protein expression. Besides the regulation by miRNA, ubiquitination of the MHC I cytoplasmic tail was described to quickly change the surface expression of MHC I proteins in immune system (van den Boomen and Lehner, 2015). This could be a way by which neurons respond to alterations in neural activity and subsequently result in formation of precise neural connections. There remain many unanswered questions regarding the regulation of MHC I in hippocampal neurons through different developmental stages and in aged animals. The answers to these questions remain very important for the field to more clearly understand the diverse roles of MHC I molecules in normal neuronal development and also in age-related cognitive dysfunction or neurodegenerative diseases.
Author Contributions
YS: contributions to the design and drafting of the manuscript and agrees to be accountable for all aspects of the work in ensuring that questions related to the accuracy or integrity of any part of the work are appropriately investigated and resolved. JZ: contributions to the revise the manuscript critically for important intellectual content. Both authors contributed to the article and approved the submitted version.
Funding
This work was supported by the National Nature Science Foundation of China (31100649 and 81571615).
Conflict of Interest
The authors declare that the research was conducted in the absence of any commercial or financial relationships that could be construed as a potential conflict of interest.
Publisher’s Note
All claims expressed in this article are solely those of the authors and do not necessarily represent those of their affiliated organizations, or those of the publisher, the editors and the reviewers. Any product that may be evaluated in this article, or claim that may be made by its manufacturer, is not guaranteed or endorsed by the publisher.
Abbreviations
CA, cornu ammonis; CNS, central nervous system; DG, dentate gyrus; CREB, cAMP response element binding protein; CIITA, class II major histocompatibility complex transactivator; GW, gestation weeks; IRF-1, interferon regulatory factor 1; KA, kainic acid; LMP, low-molecular-weight peptide; LGN, lateral geniculate nucleus; MHC, major histocompatibility complex; NF-κB, nuclear factor kappa B; NLRC5, NLR family CARD domain containing 5; RFX, regulatory factor X; RFXANK, regulatory factor X-associated ankyrin-containing protein; TAP, transporter associated with antigen processing; TTX, Tetrodotoxin.
References
Accolla, R. S., Ramia, E., Tedeschi, A., and Forlani, G. (2019). CIITA-Driven MHC Class II expressing tumor cells as antigen presenting cell performers: toward the construction of an optimal anti-tumor vaccine. Front. Immunol. 10:1806. doi: 10.3389/fimmu.2019.01806
Biswas, A., Meissner, T. B., Kawai, T., and Kobayashi, K. S. (2012). Cutting edge: impaired MHC class I expression in mice deficient for Nlrc5/class I transactivator. J. Immunol. 189, 516–520. doi: 10.4049/jimmunol.1200064
Boulanger, L. M. (2009). Immune proteins in brain development and synaptic plasticity. Neuron 64, 93–109. doi: 10.1016/j.neuron.2009.09.001
Boulanger, L. M., and Shatz, C. J. (2004). Immune signalling in neural development, synaptic plasticity and disease. Nat. Rev. Neurosci. 5, 521–531. doi: 10.1038/nrn1428
Cano, F., Bye, H., Duncan, L. M., Buchet-Poyau, K., Billaud, M., Wills, M. R., et al. (2012). The RNA-binding E3 ubiquitin ligase MEX-3C links ubiquitination with MHC-I mRNA degradation. EMBO J. 31, 3596–3606. doi: 10.1038/emboj.2012.218
Castillo, M. D., Trzaska, K. A., Greco, S. J., Ponzio, N. M., and Rameshwar, P. (2008). Immunostimulatory effects of mesenchymal stem cell-derived neurons: implications for stem cell therapy in allogeneic transplantations. Clin. Transl. Sci. 1, 27–34. doi: 10.1111/j.1752-8062.2008.00018.x
Corriveau, R. A., Huh, G. S., and Shatz, C. J. (1998). Regulation of class I MHC gene expression in the developing and mature CNS by neural activity. Neuron 21, 505–520. doi: 10.1016/s0896-6273(00)80562-0
Dixon-Salazar, T. J., Fourgeaud, L., Tyler, C. M., Poole, J. R., Park, J. J., and Boulanger, L. M. (2014). MHC class I limits hippocampal synapse density by inhibiting neuronal insulin receptor signaling. J. Neurosci. 34, 11844–11856. doi: 10.1523/JNEUROSCI.4642-12.2014
Elmer, B. M., and McAllister, A. K. (2012). Major histocompatibility complex class I proteins in brain development and plasticity. Trends Neurosci. 35, 660–670. doi: 10.1016/j.tins.2012.08.001
Fourgeaud, L., and Boulanger, L. M. (2010). Role of immune molecules in the establishment and plasticity of glutamatergic synapses. Eur. J. Neurosci. 32, 207–217. doi: 10.1111/j.1460-9568.2010.07342.x
Fourgeaud, L., Davenport, C. M., Tyler, C. M., Cheng, T. T., Spencer, M. B., and Boulanger, L. M. (2010). MHC class I modulates NMDA receptor function and AMPA receptor trafficking. Proc. Natl. Acad. Sci. U.S.A. 107, 22278–22283. doi: 10.1073/pnas.0914064107
Gainey, M. A., Tatavarty, V., Nahmani, M., Lin, H., and Turrigiano, G. G. (2015). Activity-dependent synaptic GRIP1 accumulation drives synaptic scaling up in response to action potential blockade. Proc. Natl. Acad. Sci. U.S.A. 112, E3590–E3599. doi: 10.1073/pnas.1510754112
Glynn, M. W., Elmer, B. M., Garay, P. A., Liu, X. B., Needleman, L. A., El-Sabeawy, F., et al. (2011). MHCI negatively regulates synapse density during the establishment of cortical connections. Nat. Neurosci. 14, 442–451. doi: 10.1038/nn.2764
Goddard, C. A., Butts, D. A., and Shatz, C. J. (2007). Regulation of CNS synapses by neuronal MHC class I. Proc. Natl. Acad. Sci. U.S.A. 104, 6828–6833. doi: 10.1073/pnas.0702023104
Howcroft, T. K., Weissman, J. D., Gegonne, A., and Singer, D. S. (2005). A T lymphocyte-specific transcription complex containing RUNX1 activates MHC class I expression. J. Immunol. 174, 2106–2115. doi: 10.4049/jimmunol.174.4.2106
Hu, Y., Pei, W., Hu, Y., Li, P., Sun, C., Du, J., et al. (2020). MiR34a regulates neuronal MHC class I molecules and promotes primary hippocampal neuron dendritic growth and branching. Front. Cell. Neurosci. 14:573208. doi: 10.3389/fncel.2020.573208
Huh, G. S., Boulanger, L. M., Du, H., Riquelme, P. A., Brotz, T. M., and Shatz, C. J. (2000). Functional requirement for class I MHC in CNS development and plasticity. Science 290, 2155–2159. doi: 10.1126/science.290.5499.2155
Joseph, A., and Turrigiano, G. G. (2017). All for one but not one for all: excitatory synaptic scaling and intrinsic excitability are coregulated by CaMKIV, whereas inhibitory synaptic scaling is under independent control. J. Neurosci. 37, 6778–6785. doi: 10.1523/JNEUROSCI.0618-17.2017
Josselyn, S. A., and Nguyen, P. V. (2005). CREB, synapses and memory disorders: past progress and future challenges. Curr. Drug Targets CNS Neurol. Disord. 4, 481–497. doi: 10.2174/156800705774322058
Kulkarni, S., Qi, Y., O’HUigin, C., Pereyra, F., Ramsuran, V., McLaren, P., et al. (2013). Genetic interplay between HLA-C and MIR148A in HIV control and Crohn disease. Proc. Natl. Acad. Sci. U.S.A. 110, 20705–20710. doi: 10.1073/pnas.1312237110
Kulkarni, S., Savan, R., Qi, Y., Gao, X., Yuki, Y., Bass, S. E., et al. (2011). Differential microRNA regulation of HLA-C expression and its association with HIV control. Nature 472, 495–498. doi: 10.1038/nature09914
Lazarczyk, M. J., Kemmler, J. E., Eyford, B. A., Short, J. A., Varghese, M., Sowa, A., et al. (2016). Major Histocompatibility Complex class I proteins are critical for maintaining neuronal structural complexity in the aging brain. Sci. Rep. 6:26199. doi: 10.1038/srep26199
Lee, H., Brott, B. K., Kirkby, L. A., Adelson, J. D., Cheng, S., Feller, M. B., et al. (2014). Synapse elimination and learning rules co-regulated by MHC class I H2-Db. Nature 509, 195–200. doi: 10.1038/nature13154
Lee, N., Iyer, S. S., Mu, J., Weissman, J. D., Ohali, A., Howcroft, T. K., et al. (2010). Three novel downstream promoter elements regulate MHC class I promoter activity in mammalian cells. PLoS One 5:e15278. doi: 10.1371/journal.pone.0015278
Lee, T. S., Li, A. Y., Rapuano, A., Mantis, J., Eid, T., Seyfried, T. N., et al. (2021). Gene expression in the epileptic (EL) mouse hippocampus. Neurobiol. Dis. 147:105152. doi: 10.1016/j.nbd.2020.105152
Li, P., Shen, Y., Cui, P., Hu, Y., Zhang, Y., Miao, F., et al. (2020). Neuronal NLRC5 regulates MHC class I expression in Neuro-2a cells and also during hippocampal development. J. Neurochem. 152, 182–194. doi: 10.1111/jnc.14876
Liu, J., Shen, Y., Li, M., Shi, Q., Zhang, A., Miao, F., et al. (2013). The expression pattern of classical MHC class I molecules in the development of mouse central nervous system. Neurochem. Res. 38, 290–299. doi: 10.1007/s11064-012-0920-0
Lv, D., Shen, Y., Peng, Y., Liu, J., Miao, F., and Zhang, J. (2015). Neuronal MHC Class I expression is regulated by activity driven calcium signaling. PLoS One 10:e0135223. doi: 10.1371/journal.pone.0135223
Martin, S. J., Grimwood, P. D., and Morris, R. G. (2000). Synaptic plasticity and memory: an evaluation of the hypothesis. Annu. Rev. Neurosci. 23, 649–711. doi: 10.1146/annurev.neuro.23.1.649
McAllister, A. K. (2014). Major histocompatibility complex I in brain development and schizophrenia. Biol. Psychiatry 75, 262–268. doi: 10.1016/j.biopsych.2013.10.003
Meissner, T. B., Li, A., Biswas, A., Lee, K. H., Liu, Y. J., Bayir, E., et al. (2010). NLR family member NLRC5 is a transcriptional regulator of MHC class I genes. Proc. Natl. Acad. Sci. U.S.A. 107, 13794–13799. doi: 10.1073/pnas.1008684107
Meissner, T. B., Liu, Y. J., Lee, K. H., Li, A., Biswas, A., van Eggermond, M. C., et al. (2012). NLRC5 cooperates with the RFX transcription factor complex to induce MHC class I gene expression. J. Immunol. 188, 4951–4958. doi: 10.4049/jimmunol.1103160
Milner, C. M., and Campbell, R. D. (2001). Genetic organization of the human MHC class III region. Front. Biosci. 6:D914–D926. doi: 10.2741/milner
Muhlethaler-Mottet, A., Otten, L. A., Steimle, V., and Mach, B. (1997). Expression of MHC class II molecules in different cellular and functional compartments is controlled by differential usage of multiple promoters of the transactivator CIITA. EMBO J. 16, 2851–2860. doi: 10.1093/emboj/16.10.2851
Murphy, C., Nikodem, D., Howcroft, K., Weissman, J. D., and Singer, D. S. (1996). Active repression of major histocompatibility complex class I genes in a human neuroblastoma cell line. J. Biol. Chem. 271, 30992–30999. doi: 10.1074/jbc.271.48.30992
Needleman, L. A., Liu, X. B., El-Sabeawy, F., Jones, E. G., and McAllister, A. K. (2010). MHC class I molecules are present both pre- and postsynaptically in the visual cortex during postnatal development and in adulthood. Proc. Natl. Acad. Sci. U.S.A. 107, 16999–17004. doi: 10.1073/pnas.1006087107
Neumann, H., Cavalie, A., Jenne, D. E., and Wekerle, H. (1995). Induction of MHC class I genes in neurons. Science 269, 549–552. doi: 10.1126/science.7624779
Neumann, H., Schmidt, H., Cavalie, A., Jenne, D., and Wekerle, H. (1997). Major histocompatibility complex (MHC) class I gene expression in single neurons of the central nervous system: differential regulation by interferon (IFN)-gamma and tumor necrosis factor (TNF)-alpha. J. Exp. Med. 185, 305–316. doi: 10.1084/jem.185.2.305
Patel, S. S., Nota, S. P., Sabbatino, F., Nielsen, G. P., Deshpande, V., Wang, X., et al. (2020). Defective HLA class I expression and patterns of lymphocyte infiltration in chordoma tumors. Clin. Orthop. Relat. Res. 479, 1373–1382. doi: 10.1097/CORR.0000000000001587
Reches, A., Nachmani, D., Berhani, O., Duev-Cohen, A., Shreibman, D., Ophir, Y., et al. (2016). HNRNPR regulates the expression of classical and nonclassical MHC class I proteins. J. Immunol. 196, 4967–4976. doi: 10.4049/jimmunol.1501550
Ribic, A., Zhang, M., Schlumbohm, C., Matz-Rensing, K., Uchanska-Ziegler, B., Flugge, G., et al. (2010). Neuronal MHC class I molecules are involved in excitatory synaptic transmission at the hippocampal mossy fiber synapses of marmoset monkeys. Cell. Mol. Neurobiol. 30, 827–839. doi: 10.1007/s10571-010-9510-3
Rolleke, U., Flugge, G., Plehm, S., Schlumbohm, C., Armstrong, V. W., Dressel, R., et al. (2006). Differential expression of major histocompatibility complex class I molecules in the brain of a New World monkey, the common marmoset (Callithrix jacchus). J. Neuroimmunol. 176, 39–50. doi: 10.1016/j.jneuroim.2006.04.015
Shatz, C. J. (2009). MHC class I: an unexpected role in neuronal plasticity. Neuron 64, 40–45. doi: 10.1016/j.neuron.2009.09.044
Smith, L. K., He, Y., Park, J. S., Bieri, G., Snethlage, C. E., Lin, K., et al. (2015). beta2-microglobulin is a systemic pro-aging factor that impairs cognitive function and neurogenesis. Nat. Med. 21, 932–937. doi: 10.1038/nm.3898
Staehli, F., Ludigs, K., Heinz, L. X., Seguin-Estevez, Q., Ferrero, I., Braun, M., et al. (2012). NLRC5 deficiency selectively impairs MHC class I- dependent lymphocyte killing by cytotoxic T cells. J. Immunol. 188, 3820–3828. doi: 10.4049/jimmunol.1102671
Tetruashvily, M. M., Melson, J. W., Park, J. J., Peng, X., and Boulanger, L. M. (2016). Expression and alternative splicing of classical and nonclassical MHCI genes in the hippocampus and neuromuscular junction. Mol. Cell. Neurosci. 72, 34–45. doi: 10.1016/j.mcn.2016.01.005
van den Boomen, D. J., and Lehner, P. J. (2015). Identifying the ERAD ubiquitin E3 ligases for viral and cellular targeting of MHC class I. Mol. Immunol. 68(2 Pt A), 106–111. doi: 10.1016/j.molimm.2015.07.005
VanGuilder Starkey, H. D., Van Kirk, C. A., Bixler, G. V., Imperio, C. G., Kale, V. P., Serfass, J. M., et al. (2012). Neuroglial expression of the MHCI pathway and PirB receptor is upregulated in the hippocampus with advanced aging. J. Mol. Neurosci. 48, 111–126. doi: 10.1007/s12031-012-9783-8
Weissman, J. D., and Singer, D. S. (1991). A complex regulatory DNA element associated with a major histocompatibility complex class I gene consists of both a silencer and an enhancer. Mol. Cell. Biol. 11, 4217–4227. doi: 10.1128/mcb.11.8.4217-4227.1991
Wu, Z. P., Washburn, L., Bilousova, T. V., Boudzinskaia, M., Escande-Beillard, N., Querubin, J., et al. (2011). Enhanced neuronal expression of major histocompatibility complex class I leads to aberrations in neurodevelopment and neurorepair. J. Neuroimmunol. 232, 8–16. doi: 10.1016/j.jneuroim.2010.09.009
Keywords: hippocampus, neuron, gene expression regulation, MHC class I, mice
Citation: Shen Y and Zhang J (2021) Tight Regulation of Major Histocompatibility Complex I for the Spatial and Temporal Expression in the Hippocampal Neurons. Front. Cell. Neurosci. 15:739136. doi: 10.3389/fncel.2021.739136
Received: 10 July 2021; Accepted: 06 September 2021;
Published: 01 October 2021.
Edited by:
Takeshi Kawauchi, Foundation for Biomedical Research and Innovation, JapanReviewed by:
Karen Litwa, East Carolina University, United StatesMan Jiang, Huazhong University of Science and Technology, China
Copyright © 2021 Shen and Zhang. This is an open-access article distributed under the terms of the Creative Commons Attribution License (CC BY). The use, distribution or reproduction in other forums is permitted, provided the original author(s) and the copyright owner(s) are credited and that the original publication in this journal is cited, in accordance with accepted academic practice. No use, distribution or reproduction is permitted which does not comply with these terms.
*Correspondence: Yuqing Shen, eXVxaW5nc2hlbnNldUBob3RtYWlsLmNvbQ==; orcid.org/0000-0002-1307-7360