- 1Department of Biophysics, Center for Integrative Physiology and Molecular Medicine (CIPMM), School of Medicine, Saarland University, Homburg, Germany
- 2Department of Otorhinolaryngology, Saarland University, Homburg, Germany
Inner hair cell (IHC) Cav1.3 Ca2+ channels are multifunctional channels mediating Ca2+ influx for exocytosis at ribbon synapses, the generation of Ca2+ action potentials in pre-hearing IHCs and gene expression. IHCs of deaf systemic Cav1.3-deficient (Cav1.3-/-) mice stay immature because they fail to up-regulate voltage- and Ca2+-activated K+ (BK) channels but persistently express small conductance Ca2+-activated K+ (SK2) channels. In pre-hearing wildtype mice, cholinergic neurons from the superior olivary complex (SOC) exert efferent inhibition onto spontaneously active immature IHCs by activating their SK2 channels. Because Cav1.3 plays an important role for survival, health and function of SOC neurons, SK2 channel persistence and lack of BK channels in systemic Cav1.3-/- IHCs may result from malfunctioning neurons of the SOC. Here we analyze cochlea-specific Cav1.3 knockout mice with green fluorescent protein (GFP) switch reporter function, Pax2::cre;Cacna1d-eGFPflex/flex and Pax2::cre;Cacna1d-eGFPflex/-. Profound hearing loss, lack of BK channels and persistence of SK2 channels in Pax2::cre;Cacna1d-eGFPflex/- mice recapitulated the phenotype of systemic Cav1.3-/- mice, indicating that in wildtype mice, regulation of SK2 and BK channel expression is independent of Cav1.3 expression in SOC neurons. In addition, we noticed dose-dependent GFP toxicity leading to death of basal coil IHCs of Pax2::cre;Cacna1d-eGFPflex/flex mice, likely because of high GFP concentration and small repair capacity. This and the slower time course of Pax2-driven Cre recombinase in switching two rather than one Cacna1d-eGFPflex allele lead us to study Pax2::cre;Cacna1d-eGFPflex/- mice. Notably, control Cacna1d-eGFPflex/- IHCs showed a significant reduction in Cav1.3 channel cluster sizes and currents, suggesting that the intronic construct interfered with gene translation or splicing. These pitfalls are likely to be a frequent problem of many genetically modified mice with complex or multiple gene-targeting constructs or fluorescent proteins. Great caution and appropriate controls are therefore required.
Introduction
The L-type calcium (Ca2+) channel Cav1.3 is the main voltage-gated Ca2+ channel (VGCC) in inner hair cells (IHCs) and essential for hearing (Platzer et al., 2000; Baig et al., 2011). In both pre-hearing and mature IHCs, voltage-activated Cav1.3 channels trigger glutamate release resulting in signal transmission to the auditory nerve (Brandt et al., 2003). Before the onset of hearing at postnatal day 12 in mice, IHCs produce spontaneous Ca2+-action potentials (Kros et al., 1998; Platzer et al., 2000; Marcotti et al., 2003) required for the terminal differentiation of IHCs (Brandt et al., 2003; Nemzou et al., 2006; Johnson et al., 2013a) and maturation of the auditory brainstem (Tritsch and Bergles, 2010; Clause et al., 2014; Babola et al., 2018). IHC spontaneous activity is modulated by transient efferent input originating in the superior olivary complex (SOC), which activates small-conductance SK2 potassium (K+) channels and thereby causes hyperpolarization of the IHC membrane potential (Glowatzki and Fuchs, 2000; Oliver et al., 2000). Around the onset of hearing, IHCs loose their efferent input (Simmons, 2002), SK2 channels are down-regulated (Marcotti et al., 2004) and spontaneous activity ends with the up-regulation of BK and KCNQ4 K+ channels (Kros et al., 1998; Oliver et al., 2003). IHCs of systemic Cav1.3-/- mice fail to acquire a mature composition of K+ channels (Brandt et al., 2003; Nemzou et al., 2006), which is likely caused by lack of spontaneous activity and impaired Ca2+-dependent transcriptional regulation. However, altered efferent modulation due to lack of Cav1.3 in brainstem nuclei might add to the phenotype. Cav1.3 plays an intrinsic role for development and function of SOC neurons (Hirtz et al., 2011, 2012; Satheesh et al., 2012) and is therefore regarded not only as a peripheral but also a central deafness gene (Willaredt et al., 2014).
Here, the effects of cochlea-specific ablation of Cav1.3 channels before birth on the electrophysiological and molecular phenotype of IHCs as well as hearing function were investigated. To this end, Cacna1d-eGFPflex mice were used, in which the ablation of Cacna1d encoding Cav1.3 channels is directly coupled to the expression of the reporter gene eGFP via Cre-induced inversion (“switch”) of the floxed allele (Satheesh et al., 2012). They were crossed with Pax2::cre mice (Ohyama and Groves, 2004), where Cre expression is initiated at E9.5 in the otic vesicle (Lawoko-Kerali et al., 2001; Burton et al., 2004) and found in the mature organ of Corti and spiral ganglion neurons (SGN) but not in the nuclei that are part of the afferent-efferent feedback loop onto hair cells, i.e., ventral cochlear nucleus and the SOC (Zuccotti et al., 2012).
Materials and Methods
Animals
Cacna1d-eGFPflex mice were generated within the CavNET consortium (EU-CAVNET MRTN-CT-2006-035367) by Katrin Bartels née Kunert, Kai Schönig and Dusan Bartsch, Central Institute of Mental Health, Mannheim, Germany (Satheesh et al., 2012). They were cross-bred with Cacna1d-/- mice (Platzer et al., 2000) and Pax2::cre mice (Ohyama and Groves, 2004; Zuccotti et al., 2012). To reduce the risk of unwanted effects caused by Cre expression, only mice heterozygous for Pax2::cre were used (Jae Huh et al., 2010; Janbandhu et al., 2014). Animals were housed with free access to food and water at an average temperature of 22°C and a 12 h light-dark cycle. Mice of either sex were sacrificed by decapitation under isoflurane anesthesia and their cochleae were dissected from the temporal bones. All experiments were carried out in accordance with the European Communities Council Directive (86/609/EEC) and approved by the regional board for scientific animal experiments of the Saarland, Germany. Additional ethics approval was not required according to the local and national guidelines.
Genotyping
The Pax2::cre allele was genotyped using primers detecting Cre: 5′-GCC TGC ATT ACC GGT CGA TGC AAC GA-3′ and 5′-GTG GCA GAT GGC GCG GCA ACA CCA TT-3′ (product size: 726 bp). The Cacna1d-eGFPflex (“flex”) allele was identified using: 5′-TTC AAG GAC GAC GGC AAC TAC AAG-3′ and 5′-CGG CGG CGG TCA CGA ACT CC-3′ (product size: 380 bp). To exclude accidental occurrence of unwanted embryonal or germline recombination of the flex allele in pups without Cre, we regularly used the following primers: “Flex A” (5′-GGA GTT GTG TAT ATC TGT TAA GCC ATG-3′), “Flex B” (5′-GCT GTT GGG CTG AGA AGT TGG T-3′) and “Flex C” (5′-CCA GAA GAT TCC ACT AAA GGT CAT-3′), detecting wildtype (A-B band, ∼450 bp), intact flex (A-B band, ∼600 bp) and switched flex allele (B-C band, ∼700 bp) (Bartels, 2009). The Cacna1d- (“Cav1.3-”) allele was genotyped with the primers: “Cav1.3 sense (s)” (5′-GCA AAC TAT GCA AGA GGC ACC AGA-3′), “Cav1.3 antisense (as)” (5′-TAC TTC CAT TCC ACT ATA CTA ATG CAG GCT-3′) and “Cav1.3 neosense (ns)” (5′-TTC CAT TTG TCA CGT CCT GCA CCA-3′) yielding a wildtype (s-as, ∼300 bp) and/or a knockout band (s-ns, ∼450 bp).
Hearing Measurements
Auditory brainstem responses (ABR) and distortion product otoacoustic emissions (DPOAE) were recorded in anesthetized mice aged 4–6 weeks as described in Fell et al. (2016). Growth functions of ABR waves I to IV in response to click stimuli were analyzed for peak-to-peak amplitudes and latencies between the click stimulus delivered at t = 0 and the time point of the negative peak of the respective wave.
Electrophysiological Recordings
Apical-turn organs of Corti were acutely dissected from young adult mice (P17–P23) in solution containing (in mM): 70 lactobionate⋅NaOH, 83 NaCl, 10 HEPES, 5.8 KCl, 5.3 glucose, 1.3 CaCl2, 0.95 MgCl2, 0.7 NaH2PO4. For Ba2+ current recordings, the bath solution contained (in mM): 72.5 lactobionate⋅NaOH, 40 NaCl, 35 TEA, 15 4-AP, 10 BaCl2, 10 HEPES, 5.6 glucose, 0.9 MgCl2. Both solutions were adjusted to pH 7.35, 320 mosmol kg-1. Quartz pipettes were used and filled with (in mM): 112 Cs+ methane sulfonate, 20 CsCl, 10 Na+ phosphocreatine, 5 HEPES, 1 EGTA, 4 MgCl2, 4 Na2ATP, 0.3 GTP, 0.1 CaCl2. Pipette solution was adjusted to pH 7.36, 305 mosmol kg-1.
Before performing whole-cell patch clamp recordings using an Optopatch (Cairn Research, United Kingdom) or an Axopatch 200B amplifier (Molecular Devices, United States), green fluorescent protein (GFP) fluorescence of the specimen was assessed with an epifluorescence system consisting of a UV lamp and FITC fluorescence filters attached to the patch microscope (Olympus BX51WI with a 40 x water immersion objective, Germany) and a CCD camera (Scientifica, United Kingdom). Ba2+ currents were elicited by depolarizing the cells for 8 ms from –98 to +48 mV in 5 mV increments. Uncompensated series resistance was corrected by 70–80%. Analysis, including off-line linear leak subtraction and correction of the currents by subtracting the liquid junction potential of 8 mV, was performed using Igor Pro software (Wavemetrics, United States). I–V relations were calculated as the average current taken from the last ms of the voltage step as a function of the respective voltage.
I–V curves of Ba2+ currents were fitted to a second-order Boltzmann function times Goldman-Hodgkin-Katz driving force to determine parameters of activation, the voltage of half-maximum activation, Vh, and the voltage sensitivity of activation determined by the slope factor k, according to
where I is IBa at the time point the I–V was calculated (average over 7–8 ms after depolarization); Pmax the maximum permeability; ν = zFV/(RT), with z being 2, F the Faraday constant, R the universal gas constant, T the absolute temperature, V the membrane potential. [Ba]i (set at 50 nM) and [Ba]o denote the intra- and extracellular Ba2+ concentration, respectively.
Immunohistochemistry
Immunolabeling was performed on whole-mount organs of Corti of 4–6 week-old mice as described in Fell et al. (2016) using Zamboni’s fixative for 8 min on ice. Specimens were labeled using antibodies against Cav1.3 (rabbit polyclonal, Alomone Labs, Israel, 1:500), BKα (rabbit polyclonal, Alomone Labs, Israel, 1:500; mouse monoclonal, antibodies-online, Germany, 1:500), GFP (goat polyclonal, Rockland, United States, 1:500), CtBP2/RIBEYE (mouse monoclonal, BD Transduction Laboratories, Germany, 1:100 – 1:200), SK2 (rabbit polyclonal, Sigma-Aldrich, Germany, 1:400), and calbindin (rabbit polyclonal, Swant Inc., Switzerland, 1:400). Primary antibodies were detected with Cy3-conjugated (donkey anti-rabbit: Jackson Immuno Research Laboratories, United Kingdom, 1:1500; donkey anti-goat: Abcam, United Kingdom, 1:1500) or Alexa 488-conjugated (anti-mouse: Invitrogen, United Kingdom, 1:500; anti-goat: Abcam, United Kingdom, 1:500) secondary antibodies. For immunolabeling experiments, at least three specimens of ≥3 animals were analyzed. z-stacks of fluorescence images were acquired using a confocal laser scanning microscope LSM710 (Carl Zeiss Microscopy GmbH, Germany). Images were analyzed with Fiji (Schindelin et al., 2012).
For quantification of Cav1.3 clusters and RIBEYE-positive ribbons, images of 67.5 μm × 38.9 μm size covering eight IHCs were acquired at equal laser and gain settings, and maximum intensity projections (MIPs) were calculated. The channel of interest of a MIP image was background subtracted. A thresholded binary image was created (0 below threshold; 1 above threshold) with thresholds of 10% of the maximum intensity of the green color channel (RIBEYE) and 17% of the red color channel (Cav1.3). Fluorescent dots < 0.05 μm2 were discarded. Size and number of clusters were analyzed using the particle count routine in Fiji and normalized to one IHC.
Statistics
Data are provided as mean ± SD, unless otherwise stated. Depending on the distribution of the data, Ba2+ current properties, as well as size and number of Cav1.3 clusters and ribbons were statistically analyzed using Student’s t-test or Mann–Whitney U test (MWU test; comparison of two groups) or using one-way ANOVA followed by Tukey post hoc test or Kruskal–Wallis test followed by Dunn-Holland-Wolfe post hoc test (comparison of > 2 groups) with Igor Pro software (WaveMetrics, United States) and SPSS statistics (IBM, Germany).
Statistical analysis of hearing measurements was performed with SPSS. Click ABR thresholds were analyzed using a one-way ANOVA, DPOAE amplitudes with a Kruskal–Wallis test and frequency-dependent ABR thresholds with a two-way ANOVA; all tests were followed by a Bonferroni post hoc test. ABR growth functions of amplitudes and latencies could not be tested by a two-way ANOVA due to unequal variances. Instead, a regression analysis was performed, and the parameters of the resulting regression lines (slope and y-axis intercept) were tested for differences using Student’s t-test or MWU test according to Sachs (1999).
Results
Cochlea-Specific Deletion of the Cav1.3 Channel Using Cav1.3-flex Mice With Cre Expression Under the Pax2 Promoter
In order to assess the phenotype of mice with cochlea-specific ablation of Cacna1d before birth, we analyzed GFP signals and whole-cell Ba2+ currents through Cav1.3 channels in IHCs of conditional knockout (cKO) Pax2::cre;Cacna1d-eGFPflex/flex mice, in short cKO-Cav1.3flex/flex. GFP fluorescence of two distinct intensity levels was present in IHCs of the apical cochlear turn acutely dissected from 3-week-old cKO-Cav1.3flex/flex mice (Figure 1B) but not in wildtype IHCs (Figure 1A). Analysis of Ba2+ currents (IBa) using 10 mM Ba2+ as a charge carrier in response to 8 ms step depolarizations revealed lack of IBa exclusively in those IHCs with a strong GFP signal (Figure 1D, green trace). In contrast, IBa was present in one IHC of cKO-Cav1.3flex/flex mice with weak (blue trace), one IHC without GFP fluorescence (gray trace, Figure 1D) and a wildtype IHC (Figure 1C). Corresponding individual peak I–V relations show that IBa was abolished in the IHC with strong GFP fluorescence whereas it was present in the two cKO-Cav1.3flex/flex IHCs with weak or no GFP fluorescence and in the wildtype IHC (Figure 1E). Averaged peak IBa from cKO-Cav1.3flex/flex IHCs with strong GFP (–7.9 ± 1.9 pA; n = 4) was significantly reduced compared with IHCs showing weak GFP fluorescence (–101.6 ± 14.2 pA; n = 3; P = 0.0262, MWU test, Figure 1F). We concluded that only those IHCs with strong fluorescence represented true knockout cells with two switched flex alleles whereas IHCs with weak fluorescence represented cells with one switched and one intact flex allele.
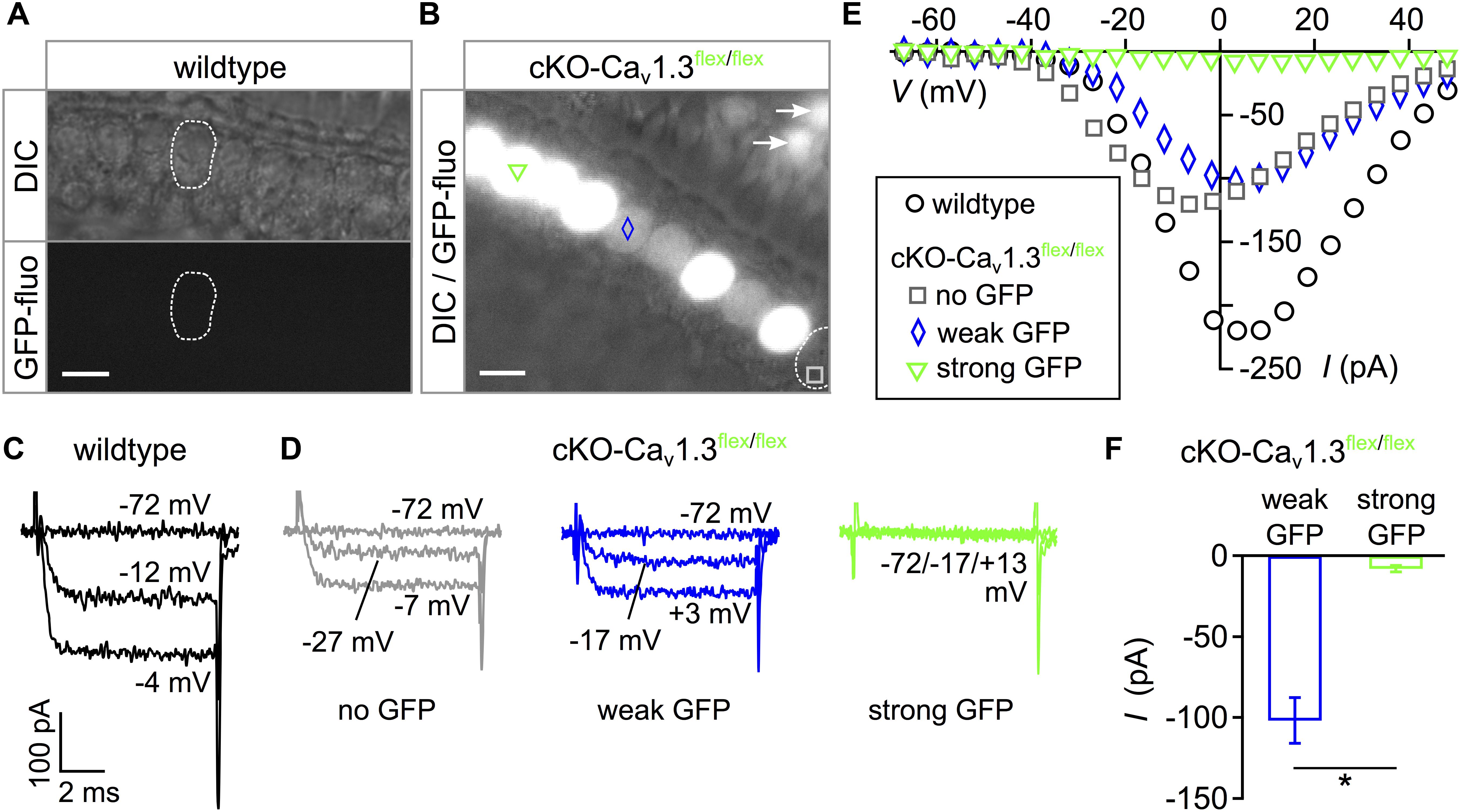
Figure 1. Intensity of eGFP fluorescence reflects success of Cav1.3 ablation in cKO-Cav1.3flex/flex mice. (A) Apical turn IHCs of a whole-mount organ of Corti from a 3-week-old wildtype mouse did not show eGFP fluorescence. Top: DIC, bottom: GFP fluorescence. (B) Heterogeneous eGFP fluorescence (merged with DIC image) in IHCs of a 3-week-old cKO-Cav1.3flex/flex mouse with either strong (green triangle), weak (blue diamond) or no (gray square) fluorescence, respectively. Arrows: eGFP-positive OHCs. Scale bar in panels (A,B): 10 μm. (C,D) Representative Ba2+ currents (IBa) in response to 8-ms step depolarizations to the voltages indicated of a wildtype (C) and three cKO-Cav1.3flex/flex IHCs (D) with no (gray), weak (blue) or strong (green) eGFP fluorescence. (E) Corresponding individual I–V curves averaged during the last ms of the depolarizing step. (F) Peak IBa ± SD averaged from IHCs with weak (blue; n = 3) and strong GFP fluorescence (green; n = 4) of cKO-Cav1.3flex/flex mice (MWU test, ∗P = 0.0262).
In order to increase the ratio of “true knockout” IHCs without remaining intact flex alleles, cKO-Cav1.3flex/flex mice were crossbred with Cav1.3-/- mice resulting in cKO-Cav1.3flex/- mice, in which Cre needed to cut and switch only one flex allele per cell. Here, GFP fluorescence was uniform in IHCs of acutely dissected organs of Corti from 3-week-old cKO-Cav1.3flex/- mice (Figure 2A). Typical IBa traces (Figures 2B,C) and corresponding I–V curves (Figure 2D) show that IBa was abolished in GFP-positive IHCs of cKO-Cav1.3flex/- mice (Figure 2C) but retained in control IHCs of Cav1.3flex/- mice (Figure 2B) where the flex allele was not switched due to absence of Cre. I–V curves further showed a reduction of IBa in control Cav1.3flex/- (gray) compared with wildtype IHCs (black, Figure 2D). The average peak IBa from cKO-Cav1.3flex/- IHCs was significantly reduced compared with control Cav1.3flex/- IHCs (Figure 2E, bars; MWU test, P < 0.001). Peak IBa from individual cKO-Cav1.3flex/flex IHCs demonstrate that the IBa amplitude of IHCs with strong GFP fluorescence (cf. Figure 1) resembled that of GFP-positive IHCs of cKO-Cav1.3flex/- mice whereas IBa values of IHCs with weak or no GFP fluorescence were similar to those of Cav1.3flex/- IHCs (Figure 2E, right). The lack of IBa was accompanied by a reduction in cell size as evident by a significantly reduced membrane capacitance (P < 0.001, Kruskal–Wallis Test) in cKO-Cav1.3flex/- mice (6.7 ± 0.6 pF; n = 15; P = 0.001, effect of genotype) but not in control Cav1.3flex/- mice (9.5 ± 1.4 pF; n = 10) compared with the wildtype (8.7 ± 1.0 pF; n = 10).
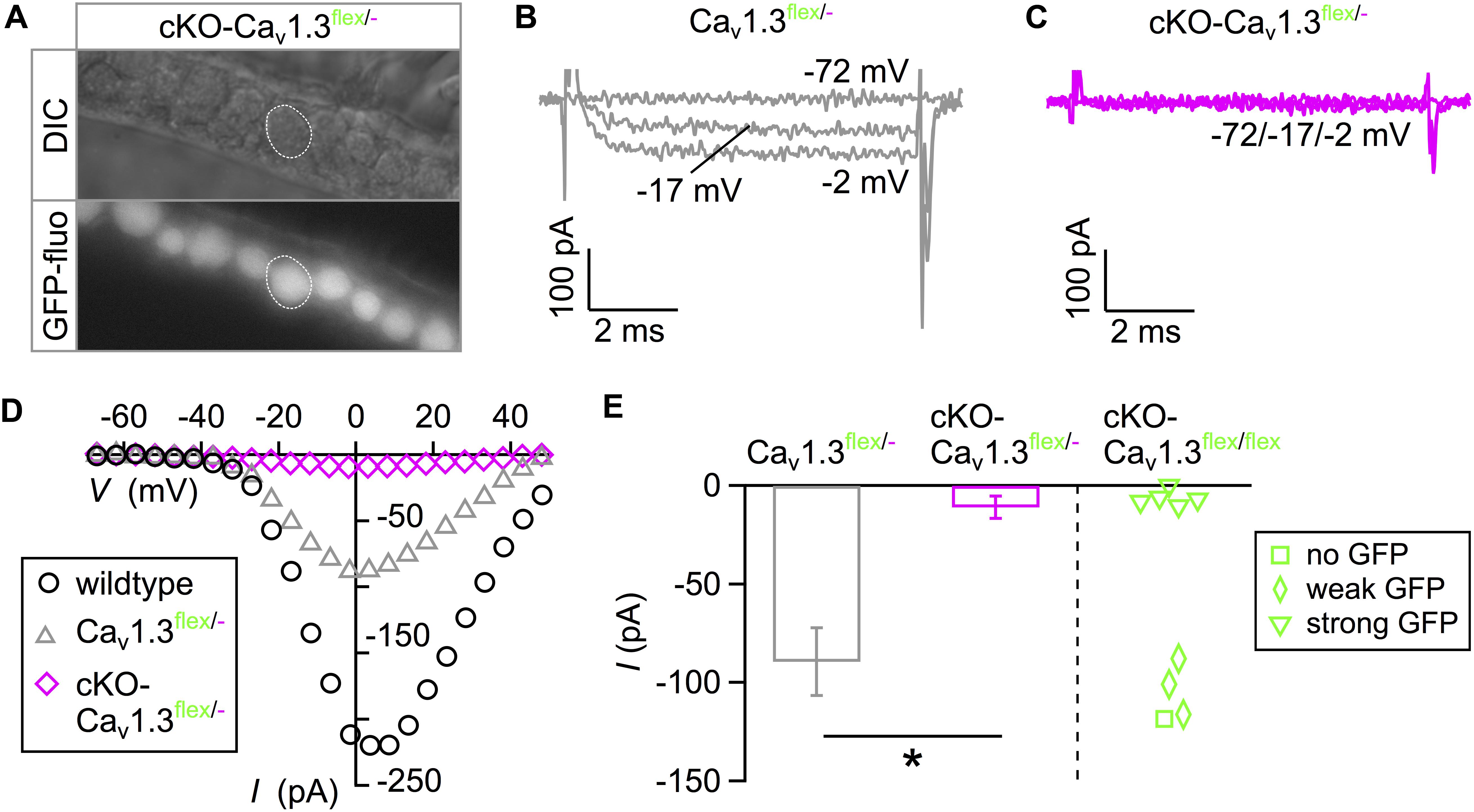
Figure 2. eGFP fluorescence faithfully reflects IBa ablation in IHCs of cKO-Cav1.3flex/- mice. (A) Uniform eGFP fluorescence (bottom) in IHCs (top: DIC image) from an apical cochlear turn of a 3-week-old cKO-Cav1.3flex/- mouse. (B,C) Representative IBa responses to 8-ms step depolarizations of a Cav1.3flex/- control (B) and an eGFP-positive cKO-Cav1.3flex/- IHC (C) to the voltages indicated. (D) I–V curves corresponding to panels (B) and (C) and a wildtype IHC (Figure 1C). (E) Left: Average peak IBa ± SD of 10 Cav1.3flex/- and 15 cKO-Cav1.3flex/- IHCs (MWU test, ∗∗∗P < 0.001). Right: IBa of individual IHCs with no (square; n = 1), weak (diamonds; n = 3) or strong (triangles; n = 5) eGFP fluorescence from cKO-Cav1.3flex/flex mice.
In summary, heterogeneous GFP expression and persistence of IBa in IHCs with weak GFP fluorescence of cKO-Cav1.3flex/flex mice show that (i) Cre did not faithfully switch both flex alleles at 3 weeks of age and (ii) GFP fluorescence is no reliable marker for deletion of Cav1.3 channels in IHCs of cKO-Cav1.3flex/flex mice. In contrast, in IHCs of cKO-Cav1.3flex/- mice containing only one flex allele, GFP fluorescence unequivocally indicated a cellular knockout genotype.
GFP Toxicity in IHCs of cKO-Cav1.3flex/flex Mice
In Cav1.3-/- mice, mild degeneration of IHCs has been reported in the apical cochlear turn starting after P20 and in the basal cochlear turn after P35 (Platzer et al., 2000; Glueckert et al., 2003; Nemzou et al., 2006). Degeneration of IHCs after cochlea-specific deletion of Cav1.3 was analyzed in organs of Corti of 4–5 week-old cKO-Cav1.3flex/flex and cKO-Cav1.3flex/- compared with Cav1.3-/- mice, which were double-immunolabeled for GFP and the hair-cell marker calbindin (Figure 3 and Table 1).
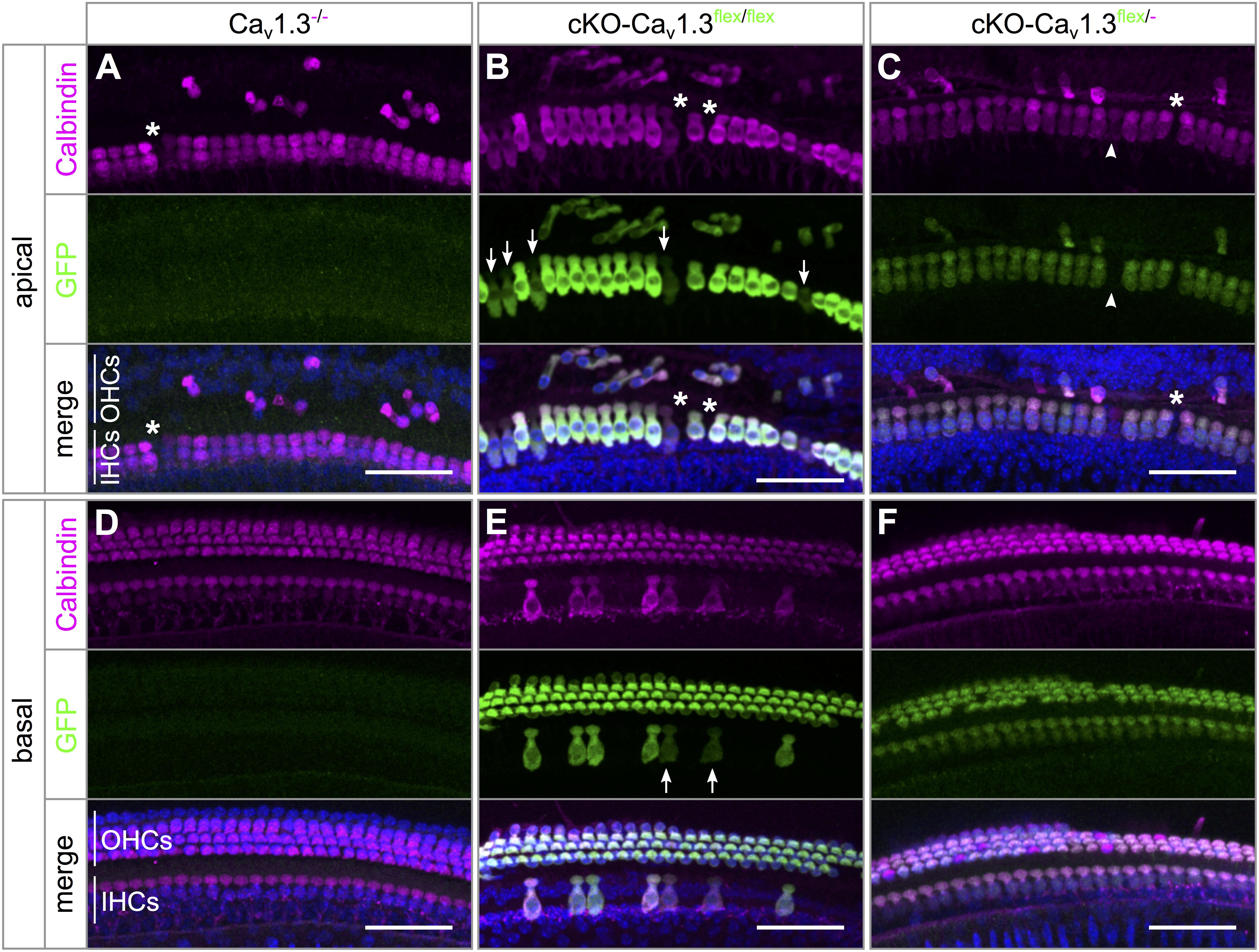
Figure 3. Pattern of eGFP immunolabeling and degree of basal-turn degeneration differ between IHCs of cKO-Cav1.3flex/flex and cKO-Cav1.3flex/- mice. Maximum intensity projections (MIP) of confocal stacks of whole-mount organs of Corti from 4 to 5 week-old mice. Stretches of ∼25 IHCs and adjacent OHCs from apical (A–C) and basal (D–F) cochlear turns of a Cav1.3-/- (A,D), a cKO-Cav1.3flex/flex (B,E) and a cKO-Cav1.3flex/- mouse (C,F) were co-immunolabeled for the hair cell marker calbindin (magenta, top) and for GFP (green, middle). Merged colors are shown below with DAPI-stained nuclei (blue). (A–C) GFP labeling was irregular among IHCs of a cKO-Cav1.3flex/flex mouse (arrows: IHCs with weak GFP labeling), but regular among IHCs of a cKO-Cav1.3flex/- mouse (arrowhead: IHC without GFP signal). Independently of the genotype, Cav1.3-deficiency in the apical cochlear turn resulted in degeneration of few IHCs [asterisks in panels (A–C)], whereas the majority of OHCs were degenerated. (D) No degeneration of IHCs or OHCs in the basal cochlear turn of a Cav1.3-/- mouse. (E) In a cKO-Cav1.3flex/flex mouse, the majority of IHCs was degenerated, with the remaining IHCs showing heterogeneous GFP labeling (arrows: weak GFP signal). (F) Uniform GFP labeling in IHCs and lack of hair cell degeneration in the basal turn of a cKO-Cav1.3flex/- mouse. Scale bar: 50 μm.
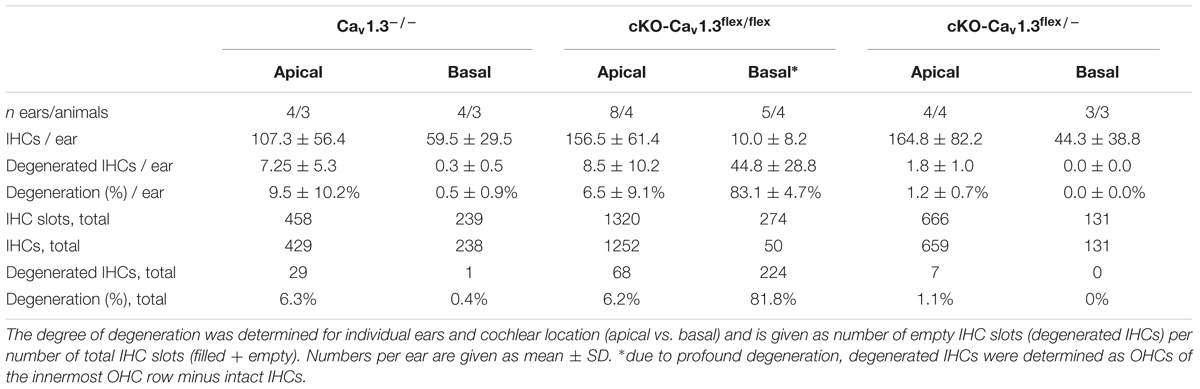
Table 1. Degeneration of IHCs in the apical and basal cochlear turn of mice with systemic (Cav1.3-/-) and cochlea-specific deletion of Cav1.3 with GFP reporter function (cKO-Cav1.3flex/flex and cKO-Cav1.3flex/-).
IHCs of all three genotypes showed mild IHC loss of ≤6.3% in the apical turn (Figures 3A–C and Table 1). In contrast, the majority (81.3%) of basal-turn IHCs of cKO-Cav1.3flex/flex mice was missing (Figure 3E and Table 1). This pronounced degeneration was not caused by lack of Cav1.3 because basal-turn IHCs of Cav1.3-/- and cKO-Cav1.3flex/- mice did not show any degeneration (<0.5%; Figures 3D,F and Table 1). We conclude that high expression levels of GFP caused by two functional flex alleles in cKO-Cav1.3flex/flex mice (cf. Figure 1A) resulted in a toxic effect of GFP on basal IHCs. The lack of IHC degeneration in the basal cochlea from cKO-Cav1.3flex/- mice suggests a dose-dependent toxicity of GFP that requires more than one functional GFP allele.
The majority of outer hair cells (OHCs) from the apical (Figures 3A–C) but not basal cochlear turn (Figures 3D–F) were degenerated, as described before for Cav1.3-/- mice (Platzer et al., 2000; Glueckert et al., 2003; Engel et al., 2006). Thus, cochlea-specific deletion of Cav1.3 channels coupled to GFP expression resulted in a similar degeneration of apical turn OHCs as observed in systemic Cav1.3-/- mice.
The patterns of IHC GFP labeling in either cKO-Cav1.3flex/flex (Figures 3B,E) or cKO-Cav1.3flex/- mice (Figures 3C,F) were similar to the eGFP fluorescence patterns in acutely dissected organs of Corti from these genotypes (Figures 1A, 2A). GFP labeling intensity was heterogeneous between individual IHCs of cKO-Cav1.3flex/flex mice with either intense or weak (arrows) labeling (Figures 3B,E) but uniform in IHCs of cKO-Cav1.3flex/- mice (Figures 3C,F), with few individual IHCs not being labeled (Figure 3C, arrowheads).
The rate of true knockout IHCs was assessed by quantification of apical-turn organs of Corti immunolabeled for GFP, Cav1.3 and/or BK channels (Table 2). In cKO-Cav1.3flex/- mice, the knockout rate was 89.2%, which was only slightly higher than the knockout rate of 87.4% in cKO-Cav1.3flex/flex mice at 4–5 weeks of age. Although replacing one flex allele by a knockout (–) allele increased the success rate of Cre in switching one flex allele at 3 weeks of age (cf. Figures 1A,B, 2A), Cre caught up in switching both flex alleles in cKO-Cav1.3flex/flex mice 2 weeks later.
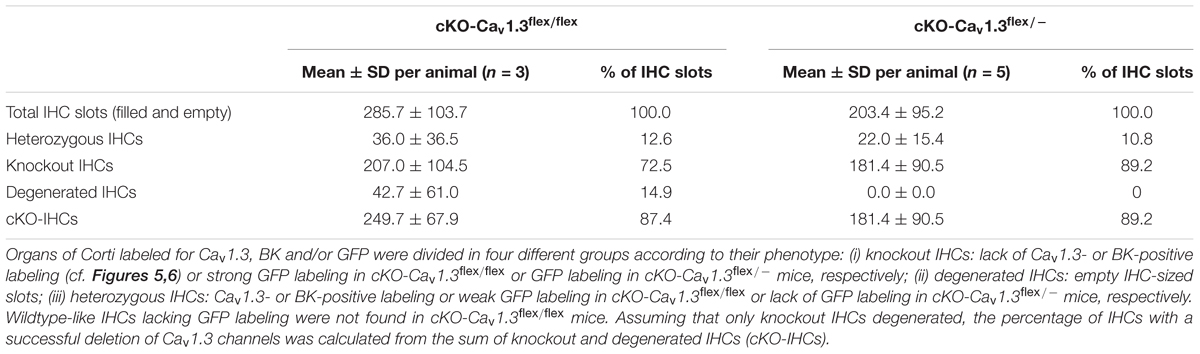
Table 2. Rate of successful flex switch in IHCs from the apical cochlear turn of cKO-Cav1.3flex/flex and cKO-Cav1.3flex/-mice at 4–6 weeks of age.
In conclusion, the percentage of knockout IHCs finally was not increased by replacement of one flex allele with a knockout (–) allele to obtain cKO-Cav1.3flex/- mice. However, we found that in cKO-Cav1.3flex/flex mice (i) basal-turn IHCs degenerated, most likely due to dose-dependent GFP toxicity and (ii) GFP expression in IHCs was not unequivocally associated with deletion of Cav1.3 channels, thus leading us to further use cKO-Cav1.3flex/- instead of cKO-Cav1.3flex/flex mice.
Similar Phenotypes of IHCs From cKO-Cav1.3flex/- and Systemic Cav1.3-/- Mice
In wildtype mice, up-regulation of BK K+ channels around the onset of hearing (P12) and down-regulation of neonatal SK2 K+ channels mark the end of terminal maturation and the onset of the mature function of IHCs (Figure 4A) (Kros et al., 1998; Marcotti et al., 2004). In systemic Cav1.3-/- mice, IHCs maintain an immature-like ion channel composition with persistent expression of SK2 but lack of BK K+ channels (Brandt et al., 2003; Engel et al., 2006; Nemzou et al., 2006). The failure of acquiring a mature composition of K+ channels may have been caused by lack of Cav1.3 currents (i) in the IHC itself or (ii) in brainstem nuclei causing an altered efferent input on the IHC (Hirtz et al., 2011, 2012; Satheesh et al., 2012). Precise timing and patterning of Ca2+ action potentials generated by IHCs during a critical period before the onset of hearing are crucial for their maturation (Johnson et al., 2013a). Altered neuronal activity of the efferent input onto neonatal IHCs therefore might also affect their Ca2+ action potentials and hence their development.
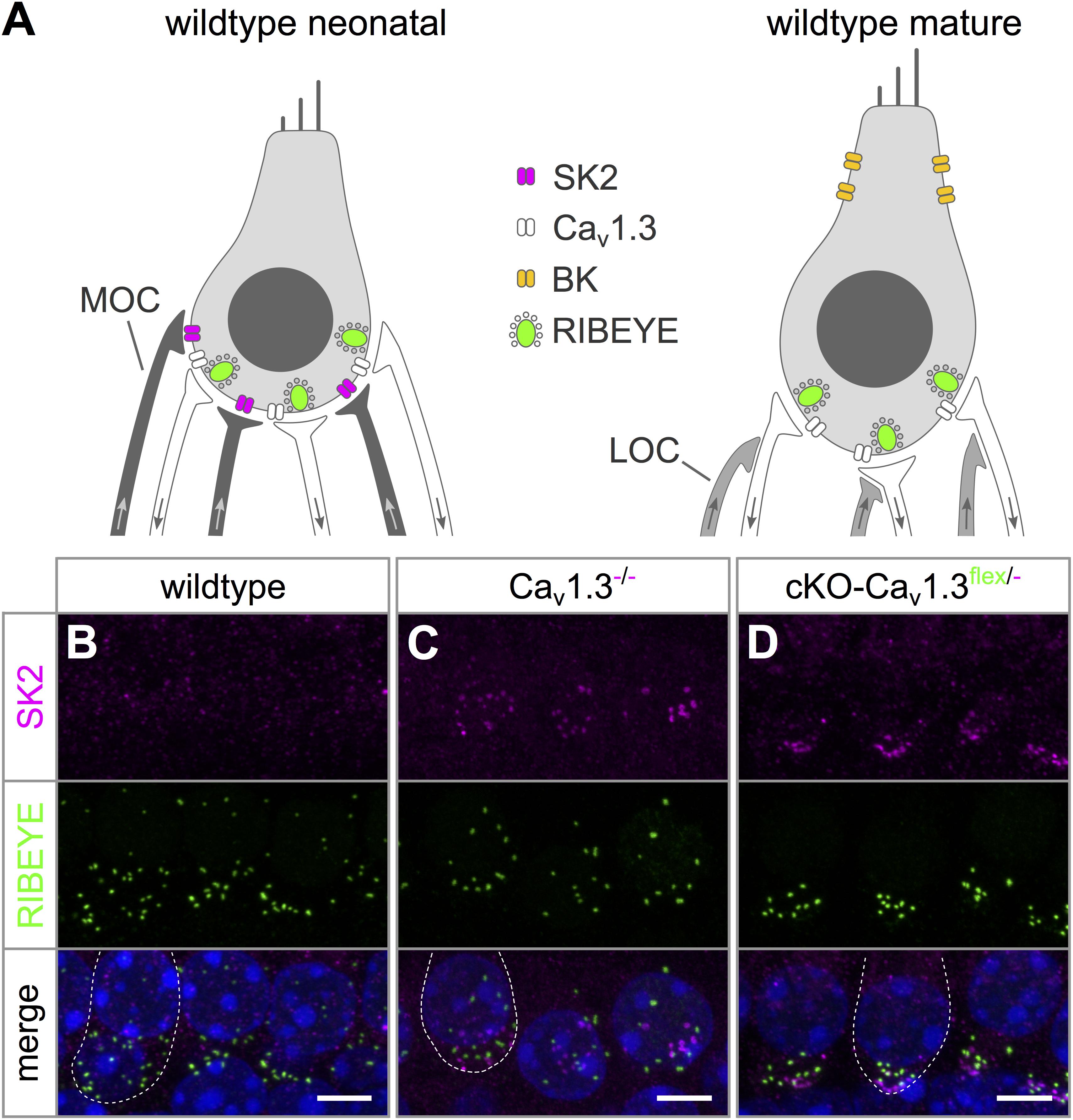
Figure 4. Persistence of SK2 channels in IHCs of cKO-Cav1.3flex/- mice. (A) Sketch of a wildtype IHC before (left, neonatal) and after the onset of hearing (right, mature) depicting differences in shape, ion channel composition and innervation. Immature IHCs express SK2 channels (magenta) at the basal pole, which are down-regulated after the onset of hearing, and do not possess BK channels. At the onset of hearing, BK channels are up-regulated and localize mainly to the neck of mature IHCs. Medial olivocochlear (MOC) efferent fibers of the SOC (dark gray) innervate IHCs of neonatal mice. Whereas mature IHCs lack direct efferent innervation, their afferent fibers receive lateral olivocochlear (LOC) efferent fibers (light gray). (B) SK2 labeling (top, magenta) was absent from apical-turn wildtype IHCs at 4–6 weeks of age. (C,D) Dot-like SK2 labeling was present at the basal pole, but not co-localized with synaptic ribbons (RIBEYE, middle, green) of apical-turn IHCs of a Cav1.3-/-(C) and a cKO-Cav1.3flex/- mouse at the age of 4–6 weeks. The merged image is shown with nuclei stained in blue with DAPI. Scale bars: 5 μm.
SK2 immunolabeling was localized at the basolateral pole apart from synaptic ribbons (RIBEYE) of apical turn IHCs from 4 to 5 week-old cKO-Cav1.3flex/- and Cav1.3-/- mice (Figures 4C,D) indicating an immature phenotype. In contrast, no SK2 labeling was found at the basolateral pole of wildtype IHCs (Figure 4B).
BK channel expression was assessed in wildtype and Cav1.3-/- IHCs co-labeled with calbindin (Figures 5A–C) and in Cav1.3flex/- controls, cKO-Cav1.3flex/flex and cKO-Cav1.3flex/- IHCs co-labeled with GFP (Figures 5D–F). BK channels, which are indicators of a mature IHC phenotype, were present at the neck of wildtype IHCs (Figure 5A). In Cav1.3-/- mice, BK labeling was absent from apical-turn IHCs (Figure 5B), whereas sparse and faint labeling was found in basal-turn IHCs (Figure 5C). In Cav1.3flex/- control IHCs, normal BK labeling was found at the neck of IHCs (Figure 5D). In true cKO IHCs i.e., IHCs with strong GFP labeling in cKO-Cav1.3flex/flex mice (Figure 5E) and with GFP labeling in cKO-Cav1.3flex/- mice (Figure 5F), BK labeling was missing. Unexpectedly, BK immunolabeling in heterozygous IHCs of both cKO genotypes, i.e., IHCs with weak (cKO-Cav1.3flex/flex, Figure 5E) or no GFP labeling (cKO-Cav1.3flex/-, Figure 5F), which appeared as small dots at the neck, clearly differed from the large BK patches in control Cav1.3flex/- (Figure 5D) or wildtype IHCs (Figure 5A). The GFP-negative IHCs of cKO-Cav1.3flex/- mice containing a non-switched flex allele should, however, have the same phenotype and thus the same BK labeling pattern as IHCs of control Cav1.3flex/- mice.
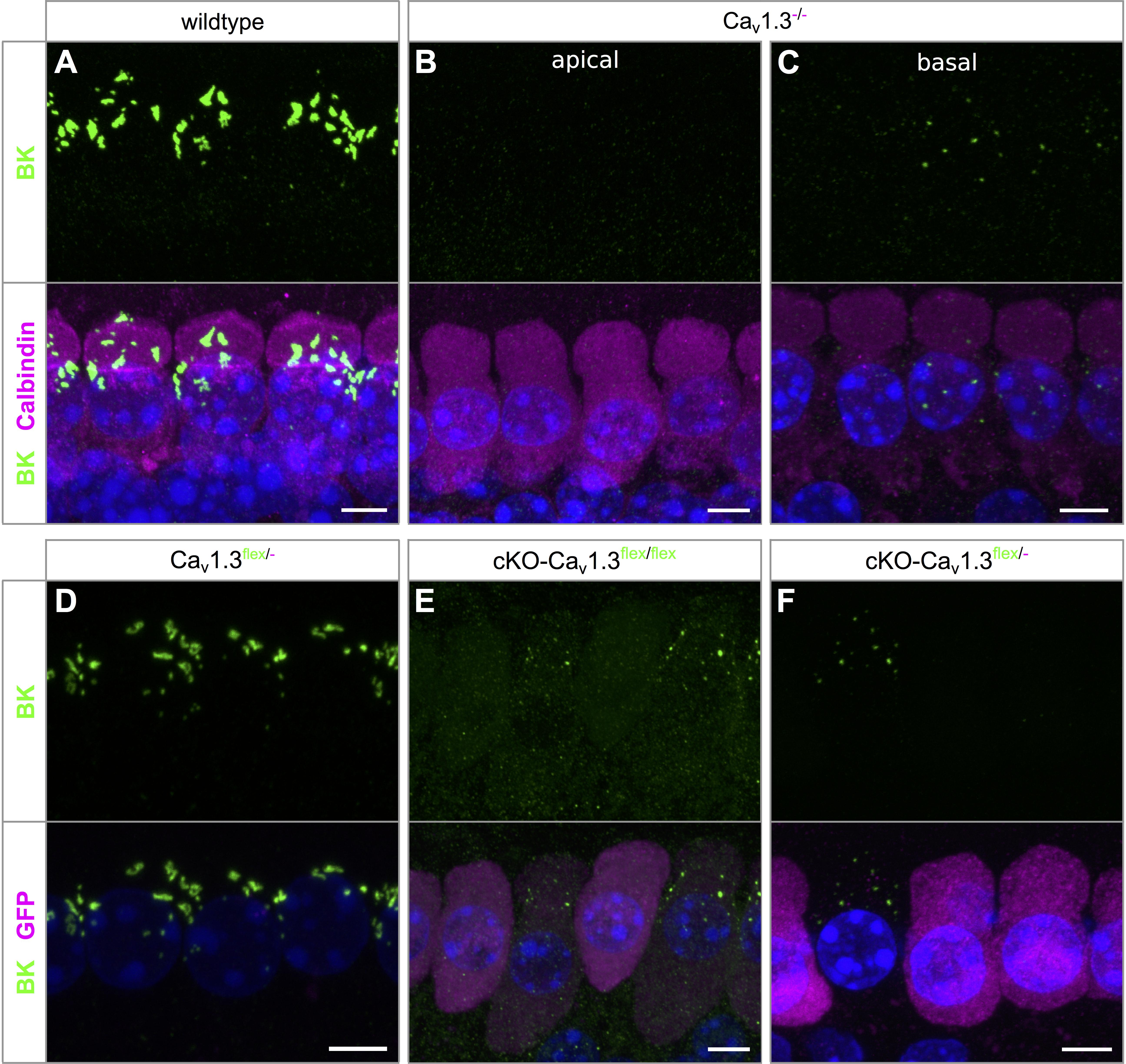
Figure 5. BK channel expression is related to reduced GFP labeling in cKO-Cav1.3flex/flex and cKO-Cav1.3flex/- IHCs. (A–C) MIP of confocal stacks of IHCs labeled for BK (green) and calbindin (magenta) of a wildtype (A) and a Cav1.3-/- mouse (B,C) at the age of 4–5 weeks. (A) Large spots of BK immunoreactivity at the neck of wildtype IHCs. (B,C) In Cav1.3-/- IHCs, BK immunolabeling was absent from apical-turn IHCs (B) and vastly reduced in IHCs of the basal turn (C) compared with apical-turn wildtype IHCs (A). (D–F) Co-labeling for BK channels (green) and GFP (magenta) in apical-turn IHCs of a control Cav1.3flex/- (D), a cKO-Cav1.3flex/flex (E) and a cKO-Cav1.3flex/- mouse (F). (D) Normal, wildtype-like BK labeling at the neck in control Cav1.3flex/- IHCs. (E,F) BK labeling was absent from those IHCs of the cKO-Cav1.3flex/flex mouse with strong GFP labeling (E) and from GFP-positive IHCs of the cKO-Cav1.3flex/- mouse (F). Note dot-like BK labeling in those IHCs of the cKO-Cav1.3flex/flex mouse with weak GFP expression (E) and in a GFP-negative IHC of the cKO-Cav1.3flex/- mouse (F), both indicative of a non-switched flex allele. Scale bar: 5 μm.
Reduced Ba2+ Currents and Cav1.3 Protein Clusters in Control Cav1.3flex/- IHCs
We noticed that expression of one or two flex alleles without Cre resulted in smaller IHC Ba2+ currents compared with wildtype IHCs (cf. Figures 1D,E, 2B–E). However, in conditional mouse lines, the function of the target gene should remain unaltered unless it is deleted or manipulated by Cre or other recombinases. For generating the conditional Cacna1d construct, loxP sites were inserted in intronic regions flanking exon 2 of the Cacna1d gene, which should not impair its function (Satheesh et al., 2012). To determine the side effect of the construct in the Cacna1d-eGFPflex allele, we measured IBa in IHCs of mice with different combinations of wildtype (+), flex and knockout (-) alleles, i.e., Cav1.3+/-, Cav1.3+/flex, Cav1.3flex/flex and Cav1.3flex/- compared with wildtype mice (Figure 6). Averaged peak IBa amplitudes of IHCs from Cav1.3flex/flex (–102.7 ± 16.4 pA; n = 10) and Cav1.3flex/- mice (–89.5 ± 17.2 pA; n = 10) were significantly reduced compared with wildtype (–212.4 ± 48.2 pA; n = 10; P < 0.001, Kruskal–Wallis Test; Figure 6A). IBa normalized to the wildtype (100%) was reduced to 48.4% in Cav1.3flex/flex and 42.1% in Cav1.3flex/- IHCs, respectively (Figure 6B). In mice with only one wildtype (+) allele, IBa was slightly but not significantly reduced to –171.6 ± 50.9 pA or 80.9% (Cav1.3+/-; n = 10) and –149.5 ± 36.5 pA or 70.4% (Cav1.3+/flex; n = 7; Figures 6A,B), respectively. In contrast, IBa was reduced to 5.1% in IHCs of cKO-Cav1.3flex/- mice indicating a complete loss of Cav1.3 channels leaving a small residual Ca2+ current that has been described before in the systemic knockout (Platzer et al., 2000; Brandt et al., 2003; Dou et al., 2004). Additionally, Cre expression in the cochlea did not affect IBa in IHCs of Pax2::cre control mice (–216.4 ± 53.1 pA; n = 10; Figure 6B). Analysis of gating properties by fitting the I–V curves to a second-order Boltzmann function times Goldman-Hodgkin-Katz driving force yielded a small but significant shift of Vh by –2.5 mV in Cav1.3flex/- (–12.6 ± 2.0 mV; n = 10) versus wildtype IHCs (–10.1 ± 2.3 mV; n = 10; P = 0.019, MWU test), whereas the voltage sensitivity of activation determined by the slope factor k was unaffected (Cav1.3flex/-: 11.22 ± 0.97 mV; wildtype: 11.26 ± 0.30 mV; P = 0.762, MWU test).
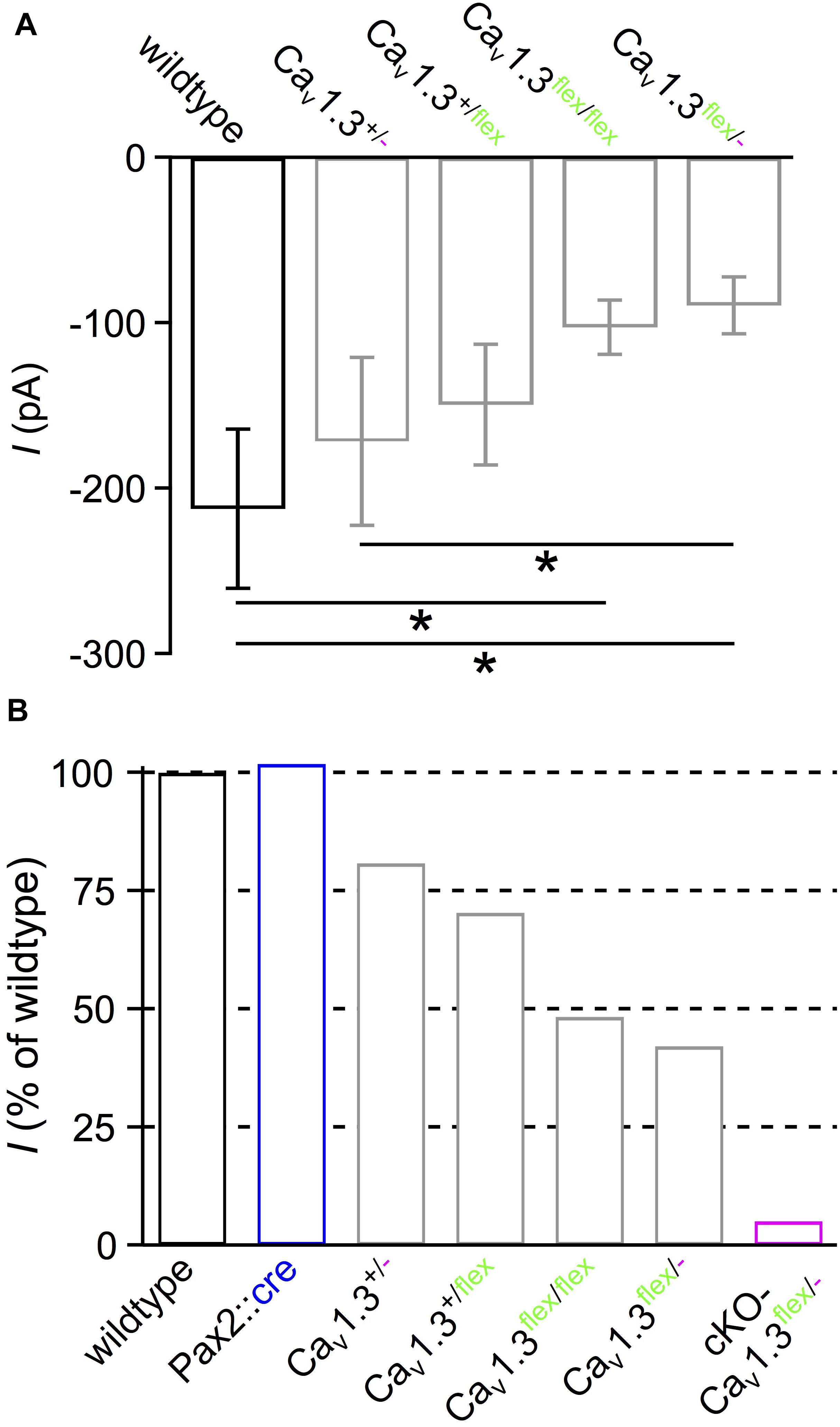
Figure 6. Reduced IBa amplitude in IHCs with unswitched flex alleles. (A) Averaged peak IBa ± SD of 10 wildtype, 10 Cav1.3+/-, 7 Cav1.3+/flex, 10 Cav1.3flex/flex and 10 Cav1.3flex/- IHCs reveal that IBa was reduced when at least one wildtype allele was replaced by a flex allele (∗ effect of genotype; P < 0.001, Kruskal–Wallis Test). (B) Percentage of average peak IBa of IHC groups from panel (A), 10 Pax2::cre controls and 15 cKO-Cav1.3flex/- IHCs (Figure 2E), all normalized to the wildtype (100%).
In summary, reduction of IBa amplitude in IHCs of control Cav1.3flex/flex and Cav1.3flex/- mice, as well as altered gating properties in Cav1.3flex/- control IHCs demonstrate that the unswitched Cacna1d flex allele functionally does not fully replace the wildtype allele.
The functional reduction of Cav1.3 channels might be caused by a reduced amount of Cav1.3 channel protein in the IHC membrane or by a reduced function of Cav1.3 channels in Cav1.3flex/- mice. The abundance of Cav1.3 channel protein was assessed by co-immunolabeling for Cav1.3 (magenta) and synaptic ribbons (RIBEYE, green, Figure 7). Cav1.3 clusters were localized at the synaptic ribbons of wildtype IHCs (Figure 7A,a) and at the majority of ribbons of IHCs from Cav1.3flex/- control mice (Figure 7C,c). In contrast, no specific Cav1.3 labeling was found at the synapses of Cav1.3-/- IHCs (Figure 7B,b) and most, but not all IHCs of cKO-Cav1.3flex/- mice (Figure 7D,d′). In part of the IHCs from cKO-Cav1.3flex/- mice, Cav1.3 labeling was still present at the synaptic ribbons (Figure 7D,d″) indicating that the flex allele was not switched in these cells. Synaptic ribbons (RIBEYE) of Cav1.3-deficient IHCs from Cav1.3-/- and cKO-Cav1.3flex/- mice were agglomerated and localized closer to the nucleus (Figures 7B,D) as described before (Nemzou et al., 2006).
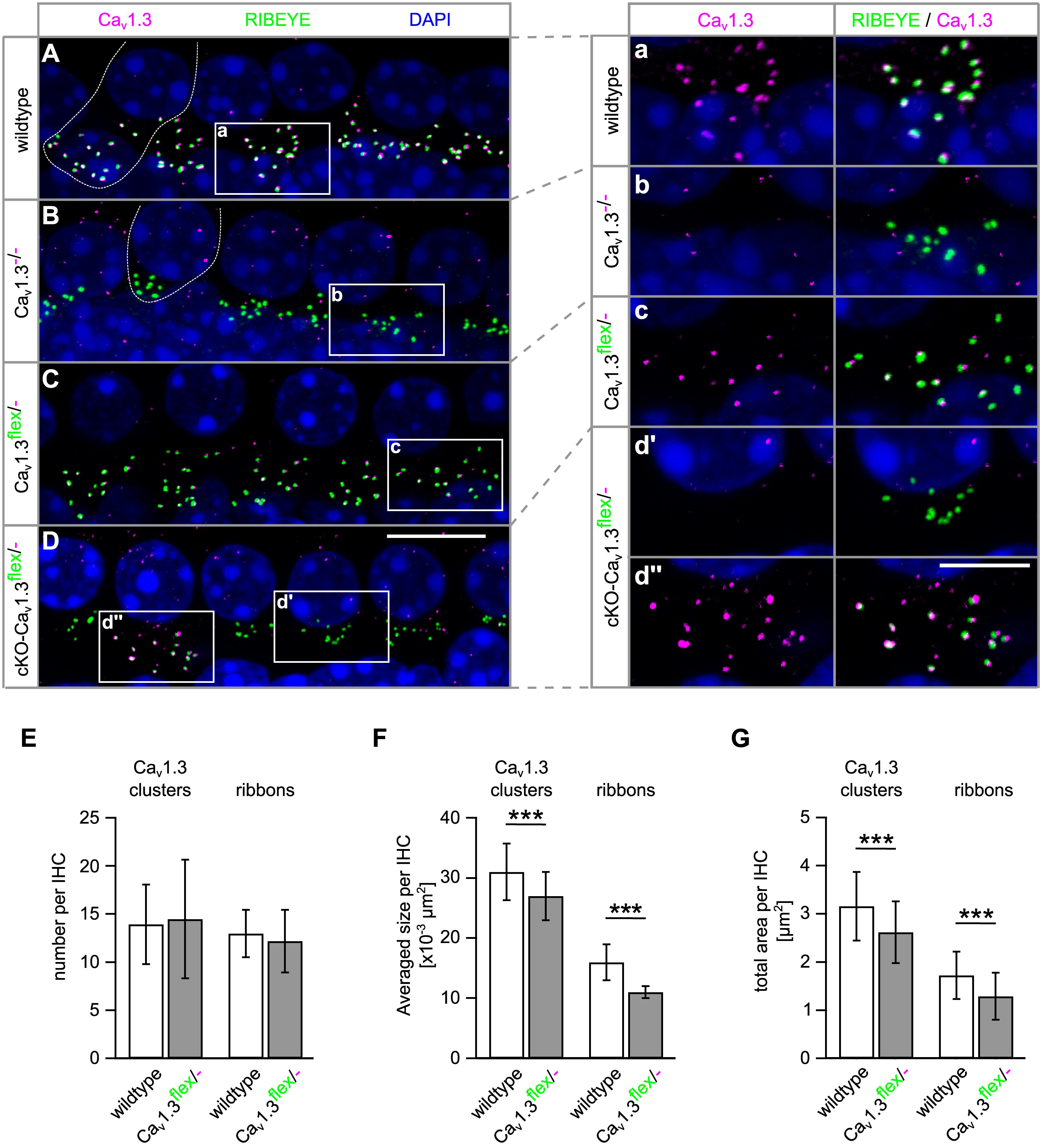
Figure 7. Presynaptic Cav1.3 clusters are missing at the IHC synapse of cKO-Cav1.3flex/- mice and are smaller at IHC synapses of Cav1.3flex/- control mice. (A–D) MIP of confocal stacks of whole-mount preparations of apical turn organs of Corti co-immunolabeled for Cav1.3 and RIBEYE at 4 weeks of age. Stretches with four to five IHCs of a wildtype (A), a Cav1.3-/- (B), a Cav1.3flex/- control (C) and a cKO-Cav1.3flex/- mouse (D) labeled with anti-Cav1.3 (magenta) and anti- RIBEYE (green) are shown, with one wildtype and one Cav1.3-/- IHC indicated by a dashed line in panels (A) and (B). (a–d″) Enlarged synaptic poles of IHCs from boxes in panels (A–D) shown with split color channels and as merge. (E–G) Quantitative analysis of the Cav1.3 clusters and RIBEYE-labeled ribbons in IHCs of Cav1.3flex/- control and wildtype mice. (E) Number of Cav1.3 clusters (P = 0.69) and ribbons per IHC (P = 0.074) were unaffected in Cav1.3flex/- control mice (MWU test). (F) Average sizes per IHC of both, Cav1.3 clusters and ribbons were reduced in Cav1.3flex/- control mice (∗∗∗P < 0.001, MWU test). (G) This reduction also applied to the total area of Cav1.3 clusters and of ribbons per IHC in Cav1.3flex/- control mice (∗∗∗P < 0.001, Student’s t-test). Number of ears/animals/images analyzed (with eight IHCs per image), respectively: wildtype, 4/2/56; Cav1.3flex/- control mice: 5/3/100; scale bars: (A–D), 10 μm; (a–d″), 5 μm.
To elucidate the cause of the reduced IBa amplitude (42% of wildtype, Figures 6A,B) in IHCs of Cav1.3flex/- control mice, a quantitative analysis of the size and number of Cav1.3 clusters and synaptic ribbons was performed (Figures 7E–G). Whereas the number of Cav1.3 clusters and ribbons was unchanged, the average size of both Cav1.3 clusters and synaptic ribbons was significantly reduced to 73 and 89% in Cav1.3flex/- control IHCs compared with wildtype (Figure 7F). This reduction in size also applied to the total area of Cav1.3 clusters to 75% and of ribbons to 83% of the total areas in wildtype, respectively (Figure 7G). In conclusion, less Cav1.3 protein was produced in IHCs of Cav1.3flex/- mice evident by reduced IBa amplitudes and smaller Cav1.3 channel clusters, which was accompanied by smaller ribbons.
Profound Hearing Loss in cKO-Cav1.3flex/- and Mild Hearing Impairment in Control Cav1.3flex/- Mice
Next, we assessed how the loss/reduction of Cav1.3 channels affected the auditory function of cKO-Cav1.3flex/- and control mice. In 4–6 week-old cKO-Cav1.3flex/- mice, click-evoked ABR thresholds (Figure 8A) were absent (threshold > 100 dB SPL) in 4/3 ears/animals and significantly elevated in the remaining 12/7 out of 16/8 ears/animals (81.3 ± 8.0 dB SPL; P < 0.001) compared with wildtype mice (17.9 ± 6.7 dB SPL, 14/7 ears/animals). In contrast, click ABR thresholds were unaffected in all control groups (Pax2::cre: 13.8 ± 7.6 dB SPL, 16/8 ears/animals; Cav1.3+/flex: 16.3 ± 4.3 dB SPL, 12/6 ears/animals; Cav1.3flex/-: 20.4 ± 4.1 dB SPL; 14/7 ears/animals; P > 0.05; one-way ANOVA with Bonferroni post hoc test, Figure 8A). Frequency-dependent ABR (f-ABR) thresholds (Figure 8B) could only be measured in part of the cKO-Cav1.3flex/- mice analyzed (16/8 ears/animals, single data points) and were thus not included in the statistical analysis. f-ABR thresholds of Pax2::cre (15/8 ears/animals) and Cav1.3flex/- (14/7 ears/animals) significantly differed from wildtype mice (14/7 ears/animals; two-way ANOVA with Bonferroni post hoc test, effect of genotype: P < 0.001). Specifically, thresholds were increased in Cav1.3flex/- control mice (P < 0.001), reaching significance at all frequencies except at 22.6 kHz; and slightly reduced in Pax2::cre mice (P = 0.001), reaching significance at 2 and 8 kHz.
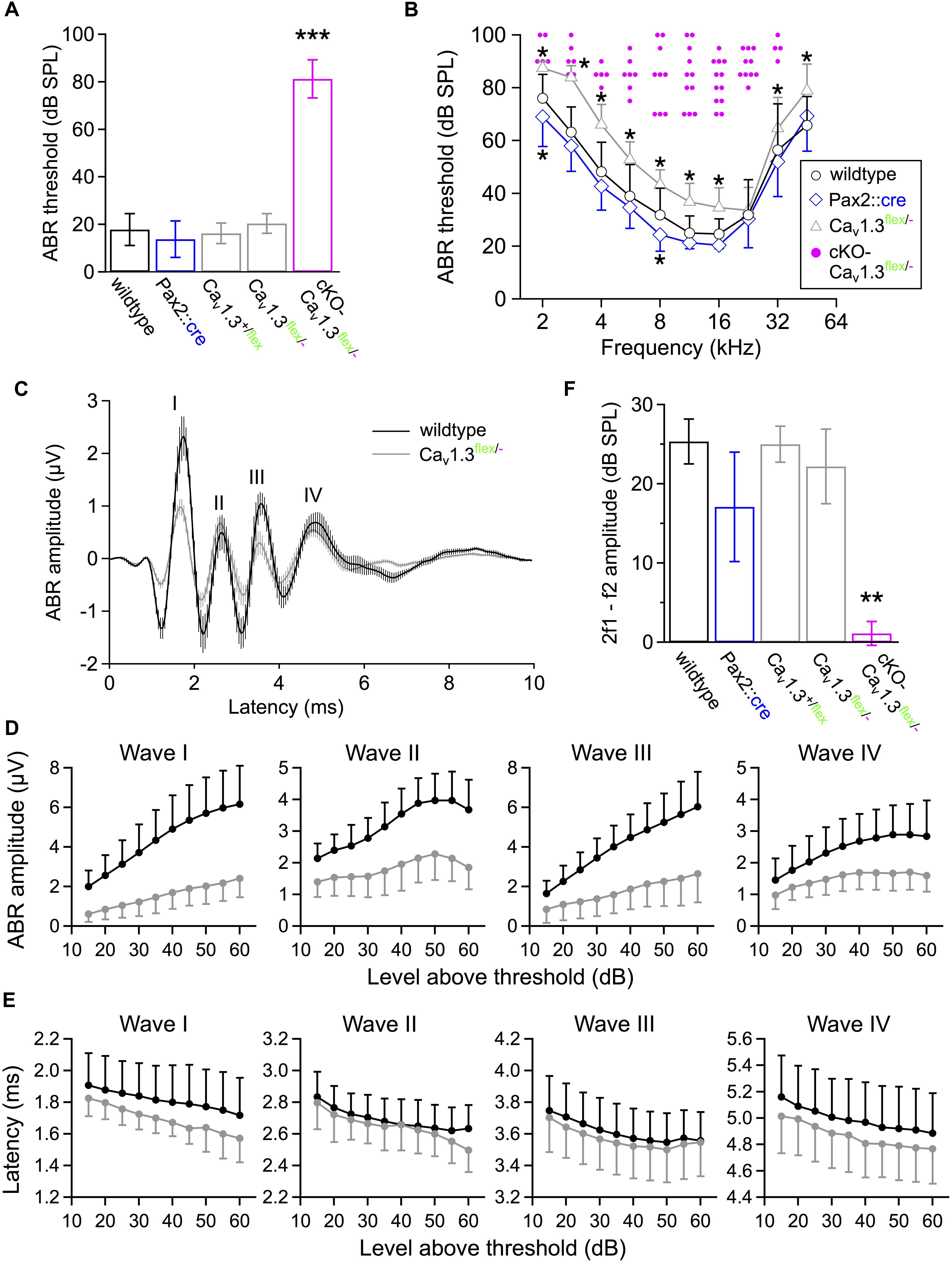
Figure 8. Hearing function of 4–6 week-old cKO-Cav1.3flex/- and control mice assessed by measurements of auditory brainstem responses (ABR) and distortion product otoacoustic emissions (DPOAE). (A) Click-evoked ABR thresholds (mean ± SD) were measured when ≤100 dB SPL in 12/7 out of 16/8 ears/animals of cKO-Cav1.3flex/- mice. They were significantly increased compared with wildtype (14/7 ears/animals) and controls (Pax2::cre, 16/8 ears/animals; Cav1.3+/flex, 12/6 ears/animals; Cav1.3flex/-, 14/7 ears/animals, ∗∗∗P < 0.001, one-way ANOVA with Bonferroni post hoc test). (B) Frequency-specific ABR thresholds of cKO-Cav1.3flex/- mice (single data, pink) could be determined in ears/animals: 2 kHz, 5/3; 2.8 kHz, 6/3; 4 kHz, 6/3; 5.6 kHz, 7/4; 8 kHz, 10/5; 11.3 kHz, 12/7; 16 kHz, 12/6; 22.6 kHz, 10/6; 32 kHz, 4/4; 45.2 kHz, 0/0; out of a total of 16/8 ears/animals recorded. Frequency-dependent ABR thresholds were increased in Cav1.3flex/- control mice (14/7 ears/animals; group effect of two-way ANOVA with Bonferroni post hoc test, P < 0.001; effect of genotype at 2, 2.8, 4, 5.6, 8, 11.3, 16, 32 and 45.2 kHz) and reduced in Pax2::cre control mice (15/8 ears/animals; group effect of two-way ANOVA with Bonferroni post hoc test, P < 0.001; ∗ effect of genotype at 2 and 8 kHz) compared with wildtype (14/7 ears/animals). (C) Averaged ABR waveforms to click stimuli at 40 dB above threshold (mean ± SEM) for 14/7 wildtype ears/animals (black) and 14/7 Cav1.3flex/- control ears/animals (gray). (D) Mean DPOAE maximum amplitudes ± SD with f1 starting at 10.0 kHz, L1 = 55 dB SPL, f2 = 1.2 × f1 averaged over 10–18 kHz in 0.5 kHz steps, and L2 = 45 dB SPL were reduced in cKO-Cav1.3flex/- mice (14/7 ears/animals) compared with wildtype (14/7 ears/animals) and controls (Pax2::cre, 16/8 ears/animals; Cav1.3+/flex, 12/6 ears/animals; Cav1.3flex/-, 14/7 ears/animals; Kruskal–Wallis with Bonferroni post hoc test, ∗∗P ≤ 0.008). (E,F) Growth functions of amplitudes and latencies of waves I to IV (mean ± SD) revealed reduced mean amplitude values for all four waves and all stimulus levels in Cav1.3flex/- control (gray; n = 14/7 ears/animal) compared with wildtype mice (black; n = 12–14/6–7 ears/animals). For clarity, the SD is plotted in one direction only (+SD or -SD).
Averaged ABR waveforms of Cav1.3flex/- controls had smaller amplitudes than in wildtype mice for click stimuli 40 dB above threshold (Figure 8C). Growth functions of peak-to-peak amplitudes showed a significant reduction of all waves in Cav1.3flex/- control (14/7 ears/animals) compared with wildtype (wave I, IV: 14/7 ears/animals; wave II, III: 12/6 ears/animals, Figure 8D), revealed by a regression analysis of the smaller slopes of fits to the amplitudes as a function of level above threshold of wave I to IV (wave I, P < 0.001; wave II, P = 0.015; wave III, P < 0.001; wave IV, P = 0.027; MWU test) and a smaller y-axis intercept value of wave I (MWU test, P = 0.002). Growth functions of latencies, calculated as time between stimulus application and the negative peak of the respective wave, were not significantly altered for all waves (I – IV) in Cav1.3flex/- control mice compared with the wildtype (Figure 8E).
Finally, we tested the function of the cochlear amplifier including OHC electromotility by measuring DPOAEs (Figure 8F). Mean 2f1–f2 DPOAE maximum amplitudes averaged between 10 and 18 kHz in 0.5 kHz steps were strongly reduced in cKO-Cav1.3flex/- mice (12/7 ears/animals, P < 0.001, Kruskal–Wallis Test, effect of genotype) but unaffected in all control groups (wildtype: 14/7 ears/animals; Pax2::cre: 16/8 ears/animals; Cav1.3+/flex: 12/6 ears/animals; Cav1.3flex/-: 14/7 ears/animals).
In summary, cochlea-specific deletion of Cav1.3 in cKO-Cav1.3flex/- mice resulted in highly elevated ABR thresholds and strongly reduced DPOAEs, reflecting profound hearing loss. Moreover, in Cav1.3flex/- control mice the reduction of mean IHC IBa amplitude to 42% and of mean Cav1.3 cluster size to 73% is accompanied by increases in f-ABR thresholds up to 10 - 20 dB and strongly reduced amplitudes of ABR waves I to IV. Notably, click-ABR thresholds and DPOAEs were not affected in Cav1.3flex/- control mice.
Discussion
Here we show that cochlea-specific ablation of Cav1.3 channels resulted in an IHC phenotype replicating that of systemic Cav1.3-/- mice. For obtaining a cochlea-specific Cav1.3 knockout mouse, we started with a conditional Cav1.3flex/flex mouse model that was crossed with a cochlea-specific Cre line, Pax2::cre. When determining Ca2+ channel currents through Cav1.3 channels we found inefficient switch of both flex alleles in mice aged 3 weeks. In addition, IHCs of the basal cochlear turn from cKO-Cav1.3flex/flex mice were largely degenerated at 4–6 weeks of age, but not in cKO-Cav1.3flex/- mice. Moreover, it turned out that the flex allele itself had an impact on Cav1.3 expression in IHCs. These obstacles demanded an in-depth analysis of the problems of the conditional mouse models used before drawing conclusions on the auditory phenotype caused by cochlea-specific deletion of Cav1.3.
Conditional Tissue-Specific Knockout Mice – Benefits and Pitfalls
Satheesh et al. (2012) were the first to analyze a conditional Cav1.3 knockout mouse model with deletion in the auditory brainstem, the Egr2::cre;Cacna1d-eGFPflex/flex mouse. There, eGFP fluorescence was not detectable in unfixed brain tissue (Bartels, 2009), most likely due to the much lower abundance of eGFP/Cav1.3 in these brainstem nuclei compared with IHCs. In the present study, the flex allele had the advantage that successful deletion of Cav1.3 in IHCs resulted in eGFP fluorescence that could be judged semi-quantitatively at the cellular level. Moreover, the presence of individual IHCs where the flex allele was not switched enabled us to use these cells as positive controls, e.g., for Cav1.3 immunolabeling within one specimen.
An unwanted side effect of transgenic animals is unexpected germline expression of Cre recombinase resulting in embryonal recombination of loxP sites that might even occur in Cre-negative offspring carrying a flex or lox allele (Song and Palmiter, 2018). This can be monitored (i) by adapting the genotyping protocol to recognize excised or switched lox or flex alleles and (ii) in the flex switch system as eGFP expression in cells of Cre-negative flex control mice with one or two flex alleles.
Without the GFP reporter function, we might not have detected the incomplete recombination of flex alleles in IHCs. Partial recombination of floxed alleles, resulting in a mixture of cells with recombination of both, one or even no allele, is a frequent problem in conditional knockout mice (Saam and Gordon, 1999; Schulz et al., 2007; Weis et al., 2010). Lack of knowledge about the amount of successful cellular deletion events may lead to wrong conclusions caused by residual functions contributed by non-knockout cells. In this study, about 10% of the IHCs in cKO-Cav1.3flex/- mice carried an unswitched flex allele resulting in residual hearing function compared with complete deafness of Cav1.3-/- mice (Platzer et al., 2000; Dou et al., 2004).
Our attempt to increase the success rate of Cre in switching the flex alleles by replacing one flex by a constitutive knockout (“-”) allele resulted in a higher ratio of true knockout IHCs in cKO-Cav1.3flex/- compared with cKO-Cav1.3flex/flex mice (cf. Figures 1, 2) at 3 weeks of age. However, this difference was no longer present 2 weeks later (Table 2), indicating that Cre managed to switch most flex alleles by this time point. An alternative approach to increase the recombination rate of loxP sites would be to increase expression of Cre recombinase (Schnütgen et al., 2003; Schulz et al., 2007) using Pax2::cre/cre instead of Pax2::cre/+ mice. However, high Cre expression levels on the other hand might increase the risk of possible side effects. High levels of Cre expression in α-myosin heavy chain-Cre mice have for example been demonstrated to be cardiotoxic causing altered cardiac function, DNA damage and inflammation (Bhandary and Robbins, 2015; Pugach et al., 2015). A dose dependence of Cre toxicity has been confirmed in cell culture titration experiments (Loonstra et al., 2001; Baba et al., 2005).
In summary, due to ambiguous eGFP expression in cKO-Cav1.3flex/flex mice when only one flex allele was switched resulting in heterozygous IHCs that still produced Cav1.3, we decided to further use cKO-Cav1.3flex/- mice, where eGFP expression was a reliable marker of Cav1.3 ablation. Since incomplete recombination of both flex (or lox) alleles is likely to be a general problem in conditional mice, a combination with a systemic knockout allele (flex/– or lox/–) should be used if possible.
Toxicity of Excessive eGFP
Degeneration of IHCs in the basal cochlear turn as early as P25 in cKO-Cav1.3flex/flex but not Cav1.3-/- mice suggests toxicity of excessive eGFP. Furthermore, direct fluorescence of eGFP can be seen in non-fixed IHCs (this study) but not in the auditory brainstem (Bartels, 2009), further indicating particularly high expression of Cav1.3 in wildtype and eGFP in cKO-Cav1.3flex/flex IHCs, respectively. GFP toxicity has been demonstrated in cell lines where its expression induced apoptosis (Liu et al., 1999) or inhibited polyubiquitination (Baens et al., 2006). In mice, neuronal expression of yellow fluorescent protein induced multiple dose-dependent stress responses (Comley et al., 2011). A possible cause for these damaging effects is that the pre-mature, colorless form of eGFP, which is present in variable proportions in GFP-expressing cells, produces the free radical O2●- and hydrogen peroxide (H2O2) under consumption of NAD(P)H (Ganini et al., 2017). Such a GFP-induced oxidative stress may explain why only IHCs of cKO-Cav1.3flex/flex mice but not of cKO-Cav1.3flex/- degenerated because of the higher dose of eGFP produced by two flex alleles.
Side Effects of Gene-Targeted Alleles Without Gene Deletion by Cre Recombinase
In conditional models, the modifications of the target gene should not affect its function unless recombined by Cre. In the study of Satheesh et al. (2012), who first described the Cav1.3-flex model, Ca2+ currents were not analyzed. Normal ABR thresholds of Cav1.3flex/flex control mice led the authors to the conclusion that unswitched flex alleles did not affect Cav1.3 channel function. Since Cav1.3 channels mediate the majority of Ca2+ current in IHCs, the present study provided the unique opportunity to analyze potential side effects of the flex construct in detail by measuring Ca2+ channel currents and quantitatively analyzing Cav1.3 protein clusters. We found reduced IBa amplitudes and a lower amount of Cav1.3 protein in IHCs of cre-negative Cav1.3flex/flex and Cav1.3flex/- control mice demonstrating that the unswitched flex allele did not fully replace the wildtype function. As has been shown before, a considerable reduction or increase in peak Ca2+ current amplitudes of IHCs has only minor effects on click ABR thresholds (Scharinger et al., 2015; Fell et al., 2016), which can be misleading when used as the only method to assess the function of IHCs. Here, frequency-dependent ABR thresholds were increased by 10–20 dB at most frequencies in Cav1.3flex/- control mice upon reduction of IBa to 42% of the wildtype value, which is in accordance to threshold increases of 5 – 20 dB in null mutants of the auxiliary α2δ2 Ca2+ channel subunit causing reductions of IBa to 60–70% (Fell et al., 2016). The most prominent consequence of IBa reduction with respect to hearing function of Cav1.3flex/- control mice are the reduced growth functions of peak-to-peak amplitudes of the ABR waves, especially wave I, indicating strongly reduced IHC output at all levels above threshold. It should be kept in mind that both click and frequency-specific ABR thresholds are determined by only one afferent fiber type, the low threshold, high spontaneous rate fibers, whereas growth functions of ABR amplitudes cover the activity of all (high, medium and low spontaneous rate) afferent fiber types (Kiang et al., 1965; Liberman, 1978, 1982; Petitpré et al., 2018; Shrestha et al., 2018; Sun et al., 2018).
The question arises as to why IBa was reduced in IHCs of Cre-negative mice containing at least one flex allele (Figure 6). For generating the conditional Cav1.3 mouse, the Cacna1d-eGFPflex construct was placed outside and a few hundred base pairs up- and downstream of the coding regions of exon 2 in the Cacna1d gene to avoid unintended manipulation of regulatory elements flanking the exon (Bartels, 2009). This insertion might have disrupted unknown regulatory elements and thus reduced the expression level of the channel. Moreover, IBa gating properties were altered in Cav1.3flex/- control mice, suggesting that insertion of the flex construct might have affected splicing of Cacna1d mRNA (Bock et al., 2011; Scharinger et al., 2015).
The IHC and Auditory Phenotype Following Systemic Versus Cochlea-Specific Deletion of Cav1.3 Channels
The ablation of Cav1.3 channels before birth in cKO-Cav1.3flex/- mice caused an IHC phenotype similar to that of Cav1.3-/- mice, including persistent expression of SK2, lack of BK expression in apical-turn IHCs, and a reduced cell size (Brandt et al., 2003; Glueckert et al., 2003; Nemzou et al., 2006).
Until the onset of hearing, SK2 channels mediate efferent inhibition of IHCs via α9α10 Ca2+-permeable nicotinic acetylcholine receptors (nAChRs) (Oliver et al., 2000; Elgoyhen et al., 2001), The origin of these efferent fibers lies in cholinergic neurons in the SOC. Shortly after birth, neurons of the auditory brainstem are spontaneously active and undergo a developmental program including synaptic pruning and establishment of tonotopy (Blankenship and Feller, 2010; Clause et al., 2014). The spiking pattern of SOC neurons is modulated by ascending information from the cochlea, where IHCs produce spontaneous Ca2+ action potentials, which are synchronized by Ca2+ waves in the transient Kölliker’s organ (Tritsch and Bergles, 2010; Johnson et al., 2011, 2017; Sendin et al., 2014; Eckrich et al., 2018; Mammano and Bortolozzi, 2018). In turn, efferent inhibition from the SOC closes a feedback loop by shaping the spontaneous activity of IHCs (Guinan, 2006; Frank and Goodrich, 2018). In the systemic Cav1.3 knockout mouse both SOC neurons and IHCs lack Cav1.3 currents (Platzer et al., 2000; Hirtz et al., 2011), the latter of which as a consequence cannot produce action potentials (Brandt et al., 2003). In the SOC of Cav1.3-/- mice, depolarization-induced spiking of lateral superior olive (LSO) neurons was changed from a single to a multiple firing pattern due to a reduction in Kv1.2 channels (Hirtz et al., 2011). This was most likely caused by the specific lack of Cav1.3 channels in brainstem neurons despite intact cochlear expression as confirmed in brainstem-specific Cav1.3 knockout mice (Satheesh et al., 2012). In the present study with SOC neurons expressing Cav1.3 channels, the phenotype of Cav1.3-deficient IHCs from cKO-Cav1.3flex/- mice (lack of BK channels, persistence of SK2 channels, smaller cell size) was very similar to that of systemic Cav1.3-/- mice. Therefore a potentially altered feedback signaling by Cav1.3-deficient SOC neurons onto IHCs cannot be causative for the IHC phenotype of Cav1.3-/- mice. Nevertheless, the spiking pattern of SOC neurons and, thus, efferent signaling back to immature IHCs might still be altered due to the loss of afferent activation by IHCs. In α9- and α10-nAChR knockout mice, maturation of IHC K+ channels was normal despite the complete lack of cholinergic efferent input from SOC neurons (Gomez-Casati et al., 2009; Johnson et al., 2013b). In summary, maturation of the IHC’s K+ channel composition is mainly controlled by intrinsic Ca2+ signaling within the IHC and does not depend on Cav1.3 expression in the SOC exerting efferent feedback.
BK Channel Expression in Basal-Turn IHCs of Cav1.3-/- and Cre-Negative IHCs of cKO-Cav1.3flex/- Mice
We found residual BK labeling in IHCs of the basal but not the apical cochlear turn of Cav1.3-/- mice. So far it is unknown why BK protein is missing in IHCs of Cav1.3-/- mice along most of the cochlear length (Brandt et al., 2003) despite expression of the respective Kcnma1 mRNA (Nemzou et al., 2006).
The faint and dot-like BK labeling in GFP-negative IHCs of cKO-Cav1.3flex/- mice clearly differed from the large BK patches found in IHCs of wildtype and control Cav1.3flex/- mice (Figure 5F). Assuming that Cre is not active in these IHCs their phenotype should be the same as that of Cav1.3flex/- controls (Figure 5D) but this was not the case. We can thus exclude that the reduced BK expression was caused by the incomplete wildtype function of the unswitched flex allele. But what are the differences between IHCs of Cav1.3flex/- controls and GFP-negative IHCs of cKO-Cav1.3flex/- mice? Differences intrinsic to the IHCs are: (i) Presence of the Pax2::cre allele at an unknown location in the genome, which might interfere with modulatory sequences affecting BK expression; (ii) Cre might be expressed in these IHCs without switching the flex allele, but it could still interfere with BK expression. Alternatively, a factor extrinsic to the IHC might be causing the reduced BK expression. GFP-negative IHCs of cKO-Cav1.3flex/- mice are surrounded by Cav1.3-deficient, electrically silent IHCs, whereas neonatal IHCs produce Ca2+ action potentials in wildtype and presumably Cav1.3flex/- mice (Brandt et al., 2003). This activity causes periodic efflux of K+ ions from the IHCs, which depolarizes neighboring phalangeal cells and IHCs, thereby amplifying and synchronizing Ca2+ AP activity (Wang et al., 2015; Eckrich et al., 2018). In summary, impaired expression of BK channels in solitary GFP-negative IHCs surrounded by true Cav1.3 knockout IHCs of cKO-Cav1.3flex/- mice may result from a lack of mutual activation and synchronization of Ca2+ AP activity among IHCs during the critical developmental period. It would be interesting to analyze whether the Ca2+ action potential activity in GFP-negative IHCs of cKO-Cav1.3flex/- is altered compared to Cav1.3flex/- controls.
Data Availability
The datasets generated for this study are available on request to the corresponding author.
Ethics Statement
All experiments were carried out in accordance with the European Communities Council Directive (86/609/EEC) and approved by the regional board for scientific animal experiments of the Saarland, Germany. Additional ethics approval was not required according to the local and national guidelines.
Author Contributions
SE and JE conceived and designed the study. SE, DH, KS, KB, KF, and SM acquired the data. SE, JE, DH, KB, SM, GW, and BS drafted the article. JE contributed to funding acquisition and project administration. All authors analyzed and interpreted the data.
Funding
This work was supported by DFG SFB 894 (A8 to JE), EU-CAVNET MRTN-CT-2006-035367 (to JE), DFG Priority Program 1608 “Ultrafast and Temporally Precise Information Processing: Normal and Dysfunctional Hearing” (En 294/5-2 to JE), DFG EC 488/1-1 (to SE), and Saarland University.
Conflict of Interest Statement
The authors declare that the research was conducted in the absence of any commercial or financial relationships that could be construed as a potential conflict of interest.
Acknowledgments
We thank Jennifer Ihl, Angela Di Turi, and Barbara Fell for excellent technical assistance and Kai Schönig and Dušan Bartsch for discussion of the data.
References
Baba, Y., Nakano, M., Yamada, Y., Saito, I., and Kanegae, Y. (2005). Practical range of effective dose for cre recombinase-expressing recombinant adenovirus without cell toxicity in mammalian cells. Microbiol. Immunol. 49, 559–570. doi: 10.1111/j.1348-0421.2005.tb03753.x
Babola, T. A., Li, S., Gribizis, A., Lee, B. J., Issa, J. B., Wang, H. C., et al. (2018). Homeostatic control of spontaneous activity in the developing auditory system. Neuron 99, 511.e5–524.e5. doi: 10.1016/j.neuron.2018.07.004
Baens, M., Noels, H., Broeckx, V., Hagens, S., Fevery, S., Billiau, A. D., et al. (2006). The dark side of EGFP: defective polyubiquitination. PLoS One 1:e54. doi: 10.1371/journal.pone.0000054
Baig, S. M., Koschak, A., Lieb, A., Gebhart, M., Dafinger, C., Nürnberg, G., et al. (2011). Loss of Cav1.3 (CACNA1D) function in a human channelopathy with bradycardia and congenital deafness. Nat. Neurosci. 14, 77–84. doi: 10.1038/nn.2694
Bartels, K. (2009). Conditional Knockout of the L-Type Voltage-Gated Calcium Channel CaV1.3 via the FLEX Switch. Thesis. Heidelberg: Ruprecht-Karls-Universität Heidelberg, doi: 10.11588/heidok.00010021
Bhandary, B., and Robbins, J. (2015). Giving credence to controls: avoiding the false phenotype. J. Mol. Cell. Cardiol. 86, 136–137. doi: 10.1016/j.yjmcc.2015.07.007
Blankenship, A. G., and Feller, M. B. (2010). Mechanisms underlying spontaneous patterned activity in developing neural circuits. Nat. Rev. Neurosci. 11, 18–29. doi: 10.1038/nrn2759
Bock, G., Gebhart, M., Scharinger, A., Jangsangthong, W., Busquet, P., Poggiani, C., et al. (2011). Functional properties of a newly identified c-terminal splice variant of Cav1.3 L-type Ca2+ channels. J. Biol. Chem. 286, 42736–42748. doi: 10.1074/jbc.M111.269951
Brandt, A., Striessnig, J., and Moser, T. (2003). CaV1.3 channels are essential for development and presynaptic activity of cochlear inner hair cells. J. Neurosci. 23, 10832–10840. doi: 10.1523/JNEUROSCI.23-34-10832.2003
Burton, Q., Cole, L. K., Mulheisen, M., Chang, W., and Wu, D. K. (2004). The role of Pax2 in mouse inner ear development. Dev. Biol. 272, 161–175. doi: 10.1016/j.ydbio.2004.04.024
Clause, A., Kim, G., Sonntag, M., Weisz, C. J. C., Vetter, D. E., Rübsamen, R., et al. (2014). The precise temporal pattern of prehearing spontaneous activity is necessary for tonotopic map refinement. Neuron 82, 822–835. doi: 10.1016/j.neuron.2014.04.001
Comley, L. H., Wishart, T. M., Baxter, B., Murray, L. M., Nimmo, A., Thomson, D., et al. (2011). Induction of cell stress in neurons from transgenic mice expressing yellow fluorescent protein: implications for neurodegeneration research. PLoS One 6:e17639. doi: 10.1371/journal.pone.0017639
Dou, H., Vazquez, A. E., Namkung, Y., Chu, H., Cardell, E. L., Nie, L., et al. (2004). Null mutation of alpha1D Ca2+ channel gene results in deafness but no vestibular defect in mice. J. Assoc. Res. Otolaryngol. 5, 215–226. doi: 10.1007/s10162-003-4020-3
Eckrich, T., Blum, K., Milenkovic, I., and Engel, J. (2018). Fast Ca2+ transients of inner hair cells arise coupled and uncoupled to Ca2+ waves of inner supporting cells in the developing mouse cochlea. Front. Mol. Neurosci. 11:264. doi: 10.3389/fnmol.2018.00264
Elgoyhen, A. B., Vetter, D. E., Katz, E., Rothlin, C. V., Heinemann, S. F., and Boulter, J. (2001). α10: a determinant of nicotinic cholinergic receptor function in mammalian vestibular and cochlear mechanosensory hair cells. Proc. Natl. Acad. Sci. U.S.A. 98, 3501–3506. doi: 10.1073/pnas.051622798
Engel, J., Braig, C., Ruttiger, L., Kuhn, S., Zimmermann, U., Blin, N., et al. (2006). Two classes of outer hair cells along the tonotopic axis of the cochlea. Neuroscience 143, 837–849. doi: 10.1016/j.neuroscience.2006.08.060
Fell, B., Eckrich, S., Blum, K., Eckrich, T., Hecker, D., Obermair, G. J., et al. (2016). α2δ2 controls the function and trans-synaptic coupling of Cav1.3 channels in mouse inner hair cells and is essential for normal hearing. J. Neurosci. 36, 11024–11036. doi: 10.1523/JNEUROSCI.3468-14.2016
Frank, M. M., and Goodrich, L. V. (2018). Talking back: development of the olivocochlear efferent system. Wiley Interdiscip. Rev. Dev. Biol. 7:e324. doi: 10.1002/wdev.324
Ganini, D., Leinisch, F., Kumar, A., Jiang, J., Tokar, E. J., Malone, C. C., et al. (2017). Fluorescent proteins such as eGFP lead to catalytic oxidative stress in cells. Redox Biol. 12, 462–468. doi: 10.1016/j.redox.2017.03.002
Glowatzki, E., and Fuchs, P. A. (2000). Cholinergic synaptic inhibition of inner hair cells in the neonatal mammalian cochlea. Science 288, 2366–2368. doi: 10.1126/science.288.5475.2366
Glueckert, R., Wietzorrek, G., Kammen-Jolly, K., Scholtz, A., Stephan, K., Striessnig, J., et al. (2003). Role of class D L-type Ca2+ channels for cochlear morphology. Hear. Res. 178, 95–105. doi: 10.1016/S0378-5955(03)00054-6
Gomez-Casati, M. E., Wedemeyer, C., Taranda, J., Lipovsek, M., Dalamon, V., Elgoyhen, A. B., et al. (2009). Electrical properties and functional expression of ionic channels in cochlear inner hair cells of mice lacking the alpha10 nicotinic cholinergic receptor subunit. J. Assoc. Res. Otolaryngol. 10, 221–232. doi: 10.1007/s10162-009-0164-0
Guinan, J. J. (2006). Olivocochlear efferents: anatomy, physiology, function, and the measurement of efferent effects in humans. Ear Hear. 27, 589–607. doi: 10.1097/01.aud.0000240507.83072.e7
Hirtz, J. J., Boesen, M., Braun, N., Deitmer, J. W., Kramer, F., Lohr, C., et al. (2011). Cav1.3 calcium channels are required for normal development of the auditory brainstem. J. Neurosci. 31, 8280–8294. doi: 10.1523/JNEUROSCI.5098-10.2011
Hirtz, J. J., Braun, N., Griesemer, D., Hannes, C., Janz, K., Löhrke, S., et al. (2012). Synaptic refinement of an inhibitory topographic map in the auditory brainstem requires functional CaV1.3 calcium channels. J. Neurosci. 32, 14602–14616. doi: 10.1523/JNEUROSCI.0765-12.2012
Jae Huh, W., Mysorekar, I. U., and Mills, J. C. (2010). Inducible activation of Cre recombinase in adult mice causes gastric epithelial atrophy, metaplasia, and regenerative changes in the absence of “floxed” alleles. Am. J. Physiol. Gastrointest. Liver Physiol. 299, G368–G380. doi: 10.1152/ajpgi.00021.2010
Janbandhu, V., Moik, D., and Fässler, R. (2014). Cre recombinase induces DNA damage and tetraploidy in the absence of LoxP sites. Cell Cycle 13, 462–470. doi: 10.4161/cc.27271
Johnson, S. L., Ceriani, F., Houston, O., Polishchuk, R., Polishchuk, E., Crispino, G., et al. (2017). Connexin-mediated signaling in nonsensory cells is crucial for the development of sensory inner hair cells in the mouse cochlea. J. Neurosci. 37, 258–268. doi: 10.1523/JNEUROSCI.2251-16.2016
Johnson, S. L., Eckrich, T., Kuhn, S., Zampini, V., Franz, C., Ranatunga, K. M., et al. (2011). Position-dependent patterning of spontaneous action potentials in immature cochlear inner hair cells. Nat. Neurosci. 14, 711–717. doi: 10.1038/nn.2803
Johnson, S. L., Kuhn, S., Franz, C., Ingham, N., Furness, D. N., Knipper, M., et al. (2013a). Presynaptic maturation in auditory hair cells requires a critical period of sensory-independent spiking activity. Proc. Natl. Acad. Sci. U.S.A. 110, 8720–8725. doi: 10.1073/pnas.1219578110
Johnson, S. L., Wedemeyer, C., Vetter, D. E., Adachi, R., Holley, M. C., Elgoyhen, A. B., et al. (2013b). Cholinergic efferent synaptic transmission regulates the maturation of auditory hair cell ribbon synapses. Open Biol. 3:130163. doi: 10.1098/rsob.130163
Kiang, N. Y., Pfeiffer, R. R., Warr, W. B., and Backus, A. S. (1965). Stimulus coding in the cochlear nucleus. Trans. Am. Otol. Soc. 53, 35–58.
Kros, C. J., Ruppersberg, J. P., and Rüsch, A. (1998). Expression of a potassium current in inner hair cells during development of hearing in mice. Nature 394, 281–284. doi: 10.1038/28401
Lawoko-Kerali, G., Rivolta, M. N., and Holley, M. C. (2001). Expression of the transcription factors GATA3 and Pax2 during development of the mammalian inner ear. J. Comp. Neurol. 442, 378–391. doi: 10.1002/cne.10088
Liberman, M. C. (1978). Auditory-nerve response from cats raised in a low-noise chamber. J. Acoust. Soc. Am. 63, 442–455. doi: 10.1121/1.381736
Liberman, M. C. (1982). Single-neuron labeling in the cat auditory nerve. Science 216, 1239–1241. doi: 10.1126/science.7079757
Liu, H.-S., Jan, M.-S., Chou, C.-K., Chen, P.-H., and Ke, N.-J. (1999). Is green fluorescent protein toxic to the living cells? Biochem. Biophys. Res. Commun. 260, 712–717. doi: 10.1006/bbrc.1999.0954
Loonstra, A., Vooijs, M., Beverloo, H. B., Allak, B. A., Drunen, E., van, Kanaar, R., et al. (2001). Growth inhibition and DNA damage induced by Cre recombinase in mammalian cells. Proc. Natl. Acad. Sci. 98, 9209–9214. doi: 10.1073/pnas.161269798
Mammano, F., and Bortolozzi, M. (2018). Ca2+ signaling, apoptosis and autophagy in the developing cochlea: milestones to hearing acquisition. Cell Calcium 70, 117–126. doi: 10.1016/j.ceca.2017.05.006
Marcotti, W., Johnson, S. L., and Kros, C. J. (2004). A transiently expressed SK current sustains and modulates action potential activity in immature mouse inner hair cells. J. Physiol. 560, 691–708. doi: 10.1113/jphysiol.2004.072868
Marcotti, W., Johnson, S. L., Rusch, A., and Kros, C. J. (2003). Sodium and calcium currents shape action potentials in immature mouse inner hair cells. J. Physiol. 552, 743–761. doi: 10.1113/jphysiol.2003.043612
Nemzou, N. R. M., Bulankina, A. V., Khimich, D., Giese, A., and Moser, T. (2006). Synaptic organization in cochlear inner hair cells deficient for the CaV1.3 (α1D) subunit of L-type Ca2+ channels. Neuroscience 141, 1849–1860. doi: 10.1016/j.neuroscience.2006.05.057
Ohyama, T., and Groves, A. K. (2004). Generation of Pax2-Cre mice by modification of a Pax2 bacterial artificial chromosome. Genesis 38, 195–199. doi: 10.1002/gene.20017
Oliver, D., Klocker, N., Schuck, J., Baukrowitz, T., Ruppersberg, J. P., and Fakler, B. (2000). Gating of Ca2+-activated K+ channels controls fast inhibitory synaptic transmission at auditory outer hair cells. Neuron 26, 595–601. doi: 10.1016/S0896-6273(00)81197-6
Oliver, D., Knipper, M., Derst, C., and Fakler, B. (2003). Resting potential and submembrane calcium concentration of inner hair cells in the isolated mouse cochlea are set by KCNQ-type potassium channels. J. Neurosci. 23, 2141–2149. doi: 10.1523/JNEUROSCI.23-06-02141.2003
Petitpré, C., Wu, H., Sharma, A., Tokarska, A., Fontanet, P., Wang, Y., et al. (2018). Neuronal heterogeneity and stereotyped connectivity in the auditory afferent system. Nat. Commun. 9:3691. doi: 10.1038/s41467-018-06033-3
Platzer, J., Engel, J., Schrott-Fischer, A., Stephan, K., Bova, S., Chen, H., et al. (2000). Congenital deafness and sinoatrial node dysfunction in mice lacking class D L-type Ca2+ channels. Cell 102, 89–97. doi: 10.1016/s0092-8674(00)00013-1
Pugach, E. K., Richmond, P. A., Azofeifa, J. G., Dowell, R. D., and Leinwand, L. A. (2015). Prolonged Cre expression driven by the α-myosin heavy chain promoter can be cardiotoxic. J. Mol. Cell. Cardiol. 86, 54–61. doi: 10.1016/j.yjmcc.2015.06.019
Saam, J. R., and Gordon, J. I. (1999). Inducible gene knockouts in the small intestinal and colonic epithelium. J. Biol. Chem. 274, 38071–38082. doi: 10.1074/jbc.274.53.38071
Satheesh, S. V., Kunert, K., Rüttiger, L., Zuccotti, A., Schönig, K., Friauf, E., et al. (2012). Retrocochlear function of the peripheral deafness gene Cacna1d. Hum. Mol. Genet. 21, 3896–3909. doi: 10.1093/hmg/dds217
Scharinger, A., Eckrich, S., Vandael, D. H., Schönig, K., Koschak, A., Hecker, D., et al. (2015). Cell-type-specific tuning of Cav1.3 Ca2+-channels by a C-terminal automodulatory domain. Front. Cell. Neurosci. 9:309. doi: 10.3389/fncel.2015.00309
Schindelin, J., Arganda-Carreras, I., Frise, E., Kaynig, V., Longair, M., Pietzsch, T., et al. (2012). Fiji: an open-source platform for biological-image analysis. Nat. Methods 9, 676–682. doi: 10.1038/nmeth.2019
Schnütgen, F., Doerflinger, N., Calléja, C., Wendling, O., Chambon, P., and Ghyselinck, N. B. (2003). A directional strategy for monitoring Cre-mediated recombination at the cellular level in the mouse. Nat. Biotechnol. 21, 562–565. doi: 10.1038/nbt811
Schulz, T. J., Glaubitz, M., Kuhlow, D., Thierbach, R., Birringer, M., Steinberg, P., et al. (2007). Variable expression of cre recombinase transgenes precludes reliable prediction of tissue-specific gene disruption by tail-biopsy genotyping. PLoS One 2:e1013. doi: 10.1371/journal.pone.0001013
Sendin, G., Bourien, J., Rassendren, F., Puel, J.-L., and Nouvian, R. (2014). Spatiotemporal pattern of action potential firing in developing inner hair cells of the mouse cochlea. Proc. Natl. Acad. Sci. U.S.A. 111, 1999–2004. doi: 10.1073/pnas.1319615111
Shrestha, B. R., Chia, C., Wu, L., Kujawa, S. G., Liberman, M. C., and Goodrich, L. V. (2018). Sensory neuron diversity in the inner ear is shaped by activity. Cell 174, 1229.e17–1246.e17. doi: 10.1016/j.cell.2018.07.007
Simmons, D. D. (2002). Development of the inner ear efferent system across vertebrate species. J. Neurobiol. 53, 228–250. doi: 10.1002/neu.10130
Song, A. J., and Palmiter, R. D. (2018). Detecting and avoiding problems when using the Cre–lox system. Trends Genet. 34, 333–340. doi: 10.1016/j.tig.2017.12.008
Sun, S., Babola, T., Pregernig, G., So, K. S., Nguyen, M., Su, S.-S. M., et al. (2018). Hair cell mechanotransduction regulates spontaneous activity and spiral ganglion subtype specification in the auditory system. Cell 174, 1247.e–1263.e. doi: 10.1016/j.cell.2018.07.008
Tritsch, N. X., and Bergles, D. E. (2010). Developmental regulation of spontaneous activity in the Mammalian cochlea. J. Neurosci. 30, 1539–1550. doi: 10.1523/JNEUROSCI.3875-09.2010
Wang, H. C., Lin, C.-C., Cheung, R., Zhang-Hooks, Y., Agarwal, A., Ellis-Davies, G., et al. (2015). Spontaneous activity of cochlear hair cells triggered by fluid secretion mechanism in adjacent support cells. Cell 163, 1348–1359. doi: 10.1016/j.cell.2015.10.070
Weis, B., Schmidt, J., Lyko, F., and Linhart, H. G. (2010). Analysis of conditional gene deletion using probe based real-time PCR. BMC Biotechnol. 10:75. doi: 10.1186/1472-6750-10-75
Willaredt, M. A., Ebbers, L., and Nothwang, H. G. (2014). Central auditory function of deafness genes. Hear. Res. 312, 9–20. doi: 10.1016/j.heares.2014.02.004
Keywords: inner hair cell, Ca2+ channel, Cav1.3, BK, conditional knockout, flex switch, GFP toxicity, hearing
Citation: Eckrich S, Hecker D, Sorg K, Blum K, Fischer K, Münkner S, Wenzel G, Schick B and Engel J (2019) Cochlea-Specific Deletion of Cav1.3 Calcium Channels Arrests Inner Hair Cell Differentiation and Unravels Pitfalls of Conditional Mouse Models. Front. Cell. Neurosci. 13:225. doi: 10.3389/fncel.2019.00225
Received: 07 March 2019; Accepted: 03 May 2019;
Published: 22 May 2019.
Edited by:
Josef Bischofberger, Universität Basel, SwitzerlandReviewed by:
Petronel Tuluc, University of Innsbruck, AustriaJoshua Singer, University of Maryland, College Park, United States
Copyright © 2019 Eckrich, Hecker, Sorg, Blum, Fischer, Münkner, Wenzel, Schick and Engel. This is an open-access article distributed under the terms of the Creative Commons Attribution License (CC BY). The use, distribution or reproduction in other forums is permitted, provided the original author(s) and the copyright owner(s) are credited and that the original publication in this journal is cited, in accordance with accepted academic practice. No use, distribution or reproduction is permitted which does not comply with these terms.
*Correspondence: Jutta Engel, jutta.engel@uni-saarland.de