- 1Aix Marseille Univ, INSERM, INS, Institut de Neurosciences des Systèmes, Marseille, France
- 2Inserm, CHU Lille, LabEx DISTALZ, UMR-S 1172 - JPArc, Université de Lille, Lille, France
Psychoactive drugs used during pregnancy can affect the development of the brain of offspring, directly triggering neurological disorders or increasing the risk for their occurrence. Caffeine is the most widely consumed psychoactive drug, including during pregnancy. In Wild type mice, early life exposure to caffeine renders offspring more susceptible to seizures. Here, we tested the long-term consequences of early life exposure to caffeine in THY-Tau22 transgenic mice, a model of Alzheimer’s disease-like Tau pathology. Caffeine exposed mutant offspring developed cognitive earlier than water treated mutants. Electrophysiological recordings of hippocampal CA1 pyramidal cells in vitro revealed that early life exposure to caffeine changed the way the glutamatergic and GABAergic drives were modified by the Tau pathology. We conclude that early-life exposure to caffeine affects the Tau phenotype and we suggest that caffeine exposure during pregnancy may constitute a risk-factor for early onset of Alzheimer’s disease-like pathology.
Introduction
Adult brain structure is primarily established in early life (Lenroot and Giedd, 2006). Disturbances in anatomical (number and connectivity of neurons) and functional (ability to engage alternative brain networks) development can interfere with crucial processes, including cell proliferation, neuronal migration (Crandall et al., 2004) and post-migration cortical connectivity (Guerrini and Dobyns, 2014). Disturbances can be genetic (e.g., a mutation) and environmental (e.g., maternal separation), resulting in increased risk to develop pathologies during early life such as cortical malformations, developmental delay, epilepsy, and/or autism (Barkovich et al., 2012; Guerrini and Dobyns, 2014), as well as during adulthood/aging, leading to dementia such as Alzheimer’s Disease (AD)-related pathology (Borenstein et al., 2006; Whalley et al., 2006; Stern, 2012; Seifan et al., 2015).
Neuropathologically, AD is defined by the extracellular accumulation of amyloid beta (Aß) peptides into amyloid plaques, and the presence of intraneuronal fibrillar aggregates of hyper- and abnormally-phosphorylated tau proteins (Masters et al., 1985; Sergeant et al., 2008). Tau pathology is observed early in the brain stem and entorhinal cortex (Braak et al., 2011) and its progression from entorhinal cortex, to the hippocampus, and finally neocortex corresponds to the progression of the symptoms in AD (Duyckaerts et al., 1997; Grober et al., 1999) supporting a pivotal role of Tau pathology in AD-related memory impairments. Various genetic and environmental risk factors are associated with dementia and/or AD (Reitz et al., 2011). Most of these environmental and lifestyle-related factors also impact AD lesions, and in particular Tau pathology. For instance, physical exercise (Belarbi et al., 2011), anesthetics (Le Freche et al., 2012; Whittington et al., 2013), or obesity/diabetes (Leboucher et al., 2013; Papon et al., 2013) modulate Tau pathology and associated memory disturbances.
Among the numerous existing environmental risk factors, those occurring during pregnancy and lactation can have important functional consequences. Exposure to psychoactive substances in utero can alter fetal brain development, leading to pathological states later in life for the offspring, including psychiatric disorders (Marroun et al., 2015; Skorput et al., 2015). Caffeine is the most frequently consumed psychoactive substance, including during pregnancy (Mandel, 2002; Greenwood et al., 2014). In mice, caffeine exposure during pregnancy and until weaning delays the migration and integration of GABA neurons, enhances seizure susceptibility, as well as alters brain rhythms and hippocampus-dependent memory function in the offspring (Silva et al., 2013; Fazeli et al., 2017). Although it is difficult to generalize rodent studies to humans, a study in mother–child pairs showed an association between caffeine exposure during pregnancy and impaired cognitive development (Galéra et al., 2015). Guidelines for pregnant women recommend to limit the amount of caffeine consumption to 200–300 mg/kg (American College of Obstetricians and Gynecologists, 2010). Whether early life exposure to caffeine may prime exposed offsprings to the development of neurodegenerative disorders later in life remains unknown. In the present study, we specifically aimed at determining whether Tau pathology related pathological traits would appear sooner in animals exposed to caffeine during brain development. To address this question, we evaluated the effects of early life caffeine exposure in offspring of the THY-Tau22 transgenic mouse model that progressively develops AD-like hippocampal Tau pathology, with ongoing deficits at 6–8 months of age and a full pathology and memory impairments occurring at 12 months of age (Van der Jeugd et al., 2013).
Materials and Methods
Animals
Male mice were group housed to reduce stress (Manouze et al., 2019), in standard mouse cages under conventional laboratory conditions (12 h/12 h dark-light cycle, constant temperature, constant humidity, and food and water ad libitum). Animal care and experimental procedures were conducted in accordance with institutional guidelines and with the European Communities Council Directive 86/609/EEC and were approved by the Aix-Marseille University Chancellor’s Animal Research Committee, Marseille (France). Since age is a highly important factor in studies related to Tauophaties as a major determinant of phenotype and disease progression, experiments were performed in 8 and 12 month-old mice.
Caffeine Treatment
This study is designed to mimic the situation in pregnant women consuming caffeine before and during their entire pregnancy until full-term birth, both in terms of time span of caffeine exposure and caffeine concentration. In the caffeine groups, female mice received caffeine via their drinking water. Caffeine treatment was started 2 weeks before mating — reflecting the situation in women who usually do not start consuming caffeine with the onset of pregnancy but did consume caffeine beforehand — and was continued up until postnatal day 15. This end point of the treatment period was chosen based on the assumption that, from a developmental point of view, term birth in humans (= end of gestational week 40) is approximately comparable to postnatal days 12/13 in rodents (Romijn et al., 1991; Clancy et al., 2001). Therefore, caffeine exposure during the entire pregnancy up until postnatal day 15 in mice would approximately reflect pregnancy until term birth in humans. Caffeine powder was dissolved in tap water at a concentration of 0.3 g/l and given as drinking water to the dam. This concentration was chosen based on previous findings that exposure to 0.3 g/l caffeine via drinking water results in a plasma caffeine concentration in rat dams that is similar to that found in the blood of humans drinking three to four cups of coffee per day (Adén et al., 2000) and in a plasma concentration of caffeine in rat pups at P7 similar to that found in the umbilical cord of human neonates whose mothers consume moderate amounts of coffee (up to three cups per day) (Björklund et al., 2008). In a previous study, we confirmed that this caffeine concentration in mice results in a serum concentration comparable to the one previously reported in rats (Silva et al., 2013). Also, we showed that this treatment regimen only affects maternal water intake on the first day of treatment, but not in the following time span (Silva et al., 2013), suggesting that 2 weeks of treatment prior to mating should be enough to reach stable caffeine concentrations in the dam at the onset of pregnancy.
From postnatal day 15 on, dams and offspring received pure drinking water. The water groups was never exposed to caffeine and received pure drinking water at all times.
Electrophysiology in Hippocampal Slices
The barrage of synaptic inputs received by neurons can characterize the functional state of neuronal networks. To quantify the excitatory and inhibitory inputs received by CA1 hippocampal pyramidal cells, we measured the frequency and amplitude of spontaneous and miniature excitatory and inhibitory post-synaptic currents in acute slices from wild type, wild type caffeine, Tau water, and Tau caffeine mice.
Number of animals per group: n = 8 cells, 8 slices, from 5 Wild type water mice vs. n = 8 cells, 8 slices, from 5 Wild type caffeine-exposed mice, vs. n = 9 cells, 9 slices, from 5 Tau water mice vs. n = 9 cells, 9 slices, from 5 Tau caffeine-exposed mice (8 months) and n = 8 cells, 8 slices, from 5 Wild type water mice vs. n = 8 cells, 8 slices, from 5 Wild type caffeine-exposed mice, vs. n = 7 cells, 7 slices, from 4 Tau water mice vs. n = 9 cells, 9 slices, from 5 Tau caffeine-exposed mice (12 months). Transverse cortical slices (350 μm) were prepared with a vibroslicer Leica VT 1200S in a cold (lower than 4°C) cutting solution containing 140.0 mM potassium gluconate, 10.0 mM HEPES, 15.0 mM sodium gluconate, 0.2 mM EGTA, 4.0 mM NaCl, pH 7.2. After 20 min recovery in a preincubation solution (110 mM Choline chloride, 2.5 mM KCl, 1.25 mM NaH2PO4, 10 mM MgCl2, 0.5 mM CaCl2, 25 mM NaHCO3, 10 mM D-glucose, 5 mM sodium pyruvate equilibrated with 5% CO2 in 95% O2 at room temperature), slices were perfused for at least 1 h with aCSF containing 126.0 mM NaCl, 25.0 mM NaHCO3, 10.0 mM D-glucose, 3.5 mM KCl, 2.0 mM CaCl2, 1.3 mM MgCl2.6H2O, and 1.2 mM NaH2PO4 equilibrated with 5% CO2 in 95% O2 at room temperature and transferred to a chamber containing the same aCSF, kept at a temperature between 33 and 35°C. Cells were recorded under visual control (Nikon FN1 microscope – Scientifica Patch Star manipulators) with an Multiclamp 700B amplifier and Digidata 1322 interface (Axon Instruments). Healthy-looking (based on infrared images) cells were selected. Although we do not know how cells containing neurofibrillary tangles would appear visually under the microscope, there is a possibility that the sampled cells may not be pathological, i.e., containing neurofibrillary tangles. PSCs were sampled at 10 kHz and low-pass filtered at 2 kHz. Currents were recorded using an internal pipette solution of 120.0 mM CsGluconate, 20.0 mM CsCl, 1.1 mM EGTA, 0.1 mM CaCl2.2H2O, 10.0 mM HEPES, 2.0 mM Mg-ATP, 0.4 mM Na-GTP, 2 mM MgCl2.6H2O, CsOH.H2O to adjust pH (pH 7.3, 280 mOsM). Inhibitory Post-Synaptic Currents (IPSCs) were recorded at a holding potential of +10 mV, the reversal potential for glutamatergic events; Excitatory PSCs (EPSCs) were recorded at −60 mV, the reversal potential for GABAergic events (Cossart et al., 2001). We also measured miniature excitatory post-synaptic currents (mEPSCs) and miniature inhibitory post-synaptic currents (mIPSCs) in acute slices from the four groups at 8 and 12 months, after the addition of TTX (1 μM). Average resistance series (RS) in neurons from 8 months wild type, wild type caffeine, Tau water and Tau caffeine mice were 35.3 ± 3.6, 34.91 ± 2.6, 23.58 ± 2.8, and 20.70 ± 3.1 MΩ, respectively (P < 0.05 Wild type vs. Tau water and Tau caffeine, P < 0.01 Wild type caffeine vs. Tau caffeine); in neurons from 12 months Wild type, Wild type caffeine, Tau water and Tau caffeine mice were 31.0 ± 2.9, 22.88 ± 3.0, 27.32 ± 3.6, and 24.34 ± 3.0 MΩ, respectively (P > 0.05). Although RS is in the range of that found in old control animals, we do not know why it is lower in Tau mice. A lower RS should improve the detection of small amplitude currents. Hence, the increase in frequency we find in Tau mice may be partly due to improved detection of synaptic events. We speculate that this would involve a minority of events, whilst the changes in frequency we describe are large. When the RS changed during the recording by more than 20%, the recording was terminated. Following break-in, the cells were allowed to dialyze and stabilize for 5 min. Cells were voltage clamped at −60 mV and recorded at that potential during 5 min. Then, the cells were voltage clamped at +10 mV, and after stabilization of the holding current, the cells were recorded during 10 min. The ACSF solution was then switched to one containing 1 μM of TTX. After 20 min of wash in recordings resumed first at +10 mV and then at −60 mV (5 min each). Recordings with a stable baseline were transferred into the Mini-Analysis software (Synaptosoft). The settings of the Mini-Analysis Program for the detection of synaptic events were set to conservative values: threshold amplitude (8 pA for s/mEPSCs, 10 pA for s/mIPSCs); area threshold (>10 pA.ms); rise time (<10 ms); and average baseline before onset (> 10 ms). These settings led to the detection of numerous false positives, including electrical artifacts, double detection of the same event, and multiple synaptic events occurring without a return to baseline. In the latter case, the events were kept for the inter-event interval distribution, but they were not used for amplitude analysis. All detected events were checked manually and false positives removed. All inter-interval and amplitude values in all conditions can be found in Table 1. All recorded cells were filled with biocytin for post hoc morphological identification, which was performed according to a previously described protocol (Esclapez et al., 1999). We did not notice obvious morphological differences between the different conditions. The two-sample Kolmogorov–Smirnov test was used for statistical analyses between groups. The level of significance was set at P < 0.05.
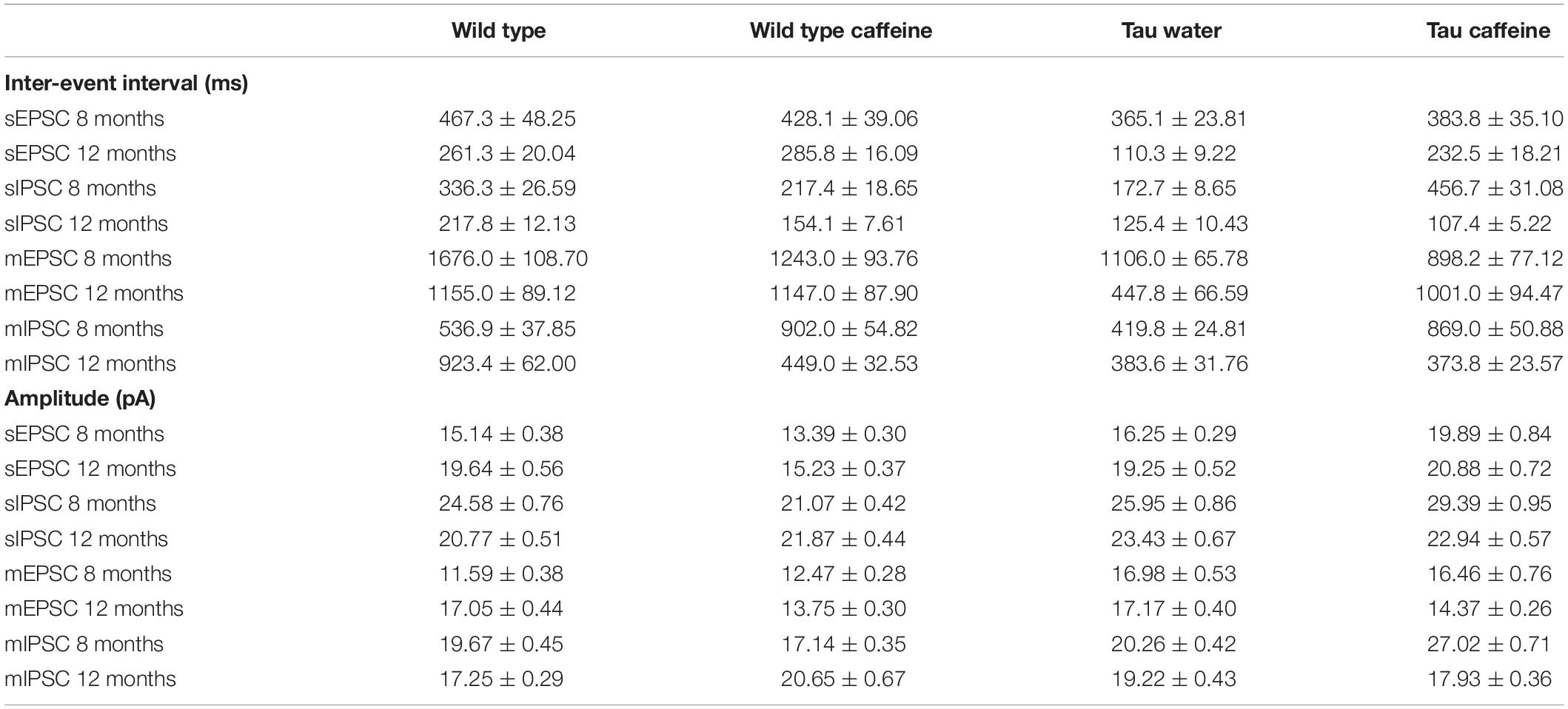
Table 1. Characteristics (inter-event intervals and amplitudes) of spontaneous and miniature glutamatergic and GABAergic currents recorded in vitro in CA1 pyramidal cells in 8 and 12 month-old Wild type and Tau mice exposed or not to caffeine during early life.
Barnes Maze
Barnes maze was used to compare cognitive deficits in learning and memory of 8- and 12-months Tau caffeine (n = 13 and 10), Tau water (n = 11 and 4) mice to the Wild type caffeine (n = 13 and 10) and Wild type (n = 11 and 8) groups. The maze was made from a circular, 13-mm thick, white PVC slab with a diameter of 1.2 m. Twenty holes with a diameter of 44.4 mm were made on the perimeter at a distance of 25.4 mm from the edge. This circular platform was then mounted on top of a rotating stool, 0.89 m above the ground and balanced. The escape cage was made by using a mouse cage and assembling a platform and ramp 25.4 mm below the surface of the maze. The maze was rotated clockwise after every three mice to avoid intra-maze odor or visual cues. All sessions were recorded using COP Security Monochrome CCD Camera (Model 15-CC20) and MyTV/x software (Eskape Labs).
The animals interacted with the Barnes maze in three phases: habituation (1 day), training (3 days), probes (2 days: 1 day after 1 week from the last trial day = Probe 1; 1 day after 2 weeks from the last trial day = Probe 2). Before starting each experiment, mice were acclimated to the testing room for 1 h. Then all mice (n = 2–4) from one cage were placed in individual holding cages where they remained until the end of their testing sessions. On the habituation day, the mice were placed in the center of the maze underneath a clear 3,500-ml glass beaker for 30 s while white noise was played through a sound system. Then, the mice were guided slowly by moving the glass beaker, over 10–15 s to the target hole that leads to the escape cage. The mice were then given 3 min to independently enter through the target hole into the escape cage. If they did not enter on their own during that time, they were nudged with the beaker to enter. The mice were allowed to stay in the escape cage for 1 min before being returned to the holding cage. Once all animals had completed the 1-session habituation, they were all returned to their home cage. In the training phase, mice were placed inside an opaque cardboard cylinder, 25.4 cm tall and 17.8 cm in diameter, in the center of the Barnes maze for 15 s. At the end of the holding period, a buzzer was turned on, the cylinder was removed, and the mice were allowed to explore the maze for 2 min. If a mouse found the target hole and entered the escape cage during that time, the end-point of the trial, it was allowed to stay in the escape cage for 1 min before being returned to the holding cage. If it did not find the target hole, the mouse was guided to the escape hole using the glass beaker. If a mouse still did not enter the escape cage after 1 min of nudging, it was picked up and manually put on the platform in the escape cage. This process typically took 5–7 min per mouse and was done with four mice at a time, providing a 20–30 min inter-trial interval. The total number of trials used was three trials on training days 1, 2, and 3. During the training phase, measures of primary latency were recorded. Primary latency was defined as the time to identify the target hole the first time, as mice did not always enter the hole upon first identifying it. On the probe day, 7 and 14 days after the last training day, the escape cage was removed. Each mouse was given 2 min to explore the maze. During the probe phase, measures of time spent per quadrant and holes searched (HS) per quadrant were recorded. HS was defined as nose pokes and head deflections over any hole. Primary HS was defined as the HS before identifying the target hole for the first time. For these analyses, the maze was divided into quadrants consisting of 5 holes with the target hole (goal) in the center of the target quadrant (goal zone). The other quadrants going clockwise from the goal zone were labeled: right, opposite, and left zone; all the data collected out of the goal zone are expressed as “other zone.” Data are shown as means ± standard error of the mean (SEM). Two-way repeated measures ANOVA followed by Bonferroni post hoc analysis were used for probe day and training trials data, respectively. The level of significance was set at P < 0.01.
Biochemical and Molecular Evaluations
Animals were sacrificed at 6 months of age by cervical dislocation, brains harvested, left and right hippocampi dissected out using a coronal acrylic slicer (Delta Microscopies) at 4°C and stored at −80°C for biochemical and mRNA analyses. For Tau biochemistry, tissue was homogenized in 200 μl Tris buffer (pH 7.4) containing 10% sucrose and protease inhibitors (Complete; Roche Diagnostics), sonicated and kept at −80°C until use. Protein amounts were quantified using the BCA assay (Pierce), and samples diluted with lithium dodecyl sulfate buffer (2) supplemented with reducing agents (Invitrogen) and then separated on 4–12% NuPAGE Novex gels (Invitrogen). Proteins were transferred to nitrocellulose membranes, which were then saturated with 5% non-fat dried milk or 5% bovine serum albumin in TNT (Tris 15 mM pH 8, NaCl 140 mM, 0.05% Tween) and incubated at 4°C for 24 h with the primary antibodies. All primary antibodies used are described in Table 2. Appropriate HRP-conjugated secondary antibodies (anti-mouse PI-2000 et anti-rabbit PI-1000, Vector Laboratories) were incubated for 1 h at room temperature and signals were visualized using chemoluminescence kits (ECL, Amersham Bioscience) and a LAS4000 imaging system (Fujifilm). Results were normalized to actin or GAPDH and quantifications were performed using ImageJ software (Scion Software).
Neuroinflammatory markers were studied using quantitative PCR. Total RNA was extracted from hippocampi and purified using the RNeasyLipid Tissue Mini Kit (Qiagen). One microgram of total RNA was reverse-transcribed using the HighCapacity cDNA reverse transcription kit (Applied Biosystems). Quantitative real-time polymerase chain reaction (qPCR) analysis was performed on an Applied BiosystemsTM StepOnePlusTM Real-Time PCR Systems using Power SYBRGreen PCR Master Mix (Applied Biosystems) or TaqManTM Gene Expression Master Mix (Applied BiosystemsTM). The thermal cycler conditions were as follows: 95°C for 10 min, then 40 cycles at 95°C for 15 s and 60°C for 25 s for SYBRGreen; and 95°C for 10 min, then 40 cycles at 95°C for 15 s and 60°C for 1 min for Taqman. Sequences of primers used in this study are given in Table 3. Cyclophilin A was used as a reference housekeeping gene for normalization. Amplifications were carried out in duplicate and the relative expression of target genes was determined by the ΔΔCt method.
Results
Increased Synaptic Drive in Tau Water as Compared to Wild Type Control Mice at 8 Months
The excitatory and inhibitory synaptic drives received by CA1 pyramidal cells have not been characterized in Tau transgenic mice. We first characterized them in comparison with littermate wild type mice. We found that the frequency of sEPSCs and sIPSCs was increased by 34% (P = 0.0285) and 98% (P = 0.0135), respectively (Figures 1A,E), in Tau water (n = 9 cells, 9 slices, from 5 mice) as compared to wild type controls (n = 8 cells, 8 slices, from 5 mice). The distribution of sEPSC amplitudes was not different but sIPSCs showed a slight (5%) but significant (P = 0.0464) increase in amplitude in Tau mice as compared to wild type controls (Figures 1B,F). All inter-interval and amplitude values in all conditions are listed in Table 1. Since spontaneous currents are a mixture of action potential-dependent and independent (miniature) events, we measured miniature currents in the same cells in the presence of TTX (1 μM) to block action potentials. We found that the frequency of miniature EPSCs (mEPSCs) and IPSCs (mIPSCs) was increased by 68% (P = 0.0270) and 44% (P = 0.0263), respectively (Figures 2A,E), in Tau water (n = 9 cells, 9 slices, from 5 mice) as compared to wild type controls (n = 8 cells, 8 slices, from 5 mice). Although the amplitude of mIPSCs was not modified in Tau mice, the amplitude of mEPSCs was increased by 47% (P = 0.0253) as compared to Wild type controls (Figures 2B,F). The overall synaptic drive received by a neuron is a combination of action potential dependent and independent EPSCs and IPSCs. In order to assess their respective contributions, we calculated the frequency ratio for miniature/spontaneous and EPSC/IPSC events in each recorded cell. In Wild type controls the contribution of miniature events was around 20% and the contribution of EPSCs vs. IPSCs was also around 20%, ratios that were not statistically different in Tau water (Figures 3A–D). At 8 months, the data suggests that there is a general increase in the overall synaptic drive received by CA1 pyramidal cells in Tau mice, whilst both miniature/spontaneous and EPSC/IPSC ratios are not modified (Figures 3E–H).
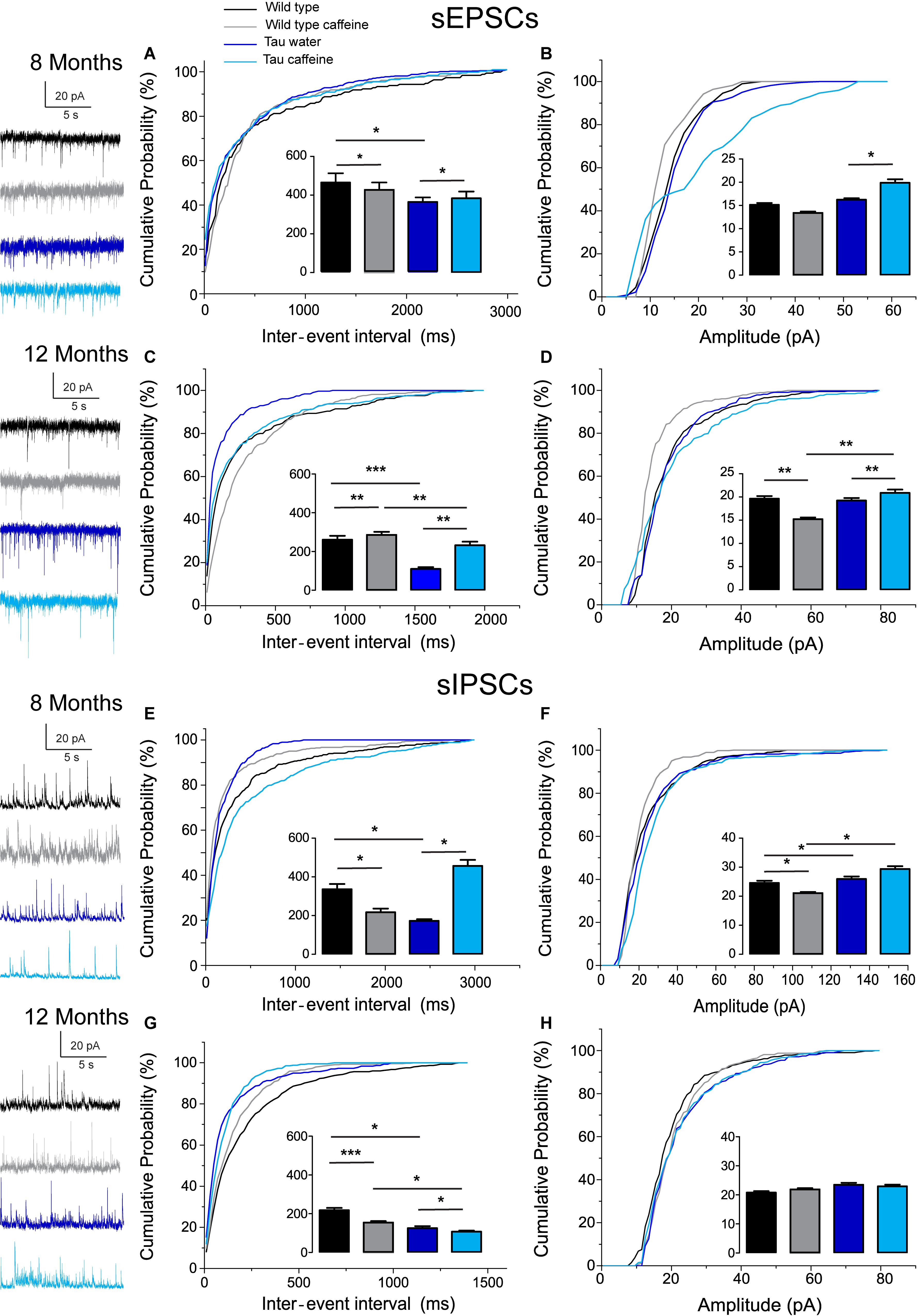
Figure 1. Investigation of chronic caffeine exposure on spontaneous glutamatergic and GABAergic in vitro activities in 8 and 12 month-old Wild type and Tau mice. Representative traces of sEPSCs and sIPSCs recorded in CA1 hippocampal pyramidal cells of Wild type water (black), Wild type caffeine-treated (gray), Tau water (blue), and Tau caffeine-treated (light blue) mice are depicted on the left of the cumulative probability histograms [8 month-old sEPSCs: (A,B); 12 month-old sEPSCs: (C,D); 8 month-old sIPSCs: (E,F); 12 month-old sIPSCs: (G,H)]. Comparison between cumulative probability distributions was made using the Kolmogorov–Smirnov test (∗P < 0.05; ∗∗P < 0.01; ∗∗∗P < 0.001); bar graphs represent mean ± standard error. n = 8 cells, 8 slices, from 5, 8 month-old Wild type water mice vs. n = 8 cells, 8 slices, from 5, 8 month-old Wild type caffeine-treated mice; n = 8 cells, 8 slices, from 5, 8 month-old Wild type water mice vs. n = 9 cells, 9 slices, from 5, 8 month-old Tau water mice; n = 9 cells, 9 slices, from 5, 8 month-old Tau water mice vs. n = 9 cells, 9 slices, from 5, 8 month-old Tau caffeine-treated mice; n = 8 cells, 8 slices, from 5, 8 month-old Wild type caffeine-treated mice vs. n = 9 cells, 9 slices, from 5, 8 month-old Tau caffeine-treated mice; n = 8 cells, 8 slices, from 5, 12 month-old Wild type water mice vs. n = 8 cells, 8 slices, from 5, 12 month-old Wild type caffeine-treated mice; n = 8 cells, 8 slices, from 5, 12 month-old Wild type water mice vs. n = 7 cells, 7 slices, from 4, 12 month-old Tau water mice; n = 7 cells, 7 slices, from 4, 12 month-old Tau water mice vs. n = 9 cells, 9 slices, from 5, 12 month-old Tau caffeine-treated mice; n = 8 cells, 8 slices, from 5, 12 month-old Wild type caffeine-treated mice vs. n = 9 cells, 9 slices, from 5, 12 month-old Tau caffeine-treated mice.
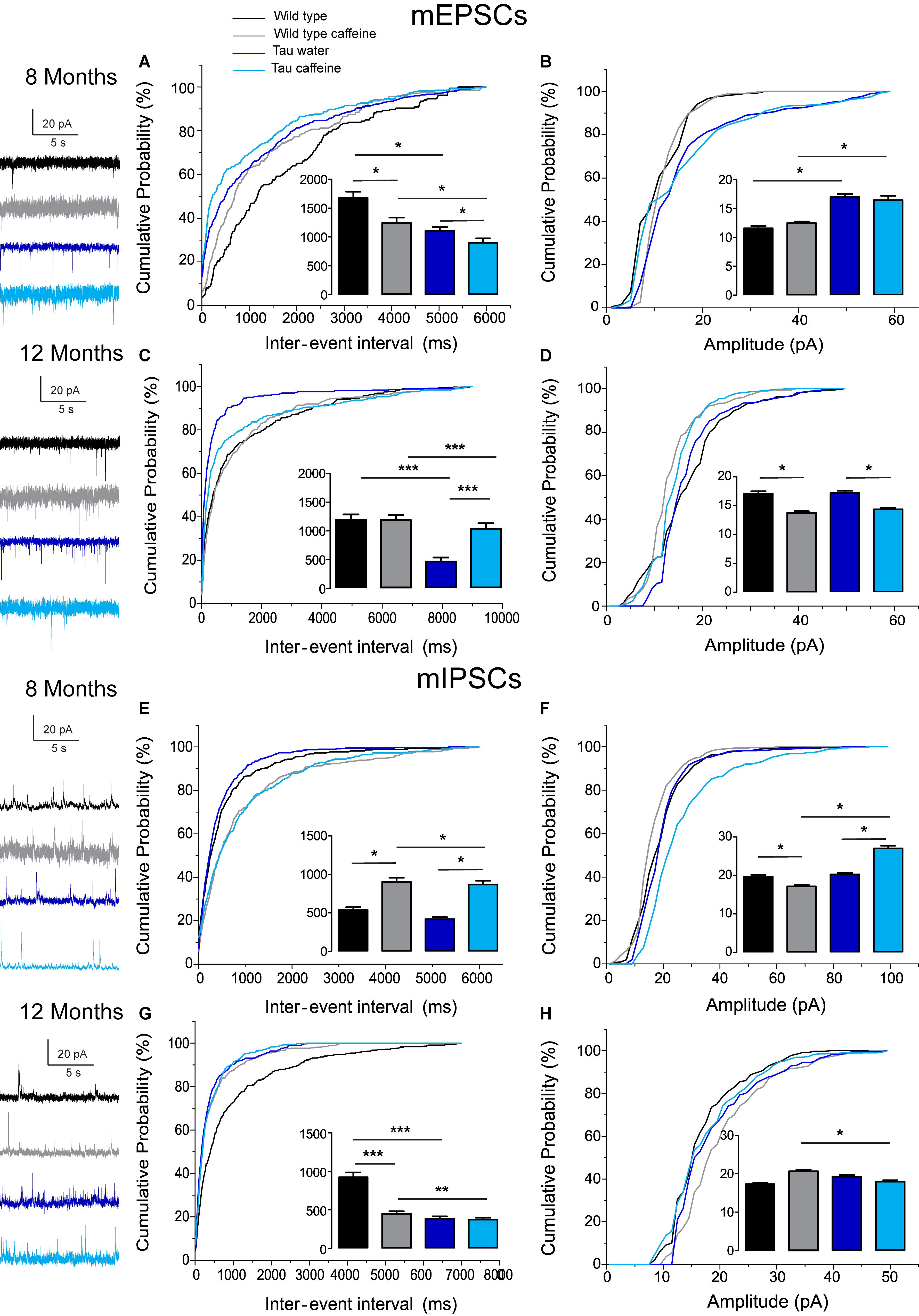
Figure 2. Investigation of chronic caffeine exposure on miniature glutamatergic and GABAergic in vitro activities in 8 and 12 month-old Wild type and Tau mice. Representative traces of mEPSCs and mIPSCs recorded in CA1 hippocampal pyramidal cells of Wild type water (black), Wild type caffeine-treated (gray), Tau water (blue), and Tau caffeine-treated (light blue) mice are depicted on the left of the cumulative probability histograms [8 month-old mEPSCs: (A,B); 12 month-old mEPSCs: (C,D); 8 month-old mIPSCs: (E,F); 12 month-old mIPSCs: (G,H)]. Comparison between cumulative probability distributions was made using the Kolmogorov–Smirnov test (∗P < 0.05; ∗∗P < 0.01; ∗∗∗P < 0.001); bar graphs represent mean ± standard error. n = 8 cells, 8 slices, from 5, 8 month-old Wild type water mice vs. n = 8 cells, 8 slices, from 5, 8 month-old Wild type caffeine-treated mice; n = 8 cells, 8 slices, from 5, 8 month-old Wild type water mice vs. n = 9 cells, 9 slices, from 5, 8 month-old Tau water mice; n = 9 cells, 9 slices, from 5, 8 month-old Tau water mice vs. n = 9 cells, 9 slices, from 5, 8 month-old Tau caffeine-treated mice; n = 8 cells, 8 slices, from 5, 8 month-old Wild type caffeine-treated mice vs. n = 9 cells, 9 slices, from 5, 8 month-old Tau caffeine-treated mice; n = 8 cells, 8 slices, from 5, 12 month-old Wild type water mice vs. n = 8 cells, 8 slices, from 5, 12 month-old Wild type caffeine-treated mice; n = 8 cells, 8 slices, from 5, 12 month-old Wild type water mice vs. n = 7 cells, 7 slices, from 4, 12 month-old Tau water mice; n = 7 cells, 7 slices, from 4, 12 month-old Tau water mice vs. n = 9 cells, 9 slices, from 5, 12 month-old Tau caffeine-treated mice; n = 8 cells, 8 slices, from 5, 12 month-old Wild type caffeine-treated mice vs. n = 9 cells, 9 slices, from 5, 12 month-old Tau caffeine-treated mice.
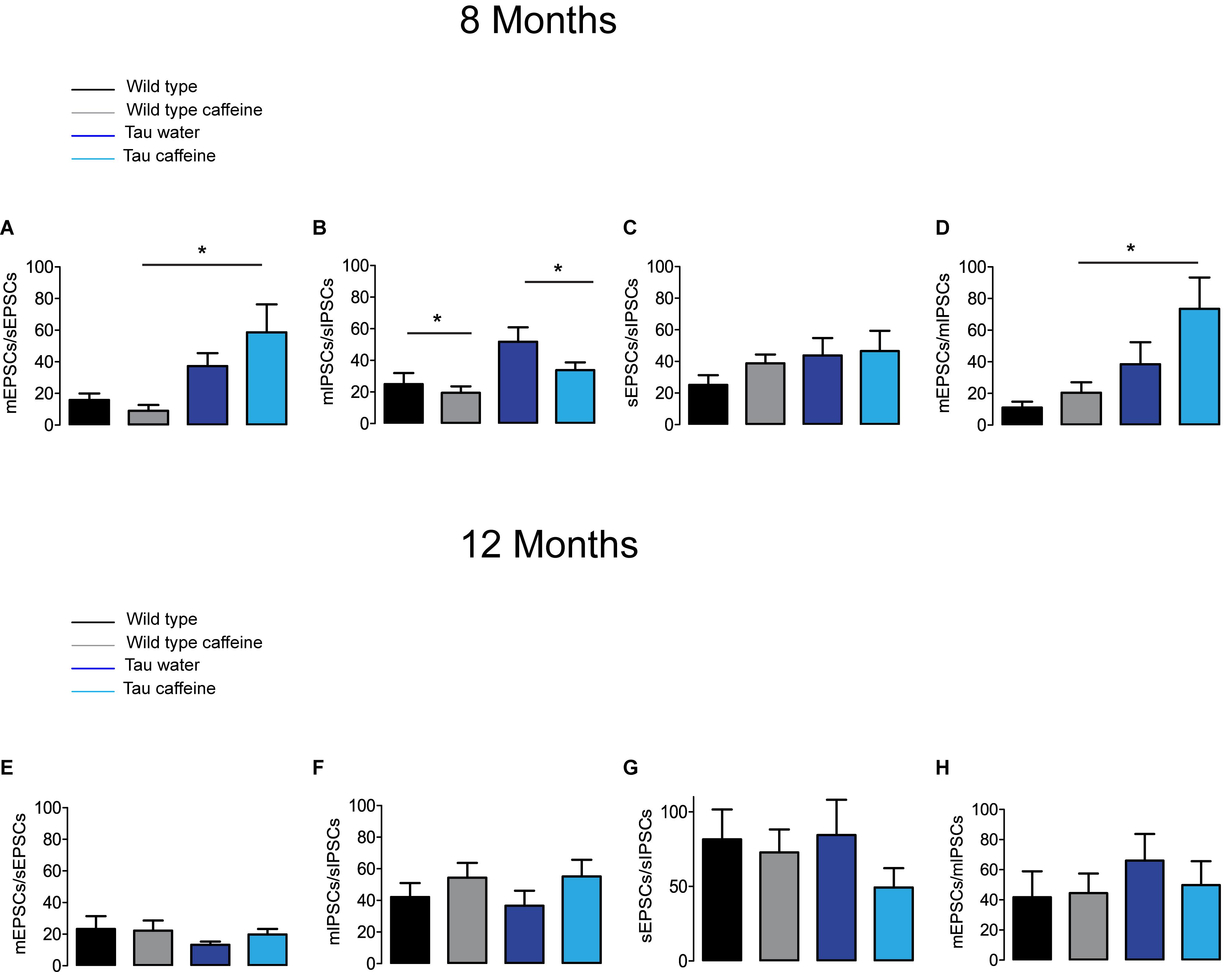
Figure 3. Investigation of chronic caffeine exposure on miniature/spontaneous EPSC/IPSC ratios in 8 and 12 month-old mice. The ratios of the frequencies of mEPSCs to sEPSCs (A,E), mIPSCs to sIPSCs (B,F), sEPSCs to sIPSCs (C,G) and mEPSCs to mIPSCs (D,H) were analyzed for Wild type water (black), Wild type caffeine-treated (gray), Tau water (blue), and Tau caffeine-treated (light blue) 8 and 12 month-old mice. Bar graphs represent mean ± standard error; comparison between ratios was made using One way ANOVA Tukey–Kramer post doc test (∗P < 0.05) n = 8 cells, 8 slices, from 5, 8 month-old Wild type water mice vs. n = 8 cells, 8 slices, from 5, 8 month-old Wild type caffeine-treated mice; n = 9 cells, 9 slices, from 5, 8 month-old Tau water mice vs. n = 9 cells, 9 slices, from 5, 8 month-old Tau caffeine-treated mice; n = 8 cells, 8 slices, from 5, 8 month-old Wild type caffeine-treated mice vs. n = 9 cells, 9 slices, from 5, 8 month-old Tau caffeine-treated mice; n = 8 cells, 8 slices, from 5, 12 month-old Wild type water mice vs. n = 8 cells, 8 slices, from 5, 12 month-old Wild type caffeine-treated mice; n = 9 cells, 9 slices, from 5, 12 month-old Tau water mice vs. n = 9 cells, 9 slices, from 5, 12 month-old Tau caffeine-treated mice; n = 8 cells, 8 slices, from 5, 12 month-old Wild type caffeine-treated mice vs. n = 9 cells, 9 slices, from 5, 12 month-old Tau caffeine-treated mice.
Effects of Early Life Caffeine Exposure in Wild Type Mice Evaluated at 8 Months
We found that the frequency of sEPSCs and sIPSCs was increased by 17% (P = 0.0385) and 54% (P = 0.0144), respectively (Figures 1A,E), in Wild type caffeine (n = 8 cells, 8 slices, from 5 mice) as compared to wild type controls (n = 8 cells, 8 slices, from 5 mice). Caffeine exposure did not affect the amplitude of sEPSCs but decreased the amplitude of sIPSCs by 14% (P = 0.0262) (Figures 1B,F). The frequency of mEPSCs was increased by 42% (P = 0.0157) whilst the frequency of mIPSCs was decreased by 41% (P = 0.0169) as compared to Wild type (Figures 2A,E). As for sEPSCs and sIPSCs, caffeine did not affect the amplitude of mEPSCs, but decreased mIPSC amplitude by 12.8% (P = 0.0216) as compared to Wild type (Figures 2B,F). Caffeine treatment did not affect the miniature/spontaneous and EPSC/IPSC ratios (Figures 3A–D). Thus, as reported in 3 months-old offspring of caffeine-exposed mice, there is an hyperactivity of GABAergic networks (Silva et al., 2013). However, we did not find hypoactivity of glutamatergic networks found in 3 months-old offspring (Silva et al., 2013), perhaps reflecting the time-dependent reorganization of hippocampal networks.
Effects of Early Life Caffeine Exposure in Tau Mice Evaluated at 8 Months
Tau mice exposed to caffeine during gestation and lactation (n = 9 cells, 9 slices, from 5 mice) showed a small decrease in sEPSC frequency by 10% (P = 0,0244) and a large decrease in sIPSC frequency by 62% (P = 0.0165) as compared to Tau water mice (Figures 1A,E). Therefore, although caffeine exposure resulted in increase of sIPSC frequency in wild type mice, it produced a decrease in Tau mice. Although caffeine exposure results in a decreased amplitude of sIPSCs in Wild type mice, the amplitude was not changed in Tau mice (Figure 1F). In contrast, the amplitude of sEPSCs was increased by 23% (P = 0.0408) in Tau caffeine mice as compared to Tau water mice (Figure 1B). As compared to the Tau water group, Tau mice exposed to caffeine during gestation and lactation showed a 38% (P = 0.0135) increase in mEPSC frequency and a decrease in mIPSCs frequency by 52% (P = 0.0256) (Figures 2A,E). In Tau caffeine, the amplitude of mEPSCs was not modified but the amplitude of mIPSCs was increased by 14% (P = 0.0285) as compared to Tau water mice (Figures 2B,F). Caffeine treatment did not affect the miniature/spontaneous and EPSC/IPSC ratios (Figures 3A–D).
Effects of Early Life Caffeine Exposure in Wild Type Mice Evaluated at 12 Months
Although early life exposure to caffeine resulted in a slight increase of sEPSC frequency at 8 months, it produced a decrease of sEPSC frequency by 19% (P = 0.0025) as compared to Wild type on water at 12 months (Figure 1C). The frequency of sIPSCs was increased by 59% (P = 1.1151e–8) in Wild type caffeine mice, similarly to what we observed at 8 months. Caffeine treatment did not affect the amplitudes of sIPSCs but produced a decrease of sEPSC amplitude by 22% (P = 0.0026) (Figures 1D,H). Caffeine did not affect mEPSC frequency but increased mIPSC frequency by 106% (P = 8.2161e–6) as compared to Wild type on water (Figures 2C,G), in contrast to that observed at 8 months. Caffeine exposure resulted in a decrease of mEPSC amplitude by 19% (P = 0.0391) but did not affect that of mIPSCs (Figures 2D,H). Therefore the most striking effect of early life exposure to caffeine in Wild type animals is the age-dependent large increase in GABAergic activity received by CA1 pyramidal cells.
Effects of Early Life Caffeine Exposure in Tau Mice Evaluated at 12 Months
In Tau caffeine mice (n = 9 cells, 9 slices, from 5 mice) we found a large decrease in sEPSC frequency by 53% (P = 0.0013) as compared to Tau on water mice (Figure 1C), in keeping with the effect of caffeine on sEPSC frequency described above in wild type mice exposed to caffeine. Although there was an increase in sEPSC at 8 and 12 months in Tau water mice, caffeine treatment prevented such increase. Although caffeine treatment results in a decreased sIPSC frequency at 8 months, we found an increase in sIPSC frequency by 17% (P = 0.0216) at 12 months as compared to Tau on water (Figure 1G). Caffeine exposure resulted in a slight increase of sEPSC amplitude by 9% (P = 0.0191) but did not change sIPSC amplitude as compared to Tau on water (Figures 1C,G). In Tau mice exposed to caffeine there was a large decrease in mEPSC frequency by 55% (P = 4.99691e–50), while mIPSC frequency was not modified (Figures 2D,H). The amplitude of mEPSCs (but not that of mIPSCs) was decreased by 16% (P = 0.0391) as compared to Tau on water (Figures 2D,H). Thus, during aging, early exposure to caffeine exacerbates the increase in GABAergic drive received by CA1 pyramidal cells in Tau mice (Figures 3E–H).
Reorganization During Aging: Differences Between 8 Month- and 12 Month-Old Wild Type and Tau Mice on Water
Since our hypothesis is that early-life exposure to caffeine is accelerating the occurrence of phenotypic traits, we now compare the most striking modifications between 8 month- and 12 month-old animals. In both Wild type (n = 8 cells, 8 slices, from 5 mice) and Tau mice on water (n = 7 cells, 7 slices, from 4 mice) we found a large increase in sEPSC frequency by 87 and 231%, respectively, as well as in sIPSC frequency by 55 and 38%, respectively (Figures 1A,C,E,G). The amplitude of sEPSCs increased by 30 and 19%, respectively, in Wild type and Tau mice on water, while for sIPSCs we found a decrease by 20 and 26%, respectively (Figures 1B,D,F,H). The frequency of mEPSCs also increased at 8 months in both strains (81 and 72% respectively), but the frequency of mIPSCs decreased (42%) in Wild type whilst it increased (10%) in Tau mice (Figures 2A,C,E,G). The amplitude of mEPSCs increased by 47 and 1% in Wild type and Tau mice on water, respectively, while for mIPSCs it decreased by 13 and 5%, respectively (Figures 2B,D,F,H). During aging, at least for the two time points considered in this study, there is a global increase of glutamatergic and GABAergic activity received in CA1 pyramidal cells. Interestingly, the fraction of action potential-dependent events is largely increased in 12 month-old wild type as compared to 8 months (since the contribution of mIPSCs is decreased).
Effects of Early-Life Exposure to Caffeine on Learning and Memory Performance
Hippocampal dependent memory was assessed using the Barnes maze test (Koopmans et al., 2003) in 8 month-old mice (n = 11 wild type water, 13 wild type caffeine-treated, 11 TAU water, 13 TAU caffeine-treated mice). Training was performed for 3 days (D1, D2, D3), with three trials (T1, T2, T3) per day. We found the typical learning curve in wild type mice on water as assessed by the latency to the target (Figure 4G). The learning curve was similar in Wild type caffeine mice, while a delay in learning was found in both Tau groups (treated or not with caffeine) with a significant difference at D1T2 and D2T1 between Wild Type and Tau mice (Figure 4G). No difference was found from D2T2. Thus, caffeine exposure by itself does not affect the learning curve, but the underlying Tau pathology does.
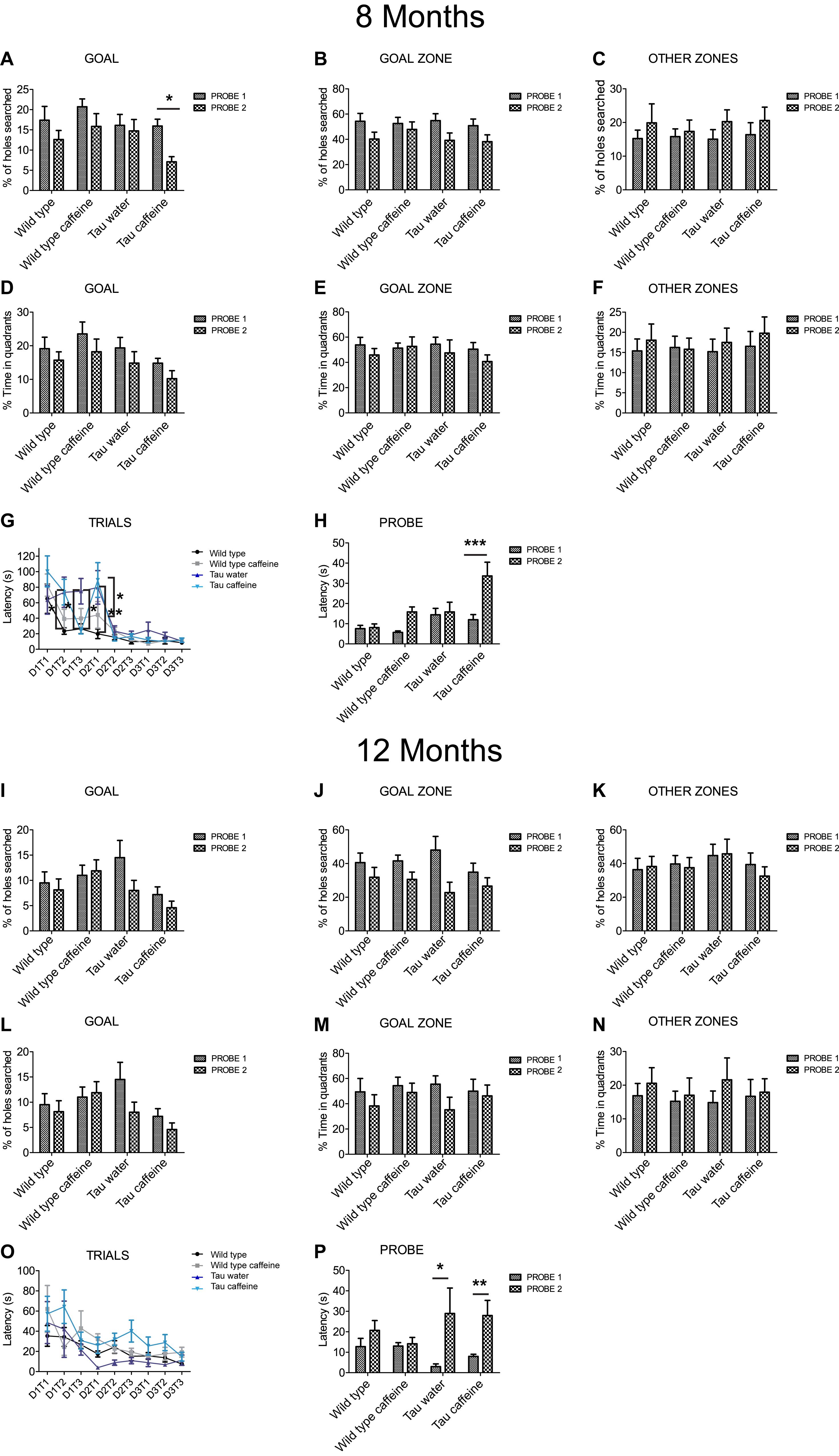
Figure 4. Investigation of early-life exposure to caffeine on learning and memory performance. Barnes maze test was performed in 8 (A–G) and 12 (I–O) month-old mice. Training was performed for 3 days (D1, D2, D3), with three trials (T1, T2, T3) per day. For 8 and 12 month-old mice, the% of holes searched in GOAL (A,I), GOAL ZONE (B,J) and OTHER ZONES (C,K), the% time in quadrants (D,L), GOAL ZONE (E,M) and OTHER ZONES (F,N) and the latency (G,O) were analyzed. To assess memory retention 1 week after the learning part, a probe test (P1) was performed and a second one, 1 week later (P2) on 8 and 12 month-old mice (H,P). Two way ANOVA followed by Bonferroni post hoc test (∗P < 0.05; ∗∗P < 0.01; ∗∗∗P < 0.001). n = 11 Wild type water 8 month-old mice vs. 11 Tau water 8 month-old mice at D1T2; n = 11 Wild type water 8 month-old mice vs. 11 Tau water 8 month-old mice at D2T1; n = 11 Tau water 8 month-old mice vs. 11 Tau caffeine-treated 8 month-old mice at D1T3; n = 11 Tau water 8 month-old mice vs. 11 Tau caffeine-treated 8 month-old mice at D2T1; n = 13 Tau caffeine-treated 8 month-old mice at P1 vs. n = 13 Tau caffeine-treated 8 month-old mice at P2; n = 4 Tau water 12 month-old mice at P1 vs. n = 4 Tau water 12 month-old mice at P2; n = 10 Tau caffeine-treated 12 month-old mice at P1 vs. n = 10 Tau caffeine-treated 12 month-old mice at P2.
One week after the learning part, we performed a probe test (P1) and a second one, 1 week later (P2) to assess memory retention. The caffeine exposed Tau group showed a significant decrease in the number of holes searched in the GOAL area during P2 as compared to P1 (56%, P = 0.0383), which shows an impairment to remember the general location of the escape hole in Tau mice exposed to caffeine (Figure 4A). The latency to the escape hole was increased by 181% (P = 0.0003) in Tau caffeine mice at P2 (Figure 4H). The same trends were found in Wild type animals exposed to caffeine but it was not significant. Thus, caffeine exposed Tau mice show memory deficits. No difference was found in the number of holes searched in the GOAL ZONE and in the OTHER ZONE (Figures 4B,C), nor in the time in the quadrants in the GOAL, GOAL ZONE and OTHER ZONE (Figures 4D–F) for the 4 groups at P1 and P2.
We performed the same study in another series of 12 month-old animals (n = 8 wild type water, 10 wild type caffeine-treated, 4 TAU water, 10 TAU caffeine-treated mice). Surprisingly, in contrast to 8 month-old animals, the learning curves were similar in the four groups, although the TAU caffeine group showed a tendency for slower learning (Figure 4O). Both Tau water and Tau caffeine groups showed a decrease in the number of holes searched in the GOAL area at P2, by 45 and 36% respectively, but the decrease was not significant (Figure 4I). However, the latency at P2 showed an increase by 877.5% (P = 0.0122) and 249.9% (P = 0.0012) for Tau water and Tau caffeine, respectively (Figure 4P), confirming an impairment in memory retention for Tau mice exposed to an early caffeine consumption and the appearance of the same deficit in the Tau control group. This suggests that caffeine exposure in Tau mice enabled an earlier expression of memory deficits at 8 months. No difference was found in the number of holes searched in the GOAL ZONE and in the OTHER ZONE (Figures 4J,K), nor in the time in the quadrants in the GOAL, GOAL ZONE and OTHER ZONE (Figures 4L–N) for the 4 groups at P1 and P2.
Impact of Early Life Caffeine Consumption on Hippocampal Tau Phosphorylation and Related Neuroinflammatory Markers
THY-Tau22 mice exhibit progressive memory impairments in parallel with the development of hippocampal Tau hyperphosphorylation and neuroinflammation (Van der Jeugd et al., 2013; Laurent et al., 2017). At 6–8 months of age, Tau pathology and neuroinflammation are ongoing in the hippocampus of THY-Tau22 mice. The potentiation of memory deficits by early-life caffeine in Tau mice opened the possibility that Tau pathology itself or neuroinflammation might have been advanced, therefore we performed biochemical and qPCR experiments in an additional group of animals at the age of 6 months. Using sodium dodecyl sulfate-polyacrylamide gel electrophoresis, we evaluated Tau phosphorylation in both Tau experimental groups using antibodies raised against several Tau phosphoepitopes. None of the epitope studied was modified by early life exposure to caffeine (Figure 5A). As caffeine modulates neuroinflammation (Brothers et al., 2010; Laurent et al., 2014), we checked several neuroinflammatory markers previously described to be early or lately upregulated in the hippocampus of THY-Tau22 animals (Laurent et al., 2017). In general, in accordance with the absence of impact on Tau phosphorylation, early caffeine treatment did not modulate any of the neuroinflammatory markers studied. We could only evidence a slight reduction of the expression of the microglia CD68 markers in TAU mice treated with caffeine vs. Tau water animals (Figure 5B).
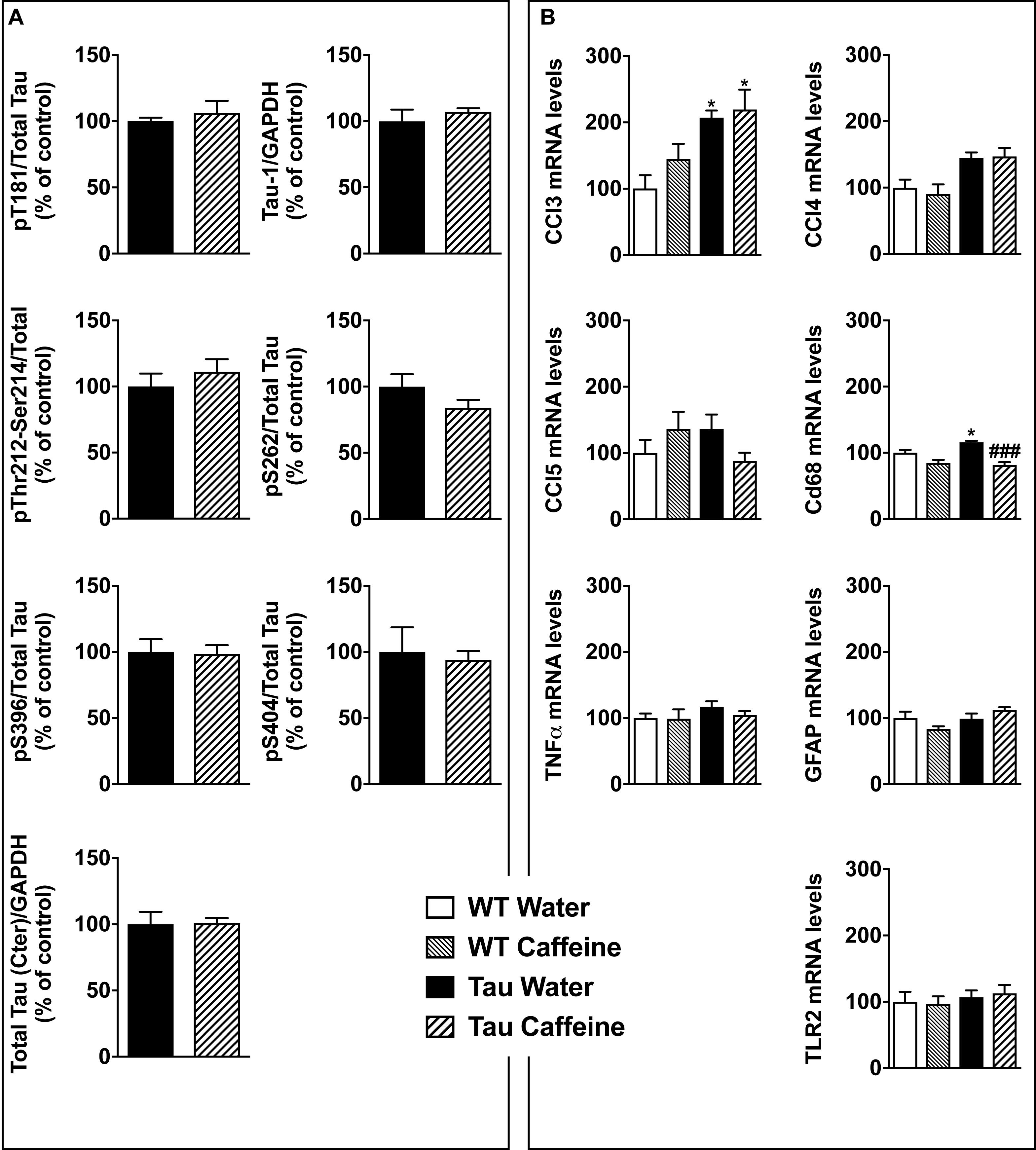
Figure 5. Investigation of early-life exposure to caffeine on hippocampal Tau phosphorylation and associated neuroinflammatory markers. (A) Western blot analysis of tau phosphorylation in water or caffeine exposed THY-Tau22 offsprings using antibodies targeting physiological (pThr181, Tau-1, pSer262, pSer396, pSer404) and pathologic (pThr212/Ser214) Tau epitopes. Quantifications were performed over total tau levels (Cter). Total tau levels were quantified versus GAPDH, used as loading control (n = 7/group). (B) qPCR analysis of hippocampal neuroinflammatory markers associated with Tau pathology in the THY-Tau22 model (n = 6–10 per group).
Discussion
Psychoactive drugs ingested during pregnancy can have widespread deleterious effects in the fetus, in particular in the brain (Salisbury et al., 2009). Substances of abuse, such as alcohol, cannabis and cocaine, can directly alter the construction of the brain, in particular GABAergic circuits (Miller, 1986; Berghuis et al., 2007; Navi-Goffer and Mulder, 2009; Thompson et al., 2009). As the most widely consumed psychoactive drug, including during pregnancy, caffeine can also have body-wide effects (Temple et al., 2017). Previous works in control GIN (GFP-expressing Inhibitory Neurons) mice have shown that caffeine slows down the migration of GABA neurons resulting in a delayed insertion in the circuitry, both in the hippocampus and the cortex during the first postnatal week (Silva et al., 2013; Fazeli et al., 2017). Such alterations may have a direct impact on hippocampal network activity and performance during development (Salesse et al., 2011). Indeed, they are associated with enhanced sensitivity to epilepsy, hyperactivity in vivo and cognitive deficits later in life in offspring (Silva et al., 2013; Fazeli et al., 2017). Based on these studies, we hypothesized that the modifications produced by early life exposure to caffeine (acting as a first hit) would leave a long-term trace in the circuits, rendering them more vulnerable to a second hit, in line with the diathesis-disease theory (Bernard, 2016). This theory has been validated in the context of stress-induced vulnerability to epilepsy, depression and cognitive deficits, with the demonstration that social defeat (first hit) induces a state of vulnerability in some rats; a second hit being necessary to trigger a phenotype in the vulnerable population (Blugeot et al., 2011; Becker et al., 2015, 2019; Bouvier et al., 2016). If early-life exposure to caffeine constitutes a first hit and induces a state of vulnerability to a tauopathy, we reasoned that Tau mutant offspring exposed to caffeine would express phenotypic traits earlier than Tau mutants on water, in particular the cognitive deficits and biochemical alterations known to occur in this mouse model. Since we wanted to bridge behavioral and molecular levels, we looked at the circuit level, focusing on the CA1 region, which is characterized by strong alterations in the GABAergic and glutamatergic circuits in caffeine exposed WT mice (Silva et al., 2013; Fazeli et al., 2017). Our ultimate goal was to be able to provide a coherent picture, linking all levels of analysis. However, the three levels of analysis do not provide a coherent picture.
The most salient feature of the results is the occurrence of deficits in spatial memory and learning already at 8 months in caffeine exposed Tau mice. These deficits occurred at 12 months in water exposed Tau mice and were still present at that age in caffeine Tau mice. In the present work, we used the Barnes maze, which is a terrestrial version of the Morris water maze and which is less stressful to mice than the Morris water maze (Harrison et al., 2009). We did not use other tests (such as anxiety and novel object recognition) to prevent interference when using different behavioral tests in succession. Interestingly, caffeine treatment did not alter spatial memory as assessed with the Barnes maze in Wild type mice, whilst caffeine-treated WT mice showed deficits in the object-location memory task (Silva et al., 2013). This suggests that specific types of memories are affected. The Barnes maze test is particularly useful in our case as WT mice exposed to caffeine do not display deficits, which would have added an independent variable, rendering the interpretation of the results more difficult. We can thus propose that early life exposure to caffeine accelerates the occurrence of the behavioral phenotype (with the caveat that we only looked at two time points). Humans have different genetic backgrounds and go through different life experiences, which will determine their sensitivity to the development of diseases, in particular neurological disorders (Bernard, 2016). The fact that caffeine exposure during early life produces an earlier occurrence of cognitive deficits in the Tau model used here may be relevant to a subset of human individuals. Said differently, exposure to caffeine during pregnancy may sensitize some but not all offsprings. Future studies will need to investigate other time points during aging, other cognitive tests and other models of AD. The experimental protocol is however highly time-consuming. We cannot rely on established aging colonies, since females need to be exposed to caffeine in the drinking water before mating until weaning. Then, it is necessary to wait until offspring reach the appropriate age.
Multiple mechanisms can be proposed to explain cognitive deficits in the Barnes maze (activation of the HPA axis, inflammation, metabolic defect, cell death, synaptopathy, channelopathy, epigenetic modifications, to name but a few). In THY-Tau22 mice, cognitive deficits have been associated with Tau pathological load and neuroinflammation (Van der Jeugd et al., 2013; Laurent et al., 2016, 2017, but see Burlot et al., 2015; Chatterjee et al., 2018). However, analysis of cardinal pTau and neuroinflammatory markers did not correlate with memory deficits in offspring exposed to caffeine. This result is in keeping with the proposal that cognitive decline may occur before the expression of molecular phenotypic traits in patients with AD (Jessen et al., 2014).
Since the hippocampus plays a key role is spatial memory, we were expecting circuit alterations in this region. To assess them, we measured glutamatergic and GABAergic synaptic currents received by hippocampal CA1 pyramidal cells. The results are very difficult to interpret because of the presence of three independent variables imposed by the experimental protocol. The first two independent variables are caffeine and Tau, both of which result in morpho-physiological alterations. The third independent variable is age, since we are considering two time points, 8 and 12 months. Yet, the in vitro approach provides interesting results in their complexity. Since our hypothesis was that caffeine exposure accelerates the occurrence of the phenotype, we start to discuss the age factor. In a given condition (WT mice or Tau mice), we were expecting to find whichever properties identified at 12 months in water treated animals at 8 months in caffeine exposed animals. In WT mice on water, we found an increase in both glutamatergic and GABAergic drives between 8 and 12 months. We can speculate that during normal aging, there is a gradual increase of the barrage of excitatory and inhibitory synaptic events received by CA1 pyramidal cells. More time points should be investigated to test this hypothesis. Several non-excluding mechanisms can explain such a rise, including an increase in the number of synapses, more active presynaptic cells, and a greater release probability from the presynaptic terminals. This result is consistent with that reported in the prefrontal cortex during aging (using later time points) for animals preserving their cognitive performances (Bories et al., 2013). Interestingly, in Tau mice on water, both glutamatergic and GABAergic drives were increased at 8 and 12 months as compared to their Wild type counterparts. In keeping with our results, most studies using Tau or Aß models report an increase in the excitatory drive as compared to Wild Type (Crimins et al., 2011; Dalby et al., 2014; Ovsepian et al., 2017), but see Rocher et al. (2008). Less data is available for the GABAergic drive. In the cortex of a different Tau model, there was no difference in sIPSC frequency at 9 months as compared to Wild Type (Crimins et al., 2011). The discrepancy with our results may stem from the type of mutant used and the brain region selected. Together, our results raise the intriguing possibility that the Tau mutation accelerates the aging process as assessed with the glutamatergic and GABAergic drives received by CA1 pyramidal cells.
Early life exposure to caffeine changed the apparent co-variance relationship between the two age and Tau independent variables. At 8 months, both GABAergic and glutamatergic drives were decreased in Tau caffeine mice as compared to Tau water mice. However, at 12 months, although the excitatory drive was decreased as compared to that measured in Tau water mice, the inhibitory drive was increased. Hence, contrary to our hypothesis, caffeine exposure does not accelerate the increase in synaptic barrage received by CA1 pyramidal cells that occurs in water exposed animal. We propose that the three variables tau-caffeine-age interact in non-linear fashion, thus constituting a complex system (the global behavior cannot be predicted from the observation of its independent components). This exemplifies the difficulty in interpreting the in vitro data. However, our data emphasize that caffeine exposure disrupts the effect of the pathogenic process characteristic of the Tau phenotype in CA1 pyramidal cells. If there is a correlation between cognitive deficits and electrophysiological alterations at the circuit level, more parameters need to be measured (e.g., ion channels or metabolism). The possibility also exists that the CA1 region is not the most appropriate to establish such a correlation. In vivo recordings may also provide a different entry point into the underlying mechanisms, in particular measuring the properties of brain rhythms (theta, gamma) and sleep patterns.
Conclusion
We propose that early caffeine exposure produces physiological and cognitive alterations in a Tau pathological context, supporting our hypothesis that caffeine consumption during pregnancy may constitute a risk-factor for an earlier development of AD-related phenotypes. Future studies are needed using other mouse models, and, as importantly, to determine whether re-exposure to caffeine during adulthood is protective.
Data Availability Statement
The datasets generated for this study are available on request to the corresponding author.
Ethics Statement
The animal study was reviewed and approved by Aix-Marseille University Animal Care and Use Committee.
Author Contributions
SZ, EF, AG, and SC performed the experiments and analyzed the data. DB, ME, and CB designed the project. CB managed the project. All authors contributed to the writing of the manuscript.
Funding
This work was supported by a grant from Association France Alzheimer, Project No. AAP SM 2016/1567 and Aix-Marseille University. INSERM UMR-S1172 is supported by grants from Hauts-de-France (PARTEN-AIRR, COGNADORA), ANR (ADORATAU and ADOSTAsTRAU to DB; GRAND and TONIC to LB) and Programs d’Investissements d’Avenir LabEx (excellence laboratory) DISTALZ (Development of Innovative Strategies for a Transdisciplinary approach to ALZheimer’s disease), Fondation pour la Recherche Médicale, France Alzheimer/Fondation de France, FHU VasCog research network (Lille, France), Fondation Vaincre Alzheimer, Fondation Plan Alzheimer, INSERM, CNRS, Université de Lille, Lille Métropole Communauté Urbaine, DN2M.
Conflict of Interest
The authors declare that the research was conducted in the absence of any commercial or financial relationships that could be construed as a potential conflict of interest.
Acknowledgments
We thank the Animal Facility (Lille, France) and Mélanie Besegher, Cyrille Degraeve, Caroline Declerck, Kim Letten, Yann Lepage, Benjamin Guerrin, Didier Montignies, Christian Meunier, Quentin Dekeyser, and Romain Dehaynin for animal care.
References
Adén, U., Herlenius, E., Tang, L. Q., and Fredholm, B. B. (2000). Maternal caffeine intake has minor effects on adenosine receptor ontogeny in the rat brain. Pediatr. Res. 48, 177–183. doi: 10.1203/00006450-200008000-00010
American College of Obstetricians and Gynecologists, (2010). Moderate caffeine consumption during pregnancy. Obstet. Gynecol. 116(2 Pt 1), 467–468. doi: 10.1097/aog.0b013e3181eeb2a1
Barkovich, A. J., Guerrini, R., Kuzniecky, R. I., Jackson, G. D., and Dobyns, W. B. (2012). A developmental and genetic classification for malformations of cortical development: update 2012. Brain 135, 1348–1369. doi: 10.1093/brain/aws019
Becker, C., Bouvier, E., Ghestem, A., Siyoucef, S., Claverie, D., Camus, F., et al. (2015). Predicting and treating stress-induced vulnerability to epilepsy and depression. Ann. Neurol. 78, 128–136. doi: 10.1002/ana.24414
Becker, C., Mancic, A., Ghestem, A., Poillerat, V., Claverie, D., Bartolomei, F., et al. (2019). Antioxidant treatment after epileptogenesis onset prevents comorbidities in rats sensitized by a past stressful event. Epilepsia 60, 648–655. doi: 10.1111/epi.14692
Belarbi, K., Burnouf, S., Fernandez-Gomez, F. J., Laurent, C., Lestavel, S., Figeac, M., et al. (2011). Beneficial effects of exercise in a transgenic mouse model of Alzheimer’s disease-like tau pathology. Neurobiol. Dis 42, 486–494. doi: 10.1016/j.nbd.2011.04.022
Berghuis, P., Rajnicek, A. M., Morozov, Y. M., Ross, R. A., Mulder, J., Urban, G. M., et al. (2007). Hardwiring the brain: endocannabinoids shape neuronal connectivity. Science 316, 1212–1216. doi: 10.1126/science.1137406
Bernard, C. (2016). The diathesis-epilepsy model: how past events impact the development of epilepsy and comorbidities. Cold Spring Harb. Perspect. Med. 6, a022418. doi: 10.1101/cshperspect.a022418
Björklund, O., Kahlstrom, J., Salmi, P., and Fredholm, B. B. (2008). Perinatal caffeine, acting on maternal adenosine A1 receptors, causes long-lasting behavioral changes in mouse offspring. PLoS One 3:e3977. doi: 10.1371/journal.pone.0003977
Blugeot, A., Rivat, C., Bouvier, E., Molet, J., Mouchard, A., Zeau, B., et al. (2011). Vulnerability to depression: from brain neuroplasticity to identification of biomarkers. J. Neurosci. 31, 12889–12899. doi: 10.1523/JNEUROSCI.1309-11.2011
Borenstein, A. R., Copenhaver, C. I., and Mortimer, J. A. (2006). Early-life risk factors for Alzheimer disease. Alzheimer Dis. Assoc. Disord. 2006, 63–72. doi: 10.1097/01.wad.0000201854.62116.d7
Bories, C., Husson, Z., Guitton, M. J., and De Koninck, Y. (2013). Differential balance of prefrontal synaptic activity in successful versus unsuccessful cognitive aging. J. Neurosci. 33, 1344–1356. doi: 10.1523/JNEUROSCI.3258-12.2013
Bouvier, E., Brouillard, F., Molet, J., Claverie, D., Cabungcal, J. H., Cresto, N., et al. (2016). Nrf2-dependent persistent oxidative stress results in stress-induced vulnerability to depression. Mol. Psychiatry. 22:1795. doi: 10.1038/mp.2016.211
Braak, H., Thal, D. R., Ghebremedhin, E., and Del Tredici, K. (2011). Stages of the pathologic process in Alzheimer disease: age categories from 1 to 100 years. J. Neuropathol. Exp. Neurol 70, 960–969. doi: 10.1097/NEN.0b013e318232a379
Brothers, H. M., Marchalant, Y., and Wenk, G. L. (2010). Caffeine attenuates lipopolysaccharide-induced neuroinflammation. Neurosci. Lett. 480, 97–100. doi: 10.1016/j.neulet.2010.06.013
Burlot, M. A., Braudeau, J., Michaelsen-Preusse, K., Potier, B., Ayciriex, S., Varin, J., et al. (2015). Cholesterol 24-hydroxylase defect is implicated in memory impairments associated with Alzheimer-like Tau pathology. Hum. Mol. Genet. 24, 5965–5976. doi: 10.1093/hmg/ddv268
Chatterjee, S., Cassel, R., Schneider-Anthony, A., Merienne, K., Cosquer, B., Tzeplaeff, L., et al. (2018). Reinstating plasticity and memory in a tauopathy mouse model with an acetyltransferase activator. EMBO Mol. Med. 10:e8587. doi: 10.15252/emmm.201708587
Clancy, B., Darlington, R. B., and Finlay, B. L. (2001). Translating developmental time across mammalian species. Neuroscience 105, 7–17. doi: 10.1016/s0306-4522(01)00171-3
Cossart, R., Dinocourt, C., Hirsch, J. C., Merchan-Perez, A., De, F. J., Ben-Ari, Y., et al. (2001). Dendritic but not somatic GABAergic inhibition is decreased in experimental epilepsy. Nat. Neurosci. 4, 52–62. doi: 10.1038/82900
Crandall, J. E., Hackett, H. E., Tobet, S. A., Kosofsky, B. E., and Bhide, P. G. (2004). Cocaine exposure decreases GABA neuron migration from the ganglionic eminence to the cerebral cortex in embryonic mice. Cereb. Cortex 14, 665–675. doi: 10.1093/cercor/bhh027
Crimins, J. L., Rocher, A. B., Peters, A., Shultz, P., Lewis, J., and Luebke, J. I. (2011). Homeostatic responses by surviving cortical pyramidal cells in neurodegenerative tauopathy. Acta Neuropathol. 122, 551–564. doi: 10.1007/s00401-011-0877-0
Dalby, N. O., Volbracht, C., Helboe, L., Larsen, P. H., Jensen, H. S., Egebjerg, J., et al. (2014). Altered function of hippocampal CA1 pyramidal neurons in the rTg4510 mouse model of tauopathy. J. Alzheimers. Dis. 40, 429–442. doi: 10.3233/JAD-131358
Duyckaerts, C., Bennecib, M., Grignon, Y., Uchihara, T., He, Y., Piette, F., et al. (1997). Modeling the relation between neurofibrillary tangles and intellectual status. Neurobiol. Aging 18, 267–273. doi: 10.1016/s0197-4580(97)80306-5
Esclapez, M., Hirsch, J. C., Ben-Ari, Y., and Bernard, C. (1999). Newly formed excitatory pathways provide a substrate for hyperexcitability in experimental temporal lobe epilepsy. J. Comp. Neurol 408, 449–460. doi: 10.1002/(sici)1096-9861(19990614)408:4<449::aid-cne1>3.0.co;2-r
Fazeli, W., Zappettini, S., Marguet, S. L., Grendel, J., Esclapez, M., Bernard, C., et al. (2017). Early-life exposure to caffeine affects the construction and activity of cortical networks in mice. Exp. Neurol. 295, 88–103. doi: 10.1016/j.expneurol.2017.05.013
Galéra, C., Bernard, J. Y., van der Waerden, J., Bouvard, M.-P., Lioret, S., Forhan, A., et al. (2015). Prenatal caffeine exposure and child intelligence quotient at age 5.5 years: the Eden mother-child cohort. Biol. Psychiatry 80, 720–726. doi: 10.1016/j.biopsych.2015.08.034
Greenwood, D. C., Thatcher, N. J., Ye, J., Garrard, L., Keogh, G., King, L. G., et al. (2014). Caffeine intake during pregnancy and adverse birth outcomes: a systematic review and dose-response meta-analysis. Eur. J. Epidemiol 29, 725–734. doi: 10.1007/s10654-014-9944-x
Grober, E., Dickson, D., Sliwinski, M. J., Buschke, H., Katz, M., Crystal, H., et al. (1999). Memory and mental status correlates of modified Braak staging. Neurobiol. Aging 20, 573–579. doi: 10.1016/s0197-4580(99)00063-9
Guerrini, R., and Dobyns, W. B. (2014). Malformations of cortical development: clinical features and genetic causes. Lancet Neurol. 13, 710–726. doi: 10.1016/S1474-4422(14)70040-7
Harrison, F. E., Hosseini, A. H., and McDonald, M. P. (2009). Endogenous anxiety and stress responses in water maze and Barnes maze spatial memory tasks. Behav. Brain Res. 198, 247–251. doi: 10.1016/j.bbr.2008.10.015
Jessen, F., Amariglio, R. E., van Boxtel, M., Breteler, M., Ceccaldi, M., Chetelat, G., et al. (2014). A conceptual framework for research on subjective cognitive decline in preclinical Alzheimer’s disease. Alzheimers Dement 10, 844–852. doi: 10.1016/j.jalz.2014.01.001
Koopmans, G., Blokland, A., van Nieuwenhuijzen, P., and Prickaerts, J. (2003). Assessment of spatial learning abilities of mice in a new circular maze. Physiol. Behav. 79, 683–693. doi: 10.1016/s0031-9384(03)00171-9
Laurent, C., Burnouf, S., Ferry, B., Batalha, V. L., Coelho, J. E., Baqi, Y., et al. (2016). A2A adenosine receptor deletion is protective in a mouse model of Tauopathy. Mol. Psychiatry. 21:97–107. doi: 10.1038/mp.2015.115
Laurent, C., Dorothee, G., Hunot, S., Martin, E., Delarasse, C., Buee, L., et al. (2017). [Tau and cognitive disorders: a role for T lymphocytes]. Med. Sci. 33, 817–819. doi: 10.1051/medsci/20173310002
Laurent, C., Eddarkaoui, S., Derisbourg, M., Leboucher, A., Demeyer, D., Carrier, S., et al. (2014). Beneficial effects of caffeine in a transgenic model of Alzheimer’s disease-like tau pathology. Neurobiol. Aging 35, 2079–2090. doi: 10.1016/j.neurobiolaging.2014.03.027
Le Freche, H., Brouillette, J., Fernandez-Gomez, F. J., Patin, P., Caillierez, R., Zommer, N., et al. (2012). Tau phosphorylation and sevoflurane anesthesia: an association to postoperative cognitive impairment. Anesthesiology 116, 779–787. doi: 10.1097/ALN.0b013e31824be8c7
Leboucher, A., Laurent, C., Fernandez-Gomez, F. J., Burnouf, S., Troquier, L., Eddarkaoui, S., et al. (2013). Detrimental effects of diet-induced obesity onspathology are independent of insulin resistance instransgenic mice. Diabetes Metab. Res. Rev. 62, 1681–1688. doi: 10.2337/db12-0866
Lenroot, R. K., and Giedd, J. N. (2006). Brain development in children and adolescents: insights from anatomical magnetic resonance imaging. Neurosci. Biobehav. Rev. 30, 718–729. doi: 10.1016/j.neubiorev.2006.06.001
Mandel, H. G. (2002). Update on caffeine consumption, disposition and action. Food Chem. Toxicol. 40, 1231–1234. doi: 10.1016/s0278-6915(02)00093-5
Manouze, H., Ghestem, A., Poillerat, V., Bennis, M., Ba-M’hamed, S., Benoliel, J. J., et al. (2019). Effects of single cage housing on stress, cognitive, and seizure parameters in the rat and mouse pilocarpine models of epilepsy. eNeuro 6. doi: 10.1523/ENEURO.0179-18.2019
Marroun, H. E., Tiemeier, H., Franken, I. H. A., Jaddoe, V. W. V., Van Der Lugt, A., Verhulst, F. C., et al. (2015). Prenatal cannabis and tobacco exposure in relation to brain morphology: a prospective neuroimaging study in young children. Biol. Psychiatry 79, 1–9. doi: 10.1016/j.biopsych.2015.08.024
Masters, C. L., Simms, G., Weinman, N. A., Multhaup, G., McDonald, B. L., and Beyreuther, K. (1985). Amyloid plaque core protein in Alzheimer disease and down syndrome. Proc. Natl. Acad. Sci. U.S.A 82, 4245–4249. doi: 10.1073/pnas.82.12.4245
Miller, M. W. (1986). Effects of alcohol on the generation and migration of cerebral cortical neurons. Science 233, 1308–1311. doi: 10.1126/science.3749878
Navi-Goffer, S., and Mulder, J. (2009). The polarised life of the endocannabinoid system in CNS development. Chembiochem 10, 1591–1598. doi: 10.1002/cbic.200800827
Ovsepian, S. V., Blazquez-Llorca, L., Freitag, S. V., Rodrigues, E. F., and Herms, J. (2017). Ambient glutamate promotes paroxysmal hyperactivity in cortical pyramidal neurons at amyloid plaques via presynaptic mGluR1 receptors. Cereb. Cortex 27, 4733–4749. doi: 10.1093/cercor/bhw267
Papon, M. A., El Khoury, N. B., Marcouiller, F., Julien, C., Morin, F., Bretteville, A., et al. (2013). Deregulation of protein phosphatase 2A and hyperphosphorylation ofsprotein following onset of diabetes in NOD mice. Diabetes Metab. Res. Rev. 62, 609–617. doi: 10.2337/db12-0187
Reitz, C., Brayne, C., and Mayeux, R. (2011). Epidemiology of Alzheimer disease. Nat. Rev. Neurol. 7, 137–152. doi: 10.1038/nrneurol.2011.2
Rocher, A. B., Kinson, M. S., and Luebke, J. I. (2008). Significant structural but not physiological changes in cortical neurons of 12-month-old Tg2576 mice. Neurobiol. Dis. 32, 309–318. doi: 10.1016/j.nbd.2008.07.014
Romijn, H. J., Hofman, M. A., and Gramsbergen, A. (1991). At what age is the developing cerebral cortex of the rat comparable to that of the full-term newborn human baby? Early Hum. Dev. 26, 61–67. doi: 10.1016/0378-3782(91)90044-4
Salesse, C., Mueller, C. L., Chamberland, S., and Topolnik, L. (2011). Age-dependent remodelling of inhibitory synapses onto hippocampal CA1 oriens-lacunosum moleculare interneurons. J. Physiol. 589, 4885–4901. doi: 10.1113/jphysiol.2011.215244
Salisbury, A. L., Ponder, K. L., Padbury, J. F., and Lester, B. M. (2009). Fetal effects of psychoactive drugs. Clin. Perinatol. 36, 595–619. doi: 10.1016/j.clp.2009.06.002
Seifan, A., Schelke, M., Obeng-Aduasare, Y., and Isaacson, R. (2015). Early life epidemiology of Alzheimer’s disease – a critical review. Neuroepidemiology 45, 237–254. doi: 10.1159/000439568
Sergeant, N., Bretteville, A., Hamdane, M., Caillet-Boudin, M. L., Grognet, P., Bombois, S., et al. (2008). Biochemistry of tau in Alzheimer’s disease and related neurological disorders. Expert Rev. Proteomics 5, 207–224.
Silva, C. G., Metin, C., Fazeli, W., Machado, N. J., Darmopil, S., Launay, P.-S., et al. (2013). Adenosine receptor antagonists including caffeine alter fetal brain development in mice. Sci. Transl. Med. 5:197ra104. doi: 10.1126/scitranslmed.3006258
Skorput, A. G., Gupta, V. P., Yeh, P. W. L., and Yeh, H. H. (2015). Persistent interneuronopathy in the prefrontal cortex of young adult offspring exposed to ethanol in utero. J. Neurosci. 35, 10977–10988. doi: 10.1523/JNEUROSCI.1462-15.2015
Stern, Y. (2012). Cognitive reserve in ageing and Alzheimer’s disease. Lancet Neurol. 11, 1006–1012. doi: 10.1016/S1474-4422(12)70191-6
Temple, J. L., Bernard, C., Lipshultz, S. E., Czachor, J. D., Westphal, J. A., and Mestre, M. A. (2017). The safety of ingested caffeine: a comprehensive review. Front. Psychiatry 8:80. doi: 10.3389/fpsyt.2017.00080
Thompson, B. L., Levitt, P., and Stanwood, G. D. (2009). Prenatal exposure to drugs: effects on brain development and implications for policy and education. Nat. Rev. Neurosci. 10, 303–312. doi: 10.1038/nrn2598
Van der Jeugd, A., Vermaercke, B., Derisbourg, M., Lo, A. C., Hamdane, M., Blum, D., et al. (2013). Progressive age-related cognitive decline in tau mice. J. Alzheimers. Dis. 37, 777–788. doi: 10.3233/JAD-130110
Whalley, L. J., Dick, F. D., and McNeill, G. (2006). A life-course approach to the aetiology of late-onset dementias. Lancet Neurol. 5, 87–96. doi: 10.1016/s1474-4422(05)70286-6
Keywords: caffeine, development, Alzheimer, tauopathy, hippocampus, memory, learning, synaptic currents
Citation: Zappettini S, Faivre E, Ghestem A, Carrier S, Buée L, Blum D, Esclapez M and Bernard C (2019) Caffeine Consumption During Pregnancy Accelerates the Development of Cognitive Deficits in Offspring in a Model of Tauopathy. Front. Cell. Neurosci. 13:438. doi: 10.3389/fncel.2019.00438
Received: 18 July 2019; Accepted: 13 September 2019;
Published: 01 October 2019.
Edited by:
Sonia Gasparini, LSU Health Sciences Center New Orleans, United StatesReviewed by:
Haruyuki Kamiya, Hokkaido University, JapanKelly Dougherty, Rhodes College, United States
Copyright © 2019 Zappettini, Faivre, Ghestem, Carrier, Buée, Blum, Esclapez and Bernard. This is an open-access article distributed under the terms of the Creative Commons Attribution License (CC BY). The use, distribution or reproduction in other forums is permitted, provided the original author(s) and the copyright owner(s) are credited and that the original publication in this journal is cited, in accordance with accepted academic practice. No use, distribution or reproduction is permitted which does not comply with these terms.
*Correspondence: Christophe Bernard, Y2hyaXN0b3BoZS5iZXJuYXJkQHVuaXYtYW11LmZy