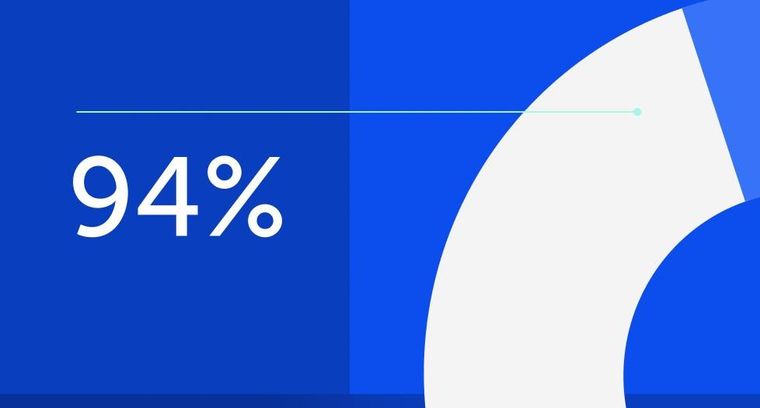
94% of researchers rate our articles as excellent or good
Learn more about the work of our research integrity team to safeguard the quality of each article we publish.
Find out more
MINI REVIEW article
Front. Cell. Neurosci., 31 August 2021
Sec. Cellular Neuropathology
Volume 15 - 2021 | https://doi.org/10.3389/fncel.2021.718324
This article is part of the Research TopicMultifaceted interactions between immunity and the diseased brainView all 9 articles
In the central nervous system (CNS), glial cells, such as microglia and astrocytes, are normally associated with support roles including contributions to energy metabolism, synaptic plasticity, and ion homeostasis. In addition to providing support for neurons, microglia and astrocytes function as the resident immune cells in the brain. The glial function is impacted by multiple aspects including aging and local CNS changes caused by neurodegeneration. During aging, microglia and astrocytes display alterations in their homeostatic functions. For example, aged microglia and astrocytes exhibit impairments in the lysosome and mitochondrial function as well as in their regulation of synaptic plasticity. Recent evidence suggests that glia can also alter the pathology associated with many neurodegenerative disorders including Alzheimer’s disease (AD) and Parkinson’s disease (PD). Shifts in the microbiome can impact glial function as well. Disruptions in the microbiome can lead to aberrant microglial and astrocytic reactivity, which can contribute to an exacerbation of disease and neuronal dysfunction. In this review, we will discuss the normal physiological functions of microglia and astrocytes, summarize novel findings highlighting the role of glia in aging and neurodegenerative diseases, and examine the contribution of microglia and astrocytes to disease progression.
The majority of cells that comprise the central nervous system (CNS) lie within two broad classifications: neurons and glia. Glial cells can further be broken down into subtypes including microglia and macroglia, with the latter group consisting of astrocytes, oligodendrocytes, and ependymal cells. Unlike microglia, which originate from the mesoderm and are derived from primitive macrophages in the yolk sac, macroglia have a common neuroectodermal embryonic origin (Curtis et al., 1988; Takahashi et al., 1989; Kaur et al., 2001; Butt, 2009; Ginhoux et al., 2010; Gomez Perdiguero et al., 2015). Among these glial subpopulations, microglia and astrocytes serve as crucial regulators of the innate immune response in the brain (Aloisi, 1999; Raivich et al., 1999; Olson and Miller, 2004; Jack et al., 2005; Choi et al., 2014). Microglia provide a variety of support roles including: synaptic pruning and remodeling in development, parenchymal surveillance to clear metabolic products and deteriorated tissue components in the normal CNS, and degrading pathogenic substances such as amyloid-β (Aβ) plaques or harmful viruses and bacteria in diseased states (Nimmerjahn et al., 2005; Lim et al., 2013; Heneka et al., 2015; Weinhard et al., 2018; Cangalaya et al., 2020; Favuzzi et al., 2021). Additionally, microglia play important roles in regulating myelination, controlling and maintaining vascular integrity, neurogenesis, and astrogliogenesis (Antony et al., 2011; Lampron et al., 2015; Halder and Milner, 2019; Diaz-Aparicio et al., 2020; Dudiki et al., 2020; Hughes and Appel, 2020). Like microglia, astrocytes are immunocompetent, possessing the capability to detect danger signals, responding through the release of chemokines and cytokines, and mounting an immune response (Cornet et al., 2000; Gimsa et al., 2013; Wang et al., 2021). Astrocytes also execute essential functions regulating synaptogenesis, providing metabolic support to neurons, and supporting the integrity of the blood-brain barrier in development and in the normal CNS (Pellerin and Magistretti, 1994; Isobe et al., 1996; Kucukdereli et al., 2011; Sultan et al., 2015; Heithoff et al., 2020). Moreover, astrocytes demonstrate the ability to regulate Aβ as well as α-synuclein (α-syn) pathology and maintain synaptic integrity in neurodegenerative disease (Morales et al., 2017; Katsouri et al., 2020; Saha et al., 2020; Tsunemi et al., 2020).
Previous findings reveal that glial cells are heterogenous, varying across species as well as regionally and spatially within the brain (Lawson et al., 1990; Ko et al., 2013; Herculano-Houzel, 2014). Similarly, glia within the same brain can be differentially reactive or functionally distinct depending on their microenvironment (Lawson et al., 1990; Ko et al., 2013; Boisvert et al., 2018; Lanjakornsiripan et al., 2018). Aging also impacts glia, hindering their normal physiological functions. For instance, microglia in aged mice have a slower, less robust response to insults while aging astrocytes display increased mitochondrial oxidative metabolism that disrupts the cell’s ability to provide neuronal support (Hefendehl et al., 2014; Jiang and Cadenas, 2014). Just as aging impacts glial function, it also affects the composition of one’s microbiome and systemic metabolism, which can, in turn, modulate glial cell activity and contribute to age-associated diseases (Fukagawa et al., 1990; Vogt et al., 2017, 2018; Rash et al., 2018; Spencer et al., 2019; Xu et al., 2019; Lee et al., 2020; Wilmanski et al., 2021).
Furthermore, glial cells have been shown to impact the pathology of neurodegenerative diseases though their roles are complex and can be both detrimental and beneficial. In the context of Alzheimer’s disease (AD), microglial activation has been shown to precede amyloid-β (Aβ) plaque deposition and the formation of tau tangles and may result in enhanced pathology (Yoshiyama et al., 2007; Heneka et al., 2013; Wright et al., 2013; Leyns et al., 2017; Venegas et al., 2017; Ising et al., 2019; Shippy et al., 2020). In contrast, in vivo and in vitro studies using AD mice and human samples show that microglia are instrumental for Aβ plaque clearance, which could slow AD progression (Wang et al., 2015, 2016; Richter et al., 2020; Zhou et al., 2020). The role of astrocytes in AD pathology is also very complex but not fully understood to date. In an AD mouse model, the inhibition or deletion of astrocyte-specific Stat3, a transcription factor that is required for astrogliosis, ameliorated AD pathology, reducing plaque load and dystrophic or deteriorating neurites (Reichenbach et al., 2019). This study suggests that astrocytic Stat3 signaling could exacerbate AD progression. Conversely, in vivo and in vitro studies using AD mice or astrocyte-neuron co-cultures illustrate that astrocytes play a neuroprotective role in AD as in their absence, Aβ oligomers were more likely to bind dendrites and result in synaptotoxicity (Pitt et al., 2017; Katsouri et al., 2020). Additionally, there was enhanced Aβ aggregation, synapse deterioration, and memory loss without astrocyte signaling, which would increase AD pathogenicity (Pitt et al., 2017; Katsouri et al., 2020). Astrocytes also appear to play a similarly multifaceted role in the context of other neurodegenerative diseases such as Parkinson’s disease (PD), addressed later in this review.
Here, we discuss current literature addressing the physiological function of microglia and astrocytes. We also review how microglial and astrocyte functions are altered by changes associated with aging and microbiome alterations as well as assess each cell’s contribution to the progression of neurodegenerative diseases. Since mice serve as a primary model for many aspects of neurobiology, this review will primarily focus on data from mouse models and will address how those findings confirm or complement studies in humans.
Among the glial cells in the CNS, microglia, and astrocytes are primarily responsible for mounting an immune response through the production of cytokines and chemokines (Aloisi, 1999; Raivich et al., 1999; Cornet et al., 2000). In an effort to maintain or return to homeostasis, microglia and astrocytes can repair damage after injury and fight off infections (Figure 1; Myer, 2006; Rasley et al., 2006; Choi et al., 2020; Moseman et al., 2020; Sariol et al., 2020).
Figure 1. Role of microglia and astrocytes in health, age, and neurodegenerative disease. Microglia and astrocytes release cytokines and chemokines in the neuronal milieu, adopting different responses depending on their surrounding environment. Their responses modulate each other’s activity as well as the activity of neurons and other nearby cell types. In this schematic, the contributions of microglia and astrocytes as well as their influence on neuronal outcomes are identified in health, aging, and neurogenerative disease. Microglial and astrocyte influences in neuropathology are also indicated as pathological (orange) or neuroprotective (blue). OPC, oligodendrocyte progenitor cells; BBB, blood-brain barrier; CNS, central nervous system; LTP, long-term potentiation; Aβ, amyloid-β; AD, Alzheimer’s disease; α-syn, α-synuclein; PD, Parkinson’s disease. *Created with Biorender.com.
Microglia continuously sample their microenvironments within the brain parenchyma by extending and retracting their processes, detecting complement fragments, immunoglobulins, and other inflammatory stimuli e.g., cytokines, chemokines, danger-associated molecular patterns (DAMPs), and pathogen-associated molecular patterns (PAMPs; Raivich et al., 1999; Nimmerjahn et al., 2005). Microglia detect DAMPs and PAMPs using pattern recognition receptors (PRRs) including toll-like receptors (TLRs), nucleotide-binding and oligomerization domain (NOD)-like receptors (NLRs), and absent in melanoma 2 (AIM2)-like receptors among others (Olson and Miller, 2004; Freeman et al., 2017; Ma et al., 2021). Following detection of a DAMP or PAMP, microglia produce and release an array of cytokines and chemokines and become reactive, allowing them to respond to their microenvironment following injury or pathological events (Figure 1; Hammond et al., 2019; Masuda et al., 2019; Wang et al., 2021). In addition to their immune functions, microglia play other key homeostatic roles in the healthy adult brain. First and foremost, microglia serve as the brain’s primary phagocytes, removing dead cells, cell debris, and other harmful, inflammatory stimuli in the healthy brain (Nimmerjahn et al., 2005; Damisah et al., 2020). In adulthood microglial phagocytosis is critical for the removal of new granule cells and the maintenance of long-term hippocampal neurogenesis (Sierra et al., 2010; Diaz-Aparicio et al., 2020). Microglial phagocytosis is also important after brain injury for the removal of cell debris, which prevents secondary neuronal death and reduces additional brain atrophy (Herzog et al., 2019; Wen et al., 2020). Furthermore, microglia modulate neuronal plasticity by monitoring and pruning synapses in the adult brain just as they do in development (Tremblay et al., 2010; Schafer et al., 2012; Ji et al., 2013; Miyamoto et al., 2016; Weinhard et al., 2018). Microglial regulation of synapses can facilitate adaptation to the environment by promoting learning through memory synapse formation or remodeling (Ehninger, 2003; Parkhurst et al., 2013; Nguyen et al., 2020; Wang et al., 2020). Microglia are also active near the brain’s vasculature and are important for maintaining CNS vascular and blood-brain barrier integrity, contributing to the perivascular glia limitans, and regulating fine-scale vasculature remodeling (Lassmann et al., 1991; Halder and Milner, 2019; Haruwaka et al., 2019; Joost et al., 2019; Mondo et al., 2020). More recently, microglia have been cited for playing roles in regulating oligodendrocyte progenitor cell differentiation and myelin homeostasis (Hagemeyer et al., 2017).
Astrocytes also play important immune and homeostatic roles including maintaining ion levels, regulating synaptogenesis, and providing metabolic support to neurons among others (Pellerin and Magistretti, 1994; Kucukdereli et al., 2011). Astrocytes interact with their microenvironment in ways analogous to microglia and express TLRs allowing them to detect tissue injuries, bacteria, viruses, or other pathogenic agents including bacterial products (Bowman et al., 2003; Park et al., 2006). Upon TLR engagement, astrocytes, like microglia, produce and release cytokines and chemokines that serve as mediators of cell migration and communication (Jack et al., 2005; Cordiglieri and Farina, 2010; Choi et al., 2014). Astrocytes are also responsible for glutamate synthesis, uptake, and recycling, a function that has been shown to protect neurons from glutamate-induced excitotoxicity (Shank et al., 1985; Rothstein et al., 1996). Similar to microglia, astrocytes are involved in synaptic pruning and regulating hippocampal neurogenesis, two roles that are both crucial for maintaining circuit connectivity and neural plasticity (Song et al., 2002; Wilhelmsson et al., 2012, 2019; Chung et al., 2013; Magnusson et al., 2020; Lee et al., 2021). Furthermore, astrocytes play a role in maintaining the integrity of the blood-brain barrier (BBB), though this finding has been disputed in some disease contexts (Eugenin et al., 2011; Heithoff et al., 2020). Astrocyte-secreted factors such as hevin, a synaptic glycoprotein, and secreted protein acidic and rich in cysteine (SPARC), an antagonist of hevin’s synaptogenic function, are crucial in excitatory and inhibitory synaptogenesis, which implicates their role in neural plasticity and circuit formation (Hughes et al., 2010; Kucukdereli et al., 2011). Additionally, to ensure the metabolic needs of neurons are met, astrocytes provide them with energetic support through direct astrocyte-neuron metabolic-coupling interactions that can impact long–term memory formation and have neuroprotective effects (Pellerin and Magistretti, 1994; Suzuki et al., 2011; Ioannou et al., 2019).
While microglia and astrocytes each have important functions, these cells do not operate in isolation. Recently, an emerging role for the interplay between astrocytes and microglia in brain homeostasis has been demonstrated, though these examples are not extensive due to technical limitations (Tremblay et al., 2010; Pascual et al., 2012; Damisah et al., 2020). Much of the current knowledge presented about the microglia-astrocyte-neuron interactions have developed through the collective knowledge of how particular cellular crosstalk impacts a cell type, involving considerable speculation, and not through real-time observation (Cerbai et al., 2012; Lian et al., 2016; Liddelow et al., 2017; Shi et al., 2020; Xie et al., 2020). However, using a novel 2Phatal technique, which enables observation of the kinetics of cell death and engulfment through live imaging in vivo, distinct roles for microglia and astrocytes were illustrated in neuronal corpse removal in the adult mouse (Damisah et al., 2020). Over various modes of cell death, microglia engulfed cell bodies and proximal dendrites while astrocytes preferentially engulfed distal processes and diffuse neuritic debris during early postnatal development (Damisah et al., 2020). This method also showed that apoptotic cell corpse removal was markedly delayed in 26-month-old aged mice compared to 4-month-old adult mice, which is suggestive of altered glial function in aging (Damisah et al., 2020).
As the brain ages, it is known that cellular and molecular processes are altered causing mitochondrial dysfunction, higher levels of oxidative stress and damage, dysfunctional autophagy mechanisms, and dysregulated stress and inflammatory responses (Mattson and Arumugam, 2018). The normal physiological functions and support roles provided by microglia and astrocytes are also vulnerable to aging. Microglial immune responses following an intraperitoneal injection of lipopolysaccharide (LPS) differ in mice considered middle aged (9–15 months), suggesting that the central nervous system (CNS) may be more vulnerable to age-dependent changes earlier than initially thought (Nikodemova et al., 2016; Keane et al., 2021). Thus, observing how microglial and astrocytic responses change in age is imperative for understanding their contribution to age-associated pathologies.
Aging microglia in both mice and humans have a distinct transcriptome profile, exhibiting an upregulation of gene transcripts associated with the cell stress response, brain inflammation, and age-related diseases such as AD (Lukiw, 2004; Sierra et al., 2007; Galatro et al., 2017; Olah et al., 2018; Bonham et al., 2019). In aging mice, microglia undergo morphological changes, exhibit a less ramified cell morphology, reduced process length, and increased soma volume (Sierra et al., 2007; Tremblay et al., 2012; Hefendehl et al., 2014). These age-related morphological alterations vary across brain regions and have further been correlated with shifts in microglial function (Hart et al., 2012). For example, microglia display an age-diminished response to laser injury, reacting with a drastic decrease in process motility compared to their younger counterparts (Hefendehl et al., 2014). Microglia in aged mice also display increased mitochondrial activity, which may impact neuronal function in aging (Ye and Johnson, 1999; Njie et al., 2012; Ritzel et al., 2015). Aging additionally impaired microglial phagocytic capabilities and lysosome function as illustrated through the accumulation of lipofuscin-like lysosomal inclusions (Njie et al., 2012; Ritzel et al., 2015; Safaiyan et al., 2016). Likewise, microglia can accumulate lipid droplets in age, which is accompanied by defective microglial phagocytosis and an increased production of toxic cell byproducts including reactive oxygen species and pro-inflammatory cytokines (Shimabukuro et al., 2016; Marschallinger et al., 2020; Loving et al., 2021). Microglia that have become dysfunctional, such as dystrophic or senescent microglia, increase with age, have shorter telomeres, and less complex, fragmented processes (Streit et al., 2004; Flanary et al., 2007; Lopes et al., 2008).
Astrocytes in aged mice also display elevated levels of inflammatory and oxidative stress genes (Jiang and Cadenas, 2014; Boisvert et al., 2018; Clarke et al., 2018; Habib et al., 2020). An elevation in genes associated with neuroinflammation accompanied by the loss of an astrocyte’s other normal functions such as synapse regulation characterize reactive astrocytes, which increase with age and can influence the outcomes of brain injury or neurodegenerative diseases (Myer, 2006; Boisvert et al., 2018; Clarke et al., 2018; Reichenbach et al., 2019). In addition, astrocytes isolated from aged mice expressed increased levels of genes in synapse elimination pathways as well as those linked with age-related diseases including Snca and Sncg, two genes associated with PD (Boisvert et al., 2018; Early et al., 2020; Pan et al., 2020). Similar to microglia, cultured astrocytes isolated from aged mice display an increase in mitochondrial activity, which limits the substrate supply from astrocytes to neurons and can contribute to age-related cognitive decline (Jiang and Cadenas, 2014). Astrocytes in aged mice also mirror aged microglia in that they undergo region-specific morphological changes, demonstrating variable changes in cell complexity, a reduced domain size with short, stubby processes, and decreased astrocyte coupling through gap junctions (Rodríguez et al., 2014; Jyothi et al., 2015; Bondi et al., 2021; Popov et al., 2021). Age-dependent morphological changes were also concomitant with deficiencies in astrocytic physiology, specifically in potassium buffering and glutamate clearance, which impaired synaptic plasticity and hippocampal long–term potentiation (Popov et al., 2021). Taken together, these findings suggest that changes in microglia and astrocyte function due to aging may alter outcomes following brain injury or impact age-associated disease progression, though this continues to be a topic of debate.
In AD microglia and astrocytes undergo functional change, enhancing their neuropathological or neuroprotective influences in disease progression depending on their microenvironment. For example, in human patients with mild cognitive impairment and AD mouse models, microglia can release pro-inflammatory cytokines that induce neuroinflammation, which can exacerbate AD pathology resulting in increased Aβ plaque deposition, neuronal tau accumulation, and synapse loss (Tarkowski, 2003; Yoshiyama et al., 2007; Wright et al., 2013). In depletion studies elimination of microglia through the inhibition of colony-stimulating factor 1 receptor (CSF1R) in 5xFAD mice, an AD mouse model that overexpresses amyloid-β (Aβ) and develops plaque pathology, there was impaired Aβ plaque formation, reduced inflammation, and neuronal loss (Spangenberg et al., 2016, 2019). These results suggest microglia contribute to the development and progression of AD pathology by causing neuronal atrophy and initiating plaque formation among other roles (Spangenberg et al., 2016, 2019). Microglia can also activate the NOD, leucine-rich repeat (LRR), and pyrin-domain containing 3 (Nlrp3) inflammasome, which results in the production of Asc specs that cross-seed Aβ plaques and enhance both amyloid and tau-associated pathology in AD (Heneka et al., 2013; Venegas et al., 2017; Ising et al., 2019). Inhibition of the Nlrp3 inflammasome in AD mouse models was recently shown to reduce Asc speck formation, plaque pathology, and microgliosis, suggesting that Nlrp3 inflammasome activation in microglia could be driving its neurotoxic effects (Lonnemann et al., 2020; Shippy et al., 2020). In contrast, microglia also appear to be neuroprotective early on in AD pathology (Condello et al., 2015; Maphis et al., 2015; Hong et al., 2016; Wang et al., 2016; Yuan et al., 2016). Microglia have been shown to act as a physical barrier between neurotoxic plaques and the surrounding CNS, thereby protecting neurons from damage (Condello et al., 2015; Wang et al., 2016; Yuan et al., 2016). The beneficial contributions of microglia in disease were further highlighted when observing triggering receptors expressed on myeloid cells (TREM2) deficient 5xFAD mice or mice that lack an important microglial receptor (Wang et al., 2015, 2016; Ulland et al., 2017). Microglial deficiencies in TREM2 are associated with increased AD risk in humans and result in diminished microglial responses to amyloidosis in 5xFAD mice (Guerreiro et al., 2013; Jonsson et al., 2013; Wang et al., 2015, 2016; Keren-Shaul et al., 2017; Kleinberger et al., 2017; Krasemann et al., 2017; Zhou et al., 2020). In TREM2 deficient 5xFAD mice, total microglial cell counts were lower and microglia were less likely to associate with and internalize Aβ plaques (Wang et al., 2015, 2016). Microglia in 5xFAD TREM2 knock-out mice also accumulated more autophagic-like vesicles compared to 5xFAD microglia, a trend also verified in human AD patients (Ulland et al., 2017). This microglial deficit was partially rescued through dietary cyclocreatine supplementation, resulting in normalized microgliosis as well as microglial plaque clustering and significantly reduced plaque-associated neurite dystrophy (Ulland et al., 2017). Thus, functional microglia are crucial for eliciting a proper immune response in AD and can either exacerbate or help attenuate associated disease pathology.
Like microglia, astrocytes have also been implicated in roles that exacerbate or are neuroprotective in AD. Astrocytic ApoE4 expression, a strong genetic risk factor for late-onset AD, potentiates neuronal tau aggregation in multiple in vitro and in vivo tau models of AD, prompting AD development (Jablonski et al., 2021). Similarly, astrocytes in primary co-cultures with rat embryo neurons were demonstrated to exacerbate Aβ-induced neurotoxicity, caspase-3 activation, and the production of caspase-3-cleaved tau, which is more likely to aggregate (Garwood et al., 2011). However, the administration of an anti-inflammatory agent, minocycline, reduced tau phosphorylation and other astrocytic inflammatory responses as well as associated neuronal loss, further illustrating the pathogenic contributions of astrocytes to AD (Garwood et al., 2011). Astrocytes also exacerbate AD pathology through their production of hydrogen peroxide and their dysregulated metabolic processes resulting in compromised neuronal support (Oksanen et al., 2017; Chun et al., 2020). In parallel, reactive astrocytes are elevated in localized regions associated with neurodegeneration in human AD patient post-mortem tissue including the hippocampus and prefrontal cortex (Liddelow et al., 2017). This finding mirrors trends seen in other research using human tissue, noting an accumulation of Aβ42, a pathogenic form of amyloid-β peptides, in astrocytes within the entorhinal cortex of clinically diagnosed sporadic AD patients (Nagele et al., 2003). The amount of Aβ42 accumulation in reactive astrocytes was directly correlated to the extent of AD pathology, suggesting that astrocytes contribute to the local inflammatory response (Nagele et al., 2003). In contrast, previous literature has demonstrated that astrocytes have neuroprotective effects in AD. This notion was illustrated through the ablation of reactive proliferating astrocytes in a transgenic AD mouse, which resulted in elevated cortical Aβ pathology and increased levels of monomeric Aβ in brain homogenates (Katsouri et al., 2020). In addition, astrocyte ablation reduced hippocampal neuronal and synaptic density, which was accompanied by increased neuroinflammation and spatial memory deficits (Katsouri et al., 2020). Though astrocytes are known to produce neurotoxic products following systemic injection of LPS or induced ischemia, cytokines released from astrocytes such as TIMP-1 have also been coupled with neuroprotective effects (Zamanian et al., 2012; Liddelow et al., 2017; Saha et al., 2020). For instance, in a well-characterized Aβ-infused rat model of AD intra-cerebroventricular TIMP-1 injection resulted in a reduction in Aβ load and Aβ-induced apoptosis in the hippocampus and cortex (Saha et al., 2020). Furthermore, TIMP-1 treated rats displayed restored synaptic integrity and showed an improvement in memory including associative learning (Saha et al., 2020). Additionally, a subset of reactive astrocytes expressing higher levels of GLT-1, an abundant glutamate transporter, was identified in AD patients that had neuropathological changes consistent with AD but without dementia, unlike AD patients who had dementia (Kobayashi et al., 2018). This finding suggests GLT-1 expressing reactive astrocytes could help preserve cognitive function (Kobayashi et al., 2018). Together, these results indicate that astrocytes are important mediators of neuroinflammation and, depending on the context, can contribute to neurotoxic or neuroprotective responses in AD models.
Similar trends associating glial cells with beneficial and detrimental effects were noted within the context of PD. Microglial reactivity in PD was associated with similar pathological features as those identified in AD such as increased neuroinflammation, which precedes α-syn pathology in PD mice (Marinova-Mutafchieva et al., 2009; Izco et al., 2021). Another study targeting metabotropic glutamate receptor 5 (mGluR5), a G-coupled protein receptor that specifically inhibits the microglial inflammatory response, showed that mGluR5 activation partially inhibits microglial reactivity in vitro and had an anti-inflammatory effect in vivo, partly protecting neurons from neurotoxicity induced by microglial reactivity (Zhang et al., 2021). Like in aging, microglia in the substantia nigra, a brain region severely affected by PD, of postmortem PD patient brains have been shown to accumulate more neutral lipids or triglycerides compared to healthy controls (Brekk et al., 2020). Brains from patients with PD also contained a higher abundance of microglia in the substantia nigra compared to healthy subjects, suggesting microglia could be reactive (Brekk et al., 2020). Though microglial pathogenicity was not specifically measured in this study, lipid- rich microglia have been associated with functional deficits in microglia that suggest they exacerbate neurodegeneration (Marschallinger et al., 2020). Microglia are also associated with neuroprotective roles in PD, clearing neuronal α-syn and mitigating neuronal degeneration (Choi et al., 2020). Specifically, transgenic PD mice demonstrated that microglia exhibit synucleinphagy in PD, which is the microglial engulfment of α-syn into autophagosomes for degradation through selective autophagy (Choi et al., 2020). When microglial autophagy was disrupted, α-syn mediated neurotoxicity and neurodegeneration were enhanced, demonstrating a protective role for microglia in PD (Choi et al., 2020). Furthermore, in a 1-methyl-4-phenyl-1,2,3,6-tetrahydropyridine (MPTP) induced mouse model of PD, microglial depletion through CSFR1 inhibition aggravated MPTP-induced neurotoxicity, resulting in locomotor impairment and the loss of dopaminergic neurons (Yang et al., 2018). Additionally, the knockdown of microglia-specific Cav1.2, a voltage-dependent calcium channel, in MPTP-induced PD mice resulted in severe degeneration of dopaminergic neurons and accompanying motor deficits, further suggesting microglia help mitigate disease progression (Wang et al., 2019). In all, these and past studies demonstrate that microglia play dual roles in PD, either contributing to or counteracting PD progression (Toku et al., 1998; Zhang et al., 2005).
In PD, astrocytes also play a dual role. For instance, in an α-syn preformed fibril PD mouse model inhibition of reactive astrocytes was neuroprotective (Yun et al., 2018). Specifically, the inhibition of reactive astrocytes protected against dopaminergic neuron loss and associated behavioral deficits in vivo (Yun et al., 2018). Likewise, when applied to primary rat astrocyte cultures multiple forms of α-syn, including monomeric, oligomeric, and fibrillar forms, can cause astrocytes to become reactive (Chavarría et al., 2018). Astrocyte reactivity was accompanied by increased levels of intracellular oxidants and pro-inflammatory cytokine release (Chavarría et al., 2018). In co-culture with hippocampal neurons, astrocytes exposed to different α-syn species increased cytotoxicity, provoking neuronal death (Chavarría et al., 2018). Additionally, selective expression of A53T mutant α-syn, a genetic mutation in α-syn linked with increased PD risk, in astrocytes of transgenic PD mice compromised their normal functions as seen through a downregulation of GLAST1 and GLT1, two proteins associated with glutamate transport (Gu et al., 2010). Astrocytic A53T α-syn expression was also associated with neurological dysfunction, a shortened lifespan, and neurodegeneration (Gu et al., 2010). Furthermore, some of these pathological trends were exhibited in induced pluripotent stem cells (iPSC)-derived astrocytes from PD patients with a G2019S mutation in the Leucine Rich Repeat Kinase 2 (LRRK2) gene, which is the most common cause of familial PD (Ramos-Gonzalez et al., 2021). iPSC astrocyte cultures from these patients demonstrated decreased homeostatic support to neurons and elevated oxidative stress, an observation that has previously been linked with enhanced neurodegeneration both in vitro and in vivo (Hashioka et al., 2009; Gu et al., 2010; Chavarría et al., 2018; Ramos-Gonzalez et al., 2021). RNA sequencing of LRRK2 G2019S iPSC-derived astrocytes also revealed a downregulation of transforming growth factor beta 1 (TGFB1) and matrix metallopeptidase 2 (MMP2), which are both involved in regulating the extracellular matrix, a role that can profoundly alter an astrocyte’s response to inflammatory stimuli (Johnson et al., 2015; Booth et al., 2019). Therefore, astrocytes carrying the LRRK2 G2019S mutation have a reduced capacity to perform roles contributing to neuroprotection and thus, support PD pathogenesis. Astrocytes in PD have also been associated with providing a neuroprotective potential. Astrocyte-specific overexpression of human DJ-1 also known as Parkinson Disease Protein 7 (PARK7), a redox sensitive protein with multiple reported functions crucial for mitochondrial physiology and protein transcription, was shown to mitigate neurotoxicity in a PD rat model (De Miranda et al., 2018). In rats overexpressing astrocytic DJ-1 there was a reduction in mitochondrial dysfunction, α-syn accumulation and phosphorylation, astrocyte-induced neuroinflammation, and neurodegeneration in dopaminergic neurons in the substantia nigra (De Miranda et al., 2018). Additionally, following the MPTP challenge, mice with astrocytes lacking Kir6.1, an ATP-sensitive potassium channel, exhibited increased dopaminergic neuron loss in the substantia nigra compacta and more severe motor dysfunction compared to controls (Hu et al., 2019). Similarly, astrocytic Kir6.1 deletion resulted in increased production of mitochondrial reactive oxygen species, astrocyte-mediated neuroinflammation, and decreased mitophagy in astrocytes (Hu et al., 2019). Another study using rats injected with 6-hydroxydopamine, which induces dopaminergic denervation of the striatum, revealed that striatal astrocytes possess physiological phagocytic properties (Morales et al., 2017). While this functional response is more typical of microglia, this role in astrocytes may be critical for clearing striatal debris at the onset of PD (Morales et al., 2017). In parallel, iPSC-derived astrocytes from PD patients carrying an ATP13A2 mutation, which affects lysosome function, were protective, resulting in rapid phagocytosis of α-syn and higher lysosomal degradation rates (Tsunemi et al., 2020). When co-cultured with iPSC-derived dopaminergic neurons, the iPSC-derived astrocytes further prevented neuronal α-syn accumulation and inter-neuronal α-syn transfer (Tsunemi et al., 2020). Collectively, these studies reveal that astrocytes, like microglia, can be either toxic or beneficial in PD pathology.
Increasingly the bidirectional communication between the brain and the gut, termed the gut-brain axis, has been recognized as playing an important role in brain health and disease. The gut microbiome is predominantly composed of bacteria but it can also contain viruses, fungi, archaea, and helminths (Vemuri et al., 2020). The gut microbiome composition varies across individuals as well as species and is influenced by a variety of factors including age, diet, and disease among others (Figure 2; The Human Microbiome Project Consortium, 2012; David et al., 2014; Nagpal et al., 2018; Carmody et al., 2019; Xu et al., 2019; Manor et al., 2020; Kundu et al., 2021; Wilmanski et al., 2021). Microbial metabolites produced by gut bacteria in humans have been shown to impact host health by directly interacting with immune cells and intestinal epithelial cells, which allows for selective penetration of nutrients while preventing the passage of harmful stimuli such as toxins in subjects with healthy gut barrier function (Figure 2; Cabinian et al., 2018; James et al., 2020; Ghosh et al., 2021). As gut permeability increases with age, certain individual differences in the microbiome may also be causative in exacerbating or ameliorating disease as differentially abundant microbiota and gut metabolites such as trimethylamine N-oxide (TMAO) have been associated with increased risk of AD and other neurogenerative diseases (Man et al., 2015; Qi et al., 2017; Vogt et al., 2017, 2018; Brunt et al., 2021; Romano et al., 2021). In humans, the gut microbiome has been implicated in modulating immune function both systemically and in the CNS, suggesting that the microbiome can influence microglial and astrocyte function (Erny et al., 2015; Buford et al., 2018; Schluter et al., 2020; Sanmarco et al., 2021).
Figure 2. Microbiome influence on microglia and astrocyte function in health, age, and neuropathology. Multiple factors affect an individual’s microbiome composition including diet, age, and disease. In the distal intestine, gut metabolites can cross the gut epithelium and modulate the immune system, which can influence the function of microglia and astrocytes in health, age, and neurodegenerative diseases. Functions of microglia and astrocytes that are altered in mice who have had their microbiome altered, contain a microbiome or lack thereof have been identified. Aβ, amyloid-β; GF, germ-free; ABX, antibiotic; AD, Alzheimer’s disease; EAE, experimental autoimmune encephalomyelitis; CNS, central nervous system; DC, dendritic cell; HF, high-fat; TMAO, trimethylamine N-oxide; AHR, aryl hydrocarbon receptor. *Created with Biorender.com.
Though the timing of fetal microbiome development is highly debated, convincing evidence suggests the fetus begins developing its microbiome during birth in the mouse and human and is generally compositionally similar to the maternal microbiome (Jiménez et al., 2008; Ferretti et al., 2018; Younge et al., 2019; Kennedy et al., 2021). During early life, the microbiome is critical for postnatal innate immune development as well as microglial maturation, which could affect how microglia respond in physiological and pathological conditions in adulthood (Erny et al., 2015; Gomez de Aguero et al., 2016; Matcovitch-Natan et al., 2016; Thion et al., 2018). The microbiome also plays a role in postnatal microglial synaptic pruning, affecting neurodevelopment outcomes (Lebovitz et al., 2019; Luck et al., 2020). Broadly, the host microbiota is a critical component for shaping microglial function (Erny et al., 2015). Compared to conventionally colonized specific pathogen free (SPF) mice, germ-free (GF) mice lacking a microbiota contain microglia with a more complex morphology and an immature phenotype (Erny et al., 2015). As a result, microglia in GF mice are more prone to functional deficits including a diminished immune response, a finding mirrored in aged mice (Hefendehl et al., 2014; Erny et al., 2015; Ritzel et al., 2015; Galatro et al., 2017). Particularly, Bifidobacteria species and Lactobacillus murinus HU-1 can normalize dystrophic microglia, indicating that microglial function could be modulated by a specific type of bacteria in the postnatal period (Lebovitz et al., 2019; Luck et al., 2020). To date, the impact of the microbiome on astrocyte function in postnatal development remains to be explored. However, there is one study that demonstrated maternal probiotic supplementation with Lactobacillus acidophilus and Bifidobacterium infantis attenuates astrocyte reactivity in postnatal offspring (Lu et al., 2020). Taken together, these studies establish that microbiome influences on brain function occur early on and could contribute to neurological outcomes in age or disease through adulthood.
In mature animals, the microbiome also influences the homeostatic roles of microglia and astrocyte function in health. The importance of the microbiome in the normal microglial immune response is illustrated by the fact that in GF mice, microglia exhibit a diminished response to bacterial and viral infections, compared to microglia from SPF mice (Erny et al., 2015; Brown et al., 2019). Furthermore, the depletion of the microbiome using broad-spectrum antibiotics in 6–10 week old SPF mice significantly changed microglial morphology (Erny et al., 2015). After antibiotic administration, microglia in SPF mice resembled malformed microglia similar to those identified in GF mice, suggesting that the microbiome is critical for microglial homeostasis in physiological conditions (Erny et al., 2015). Although there is limited research illustrating how the microbiome directly modulates other functional roles of microglia, one study links isoflavones and lignans, two classes of highly gut and BBB permeable polyphenol microbial metabolites, with decreased nitric oxide and pro-inflammatory cytokine levels in murine microglia (Johnson et al., 2019). This finding indicates that gut-microbial-derived-metabolites can dampen microglia-specific neuroinflammation and could be beneficial to administer as a therapeutic for neurodegenerative diseases.
In addition to microglial changes, the microbiome was also shown to be important for maintaining astrocyte function. Following gut microbiota perturbations, an upregulation in genes associated with astrocyte-neuron metabolic coupling was detected within the mouse hippocampus (Margineanu et al., 2020). Specifically, there was an upregulation in Pfkfb3 and Atp1a2, two astrocytic proteins responsible for regulating glycolysis and glutamate reuptake, respectively, indicating that the microbiome can affect astrocytic contributions to brain energy metabolism (Margineanu et al., 2020). Additionally, the application of neuroprotective metabolites including sodium butyrate and indole-3-propionic acid to human primary astrocyte cultures showed that both bacterial metabolites could prevent LPS-induced inflammation and mitigate pro-inflammatory cytokine release following an LPS challenge (Garcez et al., 2020). There is also some evidence suggesting that the microbiome impacts processes that microglia and astrocytes are associated with, including adult hippocampal neurogenesis (Ogbonnaya et al., 2015). Likewise, the microbiome influences pro-inflammatory mechanisms within the hippocampus, fear extinction learning, and BBB permeability, all of which microglia or astrocytes are implicated in, through the immune system via monocytes or other microbiota-derived signals (Braniste et al., 2014; Campos et al., 2016; Möhle et al., 2016; Chu et al., 2019). Taken together, these findings demonstrate that the microbiome is important for maintaining the homeostatic functions of microglia and astrocytes.
The microbiome also displays compositional differences with age but work exploring the effects of an aging microbiome, outside of a disease context, on glia remains limited. However, the effects of a short-term high-fat diet on microglia in the amygdala given to young and old rats was recently investigated, paralleling microglial changes identified in aging studies alone (Spencer et al., 2019). Upon receiving the high-fat diet, microglia in the amygdala in aged rats displayed reduced microglial complexity and suppressed phagocytic capacity compared to their younger counterparts (Spencer et al., 2019). Additionally, transcriptional analysis on microglia isolated from aged mouse brains with or without antibiotic-induced gut dysbiosis revealed that aged groups in both treatment conditions were enriched with gene pathways involved in dendritic cell interaction and macrophage cytokine production (Golomb et al., 2020). Microglia from aged mice in both cohorts also exhibited an upregulation of mitochondrial genes and a decreased expression of microglial homeostatic genes such as Trem2 (Golomb et al., 2020). Though there were similar trends in both the antibiotic-treated and non-antibiotic-treated aged cohorts, microglia with these properties were slightly more prevalent in aged mice receiving antibiotics (Golomb et al., 2020). Together, this data suggests that gut dysbiosis increases the likelihood of developing neuroinflammation during aging.
Similarly, shifts in astrocyte function have been linked with microbiome modulations in aged mice and humans. In a recent study exploring the potential role of TMAO in modulating neuroinflammation and cognitive function, both middle-aged to older adults (65 ± 7 years old) and aged mouse plasma TMAO levels were higher compared to their younger counterparts (Brunt et al., 2021). Likewise, circulating plasma TMAO levels were inversely correlated with working and episodic memory as well as fluid cognition in humans as measured through the NIH Toolbox Cognition Battery Test (Brunt et al., 2021). TMAO levels in 27-month-old mice were also increased in the brain compared to 6-month-old mice, suggesting that TMAO crosses the BBB, and is associated with increased neuroinflammation as well as astrocyte reactivity (Brunt et al., 2021). Furthermore, young mice supplemented with TMAO exhibited similar impairments in memory and spatial learning as observed in aging compared to young control mice (Brunt et al., 2021). In total, these findings suggest elevated TMAO levels that accompany aging are correlated with astrocyte reactivity and increased neuroinflammation associated with cognitive impairments (Brunt et al., 2021). Another approach isolated the microbiome in young and aged mice to broadly explore the effects of age on the microbiome and brain (Lee et al., 2020). In this study, fecal transplant gavages from aged or young SPF mice into young GF mice revealed that the aged microbiome alone was sufficient to produce a cognitive decline in the recipient mice who exhibited depressive-like behavior as well as impaired short–term and spatial memory (Lee et al., 2020). Though this approach did not directly implicate microglia or astrocytes specifically, it was an elegant study design illustrating that the aging gut microbiome impacts the brain and can influence cognitive performance. More research needs to be done to better understand how an aging microbiome directly or indirectly impacts both microglia and astrocyte functions and what that may mean for human health.
The microbiome can also modulate microglia and astrocyte function in neurodegenerative disease as explored in the context of AD and PD mouse models. For example, comparisons of gut microbiota in APP/PS1, an early-onset AD mouse model with Aβ pathology, to wild-type mice spanning 1–9 months old demonstrated distinct changes in bacterial taxa that preceded the development of key features indicative of AD pathology (Chen et al., 2020). The divergence in microbiota composition between wild-type and APP/PS1 mice was noted at 1–3 months of age, before amyloid deposition and plaque-localized microglial activation are apparent (Chen et al., 2020). In addition to reporting decreased microbial richness and diversity in older mice, this study also demonstrated that 6 and 9 months old mice had increased abundances of inflammation-related bacterial taxa (Chen et al., 2020). Microbial products such as short chain fatty acids were also recently identified as modulators of microglial function in vivo, increasing microglial reactivity and hindering microglial phagocytosis (Colombo et al., 2021). Likewise, microbiome modulations in 5xFAD mice were shown to differentially control microglial Aβ clearance mechanisms and influence plaque deposition (Mezö et al., 2020). In both GF and antibiotic-treated 5xFAD mice, there was less microglial accumulation around compact Aβ plaques and attenuated plaque pathology in the hippocampi compared to SPF 5xFAD controls (Mezö et al., 2020). These findings are congruent with previous studies that administered an antibiotic cocktail to transgenic APP/PS1 AD mice and showed a reduction in Aβ plaque pathology and plaque-associated microgliosis as well as increased cytokine and chemokine circulation (Minter et al., 2016, 2017; Harach et al., 2017; Dodiya et al., 2019). Though recent literature notes that the reduced plaque deposition trends previously established in two transgenic APP/PS1 mice were only replicable when administered an antibiotic cocktail and that individual antibiotic did not affect cerebral Aβ amyloidosis (Dodiya et al., 2020). AD pathology was also curtailed in 5xFAD mice through the dietary supplementation of β-hydroxybutyrate (BHB), a ketone body produced during ketogenesis, which reduced Aβ plaque formation, microgliosis, and Asc speck formation (Shippy et al., 2020). Similarly, the colonization of a curli-producing gut bacteria, Escherichia coli (E. Coli), in mice overexpressing α-synuclein, a hallmark of synucleinopathies such as PD, was demonstrated to exacerbate disease progression (Chen et al., 2016; Sampson et al., 2020). Curli are microbial amyloid proteins expressed specifically by gut bacteria, which is not to be confused with amyloidogenic proteins found within the brain such as amyloid-β found in AD plaques (Barnhart and Chapman, 2006). Gut-associated microbial amyloid produced by Pseudomonas aeruginosa was recently shown to exacerbate Aβ amyloidosis (Javed et al., 2020). For instance, mice receiving a mono-colonization of amyloid-producing E. Coli showed significantly impaired motor and gastrointestinal performance compared to mice with a complex microbiota lacking the amyloid-producing strain (Sampson et al., 2020). Morphometric analysis of midbrain microglia in mice harboring the amyloid-producing strain further displayed concomitant alterations indicative of increased inflammation in the midbrain (Sampson et al., 2020). Using in vitro assays, another study illustrated that chlorogenic acid (CGA), a coffee component with antioxidant properties, significantly suppressed the release of inflammatory products from primary microglia and prevented microglia-induced neurotoxicity in dopaminergic neurons (Shen et al., 2012). Collectively, these studies suggest that microbiome products or perturbations can alter microglia and therefore, neurological outcomes in a disease.
While the impact of the gut microbiome on astrocyte function in neurodegenerative disease is not well understood, a few reports using Multiple sclerosis (Ms) and PD mouse models have begun to establish this connection. In experimental autoimmune encephalomyelitis (EAE), a common mouse model of Ms, gut metabolites derived from dietary tryptophan (Trp) were shown to activate astrocytic aryl hydrocarbon receptor (AHR) signaling, which limits NF-κB signaling and suppresses CNS inflammation (Rothhammer et al., 2016). The favorable effects of dietary Trp were observed in wild-type and GFAP-AHR knock-out EAE mice fed a tryptophan-depleted diet (TDD), which both had increased EAE clinical scores, indicative of disease progression (Rothhammer et al., 2016). However, EAE scores could be improved through Trp supplementation in wild-type but not GFAP-AHR knock-out EAE mice, suggesting that Trp-derived ligands of AHR signaling function through astrocytes and serve a beneficial role in hindering disease progression (Rothhammer et al., 2016). AHR signaling mechanisms established in the EAE model were also verified in human brain samples from individuals with Ms, suggesting this signaling mechanism has some clinical relevance and could contribute to Ms pathogenesis (Rothhammer et al., 2016). Microbial-derived tryptophan metabolites were also shown to influence astrocyte function indirectly through microglia (Rothhammer et al., 2018). Specifically, administration of a TDD modulated AHR signaling in microglia, impacting microglial VEGF-B and TGFα expression, which in turn regulated astrocytic pathogenic responses in EAE via NF-κB signaling (Rothhammer et al., 2018). Microglial control of astrocytes via VEGF-B and TGFα were further verified in human samples and found to contribute to Ms pathogenesis (Rothhammer et al., 2018). Indirect effects of the microbiome on astrocytes have also been observed in a subset of astrocytes expressing TRAIL, a gene that can induce the apoptosis of other cells (Sanmarco et al., 2021). Gut microbiota depletion via antibiotics in SPF IfngEYFP reporter mice, a mouse model that fluorescently labels the pro-inflammatory cytokine interferon gamma (IFN-Γ), reduced TRAIL+ astrocytes relative to mice receiving a vehicle or an antibiotic treatment followed by a fecal microbiota transfer (Sanmarco et al., 2021). With lower TRAIL+ astrocytes, the reporter mice were unable to limit CNS inflammation and therefore, succumbed faster to EAE (Sanmarco et al., 2021). Additionally, PD mice receiving a fecal microbiota transplant (FMT) from healthy control mice exhibited less astrocyte and microglial reactivity (Sun et al., 2018). PD mice that regularly received coffee components, caffeic, acid and CGA, also displayed enhanced antioxidative properties in striatal astrocytes and exhibited less neurodegeneration of dopaminergic and intestinal enteric neurons (Miyazaki et al., 2019). Though the current literature is limited and the results here do not comprehensively explore the impact of the microbiome on glia in neurodegenerative diseases, these data indicate that the microbiome can favorably or detrimentally modulate neurodegeneration. This data also presents a promising new frontier to explore therapeutic interventions that hinder disease progression through the modification of the microbiome.
Overall, microglia and astrocytes, two immunocompetent glial cells that normally perform vital support roles in the brain, are crucial for responding to injury or pathogenic insults. However, factors including one’s microbiome composition, age, and neurodegenerative disease can alter their physiological functions. By exploring how inflammatory processes or functions of microglia and astrocytes can be manipulated or altered through the microbiome, during aging, and in neurogenerative diseases, we can gain more insight into potential disease mechanisms and discover methods to better regulate age-and disease-associated pathology.
Though glial cells were initially identified in the very early years of neuroscience research, we have only recently begun to grasp the full scope of glial contribution to brain health, aging, and disease. Although the function of both microglia and astrocytes are dramatically impacted in aging and neurodegenerative disease, it remains unclear whether these functional changes are detrimental or beneficial. Complex interactions between the gut microbiome and brain in health, aging, and disease have also been shown to modulate glial function although literature addressing how the microbiome affects astrocyte function remains especially limited. To better understand the contribution of glia to age and disease, new in vivo and in vitro techniques need to be employed. Novel, promising approaches including 2Phatal and the use of tri-cultures supporting neurons, microglia, and astrocytes may enable such opportunities, allowing multi-cellular interactions to be observed in vivo and in vitro (Goshi et al., 2020; Guttikonda et al., 2021). Furthermore, the recent development of an in vitro all-human physiomimetic model has exciting implications for how we understand neurodegenerative diseases, broadening the questions that can be asked about how microglia and astrocytes are impacted by or contribute to aging and disease (Trapecar et al., 2021). This model links three complex microphysiological systems including the gut/immune, liver/immune, and cerebral/immune systems in a common culture media containing circulating immune cells in continuous coculture (Trapecar et al., 2021). Using an in vitro human physiomimetic model enables a controlled systems approach that will allow organ-organ and organ-immune system interactions mimicking in vivo like behavior to be explored. These techniques utilizing multiple cells and systems can more realistically recapitulate in vivo inflammatory responses that will allow us to further expand the questions that can be asked and knowledge that can be collected about microglia and astrocytes in the next decade.
KH conceived the review. KH, KM, and TU discussed and contributed to the writing of this review. All authors contributed to the article and approved the submitted version.
This work was supported by 1R01AG070973 from the NIA at the NIH (to TKU and others), 1R21AG068652 from the NIA at the NIH (to TKU), 3R01HL142752-02 from the NHLBI and NIA at the NIH (to TKU and others), UWADRCDP (subawarded to TKU and others from P30-AG534255 from the NIA at the NIH to Wisconsin ADRC), and 233AAH8848 from the University of Wisconsin Medical Foundation (to TKU and others).
The authors declare that the research was conducted in the absence of any commercial or financial relationships that could be construed as a potential conflict of interest.
All claims expressed in this article are solely those of the authors and do not necessarily represent those of their affiliated organizations, or those of the publisher, the editors and the reviewers. Any product that may be evaluated in this article, or claim that may be made by its manufacturer, is not guaranteed or endorsed by the publisher.
Aloisi, F. (1999). “The Role of Microglia and Astrocytes in Cns Immune Surveillance and Immunopathology,” in The Functional Roles of Glial Cells in Health and Disease Advances in Experimental Medicine and Biology, eds R. Matsas, and M. Tsacopoulos (Boston, MA: Springer US), 123–133.
Antony, J. M., Paquin, A., Nutt, S. L., Kaplan, D. R., and Miller, F. D. (2011). Endogenous microglia regulate development of embryonic cortical precursor cells. J. Neurosci. Res. 89, 286–298. doi: 10.1002/jnr.22533
Barnhart, M. M., and Chapman, M. R. (2006). Curli biogenesis and function. Annu. Rev. Microbiol. 60, 131–147. doi: 10.1146/annurev.micro.60.080805.142106
Boisvert, M. M., Erikson, G. A., Shokhirev, M. N., and Allen, N. J. (2018). The aging astrocyte transcriptome from multiple regions of the mouse brain. Cell Rep. 22, 269–285. doi: 10.1016/j.celrep.2017.12.039
Bondi, H., Bortolotto, V., Canonico, P. L., and Grilli, M. (2021). Complex and regional-specific changes in the morphological complexity of GFAP+ astrocytes in middle-aged mice. Neurobiol. Aging 100, 59–71. doi: 10.1016/j.neurobiolaging.2020.12.018
Bonham, L. W., Sirkis, D. W., and Yokoyama, J. S. (2019). The transcriptional landscape of microglial genes in aging and neurodegenerative disease. Front. Immunol. 10:17. doi: 10.3389/fimmu.2019.01170
Booth, H. D. E., Wessely, F., Connor-Robson, N., Rinaldi, F., Vowles, J., Browne, C., et al. (2019). RNA sequencing reveals MMP2 and TGFB1 downregulation in LRRK2 G2019S Parkinson’s iPSC-derived astrocytes. Neurobiol. Dis. 129, 56–66. doi: 10.1016/j.nbd.2019.05.006
Bowman, C. C., Rasley, A., Tranguch, S. L., and Marriott, I. (2003). Cultured astrocytes express toll-like receptors for bacterial products. Glia 43, 281–291. doi: 10.1002/glia.10256
Braniste, V., Al-Asmakh, M., Kowal, C., Anuar, F., Abbaspour, A., Toth, M., et al. (2014). The gut microbiota influences blood-brain barrier permeability in mice. Sci. Transl. Med. 6:263ra158. doi: 10.1126/scitranslmed.3009759
Brekk, O. R., Honey, J. R., Lee, S., Hallett, P. J., and Isacson, O. (2020). Cell type-specific lipid storage changes in Parkinson’s disease patient brains are recapitulated by experimental glycolipid disturbance. Proc. Natl. Acad. Sci. U S A 117, 27646–27654. doi: 10.1073/pnas.2003021117
Brown, D. G., Soto, R., Yandamuri, S., Stone, C., Dickey, L., Gomes-Neto, J. C., et al. (2019). The microbiota protects from viral-induced neurologic damage through microglia-intrinsic TLR signaling. eLife 8:e47117. doi: 10.7554/eLife.47117
Brunt, V. E., LaRocca, T. J., Bazzoni, A. E., Sapinsley, Z. J., Miyamoto-Ditmon, J., Gioscia-Ryan, R. A., et al. (2021). The gut microbiome-derived metabolite trimethylamine N-oxide modulates neuroinflammation and cognitive function with aging. GeroScience 43, 377–394. doi: 10.1007/s11357-020-00257-2
Buford, T. W., Carter, C. S., VanDerPol, W. J., Chen, D., Lefkowitz, E. J., Eipers, P., et al. (2018). Composition and richness of the serum microbiome differ by age and link to systemic inflammation. GeroScience 40, 257–268. doi: 10.1007/s11357-018-0026-y
Butt, A. M. (2009). “Macroglial Lineages,” in Encyclopedia of Neuroscience, (Amsterdam, Netherlands: Elsevier), 597–599.
Cabinian, A., Sinsimer, D., Tang, M., Jang, Y., Choi, B., Laouar, Y., et al. (2018). Gut symbiotic microbes imprint intestinal immune cells with the innate receptor SLAMF4 which contributes to gut immune protection against enteric pathogens. Gut 67, 847–859. doi: 10.1136/gutjnl-2016-313214
Campos, A. C., Rocha, N. P., Nicoli, J. R., Vieira, L. Q., Teixeira, M. M., Teixeira, A. L., et al. (2016). Absence of gut microbiota influences lipopolysaccharide-induced behavioral changes in mice. Behav. Brain Res. 312, 186–194. doi: 10.1016/j.bbr.2016.06.027
Cangalaya, C., Stoyanov, S., Fischer, K.-D., and Dityatev, A. (2020). Light-induced engagement of microglia to focally remodel synapses in the adult brain. eLife 9:e58435. doi: 10.7554/eLife.58435
Carmody, R. N., Bisanz, J. E., Bowen, B. P., Maurice, C. F., Lyalina, S., Louie, K. B., et al. (2019). Cooking shapes the structure and function of the gut microbiome. Nat. Microbiol. 4, 2052–2063. doi: 10.1038/s41564-019-0569-4
Cerbai, F., Lana, D., Nosi, D., Petkova-Kirova, P., Zecchi, S., Brothers, H. M., et al. (2012). The neuron-astrocyte-microglia triad in normal brain ageing and in a model of neuroinflammation in the rat hippocampus. PLoS One 7:e45250. doi: 10.1371/journal.pone.0045250
Chavarría, C., Rodríguez-Bottero, S., Quijano, C., Cassina, P., and Souza, J. M. (2018). Impact of monomeric, oligomeric and fibrillar alpha-synuclein on astrocyte reactivity and toxicity to neurons. Biochem. J. 475, 3153–3169. doi: 10.1042/BCJ20180297
Chen, S. G., Stribinskis, V., Rane, M. J., Demuth, D. R., Gozal, E., Roberts, A. M., et al. (2016). Exposure to the functional bacterial amyloid protein curli enhances alpha-synuclein aggregation in aged fischer 344 rats and Caenorhabditis elegans. Sci. Rep. 6:34477. doi: 10.1038/srep34477
Chen, Y., Fang, L., Chen, S., Zhou, H., Fan, Y., Lin, L., et al. (2020). Gut microbiome alterations precede cerebral amyloidosis and microglial pathology in a mouse model of Alzheimer’s disease. BioMed Res. Int. 2020, 1–15. doi: 10.1155/2020/8456596
Choi, I., Zhang, Y., Seegobin, S. P., Pruvost, M., Wang, Q., Purtell, K., et al. (2020). Microglia clear neuron-released α-synuclein via selective autophagy and prevent neurodegeneration. Nat. Commun. 11:1386. doi: 10.1038/s41467-020-15119-w
Choi, S. S., Lee, H. J., Lim, I., Satoh, J., and Kim, S. U. (2014). Human astrocytes: secretome profiles of cytokines and chemokines. PLoS One 9:e92325. doi: 10.1371/journal.pone.0092325
Chu, C., Murdock, M. H., Jing, D., Won, T. H., Chung, H., Kressel, A. M., et al. (2019). The microbiota regulate neuronal function and fear extinction learning. Nature 574, 543–548. doi: 10.1038/s41586-019-1644-y
Chun, H., Im, H., Kang, Y. J., Kim, Y., Shin, J. H., Won, W., et al. (2020). Severe reactive astrocytes precipitate pathological hallmarks of Alzheimer’s disease via H2O2− production. Nat. Neurosci. 23, 1555–1566. doi: 10.1038/s41593-020-00735-y
Chung, W.-S., Clarke, L. E., Wang, G. X., Stafford, B. K., Sher, A., Chakraborty, C., et al. (2013). Astrocytes mediate synapse elimination through MEGF10 and MERTK pathways. Nature 504, 394–400. doi: 10.1038/nature12776
Clarke, L. E., Liddelow, S. A., Chakraborty, C., Münch, A. E., Heiman, M., and Barres, B. A. (2018). Normal aging induces A1-like astrocyte reactivity. Proc. Natl. Acad. Sci. U S A 115, E1896–E1905. doi: 10.1073/pnas.1800165115
Colombo, A. V., Sadler, R. K., Llovera, G., Singh, V., Roth, S., Heindl, S., et al. (2021). Microbiota-derived short chain fatty acids modulate microglia and promote Aβ plaque deposition. eLife 10:e59826. doi: 10.7554/eLife.59826
Condello, C., Yuan, P., Schain, A., and Grutzendler, J. (2015). Microglia constitute a barrier that prevents neurotoxic protofibrillar Aβ42 hotspots around plaques. Nat. Commun. 6:6176. doi: 10.1038/ncomms7176
Cordiglieri, C., and Farina, C. (2010). Astrocytes exert and control immune responses in the brain. Curr. Immunol. Rev. 6, 150–159. doi: 10.2174/157339510791823655
Cornet, A., Bettelli, E., Oukka, M., Cambouris, C., Avellana-Adalid, V., Kosmatopoulos, K., et al. (2000). Role of astrocytes in antigen presentation and naive T-cell activation. J. Neuroimmunol. 106, 69–77. doi: 10.1016/s0165-5728(99)00215-5
Curtis, R., Cohen, J., Fok-Seang, J., Hanley, M. R., Gregson, N. A., Reynolds, R., et al. (1988). Development of macroglial cells in rat cerebellum. I. Use of antibodies to follow early in vivo development and migration of oligodendrocytes. J. Neurocytol. 17, 43–54. doi: 10.1007/BF01735376
Damisah, E. C., Hill, R. A., Rai, A., Chen, F., Rothlin, C. V., Ghosh, S., et al. (2020). Astrocytes and microglia play orchestrated roles and respect phagocytic territories during neuronal corpse removal in vivo. Sci. Adv. 6:eaba3239. doi: 10.1126/sciadv.aba3239
David, L. A., Maurice, C. F., Carmody, R. N., Gootenberg, D. B., Button, J. E., Wolfe, B. E., et al. (2014). Diet rapidly and reproducibly alters the human gut microbiome. Nature 505, 559–563. doi: 10.1038/nature12820
De Miranda, B. R., Rocha, E. M., Bai, Q., El Ayadi, A., Hinkle, D., Burton, E. A., et al. (2018). Astrocyte-specific DJ-1 overexpression protects against rotenone-induced neurotoxicity in a rat model of Parkinson’s disease. Neurobiol. Dis. 115, 101–114. doi: 10.1016/j.nbd.2018.04.008
Diaz-Aparicio, I., Paris, I., Sierra-Torre, V., Plaza-Zabala, A., Rodríguez-Iglesias, N., Márquez-Ropero, M., et al. (2020). Microglia actively remodel adult hippocampal neurogenesis through the phagocytosis secretome. J. Neurosci. 40, 1453–1482. doi: 10.1523/JNEUROSCI.0993-19.2019
Dodiya, H. B., Frith, M., Sidebottom, A., Cao, Y., Koval, J., Chang, E., et al. (2020). Synergistic depletion of gut microbial consortia, but not individual antibiotics, reduces amyloidosis in APPP S1–21 Alzheimer’s transgenic mice. Sci. Rep. 10:8183. doi: 10.1038/s41598-020-64797-5
Dodiya, H. B., Kuntz, T., Shaik, S. M., Baufeld, C., Leibowitz, J., Zhang, X., et al. (2019). Sex-specific effects of microbiome perturbations on cerebral Aβ amyloidosis and microglia phenotypes. J. Exp. Med. 216, 1542–1560. doi: 10.1084/jem.20182386
Dudiki, T., Meller, J., Mahajan, G., Liu, H., Zhevlakova, I., Stefl, S., et al. (2020). Microglia control vascular architecture via a TGFβ1 dependent paracrine mechanism linked to tissue mechanics. Nat. Commun. 11:986. doi: 10.1038/s41467-020-14787-y
Early, A. N., Gorman, A. A., Van Eldik, L. J., Bachstetter, A. D., and Morganti, J. M. (2020). Effects of advanced age upon astrocyte-specific responses to acute traumatic brain injury in mice. J. Neuroinflammation 17:115. doi: 10.1186/s12974-020-01800-w
Ehninger, D. (2003). Regional effects of wheel running and environmental enrichment on cell genesis and microglia proliferation in the adult murine neocortex. Cereb. Cortex 13, 845–851. doi: 10.1093/cercor/13.8.845
Erny, D., Hrabě de Angelis, A. L., Jaitin, D., Wieghofer, P., Staszewski, O., David, E., et al. (2015). Host microbiota constantly control maturation and function of microglia in the CNS. Nat. Neurosci. 18, 965–977. doi: 10.1038/nn.4030
Eugenin, E. A., Clements, J. E., Zink, M. C., and Berman, J. W. (2011). Human immunodeficiency virus infection of human astrocytes disrupts blood-brain barrier integrity by a gap junction-dependent mechanism. J. Neurosci. 31, 9456–9465. doi: 10.1523/JNEUROSCI.1460-11.2011
Favuzzi, E., Ibrahim, L. A., Saldi, G. A., Cao, Y., Fernández-Otero, M., Zeine, A., et al. (2021). GABA-receptive microglia selectively sculpt developing inhibitory circuits. Cell 184, 4048–4063. doi: 10.1016/j.cell.2021.06.018
Ferretti, P., Pasolli, E., Tett, A., Asnicar, F., Gorfer, V., Fedi, S., et al. (2018). Mother-to-infant microbial transmission from different body sites shapes the developing infant gut microbiome. Cell Host Microbe 24, 133–145.e5. doi: 10.1016/j.chom.2018.06.005
Flanary, B. E., Sammons, N. W., Nguyen, C., Walker, D., and Streit, W. J. (2007). Evidence that aging and amyloid promote microglial cell senescence. Rejuvenation Res. 10, 61–74. doi: 10.1089/rej.2006.9096
Freeman, L., Guo, H., David, C. N., Brickey, W. J., Jha, S., and Ting, J. P.-Y. (2017). NLR members NLRC4 and NLRP3 mediate sterile inflammasome activation in microglia and astrocytes. J. Exp. Med. 214, 1351–1370. doi: 10.1084/jem.20150237
Fukagawa, N. K., Bandini, L. G., and Young, J. B. (1990). Effect of age on body composition and resting metabolic rate. Am. J. Physiol. Endocrinol. Metab. 259, E233–E238.
Galatro, T. F., Holtman, I. R., Lerario, A. M., Vainchtein, I. D., Brouwer, N., Sola, P. R., et al. (2017). Transcriptomic analysis of purified human cortical microglia reveals age-associated changes. Nat. Neurosci. 20, 1162–1171. doi: 10.1038/nn.4597
Garcez, M. L., Tan, V. X., Heng, B., and Guillemin, G. J. (2020). Sodium butyrate and indole-3-propionic acid prevent the increase of cytokines and kynurenine levels in LPS-induced human primary astrocytes. Int. J. Tryptophan Res. 13:1178646920978404. doi: 10.1177/1178646920978404
Garwood, C. J., Pooler, A. M., Atherton, J., Hanger, D. P., and Noble, W. (2011). Astrocytes are important mediators of Aβ-induced neurotoxicity and tau phosphorylation in primary culture. Cell Death Dis. 2: e167. doi: 10.1038/cddis.2011.50
Ghosh, S., Whitley, C. S., Haribabu, B., and Jala, V. R. (2021). Regulation of intestinal barrier function by microbial metabolites. Cell. Mol. Gastroenterol. Hepatol. 11, 1463–1482. doi: 10.1016/j.jcmgh.2021.02.007
Gimsa, U., Mitchison, N. A., and Brunner-Weinzierl, M. C. (2013). Immune privilege as an intrinsic CNS property: astrocytes protect the CNS against T-cell-mediated neuroinflammation. Mediators Inflamm. 2013, 1–11. doi: 10.1155/2013/320519
Ginhoux, F., Greter, M., Leboeuf, M., Nandi, S., See, P., Gokhan, S., et al. (2010). Fate mapping analysis reveals that adult microglia derive from primitive macrophages. Science 330, 841–845. doi: 10.1126/science.1194637
Golomb, S. M., Guldner, I. H., Zhao, A., Wang, Q., Palakurthi, B., Aleksandrovic, E. A., et al. (2020). Multi-modal single-cell analysis reveals brain immune landscape plasticity during aging and gut microbiota dysbiosis. Cell Rep. 33:108438. doi: 10.1016/j.celrep.2020.108438
Gomez de Aguero, M., Ganal-Vonarburg, S. C., Fuhrer, T., Rupp, S., Uchimura, Y., Li, H., et al. (2016). The maternal microbiota drives early postnatal innate immune development. Science 351, 1296–1302. doi: 10.1126/science.aad2571
Gomez Perdiguero, E., Klapproth, K., Schulz, C., Busch, K., Azzoni, E., Crozet, L., et al. (2015). Tissue-resident macrophages originate from yolk-sac-derived erythro-myeloid progenitors. Nature 518, 547–551. doi: 10.1038/nature13989
Goshi, N., Morgan, R. K., Lein, P. J., and Seker, E. (2020). A primary neural cell culture model to study neuron, astrocyte and microglia interactions in neuroinflammation. J. Neuroinflammation 17:155. doi: 10.1186/s12974-020-01819-z
Gu, X.-L., Long, C.-X., Sun, L., Xie, C., Lin, X., and Cai, H. (2010). Astrocytic expression of Parkinson’s disease-related A53T α-synuclein causes neurodegeneration in mice. Mol. Brain 31:6. doi: 10.1186/1756-6606-3-12
Guerreiro, R., Wojtas, A., Bras, J., Carrasquillo, M., Rogaeva, E., Majounie, E., et al. (2013). TREM2 variants in Alzheimer’s disease. N. Engl. J. Med. 368, 117–127. doi: 10.1056/NEJMoa1211851
Guttikonda, S. R., Sikkema, L., Tchieu, J., Saurat, N., Walsh, R. M., Harschnitz, O., et al. (2021). Fully defined human pluripotent stem cell-derived microglia and tri-culture system model C3 production in Alzheimer’s disease. Nat. Neurosci. 24, 343–354. doi: 10.1038/s41593-020-00796-z
Habib, N., McCabe, C., Medina, S., Varshavsky, M., Kitsberg, D., Dvir-Szternfeld, R., et al. (2020). Disease-associated astrocytes in Alzheimer’s disease and aging. Nat. Neurosci. 23, 701–706. doi: 10.1038/s41593-020-0624-8
Hagemeyer, N., Hanft, K.-M., Akriditou, M.-A., Unger, N., Park, E. S., Stanley, E. R., et al. (2017). Microglia contribute to normal myelinogenesis and to oligodendrocyte progenitor maintenance during adulthood. Acta Neuropathol. 134, 441–458. doi: 10.1007/s00401-017-1747-1
Halder, S. K., and Milner, R. (2019). A critical role for microglia in maintaining vascular integrity in the hypoxic spinal cord. Proc. Natl. Acad. Sci. U S A 116, 26029–26037. doi: 10.1073/pnas.1912178116
Hammond, T. R., Dufort, C., Dissing-Olesen, L., Giera, S., Young, A., Wysoker, A., et al. (2019). Single-cell RNA sequencing of microglia throughout the mouse lifespan and in the injured brain reveals complex cell-state changes. Immunity 50, 253–271.e6. doi: 10.1016/j.immuni.2018.11.004
Harach, T., Marungruang, N., Duthilleul, N., Cheatham, V., Mc Coy, K. D., Frisoni, G., et al. (2017). Reduction of Abeta amyloid pathology in APPPS1 transgenic mice in the absence of gut microbiota. Sci. Rep. 7:41802. doi: 10.1038/srep41802
Hart, A. D., Wyttenbach, A., Hugh Perry, V., and Teeling, J. L. (2012). Age related changes in microglial phenotype vary between CNS regions: gray versus white matter differences. Brain Behav. Immun. 26, 754–765. doi: 10.1016/j.bbi.2011.11.006
Haruwaka, K., Ikegami, A., Tachibana, Y., Ohno, N., Konishi, H., Hashimoto, A., et al. (2019). Dual microglia effects on blood brain barrier permeability induced by systemic inflammation. Nat. Commun. 10:5816. doi: 10.1038/s41467-019-13812-z
Hashioka, S., Klegeris, A., Schwab, C., and McGeer, P. L. (2009). Interferon-γ-dependent cytotoxic activation of human astrocytes and astrocytoma cells. Neurobiol. Aging 30, 1924–1935. doi: 10.1016/j.neurobiolaging.2008.02.019
Hefendehl, J. K., Neher, J. J., Sühs, R. B., Kohsaka, S., Skodras, A., and Jucker, M. (2014). Homeostatic and injury-induced microglia behavior in the aging brain. Aging Cell 13, 60–69. doi: 10.1111/acel.12149
Heithoff, B. P., George, K. K., Phares, A. N., Zuidhoek, I. A., Munoz-Ballester, C., and Robel, S. (2020). Astrocytes are necessary for blood-brain barrier maintenance in the adult mouse brain. Glia 69, 436–472. doi: 10.1002/glia.23908
Heneka, M. T., Golenbock, D. T., and Latz, E. (2015). Innate immunity in Alzheimer’s disease. Nat. Immunol. 16, 229–236. doi: 10.1038/ni.3102
Heneka, M. T., Kummer, M. P., Stutz, A., Delekate, A., Schwartz, S., Vieira-Saecker, A., et al. (2013). NLRP3 is activated in Alzheimer’s disease and contributes to pathology in APP/PS1 mice. Nature 493, 674–678. doi: 10.1038/nature11729
Herculano-Houzel, S. (2014). The glia/neuron ratio: how it varies uniformly across brain structures and species and what that means for brain physiology and evolution: the glia/neuron ratio. Glia 62, 1377–1391. doi: 10.1002/glia.22683
Herzog, C., Pons Garcia, L., Keatinge, M., Greenald, D., Moritz, C., Peri, F., et al. (2019). Rapid clearance of cellular debris by microglia limits secondary neuronal cell death after brain injury in vivo. Development 146:dev174698. doi: 10.1242/dev.174698
Hong, S., Beja-Glasser, V. F., Nfonoyim, B. M., Frouin, A., Li, S., Ramakrishnan, S., et al. (2016). Complement and microglia mediate early synapse loss in Alzheimer mouse models. Science 352, 712–716. doi: 10.1126/science.aad8373
Hu, Z.-L., Sun, T., Lu, M., Ding, J.-H., Du, R.-H., and Hu, G. (2019). Kir6.1/K-ATP channel on astrocytes protects against dopaminergic neurodegeneration in the MPTP mouse model of Parkinson’s disease via promoting mitophagy. Brain Behav. Immun. 81, 509–522. doi: 10.1016/j.bbi.2019.07.009
Hughes, A. N., and Appel, B. (2020). Microglia phagocytose myelin sheaths to modify developmental myelination. Nat. Neurosci. 23, 1055–1066 doi: 10.1038/s41593-020-0654-2
Hughes, E. G., Elmariah, S. B., and Balice-Gordon, R. J. (2010). Astrocyte secreted proteins selectively increase hippocampal GABAergic axon length, branching and synaptogenesis. Mol. Cell. Neurosci. 43, 136–145. doi: 10.1016/j.mcn.2009.10.004
Ioannou, M. S., Jackson, J., Sheu, S.-H., Chang, C.-L., Weigel, A. V., Liu, H., et al. (2019). Neuron-astrocyte metabolic coupling protects against activity-induced fatty acid toxicity. Cell 177, 1522–1535.e14. doi: 10.1016/j.cell.2019.04.001
Ising, C., Venegas, C., Zhang, S., Scheiblich, H., Schmidt, S. V., Vieira-Saecker, A., et al. (2019). NLRP3 inflammasome activation drives tau pathology. Nature 575, 669–673. doi: 10.1038/s41586-019-1769-z
Isobe, I., Watanabe, T., Yotsuyanagi, T., Hazemoto, N., Yamagata, K., Ueki, T., et al. (1996). Astrocytic contributions to blood-brain barrier (BBB) formation by endothelial cells: a possible use of aortic endothelial cell for in vitro BBB model. Neurochem. Int. 28, 523–533. doi: 10.1016/0197-0186(95)00142-5
Izco, M., Blesa, J., Verona, G., Cooper, J. M., and Alvarez-Erviti, L. (2021). Glial activation precedes alpha-synuclein pathology in a mouse model of Parkinson’s disease. Neurosci. Res. 170, 330–340. doi: 10.1016/j.neures.2020.11.004
Jablonski, A. M., Warren, L., Usenovic, M., Zhou, H., Sugam, J., Parmentier-Batteur, S., et al. (2021). Astrocytic expression of the Alzheimer’s disease risk allele, ApoEε4, potentiates neuronal tau pathology in multiple preclinical models. Sci. Rep. 11:3438. doi: 10.1038/s41598-021-82901-1
Jack, C. S., Arbour, N., Manusow, J., Montgrain, V., Blain, M., McCrea, E., et al. (2005). TLR signaling tailors innate immune responses in human microglia and astrocytes. J. Immunol. 175, 4320–4330. doi: 10.4049/jimmunol.175.7.4320
James, K. R., Gomes, T., Elmentaite, R., Kumar, N., Gulliver, E. L., King, H. W., et al. (2020). Distinct microbial and immune niches of the human colon. Nat. Immunol. 21, 343–353. doi: 10.1038/s41590-020-0602-z
Javed, I., Zhang, Z., Adamcik, J., Andrikopoulos, N., Li, Y., Otzen, D. E., et al. (2020). Accelerated amyloid beta pathogenesis by bacterial amyloid FapC. Adv. Sci. 7:2001299. doi: 10.1002/advs.202001299
Ji, K., Akgul, G., Wollmuth, L. P., and Tsirka, S. E. (2013). Microglia actively regulate the number of functional synapses. PLoS One 8:e56293. doi: 10.1371/journal.pone.0056293
Jiang, T., and Cadenas, E. (2014). Astrocytic metabolic and inflammatory changes as a function of age. Aging Cell 13, 1059–1067. doi: 10.1111/acel.12268
Jiménez, E., Marín, M. L., Martín, R., Odriozola, J. M., Olivares, M., Xaus, J., et al. (2008). Is meconium from healthy newborns actually sterile? Res. Microbiol. 159, 187–193. doi: 10.1016/j.resmic.2007.12.007
Johnson, K. M., Milner, R., and Crocker, S. J. (2015). Extracellular matrix composition determines astrocyte responses to mechanical and inflammatory stimuli. Neurosci. Lett. 600, 104–109. doi: 10.1016/j.neulet.2015.06.013
Johnson, S. L., Kirk, R. D., DaSilva, N. A., Ma, H., Seeram, N. P., and Bertin, M. J. (2019). Polyphenol microbial metabolites exhibit gut and blood-brain barrier permeability and protect murine microglia against LPS-induced inflammation. Metabolites 9:78. doi: 10.3390/metabo9040078
Jonsson, T., Stefansson, H., Steinberg, S., Jonsdottir, I., Jonsson, P. V., Snaedal, J., et al. (2013). Variant of TREM2 associated with the risk of Alzheimer’s disease. N. Engl. J. Med. 368, 107–116. doi: 10.1056/NEJMoa1211103
Joost, E., Jordão, M. J. C., Mages, B., Prinz, M., Bechmann, I., and Krueger, M. (2019). Microglia contribute to the glia limitans around arteries, capillaries and veins under physiological conditions, in a model of neuroinflammation and in human brain tissue. Brain Struct. Funct. 224, 1301–1314. doi: 10.1007/s00429-019-01834-8
Jyothi, H. J., Vidyadhara, D. J., Mahadevan, A., Philip, M., Parmar, S. K., Manohari, S. G., et al. (2015). Aging causes morphological alterations in astrocytes and microglia in human substantia nigra pars compacta. Neurobiol. Aging 36, 3321–3333. doi: 10.1016/j.neurobiolaging.2015.08.024
Katsouri, L., Birch, A. M., Renziehausen, A. W. J., Zach, C., Aman, Y., Steeds, H., et al. (2020). Ablation of reactive astrocytes exacerbates disease pathology in a model of Alzheimer’s disease. Glia 68, 1017–1030. doi: 10.1002/glia.23759
Kaur, C., Hao, A.-J., Wu, C.-H., and Ling, E.-A. (2001). Origin of microglia. Microsc. Res. Tech. 54, 2–9. doi: 10.1002/jemt.1114
Keane, L., Antignano, I., Riechers, S.-P., Zollinger, R., Dumas, A. A., Offermann, N., et al. (2021). mTOR-dependent translation amplifies microglia priming in aging mice. J. Clin. Invest. 131:e132727. doi: 10.1172/JCI132727
Kennedy, K. M., Gerlach, M. J., Adam, T., Heimesaat, M. M., Rossi, L., Surette, M. G., et al. (2021). Fetal meconium does not have a detectable microbiota before birth. Nat. Microbiol. 6, 865–873 doi: 10.1038/s41564-021-00904-0
Keren-Shaul, H., Spinrad, A., Weiner, A., Matcovitch-Natan, O., Dvir-Szternfeld, R., Ulland, T. K., et al. (2017). A unique microglia type associated with restricting development of Alzheimer’s disease. Cell 169, 1276–1290.e17. doi: 10.1016/j.cell.2017.05.018
Kleinberger, G., Brendel, M., Mracsko, E., Wefers, B., Groeneweg, L., Xiang, X., et al. (2017). The FTD-like syndrome causing TREM 2 T66M mutation impairs microglia function, brain perfusion and glucose metabolism. EMBO J. 36, 1837–1853. doi: 10.15252/embj.201796516
Ko, Y., Ament, S. A., Eddy, J. A., Caballero, J., Earls, J. C., Hood, L., et al. (2013). Cell type-specific genes show striking and distinct patterns of spatial expression in the mouse brain. Proc. Natl. Acad. Sci. U S A 110, 3095–3100. doi: 10.1073/pnas.1222897110
Kobayashi, E., Nakano, M., Kubota, K., Himuro, N., Mizoguchi, S., Chikenji, T., et al. (2018). Activated forms of astrocytes with higher GLT-1 expression are associated with cognitive normal subjects with Alzheimer pathology in human brain. Sci. Rep. 8:1712. doi: 10.1038/s41598-018-19442-7
Krasemann, S., Madore, C., Cialic, R., Baufeld, C., Calcagno, N., El Fatimy, R., et al. (2017). The TREM2-APOE pathway drives the transcriptional phenotype of dysfunctional microglia in neurodegenerative diseases. Immunity 47, 566–581.e9. doi: 10.1016/j.immuni.2017.08.008
Kucukdereli, H., Allen, N. J., Lee, A. T., Feng, A., Ozlu, M. I., Conatser, L. M., et al. (2011). Control of excitatory CNS synaptogenesis by astrocyte-secreted proteins Hevin and SPARC. Proc. Natl. Acad. Sci. U S A 108, E440–E449. doi: 10.1073/pnas.1104977108
Kundu, P., Torres, E. R. S., Stagaman, K., Kasschau, K., Okhovat, M., Holden, S., et al. (2021). Integrated analysis of behavioral, epigenetic and gut microbiome analyses in AppNL-G-F, AppNL-F and wild type mice. Sci. Rep. 11:4678. doi: 10.1038/s41598-021-83851-4
Lampron, A., Larochelle, A., Laflamme, N., Préfontaine, P., Plante, M.-M., Sánchez, M. G., et al. (2015). Inefficient clearance of myelin debris by microglia impairs remyelinating processes. J. Exp. Med. 212, 481–495. doi: 10.1084/jem.20141656
Lanjakornsiripan, D., Pior, B.-J., Kawaguchi, D., Furutachi, S., Tahara, T., Katsuyama, Y., et al. (2018). Layer-specific morphological and molecular differences in neocortical astrocytes and their dependence on neuronal layers. Nat. Commun. 9:1623. doi: 10.1038/s41467-018-03940-3
Lassmann, H., Zimprich, F., Vass, K., and Hickey, W. F. (1991). Microglial cells are a component of the perivascular glia limitans. J. Neurosci. Res. 28, 236–243. doi: 10.1002/jnr.490280211
Lawson, L. J., Perry, V. H., Dri, P., and Gordon, S. (1990). Heterogeneity in the distribution and morphology of microglia in the normal adult mouse brain. Neuroscience 39, 151–170. doi: 10.1016/0306-4522(90)90229-w
Lebovitz, Y., Kowalski, E. A., Wang, X., Kelly, C., Lee, M., McDonald, V., et al. (2019). Lactobacillus rescues postnatal neurobehavioral and microglial dysfunction in a model of maternal microbiome dysbiosis. Brain Behav. Immun. 81, 617–629. doi: 10.1016/j.bbi.2019.07.025
Lee, J., Venna, V. R., Durgan, D. J., Shi, H., Hudobenko, J., Putluri, N., et al. (2020). Young versus aged microbiota transplants to germ-free mice: increased short-chain fatty acids and improved cognitive performance. Gut Microbes 12:1814107. doi: 10.1080/19490976.2020.1814107
Lee, J.-H., Kim, J., Noh, S., Lee, H., Lee, S. Y., Mun, J. Y., et al. (2021). Astrocytes phagocytose adult hippocampal synapses for circuit homeostasis. Nature 590, 612–617. doi: 10.1038/s41586-020-03060-3
Leyns, C. E. G., Ulrich, J. D., Finn, M. B., Stewart, F. R., Koscal, L. J., Remolina Serrano, J., et al. (2017). TREM2 deficiency attenuates neuroinflammation and protects against neurodegeneration in a mouse model of tauopathy. Proc. Natl. Acad. Sci. U S A 114, 11524–11529. doi: 10.1073/pnas.1710311114
Lian, H., Litvinchuk, A., Chiang, A. C.-A., Aithmitti, N., Jankowsky, J. L., and Zheng, H. (2016). Astrocyte-microglia cross talk through complement activation modulates amyloid pathology in mouse models of Alzheimer’s disease. J. Neurosci. 36, 577–589. doi: 10.1523/JNEUROSCI.2117-15.2016
Liddelow, S. A., Guttenplan, K. A., Clarke, L. E., Bennett, F. C., Bohlen, C. J., Schirmer, L., et al. (2017). Neurotoxic reactive astrocytes are induced by activated microglia. Nature 541, 481–487. doi: 10.1038/nature21029
Lim, S.-H., Park, E., You, B., Jung, Y., Park, A.-R., Park, S. G., et al. (2013). Neuronal synapse formation induced by microglia and interleukin 10. PLoS One 8:e81218. doi: 10.1371/journal.pone.0081218
Lonnemann, N., Hosseini, S., Marchetti, C., Skouras, D. B., Stefanoni, D., D’Alessandro, A., et al. (2020). The NLRP3 inflammasome inhibitor OLT1177 rescues cognitive impairment in a mouse model of Alzheimer’s disease. Proc. Natl. Acad. Sci. U S A 117, 32145–32154. doi: 10.1073/pnas.2009680117
Lopes, K. O., Sparks, D. L., and Streit, W. J. (2008). Microglial dystrophy in the aged and Alzheimer’s disease brain is associated with ferritin immunoreactivity. Glia 56, 1048–1060. doi: 10.1002/glia.20678
Loving, B. A., Tang, M., Neal, M. C., Gorkhali, S., Murphy, R., Eckel, R. H., et al. (2021). Lipoprotein lipase regulates microglial lipid droplet accumulation. Cells 10:198. doi: 10.3390/cells10020198
Lu, J., Lu, L., Yu, Y., Baranowski, J., and Claud, E. C. (2020). Maternal administration of probiotics promotes brain development and protects offspring’s brain from postnatal inflammatory insults in C57/BL6J mice. Sci. Rep. 10:8178. doi: 10.1038/s41598-020-65180-0
Luck, B., Engevik, M. A., Ganesh, B. P., Lackey, E. P., Lin, T., Balderas, M., et al. (2020). Bifidobacteria shape host neural circuits during postnatal development by promoting synapse formation and microglial function. Sci. Rep. 10:7737. doi: 10.1038/s41598-020-64173-3
Lukiw, W. J. (2004). Gene expression profiling in fetal, aged and Alzheimer hippocampus: a continuum of stress-related signaling. Neurochem. Res. 29, 1287–1297. doi: 10.1023/b:nere.0000023615.89699.63
Ma, C., Li, S., Hu, Y., Ma, Y., Wu, Y., Wu, C., et al. (2021). AIM2 controls microglial inflammation to prevent experimental autoimmune encephalomyelitis. J. Exp. Med. 218:e20201796. doi: 10.1084/jem.20201796
Magnusson, J. P., Zamboni, M., Santopolo, G., Mold, J. E., Barrientos-Somarribas, M., Talavera-Lopez, C., et al. (2020). Activation of a neural stem cell transcriptional program in parenchymal astrocytes. eLife 9:e59733. doi: 10.7554/eLife.59733
Man, A. L., Bertelli, E., Rentini, S., Regoli, M., Briars, G., Marini, M., et al. (2015). Age-associated modifications of intestinal permeability and innate immunity in human small intestine. Clin. Sci. 129, 515–527. doi: 10.1042/CS20150046
Manor, O., Dai, C. L., Kornilov, S. A., Smith, B., Price, N. D., Lovejoy, J. C., et al. (2020). Health and disease markers correlate with gut microbiome composition across thousands of people. Nat. Commun. 11:5206. doi: 10.1038/s41467-020-18871-1
Maphis, N., Xu, G., Kokiko-Cochran, O. N., Jiang, S., Cardona, A., Ransohoff, R. M., et al. (2015). Reactive microglia drive tau pathology and contribute to the spreading of pathological tau in the brain. Brain 138, 1738–1755. doi: 10.1093/brain/awv081
Margineanu, M. B., Sherwin, E., Golubeva, A., Peterson, V., Hoban, A., Fiumelli, H., et al. (2020). Gut microbiota modulates expression of genes involved in the astrocyte-neuron lactate shuttle in the hippocampus. Eur. Neuropsychopharmacol. 41, 152–159. doi: 10.1016/j.euroneuro.2020.11.006
Marinova-Mutafchieva, L., Sadeghian, M., Broom, L., Davis, J. B., Medhurst, A. D., and Dexter, D. T. (2009). Relationship between microglial activation and dopaminergic neuronal loss in the substantia nigra: a time course study in a 6-hydroxydopamine model of Parkinson’s disease. J. Neurochem. 110, 966–975. doi: 10.1111/j.1471-4159.2009.06189.x
Marschallinger, J., Iram, T., Zardeneta, M., Lee, S. E., Lehallier, B., Haney, M. S., et al. (2020). Lipid-droplet-accumulating microglia represent a dysfunctional and proinflammatory state in the aging brain. Nat. Neurosci. 23, 194–208. doi: 10.1038/s41593-019-0566-1
Masuda, T., Sankowski, R., Staszewski, O., Böttcher, C., Amann, L., Scheiwe, C., et al. (2019). Spatial and temporal heterogeneity of mouse and human microglia at single-cell resolution. Nature 566, 388–392. doi: 10.1038/s41586-019-0924-x
Matcovitch-Natan, O., Winter, D. R., Giladi, A., Vargas Aguilar, S., Spinrad, A., Sarrazin, S., et al. (2016). Microglia development follows a stepwise program to regulate brain homeostasis. Science 353:aad8670. doi: 10.1126/science.aad8670
Mattson, M. P., and Arumugam, T. V. (2018). Hallmarks of brain aging: adaptive and pathological modification by metabolic states. Cell Metab. 27, 1176–1199. doi: 10.1016/j.cmet.2018.05.011
Mezö, C., Dokalis, N., Mossad, O., Staszewski, O., Neuber, J., Yilmaz, B., et al. (2020). Different effects of constitutive and induced microbiota modulation on microglia in a mouse model of Alzheimer’s disease. Acta Neuropathol. Commun. 8:119. doi: 10.1186/s40478-020-00988-5
Minter, M. R., Hinterleitner, R., Meisel, M., Zhang, C., Leone, V., Zhang, X., et al. (2017). Antibiotic-induced perturbations in microbial diversity during post-natal development alters amyloid pathology in an aged APPSWE/PS1ΔE9 murine model of Alzheimer’s disease. Sci. Rep. 7:10411. doi: 10.1038/s41598-017-11047-w
Minter, M. R., Zhang, C., Leone, V., Ringus, D. L., Zhang, X., Oyler-Castrillo, P., et al. (2016). Antibiotic-induced perturbations in gut microbial diversity influences neuro-inflammation and amyloidosis in a murine model of Alzheimer’s disease. Sci. Rep. 6:30028. doi: 10.1038/srep30028
Miyamoto, A., Wake, H., Ishikawa, A. W., Eto, K., Shibata, K., Murakoshi, H., et al. (2016). Microglia contact induces synapse formation in developing somatosensory cortex. Nat. Commun. 7:12540. doi: 10.1038/ncomms12540
Miyazaki, I., Isooka, N., Wada, K., Kikuoka, R., Kitamura, Y., and Asanuma, M. (2019). Effects of enteric environmental modification by coffee components on neurodegeneration in rotenone-treated mice. Cells 8:221. doi: 10.3390/cells8030221
Möhle, L., Mattei, D., Heimesaat, M. M., Bereswill, S., Fischer, A., Alutis, M., et al. (2016). Ly6Chi monocytes provide a link between antibiotic-induced changes in gut microbiota and adult hippocampal neurogenesis. Cell Rep. 15, 1945–1956. doi: 10.1016/j.celrep.2016.04.074
Mondo, E., Becker, S. C., Kautzman, A. G., Schifferer, M., Baer, C. E., Chen, J., et al. (2020). A developmental analysis of juxtavascular microglia dynamics and interactions with the vasculature. J. Neurosci. 40, 6503–6521. doi: 10.1523/JNEUROSCI.3006-19.2020
Morales, I., Sanchez, A., Rodriguez-Sabate, C., and Rodriguez, M. (2017). Striatal astrocytes engulf dopaminergic debris in Parkinson’s disease: a study in an animal model. PLoS One 12:e0185989. doi: 10.1371/journal.pone.0185989
Moseman, E. A., Blanchard, A. C., Nayak, D., and McGavern, D. B. (2020). T cell engagement of cross-presenting microglia protects the brain from a nasal virus infection. Sci. Immunol. 5:eabb1817. doi: 10.1126/sciimmunol.abb1817
Myer, D. J. (2006). Essential protective roles of reactive astrocytes in traumatic brain injury. Brain 129, 2761–2772. doi: 10.1093/brain/awl165
Nagele, R. G., D’Andrea, M. R., Lee, H., Venkataraman, V., and Wang, H.-Y. (2003). Astrocytes accumulate Ab42 and give rise to astrocytic amyloid plaques in Alzheimer disease brains. Brain Res. 13:197. doi: 10.1016/s0006-8993(03)02361-8
Nagpal, R., Wang, S., Solberg Woods, L. C., Seshie, O., Chung, S. T., Shively, C. A., et al. (2018). Comparative microbiome signatures and short-chain fatty acids in mouse, rat, non-human primate and human feces. Front. Microbiol. 9:2897. doi: 10.3389/fmicb.2018.02897
Nguyen, P. T., Dorman, L. C., Pan, S., Vainchtein, I. D., Han, R. T., Nakao-Inoue, H., et al. (2020). Microglial remodeling of the extracellular matrix promotes synapse plasticity. Cell 182, 388–403.e15. doi: 10.1016/j.cell.2020.05.050
Nikodemova, M., Small, A. L., Kimyon, R. S., and Watters, J. J. (2016). Age-dependent differences in microglial responses to systemic inflammation are evident as early as middle age. Physiol. Genom. 48, 336–344. doi: 10.1152/physiolgenomics.00129.2015
Nimmerjahn, A., Kirchhoff, F., and Helmchen, F. (2005). Resting microglial cells are highly dynamic surveillants of brain parenchyma in vivo. Science 308:5. doi: 10.1126/science.1110647
Njie, E. G., Boelen, E., Stassen, F. R., Steinbusch, H. W. M., Borchelt, D. R., and Streit, W. J. (2012). Ex vivo cultures of microglia from young and aged rodent brain reveal age-related changes in microglial function. Neurobiol. Aging 33, 195e1–e12. doi: 10.1016/j.neurobiolaging.2010.05.008
Ogbonnaya, E. S., Clarke, G., Shanahan, F., Dinan, T. G., Cryan, J. F., and O’Leary, O. F. (2015). Adult hippocampal neurogenesis is regulated by the microbiome. Biol. Psychiatry 78, e7–9. doi: 10.1016/j.biopsych.2014.12.023
Oksanen, M., Petersen, A. J., Naumenko, N., Puttonen, K., Lehtonen, Š., Gubert Olivé, M., et al. (2017). PSEN1 mutant iPSC-derived model reveals severe astrocyte pathology in Alzheimer’s disease. Stem Cell Rep. 9, 1885–1897. doi: 10.1016/j.stemcr.2017.10.016
Olah, M., Patrick, E., Villani, A.-C., Xu, J., White, C. C., Ryan, K. J., et al. (2018). A transcriptomic atlas of aged human microglia. Nat. Commun. 9:539. doi: 10.1038/s41467-018-02926-5
Olson, J. K., and Miller, S. D. (2004). Microglia initiate central nervous system innate and adaptive immune responses through multiple TLRs. J. Immunol. 173, 3916–3924. doi: 10.4049/jimmunol.173.6.3916
Pan, J., Ma, N., Yu, B., Zhang, W., and Wan, J. (2020). Transcriptomic profiling of microglia and astrocytes throughout aging. J. Neuroinflammation 17:97. doi: 10.1186/s12974-020-01774-9
Park, C., Lee, S., Cho, I.-H., Lee, H. K., Kim, D., Choi, S.-Y., et al. (2006). TLR3-mediated signal induces proinflammatory cytokine and chemokine gene expression in astrocytes: differential signaling mechanisms of TLR3-induced IP-10 and IL-8 gene expression. Glia 53, 248–256. doi: 10.1002/glia.20278
Parkhurst, C. N., Yang, G., Ninan, I., Savas, J. N., Yates, J. R., Lafaille, J. J., et al. (2013). Microglia promote learning-dependent synapse formation through brain-derived neurotrophic factor. Cell 155, 1596–1609. doi: 10.1016/j.cell.2013.11.030
Pascual, O., Ben Achour, S., Rostaing, P., Triller, A., and Bessis, A. (2012). Microglia activation triggers astrocyte-mediated modulation of excitatory neurotransmission. Proc. Natl. Acad. Sci. U S A 109, E197–E205. doi: 10.1073/pnas.1111098109
Pellerin, L., and Magistretti, P. J. (1994). Glutamate uptake into astrocytes stimulates aerobic glycolysis: a mechanism coupling neuronal activity to glucose utilization. Proc. Natl. Acad. Sci. U S A 91, 10625–10629. doi: 10.1073/pnas.91.22.10625
Pitt, J., Wilcox, K. C., Tortelli, V., Diniz, L. P., Oliveira, M. S., Dobbins, C., et al. (2017). Neuroprotective astrocyte-derived insulin/insulin-like growth factor 1 stimulates endocytic processing and extracellular release of neuron-bound Aβ oligomers. Mol. Biol. Cell 28, 2623–2636. doi: 10.1091/mbc.E17-06-0416
Popov, A., Brazhe, A., Denisov, P., Sutyagina, O., Li, L., Lazareva, N., et al. (2021). Astrocyte dystrophy in ageing brain parallels impaired synaptic plasticity. Aging Cell 20:e13334. doi: 10.1111/acel.13334
Qi, Y., Goel, R., Kim, S., Richards, E. M., Carter, C. S., Pepine, C. J., et al. (2017). Intestinal permeability biomarker zonulin is elevated in healthy aging. J. Am. Med .Dir. Assoc. 18, 810.e1–810.e4. doi: 10.1016/j.jamda.2017.05.018
Raivich, G., Bohatschek, M., Kloss, C. U. A., Werner, A., Jones, L. L., and Kreutzberg, G. W. (1999). Neuroglial activation repertoire in the injured brain: graded response, molecular mechanisms and cues to physiological function. Brain Res. Rev. 30, 77–105. doi: 10.1016/s0165-0173(99)00007-7
Ramos-Gonzalez, P., Mato, S., Chara, J. C., Verkhratsky, A., Matute, C., and Cavaliere, F. (2021). Astrocytic atrophy as a pathological feature of Parkinson’s disease with LRRK2 mutation. NPJ Parkinsons Dis. 7:31. doi: 10.1038/s41531-021-00175-w
Rash, B. G., Micali, N., Huttner, A. J., Morozov, Y. M., Horvath, T. L., and Rakic, P. (2018). Metabolic regulation and glucose sensitivity of cortical radial glial cells. Proc. Natl. Acad. Sci. U S A 115, 10142–10147. doi: 10.1073/pnas.1808066115
Rasley, A., Tranguch, S. L., Rati, D. M., and Marriott, I. (2006). Murine glia express the immunosuppressive cytokine, interleukin-10, following exposure toBorrelia burgdorferi orNeisseria meningitidis. Glia 53, 583–592. doi: 10.1002/glia.20314
Reichenbach, N., Delekate, A., Plescher, M., Schmitt, F., Krauss, S., Blank, N., et al. (2019). Inhibition of Stat3-mediated astrogliosis ameliorates pathology in an Alzheimer’s disease model. EMBO Mol. Med. 11:e9665. doi: 10.15252/emmm.201809665
Richter, M., Vidovic, N., Biber, K., Dolga, A., Culmsee, C., Dodel, R., et al. (2020). The neuroprotective role of microglial cells against amyloid beta-mediated toxicity in organotypic hippocampal slice cultures. Brain Pathol. 30, 589–602. doi: 10.1111/bpa.12807
Ritzel, R. M., Patel, A. R., Pan, S., Crapser, J., Hammond, M., Jellison, E., et al. (2015). Age- and location-related changes in microglial function. Neurobiol. Aging 36, 2153–2163. doi: 10.1016/j.neurobiolaging.2015.02.016
Rodríguez, J. J., Yeh, C.-Y., Terzieva, S., Olabarria, M., Kulijewicz-Nawrot, M., Verkhratsky, A., et al. (2014). Complex and region-specific changes in astroglial markers in the aging brain. Neurobiol. Aging 35, 15–23. doi: 10.1002/ajb2.1693
Romano, S., Savva, G. M., Bedarf, J. R., Charles, I. G., Hildebrand, F., Narbad, A., et al. (2021). Meta-analysis of the Parkinson’s disease gut microbiome suggests alterations linked to intestinal inflammation. NPJ Parkinsons Dis. 7:27. doi: 10.1038/s41531-021-00156-z
Rothhammer, V., Borucki, D. M., Tjon, E. C., Takenaka, M. C., Chao, C.-C., Ardura-Fabregat, A., et al. (2018). Microglial control of astrocytes in response to microbial metabolites. Nature 557, 724–728. doi: 10.1038/s41586-018-0119-x
Rothhammer, V., Mascanfroni, I. D., Bunse, L., Takenaka, M. C., Kenison, J. E., Mayo, L., et al. (2016). Type I interferons and microbial metabolites of tryptophan modulate astrocyte activity and central nervous system inflammation via the aryl hydrocarbon receptor. Nat. Med. 22, 586–597. doi: 10.1038/nm.4106
Rothstein, J. D., Dykes-Hoberg, M., Pardo, C. A., Bristol, L. A., Jin, L., Kuncl, R. W., et al. (1996). Knockout of glutamate transporters reveals a major role for astroglial transport in excitotoxicity and clearance of glutamate. Neuron 16, 675–686. doi: 10.1016/s0896-6273(00)80086-0
Safaiyan, S., Kannaiyan, N., Snaidero, N., Brioschi, S., Biber, K., Yona, S., et al. (2016). Age-related myelin degradation burdens the clearance function of microglia during aging. Nat. Neurosci. 19, 995–998. doi: 10.1038/nn.4325
Saha, P., Sarkar, S., Paidi, R. K., and Biswas, S. C. (2020). TIMP-1: a key cytokine released from activated astrocytes protects neurons and ameliorates cognitive behaviours in a rodent model of Alzheimer’s disease. Brain Behav. Immun. 87, 804–819. doi: 10.1016/j.bbi.2020.03.014
Sampson, T. R., Challis, C., Jain, N., Moiseyenko, A., Ladinsky, M. S., Shastri, G. G., et al. (2020). A gut bacterial amyloid promotes α-synuclein aggregation and motor impairment in mice. eLife 9:e53111. doi: 10.7554/eLife.53111
Sanmarco, L. M., Wheeler, M. A., Gutiérrez-Vázquez, C., Polonio, C. M., Linnerbauer, M., Pinho-Ribeiro, F. A., et al. (2021). Gut-licensed IFNγ+ NK cells drive LAMP1+TRAIL+ anti-inflammatory astrocytes. Nature 590, 473–479. doi: 10.1038/s41586-020-03116-4
Sariol, A., Mackin, S., Allred, M.-G., Ma, C., Zhou, Y., Zhang, Q., et al. (2020). Microglia depletion exacerbates demyelination and impairs remyelination in a neurotropic coronavirus infection. Proc. Natl. Acad. Sci. U S A 117, 24464–24474. doi: 10.1073/pnas.2007814117
Schafer, D. P., Lehrman, E. K., Kautzman, A. G., Koyama, R., Mardinly, A. R., Yamasaki, R., et al. (2012). Microglia sculpt postnatal neural circuits in an activity and complement-dependent manner. Neuron 74, 691–705. doi: 10.1016/j.neuron.2012.03.026
Schluter, J., Peled, J. U., Taylor, B. P., Markey, K. A., Smith, M., Taur, Y., et al. (2020). The gut microbiota is associated with immune cell dynamics in humans. Nature 588, 303–307. doi: 10.1038/s41586-020-2971-8
Shank, R. P., Bennett, G. S., Freytag, S. O., and Campbell, G. L. (1985). Pyruvate carboxylase: an astrocyte-specific enzyme implicated in the replenishment of amino acid neurotransmitter pools. Brain Res. 329, 364–367. doi: 10.1016/0006-8993(85)90552-9
Shen, W., Qi, R., Zhang, J., Wang, Z., Wang, H., Hu, C., et al. (2012). Chlorogenic acid inhibits LPS-induced microglial activation and improves survival of dopaminergic neurons. Brain Res. Bull. 88, 487–494. doi: 10.1016/j.brainresbull.2012.04.010
Shi, S. X., Li, Y.-J., Shi, K., Wood, K., Ducruet, A. F., and Liu, Q. (2020). IL (Interleukin)-15 bridges astrocyte-microglia crosstalk and exacerbates brain injury following intracerebral hemorrhage. Stroke 51, 967–974. doi: 10.1161/STROKEAHA.119.028638
Shimabukuro, M. K., Langhi, L. G. P., Cordeiro, I., Brito, J. M., Batista, C. M. de C., Mattson, M. P., et al. (2016). Lipid-laden cells differentially distributed in the aging brain are functionally active and correspond to distinct phenotypes. Sci. Rep. 6:23795. doi: 10.1038/srep23795
Shippy, D. C., Wilhelm, C., Viharkumar, P. A., Raife, T. J., and Ulland, T. K. (2020). β-Hydroxybutyrate inhibits inflammasome activation to attenuate Alzheimer’s disease pathology. J. Neuroinflammation 17:280. doi: 10.1186/s12974-020-01948-5
Sierra, A., Encinas, J. M., Deudero, J. J. P., Chancey, J. H., Enikolopov, G., Overstreet-Wadiche, L. S., et al. (2010). Microglia shape adult hippocampal neurogenesis through apoptosis-coupled phagocytosis. Cell Stem Cell 7, 483–495. doi: 10.1016/j.stem.2010.08.014
Sierra, A., Gottfried-Blackmore, A. C., McEwen, B. S., and Bulloch, K. (2007). Microglia derived from aging mice exhibit an altered inflammatory profile. Glia 55, 412–424. doi: 10.1002/glia.20468
Song, H., Stevens, C. F., and Gage, F. H. (2002). Astroglia induce neurogenesis from adult neural stem cells. Nature 417, 39–44. doi: 10.1038/417039a
Spangenberg, E. E., Lee, R. J., Najafi, A. R., Rice, R. A., Elmore, M. R. P., Blurton-Jones, M., et al. (2016). Eliminating microglia in Alzheimer’s mice prevents neuronal loss without modulating amyloid-β pathology. Brain 139, 1265–1281. doi: 10.1093/brain/aww016
Spangenberg, E., Severson, P. L., Hohsfield, L. A., Crapser, J., Zhang, J., Burton, E. A., et al. (2019). Sustained microglial depletion with CSF1R inhibitor impairs parenchymal plaque development in an Alzheimer’s disease model. Nat. Commun. 10:3758. doi: 10.1038/s41467-019-11674-z
Spencer, S. J., Basri, B., Sominsky, L., Soch, A., Ayala, M. T., Reineck, P., et al. (2019). High-fat diet worsens the impact of aging on microglial function and morphology in a region-specific manner. Neurobiol. Aging 74, 121–134. doi: 10.1016/j.neurobiolaging.2018.10.018
Streit, W. J., Sammons, N. W., Kuhns, A. J., and Sparks, D. L. (2004). Dystrophic microglia in the aging human brain. Glia 45, 208–212. doi: 10.1002/glia.10319
Sultan, S., Li, L., Moss, J., Petrelli, F., Cassé, F., Gebara, E., et al. (2015). Synaptic integration of adult-born hippocampal neurons is locally controlled by astrocytes. Neuron 88, 957–972. doi: 10.1016/j.neuron.2015.10.037
Sun, M.-F., Zhu, Y.-L., Zhou, Z.-L., Jia, X.-B., Xu, Y.-D., Yang, Q., et al. (2018). Neuroprotective effects of fecal microbiota transplantation on MPTP-induced Parkinson’s disease mice: Gut microbiota, glial reaction and TLR4/TNF-α signaling pathway. Brain Behav. Immun. 70, 48–60. doi: 10.1016/j.bbi.2018.02.005
Suzuki, A., Stern, S. A., Bozdagi, O., Huntley, G. W., Walker, R. H., Magistretti, P. J., et al. (2011). Astrocyte-neuron lactate transport is required for long-term memory formation. Cell 144, 810–823. doi: 10.1016/j.cell.2011.02.018
Takahashi, K., Yamamura, F., and Naito, M. (1989). Differentiation, maturation and proliferation of macrophages in the mouse yolk sac: a light-microscopic, enzyme-cytochemical, immunohistochemical and ultrastructural study. J. Leukoc. Biol. 45, 87–96. doi: 10.1002/jlb.45.2.87
Tarkowski, E. (2003). Intrathecal inflammation precedes development of Alzheimer’s disease. J. Neurol. Neurosurg. Psychiatry 74, 1200–1205. doi: 10.1136/jnnp.74.9.1200
The Human Microbiome Project Consortium (2012). Structure, function and diversity of the healthy human microbiome. Nature 486, 207–214. doi: 10.1038/nature11234
Thion, M. S., Low, D., Silvin, A., Chen, J., Grisel, P., Schulte-Schrepping, J., et al. (2018). Microbiome influences prenatal and adult microglia in a sex-specific manner. Cell 172, 500–516.e16. doi: 10.1016/j.cell.2017.11.042
Toku, K., Tanaka, J., Yano, H., Desaki, J., Zhang, B., Yang, L., et al. (1998). Microglial cells prevent nitric oxide-induced neuronal apoptosis in vitro. J. Neurosci. Res. 53:11. doi: 10.1002/(SICI)1097-4547(19980815)53:4<415::AID-JNR3>3.0.CO;2-9
Trapecar, M., Wogram, E., Svoboda, D., Communal, C., Omer, A., Lungjangwa, T., et al. (2021). Human physiomimetic model integrating microphysiological systems of the gut, liver and brain for studies of neurodegenerative diseases. Sci. Adv. 7:eabd1707. doi: 10.1126/sciadv.abd1707
Tremblay, M.-È., Lowery, R. L., and Majewska, A. K. (2010). Microglial interactions with synapses are modulated by visual experience. PLoS Biol 8:e1000527. doi: 10.1371/journal.pbio.1000527
Tremblay, M.-È., Zettel, M. L., Ison, J. R., Allen, P. D., and Majewska, A. K. (2012). Effects of aging and sensory loss on glial cells in mouse visual and auditory cortices. Glia 60, 541–558. doi: 10.1002/glia.22287
Tsunemi, T., Ishiguro, Y., Yoroisaka, A., Valdez, C., Miyamoto, K., Ishikawa, K., et al. (2020). Astrocytes protect human dopaminergic neurons from α-synuclein accumulation and propagation. J. Neurosci. 40, 8618–8628. doi: 10.1523/JNEUROSCI.0954-20.2020
Ulland, T. K., Song, W. M., Huang, S. C.-C., Ulrich, J. D., Sergushichev, A., Beatty, W. L., et al. (2017). TREM2 maintains microglial metabolic fitness in Alzheimer’s disease. Cell 170, 649–663.e13. doi: 10.1016/j.cell.2017.07.023
Vemuri, R., Shankar, E. M., Chieppa, M., Eri, R., and Kavanagh, K. (2020). Beyond just bacteria: functional biomes in the gut ecosystem including virome, mycobiome, archaeome and helminths. Microorganisms 8:483. doi: 10.3390/microorganisms8040483
Venegas, C., Kumar, S., Franklin, B. S., Dierkes, T., Brinkschulte, R., Tejera, D., et al. (2017). Microglia-derived ASC specks cross-seed amyloid-β in Alzheimer’s disease. Nature 552, 355–361. doi: 10.1038/nature25158
Vogt, N. M., Kerby, R. L., Dill-McFarland, K. A., Harding, S. J., Merluzzi, A. P., Johnson, S. C., et al. (2017). Gut microbiome alterations in Alzheimer’s disease. Sci. Rep. 7:13537. doi: 10.1038/s41598-017-13601-y
Vogt, N. M., Romano, K. A., Darst, B. F., Engelman, C. D., Johnson, S. C., Carlsson, C. M., et al. (2018). The gut microbiota-derived metabolite trimethylamine N-oxide is elevated in Alzheimer’s disease. Alzheimers Res. Ther. 10:124. doi: 10.1186/s13195-018-0451-2
Wang, C., Yue, H., Hu, Z., Shen, Y., Ma, J., Li, J., et al. (2020). Microglia mediate forgetting via complement-dependent synaptic elimination. Science 367, 688–694. doi: 10.1126/science.aaz2288
Wang, X., Saegusa, H., Huntula, S., and Tanabe, T. (2019). Blockade of microglial Cav1.2 Ca2+ channel exacerbates the symptoms in a Parkinson’s disease model. Sci. Rep. 9:9138. doi: 10.1038/s41598-019-45681-3
Wang, Y., Cella, M., Mallinson, K., Ulrich, J. D., Young, K. L., Robinette, M. L., et al. (2015). TREM2 lipid sensing sustains the microglial response in an Alzheimer’s disease model. Cell 160, 1061–1071. doi: 10.1016/j.cell.2015.01.049
Wang, Y., Ulland, T. K., Ulrich, J. D., Song, W., Tzaferis, J. A., Hole, J. T., et al. (2016). TREM2-mediated early microglial response limits diffusion and toxicity of amyloid plaques. J. Exp. Med. 213, 667–675. doi: 10.1084/jem.20151948
Wang, Y.-J., Monteagudo, A., Downey, M. A., Ashton-Rickardt, P. G., and Elmaleh, D. R. (2021). Cromolyn inhibits the secretion of inflammatory cytokines by human microglia (HMC3). Sci. Rep. 11:8054. doi: 10.1038/s41598-021-85702-8
Weinhard, L., di Bartolomei, G., Bolasco, G., Machado, P., Schieber, N. L., Neniskyte, U., et al. (2018). Microglia remodel synapses by presynaptic trogocytosis and spine head filopodia induction. Nat. Commun. 9:1228. doi: 10.1038/s41467-018-03566-5
Wen, R., Shen, H., Huang, S., Wang, L., Li, Z., Peng, P., et al. (2020). P2Y6 receptor inhibition aggravates ischemic brain injury by reducing microglial phagocytosis. CNS Neurosci. Ther. 26, 416–429. doi: 10.1111/cns.13296
Wilhelmsson, U., Faiz, M., de Pablo, Y., Sjöqvist, M., Andersson, D., Widestrand, Å., et al. (2012). Astrocytes negatively regulate neurogenesis through the jagged1-mediated notch pathway: astrocytes regulate neurogenesis. Stem Cells 30, 2320–2329. doi: 10.1002/stem.1196
Wilhelmsson, U., Lebkuechner, I., Leke, R., Marasek, P., Yang, X., Antfolk, D., et al. (2019). Nestin regulates neurogenesis in mice through notch signaling from astrocytes to neural stem cells. Cereb. Cortex 29, 4050–4066. doi: 10.1093/cercor/bhy284
Wilmanski, T., Diener, C., Rappaport, N., Patwardhan, S., Wiedrick, J., Lapidus, J., et al. (2021). Gut microbiome pattern reflects healthy ageing and predicts survival in humans. Nat. Metab. 3, 274–286. doi: 10.1038/s42255-021-00348-0
Wright, A. L., Zinn, R., Hohensinn, B., Konen, L. M., Beynon, S. B., Tan, R. P., et al. (2013). Neuroinflammation and neuronal loss precede Aβ plaque deposition in the hAPP-J20 mouse model of Alzheimer’s disease. PLoS One 8:e59586. doi: 10.1371/journal.pone.0059586
Xie, L., Zhang, N., Zhang, Q., Li, C., Sandhu, A. F., Iii, G. W., et al. (2020). Inflammatory factors and amyloid β-induced microglial polarization promote inflammatory crosstalk with astrocytes. Aging 12, 22538–22549. doi: 10.18632/aging.103663
Xu, C., Zhu, H., and Qiu, P. (2019). Aging progression of human gut microbiota. BMC Microbiol. 19:10. doi: 10.1186/s12866-019-1616-2
Yang, X., Ren, H., Wood, K., Li, M., Qiu, S., Shi, F.-D., et al. (2018). Depletion of microglia augments the dopaminergic neurotoxicity of MPTP. FASEB J. 32, 3336–3345. doi: 10.1096/fj.201700833RR
Ye, S.-M., and Johnson, R. W. (1999). Increased interleukin-6 expression by microglia from brain of aged mice. J. Neuroimmunol. 93, 139–148. doi: 10.1016/s0165-5728(98)00217-3
Yoshiyama, Y., Higuchi, M., Zhang, B., Huang, S.-M., Iwata, N., Saido, T. C., et al. (2007). Synapse loss and microglial activation precede tangles in a P301S tauopathy mouse model. Neuron 53, 337–351. doi: 10.1016/j.neuron.2007.01.010
Younge, N., McCann, J. R., Ballard, J., Plunkett, C., Akhtar, S., Araújo-Pérez, F., et al. (2019). Fetal exposure to the maternal microbiota in humans and mice. JCI Insight 4:e127806. doi: 10.1172/jci.insight.127806
Yuan, P., Condello, C., Keene, C. D., Wang, Y., Bird, T. D., Paul, S. M., et al. (2016). TREM2 haplodeficiency in mice and humans impairs the microglia barrier function leading to decreased amyloid compaction and severe axonal dystrophy. Neuron 92, 252–264. doi: 10.1016/j.neuron.2016.09.016
Yun, S. P., Kam, T.-I., Panicker, N., Kim, S., Oh, Y., Park, J.-S., et al. (2018). Block of A1 astrocyte conversion by microglia is neuroprotective in models of Parkinson’s disease. Nat. Med. 24, 931–938. doi: 10.1038/s41591-018-0051-5
Zamanian, J. L., Xu, L., Foo, L. C., Nouri, N., Zhou, L., Giffard, R. G., et al. (2012). Genomic analysis of reactive astrogliosis. J. Neurosci. 32, 6391–6410. doi: 10.1523/JNEUROSCI.6221-11.2012
Zhang, W., Wang, T., Pei, Z., Miller, D. S., Wu, X., Block, M. L., et al. (2005). Aggregated α-synuclein activates microglia: a process leading to disease progression in Parkinson’s disease. FASEB J. 19, 533–542. doi: 10.1096/fj.04-2751com
Zhang, Y.-N., Fan, J.-K., Gu, L., Yang, H.-M., Zhan, S.-Q., and Zhang, H. (2021). Metabotropic glutamate receptor 5 inhibits α-synuclein-induced microglia inflammation to protect from neurotoxicity in Parkinson’s disease. J. Neuroinflammation 18:23. doi: 10.1186/s12974-021-02079-1
Keywords: astrocytes, microglia, aging, neurodegeneration, glia, Alzheimer’s disease, microbiome, Parkinson’s disease
Citation: Hanslik KL, Marino KM and Ulland TK (2021) Modulation of Glial Function in Health, Aging, and Neurodegenerative Disease. Front. Cell. Neurosci. 15:718324. doi: 10.3389/fncel.2021.718324
Received: 31 May 2021; Accepted: 27 July 2021;
Published: 31 August 2021.
Edited by:
Sandro Dá Mesquita, Mayo Clinic Florida, United StatesReviewed by:
Fernanda Marques, , University of Minho, PortugalCopyright © 2021 Hanslik, Marino and Ulland. This is an open-access article distributed under the terms of the Creative Commons Attribution License (CC BY). The use, distribution or reproduction in other forums is permitted, provided the original author(s) and the copyright owner(s) are credited and that the original publication in this journal is cited, in accordance with accepted academic practice. No use, distribution or reproduction is permitted which does not comply with these terms.
*Correspondence: Tyler K. Ulland, dHVsbGFuZEB3aXNjLmVkdQ==
Disclaimer: All claims expressed in this article are solely those of the authors and do not necessarily represent those of their affiliated organizations, or those of the publisher, the editors and the reviewers. Any product that may be evaluated in this article or claim that may be made by its manufacturer is not guaranteed or endorsed by the publisher.
Research integrity at Frontiers
Learn more about the work of our research integrity team to safeguard the quality of each article we publish.