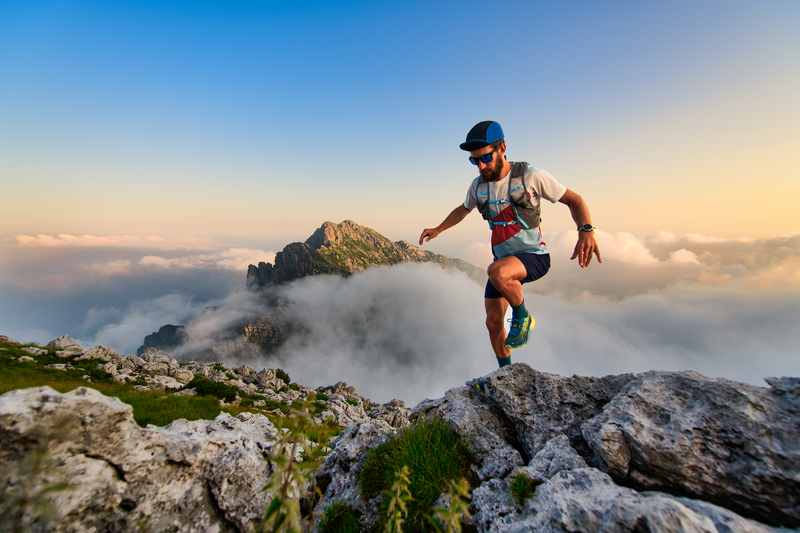
94% of researchers rate our articles as excellent or good
Learn more about the work of our research integrity team to safeguard the quality of each article we publish.
Find out more
REVIEW article
Front. Cell. Neurosci. , 26 April 2021
Sec. Cellular Neurophysiology
Volume 15 - 2021 | https://doi.org/10.3389/fncel.2021.667590
The ability to reliably repair spinal cord injuries (SCI) will be one of the greatest human achievements realized in regenerative medicine. Until recently, the cellular path to this goal has been challenging. However, as detailed developmental principles are revealed in mouse and human models, their application in the stem cell community brings trunk and spine embryology into efforts to advance human regenerative medicine. New models of posterior embryo development identify neuromesodermal progenitors (NMPs) as a major bifurcation point in generating the spinal cord and somites and is leading to production of cell types with the full range of axial identities critical for repair of trunk and spine disorders. This is coupled with organoid technologies including assembloids, circuitoids, and gastruloids. We describe a paradigm for applying developmental principles towards the goal of cell-based restorative therapies to enable reproducible and effective near-term clinical interventions.
For the entirety of recorded human medical history, injury to the trunk and spinal cord has carried with it the potential for untreatable loss of functional modalities, and severely compromised quality and duration of life (Silva et al., 2014). Human stem cell research has reignited the conviction that meaningful functional recovery is achievable. With recent discoveries and technological improvements, new strategies to advance the application of human stem cells to model or repair the trunk and spine are being rapidly implemented. Previous embryonic models based largely on amphibian developmental biology, resulted in the use of non-ideal differentiation strategies that failed to generate cells with the complete range of axial identities needed along the rostral-caudal neuraxis. One major advance was the identification of a pool of caudal axial progenitor cells in vivo, referred to as a neuromesodermal progenitors (NMPs; Tzouanacou et al., 2009), and the in vitro implementation to derive and cultivate this cell type from human pluripotent stem cells (hPSCs; Gouti et al., 2014). Suddenly, with NMPs as a starting point, the in vitro recapitulation of phenotypes along the entire neuraxis became feasible. This developmental discovery in human embryology is opening the door to an astounding degree of progress for generating and understanding cell types of the trunk and spine. In animal models, these cells applied therapeutically will bring anatomical and physiological matching that is expected to remove or overcome previous barriers to cellular repair including early integration events and host interconnectivity by expressing the appropriate targets. Here we discuss advances in human stem cell biology that is an integral component of human developmental neurotechnologies. We describe the clinical potential of developmental neurotechnologies as they relate to injury and disease processes of the trunk and spine.
NMP models that give rise to the trunk and spine including the central and peripheral nervous systems (CNS, PNS) differ strikingly from the organization of the brain into its interacting systems, such as development of the telencephalon and neocortical regions reviewed elsewhere (Molyneaux et al., 2007; Greig et al., 2013). NMPs are thought to functionally bifurcate into separable neuroectodermal and mesodermal lineages, as well as into neural crest cells (NCCs). In vitro, NMPs are being used to generate anatomically matched neural cells for injury repair and applied in advanced culture systems such as multi-lineage gastruloids, circuit organoids, and cortico-motor assembloids. These living human cell platforms allow the interrogation of previously unobtainable stages of human development and the earliest manifestations of disease with unprecedented detail. By highlighting recent advances in our understanding of developmental stages of the spinal cord and the metameric segmentation (Diaz-Cuadros et al., 2020; Matsuda et al., 2020), we reveal how the application of such developmental principles to stem cell research and therapies will benefit clinical outcomes for example in congenital disorders, neuromuscular disorders, and adult spinal cord trauma.
Below we discuss the discoveries from animal models that formed the basis for insights into human development, from anterior to posterior CNS formation, the discovery of NMPs, ventral and dorsal spinal cord patterning, the design potential of NCCs, and the integral roles of glia.
In the 1950’s, amphibian studies by Nieuwkoop (Nieuwkoop, 1952; Nieuwkoop and Nigtevecht, 1952) provided the earliest model of nervous system development. In this model, a single pool of neural stem cells (NSCs) arises from early epiblasts in the anterior neural plate and becomes transformed along the anterior-posterior body length by caudalizing, or posteriorizing, signals. This generates the gamut of the rostral-caudal neuraxis from forebrain to spinal cord (Stern, 2001; Andoniadou and Martinez-Barbera, 2013). Foundational vertebrate stem cell differentiation protocols often paralleled this model to produce diverse therapeutic CNS cells for brain and spinal cord applications. However, as a matter of longstanding debate (Handrigan, 2003; Stern, 2006), more recent studies support an extension of the Nieuwkoop model in which the vertebrate brain and spinal cord are instead realized to have independent developmental origins and arise from distinct populations of NSCs (Tzouanacou et al., 2009; Henrique et al., 2015). Accordingly, a foundational study by Metzis et al. (2018) revealed genome-wide chromatin-remodeling events that allow expression of genes for directing “primary axial regionalization.” This revealed an important distinction by regulated expression to specify regionally separate pools of epiblast cells that will be allocated to anterior (forebrain) or posterior (spinal cord) positions in the developing nervous system prior to neural induction. In this revised model for vertebrates, NSCs in the anterior neural plate generate cranial neurons in the brain and descending white matter tracts, whereas induction of distinct posterior axial stem zones begins the generation and patterning of the spinal cord (Figure 1). NMPs are thought to be the common origin of the posterior CNS and its associated musculoskeletal system (Cambray and Wilson, 2002; Wilson et al., 2009) and trigger a cascade of downstream developmental events and pathways. The principle behind therapeutic intervention with human cells derived by stem cell technology is to match cells as accurately as possible to the anatomical site of injury to favor microenvironment integration. For spinal cord injuries (SCI) cell-based therapies, the repair and restoration of function should apply vertebrate posterior developmental processes. Previous use of developmentally-mismatched anterior NSCs for SCI therapy that by default are programmed for the brain creates additional challenges for these cells that must re-align gene expression to adapt to new neurophysiological signaling cues in the spine in a complex cytokine injury microenvironment. NMPs help to remove this barrier.
Figure 1. Overview of vertebrate ontogeny of cell types in the head vs. trunk and spine. Anterior/rostral development from neuroectoderm vs. posterior/caudal development from neuromesodermal progenitor cells. Lineage ontogeny from distinct stem and progenitor pools is detailed. General cell sources (left column) and general cell types produced by their differentiation (middle column) along with resulting general tissues (right column) are listed. Differentiation potential varies along the anterior–posterior neuraxis such as in cell types of the neural crest that can otherwise behave similarly.NSPCs, neural stem/progenitor cells; NCCs, neural crest cells; Hox PG, Hox paralogous group; CSF, cerebrospinal fluid; BBB, blood-brain barrier; PA, pharyngeal arch.
Human NMPs constitute a cellular pool with a bipotent fate map for spinal cord and somite development. Their identification reveals that a possible common mechanism in vertebrate embryos investigated from fish to human (Kimelman, 2016). This cell population has not yet been identified in amphibian, which may account for the different findings of Nieuwkoop using the amphibian embryo model system (Nieuwkoop, 1952; Nieuwkoop and Nigtevecht, 1952). NMPs are present within a region called the node streak border (NSB) and from this location contribute to axial elongation of early, developing embryos (Cambray and Wilson, 2002; Tzouanacou et al., 2009; Wilson et al., 2009), sustainably sourcing new neural and paraxial mesodermal tissues (Figure 2). In contrast to Nieuwkoop’s model, in which caudalization of anterior NSCs is sufficient to produce the entire rostral-caudal neuraxis, NMPs appear to contribute predominately to hindbrain and anterior spinal cord, posterior spinal cord, and paraxial mesoderm rather than forebrain and midbrain structures in animal and human models. Thus, NMPs are described as multipotent building blocks of the posterior body (Figures 2A,B) and are reviewed elsewhere (Henrique et al., 2015). In an alternative model that is not necessarily exclusionary, NMPs may also contribute an important role to form a barrier protecting the interface between two developing stem cell zones (Figure 2C; Wood et al., 2020). Molecularly, NMPs are identified by co-expression of mesodermal transcription factor brachyury (T/Bra) and the NSC marker SOX2. Expression of the latter is driven by a unique N1 enhancer element. This enhancer is distinct from the N2 enhancer element that regulates Sox2 expression in forebrain and in pluripotent stem cells (Uchikawa et al., 2003; Iwafuchi-Doi et al., 2011, 2012). The transition from N2 to N1 enhancer activity correlates with cells that will generate the posterior CNS (Takemoto et al., 2006).
Figure 2. Neuromesodermal progenitor (NMP) models of human trunk and spine development. (A) NMPs act upstream as multipotent building blocks to generate the trunk and spine. (B) Putative gene regulatory network (GRN) of NMP differentiation (Gouti-Briscoe model). RA (retinoic acid), NSPC (neural stem/progenitor cell). (C) Two proposed NMP developmental models. NMP model 1: NMPs in the caudolateral epiblast (CLE)/node streak border (NSB) act as caudal axial stem cells, that give rise to NPCs and the presomitic mesoderm (PSM) that undergoes somitogenesis in waves under a segmentation clock (Henrique et al., 2015). NMP model 2: NMPs form a barrier between distinct neural stem and mesoderm stem zones (Wood et al., 2020). Both models produce the spinal cord and paraxial mesoderm/somites from caudal stem cell pools. SOX2/Bra spatial heterogeneity in NMPs correlates with differential migratory velocity and PSM vs. spinal cord lineage commitment (Romanos et al., 2021). NCC, neural crest cell; aPSM/pPSM, anterior/posterior presomitic mesoderm; PNS, peripheral nervous system; CNS, central nervous system.
NMPs are transient cell types whose maintenance and differentiation into either presomitic mesoderm (PSM) or into caudal neural progenitors is thought to depend on the spatiotemporal distribution of signaling cues. In particular, inputs of the secretory glycoprotein, Wingless-type integration site protein (Wnt), and fibroblast growth factor (FGF) are interfaced within an intrinsic, complex gene regulatory network (GRN) that we refer to as the Gouti-Briscoe model (Figures 2A,B; Gouti et al., 2017). Briefly, Wnt and FGF signaling are provided by the primitive streak and caudolateral epiblast (CLE) that acts as a caudal neural plate. Here they induce the characteristic SOX2 (N1)/Bra co-expression phenotype. SOX2 levels finely tune this process. Low SOX2 expression enables low-affinity SOX2 genomic binding sites to be occupied by T/Bra and CDX-2 in NMPs (Blassberg et al., 2020). Wnt and FGF also act synergistically to induce Fgf8 transcription. This further drives a Wnt/FGF positive feedback loop in the posterior embryo (Aulehla and Pourquié, 2008; Wilson et al., 2009). In turn, Wnt signaling activates CDX-2, the upstream master regulator of posterior Hox genes that specify body patterning and segmentation (Nordström et al., 2006). The NMP induction is restricted by bone morphogenetic protein (BMP) signaling and by Bra repression of Sox2 to within the CLE and NSB (Takemoto et al., 2006). Continued exposure to Wnt biases towards mesodermal fate (Garriock et al., 2015), without inhibiting neural cell fates, and drives the further induction of Hox genes. The expression of the Hox genes is essential to caudal neural development, establishment of neuronal diversity (Sagner and Briscoe, 2019), and ultimately connectivity and circuit organization (Philippidou and Dasen, 2013).
Segmentation into somites from the presomitic mesoderm (PSM) uses the mesodermal transcription factor TBX6 that suppresses Sox2 via the N1 enhancer (Takemoto et al., 2011). Additionally, pairs of somites are rhythmically produced by further control via an innate segmentation clock oscillator (Hubaud and Pourquié, 2014). By these mechanisms in zebrafish and mouse vertebrate models, segmented somites form simultaneously and in close relation to the neural tube and as a precursor to functional neuromuscular connectivity. In a recent exciting discovery, Diaz-Cuadros et al. (2020) utilized human stem cell models to demonstrate the existence of this segmentation clock in vitro for the first time, which is similarly regulated by Wnt and FGF signaling pathways (Diaz-Cuadros et al., 2020). This segmentation clock was exploited in vitro to recapitulate somitogenesis using human PSCs (Matsuda et al., 2020). As somites develop, they express the enzyme retinaldehyde dehydrogenase two that catalyzes the production of retinoic acid (RA; Molotkova et al., 2005). RA gradients are important in defining neuroanatomical regions. By repressing Wnt and FGF-mediated signaling pathways, RA gradients halt the collinear activation of Hox genes within the neural tube. This may contribute to neural cell fate commitment with precisely defined positional identity along the rostral-caudal neuraxis during elongation (Diez del Corral et al., 2003; Lippmann et al., 2015). In a new study, it was shown that the NMP population comprising the progenitor zone is spatially heterogenous with differential stochastic expression of SOX2 vs. Bra (Romanos et al., 2021). By in silico modeling and in vivo validation of the model in quail embryos, it was shown that higher SOX2 expression biases to spinal cord fate while higher Bra expression biases to PSM, where PSM-biased cells have higher migration rates vs. SOX2-biased cells (Figure 2C).
The Hox code and RA signaling gradients direct rostral-caudal elements of spinal organization (Philippidou and Dasen, 2013). Coincidentally, the regional identity along the dorsal-ventral axis must also be specified and is done so by opposing morphogen signaling gradients that guide neuronal subtype diversification along the dorsal-ventral axis (reviewed in detail: Tao and Zhang, 2016; Sagner and Briscoe, 2019). In the developing embryo, opposing morphogen gradients have long been known to be key concentration-dependent patterning effectors (Turing, 1953; Christian, 2012), and similarly are critical in directing cells of the ventral and dorsal spinal cord to be functionally distinct. The inductive ventralizing Sonic hedgehog (Shh) morphogen is a glycoprotein and growth factor with well-established roles in neural patterning in mouse embryogenesis. Shh is secreted by the notochord at a position that is immediately ventral to the developing neural tube, and later by the floor plate. A signaling gradient is generated that is most highly concentrated at the ventral midline and which diminishes along the ventral-dorsal axis to reliably establish five ventral neural progenitor domains (ventral to dorsal: p3, pMN, p2, p1, p0). These five domains give rise to six functionally distinct neuronal subtypes V3, MN, V2b, V2a, V1, and V0, respectively (Marti et al., 1995; Roelink et al., 1995). Of therapeutic interest for SCI are motor neurons generated from motor neuron progenitors (MNPs) and interneurons that vary in primary neurotransmitter phenotype (broadly, excitatory or inhibitory). Spinal motor neurons (SMNs) are specialized along the rostral-caudal neuraxis into columns. Smaller SMN pools innervate compartments of skeletal muscle or contribute to the autonomic nervous system (Philippidou and Dasen, 2013). Each spinal cord progenitor domain is characterized by a unique combination of transcription factors (Sathyamuarthy et al., 2018; Sagner and Briscoe, 2019), albeit partially overlapping, that provides a molecular signature. Since different transcription factor hetero-complexes activate neuronal subtype-specific genes, how they interact in complex gene regulatory networks and repress alternative transcriptomic developmental pathways is of broad interest. The neural transcription factor code, originally described in nematode (Hobert, 2005–2018), is believed to be critical in vertebrates as well for guiding and specifying neuronal fate (Shirasaki and Pfaff, 2002). Because of the complexity of morphogen gradients and transcription factor codes, the field has not yet linked gene expression molecular profiles with all functionally defined cell types, that includes the full scope of neuronal subtype diversity. The quest for molecular signatures therefore remains a highly active area of research investigation with far-reaching applications therapeutically to provide the most appropriate cell types and supportive networks.
Whereas SMNs reside in the ventral spinal cord, the dorsal spinal cord receives signals from sensory neurons. In a process analogous to ventral spinal cord specification, dorsal spinal cord identity and progenitor domains are also specified by inductive morphogen gradients. Two of these signaling ligand classes, BMP and Wnt, are secreted by the neural tube roof plate (Liem et al., 1997; Lee et al., 2000; Muroyama et al., 2002). They function in opposition to ventral Shh inductive signaling. By short-range interactions between neighboring cells, reliable patterning of region-specific progenitor domains is achieved to ensure high fidelity nervous system connectivity and function. Six dorsal progenitor domains (dorsal to ventral: dp1–dp6) become distinguished through a transcription factor code (Lai et al., 2016; Sagner and Briscoe, 2019). Dorsal progenitor (dp) domains give rise to dorsal interneuron subtypes dI1–6 that use excitatory or inhibitory neurotransmission. This process is thought to be regulated in part by Notch signaling (Mizuguchi et al., 2006). Information flow from the brain via descending CNS white matter tracts to the PNS is modulated by synaptic interactions of dorsal interneurons with ventral interneurons and SMNs. The reversed flow of information to the brain from the somatic and autonomic nervous systems through the PNS and spinal cord also relies on interneurons and ascending white matter tracts. Interneurons are core components of sensorimotor neural circuits required for higher-level network integration and behavior. As well, propriospinal neurons that originate peripherally and terminate in the spinal cord and brainstem have been shown to be central for restoring lost locomotor function after SCI (Formento et al., 2018).
NCCs constitute a remarkably diverse set of cell lineages that originate at the border of neural ectoderm and non-neural ectoderm in the embryo. The majority of our knowledge about NCCs comes from avian, mouse, and zebrafish models. Unique to vertebrates, NCCs are induced from neuroepithelium at this border and migrate to distant sites throughout the embryo where they differentiate through complex competing pathways and cell state biases into a plethora of mature cell lineages (Etchevers et al., 2019). They are the developmental source of PNS sensory neurons and Schwann cell glia, the enteric nervous system (ENS), the autonomic nervous system, chondrocytes, osteocytes, smooth muscle, melanocytes, and other mesenchymal tissues (Mayor and Theveneau, 2013). Since the first description of single-cell RNA-sequencing (Tang et al., 2009) through to current highly-parallel single-cell RNA-Seq and genome-editing strategies, the many genes and regulatory networks that direct NCC fate specification, epithelial-to-mesenchymal transition (EMT), migration, and differentiation are beginning to be described in context with functional events (Soldatov et al., 2019). Wnt, BMP, and FGF signals mediate the cross-talk between neural and non-neural ectoderm at the neural plate border to specify this region. NCC precursors are distinguishable from neuroectoderm and ectoderm by a suite of neural crest specifier transcription factors that allow molecular lineage tracing. Their position at the edges of the early neural folds changes as the dorsal neural tube closes, and is followed by an EMT that imparts migration capabilities to NCCs to move temporally and peripherally throughout the developing embryo. The ability to recapitulate some of these events in NCC migration and downstream events linking CNS, PNS and enteric nervous systems was realized for the first time, in complex human elongating-multilineage-organized (EMLO) gastruloids (Olmsted and Paluh, 2020).
Migratory NCCs host competing transcriptional programs that are eventually biased to a particular lineage through a series of binary decisions (Soldatov et al., 2019). In mouse, the first of these decisions dictates between sensory and non-sensory fate while subsequent decisions regulate mesenchymal vs. autonomic fate. This study also reported the differential lineage potential of NCCs along the rostral-caudal neuraxis, varying with axial identity. That is, the trunk NCCs have neuronal bias to pattern the PNS while cranial NCCs have mesenchymal bias to produce craniofacial compartments. Strikingly, it was recently shown that cranial NCCs undergo an in vivo reprogramming event wherein OCT4 and Nanog are reactivated following ectodermal commitment to then further generate the array of other cell lineages such as mesenchyme (Zalc et al., 2021). This impressive array of known diverse cell types and emerging fate maps emphasize, how important NCC roles are in multiple aspects of neurodevelopment of the PNS. Indeed, non-lethal defects in these processes underlie a spectrum of human deformations, such as in Hirschsprung’s disease, that are deemed neurocristopathies (Etchevers et al., 2019).
Efforts toward neuro-restoration following injury also consider roles of assisting oligodendrocyte and astrocyte neural cells. The familiar myelin sheath, formed by the interactions of oligodendrocytes with neuronal axons, facilitates signal propagation in neurons, while multiple astrocyte-neuron interactions enable metabolic and neurotransmission homeostatic support and synaptic regulation as part of the described tripartite synapse (Farhy-Tselnicker and Allen, 2018). Studies in animal models reveal that gliogenesis occurs in temporal waves subsequent to early neurogenesis (Rowitch, 2004; Rowitch and Kriegstein, 2010). Oligodendrocyte progenitor cells (OPCs) in mice are produced in two embryonic waves and one postnatal wave. In the first embryonic wave (E12.5 in mice), ventral Shh drives expression of homeobox genes Nkx-6.1 and Nkx-6.2 in a gene regulatory network with oligodendrogenic transcription factors Olig1 and Olig2 (Vallstedt et al., 2005). Dorsal OPCs are produced in a second, Shh-independent wave (Cai et al., 2005; Vallstedt et al., 2005) that requires BMP and FGF signaling. Oligodendrocytes from dorsal OPCs are retained predominately in the dorsal white matter (Fogarty et al., 2005). In humans, maturation of OPCs to myelinating oligodendrocytes in the CNS continues after birth through the first decades of life, and likely throughout life, and may remyelinate denuded axons (Franklin and Ffrench-Constant, 2008).
Astroglial populations similarly develop in both the brain and spinal cord as heterogenous cell populations (Bayraktar et al., 2015). During the switch from neuronal to glial production in the spinal cord, the transcription factor stem cell leukemia (SCL) expressed in the ventral p2 domain represses OLIG2-mediated oligodendrocyte production to promote astrogenesis. In p1-p3 ventral progenitor domains, the PAX6/NKX-6.1 homeodomain transcription factor code (Hochstim et al., 2008) specifies three subpopulations of GFAP+ astrocytes that vary in molecular profiles. Positional identity is speculated to be coincident with the organizing process underlying functional subtype diversification. Astroglial cells migrate into white matter as VA1–3 populations corresponding to p1–3 progenitor domains along the dorsal-ventral axis. BMP signaling has also been shown to modulate astrocyte development in the spinal cord (Agius et al., 2010). The balance of neurons and glia is therefore necessary in development, and likely also in repair, to support maintenance, maturation, and neural circuitry demands in specific physiological domains for high-fidelity nervous system function. As well, interesting cross-regulation of shared factors like SCL point to additional physiological events that are occurring. Stem cell differentiation models in vitro are driving the production of cell types with relevant regional identity needed for optimal functional host interconnectivity after in vivo transplantation.
Early in human development, the formation of the head, trunk and spine requires the interaction of a multitude of cell types. Despite complex multicellular organization, the spatiotemporal processes underlying the growth and patterning of these regions can be separately replicated in part by stem cell differentiation protocols. The convergence of developmental biology, in vitro stem cell differentiation, bioengineering technologies, and in vivo clinical relevance of trunk and spinal cord diverse cell types together form a strong foundation for developmental neurotechnologies. Multiple previous reviews provide comprehensive descriptions of unique, focused aspects of trunk and spine development (Philippidou and Dasen, 2013; Stifani, 2014; Green et al., 2015; Henrique et al., 2015; Sagner and Briscoe, 2019) and neurotechnologies (Vázquez-Guardado et al., 2020). In the remainder of this review, we describe how developmental principles are being applied to human stem cell research and incorporated with neurotechnologies to advance human therapies.
Since 2014, in vitro studies using mouse and human ESCs have contributed to our understanding of NMP biology. A fortified, extended developmental model for neural induction from separable posterior and anterior stem cell pools is now established (Gouti et al., 2014; Tsakiridis et al., 2014; Turner et al., 2014; Henrique et al., 2015; Lippmann et al., 2015). Gouti et al. (2014) performed one of the most foundational studies on NMPs using human stem cells. The differentiation of mESCs and hESCs to NMPs, done in parallel, as well as findings from Turner et al. (2014) and others (Tsakiridis and Wilson, 2015) demonstrated the bipotential nature of these cells co-expressing SOX2/Bra to produce neuroectodermal and mesodermal cell types in vitro. These developmental principles provide a strong foundation for future stem cell therapies to advance repair of SCI with regionally-matched cells (Figure 3A).
Figure 3. Developmental principles applied to stem cell differentiation. (A) Overview flow chart of human pluripotent stem cell (hPSC) differentiation through NMPs. Key differentiation factors and biomarkers are provided. It should be noted that induced protocols with forced transcription factor expression are also used. Variations in differentiation factors for each lineage have been performed in the literature. (B) Two routes to caudal neural crest cells (NCCs). Route 1 is to caudalize anterior neural crest progenitors (Hox negative) using retinoic acid (RA) to produce posterior cranial, vagal, and cardiac NCCs (Hox1–5). Route 2 is to first generate an NMP intermediate that can in turn produce trunk NCCs with broad axial identity. (C) Hox code of spinal cord motor columns along the neuraxis (Philippidou and Dasen, 2013). R (rostral), C (caudal) directions. (D) Recapitulating the in vivo somite segmentation clock in vitro (Diaz-Cuadros et al., 2020; Matsuda et al., 2020). (E) SMN co-culture with skeletal myotubes to model NMJ function and dysfunction. (F) Neural circuit signal propagation efficiency depends on multicellular interactions between neurons (blue/pink), oligodendrocytes (green), and astrocytes (purple). (G) Tripartite synapse model wherein astrocytes (purple) assist synapse formation, function, and homeostasis.
In all studies, Wnt agonism by the small molecule CHIR 99021 and soluble recombinant FGF signaling are necessary and sufficient to produce high-yield NMPs. Next generation sequencing technologies are revealing new human temporal information by applying strategies that enable lineage tracing of cell identity during differentiation in vitro and comparing this to transitional developmental events (morphological and functional) to capture evolving gene regulatory networks (Figure 2B; Gouti et al., 2014, 2017; Verrier et al., 2018). NMPs are generated by use of CHIR 99021-mediated Wnt agonism plus FGF2 and/or FGF8b in protocols that may include simultaneous BMP, TGF-β, and Notch signaling inhibition for further lineage restriction (Lippmann et al., 2015). This allows the maintenance of cells in an intermediate stage and establishment of stable NSC lines retaining multipotency and spinal cord identity (scNSCs) over multiple passages (Kumamaru et al., 2018). As well, NMPs derived in vitro provide a physiologically relevant starting material for production of axial NCCs with trunk identity (Frith et al., 2018).
Two pathways to neuroectoderm have recently been defined in mammalian models that define brain and spinal cord lineages of anterior neuroepithelium vs. posterior NMPs. A key advance towards this goal for in vitro neural differentiation was the advent of a dual SMAD inhibition protocol utilizing small molecules LDN193189 and SB431542 to inhibit BMP and TGF-β/Activin/Nodal signaling pathways (Chambers et al., 2009, 2012). In vivo, the inhibition of pluripotency maintenance pathways involving TGF-β and FGF induces infoldings of the neural plate to form the neural tube. By applying dual SMAD inhibition, NSC generation was both optimized and accelerated due to the repression of stem cell differentiation into non-neural lineages. This produces abundant “neural rosettes” that are approximate 2D representations of the 3D developing neural tube (Conti and Cattaneo, 2010). In vitro, this method by default yields NSCs with anterior (forebrain) identity (Pankratz et al., 2007; Rowland et al., 2011). Attempts to subsequently caudalize such NSCs with RA, yields transcriptomic profiles exhibiting activation of 3’ Hox genes, that correspond to the hindbrain and cervical spinal cord, but importantly fail to recapitulate the entire rostral-caudal neuraxis (brachial, thoracic, lumbosacral vertebral levels). By applying a method that instead proceeds through an NMP differentiation intermediate in vitro, it was shown that caudalization of NSCs achieves more complete Hox gene collinearity with expression of 5’ Hox genes HoxD10–12 (Lippmann et al., 2015; Kumamaru et al., 2018; Verrier et al., 2018). This method gained traction in the stem cell field, and was further extended to lumbosacral phenotypes by inclusion of the TGF-β ligand, GDF11 (Lippmann et al., 2015). In the absence of Wnt agonism, the anterior NSC phenotype again predominates (Gouti et al., 2014). In general, longer exposure to graded CHIR 99021 and FGF signaling yields more posterior Hox gene induction. RA addition during regionalization in vitro is sufficient to arrest this process yielding a population of cells with fixed rostral-caudal identity (Figure 3C; Mazzoni et al., 2013; Lippmann et al., 2015). When exposed to RA signaling, the NMP transcription factor Bra that otherwise biases towards mesoderm is downregulated. This allows entry into neuroectoderm and subsequent neuronal differentiation (Maury et al., 2015). These approaches mirror in vivo developmental signaling and outcomes.
One core principle emerging from NMP induction and patterning of the posterior CNS is the requirement for caudalization prior to or in conjunction with differentiation and lineage commitment (Metzis et al., 2018). Throughout elongation of the embryo, RA production by adjacent somites in animals works to establish the Hox code for body patterning and segmentation (Diez del Corral et al., 2003; Molotkova et al., 2005; Wilson et al., 2009). For in vitro studies to be relevant for in vivo use, the requirement for sufficient regionalization prior to neural differentiation must be mimicked. Recently, the in vivo action of a segmentation clock associated with waves of somite production was recapitulated in vitro using human stem cells (Figure 3D) (Diaz-Cuadros et al., 2020; Matsuda et al., 2020). Together, these multiple important refinements in differentiation protocols enable us now to achieve the production of regionally-specified neuronal and mesodermal subtypes. Spinal cells generated in this manner are expected to be better primed for rapid spinal cord integration in regenerative cell therapies and will be more accurate for in vitro disease modeling.
The extent of neuronal diversity in the human spinal cord is unknown but expected to be extensive. Among the most highly refined information in this regard comes from stem cell differentiation protocols to SMNs (reviewed in: Davis-Dusenbery et al., 2014; Sances et al., 2016; Trawczynski et al., 2019) and include SMN subtype- and regional-specification (Stifani, 2014; Patani, 2016; Tao and Zhang, 2016). SMN somata residing in the spinal cord ventral horn project axons via peripheral nerve conduits to innervate muscle tissue or contribute to the autonomic nervous system (Figure 3E). In contrast, cranial, or upper MNs (UMNs) are those that develop in the neocortex and synapse with SMNs and interneurons via descending white matter tracts. In seminal work with mESCs, SMN differentiation was induced through a committed MNP intermediate by withdrawal of leukemia inhibitory factor (LIF) and subsequent stimulation of RA and Shh signaling pathways (Wichterle et al., 2002), a strategy also applied successfully to human ESCs (Li et al., 2005). A more robust protocol is now available that potentiates neuroectodermal programs by combining dual SMAD inhibition to prevent mesodermal and endodermal differentiating contaminants (Chambers et al., 2009, 2012). Currently, dual SMAD inhibition uses LDN 193189 (BMP pathway inhibitor by ALK1, 2, 3, 6 inhibition) and SB 431542 (Activin/BMP/TGF-β pathway inhibitor by ALK4, 5, 7 inhibition). Progress has also been made to further refine SMN subtypes generated by additional perturbation of RA and Shh signaling pathways.
Therapeutically, the ability to ideally match neuron type with physiological need is an exciting and critical advancement. For example, generating SMNs with lateral motor column (LMC) identity that in vivo innervate skeletal muscles of the limbs to assist in locomotion (Amoroso et al., 2013). MNP protocols have also extended to human iPSCs (Dimos et al., 2008; Hu et al., 2010), and it is now possible to generate highly pure cultures of MNPs across stem cell models (Qu et al., 2014; Du et al., 2015). An excellent summary of SMN differentiation protocols used across many studies, is detailed in Sances et al. (2016). Most previous transplantation studies with human NSCs have used dual SMAD inhibition alone. This generates NSCs with anterior forebrain identity that must be subsequently caudalized, whereas the recent redirection to first generate spinal cord NSCs through NMPs is a more developmentally matched strategy.
The expectation is that anatomic regional matching will improve functional recovery. This is being done to favor host integration of transplanted cells and to advance in vitro disease modeling and drug screening. A clear example is seen with disease progression of amyotrophic lateral sclerosis (ALS), wherein distinct SMN pools are selectively vulnerable to neurodegeneration (e.g., lumbar ventral horn neurons), while others are resistant (e.g., brainstem oculomotor neurons) (Nijssen et al., 2017). This critical finding is recapitulated by developmentally matched differentiation protocols (Allodi et al., 2019). In addition to traditional cell culture and signaling pathway manipulation, alternative protocols with forced viral or mRNA-mediated expression of motor neurogenic transcription factors, and direct conversion from somatic cells, are being explored (Hester et al., 2011; Son et al., 2011). The goal of these trans-differentiation protocols is to achieve high-yield production of functional SMNs in a protracted timeframe. RNA-Seq remains a key strategy to initially compare gene expression profiles of cells generated by these alternative methods before functional testing in vivo. In this regard, one substantial barrier in the SCI field is the ability to rapidly access the outcomes of transplanted cells. New technologies such as intravital windows, improved biomarkers for connectivity mapping, and electrical stimulation and readouts are desperately needed to rapidly assess successes or failures and to accelerate the pace to therapeutic intervention.
Ventral and dorsal interneurons are components of the precise neuronal circuits needed for spinal cord repair (Francius et al., 2013). These cells have remained understudied, not for lack of importance, but due to difficulties in specifying pure interneuron subtypes. However, by applying NMPs, the ability to begin to model circuit formation event in vitro is expanding (Nedelec and Martinez-Arias, 2021). Recent advancements out of the Sakiyama-Elbert lab have enabled new protocols to produce V3 excitatory commissural interneurons using mESCs (Xu and Sakiyama-Elbert, 2015), and V2a excitatory interneurons both from mESCs (Brown et al., 2014) and human PSCs (Butts et al., 2019). V3 interneuron (NKX-2.2 + /SIM1+) differentiation was similar to SMN protocols but modified to decrease RA concentration (10 nM) and lengthen the exposure to the potent Shh agonist, SAG (~18% efficiency NKX-2.2 cells). V2 interneurons originate from the p2 progenitor domain that further subdivides into excitatory (V2a, CHX10+) and inhibitory (V2b, GATA3+) regions as neurons specialize. Notch signaling promotes the inhibitory V2b subtype, but directly inhibits the generation of excitatory V2a neurons. In a recent protocol (Butts et al., 2019), the γ-secretase inhibitor DAPT was added early in neural induction to inhibit Notch, and along with dual SMAD inhibition and optimized RA/Shh concentrations was sufficient to bias towards V2a excitatory interneuron fate. These advancements begin to open up studies and therapeutic applications of interneurons.
As with ventral interneurons, detailed methodologies for dorsal interneurons have lagged behind SMN advances, but are beginning to emerge. These protocols similarly co-opt developmental principles governing dorsal spinal cord patterning into distinct progenitor domains dp1-dp6, and specialization into laminae containing the corresponding interneurons dI1-dI6. The first protocol for generating dorsal spinal interneurons was published in 2018 (Gupta et al., 2018). Gupta et al. (2018) leveraged BMP4 and RA signaling to dorsalize and caudalize neural progenitors, respectively. This method generates dorsal interneurons of three classes that are dI1, dI2 (proprioceptive) and dI3 (mechanosensory). A critical feature of this method was the requirement for optimal timing of BMP4 exposure in a temporally-restricted window to guide neural progenitors towards dI1 and dI3 fates. Unexpectedly, dI2 neurons were only observed in RA control cultures and were suppressed by exposure to BMP4. This protocol was applied to both hESC and hiPSC models demonstrating a similar developmental program and timeline for dorsal sensory interneurons. Dorsal interneuron production has also now been extended to 3D organoid culture platforms (Ogura et al., 2018; Duval et al., 2019). Neural organoid exposure to BMP4 was sufficient to recapitulate characteristic arrangements of dorsal neural tube cells which are capable of differentiating into patterned subsets of dorsal interneurons using both mouse and human stem cell models (Duval et al., 2019). As these protocols are refined, cell replacement strategies in SCI, where acute injury can impact both motor and sensory systems, will benefit. Propriospinal neurons remain of particular interest since they convey and integrate positional feedback information, and are essential for restoring behavioral locomotor function in animals and humans (Formento et al., 2018).
Multipotent NCCs share transcriptomic similarities to PSCs and have an impressive capacity to produce a diverse array of adult cell types such as peripheral sensory and autonomic neurons, Schwann cells, enteric ganglia, bone and cartilage, pigmenting melanocytes, sympathoadrenal cells, smooth muscle, and other cells of the mesenchyme in the trunk and craniofacial anatomic compartments (Srinivasan and Toh, 2019). Given the multipotent nature of NCCs, differentiation protocols for generating terminal cell types through NCC intermediates are numerous. While early protocols required stromal feeder layers (Pomp et al., 2005; Lee et al., 2007) or employed suspension neurospheres (Pomp et al., 2008), these strategies helped to enable differentiation to PNS sensory and sympathetic neurons (Pomp et al., 2005; Brokhman et al., 2008). It is now more common to use fully defined human differentiation protocols to generate NCCs and downstream cells (Menendez et al., 2011; Mica et al., 2013; Fattahi et al., 2016). Exposure of NCCs to BMP2/4, Shh, or FGF can drive the production of roof plate, floor plate, and neuroepithelial tissue, respectively (Denham et al., 2015). The caudalization by RA is employed when patterning more posterior trunk NCCs as opposed to those with anterior craniofacial identity (Huang et al., 2016), reflecting lineage biases that differ between trunk and craniofacial compartments (Soldatov et al., 2019). Direct transcription factor-based reprograming methods to generate induced NCCs, using Sox10 or FoxD3 forced expression have also been developed (Kim et al., 2014). Continued refinement of protocols for NCCs that are regionally patterned along the rostral-caudal neuraxis will be critical to complex regenerative repair of spinal damage. Damage that extends beyond CNS tissue will also require new bone, cartilage, tendon, peripheral nerves, autonomic neurons, and myelinating Schwann cells (Srinivasan and Toh, 2019).
The axial positions of NCCs are reflected in the Hox gene code and correspond to differential capacities in lineage specification (Frith et al., 2018). While Wnt signaling dictates the cranial vs. trunk decision, FGF signaling modulates axial identity (Hackland et al., 2019). In vitro NMPs have been used to produce sympathoadrenal progenitors and sympathetic neurons (Kirino et al., 2018; Saito-Diaz et al., 2019), as well as enteric neural progenitors and neurons (Frith et al., 2020). Knowledge of how to differentiate NCCs with a given axial fingerprint is advancing. NCCs by default, without signaling interventions, produce primarily anterior cranial NCCs that are Hox negative. Similar to the situation in the CNS, two routes exist to produce NCCs with more caudal identity (Figure 3B). The first uses RA to caudalize anterior cells to posterior cranial (Hox1–3), vagal and cardiac (Hox3–5), but not trunk, NCCs. The second route is through caudal NMPs to produce NCCs with trunk identity (Hox6–9). At present, this approach seems to be the sole method of generating trunk phenotypes and is paralleled in CNS protocols. The recognition of the central role of NMPs even in regards to NCCs has therefore been a major turning point in understanding and treating the trunk and spine.
Sensory neurons in the PNS are broadly specialized for sensing noxious, thermal, mechanical, or proprioceptive stimuli and relay this information to the CNS directly and through connections with dorsal spinal interneurons (Lai et al., 2016). Sensory neurons are characterized based on electrophysiological phenotypes, axon diameters, and surface receptor expression, and are also categorized by sensory modality. Early strategies to produce nociceptive sensory neurons again used neuroectodermal induction through dual SMAD inhibition followed by nociceptor induction by inhibitory small molecules CHIR 99021, SU 5402, and DAPT (Chambers et al., 2012; Clark et al., 2017). More recently, strategies aimed at achieving a closer adherence to the developmental origin of the PNS apply NCC intermediates from hPSCs to generate diverse sensory neuronal subtypes found within the dorsal root ganglion (Denham et al., 2015; Alshawaf et al., 2018). Alshawaf et al. (2018) optimized the temporal addition of inhibitors and soluble ligands to produce sensory neurons that respond to noxious, thermal, and mechanical stimuli.
SCI cellular repair may require a balance between retaining existing neurons and the need to replace lost neurons. Oligodendrocytes play critical support and structural roles for CNS neurons, enabling saltatory conduction, trophic support and axonal stability (Figures 3F,G; reviewed in Li and Leung, 2015). They are the myelinating cells for CNS axons within the subpial white matter, whereas neurons with axons directed distally to muscles utilize specialized Schwann cells for myelination. Studies in animal models following SCI demonstrate acute (Grossman et al., 2001) and chronic (Totoiu and Keirstead, 2005; Lytle and Wrathall, 2007) loss of CNS myelination. Demyelination following SCI trauma and the challenge for host oligodendrocyte progenitor cells (OPCs) to activate and fulfill the massive post-traumatic remyelination needs has led to a desire for therapeutic strategies that generate and co-transplant OPCs with other spinal cell types to protect against chronic die-back of axons and facilitate regeneration (Li and Leung, 2015). In 2005, the high-efficiency production of OPCs from hESCs in vitro and ability to promote remyelination with OPC transplants with improved locomotion in rodent models of SCI demonstrated one strategy to further optimize cell-based regenerative therapies for SCI (Keirstead et al., 2005; Nistor et al., 2005). This foundational work formed the basis for the first human clinical hESC trial for SCI by Geron (Geron Corporation, 2009; Scott and Magnus, 2014). Although the Geron study was discontinued, in part due to costs, in subsequent years advances in the in vitro production of OPCs and oligodendrocytes have continued (reviewed in Goldman and Kuypers, 2015). This is now being coupled with significant advances in generating homotypic spinal cell types relevant to the site of injury that together with OPC studies have invoked a new era of near term optimism for the clinical use and success of stem cell based neural therapies.
In hESCs, research has shown that the vertebrate developmental signaling pathways to sequentially activate oligodendrogenic transcription factors, bHLH-type OLIG2/NKX-2.2/SOX10 via Shh can be replicated, and along with other factors drive OPC maturation and myelinating potential. Initially, FGF2 is used to stimulate pre-OPC induction and expansion, but then later must be removed since it acts to inhibit further differentiation to oligodendrocytes by repressing the OLIG2 and NKX-2.2 expression induced by Shh (Hu et al., 2009). Oligodendrocyte protocols are now substantially refined by many groups (Goldman and Kuypers, 2015) and incorporate common principles including temporal exposure to FGF/RA conditions prior to stimulating the Shh pathway. In addition to FGF2, platelet derived growth factor (PDGF) exposure also helps to drive expansion of OPCs in vitro while insulin-like growth factor 1 (IGF-1) and thyroid hormone are added to promote maturation to myelinating oligodendrocytes. Three key articles demonstrate that successful sequential developmental events in fully defined systems to produce OPCs and oligodendrocytes in vitro that are capable of functional activity in rodent myelination models (Wang et al., 2013; Douvaras et al., 2014; Piao et al., 2015). These protocols are detailed in the literature (Douvaras and Fossati, 2015). Both hESC- and hiPSC-derived OPC populations have been generated at ~70–80% efficiency as validated by a panel of biomarkers. Protocols have also been developed that generate induction by small molecules to mimic normal development. These strategies are all currently time-inefficient however, requiring up to 150 days to produce functioning oligodendrocytes. This may reflect the natural time course of oligodendrocyte development in human gestation in oligodendrogenic waves. An alternate approach by Yang et al. (2013) instead applied direct lineage conversion of fibroblasts to oligodendrocytes by forced expression of SOX10, OLIG2, and ZFP536 in a protracted time course. To study oligodendrocyte maturation along with interactions with other neural cell types in vitro over extended time periods, a defined 3D spheroid culture and differentiation protocol was recently established (Marton et al., 2019). These oligodendrocytes shared electrical properties with mature in vivo counterparts including the firing of glial action potentials. A single oligodendrocyte can myelinate up to seven neurons. The use of in vitro models along with continued animal studies will better define the number of OPCs needed to transplant in context with the SCI features for therapeutic recovery.
As in the brain, astrocytes are important for neural homeostasis in the spinal cord. Brain astrocytes were first produced from hESCs in 2011 (Krencik and Zhang, 2011), requiring 6 months to generate pure cultures. These lengthy initial protocols yielded cells with immature reactive phenotypes, generated from anterior NSCs. A few years later Roybon et al. (2013) detailed a method for inducing astrocytes with spinal cord identity from mESCs, hESCs and hiPSCs with the ability to transition between immature reactive and mature quiescent phenotypes. Following developmental principles, RA-mediated caudalization and ventralization in the context of dual SMAD inhibition was exploited with the addition of ascorbic acid. Notably, FGF1 or FGF2 was sufficient to induce a mature, quiescent phenotype while pro-inflammatory cytokines TNF-α and IL-1β induced reactivity, marking a key advancement in the study of dynamic and extrinsically-influenced spinal astroglial phenotypes in vitro.
Since the CRISPR/Cas9 system was adapted to mammalian cell types in 2013 (Cong et al., 2013), there has been an explosion in the ability to edit genomes to developmental and therapeutic ends including for the CNS (Zavvarian et al., 2020). The application of CRISPR and other genetic and optogenetic approaches for SCI treatment was recently expertly reviewed (Paschon et al., 2020). Beyond error-correction for diseases, the power of CRISPR is in setting up biosensors to address the critical need in the SCI field to identify the legitimate integration between host tissue and transplanted therapeutic cells, as well as function such as by calcium-encoded readouts, neuronal activity such as cFos, and synaptogenesis. Towards this end, CRISPR along with optogenetics and other tools will bring reproducibility and analysis to the field. In addition to gene editing, further linking cellular phenotypes and functions to gene expression has long been a driving force in biology, and in recent decades the development of next generation sequencing tools has ushered in an explosion of information as well as of methodologies and analysis platforms to manage whole-transcriptome data. Initially, differentiating stem cell cultures were characterized using bulk RNA-Seq in which a library of RNA is prepared from an ensemble of heterogenous cells. The resulting picture is a one of a population average of global gene expression levels. Although extremely valuable, this technique does not benefit from single-cell resolution and therefore fails to detect stochastic variation in gene expression even between two cells that are otherwise considered identical. Developmental biology and stem cell fields have been revolutionized by the advent of single-cell RNA-Seq techniques that enable the elucidation of cell identity, transition states, and heterogeneity over time during development, reprogramming and differentiation (Kulkarni et al., 2019), otherwise known as fate mapping. Highly-parallel single-cell RNA-Seq permits the detection of rare cell populations in a differentiating culture and also identifies key transitional phenotypes that are critical to cell fate determination and ultimately to terminal differentiation potential. These methods help to delineate the identities and proportions of diverse cell types in a given heterogenous population by cluster analysis, and have been particularly applicable to organoid studies in which complex cytoarchitectures self-organize as models of or precursors to functional tissues. Further analysis by ATAC-Seq (assay for transposase-accessible chromatin using sequencing) or ChIP-Seq (chromatin immunoprecipitation sequencing) informs on chromatin states such as accessibility to transcription factors and identifies binding sites for proteins that complex with nucleic acids, respectively (Yan et al., 2020). By combining single-cell RNA-Seq with in situ cell-omics of intact tissues (e.g., MERFISH, multiplexed error-robust fluorescence in situ hybridization), spatial resolution of single cell transcriptome profiles with intact cultures and tissues can be achieved while retaining structural information (Mayr et al., 2019). In one key human study (Ho et al., 2016), single-cell RNA-Seq was used to understand and compare human motor neuron differentiation from iPSCs to fetal tissue counterparts during development. This elegant work resolved gene regulatory networks and pathways at play in differentiating human motor neurons as they mature and age. Ho et al. (2016) also established a framework by which to assess motor neuron functional maturity that contributes to the refinement of differentiation protocols and in vitro culture practices. Such studies are expected elucidate key developmental mechanisms that can be mirrored or perturbed in stem cell differentiation to achieve functional maturity. Advances in gene editing technologies and single cell sequencing with retained structural information are seemingly advancing by the day.
The extraordinary capability of pluripotent and multipotent stem cells to self-organize as 3D aggregates that recapitulate mammalian tissues, sub-organ microenvironments, and developmental stages is astonishing and is advancing strategies for medical treatments. The differentiation and maturation of these stem cell aggregates that respond to internal and external cues, such as described in this review, yields so-called “organoids” that can be coaxed into increasingly more complex and functional models. Early developmental events that underlying normal physiology and disease processes are advancing rapidly (Figure 4; expertly reviewed in Amin and Paşca, 2018). For the maturation of multiple neural and non-neural cell types, long-term survival of organoids in suspension (>2 years) has been achieved. Most exciting is the ability to interrogate complex cell-cell interactions in a microenvironment approximating embryological and postnatal events. A fundamental challenge to generating larger organoids beyond millimeter scale, and eventually organs or multi-organ systems, is a distinct lack of vascularization and the homeostatic and signaling benefits that vascular supply can provide. Beyond methods such as transplantation of organoids into mouse models to achieve in vivo vascularization and maturation, multiple groups are addressing this in vitro by generation of endothelial cells in organoids. Cakir et al. (2019) expressed a gene for the transcription factor master regulator, ETS variant 2 (ETV2), in human cortical organoids to achieve vascular-like networks (Cakir et al., 2019; Grebenyuk and Ranga, 2019). However, whether this is sufficient to recapitulate the dynamic spatiotemporal developmental interplay along with multiple other differentiation events in tissue specific context is unclear (Grebenyuk and Ranga, 2019), and organoids still expand to large millimeter-scale dimensions without a vascular contribution.
Figure 4. 3D heterogenous cell culture developmental models and biotechnologies. Overview of stem cell integration with biotechnologies to produce developmental neurotechnologies. In vitro 3D heterogenous cell culture systems include gastruloids, organoids, and assembloids that can be merged with biomaterials, microfluidics, and devices. mESC, mouse embryonic stem cell; hiPSC, human induced pluripotent stem cell; hESC, human embryonic stem cell; NMJ, neuromuscular junction.
During embryonic development, the earliest stages of organogenesis that may be helpful for understanding morphogenetic patterning and regeneration in the human trunk and spine are morally challenging to study. In 2014, remarkable progress was made using mESCs to recapitulate 3D neural tube patterning (Meinhardt et al., 2014), symmetry breaking, and axial organization in aggregates (van den Brink et al., 2014; Figure 4). In the later study, the use of small cell number aggregates (300–400 cells) yielded symmetry-breaking stochastic events and subsequent non-linear polarized growth (van den Brink et al., 2014; Baillie-Johnson et al., 2015), critical embryonic development events. Amazingly, self-organized “gastruloids” form multiple germ layers and in the absence of extra-embryonic tissues (Turner et al., 2017; Beccari et al., 2018; Rossi et al., 2021). Gastruloids have enabled our ability to study embryonic mechanisms for anterior–posterior polarization, axial elongation, and compartmentalized signaling without the use of embryos. Attention to developmental signaling events created improved protocols, and along with Matrigel embedding, coaxed formation of trunk-like structures containing a neural tube with segmented mesodermal somites (Veenvliet et al., 2020) as well as extended somitogenesis in gastruloids (van den Brink et al., 2020). The ability to use gastruloids to integrate central spinal cord and peripheral NCC-derived neurons was recently demonstrated in elongating multilineage organized (EMLO) gastruloids (Olmsted and Paluh, 2020). The EMLO gastruloids are formed from SOX2/FOXA2 mesendoderm and SOX2/Bra NMP starting cells and encompass features of both central and peripheral nervous systems including the enteric nervous system. This novel platform enables the multi-lineage study of nervous system development, NCC ontogeny, and neuromodulators. In the trunk and spinal fields, gastruloids now represent a powerful embryo-like system in which to study fundamental human multi-lineage developmental processes with tremendously broad neurotechnology implications.
Heterogenous 3D tissue models also enable the interrogation of neural circuitry. Rhythmically-active spinal circuits have been generated within 3D aggregates. Sternfeld et al. (2017) incorporated mESC-derived spinal motor and interneurons into aggregates, deemed “circuitoids,” for the dissection of neural circuit formation and function. Human stem cell based models that are 3D suspension cultures provides a method to drive the maturation of multiple neuronal types, particularly of motor neurons (Rigamonti et al., 2016). Dorsal or ventral morphogen patterning of 3D aggregates was shown to be sufficient to spontaneously form distinct human progenitor spinal domains prior to interneuron differentiation (Ogura et al., 2018; Duval et al., 2019). Andersen et al. (2020) recently recapitulated the functional human cortico-motor tract by the fusion of three regionally-patterned spheroids into sophisticated “assembloids.” As well, the assembloid approach was used in enteric nervous system development by seeding human intestinal organoids with hPSC-derived NCCs to produce a rhythmically-active gut-neural assembloid (Workman et al., 2017). By use of in vivo grafting further maturation of the gut-neural assembloid was achieved, including generation of neuroglial structures resembling myenteric and submucosal plexuses as well as functional interstitial cells of Cajal, and electromechanical coupling to regulate contraction wave propagation. This ability in multiple mouse and human models to address neural circuitry, CNS and PNS co-development and interplay, and multi-lineage organogenesis will be extremely beneficial to help direct therapeutic cell types and regenerate functional networks. These studies also raise interest in approaches to trunk and spine therapy in regards to optimal transplanted cellular content. Different considerations include NSCs, directed spine neural progenitors, or more complex anatomically matched cells and circuitry to accelerate repair and reproducible recovery. Along with functional recovery, the ability comes to regulate spinal mechanisms of pain, recently achieved by transplantation of inhibitory interneurons (Manion et al., 2020).
Functionally-active neuromuscular trunk organoids from hPSC-derived NMPs were recently achieved (Faustino Martins et al., 2020), that build on previous key studies (Gouti et al., 2014; Warmflash et al., 2014). These informative neuromuscular trunk organoids contain an astonishing myriad of differentiating cells types and their progenitors, including SMNs, spinal interneurons, skeletal muscle, myelinating glia, astrocytes, and neural crest-derived tissues such as sclerotome/cartilage cells and Schwann cells. The neuroectodermal and mesodermal tissues self-organize into distinct functionally connected compartments. The trunk organoids exhibit posterior Hox profiles (HoxC9–10) by single-cell RNA-Seq, and mature structural features like myelinated neuronal fibers and functional neuromuscular junctions (NMJs). Central pattern generator-like circuits formed spontaneously, with skeletal contractions driven by electrically active neurons. These organoids are being used to model pathophysiological aspects of myasthenia gravis, a neuromuscular disease that later manifests in muscle weakness in walking and facial movements. Human disease lines generated from patient iPSCs will be particularly useful in modeling CNS or neuromuscular diseases with organoids, gastruloids, assembloids and circuitoids. This approach has already revealed novel mechanisms in disease pathology for spinal muscular atrophy and familial amyotrophic lateral sclerosis (ALS; Sances et al., 2016; Adami and Bottai, 2019).
Beginning with simpler systems and integrating human stem cell organoid technologies (Figure 4), spinal and neuromuscular physiology is benefiting from bioengineering. This includes multilineage tissue engineering for multiple sclerosis (Maffioletti et al., 2018) and neuron optogenetics for ALS and the neuromuscular junction (Osaki et al., 2018; Bakooshli et al., 2019). Engineered devices enable the cost-effective, reproducible, customizable, and scalable high-throughput analysis of reductive cell–cell interactions in defined systems. These can be further integrated with optogenetic or electrical controls (Steinbeck et al., 2016). Kawada et al. (2017) used patterned microchannel devices to generate motor nerve organoids that project a unidirectional fascicle of axons. Tissue engineered in vitro platforms are also useful in modeling complex injury environments, such as with SCI, wherein highly parallel experiments can be conducted to refine the conditions translated to cost-intensive animal studies. These platforms can be fully reconstituted from in vitro components, or can incorporate spinal cord explant slices as ex vivo models of injury (Weightman et al., 2014). In a recent study, Giandomenico et al. (2019) integrated human organoids and mouse ex vivo spinal explants. The cerebral cortical organoids formed at the air-liquid interface projected extracortical pyramidal-like tracts that innervated spinal explants to induce muscle contractions. In effect, this study demonstrated recreation of the entire cortical-spinal-muscular circuit containing human upper motors neurons and mouse spinal motor and interneurons. This effort was also successfully demonstrated in a fully human stem cell system (Andersen et al., 2020). Such studies demonstrate the incredible power and pace at which in vitro systems that can be combined with biomaterials to impart higher organization in customizable geometries for in vivo transplantation and restoration of function after injury.
The vertebrate CNS has historically been regarded as a non-regenerating organ system, and spinal cord injury “an ailment not to be treated.” The history of SCI treatments has been reviewed elsewhere (Silver, 2005; Donovan, 2007). Seminal early research to graft intact fetal or adult tissue into the injured CNS provided foundational evidence that restoration of damaged circuitry by exogenous therapeutic cells is feasible (Das, 1990; Ramon y Cajal et al., 1991). Prior to the late 21st century, these models were applied to advance basic neuroscience studies. This included the investigation of intrinsic and extrinsic barriers to spontaneous regeneration of CNS neurons, high-fidelity axon path finding and target innervation, the wiring and re-wiring of the CNS, and interactions with ECM and other signaling ligands that influence these processes. The concept of “bridges” was also explored, in which a nerve graft was transplanted in a rat with intact spinal cord to attempt to link medullary and thoracic regions (David and Aguayo, 1981). This model system allowed transplant-host bridge junctions to be tracked with relative ease. Host spinal axon extension into the peripheral nerve grafts within the CNS was observed, but the study also revealed possible inhibitory effects from the host injury environment. Early transplant work also assessed the viability and survival of fetal grafts in the injured CNS in rodent models. Beginning in the 1980s, Bregman and Reier (1986) demonstrated that fetal spinal cord grafted into the mid-thoracic region of <72 h postnatal rats can retain some degree of viability, differentiate and integrate to establish graft-host synaptic connectivity (Reier et al., 1986; Bregman et al., 1993). In 2001, fetal grafts were shown to be safe and feasible in humans (Wirth et al., 2001) in a Phase 1 study. However, ethical concerns limit broad use of such therapies. The emergence of the mouse stem cell field in 1981 (reviewed in Evans, 2011) and later in human stem cells in 1998 (Thomson et al., 1998) has refocused efforts on stem cell based therapies.
Initial successes influenced a transition to cellular-based therapies in SCI (reviewed in Assinck et al., 2017) to promote neuroregeneration, neuro-restoration and functional improvement. Mesenchymal stem cells (MSCs) have a long history in transplantation (reviewed in Cofano et al., 2019), as well as other primary candidate cell types such as olfactory ensheathing cells and Schwann cells. More recently OPCs, NSPCs, lineage-restricted progenitors, and in some instances, postmitotic neurons have seen increased use (Assinck et al., 2017; Lee et al., 2020). In 2006, the advent of reprogramming to induce pluripotency (Takahashi and Yamanaka, 2006) and improved differentiation protocols accelerated human neural stem cell endeavors, as it became possible to establish patient-specific, renewable cell resources for grafting that have tailored neural identities and reduced immunogenicity concerns (Trawczynski et al., 2019). In order to make clinically relevant cellular therapies more readily accessible and to more accurately model disease, stable population-diverse iPSC lines were derived from donors of numerous self-reported ethnicities (Chang et al., 2015; Tomov et al., 2016). Genome-wide association (GWAS) studies are assisting in elucidating the underlying genetics (Gurdasani et al., 2019). An expansive knowledge base of stem cell therapies with a variety of cell types, injuries, and animal models for SCI has been generated, particularly over the past 20 years. Never before has the SCI field been better poised to develop meaningful and reproducible therapeutic regimens with a revitalized expectation that partial or full functional recovery and restoration of lost modalities is achievable. Meanwhile, specific methodological, technical, and pathophysiological barriers continue to be addressed to refine new approaches.
The clinical relevance of cell therapies to SCI has remained limited due to a number of pathophysiological barriers inherent to SCI (Kraus, 1996) as well as technical barriers requiring improved methodologies for CNS cell delivery and retention at the injury site. At present, the restoration of spinal cytoarchitectures to a pre-injury state, such as restoring neural circuits, has not been achieved except by a limited extent in pre-clinical animal studies. Neuronal relays that connect rostral, injured axons to caudal targets by use of novel intermediary graft architectures have also been described in numerous studies. However, efficient and accurate transmission of information within a new, non-specific network is challenging, including reversal of signals from excitatory to inhibitory, misdirected, or prematurely dissipated signals. A recent variation on this strategy is generation de novo from human stem cells a transplantable neuronal network provided in degradable alginate neural ribbons (Olmsted et al., under review). To obtain physical continuity of any graft, the type of parameters to be optimized has remained fairly constant, but the means with which they are characterized has been extended with additional complexity. Therapeutic neural cell delivery requires region-specified neural cell type(s) and ratios, use of highly-characterized cell sources that include transcriptomics, and appropriate cell dose as well as delivery mechanisms for cell positioning, alignment, and retention at the injury site. Directed differentiation or maturation must be tracked to distinguish transplanted cells from host cells and the degree of integration with host tissue. Earlier studies established precedent that injection of unprotected cells in suspension into the injury site resulted in significant loss of those cells due to the immune cytotoxicity and lost support cells of the microenvironment, and that age of the injury as acute, subacute or chronic was also relevant.
Astrocyte hypertrophy-hyperplasia further fuels the establishment of the glial “scar” that is inhibitory to axon regeneration, although pro-regenerative features of the response mechanism are being revealed (summarized in Bradbury and Burnside, 2019; Yang et al., 2020). The proliferative ability of grafts is a double-edged sword, desired to ensure that sufficient cells are available to heal the injury site, accounting for some cell loss, while avoiding tumorigenicity, such as in neuromas, can occur with NSCs. Tumorigenicity appears reduced in non-human primates vs. rodents (Rosenzweig et al., 2017), and is affected by the maturation state of the graft. With the capability now of human stem cell technologies to provide injury-matched SMNs and support cells, the focus can move to refining biomaterials, microenvironment regulators, and biosensors to overcome pathophysiological barriers. This is expected to provide greater reproducibility and predictive responses that is needed to propel the SCI field into a new realm of opportunities for therapeutic advancement.
The developmental pathways to precisely generate in vitro specific cells and tissues of the spine as defined physiologically, functionally and by transcriptomics are in hand and expected to work their way into the clinic. More recent extensions of these technologies include assembloids and gastruloids, as well as possible small molecule interventions to avoid transplantation altogether. The fundamental in vivo pathophysiological mechanisms underlying SCI are also being targeted therapeutically to promote regeneration. It appears now as a question not of if, but when, full restoration of lost functional modalities after injury will be achievable. The merger of therapeutic cells with biomaterial scaffolds, neurotrophins and growth factors, and injury site enhancers such as the bacterial enzyme chondroitinase ABC are summarized (Katoh et al., 2019).
The in vivo direct lineage conversion and activation of endogenous NSCs is an alternative to the replacement of injured cells in the CNS. One approach applies recombinant cytokines to promote host tissue regeneration by stimulating endogenous stem cells pharmacologically (Lowry and Temple, 2007; Hachem et al., 2020). Alternatively the in vivo direct lineage conversion of somatic cells to neurons by forced expression of transcription factors could overcome endogenous NSC limitations. In situ reprogramming to neurons and oligodendroglia from astrocytes in the injured CNS has been demonstrated and reviewed in the literature (Li and Chen, 2016). This approach benefits from minimally-invasive delivery and bypasses immune-rejection concerns, though will also require cGMP-grade production of viral expression vectors. Such approaches rely on what is typically seen in vitro with organoid research, which is the inherent ability of cells to differentiate and organize effectively given the needed cues to bypass developmental pathways but achieve sufficient terminal cell identity.
Successful outcomes in the treatment of SCI in rodents and other small animal models that include partial restoration in transected spinal cords has led to the progression to large animal studies (reviewed in Gabel et al., 2017) and early clinical interventions in humans. An exciting combinatorial approach by Lai et al. (2019) applies an acute model of SCI that is a complete thoracic transection using a preconstructed neural network established in collagen scaffolds as a bridge. The neural networks are formed in vitro from cells obtained from newborn beagles that are hippocampus-derived NSCs and differentiated into neurons as well as with isolated Schwann cells. This approach encompasses neurotechnologies and scaffolds in an advanced approach that realized repair outcomes in vivo. Subsequent transplantation into the SCI site of 6–8 month old canines resulted in significant motor recovery of paralyzed pelvic limbs, where there storation of neural relays restored information to the paralyzed hind limbs (Lai et al., 2019). New stem cell neurotechnologies can be adopted to refine this approach by instead producing the precise therapeutic cells in vitro, similar to neural ribbons (Olmsted et al., 2020, under review). Syngeneic and allogeneic iPSC-derived NSPCs were shown to survive after transplantation into the spinal cord of minipigs (Strnadel et al., 2018). In a first-in-human Phase 1 SCI clinical trial for chronic SCI, clinical grade NSCs from line NSI-566 were transplanted as a part of a safety analysis for treatment of clinically complete thoracic transections (Curtis et al., 2018). An alternate Phase 1 trial using autologous adipose tissue-derived MSCs for traumatic SCI was also reported by the Mayo Clinic (Bydon et al., 2019). In these two recent works, the stem cell therapies were deemed safe, but meaningful clinical improvement in function was not observed. Interestingly, by incorporating collagen scaffolds adsorbed with patient autologous MSCs into the transplantation site, two traumatic SCI patients regained motor, sensory, and autonomic functions one year later in a separate study (Xiao et al., 2018). Currently, a variety of stem cell based sources of cells and protocols are being used, with varying degrees of success. The field may benefit by unifying efforts to focus on those most promising neural or MSC therapies. It is also evident that a combination of biomaterials with stem cell-derived neural cells adds further benefit to rehabilitation regimens (Harkema et al., 2011) along with spinal cord stimulation to drive neuroplasticity after transplantation (Formento et al., 2018). Human SCI clinical trials with cell-based therapies were recently reviewed (Silvestro et al., 2020; Yamazaki et al., 2020), along with a protocol in place for systematic review and meta-analysis of efficacy (Tang et al., 2020). An excellent report on the requirements for translation of stem cell therapies to the clinic for CNS repair including industry and regulatory considerations is provided by Aboody et al. (2011).
The first decade of the 21st century has seen a new revolution in human developmental neurotechnologies that would not be possible without mouse and now human stem cell advances that continue to accelerate (Figure 5; Thomson et al., 1998; Takahashi and Yamanaka, 2006; Cong et al., 2013; Mayr et al., 2019). It is now primarily the temporal nature of clinical trials, cGMP, and natural healing that will determine the timeframe to therapeutic advancements. For Parkinson’s disease, clinical trials by GForce-PD cell replacement therapies have recently begun (Barker et al., 2017). Not only the stem cell based models are bringing expanded understanding of fundamental elements of human disease processes, but also the temporal scope around disease initiation and progression, while also doubling as treatments testing “bench-to-therapy” platforms. Stem cell neurotechnologies for injury and trauma have advanced in part through more complex integration with biomaterials, optogenetics, biosensors, and electronics and are pushing the boundaries in research discovery and treatment. Specifically for human therapies of the trunk and spine (Figures 4, 5), information arising from developmental neurotechnologies, including embryology, can now be analyzed to inform and improve treatments beyond trauma. Most recently, this includes neuromuscular disorders such as ALS and myasthenia gravis (reviewed in Sances et al., 2016; Faustino Martins et al., 2020), neural tube defects such as spina bifida that can be related to the Pax3 gene (Sudiwala et al., 2019), neurocristopathies such as Hirschsprung’s disease resulting from NCC migration defects (Fattahi et al., 2016; Workman et al., 2017), neurodegeneration or demyelinating process originating in the CNS (Garden and La Spada, 2012), and sensory disorders such as neuropathic pain (Manion et al., 2020). We expect that human stem cell developmental neurotechnologies will continue to play an essential role in understanding and treating these disorders through the elementary discovery of fundamental processes, in vitro models, and scalable high throughput platforms for drug testing, as well as by supplying an unlimited customized cell resource for replacement therapies. A summary of select recent stem cell models applied to disease and therapeutic studies is provided (Table 1). Developmental neurotechnologies are further benefiting by the use of population diverse hiPSC lines (Chang et al., 2015; Tomov et al., 2016; Olmsted and Paluh, 2020) to more accurately represent global ethnicities and genomes (Laurent et al., 2010; Mosher et al., 2010; Gurdasani et al., 2019).
Figure 5. Clinical potential of stem cells and developmental neurotechnologies. (A) Conditions of the human trunk and spine that will benefit from developmental neurotechnologies. Examples include trauma and spinal cord injury (SCI), neuromuscular disorders such as amyotrophic lateral sclerosis (ALS) and myasthenia gravis, neural tube closure defects such as spina bifida that relate to PAX3, neurocristopathies such as Hirschsprung’s disease that result from NCC migration defects, CNS neurodegeneration, demyelinating disorders such as multiple sclerosis, and sensory disorders such as neuropathic pain. (B) Clinical stages of developmental neurotechnology discovery and testing from pre-clinical through to current good manufacturing processes (cGMP). (C) The developmental neurotechnology paradigm to generate clinical therapies and disease models by merging stem cell developmental principles with neurotechnologies.
Table 1. Stem cell technologies for trunk and spine disease modeling and therapy (select publications).
Towards the goal of reproducible human SCI transplantation therapy, the field is embracing regional matching of therapeutic cells.This is now possible by the wealth of studies with stem cell-derived neural cells, such as described in this review, that reveal a comprehensive understanding of key signaling pathways and complex, intrinsic gene regulatory networks. Organoid, gastruloid, assembloid, and circuitoid technologies reveal the inherent ability of cells to adapt into functional complexes. Matched therapeutic cells by definition should interface more appropriately and efficiently with host. Biomaterial platforms that facilitate better cell–cell interactions and favor network connectivity in vitro are an elegant approach to bridge in vitro design with in vivo transplantation (Lai et al., 2019) and are continuing to evolve (Olmsted et al., under review). Neuronal maturation, synaptogenesis, and plasticity are also driven by network synchronous-firing activity (Kirkby et al., 2013) i.e., optimal in 3D vs. adherent neuronal cultures. Neuromodulation by use of programmed, electrical frequency-specific stimulation of the dorsal columns has been demonstrated to be critical for managing chronic pain patients that are refractory to medical management (Staudt et al., 2020), and may be needed in developing more complex in vitro systems to drive appropriate network formation and synaptic plasticity mechanisms for motor recovery. Continually evolving and integrating methodologies and tools to elucidate, and exploit natural mechanisms required for functional maturation will continue to position the field within an exciting era of innovative reproducible treatments.
In closing, stem cell developmental neurotechnologies will continue to be cross-disciplinary, bringing together additional fields beyond stem cell biology, neurodevelopment, multi-omics, and bioengineering. To this end, recent advancements in genetic engineering of human cell lines enabled by CRISPR/Cas gene-editing technologies are advancing differentiation, enrichment, subtype purification, comparison of cell types in normal and disease states, and assessment of functional graft integration (Cota-Coronado et al., 2019; Tian et al., 2019; Paschon et al., 2020). Additionally, 3D organoid and complex co-culture platforms have improved context-specific maturation of multiple neural cell types. The use of pharmacological agents, targeting the cytoskeleton and consideration of innate circadian clocks have also contributed to an understanding of in vitro maturation in the stem cell field (Alvarez-Dominguez et al., 2020; Hogrebe et al., 2020) and are now extending to protocols using NMPs. Furthermore, sophisticated co-cultures of PSC-derived neurons with astrocytes have produced marked improvement in population-level connectivity and synaptogenesis in addition to more hyperpolarized resting membrane potentials (Taga et al., 2019). The ability to generate functionally mature neurons in vitro along with other cell types that are indistinguishable from in vivo counterparts is unlikely to be required for all therapeutic success, particularly for simpler injuries and microenvironments that allow cells to mature quickly after transplantation. However, it is likely necessary in vitro to accurately model disease mechanisms, and will benefit pharmaceutical development to ultimately guide appropriate in vivo transplantation and non-transplantation therapeutic interventions alike.
ZO composed figures. All authors contributed to the article and approved the submitted version.
This work was funded by the New York State Department of Health (NYS DOH)—Wadsworth Center, New York State Spinal Cord Injury Research Board (NYSCIRB), and Projects to Accelerate Research Translation (PART) award C33278GG.
The authors declare that the research was conducted in the absence of any commercial or financial relationships that could be construed as a potential conflict of interest.
We would like to sincerely apologize to the authors of work which we were unable to cite due to space restrictions. Figures were made with a combination of Adobe Illustrator, Keynote, and BioRender.com.
Aboody, K., Capela, A., Niazi, N., Stern, J. H., and Temple, S. (2011). Translating stem cell studies to the clinic for CNS repair: current state of the art and the need for a Rosette stone. Neuron 70, 597–613. doi: 10.1016/j.neuron.2011.05.007
Adami, R., and Bottai, D. (2019). Spinal muscular atrophy modeling and treatment advances by induced pluripotent stem cell studies. Stem Cell Rev. Rep. 15, 795–813. doi: 10.1007/s12015-019-09910-6
Agius, E., Decker, Y., Soukkarieh, C., Soula, C., and Cochard, P. (2010). Role of BMPs in controlling the spatial and temporal origin of GFAP astrocytes in the embryonic spinal cord. Dev. Biol. 344, 611–620. doi: 10.1016/j.ydbio.2010.05.017
Allodi, I., Nijssen, J., Benitez, J. A., Schweingruber, C., Fuchs, A., Bonvicini, G., et al. (2019). Modeling motor neuron resilience in ALS using stem cells. Stem Cell Reports 12, 1329–1341. doi: 10.1016/j.stemcr.2019.04.009
Alshawaf, A. J., Viventi, S., Qiu, W., D’Abaco, G., Nayagam, B., Erlichster, M., et al. (2018). Phenotypic and functional characterization of peripheral sensory neurons derived from human embryonic stem cells. Sci. Rep. 8:603. doi: 10.1038/s41598-017-19093-0
Alvarez-Dominguez, J. R., Donaghey, J., Rasouli, N., Kenty, J. H. R., Helman, A., Charlton, J., et al. (2020). Circadian entrainment triggers maturation of human in vitro islets. Cell Stem Cell 26, 108–122. doi: 10.1016/j.stem.2019.11.011
Amin, N. D., and Paşca, S. P. (2018). Building models of brain disorders with three-dimensional organoids. Neuron 100, 389–405. doi: 10.1016/j.neuron.2018.10.007
Amoroso, M. W., Croft, G. F., Williams, D. J., O’Keeffe, S., Carrasco, M. A., Favis, A. R., et al. (2013). Accelerated high-yield generation of limb-innervating motor neurons from human stem cells. J. Neurosci. 33, 574–586. doi: 10.1523/JNEUROSCI.0906-12.2013
Andersen, J., Revah, O., Miura, Y., Thom, N., Amin, N. D., Kelley, K. W., et al. (2020). Generation of functional human 3D cortico-motor assembloids. Cell 183, 1913.e26–1929.e26. doi: 10.1016/j.cell.2020.11.017
Andoniadou, C. L., and Martinez-Barbera, J. P. (2013). Developmental mechanisms directing early anterior forebrain specification in vertebrates. Cell. Mol. Life Sci. 70, 3739–3752. doi: 10.1007/s00018-013-1269-5
Assinck, P., Duncan, G. J., Hilton, B. J., Plemel, J. R., and Tetzlaff, W. (2017). Cell transplantation therapy for spinal cord injury. Nat. Neurosci. 20, 637–647. doi: 10.1038/nn.4541
Aulehla, A., and Pourquié, O. (2008). Oscillating signaling pathways during embryonic development. Curr. Opin. Cell Biol. 20, 632–637. doi: 10.1016/j.ceb.2008.09.002
Baillie-Johnson, P., van den Brink, S. C., Balayo, T., Turner, D. A., and Arias, A. M. (2015). Generation of aggregates of mouse embryonic stem cells that show symmetry breaking, polarization and emergent collective behavior in vitro. J. Vis. Exp. 105:53252. doi: 10.3791/53252
Bakooshli, M. A., Lippmann, E. S., Mulchay, B., Iyer, N., Nguyen, C. T., Tung, K., et al. (2019). A 3D culture model of innervated human skeletal muscle enables studies of the adult neuromuscular junction. eLife 8:e44530. doi: 10.7554/eLife.44530
Barker, R. A., Parmer, M., Studer, L., and Takahashi, J. (2017). Human trials of stem cell-derived dopamine neurons for Parkinson’s disease: dawn of a new era. Cell Stem Cell 21, 569–573. doi: 10.1016/j.stem.2017.09.014
Bayraktar, O. A., Fuentealba, L. C., Alvarez-Buylla, A., and Rowitch, D. H. (2015). Astrocyte development and heterogeneity. Cold Spring Harb. Perspect. Biol. 7:a020362. doi: 10.1101/cshperspect.a020362
Beccari, L., Moris, N., Girgin, M., Turner, D. A., Baillie-Johnson, P., Cossy, A.-C., et al. (2018). Multi-axial self-organization properties of mouse embryonic stem cells into gastruloids. Nature 562, 272–276. doi: 10.1038/s41586-018-0578-0
Blassberg, R., Patel, H., Watson, T., Gouti, M., Metzis, V., Delas, M. J., et al. (2020). Sox2 levels configure the WNT response of epiblast progenitors responsible for vertebrate body formation. bioRxiv [Preprint]. doi: 10.1101/2020.12.29.424684
Bradbury, E. J., and Burnside, E. R. (2019). Moving beyond the glial scar for spinal cord repair. Nat. Commun. 10:3879. doi: 10.1038/s41467-019-11707-7
Bregman, B. S., Kunkel-Bagden, E., Reier, P. J., Dai, H. N., McAtee, M., and Gao, D. (1993). Recovery of function after spinal cord injury: mechanisms underlying transplant-mediated recovery of function differ after spinal cord injury in newborn and adult rats. Exp. Neurol. 123, 3–16. doi: 10.1006/exnr.1993.1136
Bregman, B. S., and Reier, P. J. (1986). Neural tissue transplants rescue axotomized rubrospinal cells from retrograde death. J. Comp. Neurol. 244, 86–95. doi: 10.1002/cne.902440107
Brokhman, I., Gamarnik-Ziegler, L., Pomp, O., Aharonowiz, M., Reubinoff, B. E., and Goldstein, R. S. (2008). Peripheral sensory neurons differentiate from neural precursors derived from human embryonic stem cells. Differentiation 76, 145–155. doi: 10.1111/j.1432-0436.2007.00196.x
Brown, C. R., Butts, J. C., McCreedy, D. A., and Sakiyama-Elbert, S. E. (2014). Generation of V2a interneurons from mouse embryonic stem cells. Stem Cells Dev. 23, 1765–1776. doi: 10.1089/scd.2013.0628
Bryson, J. B., Machado, C. B., Crossley, M., Stevenson, D., Bros-Facer, V., Burrone, J., et al. (2014). Optical control of muscle function by transplantation of stem cell-derived motor neurons in mice. Science 344, 94–97. doi: 10.1126/science.1248523
Butts, J. C., Iyer, N., White, N., Thompson, R., Sakiyama-Elbert, S., and McDevitt, T. D. (2019). V2a interneuron differentiation from mouse and human pluripotent stem cells. Nat. Protoc. 14, 3033–3058. doi: 10.1038/s41596-019-0203-1
Bydon, M., Dietz, A. B., Goncalves, S., Moinuddin, F. M., Alvi, M. A., Goyal, A., et al. (2019). CELLTOP clinical trial: first report from a phase 1 trial of autologous adipose tissue-derived mesenchymal stem cells in the treatment of paralysis due to traumatic spinal cord injury. Mayo Clin. Proc. 95, 406–414. doi: 10.1016/j.mayocp.2019.10.008
Cai, J., Qi, Y., Hu, X., Tan, M., Liu, Z., Zhang, J., et al. (2005). Generation of oligodendrocyte precursor cells from mouse dorsal spinal cord independent of Nkx6 regulation and Shh signaling. Neuron 45, 41–53. doi: 10.1016/j.neuron.2004.12.028
Cakir, B., Xiang, Y., Tanaka, Y., Kural, M. H., Parent, M., Kang, Y.-J., et al. (2019). Engineering of human brain organoids with a functional vascular-like system. Nat. Methods 16, 1169–1175. doi: 10.1038/s41592-019-0586-5
Cambray, N., and Wilson, V. (2002). Axial progenitors with extensive potency are localized to the mouse chordoneural hinge. Development 129, 4855–4866.
Chal, J., Oginuma, M., Tanoury, Z. A., Gobert, B., Sumara, O., Hick, A., et al. (2015). Differentiation of pluripotent stem cells to muscle fiber to model Duchenne muscular dystrophy. Nat. Biotechnol. 33, 962–969. doi: 10.1038/nbt.3297
Chambers, S. M., Fasano, C. A., Papapetrou, E. P., Tomishima, M., Sadelain, M., and Studer, L. (2009). Highly efficient neural conversion of human ES and iPS cells by dual inhibition of SMAD signaling. Nat. Biotechnol. 27, 275–280. doi: 10.1038/nbt.1529
Chambers, S. M., Qi, Y., Mica, Y., Lee, G., Zhang, X.-J., Niu, L., et al. (2012). Combined small molecule inhibition accelerates developmental timing and converts human pluripotent stem cells in nociceptors. Nat. Biotechnol. 30, 715–720. doi: 10.1038/nbt.2249
Chang, E. A., Tomov, M. L., Suhr, S. T., Luo, J., Olmsted, Z. T., Paluh, J. L., et al. (2015). Derivation of ethnically diverse human induced pluripotent stem cell lines. Sci. Rep. 5:15234. doi: 10.1038/srep15234
Chen, H., Qian, K., Du, Z., Cao, J., Petersen, A., Liu, H., et al. (2014). Modeling ALS with iPSCs reveals that mutant SOD1 misregulates neurofilament balance in motor neurons. Cell Stem Cell 14, 796–809. doi: 10.1016/j.stem.2014.02.004
Christian, J. L. (2012). Morphogen gradients in development: from form to function. Wiley Interdiscip. Rev. Dev. Biol. 1, 3–15. doi: 10.1002/wdev.2
Clark, A. J., Kaller, M. S., Galino, J., Willison, H. J., Rinaldi, S., and Bennett, D. L. H. (2017). Co-cultures with stem cell-derived human sensory neurons reveal regulators of peripheral myelination. Brain 140, 898–913. doi: 10.1093/brain/awx012
Cofano, F., Boido, M., Monticelli, M., Zenga, F., Ducati, A., Vercelli, A., et al. (2019). Mesenchymal stem cells for spinal cord injury: current options, limitations, and future of cell therapy. Int. J. Mol. Sci. 20:2698. doi: 10.3390/ijms20112698
Cong, L., Ran, F. A., Cox, D., Lin, S., Barretto, R., Habib, N., et al. (2013). Multiplex genome engineering using CRISPR/Cas systems. Science 339, 819–823. doi: 10.1126/science.1231143
Conti, L., and Cattaneo, E. (2010). Neural stem cell systems: physiological players or in vitro entities? Nat. Rev. Neurosci. 11, 176–187. doi: 10.1038/nrn2761
Cota-Coronado, A., Díaz-Martínez, N. F., Padilla-Camberos, E., and Díaz-Martinez, N. E. (2019). Editing the central nervous system through CRISPR/Cas9 systems. Front. Mol. Neurosci. 12:110. doi: 10.3389/fnmol.2019.00110
Curtis, E., Martin, J. R., Gabel, B., Sidhu, N., Rzesiewicz, T. K., Mandeville, R., et al. (2018). A first-in-human, phase 1 study of neural stem cell transplantation for chronic spinal cord injury. Cell Stem Cell 22, 941.e6–950.e6. doi: 10.1016/j.stem.2018.05.014
Das, G. D. (1990). Neural transplantation: an historical perspective. Neurosci. Biobehav. Rev. 14, 389–401. doi: 10.1016/s0149-7634(05)80061-2
David, S., and Aguayo, A. J. (1981). Axonal elongation into peripheral nervous system “bridges” after central nervous system injury in adult rats. Science 214, 931–933. doi: 10.1126/science.6171034
Davis-Dusenbery, B. N., Williams, L. A., Klim, J. R., and Eggan, K. (2014). How to make spinal motor neurons. Development 141, 491–501. doi: 10.1242/dev.097410
Denham, M., Hasegawa, K., Menhenoitt, T., Rollo, B., Zhang, D., Hough, S., et al. (2015). Multipotent caudal neural progenitors derived from human pluripotent stem cells that give rise to lineages of the central and peripheral nervous system. Stem Cells 33, 1759–1770. doi: 10.1002/stem.1991
Devlin, A.-C., Burr, K., Borooah, S., Foster, J. D., Cleary, E. M., Geti, I., et al. (2015). Human iPSC-derived motoneurons harbouring TARDBP or C9ORF72 ALS mutations are dysfunctional despite maintaining viability. Nat. Commun. 6:5999. doi: 10.1038/ncomms6999
Diaz-Cuadros, M., Wagner, D. E., Budjan, C., Hubaud, A., Tarazona, O. A., Donelly, S., et al. (2020). In vitro characterization of the human segmentation clock. Nature 580, 113–118. doi: 10.1038/s41586-019-1885-9
Diez del Corral, R., Olivera-Martinez, I., Goriely, A., Gale, E., Maden, M., and Storey, K. (2003). Opposing FGF and retinoid pathways control ventral neural pattern, neuronal differentiation, and segmentation during body axis extension. Neuron 40, 65–79. doi: 10.1016/s0896-6273(03)00565-8
Dimos, J. T., Rodolfa, K. T., Niakan, K. K., Weisenthal, L. M., Mitsumoto, H., Chung, W., et al. (2008). Induced pluripotent stem cells generated from patients with ALS can be differentiated into motor neurons. Science 321, 1218–1221. doi: 10.1126/science.1158799
Dionisi, C., Rai, M., Chazalon, M., Schiffmann, S. N., and Pandolfo, M. (2020). Induced pluripotent stem cell-derived primary proprioceptive neurons as Friedreich ataxia cell model. bioRxiv [Preprint]. doi: 10.1101/829358
Donovan, W. H. (2007). Spinal cord injury—past, present, and future. J. Spinal Cord Med. 30, 85–100. doi: 10.1080/10790268.2007.11753918
Douvaras, P., and Fossati, V. (2015). Generation and isolation of oligodendrocyte progenitor cells from human pluripotent stem cells. Nat. Protoc. 10, 1143–1154. doi: 10.1038/nprot.2015.075
Douvaras, P., Wang, J., Zimmer, M., Hanchuk, S., O’Bara, M. A., Sadiq, S., et al. (2014). Efficient generation of myelinating oligodendrocytes from primary progressive multiple sclerosis patients by induced pluripotent stem cells. Stem Cell Rep. 3, 250–259. doi: 10.1016/j.stemcr.2014.06.012
Du, Z.-W., Chen, H., Liu, H., Lu, J., Qian, K., Huang, C.-L., et al. (2015). Generation and expansion of highly pure motor neuron progenitors from human pluripotent stem cells. Nat. Commun. 6:6626. doi: 10.1038/ncomms7626
Duval, N., Vaslin, C., Barata, T. C., Frama, Y., Contremoulins, V., Baudin, X., et al. (2019). BMP4 patterns Smad activity and generates stereotyped cell fate organization in spinal organoids. Development 146:dev175430. doi: 10.1242/dev.175430
Etchevers, H. C., Dupin, E., and Le Douarin, N. M. (2019). The diverse neural crest: from embryology to human pathology. Development 146:dev169821. doi: 10.1242/dev.169821
Evans, M. (2011). Discovering pluripotency: 30 years of mouse embryonic stem cells. Nat. Rev. Mol. Cell Biol. 12, 680–686. doi: 10.1038/nrm3190
Fandel, T. M., Trivedi, A., Nicholas, C. R., Zhang, H., Chen, J., Martinez, A. F., et al. (2016). Transplanted human stem cell-derived interneuron precursors mitigate mouse bladder dysfunction and central neuropathic pain after spinal cord injury. Cell Stem Cell 19, 544–557. doi: 10.1016/j.stem.2016.08.020
Farhy-Tselnicker, I., and Allen, N. J. (2018). Astrocytes, neurons, synapses: a tripartite view on cortical circuit development. Neural Dev. 13:7. doi: 10.1186/s13064-018-0104-y
Fattahi, F., Steinbeck, J. A., Kriks, S., Tchieu, J., Zimmer, B., Kishinevsky, S., et al. (2016). Deriving human ENS lineages for cell therapy and drug discovery in Hirschsprung disease. Nature 531, 105–109. doi: 10.1038/nature16951
Faustino Martins, J. M., Fischer, C., Urzi, A., Vidal, R., Kunz, S., Ruffault, P.-L., et al. (2020). Self-organizing 3D human trunk neuromuscular organoids. Cell Stem Cell 26, 172–186. doi: 10.1016/j.stem.2019.12.007
Fogarty, M., Richardson, W. D., and Kessaris, N. (2005). A subset of oligodendrocytes generated from radial glia in the dorsal spinal cord. Development 132, 1951–1959. doi: 10.1242/dev.01777
Formento, E., Minassian, K., Wagner, F., Mignardot, J. B., Le Goff-Mignardot, C. G., Rowald, A., et al. (2018). Electrical spinal cord stimulation must preserve proprioception to enable locomotion in humans with spinal cord injury. Nat. Neurosci. 21, 1728–1741. doi: 10.1038/s41593-018-0262-6
Francius, C., Harris, A., Rucchin, V., Hendricks, T. J., Stam, F. J., Barber, M., et al. (2013). Identification of multiple subsets of ventral interneurons and differential distribution along the rostrocaudal axis of the developing spinal cord. PLoS One 8:e70325. doi: 10.1371/journal.pone.0070325
Franklin, R. J. M., and Ffrench-Constant, C. (2008). Remyelination in the CNS: from biology to therapy. Nat. Rev. Neurosci. 9, 839–855. doi: 10.1038/nrn2480
Frith, T. Jr., Gogolou, A., Hackland, J. O. S., Hewitt, Z. A., Moore, H. D., Barbaric, I., et al. (2020). Retinoic acid accelerates the specification of enteric neural progenitors from in-vitro-derived neural crest. Stem Cell Rep. 15, 557–565. doi: 10.1016/j.stemcr.2020.07.024
Frith, T. Jr., Granata, I., Wind, M., Stout, E., Thompson, O., Neumann, K., et al. (2018). Human axial progenitors generate trunk neural crest cells in vitro. eLife 7:e35786. doi: 10.7554/eLife.35786
Gabel, B. C., Curtis, E. I., Marsala, M., and Ciacci, J. D. (2017). A review of stem cell therapy for spinal cord injury: large animal models and the frontier in humans. World Neurosurg. 98, 438–443. doi: 10.1016/j.wneu.2016.11.053
Garden, G. A., and La Spada, A. R. (2012). Intercellular (mis)communication in neurodegenerative disease. Neuron 73, 886–901. doi: 10.1016/j.neuron.2012.02.017
Garriock, R. J., Chalamalasetty, R. B., Kennedy, M. W., Canizales, L. C., Lewandoski, M., and Yamaguchi, T. P. (2015). Lineage tracing of neuromesodermal progenitors reveals novel Wnt-dependent roles in trunk posterior cell maintenance and differentiation. Development 142, 1628–1638. doi: 10.1242/dev.111922
Geron Corporation. (2009). World’s first clinical trial of human embryonic stem cell therapy cleared. Regen. Med. 4:161. doi: 10.2217/17460751.4.2.159
Giandomenico, S. L., Mierau, S. B., Gibbons, G. M., Wenger, L. M. D., Masullo, L., Sit, T., et al. (2019). Cerebral organoids at the air-liquid interface generate diverse nerve tracts with functional output. Nat. Neurosci. 22, 669–679. doi: 10.1038/s41593-019-0350-2
Goldman, S. A., and Kuypers, N. J. (2015). How to make an oligodendrocyte. Development 142, 3983–3995. doi: 10.1242/dev.126409
Gouti, M., Delile, J., Stamataki, D., Wymeersch, F., Huang, Y., Kleinjung, J., et al. (2017). A gene regulatory network balances neural and mesoderm specification during vertebrate trunk development. Dev. Cell 41, 243.e7–261.e7. doi: 10.1016/j.devcel.2017.04.002
Gouti, M., Tsakiridis, A., Wymeersch, F. J., Huang, Y., Kleinjung, J., Wilson, V., et al. (2014). In vitro generation of neuromesodermal progenitors reveals distinct roles for Wnt signaling in the specification of spinal cord and paraxial mesoderm identity. PLoS Biol. 12:e1001937. doi: 10.1371/journal.pbio.1001937
Grebenyuk, S., and Ranga, A. (2019). Engineering organoid vascularization. Front. Bioeng. Biotechnol. 7:39. doi: 10.3389/fbioe.2019.00039
Green, S. A., Simoes-Costa, M., and Bronner, M. E. (2015). Evolution of vertebrates as viewed from the neural crest. Nature 520, 474–482. doi: 10.1038/nature14436
Greig, L. C., Woodworth, M. B., Galazo, M. J., Padmanabhan, H., and Macklis, J. D. (2013). Molecular logic of neocortical projection neuron specification, development and diversity. Nat. Rev. Neurosci. 14, 755–769. doi: 10.1038/nrn3586
Grossman, S. D., Rosenberg, L. J., and Wrathall, J. R. (2001). Temporal-spatial pattern of acute neuronal and glial loss after spinal cord contusion. Exp. Neurol. 168, 273–282. doi: 10.1006/exnr.2001.7628
Gupta, S., Sivalingam, D., Hain, S., Makkar, C., Sosa, E., Clark, A., et al. (2018). Deriving dorsal spinal sensory interneurons from human pluripotent stem cells. Stem Cell Rep. 10, 390–405. doi: 10.1016/j.stemcr.2017.12.012
Gurdasani, D., Castensen, T. C., Fatumo, S., Chen, G., Franklin, C. S., Prado-Martinez, J., et al. (2019). Uganda genome resource enables insights into population history and genomic discovery in Africa. Cell 179, 984–1002. doi: 10.1016/j.cell.2019.10.004
Hachem, L. D., Mothe, A. J., and Tator, C. H. (2020). Unlocking the paradoxical endogenous stem cell response after spinal cord injury. Stem Cells 38, 187–194. doi: 10.1002/stem.3107
Hackland, J. O. S., Shelar, P. B., Sandhu, N., Prasad, M. S., Charney, R. M., Gomez, G. A., et al. (2019). FGF modulates the axial identity of trunk hPSC-derived neural crest but not the cranial-trunk decision. Stem Cell Rep. 12, 920–933. doi: 10.1016/j.stemcr.2019.04.015
Handrigan, G. R. (2003). Concordia discors: duality in the origin of the vertebrate tail. J. Anat. 202, 255–267. doi: 10.1046/j.1469-7580.2003.00163.x
Harkema, S., Gerasimenko, Y., Hodes, J., Burdick, J., Angeli, C., Chen, Y., et al. (2011). Effect of epidural stimulation of the lumbosacral spinal cord on voluntary movement, standing and assisted stepping after motor complete paraplegia: a case study. Lancet 377, 1938–1947. doi: 10.1016/S0140-6736(11)60547-3
Henrique, D., Abranches, E., Verrier, L., and Storey, K. G. (2015). Neuromesodermal progenitors and the making of the spinal cord. Development 142, 2864–2875. doi: 10.1242/dev.119768
Hester, M. E., Murtha, M. J., Song, S. W., Rao, M., Miranda, C. J., Meyer, K., et al. (2011). Rapid and efficient generation of functional motor neurons from human pluripotent stem cells using gene delivered transcription factor codes. Mol. Ther. 19, 1905–1912. doi: 10.1038/mt.2011.135
Ho, R., Sances, S., Gowing, G., Amoroso, M. W., O’Rourke, J. G., Sahabian, A., et al. (2016). ALS disrupts spinal motor neuron maturation and aging pathways within gene co-expression networks. Nat. Neurosci. 19, 1256–1267. doi: 10.1038/nn.4345
Hobert, O. (2005–2018). “Neurogenesis in the nematode Caenorhabditis elegans”, in WormBook: The Online Review of C. elgans Biology. Available online at: https://www.ncbi.nlm.nih.gov/books/NBK116086/.
Hochstim, C., Deneen, B., Lukaszewicz, A., Zhou, Q., and Anderson, J. (2008). Identification of positionally distinct astrocyte subtypes whose identities are specified by a homeodomain code. Cell 133, 510–522. doi: 10.1016/j.cell.2008.02.046
Hogrebe, N. J., Augsornworawat, P., Maxwell, K. G., Velazco-Cruz, L., and Millman, J. R. (2020). Targeting the cytoskeleton to direct pancreatic differentiation of human pluripotent stem cells. Nat. Biotechnol. 38, 460–470. doi: 10.1038/s41587-020-0430-6
Hor, J. H., Soh, E. S.-Y., Tan, L. Y., Lim, V. J. W., Santosa, M. M., Winanto, ., et al. (2018). Cell cycle inhibitors protect motor neurons in an organoid model of spinal muscular atrophy. Cell Death Dis. 9:1100. doi: 10.1038/s41419-018-1081-0
Hu, B.-Y., Du, Z.-W., Li, X.-J., Ayala, M., and Zhang, S.-C. (2009). Human oligodendrocytes from embryonic stem cells: conserved SHH signaling networks and divergent FGF effects. Development 136, 1443–1452. doi: 10.1242/dev.029447
Hu, B.-Y., Weick, J. P., Yu, J., Ma, L.-X., Zhang, X.-Q., Thomson, J. A., et al. (2010). Neural differentiation of human induced pluripotent stem cells follows developmental principles but with variable potency. Proc. Natl. Acad. Sci. U S A 107, 4335–4340. doi: 10.1073/pnas.0910012107
Huang, M., Miller, M. L., McHenry, L. K., Zheng, T., Zhen, Q., Ilkhanizadeh, S., et al. (2016). Generating trunk neural crest from human pluripotent stem cells. Sci. Rep. 6:19727. doi: 10.1038/srep19727
Hubaud, A., and Pourquié, O. (2014). Signaling dynamics in vertebrate segmentation. Nat. Rev. Mol. Cell Biol. 15, 709–721. doi: 10.1038/nrm3891
Iwafuchi-Doi, M., Matsuda, K., Murakami, K., Niwa, H., Tesar, P. J., Aruga, J., et al. (2012). Transcriptional regulatory networks in epiblast cells and during anterior neural plate development as modeled in epiblast stem cells. Development 139, 3926–3937. doi: 10.1242/dev.085936
Iwafuchi-Doi, M., Yoshida, Y., Onichtchouk, D., Leichsenring, M., Driever, W., Takemoto, T., et al. (2011). The Pou5f1/Pou3f-dependent but SoxB-independent regulation of conserved enhancer N2 initiates Sox2 expression during epiblast to neural plate stages in vertebrates. Dev. Biol. 352, 354–366. doi: 10.1016/j.ydbio.2010.12.027
Katoh, H., Yokota, K., and Fehlings, M. G. (2019). Regeneration of spinal cord connectivity through stem cell transplantation and biomaterial scaffolds. Front. Cell. Neurosci. 13:248. doi: 10.3389/fncel.2019.00248
Kawada, J., Kaneda, S., Kirihara, T., Maroof, A., Levi, T., Eggan, K., et al. (2017). Generation of a motor nerve organoid with human stem cell-derived neurons. Stem Cell Rep. 9, 1441–1449. doi: 10.1016/j.stemcr.2017.09.021
Keirstead, H. S., Nistor, G., Bernal, G., Totoiu, M., Cloutier, F., Sharp, K., et al. (2005). Human embryonic stem cell-derived oligodendrocyte progenitor cell transplants remyelinate and restore locomotion after spinal cord injury. J. Neurosci. 25, 4694–4705. doi: 10.1523/JNEUROSCI.0311-05.2005
Kim, Y. J., Lim, H., Li, Z., Oh, Y., Kovlyagina, I., Choi, I. Y., et al. (2014). Generation of multipotent induced neural crest by direct reprogramming of human postnatal fibroblasts with a single transcription factor. Cell Stem Cell 15, 497–506. doi: 10.1016/j.stem.2014.07.013
Kimelman, D. (2016). Tales of tails (and trunks): forming the posterior body in vertebrate embryos. Curr. Top. Dev. Biol. 116, 517–536. doi: 10.1016/bs.ctdb.2015.12.008
Kirino, K., Nakahata, T., Taguchi, T., and Saito, M. K. (2018). Efficient derivation of sympathetic neurons from human pluripotent stem cells with a defined condition. Sci. Rep. 8:12865. doi: 10.1038/s41598-018-31256-1
Kirkby, L. A., Sack, G. S., Firl, A., and Feller, M. B. (2013). A role for correlated spontaneous activity in the assembly of neural circuits. Neuron 80, 1129–1144. doi: 10.1016/j.neuron.2013.10.030
Kiskinis, E., Sandoe, J., Williams, L. A., Boulting, G. L., Moccia, R., Wainger, B. J., et al. (2014). Pathways disrupted in human ALS motor neurons identified through genetic correction of mutant SOD1. Cell Stem Cell 14, 781–795. doi: 10.1016/j.stem.2014.03.004
Kraus, K. H. (1996). The pathophysiology of spinal cord injury and its clinical implications. Semin. Vet. Med. Surg. Small Anim. 11, 201–207. doi: 10.1016/s1096-2867(96)80013-2
Krencik, R., and Zhang, S.-C. (2011). Directed differentiation of functional astroglial subtypes from human pluripotent stem cells. Nat. Protoc. 6, 1710–1717. doi: 10.1038/nprot.2011.405
Kulkarni, A., Anderson, A. G., Merullo, D. P., and Konopka, G. (2019). Beyond bulk: a review of single cell transcriptomics methodologies and applications. Curr. Opin. Biotechnol. 58, 129–136. doi: 10.1016/j.copbio.2019.03.001
Kumamaru, H., Kadoya, K., Adler, A. F., Takashima, Y., Graham, L., Coppola, G., et al. (2018). Generation and post-injury integration of human spinal cord neural stem cells. Nat. Methods 15, 723–731. doi: 10.1038/s41592-018-0074-3
Lai, B.-Q., Che, M.-T., Feng, B., Bai, Y.-R., Ma, Y.-H., Hang, L.-J., et al. (2019). Tissue-engineered neural network graft relays excitatory signal in the completely transected canine spinal cord. Adv. Sci. 6:1901240. doi: 10.1002/advs.201901240
Lai, H. C., Seal, R. P., and Johnson, J. E. (2016). Making sense out of spinal cord somatosensory development. Development 143, 3434–3448. doi: 10.1242/dev.139592
Laurent, L. C., Nievergelt, C. M., Lynch, C., Fakunle, E., Harness, J. V., Schmidt, U., et al. (2010). Restricted ethnic diversity in human embryonic stem cell lines. Nat. Methods 7, 6–7. doi: 10.1038/nmeth0110-06
Lee, K. J., Dietrich, P., and Jessell, T. M. (2000). Genetic ablation reveals that the roof plate is essential for dorsal interneuron specification. Nature 403, 734–740. doi: 10.1038/35001507
Lee, G., Kim, G., Elkabetz, Y., Shamy, G. A., Panagiotakos, G., Barberi, T., et al. (2007). Isolation and directed differentiation of of neural crest stem cells derived from human embryonic stem cells. Nat. Biotechnol. 25, 1468–1475. doi: 10.1038/nbt1365
Lee, H., Lee, H. Y., Lee, B. E., Gerovska, D., Park, S. Y., Zaehres, H., et al. (2020). Sequentially induced motor neurons from human fibroblasts facilitate locomotor recovery in a rodent spinal cord injury model. eLife 9:e52069. doi: 10.7554/eLife.52069
Lee, G., Ramirez, C. N., Kim, H., Zeltner, N., Liu, B., Radu, C., et al. (2012). Large-scale screening using familial dysautonomia induced pluripotent stem cells identifies compounds that rescue IKBKAP expression. Nat. Biotechnol. 30, 1244–1248. doi: 10.1038/nbt.2435
Li, H., and Chen, G. (2016). In vivo reprogramming for CNS repair: regenerating neurons from endogenous glial cells. Neuron 91, 728–738. doi: 10.1016/j.neuron.2016.08.004
Li, X.-J., Du, Z.-W., Zarnowska, E. D., Pankratz, M., Hansen, L. O., Pearce, R., et al. (2005). Specification of motorneurons from human embryonic stem cells. Nat. Biotechnol. 23, 215–221. doi: 10.1038/nbt1063
Li, N., and Leung, G. K. K. (2015). Oligodendrocyte precursor cells in spinal cord injury: a review and update. BioMed Res. Int. 2015:235195. doi: 10.1155/2015/235195
Liem, K. F. Jr., Tremml, G., and Jessell, T. M. (1997). A role for the roof plate and its resident TGFb-related proteins in neuronal patterning in the dorsal spinal cord. Cell 91, 127–138. doi: 10.1016/s0092-8674(01)80015-5
Lippmann, E. S., Williams, C. E., Ruhl, D. A., Estevez-Silva, M., Chapman, E. R., Coon, J. J., et al. (2015). Deterministic HOX patterning in human pluripotent stem cell-derived neuroectoderm. Stem Cell Rep. 4, 632–644. doi: 10.1016/j.stemcr.2015.02.018
Lowry, N. A., and Temple, S. (2007). Making human neurons from stem cells after spinal cord injury. PLoS Med. 4:e48. doi: 10.1371/journal.pmed.0040048
Lytle, J. M., and Wrathall, J. R. (2007). Glial cell loss, proliferation and replacement in the contused murine spinal cord. Eur. J. Neurosci. 25, 1711–1724. doi: 10.1111/j.1460-9568.2007.05390.x
Maffioletti, M., Sarcar, S., Henderson, A. B. H., Mannhardt, I., Pinton, L., Moyle, L. A., et al. (2018). Three-dimensional human iPSC-derived artificial skeletal muscles model muscular dystrophies and enable multilineage tissue engineering. Cell Rep. 23, 899–908. doi: 10.1016/j.celrep.2018.03.091
Manion, J., Khoung, T., Harney, D., Littleboy, J. B., Ruan, T., Loo, L., et al. (2020). Human induced pluripotent stem cell-derived GABAergic interneuron transplants attenuate neuropathic pain. Pain 161, 379–387. doi: 10.1097/j.pain.0000000000001733
Marti, E., Bumcrot, D. A., Takada, R., and McMahon, A. P. (1995). Requirement of 19K form of Sonic hedgehog for induction of distinct ventral cell types in CNS explants. Nature 375, 322–325. doi: 10.1038/375322a0
Marton, R. M., Miura, Y., Sloan, S. A., Li, Q., Revah, O., Levy, R. J., et al. (2019). Differentiation and maturation of oligodendrocytes in human three-dimensional neural cultures. Nat. Neurosci. 22, 484–491. doi: 10.1038/s41593-018-0316-9
Matsuda, M., Yamanaka, Y., Uemura, M., Osawa, M., Saito, M. K., Nagahashi, A., et al. (2020). Recapitulating the human segmentation clock with pluripotent stem cells. Nature 580, 124–129. doi: 10.1038/s41586-020-2144-9
Maury, Y., Côme, J., Piskorowski, R. A., Salah-Mohellibi, N., Chevaleyre, V., Peschanski, M., et al. (2015). Combinatorial analysis of developmental cues efficiently converts human pluripotent stem cells into multiple neuronal subtypes. Nat. Biotechnol. 33, 89–96. doi: 10.1038/nbt.3049
Mayor, R., and Theveneau, E. (2013). The neural crest. Development 140, 2247–2251. doi: 10.1242/dev.091751
Mayr, U., Serra, D., and Liberali, P. (2019). Exploring single cells in space and time during tissue development, homeostasis and regeneration. Development 146:dev176727. doi: 10.1242/dev.176727
Mazzara, P. G., Muggeo, S., Luoni, M., Massimino, L., Zaghi, M., Valverde, T.-T., et al. (2020). Frataxin gene editing rescues Friedreich’s ataxia pathology in dorsal root ganglia organoid-derived sensory neurons. Nat. Commun. 11:4178. doi: 10.1038/s41467-020-17954-3
Mazzoni, E. O., Mahony, S., Peljto, M., Patel, T., Thornton, S. R., McCuine, S., et al. (2013). Saltatory remodeling of Hox chromatin in response to rostro-caudal patterning signals. Nat. Neurosci. 16, 1191–1198. doi: 10.1038/nn.3490
Meinhardt, A., Eberle, D., Tazaki, A., Ranga, A., Niesche, M., Wilsch-Brauninger, M., et al. (2014). 3D reconstitution of the patterned neural tube from embryonic stem cells. Stem Cell Rep. 3, 987–999. doi: 10.1016/j.stemcr.2014.09.020
Menendez, L., Yatskievych, T. A., Antin, P. B., and Dalton, S. (2011). Wnt signaling and a Smad pathway blockade direct the differentiation of human pluripotent stem cells to multipotent neural crest cells. Proc. Natl. Acad. Sci. U S A 108, 19240–19245. doi: 10.1073/pnas.1113746108
Metzis, V., Steinhauser, S., Pakanavicius, E., Gouti, M., Stamataki, D., Ivanovitch, K., et al. (2018). Nervous system regionalization entails axial allocation before neural differentiation. Cell 175, 1105–1118. doi: 10.1016/j.cell.2018.09.040
Mica, Y., Lee, G., Chambers, S. M., Tomishima, M. J., and Studer, L. (2013). Modeling neural crest induction, melanocyte specification and disease-related pigmentation defects in hESCs and patient-specific iPSCs. Cell Rep. 3, 1140–1152. doi: 10.1016/j.celrep.2013.03.025
Mizuguchi, R., Kriks, S., Cordes, R., Gossler, A., Ma, Q., and Goulding, M. (2006). Ascl1 and Gsh1/2 control inhibitory and excitatory cell fate in spinal sensory interneurons. Nat. Neurosci. 9, 770–778. doi: 10.1038/nn1706
Molotkova, N., Molotkov, A., Sirbu, I. O., and Duester, G. (2005). Requirement of mesodermal retinoic acid generated by Raldh2 for posterior neural transformation. Mech. Dev. 122, 145–155. doi: 10.1016/j.mod.2004.10.008
Molyneaux, B. J., Arlotta, P., Menezes, J. R., and Macklis, J. D. (2007). Neuronal subtype specification in the cerebral cortex. Nat. Rev. Neurosci. 8, 427–437. doi: 10.1038/nrn2151
Mosher, J. T., Pemberton, T. J., Harter, K., Wang, C., Buzbas, E. O., Dvorak, P., et al. (2010). Lack of population diversity in commonly used human embryonic stem cell lines. N. Engl. J. Med. 362, 185–186. doi: 10.1056/NEJMc0910371
Muroyama, Y., Fujihara, M., Ikeya, M., Kondoh, H., and Takada, S. (2002). Wnt signaling plays an essential role in neuronal specification of the dorsal spinal cord. Genes Dev. 16, 548–553. doi: 10.1101/gad.937102
Nedelec, S., and Martinez-Arias, A. (2021). In vitro models of spinal motor circuit’s development in mammals: achievements and challenges. Curr. Opin. Neurobiol. 66, 240–249. doi: 10.1016/j.conb.2020.12.002
Nieuwkoop, P. D. (1952). Activation and organization of the central nervous system in amphibians. Part III. Synthesis of a new working hypothesis. J. Exp. Zool. 120, 83–108. doi: 10.1002/jez.1401200104
Nieuwkoop, P. D., and Nigtevecht, G. V. (1952). Neural activation and transformation in explants of competent ectoderm under the influence of fragments of anterior notochord in urodeles. Development 2, 175–193.
Nijssen, J., Comley, L. H., and Hedlund, E. (2017). Motor neuron vulnerability and resistance in amyotrophic lateral sclerosis. Acta Neuropathol. 133, 863–885. doi: 10.1007/s00401-017-1708-8
Nistor, G. I., Totoiu, M. O., Haque, N., Carpenter, M. K., and Keirstead, H. S. (2005). Human embryonic stem cells differentiate into oligodendrocytes in high purity and myelinate after spinal cord transplantation. Glia 49, 385–396. doi: 10.1002/glia.20127
Nordström, U., Maier, E., Jessell, T. M., and Edlund, T. (2006). An early role for Wnt signaling in specifying neural patterns of Cdx and Hox gene expression and motor neuron subtype identity. PLoS Biol. 4:e252. doi: 10.1371/journal.pbio.0040252
Ogura, T., Sakaguchi, H., Miyamoto, S., and Takahashi, J. (2018). Three-dimensional induction of dorsal, intermediate and ventral spinal cord tissues from human pluripotent stem cells. Development 145:dev162214. doi: 10.1242/dev.162214
Olmsted, Z. T., and Paluh, J. L. (2020). Integrating central and peripheral neurons in elongating multi-lineage-organized gastruloids. bioRxiv [Preprint]. doi: 10.1101/2020.12.29.424774
Olmsted, Z. T., Stigliano, C., Badri, A., Zhang, F., Williams, A., Koffas, M. A. G., et al. (2020). Fabrication of homotypic neural ribbons as a multiplex platform optimized for spinal cord delivery. Sci. Rep. 10:12939. doi: 10.1038/s41598-020-69274-7
Osaki, T., Uzel, S. G. M., and Kamm, R. D. (2018). Microphysiological 3D model of amyotrophic lateral sclerosis (ALS) from human iPS-derived muscle cells and optogenetic motor neurons. Sci. Adv. 4:eaat5847. doi: 10.1126/sciadv.aat5847
Pankratz, M. T., Li, X.-J., Lavaute, T. M., Lyons, E. A., Chen, X., and Zhang, S. (2007). Directed neural differentiation of human embryonic stem cells via an obligated primitive anterior stage. Stem Cells 25, 1511–1520. doi: 10.1634/stemcells.2006-0707
Paschon, V., Correia, F. F., Morena, B. C., da Silva, V. A., Dos Santos, G. B., da Silva, M. C. C., et al. (2020). CRISPR, prime editing, optogenetics, and DREADDs: new therapeutic approaches provided by emerging technologies in the treatment of spinal cord injury. Molec. Neurobiol. 57, 2085–2100. doi: 10.1007/s12035-019-01861-w
Patani, R. (2016). Generating diverse spinal motor neuron subtypes from human pluripotent stem cells. Stem Cells Int. 2016:1036974. doi: 10.1155/2016/1036974
Philippidou, P., and Dasen, J. S. (2013). Hox genes: choreographers in neural development, architects of circuit organization. Neuron 80, 12–34. doi: 10.1016/j.neuron.2013.09.020
Piao, J., Major, T., Auyeung, G., Policarpio, E., Menon, J., Droms, L., et al. (2015). Human embryonic stem cell-derived oligodendrocyte progenitors remyelinate the brain and rescue behavioral deficits following radiation. Cell Stem Cell 16, 198–210. doi: 10.1016/j.stem.2015.01.004
Pomp, O., Brokhman, I., Ben-Dor, I., Reubinoff, B., and Goldstein, R. S. (2005). Generation of peripheral sensory and sympathetic neurons and neural crest cells from human embryonic stem cells. Stem Cells 23, 923–930. doi: 10.1634/stemcells.2005-0038
Pomp, O., Brokhman, I., Ziegler, L., Almong, M., Korngreen, A., Tavian, M., et al. (2008). PA6-induced human embryonic stem cell-derived neurospheres: a new source of human peripheral sensory neurons and neural crest cells. Brain Res. 1230, 50–60. doi: 10.1016/j.brainres.2008.07.029
Qu, Q., Li, D., Louis, K. R., Li, X., Yang, H., Sun, Q., et al. (2014). High-efficiency motor neuron differentiation from human pluripotent stem cells and the function of Islet-1. Nat. Commun. 5:3449. doi: 10.1038/ncomms4449
Ramon y Cajal, S., DeFelipe, J., and Jones, E. G. (1991). Cajal’s Degeneration and Regeneration in the Nervous System. New York, NY: Oxford University Press.
Reier, P. J., Bregman, B. S., and Wujek, J. R. (1986). Intraspinal transplantation of embryonic spinal cord tissue in neonatal and adult rats. J. Comp. Neurol. 247, 275–296. doi: 10.1002/cne.902470302
Rigamonti, A., Repetti, G. G., Sun, C., Price, F. D., Reny, D. C., Rapino, F., et al. (2016). Large-scale production of mature neurons from human pluripotent stem cells in a three-dimensional suspension culture system. Stem Cell Rep. 6, 993–1008. doi: 10.1016/j.stemcr.2016.05.010
Roelink, H., Porter, J. A., Chiang, C., Tanabe, Y., Chang, D. T., Beachy, P. A., et al. (1995). Floor plate and motor neuron induction by different concentrations of the amino-terminal cleavage product of sonic hedgehog autoproteolysis. Cell 81, 445–455. doi: 10.1016/0092-8674(95)90397-6
Romanos, M., Allio, G., Combres, L., Medevielle, F., Escalas, N., Soula, C., et al. (2021). Cell-to-cell heterogeneity in Sox2 and Brachyury expression ratio guides progenitor destiny by controlling their motility. bioRxiv [Preprint]. doi: 10.1101/2020.11.18.388611
Rosenzweig, E. S., Brock, J. H., Lu, P., Kumamaru, H., Salegio, E. A., Kadoya, K., et al. (2017). Restorative effects of human neural stem cell grafts on the primate spinal cord. Nat. Med. 24, 484–490. doi: 10.1038/nm.4502
Rossi, G., Broguiere, N., Miyamoto, M., Boni, A., Guiet, R., Girgin, M., et al. (2021). Capturing cardiogenesis in gastruloids. Cell Stem Cell 28, 230.e6–240.e6. doi: 10.1016/j.stem.2020.10.013
Rowitch, D. H. (2004). Glial specification in the vertebrate neural tube. Nat. Rev. Neurosci. 5, 409–419. doi: 10.1038/nrn1389
Rowitch, D. H., and Kriegstein, A. R. (2010). Developmental genetics of vertebrate glial-cell specification. Nature 468, 214–222. doi: 10.1038/nature09611
Rowland, J. W., Lee, J. J., Salewski, R. P., Eftekharpour, E., van der Kooy, D., and Fehlings, M. G. (2011). Generation of neural stem cells from embryonic stem cells using the default mechanism: in vitro and in vivo characterization. Stem Cells Dev. 20, 1829–1845. doi: 10.1089/scd.2011.0214
Roybon, L., Lamas, N. J., Garcia, A. D., Yang, E. J., Sattler, R., Lewis, V. J., et al. (2013). Human stem cell-derived spinal cord astrocytes with defined mature or reactive phenotypes. Cell Rep. 4, 1035–1048. doi: 10.1016/j.celrep.2013.06.021
Sagner, A., and Briscoe, J. (2019). Establishing neuronal diversity in the spinal cord: a time and a place. Development 146:dev182154. doi: 10.1242/dev.182154
Saito-Diaz, K., Wu, H. F., and Zeltner, N. (2019). Autonomic neurons with sympathetic character derived from human pluripotent stem cells. Curr. Protoc. Stem Cell Biol. 49:e78. doi: 10.1002/cpsc.78
Sances, S., Bruijn, L. I., Chandran, S., Eggan, K., Ho, R., Klim, J. R., et al. (2016). Modeling ALS with motor neurons derived from human induced pluripotent stem cells. Nat. Neurosci. 19, 542–553. doi: 10.1038/nn.4273
Sareen, D., O’Rourke, J. G., Meera, P., Muhammad, A. K. M. G., Grant, S., Simpkinson, M., et al. (2013). Targeting RNA focus in iPSC-derived motor neurons from ALS patients with a C9ORF72 repeat expansion. Sci. Transl. Med. 5:208ra149. doi: 10.1126/scitranslmed.3007529
Sathyamuarthy, A., Johnson, K. R., Matson, K. J. E., Dobrott, C. I., Li, L., Ryba, A. R., et al. (2018). Massively parallel single nucleus transcriptional profiling defines spinal cord neurons and their activity during behavior. Cell Rep. 22, 2216–2225. doi: 10.1016/j.celrep.2018.02.003
Scott, C. T., and Magnus, D. (2014). Wrongful termination: lessons from the Geron clinical trial. Stem Cells Transl. Med. 3, 1398–1401. doi: 10.5966/sctm.2014-0147
Shirasaki, R., and Pfaff, S. L. (2002). Transcriptional codes and the control of neuronal identity. Annu. Rev. Neurosci. 25, 251–281. doi: 10.1146/annurev.neuro.25.112701.142916
Silva, N. A., Sousa, N., Reis, R. L., and Salgado, A. J. (2014). From basics to clinical: a comprehensive review of spinal cord injury. Prog. Neurobiol. 114, 25–57. doi: 10.1016/j.pneurobio.2013.11.002
Silver, J. R. (2005). History of the treatment of spinal injuries. Postgrad. Med. J. 81, 108–114. doi: 10.1136/pgmj.2004.019992
Silvestro, S., Bramanti, P., Trubiani, O., and Mazzon, E. (2020). Stem cell therapy for spinal cord injury: an overview of clinical trials. Int. J. Mol. Sci. 21:659. doi: 10.3390/ijms21020659
Soldatov, R., Kaucka, M., Kastriti, M. E., Petersen, J., Chontorotzea, T., Englmaier, L., et al. (2019). Spatiotemporal structure of cell fate decisions in murine neural crest. Science 364:eaas9536. doi: 10.1126/science.aas9536
Son, E. Y., Ichida, J. K., Wainger, B. K., Toma, J. S., Rafuse, V. F., Woolf, C. J., et al. (2011). Conversion of mouse and human fibroblasts into functional spinal motor neurons. Cell Stem Cell 9, 205–218. doi: 10.1016/j.stem.2011.07.014
Srinivasan, A., and Toh, Y.-C. (2019). Human pluripotent stem cell-derived neural crest cells for tissue regeneration and disease modeling. Front. Mol. Neurosci. 12:39. doi: 10.3389/fnmol.2019.00039
Staudt, M. D., Patel, S., Hellman, A., Platanitis, K., DiMarzio, M., Khazen, O., et al. (2020). Efficacy of simultaneous usage of spinal cord stimulation and intrathecal therapy for nonmalignant chronic neuropathic pain. World Neurosurg. 143, e443–e449. doi: 10.1016/j.wneu.2020.07.187
Steinbeck, J. A., Jaiswal, M. K., Calder, E. L., Kishinevsky, S., Weishaupt, A., Toyka, K. V., et al. (2016). Functional connectivity under optogenetic control allows modeling of human neuromuscular disease. Cell Stem Cell 18, 134–143. doi: 10.1016/j.stem.2015.10.002
Stern, C. D. (2001). Initial patterning of the central nervous system: how many organizers? Nat. Rev. Neurosci. 2, 92–98. doi: 10.1038/35053563
Stern, C. D. (2006). Neural induction: 10 years on since the ‘default model’. Curr. Opin. Cell Biol. 18, 692–697. doi: 10.1016/j.ceb.2006.09.002
Sternfeld, M. J., Hinckley, C. A., Moore, N. J., Pankratz, M. T., Hilde, K. L., Driscoll, S. P., et al. (2017). Speed and segmentation control mechanisms characterized in rhythmically-active circuits created from spinal neurons produced from genetically-tagged embryonic stem cells. eLife 6:e21540. doi: 10.7554/eLife.21540
Stifani, N. (2014). Motor neurons and the generation of spinal motor neuron diversity. Front. Cell. Neurosci. 8:293. doi: 10.3389/fncel.2014.00293
Strnadel, J., Carromeu, C., Bardy, C., Navarro, M., Platoshyn, O., Glud, A. N., et al. (2018). Survival of syngeneic and allogeneic iPSC-derived neural precursors after spinal grafting in minipigs. Sci. Transl. Med. 10:eaam6651. doi: 10.1126/scitranslmed.aam6651
Sudiwala, S., Palmer, A., Massa, V., Burns, A. J., Dunlevy, L. P. E., de Castro, S. C. P., et al. (2019). Cellular mechanisms underlying Pax3-related neural tube defects and their prevention by folic acid. Dis. Model Mech. 12:dmm042234. doi: 10.1242/dmm.042234
Taga, A., Dastgheyb, R., Habela, C., Joseph, J., Richard, J.-P., Gross, S. K., et al. (2019). Role of human-induced pluripotent stem cell-derived spinal cord astrocytes in the functional maturation of motor neurons in a multielectrode array system. Stem Cells Transl. Med. 8, 1272–1285. doi: 10.1002/sctm.19-0147
Takahashi, K., and Yamanaka, S. (2006). Induction of pluripotent stem cells from mouse embryonic and adult fibroblast cultures by defined factors. Cell 126, 663–676. doi: 10.1016/j.cell.2006.07.024
Takemoto, T., Uchikawa, M., Kamachi, Y., and Kondoh, H. (2006). Convergence of Wnt and FGF signals in the genesis of posterior neural plate through activation of the Sox2 enhancer N-1. Development 133, 297–306. doi: 10.1242/dev.02196
Takemoto, T., Uchikawa, M., Yoshida, M., Bell, D. M., Lovell-Badge, R., Papaioannou, V. E., et al. (2011). Tbx6-dependent Sox2 regulation determines neural vs. mesodermal fate in axial stem cells. Nature 470, 394–398. doi: 10.1038/nature09729
Tang, F., Barbacioru, C., Wang, Y., Nordman, E., Lee, C., Xu, N., et al. (2009). mRNA-Seq whole-transcriptome analysis of a single cell. Nat. Methods 6, 377–382. doi: 10.1038/nmeth.1315
Tang, H.-Y., Li, Y.-Z., Tang, Z.-C., Wang, L.-Y., Wang, T.-S., and Araujo, F. (2020). Efficacy of neural stem cell transplantation for the treatment of patients with spinal cord injury. Medicine 99:e20169. doi: 10.1097/MD.0000000000020169
Tao, Y., and Zhang, S.-C. (2016). Neural subtype specification from human pluripotent stem cells. Cell Stem Cell 19, 573–586. doi: 10.1016/j.stem.2016.10.015
Tao, Y., Vermilyea, S. C., Zammit, M., Lu, J., Olsen, M., Metzger, J. M., et al. (2021). Autologous transplant therapy alleviates motor and depressive behaviors in parkinsonian monkeys. Nat. Med. doi: 10.1038/s41591-021-01257-1 [Epub ahead of print].
Thomson, J. A., Itskovitz-Eldor, J., Shapiro, S. S., Waknitz, M. A., Swiergiel, J. J., Marshall, V. S., et al. (1998). Embryonic stem cell lines derived from human blastocysts. Science 282, 1145–1147. doi: 10.1126/science.282.5391.1145
Tian, R., Gachedchiladze, M. A., Ludwig, C. H., Laurie, M. T., Hong, J. Y., Nathaniel, D., et al. (2019). CRISPR interface-based platform for multimodal genetic screens in human iPSC-derived neurons. Neuron 104, 239–255. doi: 10.1016/j.neuron.2019.07.014
Tomov, M. L., Olmsted, Z. T., Dogan, H., Gongorurler, E., Tsompana, M., Out, H. H., et al. (2016). Distinct and shared determinants of cardiomyocyte contractility in multi-lineage competent ethnically diverse human iPSCs. Sci. Rep. 6:37637. doi: 10.1038/srep37637
Totoiu, M. O., and Keirstead, H. S. (2005). Spinal cord injury is accompanied by chronic progressive demyelination. J. Comp. Neurol. 486, 373–383. doi: 10.1002/cne.20517
Trawczynski, M., Liu, G., David, B. T., and Fessler, R. G. (2019). Restoring motor neurons in spinal cord injury with induced pluripotent stem cells. Front. Cell. Neurosci. 13:369. doi: 10.3389/fncel.2019.00369
Tsakiridis, A., Huang, Y., Blin, G., Skylaki, S., Wymeersch, F., Osorno, R., et al. (2014). Distinct Wnt-driven primitive streak-like populations reflect in vivo lineage precursors. Development 141, 1208–1221. doi: 10.1242/dev.101014
Tsakiridis, A., and Wilson, V. (2015). Assessing the bipotency of in vitro-derived neuromesodermal progenitors. F1000Res. 4:100. doi: 10.12688/f1000research.6345.2
Turing, A. M. (1953). The chemical basis of morphogenesis. 1953. Bull. Math. Biol. 52, 153–197. doi: 10.1007/BF02459572
Turner, D. A., Girgin, M., Alonso-Crisotomo, L., Trivedi, V., Baillie-Johnson, P., Glodowski, C. R., et al. (2017). Anteroposterior polarity and elongation in the absence of extra-embryonic tissues and spatially localized signaling in gastruloids: mammalian embryonic organoids. Development 144, 3894–3906. doi: 10.1242/dev.150391
Turner, D. A., Hayward, P. C., Baillie-Johnson, P., Rue, P., Broome, R., Faunes, F., et al. (2014). Wnt/b-catenin and FGF signaling direct the specification and maintenance of a neuromesodermal axial progenitor in ensembles of mouse embryonic stem cells. Development 141, 4243–4253. doi: 10.1242/dev.112979
Tzouanacou, E., Wegener, A., Wymeersch, F. J., Wilson, V., and Nicolas, J. F. (2009). Redefining the progression of lineage segregations during mammalian embryogenesis by clonal analysis. Dev. Cell 17, 365–376. doi: 10.1016/j.devcel.2009.08.002
Uchikawa, M., Ishida, Y., Takemoto, T., Kamachi, Y., and Kondoh, H. (2003). Functional analysis of chicken Sox2 enhancers highlights an array of diverse regulatory elements that are conserved in mammals. Dev. Cell 4, 509–519. doi: 10.1016/s1534-5807(03)00088-1
Vallstedt, A., Klos, J. M., and Ericson, J. (2005). Multiple dorsoventral origins of oligodendrocyte generation in the spinal cord and hindbrain. Neuron 45, 55–67. doi: 10.1016/j.neuron.2004.12.026
van den Brink, S. C., Alemany, A., van Batenburg, V., Moris, N., Blotenburg, M., Vivie, J., et al. (2020). Single-cell spatial transcriptomics reveal somitogenesis in gastruloids. Nature 582, 405–409. doi: 10.1038/s41586-020-2024-3
van den Brink, S. C., Baillie-Johnson, P., Balayo, T., Hadjantonakis, A.-K., Nowotschin, S., Turner, D. A., et al. (2014). Symmetry breaking, germ layer specification and axial organization in aggregates of mouse embryonic stem cells. Development 141, 4231–4242. doi: 10.1242/dev.113001
Vázquez-Guardado, A., Yang, Y., Bandodkar, A. J., and Rogers, J. A. (2020). Recent advances in neurotechnologies with broad potential for neuroscience research. Nat. Neurosci. 23, 1522–1536. doi: 10.1038/s41593-020-00739-8
Veenvliet, J. V., Bolondi, A., Kretzmer, H., Haut, L., Scholze-Wittler, M., Schifferl, D., et al. (2020). Mouse embryonic stem cells self-organize intro trunk-like structures with neural tube and somites. Science 370:eaba4937. doi: 10.1126/science.aba4937
Verrier, L., Davidson, L., Gierliński, M., Dady, A., and Storey, K. G. (2018). Neural differentiation, selection and transcriptomic profiling of human neuromesodermal progenitor-like cells in vitro. Development 145:dev166215. doi: 10.1242/dev.166215
Wainger, B. J., Kiskinis, E., Mellin, C., Wiskow, O., Han, S. S. W., Sandoe, J., et al. (2014). Intrinsic membrane hyperexcitability of amyotrophic lateral sclerosis patient-derived motor neurons. Cell Rep. 7, 1–11. doi: 10.1016/j.celrep.2014.03.019
Wang, S., Bates, J., Li, X., Schanz, S., Chandler-Militello, D., Levine, C., et al. (2013). Human iPSC-derived oligodendrocyte progenitor cells can myelinate and rescue a mouse model of congenital hypomyelination. Cell Stem Cell 12, 252–264. doi: 10.1016/j.stem.2012.12.002
Warmflash, A., Sorre, B., Etoc, F., Siggia, E. D., and Brivanlou, A. H. (2014). A method to recapitulate early embryonic spatial patterning in human embryonic stem cells. Nat. Methods 11, 847–854. doi: 10.1038/nmeth.3016
Weightman, A. P., Pickard, M. R., Yang, Y., and Chari, D. M. (2014). An in vitro spinal cord injury model to screen neuroregenerative materials. Biomaterials 35, 3756–3765. doi: 10.1016/j.biomaterials.2014.01.022
Wichterle, H., Lieberam, I., Porter, J. A., and Jessell, T. M. (2002). Directed differentiation of embryonic stem cells into motor neurons. Cell 110, 385–397. doi: 10.1016/s0092-8674(02)00835-8
Wilson, V., Olivera-Martinez, I., and Storey, K. G. (2009). Stem cells, signals and vertebrate body axis elongation. Development 136, 1591–1604. doi: 10.1242/dev.021246
Wirth, E. D. III., Reier, P. J., Fessler, R. G., Thompson, F. J., Uthman, B., Behrman, A., et al. (2001). Feasibility and safety of neural tissue transplantation in patients with syringomyelia. J. Neurotrauma 18, 911–929. doi: 10.1089/089771501750451839
Wood, T. R., Kyrsting, A., Stegmaier, J., Kucinski, I., Kaminski, C. F., Mikut, R., et al. (2020). Neuromesodermal progenitors separate the axial zones while producing few single- and dual-fated descendants. bioRxiv [Preprint]. doi: 10.1101/622571
Workman, M. J., Mahe, M. M., Trisno, S., Poling, H. M., Watson, C. L., Sundaram, N., et al. (2017). Engineering human pluripotent-stem-cell-derived intestinal tissues with a functional enteric nervous system. Nat. Med. 23, 49–59. doi: 10.1038/nm.4233
Xiao, Z., Tang, F., Zhao, Y., Han, G., Yin, N., Li, X., et al. (2018). Significant improvement of acute complete spinal cord injury patients diagnosed by a combined criteria implanted with NeuroRegen scaffolds and mesenchymal stem cells. Cell Transpl. 27, 907–915. doi: 10.1177/0963689718766279
Xiong, M., Tao, Y., Gao, Q., Feng, B., Yan, W., Zhou, Y., et al. (2021). Human stem cell-derived neurons repair circuits and restore neural function. Cell Stem Cell 28, 112–126. doi: 10.1016/j.stem.2020.08.014
Xu, H., and Sakiyama-Elbert, S. E. (2015). Directed differentiation of V3 interneurons from mouse embryonic stem cells. Stem Cells Dev. 24, 2723–2732. doi: 10.1089/scd.2015.0122
Yamazaki, K., Kawabori, M., Seki, T., and Houkin, K. (2020). Clinical trials of stem cell treatment for spinal cord injury. Int. J. Mol. Sci. 21:3994. doi: 10.3390/ijms21113994
Yan, F., Powell, D., Curtis, D. J., and Wong, N. C. (2020). From reads to insight: a hitchhiker’s guide to ATAC-seq data analysis. Genome Biol. 21:22. doi: 10.1186/s13059-020-1929-3
Yang, T., Dai, Y., Chen, G., and Cui, S. (2020). Dissecting the dual role of the glial scar and scar-forming astrocytes in spinal cord injury. Front. Cell. Neurosci. 14:78. doi: 10.3389/fncel.2020.00078
Yang, N., Zuchero, J. B., Ahlenius, H., Marro, S., Ng, Y. H., Vierbuchen, T., et al. (2013). Generation of oligodendroglial cells by direct lineage conversion. Nat. Biotechnol. 31, 434–439. doi: 10.1038/nbt.2564
Zalc, A., Sinha, R., Gulati, G. S., Wseche, D. J., Daszczuk, P., Swigut, T., et al. (2021). Reactivation of the pluripotency program precedes formation of cranial neural crest. Science 371:eabb4776. doi: 10.1126/science.abb4776
Keywords: stem cells, differentiation, trunk development, spine development, organoids, gastruloids, assembloids, cell therapy
Citation: Olmsted ZT and Paluh JL (2021) Stem Cell Neurodevelopmental Solutions for Restorative Treatments of the Human Trunk and Spine. Front. Cell. Neurosci. 15:667590. doi: 10.3389/fncel.2021.667590
Received: 13 February 2021; Accepted: 29 March 2021;
Published: 26 April 2021.
Edited by:
Haruyuki Kamiya, Hokkaido University, JapanReviewed by:
Bin Jiang, Sun Yat-Sen University, ChinaCopyright © 2021 Olmsted and Paluh. This is an open-access article distributed under the terms of the Creative Commons Attribution License (CC BY). The use, distribution or reproduction in other forums is permitted, provided the original author(s) and the copyright owner(s) are credited and that the original publication in this journal is cited, in accordance with accepted academic practice. No use, distribution or reproduction is permitted which does not comply with these terms.
*Correspondence: Janet L. Paluh, anBhbHVoQHN1bnlwb2x5LmVkdQ==
† These authors have contributed equally to this work
Disclaimer: All claims expressed in this article are solely those of the authors and do not necessarily represent those of their affiliated organizations, or those of the publisher, the editors and the reviewers. Any product that may be evaluated in this article or claim that may be made by its manufacturer is not guaranteed or endorsed by the publisher.
Research integrity at Frontiers
Learn more about the work of our research integrity team to safeguard the quality of each article we publish.