- 1Laboratory of Neurobiology, Achucarro Basque Center for Neuroscience, Leioa, Spain
- 2Department of Neurosciences, University of the Basque Country (UPV/EHU), Leioa, Spain
- 3Centro de Investigación Biomédica en Red de Enfermedades Neurodegenerativas (CIBERNED), Leioa, Spain
- 4Laboratorio de Neurofisiología Celular, Instituto de Neurobiología, Universidad Nacional Autónoma de México, Juriquilla, Mexico
- 5Department of Neurology, Mitchell Center for Neurodegenerative Diseases, University of Texas Medical Branch, Galveston, TX, United States
Myelin facilitates the fast transmission of nerve impulses and provides metabolic support to axons. Differentiation of oligodendrocyte progenitor cells (OPCs) and Schwann cell (SC) precursors is critical for myelination during development and myelin repair in demyelinating disorders. Myelination is tightly controlled by neuron-glia communication and requires the participation of a wide repertoire of signals, including neurotransmitters such as glutamate, ATP, adenosine, or γ-aminobutyric acid (GABA). GABA is the main inhibitory neurotransmitter in the central nervous system (CNS) and it is also present in the peripheral nervous system (PNS). The composition and function of GABA receptors (GABARs) are well studied in neurons, while their nature and role in glial cells are still incipient. Recent studies demonstrate that GABA-mediated signaling mechanisms play relevant roles in OPC and SC precursor development and function, and stand out the implication of GABARs in oligodendrocyte (OL) and SC maturation and myelination. In this review, we highlight the evidence supporting the novel role of GABA with an emphasis on the molecular identity of the receptors expressed in these glial cells and the possible signaling pathways involved in their actions. GABAergic signaling in myelinating cells may have potential implications for developing novel reparative therapies in demyelinating diseases.
Introduction
Glial cells express a vast repertoire of receptors and transporters for neurotransmitters and neuromodulators and respond to axonal signals, being key and active elements of the nervous system (Allen and Lyons, 2018). In vertebrates, oligodendrocytes (OLs) and Schwann cells (SCs) are the myelin-forming glia of the central nervous system (CNS) and peripheral nervous system (PNS), respectively. These cells are responsible for myelin building and maintenance, a function highly regulated by neuronal activity (Gibson et al., 2014; Mitew et al., 2018). Myelin speeds up nerve impulse propagation and provides metabolic and trophic support to axons (Nave and Trapp, 2008; Kidd et al., 2013; Philips and Rothstein, 2017). Thus, myelination represents the major function of these cells, although they carry it out with some differences; while OLs can myelinate multiple axons simultaneously, each SC wraps one single axon (Jessen and Mirsky, 2005; Nave and Trapp, 2008). Regarding their specific characteristics, oligodendroglial cells represent a highly diverse and specialized cell population (Marques et al., 2016). Mature myelinating OLs develop from glial precursors named oligodendrocyte progenitor cells (OPCs), which constitute the main proliferating cell type in the adult CNS (Dawson et al., 2003). On the other hand, SCs derive from SC precursors, which differentiate into immature SCs. These immature SCs can generate both myelinating and non-myelinating SCs (or Remak glia) according to PNS requirements, like the presence of specific signals in the microenvironment and the diameter of axons in their vicinity (Jessen and Mirsky, 2005, 2019; Kidd et al., 2013).
Differentiation of OPCs and SC precursors is necessary for remyelination in demyelinating diseases like multiple sclerosis (MS) and myelin formation in dysmyelinating diseases such as leukodystrophies in the CNS or Charcot-Marie Tooth in the PNS. In this regard, understanding the mechanisms of action involved in this complex neuron-glia crosstalk will help us in the search for new therapeutic approaches in these pathologies.
Neuronal activity and several signals such as transcriptional and growth factors, axonal ligands, hormones, extracellular matrix components or neurotransmitters regulate OPC/SC precursor differentiation and myelination. Among them, GABAergic signaling has attracted great interest in the last years (Procacci et al., 2013; Zonouzi et al., 2015; Arellano et al., 2016; Hamilton et al., 2017; Serrano-Regal et al., 2020).
GABA, which is present both in the CNS and PNS, exerts an excitatory role during development to modulate neuronal growth and synapse formation (Ben-Ari, 2002). It acts mostly through ionotropic GABAA (GABAARs) and metabotropic GABAB receptors (GABABRs) that are well described in neurons but not yet fully characterized in myelinating cells. Although the expression of GABA receptors (GABARs) in OL/SC precursor lineages is widely documented (von Blankenfeld et al., 1991; Williamson et al., 1998; Magnaghi et al., 2004; Luyt et al., 2007; Arellano et al., 2016; Serrano-Regal et al., 2020), their role in differentiation and myelination is a matter of ongoing research.
In this review, we recapitulate recent evidence about GABAA and GABAB receptor expression and function in oligodendroglial and SCs, together with the implication of the GABAergic signaling in OPC/SC differentiation and myelination. Furthermore, we discuss possible signaling pathways involved in these events and their relevance to develop new therapies to treat demyelinating and dysmyelinating diseases.
Expression of GABARs in Oligodendroglial and Schwann Cells
GABAA Receptors
GABAARs are integral membrane ion channels—permeable to Cl− and HCO3− anions—composed of five subunits that mediate the major form of fast inhibitory neurotransmission in the CNS (Olsen and Sieghart, 2008; Doyon et al., 2016). There are, at least, 19 distinct GABAAR subunit genes, which include 6 α (α1-α6), 3 β (β1-β3), 3 γ (γ1-γ3), 3 ρ (ρ1-ρ3), and 1 gene of the respective δ, ε, θ, and π subunits (Sieghart and Savić, 2018). This diversity results in different homomeric or heteromeric subunit combinations that may have specific locations in the CNS, particular pharmacology, and, consequently, distinctive functional characteristics (Vogt, 2015). The subunit profile that forms GABAARs depends on several factors including brain region, cell type, developmental stage, and physiological or pathophysiological conditions (Levitan et al., 1988; Seeburg et al., 1990; Waldvogel and Faull, 2015). Currently, 11 GABAAR subtypes with different subunit combinations have been identified, being most of them heteromeric receptors formed by αxβxγx or αxβxδ (where x represents any subtype of a given subunit; Figure 1A), whereas others are homomeric receptors formed by ρ subunits (Barnard et al., 1998).
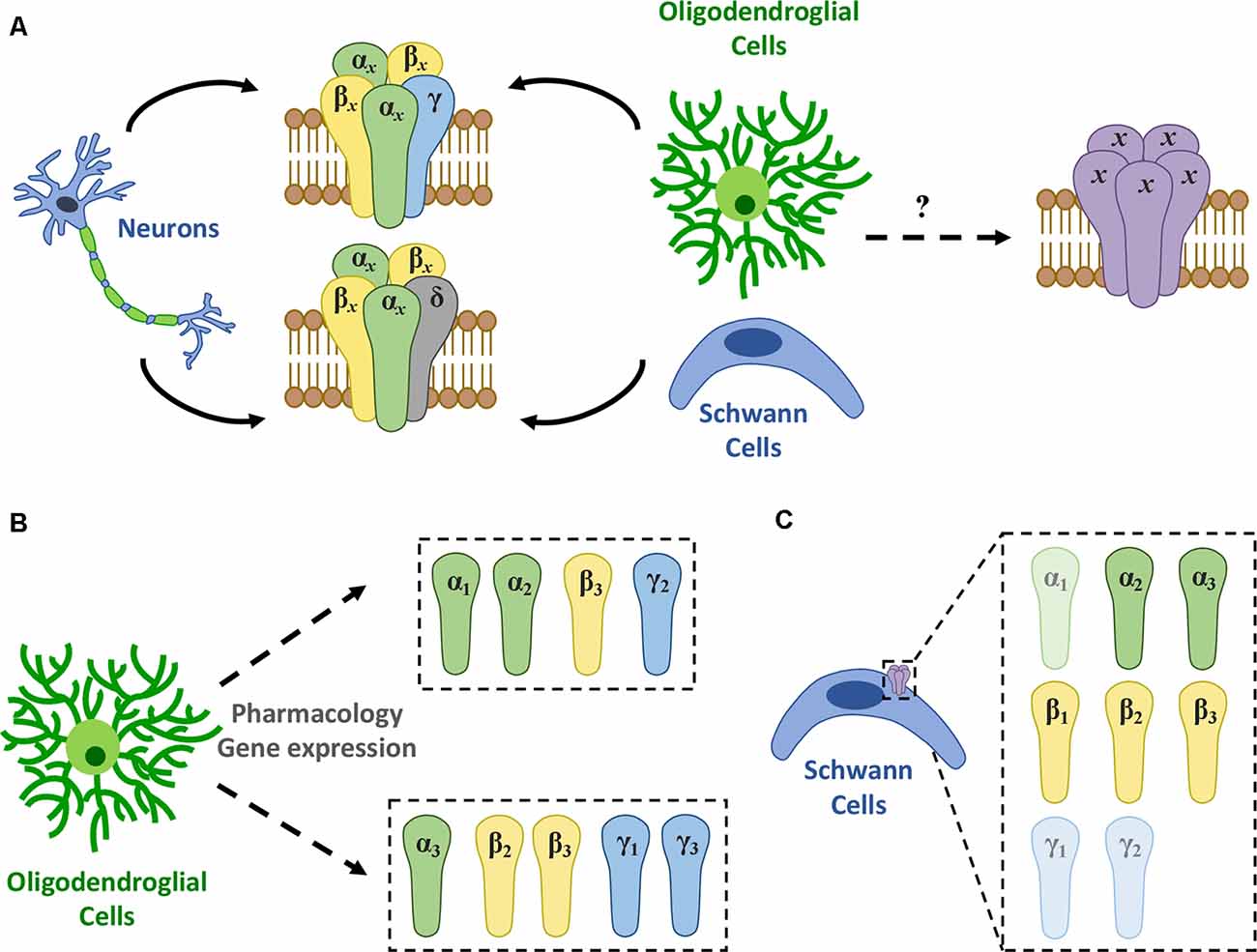
Figure 1. GABAAR expression in myelinating cells. (A) There are 11 different GABAAR subtypes described, mainly heteromeric receptors with αxβxγx or αxβxδ (where x represents any subtype of a given subunit) stoichiometry (Olsen and Sieghart, 2008). Among them, a single type of GABAAR appears to be present in oligodendrocytes though its molecular nature remains elusive. (B) Recent evidence using gene expression and pharmacology shows that GABAARs in oligodendroglia are heterogeneous and may change in subunit composition during differentiation. Thus, at least two novel subtypes were identified: one formed by a combination of α1 or α2, β3, and γ2 subunits (Passlick et al., 2013) and another made up by α3, β2 or β3, and γ1 or γ3 subunits (Arellano et al., 2016). (C) In turn, studies using RT-PCR and/or immunohistochemistry conclude that SCs express high levels of α2, α3, β1, β2, and β3, while α1, γ1, and γ2 levels are relatively low in these cells (Magnaghi et al., 2006).
Oligodendroglial Cells
Activation of GABAARs is relevant for the modulation of myelinating cell physiology (Magnaghi, 2007; Vélez-Fort et al., 2012), however, the specific subunit composition of GABAARs expressed in these cells remains unknown. Electrophysiological recordings in OPCs and OLs reveal differences between the response of the GABAAR expressed in these cells and those expressed in neurons and astrocytes (Table 1), suggesting the presence of a novel GABAAR subtype with unique stoichiometry in the oligodendroglial lineage (von Blankenfeld et al., 1991; Williamson et al., 1998; Vélez-Fort et al., 2012; Arellano et al., 2016).
von Blankenfeld et al. (1991) suggested that GABAARs in murine OPCs and OLs carry a γ subunit required to form the benzodiazepine binding site, as they observed potentiation of the GABA response in these cells with classic benzodiazepines. Moreover, the inverse agonist β-carboline methyl 4-ethyl-6,7-dimethoxy-9H-β-carboline-3-carboxylate (DMCM) reduced the GABA-induced current responses in oligodendroglial cells, unlike what happens in astrocytes. Contrary to that, Williamson et al. (1998) reported no influence of flunitrazepam or DMCM in the response elicited by GABA in rat-derived OPCs, indicating an absence of the γ subunit in the GABAARs expressed by these cells. This observation was supported by the inhibitory effect of Zn2+, which is characteristic of receptors that lack the γ2 subunit. RT-PCR analyses conducted in the same study did not find expression of γ2, α1, α6, and δ subunit mRNAs in OPCs. Although amplification of other subunits was demonstrated, the results were interpreted with caution since the preparation was 85% pure for OPCs and the presence of GABAARs from other cell types could not be excluded. In a third study conducted by Bronstein et al. (1998), the GABA response of an immortalized murine glial cell line that expresses mature myelin proteins was insensitive to diazepam and sensitive to Zn2+, reinforcing the idea of γ-subunit absence.
Later, Passlick et al. (2013) reported the expression of two types of GABAARs in hippocampal NG2 cells from juvenile mice by functional and pharmacological analyses and single-cell RT-PCR. NG2 cells from this brain area express, on the one hand, postsynaptic GABAARs comprised of a combination of α1, α2, β3, γ1, and γ2 subunits and, on the other hand, they have extrasynaptic GABAARs mostly lacking the γ2 subunit (Figure 1B).
Our studies of GABAAR responses conducted in cultured immature OLs from the rat forebrain and mature OLs from the optic nerve showed that these cells are diazepam-sensitive, suggesting once more the presence of a γ subunit (Arellano et al., 2016). This positive modulation by benzodiazepines was observed when using low GABA concentrations (≤EC30), which may explain the discrepancies with previous studies. Concerning the specific subtype of γ subunit, two observations indicate that γ2 may not contribute to oligodendroglial GABAARs: (1) Zn2+ blocks GABA responses; and (2) indiplon, a positive allosteric modulator acting on γ2 subunit-containing receptors, does not modulate GABA currents. Therefore, these receptors likely contain either γ1 or γ3 subunit (Arellano et al., 2016).
Regarding β subunits, potentiation of the GABA response by loreclezole suggests the presence of β2 or β3 subunits, since β1 subunit-containing receptors are insensitive to this drug (Arellano et al., 2016). Finally, concerning α subunits, α3 is the most likely candidate because it forms receptors with low sensitivity to GABA (Karim et al., 2013), as it is the case of OLs (EC50 between 70 and 100 μM).
Together, these pharmacological studies suggest that the composition of the GABAAR expressed in rat-derived oligodendroglial cells is a combination of the α3, β2 or β3, and γ1 or γ3 subunits (Figure 1B). Also, we confirmed the expression of the α3 subunit by immunocytochemistry in cultured OLs (Arellano et al., 2016). These observations are supported by previous functional genomic analyses performed in OLs (Cahoy et al., 2008). Moreover, RNA sequencing (RNA-Seq) transcriptional analyses of purified NG2 cells obtained from P17 mice revealed that the α3 subunit is the most expressed α-subtype, while among β subunits, β3 and β2 are much more abundant than β1. Lastly, γ1 is expressed at much higher levels than γ2 and γ3 (Larson et al., 2016).
An important factor for the diversity of GABAARs expressed and the subunits involved in their conformation will undoubtedly be the species. For example, regarding humans, a recent study of the GABAAR-subunit expression in OPCs isolated from the middle temporal gyrus of healthy adults—based on the single-nucleus RNA-Seq analysis by Hodge et al. (2019)—showed that OPCs from this brain area express high mRNA levels of α3, all β subunits, γ2 and, interestingly, the ε subunit (Figure 2). These mRNAs, if translated and incorporated into functional receptors, would increase the variety of potential configurations and may have important functional and pharmacological consequences (Jones and Henderson, 2007; Bollan et al., 2008; Belujon et al., 2009).
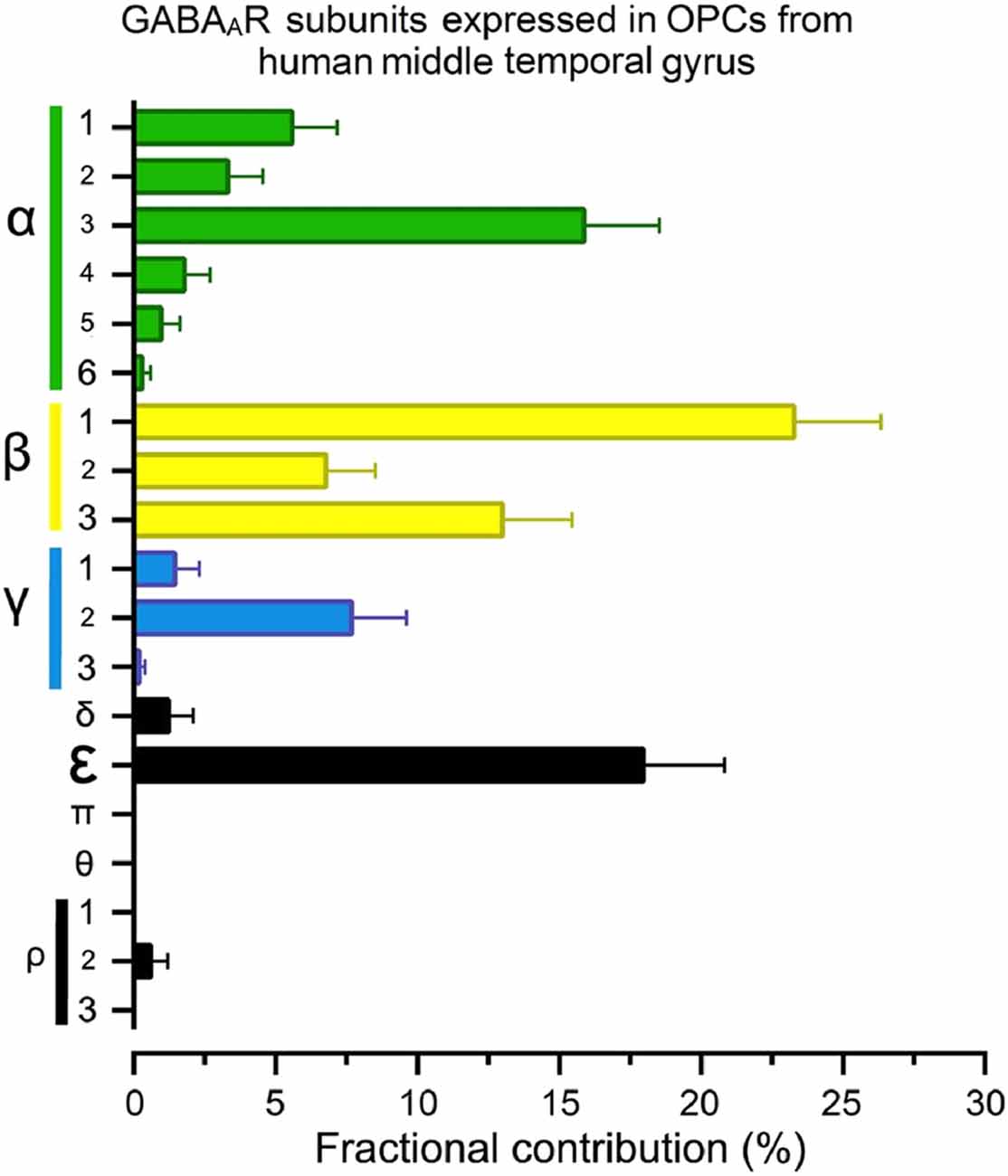
Figure 2. The fractional contribution (FC) of GABAAR subunits in human oligodendrocyte progenitor cells (OPCs). Normalized gene expression levels for all 19 GABAAR subunits expressed as a percentage (mean ± S.E.M.) of the total available pool of mRNA for GABAARs in OPCs (PDGFRα+ cells) from human brains, estimated from publicly available datasets (Hodge et al., 2019; https://celltypes.brain-map.org/rnaseq). The single-nucleus analysis used normalized RNA-Seq datasets from the middle temporal gyrus isolated from six subjects with no known neuropsychiatry or neuropathological history (three males and three females; 35–66 years old). Gene expression level in each dataset was transformed into FC (Sequeira et al., 2019). FC is defined as the percentage of the expression level of each subunit gene (signaled in the “y” axis) to the sum of the 19 genes for GABAARs subunits within each human/cell. Detailed demographic characteristics, as well as technical white papers for data processing and quality control, can be downloaded from the same site. Confirmatory analysis of OPC markers enrichment and lack of neuronal markers were performed for all datasets.
Schwann Cells
GABAA-type receptors are relevant to SC physiology (Magnaghi et al., 2001, 2006). However, their pharmacological and functional properties, as well as their molecular identity, are not clear. GABAAR subunit composition in rat-derived cultured SCs includes α2 and α3, as well as the three β subunits, while mRNAs of α1 and γ2 subunits have been found at much lower levels (Magnaghi et al., 2006; Figure 1C). Moreover, the presence of α2, α3, and β3 proteins were confirmed by immunocytochemistry. Alpha-2 and β3 subunits are also expressed in SC-like adult stem cells derived from bone marrow or adipose tissue, as their levels are upregulated following SC differentiation in vitro (Faroni et al., 2012). Also, GABAAR stimulation with muscimol increases the proliferation rate of SCs, meaning that GABAergic signaling has an important role in these cells (Magnaghi et al., 2006). However, despite these important findings, there is still little knowledge about the specific composition of GABAARs expressed in differentiated SCs.
GABAB Receptors
GABABRs are G-protein coupled receptors (GPCRs) responsible for the slower and prolonged GABA-mediated inhibitory transmission. They were first described pharmacologically as bicuculline-insensitive metabotropic receptors that were activated by the GABA analog baclofen (Bowery and Hudson, 1979; Hill and Bowery, 1981). Functional GABABRs are heterodimers constituted by two receptor subunits, GABAB1 and GABAB2, that cooperate to perform signal activation (Kaupmann et al., 1998; Kuner et al., 1999). GABAB1 is responsible for ligand binding, while GABAB2 contains binding sites for allosteric modulators (Galvez et al., 2001; Binet et al., 2004), couples with Gi/o-protein, and is necessary for trafficking the heterodimer to the cell surface, where the receptor becomes active (Calver et al., 2000; Couve et al., 2000). Among the effector elements involved in GABABR signaling pathways in neurons are voltage-gated Ca2+ channels (VGCC), inwardly-rectifying potassium channels (Kir) and adenylyl cyclase (AC; Bowery et al., 2002; Bettler et al., 2004; Figure 3). However, the specific coupling of GABABRs to the molecular effector may differ depending on the cell type and region analyzed (Booker et al., 2018).
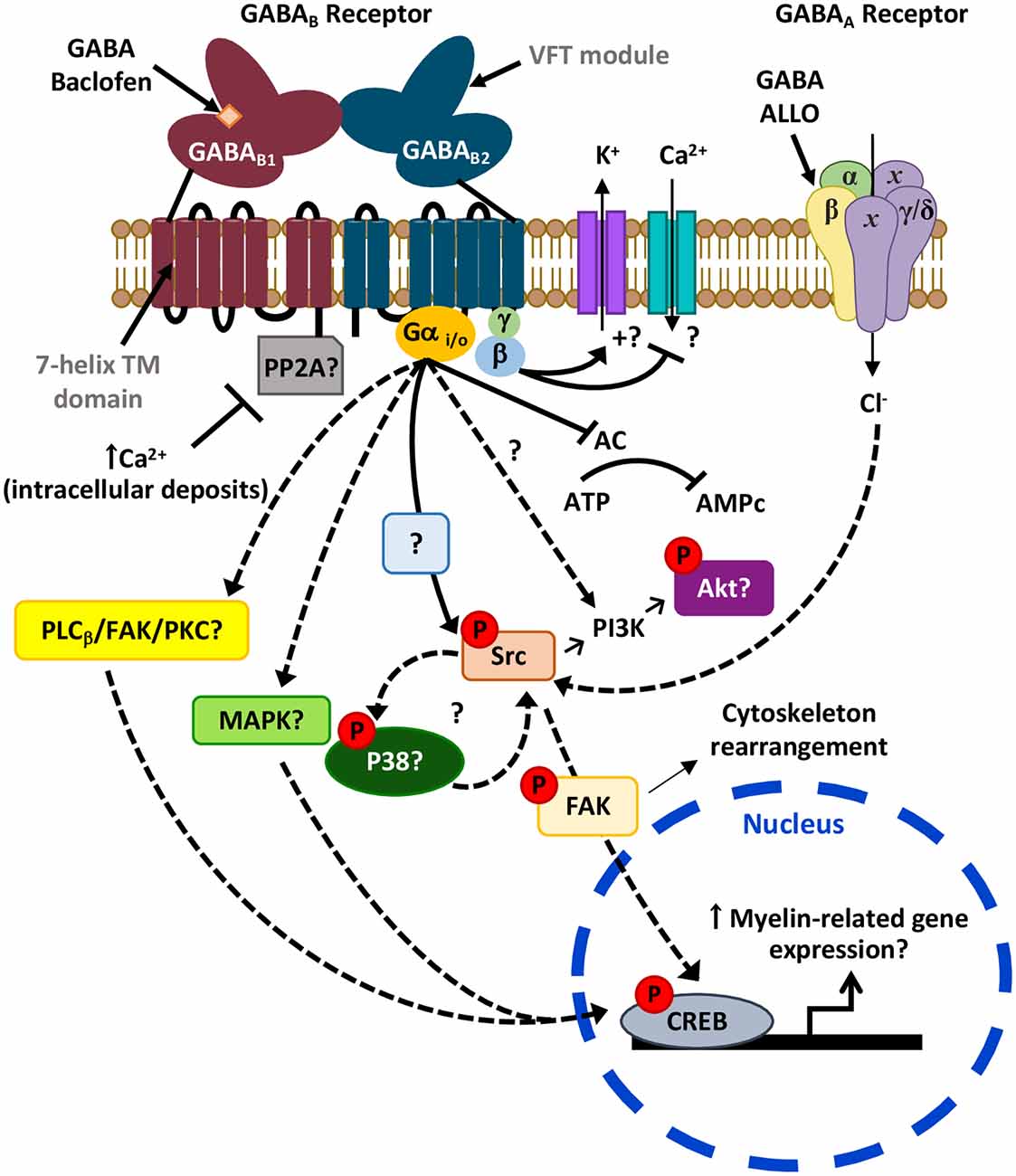
Figure 3. Possible signaling pathways downstream GABARs leading to myelination. Activation of the Gi/o-linked GABABR may reduce CREB phosphorylation as it is negatively coupled to adenylyl cyclase (AC; Luyt et al., 2007). Alternatively, it may also induce CREB phosphorylation possibly via activation of PLCβ/FAK/PKC or MAPK cascades, as observed in neurons (Carlezon et al., 2005; Zhang et al., 2015). Moreover, activation of GABABR leads to Src phosphorylation (Serrano-Regal et al., 2020) that could ultimately induce CREB activation. Phosphorylation of Src may also lead to Akt phosphorylation via PI3K as observed earlier (Barati et al., 2015), and contribute to a positive feedback loop with p38MAPK (Mugabe et al., 2010; Lin et al., 2015), which is involved in myelination (Fragoso et al., 2003, 2007). On the other hand, the GABAB1 subunit of GABABR might be sequestered by phosphatases like PP2A (as occurs in some neurons), a mechanism that blocks its activity and can be reverted by high intracellular Ca2+ levels (Li et al., 2020). Finally, the GABAAR allosteric modulator ALLO regulates Schwann cell (SC) myelination via Src-FAK signaling, involving cytoskeleton reorganization (Melfi et al., 2017).
Oligodendroglial Cells
Myelinating cells express both subunits of GABABRs, which are negatively-coupled to AC (Magnaghi et al., 2004; Luyt et al., 2007). However, their functional characteristics are not as well known as in the case of neurons. Regarding oligodendroglial cells, we recently confirmed by immunocytochemistry and RT-qPCR the expression of GABAB1 and GABAB2 subunits in OPCs and OLs from the rat cerebral cortex and in OLs from the optic nerve (Serrano-Regal et al., 2020). We also performed calcium imaging assays and electrophysiological recordings in these cells and observed that baclofen does not modify their response to KCl 50 mM, calcium influx, and Kir currents. These results strongly suggest that GABABRs in oligodendroglial cells are not coupled to Ca2+ and Kir channels in the same manner as in other cell types (Serrano-Regal et al., 2020). Likewise, GABABRs from CA1 somatostatin interneurons, unlike pyramidal neurons, are not coupled to the canonical Kir3 signaling cascade (Booker et al., 2018), which suggests a functional diversity of downstream effectors depending on cell type and location, and warrants the need of exploring these features in OLs as well as in SCs.
Charles et al. (2003) did not find any colocalization of GABAB1 subunit and MBP expression in myelinating OLs in the white matter of the rat spinal cord and suggested that GABABR expression in developing OLs decreases during differentiation. Following this, Luyt et al. (2007) observed downregulation of the GABAB1 subunit in mature OLs from the mouse periventricular white matter, while the expression of GABAB2 remained constant. As they reported changes in GABAB1/GABAB2 ratios in mature OLs, they raised the possibility that one subunit alone or in combination with another protein could make GABABR functional in different cell types (Calver et al., 2000; Luyt et al., 2007). In contrast, our recent results show that cultured oligodendroglial cells from the rat forebrain and mature OLs from the optic nerve express GABAB1 and GABAB2 subunits at different stages of maturation, as well as mature OLs from the juvenile and adult rodent corpus callosum in vivo (Serrano-Regal et al., 2020). These discrepancies can be explained by the different regions analyzed, as GABAB1/GABAB2 expression exhibits important regional variations (Luyt et al., 2007). Since oligodendroglial cells are extremely diversified, the different cells targeted in these studies may correspond to distinct oligodendroglial and OPC subpopulations (Marques et al., 2016; Spitzer et al., 2019; Marisca et al., 2020).
Schwann Cells
SCs also express different isoforms of the GABABR, such as -1a, -1b, -1c, and -2 (Magnaghi et al., 2004, 2006).
Downregulation of GABABR expression occurs in pre- and non-myelinating SCs (Corell et al., 2015). Neurosteroids modulate the expression of GABABR subunits in cultured SCs and, as GABABR is downregulated with age in the PNS, the GABA synthesized in the adult sciatic nerve acts through the ionotropic GABAAR, present both in neurons and SCs (Magnaghi et al., 2006). Interestingly, the conditional knockout of the GABAB1 subunit in SCs changes the expression of GABAAR subunits α3, α4, β1, and δ (Faroni et al., 2019), suggesting that GABABRs in these cells regulate somehow the expression of GABAARs and/or their subunits.
Overall, more detailed analyses such as single-cell RNA-seq would help to better figure out the expression of GABAB1 and GABAB2 subunits along the oligodendroglial and SC lineages.
Potential GABA Synthesis and Release in Myelinating Cells
Although GABAergic neurons are the main source of GABA (especially in the CNS), GABA synthesis also occurs in glial cells (Seiler et al., 1979; Angulo et al., 2008; Héja et al., 2012). Two potential pathways for GABA synthesis have been described in brain-derived glial cells. GABA is mainly produced through the classical pathway as a result of glutamate decarboxylation by the glutamic acid decarboxylase (GAD) enzymes (Roberts and Frankel, 1950). In neurons, the two isoforms of GAD—GAD65 and GAD67—differ in their catalytic and kinetic properties and their subcellular distribution (Kaufman et al., 1991). Also, GABA can be synthesized from the monoacetylation of putrescine with the participation of the monoamine oxidase B (MAOB) enzyme in the non-classical pathway (Seiler et al., 1973).
Consistent with an RNA-seq transcriptome and splicing database (Zhang et al., 2014), oligodendroglial cells express gad1, gad2, and maob mRNAs. GAD67 mRNA (gad1) is greatly expressed by OPCs, although its levels decrease notably as they differentiate into mature myelinating OLs. However, gad2 is expressed to a lesser extent throughout the oligodendroglial lineage. Regarding maob, OPCs and myelinating OLs express higher levels than newly formed or immature OLs (Zhang et al., 2014). Accordingly, we confirmed the presence of GAD65/67 by immunocytochemistry and western blot in rat-derived cortical oligodendroglial cells at 1, 3, and 6 days in vitro (Serrano-Regal et al., 2020). Similarly, we verified the expression of the MAOB enzyme in these cells at the same time points. These results indicate that cultured oligodendroglial cells may synthesize GABA by the two alternative pathways mentioned above. Indeed, we found GABA immunostaining in cortical and optic-nerve derived oligodendroglial cells at different stages of maturation (Serrano-Regal et al., 2020). Possibly, GABA is synthesized by one pathway or another depending on the stage of maturation of the cells, as GABA may have different roles in OPCs vs. mature OLs.
GAD67 is present in SCs and its levels increase in the presence of the progesterone metabolite allopregnanolone (ALLO; Magnaghi et al., 2010). Moreover, Corell et al. (2015) demonstrated the presence of GABA and GAD65/67 in premyelinating and non-myelinating SCs. These findings show that SCs both produce and store GABA.
Together, these observations indicate that OLs and SCs synthesize and store GABA, which could be released by reversal operation of GABA transporters including GAT-1 and GAT-3, that are expressed by OLs (Fattorini et al., 2017; Serrano-Regal et al., 2020). Also, SCs are capable to take up ambient GABA at concentrations above 1 μM (Brown et al., 1979), though the nature of the transporters involved is still unknown.
GABA Receptors in OPC/SC Precursor Differentiation and Myelination
In the CNS, OPCs are the main (but not unique) source of remyelination, since they respond to white matter injury, migrate to the lesioned area and differentiate into mature OLs to produce new myelin sheaths (Franklin et al., 1997; Nait-Oumesmar et al., 1999; Hesp et al., 2015). Moreover, surviving mature OLs are also a source of remyelination (Duncan et al., 2018). SCs may also participate, although to a lesser extent, in restoring myelin in the CNS (Zawadzka et al., 2010; García-Díaz and Baron-Van Evercooren, 2020).
Differentiation of OPCs/SCs and myelination are exquisitely coordinated processes mediated by a deep dialogue between neuronal and glial cells that entail the participation of a variety of signals and intercellular communication systems, including ATP, glutamate or GABA neurotransmitter signaling (Li et al., 2013; Faroni et al., 2014; Salzer, 2015; Zonouzi et al., 2015; Arellano et al., 2016; Hamilton et al., 2017; Serrano-Regal et al., 2020), as well as neuronal activity (Wake et al., 2011; Gibson et al., 2014; Fannon et al., 2015). Specifically, GABAergic neurons establish direct synapses with OPCs throughout the CNS, indicating that this communication may control proliferation, migration, differentiation, the establishment of axonal contacts and their wrapping, OPC survival in the adult brain or myelin maintenance (Lin and Bergles, 2004; Kukley et al., 2008; Vélez-Fort et al., 2010; Orduz et al., 2015; Zonouzi et al., 2015; Balia et al., 2017; Mount et al., 2019). However, the precise function of GABA in OPC differentiation and myelination remains controversial.
GABAA Receptors
Contrary to mature neurons, activation of GABAARs in OPCs leads to depolarization and an increase in cytosolic Ca2+ levels (Kirchhoff and Kettenmann, 1992), resulting from Ca2+ influx through activated VGCC (Paez and Lyons, 2020). Thus, a rise in Ca2+ in the cytosol may regulate OPC proliferation, migration and maturation and, consequently, OL (re)myelination (Cheli et al., 2016; Santiago-González et al., 2017; Baraban et al., 2018; Krasnow et al., 2018; Marisca et al., 2020).
As oligodendroglial cells constitute a highly dynamic and heterogeneous population, the expression of GABAARs and their different subunits changes as these cells progress along their lineage, as occurs with the expression of certain ion-channels (Spitzer et al., 2019), and these changes can affect their intercellular relationship and differentiation. In line with this, we observed that oligodendroglial GABAAR expression in vitro is dependent on the close interaction between axons and OLs, as OLs cultured alone lose GABA responses with differentiation (Arellano et al., 2016). Moreover, the presence of the γ2 subunit, which is associated with a possible role in neuron-OPC synapse formation, decreases with age along with the density of GABAergic synaptic contacts in cortical NG2 cells of mature mice (Balia et al., 2015). Thus, NG2 cells may switch the expression of GABAARs from synaptic (with γ2 subunit) to extrasynaptic (without γ2 subunit) during development (Vélez-Fort et al., 2010). Surprisingly, genetic inactivation of oligodendroglial γ2 does not affect OPC proliferation and differentiation, while it causes progressive and specific depletion of the OPC pool that lacks γ2-mediated synaptic activity without affecting the oligodendrocyte production (Balia et al., 2017). These observations indicate that GABAergic communication in cortical OPCs through γ2-containing GABAARs does not play a role in oligodendrogenesis but rather modulates OPC maintenance.
GABAergic signaling regulates OPC population and OPC differentiation and myelination in the cerebellar white matter in vivo (Zonouzi et al., 2015). In turn, hypoxia causes a strong downregulation of the GABAergic synaptic input from local interneurons to OPCs (NG2 cells) as well as an increase in the proliferation of these cells and a delay in their maturation, which limits myelination. These effects are mimicked in control animals when blocking GABAARs with their antagonist bicuculline. However, they are reverted when applying tiagabine, a selective inhibitor of the GABA transporter GAT-1 that increases GABA availability in the extracellular space. Treatment with tiagabine results in a decrease of NG2 cell proliferation and an increase of myelinating OLs, reverting the hypomyelinating effect caused by perinatal hypoxia (Zonouzi et al., 2015). These findings strongly suggest that GABAergic signaling (either neuronal activity-dependent or independent) influences OPC development and differentiation and, therefore, it may help to develop novel therapies to improve OPC differentiation into damaged brain areas.
GABAergic signaling through GABAARs may also be relevant for the stronger remyelination that occurs following focal demyelination in the corpus callosum of late pregnant rats compared to virgin and postpartum ones (Kalakh and Mouihate, 2019). This pregnancy-associated promyelinating effect was lost when either the GABAAR was blocked or when 5α-reductase, the rate-limiting enzyme for the endogenous GABAAR activator ALLO, was inhibited (Kalakh and Mouihate, 2019). Moreover, N-butyl-β-carboline-3-carboxylate (β-CCB), a selective drug activating preferentially oligodendroglial GABAARs, promotes remyelination in a model of gliotoxin-induced demyelination in the rat cerebellar caudal peduncle as assessed using magnetic resonance imaging (MRI) together with myelin staining (Cisneros-Mejorado et al., 2020). Together, these results strongly suggest that GABAAR-mediated signaling promotes myelination and remyelination in OLs either directly or indirectly. However, at odds with these data, activation of GABAARs by endogenous GABA in cortical organotypic cultures reduces the number of oligodendroglial cells and myelination whereas enlarges internode length, influencing the velocity of the nerve impulse propagation (Hamilton et al., 2017). These effects may be due to GABA released by glial cells. However, we could not assess this idea as gabazine treatment of OPC cultures had no significant effect on myelin protein production (Serrano-Regal et al., 2020).
Neurosteroid therapy is another pharmacological approach to modulate GABAAR activity in the nervous system, as they act as allosteric modulators of these receptors in the nanomolar concentration range (Lambert et al., 2009). Indeed, progestin ALLO increases myelin basic protein (MBP) production in rat-derived cerebellar organotypic slices, an effect that requires GABAARs (Ghoumari et al., 2003). This observation points to these receptors as mediators of myelination. The effects of neurosteroids are of special interest in postnatal development, as they may help to prevent neurodevelopmental disorders associated with preterm birth (Shaw et al., 2019). ALLO, which is mainly synthesized in the placenta, has an important role during nervous system development. In premature neonates, ALLO concentration decreases abruptly and this decrease is associated, in part, with hypomyelination (Shaw et al., 2015). Consequently, experimental administration of the ALLO analog ganaxolone as replacement therapy in guinea pig-preterm neonates showed positive effects on myelination, through its interaction with GABAARs. Therefore, neurosteroid replacement could be a good therapeutic option to improve myelination in this condition (Shaw et al., 2019).
Neurosteroids may also enhance GABAAR function in SCs. Thus, ALLO acting via GABAA receptor can influence peripheral myelin protein 22 (PMP22) synthesis (Magnaghi et al., 2006). Moreover, ALLO modulates SC morphology, motility, and myelination in SC/dorsal root ganglia neuron (DRG) co-cultures via the Src/focal adhesion kinase (FAK) pathway, a signaling cascade that involves GABAARs and relies on actin rearrangements (Melfi et al., 2017). Therefore, neurosteroids represent a promising molecular approach for the treatment of peripheric pathologies. Together, the studies discussed in this section connect GABAAR signaling with OPC/SC differentiation and/or myelination using pharmacological approaches. However, the results observed cannot be solely attributed to the action of GABA on GABAARs. Although pharmacology may be a good strategy to enhance OPC/SC differentiation and myelination in pathological conditions, potential side-effects must also be considered. To minimize them, it would be of great interest to use more specific drugs acting on myelinating cell GABAARs and/or to use genetic approaches to specifically target the different GABAAR subunits expressed in these cells.
GABAB Receptors
An early study suggests that GABABRs may be relevant for OPC development as baclofen increases migration and proliferation in cultured OPCs derived from periventricular white matter (Luyt et al., 2007). However, more recent studies could not confirm those findings as baclofen did not change OPC proliferation or total OPC number in dissociated and organotypic cultures derived from the cortex (Hamilton et al., 2017; Serrano-Regal et al., 2020). This discrepancy could reflect the different brain areas studied. Thus, different subpopulations of OPCs may exist in white and gray matter that behave differently or have different responses to baclofen, as proposed by Luyt et al. (2007). In contrast, baclofen reduced cell proliferation of SC cultures (Magnaghi et al., 2004). However, in dissociated developing DRG primary cultures, in which SCs proliferate spontaneously in vitro, baclofen did not affect (Corell et al., 2015).
On the other hand, GABA and baclofen modulate OPC differentiation, as well as the myelination capacity of mature OLs cultured with DRG neurons, pointing out GABABRs as relevant modulators of OL maturation and myelination (Serrano-Regal et al., 2020). Consistent with these observations, GABABRs also regulate SC differentiation both in the myelinating and non-myelinating phenotypes. Thus, forskolin-induced SC differentiation in vitro correlates with a redistribution of GABAB1 and GABAB2 subunits of GABABRs. Indeed, in the cytoskeleton rearrangement that takes place during differentiation, GABABRs colocalize with f-actin on the SC elongated processes (Procacci et al., 2013).
Apart from being essential for SC commitment to a non-myelinating phenotype during development, GABABRs are key modulators of neuronal-SC interactions regarding myelination, as GABAB1 receptor total null mice showed altered levels of PMP22 and myelin protein zero (P0) as well as thinner myelin sheaths. These mice also presented fiber alterations, which causes changes in pain behavior, gait abnormalities, and motor coordination disturbances (Magnaghi et al., 2008). Together, these findings suggest a role for GABABRs in the control of SC myelination. Moreover, both GABABR subunits in addition to GABA and GAD65/67 were found at the node of Ranvier in a sub-population of myelinated sensory fibers (Corell et al., 2015). Surprisingly, GABABR expression is upregulated in SCs of injured nerves, which may be interpreted as an adaptive response for stimulating the neighboring axons to re-grow distally to the injury (Corell et al., 2015).
Finally, conditional deletion of the GABAB1 subunit in SCs altered their proliferation, migration, and myelination capacities, as well as reduced neurite length of co-cultured DRGs (Faroni et al., 2019). Furthermore, molecular and transcriptomic changes were also observed both in SCs and DRGs derived from mice lacking GABAB1 subunit in SCs (P0-GABA-B1fl/fl). Interestingly, the expression of some GABAAR subunits by SCs and DRGs was also altered, indicating a possible role of GABABRs in regulating the expression of GABAARs in these cells (Faroni et al., 2019). Similar studies using conditional deletion of GABABR subunits in oligodendroglia will help to understand the role of these receptors and their impact on myelination and pain and motor behavior.
Possible Signaling Pathways Downstream Gabars Related to Myelination
Myelination, either by OLs or SCs, involves the participation of several intracellular signaling pathways. Indeed, some of those pathways are common in the CNS and PNS. For instance, binding of neuregulins (NRGs) to ErbB receptors activates a sequence of canonical intracellular pathways downstream from many receptor tyrosine kinases (RTKs), such as phosphatidylinositol-3-kinase (PI3K)/Akt/mammalian target of rapamycin (mTOR) or mitogen-activated protein kinases (MAPK; Newbern and Birchmeier, 2010).
Intracellular 3′,5′-cyclic adenosine monophosphate (cAMP) induces cell differentiation and myelination requiring the participation of the cAMP response element-binding protein (CREB). CREB mediates the stimulation of MBP expression by cAMP in OLs (Afshari et al., 2001). Moreover, in mouse-derived cultured SCs, the combined action of cAMP/NRG1 increases the expression of myelin proteins Krox-20 and P0, through a mechanism that relies on the activity of transcription factors from the CREB family (Arthur-Farraj et al., 2011).
The Src family kinases (SFKs) are nonreceptor tyrosine kinases that integrate external signals from both integrin and growth factor receptors and transduce signals related to OL and SC development and myelination (Colognato et al., 2004; Melfi et al., 2017). In particular, signaling pathways downstream the Src-family member Fyn regulate morphological differentiation of OLs, the recruitment of cytoskeleton components, and local translation of MBP (see White and Krämer-Albers, 2014; Quintela-López et al., 2019). GABABR specific activation with baclofen induces Akt phosphorylation, which is dependent on PI3K and Src kinases, promoting chemotaxis and cytoskeletal rearrangement in rat basophilic leukemic cells (Barati et al., 2015). Accordingly, we found that Src-family kinases inhibition abrogates GABABR-induced OL differentiation (Serrano-Regal et al., 2020). This observation corroborates the role of the Src family in OL differentiation through a mechanism dependent on GABABR activation (Figure 3).
GABAARs are also linked to Src and FAK signaling. The modulation of SC development and myelination by the neurosteroid ALLO in SC/DRG co-cultures occurs via Src and FAK signaling activation, which depends on GABAARs and actin reorganization (Melfi et al., 2017). Besides, Src-family members can interact reciprocally with kinases from the MAPK family, like the serine/threonine-protein kinase p38 MAPK, as c-Src elicits p38 MAPK phosphorylation and the opposite (Mugabe et al., 2010; Lin et al., 2015; Wu et al., 2015). Therefore, it would be of great interest to investigate this kind of interactions in myelinating cells, as p38 MAPK is a key element in the initial steps of myelination in SCs (Fragoso et al., 2003), as well as in OL maturation and myelination since specific p38 inhibitors block in vitro myelination of DRGs by OLs (Fragoso et al., 2007). Also, conditional knockout of p38 in oligodendroglial cells leads to defects in myelination early in development (Chung et al., 2015). At odds with those findings, deletion of p38 in the same mouse model increases remyelination after cuprizone-induced demyelination (Chung et al., 2015), while selective deletion of p38α MAPK in OLs did not compromise myelination in a mouse model of periventricular leukomalacia (PVL; Chung et al., 2018). These conflicting pieces of evidence indicate that the precise role of p38 MAPK in SC/OL differentiation and myelination and its relation with GABARs remains to be elucidated (Figure 3).
Since GABABRs couple negatively to AC in OLs (Luyt et al., 2007), activation of CREB downstream these receptors is not expected due to decreased cAMP levels. However, other intracellular signaling cascades activated downstream G-protein coupled receptors, such as MAPK cascades, may phosphorylate CREB (see Carlezon et al., 2005). Thus, GABABR stimulation in cultured mouse cerebellar granule neurons with baclofen activates CREB via PLCβ/FAK/PKC (Zhang et al., 2015). Further clarification of the link between GABABR activation and CREB specifically in myelinating cells is a matter of ongoing study and could contribute to a better understanding of the signaling routes that control myelination and remyelination (Figure 3).
Finally, GABABR function may be modulated by its direct association to protein phosphatase 2A (PP2A), as observed in GABAergic neurons from the rodent ventral tegmental area (Li et al., 2020). Therefore, PP2A-GABABR interaction results in an increase of GABABR dephosphorylation and its subsequent internalization, an effect reverted with high intracellular Ca2+ levels. Again, it is worth exploring if these mechanisms also occur in myelinating cells and whether they are relevant to myelin pathology (Figure 3).
Discussion
GABA is among the signals that drive OLs and SCs to axon interactions. The fact that GABA acts mainly on two different types of receptors—ionotropic GABAARs and metabotropic GABABRs—makes it difficult to understand the role of this neurotransmitter in myelinating cell physiology. Moreover, OLs and SCs are highly dynamic cell lineages with different stages of maturation.
While the molecular composition of both GABARs and their mechanisms of action are well described in neurons, their properties in myelinating glial cells remain elusive. Native GABAARs are composed of multiple subunit combinations with diverse pharmacology, both of which vary regionally, adding a huge heterogeneity to their properties and functions. This diversity is also reflected somehow in GABAARs in OLs and SCs. Thus, SCs express extra-synaptic subunits (in particular the δ subunit, which is key for neurosteroid affinity), while OLs express subunits commonly found at postsynaptic densities, meaning that GABAARs of OLs and SCs have different subunit composition and, consequently, different pharmacological profiles and functional behaviors (Faroni et al., 2019). Also, there is a switch in the expression of synaptic to extrasynaptic GABAARs as OPCs progress in the lineage (Vélez-Fort et al., 2010; Balia et al., 2017).
In oligodendroglial cells, GABAAR expression goes down as they mature and acquire a myelinating phenotype (Berger et al., 1992; Arellano et al., 2016). In contrast, GABABR expression is quite stable at all stages (Serrano-Regal et al., 2020). Sustained GABAAR expression in oligodendroglial cells depends on the presence of axons, though the mechanisms driving GABAAR stabilization remain still unknown. Thus, it is likely that molecules released from neurons in an activity-independent manner may drive GABAAR expression (Arellano et al., 2016; Hamilton et al., 2017; Figure 4).
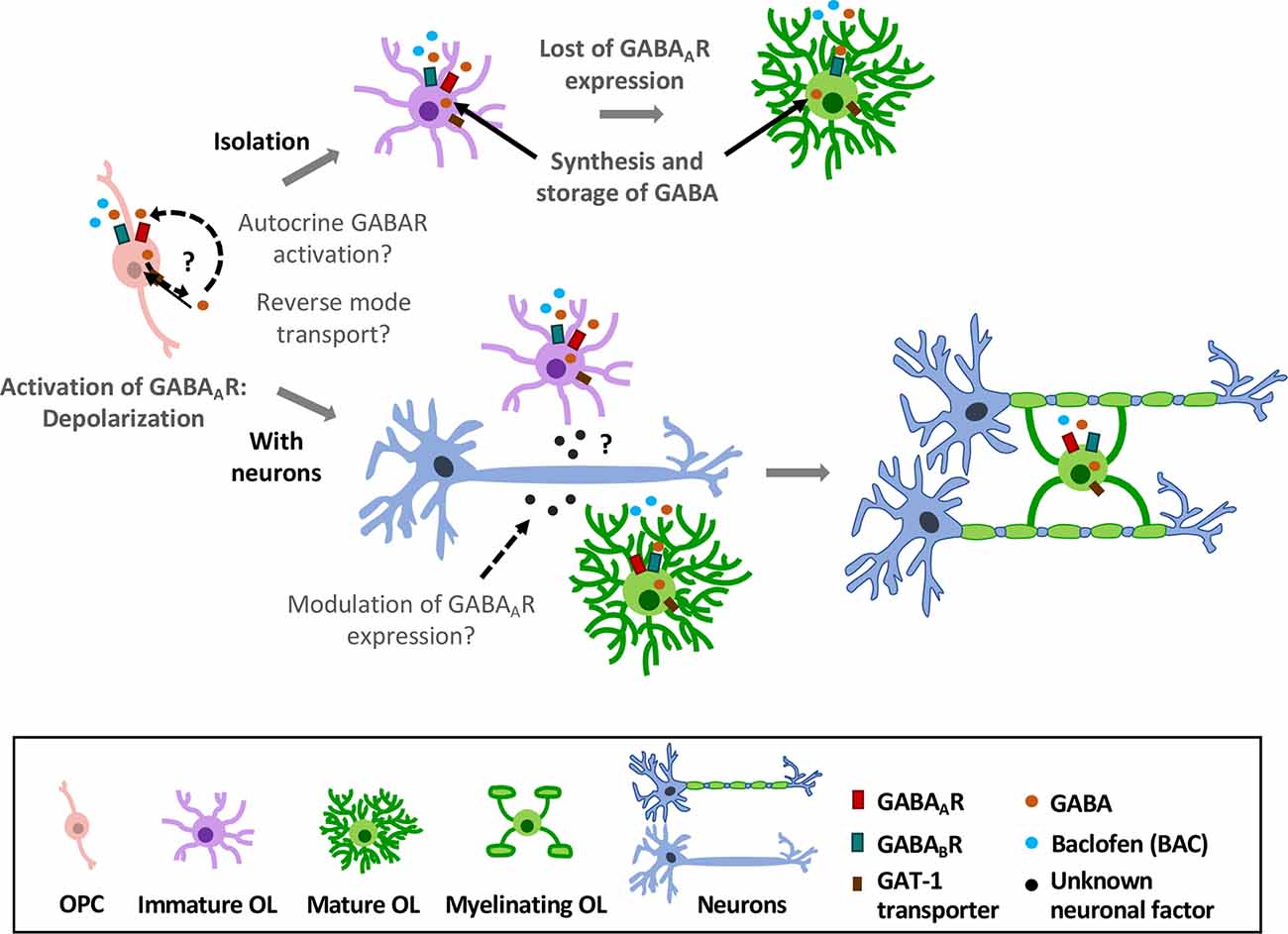
Figure 4. Effects of GABAergic signaling in oligodendroglial differentiation and myelination. OPCs express GABAA and GABABRs. Activation of GABAARs causes depolarization in these cells (Kirchhoff and Kettenmann, 1992; Baraban et al., 2018). In absence of axons (top), they lose GABAAR expression as they differentiate into mature OLs (Berger et al., 1992; Arellano et al., 2016); however, expression of GABABRs is largely stable over time (Serrano-Regal et al., 2020). In the presence of axons (low), GABAAR expression is modulated by neurons, as OPCs maintain their expression towards the myelinating stage (Arellano et al., 2016). Moreover, oligodendroglial cells express GAT-1 transporter and synthesize GABA, which may be released by reverse GAT-1 operation and activate GABA receptors (GABARs) in an autocrine manner. Exogenous GABA or baclofen promotes oligodendroglial differentiation and myelination in vitro (Serrano-Regal et al., 2020).
Also, OLs and SCs can synthesize and store GABA, and to take it up from the extracellular fluid through specific GABA transporters (Fattorini et al., 2017). It is therefore conceivable that these cells may release GABA by mechanisms including reverse functioning of the transporters, as observed in other glial cell types (Barakat and Bordey, 2002). Early experiments demonstrated that cultured satellite glial cells from DRG can release [3H]GABA in response to a depolarizing stimulus (Minchin and Iversen, 1974). Thus, GABA released by myelinating cells might act in a paracrine or autocrine way (as suggested by Magnaghi, 2007) to, ultimately, modulate their differentiation and/or their myelination capacity (Figure 4). Interestingly, this mechanism operates for instance in polysialylated forms of neural cell-adhesion molecule (PSA-NCAM) progenitor cells in the CNS, which eventually differentiate into glial cells. Thus, autocrine/paracrine loops involving neurosteroids and GABA signaling in these progenitors modulate their proliferation and differentiation (Gago et al., 2004). Synthesis of neurosteroids occurs in SCs (Chan et al., 2000), and may stimulate GABA synthesis in these cells via a rapid protein kinase A (PKA)-dependent autocrine loop (Magnaghi et al., 2010). In this way, neurosteroids provide the specific ligand for GABAAR activation (Magnaghi et al., 2010). As neurosteroids are involved in promoting SC differentiation and myelination acting through GABAARs, a possible paracrine/autocrine mechanism could underlie these processes.
GABAA and GABAB receptors may exert opposite roles on myelinating cells, as proposed for SCs in pathological conditions (Faroni and Magnaghi, 2011). Both central and peripheral myelinating cells express GABAA and GABAB receptors, however, this expression depends on the presence of surrounding axons and, as occurs with other receptors and transporters, may vary along the lineage or even depending on the nervous system area (Marques et al., 2016; Spitzer et al., 2019). Thus, depending on the developmental stage of these cells and the GABAR involved, the neurotransmitter GABA may play different physiological functions. Moreover, GABAergic signaling could potentially regulate specific subgroups of cells from the OL/SC lineages in different ways, either action-potential dependently or independently. Thus, it appears that in OPCs GABA acts mostly through GABAARs to carry out some important functions at the progenitor level (as regulation of the population size, OPC maintenance, axon-glia recognition, differentiation or myelination initiation; Zonouzi et al., 2015; Arellano et al., 2016; Balia et al., 2017; Hamilton et al., 2017; Marisca et al., 2020).
Finally, GABABRs may contribute to myelin maintenance (Figure 4). In this regard, GABARs may parallel somehow the various functions played by glutamate receptors, as AMPARs are crucial for the early stages of remyelination while NMDARs are relevant for myelin maintenance and to fuel axonal function (Lundgaard et al., 2013; Gautier et al., 2015; Saab et al., 2016).
In sum, understanding the contribution of the GABAergic signaling to OL and SC physiology may be critical to find therapeutic tools to improve remyelination in demyelinating diseases. Meanwhile, it is necessary to clarify in detail the role of GABA in OL and SC differentiation and myelination, and the mechanisms that mediate these responses. To that aim, it will be important to specifically target GABAARs and GABABRs either at the progenitor stage or the more mature stages of the myelinating cells. Drugs preferentially acting on GABARs in OLs and SCs will certainly help to successfully tackle these tasks.
Author Contributions
MS-R and RA wrote the manuscript, designed its content, and prepared the figures and table. LB-C, RO, and AL wrote the manuscript and prepared the figures and table. EG wrote the manuscript. CM and MS-G wrote the manuscript and designed its content.
Funding
This work was supported by CIBERNED (CB06/05/0076; CM) and by grants from the Ministry of Economy and Competitiveness, Government of Spain (SAF2016-75292-R and PID2019-109724RB-I00; CM), Basque Government (IT1203-19; CM), CONACYT-México (No. 252121; RA), PAPIIT- UNAM-México (IN203519; RA) and NIH (R21AG053740 and R21MH113177; AL). MS-R was hired thanks to the Gangoiti Foundation (Bilbao). LB-C and RO hold fellowships from Basque Government and CONACYT-México, respectively.
Conflict of Interest
The authors declare that the research was conducted in the absence of any commercial or financial relationships that could be construed as a potential conflict of interest.
References
Afshari, F. S., Chu, A. K., and Sato-Bigbee, C. (2001). Effect of cyclic AMP on the expression of myelin basic protein species and myelin proteolipidprotein in committed oligodendrocytes: differential involvement of the transcription factor CREB. J. Neurosci. Res. 66, 37–45. doi: 10.1002/jnr.1195
Allen, N. J., and Lyons, D. A. (2018). Glia as architects of central nervous system formation and function. Science 362, 181–185. doi: 10.1126/science.aat0473
Angulo, M. C., Le Meur, K., Kozlov, A. S., Charpak, S., and Audinat, E. (2008). GABA, a forgotten gliotransmitter. Prog. Neurobiol. 86, 297–303. doi: 10.1016/j.pneurobio.2008.08.002
Arellano, R. O., Sánchez-Gómez, M. V., Alberdi, E., Canedo-Antelo, M., Chara, J. C., Palomino, A., et al. (2016). Axon-to-glia interaction regulates GABAA receptor expression in oligodendrocytes. Mol. Pharmacol. 89, 63–74. doi: 10.1124/mol.115.100594
Arthur-Farraj, P., Wanek, K., Hantke, J., Davis, C. M., Jayakar, A., Parkinson, D. B., et al. (2011). Mouse Schwann cells need both NRG1 and cyclic AMP to myelinate. Glia 59, 720–733. doi: 10.1002/glia.21144
Balia, M., Benamer, N., and Angulo, M. C. (2017). A specific GABAergic synapse onto oligodendrocyte precursors does not regulate cortical oligodendrogenesis. Glia 65, 1821–1832. doi: 10.1002/glia.23197
Balia, M., Vélez-Fort, M., Passlick, S., Schäfer, C., Audinat, E., Steinhäuser, C., et al. (2015). Postnatal down-regulation of the GABAA receptor γ2 subunit in neocortical NG2 cells accompanies synaptic-to-extrasynaptic switch in the GABAergic transmission mode. Cereb. Cortex 25, 1114–1123. doi: 10.1093/cercor/bht309
Baraban, M., Koudelka, S., and Lyons, D. A. (2018). Ca2+ activity signatures of myelin sheath formation and growth in vivo. Nat. Neurosci. 21, 19–23. doi: 10.1038/s41593-017-0040-x
Barakat, L., and Bordey, A. (2002). GAT-1 and reversible GABA transport in Bergmann glia in slices. J. Neurophysiol. 88, 1407–1419. doi: 10.1152/jn.2002.88.3.1407
Barati, M. T., Lukenbill, J., Wu, R., Rane, M. J., and Klein, J. B. (2015). Cytoskeletal rearrangement and Src and PI-3K-dependent Akt activation control GABABR-mediated chemotaxis. Cell. Signal. 27, 1178–1185. doi: 10.1016/j.cellsig.2015.02.022
Barnard, E. A., Skolnick, P., Olsen, R. W., Mohler, H., Sieghart, W., Biggio, G., et al. (1998). International Union of Pharmacology. XV. Subtypes of gamma-aminobutyric acid A receptors: classification on the basis of subunit structure and receptor function. Pharmacol. Rev. 50, 291–313.
Baur, R., and Sigel, E. (2003). On high- and low-affinity agonist sites in GABAA receptors. J. Neurochem. 87, 325–332. doi: 10.1046/j.1471-4159.2003.01982.x
Belujon, P., Baufreton, J., Grandoso, L., Boué-Grabot, E., Batten, T. F., Ugedo, L., et al. (2009). Inhibitory transmission in locus coeruleus neurons expressing GABAA receptor epsilon subunit has a number of unique properties. J. Neurophysiol. 102, 2312–2325. doi: 10.1152/jn.00227.2009
Ben-Ari, Y. (2002). Excitatory actions of gaba during development: the nature of the nurture. Nat. Rev. Neurosci. 3, 728–739. doi: 10.1038/nrn920
Berger, T., Walz, W., Schnitzer, J., and Kettenmann, H. (1992). GABA-and glutamate-activated currents in glial cells of the mouse corpus callosum slice. J. Neurosci. Res. 31, 21–27. doi: 10.1002/jnr.490310104
Bettler, B., Kaupmann, K., Mosbacher, J., and Gassmann, M. (2004). Molecular structure and physiological functions of GABAB receptors. Physiol. Rev. 84, 835–867. doi: 10.1152/physrev.00036.2003
Binet, V., Brajon, C., Le Corre, L., Acher, F., Pin, J. P., and Prézeau, L. (2004). The heptahelical domain of GABAB2 is activated directly by CGP7930, a positive allosteric modulator of the GABAB receptor. J. Biol. Chem. 279, 29085–29091. doi: 10.1074/jbc.m400930200
Bollan, K. A., Baur, R., Hales, T. G., Sigel, E., and Connolly, C. N. (2008). The promiscuous role of the epsilon subunit in GABAA receptor biogenesis. Mol. Cell. Neurosci. 37, 610–621. doi: 10.1016/j.mcn.2007.12.011
Booker, S. A., Loreth, D., Gee, A. L., Watanabe, M., Kind, P. C., Wyllie, D. J. A., et al. (2018). Postsynaptic GABABRs inhibit L-type calcium channels and abolish long-term potentiation in hippocampal somatostatin interneurons. Cell. Rep. 22, 36–43. doi: 10.1016/j.celrep.2017.12.021
Bowery, N. G., Bettler, B., Froestl, W., Gallagher, J. P., Marshall, F., Raiteri, M., et al. (2002). International Union of Pharmacology. XXXIII. Mammalian gamma-aminobutyric AcidB receptors: structure and function. Pharmacol. Rev. 54, 247–264. doi: 10.1124/pr.54.2.247
Bowery, N. G., and Hudson, A. L. (1979). γ-aminobutyric acid reduces the evoked release of [3H]-noradrenaline from sympathetic nerve therminals [proceedings]. Br. J. Pharmacol. 66:108P.
Bronstein, J. M., Hales, T. G., Tyndale, R. F., and Charles, A. C. (1998). A conditionally immortalized glial cell line that expresses mature myelin proteins and functional GABAA receptors. J. Neurochem. 70, 483–491. doi: 10.1046/j.1471-4159.1998.70020483.x
Brown, D. A., Adams, P. R., Higgins, A. J., and Marsh, S. (1979). Distribution of gaba-receptors and gaba-carriers in the mammalian nervous system. J. Physiol. 75, 667–671.
Brown, N., Kerby, J., Bonnert, T. P., Whiting, P. J., and Wafford, K. A. (2002). Pharmacological characterization of a novel cell line expressing human α4β3δ GABAA receptors. Br. J. Pharmacol. 136, 965–974. doi: 10.1038/sj.bjp.0704796
Cahoy, J. D., Emery, B., Kaushal, A., Foo, L. C., Zamanian, J. L., Christopherson, K. S., et al. (2008). A transcriptome database for astrocytes, neurons and oligodendrocytes: a new resource for understanding brain development and function. J. Neurosci. 28, 264–278. doi: 10.1523/JNEUROSCI.4178-07.2008
Calver, A. R., Medhurst, A. D., Robbins, M. J., Charles, K. J., Evans, M. L., Harrison, D. C., et al. (2000). The expression of GABAB1 and GABAB2 receptor subunits in the CNS differs from that in peripheral tissues. Neuroscience 100, 155–170. doi: 10.1016/s0306-4522(00)00262-1
Carlezon, W. A. Jr., Duman, R. S., and Nestler, E. J. (2005). The many faces of CREB. Trends Neurosci. 28, 436–445. doi: 10.1016/j.tins.2005.06.005
Carver, C. M., Chuang, S., and Reddy, D. S. (2016). Zinc selectively blocks neurosteroid-sensitive extrasynaptic δGABA A receptors in the hippocampus. J. Neurosci. 36, 8070–8077. doi: 10.1523/jneurosci.3393-15.2016
Chan, J. R., Rodriguez-Waitkus, P. M., Ng, B. K., Liang, P., and Glaser, M. (2000). Progesterone synthesized by Schwann cells during myelin formation regulates neuronal gene expression. Mol. Biol. Cell. 11, 2283–2295. doi: 10.1091/mbc.11.7.2283
Charles, K. J., Deuchars, J., Davies, C. H., and Pangalos, M. N. (2003). GABAB receptor subunit expression in glia. Mol. Cell. Neurosci. 24, 214–223. doi: 10.1016/s1044-7431(03)00162-3
Cheli, V. T., Sántiago-González, D. A., Namgyal-Lama, T., Spreuer, V., Handley, V., Murphy, G. G., et al. (2016). Conditional deletion of the L-type calcium channel Cav1.2 in oligodendrocyte progenitor cells affects postnatal myelination in mice. J. Neurosci. 36, 10853–10869. doi: 10.1523/jneurosci.1770-16.2016
Chung, S. H., Biswas, S., Selvaraj, V., Liu, X. B., Sohn, J., Jiang, P., et al. (2015). The p38α mitogen-activated protein kinase is a key regulator of myelination and remyelination in the CNS. Cell Death Dis. 6:e1748. doi: 10.1038/cddis.2015.119
Chung, S. H., Biswas, S., Sohn, J., Jiang, P., Dehghan, S., Marzban, H., et al. (2018). The p38α MAPK deletion in oligodendroglia does not attentuate myelination defects in a mouse model of periventricular leukomalacia. Neuroscience 386, 175–181. doi: 10.1016/j.neuroscience.2018.06.037
Cisneros-Mejorado, A. J., Garay, E., Ortiz-Retana, J., Concha, L., Moctezuma, J. P., Romero, S., et al. (2020). Demyelination-remyelination of the rat caudal cerebellar peduncle evaluated with magnetic resonance imaging. Neuroscience 439, 255–267. doi: 10.1016/j.neuroscience.2019.06.042
Colognato, H., Ramachandrappa, S., Olsen, I. M., and ffrench-Constant, C. (2004). Integrins direct Src family kinases to regulate distinct phases of oligodendrocyte development. J. Cell Biol. 167, 365–375. doi: 10.1083/jcb.200404076
Corell, M., Wicher, G., Radomska, K. J., Daglikoca, E. D., Godskesen, R. E., Fredriksson, R., et al. (2015). GABA and its B-receptor are present at the node of Ranvier in a small population of sensory fibers, implicating a role in myelination. J. Neurosci. Res. 93, 285–295. doi: 10.1002/jnr.23489
Couve, A., Moss, S. J., and Pangalos, M. N. (2000). GABAB receptors: a new paradigm in G protein signaling. Mol. Cell. Neurosci. 16, 296–312. doi: 10.1006/mcne.2000.0908
Dawson, M. R., Polito, A., Levine, J. M., and Reynolds, R. (2003). NG2-expressing glial progenitor cells: an abundant and widespread population of cycling cells in the adult rat CNS. Mol. Cell. Neurosci. 24, 476–488. doi: 10.1016/s1044-7431(03)00210-0
Doyon, N., Vinay, L., Prescott, S. A., and De Koninck, Y. (2016). Chloride regulation: a dynamic equilibrium crucial for synaptic inhibition. Neuron 89, 1157–1172. doi: 10.1016/j.neuron.2016.02.030
Duncan, I. D., Radcliff, A. B., Heidari, M., Kidd, G., August, B. K., and Wierenga, L. A. (2018). The adult oligodendrocyte can participate in remyelination. Proc. Natl. Acad. Sci. U S A 115, E11807–E11816. doi: 10.1073/pnas.1808064115
Fannon, J., Tarmier, W., and Fulton, D. (2015). Neuronal activity and AMPA-type glutamate receptor activation regulates the morphological development of oligodendrocyte precursor cells. Glia 63, 1021–1035. doi: 10.1002/glia.22799
Faroni, A., and Magnaghi, V. (2011). The neurosteroid allopregnanolone modulates specific functions in central al peripheral glial cells. Front. Endocrinol. 2:103. doi: 10.3389/fendo.2011.00103
Faroni, A., Melfi, S., Castelnovo, L. F., Bonalume, V., Colleoni, D., Magni, P., et al. (2019). GABA-B1 receptor-null Schwann cells exhibit compromised in vitro myelination. Mol. Neurobiol. 56, 1461–1474. doi: 10.1007/s12035-018-1158-x
Faroni, A., Smith, R. J., Procacci, P., Castelnovo, L. F., Puccianti, E., Reid, A. J., et al. (2014). Purinergic signaling mediated by P2X7 receptors controls myelination in sciatic nerves. J. Neurosci. Res. 92, 1259–1269. doi: 10.1002/jnr.23417
Faroni, A., Terenghi, G., and Magnaghi, V. (2012). Expression of functional γ-aminobutyric acid type A receptors in Schwann-like adult stem cells. J. Mol. Neurosci. 47, 619–630. doi: 10.1007/s12031-011-9698-9
Fattorini, G., Melone, M., Sánchez-Gómez, M. V., Arellano, R. O., Bassi, S., Matute, C., et al. (2017). GAT-1 mediated GABA uptake in rat oligodendrocytes. Glia 65, 514–522. doi: 10.1002/glia.23108
Fragoso, G., Haines, J. D., Robertson, J., Pedraza, L., Mushynski, W. E., and Almazan, G. (2007). p38 mitogen-activated protein kinase is required for central nervous system myelination. Glia 55, 1531–1541. doi: 10.1002/glia.20567
Fragoso, G., Robertson, J., Athlan, E., Tam, E., Almazan, G., and Mushynski, W. E. (2003). Inhibition of p38 mitogen-activated protein kinase interferes with cell shape changes and gene expression associated with Schwann cell myelination. Exp. Neurol. 183, 34–46. doi: 10.1016/s0014-4886(03)00101-8
Franklin, R. J., Gilson, J. M., and Blakemore, W. F. (1997). Local recruitment of remyelinating cells in the repair of demyelination in the central nervous system. J. Neurosci. Res. 50, 337–344. doi: 10.1002/(sici)1097-4547(19971015)50:2<337::aid-jnr21>3.0.co;2-3
Gago, N., El-Etr, M., Sananès, N., Cadepond, F., Samuel, D., Avellana-Adalid, V., et al. (2004). 3alpha,5alpha-Tetrahydroprogesterone (allopregnanolone) and gamma-aminobutyric acid: autocrine/paracrine interactions in the control of neonatal PSA-NCAM+ progenitor proliferation. J. Neurosci. Res. 78, 770–783. doi: 10.1002/jnr.20348
Galvez, T., Duthey, B., Kniazeff, J., Blahos, J., Rovelli, G., Bettler, B., et al. (2001). Allosteric interactions between GB1 and GB2 subunits are required for optimal GABAB receptor function. EMBO J. 20, 2152–2159. doi: 10.1093/emboj/20.9.2152
García-Díaz, B., and Baron-Van Evercooren, A. (2020). Schwann cells: recuers of central demyelination. Glia doi: 10.1002/glia.23788 [Epub ahead of print].
Gautier, H. O., Evans, K. A., Volbracht, K., James, R., Sitnikov, S., Lundgaard, I., et al. (2015). Neuronal activity regulates remyelination via glutamate signalling to oligodendrocyte protenitors. Nat. Commun. 6:8518. doi: 10.1038/ncomms9518
Ghoumari, A. M., Ibanez, C., El-Etr, M., Leclerc, P., Eychenne, B., O’Malley, B. W., et al. (2003). Progesterone and its metabolites increase myelin basic protein expression in organotypic slice cultures of rat cerebellum. J. Neurochem. 86, 848–859. doi: 10.1046/j.1471-4159.2003.01881.x
Gibbs, J. W., Zhang, Y. F., Kao, C. Q., Holloway, K. L., Oh, K. S., and Coulter, D. A. (1996). Characterization of GABAA receptor function in human temporal cortical neurons. J. Neurophysiol. 75, 1458–1471. doi: 10.1152/jn.1996.75.4.1458
Gibson, E. M., Purger, D., Mount, C. W., Goldstein, A. K., Lin, G. L., Wood, L. S., et al. (2014). Neuronal activity promotes oligodendrogenesis and adaptive myelination in the mammalian brain. Science 344:1252304. doi: 10.1126/science.1252304
Goodkin, H. P., and Kapur, J. (2009). The impact of diazepam’s discovery on the treatment and understanding of status epilepticus. Epilepsia 50, 2011–2018. doi: 10.1111/j.1528-1167.2009.02257.x
Hamilton, N. B., Clarke, L. E., Arancibia-Carcamo, I. L., Kougioumtzidou, E., Matthey, M., Karadottir, R., et al. (2017). Endogenous GABA controls oligodendrocyte lineage cell number, myelination and CNS internode length. Glia 65, 309–321. doi: 10.1002/glia.23093
Héja, L., Nyitrai, G., Kékesi, O., Dobolyi, A., Szabó, P., Fiáth, R., et al. (2012). Astrocytes convert network excitation to tonic inhibition of neurons. BMC Biol. 10:26. doi: 10.1186/1741-7007-10-26
Hesp, Z. C., Goldstein, E. A., Miranda, C. J., Kaspar, B. K., McTigue, D. M., Kaspar, B. K., et al. (2015). Chronic oligodendrogenesis and remyelination after spinal cord injury in mice and rats. J. Neurosci. 35, 1274–1290. doi: 10.1523/jneurosci.1414-15.2015
Hill, D. R., and Bowery, N. G. (1981). 3H-Baclofen and 3H-GABA bind to bicuculline-insensitive GABAB sites in rat brain. Nature 290, 149–152. doi: 10.1038/290149a0
Hodge, R. D., Bakken, T. E., Miller, J. A., Smith, K. A., Barkan, E. R., Graybuck, L. T., et al. (2019). Conserved cell types with divergent features in human versus mouse cortex. Nature 573, 61–68. doi: 10.1038/s41586-019-1506-7
Hosie, A. M., Dunne, E. L., Harvey, R. J., and Smart, T. G. (2003). Zinc-mediated inhibition of GABAA receptors: discrete binding sites underlie subtype specificity. Nat. Neurosci. 6, 362–369. doi: 10.1038/nn1030
Jessen, K. R., and Mirsky, R. (2005). The origin and development of glial cells in peripheral nerves. Nat. Rev. Neurosci. 6, 671–682. doi: 10.1038/nrn1746
Jessen, K. R., and Mirsky, R. (2019). The success and failure of the Schwann cell response to nerve injury. Front. Cell. Neurosci. 13:33. doi: 10.3389/fncel.2019.00033
Jiménez-González, C., Pirttimaki, T., Cope, D. W., and Parri, H. R. (2011). Non-neuronal, slow GABA signalling in the ventrobasal thalamus targets δ-subunit- containing GABAA receptors. Eur. J. Neurosci. 33, 1471–1482. doi: 10.1111/j.1460-9568.2011.07645.x
Jones, B. L., and Henderson, L. P. (2007). Trafficking and potential assembly patterns of epsilon-containing GABAA receptors. J. Neurochem. 103, 1258–1271. doi: 10.1111/j.1471-4159.2007.04833.x
Kalakh, S., and Mouihate, A. (2019). Enhanced remyelination during late pregnancy: involvement of the GABAergic system. Sci. Rep. 9:7728. doi: 10.1038/s41598-019-44050-4
Karim, N., Wellendorph, P., Absalom, N., Johnston, G. A., Hanrahan, J. R., and Chebib, M. (2013). Potency of GABA at human recombinant GABAA receptors expressed in Xenopus oocytes: a mini review. Amino Acids 44, 1139–1149. doi: 10.1007/s00726-012-1456-y
Kaufman, D. L., Houser, C. R., and Tobin, A. J. (1991). Two forms of the gamma-aminobutyric acid synthetic enzyme glutamate decarboxylase have distinct intraneuronal distributions and cofactor interactions. J. Neurochem. 56, 720–723. doi: 10.1111/j.1471-4159.1991.tb08211.x
Kaupmann, K., Malitschek, B., Schuler, V., Heid, J., Froestl, W., Beck, P., et al. (1998). GABAB-receptor subtypes assemble into functional heteromeric complexes. Nature 396, 683–687. doi: 10.1038/25360
Kidd, G. J., Ohno, N., and Trapp, B. D. (2013). Biology of Schwann cells. Handb. Clin. Neurol. 115, 55–79. doi: 10.1016/B978-0-444-52902-2.00005-9
Kirchhoff, F., and Kettenmann, H. (1992). GABA triggers a [Ca2+]i increase in murine precursor cells of the oligodendrocyte lineage. Eur. J. Neurosci. 4, 1049–1058. doi: 10.1111/j.1460-9568.1992.tb00131.x
Krasnow, A. M., Ford, M. C., Valdivia, L. E., Wilson, S. W., and Attwell, D. (2018). Regulation of developing myelin sheath elongation by oligodendrocyte calcium transients in vivo. Nat. Neurosci. 21, 24–30. doi: 10.1038/s41593-017-0031-y
Kukley, M., Kiladze, M., Tognatta, R., Hans, M., Swandulla, D., Schramm, J., et al. (2008). Glial cells are born with synapses. FASEB J. 22, 2957–2969. doi: 10.1096/fj.07-090985
Kuner, R., Köhr, G., Grünewald, S., Eisenhardt, G., Bach, A., and Kornau, H. C. (1999). Role of the heteromer formation in GABAB receptor function. Science 283, 74–77. doi: 10.1126/science.283.5398.74
Lambert, J. J., Cooper, M. A., Simmons, R. D., Weir, C. J., and Belelli, D. (2009). Neurosteroids: endogenous allosteric modulators of GABAA receptors. Psychoneuroendocrinology 34, S48–S58. doi: 10.1016/j.psyneuen.2009.08.009
Larson, V. A., Zhang, Y., and Bergles, D. E. (2016). Electrophysiological properties of NG2+ cells: matching physiological studies with gene expression profiles. Brain Res. 1638, 138–160. doi: 10.1016/j.brainres.2015.09.010
Levitan, E. S., Schofieldh, P. R., Burt, D. R., Rhee, L. M., Wisden, W., Kohler, M., et al. (1988). Structural and functional basis for GABAA receptor heterogeneity. Nature 335, 76–79. doi: 10.1038/335076a0
Li, C., Xiao, L., Liu, X., Yang, W., Shen, W., Hu, C., et al. (2013). A functional role of NMDA receptor in regulating the differentiation of oligodendrocyte precursor cells and remyelination. Glia 61, 732–749. doi: 10.1002/glia.22469
Li, X., Terunuma, M., Deeb, T. G., Wiseman, S., Pangalos, M. N., Nairn, A. C., et al. (2020). Direct interaction of PP2A phosphatase with GABAB receptors alters functional signaling. J. Neurosci. 40, 2808–2816. doi: 10.1523/JNEUROSCI.2654-19.2020
Lin, S. C., and Bergles, D. E. (2004). Synaptic signaling between GABAergic interneurons and oligodendrocyte precursor cells in the hippocampus. Nat. Neurosci. 7, 24–32. doi: 10.1038/nn1162
Lin, C. C., Lee, I. T., Hsu, C. H., Hsu, C. K., Chi, P. L., Hsiao, L. D., et al. (2015). Sphingosine-1-phosphate mediates ICAM-1-dependent monocyte adhesion through p38 MAPK and p42/p44 MAPK-dependent Akt activation. PLoS One 10:e0118473. doi: 10.1371/journal.pone.0118473
Lundgaard, I., Luzhynskaya, A., Stockley, J. H., Wang, Z., Evans, K. A., Swire, M., et al. (2013). Neuregulin and BDNF induce a switch to NMDA receptor-dependent myelination by oligodendrocytes. PLoS Biol. 11:e1001743. doi: 10.1371/journal.pbio.1001743
Luyt, K., Slade, T. P., Dorward, J. J., Durant, C. F., Wu, Y., Shigemoto, R., et al. (2007). Developing oligodendrocytes express functional GABAB receptors that stimulate cell proliferation and migration. J. Neurochem. 100, 822–840. doi: 10.1111/j.1471-4159.2006.04255.x
Magnaghi, V. (2007). GABA and neuroactive steroid interactions in glia: new roles for old players? Curr. Neuropharmacol. 5, 47–64. doi: 10.2174/157015907780077132
Magnaghi, V., Ballabio, M., Camozzi, F., Colleoni, M., Consoli, A., Gassmann, M., et al. (2008). Altered peripheral myelination in mice lacking GABAB receptors. Mol. Cell. Neurosci. 37, 599–609. doi: 10.1016/j.mcn.2007.12.009
Magnaghi, V., Ballabio, M., Cavarretta, I. T., Froestl, W., Lambert, J. J., Zucchi, I., et al. (2004). GABAB receptors in Schwann cells influence proliferation and myelin protein expression. Eur. J. Neurosci. 19, 2641–2649. doi: 10.1111/j.0953-816x.2004.03368.x
Magnaghi, V., Ballabio, M., Consoli, A., Lambert, J. J., Roglio, I., and Melcangi, R. C. (2006). GABA receptor-mediated effects in the peripheral nervous system: a cross-interaction with neuroactive steroids. J. Mol. Neurosci. 28, 89–102. doi: 10.1385/jmn:28:1:89
Magnaghi, V., Cavarretta, I., Galbiati, M., Martini, L., and Melcangi, R. C. (2001). Neuroactive steroids and peripheral myelin proteins. Brain Res. Rev. 37, 360–371. doi: 10.1016/s0165-0173(01)00140-0
Magnaghi, V., Parducz, A., Frasca, A., Ballabio, M., Procacci, P., Racagni, G., et al. (2010). GABA synthesis in Schwann cells is induced by the neuroactive steroid allopregnanolone. J. Neurochem. 112, 980–990. doi: 10.1111/j.1471-4159.2009.06512.x
Marisca, R., Hoche, T., Agirre, E., Jane Hoodless, L., Barkey, W., Auer, F., et al. (2020). Functionally distinct subgroups of oligodendrocyte precursor cells integrate neural activity and execute myelin formation. Nat. Neurosci. 23, 363–374. doi: 10.1038/s41593-019-0581-2
Marques, S., Zeisel, A., Codeluppi, S., van Bruggen, D., Mendanha Falcão, A., Xiao, L., et al. (2016). Oligodendrocyte heterogeneity in the mouse juvenile and adult central nervous system. Science 352, 1326–1329. doi: 10.1126/science.aaf6463
Meera, P., Wallner, M., and Otis, T. S. (2011). Molecular basis for the high THIP/gaboxadol sensitivity of extrasynaptic GABAA receptors. J. Neurophysiol. 106, 2057–2064. doi: 10.1152/jn.00450.2011
Melfi, S., Montt Guevara, M. M., Bonalume, V., Ruscica, M., Colciago, A., Simoncini, T., et al. (2017). Src and phosphor-FAK kinases are activated by allopregnanolone promoting Schwann cell motility, morphology and myelination. J. Neurochem. 141, 165–178. doi: 10.1111/jnc.13951
Michelsen, S., Sánchez, C., and Ebert, B. (2007). Lack of generalisation between the GABAA receptor agonist, gaboxadol and allosteric modulators of the benzodiazepine binding site in the rat drug discrimination procedure. Psychopharmacology 193, 151–157. doi: 10.1007/s00213-007-0750-y
Minchin, M. C., and Iversen, L. L. (1974). Release of (3H)gamma-aminobutyric acid from glial cells in rat dorsal root ganglia. J. Neurochem. 23, 533–540. doi: 10.1111/j.1471-4159.1974.tb06056.x
Mitew, S., Gobius, I., Fenlon, L. R., McDougall, S. J., Hawkes, D., Xing, Y. L., et al. (2018). Pharmacogenetic stimulation of neuronal activity increases myelination in an axon-specific manner. Nat. Commun. 9:306. doi: 10.3410/f.732542465.793542118
Mortensen, M., Ebert, B., Wafford, K., and Smart, T. G. (2010). Distinct activities of GABA agonists at synaptic- and extrasynaptic-type GABAA receptors. J. Physiol. 588, 1251–1268. doi: 10.1113/jphysiol.2009.182444
Mortensen, M., Patel, B., and Smart, T. G. (2012). GABA potency at GABAAreceptors found in synaptic and extrasynaptic zones. Front. Cell. Neurosci. 6:1. doi: 10.3389/fncel.2012.00001
Mount, C. W., Yalçın, B., Cunliffe-Koehler, K., Sundaresh, S., and Monje, M. (2019). Monosynaptic tracing maps brain-wide afferent oligodendrocyte precursor cell connectivity. Elife 8:e49291. doi: 10.7554/eLife.49291
Mugabe, B. E., Yaghini, F. A., Song, C. Y., Buharalioglu, C. K., Waters, C. M., and Malik, K. U. (2010). Angiotensin II-induced migration of vascular smooth muscle cells is mediated by p38 mitogen-activated protein kinase-activated c-Src through spleen tyrosine kinase and epidermal growth factor receptor transactivation. J. Pharmacol. Exp. Ther. 332, 116–124. doi: 10.1124/jpet.109.157552
Nait-Oumesmar, B., Decker, L., Lachapelle, F., Avellana-Adalid, V., Bachelin, C., and Baron-Van Evercooren, A. (1999). Progenitor cells of the adult mouse subventricular zone proliferate, migrate and differentiate into oligodendrocytes after demyelination. Eur. J. Neurosci. 11, 4357–4366. doi: 10.1046/j.1460-9568.1999.00873.x
Nave, K. A., and Trapp, B. D. (2008). Axon-glial signaling and the glial support of axon function. Annu. Rev. Neurosci. 31, 535–561. doi: 10.1146/annurev.neuro.30.051606.094309
Newbern, J., and Birchmeier, C. (2010). Nrg1/ErbB signaling networks in Schwann cell development and myelination. Semin. Cell. Dev. Biol. 21, 922–928. doi: 10.1016/j.semcdb.2010.08.008
Olsen, R. W., and Sieghart, W. (2008). International Union of Pharmacology. LXX. Subtypes of gamma-aminobutyric AcidA receptors: classification on the basis of subunit composition, pharmacology and function. Update. Pharmacol. Rev. 60, 243–260. doi: 10.1124/pr.108.00505
Orduz, D., Maldonado, P. P., Balia, M., Vélez-Fort, M., de Sars, V., Yanagawa, Y., et al. (2015). Interneurons and oligodendrocyte progenitors form a structured synaptic network in the developing neocortex. Elife 4:e06953. doi: 10.7554/eLife.06953.021
Paez, P. M., and Lyons, D. (2020). Calcium signaling in the oligodendrocyte lineage: regulators and consequences. Annu. Rev. Neurosci. 43, 163–186. doi: 10.1146/annurev-neuro-100719-093305
Passlick, S., Grauer, M., Schafer, C., Jabs, R., Seifert, G., and Steinhauser, C. (2013). Expression of the 2-subunit distinguishes synaptic and extrasynaptic GABAA receptors in NG2 cells of the hippocampus. J. Neurosci. 33, 12030–12040. doi: 10.1523/JNEUROSCI.5562-12.2013
Peña, C., Medina, J. H., Novas, M. L., Paladini, A. C., and De Robertis, E. (1986). Isolation and identification in bovine cerebral cortex of n-butyl β-carboline-3-carboxylate, a potent benzodiazepine binding inhibitor. Proc. Natl. Acad. Sci. U S A 83, 4952–4956. doi: 10.1073/pnas.83.13.4952
Petroski, R. E., Pomeroy, J. E., Das, R., Bowman, H., Yang, W., Chen, A. P., et al. (2006). Indiplon is a high-affinity positive allosteric modulator with selectivity for alpha1 subunit-containing GABAA receptors. J. Pharmacol. Exp. Ther. 317, 369–377. doi: 10.1124/jpet.105.096701
Philips, T., and Rothstein, J. D. (2017). Oligodendroglia: metabolic supporters of neurons. J. Clin. Invest. 127, 3271–3280. doi: 10.1172/JCI90610
Procacci, P., Ballabio, M., Castelnovo, L. F., Mantovani, C., and Magnaghi, V. (2013). GABA-B receptors in the PNS have a role in Schwann cells differentiation? Front. Cell. Neurosci. 6:68. doi: 10.3389/fncel.2012.00068
Quintela-López, T., Ortiz-Sanz, C., Serrano-Regal, M. P., Gaminde-Blasco, A., Valero, J., Baleriola, J., et al. (2019). Aβ oligomers promote oligodendrocyte differentiation and maturation via integrin β1 and Fyn kinase signaling. Cell Death Dis. 10:445. doi: 10.1038/s41419-019-1636-8
Roberts, E., and Frankel, S. (1950). γ-aminobutyric acid in brain: its formation from glutamic acid. J. Biol. Chem. 187, 55–63.
Saab, A. S., Tzvetavona, I. D., Trevisiol, A., Baltan, S., Dibaj, P., Kusch, K., et al. (2016). Oligodendroglial NMDA receptors regulate glucose import and axonal energy metabolism. Neuron 91, 119–132. doi: 10.1016/j.neuron.2016.05.016
Salzer, J. (2015). Schwann cell myelination. Cold Spring Harb. Perspect. Biol. 7:a020529. doi: 10.1101/cshperspect.a020529
Santiago-González, D. A., Cheli, V. T., Zamora, N. N., Lama, T. N., Spreuer, V., Murphy, G. G., et al. (2017). Conditional deletion of the L-type calcium channel Cav1.2 in NG2-positive cells impairs remyelination in mice. J. Neurosci. 37, 10038–10051. doi: 10.1523/JNEUROSCI.1787-17.2017
Seeburg, P. H., Wisden, W., Verdoorn, T. A., Pritchett, D. B., Werner, P., Herb, A., et al. (1990). The GABAA receptor family: molecular and functional diversity. Cold Spring Harb. Symp. Quant. Biol. 55, 29–40. doi: 10.1101/sqb.1990.055.01.006
Seiler, N., al-Therib, M. J., and Kataola, K. (1973). Formation of GABA from putrescine in the brain of fish (Salmo irideus Gibb.). J. Neurochem. 20, 699–708. doi: 10.1111/j.1471-4159.1973.tb00030.x
Seiler, N., Schmidt-Glenewinkel, T., and Sarhan, S. (1979). On the formation of gamma-aminobutyric acid from putrescine in brain. J. Biochem. 86, 277–279. doi: 10.1093/oxfordjournals.jbchem.a132515
Sequeira, A., Shen, K., Gottlieb, A., and Limon, A. (2019). Human brain transcriptome analysis finds region- and subject-specific expression signatures of GABAAR subunits. Commun. Biol. 2:153. doi: 10.1038/s42003-019-0413-7
Serrano-Regal, M. P., Luengas-Escuza, I., Bayón-Cordero, L., Ibarra-Aizpurua, N., Alberdi, E., Pérez-Samartín, A., et al. (2020). Oligodendrocyte differentiation and myelination is potentiated via GABAB receptor activation. Neuroscience 439, 163–180. doi: 10.1016/j.neuroscience.2019.07.014
Shaw, J. C., Berry, M. J., Dyson, R. M., Crombie, G. K., Hirst, J. J., and Palliser, H. K. (2019). Reduced neurosteroid exposure following preterm birth and its’ contribution to neurological impairment: a novel avenue for preventative therapies. Front. Physiol. 10:599. doi: 10.3389/fphys.2019.00599
Shaw, J. C., Dyson, R. M., Palliser, H. K., Gray, C., Berry, M. J., and Hirst, J. J. (2019). Neurosteroid replacement therapy using the allopregnanolone-analogue ganaxolone following preterm birth in male guinea pigs. Pediatr. Res. 85, 86–96. doi: 10.1038/s41390-018-0185-7
Shaw, J. C., Palliser, H. K., Walker, D. W., and Hirst, J. J. (2015). Preterm birth affects GABAA receptor subunit mRNA levels during the foetal-to-neonatal transition in guinea pigs. J. Dev. Orig. Health Dis. 6, 250–260. doi: 10.1017/S2040174415000069
Sieghart, W., and Savić, M. M. (2018). International Union of Basic and Clinical Pharmacology. CVI: GABAA receptor subtype- and function-selective ligands: key issues in translation to humans. Pharmacol. Rev. 70, 836–878. doi: 10.1124/pr.117.014449
Spitzer, S. O., Sitnikov, S., Kamen, Y., Evans, K. A., Kronenberg-Versteeg, D., Dietmann, S., et al. (2019). Oligodendrocyte progenitor cells become regionally diverse and heterogeneous with age. Neuron 101, 459–471. doi: 10.1016/j.neuron.2018.12.020
Vélez-Fort, M., Audinat, E., and Angulo, M. C. (2012). Central role of GABA in neuron-glia interactions. Neuroscientist 18, 237–250. doi: 10.1177/1073858411403317
Vélez-Fort, M., Maldonado, P. P., Butt, A. M., Audinat, E., and Angulo, M. C. (2010). Postnatal switch from synaptic to extrasynaptic transmission between interneurons and NG2 cells. J. Neurosci. 30, 6921–6929. doi: 10.1523/JNEUROSCI.0238-10.2010
Vogt, K. (2015). “Diversity in GABAergic signaling,” in Diversity and Functions of GABA Receptors: a Tribute to Hanns Möhler, Part B, ed. U. Rudolph (Waltham and San Diego, USA; London and Oxford, UK: Elsevier Inc.), 203–222.
von Blankenfeld, G., Trotter, J., and Kettenmann, H. (1991). Expression and developmental regulation of a GABAA receptor in cultured murine cells of the oligodendrocyte lineage. Eur. J. Neurosci. 3, 310–316. doi: 10.111/j.1460-9568.1991.tb00817.x
Wake, H., Lee, P. R., and Fields, R. D. (2011). Control of local protein synthesis and initial events in myelination by action potentials. Science 333, 1647–1651. doi: 10.1126/science.1206998
Waldvogel, H. J., and Faull, R. L. M. (2015). “The diversity of GABAA receptor subunit distribution in the normal and Huntington’s disease human brain1,” in Diversity and Functions of GABA Receptors: a Tribute to Hanns Möhler, Part B, ed. U. Rudolph (London and Oxford, UK: Elsevier Inc.), 223–264.
Wallner, M., Hanchar, H. J., and Olsen, R. W. (2003). Ethanol enhances alpha 4 beta 3 delta and alpha 6 beta 3 delta gamma-aminobutyric acid type A receptors at low concentrations known to affect humans. Proc. Natl. Acad. Sci. U S A 100, 15218–15223. doi: 10.1073/pnas.2435171100
Walters, R. J., Hadley, S. H., Morris, K. D. W., and Amin, J. (2000). Benzodiazepines act on GABAAreceptors via two distinct and separable mechanisms. Nat. Neurosci. 3, 1274–1281. doi: 10.1038/81800
White, R., and Krämer-Albers, E. M. (2014). Axon-glia interaction and membrane traffic in myelin formation. Front. Cell. Neurosci. 7:284. doi: 10.3389/fncel.2013.00284
Williamson, A. V., Mellor, J. R., Grant, A. L., and Randall, A. D. (1998). Properties of GABAA receptors in cultured rat oligodendrocyte progenitor cells. Neuropharmacology 37, 859–873. doi: 10.1016/s0028-3908(98)00016-1
Wingrove, P. B., Wafford, K. A., Bain, C., and Whiting, P. J. (1994). The modulatory action of loreclezole at the gamma-aminobutyric acid type A receptor is determined by a single amino acid in the beta2 and beta3 subunit. Proc. Natl. Acad. Sci. U S A 91, 4569–4573. doi: 10.1073/pnas.91.10.4569
Wu, H., Shi, Y., Deng, X., Su, Y., Du, C., Wei, J., et al. (2015). Inhibition of c-Src/p38 MAPK pathway ameliorates renal tubular epithelial cells apoptosis in db/db mice. Mol. Cell. Endocrinol. 417, 27–35. doi: 10.1016/j.mce.2015.09.008
Zawadzka, M., Rivers, L. E., Fancy, S. P., Zhao, C., Tripathi, R., Jamen, F., et al. (2010). CNS-resident glial progenitors/stem cells produce Schwann cells as well as oligodendrocytes during repair of CNS demyelination. Cell Stem Cell 6, 578–590. doi: 10.1016/j.stem.2010.04.002
Zhang, Y., Chen, K., Sloan, S. A., Bennett, M. L., Scholze, A. R., O’ Keeffe, S., et al. (2014). An RNA-sequencing transcriptome and splicing database of glia, neurons and vascular cells of the cerebral cortex. J. Neurosci. 34, 11929–11947. doi: 10.1523/JNEUROSCI.1860-14.2014
Zhang, W., Xu, C., Tu, H., Wang, Y., Sun, Q., Hu, P., et al. (2015). GABAB receptor upregulates Fragile X mental retardation protein expression in neurons. Sci. Rep. 5:10468. doi: 10.1038/srep10468
Keywords: GABA, GABA receptor, oligodendrocyte, Schwann cell, differentiation, myelination
Citation: Serrano-Regal MP, Bayón-Cordero L, Ordaz RP, Garay E, Limon A, Arellano RO, Matute C and Sánchez-Gómez MV (2020) Expression and Function of GABA Receptors in Myelinating Cells. Front. Cell. Neurosci. 14:256. doi: 10.3389/fncel.2020.00256
Received: 25 May 2020; Accepted: 24 July 2020;
Published: 21 August 2020.
Edited by:
Nicola B. Hamilton-Whitaker, King’s College London, United KingdomReviewed by:
Åsa Fex-Svenningsen, University of Southern Denmark, DenmarkBeatriz Garcia-Diaz, INSERM U1127 Institut du Cerveau et de la Moelle épinière (ICM), France
Maria Cecilia Angulo, Centre National de la Recherche Scientifique (CNRS), France
Copyright © 2020 Serrano-Regal, Bayón-Cordero, Ordaz, Garay, Limon, Arellano, Matute and Sánchez-Gómez. This is an open-access article distributed under the terms of the Creative Commons Attribution License (CC BY). The use, distribution or reproduction in other forums is permitted, provided the original author(s) and the copyright owner(s) are credited and that the original publication in this journal is cited, in accordance with accepted academic practice. No use, distribution or reproduction is permitted which does not comply with these terms.
*Correspondence: Carlos Matute, Y2FybG9zLm1hdHV0ZUBlaHUuZXVz; María Victoria Sánchez-Gómez, dmlja3kuc2FuY2hlekBlaHUuZXVz
†ORCID: Mari Paz Serrano-Regal orcid.org/0000-0002-1133-7261