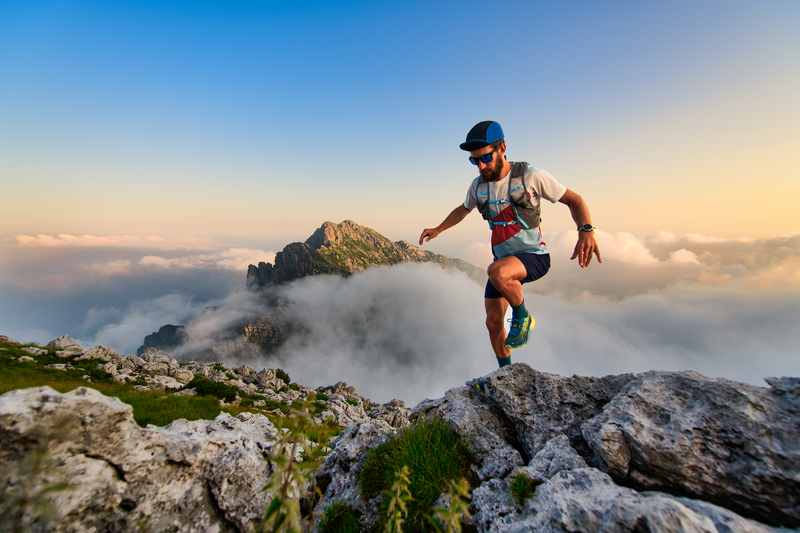
95% of researchers rate our articles as excellent or good
Learn more about the work of our research integrity team to safeguard the quality of each article we publish.
Find out more
MINI REVIEW article
Front. Cell. Neurosci. , 08 October 2021
Sec. Cellular Neurophysiology
Volume 15 - 2021 | https://doi.org/10.3389/fncel.2021.730641
This article is part of the Research Topic ASICs: Structure, Function, and Pharmacology, Volume I View all 11 articles
Acidic pH shift occurs in many physiological neuronal activities such as synaptic transmission and synaptic plasticity but also represents a characteristic feature of many pathological conditions including inflammation and ischemia. Neuroinflammation is a complex process that occurs in various neurodegenerative diseases such as Alzheimer’s disease, Parkinson’s disease, multiple sclerosis, and Huntington’s disease. Acid-sensing ion channels (ASICs) represent a widely expressed pH sensor in the brain that play a key role in neuroinflammation. On this basis, acid-sensing ion channel blockers are able to exert neuroprotective effects in different neurodegenerative diseases. In this review, we discuss the multifaceted roles of ASICs in brain physiology and pathology and highlight ASIC1a as a potential pharmacological target in neurodegenerative diseases.
The maintenance of cytosolic pH in its physiological range is required for normal neuronal activity, and even minor alterations can have serious consequences. Under normal physiological conditions, intra- and extracellular pH is maintained between 7.0 and 7.3; however, normal neuronal activity causes local pH changes (Chen and Chesler, 1992; Chesler and Kaila, 1992; Makani and Chesler, 2007; Magnotta et al., 2012). In the mammalian central nervous system (CNS), increased neuronal activity and the intense synchronous synaptic activity occurring in the synaptic plasticity process alter pH, producing alkalinization followed by acidification (DeVries, 2001; Du et al., 2014).
Under pathological conditions, including ischemic strokes, seizures, and inflammation associated with several neurodegenerative diseases, the pH shifts toward a persistent acidification that is correlated with neuronal hyperexcitability (Chiacchiaretta et al., 2017).
Neuroinflammation within the brain or spinal cord is mediated by the release of cytokines, chemokines, reactive oxygen species produced from resident microglia and astrocytes, endothelial cells, and peripherally derived immune cells (DiSabato et al., 2016). In the last decades, correlation between neuroinflammation and neurodegeneration has become increasingly solid in the light of accumulating evidence gathered on Alzheimer’s disease (AD), Parkinson’s disease (PD), and multiple sclerosis (MS) (Salter and Stevens, 2017).
Several studies in animal models and in humans have demonstrated that neurodegenerative diseases, characterized by a persistent inflammatory state, are associated with a significant reduction in the brain pH (Amor et al., 2010; Tyrtyshnaia et al., 2016; Decker et al., 2021), suggesting that neuroinflammation per se affects brain pH level. All this evidence has prompted the investigation of the role of acid-sensing ion channels (ASICs) in neurodegenerative diseases. The present work provides an overview of the possible roles of ASIC1a in neurodegenerative conditions and highlights this protein as a new potential therapeutic target.
ASICs belong to a class of voltage-insensitive cation channels, which are widely expressed in the central and peripheral nervous systems (Sherwood et al., 2012; Vullo and Kellenberger, 2020; Rook et al., 2021). Today, it is well accepted that protons (H+) can act as neurotransmitters through the specific activation of proton-sensing channels, and these channels were cloned and named for the first time as ASICs in 1997 (Waldmann et al., 1997). Thereafter, many studies have investigated their structure, expression, and localization, and many experimental ligands, to explore and understand the role and function of ASIC in physiological and pathological processes, were developed (Figure 1; Chesler, 2003; Mango and Nisticò, 2020; Rook et al., 2021). Among six different mammalian protein subunits that have been cloned (ASIC1a, ASIC1b, ASIC2a, ASIC2b, ASIC3, and ASIC4), ASIC1 and ASIC2 are predominant subunits in the CNS (Waldmann et al., 1997; Chen et al., 1998; Bässler et al., 2001). Each ASIC subunit has two transmembrane domains and a large extracellular domain enriched with acidic residues. ASICs can be assembled as either homotrimers or heterotrimers to form functional channels (Bassilana et al., 1997; Babinski et al., 1999; Jasti et al., 2007; Gonzales et al., 2009; Magnotta et al., 2012; Rook et al., 2021).
Figure 1. Acid-sensing ion channel 1a (ASIC1a) structure and the binding sites of its modulators. (A) A schematic view of the ASIC1 subunit highlighting the different domains: finger (purple), knuckle (turquoise), β-ball (orange), palm (yellow), and thumb (blue) and transmembrane domains (red). The binding sites for the different drugs are also indicated. (B) Crystal structure of an ASIC1 subunit obtained from chicken ASIC1 binding Mit-Tx (Baconguis et al., 2014). The domains are colored as in panel A. Readapted from Boscardin et al. (2016).
The most important characteristic of ASICs is the high pH sensitivity (Wemmie et al., 2013) making them the best candidates for pH sentinels in the brain that can detect and respond to small pH variations (Papalampropoulou-Tsiridou et al., 2020; Rook et al., 2021). A recent study has provided insights into the role of ASIC currents in the generation of action potential (AP) firing in neurons in relation to the speed of pH change. The authors demonstrate that moderate acidification (4–10 s) mediates AP increase, while slow acidification (>10 s) is not able to induce AP changes, suggesting a new important parameter that influences ASIC modulation of neuronal depolarization (Alijevic et al., 2020).
Most studies are focused on the most abundant form of an ASIC channel expressed in the CNS, which is represented by ASIC1a. Activation of ASIC1a plays a key role in pathological processes by promoting neuronal death via calcium (Ca2+)-mediated toxicity (Yermolaieva et al., 2004). The abundant expression of ASIC1a in discrete regions of the brain, such as the hippocampus, cortex, striatum, and amygdala (Alvarez de la Rosa et al., 2003; Wemmie et al., 2003; Coryell et al., 2009) and the characteristic to be Ca2+ permeable has suggested a role for this channel in calcium-related processes such as synaptic plasticity, learning and memory, as well as neuronal death (Wemmie et al., 2002; Gao et al., 2015; Wang et al., 2015). Accordingly, blocking ASIC1a affects multiple forms of synaptic plasticity in many brain regions including the amygdala and hippocampus (Wemmie et al., 2002, 2003; Du et al., 2014; Mango et al., 2017; Mango and Nisticò, 2020). Inhibition of ASIC1a produces a reduction in magnitude of both forms of synaptic plasticity long-term potentiation (LTP) and long-term depression (LTD) and ASIC1a KO mice show impairment of learning and memory evaluated with the Morris water maze and elevated-plus maze behavioral tests.
Moreover, the interplay between ASIC1a and NMDA, AMPA, and GABA receptors represents a potential target for acidotoxicity and exitotoxicity that occur in pathological conditions, such as ischemia (Gao et al., 2005, 2016; Chen et al., 2011; González-Inchauspe et al., 2017). Intriguingly, protons are able to modulate different synaptic receptors including AMPA and NMDA glutamate receptors (Tang et al., 1990; Traynelis and Cull-Candy, 1990; Lei et al., 2001), as well as GABAA receptors (Kaila, 1994; Dietrich and Morad, 2010), indicating that pH changes affect neuronal excitability, neurotransmission, and post-synaptic responses (Chen and Chesler, 1992; Makani and Chesler, 2007; Dietrich and Morad, 2010).
Growing evidence supports the relationship between neuroinflammation and synaptic degeneration as a common feature in neurodegenerative diseases including AD, PD, and MS (Nisticò et al., 2017; Guzman-Martinez et al., 2019). Acidosis and accumulation of protons in the extracellular space represent hallmarks of the inflammatory process (see Diaz et al., 2018). The shift to the acidic pH was observed in normal aging and in patients affected by a variety of neurodegenerative disorders and was also confirmed in experimental models of neuroinflammation (Roberts and Sick, 1996; Roberts and Chih, 1997; Friese et al., 2007; Vergo et al., 2011; Mandal et al., 2012; Tyrtyshnaia et al., 2016).
To date, there is limited experimental evidence linking ASICs to neuroinflammation. For example, NSAIDs were reported to act against ASICs by both preventing inflammation-induced increase in expression and directly inhibiting the channels (Voilley et al., 2001). Our group has published that CHF5074 (also called CSP-1103), a flurbiprofen derivative, induces inhibition of ASIC1a-mediated currents elicited by application of pH 5.5 bath medium (IC50 ∼50 nM) (Mango et al., 2014). A previous work has also demonstrated an increased expression of ASIC3 on nerve afferents supplying joints in response to inflammatory stimulus and an anti-inflammatory action exerted by ASIC3 inhibitors in animal models of rheumatoid arthritis. Notably, ASIC–/– animals manifest higher joint inflammation and destruction compared with ASIC+/+ controls (Sluka et al., 2013).
Therefore, ASICs represent the receptors on which the protons act as neurotransmitter and prolonged changes in pH shift likely contribute to the chronic inflammatory states underlying the neurodegenerative process.
AD is a major cause of dementia in the world population. AD is characterized by chronic neurodegeneration with progressive memory impairment (Chapman et al., 1999), and currently, there is limited effective treatment or cure.
Several studies highlighted a shift to acidic pH in the AD brain caused by reduced cerebral perfusion (Siesjo, 1988). Changes in pH have also been observed in cerebrospinal fluid and post-mortem brain tissue of AD patients (Liu et al., 1999; Preece and Cairns, 2003).
ASIC1a plays a key role in synaptic plasticity processes including neurotransmission, dendritic structural remodeling, learning and memory, and fear response. This has been widely demonstrated in non-clinical studies using electrophysiological and behavioral approaches in mice (Wemmie et al., 2002, 2003; Chu and Xiong, 2012; Huang et al., 2015; Mango and Nisticò, 2019).
However, the role of ASIC1a in the synaptic alterations underlying AD remains still elusive. Indeed, our group has previously demonstrated an involvement of ASIC1a in hippocampal LTD ion in two experimental models of AD (Mango and Nisticò, 2018). Specifically, blocking ASIC1a with the selective blocker psalmotoxin-1 inhibited the enhancement of mGlu receptor-dependent LTD seen in the hippocampal slices, which were either treated with Aβ oligomers or obtained from Tg2576 mouse model of AD (Mango and Nisticò, 2018). The significance of these results remains to be established, and further studies need to be conducted in order to clarify the ASIC1a involvement in AD pathophysiology.
PD is a neurodegenerative disorder characterized by progressive and selective degeneration of dopaminergic neurons localized in the substantia nigra pars compacta (SNpc) area of the brain and cytoplasmic inclusions of alpha-synuclein aggregates, called Lewy bodies (Gelders et al., 2018). The neurodegeneration is responsible for motor and non-motor symptomatology including tremor, rigidity, bradykinesia, olfactory dysfunction, sleep disturbance, and cognitive impairment (Gelders et al., 2018).
Several studies described an elevated concentration of neuroinflammatory markers in PD patients suggesting the hypothesis that inflammation plays a role in neurodegeneration (Gelders et al., 2018). Also, PD is associated with lactic acidosis, and a study performed using an animal model of PD, the MPTP-treated mouse, described an involvement of ASIC1a in neurodegeneration of dopaminergic neurons. Indeed, blocking ASIC1a with amiloride or psalmotoxin-1 preserved dopaminergic neurons of SNpc from degeneration (Arias et al., 2008). Mutations in Parkin gene associated with autosomal recessive juvenile onset of PD (Kitada et al., 1998) or lack of the Parkin gene facilitate ASIC1a currents in hippocampal neurons evoked by application of acidic extracellular solution, and increase vulnerability of dopaminergic neurons, suggesting a significant role of ASIC1a in PD pathophysiology (Joch et al., 2007).
Nevertheless, not many studies have been performed to verify the role of ASIC1a as a therapeutic target for PD.
MS is a chronic autoimmune inflammatory disease characterized by demyelination and axonal degeneration processes (Goldenberg, 2012).
Many studies investigated the involvement of ASIC1a in MS using a well-accepted animal model of MS that is the experimental autoimmune encephalomyelitis (EAE) model (Friese et al., 2007; Bjelobaba et al., 2018). Previous studies found ASIC1a upregulation in axons and oligodendrocytes in the EAE model and in MS patients (Vergo et al., 2011). In MS patients, a relationship between elevated ASIC1a expression and axon injury markers was demonstrated, and this was also confirmed in EAE mice (Vergo et al., 2011; Arun et al., 2013). Also, administration of ASIC blocker amiloride attenuated demyelination and neuronal damage in EAE animal model as well as in a cohort of MS patients (Arun et al., 2013), indicating that ASIC1a inhibition is neuroprotective and represents a promising therapeutic strategy for MS.
HD is a rare hereditary neurodegenerative disease characterized by movement and cognitive disorders and polyglutamine repeat as pathologic hallmarks (Agostinho et al., 2013). Not much evidence is present in literature linking ASIC1a to HD. The acidosis that occurs in the brain of HD patients and in mouse models of HD suggests an involvement of these channels in the pathophysiology of HD (Tsang et al., 2006; Josefsen et al., 2010). Notably, blocking ASIC1a with an amiloride derivative benzamil was able to alleviate pathology through reduction of Huntingtin–polyglutamine aggregation, the HD hallmark in the striatum of R6/2 mice model of HD (Wong et al., 2008).
Experimental evidence suggests that ASIC1a could represent a new therapeutic target for neurodegenerative diseases. Below, we present the main ligands targeting ASIC1a, and the binding sites of ASIC1a are illustrated in Figure 1.
Amiloride a potassium-sparing diuretic agent and acts as a non-specific ASIC1a inhibitor in the range of 1–100 μM, which act by binding to the ion pore (Schild et al., 1997; Leng et al., 2016). Clinical studies have detected a neuroprotective and myeloprotective effect in patients with primary progressive MS (Arun et al., 2013). Nevertheless, amiloride was not effective in reducing the brain atrophy in patients with secondary progressive multiple sclerosis (De Angelis et al., 2020). In the light of these data, it could be important to test other amiloride analogs, such as benzamil with increased potency and selectivity for ASIC1a (Leng et al., 2016).
Non-steroidal anti-inflammatory drugs (NSAIDs) are a class of drugs widely used for the treatment of inflammation, pain, and many other disorders (Voilley, 2004). Previous studies have demonstrated that flurbiprofen, ibuprofen, and derivates mediate a direct inhibition of ASIC currents acting on the β-ball domain, with a significant reduction in evoked currents by the application of acidic medium (Voilley et al., 2001; Mango et al., 2014).
Different small molecules targeting ASICs were developed and represent new pharmacological tools used to study the ASICs function. Some of these molecules are clinically tested for therapeutic use, as PPC-5650 for the irritable bowel syndrome (Dubé et al., 2009; Olesen et al., 2015).
In this class can be cited NS-383 small potent inhibitor of ASIC1a (Munro et al., 2016) and two potent allosteric antagonists of ASIC1a JNJ-799760 and JNJ-67869386, which act by binding to the acidic pocket (Liu et al., 2021). Other new potent antagonists are represented by A-317567 and 5b (Dubé et al., 2005; Buta et al., 2015).
Psalmotoxin-1 (PcTx-1) was the first toxin identified as a selective ASIC1a inhibitor, which acts by binding to an acidic pocket (Escoubas et al., 2000). Although, the structure of PcTx-1 does not allow passage through the blood–brain barrier, previous studies demonstrated its neuroprotective effect in different experimental models of neurodegenerative diseases via intrathecal or intracerebroventricular administration (Diochot et al., 2012; Baron et al., 2013; Chassagnon et al., 2017).
ASC06-IgG1 is a novel selective ASIC1a-blocking monoclonal antibody, which showed potent, sustained, and highly selective inhibition of ASIC1a (Qiang et al., 2018). This specific antibody shows a neuroprotective effect against ischemia (Qiang et al., 2018). Further studies are needed to investigate the neuroprotective effect of ASC06-IgG in experimental models of neurodegenerative diseases. Today, numerous monoclonal antibodies have been approved or are under development for neurological diseases (Sirbu et al., 2021), but many limitations, including the route of administration, as well as class-specific and target-associated risks, have limited their use (Loureiro et al., 2014, 2017; Teleanu et al., 2019).
Neurodegenerative diseases are characterized by different and multifactorial processes sharing accumulation of misfolding proteins, damage of specific neuronal populations, and chronic inflammation state neuroinflammation. Another common feature is the shift to the acidic pH in the brain, which might contribute to apoptosis, protein misfolding, excitotoxicity, and neurodegeneration (Parton et al., 1991; Bender et al., 1997; Ding et al., 2000; Liu et al., 2012; Wang et al., 2012).
Emerging evidence supports the role of ASIC activation in several physiological processes such as synaptic plasticity and memory. On the other hand, ASICs are implicated in the pathophysiology of inflammatory and neurodegenerative diseases including PD, MS, AD, and HD.
The complex molecular pathways leading to neurodegeneration makes the search for novel effective agents difficult. In this frame, the development of potent and specific blockers for individual ASIC subunits, as well as the targeting of endogenous signaling molecules modulating the function of ASICs, might represent a new approach for the treatment of neurodegenerative conditions.
DM conceived the idea and prepared the manuscript. RN reviewed the drafts. Both authors contributed to the writing and approved final version of the manuscript.
The authors declare that the research was conducted in the absence of any commercial or financial relationships that could be construed as a potential conflict of interest.
All claims expressed in this article are solely those of the authors and do not necessarily represent those of their affiliated organizations, or those of the publisher, the editors and the reviewers. Any product that may be evaluated in this article, or claim that may be made by its manufacturer, is not guaranteed or endorsed by the publisher.
Agostinho, L. A., Dos Santos, S. R., Alvarega, R. M., and Paiva, C. L. (2013). A systematic review of the intergenerational aspects and the diverse genetic profiles of Huntington’s disease. Genet. Mol. Res. 12, 1974–1981.
Alijevic, O., Bignucolo, O., Hichri, E., Peng, Z., Kucera, J. P., and Kellenberger, S. (2020). Slowing of the time course of acidification decreases the acid-sensing ion channel 1a current amplitude and modulates action potential firing in neurons. Front. Cell. Neurosci. 28:41. doi: 10.3389/fncel.2020.00041
Alvarez de la Rosa, D., Krueger, S. R., Kolar, A., Shao, D., Fitzsimonds, R. M., and Canessa, C. M. (2003). Distribution, subcellular localization and ontogeny of ASIC1 in the mammalian central nervous system. J. Physiol. 546, 77–87. doi: 10.1113/jphysiol.2002.030692
Amor, S., Puentes, F., Baker, D., and van der Valk, P. (2010). Inflammation in neurodegenerative diseases. Immunology 129, 154–169.
Arias, R. L., Sung, M. L. I., Vasylyev, D., Zhang, M. Y., Albinson, K., Kubek, K., et al. (2008). Amiloride is neuroprotective in an MPTP model of Parkinson’s disease. Neurobiol. Dis. 31, 334–341. doi: 10.1016/j.nbd.2008.05.008
Arun, T., Tomassini, V., Sbardella, E., de Ruiter, M. B., Matthews, L., Leite, M. I., et al. (2013). Targeting ASIC1 in primary progressive multiple sclerosis: evidence of neuroprotection with amiloride. Brain 136, 106–115. doi: 10.1093/brain/aws325
Babinski, K., Le, K. T., and Seguela, P. (1999). Molecular cloning and regional distribution of a human proton receptor subunit with biphasic functional properties. J. Neurochem. 72, 51–57. doi: 10.1046/j.1471-4159.1999.0720051.x
Baconguis, I., Bohlen, C. J., Goehring, A., Julius, D., and Gouaux, E. (2014). X-ray structure of acid-sensing ion channel 1-snake toxin complex reveals open state of a Na(+)-selective channel. Cell 156, 717–729. doi: 10.1016/j.cell.2014.01.011
Baron, A., Diochot, S., Salinas, M., Deval, E., Noel, J., and Lingueglia, E. (2013). Venom toxin in the exploration of molecular, physiological and pathophysiological functions of acid-sensing ion channels. Toxicon 75, 187–204. doi: 10.1016/j.toxicon.2013.04.008
Bassilana, F., Champigny, G., Waldmann, R., de Weille, J. R., Heurteaux, C., and Lazdunski, M. (1997). The acid-sensitive ionic channel subunit ASIC and the mammalian degenerin MDEG form a heteromultimeric H+-gated Na+ channel with novel properties. J. Biol. Chem. 272, 28819–28822. doi: 10.1074/jbc.272.46.28819
Bässler, E. L., Ngo-Anh, T. J., Geisler, H. S., Ruppersberg, J. P., and Gründer, S. (2001). Molecular and functional characterization of acid-sensing ion channel (ASIC) 1b. J. Biol. Chem. 276, 33782–33787. doi: 10.1074/jbc.m104030200
Bender, A. S., Young, L. P., and Norenberg, M. D. (1997). Effect of lactic acid on L-glutamate uptake in cultured astrocytes: Mediators of Inflammation mechanistic considerations. Brain Res. 1, 59–66. doi: 10.1016/s0006-8993(96)01331-5
Bjelobaba, I., Bergovic-Kupresanin, V., Pekovic, S., and Lavnja, I. (2018). Animal models of multiple sclerosis: Focus on experimental autoimmuneencephalomyelitis. J. Neurosci. Res. 96, 1021–1042. doi: 10.1002/jnr.24224
Boscardin, E., Alijevic, O., Hummler, E., Frateschi, S., and Kellenberger, S. (2016). The function and regulation of acid-sensing ion channels (ASICs) and the epithelian Na+ channel (ENaC): IUPHAR Review 19. Br. J. Pharmacol. 173, 2671–2701. doi: 10.1111/bph.13533
Buta, A., Maximyuk, O., Kovalskyy, D., Sukach, V., Vovk, M., Ievglevskyi, O., et al. (2015). Novel potent orthosteric antagonist of ASIC1a prevents NMDAR-dependent LTP induction. J. Med. Chem. 58, 4449–4461. doi: 10.1021/jm5017329
Chapman, P. F., White, G. L., Jones, M. W., Cooper-Blacketer, D., Marshall, V. J., Irizarry, M., et al. (1999). Impaired synaptic plasticity and learning in aged amyloid precursor protein transgenic mice. Nat. Neurosci. 2, 271–276. doi: 10.1038/6374
Chassagnon, I. R., McCarthy, C. A., Chin, Y. K., Pineda, S. S., Keramidasd, A., Moblie, M., et al. (2017). Potent neuroprotection after stroke afforded by a double-knot spider-venom peptide that inhibits acid-sensing ion channel 1a. Proc. Natl. Acad. Sci. U.S.A. 114, 3750–3755. doi: 10.1073/pnas.1614728114
Chen, C. C., England, S., Akopian, A. N., and Wood, J. N. (1998). A sensory neuron-specific, proton-gated ion channel. Proc. Natl. Acad. Sci. U.S.A. 95, 10240–10245. doi: 10.1073/pnas.95.17.10240
Chen, J. C., and Chesler, M. (1992). pH transients evoked by excitatory synaptic transmission are increased by inhibition of extracellular carbonic anhydrase. Proc. Natl. Acad. Sci. U.S.A 89, 7786–7790. doi: 10.1073/pnas.89.16.7786
Chen, X., Whissell, P., Orser, B. A., and MacDonald, J. F. (2011). Functional modifications of acid-sensing ion channels by ligand-gated chloride channels. PLoS One 6:e21970. doi: 10.1371/journal.pone.0021970
Chesler, M. (2003). Regulation and modulation of pH in the brain. Physiol. Rev. 83, 1183–1221. doi: 10.1152/physrev.00010.2003
Chesler, M., and Kaila, K. (1992). Modulation of pH by neuronal activity. Trends Neurosci. 15, 396–402. doi: 10.1016/0166-2236(92)90191-a
Chiacchiaretta, M., Latifi, S., Bramini, M., Fadda, M., Fassio, A., Benfenati, F., et al. (2017). Neuronal hyperactivity causes Na +/H + exchanger-induced extracellular acidification at active synapses. J. Cell Sci. 130, 1435–1449. doi: 10.1242/jcs.198564
Chu, X. P., and Xiong, Z. G. (2012). Physiological and pathological functions of Acid-sensing ion channels in the central nervous system. Curr. Drug Targets 13, 263–271. doi: 10.2174/138945012799201685
Coryell, M. W., Wunsch, A. M., Haenfler, J. M., Allen, J. E., Schnizler, M., Ziemann, A. E., et al. (2009). Acid-sensing ion channel-1a in the amygdala, a novel therapeutic target in depression-related behavior. J. Neurosci. 29, 5381–5388. doi: 10.1523/JNEUROSCI.0360-09.2009
De Angelis, F., Connick, P., Parker, R. A., Plantone, D., Doshi, A., John, N., et al. (2020). Amiloride, fluoxetine or riluzole to reduce brain volume loss in secondary progressive multiple sclerosis: the MS-SMART four-arm RCT. Effic. Mech. Eval. 7:32453521. doi: 10.3310/eme07030
Decker, Y., Németh, E., Schomburg, R., Chemla, A., Fülöp, L., Menger, M. D., et al. (2021). Decreased pH in the aging brain and Alzheimer’s disease. Neurobiol. Aging 101, 40–49. doi: 10.1016/j.neurobiolaging.2020.12.007
DeVries, S. H. (2001). Exocytosen protons feedback to suppress the Ca2+ current in mammalian cone photoreceptors. Neuron 32, 1107–1117. doi: 10.1016/s0896-6273(01)00535-9
Diaz, F. E., Dantas, E., and Geffner, J. (2018). Unrevelling the interplay between extracellular acidosis and immune cells. Mediators of Inflamm. 2018:1218297. doi: 10.1155/2018/1218297
Dietrich, C. J., and Morad, M. (2010). Synaptic acidification enhances GABAA signaling. J. Neurosci. 30, 16044–16052. doi: 10.1523/JNEUROSCI.6364-09.2010
Ding, D., Moskowitz, S. I, Li, R., Lee, S. B., Esteban, M., Tomaselli, K., et al. (2000). Acidosis induces necrosis and apoptosis of cultured hippocampal neurons. Exp. Neurol. 162, 1–12. doi: 10.1006/exnr.2000.7226
Diochot, S., Baron, A., Salinas, M., Douguet, D., Scarzello, S., Dabert-Gay, A. S., et al. (2012). Black mamba venom peptides target acid-sensing ion channels to abolish pain. Nature 490, 552–555. doi: 10.1038/nature11494
DiSabato, D. J., Quan, N., and Godbout, J. P. (2016). Neuroinflammation: the devil is in the details. J. Neurochem. 139(Suppl. 2), 136–153. doi: 10.1111/jnc.13607
Du, J., Reznikov, L. R., Price, M. P., Zha, X. M., Lu, Y., Moninger, T. O., et al. (2014). Protons are a neurotransmitter that regulates synaptic plasticity in the lateral amygdala. Proc. Natl. Acad. Sci. U.S.A. 111, 8961–8966. doi: 10.1073/pnas.1407018111
Dubé, G. R., Elagoz, A., and Mangat, H. (2009). Acid sensing ion channels and acid nociception. Curr. Pharm. Des. 15, 1750–1766. doi: 10.2174/138161209788186263
Dubé, G. R., Lehto, S. G., Breese, N. M., Baker, S. J., Wang, X., Matulenko, M. A., et al. (2005). Electrophysiological and in vivo characterization of A-317567, a novel blocker of acid sensing ion channels. Pain 117, 88–96. doi: 10.1016/j.pain.2005.05.021
Escoubas, P., de Weille, J. R., Lecoq, A., Diochot, S., Waldmann, R., Champigny, G., et al. (2000). Isolation of a tarantula toxin specific for a class of proton-gated Na+ channels. J. Biol. Chem. 33, 25116–25121. doi: 10.1074/jbc.M003643200
Friese, M. A., Craner, M. J., Etzensperger, R., Vergo, S., Wemmie, J. A., Welsh, M. J., et al. (2007). Acid-sensing ion channel-1 contributes to axonal degeneration in autoimmune inflammation of the central nervous system. Nat. Med. 13, 1483–1489. doi: 10.1038/nm1668
Gao, J., Duan, B., Wang, D. G., Deng, X. H., Zhang, G. Y., Xu, L., et al. (2005). Coupling between NMDA receptor and acid-sensing ion channel contributes to ischemic neuronal death. Neuron 48, 635–646. doi: 10.1016/j.neuron.2005.10.011
Gao, S., Yu, Y., Ma, Z., Sun, H., Zhang, Y. L., Wang, X. T., et al. (2015). NMDA-Mediated hippocampal neuronal death is exacerbated by activities of ASIC1a. Neurotox Res. 28, 122-137. doi: 10.1007/s12640-015-9530-3
Gao, S., Yu, Y., Ma, Z. Y., Sun, H., Zhang, Y. L., Wang, X. T., et al. (2016). NMDAR-mediated hippocampal neuronal death is exacerbated by activities of ASIC1a. Neurotox. Res. 28, 122–137. doi: 10.1007/s12640-015-9530-3
Gelders, G., Baekelandt, V., and Van der Perren, A. (2018). Linking neuroinflammation and neurodegeneration in Parkinson’s disease. J. Immunol. Res. 2018:4784268. doi: 10.1155/2018/4784268
Gonzales, E. B., Kawate, T., and Gouaux, E. (2009). Pore architecture and ion sites in acid- sensing ion channels and P2X receptors. Nature 460, 599–604. doi: 10.1038/nature08218
González-Inchauspe, C., Urbano, F. J., Di Guilmi, M. N., and Uchitel, O. D. (2017). Acid-Sensing ion channels activated by evoked released protons modulate synaptic transmission at the mouse calyx of held synapse. J. Neurosci. 37, 2589–2599. doi: 10.1523/JNEUROSCI.2566-16.2017
Guzman-Martinez, L., Maccioni, R. B., Andrade, V., Navarrete, L. P., Pastor, M. G., and Ramos-Escobar, N. (2019). Neuroinflammation as a common feature of neurodegenerative disorders. Front. Pharmacol. 10:1008. doi: 10.3389/fphar.2019.01008
Huang, Y., Jiang, N., Li, J., Ji, Y. H., Xiong, Z. J., and Zha, X. M. (2015). Two aspects of ASIC function: synaptic plasticity and neuronal injury. Neuropharmacology 94, 42–48. doi: 10.1016/j.neuropharm.2014.12.010
Jasti, J., Furukawa, H., Gonzales, E. B., and Gouaux, E. (2007). Structure of acid-sensing ion channel 1 at 1.9 A resolution and low pH. Nature 449, 316–323. doi: 10.1038/nature06163
Joch, M., Ase, A. R., Chen, C. X., MacDonald, P. A., Kontogiannea, M., Corera, A. T., et al. (2007). Parkin-mediated monoubiquitination of the PDZ protein PICK1 regulates the activity of acid-sensing ion channels. Mol. Biol. Cell 18, 3105–3118. doi: 10.1091/mbc.e05-11-1027
Josefsen, K., Nielsen, S. M., Campos, A., Seifert, T., Hasholt, L., Nielsen, J. E., et al. (2010). Reduced gluconeogenesis and lactate clearance in Huntington’s disease. Neurobiol. Dis. 40, 656–662. doi: 10.1016/j.nbd.2010.08.009
Kaila, K. (1994). Ionic basis of GABAA receptor channel function in the nervous system. Prog. Neurobiol. 42, 489–537. doi: 10.1016/0301-0082(94)90049-3
Kitada, T., Asakawa, S., Hattori, N., Matsumine, H., Yamamura, Y., Minoshima, S., et al. (1998). Mutations in the parkin gene cause autosomal recessive juvenile Parkinsonism. Nature 392, 605–608. doi: 10.1038/33416
Lei, S., Orser, B. A., Thatcher, G. R., Reynolds, J. N., and MacDonald, J. F. (2001). Positive allosteric modulators of AMPA receptors reduce proton-induced receptor desensitization in rat hippocampal neurons. J. Neurophysiol. 85, 2030–2038.
Leng, T. D., Si, H. F., Li, J., Yang, T., Zhu, M., Wang, B., et al. (2016). Amiloride Analogs as ASIC1a Inhibitors. CNS Neurosci. Ther. 22, 468–476.
Liu, C. H., Zhang, F., Krisrian, T., Polster, B., Fiskum, G. M., and Hu, B. (2012). “Protein aggregation and multiple organelle damage after brain ischemia,” in Translational Stroke Research eds P. Lapchak and J. Zhang (Berlin: Springer), 101–116
Liu, R. Y., Zhou, J. N., van Heerikhuize, J., Hofman, M. A., and Swaab, D. F. (1999). Decreased melatonin levels in postmortem cerebrospinal fluid in relation to aging, Alzheimer’s disease, and apolipoprotein E-epsilon4/4 genotype. J. Clin. Endocrinol. Metab. 84, 323–327. doi: 10.1210/jcem.84.1.5394
Liu, Y., Ma, J., DesJarlais, R. L., Hagan, R., Rech, J., Lin, D., et al. (2021). Molecular mechanism and structural basis of small-molecule modulation of the gating of acid-sensing ion channel 1. Commun. Biol. 4:174. doi: 10.1038/s42003-021-01678-1
Loureiro, J. A., Andrade, S., Duarte, A., Neves, A. R., Queiroz, J. F., Nunes, C., et al. (2017). Resveratrol and Grape Extract-loaded solid lipid nanoparticles for the treatment of Alzheimenr’s disease. Molecules 13, 222–277.
Loureiro, J. A., Gomes, B., Coelho, M. A., Carmo Pereira, M., and Rocha, S. (2014). Targeting nanoparticles across the blood-brain barrier with monoclonal antobodies. Nanomedicine 9, 709–722. doi: 10.2217/nnm.14.27
Magnotta, V. A., Heo, H. Y., Dlouhy, B. J., Dahdaleh, N. S., Follmer, R. L., Thedens, D. R., et al. (2012). Detecting activity- evoked pH changes in human brain. Proc. Natl. Acad. Sci. U.S.A. 109, 8270–8273. doi: 10.1073/pnas.1205902109
Makani, S., and Chesler, M. (2007). Endogenous alkaline transients boost postsynaptic NMDA receptor responses in hippocampal CA1 pyramidal neurons. J. Neurosci. 27, 7438–7446.
Mandal, P. K., Akolkar, H., and Tripathi, M. (2012). Mapping of hippocampal pH and neurochemicals from in vivo multi-voxel 31P study in healthy normal young male/female, mild cognitive impairment, and Alzheimer’s disease. J. Alzheimers Dis. 3, S75–S86.
Mango, D., and Nisticò, R. (2018). Role of ASIC1a in Aβ-induced synaptic alterations in the hippocampus. Pharmacol. Res. 131, 61–65. doi: 10.1016/j.phrs.2018.03.016
Mango, D., and Nisticò, R. (2019). Acid-Sensing ion channel 1a is involved in N-Methyl D-aspartate receptor-dependent long-term depression in the hippocampus. Front. Pharmacol. 10:555. doi: 10.3389/fphar.2019.00555
Mango, D., and Nisticò, R. (2020). Role of ASIC1a in normal and pathological synaptic plasticity. Rev. Physiol. Biochem. Pharmacol. 177, 83–100. doi: 10.1007/112_2020_45
Mango, D., Barbato, G., Piccirilli, S., Panico, M. B., Feligioni, M., Schepisi, C., et al. (2014). Electrophysiological and metabolic effects of CHF5074 in the hippocampus: protection against in vitro ischemia. Pharmacol. Res. 81, 83–90. doi: 10.1016/j.phrs.2014.02.010
Mango, D., Braksator, E., Battaglia, G., Marcelli, S., Mercuri, N. B., Feligioni, M., et al. (2017). Acid-sensing ion channel 1a is required for mGlu receptor dependent long-term depression in the hippocampus. Pharmacol. Res. 119, 12–19. doi: 10.1016/j.phrs.2017.01.028
Munro, G., Christensen, J. K., Erichsen, H. K., Dyhring, T., Demnitz, J., Dam, E., et al. (2016). NS383 selectively inhibits acid-sensing ion channels containing 1a and 3 subunits to reverse inflammatory and neuropathic hyperalgesia in rats. CNS Neurosci. Ther. 22, 135–145. doi: 10.1111/cns.12487
Nisticò, R., Salter, E., Nicolas, C., Feligioni, M., Mango, D., Bortolotto, Z. A., et al. (2017). Synaptoimmunology - roles in health and disease. Mol. Brain 10:26. doi: 10.1186/s13041-017-0308-9
Olesen, A. E., Nielsen, L. M., Larsen, I. M., and Drewes, A. M. (2015). Randomized clinical trial: efficacy and safety of PPC-5650 on experimental esophageal pain and hyperalgesia in healthy volunteer. Scand. J. Gastroenterol. 50, 138–144. doi: 10.3109/00365521.2014.966319
Papalampropoulou-Tsiridou, M., Labrecque, S., Godin, A. G., De Koninck, Y., and Wang, F. (2020). Differential expression of acid – sensing ion channels in mouse primary afferents in naïve and injured conditions. Front Cell Neurosci. 14:103. doi: 10.3389/fncel.2020.00103
Parton, R. G., Dotti, C. G., Bacallao, R., Kurtz, I., Simons, K., and Prydz, K. (1991). pH-induced microtubule-dependent redistribution of late endosomes in neuronal and epithelial cells. J. Cell Biol. 113, 261–274. doi: 10.1083/jcb.113.2.261
Preece, P., and Cairns, N. J. (2003). Quantifying mRNA in postmortem human brain: influence of gender, age at death, postmortem interval, brain pH, agonal state and inter-lobe mRNA variance. Brain Res. Mol. Brain Res. 118, 60–71. doi: 10.1016/S0169-328X(03)00337-1
Qiang, M., Dong, X., Zha, Z., Zuo, X.K., Song, X.L., Zhao, L., et al. (2018). Selectiom of an ASIC1a-blocking combinatorial antibody that protects cells from ischemic death. PNAS U.S.A. 115, E7469–E7477. doi: 10.1073/pnas.1807233115
Roberts, E. L., and Chih, C. P. (1997). The influence of age of pH regulation in hippocampal slices before, during, and after anoxia. J. Cereb. Blood Flow Metab. 17, 560–566. doi: 10.1097/00004647-199705000-00010
Roberts, E. L., and Sick, T. J. (1996). Aging impairs regulation of intracellular pH in rat hippocampal slices. Brain Res. 735, 339–342.
Rook, M. L., Musgaard, M., and MacLean, D. M. (2021). Coupling structure with function in acid-sensing ion channels: challenges in pursuit of proton sensors. J. Physiol. 599, 417–430. doi: 10.1113/JP278707
Salter, M. W., and Stevens, B. (2017). Microglia emerge as central players in brain disease. Nat. Med. 23, 1018–1027. doi: 10.1038/nm.4397
Schild, L., Schneeberger, E., Gautschi, I., and Firsov, D. (1997). Identification of amino acid residues in the alpha, beta, and gamma subunits of the epithelial sodium channel (ENaC) involved in amiloride block and ion permeation. J. Gen. Physiol. 1, 15–26.
Sherwood, T. W., Frey, E. N., and Askwith, C. C. (2012). Structure and activity of the acid-sensing ion channels. Am. J. Physiol. Cell Physiol. 303, C699–C710. doi: 10.1152/ajpcell.00188.2012
Sirbu, C. A., Ghinescu, M. C., Axelerad, A. D., Sirbu, A. M., and Ionita-Radu, F. (2021). A new era for monoclonal antibodies with applications in neurology. Exp. Ther. Med. 21:86. doi: 10.3892/etm.2020.9519
Sluka, K. A., Rasmussen, L. A., Edgar, M. M., O’Donnell, J. M., Walder, R. Y., Kolker, S. J., et al. (2013). Acid-sensing ion channel 3 deficiency increases inflammation but decreases pain behavior in murine arthritis. Arthritis Rheum. 65, 1194–1202. doi: 10.1002/art.37862
Tang, C. M., Dichter, M., and Morad, M. (1990). Modulation of the N-methyl-D- aspartate channel by extracellular H+. Proc. Natl. Acad. Sci. U.S.A. 87, 6445–6449. doi: 10.1073/pnas.87.16.6445
Teleanu, D. M., Negut, I., Grumezescu, V., Grumezescu, A. M., and Teleanu, R. I. (2019). Nanomaterials for drug delivery to the central nervous system. Nanomaterials 9:E371. doi: 10.3390/nano9030371
Traynelis, S. F., and Cull-Candy, S. G. (1990). Proton inhibition of N-methyl-D- aspartate receptors in cerebellar neurons. Nature 345, 347–350.
Tsang, T. M., Woodman, B., Mcloughlin, G. A., Griffin, J. L., Tabrizi, S. J., Bates, G. P., et al. (2006). Metabolic characterization of the R6/2 transgenic mouse model of Huntington’s disease by high-resolution MAS1H NMR spectroscopy. J. Proteome Res. 5, 483–492. doi: 10.1021/pr050244o
Tyrtyshnaia, A. A., Lysenko, L. V., Madamba, F., Manzhulo, I. V., Khotimchenko, M. Y., and Kleschevnikov, A. M. (2016). Acute neuroinflammation provokes intracellular acidification in mouse hippocampus. J. Neuroinflamm. 13:283. doi: 10.1186/s12974-016-0747-8
Vergo, S., Craner, M. J., Etzensperger, R., Attfield, K., Friese, M. A., Newcombe, J., et al. (2011). Acid-sensing ion channel 1 is involved in both axonal injury and demyelination in multiple sclerosis and its animal model. Brain 134, 571–584. doi: 10.1093/brain/awq337
Voilley, N. (2004). Acid-sensing ion channels (ASICs): new targets for the analgesic effects of non-steroid anti-inflammatory drugs (NSAIDs). Curr. Drug Targets Inflamm. Allergy 3, 71–79. doi: 10.2174/1568010043483980
Voilley, N., de Weille, J., Mamet, J., and Lazdunski, M. (2001). Nonsteroid anti-inflammatory drugs inhibit both the activity and the inflammation-induced expression of acid-sensing ion channels in nociceptors. J. Neurosci. 21, 8026–8033. doi: 10.1523/JNEUROSCI.21-20-08026.2001
Vullo, S., and Kellenberger, S. (2020). A molecular view of the function and pharmacology of acid-sensing ion channels. Pharmacol. Res. 154:104166. doi: 10.1016/j.phrs.2019.02.005
Waldmann, R., Champigny, G., Bassilana, F., Heurteaux, C., and Lazdunski, M. (1997). A proton-gated cation channel involved in acid-sensing. Nature 386, 173–177. doi: 10.1038/386173a0
Wang, J. Z., Xi, L., Zhu, G. F., Han, Y. G., Luo, Y., Wang, M., et al. (2012). The acidic pH-induced structural changes in Pin1 as revealed by spectral methodologies. Spectrochim. Acta Part A Mol. Biomol. Spectrosc. 98, 199–206. doi: 10.1016/j.saa.2012.07.105
Wang, Y. Z., Wang, J. J., Huang, Y., Liu, F., Zeng, W. Z., Li, Y., et al. (2015). Tissue acidosis induces neuronal necroptosis via ASIC1a channel independent of its ionic conduction. ELife 4:e05682. doi: 10.7554/eLife.05682.021
Wemmie, J. A., Askwith, C. C., Lamani, E., Cassell, M. D., Freeman, J. H. Jr., and Welsh, M. J. (2003). Acid-sensing ion channel 1 is localized in brain regions with high synaptic density and contributes to fear conditioning. J. Neurosci. 23, 5496–5502. doi: 10.1523/JNEUROSCI.23-13-05496.2003
Wemmie, J. A., Chen, J., Askwith, C. C., Hruska-Hageman, A. M., Price, M. P., Nolan, B. C., et al. (2002). The acid-activated ion channel ASIC contributes to synaptic plasticity, learning, and memory. Neuron 34, 463–477. doi: 10.1016/S0896-6273(02)00661-X
Wemmie, J. A., Taugher, R. J., and Kreple, C. J. (2013). Acid-sensing ion channels in pain and disease. Nat. Rev. Neurosci. 14, 461–471. doi: 10.1038/nrn3529
Wong, H. K., Bauer, P. O., Kurosawa, M., Goswami, A., Washizu, C., Machida, Y., et al. (2008). Blocking acid-sensing ion channel 1 alleviates Huntington’s disease pathology via an ubiquitin-proteasome system-dependent mechanism. Hum. Mol. Genet. 17, 3223–3235. doi: 10.1093/hmg/ddn218
Keywords: ASIC1a, neurodegenerative disease, neuroinflammation, pH shift, acidosis
Citation: Mango D and Nisticò R (2021) Neurodegenerative Disease: What Potential Therapeutic Role of Acid-Sensing Ion Channels? Front. Cell. Neurosci. 15:730641. doi: 10.3389/fncel.2021.730641
Received: 25 June 2021; Accepted: 15 September 2021;
Published: 08 October 2021.
Edited by:
Osvaldo D. Uchitel, University of Buenos Aires, ArgentinaReviewed by:
David MacLean, Medical Center, University of Rochester, United StatesCopyright © 2021 Mango and Nisticò. This is an open-access article distributed under the terms of the Creative Commons Attribution License (CC BY). The use, distribution or reproduction in other forums is permitted, provided the original author(s) and the copyright owner(s) are credited and that the original publication in this journal is cited, in accordance with accepted academic practice. No use, distribution or reproduction is permitted which does not comply with these terms.
*Correspondence: Dalila Mango, ZC5tYW5nb0BlYnJpLml0
Disclaimer: All claims expressed in this article are solely those of the authors and do not necessarily represent those of their affiliated organizations, or those of the publisher, the editors and the reviewers. Any product that may be evaluated in this article or claim that may be made by its manufacturer is not guaranteed or endorsed by the publisher.
Research integrity at Frontiers
Learn more about the work of our research integrity team to safeguard the quality of each article we publish.