- 1School of Medical Sciences, Faculty of Medicine and Health, University of Sydney, Sydney, NSW, Australia
- 2Department of Neurological Surgery, Oregon Health & Science University, Portland, OR, United States
- 3Department of Biology, SUNY Albany, Albany, NY, United States
Neurotransmitter transporters limit spillover between synapses and maintain the extracellular neurotransmitter concentration at low yet physiologically meaningful levels. They also exert a key role in providing precursors for neurotransmitter biosynthesis. In many cases, neurons and astrocytes contain a large intracellular pool of transporters that can be redistributed and stabilized in the plasma membrane following activation of different signaling pathways. This means that the uptake capacity of the brain neuropil for different neurotransmitters can be dynamically regulated over the course of minutes, as an indirect consequence of changes in neuronal activity, blood flow, cell-to-cell interactions, etc. Here we discuss recent advances in the mechanisms that control the cell membrane trafficking and biophysical properties of transporters for the excitatory, inhibitory and modulatory neurotransmitters glutamate, GABA, and dopamine.
Glutamate Transporters
In addition to being one of the most abundant amino acids and the main excitatory neurotransmitter in the brain, glutamate controls synapse formation, and maturation, acts as an energy substrate for oxidative metabolism, contributes to the antioxidant properties of glutathione, and can be used as a building block for non-ribosomal peptide synthesis and as the precursor for the biosynthesis of the inhibitory neurotransmitter GABA (Sieber and Marahiel, 2005; Brosnan and Brosnan, 2013; Martinez-Lozada et al., 2016; Walker and van der Donk, 2016). In addition, in the developing brain, glutamate guides cell proliferation, migration, differentiation, and survival of neural progenitor cells (Jansson et al., 2013). Because of the plethora of effects that glutamate can exert, its lifetime in the extracellular space needs to be finely controlled through the activity of a family of Na+- and K+-dependent secondary active transporters. Geneticists, structural biologists, physiologists and clinicians all have their preferred nomenclature to refer to the five known glutamate transporters subtypes, summarized as follows: (i) Slc1a1/SLC1A1/EAAC1/EAAT3; (ii) Slc1a2/SLC1A2/GLT1/EAAT2; (iii) Slc1a3/SLC1A3/GLAST/EAAT1; (iv) Slc1a6/SLC1A6/EAAT4; (v) Slc1a7/SLC1A7/EAAT5. The use of each terminology is physiologically meaningful, as it refers to the genes encoding each transporter in rodents (Slc1a1, Slc1a2, Slc1a3, Slc1a6, Slc1a7) and in humans (SLC1A1, SLC1A2, SLC1A3, SLC1A6, SLC1A7), or the protein product in rodents (EAAC1, GLT1, GLAST, EAAT4-5) and humans (EAAT1-5). For simplicity, in this review, we refer to them as EAAT1-5. Different subtypes of glutamate transporters are differentially expressed in neuronal and glial cells (Danbolt, 2001). Accordingly, the glutamate transporters EAAT1-2 are mostly expressed in astrocytes, whereas EAAT3-5 are mostly expressed in neurons. Two of the neuronal transporters are predominantly expressed in the cerebellum (EAAT4) or retina (EAAT5), with a low yet experimentally measurable concentration in the forebrain (Dehnes et al., 1998; Danbolt, 2001). The others (i.e., EAAT1, EAAT2, and EAAT3) are expressed broadly throughout the brain, at varying levels.
Structural information of the glutamate transporters comes from prokaryote homologs such as GltPh and GltTk which share ∼35 amino acid identity with human EAAT2 (Yernool et al., 2004; Boudker et al., 2007; Reyes et al., 2009; Verdon and Boudker, 2012; Guskov et al., 2016; Scopelliti et al., 2018) and more recent crystal and cryo-EM structures of human transporters including EAAT1, EAAT3, and ASCT2 (Canul-Tec et al., 2017; Garaeva et al., 2018; Garaeva et al., 2019; Yu et al., 2019; Wang and Boudker, 2020) (Figure 1). All members of this family appear to assemble as trimers, with each monomer capable of transporting substrate and coupled ions, generating stoichiometric and non-stoichiometric currents, independently of the two other monomers (Grewer et al., 2005; Koch et al., 2007; Leary et al., 2007). The transporters are composed of a “transport domain” which binds and transports substrate and coupled ions, and a “scaffold domain” that forms inter-protomer contacts and interacts with the lipid membrane (Boudker et al., 2007; Reyes et al., 2009). GltPh transports aspartate together with three Na+ ions into the cytoplasm using a twisting elevator mechanism (Reyes et al., 2009; Ryan and Vandenberg, 2016) and generates a stoichiometrically uncoupled Cl– conductance (Boudker et al., 2007; Ryan and Mindell, 2007; Reyes et al., 2009).
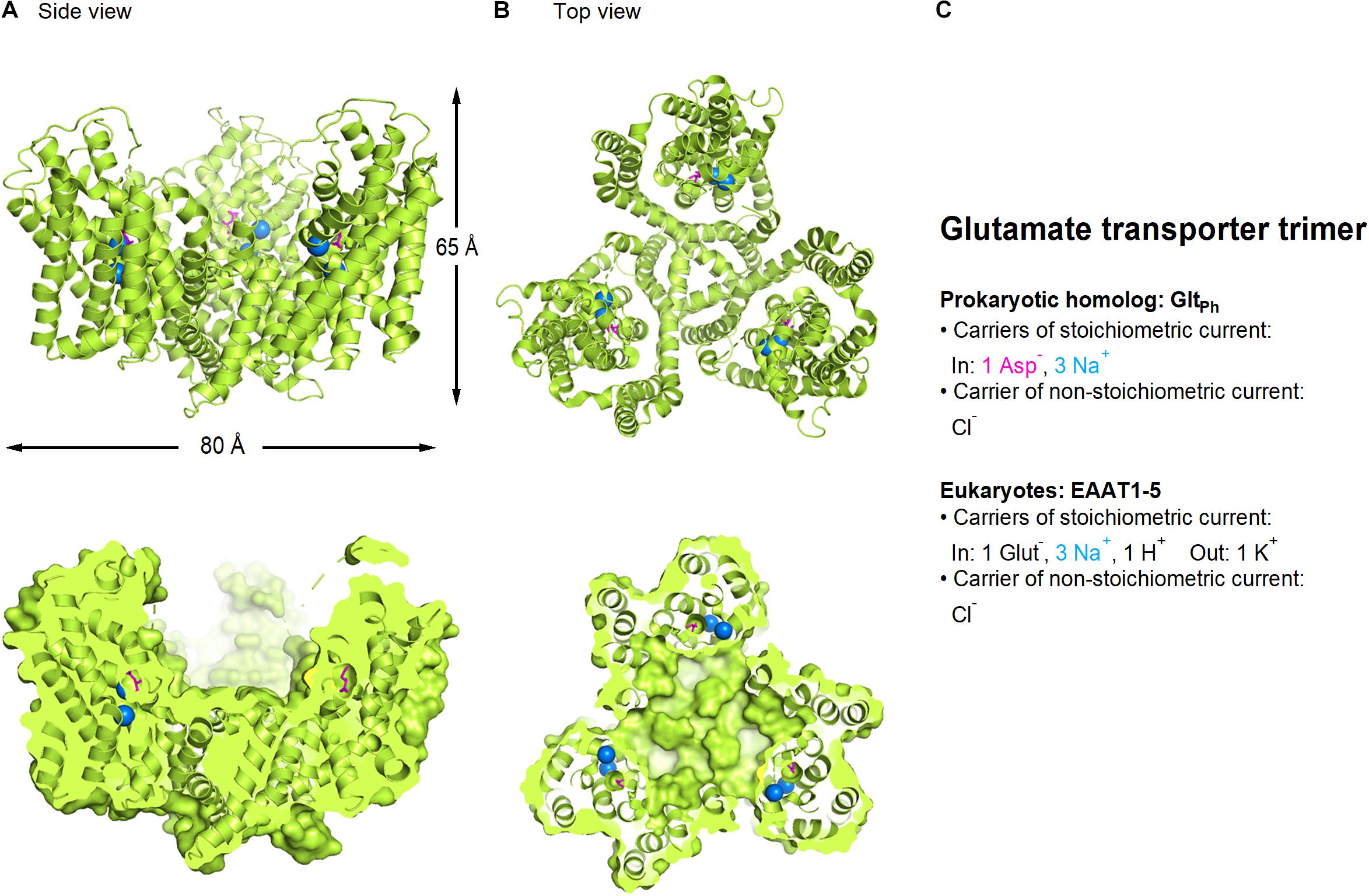
Figure 1. Crystal structure of GltPh, a bacterial homolog of glutamate transporters (PDB: 2NWX). (A) Side view of the glutamate transporter trimer parallel to the membrane, using a ribbon (top) and a surface representation sliced through the center of the transporter basin (bottom). (B) As in A, viewed from the extracellular side of the membrane. Aspartate (magenta) and sodium ions (blue) are represented as spheres. (C) Summary of the main feature of the stoichiometric and non-stoichiometric current mediated by glutamate transporters in prokaryotes and eukaryotes, respectively. Image generated using The PyMOL Molecular Graphics System (Version 2.3.2; Schrödinger, 2010).
In contrast to prokaryotes, for all eukaryotic transporters, glutamate uptake is driven by the electrochemical gradient for Na+ and H+ ions, and the rate limiting step is the counter-transport of one K+ ion across the membrane (Zerangue and Kavanaugh, 1996). The stoichiometry of the transport process is the inward movement of 1 glutamate (which carries a negative charge): 3 Na+: 1 H+, followed by the counter-transport of 1 K+, leading to the net influx of two positive charges per transport cycle (Zerangue and Kavanaugh, 1996; Levy et al., 1998). In addition, these transporters mediate a glutamate-dependent anion flux, which under physiological conditions is carried by Cl– ions (Picaud et al., 1995; Wadiche et al., 1995b; Eliasof and Jahr, 1996). The chloride channel forms at the interface of the transport and scaffold domains during the transport cycle (Ryan et al., 2004; Cater et al., 2014, 2016; Cheng et al., 2017; Kolen et al., 2020; Chen et al., 2021) and the direction of this flux is determined by the driving force for chloride (i.e., the difference between membrane potential and reversal potential for chloride). The function of the chloride current remains incompletely understood but it has been suggested that it might serve to counterbalance the influx of positive charges due to glutamate transport and prevent cell depolarization (Grewer and Rauen, 2005). This hypothesis would only hold true in the presence of a positive driving force for chloride, causing an inward movement of chloride into the cell. This occurs when the reversal potential for chloride is more hyperpolarized than the membrane potential. This condition, however, is not fulfilled in developing neurons. Here, the reversal potential for chloride is more depolarized than in mature neurons, due to a delayed expression of the potassium chloride co-transporter KCC2, and a consequently higher intracellular chloride concentration in developing compared to mature neurons (Rivera et al., 1999). Other physiological roles for the chloride conductance have been demonstrated with EAAT5 in the retina, where it regulates cell activity and neurotransmitter release (Picaud et al., 1995; Veruki et al., 2006) and in cerebellar astrocytes, where the chloride currents of EAAT1/2 affects the resting intracellular chloride concentration (Sonders and Amara, 1996; Untiet et al., 2017).
Similarities and Distinctive Biophysical Features of Different Glutamate Transporter Subtypes
The general mechanisms of substrate transport, identified through studies of uptake of radiolabeled substrates or voltage-clamp recordings of transporter currents under steady-state conditions, are roughly similar among different glutamate transporter types. For all of them, the transport efficiency is in the order of ∼50%, which means that − perhaps surprisingly − these molecules translocate into the cell cytoplasm only 50% of all glutamate molecules they bind (Tzingounis and Wadiche, 2007). The remaining 50% of glutamate molecules that unbind from the transporters are released back in the extracellular space, and ultimately bind to nearby glutamate receptors, neuronal or glial transporters. This low transport efficiency can lead to an apparently paradoxical prolongation of glutamate lifetime in the extracellular space (Scimemi et al., 2009). Since the rates of glutamate binding to receptors and transporters are relatively similar, the likelihood that a glutamate molecule unbound from a transporter will eventually bind to a receptor or another transporter depends on the relative abundance of receptors compared to that of transporters. Typically, the surface density of expression of transporters in glial membranes is four orders of magnitude higher than that of glutamate receptors in extra-synaptic neuronal membrane (Lehre and Danbolt, 1998). For this reason, the most likely ultimate fate of glutamate molecules unbound from transporters is to be bound again by other transporter molecules (Leary et al., 2011).
Non-rate-limiting partial reaction steps, obtained by perturbing the steady-state and by subsequently following the kinetics of the relaxation to a new steady-state (i.e., the pre-steady-state kinetics), are similar for EAAT1-3 (Grewer and Rauen, 2005). However, the pre-steady-state kinetics and steady-state turnover rats are significantly slower for EAAT4 (Mim et al., 2005). There are also notable differences in the steady state affinity of different transporter types (km EAAT1: 48 ± 10 μM; EAAT2: 97 ± 4 μM; EAAT3: 62 ± 8 μM; EAAT4: 0.6 μM; Wadiche and Kavanaugh, 1998; Grewer et al., 2000; Bergles et al., 2002), and in the ratio of substrate transport versus anion permeation (Arriza et al., 1994; Seal and Amara, 1999; Mim et al., 2005; Torres-Salazar and Fahlke, 2007). Interestingly, and in contrast to EAAT1-3, the apparent affinity for glutamate is voltage dependent for EAAT4 and increases with negative voltages, suggesting higher glutamate buffering capacity for EAAT4 than other glutamate transporters (Mim et al., 2005). The fact that EAAT4 has a 10-fold higher affinity for glutamate but a 10-fold slower translocation rate than other transporters has led to hypothesize that the main functional role of EAAT4 is accounted for by its ability to generate a stoichiometrically uncoupled anion current (Fairman et al., 1995; Lin et al., 1998). Others have suggested that these biophysical properties would allow EAAT4 to clear glutamate away from synapses, where its concentration is lower than at the outer boundary of the synaptic cleft (Mim et al., 2005). Consistent with this hypothesis, one of the most prominent roles of EAAT4 is to limit metabotropic glutamate receptor activation in cerebellar Purkinje cells, in sub-cellular domains where the density of expression of these receptors and EAAT4 are both high (Wadiche and Jahr, 2005).
Glutamate transport via EAAT4 has a unique voltage-dependence. Its maximum transport activity is detected at –20 mV < Vm < 0 mV and the transporter inactivates at more negative membrane potentials (Mim et al., 2005). Membrane hyperpolarization promotes glutamate transport via other glutamate transporters, which have reversal potentials of 9.3 ± 0.7 mV (EAAT1), >80 mV (EAAT2) and 38.0 ± 2.7 mV (EAAT3) (Arriza et al., 1994). At hyperpolarized potentials, not only transport, but also the anion conductance of EAAT4 is inhibited (Mim et al., 2005). This means that at membrane potentials close to the resting potential of neurons, glutamate is bound strongly to all transporters, but its transport via EAAT4 is inhibited (Mim et al., 2005).
There are differences in the sodium requirement for activation of the anion conductance between neuronal and glial glutamate transporters (Wadiche et al., 1995a; Grewer et al., 2000, 2001; Otis and Kavanaugh, 2000). For EAAT3, the anion conductance can be activated by glutamate and Na+ ions from both sides of the membrane (Watzke and Grewer, 2001). The activation of the anion conductance by sodium alone has only been demonstrated for EAAT3-4, while EAAT1-2 mediate glutamate- and sodium-independent anion conducting states (Divito et al., 2017). For EAAT4-5, the anion conductance is particularly large compared to their glutamate transport capacity (Sonders and Amara, 1996; Seal et al., 2001). Consequently, transport currents generated by EAAT1-3 are easily measured experimentally using heterologous expression systems, whereas those mediated by EAAT4-5 are relatively small (Wadiche et al., 1995a; Grewer et al., 2001; Mitrovic et al., 2001; Watzke et al., 2001).
The existence of functional differences in the properties of glutamate transporter subtypes indicates that the function of these molecules is much more complex than previously thought, and that an evaluation of the physiological implications of glutamate transporters cannot bypass an understanding of the biophysical properties of these molecules in their native environments.
The Surface Mobility and Cellular Distribution of EAAT2
Out of all glutamate transporter types, EAAT2 has the highest density of expression in the adult brain, and is responsible for the largest proportion of glutamate transport (Minelli et al., 2001). In astrocytes, 70–75% of all EAAT2 is expressed on the plasma membrane (Michaluk et al., 2020). In the hippocampus, cerebellum and neocortex, EAAT2 is expressed mostly in astrocytic processes, in the vicinity and at a distance from the synaptic cleft (Danbolt et al., 1992; Levy et al., 1993; Rothstein et al., 1994; Torp et al., 1994; Chaudhry et al., 1995; Lehre et al., 1995). EAAT2 exists in at least three splice variants which differ only in their C-terminal domain. EAAT2a is the predominant variant, and represents ∼90%, whereas EAAT2b and EAAT2c represent ∼6% and ∼1% of total EAAT2 protein, respectively (Chen et al., 2002; Rauen et al., 2004; Holmseth et al., 2009). These variants have similar regional distribution and although they are all primarily expressed in astrocytes, they have also been detected in neurons (Schmitt et al., 2002; Chen et al., 2004; Maragakis et al., 2004; Bassan et al., 2008; Gonzalez-Gonzalez et al., 2008a; Holmseth et al., 2009). EAAT2a is more abundantly expressed than EAAT2b, and is a presynaptic glutamate transporter (Berger et al., 2005; Rimmele and Rosenberg, 2016). Within the hippocampus, 14–29% of axon terminals express EAAT2a (Chen et al., 2004). Though EAAT2a and EAAT2b share similar functional properties, they differ for the sequence of their extreme intracellular C-terminus (Chen et al., 2002; Sullivan et al., 2004). Unlike EAAT2a, EAAT2b has a PDZ binding domain that makes it capable of binding to proteins like PICK1, PSD95, and DLG1 (Bassan et al., 2008; Gonzalez-Gonzalez et al., 2008a; Underhill et al., 2015). These, in turn, can alter the currents mediated by the EAAT2b transporter (Sogaard et al., 2013). EAAT2a forms heteromers with EAAT2b, which have been proposed to stabilize EAAT2a around synapses (Haugeto et al., 1996; Peacey et al., 2009).
EAAT2 is highly mobile on the plasma membrane of astrocytes (D∗ = 0.15–0.23 μm2/s) (though a lower value of D∗ = 0.039 μm2/s has been measured using quantum dots; Al Awabdh et al., 2016), and the rate with which it diffuses along the plasma membrane can be increased by glutamate binding to EAAT2 or to AMPA, NMDA and metabotropic glutamate receptors, and can be reduced by blocking glutamate binding to EAAT2 (Benediktsson et al., 2012; Murphy-Royal et al., 2015; Michaluk et al., 2020). The surface diffusion of the EAAT2 variants EAAT2a and EAAT2b is more confined at astrocytic processes proximal to synapses, especially for EAAT2b (Al Awabdh et al., 2016). Consistent with previous work, glutamate increases the surface mobility of both EAAT2 variants, whereas blocking synaptic activity reduces it (Al Awabdh et al., 2016). The more confined diffusion of EAAT2b may be attributed to the presence of the PDZ domain, which allows EAAT2b to interact with scaffolding proteins and anchor it to macromolecular complexes that astrocytes form in subcellular domains opposite to neuronal presynaptic terminals (Al Awabdh et al., 2016). There is also evidence that membrane raft association regulates the targeting and function of glutamate transporters, especially for EAAT2 (Butchbach et al., 2004).
Glutamate transporters have been suggested to become incorporated into the plasma membrane through exocytosis (Cheng et al., 2002; Chowdhury et al., 2002; Robinson, 2002; Fournier et al., 2004; Karylowski et al., 2004; Zeigerer et al., 2004), and several components of this molecular machinery have been identified in astrocytes (Parpura et al., 1995; Hepp et al., 1999; Zhang et al., 2004). Consistent with these findings, calcium-dependent exocytosis of EAAT2 has been detected using FM dyes in cultured astrocytes (Stenovec et al., 2008). The same experiments showed that EAAT2 has a punctate distribution along the astrocytic plasma membrane, suggesting that the glutamate uptake capacity of these cells varies depending on the local density of expression of glutamate transporters (Stenovec et al., 2008). Interestingly, recent work indicate that the lifetime of EAAT2 on the astrocyte plasma membrane is ∼22 s (Michaluk et al., 2020). Whereas lateral diffusion could serve as a mechanism for transporter turnover away from synapses, where the diffusivity of these molecules is higher, endo/exocytosis from/to the plasma membrane to intracellular organelles could contribute to transporter turnover in astrocytic processes nearby synapses, where the diffusivity of these molecules is lower (Michaluk et al., 2020).
Although 80–90% of EAAT2 is localized in astrocytes, there is a small proportion (5–10%) in neuronal axon terminals (Rauen and Kanner, 1994; Torp et al., 1994; Schmitt et al., 1996; Torp et al., 1997; Chen et al., 2004; Furness et al., 2008; Melone et al., 2009, 2011, 2019). In neurons, EAAT2 co-localizes with the α1 and α3 isoforms of the Na+/K+-ATPase, but this co-localization is looser than that with the α2 isoform in astrocytes, suggesting a less efficient interaction between EAAT2 and the Na+/K+-ATPase in neurons (Melone et al., 2019). Neuronal EAAT2 appears to be required to provide glutamate to synaptic mitochondria, and is therefore linked to energy metabolism (Petr et al., 2015; Fischer et al., 2018; McNair et al., 2019, 2020; Sharma et al., 2019). By contrast, astrocytic EAAT2 is crucial to ensure survival, resistance to epilepsy, and prevent cognitive decline. Loss of neuronal and astrocytic EAAT2 have both implications on long-term memory and spatial reference learning, but the time scale over which they exert these effects is different (Sharma et al., 2019). Loss of astrocytic EAAT2 leads to early deficits, whereas loss of neuronal EAAT2 leads to late-onset deficits in long-term memory and spatial reference learning (Sharma et al., 2019). These findings are important because they identify neuronal and astrocytic EAAT2 as contributing to different aspects of cognitive function and, potentially, as different therapeutic targets in cognitive decline (Petr et al., 2015; Fischer et al., 2018; McNair et al., 2019, 2020; Sharma et al., 2019).
Multiple studies have shown that there are interesting relationships between astrocytic coverage, glutamate transporter expression and synaptic size (Ventura and Harris, 1999; Genoud et al., 2006; Witcher et al., 2007; Lushnikova et al., 2009; Witcher et al., 2010; Patrushev et al., 2013; Medvedev et al., 2014; Gavrilov et al., 2018; Herde et al., 2020). For example, in the rodent hippocampus, only 40–60% of synapses have astrocytic processes, and these cover only 53% of their perimeter (Ventura and Harris, 1999; Witcher et al., 2007, 2010). The size of a spine correlates with its release probability (Schikorski and Stevens, 1997). Astrocytes are closer to smaller spines (Medvedev et al., 2014), but the overall astrocytic coverage does not change with spine size (Gavrilov et al., 2018). Although there is less EAAT2 at smaller spines, its density is higher (Herde et al., 2020). These findings have important implications for understanding how glutamate transporters control spillover at synapses with different size (Scimemi et al., 2004), and the implications of this phenomenon on synaptic and astrocyte plasticity (Wenzel et al., 1991; Matsusaki et al., 2001; Bernardinelli et al., 2014; Perez-Alvarez et al., 2014).
Transcriptional, Translational, and Post-translational Regulation of Glutamate Transporters
Despite current agreements on the presence of EAAT2 in neurons, in the 1990s, some groups failed to find EAAT2 proteins in subsets of cortical neurons (Rothstein et al., 1994; Chaudhry et al., 1995; Lehre et al., 1995), in contrast to others (Torp et al., 1994; Schmitt et al., 1996; Torp et al., 1997). The discrepancy between these findings was attributed to the existence of a post-transcriptional and post-translational control of EAAT2 expression (Gegelashvili and Schousboe, 1997; Anderson and Swanson, 2000; Plachez et al., 2000). In fact, like most membrane proteins, glutamate transporters can be regulated at the gene expression, protein targeting and trafficking, and post-translational level.
The gene structure and organization of most glutamate transporters identified in the late 1990s showed that the promoter regions are highly conserved between mouse and human (Hagiwara et al., 1996; Stoffel et al., 1996). These regions do not contain a TATA box but a GC box and, in humans, an E box (Martinez-Lozada et al., 2016). The genes encoding different glutamate transporters contain different excision/splicing sites for different exons, which can generate variant mRNA species and proteins. Accordingly, multiple studies have reported the existence of several mRNA size classes encoding EAAT1 and EAAT3 (Pines et al., 1992; Tanaka, 1993; Mukainaka et al., 1995; Nakayama et al., 1996; Palos et al., 1996; Gegelashvili and Schousboe, 1997). This might be due to differences in polyadenylation and the existence of mRNAs with different coding capacity (Gegelashvili and Schousboe, 1997).
When glutamate transporters and their human homologs were first cloned, knowledge on the elements regulating their transcription largely relied on information collected from ASCT1, a neutral amino acid transporter of the same family of high affinity transporters as glutamate transporters (Hofmann et al., 1994). ASCT1 has 39–44% amino acid sequence identity, similar hydropathy profiles, trans-membrane organization and conservation of crucial function-related motifs compared to EAAT2 (Gegelashvili and Schousboe, 1997). According to these initial reports, the promoter region of EAAT2 was thought to contain at least five consensus sequences for the Sp1 transcription factor (krox24, krox20, Egr3, NGFI-C), involved in cell differentiation (Hofmann et al., 1994; Gegelashvili and Schousboe, 1997). By the early 2000’s, cloning and bioinformatics works established that the promoter region for EAAT1-2 is highly conserved: not only does it not have a TATA box, but lacks well-defined cis-elements and contains five consensus sequences for Sp1 and GC-rich repeats, also found in humans (Su et al., 2003). The search for regulatory transcription factors has led to the identification of the Nuclear Factor of Activated T cells (NFAT), the N-myc proto-oncogene protein (N-myc), and the Nuclear Factor κB (NF-κB), and a consensus NF-κB binding sequence in the 5′-UTR region of the Slc1a2 gene in humans (Meyer et al., 1996; Su et al., 2003). The Tumor Necrosis Factor α (TNFα), a cytokine involved in the acute phase of inflammatory reactions, decreases EAAT2 mRNA expression by increasing NF-κB activation (Su et al., 2003), perhaps by promoting NF-kB interactions with other transcription factors (Sitcheran et al., 2005). The ability of transcription factors to regulate gene expression can change the sensitivity of glutamate transporter expression to other regulatory proteins. For example, TNFα regulates the activity of the Yin Yang 1 (YY1) transcription factor which, when bound to the EAAT2 promoter, changes the effect of NF-κB from activation to suppression (Karki et al., 2014). In turn, NF-κB regulates YY1 expression. These findings suggest the existence of complex interplays between NF-κB and YY1 for the transcriptional regulation of EAAT2 expression.
Over the last two decades, other groups have identified factors, cis-regulatory elements and epigenetic mechanisms regulating EAAT1-2 transcription, but many unknowns remain about the molecular mechanisms regulating EAAT3-5 expression. In Table 1, we provide a summary of current studies on modulation of glutamate transporters listed in PubMed. One can easily note that in many cases, the results are conflicting. One may argue that inconsistencies are inevitable when studying a given transporter in different cell types and animal species. However, in some cases these considerations do not allow to resolve conflicting results from different laboratories. For this reason, in this review, we limit our discussion to forms of modulation of glutamate transporters for which some consensus exists.
There is a general agreement on the fact that in cultured neurons, PACAP, cAMP, and PKA-dependent pathways increase EAAT1 expression (Martinez-Lozada et al., 2016) and uptake (Hertz et al., 1978; Gegelashvili et al., 1996). Presumably this effect is mediated by activation of the transcription factor cAMP-response element binding protein (CREB), but the transcription factors or the cis elements of the promoter responsible for this effect have not been identified (Martinez-Lozada et al., 2016). This form of transcriptional regulation may provide a pathway through which activation of G-protein membrane receptors coupled to cAMP and PKA-dependent signaling pathways indirectly affect glutamate uptake, as it has been shown in other contexts for D2 dopamine receptors or α1 and β-adrenergic receptors (Kerkerian et al., 1987).
Stable epigenetic alterations of glutamate transporter gene expression are heritable in the short term, but do not involve DNA mutations. These include methylation and histone modifications, and there are multiple CpG regions in the promoter for EAAT2 where methylation can occur (Zschocke et al., 2005). One of the consequences of methylation is that it alters the ability of glucocorticoids to change EAAT2 expression. Accordingly, in the cerebellum, where the EAAT2 promoter is hyper-methylated, glucocorticoids are unable to change EAAT2 expression (Zschocke et al., 2005). By contrast, in the forebrain, where the EAAT2 promoter is hypo-methylated, glucocorticoid up-regulate EAAT2 expression (Zschocke et al., 2005).
Phosphorylation and glycosylation are two documented forms of post-translational modifications for glutamate transporters (Gegelashvili and Schousboe, 1997). Accordingly, increasing protein kinase C (PKC) activation by phorbol esters leads to increased phosphorylation of EAAT2 at the residue Ser113 and increased glutamate uptake (Roginski et al., 1993). N-glycosylation promotes EAAT3 expression, but has no effect on EAAT1 (Conradt et al., 1995; Ferrer-Martinez et al., 1995).
Arachidonic acid, produced via activation of NMDA receptors in neurons and metabotropic glutamate receptors in astrocytes, can directly interact with different types of glutamate transporters, with different effects. For example, it reduces glutamate uptake via EAAT1, enhances glutamate uptake via EAAT2 and leads to a moderate increase of glutamate uptake via EAAT3 (Chan et al., 1983; Volterra et al., 1992; Trotti et al., 1995). Arachidonic acid can lead to the activation of PKC, promoting glutamate uptake via EAAT2. Metabolites of arachidonic acid can be a source of reactive oxygen species (ROS), which inhibit glutamate uptake but increase the transporters’ steady state affinity for glutamate (Volterra et al., 1994b).
There is a growing awareness that these forms of regulation are complex not only because they likely differ among species, cell types and brain regions, but also because they interact with one another in ways that can be difficult to reproduce in reduced preparations but that affect the function of these transporters in vivo. These currently unknowns are likely going to be addressed by using experimental approaches that allow manipulation of different regulation factor in situ, and in a cell-specific manner.
Activity-Dependent Modulation of Glutamate Transporter Trafficking
The first observation that glutamate itself can modulate its uptake came from data showing that glutamate uptake is increased in astrocyte cultures supplemented with conditioned media from neuronal cultures (Drejer et al., 1983; Voisin et al., 1993). Pure astrocytic cultures only express EAAT1, but co-culture of neurons and astrocytes increases EAAT1 expression and induces EAAT2 expression (Gegelashvili and Schousboe, 1997; Swanson et al., 1997). This effect depends on p42/44 MAP kinases activation via the tyrphostin-sensitive Receptor Tyrosine Kinase (RTK) signaling pathway, and is abolished by inhibitors of PI3K, tyrosine kinase and NF-kB (Swanson et al., 1997; Zelenaia et al., 2000). Similarly, decreased glutamate uptake via EAAT1-2 (not EAAT3) occurs in response to axotomy of glutamatergic neurons of cortical lesions (McGeer et al., 1977; Shifman, 1991; Ginsberg et al., 1995; Levy et al., 1995). Together, these forms of activity-dependent regulation of EAAT2 expression allow this transporter to be more abundant and less mobile in astrocytic processes close to active glutamatergic synapses, an effect that can provide an effective strategy to limit glutamate spillover away from the synaptic cleft.
Similarly, to glutamate, glutamate transporter substrates like D-Aspartate and ligands like L-trans-PDC and TBOA can also produce a redistribution of EAAT1 on the cell membrane (Shin et al., 2009). Consistent with these findings, treating astrocyte cultures with kainate, dbcAMP or AMPA receptor agonists increases D-aspartate uptake and EAAT1 protein expression (Gegelashvili et al., 1996). It is unclear whether these effects are due to binding to AMPA receptors or are due to release of diffusible molecules (e.g., arachidonic acid, diacylglycerol, nitric oxide) through more complex intracellular signaling cascades (Gegelashvili and Schousboe, 1997).
Physiological Roles of Glutamate Transporters
The role of glutamate transporters in regulation of phasic and tonic extracellular glutamate levels is critical for neuronal signaling and controlling excitotoxicity (Rothstein et al., 1996). Spillover of glutamate and inter-synaptic cross-talk have been associated with multiple neuropsychiatric disorders, including schizophrenia, epilepsy, addiction, depression and obsessive compulsive disorder (O’Donovan et al., 2017; Bellini et al., 2018; Malik and Willnow, 2019). Knock-out mouse models of different subtypes of glutamate transporters have revealed major motor deficits with decreased levels of glial transporters EAAT1-2 (Rothstein et al., 1996), with more subtle behavioral deficits with loss of the neuronal transporter EAAT3 (Peghini et al., 1997; Bellini et al., 2018).
In humans, decreased expression and reduced function of EAAT2 are associated with amyotrophic lateral sclerosis (ALS) (Rothstein et al., 1995; Bruijn et al., 1997; Rosenblum and Trotti, 2017). In transgenic mice expressing an N-terminal fragment of mutant huntingtin (R6/2), there is an age-dependent downregulation of EAAT2, which leads to a progressive increase in the extracellular glutamate (Behrens et al., 2002). Other glutamate transporters, however, remain unchanged, suggesting that EAAT2-mediated excitotoxicity might contribute to Huntington’s disease (Behrens et al., 2002). In the few identified polymorphisms of the gene encoding EAAT3, dicarboxylic aminoaciduria, a deficit in kidney function was prominent, as well as family linkage to schizophrenia and obsessive–compulsive disorders (OCD) (Bailey et al., 2011; Porton et al., 2013). In mice, loss or increased EAAT3 are associated with increased anxiety and OCD-like behaviors, suggesting that preserving an optimal expression of this transporter is key for the function of neuronal circuits affected by this disease (Zike et al., 2017; Bellini et al., 2018; Delgado-Acevedo et al., 2019). Significant lower levels of EAAT2 have also been reported in vitro (Scimemi et al., 2013) and in vivo, in animal models of Alzheimer’s disease (Wilson et al., 2003; Dabir et al., 2006; Mookherjee et al., 2011; Schallier et al., 2011; Hefendehl et al., 2016), as well as in humans affected by this disease (Li et al., 1997; Jacob et al., 2007; Scott et al., 2011; Leng et al., 2021), whereas promoting EAAT2 expression improves cognitive function (Fan et al., 2018). Several mutations in the gene that encodes EAAT1 have also been linked to the neurological disease Episodic Ataxia Type 6 (Choi et al., 2017; Chivukula et al., 2020). The most well-studied mutation (P290R) results in reduced glutamate transport activity and a large increase in the uncoupled anion conductance, the latter property being suggested to be responsible for the phenotype in studies using a Drosophila melanogaster model (Winter et al., 2012; Parinejad et al., 2016). Further understanding of how these transporters are regulated in specific circuits and synapses may allow for design of therapeutics that correct for specific deficits in transporter function.
GABA Transporters
In mammals, GABA transporters are classified into four subtypes, based on amino acid sequence homology and pharmacological properties. These include GAT1-3 and the betaine GABA transporter BGT1. Out of these, GAT1 and GAT3 account for the largest proportion of GABA uptake in the CNS, and for this reason, they will be the focus of our attention in this review. Structural information of the GABA transporters comes from prokaryote homologs such as LeuTAa (Figure 2; Yamashita et al., 2005). In the neocortex, GAT1 is expressed robustly in GABAergic axon terminals, astrocytic processes, oligodendrocytes and microglial cells (Fattorini et al., 2020). The expression of GAT1 is pronounced in the axon terminal of chandelier GABAergic neurons (known as “cartridges”), which provide inhibitory inputs to the axon initial segment of pyramidal cells (Woo et al., 1998). In young mice (P9), GAT1 is also transiently expressed somatically, but this somatic expression is lost in juvenile mice (P29) (Yan et al., 1997; Yan and Ribak, 1998). Presynaptic boutons in the cerebellum and hippocampus express 800–1,300 μm–2 GAT1 molecules, with a preferential perisynaptic localization (Chiu et al., 2002; Melone et al., 2015). These density values drop to 640 μm–2 GAT1 molecules along the length of the axon (Chiu et al., 2002), whereas the surface density of GAT1 in astrocytic membranes is 3.5 times higher than in axon terminals (Melone et al., 2015). GAT3 is mainly localized in peri-synaptic astrocytic processes, but has also been detected in brainstem and cortical neurons (Clark et al., 1992; Melone et al., 2003, 2005, 2015). These findings are important because they provide anatomical evidence to the fact that no GABA transporter can be described as being purely neuronal or glial, although they may have a preferential distribution in a given cell type depending on the age and brain region of different animal models.
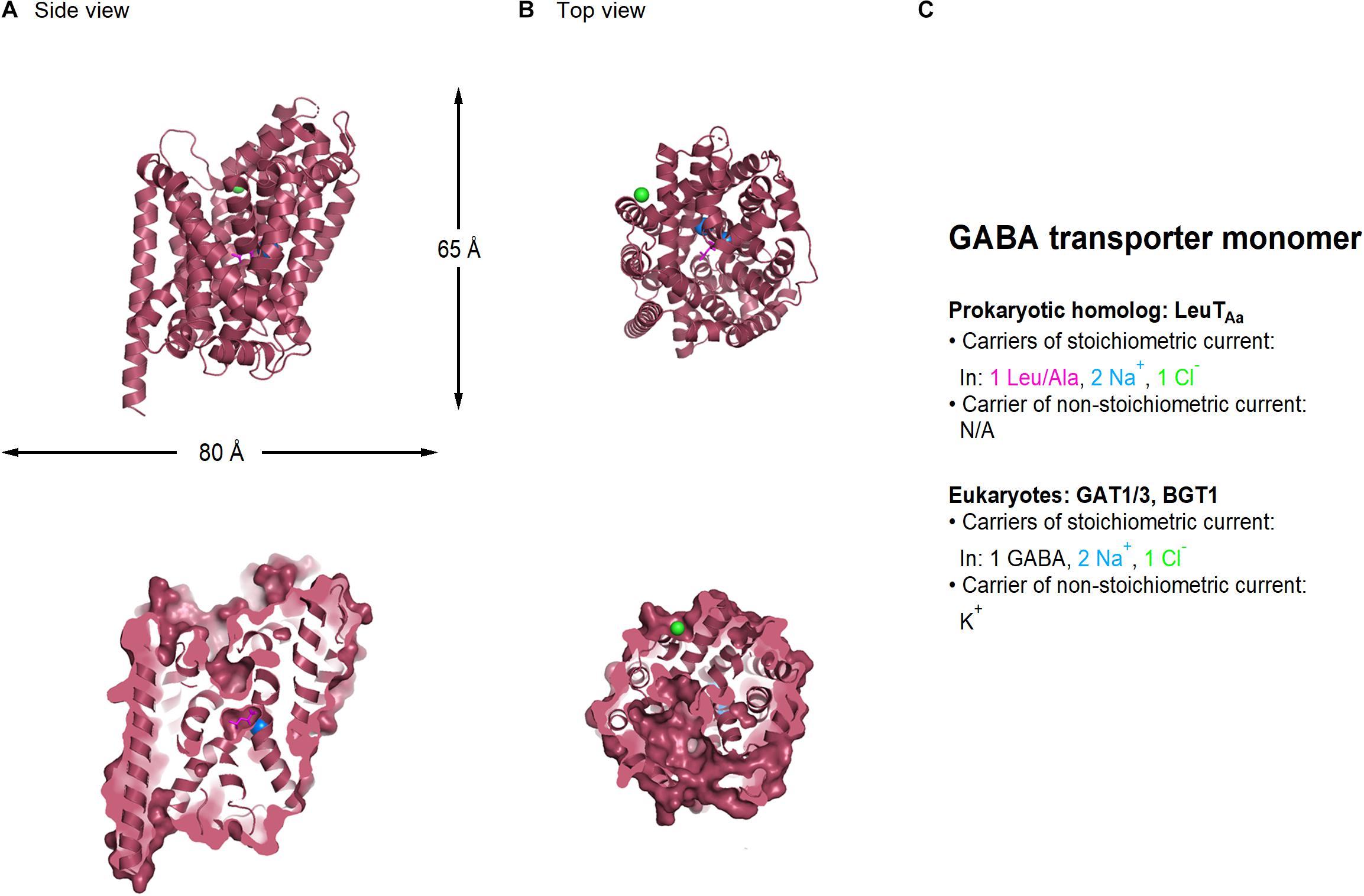
Figure 2. Crystal structure of LeuTAA, a bacterial homolog of GABA transporters (PDB: 2A65). (A) Side view of the LeuTAa transporter parallel to the membrane, using a ribbon (top) and a surface representation sliced through the center of the transporter (bottom). (B) As in (A), viewed from the extracellular side of the membrane. Leu (magenta), sodium (blue), and chloride ions (green) are represented as spheres. (C) Summary of the main feature of the stoichiometric and non-stoichiometric current mediated by Leu and GABA transporters in prokaryotes and eukaryotes, respectively. Image generated using The PyMOL Molecular Graphics System (Version 2.3.2; Schrödinger, 2010).
Both GAT1 and GAT3 transporters translocate the zwitterion GABA across the membrane by coupling its movement to the co-transport of two Na+ and one Cl– ion, leading to the net influx of one positive charge per transport cycle (1 GABA: 2 Na+: 1 Cl–) (Radian and Kanner, 1983; Keynan and Kanner, 1988). In addition to this stoichiometric current, GABA transporters mediate an agonist-independent leak current carried by alkali ions, which can be detected in mammalian expression systems but not in Xenopus laevis oocytes (Cammack and Schwartz, 1996; Eckstein-Ludwig et al., 1999; Lu and Hilgemann, 1999; MacAulay et al., 2002; Matthews et al., 2009). This current can generate a local change in membrane voltage and/or membrane resistance, which can be more or less pronounced depending on the magnitude and direction of the driving force for the permeant ions and the local density of the transporters. Changes in membrane resistance are important because they act as a local shunt capable of hampering action potential propagation and cell excitability.
As in the case of glutamate transporters, we provide a summary of current studies on modulation of GABA transporters listed in PubMed (Table 2).
The Surface Mobility and Cellular Distribution of GAT1
The GABA transporter GAT1 has a membrane pool of 61–63% (Chiu et al., 2002). Fluorescence Recovery After Photobleaching (FRAP) experiments in neuroblastoma 2a cells show that 50% of membrane GAT1 is immobile, likely due to the existence of tight interactions between GAT1 and the actin cytoskeleton, mediated by the adaptor protein ezrin (Imoukhuede et al., 2009). Accordingly, depolymerizing actin or interrupting the GAT1 PDZ-interacting domain increases the transporter mobility, whereas depolymerizing microtubules does not (Imoukhuede et al., 2009). Numerous mechanisms contribute to cycle this pool of transporter to and from the plasma membrane. Specifically, there are two regions in the C-terminal domain of GAT1 that are responsible for supporting GAT1 export from the endoplasmic reticulum and for putting GAT1 under the control of the exocyst (Farhan et al., 2004; Moss et al., 2009). In addition to these, the MAGUK protein Pals1 is co-expressed with GAT1 in COS7 cells and contributes to stabilize GAT1 on the cell membrane (McHugh et al., 2004).
Regulation of GABA Uptake
The control of GABA uptake can be expressed through changes in the rate with which GABA transporters are trafficked and redistributed in the plasma membrane, with consequences on the number of transporter molecules available for binding extracellular GABA, or by modulating the biophysical properties of GABA transporters (e.g., Vmax, km, etc.). Neurons regulate the surface expression of GAT1 in parallel with that of extracellular neurotransmitter levels. GABA transporters interact with the SNARE proteins syntaxin 1A and Munc-18 through molecular interactions modulated by PKC (Beckman et al., 1998; Deken et al., 2000; Geerlings et al., 2001; Horton and Quick, 2001). These transporters are associated with presynaptic vesicles similar to synaptic vesicles, capable of undergoing clathrin-mediated internalization and endosomal sorting (Barbaresi et al., 2001). Despite being morphologically similar to synaptic vesicles, and despite the fact that they can be released at similar rates in a calcium dependent manner, they lack synaptophysin and vesicular GABA transporters (Deken et al., 2003). It has been suggested that these GABA transporter-containing vesicles might represent a population of endocytic or exocytic vesicles acting as cargos for the assembly of synaptic domains distinct from the active zone (Deken et al., 2003).
PKC modulation is important to change the number of functional GABA transporters expressed on the plasma membrane (Corey et al., 1994; Quick et al., 1997). In Xenopus laevis oocytes, PKC activation with PMA promotes GAT1 translocation to the cell membrane at low basal GAT1 expression levels, but this effect is not detected at high levels of expression of GAT1. The rate of GABA uptake can also be altered by PKC (Corey et al., 1994; Quick et al., 1997). Accordingly, inhibiting PKC reduces GABA uptake through a reduction of Vmax (not km), whereas inhibiting protein phosphatase 2B increases it. These forms of PKC-dependent modulation differ in cultured neurons and isolated nerve terminals, where surface expression of GAT1 is decreased by PKC dependent phosphorylation (Beckman et al., 1999; Wang and Quick, 2005; Cristovao-Ferreira et al., 2009).
Another protein kinase, PKA, stimulates GABA transport via GAT1 and activates a GAT1-mediated cationic current during opioid withdrawal (Bagley et al., 2005). This enhanced PKA signaling contributes to increase neuronal action potential firing rates in opioid-sensitive PAG neurons during opioid withdrawal (Bagley et al., 2005).
In the area CA1 of the rat hippocampus, chronic stimulation of cannabinoid receptors CB1/2 reduces GAT1 gene expression (Higuera-Matas et al., 2012). Conversely, an endogenous agonist of endocannabinoid receptors, 2-arachidonoylglycerol (2-AG), increases GABA uptake (Romero et al., 1998; Venderova et al., 2005). CB1 receptors have been suggested to interact or co-localize with β2 adrenergic receptors (Hudson et al., 2010), the activation of which also increases GAT1 expression and GABA uptake (Martins et al., 2018). The ability of β2 adrenergic receptors to promote GABA uptake (and that of β1 adrenergic receptors to inhibit it) are mediated by a PKA pathway controlled by cannabinoid receptors, because the modulation of GABA update by adrenergic receptors is inhibited by the cannabinoid receptor agonist WIN55,212-2 (Martins et al., 2018).
In astrocytes, GABA uptake via GAT1, not GAT3, is modulated by neurotrophic factors like BDNF (Vaz et al., 2011). This is due to the ability of BDNF to inhibit dynamin/clathrin-dependent constitutive internalization of GAT1, effectively increasing the lifetime of GAT1 on the cell membrane. The effect of BDNF is mediated by activation of a truncated form of the TrkB receptor, is coupled to a PLC-γ/PKC-δ and ERK/MAPK pathway, and requires activation of adenosine A2A receptors (Vaz et al., 2011). The cross talk between A2A receptors and BDNF is likely due to the fact that activation of A2A receptors activates TrkB and induces its translocation to lipid rafts (Tebano et al., 2008; Assaife-Lopes et al., 2010). This type of modulation differs in neurons, where BDNF can still inhibit GABA uptake via GAT1 in conjunction with A2A receptors, but here the BDNF modulation persists in the presence of A2A receptor antagonists or upon removal of extracellular adenosine (Vaz et al., 2008).
Physiological Roles of GABA Transporters
Genetic variants in the solute carrier family 6 member 1 (SLC6A1) gene, encoding GAT1, are associated with various neurodevelopmental disorders, including epilepsy with myoclonic atonic seizures, autism spectrum disorder and intellectual disability (Bhat et al., 2020; Goodspeed et al., 2020). Knockout mouse models of GAT1, as well as GAT1 inhibitors, have shown a range of physiological effects that indicate that GAT1, through its exquisite regulation of GABA in the brain, may be an interesting target for therapies for neuropsychiatric diseases (Salat and Kulig, 2011; Egawa and Fukuda, 2013; Bhat et al., 2020). GAT1 inhibitors, such as tiagabine, NO-711 and DDPM-2571, have anti-seizure, antinociceptive, antiallodynic and anxiolytic properties (Laughlin et al., 2002; Todorov et al., 2005; Pakulska, 2007; Xu et al., 2008; Salat et al., 2017). Loss of GAT1 in the nucleus accumbens has also been observed in mice treated with chronic social defeat stress paralleling observations in patients with major depressive disorder (MDD) (Heshmati et al., 2020).
During epileptic seizures, the ionic gradient that typically supports GABA uptake from the extracellular space can be dissipated or even inverted, promoting GABA release through the reversed activity of GABA transporters like GAT3 (Raiteri et al., 2002; Wu et al., 2003; Richerson and Wu, 2004; Kinney, 2005). Under these conditions, the reversal of GABA uptake could provide a useful mechanism to curtail seizure propagation. With some exceptions (Xie et al., 2017), single nucleotide polymorphisms in SLC6A11, the gene encoding human GAT3, have been detected in patients with antiepileptic drug resistance (Kim et al., 2011). It is possible that a dysfunction of GAT3 could alter both phasic and tonic GABAergic transmission in the epileptic brain.
GATs regulate neurotransmission in other complex ways. As mentioned above, substrate transport through GAT elicits an inward current that can directly excite neurons (Bagley et al., 2005, 2011). GAT1 also generates a sodium-dependent capacitive current that can also contribute to shunting (Mager et al., 1993). Efflux of GABA into the extracellular space contributes to tonic currents mediated by GABAA receptors in hippocampal neurons (Wu et al., 2007) and glia (Barakat and Bordey, 2002). Tonic currents are critical in neurodevelopment (Egawa and Fukuda, 2013) and are increased in pathological conditions, such as inflammation (Tonsfeldt et al., 2016). Regulation of GAT activity and trafficking has a dramatic effect on tonic currents, especially those mediated by extrasynaptic GABAA receptors (Scimemi et al., 2005; Scimemi, 2014a,b). In addition to controlling integration of inputs onto GABAergic neurons, these tonic currents control the coincidence detection window of excitatory inputs onto pyramidal neurons (Sylantyev et al., 2020) and contribute to regulation of dopamine release in the dorsal striatum (Roberts et al., 2020). GAT3 activity in astrocytes regulates release of ATP and adenosine that contributes to heterosynaptic depression in the hippocampus (Boddum et al., 2016), highlighting an additional mechanism for regulating synaptic activity.
Dopamine Uptake: One Transporter, Three Currents, Multiple Regulation Sites
Dopamine uptake via the membrane transporter DAT is stoichiometrically coupled to the co-transport of two Na+ and one Cl– ion (Figure 3) (Krueger, 1990; McElvain and Schenk, 1992; Gu et al., 1994; Penmatsa et al., 2013, 2015). Voltage-clamp recordings of DAT-mediated currents in Xenopus oocytes, however, show that the mean net charge to dopamine ratio is significantly larger than the one predicted by the stoichiometric coupling ratio (Sonders et al., 1997; Ingram et al., 2002). This discrepancy can be accounted for by the fact that DAT, like glutamate and GABA transporters (as well as serotonin and norepinephrine transporters; Bruns et al., 1993; Cammack et al., 1994; Mager et al., 1994; Galli et al., 1995; Jayanthi et al., 2002), also mediates two stoichiometrically uncoupled conductances: one that requires dopamine binding to the transporter, and one that does not and is constitutively active. For simplicity, we refer to them as the uncoupled and the leak conductance, respectively.
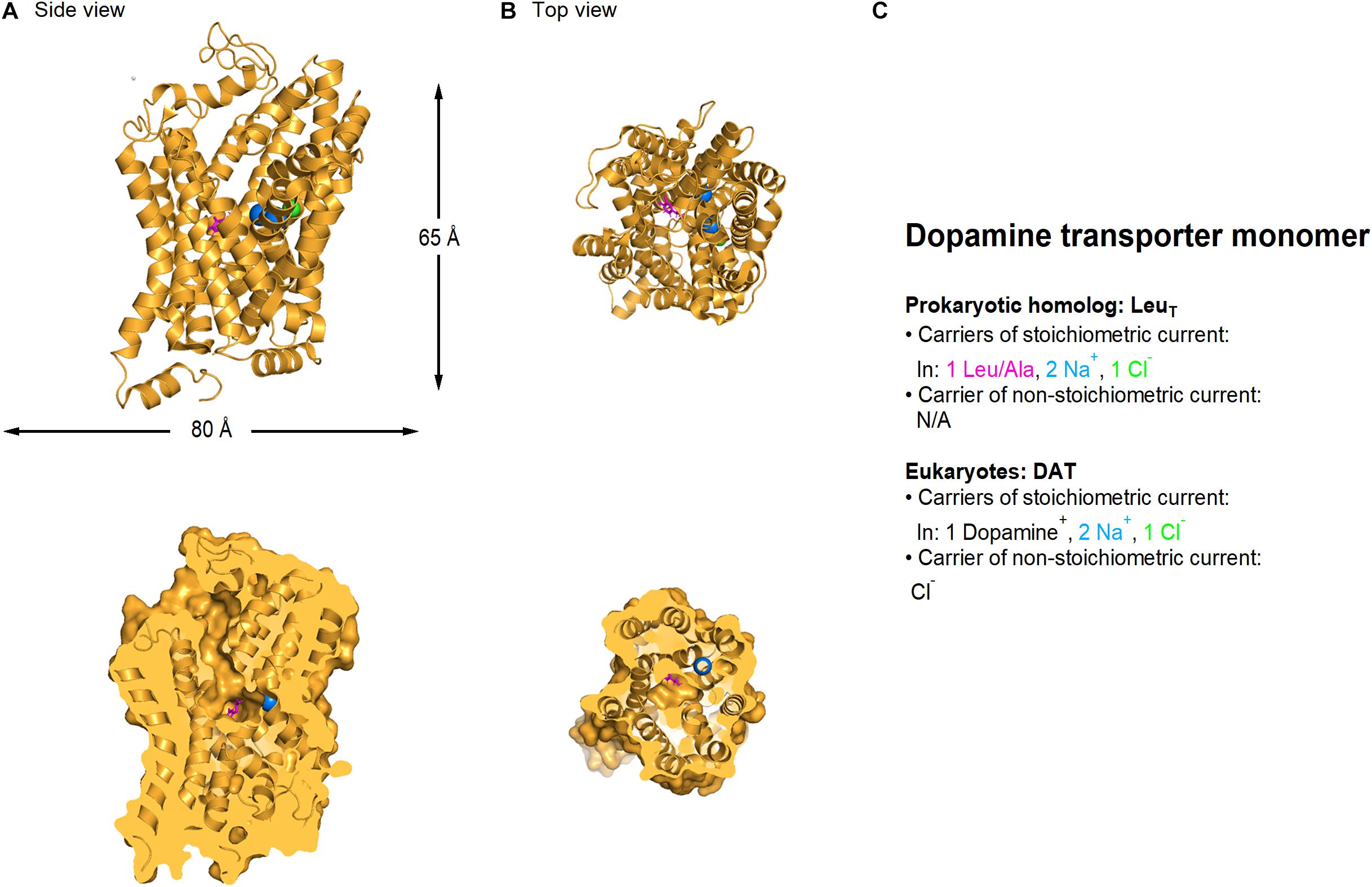
Figure 3. Crystal structure of Drosophila melanogaster dDAT dopamine transporter (PDB: 4XP1). (A) Side view of the Drosophila dopamine transporter parallel to the membrane, using a ribbon (top) and a surface representation sliced through the center of the transporter (bottom). (B) As in A, viewed from the extracellular side of the membrane. L-Dopa (magenta), sodium (blue) and chloride ions (green) are represented as spheres. (C) Summary of the main feature of the stoichiometric and non-stoichiometric current mediated by LeuT (prokaryotic dopamine transporter homolog) and DAT transporters in eukaryotes, respectively. Image generated using The PyMOL Molecular Graphics System (Version 2.3.2; Schrödinger, 2010).
It is interesting to note that the concentration of dopamine required for half-maximal transport is ∼580 nM, whereas the one required for activation of the uncoupled current is only ∼35 nM (Ingram et al., 2002). The extracellular concentration of dopamine in the brain has been known to vary on a sub-second time scale due to phasic firing of dopaminergic neurons, and to be modulated by administration of substances of abuse. Until recently, fast-scan cyclic voltammetry (FSCV) has been considered the technique that provides the best combination of temporal resolution, sensitivity and chemical selectivity (Roberts et al., 2013), but even in this case it has been traditionally used to obtain relative, as opposed to absolute values of dopamine concentrations in the brain. In 2018, the development of cellular-scale probes have enabled stable recording of sub-second extracellular dopamine oscillations (Schwerdt et al., 2018). Although the recorded levels of dopamine were specific for each mouse, they ranged between 40–450 nM (Schwerdt et al., 2018). This means that the uncoupled DAT current is likely to be a main contributor to cell excitability and neurotransmitter release in DAT-expressing neurons. The uncoupled DAT-mediated current is carried by chloride and, surprisingly, its activation increases cell excitability in cultured dopaminergic neurons (Ingram et al., 2002; Carvelli et al., 2004).
In physiological conditions, the leak current is carried by K+ and perhaps H+ ions. This leak current is voltage-dependent, outward-rectifying and reverses at ∼–10 mV (Sonders et al., 1997). Although its activation does not require ion or agonist binding to the transporter, it can be blocked by dopamine and other DAT ligands. The ability of substrates to block the leak current is not consistently observed among other members of the Na+/Cl–-dependent cotransporter family, with the exception of GAT1 (Mager et al., 1994; Cammack and Schwartz, 1996; MacAulay et al., 2002; Kanner, 2003) and the serotonin (Mager et al., 1994) and norepinephrine transporters (Galli et al., 1995). These findings identify multiple mechanisms through which dopamine transporters can change the membrane potential and the signaling properties of neurons through mechanisms that are distinct from its ability to take up dopamine from the extracellular space.
A summary of current studies on modulation of dopamine transporters listed in PubMed is provided in Table 3.
The Pharmacology of Dopamine Transporters
DAT is a target of psychostimulants like cocaine and amphetamine. Cocaine binds to DAT and increases extracellular dopamine levels by blocking its transport activity (Chen and Reith, 2000; Norregaard and Gether, 2001; Goldberg et al., 2003; Torres et al., 2003). In contrast, amphetamine is a substrate for this transporter (Kahlig et al., 2005). The inward transport of amphetamine increases the number of inward-facing transporter binding sites, which results in an increased rate of dopamine efflux through what at first sight may look like an exchange process (Sulzer et al., 1995; Kahlig et al., 2005). In reality, this phenomenon may be more complex, as evidenced by the fact that N-terminus phosphorylation of DAT alters only the amphetamine-induced dopamine efflux without altering dopamine uptake (Khoshbouei et al., 2004). Dopamine and amphetamine are both substrates for DAT, and compete with each other for binding to the transporter (Sulzer et al., 1993; Hoffman et al., 1998). They can both trigger dopamine efflux through reversed uptake, by inverting the electrochemical gradient for Na+ and Cl– (Sitte et al., 1998). Whereas dopamine inhibits the DAT channel-like behavior, amphetamine activates it. This effect is not due to differences in the ability of dopamine and amphetamine to change the intracellular Na+ concentration, because it can still be detected in outside-out patches, where the intracellular Na+ concentration is controlled (Kahlig et al., 2005).
The Surface Mobility and Cellular Distribution of Dopamine Transporters
Dopamine transporters are monoamine neurotransmitter transporters located in the pre-synaptic terminal of dopaminergic neurons, away from the synaptic area (Nirenberg et al., 1997). These cells are located in the ventral tegmental area and substantia nigra, project to the cingulate and medial pre-frontal cortex, nucleus accumbens, olfactory tubercle, lateral habenula, and striatum. Through their axonal projections, dopaminergic neurons control motivation, reward reinforcement and movement, in addition to attention, planning and memory. Therefore, the regulation of DAT function has extensive functional implications for all these behaviors. It is now evident that the role of DAT is not limited to regulating the extracellular concentration of dopamine but is also involved in the homeostatic maintenance of presynaptic function (Torres et al., 2003). Like other membrane proteins, DAT is associated with intracellular proteins that ensure the appropriate location of the transporter in specific domains of the cell membrane at a given time. Accordingly, DAT has been shown to bind to the SNARE protein complex syntaxin 1A (Lee et al., 2004), the PDZ domain protein PICK1 (Torres et al., 2001; Bjerggaard et al., 2004), CaMKII (Fog et al., 2006), and the multiple-LIM-domain-containing adaptor protein HIC-5 (Carneiro et al., 2002).
DAT is equally distributed in and out of rafts (Foster et al., 2008), nanometer-wide and temporally dynamic lipid microdomains enriched in cholesterol, sphingolipids and glycosylphosphatidylinositol (GPI)-anchored proteins (Hancock, 2006). Structural studies of the Drosophila melanogaster DAT (dDAT) reveal that cholesterol can bind in a crevice formed by transmembrane domains 5, 7, and 1a, which is thought to prevent the conformational changes required for the transporter to transition from outward-facing to inward-facing (Penmatsa et al., 2013, 2015). This is in agreement with functional studies that show DAT can be regulated by cholesterol, due to its ability to promote an outward-facing conformation of the transporter (Hong and Amara, 2010; Jones et al., 2012).
By using Fluorescence Correlation Spectroscopy (FCS) and FRAP, Adkins et al. (2007) measured the surface diffusion coefficient of DAT in two types of cells: HEK293 and N2a cells. Due to differences in laser beam waist and sampling areas between FRAP and FCS, FCS is better suited to detect fast protein movements within a confined domain, whereas FRAP allows to detect long-range diffusion between domains in the membrane (Adkins et al., 2007). In HEK293 cells, DAT diffuses with a diffusion coefficient of 3.6 × 10–9 cm2/s, consistent with a relatively freely diffusible protein. This diffusion coefficient is lower in N2a cells, where DAT is partially immobilized (D < 10–10 cm2/s). This difference is likely due to the existence of cell-specific direct or indirect interactions between DAT and cytoskeletal proteins or with membrane rafts. Accordingly, single particle tracking studies confirm that the median diffusion coefficient for DAT is 1.6 × 10–10 cm2/s in Flp-In 293 cells (Kovtun et al., 2015).
Overall, these findings indicate that DAT transporters diffuse substantially slower and in a more confined manner than the glutamate transporter GLT-1, probably because of the presence of different types of protein interactions in different membrane transporters or because of the presence/lack of specific interacting substrates (Murphy-Royal et al., 2015).
Transcriptional, Translational, and Post-translational Regulation of Dopamine Transporters
The human DAT gene was first cloned in 1991, and it is localized to chromosome 5p15.3 and has a single transcriptional start site (Kilty et al., 1991; Vandenbergh et al., 1992a,b). DAT has a half-life of about 2 days on the cell membrane, suggesting the existence of dynamic processes of transcriptional and translational regulation (Kimmel et al., 2000; Kahlig and Galli, 2003). Transcription factors like Nurr1 and Pitx3 have an expression pattern that match that of dopaminergic neurons, and are known to be crucial for the development, survival and maintenance of midbrain dopaminergic neurons (Lee et al., 2010; Rodriguez-Traver et al., 2016; Salemi et al., 2016). Accordingly, disrupting the Nurr1 gene alters the development of dopaminergic neurons (Zetterstrom et al., 1997; Castillo et al., 1998; Saucedo-Cardenas et al., 1998). Nurr1 binds with high-affinity to an NGFI-B responsive element within the promoter regions of DAT and other dopamine-related genes (Sacchetti et al., 1999). The ability of Nurr1 to increase DAT expression, however, relies on mechanisms that are independent of the NGFI-B responsive element. There are a variety of sequence motifs identified through in silico studies, which point to the fact that DAT can be regulated epigenetically via DNA methylation and histone acetylation in vitro and in vivo (Wang et al., 2007). Like other housekeeping genes, the DAT gene lacks conserved TATA and CAAT boxes (confirming that DAT is susceptible to regulation by histone acetylation), and its core promoter is GC-rich (confirming that DAT expression can be regulated via DNA methylation) (Choi and Kim, 2008).
These discoveries prompted a number of biochemical and mutagenesis studies on heterologous expression systems, which led to the identification of key structural features and functional domains of the transporter. A major breakthrough occurred when the first X-ray crystal structure of the bacterial leucine transporter LeuTAa, which shares 20–25% homology with all monoamine transporters was solved at 1.65 Å resolution (Yamashita et al., 2005). This was followed by the structure of Drosophila melanogaster DAT, which shares 50–55% homology with monoamine transporters (Penmatsa et al., 2013, 2015; Wang et al., 2015). Together, these studies suggest that all monoamine transporters have 12 α-helix spanning domains and an alternating access substrate translocation mechanism (Kristensen et al., 2011). Despite the structural similarity of the core transmembrane regions of all monoamine transporters, their extracellular loops, N- and C-termini differ significantly in length and sequence (Kristensen et al., 2011). This is important because these regions are the site of post-translational modifications and can be the site of protein–protein interactions that control transporter localization, stability and activity (Aggarwal and Mortensen, 2017). Post-translational modifications, binding partner interactions, modulation by cholesterol and membrane raft associations are all capable of modulating DAT activity and ultimately dopamine clearance from the extracellular space.
The N-terminus of the DAT protein is subject to phosphorylation and ubiquitination (Karam and Javitch, 2018). Although five serine residues at positions 2, 4, 7, 12, 13 have been identified as targets for phosphorylation by PKC, the only verified phosphorylation site is Ser7 (Moritz et al., 2013). The localization of these sites at the distal end of a long and flexible domain suggests that these residues may be also regulated by partner interactions, but these effects have not been demonstrated. A second verified phosphorylation site is Thr53, which is followed by a Pro residue, making it specific for proline-directed kinases such as ERK. Thr53 is also flanked by an SH3 domain, which is a ligand for protein scaffolding (Saksela and Permi, 2012). In between these two phosphorylation sites, Lys27 is a residue that undergoes ubiquitination catalyzed by the ubiquitin E3 ligases Nedd4-2 and Parkin. This modification is increased by PKC activation and contributes to promote DAT endocytosis (Hong and Amara, 2010; Vina-Vilaseca and Sorkin, 2010). The N-terminal domain also contains binding sites for the regulatory partners syntaxin 1 (res 1–33, which reduces dopamine uptake) and D2 dopamine receptors (res. 1–15) (Lee et al., 2007; Binda et al., 2008; Carvelli et al., 2008). On the C-terminal domain of the DAT protein, Cys580 provides an S-palmitoylation site, and a FREK motif at residues 587–590 binds the small Ras-like GTPase Rin1 and contributes to PKC-mediated endocytosis (Boudanova et al., 2008b; Navaroli et al., 2011). Other regulatory domains in the C-terminal region include binding sites for CaMK (res. 612–617) and α-synuclein (res. 606–620) (Lee et al., 2001; Moszczynska et al., 2007). The C-terminal domain also contains a PDZ domain-binding sequence, where interactions with scaffolding proteins like PICK1 occur. Notably, these interactions are neither necessary nor sufficient for surface targeting of DAT, and have yet unidentified functional consequences (Bjerggaard et al., 2004). Other members of the “DAT interactome” include PP2Ac (Baumann et al., 2000), Hic-5 (Carneiro et al., 2002), RACK (Lee et al., 2004), D2 dopamine receptors (Bolan et al., 2007), flotillin-1 (Cremona et al., 2011), Rin (Navaroli et al., 2011), and the k-opioid receptor (Kivell et al., 2014; Bermingham and Blakely, 2016).
The regulatory mechanisms described so far make it easy to spot that protein kinases like PKC, one of the best characterized regulatory proteins for DAT, can regulate dopamine uptake through a variety of processes, including an endocytotic mechanism driven by the phosphorylation of DAT accessory proteins, a kinetic down-regulation mediated by Ser7 phosphorylation, and an increased dopamine efflux mediated by altered surface transporter activity. Together, these modifications allow PKC to reduce dopamine uptake. The actions of PKC are opposite to those induced by palmitoylation, which lead to reduced DAT degradation (Chen et al., 2013). It is important to keep in mind that the primary sites of PKC-stimulated phosphorylation are the membrane rafts, the microdomains rich in cholesterol and sphingolipids. Therefore, changing PKC-mediated phosphorylation of DAT can affect its raft distribution and protein interactions. In contrast to PKC, ERK provides a tonic mechanism to increase dopamine uptake perhaps via phosphorylation of Thr53 (Foster et al., 2012). In addition to PKC and ERK, there is a host of other kinases that are capable of regulating DAT activity, including PKA, PKG, CaMKII, MAPK, PI3K/Akt and tyrosine kinases. PKCβ may also be involved in the mechanism of D2 receptor regulation of DAT by ERK (Chen et al., 2013).
Physiological Roles of Dopamine Transporters
DAT regulates extracellular concentrations of the neuromodulator dopamine and thus, subsequent activation of dopamine receptors that can enhance or inhibit neurons. DAT is the target of psychoactive and psychotherapeutic drugs such as methylphenidate and amphetamines that are used in treatment of attention deficit and hyperactivity disorder (ADHD) symptoms, as well as drugs of abuse (Schmitt et al., 2013; German et al., 2015). Genetic variants of SLC6A3, the gene encoding DAT in humans, have been identified in patients with neuropsychiatric, neurodevelopmental and neurodegenerative disorders (Mazei-Robison et al., 2005; Serretti and Mandelli, 2008; Mick and Faraone, 2009; Sakrikar et al., 2012; Bowton et al., 2014; Hansen et al., 2014; Mergy et al., 2014; Herborg et al., 2018; Campbell et al., 2019; DiCarlo et al., 2019). Studies of these variants have observed deficits in uptake, transporter-associated currents, trafficking and regulation (Gowrishankar et al., 2014). Further studies of these mutants should give more insight into the complex regulation of dopamine-modulated circuits. An intriguing new area is the role that DAT plays in transport of ligands that act on the trace amine-associated receptor subtype 1 (Taar1), an intracellularly localized G protein-coupled receptor that can, in turn, regulate internalization of both the DAT and EAAT3 in dopaminergic neurons (Underhill et al., 2014, 2019), enhancing extrasynaptic glutamate signaling (Li et al., 2017). Taar1 is known to regulate monoaminergic signaling and dysregulation of TAAR1 signaling may play important roles in neuropsychiatric disorders (Schwartz et al., 2018; Dodd et al., 2020).
Conclusion
Transporters for excitatory, inhibitory, and modulatory neurotransmitters like glutamate, GABA and dopamine are complex molecular machines that do much more than act as vacuums that clear neurotransmitters out of the extracellular space. This complexity arises in part from the core biophysical properties of these transporters, which are capable of generating different types of ionic currents. The additional levels of complexity comes from the fact that their residence time on the membrane, their trafficking from intracellular compartments and their kinetics can be modulated at different levels, including transcriptional, epigenetic, translational and post-translational levels. All these forms of modulation can change across species, cell types and brain regions. Since these regulatory mechanisms also change over time, their efficacy likely follows the metabolic state of a given neuron or astrocyte. The knowledge accumulated over the last few years and the growth of novel experimental approaches will undoubtedly provide new insights into cell-specific changes in the way the activity of neurotransmitter transporters can control cell excitability and metabolism across the brain.
Author Contributions
RMR and SLI wrote the manuscript. AS wrote the manuscript and coordinated the investigative team. All authors contributed to the article and approved the submitted version.
Funding
This work was supported by the Australian National Health and Medical Research Council Project grant APP1164494 to RMR, NIH grant R01 DA042565 to SLI, and NSF grants IOS1655365 and IOS2011998 to AS.
Conflict of Interest
The authors declare that the research was conducted in the absence of any commercial or financial relationships that could be construed as a potential conflict of interest.
Acknowledgments
We performed detailed PubMed searches to include information listed in Tables 1–3. We apologize to authors of any work we might have missed.
References
Adkins, E. M., Samuvel, D. J., Fog, J. U., Eriksen, J., Jayanthi, L. D., Vaegter, C. B., et al. (2007). Membrane mobility and microdomain association of the dopamine transporter studied with fluorescence correlation spectroscopy and fluorescence recovery after photobleaching. Biochemistry 46, 10484–10497. doi: 10.1021/bi700429z
Adolph, O., Koster, S., Rath, M., Georgieff, M., Weigt, H. U., Engele, J., et al. (2007). Rapid increase of glial glutamate uptake via blockade of the protein kinase A pathway. Glia 55, 1699–1707. doi: 10.1002/glia.20583
Aggarwal, S., and Mortensen, O. V. (2017). Overview of monoamine transporters. Curr. Protoc. Pharmacol. 79, 12.16.1–12.16.17. doi: 10.1002/cpph.32
Al Awabdh, S., Gupta-Agarwal, S., Sheehan, D. F., Muir, J., Norkett, R., Twelvetrees, A. E., et al. (2016). Neuronal activity mediated regulation of glutamate transporter GLT-1 surface diffusion in rat astrocytes in dissociated and slice cultures. Glia 64, 1252–1264. doi: 10.1002/glia.22997
Anderson, B. B., Chen, G., Gutman, D. A., and Ewing, A. G. (1998). Dopamine levels of two classes of vesicles are differentially depleted by amphetamine. Brain Res. 788, 294–301. doi: 10.1016/s0006-8993(98)00040-7
Anderson, C. M., and Swanson, R. A. (2000). Astrocyte glutamate transport: review of properties, regulation, and physiological functions. Glia 32, 1–14. doi: 10.1002/1098-1136(200010)32:1<1::aid-glia10>3.0.co;2-w
Anderson, J. G., Cooney, P. T., and Erikson, K. M. (2007). Brain manganese accumulation is inversely related to gamma-amino butyric acid uptake in male and female rats. Toxicol. Sci. 95, 188–195. doi: 10.1093/toxsci/kfl130
Anderson, J. G., Fordahl, S. C., Cooney, P. T., Weaver, T. L., Colyer, C. L., and Erikson, K. M. (2008). Manganese exposure alters extracellular GABA, GABA receptor and transporter protein and mRNA levels in the developing rat brain. Neurotoxicology 29, 1044–1053. doi: 10.1016/j.neuro.2008.08.002
Arriza, J. L., Fairman, W. A., Wadiche, J. I., Murdoch, G. H., Kavanaugh, M. P., and Amara, S. G. (1994). Functional comparisons of three glutamate transporter subtypes cloned from human motor cortex. J. Neurosci. 14, 5559–5569. doi: 10.1523/jneurosci.14-09-05559.1994
Assaife-Lopes, N., Sousa, V. C., Pereira, D. B., Ribeiro, J. A., Chao, M. V., and Sebastiao, A. M. (2010). Activation of adenosine A2A receptors induces TrkB translocation and increases BDNF-mediated phospho-TrkB localization in lipid rafts: implications for neuromodulation. J. Neurosci. 30, 8468–8480. doi: 10.1523/jneurosci.5695-09.2010
Bagley, E. E., Gerke, M. B., Vaughan, C. W., Hack, S. P., and Christie, M. J. (2005). GABA transporter currents activated by protein kinase A excite midbrain neurons during opioid withdrawal. Neuron 45, 433–445. doi: 10.1016/j.neuron.2004.12.049
Bagley, E. E., Hacker, J., Chefer, V. I., Mallet, C., McNally, G. P., Chieng, B. C., et al. (2011). Drug-induced GABA transporter currents enhance GABA release to induce opioid withdrawal behaviors. Nat. Neurosci. 14, 1548–1554. doi: 10.1038/nn.2940
Bahena-Trujillo, R., and Arias-Montano, J. A. (1999). [3H] gamma-aminobutyric acid transport in rat substantia nigra pars reticulata synaptosomes: pharmacological characterization and phorbol ester-induced inhibition. Neurosci. Lett. 274, 119–122. doi: 10.1016/s0304-3940(99)00692-8
Bailey, C. G., Ryan, R. M., Thoeng, A. D., Ng, C., King, K., Vanslambrouck, J. M., et al. (2011). Loss-of-function mutations in the glutamate transporter SLC1A1 cause human dicarboxylic aminoaciduria. J. Clin. Invest. 121, 446–453. doi: 10.1172/jci44474
Barakat, L., and Bordey, A. (2002). GAT-1 and reversible GABA transport in Bergmann glia in slices. J. Neurophysiol. 88, 1407–1419. doi: 10.1152/jn.2002.88.3.1407
Barbaresi, P., Gazzanelli, G., and Malatesta, M. (2001). gamma-Aminobutyric acid transporters in the cat periaqueductal gray: a light and electron microscopic immunocytochemical study. J. Comp. Neurol. 429, 337–354. doi: 10.1002/1096-9861(20000108)429:2<337::aid-cne12>3.0.co;2-z
Bassan, M., Liu, H., Madsen, K. L., Armsen, W., Zhou, J., Desilva, T., et al. (2008). Interaction between the glutamate transporter GLT1b and the synaptic PDZ domain protein PICK1. Eur. J. Neurosci. 27, 66–82. doi: 10.1111/j.1460-9568.2007.05986.x
Baumann, M. H., Ayestas, M. A., Dersch, C. M., Brockington, A., Rice, K. C., and Rothman, R. B. (2000). Effects of phentermine and fenfluramine on extracellular dopamine and serotonin in rat nucleus accumbens: therapeutic implications. Synapse 36, 102–113. doi: 10.1002/(sici)1098-2396(200005)36:2<102::aid-syn3>3.0.co;2-#
Beckman, M. L., Bernstein, E. M., and Quick, M. W. (1998). Protein kinase C regulates the interaction between a GABA transporter and syntaxin 1A. J. Neurosci. 18, 6103–6112. doi: 10.1523/jneurosci.18-16-06103.1998
Beckman, M. L., Bernstein, E. M., and Quick, M. W. (1999). Multiple G protein-coupled receptors initiate protein kinase C redistribution of GABA transporters in hippocampal neurons. J. Neurosci. 19:RC9.
Behrens, P. F., Franz, P., Woodman, B., Lindenberg, K. S., and Landwehrmeyer, G. B. (2002). Impaired glutamate transport and glutamate-glutamine cycling: downstream effects of the Huntington mutation. Brain 125, 1908–1922. doi: 10.1093/brain/awf180
Bellini, S., Fleming, K. E., De, M., McCauley, J. P., Petroccione, M. A., D’Brant, L. Y., et al. (2018). Neuronal glutamate transporters control dopaminergic signaling and compulsive behaviors. J. Neurosci. 38, 937–961. doi: 10.1523/jneurosci.1906-17.2017
Benediktsson, A. M., Marrs, G. S., Tu, J. C., Worley, P. F., Rothstein, J. D., Bergles, D. E., et al. (2012). Neuronal activity regulates glutamate transporter dynamics in developing astrocytes. Glia 60, 175–188. doi: 10.1002/glia.21249
Berger, U. V., DeSilva, T. M., Chen, W., and Rosenberg, P. A. (2005). Cellular and subcellular mRNA localization of glutamate transporter isoforms GLT1a and GLT1b in rat brain by in situ hybridization. J. Comp. Neurol. 492, 78–89. doi: 10.1002/cne.20737
Bergles, D. E., Tzingounis, A. V., and Jahr, C. E. (2002). Comparison of coupled and uncoupled currents during glutamate uptake by GLT-1 transporters. J. Neurosci. 22, 10153–10162. doi: 10.1523/jneurosci.22-23-10153.2002
Bermingham, D. P., and Blakely, R. D. (2016). Kinase-dependent regulation of monoamine neurotransmitter transporters. Pharmacol. Rev. 68, 888–953. doi: 10.1124/pr.115.012260
Bernabe, A., Mendez, J. A., Hernandez-Kelly, L. C., and Ortega, A. (2003). Regulation of the Na+-dependent glutamate/aspartate transporter in rodent cerebellar astrocytes. Neurochem. Res. 28, 1843–1849.
Bernardinelli, Y., Randall, J., Janett, E., Nikonenko, I., Konig, S., Jones, E. V., et al. (2014). Activity-dependent structural plasticity of perisynaptic astrocytic domains promotes excitatory synapse stability. Curr. Biol. 24, 1679–1688. doi: 10.1016/j.cub.2014.06.025
Bernstein, E. M., and Quick, M. W. (1999). Regulation of gamma-aminobutyric acid (GABA) transporters by extracellular GABA. J. Biol. Chem. 274, 889–895. doi: 10.1074/jbc.274.2.889
Berry, C. B., Hayes, D., Murphy, A., Wiessner, M., Rauen, T., and McBean, G. J. (2005). Differential modulation of the glutamate transporters GLT1, GLAST and EAAC1 by docosahexaenoic acid. Brain Res. 1037, 123–133. doi: 10.1016/j.brainres.2005.01.008
Bhat, S., El-Kasaby, A., Freissmuth, M., and Sucic, S. (2020). Functional and biochemical consequences of disease variants in neurotransmitter transporters: a special emphasis on folding and trafficking deficits. Pharmacol. Ther. 2020:107785. doi: 10.1016/j.pharmthera.2020.107785
Binda, F., Dipace, C., Bowton, E., Robertson, S. D., Lute, B. J., Fog, J. U., et al. (2008). Syntaxin 1A interaction with the dopamine transporter promotes amphetamine-induced dopamine efflux. Mol. Pharmacol. 74, 1101–1108. doi: 10.1124/mol.108.048447
Bjerggaard, C., Fog, J. U., Hastrup, H., Madsen, K., Loland, C. J., Javitch, J. A., et al. (2004). Surface targeting of the dopamine transporter involves discrete epitopes in the distal C terminus but does not require canonical PDZ domain interactions. J. Neurosci. 24, 7024–7036. doi: 10.1523/jneurosci.1863-04.2004
Boddum, K., Jensen, T. P., Magloire, V., Kristiansen, U., Rusakov, D. A., Pavlov, I., et al. (2016). Astrocytic GABA transporter activity modulates excitatory neurotransmission. Nat. Commun. 7:13572.
Bohmer, C., Philippin, M., Rajamanickam, J., Mack, A., Broer, S., Palmada, M., et al. (2004). Stimulation of the EAAT4 glutamate transporter by SGK protein kinase isoforms and PKB. Biochem. Biophys. Res. Commun. 324, 1242–1248. doi: 10.1016/j.bbrc.2004.09.193
Bolan, E. A., Kivell, B., Jaligam, V., Oz, M., Jayanthi, L. D., Han, Y., et al. (2007). D2 receptors regulate dopamine transporter function via an extracellular signal-regulated kinases 1 and 2-dependent and phosphoinositide 3 kinase-independent mechanism. Mol. Pharmacol. 71, 1222–1232. doi: 10.1124/mol.106.027763
Boudanova, E., Navaroli, D. M., and Melikian, H. E. (2008a). Amphetamine-induced decreases in dopamine transporter surface expression are protein kinase C-independent. Neuropharmacology 54, 605–612. doi: 10.1016/j.neuropharm.2007.11.007
Boudanova, E., Navaroli, D. M., Stevens, Z., and Melikian, H. E. (2008b). Dopamine transporter endocytic determinants: carboxy terminal residues critical for basal and PKC-stimulated internalization. Mol. Cell Neurosci. 39, 211–217. doi: 10.1016/j.mcn.2008.06.011
Boudker, O., Ryan, R. M., Yernool, D., Shimamoto, K., and Gouaux, E. (2007). Coupling substrate and ion binding to extracellular gate of a sodium-dependent aspartate transporter. Nature 445, 387–393. doi: 10.1038/nature05455
Bowton, E., Saunders, C., Reddy, I. A., Campbell, N. G., Hamilton, P. J., Henry, L. K., et al. (2014). SLC6A3 coding variant Ala559Val found in two autism probands alters dopamine transporter function and trafficking. Transl. Psychiatry 4:e464. doi: 10.1038/tp.2014.90
Brosnan, J. T., and Brosnan, M. E. (2013). Glutamate: a truly functional amino acid. Amino Acids 45, 413–418. doi: 10.1007/s00726-012-1280-4
Bruijn, L. I., Becher, M. W., Lee, M. K., Anderson, K. L., Jenkins, N. A., Copeland, N. G., et al. (1997). ALS-linked SOD1 mutant G85R mediates damage to astrocytes and promotes rapidly progressive disease with SOD1-containing inclusions. Neuron 18, 327–338. doi: 10.1016/s0896-6273(00)80272-x
Bruns, D., Engert, F., and Lux, H. D. (1993). A fast activating presynaptic reuptake current during serotonergic transmission in identified neurons of Hirudo. Neuron 10, 559–572. doi: 10.1016/0896-6273(93)90159-o
Bull, N. D., and Barnett, N. L. (2002). Antagonists of protein kinase C inhibit rat retinal glutamate transport activity in situ. J. Neurochem. 81, 472–480. doi: 10.1046/j.1471-4159.2002.00819.x
Butchbach, M. E., Tian, G., Guo, H., and Lin, C. L. (2004). Association of excitatory amino acid transporters, especially EAAT2, with cholesterol-rich lipid raft microdomains: importance for excitatory amino acid transporter localization and function. J. Biol. Chem. 279, 34388–34396. doi: 10.1074/jbc.m403938200
Cammack, J. N., Rakhilin, S. V., and Schwartz, E. A. (1994). A GABA transporter operates asymmetrically and with variable stoichiometry. Neuron 13, 949–960. doi: 10.1016/0896-6273(94)90260-7
Cammack, J. N., and Schwartz, E. A. (1996). Channel behavior in a gamma-aminobutyrate transporter. Proc. Natl. Acad. Sci. U.S.A. 93, 723–727. doi: 10.1073/pnas.93.2.723
Campbell, N. G., Shekar, A., Aguilar, J. I., Peng, D., Navratna, V., Yang, D., et al. (2019). Structural, functional, and behavioral insights of dopamine dysfunction revealed by a deletion in SLC6A3. Proc. Natl. Acad. Sci. U.S.A. 116, 3853–3862. doi: 10.1073/pnas.1816247116
Canul-Tec, J. C., Assal, R., Cirri, E., Legrand, P., Brier, S., Chamot-Rooke, J., et al. (2017). Structure and allosteric inhibition of excitatory amino acid transporter 1. Nature 544, 446–451. doi: 10.1038/nature22064
Carneiro, A. M., Ingram, S. L., Beaulieu, J. M., Sweeney, A., Amara, S. G., Thomas, S. M., et al. (2002). The multiple LIM domain-containing adaptor protein Hic-5 synaptically colocalizes and interacts with the dopamine transporter. J. Neurosci. 22, 7045–7054. doi: 10.1523/jneurosci.22-16-07045.2002
Carvelli, L., Blakely, R. D., and DeFelice, L. J. (2008). Dopamine transporter/syntaxin 1A interactions regulate transporter channel activity and dopaminergic synaptic transmission. Proc. Natl. Acad. Sci. U.S.A. 105, 14192–14197. doi: 10.1073/pnas.0802214105
Carvelli, L., McDonald, P. W., Blakely, R. D., and DeFelice, L. J. (2004). Dopamine transporters depolarize neurons by a channel mechanism. Proc. Natl. Acad. Sci. U.S.A. 101, 16046–16051. doi: 10.1073/pnas.0403299101
Carvelli, L., Moron, J. A., Kahlig, K. M., Ferrer, J. V., Sen, N., Lechleiter, J. D., et al. (2002). PI 3-kinase regulation of dopamine uptake. J. Neurochem. 81, 859–869. doi: 10.1046/j.1471-4159.2002.00892.x
Casado, M., Bendahan, A., Zafra, F., Danbolt, N. C., Aragon, C., Gimenez, C., et al. (1993). Phosphorylation and modulation of brain glutamate transporters by protein kinase C. J. Biol. Chem. 268, 27313–27317. doi: 10.1016/s0021-9258(19)74251-3
Castillo, S. O., Baffi, J. S., Palkovits, M., Goldstein, D. S., Kopin, I. J., Witta, J., et al. (1998). Dopamine biosynthesis is selectively abolished in substantia nigra/ventral tegmental area but not in hypothalamic neurons in mice with targeted disruption of the Nurr1 gene. Mol. Cell Neurosci. 11, 36–46. doi: 10.1006/mcne.1998.0673
Cater, R. J., Vandenberg, R. J., and Ryan, R. M. (2014). The domain interface of the human glutamate transporter EAAT1 mediates chloride permeation. Biophys. J. 107, 621–629. doi: 10.1016/j.bpj.2014.05.046
Cater, R. J., Vandenberg, R. J., and Ryan, R. M. (2016). Tuning the ion selectivity of glutamate transporter-associated uncoupled conductances. J. Gen. Physiol. 148, 13–24. doi: 10.1085/jgp.201511556
Cervinski, M. A., Foster, J. D., and Vaughan, R. A. (2005). Psychoactive substrates stimulate dopamine transporter phosphorylation and down-regulation by cocaine-sensitive and protein kinase C-dependent mechanisms. J. Biol. Chem. 280, 40442–40449. doi: 10.1074/jbc.m501969200
Chan, P. H., Kerlan, R., and Fishman, R. A. (1983). Reductions of gamma-aminobutyric acid and glutamate uptake and (Na+ + K+)-ATPase activity in brain slices and synaptosomes by arachidonic acid. J. Neurochem. 40, 309–316. doi: 10.1111/j.1471-4159.1983.tb11284.x
Chang, M. Y., Lee, S. H., Kim, J. H., Lee, K. H., Kim, Y. S., Son, H., et al. (2001). Protein kinase C-mediated functional regulation of dopamine transporter is not achieved by direct phosphorylation of the dopamine transporter protein. J. Neurochem. 77, 754–761. doi: 10.1046/j.1471-4159.2001.00284.x
Chaudhry, F. A., Lehre, K. P., van Lookeren Campagne, M., Ottersen, O. P., Danbolt, N. C., and Storm-Mathisen, J. (1995). Glutamate transporters in glial plasma membranes: highly differentiated localizations revealed by quantitative ultrastructural immunocytochemistry. Neuron 15, 711–720. doi: 10.1016/0896-6273(95)90158-2
Chen, I., Pant, S., Wu, Q., Cater, R., Sobti, M., Vandenberg, R. J., et al. (2021). Glutamate transporters contain a conserved chloride channel with two hydrophobic gates. bioRxiv [Preprint], doi: 10.1101/2020.05.25.115360
Chen, N., and Reith, M. E. (2000). Structure and function of the dopamine transporter. Eur. J. Pharmacol. 405, 329–339.
Chen, R., Daining, C. P., Sun, H., Fraser, R., Stokes, S. L., Leitges, M., et al. (2013). Protein kinase Cbeta is a modulator of the dopamine D2 autoreceptor-activated trafficking of the dopamine transporter. J. Neurochem. 125, 663–672. doi: 10.1111/jnc.12229
Chen, R., Furman, C. A., Zhang, M., Kim, M. N., Gereau, R. W. T., Leitges, M., et al. (2009). Protein kinase Cbeta is a critical regulator of dopamine transporter trafficking and regulates the behavioral response to amphetamine in mice. J. Pharmacol. Exp. Ther. 328, 912–920. doi: 10.1124/jpet.108.147959
Chen, T., Tanaka, M., Wang, Y., Sha, S., Furuya, K., Chen, L., et al. (2017). Neurosteroid dehydroepiandrosterone enhances activity and trafficking of astrocytic GLT-1 via sigma1 receptor-mediated PKC activation in the hippocampal dentate gyrus of rats. Glia 65, 1491–1503. doi: 10.1002/glia.23175
Chen, W., Aoki, C., Mahadomrongkul, V., Gruber, C. E., Wang, G. J., Blitzblau, R., et al. (2002). Expression of a variant form of the glutamate transporter GLT1 in neuronal cultures and in neurons and astrocytes in the rat brain. J. Neurosci. 22, 2142–2152. doi: 10.1523/jneurosci.22-06-02142.2002
Chen, W., Mahadomrongkul, V., Berger, U. V., Bassan, M., DeSilva, T., Tanaka, K., et al. (2004). The glutamate transporter GLT1a is expressed in excitatory axon terminals of mature hippocampal neurons. J. Neurosci. 24, 1136–1148. doi: 10.1523/jneurosci.1586-03.2004
Cheng, C., Glover, G., Banker, G., and Amara, S. G. (2002). A novel sorting motif in the glutamate transporter excitatory amino acid transporter 3 directs its targeting in Madin-Darby canine kidney cells and hippocampal neurons. J. Neurosci. 22, 10643–10652. doi: 10.1523/jneurosci.22-24-10643.2002
Cheng, M. H., Torres-Salazar, D., Gonzalez-Suarez, A. D., Amara, S. G., and Bahar, I. (2017). Substrate transport and anion permeation proceed through distinct pathways in glutamate transporters. eLife 6:e25850.
Chi, L., and Reith, M. E. (2003). Substrate-induced trafficking of the dopamine transporter in heterologously expressing cells and in rat striatal synaptosomal preparations. J. Pharmacol. Exp. Ther. 307, 729–736. doi: 10.1124/jpet.103.055095
Chiu, C. S., Jensen, K., Sokolova, I., Wang, D., Li, M., Deshpande, P., et al. (2002). Number, density, and surface/cytoplasmic distribution of GABA transporters at presynaptic structures of knock-in mice carrying GABA transporter subtype 1-green fluorescent protein fusions. J. Neurosci. 22, 10251–10266. doi: 10.1523/jneurosci.22-23-10251.2002
Chivukula, A. S., Suslova, M., Kortzak, D., Kovermann, P., and Fahlke, C. (2020). Functional consequences of SLC1A3 mutations associated with episodic ataxia 6. Hum. Mutat. 41, 1892–1905. doi: 10.1002/humu.24089
Choi, J. K., and Kim, Y. J. (2008). Epigenetic regulation and the variability of gene expression. Nat. Genet. 40, 141–147. doi: 10.1038/ng.2007.58
Choi, K. D., Jen, J. C., Choi, S. Y., Shin, J. H., Kim, H. S., Kim, H. J., et al. (2017). Late-onset episodic ataxia associated with SLC1A3 mutation. J. Hum. Genet. 62, 443–446. doi: 10.1038/jhg.2016.137
Chowdhury, H. H., Kreft, M., and Zorec, R. (2002). Rapid insulin-induced exocytosis in white rat adipocytes. Pflugers Arch. 445, 352–356. doi: 10.1007/s00424-002-0938-2
Clark, J. A., Deutch, A. Y., Gallipoli, P. Z., and Amara, S. G. (1992). Functional expression and CNS distribution of a beta-alanine-sensitive neuronal GABA transporter. Neuron 9, 337–348. doi: 10.1016/0896-6273(92)90172-a
Conradt, M., and Stoffel, W. (1997). Inhibition of the high-affinity brain glutamate transporter GLAST-1 via direct phosphorylation. J. Neurochem. 68, 1244–1251. doi: 10.1046/j.1471-4159.1997.68031244.x
Conradt, M., Storck, T., and Stoffel, W. (1995). Localization of N-glycosylation sites and functional role of the carbohydrate units of GLAST-1, a cloned rat brain L-glutamate/L-aspartate transporter. Eur. J. Biochem. 229, 682–687. doi: 10.1111/j.1432-1033.1995.0682j.x
Copeland, B. J., Vogelsberg, V., Neff, N. H., and Hadjiconstantinou, M. (1996). Protein kinase C activators decrease dopamine uptake into striatal synaptosomes. J. Pharmacol. Exp. Ther. 277, 1527–1532.
Cordeiro, J. M., Meireles, S. M., Vale, M. G., Oliveira, C. R., and Goncalves, P. P. (2000). Ca(2+) regulation of the carrier-mediated gamma-aminobutyric acid release from isolated synaptic plasma membrane vesicles. Neurosci. Res. 38, 385–395. doi: 10.1016/s0168-0102(00)00193-0
Corey, J. L., Davidson, N., Lester, H. A., Brecha, N., and Quick, M. W. (1994). Protein kinase C modulates the activity of a cloned gamma-aminobutyric acid transporter expressed in Xenopus oocytes via regulated subcellular redistribution of the transporter. J. Biol. Chem. 269, 14759–14767. doi: 10.1016/s0021-9258(17)36690-5
Cremona, M. L., Matthies, H. J., Pau, K., Bowton, E., Speed, N., Lute, B. J., et al. (2011). Flotillin-1 is essential for PKC-triggered endocytosis and membrane microdomain localization of DAT. Nat. Neurosci. 14, 469–477. doi: 10.1038/nn.2781
Cristovao-Ferreira, S., Navarro, G., Brugarolas, M., Perez-Capote, K., Vaz, S. H., Fattorini, G., et al. (2013). A1R-A2AR heteromers coupled to Gs and G i/0 proteins modulate GABA transport into astrocytes. Purinerg. Signal. 9, 433–449. doi: 10.1007/s11302-013-9364-5
Cristovao-Ferreira, S., Vaz, S. H., Ribeiro, J. A., and Sebastiao, A. M. (2009). Adenosine A2A receptors enhance GABA transport into nerve terminals by restraining PKC inhibition of GAT-1. J. Neurochem. 109, 336–347. doi: 10.1111/j.1471-4159.2009.05963.x
Dabir, D. V., Robinson, M. B., Swanson, E., Zhang, B., Trojanowski, J. Q., Lee, V. M., et al. (2006). Impaired glutamate transport in a mouse model of tau pathology in astrocytes. J. Neurosci. 26, 644–654. doi: 10.1523/jneurosci.3861-05.2006
Dall’Igna, O. P., Bobermin, L. D., Souza, D. O., and Quincozes-Santos, A. (2013). Riluzole increases glutamate uptake by cultured C6 astroglial cells. Int. J. Dev. Neurosci. 31, 482–486. doi: 10.1016/j.ijdevneu.2013.06.002
Danbolt, N. C., Storm-Mathisen, J., and Kanner, B. I. (1992). An [Na+ + K+]coupled L-glutamate transporter purified from rat brain is located in glial cell processes. Neuroscience 51, 295–310. doi: 10.1016/0306-4522(92)90316-t
Daniels, G. M., and Amara, S. G. (1999). Regulated trafficking of the human dopamine transporter. Clathrin-mediated internalization and lysosomal degradation in response to phorbol esters. J. Biol. Chem. 274, 35794–35801. doi: 10.1074/jbc.274.50.35794
Davis, K. E., Straff, D. J., Weinstein, E. A., Bannerman, P. G., Correale, D. M., Rothstein, J. D., et al. (1998). Multiple signaling pathways regulate cell surface expression and activity of the excitatory amino acid carrier 1 subtype of Glu transporter in C6 glioma. J. Neurosci. 18, 2475–2485. doi: 10.1523/jneurosci.18-07-02475.1998
Dehnes, Y., Chaudhry, F. A., Ullensvang, K., Lehre, K. P., Storm-Mathisen, J., and Danbolt, N. C. (1998). The glutamate transporter EAAT4 in rat cerebellar Purkinje cells: a glutamate-gated chloride channel concentrated near the synapse in parts of the dendritic membrane facing astroglia. J. Neurosci. 18, 3606–3619. doi: 10.1523/jneurosci.18-10-03606.1998
Deken, S. L., Beckman, M. L., Boos, L., and Quick, M. W. (2000). Transport rates of GABA transporters: regulation by the N-terminal domain and syntaxin 1A. Nat. Neurosci. 3, 998–1003. doi: 10.1038/79939
Deken, S. L., Wang, D., and Quick, M. W. (2003). Plasma membrane GABA transporters reside on distinct vesicles and undergo rapid regulated recycling. J. Neurosci. 23, 1563–1568. doi: 10.1523/jneurosci.23-05-01563.2003
Delgado-Acevedo, C., Estay, S. F., Radke, A. K., Sengupta, A., Escobar, A. P., Henriquez-Belmar, F., et al. (2019). Behavioral and synaptic alterations relevant to obsessive-compulsive disorder in mice with increased EAAT3 expression. Neuropsychopharmacology 44, 1163–1173. doi: 10.1038/s41386-018-0302-7
DiCarlo, G. E., Aguilar, J. I., Matthies, H. J., Harrison, F. E., Bundschuh, K. E., West, A., et al. (2019). Autism-linked dopamine transporter mutation alters striatal dopamine neurotransmission and dopamine-dependent behaviors. J. Clin. Invest. 129, 3407–3419. doi: 10.1172/jci127411
Divito, C. B., Borowski, J. E., Glasgow, N. G., Gonzalez-Suarez, A. D., Torres-Salazar, D., Johnson, J. W., et al. (2017). Glial and neuronal glutamate transporters differ in the Na(+) requirements for activation of the substrate-independent anion conductance. Front. Mol. Neurosci. 10:150. doi: 10.3389/fnmol.2017.00150
Dodd, S., Carvalho, A. F., Puri, B. K., Maes, M., Bortolasci, C. C., Morris, G., et al. (2020). Trace amine-associated receptor 1 (TAAR1): a new drug target for psychiatry? Neurosci. Biobehav. Rev. 120, 537–541. doi: 10.1016/j.neubiorev.2020.09.028
Doolen, S., and Zahniser, N. R. (2001). Protein tyrosine kinase inhibitors alter human dopamine transporter activity in Xenopus oocytes. J. Pharmacol. Exp. Ther. 296, 931–938.
Dowd, L. A., and Robinson, M. B. (1996). Rapid stimulation of EAAC1-mediated Na+-dependent L-glutamate transport activity in C6 glioma cells by phorbol ester. J. Neurochem. 67, 508–516. doi: 10.1046/j.1471-4159.1996.67020508.x
Drejer, J., Meier, E., and Schousboe, A. (1983). Novel neuron-related regulatory mechanisms for astrocytic glutamate and GABA high affinity uptake. Neurosci. Lett. 37, 301–306. doi: 10.1016/0304-3940(83)90448-2
Eckstein-Ludwig, U., Fei, J., and Schwarz, W. (1999). Inhibition of uptake, steady-state currents, and transient charge movements generated by the neuronal GABA transporter by various anticonvulsant drugs. Br. J. Pharmacol. 128, 92–102. doi: 10.1038/sj.bjp.0702794
Egawa, K., and Fukuda, A. (2013). Pathophysiological power of improper tonic GABA(A) conductances in mature and immature models. Front. Neural Circ. 7:170. doi: 10.3389/fncir.2013.00170
Eliasof, S., and Jahr, C. E. (1996). Retinal glial cell glutamate transporter is coupled to an anionic conductance. Proc. Natl. Acad. Sci. U.S.A. 93, 4153–4158. doi: 10.1073/pnas.93.9.4153
Eriksen, J., Rasmussen, S. G., Rasmussen, T. N., Vaegter, C. B., Cha, J. H., Zou, M. F., et al. (2009). Visualization of dopamine transporter trafficking in live neurons by use of fluorescent cocaine analogs. J. Neurosci. 29, 6794–6808. doi: 10.1523/jneurosci.4177-08.2009
Espinoza-Rojo, M., Lopez-Bayghen, E., and Ortega, A. (2000). GLAST: gene expression regulation by phorbol esters. Neuroreport 11, 2827–2832. doi: 10.1097/00001756-200008210-00043
Fairman, W. A., Vandenberg, R. J., Arriza, J. L., Kavanaugh, M. P., and Amara, S. G. (1995). An excitatory amino-acid transporter with properties of a ligand-gated chloride channel. Nature 375, 599–603. doi: 10.1038/375599a0
Fan, H. P., Fan, F. J., Bao, L., and Pei, G. (2006). SNAP-25/syntaxin 1A complex functionally modulates neurotransmitter gamma-aminobutyric acid reuptake. J. Biol. Chem. 281, 28174–28184. doi: 10.1074/jbc.m601382200
Fan, S., Xian, X., Li, L., Yao, X., Hu, Y., Zhang, M., et al. (2018). Ceftriaxone improves cognitive function and upregulates GLT-1-related glutamate-glutamine cycle in APP/PS1 mice. J. Alzheimers Dis. 66, 1731–1743. doi: 10.3233/jad-180708
Fang, H., Huang, Y., and Zuo, Z. (2006). Enhancement of substrate-gated Cl- currents via rat glutamate transporter EAAT4 by PMA. Am. J. Physiol. Cell Physiol. 290, C1334–C1340.
Fang, Q., Hu, W. W., Wang, X. F., Yang, Y., Lou, G. D., Jin, M. M., et al. (2014). Histamine up-regulates astrocytic glutamate transporter 1 and protects neurons against ischemic injury. Neuropharmacology 77, 156–166. doi: 10.1016/j.neuropharm.2013.06.012
Farhan, H., Korkhov, V. M., Paulitschke, V., Dorostkar, M. M., Scholze, P., Kudlacek, O., et al. (2004). Two discontinuous segments in the carboxyl terminus are required for membrane targeting of the rat gamma-aminobutyric acid transporter-1 (GAT1). J. Biol. Chem. 279, 28553–28563. doi: 10.1074/jbc.m307325200
Fattorini, G., Melone, M., and Conti, F. (2020). A reappraisal of GAT-1 localization in Neocortex. Front. Cell Neurosci. 14:9. doi: 10.3389/fncel.2020.00009
Ferrer-Martinez, A., Felipe, A., Nicholson, B., Casado, J., Pastor-Anglada, M., and McGivan, J. (1995). Induction of the high-affinity Na(+)-dependent glutamate transport system XAG- by hypertonic stress in the renal epithelial cell line NBL-1. Biochem. J. 310(Pt 2), 689–692. doi: 10.1042/bj3100689
Figiel, M., Maucher, T., Rozyczka, J., Bayatti, N., and Engele, J. (2003). Regulation of glial glutamate transporter expression by growth factors. Exp. Neurol. 183, 124–135. doi: 10.1016/s0014-4886(03)00134-1
Fischer, J. F., and Cho, A. K. (1979). Chemical release of dopamine from striatal homogenates: evidence for an exchange diffusion model. J. Pharmacol. Exp. Ther. 208, 203–209.
Fischer, K. D., Houston, A. C. W., Desai, R. I., Doyle, M. R., Bergman, J., Mian, M., et al. (2018). Behavioral phenotyping and dopamine dynamics in mice with conditional deletion of the glutamate transporter GLT-1 in neurons: resistance to the acute locomotor effects of amphetamine. Psychopharmacology 235, 1371–1387. doi: 10.1007/s00213-018-4848-1
Fog, J. U., Khoshbouei, H., Holy, M., Owens, W. A., Vaegter, C. B., Sen, N., et al. (2006). Calmodulin kinase II interacts with the dopamine transporter C terminus to regulate amphetamine-induced reverse transport. Neuron 51, 417–429. doi: 10.1016/j.neuron.2006.06.028
Foster, J. D., Adkins, S. D., Lever, J. R., and Vaughan, R. A. (2008). Phorbol ester induced trafficking-independent regulation and enhanced phosphorylation of the dopamine transporter associated with membrane rafts and cholesterol. J. Neurochem. 105, 1683–1699. doi: 10.1111/j.1471-4159.2008.05262.x
Foster, J. D., Yang, J. W., Moritz, A. E., Challasivakanaka, S., Smith, M. A., Holy, M., et al. (2012). Dopamine transporter phosphorylation site threonine 53 regulates substrate reuptake and amphetamine-stimulated efflux. J. Biol. Chem. 287, 29702–29712. doi: 10.1074/jbc.m112.367706
Fournier, K. M., Gonzalez, M. I., and Robinson, M. B. (2004). Rapid trafficking of the neuronal glutamate transporter, EAAC1: evidence for distinct trafficking pathways differentially regulated by protein kinase C and platelet-derived growth factor. J. Biol. Chem. 279, 34505–34513. doi: 10.1074/jbc.m404032200
Furman, C. A., Chen, R., Guptaroy, B., Zhang, M., Holz, R. W., and Gnegy, M. (2009). Dopamine and amphetamine rapidly increase dopamine transporter trafficking to the surface: live-cell imaging using total internal reflection fluorescence microscopy. J. Neurosci. 29, 3328–3336. doi: 10.1523/jneurosci.5386-08.2009
Furness, D. N., Dehnes, Y., Akhtar, A. Q., Rossi, D. J., Hamann, M., Grutle, N. J., et al. (2008). A quantitative assessment of glutamate uptake into hippocampal synaptic terminals and astrocytes: new insights into a neuronal role for excitatory amino acid transporter 2 (EAAT2). Neuroscience 157, 80–94. doi: 10.1016/j.neuroscience.2008.08.043
Galli, A., DeFelice, L. J., Duke, B. J., Moore, K. R., and Blakely, R. D. (1995). Sodium-dependent norepinephrine-induced currents in norepinephrine-transporter-transfected HEK-293 cells blocked by cocaine and antidepressants. J. Exp. Biol. 198, 2197–2212.
Gamboa, C., and Ortega, A. (2002). Insulin-like growth factor-1 increases activity and surface levels of the GLAST subtype of glutamate transporter. Neurochem. Int. 40, 397–403. doi: 10.1016/s0197-0186(01)00106-1
Ganel, R., and Crosson, C. E. (1998). Modulation of human glutamate transporter activity by phorbol ester. J. Neurochem. 70, 993–1000. doi: 10.1046/j.1471-4159.1998.70030993.x
Garaeva, A. A., Guskov, A., Slotboom, D. J., and Paulino, C. (2019). A one-gate elevator mechanism for the human neutral amino acid transporter ASCT2. Nat. Commun. 10:3427.
Garaeva, A. A., Oostergetel, G. T., Gati, C., Guskov, A., Paulino, C., and Slotboom, D. J. (2018). Cryo-EM structure of the human neutral amino acid transporter ASCT2. Nat. Struct. Mol. Biol. 25, 515–521. doi: 10.1038/s41594-018-0076-y
Garcia, B. G., Wei, Y., Moron, J. A., Lin, R. Z., Javitch, J. A., and Galli, A. (2005). Akt is essential for insulin modulation of amphetamine-induced human dopamine transporter cell-surface redistribution. Mol. Pharmacol. 68, 102–109. doi: 10.1124/mol.104.009092
Garcia-Tardon, N., Gonzalez-Gonzalez, I. M., Martinez-Villarreal, J., Fernandez-Sanchez, E., Gimenez, C., and Zafra, F. (2012). Protein kinase C (PKC)-promoted endocytosis of glutamate transporter GLT-1 requires ubiquitin ligase Nedd4-2-dependent ubiquitination but not phosphorylation. J. Biol. Chem. 287, 19177–19187. doi: 10.1074/jbc.m112.355909
Gavrilov, N., Golyagina, I., Brazhe, A., Scimemi, A., Turlapov, V., and Semyanov, A. (2018). Astrocytic coverage of dendritic spines, dendritic shafts, and axonal boutons in hippocampal neuropil. Front. Cell Neurosci. 12:248. doi: 10.3389/fncel.2018.00248
Geerlings, A., Nunez, E., Lopez-Corcuera, B., and Aragon, C. (2001). Calcium- and syntaxin 1-mediated trafficking of the neuronal glycine transporter GLYT2. J. Biol. Chem. 276, 17584–17590. doi: 10.1074/jbc.m010602200
Gegelashvili, G., Civenni, G., Racagni, G., Danbolt, N. C., Schousboe, I., and Schousboe, A. (1996). Glutamate receptor agonists up-regulate glutamate transporter GLAST in astrocytes. Neuroreport 8, 261–265. doi: 10.1097/00001756-199612200-00052
Gegelashvili, G., and Schousboe, A. (1997). High affinity glutamate transporters: regulation of expression and activity. Mol. Pharmacol. 52, 6–15. doi: 10.1124/mol.52.1.6
Genoud, C., Quairiaux, C., Steiner, P., Hirling, H., Welker, E., and Knott, G. W. (2006). Plasticity of astrocytic coverage and glutamate transporter expression in adult mouse cortex. PLoS Biol. 4:e343. doi: 10.1371/journal.pbio.0040343
German, C. L., Baladi, M. G., McFadden, L. M., Hanson, G. R., and Fleckenstein, A. E. (2015). Regulation of the dopamine and vesicular monoamine transporters: pharmacological targets and implications for disease. Pharmacol. Rev. 67, 1005–1024. doi: 10.1124/pr.114.010397
Ginsberg, S. D., Martin, L. J., and Rothstein, J. D. (1995). Regional deafferentation down-regulates subtypes of glutamate transporter proteins. J. Neurochem. 65, 2800–2803. doi: 10.1046/j.1471-4159.1995.65062800.x
Goldberg, N. R., Beuming, T., Soyer, O. S., Goldstein, R. A., Weinstein, H., and Javitch, J. A. (2003). Probing conformational changes in neurotransmitter transporters: a structural context. Eur. J. Pharmacol. 479, 3–12. doi: 10.1016/j.ejphar.2003.08.052
Goncalves, P. P., Carvalho, A. P., and Vale, M. G. (1997). Regulation of [gamma-3H]aminobutyric acid transport by Ca2 + in isolated synaptic plasma membrane vesicles. Brain Res. Mol. Brain Res. 51, 106–114. doi: 10.1016/s0169-328x(97)00223-4
Goncalves, P. P., Meireles, S. M., and Vale, M. G. (1999). Regulation of the gamma-aminobutyric acid transporter activity by protein phosphatases in synaptic plasma membranes. Neurosci. Res. 33, 41–47. doi: 10.1016/s0168-0102(98)00107-2
Gonzalez, M. I., Kazanietz, M. G., and Robinson, M. B. (2002). Regulation of the neuronal glutamate transporter excitatory amino acid carrier-1 (EAAC1) by different protein kinase C subtypes. Mol. Pharmacol. 62, 901–910. doi: 10.1124/mol.62.4.901
Gonzalez, M. I., Lopez-Colom, A. M., and Ortega, A. (1999). Sodium-dependent glutamate transport in Muller glial cells: regulation by phorbol esters. Brain Res. 831, 140–145. doi: 10.1016/s0006-8993(99)01438-9
Gonzalez-Gonzalez, I. M., Garcia-Tardon, N., Cubelos, B., Gimenez, C., and Zafra, F. (2008a). The glutamate transporter GLT1b interacts with the scaffold protein PSD-95. J. Neurochem. 105, 1834–1848. doi: 10.1111/j.1471-4159.2008.05281.x
Gonzalez-Gonzalez, I. M., Garcia-Tardon, N., Gimenez, C., and Zafra, F. (2008b). PKC-dependent endocytosis of the GLT1 glutamate transporter depends on ubiquitylation of lysines located in a C-terminal cluster. Glia 56, 963–974. doi: 10.1002/glia.20670
Goodspeed, K., Pérez-Palma, E., Iqbal, S., Cooper, D., Scimemi, A., Johannesen, K. M., et al. (2020). Current knowledge of SLC6A1-related neurodevelopmental disorders. Brain Commun. 2:fcaa170.
Gorentla, B. K., and Vaughan, R. A. (2005). Differential effects of dopamine and psychoactive drugs on dopamine transporter phosphorylation and regulation. Neuropharmacology 49, 759–768. doi: 10.1016/j.neuropharm.2005.08.011
Gowrishankar, R., Hahn, M. K., and Blakely, R. D. (2014). Good riddance to dopamine: roles for the dopamine transporter in synaptic function and dopamine-associated brain disorders. Neurochem. Int. 73, 42–48. doi: 10.1016/j.neuint.2013.10.016
Granas, C., Ferrer, J., Loland, C. J., Javitch, J. A., and Gether, U. (2003). N-terminal truncation of the dopamine transporter abolishes phorbol ester- and substance P receptor-stimulated phosphorylation without impairing transporter internalization. J. Biol. Chem. 278, 4990–5000. doi: 10.1074/jbc.m205058200
Grewer, C., Balani, P., Weidenfeller, C., Bartusel, T., Tao, Z., and Rauen, T. (2005). Individual subunits of the glutamate transporter EAAC1 homotrimer function independently of each other. Biochemistry 44, 11913–11923. doi: 10.1021/bi050987n
Grewer, C., Madani Mobarekeh, S. A., Watzke, N., Rauen, T., and Schaper, K. (2001). Substrate translocation kinetics of excitatory amino acid carrier 1 probed with laser-pulse photolysis of a new photolabile precursor of D-aspartic acid. Biochemistry 40, 232–240. doi: 10.1021/bi0015919
Grewer, C., and Rauen, T. (2005). Electrogenic glutamate transporters in the CNS: molecular mechanism, pre-steady-state kinetics, and their impact on synaptic signaling. J. Membr. Biol. 203, 1–20. doi: 10.1007/s00232-004-0731-6
Grewer, C., Watzke, N., Wiessner, M., and Rauen, T. (2000). Glutamate translocation of the neuronal glutamate transporter EAAC1 occurs within milliseconds. Proc. Natl. Acad. Sci. U.S.A. 97, 9706–9711. doi: 10.1073/pnas.160170397
Gu, H., Wall, S. C., and Rudnick, G. (1994). Stable expression of biogenic amine transporters reveals differences in inhibitor sensitivity, kinetics, and ion dependence. J. Biol. Chem. 269, 7124–7130. doi: 10.1016/s0021-9258(17)37256-3
Guillet, B. A., Velly, L. J., Canolle, B., Masmejean, F. M., Nieoullon, A. L., and Pisano, P. (2005). Differential regulation by protein kinases of activity and cell surface expression of glutamate transporters in neuron-enriched cultures. Neurochem. Int. 46, 337–346. doi: 10.1016/j.neuint.2004.10.006
Gulley, J. M., Doolen, S., and Zahniser, N. R. (2002). Brief, repeated exposure to substrates down-regulates dopamine transporter function in Xenopus oocytes in vitro and rat dorsal striatum in vivo. J. Neurochem. 83, 400–411. doi: 10.1046/j.1471-4159.2002.01133.x
Guskov, A., Jensen, S., Faustino, I., Marrink, S. J., and Slotboom, D. J. (2016). Coupled binding mechanism of three sodium ions and aspartate in the glutamate transporter homologue GltTk. Nat. Commun. 7:13420.
Hagiwara, T., Tanaka, K., Takai, S., Maeno-Hikichi, Y., Mukainaka, Y., and Wada, K. (1996). Genomic organization, promoter analysis, and chromosomal localization of the gene for the mouse glial high-affinity glutamate transporter Slc1a3. Genomics 33, 508–515. doi: 10.1006/geno.1996.0226
Hancock, J. F. (2006). Lipid rafts: contentious only from simplistic standpoints. Nat. Rev. Mol. Cell Biol. 7, 456–462. doi: 10.1038/nrm1925
Hansen, F. H., Skjørringe, T., Yasmeen, S., Arends, N. V., Sahai, M. A., Erreger, K., et al. (2014). Missense dopamine transporter mutations associate with adult parkinsonism and ADHD. J. Clin. Invest. 124, 3107–3120. doi: 10.1172/jci73778
Harsing, L. G. Jr., Solyom, S., and Salamon, C. (2001). The role of glycineB binding site and glycine transporter (GlyT1) in the regulation of [3H]GABA and [3H]glycine release in the rat brain. Neurochem. Res. 26, 915–923.
Haugeto, O., Ullensvang, K., Levy, L. M., Chaudhry, F. A., Honore, T., Nielsen, M., et al. (1996). Brain glutamate transporter proteins form homomultimers. J. Biol. Chem. 271, 27715–27722. doi: 10.1074/jbc.271.44.27715
Hefendehl, J. K., LeDue, J., Ko, R. W., Mahler, J., Murphy, T. H., and MacVicar, B. A. (2016). Mapping synaptic glutamate transporter dysfunction in vivo to regions surrounding Abeta plaques by iGluSnFR two-photon imaging. Nat. Commun. 7:13441.
Hepp, R., Perraut, M., Chasserot-Golaz, S., Galli, T., Aunis, D., Langley, K., et al. (1999). Cultured glial cells express the SNAP-25 analogue SNAP-23. Glia 27, 181–187. doi: 10.1002/(sici)1098-1136(199908)27:2<181::aid-glia8>3.0.co;2-9
Herborg, F., Andreassen, T. F., Berlin, F., Loland, C. J., and Gether, U. (2018). Neuropsychiatric disease-associated genetic variants of the dopamine transporter display heterogeneous molecular phenotypes. J. Biol. Chem. 293, 7250–7262. doi: 10.1074/jbc.RA118.001753
Herde, M. K., Bohmbach, K., Domingos, C., Vana, N., Komorowska-Muller, J. A., Passlick, S., et al. (2020). Local efficacy of glutamate uptake decreases with synapse size. Cell Rep. 32:108182. doi: 10.1016/j.celrep.2020.108182
Hertz, L., Bock, E., and Schousboe, A. (1978). GFA content, glutamate uptake and activity of glutamate metabolizing enzymes in differentiating mouse astrocytes in primary cultures. Dev. Neurosci. 1, 226–238. doi: 10.1159/000112577
Heshmati, M., Christoffel, D. J., LeClair, K., Cathomas, F., Golden, S. A., Aleyasin, H., et al. (2020). Depression and social defeat stress are associated with inhibitory synaptic changes in the nucleus accumbens. J. Neurosci. 40, 6228–6233. doi: 10.1523/jneurosci.2568-19.2020
Higuera-Matas, A., Miguens, M., Coria, S. M., Assis, M. A., Borcel, E., del Olmo, N., et al. (2012). Sex-specific disturbances of the glutamate/GABA balance in the hippocampus of adult rats subjected to adolescent cannabinoid exposure. Neuropharmacology 62, 1975–1984. doi: 10.1016/j.neuropharm.2011.12.028
Hoffman, A. F., Lupica, C. R., and Gerhardt, G. A. (1998). Dopamine transporter activity in the substantia nigra and striatum assessed by high-speed chronoamperometric recordings in brain slices. J. Pharmacol. Exp. Ther. 287, 487–496.
Hofmann, K., Duker, M., Fink, T., Lichter, P., and Stoffel, W. (1994). Human neutral amino acid transporter ASCT1: structure of the gene (SLC1A4) and localization to chromosome 2p13-p15. Genomics 24, 20–26. doi: 10.1006/geno.1994.1577
Holmseth, S., Scott, H. A., Real, K., Lehre, K. P., Leergaard, T. B., Bjaalie, J. G., et al. (2009). The concentrations and distributions of three C-terminal variants of the GLT1 (EAAT2; slc1a2) glutamate transporter protein in rat brain tissue suggest differential regulation. Neuroscience 162, 1055–1071. doi: 10.1016/j.neuroscience.2009.03.048
Holton, K. L., Loder, M. K., and Melikian, H. E. (2005). Nonclassical, distinct endocytic signals dictate constitutive and PKC-regulated neurotransmitter transporter internalization. Nat. Neurosci. 8, 881–888. doi: 10.1038/nn1478
Hong, W. C., and Amara, S. G. (2010). Membrane cholesterol modulates the outward facing conformation of the dopamine transporter and alters cocaine binding. J. Biol. Chem. 285, 32616–32626. doi: 10.1074/jbc.m110.150565
Hong, W. C., and Amara, S. G. (2013). Differential targeting of the dopamine transporter to recycling or degradative pathways during amphetamine- or PKC-regulated endocytosis in dopamine neurons. FASEB J. 27, 2995–3007. doi: 10.1096/fj.12-218727
Hoover, B. R., Everett, C. V., Sorkin, A., and Zahniser, N. R. (2007). Rapid regulation of dopamine transporters by tyrosine kinases in rat neuronal preparations. J. Neurochem. 101, 1258–1271. doi: 10.1111/j.1471-4159.2007.04522.x
Horton, N., and Quick, M. W. (2001). Syntaxin 1A up-regulates GABA transporter expression by subcellular redistribution. Mol. Membr. Biol. 18, 39–44. doi: 10.1080/09687680010029383
Hu, J., Fei, J., Reutter, W., and Fan, H. (2011). Involvement of sialic acid in the regulation of gamma–aminobutyric acid uptake activity of gamma-aminobutyric acid transporter 1. Glycobiology 21, 329–339. doi: 10.1093/glycob/cwq166
Hu, J., and Quick, M. W. (2008). Substrate-mediated regulation of gamma-aminobutyric acid transporter 1 in rat brain. Neuropharmacology 54, 309–318. doi: 10.1016/j.neuropharm.2007.09.013
Huang, H. T., Liao, C. K., Chiu, W. T., and Tzeng, S. F. (2017). Ligands of peroxisome proliferator-activated receptor-alpha promote glutamate transporter-1 endocytosis in astrocytes. Int. J. Biochem. Cell Biol. 86, 42–53. doi: 10.1016/j.biocel.2017.03.008
Hudson, B. D., Hebert, T. E., and Kelly, M. E. (2010). Physical and functional interaction between CB1 cannabinoid receptors and beta2-adrenoceptors. Br. J. Pharmacol. 160, 627–642. doi: 10.1111/j.1476-5381.2010.00681.x
Huff, R. A., Vaughan, R. A., Kuhar, M. J., and Uhl, G. R. (1997). Phorbol esters increase dopamine transporter phosphorylation and decrease transport Vmax. J. Neurochem. 68, 225–232. doi: 10.1046/j.1471-4159.1997.68010225.x
Imoukhuede, P. I., Moss, F. J., Michael, D. J., Chow, R. H., and Lester, H. A. (2009). Ezrin mediates tethering of the gamma-aminobutyric acid transporter GAT1 to actin filaments via a C-terminal PDZ-interacting domain. Biophys. J. 96, 2949–2960. doi: 10.1016/j.bpj.2008.11.070
Ingram, S. L., Prasad, B. M., and Amara, S. G. (2002). Dopamine transporter-mediated conductances increase excitability of midbrain dopamine neurons. Nat. Neurosci. 5, 971–978. doi: 10.1038/nn920
Jacob, C. P., Koutsilieri, E., Bartl, J., Neuen-Jacob, E., Arzberger, T., Zander, N., et al. (2007). Alterations in expression of glutamatergic transporters and receptors in sporadic Alzheimer’s disease. J. Alzheimers Dis. 11, 97–116. doi: 10.3233/jad-2007-11113
Jansson, L. C., Louhivuori, L., Wigren, H. K., Nordstrom, T., Louhivuori, V., Castren, M. L., et al. (2013). Effect of glutamate receptor antagonists on migrating neural progenitor cells. Eur. J. Neurosci. 37, 1369–1382. doi: 10.1111/ejn.12152
Jayanthi, L. D., Vargas, G., and DeFelice, L. J. (2002). Characterization of cocaine and antidepressant-sensitive norepinephrine transporters in rat placental trophoblasts. Br. J. Pharmacol. 135, 1927–1934. doi: 10.1038/sj.bjp.0704658
Ji, Y. F., Xu, S. M., Zhu, J., Wang, X. X., and Shen, Y. (2011). Insulin increases glutamate transporter GLT1 in cultured astrocytes. Biochem. Biophys. Res. Commun. 405, 691–696. doi: 10.1016/j.bbrc.2011.01.105
Ji, Y. F., Zhou, L., Xie, Y. J., Xu, S. M., Zhu, J., Teng, P., et al. (2013). Upregulation of glutamate transporter GLT-1 by mTOR-Akt-NF-small ka, CyrillicB cascade in astrocytic oxygen-glucose deprivation. Glia 61, 1959–1975. doi: 10.1002/glia.22566
Jiang, N. W., Wang, D. J., Xie, Y. J., Zhou, L., Su, L. D., Li, H., et al. (2016). Downregulation of glutamate transporter EAAT4 by conditional knockout of rheb1 in Cerebellar Purkinje cells. Cerebellum 15, 314–321. doi: 10.1007/s12311-015-0701-9
Johnson, L. A., Furman, C. A., Zhang, M., Guptaroy, B., and Gnegy, M. E. (2005a). Rapid delivery of the dopamine transporter to the plasmalemmal membrane upon amphetamine stimulation. Neuropharmacology 49, 750–758. doi: 10.1016/j.neuropharm.2005.08.018
Johnson, L. A., Guptaroy, B., Lund, D., Shamban, S., and Gnegy, M. E. (2005b). Regulation of amphetamine-stimulated dopamine efflux by protein kinase C beta. J. Biol. Chem. 280, 10914–10919. doi: 10.1074/jbc.m413887200
Jones, K. T., Zhen, J., and Reith, M. E. (2012). Importance of cholesterol in dopamine transporter function. J. Neurochem. 123, 700–715. doi: 10.1111/jnc.12007
Jones, S. R., Gainetdinov, R. R., Wightman, R. M., and Caron, M. G. (1998). Mechanisms of amphetamine action revealed in mice lacking the dopamine transporter. J. Neurosci. 18, 1979–1986. doi: 10.1523/jneurosci.18-06-01979.1998
Jones, S. R., Joseph, J. D., Barak, L. S., Caron, M. G., and Wightman, R. M. (1999). Dopamine neuronal transport kinetics and effects of amphetamine. J. Neurochem. 73, 2406–2414. doi: 10.1046/j.1471-4159.1999.0732406.x
Kahlig, K. M., Binda, F., Khoshbouei, H., Blakely, R. D., McMahon, D. G., Javitch, J. A., et al. (2005). Amphetamine induces dopamine efflux through a dopamine transporter channel. Proc. Natl. Acad. Sci. U.S.A. 102, 3495–3500. doi: 10.1073/pnas.0407737102
Kahlig, K. M., and Galli, A. (2003). Regulation of dopamine transporter function and plasma membrane expression by dopamine, amphetamine, and cocaine. Eur. J. Pharmacol. 479, 153–158. doi: 10.1016/j.ejphar.2003.08.065
Kahlig, K. M., Javitch, J. A., and Galli, A. (2004). Amphetamine regulation of dopamine transport. Combined measurements of transporter currents and transporter imaging support the endocytosis of an active carrier. J. Biol. Chem. 279, 8966–8975.
Kalandadze, A., Wu, Y., and Robinson, M. B. (2002). Protein kinase C activation decreases cell surface expression of the GLT-1 subtype of glutamate transporter. Requirement of a carboxyl-terminal domain and partial dependence on serine 486. J. Biol. Chem. 277, 45741–45750. doi: 10.1074/jbc.m203771200
Kang, M., Ryu, J., Kim, J. H., Na, H., Zuo, Z., and Do, S. H. (2010). Corticosterone decreases the activity of rat glutamate transporter type 3 expressed in Xenopus oocytes. Steroids 75, 1113–1118. doi: 10.1016/j.steroids.2010.07.003
Kanner, B. I. (2003). Transmembrane domain I of the gamma-aminobutyric acid transporter GAT-1 plays a crucial role in the transition between cation leak and transport modes. J. Biol. Chem. 278, 3705–3712. doi: 10.1074/jbc.m210525200
Karam, C. S., and Javitch, J. A. (2018). Phosphorylation of the amino terminus of the dopamine transporter: regulatory mechanisms and implications for amphetamine action. Adv. Pharmacol. 82, 205–234. doi: 10.1016/bs.apha.2017.09.002
Karatas-Wulf, U., Koepsell, H., Bergert, M., Sonnekes, S., and Kugler, P. (2009). Protein kinase C-dependent trafficking of glutamate transporters excitatory amino acid carrier 1 and glutamate transporter 1b in cultured cerebellar granule cells. Neuroscience 161, 794–805. doi: 10.1016/j.neuroscience.2009.04.017
Karki, P., Webb, A., Smith, K., Johnson, J. Jr., Lee, K., Son, D. S., et al. (2014). Yin Yang 1 is a repressor of glutamate transporter EAAT2, and it mediates manganese-induced decrease of EAAT2 expression in astrocytes. Mol. Cell Biol. 34, 1280–1289. doi: 10.1128/mcb.01176-13
Karylowski, O., Zeigerer, A., Cohen, A., and McGraw, T. E. (2004). GLUT4 is retained by an intracellular cycle of vesicle formation and fusion with endosomes. Mol. Biol. Cell 15, 870–882. doi: 10.1091/mbc.e03-07-0517
Kerkerian, L., Dusticier, N., and Nieoullon, A. (1987). Modulatory effect of dopamine on high-affinity glutamate uptake in the rat striatum. J. Neurochem. 48, 1301–1306. doi: 10.1111/j.1471-4159.1987.tb05661.x
Keynan, S., and Kanner, B. I. (1988). gamma-Aminobutyric acid transport in reconstituted preparations from rat brain: coupled sodium and chloride fluxes. Biochemistry 27, 12–17. doi: 10.1021/bi00401a003
Khoshbouei, H., Sen, N., Guptaroy, B., Johnson, L., Lund, D., Gnegy, M. E., et al. (2004). N-terminal phosphorylation of the dopamine transporter is required for amphetamine-induced efflux. PLoS Biol. 2:E78. doi: 10.1371/journal.pbio.0020078
Kilty, J. E., Lorang, D., and Amara, S. G. (1991). Cloning and expression of a cocaine-sensitive rat dopamine transporter. Science 254, 578–579. doi: 10.1126/science.1948035
Kim, D. U., Kim, M. K., Cho, Y. W., Kim, Y. S., Kim, W. J., Lee, M. G., et al. (2011). Association of a synonymous GAT3 polymorphism with antiepileptic drug pharmacoresistance. J. Hum. Genet. 56, 640–646. doi: 10.1038/jhg.2011.73
Kim, S., Westphalen, R., Callahan, B., Hatzidimitriou, G., Yuan, J., and Ricaurte, G. A. (2000). Toward development of an in vitro model of methamphetamine-induced dopamine nerve terminal toxicity. J. Pharmacol. Exp. Ther. 293, 625–633.
Kimmel, H. L., Carroll, F. I., and Kuhar, M. J. (2000). Dopamine transporter synthesis and degradation rate in rat striatum and nucleus accumbens using RTI-76. Neuropharmacology 39, 578–585. doi: 10.1016/s0028-3908(99)00160-4
Kinney, G. A. (2005). GAT-3 transporters regulate inhibition in the neocortex. J, Neurophysiol. 94, 4533–4537. doi: 10.1152/jn.00420.2005
Kitayama, S., Dohi, T., and Uhl, G. R. (1994). Phorbol esters alter functions of the expressed dopamine transporter. Eur. J. Pharmacol. 268, 115–119. doi: 10.1016/0922-4106(94)90180-5
Kivell, B., Uzelac, Z., Sundaramurthy, S., Rajamanickam, J., Ewald, A., Chefer, V., et al. (2014). Salvinorin A regulates dopamine transporter function via a kappa opioid receptor and ERK1/2-dependent mechanism. Neuropharmacology 86, 228–240. doi: 10.1016/j.neuropharm.2014.07.016
Koch, H. P., Brown, R. L., and Larsson, H. P. (2007). The glutamate-activated anion conductance in excitatory amino acid transporters is gated independently by the individual subunits. J. Neurosci. 27, 2943–2947. doi: 10.1523/jneurosci.0118-07.2007
Kolen, B., Kortzak, D., Franzen, A., and Fahlke, C. (2020). An amino-terminal point mutation increases EAAT2 anion currents without affecting glutamate transport rates. J. Biol. Chem. 295, 14936–14947. doi: 10.1074/jbc.ra120.013704
Kovtun, O., Sakrikar, D., Tomlinson, I. D., Chang, J. C., Arzeta-Ferrer, X., Blakely, R. D., et al. (2015). Single-quantum-dot tracking reveals altered membrane dynamics of an attention-deficit/hyperactivity-disorder-derived dopamine transporter coding variant. ACS Chem. Neurosci. 6, 526–534. doi: 10.1021/cn500202c
Kristensen, A. S., Andersen, J., Jorgensen, T. N., Sorensen, L., Eriksen, J., Loland, C. J., et al. (2011). SLC6 neurotransmitter transporters: structure, function, and regulation. Pharmacol. Rev. 63, 585–640.
Krueger, B. K. (1990). Kinetics and block of dopamine uptake in synaptosomes from rat caudate nucleus. J. Neurochem. 55, 260–267. doi: 10.1111/j.1471-4159.1990.tb08847.x
Laughlin, T. M., Tram, K. V., Wilcox, G. L., and Birnbaum, A. K. (2002). Comparison of antiepileptic drugs tiagabine, lamotrigine, and gabapentin in mouse models of acute, prolonged, and chronic nociception. J. Pharmacol. Exp. Ther. 302, 1168–1175. doi: 10.1124/jpet.302.3.1168
Law, R. M., Stafford, A., and Quick, M. W. (2000). Functional regulation of gamma-aminobutyric acid transporters by direct tyrosine phosphorylation. J. Biol. Chem. 275, 23986–23991. doi: 10.1074/jbc.m910283199
Leary, G. P., Holley, D. C., Stone, E. F., Lyda, B. R., Kalachev, L. V., and Kavanaugh, M. P. (2011). The central cavity in trimeric glutamate transporters restricts ligand diffusion. Proc. Natl. Acad. Sci. U.S.A. 108, 14980–14985. doi: 10.1073/pnas.1108785108
Leary, G. P., Stone, E. F., Holley, D. C., and Kavanaugh, M. P. (2007). The glutamate and chloride permeation pathways are colocalized in individual neuronal glutamate transporter subunits. J. Neurosci. 27, 2938–2942. doi: 10.1523/jneurosci.4851-06.2007
Lee, F. J., Liu, F., Pristupa, Z. B., and Niznik, H. B. (2001). Direct binding and functional coupling of alpha-synuclein to the dopamine transporters accelerate dopamine-induced apoptosis. FASEB J. 15, 916–926. doi: 10.1096/fsb2fj000334com
Lee, F. J., Pei, L., Moszczynska, A., Vukusic, B., Fletcher, P. J., and Liu, F. (2007). Dopamine transporter cell surface localization facilitated by a direct interaction with the dopamine D2 receptor. EMBO J. 26, 2127–2136. doi: 10.1038/sj.emboj.7601656
Lee, G., Huang, Y., Washington, J. M., Briggs, N. W., and Zuo, Z. (2005). Carbamazepine enhances the activity of glutamate transporter type 3 via phosphatidylinositol 3-kinase. Epilepsy Res. 66, 145–153. doi: 10.1016/j.eplepsyres.2005.08.003
Lee, H. S., Bae, E. J., Yi, S. H., Shim, J. W., Jo, A. Y., Kang, J. S., et al. (2010). Foxa2 and Nurr1 synergistically yield A9 nigral dopamine neurons exhibiting improved differentiation, function, and cell survival. Stem Cells 28, 501–512.
Lee, K. H., Kim, M. Y., Kim, D. H., and Lee, Y. S. (2004). Syntaxin 1A and receptor for activated C kinase interact with the N-terminal region of human dopamine transporter. Neurochem. Res. 29, 1405–1409. doi: 10.1023/b:nere.0000026404.08779.43
Lehre, K. P., and Danbolt, N. C. (1998). The number of glutamate transporter subtype molecules at glutamatergic synapses: chemical and stereological quantification in young adult rat brain. J. Neurosci. 18, 8751–8757. doi: 10.1523/jneurosci.18-21-08751.1998
Lehre, K. P., Levy, L. M., Ottersen, O. P., Storm-Mathisen, J., and Danbolt, N. C. (1995). Differential expression of two glial glutamate transporters in the rat brain: quantitative and immunocytochemical observations. J. Neurosci. 15, 1835–1853. doi: 10.1523/jneurosci.15-03-01835.1995
Leng, K., Li, E., Eser, R., Piergies, A., Sit, R., Tan, M., et al. (2021). Molecular characterization of selectively vulnerable neurons in Alzheimer’s disease. Nat. Neurosci. 24, 276–287.
Leonova, J., Thorlin, T., Aberg, N. D., Eriksson, P. S., Ronnback, L., and Hansson, E. (2001). Endothelin-1 decreases glutamate uptake in primary cultured rat astrocytes. Am. J. Physiol. Cell Physiol. 281, C1495–C1503.
Levy, L., Lehre, K., Walaas, S., Storm-Mathisen, J., and Danbolt, N. (1995). Down-regulation of glial glutamate transporters after glutamatergic denervation in the rat brain. Eur. J. Neurosci. 7, 2036–2041. doi: 10.1111/j.1460-9568.1995.tb00626.x
Levy, L. M., Lehre, K. P., Rolstad, B., and Danbolt, N. C. (1993). A monoclonal antibody raised against an [Na(+)+K+]coupled L-glutamate transporter purified from rat brain confirms glial cell localization. FEBS Lett. 317, 79–84. doi: 10.1016/0014-5793(93)81495-l
Levy, L. M., Warr, O., and Attwell, D. (1998). Stoichiometry of the glial glutamate transporter GLT-1 expressed inducibly in a Chinese hamster ovary cell line selected for low endogenous Na+-dependent glutamate uptake. J. Neurosci. 18, 9620–9628. doi: 10.1523/jneurosci.18-23-09620.1998
Li, L. B., Toan, S. V., Zelenaia, O., Watson, D. J., Wolfe, J. H., Rothstein, J. D., et al. (2006). Regulation of astrocytic glutamate transporter expression by Akt: evidence for a selective transcriptional effect on the GLT-1/EAAT2 subtype. J. Neurochem. 97, 759–771. doi: 10.1111/j.1471-4159.2006.03743.x
Li, M. H., Underhill, S. M., Reed, C., Phillips, T. J., Amara, S. G., and Ingram, S. L. (2017). Amphetamine and methamphetamine increase NMDAR-GluN2B synaptic currents in midbrain dopamine neurons. Neuropsychopharmacology 42, 1539–1547. doi: 10.1038/npp.2016.278
Li, S., Mallory, M., Alford, M., Tanaka, S., and Masliah, E. (1997). Glutamate transporter alterations in Alzheimer disease are possibly associated with abnormal APP expression. J. Neuropathol. Exp. Neurol. 56, 901–911. doi: 10.1097/00005072-199708000-00008
Lim, S. K., Park, M. J., Jung, H. K., Park, A. Y., Kim, D. I., Kim, J. C., et al. (2008). Bradykinin stimulates glutamate uptake via both B1R and B2R activation in a human retinal pigment epithelial cells. Life Sci. 83, 761–770. doi: 10.1016/j.lfs.2008.09.014
Lin, C. L., Tzingounis, A. V., Jin, L., Furuta, A., Kavanaugh, M. P., and Rothstein, J. D. (1998). Molecular cloning and expression of the rat EAAT4 glutamate transporter subtype. Brain Res. Mol. Brain Res. 63, 174–179. doi: 10.1016/s0169-328x(98)00256-3
Loder, M. K., and Melikian, H. E. (2003). The dopamine transporter constitutively internalizes and recycles in a protein kinase C-regulated manner in stably transfected PC12 cell lines. J. Biol. Chem. 278, 22168–22174. doi: 10.1074/jbc.m301845200
Lu, C.-C., and Hilgemann, D. W. (1999). GAT1 (GABA: Na+: Cl–) cotransport function: steady state studies in giant Xenopus oocyte membrane patches. J. Gen. Physiol. 114, 429–444. doi: 10.1085/jgp.114.3.429
Lushnikova, I., Skibo, G., Muller, D., and Nikonenko, I. (2009). Synaptic potentiation induces increased glial coverage of excitatory synapses in CA1 hippocampus. Hippocampus 19, 753–762. doi: 10.1002/hipo.20551
Lute, B. J., Khoshbouei, H., Saunders, C., Sen, N., Lin, R. Z., Javitch, J. A., et al. (2008). PI3K signaling supports amphetamine-induced dopamine efflux. Biochem. Biophys. Res. Commun. 372, 656–661. doi: 10.1016/j.bbrc.2008.05.091
MacAulay, N., Zeuthen, T., and Gether, U. (2002). Conformational basis for the Li(+)-induced leak current in the rat gamma-aminobutyric acid (GABA) transporter-1. J. Physiol. 544, 447–458. doi: 10.1113/jphysiol.2002.022897
Mager, S., Min, C., Henry, D. J., Chavkin, C., Hoffman, B. J., Davidson, N., et al. (1994). Conducting states of a mammalian serotonin transporter. Neuron 12, 845–859. doi: 10.1016/0896-6273(94)90337-9
Mager, S., Naeve, J., Quick, M., Labarca, C., Davidson, N., and Lester, H. A. (1993). Steady states, charge movements, and rates for a cloned GABA transporter expressed in Xenopus oocytes. Neuron 10, 177–188. doi: 10.1016/0896-6273(93)90309-f
Malik, A. R., and Willnow, T. E. (2019). Excitatory amino acid transporters in physiology and disorders of the central nervous system. Int. J. Mol. Sci. 20:5671. doi: 10.3390/ijms20225671
Maragakis, N. J., Dykes-Hoberg, M., and Rothstein, J. D. (2004). Altered expression of the glutamate transporter EAAT2b in neurological disease. Ann. Neurol. 55, 469–477. doi: 10.1002/ana.20003
Martinez-Lozada, Z., Guillem, A. M., and Robinson, M. B. (2016). Transcriptional regulation of glutamate transporters: from extracellular signals to transcription factors. Adv. Pharmacol. 76, 103–145.
Martins, R. S., de Freitas, I. G., Sathler, M. F., Martins, V., Schitine, C. S., da Silva Sampaio, L., et al. (2018). Beta-adrenergic receptor activation increases GABA uptake in adolescent mice frontal cortex: Modulation by cannabinoid receptor agonist WIN55,212-2. Neurochem. Int. 120, 182–190. doi: 10.1016/j.neuint.2018.08.011
Mason, J. N., Farmer, H., Tomlinson, I. D., Schwartz, J. W., Savchenko, V., DeFelice, L. J., et al. (2005). Novel fluorescence-based approaches for the study of biogenic amine transporter localization, activity, and regulation. J. Neurosci. Methods 143, 3–25. doi: 10.1016/j.jneumeth.2004.09.028
Matsusaki, M., Kishida, A., Stainton, N., Ansell, C. W. G., and Akashi, M. (2001). Synthesis and characterization of novel biodegradable polymers composed of hydroxycinnamic acid and D,L-lactic acid. J. Appl. Polym. Sci. 82, 2357–2364. doi: 10.1002/app.2085
Matthews, E. Jr., Rahnama-Vaghef, A., and Eskandari, S. (2009). Inhibitors of the γ-aminobutyric acid transporter 1 (GAT1) do not reveal a channel mode of conduction. Neurochem. Intern. 55, 732–740. doi: 10.1016/j.neuint.2009.07.005
Mazei-Robison, M. S., Couch, R. S., Shelton, R. C., Stein, M. A., and Blakely, R. D. (2005). Sequence variation in the human dopamine transporter gene in children with attention deficit hyperactivity disorder. Neuropharmacology 49, 724–736. doi: 10.1016/j.neuropharm.2005.08.003
McElvain, J. S., and Schenk, J. O. (1992). A multisubstrate mechanism of striatal dopamine uptake and its inhibition by cocaine. Biochem. Pharmacol. 43, 2189–2199. doi: 10.1016/0006-2952(92)90178-l
McGeer, P., McGeer, E., Scherer, U., and Singh, K. (1977). A glutamatergic corticostriatal path? Brain Res. 128, 369–373. doi: 10.1016/0006-8993(77)91003-4
McHugh, E. M., Zhu, W., Milgram, S., and Mager, S. (2004). The GABA transporter GAT1 and the MAGUK protein Pals1: interaction, uptake modulation, and coexpression in the brain. Mol. Cell Neurosci. 26, 406–417. doi: 10.1016/j.mcn.2004.03.006
McNair, L. F., Andersen, J. V., Aldana, B. I., Hohnholt, M. C., Nissen, J. D., Sun, Y., et al. (2019). Deletion of neuronal GLT-1 in mice reveals its role in synaptic glutamate homeostasis and mitochondrial function. J. Neurosci. 39, 4847–4863. doi: 10.1523/jneurosci.0894-18.2019
McNair, L. F., Andersen, J. V., Nissen, J. D., Sun, Y., Fischer, K. D., Hodgson, N. W., et al. (2020). Conditional knockout of GLT-1 in neurons leads to alterations in aspartate homeostasis and synaptic mitochondrial metabolism in Striatum and Hippocampus. Neurochem. Res. 45, 1420–1437. doi: 10.1007/s11064-020-03000-7
Medvedev, N., Popov, V., Henneberger, C., Kraev, I., Rusakov, D. A., and Stewart, M. G. (2014). Glia selectively approach synapses on thin dendritic spines. Philos. Trans. R. Soc. Lond. B Biol. Sci. 369:20140047. doi: 10.1098/rstb.2014.0047
Melikian, H. E., and Buckley, K. M. (1999). Membrane trafficking regulates the activity of the human dopamine transporter. J. Neurosci. 19, 7699–7710. doi: 10.1523/jneurosci.19-18-07699.1999
Melone, M., Barbaresi, P., Fattorini, G., and Conti, F. (2005). Neuronal localization of the GABA transporter GAT-3 in human cerebral cortex: a procedural artifact? J. Chem. Neuroanat. 30, 45–54. doi: 10.1016/j.jchemneu.2005.04.002
Melone, M., Bellesi, M., and Conti, F. (2009). Synaptic localization of GLT-1a in the rat somatic sensory cortex. Glia 57, 108–117. doi: 10.1002/glia.20744
Melone, M., Bellesi, M., Ducati, A., Iacoangeli, M., and Conti, F. (2011). Cellular and synaptic localization of EAAT2a in human cerebral cortex. Front. Neuroanat. 4:151. doi: 10.3389/fnana.2010.00151
Melone, M., Ciappelloni, S., and Conti, F. (2015). A quantitative analysis of cellular and synaptic localization of GAT-1 and GAT-3 in rat neocortex. Brain Struct. Funct. 220, 885–897. doi: 10.1007/s00429-013-0690-8
Melone, M., Ciriachi, C., Pietrobon, D., and Conti, F. (2019). Heterogeneity of Astrocytic and neuronal GLT-1 at cortical excitatory synapses, as revealed by its colocalization with Na+/K+-ATPase alpha isoforms. Cereb. Cortex 29, 3331–3350. doi: 10.1093/cercor/bhy203
Melone, M., Cozzi, A., Pellegrini-Giampietro, D. E., and Conti, F. (2003). Transient focal ischemia triggers neuronal expression of GAT-3 in the rat perilesional cortex. Neurobiol. Dis. 14, 120–132. doi: 10.1016/s0969-9961(03)00042-1
Mergy, M. A., Gowrishankar, R., Davis, G. L., Jessen, T. N., Wright, J., Stanwood, G. D., et al. (2014). Genetic targeting of the amphetamine and methylphenidate-sensitive dopamine transporter: on the path to an animal model of attention-deficit hyperactivity disorder. Neurochem. Int. 73, 56–70. doi: 10.1016/j.neuint.2013.11.009
Metzger, R. R., Haughey, H. M., Wilkins, D. G., Gibb, J. W., Hanson, G. R., and Fleckenstein, A. E. (2000). Methamphetamine-induced rapid decrease in dopamine transporter function: role of dopamine and hyperthermia. J. Pharmacol. Exp. Ther. 295, 1077–1085.
Meyer, T., Speer, A., Meyer, B., Sitte, W., Kuther, G., and Ludolph, A. C. (1996). The glial glutamate transporter complementary DNA in patients with amyotrophic lateral sclerosis. Ann. Neurol. 40, 456–459. doi: 10.1002/ana.410400317
Michaluk, P. H., Heller, J., and Rusakov, D. A. (2020). Rapid recycling of glutamate transporters on the astroglial surface. bioRxiv [Preprint], doi: 10.1101/2020.11.08.373233v1
Mick, E., and Faraone, S. V. (2009). Family and genetic association studies of bipolar disorder in children. Child Adolesc. Psychiatr. Clin. N. Am. 18, 441–453. doi: 10.1016/j.chc.2008.11.008
Mim, C., Balani, P., Rauen, T., and Grewer, C. (2005). The glutamate transporter subtypes EAAT4 and EAATs 1-3 transport glutamate with dramatically different kinetics and voltage dependence but share a common uptake mechanism. J. Gen. Physiol. 126, 571–589. doi: 10.1085/jgp.200509365
Minelli, A., Barbaresi, P., Reimer, R. J., Edwards, R. H., and Conti, F. (2001). The glial glutamate transporter GLT-1 is localized both in the vicinity of and at distance from axon terminals in the rat cerebral cortex. Neuroscience 108, 51–59. doi: 10.1016/s0306-4522(01)00375-x
Miranda, M., Dionne, K. R., Sorkina, T., and Sorkin, A. (2007). Three ubiquitin conjugation sites in the amino terminus of the dopamine transporter mediate protein kinase C-dependent endocytosis of the transporter. Mol. Biol. Cell 18, 313–323. doi: 10.1091/mbc.e06-08-0704
Mitrovic, A. D., Plesko, F., and Vandenberg, R. J. (2001). Zn(2+) inhibits the anion conductance of the glutamate transporter EEAT4. J. Biol. Chem. 276, 26071–26076. doi: 10.1074/jbc.m011318200
Mookherjee, P., Green, P. S., Watson, G. S., Marques, M. A., Tanaka, K., Meeker, K. D., et al. (2011). GLT-1 loss accelerates cognitive deficit onset in an Alzheimer’s disease animal model. J. Alzheimers Dis. 26, 447–455. doi: 10.3233/jad-2011-110503
Moritz, A. E., Foster, J. D., Gorentla, B. K., Mazei-Robison, M. S., Yang, J. W., Sitte, H. H., et al. (2013). Phosphorylation of dopamine transporter serine 7 modulates cocaine analog binding. J. Biol. Chem. 288, 20–32. doi: 10.1074/jbc.m112.407874
Moron, J. A., Zakharova, I., Ferrer, J. V., Merrill, G. A., Hope, B., Lafer, E. M., et al. (2003). Mitogen-activated protein kinase regulates dopamine transporter surface expression and dopamine transport capacity. J. Neurosci. 23, 8480–8488. doi: 10.1523/jneurosci.23-24-08480.2003
Moss, F. J., Imoukhuede, P. I., Scott, K., Hu, J., Jankowsky, J. L., Quick, M. W., et al. (2009). GABA transporter function, oligomerization state, and anchoring: correlates with subcellularly resolved FRET. J. Gen. Physiol. 134, 489–521. doi: 10.1085/jgp.200910314
Moszczynska, A., Saleh, J., Zhang, H., Vukusic, B., Lee, F. J., and Liu, F. (2007). Parkin disrupts the alpha-synuclein/dopamine transporter interaction: consequences toward dopamine-induced toxicity. J. Mol. Neurosci. 32, 217–227. doi: 10.1007/s12031-007-0037-0
Mukainaka, Y., Tanaka, K., Hagiwara, T., and Wada, K. (1995). Molecular cloning of two glutamate transporter subtypes from mouse brain. Biochim. Biophys. Acta 1244, 233–237. doi: 10.1016/0304-4165(95)00062-g
Mundorf, M. L., Hochstetler, S. E., and Wightman, R. M. (1999). Amine weak bases disrupt vesicular storage and promote exocytosis in chromaffin cells. J. Neurochem. 73, 2397–2405. doi: 10.1046/j.1471-4159.1999.0732397.x
Murphy-Royal, C., Dupuis, J. P., Varela, J. A., Panatier, A., Pinson, B., Baufreton, J., et al. (2015). Surface diffusion of astrocytic glutamate transporters shapes synaptic transmission. Nat. Neurosci. 18, 219–226. doi: 10.1038/nn.3901
Nakayama, T., Kawakami, H., Tanaka, K., and Nakamura, S. (1996). Expression of three glutamate transporter subtype mRNAs in human brain regions and peripheral tissues. Brain Res. Mol. Brain Res. 36, 189–192. doi: 10.1016/0169-328x(95)00297-6
Navaroli, D. M., Stevens, Z. H., Uzelac, Z., Gabriel, L., King, M. J., Lifshitz, L. M., et al. (2011). The plasma membrane-associated GTPase Rin interacts with the dopamine transporter and is required for protein kinase C-regulated dopamine transporter trafficking. J. Neurosci. 31, 13758–13770. doi: 10.1523/jneurosci.2649-11.2011
Nirenberg, M. J., Chan, J., Pohorille, A., Vaughan, R. A., Uhl, G. R., Kuhar, M. J., et al. (1997). The dopamine transporter: comparative ultrastructure of dopaminergic axons in limbic and motor compartments of the nucleus accumbens. J. Neurosci. 17, 6899–6907. doi: 10.1523/jneurosci.17-18-06899.1997
Norregaard, L., and Gether, U. (2001). The monoamine neurotransmitter transporters: structure, conformational changes and molecular gating. Curr. Opin. Drug Discov. Dev. 4, 591–601.
O’Donovan, S. M., Sullivan, C. R., and McCullumsmith, R. E. (2017). The role of glutamate transporters in the pathophysiology of neuropsychiatric disorders. NPJ Schizophr. 3:32.
Otis, T. S., and Kavanaugh, M. P. (2000). Isolation of current components and partial reaction cycles in the glial glutamate transporter EAAT2. J. Neurosci. 20, 2749–2757. doi: 10.1523/jneurosci.20-08-02749.2000
Padovano, V., Massari, S., Mazzucchelli, S., and Pietrini, G. (2009). PKC induces internalization and retention of the EAAC1 glutamate transporter in recycling endosomes of MDCK cells. Am. J. Physiol. Cell Physiol. 297, C835–C844.
Page, G., Barc-Pain, S., Pontcharraud, R., Cante, A., Piriou, A., and Barrier, L. (2004). The up-regulation of the striatal dopamine transporter’s activity by cAMP is PKA-, CaMK II- and phosphatase-dependent. Neurochem. Int. 45, 627–632. doi: 10.1016/j.neuint.2004.04.002
Pakulska, W. (2007). Influence of tiagabine on the antinociceptive action of morphine, metamizole and indomethacin in mice. Acta Pol. Pharm. 64, 263–270.
Palos, T. P., Ramachandran, B., Boado, R., and Howard, B. D. (1996). Rat C6 and human astrocytic tumor cells express a neuronal type of glutamate transporter. Brain Res. Mol. Brain Res. 37, 297–303. doi: 10.1016/0169-328x(95)00331-l
Parinejad, N., Peco, E., Ferreira, T., Stacey, S. M., and van Meyel, D. J. (2016). Disruption of an EAAT-mediated chloride channel in a Drosophila model of Ataxia. J. Neurosci. 36, 7640–7647. doi: 10.1523/jneurosci.0197-16.2016
Park, H. Y., Kim, J. H., Zuo, Z., and Do, S. H. (2008). Ethanol increases the activity of rat excitatory amino acid transporter type 4 expressed in Xenopus oocytes: role of protein kinase C and phosphatidylinositol 3-kinase. Alcohol. Clin. Exp. Res. 32, 348–354. doi: 10.1111/j.1530-0277.2007.00577.x
Park, S. J., Shin, H. J., Gu, B. W., Woo, K. I., Zuo, Z., Do, S. H., et al. (2015). Desflurane increased the activity of excitatory amino-acid carrier 1 (EAAC1) expressed in Xenopus oocytes. Eur. J. Pharmacol. 757, 84–89. doi: 10.1016/j.ejphar.2015.03.058
Parker, E. M., and Cubeddu, L. X. (1988). Comparative effects of amphetamine, phenylethylamine and related drugs on dopamine efflux, dopamine uptake and mazindol binding. J. Pharmacol. Exp. Ther. 245, 199–210.
Parpura, V., Fang, Y., Basarsky, T., Jahn, R., and Haydon, P. G. (1995). Expression of synaptobrevin II, cellubrevin and syntaxin but not SNAP-25 in cultured astrocytes. FEBS Lett. 377, 489–492. doi: 10.1016/0014-5793(95)01401-2
Patrushev, I., Gavrilov, N., Turlapov, V., and Semyanov, A. (2013). Subcellular location of astrocytic calcium stores favors extrasynaptic neuron-astrocyte communication. Cell Calc. 54, 343–349. doi: 10.1016/j.ceca.2013.08.003
Peacey, E., Miller, C. C., Dunlop, J., and Rattray, M. (2009). The four major N- and C-terminal splice variants of the excitatory amino acid transporter GLT-1 form cell surface homomeric and heteromeric assemblies. Mol. Pharmacol. 75, 1062–1073. doi: 10.1124/mol.108.052829
Peghini, P., Janzen, J., and Stoffel, W. (1997). Glutamate transporter EAAC-1-deficient mice develop dicarboxylic aminoaciduria and behavioral abnormalities but no neurodegeneration. EMBO J. 16, 3822–3832. doi: 10.1093/emboj/16.13.3822
Penmatsa, A., Wang, K. H., and Gouaux, E. (2013). X-ray structure of dopamine transporter elucidates antidepressant mechanism. Nature 503, 85–90. doi: 10.1038/nature12533
Penmatsa, A., Wang, K. H., and Gouaux, E. (2015). X-ray structures of Drosophila dopamine transporter in complex with Nisoxetine and Reboxetine. Nat. Struct. Mol. Biol. 22, 506–508. doi: 10.1038/nsmb.3029
Perego, C., Di Cairano, E. S., Ballabio, M., and Magnaghi, V. (2012). Neurosteroid allopregnanolone regulates EAAC1-mediated glutamate uptake and triggers actin changes in Schwann cells. J. Cell Physiol. 227, 1740–1751. doi: 10.1002/jcp.22898
Perez-Alvarez, A., Navarrete, M., Covelo, A., Martin, E. D., and Araque, A. (2014). Structural and functional plasticity of astrocyte processes and dendritic spine interactions. J. Neurosci. 34, 12738–12744. doi: 10.1523/jneurosci.2401-14.2014
Petr, G. T., Sun, Y., Frederick, N. M., Zhou, Y., Dhamne, S. C., Hameed, M. Q., et al. (2015). Conditional deletion of the glutamate transporter GLT-1 reveals that astrocytic GLT-1 protects against fatal epilepsy while neuronal GLT-1 contributes significantly to glutamate uptake into synaptosomes. J. Neurosci. 35, 5187–5201. doi: 10.1523/jneurosci.4255-14.2015
Piani, D., Frei, K., Pfister, H. W., and Fontana, A. (1993). Glutamate uptake by astrocytes is inhibited by reactive oxygen intermediates but not by other macrophage-derived molecules including cytokines, leukotrienes or platelet-activating factor. J. Neuroimmunol. 48, 99–104. doi: 10.1016/0165-5728(93)90063-5
Picaud, S., Larsson, H. P., Wellis, D. P., Lecar, H., and Werblin, F. (1995). Cone photoreceptors respond to their own glutamate release in the tiger salamander. Proc. Natl. Acad. Sci. U.S.A. 92, 9417–9421. doi: 10.1073/pnas.92.20.9417
Pines, G., Danbolt, N. C., Bjoras, M., Zhang, Y., Bendahan, A., Eide, L., et al. (1992). Cloning and expression of a rat brain L-glutamate transporter. Nature 360, 464–467.
Plachez, C., Danbolt, N. C., and Recasens, M. (2000). Transient expression of the glial glutamate transporters GLAST and GLT in hippocampal neurons in primary culture. J. Neurosci. Res. 59, 587–593. doi: 10.1002/(sici)1097-4547(20000301)59:5<587::aid-jnr1>3.0.co;2-l
Pogun, S., Dawson, V., and Kuhar, M. J. (1994). Nitric oxide inhibits 3H-glutamate transport in synaptosomes. Synapse 18, 21–26. doi: 10.1002/syn.890180104
Pogun, S., and Kuhar, M. J. (1994). Regulation of neurotransmitter reuptake by nitric oxide. Ann. N.Y. Acad. Sci. 738, 305–315. doi: 10.1111/j.1749-6632.1994.tb21816.x
Porton, B., Greenberg, B. D., Askland, K., Serra, L. M., Gesmonde, J., Rudnick, G., et al. (2013). Isoforms of the neuronal glutamate transporter gene, SLC1A1/EAAC1, negatively modulate glutamate uptake: relevance to obsessive-compulsive disorder. Transl. Psychiatry 3:e259. doi: 10.1038/tp.2013.35
Pristupa, Z. B., McConkey, F., Liu, F., Man, H. Y., Lee, F. J., Wang, Y. T., et al. (1998). Protein kinase-mediated bidirectional trafficking and functional regulation of the human dopamine transporter. Synapse 30, 79–87. doi: 10.1002/(sici)1098-2396(199809)30:1<79::aid-syn10>3.0.co;2-k
Quick, M. W., Corey, J. L., Davidson, N., and Lester, H. A. (1997). Second messengers, trafficking-related proteins, and amino acid residues that contribute to the functional regulation of the rat brain GABA transporter GAT1. J. Neurosci. 17, 2967–2979. doi: 10.1523/jneurosci.17-09-02967.1997
Radian, R., and Kanner, B. I. (1983). Stoichiometry of sodium- and chloride-coupled gamma-aminobutyric acid transport by synaptic plasma membrane vesicles isolated from rat brain. Biochemistry 22, 1236–1241. doi: 10.1021/bi00274a038
Raiteri, L., Stigliani, S., Zedda, L., Raiteri, M., and Bonanno, G. (2002). Multiple mechanisms of transmitter release evoked by “pathologically” elevated extracellular [K+]: involvement of transporter reversal and mitochondrial calcium. J. Neurochem. 80, 706–714. doi: 10.1046/j.0022-3042.2001.00750.x
Raiteri, M., Cerrito, F., Cervoni, A. M., and Levi, G. (1979). Dopamine can be released by two mechanisms differentially affected by the dopamine transport inhibitor nomifensine. J. Pharmacol. Exp. Ther. 208, 195–202.
Rauen, T., and Kanner, B. I. (1994). Localization of the glutamate transporter GLT-1 in rat and macaque monkey retinae. Neurosci. Lett. 169, 137–140. doi: 10.1016/0304-3940(94)90375-1
Rauen, T., Wiessner, M., Sullivan, R., Lee, A., and Pow, D. V. (2004). A new GLT1 splice variant: cloning and immunolocalization of GLT1c in the mammalian retina and brain. Neurochem. Int. 45, 1095–1106. doi: 10.1016/j.neuint.2004.04.006
Reyes, N., Ginter, C., and Boudker, O. (2009). Transport mechanism of a bacterial homologue of glutamate transporters. Nature 462, 880–885. doi: 10.1038/nature08616
Richerson, G. B., and Wu, Y. (2004). Role of the GABA transporter in epilepsy. Adv. Exp. Med. Biol. 548, 76–91. doi: 10.1007/978-1-4757-6376-8_6
Rimmele, T. S., and Rosenberg, P. A. (2016). GLT-1: the elusive presynaptic glutamate transporter. Neurochem. Int. 98, 19–28. doi: 10.1016/j.neuint.2016.04.010
Rivera, C., Voipio, J., Payne, J. A., Ruusuvuori, E., Lahtinen, H., Lamsa, K., et al. (1999). The K+/Cl- co-transporter KCC2 renders GABA hyperpolarizing during neuronal maturation. Nature 397, 251–255. doi: 10.1038/16697
Roberts, B. M., Doig, N. M., Brimblecombe, K. R., Lopes, E. F., Siddorn, R. E., Threlfell, S., et al. (2020). GABA uptake transporters support dopamine release in dorsal striatum with maladaptive downregulation in a parkinsonism model. Nat. Commun. 11:4958.
Roberts, J. G., Lugo-Morales, L. Z., Loziuk, P. L., and Sombers, L. A. (2013). Real-time chemical measurements of dopamine release in the brain. Methods Mol. Biol. 964, 275–294. doi: 10.1007/978-1-62703-251-3_16
Robinson, M. B. (2002). Regulated trafficking of neurotransmitter transporters: common notes but different melodies. J. Neurochem. 80, 1–11. doi: 10.1046/j.0022-3042.2001.00698.x
Rodriguez-Traver, E., Solis, O., Diaz-Guerra, E., Ortiz, O., Vergano-Vera, E., Mendez-Gomez, H. R., et al. (2016). Role of Nurr1 in the generation and differentiation of dopaminergic neurons from stem cells. Neurotox Res. 30, 14–31. doi: 10.1007/s12640-015-9586-0
Roginski, R., Choudhury, K., Marone, M., and Geller, H. (1993). Molecular characterization and expression of a rat-brain glutamate transporter CDNA. Anesthesiology 79:A787.
Romero, J., de Miguel, R., Ramos, J. A., and Fernandez-Ruiz, J. J. (1998). The activation of cannabinoid receptors in striatonigral GABAergic neurons inhibited GABA uptake. Life Sci. 62, 351–363. doi: 10.1016/s0024-3205(97)01117-x
Rosenblum, L. T., and Trotti, D. (2017). EAAT2 and the molecular signature of amyotrophic lateral sclerosis. Adv. Neurobiol. 16, 117–136. doi: 10.1007/978-3-319-55769-4_6
Rothstein, J. D., Dykes-Hoberg, M., Pardo, C. A., Bristol, L. A., Jin, L., Kuncl, R. W., et al. (1996). Knockout of glutamate transporters reveals a major role for astroglial transport in excitotoxicity and clearance of glutamate. Neuron 16, 675–686. doi: 10.1016/s0896-6273(00)80086-0
Rothstein, J. D., Martin, L., Levey, A. I., Dykes-Hoberg, M., Jin, L., Wu, D., et al. (1994). Localization of neuronal and glial glutamate transporters. Neuron 13, 713–725. doi: 10.1016/0896-6273(94)90038-8
Rothstein, J. D., Van Kammen, M., Levey, A. I., Martin, L. J., and Kuncl, R. W. (1995). Selective loss of glial glutamate transporter GLT-1 in amyotrophic lateral sclerosis. Ann. Neurol. 38, 73–84. doi: 10.1002/ana.410380114
Ryan, R. M., and Mindell, J. A. (2007). The uncoupled chloride conductance of a bacterial glutamate transporter homolog. Nat. Struct. Mol. Biol. 14, 365–371. doi: 10.1038/nsmb1230
Ryan, R. M., Mitrovic, A. D., and Vandenberg, R. J. (2004). The chloride permeation pathway of a glutamate transporter and its proximity to the glutamate translocation pathway. J. Biol. Chem. 279, 20742–20751. doi: 10.1074/jbc.m304433200
Ryan, R. M., and Vandenberg, R. J. (2016). Elevating the alternating-access model. Nat. Struct. Mol. Biol. 23, 187–189. doi: 10.1038/nsmb.3179
Sacchetti, P., Brownschidle, L. A., Granneman, J. G., and Bannon, M. J. (1999). Characterization of the 5′-flanking region of the human dopamine transporter gene. Brain Res. Mol. Brain Res. 74, 167–174. doi: 10.1016/s0169-328x(99)00275-2
Sakrikar, D., Mazei-Robison, M. S., Mergy, M. A., Richtand, N. W., Han, Q., Hamilton, P. J., et al. (2012). Attention deficit/hyperactivity disorder-derived coding variation in the dopamine transporter disrupts microdomain targeting and trafficking regulation. J. Neurosci. 32, 5385–5397. doi: 10.1523/jneurosci.6033-11.2012
Saksela, K., and Permi, P. (2012). SH3 domain ligand binding: what’s the consensus and where’s the specificity? FEBS Lett. 586, 2609–2614. doi: 10.1016/j.febslet.2012.04.042
Salat, K., and Kulig, K. (2011). GABA transporters as targets for new drugs. Future Med. Chem. 3, 211–222. doi: 10.4155/fmc.10.298
Salat, K., Podkowa, A., Malikowska, N., Kern, F., Pabel, J., Wojcieszak, E., et al. (2017). Novel, highly potent and in vivo active inhibitor of GABA transporter subtype 1 with anticonvulsant, anxiolytic, antidepressant and antinociceptive properties. Neuropharmacology 113, 331–342. doi: 10.1016/j.neuropharm.2016.10.019
Salemi, S., Baktash, P., Rajaei, B., Noori, M., Amini, H., Shamsara, M., et al. (2016). Efficient generation of dopaminergic-like neurons by overexpression of Nurr1 and Pitx3 in mouse induced pluripotent stem cells. Neurosci. Lett. 626, 126–134. doi: 10.1016/j.neulet.2016.05.032
Sandoval, V., Riddle, E. L., Ugarte, Y. V., Hanson, G. R., and Fleckenstein, A. E. (2001). Methamphetamine-induced rapid and reversible changes in dopamine transporter function: an in vitro model. J. Neurosci. 21, 1413–1419. doi: 10.1523/jneurosci.21-04-01413.2001
Saucedo-Cardenas, O., Quintana-Hau, J. D., Le, W. D., Smidt, M. P., Cox, J. J., De Mayo, F., et al. (1998). Nurr1 is essential for the induction of the dopaminergic phenotype and the survival of ventral mesencephalic late dopaminergic precursor neurons. Proc. Natl. Acad. Sci. U.S.A. 95, 4013–4018. doi: 10.1073/pnas.95.7.4013
Saunders, C., Ferrer, J. V., Shi, L., Chen, J., Merrill, G., Lamb, M. E., et al. (2000). Amphetamine-induced loss of human dopamine transporter activity: an internalization-dependent and cocaine-sensitive mechanism. Proc. Natl. Acad. Sci. U.S.A. 97, 6850–6855. doi: 10.1073/pnas.110035297
Schallier, A., Smolders, I., Van Dam, D., Loyens, E., De Deyn, P. P., Michotte, A., et al. (2011). Region- and age-specific changes in glutamate transport in the AbetaPP23 mouse model for Alzheimer’s disease. J. Alzheimers Dis. 24, 287–300. doi: 10.3233/jad-2011-101005
Schikorski, T., and Stevens, C. F. (1997). Quantitative ultrastructural analysis of hippocampal excitatory synapses. J. Neurosci. 17, 5858–5867. doi: 10.1523/jneurosci.17-15-05858.1997
Schitine, C. S., Mendez-Flores, O. G., Santos, L. E., Ornelas, I., Calaza, K. C., Perez-Toledo, K., et al. (2015). Functional plasticity of GAT-3 in avian Muller cells is regulated by neurons via a glutamatergic input. Neurochem. Int. 82, 42–51. doi: 10.1016/j.neuint.2015.02.004
Schmitt, A., Asan, E., Lesch, K. P., and Kugler, P. (2002). A splice variant of glutamate transporter GLT1/EAAT2 expressed in neurons: cloning and localization in rat nervous system. Neuroscience 109, 45–61. doi: 10.1016/s0306-4522(01)00451-1
Schmitt, A., Asan, E., Puschel, B., Jons, T., and Kugler, P. (1996). Expression of the glutamate transporter GLT1 in neural cells of the rat central nervous system: non-radioactive in situ hybridization and comparative immunocytochemistry. Neuroscience 71, 989–1004. doi: 10.1016/0306-4522(95)00477-7
Schmitt, K. C., Rothman, R. B., and Reith, M. E. (2013). Nonclassical pharmacology of the dopamine transporter: atypical inhibitors, allosteric modulators, and partial substrates. J. Pharmacol. Exp. Ther. 346, 2–10. doi: 10.1124/jpet.111.191056
Schoffelmeer, A. N., Drukarch, B., De Vries, T. J., Hogenboom, F., Schetters, D., and Pattij, T. (2011). Insulin modulates cocaine-sensitive monoamine transporter function and impulsive behavior. J. Neurosci. 31, 1284–1291. doi: 10.1523/jneurosci.3779-10.2011
Schwartz, M. D., Canales, J. J., Zucchi, R., Espinoza, S., Sukhanov, I., and Gainetdinov, R. R. (2018). Trace amine-associated receptor 1: a multimodal therapeutic target for neuropsychiatric diseases. Expert Opin. Ther. Targets 22, 513–526. doi: 10.1080/14728222.2018.1480723
Schwerdt, H. N., Zhang, E., Kim, M. J., Yoshida, T., Stanwicks, L., Amemori, S., et al. (2018). Cellular-scale probes enable stable chronic subsecond monitoring of dopamine neurochemicals in a rodent model. Commun. Biol. 1:144.
Scimemi, A. (2014a). Plasticity of GABA transporters: an unconventional route to shape inhibitory synaptic transmission. Front. Cell Neurosci. 8:128. doi: 10.3389/fncel.2014.00128
Scimemi, A. (2014b). Structure, function, and plasticity of GABA transporters. Front. Cell. Neurosci. 8:161. doi: 10.3389/fncel.2014.00161
Scimemi, A., Fine, A., Kullmann, D. M., and Rusakov, D. A. (2004). NR2B-containing receptors mediate cross talk among hippocampal synapses. J. Neurosci. 24, 4767–4777. doi: 10.1523/jneurosci.0364-04.2004
Scimemi, A., Meabon, J. S., Woltjer, R. L., Sullivan, J. M., Diamond, J. S., and Cook, D. G. (2013). Amyloid-beta1-42 slows clearance of synaptically released glutamate by mislocalizing astrocytic GLT-1. J. Neurosci. 33, 5312–5318. doi: 10.1523/jneurosci.5274-12.2013
Scimemi, A., Semyanov, A., Sperk, G., Kullmann, D. M., and Walker, M. C. (2005). Multiple and plastic receptors mediate tonic GABA(A) receptor currents in the hippocampus. J. Neurosci. 25, 10016–10024. doi: 10.1523/jneurosci.2520-05.2005
Scimemi, A., Tian, H., and Diamond, J. S. (2009). Neuronal transporters regulate glutamate clearance, NMDA receptor activation, and synaptic plasticity in the hippocampus. J. Neurosci. 29, 14581–14595. doi: 10.1523/jneurosci.4845-09.2009
Scopelliti, A. J., Font, J., Vandenberg, R. J., Boudker, O., and Ryan, R. M. (2018). Structural characterisation reveals insights into substrate recognition by the glutamine transporter ASCT2/SLC1A5. Nat. Commun. 9:38.
Scott, H. A., Gebhardt, F. M., Mitrovic, A. D., Vandenberg, R. J., and Dodd, P. R. (2011). Glutamate transporter variants reduce glutamate uptake in Alzheimer’s disease. Neurobiol. Aging 32, 553.e551–e511.
Seal, R. P., and Amara, S. G. (1999). Excitatory amino acid transporters: a family in flux. Annu. Rev. Pharmacol. Toxicol. 39, 431–456. doi: 10.1146/annurev.pharmtox.39.1.431
Seal, R. P., Shigeri, Y., Eliasof, S., Leighton, B. H., and Amara, S. G. (2001). Sulfhydryl modification of V449C in the glutamate transporter EAAT1 abolishes substrate transport but not the substrate-gated anion conductance. Proc. Natl. Acad. Sci. U.S.A. 98, 15324–15329. doi: 10.1073/pnas.011400198
Serretti, A., and Mandelli, L. (2008). The genetics of bipolar disorder: genome ‘hot regions,’ genes, new potential candidates and future directions. Mol. Psychiatry 13, 742–771. doi: 10.1038/mp.2008.29
Sharma, A., Kazim, S. F., Larson, C. S., Ramakrishnan, A., Gray, J. D., McEwen, B. S., et al. (2019). Divergent roles of astrocytic versus neuronal EAAT2 deficiency on cognition and overlap with aging and Alzheimer’s molecular signatures. Proc. Natl. Acad. Sci. U.S.A. 116, 21800–21811. doi: 10.1073/pnas.1903566116
Sheldon, A. L., Gonzalez, M. I., Krizman-Genda, E. N., Susarla, B. T., and Robinson, M. B. (2008). Ubiquitination-mediated internalization and degradation of the astroglial glutamate transporter, GLT-1. Neurochem. Int. 53, 296–308. doi: 10.1016/j.neuint.2008.07.010
Shifman, M. (1991). The effect of gangliosides upon recovery of aspartate/glutamatergic synapses in striatum after lesions of the rat sensorimotor cortex. Brain Res. 568, 323–324. doi: 10.1016/0006-8993(91)91419-2
Shin, J. W., Nguyen, K. T., Pow, D. V., Knight, T., Buljan, V., Bennett, M. R., et al. (2009). Distribution of glutamate transporter GLAST in membranes of cultured astrocytes in the presence of glutamate transport substrates and ATP. Neurochem. Res. 34, 1758–1766. doi: 10.1007/s11064-009-9982-z
Sieber, S. A., and Marahiel, M. A. (2005). Molecular mechanisms underlying nonribosomal peptide synthesis: approaches to new antibiotics. Chem. Rev. 105, 715–738. doi: 10.1021/cr0301191
Sims, K. D., Straff, D. J., and Robinson, M. B. (2000). Platelet-derived growth factor rapidly increases activity and cell surface expression of the EAAC1 subtype of glutamate transporter through activation of phosphatidylinositol 3-kinase. J. Biol. Chem. 275, 5228–5237. doi: 10.1074/jbc.275.7.5228
Sitcheran, R., Gupta, P., Fisher, P. B., and Baldwin, A. S. (2005). Positive and negative regulation of EAAT2 by NF-kappaB: a role for N-myc in TNFalpha-controlled repression. EMBO J. 24, 510–520. doi: 10.1038/sj.emboj.7600555
Sitte, H. H., Huck, S., Reither, H., Boehm, S., Singer, E. A., and Pifl, C. (1998). Carrier-mediated release, transport rates, and charge transfer induced by amphetamine, tyramine, and dopamine in mammalian cells transfected with the human dopamine transporter. J. Neurochem. 71, 1289–1297. doi: 10.1046/j.1471-4159.1998.71031289.x
Sogaard, R., Borre, L., Braunstein, T. H., Madsen, K. L., and MacAulay, N. (2013). Functional modulation of the glutamate transporter variant GLT1b by the PDZ domain protein PICK1. J. Biol. Chem. 288, 20195–20207. doi: 10.1074/jbc.m113.471128
Sonders, M. S., and Amara, S. G. (1996). Channels in transporters. Curr. Opin. Neurobiol. 6, 294–302.
Sonders, M. S., Zhu, S. J., Zahniser, N. R., Kavanaugh, M. P., and Amara, S. G. (1997). Multiple ionic conductances of the human dopamine transporter: the actions of dopamine and psychostimulants. J. Neurosci. 17, 960–974. doi: 10.1523/jneurosci.17-03-00960.1997
Sorkina, T., Doolen, S., Galperin, E., Zahniser, N. R., and Sorkin, A. (2003). Oligomerization of dopamine transporters visualized in living cells by fluorescence resonance energy transfer microscopy. J. Biol. Chem. 278, 28274–28283. doi: 10.1074/jbc.m210652200
Sorkina, T., Hoover, B. R., Zahniser, N. R., and Sorkin, A. (2005). Constitutive and protein kinase C-induced internalization of the dopamine transporter is mediated by a clathrin-dependent mechanism. Traffic 6, 157–170. doi: 10.1111/j.1600-0854.2005.00259.x
Stenovec, M., Kreft, M., Grilc, S., Pangrsic, T., and Zorec, R. (2008). EAAT2 density at the astrocyte plasma membrane and Ca(2 +)-regulated exocytosis. Mol. Membr. Biol. 25, 203–215. doi: 10.1080/09687680701790925
Stoffel, W., Sasse, J., Duker, M., Muller, R., Hofmann, K., Fink, T., et al. (1996). Human high affinity, Na(+)-dependent L-glutamate/L-aspartate transporter GLAST-1 (EAAT-1): gene structure and localization to chromosome 5p11-p12. FEBS Lett. 386, 189–193. doi: 10.1016/0014-5793(96)00424-3
Su, Z. Z., Leszczyniecka, M., Kang, D. C., Sarkar, D., Chao, W., Volsky, D. J., et al. (2003). Insights into glutamate transport regulation in human astrocytes: cloning of the promoter for excitatory amino acid transporter 2 (EAAT2). Proc. Natl. Acad. Sci. U.S.A. 100, 1955–1960. doi: 10.1073/pnas.0136555100
Sullivan, R., Rauen, T., Fischer, F., Wiessner, M., Grewer, C., Bicho, A., et al. (2004). Cloning, transport properties, and differential localization of two splice variants of GLT-1 in the rat CNS: implications for CNS glutamate homeostasis. Glia 45, 155–169. doi: 10.1002/glia.10317
Sulzer, D., Chen, T. K., Lau, Y. Y., Kristensen, H., Rayport, S., and Ewing, A. (1995). Amphetamine redistributes dopamine from synaptic vesicles to the cytosol and promotes reverse transport. J. Neurosci. 15, 4102–4108. doi: 10.1523/jneurosci.15-05-04102.1995
Sulzer, D., Maidment, N. T., and Rayport, S. (1993). Amphetamine and other weak bases act to promote reverse transport of dopamine in ventral midbrain neurons. J. Neurochem. 60, 527–535. doi: 10.1111/j.1471-4159.1993.tb03181.x
Susarla, B. T., and Robinson, M. B. (2003). Rottlerin, an inhibitor of protein kinase Cdelta (PKCdelta), inhibits astrocytic glutamate transport activity and reduces GLAST immunoreactivity by a mechanism that appears to be PKCdelta-independent. J. Neurochem. 86, 635–645. doi: 10.1046/j.1471-4159.2003.01886.x
Susarla, B. T., and Robinson, M. B. (2008). Internalization and degradation of the glutamate transporter GLT-1 in response to phorbol ester. Neurochem. Int. 52, 709–722. doi: 10.1016/j.neuint.2007.08.020
Susarla, B. T., Seal, R. P., Zelenaia, O., Watson, D. J., Wolfe, J. H., Amara, S. G., et al. (2004). Differential regulation of GLAST immunoreactivity and activity by protein kinase C: evidence for modification of amino and carboxyl termini. J. Neurochem. 91, 1151–1163. doi: 10.1111/j.1471-4159.2004.02791.x
Swanson, R. A., Liu, J., Miller, J. W., Rothstein, J. D., Farrell, K., Stein, B. A., et al. (1997). Neuronal regulation of glutamate transporter subtype expression in astrocytes. J. Neurosci. 17, 932–940. doi: 10.1523/jneurosci.17-03-00932.1997
Sylantyev, S., Savtchenko, L. P., O’Neill, N., and Rusakov, D. A. (2020). Extracellular GABA waves regulate coincidence detection in excitatory circuits. J. Physiol. 598, 4047–4062. doi: 10.1113/jp279744
Tai, Y. H., Wang, Y. H., Tsai, R. Y., Wang, J. J., Tao, P. L., Liu, T. M., et al. (2007). Amitriptyline preserves morphine’s antinociceptive effect by regulating the glutamate transporter GLAST and GLT-1 trafficking and excitatory amino acids concentration in morphine-tolerant rats. Pain 129, 343–354. doi: 10.1016/j.pain.2007.01.031
Tan, J., Zelenaia, O., Correale, D., Rothstein, J. D., and Robinson, M. B. (1999). Expression of the GLT-1 subtype of Na+-dependent glutamate transporter: pharmacological characterization and lack of regulation by protein kinase C. J. Pharmacol. Exp. Ther. 289, 1600–1610.
Tanaka, K. (1993). Cloning and expression of a glutamate transporter from mouse brain. Neurosci. Lett. 159, 183–186. doi: 10.1016/0304-3940(93)90829-a
Tebano, M. T., Martire, A., Potenza, R. L., Gro, C., Pepponi, R., Armida, M., et al. (2008). Adenosine A(2A) receptors are required for normal BDNF levels and BDNF-induced potentiation of synaptic transmission in the mouse hippocampus. J. Neurochem. 104, 279–286.
Tian, Y., Kapatos, G., Granneman, J. G., and Bannon, M. J. (1994). Dopamine and gamma-aminobutyric acid transporters: differential regulation by agents that promote phosphorylation. Neurosci. Lett. 173, 143–146. doi: 10.1016/0304-3940(94)90169-4
Todorov, A. A., Kolchev, C. B., and Todorov, A. B. (2005). Tiagabine and gabapentin for the management of chronic pain. Clin. J. Pain 21, 358–361. doi: 10.1097/01.ajp.0000110637.14355.77
Tonsfeldt, K. J., Suchland, K. L., Beeson, K. A., Lowe, J. D., Li, M. H., and Ingram, S. L. (2016). Sex Differences in GABAA signaling in the periaqueductal gray induced by persistent inflammation. J. Neurosci. 36, 1669–1681. doi: 10.1523/jneurosci.1928-15.2016
Torp, R., Danbolt, N. C., Babaie, E., Bjoras, M., Seeberg, E., Storm-Mathisen, J., et al. (1994). Differential expression of two glial glutamate transporters in the rat brain: an in situ hybridization study. Eur. J. Neurosci. 6, 936–942. doi: 10.1111/j.1460-9568.1994.tb00587.x
Torp, R., Hoover, F., Danbolt, N. C., Storm-Mathisen, J., and Ottersen, O. P. (1997). Differential distribution of the glutamate transporters GLT1 and rEAAC1 in rat cerebral cortex and thalamus: an in situ hybridization analysis. Anat. Embryol. 195, 317–326. doi: 10.1007/s004290050051
Torres, G. E., Gainetdinov, R. R., and Caron, M. G. (2003). Plasma membrane monoamine transporters: structure, regulation and function. Nat. Rev. Neurosci. 4, 13–25. doi: 10.1038/nrn1008
Torres, G. E., Yao, W. D., Mohn, A. R., Quan, H., Kim, K. M., Levey, A. I., et al. (2001). Functional interaction between monoamine plasma membrane transporters and the synaptic PDZ domain-containing protein PICK1. Neuron 30, 121–134. doi: 10.1016/s0896-6273(01)00267-7
Torres-Salazar, D., and Fahlke, C. (2007). Neuronal glutamate transporters vary in substrate transport rate but not in unitary anion channel conductance. J. Biol. Chem. 282, 34719–34726. doi: 10.1074/jbc.m704118200
Trotti, D., Peng, J. B., Dunlop, J., and Hediger, M. A. (2001). Inhibition of the glutamate transporter EAAC1 expressed in Xenopus oocytes by phorbol esters. Brain Res. 914, 196–203. doi: 10.1016/s0006-8993(01)02802-5
Trotti, D., Volterra, A., Lehre, K. P., Rossi, D., Gjesdal, O., Racagni, G., et al. (1995). Arachidonic acid inhibits a purified and reconstituted glutamate transporter directly from the water phase and not via the phospholipid membrane. J. Biol. Chem. 270, 9890–9895. doi: 10.1074/jbc.270.17.9890
Tzingounis, A. V., and Wadiche, J. I. (2007). Glutamate transporters: confining runaway excitation by shaping synaptic transmission. Nat. Rev. Neurosci. 8, 935–947. doi: 10.1038/nrn2274
Underhill, S. M., Ingram, S. L., Ahmari, S. E., Veenstra-VanderWeele, J., and Amara, S. G. (2019). Neuronal excitatory amino acid transporter EAAT3: emerging functions in health and disease. Neurochem. Int. 123, 69–76. doi: 10.1016/j.neuint.2018.05.012
Underhill, S. M., Wheeler, D. S., and Amara, S. G. (2015). Differential regulation of two isoforms of the glial glutamate transporter EAAT2 by DLG1 and CaMKII. J. Neurosci. 35, 5260–5270. doi: 10.1523/jneurosci.4365-14.2015
Underhill, S. M., Wheeler, D. S., Li, M., Watts, S. D., Ingram, S. L., and Amara, S. G. (2014). Amphetamine modulates excitatory neurotransmission through endocytosis of the glutamate transporter EAAT3 in dopamine neurons. Neuron 83, 404–416. doi: 10.1016/j.neuron.2014.05.043
Untiet, V., Kovermann, P., Gerkau, N. J., Gensch, T., Rose, C. R., and Fahlke, C. (2017). Glutamate transporter-associated anion channels adjust intracellular chloride concentrations during glial maturation. Glia 65, 388–400. doi: 10.1002/glia.23098
Vandenbergh, D. J., Persico, A. M., Hawkins, A. L., Griffin, C. A., Li, X., Jabs, E. W., et al. (1992a). Human dopamine transporter gene (DAT1) maps to chromosome 5p15.3 and displays a VNTR. Genomics 14, 1104–1106. doi: 10.1016/s0888-7543(05)80138-7
Vandenbergh, D. J., Persico, A. M., and Uhl, G. R. (1992b). A human dopamine transporter cDNA predicts reduced glycosylation, displays a novel repetitive element and provides racially-dimorphic TaqI RFLPs. Brain Res. Mol. Brain Res. 15, 161–166. doi: 10.1016/0169-328x(92)90165-8
Vaughan, R. A., Huff, R. A., Uhl, G. R., and Kuhar, M. J. (1997). Protein kinase C-mediated phosphorylation and functional regulation of dopamine transporters in striatal synaptosomes. J. Biol. Chem. 272, 15541–15546. doi: 10.1074/jbc.272.24.15541
Vaz, S. H., Cristovao-Ferreira, S., Ribeiro, J. A., and Sebastiao, A. M. (2008). Brain-derived neurotrophic factor inhibits GABA uptake by the rat hippocampal nerve terminals. Brain Res. 1219, 19–25. doi: 10.1016/j.brainres.2008.04.008
Vaz, S. H., Jorgensen, T. N., Cristovao-Ferreira, S., Duflot, S., Ribeiro, J. A., Gether, U., et al. (2011). Brain-derived neurotrophic factor (BDNF) enhances GABA transport by modulating the trafficking of GABA transporter-1 (GAT-1) from the plasma membrane of rat cortical astrocytes. J. Biol. Chem. 286, 40464–40476. doi: 10.1074/jbc.m111.232009
Venderova, K., Brown, T. M., and Brotchie, J. M. (2005). Differential effects of endocannabinoids on [(3)H]-GABA uptake in the rat Globus pallidus. Exp. Neurol. 194, 284–287. doi: 10.1016/j.expneurol.2005.02.012
Ventura, R., and Harris, K. M. (1999). Three-dimensional relationships between hippocampal synapses and astrocytes. J. Neurosci. 19, 6897–6906. doi: 10.1523/jneurosci.19-16-06897.1999
Verdon, G., and Boudker, O. (2012). Crystal structure of an asymmetric trimer of a bacterial glutamate transporter homolog. Nat. Struct. Mol. Biol. 19, 355–357. doi: 10.1038/nsmb.2233
Veruki, M. L., Morkve, S. H., and Hartveit, E. (2006). Activation of a presynaptic glutamate transporter regulates synaptic transmission through electrical signaling. Nat. Neurosci. 9, 1388–1396. doi: 10.1038/nn1793
Vina-Vilaseca, A., and Sorkin, A. (2010). Lysine 63-linked polyubiquitination of the dopamine transporter requires WW3 and WW4 domains of Nedd4-2 and UBE2D ubiquitin-conjugating enzymes. J. Biol. Chem. 285, 7645–7656. doi: 10.1074/jbc.m109.058990
Voisin, P., Viratelle, O., Girault, J. M., Morrison-Bogorad, M., and Labouesse, J. (1993). Plasticity of astroglial glutamate and γ-Aminobutyric acid uptake in cell cultures derived from postnatal mouse cerebellum. J. Neurochem. 60, 114–127. doi: 10.1111/j.1471-4159.1993.tb05829.x
Volterra, A., Trotti, D., Cassutti, P., Tromba, C., Galimberti, R., Lecchi, P., et al. (1992). A role for the arachidonic acid cascade in fast synaptic modulation: ion channels and transmitter uptake systems as target proteins. Adv. Exp. Med. Biol. 318, 147–158. doi: 10.1007/978-1-4615-3426-6_13
Volterra, A., Trotti, D., and Racagni, G. (1994a). Glutamate uptake is inhibited by arachidonic acid and oxygen radicals via two distinct and additive mechanisms. Mol. Pharmacol. 46, 986–992.
Volterra, A., Trotti, D., Tromba, C., Floridi, S., and Racagni, G. (1994b). Glutamate uptake inhibition by oxygen free radicals in rat cortical astrocytes. J. Neurosci. 14, 2924–2932. doi: 10.1523/jneurosci.14-05-02924.1994
Voutsinos, B., Dutuit, M., Reboul, A., Fevre-Montange, M., Bernard, A., Trouillas, P., et al. (1998). Serotoninergic control of the activity and expression of glial GABA transporters in the rat cerebellum. Glia 23, 45–60. doi: 10.1002/(sici)1098-1136(199805)23:1<45::aid-glia5>3.0.co;2-3
Wadiche, J. I., Amara, S. G., and Kavanaugh, M. P. (1995a). Ion fluxes associated with excitatory amino acid transport. Neuron 15, 721–728. doi: 10.1016/0896-6273(95)90159-0
Wadiche, J. I., Arriza, J. L., Amara, S. G., and Kavanaugh, M. P. (1995b). Kinetics of a human glutamate transporter. Neuron 14, 1019–1027. doi: 10.1016/0896-6273(95)90340-2
Wadiche, J. I., and Jahr, C. E. (2005). Patterned expression of Purkinje cell glutamate transporters controls synaptic plasticity. Nat. Neurosci. 8, 1329–1334. doi: 10.1038/nn1539
Wadiche, J. I., and Kavanaugh, M. P. (1998). Macroscopic and microscopic properties of a cloned glutamate transporter/chloride channel. J. Neurosci. 18, 7650–7661. doi: 10.1523/jneurosci.18-19-07650.1998
Walker, M. C., and van der Donk, W. A. (2016). The many roles of glutamate in metabolism. J. Ind. Microbiol. Biotechnol. 43, 419–430. doi: 10.1007/s10295-015-1665-y
Wang, D., Deken, S. L., Whitworth, T. L., and Quick, M. W. (2003). Syntaxin 1A inhibits GABA flux, efflux, and exchange mediated by the rat brain GABA transporter GAT1. Mol. Pharmacol. 64, 905–913. doi: 10.1124/mol.64.4.905
Wang, Z., Li, W., Mitchell, C. K., and Carter-Dawson, L. (2003). Activation of protein kinase C reduces GLAST in the plasma membrane of rat Muller cells in primary culture. Vis. Neurosci. 20, 611–619. doi: 10.1017/s0952523803206039
Wang, D., and Quick, M. W. (2005). Trafficking of the plasma membrane gamma-aminobutyric acid transporter GAT1. Size and rates of an acutely recycling pool. J. Biol. Chem. 280, 18703–18709. doi: 10.1074/jbc.m500381200
Wang, J., Michelhaugh, S. K., and Bannon, M. J. (2007). Valproate robustly increases Sp transcription factor-mediated expression of the dopamine transporter gene within dopamine cells. Eur. J. Neurosci. 25, 1982–1986. doi: 10.1111/j.1460-9568.2007.05460.x
Wang, K. H., Penmatsa, A., and Gouaux, E. (2015). Neurotransmitter and psychostimulant recognition by the dopamine transporter. Nature 521, 322–327. doi: 10.1038/nature14431
Wang, X., and Boudker, O. (2020). Large domain movements through the lipid bilayer mediate substrate release and inhibition of glutamate transporters. eLife 9:e58417.
Wang, Y., Lu, S., Qu, Z., Wu, L., and Wang, Y. (2017). Sonic hedgehog induces GLT-1 degradation via PKC delta to suppress its transporter activities. Neuroscience 365, 217–225. doi: 10.1016/j.neuroscience.2017.09.051
Watzke, N., Bamberg, E., and Grewer, C. (2001). Early intermediates in the transport cycle of the neuronal excitatory amino acid carrier EAAC1. J. Gen. Physiol. 117, 547–562. doi: 10.1085/jgp.117.6.547
Watzke, N., and Grewer, C. (2001). The anion conductance of the glutamate transporter EAAC1 depends on the direction of glutamate transport. FEBS Lett. 503, 121–125. doi: 10.1016/s0014-5793(01)02715-6
Wenzel, J., Lammert, G., Meyer, U., and Krug, M. (1991). The influence of long-term potentiation on the spatial relationship between astrocyte processes and potentiated synapses in the dentate gyrus neuropil of rat brain. Brain Res. 560, 122–131. doi: 10.1016/0006-8993(91)91222-m
Wheeler, D. S., Ebben, A. L., Kurtoglu, B., Lovell, M. E., Bohn, A. T., Jasek, I. A., et al. (2017). Corticosterone regulates both naturally occurring and cocaine-induced dopamine signaling by selectively decreasing dopamine uptake. Eur. J. Neurosci. 46, 2638–2646. doi: 10.1111/ejn.13730
Wheeler, D. S., Underhill, S. M., Stolz, D. B., Murdoch, G. H., Thiels, E., Romero, G., et al. (2015). Amphetamine activates Rho GTPase signaling to mediate dopamine transporter internalization and acute behavioral effects of amphetamine. Proc. Natl. Acad. Sci. U.S.A. 112, E7138–E7147.
Whitworth, T. L., and Quick, M. W. (2001). Upregulation of gamma-aminobutyric acid transporter expression: role of alkylated gamma-aminobutyric acid derivatives. Biochem. Soc. Trans. 29, 736–741. doi: 10.1042/bst0290736
Wieczorek, W. J., and Kruk, Z. L. (1994). Differential action of (+)-amphetamine on electrically evoked dopamine overflow in rat brain slices containing corpus striatum and nucleus accumbens. Br. J. Pharmacol. 111, 829–836. doi: 10.1111/j.1476-5381.1994.tb14813.x
Williams, J. M., Owens, W. A., Turner, G. H., Saunders, C., Dipace, C., Blakely, R. D., et al. (2007). Hypoinsulinemia regulates amphetamine-induced reverse transport of dopamine. PLoS Biol. 5:e274. doi: 10.1371/journal.pbio.0050274
Wilson, J. M., Khabazian, I., Pow, D. V., Craig, U. K., and Shaw, C. A. (2003). Decrease in glial glutamate transporter variants and excitatory amino acid receptor down-regulation in a murine model of ALS-PDC. Neuromolecul. Med. 3, 105–118. doi: 10.1385/nmm:3:2:105
Winter, N., Kovermann, P., and Fahlke, C. (2012). A point mutation associated with episodic ataxia 6 increases glutamate transporter anion currents. Brain 135, 3416–3425. doi: 10.1093/brain/aws255
Witcher, M. R., Kirov, S. A., and Harris, K. M. (2007). Plasticity of perisynaptic astroglia during synaptogenesis in the mature rat hippocampus. Glia 55, 13–23. doi: 10.1002/glia.20415
Witcher, M. R., Park, Y. D., Lee, M. R., Sharma, S., Harris, K. M., and Kirov, S. A. (2010). Three-dimensional relationships between perisynaptic astroglia and human hippocampal synapses. Glia 58, 572–587.
Woo, T. U., Whitehead, R. E., Melchitzky, D. S., and Lewis, D. A. (1998). A subclass of prefrontal gamma-aminobutyric acid axon terminals are selectively altered in schizophrenia. Proc. Natl. Acad. Sci. U.S.A. 95, 5341–5346. doi: 10.1073/pnas.95.9.5341
Wu, X., Kihara, T., Akaike, A., Niidome, T., and Sugimoto, H. (2010). PI3K/Akt/mTOR signaling regulates glutamate transporter 1 in astrocytes. Biochem. Biophys. Res. Commun. 393, 514–518. doi: 10.1016/j.bbrc.2010.02.038
Wu, Y., Wang, W., Diez-Sampedro, A., and Richerson, G. B. (2007). Nonvesicular inhibitory neurotransmission via reversal of the GABA transporter GAT-1. Neuron 56, 851–865. doi: 10.1016/j.neuron.2007.10.021
Wu, Y., Wang, W., and Richerson, G. B. (2003). Vigabatrin induces tonic inhibition via GABA transporter reversal without increasing vesicular GABA release. J. Neurophysiol. 89, 2021–2034. doi: 10.1152/jn.00856.2002
Xie, Y. Y., Qu, J., Zhou, L., Lv, N., Gong, J. E., Cao, Y. Z., et al. (2017). Lack of association between SLC6A11 genetic polymorphisms and drug resistant epilepsy in chinese han population. Clin. Lab. 63, 1113–1120.
Xu, Y. F., Cai, Y. Q., Cai, G. Q., Jiang, J., Sheng, Z. J., Wang, Z. G., et al. (2008). Hypoalgesia in mice lacking GABA transporter subtype 1. J. Neurosci. Res. 86, 465–470. doi: 10.1002/jnr.21499
Yamashita, A., Singh, S. K., Kawate, T., Jin, Y., and Gouaux, E. (2005). Crystal structure of a bacterial homologue of Na+/Cl–dependent neurotransmitter transporters. Nature 437, 215–223. doi: 10.1038/nature03978
Yan, X., Yadav, R., Gao, M., and Weng, H. R. (2014). Interleukin-1 beta enhances endocytosis of glial glutamate transporters in the spinal dorsal horn through activating protein kinase C. Glia 62, 1093–1109. doi: 10.1002/glia.22665
Yan, X. X., Cariaga, W. A., and Ribak, C. E. (1997). Immunoreactivity for GABA plasma membrane transporter, GAT-1, in the developing rat cerebral cortex: transient presence in the somata of neocortical and hippocampal neurons. Brain Res. Dev. Brain Res. 99, 1–19. doi: 10.1016/s0165-3806(96)00192-7
Yan, X. X., and Ribak, C. E. (1998). Developmental expression of gamma-aminobutyric acid transporters (GAT-1 and GAT-3) in the rat cerebellum: evidence for a transient presence of GAT-1 in Purkinje cells. Brain Res. Dev. Brain Res. 111, 253–269. doi: 10.1016/s0165-3806(98)00144-8
Yang, Y., Kinney, G. A., Spain, W. J., Breitner, J. C., and Cook, D. G. (2004). Presenilin-1 and intracellular calcium stores regulate neuronal glutamate uptake. J. Neurochem. 88, 1361–1372. doi: 10.1046/j.1471-4159.2003.02279.x
Yernool, D., Boudker, O., Jin, Y., and Gouaux, E. (2004). Structure of a glutamate transporter homologue from Pyrococcus horikoshii. Nature 431, 811–818. doi: 10.1038/nature03018
Yoo, S. Y., Kim, J. H., Do, S. H., and Zuo, Z. (2008). Inhibition of the activity of excitatory amino acid transporter 4 expressed in Xenopus oocytes after chronic exposure to ethanol. Alcohol. Clin. Exp. Res. 32, 1292–1298. doi: 10.1111/j.1530-0277.2008.00697.x
Yu, X., Plotnikova, O., Bonin, P. D., Subashi, T. A., McLellan, T. J., Dumlao, D., et al. (2019). Cryo-EM structures of the human glutamine transporter SLC1A5 (ASCT2) in the outward-facing conformation. eLife 8:e48120.
Zeigerer, A., McBrayer, M. K., and McGraw, T. E. (2004). Insulin stimulation of GLUT4 exocytosis, but not its inhibition of endocytosis, is dependent on RabGAP AS160. Mol. Biol. Cell 15, 4406–4415. doi: 10.1091/mbc.e04-04-0333
Zelenaia, O., Schlag, B. D., Gochenauer, G. E., Ganel, R., Song, W., Beesley, J. S., et al. (2000). Epidermal growth factor receptor agonists increase expression of glutamate transporter GLT-1 in astrocytes through pathways dependent on phosphatidylinositol 3-kinase and transcription factor NF-kappaB. Mol. Pharmacol. 57, 667–678. doi: 10.1124/mol.57.4.667
Zerangue, N., Arriza, J. L., Amara, S. G., and Kavanaugh, M. P. (1995). Differential modulation of human glutamate transporter subtypes by Arachidonic acid. J. Biol. Chem. 270, 6433–6435. doi: 10.1074/jbc.270.12.6433
Zerangue, N., and Kavanaugh, M. P. (1996). Flux coupling in a neuronal glutamate transporter. Nature 383, 634–637. doi: 10.1038/383634a0
Zestos, A. G., Mikelman, S. R., Kennedy, R. T., and Gnegy, M. E. (2016). PKCbeta inhibitors attenuate amphetamine-stimulated dopamine efflux. ACS Chem. Neurosci. 7, 757–766. doi: 10.1021/acschemneuro.6b00028
Zetterstrom, R. H., Solomin, L., Jansson, L., Hoffer, B. J., Olson, L., and Perlmann, T. (1997). Dopamine neuron agenesis in Nurr1-deficient mice. Science 276, 248–250. doi: 10.1126/science.276.5310.248
Zhang, H., Li, S., Wang, M., Vukusic, B., Pristupa, Z. B., and Liu, F. (2009). Regulation of dopamine transporter activity by carboxypeptidase E. Mol. Brain 2:10. doi: 10.1186/1756-6606-2-10
Zhang, L., Coffey, L. L., and Reith, M. E. (1997). Regulation of the functional activity of the human dopamine transporter by protein kinase C. Biochem. Pharmacol. 53, 677–688. doi: 10.1016/s0006-2952(96)00898-2
Zhang, L., Li, L., Wang, B., Qian, D. M., Song, X. X., and Hu, M. (2014). HCMV induces dysregulation of glutamate uptake and transporter expression in human fetal astrocytes. Neurochem. Res. 39, 2407–2418. doi: 10.1007/s11064-014-1445-5
Zhang, Q., Fukuda, M., Van Bockstaele, E., Pascual, O., and Haydon, P. G. (2004). Synaptotagmin IV regulates glial glutamate release. Proc. Natl. Acad. Sci. U.S.A. 101, 9441–9446. doi: 10.1073/pnas.0401960101
Zhou, J., and Sutherland, M. L. (2004). Glutamate transporter cluster formation in astrocytic processes regulates glutamate uptake activity. J. Neurosci. 24, 6301–6306. doi: 10.1523/jneurosci.1404-04.2004
Zhu, S. J., Kavanaugh, M. P., Sonders, M. S., Amara, S. G., and Zahniser, N. R. (1997). Activation of protein kinase C inhibits uptake, currents and binding associated with the human dopamine transporter expressed in Xenopus oocytes. J. Pharmacol. Exp. Ther. 282, 1358–1365.
Zike, I. D., Chohan, M. O., Kopelman, J. M., Krasnow, E. N., Flicker, D., Nautiyal, K. M., et al. (2017). OCD candidate gene SLC1A1/EAAT3 impacts basal ganglia-mediated activity and stereotypic behavior. Proc. Natl. Acad. Sci. U.S.A. 114, 5719–5724. doi: 10.1073/pnas.1701736114
Keywords: glutamate, GABA, dopamine, transporter, uptake, surface mobility
Citation: Ryan RM, Ingram SL and Scimemi A (2021) Regulation of Glutamate, GABA and Dopamine Transporter Uptake, Surface Mobility and Expression. Front. Cell. Neurosci. 15:670346. doi: 10.3389/fncel.2021.670346
Received: 21 February 2021; Accepted: 15 March 2021;
Published: 13 April 2021.
Edited by:
Fiorenzo Conti, Marche Polytechnic University, ItalyReviewed by:
Janosch P. Heller, Dublin City University, IrelandMichael B. Robinson, Children’s Hospital of Philadelphia Research Institute, United States
Copyright © 2021 Ryan, Ingram and Scimemi. This is an open-access article distributed under the terms of the Creative Commons Attribution License (CC BY). The use, distribution or reproduction in other forums is permitted, provided the original author(s) and the copyright owner(s) are credited and that the original publication in this journal is cited, in accordance with accepted academic practice. No use, distribution or reproduction is permitted which does not comply with these terms.
*Correspondence: Annalisa Scimemi, c2NpbWVtaWFAZ21haWwuY29t; YXNjaW1lbWlAYWxiYW55LmVkdQ==