- 1Normandy University, UNIROUEN, INSERM U1245, Rouen, France
- 2Department of Genetics and Reference Center for Developmental Disorders, Normandie University, UNIROUEN, INSERM U1245 and Rouen University Hospital, Rouen, France
- 3Department of Neurosurgery, Normandy University, UNIROUEN, INSERM U1245 and Rouen University Hospital, Rouen, France
Introduction
Hydrocephalus is the most common cause of pediatric surgical intervention (Lim et al., 2018), occurring about once per 1,000 births in the US (Kahle et al., 2016). Current treatments are invasive, involving either CSF drainage by ventriculo-peritoneal shunt (VPS), ventriculo-atrial shunt, and lumbo-peritoneal shunt, or endoscopic third ventriculostomy (ETV). Shunting entails increased rates of mortality and morbidity due to intraparenchymal hematoma during implantation and device failure or infection (Christian et al., 2016; Kahle et al., 2016; Riva-Cambrin et al., 2016). The hospitalization required for these procedures costs annually more than 2 billion dollars (Stein and Guo, 2008). CSF drainage from the ventricles to the peritoneum, the prevalent treatment for hydrocephalus, requires multiple revisions during the patients' lifetimes, at an average interval of 14.1 years (Reddy et al., 2014). Ultimately, surgical treatment is palliative and does not address the underlying cause of hydrocephalus. These exigencies provide the rationale for the implementation of non-invasive approaches in place of or in support of invasive treatments. To date, no effective non-invasive treatment has been reported.
Shunt implantation facilitates sampling and analysis of the CSF for early detection of infection (Khalil et al., 2016). It could conceivably be analyzed also for evaluating parenchymal health. If suitable markers are identified, their overabundance in the CSF of hydrocephalic patients could potentially serve as biomarkers of case-specific injuries. Furthermore, the composition of the CSF could provide information on the brain's response to the elevated intra-cranial pressure exerted on the parenchyma as a result of ventriculomegaly. Delineation of the molecular pathways employed by the brain to this end could be used in principle to guide future pharmacological approaches and identify new druggable targets.
We had published comparative proteomics between the CSF of healthy and hydrocephalus-harboring Mpdz KO mice (Yang et al., 2019). The Mpdz knockout mouse model phenocopies similar loss-of-function mutations in the human ortholog, MPDZ (Al-Dosari et al., 2013; Saugier-Veber et al., 2017). Here, we compare the Yang et al. data on murine CSF to proteomic analyses of human CSF to gauge their similarity and, consequently, the validity of drawing diagnostic and therapeutic inferences collectively by pooling all the available data.
Analysis
Inventory of Relevant Proteomic Studies on Human CSF
The number of comparative proteomic analyses of CSF of hydrocephalic patients to normal subjects reported to date is surprisingly small. We identified only three studies that had analyzed the CSF of hydrocephalus-harboring patients (Li et al., 2006; Scollato et al., 2010; Waybright et al., 2010). Out of these, only Li et al. compared the CSF of adult patients with idiopathic normal pressure hydrocephalus (INPH) to normal adult subjects (15 vs. 12 subjects, respectively). The CSF was resolved by 2D SDS-PAGE before undergoing mass-spectroscopy, reducing substantially the sensitivity of the assay and the total number of quantified proteins. Among the nine proteins they reported on, the intensity of the 2D ApoD spot was 2.3-fold higher in the CSF of INPH patients. The remaining eight proteins were not detected in our and others' proteomic studies (see below). Scollato et al. also used 2D SDS-PAGE followed by mass-spectroscopy to analyze the CSF of 17 adult patients with NPH. They identified 12 proteins but did not compare the proteome of their CSF to that of normal subjects. Waybright et al. analyzed the CSF of nine infants, out of which one harbored congenital hydrocephalus, one underwent shunt revision, and the remaining seven acquired hydrocephalus caused by several types of CSF flow obstructions (Waybright et al., 2010). Unlike the previous studies, the CSF was processed by microcapillary liquid chromatography followed by mass spectrometry, resulting in higher sensitivity and culminating in the detection of 3,336 proteins
Based on their attributes, a subgroup of 18 proteins out of the 23 reported by Yang et al. was classified into five functional categories (from top to bottom in Table 1): extracellular matrix (ECM), complement factors, lipoproteins, cytokine binding proteins, and proteinase inhibitors. The 18 proteins were considered either as reporters of the injuries inflicted by ventriculomegaly on the parenchyma, or as components of autoregulatory pathways activated by the brain to counter these injuries. These classifications reflect literature searches on the associations between these proteins in the CSF of Mpdz KO mice (Yang et al., 2019) on one hand, and CNS pathologies that injured the parenchyma and affected CSF production, on the other. Five out of the original 23 overabundant proteins were excluded because we opted not to address the immune response, or because we did not find evidence in previous studies for their involvement in neuronal injury.
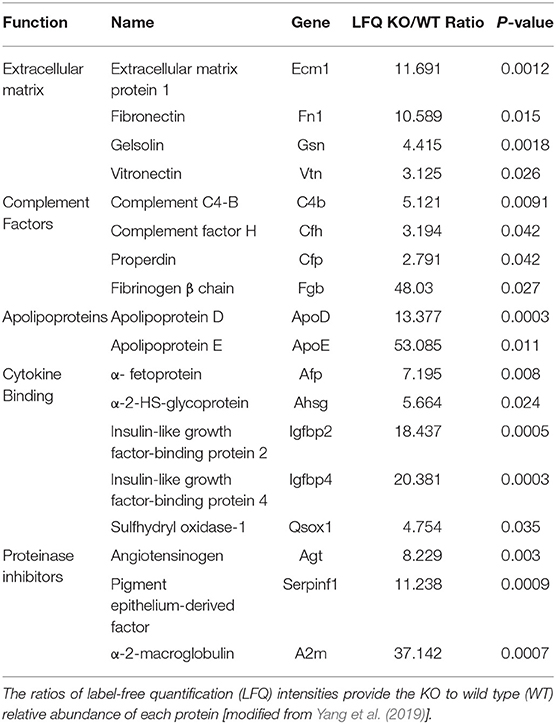
Table 1. List of proteins that were at least twice overabundant in the CSF of Mpdz KO mice relative to the CSF of healthy mice with a null hypothesis probability (P) of ≤ 0.05 (Yang et al., 2019).
Clearly, none of the previous studies affords comparison with the comparative proteomics we undertook (Yang et al., 2019). Similarly, we were unable to find published information on the specific functions of any of the 18 overabundant proteins in the etiology of congenital, or, for that matter, any type of hydrocephalus. At most, the murine CSF proteome can be compared to the human one. To date, at least 31 studies undertook proteomics analysis of human CSF (see https://proteomics.uib.no/csf-pr/). We are aware only of the single aforementioned study by Waybright et al. for proteomic analysis of the CSF of hydrocephalic infants.
Out of the 18 overabundant proteins, 17 (except α-fetoprotein) were detected in the CSF of hydrocephalic patients (Waybright et al., 2010). Their majority (15) was among the top 100 (out of a total exceeding 3,300) most abundant CSF proteins identified by Waybright et al. To further match the murine and human CSF proteomes, we searched the 18 proteins in the proteomic data sets from two studies on either 21 (Schutzer et al., 2010) or 38 (Lleo et al., 2019) CSF samples from normal adults. Either 16 (except fibronectin and α-fetoprotein) or 17 (except α-fetoprotein) proteins, respectively, were abundant in human CSF samples.
Biomarkers of Brain Injury
The overabundant ECM proteins, complement factors and apolipoproteins (Table 1), were associated with mechanisms of ventriculomegaly-inflicted injuries based on the following evidence: ECM1 was linked to neuroinflammation, whereby it ameliorated the demyelination caused by T helper cells (Su et al., 2016). Plasma fibronectin, the soluble isoform detected in our previous study (Yang et al., 2019), supported neuronal survival and reduced brain injury following cerebral ischemia (Sakai et al., 2001). Extracellular gelsolin ameliorated inflammation (Bucki et al., 2008) and had a neuroprotective effect during stroke (Endres et al., 1999). Vitronectin activated microglia (Milner and Campbell, 2002), the primary immune effector cell of the CNS.
The complement system had a neuroprotective role in CNS neurodegenerative diseases (Bonifati and Kishore, 2007). Specifically, complement C4-B was produced by microglial cells in response to ischemia and inflammation (D'ambrosio et al., 2001). Complement factor H was produced by neurons and had a protective function in neuroinflammation (Griffiths et al., 2009). Properdin (complement factor P), activated microglia and contributed to inflammation in response to ischemia (Sisa et al., 2019).
ApoE is produced by the choroid plexus (Xu et al., 2006); it protected the brain against neuronal injury and promoted neuronal survival (Beffert et al., 2006). ApoD had a similar neuroprotective role in CNS degeneration (Navarro et al., 2013). Lastly, α-2-macroglobulin was identified as a marker of neuronal injury (Varma et al., 2017).
Collectively, the overabundance of the aforementioned proteins reveals that severe hydrocephalus inflicted a set of injuries on the parenchyma, including neuroinflammation, demyelination, and impaired perfusion. Activation of CNS immune defense by microglial cells accompanied these processes and possibly aggravated the inflammation. This injury pattern conforms to the empirical picture of neonatal hydrocephalus (Mcallister, 2012).
Biomarkers of Autoregulatory Pathways
The IGF-binding proteins (IGFBPs) are produced in the CNS, including the choroid plexus (Tseng et al., 1989). Specifically, IGFPB2 and IGFBP4, which were overabundant in the CSF of Mpdz KO mice (Yang et al., 2019), are secreted by astrocytes in response to brain injury (Lewitt and Boyd, 2019). While the differential functions of each IGFPB are not fully understood, they are thought to stabilize IGF, extend its half-life (Zapf et al., 1986), and modulate its activity (Firth and Baxter, 2002). α-2-HS-glycoprotein, which binds to and modulates the signaling of the IGF receptor (Mathews et al., 2000), α-fetoprotein, a cytokine-binding plasma component (Mori et al., 2011), and sulfhydryl oxidase-1 participate in IGF uptake and transport by IGFPBs (Jassal et al., 2020). IGF and the IGFPBs are produced in choroid plexus epithelial cells (Stylianopoulou et al., 1988; Holm et al., 1994). The secretion of IGF into the CSF is increased in response to injury (Walter et al., 1999). Remarkably, all the five proteins in this group (Table 1) regulate multiple facets of IGF secretion and signaling. IGF protected neurons subjected to ischemia (Wang et al., 2013) and reduced microglia activation (Rodriguez-Perez et al., 2016).
Two of the overabundant proteins are the serpins angiotensinogen and PEDF (Table 1). Angiotensinogen is the precursor of several cleaved derivatives and peptides. One of the latter, angiotensin-2, reduced blood flow to the choroid plexus (Maktabi et al., 1990) and CSF production (Maktabi et al., 1993). However, its cleaved derivatives were antiangiogenic (Celerier et al., 2002). The anti-angiogenic effect is shared by PEDF (Dawson et al., 1999), which is secreted by ependymal cells in the subventricular zone (Ramirez-Castillejo et al., 2006). It appears, therefore, that serine protease inhibitors may reduce CSF production by pruning the dense capillary network that surrounds the choroid plexus vessels (Zagorska-Swiezy et al., 2008).
Discussion
Few previous studies had analyzed the diagnostic potential of the CSF. Developmental defects originating from attenuated cycling of germinal matrix cells in the brains of hydrocephalus-harboring rats had been attributed to alteration in the composition of the CSF relative to healthy subjects, but the putative difference between the CSF of healthy and hydrocephalic rats had not been identified (Owen-Lynch et al., 2003). Comparison of select proteins in the CSF of patients with idiopathic normal pressure hydrocephalus before VPS implantation and of healthy subject was used to diagnose the damage to the patients' parenchyma. Subsequently, the concentrations of the same proteins after VPS shunting were used to gauge if the shunt ameliorated the damage (Jeppsson et al., 2013). We did not find, however, published comparisons between the CSF proteomics of normal subjects and hydrocephalus-harboring patients that could be used for diagnostic or therapeutic purposes.
Potential Diagnostic Implications
The interpretation of the overabundance of ECM proteins, complement factors, and apolipoproteins present in the subgroup of 18 proteins reported by Yang et al. as biomarkers of brain injuries is supported by previous studies (Mcallister, 2012). Their individual or collective overabundances could be potentially measured in the CSF collected during VP shunting or ETV of hydrocephalic patients. Beyond verifying the occurrence of hydrocephalus, such measurements would highlight case-specific attributes, such as the presence of demyelination, revealed by the overabundance of ECM1, or of an aggressive immune response indicated by the overabundances of vitronectin and complement factors.
Potential Therapeutic Implications
The combined overabundance of all the five cytokine-binding proteins present in the above subgroup suggests that IGF signaling is a major autoregulatory pathway employed by the brain, possibly to counteract the neuronal injury caused by ventriculomegaly (Johnston et al., 1996). Similarly, the concurrence of the overabundances of angiotensinogen and PEDF indicates that angiogenesis is suppressed as a negative feedback loop to counter the expansion of the ventricles by a reduction in CSF production. Each of these pathways could potentially be exploited for non-invasive therapies in place of or as adjuvants to the current invasive approaches. Finally, the marked overabundances of ApoE and ApoD suggest that these apolipoproteins may be used directly for neuroprotection of the parenchyma.
Author Contributions
AH initiated the proteomics data analysis. All authors contributed to the writing of the manuscript.
Conflict of Interest
The authors declare that the research was conducted in the absence of any commercial or financial relationships that could be construed as a potential conflict of interest.
Acknowledgments
The manuscript of this publication was released and posted on the bioRxiv preprint server (https://www.biorxiv.org/content/10.1101/2020.05.26.117457v4).
Abbreviations
Apo, apolipoprotein; CNS, central nervous system; ECM, extracellular matrix; ETV, endoscopic third ventriculostomy; ICP, intracranial pressure; IGF, insulin-like growth factor; IGFBP, IGF-binding protein; KO, knockout; LFQ, label-free quantification; Mpdz, multi PDZ; PDZ, PSD-95 (= postsynaptic density 95), Dlg (= disks large), ZO-1 (= zonula occludens-1); PEDF, pigment epithelium-derived factor; VP, ventriculoperitoneal; WT, wild type.
References
Al-Dosari, M. S., Al-Owain, M., Tulbah, M., Kurdi, W., Adly, N., Al-Hemidan, A., et al. (2013). Mutation in MPDZ causes severe congenital hydrocephalus. J. Med. Genet. 50, 54–58. doi: 10.1136/jmedgenet-2012-101294
Beffert, U., Nematollah Farsian, F., Masiulis, I., Hammer, R.E., Yoon, S.O., Giehl, K.M., et al. (2006). ApoE receptor 2 controls neuronal survival in the adult brain. Curr. Biol. 16, 2446–2452. doi: 10.1016/j.cub.2006.10.029
Bonifati, D. M., and Kishore, U. (2007). Role of complement in neurodegeneration and neuroinflammation. Mol. Immunol. 44, 999–1010. doi: 10.1016/j.molimm.2006.03.007
Bucki, R., Byfield, F. J., Kulakowska, A., Mccormick, M. E., Drozdowski, W., Namiot, Z., et al. (2008). Extracellular gelsolin binds lipoteichoic acid and modulates cellular response to proinflammatory bacterial wall components. J. Immunol. 181, 4936–4944. doi: 10.4049/jimmunol.181.7.4936
Celerier, J., Cruz, A., Lamande, N., Gasc, J. M., and Corvol, P. (2002). Angiotensinogen and its cleaved derivatives inhibit angiogenesis. Hypertension 39, 224–228. doi: 10.1161/hy0202.103441
Christian, E. A., Jin, D. L., Attenello, F., Wen, T., Cen, S., Mack, W. J., et al. (2016). Trends in hospitalization of preterm infants with intraventricular hemorrhage and hydrocephalus in the United States, 2000-2010. J. Neurosurg. Pediatr. 17, 260–269. doi: 10.3171/2015.7.PEDS15140
D'ambrosio, A. L., Pinsky, D. J., and Connolly, E. S. (2001). The role of the complement cascade in ischemia/reperfusion injury: implications for neuroprotection. Mol. Med. 7, 367–382. doi: 10.1007/BF03402183
Dawson, D. W., Volpert, O. V., Gillis, P., Crawford, S. E., Xu, H., Benedict, W., et al. (1999). Pigment epithelium-derived factor: a potent inhibitor of angiogenesis. Science 285, 245–248. doi: 10.1126/science.285.5425.245
Endres, M., Fink, K., Zhu, J., Stagliano, N. E., Bondada, V., Geddes, J. W., et al. (1999). Neuroprotective effects of gelsolin during murine stroke. J. Clin. Invest 103, 347–354. doi: 10.1172/JCI4953
Firth, S. M., and Baxter, R. C. (2002). Cellular actions of the insulin-like growth factor binding proteins. Endocr. Rev. 23, 824–854. doi: 10.1210/er.2001-0033
Griffiths, M. R., Neal, J. W., Fontaine, M., Das, T., and Gasque, P. (2009). Complement factor H, a marker of self protects against experimental autoimmune encephalomyelitis. J. Immunol. 182, 4368–4377. doi: 10.4049/jimmunol.0800205
Holm, N. R., Hansen, L. B., Nilsson, C., and Gammeltoft, S. (1994). Gene expression and secretion of insulin-like growth factor-II and insulin-like growth factor binding protein-2 from cultured sheep choroid plexus epithelial cells. Brain Res. Mol. Brain Res. 21, 67–74. doi: 10.1016/0169-328X(94)90379-4
Jassal, B., Matthews, L., Viteri, G., Gong, C., Lorente, P., Fabregat, A., et al. (2020). The reactome pathway knowledgebase. Nucleic Acids Res. 48, D498–D503. doi: 10.1093/nar/gkz1031
Jeppsson, A., Zetterberg, H., Blennow, K., and Wikkelso, C. (2013). Idiopathic normal-pressure hydrocephalus: pathophysiology and diagnosis by CSF biomarkers. Neurology 80, 1385–1392. doi: 10.1212/WNL.0b013e31828c2fda
Johnston, B. M., Mallard, E. C., Williams, C. E., and Gluckman, P. D. (1996). Insulin-like growth factor-1 is a potent neuronal rescue agent after hypoxic-ischemic injury in fetal lambs. J. Clin. Invest 97, 300–308. doi: 10.1172/JCI118416
Kahle, K. T., Kulkarni, A. V., Limbrick, D. D. Jr., and Warf, B. C. (2016). Hydrocephalus in children. Lancet 387, 788–799. doi: 10.1016/S0140-6736(15)60694-8
Khalil, A., Mandiwanza, T., Zakaria, Z., and Crimmins, D. (2016). Routine cerebrospinal fluid analysis during 'de novo' ventriculoperitoneal shunt insertion: single Institution Experience. Br. J. Neurosurg. 30, 427–428. doi: 10.3109/02688697.2015.1119235
Lewitt, M. S., and Boyd, G. W. (2019). The role of insulin-like growth factors and insulin-like growth factor-binding proteins in the nervous system. Biochem. Insights 12, 1178626419842176. doi: 10.1177/1178626419842176
Li, X., Miyajima, M., Mineki, R., Taka, H., Murayama, K., and Arai, H. (2006). Analysis of potential diagnostic biomarkers in cerebrospinal fluid of idiopathic normal pressure hydrocephalus by proteomics. Acta Neurochir. 148, 859–864; discussion 864. doi: 10.1007/s00701-006-0787-4
Lim, J., Tang, A. R., Liles, C., Hysong, A. A., Hale, A. T., Bonfield, C. M., et al. (2018). The cost of hydrocephalus: a cost-effectiveness model for evaluating surgical techniques. J. Neurosurg. Pediatr 23, 109–118. doi: 10.3171/2018.6.PEDS17654
Lleo, A., Nunez-Llaves, R., Alcolea, D., Chiva, C., Balateu-Panos, D., Colom-Cadena, M., et al. (2019). Changes in synaptic proteins precede neurodegeneration markers in preclinical Alzheimer's disease cerebrospinal fluid. Mol. Cell. Proteomics 18, 546–560. doi: 10.1074/mcp.RA118.001290
Maktabi, M. A., Heistad, D. D., and Faraci, F. M. (1990). Effects of angiotensin II on blood flow to choroid plexus. Am. J. Physiol. 258, H414–418. doi: 10.1152/ajpheart.1990.258.2.H414
Maktabi, M. A., Stachovic, G. C., and Faraci, F. M. (1993). Angiotensin II decreases the rate of production of cerebrospinal fluid. Brain Res. 606, 44–49. doi: 10.1016/0006-8993(93)91567-C
Mathews, S. T., Chellam, N., Srinivas, P. R., Cintron, V. J., Leon, M. A., Goustin, A. S., et al. (2000). Alpha2-HSG, a specific inhibitor of insulin receptor autophosphorylation, interacts with the insulin receptor. Mol. Cell. Endocrinol. 164, 87–98. doi: 10.1016/S0303-7207(00)00237-9
Mcallister, J. P. 2nd. (2012). Pathophysiology of congenital and neonatal hydrocephalus. Semin. Fetal Neonatal. Med. 17, 285–294. doi: 10.1016/j.siny.2012.06.004
Milner, R., and Campbell, I. L. (2002). Cytokines regulate microglial adhesion to laminin and astrocyte extracellular matrix via protein kinase C-dependent activation of the alpha6beta1 integrin. J. Neurosci. 22, 1562–1572. doi: 10.1523/JNEUROSCI.22-05-01562.2002
Mori, K., Emoto, M., and Inaba, M. (2011). Fetuin-A: a multifunctional protein. Recent Pat. Endocr. Metab. Immune Drug Discov. 5, 124–146. doi: 10.2174/187221411799015372
Navarro, A., Mendez, E., Diaz, C., Del Valle, E., Martinez-Pinilla, E., Ordonez, C., et al. (2013). Lifelong expression of apolipoprotein D in the human brainstem: correlation with reduced age-related neurodegeneration. PLoS ONE 8:e77852. doi: 10.1371/journal.pone.0077852
Owen-Lynch, P. J., Draper, C. E., Mashayekhi, F., Bannister, C. M., and Miyan, J. A. (2003). Defective cell cycle control underlies abnormal cortical development in the hydrocephalic Texas rat. Brain 126, 623–631. doi: 10.1093/brain/awg058
Ramirez-Castillejo, C., Sanchez-Sanchez, F., Andreu-Agullo, C., Ferron, S. R., Aroca-Aguilar, J. D., Sanchez, P., et al. (2006). Pigment epithelium-derived factor is a niche signal for neural stem cell renewal. Nat. Neurosci. 9, 331–339. doi: 10.1038/nn1657
Reddy, G. K., Bollam, P., and Caldito, G. (2014). Long-term outcomes of ventriculoperitoneal shunt surgery in patients with hydrocephalus. World Neurosurg. 81, 404–410. doi: 10.1016/j.wneu.2013.01.096
Riva-Cambrin, J., Kestle, J. R., Holubkov, R., Butler, J., Kulkarni, A. V., Drake, J., et al. (2016). Risk factors for shunt malfunction in pediatric hydrocephalus: a multicenter prospective cohort study. J. Neurosurg. Pediatr. 17, 382–390. doi: 10.3171/2015.6.PEDS14670
Rodriguez-Perez, A. I., Borrajo, A., Diaz-Ruiz, C., Garrido-Gil, P., and Labandeira-Garcia, J. L. (2016). Crosstalk between insulin-like growth factor-1 and angiotensin-II in dopaminergic neurons and glial cells: role in neuroinflammation and aging. Oncotarget 7, 30049–30067. doi: 10.18632/oncotarget.9174
Sakai, T., Johnson, K. J., Murozono, M., Sakai, K., Magnuson, M. A., Wieloch, T., et al. (2001). Plasma fibronectin supports neuronal survival and reduces brain injury following transient focal cerebral ischemia but is not essential for skin-wound healing and hemostasis. Nat. Med. 7, 324–330. doi: 10.1038/85471
Saugier-Veber, P., Marguet, F., Lecoquierre, F., Adle-Biassette, H., Guimiot, F., Cipriani, S., et al. (2017). Hydrocephalus due to multiple ependymal malformations is caused by mutations in the MPDZ gene. Acta Neuropathol. Commun. 5:36. doi: 10.1186/s40478-017-0438-4
Schutzer, S. E., Liu, T., Natelson, B. H., Angel, T. E., Schepmoes, A. A., Purvine, S. O., et al. (2010). Establishing the proteome of normal human cerebrospinal fluid. PLoS ONE 5:e10980. doi: 10.1371/journal.pone.0010980
Scollato, A., Terreni, A., Caldini, A., Salvadori, B., Gallina, P., Francese, S., et al. (2010). CSF proteomic analysis in patients with normal pressure hydrocephalus selected for the shunt: CSF biomarkers of response to surgical treatment. Neurol. Sci. 31, 283–291. doi: 10.1007/s10072-009-0181-0
Sisa, C., Agha-Shah, Q., Sanghera, B., Carno, A., Stover, C., and Hristova, M. (2019). Properdin: a novel target for neuroprotection in neonatal hypoxic-ischemic brain injury. Front. Immunol. 10:2610. doi: 10.3389/fimmu.2019.02610
Stein, S. C., and Guo, W. (2008). Have we made progress in preventing shunt failure? A critical analysis. J. Neurosurg. Pediatr. 1, 40–47. doi: 10.3171/PED-08/01/040
Stylianopoulou, F., Herbert, J., Soares, M. B., and Efstratiadis, A. (1988). Expression of the insulin-like growth factor II gene in the choroid plexus and the leptomeninges of the adult rat central nervous system. Proc. Natl. Acad. Sci. U.S.A. 85, 141–145. doi: 10.1073/pnas.85.1.141
Su, P., Chen, S., Zheng, Y. H., Zhou, H. Y., Yan, C. H., Yu, F., et al. (2016). Novel Function of Extracellular Matrix Protein 1 in Suppressing Th17 Cell Development in Experimental Autoimmune Encephalomyelitis. J. Immunol. 197, 1054–1064. doi: 10.4049/jimmunol.1502457
Tseng, L. Y., Brown, A. L., Yang, Y. W., Romanus, J. A., Orlowski, C. C., Taylor, T., et al. (1989). The fetal rat binding protein for insulin-like growth factors is expressed in the choroid plexus and cerebrospinal fluid of adult rats. Mol. Endocrinol. 3, 1559–1568. doi: 10.1210/mend-3-10-1559
Varma, V. R., Varma, S., An, Y., Hohman, T. J., Seddighi, S., Casanova, R., et al. (2017). Alpha-2 macroglobulin in Alzheimer's disease: a marker of neuronal injury through the RCAN1 pathway. Mol. Psychiatry 22, 13–23. doi: 10.1038/mp.2016.206
Walter, H. J., Berry, M., Hill, D. J., Cwyfan-Hughes, S., Holly, J. M., and Logan, A. (1999). Distinct sites of insulin-like growth factor (IGF)-II expression and localization in lesioned rat brain: possible roles of IGF binding proteins (IGFBPs) in the mediation of IGF-II activity. Endocrinology 140, 520–532. doi: 10.1210/endo.140.1.6463
Wang, J., Tang, Y., Zhang, W., Zhao, H., Wang, R., Yan, Y., et al. (2013). Insulin-like growth factor-1 secreted by brain microvascular endothelial cells attenuates neuron injury upon ischemia. FEBS J. 280, 3658–3668. doi: 10.1111/febs.12359
Waybright, T., Avellino, A. M., Ellenbogen, R. G., Hollinger, B. J., Veenstra, T. D., and Morrison, R. S. (2010). Characterization of the human ventricular cerebrospinal fluid proteome obtained from hydrocephalic patients. J. Proteomics 73, 1156–1162. doi: 10.1016/j.jprot.2010.02.004
Xu, Q., Bernardo, A., Walker, D., Kanegawa, T., Mahley, R. W., and Huang, Y. (2006). Profile and regulation of apolipoprotein E (ApoE) expression in the CNS in mice with targeting of green fluorescent protein gene to the ApoE locus. J. Neurosci. 26, 4985–4994. doi: 10.1523/JNEUROSCI.5476-05.2006
Yang, J., Simonneau, C., Kilker, R., Oakley, L., Byrne, M. D., Nichtova, Z., et al. (2019). Murine MPDZ-linked hydrocephalus is caused by hyperpermeability of the choroid plexus. EMBO Mol. Med. 11:40. doi: 10.15252/emmm.201809540
Zagorska-Swiezy, K., Litwin, J. A., Gorczyca, J., Pitynski, K., and Miodonski, A. J. (2008). The microvascular architecture of the choroid plexus in fetal human brain lateral ventricle: a scanning electron microscopy study of corrosion casts. J. Anat. 213, 259–265. doi: 10.1111/j.1469-7580.2008.00941.x
Keywords: hydrocephalus, proteomics, choroid plexus, biomarkers, cerebrospinal fluid
Citation: Horowitz A, Saugier-Veber P and Gilard V (2020) Inference of Diagnostic Markers and Therapeutic Targets From CSF Proteomics for the Treatment of Hydrocephalus. Front. Cell. Neurosci. 14:576028. doi: 10.3389/fncel.2020.576028
Received: 25 June 2020; Accepted: 08 September 2020;
Published: 21 October 2020.
Edited by:
Youichi Shinozaki, University of Yamanashi, JapanReviewed by:
Ryo Yamasaki, Kyushu University, JapanCopyright © 2020 Horowitz, Saugier-Veber and Gilard. This is an open-access article distributed under the terms of the Creative Commons Attribution License (CC BY). The use, distribution or reproduction in other forums is permitted, provided the original author(s) and the copyright owner(s) are credited and that the original publication in this journal is cited, in accordance with accepted academic practice. No use, distribution or reproduction is permitted which does not comply with these terms.
*Correspondence: Arie Horowitz, YXJpZS5ob3Jvd2l0eiYjeDAwMDQwO2luc2VybS5mcg==