- 1Instituto de Investigaciones Bioquímicas de La Plata (INIBIOLP), Centro Científico Tecnológico – La Plata, Consejo Nacional de Investigaciones Científicas y Técnicas (CONICET), La Plata, Argentina
- 2Facultad de Ciencias Exactas, Universidad Nacional de La Plata (UNLP), La Plata, Argentina
- 3Facultad de Ciencias Médicas, Universidad Nacional de La Plata (UNLP), La Plata, Argentina
Fatty acids (FAs) are typically associated with structural and metabolic roles, as they can be stored as triglycerides, degraded by β-oxidation or used in phospholipids’ synthesis, the main components of biological membranes. It has been shown that these lipids exhibit also regulatory functions in different cell types. FAs can serve as secondary messengers, as well as modulators of enzymatic activities and substrates for cytokines synthesis. More recently, it has been documented a direct activity of free FAs as ligands of membrane, cytosolic, and nuclear receptors, and cumulative evidence has emerged, demonstrating its participation in a wide range of physiological and pathological conditions. It has been long known that the central nervous system is enriched with poly-unsaturated FAs, such as arachidonic (C20:4ω-6) or docosohexaenoic (C22:6ω-3) acids. These lipids participate in the regulation of membrane fluidity, axonal growth, development, memory, and inflammatory response. Furthermore, a whole family of low molecular weight compounds derived from FAs has also gained special attention as the natural ligands for cannabinoid receptors or key cytokines involved in inflammation, largely expanding the role of FAs as precursors of signaling molecules. Nutritional deficiencies, and alterations in lipid metabolism and lipid signaling have been associated with developmental and cognitive problems, as well as with neurodegenerative diseases. The molecular mechanism behind these effects still remains elusive. But in the last two decades, different families of proteins have been characterized as receptors mediating FAs signaling. This review focuses on different receptors sensing and transducing free FAs signals in neural cells: (1) membrane receptors of the family of G Protein Coupled Receptors known as Free Fatty Acid Receptors (FFARs); (2) cytosolic transport Fatty Acid-Binding Proteins (FABPs); and (3) transcription factors Peroxisome Proliferator-Activated Receptors (PPARs). We discuss how these proteins modulate and mediate direct regulatory functions of free FAs in neural cells. Finally, we briefly discuss the advantages of evaluating them as potential targets for drug design in order to manipulate lipid signaling. A thorough characterization of lipid receptors of the nervous system could provide a framework for a better understanding of their roles in neurophysiology and, potentially, help for the development of novel drugs against aging and neurodegenerative processes.
Introduction
The central nervous system (CNS) is an intricate network of a variety of cell types, with a wide range of distinct properties and functions. Notoriously, lipids represent a larger proportion of mass than in most other tissues, second only after adipose tissue (Etschmaier et al., 2011). The main difference is that, whereas in adipocytes lipids are mainly stored as energy reserve in lipid droplets, in the CNS lipids fulfill multiple functions; such as forming large extensions of membranes necessary for crosstalk between neural cells. This is not limited only to the inner and plasma membranes of neurons, comprising axons, dendrites, and spines; astrocytes, oligodendrocytes, and microglia also have highly complex cellular shapes and, hence, extensive surface areas defined largely by lipids, and their functions in the healthy brain are integrated both physically and metabolically (von Bernhardi et al., 2016). Brain membranes are constituted by proteins, cholesterol (on average, 21.5% mol/mol lipids), sphingomyelin (1.9%), gangliosides (1.4%) and phospholipids (75.1%) (Scandroglio et al., 2008), where the lipids were typically constrained to structural or support functions for the first ones. Lipid distribution is not equivalent for all cell types or brain regions, and neurons are usually enriched in polyunsaturated glycerolipids and cholesterol (Scandroglio et al., 2008; Chan et al., 2012; Arai et al., 2015; Ingolfsson et al., 2017). These lipids are essential for many cellular processes in CNS. For example, the brain is the richest tissue in cholesterol with up to 20 mg/g of tissue, 10-time more than the rest, and it is mainly synthesized in situ (Dietschy and Turley, 2001, 2004). Therefore, its levels are independent from circulating cholesterol, highlighting its pivotal role in multiple brain functions such as signal transduction, synaptic transmission, and cell differentiation by modulation of lipid rafts organization and segregation of membrane proteins, as well as in several pathological conditions, either directly by its self or through its metabolism into neurosteroids and oxysterols (Reddy, 2010; Leoni and Caccia, 2011; Orth and Bellosta, 2012; Vance, 2012).
Lipid signaling has attracted increasing attention as different families of lipids have been shown to exhibit important regulatory functions through highly specific receptors (Fernandis and Wenk, 2007; Sunshine and Iruela-Arispe, 2017). Polyunsaturated fatty acids (PUFAs) are of particular relevance because they can also be transformed into much more potent derivatives, such as eicosanoids and docosanoids, from arachidonic (C20:4ω-6, ARA) and docosahexanoic (C22:6ω-3, DHA) acids, respectively (Serhan, 2014; Dennis and Norris, 2015). In the last decades, different families of proteins have been identified and characterized to mediate signaling processes triggered directly by lipids per se, including free FAs (Im, 2004; Kostenis, 2004; Wolfrum, 2007). The interest on how these proteins mediate FAs regulatory functions increased as they have been demonstrated to be targets and/or essential carriers for the therapeutic actions of certain drugs (Wolfrum et al., 2001; Landrier et al., 2004; Martin et al., 2013).
Fatty acids regulatory functions are most probably defined by the group of specific receptors expressed in a particular cell-type and, therefore, they are dynamically modulated throughout the cell life-span, the developmental stage, and its differentiation process. This review resumes part of the present understanding on FAs regulatory functions in pathophysiology of neural tissues and three families of proteins involved in these roles. Noteworthy, other proteins involved in lipid signaling with more promiscuous selectivity of ligands, such as FA Translocase (FAT/CD36) or Toll-like Receptor 4 (TLR4), were not included in this manuscript, and their roles could be consulted elsewhere (Pepino et al., 2014; Magnan et al., 2015; Rocha et al., 2016).
Physiological Functions and Participation of Fatty Acids in Neuronal Pathologies
Fatty acids are typically considered as a source of energy through β-oxidation, and, as part of phospholipids, either as membrane building blocks or reservoir of second messengers and substrates for cytokines synthesis. They can also induce multiple cellular responses, ranging from cell motility and changes in cell morphology (Kamata et al., 2007; Brown et al., 2014), to regulation of gene expression (Kitajka et al., 2004), modulation of hormones secretion or their effects (Wang and Chan, 2015). But several distinctions must be made when referring to FAs in general, because saturated FAs (SFAs) have a clear different origin, metabolism, and functions compared to essential ω-3 and ω-6 PUFAs. Brain SFAs, such as palmitate (16:0, PA) or stearic (18:0, SA), are known to be generated in situ as much as being imported into the brain, whereas PUFAs are elongated and further unsaturated mainly in the liver and then transported through the bloodstream and imported into the neural tissue as non-esterified FAs (Edmond et al., 1998; Chen et al., 2008). Only a small fraction of PUFAs is actually synthesized locally from linoleic (C18:2ω-6) and α-linolenic (C18:3ω-3, ALA) acids; and, due to enzymatic restrictions and competition between ω-3 and ω-6 PUFAS for the same enzymes, less than 5% of total ALA assimilated from diet can be transformed into DHA (Dyall and Michael-Titus, 2008). Both, endogenous and blood-derived PUFAs, accumulate preferentially in neurons as part of phospholipids.
Neurogenesis includes three contiguous phases, namely proliferation, migration and differentiation, and maturation and integration of the precursor cells; with PUFAs having inherence in all of these stages (Chalon, 2006). Therefore, PUFAs are critical for pre- and post-natal brain development, as well as in adulthood or during natural aging (Uauy and Dangour, 2006; Rombaldi Bernardi et al., 2012). For example, ALA maternal restriction during gestation and lactation impairs hippocampal neuronal differentiation, thus compromising neuronal maturation and related brain functions, such as learning and memory (Bhatia et al., 2011; Niculescu et al., 2011). DHA is the most abundant PUFA and accumulates in the immature brain during perinatal life all through the grey matter expansion; and it can reach over 10% of total FAs in human adult brains (McNamara and Carlson, 2006). Actually, preterm-born adolescents, who skipped the fetal DHA accumulation in the CNS during the last weeks of gestation and usually also lactation during the early life, exhibit deficits in cognitive functions associated to attention, including increased risk for attention-deficit/hyperactivity disorder (ADHD) and schizophrenia (McNamara and Carlson, 2006). That is why dietary deficiencies commonly stimulate a more tightened retention of essential FAs in the brain.
Docosahexanoic acid is particularly important for proper neuronal development in cerebral cortex, retina, and hippocampus, where it promotes neurogenesis and neuronal differentiation (Cao et al., 2009; Gharami et al., 2015). It also boosts synaptogenesis by promoting neurite outgrowth and synapsis formation, accompanied by an increase in the expression of neuronal and synaptic proteins. Particularly, in rat neuronal stem cells, DHA stimulates neuronal differentiation by two mechanisms: (1) a decrease in expression levels of basic Helix-loop-Helix transcription factors (NeuroD, Mash1, Hes1, etc.); and (2) an extension of the expression of cyclin-dependent kinase inhibitor p27 (kip1). This results in an increase in the number of positive cells for the neuronal markers TUBB3 and MAP2 and in a reduction of the percentage of cells in S-phase, suggesting an exit from the cell cycle (Katakura et al., 2009). In aged mice, DHA also prevents neuroinflammation and apoptosis, whereas improving memory (Labrousse et al., 2012). DHA can prevent many of the lipopolysaccharide (LPS) deleterious effects on both neurons and microglia, including loss of dendritic spines or production of nitric oxide as biomarkers of neuroinflammation (Chang et al., 2015). Actually, multiple animal and in vitro models of neuroinflammation consistently show a marked anti-inflammatory effect of DHA and other ω-3 PUFAs, presumably by reducing the production of proinflammatory cytokines and/or promoting the secretion of anti-inflammatory cytokines (reviewed in Orr et al., 2013). PUFAs can also directly modify neurotransmitter production, accumulation, release, and re-uptake. Dopamine’s content, storage in presynaptic vesicles and tyramine-stimulated release, for example, significantly decrease in rat frontal cortex after chronic ω-3 PUFAs deficiency (Delion et al., 1996; Zimmer et al., 1998, 2000). Contrarily, supplementation with ω-3 PUFAs increases dopamine levels in the same area (Chalon et al., 1998).
Fatty acids have been implicated in neuropathological conditions, including neurodegenerative diseases, mental disorders, stroke, and trauma. Severe dietary restriction of essential FAs usually correlates with anxiety-like behavior and deficit in cognitive functions, including memory and learning. However, imbalance between ω-3 and ω-6 PUFAs during gestation also leads to alterations in brain development. In adults, an imbalance in the PUFAs ratio in brain membranes is believed to be a risk factor active during the pathogenesis of neurological and psychiatric disorders (Chalon, 2006). For example, an increase in ARA is known to promote α-Synuclein aggregation. However, it is not clear which is the most relevant cause affecting secretion of dopamine and serotonin, the greater availability of ARA, the deficiency of DHA or the imbalance between them (Chalon, 2006). During normal aging, there is a common cognitive decline and an increasing risk of dementia. High ω-3 PUFAs intake slows down this decline (Johnson and Schaefer, 2006; van Gelder et al., 2007), suggesting that they may have neuroprotective action in the aging brain and even therapeutic potential. Aging is also characterized by increased oxidative stress and neuroinflammation together with altered energy metabolism (reviewed in Prolla and Mattson, 2001). Beside changes in FA composition that favor monounsaturated FAs (MUFAs) over ω-3 and ω-6 PUFAs with time in rodents, the aging brain is also prone to lipid oxidation due to its large content of PUFAs (Favrelere et al., 2000). In humans, the decrease of PUFAs, though subtler during normal aging, is quite evident in neurodegenerative pathologies, including Alzheimer Disease (AD), Parkinson’s Disease (PD), Schizophrenia and depression (reviewed in Hussain et al., 2013). Nevertheless, PUFAs are known to promote α-Synuclein pathological aggregation (Yakunin et al., 2012). On the other hand, the anterior cingulate cortex of depressive patients shows reduced quantities of SFAs and PUFAs (Conklin et al., 2010). The deficiency of ω-3 PUFAs also impairs the normal signaling of endocannabinoid in prelimbic prefrontal cortex and accumbens, leading to abnormal emotional behavior (Lafourcade et al., 2011). The reduction in total PUFAs in erythrocyte’s membranes of patients with early onset schizophrenia correlates with the degree of demyelination in brain white matter (Peters et al., 2009). Finally, PUFAs also serve as anti-depressants and anti-convulsants, confer protection against traumatic insults, and enhance repairing processes. For example, numerous animal models of epilepsy support the anticonvulsant properties of PUFAs, like the greater resistance to pentylenetetrazol-induced seizures in ω-3 PUFAs fed rats (Taha et al., 2009).
Arachidonic acid is particularly enriched in phosphatidylinositol (PI), whereas DHA is a major component of brain phosphatidylethanolamine (PE) and phosphatidylserine (PS). Both PUFAs are highly enriched in the phospholipids of the synaptic plasma membrane and synaptic vesicles (Glomset, 2006). ARA is also particularly rich in membranes of leukocytes, presumably because it serves as a precursor of a myriad of cytokines (Innes and Calder, 2018). PUFAs are almost exclusively found in position 2 of the glycerol moiety within any phospholipid and, therefore, their release is catalyzed by phospholipases A2 (PLA2). Noteworthy, ARA and eicosapentaenoic acid (20:5ω-3, EPA) are released preferentially by cytoplasmic PLA2 (cPLA2), whereas DHA is released by Ca+2-independent PLA2 (iPLA2) (Dyall and Michael-Titus, 2008). These three PUFAs can be converted into more potent cytokines than the free FAs, including prostaglandins, leukotrienes, thromboxanes, protectins and resolvins, and their effects differ from one other (Calder, 2015; Dyall, 2017).
Neuroinflammation is a common denominator for several neuropathologies. SFAs are known to promote the inflammatory phenotype of microglia, stimulating the secretion of TNF-α and IL-6 through TLR4. Furthermore, PA exposure of astrocytes leads to Caspase-3 activation and alters Bax/Bcl-2 ratio, both effects promoting apoptosis (Gupta et al., 2012). On the contrary, ω-3 PUFAs usually have a marked antiinflammatory effect by blocking microglia activation and stimulating the secretion of neurotrophic factors. However, some deleterious effects have also been reported, such as the worsening of neuritic injury and astrocytosis in PD-mice model (Hussain et al., 2013). ARA is the precursor for potent proinflammatory eicosanoids. On the other hand, compounds derived from DHA and EPA, known as resolvins and protectins, show strong anti-inflammatory effects and mediate the end of an ongoing inflammatory response (Hussain et al., 2013). These compounds are just an example of those derived from FAs that have important roles in brain pathophysiology. A special mention should be given to endocannabinoids, such as arachidonoylethanolamine (also known as anandamide), arachidonylglycerol or oleoylethanolamine, just to name a few (Freitas et al., 2017). Nevertheless, the role of free FAs has a renewed interest since the identification of multiple receptors that can couple their signaling to diverse cellular responses. The next section focusses on three families of proteins that recognize FAs and could be modulating FAs regulatory functions.
Receptors for Fatty Acids Signaling
Originally, lipid signaling was limited to steroid hormones and its binding to cytosolic receptors, or to cytokines derived from ARA (leukotrienes and thromboxanes) that bind to specific membrane receptors. As of today, three families of proteins have been identified to be able to sense the presence and type of FAs whether in the extracellular medium, the cytosol or the nuclear matrix. At the plasma membrane, FAs can activate G Protein-coupled receptors known as Free Fatty Acid Receptors (FFARs) (Hara et al., 2013; Offermanns, 2013); while in the cytosol they can be taken by Fatty Acid Binding Proteins (FABPs) and targeted to specific subcellular structures or metabolic pathways (Veerkamp and Zimmerman, 2001; Storch and Corsico, 2008). Finally, nuclear receptors Peroxisome Proliferator-Activated Receptors (PPARs) mediate FAs regulatory functions in the nucleus (Zolezzi et al., 2017). The specific spatio-temporal pattern of expression and the co-expression of more than one isoform from each family of proteins in a single cell suggest a platform for sensing and modulating the cellular response to the bioavailability of the different FAs, for example, adapting the cell to developmental or functional requirements. Therefore, the regulatory and signaling roles of free FAs are gaining importance in physiological and pathological processes as these receptors are better characterized.
We describe bellow some of the known characteristics of each family of FAs receptors selected for this review, including expression patterns, structural features, and specific functions in the CNS. Noteworthy, when the source of expression data is not specified, it was taken from The Human Protein Atlas website1 (Uhlen et al., 2015), based on Genotype-Tissue Expression (GTEx) project (The GTEx Consortium, 2013), or from the Allen Mouse Brain Atlas2 (Lein et al., 2007).
Plasma Membrane Receptors
A large number of putative genes coding for GPCRs was identified based on genome sequences, and their deorphanization is still in progress (Civelli, 2005; Diaz et al., 2018; Laschet et al., 2018). Particularly, a cluster of 4 sequences (GPR40, GPR41, GPR42 and GPR43) was identified in chromosome 19 (Sawzdargo et al., 1997) and FAs were proved to work as their specific endogenous ligands (Briscoe et al., 2003; Brown et al., 2003), dubbing this subfamily of GPCRs as FFARs (Offermanns, 2013). Later on, two other receptors activated by FAs were described, GPR84 and GPR120, located in chromosome 12 and 10, respectively; as well as many others activated by diverse lipids derived from FAs, including lysophosphatidic acid (termed LPARs), endocannabinoids (CBs) and hydroxycarboxylic acids (HCAs) (Offermanns, 2013; Audet and Stevens, 2019).
Within the superfamily of GPCRs, FFARs belong to the largest subfamily of Class A/1 (rhodopsin-like) receptors, constituted by a motif of 7 transmembrane segments (TMs) and at least one longer cytosolic domain that serves as binding site for signaling machinery assembly (Dorsam and Gutkind, 2007; Heldin et al., 2016). The ligand recognition site is defined within the transmembrane helix bundle (Figure 1A). The original cluster of FFARs was deorphanized by heterologous expression and ligand screening through monitoring cytosolic Ca+2 levels (Briscoe et al., 2003). FAs-triggered Ca+2 release from the endoplasmic reticulum is PI3K-dependent. However, some of these receptors can also transduce FAs signal through inhibition of cAMP synthesis, CREB, Akt/PKB, and/or Erk phosphorylation, as well as by β-Arrestin recruitment (Kimura et al., 2011; Zamarbide et al., 2014). Each FFAR shows specific patterns of expression and distinctive ligand selectivity. Their functions have been better studied in peripheral tissues, including the promotion of hormone secretion by the pancreas, lipid taste sensing and the activation of immune cells as a preliminary response toward a putative growing infection. Their participation in the CNS had less attention, although recent results suggest that some isoforms could participate in brain development, neuronal differentiation (Ma et al., 2010) and could be potential drug targets against several neuropathies, such as neuropathic pain and epilepsy (Yoshimura et al., 2015; Yang et al., 2018).
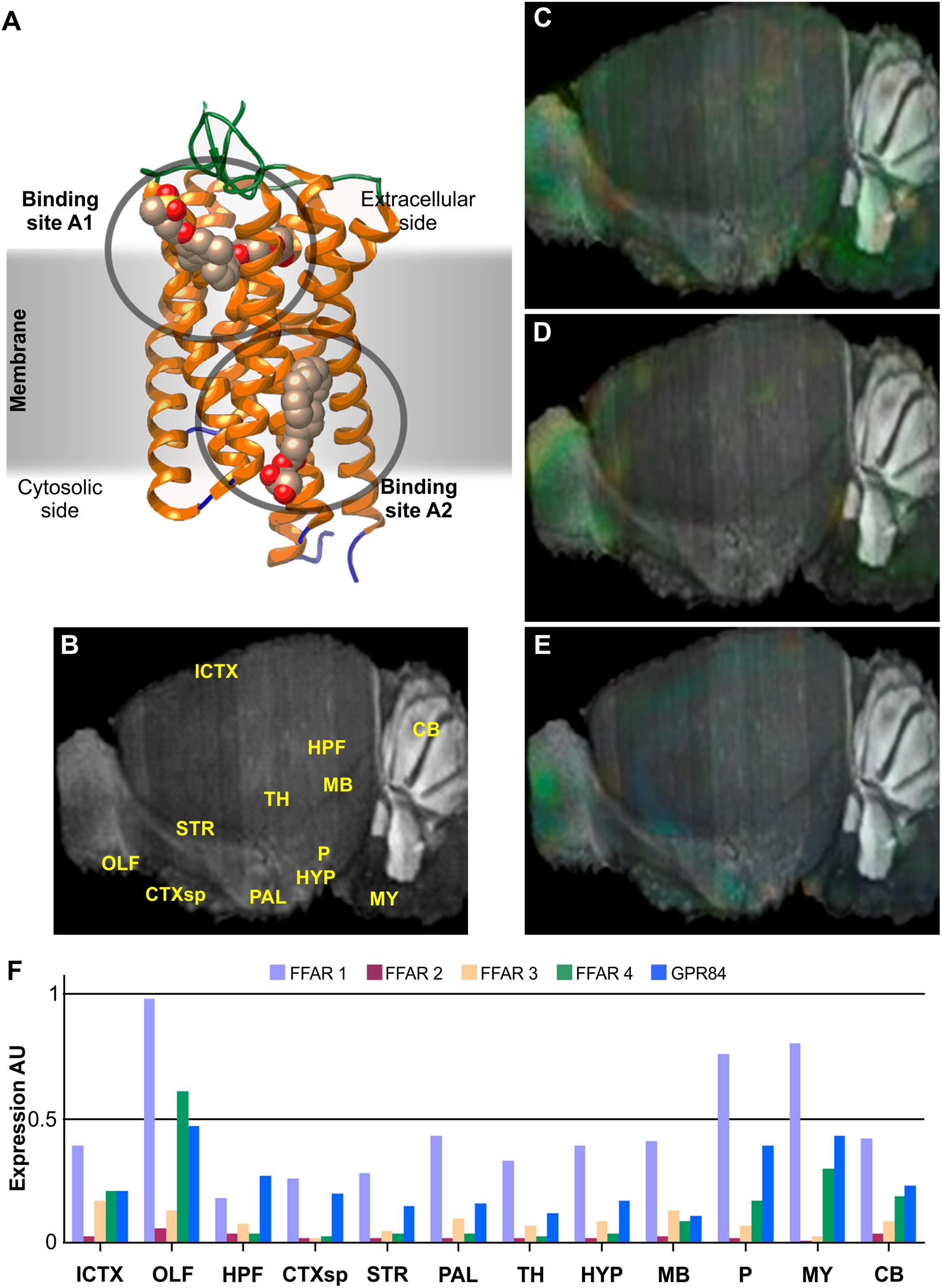
Figure 1. Structure and brain expression patterns of FFARs. (A) Cartoon based on FFAR1 crystal structure (PDB-ID: 4PHU) (Srivastava et al., 2014), highlighting membrane orientation and two ligand binding sites in opposite sides of the membrane. (B) Central brain section of 8-weeks, male mouse (C57BL/6J) indicating reference regions. (C–E) Brain left hemisphere sagittal projection showing expression of FFAR1, FFAR4 and GPR84, respectively, based on in situ hybridization (ISH) data. FFAR2 and FFAR3 are not shown due to its lower expression levels. (F) Quantification of relative expression from ISH data for all FFARs isoforms. AU: Arbitrary units; ICTX: Isocortex; OLF: Olfactory areas; HPF: Hyppocampal formation; CTXsp: Cortical subplate; STR: Striatum; PAL: Pallidum; TH: Thalamus; HYP: Hypothalamus; MB: Midbrain; P: Pons; MY: Medulla; and CB: Cerebellum. Image credit for panels C–E: Allen Institute © 2007 Allen Institute for Brain Science. Allen Mouse Brain Atlas. Available from: http://mouse.brain-map.org/search/. Panels B and F were constructed from data available through Allen Mouse Brain Atlas website.
Each isoform presents distinct body expression profiles and different affinity for FAs (Brown et al., 2005; Hara et al., 2013; Offermanns, 2013). There are two FFARs with prominent levels of expression in neural tissues: FFAR1 (also known as GPR40) and FFAR4 (GPR120 or O3FAR). Nevertheless, all isoforms have been detected, though weakly, in some restricted brain region. We review bellow some of the most recent findings about this novel family of GPCRs activated by FAs.
FFAR1 (GPR40)
The genes that code for FFAR1, FFAR2 and FFAR3, originally named GPR40, GPR43 and GPR41, respectively, were identified in 1997 as a group of tandemly encoded genes located on human chromosome 19q13.1. FFAR1 is a 300 amino acids membrane protein of 31.45 kDa. As the product of the single exon gene GPR40, it is most abundantly expressed in pancreas and brain of primates (Briscoe et al., 2003; Brown et al., 2005). Other tissues with significant expression include small intestine, spleen, testis, ovaries, and Fallopian tubes. According to GTEx data, within the CNS, higher expression levels of FFAR1 mRNA are found in hippocampus, caudate nucleus, hypothalamus, and cerebral cortex, presumably mainly in neurons but also in glial cells. On the other hand, qPCR targeted experiments showed a wider expression of FFAR1 in neuronal tissues, with higher expression levels in medulla oblongata, substantia nigra and spinal cord, followed by putamen, locus cereleus, globus palidus, and amygdala (Briscoe et al., 2003), while western blot analysis showed higher expression in pons, dentate gyrus, pituitary gland, substantia nigra and spinal cord, followed by subgranular and subventricular zones, CA1, medulla oblongata, and cerebral cortex, with minor expression in cerebellum (Ma et al., 2007). In situ hybridization (ISH) of mice brain sagittal sections indicated discrete preferential FFAR1 expression in olfactory bulb, pons, and medulla (Figures 1C,F), while coronal sections highlighted hippocampus and cerebral cortex (Zamarbide et al., 2014). Its levels are weaker than in primates and, hence, it has been suggested that is not essential. Actually, FFAR1-KO mice manifested no evident alteration of social or motor behavior, although they showed a reduction anxiety-like responses (Mancini et al., 2015). An increase in noradrenaline was also observed in FFAR1-KO mice brain regions were the expression of the receptor was shown to be higher, suggesting a role in anxiety and depression symptoms.
This receptor is the only FFAR with solved crystal structures (PDB-ID: 5KW2, 4PHU, 5TZR and 5TZY), as different fusions to Lysozyme, and in complex with different partial and full synthetic agonists, such as TAK-875, MK-8666 or the novel compound (3∼{S})-3-cyclopropyl-3-[2-[1-[2-[2,2-dimethylpropyl-(6-methylpyridin-2-yl) carbamoyl]-5-methoxy-phenyl]piperidin-4-yl]-1-benzofuran-6-yl] propanoic acid (Srivastava et al., 2014; Lu et al., 2017; Ho et al., 2018). Noteworthy, the different structures identified two putative binding sites in opposite sides of the transmembrane region, A1 in the extracellular side and A2 in the cytosolic one (Figure 1A). Only the occupancy of the latter by synthetic or natural ligands promotes full agonistic response and structure of intracellular loop IC2 located between transmembrane regions TM3 and TM4, which is believed to be necessary for G-protein interaction. The extracellular loop located between TM4 and TM5 is stabilized by a disulfide bond formed between Cys79 and Cys170. Residues participating in ligand recognition have been mapped to include Arg183, Asn244 and Arg258 that work as anchors for the carboxylic head of the FAs. Residues Tyr12, Tyr91, His137, and Leu186 were also identified as relevant for receptor activation; while His86, Tyr91, His137 and the anchoring positions seem to be critical for the binding and activation by the synthetic agonist GW9508 (Sum et al., 2007; Tikhonova et al., 2007).
FFAR1 has approximately the same high affinity (EC50 5-60 μM) for medium and long chain saturated FAs ranging from C6 (caproic acid) to C23 (tricosanoic acid), with FAs between C10 to C18 being the preferred endogenous agonists; but it is not active in the presence of acetate, propionate, butyrate, or pentanoate (valerate). It also shows the same range of high affinities for both MUFAs and PUFAs, with ARA (C20:4ω-6), EPA (C22:5ω-3), and DHA (22:6ω-3) exhibiting the highest potencies (Briscoe et al., 2003; Offermanns, 2013). Noteworthy, retinoids can also activate FFAR1 with similar affinities, and, particularly, all-trans-retinoic acid, a well-known factor required for neuronal differentiation (Yu et al., 2012; Janesick et al., 2015), shows one of the highest affinities, EC50 < 5 μM (Briscoe et al., 2003).
In pancreas, its activation stimulates insulin secretion from β-cells (Itoh et al., 2003), involving signaling by both Gαq/11 subunit and β-Arrestin2 (Mancini et al., 2015), what does have an impact on systemic metabolism control. Diverse functions have been proposed for FFAR1 in the CNS or the peripheral sensory system. Cartoni et al. (2010) described the expression of FFAR1 in type I cells of the taste buds, along with FFAR4 in type II cells, both participating in the oral perception of fats. FFAR1 has also been associated to neurogenesis and linked to cognitive functions such as memory, space orientation and learning (Ma et al., 2008; Yamashima, 2008; Boneva et al., 2011; Zamarbide et al., 2014). Although these effects are probably mediated mainly by phosphorylation of CREB, its signaling pathway is still not well understood. Since Gαs proteins do not seem to be activated by FFAR1, an alternative phosphorylation of CERB via Erk has been proposed (Yamashima, 2012). Differentiation and maturation of cultures of rat neuronal stem cells overexpressing FFAR1 can be stimulated by DHA, promoting neurite outgrowth and branching, even at concentrations as low as 1,5 μM (Ma et al., 2010). FFAR1 has also been proved to mediate the antinociceptive activities of DHA via induction of secretion of μ and δ opioid peptides, but not κ opioid peptides (Nakamoto et al., 2012). In the hypothalamus FFAR1 is expressed in NPY and POMC expressing neurons linked to satiety and control of food intake, and its activation by its synthetic agonist TUG905 increase POMC (anorexigenic precursor) secretion that leads to body weight loss (Dragano et al., 2017).
High fat diet in mice and PA-albumin complex in neuroblastome derived cells in culture were shown to stimulate synthesis of amyloid precursor protein (APP) and β-site APP cleaving enzyme 1 (BACE1), promoting the release of Aβ peptides in hippocampus and brain cortex, similarly to Akt pathways activation by GW9508 agonist of FFAR1 (Kim et al., 2017). This effect can be blocked in SK-N-MC cells in vitro if FFAR1 is pharmacologically inhibited with GW1100, knocked down by siRNA or delocalized from lipid rafts when membranes are depleted from cholesterol with methyl-β-cyclodextrin. However, GW9508 activation of FFAR1 in a mouse model of AD, based on Aβ intracerebroventricular (icv) injection, leads to significant improvement in cognitive and behavioral tests, what seems to be mediated by CREB phosphorylation and the concomitant increase in expression of NGF and BDNF neurotrophic factors. These effects were not observed when the inhibitor GW1100 was co-administered with the synthetic agonist (Khan et al., 2016). As these opposing effects originate from divergent signaling pathways from a single receptor, exploitation of FFAR1 synthetic agonists or inhibitors for treating neuropathologies may be a task worth pursuing. But first, it is imperative to fully characterize the signal transduction mechanisms active in the CNS, as well as to develop specific drugs that can selectively activate only those promoting neuroprotection, neuronal differentiation, and maturation. During the last decades, an increasing amount of evidence was collected linking the etiology and pathology of AD with Type-2 Diabetes Mellitus (Benedict and Grillo, 2018; Ferreira et al., 2018; Chornenkyy et al., 2019). Considering the wide range of synthetic agonist of FFAR1 being developed to treat Diabetes, it became an interesting possibility to evaluate their repurposing for treating AD as well (Li et al., 2016; Chen et al., 2019). For example, two different mouse models of diabesity (diabetes and obesity), based on high fat diet or db/db mice, present a decrease in FFAR1 and BDNF expression in the hippocampus and brain cortex, that can be reverted by chronic administration of DHA or GW9608 (Sona et al., 2018). Since it can be inhibited by Erk and MAPK inhibitors, BDNF expression depends on FFAR1-pErk pathway.
FFAR2 (GPR43)
Although the gene structure of GPR43 is not yet fully defined, FFAR2 was described to be encoded as a 330 amino acids protein (37.14 kDa) in a single exon. Its structure is predicted to have a topology very similar to FFAR1, where the extra 30 amino acids would correspond mainly to a longer C-terminal tail. However, FFAR2 preferential endogenous ligands are limited to acetate, propionate, butyrate, and pentanoate (with EC50 somewhat an order of magnitude larger than those for FFAR1), and this receptor does not respond to ligands with chain length above C6 (Brown et al., 2003; Offermanns, 2013). Its activation has been describe to be coupled both to pertussis toxin sensitive (Gαi) and insensitive (Gαq) proteins (Brown et al., 2003; Stoddart et al., 2008). In the first pathway, FFAR2 activation leads to a decrease in cAMP production by adenylate cyclase (AC) and, therefore, to a reduction in protein kinase cAMP-activated (PKA) activity. On the other hand, Gαq activates phospholipase C (PLC) that cleaves phosphatidylinositol bisphosphates (PIP2) into inositol-triphosphate (IP3) and diacylglycerol. IP3 binds to IP3R receptor in the endoplasmic reticulum and induces the internal release of Ca+2 to the cytosol, which then activates Ca+2-dependent protein kinase C (PKC). DAG attracts certain PKC isoforms to the plasma membrane and helps to direct its activity to a subset of potential target substrates.
FFAR2 is most abundantly expressed in spleen, bone marrow, and lung, followed by adipose tissue, breast, and all the digestive tract. Its expression is mainly consistent with a leukocyte markers expression pattern, with higher presence in macrophages and leukocyte germ line (Brown et al., 2003). The low level, but widespread FFAR2 expression may be due to its presence in immune cells, such as infiltrating neutrophils and macrophages. Its expression in CNS is rather low compared to other FFARs (Figure 1F) and limited to glia and neurons of the caudate, but FFAR2 can also be detected in cortical neurons and pituitary gland. However, the participation of FFAR2 in neuronal processes still needs further analysis.
FFAR3 (GPR41)
FFAR3 is the product of the gene GPR41, only 6.62 kb downstream of GPR40 promoter, but it lacks a formal promoter of its own. Although 3 sites of transcription initiation can be predicted in putative exons upstream GPR41, none of them could be confirmed. Contrarily, GPR41 may be expressed as the result of the skipping of a termination sequence immediately downstream FFAR1 stop codon. Bahar Halpern et al. (2012) demonstrated that GPR40 and GPR41 are transcribed as a single bicistronic mRNA thanks to the action of an H2R enhancer, and that a tissue-specific internal ribosome entry site controls the translation of GPR41 into FFAR3 in pancreatic β-cells. FFAR3 is also coded in a single exon, as a 346 amino acids protein (38.,65 kDa). GPR40-common origin is inferred from the relatively high sequence similarity/identity (31/34%), compared to other GPCRs. Like FFAR2, its structure is believed to be similar to FFAR1, with the extra 46 amino acids mainly corresponding to an extension of the cytosolic C-terminal tail; and its activation is mainly due to the binding of the same short chain FAs (Brown et al., 2003). The difference is that pentanoate is more potent than acetate for FFAR3, while it is quite the opposite for FFAR2. FFAR3 activation has been described to be coupled only to Gαi subunits. Its activation by β-hydroxy-butirate negatively regulates the activity of Cav2.2 N-type Ca+2 channel (Won et al., 2013; Colina et al., 2018).
High-throughput RNAseq screening analysis of FFAR3 expression indicated a widespread, but weak pattern, with higher levels observed in adipose tissue, breast, spleen, and digestive tract. Neural expression is scarce (Figure 1F) and only very weakly detected in the pituitary gland in GTEx (The GTEx Consortium, 2013). A GPR41-mRFP transgenic mice model published by Nohr et al. (2013) showed FFAR3 expression in the digestive tract intimately associated to the enteroendocrine system, mainly in enteric neurons of the submucosal and myenteric ganglia, and in several of the postganglionic sympathetic and sensory neurons, both in autonomic and somatic peripheral nervous system (PNS). But this model showed no expression in the brain or spinal cord. FFAR3 enteroendocrine expression links signaling of short FAs from the microbiota with enteric hormone secretion (Samuel et al., 2008). Its activation by short chain FAs and β-hydroxy-butirate in sympathetic neurons also inhibited CaV2.2 currents, linking the functions of the PNS to the metabolic state (Won et al., 2013).
FFAR4 (GPR120)
The gene that codes for FFAR4, originally called GPR120, was described in 2003 to have four exons that are located on human chromosome 10q23.33 (Fredriksson et al., 2003). It is expressed, at least, as two variants generated by alternative splicing that leads to exon 3 skipping, GPR120S (coding for a 361 amino acids peptide, 40,49 kDa) and GPR120L (377 amino acids, 42,24 kDa). Predicted transmembrane regions leave N- and C-terminal segments longer than in previous FFARs, but the most notorious difference is the cytoplasmic loop between TM5 and TM6, where the GPR120S lacks 16 amino acids (from 233 to 248) compared to the longer variant GPR120L. Activation of FFAR4 signals through Gαq/11.
Highest expression of FFAR4 includes lower digestive tract, brain, adipose tissue, lung, testis, breast, and adrenal glands. Within the CNS, FFAR4 is found mainly in glial and neuronal cells of hippocampus, cerebral cortex, hypothalamus, and cerebellum (only granular layer and Purkinje cells), with a particularly high expression in pituitary gland (The GTEx Consortium, 2013). ISH of mice brain sections show FFAR4 preferential expression in medulla, pons and olfactory bulb, followed by hypothalamus and cerebellum (Figures 1D,F).
FFAR4 natural ligands include saturated FAs of C14, C16 and C18, with affinities around EC50 = 30, 52 and 18 μM, respectively. When overexpressed in HEK293 cells, both agonist-stimulated GPR120S and GPR120L receptors recruit β-Arrestin2 and undergo internalization, but the longer variant shows much less Ca+2 mobilization and average cellular response (Watson et al., 2012). Two Arg residues on the outer edge of TM2 (Arg99) and TM4 (Arg178) have been mapped to be responsible for FAs binding (Watson et al., 2012), unlike the other FFARs members that have two conserved Arg residues on the outer edge of TM5 and TM7 (Sum et al., 2007; Stoddart et al., 2008), showing once more the evolutionary divergence of FFAR4 from the rest of the GPCRs activated by FAs. Other residues involved in ligand binding and activation of the receptor included four aromatic residues Phe115, Phe211, Trp277, and Phe304 that, when mutated, essentially eliminated responsiveness to agonists (Hudson et al., 2014).
FFAR4 has been more thoroughly studied in adipocytes and intestine where it promotes insulin sensitizing and anti-inflammatory effects, respectively (Song et al., 2017). In enteroendocrine cells it helps controlling the secretion of hormones, promoting glucagon-like peptide 1 release but inhibiting ghrelin secretion (Hirasawa et al., 2005; Engelstoft et al., 2013); whereas, in adipocytes, oleic acid stimulates lipid droplet formation by activation of this receptor, which signals through Gαq, PI3K-Akt, and PLC pathways (Rohwedder et al., 2014; Villegas-Comonfort et al., 2017). However, it has a greater affinity for PUFAs, particularly those from the ω-3 series. In Caco-2 cells, EPA, DHA, and ARA elicit the same signaling events through FFAR4, but with different kinetics and efficiency (Mobraten et al., 2013). Basal and heterologous phosphorylation of Thr347, Ser350, and Ser357 in the C-terminal tail (GPR120S) mediates receptor internalization (Burns et al., 2014; Sanchez-Reyes et al., 2014). Hypothalamic expression of FFAR4 corresponds to microglia and its activation ameliorates neuroinflammatory response by decreasing the production of proinflammatory cytokines (TNFα and IL-1β) and promoting those with anti-inflammatory action (IL-6 and IL-10) (Dragano et al., 2017).
GPR84
This isoform was identified, through an EST library, in B cells encoded in a single exon (exon2) (Wittenberger et al., 2001), but it was the last one to be deorphanized (Wang et al., 2006). Therefore, GPR84 is the least studied isoform of the FFARs and has not yet been assigned with the “FFAR5” name. Its expression was confirmed by Northern-blot in brain, colon, thymus, spleen, kidney, liver, intestine, placenta, lung, leukocytes, heart, and muscle. Regarding CNS expression, it is most abundantly found in medulla and spinal cord, but also significantly in amygdala, substantia nigra, thalamus, and corpus callosum, whereas only weakly detected in other brain regions such as the cerebellum (Wittenberger et al., 2001) (Figures 1E,F). GPR84 is activated only by medium length acyl chain FAs (C9 to C14) and does not recognize longer or shorter chain carboxylic acids, promoting Ca+2 mobilization and inhibiting cAMP production, mainly through the activation of Gαi/o (Wang et al., 2006). Its expression is augmented in monocytes by incubation with LPS, and its activation by medium chain FAs exacerbates the production of proinflammatory cytokines, highlighting GPR84 role in immune responses (Wang et al., 2006; Muller et al., 2017). Alternatively to endogenous ligands, GPR84 can also be activated by hidroxylated medium chain FAs and synthetic drugs like ZQ16 (2-(hexylthio)pyrimidine-4,6-diol), diindolylmethane or 6-n-octylaminouracil (Suzuki et al., 2013; Nikaido et al., 2015; Zhang et al., 2016).
When activated, GPR84 expressed in mouse primary cultures of microglia stimulates membrane ruffling, modifies its morphology, and promotes motility, but it does not promote an inflammatory response, including the secretion proinflammatory cytokines (Wei et al., 2017). Different FAs are known to be released after diverse brain injuries, from traumatic lesions to neurodegenerative diseases and neuroinflammatory conditions, and they may function as chemo-attractants for microglia, especially short and middle chain FAs that exhibit higher solubility and that can be recognized by FFAR2, FFAR3, and GPR84. This suggests that GPR84 could be a valid therapeutic target in microglia-associated diseases, such as AD or Multiple Sclerosis.
Citosolic Receptors
Due to their low solubility, free FAs need to be bound to proteins in order to diffuse through the aqueous medium of the cytosol. Since the 1970s, the proteins known as FABPs have been studied and proved to help overcome this problem. They are small (15 kDa) intracellular soluble proteins that reversibly bind FAs and other hydrophobic ligands, trafficking them to different intracellular compartments, such as mitochondria, peroxisome, endoplasmic reticulum or the nucleus. Even with as low as 30% identity of amino acids sequence, they share a highly conserved structure (Figures 2A,B), consisting in a barrel of 10 β-strands enclosing the binding cavity, and a helix-turn-helix motif capping this barrel. The latter regulates both ligand entry and exit, as well as origin and destiny points of traffic (Banaszak et al., 1994; Thompson et al., 1999; Storch and Corsico, 2008; Storch and Thumser, 2010). These proteins have been extensively characterized in vitro, including its three-dimensional structures by NMR and X-ray Crystallography, with and without any cargo, natural or synthetic (Sacchettini et al., 1987; Xu et al., 1991; Ory et al., 1997; Balendiran et al., 2000; Hanhoff et al., 2002; Rademacher et al., 2002; Reese and Banaszak, 2004). They can uptake FAs from model phospholipid vesicles and transfer its cargo to other membranes, according to the properties of each isoform (Herr et al., 1996; Storch and Thumser, 2000; Veerkamp and Zimmerman, 2001; Liou et al., 2002; Storch et al., 2002; Falomir-Lockhart et al., 2006). It has been demonstrated that some isoforms interact better with membranes in the apo-form, presumably looking for ligand uptake; whereas others are dominated mainly by electrostatic interaction between the holo-protein and the phospholipid’s headgroups (Falomir-Lockhart et al., 2006, 2011).
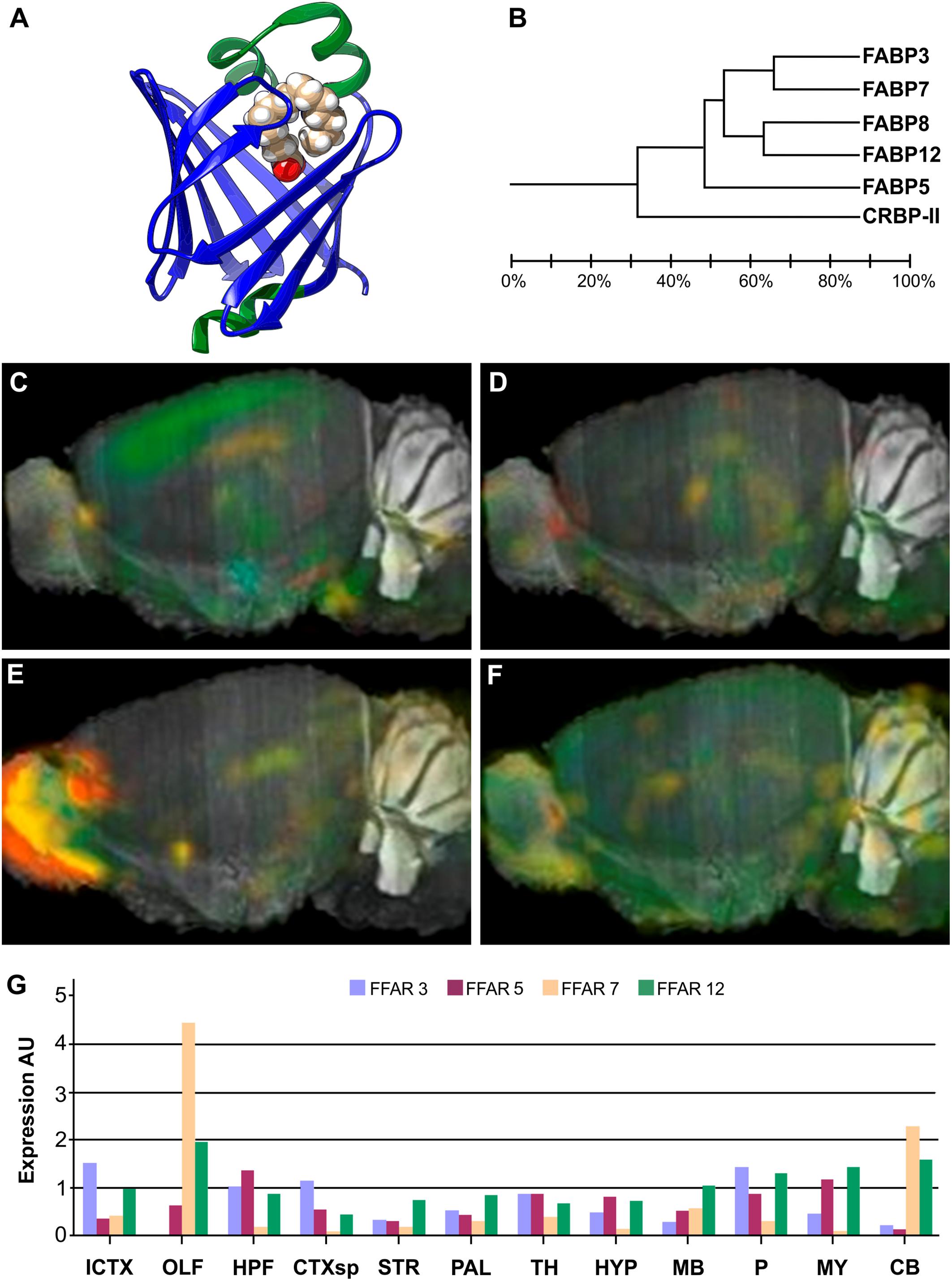
Figure 2. Structure and brain expression patterns of FABPs. (A) Cartoon based on crystal structure of human FABP3 complex with palmitic acid (PDB-ID: 6AQ1) (Yan et al., 2018). The β-barrel, array of 10 antiparallel β-strands, is shown in blue, and the helix-tur-helix motif cap is shown in green, with the palmitic acid (in filled-balls model) adopting a U-shaped conformation inside. (B) Dendrogram showing relationship between identity of sequence between FABPs expressed in neural tissues. Also belonging to the same folding, CRBP-II was included as and outlier. (C–F) Brain left hemisphere sagittal projection showing expression of FABP3, FABP5, FABP7 and FABP12, respectively, based on ISH data. FABP8 expression in mice brain is not significant. (G) Quantification of relative brain expression from ISH data for neural FABPs isoforms. AU: Arbitrary units. For brain regions references see legend of Figure 1. Image credit for panels C–F: Allen Institute. © 2007 Allen Institute for Brain Science. Allen Mouse Brain Atlas. Available from: http://mouse.brain-map.org/search/. Panel G was constructed from data available through Allen Mouse Brain Atlas website.
FABPs were originally named after the tissue where each isoform was firstly identified, and intestinal enterocytes were the exception that expressed large quantities of two isoforms, FABP1 and FABP2. Nowadays, the numbered nomenclature is preferred over tissue-related names in order to avoid misinterpretations derived from the fact that most cell types can express more than one member of the FABP family, and their level of expression responds to metabolic conditions, external stimuli, or development stages. Therefore, most FABPs show distinctive expression patterns in different organs and cell types. No isoform shows a high degree of selectivity for a particular FA, although it is usually found a higher affinity for saturated compared to unsaturated FAs (Richieri et al., 2000). The affinity for FAs of each isoform depends on chain length and number of double bonds, but isoforms FABP1 and FABP5 show significant affinity for a wider range of hydrophobic ligands. A total of 9 FABP isoforms with high affinity for long chain FAs (between C12 and C22) are described in mammals, plus 4 more members that show specificity for retinoid ligands (CRBP-I, CRBP-II, CRABP1 and CRABP2) and one extra for bile acids (FABP6, I-BABP or ILBP).
There are five FABP isoforms with relevant levels of expression in neural tissues: FABP3 (commonly known also as Heart-FABP), FABP5 (Epidermal-FABP), FABP7 (Brain-FABP), FABP8 (Myelin-FABP or PMP2), and FABP12 (Retinal-FABP). Each of them presents distinct spatio-temporal expression patterns (Owada et al., 1996; Liu et al., 2000). Many of them are significantly expressed in the brain, whereas FABP8 is apparently exclusive of PNS. Bellow there is a resume of the most prominent information available about each neural isoform.
FABP3
Significant expression of FABP3 is not detected in embryonic stages of rodent or in fetal human brains (Cheon et al., 2003; Saino-Saito et al., 2009). Perinatal FABP3 expression increases gradually, mainly confined to gray matter in rodents. In adult individuals, FABP3 is stably expressed in the neuronal layers of hippocampus, the cerebral neocortex, interneurons of the retina and the olfactory mitral cell layer, particularly in CA1 and CA2 portions (Owada, 2008; Saino-Saito et al., 2009). Its mRNA can also be detected in Purkinje and granulate cells of the cerebellum (Owada, 2008). ISH of mice brain slices localizes FABP3 expression in isocortex, cortex, pons and hippocampus (Figures 2C,G).
FABP3 shows somewhat preferential binding of ω-6 PUFAs, but affinities for all FAs range from 0.8 to 5 μM. FABP3 is thought to help to consolidate and maintain the differentiated status of neurons in adult brains through the utilization of PUFAs. Actually, brain ARA assimilation and its incorporation into phospholipids correlate with FABP3 expression levels, which is also necessary to maintain an optimal balance between ω-6 and ω-3 PUFAs in adult neurons (Murphy et al., 2005). FABP3 could also modulate dopamine signaling, since it is highly expressed in acetylcholinergic and terminals of glutamatergic neurons of dorsal striatum, and it can interact physically and modulate dopamine D2 receptors (D2R) in mice (Shioda et al., 2010). This was confirmed by dysfunctional response of D2R to amphetamines and to D2R-specific agonist Haloperidol in FABP3-/- mice; whereas response to D1R-specific agonist SCH23390 was not impaired. The expression of FABP3 in dopaminergic neurons is still controversial, even between the same authors (Shioda et al., 2010, 2014). On the other hand, FABP3 can be found in the cochlea, as well as FABP7, not involved in sensing but present in different support cells, suggesting a non-redundant function of these proteins in modulation of the hearing process (Saino-Saito et al., 2010).
Regarding pathological conditions, FABP3 was found to be significantly decreased in frontal, occipital, and parietal cortices of patients suffering Down Syndrome; and it was also elevated in temporal cortex of patients with AD (Cheon et al., 2003; Sanchez-Font et al., 2003; Watanabe et al., 2007). FABP3 expression in dopaminergic neurons may promote MPTP and ARA-induced α-Synuclein aggregation (Shioda et al., 2014), the main component of the characteristic Lewy Bodies present in PD and related neurodegenerative diseases. Furthermore, aberrant expression of FABP3 may affect PUFA enrichment and alter membrane fluidity and signal transduction. Consequently, this deficiency could lead to cellular dysfunction in neurodegenerative disorders.
FABP5
FABP5 is the most ubiquitously expressed FABP. During midterm embryonic stages, it is particularly present in the ventricular germinal zone and the cerebral cortex, as well as in the stem cells differentiating into motor neurons or astrocytes. But its expression progressively decreases after birth until it is only weakly detected in the adult brain (Liu et al., 2010). This moderate expression is shared, with varying intensity in different brain regions, by neurons and glia (Figures 2D,G). Regarding mouse retina, FABP5 is found in the retinal ganglion cells, up to E14 strictly localized in the soma, but progressively migrating into axons of optic nerve by E18 and until P10 (Allen et al., 2001; Saino-Saito et al., 2009). This suggests that FABP5 may have a role in neurite outgrowth and axon development by supplying LCFA for phospholipid synthesis. FABP5 also shows high affinity for retinoic acid (RA) and, therefore, it is associated to neuronal survival and differentiation by activation of PPARβ/δ, not only through FAs but RA signaling as well. Actually, the latter depends on the distribution ratio between CRABP2 and FABP5, and it may be as well modulated by displacement from either protein by FAs, and particularly by DHA (Schug et al., 2007, 2008; Yu et al., 2012). Recent publications also link FABP5 to regulatory functions of estrogen receptors (Senga et al., 2018), which could be related to its heterodimerization to Retinoid X Receptors (RXRs), similarly to PPARs.
FABP5 expression is induced under pathological or stress conditions, for example, after axonal injury in peripheral nerves (De Leon et al., 1996), but not in Down Syndrome, AD or Bipolar Disorder (Cheon et al., 2003). Under oxidative stress conditions, FABP5 has been proved to work as a scavenging system of 4-Hydroxynonenal lipid peroxidation subproduct (Bennaars-Eiden et al., 2002). Altogether, we can summarize that FABP5 is expressed when the fate of the neural cell differentiation must be decided or when it has to adapt to stress or pathological conditions.
FABP7
In embryonic brains, FABP7 is highly expressed in the radial glia, in the ventricular and subventricular zones. After birth, the expression remains strongly positive in gray and white matter (Kurtz et al., 1994). In the neonatal brain, its expression is more evident in the olfactory nerve fiber layers, hippocampal dentate gyrus, and the cerebellar Purkinje cell layer. Finally, in adulthood, the expression decreases although it remains in the Schwann cells of olfactory nerve, in the radial glia of dentate gyrus, and in the glial cells located adjacent to the cerebellar Purkinje cells (Kurtz et al., 1994). Its mRNA expression in 8 weeks-old mice is evident only in olfactory bulb and the cerebellum (Figures 2E,G). FABP7 was proposed to be downstream regulator of Pax6 participating in the maintenance of pre-neurogenesis neuroepithelia, while its knock-down promotes neuronal differentiation (Arai et al., 2005). FABP7 and FABP3 are usually expressed in the same regions of the brain, with the later usually showing 10-time higher levels, although local concentration may vary (Pelsers et al., 2004). As mentioned before, both proteins are present in different support cells of the cochlea, suggesting a regulatory function of hearing signals (Saino-Saito et al., 2010). FABP7 is also expressed by glial progenitor cells located in the foregut and midgut during enteric nervous system development (Sasselli et al., 2012).
FABP7 preferentially binds ω-3 PUFAs and oleic acid (18:1n-9, OA) over ω-6 PUFAs, and shows lower affinity for SFAs (Xu et al., 1996; Balendiran et al., 2000; Hanhoff et al., 2002). Therefore, null mice display decreased DHA incorporation into PLs, with an increase in AA and PA instead (Owada, 2008). Its up-regulation during embryonic stages of development (and, probably, FABP5’s as well) is likely related to the proliferation and initial differentiation of neural stem cells and progenitorse, with an increasing demand of PUFAs, rather than to their maturation and integration (Liu et al., 2010). Actually, the fluorescent probe CDr3 was identified as a specific ligand for FABP7 in neural stem cells (Yun et al., 2012) and successfully employed for its flow cytometry isolation from both adult and embryonic mouse brains (Leong et al., 2013).
Regarding pathological conditions, FABP7 has been shown to be significantly increased in occipital cortex of patients with Down Syndrome, although no changes were detected in fetal brain (Cheon et al., 2003). Gene allele association studies showed correlation of FABP7 expression with schizophrenia and bipolar disorder (Saino-Saito et al., 2010).
FABP8
FABP8 can be abundantly and exclusively found, although unevenly distributed, in the myelin sheaths and Schwann cells of peripheral nerves, for example, in the human nervus suralis. It can represent up to 15% of total protein of bovine PNS myelin (Greenfield et al., 1973). In mice, mRNA levels increase gradually and the protein is detectable after P4 (Zenker et al., 2014). FABP8 is present only in minor amounts in CNS white matter, being more abundant in spinal cord and brain stem myelin. The expression levels of FABP8 vary both in intensity and distribution between different regions of a single nerve as well as between nerves (DeArmond et al., 1980).
FABP8 has a unique function in the organization and stabilization of myelin multilayers as it is capable of stacking phospholipid membranes synergistically with Myelin Basic Protein (Greenfield et al., 1973). Its higher expression during the early postnatal life also suggests a role in FAs uptake and lipid metabolism toward myelination of axons (Zenker et al., 2014). Notably, knock-out of one or both proteins does not affect myelin structure, and its only consequence is a minor retardation in motor nerve conduction (Zenker et al., 2014). However, several point mutations of FABP8 have been associated to the inherited neuropathy known as Charcot-Marie-Tooth disease (Motley et al., 2016; Punetha et al., 2018).
FABP12
The FABP12 gene was the last one to be identified to code for a member of this family of proteins (Liu et al., 2008) and, hence, has not yet been thoroughly studied like other members. Its mRNA was found at high levels mainly in the retina and testis, and to a lesser extent in the cerebral cortex, kidney and epididymis of rat and mouse tissues (Smathers and Petersen, 2011). ISH of mouse brain sections shows a ubiquitous expression (Figures 2F,G). Under oxidative stress conditions, FABP12 protects retinal rod cells from peroxidation mediated by LCFA hydroperoxides (Guajardo et al., 2002).
Nuclear Receptors-Transcription Factors
In many tissues, FAs induce changes in gene expression through nuclear receptors of the PPARs family of transcription factors, ligand-activated nuclear receptors subfamily 1 group C (Capelli et al., 2016). PPARs structure consists of a variable N-terminal region, a conserved DNA binding domain, a variable hinge region, a conserved ligand binding domain, and a variable C-terminal region (Mangelsdorf et al., 1995). Heterodimerization of PPARs with Retinoid-X Receptor (RXR) depends on ligand and DNA binding, being affected also by posttranslational modifications (Berrabah et al., 2011; Anbalagan et al., 2012; Brunmeir and Xu, 2018). The DNA binding domain includes two segments with Zn-fingers motifs, which recognizes its target sequence or Response Element (RE). The N- and C-terminal regions include the Activation-Function 1 (AF1) and 2 (AF2), respectively, essential for interaction with coregulators and transcription modulation functions (Figure 3A). PPAR–RXR complexes recognize a direct repeat of the consensus sequence AGGTCA with a single nucleotide in between, also referred to as PPRE: AGGTCA-N1-AGGTCA. These features have been corroborated by the crystal structures available (PDB-IDs: 3E00, 3DZU, 3DZY) (Figure 3A) (Chandra et al., 2008). PPARs are thought to be permanently bound to PPREs as heterodimers with one of the three RXR isoforms (RXRα, RXRβ, or RXRγ). The lack of ligand bound to them promotes their repressor activity through association with corepressors, such as Nuclear Receptor Corepressor (NCoR) or the Silencing Mediator of Retinoid and Thyroid hormone receptor (SMART). Upon ligand binding, corepressors are released and co-activators recruited, including p300, CREB-binding protein, or Steroid Receptor Coactivator 1 (SRC1); promoting transcriptional activation of their target genes (Zoete et al., 2007).
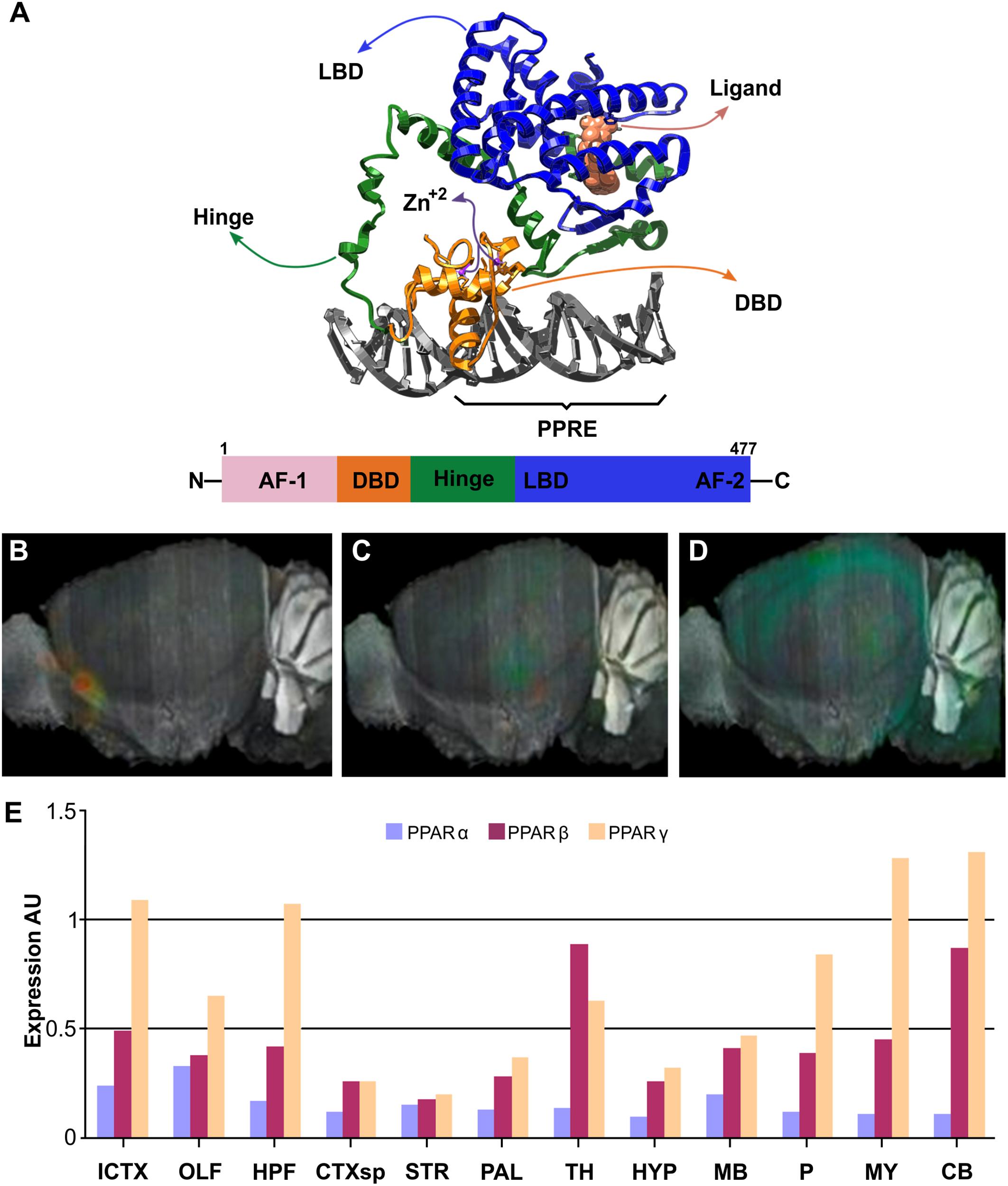
Figure 3. Structure and brain expression patterns of PPARs. (A) Cartoon based on crystal structure (PDB-ID: 3DZY) of the full-length PPARγ in a complex with RXRα and two accessory 13-amino acids peptides of Nuclear receptor coactivator 2 and a dsDNA containing the PPRE (Chandra et al., 2008). For clarity, only PPARγ, its synthetic agonist Rosiglitazone and the dsDNA are shown. N-terminal region of PPARγ is not visible because it was not determined due to high flexibility. (B–D) Brain left hemisphere sagittal projection showing expression of PPARα, PPARβ and PPARγ, respectively based on ISH data. (E) Quantification of relative brain expression from ISH data for neural FABPs isoforms. AU: Arbitrary units. For brain regions references see legend of Figure 1. Image credit for panels B–D: Allen Institute. © 2007 Allen Institute for Brain Science. Allen Mouse Brain Atlas. Available from: http://mouse.brain-map.org/search/. Panel E was constructed from data available through Allen Mouse Brain Atlas website.
PPARα was first identified as a new member of the steroid hormone receptor superfamily and proved to mediate the effects of hypolipidemic drugs commonly used in hyperlipidemias. These drugs were known as peroxisome proliferators, dubbing this receptor PPARs (Issemann and Green, 1990). Parallel identification of multiple isoforms in mammals and Xenopus sp. led to some controversy regarding their nomenclature, but nowadays it is accepted that three isoforms are present, comprising PPARα, PPARβ/δ, and PPARγ (Echeverria et al., 2016; Brunmeir and Xu, 2018). Besides FAs, PPARs have also been reported to be activated by other endogenous lipidic compounds, such as endocannabinoids (oleylethanolamide, arachidonylethanolamide, 2-arachidonyl-glycerol, etc.) and by RA (O’Sullivan, 2007; Schug et al., 2007). Unfortunately, little progress has been made identifying natural endogenous ligands which are specific or preferential for any of the PPARs isoforms due to their rather low affinity and similar specificities.
As mentioned above, the activation of PPARs modulates directly the expression of genes near the bound PPER. But a second mechanism of action has been proposed for certain isoforms, blocking or preventing the interaction of other transcription factors. The better studied example corresponds to the ligand-dependent SUMOylation of activated PPARγ that gets displaced from its PPRE and blocks NFκB interaction with its own response elements and, hence, limiting the inflammatory response (Ricote and Glass, 2007; Glass and Saijo, 2010). This mechanism is known as transrepression and may be a putative target for handling neuroinflmammation.
All PPARs isoforms were detected in the CNS employing multiple techniques, such as reverse transcription-quantitative PCR (RT-qPCR), immunohistochemistry (IHC), and ISH (Kainu et al., 1994; Braissant et al., 1996; Cullingford et al., 1998; Warden et al., 2016). However, despite the vast literature available, there is still some controversy regarding PPARs expression patterns. They are essential for embryonic and fetal development in mammals and, therefore, drug-inducible, tissue specific knockout models have been developed to study their specific roles, particularly for PPARγ null mice which is lethal (Gray et al., 2005; Wang et al., 2013; Rautureau et al., 2017). Double neuronal conditional knockout of PPARβ/δ and PPARγ exacerbates cytotoxicity of 1-methyl-4-phenyl-1,2,3,6-tetrahydropyridine (MPTP) on striatum dopaminergic neurons (Mounsey et al., 2015). Noteworthy, it is not rare to find co-expression of PPARs within a single cell. Some details are offered below about protein expression levels, together with mRNA and protein variants, and the connections of PPARs with brain pathophysiology.
PPARα
Human PPARA (or NR1C1) gene is located in chromosome 22q12-q13.1 and contains at least 9 exons, with PPARα encoded as a 468 amino acid protein (52.23 kDa). A shorter variant is also described by alternative splicing that introduces an early stop codon after the DNA binding domain and, therefore, is not ligand-sensitive. PPARα shows a wide and similar expression in all tissues, with more prominent levels in liver, intestine, kidney, heart, and skeletal muscle; and its presence is also reported in brown adipose tissue. The higher expression levels of PPARα in these tissues correlate with their high capacities for FAs oxidation, and its main function seems to be related to the control of energy expenditure through lipid catabolism and the adaptation to different nutritional states, such as the postprandial period or fasting. Regarding CNS expression, the GTEx project detected similar levels of PPARA mRNA in caudate, pituitary gland, hypothalamus, hippocampus, cerebral cortex, and cerebellum. Furthermore, ISH of brain section showed very similar expression throughout regions and weaker than other isoforms (Figures 3B,E). Targeted expression analysis of PPARα reported it in olfactory bulb, retina, cerebellum, and hippocampus (Braissant et al., 1996; Moreno et al., 2004; Rivera et al., 2014). PPARα has been localized in neurons (Cristiano et al., 2001; Santos et al., 2005), oligodendrocytes (Kainu et al., 1994), microglia (Warden et al., 2016), and specially in astrocytes (Cristiano et al., 2001; Chistyakov et al., 2015).
PPARα shows relatively higher affinities for FAs compared to alternative endogenous agonists (eicosanoids and endocannabinoids), and it can be activated also by hypolimidemic drugs, but generally not or only weakly by non-steroidal anti-inflammatory drugs (NSAIDs) and anti-diabetic thiazolidinediones (Corton et al., 2000). PPARα functions in the CNS are still unclear. Its proposed functions include the regulation of sleep process (Murillo-Rodriguez, 2017), also have an impact on learning and memory consolidation. Nevertheless, it has been shown that PPARα agonists such as Wy-14643 reduce Aβ-derived oxidative damage by increasing catalase activity, and activate the Wnt/β-Catenin survival pathway (Santos et al., 2005). Furthermore, PPARα activation by statins is responsible for boosting BDNF production, that mediates cognitive improvement in another mouse mode of AD (Roy and Pahan, 2015).
PPARβ/δ
Gene structure of PPARD (NR1C2) gene in chromosome 6p21.2-21.1 consists of 11 exons, with the ORF spanning from exon 4 to exon 9 and coding for a 441 amino acids protein termed PPARδ1 (49,09 kDa). Alternative splicing gives rise to shorter isoforms: 80 amino acids shorter isoform 2 (PPARδ2) is the product of an early stop signal due to overtranscription of the 3’-end of exon 8 that introduces an in-frame non-sense codon; variant 4 lacks 98 amino acids (44 to 141) due to exon skipping; while variant 3 is the result of an alternative transcription initiation site with three new N-terminal amino acids instead of the first 43 residues of PPARδ1 (coded by exon 4). Another four alternative transcription initiation sites may be active in PPARD downstream to exon 1 and associated to alternative exon 2 (2a′, 2c, 2c′ and 2e) (Lundell et al., 2007). These mRNA 5′-UTR variants do not alter the final ORF but probably are related to translational regulation of PPARβ/δ. The different isoforms are suspected to show distinctive spatio-temporal expression patterns and/or specific functions. For example, PPARδ2 has been proposed to act as a dominant negative form of PPARδ1 (Dickey et al., 2016).
PPARD expression is broad and is particularly high in tissues associated with FAs metabolism, such as the gastrointestinal tract, heart, kidney, skeletal muscle, fat, and skin. Its systemic physiological function is to coordinate and balance the usage levels of FAs and glucose in muscle and liver. However, PPARβ/δ is also the most predominant PPARs isoform in the human CNS and has a ubiquitous expression in the rat brain (Figures 3C,E). Despite, all PPARs are co-expressed in developing CNS, PPARβ/δ remains with high expression levels in adult rats (Braissant et al., 1996), suggesting a role in brain development, mielynation, and neuronal function. It was localized in olfactory bulb, cerebral cortex, basal ganglia, hippocampus, hypothalamus, cerebellum, and spinal cord, among others brain areas (Xing et al., 1995; Braissant et al., 1996; Moreno et al., 2004). In mouse models, PPARβ/δ is widely distributed and has been found in neurons of the prefrontal cortex, nucleus accumbens, amydala, cerebellum, hypothalamus, and spinal cord, at mRNA and protein expression levels (Woods et al., 2003; Warden et al., 2016). Regarding cell types, PPARβ/δ is mainly expressed in neurons (Hall et al., 2008), but it has also been found in oligondendrocytes of the corpus callosum (Moreno et al., 2004), and in primary cultures of cortical and cerebral astrocytes (Cristiano et al., 2001). Among the glial cells, PPARβ/δ only co-localized with oligondendrocytes of the corpus callosum (Woods et al., 2003), but no with astrocytes and microglia. In vitro, agonists of PPARβ/δ induce the differentiation of SH-SY5Y cells (Di Loreto et al., 2007).
PPARβ/δ shows preferentially affinities for PUFAs, compared to other FAs and eicosanoids, and is selectively activated by bezafibrate (hypolipidemic drug), GW2433 and L-65041 (NSAIDs) among synthetic agonists (Corton et al., 2000). Regarding its pathological links, PPARβ/δ is repressed in patients with Huntington Disease (HD) and its pharmacological activation improves motor function, reduces neurodegeneration, and increases neuronal survival in a HD mice and cellular models (Dickey et al., 2016). The novel PPARβ/δ agonist gemfibrozil is believed to promote oligodentrocyte differentiation by increasing the expression of genes required for myelin formation (Jana et al., 2012) and could potentially be employed against demyelination-related pathologies.
PPARγ
PPARG (NR1C3) gene is located in chromosome 3p25. At least four transcriptional start sites actively transcribe different mRNAs with functional cDNAs coding for PPARγ isoforms. While variants 1, 3 and 4 code for the same PPARγ1 isoform (477 amino acids, 54.68 kDa), mRNA variant 2 codes for PPARγ2 which has an additional 28 amino acids at the N-terminus (505 amino acids, 57,62 kDA). PPARγ1 is expressed in a broad variety of cells including immune and brain cells, whereas PPARγ2 is highly abundant in adipose tissue and is considered the master regulator of adipocyte differentiation, where it controls FAs uptake and storage in lipid droplets as triglycerides. Within the CNS, PPARγ was found to show a more discrete pattern of expression than PPARβ/δ, and to be slightly enriched in the hippocampus, being identified both in neurons and glial cells, including microglia, (Bernardo et al., 2000; Roth et al., 2003; Heneka and Landreth, 2007; Sarruf et al., 2009; Warden et al., 2016; Villapol, 2018). Expression analysis in mice brain by ISH showed hippocampus, isocortex, cerebellum and medulla higher levels of PPARG mRNAs (Figures 3D,E). In the neuroblastome cell line SH-SY5Y, the activation of PPARγ by synthetic agonists promotes neurite outgrowth and neuronal differentiation (Miglio et al., 2009).
PPARγ shows higher affinities for PUFAs than for MUFAs, and it cannot be activated by SFAs, and its response to eicosanoids, hipolipidemic and NSAIDs is diverse, but it is generally activated by thiazolidinediones (Corton et al., 2000). PPARγ is probably the most studied isoform regarding its functions in immune cells, and its interest in neuropathological processes points toward its strong anti-inflammatory effects (Corona and Duchen, 2015). PPARγ activation can induce differentiation of oligodendrocytes and protects them from TNFα toxicity (Bernardo et al., 2009, 2017; De Nuccio et al., 2015). Furthermore, natural and synthetic agonists PPARγ can control brain inflammation processes by shutting-down proinflammatory phenotype of activated microglia, and inhibiting the expression of surface antigens or the synthesis of proinflammatory signals, such as prostaglandins and nitric oxide (Bernardo and Minghetti, 2006). PPARγ can be either positively or negatively modulated by phosphorylation, according to the residue that is modified (Shao et al., 1998; Anbalagan et al., 2012; Brunmeir and Xu, 2018), and can be sent for degradation via the Ubiquitine-Proteasome pathway. PPARγ ability to transrepress NFκB inhibits, or at least limits, the inflammatory response, a regulatory mechanism activated by SUMOylation of its N-terminal region (Ricote and Glass, 2007; Diezko and Suske, 2013; Glass and Saijo, 2010). This could be a putative target for minimizing the neuroinflammatory condition characteristic of many neurodegenerative diseases, such as PAD and AD. Actually, multiple drugs designed as agonist of PPARγ have been and tested in AD models showed to reduce the β-amyloid accumulation, its cytotoxicity and stimulation of inflammatory cytokines (Combs et al., 2000; Sastre et al., 2006; Zolezzi and Inestrosa, 2013; Bonet-Costa et al., 2016).
Future Perspectives
Fatty acids participate in essential and diverse cellular processes involving neurons and glial cells, ranging from embryonic and perinatal development of tissues, including the CNS, to cognitive functions such as memory and learning. Therefore, they are unavoidably involved in neuropathological processes, including traumatic brain injury and neurodegenerative diseases. But, due to its rather simple chemical structures and low solubility, FAs require specific proteins that could recognize them and mediate its regulatory or signaling functions. Membrane receptors FFARs, cytosolic transport proteins FABPs and nuclear transcription factors PPARs are the preferential mediators of FAs functions. Taken together, and considering that almost all cell types express at least one member of each of these three families of proteins, we could hypothesize that they may be working as a complex, but coordinated sensory system for FAs. Actually, functional interaction of FABPs and PPARs has already been proved in hepatocytes and adipocytes (Smith et al., 2008; Hostetler et al., 2009). The identification of two possible ligand binding sites in FFAR1 structures in opposite sides of the membrane opens the possibility that they could be regulated and/or activated by intracellular lipids as well as by external lipokines, for example released by PLA2 enzymes, but also by interaction with FABPs. FABP-FFAR interaction, though not yet proved, could be understood either by unloading FAs cargo from the FABPs to activate the FFARs, or as a termination mechanism of FFAR signaling by retrieval of ligands by the FABPs from the FFARs. This is an interesting possibility that would integrate all FAs receptors (Figure 4), and its better understanding would definitely help in the design of new drugs with increased specificity and selectivity for its primary targets, avoiding undesired adverse effects.
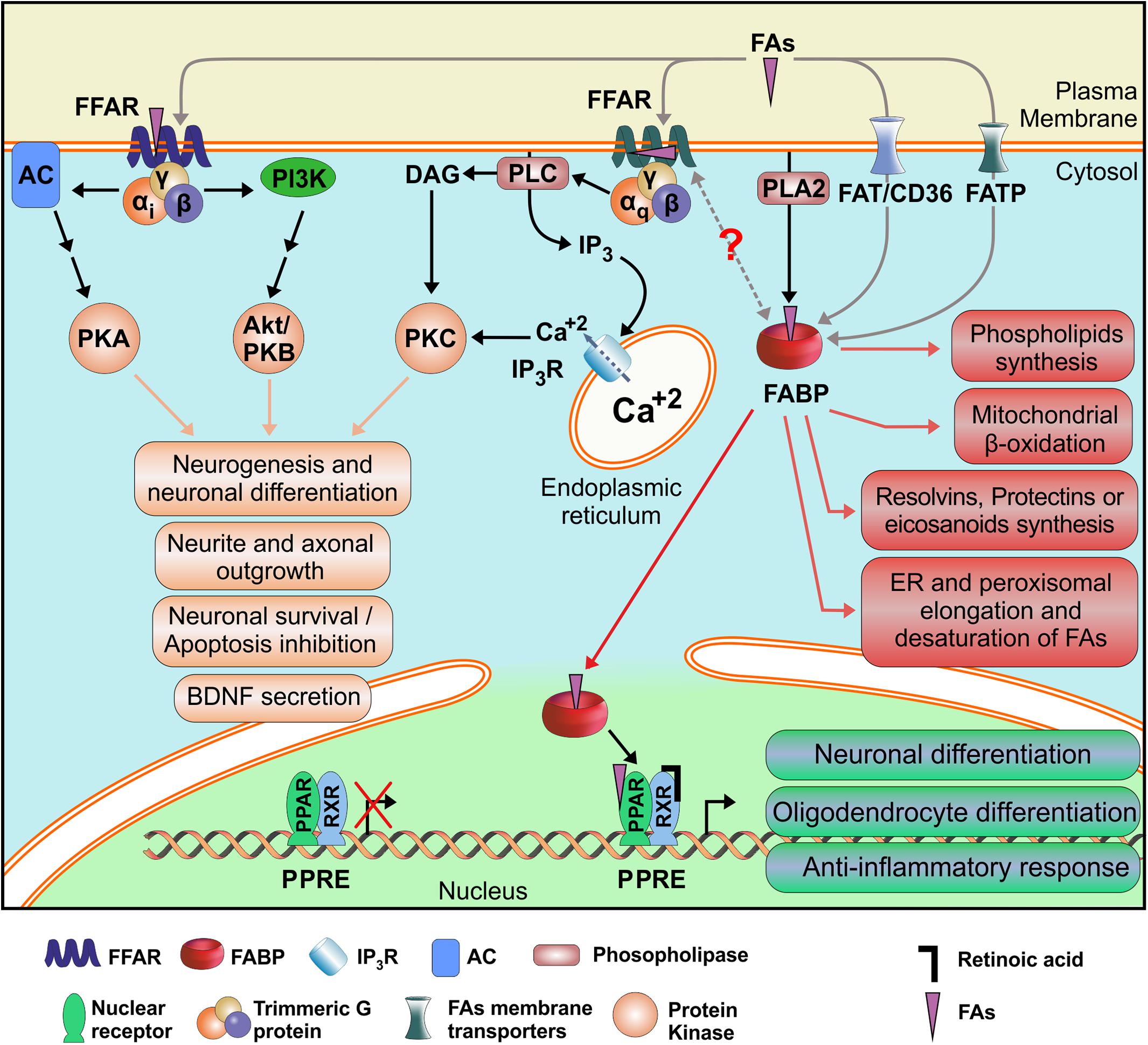
Figure 4. Schematic mechanism proposed for integration of the FAs Receptors System. Alternative FAs receptors are shown along with their demonstrated or putative interactions and their better characterized cellular effects. FFAR: Free Fatty Acid Receptor; FABP: Fatty Acid Binding Protein; PPAR: Peroxisome Proliferator-Activated Receptor; RXR: Retinoid X Receptor; FA: Fatty Acid; PPRE: PPAR Response Element. AC: Adenylate Cyclase; DAG: Diacylglycerol; FATP: FAT/CD36: Fatty Acid Translocase; Fatty Acid Transport Protein; IP3: Inositol (1,4,5)-Trisphosphate; IP3R: Inositol trisphosphate Receptor; PI3K: Phosphatidyl Inositol (3,4,5)-Trisphosphate Kinase; PLA2: Phospholipase A2; PLC: Phospholipase C; PKA: Protein Kinase A; PKAB/Akt: Protein Kinase B; PKC: Protein Kinase C.
Fatty acids signaling effects are usually mixed with those displayed by their multiple derivatives, adding a new layer of complexity to distinguish direct cellular responses free FAs signaling. Although specific receptors have been identified for endocannabinoids, eicosanoids, resolvins, protectines, lysophasphatidic acids, monoacylglycerols, N-acylethanolamines, and so on, crosstalk between the different receptors should not be neglected or discarded. Cellular responses are the result of the integration of a myriad of external and internal signals, as evidenced by the convergence of signaling pathways. Particularly, a functional interaction between FFAR2 and FFAR3 to form an heteromeric receptor has been proposed in primary monocytes and macrophages, and during heterologous expression in HEK293 cells (Ang et al., 2018). Considering that the origin of the short chain carboxylic acids available in mammals’ bloodstream is exclusive from the microbiota colonizing their body, FFAR2 and FFAR3 may act as a remote sensory system to prevent infections, activating the proliferation and modulating the reactivity of immune cells in preparation against a growing focus of bacteria (Ulven, 2012).
Signaling of PUFAs is intimately related to RA signaling, as mentioned above by the similar activation of FFAR1. Furthermore, and similarly to what happens with FABP5 and CRABP2, PPARγ has been described to be activated by RA, as well as RXRs by DHA. In this case, FABP5 translocate its ligand, either DHA or RA, to the nucleus and unload it to the PPARβ/δ (Noy, 2016). Similarly, CRABP2 would do the same to RXR. Therefore, FABP/CRABP2 biological interaction with PPAR/RXXR is not only direct but they also compete for the same ligands, increasing the possibilities of diverse responses mounted and the subset of target genes affected. Regarding this, one must remember that many nuclear receptors heterodimerize with RXRs and, therefore the final cellular response observed is also affected by their presence and ligands (Zhang et al., 2015).
Another utility for FABPs is their detection as early biomarkers of tissue injuries, and increasing evidence is being recorded for the early or differential diagnosis of diverse pathologies (Pelsers et al., 2004, 2005; Wunderlich et al., 2005). Their main advantages are related to their fast release, as close as 30 min to the injurious event, and their relatively fast clearance. Consequently, FABPs presence in plasma or cephaloraquidean liquid reports for recent trauma, allowing for the differentiation of consecutive events separated by less than 24 h. Neural FABPs detection has been correlated with traumatic brain injury and certain gliomas overexpressing FABP5 or FABP7 (De Rosa et al., 2012; Walder et al., 2013).
Finally, the increasing complexity of the lipid-sensing system available in humans raises the question if the regulatory and signaling functions of FAs can be individually discriminated and specifically targeted for the treatment of pathological conditions. Significant effort is being invested in the development of new drugs that would allow for their precise manipulation and the better treatment of brain injuries, neurological or neurodegenerative diseases by taking advantage of the neuroprotective and anti-inflammatory properties, particularly of PUFAs, employing either FFARs, FABPs, or PPARs as drug targets (Holliday et al., 2012; Wang et al., 2016; Zhou et al., 2016; Li et al., 2018a,b,c, 2019; Shang et al., 2018). Specificity will come together with our better understanding of how these proteins work. For example, an important step forward in this direction is the recent variation analysis of the PPRE sequence for PPARα/RXRα complex that resulted in the ideal sequence WAWVT-RGGBBA-H-RGKTYA (where W = A or T; V = not T; R = A or G; B = not A; H = not G; K = G or T; Y = T or C) as an optimized DNA sequence for PPARα (underlined) and RXRα binding (not underlined) (Tzeng et al., 2015). Noteworthy, although stronger DNA binding of RXRα to PPRE led to higher transcription rates, this is not always the case for PPARα. New candidate target genes have been identified employing this optimized sequence to screen genomic databases as being regulated by PPARα, and that could not be recognized by the consensus sequence for PPRE, improving our understanding of PPARs isoform specific functions. This could also help to development of drugs that could selectively affect only one PPAR isoform and/or only its functions regarding a certain subset of genes under its control.
Another elements that must be considered are non-coding microRNAs (miRs), which act usually as negative regulators of gene expression in the CNS, and several of them have been reported to participate in the regulation exhibited by lipids (Wnuk and Kajta, 2017). For example, the expression levels of miR-21 are decreased by DHA treatment of SH-SY5Y neuroblastome cells, showing an inverse correlation with PPARα levels (Fu et al., 2017). The miR-21 is thought to destabilize PPARα mRNAs and reduce its translation (Chen et al., 2017). Treatment with DHA plus salicylic acid promotes PPARα-RXRα heterodimer formation that correlates with a reduction of miR-21. Together, they promote PSD-95, BDNF and GDNF neuronal differentiation markers and reduce NFκB, COX-2, caspase 3 levels proinflammatory markers (Fu et al., 2017). Similarly, miR-499a negatively regulates the level of expression of PPARγ in microglia cells, promoting the expression of proinflammatory markers, such as iba-1, TNFα and IL-1β (Yu et al., 2018). Finally, PPARs can be activated by valproic acid and its short/medium-chain derivatives, a long time used family of anticonvulsants to treat epilepsy, that has also showed beneficial effects against autism, maniac and bipolar disorders (Lampen et al., 2001; Hunsberger et al., 2013; Hirsch et al., 2018). Its beneficial effects as a promoter of neuroregeneration, neurodifferentiation and neuroprotection (Liu et al., 2012; Foti et al., 2013; Oikawa and Sng, 2016) may come from the modulation of the expression of multiple miRs through PPARs, either by their transrepression or transactivation (Dharap et al., 2015). FFARs may also participate of miRs expression, such as miR-143 that is controlled in adipocytes by both PPARγ and FFAR4 activation (Bae et al., 2017). These line of evidence has recently pushed miRs to be evaluated as novel therapeutic targets against neurodegenerative diseases, such as PD o AD.
Drug discovery strategies focused on FFARs, FABPs and PPARs have already started aiming at neurological and also other pathologies like diabetes, obesity, leukemia, cancer, and so on (Kaczocha et al., 2014; Nikaido et al., 2015; Corona and Duchen, 2016; Wang et al., 2016; Cheng et al., 2019), paving the way for new developments and facilitating drug repurposing, what may save years of research and development. Noteworthy, in the case of FFARs with multiple signal transduction pathways, the concept of biased signaling is of particular interest, where a partial agonistic ligand may only and selectively activate a single pathway over the others available for that receptor. For example, FFARs partial agonist could activate β-Arrestin-mediated but not G protein-mediated signaling (Mancini et al., 2015). This kind of developments has attracted substantial attention for its potential to model molecular differences in receptors functionality that could yield enhanced therapeutic strategies, with improved efficacy and reduced adverse effects (Whalen et al., 2011; Kenakin and Christopoulos, 2013; Correll and McKittrick, 2014).
Author Contributions
All authors listed have made a substantial, direct and intellectual contribution to the work, and approved it for publication.
Funding
This work was supported by funds from the Consejo Nacional de Investigaciones Científicas y Tecnológicas (CONICET, Argentina, grant PIP2014-618), Agencia Nacional de Promoción Científica y Tecnológica (ANPCyT, Argentina, grants PICT2014-2019 and PICT2016-3776) to LJF-L. AMT was recipient of CONICET postdoctoral fellowship. EG was recipient of CONICET doctoral fellowship and of Bunge & Born Foundation-Max Planck Society-Williams Foundations doctoral research fellowship. GFC was recipient of Consejo Interuniversitario Nacional (CIN, Argentina) undergraduate and of Universidad Nacional de La Plata (UNLP, Argentina) doctoral fellowships.
Conflict of Interest Statement
The authors declare that the research was conducted in the absence of any commercial or financial relationships that could be construed as a potential conflict of interest.
Acknowledgments
We are grateful with Rosana del Cid for use-of-English revision, and with Mario R. Ramos for graphic design support. We apologize to all researchers whose work is also relevant to this manuscript but, in interest of space, was not included.
Footnotes
- ^ www.proteinatlas.org (accessed January 3, 2019)
- ^ http://portal.brain-map.org (accessed January 3, 2019)
References
Allen, G. W., Liu, J., Kirby, M. A., and De Leon, M. (2001). Induction and axonal localization of epithelial/epidermal fatty acid-binding protein in retinal ganglion cells are associated with axon development and regeneration. J. Neurosci. Res. 66, 396–405. doi: 10.1002/jnr.1232
Anbalagan, M., Huderson, B., Murphy, L., and Rowan, B. G. (2012). Post-translational modifications of nuclear receptors and human disease. Nucl. Recept. Signal. 10:e001. doi: 10.1621/nrs.10001
Ang, Z., Xiong, D., Wu, M., and Ding, J. L. (2018). FFAR2-FFAR3 receptor heteromerization modulates short-chain fatty acid sensing. FASEB J. 32, 289–303. doi: 10.1096/fj.201700252RR
Arai, Y., Funatsu, N., Numayama-Tsuruta, K., Nomura, T., Nakamura, S., and Osumi, N. (2005). Role of Fabp7, a downstream gene of Pax6, in the maintenance of neuroepithelial cells during early embryonic development of the rat cortex. J. Neurosci. 25, 9752–9761. doi: 10.1523/JNEUROSCI.2512-05.2005
Arai, Y., Sampaio, J. L., Wilsch-Brauninger, M., Ettinger, A. W., Haffner, C., and Huttner, W. B. (2015). Lipidome of midbody released from neural stem and progenitor cells during mammalian cortical neurogenesis. Front. Cell. Neurosci. 9:325. doi: 10.3389/fncel.2015.00325
Audet, M., and Stevens, R. C. (2019). Emerging structural biology of lipid G protein-coupled receptors. Protein Sci. 28, 292–304. doi: 10.1002/pro.3509
Bae, I.-S., Park, P. J., Lee, J. H., Cho, E.-G., Lee, T. R., and Kim, S. H. (2017). PPARgamma-mediated G-protein coupled receptor 120 signaling pathway promotes transcriptional activation of miR-143 in adipocytes. Gene 626, 64–69. doi: 10.1016/j.gene.2017.05.016
Bahar Halpern, K., Veprik, A., Rubins, N., Naaman, O., and Walker, M. D. (2012). GPR41 gene expression is mediated by internal ribosome entry site (IRES)-dependent translation of bicistronic mRNA encoding GPR40 and GPR41 proteins. J. Biol. Chem. 287, 20154–20163. doi: 10.1074/jbc.M112.358887
Balendiran, G. K., Schnutgen, F., Scapin, G., Borchers, T., Xhong, N., Lim, K., et al. (2000). Crystal structure and thermodynamic analysis of human brain fatty acid-binding protein. J. Biol. Chem. 275, 27045–27054. doi: 10.1074/jbc.M003001200
Banaszak, L., Winter, N., Xu, Z. H., Bernlohr, D. A., Cowan, S., and Jones, T. A. (1994). Lipid-binding proteins - a family of fatty-acid and retinoid transport proteins. Adv. Protein Chem. 45, 89–151. doi: 10.1016/S0065-3233(08)60639-7
Benedict, C., and Grillo, C. A. (2018). Insulin resistance as a therapeutic target in the treatment of Alzheimer’s disease: a state-of-the-art review. Front. Neurosci. 12:215. doi: 10.3389/fnins.2018.00215
Bennaars-Eiden, A., Higgins, L., Hertzel, A. V., Kapphahn, R. J., Ferrington, D. A., and Bernlohr, D. A. (2002). Covalent modification of epithelial fatty acid-binding protein by 4-hydroxynonenal in vitro and in vivo. Evidence for a role in antioxidant biology. J. Biol. Chem. 277, 50693–50702. doi: 10.1074/jbc.M209493200
Bernardo, A., Bianchi, D., Magnaghi, V., and Minghetti, L. (2009). Peroxisome proliferator-activated receptor-gamma agonists promote differentiation and antioxidant defenses of oligodendrocyte progenitor cells. J. Neuropathol. Exp. Neurol. 68, 797–808. doi: 10.1097/NEN.0b013e3181aba2c1
Bernardo, A., Giammarco, M. L., De Nuccio, C., Ajmone-Cat, M. A., Visentin, S., De Simone, R., et al. (2017). Docosahexaenoic acid promotes oligodendrocyte differentiation via PPAR-gamma signalling and prevents tumor necrosis factor-alpha-dependent maturational arrest. Biochim. Biophys. Acta 1862, 1013–1023. doi: 10.1016/j.bbalip.2017.06.014
Bernardo, A., Levi, G., and Minghetti, L. (2000). Role of the peroxisome proliferator-activated receptor-gamma (PPAR-gamma) and its natural ligand 15-deoxy-Delta12, 14-prostaglandin J2 in the regulation of microglial functions. Eur. J. Neurosci. 12, 2215–2223. doi: 10.1046/j.1460-9568.2000.00110.x
Bernardo, A., and Minghetti, L. (2006). PPAR-gamma agonists as regulators of microglial activation and brain inflammation. Curr. Pharm. Des. 12, 93–109. doi: 10.2174/138161206780574579
Berrabah, W., Aumercier, P., Lefebvre, P., and Staels, B. (2011). Control of nuclear receptor activities in metabolism by post-translational modifications. FEBS Lett. 585, 1640–1650. doi: 10.1016/j.febslet.2011.03.066
Bhatia, H. S., Agrawal, R., Sharma, S., Huo, Y.-X., Ying, Z., and Gomez-Pinilla, F. (2011). Omega-3 fatty acid deficiency during brain maturation reduces neuronal and behavioral plasticity in adulthood. PLoS One 6:e28451. doi: 10.1371/journal.pone.0028451
Bonet-Costa, V., Herranz-Perez, V., Blanco-Gandia, M., Mas-Bargues, C., Ingles, M., Garcia-Tarraga, P., et al. (2016). Clearing amyloid-beta through PPARgamma/ApoE activation by Genistein is a treatment of experimental Alzheimer’s disease. J. Alzheimers Dis. 51, 701–711. doi: 10.3233/JAD-151020
Boneva, N. B., Kaplamadzhiev, D. B., Sahara, S., Kikuchi, H., Pyko, I. V., Kikuchi, M., et al. (2011). Expression of fatty acid-binding proteins in adult hippocampal neurogenic niche of postischemic monkeys. Hippocampus 21, 162–171. doi: 10.1002/hipo.20732
Braissant, O., Foufelle, F., Scotto, C., Dauca, M., and Wahli, W. (1996). Differential expression of peroxisome proliferator-activated receptors (PPARs): tissue distribution of PPAR-alpha, -beta, and -gamma in the adult rat. Endocrinology 137, 354–366. doi: 10.1210/endo.137.1.8536636
Briscoe, C. P., Tadayyon, M., Andrews, J. L., Benson, W. G., Chambers, J. K., Eilert, M. M., et al. (2003). The orphan G protein-coupled receptor GPR40 is activated by medium and long chain fatty acids. J. Biol. Chem. 278, 11303–11311. doi: 10.1074/jbc.M211495200
Brown, A. J., Goldsworthy, S. M., Barnes, A. A., Eilert, M. M., Tcheang, L., Daniels, D., et al. (2003). The Orphan G protein-coupled receptors GPR41 and GPR43 are activated by propionate and other short chain carboxylic acids. J. Biol. Chem. 278, 11312–11319. doi: 10.1074/jbc.M211609200
Brown, A. J., Jupe, S., and Briscoe, C. P. (2005). A family of fatty acid binding receptors. DNA Cell Biol. 24, 54–61. doi: 10.1089/dna.2005.24.54
Brown, M., Roulson, J.-A., Hart, C. A., Tawadros, T., and Clarke, N. W. (2014). Arachidonic acid induction of Rho-mediated transendothelial migration in prostate cancer. Br. J. Cancer 110, 2099–2108. doi: 10.1038/bjc.2014.99
Brunmeir, R., and Xu, F. (2018). Functional regulation of PPARs through post-translational modifications. Int. J. Mol. Sci. 19:E1738. doi: 10.3390/ijms19061738
Burns, R. N., Singh, M., Senatorov, I. S., and Moniri, N. H. (2014). Mechanisms of homologous and heterologous phosphorylation of FFA receptor 4 (GPR120): GRK6 and PKC mediate phosphorylation of Thr(3)(4)(7), Ser(3)(5)(0), and Ser(3)(5)(7) in the C-terminal tail. Biochem. Pharmacol. 87, 650–659. doi: 10.1016/j.bcp.2013.12.016
Calder, P. C. (2015). Marine omega-3 fatty acids and inflammatory processes: effects, mechanisms and clinical relevance. Biochim. Biophys. Acta 1851, 469–484. doi: 10.1016/j.bbalip.2014.08.010
Cao, D., Kevala, K., Kim, J., Moon, H.-S., Jun, S. B., Lovinger, D., et al. (2009). Docosahexaenoic acid promotes hippocampal neuronal development and synaptic function. J. Neurochem. 111, 510–521. doi: 10.1111/j.1471-4159.2009.06335.x
Capelli, D., Cerchia, C., Montanari, R., Loiodice, F., Tortorella, P., Laghezza, A., et al. (2016). Structural basis for PPAR partial or full activation revealed by a novel ligand binding mode. Sci. Rep. 6:34792. doi: 10.1038/srep34792
Cartoni, C., Yasumatsu, K., Ohkuri, T., Shigemura, N., Yoshida, R., Godinot, N., et al. (2010). Taste preference for fatty acids is mediated by GPR40 and GPR120. J. Neurosci. 30, 8376–8382. doi: 10.1523/JNEUROSCI.0496-10.2010
Chalon, S. (2006). Omega-3 fatty acids and monoamine neurotransmission. Prostaglandins Leukot. Essent. Fatty Acids 75, 259–269. doi: 10.1016/j.plefa.2006.07.005
Chalon, S., Delion-Vancassel, S., Belzung, C., Guilloteau, D., Leguisquet, A. M., Besnard, J. C., et al. (1998). Dietary fish oil affects monoaminergic neurotransmission and behavior in rats. J. Nutr. 128, 2512–2519. doi: 10.1093/jn/128.12.2512
Chan, R. B., Oliveira, T. G., Cortes, E. P., Honig, L. S., Duff, K. E., Small, S. A., et al. (2012). Comparative lipidomic analysis of mouse and human brain with Alzheimer disease. J. Biol. Chem. 287, 2678–2688. doi: 10.1074/jbc.M111.274142
Chandra, V., Huang, P., Hamuro, Y., Raghuram, S., Wang, Y., Burris, T. P., et al. (2008). Structure of the intact PPAR-gamma-RXR- nuclear receptor complex on DNA. Nature 456, 350–356. doi: 10.1038/nature07413
Chang, P. K.-Y., Khatchadourian, A., McKinney, R. A., and Maysinger, D. (2015). Docosahexaenoic acid (DHA): a modulator of microglia activity and dendritic spine morphology. J. Neuroinflammation 12:34. doi: 10.1186/s12974-015-0244-5
Chen, C. T., Green, J. T., Orr, S. K., and Bazinet, R. P. (2008). Regulation of brain polyunsaturated fatty acid uptake and turnover. Prostaglandins Leukot. Essent. Fatty Acids 79, 85–91. doi: 10.1016/j.plefa.2008.09.003
Chen, J.-J., Gong, Y.-H., and He, L. (2019). Role of GPR40 in pathogenesis and treatment of Alzheimer’s disease and type 2 diabetic dementia. J. Drug Target. 27, 347–354. doi: 10.1080/1061186X.2018.1491979
Chen, Q., Qiu, F., Zhou, K., Matlock, H. G., Takahashi, Y., Rajala, R. V. S., et al. (2017). Pathogenic role of microRNA-21 in diabetic retinopathy through downregulation of PPARalpha. Diabetes 66, 1671–1682. doi: 10.2337/db16-1246
Cheng, A., Shinoda, Y., Yamamoto, T., Miyachi, H., and Fukunaga, K. (2019). Development of FABP3 ligands that inhibit arachidonic acid-induced alpha-synuclein oligomerization. Brain Res. 1707, 190–197. doi: 10.1016/j.brainres.2018.11.036
Cheon, M. S., Kim, S. H., Fountoulakis, M., and Lubec, G. (2003). “Heart type fatty acid binding protein (H-FABP) is decreased in brains of patients with Down syndrome and Alzheimer’s disease,” in Advances in Down Syndrome Research. Journal of Neural Transmission Supplement 67, ed. G. Lubec (Vienna: Springer), 225–234. doi: 10.1007/978-3-7091-6721-2_20
Chistyakov, D. V., Aleshin, S. E., Astakhova, A. A., Sergeeva, M. G., and Reiser, G. (2015). Regulation of peroxisome proliferator-activated receptors (PPAR) α and -γ Of rat brain astrocytes in the course of activation by toll-like receptor agonists. J. Neurochem. 134, 113–124. doi: 10.1111/jnc.13101
Chornenkyy, Y., Wang, W.-X., Wei, A., and Nelson, P. T. (2019). Alzheimer’s disease and type 2 diabetes mellitus are distinct diseases with potential overlapping metabolic dysfunction upstream of observed cognitive decline. Brain Pathol. 29, 3–17. doi: 10.1111/bpa.12655
Civelli, O. (2005). GPCR deorphanizations: the novel, the known and the unexpected transmitters. Trends Pharmacol. Sci. 26, 15–19. doi: 10.1016/j.tips.2004.11.005
Colina, C., Puhl, H. L. III, and Ikeda, S. R. (2018). Selective tracking of FFAR3-expressing neurons supports receptor coupling to N-type calcium channels in mouse sympathetic neurons. Sci. Rep. 8:17379. doi: 10.1038/s41598-018-35690-z
Combs, C. K., Johnson, D. E., Karlo, J. C., Cannady, S. B., and Landreth, G. E. (2000). Inflammatory mechanisms in Alzheimer’s disease: inhibition of beta-amyloid-stimulated proinflammatory responses and neurotoxicity by PPARgamma agonists. J. Neurosci. 20, 558–567. doi: 10.1523/JNEUROSCI.20-02-00558.2000
Conklin, S. M., Runyan, C. A., Leonard, S., Reddy, R. D., Muldoon, M. F., and Yao, J. K. (2010). Age-related changes of n-3 and n-6 polyunsaturated fatty acids in the anterior cingulate cortex of individuals with major depressive disorder. Prostaglandins Leukot. Essent. Fatty Acids 82, 111–119. doi: 10.1016/j.plefa.2009.12.002
Corona, J. C., and Duchen, M. R. (2015). PPAR c and PGC-1 a as Therapeutic Targets in Parkinson ’ s. Neurochem. Res. 40, 308–316. doi: 10.1007/s11064-014-1377-0
Corona, J. C., and Duchen, M. R. (2016). PPARgamma as a therapeutic target to rescue mitochondrial function in neurological disease. Free Radic. Biol. Med. 100, 153–163. doi: 10.1016/j.freeradbiomed.2016.06.023
Correll, C. C., and McKittrick, B. A. (2014). Biased ligand modulation of seven transmembrane receptors (7TMRs): functional implications for drug discovery. J. Med. Chem. 57, 6887–6896. doi: 10.1021/jm401677g
Corton, J. C., Anderson, S. P., and Stauber, A. (2000). Central role of peroxisome proliferator-activated receptors in the actions of peroxisome proliferators. Annu. Rev. Pharmacol. Toxicol. 40, 491–518. doi: 10.1146/annurev.pharmtox.40.1.491
Cristiano, L., Bernardo, A., and Cerù, M. P. (2001). Peroxisome proliferator-activated receptors (PPARs) and peroxisomes in rat cortical and cerebellar astrocytes. J. Neurocytol. 30, 671–683. doi: 10.1023/A:1016525716209
Cullingford, T. E., Bhakoo, K., Peuchen, S., Dolphin, C. T., Patel, R., and Clark, J. B. (1998). Distribution of mRNAs encoding the peroxisome proliferator-activated receptor alpha, beta, and gamma and the retinoid X receptor α, β, and γ in rat central nervous system. J. Neurochem. 70, 1366–1375. doi: 10.1046/j.1471-4159.1998.70041366.x
De Leon, M., Welcher, A. A., Nahin, R. H., Liu, Y., Ruda, M. A., Shooter, E. M., et al. (1996). Fatty acid binding protein is induced in neurons of the dorsal root ganglia after peripheral nerve injury. J. Neurosci. Res. 44, 283–292. doi: 10.1002/(sici)1097-4547(19960501)44:3<283::aid-jnr9>3.0.co;2-c
De Nuccio, C., Bernardo, A., Cruciani, C., De Simone, R., Visentin, S., and Minghetti, L. (2015). Peroxisome proliferator activated receptor-gamma agonists protect oligodendrocyte progenitors against tumor necrosis factor-alpha-induced damage: effects on mitochondrial functions and differentiation. Exp. Neurol. 271, 506–514. doi: 10.1016/j.expneurol.2015.07.014
De Rosa, A., Pellegatta, S., Rossi, M., Tunici, P., Magnoni, L., Speranza, M. C., et al. (2012). A radial glia gene marker, fatty acid binding protein 7 (FABP7), is involved in proliferation and invasion of glioblastoma cells. PLoS One 7:e52113. doi: 10.1371/journal.pone.0052113PONE-D-12-11234
DeArmond, S. J., Deibler, G. E., Bacon, M., Kies, M. W., and Eng, L. F. (1980). A neurochemical and immunocytochemical study of P2 protein in human and bovine nervous systems. J. Histochem. Cytochem. 28, 1275–1285. doi: 10.1177/28.12.6785343
Delion, S., Chalon, S., Guilloteau, D., Besnard, J. C., and Durand, G. (1996). alpha-Linolenic acid dietary deficiency alters age-related changes of dopaminergic and serotoninergic neurotransmission in the rat frontal cortex. J. Neurochem. 66, 1582–1591. doi: 10.1046/j.1471-4159.1996.66041582.x
Dennis, E. A., and Norris, P. C. (2015). Eicosanoid storm in infection and inflammation. Nat. Rev. Immunol. 15, 511–523. doi: 10.1038/nri3859
Dharap, A., Pokrzywa, C., Murali, S., Kaimal, B., and Vemuganti, R. (2015). Mutual induction of transcription factor PPARgamma and microRNAs miR-145 and miR-329. J. Neurochem. 135, 139–146. doi: 10.1111/jnc.13220
Di Loreto, S., D’Angelo, B., D’Amico, M. A., Benedetti, E., Cristiano, L., Cinque, B., et al. (2007). PPARbeta agonists trigger neuronal differentiation in the human neuroblastoma cell line SH-SY5Y. J. Cell. Physiol. 211, 837–847. doi: 10.1002/jcp.20996
Diaz, C., Angelloz-Nicoud, P., and Pihan, E. (2018). Modeling and Deorphanization of Orphan GPCRs. Methods Mol. Biol. 1705, 413–429. doi: 10.1007/978-1-4939-7465-8_21
Dickey, A. S., Pineda, V. V., Tsunemi, T., Liu, P. P., Miranda, H. C., Gilmore-Hall, S. K., et al. (2016). PPAR-delta is repressed in Huntington’s disease, is required for normal neuronal function and can be targeted therapeutically. Nat. Med. 22, 37–45. doi: 10.1038/nm.4003
Dietschy, J. M., and Turley, S. D. (2001). Cholesterol metabolism in the brain. Curr. Opin. Lipidol. 12, 105–112.
Dietschy, J. M., and Turley, S. D. (2004). Thematic review series: brain Lipids. Cholesterol metabolism in the central nervous system during early development and in the mature animal. J. Lipid Res. 45, 1375–1397. doi: 10.1194/jlr.R400004-JLR200
Diezko, R., and Suske, G. (2013). Ligand binding reduces SUMOylation of the peroxisome proliferator-activated receptor gamma (PPARgamma) activation function 1 (AF1) domain. PLoS One 8:e66947. doi: 10.1371/journal.pone.0066947
Dorsam, R. T., and Gutkind, J. S. (2007). G-protein-coupled receptors and cancer. Nat. Rev. Cancer 7, 79–94. doi: 10.1038/nrc2069
Dragano, N. R. V., Solon, C., Ramalho, A. F., de Moura, R. F., Razolli, D. S., Christiansen, E., et al. (2017). Polyunsaturated fatty acid receptors, GPR40 and GPR120, are expressed in the hypothalamus and control energy homeostasis and inflammation. J. Neuroinflammation 14:91. doi: 10.1186/s12974-017-0869-7
Dyall, S. C. (2017). Interplay between n-3 and n-6 long-chain polyunsaturated fatty acids and the endocannabinoid system in brain protection and repair. Lipids 52, 885–900. doi: 10.1007/s11745-017-4292-8
Dyall, S. C., and Michael-Titus, A. T. (2008). Neurological benefits of omega-3 fatty acids. Neuromolecular Med. 10, 219–235. doi: 10.1007/s12017-008-8036-z
Echeverria, F., Ortiz, M., Valenzuela, R., and Videla, L. A. (2016). Long-chain polyunsaturated fatty acids regulation of PPARs, signaling: Relationship to tissue development and aging. Prostaglandins Leukot. Essent. Fatty Acids 114, 28–34. doi: 10.1016/j.plefa.2016.10.001
Edmond, J., Higa, T. A., Korsak, R. A., Bergner, E. A., and Lee, W. N. (1998). Fatty acid transport and utilization for the developing brain. J. Neurochem. 70, 1227–1234. doi: 10.1046/j.1471-4159.1998.70031227.x
Engelstoft, M. S., Park, W.-M., Sakata, I., Kristensen, L. V., Husted, A. S., Osborne-Lawrence, S., et al. (2013). Seven transmembrane G protein-coupled receptor repertoire of gastric ghrelin cells. Mol. Metab. 2, 376–392. doi: 10.1016/j.molmet.2013.08.006
Etschmaier, K., Becker, T., Eichmann, T. O., Schweinzer, C., Scholler, M., Tam-Amersdorfer, C., et al. (2011). Adipose triglyceride lipase affects triacylglycerol metabolism at brain barriers. J. Neurochem. 119, 1016–1028. doi: 10.1111/j.1471-4159.2011.07498.x
Falomir-Lockhart, L. J., Franchini, G. R., Guerbi, M. X., Storch, J., and Córsico, B. (2011). Interaction of enterocyte FABPs with phospholipid membranes: clues for specific physiological roles. Biochim. Biophys. Acta 1811, 452–459. doi: 10.1016/j.bbalip.2011.04.005
Falomir-Lockhart, L. J., Laborde, L., Kahn, P. C., Storch, J., Córsico, B., Falomir Lockhart, L. J., et al. (2006). Protein-membrane interaction and fatty acid transfer from intestinal fatty acid-binding protein to membranes. Support for a multistep process. J. Biol. Chem. 281, 13979–13989. doi: 10.1074/jbc.M511943200
Favrelere, S., Stadelmann-Ingrand, S., Huguet, F., De Javel, D., Piriou, A., Tallineau, C., et al. (2000). Age-related changes in ethanolamine glycerophospholipid fatty acid levels in rat frontal cortex and hippocampus. Neurobiol. Aging 21, 653–660. doi: 10.1016/S0197-4580(00)00170-6
Fernandis, A. Z., and Wenk, M. R. (2007). Membrane lipids as signaling molecules. Curr. Opin. Lipidol. 18, 121–128. doi: 10.1097/MOL.0b013e328082e4d5
Ferreira, L. S. S., Fernandes, C. S., Vieira, M. N. N., and De Felice, F. G. (2018). Insulin Resistance in Alzheimer’s Disease. Front. Neurosci. 12:830. doi: 10.3389/fnins.2018.00830
Foti, S. B., Chou, A., Moll, A. D., and Roskams, A. J. (2013). HDAC inhibitors dysregulate neural stem cell activity in the postnatal mouse brain. Int. J. Dev. Neurosci. 31, 434–447. doi: 10.1016/j.ijdevneu.2013.03.008
Fredriksson, R., Höglund, P. J., Gloriam, D. E., Lagerström, M. C., and Schiöth, H. B. (2003). Seven evolutionarily conserved human rhodopsin G protein-coupled receptors lacking close relatives. FEBS Lett. 554, 381–388. doi: 10.1016/S0014-5793(03)01196-7
Freitas, H. R., Isaac, A. R., Malcher-Lopes, R., Diaz, B. L., Trevenzoli, I. H., and De Melo Reis, R. A. (2017). Polyunsaturated fatty acids and endocannabinoids in health and disease. Nutr. Neurosci. 21, 695–714. doi: 10.1080/1028415X.2017.1347373
Fu, Y., Zhen, J., and Lu, Z. (2017). Synergetic neuroprotective effect of docosahexaenoic acid and aspirin in SH-Y5Y by inhibiting miR-21 and activating RXRalpha and PPARalpha. DNA Cell Biol. 36, 482–489. doi: 10.1089/dna.2017.3643
Gharami, K., Das, M., and Das, S. (2015). Essential role of docosahexaenoic acid towards development of a smarter brain. Neurochem. Int. 89, 51–62. doi: 10.1016/j.neuint.2015.08.014
Glass, C. K., and Saijo, K. (2010). Nuclear receptor transrepression pathways that regulate inflammation in macrophages and T cells. Nat. Rev. Immunol. 10, 365–376. doi: 10.1038/nri2748
Glomset, J. A. (2006). Role of docosahexaenoic acid in neuronal plasma membranes. Sci. STKE 2006:pe6. doi: 10.1126/stke.3212006pe6
Gray, S. L., Dalla Nora, E., and Vidal-Puig, A. J. (2005). Mouse models of PPAR-gamma deficiency: dissecting PPAR-gamma’s role in metabolic homoeostasis. Biochem. Soc. Trans. 33, 1053–1058. doi: 10.1042/BST20051053
Greenfield, S., Brostoff, S., Eylar, E. H., and Morell, P. (1973). Protein composition of myelin of the peripheral nervous system. J. Neurochem. 20, 1207–1216. doi: 10.1111/j.1471-4159.1973.tb00089.x
Guajardo, M. H., Terrasa, A. M., and Catala, A. (2002). Retinal fatty acid binding protein reduce lipid peroxidation stimulated by long-chain fatty acid hydroperoxides on rod outer segments. Biochim. Biophys. Acta 1581, 65–74. doi: 10.1016/S1388-1981(02)00121-X
Gupta, S., Knight, A. G., Gupta, S., Keller, J. N., and Bruce-Keller, A. J. (2012). Saturated long-chain fatty acids activate inflammatory signaling in astrocytes. J. Neurochem. 120, 1060–1071. doi: 10.1111/j.1471-4159.2012.07660.x
Hall, M. G., Quignodon, L., and Desvergne, B. (2008). Peroxisome proliferator-activated receptor β/δ in the brain: Facts and hypothesis. PPAR Res. 2008:780452. doi: 10.1155/2008/780452
Hanhoff, T., Lücke, C., and Spener, F. (2002). Insights into binding of fatty acids by fatty acid binding proteins. Mol. Cell. Biochem. 239, 45–54. doi: 10.1023/A:1020502624234
Hara, T., Kimura, I., Inoue, D., Ichimura, A., and Hirasawa, A. (2013). Free fatty acid receptors and their role in regulation of energy metabolism. Rev. Physiol. Biochem. Pharmacol. 164, 77–116. doi: 10.1007/112_2013_13
Heldin, C., Lu, B., Evans, R., and Gutkind, J. S. (2016). Signals and receptors. Cold Spring Harb. Perspect. Biol. 8:a005900. doi: 10.1101/cshperspect.a005900
Heneka, M. T., and Landreth, G. E. (2007). PPARs in the brain. Biochim. Biophys. Acta 1771, 1031–1045. doi: 10.1016/j.bbalip.2007.04.016
Herr, F. M., Aronson, J., and Storch, J. (1996). Role of portal region lysine residues in electrostatic interactions between heart fatty acid binding protein and phospholipid membranes. Biochemistry 35, 1296–1303. doi: 10.1021/bi952204b
Hirasawa, A., Tsumaya, K., Awaji, T., Katsuma, S., Adachi, T., Yamada, M., et al. (2005). Free fatty acids regulate gut incretin glucagon-like peptide-1 secretion through GPR120. Nat. Med. 11, 90–94. doi: 10.1038/nm1168
Hirsch, M. M., Deckmann, I., Fontes-Dutra, M., Bauer-Negrini, G., Della-Flora Nunes, G., Nunes, W., et al. (2018). Behavioral alterations in autism model induced by valproic acid and translational analysis of circulating microRNA. Food Chem. Toxicol. 115, 336–343. doi: 10.1016/j.fct.2018.02.061
Ho, J. D., Chau, B., Rodgers, L., Lu, F., Wilbur, K. L., Otto, K. A., et al. (2018). Structural basis for GPR40 allosteric agonism and incretin stimulation. Nat. Commun. 9:1645. doi: 10.1038/s41467-017-01240-w
Holliday, N. D., Watson, S.-J., and Brown, A. J. H. (2012). Drug discovery opportunities and challenges at g protein coupled receptors for long chain free fatty acids. Front. Endocrinol. 2:112. doi: 10.3389/fendo.2011.00112
Hostetler, H. A., McIntosh, A. L., Atshaves, B. P., Storey, S. M., Payne, H. R., Kier, A. B., et al. (2009). L-FABP directly interacts with PPAR alpha in cultured primary hepatocytes. J. Lipid Res. 50, 1663–1675. doi: 10.1194/jlr.M900058-JLR200
Hudson, B. D., Shimpukade, B., Milligan, G., and Ulven, T. (2014). The molecular basis of ligand interaction at free fatty acid receptor 4 (FFA4/GPR120). J. Biol. Chem. 289, 20345–20358. doi: 10.1074/jbc.M114.561449
Hunsberger, J. G., Fessler, E. B., Chibane, F. L., Leng, Y., Maric, D., Elkahloun, A. G., et al. (2013). Mood stabilizer-regulated miRNAs in neuropsychiatric and neurodegenerative diseases: identifying associations and functions. Am. J. Transl. Res. 5, 450–464.
Hussain, G., Schmitt, F., Loeffler, J.-P., and de Aguilar, J.-L. G. (2013). Fatting the brain: a brief of recent research. Front. Cell. Neurosci. 7:144. doi: 10.3389/fncel.2013.00144
Im, D.-S. (2004). Discovery of new G protein-coupled receptors for lipid mediators. J. Lipid Res. 45, 410–418. doi: 10.1194/jlr.R300006-JLR200
Ingolfsson, H. I., Carpenter, T. S., Bhatia, H., Bremer, P.-T., Marrink, S. J., and Lightstone, F. C. (2017). Computational lipidomics of the neuronal plasma membrane. Biophys. J. 113, 2271–2280. doi: 10.1016/j.bpj.2017.10.017
Innes, J. K., and Calder, P. C. (2018). Omega-6 fatty acids and inflammation. Prostaglandins Leukot. Essent. Fatty Acids 132, 41–48. doi: 10.1016/j.plefa.2018.03.004
Issemann, I., and Green, S. (1990). Activation of a member of the steroid hormone receptor superfamily by peroxisome proliferators. Nature 347, 645–650. doi: 10.1038/347645a0
Itoh, Y., Kawamata, Y., Harada, M., Kobayashi, M., Fujii, R., Fukusumi, S., et al. (2003). Free fatty acids regulate insulin secretion from pancreatic beta cells through GPR40. Nature 422, 173–176. doi: 10.1038/nature01478
Jana, M., Mondal, S., Gonzalez, F. J., and Pahan, K. (2012). Gemfibrozil, a lipid-lowering drug, increases myelin genes in human oligodendrocytes via peroxisome proliferator-activated receptor-beta. J. Biol. Chem. 287, 34134–34148. doi: 10.1074/jbc.M112.398552
Janesick, A., Wu, S. C., and Blumberg, B. (2015). Retinoic acid signaling and neuronal differentiation. Cell. Mol. Life Sci. 72, 1559–1576. doi: 10.1007/s00018-014-1815-9
Johnson, E. J., and Schaefer, E. J. (2006). Potential role of dietary n-3 fatty acids in the prevention of dementia and macular degeneration. Am. J. Clin. Nutr. 83, 1494S–1498S. doi: 10.1093/ajcn/83.6.1494S
Kaczocha, M., Rebecchi, M. J., Ralph, B. P., Teng, Y.-H. G., Berger, W. T., Galbavy, W., et al. (2014). Inhibition of fatty acid binding proteins elevates brain anandamide levels and produces analgesia. PLoS One 9:e94200. doi: 10.1371/journal.pone.0094200
Kainu, T., Wikstrom, A. C., Gustafsson, J. A., and Pelto-Huikko, M. (1994). Localization of the peroxisome proliferator-activated receptor in the brain. Neuroreport 5, 2481–2485.
Kamata, Y., Shiraga, H., Tai, A., Kawamoto, Y., and Gohda, E. (2007). Induction of neurite outgrowth in PC12 cells by the medium-chain fatty acid octanoic acid. Neuroscience 146, 1073–1081. doi: 10.1016/j.neuroscience.2007.03.001
Katakura, M., Hashimoto, M., Shahdat, H. M., Gamoh, S., Okui, T., Matsuzaki, K., et al. (2009). Docosahexaenoic acid promotes neuronal differentiation by regulating basic helix-loop-helix transcription factors and cell cycle in neural stem cells. Neuroscience 160, 651–660. doi: 10.1016/j.neuroscience.2009.02.057
Kenakin, T., and Christopoulos, A. (2013). Signalling bias in new drug discovery: detection, quantification and therapeutic impact. Nat. Rev. Drug Discov. 12, 205–216. doi: 10.1038/nrd3954
Khan, M. Z., Zhuang, X., and He, L. (2016). GPR40 receptor activation leads to CREB phosphorylation and improves cognitive performance in an Alzheimer’s disease mouse model. Neurobiol. Learn. Mem. 131, 46–55. doi: 10.1016/j.nlm.2016.03.006
Kim, J. Y., Lee, H. J., Lee, S.-J., Jung, Y. H., Yoo, D. Y., Hwang, I. K., et al. (2017). Palmitic acid-BSA enhances amyloid-beta production through GPR40-mediated dual pathways in neuronal cells: Involvement of the Akt/mTOR/HIF-1alpha and Akt/NF-kappaB pathways. Sci. Rep. 7:4335. doi: 10.1038/s41598-017-04175-w
Kimura, I., Inoue, D., Maeda, T., Hara, T., Ichimura, A., Miyauchi, S., et al. (2011). Short-chain fatty acids and ketones directly regulate sympathetic nervous system via G protein-coupled receptor 41 (GPR41). Proc. Natl. Acad. Sci. U.S.A. 108, 8030–8035. doi: 10.1073/pnas.1016088108
Kitajka, K., Sinclair, A. J., Weisinger, R. S., Weisinger, H. S., Mathai, M., Jayasooriya, A. P., et al. (2004). Effects of dietary omega-3 polyunsaturated fatty acids on brain gene expression. Proc. Natl. Acad. Sci. U.S.A. 101, 10931–10936. doi: 10.1073/pnas.0402342101
Kostenis, E. (2004). A glance at G-protein-coupled receptors for lipid mediators: a growing receptor family with remarkably diverse ligands. Pharmacol. Ther. 102, 243–257. doi: 10.1016/j.pharmthera.2004.04.005
Kurtz, A., Zimmer, A., Schnutgen, F., Bruning, G., Spener, F., and Muller, T. (1994). The expression pattern of a novel gene encoding brain-fatty acid binding protein correlates with neuronal and glial cell development. Development 120, 2637–2649.
Labrousse, V. F., Nadjar, A., Joffre, C., Costes, L., Aubert, A., Gregoire, S., et al. (2012). Short-term long chain omega3 diet protects from neuroinflammatory processes and memory impairment in aged mice. PLoS One 7:e36861. doi: 10.1371/journal.pone.0036861
Lafourcade, M., Larrieu, T., Mato, S., Duffaud, A., Sepers, M., Matias, I., et al. (2011). Nutritional omega-3 deficiency abolishes endocannabinoid-mediated neuronal functions. Nat. Neurosci. 14, 345–350. doi: 10.1038/nn.2736
Lampen, A., Carlberg, C., and Nau, H. (2001). Peroxisome proliferator-activated receptor delta is a specific sensor for teratogenic valproic acid derivatives. Eur. J. Pharmacol. 431, 25–33. doi: 10.1016/s0014-2999(01)01423-6
Landrier, J.-F., Thomas, C., Grober, J., Duez, H., Percevault, F., Souidi, M., et al. (2004). Statin induction of liver fatty acid-binding protein (L-FABP) gene expression is peroxisome proliferator-activated receptor-alpha-dependent. J. Biol. Chem. 279, 45512–45518. doi: 10.1074/jbc.M407461200
Laschet, C., Dupuis, N., and Hanson, J. (2018). The G protein-coupled receptors deorphanization landscape. Biochem. Pharmacol. 153, 62–74. doi: 10.1016/j.bcp.2018.02.016
Lein, E. S., Hawrylycz, M. J., Ao, N., Ayres, M., Bensinger, A., Bernard, A., et al. (2007). Genome-wide atlas of gene expression in the adult mouse brain. Nature 445, 168–176. doi: 10.1038/nature05453
Leong, C., Zhai, D., Kim, B., Yun, S.-W., and Chang, Y.-T. (2013). Neural stem cell isolation from the whole mouse brain using the novel FABP7-binding fluorescent dye, CDr3. Stem Cell Res. 11, 1314–1322. doi: 10.1016/j.scr.2013.09.002
Leoni, V., and Caccia, C. (2011). Oxysterols as biomarkers in neurodegenerative diseases. Chem. Phys. Lipids 164, 515–524. doi: 10.1016/j.chemphyslip.2011.04.002
Li, Z., Chen, Y., Zhang, Y., Jiang, H., Liu, Y., Chen, Y., et al. (2018a). Structure-based design of free fatty acid receptor 1 agonists bearing non-biphenyl scaffold. Bioorg. Chem. 80, 296–302. doi: 10.1016/j.bioorg.2018.06.039
Li, Z., Chen, Y., Zhou, Z., Deng, L., Xu, Y., Hu, L., et al. (2019). Discovery of first-in-class thiazole-based dual FFA1/PPARdelta agonists as potential anti-diabetic agents. Eur. J. Med. Chem. 164, 352–365. doi: 10.1016/j.ejmech.2018.12.069
Li, Z., Qiu, Q., Geng, X., Yang, J., Huang, W., and Qian, H. (2016). Free fatty acid receptor agonists for the treatment of type 2 diabetes: drugs in preclinical to phase II clinical development. Expert Opin. Investig. Drugs 25, 871–890. doi: 10.1080/13543784.2016.1189530
Li, Z., Xu, X., Hou, J., Wang, S., Jiang, H., and Zhang, L. (2018b). Structure-based optimization of free fatty acid receptor 1 agonists bearing thiazole scaffold. Bioorg. Chem. 77, 429–435. doi: 10.1016/j.bioorg.2018.01.039
Li, Z., Zhou, Z., Deng, F., Li, Y., Zhang, D., and Zhang, L. (2018c). Design, synthesis, and biological evaluation of novel pan agonists of FFA1, PPARgamma and PPARdelta. Eur. J. Med. Chem. 159, 267–276. doi: 10.1016/j.ejmech.2018.09.071
Liou, H. L., Kahn, P. C., and Storch, J. (2002). Role of the helical domain in fatty acid transfer from adipocyte and heart fatty acid-binding proteins to membranes - Analysis of chimeric proteins. J. Biol. Chem. 277, 1806–1815. doi: 10.1074/jbc.M107987200
Liu, R.-Z., Li, X., and Godbout, R. (2008). A novel fatty acid-binding protein (FABP) gene resulting from tandem gene duplication in mammals: transcription in rat retina and testis. Genomics 92, 436–445. doi: 10.1016/j.ygeno.2008.08.003
Liu, R. Z., Mita, R., Beaulieu, M., Gao, Z., and Godbout, R. (2010). Fatty acid binding proteins in brain development and disease. Int. J. Dev. Biol. 54, 1229–1239. doi: 10.1387/ijdb.092976rl092976rl
Liu, X. S., Chopp, M., Kassis, H., Jia, L. F., Hozeska-Solgot, A., Zhang, R. L., et al. (2012). Valproic acid increases white matter repair and neurogenesis after stroke. Neuroscience 220, 313–321. doi: 10.1016/j.neuroscience.2012.06.012
Liu, Y., Longo, L. D., and De Leon, M. (2000). In situ and immunocytochemical localization of E-FABP mRNA and protein during neuronal migration and differentiation in the rat brain. Brain Res. 852, 16–27. doi: 10.1016/s0006-8993(99)02158-7
Lu, J., Byrne, N., Wang, J., Bricogne, G., Brown, F. K., Chobanian, H. R., et al. (2017). Structural basis for the cooperative allosteric activation of the free fatty acid receptor GPR40. Nat. Struct. Mol. Biol. 24, 570–577. doi: 10.1038/nsmb.3417
Lundell, K., Thulin, P., Hamsten, A., and Ehrenborg, E. (2007). Alternative splicing of human peroxisome proliferator-activated receptor delta (PPAR delta): effects on translation efficiency and trans-activation ability. BMC Mol. Biol. 8:70. doi: 10.1186/1471-2199-8-70
Ma, D., Lu, L., Boneva, N. B., Warashina, S., Kaplamadzhiev, D. B., Mori, Y., et al. (2008). Expression of free fatty acid receptor GPR40 in the neurogenic niche of adult monkey hippocampus. Hippocampus 18, 326–333. doi: 10.1002/hipo.20393
Ma, D., Tao, B., Warashina, S., Kotani, S., Lu, L., Kaplamadzhiev, D. B., et al. (2007). Expression of free fatty acid receptor GPR40 in the central nervous system of adult monkeys. Neurosci. Res. 58, 394–401. doi: 10.1016/j.neures.2007.05.001
Ma, D., Zhang, M., Larsen, C. P., Xu, F., Hua, W., Yamashima, T., et al. (2010). DHA promotes the neuronal differentiation of rat neural stem cells transfected with GPR40 gene. Brain Res. 1330, 1–8. doi: 10.1016/j.brainres.2010.03.002
Magnan, C., Levin, B. E., and Luquet, S. (2015). Brain lipid sensing and the neural control of energy balance. Mol. Cell. Endocrinol. 418(Pt. 1), 3–8. doi: 10.1016/j.mce.2015.09.019
Mancini, A. D., Bertrand, G., Vivot, K., Carpentier, E., Tremblay, C., Ghislain, J., et al. (2015). beta-Arrestin recruitment and biased agonism at free fatty acid receptor 1. J. Biol. Chem. 290, 21131–21140. doi: 10.1074/jbc.M115.644450
Mangelsdorf, D. J., Thummel, C., Beato, M., Herrlich, P., Schutz, G., Umesono, K., et al. (1995). The nuclear receptor superfamily: the second decade. Cell 83, 835–839. doi: 10.1016/0092-8674(95)90199-X
Martin, G. G., McIntosh, A. L., Huang, H., Gupta, S., Atshaves, B. P., Landrock, K. K., et al. (2013). The human liver fatty acid binding protein T94A variant alters the structure, stability, and interaction with fibrates. Biochemistry 52, 9347–9357. doi: 10.1021/bi401014k
McNamara, R. K., and Carlson, S. E. (2006). Role of omega-3 fatty acids in brain development and function: potential implications for the pathogenesis and prevention of psychopathology. Prostaglandins Leukot. Essent. Fatty Acids 75, 329–349. doi: 10.1016/j.plefa.2006.07.010
Miglio, G., Rattazzi, L., Rosa, A. C., and Fantozzi, R. (2009). PPARgamma stimulation promotes neurite outgrowth in SH-SY5Y human neuroblastoma cells. Neurosci. Lett. 454, 134–138. doi: 10.1016/j.neulet.2009.03.014
Mobraten, K., Haug, T. M., Kleiveland, C. R., and Lea, T. (2013). Omega-3 and omega-6 PUFAs induce the same GPR120-mediated signalling events, but with different kinetics and intensity in Caco-2 cells. Lipids Health Dis. 12:101. doi: 10.1186/1476-511X-12-101
Moreno, S., Farioli-vecchioli, S., and Cerù, M. P. (2004). Immunolocalization of peroxisome proliferator-activated receptors and retinoid X receptors in the adult rat CNS. Neuroscience 123, 131–145. doi: 10.1016/j.neuroscience.2003.08.064
Motley, W. W., Palaima, P., Yum, S. W., Gonzalez, M. A., Tao, F., Wanschitz, J. V., et al. (2016). De novo PMP2 mutations in families with type 1 Charcot-Marie-Tooth disease. Brain 139, 1649–1656. doi: 10.1093/brain/aww055
Mounsey, R. B., Martin, H. L., Nelson, M. C., Evans, R. M., and Teismann, P. (2015). The effect of neuronal conditional knock-out of peroxisome proliferator-activated receptors in the MPTP mouse model of Parkinson’s disease. Neuroscience 300, 576–584. doi: 10.1016/j.neuroscience.2015.05.048
Muller, M. M., Lehmann, R., Klassert, T. E., Reifenstein, S., Conrad, T., Moore, C., et al. (2017). Global analysis of glycoproteins identifies markers of endotoxin tolerant monocytes and GPR84 as a modulator of TNFalpha expression. Sci. Rep. 7:838. doi: 10.1038/s41598-017-00828-y
Murillo-Rodriguez, E. (2017). The role of nuclear receptor pparalpha in the sleep-wake cycle modulation. A tentative approach for treatment of sleep disorders. Curr. Drug Deliv. 14, 473–482. doi: 10.2174/1567201814666161109123803
Murphy, E. J., Owada, Y., Kitanaka, N., Kondo, H., and Glatz, J. F. C. (2005). Brain arachidonic acid incorporation is decreased in heart fatty acid binding protein gene-ablated mice. Biochemistry 44, 6350–6360. doi: 10.1021/bi047292r
Nakamoto, K., Nishinaka, T., Matsumoto, K., Kasuya, F., Mankura, M., Koyama, Y., et al. (2012). Involvement of the long-chain fatty acid receptor GPR40 as a novel pain regulatory system. Brain Res. 1432, 74–83. doi: 10.1016/j.brainres.2011.11.012
Niculescu, M. D., Lupu, D. S., and Craciunescu, C. N. (2011). Maternal alpha-linolenic acid availability during gestation and lactation alters the postnatal hippocampal development in the mouse offspring. Int. J. Dev. Neurosci. 29, 795–802. doi: 10.1016/j.ijdevneu.2011.09.006
Nikaido, Y., Koyama, Y., Yoshikawa, Y., Furuya, T., and Takeda, S. (2015). Mutation analysis and molecular modeling for the investigation of ligand-binding modes of GPR84. J. Biochem. 157, 311–320. doi: 10.1093/jb/mvu075
Nohr, M. K., Pedersen, M. H., Gille, A., Egerod, K. L., Engelstoft, M. S., Husted, A. S., et al. (2013). GPR41/FFAR3 and GPR43/FFAR2 as cosensors for short-chain fatty acids in enteroendocrine cells vs FFAR3 in enteric neurons and FFAR2 in enteric leukocytes. Endocrinology 154, 3552–3564. doi: 10.1210/en.2013-1142
Noy, N. (2016). Non-classical transcriptional activity of retinoic acid. Subcell. Biochem. 81, 179–199. doi: 10.1007/978-94-024-0945-1_7
Offermanns, S. (2013). Free fatty acid (FFA) and hydroxy carboxylic acid (HCA) receptors. Annu. Rev. Pharmacol. Toxicol. 54, 407–434. doi: 10.1146/annurev-pharmtox-011613-135945
Oikawa, H., and Sng, J. C. G. (2016). Valproic acid as a microRNA modulator to promote neurite outgrowth. Neural Regen. Res. 11, 1564–1565. doi: 10.4103/1673-5374.193227
Orr, S. K., Trepanier, M. O., and Bazinet, R. P. (2013). n-3 Polyunsaturated fatty acids in animal models with neuroinflammation. Prostaglandins Leukot. Essent. Fatty Acids 88, 97–103. doi: 10.1016/j.plefa.2012.05.008
Orth, M., and Bellosta, S. (2012). Cholesterol: its regulation and role in central nervous system disorders. Cholesterol 2012:292598. doi: 10.1155/2012/292598
Ory, J., Kane, C. D., Simpson, M. A., Banaszak, L. J., and Bernlohr, D. A. (1997). Biochemical and crystallographic analyses of a portal mutant of the adipocyte lipid-binding protein. J. Biol. Chem. 272, 9793–9801. doi: 10.1074/jbc.272.15.9793
O’Sullivan, S. E. (2007). Cannabinoids go nuclear: evidence for activation of peroxisome proliferator-activated receptors. Br. J. Pharmacol. 152, 576–582. doi: 10.1038/sj.bjp.0707423
Owada, Y. (2008). Fatty acid binding protein: localization and functional significance in the brain. Tohoku J. Exp. Med. 214, 213–220. doi: 10.1620/tjem.214.213
Owada, Y., Yoshimoto, T., and Kondo, H. (1996). Spatio-temporally differential expression of genes for three members of fatty acid binding proteins in developing and mature rat brains. J. Chem. Neuroanat. 12, 113–122. doi: 10.1016/s0891-0618(96)00192-5
Pelsers, M. M., Hanhoff, T., Van Der Voort, D., Arts, B., Peters, M., Ponds, R., et al. (2004). Brain- and heart-type fatty acid-binding proteins in the brain: Tissue distribution and clinical utility. Clin. Chem. 50, 1568–1575. doi: 10.1373/clinchem.2003.030361
Pelsers, M. M., Hermens, W. T., and Glatz, J. F. C. (2005). Fatty acid-binding proteins as plasma markers of tissue injury. Clin. Chim. Acta 352, 15–35. doi: 10.1016/j.cccn.2004.09.001
Pepino, M. Y., Kuda, O., Samovski, D., and Abumrad, N. A. (2014). Structure-function of CD36 and importance of fatty acid signal transduction in fat metabolism. Annu. Rev. Nutr. 34, 281–303. doi: 10.1146/annurev-nutr-071812-161220
Peters, B. D., Duran, M., Vlieger, E. J., Majoie, C. B., den Heeten, G. J., Linszen, D. H., et al. (2009). Polyunsaturated fatty acids and brain white matter anisotropy in recent-onset schizophrenia: a preliminary study. Prostaglandins Leukot. Essent. Fatty Acids 81, 61–63. doi: 10.1016/j.plefa.2009.04.007
Prolla, T. A., and Mattson, M. P. (2001). Molecular mechanisms of brain aging and neurodegenerative disorders: lessons from dietary restriction. Trends Neurosci. 24, S21–S31. doi: 10.1016/S0166-2236(01)00005-4
Punetha, J., Mackay-Loder, L., Harel, T., Coban-Akdemir, Z., Jhangiani, S. N., Gibbs, R. A., et al. (2018). Identification of a pathogenic PMP2 variant in a multi-generational family with CMT type 1: clinical gene panels versus genome-wide approaches to molecular diagnosis. Mol. Genet. Metab. 125, 302–304. doi: 10.1016/j.ymgme.2018.08.005
Rademacher, M., Zimmerman, A. W., Ruterjans, H., Veerkamp, J. H., and Lucke, C. (2002). Solution structure of fatty acid-binding protein from human brain. Mol. Cell. Biochem. 239, 61–68. doi: 10.1023/A:1020566909213
Rautureau, Y., Paradis, P., and Schiffrin, E. L. (2017). Generation of a mouse model with smooth muscle cell specific loss of the expression of PPARgamma. Methods Mol. Biol. 1527, 381–407. doi: 10.1007/978-1-4939-6625-7_30
Reddy, D. S. (2010). Neurosteroids: endogenous role in the human brain and therapeutic potentials. Prog. Brain Res. 186, 113–137. doi: 10.1016/B978-0-444-53630-3.00008-7
Reese, A. J., and Banaszak, L. J. (2004). Specificity determinants for lipids bound to beta-barrel proteins. J. Lipid Res. 45, 232–243. doi: 10.1194/jlr.M300113-JLR200
Richieri, G. V., Ogata, R. T., Zimmerman, A. W., Veerkamp, J. H., and Kleinfeld, A. M. (2000). Fatty acid binding proteins from different tissues show distinct patterns of fatty acid interactions. Biochemistry 39, 7197–7204. doi: 10.1021/bi000314z
Ricote, M., and Glass, C. K. (2007). PPARs and molecular mechanisms of transrepression. Biochim. Biophys. Acta 1771, 926–935. doi: 10.1016/j.bbalip.2007.02.013
Rivera, P., Arrabal, S., Vargas, A., Blanco, E., Serrano, A., Pavon, F. J., et al. (2014). Localization of peroxisome proliferator-activated receptor alpha (PPARα) and N-acyl phosphatidylethanolamine phospholipase D (NAPE-PLD) in cells expressing the Ca2+-binding proteins calbindin, calretinin, and parvalbumin in the adult rat hippocampus. Front. Neuroanat. 8:12. doi: 10.3389/fnana.2014.00012
Rocha, D. M., Caldas, A. P., Oliveira, L. L., Bressan, J., and Hermsdorff, H. H. (2016). Saturated fatty acids trigger TLR4-mediated inflammatory response. Atherosclerosis 244, 211–215. doi: 10.1016/j.atherosclerosis.2015.11.015
Rohwedder, A., Zhang, Q., Rudge, S. A., and Wakelam, M. J. O. (2014). Lipid droplet formation in response to oleic acid in Huh-7 cells is mediated by the fatty acid receptor FFAR4. J. Cell Sci. 127, 3104–3115. doi: 10.1242/jcs.145854
Rombaldi Bernardi, J., de Souza Escobar, R., Ferreira, C. F., and Pelufo Silveira, P. (2012). Fetal and neonatal levels of omega-3: effects on neurodevelopment, nutrition, and growth. ScientificWorldJournal 2012:202473. doi: 10.1100/2012/202473
Roth, A. D., Leisewitz, A. V., Jung, J. E., Cassina, P., Barbeito, L., Inestrosa, N. C., et al. (2003). PPAR γ activators induce growth arrest and process extension in B12 oligodendrocyte-like cells and terminal differentiation of cultured oligodendrocytes. J. Neurosci. Res. 72, 425–435. doi: 10.1002/jnr.10596
Roy, A., and Pahan, K. (2015). PPARalpha signaling in the hippocampus: crosstalk between fat and memory. J. Neuroimmune Pharmacol. 10, 30–34. doi: 10.1007/s11481-014-9582-9
Sacchettini, J. C., Meininger, T. A., Lowe, J. B., Gordon, J. I., and Banaszak, L. J. (1987). Crystallization of rat intestinal fatty-acid binding protein - preliminary-x-ray data obtained from protein expressed in Escherichia-coli. J. Biol. Chem. 262, 5428–5430.
Saino-Saito, S., Nourani, R. M., Iwasa, H., Kondo, H., and Owada, Y. (2009). Discrete localization of various fatty-acid-binding proteins in various cell populations of mouse retina. Cell Tissue Res. 338, 191–201. doi: 10.1007/s00441-009-0862-2
Saino-Saito, S., Suzuki, R., Tokuda, N., Abe, H., Kondo, H., and Owada, Y. (2010). Localization of fatty acid binding proteins (FABPs) in the cochlea of mice. Ann. Anat. 192, 210–214. doi: 10.1016/j.aanat.2010.06.007
Samuel, B. S., Shaito, A., Motoike, T., Rey, F. E., Backhed, F., Manchester, J. K., et al. (2008). Effects of the gut microbiota on host adiposity are modulated by the short-chain fatty-acid binding G protein-coupled receptor, Gpr41. Proc. Natl. Acad. Sci. U.S.A. 105, 16767–16772. doi: 10.1073/pnas.0808567105
Sanchez-Font, M. F., Bosch-Comas, A., Gonzalez-Duarte, R., and Marfany, G. (2003). Overexpression of FABP7 in Down syndrome fetal brains is associated with PKNOX1 gene-dosage imbalance. Nucleic Acids Res. 31, 2769–2777. doi: 10.1093/nar/gkg396
Sanchez-Reyes, O. B., Romero-Avila, M. T., Castillo-Badillo, J. A., Takei, Y., Hirasawa, A., Tsujimoto, G., et al. (2014). Free fatty acids and protein kinase C activation induce GPR120 (free fatty acid receptor 4) phosphorylation. Eur. J. Pharmacol. 723, 368–374. doi: 10.1016/j.ejphar.2013.11.003
Santos, M. J., Quintanilla, R. A., Toro, A., Grandy, R., Dinamarca, M. C., Godoy, J. A., et al. (2005). Peroxisomal proliferation protects from beta-amyloid neurodegeneration. J. Biol. Chem. 280, 41057–41068. doi: 10.1074/jbc.M505160200
Sarruf, D. A., Yu, F., Nguyen, H. T., Williams, D. L., Printz, R. L., Niswender, K. D., et al. (2009). Expression of peroxisome proliferator-activated receptor-γ in key neuronal subsets regulating glucose metabolism and energy homeostasis. Endocrinology 150, 707–712. doi: 10.1210/en.2008-0899
Sasselli, V., Pachnis, V., and Burns, A. J. (2012). The enteric nervous system. Dev. Biol. 366, 64–73. doi: 10.1016/j.ydbio.2012.01.012
Sastre, M., Klockgether, T., and Heneka, M. T. (2006). Contribution of inflammatory processes to Alzheimer’s disease: molecular mechanisms. Int. J. Dev. Neurosci. 24, 167–176. doi: 10.1016/j.ijdevneu.2005.11.014
Sawzdargo, M., George, S. R., Nguyen, T., Xu, S., Kolakowski, L. F., and O’dowd, B. F. (1997). A cluster of four novel human g protein-coupled receptor genes occurring in close proximity to CD22 gene on chromosome 19q13.1. Biochem. Biophys. Res. Commun. 239, 543–547. doi: 10.1006/bbrc.1997.7513
Scandroglio, F., Venkata, J. K., Loberto, N., Prioni, S., Schuchman, E. H., Chigorno, V., et al. (2008). Lipid content of brain, brain membrane lipid domains, and neurons from acid sphingomyelinase deficient mice. J. Neurochem. 107, 329–338. doi: 10.1111/j.1471-4159.2008.05591.x
Schug, T. T., Berry, D. C., Shaw, N. S., Travis, S. N., and Noy, N. (2007). Opposing effects of retinoic acid on cell growth result from alternate activation of two different nuclear receptors. Cell 129, 723–733. doi: 10.1016/j.cell.2007.02.050
Schug, T. T., Berry, D. C., Toshkov, I. A., Cheng, L., Nikitin, A. Y., and Noy, N. (2008). Overcoming retinoic acid-resistance of mammary carcinomas by diverting retinoic acid from PPARbeta/delta to RAR. Proc. Natl. Acad. Sci. U.S.A. 105, 7546–7551. doi: 10.1073/pnas.0709981105
Senga, S., Kawaguchi, K., Kobayashi, N., Ando, A., and Fujii, H. (2018). A novel fatty acid-binding protein 5-estrogen-related receptor alpha signaling pathway promotes cell growth and energy metabolism in prostate cancer cells. Oncotarget 9, 31753–31770. doi: 10.18632/oncotarget.25878
Serhan, C. N. (2014). Pro-resolving lipid mediators are leads for resolution physiology. Nature 510, 92–101. doi: 10.1038/nature13479
Shang, J., Brust, R., Mosure, S. A., Bass, J., Munoz-Tello, P., Lin, H., et al. (2018). Cooperative cobinding of synthetic and natural ligands to the nuclear receptor PPARgamma. eLife 7:e43320. doi: 10.7554/eLife.43320
Shao, D., Rangwala, S. M., Bailey, S. T., Krakow, S. L., Reginato, M. J., and Lazar, M. A. (1998). Interdomain communication regulating ligand binding by PPAR-gamma. Nature 396, 377–380. doi: 10.1038/24634
Shioda, N., Yabuki, Y., Kobayashi, Y., Onozato, M., Owada, Y., and Fukunaga, K. (2014). FABP3 protein promotes alpha-synuclein oligomerization associated with 1-methyl-1,2,3,6-tetrahydropiridine-induced neurotoxicity. J. Biol. Chem. 289, 18957–18965. doi: 10.1074/jbc.M113.527341
Shioda, N., Yamamoto, Y., Watanabe, M., Binas, B., Owada, Y., and Fukunaga, K. (2010). Heart-type fatty acid binding protein regulates dopamine D2 receptor function in mouse brain. J. Neurosci. 30, 3146–3155. doi: 10.1523/JNEUROSCI.4140-09.2010
Smathers, R. L., and Petersen, D. R. (2011). The human fatty acid-binding protein family: evolutionary divergences and functions. Hum. Genomics 5, 170–191.
Smith, A. J., Sanders, M. A., Juhlmann, B. E., Hertzel, A. V., and Bernlohr, D. A. (2008). Mapping of the hormone-sensitive lipase binding site on the adipocyte fatty acid-binding protein (AFABP). Identification of the charge quartet on the AFABP/aP2 helix-turn-helix domain. J. Biol. Chem. 283, 33536–33543. doi: 10.1074/jbc.M806732200
Sona, C., Kumar, A., Dogra, S., Kumar, B. A., Umrao, D., and Yadav, P. N. (2018). Docosahexaenoic acid modulates brain-derived neurotrophic factor via GPR40 in the brain and alleviates diabesity-associated learning and memory deficits in mice. Neurobiol. Dis. 118, 94–107. doi: 10.1016/j.nbd.2018.07.002
Song, T., Yang, Y., Zhou, Y., Wei, H., and Peng, J. (2017). GPR120: a critical role in adipogenesis, inflammation, and energy metabolism in adipose tissue. Cell. Mol. Life Sci. 74, 2723–2733. doi: 10.1007/s00018-017-2492-2
Srivastava, A., Yano, J., Hirozane, Y., Kefala, G., Gruswitz, F., Snell, G., et al. (2014). High-resolution structure of the human GPR40 receptor bound to allosteric agonist TAK-875. Nature 513, 124–127. doi: 10.1038/nature13494
Stoddart, L. A., Smith, N. J., Jenkins, L., Brown, A. J., and Milligan, G. (2008). Conserved polar residues in transmembrane domains V, VI, and VII of free fatty acid receptor 2 and free fatty acid receptor 3 are required for the binding and function of short chain fatty acids. J. Biol. Chem. 283, 32913–32924. doi: 10.1074/jbc.M805601200
Storch, J., and Corsico, B. (2008). The emerging functions and mechanisms of mammalian fatty acid-binding proteins. Annu. Rev. Nutr. 28, 73–95. doi: 10.1146/annurev.nutr.27.061406.093710
Storch, J., and Thumser, A. E. (2010). Tissue-specific functions in the fatty acid-binding protein family. J. Biol. Chem. 285, 32679–32683. doi: 10.1074/jbc.R110.135210
Storch, J., and Thumser, A. E. A. (2000). The fatty acid transport function of fatty acid-binding proteins. Biochim. Biophys. Acta 1486, 28–44. doi: 10.1016/S1388-1981(00)00046-9
Storch, J., Veerkamp, J. H., and Hsu, K. T. (2002). Similar mechanisms of fatty acid transfer from human anal rodent fatty acid-binding proteins to membranes: liver, intestine, heart muscle, and adipose tissue FABPs. Mol. Cell. Biochem. 239, 25–33. doi: 10.1023/A:1020546321508
Sum, C. S., Tikhonova, I. G., Neumann, S., Engel, S., Raaka, B. M., Costanzi, S., et al. (2007). Identification of residues important for agonist recognition and activation in GPR40. J. Biol. Chem. 282, 29248–29255. doi: 10.1074/jbc.M705077200
Sunshine, H., and Iruela-Arispe, M. L. (2017). Membrane lipids and cell signaling. Curr. Opin. Lipidol. 28, 408–413. doi: 10.1097/MOL.0000000000000443
Suzuki, M., Takaishi, S., Nagasaki, M., Onozawa, Y., Iino, I., Maeda, H., et al. (2013). Medium-chain fatty acid-sensing receptor, GPR84, is a proinflammatory receptor. J. Biol. Chem. 288, 10684–10691. doi: 10.1074/jbc.M112.420042
Taha, A. Y., Filo, E., Ma, D. W. L., and McIntyre Burnham, W. (2009). Dose-dependent anticonvulsant effects of linoleic and alpha-linolenic polyunsaturated fatty acids on pentylenetetrazol induced seizures in rats. Epilepsia 50, 72–82. doi: 10.1111/j.1528-1167.2008.01731.x
The GTEx Consortium (2013). The genotype-tissue expression (GTEx) project. Nat. Genet. 45, 580–585. doi: 10.1038/ng.2653
Thompson, J., Reese-Wagoner, A., and Banaszak, L. (1999). Liver fatty acid binding protein: species variation and the accommodation of different ligands. Biochim. Biophys. Acta 1441, 117–130. doi: 10.1016/S1388-1981(99)00146-8
Tikhonova, I. G., Sum, C. S., Neumann, S., Thomas, C. J., Raaka, B. M., Costanzi, S., et al. (2007). Bidirectional, iterative approach to the structural delineation of the functional “chemoprint” in GPR40 for agonist recognition. J. Med. Chem. 50, 2981–2989. doi: 10.1021/jm0614782
Tzeng, J., Byun, J., Park, J. Y., Yamamoto, T., Schesing, K., Tian, B., et al. (2015). An Ideal PPAR response element bound to and activated by PPARalpha. PLoS One 10:e0134996. doi: 10.1371/journal.pone.0134996
Uauy, R., and Dangour, A. D. (2006). Nutrition in brain development and aging: role of essential fatty acids. Nutr. Rev. 64, S24–S33. doi: 10.1111/j.1753-4887.2006.tb00242.x
Uhlen, M., Fagerberg, L., Hallstrom, B. M., Lindskog, C., Oksvold, P., Mardinoglu, A., et al. (2015). Proteomics. Tissue-based map of the human proteome. Science 347:1260419. doi: 10.1126/science.1260419
Ulven, T. (2012). Short-chain free fatty acid receptors FFA2/GPR43 and FFA3/GPR41 as new potential therapeutic targets. Front. Endocrinol. 3:111. doi: 10.3389/fendo.2012.00111
van Gelder, B. M., Tijhuis, M., Kalmijn, S., and Kromhout, D. (2007). Fish consumption, n-3 fatty acids, and subsequent 5-y cognitive decline in elderly men: the Zutphen Elderly Study. Am. J. Clin. Nutr. 85, 1142–1147. doi: 10.1093/ajcn/85.4.1142
Vance, J. E. (2012). Dysregulation of cholesterol balance in the brain: contribution to neurodegenerative diseases. Dis. Model. Mech. 5, 746–755. doi: 10.1242/dmm.010124
Veerkamp, J. H., and Zimmerman, A. W. (2001). Fatty acid-binding proteins of nervous tissue. J. Mol. Neurosci. 16, 133–137.
Villapol, S. (2018). Roles of peroxisome proliferator-activated receptor gamma on brain and peripheral inflammation. Cell. Mol. Neurobiol. 38, 121–132. doi: 10.1007/s10571-017-0554-5
Villegas-Comonfort, S., Takei, Y., Tsujimoto, G., Hirasawa, A., and García-Sáinz, J. A. (2017). Effects of arachidonic acid on FFA4 receptor: signaling, phosphorylation and internalization. Prostaglandins Leukot. Essent. Fat. Acids 117, 1–10. doi: 10.1016/j.plefa.2017.01.013
von Bernhardi, R., Eugenin-von Bernhardi, J., Flores, B., and Eugenin Leon, J. (2016). Glial cells and integrity of the nervous system. Adv. Exp. Med. Biol. 949, 1–24. doi: 10.1007/978-3-319-40764-7_1
Walder, B., Robin, X., Rebetez, M. M. L., Copin, J.-C., Gasche, Y., Sanchez, J.-C., et al. (2013). The prognostic significance of the serum biomarker heart-fatty acidic binding protein in comparison with s100b in severe traumatic brain injury. J. Neurotrauma 30, 1631–1637. doi: 10.1089/neu.2012.2791
Wang, F., Mullican, S. E., DiSpirito, J. R., Peed, L. C., and Lazar, M. A. (2013). Lipoatrophy and severe metabolic disturbance in mice with fat-specific deletion of PPARgamma. Proc. Natl. Acad. Sci. U.S.A. 110, 18656–18661. doi: 10.1073/pnas.1314863110
Wang, J., Wu, X., Simonavicius, N., Tian, H., and Ling, L. (2006). Medium-chain fatty acids as ligands for orphan G protein-coupled receptor GPR84. J. Biol. Chem. 281, 34457–34464. doi: 10.1074/jbc.M608019200
Wang, X., and Chan, C. B. (2015). n-3 polyunsaturated fatty acids and insulin secretion. J. Endocrinol. 224, R97–R106. doi: 10.1530/JOE-14-0581
Wang, Y.-T., Liu, C.-H., and Zhu, H.-L. (2016). Fatty acid binding protein (FABP) inhibitors: a patent review (2012-2015). Expert Opin. Ther. Pat. 26, 767–776. doi: 10.1080/13543776.2016.1182500
Warden, A., Truitt, J., Merriman, M., Ponomareva, O., Jameson, K., Ferguson, L. B., et al. (2016). Localization of PPAR isotypes in the adult mouse and human brain. Sci. Rep. 6:27618. doi: 10.1038/srep27618
Watanabe, A., Toyota, T., Owada, Y., Hayashi, T., Iwayama, Y., Matsumata, M., et al. (2007). Fabp7 maps to a quantitative trait locus for a schizophrenia endophenotype. PLoS Biol. 5:e297. doi: 10.1371/journal.pbio.0050297
Watson, S.-J., Brown, A. J. H., and Holliday, N. D. (2012). Differential signaling by splice variants of the human free fatty acid receptor GPR120. Mol. Pharmacol. 81, 631–642. doi: 10.1124/mol.111.077388
Wei, L., Tokizane, K., Konishi, H., Yu, H.-R., and Kiyama, H. (2017). Agonists for G-protein-coupled receptor 84 (GPR84) alter cellular morphology and motility but do not induce pro-inflammatory responses in microglia. J. Neuroinflammation 14:198. doi: 10.1186/s12974-017-0970-y
Whalen, E. J., Rajagopal, S., and Lefkowitz, R. J. (2011). Therapeutic potential of beta-arrestin- and G protein-biased agonists. Trends Mol. Med. 17, 126–139. doi: 10.1016/j.molmed.2010.11.004
Wittenberger, T., Schaller, H. C., and Hellebrand, S. (2001). An expressed sequence tag (EST) data mining strategy succeeding in the discovery of new G-protein coupled receptors. J. Mol. Biol. 307, 799–813. doi: 10.1006/jmbi.2001.4520
Wnuk, A., and Kajta, M. (2017). Steroid and xenobiotic receptor signalling in apoptosis and autophagy of the nervous system. Int. J. Mol. Sci. 18:E2394. doi: 10.3390/ijms18112394
Wolfrum, C. (2007). Cytoplasmic fatty acid binding protein sensing fatty acids for peroxisome proliferator activated receptor activation. Cell. Mol. Life Sci. 64, 2465–2476. doi: 10.1007/s00018-007-7279-4
Wolfrum, C., Borrmann, C. M., Borchers, T., and Spener, F. (2001). Fatty acids and hypolipidemic drugs regulate peroxisome proliferator-activated receptors alpha - and gamma-mediated gene expression via liver fatty acid binding protein: a signaling path to the nucleus. Proc. Natl. Acad. Sci. U.S.A. 98, 2323–2328. doi: 10.1073/pnas.051619898
Won, Y.-J., Lu, V. B., Puhl, H. L. III, and Ikeda, S. R. (2013). beta-Hydroxybutyrate modulates N-type calcium channels in rat sympathetic neurons by acting as an agonist for the G-protein-coupled receptor FFA3. J. Neurosci. 33, 19314–19325. doi: 10.1523/JNEUROSCI.3102-13.2013
Woods, J. W., Tanen, M., Figueroa, D. J., Biswas, C., Zycband, E., Moller, D. E., et al. (2003). Localization of PPARδ in murine central nervous system: expression in oligodendrocytes and neurons. Brain Res. 975, 10–21. doi: 10.1016/S0006-8993(03)02515-0
Wunderlich, M. T., Hanhoff, T., Goertler, M., Spener, F., Glatz, J. F. C., Wallesch, C. W., et al. (2005). Release of brain-type and heart-type fatty acid-binding proteins in serum after acute ischaemic stroke. J. Neurol. 252, 718–724. doi: 10.1007/s00415-005-0725-z
Xing, G., Zhang, L., Zhang, L., Heynen, T., Yoshikawa, T., Smith, M., et al. (1995). Rat PPARgama contains A CGG triplet repeat and is prminently expressed in the thalamic nuclei. Biochem. Biophys. Res. Commun. 217, 1015–1025. doi: 10.1006/bbrc.1995.2871
Xu, L. Z., Sanchez, R., Sali, A., and Heintz, N. (1996). Ligand specificity of brain lipid-binding protein. J. Biol. Chem. 271, 24711–24719. doi: 10.1074/jbc.271.40.24711
Xu, Z. H., Buelt, M. K., Banaszak, L. J., and Bernlohr, D. A. (1991). Expression, purification, and crystallization of the adipocyte lipid-binding protein. J. Biol. Chem. 266, 14367–14370.
Yakunin, E., Loeb, V., Kisos, H., Biala, Y., Yehuda, S., Yaari, Y., et al. (2012). Alpha-synuclein neuropathology is controlled by nuclear hormone receptors and enhanced by docosahexaenoic acid in a mouse model for Parkinson’s disease. Brain Pathol. 22, 280–294. doi: 10.1111/j.1750-3639.2011.00530.x
Yamashima, T. (2008). A putative link of PUFA, GPR40 and adult-born hippocampal neurons for memory. Prog. Neurobiol. 84, 105–115. doi: 10.1016/j.pneurobio.2007.11.002
Yamashima, T. (2012). PUFA-GPR40-CREB signaling hypothesis for the adult primate neurogenesis. Prog. Lipid Res. 51, 221–231. doi: 10.1016/j.plipres.2012.02.001
Yan, S., Elmes, M. W., Tong, S., Hu, K., Awwa, M., Teng, G. Y. H., et al. (2018). SAR studies on truxillic acid mono esters as a new class of antinociceptive agents targeting fatty acid binding proteins. Eur. J. Med. Chem. 154, 233–252. doi: 10.1016/j.ejmech.2018.04.050
Yang, Y., Tian, X., Xu, D., Zheng, F., Lu, X., Zhang, Y., et al. (2018). GPR40 modulates epileptic seizure and NMDA receptor function. Sci. Adv. 4:eaau2357. doi: 10.1126/sciadv.aau2357
Yoshimura, M., Miyata, A., Arita, K., Kurihara, T., Shioda, S., Oyoshi, T., et al. (2015). Attenuation of inflammatory and neuropathic pain behaviors in mice through activation of free fatty acid receptor GPR40. Mol. Pain 11:6. doi: 10.1186/s12990-015-0003-8
Yu, S., Levi, L., Siegel, R., and Noy, N. (2012). Retinoic acid induces neurogenesis by activating both retinoic acid receptors (RARs) and peroxisome proliferator-activated receptor beta/delta (PPARbeta/delta). J. Biol. Chem. 287, 42195–42205. doi: 10.1074/jbc.M112.410381
Yu, Y., Zhu, M., Zhao, Y., Xu, M., and Qiu, M. (2018). Overexpression of TUSC7 inhibits the inflammation caused by microglia activation via regulating miR-449a/PPAR-gamma. Biochem. Biophys. Res. Commun. 503, 1020–1026. doi: 10.1016/j.bbrc.2018.06.111
Yun, S. W., Leong, C., Zhai, D., Tan, Y. L., Lim, L., Bi, X., et al. (2012). Neural stem cell specific fluorescent chemical probe binding to FABP7. Proc. Natl. Acad. Sci. U.S.A. 109, 10214–10217. doi: 10.1073/pnas.12008171091200817109
Zamarbide, M., Etayo-Labiano, I., Ricobaraza, A., Martinez-Pinilla, E., Aymerich, M. S., Lanciego, J. L., et al. (2014). GPR40 activation leads to CREB and ERK phosphorylation in primary cultures of neurons from the mouse CNS and in human neuroblastoma cells. Hippocampus 24, 733–739. doi: 10.1002/hipo.22263
Zenker, J., Stettner, M., Ruskamo, S., Domenech-Estevez, E., Baloui, H., Medard, J.-J., et al. (2014). A role of peripheral myelin protein 2 in lipid homeostasis of myelinating Schwann cells. Glia 62, 1502–1512. doi: 10.1002/glia.22696
Zhang, Q., Yang, H., Li, J., and Xie, X. (2016). Discovery and characterization of a novel small-molecule agonist for medium-chain free fatty acid receptor G protein-coupled receptor 84. J. Pharmacol. Exp. Ther. 357, 337–344. doi: 10.1124/jpet.116.232033
Zhang, R., Wang, Y., Li, R., and Chen, G. (2015). Transcriptional factors mediating retinoic acid signals in the control of energy metabolism. Int. J. Mol. Sci. 16, 14210–14244. doi: 10.3390/ijms160614210
Zhou, Y., Nie, T., Zhang, Y., Song, M., Li, K., Ding, M., et al. (2016). The discovery of novel and selective fatty acid binding protein 4 inhibitors by virtual screening and biological evaluation. Bioorg. Med. Chem. 24, 4310–4317. doi: 10.1016/j.bmc.2016.07.022
Zimmer, L., Delpal, S., Guilloteau, D., Aioun, J., Durand, G., and Chalon, S. (2000). Chronic n-3 polyunsaturated fatty acid deficiency alters dopamine vesicle density in the rat frontal cortex. Neurosci. Lett. 284, 25–28. doi: 10.1016/s0304-3940(00)00950-2
Zimmer, L., Hembert, S., Durand, G., Breton, P., Guilloteau, D., Besnard, J. C., et al. (1998). Chronic n-3 polyunsaturated fatty acid diet-deficiency acts on dopamine metabolism in the rat frontal cortex: a microdialysis study. Neurosci. Lett. 240, 177–181. doi: 10.1016/s0304-3940(97)00938-5
Zoete, V., Grosdidier, A., and Michielin, O. (2007). Peroxisome proliferator-activated receptor structures: ligand specificity, molecular switch and interactions with regulators. Biochim. Biophys. Acta 1771, 915–925. doi: 10.1016/j.bbalip.2007.01.007
Zolezzi, J. M., and Inestrosa, N. C. (2013). Peroxisome proliferator-activated receptors and Alzheimer’s disease: hitting the blood-brain barrier. Mol. Neurobiol. 48, 438–451. doi: 10.1007/s12035-013-8435-5
Keywords: lipid sensing, neuronal differentiation and development, signal transduction, free fatty acid receptor, fatty acid binding protein, peroxisome proliferator activated receptor, docosahexaenoic acid, arachidonic acid
Citation: Falomir-Lockhart LJ, Cavazzutti GF, Giménez E and Toscani AM (2019) Fatty Acid Signaling Mechanisms in Neural Cells: Fatty Acid Receptors. Front. Cell. Neurosci. 13:162. doi: 10.3389/fncel.2019.00162
Received: 15 January 2019; Accepted: 08 April 2019;
Published: 24 April 2019.
Edited by:
Gabriela Alejandra Salvador, Universidad Nacional del Sur, ArgentinaReviewed by:
Sharon DeMorrow, The University of Texas at Austin, United StatesTatsuro Mutoh, Fujita Health University, Japan
Copyright © 2019 Falomir-Lockhart, Cavazzutti, Giménez and Toscani. This is an open-access article distributed under the terms of the Creative Commons Attribution License (CC BY). The use, distribution or reproduction in other forums is permitted, provided the original author(s) and the copyright owner(s) are credited and that the original publication in this journal is cited, in accordance with accepted academic practice. No use, distribution or reproduction is permitted which does not comply with these terms.
*Correspondence: Lisandro Jorge Falomir-Lockhart, bGZhbG9taXJAY29uaWNldC5nb3YuYXI=; bGpmYWxvbWlyQGJpb2wudW5scC5lZHUuYXI=