- Vinberg Lab, Department of Ophthalmology and Visual Science, John A. Moran Center, University of Utah, Salt Lake City, UT, United States
Sensing changes in the environment is crucial for survival. Animals from invertebrates to vertebrates use both visual and olfactory stimuli to direct survival behaviors including identification of food sources, finding mates, and predator avoidance. In primary sensory neurons there are signal transduction mechanisms that convert chemical or light signals into an electrical response through ligand binding or photoactivation of a receptor, that can be propagated to the olfactory and visual centers of the brain to create a perception of the odor and visual landscapes surrounding us. The fundamental principles of olfactory and phototransduction pathways within vertebrates are somewhat analogous. Signal transduction in both systems takes place in the ciliary sub-compartments of the sensory cells and relies upon the activation of G protein-coupled receptors (GPCRs) to close cyclic nucleotide-gated (CNG) cation channels in photoreceptors to produce a hyperpolarization of the cell, or in olfactory sensory neurons open CNG channels to produce a depolarization. However, while invertebrate phototransduction also involves GPCRs, invertebrate photoreceptors can be either ciliary and/or microvillar with hyperpolarizing and depolarizing responses to light, respectively. Moreover, olfactory transduction in invertebrates may be a mixture of metabotropic G protein and ionotropic signaling pathways. This review will highlight differences of the visual and olfactory transduction mechanisms between vertebrates and invertebrates, focusing on the implications to the gain of the transduction processes, and how they are modulated to allow detection of small changes in odor concentration and light intensity over a wide range of background stimulus levels.
Introduction
Phototransduction and olfaction have been widely investigated in both invertebrate (Hardie and Raghu, 2001; Touhara and Vosshall, 2009; Yau and Hardie, 2009; Hardie and Juusola, 2015; Honkanen et al., 2017; Fleischer et al., 2018; Schmidt and Benton, 2020) and vertebrate (Schild and Restrepo, 1998; Burns and Baylor, 2001; Fain et al., 2001; Arshavsky et al., 2002; Luo et al., 2008; Kaupp, 2010; Pifferi et al., 2010; Vinberg et al., 2018) species. Signal transduction in vertebrate olfactory sensory neurons (OSNs) and photoreceptors (PRs) both rely upon G protein signaling cascades, while using distinct classes of G and effector proteins: A G-protein-coupled receptor (GPCR) detects a stimulus and modulates the open probability of cyclic nucleotide-gated (CNG) ion channels via classical GPCR signaling cascades involving the activation of an effector enzyme (E), adenylyl cyclase (AC) in the OSN and phosphodiesterase (PDE) in photoreceptors. This leads to a change in the membrane potential (Vm) of the primary sensory neuron that can be propagated to the sensory centers of the brain. Invertebrates appear to use a wider range of signaling cascades to detect odors or light. Their phototransduction is mostly mediated by a Gq-coupled pathway controlling transient receptor potential (TRP) and transient receptor potential like (TRPL) channels in the plasma membrane of the microvillar compartments of the photoreceptor neuron. However, there are some invertebrate species that also utilize the release of Ca2+ from intracellular endoplasmic reticulum (ER) stores via inositol triphosphate (IP3) pathway, or the same phototransduction pathway found in vertebrates. Some details of invertebrate phototransduction are unknown, most notably the second messenger gating their transduction channels remains to be resolved. Olfactory signal transduction in invertebrates is not understood in detail, however, it is often mediated by ligand-gated odor receptor (OR)/co-receptor (Orco) heteromer that may also be regulated by metabotropic pathway(s) (Wicher et al., 2008; Nakagawa and Vosshall, 2009; Hansson et al., 2010). In addition, some odors are detected via a purely ionotropic receptors (IRs) reminiscent of ionotropic glutamate receptors widely used in chemical synaptic transmission between neurons. In this review we will describe visual and olfactory transduction in vertebrates and invertebrates, focusing on how their differences contribute to the gain of the visual and odor transduction in vertebrates and invertebrates as well as how this gain is modulated to allow adaptation of vision and olfaction over a wide range of background light and odorant levels.
Transduction Cascades In the Primary Sensory Neurons of Olfactory and Visual Systems
Specialized light-detecting neurons are present in nearly all animals and can be classified into two types: ciliary and microvillar PRs (Fain et al., 2010). Ciliary photoreceptors are formed through membrane invaginations in the outer segment, resulting in compact disk-like structures (Figure 1A, inset). These disks create a large surface area with the membranes containing a high concentration of visual pigments and phototransduction cascade proteins (Sjöstrand and Kreman, 1978; Gilliam et al., 2012). Rhabdomeric photoreceptors form microvillar evaginations containing the photopigment and phototransduction machinery (Figure 1B, inset). Almost all vertebrates use ciliary PRs for their image-forming vision whereas microvillar PRs are found in most invertebrates. However, some invertebrate species, such as box jellyfish or scallops, also have ciliary PRs (Arendt et al., 2004; Fain et al., 2010).
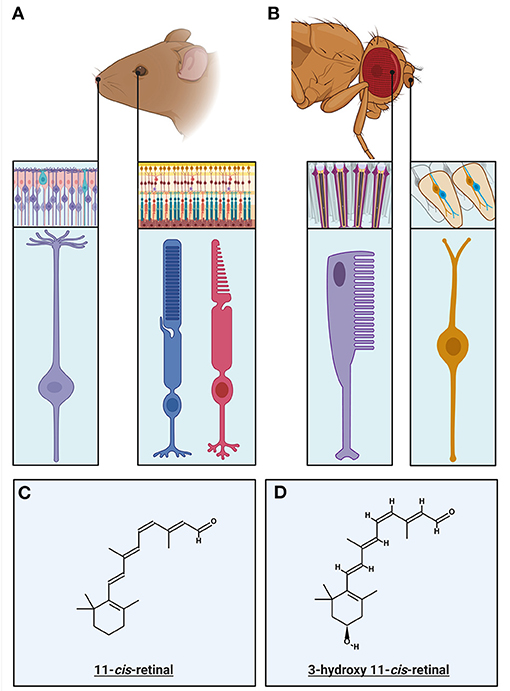
Figure 1. Primary Sensory Neurons of the invertebrate and vertebrate visual and olfactory sensory systems: (A) The primary sensory neurons in the vertebrate olfactory and visual systems and their respective circuitry. Rods (blue) and cones (magenta) mediate vision at different light intensities. Rods mediate low light vision and having specializations enabling this, detailed within this review. Cones mediate daylight color vision and have specific specializations that mediate this. The olfactory sensory neurons (purple) have multiple cilia protruding from the end of the neuron into the olfactory lumen, in which the olfactory transduction machinery, including olfactory receptors, are localized. (B) The primary sensory neurons in the invertebrate (Drosophila) olfactory and visual systems, within their respective circuitry. The invertebrate ommatidium is the structure in which invertebrate photoreceptors (rhabdomeres) are coupled with the support and pigment cells. The rhabdomere (purple) is primary sensory neuron in which the visual transduction machinery is localized within the villi. The olfactory sensory neurons (OSNs) are located within sensory hairs, sensilla. OSNs (orange) project dendrites into the sensillum, with the olfactory receptors expressed on the surface. (C) The chemical structure of the chromophore responsible for vertebrate visual transduction, 11-cis-retinal. (D) The chemical structure of the chromophore present in Microvillar photoreceptors, 3-hydroxy-11-cis-retinal.
In mammals, the primary sensory neurons of the olfactory system are clustered in the olfactory epithelium at the back of the nasal cavity (Buck and Axel, 1991; Mombaerts, 1999). These are bipolar neurons, with an axon that extends to the olfactory bulb, and a dendrite with several cilia which extend into the olfactory lumen (Figure 1A, inset; Menco, 1997). These cilia are the location of the olfactory receptors (ORs) as well as the concentrated localization of the components of olfactory transduction. The vomeronasal organ (VNO) is located at the base of the nasal septum, and is primarily thought to be involved in sensing of pheromones (for review see Dulac and Torello, 2003). Since its' neurons do not project to the main olfactory bulb and contain vomeronasal receptors that are distinct to the olfactory receptors, the VNO is considered part of the Accessory Olfactory System (AOS). Interestingly there is evidence that the vomeronasal system is no longer functional in primates (Meredith, 2001; Zhang and Webb, 2003). For simplicity, this review will primarily focus on transduction in olfactory receptor neurons projecting to the main olfactory bulb. In insects, the OSNs are located on sensory hairs called sensilla on the antennae and maxillary palp (Figure 1B, inset; Pellegrino and Nakagawa, 2009).
In contrast to the graded potentials transmitted by most PRs, both vertebrate and invertebrate OSNs fire action potentials in response to the depolarization caused by the activation of olfactory transduction in the cilia. However, there are some invertebrates with photoreceptor axons projecting directly to the visual centers of the brain, generating regenerative action potentials to enable signal transmission without attenuation (Hartline, 1938). This review will focus on the photoreceptors that do not generate action potentials.
Below, we will describe transduction activation and inactivation as well as adaptation mechanisms in PRs and OSNs of vertebrates and invertebrates. In addition, we will compare the contribution of these mechanisms to the gain of transduction cascades and how that gain is modulated via various feedback mechanisms to enable detection of changes in odorant concentration or light intensity over a wide range of background stimulus levels.
Vertebrate Transduction Cascades
There are several reviews describing vertebrate phototransduction (Fu and Yau, 2007; Yau and Hardie, 2009; Fain et al., 2010; Vinberg et al., 2018; Lamb, 2020), and olfactory transduction cascade in detail (Schild and Restrepo, 1998; Touhara and Vosshall, 2009; Kaupp, 2010; Pifferi et al., 2010; Antunes and Simoes De Souza, 2016; Boccaccio et al., 2021). Here, we will summarize the latest findings and highlight some of the gaps in knowledge. Important components of the prototypical transduction cascades are compiled in Table 1 and shown in Figures 2, 3.
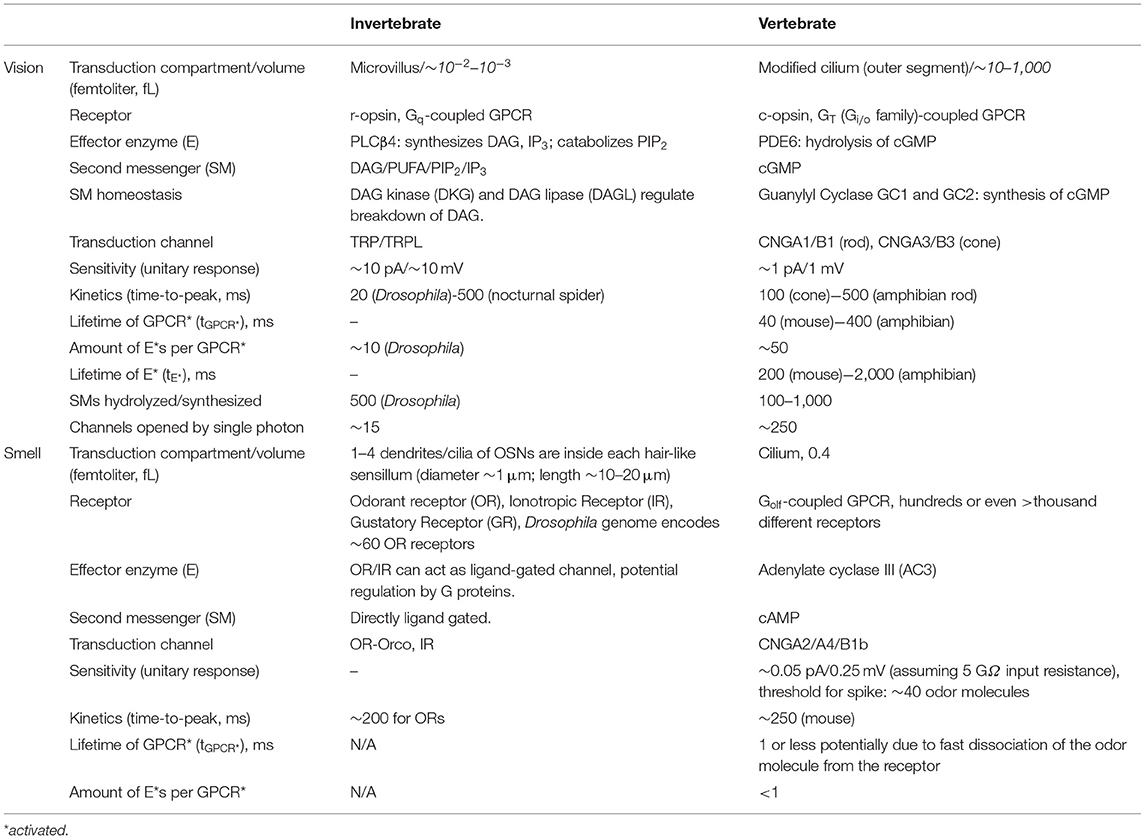
Table 1. Overview and comparison table of the transduction cascades in vertebrates and invertebrates in vision and olfaction.
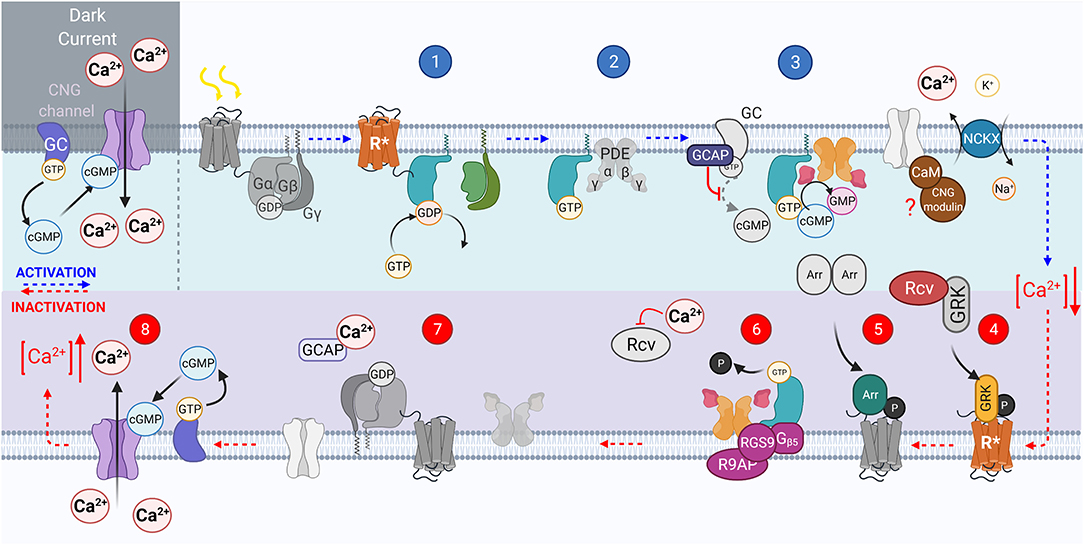
Figure 2. The vertebrate phototransduction cascade, inactivation, and adaptation mechanisms. The key steps within the vertebrate phototransduction cascade (blue numbering from top left to right) and its subsequent inactivation (red numbering from bottom right to left). In the dark, the CNG channel maintains a dark current, in which Ca2+ ions influx through open CNG channels as the Guanyl Cyclase enzyme is constituently active. Upon absorption of a photon, the chromophore 11-cis-retinal is isomerized to all-trans-retinal, causing the receptor to become activated (R*). In turn, the associated G protein, transducin, is activated with the exchange of GDP for GTP, (step 1). then displaces PDEβγ subunits (step 2). The displaced PDEγ subunit allows cGMP to be reduced to GMP, reducing the concentration of cGMP, closing CNG channels, causing a hyperpolarization of the photoreceptor (step 3). The activated receptor, R*, is inactivated by GRK mediated phosphorylation (step 4). Followed by Arrestin binding (step 5). The GAP protein complex formed of RGS9, R9AP, and Gβ5 bound to both PDEγ and activated causes hydrolysis of GTP on the GTα to GDP (step 6). GTα then dissociates from PDE, reassociating to the βγ subunits, and PDEγ also inhibits the PDEαβ to prevent cGMP hydrolysis (step 7). Intracellular Ca2+ concentration is raised as CNG channels reopen in response to the increasing cGMP concentration, reestablishing the dark current (step 8).
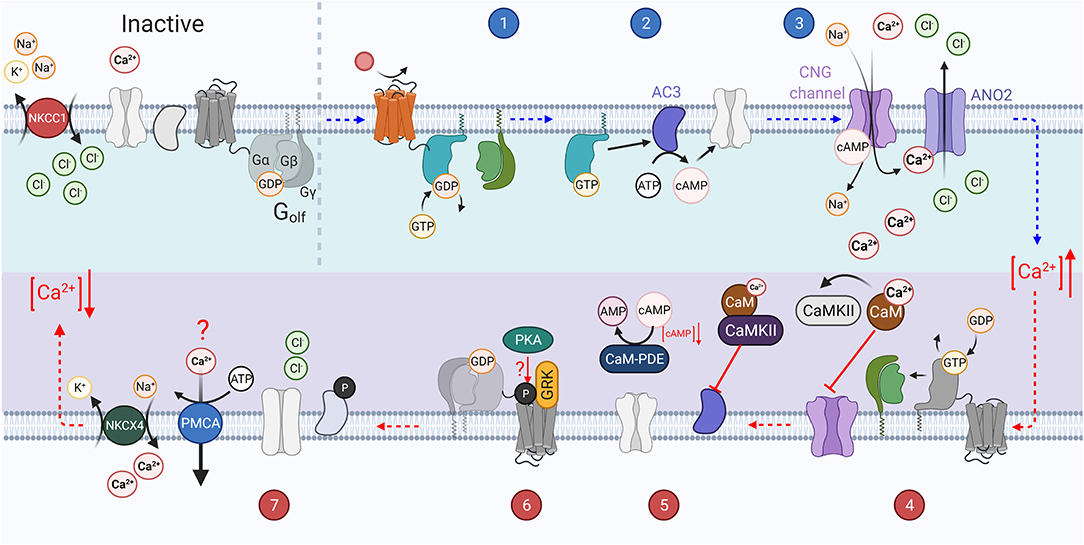
Figure 3. The vertebrate olfactory transduction cascade, inactivation, and adaptation mechanisms: OSNs maintain a high intracellular Cl− ion concentration through the constitutive activity of the NKCC1 transporter, which transports Cl− ions into the cell using the energy from the export of Na+ and K+ ions. An odorant binds to the G-protein coupled olfactory receptor (OR) on the cilium of the sensory neuron. The activated receptor in turn activates Golf (step 1). The Gαolf subunits then activate adenylyl cyclase III (AC3) which catalyzes the conversion of ATP to cAMP (step 2). The increasing concentration of cAMP causes the opening of CNG channels on the membrane. This causes an influx of Ca2+ ions. The increasing Ca2+ concentration also causes the opening of the calcium activated chloride ion channel, ANO2, which further depolarizes the cell as the increased intracellular chloride gradient causes an efflux of Cl−. The increased Ca2+ concentration leads to several inactivation mechanisms (step 3). GTP bound to the active Gαolf subunit is hydrolyzed to GDP, inactivating it, and causing it to re-associate with the βγ subunits. Ca2+ binds to Calmodulin (CaM) which in turn activates CaMKII. Calmodulin potentially reduces CNG channel affinity for cAMP through interaction with the A-subunit of the channel (step 4). CaMKII inhibits the activity of AC3 by phosphorylating it, preventing the generation of cAMP. PDE1C is also activated by Ca2+ bound CaM and accelerates the hydrolysis of cAMP to AMP (step 5). GRK mediated phosphorylation of the OR (step 6). Intracellular Ca2+ concentration is re-established through the activity of ion exchangers (NCKX4 and possibly PMCAs), and the high intracellular Cl− concentration is reestablished through NKCC1 activity and the closure of ANO2 channels (step 7).
Both olfaction and phototransduction in vertebrates relies upon G protein-coupled receptors, involve divergent classes of transduction components. In phototransduction the absorption of a photon causes isomerization of chromophore (see Figure 1C), activating rhodopsin to Metarhodopsin II (R*). Active rhodopsin can activate several G proteins, transducins (GT), by catalyzing the exchange of GDP for GTP on its α subunit (Figure 2, step 1; Shichida and Morizumi, 2007). In olfactory transduction, an odorant binds to the G protein-coupled olfactory receptor (Figure 3, step 1; Buck and Axel, 1991; Mombaerts, 1999; Touhara, 2002), which then activates the G-protein, Golf (Jones and Reed, 1989; Belluscio et al., 1998). The activated Gα subunits act upon different effector enzymes within the cells: in phototransduction PDEγ is displaced (Figure 2, step 2) thus decreasing levels of cGMP by increasing its' hydrolysis by active PDE*. This lowered level of cGMP in the PR outer segment closes CNG channels (Figure 2, step 3; Pugh and Lamb, 1990). Conversely in olfactory transduction, Gαolf activates adenylyl cyclase III (AC3, Figure 3, step 2; Pace et al., 1985) which catalyzes the generation of cAMP from ATP (Bakalyar and Reed, 1990; Wong et al., 2000), opening CNG channels (Figure 3, step 3; Nakamura and Gold, 1987; Brunet et al., 1996).
Interestingly, neurons in the VNO also utilize G protein-coupled receptors to detect pheromones. These can be divided into two sub-groups: Basal neurons express the Gαo, the receptors sharing sequence homology with metabotropic glutamate receptors (Matsunami and Buck, 1997; Ryba and Tirindelli, 1997). Whereas, the apical neurons express Gαi2, and the receptors share sequence homology with T2R bitter taste receptors (Dulac and Axel, 1995). Unlike both olfaction and phototransduction, VNO neurons rely on Trp2 channels in their signaling cascade (Leypold et al., 2002; Stowers et al., 2002).
One of the most notable differences between vertebrate PR and OSN transduction is that their activation leads either to a hyperpolarizing response in photoreceptors or a depolarizing response in olfactory neurons. This is due to the classes of G-protein and effector enzymes they express. Photoreceptor GT protein, which is coupled to phosphodiesterase, belongs to the inhibitory Gi family whereas Golf belongs to Gs subfamily of G-proteins that couple to adenylate cyclases (Roof et al., 1985; Gilman, 1987; Jones and Reed, 1989). While OSNs are relatively hyperpolarized in the absence of odors, with a range of reported Vm values from −90 to −30 mV (Schild and Restrepo, 1998), vertebrate photoreceptors are relatively depolarized in darkness (Vm ~-40 mV). Therefore, vertebrate photoreceptors need to maintain a large inward cation current in darkness, which dominates their energy consumption. As light closes CNG channels, increasing ambient illumination decreases the energy consumption of photoreceptors (Linsenmeier and Braun, 1992; Okawa et al., 2008; Ingram et al., 2020) whereas higher concentration of odors would be expected to increase energy expenditure in OSNs.
A hallmark of vertebrate phototransduction is its large gain achieved via multiple steps of amplification: (1) one R* activates many G*s, (2) PDE* hydrolyzes many cGMP molecules, and (3) co-operative binding of cGMP to the CNG channels (see numerical values in Table 1). This large amplification allows rod photoreceptors to detect single photons (Baylor et al., 1979). As vertebrate (rod) phototransduction became the model for GPCR signaling cascade, it was previously assumed that high amplification is a general feature of G-protein signaling. However, it is now known that an individual activated olfactory GPCR activates < <1 Golf proteins, requiring tens of odor molecules to generate an action potential in a mouse OSN (Bhandawat et al., 2005; Ben-Chaim et al., 2011). This lower amplification is still true for vertebrate olfactory neurons despite the additional amplification mechanism unrelated to the GPCR signaling cascade. Namely, the influx of Ca2+ through open CNG channels also opens the calcium activated chloride ion channel ANO2 (Figure 3, step 3). This leads to chloride efflux and further depolarization of the cell due to the higher Cl− concentration in OSNs compared to a typical mature neuron (Kaneko et al., 2004).
Interestingly, photoreceptors also express ANO2 channels that are expected to mediate a depolarizing current (i.e., Cl− efflux) (Thoreson et al., 2003; Cia et al., 2005; Stöhr et al., 2009). Since light activation of photoreceptors leads to a hyperpolarizing response and closing of ANO2 channels, this chloride current would effectively promote light-induced Vm hyperpolarization, i.e., act as a positive feedback mechanism. However, Cl− efflux has been shown to inhibit Ca2+ channels in photoreceptor terminals which is expected to provide a negative feedback in response to Vm depolarization (Thoreson et al., 2000). Indeed, a Ca2+-activated chloride current has been shown to mediate negative feedback in monkey cones to prevent a regenerative depolarization by Ca2+ influx in the terminal (Yagi and Macleish, 1994).
Whereas the rod and cone CNG channels are highly selective for cGMP (Fesenko et al., 1985; Yu et al., 1996), the olfactory CNG channel has similar affinity to both cGMP and cAMP (Nakamura and Gold, 1987; Zufall et al., 1994). These differences in the affinity to cyclic nucleotides are naturally important for efficient transduction of light and odorant signals that are mediated by cGMP and cAMP, respectively. However, since olfactory channels are sensitive to both cGMP and cAMP, they also have the potential to be regulated by cGMP. Indeed, a subset of OSNs use also a cGMP-mediated signaling pathway (Meyer et al., 2000), exemplifying that odorant transduction may be more diverse than phototransduction in vertebrates. This is not surprising considering the multitude of genes encoding ORs detecting a wide variety of odorants (Niimura and Nei, 2007) compared to typically only 2–5 different genes encoding visual pigment molecules tuned to detect different several wavelengths of light (Table 1).
The phototransduction pathway is generally well-conserved in vertebrates. However, ciliary PRs come in two forms, highly sensitive rods that can mediate dim light vision and less sensitive cones that rarely saturate and can mediate daytime color vision. It is thought that early primitive metazoans had cone-like ciliary photoreceptors (Fain et al., 2010; Lamb, 2013, 2020), making these the evolutionarily older cell type. Unlike most invertebrates that adopted rhabdomeric PRs with high sensitivity and a wide dynamic range (see below), vertebrates further developed highly sensitive ciliary rod photoreceptors by tuning the molecular properties and expression levels of the visual pigment and transduction components (Ingram et al., 2016; Lamb, 2020). Rod photoreceptors enable vision in dim light and are able to mediate energy-efficient vision (Fain et al., 2010) together with cones in the classical vertebrate duplex rod/cone retina, from single photons up to bright daylight (~107 photons μm−2 s−1, Rodieck, 1998).
Invertebrate Sensory Transduction Cascades
The invertebrate phototransduction cascade has also been reviewed extensively (Hardie, 2012; Montell, 2012; Hardie and Juusola, 2015), while olfactory transduction is still under active investigation (Touhara and Vosshall, 2009; Schmidt and Benton, 2020). In this review we will focus on Drosophila olfactory and visual transduction for simplicity, however several studies have identified many differences between invertebrate species including different receptors and transduction signaling mechanisms (for recent reviews, see Honkanen et al., 2017; Schmidt and Benton, 2020). The main steps of the transduction are shown in Figures 4, 5, and described below, with the main components listed in Table 1 for comparison. It should be noted that these Figures show pathways for which there is evidence in literature although the physiological relevance of some of them remain controversial. Furthermore, for the sake of simplicity they do not show all discovered pathways. Specifically, olfactory transduction in invertebrates appears to be extremely diverse as will be briefly discussed below.
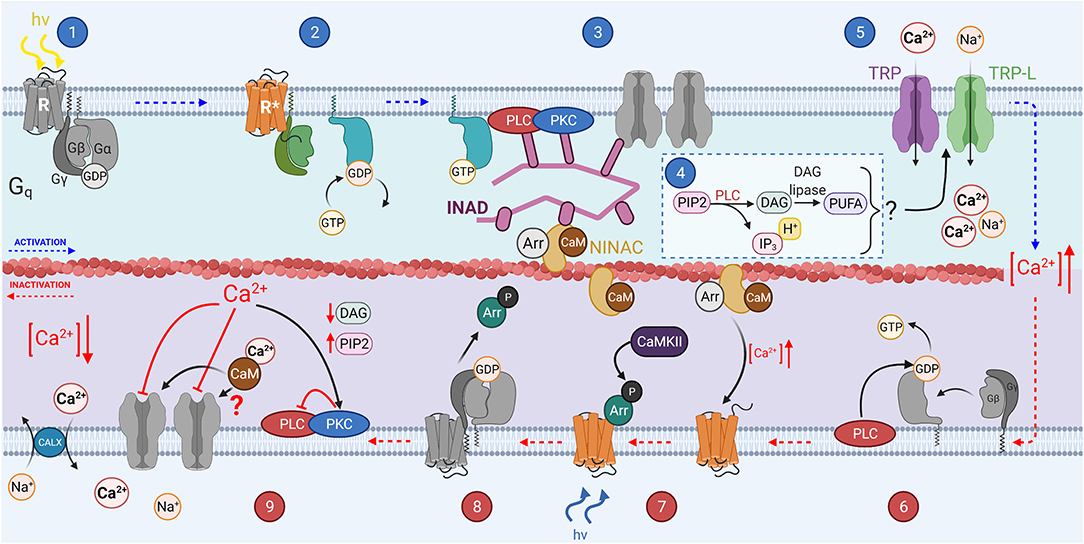
Figure 4. The invertebrate phototransduction cascade, inactivation, and adaptation mechanisms: A photon causes isomerization of the chromophore 3-hydroxy 11-cis-retinal to 3-hydroxy all-trans-retinal (step 1). This activates the opsin (step 2), which also activates the Gqα subunit through GDP-GTP exchange. The Gqα subunit then activates membrane bound PLC (step 3), which hydrolyzes PIP2 to produce IP3, DAG and H+ (step 4), and potentially DAG is further catalyzed to produce PUFAs. Some or all the products from step 4 activate the membrane bound TRP and TRP-L channels in step 5, causing an influx of Ca2+ and Na+ ions, depolarizing the microvillus. PLC can act as a GTPase, hydrolyzing the GTP bound to Gα to GDP (step 6). Invertebrate chromophore is bistable and is converted back from 3-hydroxy 11-cis retinal to 3-hydroxy-all-trans retinal at via the absorption of a second photon (step 7). CaMKII phosphorylates Arrestin 2 allowing it to dissociate from R* after the chromophore returns to its inactive state (step 8).
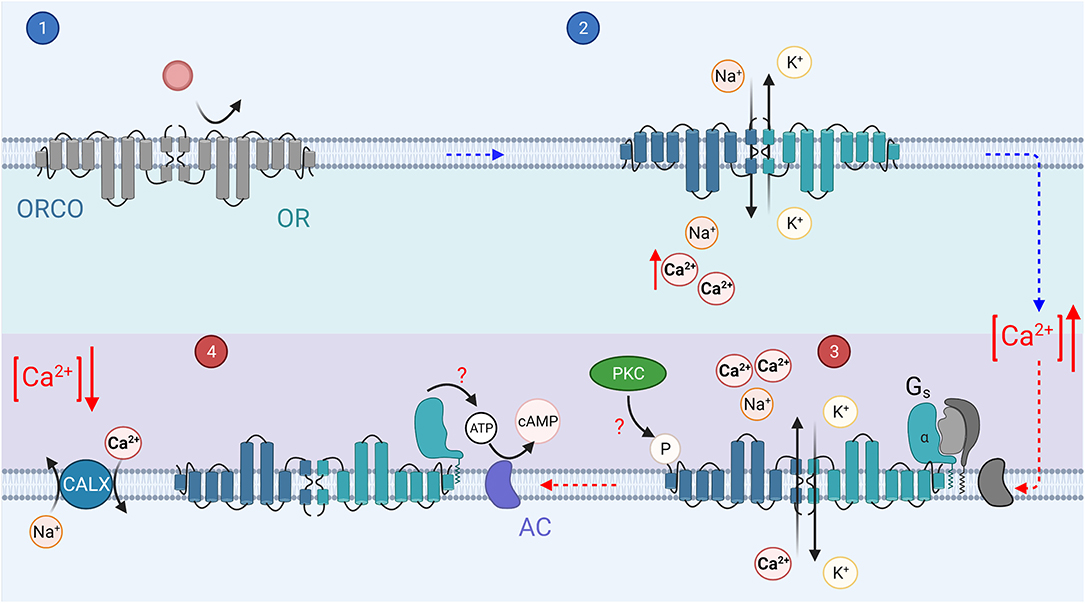
Figure 5. The invertebrate olfactory transduction cascade: In invertebrates, the ORs are 7 transmembrane domain receptors, which associate with a co-receptor, Orco. Upon binding of an odorant (step 1), the OR/Orco co-receptor channel pore opens, allowing the influx of cations, Ca2+ and Na+ (step 2). It is possible that a G protein mediated pathway regulates the sensitivity of the channels, odorant binding activates Gs (step 3). The Gsα subunit then in turn activates adenylyl cyclase to create cAMP from ATP, which may mediate sensitization of the odorant response (step 4). Phosphorylation by PKC of the Orco receptor may mediate the olfactory neuron sensitization (step 3).
Phototransduction is initiated when light induces a conformational change in the chromophore attached to an opsin (see Figure 1D for chromophore structure Figure 4, step 1; Paulsen and Schwemer, 1972). Unlike in vertebrates, the chromophore remains bound to opsin. Upon activation, rhodopsin is isomerized to metarhodopsin which activates the heterotrimeric Gq protein through GDP-GTP exchange, releasing the Gqα subunit (Figure 4, step 2; Scott et al., 1995). Gqα activates membrane-bound phospholipase C (PLC, Figure 4 step 3; Bloomquist et al., 1988; Estacion et al., 2001). PLC in turn hydrolyzes phosphatidylinositol 4,5-bisphophate (PIP2) to produce inositol 1,4,5-trisphosphate (IP3), diacylglycerol (DAG), and H+ in a classical second messenger pathway mediating a multitude of functions across multiple species (Figure 4, step 4; Litosch, 2015; Machaty, 2016; Martin-Aragon Baudel et al., 2020). Despite extensive efforts, the second messenger(s) gating Ca2+ permeable transient receptor potential (TRP) and transient receptor potential-like (TRP-L) cation channels in vivo remain unknown (Figure 4, step 5; Montell and Rubin, 1989; Hardie and Minke, 1992; Phillips et al., 1992; Huang et al., 2010). DAG itself is a well-known TRP channel activator and has been shown to activate recombinant TRPL channels (Estacion et al., 2001). However, DAG can also be converted into polyunsaturated fatty acids (PUFAs) by DAG lipase, and PUFAs are effective activators of both TRP and TRPL channels in intact Drosophila cells (see Figure 4, step 4; Chyb et al., 1999; Estacion et al., 2001). There is also significant evidence supporting a model in which activation of PLC leads to depletion of PIP2 in the phospholipid bilayer of microvilli, generating a mechanical force that leads to opening of TRP and TRPL (Hardie and Franze, 2012). Since DAG appears to be an ineffective activator of the native invertebrate TRP channels, it is most likely that either PIP2 depletion and/or PUFAs mediate the gating of invertebrate phototransduction channels in response to light. IP3 can also mediate Ca2+ release from ER but this mechanism does not contribute to Drosophila phototransduction (Acharya et al., 1997; Raghu et al., 2000). Invertebrate phototransduction has an amplification step not present in vertebrates which contributes to the very high sensitivity of rhabdomeric photoreceptors when compared even to rods (Hardie and Raghu, 2001; Fain et al., 2010). Once enough second messenger has accumulated during the variable latent period (15–100 ms) and the first TRP channel opens, the influx of Ca2+ mediates sensitization of the remaining TRP channels to their gating substance leading to the majority of the TRP channels in an individual microvillus opening even in response to a single photon (Henderson et al., 2000).
Invertebrate olfactory transduction appears to use a diverse set of pathways across species, within a single species, and even within a single OSN (for a recent review, see Schmidt and Benton, 2020). The receptors can be divided into two different classes; (1) seven transmembrane domain odorant receptors (ORs) (Clyne et al., 1999) and (2) three transmembrane domain ionotropic receptors (IRs, see Table 1; Benton et al., 2009). IRs have some homology with ionotropic glutamate receptors but will not be discussed extensively in this review. This diverse set of olfactory receptors may help animals to detect a large amount of different odorant molecules with high temporal resolution or high sensitivity across a wide range of background odorant levels. Drosophila odorant receptors (ORs) are odorant specific proteins (Clyne et al., 1999) with no obvious sequence homology to GPCRs (Benton et al., 2006) that form heteromers with a co-receptor, Orco [previously OR83b (Larsson et al., 2004; Butterwick et al., 2018)]. These OR/Orco complexes form ligand-gated cation channels (Figure 5, step 2; Sato et al., 2008; Smart et al., 2008; Wicher et al., 2008; Nakagawa and Vosshall, 2009). However, this type of ionotropic pathway is typically assumed to be relatively insensitive and not sufficient to mediate highly sensitive olfaction in flying insects. As a resolution to this apparent contradiction, odorant-binding was suggested to activate Gs and consequently induce production of cAMP molecules that presumably gate the Orco channel (Figure 5, step 4; Wicher et al., 2008; Miazzi et al., 2016). Indeed, as an example, vertebrate taste receptors can utilize either metabotropic GPCRs or ionotropic ligand-gated channels depending on the taste molecules (Kinnamon and Finger, 2019). However, experiments by Wicher et al. relied upon the HEK293 expression system, not directly demonstrating that Gs-mediated signaling plays a role in intact insect OSNs. Indeed, a subsequent study by Andrea Yao and Carlson (2010) did not find a significant role for any GPCR signaling in Drosophila OR-mediated transduction. On the other hand, several other studies have lent support to the contribution of various metabotropic pathways to Drosophila olfactory transduction in vivo (Gomez-Diaz et al., 2004; Kain et al., 2008; Chatterjee et al., 2009; Deng et al., 2011; Getahun et al., 2013). Thus, the evidence taken as a whole suggests that invertebrate OR/Orco receptors mediate fast ionotropic transduction, but slower and potentially more sensitive metabotropic G protein coupled signaling cascade reminiscent of vertebrate olfactory transduction described above probably mediates the regulation of transduction (further described below) (Nakagawa and Vosshall, 2009; Hansson et al., 2010; Miazzi et al., 2016). Thus, the role of G protein mediated signaling in the invertebrate olfactory transduction is most likely to be a minor secondary one, perhaps solely regulatory.
Interestingly, an alternative way to increase the sensitivity of the invertebrate OSNs was suggested recently: a sodium channel (Pickpocket 25, PPK25) was shown to mediate Ca2+/calmodulin-dependent amplification of odorant responses in certain olfactory receptor neurons in Drosophila (Ng et al., 2019). This mechanism is analogous to vertebrate OSNs in which odorant induced Ca2+ influx activates ANO2 channels to promote depolarization (see above). In addition, a recent study showed that the OR/Orco complex can also be activated by odor-induced Ca2+ influx via a calmodulin mediated pathway in Drosophila olfactory neurons (Jain et al., 2021). This is analogous to invertebrate phototransduction where the signal is amplified via Ca2+ feedback (see above) and maybe critical for increasing the sensitivity of the OR-mediated olfactory transduction. In summary, it seems plausible that a combination of ionotropic and metabotropic signaling together with Ca2+ feedbacks and PPK25 channel can promote both fast and sensitive olfaction that is critical specifically for flying insects (Getahun et al., 2012, 2013).
Inactivation of Signal Transduction
Vertebrate
Timely inactivation of transduction is key to maintaining the temporal relevance of information from primary sensory neurons. Each activated component of the signaling pathway needs to be inactivated and the concentration of secondary messengers restored to pre-stimulus levels to enable rapid and repeated detection of sensory stimuli. In this section we will focus on the inactivation of receptors and Gα/effector complex in vertebrate PR and OSN cilia since these processes are required for the cessation of the electrical PR and OSN response. Mechanisms that accelerate response recovery and/or regulate the sensitivity of the receptor cells to odors/light by modulating the homeostasis of second messengers and the affinity of cyclic nucleotides to CNG channels will be discussed below in the context of adaptation.
In vertebrate phototransduction, the active receptor, R*, is inactivated through two processes: rapid initial phosphorylation through the activity of GPCR kinase 1 (GRK1) (Bownds et al., 1972; Cideciyan et al., 1998; Lyubarsky et al., 2000; Sakurai et al., 2015) reducing R*'s catalytic activity (Figure 2, step 4; Xu et al., 1997), and rapid arrestin binding (Figure 2, step 5; Kuhn, 1978; Wilden, 1995; Kennedy et al., 2001). Rods, in mice, use only Arr1 whereas cones use both Arr1 and Arr4, however the functional significance of having two different isoforms in cones is not clear since deletion of either one of these arrestins alone does not have a significant physiological phenotype in mice (Lyubarsky et al., 2002; Shi et al., 2007; Nikonov et al., 2008). There is evidence that vertebrate olfactory receptors are also desensitized by GPCR kinase (GRK3, Figure 3, step 6) and that this is important for fast recovery of the olfactory response (Schleicher et al., 1993; Boekhoff et al., 1994; Peppel et al., 1997). However, the significance of GRK3 in modulating OSN desensitization has been called into question recently (Kato et al., 2014). In addition to GPCR kinase, second messenger activated kinase (PKA) is thought to play a role in rapid olfactory transduction termination potentially by phosphorylating the olfactory receptor (Figure 3, step 6; Boekhoff et al., 1992). However, if olfactory receptor phosphorylation plays a physiological role in terminating the olfactory response in vertebrates remains unclear since although Boekhoff et al. presented data supporting the role of PKA in fast response termination, their experiments did not confirm that PKA targets the receptor.
The active effector/GTα complex in the photoreceptors is inactivated through hydrolysis of bound GTP by GTPase-accelerating protein (GAP) complex (Figure 2, steps 6 and 7; Chen et al., 2000). Inactivation of the GTα/PDE* complex is the slowest step in mouse rod phototransduction termination and therefore rate-limits light response recovery after stimulation (Krispel et al., 2006). However, in amphibian cone photoreceptors inactivation of the receptor rather than PDE* appears to rate-limit the light response recovery (Matthews and Sampath, 2010; Zang and Matthews, 2012). The rate-limiting step of mammalian cone phototransduction termination remains unknown. The active effector enzyme of olfactory transduction, AC3, is phosphorylated by calmodulin kinase II (CaMKII) to inhibit cAMP production (Wei et al., 1996). This inhibition is thought to contribute to the termination of olfactory transduction after odorant stimulation (Wayman et al., 1995; Wei et al., 1996, 1998). The mechanism that rate-limits the recovery of the electrical response of vertebrate OSN cilia appears to be mediated by the inactivation of the Ca2+-activated Cl− channels, rather than any step of the GPCR signaling cascade, following the kinetics of Ca2+ extrusion from the OSN cilia (Reisert and Matthews, 2001; Antolin et al., 2010). Regulation of Ca2+ and potential modulation of the receptor or effector inactivation in PRs and OSNs by Ca2+ feedback will be discussed below.
Invertebrate
Like in vertebrate phototransduction, inactivation of the active receptor (R*) in invertebrates involves Arrestins, two of which have been identified in Drosophila, Arr1 and Arr2 (Smith et al., 1990). However, unlike vertebrates, phosphorylation of rhodopsin is not necessary for termination of phototransduction or the binding of Arr2 in invertebrates (Plangger et al., 1994; Vinos et al., 1997). The Gqα subunit itself may facilitate the binding of Arr2 to R* (Hu et al., 2012). Binding of Arr2 displaces the Gqα subunit from R*, reducing its activity (Figure 4, step 7). Interestingly CaMKII, a kinase that inhibits AC3 in vertebrate OSN, phosphorylates Arr2 in Drosophila (Matsumoto et al., 1994). However, this phosphorylation is not necessary for binding of arrestin to rhodopsin, but rather enables arrestin to dissociate from rhodopsin once it returns back to the ground state via absorption of a long-wavelength photon (Alloway and Dolph, 1999).
As in vertebrate phototransduction, the effector enzyme PLC functions as a GTPase activating protein (GAP) for Gqα, mediating the termination of its activity (Figure 4, step 6; Cook et al., 2000). Inactivation of invertebrate phototransduction is significantly accelerated by Ca2+ feedback mechanisms which will be discussed below. As discussed earlier, invertebrate OSNs rely largely on GPCR-independent transduction where the receptor acts as an odor-gated channel. This allows fast inactivation of the odor-induced signal without the requirement for complicated enzymatic reactions to inactivate the multiple components involved. This strategy provides fast odor detection while conserving energy and can therefore be particularly advantageous for an insect with multiple predators and potentially scarce food sources. Though, specifically flying insects have also very sensitive olfaction that could be mediated by regulation of the OR/Orco complex by GPCR signaling cascade(s) as discussed above. However, the molecular details of these metabotropic pathways remain unclear and controversial.
Adaptation Mechanisms in Invertebrate and Vertebrate Olfactory and Phototransduction Cascades
Animals live in environments where sensory exposure levels can vary dramatically, for example from the low light levels of shaded forests and underground burrows to the bright sunlight of a field at noon. The ability to adapt to these very dramatic differences in light levels is crucial for survival. Adaptation is a universal feature of all sensory systems, including vision and olfaction (Torre et al., 1995; Zufall and Leinders-Zufall, 2000); it is important to permit detection of novel stimuli in the presence of persistent activation. For vision, two crucial types of adaptation occur, light and dark adaptation. Here we define light adaptation as a deterministic and reversible process where the gain of the transduction can be adjusted up or down in response to increase or decrease in ambient light, respectively, to enable detection of increments or decrements of stimuli around the background stimulus level. Dark adaptation refers here specifically to visual adaptation to a large drop in ambient light following a bright light exposure that has bleached a significant fraction of the visual pigment molecules (Lamb and Pugh, 2004). This process involves regeneration of the visual pigment molecules, a process that can be quite slow specifically in vertebrate rod photoreceptors. This slow regeneration is responsible for the temporary inability to see you may have experienced when stepping into a dark movie theater. Both adaptation processes are critical for our ability to see over a wide range of light conditions, as well as adapt quickly to changes in ambient light during our everyday lives. Although both vertebrates and invertebrates can see over an impressive range of illumination conditions, they have adopted very different strategies to do so. Additionally, whereas increasing background light intensity decreases the sensitivity of photoreceptors based on Weber law, invertebrate olfactory receptors can be also sensitized by repetitive sub-threshold stimulation (Getahun et al., 2013; Mukunda et al., 2016). These differences will be highlighted below in terms of molecular mechanisms that control the gain and kinetics of the vertebrate and invertebrate phototransduction. Table 2 summarizes some of the important Ca2+ feedback mechanisms and adaptation characteristics across all four transduction modalities discussed in this review.
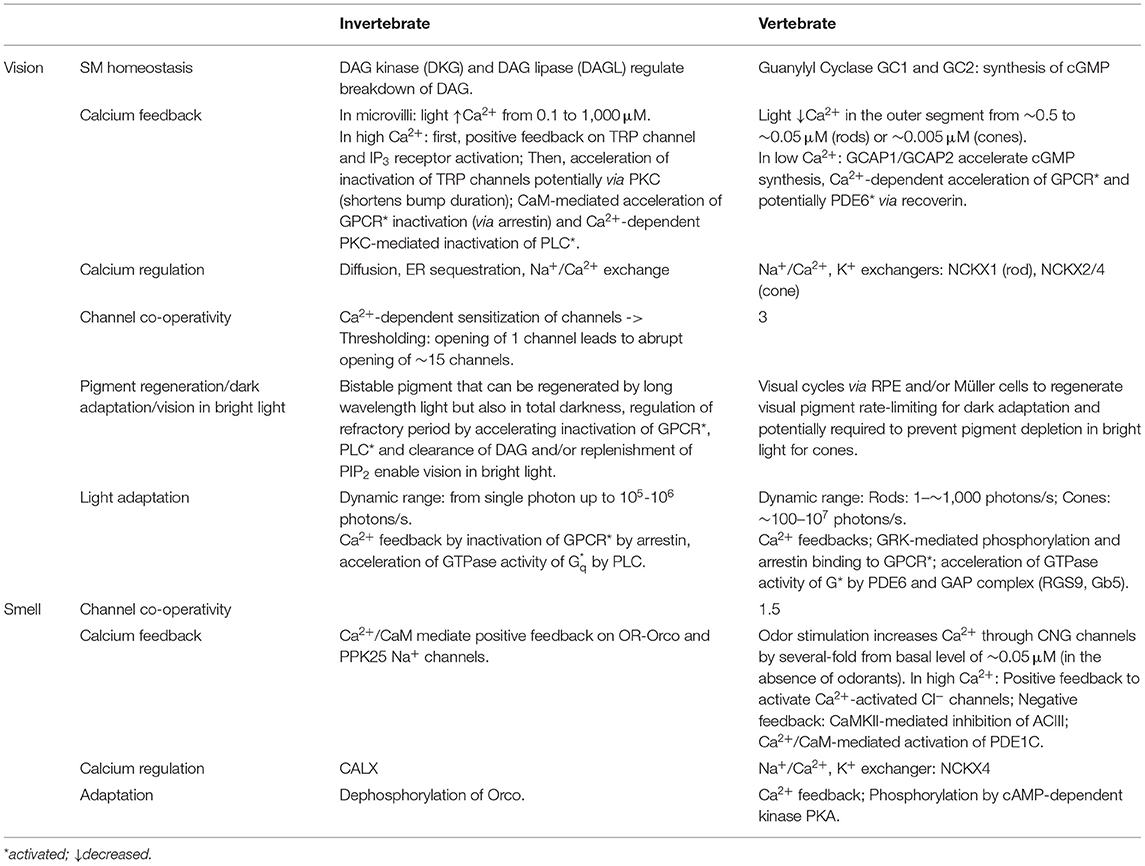
Table 2. Overview and comparison of the adaptation and feedback mechanisms in invertebrate and vertebrate phototransduction and olfactory transduction cascades.
Light/Odor Adaptation
Regulation of Ca2+ in the Transduction Compartments
Calcium ions (Ca2+) play a critical role in both light and odor adaptation. Thus, it is important to first understand how Ca2+ is regulated in the cilia or microvilli of the photoreceptors and OSNs in response to sensory stimulus. The channels mediating the influx of Ca2+ into vertebrate PR and OSN cilia are CNG channels which are not only permeable to Na+ but also to Ca2+ (Yu et al., 1996). In invertebrates, the transduction channels, TRP and OR/Orco receptor channels, also mediate Ca2+ influx into the PR and OSN transduction compartments (Chu et al., 2013). A sensory stimulus leads to increases in cytosolic Ca2+ in all OSNs and in invertebrate PRs (i.e., activation = increase in neuronal Ca2+). However, vertebrate photoreceptors are a notable exception to this rule, their CNG channels are closed by light, leading to a decline in the outer segment [Ca2+]. Even if the details of how Ca2+ is regulated in these different transduction compartments may differ, the general principle of using the transduction channel to regulate Ca2+ influx seems to be a conserved strategy. This way Ca2+ level in the cilia or microvilli can be proportional to sensory stimulus level, an important feature for its ability to adjust the transduction gain in response to changes in ambient light or odorant level.
In vertebrate PR outer segments [Ca2+] is ~0.5 μM in darkness and in bright light decreases 10-fold in rods and 100-fold in cones (see Table 2, Sampath et al., 1998, 1999; Woodruff et al., 2002). In the small microvilli of the invertebrate PR [Ca2+] can quickly increase 10,000-fold upon light stimulation (Asteriti et al., 2017), a several log-units larger dynamic range compared to vertebrate rods and cones (See Table 2 for values). Additionally, the global Ca2+ levels in PR cells can range from ~0.1 μM in darkness up to 10 μM in bright light (Oberwinkler and Stavenga, 2000). This could play a role in the larger adaptation capacity of individual invertebrate microvillar photoreceptors compared to ciliary photoreceptors found mainly in vertebrates. The basal Ca2+ level in vertebrate olfactory neurons is ~0.05 μM (Leinders-Zufall et al., 1997), and can increase several-fold in response to odor stimulation (Antolin et al., 2010). However, this increase is not as large as in photoreceptors, which are known for their remarkable ability to adapt. Permeability of the transduction channels to Ca2+ and particularly the fraction of inward current carried by Ca2+ may play an important role for the amplitude of Ca2+ signals. For example, Ca2+ ions carry a significantly smaller fraction of the transduction channel current in rods than in cones (Picones and Korenbrot, 1995), microvillar photoreceptors (Chu et al., 2013) or vertebrate OSN CNG channels (Frings et al., 1995). This can contribute to the higher fluxes of Ca2+ across the plasma membrane to promote faster and larger Ca2+ signals and adaptation in vertebrate cones or fly photoreceptors compared to rods (Sampath et al., 1998, 1999).
In addition to transduction channels, an important part of Ca2+ homeostasis is its cytosolic clearance. Visual and olfactory transduction in both vertebrates and invertebrates all use plasma membrane Na+/Ca2+ exchangers to extrude Ca2+. Na+/Ca2+ exchangers have significantly higher capacity compared to PMCAs. This enables faster regulation of Ca2+, however, the affinity of Na+/Ca2+ exchangers to Ca2+ is rather low and they are not able to drive Ca2+ to extremely low levels. In some cases, plasma membrane ATPases (PMCAs) (Weeraratne et al., 2006; Castillo et al., 2007) as well as intracellular stores (ER and mitochondria) (Molnar et al., 2012; Bisbach et al., 2020; Hutto et al., 2020; Liu et al., 2020) can also contribute to cytosolic Ca2+ homeostasis in sensory cilia or microvilli, but the contribution of these mechanisms to the overall Ca2+ homeostasis and transduction is not well-understood.
In vertebrate photoreceptors extrusion of Ca2+ is directly related to the kinetics of response termination and light adaptation, as it mediates the reduction of Ca2+ required for critical feedback mechanisms that accelerate response termination and desensitize the photoreceptors. Extrusion of Ca2+ from the vertebrate OSN cilium directly controls inactivation of the Ca2+-activated Cl− channels and thus rate-limits the recovery of the electrical response of olfactory neurons (Antolin et al., 2010). On the other hand, in both invertebrate phototransduction and olfactory transduction, Ca2+ extrusion plays a role in Ca2+ homeostasis rather than directly contributing to adaptation kinetics in response to increases in ambient light or odorant levels.
Vertebrate PR and OSN cilia use Na+/Ca2+, K+ exchangers (NCKXs, for a recent review Jalloul et al., 2020) to extrude Ca2+. These exchangers use energy derived from the driving force of both Na+ and K+ to extrude Ca2+ and therefore, can promote fast Ca2+ extrusion, response recovery or adaptation. Their role in vertebrate rod and cone photoreceptors was recently reviewed in Vinberg et al. (2018). Rod photoreceptor outer segments rely almost exclusively on NCKX1 for Ca2+ extrusion (Reilander et al., 1992; Vinberg et al., 2018) although some other mechanism(s) may also have a small contribution to Ca2+ homeostasis in the mouse rod outer segment (Vinberg et al., 2015; Bisbach et al., 2020). Cones were originally thought to rely exclusively on NCKX2, however, a recent study showed that vertebrate cones (including primate cones) also express NCKX4 in their outer segments and that NCKX4 is important for the fast termination and light adaptation of mouse cones (Vinberg et al., 2017). It has been speculated that cones use different exchangers and/or two exchangers to fulfill their requirement for faster signaling and wider operating range. However, a recent study did not find any significant biophysical differences between different NCKX isoforms (Jalloul et al., 2016). A more relevant question might be why rods acquired NCKX1 instead of NCKX2 and NCKX4, which are used by the evolutionarily older cone photoreceptors. It is possible that it could be related to how these exchangers can interact with CNG channels. It is known that NCKX1 forms heteromers with the rod CNG channel (Bauer and Drechsler, 1992), and the same may be true also for the cone channels and exchangers (Kang et al., 2003). Interestingly, NCKX4 is also important for termination and adaptation of the mouse OSN response (Stephan et al., 2011) although OSNs also express functionally important PMCA2 and PMCA4 in their cilia (Weeraratne et al., 2006; Castillo et al., 2007).
The identity of the plasma membrane Na+/Ca2+ exchangers in the Drosophila PR and OSN cilia and microvilli is also known. Interestingly, both of these sensory neurons appear to rely on Na+/Ca2+ exchanger CALX, a homolog of vertebrate exchanger NCX (Wang et al., 2005; Halty-Deleon et al., 2018). However, CALX has a unique property of being inhibited by high cytosolic Ca2+ (Hryshko et al., 1996), a property that is puzzling considering its presumed role in restoring low Ca2+ after stimulus-induced increase of [Ca2+]i. A recent study found that CALX is also expressed in the ER of Drosophila photoreceptors and contributes to increased ciliary Ca2+ after light stimulation by transporting Ca2+ from ER stores (Liu et al., 2020). Although it might be expected that invertebrate microvillar photoreceptors need extremely efficient mechanisms to extrude Ca2+ due to their fast response kinetics and wide operating range, it should be pointed out that invertebrate phototransduction is also compartmentalized to extremely small microvilli, where the influx/efflux of just one calcium ion can raise or decrease [Ca2+]i by 1 μM. Thus, the large membrane surface area to volume ratio may be more important for fast and efficient Ca2+ signaling than the biophysical properties of the exchanger protein itself.
Regulation of Receptor Inactivation
Both vertebrate and invertebrate phototransduction employs Ca2+ feedback to accelerate inactivation of the active receptor, R*. However, the mechanisms and their relative contributions to the lifetime of R* and light response termination are different. The lifetime of R* is ~40 ms in mammalian (Gross and Burns, 2010) and ~400 ms in amphibian dark-adapted photoreceptors (Nikonov et al., 1998). The mechanisms that contribute to these relatively short lifetimes: phosphorylation by GRK1 and binding of arrestin have already been discussed in this review. R* lifetime is further shortened by background light via Ca2+ feedback mediated by recoverin in both rods and cones (Xu et al., 1997; Cideciyan et al., 1998; Lyubarsky et al., 2000; Sakurai et al., 2015). In the dark state, where intracellular calcium is high, Ca2+ bound recoverin inhibits the activity of GRK1 (Kawamura, 1993; Chen et al., 1995; Klenchin et al., 1995). Once light closes CNG channels, and intracellular calcium is reduced, recoverin no longer binds calcium and thus releases inhibition of GRK allowing it to phosphorylate R*. Thus, increasing light intensity is expected to decrease the lifetime of R*, and the subsequent gain of the phototransduction. Since the lifetime of R* is not rate-limiting for the response recovery in mouse rods, its role in shaping the light responses is not obvious, but it regulates kinetics of mouse rod bright flash responses (Makino et al., 2004) and light sensitivity of cones (Sakurai et al., 2015). Moreover, the contribution of recoverin to light adaptation at least in rod photoreceptors is minor, affecting only adaptation kinetics but not the steady state adaptation (Chen et al., 2010; Morshedian et al., 2018).
Although phosphorylation of the R* in invertebrate PRs is less important, arrestins also have a critical role in inactivating the R* in microvillar photoreceptors as described above. Whereas, Arr1 and Arr4 in vertebrate photoreceptors are not known to be regulated by any Ca2+-dependent mechanism, Arr2 is regulated by Ca2+/calmodulin in Drosophila (Kahn and Matsumoto, 1997). In low calcium solutions inactivation of R* is slowed down by 10-fold, and Ca2+ dependent inactivation is abolished when calmodulin (CaM) or CaM-binding myosin III (NINAC) is mutated (Li et al., 1998; Liu et al., 2008), indicating that Ca2+ influx accelerates the binding of Arr2 to R*via CaM, and NINAC sequesters Arr2 in low Ca2+. This suggests that whereas Ca2+ feedback on R* inactivation has minimal significance in vertebrate photoreceptors, it has a major role in accelerating light responses in Drosophila. This difference could be contributing to the faster light responses and vision in insects as compared to vertebrates.
The lifetime of active olfactory receptor in vertebrates is very transient, potentially as short as 0.1 ms. This has important implications for the gain and sensitivity of olfactory transduction which, unlike phototransduction, is not capable of transducing single odor molecule events (Ben-Chaim et al., 2011). Although there is some evidence that phosphorylation of the OR in vertebrates by GRK3 may be important for the fast inactivation of the activated receptor (see above), it is not known if any Ca2+ feedback mechanisms exist to regulate the lifetime of the active ORs. It may not be necessary since the baseline lifetime is already extremely short and determined by the short lifetime of the odorant-OR complex rather than by any enzymatic reaction (Bhandawat et al., 2005). As described above, sensitivity of invertebrate OR/Orco receptor is modulated by phosphorylation or dephosphorylation. Furthermore, as described above the sensitivity of the OR/Orco complex can be increased by Ca2+-calmodulin to mediate sensitization of the OSNs in response to weak stimulation (Mukunda et al., 2016; Jain et al., 2021).
Regulation of Effector Enzyme Inactivation
Inactivation of the GTα/PDE* in vertebrate PRs is significantly accelerated by a GAP complex (see above). Initially, the lifetime of PDE* was thought to be independent of background light level or Ca2+ (Nikonov et al., 1998). However, it has been shown in salamander and mouse rods that the lifetime of PDE* shortens under increasing background light intensity, and experiments in mice suggest that this is mediated by recoverin (Nikonov et al., 1998, 2000; Chen et al., 2015; Morshedian et al., 2018). These results suggests that recoverin can mediate a Ca2+-dependent acceleration of R* and PDE* inactivation, though the contribution of this regulation to light adaptation capacity of vertebrate rod photoreceptors is very small.
In invertebrate PRs the inactivation of effector enzyme PLC is also mediated by Ca2+, but via different mechanisms than in vertebrates (Smith et al., 1991). In Drosophila PRs, light-induced increase of Ca2+ inhibits PLC through the kinase activity of PKC, slowing down depletion of PIP2 and synthesis of DAG (Figure 4, step 9; Shieh et al., 1997; Gu et al., 2005). This mechanism is expected to contribute to acceleration of the light response termination and desensitization of the microvillar photoreceptors upon increased ambient light, i.e., adaptation.
The effector enzyme in vertebrate OSNs is cAMP-synthesizing enzyme AC3, which is activated by Gαolf as described earlier. Restoration of resting state levels of cAMP after odor-induced increases is accelerated by inhibition of AC3 via a Ca2+/calmodulin kinase II mediated phosphorylation (Wei et al., 1996, 1998). Thus, inhibition of AC3 causes the accelerated termination of the electrical odor response, and the OSN is desensitized in response to increased Ca2+ concentration triggered by increased background odorant levels.
Regulation of the Second Messenger Homeostasis
In addition to inactivating the effector enzyme of the GPCR signal cascade, the restoration of pre-stimulus second messenger concentration requires enzymes that either reduce the excess cAMP in vertebrate OSNs (Boekhoff and Breer, 1992) or DAG/PUFA in invertebrate PRs, respectively, or synthesize cGMP in vertebrate PRs or PIP2 in invertebrate PRs. Whereas, these enzymes are not directly part of the GPCR signaling cascade, some of them are known to play an important role in transduction termination and light adaptation. Indeed, regulation of cGMP synthesis by guanylyl cyclases (GC1 and GC2 in mice) is the dominant mechanism for regulating sensitivity in vertebrate rods and cones (Mendez et al., 2001; Sakurai et al., 2011). In darkness when Ca2+ is high, Guanylyl Cyclase Activating Proteins (GCAP1 and GCAP2 in mice) have Ca2+ bound to them and they cannot activate GCs. With light-induced decreases of Ca2+, Mg2+ replaces Ca2+ in GCAP proteins, and the Mg2+-GCAPs increase cGMP synthesis by activating GCs (Peshenko and Dizhoor, 2007). GCAP1 and GCAP2 have different affinity to Ca2+ so that GCAP1 is disinhibited and can activate GCs with smaller decline of Ca2+ in dim light whereas GCAP2 becomes more important under bright light conditions with larger decrease in Ca2+ (Makino et al., 2012). Invertebrate phototransduction is more complicated and as the identity of second messenger is still unclear, the contribution of its degradation/synthesis regulation to the light adaptation capacity is also not known. As described above the effector enzyme PLC plays an important role in invertebrate phototransduction since it releases DAG from PIP2. Whether enzymes like DAG lipase that convert DAG into PUFAs, or those involved in PUFA metabolism or PIP2 synthesis are regulated by light-induced increases of Ca2+ remain open questions.
Olfactory neurons in vertebrates express cAMP-hydrolyzing enzyme PDE1C specifically in their cilia (Yan et al., 1995), and PDE4A throughout the OSN excluding the cilium (Juilfs et al., 1997) which balance the synthesis of cAMP by AC3. Although PDE4A is not expressed in the OSN cilia, termination of mouse OSN response is only slowed when both PDE1C and PDE4A are knocked out (Cygnar and Zhao, 2009). However, odorant adaptation in mice lacking PDE1C alone is compromised, demonstrating a role for PDE1C in regulating the gain of the olfactory transduction in response to increased background levels of odorants (Cygnar and Zhao, 2009). PDE1C is known to be activated by Ca2+-bound calmodulin (Yan et al., 1995). Thus, it is expected that odorant-induced increase of Ca2+ activates PDE1C via Ca2+/calmodulin to accelerate hydrolysis of cAMP, explaining how PDE1C can contribute to adaptation.
In invertebrate olfactory transduction, increasing evidence has demonstrated that although G protein mediated signaling does not have a primary role in olfactory transduction, it is likely to be involved in the adaptation mechanisms. Odorant-binding has been suggested to activate Gs and consequently induce production of cAMP molecules that presumably gate Orco channel, perhaps as a mechanism to mediate the sensitization of the neurons (Figure 5, steps 3 and 4; Wicher et al., 2008; Miazzi et al., 2016).
Regulation of the Transduction Channel
All transduction channels discussed in this review, including the ionotropic OR/Orco receptor channels, have been suggested to be regulated in response to changes in background sensory stimulus level. The CNG channels used by vertebrate PRs and OSNs as well as TRP channels in the invertebrate photoreceptors all have a binding domain for calmodulin. Indeed, in vitro studies suggested that the affinity of vertebrate PR and OSN CNG channels to second messengers is regulated by calmodulin mediated Ca2+ feedback (Hsu and Molday, 1994; Liu et al., 1994; Bradley et al., 2001; Song et al., 2008; Nache et al., 2016). However, Ca2+/calmodulin modulation may not be critical for vertebrate photoreceptor (Haynes and Stotz, 1997; Chen et al., 2010) or OSN adaptation (Song et al., 2008). The odor receptor-channel in invertebrates can also be regulated in response to increased background levels of odorants. Interestingly, phosphorylation (Sargsyan et al., 2011; Getahun et al., 2013) and dephosphorylation (Guo et al., 2017) of the Orco co-receptor sensitizes and desensitizes the Drosophila olfactory transduction in response to OSN activation, respectively. Phosphorylation is mediated by PKC but the molecular mechanism of dephosphorylation is not known. These results indicate that phosphorylation of the ORs by PKC mediates the sensitization of the ORNs to sub-threshold stimulation whereas dephosphorylation in response to stronger background odorant levels would mediate desensitization of the ORNs. These mechanisms together can contribute to widen the operating range of the insect olfaction (Jain et al., 2021). It is possible that calmodulin independent Ca2+ feedbacks on the CNG channels exist in vertebrate photoreceptors. For example, in catfish cone photoreceptors calmodulin does not modulate cone CNG channel activity (Haynes and Stotz, 1997). On the other hand, striped bass cones express CNG modulin that may be responsible for the Ca2+ induced modulation of CNG channel activity in vertebrate cones (Rebrik et al., 2012). The mammalian homolog to CNG modulin is echinoderm microtubule-associated protein-like 1 (Eml1) which is expressed in the human and mouse retina (Rebrik et al., 2012). It remains to be determined whether it plays any role in the mammalian cone or rod phototransduction. As described above, termination of the vertebrate olfactory transduction requires also closure of Ca2+-activated Cl− channels. Gating of the Ca2+-activated Cl− channel appears to be modulated via a Ca2+-dependent pathway mediated by calmodulin (Yang et al., 2014). Both TRP and TRP-L channels in invertebrate photoreceptors have CaM binding sites (Phillips et al., 1992; Chevesich et al., 1997), and have been hypothesized to contribute to light-adaptation. In summary, although a lot of in vitro evidence supporting Ca2+-dependent modulation of the transduction channels in vertebrate and invertebrate sensory transductions exist, its physiological significance in vivo is either minor or remains to be determined.
Dark Adaptation and Pigment Regeneration in Vertebrate and Invertebrate Photoreceptors
Maintenance of vision during sustained background light or adaptation to darkness after bright light exposure requires a mechanism to reset the activated visual pigment back to its ground state. Invertebrate rhabdomeric photoreceptors can regenerate photopigment in a light-dependent manner. Their rhodopsin is bistable as the chromophore remains bound to opsin after the absorption of an initial photon, so that a further absorption of a long-wavelength photon converts the activated rhodopsin back to the ground state (see Figure 6B). This may imply that a separate pigment regeneration pathway as described below for vertebrates is not required for invertebrate vision in bright light. However, in case of limited nutritional intake, or when rhodopsin is endocytosed, a pigment regeneration pathway exists to allow the maintenance of normal chromophore (Wang et al., 2010). Indeed, a retinal dehydrogenase present in the retinal pigment cells that surround the photoreceptors, was found to promote synthesis of 3-OH-11-cis-retinal (Wang et al., 2012). This pathway could also be important for dark adaptation or under short-wavelength illumination when the photons for converting cis-retinal back to trans-retinal are not available.
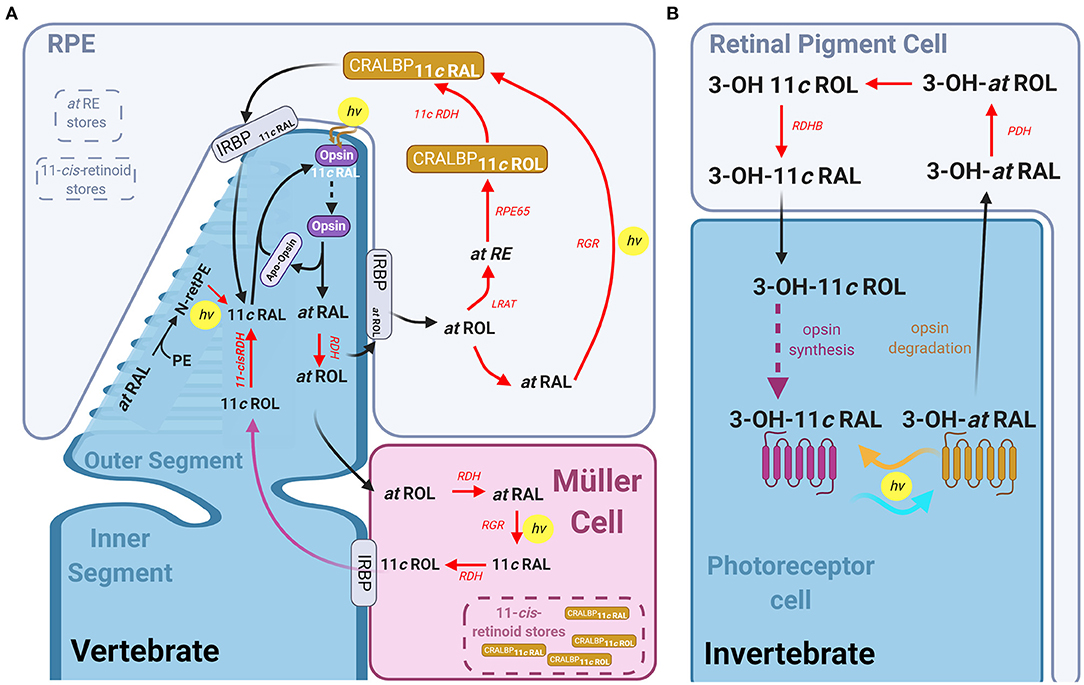
Figure 6. The visual pigment cycle reactions in vertebrate and invertebrate photoreceptors: (A) Pathways for visual pigment regeneration in the vertebrate retina, including both the classical and non-classical pathways. A cone photoreceptor (dark blue) and its' interactions with the muller glial cells (magenta) and Retinal pigment epithelium (RPE, light blue). Red arrows with red text annotation indicate enzyme mediated reactions and their respective enzymes. The classical pathway occurs via the RPE, all-trans-retinol is taken up by IRBP into the RPE, where LRAT, RPE65, and 11-cis-RDH mediates its conversion to 11-cis-retinal which is then taken up by the photoreceptor via IRBP again, which reassociated with the apo-opsin. The intrinsic light mediated pathway combining all-trans-RAL with phosphatidylethanolamine (PE) to form all-trans-N-retinyl-PE (N-retPE), which is then converted to 11-cis-retinal via a blue photon. Light mediated pigment regeneration can occur both in Müller cells and RPE, whereby all-trans retinal is converted to 11-cis retinal mediated by RGR opsin and the absorption of a photon. Stores of 11-cis-retinoids as well as all-trans-RE are present in both the RPE and Müller cells. (B) Summary of the visual pigment cycle in invertebrates. The invertebrate visual pigment, 3-OH 11-cis retinal is a bistable pigment, and remains associated to the opsin, upon absorption of a second photon, it can convert back from 3-OH all-trans retinal to 3-OH 11-cis retinal. An external pathway is only required in conditions in which the animal is calorie restricted. This pathway is mediated by the retinal pigment cells that surround the rhabdomeres and involves the Photoreceptor retinol dehydrogenase (PDH) and retinol dehydrogenase B (RDHB) enzymes.
Unlike the r-opsins in rhabdomeric photoreceptors, the c-opsins in ciliary photoreceptors are not thought to be bistable. Thus, pathway(s) to regenerate visual pigment are critical for vertebrate photoreceptors to escape saturation under continuous illumination or to restore full sensitivity after bright light exposure. Absorption of a photon by 11-cis-retinal (11cRAL) causes several conformational changes in rhodopsin converting it to a physiologically active meta II form (or R*) which eventually decays to free opsin and all-trans retinal (Kolesnikov et al., 2003; Lamb and Pugh, 2004). This process takes minutes in rods, but only seconds in cones (Shi et al., 2007; Estevez et al., 2009). Although formation of free opsin is a prerequisite for the pigment regeneration, restoration of light sensitivity after bright light exposure is thought to be rate-limited by the 11-cis-retinoid supply to the photoreceptors (Lamb and Pugh, 2004). Several pathways to deliver 11-cis-retinoids to rods or cones are now known to take place in retinal pigment epithelium (RPE), Müller and photoreceptor cells. These pathways have been reviewed previously (Lamb and Pugh, 2004; Wang and Kefalov, 2011; Kiser et al., 2014; Palczewski and Kiser, 2020) and are summarized in the following paragraphs and in Figure 6A.
Recycling of atRAL according to the canonical pathway occurs outside the photoreceptor, in the retinal pigment epithelium (RPE) monolayer (Dowling, 1960). Following activation, atRAL is reduced to all-trans-retinol (atROL) by retinol dehydrogenase (RDH) and transported to RPE by interphotoreceptor retinoid binding protein (IRBP). Lecithin:retinol acyltransferase (LRAT) esterifies the atROL to all-trans-retinyl-ester (atRE) (Macdonald and Ong, 1988; Saari and Bredberg, 1989). These esters are then hydrolyzed and isomerized to 11-cis-ROL (11cROL) by retinoid Isomerohydrolase (RPE65, Gollapalli and Rando, 2003; Moiseyev et al., 2003; Mata et al., 2004), and oxidized to 11cRAL by 11-cis-RDH. This 11cRAL is then transported into the photoreceptors by IRBP and re-associates with the apo-opsin to complete the cycle.
Cone-Specific Pigment Regeneration Pathway Mediate by CRALBP
Critically, the canonical pigment regeneration pathway is too slow to resupply cones with sufficient pigment to remain responsive under continuous daylight conditions. In fact, several studies in multiple vertebrates found that cone sensitivity and pigment regeneration is maintained in the absence of RPE (Goldstein, 1970; Bruch Goldstein and Wolf, 1973). Thus, evidence overwhelmingly supports the existence of a cone-specific intra-retinal pigment regeneration pathway that supplies chromophore exclusively to cones. Studies in cultures of Müller cells from chicken found that they were able to synthesize 11cROL and 11-cis retinyl palmitate from atROL (Das et al., 1992), and CRALBP stimulates this synthesis (Mata et al., 2002). This is significant as it suggests a second population of cells in the retina that has isomerase activity (like RPE65 in the RPE) and retinyl ester synthase activity (Das et al., 1992). Pharmacological ablation of Müller cells in salamander, mouse, macaque or human retinas eliminates the ability of their cone photoreceptors to dark adapt following a bright light exposure in the absence of RPE (Wang and Kefalov, 2009; Wang et al., 2009). A later study demonstrated that CRALBP in Müller cells is critical for complete cone dark adaptation in isolated mouse retinas (Xue et al., 2015). It was initially proposed that CRALBP required interaction with Des1 in Müller cells to regenerate pigment in cones (Kaylor et al., 2014), however, deletion of Des1 in the mouse Müller cells had no effect on cone dark adaptation in isolated retinas (Kiser et al., 2019). One hypothesis, proposed by Kiser et al. (2018), is that 11-cis retinoids bound to CRALBP in Müller cells form a 11-cis retinoid storage that can be quickly used for rapid pigment regeneration and dark adaptation of cones.
Photic Pigment Regeneration Pathways
Recent work has potentially identified a light-dependent pathway for pigment regeneration in ciliary photoreceptors that depends on blue light. Much like the bistable pigment regeneration in rhabdomeres, this mechanism is independent of enzyme activity, and occurs in the outer segment membrane. After atRAL condenses with phosphatidylethanolamine (PE) to form all-trans-N-retinyl-PE in the lipid membrane, and a blue photon then converts this to 11-cis-N-retinyl-PE, which spontaneously hydrolyses to 11cRAL. Exposure of retinas to light around 450 nm wavelength after a bleach produced a higher regeneration of pigment than retinas left to regenerate in the dark (Kaylor et al., 2017). This mechanism also contributed to mouse photoreceptor pigment regeneration in vivo and is expected to be functional in both rods and cones.
Recent studies have also demonstrated a light-dependent pigment regeneration pathway mediated by Retinal G protein-coupled opsin (RGR) in RPE (Zhang et al., 2019) and Müller cells (Morshedian et al., 2019). RGR opsin is a non-signaling opsin, expressed in human, bovine and chicken Müller cells as well as in RPE cells (Pandey et al., 1994; Trifunovic et al., 2008; Diaz et al., 2017). Knocking out Rgr in the mouse retina results in faster sensitivity loss in cones under constant bright background illumination. Morshedian et al. (2019) found that this light-dependent pigment regeneration is also dependent on the activity of Rdh10 in Müller glia cells. In their model atROL is taken up by Müller cells, and RDH10 and RGR opsin together convert it to 11cROL in the presence of blue light. The alcohol form of 11-cis retinoid can then be used by cones but not rods for pigment regeneration. The identity of the RDH enzyme expressed in cones but not in rods catalyzing the oxidation of 11cROL to 11cRAL remains unknown. The ability of cones to maintain sensitivity under conditions of continuous pigment bleaching is expected to be promoted by these light-dependent visual cycles mediated by RGR opsin in both RPE and Müller cells. The contribution of CRALBP- and RGR opsin-mediated as well as non-enzymatic pigment regeneration and canonical visual cycle to the bright light vision and dark adaptation in different species are not known and remains an interesting question to study.
Concluding Remarks
Vertebrate visual and olfactory transduction and invertebrate phototransduction rely on prototypical but different GPCR signaling cascades whereas invertebrate olfaction uses a more diverse signaling toolbox including fast ionotropic as well as potentially more sensitive metabotropic mechanisms for odorant detection.
Rhabdomeric phototransduction has extremely high amplification and fast response kinetics largely due to Ca2+-dependent amplification and feedback mechanisms together with compartmentalization of the transduction into a very small volume (microvilli). This allows invertebrates to detect single photons, and due to the large number of microvilli and bistable visual pigment, they can also sustain vision into bright daylight. Vertebrates developed an alternative strategy to see over a similar range of ambient light. During evolution, the molecular properties of ciliary cone photoreceptors became tuned to generate more sensitive rod phototransduction with high amplification, slower response termination and extremely stable visual pigment to enable single photon detection and vision at night. With a duplex rod/cone retina, vertebrates can also see both at night and in bright sun light in a way that is perhaps more energy efficient compared the invertebrate retina (Fain et al., 2010).
Unlike r-opsins in rhabdomeric photoreceptors, c-opsins in ciliary photoreceptors are not thought to be bistable and several enzymatic pathways to regenerate rod and cone visual pigments exist. The rod pigment is extremely stable with a low rate of spontaneous activation, and once activated it is also very slow to decay back to free opsin and all-trans-retinal compared to cone pigments. In addition, cones can use several different visual cycles for faster and more efficient pigment regeneration, enabling vision in very bright light. Most of our information about the function of cones from the past 2–3 decades has been taken from mice, a nocturnal animal with rod-dominant retina. More details about biology of non-human primate and human fovea and para-/perifovea have emerged, but significant gaps in our knowledge remain. For example, the contribution or existence of the different pigment regeneration pathways for bright light vision in the human fovea and macula, specialized to promote high-acuity color vision in bright daylight conditions, are not known. Then again, significant gaps in knowledge about invertebrate phototransduction also remain, where even the identity of second messengers remains controversial.
Although olfactory transduction in vertebrates also uses GPCR signaling cascade to transduce odorant signals, it has much lower amplification compared to phototransduction in either vertebrates or invertebrates. Indeed, the probability for activated olfactory receptor to activate Golf is very low and, whereas rods and rhabdomeric PRs can detect single photons, ~40 odor molecules are required for olfactory sensation in mice. Where vision seems to have evolved in humans to enable high-acuity color vision during the day, mice seem to have retained a significantly larger number of functional olfactory receptors ~1,000, compared to ~400 in humans (Niimura and Nei, 2007).
Author Contributions
All authors contributed to the writing of this review article to the work and approved it for publication. Figures were generated by FA.
Funding
This work was supported by NIH grants EY026651 and R01EY031706, the International Retinal Research Foundation, and Research to Prevent Blindness/Dr. H. James and Carole Free Career Development Awards (to FV) as well as from an RPB unrestricted grant to the department of Ophthalmology and Visual Sciences, University of Utah.
Conflict of Interest
The authors declare that the research was conducted in the absence of any commercial or financial relationships that could be construed as a potential conflict of interest.
Acknowledgments
Thanks to Silke Becker and Nguyen Pham for discussions. Figures created with BioRender.com.
References
Acharya, J. K., Jalink, K., Hardy, R. W., Hartenstein, V., and Zuker, C. S. (1997). InsP3 receptor is essential for growth and differentiation but not for vision in Drosophila. Neuron 18, 881–887. doi: 10.1016/S0896-6273(00)80328-1
Alloway, P. G., and Dolph, P. J. (1999). A role for the light-dependent phosphorylation of visual arrestin. Proc. Natl. Acad. Sci. U.S.A. 96, 6072–6077. doi: 10.1073/pnas.96.11.6072
Andrea Yao, C., and Carlson, J. R. (2010). Role of G-proteins in odor-sensing and CO2-sensing neurons in Drosophila. J. Neurosci. 30, 4562–4572. doi: 10.1523/JNEUROSCI.6357-09.2010
Antolin, S., Reisert, J., and Matthews, H. R. (2010). Olfactory response termination involves Ca2+-ATPase in vertebrate olfactory receptor neuron cilia. J. Gen. Physiol. 135, 367–378. doi: 10.1085/jgp.200910337
Antunes, G., and Simoes De Souza, F. M. (2016). Olfactory receptor signaling. Methods Cell Biol 132, 127–145. doi: 10.1016/bs.mcb.2015.11.003
Arendt, D., Tessmar-Raible, K., Snyman, H., Dorresteijn, A. W., and Wittbrodt, J. (2004). Ciliary photoreceptors with a vertebrate-type opsin in an invertebrate brain. Science 306, 869–871. doi: 10.1126/science.1099955
Arshavsky, V. Y., Lamb, T. D., and Pugh, E. N. Jr. (2002). G proteins and phototransduction. Annu. Rev. Physiol. 64, 153–187. doi: 10.1146/annurev.physiol.64.082701.102229
Asteriti, S., Liu, C. H., and Hardie, R. C. (2017). Calcium signalling in Drosophila photoreceptors measured with GCaMP6f. Cell Calcium 65, 40–51. doi: 10.1016/j.ceca.2017.02.006
Bakalyar, H., and Reed, R. (1990). Identification of a specialized adenylyl cyclase that may mediate odorant detection. Science 250, 1403–1406. doi: 10.1126/science.2255909
Bauer, P. J., and Drechsler, M. (1992). Association of cyclic GMP-gated channels and Na(+)-Ca(2+)-K+ exchangers in bovine retinal rod outer segment plasma membranes. J. Physiol. 451, 109–131. doi: 10.1113/jphysiol.1992.sp019156
Baylor, D. A., Lamb, T. D., and Yau, K. W. (1979). Responses of retinal rods to single photons. J. Physiol. (Lond) 288, 613–634.
Belluscio, L., Gold, G. H., Nemes, A., and Axel, R. (1998). Mice deficient in golf are anosmic. Neuron 20, 69–81. doi: 10.1016/S0896-6273(00)80435-3
Ben-Chaim, Y., Cheng, M. M., and Yau, K. W. (2011). Unitary response of mouse olfactory receptor neurons. Proc. Natl. Acad. Sci. U.S.A. 108, 822–827. doi: 10.1073/pnas.1017983108
Benton, R., Sachse, S., Michnick, S. W., and Vosshall, L. B. (2006). Atypical membrane topology and heteromeric function of Drosophila odorant receptors in vivo. PLoS Biol. 4:e20. doi: 10.1371/journal.pbio.0040020
Benton, R., Vannice, K. S., Gomez-Diaz, C., and Vosshall, L. B. (2009). Variant ionotropic glutamate receptors as chemosensory receptors in Drosophila. Cell 136, 149–162. doi: 10.1016/j.cell.2008.12.001
Bhandawat, V., Reisert, J., and Yau, K. W. (2005). Elementary response of olfactory receptor neurons to odorants. Science 308, 1931–1934. doi: 10.1126/science.1109886
Bisbach, C. M., Hutto, R. A., Poria, D., Cleghorn, W. M., Abbas, F., Vinberg, F., et al. (2020). Mitochondrial Calcium Uniporter (MCU) deficiency reveals an alternate path for Ca(2+) uptake in photoreceptor mitochondria. Sci. Rep. 10:16041. doi: 10.1038/s41598-020-72708-x
Bloomquist, B. T., Shortridge, R. D., Schneuwly, S., Perdew, M., Montell, C., Steller, H., et al. (1988). Isolation of a putative phospholipase C gene of Drosophila, norpA, and its role in phototransduction. Cell 54, 723–733. doi: 10.1016/S0092-8674(88)80017-5
Boccaccio, A., Menini, A., and Pifferi, S. (2021). The cyclic AMP signaling pathway in the rodent main olfactory system. Cell Tissue Res. 383, 429–443. doi: 10.1007/s00441-020-03391-7
Boekhoff, I., and Breer, H. (1992). Termination of second messenger signaling in olfaction. Proc. Natl. Acad. Sci. U.S.A. 89, 471–474. doi: 10.1073/pnas.89.2.471
Boekhoff, I., Inglese, J., Schleicher, S., Koch, W. J., Lefkowitz, R. J., and Breer, H. (1994). Olfactory desensitization requires membrane targeting of receptor kinase mediated by beta gamma-subunits of heterotrimeric G proteins. J. Biol. Chem. 269, 37–40. doi: 10.1016/S0021-9258(17)42306-4
Boekhoff, I., Schleicher, S., Strotmann, J., and Breer, H. (1992). Odor-induced phosphorylation of olfactory cilia proteins. Proc. Natl. Acad. Sci. U.S.A. 89, 11983–11987. doi: 10.1073/pnas.89.24.11983
Bownds, D., Dawes, J., Miller, J., and Stahlman, M. (1972). Phosphorylation of frog photoreceptor membranes induced by light. Nat. New Biol. 237, 125–127. doi: 10.1038/newbio237125a0
Bradley, J., Reuter, D., and Frings, S. (2001). Facilitation of calmodulin-mediated odor adaptation by cAMP-gated channel subunits. Science 294, 2176–2178. doi: 10.1126/science.1063415
Bruch Goldstein, E., and Wolf, B. M. (1973). Regeneration of the green-rod pigment in the isolated frog retina. Vision Res. 13, 527–534. doi: 10.1016/0042-6989(73)90022-9
Brunet, L. J., Gold, G. H., and Ngai, J. (1996). General anosmia caused by a targeted disruption of the mouse olfactory cyclic nucleotide-gated cation channel. Neuron 17, 681–693. doi: 10.1016/S0896-6273(00)80200-7
Buck, L., and Axel, R. (1991). A novel multigene family may encode odorant receptors: a molecular basis for odor recognition. Cell 65, 175–187. doi: 10.1016/0092-8674(91)90418-X
Burns, M. E., and Baylor, D. A. (2001). Activation, deactivation, and adaptation in vertebrate photoreceptor cells. Annu. Rev. Neurosci. 24, 779–805. doi: 10.1146/annurev.neuro.24.1.779
Butterwick, J. A., Del Mármol, J., Kim, K. H., Kahlson, M. A., Rogow, J. A., Walz, T., et al. (2018). Cryo-EM structure of the insect olfactory receptor Orco. Nature 560, 447–452. doi: 10.1038/s41586-018-0420-8
Castillo, K., Delgado, R., and Bacigalupo, J. (2007). Plasma membrane Ca(2+)-ATPase in the cilia of olfactory receptor neurons: possible role in Ca(2+) clearance. Eur. J. Neurosci. 26, 2524–2531. doi: 10.1111/j.1460-9568.2007.05863.x
Chatterjee, A., Roman, G., and Hardin, P. E. (2009). Go contributes to olfactory reception in Drosophila melanogaster. BMC Physiol. 9:22. doi: 10.1186/1472-6793-9-22
Chen, C. K., Burns, M. E., He, W., Wensel, T. G., Baylor, D. A., and Simon, M. I. (2000). Slowed recovery of rod photoresponse in mice lacking the GTPase accelerating protein RGS9-1. Nature 403, 557–560. doi: 10.1038/35000601
Chen, C. K., Inglese, J., Lefkowitz, R. J., and Hurley, J. B. (1995). Ca(2+)-dependent interaction of recoverin with rhodopsin kinase. J. Biol. Chem. 270, 18060–18066. doi: 10.1074/jbc.270.30.18060
Chen, C. K., Woodruff, M. L., and Fain, G. L. (2015). Rhodopsin kinase and recoverin modulate phosphodiesterase during mouse photoreceptor light adaptation. J. Gen. Physiol. 145, 213–224. doi: 10.1085/jgp.201411273
Chen, J., Woodruff, M. L., Wang, T., Concepcion, F. A., Tranchina, D., and Fain, G. L. (2010). Channel modulation and the mechanism of light adaptation in mouse rods. J. Neurosci. 30, 16232–16240. doi: 10.1523/JNEUROSCI.2868-10.2010
Chevesich, J., Kreuz, A. J., and Montell, C. (1997). Requirement for the PDZ domain protein, INAD, for localization of the TRP store-operated channel to a signaling complex. Neuron 18, 95–105. doi: 10.1016/S0896-6273(01)80049-0
Chu, B., Postma, M., and Hardie, R. C. (2013). Fractional Ca(2+) currents through TRP and TRPL channels in Drosophila photoreceptors. Biophys. J. 104, 1905–1916. doi: 10.1016/j.bpj.2013.03.047
Chyb, S., Raghu, P., and Hardie, R. C. (1999). Polyunsaturated fatty acids activate the Drosophila light-sensitive channels TRP and TRPL. Nature 397, 255–259. doi: 10.1038/16703
Cia, D., Bordais, A., Varela, C., Forster, V., Sahel, J. A., Rendon, A., et al. (2005). Voltage-gated channels and calcium homeostasis in mammalian rod photoreceptors. J. Neurophysiol. 93, 1468–1475. doi: 10.1152/jn.00874.2004
Cideciyan, A. V., Zhao, X., Nielsen, L., Khani, S. C., Jacobson, S. G., and Palczewski, K. (1998). Null mutation in the rhodopsin kinase gene slows recovery kinetics of rod and cone phototransduction in man. Proc. Natl. Acad. Sci. U.S.A. 95, 328–333. doi: 10.1073/pnas.95.1.328
Clyne, P. J., Warr, C. G., Freeman, M. R., Lessing, D., Kim, J., and Carlson, J. R. (1999). A novel family of divergent seven-transmembrane proteins: candidate odorant receptors in Drosophila. Neuron 22, 327–338. doi: 10.1016/S0896-6273(00)81093-4
Cook, B., Bar-Yaacov, M., Cohen Ben-Ami, H., Goldstein, R. E., Paroush, Z., Selinger, Z., et al. (2000). Phospholipase C and termination of G-protein-mediated signalling in vivo. Nat. Cell Biol. 2, 296–301. doi: 10.1038/35010571
Cygnar, K. D., and Zhao, H. (2009). Phosphodiesterase 1C is dispensable for rapid response termination of olfactory sensory neurons. Nat. Neurosci. 12, 454–462. doi: 10.1038/nn.2289
Das, S. R., Bhardwaj, N., Kjeldbye, H., and Gouras, P. (1992). Muller cells of chicken retina synthesize 11-cis-retinol. Biochem. J. 285(Pt 3), 907–913. doi: 10.1042/bj2850907
Deng, Y., Zhang, W., Farhat, K., Oberland, S., Gisselmann, G., and Neuhaus, E. M. (2011). The stimulatory Galpha(s) protein is involved in olfactory signal transduction in Drosophila. PLoS ONE 6:e18605. doi: 10.1371/journal.pone.0018605
Diaz, N. M., Morera, L. P., Tempesti, T., and Guido, M. E. (2017). The visual cycle in the inner retina of chicken and the involvement of retinal G-protein-coupled receptor (RGR). Mol. Neurobiol. 54, 2507–2517. doi: 10.1007/s12035-016-9830-5
Dowling, J. E. (1960). Chemistry of visual adaptation in the rat. Nature 188, 114–118. doi: 10.1038/188114a0
Dulac, C., and Axel, R. (1995). A novel family of genes encoding putative pheromone receptors in mammals. Cell 83, 195–206. doi: 10.1016/0092-8674(95)90161-2
Dulac, C., and Torello, A. T. (2003). Molecular detection of pheromone signals in mammals: from genes to behaviour. Nat. Rev. Neurosci. 4, 551–562. doi: 10.1038/nrn1140
Estacion, M., Sinkins, W. G., and Schilling, W. P. (2001). Regulation of Drosophila transient receptor potential-like (TrpL) channels by phospholipase C-dependent mechanisms. J. Physiol. 530, 1–19. doi: 10.1111/j.1469-7793.2001.0001m.x
Estevez, M. E., Kolesnikov, A. V., Ala-Laurila, P., Crouch, R. K., Govardovskii, V. I., and Cornwall, M. C. (2009). The 9-methyl group of retinal is essential for rapid Meta II decay and phototransduction quenching in red cones. J. Gen. Physiol. 134, 137–150. doi: 10.1085/jgp.200910232
Fain, G. L., Hardie, R., and Laughlin, S. B. (2010). Phototransduction and the evolution of photoreceptors. Curr. Biol. 20, R114–124. doi: 10.1016/j.cub.2009.12.006
Fain, G. L., Matthews, H. R., Cornwall, M. C., and Koutalos, Y. (2001). Adaptation in vertebrate photoreceptors. Physiol. Rev. 81, 117–151. doi: 10.1152/physrev.2001.81.1.117
Fesenko, E. E., Kolesnikov, S. S., and Lyubarsky, A. L. (1985). Induction by cyclic GMP of cationic conductance in plasma membrane of retinal rod outer segment. Nature 313, 310–313. doi: 10.1038/313310a0
Fleischer, J., Pregitzer, P., Breer, H., and Krieger, J. (2018). Access to the odor world: olfactory receptors and their role for signal transduction in insects. Cell. Mol. Life Sci. 75, 485–508. doi: 10.1007/s00018-017-2627-5
Frings, S., Seifert, R., Godde, M., and Kaupp, U. B. (1995). Profoundly different calcium permeation and blockage determine the specific function of distinct cyclic nucleotide-gated channels. Neuron 15, 169–179. doi: 10.1016/0896-6273(95)90074-8
Fu, Y., and Yau, K. W. (2007). Phototransduction in mouse rods and cones. Pflugers Arch. 454, 805–819. doi: 10.1007/s00424-006-0194-y
Getahun, M. N., Olsson, S. B., Lavista-Llanos, S., Hansson, B. S., and Wicher, D. (2013). Insect odorant response sensitivity is tuned by metabotropically autoregulated olfactory receptors. PLoS ONE 8:e58889. doi: 10.1371/journal.pone.0058889
Getahun, M. N., Wicher, D., Hansson, B. S., and Olsson, S. B. (2012). Temporal response dynamics of Drosophila olfactory sensory neurons depends on receptor type and response polarity. Front. Cell. Neurosci. 6:54. doi: 10.3389/fncel.2012.00054
Gilliam, J. C., Chang, J. T., Sandoval, I. M., Zhang, Y., Li, T., Pittler, S. J., et al. (2012). Three-dimensional architecture of the rod sensory cilium and its disruption in retinal neurodegeneration. Cell 151, 1029–1041. doi: 10.1016/j.cell.2012.10.038
Gilman, A. G. (1987). G proteins: transducers of receptor-generated signals. Annu. Rev. Biochem. 56, 615–649. doi: 10.1146/annurev.bi.56.070187.003151
Goldstein, E. B. (1970). Cone pigment regeneration in the isolated frog retina. Vision Res. 10, 1065–1068. doi: 10.1016/0042-6989(70)90082-9
Gollapalli, D. R., and Rando, R. R. (2003). All-trans-retinyl esters are the substrates for isomerization in the vertebrate visual cycle. Biochemistry 42, 5809–5818. doi: 10.1021/bi0341004
Gomez-Diaz, C., Martin, F., and Alcorta, E. (2004). The cAMP transduction cascade mediates olfactory reception in Drosophila melanogaster. Behav. Genet. 34, 395–406. doi: 10.1023/B:BEGE.0000023645.02710.fe
Gross, O. P., and Burns, M. E. (2010). Control of rhodopsin's active lifetime by arrestin-1 expression in mammalian rods. J. Neurosci. 30, 3450–3457. doi: 10.1523/JNEUROSCI.5391-09.2010
Gu, Y., Oberwinkler, J., Postma, M., and Hardie, R. C. (2005). Mechanisms of light adaptation in Drosophila photoreceptors. Curr. Biol. 15, 1228–1234. doi: 10.1016/j.cub.2005.05.058
Guo, H., Kunwar, K., and Smith, D. (2017). Odorant receptor sensitivity modulation in Drosophila. J. Neurosci. 37, 9465–9473. doi: 10.1523/JNEUROSCI.1573-17.2017
Halty-Deleon, L., Hansson, B. S., and Wicher, D. (2018). The Drosophila melanogaster Na(+)/Ca(2+) exchanger CALX controls the Ca(2+) level in olfactory sensory neurons at rest and after odorant receptor activation. Front. Cell. Neurosci. 12:186. doi: 10.3389/fncel.2018.00186
Hansson, B. S., Knaden, M., Sachse, S., Stensmyr, M. C., and Wicher, D. (2010). Towards plant-odor-related olfactory neuroethology in Drosophila. Chemoecology 20, 51–61. doi: 10.1007/s00049-009-0033-7
Hardie, R. C. (2012). Phototransduction mechanisms in Drosophila microvillar photoreceptors. Wiley Interdiscipl. Rev. Membrane Transport Signal. 1, 162–187. doi: 10.1002/wmts.20
Hardie, R. C., and Franze, K. (2012). Photomechanical responses in Drosophila photoreceptors. Science 338, 260–263. doi: 10.1126/science.1222376
Hardie, R. C., and Juusola, M. (2015). Phototransduction in Drosophila. Curr. Opin. Neurobiol. 34, 37–45. doi: 10.1016/j.conb.2015.01.008
Hardie, R. C., and Minke, B. (1992). The trp gene is essential for a light-activated Ca2+ channel in Drosophila photoreceptors. Neuron 8, 643–651. doi: 10.1016/0896-6273(92)90086-S
Hardie, R. C., and Raghu, P. (2001). Visual transduction in Drosophila. Nature 413, 186–193. doi: 10.1038/35093002
Hartline, H. K. (1938). The response of single optic nerve fibers of the vertebrate eye to illumination of the retina. Am. J. Physiol. Legacy Content 121, 400–415. doi: 10.1152/ajplegacy.1938.121.2.400
Haynes, L. W., and Stotz, S. C. (1997). Modulation of rod, but not cone, cGMP-gated photoreceptor channels by calcium-calmodulin. Vis. Neurosci. 14, 233–239. doi: 10.1017/S0952523800011378
Henderson, S. R., Reuss, H., and Hardie, R. C. (2000). Single photon responses in Drosophila photoreceptors and their regulation by Ca2+. J. Physiol. 524(Pt 1), 179–194. doi: 10.1111/j.1469-7793.2000.00179.x
Honkanen, A., Immonen, E. V., Salmela, I., Heimonen, K., and Weckstrom, M. (2017). Insect photoreceptor adaptations to night vision. Philos. Trans. R. Soc. Lond. B. Biol. Sci. 372. doi: 10.1098/rstb.2016.0077
Hryshko, L. V., Matsuoka, S., Nicoll, D. A., Weiss, J. N., Schwarz, E. M., Benzer, S., et al. (1996). Anomalous regulation of the Drosophila Na(+)-Ca2+ exchanger by Ca2+. J. Gen. Physiol. 108, 67–74. doi: 10.1085/jgp.108.1.67
Hsu, Y. T., and Molday, R. S. (1994). Interaction of calmodulin with the cyclic GMP-gated channel of rod photoreceptor cells. Modulation of activity, affinity purification, and localization. J. Biol. Chem. 269, 29765–29770. doi: 10.1016/S0021-9258(18)43947-6
Hu, W., Wan, D., Yu, X., Cao, J., Guo, P., Li, H. S., et al. (2012). Protein Gq modulates termination of phototransduction and prevents retinal degeneration. J. Biol. Chem. 287, 13911–13918. doi: 10.1074/jbc.M112.339895
Huang, J., Liu, C. H., Hughes, S. A., Postma, M., Schwiening, C. J., and Hardie, R. C. (2010). Activation of TRP channels by protons and phosphoinositide depletion in Drosophila photoreceptors. Curr. Biol. 20, 189–197. doi: 10.1016/j.cub.2009.12.019
Hutto, R. A., Bisbach, C. M., Abbas, F., Brock, D. C., Cleghorn, W. M., Parker, E. D., et al. (2020). Increasing Ca(2+) in photoreceptor mitochondria alters metabolites, accelerates photoresponse recovery, and reveals adaptations to mitochondrial stress. Cell Death Differ. 27, 1067–1085. doi: 10.1038/s41418-019-0398-2
Ingram, N. T., Fain, G. L., and Sampath, A. P. (2020). Elevated energy requirement of cone photoreceptors. Proc. Natl. Acad. Sci. U.S.A. 117, 19599–19603. doi: 10.1073/pnas.2001776117
Ingram, N. T., Sampath, A. P., and Fain, G. L. (2016). Why are rods more sensitive than cones? J. Physiol. 594, 5415–5426. doi: 10.1113/JP272556
Jain, K., Lavista-Llanos, S., Grabe, V., Hansson, B. S., and Wicher, D. (2021). Calmodulin regulates the olfactory performance in Drosophila melanogaster. Sci. Rep. 11:3747. doi: 10.1038/s41598-021-83296-9
Jalloul, A. H., Szerencsei, R. T., Rogasevskaia, T. P., and Schnetkamp, P. P. M. (2020). Structure-function relationships of K(+)-dependent Na(+)/Ca(2+) exchangers (NCKX). Cell Calcium 86:102153. doi: 10.1016/j.ceca.2019.102153
Jalloul, A. H., Szerencsei, R. T., and Schnetkamp, P. P. (2016). Cation dependencies and turnover rates of the human K(+)-dependent Na(+)-Ca(2)(+) exchangers NCKX1, NCKX2, NCKX3 and NCKX4. Cell Calcium 59, 1–11. doi: 10.1016/j.ceca.2015.11.001
Jones, D. T., and Reed, R. R. (1989). Golf: an olfactory neuron specific-G protein involved in odorant signal transduction. Science 244, 790–795. doi: 10.1126/science.2499043
Juilfs, D. M., Fulle, H. J., Zhao, A. Z., Houslay, M. D., Garbers, D. L., and Beavo, J. A. (1997). A subset of olfactory neurons that selectively express cGMP-stimulated phosphodiesterase (PDE2) and guanylyl cyclase-D define a unique olfactory signal transduction pathway. Proc. Natl. Acad. Sci. U.S.A. 94, 3388–3395. doi: 10.1073/pnas.94.7.3388
Kahn, E. S., and Matsumoto, H. (1997). Calcium/calmodulin-dependent kinase II phosphorylates Drosophila visual arrestin. J. Neurochem. 68, 169–175. doi: 10.1046/j.1471-4159.1997.68010169.x
Kain, P., Chakraborty, T. S., Sundaram, S., Siddiqi, O., Rodrigues, V., and Hasan, G. (2008). Reduced odor responses from antennal neurons of G(q)alpha, phospholipase Cbeta, and rdgA mutants in Drosophila support a role for a phospholipid intermediate in insect olfactory transduction. J. Neurosci. 28, 4745–4755. doi: 10.1523/JNEUROSCI.5306-07.2008
Kaneko, H., Putzier, I., Frings, S., Kaupp, U. B., and Gensch, T. (2004). Chloride accumulation in mammalian olfactory sensory neurons. J. Neurosci. 24, 7931–7938. doi: 10.1523/JNEUROSCI.2115-04.2004
Kang, K., Bauer, P. J., Kinjo, T. G., Szerencsei, R. T., Bonigk, W., Winkfein, R. J., et al. (2003). Assembly of retinal rod or cone Na(+)/Ca(2+)-K(+) exchanger oligomers with cGMP-gated channel subunits as probed with heterologously expressed cDNAs. Biochemistry 42, 4593–4600. doi: 10.1021/bi027276z
Kato, A., Reisert, J., Ihara, S., Yoshikawa, K., and Touhara, K. (2014). Evaluation of the role of g protein-coupled receptor kinase 3 in desensitization of mouse odorant receptors in a Mammalian cell line and in olfactory sensory neurons. Chem. Senses 39, 771–780. doi: 10.1093/chemse/bju050
Kaupp, U. B. (2010). Olfactory signalling in vertebrates and insects: differences and commonalities. Nat. Rev. Neurosci. 11, 188–200. doi: 10.1038/nrn2789
Kawamura, S. (1993). Rhodopsin phosphorylation as a mechanism of cyclic GMP phosphodiesterase regulation by S-modulin. Nature 362, 855–857. doi: 10.1038/362855a0
Kaylor, J. J., Cook, J. D., Makshanoff, J., Bischoff, N., Yong, J., and Travis, G. H. (2014). Identification of the 11-cis-specific retinyl-ester synthase in retinal Muller cells as multifunctional O-acyltransferase (MFAT). Proc. Natl. Acad. Sci. U.S.A. 111, 7302–7307. doi: 10.1073/pnas.1319142111
Kaylor, J. J., Xu, T., Ingram, N. T., Tsan, A., Hakobyan, H., Fain, G. L., et al. (2017). Blue light regenerates functional visual pigments in mammals through a retinyl-phospholipid intermediate. Nat. Commun. 8:16. doi: 10.1038/s41467-017-00018-4
Kennedy, M. J., Lee, K. A., Niemi, G. A., Craven, K. B., Garwin, G. G., Saari, J. C., et al. (2001). Multiple phosphorylation of rhodopsin and the in vivo chemistry underlying rod photoreceptor dark adaptation. Neuron 31, 87–101. doi: 10.1016/S0896-6273(01)00340-3
Kinnamon, S. C., and Finger, T. E. (2019). Recent advances in taste transduction and signaling. F1000Research 8. doi: 10.12688/f1000research.21099.1
Kiser, P. D., Golczak, M., and Palczewski, K. (2014). Chemistry of the retinoid (visual) cycle. Chem. Rev. 114, 194–232. doi: 10.1021/cr400107q
Kiser, P. D., Kolesnikov, A. V., Kiser, J. Z., Dong, Z., Chaurasia, B., Wang, L., et al. (2019). Conditional deletion of Des1 in the mouse retina does not impair the visual cycle in cones. FASEB J. 33, 5782–5792. doi: 10.1096/fj.201802493R
Kiser, P. D., Zhang, J., Sharma, A., Angueyra, J. M., Kolesnikov, A. V., Badiee, M., et al. (2018). Retinoid isomerase inhibitors impair but do not block mammalian cone photoreceptor function. J. Gen. Physiol. 150, 571–590. doi: 10.1085/jgp.201711815
Klenchin, V. A., Calvert, P. D., and Bownds, M. D. (1995). Inhibition of rhodopsin kinase by recoverin. Further evidence for a negative feedback system in phototransduction. J. Biol. Chem. 270, 16147–16152. doi: 10.1074/jbc.270.27.16147
Kolesnikov, A. V., Golobokova, E. Y., and Govardovskii, V. I. (2003). The identity of metarhodopsin III. Vis. Neurosci. 20, 249–265. doi: 10.1017/S0952523803203047
Krispel, C. M., Chen, D., Melling, N., Chen, Y. J., Martemyanov, K. A., Quillinan, N., et al. (2006). RGS expression rate-limits recovery of rod photoresponses. Neuron 51, 409–416. doi: 10.1016/j.neuron.2006.07.010
Kuhn, H. (1978). Light-regulated binding of rhodopsin kinase and other proteins to cattle photoreceptor membranes. Biochemistry 17, 4389–4395. doi: 10.1021/bi00614a006
Lamb, T. D. (2013). Evolution of phototransduction, vertebrate photoreceptors and retina. Prog. Retin. Eye Res. 36, 52–119. doi: 10.1016/j.preteyeres.2013.06.001
Lamb, T. D. (2020). Evolution of the genes mediating phototransduction in rod and cone photoreceptors. Prog. Retin. Eye Res. 76:100823. doi: 10.1016/j.preteyeres.2019.100823
Lamb, T. D., and Pugh, E. N. Jr. (2004). Dark adaptation and the retinoid cycle of vision. Prog. Retin. Eye Res. 23, 307–380. doi: 10.1016/j.preteyeres.2004.03.001
Larsson, M. C., Domingos, A. I., Jones, W. D., Chiappe, M. E., Amrein, H., and Vosshall, L. B. (2004). Or83b encodes a broadly expressed odorant receptor essential for Drosophila olfaction. Neuron 43, 703–714. doi: 10.1016/j.neuron.2004.08.019
Leinders-Zufall, T., Rand, M. N., Shepherd, G. M., Greer, C. A., and Zufall, F. (1997). Calcium entry through cyclic nucleotide-gated channels in individual cilia of olfactory receptor cells: spatiotemporal dynamics. J. Neurosci. 17, 4136–4148. doi: 10.1523/JNEUROSCI.17-11-04136.1997
Leypold, B. G., Yu, C. R., Leinders-Zufall, T., Kim, M. M., Zufall, F., and Axel, R. (2002). Altered sexual and social behaviors in trp2 mutant mice. Proc. Natl. Acad. Sci. U.S.A. 99, 6376–6381. doi: 10.1073/pnas.082127599
Li, H. S., Porter, J. A., and Montell, C. (1998). Requirement for the NINAC kinase/myosin for stable termination of the visual cascade. J. Neurosci. 18, 9601–9606. doi: 10.1523/JNEUROSCI.18-23-09601.1998
Linsenmeier, R. A., and Braun, R. D. (1992). Oxygen distribution and consumption in the cat retina during normoxia and hypoxemia. J. Gen. Physiol. 99, 177–197. doi: 10.1085/jgp.99.2.177
Litosch, I. (2015). Regulating G protein activity by lipase-independent functions of phospholipase C. Life Sci. 137, 116–124. doi: 10.1016/j.lfs.2015.07.025
Liu, C. H., Chen, Z., Oliva, M. K., Luo, J., Collier, S., Montell, C., et al. (2020). Rapid release of Ca(2+) from endoplasmic reticulum mediated by Na(+)/Ca(2+) exchange. J. Neurosci. 40, 3152–3164. doi: 10.1523/JNEUROSCI.2675-19.2020
Liu, C. H., Satoh, A. K., Postma, M., Huang, J., Ready, D. F., and Hardie, R. C. (2008). Ca2+-dependent metarhodopsin inactivation mediated by calmodulin and NINAC myosin III. Neuron 59, 778–789. doi: 10.1016/j.neuron.2008.07.007
Liu, M., Chen, T. Y., Ahamed, B., Li, J., and Yau, K. W. (1994). Calcium-calmodulin modulation of the olfactory cyclic nucleotide-gated cation channel. Science 266, 1348–1354. doi: 10.1126/science.266.5189.1348
Luo, D. G., Xue, T., and Yau, K. W. (2008). How vision begins: an odyssey. Proc. Natl. Acad. Sci. U.S.A. 105, 9855–9862. doi: 10.1073/pnas.0708405105
Lyubarsky, A. L., Chen, C., Simon, M. I., and Pugh, E. N. Jr. (2000). Mice lacking G-protein receptor kinase 1 have profoundly slowed recovery of cone-driven retinal responses. J. Neurosci. 20, 2209–2217. doi: 10.1523/JNEUROSCI.20-06-02209.2000
Lyubarsky, A. L., Lem, J., Chen, J., Falsini, B., Iannaccone, A., and Pugh, E. N. Jr. (2002). Functionally rodless mice: transgenic models for the investigation of cone function in retinal disease and therapy. Vision Res. 42, 401–415. doi: 10.1016/S0042-6989(01)00214-0
Macdonald, P. N., and Ong, D. E. (1988). Evidence for a lecithin-retinol acyltransferase activity in the rat small intestine. J. Biol. Chem. 263, 12478–12482. doi: 10.1016/S0021-9258(18)37779-2
Machaty, Z. (2016). Signal transduction in mammalian oocytes during fertilization. Cell Tissue Res. 363, 169–183. doi: 10.1007/s00441-015-2291-8
Makino, C. L., Dodd, R. L., Chen, J., Burns, M. E., Roca, A., Simon, M. I., et al. (2004). Recoverin regulates light-dependent phosphodiesterase activity in retinal rods. J. Gen. Physiol. 123, 729–741. doi: 10.1085/jgp.200308994
Makino, C. L., Wen, X. H., Olshevskaya, E. V., Peshenko, I. V., Savchenko, A. B., and Dizhoor, A. M. (2012). Enzymatic relay mechanism stimulates cyclic GMP synthesis in rod photoresponse: biochemical and physiological study in guanylyl cyclase activating protein 1 knockout mice. PLoS ONE 7:e47637. doi: 10.1371/journal.pone.0047637
Martin-Aragon Baudel, M. A. S., Shi, J., Large, W. A., and Albert, A. P. (2020). Obligatory role for PKCdelta in PIP2 -mediated activation of store-operated TRPC1 channels in vascular smooth muscle cells. J. Physiol. 598, 3911–3925. doi: 10.1113/JP279947
Mata, N. L., Moghrabi, W. N., Lee, J. S., Bui, T. V., Radu, R. A., Horwitz, J., et al. (2004). Rpe65 is a retinyl ester binding protein that presents insoluble substrate to the isomerase in retinal pigment epithelial cells. J. Biol. Chem. 279, 635–643. doi: 10.1074/jbc.M310042200
Mata, N. L., Radu, R. A., Clemmons, R. C., and Travis, G. H. (2002). Isomerization and oxidation of vitamin a in cone-dominant retinas: a novel pathway for visual-pigment regeneration in daylight. Neuron 36, 69–80. doi: 10.1016/S0896-6273(02)00912-1
Matsumoto, H., Kurien, B. T., Takagi, Y., Kahn, E. S., Kinumi, T., Komori, N., et al. (1994). Phosrestin I undergoes the earliest light-induced phosphorylation by a calcium/calmodulin-dependent protein kinase in drosophila photoreceptors. Neuron 12, 997–1010. doi: 10.1016/0896-6273(94)90309-3
Matsunami, H., and Buck, L. B. (1997). A multigene family encoding a diverse array of putative pheromone receptors in mammals. Cell 90, 775–784. doi: 10.1016/S0092-8674(00)80537-1
Matthews, H. R., and Sampath, A. P. (2010). Photopigment quenching is Ca2+ dependent and controls response duration in salamander L-cone photoreceptors. J. Gen. Physiol. 135, 355–366. doi: 10.1085/jgp.200910394
Menco, B. P. (1997). Ultrastructural aspects of olfactory signaling. Chem. Senses 22, 295–311. doi: 10.1093/chemse/22.3.295
Mendez, A., Burns, M. E., Sokal, I., Dizhoor, A. M., Baehr, W., Palczewski, K., et al. (2001). Role of guanylate cyclase-activating proteins (GCAPs) in setting the flash sensitivity of rod photoreceptors. Proc. Natl. Acad. Sci. U.S.A. 98, 9948–9953. doi: 10.1073/pnas.171308998
Meredith, M. (2001). Human vomeronasal organ function: a critical review of best and worst cases. Chem. Senses 26, 433–445. doi: 10.1093/chemse/26.4.433
Meyer, M. R., Angele, A., Kremmer, E., Kaupp, U. B., and Muller, F. (2000). A cGMP-signaling pathway in a subset of olfactory sensory neurons. Proc. Natl. Acad. Sci. U.S.A. 97, 10595–10600. doi: 10.1073/pnas.97.19.10595
Miazzi, F., Hansson, B. S., and Wicher, D. (2016). Odor-induced cAMP production in Drosophila melanogaster olfactory sensory neurons. J. Exp. Biol. 219, 1798–1803. doi: 10.1242/jeb.137901
Moiseyev, G., Crouch, R. K., Goletz, P., Oatis, J. Jr., Redmond, T. M., and Ma, J. X. (2003). Retinyl esters are the substrate for isomerohydrolase. Biochemistry 42, 2229–2238. doi: 10.1021/bi026911y
Molnar, T., Barabas, P., Birnbaumer, L., Punzo, C., Kefalov, V., and Krizaj, D. (2012). Store-operated channels regulate intracellular calcium in mammalian rods. J. Physiol. 590, 3465–3481. doi: 10.1113/jphysiol.2012.234641
Mombaerts, P. (1999). Molecular biology of odorant receptors in vertebrates. Annu. Rev. Neurosci. 22, 487–509. doi: 10.1146/annurev.neuro.22.1.487
Montell, C. (2012). Drosophila visual transduction. Trends Neurosci. 35, 356–363. doi: 10.1016/j.tins.2012.03.004
Montell, C., and Rubin, G. M. (1989). Molecular characterization of the Drosophila trp locus: a putative integral membrane protein required for phototransduction. Neuron 2, 1313–1323. doi: 10.1016/0896-6273(89)90069-X
Morshedian, A., Kaylor, J. J., Ng, S. Y., Tsan, A., Frederiksen, R., Xu, T., et al. (2019). Light-driven regeneration of cone visual pigments through a mechanism involving RGR opsin in muller glial cells. Neuron 102, 1172–1183 e1175. doi: 10.1016/j.neuron.2019.04.004
Morshedian, A., Woodruff, M. L., and Fain, G. L. (2018). Role of recoverin in rod photoreceptor light adaptation. J. Physiol. 596, 1513–1526. doi: 10.1113/JP275779
Mukunda, L., Miazzi, F., Sargsyan, V., Hansson, B. S., and Wicher, D. (2016). Calmodulin affects sensitization of Drosophila melanogaster odorant receptors. Front. Cell. Neurosci. 10:28. doi: 10.3389/fncel.2016.00028
Nache, V., Wongsamitkul, N., Kusch, J., Zimmer, T., Schwede, F., and Benndorf, K. (2016). Deciphering the function of the CNGB1b subunit in olfactory CNG channels. Sci. Rep. 6:29378. doi: 10.1038/srep29378
Nakagawa, T., and Vosshall, L. B. (2009). Controversy and consensus: noncanonical signaling mechanisms in the insect olfactory system. Curr. Opin. Neurobiol. 19, 284–292. doi: 10.1016/j.conb.2009.07.015
Nakamura, T., and Gold, G. H. (1987). A cyclic nucleotide-gated conductance in olfactory receptor cilia. Nature 325, 442–444. doi: 10.1038/325442a0
Ng, R., Salem, S. S., Wu, S. T., Wu, M., Lin, H. H., Shepherd, A. K., et al. (2019). Amplification of drosophila olfactory responses by a DEG/ENaC channel. Neuron 104, 947–959 e945. doi: 10.1016/j.neuron.2019.08.041
Niimura, Y., and Nei, M. (2007). Extensive gains and losses of olfactory receptor genes in mammalian evolution. PLoS ONE 2:e708. doi: 10.1371/journal.pone.0000708
Nikonov, S., Engheta, N., and Pugh, E. N. Jr. (1998). Kinetics of recovery of the dark-adapted salamander rod photoresponse. J. Gen. Physiol. 111, 7–37. doi: 10.1085/jgp.111.1.7
Nikonov, S., Lamb, T. D., and Pugh, E. N. Jr. (2000). The role of steady phosphodiesterase activity in the kinetics and sensitivity of the light-adapted salamander rod photoresponse. J. Gen. Physiol. 116, 795–824. doi: 10.1085/jgp.116.6.795
Nikonov, S. S., Brown, B. M., Davis, J. A., Zuniga, F. I., Bragin, A., Pugh, E. N. Jr., et al. (2008). Mouse cones require an arrestin for normal inactivation of phototransduction. Neuron 59, 462–474. doi: 10.1016/j.neuron.2008.06.011
Oberwinkler, J., and Stavenga, D. G. (2000). Calcium transients in the rhabdomeres of dark- and light-adapted fly photoreceptor cells. J. Neurosci. 20, 1701–1709. doi: 10.1523/JNEUROSCI.20-05-01701.2000
Okawa, H., Sampath, A. P., Laughlin, S. B., and Fain, G. L. (2008). ATP consumption by mammalian rod photoreceptors in darkness and in light. Curr. Biol. 18, 1917–1921. doi: 10.1016/j.cub.2008.10.029
Pace, U., Hanski, E., Salomon, Y., and Lancet, D. (1985). Odorant-sensitive adenylate cyclase may mediate olfactory reception. Nature 316, 255–258. doi: 10.1038/316255a0
Palczewski, K., and Kiser, P. D. (2020). Shedding new light on the generation of the visual chromophore. Proc. Natl. Acad. Sci. U.S.A. 117, 19629–19638. doi: 10.1073/pnas.2008211117
Pandey, S., Blanks, J. C., Spee, C., Jiang, M., and Fong, H. K. (1994). Cytoplasmic retinal localization of an evolutionary homolog of the visual pigments. Exp. Eye Res. 58, 605–613. doi: 10.1006/exer.1994.1055
Paulsen, R., and Schwemer, J. (1972). Studies on the insect visual pigment sensitive to ultraviolet light: retinal as the chromophoric group. Biochim. Biophys. Acta Bioenerget. 283, 520–529. doi: 10.1016/0005-2728(72)90268-X
Pellegrino, M., and Nakagawa, T. (2009). Smelling the difference: controversial ideas in insect olfaction. J. Exp. Biol. 212, 1973–1979. doi: 10.1242/jeb.023036
Peppel, K., Boekhoff, I., Mcdonald, P., Breer, H., Caron, M. G., and Lefkowitz, R. J. (1997). G protein-coupled receptor kinase 3 (GRK3) gene disruption leads to loss of odorant receptor desensitization. J. Biol. Chem. 272, 25425–25428. doi: 10.1074/jbc.272.41.25425
Peshenko, I. V., and Dizhoor, A. M. (2007). Activation and inhibition of photoreceptor guanylyl cyclase by guanylyl cyclase activating protein 1 (GCAP-1): the functional role of Mg2+/Ca2+ exchange in EF-hand domains. J. Biol. Chem. 282, 21645–21652. doi: 10.1074/jbc.M702368200
Phillips, A. M., Bull, A., and Kelly, L. E. (1992). Identification of a Drosophila gene encoding a calmodulin-binding protein with homology to the trp phototransduction gene. Neuron 8, 631–642. doi: 10.1016/0896-6273(92)90085-R
Picones, A., and Korenbrot, J. I. (1995). Permeability and interaction of Ca2+ with cGMP-gated ion channels differ in retinal rod and cone photoreceptors. Biophys. J. 69, 120–127. doi: 10.1016/S0006-3495(95)79881-2
Pifferi, S., Menini, A., and Kurahashi, T. (2010). “Signal transduction in vertebrate olfactory cilia,” in The Neurobiology of Olfaction, ed A. Menini (Boca Raton, FL: CRC Press/Taylor & Francis), p. 203–224.
Plangger, A., Malicki, D., Whitney, M., and Paulsen, R. (1994). Mechanism of arrestin 2 function in rhabdomeric photoreceptors. J. Biol. Chem. 269, 26969–26975. doi: 10.1016/S0021-9258(18)47113-X
Pugh, E. N. Jr., and Lamb, T. D. (1990). Cyclic GMP and calcium: the internal messengers of excitation and adaptation in vertebrate photoreceptors. Vision Res. 30, 1923–1948. doi: 10.1016/0042-6989(90)90013-B
Raghu, P., Colley, N. J., Webel, R., James, T., Hasan, G., Danin, M., et al. (2000). Normal phototransduction in Drosophila photoreceptors lacking an InsP(3) receptor gene. Mol. Cell. Neurosci. 15, 429–445. doi: 10.1006/mcne.2000.0846
Rebrik, T. I., Botchkina, I., Arshavsky, V. Y., Craft, C. M., and Korenbrot, J. I. (2012). CNG-modulin: a novel Ca-dependent modulator of ligand sensitivity in cone photoreceptor cGMP-gated ion channels. J. Neurosci. 32, 3142–3153. doi: 10.1523/JNEUROSCI.5518-11.2012
Reilander, H., Achilles, A., Friedel, U., Maul, G., Lottspeich, F., and Cook, N. J. (1992). Primary structure and functional expression of the Na/Ca,K-exchanger from bovine rod photoreceptors. EMBO J. 11, 1689–1695. doi: 10.1002/j.1460-2075.1992.tb05219.x
Reisert, J., and Matthews, H. R. (2001). Simultaneous recording of receptor current and intraciliary Ca2+ concentration in salamander olfactory receptor cells. J. Physiol. 535, 637–645. doi: 10.1111/j.1469-7793.2001.00637.x
Roof, D. J., Applebury, M. L., and Sternweis, P. C. (1985). Relationships within the family of GTP-binding proteins isolated from bovine central nervous system. J. Biol. Chem. 260, 16242–16249. doi: 10.1016/S0021-9258(17)36227-0
Ryba, N. J., and Tirindelli, R. (1997). A new multigene family of putative pheromone receptors. Neuron 19, 371–379. doi: 10.1016/S0896-6273(00)80946-0
Saari, J. C., and Bredberg, D. L. (1989). Lecithin:retinol acyltransferase in retinal pigment epithelial microsomes. J. Biol. Chem. 264, 8636–8640. doi: 10.1016/S0021-9258(18)81839-7
Sakurai, K., Chen, J., and Kefalov, V. J. (2011). Role of guanylyl cyclase modulation in mouse cone phototransduction. J. Neurosci. 31, 7991–8000. doi: 10.1523/JNEUROSCI.6650-10.2011
Sakurai, K., Chen, J., Khani, S. C., and Kefalov, V. J. (2015). Regulation of mammalian cone phototransduction by recoverin and rhodopsin kinase. J. Biol. Chem. 290, 9239–9250. doi: 10.1074/jbc.M115.639591
Sampath, A. P., Matthews, H. R., Cornwall, M. C., Bandarchi, J., and Fain, G. L. (1999). Light-dependent changes in outer segment free-Ca2+ concentration in salamander cone photoreceptors. J. Gen. Physiol. 113, 267–277. doi: 10.1085/jgp.113.2.267
Sampath, A. P., Matthews, H. R., Cornwall, M. C., and Fain, G. L. (1998). Bleached pigment produces a maintained decrease in outer segment Ca2+ in salamander rods. J. Gen. Physiol. 111, 53–64. doi: 10.1085/jgp.111.1.53
Sargsyan, V., Getahun, M. N., Llanos, S. L., Olsson, S. B., Hansson, B. S., and Wicher, D. (2011). Phosphorylation via PKC regulates the function of the drosophila odorant co-receptor. Front. Cell. Neurosci. 5:5. doi: 10.3389/fncel.2011.00005
Sato, K., Pellegrino, M., Nakagawa, T., Nakagawa, T., Vosshall, L. B., and Touhara, K. (2008). Insect olfactory receptors are heteromeric ligand-gated ion channels. Nature 452, 1002–1006. doi: 10.1038/nature06850
Schild, D., and Restrepo, D. (1998). Transduction mechanisms in vertebrate olfactory receptor cells. Physiol. Rev. 78, 429–466. doi: 10.1152/physrev.1998.78.2.429
Schleicher, S., Boekhoff, I., Arriza, J., Lefkowitz, R. J., and Breer, H. (1993). A beta-adrenergic receptor kinase-like enzyme is involved in olfactory signal termination. Proc. Natl. Acad. Sci. U.S.A. 90, 1420–1424. doi: 10.1073/pnas.90.4.1420
Schmidt, H. R., and Benton, R. (2020). Molecular mechanisms of olfactory detection in insects: beyond receptors. Open Biol. 10:200252. doi: 10.1098/rsob.200252
Scott, K., Becker, A., Sun, Y., Hardy, R., and Zuker, C. (1995). Gqα protein function in vivo: genetic dissection of its role in photoreceptor cell physiology. Neuron 15, 919–927. doi: 10.1016/0896-6273(95)90182-5
Shi, G., Yau, K. W., Chen, J., and Kefalov, V. J. (2007). Signaling properties of a short-wave cone visual pigment and its role in phototransduction. J. Neurosci. 27, 10084–10093. doi: 10.1523/JNEUROSCI.2211-07.2007
Shichida, Y., and Morizumi, T. (2007). Mechanism of G-protein activation by rhodopsin. Photochem. Photobiol. 83, 70–75. doi: 10.1562/2006-03-22-IR-854
Shieh, B.-H., Zhu, M.-Y., Lee, J. K., Kelly, I. M., and Bahiraei, F. (1997). Association of INAD with NORPA is essential for controlled activation and deactivation of Drosophila phototransduction in vivo. Proc. Natl. Acad. Sci. U.S.A. 94, 12682–12687. doi: 10.1073/pnas.94.23.12682
Sjöstrand, F. S., and Kreman, M. (1978). Molecular structure of outer segment disks in photoreceptor cells. J. Ultrastruct. Res. 65, 195–226. doi: 10.1016/S0022-5320(78)80059-8
Smart, R., Kiely, A., Beale, M., Vargas, E., Carraher, C., Kralicek, A. V., et al. (2008). Drosophila odorant receptors are novel seven transmembrane domain proteins that can signal independently of heterotrimeric G proteins. Insect Biochem. Mol. Biol. 38, 770–780. doi: 10.1016/j.ibmb.2008.05.002
Smith, D. P., Ranganathan, R., Hardy, R. W., Marx, J., Tsuchida, T., and Zuker, C. S. (1991). Photoreceptor deactivation and retinal degeneration mediated by a photoreceptor-specific protein kinase C. Science 254, 1478–1484. doi: 10.1126/science.1962207
Smith, D. P., Shieh, B. H., and Zuker, C. S. (1990). Isolation and structure of an arrestin gene from Drosophila. Proc. Natl. Acad. Sci. U.S.A. 87, 1003–1007. doi: 10.1073/pnas.87.3.1003
Song, Y., Cygnar, K. D., Sagdullaev, B., Valley, M., Hirsh, S., Stephan, A., et al. (2008). Olfactory CNG channel desensitization by Ca2+/CaM via the B1b subunit affects response termination but not sensitivity to recurring stimulation. Neuron 58, 374–386. doi: 10.1016/j.neuron.2008.02.029
Stephan, A. B., Tobochnik, S., Dibattista, M., Wall, C. M., Reisert, J., and Zhao, H. (2011). The Na(+)/Ca(2+) exchanger NCKX4 governs termination and adaptation of the mammalian olfactory response. Nat. Neurosci. 15, 131–137. doi: 10.1038/nn.2943
Stöhr, H., Heisig, J. B., Benz, P. M., Schoberl, S., Milenkovic, V. M., Strauss, O., et al. (2009). TMEM16B, a novel protein with calcium-dependent chloride channel activity, associates with a presynaptic protein complex in photoreceptor terminals. J. Neurosci. 29, 6809–6818. doi: 10.1523/JNEUROSCI.5546-08.2009
Stowers, L., Holy, T. E., Meister, M., Dulac, C., and Koentges, G. (2002). Loss of sex discrimination and male-male aggression in mice deficient for TRP2. Science 295, 1493–1500. doi: 10.1126/science.1069259
Thoreson, W. B., Bryson, E. J., and Rabl, K. (2003). Reciprocal interactions between calcium and chloride in rod photoreceptors. J. Neurophysiol. 90, 1747–1753. doi: 10.1152/jn.00932.2002
Thoreson, W. B., Nitzan, R., and Miller, R. F. (2000). Chloride efflux inhibits single calcium channel open probability in vertebrate photoreceptors: chloride imaging and cell-attached patch-clamp recordings. Vis. Neurosci. 17, 197–206. doi: 10.1017/S0952523800172025
Torre, V., Ashmore, J. F., Lamb, T. D., and Menini, A. (1995). Transduction and adaptation in sensory receptor cells. J. Neurosci. 15, 7757–7768. doi: 10.1523/JNEUROSCI.15-12-07757.1995
Touhara, K. (2002). Odor discrimination by G protein-coupled olfactory receptors. Microsc. Res. Tech. 58, 135–141. doi: 10.1002/jemt.10131
Touhara, K., and Vosshall, L. B. (2009). Sensing odorants and pheromones with chemosensory receptors. Annu. Rev. Physiol. 71, 307–332. doi: 10.1146/annurev.physiol.010908.163209
Trifunovic, D., Karali, M., Camposampiero, D., Ponzin, D., Banfi, S., and Marigo, V. (2008). A high-resolution RNA expression atlas of retinitis pigmentosa genes in human and mouse retinas. Invest. Ophthalmol. Vis. Sci. 49, 2330–2336. doi: 10.1167/iovs.07-1513
Vinberg, F., Chen, J., and Kefalov, V. J. (2018). Regulation of calcium homeostasis in the outer segments of rod and cone photoreceptors. Prog. Retin. Eye Res. 67, 87–101. doi: 10.1016/j.preteyeres.2018.06.001
Vinberg, F., Wang, T., De Maria, A., Zhao, H., Bassnett, S., Chen, J., et al. (2017). The Na(+)/Ca(2+), K(+) exchanger NCKX4 is required for efficient cone-mediated vision. Elife 6:e20975. doi: 10.7554/eLife.24550.015
Vinberg, F., Wang, T., Molday, R. S., Chen, J., and Kefalov, V. J. (2015). A new mouse model for stationary night blindness with mutant Slc24a1 explains the pathophysiology of the associated human disease. Hum. Mol. Genet. 24, 5915–5929. doi: 10.1093/hmg/ddv319
Vinos, J., Jalink, K., Hardy, R. W., Britt, S. G., and Zuker, C. S. (1997). A G protein-coupled receptor phosphatase required for rhodopsin function. Science 277, 687–690. doi: 10.1126/science.277.5326.687
Wang, J. S., Estevez, M. E., Cornwall, M. C., and Kefalov, V. J. (2009). Intra-retinal visual cycle required for rapid and complete cone dark adaptation. Nat. Neurosci. 12, 295–302. doi: 10.1038/nn.2258
Wang, J. S., and Kefalov, V. J. (2009). An alternative pathway mediates the mouse and human cone visual cycle. Curr. Biol. 19, 1665–1669. doi: 10.1016/j.cub.2009.07.054
Wang, J. S., and Kefalov, V. J. (2011). The cone-specific visual cycle. Prog. Retin. Eye Res. 30, 115–128. doi: 10.1016/j.preteyeres.2010.11.001
Wang, T., Xu, H., Oberwinkler, J., Gu, Y., Hardie, R. C., and Montell, C. (2005). Light activation, adaptation, and cell survival functions of the Na+/Ca2+ exchanger CalX. Neuron 45, 367–378. doi: 10.1016/j.neuron.2004.12.046
Wang, X., Wang, T., Jiao, Y., Von Lintig, J., and Montell, C. (2010). Requirement for an enzymatic visual cycle in Drosophila. Curr. Biol. 20, 93–102. doi: 10.1016/j.cub.2009.12.022
Wang, X., Wang, T., Ni, J. D., Von Lintig, J., and Montell, C. (2012). The Drosophila visual cycle and de novo chromophore synthesis depends on rdhB. J. Neurosci. 32, 3485–3491. doi: 10.1523/JNEUROSCI.5350-11.2012
Wayman, G. A., Impey, S., and Storm, D. R. (1995). Ca2+ inhibition of type III adenylyl cyclase in vivo(*). J. Biol. Chem. 270, 21480–21486. doi: 10.1074/jbc.270.37.21480
Weeraratne, S. D., Valentine, M., Cusick, M., Delay, R., and Van Houten, J. L. (2006). Plasma membrane calcium pumps in mouse olfactory sensory neurons. Chem. Senses 31, 725–730. doi: 10.1093/chemse/bjl014
Wei, J., Wayman, G., and Storm, D. R. (1996). Phosphorylation and inhibition of type III adenylyl cyclase by calmodulin-dependent protein kinase II in vivo. J. Biol. Chem. 271, 24231–24235. doi: 10.1074/jbc.271.39.24231
Wei, J., Zhao, A. Z., Chan, G. C., Baker, L. P., Impey, S., Beavo, J. A., et al. (1998). Phosphorylation and inhibition of olfactory adenylyl cyclase by CaM kinase II in neurons: a mechanism for attenuation of olfactory signals. Neuron 21, 495–504. doi: 10.1016/S0896-6273(00)80561-9
Wicher, D., Schafer, R., Bauernfeind, R., Stensmyr, M. C., Heller, R., Heinemann, S. H., et al. (2008). Drosophila odorant receptors are both ligand-gated and cyclic-nucleotide-activated cation channels. Nature 452, 1007–1011. doi: 10.1038/nature06861
Wilden, U. (1995). Duration and amplitude of the light-induced cGMP hydrolysis in vertebrate photoreceptors are regulated by multiple phosphorylation of rhodopsin and by arrestin binding. Biochemistry 34, 1446–1454. doi: 10.1021/bi00004a040
Wong, S. T., Trinh, K., Hacker, B., Chan, G. C., Lowe, G., Gaggar, A., et al. (2000). Disruption of the type III adenylyl cyclase gene leads to peripheral and behavioral anosmia in transgenic mice. Neuron 27, 487–497. doi: 10.1016/S0896-6273(00)00060-X
Woodruff, M. L., Sampath, A. P., Matthews, H. R., Krasnoperova, N. V., Lem, J., and Fain, G. L. (2002). Measurement of cytoplasmic calcium concentration in the rods of wild-type and transducin knock-out mice. J. Physiol. 542, 843–854. doi: 10.1113/jphysiol.2001.013987
Xu, J., Dodd, R. L., Makino, C. L., Simon, M. I., Baylor, D. A., and Chen, J. (1997). Prolonged photoresponses in transgenic mouse rods lacking arrestin. Nature 389, 505–509. doi: 10.1038/39068
Xue, Y., Shen, S. Q., Jui, J., Rupp, A. C., Byrne, L. C., Hattar, S., et al. (2015). CRALBP supports the mammalian retinal visual cycle and cone vision. J. Clin. Investig. 125, 727–738. doi: 10.1172/JCI79651
Yagi, T., and Macleish, P. R. (1994). Ionic conductances of monkey solitary cone inner segments. J. Neurophysiol. 71, 656–665. doi: 10.1152/jn.1994.71.2.656
Yan, C., Zhao, A. Z., Bentley, J. K., Loughney, K., Ferguson, K., and Beavo, J. A. (1995). Molecular cloning and characterization of a calmodulin-dependent phosphodiesterase enriched in olfactory sensory neurons. Proc. Natl. Acad. Sci. U.S.A. 92, 9677–9681. doi: 10.1073/pnas.92.21.9677
Yang, T., Hendrickson, W. A., and Colecraft, H. M. (2014). Preassociated apocalmodulin mediates Ca2+-dependent sensitization of activation and inactivation of TMEM16A/16B Ca2+-gated Cl- channels. Proc. Natl. Acad. Sci. U.S.A. 111, 18213–18218. doi: 10.1073/pnas.1420984111
Yau, K. W., and Hardie, R. C. (2009). Phototransduction motifs and variations. Cell 139, 246–264. doi: 10.1016/j.cell.2009.09.029
Yu, W. P., Grunwald, M. E., and Yau, K. W. (1996). Molecular cloning, functional expression and chromosomal localization of a human homolog of the cyclic nucleotide-gated ion channel of retinal cone photoreceptors. FEBS Lett. 393, 211–215. doi: 10.1016/0014-5793(96)00889-7
Zang, J., and Matthews, H. R. (2012). Origin and control of the dominant time constant of salamander cone photoreceptors. J. Gen. Physiol. 140, 219–233. doi: 10.1085/jgp.201110762
Zhang, J., Choi, E. H., Tworak, A., Salom, D., Leinonen, H., Sander, C. L., et al. (2019). Photic generation of 11-cis-retinal in bovine retinal pigment epithelium. J. Biol. Chem. 294, 19137–19154. doi: 10.1074/jbc.RA119.011169
Zhang, J., and Webb, D. M. (2003). Evolutionary deterioration of the vomeronasal pheromone transduction pathway in catarrhine primates. Proc. Natl. Acad. Sci. U.S.A. 100, 8337–8341. doi: 10.1073/pnas.1331721100
Zufall, F., Firestein, S., and Shepherd, G. M. (1994). Cyclic nucleotide-gated ion channels and sensory transduction in olfactory receptor neurons. Annu. Rev. Biophys. Biomol. Struct. 23, 577–607. doi: 10.1146/annurev.bb.23.060194.003045
Keywords: adaptation, olfaction, activation, inactivation, phototransduction cascade
Citation: Abbas F and Vinberg F (2021) Transduction and Adaptation Mechanisms in the Cilium or Microvilli of Photoreceptors and Olfactory Receptors From Insects to Humans. Front. Cell. Neurosci. 15:662453. doi: 10.3389/fncel.2021.662453
Received: 01 February 2021; Accepted: 02 March 2021;
Published: 01 April 2021.
Edited by:
Wallace B. Thoreson, University of Nebraska Medical Center, United StatesReviewed by:
Gordon Fain, University of California, Los Angeles, United StatesDieter Wicher, Max Planck Institute for Chemical Ecology, Germany
Copyright © 2021 Abbas and Vinberg. This is an open-access article distributed under the terms of the Creative Commons Attribution License (CC BY). The use, distribution or reproduction in other forums is permitted, provided the original author(s) and the copyright owner(s) are credited and that the original publication in this journal is cited, in accordance with accepted academic practice. No use, distribution or reproduction is permitted which does not comply with these terms.
*Correspondence: Fatima Abbas, ZmF0aW1hLmFiYmFzQHV0YWguZWR1; Frans Vinberg, ZnJhbnMudmluYmVyZ0B1dGFoLmVkdQ==