- 1Victor Chang Cardiac Research Institute, Darlinghurst, NSW, Australia
- 2St. Vincent’s Clinical School, University of New South Wales, Darlinghurst, NSW, Australia
- 3Division of Psychology, School of Medicine, University of Tasmania, Launceston, TAS, Australia
- 4Department of Psychiatry, Center for the Study of Traumatic Stress, Uniformed Services University of the Health Sciences, Bethesda, MD, United States
- 5School of Biomedical Sciences, Institute of Health and Biomedical Innovation (IHBI), Queensland University of Technology, Brisbane, QLD, Australia
- 6Prince Charles Hospital Northside Clinical Unit, School of Clinical Medicine, The University of Queensland, Brisbane, QLD, Australia
- 7Translational Research Institute, Woolloongabba, QLD, Australia
An increase in post-synaptic Ca2+ conductance through activation of the ionotropic N-methyl-D-aspartate receptor (NMDAR) and concomitant structural changes are essential for the initiation of long-term potentiation (LTP) and memory formation. Memories can be initiated by coincident events, as occurs in classical conditioning, where the NMDAR can act as a molecular coincidence detector. Binding of glutamate and glycine, together with depolarization of the postsynaptic cell membrane to remove the Mg2+ channel pore block, results in NMDAR opening for Ca2+ conductance. Accumulating evidence has implicated both force-from-lipids and protein tethering mechanisms for mechanosensory transduction in NMDAR, which has been demonstrated by both, membrane stretch and application of amphipathic molecules such as arachidonic acid (AA). The contribution of mechanosensitivity to memory formation and consolidation may be to increase activity of the NMDAR leading to facilitated memory formation. In this review we look back at the progress made toward understanding the physiological and pathological role of NMDA receptor channels in mechanobiology of the nervous system and consider these findings in like of their potential functional implications for memory formation. We examine recent studies identifying mechanisms of both NMDAR and other mechanosensitive channels and discuss functional implications including gain control of NMDA opening probability. Mechanobiology is a rapidly growing area of biology with many important implications for understanding form, function and pathology in the nervous system.
Introduction
A key feature of the brain is its remarkable ability to form associations between coincident, or simultaneously linked events, allowing organisms to learn from the past and thereby predict the future. When individual neurons are coincidently active, they will promote a strengthening of synaptic connections, which forms the basis of the synaptic theory of associative memory (Langille and Brown, 2018). The later identification of associative long-term potentiation (LTP) has provided a cellular mechanism for Hebb’s first postulate on memory formation. This theory has proved tremendously informative, and extensive evidence (Johnson et al., 2008, 2009; Sah and Westbrook, 2008; Johansen et al., 2014) suggests that Hebb’s first postulate is a likely mechanism of learning and memory at the level of the individual neuron (Hebb, 1949). Since Hebb’s landmark theory, an extensive body of research has identified some of the key cellular and receptor mechanisms that underlie the formation of LTP. Memories are formed by plasticity at excitatory glutamatergic synapses in neural circuits, a process which depends on activation of ionotropic N-methyl-D-aspartate receptors (NMDAR). Activation of NMDAR is an essential step for memory acquisition leading to consolidation, which indicates that it is a crucial receptor for neural network activity and network plasticity. It is also well suited for coincidence detection because of its open channel block by Mg2+ that must be simultaneously removed in conjunction with its activation by the endogenous synaptic ligands, glutamate and glycine. In addition, the NMDAR plays an important role in intra-neural network activity, where propagating and feedback circuits also can undergo memory related plasticity (Nakazawa et al., 2002; Johnson et al., 2008, 2009; Prager and Johnson, 2009).
Starting with the original study by Paoletti and Ascher (1994), an increasing body of work indicates that NMDAR exhibits mechanosensitivity comparable to inherently mechanosensitive ion channels (Cox et al., 2019). Both prokaryotic and eukaryotic cells have been shown to have mechanosensitive (MS) channels, which play roles in physical signal transduction. In prokaryotes, mechanical signal transduction can be mediated through the force-from-lipids mechanism by protein-lipid interactions directly at the membrane bilayer, whereas in eukaryotes, both “force from lipids” and “force from filament” transduced by protein tethering to the cytoskeleton has been demonstrated (Khairallah et al., 2012; Martinac, 2014; Cox et al., 2019). A growing body of evidence indicates that, in addition to voltage, membrane tension and/or curvature can also remove Mg2+ channel block allowing for activation of NMDA receptor channels (Kloda et al., 2007a, b; Parnas et al., 2009; Maneshi et al., 2017). Moreover, mechanosensitive ion channels expressed in neocortical and hippocampal neurons have been shown to exert surprisingly powerful influences on neuronal activities (Nikolaev et al., 2015). This growing evidence invites further exploration of the brain as a “mechanically sensitive organ” and the role mechanical forces play in its function.
NMDAR in Memory
N-methyl-D-aspartate receptor is essential for normal memory formation. Blockade of NMDAR leads to memory impairment and pathologies which is increasingly being linked to a range of memory related neurological disorders (Newcomer et al., 2000; Morris et al., 2013). NMDAR antagonists have been shown to reduce different types of memories including hippocampal dependent spatial memories and amygdala dependent conditioned memories (Fanselow and Kim, 1994; Mchugh et al., 1996; Morris, 2013). Importantly, NMDAR antagonists impact the formation of long-term consolidated memories and do not impact the retrieval of already established memories. These effects on memory have been demonstrated in experimental in vivo models and in vitro LTP models of synaptic plasticity (Morris, 2013).
Seminal pharmacological studies linking NMDAR to memory mechanism investigated hippocampal dependent spatial memory using the water maze. Synthesis of the NMDAR specific antagonist D-2-amino-5-phosphonovalerate (APV) enabled investigations into the essential role of NMDAR in memory formation. In comparison to control animals, APV-treated animals failed to form spatial memories of platform location. Control studies confirmed that APV did not affect visual or other sensory systems and the effects were specific to the formation of memory (Morris, 2013). In addition a genetic study, carried out by Wilson and colleagues (Mchugh et al., 1996), also showed that mice whose NMDAR receptors were knocked out in pyramidal cells of the CA1 subregion of the hippocampus suffered deficits in spatial specificity, resulting in navigational learning impairments. Furthermore, a very large body of work has also investigated the role of NMDAR in associative memories, using classical (or Pavlovian) fear conditioning as series of studies investigated the role of associative memories whereby shocks are paired with a specific context or with a specific sound (Ledoux, 2000). This conditioning process leads to the formation of long-term memories that are NMDAR dependent and blocked by APV (Fanselow and Kim, 1994; Maren, 1999; Ledoux, 2000).
The NMDAR ion channel and the subunits contributing to the functional ion channel (see below for subunit details) have been localized to postsynaptic afferent sites as well as to internal excitatory circuits of the hippocampus and amygdala, by electrophysiological and electron microscopy studies. Recurrent circuits necessary for spatial memory pattern recognition in the hippocampus CA3 regions are mediated by NMDA receptors (Nakazawa et al., 2000). Likewise, circuits necessary for Pavlovian fear memory, thalamo-amygdala circuits, as well as amygdala recurrent circuits are mediated by NMDAR (Radley et al., 2007; Johnson et al., 2008, 2009). The thalamo-amygdala afferents target NMDAR containing GluN1 and GluN2B subunits of the NMDAR (Radley et al., 2007). These data identify the presence of NMDAR and especially the GluN2B subunits in circuits for established memory models. The GluN2B unit has also been shown to be essential for LTP formation and mechanosensitivity of the NMDAR (Foster et al., 2010; Singh et al., 2012).
NMDAR in Long Term Potentiation (LTP)
Memory mechanisms dependent on NMDAR have also been extensively studied using in vitro and in vivo models of synaptic plasticity. A leading model for this process is LTP of excitatory currents at potentiated synapses. LTP can be induced by high frequency (100 Hz) stimulation of afferent synapses (Bliss and Lomo, 1973), and lower frequency stimulation combined with direct postsynaptic depolarization can also induce LTP (Weisskopf and Ledoux, 1999). Both approaches suggest that LTP needs depolarization activity in the postsynaptic dendrites or spines, induced by repeated high frequency stimulation or by direct depolarization, in combination with presynaptic activity. These findings suggest a role for excitatory synapses and coincidence detection for the formation of LTP (Bliss and Collingridge, 2013).
Several seminal studies of LTP mechanisms have identified the essential role of NMDAR, influx of Ca2+ via NMDAR, and the essential role of removal of the Mg2+ block from the NMDAR pore, for LTP induction. Collingridge and colleagues identified that LTP induction is dependent upon NMDAR (Collingridge, 1987). This discovery was enabled in part by the synthesis of the NMDAR specific antagonist APV (Bliss and Collingridge, 2013). Injection of the Ca2+ chelator EGTA into the post synaptic cell prior to LTP induction blocked the LTP, suggesting an essential role for Ca2+ influx in LTP mechanisms (Lynch et al., 1983). Interestingly, the NMDAR was also shown to have a low conductance at rest and needed depolarization for increased conduction. This conduction block was mediated by Mg2+ in the channel pore [see (Bliss and Collingridge, 2013)]. These findings show that Mg2+ block of the NMDAR channel pore can also be removed by mechanical activation, indicating that mechanosensitivity, next to ligand binding and voltage dependence, is another modality of the NMDAR (Kloda et al., 2007a; Maneshi et al., 2017), which opens new lines of enquiry into LTP induction mechanisms.
The contribution and necessity of NMDAR subunits to LTP and memory has also been identified.
Long-term potentiation studies have focused on the necessity for the GluN2B subunit for LTP to be induced and maintained (Gardoni et al., 2009; Foster et al., 2010). Both pharmacological and subunit gene knockout studies have established the importance of the GluN2B subunit. The essential role of this subunit is supported by studies in memory reconsolidation of Pavlovian fear memories, where it was identified within lateral amygdala to be necessary and associated with memory reconsolidation (Nader, 2015). The importance of the GluN2B subunit to memory and its role in the mechanosensitivity of the subunit is further discussed below.
NMDAR in Memory Related Pathologies
Changes in NMDAR function have been hypothesized to underlie major neurological dysfunctions and psychopathologies. These pathologies potentially include learning and memory deficits, psychosis and excitotoxicity related to cerebral vascular accidence (CVA) and traumatic brain injury (TBI) (Newcomer et al., 2000). Pathological changes in NMDAR may be driven by receptor hypofunction. In age related declines in learning and memory, age has been shown in experimental animal models to be a factor in the decline in NMDAR number and some age-related variations in NMDAR glycine binding sites (see below) (Newcomer et al., 2000). For psychosis, antagonist induced NMDAR hypofunction, driven by subanesthetic doses of ketamine, or more potently, phencyclidine (PCP), can induce a transient psychosis like state (Newcomer et al., 2000). Recently, acute depression has been shown to be responsive to low doses of ketamine suggesting another psychopathology linked to NMDAR hypofunction (Lener et al., 2017a, b). In contrast, hyperfunction of NMDAR, which can be induced by CVA or TBI, can trigger a cascade of excitotoxicity, whereby physical force induced NMDAR activation leads to excessive Ca2+ entry triggering neuronal hyperexcitability and cell death, including a cascade of hyperexcitability from the original site of injury to other brain regions (Newcomer et al., 2000). These potential pathologies of NMDAR function highlight the essential need in understanding drivers of NMDAR function including mechanical stimuli, the lipid environment and physical sensation. Thus, all regulators of NMDAR are important for understanding memory associated pathologies including fear, phobias, PTSD, psychoses, and injury.
Structure of NMDAR
N-methyl-D-aspartate receptor is a heterotetrameric cation channel assembled as dimer of GluN1/GluN2 heterodimers (Lee and Gouaux, 2011; Karakas and Furukawa, 2014) that mediates LTP, synaptic plasticity and neuro-degeneration via conditional Ca2+ signaling (Marambaud et al., 2009). The structure of the GluN1/GluN2B NMDA receptor ion channel has been solved by X-ray crystallography at 4 Å resolution (Figure 1A; Karakas and Furukawa, 2014). The complexity of NMDA receptors arises from multiple genes encoding different subunits of the channels and alternative splicing of mRNA, which determines the variability in subunit composition, as well as functional heterogeneity (Sugihara et al., 1992; Bradley et al., 2006; Kohr, 2006; Maneshi et al., 2017). Functional channels are comprised of seven known subunits: GluN1, GluN2A-D, and GluN3A-B. The ubiquitously expressed GluN1 subunit is a product of a single gene encoding eight splice variants, whereas four GluN2 subunits (GluN2A–GluN2D) and two GluN3 (GluN3A, GluN3B) subunits are encoded by distinct genes and their products are differentially distributed throughout the central nervous system. The transmembrane topology of NMDAR proteins shows an extracellular N-terminus, followed by three transmembrane domains (TM1–TM3) and intracellular C-terminus. A cytoplasmic re-entrant loop (P) lines the channel pore, whereas an extracellular loop links the TM2 and TM3 domains. In the GluN1 subunit the cytoplasmic C-terminus is the subject of alternative splicing which differentially affects its interaction with intracellular proteins and phospholipids.
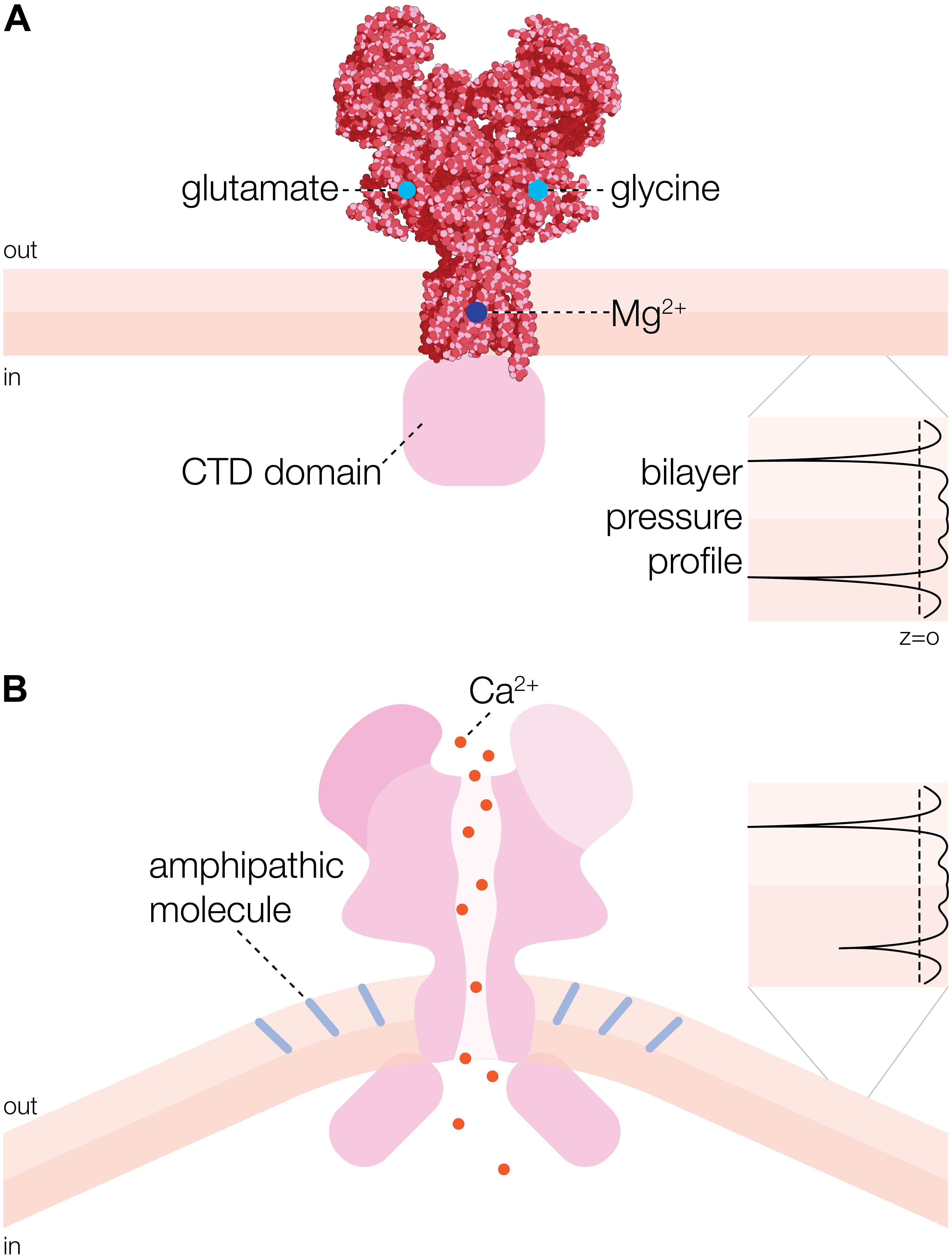
Figure 1. Overall structure and activation mechanism of N-methyl-D-aspartate receptor (NMDAR) ion channel. (A) 3D structure of heterotetrameric NMDAR channel consisting of two GluN1a and two GluN2B subunits [adapted from Karakas and Furukawa (2014)]. Glycine and glutamate binding sites are indicated on the GluN1a and GluN2B subunits, respectively. Mg2+ binding site is represented by a dark blue circle. CTD refers to cytoplasmic domain. (B) Activation of NMDAR channel by insertion of an amphipathic molecule (e.g., arachidonic acid (AA) indicated by light blue rods) into the extracellular leaflet of the membrane bilayer (Casado and Ascher, 1998). One-sided insertion of AA is curving the bilayer, which changes the lateral pressure in the bilayer (black profile inset) causing the channel to open (Bavi O. et al., 2016).
Mechanisms of NMDAR Activation #1: Ligand Binding
Channel activation requires binding of glycine and glutamate to GluN1 and GluN2 subunits, respectively (Hansen et al., 2013), as well as the voltage dependent removal of Mg2+ to allow postsynaptic Ca2+ influx. This requirement for activation contributes to their role as coincidence detectors and their central role in learning and memory. Importantly, other mechanisms of NMDAR activation and modulation have also been reported, including mechanical stimuli such as membrane tension (Kloda et al., 2007a) and shear force (Maneshi et al., 2017), which is central to this review.
Mechanisms of NMDAR Activation: #2 Voltage
Voltage-dependent properties of the NMDA receptor that result from a voltage pulse depolarizing the cell membrane combined with the removal of the Mg2+-block by depolarization of the cell membrane through the activation of α-amino-3-hydroxy-5-methyl-4-isoxazole-propionic acid (AMPA) receptors, provide a satisfying explanation for the function of this ligand-gated ion channel as a molecular coincidence detector in LTP and memory (Bliss and Collingridge, 1993). Mg2+ blocks the NMDA receptor channel from both extracellular and intracellular side with each side having a different Mg2+ un-binding rate, suggesting two distinct binding sites, which implies that the NMDA receptor acts as a bidirectional rectifier during synaptic transmission (Johnson and Ascher, 1990). It is the voltage-dependent Mg2+-block at the extracellular side of the channel (Zito and Scheuss, 2009), which controls Ca2+ permeation and requires removal of Mg2+ from a narrow constriction of the selectivity filter by membrane depolarization for LTP and memory formation. This is because high-frequency synaptic transmission in hippocampus, where LTP is mediated via NMDAR channels, leads to increased excitation due to summation of AMPA receptor-mediated excitatory post-synaptic potentials. The concomitant reduction in GABAA receptor-mediated neuronal inhibition during high-frequency synaptic transmission potentiates further the effect of NMDAR activity. Given that the NMDAR permeability to Ca2+ contributes to depolarization of the postsynaptic membrane this leads to further reduction of the Mg2+ block and thus to strengthening of LTP and memory function (Bliss and Collingridge, 1993; Bliss and Richter-Levin, 1993).
Mechanisms of NMDAR Activation #3: Mechanosensitivity
A long-standing and increasing body of work - from different laboratories worldwide - have identified mechanosensitivity properties in NMDAR. In contrast to other ionotropic glutamate receptors NMDAR have been repeatedly reported to have mechanical force regulated gating. Why NMDAR and not AMPA or Kainate receptors have reported mechanical sensing properties is not fully known, here we detail both force from lipids and force from filament mechanisms that may regulate the NMDAR mechanosensitivity. As early as 1992, NMDAR channels have been recognized for potential direct physical interactions impacting on their gating properties (Table 1). By measuring NMDAR current in cerebellar granule neurons, (Miller et al., 1992) identified that arachidonic acid (AA) potentiates these currents. Working in cultured neurons from mouse Paoletti and Ascher (1994) identified that changes in osmolarity and consequent membrane stretch modulated NMDAR currents where reduced osmolarity increased NMDAR currents (Paoletti and Ascher, 1994). Similarly, using cortical and diencephalic cultured neurons, Casado and Ascher (1998) identified that both Lysophospholipids and AA potentiated NMDAR currents (Casado and Ascher, 1998). Working in Dorsal Root Ganglion (DRG) neurons, Chaban et al. (2004) identified that physical poke increased NMDAR dependent Ca2+ signals suggesting a mechanical component to the NMDAR mediated Ca2+ influx. In 2007 Kloda, Adams, and Martinac using an artificial bilayer preparation found that both membrane stretch and AA could potentiate NMDAR currents (Kloda et al., 2007a). Further studies have identified NMDAR mechanosensitivity properties in a variety of cell types and systems. In 2008, Ma and colleagues, working in renal afferent nerve found that pharmacological modulation of NMDAR and endogenous pressure could modulate urine output. Moreover, they identified the GLuN1 subunit (zeta-1 isoform) in renal nerve (Ma et al., 2008). In 2009, Parnas and Minke, working in both photo receptors and hippocampal CAI neurons found that linoleic acid modulates NMDAR currents and that this lipid modulation could remove the Mg2+ open channel block (Parnas et al., 2009).
Recent studies have not only confirmed the mechanosensitivity of NMDAR but also identified key subunits in the receptor associated with the mechano-properties. In 2012, Meaney and colleagues, working in HEK293 cells identified that physical stretch increased Ca2+ entry measured with imaging. Moreover, this group also found that the stretch sensitivity is mediated by the GluN2B subunit and that the C-terminal sequence at Ser-1323 was critical for NMDAR mechanosensitivity (Singh et al., 2012). More recently, Maneshi et al. (2017) working in rat astrocytes found that shear stress increased Ca2+ entry and that this effect is NMDAR dependent (Maneshi et al., 2017). Collectively, all these studies provide a substantial and increasing body of work, in different systems and with force applied using different approaches, that collectively identify mechanosensitivity of NMDAR.
Other studies have identified functionally important interactions between cytoskeleton associated proteins and NMDAR that appear necessary for normal NMDAR function and gating and may also be necessary for mechanosensitivity. For example, Rosenmund and Westbrook (1993) identified that actin filaments can directly regulate NMDAR activity. Moreover, calcium and ATP activity can feedback to alter NMDAR activity by directly regulating actin polymerization. Further studies, including immunoprecipitation studies by Sheng and colleagues (Wyszynski et al., 1997) of NMDAR linkages to the postsynaptic cytoskeleton, indicate strong interactions between PSD95 and α-Actinin-2. Importantly, they identified that α-Actinin-2 binds to the cytoplasmic tail of NMDAR subunits including GluN1 and GluN2B (Wyszynski et al., 1997). This is of note as the GluN2B subunit was identified by Singh et al. (2012) as being necessary for NMDAR mechanosensitivity. However, these data suggest actin tethering may be an important mechanism in mechanosensitive properties of NMDAR. NMDAR protein tethering and force from lipids mechanism are not necessarily mutually exclusive, as cytoskeletal tension can interact with bilayer tension (Cox et al., 2016), as shown for Piezo1 channels (Cox et al., 2019). This suggests that, similar to Piezo1 channels, NMDAR mechanosensitivity is driven by a combination of force from lipids and force from filament via cytoskeletal interactions with the NMDAR cytoplasmic domain.
The central question of the degree to which NMDAR depends on force from filament via cytoskeletal interactions remains unknown as different studies have reported apparently contradictory findings. For example, a classic study by Casado and Ascher (1998) found that GluN1 and GluN2 C-terminus deletions did not affect mechanosensitivity using out-side out and cell attached patch clamp recordings. In contrast in an influential study of NMDAR mechanosensitivity, Meaney and colleagues (Singh et al., 2012) found that mechanosensitivity is governed by the GluN2B subunit. Specifically, they identified a PKC phosphorylation site, Ser-1323 on GluN2B, as a regulator of mechanosensitivity as measured by Ca2+ imaging. Explanations for these differences in findings could be dependent on the critical locus of the point mutation on the GluNR2 subunit in confirming mechanical sensitivity and regulating the extent of mechanical sensitivity.
Traumatic brain injury is linked to an increase in intracellular Ca2+ leading to excitotoxicity that can cause cell death in both neuronal and glial cells and NMDAR channels have been implicated in this process as well (Hardingham and Bading, 2010). Interestingly, it has been reported that the voltage-dependent Mg2+ block of NMDAR current was reduced in mechanically injured neurons (Zhang et al., 1996) suggesting that mechanosensitivity of NMDARs may play a role in TBI. Indeed, a recent study showed that NMDAR channels were mechanically responsive to fluid shear stress in the absence of agonists (Maneshi et al., 2017), which seems also to indicate a physiological role for NMDAR in the glymphatic clearance pathway in the brain (Jessen and Kotz, 2015; Cox et al., 2019; Martinac and Bilston, 2019). Moreover, like mechanosensitive MscS, MscL, TREK-1, TREK-2, TRAAK, Piezo1, and OSCA channels (Kloda et al., 2007a; Brohawn et al., 2014; Cox et al., 2016; Syeda et al., 2016; Murthy et al., 2018), the NMDAR channel protein could be reconstituted into liposomes and activated by stretching liposome patches (Kloda et al., 2007a), which indicates that NMDAR is inherently mechanosensitive meaning that lipid-protein interactions are sufficient for the channel activation by mechanical force.
Importance of Membrane Lipids for NMDAR Mechanosensitivity
The mammalian brain consists of about 60% fat with fatty acids constituting the major components (Chang et al., 2009). They control many processes essential for the physiology of neuronal and glial cells, including protein trafficking, recognition between cells and production of large numbers of signaling molecules trafficking within and across cells. Long-standing data has shown the potentiating effects of AA on NMDAR conductance through increases in channel open probability. This effect has been suspected to be mediated by modifications to the NMDAR lipid environment. Indeed, released from membrane phospholipids of neuronal and glial cells through the action of cellular phospholipases fatty acids such as AA, phosphatidic acid (PA) and decosahexanoic acid (DHA), play an important role in regulating and modulating activity of membrane receptors, including ion channels. Moreover, the LTP increase in synaptic transmission could be prevented by inhibition of phospholipase A2 (PLA2), since PLA2 hydrolyzes the sn-2 acyl bond of phospholipids causing release of AA (Okada and Miyamoto, 1989) and AA was shown to enhance NMDA receptor-mediated Ca2+ entry into hippocampal neurons (Casado and Ascher, 1998; Richards et al., 2003; Table 1). Given that AA is a bioactive amphipathic molecule exerting a direct effect on the membrane lipid bilayer, this result indicates that NMDA receptor channel is able to detect changes in biophysical properties of the membrane bilayer, similar to inherently mechanosensitive bacterial channels MscS and MscL (Martinac et al., 1990; Nomura et al., 2012), which comply with the force-from-lipids paradigm of MS channel gating (Figure 1B; Martinac et al., 1990; Teng et al., 2015). Importantly, insertion of fatty acids into membrane phospholipids can influence membrane fluidity, which has also been shown to affect activity of both MscL and MscS (Ridone et al., 2018) as well as Piezo1 ion channels (Ridone et al., 2019; Romero et al., 2019). Together, these results have firmly established NMDAR as an inherently mechanosensitive ion channel, whose activity in addition to glutamate/glycine, Mg2+ and voltage can be modulated directly by mechanical force. More recent data has also implicated intracellular C-terminus of the GluN2B subunit of NMDAR to be essential for its mechanosensitivity (Dela Cruz et al., 2012; Singh et al., 2012). PKC activity on this subunit also appears to be important for mechanochemistry in NMDAR (Singh et al., 2012; Bu et al., 2015).
In addition to fatty acids, lateral membrane heterogeneity in the X-Y direction in the form of the highly ordered and dynamic “raft domains”, also referred to as lipid microdomains, existing on the nanoscale alongside less organized and more fluid regions of the cell membrane (Nicolson, 2014), can modulate the function of NMDAR ion channels (Schrattenholz and Soskic, 2006). These lipid microdomains [which are enriched with cholesterol, sphingolipids and heparan sulfate proteoglycans (HSPG)] have been found to play a critical role for maintenance of synaptic transmission (Hering et al., 2003). Their effect on the function of NMDAR ion channels results most likely from their distinct lipid composition, which is directly linked to biophysical properties of the lipid bilayer (Cox et al., 2019). Consequently, by modulating NMDAR activity lipid microdomains apparently promote LTP and synaptogenesis (Washbourne et al., 2004). Given that amyloid-beta, a neurotoxic peptide characteristic of Alzheimer’s disease, binds to HSPG, it may affect the fluidity of neuronal membrane (Verdier and Penke, 2004; Verdier et al., 2004) and thus cause over-activation of NMDA receptors leading to neuronal excitotoxicity. Also, cholesterol plays an important role in NMDA-dependent Ca2+ currents in hippocampal neurons and cerebellar granule cells since its depletion was shown to have a dramatic effect on neuronal excitability (Frank et al., 2004; Korinek et al., 2015), which strikingly resembles the effect of cholesterol depletion on the activity of Piezo1 channels (Cox et al., 2019; Ridone et al., 2019). This is because the cholesterol enriched lipid domains act as “stiffened platforms” for the efficient transmission of mechanical force (Anishkin and Kung, 2013). Since cholesterol is known to affect the bilayer thickness, stiffness, and lateral pressure profile, which have been shown to have a significant effect on the gating of bacterial MscL and MscS channels (Nomura et al., 2012), it may also similarly affect the activation of NMDAR. Nevertheless, the exact mechanism of how lipid microdomains and cholesterol affect NMDAR function and influence neuronal excitability remains unclear and requires further studies of the role lipid-protein interactions play for NMDAR mechanosensitivity.
Membrane Curvature as a Possible Mechanism of NMDAR Activation by Force-From-Lipids
Curvature can be generated by asymmetric incorporation of amphipathic compounds into lipid bilayers, which depending on the structure of a membrane protein such as an ion channel, can either activate or inhibit the protein function (Cox et al., 2019). The effect of curvature generation on the activity of MS ion channels has been indicated experimentally (Martinac et al., 1990; Perozo et al., 2002; Nomura et al., 2012; Mukherjee et al., 2014; Maneshi et al., 2017) as well as computationally and theoretically (Meyer et al., 2006; Yoo and Cui, 2009; Bavi O. et al., 2016; Kheyfets et al., 2016). Importantly, only local bilayer curvatures of ≤50 nm in radius comparable to a size of an average MS channel oligomer, can generate a change of ∼5–10 mN/m in the transbilayer pressure profile along the membrane bilayer thickness (Bavi O. et al., 2016; Ridone et al., 2018) sufficient to affect the activity of MS channels (Bavi N. et al., 2017), including NMDAR (Kloda et al., 2007a). It is important to note here that the transbilayer pressure profile is an intrinsic property of the membrane lipid bilayer characterized by large stress heterogeneity across the bilayer thickness (Cantor, 1999). This stress heterogeneity is affected by a local bilayer curvature of such dimensions, which is formed in the cell membrane upon activation of phospholipase C (PLC) that cleaves PIP2 in the inner bilayer leaflet resulting in generation of IP3 and diacylglycerol (DAG). This is because upon removal of the large inositol phosphate head by PLC a small molecule of DAG is left in the inner leaflet of the bilayer that causes the bilayer to curve locally. The change in the local membrane curvature has been proposed to induce membrane stress large enough to open TRPC6 ion channels, for example (Spassova et al., 2006; Nikolaev et al., 2019). Similarly, the addition of lysophosphatidylcholine (LPC) is thought to generate a local membrane curvature sufficient to activate different MS channels, including MscL, MscS, TREK-1, TREK-2, and TRAAK (Patel and Honoré, 2001; Patel et al., 2001; Nomura et al., 2012). Curving of the lipid bilayer by LPC was demonstrated computationally by molecular dynamics simulations (Yoo and Cui, 2009). The idea that local membrane curvature can be a relevant stimulus affecting MS channel activity is supported by recent determination of the 3D structure of Piezo1 by cryo-electronmicroscopy (cryo-EM) (Guo and Mackinnon, 2017). The cryo-EM structure shows that the shape of the Piezo1 trimer is curving the membrane locally into a dome. When the local dome-shaped deformation becomes flatter upon stretching cell membrane, Piezo1 channel opens by becoming co-planar with the membrane. Together, all the present experimental, theoretical and computational evidence supports the view that curvature is a way to amplify local membrane forces (Cox et al., 2019) to the extent sufficient for activation of MS channels, including NMDAR.
What is the consequence of these findings for the mechanosensitivity of NMDA receptors? The bonus of these findings is that local curvature on the scale of tens of nanometers can be influenced by a host of proteins, lipid types and unilateral insertion of physiologically active amphipathic molecules, such as arachidonic or lysophosphatidic acid (Figure 1; Zimmerberg and Kozlov, 2006; Kloda et al., 2007b; Frisca et al., 2012; Syeda et al., 2016). Moreover, the list of amphipathic molecules produced and released in physiological settings that could modify NMDAR channel function is potentially very long. In addition to unilateral insertion of such amphipaths into the lipid bilayer NMDAR has been shown to be affected by poly-unsaturated fatty acids (PUFAs) (Calon et al., 2005; Keleshian et al., 2014; Islam et al., 2017), which have also been reported to have significant effect on the transbilayer pressure profile and MS channel activity (Ridone et al., 2018). Consequently, we propose that asymmetric insertion of amphipathic compounds into lipid bilayers made of lipids of different poly-unsaturation can be physiologically relevant for the activity of NMDA receptors. Thus, NMDAR channel activation by membrane stretch may be just an epiphenomenon resulting from the fact that membrane tension also affects the transbilayer pressure profile (Bavi N. et al., 2016), which has been postulated to be the common denominator of any mechanical stimuli acting at the membrane interface via cytoskeleton and/or extracellular matrix (Cox et al., 2019). However, it is yet to be conclusively shown how TBI, for example, may perturb the transbilayer pressure profile and thus alter the voltage-dependent Mg2+-block and trigger Ca2+ influx through NMDAR ion channels to cause neuronal excitotoxicity.
In conclusion, an accumulating body of evidence demonstrates mechanosensing properties of NMDAR; however, more work is needed to fully understand the origin and mechanism of the NMDAR mechanosensitivity and how it influences the key neuronal functions of this receptor channel. Under periods of mechanical force including, spine growth, heart rate, and interstitial cerebral-spinal fluid movement, forces can be applied to cell membrane and through combined force from lipids and force from filament to NMDAR, potentially modify memory acquisition and consolidation, thus impacting on both normal and pathological behavior.
The Function of Mechanosensitivity in NMDAR
The function of NMDAR mechanosensitivity in neurons, and ultimately its role in brain function and behavior, is an important question to explore and identify. Several functional implications for NMDAR mechanosensitivity have been proposed (Miller et al., 1992; Paoletti and Ascher, 1994; Casado and Ascher, 1998; Chaban et al., 2004; Kloda et al., 2007a, b; Ma et al., 2008; Parnas et al., 2009; Kloda and Martinac, 2012; Singh et al., 2012; Maneshi et al., 2017) and here we propose NMDAR gain control mediated by NMDAR mechanosensitivity. Previous proposed functions of NMDAR mechanosensitivity include: (i) amplification of glutamate signaling via phospholipase A2 induced AA release was proposed in the early studies of Atwell and colleagues (Miller et al., 1992); (ii) modulation of NMDAR activity in dynamic dendritic spines and growth cones (Paoletti and Ascher, 1994); (iii) NMDAR mechanosensitivity may play a role in TBI when there is clear mechanical force applied to brain (Singh et al., 2012). Additionally, proposed is the role of mechanical modulation of open channel block in NMDAR as an alternative or adjunct to voltage modulation (Kloda et al., 2007a; Parnas et al., 2009). More recently, mechanical force induced during brain development and also the force through ongoing perfusion in the glymphatic system has been proposed as an endogenous modulation of NMDAR mechanosensitivity, where periods of force may interact with the mechanosensitivity of NMDA receptors (Maneshi et al., 2017).
The micro-anatomical structure of neurons can undergo regular physical transformation. Micro- structural plasticity is proposed as a major physical representation of memory (Blumenfeld-Katzir et al., 2011). During memory formation, dendritic spines containing NMDA and AMPA receptors on dendritic spine heads, likely undergo structural modification or enlargement. In addition, structural plasticity underlying memory formation can also include formation of new spines which form synaptic contacts with passing of changing axons (Paoletti and Ascher, 1994; Lendvai et al., 2000; Komiyama et al., 2010; Ozcan, 2017). The formation of new spines is driven by the movement of philopodia, when these membranous structures can protrude from the dendrite on a timescale from seconds and minutes, whereby they may develop into NMDAR and AMPA containing dendrites contributing to the encoding of a memory and also its ongoing stabilization and updating. Furthermore, as highlighted by Paoletti and Ascher (1994), growth cones undergo considerable membrane tension during extension and retraction. NMDAR are also present in these growth cones and direct tension on the membrane in the growth cones will regulate Ca2+ entry and axon formation (Paoletti and Ascher, 1994). These plastic, and at times rapid changes to the small micro-structure of neurons, will induce regular membrane shape transformations, which are now known to have large regulatory effects on the NMDAR opening probabilities. Thus, structural plasticity of neurons will induce modification and plasticity to the behavior of NMDAR themselves, which will in turn drive additional plasticity mechanisms.
If NMDAR mechanosensitivity leads to modulation of behavior, a key candidate mechanism is modulation of memory systems in the brain. Moreover, pathology in these mechanisms may lead to altered memory functions as is observed in memory related disorders including PTSD, dementias and TBI. NMDAR mechanosensitivity may be related to memory function through several mechanisms. Early studies by Atwell and colleagues (Miller et al., 1992; Kovalchuk et al., 1994) suggested changes in lipid environment could change thresholds for LTP. Thus, one functional possibility is that mechanosensitivity may serve as a gain control mechanism, whereby increase in AA or other lipid changes exert a continuous force from lipids (Cox et al., 2019) during memory formation that alter thresholds for LTP and memory induction. Further critical studies are needed to investigate the intricate relationship between force from lipids and memory formation.
Author Contributions
LJ and BM planned and wrote the manuscript. AB prepared the figure. All authors edited the manuscript.
Funding
This work was supported by a grant APP1135076 and a Principal Research Fellowship APP1135974 to BM from the National Health and Medical Research Council (NHMRC) of Australia, and research support from the University of Tasmania School of Medicine to LJ.
Conflict of Interest
The authors declare that the research was conducted in the absence of any commercial or financial relationships that could be construed as a potential conflict of interest.
Acknowledgments
We thank Dr. Yury A. Nikolaev for designing Figure 1 as well as for his comments and proofreading the manuscript. AB would like to acknowledge the UA-DAAD Australia-Germany Joint Research Co-operation Scheme for funding.
References
Anishkin, A., and Kung, C. (2013). Stiffened lipid platforms at molecular force foci. Proc. Natl. Acad. Sci. U.S.A. 110, 4886–4892. doi: 10.1073/pnas.1302018110
Bavi, N., Bavi, O., Vossoughi, M., Naghdabadi, R., Hill, A. P., Martinac, B., et al. (2017). Nanomechanical properties of MscL alpha helices: a steered molecular dynamics study. Channels 11, 209–223. doi: 10.1080/19336950.2016.1249077
Bavi, N., Cortes, D. M., Cox, C. D., Rohde, P. R., Liu, W., Deitmer, J. W., et al. (2016). The role of MscL amphipathic N terminus indicates a blueprint for bilayer-mediated gating of mechanosensitive channels. Nat. Commun. 7:11984. doi: 10.1038/ncomms11984
Bavi, O., Cox, C. D., Vossoughi, M., Naghdabadi, R., Jamali, Y., and Martinac, B. (2016). Influence of global and local membrane curvature on mechanosensitive ion channels: a finite element approach. Membranes 6:E14. doi: 10.3390/membranes6010014
Bliss, T. V., and Collingridge, G. L. (1993). A synaptic model of memory: long-term potentiation in the hippocampus. Nature 361, 31–39. doi: 10.1038/361031a0
Bliss, T. V., and Collingridge, G. L. (2013). Expression of NMDA receptor-dependent LTP in the hippocampus: bridging the divide. Mol. Brain 6:5. doi: 10.1186/1756-6606-6-5
Bliss, T. V., and Lomo, T. (1973). Long-lasting potentiation of synaptic transmission in the dentate area of the anaesthetized rabbit following stimulation of the perforant path. J. Physiol. 232, 331–356. doi: 10.1113/jphysiol.1973.sp010273
Bliss, T. V., and Richter-Levin, G. (1993). Spatial learning and the saturation of long-term potentiation. Hippocampus 3, 123–125. doi: 10.1002/hipo.450030203
Blumenfeld-Katzir, T., Pasternak, O., Dagan, M., and Assaf, Y. (2011). Diffusion MRI of structural brain plasticity induced by a learning and memory task. PLoS One 6:e20678. doi: 10.1371/journal.pone.0020678
Bradley, J., Carter, S. R., Rao, V. R., Wang, J., and Finkbeiner, S. (2006). Splice variants of the NR1 subunit differentially induce NMDA receptor-dependent gene expression. J. Neurosci. 26, 1065–1076. doi: 10.1523/jneurosci.3347-05.2006
Brohawn, S. G., Campbell, E. B., and Mackinnon, R. (2014). Physical mechanism for gating and mechanosensitivity of the human TRAAK K+ channel. Nature 516, 126–130. doi: 10.1038/nature14013
Bu, F., Tian, H., Gong, S., Zhu, Q., Xu, G. Y., Tao, J., et al. (2015). Phosphorylation of NR2B NMDA subunits by protein kinase C in arcuate nucleus contributes to inflammatory pain in rats. Sci. Rep. 5:15945. doi: 10.1038/srep15945
Calon, F., Lim, G. P., Morihara, T., Yang, F., Ubeda, O., Salem, N., et al. (2005). Dietary n-3 polyunsaturated fatty acid depletion activates caspases and decreases NMDA receptors in the brain of a transgenic mouse model of Alzheimer’s disease. Eur. J. Neurosci. 22, 617–626. doi: 10.1111/j.1460-9568.2005.04253.x
Cantor, R. S. (1999). Lipid composition and the lateral pressure profile in bilayers. Biophys. J. 76, 2625–2639. doi: 10.1016/s0006-3495(99)77415-1
Casado, M., and Ascher, P. (1998). Opposite modulation of NMDA receptors by lysophospholipids and arachidonic acid: common features with mechanosensitivity. J. Physiol. 513(Pt 2), 317–330. doi: 10.1111/j.1469-7793.1998.317bb.x
Chaban, V. V., Li, J., Ennes, H. S., Nie, J., Mayer, E. A., and McRoberts, J. A. (2004). N-methyl-D-aspartate receptors enhance mechanical responses and voltage-dependent Ca2+ channels in rat dorsal root ganglia neurons through protein kinase C. Neuroscience 128, 347–357. doi: 10.1016/j.neuroscience.2004.06.051
Chang, C. Y., Ke, D. S., and Chen, J. Y. (2009). Essential fatty acids and human brain. Acta Neurol. Taiwan 18, 231–241.
Collingridge, G. (1987). Synaptic plasticity. The role of NMDA receptors in learning and memory. Nature 330, 604–605.
Cox, C. D., Bae, C., Ziegler, L., Hartley, S., Nikolova-Krstevski, V., Rohde, P. R., et al. (2016). Removal of the mechanoprotective influence of the cytoskeleton reveals PIEZO1 is gated by bilayer tension. Nat. Commun. 7:10366. doi: 10.1038/ncomms10366
Cox, C. D., Bavi, N., and Martinac, B. (2019). Biophysical principles of ion-channel-mediated mechanosensory transduction. Cell Rep. 29, 1–12. doi: 10.1016/j.celrep.2019.08.075
Dela Cruz, J. A., Bae, V. S., Icaza-Cukali, D., Sampson, C., Bamshad, D., Samra, A., et al. (2012). Critical role of NMDA but not opioid receptors in the acquisition of fat-conditioned flavor preferences in rats. Neurobiol. Learn. Mem. 98, 341–347. doi: 10.1016/j.nlm.2012.10.007
Fanselow, M. S., and Kim, J. J. (1994). Acquisition of contextual Pavlovian fear conditioning is blocked by application of an NMDA receptor antagonist D,L-2-amino-5-phosphonovaleric acid to the basolateral amygdala. Behav. Neurosci. 108, 210–212. doi: 10.1037/0735-7044.108.1.210
Foster, K. A., Mclaughlin, N., Edbauer, D., Phillips, M., Bolton, A., Constantine-Paton, M., et al. (2010). Distinct roles of NR2A and NR2B cytoplasmic tails in long-term potentiation. J. Neurosci. 30, 2676–2685. doi: 10.1523/JNEUROSCI.4022-09.2010
Frank, C., Giammarioli, A. M., Pepponi, R., Fiorentini, C., and Rufini, S. (2004). Cholesterol perturbing agents inhibit NMDA-dependent calcium influx in rat hippocampal primary culture. FEBS Lett. 566, 25–29. doi: 10.1016/s0014-5793(04)00428-4
Frisca, F., Sabbadini, R. A., Goldshmit, Y., and Pebay, A. (2012). Biological effects of lysophosphatidic acid in the nervous system. Int. Rev. Cell Mol. Biol. 296, 273–322. doi: 10.1016/b978-0-12-394307-1.00005-9
Gardoni, F., Mauceri, D., Malinverno, M., Polli, F., Costa, C., Tozzi, A., et al. (2009). Decreased NR2B subunit synaptic levels cause impaired long-term potentiation but not long-term depression. J. Neurosci. 29, 669–677. doi: 10.1523/JNEUROSCI.3921-08.2009
Guo, Y. R., and Mackinnon, R. (2017). Structure-based membrane dome mechanism for Piezo mechanosensitivity. eLife 6:e33660. doi: 10.7554/eLife.33660
Hansen, K. B., Tajima, N., Risgaard, R., Perszyk, R. E., Jorgensen, L., Vance, K. M., et al. (2013). Structural determinants of agonist efficacy at the glutamate binding site of N-methyl-D-aspartate receptors. Mol. Pharmacol. 84, 114–127. doi: 10.1124/mol.113.085803
Hardingham, G. E., and Bading, H. (2010). Synaptic versus extrasynaptic NMDA receptor signalling: implications for neurodegenerative disorders. Nat. Rev. Neurosci. 11, 682–696. doi: 10.1038/nrn2911
Hering, H., Lin, C. C., and Sheng, M. (2003). Lipid rafts in the maintenance of synapses, dendritic spines, and surface AMPA receptor stability. J. Neurosci. 23, 3262–3271. doi: 10.1523/jneurosci.23-08-03262.2003
Islam, R., Trepanier, M. O., Milenkovic, M., Horsfall, W., Salahpour, A., Bazinet, R. P., et al. (2017). Vulnerability to omega-3 deprivation in a mouse model of NMDA receptor hypofunction. NPJ Schizophr. 3:12. doi: 10.1038/s41537-017-0014-8
Jessen, S., and Kotz, S. A. (2015). Affect differentially modulates brain activation in uni- and multisensory body-voice perception. Neuropsychologia 66, 134–143. doi: 10.1016/j.neuropsychologia.2014.10.038
Johansen, J. P., Diaz-Mataix, L., Hamanaka, H., Ozawa, T., Ycu, E., Koivumaa, J., et al. (2014). Hebbian and neuromodulatory mechanisms interact to trigger associative memory formation. Proc. Natl. Acad. Sci. U.S.A. 111, E5584–E5592. doi: 10.1073/pnas.1421304111
Johnson, J. W., and Ascher, P. (1990). Voltage-dependent block by intracellular Mg2+ of N-methyl-D-aspartate-activated channels. Biophys. J. 57, 1085–1090. doi: 10.1016/s0006-3495(90)82626-6
Johnson, L. R., Hou, M., Ponce-Alvarez, A., Gribelyuk, L. M., Alphs, H. H., Albert, L., et al. (2008). A recurrent network in the lateral amygdala: a mechanism for coincidence detection. Front. Neural. Circuits 2:3. doi: 10.3389/neuro.04.003.2008
Johnson, L. R., Ledoux, J. E., and Doyere, V. (2009). Hebbian reverberations in emotional memory micro circuits. Front. Neurosci. 3:198–205. doi: 10.3389/neuro.01.027.2009
Karakas, E., and Furukawa, H. (2014). Crystal structure of a heterotetrameric NMDA receptor ion channel. Science 344, 992–997. doi: 10.1126/science.1251915
Keleshian, V. L., Kellom, M., Kim, H. W., Taha, A. Y., Cheon, Y., Igarashi, M., et al. (2014). Neuropathological responses to chronic NMDA in rats are worsened by dietary n-3 PUFA deprivation but are not ameliorated by fish oil supplementation. PLoS One 9:e95318. doi: 10.1371/journal.pone.0095318
Khairallah, R. J., Shi, G., Sbrana, F., Prosser, B. L., Borroto, C., Mazaitis, M. J., et al. (2012). Microtubules underlie dysfunction in duchenne muscular dystrophy. Sci. Signal. 5:ra56. doi: 10.1126/scisignal.2002829
Kheyfets, B., Galimzyanov, T., Drozdova, A., and Mukhin, S. (2016). Analytical calculation of the lipid bilayer bending modulus. Phys. Rev. E 94:042415.
Kloda, A., Lua, L., Hall, R., Adams, D. J., and Martinac, B. (2007a). Liposome reconstitution and modulation of recombinant N-methyl-D-aspartate receptor channels by membrane stretch. Proc. Natl. Acad. Sci. U.S.A. 104, 1540–1545. doi: 10.1073/pnas.0609649104
Kloda, A., Martinac, B., and Adams, D. J. (2007b). Polymodal regulation of NMDA receptor channels. Channels 1, 334–343. doi: 10.4161/chan.5044
Kloda, A., and Martinac, B. (2012). “Smart fats”, healthy brain and function of lipid-sensing NMDA receptors. Adv. Biol. Chem. 2, 106–114. doi: 10.4236/abc.2012.22013
Kohr, G. (2006). NMDA receptor function: subunit composition versus spatial distribution. Cell Tissue Res. 326, 439–446. doi: 10.1007/s00441-006-0273-6
Komiyama, T., Sato, T. R., O’connor, D. H., Zhang, Y. X., Huber, D., Hooks, B. M., et al. (2010). Learning-related fine-scale specificity imaged in motor cortex circuits of behaving mice. Nature 464, 1182–1186. doi: 10.1038/nature08897
Korinek, M., Vyklicky, V., Borovska, J., Lichnerova, K., Kaniakova, M., Krausova, B., et al. (2015). Cholesterol modulates open probability and desensitization of NMDA receptors. J. Physiol. 593, 2279–2293. doi: 10.1113/jphysiol.2014.288209
Kovalchuk, Y., Miller, B., Sarantis, M., and Attwell, D. (1994). Arachidonic acid depresses non-NMDA receptor currents. Brain Res. 643, 287–295. doi: 10.1016/0006-8993(94)90035-3
Langille, J. J., and Brown, R. E. (2018). The synaptic theory of memory: a historical survey and reconciliation of recent opposition. Front. Syst. Neurosci. 12:52. doi: 10.3389/fnsys.2018.00052
Ledoux, J. E. (2000). Emotion circuits in the brain. Annu. Rev. Neurosci. 23, 155–184. doi: 10.1146/annurev.neuro.23.1.155
Lee, C. H., and Gouaux, E. (2011). Amino terminal domains of the NMDA receptor are organized as local heterodimers. PLoS One 6:e19180. doi: 10.1371/journal.pone.0019180
Lendvai, B., Stern, E. A., Chen, B., and Svoboda, K. (2000). Experience-dependent plasticity of dendritic spines in the developing rat barrel cortex in vivo. Nature 404, 876–881. doi: 10.1038/35009107
Lener, M. S., Kadriu, B., and Zarate, C. A. Jr. (2017a). Ketamine and beyond: investigations into the potential of glutamatergic agents to treat depression. Drugs 77, 381–401. doi: 10.1007/s40265-017-0702-8
Lener, M. S., Niciu, M. J., Ballard, E. D., Park, M., Park, L. T., Nugent, A. C. Jr., et al. (2017b). Glutamate and gamma-aminobutyric acid systems in the pathophysiology of major depression and antidepressant response to ketamine. Biol. Psychiatry 81, 886–897. doi: 10.1016/j.biopsych.2016.05.005
Lynch, G., Larson, J., Kelso, S., Barrionuevo, G., and Schottler, F. (1983). Intracellular injections of EGTA block induction of hippocampal long-term potentiation. Nature 305, 719–721. doi: 10.1038/305719a0
Ma, M. C., Huang, H. S., Chen, Y. S., and Lee, S. H. (2008). Mechanosensitive N-methyl-D-aspartate receptors contribute to sensory activation in the rat renal pelvis. Hypertension 52, 938–944. doi: 10.1161/HYPERTENSIONAHA.108.114116
Maneshi, M. M., Maki, B., Gnanasambandam, R., Belin, S., Popescu, G. K., Sachs, F., et al. (2017). Mechanical stress activates NMDA receptors in the absence of agonists. Sci. Rep. 7:39610. doi: 10.1038/srep39610
Marambaud, P., Dreses-Werringloer, U., and Vingtdeux, V. (2009). Calcium signaling in neurodegeneration. Mol. Neurodegener. 4:20. doi: 10.1186/1750-1326-4-20
Maren, S. (1999). Long-term potentiation in the amygdala: a mechanism for emotional learning and memory. Trends Neurosci. 22, 561–567. doi: 10.1016/s0166-2236(99)01465-4
Martinac, A. D., and Bilston, L. (2019). Computational modelling of fluid and solute transport in the brain. Biomech. Model. Mechanobiol. doi: 10.1007/s10237-019-01253-y [Epub ahead of print].
Martinac, B. (2014). The ion channels to cytoskeleton connection as potential mechanism of mechanosensitivity. Biochim. Biophys. Acta 1838, 682–691. doi: 10.1016/j.bbamem.2013.07.015
Martinac, B., Adler, J., and Kung, C. (1990). Mechanosensitive ion channels of E. coli activated by amphipaths. Nature 348, 261–263. doi: 10.1038/348261a0
Mchugh, T. J., Blum, K. I., Tsien, J. Z., Tonegawa, S., and Wilson, M. A. (1996). Impaired hippocampal representation of space in CA1-specific NMDAR1 knockout mice. Cell 87, 1339–1349. doi: 10.1016/s0092-8674(00)81828-0
Meyer, G. R., Gullingsrud, J., Schulten, K., and Martinac, B. (2006). Molecular dynamics study of MscL interactions with a curved lipid bilayer. Biophys. J. 91, 1630–1637. doi: 10.1529/biophysj.106.080721
Miller, B., Sarantis, M., Traynelis, S. F., and Attwell, D. (1992). Potentiation of NMDA receptor currents by arachidonic acid. Nature 355, 722–725. doi: 10.1038/355722a0
Morris, R. G. (2013). NMDA receptors and memory encoding. Neuropharmacology 74, 32–40. doi: 10.1016/j.neuropharm.2013.04.014
Morris, R. G., Steele, R. J., Bell, J. E., and Martin, S. J. (2013). N-methyl-d-aspartate receptors, learning and memory: chronic intraventricular infusion of the NMDA receptor antagonist d-AP5 interacts directly with the neural mechanisms of spatial learning. Eur. J. Neurosci. 37, 700–717. doi: 10.1111/ejn.12086
Mukherjee, N., Jose, M. D., Birkner, J. P., Walko, M., Ingolfsson, H. I., Dimitrova, A., et al. (2014). The activation mode of the mechanosensitive ion channel, MscL, by lysophosphatidylcholine differs from tension-induced gating. FASEB J. 28, 4292–4302. doi: 10.1096/fj.14-251579
Murthy, S. E., Dubin, A. E., Whitwam, T., Jojoa-Cruz, S., Cahalan, S. M., Mousavi, S. A. R., et al. (2018). OSCA/TMEM63 are an evolutionarily conserved family of mechanically activated ion channels. eLife 7:e41844. doi: 10.7554/eLife.41844
Nader, K. (2015). Reconsolidation and the dynamic nature of memory. Cold Spring Harb. Perspect. Biol. 7:a021782. doi: 10.1101/cshperspect.a021782
Nakazawa, K., Granata, A. R., and Cohen, M. I. (2000). Synchronized fast rhythms in inspiratory and expiratory nerve discharges during fictive vocalization. J. Neurophysiol. 83, 1415–1425. doi: 10.1152/jn.2000.83.3.1415
Nakazawa, K., Quirk, M. C., Chitwood, R. A., Watanabe, M., Yeckel, M. F., Sun, L. D., et al. (2002). Requirement for hippocampal CA3 NMDA receptors in associative memory recall. Science 297, 211–218. doi: 10.1126/science.1071795
Newcomer, J. W., Farber, N. B., and Olney, J. W. (2000). NMDA receptor function, memory, and brain aging. Dialogues Clin. Neurosci. 2, 219–232.
Nicolson, G. L. (2014). The fluid-mosaic model of membrane structure: still relevant to understanding the structure, function and dynamics of biological membranes after more than 40 years. Biochim. Biophys. Acta 1838, 1451–1466. doi: 10.1016/j.bbamem.2013.10.019
Nikolaev, Y. A., Cox, C. D., Ridone, P., Rohde, P. R., Cordero-Morales, J. F., Vásquez, V., et al. (2019). Mammalian TRP ion channels are insensitive to membrane stretch. J. Cell Sci. 238360. doi: 10.1242/jcs.238360 [Epub ahead of print].
Nikolaev, Y. A., Dosen, P. J., Laver, D. R., Van Helden, D. F., and Hamill, O. P. (2015). Single mechanically-gated cation channel currents can trigger action potentials in neocortical and hippocampal pyramidal neurons. Brain Res. 1608, 1–13. doi: 10.1016/j.brainres.2015.02.051
Nomura, T., Cranfield, C. G., Deplazes, E., Owen, D. M., Macmillan, A., Battle, A. R., et al. (2012). Differential effects of lipids and lyso-lipids on the mechanosensitivity of the mechanosensitive channels MscL and MscS. Proc. Natl. Acad. Sci. U.S.A. 109, 8770–8775. doi: 10.1073/pnas.1200051109
Okada, Y., and Miyamoto, T. (1989). Formation of long-term potentiation in superior colliculus slices from the guinea pig. Neurosci. Lett. 96, 108–113. doi: 10.1016/0304-3940(89)90251-6
Ozcan, A. S. (2017). Filopodia: a rapid structural plasticity substrate for fast learning. Front. Synaptic Neurosci. 9:12. doi: 10.3389/fnsyn.2017.00012
Paoletti, P., and Ascher, P. (1994). Mechanosensitivity of NMDA receptors in cultured mouse central neurons. Neuron 13, 645–655. doi: 10.1016/0896-6273(94)90032-9
Parnas, M., Katz, B., Lev, S., Tzarfaty, V., Dadon, D., Gordon-Shaag, A., et al. (2009). Membrane lipid modulations remove divalent open channel block from TRP-like and NMDA channels. J. Neurosci. 29, 2371–2383. doi: 10.1523/JNEUROSCI.4280-08.2009
Patel, A. J., and Honoré, E. (2001). Properties and modulation of mammalian 2P domain K+ channels. Trends Neurosci. 24, 339–346. doi: 10.1016/s0166-2236(00)01810-5
Patel, A. J., Lazdunski, M., and Honoré, E. (2001). Lipid and mechano-gated 2P domain K(+) channels. Curr. Opin. Cell Biol. 13, 422–428. doi: 10.1016/s0955-0674(00)00231-3
Perozo, E., Kloda, A., Cortes, D. M., and Martinac, B. (2002). Physical principles underlying the transduction of bilayer deformation forces during mechanosensitive channel gating. Nat. Struct. Biol. 9, 696–703. doi: 10.1038/nsb827
Prager, E. M., and Johnson, L. R. (2009). Stress at the synapse: signal transduction mechanisms of adrenal steroids at neuronal membranes. Sci. Signal. 2:re5. doi: 10.1126/scisignal.286re5
Radley, J. J., Farb, C. R., He, Y., Janssen, W. G., Rodrigues, S. M., Johnson, L. R., et al. (2007). Distribution of NMDA and AMPA receptor subunits at thalamo-amygdaloid dendritic spines. Brain Res. 1134, 87–94. doi: 10.1016/j.brainres.2006.11.045
Richards, D. A., Bliss, T. V., and Richards, C. D. (2003). Differential modulation of NMDA-induced calcium transients by arachidonic acid and nitric oxide in cultured hippocampal neurons. Eur. J. Neurosci. 17, 2323–2328. doi: 10.1046/j.1460-9568.2003.02671.x
Ridone, P., Grage, S. L., Patkunarajah, A., Battle, A. R., Ulrich, A. S., and Martinac, B. (2018). “Force-from-lipids” gating of mechanosensitive channels modulated by PUFAs. J. Mech. Behav. Biomed. Mater 79, 158–167. doi: 10.1016/j.jmbbm.2017.12.026
Ridone, P., Pandzic, E., Vassalli, M., Cox, C. D., Macmillan, A. M., Gottlieb, P. A., et al. (2019). Cholesterol-dependent Piezol clusters are essential for efficient cellular mechanotransduction. Biophys. J. 116, 377A–377A.
Romero, L. O., Massey, A. E., Mata-Daboin, A. D., Sierra-Valdez, F. J., Chauhan, S. C., Cordero-Morales, J. F., et al. (2019). Dietary fatty acids fine-tune Piezo1 mechanical response. Nat. Commun. 10:1200. doi: 10.1038/s41467-019-09055-7
Rosenmund, C., and Westbrook, G. L. (1993). Calcium-induced actin depolymerization reduces NMDA channel activity. Neuron 10, 805–814. doi: 10.1016/0896-6273(93)90197-y
Sah, P., and Westbrook, R. F. (2008). Behavioural neuroscience: the circuit of fear. Nature 454, 589–590.
Schrattenholz, A., and Soskic, V. (2006). NMDA receptors are not alone: dynamic regulation of NMDA receptor structure and function by neuregulins and transient cholesterol-rich membrane domains leads to disease-specific nuances of glutamate-signalling. Curr. Top. Med. Chem. 6, 663–686. doi: 10.2174/156802606776894519
Singh, P., Doshi, S., Spaethling, J. M., Hockenberry, A. J., Patel, T. P., Geddes-Klein, D. M., et al. (2012). N-methyl-D-aspartate receptor mechanosensitivity is governed by C terminus of NR2B subunit. J. Biol. Chem. 287, 4348–4359. doi: 10.1074/jbc.M111.253740
Spassova, M. A., Hewavitharana, T., Xu, W., Soboloff, J., and Gill, D. L. (2006). A common mechanism underlies stretch activation and receptor activation of TRPC6 channels. Proc. Natl. Acad. Sci. U.S.A. 103, 16586–16591. doi: 10.1073/pnas.0606894103
Sugihara, H., Moriyoshi, K., Ishii, T., Masu, M., and Nakanishi, S. (1992). Structures and properties of seven isoforms of the NMDA receptor generated by alternative splicing. Biochem. Biophys. Res. Commun. 185, 826–832. doi: 10.1016/0006-291x(92)91701-q
Syeda, R., Santos, J. S., and Montal, M. (2016). The sensorless pore module of voltage-gated K+ channel family 7 embodies the target site for the anticonvulsant retigabine. J. Biol. Chem. 291, 2931–2937. doi: 10.1074/jbc.M115.683185
Teng, J., Loukin, S., Anishkin, A., and Kung, C. (2015). The force-from-lipid (FFL) principle of mechanosensitivity, at large and in elements. Pflugers. Arch. 467, 27–37. doi: 10.1007/s00424-014-1530-2
Verdier, Y., and Penke, B. (2004). Binding sites of amyloid beta-peptide in cell plasma membrane and implications for Alzheimer’s disease. Curr. Protein Pept. Sci. 5, 19–31. doi: 10.2174/1389203043486937
Verdier, Y., Zarandi, M., and Penke, B. (2004). Amyloid beta-peptide interactions with neuronal and glial cell plasma membrane: binding sites and implications for Alzheimer’s disease. J. Pept. Sci. 10, 229–248. doi: 10.1002/psc.573
Washbourne, P., Liu, X. B., Jones, E. G., and Mcallister, A. K. (2004). Cycling of NMDA receptors during trafficking in neurons before synapse formation. J. Neurosci. 24, 8253–8264. doi: 10.1523/jneurosci.2555-04.2004
Weisskopf, M. G., and Ledoux, J. E. (1999). Distinct populations of NMDA receptors at subcortical and cortical inputs to principal cells of the lateral amygdala. J. Neurophysiol. 81, 930–934. doi: 10.1152/jn.1999.81.2.930
Wyszynski, M., Lin, J., Rao, A., Nigh, E., Beggs, A. H., Craig, A. M., et al. (1997). Competitive binding of alpha-actinin and calmodulin to the NMDA receptor. Nature 385, 439–442. doi: 10.1038/385439a0
Yoo, J., and Cui, Q. (2009). Curvature generation and pressure profile modulation in membrane by lysolipids: insights from coarse-grained simulations. Biophys. J. 97, 2267–2276. doi: 10.1016/j.bpj.2009.07.051
Zhang, L., Rzigalinski, B. A., Ellis, E. F., and Satin, L. S. (1996). Reduction of voltage-dependent Mg2+ blockade of NMDA current in mechanically injured neurons. Science 274, 1921–1923. doi: 10.1126/science.274.5294.1921
Zimmerberg, J., and Kozlov, M. M. (2006). How proteins produce cellular membrane curvature. Nat. Rev. Mol. Cell Biol. 7, 9–19. doi: 10.1038/nrm1784
Keywords: memory, learning, NMDA, mechanobiology, amygdala, force, lipids
Citation: Johnson LR, Battle AR and Martinac B (2019) Remembering Mechanosensitivity of NMDA Receptors. Front. Cell. Neurosci. 13:533. doi: 10.3389/fncel.2019.00533
Received: 27 July 2019; Accepted: 18 November 2019;
Published: 05 December 2019.
Edited by:
Dirk Feldmeyer, Julich Research Centre, GermanyReviewed by:
Steven James Mennerick, Washington University in St. Louis, United StatesPierre Paoletti, Institut National de la Santé et de la Recherche Médicale (INSERM), France
Copyright © 2019 Johnson, Battle and Martinac. This is an open-access article distributed under the terms of the Creative Commons Attribution License (CC BY). The use, distribution or reproduction in other forums is permitted, provided the original author(s) and the copyright owner(s) are credited and that the original publication in this journal is cited, in accordance with accepted academic practice. No use, distribution or reproduction is permitted which does not comply with these terms.
*Correspondence: Boris Martinac, Yi5tYXJ0aW5hY0B2aWN0b3JjaGFuZy5lZHUuYXU=