- 1Program in Neuroscience, Florida State University, Tallahassee, FL, United States
- 2Cell and Molecular Biology Program, Department of Biological Science, Florida State University, Tallahassee, FL, United States
- 3Institute of Molecular Biophysics, Florida State University, Tallahassee, FL, United States
Neuromodulation influences neuronal processing, conferring neuronal circuits the flexibility to integrate sensory inputs with behavioral states and the ability to adapt to a continuously changing environment. In this original research report, we broadly discuss the basis of neuromodulation that is known to regulate intrinsic firing activity, synaptic communication, and voltage-dependent channels in the olfactory bulb. Because the olfactory system is positioned to integrate sensory inputs with information regarding the internal chemical and behavioral state of an animal, how olfactory information is modulated provides flexibility in coding and behavioral output. Herein we discuss how neuronal microcircuits control complex dynamics of the olfactory networks by homing in on a special class of local interneurons as an example. While receptors for neuromodulation and metabolic peptides are widely expressed in the olfactory circuitry, centrifugal serotonergic and cholinergic inputs modulate glomerular activity and are involved in odor investigation and odor-dependent learning. Little is known about how metabolic peptides and neuromodulators control specific neuronal subpopulations. There is a microcircuit between mitral cells and interneurons that is comprised of deep-short-axon cells in the granule cell layer. These local interneurons express pre-pro-glucagon (PPG) and regulate mitral cell activity, but it is unknown what initiates this type of regulation. Our study investigates the means by which PPG neurons could be recruited by classical neuromodulators and hormonal peptides. We found that two gut hormones, leptin and cholecystokinin, differentially modulate PPG neurons. Cholecystokinin reduces or increases spike frequency, suggesting a heterogeneous signaling pathway in different PPG neurons, while leptin does not affect PPG neuronal firing. Acetylcholine modulates PPG neurons by increasing the spike frequency and eliciting bursts of action potentials, while serotonin does not affect PPG neuron excitability. The mechanisms behind this diverse modulation are not known, however, these results clearly indicate a complex interplay of metabolic signaling molecules and neuromodulators that may fine-tune neuronal microcircuits.
Introduction
When neurotransmitters are released from synaptic termini, information transfer takes place. This simple mechanism is the foundation of how we make decisions, learn, process emotions, or use our senses to interpret and navigate our external environments. By changing these parameters, or even factors regulating the likelihood of neurotransmitter release, our global behavioral state can impact how information is processed. This is the field of neuromodulation, the means by which our physiological state dynamically influences aspects of synaptic activity, neural excitability, and gene expression (Florey, 1967). Neuromodulatory mechanisms are numerous and target different aspects of neuronal activity to produce diverse effects, but ultimately each fine-tunes the information being transferred (Figure 1). The largest group of neuromodulators bind to GPCRs and activate G proteins that initiate intracellular signaling cascades via second messengers (Chen et al., 2006; Newton et al., 2016; Byczkowicz et al., 2019; Moro et al., 2020). Subsequent to GPCR binding, effects include changes in gene expression (Fukuchi et al., 2015), ion channel properties that impact action potential propagation (Burke and Bender, 2019), and even interaction of Gβγ with the soluble NSF attachment protein REceptor, or SNARE complex, inhibiting neurotransmitter release (Zurawski et al., 2019; Hamm and Alford, 2020). Useful in vivo techniques are emerging to study neuromodulatory signaling including a mouse model allowing for real time cAMP visualization (Kim et al., 2014; Wu et al., 2015; Muntean et al., 2018), and fluorescent biosensors for several neurotransmitters (Leopold et al., 2019).
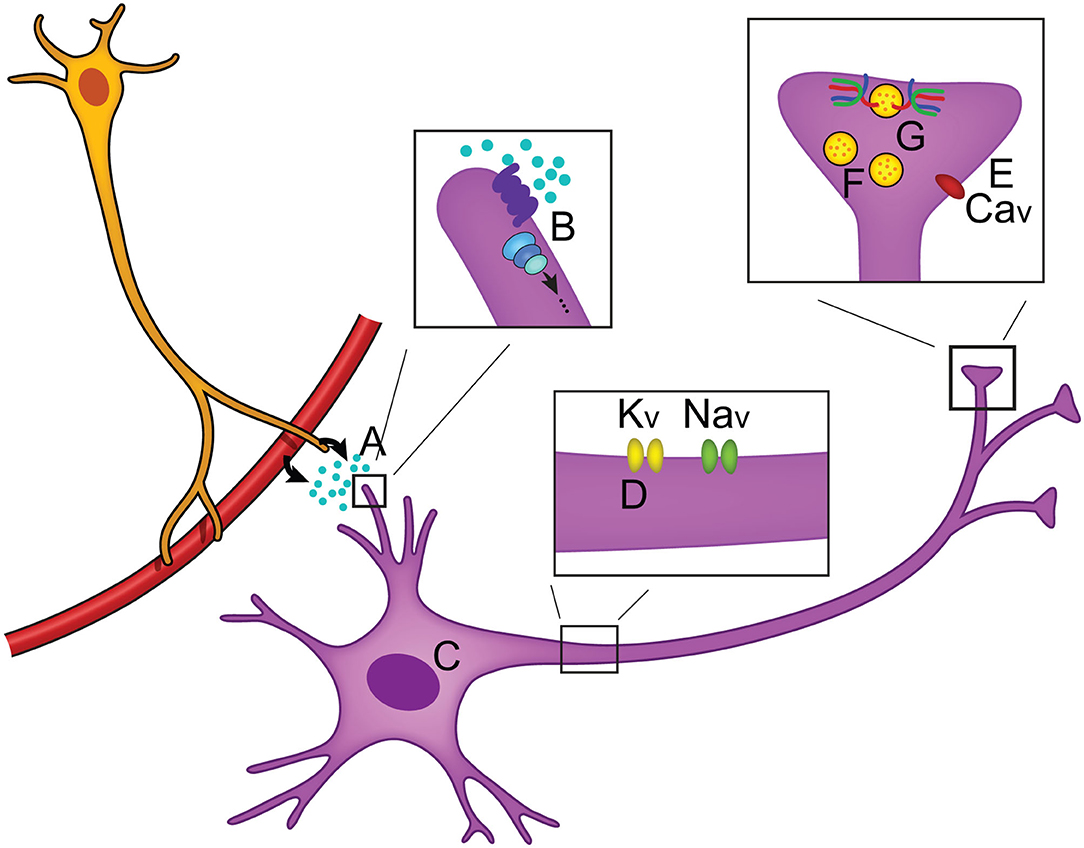
Figure 1. Overview of neuromodulation. (A) Neuromodulators can be released locally or circulated from the periphery and often bind to GPCRs (B) to activate intracellular signaling cascades. Intracellular effects include changes in (C) gene expression, (D) axonal sodium and potassium ion channel activity, and (E) changes in calcium ion channel activity (Zhou et al., 2007; Gray et al., 2017; Williams et al., 2018). (F) Neuronal activity can be modulated by changing the number of vesicles that are in the reserve pool, readily releasable pool, or docked at the synaptic terminal, thus changing the likelihood of neurotransmitter release (Rosenmund and Stevens, 1996; Logsdon et al., 2006; Taschenberger et al., 2016). (G) Modulation of the SNARE complex activity has been found to regulate neurotransmitter release (Sakisaka et al., 2008; Liu et al., 2018).
We have been exploring neuromodulation and information processing within the olfactory bulb, the first relay center for olfactory signals. Our intention is to understand how the physiological states of satiety, fasting, or over-nutrition can perturb or modulate transmission of olfactory information that ultimately can change eating behaviors (Palouzier-Paulignan et al., 2012; Julliard et al., 2017; Kolling and Fadool, 2020). In this topical issue, several authors have presented the functional synaptic activities of the known olfactory bulb circuitry (Ackels et al., 2020; Egger and Diamond, 2020; Imamura et al., 2020), so readers are directed to those works as an overview of the comprehensive neural circuit. The olfactory field is rich with investigations of synaptic interactions that drive an understanding of anatomical relationships and physiological mechanisms that ultimately modulate mitral/tufted (M/TC) cell output and subsequent olfactory behavior or detection (i.e., Shepherd, 1972; Jahr and Nicoll, 1980; Orona et al., 1984; Ezeh et al., 1993; Isaacson and Strowbridge, 1998; Aungst et al., 2003; Hayar et al., 2004a,b; Hayar et al., 2005; Zhou and Belluscio, 2008; Abraham et al., 2010; Huang et al., 2013; Banerjee et al., 2015; Najac et al., 2015; Liu et al., 2016; Burton, 2017; Pressler and Strowbridge, 2017; Harvey and Heinbockel, 2018; Jones et al., 2020). Herein, as schematized in Figure 2, we wish to home in on interneurons within the olfactory bulb that can provide neuromodulation of contrast and gain of the mitral/tufted (M/TC) cell output. These interneurons include those within the glomerular layer (GML), those within the external plexiform layer (EPL), and those centrally in the granule cell layer (GCL). It is also important to note that olfactory circuits do not solely rely on a linear feedforward transmission to interpret the external chemical environment - higher processing centers of the brain also present reciprocal connections with the olfactory bulb to modulate activity. These reciprocal connections mainly target GABAergic interneurons to modulate contrast and gain of M/TC output (Price and Powell, 1970b; Engel et al., 2001; Arevian et al., 2008; Fukunaga et al., 2012; Nagayama et al., 2014; Padmanabhan et al., 2018).
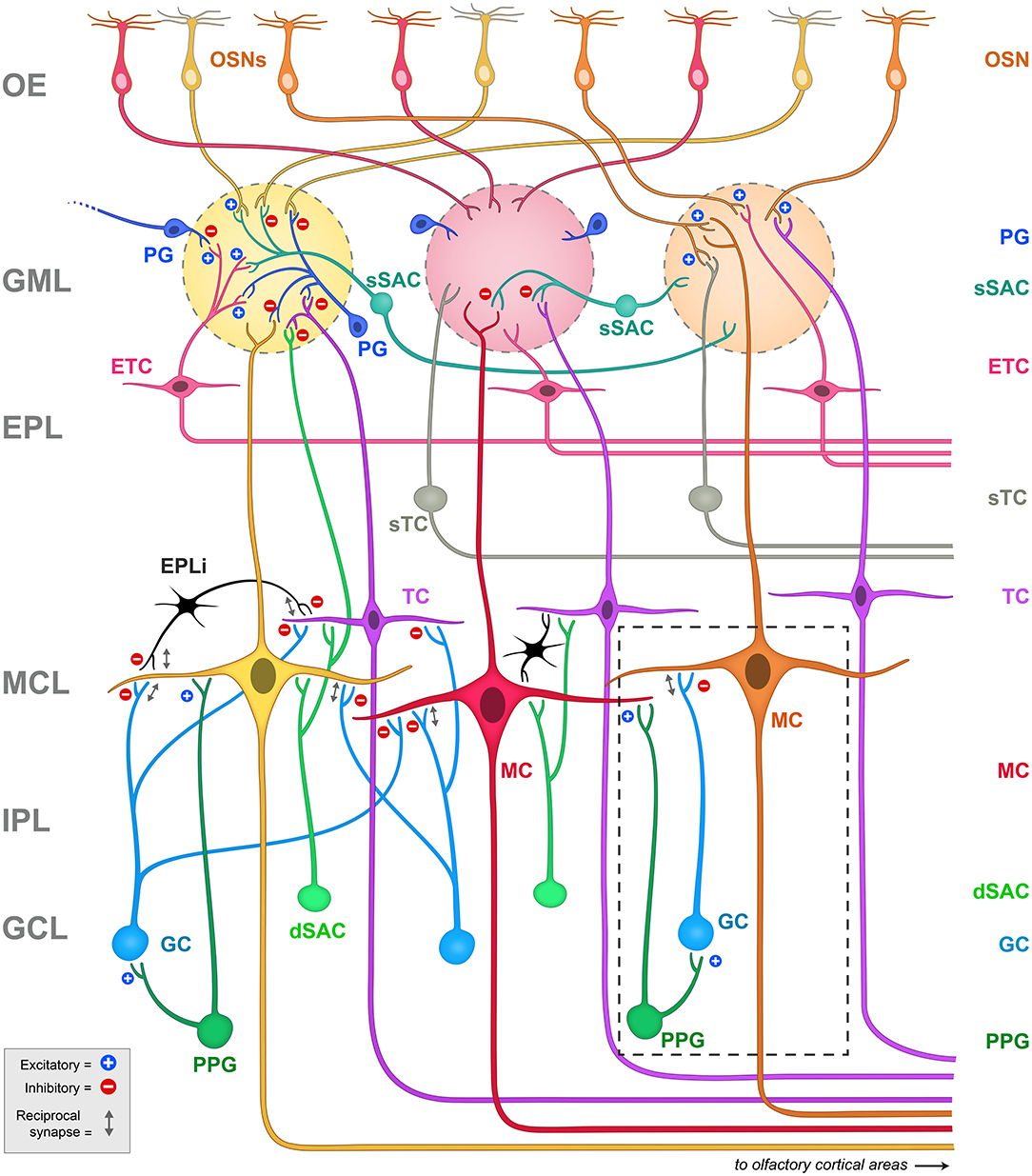
Figure 2. Schematic representation of the principal projection neurons and interneurons in the olfactory bulb, including the synapses between them. Blue plus signs (+) represent excitatory synapses, while red minus signs (-) represent inhibitory synapses. Reciprocal synapses are indicated by a gray double arrow. Note the highly laminated organization of the region that has been amendable for physiological and anatomical studies since first explored by Ramon y Cajal. The glomeruli are considered the first sensory processing station along the olfactory pathway where the olfactory signal is transferred from OSNs to glutamatergic output neurons, called mitral (MC) and tufted (TC) cells (Nagayama et al., 2014). Mitral and tufted cells' axons project to the olfactory cortex and higher order cortical structures conveying the information to the central nervous system (Ghosh et al., 2011; Nagayama et al., 2014). Both mitral and tufted cells (M/TCs) send an apical dendrite into a defined glomerulus, where they establish reciprocal synapses with OSNs and with a heterogeneous population of juxtaglomerular (JG) cells that include periglomerular (PG) neurons, external tufted cells (ETCs) and short axon cells (SACs). ETC have been shown to form an intrabulbar network of isofunctional columns and as such do not project out the of OB (Belluscio et al., 2002; Lodovichi et al., 2003; Zhou and Belluscio, 2008). MCs lateral dendrites form reciprocal synapses with granule cell (GC) dendrites whose cell bodies are located in the granule cell layer (GCL). The GCL also contains other types of neurons including deep short axon cells (dSACs) and several non-GCs populations (Ramon y Cajal, 1911; Price and Powell, 1970a; Schneider and Macrides, 1978). Very little is known regarding the role of these non-GC neurons and the modulatory afferents they receive. Dashed box = schematic representation of the PPG>MC>GC microcircuit. Note that the PPG neuron (forest green) is a subset of the dSAC variety (kelly green) located within the GCL. It has excitatory synaptic connections with both the GC and the MC (blue plus signs), following which, the GC has a classical dendro-dendritic reciprocal synapse onto MCs (gray double arrow) where it can exert inhibition (red minus sign). OE, olfactory epithelium; GML, glomerular layer; EPL, external plexiform layer; MCL, mitral cell layer; IPL, internal plexiform layer; GCL, granule cell layer; OSNs, olfactory sensory neurons; PG, periglomerular cell; sSAC, superficial short axon cell; ETC, external tufted cell; sTC, superficial tufted cell; TC, tufted cell; MC, mitral cell; dSAC, deep short axon cell; GC, granule cell; PPG, preproglucagon neuron; EPLi, interneuron of the external plexiform layer.
Due to the complexity of the neurolamina and diversity of the interneurons in the olfactory bulb, discovery of the mechanisms of neuromodulation of the olfactory output remains an ongoing process. This is particularly true for the largest neurolamina of the bulb, the granule cell layer (GCL), where much is known regarding the inhibitory network of granule cells (GC), yet the heterogeneity of non-GCs types in this region (Ramon y Cajal, 1911; Price and Powell, 1970a; Schneider and Macrides, 1978; Nagayama et al., 2014) does not afford a clear or completed picture of synaptic communication. A population of pre-proglucagon (PPG) neurons in the GCL has been discovered (Merchenthaler et al., 1999; Thiebaud et al., 2016) to project axons to the internal plexiform layer (IPL) and the mitral cell layer (MCL), and are speculated to release glucagon-like peptide 1, or GLP-1 (Thiebaud et al., 2016, 2019). The PPG neurons are a specialized type of deep short-axon cell (dSAC) (Eyre et al., 2008) and present stellate dendrites with abundant dendritic spines (Thiebaud et al., 2016, 2019; Burton et al., 2017). Stimulating PPG neurons can produce an excitatory or an inhibitory response on MCs due to a multi-synaptic interaction: PPG neurons form dendrodendritic synapses with MCs (PPG-MC) and with granule cells (PPG-GC). These three cell types therefore form a PPG neuron>MC>GC microcircuit (Figure 2, dashed box). Both synapses are usually excitatory, but stimulating GCs results in an inhibition of MCs through the release of GABA (Thiebaud et al., 2019). The functional significance of the microcircuit they hence establish, as a unique excitatory class of glutaminergic interneuron, remains incompletely known. Previous research on PPG neurons in the nucleus tractus solitarius (NTS) has shown that these neurons could be modulated by metabolic-related hormones such as cholecystokinin (CCK) (Hisadome et al., 2011) and leptin (Hisadome et al., 2010). These NTS PPG neurons have been suggested to provide a link between the energy state of an individual and their response to stress (Maniscalco et al., 2015). A negative energy balance induced by overnight fast was shown to block neural and behavioral responses to acute stress through inhibiting the activity of the NTS PPG neurons (Maniscalco et al., 2015). By comparison, PPG neurons in the olfactory bulb could act as a link between the individual's energy/nutritional state and their olfactory response. The expression of a variety of metabolic hormones such as ghrelin, orexins, leptin, insulin, CCK and their receptors (Palouzier-Paulignan et al., 2012) would allow the olfactory bulb to detect metabolic state while simultaneously modulating olfactory information processing.
Gut peptides such as GLP-1, CCK and leptin have been well-demonstrated to modulate olfactory circuit dynamics and could serve as plausible neuromodulators of PPG neurons (Ravel et al., 1990; Lemaire et al., 1994a,b; Prud'homme et al., 2009; Palouzier-Paulignan et al., 2012; Ma et al., 2013; Thiebaud et al., 2016, 2019; Sun et al., 2019) (see Table 1). Indeed, in the nucleus of the solitary tract, GLP-1-expressing neurons are modulated by CCK and leptin (Hisadome et al., 2010, 2011). It is not known whether the analogous PPG neurons in the olfactory bulb are also modulated by leptin and CCK. CCK was first reported in the gastrointestinal tract and later in the CNS (Vanderhaeghen et al., 1975). It represents the most abundant neuropeptide in the CNS, being found in the amygdala, cerebral cortex, hypothalamus, and olfactory system. Specifically within the olfactory system, CCK is expressed in the olfactory bulb, the olfactory tubercle and the piriform cortex (Beinfeld et al., 1981; Dupont et al., 1982; Ekstrand et al., 2001; Gutiérrez-Mecinas et al., 2005). Within the olfactory bulb, CCK immunoreactivity is detected in the superficial tufted cells and in the IPL (Marks et al., 2006; Kosaka and Kosaka, 2007) while the CCK receptors are located in the IPL, juxtaglomerular and MCL (Mercer and Beart, 1997). Leptin, alternatively, is produced by peripheral adipocytes and is involved in the regulation of body weight and food intake depending upon the nutritional state (Friedman and Halaas, 1998; Baly et al., 2007). Several studies support peripheral and central production of leptin (Morash et al., 1999). Leptin is capable of crossing the blood brain barrier using a saturable receptor-mediated mechanism (Banks, 2001). Leptin receptors are found in the central nervous system including the hypothalamus and the olfactory bulb (Guan et al., 1997; Elmquist et al., 1998). Fasting increases the transcription of leptin mRNA. Specifically within the olfactory system, leptin receptors have been shown to modulate spontaneous and odor-evoked electric activity in olfactory sensory neurons and to decrease the spontaneous firing of MCs (Baly et al., 2007; Savigner et al., 2009). In vivo experiments indicate that leptin inhibits odor-evoked oscillations (Sun et al., 2019) and decreases olfactory sensitivity (Julliard et al., 2007; Alkam et al., 2011; Sun et al., 2019).
Although it is not known if CCK or leptin have the capacity to modulate neural activity of the olfactory PPG neurons, as mentioned above, the interneurons in the GCL of the olfactory bulb additionally receive multiple centrifugal projections from higher brain areas including serotonergic, noradrenergic, cholinergic, and cortical feedback fibers. These centrifugal projections are believed to modulate olfactory information processing depending upon an animals' metabolic state.
Afferent serotonergic fibers that originate from the dorsal and medial raphe nuclei innervate all layers of the olfactory bulb, and thus the transmitter could serve as a neuromodulator of PPG neurons. One important mechanism underlying the ability to achieve diverse serotonergic modulation in the olfactory bulb is the broad expression of serotonin (5-HT) receptor subtypes (I to III). Indeed both in vitro and in vivo studies have shown diverse effects on different targets in the olfactory bulb by serotonergic modulation (Hardy et al., 2005b; Dugue and Mainen, 2009; Petzold et al., 2009; Liu et al., 2012; Schmidt and Strowbridge, 2014; Brill et al., 2016; Gaudry, 2018; Sizemore et al., 2020).
Similar to that of widespread serotoninergic innervation, centrifugal cholinergic fibers from the horizontal limb of the diagonal band of Broca innervate all layers of the olfactory bulb (Macrides et al., 1981; Záborszky et al., 1986). Bulbar neurons express nicotinic (nAChR) and muscarinic (mAChR) acetylcholine receptors (Castillo et al., 1999; Ghatpande et al., 2006; Pressler et al., 2007), conferring the cholinergic system the capacity to modulate specific synapses involved in olfactory information processing. At a circuit level, cholinergic modulation has been shown to target the glomerular microcircuit and modulate reciprocal, dendrodendritic synapses between MCs and GCs (Castillo et al., 1999; Ghatpande et al., 2006; Pressler et al., 2007). Behavioral studies indicate that the cholinergic system is involved in the regulation of several olfactory-guided behaviors in mice including odor discrimination (Doty et al., 1999; Chaudhury et al., 2009; D'Souza and Vijayaraghavan, 2014; Smith et al., 2015), short-term olfactory memory and fine tuning of MC activity (Devore and Linster, 2012), and olfactory perceptual learning (Fletcher and Wilson, 2002; Wilson et al., 2004).
All these neuromodulatory studies indicate that the increased excitatory drive in GCs might shift the excitatory/inhibitory (E/I) balance in MCs toward inhibition, ultimately influencing MCs input-output relationship. Therefore, acetylcholine (ACh) influences the final message to the olfactory cortex enhancing specificity and temporal precision of odor-evoked responses in MCs. Serotonergic and cholinergic modulation within the olfactory bulb is summarized in Table 2.
In summary, given the paucity of information regarding the purpose of this newly discovered microcircuit, we thereby focused our study upon what might modulate PPG neuron activity by testing suspected metabolic hormones or neurotransmitters well-studied in the olfactory bulb, and whose receptors were known to be expressed in this lamina. A series of ex vivo slice electrophysiology experiments were performed to determine the basal membrane properties of these neurons and identify possible changes in excitability induced by neurotransmitters or metabolic-related hormones that are common signaling molecules in the olfactory bulb. Less is known about how metabolic peptides and neuromodulators control specific neuronal subpopulations. Such a PGG>MC>GC microcircuit has the potential to be recruited to provide neuromodulation during ever changing metabolic states induced by feeding and fasting.
Materials and Methods
Ethical Approval
All animal experiments were approved by the Florida State University (FSU) Institutional Animal Care and Use Committee (IACUC) under protocol #1427 and were conducted in accordance with the American Veterinary Medicine Association (AVMA) and the National Institutes of Health (NIH). In preparation for olfactory slice electrophysiology, mice were anesthetized with isoflurane (Aerrane; Baxter, Deerfield, IL, USA) using the IACUC-approved drop method and were then sacrificed by decapitation (Leary, 2020).
Animal Care
Detection of pre-proglucagon (PPG) neurons expressing a red fluorescent protein (RFP) was achieved by crossing Rosa26-tandem-dimer red fluorescent protein (tdRFP) reporter mice (Luche et al., 2007) with mice expressing Cre recombinase under the control of the pre-proglucagon promoter (GLU-Cre12 mice) (Parker et al., 2012). For simplification, homozygous progeny resulting from the breeding of GLU-Cre12 and Rosa26 tdRFP mice are referred to as PPG-Cre-RFP mice (Thiebaud et al., 2019). All mice were housed in the Florida State University vivarium on a standard 12 h/12 h light/dark cycle and were allowed ad libitum access to 5001 Purina Chow (Purina, Richmond, VA, USA) and water. Mice of both sexes at post-natal day 20–45 were used for slice electrophysiology experiments.
Solutions and Reagents
Artificial cerebral spinal fluid (ACSF) contained (in mM): 119 NaCl, 26.2 NaHCO3, 2.5 KCl, 1 NaH2PO4, 1.3 MgCl2, 2.5 CaCl2, 22 glucose; 305–310 mOsm, pH 7.3-7.4. Sucrose-modified artificial cerebral spinal fluid (sucrose ACSF) contained (in mM): 83 NaCl, 26.2 NaHCO3, 1 NaH2PO4, 3.3 MgCl2, 0.5 CaCl2, 72 sucrose, 22 glucose, 5 sodium ascorbate, 2 thiourea, 3 sodium pyruvate; 315–325 mOsm, pH 7.3–7.4. The intracellular pipette solution contained (in mM): 135 K gluconate, 10 KCl, 10 HEPES, 10 MgCl2, 2 Na-ATP, 0.4 Na-GTP; 280–290 mOsm, pH 7.3–7.4. All salts and sugars were purchased from Sigma-Aldrich (St. Louis, MO, USA) or Fisher Scientific (Pittsburgh, PA, USA). The synaptic blockers 2,3-dihydroxy-6-nitro-7-sulfamoyl-benzo[f]quinoxaline (NBQX), D-(-)-2-amino-5-phosphonopentanoic acid (APV), and 2-(3-carboxypropyl)-3-amino-6-(4 methoxyphenyl) pyridazinium bromide (gabazine) were purchased from Ascent Scientific (Princeton, NJ, USA). All synaptic blockers were prepared as stock solutions (NBQX 5 mM, APV 25 mM, gabazine 6 mM) in Milli-Q water and stored at −20°C. They were diluted to working concentrations (NBQX 5 μM, APV 50 μM, gabazine 6 μM) in ACSF on the day of use. All pharmacological agents were introduced to the olfactory bulb slices through the bath chamber using ACSF as the control vehicle.
Serotonin hydrochloride (5-HT, H9523–100 mg, Sigma) was prepared at stock concentration (0.8 mM) in ACSF and was diluted to working concentrations (40 μM) in ACSF on the day of use. Stock solutions were prepared in Milli-Q water for the following drugs that were then diluted in ACSF to working concentrations on the day of use: 5 mM acetylcholine chloride (ACh, A6625-10 mg, Sigma), 0.2 mM cholecystokinin octapeptide (sulfated) ammonium salt (CCK, H2080-1 mg, Bachem Americas, Inc., Torrance, CA), 0.1 mM leptin (116–130) amide (mouse) trifluoroacetate salt (Leptin, H3966-1 mg, Bachem).
Olfactory Bulb Slice Electrophysiology
Mice were anesthetized by inhalation of isoflurane (see Ethical Approval section), quickly decapitated, and then the olfactory bulbs were exposed by removing the dorsal and lateral portions of the skull between the lambda suture and the cribriform plate. The olfactory bulbs were harvested and prepared for slice electrophysiology as described previously (Fadool et al., 2011). Briefly, after removing the dura, a portion of forebrain attached with the olfactory bulbs were cut and quickly glued to a sectioning block with Superglue (Lowe's Home Improvement, USA), and submerged in oxygenated (95%O2 / 5%CO2), ice-cold, sucrose-modified ACSF for ~2 minutes (min) prior to vibratome sectioning (Vibratome/Leica Model 1000, Wetzlar, Germany). Coronal sections were made at a thickness of 300 μM and then allowed to recover in an interface chamber (Krimer and Goldman-Rakic, 1997) for 20–30 min at ~33°C containing oxygenated ACSF. The slices were then maintained at room temperature (~23°C) for about 60 min before recording. Olfactory bulb slices were recorded in a continuously-perfused (Ismatec; 1–2 ml/min), submerged-slice recording chamber (RC-26, Warner Instruments, Hamden, CT) with ACSF at room temperature. Slices were visualized at 10× and 40× using an Axioskop 2FS Plus microscope (Carl Zeiss Microimaging, Inc., Thornwood, NY) equipped with infrared detection capability (Dage MTI, CCD100, Michigan, IN). Electrodes were fabricated from borosilicate glass (Hilgenberg #1405002, Malsfeld, Germany) to a pipette resistance ranging from 9 to 15 MΩ. Positive pressure was retained while navigating through the olfactory bulb laminae until a slight increase in the pipette resistance (typically 0.1–0.2 MΩ) was observed; indicating that the pipette tip had made contact with the cell. A giga-ohm seal (Re = 2.0–16.4 GΩ) was achieved by releasing positive pressure and simultaneously applying a light suction. The whole-cell configuration was established by applying a rapid but strong suction to the lumen of the pipette while monitoring resistance.
After establishing a whole-cell configuration, PPG neurons were first sampled for adequate resting potential (< –70 mV) and proper series resistance (<60 MΩ) prior to initiating a series of current-clamp recordings. Perithreshold current levels were determined by incrementally injecting 1,200 milliseconds (ms)-long, 25 pA steps of current every 10 s, starting at −100 pA. Following the determination of spike threshold, cells were then stimulated with a long, perithreshold current step of 5,000 ms duration (typically ranging from 5 to 50 pA) every 18 s to acquire spike frequency data.
Data Acquisition and Statistical Analysis
Current-clamp experiments were performed using a Multiclamp 700B amplifier (Axon Instruments, Molecular Devices, Sunnyvale, CA). The analog signal was filtered at 10 kHz and minimally digitally sampled every 100 μs. The signals were digitized with a Digidata 1440A digitizer (Axon Instruments, Molecular Devices). The pipette capacitance was electrically compensated through the capacitance neutralization circuit of the Multiclamp 700B amplifier. Resting membrane potentials were corrected for a calculated −14 mV junction potential offset. Membrane capacitance and input resistance were acquired from the membrane test function of Clampex 10.3 (Axon Instruments). Data were analyzed using Clampfit 10.3 (Axon CNS), in combination with the analysis packages Origin 8.0 (MicroCal Software, Northampton, MA), and Igor Pro 6.0.2 (Wavemetrics Inc., Portland, OR) with the NeuroMatics 2.02 plugin (written by Jason Rothman). Baseline, treatment, and washout values were calculated from the mean of at least 10 consecutive traces. Statistical significance was determined between baseline biophysical property and that following the modulator using a two-tailed, paired t-test or a one-way repeated measures (RM) analysis of variance (ANOVA) at the 95% confidence level (α = 0.05). All sampled populations were analyzed using Prism 6 (GraphPad Software Inc., CA, USA). All reported values are mean (standard deviation -SD) unless otherwise noted.
Results
Electrophysiological Properties of PPG Neurons
Under our recording conditions nearly all PPG neurons lacked spontaneous firing at rest. Once an adequate resting membrane potential (< −70 mV) was sampled, perithreshold current levels were determined by incrementally injecting 1,200 milliseconds (ms)-long, 25 pA steps of current every 10 s, starting at −100 pA (Figure 3). All PPG neurons showed a “sag” potential at a hyperpolarized state. The “sag” potential is associated with hyperpolarization-activated, cyclic nucleotide-gated (HCN) channels (He et al., 2014) and is defined as the membrane potential difference between the peak potential and the tail potential. Basic electrophysiological properties of PPG neurons are tabled for a population of 21 neurons along with a representative recording and summary graph of action potential firing frequency vs. injected current (input-output) in Figure 3.
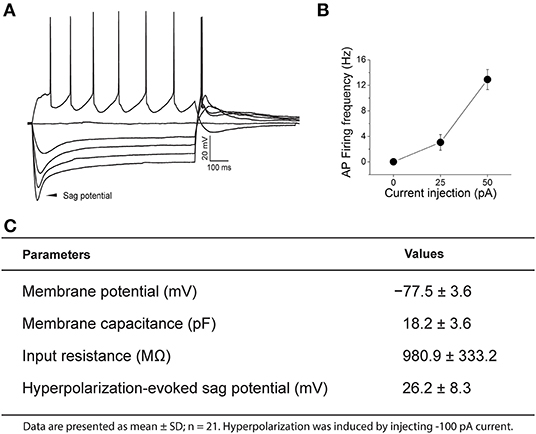
Figure 3. Representative current-clamp recording of a pre-proglucagon (PPG) neuron. (A) Perithreshold current levels were determined by incrementally injecting 1,200 ms-long, 25 pA steps of current every 10 s, starting at −100 pA. Notice the rebound firing after hyperpolarization and the degree of adaptation in action potential (AP) firing frequency. (B) Plot of injected current vs. mean action potential firing frequency (input-output relationship) for 21 sampled PPG neurons. (C) Basic intrinsic properties of a sampled population of PPG neurons are listed in the chart.
The Regulation of PPG Neurons by Centrifugal Projections
Because the olfactory bulb receives multiple centrifugal projections from higher brain areas including serotonergic, cholinergic, and noradrenergic afferents, we first examined the possible top-down regulation of PPG neurons by these centrifugal projections. Despite widespread serotonin fiber innervation, bath application of serotonin (40 μM, n = 4) had no effect on PPG neuron evoked action potential firing frequency (Figures 4A,B, paired t-test, p > 0.05). Bath application of acetylcholine (ACh; 100 μM), however, caused increased excitation of PPG neurons (Figure 5). Recording in the current-clamp mode, bath application of ACh resulted in the development of a spike train with prominent spike adaptation over the course of the burst (Figures 5A,B). With continued ACh application, spike trains ceased over the course of 2–3 min and an increase in spike frequency remained (Figure 5C). The mean spike frequency was significantly increased (1.9 ± 0.6-fold; n = 21, 1-way RM ANOVA, Tukey's post-hoc test, p < 0.001, Figure 5D), compared with that of pre-stimulation and post-stimulation (wash). In an additional two cells, ACh delayed the latency to the first spike (control: 253 ± 30 ms, ACh: 396 ± 4 ms) but did not modify spike frequency.
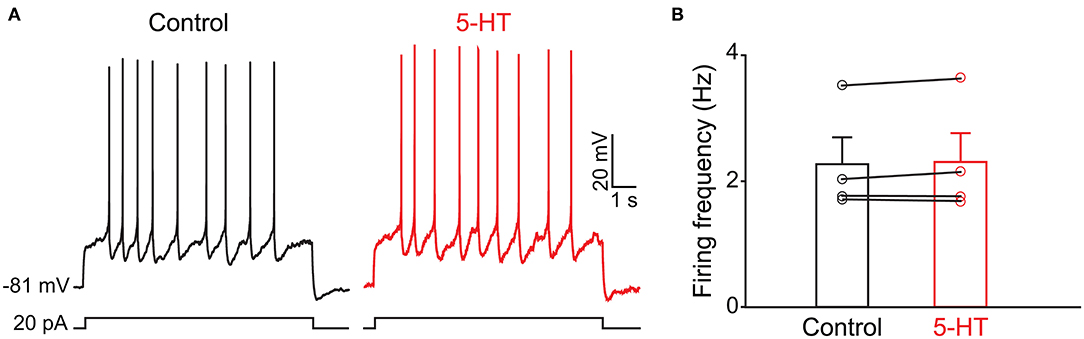
Figure 4. The activity of PPG neurons is not modulated by serotonin (5-HT) (A) Representative current-clamp recording elicited by injecting a perithreshold current of 25 pA with a pulse duration of 5 s in 18 s intervals. A baseline recording of 15 min was acquired before switching to bath application of 5-HT for an additional 30 min. (B) Bar/scatter plot of the mean AP firing frequency under baseline then 5-HT stimulation conditions in 4 cells, not significantly different, paired t-test, p > 0.05.
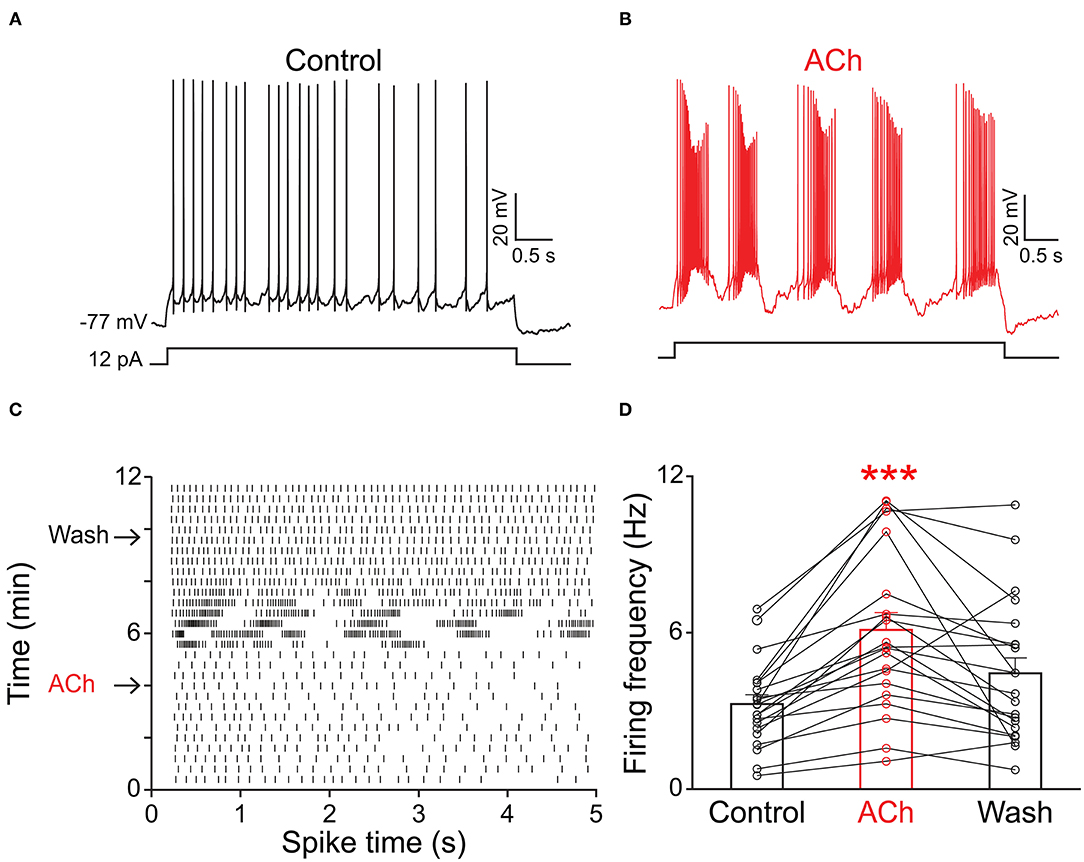
Figure 5. PPG neurons were excited by acetylcholine (ACh). Representative current-clamp recording elicited by injecting a perithreshold current of 12 pA with a pulse duration of 5 s in 18 s intervals. (A) A baseline recording of 5 min was acquired before (B) switching to bath application of ACh for 5 min, followed by a wash out. Note development of bursting activity with prominent spike adaptation within the burst. (C) Example raster plot of the cell in A-B indicating 5 s pulse duration vs. time of recording period (12 min). Arrows indicate the times when ACh was introduced or washed from the bath, respectively. (D) Bar/line graph of the mean spike frequency changes for 21 sampled PPG neurons under baseline, ACh, and wash conditions. ***Significantly-different from baseline, one-way RM ANOVA with Tukey's post-hoc test, p < 0.001.
The Regulation of PPG Neurons by Metabolic-Related Signals
Previous evidence has shown that PPG neurons in the NTS can be modulated by metabolic-related hormones such as leptin or cholecystokinin (CCK) (Hisadome et al., 2010, 2011). Bath application of leptin did not significantly modulate action potential firing frequency of PPG neurons (Figures 6A,B, paired t-test, p > 0.05). Bath application of CCK (0.8 μM), however, led to either a significant increase in firing in 52 percent of the recorded neurons (1.7 ± 0.4-fold; n = 11, 1-way RM ANOVA, Tukey's post-hoc test, p < 0.01, Figures 7A–D) or cessation of firing (n = 10, Figures 7E–H) in 48% of the recorded neurons, where a majority of these inhibited neurons (8 of 10) did not recover following washout. Following the ingestion of a meal, another altered signal other than satiety hormones can be glucose availability. We were curious as to whether PPG neurons might be glucose sensitive as we previously reported for that of MCs (Tucker et al., 2013). PPG neurons were thus stimulated with a peri-stimulus evoked current intensity (40 pA) and then bath application of the standard ACSF (22 mM glucose) was switched to a modified ACSF balanced osmotically with mannitol (1 mM glucose). A subset of PPG neurons (6 of 16 cells; 38%) showed a modest increase in action potential firing frequency (1.2 ± 0.4-fold) that was not significantly different than that of baseline (paired t-test, p = 0.13) and was accompanied by a 1–2 mV depolarization (Figure 8).
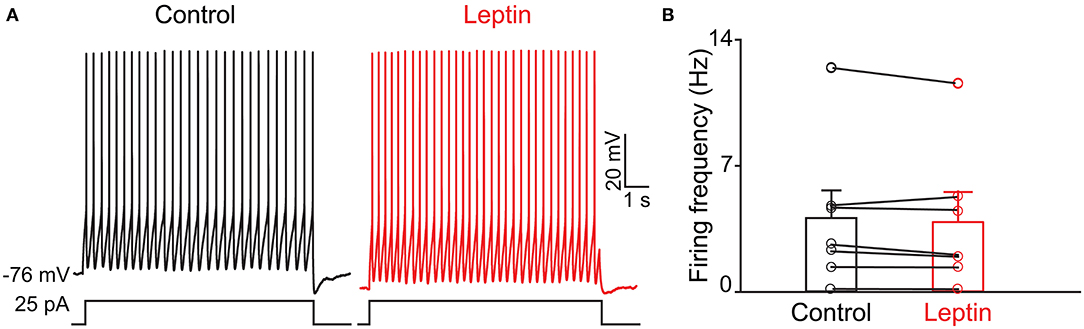
Figure 6. The activity of PPG neurons is not modulation by leptin. (A) Representative current-clamp recording and (B) bar/scatter plot of the mean AP firing frequency as in Figure 4, but for leptin, n = 7, not significantly different from baseline, paired t-test, p > 0.05.
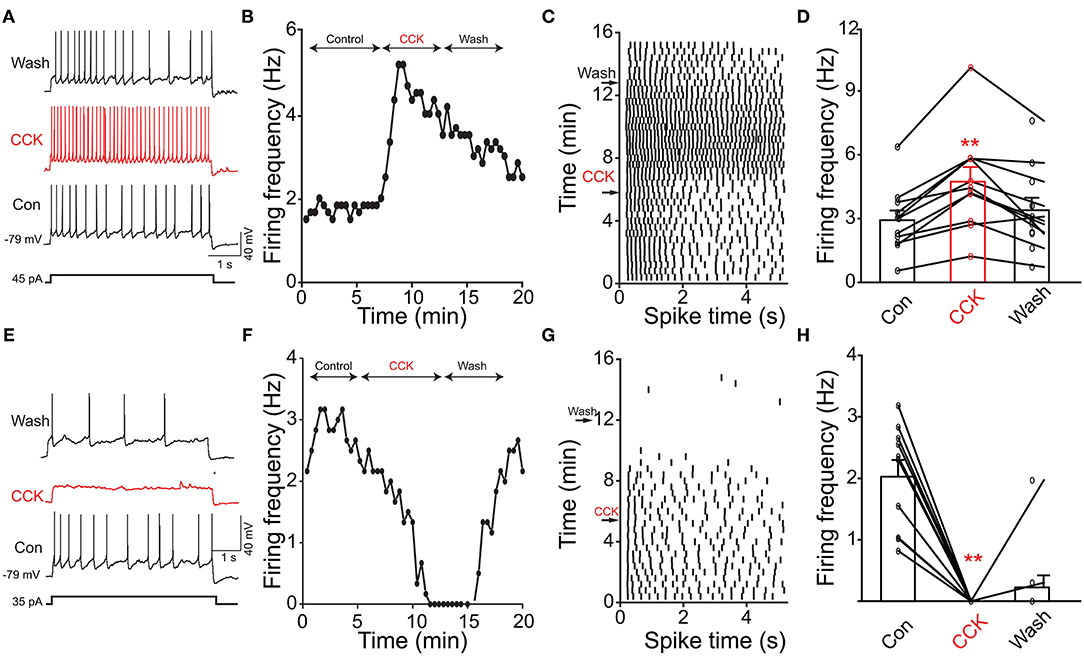
Figure 7. Cholecystokinin (CCK) evoked increased or decreased excitability of PPG neurons. (A) Representative action potentials elicited under baseline (Con), neuromodulator (CCK), and washout (Wash) conditions. (B) Line graph of the recording in A where AP firing frequency vs. time of the recording is plotted. (C) Example raster plot of the cell in (A,B). (D) Bar/line graph of the mean spike frequency changes for 11 sampled PPG neurons. **Significantly-different from baseline and wash, one-way RM ANOVA with Tukey's post-hoc test, p < 0.01. (E–H) Same as top panels but for 10 sampled PPG neurons that were inhibited by CCK.
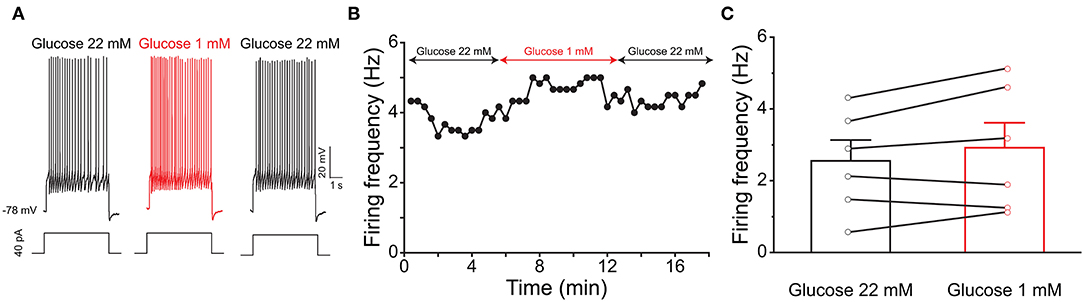
Figure 8. PPG neurons are not glucose sensitive. (A) Representative current-clamp recording elicited by injecting a perithreshold current of 40 pA with a pulse duration of 5 s in 18 s intervals. A baseline recording of 6 min was acquired for the PPG neuron under control ASCF conditions (22 mM) before switching to a bath application of low glucose (1 mM) for 6 min, followed by a return to original control ASCF for 6 min. (B) Line graph of the cell in (A) plotting action potential firing frequency over time. (C) Bar/line graph of the mean spike frequency changes for 6 of 16 sampled PPG neurons that had a change of at least 0.5 Hz following low glucose. Not significantly-different from baseline and wash, one-way RM ANOVA with Tukey's post-hoc test, p >0.05.
Discussion
Performing ex vivo olfactory bulb slice experiments allowed us to understand the extent of neuromodulation of PPG neurons, a unique excitatory interneuron that is part of a recently discovered microcircuit. We discovered that these PPG neurons exhibit enhanced bursting and firing frequency in the presence of the neurotransmitter ACh yet are unmodulated by serotonin. Given that the olfactory bulb integrates both intrinsic and extrinsic regulatory feedback to shape MC and TC excitability before conveying the olfactory information to the piriform cortex (Cleland and Linster, 2005; Devore and Linster, 2012; Igarashi et al., 2012; Linster and Cleland, 2016; Lizbinski and Dacks, 2017), it appears that extrinsic sources of modulation that have been richly studied in the olfactory system (McLean and Shipley, 1987a,b; Mandairon et al., 2006; Matsutani and Yamamoto, 2008; Fletcher and Chen, 2010; Devore and Linster, 2012; Lizbinski and Dacks, 2017; Brunert and Rothermel, 2021) could significantly impact the function of PPG neurons (Figure 9). Extrinsic neuromodulation is thought to provide contextual information regarding the behavioral and chemical state of an animal and to influence olfactory sensitivity and olfactory-based behaviors. We also found that these PPG neurons could be differentially modulated by the metabolic-related hormone CCK but were not responsive to leptin. Metabolic peptides, neuropeptides, and hormones represent an extra source of extrinsic modulation in the olfactory system (Palouzier-Paulignan et al., 2012).
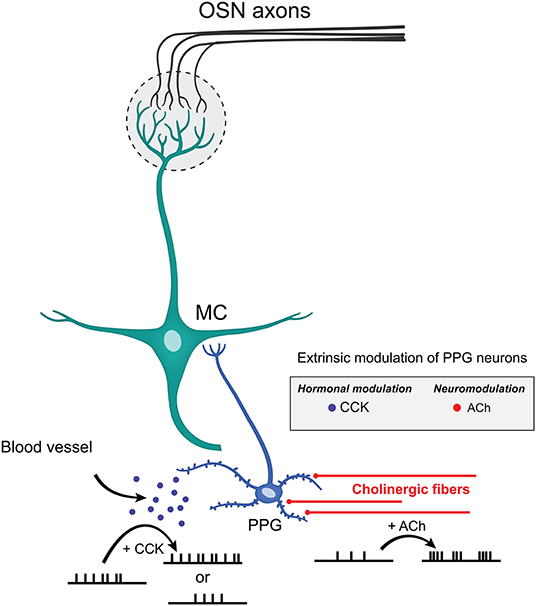
Figure 9. Extrinsic modulation of PPG neurons. Schematic diagram summarizing the effects mediated by CCK and ACh on PPG neuronal firing. CCK release by the blood vessels may either reduce or enhance AP firing frequency while ACh released by cholinergic fibers increases action potential firing frequency while eliciting bursting behavior. Such diversity of modulation of PPG neurons within a PPG>MC>GC microcircuit could allow great dynamics of outputted information by MCs to the higher olfactory cortical areas.
In defining the intrinsic properties of PPG neurons, the resting potential was more negative than that of GCs in general, but the input resistance was very much in keeping with values reported for GCs (Wellis and Kauer, 1994; Hall and Delaney, 2002; Pinato and Midtgaard, 2003). All PPG neurons exhibited a significant hyperpolarization-induced “sag” potential. The “sag” potential is attributed to a hyperpolarization-activated, cyclic nucleotide-gated (HCN) channel (He et al., 2014). In mammals there are four subtypes of this channel (HCN1-4) expressed widely throughout the CNS (Notomi and Shigemoto, 2004). Although all isoforms have been immunocytochemically identified in the olfactory bulb, HCN3-expressing axon bundles have been noted to be dispersed across the GCL (Notomi and Shigemoto, 2004). Activation of HCN channels leads to increased permeability of potassium and sodium ions, producing an inward, Ih current (Biel et al., 2009). Ih current is known to play important roles in stabilizing the resting membrane potential (Llinas and Jahnsen, 1982; Lupica et al., 2001) and integrating the synaptic inputs (Magee, 1998). Ih current has been implicated in a variety of physiological processes including learning and memory, sleep and wakefulness, sensation, and perception (Robinson and Siegelbaum, 2003). It has been shown that Ih currents are involved in adjusting sensory signal transduction and perceiving environmental stimuli (Orio et al., 2009; Zhou et al., 2010). In the visual system, Ih current has been well-characterized in photoreceptor cells where it has been shown to contribute to visual adaptation to bright light (Bader et al., 1979; Attwell and Wilson, 1980). In the taste system, HCN channels generate the sensory receptor potential to mediate sour taste response (Stevens et al., 2001). In general, HCN channels are regulated by wide-ranging cellular signals and their dysregulation has been shown to involve multiple pathological conditions such as epilepsy, neuropathic pain, parkinsonian disease (He et al., 2014). Interestingly ACh can both inhibit (Heys et al., 2010) and upregulate (Pian et al., 2007) HCN channels. It will be interesting to examine whether the modulation of PPG neurons by ACh is targeting Ih current, which may adjust olfactory signal transduction and eventually lead to changes in the olfactory perception.
The important role of cholinergic modulation of olfactory acuity has been long established (Fletcher and Wilson, 2002; Wilson et al., 2004; Chaudhury et al., 2009; Devore et al., 2014; D'Souza and Vijayaraghavan, 2014; Smith et al., 2015; Linster and Cleland, 2016; Cho and Linster, 2020). More specifically, odor response tuning of M/TCs is sharpened by the cholinergic input, thereby facilitating contrast enhancement (Castillo et al., 1999; Ma and Luo, 2012). The olfactory bulb receives cholinergic input from the horizontal limb of the diagonal band of Broca (HDB) of the basal forebrain (Záborszky et al., 1986; Kasa et al., 1995). Interestingly, this basal forebrain cholinergic system also projects to the hypothalamus and has been shown to modulate appetite-related synapses in lateral hypothalamic slices (Jo et al., 2005). A recent study has shown that the basal forebrain to hypothalamus cholinergic circuit plays an important role in regulating feeding behavior (Herman et al., 2016). When the cholinergic signaling was impaired either by ablating cholinergic neurons or knockdown of the transmitter's degradation enzyme, acetylcholine transferase, animals showed increased food intake leading to severe obesity. Alternatively, enhanced cholinergic signaling led to decreased food intake. Analogous to these studies, a link between satiation/positive energy state and altered olfactory processes could be constructed. Through unknown mechanisms, feeding activates the basal forebrain cholinergic neurons (Herman et al., 2016), which, in turn, will act on hypothalamic targets to exert their appetite suppression effect. One could speculate that this could simultaneously modulate the activity of PPG neurons in the olfactory bulb to alter olfactory processes.
Hormones and nutritionally important molecules that govern our state of satiety and hunger are classically defined as either orexigenic or anorexigenic signals, meaning those that stimulate or inhibit food intake, respectively. These molecules are produced by the gastrointestinal tract, adipose tissue, and the pancreas, and serve as an additional source of extrinsic modulation to the olfactory system, and, in particular, the olfactory bulb (Palouzier-Paulignan et al., 2012; Julliard et al., 2017; Kolling and Fadool, 2020). Receptors for orexigenic signaling pathways (i.e., ghrelin, neuropeptide Y, endocannabinoids, orexin, somatostatin) and anorexigenic pathways (i.e., insulin, GLP-1, leptin, and CCK) are expressed throughout the neurolamina of the olfactory bulb [see detailed reviews - Palouzier-Paulignan et al. (2012) and Julliard et al. (2017)].
Leptin and CCK are two anorectic hormones that we examined as neuromodulators of PPG neurons. Both are synthesized in the periphery and curb hunger. Removal of leptin or leptin receptors in mice causes an increase in olfactory performance in hidden odor tasks, which is decreased to control levels when the hormone is restored (Getchell et al., 2006). Central injections of leptin into fasted rats causes a dose-dependent decrease in olfactory detection (Julliard et al., 2007). Leptin receptors are found in the GML and on MCs (Shioda et al., 1998; Prud'homme et al., 2009), and also on astrocytes within the GCL, rather than on neurons (Prud'homme et al., 2009). This astrocytic pattern of GCL expression of the hormone receptor may be consistent with our lack of direct modulation of the PPG neurons in this lamina. In contrast, PPG neurons had differential responses to CCK: some neurons were excited, and some were inhibited. Such a heterogeneous response might suggest different subtypes of PPG neurons that express different CCK receptors or different activation of downstream intracellular signaling pathways. As a whole, few functional studies have examined CCK modulation in the olfactory bulb. Ex vivo recordings have indicated that CCK modulates MCs excitability by increasing action potential frequency (Ma et al., 2013) and behavioral studies have shown that activation of CCK receptors (CCK-A and CCK-B) modulate olfactory recognition and memory retention in rodents (Lemaire et al., 1994a,b).
Finally, the blood brain barrier surrounding the olfactory bulb is more permeable than other brain regions (Ueno et al., 1991, 1996) and it has been suggested that metabolic molecules can easily penetrate and bind to receptors for hormones broadly expressed in the olfactory system to modulate the electrical activity of olfactory networks (Fadool et al., 2000, 2011; Apelbaum et al., 2005; Hardy et al., 2005a; Lacroix et al., 2008; Savigner et al., 2009; Kuczewski et al., 2014). For example, insulin and glucose modulate the firing activity of MCs through post-translational modifications and other interactions with the voltage-gated potassium ion channel, Kv1.3 (Fadool et al., 2000, 2011; Savigner et al., 2009; Kuczewski et al., 2014). Despite this, we did not observe any significant glucose sensitivity of PPG neurons under our recording conditions. It may be that a combined environment where there are changes in both neurotransmission and metabolic factors, is required to produce synergistic changes for modulation of PPG neuronal excitability. It would be interesting in future investigations to explore cholinergic modulation, for example, while modifying glucose availability.
In summary, our study has furthered our biophysical understanding of a novel class of dSACs called PPG neurons that define a microcircuit within the olfactory bulb to modulate MC outputs. Future experiments need to probe olfactory behavioral changes in response to loss or gain of PPG neuron function. Because both central and peripheral effects of GLP-1 have demonstrated reduction in food intake (Williams, 2009), links between olfactory and ingestive behaviors should be sought. Due to the fact that GLP-1 is secreted after meal ingestion, it's possible that the GLP-1 system in the olfactory bulb could link weaker odor sensing to satiety state to inhibit food intake.
Data Availability Statement
The original contributions presented in the study are included in the article/supplementary material, further inquiries can be directed to the corresponding author.
Author Contributions
DF, RT, AL, and ZH: conceptualization. ZH: electrophysiological data collection and analysis. RT and DL: figure preparation. AL and RT: lead citation research and compiling. ZH, AL, RT, and DL: writing an original draft section. DF: writing—review and editing, supervision, project administration, and funding acquisition. All authors contributed to the article, written revisions, and approval of the submitted version.
Funding
This work was supported by NIH Chemosensory Training Grant T32DC000044 and R01DC0013080 from the NIDCD, funding from the Robinson Family Foundation at Tallahassee Memorial Hospital, the Latin-American Caribbean Scholarship, and a bridge award from the FSU Research Foundation.
Conflict of Interest
The authors declare that the research was conducted in the absence of any commercial or financial relationships that could be construed as a potential conflict of interest.
Acknowledgments
We would like to thank Ms. Carley Huffstetler for assistance with citation management of our manuscript. We are appreciative of excellent laboratory management and animal husbandry provided by Ms. Abigail Thomas and Carley Huffstetler. We are most grateful for artwork of our figures provided by Mr. Charles Badland, graphic artist for our FSU Program in Neuroscience.
Abbreviations
Ach, acetylcholine; AVMA, American Veterinary Medicine Association; ANOVA, analysis of variance; AON, anterior olfactory nucleus; aPCx, anterior piriform cortex; ACSF, artificial cerebrospinal fluid; CCK, cholecystokinin; cAMP, cyclic adenosine monophosphate; dSAC, deep short axon cell; EPL, external plexiform layer; EPLi, external plexiform layer interneuron; ETC, external tufted cell; FSU, Florida State University; GPCR, G-protein-coupled receptor; GML, glomerular layer; GLP-1, glucagon-like peptide-1; GC, granule cell; GCL, granule cell layer; HDB, horizontal limb of the diagonal band of Broca; HCN, hyperpolarization-activated, cyclic nucleotide-gated ion channel; IACUC, Institutional Animal Care and Use Committee; IPL, internal plexiform layer; JG, juxtaglomerular; MC, mitral cell; MCL, mitral cell layer; M/TC, mitral/tufted cell; mAChR, muscarinic acetylcholine receptor; NIH, National Institute of Health; nAChR, nicotinic acetylcholine receptor; NTS, nucleus tractus solitarius; OB, olfactory bulb; OSN, olfactory sensory neuron; PG, periglomerular; PGC, periglomerular cell; PPG, pre-pro-glucagon; RFP, red fluorescent protein; RM, repeated measures; SAC, short axon cell; sSAC, superficial short axon cell; STC, superficial tufted cell; SNARE, soluble NSF attachment protein receptor; sTC, superficial tufted cell; tdRFP, tandem-dimer red fluorescent protein; TC, tufted cell.
References
Abraham, N. M., Egger, V., Shimshek, D. R., Renden, R., Fukunaga, I., Sprengel, R., et al. (2010). Synaptic inhibition in the olfactory bulb accelerates odor discrimination in mice. Neuron 65, 399–411. doi: 10.1016/j.neuron.2010.01.009
Ackels, T., Jordan, R., Schaefer, A. T., and Fukunaga, I. (2020). Respiration-locking of olfactory receptor and projection neurons in the mouse olfactory bulb and its modulation by brain state. Front. Cell Neurosci. 14:220. doi: 10.3389/fncel.2020.00220
Alkam, T., Hiramatsu, M., Mamiya, T., Aoyama, Y., Nitta, A., Yamada, K., et al. (2011). Evaluation of object-based attention in mice. Behav. Brain Res. 220, 185–193. doi: 10.1016/j.bbr.2011.01.039
Apelbaum, A., Perrut, A., and Chaput, M. (2005). Orexin A effects on the olfactory bulb spontaneous activity and odor responsiveness in freely breathing rats. Regul. Pept. 129, 49–61. doi: 10.1016/j.regpep.2005.01.003
Arevian, A. C., Kapoor, V., and Urban, N. N. (2008). Activity-dependent gating of lateral inhibition in the mouse olfactory bulb. Nat. Neurosci. 11, 80–87. doi: 10.1038/nn2030
Attwell, D., and Wilson, M. (1980). Behaviour of the rod network in the tiger salamander retina mediated by membrane properties of individual rods. J. Physiol. 309, 287–315. doi: 10.1113/jphysiol.1980.sp013509
Aungst, J. L., Heyward, P. M., Puche, A. C., Karnup, S. V., Hayar, A., Szabo, G., et al. (2003). Centre-surround inhibition among olfactory bulb glomeruli. Nature 426, 623–629. doi: 10.1038/nature02185
Bader, C. R., Macleish, P. R., and Schwartz, E. A. (1979). A voltage-clamp study of the light response in solitary rods of the tiger salamander. J. Physiol. 296, 1–26. doi: 10.1113/jphysiol.1979.sp012988
Baly, C., Aioun, J., Badonnel, K., Lacroix, M. C., Durieux, D., Schlegel, C., et al. (2007). Leptin and its receptors are present in the rat olfactory mucosa and modulated by the nutritional status. Brain Res. 1129, 130–141. doi: 10.1016/j.brainres.2006.10.030
Banerjee, A., Marbach, F., Anselmi, F., Koh, M. S., Davis, M. B., Garcia da, S. P., et al. (2015). An interglomerular circuit gates glomerular output and implements gain control in the mouse olfactory bulb. Neuron 87, 193–207. doi: 10.1016/j.neuron.2015.06.019
Banks, W. A. (2001). Leptin transport across the blood-brain barrier: implications for the cause and treatment of obesity. Curr. Pharm. Des. 7, 125–133. doi: 10.2174/1381612013398310
Beinfeld, M. C., Meyer, D. K., Eskay, R. L., Jensen, R. T., and Brownstein, M. J. (1981). The distribution of cholecystokinin immunoreactivity in the central nervous system of the rat as determined by radioimmunoassay. Brain Res. 212, 51–57. doi: 10.1016/0006-8993(81)90031-7
Belluscio, L., Lodovichi, C., Feinstein, P., Mombaerts, P., and Katz, L. C. (2002). Odorant receptors instruct functional circuitry in the mouse olfactory bulb. Nature 419, 296–300. doi: 10.1038/nature01001
Biel, M., Wahl-Schott, C., Michalakis, S., and Zong, X. (2009). Hyperpolarization-activated cation channels: from genes to function. Physiol. Rev. 89, 847–885. doi: 10.1152/physrev.00029.2008
Brill, J., Shao, Z., Puche, A. C., Wachowiak, M., and Shipley, M. T. (2016). Serotonin increases synaptic activity in olfactory bulb glomeruli. J. Neurophysiol. 115, 1208–1219. doi: 10.1152/jn.00847.2015
Brunert, D., and Rothermel, M. (2021). Extrinsic neuromodulation in the rodent olfactory bulb. Cell Tissue Res. 383, 507–524. doi: 10.1007/s00441-020-03365-9
Burke, K. J. Jr., and Bender, K. J. (2019). Modulation of ion channels in the axon: mechanisms and function. Front. Cell. Neurosci. 13:221. doi: 10.3389/fncel.2019.00221
Burton, S. D. (2017). Inhibitory circuits of the mammalian main olfactory bulb. J. Neurophysiol. 118, 2034–2051. doi: 10.1152/jn.00109.2017
Burton, S. D., LaRocca, G., Liu, A., Cheetham, C. E., and Urban, N. N. (2017). Olfactory bulb deep short-axon cells mediate widespread inhibition of tufted cell apical dendrites. J. Neurosci. 37, 1117–1138. doi: 10.1523/JNEUROSCI.2880-16.2016
Byczkowicz, N., Eshra, A., Montanaro, J., Trevisiol, A., Hirrlinger, J., Kole, M. H., et al. (2019). HCN channel-mediated neuromodulation can control action potential velocity and fidelity in central axons. eLife 8:e42766. doi: 10.7554/eLife.42766
Castillo, P. E., Carleton, A., Vincent, J. D., and Lledo, P. M. (1999). Multiple and opposing roles of cholinergic transmission in the main olfactory bulb. J. Neurosci. 19, 9180–9191. doi: 10.1523/JNEUROSCI.19-21-09180.1999
Chaudhury, D., Escanilla, O., and Linster, C. (2009). Bulbar acetylcholine enhances neural and perceptual odor discrimination. J. Neurosci. 29, 52–60. doi: 10.1523/JNEUROSCI.4036-08.2009
Chen, Y., Yu, F. H., Surmeier, D. J., Scheuer, T., and Catterall, W. A. (2006). Neuromodulation of Na+ channel slow inactivation via CAMP-dependent protein kinase and protein kinase C. Neuron 49, 409–420. doi: 10.1016/j.neuron.2006.01.009
Cho, C., and Linster, C. (2020). Experience enhances certainty about olfactory stimuli under bulbar cholinergic control. Learn Mem. 27, 414–417. doi: 10.1101/lm.051854.120
Cleland, T. A., and Linster, C. (2005). Computation in the olfactory system. Chem. Senses 30, 801–813. doi: 10.1093/chemse/bji072
Crawley, J. N. (1985). Comparative distribution of cholecystokinin and other neuropeptides. Ann. N. Y. Acad. Sci. 448, 1–8. doi: 10.1111/j.1749-6632.1985.tb29900.x
Crespo, C., Blasco-Ibáñez, J. M., Briñón, J. G., Alonso, J. R., Domínguez, M. I., and Martínez-Guijarro, F. J. (2000). Subcellular localization of m2 muscarinic receptors in GABAergic interneurons of the olfactory bulb. Eur. J. Neurosci. 12, 3963–3974. doi: 10.1046/j.1460-9568.2000.00289.x
Devore, S., de Almeida, L., and Linster, C. (2014). Distinct roles of bulbar muscarinic and nicotinic receptors in olfactory discrimination learning. J. Neurosci. 34, 11244–11260. doi: 10.1523/JNEUROSCI.1499-14.2014
Devore, S., and Linster, C. (2012). Noradrenergic and cholinergic modulation of olfactory bulb sensory processing. Front. Behav. Neurosci. 6:52. doi: 10.3389/fnbeh.2012.00052
Doty, R. L., Bagla, R., and Kim, N. (1999). Physostigmine enhances performance on an odor mixture discrimination test. Physiol. Behav. 65, 801–804. doi: 10.1016/S0031-9384(98)00238-8
D'Souza, R. D., Parsa, P. V., and Vijayaraghavan, S. (2013). Nicotinic receptors modulate olfactory bulb external tufted cells via an excitation-dependent inhibitory mechanism. J. Neurophysiol. 110, 1544–1553. doi: 10.1152/jn.00865.2012
D'Souza, R. D., and Vijayaraghavan, S. (2012). Nicotinic receptor-mediated filtering of mitral cell responses to olfactory nerve inputs involves the 3 4 subtype. J. Neurosci. 32, 3261–3266. doi: 10.1523/JNEUROSCI.5024-11.2012
D'Souza, R. D., and Vijayaraghavan, S. (2014). Paying attention to smell: cholinergic signaling in the olfactory bulb. Front. Synaptic Neurosci. 6:21. doi: 10.3389/fnsyn.2014.00021
Dugue, G. P., and Mainen, Z. F. (2009). How serotonin gates olfactory information flow. Nat. Neurosci. 12, 673–675. doi: 10.1038/nn0609-673
Dupont, A., Merand, Y., Savard, P., Leblanc, J., and Dockray, G. J. (1982). Evidence that cholecystokinin is a neurotransmitter of olfaction in nucleus olfactorius anterior. Brain Res. 250, 386–390. doi: 10.1016/0006-8993(82)90437-1
Egger, V., and Diamond, J. S. (2020). A17 Amacrine cells and olfactory granule cells: Parallel processors of early sensory information. Front. Cell Neurosci. 14:600537. doi: 10.3389/fncel.2020.600537
Ekstrand, J. J., Domroese, M. E., Feig, S. L., Illig, K. R., and Haberly, L. B. (2001). Immunocytochemical analysis of basket cells in rat piriform cortex. J. Comp. Neurol. 434, 308–328. doi: 10.1002/cne.1179
Elmquist, J. K., Bjørbaek, C., Ahima, R. S., Flier, J. S., and Saper, C. B. (1998). Distributions of leptin receptor mRNA isoforms in the rat brain. J. Comp. Neurol. 395, 535–547. doi: 10.1002/(SICI)1096-9861(19980615)395:4<535::AID-CNE9>3.0.CO;2-2
Engel, A. K., Fries, P., and Singer, W. (2001). Dynamic predictions: oscillations and synchrony in top-down processing. Nat. Rev. Neurosci. 2, 704–716. doi: 10.1038/35094565
Eyre, M. D., Antal, M., and Nusser, Z. (2008). Distinct deep short-axon cell subtypes of the main olfactory bulb provide novel intrabulbar and extrabulbar GABAergic connections. J. Neurosci. 28, 8217–8229. doi: 10.1523/JNEUROSCI.2490-08.2008
Ezeh, P. I., Wellis, D. P., and Scott, J. W. (1993). Organization of inhibition in the rat olfactory bulb external plexiform layer. J. Neurophysiol. 70, 263–274. doi: 10.1152/jn.1993.70.1.263
Fadool, D. A., Tucker, K., and Pedarzani, P. (2011). Mitral cells of the olfactory bulb perform metabolic sensing and are disrupted by obesity at the level of the Kv1.3 ion channel. PLoS ONE 6:e24921. doi: 10.1371/journal.pone.0024921
Fadool, D. A., Tucker, K., Phillips, J. J., and Simmen, J. A. (2000). Brain insulin receptor causes activity-dependent current suppression in the olfactory bulb through multiple phosphorylation of Kv1.3. J. Neurophysiol. 83, 2332–2348. doi: 10.1152/jn.2000.83.4.2332
Fletcher, M. L., and Chen, W. R. (2010). Neural correlates of olfactory learning: critical role of centrifugal neuromodulation. Learn. Mem. 17, 561–570. doi: 10.1101/lm.941510
Fletcher, M. L., and Wilson, D. A. (2002). Experience modifies olfactory acuity: acetylcholine-dependent learning decreases behavioral generalization between similar odorants. J. Neurosci. 22:RC201. doi: 10.1523/JNEUROSCI.22-02-j0005.2002
Florey, E. (1967). Neurotransmitters and modulators in the animal kingdom. Fed. Proc. 26, 1164–1178.
Friedman, J. M., and Halaas, J. L. (1998). Leptin and the regulation of body weight in mammals. Nature 395, 763–770. doi: 10.1038/27376
Fukuchi, M., Tabuchi, A., Kuwana, Y., Watanabe, S., Inoue, M., Takasaki, I., et al. (2015). Neuromodulatory effect of Gαs - or Gαq -coupled G-protein-coupled receptor on NMDA receptor selectively activates the NMDA receptor/Ca2+/calcineurin/cAMP response element-binding protein-regulated transcriptional coactivator 1 pathway to effectively induce brain-derived neurotrophic factor expression in neurons. J. Neurosci. 35, 5606–5624. doi: 10.1523/JNEUROSCI.3650-14.2015
Fukunaga, I., Berning, M., Kollo, M., Schmaltz, A., and Schaefer, A. T. (2012). Two distinct channels of olfactory bulb output. Neuron 75, 320–329. doi: 10.1016/j.neuron.2012.05.017
Gaudry, Q. (2018). Serotonin modulation of olfaction in rodents and insects. Yale J. Biol. Med. 91, 23–32.
Getchell, T. V., Kwong, K., Saunders, C. P., Stromberg, A. J., and Getchell, M. L. (2006). Leptin regulates olfactory-mediated behavior in ob/ob mice. Physiol. Behav. 87, 848–856. doi: 10.1016/j.physbeh.2005.11.016
Ghatpande, A. S., Sivaraaman, K., and Vijayaraghavan, S. (2006). Store calcium mediates cholinergic effects on MIPSCs in the rat main olfactory bulb. J. Neurophysiol. 95, 1345–1355. doi: 10.1152/jn.00757.2005
Ghosh, S., Larson, S. D., Hefzi, H., Marnoy, Z., Cutforth, T., Dokka, K., et al. (2011). Sensory maps in the olfactory cortex defined by long-range viral tracing of single neurons. Nature 472, 217–220. doi: 10.1038/nature09945
Gómez, C., Briñón, J. G., Barbado, M. V., Weruaga, E., Valero, J., and Alonso, J. R. (2005). Heterogeneous targeting of centrifugal inputs to the glomerular layer of the main olfactory bulb. J. Chem. Neuroanat. 29, 238–254. doi: 10.1016/j.jchemneu.2005.01.005
Gray, M., Daudelin, D. H., and Golowasch, J. (2017). Activation mechanism of a neuromodulator-gated pacemaker ionic current. J. Neurophysiol. 118, 595–609. doi: 10.1152/jn.00743.2016
Guan, X. M., Hess, J. F., Yu, H., Hey, P. J., and van der Ploeg, L. H. (1997). Differential expression of mRNA for leptin receptor isoforms in the rat brain. Mol. Cell. Endocrinol. 133, 1–7. doi: 10.1016/S0303-7207(97)00138-X
Gutiérrez-Mecinas, M., Crespo, C., Blasco-Ibanez, J. M., Gracia-Llanes, F. J., Marques-Mari, A. I., and Martinez-Guijarro, F. J. (2005). Characterization of somatostatin- and cholecystokinin-immunoreactive periglomerular cells in the rat olfactory bulb. J. Comp. Neurol. 489, 467–479. doi: 10.1002/cne.20649
Hall, B. J., and Delaney, K. R. (2002). Contribution of a calcium-activated non-specific conductance to NMDA receptor-mediated synaptic potentials in granule cells of the frog olfactory bulb. J. Physiol. 543, 819–834. doi: 10.1113/jphysiol.2002.024638
Hamm, H. E., and Alford, S. T. (2020). Physiological roles for neuromodulation via Gi/o GPCRs working through G-βγ -SNARE interaction. Neuropsychopharmacology 45:221. doi: 10.1038/s41386-019-0497-2
Hardy, A. B., Aioun, J., Baly, C., Julliard, K., Caillol, M., Salesse, R., et al. (2005a). Orexin A modulates mitral cell activity in the rat olfactory bulb: patch-clamp study on slices and immunocytochemical localization of orexin receptors. Endocrinology 146, 4042–4053. doi: 10.1210/en.2005-0020
Hardy, A. B., Palouzier-Paulignan, B., Duchamp, A., Royet, J. P., and Duchamp-Viret, P. (2005b). 5-Hydroxytryptamine action in the rat olfactory bulb: in vitro electrophysiological patch-clamp recordings of juxtaglomerular and mitral cells. Neuroscience 131, 717–731. doi: 10.1016/j.neuroscience.2004.10.034
Harvey, J. D., and Heinbockel, T. (2018). Neuromodulation of synaptic transmission in the main olfactory bulb. Int. J. Environ. Res. Public Health 15:2194. doi: 10.3390/ijerph15102194
Hayar, A., Karnup, S., Ennis, M., and Shipley, M. T. (2004a). External tufted cells: a major excitatory element that coordinates glomerular activity. J. Neurosci. 24, 6676–6685. doi: 10.1523/JNEUROSCI.1367-04.2004
Hayar, A., Karnup, S., Shipley, M. T., and Ennis, M. (2004b). Olfactory bulb glomeruli: external tufted cells intrinsically burst at theta frequency and are entrained by patterned olfactory input. J. Neurosci. 24, 1190–1199. doi: 10.1523/JNEUROSCI.4714-03.2004
Hayar, A., Shipley, M. T., and Ennis, M. (2005). Olfactory bulb external tufted cells are synchronized by multiple intraglomerular mechanisms. J. Neurosci. 25, 8197–8208. doi: 10.1523/JNEUROSCI.2374-05.2005
He, C., Chen, F., Li, B., and Hu, Z. (2014). Neurophysiology of HCN channels: from cellular functions to multiple regulations. Prog. Neurobiol. 112, 1–23. doi: 10.1016/j.pneurobio.2013.10.001
Herman, A. M., Ortiz-Guzman, J., Kochukov, M., Herman, I., Quast, K. B., Patel, J. M., et al. (2016). A cholinergic basal forebrain feeding circuit modulates appetite suppression. Nature 538, 253–256. doi: 10.1038/nature19789
Heys, J. G., Giocomo, L. M., and Hasselmo, M. E. (2010). Cholinergic modulation of the resonance properties of stellate cells in layer II of medial entorhinal cortex. J. Neurophysiol. 104, 258–270. doi: 10.1152/jn.00492.2009
Hisadome, K., Reimann, F., Gribble, F. M., and Trapp, S. (2010). Leptin directly depolarizes preproglucagon neurons in the nucleus tractus solitarius: electrical properties of glucagon-like peptide 1 neurons. Diabetes 59, 1890–1898. doi: 10.2337/db10-0128
Hisadome, K., Reimann, F., Gribble, F. M., and Trapp, S. (2011). CCK stimulation of GLP-1 neurons involves alpha1-adrenoceptor-mediated increase in glutamatergic synaptic inputs. Diabetes 60, 2701–2709. doi: 10.2337/db11-0489
Huang, L., Garcia, I., Jen, H. I., and Arenkiel, B. R. (2013). Reciprocal connectivity between mitral cells and external plexiform layer interneurons in the mouse olfactory bulb. Front. Neural Circuits 7:32. doi: 10.3389/fncir.2013.00032
Huang, Z., Thiebaud, N., and Fadool, D. A. (2017). Differential serotonergic modulation across the main and accessory olfactory bulbs. J. Physiol. 595, 3515–3533. doi: 10.1113/JP273945
Igarashi, K. M., Ieki, N., An, M., Yamaguchi, Y., Nagayama, S., Kobayakawa, K., et al. (2012). Parallel mitral and tufted cell pathways route distinct odor information to different targets in the olfactory cortex. J. Neurosci. 32, 7970–7985. doi: 10.1523/JNEUROSCI.0154-12.2012
Imamura, F., Ito, A., and LaFever, B. J. (2020). Subpopulations of projection neurons in the olfactory bulb. Front. Neural Circuits 14:561822. doi: 10.3389/fncir.2020.561822
Isaacson, J. S., and Strowbridge, B. W. (1998). Olfactory reciprocal synapses: dendritic signaling in the CNS. Neuron 20, 749–761. doi: 10.1016/S0896-6273(00)81013-2
Jahr, C. E., and Nicoll, R. A. (1980). Dendrodendritic inhibition: demonstration with intracellular recording. Science 207, 1473–1475. doi: 10.1126/science.7361098
Jo, Y. H., Wiedl, D., and Role, L. W. (2005). Cholinergic modulation of appetite-related synapses in mouse lateral hypothalamic slice. J. Neurosci. 25, 11133–11144. doi: 10.1523/JNEUROSCI.3638-05.2005
Jones, S., Zylberberg, J., and Schoppa, N. (2020). Cellular and synaptic mechanisms that differentiate mitral cells and superficial tufted cells into parallel output channels in the olfactory bulb. Front. Cell. Neurosci. 14:614377. doi: 10.3389/fncel.2020.614377
Julliard, A. K., Al Koborssy, D., Fadool, D. A., and Palouzier-Paulignan, B. (2017). Nutrient sensing: another chemosensitivity of the olfactory system. Front. Physiol. 8:468. doi: 10.3389/fphys.2017.00468
Julliard, A. K., Chaput, M. A., Apelbaum, A., Aime, P., Mahfouz, M., and Duchamp-Viret, P. (2007). Changes in rat olfactory detection performance induced by orexin and leptin mimicking fasting and satiation. Behav. Brain Res. 183, 123–129. doi: 10.1016/j.bbr.2007.05.033
Kapoor, V., Provost, A. C., Agarwal, P., and Murthy, V. N. (2016). Activation of raphe nuclei triggers rapid and distinct effects on parallel olfactory bulb output channels. Nat. Neurosci. 19, 271–282. doi: 10.1038/nn.4219
Kasa, P., Hlavati, I., Dobo, E., Wolff, A., Joo, F., and Wolff, J. R. (1995). Synaptic and non-synaptic cholinergic innervation of the various types of neurons in the main olfactory bulb of adult rat: immunocytochemistry of choline acetyltransferase. Neuroscience 67, 667–677. doi: 10.1016/0306-4522(95)00031-D
Kim, Y. S., Chu, Y., Han, L., Li, M., Li, Z., LaVinka, P. C., et al. (2014). Central terminal sensitization of TRPV1 by descending serotonergic facilitation modulates chronic pain. Neuron 81, 873–887. doi: 10.1016/j.neuron.2013.12.011
Kolling, L. J., and Fadool, D. A. (2020). “Role of olfaction for eating behavior,” in The Senses: A Comprehensive Reference, 2nd Edn., Vol. 3, eds B. Fritzsch, and W. Meyerhof (Cambridge, MA: Elsevier; Academic Press), 675–716. doi: 10.1016/B978-0-12-809324-5.23770-X
Kosaka, T., and Kosaka, K. (2007). Heterogeneity of nitric oxide synthase-containing neurons in the mouse main olfactory bulb. Neurosci. Res. 57, 165–178. doi: 10.1016/j.neures.2006.10.005
Krimer, L. S., and Goldman-Rakic, P. S. (1997). An interface holding chamber for anatomical and physiological studies of living brain slices. J. Neurosci. Methods 75, 55–58. doi: 10.1016/S0165-0270(97)02265-6
Kuczewski, N., Fourcaud-Trocme, N., Savigner, A., Thevenet, M., Aime, P., Garcia, S., et al. (2014). Insulin modulates network activity in olfactory bulb slices: impact on odour processing. J. Physiol. 592, 2751–2769. doi: 10.1113/jphysiol.2013.269639
Lacroix, M. C., Badonnel, K., Meunier, N., Tan, F., Schlegel-Le Poupon, C., Durieux, D., et al. (2008). Expression of insulin system in the olfactory epithelium: first approaches to its role and regulation. J. Neuroendocrinol. 20, 1176–1190. doi: 10.1111/j.1365-2826.2008.01777.x
Le Jeune, H., Aubert, I., Jourdan, F., and Quirion, R. (1995). Comparative laminar distribution of various autoradiographic cholinergic markers in adult rat main olfactory bulb. J. Chem. Neuroanatomy 9, 99–112. doi: 10.1016/0891-0618(95)00070-N
Leary, S. (2020). AVMA Guidelines for the Euthanasia of Animals, Vol 2020.0.1. Schaumburg: American Veterinary Medical Association.
Lee, G.-H., Proenca, R., Montez, J. M., Carroll, K. M., Darvishzadeh, J. G., Lee, J. I., et al. (1996). Abnormal splicing of the leptin receptor in diabetic mice. Nature 379, 632–635. doi: 10.1038/379632a0
Lemaire, M., Barneoud, P., Bohme, G. A., Piot, O., Haun, F., Roques, B. P., et al. (1994a). CCK-A and CCK-B receptors enhance olfactory recognition via distinct neuronal pathways. Learn. Mem. 1, 153–164.
Lemaire, M., Bohme, G. A., Piot, O., Roques, B. P., and Blanchard, J. C. (1994b). CCK-A and CCK-B selective receptor agonists and antagonists modulate olfactory recognition in male rats. Psychopharmacology 115, 435–440. doi: 10.1007/BF02245565
Leopold, A. V., Shcherbakova, D. M., and Verkhusha, V. V. (2019). Fluorescent biosensors for neurotransmission and neuromodulation: engineering and applications. Front. Cell. Neurosci. 13:474. doi: 10.3389/fncel.2019.00474
Linster, C., and Cleland, T. A. (2016). Neuromodulation of olfactory transformations. Curr. Opin. Neurobiol. 40, 170–177. doi: 10.1016/j.conb.2016.07.006
Liu, P., Khvotchev, M., Li, Y. C., Chanaday, N. L., and Kavalali, E. T. (2018). Copine-6 binds to SNAREs and selectively suppresses spontaneous neurotransmission. J. Neurosci. 38, 5888–5899. doi: 10.1523/JNEUROSCI.0461-18.2018
Liu, S., Aungst, J. L., Puche, A. C., and Shipley, M. T. (2012). Serotonin modulates the population activity profile of olfactory bulb external tufted cells. J. Neurophysiol. 107, 473–483. doi: 10.1152/jn.00741.2011
Liu, S., Puche, A. C., and Shipley, M. T. (2016). The interglomerular circuit potently inhibits olfactory bulb output neurons by both direct and indirect pathways. J. Neurosci. 36, 9604–9617. doi: 10.1523/JNEUROSCI.1763-16.2016
Liu, X., and Liu, S. (2018). Cholecystokinin selectively activates short axon cells to enhance inhibition of olfactory bulb output neurons: CCK selectively activates olfactory bulb short axon cells. J. Physiol. 596, 2185–2207. doi: 10.1113/JP275511
Lizbinski, K. M., and Dacks, A. M. (2017). Intrinsic and extrinsic neuromodulation of olfactory processing. Front. Cell. Neurosci. 11:424. doi: 10.3389/fncel.2017.00424
Llinas, R., and Jahnsen, H. (1982). Electrophysiology of mammalian thalamic neurones in vitro. Nature 297, 406–408. doi: 10.1038/297406a0
Lodovichi, C., Belluscio, L., and Katz, L. C. (2003). Functional topography of connections linking mirror-symmetric maps in the mouse olfactory bulb. Neuron 38, 265–276. doi: 10.1016/S0896-6273(03)00194-6
Logsdon, S., Johnstone, A. F., Viele, K., and Cooper, R. L. (2006). Regulation of synaptic vesicles pools within motor nerve terminals during short-term facilitation and neuromodulation. J. Appl. Physiol. 100, 662–671. doi: 10.1152/japplphysiol.00580.2005
Luche, H., Weber, O., Nageswara, R. T., Blum, C., and Fehling, H. J. (2007). Faithful activation of an extra-bright red fluorescent protein in “knock-in” cre-reporter mice ideally suited for lineage tracing studies. Eur. J. Immunol. 37, 43–53. doi: 10.1002/eji.200636745
Lupica, C. R., Bell, J. A., Hoffman, A. F., and Watson, P. L. (2001). Contribution of the hyperpolarization-activated current (I(h)) to membrane potential and GABA release in hippocampal interneurons. J. Neurophysiol. 86, 261–268. doi: 10.1152/jn.2001.86.1.261
Ma, J., Dankulich-Nagrudny, L., and Lowe, G. (2013). Cholecystokinin: an excitatory modulator of mitral/tufted cells in the mouse olfactory bulb. PLoS ONE 8:e64170. doi: 10.1371/journal.pone.0064170
Ma, M., and Luo, M. (2012). Optogenetic activation of basal forebrain cholinergic neurons modulates neuronal excitability and sensory responses in the main olfactory bulb. J. Neurosci. 32, 10105–10116. doi: 10.1523/JNEUROSCI.0058-12.2012
Macrides, F., Davis, B. J., Youngs, W. M., Nadi, N. S., and Margolis, F. L. (1981). Cholinergic and catecholaminergic afferents to the olfactory bulb in the hamster: a neuroanatomical, biochemical, and histochemical investigation. J. Comp. Neurol. 203, 495–514. doi: 10.1002/cne.902030311
Magee, J. C. (1998). Dendritic hyperpolarization-activated currents modify the integrative properties of hippocampal CA1 pyramidal neurons. J. Neurosci. 18, 7613–7624. doi: 10.1523/JNEUROSCI.18-19-07613.1998
Mandairon, N., Ferretti, C. J., Stack, C. M., Rubin, D. B., Cleland, T. A., and Linster, C. (2006). Cholinergic modulation in the olfactory bulb influences spontaneous olfactory discrimination in adult rats. Eur. J. Neurosci. 24, 3234–3244. doi: 10.1111/j.1460-9568.2006.05212.x
Maniscalco, J. W., Zheng, H., Gordon, P. J., and Rinaman, L. (2015). Negative energy balance blocks neural and behavioral responses to acute stress by “silencing” central glucagon-like peptide 1 signaling in rats. J. Neurosci. 35, 10701–10714. doi: 10.1523/JNEUROSCI.3464-14.2015
Marks, C. A., Cheng, K., Cummings, D. M., and Belluscio, L. (2006). Activity-dependent plasticity in the olfactory intrabulbar map. J. Neurosci. 26, 11257–11266. doi: 10.1523/JNEUROSCI.2805-06.2006
Matsutani, S., and Yamamoto, N. (2008). Centrifugal innervation of the mammalian olfactory bulb. Anat. Sci. Int. 83, 218–227. doi: 10.1111/j.1447-073X.2007.00223.x
McLean, J. H., Darby-King, A., and Hodge, E. (1996). 5-HT2 receptor involvement in conditioned olfactory learning in the neonate rat pup. Behav. Neurosci. 110:1426. doi: 10.1037/0735-7044.110.6.1426
McLean, J. H., Darby-King, A., and Paterno, G. D. (1995). Localization of 5-HT2A receptor mRNA by in situ hybridization in the olfactory bulb of the postnatal rat. J. Comp. Neurol. 353, 371–378. doi: 10.1002/cne.903530305
McLean, J. H., and Shipley, M. T. (1987a). Serotonergic afferents to the rat olfactory bulb: I. Origins and laminar specificity of serotonergic inputs in the adult rat. J. Neurosci. 7, 3016–3028. doi: 10.1523/JNEUROSCI.07-10-03016.1987
McLean, J. H., and Shipley, M. T. (1987b). Serotonergic afferents to the rat olfactory bulb: II. Changes in fiber distribution during development. J. Neurosci. 7, 3029–3039. doi: 10.1523/JNEUROSCI.07-10-03029.1987
Mercer, L. D., and Beart, P. M. (1997). Histochemistry in rat brain and spinal cord with an antibody directed at the cholecystokinin-A receptor. Neurosci. Lett. 225, 97–100. doi: 10.1016/S0304-3940(97)00197-3
Mercer, L. D., Le, V. Q., Nunan, J., Jones, N. M., and Beart, P. M. (2000). Direct visualization of cholecystokinin subtype2 receptors in rat central nervous system using anti-peptide antibodies. Neurosci. Lett. 293, 167–170. doi: 10.1016/S0304-3940(00)01504-4
Merchenthaler, I., Lane, M., and Shughrue, P. (1999). Distribution of pre-pro-glucagon and glucagon-like peptide-1 receptor messenger RNAs in the rat central nervous system. J. Comp Neurol. 403, 261–280. doi: 10.1002/(SICI)1096-9861(19990111)403:2<261::AID-CNE8>3.0.CO;2-5
Morash, B., Li, A., Murphy, P. R., Wilkinson, M., and Ur, E. (1999). Leptin gene expression in the brain and pituitary gland. Endocrinology 140, 5995–5998. doi: 10.1210/endo.140.12.7288
Moro, A., van Woerden, G. M., Toonen, R. F., and Verhage, M. (2020). CaMKII controls neuromodulation via neuropeptide gene expression and axonal targeting of neuropeptide vesicles. PLoS Biol. 18:e3000826. doi: 10.1371/journal.pbio.3000826
Muntean, B. S., Zucca, S., MacMullen, C. M., Dao, M. T., Johnston, C., Iwamoto, H., et al. (2018). Interrogating the spatiotemporal landscape of neuromodulatory GPCR signaling by real-time imaging of camp in intact neurons and circuits. Cell Rep. 24, 1081–1084. doi: 10.1016/j.celrep.2018.07.031
Nagayama, S., Homma, R., and Imamura, F. (2014). Neuronal organization of olfactory bulb circuits. Front. Neural Circuits. 8:98. doi: 10.3389/fncir.2014.00098
Najac, M., Sanz, D. A., Kumar, A., Benito, N., Charpak, S., and De Saint, J. D. (2015). Intraglomerular lateral inhibition promotes spike timing variability in principal neurons of the olfactory bulb. J. Neurosci. 35, 4319–4331. doi: 10.1523/JNEUROSCI.2181-14.2015
Newton, A. C., Bootman, M. D., and Scott, J. D. (2016). Second messengers. Cold Spring Harb. Perspect. Biol. 8:a005926. doi: 10.1101/cshperspect.a005926
Notomi, T., and Shigemoto, R. (2004). Immunohistochemical localization of Ih channel subunits, HCN1-4, in the rat brain. J. Comp. Neurol. 471, 241–276. doi: 10.1002/cne.11039
Orio, P., Madrid, R., de la Pena, E., Parra, A., Meseguer, V., Bayliss, D. A., et al. (2009). Characteristics and physiological role of hyperpolarization activated currents in mouse cold thermoreceptors. J. Physiol. 587, 1961–1976. doi: 10.1113/jphysiol.2008.165738
Orona, E., Rainer, E. C., and Scott, J. W. (1984). Dendritic and axonal organization of mitral and tufted cells in the rat olfactory bulb. J. Comp. Neurol. 226, 346–356. doi: 10.1002/cne.902260305
Padmanabhan, K., Osakada, F., Tarabrina, A., Kizer, E., Callaway, E. M., Gage, F. H., et al. (2018). Centrifugal inputs to the main olfactory bulb revealed through whole brain circuit-mapping. Front. Neuroanat. 12:115. doi: 10.3389/fnana.2018.00115
Palouzier-Paulignan, B., Lacroix, M. C., Aime, P., Baly, C., Caillol, M., Congar, P., et al. (2012). Olfaction under metabolic influences. Chem. Senses 37, 769–797. doi: 10.1093/chemse/bjs059
Parker, H. E., Adriaenssens, A., Rogers, G., Richards, P., Koepsell, H., Reimann, F., et al. (2012). Predominant role of active versus facilitative glucose transport for glucagon-like peptide-1 secretion. Diabetologia 55, 2445–2455. doi: 10.1007/s00125-012-2585-2
Pavesi, E., Gooch, A., Lee, E., and Fletcher, M. L. (2012). Cholinergic modulation during acquisition of olfactory fear conditioning alters learning and stimulus generalization in mice. Learn. Mem. 20, 6–10. doi: 10.1101/lm.028324.112
Petzold, G. C., Hagiwara, A., and Murthy, V. N. (2009). Serotonergic modulation of odor input to the mammalian olfactory bulb. Nat. Neurosci. 12, 784–791. doi: 10.1038/nn.2335
Pian, P., Bucchi, A., Decostanzo, A., Robinson, R. B., and Siegelbaum, S. A. (2007). Modulation of cyclic nucleotide-regulated HCN channels by PIP(2) and receptors coupled to phospholipase C. Pflugers. Arch. 455, 125–145. doi: 10.1007/s00424-007-0295-2
Pinato, G., and Midtgaard, J. (2003). Regulation of granule cell excitability by a low-threshold calcium spike in turtle olfactory bulb. J. Neurophysiol. 90, 3341–3351. doi: 10.1152/jn.00560.2003
Plassat, J. L., Boschert, U., Amlaiky, N., and Hen, R. (1992). The mouse 5HT5 receptor reveals a remarkable heterogeneity within the 5HT1D receptor family. EMBO J. 11, 4779–4786. doi: 10.1002/j.1460-2075.1992.tb05583.x
Pompeiano, M., Palacios, J. M., and Mengod, G. (1992). Distribution and cellular localization of mRNA coding for 5-HT1A receptor in the rat brain: correlation with receptor binding. J. Neurosci. 12, 440–453. doi: 10.1523/JNEUROSCI.12-02-00440.1992
Pressler, R. T., Inoue, T., and Strowbridge, B. W. (2007). Muscarinic receptor activation modulates granule cell excitability and potentiates inhibition onto mitral cells in the rat olfactory bulb. J. Neurosci. 27, 10969–10981. doi: 10.1523/JNEUROSCI.2961-07.2007
Pressler, R. T., and Strowbridge, B. W. (2017). Direct recording of dendrodendritic excitation in the olfactory bulb: divergent properties of local and external glutamatergic inputs govern synaptic integration in granule cells. J. Neurosci. 37, 11774–11788. doi: 10.1523/JNEUROSCI.2033-17.2017
Price, J. L., and Powell, T. P. (1970a). The morphology of the granule cells of the olfactory bulb. J. Cell. Sci. 7, 91–123. doi: 10.1242/jcs.7.1.91
Price, J. L., and Powell, T. P. (1970b). The synaptology of the granule cells of the olfactory bulb. J. Cell. Sci. 7, 125–155. doi: 10.1242/jcs.7.1.125
Prud'homme, M. J., Lacroix, M. C., Badonnel, K., Gougis, S., Baly, C., Salesse, R., et al. (2009). Nutritional status modulates behavioural and olfactory bulb Fos responses to isoamyl acetate or food odour in rats: roles of orexins and leptin. Neuroscience 162, 1287–1298. doi: 10.1016/j.neuroscience.2009.05.043
Ramon y Cajal, S. (1911). Histologie du Système Nerveux de 1'homme et des Vertebrés. Paris: Maloine.
Ravel, N., Akaoka, H., Gervais, R., and Chouvet, G. (1990). The effect of acetylcholine on rat olfactory bulb unit activity. Brain Res. Bull. 24, 151–155. doi: 10.1016/0361-9230(90)90199-A
Robinson, R. B., and Siegelbaum, S. A. (2003). Hyperpolarization-activated cation currents: from molecules to physiological function. Annu. Rev. Physiol. 65, 453–480. doi: 10.1146/annurev.physiol.65.092101.142734
Rosenmund, C., and Stevens, C. F. (1996). Definition of the readily releasable pool of vesicles at hippocampal synapses. Neuron 16, 1197–1207. doi: 10.1016/S0896-6273(00)80146-4
Ross, J. M., Bendahmane, M., and Fletcher, M. L. (2019). Olfactory bulb muscarinic acetylcholine type 1 receptors are required for acquisition of olfactory fear learning. Front. Behav. Neurosci. 13:164. doi: 10.3389/fnbeh.2019.00164
Sakisaka, T., Yamamoto, Y., Mochida, S., Nakamura, M., Nishikawa, K., Ishizaki, H., et al. (2008). Dual inhibition of SNARE complex formation by tomosyn ensures controlled neurotransmitter release. J. Cell. Biol. 183, 323–337. doi: 10.1083/jcb.200805150
Savigner, A., Duchamp-Viret, P., Grosmaitre, X., Chaput, M., Garcia, S., Ma, M., et al. (2009). Modulation of spontaneous and odorant-evoked activity of rat olfactory sensory neurons by two anorectic peptides, insulin and leptin. J. Neurophysiol. 101, 2898–2906. doi: 10.1152/jn.91169.2008
Schiffmann, S. N., and Vanderhaeghen, J. J. (1991). Distribution of cells containing mRNA encoding cholecystokinin in the rat central nervous system. J. Comp. Neurol. 304, 219–233. doi: 10.1002/cne.903040206
Schmidt, L. J., and Strowbridge, B. W. (2014). Modulation of olfactory bulb network activity by serotonin: synchronous inhibition of mitral cells mediated by spatially localized GABAergic microcircuits. Learn. Mem. 21, 406–416. doi: 10.1101/lm.035659.114
Schneider, S. P., and Macrides, F. (1978). Laminar distributions of interneurons in the main olfactory bulb of the adult hamster. Brain Res. Bull. 3, 73–82. doi: 10.1016/0361-9230(78)90063-1
Seroogy, K. B., Brecha, N., and Gall, C. (1985). Distribution of cholecystokinin-like immunoreactivity in the rat main olfactory bulb. J. Comp. Neurol. 239, 373–383. doi: 10.1002/cne.902390403
Shepherd, G. M. (1972). Synaptic organization of the mammalian olfactory bulb. Physiol. Rev. 52, 864–917. doi: 10.1152/physrev.1972.52.4.864
Shioda, S., Funahashi, H., Nakajo, S., Yada, T., Maruta, O., and Nakai, Y. (1998). Immunohistochemical localization of leptin receptor in the rat brain. Neurosci. Lett. 243, 41–44. doi: 10.1016/S0304-3940(98)00082-2
Sizemore, T. R., Hurley, L. M., and Dacks, A. M. (2020). Serotonergic modulation across sensory modalities. J. Neurophysiol. 123, 2406–2425. doi: 10.1152/jn.00034.2020
Smith, R. S., Hu, R., DeSouza, A., Eberly, C. L., Krahe, K., Chan, W., et al. (2015). Differential muscarinic modulation in the olfactory bulb. J. Neurosci. 35, 10773–10785. doi: 10.1523/JNEUROSCI.0099-15.2015
Steinfeld, R., Herb, J. T., Sprengel, R., Schaefer, A. T., and Fukunaga, I. (2015). Divergent innervation of the olfactory bulb by distinct raphe nuclei. J. Comp. Neurol. 523, 805–813. doi: 10.1002/cne.23713
Stevens, D. R., Seifert, R., Bufe, B., Muller, F., Kremmer, E., Gauss, R., et al. (2001). Hyperpolarization-activated channels HCN1 and HCN4 mediate responses to sour stimuli. Nature 413, 631–635. doi: 10.1038/35098087
Sun, C., Tang, K., Wu, J., Xu, H., Zhang, W., Cao, T., et al. (2019). Leptin modulates olfactory discrimination and neural activity in the olfactory bulb. Acta Physiol. 227:e13319. doi: 10.1111/apha.13319
Tartaglia, L. A., Weng, X., Devos, R., Richards, J., Arthur, L., Clark, T., et al. (1995). Identification and expression cloning of a leptin receptor, OB-R. Cell 83, 1263–1271. doi: 10.1016/0092-8674(95)90151-5
Taschenberger, H., Woehler, A., and Neher, E. (2016). Superpriming of synaptic vesicles as a common basis for intersynapse variability and modulation of synaptic strength. Proc. Natl. Acad. Sci. U. S. A. 113, 4548–4557. doi: 10.1073/pnas.1606383113
Tecott, L. H., Maricq, A. V., and Julius, D. (1993). Nervous system distribution of the serotonin 5-HT3 receptor mRNA. Proc. Natl. Acad. Sci. U.S.A. 90, 1430–1434. doi: 10.1073/pnas.90.4.1430
Thiebaud, N., Gribble, F., Reimann, F., Trapp, S., and Fadool, D. A. (2019). A unique olfactory bulb microcircuit driven by neurons expressing the precursor to glucagon-like peptide 1. Sci. Rep. 9:15542. doi: 10.1038/s41598-019-51880-9
Thiebaud, N., Llewellyn-Smith, I. J., Gribble, F., Reimann, F., Trapp, S., and Fadool, D. A. (2016). The incretin hormone glucagon-like peptide 1 increases mitral cell excitability by decreasing conductance of a voltage-dependent potassium channel. J. Physiol. 594, 2607–2628. doi: 10.1113/JP272322
Tucker, K., Cho, S., Thiebaud, N., Henderson, M. X., and Fadool, D. A. (2013). Glucose sensitivity of mouse olfactory bulb neurons is conveyed by a voltage-gated potassium channel. J. Physiol. 591, 2541–2561. doi: 10.1113/jphysiol.2013.254086
Ueno, M., Akiguchi, I., Naiki, H., Fujibayashi, Y., Fukuyama, H., Kimura, J., et al. (1991). The persistence of high uptake of serum albumin in the olfactory bulbs of mice throughout their adult lives. Arch. Gerontol. Geriatr. 13, 201–209. doi: 10.1016/0167-4943(91)90062-U
Ueno, M., Dobrogowska, D. H., and Vorbrodt, A. W. (1996). Immunocytochemical evaluation of the blood-brain barrier to endogenous albumin in the olfactory bulb and pons of senescence-accelerated mice (SAM). Histochem. Cell. Biol. 105, 203–212. doi: 10.1007/BF01462293
Vanderhaeghen, J. J., Signeau, J. C., and Gepts, W. (1975). New peptide in the vertebrate CNS reacting with antigastrin antibodies. Nature 257, 604–605. doi: 10.1038/257604a0
Wellis, D. P., and Kauer, J. S. (1994). GABAergic and glutamatergic synaptic input to identified granule cells in salamander olfactory bulb. J Physiol 475, 419–430. doi: 10.1113/jphysiol.1994.sp020082
Williams, D. L. (2009). Minireview: finding the sweet spot: peripheral versus central glucagon-like peptide 1 action in feeding and glucose homeostasis. Endocrinology 150, 2997–3001. doi: 10.1210/en.2009-0220
Williams, P. D. E., Zahratka, J. A., Rodenbeck, M., Wanamaker, J., Linzie, H., and Bamber, B. A. (2018). Serotonin disinhibits a caenorhabditis elegans sensory neuron by suppressing Ca (2+)-dependent negative feedback. J. Neurosci. 38, 2069–2080. doi: 10.1523/JNEUROSCI.1908-17.2018
Wilson, D. A., Fletcher, M. L., and Sullivan, R. M. (2004). Acetylcholine and olfactory perceptual learning. Learn. Mem. 11, 28–34. doi: 10.1101/lm.66404
Wu, A., Dvoryanchikov, G., Pereira, E., Chaudhari, N., and Roper, S. D. (2015). Breadth of tuning in taste afferent neurons varies with stimulus strength. Nat. Commun. 6:8171. doi: 10.1038/ncomms9171
Yuan, Q., Harley, C. W., and McLean, J. H. (2003). Mitral cell beta 1 and 5-HT2A receptor colocalization and cAMP coregulation: a new model of norepinephrine-induced learning in the olfactory bulb. Learn. Mem. 10, 5–15. doi: 10.1101/lm.54803
Záborszky, L., Carlsen, J., Brashear, H. R., and Heimer, L. (1986). Cholinergic and GABAergic afferents to the olfactory bulb in the rat with special emphasis on the projection neurons in the nucleus of the horizontal limb of the diagonal band. J. Comp. Neurol. 243, 488–509. doi: 10.1002/cne.902430405
Zarbin, M., Innis, R., Wamsley, J., Snyder, S., and Kuhar, M. (1983). Autoradiographic localization of cholecystokinin receptors in rodent brain. J. Neurosci. 3, 877–906. doi: 10.1523/JNEUROSCI.03-04-00877.1983
Zhou, L., Zhao, S., and Nadim, F. (2007). Neuromodulation of short-term synaptic dynamics examined in a mechanistic model based on kinetics of calcium currents. Neurocomputing 70, 2050–2054. doi: 10.1016/j.neucom.2006.10.084
Zhou, Y. H., Sun, L. H., Liu, Z. H., Bu, G., Pang, X. P., Sun, S. C., et al. (2010). Functional impact of the hyperpolarization-activated current on the excitability of myelinated A-type vagal afferent neurons in the rat. Clin. Exp. Pharmacol. Physiol. 37, 852–861. doi: 10.1111/j.1440-1681.2010.05396.x
Zhou, Z., and Belluscio, L. (2008). Intrabulbar projecting external tufted cells mediate a timing-based mechanism that dynamically gates olfactory bulb output. J. Neurosci. 28, 9920–9928. doi: 10.1523/JNEUROSCI.3082-08.2008
Keywords: olfactory bulb, cholecystokinin, leptin, glucagon-like peptide 1, GLP-1, acetylcholine, mitral cell, CCK
Citation: Huang Z, Tatti R, Loeven AM, Landi Conde DR and Fadool DA (2021) Modulation of Neural Microcircuits That Control Complex Dynamics in Olfactory Networks. Front. Cell. Neurosci. 15:662184. doi: 10.3389/fncel.2021.662184
Received: 31 January 2021; Accepted: 10 May 2021;
Published: 22 June 2021.
Edited by:
Lisa Mapelli, University of Pavia, ItalyReviewed by:
Markus Rothermel, University of Veterinary Medicine Hannover, GermanyAnna D'Errico, Goethe University Frankfurt, Germany
Copyright © 2021 Huang, Tatti, Loeven, Landi Conde and Fadool. This is an open-access article distributed under the terms of the Creative Commons Attribution License (CC BY). The use, distribution or reproduction in other forums is permitted, provided the original author(s) and the copyright owner(s) are credited and that the original publication in this journal is cited, in accordance with accepted academic practice. No use, distribution or reproduction is permitted which does not comply with these terms.
*Correspondence: Debra Ann Fadool, ZGZhZG9vbCYjeDAwMDQwO2ZzdS5lZHU=