- 1Department of Zoology, University of Cambridge, Cambridge, United Kingdom
- 2Neural Circuits and Evolution Laboratory, The Francis Crick Institute, London, United Kingdom
- 3Neurobiology Division, MRC Laboratory of Molecular Biology, Cambridge, United Kingdom
- 4Centre for Organismal Studies, Heidelberg University, Heidelberg, Germany
Neurons utilize plasticity of dendritic arbors as part of a larger suite of adaptive plasticity mechanisms. This explicitly manifests with motoneurons in the Drosophila embryo and larva, where dendritic arbors are exclusively postsynaptic and are used as homeostatic devices, compensating for changes in synaptic input through adapting their growth and connectivity. We recently identified reactive oxygen species (ROS) as novel plasticity signals instrumental in this form of dendritic adjustment. ROS correlate with levels of neuronal activity and negatively regulate dendritic arbor size. Here, we investigated NADPH oxidases as potential sources of such activity-regulated ROS and implicate Dual Oxidase (but not Nox), which generates hydrogen peroxide extracellularly. We further show that the aquaporins Bib and Drip, but not Prip, are required for activity-regulated ROS-mediated adjustments of dendritic arbor size in motoneurons. These results suggest a model whereby neuronal activity leads to activation of the NADPH oxidase Dual Oxidase, which generates hydrogen peroxide at the extracellular face; aquaporins might then act as conduits that are necessary for these extracellular ROS to be channeled back into the cell where they negatively regulate dendritic arbor size.
Introduction
Neurons are inherently plastic and their ability to respond to changes in synaptic transmission or activity patterns is central to many processes, from learning and memory (Martin et al., 2000; Stuchlik, 2014) to homeostatic adjustments that stabilize circuit function (Turrigiano and Nelson, 2000; Pozo and Goda, 2010; Wefelmeyer et al., 2016; Frank et al., 2020). We recently identified reactive oxygen species (ROS) as novel signals required for activity-regulated plasticity. ROS have long been known to affect neuronal development and function. Commonly associated with pathological conditions, ageing and disease, the roles of ROS as signalling molecules under normal physiological conditions are much less understood (Milton et al., 2011; Oswald et al., 2018b; Peng et al., 2019). At present, hydrogen peroxide (H2O2) is thought to be the species predominantly required for homeostatic maintenance of synaptic transmission at the neuromuscular junction in Drosophila larvae, and for adaptive structural changes of synaptic terminal arbors following periods of over-activation. H2O2 is also sufficient to induce structural changes that largely phenocopy effects of over-activation, suggesting that ROS mediate plastic adjustments downstream of neuronal activity (Oswald et al., 2018a). Given that neuronal activity has a high energetic cost, correlating with metabolic demand (Attwell and Laughlin, 2001; Zhu et al., 2012), a simplistic working model has been proposed whereby ROS, largely generated as obligate by-products of aerobic metabolism (Halliwell, 1992), regulate plasticity by providing ongoing feedback on a neuron’s activation status (Hongpaisan et al., 2003, 2004; Oswald et al., 2018a).
In addition to mitochondria, NADPH oxidases are another well documented source of activity-generated ROS (Hongpaisan et al., 2004; Massaad and Klann, 2011; Baxter and Hardingham, 2016; Hidalgo and Arias-Cavieres, 2016; Terzi and Suter, 2020; Terzi et al., 2021). Here, we focus on NADPH oxidases, a family of differentially expressed multisubunit enzymes (Nox 1–5 and Dual Oxidase 1–2) that catalyse the transfer of an electron from cytosolic NADPH to oxygen to generate ROS at the extracellular face of the plasma membrane (Lambeth, 2002; Panday et al., 2015). NADPH oxidases are commonly associated with immune responses, but more recently have also been shown to regulate aspects of nervous system development such as neuronal polarity (Wilson et al., 2015), growth cone dynamics (Munnamalai and Suter, 2009; Munnamalai et al., 2014; Terzi et al., 2021), and intriguingly, synaptic plasticity (Tejada-Simon et al., 2005).
Using the Drosophila locomotor network as an experimental model, we focused on the regulation of dendritic growth of identified motoneurons; a sensitive assay of structural plasticity, whereby neuronal activity and associated ROS reduce dendritic arbor size (Oswald et al., 2018a). Based on single cell-specific targeting of RNAi knockdown constructs, our data suggest that within the somato-dendritic compartment the NADPH oxidase Dual Oxidase (Duox), but not Nox, is required for activity-regulated generation of ROS. Because Duox generates H2O2 at the outer face of the plasma membrane, we tested a requirement for aquaporin channels as conduits transporting extracellular ROS into the cytoplasm, as had been shown in other systems (Miller et al., 2010; Bertolotti et al., 2013; Chakrabarti and Visweswariah, 2020). Indeed, we found a requirement for two of three characterised Drosophila aquaporins, Bib and Drip, in activity-dependent regulation of dendritic arbor size, but not for the aquaporin Prip. Overall, our data suggest that neuronal activity promotes Duox-mediated generation of extracellular H2O2, which may be returned into the cytoplasm via aquaporin channels, where it inhibits dendritic growth. Moreover, our findings imply that Duox-generated H2O2 additionally acts non-autonomously on neighbouring synaptic terminals.
Materials and Methods
Fly Genetics
Drosophila melanogaster strains were maintained on a standard apple juice-based agar medium at 25°C. The following fly strains were used: OregonR (#2376, Bloomington Drosophila Stock Center), UAS-dTrpA1 in attP16 (Hamada et al., 2008; FBtp0089791), UAS-Duox.RNAi (#32903, BDSC; FBtp0064955), UAS-Nox.RNAi (Ha et al., 2005a; FBal0191562), UAS-bib.RNAi (I) (#57493, BDSC; FBtp0096443), UAS-bib.RNAi (II) (#27691 BDSC; FBtp0052515), UAS-Drip.RNAi (I) (#44661, BDSC; FBtp0090566), UAS-Drip.RNAi (II) (#106911, Vienna Drosophila Resource Centre; FBtp0045814), UAS-Prip.RNAi (I) (#50695, BDSC; FBtp0090659), UAS-Prip.RNAi (II) (#44464, BDSC; FBtp0090258), and UAS-secreted human-catalase (FBal0190351; Ha et al., 2005b; Fogarty et al., 2016). Transgene expression was targeted to 1–3 RP2 motoneurons per nerve cord using a stochastic FLPout strategy, as detailed previously (Fujioka et al., 2003; Ou et al., 2008). The GAL4 expression stock, termed “RP2-FLP-GAL4 > YPet”, contains the following transgenes: RN2-FLP, tub84B-FRT-CD2-FRT-GAL4, and 10XUAS-IVS-myr::YPet in attP2. Briefly, yeast Flippase expression is directed to RP2 motoneurons at embryonic stages only (at a lower level also to the aCC motoneuron and pCC interneuron) via RN2-FLP (Fujioka et al., 2003; Ou et al., 2008), then initiates permanent GAL4 expression in a subset of RP2 motoneurons (as well as occasional aCC motoneurons) via FLP-conditional GAL4, tub84B-FRT-CD2-FRT-GAL4 (Pignoni and Zipursky, 1997); this in turn initiates expression of the membrane targeted myristoylated YPet morphological reporter plus any additional UAS responder transgene. 10XUAS-IVS-myr::YPet in attP2 was generated by subcloning YPet (Nguyen and Daugherty, 2005) into pJFRC12-10XUAS-IVS-myr::GFP, directly replacing GFP.
Larval Staging and Dissection
Eggs were collected at 25°C over an 8 h period on an apple juice-based agar medium supplemented with a thin film of yeast paste and, following continued incubation at 25°C, were subsequently screened for freshly hatched larvae, selected against the presence of fluorescently marked (deformed-GMR-YFP) balancer chromosomes. Larvae were transferred to a fresh agar plate with yeast paste, incubated at 25°C (aquaporin/catalase experiments) or 27°C (NADPH oxidase experiments) and allowed to develop for 48 h to the third instar stage, followed by dissection in external saline (pH 7.15) (Marley and Baines, 2011). Nerve cords were transferred with a BSA coated glass capillary onto a poly-L-lysine coated (Sigma-Aldrich) cover glass (22 × 22 mm), positioned dorsal side up. A clean cover glass was placed on top with two strips of electrical tape used as spacers.
Image Acquisition
Nerve cords were imaged within a 5 min window from dissection using a custom-built spinning disk confocal microscope consisting of a CSU-22 field scanner (Yokagawa), mounted on a fixed stage upright Olympus microscope frame (BX51-WI), equipped with a single objective piezo focusing device (Physik Instruments), a 60×/1.2 NA water immersion objective (Olympus), external filter wheel (Sutter) and programmable XY stage (Prior). Images were acquired at an effective voxel size of 0.217 × 0.217 × 0.3 μm using a back-thinned Evolve EMCCD camera (Photometrics), operated via MetaMorph software (Molecular Devices).
Neuron Reconstruction
Dendritic arbor reconstructions were carried out in Amira 6.5 (FEI). A deconvolution algorithm was used to reassign photons from out-of-focus optical sections to their points of origin, thus improving the signal-to-noise ratio of the image stack. Subsequently, thresholding of voxel grey values was used to segment the fluorescent arbor from background. Structures, which did not require reconstruction, i.e., the cell body and primary neurite, were manually removed. Post segmentation, the Amira automatic reconstruction algorithm was used to convert the centrelines of the user-defined segmentation into a spatial graph structure. This structure was manually reviewed and edited to correct for “loops” and other artefacts of the automatic reconstruction process. Quantification of cell body area was conducted in ImageJ (National Institutes of Health) by manually tracing around individual cell bodies.
Data Handling and Statistical Analysis
All data handling and statistical analyses were carried out in R. A Shapiro-Wilk test was used to confirm normality of all dendritic arbor reconstruction data presented. A one-way analysis of variance (ANOVA) followed by Tukey’s multiple comparisons test was used to compare experimental manipulations to the controls where ∗p < 0.05; ∗∗p < 0.01; ∗∗∗p < 0.001; and ****p < 0.0001.
Results
Reactive Oxygen Species Generated by Dual Oxidase Are Required for Activity-Dependent Adjustments of Dendritic Arbor Size and Geometry
The postsynaptic dendritic arbor of many neuron types can operate as a homeostatic device, adjusting its size and geometry, as well as sensitivity of postsynaptic fields and connectivity, in an activity-dependent manner, so as to maintain appropriate levels of stimulation (reviewed in Yin and Yuan, 2014; Wefelmeyer et al., 2016). In Drosophila, we demonstrated such structural homeostatic plasticity of dendrites in both embryonic (Tripodi et al., 2008) and larval motoneurons (Oswald et al., 2018a), while others demonstrated this a general principle, by showing that such activity-regulated structural adjustments of dendrites and connectivity in the visual system lead to corresponding compensatory changes in physiological output (Yuan et al., 2011; Sheng et al., 2018; Dombrovski et al., 2019). Moreover, we identified ROS signaling as necessary and sufficient for this structural remodelling to occur (Oswald et al., 2018a). That work suggested that neuronal activity leads to the production of mitochondrial ROS, generated as byproducts of oxidative phosphorylation. Here we set out to investigate the involvement of a second source of ROS, generated at the plasma membrane by NADPH oxidases, during activity-regulated structural plasticity. Drosophila codes for only two NADPH oxidases; dDuox, an orthologue of vertebrate dual oxidase, and dNox, which is closely related to human Nox5 (Kawahara et al., 2007). To investigate if either or both contributed to activity-regulated structural plasticity of dendrites, we targeted the expression of previously tested RNAi constructs designed to knockdown dDuox or dNox (Ha et al., 2005a; Fogarty et al., 2016; Fujisawa et al., 2020) to the well-characterized “RP2” motoneuron, with and without concomitant overactivation (Sink and Whitington, 1991; Baines et al., 1999; Landgraf et al., 2003). We then analysed RP2 dendritic arbors morphometrically to quantify the extent to which these manipulations impacted their development.
Expression of UAS-dDuox.RNAi or UAS-dNox.RNAi transgenes alone under endogenous activity conditions, i.e., in the absence of dTrpA1 manipulation, did not produce significant differences in arbor characteristics (Figure 1A–C); though expression of UAS-dDuox.RNAi caused abnormal cell body morphology with supernumerary filopodial protrusions (Figure 1A). In accordance with previous findings (Oswald et al., 2018a), neuronal overactivation by targeted dTrpA1 misexpression in individual RP2 motoneurons resulted in significantly smaller dendritic arbors with reduced dendritic length and branch point number, as compared to non-manipulated controls (Figure 1A–C). Co-expression of UAS-dDuox.RNAi along with UAS-dTrpA1 significantly attenuated the activity-induced reduction of total dendritic length and branch point number. In contrast, RNAi-mediated knockdown of dNox had no discernible effect on arbor morphology.
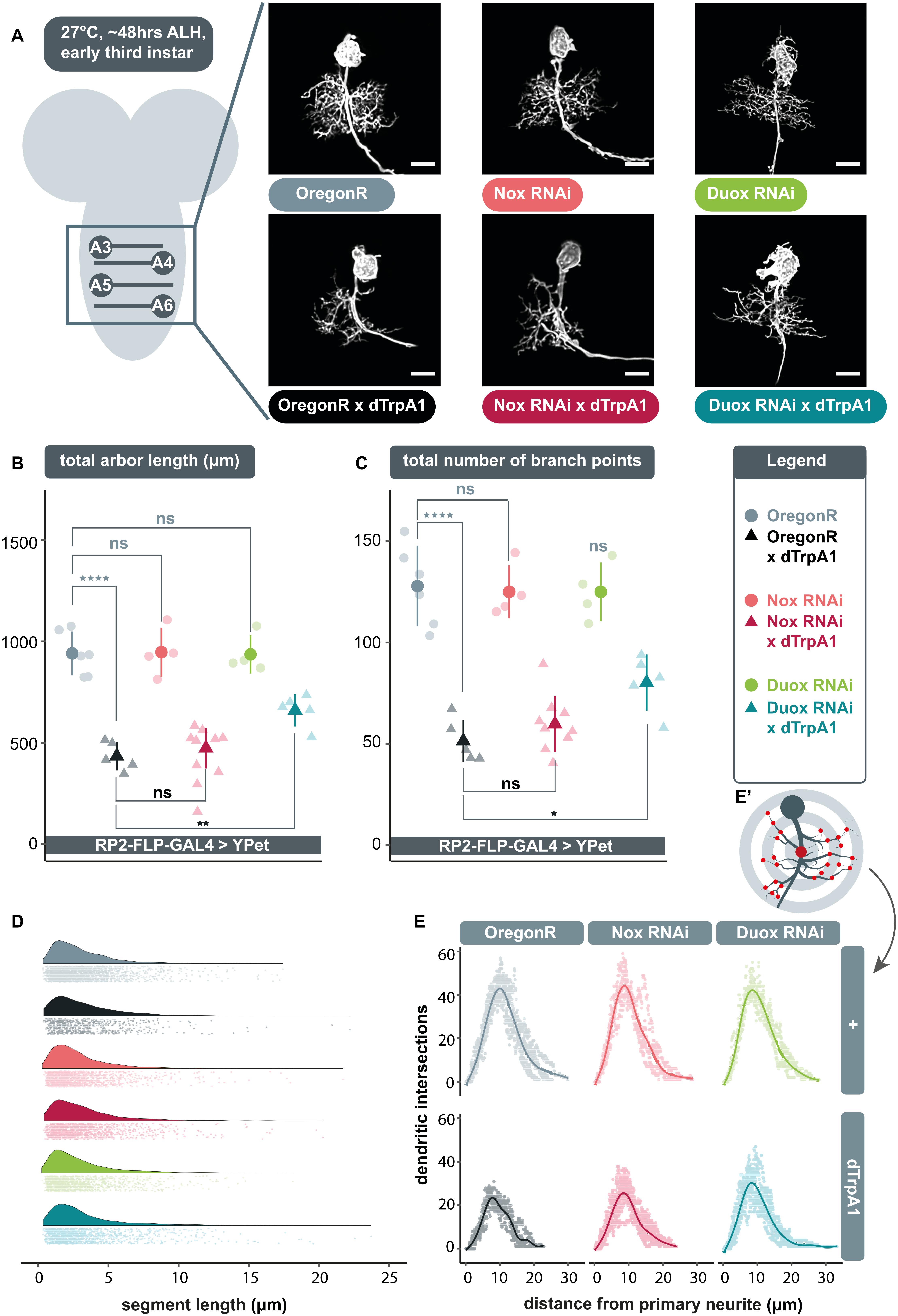
Figure 1. Extracellular reactive oxygen species (ROS) generated by dDuox, but not dNox, are required for homeostatic structural plasticity in response to increased neuronal activity. (A) Maximum intensity z-projections of representative RP2 motoneurons located within abdominal segments A3-6 in the ventral nerve cord, from young third instar larvae raised at 27°C and dissected 48 h after larval hatching. GAL4 expression was elicited and maintained in individual RP2 motoneurons by crossing RP2-FLP-GAL4 > YPet males containing the transgenes RN2-FLP, tub84B-FRT-CD2-FRT-GAL4, 10xUAS-IVS-myr::YPet (a membrane targeted YPet fluorophore) to virgins from wild type Oregon-Red (OregonR) flies (= controls; non-manipulated neurons) or from stocks containing UAS-dTrpA1 and/or UAS-dDuox.RNAi or UAS-dNox.RNAi transgenes. Note that cell body morphology is affected by expression of UAS-dDuox.RNAi, causing filopodial growth from the soma. Scale bars: 15 μm. (B,C) Targeted expression of dTrpA1, known to cause neuronal over-activation at temperatures ≥ 25°C leads to reduced dendritic arbor size. Co-expression of UAS-dDuox.RNAi, but not UAS-dNox.RNAi, significantly suppresses this activity-induced reduction in total dendritic arbor length and number of branch points relative to UAS-dTrpA1 manipulated motoneurons. In absence of UAS-dTrpA1, RP2 dendrites expressing these RNAi constructs are comparable to controls [analysis of variance (ANOVA), ns, not significant; *p < 0.05; **p < 0.01; ****p < 0.0001]. Comparisons with non-manipulated controls are shown directly above data points (light grey) and comparisons with the UAS-dTrpA1 overactivation condition are shown directly below (black). (D) Irrespective of neuronal activity regime, expression of UAS-dDuox.RNAi or UAS-dNox.RNAi does not alter the frequency distribution of dendritic segment lengths. A segment is defined as the distance between two branch points, or, in the case of a terminal neurite, a branch point and the tip. Frequency density plot shown in darker color, with all individual data points plotted below in a corresponding lighter shade. (E) Sholl analyses indicate that genetic manipulations do not obviously change the relative distribution of dendrites in 3D space. Mean dendritic intersections as a function of distance from the midpoint of the primary neurite (E’) are shown as a solid coloured line, all individual data points are shown in a corresponding lighter shade.
Next, we asked what changes in arbor structure might lead to these activity-regulated differences. We considered two principal possibilities, namely changes in the pattern of growth versus changes in the number of dendritic segments generated. Our analyses of branch point number and dendritic segment length frequency distribution point to the latter. Changes in RP2 motoneuron dendritic arbor size are associated with corresponding changes in the number of branch points (Figure 1C), indicative of segment number, while across genotypes, segment lengths were unimodally distributed with a peak at ∼2 μm (Figure 1D).
We also examined dendritic topography via Sholl analysis to see if changes in arbor length might impact on the ability to invade different regions of the neuropil. The origin of the concentric Sholl spheres was defined as the midpoint on the primary neurite (from which all dendrites arise) between the first and last branch points (Figure 1E’). Irrespective of genotype, the majority of arbor length was concentrated just under halfway along the arbor’s expanse with respect to the primary neurite (Figure 1E). This suggests that the genetic manipulations conducted do not obviously lead RP2 motoneurons to alter the placement or density of their dendritic segments. This is in agreement with earlier findings of motoneurons being specified by genetically separable programmes for dendritic growth and dendritic positioning (Ou et al., 2008).
In summary, these data implicate dDuox, but not dNox, as necessary for structural plasticity of dendritic arbors in response to elevated activity.
Aquaporin Channel Proteins Bib and Drip Regulate Dendritic Growth, Potentially by Functioning as Conduits for Extracellular ROS
NADPH oxidases generate ROS extracellularly, which poses the question of how such NADPH-generated ROS affect dendritic growth. Do they do so by modifying extracellular components or via intracellular events? The latter would require entry into the cell, and we focused on investigating this scenario. Owing to the large dipole moments of key ROS, like H2O2, simple diffusion across the hydrophobic plasma membrane, as seen with small and non-polar molecules, is limited. Instead, evidence from other experimental systems points towards a model of facilitated diffusion involving aquaporins (Bienert et al., 2007; Miller et al., 2010; Bertolotti et al., 2013; Chakrabarti and Visweswariah, 2020). Classically, the importance of these channel proteins has been stressed in the process of transmembrane fluid transport. However, some lines of research suggest that aquaporins can regulate the downstream signaling pathways that rely on ROS as a second messenger, by controlling entry of ROS into the cytosol (Miller et al., 2010; Bertolotti et al., 2013; Chakrabarti and Visweswariah, 2020).
We postulated that following neuronal overactivation extracellular ROS generated by Duox are brought into the cell via aquaporin channels, where they can then trigger compensatory structural changes in dendritic arbor size. To test this model, we overactivated individual RP2 motoneurons by targeted expression of dTrpA1 whilst simultaneously expressing RNAi constructs designed to knockdown genes that encode aquaporin channels: big brain (bib), Drosophila intrinsic proteins (Drip), or Pyrocoelia rufa integral proteins (Prip). For each aquaporin encoding gene we used two independently generated UAS-RNAi constructs, with at least one having previously been shown to have specific effects. Under conditions of cell-autonomous neuronal overactivation, targeted co-expression of UAS-bib.RNAi (Djiane et al., 2013) or UAS-Drip.RNAi (Bergland et al., 2012) transgenes resulted in a significant abrogation of activity-induced arbor reduction, similar to co-expression of UAS-dDuox.RNAi (Figures 2A–C). In contrast, targeted co-expression of UAS-Prip.RNAi (Chakrabarti and Visweswariah, 2020) transgenes did not affect the UAS-dTrpA1 mediated reduction of dendritic arbors, nor did UAS-Prip.RNAi expression by itself have a measurable impact on dendritic development in absence of TrpA1-mediated over-activation. In contrast, mis-expression of UAS-bib.RNAi or UAS-Drip.RNAi alone, without concomitant dTrpA1 activity manipulation, was not phenotypically neutral, but produced a dendritic overgrowth phenotype, of increased dendritic length (Figure 2B) and branching complexity (Figure 2C) in the ventral part of the arbor. Throughout these manipulations, changes in dendritic growth appear to result from changes in the number rather than length of dendritic segments (Figure 2E). Sholl analyses suggest that RP2 motoneurons target their normal neuropil territories irrespective of aquaporin knockdown manipulation (Figure 2F).
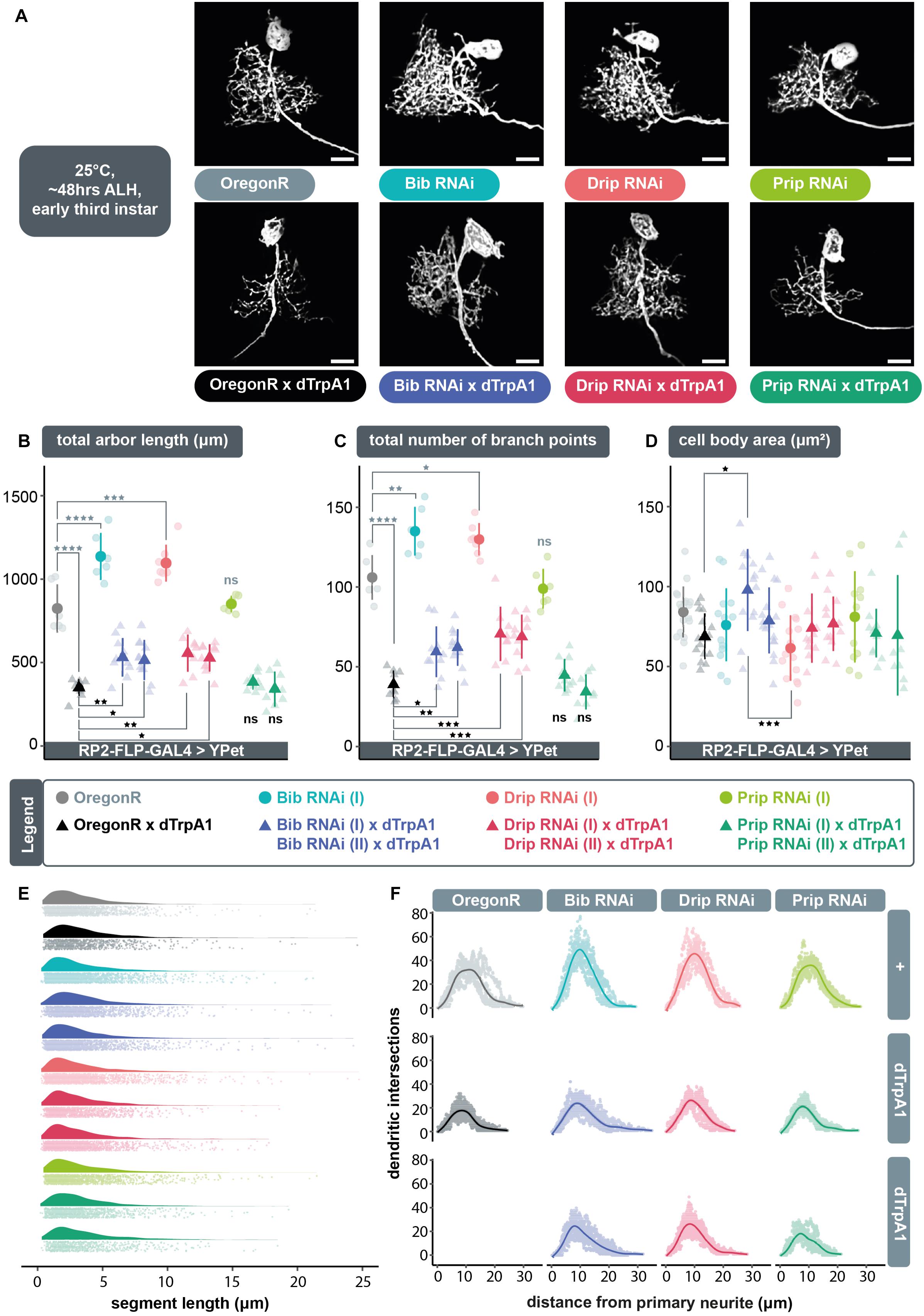
Figure 2. The aquaporins encoded by bib and Drip, but not Prip, are necessary for ROS and activity-induced dendritic plasticity. (A) Maximum intensity z-projections of representative RP2 motoneurons from young third instar larvae raised at 25°C and dissected 48 h after larval hatching, expressing GAL4 and the membrane-targeted cell morphology reporter, 10xUAS-IVS-myr::YPet. Controls were from crosses of RP2-FLP-GAL4 > YPet males containing the transgenes RN2-FLP, tub84B-FRT-CD2-FRT-GAL4, 10xUAS-IVS-myr::YPet (a membrane targeted YPet fluorophore) to virgins from wild type Oregon-Red (OregonR) flies (= controls; non-manipulated neurons) or from stocks containing UAS-dTrpA1 and/or UAS-aquaporin.RNAi transgenes. Expression of UAS-bib.RNAi or UAS-Drip.RNAi, but not UAS-Prip.RNAi, without concomitant dTrpA1 manipulation causes dendritic over-growth in RP2 motoneurons. Under conditions of cell-selective neuronal over-activation by UAS-dTrpA1 co-expression of UAS-bib.RNAi or UAS-Drip.RNAi, but not UAS-Prip.RNAi, leads to significant attenuation of dTrpA1-induced dendritic under-growth in RP2 motoneurons. Scale bars: 15 μm. (B,C) Targeted expression of UAS-bib.RNAi or UAS-Drip.RNAi, but not UAS-Prip.RNAi in RP2 motoneurons significantly attenuates the reduction in dendritic arbor length and number of branch points caused by UAS-dTrpA1-mediated neuronal overaction. Two independently generated RNAi constructs were tested for each aquaporin. Expression of UAS-bib.RNAi or UAS-Drip.RNAi without concomitant dTrpA1 manipulation produces a dendritic overgrowth phenotype characterized by increased arbor length and branching complexity relative to non-manipulated controls. (D) Quantification of cell body size, measured as the maximum area of the 2D cross-section through the centre of the soma, shows no consistent differences between controls and UAS-aquaporin.RNAi manipulations. (B–D) ANOVA, ns, not significant; *p < 0.05; **p < 0.01; ***p < 0.001; ****p < 0.0001. Comparisons with non-manipulated controls are shown directly above data points (light grey) and comparisons with overactivated controls are directly below (black). (E) Expression of RNAi transgenes designed to knock down specific aquaporins does not alter fundamental arbor structure under conditions of endogenous activity or chronic overactivation. Frequency density plot of dendritic segment lengths shown in darker color, with all individual data points plotted below in a corresponding lighter shade. (F) The branching topology of RP2 motoneurons is not affected by UAS-aquaporin-RNAi manipulations. Mean dendritic intersections as a function of distance from the midpoint of the primary neurite are shown as a solid coloured line, all individual data points are shown in a corresponding lighter shade.
We wondered whether UAS-bib.RNAi or UAS-Drip.RNAi induced dendritic overgrowth might be caused by increased internal osmotic pressure, as a result of impaired aquaporin function, which in turn may stimulate mechanically sensitive proteins (Kerstein et al., 2015). However, we could not detect evidence for cell body dilation as would be expected if expression of UAS-aquaporin.RNAi transgenes were to increase internal osmotic pressure (Figure 2D).
In summary, these data suggest a requirement for the aquaporin channels Bib and Drip, but not Prip, in regulating dendritic arbor size. However, since mis-expression of UAS-bib.RNAi or UAS-Drip.RNAi alone, in the absence of dTrpA1-mediated overactivation, leads to a dendritic overgrowth phenotype, it is unclear if aquaporins and neuronal activity act in the same pathway or in parallel pathways with opposite effects on dendritic growth.
Extracellular ROS Act as Negative Regulators of Dendritic Growth
The above data suggest that extracellular ROS could provide a negative feedback signal to reduce dendritic arbor size, if those were channeled via aquaporins into the cytoplasm. To test this idea, we expressed in single RP2 motoneurons a secreted, extracellular form of catalase, to quench extracellular H2O2. Compatible with the hypothesis, we found that expression of UAS-human-secreted-catalase (Ha et al., 2005b; Fogarty et al., 2016) produced a dendritic overgrowth phenotype comparable to that caused by expression of UAS-bib.RNAi or UAS-Drip.RNAi (Figures 2A, 3A). This overgrowth was characterized by dense dendritic arbors with significantly larger total arbor length as compared to non-manipulated controls (Figure 3B). As with the other manipulations above, here too the relative distribution of dendritic segments and arbor topography remained comparable to controls (Figures 3C–E). Somewhat unexpected though, our analysis indicates no change in branch point number despite the increase in arbor size (Figure 3C). This is counter-intuitive and we think the most parsimonious explanation for that this is an artefactual under-representation of segment number, caused by the high density of dendritic segments in these overgrown neurons, leading to failure of resolving all branch points during the reconstruction process.
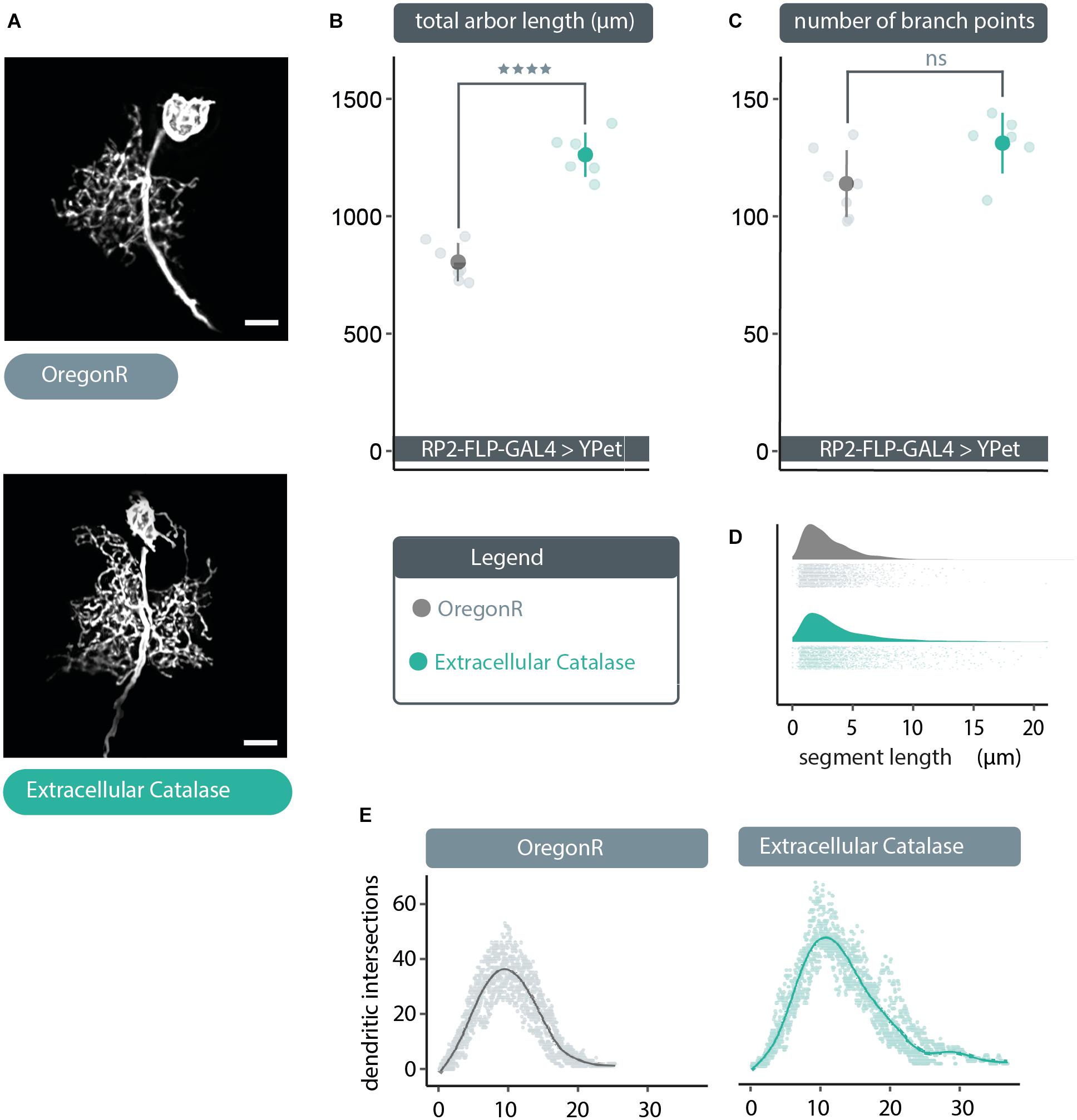
Figure 3. Cell-specific expression of extracellular catalase produces a dendritic overgrowth phenotype comparable to that produced by UAS-bib.RNAi or UAS-Drip.RNAi expression. (A) Maximum intensity z-projections of representative RP2 motoneurons, from young third instar larvae raised at 25°C and dissected 48 h after larval hatching. To target GAL4 expression to RP2 motoneurons RP2-FLP-GAL4 > YPet males were crossed to virgins from wild type Oregon-Red (OregonR) flies (= controls; non-manipulated neurons) or flies containing UAS-human secreted catalase, here termed “extracellular Catalase”. Scale bars: 15 μm. (B,C) Targeted expression of UAS-human secreted catalase in RP2 motoneurons results in a significant increase or “overgrowth” of dendritic arbor length relative to otherwise non-manipulated neurons, but does not change the total number of branch points (unexpected and likely caused by poor signal-to-noise ratio resulting from very dense branching of these enlarged arbors) (ANOVA, ns, not significant; ****p < 0.0001). (D) UAS-human secreted catalase expression in RP2 motoneurons does not change the frequency distribution of dendritic segment lengths relative to overactivated or non-manipulated controls Frequency density plot shown in darker color, with all individual data points plotted below in a corresponding lighter shade. (E) Sholl analyses indicate that UAS-human secreted catalase expression does not obviously cause RP2 motoneurons to alter the placement or density of their dendrites. Mean dendritic intersections as a function of distance from the midpoint of the primary neurite are shown as a solid coloured line, all individual data points are shown in a corresponding lighter shade.
In conclusion, in this study we identified a selective requirement for the NADPH oxidase, Duox (but not Nox), in activity-regulated adjustment of dendritic arbor growth during larval nervous system development. Thus generated extracellular ROS could signal to neighbouring cells, as well as mediate autocrine signaling. We also identified a role for the aquaporins Bib and Drip (but not Prip), which we propose serve as conduits for channeling extracellular H2O2 back into the cell, from adjacent cells but likely also mediating autocrine signaling. Overall, extracellular ROS act as negative feedback signals that mediate homeostatic adjustment of dendritic arbor size. The data suggest this process operates at physiological activity levels since manipulations where we expressed secreted Catalase to quench extracellular ROS in the immediate vicinity of a neuron lead to dendritic overgrowth that is indistinguishable from overgrowth phenotypes caused by cell-autonomously targeting the aquaporins Bib and Drip for RNAi knockdown. Such enlarged dendritic arbors would be predicted by our model (Figure 4), as a consequence of reduced influx of ROS, which act as a brake on dendritic growth.
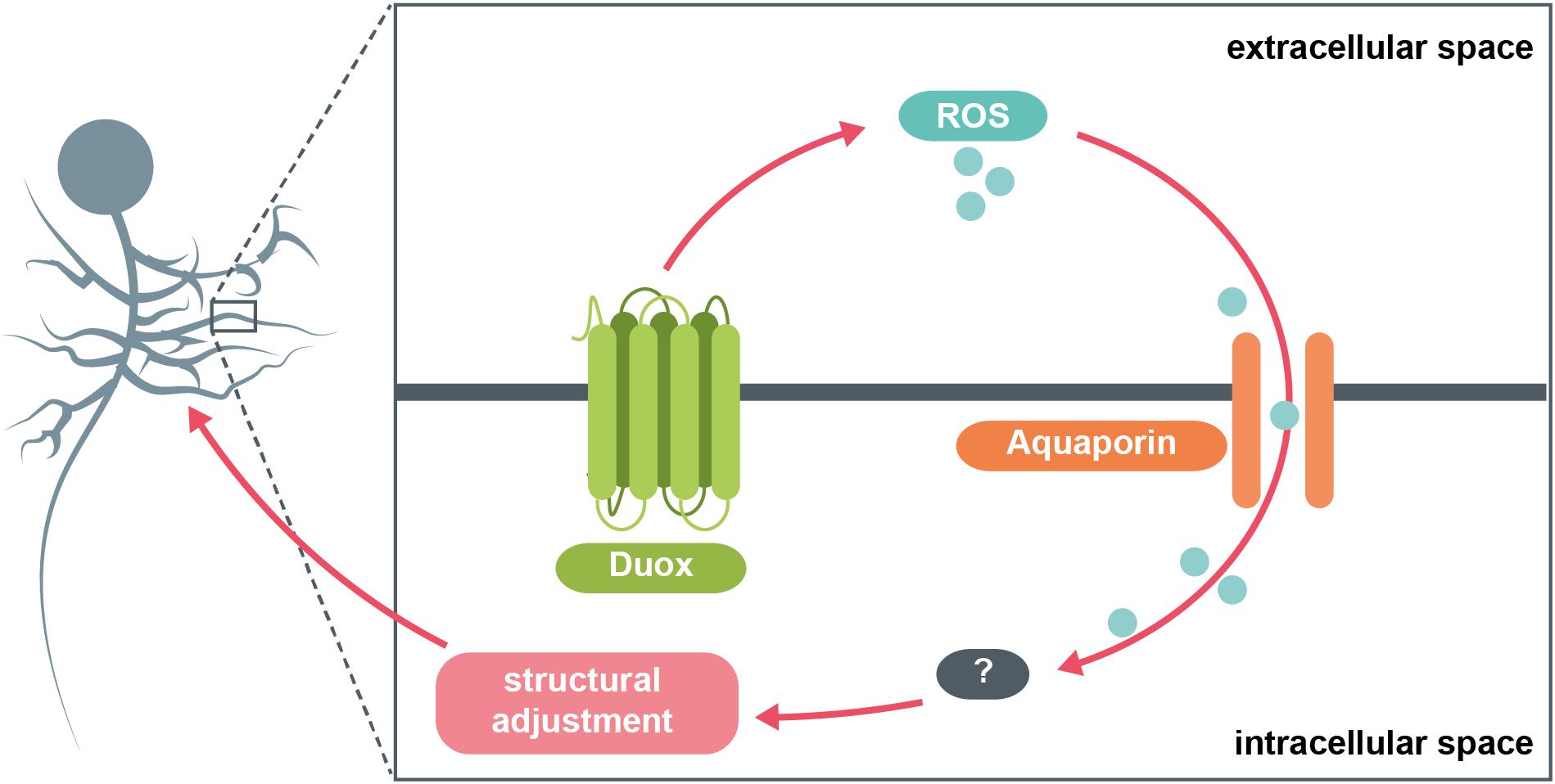
Figure 4. Model summary. The NADPH oxidase Duox generates ROS at the extracellular face of the plasma membrane in response to increases in neuronal activity. These ROS are brought back into the cytosol via specific aquaporin channel proteins. Here, they interact with various intracellular pathways, potentially involving the redox-sensitive dimer DJ-1β, which in turn mediate adaptive reductions in dendritic arbor size.
Discussion
A prerequisite of flexible yet stable neural circuitry is the ability to detect and appropriately respond to changes in activity, particularly perturbations that push activity towards extremes of quiescence or saturation (Turrigiano and Nelson, 2004; Yin and Yuan, 2014). Here, we focused on structural plasticity of dendrites in response to increased neuronal activation. Seminal comparative studies in mammals showed that the size and complexity of dendritic arbors correlates with the range and amount of synaptic input received (Purves and Lichtman, 1985; Ivanov and Purves, 1989). Similarly, in the Drosophila larval locomotor network we demonstrated that during development the dramatic growth of motoneuron dendritic arbors, which scales with overall body growth, facilitates increases in presynaptic input and thus also of the amount of synaptic drive necessary for appropriate levels of muscle activation (Zwart et al., 2013). This kind of structural plasticity is not limited to periods of growth, but also evident following activity manipulations. For example, changes in the number of active presynaptic sites are compensated for by complementary changes in postsynaptic dendritic arbor size, suggesting that neurons use their dendritic arbors as structural homeostatic devices (Tripodi et al., 2008). Similar structural homeostatic adjustments have also been documented in the developing visual system (Yuan et al., 2011; Sheng et al., 2018; Dombrovski et al., 2019). ROS are necessary for this plasticity to occur, with ROS acting as brakes on dendritic arbor growth (Oswald et al., 2018a). Here, we identify the NADPH oxidase, Duox, as a source of such activity-generated ROS. The topography of Duox, which is known to reside within the plasma membrane (Morand et al., 2009), is such that it generates H2O2 into extracellular space (Fogarty et al., 2016). We previously showed that H2O2 is required intracellularly, the effects of cell-specific over-activation rescued cell-autonomously by co-expression of cytoplasmic Catalase (Oswald et al., 2018a). This raised the question of how such extracellular ROS re-enter the neuronal cytoplasm. Our findings suggest that the aquaporin channels Bib and Drip, but not Prip, might function as conduits for extracellular ROS, necessary for activity-regulated dendritic structural remodelling (Figure 4). Specifically, targeting bib or Drip for RNAi-mediated knockdown in individual RP2 motoneurons significantly attenuates the overactivation phenotype of smaller dendritic arbors. These observations suggest that specific aquaporins located in the somato-dendritic compartment of motoneurons act as conduits that facilitate entry of extracellular H2O2 into the cytoplasm, where it can modulate dendritic growth pathways. This model is similar to one recently proposed by Chakrabarti and Visweswariah (2020) in Drosophila hemocytes, where in response to wounding Duox is activated, generates extracellular H2O2, which partly signals in an autocrine fashion with import back into the hemocyte cytoplasm facilitated by the aquaporin Prip. Moreover, expression of a secreted Catalase, IRC, modulates such H2O2 signaling (Chakrabarti and Visweswariah, 2020).
Based on our previous observations, we speculate that within the neuron H2O2 acts, amongst others, on the cytoplasmic redox-sensitive dimer DJ-1β, which we previously showed necessary for structural and physiological changes in response to activity-generated ROS (Oswald et al., 2018a). Dendritic overgrowth, as caused by expression of a secreted form of Catalase, known to scavenge extracellular H2O2 (Ha et al., 2005b; Fogarty et al., 2016; Chakrabarti and Visweswariah, 2020), suggests that extracellular ROS from adjacent cells might contribute to structural plasticity regulation. Expression of a cytoplasmic Catalase, in contrast, did not have such an effect, at least not in aCC motoneurons at an earlier stage of 24 h after larval hatching (Oswald et al., 2018a). This might suggest stage-specific differences in levels of ROS or differences in the efficacy of these two transgenes, either due to expression levels or difficulty of cytoplasmic Catalase to diffuse into small diameter dendritic branches.
The partial penetrance of RNAi knockdown phenotypes, combined with the observation that two distinct aquaporin encoding genes are involved, suggests that there may be functional redundancy between these aquaporins. Such redundancy has previously been observed in the tsetse fly, where simultaneous downregulation of multiple aquaporins exacerbates the negative effects on female fecundity produced by individual aquaporin knockdowns (Benoit et al., 2014).
Extracellular Duox-Generated ROS as a Potential Means for Coordinating Network-Wide Homeostatic Structural Adjustments
Whilst ROS typically operate as intracellular second messengers (Forman et al., 2014; Schieber and Chandel, 2014), their long-range effects have also been observed during paracrine H2O2 signaling in vertebrate and invertebrate inflammatory responses (Niethammer et al., 2009; Moreira et al., 2010). In light of this, our finding that extracellular, activity-generated ROS are necessary for structural plasticity raises the intriguing possibility of an intercellular redox-based communication network that coordinates homeostatic structural adjustments more widely, potentially within local volumes. Synaptic clefts in Drosophila are approximately 10–20 nm in width (Prokop and Meinertzhagen, 2006). ROS such as O2– and H2O2 have been reported to travel distances in the order of several micrometers within living tissue (Cuypers et al., 2016; Krumova and Cosa, 2016). It follows that extracellular ROS generated by Duox in postsynaptic dendrites could traverse the synaptic cleft and act retrogradely on the surface of presynaptic partner terminals, e.g., on ion channels (Sah et al., 2002; Sesti et al., 2010; Sahoo et al., 2014). Such ROS could further enter the cytosol of presynaptic partners via aquaporin channels and trigger compensatory structural remodelling. In addition, in analogy to retrograde nitric oxide signaling (Hardingham et al., 2013), Duox generated extracellular ROS have the potential to modify pre- and postsynaptic terminals within a local volume of the neuropil, thus acting as regional activity-triggered modulators even between non-synaptic neurons. Perhaps, such ROS may even act on adjacent glia, potentially via redox-sensitive glial proteins such as transient receptor potential melastatin 2 (TRPM2), which have been shown to modulate synaptic plasticity (Wang et al., 2016; Turlova et al., 2018).
Downstream Effector Pathways of ROS and Aquaporin-Dependent Structural Plasticity
Reactive oxygen species can regulate the activity of several protein kinases, including those implicated in canonical neurodevelopmental pathways, either via modification of reactive amino acid residues on kinases or, indirectly, by redox-mediated inhibition of counteracting phosphatases (Finkel and Holbrook, 2000; Corcoran and Cotter, 2013; Holmström and Finkel, 2014). Notably, previous work has implicated aquaporin channels in modulating the efficacy of ROS-regulated protein kinase signaling. By altering a cell’s permeability to extracellularly-generated ROS, aquaporins can amplify or diminish the strength of redox-dependent downstream pathways (Miller et al., 2010; Bertolotti et al., 2013). For instance, mammalian Aquaporin 8, which shares ∼33% of amino acid sequence identity with the Drosophila aquaporin channels, controls the entry of NADPH-oxidase derived H2O2 to increase growth factor signaling in human leukaemia B-cells (Vieceli Dalla Sega et al., 2017). Of particular interest are CaMKII and PKA signaling, which can be enhanced by elevated cytosolic ROS (Humphries et al., 2007; Anderson, 2011), and which both pathways act to limit the elaboration of dendritic arbors in an activity-dependent manner. For example, targeted inhibition of CaMKII or PKA in otherwise non-manipulated neurons results in dendritic over-growth and an increase in arbor size and complexity (Wu and Cline, 1998; Zou and Cline, 1999; Tripodi et al., 2008). This is similar to what we have seen following quenching of extracellular H2O2 or knockdown of the aquaporins Bib and Drip, suggesting that either CaMKII or PKA signaling might be downstream effectors of activity regulated, Duox-generated extracellular H2O2.
Given the increasing number of signals involved in anterograde and retrograde signaling between neurons one might ask how ROS contribute to these signaling pathways. It is possible that Duox acts as an integrator of multiple signaling pathways, in that its activity is regulated by a number of pathways, including the Rho GTPase Rac1 (Hordijk Peter, 2006) and calcium, via its EF-hands (Kawahara et al., 2007). It will be interesting to determine the range and temporal dynamics of Duox activity following neuronal activation; whether Duox reports on low, medium or high levels of neuronal activation, brief bursts or only following prolonged activation. Thus, it is conceivable that different inter-neuronal signaling pathways are utilised for distinct contexts, in terms of their activation pattern and, equally, their spatio-temporal dynamics of signaling.
Data Availability Statement
The raw data supporting the conclusions of this article will be made available by the authors, without undue reservation.
Author Contributions
SD designed and executed the experiments, analysed the data, and wrote the manuscript. PM carried out experiments. DMD, ADO and JFE generated reagents. ML conceived of the study and wrote the manuscript. All authors contributed to the article and approved the submitted version.
Funding
This work was supported by funding from the Biotechnology and Biological Sciences Research Council to ML (BB/R016666/1) and from the DFG to JFE (EV198/1-1). The work benefited from the Imaging Facility, Department of Zoology, supported by Matt Wayland and funds from a Wellcome Trust Equipment Grant (WT079204) with contributions by the Sir Isaac Newton Trust in Cambridge, including Research Grant [18.07ii(c)].
Conflict of Interest
The authors declare that the research was conducted in the absence of any commercial or financial relationships that could be construed as a potential conflict of interest.
Acknowledgments
The authors would like to thank members of the Landgraf lab for feedback on the manuscript. The authors are grateful to Andreas Bergmann, Paul Garrity, Won-Jae Lee, Paul Martin, Sean Sweeney, Helen Weavers, and Will Wood, as well as the Bloomington Drosophila Stock Center and Vienna Drosophila Resource Centre for generously providing fly stocks.
References
Anderson, M. E. (2011). Pathways for CaMKII activation in disease. Heart Rhythm 8, 1501–1503. doi: 10.1016/j.hrthm.2011.04.027
Attwell, D., and Laughlin, S. B. (2001). An energy budget for signaling in the grey matter of the brain. J. Cereb. Blood Flow Metab. 21, 1133–1145. doi: 10.1097/00004647-200110000-00001
Baines, R. A., Robinson, S. G., Fujioka, M., Jaynes, J. B., and Bate, M. (1999). Postsynaptic expression of tetanus toxin light chain blocks synaptogenesis in Drosophila. Curr. Biol. 9, 1267–1270. doi: 10.1016/s0960-9822(99)80510-7
Baxter, P. S., and Hardingham, G. E. (2016). Adaptive regulation of the brain’s antioxidant defences by neurons and astrocytes. Free Radic. Biol. Med. 100, 147–152. doi: 10.1016/j.freeradbiomed.2016.06.027
Benoit, J. B., Hansen, I. A., Attardo, G. M., Michalková, V., Mireji, P. O., Bargul, J. L., et al. (2014). Aquaporins are critical for provision of water during lactation and intrauterine progeny hydration to maintain tsetse fly reproductive success. PLoS Negl. Trop. Dis. 8:e2517. doi: 10.1371/journal.pntd.0002517
Bergland, A. O., Chae, H. S., Kim, Y. J., and Tatar, M. (2012). Fine-scale mapping of natural variation in fly fecundity identifies neuronal domain of expression and function of an aquaporin. PLoS Genet. 8:e1002631. doi: 10.1371/journal.pgen.1002631
Bertolotti, M., Bestetti, S., García-Manteiga, J. M., Medraño-Fernandez, I., Dal Mas, A., Malosio, M. L., et al. (2013). Tyrosine kinase signal modulation: a matter of H2O2 membrane permeability? Antioxid. Redox Signal. 19, 1447–1451. doi: 10.1089/ars.2013.5330
Bienert, G. P., Møller, A. L. B., Kristiansen, K. A., Schulz, A., Møller, I. M., Schjoerring, J. K., et al. (2007). Specific aquaporins facilitate the diffusion of hydrogen peroxide across membranes. J. Biol. Chem. 282, 1183–1192. doi: 10.1074/jbc.m603761200
Chakrabarti, S., and Visweswariah, S. S. (2020). Intramacrophage ROS primes the innate immune system via JAK/STAT and toll activation. Cell Rep. 33:108368. doi: 10.1016/j.celrep.2020.108368
Corcoran, A., and Cotter, T. G. (2013). Redox regulation of protein kinases. FEBS J. 280, 1944–1965. doi: 10.1111/febs.12224
Cuypers, A., Hendrix, S., Amaral Dos Reis, R., De Smet, S., Deckers, J., Gielen, H., et al. (2016). Hydrogen peroxide, signaling in disguise during metal phytotoxicity. Front. Plant Sci. 7:470.
Djiane, A., Krejci, A., Bernard, F., Fexova, S., Millen, K., and Bray, S. J. (2013). Dissecting the mechanisms of Notch induced hyperplasia. EMBO J. 32, 60–71. doi: 10.1038/emboj.2012.326
Dombrovski, M., Kim, A., Poussard, L., Vaccari, A., Acton, S., Spillman, E., et al. (2019). A Plastic visual pathway regulates cooperative behavior in Drosophila Larvae. Current Biology 29, 1866.e–1876.e. doi: 10.1016/j.cub.2019.04.060
Finkel, T., and Holbrook, N. J. (2000). Oxidants, oxidative stress and the biology of ageing. Nature 408, 239–247. doi: 10.1038/35041687
Fogarty, C. E., Diwanji, N., Lindblad, J. L., Tare, M., Amcheslavsky, A., Makhijani, K., et al. (2016). Extracellular Reactive Oxygen Species Drive Apoptosis-Induced Proliferation via Drosophila Macrophages. Curr. Biol. 26, 575–584. doi: 10.1016/j.cub.2015.12.064
Forman, H. J., Ursini, F., and Maiorino, M. (2014). An overview of mechanisms of redox signaling. J. Mol. Cell. Cardiol. 73, 2–9.
Frank, C. A., James, T. D., and Müller, M. (2020). Homeostatic control of Drosophila neuromuscular junction function. Synapse 74, e22133.
Fujioka, M., Lear, B. C., Landgraf, M., Yusibova, G. L., Zhou, J., Riley, K. M., et al. (2003). Even-skipped, acting as a repressor, regulates axonal projections in Drosophila. Development 130, 5385–5400. doi: 10.1242/dev.00770
Fujisawa, Y., Shinoda, N., Chihara, T., and Miura, M. (2020). ROS Regulate Caspase-Dependent Cell Delamination without Apoptosis in the Drosophila Pupal Notum. iScience 23, 101413. doi: 10.1016/j.isci.2020.101413
Ha, E.-M., Oh, C.-T., Bae, Y. S., and Lee, W.-J. (2005a). A direct role for dual oxidase in Drosophila gut immunity. Science 310, 847–850. doi: 10.1126/science.1117311
Ha, E.-M., Oh, C.-T., Ryu, J.-H., Bae, Y. S., Kang, S. W., Jang, I.-H., et al. (2005b). An antioxidant system required for host protection against gut infection in Drosophila. Developmental Cell 8, 125–132. doi: 10.1016/j.devcel.2004.11.007
Halliwell, B. (1992). Reactive oxygen species and the central nervous system. J. Neurochem. 59, 1609–1623. doi: 10.1111/j.1471-4159.1992.tb10990.x
Hamada, F. N., Rosenzweig, M., Kang, K., Pulver, S. R., Ghezzi, A., Jegla, T. J., et al. (2008). An internal thermal sensor controlling temperature preference in Drosophila. Nature 454, 217–220. doi: 10.1038/nature07001
Hardingham, N., Dachtler, J., and Fox, K. (2013). The role of nitric oxide in pre-synaptic plasticity and homeostasis. Front. Cell. Neurosci. 7:190.
Hidalgo, C., and Arias-Cavieres, A. (2016). Calcium, Reactive Oxygen Species, and Synaptic Plasticity. Physiology 31, 201–215. doi: 10.1152/physiol.00038.2015
Holmström, K. M., and Finkel, T. (2014). Cellular mechanisms and physiological consequences of redox-dependent signalling. Nat. Rev. Mol. Cell Biol. 15, 411–421. doi: 10.1038/nrm3801
Hongpaisan, J., Winters, C. A., and Andrews, S. B. (2003). Calcium-dependent mitochondrial superoxide modulates nuclear CREB phosphorylation in hippocampal neurons. Mol. Cell. Neurosci. 24, 1103–1115. doi: 10.1016/j.mcn.2003.09.003
Hongpaisan, J., Winters, C. A., and Andrews, S. B. (2004). Strong calcium entry activates mitochondrial superoxide generation, upregulating kinase signaling in hippocampal neurons. J. Neurosci. 24, 10878–10887. doi: 10.1523/jneurosci.3278-04.2004
Hordijk Peter, L. (2006). Regulation of NADPH Oxidases. Circ. Res. 98, 453–462. doi: 10.1161/01.res.0000204727.46710.5e
Humphries, K. M., Pennypacker, J. K., and Taylor, S. S. (2007). Redox regulation of cAMP-dependent protein kinase signaling: kinase versus phosphatase inactivation. J. Biol. Chem. 282, 22072–22079. doi: 10.1074/jbc.m702582200
Ivanov, A., and Purves, D. (1989). Ongoing electrical activity of superior cervical ganglion cells in mammals of different size. J. Comp. Neurol. 284, 398–404. doi: 10.1002/cne.902840307
Kawahara, T., Quinn, M. T., and Lambeth, J. D. (2007). Molecular evolution of the reactive oxygen-generating NADPH oxidase (Nox/Duox) family of enzymes. BMC Evol. Biol. 7:109. doi: 10.1186/1471-2148-7-109
Kerstein, P. C., Nichol, R. H. IV, and Gomez, T. M. (2015). Mechanochemical regulation of growth cone motility. Front. Cell. Neurosci. 9:244.
Lambeth, J. D. (2002). Nox/Duox family of nicotinamide adenine dinucleotide (phosphate) oxidases. Curr. Opin. Hematol. 9, 11–17. doi: 10.1097/00062752-200201000-00003
Landgraf, M., Jeffrey, V., Fujioka, M., Jaynes, J. B., and Bate, M. (2003). Embryonic origins of a motor system: motor dendrites form a myotopic map in Drosophila. PLoS Biol. 1:E41.
Marley, R., and Baines, R. A. (2011). Dissection of Third-Instar Drosophila Larvae for Electrophysiological Recording from Neurons. Cold Spring Harb. Protoc. 2011, db.rot065656. ∗,
Martin, S. J., Grimwood, P. D., and Morris, R. G. M. (2000). Synaptic Plasticity and Memory: an Evaluation of the Hypothesis. Annu. Rev. Neurosci. 23, 649–711. doi: 10.1146/annurev.neuro.23.1.649
Massaad, C. A., and Klann, E. (2011). Reactive oxygen species in the regulation of synaptic plasticity and memory. Antioxid. Redox Signal. 14, 2013–2054. doi: 10.1089/ars.2010.3208
Miller, E. W., Dickinson, B. C., and Chang, C. J. (2010). Aquaporin-3 mediates hydrogen peroxide uptake to regulate downstream intracellular signaling. Proc. Natl. Acad. Sci. U. S. A. 107, 15681–15686. doi: 10.1073/pnas.1005776107
Milton, V. J., Jarrett, H. E., Gowers, K., Chalak, S., Briggs, L., Robinson, I. M., et al. (2011). Oxidative stress induces overgrowth of the Drosophila neuromuscular junction. Proc. Natl. Acad. Sci. U. S. A. 108, 17521–17526. doi: 10.1073/pnas.1014511108
Morand, S., Ueyama, T., Tsujibe, S., Saito, N., Korzeniowska, A., and Leto, T. L. (2009). Duox maturation factors form cell surface complexes with Duox affecting the specificity of reactive oxygen species generation. FASEB J. 23, 1205–1218. doi: 10.1096/fj.08-120006
Moreira, S., Stramer, B., Evans, I., Wood, W., and Martin, P. (2010). Prioritization of competing damage and developmental signals by migrating macrophages in the Drosophila embryo. Curr. Biol. 20, 464–470. doi: 10.1016/j.cub.2010.01.047
Munnamalai, V., and Suter, D. M. (2009). Reactive oxygen species regulate F-actin dynamics in neuronal growth cones and neurite outgrowth. J. Neurochem. 108, 644–661. doi: 10.1111/j.1471-4159.2008.05787.x
Munnamalai, V., Weaver, C. J., Weisheit, C. E., Venkatraman, P., Agim, Z. S., Quinn, M. T., et al. (2014). Bidirectional interactions between NOX2-type NADPH oxidase and the F-actin cytoskeleton in neuronal growth cones. J. Neurochem. 130, 526–540. doi: 10.1111/jnc.12734
Nguyen, A. W., and Daugherty, P. S. (2005). Evolutionary optimization of fluorescent proteins for intracellular FRET. Nature Biotechnology 23, 355–360. doi: 10.1038/nbt1066
Niethammer, P., Grabher, C., Look, A. T., and Mitchison, T. J. (2009). A tissue-scale gradient of hydrogen peroxide mediates rapid wound detection in zebrafish. Nature 459, 996–999. doi: 10.1038/nature08119
Oswald, M. C., Brooks, P. S., Zwart, M. F., Mukherjee, A., West, R. J., Giachello, C. N., et al. (2018a). Reactive oxygen species regulate activity-dependent neuronal plasticity in Drosophila. Elife 7, doi: 10.7554/eLife.39393 ∗,
Oswald, M. C. W., Garnham, N., Sweeney, S. T., and Landgraf, M. (2018b). Regulation of neuronal development and function by ROS. FEBS Lett. 592, 679–691. doi: 10.1002/1873-3468.12972
Ou, Y., Chwalla, B., Landgraf, M., and van Meyel, D. J. (2008). Identification of genes influencing dendrite morphogenesis in developing peripheral sensory and central motor neurons. Neural Dev. 3, 16. doi: 10.1186/1749-8104-3-16
Panday, A., Sahoo, M. K., Osorio, D., and Batra, S. (2015). NADPH oxidases: an overview from structure to innate immunity-associated pathologies. Cell. Mol. Immunol. 12, 5–23. doi: 10.1038/cmi.2014.89
Peng, J.-J., Lin, S.-H., Liu, Y.-T., Lin, H.-C., Li, T.-N., and Yao, C.-K. (2019). A circuit-dependent ROS feedback loop mediates glutamate excitotoxicity to sculpt the Drosophila motor system. Elife 8, e47372.
Pignoni, F., and Zipursky, S. L. (1997). Induction of Drosophila eye development by decapentaplegic. Development 124, 271–278. doi: 10.1242/dev.124.2.271
Pozo, K., and Goda, Y. (2010). Unraveling mechanisms of homeostatic synaptic plasticity. Neuron 66, 337–351. doi: 10.1016/j.neuron.2010.04.028
Prokop, A., and Meinertzhagen, I. A. (2006). Development and structure of synaptic contacts in Drosophila. Semin. Cell Dev. Biol. 17, 20–30. doi: 10.1016/j.semcdb.2005.11.010
Purves, D., and Lichtman, J. W. (1985). Geometrical differences among homologous neurons in mammals. Science 228, 298–302. doi: 10.1126/science.3983631
Sah, R., Galeffi, F., Ahrens, R., Jordan, G., and Schwartz-Bloom, R. D. (2002). Modulation of the GABA(A)-gated chloride channel by reactive oxygen species. J. Neurochem. 80, 383–391. doi: 10.1046/j.0022-3042.2001.00706.x
Sahoo, N., Hoshi, T., and Heinemann, S. H. (2014). Oxidative modulation of voltage-gated potassium channels. Antioxid. Redox Signal. 21, 933–952. doi: 10.1089/ars.2013.5614
Schieber, M., and Chandel, N. S. (2014). ROS function in redox signaling and oxidative stress. Curr. Biol. 24, R453–R462.
Sesti, F., Liu, S., and Cai, S.-Q. (2010). Oxidation of potassium channels by ROS: a general mechanism of aging and neurodegeneration? Trends Cell Biol. 20, 45–51. doi: 10.1016/j.tcb.2009.09.008
Sheng, C., Javed, U., Gibbs, M., Long, C., Yin, J., Qin, B., et al. (2018). Experience-dependent structural plasticity targets dynamic filopodia in regulating dendrite maturation and synaptogenesis. Nat. Commun. 9, 3362.
Sink, H., and Whitington, P. M. (1991). Location and connectivity of abdominal motoneurons in the embryo and larva of Drosophila melanogaster. J. Neurobiol. 22, 298–311. doi: 10.1002/neu.480220309
Stuchlik, A. (2014). Dynamic learning and memory, synaptic plasticity and neurogenesis: an update. Front. Behav. Neurosci. 8:106.
Tejada-Simon, M. V., Serrano, F., Villasana, L. E., Kanterewicz, B. I., Wu, G.-Y., Quinn, M. T., et al. (2005). Synaptic localization of a functional NADPH oxidase in the mouse hippocampus. Mol. Cell. Neurosci. 29, 97–106. doi: 10.1016/j.mcn.2005.01.007
Terzi, A., Roeder, H., Weaver, C. J., and Suter, D. M. (2021). Neuronal NADPH oxidase 2 regulates growth cone guidance downstream of slit2/robo2. Developmental Neurobiology 81, 3–21. doi: 10.1002/dneu.22791
Terzi, A., and Suter, D. M. (2020). The role of NADPH oxidases in neuronal development. Free Radical Biology & Medicine 154, 33–47. doi: 10.1016/j.freeradbiomed.2020.04.027
Tripodi, M., Evers, J. F., Mauss, A., Bate, M., and Landgraf, M. (2008). Structural homeostasis: compensatory adjustments of dendritic arbor geometry in response to variations of synaptic input. PLoS Biol. 6:e260. doi: 10.1371/journal.pbio.0060260
Turlova, E., Feng, Z.-P., and Sun, H.-S. (2018). The role of TRPM2 channels in neurons, glial cells and the blood-brain barrier in cerebral ischemia and hypoxia. Acta Pharmacol. Sin. 39, 713–721. doi: 10.1038/aps.2017.194
Turrigiano, G. G., and Nelson, S. B. (2000). Hebb and homeostasis in neuronal plasticity. Curr. Opin. Neurobiol. 10, 358–364. doi: 10.1016/s0959-4388(00)00091-x
Turrigiano, G. G., and Nelson, S. B. (2004). Homeostatic plasticity in the developing nervous system. Nat. Rev. Neurosci. 5, 97–107. doi: 10.1038/nrn1327
Vieceli Dalla Sega, F., Prata, C., Zambonin, L., Angeloni, C., Rizzo, B., Hrelia, S., et al. (2017). Intracellular cysteine oxidation is modulated by aquaporin-8-mediated hydrogen peroxide channeling in leukaemia cells. Biofactors 43, 232–242. doi: 10.1002/biof.1340
Wang, J., Jackson, M. F., and Xie, Y.-F. (2016). Glia and TRPM2 Channels in Plasticity of Central Nervous System and Alzheimer’s Diseases. Neural Plast. 2016, 1680905.
Wefelmeyer, W., Puhl, C. J., and Burrone, J. (2016). Homeostatic Plasticity of Subcellular Neuronal Structures: From Inputs to Outputs. Trends Neurosci. 39, 656–667. doi: 10.1016/j.tins.2016.08.004
Wilson, C., Núñez, M. T., and González-Billault, C. (2015). Contribution of NADPH oxidase to the establishment of hippocampal neuronal polarity in culture. J. Cell Sci. 128, 2989–2995.
Wu, G. Y., and Cline, H. T. (1998). Stabilization of dendritic arbor structure in vivo by CaMKII. Science 279, 222–226. doi: 10.1126/science.279.5348.222
Yin, J., and Yuan, Q. (2014). Structural homeostasis in the nervous system: a balancing act for wiring plasticity and stability. Front. Cell. Neurosci. 8:439.
Yuan, Q., Xiang, Y., Yan, Z., Han, C., Jan, L. Y., and Jan, Y. N. (2011). Light-Induced Structural and Functional Plasticity in Drosophila Larval Visual System. Science 333, 1458–1462. doi: 10.1126/science.1207121
Zhu, X.-H., Qiao, H., Du, F., Xiong, Q., Liu, X., Zhang, X., et al. (2012). Quantitative imaging of energy expenditure in human brain. Neuroimage 60, 2107–2117. doi: 10.1016/j.neuroimage.2012.02.013
Zou, D. J., and Cline, H. T. (1999). Postsynaptic calcium/calmodulin-dependent protein kinase II is required to limit elaboration of presynaptic and postsynaptic neuronal arbors. J. Neurosci. 19, 8909–8918. doi: 10.1523/jneurosci.19-20-08909.1999
Keywords: reactive oxygen species, aquaporins, NADPH oxidases, dendrites, Drosophila, plasticity
Citation: Dhawan S, Myers P, Bailey DMD, Ostrovsky AD, Evers JF and Landgraf M (2021) Reactive Oxygen Species Mediate Activity-Regulated Dendritic Plasticity Through NADPH Oxidase and Aquaporin Regulation. Front. Cell. Neurosci. 15:641802. doi: 10.3389/fncel.2021.641802
Received: 15 December 2020; Accepted: 02 June 2021;
Published: 05 July 2021.
Edited by:
Chun Han, Cornell University, United StatesReviewed by:
Dion Dickman, University of Southern California, Los Angeles, United StatesJay Z. Parrish, University of Washington, United States
Copyright © 2021 Dhawan, Myers, Bailey, Ostrovsky, Evers and Landgraf. This is an open-access article distributed under the terms of the Creative Commons Attribution License (CC BY). The use, distribution or reproduction in other forums is permitted, provided the original author(s) and the copyright owner(s) are credited and that the original publication in this journal is cited, in accordance with accepted academic practice. No use, distribution or reproduction is permitted which does not comply with these terms.
*Correspondence: Matthias Landgraf, bWwxMDAwNkBjYW0uYWMudWs=