- 1Med-X Research Institute and School of Biomedical Engineering, Shanghai Jiao Tong University, Shanghai, China
- 2Department of Otorhinolaryngology, Shanghai Sixth People’s Hospital Affiliated to Shanghai Jiao Tong University, Shanghai, China
- 3Department of Neurology, Shanghai Fifth People’s Hospital, Fudan University, Shanghai, China
Cumulating evidence has indicated NAD+ deficiency as a common central pathological factor of multiple diseases and aging. NAD+ supplement is highly protective in various disease and aging models, while two key questions have remained unanswered: (1) Does extracellular NAD+ also produce its effects through its degradation product adenosine? (2) Does extracellular NAD+ produce the protective effects by affecting cells under pathological insults only, or by affecting both normal cell and the cells under pathological insults? Since extracellular NAD+ can be degraded into adenosine, and endogenous adenosine levels are in the nanomolar range under physiological conditions, extracellular NAD+ may produce its effects through its degradation into adenosine. In this study we used BV2 microglia as a cellular model to test our hypothesis that NAD+ treatment can increase the intracellular adenylate pool under basal conditions through its extracellular degradation into adenosine. Our study has shown that extracellular NAD+ is degraded into adenosine extracellularly, which enters BV2 microglia through equilibrative nucleoside transporters under basal conditions. The intracellular adenosine is converted to AMP by adenosine kinase, which increases the intracellular ATP levels by both activating AMPK and increasing the intracellular adenylate pool. Collectively, our study has suggested a novel mechanism underlying the protective effects of NAD+ administration, which is mediated by extracellular NAD+ degradation into adenosine as well as the activities of adenosine kinase and AMPK. Our findings have also suggested that NAD+ administration in various disease and aging models may also produce its effects by affecting the microglia that are not under pathological insults.
Introduction
NAD+ plays critical roles in a number of biological functions including energy metabolism, mitochondrial activity, DNA repair, and cell death (Ying, 2008). Accumulating evidence has also suggested that there are significant decreases in the NAD+ levels in the models of aging and a number of diseases (Ying, 2008; Verdin, 2015; Zhang and Ying, 2018). Supplement with NAD+ can produce profound beneficial effects in animal models of aging and multiple diseases (Ying et al., 2007; Yoshino et al., 2011; Mills et al., 2016; Zhang et al., 2016; Das et al., 2018). Collectively, these findings have indicated that NAD+ deficiency is a common central pathological factor of a number of diseases and aging (Ying, 2008; Imai and Guarente, 2014; Verdin, 2015; Zhang and Ying, 2018).
While NAD+ supplement can produce beneficial effects in various models of diseases and aging (Ying et al., 2007; Yoshino et al., 2011; Mills et al., 2016; Zhang et al., 2016; Das et al., 2018), two key questions regarding the mechanisms underlying the protective effects remains unanswered: (1) Does extracellular NAD+ produce its effects through its degradation products such as adenosine and nicotinamide? (2) Does extracellular NAD+ produce its protective effects by affecting cells under pathological insults only, or by affecting both normal cells and the cells under pathological insults?
Some previous findings have provided answers to the first question: intranasal administration of NAD+, but not nicotinamide, can profoundly decrease ischemic brain injury (Ying et al., 2007) and traumatic brain injury (Won et al., 2012), which argues against the possibility that extracellular NAD+ produces its protective effects via nicotinamide – one of its degradation products. However, extracellular NAD+ can also be degraded into adenosine by multiple potential mechanisms (Moreschi et al., 2006; Klein et al., 2009; Okuda et al., 2010; Adriouch et al., 2012). While it has been reported that the radiolabeled adenosine moiety was observed in astrocytes within 10 min of treatment of the cells with radiolabeled NAD+ (Okuda et al., 2010), there has been no report that provides answers to the following question: does extracellular NAD+ produce its effects through adenosine? Since, it is established that adenosine can produce beneficial effects under multiple pathological conditions (Ely and Berne, 1992; Zhang et al., 1993; Yap and Lee, 2012), in our current study we used BV2 microglia as a cellular model to test our hypothesis that extracellular NAD+ produces its biological effects on cells through its extracellular degradation into adenosine.
Regarding the second key question, there are studies suggesting that extracellular NAD+ produces its protective effects at least partially by directly entering cells to decrease the damage of the cells under pathological insults: first, because such insults as oxidative stress can lead to rapid decreases in the cytosolic NAD+ concentrations by activating PARP-1 (Ying et al., 2003; Alano et al., 2004, 2010; Pillai et al., 2005), it is reasonable to propose that extracellular NAD+ may enter cells via P2X7 receptors (Ying et al., 2003; Alano et al., 2004, 2010; Pillai et al., 2005) or Connexin 43 hemichannels (Bruzzone et al., 2001) by gradient-dependent mechanisms; second, NAD+ administration can significantly enhance the NAD+ levels of the tissues under various pathological insults (Ying, 2008; Imai and Guarente, 2014; Verdin, 2015; Zhang and Ying, 2018); and third, numerous studies have reported that NAD+ treatment can decrease the death of various types of cells exposed to oxidative stress, DNA alkylating agents, or excitotoxins (Ying et al., 2003; Alano et al., 2004, 2010; Pillai et al., 2005). The major mechanisms underlying these beneficial effects include improvement of glycolysis by restoring the activity of the NAD+-dependent enzyme glyceraldehyde-3-phosphate dehydrogenase (GAPDH), prevention of mitochondrial depolarization and mitochondrial permeability (MPT), activation of SIRT1 and SIRT3, and promotion of DNA repair (Ying et al., 2003; Alano et al., 2004, 2010; Pillai et al., 2005; Ying, 2008; Zhang and Ying, 2018).
However, there has been little information suggesting that the NAD+ administration may also decrease tissue damage by enhancing the defensive potential of normal cells that have not been attacked at the time of exposures to the administered NAD+. Since the intracellular NAD+ concentrations in normal cells range from 1 to 10 mM (Ying, 2008), it is unlikely that the NAD+ administration, usually at the doses between 10 mg and 20 mg/kg, may produce NAD+ concentrations in the blood which surpasses 1 mM. Therefore, it is unlikely that extracellular NAD+ may directly enter normal cells to produce its effects in these models. However, since endogenous adenosine levels are in the nanomolar range under physiological conditions (Latini and Pedata, 2001), it is possible that the adenosine that is generated from extracellular NAD+ degradation may enter cells under basal conditions through equilibrative nucleoside transporters (ENTs) to produce various biological effects.
There have been few studies regarding the effects of NAD+ treatment on cellular properties under basal conditions. Our recent study has shown that NAD+ treatment can induce significant increases in the intracellular ATP levels of BV2 microglia under basal conditions, while the mechanisms underlying this effect of NAD+ require future studies (Zhang et al., 2018). Because intracellular ADP and AMP are closely related with intracellular ATP (Stryer, 1995), we propose that NAD+ treatment can significantly increase the intracellular adenylate pool of BV2 microglia under basal conditions.
Increasing evidence has indicated that inflammation plays critical pathological roles in multiple neurodegenerative disorders (Frank-Cannon et al., 2009; Hirsch and Hunot, 2009). Microglial activation is a key event of neuroinflammation in neurodegenerative diseases, which can generate oxidative stress and cytokines thus impairing neuronal survival (Frank-Cannon et al., 2009; Hirsch and Hunot, 2009). Elucidation of the mechanisms underlying the regulation of functions and survival of both activated microglia and microglia under resting conditions is of critical significance for understanding the roles of microglia in neurodegenerative disorders and brain aging. Previous studies have indicated that ATP plays critical roles in both microglia under resting conditions and activated microglia: basal ATP release is necessary for its survival in microglia; and extracellular ATP induces migration, chemotaxis, and ramification of microglia (Honda et al., 2001; Wollmer et al., 2001; Duan et al., 2009; Ma et al., 2014). Therefore, studies on the effects of NAD+ treatment on the intracellular adenylate levels of microglia may significantly enhance our understanding on the mechanisms underlying the effects of NAD+ treatment in various models of neurological diseases and aging.
In this study we used BV2 microglia as a cellular model to test our hypothesis that extracellular NAD+ degradation into adenosine mediates the effects of NAD+ treatment on cells under basal conditions. Our study has provided multiple lines of evidence suggesting that extracellular NAD+ produces increased adenylate pool of BV2 microglia under basal conditions by such key factors as adenosine kinase, AMPK, and extracellular NAD+ degradation into adenosine.
Materials and Methods
Reagents
NADH (N4505), NAD+ (N0632), dorsomorhpin (P5499), Adenosine 5′-monophosphate (AMP, 01930), pyridoxyl phosphate-6-azophenyl-2′,4′-disulfonic acid (PPADS, P178), 5-Iodotubercidin (I-100) and dipyridamole (DPR, D9766) were purchased from Sigma Aldrich (St. Louis, MO, United States). AMPK siRNAs and control siRNA were purchased from GenePharma (Shanghai, China).
Cell Culture
BV2 microglia was obtained from Institute of Neurology, Ruijin Hospital (Shanghai, China). The cells were plated into 24-well culture plates at the initial density of 6 × 105 cells/mL in Dulbecco’s modified Eagle medium (HyClone, Logan, UT, United States) containing 5% fetal bovine serum (Gibco, Carlsbad, CA, United States), 100 units/ml penicillin and 100 μg/ml streptomycin. The cells were maintained at 37°C in 5% CO2 incubator.
RNA Interference
BV2 microglia were transfected with either AMPKα2 siRNA oligonucleotides (5′ GAGAAGCAGAAGCACGACGTT 3′) or control siRNA oligonucleotides (5′ UUCUCCGAACGUGUCACGUTT 3′) (GenePharma, Shanghai, China), when the cells were approximately 50% confluent. Lipofectamine 2000 (Invitrogen, Carlsbad, CA, United States) was used for the transfection according to the manufacturer’s instructions. For each well of a 24-well plate, 100 μl Opti-MEM containing 0.06 nmol of the siRNA oligonucleotides and 2 μl lipofectamine 2000 was added into 500 μl culture media of the cells. The AMPK protein level was determined by Western Blot 18 h after the transfection.
Western Blot Assays
BV2 microglia was washed by cold PBS and lysed in RIPA buffer (Millipore, Temecula, CA, United States) containing Complete Protease Inhibitor Cocktail (Roche Diagnostics, Mannheim, Germany) and 1 mM PMSF. BCA Protein Assay Kit (Pierce Biotechonology, Rockford, IL, United States) was used to determine the concentrations of the protein samples. Thirty μg of total protein was electrophoresed through a 10% SDS-PAGE gel, and then transferred to 0.45 μm nitrocellulose membranes. The membranes were incubated with 5% (v/v) BSA for 2 h. The blots were incubated overnight at 4°C with rabbit-derived anti-AMPKα antibody (2532, Cell Signaling Technology, Danvers, MA, United States), anti-phospho-AMPKα (Thr172) antibody (2535, Cell Signaling Technology), anti-phospho-Acetyl-CoA Carboxylase (Ser79) antibody (11818, Cell Signaling Technology), anti-acetyl-CoA Carboxylase antibody (3676, Cell Signaling Technology) or goat-derived anti-actin antibody (sc58673, Santa Cruz Biotechnology, Santa Cruz, CA, United States), then incubated with HRP-conjugated secondary antibody (Epitomics, Hangzhou, China). Protein signals were visualized using the ECL detection reagent (Pierce Biotechnology, Rockford, IL, United States). The intensities of the bands were analyzed by densitometry using computer-based Image-Pro Plus program.
Assays of ATP, ADP, and AMP
Adenine nucleotides were determined by a luciferin-luciferase assay adapted from Spielmann et al. (1981). In brief, cells in 24-well plates were harvested with 200 μL Tris-EDTA buffer (0.1 M Tris-HCl, pH-7.75, and 1 mM EDTA) and heated at 95°C for 5 min. For the determination of ATP, 25 μL samples were mixed with 25 μL PEP buffer (5 mM PEP, 9 mM MgCl2, 5 mM KCl in 0.1 M Tris-EDTA buffer, PH 7.75). For the determination of ADP + ATP, 25 μL samples were mixed with 25 μL PEP buffer containing pyruvate kinase (100 units/ml). For the determination of AMP + ADP + ATP, 25 μL samples were mixed with 25 μL PEP buffer (5 mM PEP, 9 mM MgCl2, 5 mM KCl in 0.1 M Tris-EDTA buffer, PH 7.75) containing pyruvate kinase (100 units/ml) and myokinase (200 units/ml). The sample and PEP-buffer were mixed and incubated for 30 min at room temperature. Then 50 μL of luciferase reagent (Roche Applied Science, Mannheim, Germany) were added and the luminescence was measured by a plate reader (Synergy 2; Biotek, Winooski, VT, United States). The amount of ADP was obtained by subtracting the ATP value from the (ATP + ADP) value, and the amount of AMP was calculated from the difference between (ATP + ADP) content and the (ATP + AMP + ADP) content. All samples were normalized to the protein concentrations.
Adenosine Assay
The adenosine level was measured by adenosine assay kit (Biovision, Milpitas, CA, United States) according to the manufacturer’s protocol. Cells were collected, resuspended in PBS buffer and went through three freeze-thaw cycles. After centrifugation at 3000 RPM for 20 min at 4°C, the supernatant was collected for use. Supernatants were mixed with Reaction Mix and incubated for 20 min and the fluorescent intensity was detected at Ex/Em = 535/587 nm. To determine the extracellular adenosine, the extracellular media was diluted mixed with the Reaction Mix and incubated for 20 min and the fluorescence was detected at Ex/Em = 535/587 nm.
NAD+ Assay
NAD+ concentrations were measured by recycling assay as described previously (Ying et al., 2003). Briefly, cells were washed with cold PBS for once and extracted in 0.5N cold perchloric acid. After centrifugation at 12,000 RPM for 5 min at 4°C, the supernatant was neutralized to pH 7.2 using 3N KOH and 1 M potassium phosphate buffer. After centrifugation at 12,000 RPM for 5 min at 4°C, 50 μl supernatant was mixed with 100 μl reaction medium containing 0.0425 mg/ml 3-[4,5-dimethylthiazol-2-yl]-2, 5-diphenyl-tetrazolium bromide (MTT), 0.0296 mg/ml phenazine methosulfate, 0.0325 mg/ml alcohol dehydrogenase, 12.21 mg/ml nicotinamide, and 6% (v/v) ethanol in Gly–Gly buffer (65 mM, pH 7.4). After 10 min, the optical absorbance of the samples at 556 nm was measured with a plate reader.
Mitochondrial Membrane Potential Assay
Mitochondrial membrane potential (Δψm) was determined by flow cytometry-based JC-1 (5,5′,6,6′-tetrachloro-1,1′,3,3′-tetraethyl-benzimidazolylcarbocyanine iodide) assay according to the manufacturer’s instruction. Cells were harvested by 0.25% trypsin-EDTA, and incubated in cell media containing 5 μg/mL JC-1 (Enzo Life Sciences, Farmingdale, NY, United States) for 15 min at 37°C. After washed once with PBS, samples was analyzed by a flow cytometer (FACSAria II, BD Biosciences, Franklin Lakes, NJ, United States) using the excitation wavelength of 488 nm and the emission wavelengths of 525 nm for green fluorescence, or the emission wavelengths of 575 nm for orange-red fluorescence. The Δψm of each cell was calculated by the ratio of red fluorescence intensity to green fluorescence intensity. For each sample, the ratio of the cells with low Δψm in total cell population was reported by the flow cytometer (FACSAria II, BD Biosciences, Franklin Lakes, NJ, United States).
Glucose Uptake Assay
2-NBDG (2-(N-(7-Nitrobenz-2-oxa-1,3-diazol-4-yl)Amino)-2-Deoxyglucose) is a fluorescent glucose analog that has been used to monitor glucose uptake in live cells. BV2 microglia were incubated in media containing 100 μM 2-NBDG (Invitrogen, Carlsbad, CA, United States) for 1 h in 37°C, then harvested by 0.25% trypsin-EDTA. After washed once with PBS, BV2 microglia was analyzed by a flow cytometer r (FACSAria II, BD Biosciences, Franklin Lakes, NJ, United States), which detected emission fluorescence at the wavelength of 518 nm (FITC-A) with excitation wavelength of 488 nm.
Statistical Analysis
Data were presented as mean ± SEM and analyzed by ANOVA followed by Student-Newman-Keuls post hoc test. P-values less than 0.05 were considered statistically significant.
Results
NAD+ Treatment Can Increase the Intracellular Levels of ATP, ADP, and AMP Without Altering the AMP/ADP and ADP/ATP Ratio of BV2 Microglia Under Basal Conditions
We determined the effects of NAD+ on the intracellular adenylate levels of BV2 microglia, showing that treatment of 10, 100, and 500 μM NAD+ can significantly increase the intracellular levels of ATP, ADP, and AMP of the cells (Figures 1A–C). NAD+ treatment can also increase the intracellular levels of NAD+ (Figure 1D). The time-course study regarding the effects of NAD+ treatment on ATP, ADP, and AMP levels showed that 500 μM NAD+ is able to increase intracellular ATP, ADP, and AMP after 10 min of the NAD+ treatment (Figures 1E–G). We also determined the effect of NAD+ treatment on both the ADP/ATP ratio and AMP/ATP ratio, showing that the NAD+ treatment was incapable of altering the ratios of ADP/ATP and AMP/ATP (Figures 1H,I).
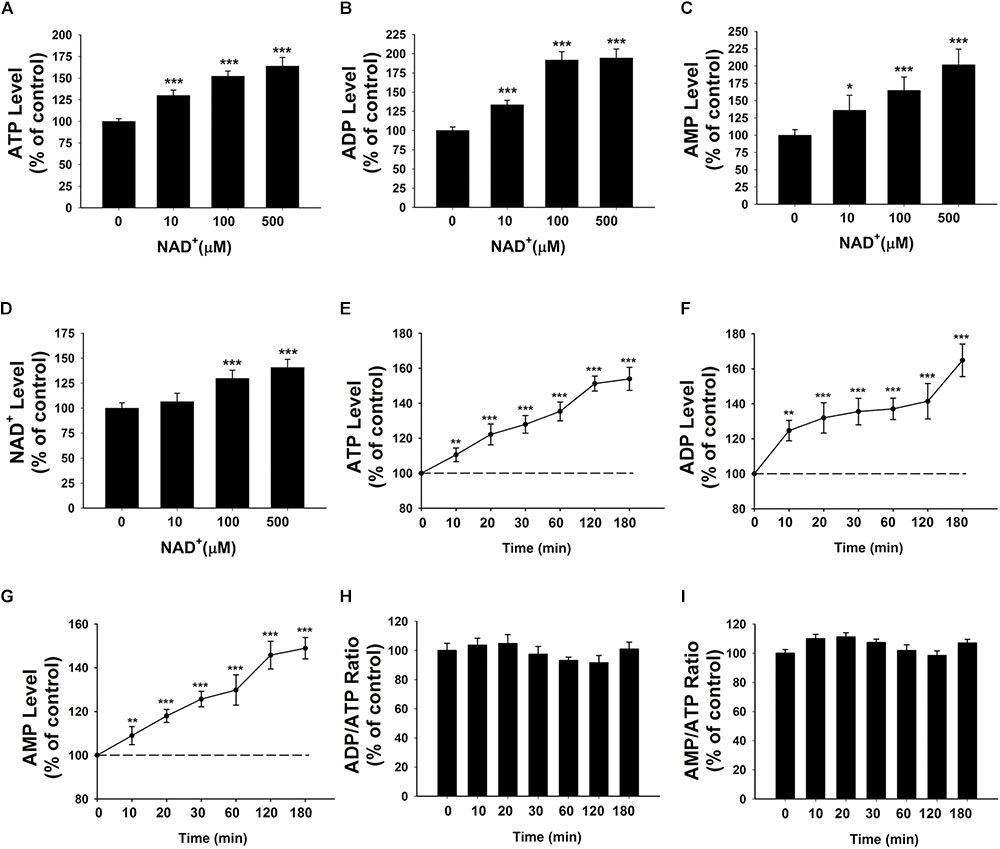
FIGURE 1. Effects of NAD+ treatment on the intracellular levels of ATP, ADP, and AMP of BV2 microglia under basal conditions. (A) NAD+ treatment significantly increased the intracellular ATP level of the cells. (B) NAD+ treatment significantly increased the intracellular ADP level of the cells. (C) NAD+ treatment significantly increased the intracellular AMP level of the cells. (D) NAD+ treatment significantly increased the intracellular NAD+ level of the cells. (E) NAD+ (500 μM) time-dependently increased the intracellular ATP level of the cells. (F) NAD+ (500 μM) time-dependently increased the intracellular ADP level of the cells. (G) NAD+ (500 μM) time-dependently increased the intracellular AMP level of the cells. (H) NAD+ (500 μM) treatment did not alter the ADP/ATP ratio. (I) NAD+ (500 μM) did not alter the AMP/ATP ratio. For (A–C), BV2 cells were treated with NAD+ for 3 h before the assays were conducted. N = 16. The data were pooled from four independent experiments. ∗P < 0.05; ∗∗P < 0.01; ∗∗∗P < 0.001.
Roles of Glucose Uptake, Mitochondrial Membrane Potential and SIRT1 in the NAD+ Treatment-Induced Increases in the Adenylate Pool of BV2 Microglia Under Basal Conditions
Glycolysis and mitochondrial oxidative phosphorylation (OXPHOS) are major pathways for ATP production, in which NAD+ plays significant roles (Stryer, 1995). Previous studies have suggested that NAD+ treatment decreases cell death induced by oxidative stress, alkylating agents, and excitotoxins by such mechanisms as improving glycolysis, preventing mitochondrial depolarization, and activating SIRT1 (Ying et al., 2003; Alano et al., 2004, 2010; Pillai et al., 2005; Ying, 2008; Zhang and Ying, 2018). In order to determine if improving glycolysis and preventing mitochondrial depolarization are also major mechanisms underlying the NAD+ treatment-produced increases in the adenylate pool of BV2 microglia under basal conditions, we determined the effects of NAD+ treatment on the glucose uptake and mitochondrial membrane potential of the cells under basal conditions: NAD+ treatment did not significantly affect the glucose uptake (Supplementary Figure S1A) or the mitochondrial membrane potential (Supplementary Figures S1B,C) of the cells under basal conditions. We further found that the SIRT1 inhibitor EX527 was incapable of affecting the NAD+-induced increases in the intracellular ATP levels of the cells (Supplementary Figure S2), thus arguing against the possibility that SIRT1 mediates the NAD+ treatment-induced increases in the adenylate pool in our study.
Contribution of Adenosine Transport to the NAD+-Induced Increases in the Intracellular Adenylate Levels of BV2 Microglia Under Basal Conditions
It has been reported that extracellular NAD+ can be degraded into adenosine and nicotinamide (Okuda et al., 2010). Therefore, we determined the extracellular adenosine level after the NAD+ treatment. We found that treatment of 10, 100, or 500 μM NAD+ significantly increased the extracellular levels of adenosine (Figure 2A). Our time-course study showed that extracellular adenosine levels increased by threefold after only 10 min of the NAD+ treatment (Figure 2B).
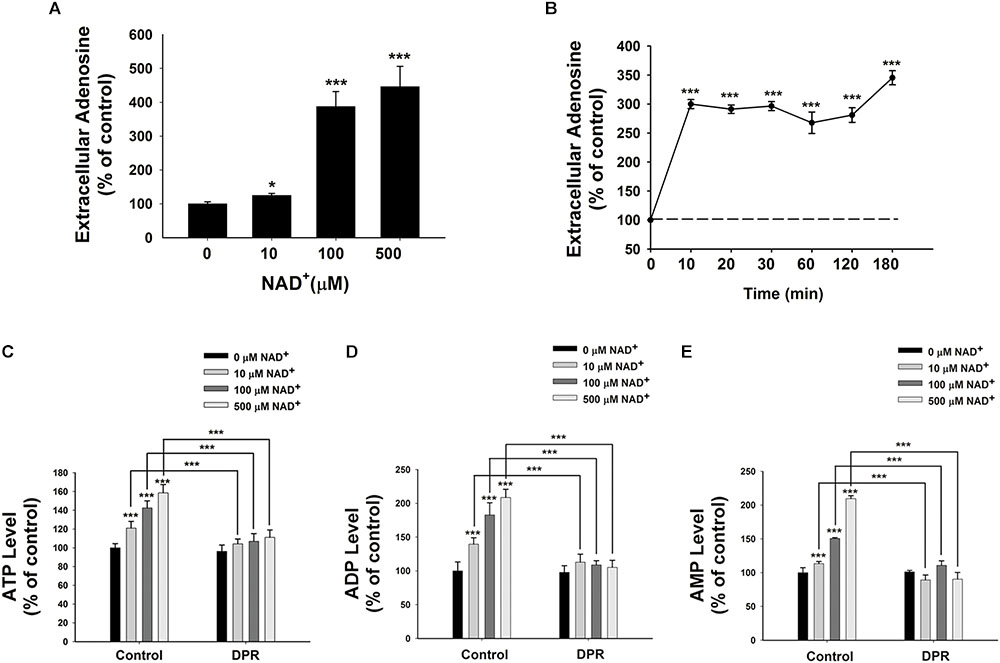
FIGURE 2. Adenosine uptake plays a key role in the NAD+-induced increases in the intracellular levels of ATP, ADP, and AMP of BV2 microglia under basal conditions. (A) NAD+ dose-dependently increased the extracellular adenosine level of BV2 cells. The cells were treated with NAD+ for 3 h. (B) Time course of 500 μM NAD+-induced increases in the extracellular adenosine levels of BV2 cells. (C) Treatment of the cells with 0.5 μM DPR completely blocked the NAD+-induced increases in the intracellular ATP levels of the cells. (D) Treatment of the cells with 0.5 μM DPR completely blocked the NAD+-induced increases in the intracellular ADP levels of the cells. (E) Treatment of the cells with 0.5 μM DPR completely blocked the NAD+-induced increases in the intracellular AMP levels of the cells. The cells were co-treated with NAD+ and DPR for 3 h. Subsequently, the assays on intracellular ATP, ADP, and AMP levels were conducted. N = 16. The data were pooled from four independent experiments. ∗P < 0.05; ∗∗P < 0.01; ∗∗∗P < 0.001.
Adenosine can be transported by ENTs into cells (Latini and Pedata, 2001), which may be used for synthesis of AMP, ADP, and ATP (Rapaport and Zamecnik, 1978). Due to the relatively high activity of intracellular adenosine kinase and the normally low intracellular adenosine levels, the net flux through ENTs is inwardly directed under normal conditions (Latini and Pedata, 2001). To test our hypothesis that extracellular NAD+ may lead to increased intracellular adenylate pool by its extracellular degradation into adenosine that is subsequently transported into cells, we determined the effects of dipyridamole (DPR), an inhibitor of ENTs (Wang et al., 2013), on the NAD+-induced increases in the intracellular ATP levels. We found that DPR blocked the NAD+-induced increases in the intracellular levels of ATP, ADP, and AMP (Figures 2C–E).
To exclude the possibility that NAD+ produced the effects on the adenylate pool by producing nicotinamide – another degradation product of NAD+, we determined if nicotinamide may affect the intracellular ATP levels. Neither 10 nor 100 μM nicotinamide affected the intracellular ATP levels, while 500 μM nicotinamide increased the intracellular ATP levels by merely 10% (Supplementary Figure S3). These observations argued against the possibility that the nicotinamide moiety is responsible for the NAD+-induced increases in the adenylate levels.
The next question to address is as to whether extracellular NAD+-induced increases in intracellular ATP is mediated by adenosine receptors. We determined if theophylline, a broad inhibitor of adenosine receptors may attenuate the NAD+-induced increases in intracellular ATP. We found that theophylline failed to block NAD+-induced increases in intracellular ATP level (Supplementary Figure S4). Combined with the fact that ENTs inhibitor completely blocked NAD+-induced increases in intracellular ATP level, these results ruled out the possibility for the implication of adenosine receptors in extracellular NAD+-induced increases in intracellular ATP level.
Extracellular Adenosine Is Capable of Increasing the Intracellular Adenylate Pool of BV2 Microglia Under Basal Conditions
To further test our proposal that the extracellular adenosine generated from extracellular NAD+ degradation can produce increased adenylate levels of BV2 microglia under basal conditions, we determined the effects of 10, 100, and 500 μM adenosine treatment on the intracellular ATP, ADP, and AMP levels of BV2 microglia under basal conditions. Our study showed that the adenosine at all of these concentrations was capable of significantly increasing the intracellular adenylate levels of the cells without changing the AMP/ATP ratio (Figures 3A–D).
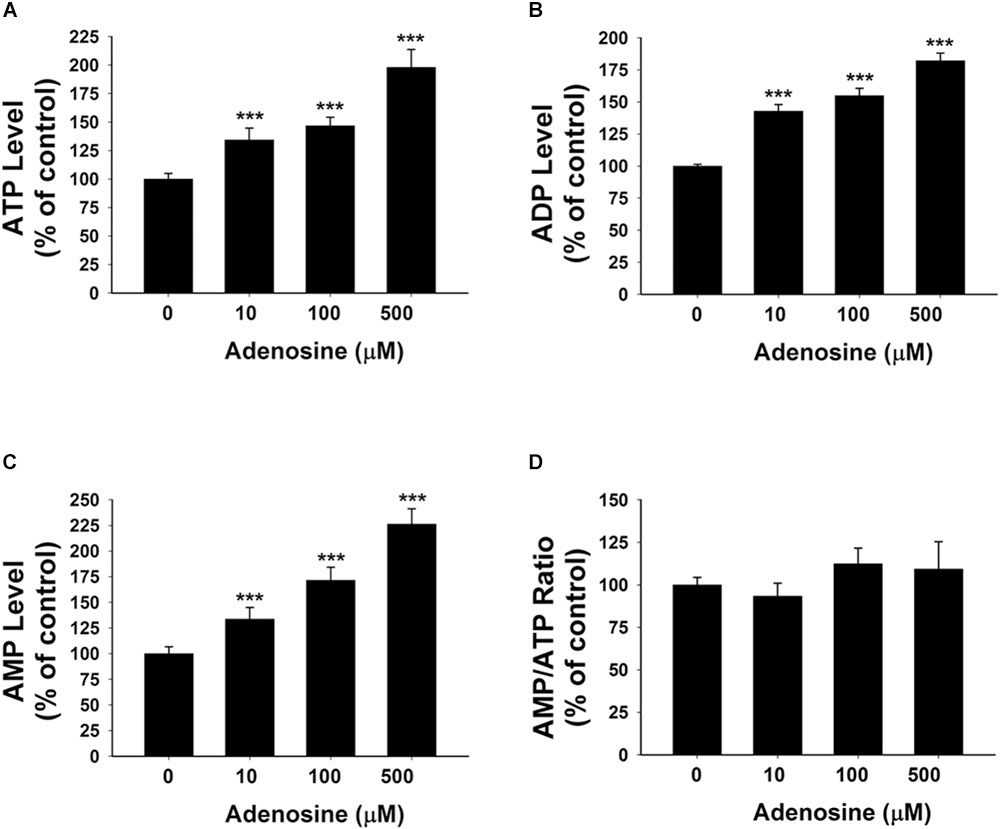
FIGURE 3. Adenosine treatment led to significant increases in the intracellular levels of ATP, ADP, and AMP of BV2 microglia under basal conditions. (A) Treatment of the cells with adenosine significantly increased the intracellular ATP levels of the cells. (B) Treatment of the cells with adenosine significantly increased the intracellular ADP levels of the cells. (C) Treatment of the cells with adenosine significantly increased the intracellular AMP levels of the cells. (D) Treatment of the cells with adenosine did not affect the AMP/ATP ratio. N = 16. The data were pooled from four independent experiments. ∗P < 0.05; ∗∗P < 0.01; ∗∗∗P < 0.001.
Roles of Adenosine Kinase in the NAD+-Induced Increases in the Adenylate Levels of BV2 Microglia Under Basal Conditions
Because endogenous adenosine levels are in the nanomolar range under normal physiological conditions (Latini and Pedata, 2001), it is possible that the concentrations of the adenosine generated from the degradation of 10–500 μM NAD+ in our study may surpass the intracellular adenosine concentrations, thus leading to entrance of the extracellular adenosine into the cells. We found that treatment of BV2 microglia with 10, 100, and 500 μM NAD+ significantly increased the intracellular adenosine levels (Figure 4A). Time-course study showed that NAD+ treatment time-dependently increased intracellular adenosine levels of BV2 cells (Figure 4B).
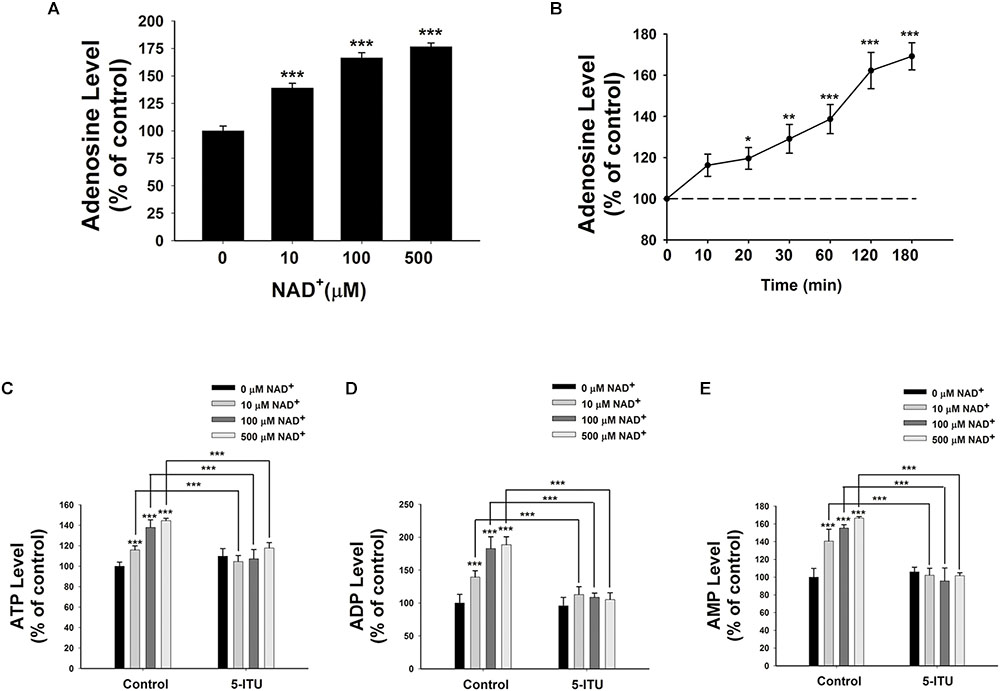
FIGURE 4. Adenosine kinase mediates the NAD+-induced increases in the intracellular levels of ATP, ADP, and AMP of BV2 microglia under basal conditions. (A) NAD+ treatment dose-dependently increased the intracellular adenosine level of the cells. (B) 500 μM NAD+ time-dependently increased the intracellular adenosine level of BV2 cells. (C) Treatment of the cells with 5 μM 5-ITU blocked the NAD+-induced increases in the intracellular ATP levels of the cells. (D) Treatment of the cells with 5 μM 5-ITU blocked the NAD+- induced increases in the intracellular ADP levels of the cells. (E) Treatment of the cells with 5 μM 5-ITU blocked the NAD+-induced increases in the intracellular AMP levels of the cells. The cells were co-treated with 5 μM 5-ITU and NAD+ for 3 h before the assays were conducted. N = 16. The data were pooled from four independent experiments. ∗∗∗P < 0.001.
It has been reported that adenosine can be converted to AMP, which is mediated by adenosine kinase (Miller et al., 1979), leading to AMPK activation (da Silva et al., 2006). Therefore, we applied the adenosine kinase inhibitor 5-Iodotubercidin (5-ITU) to test our hypothesis that the extracellular NAD+ can produce increased intracellular AMP, ADP, and ATP by increasing intracellular adenosine that is converted to AMP by adenosine kinase. We found that treatment of the cells with 5-ITU virtually completely blocked the NAD+-induced increases in the intracellular AMP, ADP, and ATP levels of BV2 microglia under basal conditions (Figures 4C–E).
Roles of AMPK in NAD+-Induced Increases in the Adenylate Levels of BV2 Microglia Under Basal Conditions
Since AMPK is a crucial regulator of energy metabolism, which can be regulated by such factors as AMP/ATP ratios (Hardie, 2015), we determined if AMPK is involved in the NAD+-induced increases in the intracellular ATP levels of BV2 cells by assessing the effects of NAD+ treatment on AMPK phosphorylation. Both 100 and 500 μM NAD+ led to increased AMPK phosphorylation at Thr172 (Figure 5A), which was associated with AMPK activation. Active AMPK phosphorylates its downstream targets, including acetyl-CoA Carboxylase (ACC). Our Western blot analysis showed that NAD+ treatment enhanced phosphorylation of ACC (Figure 5B), indicating that AMPK was activated by NAD+ treatment. Adenosine kinase inhibitor 5-ITU blocked NAD+-induced AMPK phosphorylation (Figure 5C). Moreover, the NAD+ treatment did not affect the AMP/ATP ratios (Figures 1I, 5D), thus arguing against the possibility that the NAD+ treatment led to increased AMPK activity by increasing AMP/ATP ratios.
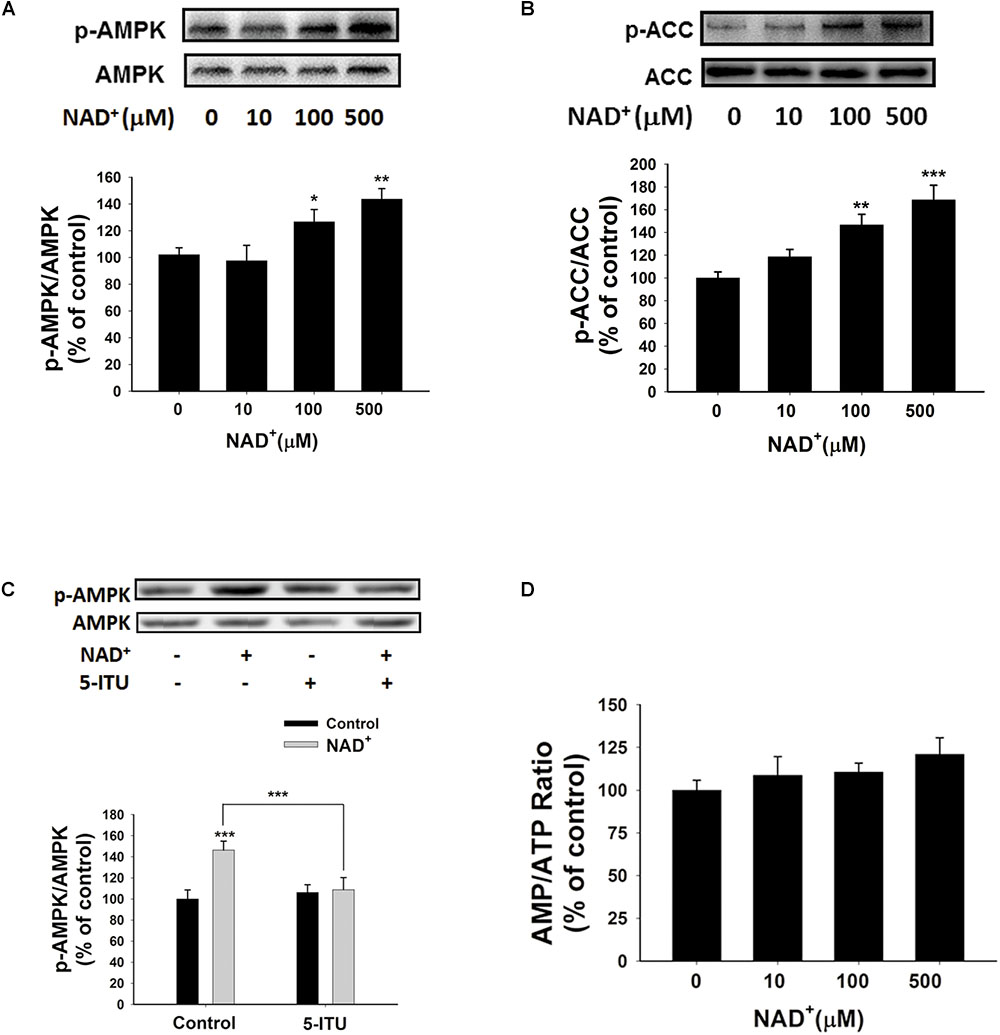
FIGURE 5. NAD+ treatment increased AMPK phosphorylation of BV2 microglia under basal conditions. (A) Both 100 and 500 μM NAD+ increased AMPK phosphorylation. (B) NAD+ treatment increased ACC phosphorylation. (C) 5 μM 5-ITU blocked the NAD+-induced AMPK phosphorylation in BV2 microglia. (D) NAD+ treatment did not affect the AMP/ATP ratios of the cells. The cells were treated with NAD+ for 0.5 h. N = 12. The data were pooled from four independent experiments. ∗P < 0.05; ∗∗P < 0.01; ∗∗∗P < 0.001.
We further determined the roles of AMPK in the NAD+-induced increases in the intracellular ATP levels, showing that the AMPK inhibitor dorsomorphin blocked the NAD+-induced increases in the ATP levels (Figure 6A). Moreover, treatment of the cells with AMPK siRNA, which led to a significant decrease in the AMPK levels of the cells (Figure 6B), also produced significant attenuation of the NAD+-induced increases in the intracellular ATP levels (Figure 6C).
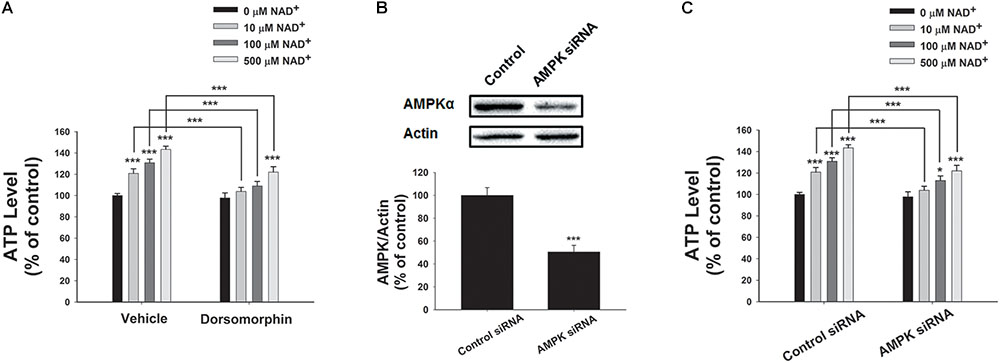
FIGURE 6. AMPK mediated the NAD+-induced increases in the intracellular ATP levels of BV2 microglia under basal conditions. (A) Dorsomorphin, an AMPK inhibitor, prevented NAD+- induced ATP increases in BV2 cells. The cells were co-treated with 15 μM dorsomorphin and NAD+ for 3 h before the ATP assay were conducted. (B) AMPK siRNA treatment led to a significant decrease in the AMPK protein level of BV2 cells. (C) AMPK siRNA significantly attenuated the NAD+-induced ATP increases of BV2 cells. The cells were pretreated with AMPK siRNA for 24 h. Subsequently, the cells were treated with NAD+ for 3 h. N = 12. The data were pooled from four independent experiments. ∗P < 0.05; ∗∗P < 0.01; ∗∗∗P < 0.001.
AMP Treatment Can Increase Both the Intracellular Adenylate Levels and the AMPK Activity of BV2 Microglia Under Basal Conditions
Our study has indicated the extracellular NAD+ can increase the intracellular AMP levels by enhancing the levels of intracellular adenosine that can be converted by adenosine kinase. We propose that the increased AMP may lead to increased levels of ATP and ADP as well as increased AMPK activity. To test the validity of this proposal, we determined the effects of AMP treatment, that has been reported to increase intracellular AMP levels (Brenner and Gorin, 1978), on the intracellular levels of ATP and ADP as well as the AMPK activity of BV2 microglia under basal conditions. Our study showed that AMP treatment led to a significant increase in the intracellular AMP levels of the cells (Figure 7A). The AMP treatment also produced significant increases in the intracellular levels of ATP and ADP (Figures 7B,C). Moreover, the AMP treatment significantly increased AMPK phosphorylation and ACC phosphorylation without affecting the intracellular AMP/ATP ratios of the cells (Figures 7D–F).
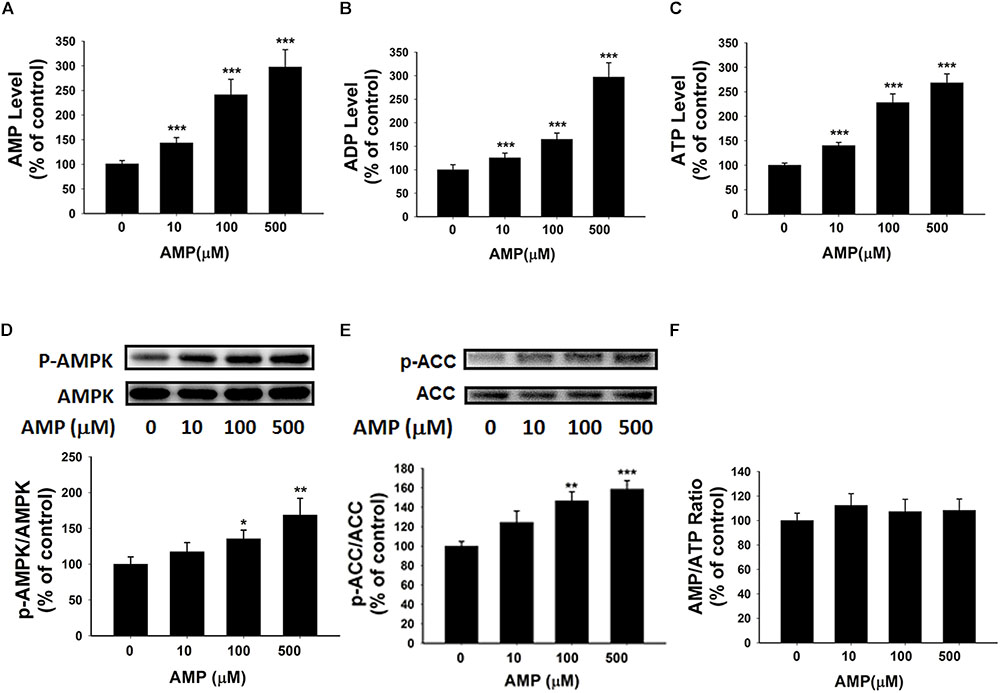
FIGURE 7. AMP treatment significantly increased the intracellular levels of ATP, ADP, and AMP of BV2 microglia under basal conditions. (A) AMP treatment significantly increased the intracellular ATP level of the cells. (B) AMP treatment significantly increased the intracellular ADP level of the cells. (C) AMP treatment significantly increased the intracellular AMP level of the cells. (D) AMP treatment dose-dependently increased the AMPK phosphorylation of the cells. (E) AMP treatment significantly increased ACC phosphorylation. (F) AMP treatment did not influence the intracellular AMP/ATP ratios. The cells were treated with AMP for 3 h. Subsequently, the assays were conducted. N = 16. The data were pooled from four independent experiments. ∗P < 0.05; ∗∗P < 0.01; ∗∗∗P < 0.001.
NAD+ Treatment Reduced Hydrogen Peroxide-Induced Cytotoxicity in BV2 Cells
To investigate whether NAD+ treatment influence cells’ defensive potential against pathological insults, we treated the cells with hydrogen peroxide. We found that NAD+ treatment blocked hydrogen peroxide-induced decrease in cell survival and mitochondrial membrane potential (Supplementary Figure S5). These results, together with previous studies about the protective effects of NAD+ treatment (Liu et al., 2014; Caito and Aschner, 2016), highlights the nutritional and therapeutic potential of NAD+.
Discussion
The major findings of our current study includes: first, NAD+ treatment can significantly increase the intracellular levels of ATP, ADP, and AMP of BV2 microglia under basal conditions. Second, our study has provided evidence arguing against the possibility that the NAD+ treatment increased the intracellular adenylate pool by affecting SIRT1, glycolytic rate or mitochondrial membrane potential of BV2 microglia under basal conditions. Third, NAD+ treatment can significantly increase the extracellular adenosine levels, while blockage of adenosine uptake by ENTs inhibitor can prevent the NAD+-induced increases in the intracellular adenylate pool of the cells. Fourth, NAD+ treatment can significantly increase the intracellular adenosine levels of the cells. Fifth, extracellular adenosine can increase intracellular adenylate levels of the cells. Sixth, adenosine kinase mediates the effects of NAD+ on the intracellular adenylate pool of the cells. Seventh, AMPK also mediates the NAD+-induced increases in the intracellular ATP.
Previous studies have indicated that extracellular NAD+ significantly decreases the damage of the cells exposed to various pathological insults by such mechanisms as improving glycolysis, preventing mitochondrial depolarization, and activating SIRT1 (Ying et al., 2003; Alano et al., 2004, 2010; Pillai et al., 2005; Ying, 2008; Zhang and Ying, 2018). However, our study has shown that the treatment of BV2 microglia with 0.01–0.5 μM NAD+ did not significantly increase the glycose uptake and mitochondrial membrane potential of BV2 microglia under basal conditions. Moreover, our study did not show that SIRT1 inhibition can affect the NAD+-produced increases in the intracellular adenylate pool of the cells under basal condition. Therefore, our study has indicated that the mechanisms underlying the NAD+ treatment-produced effects on the cells under pathological insults are not applicable to the cells under basal conditions. These observations are not surprising for the following reasons: the cytosolic NAD+ concentrations are normally in the ranges between 1 and 10 mM (Ying, 2008). Because NAD+ enters cells by gradient-driven transport (Alano et al., 2010), the NAD+ at the concentrations between 0.01 and 0.5 mM, which was used in our study, should not be able to enter the cells to influence the adenylate pools of BV2 microglia under basal conditions.
Our current study has indicated that extracellular NAD+ produces its biological effects on cells under basal conditions through mechanisms that are distinctly different from the mechanisms found in the cells exposed to various pathological insults. Our study has indicated that extracellular NAD+ can produce significant biological effects on BV2 microglia under basal conditions through the following mechanisms (Figure 8): extracellular NAD+ is degraded into adenosine extracellularly, that enters the cells through ENTs, which is converted to AMP by adenosine kinase. Increased AMP can lead to both increased AMPK activity and increased intracellular ADP levels, which jointly produce the increased intracellular ATP levels of BV2 cells under basal conditions.
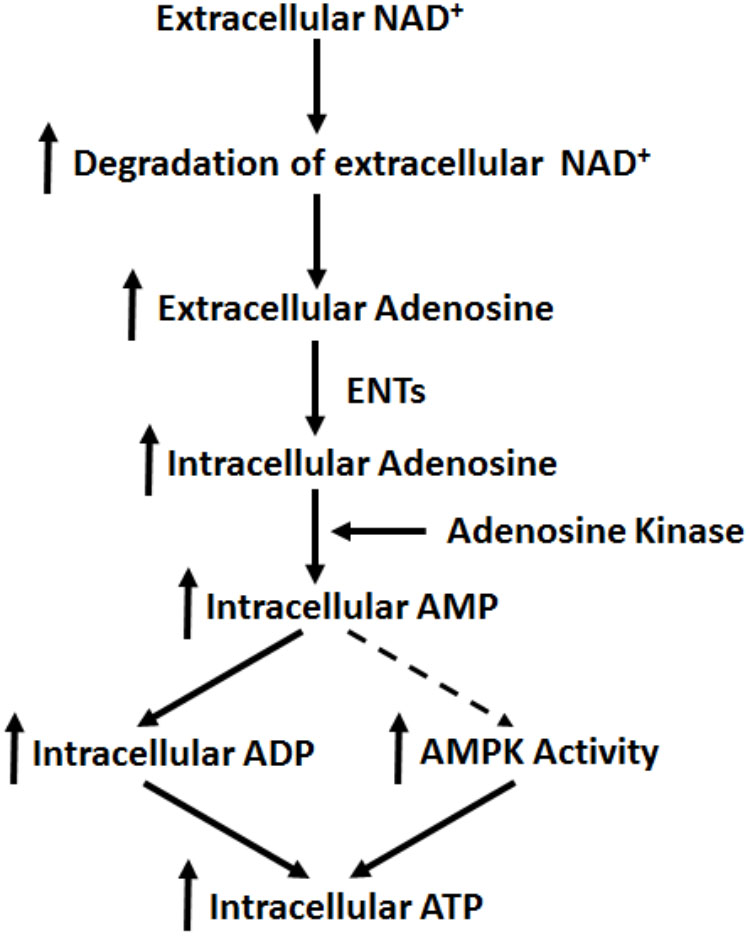
FIGURE 8. Diagrammatic presentation of the mechanisms underlying the effects of NAD+ on the intracellular adenylate pool of BV2 microglia under basal conditions.
Although the NAD+ at the concentrations between 0.01 and 0.5 mM cannot directly enter cells to increase intracellular NAD+ levels, we still found that the NAD+ can significantly increase the intracellular levels of NAD+ and adenylate of BV2 microglia through extracellular degradation into adenosine. Since intracellular adenosine levels are normally in the nanomolar range (Latini and Pedata, 2001), the extracellular NAD+-generated adenosine can enter cells to increase intracellular adenosine levels thus increasing the intracellular adenylate levels through the activities of adenosine kinase and AMPK. In other words, the exceedingly low intracellular adenosine levels under normal physiological conditions is an unique ‘attractor’ and base for the relatively low concentrations of extracellular NAD+ to produce its significant biological effects on cells. While a previous study reported that extracellular NAD+ can be degraded into adenosine that is transported into astrocytes through ENTs (Latini and Pedata, 2001), to our knowledge, our current study has provided the first direct evidence showing that extracellular adenosine generated from extracellular NAD+ degradation mediates the biological effects of exogenous NAD+ by adenosine kinase- and AMPK-mediated pathways. Our study also excluded the possibility that exogenous NAD+-induced increase in intracellular ATP level was mediated by adenosine receptors, since theophylline did not reverse the increase of intracellular ATP levels induced by NAD+ treatment.
Our study has shown that relatively low concentrations of NAD+ can increase both extracellular and intracellular adenosine levels, the AMPK activity and intracellular adenylate pools, all of these factors have been shown to enhance defensive potential of both normal cells and stressed cells against toxic insults: AMPK activation has been reported to produce multiple beneficial effects for both normal and stressed cells and tissues, for examples, AMPK activation can expand the lifespan in multiple model organisms (Apfeld et al., 2004; Stenesen et al., 2013); and AICAR, an AMPK activator, protects the liver from fatty changes associated with chronic alcohol use in rats (Tomita et al., 2005); adenosine can produce beneficial effects under multiple pathological conditions, including myocardial ischemia, seizure, and acute kidney injury (Ely and Berne, 1992; Zhang et al., 1993; Yap and Lee, 2012); an increase in the adenylate pool and absolute ATP concentrations could enhance the potential of cells to fulfill useful work (Ataullakhanov and Vitvitsky, 2002), and an intracellular ATP level is a switch for the decision between apoptosis and necrosis (Leist et al., 1997). Our current study has also shown that NAD+ administration enhance the defensive potential of BV2 microglia to oxidative stress (Supplementary Figure S5). Collectively, our current study has suggested a novel mechanism to account for the profound protective effects of NAD+ administration in the animal models of a number of diseases and aging (Liu et al., 2014; Scheibye-Knudsen et al., 2014; Fang et al., 2016): in addition to its reported capacity to enhance the capacity of the cells under pathological insults to defend against the cell death-inducing insults, the extracellular NAD+ may also increase the defensive potential of the normal cells that have not been attacked yet at the time of the exposures to exogenous NAD+ by increasing both extracellular and intracellular adenosine levels, the activities of adenosine kinase and AMPK, and intracellular adenylate pools.
It is noteworthy that the intravenous NAD+ administration in all of the animal studies should lead to the NAD+ concentrations that are well below milimolar range (Ying et al., 2007; Wang et al., 2014; Zhang et al., 2016; Xie et al., 2017). Since the cytosolic concentration of NAD+ is normally in the range between 1 and 10 mM, it is usually assumed that the NAD+ administration-produced NAD+ concentrations in the blood should not be able to enter cells to produce biological effects. However, our study has shown that as low as 10 μM NAD+ can lead to significant increases in the ATP levels of all of the cell types we have studied on this topic, including BV2 microglia, PC12 cells, and C6 glioma cells (Zhang et al., 2018). Therefore, our findings have general value for understanding the mechanisms underlying the biological effects of NAD+ administration in models of diseases, aging or healthy controls: the relatively low concentrations of extracellular NAD+ can still produce its profound effects on cells through its extracellular degradation into adenosine, which may lead to increased intracellular levels of adenosine, AMP, ADP, and ATP on the basis of the activities of ENTs, adenosine kinase and AMPK.
ATP plays critical biological roles in microglia: basal ATP release is a critical biological signal of microglia; and extracellular ATP induces migration, chemotaxis, and ramification of microglia (Honda et al., 2001; Wollmer et al., 2001; Duan et al., 2009; Ma et al., 2014). Therefore, our findings regarding the capacity of NAD+ treatment to enhance the intracellular ATP levels of BV2 microglia under basal conditions also have the following implications: NAD+ administration could produce significant biological impact on microglial activities under both pathological conditions and normal physiological conditions.
Author Contributions
WY has provided general design of the project and general management of the project. He has also played a major role in revising the article. JZ played the major role in conducting the experiments, designing the details of experiments, and writing of the draft of the paper. CW has been involved in conducting some experiments of the study. HS has been involved in general design of the article and revision of the article. DW has been involved in the general design of the study and revisions of the paper.
Funding
This study was supported by Major Special Program Grant of Shanghai Municipality (Grant No. 2017SHZDZX01 to WY), Major Basic Research Grants from Science and Technology Commission of Shanghai Municipality (Grant Nos. 16JC1400500 and 16JC1400502 to WY), and National Natural Science Foundation of China (Grant No. 81271305 to WY).
Conflict of Interest Statement
The authors declare that the research was conducted in the absence of any commercial or financial relationships that could be construed as a potential conflict of interest.
Supplementary Material
The Supplementary Material for this article can be found online at: https://www.frontiersin.org/articles/10.3389/fncel.2018.00343/full#supplementary-material
FIGURE S1 | NAD+ treatment did not significantly affect the glycolytic rate or mitochondrial membrane potential of BV2 microglia under basal conditions. (A) 2-NBDG uptake assay did not show that the NAD+ treatment significantly affected the glycolytic rate of BV2 cells. (B) FACS-based JC-1 assay did not show that the NAD+ treatment affected the mitochondrial membrane potential of the cells. (C) Quantifications of the results from the FACS-based JC-1 assay did not show that the NAD+ treatment significantly affected the mitochondrial membrane potential of the cells. The cells were treated with NAD+ for 3 h. Subsequently, the assays were conducted. N = 12. The data were pooled from four independent experiments.
FIGURE S2 | SIRT1 was not involved in the NAD+-induced increases of intracellular ATP levels. EX527, a selective SIRT1 inhibitor, did not affect the AMPK phosphorylation induced by NAD+ of BV2 microglia under basal condition. The cells were co-treated with 500 μM NAD+ and 10 μM EX527 for 3 h. Subsequently, Western blot assays were conducted. N = 12. The data were pooled from three independent experiments. ∗P < 0.05; ∗∗P < 0.01; ∗∗∗P < 0.001.
FIGURE S3 | Nicotinamide treatment did not affect the intracellular ATP levels of BV2 microglia under basal condition. Treatment of the cells with 10 or 100 μM nicotinamide did not affect the intracellular ATP levels, while treatment of the cells with 500 μM nicotinamide slightly increased the intracellular ATP levels. The cells were treated with nicotinamide for 3 h. Subsequently, ATP assays were conducted. N = 12. The data were pooled from three independent experiments. ∗P < 0.05.
FIGURE S4 | No implication of adenosine receptors in extracellular NAD+-induced increases in intracellular ATP level. Cells were co-treated with 1 μM and 0.5 mM NAD+ for 3 h. ∗∗∗P < 0.001.
FIGURE S5 | NAD+ treatment reduced hydrogen peroxide-induced cytotoxicity in BV2 cells. (A) Intracellular LDH assay showed that NAD+ treatment reduced H2O2 induced decrease in cell survival. (B) Flow cytometer based JC-1 assay showed that NAD+ treatment attenuated 1 mM H2O2 induced decrease in mitochondrial membrane potential. Cells were pretreated with 0.5 mM NAD+ for 3 h and then treated with 1 mM H2O2 for 1 h. ∗∗P < 0.01; ∗∗∗P < 0.001.
References
Adriouch, S., Haag, F., Boyer, O., Seman, M., and Koch-Nolte, F. (2012). Extracellular NAD + a danger signal hindering regulatory T cells. Microbes Infect. 14, 1284–1292. doi: 10.1016/j.micinf.2012.05.011
Alano, C. C., Garnier, P., Ying, W., Higashi, Y., Kauppinen, T. M., and Swanson, R. A. (2010). NAD + depletion is necessary and sufficient for poly(ADP-ribose) polymerase-1-mediated neuronal death. J. Neurosci. 30, 2967–2978. doi: 10.1523/JNEUROSCI.5552-09.2010
Alano, C. C., Ying, W., and Swanson, R. A. (2004). Poly(ADP-ribose) polymerase-1-mediated cell death in astrocytes requires NAD + depletion and mitochondrial permeability transition. J. Biol. Chem. 279, 18895–18902. doi: 10.1074/jbc.M313329200
Apfeld, J., O’connor, G., Mcdonagh, T., Distefano, P. S., and Curtis, R. (2004). The AMP-activated protein kinase AAK-2 links energy levels and insulin-like signals to lifespan in C. elegans. Genes Dev. 18, 3004–3009. doi: 10.1101/gad.1255404
Ataullakhanov, F. I., and Vitvitsky, V. M. (2002). What determines the intracellular ATP concentration. Biosci. Rep. 22, 501–511. doi: 10.1023/A:1022069718709
Brenner, T., and Gorin, E. (1978). Metabolic fate of exogenous 5’-AMP, cyclic AMP, and cyclic GMP in rats. Biochem. Med. 20, 160–166. doi: 10.1016/0006-2944(78)90063-7
Bruzzone, S., Guida, L., Zocchi, E., Franco, L., and De Flora, A. (2001). Connexin 43 hemi channels mediate Ca2 + -regulated transmembrane NAD + fluxes in intact cells. FASEB J. 15, 10–12. doi: 10.1096/fj.00-0566fje
Caito, S. W., and Aschner, M. (2016). NAD + supplementation attenuates methylmercury dopaminergic and mitochondrial toxicity in Caenorhabditis elegans. Toxicol. Sci. 151, 139–149. doi: 10.1093/toxsci/kfw030
da Silva, C. G., Jarzyna, R., Specht, A., and Kaczmarek, E. (2006). Extracellular nucleotides and adenosine independently activate AMP-activated protein kinase in endothelial cells: involvement of P2 receptors and adenosine transporters. Circ. Res. 98, e39–e47.
Das, A., Huang, G. X., Bonkowski, M. S., Longchamp, A., Li, C., Schultz, M. B., et al. (2018). Impairment of an endothelial NAD + -H2S signaling network is a reversible cause of vascular aging. Cell 173:e20. doi: 10.1016/j.cell.2018.02.008
Duan, Y., Sahley, C. L., and Muller, K. J. (2009). ATP and NO dually control migration of microglia to nerve lesions. Dev. Neurobiol. 69, 60–72. doi: 10.1002/dneu.20689
Ely, S. W., and Berne, R. M. (1992). Protective effects of adenosine in myocardial ischemia. Circulation 85, 893–904. doi: 10.1161/01.CIR.85.3.893
Fang, E. F., Kassahun, H., Croteau, D. L., Scheibye-Knudsen, M., Marosi, K., Lu, H., et al. (2016). NAD + replenishment improves lifespan and healthspan in ataxia telangiectasia models via mitophagy and DNA repair. Cell Metab. 24, 566–581. doi: 10.1016/j.cmet.2016.09.004
Frank-Cannon, T. C., Alto, L. T., Mcalpine, F. E., and Tansey, M. G. (2009). Does neuroinflammation fan the flame in neurodegenerative diseases? Mol. Neurodegener. 4:47. doi: 10.1186/1750-1326-4-47
Hardie, D. G. (2015). AMPK: positive and negative regulation, and its role in whole-body energy homeostasis. Curr. Opin. Cell Biol. 33, 1–7. doi: 10.1016/j.ceb.2014.09.004
Hirsch, E. C., and Hunot, S. (2009). Neuroinflammation in Parkinson’s disease: a target for neuroprotection? Lancet Neurol. 8, 382–397. doi: 10.1016/S1474-4422(09)70062-6
Honda, S., Sasaki, Y., Ohsawa, K., Imai, Y., Nakamura, Y., Inoue, K., et al. (2001). Extracellular ATP or ADP induce chemotaxis of cultured microglia through Gi/o-coupled P2Y receptors. J. Neurosci. 21, 1975–1982. doi: 10.1523/JNEUROSCI.21-06-01975.2001
Imai, S., and Guarente, L. (2014). NAD + and sirtuins in aging and disease. Trends Cell Biol. 24, 464–471. doi: 10.1016/j.tcb.2014.04.002
Klein, C., Grahnert, A., Abdelrahman, A., Muller, C. E., and Hauschildt, S. (2009). Extracellular NAD + induces a rise in [Ca2 +]i in activated human monocytes via engagement of P2Y1 and P2Y11 receptors. Cell Calcium 46, 263–272. doi: 10.1016/j.ceca.2009.08.004
Latini, S., and Pedata, F. (2001). Adenosine in the central nervous system: release mechanisms and extracellular concentrations. J. Neurochem. 79, 463–484. doi: 10.1046/j.1471-4159.2001.00607.x
Leist, M., Single, B., Castoldi, A. F., Kuhnle, S., and Nicotera, P. (1997). Intracellular adenosine triphosphate (ATP) concentration: a switch in the decision between apoptosis and necrosis. J. Exp. Med. 185, 1481–1486. doi: 10.1084/jem.185.8.1481
Liu, L., Wang, P., Liu, X., He, D., Liang, C., and Yu, Y. (2014). Exogenous NAD + supplementation protects H9c2 cardiac myoblasts against hypoxia/reoxygenation injury via Sirt1-p53 pathway. Fundam. Clin. Pharmacol. 28, 180–189. doi: 10.1111/fcp.12016
Ma, Y., Cao, W., Wang, L., Jiang, J., Nie, H., Wang, B., et al. (2014). Basal CD38/cyclic ADP-ribose-dependent signaling mediates ATP release and survival of microglia by modulating connexin 43 hemichannels. Glia 62, 943–955. doi: 10.1002/glia.22651
Miller, R. L., Adamczyk, D. L., Miller, W. H., Koszalka, G. W., Rideout, J. L., Beacham, L. M., et al. (1979). Adenosine kinase from rabbit liver. II. Substrate and inhibitor specificity. J. Biol. Chem. 254, 2346–2352.
Mills, K. F., Yoshida, S., Stein, L. R., Grozio, A., Kubota, S., Sasaki, Y., et al. (2016). Long-term administration of nicotinamide mononucleotide mitigates age-associated physiological decline in mice. Cell Metab. 24, 795–806. doi: 10.1016/j.cmet.2016.09.013
Moreschi, I., Bruzzone, S., Nicholas, R. A., Fruscione, F., Sturla, L., Benvenuto, F., et al. (2006). Extracellular NAD + is an agonist of the human P2Y11 purinergic receptor in human granulocytes. J. Biol. Chem. 281, 31419–31429. doi: 10.1074/jbc.M606625200
Okuda, H., Higashi, Y., Nishida, K., Fujimoto, S., and Nagasawa, K. (2010). Contribution of P2X7 receptors to adenosine uptake by cultured mouse astrocytes. Glia 58, 1757–1765. doi: 10.1002/glia.21046
Pillai, J. B., Isbatan, A., Imai, S., and Gupta, M. P. (2005). Poly(ADP-ribose) polymerase-1-dependent cardiac myocyte cell death during heart failure is mediated by NAD + depletion and reduced Sir2alpha deacetylase activity. J. Biol. Chem. 280, 43121–43130. doi: 10.1074/jbc.M506162200
Rapaport, E., and Zamecnik, P. C. (1978). Increased incorporation of adenosine into adenine nucleotide pools in serum-deprived mammalian cells. Proc. Natl. Acad. Sci. U.S.A. 75, 1145–1147. doi: 10.1073/pnas.75.3.1145
Scheibye-Knudsen, M., Mitchell, S. J., Fang, E. F., Iyama, T., Ward, T., Wang, J., et al. (2014). A high-fat diet and NAD + activate Sirt1 to rescue premature aging in cockayne syndrome. Cell Metab. 20, 840–855. doi: 10.1016/j.cmet.2014.10.005
Spielmann, H., Jacob-Muller, U., and Schulz, P. (1981). Simple assay of 0.1-1.0 pmol of ATP, ADP, and AMP in single somatic cells using purified luciferin luciferase. Anal. Biochem. 113, 172–178. doi: 10.1016/0003-2697(81)90061-0
Stenesen, D., Suh, J. M., Seo, J., Yu, K., Lee, K. S., Kim, J. S., et al. (2013). Adenosine nucleotide biosynthesis and AMPK regulate adult life span and mediate the longevity benefit of caloric restriction in flies. Cell Metab. 17, 101–112. doi: 10.1016/j.cmet.2012.12.006
Tomita, K., Tamiya, G., Ando, S., Kitamura, N., Koizumi, H., Kato, S., et al. (2005). AICAR, an AMPK activator, has protective effects on alcohol-induced fatty liver in rats. Alcohol. Clin. Exp. Res. 29, 240S–245S. doi: 10.1097/01.alc.0000191126.11479.69
Verdin, E. (2015). NAD + in aging, metabolism, and neurodegeneration. Science 350, 1208–1213. doi: 10.1126/science.aac4854
Wang, B., Ma, Y., Kong, X., Ding, X., Gu, H., Chu, T., et al. (2014). NAD + administration decreases doxorubicin-induced liver damage of mice by enhancing antioxidation capacity and decreasing DNA damage. Chem. Biol. Interact. 212, 65–71. doi: 10.1016/j.cbi.2014.01.013
Wang, C., Lin, W., Playa, H., Sun, S., Cameron, K., and Buolamwini, J. K. (2013). Dipyridamole analogs as pharmacological inhibitors of equilibrative nucleoside transporters. Identification of novel potent and selective inhibitors of the adenosine transporter function of human equilibrative nucleoside transporter 4 (hENT4). Biochem. Pharmacol. 86, 1531–1540. doi: 10.1016/j.bcp.2013.08.063
Wollmer, M. A., Lucius, R., Wilms, H., Held-Feindt, J., Sievers, J., and Mentlein, R. (2001). ATP and adenosine induce ramification of microglia in vitro. J. Neuroimmunol. 115, 19–27. doi: 10.1016/S0165-5728(01)00257-0
Won, S. J., Choi, B. Y., Yoo, B. H., Sohn, M., Ying, W., Swanson, R. A., et al. (2012). Prevention of traumatic brain injury-induced neuron death by intranasal delivery of nicotinamide adenine dinucleotide. J. Neurotrauma 29, 1401–1409. doi: 10.1089/neu.2011.2228
Xie, L., Wang, Z., Li, C., Yang, K., and Liang, Y. (2017). Protective effect of nicotinamide adenine dinucleotide (NAD +) against spinal cord ischemia-reperfusion injury via reducing oxidative stress-induced neuronal apoptosis. J. Clin. Neurosci. 36, 114–119. doi: 10.1016/j.jocn.2016.10.038
Yap, S. C., and Lee, H. T. (2012). Adenosine and protection from acute kidney injury. Curr. Opin. Nephrol. Hypertens. 21, 24–32. doi: 10.1097/MNH.0b013e32834d2ec9
Ying, W. (2008). NAD + /NADH and NADP + /NADPH in cellular functions and cell death: regulation and biological consequences. Antioxid. Redox Signal. 10, 179–206. doi: 10.1089/ars.2007.1672
Ying, W., Garnier, P., and Swanson, R. A. (2003). NAD + repletion prevents PARP-1-induced glycolytic blockade and cell death in cultured mouse astrocytes. Biochem. Biophys. Res. Commun. 308, 809–813. doi: 10.1016/S0006-291X(03)01483-9
Ying, W., Wei, G., Wang, D., Wang, Q., Tang, X., Shi, J., et al. (2007). Intranasal administration with NAD + profoundly decreases brain injury in a rat model of transient focal ischemia. Front. Biosci. 12, 2728–2734. doi: 10.2741/2267
Yoshino, J., Mills, K. F., Yoon, M. J., and Imai, S. (2011). Nicotinamide mononucleotide, a key NAD + intermediate, treats the pathophysiology of diet- and age-induced diabetes in mice. Cell Metab. 14, 528–536. doi: 10.1016/j.cmet.2011.08.014
Zhang, G., Franklin, P. H., and Murray, T. F. (1993). Manipulation of endogenous adenosine in the rat prepiriform cortex modulates seizure susceptibility. J. Pharmacol. Exp. Ther. 264, 1415–1424.
Zhang, J., Wang, C. X., and Ying, W. H. (2018). SIRT2 and Akt mediate NAD + -induced and NADH-induced increases in the intracellular ATP levels of BV2 microglia under basal conditions. Neuroreport 29, 65–70. doi: 10.1097/WNR.0000000000000876
Zhang, M. C., and Ying, W. H. (2018). NAD + deficiency is a common central pathological factor of a number of diseases and aging: mechanisms and therapeutic implications. Antioxid. Redox Signal. doi: 10.1089/ars.2017.7445 [Epub ahead of print].
Keywords: NAD+, microglia, adenylate pool, adenosine, AMPK
Citation: Zhang J, Wang C, Shi H, Wu D and Ying W (2018) Extracellular Degradation Into Adenosine and the Activities of Adenosine Kinase and AMPK Mediate Extracellular NAD+-Produced Increases in the Adenylate Pool of BV2 Microglia Under Basal Conditions. Front. Cell. Neurosci. 12:343. doi: 10.3389/fncel.2018.00343
Received: 30 May 2018; Accepted: 18 September 2018;
Published: 18 October 2018.
Edited by:
Rocío Martínez De Pablos, Universidad de Sevilla, SpainReviewed by:
Jianbin Tong, Central South University, ChinaValerie Vingtdeux, Université de Lille, France
Copyright © 2018 Zhang, Wang, Shi, Wu and Ying. This is an open-access article distributed under the terms of the Creative Commons Attribution License (CC BY). The use, distribution or reproduction in other forums is permitted, provided the original author(s) and the copyright owner(s) are credited and that the original publication in this journal is cited, in accordance with accepted academic practice. No use, distribution or reproduction is permitted which does not comply with these terms.
*Correspondence: Weihai Ying, d2VpaGFpeUBzanR1LmVkdS5jbg==