- 1University of Bordeaux, INSERM, Neurocentre Magendie, U1215, Bordeaux, France
- 2University of Bordeaux, CNRS, Interdisciplinary Institute for Neuroscience, IINS, UMR 5297, Bordeaux, France
- 3ShanghaiTech University, Shanghai, China
- 4Faculty of Science, Toho University, Funabashi, Japan
- 5RIKEN CLST, Kobe, Japan
Astrocytes are sensitive to ongoing neuronal/network activities and, accordingly, regulate neuronal functions (synaptic transmission, synaptic plasticity, behavior, etc.) by the context-dependent release of several gliotransmitters (e.g., glutamate, glycine, D-serine, ATP). To sense diverse input, astrocytes express a plethora of G-protein coupled receptors, which couple, via Gi/o and Gq, to the intracellular Ca2+ release channel IP3-receptor (IP3R). Indeed, manipulating astrocytic IP3R-Ca2+ signaling is highly consequential at the network and behavioral level: Depleting IP3R subtype 2 (IP3R2) results in reduced GPCR-Ca2+ signaling and impaired synaptic plasticity; enhancing IP3R-Ca2+ signaling affects cognitive functions such as learning and memory, sleep, and mood. However, as a result of discrepancies in the literature, the role of GPCR-IP3R-Ca2+ signaling, especially under physiological conditions, remains inconclusive. One primary reason for this could be that IP3R2 has been used to represent all astrocytic IP3Rs, including IP3R1 and IP3R3. Indeed, IP3R1 and IP3R3 are unique Ca2+ channels in their own right; they have unique biophysical properties, often display distinct distribution, and are differentially regulated. As a result, they mediate different physiological roles to IP3R2. Thus, these additional channels promise to enrich the diversity of spatiotemporal Ca2+ dynamics and provide unique opportunities for integrating neuronal input and modulating astrocyte–neuron communication. The current review weighs evidence supporting the existence of multiple astrocytic-IP3R isoforms, summarizes distinct sub-type specific properties that shape spatiotemporal Ca2+ dynamics. We also discuss existing experimental tools and future refinements to better recapitulate the endogenous activities of each IP3R isoform.
Introduction
Over the last three decades, Ca2+ imaging has revealed new roles for astrocytes. Indeed, astrocytic Ca2+ signaling was shown to regulate synaptic transmission, synaptic plasticity, and to influence behavior (Araque et al., 2014; Park and Lee, 2020). Inositol 1,4,5-trisphosphate receptors (IP3Rs) mediated Ca2+ signaling (IP3R-Ca2+) is regarded a primary generator of astrocytic Ca2+ signaling. Upon activation of Gq-GPCRs, the main input pathway of astrocytes, phospholipase C breaks down PIP2 into DAG and IP3, activating IP3R predominantly located on the membrane of endoplasmic reticulum (ER) resulting in Ca2+ release (Bootman et al., 2001). This IP3R-mediated Ca2+ signaling is considered to trigger the activity-dependent and selective release of chemical transmitters (gliotransmitters) such as glutamate, D-serine, and ATP, which have distinct influences over neuronal activity. Initially, IP3R subtype 2 (IP3R2) was the only recognized Ca2+ channel in astrocytes; However, advanced Ca2+ imaging techniques have since identified novel Ca2+ sources, including mitochondria (Agarwal et al., 2017), transient receptor potential ankyrin 1 (Shigetomi et al., 2012, 2013b), L-type voltage gated Ca2+ channels (Letellier et al., 2016), sodium/calcium exchanger (Kirischuk et al., 1997; Boddum et al., 2016; Rose et al., 2020), and transient receptor potential canonical (Shiratori-Hayashi et al., 2020) amongst others, thereby expanding the known Ca2+ signaling toolkit of astrocytes. Doubtlessly additional Ca2+ channels and sources will emerge in the future.
While the field’s focus has moved on from understanding IP3Rs to identifying new Ca2+ sources, understanding IP3R signaling in astrocytes remains highly relevant. Indeed, IP3Rs are the primary target for manipulating astrocytic activity, and such manipulations have proven to be very consequential in many studies. Since most of these manipulations indiscriminately influence all IP3R subtypes, this could reflect the key role played by IP3R subtypes other than IP3R2, namely IP3R1 and IP3R3, which were mostly overlooked. In this review, we summarize the evidence for different subtypes of IP3R and discuss how we can better study the role of IP3R-Ca2+ signaling in astrocytes which is one of the core issues in understanding astrocyte physiology.
Evidence for Three Subtypes
The Dogma: IP3R2 the Sole Functional Astrocytic IP3R
There are three mammalian IP3R subtypes, i.e., IP3R1 (Furuichi et al., 1989; Mignery et al., 1989; Yamada et al., 1994), IP3R2 (Mignery et al., 1990; Südhof et al., 1991; Yamamoto-Hino et al., 1994; Iwai et al., 2005), and IP3R3 (Blondel et al., 1993; Yamamoto-Hino et al., 1994; Iwai et al., 2005). Among them, IP3R2 has widely been accepted as the only functional IP3R subtype present in astrocytes, and consequently, the IP3R2KO model mouse has been at the center of numerous important studies (Agulhon et al., 2010; Takata et al., 2011; Chen et al., 2012; Navarrete et al., 2012, 2019; Cao et al., 2013; Perez-Alvarez et al., 2014; Petravicz et al., 2014; Gómez-Gonzalo et al., 2015, 2017; Mariotti et al., 2016; Monai et al., 2016; Perea et al., 2016; Martin-Fernandez et al., 2017; Tanaka et al., 2017). Although an important model in the astrocyte field, the belief that knocking out IP3R type-2 abolishes IP3 induced Ca2+ release (IICR) entirely appears to need remedying. This section considers the historical data from which the dogmatic view of astrocytic-IP3R2 has flowed and reviews the evidence for other IP3R subtypes.
Astrocyte Proteome
Several studies explored IP3Rs using immunohistochemistry which provided a consensus over the expression of IP3R2 in hippocampal/cortical astrocytes and Bergmann glia (Sharp et al., 1999; Holtzclaw et al., 2002; Hertle and Yeckel, 2007; Takata et al., 2011; Chen et al., 2012). While there are some conflicting reports over the immunoreactivity of IP3R3 in astrocytes and Bergmann glia (Sugiyama et al., 1994; Yamamoto-Hino et al., 1995; Hamada et al., 1999; Sharp et al., 1999; Holtzclaw et al., 2002), IP3R1 immunoreactivity was not initially observed in glia (Nakanishi et al., 1991; Dent et al., 1996; Hamada et al., 1999; Sharp et al., 1999; Holtzclaw et al., 2002; Hertle and Yeckel, 2007). These findings supported the view that IP3R2 is the predominant astrocytic IP3R. However, these results may also reflect limitations of the available IP3R antibodies or the difficulty of accurately assigning proteins located within ultrathin astrocyte processes, which are below the resolution limit of conventional microscopy (Panatier et al., 2014; Arizono et al., 2020) and buried amongst neuronal dendrites.
IP3R1 immunoreactivity was recently detected in spinal dorsal horn astrocytes (Shiratori-Hayashi et al., 2020) and, albeit with low stringency, in isolated astrocytes from adult mice (Chai et al., 2017). Using a state of the art TurboID construct to biotinylate proteins in the immediate proximity of tripartite synapses, Takano et al. (2020) report enrichment of IP3R1 protein in the peri-synaptic astrocytic compartment (Takano et al., 2020). This finding, however, should be interpreted with some caution as identified proteins were assigned to astrocytes based on published mRNA datasets (Zhang et al., 2014, 2016). Nevertheless, IP3R1 protein enrichment in fine astrocytic processes is consistent with Ca2+ imaging studies (Sherwood et al., 2017) and could account for the poor detection in various assays which favor detection in large subcellular compartments, i.e., major processes and soma of astrocytes. Notably, IP3R2, which is reported to be in the soma and main branches (Chen et al., 2012), was not enriched in peri-synaptic astrocytic compartments (Takano et al., 2020), likely reflecting the different subcellular distribution of IP3R1 and IP3R2 (Figure 1A and Table 1).
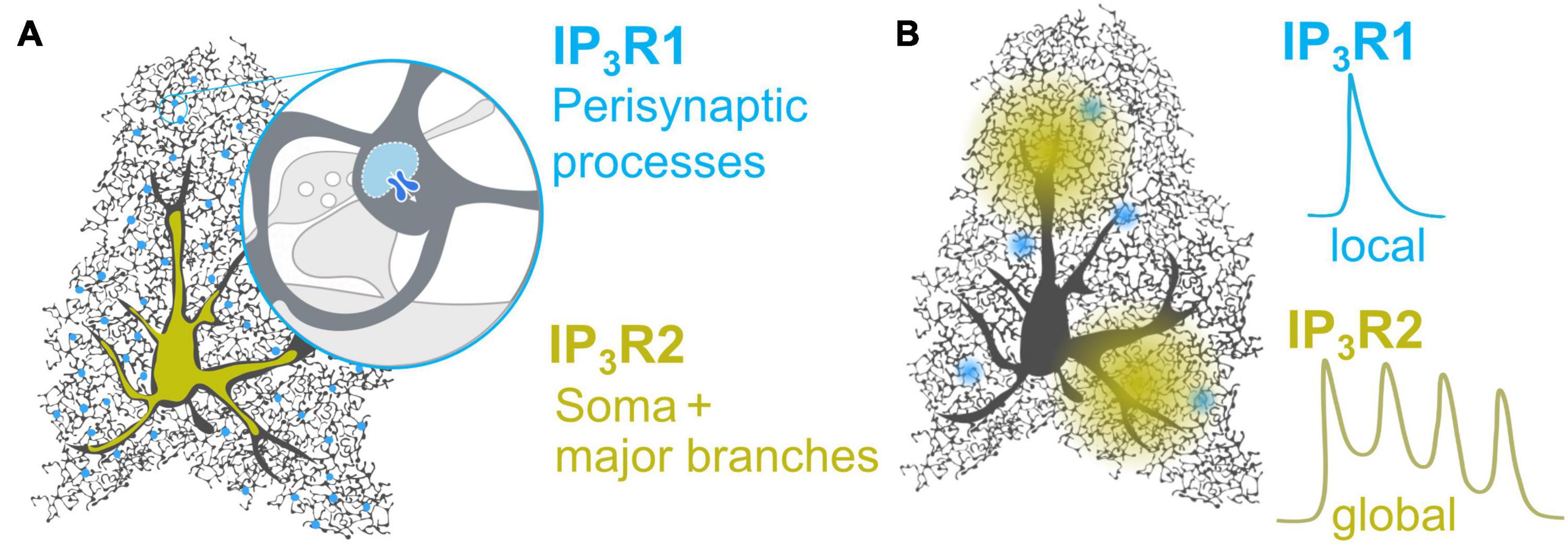
Figure 1. Proposed distribution and Ca2+ profiles of IP3R1 and IP3R2 in astrocytes. (A) While IP3R2 is distributed in the soma and major branches (Takata et al., 2011; Chen et al., 2012; Petravicz et al., 2014), IP3R1 may be enriched in the peri-synaptic processes (Sherwood et al., 2017; Takano et al., 2020), for instance in the nodes which were recently shown to host 2-APB-sensitive local Ca2+ signals at the tripartite synapse (Arizono et al., 2020). (B) IP3R2 generates global Ca2+ waves and oscillations in both astrocytes (Srinivasan et al., 2015; Sherwood et al., 2017) and model cells (Miyakawa, 1999). IP3R1 mediated Ca2+ dynamics in astrocytes have not been observed directly, but may resemble IP3R1-dependent monophasic Ca2+ transients observed in model cells (Miyakawa, 1999) and dendritic spines of pyramidal neurons (Holbro et al., 2009).
Astrocyte Transcriptome
IP3R1, IP3R2, and IP3R3 are encoded by the respective genes ITPR1, ITPR2, and ITPR3. Notably, mRNA for all three genes have been detected in astrocytes isolated from young and aged mouse brain (Cahoy et al., 2008; Zhang et al., 2014; Chai et al., 2017; Clarke et al., 2018) as well as humans (Zhang et al., 2016). Furthermore, ITPR1 and ITPR2 are actively translated in astrocytes of adult mice (Srinivasan et al., 2016; Chai et al., 2017; Clarke et al., 2018; Yu et al., 2018) indicating that their proteins are produced in astrocytes. In astrocytes the isoform transcript abundance is generally ITPR2 > ITPR1 >>> ITPR3 (ITPR3 mRNA is present in very small amounts and may be negligible). However, these transcripts are developmentally and differentially regulated across brain regions and in some instances ITPR1 mRNA appears to be more abundant than ITPR2 mRNA (Yu et al., 2018; Table 1).
Ca2+ Imaging
Over the last decade, studies show that deletion of IP3R2 does not abolish Ca2+ signaling in astrocytes. It is now generally agreed that bulk/somatic cytosolic Ca2+ responses are hard to detect in IP3R2KO astrocytes (Petravicz et al., 2008, 2014; Agulhon et al., 2010, 2013; Takata et al., 2011, 2013; Nizar et al., 2013). However, with a detailed examination, rich Ca2+ activity can be observed within fine astrocyte processes (Di Castro et al., 2011; Haustein et al., 2014; Kanemaru et al., 2014; Srinivasan et al., 2015; Rungta et al., 2016; Agarwal et al., 2017; Sherwood et al., 2017). Non-IP3R2 Ca2+ activity has largely been interpreted as evidence for non-IP3R Ca2+ stores, nevertheless, these activities could equally arise from IP3R1 and or IP3R3 (Tamamushi et al., 2012; Sherwood et al., 2017). Indeed, Ca2+ release from the ER was recently reported for IP3R2KO astrocytes (Okubo et al., 2019). In Bergmann glia, while IP3R2 was shown to be a dominant subtype, IP3R1 and IP3R3 were also shown to contribute to rapid Ca2+ events in the processes (Tamamushi et al., 2012). Similarly, using IP3R2KO and IP3R2/3 double KO mice, we found that IP3R2 is involved in global Ca2+ release, whereas local Ca2+ signals involve IP3R1 and IP3R3 (Figure 1B) (Sherwood et al., 2017) in hippocampal astrocytes. Knock-down of IP3R1 in dorsal spinal cord astrocytes of IP3R2KO mice also unmasked IP3R1 mediated Ca2+ signals (Shiratori-Hayashi et al., 2020). Furthermore, the selective activation of Gq-GPCR/IP3R/Ca2+ signaling in astrocytes, using DREADDs (designer receptor exclusively activated by designer drug, see section “Activation of IP3 Induced Ca2+ Release in Astrocytes”), triggered Ca2+ events in the astrocytic processes of IP3R2KO mice (Wang et al., 2021) indicating the presence of IP3R1/3.
2-APB was introduced as an antagonist of IP3Rs (Maruyama et al., 1997) and has been widely used to investigate the contribution of IP3Rs to cellular Ca2+ signaling. 2-APB appears to preferentially block IP3R1 and IP3R3, whereas cells predominantly expressing IP3R2 seem largely insensitive (Kukkonen et al., 2001; Bootman et al., 2002; Saleem et al., 2014). Thus, the fact that 2-APB reduces astrocyte Ca2+ amplitude and responsiveness in diverse brain regions (Sul et al., 2004; Young et al., 2010; Tamura et al., 2014; Tang et al., 2015; Arizono et al., 2020) and spinal dorsal horn (Shiratori-Hayashi et al., 2020) may support the presence of functional IP3R1 and IP3R3 in astrocytes. The functional role of non-IP3R2 in astrocytic Ca2+ signaling remains to be determined, it is possible that some membrane receptors are functionally coupled solely to IP3R1 or IP3R3 and generate local Ca2+ events, which may or may not be involved in triggering IP3R2 dependent Ca2+ waves (Petravicz et al., 2008). Alternatively, it is possible that IP3Rs exist as heterotetramers (Wojcikiewicz and He, 1995; Nucifora et al., 1996).
Phenotypic Comparison
Comparison of WT and total IP3R2KO mice has revealed some important physiological roles of IP3R2 signaling in astrocytes, i.e., motor learning (Padmashri et al., 2015) modulating depressive-like behaviors (Cao et al., 2013) but this remains controversial (Petravicz et al., 2014). The possible roles of astrocytic IP3R1 and IP3R3 may be gleaned by comparing WT and IP3R2KOs with manipulations that impair all IP3R subtypes. For example, while IP3R2KO has no impact on sleep (Cao et al., 2013) overexpressing a transgene for the IP3 hydrolyzing enzyme, IP3-5-phosphatase, in astrocytes, suppresses Ca2+ release from all IP3R subtypes and disrupts sleep (Foley et al., 2017). Similarly, although IP3R2KO had no impact on hippocampal LTP (Agulhon et al., 2010), we showed that loading a single astrocyte with the membrane-impermeable pan-IP3R antagonist, heparin, blocks long-term synaptic potentiation (Sherwood et al., 2017). These studies, taken together, indicate that non-IP3R2 IP3Rs regulate sleep and hippocampal LTP.
Summary
The expression of multiple IP3R subtypes in astrocytes has a wide-reaching implication in the field. Studies using IP3R2KO as a model for blocked IICR would need to be re-assessed to include the possibility of IICR mediated by IP3R1 and IP3R3.
Prospectus/Advantage of Multiple Ip3R Isoforms
Different Properties of IP3R Subtypes
The three IP3R subtypes share only 65–85% homology accounting for many of the subtype-specific properties leading to particular spatiotemporal features of Ca2+ responses. Although high homology is observed in regions critical for forming the IP3−gated Ca2+ channel, each subtype has a different IP3 affinity; IP3R2 > IP3R1 > IP3R3 (Zhang et al., 2011). High IP3 affinity of IP3R2 has been associated with slower kinetics and more prolonged duration of IP3R2-mediated Ca2+ microdomains, or Ca2+ puffs (Mataragka and Taylor, 2018).
Importantly, IP3R channel activity is not only regulated by IP3 but also by Ca2+ (Finch et al., 1991). The synergy between IP3 and Ca2+ creates repetitive IP3R activation and inhibition, resulting in Ca2+ oscillations. Such oscillations are crucial to protect cells from extended Ca2+ elevation that can often be toxic to the cell (Berridge et al., 2000). The oscillatory pattern is considered necessary for many cellular processes, such as fertilization (Miyazaki et al., 1992). IP3R2 mediates long-lasting, regular Ca2+ oscillations, whereas IP3R1 or IP3R3 tend to exhibit mono-phasic transients or very rapidly dampened Ca2+ oscillations (Miyakawa, 1999).
The difference in IP3R subtype properties is further characterized by various binding partners, including kinase and phosphatases, which can further fine-tune Ca2+ profiles. Interestingly, while there are many interacting partners common to all three IP3R subtypes, the nature of their regulation can be subtype-specific. For instance, protein kinase C, depending on the IP3R subtype, can either be stimulatory or inhibitory; this difference likely reflects isoform-specific phosphorylation sites. Further detailed biochemical study (Hamada et al., 2017) is required to investigate how various IP3R binding molecules specifically regulate each IP3R isoform (Hamada and Mikoshiba, 2020).
Different Distribution and Role of IP3R Subtypes Within Various Tissues
One important feature that defines the subtype-specific role of IP3Rs in vivo is the tissue distribution patterns. While IP3R1 is mostly expressed in the central nervous system, IP3R2 and IP3R3 are broadly expressed in various organs such as the heart, pancreas, liver, and salivary glands (Hisatsune and Mikoshiba, 2017). This distribution pattern is tightly linked to the physiological role of IP3R subtypes. Reflecting its rich expression in Purkinje cells, mice lacking IP3R1 exhibit severe cerebellar ataxia, a seizure−like posture, impaired cerebellar LTD. (Inoue et al., 1998), and die within 3–4 weeks of birth (Matsumoto et al., 1996). The dysregulation of IP3R1 is linked with other brain disorders such as Huntington’s disease and Alzheimer’s disease (Hisatsune and Mikoshiba, 2017). IP3R2 is associated with sweating (Klar et al., 2014), bone formation (Kuroda et al., 2008), and heart hypertrophy (Nakayama et al., 2010; Drawnel et al., 2012; Vervloessem et al., 2015). IP3R3 plays a role in taste sensing (Hisatsune et al., 2007) and hair cycle (Sato-Miyaoka et al., 2012). IP3R2 and IP3R3 together are associated with the heart’s development (Uchida et al., 2010, 2016) and secretion of saliva and tears (Futatsugi, 2005; Inaba et al., 2014).
Different Distribution and Role of IP3R Subtypes Within a Cell
Some cells express multiple IP3R subtypes, enabling each subtype to uniquely contribute to Ca2+ profiles and cellular functions. For instance, in HeLa cells, knock-down of IP3R1 terminates Ca2+ oscillations, whereas knock-down of IP3R3 results in more robust and long-lasting Ca2+ oscillations (Hattori et al., 2004). The specific contribution of IP3R subtypes can also depend on their distinct subcellular distribution, as seen in pancreatic acinar cells (Lur et al., 2011), COS cells (Pantazaka and Taylor, 2011), and DT40 cells (Bartok et al., 2019). In Bergmann glia, knocking out IP3R2 resulted in decreased agonist-induced Ca2+ release while knocking out IP3R1 and IP3R3 resulted in delaying the peak of agonist-induced Ca2+ release specifically in astrocytic processes (Tamamushi et al., 2012), suggesting their subtype-specific distribution. It is likely that astrocytes, which express multiple IP3R subtypes, also take advantage of subtype-specific distribution.
In astrocytes, IP3Rs are predominantly located on thapsigargin sensitive ER Ca2+ store. The ER in astrocytes may be found throughout the cell in the soma, major processes (Okubo et al., 2019, 2020), and peri-synaptic astrocytic processes (Bergersen et al., 2012), in close association with adhesion junctions (puncta adherentia) between dendritic spines and astrocytic processes (Spacek and Harris, 1998). Although recent studies indicate that ER and other membrane-bound organelles are absent from peri-synaptic processes (Patrushev et al., 2013) this likely reflects their sensitivity to chemical fixation (Korogod et al., 2015). The ER store in astrocytes is heterogeneous and organized into sub-compartments that can release Ca2+ independently (Golovina and Blaustein, 1997, 2000). It would be fascinating to see if IP3R subtypes are located to specific functional domains, e.g., signaling domains of membrane receptors, ER-mitochondria contacts (Bartok et al., 2019), and ER-plasma membrane junctions (Thillaiappan et al., 2017). In addition to the ER, astrocytic IP3Rs can also be found on other Ca2+ stores, with unique properties, i.e., the large dense-core vesicles (Hur et al., 2010), which may be comparable to the thapsigargin insensitive, Bafilomycin A1 sensitive, acidic Ca2+ stores previously described in secretory cells (Gerasimenko et al., 1996, 2009, 2011; Hur et al., 2010). Notably, compared to the ER, acidic Ca2+ stores can exhibit enhanced sensitivity to IP3 (Yoo, 2010). Other potential IP3-sensitive Ca2+ stores include the nuclear envelope (Gerasimenko et al., 1995; Petersen et al., 1998), nucleoplasm (Echevarría et al., 2003), Golgi (Pinton et al., 1998), and plasma membrane (Dellis et al., 2006).
Summary
While IP3R subtypes are regulated by IP3 and Ca2+ and have many common interacting partners, they differ in how they are affected by these regulators. Such differences enrich the diversity of spatio-temporal Ca2+ profiles created by IP3Rs. The IP3R subtype expression pattern, in vivo, is tissue specific and their subcellular localization is highly variable and dependent on cell types, and this carries important functional implications. Together with recent reports showing the distinct role of IP3R1 and IP3R3 in Bergmann glia and astrocytes, these facts support the view that IP3R isoforms 1 and 3 are unique Ca2+ channels that need to be addressed independently of IP3R2.
Tools to Dissect the Role of Ip3R Isoforms
Experimental and Analytical Tools
To understand the role of the various Ca2+ signals in astrocyte physiology, it will be necessary to make quantitative measurements (Neher, 2008). Progress in this direction has been frustrated by the unique astrocyte morphology and difficulties in interpreting recorded Ca2+-dependent fluorescent signals (Rusakov, 2015). To accurately capture Ca2+ dynamics in sub-cellular compartments, there is a need to adopt imaging techniques with improved resolution and to develop tools for efficient analysis in three-dimensional (Bindocci et al., 2017; Romanos et al., 2019). Because of our poor understanding of functional compartments, analysis of astrocytic Ca2+ dynamics has been moving toward state-of-the-art event-based analysis (Romanos et al., 2019; Wang et al., 2019; Bjørnstad et al., 2021), nevertheless ROI (region-of-interest) based analysis, informed by cellular anatomy (functional compartments) and molecular architecture, has been critical for understanding neuron physiology (e.g., spines and boutons). To this end, the identification of morphologically distinct subcellular compartments are promising targets for classical ROI based analysis, i.e., “glial microdomains” on Bergmann glial processes (Grosche et al., 1999) and “astrocytic compartments” on major branches (Panatier et al., 2011), both revealed using confocal microscopy, and astrocytic nodes and shafts within the spongiform structure visualized using live STED microscopy (Arizono et al., 2020). The ultimate goal of extracting quantitative Ca2+ dynamics from fluorescent data is non-trivial but achievable using realistic biophysical cell models (Rusakov, 2015; Denizot et al., 2019), a task simplified by the recent development of the open-source flexible model builder ASTRO (Savtchenko et al., 2018). For an accurate understanding of Ca2+ dynamics, it will be necessary to constrain models further using empirically determined details, e.g., receptor kinetics, expression patterns, endogenous Ca2+ buffering, etc.
Pharmacological Tools
It is difficult to disentangle the physiological roles of IP3R subtypes in cells that typically express complex mixtures of homo- and hetero-tetrameric IP3Rs. There are no ligands that usefully distinguish among IP3R subtypes (Saleem et al., 2013a,b) and nor are there effective antagonists that lack serious side effects (Michelangeli et al., 1995). Of the available antagonists, heparin is currently the most useful. Heparin is a membrane impermeant pan-IP3R inhibitor that may be selectively loaded into astrocytes using a whole-cell patch-pipette (Sherwood et al., 2017). Recent developments report small impermeant competitive antagonists of IP3R1, which, compared to heparin, are likely to have fewer off-targets (Konieczny et al., 2016). Well-characterized function-blocking monoclonal antibodies are powerful tools to specifically inhibit IP3R subtypes (Miyazaki et al., 1992; Inoue et al., 1998; Nishiyama et al., 2000; Gerasimenko et al., 2009). This technology has not yet been applied to astrocytes.
Genetic Tools
Inhibition of IP3 Induced Ca2+ Release
IP3R2KO and conditional-KO (cKO) mice (Petravicz et al., 2014; Padmashri et al., 2015; Wang et al., 2021) are widely used, however, as highlighted above, deletion of IP3R2 does not abolish IICR. IICR can be suppressed, irrespective of the underlying receptor, using an IP3-sponge to buffer IP3 (Xie et al., 2010; Tanaka et al., 2013), or an IP3-5′-phospatase transgene to enhance IP3 metabolism (Kanemaru et al., 2007; Foley et al., 2017). To study the physiological role of IP3R subtypes it is necessary to develop inducible cKO for IP3R1 (Sugawara et al., 2013) and IP3R3. Specific knock-down of IP3R subtypes can also be achieved using viruses to introduce short hairpin RNA into astrocytes (Shiratori-Hayashi et al., 2020).
Activation of IP3 Induced Ca2+ Release in Astrocytes
Pharmacogenetics
DREADDs (designer receptor exclusively activated by designer drug) enable the selective activation of GPCR-IP3R-Ca2+ signaling in astrocytes. The most used DREADDs are the excitatory Gq or inhibitory Gi-coupled receptors, hM3Dq and hM4Di, respectively (derived from human M3/M4 muscarinic receptor). Both receptors are activated by a pharmacologically inert but bioavailable ligand clozapine-N-oxide (CNO) while being non-responsive to endogenous GPCR ligands (Agulhon et al., 2013). hM3Dq has been used to demonstrate an astrocytic role in behaviors such as food intake (Yang et al., 2015), fear response (Martin-Fernandez et al., 2017), and memory recall (Adamsky et al., 2018). While the DREADDs enables selective activation of astrocytes, they have two major drawbacks: Firstly, the exogenous receptors have not been targeted to specific signaling domains and are likely to be spatially uncoupled from signaling nanodomains critical to IP3R physiology (Bootman et al., 2001); Secondly, because of the sustained (hour-long) activation by exogenous ligands (Iwai et al., 2021), temporal features of astrocyte signaling are lost. While perhaps mimicking global Ca2+ surges, the available DREADDs are unlikely to recapitulate many of the local Ca2+ transients typically observed within fine astrocytic processes (Shigetomi et al., 2013a; Arizono et al., 2020).
Optogenetics
To achieve temporal control, an optogenetic approach has been developed for the reliable stimulation of endogenous GPCR-IP3R-Ca2+ signaling cascade using light. Light activation has been achieved by introducing to astrocytes either a mammalian light-sensitive Gq/Gi/o-protein-coupled photopigment, Melanopsin (Panda, 2005; Bailes and Lucas, 2013; Mederos et al., 2019), or light-activated chimeric GPCRs, termed OptoXRs. OptoXRs are generated by replacing the intracellular loops of a light-sensitive GPCR, e.g., rhodopsin, with those of a donor GPCR, e.g., Gq-coupled human adrenergic receptor α1a (Airan et al., 2009; Figueiredo et al., 2014; Tang et al., 2014; Adamsky et al., 2018; Iwai et al., 2021).
Summary – Future Developments
While having great potential for controlling astrocytic activation, a central question is to what extent do the chimeras mimic the signaling of wild-type receptors. GPCRs can have multiple signaling axis, e.g., multiple G-protein axes, β-arrestins, wnt-frizzled, or the hedgehog-smoothened axes (Bailes and Lucas, 2013; Tichy et al., 2019), and the signaling axes bias is often not characterized but can have profound side effects on physiology (Agulhon et al., 2013; Tichy et al., 2019). Indeed, the functional outcome of activating Gq in astrocytes using different exogenous receptor (i.e., hM3Dq and MrgA1) is not reproducible (Agulhon et al., 2010; Adamsky et al., 2018). While multiple signaling axis could confuse the role of IICR, they may be required to obtain an optimal IP3R response (Konieczny et al., 2017). Another primary concern is that DREADDs and optoXRs likely activate IP3Rs from cellular compartments distinct from those of the endogenous receptors, limiting their ability to recreate physiologically relevant Ca2+ profiles. To address this, next-generation activation tools are being engineered to mimic the subcellular distribution of endogenous receptors (Oh et al., 2010; Masseck et al., 2014; Spoida et al., 2014; Tichy et al., 2019).
In the last decade, substantial progress has been made revealing diverse spatio-temporal Ca2+ signaling in astrocytes. Understanding the subtleties of these signals will require detailed knowledge of the astrocytic Ca2+ signaling toolbox along with the generation and characterization of more sophisticated tools to control and accurately recapitulate the physiologically relevant Ca2+ signals.
Author Contributions
MS and MA performed the literature survey, wrote the manuscript, and prepared the figures and tables. MS, MA, AP, KM, and SHRO reviewed, finalized, and approved the final version.
Funding
This review was funded by the CNRS, INSERM, LabEx BRAIN, and the Agence Nationale de la Recherche, grant numbers ANR-17-CE16-0002 to SHRO, and ANR-16-CE16-0001-0 to AP. MS was supported by a Takeda Science Foundation fellowship (Japan), and MA was supported by postdoctoral fellowships from RIKEN and JSPS (Japan). KM was supported by Scientific Research S (25221002) of JSPS and ICORP; ICORP-SORST of Japan Science and Technology Agency.
Conflict of Interest
The authors declare that the research was conducted in the absence of any commercial or financial relationships that could be construed as a potential conflict of interest.
Publisher’s Note
All claims expressed in this article are solely those of the authors and do not necessarily represent those of their affiliated organizations, or those of the publisher, the editors and the reviewers. Any product that may be evaluated in this article, or claim that may be made by its manufacturer, is not guaranteed or endorsed by the publisher.
References
Adamsky, A., Kol, A., Kreisel, T., Doron, A., Ozeri-Engelhard, N., Melcer, T., et al. (2018). Astrocytic activation generates de novo neuronal potentiation and memory enhancement. Cell 174, 59–71.e14. doi: 10.1016/j.cell.2018.05.002
Agarwal, A., Wu, P.-H., Hughes, E. G., Fukaya, M., Tischfield, M. A., Langseth, A. J., et al. (2017). Transient opening of the mitochondrial permeability transition pore induces microdomain calcium transients in astrocyte processes. Neuron 93, 587–605.e7. doi: 10.1016/j.neuron.2016.12.034
Agulhon, C., Boyt, K. M., Xie, A. X., Friocourt, F., Roth, B. L., and McCarthy, K. D. (2013). Modulation of the autonomic nervous system and behaviour by acute glial cell G q protein-coupled receptor activation in vivo: Glial GPCR signalling in physiology and behaviour in vivo. J. Physiol. 591, 5599–5609. doi: 10.1113/jphysiol.2013.261289
Agulhon, C., Fiacco, T. A., and McCarthy, K. D. (2010). hippocampal short- and long-term plasticity are not modulated by astrocyte Ca2+ signaling. Science 327, 1250–1254. doi: 10.1126/science.1184821
Airan, R. D., Thompson, K. R., Fenno, L. E., Bernstein, H., and Deisseroth, K. (2009). Temporally precise in vivo control of intracellular signalling. Nature 458, 1025–1029. doi: 10.1038/nature07926
Araque, A., Carmignoto, G., Haydon, P. G., Oliet, S. H. R., Robitaille, R., and Volterra, A. (2014). Gliotransmitters travel in time and space. Neuron 81, 728–739. doi: 10.1016/j.neuron.2014.02.007
Arizono, M., Inavalli, V. V. G. K., Panatier, A., Pfeiffer, T., Angibaud, J., Levet, F., et al. (2020). Structural basis of astrocytic Ca2+ signals at tripartite synapses. Nat. Commun. 11:1906. doi: 10.1038/s41467-020-15648-4
Bailes, H. J., and Lucas, R. J. (2013). Human melanopsin forms a pigment maximally sensitive to blue light (λ max ≈ 479 nm) supporting activation of G q/11 and G i/o signalling cascades. Proc. R. Soc. B Biol. Sci. 280:20122987. doi: 10.1098/rspb.2012.2987
Bartok, A., Weaver, D., Golenár, T., Nichtova, Z., Katona, M., Bánsághi, S., et al. (2019). IP3 receptor isoforms differently regulate ER-mitochondrial contacts and local calcium transfer. Nat. Commun. 10:3726. doi: 10.1038/s41467-019-11646-3
Bergersen, L. H., Morland, C., Ormel, L., Rinholm, J. E., Larsson, M., Wold, J. F. H., et al. (2012). Immunogold detection of L-glutamate and D-serine in small synaptic-like microvesicles in adult hippocampal astrocytes. Cereb. Cortex 22, 1690–1697. doi: 10.1093/cercor/bhr254
Berridge, M. J., Lipp, P., and Bootman, M. D. (2000). The versatility and universality of calcium signalling. Nat. Rev. Mol. Cell Biol. 1, 11–21. doi: 10.1038/35036035
Bindocci, E., Savtchouk, I., Liaudet, N., Becker, D., Carriero, G., and Volterra, A. (2017). Three-dimensional Ca 2+ imaging advances understanding of astrocyte biology. Science 356:eaai8185. doi: 10.1126/science.aai8185
Bjørnstad, D. M., Åbjørsbråten, K. S., Hennestad, E., Cunen, C., Hermansen, G. H., Bojarskaite, L., et al. (2021). Begonia-A two-photon imaging analysis pipeline for astrocytic Ca2+ signals. Front. Cell. Neurosci. 15:681066. doi: 10.3389/fncel.2021.681066
Blondel, O., Takeda, J., Janssen, H., Seino, S., and Bell, G. I. (1993). Sequence and functional characterization of a third inositol trisphosphate receptor subtype, IP3R-3, expressed in pancreatic islets, kidney, gastrointestinal tract, and other tissues. J. Biol. Chem. 268, 11356–11363. doi: 10.1016/s0021-9258(18)82132-9
Boddum, K., Jensen, T. P., Magloire, V., Kristiansen, U., Rusakov, D. A., Pavlov, I., et al. (2016). Astrocytic GABA transporter activity modulates excitatory neurotransmission. Nat. Commun. 7:13572. doi: 10.1038/ncomms13572
Bonder, D. E., and McCarthy, K. D. (2014). Astrocytic Gq-GPCR-linked IP3R-dependent Ca2+ signaling does not mediate neurovascular coupling in mouse visual cortex in vivo. J. Neurosci. 34, 13139–13150. doi: 10.1523/JNEUROSCI.2591-14.2014
Bootman, M. D., Collins, T. J., Mackenzie, L., Roderick, H. L., Berridge, M. J., and Peppiatt, C. M. (2002). 2-Aminoethoxydiphenyl borate (2-APB) is a reliable blocker of store-operated Ca 2+ entry but an inconsistent inhibitor of InsP 3 -induced Ca 2+ release. FASEB J. 16, 1145–1150. doi: 10.1096/fj.02-0037rev
Bootman, M. D., Lipp, P., and Berridge, M. J. (2001). The organisation and functions of local Ca(2+) signals. J. Cell Sci. 114, 2213–2222. doi: 10.1242/jcs.114.12.2213
Cahoy, J. D., Emery, B., Kaushal, A., Foo, L. C., Zamanian, J. L., Christopherson, K. S., et al. (2008). A transcriptome database for astrocytes, neurons, and oligodendrocytes: a new resource for understanding brain development and function. J. Neurosci. 28, 264–278. doi: 10.1523/JNEUROSCI.4178-07.2008
Cao, X., Li, L.-P., Wang, Q., Wu, Q., Hu, H.-H., Zhang, M., et al. (2013). Astrocyte-derived ATP modulates depressive-like behaviors. Nat. Med. 19, 773–777. doi: 10.1038/nm.3162
Chai, H., Diaz-Castro, B., Shigetomi, E., Monte, E., Octeau, J. C., Yu, X., et al. (2017). Neural circuit-specialized astrocytes: transcriptomic, proteomic, morphological, and functional evidence. Neuron 95, 531–549.e9. doi: 10.1016/j.neuron.2017.06.029
Chen, N., Sugihara, H., Sharma, J., Perea, G., Petravicz, J., Le, C., et al. (2012). Nucleus basalis-enabled stimulus-specific plasticity in the visual cortex is mediated by astrocytes. Proc. Natl. Acad. Sci. U.S.A. 109, E2832–E2841. doi: 10.1073/pnas.1206557109
Clarke, L. E., Liddelow, S. A., Chakraborty, C., Münch, A. E., Heiman, M., and Barres, B. A. (2018). Normal aging induces A1-like astrocyte reactivity. Proc. Natl. Acad. Sci. U.S.A. 115, E1896–E1905. doi: 10.1073/pnas.1800165115
Dellis, O., Dedos, S. G., Tovey, S. C., Taufiq-Ur-Rahman, Dubel, S. J., and Taylor, C. W. (2006). Ca2+ entry through plasma membrane IP3 receptors. Science 313, 229–233. doi: 10.1126/science.1125203
Denizot, A., Arizono, M., Nägerl, U. V., Soula, H., and Berry, H. (2019). Simulation of calcium signaling in fine astrocytic processes: effect of spatial properties on spontaneous activity. PLoS Comput. Biol. 15:e1006795. doi: 10.1371/journal.pcbi.1006795
Dent, M. A., Raisman, G., and Lai, F. A. (1996). Expression of type 1 inositol 1,4,5-trisphosphate receptor during axogenesis and synaptic contact in the central and peripheral nervous system of developing rat. Dev. Camb. Engl. 122, 1029–1039. doi: 10.1242/dev.122.3.1029
Di Castro, M. A., Chuquet, J., Liaudet, N., Bhaukaurally, K., Santello, M., Bouvier, D., et al. (2011). Local Ca2+ detection and modulation of synaptic release by astrocytes. Nat. Neurosci. 14, 1276–1284. doi: 10.1038/nn.2929
Drawnel, F. M., Wachten, D., Molkentin, J. D., Maillet, M., Aronsen, J. M., Swift, F., et al. (2012). Mutual antagonism between IP3RII and miRNA-133a regulates calcium signals and cardiac hypertrophy. J. Cell Biol. 199, 783–798. doi: 10.1083/jcb.201111095
Echevarría, W., Leite, M. F., Guerra, M. T., Zipfel, W. R., and Nathanson, M. H. (2003). Regulation of calcium signals in the nucleus by a nucleoplasmic reticulum. Nat. Cell Biol. 5, 440–446. doi: 10.1038/ncb980
Fiacco, T. A., Agulhon, C., Taves, S. R., Petravicz, J., Casper, K. B., Dong, X., et al. (2007). Selective stimulation of astrocyte calcium in situ does not affect neuronal excitatory synaptic activity. Neuron 54, 611–626. doi: 10.1016/j.neuron.2007.04.032
Figueiredo, M., Lane, S., Stout, R. F., Liu, B., Parpura, V., Teschemacher, A. G., et al. (2014). Comparative analysis of optogenetic actuators in cultured astrocytes. Cell Calcium 56, 208–214. doi: 10.1016/j.ceca.2014.07.007
Finch, E., Turner, T., and Goldin, S. (1991). Calcium as a coagonist of inositol 1,4,5-trisphosphate-induced calcium release. Science 252, 443–446. doi: 10.1126/science.2017683
Foley, J., Blutstein, T., Lee, S., Erneux, C., Halassa, M. M., and Haydon, P. (2017). Astrocytic IP3/Ca2+ signaling modulates theta rhythm and REM sleep. Front. Neural Circuits 11:3. doi: 10.3389/fncir.2017.00003
Furuichi, T., Yoshikawa, S., Miyawaki, A., Wada, K., Maeda, N., and Mikoshiba, K. (1989). Primary structure and functional expression of the inositol 1,4,5-trisphosphate-binding protein P400. Nature 342, 32–38. doi: 10.1038/342032a0
Futatsugi, A. (2005). IP3 receptor types 2 and 3 mediate exocrine secretion underlying energy metabolism. Science 309, 2232–2234. doi: 10.1126/science.1114110
Gerasimenko, J. V., Lur, G., Ferdek, P., Sherwood, M. W., Ebisui, E., Tepikin, A. V., et al. (2011). Calmodulin protects against alcohol-induced pancreatic trypsinogen activation elicited via Ca2+ release through IP3 receptors. Proc. Natl. Acad. Sci. U.S.A. 108, 5873–5878. doi: 10.1073/pnas.1016534108
Gerasimenko, J. V., Lur, G., Sherwood, M. W., Ebisui, E., Tepikin, A. V., Mikoshiba, K., et al. (2009). Pancreatic protease activation by alcohol metabolite depends on Ca2+ release via acid store IP3 receptors. Proc. Natl. Acad. Sci. U.S.A. 106, 10758–10763. doi: 10.1073/pnas.0904818106
Gerasimenko, O. V., Gerasimenko, J. V., Belan, P. V., and Petersen, O. H. (1996). Inositol trisphosphate and cyclic ADP-ribose-mediated release of Ca2+ from single isolated pancreatic zymogen granules. Cell 84, 473–480. doi: 10.1016/s0092-8674(00)81292-1
Gerasimenko, O. V., Gerasimenko, J. V., Tepikin, A. V., and Petersen, O. H. (1995). ATP-dependent accumulation and inositol trisphosphate- or cyclic ADP-ribose-mediated release of Ca2+ from the nuclear envelope. Cell 80, 439–444. doi: 10.1016/0092-8674(95)90494-8
Golovina, V. A., and Blaustein, M. P. (1997). Spatially and functionally distinct Ca2+ stores in sarcoplasmic and endoplasmic reticulum. Science 275, 1643–1648. doi: 10.1126/science.275.5306.1643
Golovina, V. A., and Blaustein, M. P. (2000). Unloading and refilling of two classes of spatially resolved endoplasmic reticulum Ca(2+) stores in astrocytes. Glia 31, 15–28. doi: 10.1002/(sici)1098-1136(200007)31:1<15::aid-glia20>3.0.co;2-h
Gómez-Gonzalo, M., Martin-Fernandez, M., Martínez-Murillo, R., Mederos, S., Hernández-Vivanco, A., Jamison, S., et al. (2017). Neuron-astrocyte signaling is preserved in the aging brain: neuron-astrocyte signaling in aging brain. Glia 65, 569–580. doi: 10.1002/glia.23112
Gómez-Gonzalo, M., Navarrete, M., Perea, G., Covelo, A., Martín-Fernández, M., Shigemoto, R., et al. (2015). Endocannabinoids induce lateral long-term potentiation of transmitter release by stimulation of gliotransmission. Cereb. Cortex 25, 3699–3712. doi: 10.1093/cercor/bhu231
Grosche, J., Matyash, V., Möller, T., Verkhratsky, A., Reichenbach, A., and Kettenmann, H. (1999). Microdomains for neuron-glia interaction: parallel fiber signaling to Bergmann glial cells. Nat. Neurosci. 2, 139–143. doi: 10.1038/5692
Hamada, K., and Mikoshiba, K. (2020). IP 3 receptor plasticity underlying diverse functions. Annu. Rev. Physiol. 82, 151–176. doi: 10.1146/annurev-physiol-021119-034433
Hamada, K., Miyatake, H., Terauchi, A., and Mikoshiba, K. (2017). IP 3 -mediated gating mechanism of the IP 3 receptor revealed by mutagenesis and X-ray crystallography. Proc. Natl. Acad. Sci. U.S.A. 114, 4661–4666. doi: 10.1073/pnas.1701420114
Hamada, T., Niki, T., Ziging, P., Sugiyama, T., Watanabe, S., Mikoshiba, K., et al. (1999). Differential expression patterns of inositol trisphosphate receptor types 1 and 3 in the rat suprachiasmatic nucleus. Brain Res. 838, 131–135. doi: 10.1016/s0006-8993(99)01719-9
Hattori, M., Suzuki, A. Z., Higo, T., Miyauchi, H., Michikawa, T., Nakamura, T., et al. (2004). Distinct roles of inositol 1,4,5-Trisphosphate receptor types 1 and 3 in Ca2+ signaling. J. Biol. Chem. 279, 11967–11975. doi: 10.1074/jbc.M311456200
Haustein, M. D., Kracun, S., Lu, X.-H., Shih, T., Jackson-Weaver, O., Tong, X., et al. (2014). Conditions and constraints for astrocyte calcium signaling in the hippocampal mossy fiber pathway. Neuron 82, 413–429. doi: 10.1016/j.neuron.2014.02.041
Hertle, D. N., and Yeckel, M. F. (2007). Distribution of inositol-1,4,5-trisphosphate receptor isotypes and ryanodine receptor isotypes during maturation of the rat hippocampus. Neuroscience 150, 625–638. doi: 10.1016/j.neuroscience.2007.09.058
Hisatsune, C., and Mikoshiba, K. (2017). IP 3 receptor mutations and brain diseases in human and rodents. J. Neurochem. 141, 790–807. doi: 10.1111/jnc.13991
Hisatsune, C., Yasumatsu, K., Takahashi-Iwanaga, H., Ogawa, N., Kuroda, Y., Yoshida, R., et al. (2007). Abnormal taste perception in mice lacking the Type 3 Inositol 1,4,5-trisphosphate receptor. J. Biol. Chem. 282, 37225–37231. doi: 10.1074/jbc.M705641200
Holbro, N., Grunditz, A., and Oertner, T. G. (2009). Differential distribution of endoplasmic reticulum controls metabotropic signaling and plasticity at hippocampal synapses. Proc. Natl. Acad. Sci. U.S.A. 106, 15055–15060. doi: 10.1073/pnas.0905110106
Holtzclaw, L. A., Pandhit, S., Bare, D. J., Mignery, G. A., and Russell, J. T. (2002). Astrocytes in adult rat brain express type 2 inositol 1,4,5-trisphosphate receptors. Glia 39, 69–84. doi: 10.1002/glia.10085
Hur, Y. S., Kim, K. D., Paek, S. H., and Yoo, S. H. (2010). Evidence for the existence of secretory granule (dense-core vesicle)-based inositol 1,4,5-trisphosphate-dependent Ca2+ signaling system in astrocytes. PLoS One 5:e11973. doi: 10.1371/journal.pone.0011973
Inaba, T., Hisatsune, C., Sasaki, Y., Ogawa, Y., Ebisui, E., Ogawa, N., et al. (2014). Mice lacking inositol 1,4,5-trisphosphate receptors exhibit dry eye. PLoS One 9:e99205. doi: 10.1371/journal.pone.0099205
Inoue, T., Kato, K., Kohda, K., and Mikoshiba, K. (1998). Type 1 inositol 1,4,5-trisphosphate receptor is required for induction of long-term depression in cerebellar purkinje neurons. J. Neurosci. 18, 5366–5373. doi: 10.1523/JNEUROSCI.18-14-05366.1998
Iwai, M., Tateishi, Y., Hattori, M., Mizutani, A., Nakamura, T., Futatsugi, A., et al. (2005). Molecular cloning of mouse type 2 and type 3 inositol 1,4,5-trisphosphate receptors and identification of a novel type 2 receptor splice variant. J. Biol. Chem. 280, 10305–10317. doi: 10.1074/jbc.M413824200
Iwai, Y., Ozawa, K., Yahagi, K., Mishima, T., Akther, S., Vo, C. T., et al. (2021). Transient astrocytic Gq signaling underlies remote memory enhancement. Front. Neural. Circuits, 15:658343 doi: 10.3389/fncir.2021.658343
Kanemaru, K., Okubo, Y., Hirose, K., and Iino, M. (2007). Regulation of neurite growth by spontaneous Ca2+ oscillations in astrocytes. J. Neurosci. 27, 8957–8966. doi: 10.1523/JNEUROSCI.2276-07.2007
Kanemaru, K., Sekiya, H., Xu, M., Satoh, K., Kitajima, N., Yoshida, K., et al. (2014). In vivo visualization of subtle, transient, and local activity of astrocytes using an ultrasensitive Ca2+ indicator. Cell Rep. 8, 311–318. doi: 10.1016/j.celrep.2014.05.056
Kirischuk, S., Kettenmann, H., and Verkhratsky, A. (1997). Na+/Ca2+ exchanger modulates kainate-triggered Ca2+ signaling in Bergmann glial cells in situ. FASEB J. Off. Publ. Fed. Am. Soc. Exp. Biol. 11, 566–572. doi: 10.1096/fasebj.11.7.9212080
Klar, J., Hisatsune, C., Baig, S. M., Tariq, M., Johansson, A. C. V., Rasool, M., et al. (2014). Abolished InsP3R2 function inhibits sweat secretion in both humans and mice. J. Clin. Invest. 124, 4773–4780. doi: 10.1172/JCI70720
Konieczny, V., Stefanakis, J. G., Sitsanidis, E. D., Ioannidou, N.-A. T., Papadopoulos, N. V., Fylaktakidou, K. C., et al. (2016). Synthesis of inositol phosphate-based competitive antagonists of inositol 1,4,5-trisphosphate receptors. Org. Biomol. Chem. 14, 2504–2514. doi: 10.1039/C5OB02623G
Konieczny, V., Tovey, S. C., Mataragka, S., Prole, D. L., and Taylor, C. W. (2017). Cyclic AMP recruits a discrete intracellular Ca 2+ store by unmasking hypersensitive IP 3 receptors. Cell Rep. 18, 711–722. doi: 10.1016/j.celrep.2016.12.058
Korogod, N., Petersen, C. C. H., and Knott, G. W. (2015). Ultrastructural analysis of adult mouse neocortex comparing aldehyde perfusion with cryo fixation. eLife 4:e05793. doi: 10.7554/eLife.05793
Kukkonen, J. P., Lund, P.-E., and Åkerman, K. E. O. (2001). 2-aminoethoxydiphenyl borate reveals heterogeneity in receptor-activated Ca2+discharge and store-operated Ca2+influx. Cell Calcium 30, 117–129. doi: 10.1054/ceca.2001.0219
Kuroda, Y., Hisatsune, C., Nakamura, T., Matsuo, K., and Mikoshiba, K. (2008). Osteoblasts induce Ca2+ oscillation-independent NFATc1 activation during osteoclastogenesis. Proc. Natl. Acad. Sci. U.S.A. 105, 8643–8648. doi: 10.1073/pnas.0800642105
Letellier, M., Park, Y. K., Chater, T. E., Chipman, P. H., Gautam, S. G., Oshima-Takago, T., et al. (2016). Astrocytes regulate heterogeneity of presynaptic strengths in hippocampal networks. Proc. Natl. Acad. Sci. U.S.A. 113, E2685–E2694. doi: 10.1073/pnas.1523717113
Lur, G., Sherwood, M. W., Ebisui, E., Haynes, L., Feske, S., Sutton, R., et al. (2011). InsP3 receptors and Orai channels in pancreatic acinar cells: co-localization and its consequences. Biochem. J. 436, 231–239. doi: 10.1042/BJ20110083
Mariotti, L., Losi, G., Sessolo, M., Marcon, I., and Carmignoto, G. (2016). The inhibitory neurotransmitter GABA evokes long-lasting Ca(2+) oscillations in cortical astrocytes. Glia 64, 363–373. doi: 10.1002/glia.22933
Martin-Fernandez, M., Jamison, S., Robin, L. M., Zhao, Z., Martin, E. D., Aguilar, J., et al. (2017). Synapse-specific astrocyte gating of amygdala-related behavior. Nat. Neurosci. 20, 1540–1548. doi: 10.1038/nn.4649
Maruyama, T., Kanaji, T., Nakade, S., Kanno, T., and Mikoshiba, K. (1997). 2APB, 2-aminoethoxydiphenyl borate, a membrane-penetrable modulator of Ins(1,4,5)P3-induced Ca2+ release. J. Biochem. (Tokyo) 122, 498–505. doi: 10.1093/oxfordjournals.jbchem.a021780
Masseck, O. A., Spoida, K., Dalkara, D., Maejima, T., Rubelowski, J. M., Wallhorn, L., et al. (2014). Vertebrate cone opsins enable sustained and highly sensitive rapid control of G i/o signaling in anxiety circuitry. Neuron 81, 1263–1273. doi: 10.1016/j.neuron.2014.01.041
Mataragka, S., and Taylor, C. W. (2018). All three IP 3 receptor subtypes generate Ca 2+ puffs, the universal building blocks of IP 3 -evoked Ca 2+ signals. J. Cell Sci. 131:jcs220848. doi: 10.1242/jcs.220848
Matsumoto, M., Nakagawa, T., Inoue, T., Nagata, E., Tanaka, K., Takano, H., et al. (1996). Ataxia and epileptic seizures in mice lacking type 1 inositol 1,4,5-trisphosphate receptor. Nature 379, 168–171. doi: 10.1038/379168a0
Mederos, S., Hernández-Vivanco, A., Ramírez-Franco, J., Martín-Fernández, M., Navarrete, M., Yang, A., et al. (2019). Melanopsin for precise optogenetic activation of astrocyte-neuron networks. Glia 67, 915–934. doi: 10.1002/glia.23580
Michelangeli, F., Mezna, M., Tovey, S., and Sayers, L. G. (1995). Pharmacological modulators of the inositol 1,4,5-trisphosphate receptor. Neuropharmacology 34, 1111–1122. doi: 10.1016/0028-3908(95)00053-9
Mignery, G. A., Newton, C. L., Archer, B. T., and Südhof, T. C. (1990). Structure and expression of the rat inositol 1,4,5-trisphosphate receptor. J. Biol. Chem. 265, 12679–12685. doi: 10.1016/s0021-9258(19)38397-8
Mignery, G. A., Südhof, T. C., Takei, K., and De Camilli, P. (1989). Putative receptor for inositol 1,4,5-trisphosphate similar to ryanodine receptor. Nature 342, 192–195. doi: 10.1038/342192a0
Miyakawa, T. (1999). Encoding of Ca2+ signals by differential expression of IP3 receptor subtypes. EMBO J. 18, 1303–1308. doi: 10.1093/emboj/18.5.1303
Miyazaki, S., Yuzaki, M., Nakada, K., Shirakawa, H., Nakanishi, S., Nakade, S., et al. (1992). Block of Ca2+ wave and Ca2+ oscillation by antibody to the inositol 1,4,5-trisphosphate receptor in fertilized hamster eggs. Science 257, 251–255. doi: 10.1126/science.1321497
Monai, H., Ohkura, M., Tanaka, M., Oe, Y., Konno, A., Hirai, H., et al. (2016). Calcium imaging reveals glial involvement in transcranial direct current stimulation-induced plasticity in mouse brain. Nat. Commun. 7:11100. doi: 10.1038/ncomms11100
Nakanishi, S., Maeda, N., and Mikoshiba, K. (1991). Immunohistochemical localization of an inositol 1,4,5-trisphosphate receptor, P400, in neural tissue: studies in developing and adult mouse brain. J. Neurosci. 11, 2075–2086. doi: 10.1523/JNEUROSCI.11-07-02075.1991
Nakayama, H., Bodi, I., Maillet, M., DeSantiago, J., Domeier, T. L., Mikoshiba, K., et al. (2010). The IP 3 receptor regulates cardiac hypertrophy in response to select stimuli. Circ. Res. 107, 659–666. doi: 10.1161/CIRCRESAHA.110.220038
Navarrete, M., Cuartero, M. I., Palenzuela, R., Draffin, J. E., Konomi, A., Serra, I., et al. (2019). Astrocytic p38α MAPK drives NMDA receptor-dependent long-term depression and modulates long-term memory. Nat. Commun. 10:2968. doi: 10.1038/s41467-019-10830-9
Navarrete, M., Perea, G., de Sevilla, D. F., Gómez-Gonzalo, M., Núñez, A., Martín, E. D., et al. (2012). Astrocytes mediate in vivo cholinergic-induced synaptic plasticity. PLoS Biol. 10:e1001259. doi: 10.1371/journal.pbio.1001259
Neher, E. (2008). Details of Ca2+ dynamics matter. J. Physiol. 586:2031. doi: 10.1113/jphysiol.2008.153080
Nishiyama, M., Hong, K., Mikoshiba, K., Poo, M. M., and Kato, K. (2000). Calcium stores regulate the polarity and input specificity of synaptic modification. Nature 408, 584–588. doi: 10.1038/35046067
Nizar, K., Uhlirova, H., Tian, P., Saisan, P. A., Cheng, Q., Reznichenko, L., et al. (2013). In vivo stimulus-induced vasodilation occurs without IP3 receptor activation and may precede astrocytic calcium increase. J. Neurosci. 33, 8411–8422. doi: 10.1523/JNEUROSCI.3285-12.2013
Nucifora, F. C., Sharp, A. H., Milgram, S. L., and Ross, C. A. (1996). Inositol 1,4,5-trisphosphate receptors in endocrine cells: localization and association in hetero- and homotetramers. Mol. Biol. Cell 7, 949–960. doi: 10.1091/mbc.7.6.949
Oh, E., Maejima, T., Liu, C., Deneris, E., and Herlitze, S. (2010). Substitution of 5-HT1A Receptor Signaling by a Light-activated G Protein-coupled Receptor. J. Biol. Chem. 285, 30825–30836. doi: 10.1074/jbc.M110.147298
Okubo, Y., Iino, M., and Hirose, K. (2020). Store-operated Ca2+ entry-dependent Ca2+ refilling in the endoplasmic reticulum in astrocytes. Biochem. Biophys. Res. Commun. 522, 1003–1008. doi: 10.1016/j.bbrc.2019.12.006
Okubo, Y., Kanemaru, K., Suzuki, J., Kobayashi, K., Hirose, K., and Iino, M. (2019). Inositol 1,4,5-trisphosphate receptor type 2-independent Ca 2+ release from the endoplasmic reticulum in astrocytes. Glia 67, 113–124. doi: 10.1002/glia.23531
Padmashri, R., Suresh, A., Boska, M. D., and Dunaevsky, A. (2015). Motor-Skill learning is dependent on astrocytic activity. Neural Plast. 2015:938023. doi: 10.1155/2015/938023
Panatier, A., Arizono, M., and Nägerl, U. V. (2014). Dissecting tripartite synapses with STED microscopy. Philos. Trans. R. Soc. B Biol. Sci. 369:20130597. doi: 10.1098/rstb.2013.0597
Panatier, A., Vallée, J., Haber, M., Murai, K. K., Lacaille, J.-C., and Robitaille, R. (2011). Astrocytes are endogenous regulators of basal transmission at central synapses. Cell 146, 785–798. doi: 10.1016/j.cell.2011.07.022
Panda, S. (2005). Illumination of the Melanopsin Signaling Pathway. Science 307, 600–604. doi: 10.1126/science.1105121
Pantazaka, E., and Taylor, C. W. (2011). Differential distribution, clustering, and lateral diffusion of subtypes of the inositol 1,4,5-trisphosphate receptor. J. Biol. Chem. 286, 23378–23387. doi: 10.1074/jbc.M111.236372
Park, K., and Lee, S. J. (2020). Deciphering the star codings: astrocyte manipulation alters mouse behavior. Exp. Mol. Med. 52, 1028–1038. doi: 10.1038/s12276-020-0468-z
Patrushev, I., Gavrilov, N., Turlapov, V., and Semyanov, A. (2013). Subcellular location of astrocytic calcium stores favors extrasynaptic neuron-astrocyte communication. Cell Calcium 54, 343–349. doi: 10.1016/j.ceca.2013.08.003
Perea, G., Gómez, R., Mederos, S., Covelo, A., Ballesteros, J. J., Schlosser, L., et al. (2016). Activity-dependent switch of GABAergic inhibition into glutamatergic excitation in astrocyte-neuron networks. eLife 5:e20362. doi: 10.7554/eLife.20362
Perez-Alvarez, A., Navarrete, M., Covelo, A., Martin, E. D., and Araque, A. (2014). Structural and functional plasticity of astrocyte processes and dendritic spine interactions. J. Neurosci. 34, 12738–12744. doi: 10.1523/JNEUROSCI.2401-14.2014
Petersen, O. H., Gerasimenko, O. V., Gerasimenko, J. V., Mogami, H., and Tepikin, A. V. (1998). The calcium store in the nuclear envelope. Cell Calcium 23, 87–90. doi: 10.1016/s0143-4160(98)90106-3
Petravicz, J., Boyt, K. M., and McCarthy, K. D. (2014). Astrocyte IP3R2-dependent Ca2+ signaling is not a major modulator of neuronal pathways governing behavior. Front. Behav. Neurosci. 8:384. doi: 10.3389/fnbeh.2014.00384
Petravicz, J., Fiacco, T. A., and McCarthy, K. D. (2008). Loss of IP3 receptor-dependent Ca2+ increases in hippocampal astrocytes does not affect baseline CA1 pyramidal neuron synaptic activity. J. Neurosci. 28, 4967–4973. doi: 10.1523/JNEUROSCI.5572-07.2008
Pinton, P., Pozzan, T., and Rizzuto, R. (1998). The Golgi apparatus is an inositol 1,4,5-trisphosphate-sensitive Ca2+ store, with functional properties distinct from those of the endoplasmic reticulum. EMBO J. 17, 5298–5308. doi: 10.1093/emboj/17.18.5298
Romanos, J., Thieren, L., and Santello, M. (2019). Diving into new depths of astrocyte signaling. Nat. Neurosci. 22, 1749–1750. doi: 10.1038/s41593-019-0513-1
Rose, C. R., Ziemens, D., and Verkhratsky, A. (2020). On the special role of NCX in astrocytes: translating Na+-transients into intracellular Ca2+ signals. Cell Calcium 86:102154. doi: 10.1016/j.ceca.2019.102154
Rungta, R. L., Bernier, L.-P., Dissing-Olesen, L., Groten, C. J., LeDue, J. M., Ko, R., et al. (2016). Ca 2+ transients in astrocyte fine processes occur via Ca 2+ influx in the adult mouse hippocampus: Ca 2+ Transients in Astrocyte Fine Processes. Glia 64, 2093–2103. doi: 10.1002/glia.23042
Rusakov, D. A. (2015). Disentangling calcium-driven astrocyte physiology. Nat. Rev. Neurosci. 16, 226–233. doi: 10.1038/nrn3878
Saleem, H., Tovey, S. C., Molinski, T. F., and Taylor, C. W. (2014). Interactions of antagonists with subtypes of inositol 1,4,5-trisphosphate (IP 3) receptor. Br. J. Pharmacol. 171, 3298–3312. doi: 10.1111/bph.12685
Saleem, H., Tovey, S. C., Rahman, T., Riley, A. M., Potter, B. V. L., and Taylor, C. W. (2013a). Stimulation of inositol 1,4,5-trisphosphate (IP3) receptor subtypes by analogues of IP3. PLoS One 8:e54877. doi: 10.1371/journal.pone.0054877
Saleem, H., Tovey, S. C., Riley, A. M., Potter, B. V. L., and Taylor, C. W. (2013b). Stimulation of inositol 1,4,5-trisphosphate (IP3) receptor subtypes by adenophostin A and its analogues. PLoS One 8:e58027. doi: 10.1371/journal.pone.0058027
Sato-Miyaoka, M., Hisatsune, C., Ebisui, E., Ogawa, N., Takahashi-Iwanaga, H., and Mikoshiba, K. (2012). Regulation of hair shedding by the Type 3 IP3 receptor. J. Invest. Dermatol. 132, 2137–2147. doi: 10.1038/jid.2012.141
Savtchenko, L. P., Bard, L., Jensen, T. P., Reynolds, J. P., Kraev, I., Medvedev, N., et al. (2018). Disentangling astroglial physiology with a realistic cell model in silico. Nat. Commun. 9:3554. doi: 10.1038/s41467-018-05896-w
Sharp, A. H., Nucifora, F. C., Blondel, O., Sheppard, C. A., Zhang, C., Snyder, S. H., et al. (1999). Differential cellular expression of isoforms of inositol 1,4,5-triphosphate receptors in neurons and glia in brain. J. Comp. Neurol. 406, 207–220. doi: 10.1002/(sici)1096-9861(19990405)406:2<207::aid-cne6>3.0.co;2-7
Sherwood, M. W., Arizono, M., Hisatsune, C., Bannai, H., Ebisui, E., Sherwood, J. L., et al. (2017). Astrocytic IP 3 Rs: contribution to Ca 2+ signalling and hippocampal LTP: astrocytic IP 3 Rs: Ca 2+ Signalling and LTP. Glia 65, 502–513. doi: 10.1002/glia.23107
Shigetomi, E., Bushong, E. A., Haustein, M. D., Tong, X., Jackson-Weaver, O., Kracun, S., et al. (2013a). Imaging calcium microdomains within entire astrocyte territories and endfeet with GCaMPs expressed using adeno-associated viruses. J. Gen. Physiol. 141, 633–647. doi: 10.1085/jgp.201210949
Shigetomi, E., Jackson-Weaver, O., Huckstepp, R. T., O’Dell, T. J., and Khakh, B. S. (2013b). TRPA1 channels are regulators of astrocyte basal calcium levels and long-term potentiation via constitutive D-serine release. J. Neurosci. 33, 10143–10153. doi: 10.1523/JNEUROSCI.5779-12.2013
Shigetomi, E., Tong, X., Kwan, K. Y., Corey, D. P., and Khakh, B. S. (2012). TRPA1 channels regulate astrocyte resting calcium and inhibitory synapse efficacy through GAT-3. Nat. Neurosci. 15, 70–80. doi: 10.1038/nn.3000
Shiratori-Hayashi, M., Yamaguchi, C., Eguchi, K., Shiraishi, Y., Kohno, K., Mikoshiba, K., et al. (2020). Astrocytic STAT3 activation and chronic itch require IP3R1/TRPC-dependent Ca2+ signals in mice. J. Allergy Clin. Immunol. 147, 1341–1353. doi: 10.1016/j.jaci.2020.06.039
Spacek, J., and Harris, K. M. (1998). Three-dimensional organization of cell adhesion junctions at synapses and dendritic spines in area CA1 of the rat hippocampus. J. Comp. Neurol. 393, 58–68. doi: 10.1002/(sici)1096-9861(19980330)393:1<58::aid-cne6>3.0.co;2-p
Spoida, K., Masseck, O. A., Deneris, E. S., and Herlitze, S. (2014). Gq/5-HT2c receptor signals activate a local GABAergic inhibitory feedback circuit to modulate serotonergic firing and anxiety in mice. Proc. Natl. Acad. Sci. U.S.A. 111, 6479–6484. doi: 10.1073/pnas.1321576111
Srinivasan, R., Huang, B. S., Venugopal, S., Johnston, A. D., Chai, H., Zeng, H., et al. (2015). Ca2+ signaling in astrocytes from Ip3r2-/- mice in brain slices and during startle responses in vivo. Nat. Neurosci. 18, 708–717. doi: 10.1038/nn.4001
Srinivasan, R., Lu, T.-Y., Chai, H., Xu, J., Huang, B. S., Golshani, P., et al. (2016). New transgenic mouse lines for selectively targeting astrocytes and studying calcium signals in astrocyte processes in situ and in vivo. Neuron 92, 1181–1195. doi: 10.1016/j.neuron.2016.11.030
Südhof, T. C., Newton, C. L., Archer, B. T., Ushkaryov, Y. A., and Mignery, G. A. (1991). Structure of a novel InsP3 receptor. EMBO J. 10, 3199–3206. doi: 10.1002/j.1460-2075.1991.tb04882.x
Sugawara, T., Hisatsune, C., Le, T. D., Hashikawa, T., Hirono, M., Hattori, M., et al. (2013). Type 1 inositol trisphosphate receptor regulates cerebellar circuits by maintaining the spine morphology of purkinje cells in adult mice. J. Neurosci. 33, 12186–12196. doi: 10.1523/JNEUROSCI.0545-13.2013
Sugiyama, T., Furuya, A., Monkawa, T., Yamamoto-Hino, M., Satoh, S., Ohmori, K., et al. (1994). Monoclonal antibodies distinctively recognizing the subtypes of inositol 1,4,5-trisphosphate receptor: application to the studies on inflammatory cells. FEBS Lett. 354, 149–154. doi: 10.1016/0014-5793(94)01099-4
Sul, J.-Y., Orosz, G., Givens, R. S., and Haydon, P. G. (2004). Astrocytic connectivity in the hippocampus. Neuron Glia Biol. 1, 3–11. doi: 10.1017/S1740925X04000031
Takano, T., Wallace, J. T., Baldwin, K. T., Purkey, A. M., Uezu, A., Courtland, J. L., et al. (2020). Chemico-genetic discovery of astrocytic control of inhibition in vivo. Nature 588, 296–302. doi: 10.1038/s41586-020-2926-0
Takata, N., Mishima, T., Hisatsune, C., Nagai, T., Ebisui, E., Mikoshiba, K., et al. (2011). Astrocyte Calcium signaling transforms cholinergic modulation to cortical plasticity in vivo. J. Neurosci. 31, 18155–18165. doi: 10.1523/JNEUROSCI.5289-11.2011
Takata, N., Nagai, T., Ozawa, K., Oe, Y., Mikoshiba, K., and Hirase, H. (2013). Cerebral blood flow modulation by Basal forebrain or whisker stimulation can occur independently of large cytosolic Ca2+ signaling in astrocytes. PLoS One 8:e66525. doi: 10.1371/journal.pone.0066525
Tamamushi, S., Nakamura, T., Inoue, T., Ebisui, E., Sugiura, K., Bannai, H., et al. (2012). Type 2 inositol 1,4,5-trisphosphate receptor is predominantly involved in agonist-induced Ca2+ signaling in Bergmann glia. Neurosci. Res. 74, 32–41. doi: 10.1016/j.neures.2012.06.005
Tamura, A., Yamada, N., Yaguchi, Y., Machida, Y., Mori, I., and Osanai, M. (2014). Both neurons and astrocytes exhibited tetrodotoxin-resistant metabotropic glutamate receptor-dependent spontaneous Slow Ca2+ oscillations in striatum. PLoS One 9:e85351. doi: 10.1371/journal.pone.0085351
Tanaka, M., Shih, P.-Y., Gomi, H., Yoshida, T., Nakai, J., Ando, R., et al. (2013). Astrocytic Ca2+ signals are required for the functional integrity of tripartite synapses. Mol. Brain 6:6. doi: 10.1186/1756-6606-6-6
Tanaka, M., Wang, X., Mikoshiba, K., Hirase, H., and Shinohara, Y. (2017). Rearing-environment-dependent hippocampal local field potential differences in wild-type and inositol trisphosphate receptor type 2 knockout mice. J. Physiol. 595, 6557–6568. doi: 10.1113/JP274573
Tang, F., Lane, S., Korsak, A., Paton, J. F. R., Gourine, A. V., Kasparov, S., et al. (2014). Lactate-mediated glia-neuronal signalling in the mammalian brain. Nat. Commun. 5:284. doi: 10.1038/ncomms4284
Tang, W., Szokol, K., Jensen, V., Enger, R., Trivedi, C. A., Hvalby, O., et al. (2015). Stimulation-evoked Ca2+ signals in astrocytic processes at hippocampal CA3-CA1 synapses of adult mice are modulated by glutamate and ATP. J. Neurosci. 35, 3016–3021. doi: 10.1523/JNEUROSCI.3319-14.2015
Thillaiappan, N. B., Chavda, A. P., Tovey, S. C., Prole, D. L., and Taylor, C. W. (2017). Ca2+ signals initiate at immobile IP3 receptors adjacent to ER-plasma membrane junctions. Nat. Commun. 8:1505. doi: 10.1038/s41467-017-01644-8
Tichy, A.-M., Gerrard, E. J., Sexton, P. M., and Janovjak, H. (2019). Light-activated chimeric GPCRs: limitations and opportunities. Curr. Opin. Struct. Biol. 57, 196–203. doi: 10.1016/j.sbi.2019.05.006
Uchida, K., Aramaki, M., Nakazawa, M., Yamagishi, C., Makino, S., Fukuda, K., et al. (2010). Gene knock-outs of inositol 1,4,5-trisphosphate receptors types 1 and 2 result in perturbation of cardiogenesis. PLoS One 5:e12500. doi: 10.1371/journal.pone.0012500
Uchida, K., Nakazawa, M., Yamagishi, C., Mikoshiba, K., and Yamagishi, H. (2016). Type 1 and 3 inositol trisphosphate receptors are required for extra-embryonic vascular development. Dev. Biol. 418, 89–97. doi: 10.1016/j.ydbio.2016.08.007
Vervloessem, T., Yule, D. I., Bultynck, G., and Parys, J. B. (2015). The type 2 inositol 1,4,5-trisphosphate receptor, emerging functions for an intriguing Ca2+-release channel. Biochim. Biophys. Acta BBA Mol. Cell Res. 1853, 1992–2005. doi: 10.1016/j.bbamcr.2014.12.006
Wang, Q., Kong, Y., Wu, D.-Y., Liu, J.-H., Jie, W., You, Q.-L., et al. (2021). Impaired calcium signaling in astrocytes modulates autism spectrum disorder-like behaviors in mice. Nat. Commun. 12:3321. doi: 10.1038/s41467-021-23843-0
Wang, Y., DelRosso, N. V., Vaidyanathan, T. V., Cahill, M. K., Reitman, M. E., Pittolo, S., et al. (2019). Accurate quantification of astrocyte and neurotransmitter fluorescence dynamics for single-cell and population-level physiology. Nat. Neurosci. 22, 1936–1944. doi: 10.1038/s41593-019-0492-2
Wojcikiewicz, R. J., and He, Y. (1995). Type I, II and III inositol 1,4,5-trisphosphate receptor co-immunoprecipitation as evidence for the existence of heterotetrameric receptor complexes. Biochem. Biophys. Res. Commun. 213, 334–341. doi: 10.1006/bbrc.1995.2134
Xie, Y., Wang, T., Sun, G. Y., and Ding, S. (2010). Specific disruption of astrocytic Ca2+ signaling pathway in vivo by adeno-associated viral transduction. Neuroscience 170, 992–1003. doi: 10.1016/j.neuroscience.2010.08.034
Yamada, N., Makino, Y., Clark, R. A., Pearson, D. W., Mattei, M. G., Guénet, J. L., et al. (1994). Human inositol 1,4,5-trisphosphate type-1 receptor, InsP3R1: structure, function, regulation of expression and chromosomal localization. Biochem. J. 302, 781–790. doi: 10.1042/bj3020781
Yamamoto-Hino, M., Miyawaki, A., Kawano, H., Sugiyama, T., Furuichi, T., Hasegawa, M., et al. (1995). Immunohistochemical study of inositol 1,4,5-trisphosphate receptor type 3 in rat central nervous system. NeuroReport 6, 273–276. doi: 10.1097/00001756-199501000-00012
Yamamoto-Hino, M., Sugiyama, T., Hikichi, K., Mattei, M. G., Hasegawa, K., Sekine, S., et al. (1994). Cloning and characterization of human type 2 and type 3 inositol 1,4,5-trisphosphate receptors. Recept. Channels 2, 9–22.
Yang, L., Qi, Y., and Yang, Y. (2015). Astrocytes control food intake by inhibiting AGRP neuron activity via adenosine A1 receptors. Cell Rep. 11, 798–807. doi: 10.1016/j.celrep.2015.04.002
Yoo, S. H. (2010). Secretory granules in inositol 1,4,5-trisphosphate-dependent Ca2+ signaling in the cytoplasm of neuroendocrine cells. FASEB J. Off. Publ. Fed. Am. Soc. Exp. Biol. 24, 653–664. doi: 10.1096/fj.09-132456
Young, S. Z., Platel, J., Nielsen, J. V., Jensen, N., and Bordey, A. (2010). GABAA increases calcium in subventricular zone astrocyte-like cells through L- and T-type voltage-gated calcium channels. Front. Cell. Neurosci. 4:8. doi: 10.3389/fncel.2010.00008
Yu, X., Taylor, A. M. W., Nagai, J., Golshani, P., Evans, C. J., Coppola, G., et al. (2018). Reducing astrocyte calcium signaling in vivo alters striatal microcircuits and causes repetitive behavior. Neuron 99, 1170–1187.e9. doi: 10.1016/j.neuron.2018.08.015
Zhang, S., Fritz, N., Ibarra, C., and Uhlén, P. (2011). Inositol 1,4,5-trisphosphate receptor subtype-specific regulation of calcium oscillations. Neurochem. Res. 36, 1175–1185. doi: 10.1007/s11064-011-0457-7
Zhang, Y., Chen, K., Sloan, S. A., Bennett, M. L., Scholze, A. R., O’Keeffe, S., et al. (2014). An RNA-sequencing transcriptome and splicing database of glia, neurons, and vascular cells of the cerebral cortex. J. Neurosci. 34, 11929–11947. doi: 10.1523/JNEUROSCI.1860-14.2014
Keywords: astrocyte, inositol triphosphate (IP3) receptor, IP3R subtypes, calcium, GPCR, tripartite synapse, gliotransmission
Citation: Sherwood MW, Arizono M, Panatier A, Mikoshiba K and Oliet SHR (2021) Astrocytic IP3Rs: Beyond IP3R2. Front. Cell. Neurosci. 15:695817. doi: 10.3389/fncel.2021.695817
Received: 15 April 2021; Accepted: 30 June 2021;
Published: 30 July 2021.
Edited by:
Yu-Wei Wu, Academia Sinica, TaiwanReviewed by:
Dmitri A. Rusakov, University College London, United KingdomVladimir Grubišiæ, Michigan State University, United States
Copyright © 2021 Sherwood, Arizono, Panatier, Mikoshiba and Oliet. This is an open-access article distributed under the terms of the Creative Commons Attribution License (CC BY). The use, distribution or reproduction in other forums is permitted, provided the original author(s) and the copyright owner(s) are credited and that the original publication in this journal is cited, in accordance with accepted academic practice. No use, distribution or reproduction is permitted which does not comply with these terms.
*Correspondence: Mark W. Sherwood, TVdTaGVyd29vZEBwcm90b25tYWlsLmNvbQ==; Misa Arizono, YXJpem9ubzAyMDJAZ21haWwuY29t
†These authors have contributed equally to this work and share first authorship