- 1Center for Biomedical Technology, Universidad Politécnica de Madrid, Madrid, Spain
- 2Departamento de Ciencia de Materiales, Escuela Técnica Superior de Ingenieros de Caminos, Canales y Puertos, Universidad Politécnica de Madrid, Madrid, Spain
- 3Biomedical Research Networking Center in Bioengineering Biomaterials and Nanomedicine, Madrid, Spain
- 4Department of Translational Neuroscience, Instituto Cajal – Consejo Superior de Investigaciones Científicas, Madrid, Spain
- 5Neurocomputing and Neurorobotics Research Group, Faculty of Biology and Faculty of Optics, Universidad Complutense de Madrid, Madrid, Spain
- 6Neural Plasticity Research Group, Health Research Institute of the Hospital Clínico San Carlos, Madrid, Spain
- 7Departamento de Tecnología Fotónica y Bioingeniería, Escuela Técnica Superior de Ingenieros de Telecomunicación, Universidad Politécnica de Madrid, Madrid, Spain
The restitution of damaged circuitry and functional remodeling of peri-injured areas constitute two main mechanisms for sustaining recovery of the brain after stroke. In this study, a silk fibroin-based biomaterial efficiently supports the survival of intracerebrally implanted mesenchymal stem cells (mSCs) and increases functional outcomes over time in a model of cortical stroke that affects the forepaw sensory and motor representations. We show that the functional mechanisms underlying recovery are related to a substantial preservation of cortical tissue in the first days after mSCs-polymer implantation, followed by delayed cortical plasticity that involved a progressive functional disconnection between the forepaw sensory (FLs1) and caudal motor (cFLm1) representations and an emergent sensory activity in peri-lesional areas belonging to cFLm1. Our results provide evidence that mSCs integrated into silk fibroin hydrogels attenuate the cerebral damage after brain infarction inducing a delayed cortical plasticity in the peri-lesional tissue, this later a functional change described during spontaneous or training rehabilitation-induced recovery. This study shows that brain remapping and sustained recovery were experimentally favored using a stem cell-biomaterial-based approach.
Introduction
Stroke represents the leading cause of disability and a main reason for premature mortality worldwide (Benjamin et al., 2017). The early recognition of symptoms and the rapidity of medical intervention influence the clinical evolution of each patient. In ischemic stroke, the most frequent form of stroke, the intravenous injection of the tissue plasminogen activator (tPA) and surgical procedures such as endovascular thrombectomy are currently the main advanced treatments for the early reestablishment of blood flow in the occluded vessel (Saver et al., 2015). However, a minority of stroke patients can really get benefits from these treatments due to the narrow time window for administration after the onset of symptoms and the risks of complications such as intracranial hemorrhage, major systemic hemorrhage, and angioedema. Thus, most of the patients who do not receive acute reperfusion therapies show long-term disabilities. Unfortunately, we do not have therapies to target the subacute and chronic phases of ischemic stroke and efficiently repair the damaged brain promoting a satisfactory degree of functional recovery in most patients (George and Steinberg, 2015).
A well-established observation in different species, including humans, is that the brain reorganizes itself under physiological and pathological conditions (Merzenich et al., 1984; Jenkins et al., 1990; Panetsos et al., 1995; Nudo et al., 1996b; Elbert et al., 1997; Traversa et al., 1997; Jaillard et al., 2005; Schaechter et al., 2006; Brown et al., 2009). During normal brain development, the emerging circuitry is organized to represent sensory, motor and variably distributed cognitive abilities. However, the initially established topographic and functional representations are not static and change dynamically in size and location throughout adult life in response to sensory input, experience and learning (Jenkins et al., 1990; Elbert et al., 1995; Nudo et al., 1996a). Brain reorganization also occurs after central or periphery injury (Merzenich et al., 1984; Calford and Tweedale, 1988; Elbert et al., 1997; Simoes et al., 2012). Reciprocal GABA-mediated inhibitory signals between distinct representations (Jacobs and Donoghue, 1991; Jones, 1993) and the regular activity of sensory/motor fields together with the maintenance of axonal pathways (Graziano and Jones, 2009) have been proposed to contribute to the mechanism defining topographic areas and preventing the functional invasion between surrounding representations (Calford and Tweedale, 1988; Jacobs and Donoghue, 1991).
The general principles that orchestrate the nascent brain circuitry during development are believed to be similar to those forming the compensatory circuits underlying functional recovery after brain damage. Based on accumulating evidence, motor and sensory representations are modified in the affected and non-affected hemispheres after unilateral brain damage (Nudo et al., 1996b; Brown et al., 2009; Harrison et al., 2013; Tennant et al., 2015). An evolutionarily conserved program exists in mammals to sustain the reorganization of non-affected areas surrounding the damaged regions that then perform the specific functions that were lost and initially depended of the injured areas. However, under spontaneous recovery, brain remapping in the undamaged surrounding tissue does not always correlate with functional outcomes (Nudo and Milliken, 1996; Xerri et al., 1998; Schaechter et al., 2006; Nishibe et al., 2015). A direct link between the post-stroke cortical changes and the temporal pattern of functional recovery has not been directly obtained in the majority of the studies, making a cause-effect relationship difficult to establish.
Stem cell therapy constitutes a promising approach to stimulate functional recovery after stroke (Chen et al., 2001a,b; Trounson and McDonald, 2015; Wang et al., 2016). Different types of stem cells have been used as a potential source of both replacement cells and neurotrophic factors although the precise mechanisms of action and the optimal administration route are unclear (Hermann et al., 2014; Gervois et al., 2016). Compared with the systemic delivery (Prasad et al., 2014; Hess et al., 2017), cerebral implantation requires fewer cells and provides a precision graft (Yang et al., 2011; Du G. et al., 2014; Du S. et al., 2014; Borlongan, 2016). However, the cerebral route has also reached relatively modest levels of post-stroke functional recovery, which has been associated with the severe loss of grafted cells that are generally not observed in the brain for more than 1–3 weeks after transplantation, as reported in several preclinical models (Kelly et al., 2004; Bliss et al., 2010; Mora-Lee et al., 2012; George and Steinberg, 2015; Wang et al., 2016). In patients, the cerebral implantation of stem cells has been reported to be safe and clinical improvements were observed, but the small sample size and heterogeneity between subjects currently preclude the use of this specific approach in clinical practice (Chen et al., 2014; Borlongan, 2016; Steinberg et al., 2016).
The use of biomaterials in tissue engineering is booming and has provided examples of how the integration of neurotrophic cells and factors in biomaterial-based polymers results in better post-stroke functional recovery compared to the implantation of therapeutic cells or factors alone (Guan et al., 2013; Jendelova et al., 2016; Nih et al., 2016). Different natural and synthetic polymers have been used to support stem cell engraftment including hyaluronic acid, collagen, hyaluronan-methylcellulose, polyethylene glycol, PLGA, alginate and matrigel among others (González-Nieto et al., 2018).
Although improved functional outcomes have been achieved in the majority of stroke models after the implantation of stem cells or stem cells plus different biomaterials, the mechanisms of recovery are in most cases unclear, but they might be associated with the reestablishment of the destroyed circuitry in damaged regions (Taguchi et al., 2004; Ramos-Cabrer et al., 2010; Wang et al., 2016). However, other tentative benefits of stem cell therapy could be related to the reorganization of the pre-existing circuitry in peri-lesional areas of the affected hemisphere, involving the reorganization of cortical maps (Dijkhuizen et al., 2001; Brown et al., 2009; Andres et al., 2011).
In the present study, we examined the ability of bone marrow mesenchymal stem cells (mSCs) to enhance functional outcomes after cortical stroke in mice. The mSCs were intracerebrally implanted together with a silk fibroin (SF)-based hydrogel (Fernandez-Garcia et al., 2016). We report evidence of cortical plasticity linked to functional recovery occurring late after treatment. To our knowledge, this study is unique because it shows that brain remapping and sustained recovery were experimentally favored using a stem cell-biomaterial-based approach.
Materials and Methods
Other methods are found in the Supplementary Material.
Animals
In vivo therapy experiments were conducted using adult male CD-1 mice (35–40 g body weight; 8–10 weeks old). For tracking studies EGFP-expressing mSCs were obtained from adult C57BL/6-Tg(CAG-EGFP)C14-Y01-FM131Osb mice, which were generously donated by Professor Masaru Okabe (Osaka University). All mice were bred and housed in the animal facility of the Center for Biomedical Technology. Animals were housed with free access to food and water in an animal room with a controlled temperature and a natural light cycle. Daily routines were performed between 8 a.m. and 5 p.m. by authorized personnel. All procedures were performed under the Spanish Regulations for animal experimentation and use of genetically modified organisms (Laws 53/2013, 178/2004 and ECC/566/2015) with the approval of Community of Madrid (PROEX 393/15) and according to the ARRIVE (Animal Research: Reporting In Vivo Experiments) guidelines.
Silk Fibroin Extraction and Preparation of the Hydrogel
Silk fibroin was extracted from Bombyx mori cocoons, which were provided by J. L. Cenis (IMIDA, Murcia, Spain), and processed using a previously described method (Fernandez-Garcia et al., 2016). Briefly, cocoons were cut into small pieces and degummed to remove sericin. After degumming, fibroin fibers were repeatedly rinsed with distilled water and allowed to dry overnight. Dry fibers were dissolved in a lithium bromide solution under continuous stirring. The solution was dialyzed against water, subsequently centrifuged to remove impurities, frozen at -80°C and lyophilized to obtain a final SF powder. For delayed in situ gelling the SF solutions (2% fibroin in PBS) were sonicated using a Branson 450 Sonifier coupled to a 3 mm diameter Tapered Microtip (Fernandez-Garcia et al., 2016).
Striatal Injection of mSCs and Silk Fibroin Hydrogels
Animals were anesthetized with ketamine (100 mg/kg) and xylazine (10 mg/kg) prior to the stereotaxic injection of phosphate-buffered saline (PBS at pH = 7.4), a SF solution in the pre-gel state, and mSCs resuspended in PBS (control) or in SF (pre-gel state). For analgesia, 0.1 mg/kg of buprenorphine was subcutaneously injected prior to and 8 h after surgery. The mice were secured in a stereotaxic frame (David Kopf Instruments, Los Angeles, CA, United States) and a skin incision was made, the skull was exposed, and a burr hole was drilled with a 0.8 mm drill bit. The rectal temperature was maintained at a constant value of 35–37°C using a heating pad. The different solutions were infused unilaterally into the caudate putamen (striatum) of the infarcted hemisphere using a Hamilton syringe with a needle (model 701SN, 31-G/Pst3, Teknokroma). Approximately 1 × 105 mSCs in PBS solution or in SF solution for a total volume of 5 μl were injected (1 μl/min) at the following coordinates from bregma: posterior +1.0 mm, laterally +2.7 mm, and ventrally +2.3 mm. After injection, the needle was left in place for 5 min to allow the solution to diffuse into the striatum.
Surgical Implantation of Electrodes and Recordings of Evoked Potentials
Evoked potentials (EPs) were recorded as described in our previous study (Barios et al., 2016). Briefly, ketamine/xylazine-anesthetized mice were implanted with two microelectrodes in the cortex of each hemisphere (±2.0 mm lateral and -0.5 mm rostrocaudal from bregma, at a depth of 0.5 mm) to record the activity of the forelimb somatosensory (FLs1) representation. Two additional electrodes were implanted in both hemispheres to examine the activity of the forelimb motor (cFLm1) representation (±1.0 mm lateral and +0.5 mm rostrocaudal from bregma, at a depth of 0.5 mm). These coordinates were based on anatomical and functional studies in mice (Paxinos and Franklin, 2004; Ferezou et al., 2007; Tennant et al., 2011; Hira et al., 2013a, 2015; Lim et al., 2014; Alia et al., 2016). Finally, two screws were placed over the visual cortex of both hemispheres and served as the indifferent and ground electrodes (±2.0 mm lateral and -3.0 mm rostrocaudal from bregma). Recordings were obtained from both hemispheres and cortical areas after forelimb contralateral electrical stimulation. Cortical signals were amplified (×103) and filtered (bandpass, 10–2.000 Hz) using a portable electromyography (EMG)-evoked potentials (EPs) device (Micromed, Mogliano Veneto, Treviso, Italy).
Statistics
All statistical analyses were conducted using SigmaPlot (Systat, Germany). Data are expressed as the means ± standard errors of the means (SEM). The significance of the differences between groups was determined using several statistical models. In most studies, an analysis of variance (ANOVA) was performed to test the differences between two or more means and contrast the null hypothesis. When the result was statistically significant (null hypothesis rejection), other statistical tests were used (Tukey’s test) to determine which groups showed significant differences. For example, a one-way ANOVA test was applied to examine significant differences in total cortical tissue loss and total subcortical inflammation. A two-way ANOVA was applied to determine the statistical significance of differences in forepaw footslips, evoked amplitude potentials, and latencies, as well as the number of EGFP-positive cells or amount of cortical tissue loss and subcortical inflammation along the rostrocaudal axis, considering time, treatment or brain section as independent variables. When applicable, statistical significance was assessed by performing Student’s t-test for independent samples (for example to examine evoked cortical responses between distinct hemispheric areas). Correlations between variables were determined using Pearson’s product-moment correlation coefficient.
Results
Somatosensory and Motor Responses in the Non-modified and Injured Mouse Brain
Before determining the effect of the therapy itself, we characterized the electrophysiological activity in discrete regions of the somatosensory and motor cortices in non-damaged mice in response to forelimb stimulation. We performed simultaneous recordings of evoked activity in areas of the forelimb primary-somatosensory cortex (FLs1, E1) and the most caudal forelimb-motor representation (cFLm1, E2). These specific electrode locations (Figure 1A), which are based on known anatomical and functional landmarks in mice and correspond to FLs1 (Ferezou et al., 2007; Hira et al., 2013a; Lim et al., 2014; Vanni and Murphy, 2014) and cFLm1 (Tennant et al., 2011; Hira et al., 2013a,b), were chosen to identify subsequent changes in infarcted (FLs1, E1) and surrounding peri-infarcted (cFLm1, E2) tissues, according to the particular stroke model used in the present study (Supplementary Figure S1). With this cortical stroke model, the infarcted area extended throughout the FLs1 representation and partially into the cFLm1 area (Figure 1A and Supplementary Figure S1), mostly because in mice, part of cFLm1 topographically overlaps with FLs1 (Ferezou et al., 2007; Tennant et al., 2011; Hira et al., 2013a,b).
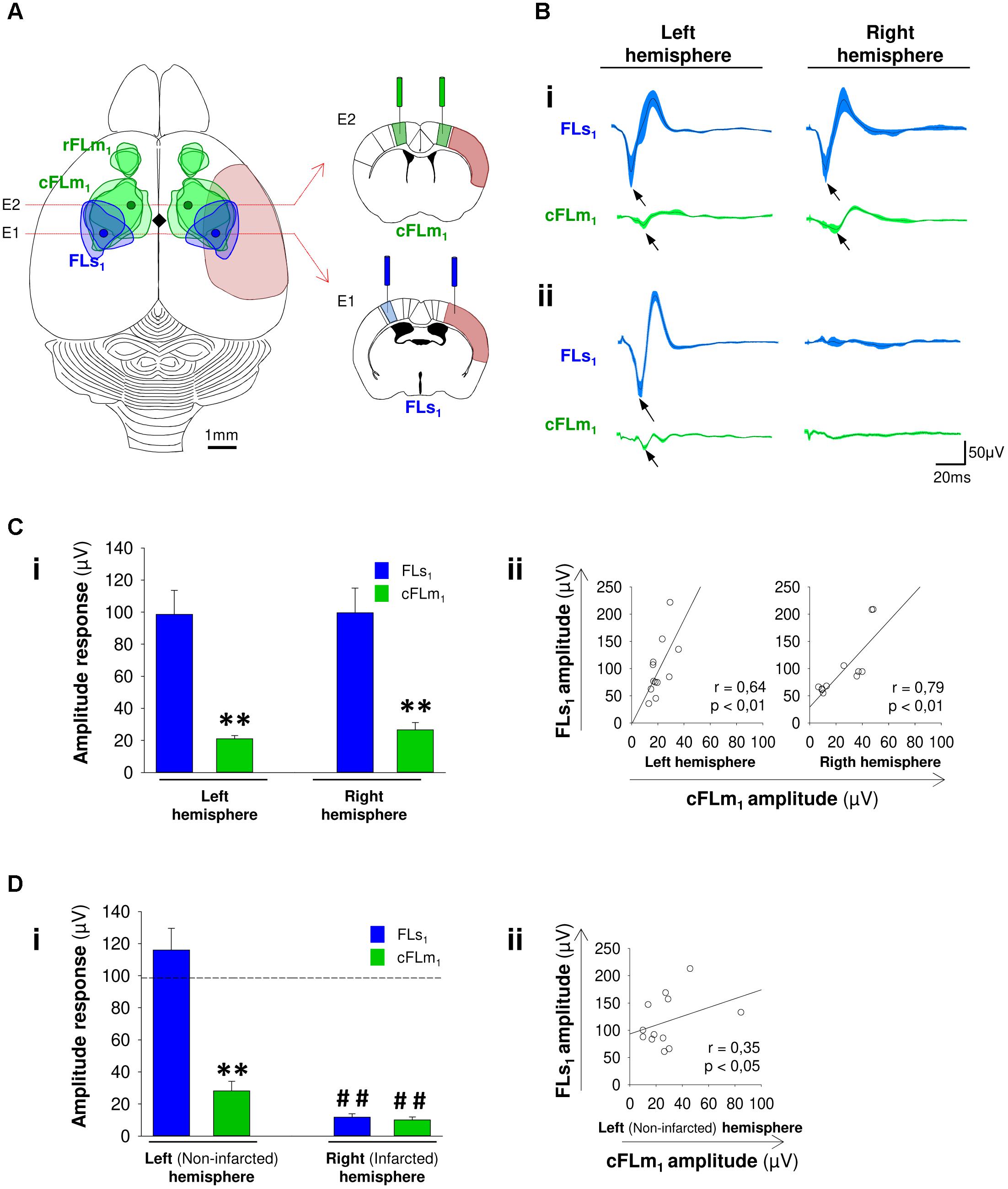
FIGURE 1. Post-stroke impairments in the functions of somatosensory and motor cortex territories. (A) Schematic showing the dorsal view of a mouse brain and the motor (green, FLm1) and the somatosensory (blue, FLs1) forelimb map territories based on intracortical microstimulation (ICMS) and voltage-sensitive dye imaging (VSD) studies performed in the mouse (Ferezou et al., 2007; Tennant et al., 2011; Hira et al., 2013a, 2015; Lim et al., 2014; Alia et al., 2016). In rodents, the forelimb motor cortex comprises a rostral (rFLm1) and a caudal (cFLm1) region. The cFLm1 region partially overlaps topographically with the somatosensory forelimb area FLs1. In the schematic, the soft red area represents the infarcted territory produced using the dMCAO approach that mostly affects FLs1 and partially affects cFLm1. The blue and green circles represent the site of electrodes implantation with respect to bregma (black diamond) that were used to record the evoked activity of discrete regions in FLs1 (infarcted site) and cFLm1 (tissue surrounding the lesion). Right panels depict a complementary scheme showing the position of electrodes in forelimb somatosensory and motor cortices of coronal brain sections (Paxinos and Franklin, 2004). (B) Evoked activity in FLs1 and cFLm1 after forelimb stimulation. Recordings are shown for both hemispheres under basal conditions (panel i) or after stroke (panel ii). In each trace the black line represents the average recordings obtained from 12 different mice. The blue or green shaded areas represent the standard errors of the means (SEM) for FLs1 and cFLm1 responses. (C, D) Amplitude of responses in FLs1 and cFLm1 for the left and right hemisphere under non-stroke (C, panel i) and stroke (D, panel i) conditions. The dashed black line in (D) represents the average amplitude of evoked potentials from FLs1 in both hemispheres before stroke. Pre-stroke (C, panel ii) and post-stroke (D, panel ii) Pearson’s correlation coefficients between the maximal responses in FLs1 and cFLm1. Data are presented as the means ± the SEM from 12 mice. Asterisks denote significant differences between FLs1 and cFLm1 responses. The hashed symbols indicate differences in pre- and post-stroke responses for each hemisphere (Student’s t-test; ∗∗p < 0.01; ##p < 0.01).
In pre-stroke conditions, a marked response of negative polarity was detected by the electrode (E1) placed in the contralateral FLs1 area in response to forelimb stimulation (Figures 1Bi,C) with maximal response amplitude occurring at approximately 17 ms after stimulation (for the right hemisphere: 17.2 ± 0.8 ms; for the left hemisphere: 17.3 ± 0.9 ms). In the same hemisphere, a small amplitude potential was concurrently detected by the electrode placed at the cFLm1 representation (Figures 1Bi,C), with the maximal response occurring at approximately 21 ms after stimulation (for the right hemisphere: 20.7 ± 0.8 ms; for the left hemisphere: 21.2 ± 0.9 ms). A significant difference in the latency of the responses between FLs1 and cFLm1 was determined for the right (3.5 ± 0.6 ms; p < 0.01) and left (3.9 ± 0.8; p < 0.01) hemispheres. This latency value (≥3.5 ms) and the anatomical location of both electrodes were consistent with the finding that cFLm1 activity is dependent on the previous response in FLs1. Consistent with the presence of an intracortical associative pathway between FLs1 and cFLm1, the maximal amplitude of recorded responses in FLs1 and cFLm1 positively correlated in each hemisphere analyzed (Figure 1C). This finding is consistent with the results of previous studies showing that sensorimotor transformation is sustained by functional communication between the primary somatosensory barrel and whisker motor cortices in rats and mice (Farkas et al., 1999; Ferezou et al., 2007; Matyas et al., 2010), or between the somatosensory and motor forepaw representations after photostimulation in mice (Lim et al., 2014). In our study, the cFLm1/FLs1 ratio, which was estimated from the maximal amplitude of responses in each recorded region, was 26.2 ± 3.4 and 24.8 ± 2.8 for the right and left hemispheres, respectively (Figure 1C). This ratio might represent to some extent the degree of functional connectivity between the recorded areas in cFLm1‘ and FLs1, respectively.
Cerebral infarction was induced in these mice by occluding the distal middle cerebral artery (MCA). This is a preferable stroke model that has been frequently used to mimic human strokes that do not spontaneously reperfuse or are refractory to treatment (Albers et al., 2011). One week after stroke, the animals showed a strong loss of cortical responsiveness in both the FLs1 and cFLm1 areas of the infarcted right hemisphere after forelimb stimulation (Figures 1Bii,D). In the non-infarcted hemisphere, a similar ratio value (26.78 ± 4.86) was obtained with respect to the pre-stroke condition, with significant correlation for the responses between cFLm1 and FLs1 (Figure 1D).
Changes in Cortical Function and Connectivity Are Linked to Functional Recovery After Treatment With mSCs Delivered via Silk Fibroin Hydrogels (mSCs-SF)
In a new study, different groups of mice were cerebrally injected with a saline solution (PBS), mSCs in PBS solution or mSCs embedded into SF. Prior to the transplant, mSCs were expanded ex vivo and functionally characterized (Supplementary Figure S2). On same day, coinciding with 72 h post-stroke, two sequential surgeries were performed to inject the cells and implant cortical electrodes (Figure 2A). In the first 48 h after stroke (before treatment), the percentage of footslips with the forelimb contralateral to the infarcted hemisphere was dramatically increased compared with the pre-stroke (baseline) condition (Figure 2B). No evidence of functional recovery was observed in mice injected with PBS or mSCs in PBS over time after treatment. However, gradual sensorimotor recovery was observed in the mice treated with mSCs-SF. Considering the temporal evolution of the mSCs-SF group, we did not observe statistically significant differences in the first weeks post-treatment compared with the other two animal groups or with the pretreatment condition, but we did observed significant differences in the interval from 6 to 10 weeks.
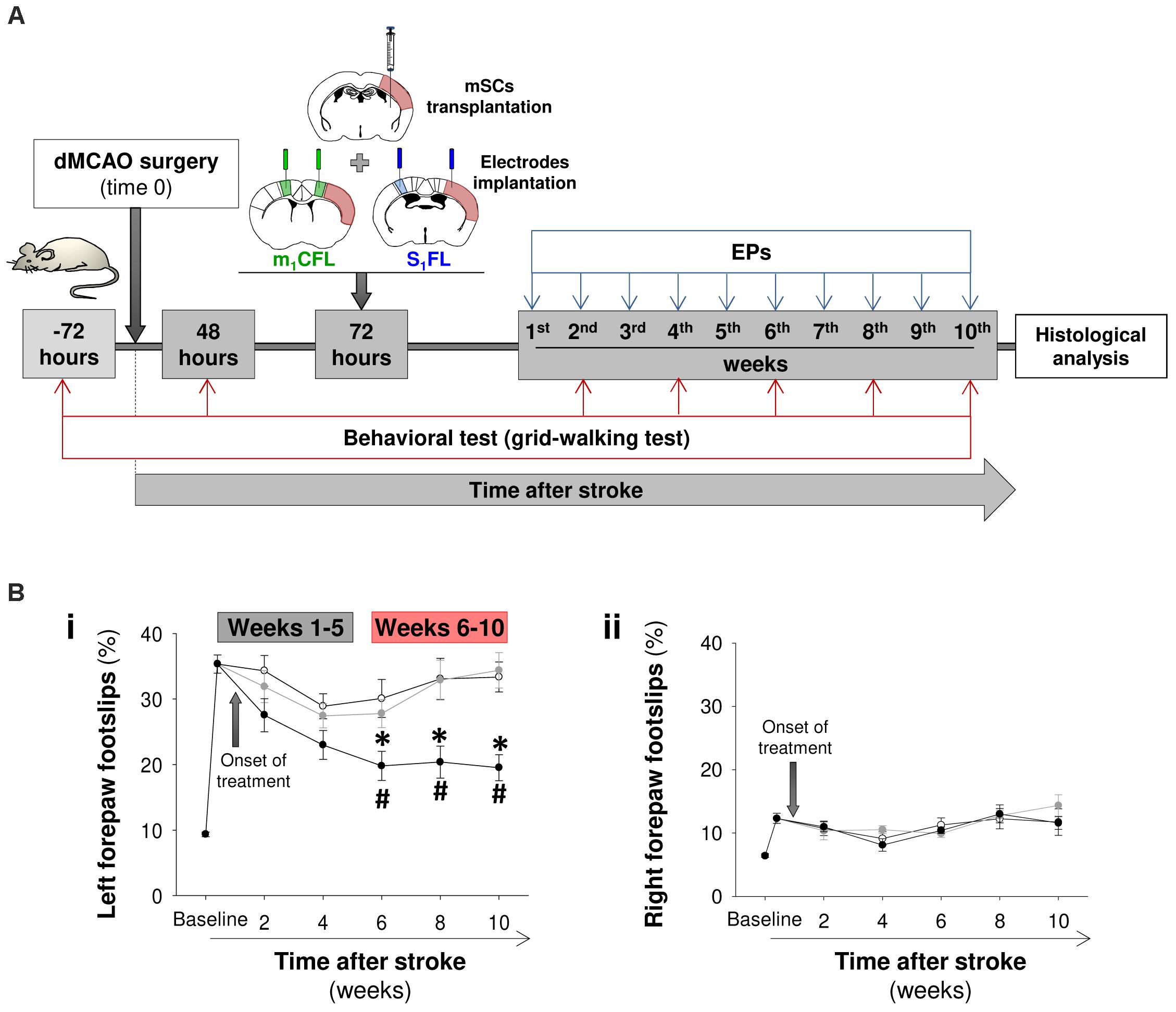
FIGURE 2. Sensorimotor recovery in stroke mice treated with mSCs-silk fibroin hydrogels. (A) Schematic of the study performed to examine the temporal progression of sensorimotor function and evoked activity in FLs1 and cFLm1 regions. The stroke animals were divided into three groups and treated with a saline solution (PBS, white circles in B), mSCs (gray circles) or mSCs encapsulated in silk fibroin hydrogels (black circles). Sensorimotor coordination was evaluated with the grid-walking test before and after stroke at different post-treatment time points. The FLs1 and cFLm1 responses were obtained for several weeks after treatment. The schematics of coronal brain slices illustrate the site of cerebral injection (treatment) and the placement of electrodes in relation to the cortical damage (soft red) induced in this specific mouse stroke model. (B) Percentages of footslips examined with the grid-walking test for the left (panel i) and right forepaws (panel ii), respectively. The left forepaw was contralateral to the infarcted (right) hemisphere. Data are presented as the means ± the SEM from nine mice per group at the onset of the study (PBS, mSCs or mSCs-Silk fibroin). Asterisks denote significant differences in data at every time point analyzed between the mSCs-SF group and the other two treated groups. The hashed symbols show statistically significant differences compared with the baseline post-stroke pretreatment values (two-way ANOVA followed by Tukey’s test; ∗p < 0.05).
The lack of sensorimotor recovery in PBS and mSCs groups paralleled the lack of evoked activity in FLs1 and cFLm1 of the infarcted hemisphere (Figures 3A,C), denoting a substantial injury of the forepaw sensory representation (FLs1), as was initially characterized in this stroke model (Figure 1). However, in the damaged hemisphere of the mSCs-SF group, a residual but still significant evoked activity was detected in both cortical representations from the first week post-treatment and during the time period analyzed (Figures 3A,C). In contrast, when we considered the non-infarcted hemisphere, clear evoked activity was observed in all studied groups (Figures 3B,D). We also examined the interval of latencies between the maximal responses detected in FLs1 and cFLm1 in both hemispheres, finding that in the mSCs-SF group the latency value in the infarcted hemisphere was reduced (at least in weeks 6–7 post-treatment) compared with the average latency estimated for the same hemisphere in non-infarcted mice (Supplementary Figure S3A).
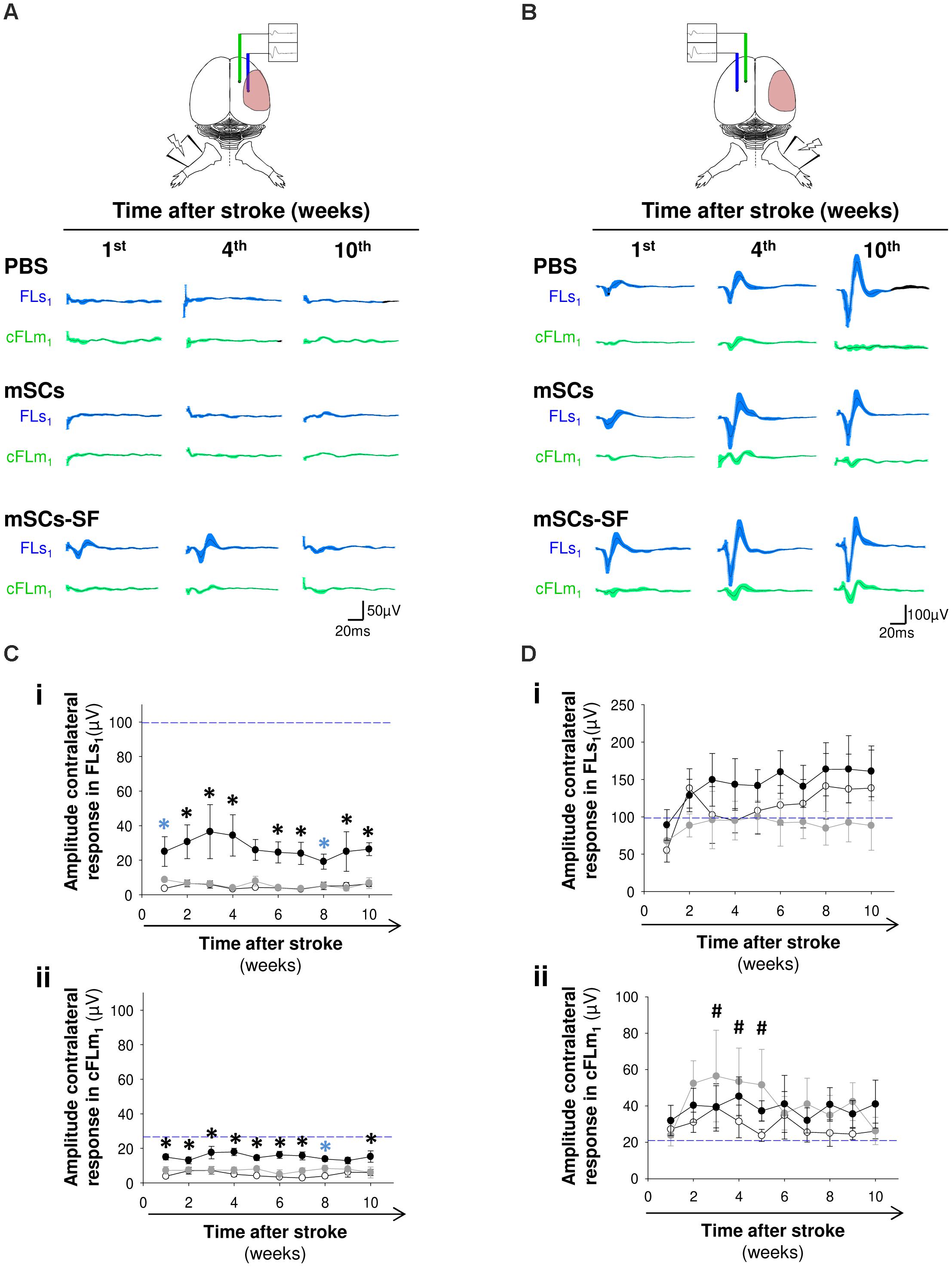
FIGURE 3. Evoked cortical activity in the infarcted and non-infarcted hemispheres of stroke mice after treatment with mSCs and silk fibroin polymers. (A) Average recordings of evoked activity in FLs1 and cFLm1 areas of the infarcted hemisphere in the group of mice treated with PBS, mSCs or mSCs-SF at discrete time points after treatment. (B) Mean recordings of cortical activity obtained for the non-infarcted hemisphere. (C,D) Amplitude of evoked activity in FLs1 and cFLm1 in the infarcted (C) and non-infarcted (D) hemispheres of mice injected with PBS (white circles), mSCs (gray circles) and mSCs encapsulated in silk fibroin (black circles) over time after treatment. In all graphs, the dashed blue lines represent the average activity in FLs1 (panels i) and cFLm1 (panels ii) under non-stroke condition. Data are presented as the means ± SEM from nine mice per group at the onset of the study. For every time point analyzed, the black asterisks denote significant differences between mSCs-SF mice and the other two studied groups, PBS and mSCs. Blue asterisks show statistically significant differences between PBS and mSCs-SF groups (two-way ANOVA followed by Tukey’s test; ∗p < 0.05). The hashed symbols denote significant differences (#p < 0.05) between the cFLm1 responses in mSCs mice compared to the pre-stroke condition (dashed blue line).
An interesting finding with the mSCs-SF group was the temporal pattern of the cFLm1/FLs1 ratio in the infarcted hemisphere, which exhibited a biphasic evolution (Figure 4A). In the first few weeks, when non-significant recovery was observed on the grid-walking test (Figure 2B), the cFLm1/FLs1 ratio remained relatively constant and did not show significant differences compared to the pre-stroke (blue dashed line) condition (Figure 4A). However, a progressive and significant increase in the cFLm1/FLs1 ratio was observed in the last few weeks after treatment (Figure 4A), the precise time at which significant sensorimotor recovery was observed in the mSCs-SF mice (Figure 2B). The increase in the cFLm1/FLs1 ratio resulted from a net decrease in FLs1 activity and an increase in the responsiveness of cFLm1 (Supplementary Figure S3B). However, no significant variation in the cFLm1/FLs1 ratio was observed in the non-infarcted hemisphere of mSCs-SF mice, with values similar to the pre-stroke condition (Figure 4A).
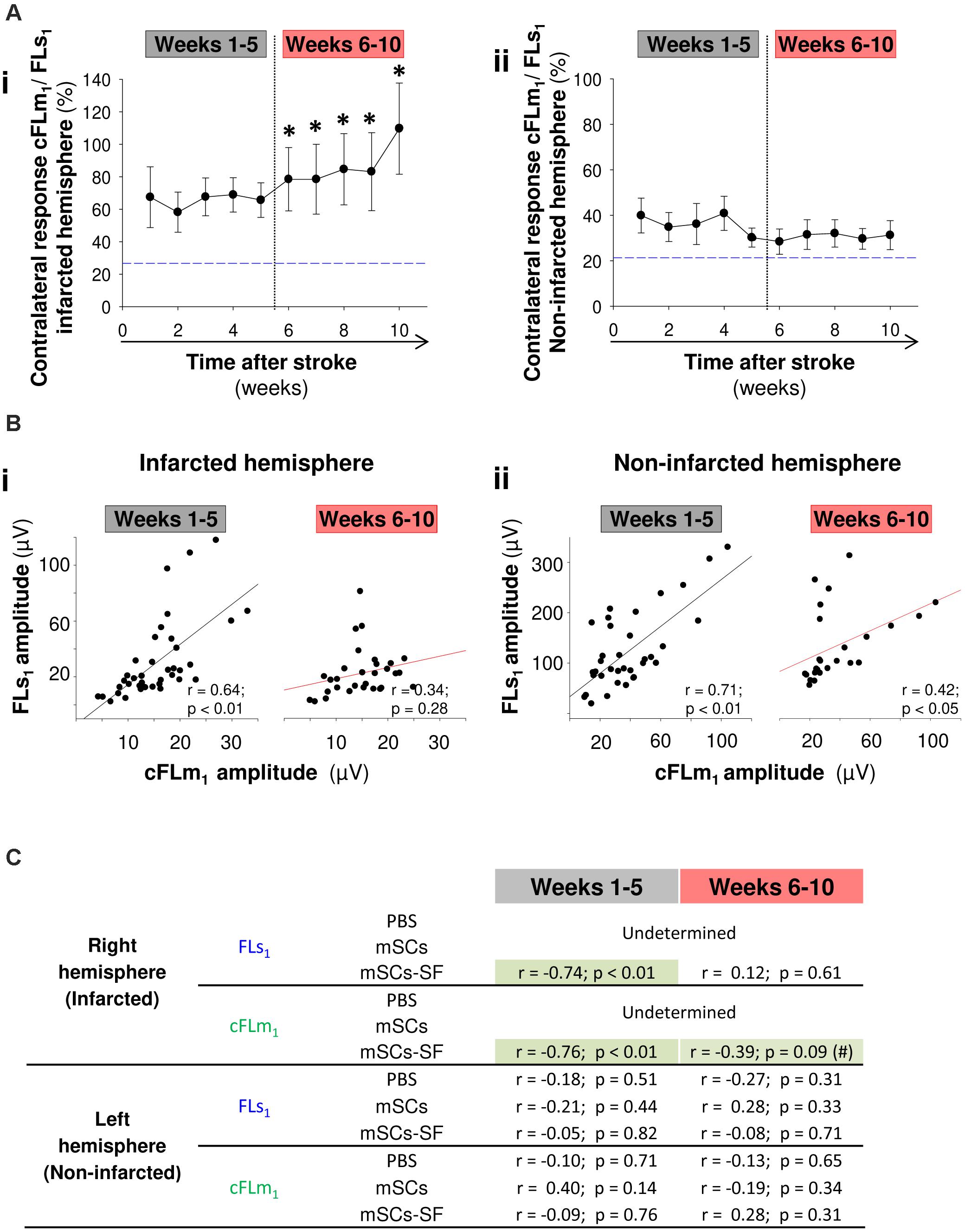
FIGURE 4. Loss of connectivity between FLs1 and cFLm1 representations underlies the functional recovery in stroke mice treated with mSCs and silk fibroin polymers. (A) cFLm1/FLs1 ratio in the infarcted (panel i) and non-infarcted (panel ii) hemispheres. The weeks corresponding with both periods of sensorimotor recovery (examined with the grid-walking test) are shown in both panels. The dashed blue lines represent the cFLm1/FLs1 ratio for both hemispheres under the non-stroke condition. (B) Pearson’s correlation coefficients for the maximal amplitude of responses in FLs1 and cFLm1 from the infarcted (panel i) and non-infarcted (panel ii) hemispheres in both intervals of recovery (weeks 1–5 and weeks 6–10). (C) Pearson’s correlation coefficients for the sensorimotor deficits examined with the grid-walking test and the maximal amplitude of responses in FLs1 and cFLm1 from the non-infarcted (left) and the infarcted (right) hemispheres for all treated mice included in the present this study. The black asterisks in the data obtained from mSCs-SF-treated mice denote significant differences between the post-stroke FLs1/cFLm1 ratio and the values obtained under the non-stroke condition (blue dashed line; one-way ANOVA; ∗p < 0.05).
In the mSCs-SF mice, the association of a progressive increase in the cFLm1/FLs1 ratio (infarcted hemisphere) with increased cFLm1 activity and decreased FLs1 responsiveness suggests an impairment of functional connectivity between FLs1 and cFLm1 when gradual sensorimotor recovery occurs. This assertion is based on the following facts. First, when we exclusively consider the non-damaged hemisphere, similar to the pre-stroke condition, a positive correlation between cFLm1 and FLs1 responses was determined independently of the two periods of sensorimotor dexterity (Figure 4B). Furthermore, in this hemisphere we did not identify any correlation between the maximal responses recorded in FLs1 and cFLm1 with the footslips of the affected paw, which was located ipsilateral to this hemisphere (Figure 4C), suggesting that the level of FLs1 and cFLm1 activity in the non-infarcted hemisphere had no effect on the evolution of sensorimotor recovery in mSCs-SF mice. Second, in the infarcted hemisphere, the maximal activation recorded in FLs1 correlated with the maximal cFLm1 response during the first weeks but not during the period of significant sensorimotor recovery (Figure 4B). During the first weeks, the footslips by the affected paw exhibited equivalent correlations with the responses recorded in both FLs1 and cFLm1 (Figure 4C); however, during weeks 6–10, only the cFLm1 responses displayed a moderate correlation with the sensorimotor deficits (p = 0.09; Figure 4C). Finally, both responses (FLs1 and cFLm1) in the non-infarcted hemisphere of PBS- or mSCs-treated animals positively correlated throughout study period (Supplementary Figure S4), suggesting that this unilateral infarction model did not impair the functional connectivity between both cortical maps in this hemisphere in any of the three studied groups.
In summary, the significant recovery observed in mSCs-SF treated mice several weeks after treatment (weeks 6–10) was apparently associated with a lack of functional connectivity between FLs1 and cFLm1 in the damaged hemisphere (Figure 6). In this late period, the activity generated in cFLm1 in response to forelimb stimulation no longer depended on the previous response in FLs1, indicating that cFLm1 probably represented the sensory afferent information of the forelimb (Figure 6). Under this condition, the activity recorded in cFLm1 moderately correlated with the degree of sensorimotor dysfunction, suggesting that the emerging sensory activity in cFLm1 was in part responsible for the recovery of sensorimotor coordination and function.
Neuroprotection Supports the Cortical Remodeling Underlying Functional Recovery
In our study, an intriguing question was related to the mechanisms underlying both: (i) the residual somatosensory activity in FLs1 that was detectable from the first days after implantation of mSCs-SF (Figure 3A) and (ii) the delayed (6–10 weeks post-treatment) emergent sensory activity in peri-infarcted areas within the cortical cFLm1 representation (Figure 4A). In order to assess these questions the loss of cortical mass after stroke as a function of the treatment was analyzed. It was found that ten weeks after treatment, the mice that had been previously injected with PBS or mSCs in PBS showed a strong loss of cortical tissue in the infarcted hemisphere compared with animals implanted with mSCs-SF (Figures 5A,B). In another set of experiments, a strong cortical loss after stroke was detected as early as 96 h after treatment, even in mice that were only injected with SF, whereas cortical damage was only significantly reduced at this time point in the group of mice implanted with mSCs encapsulated into the biomaterial (Supplementary Figure S5). The significant neuroprotective effect was favored by the inclusion of this cell population in SF, since the injection of mSCs alone did not offer a significant advantage in reducing the extent of brain damage compared with the animals that had merely been injected with PBS. We also inferred that the biomaterial exerted a potential benefit based on the results from alternative studies of intracerebral implantation of mSCs (EGFP-positive) into non-EGFP mice, where a large number of mSCs were engrafted for longer periods when this cell population was integrated into SF hydrogels (Figure 5C and Supplementary Figure S6).
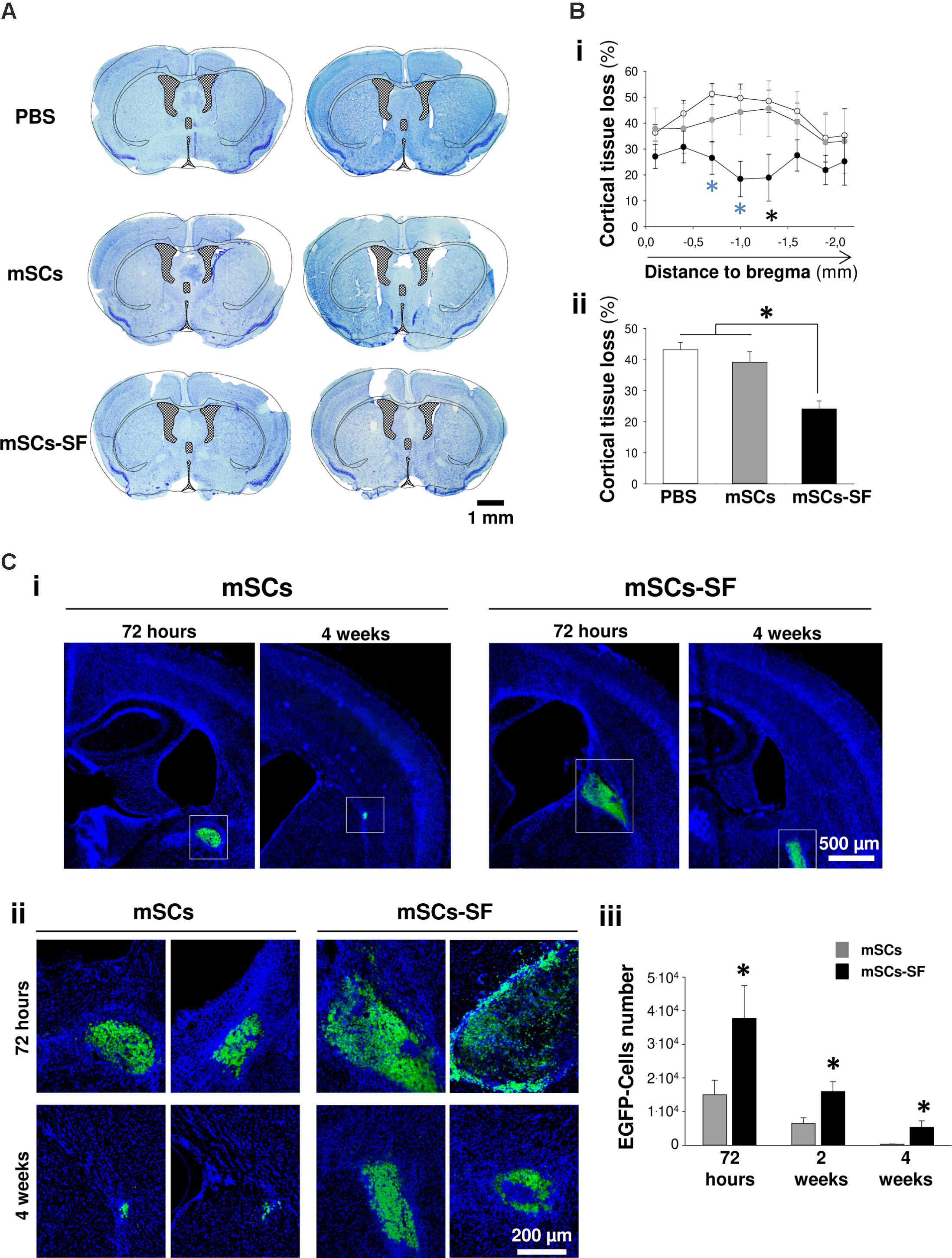
FIGURE 5. Increased survival of transplanted mSCs engrafted into silk fibroin hydrogels enhances neuroprotective effects after stroke. (A) Representative images of Nissl-stained coronal brain sections performed 10 weeks after injection of PBS, mSCs alone or mSCs encapsulated in silk fibroin hydrogels (images showed from two different mice in each group). Note how the majority of the cortical territory was preserved in stroke mice co-injected with mSCs and silk fibroin gels. (B) Panel i shows the regional distribution of the cortical tissue loss (damaged tissue) in coronal sections along the rostrocaudal axis as measured from bregma. Panel ii shows the total cortical tissue loss. (C) Representative images at low (panel i) and high (panel ii) magnification of mSCs expressing the EGF protein (EGFP) implanted into the striatum of non-EGFP-expressing mice, 72 h and 4 weeks after the injection of mSCs or mCSs-SF. Every image in panel ii corresponds to sections examined from different mice. In the figure, nuclei were stained with Hoechst dye (pseudocolored blue). Panel iii shows the quantification of EGFP-positive cells at different time points after transplantation. The asterisks in (B, panel i) show significant differences between mice treated with PBS or mSCs-SF (blue asterisks) or between mSCS-SF animals compared with the other two mice groups analyzed (black asterisk) (two-way ANOVA followed by Tukey’s test; ∗p < 0.05). The asterisks in (B, panel ii) show significant differences between groups (one-way ANOVA test followed by Tukey’s test; ∗p < 0.05). The asterisks in C (panel iii) denote statistically significant differences between groups at each time point analyzed (two-way ANOVA followed by Tukey’s test; ∗p < 0.05).
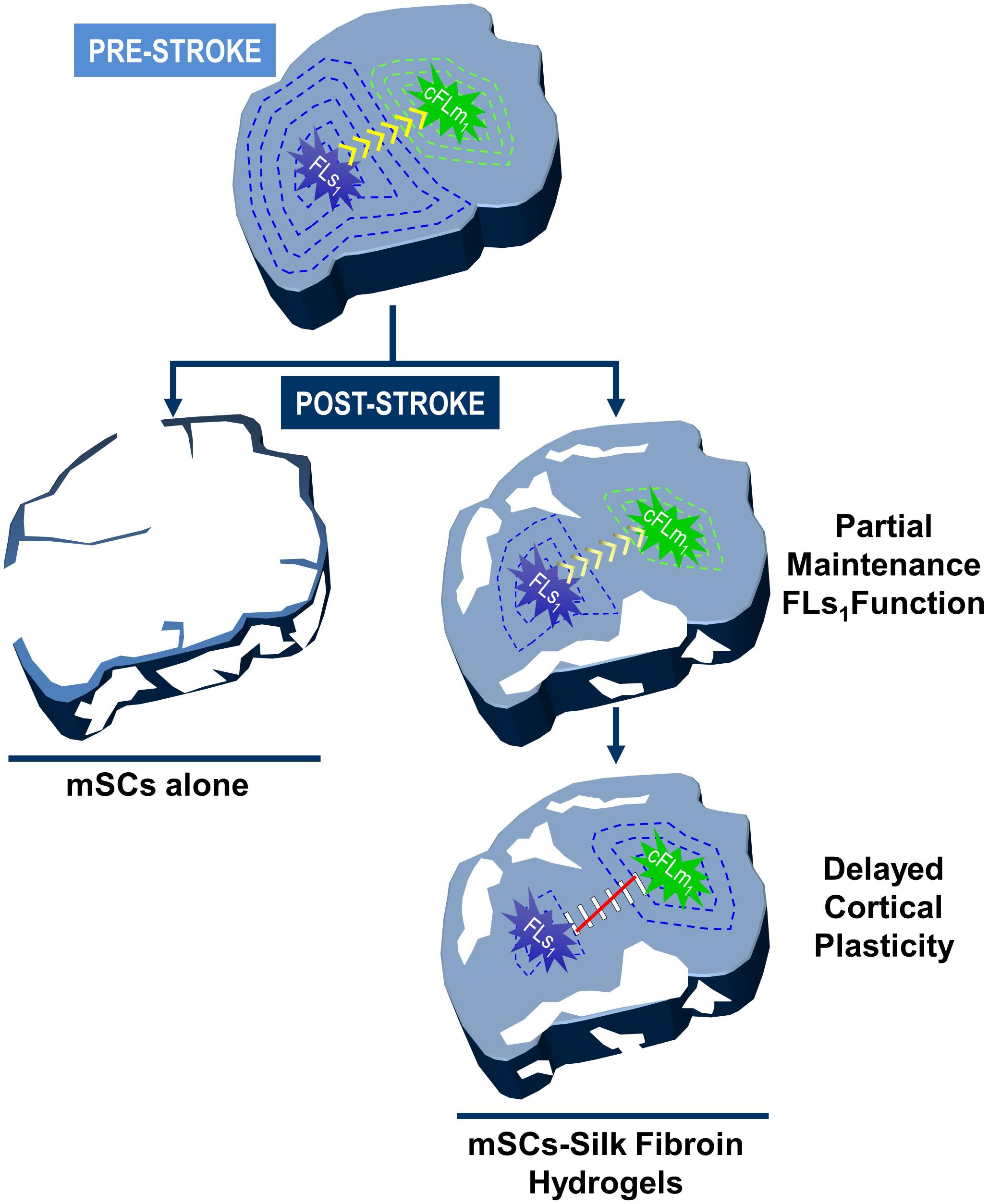
FIGURE 6. Cortical remodeling and the emergence sensory activity in cFLm1 underlies post-stroke functional recovery after transplantation of mSCs encapsulated in silk fibroin hydrogels. Model showing the two steps of mSCs-SF-based therapeutic action determined in the present study: neuroprotection and cortical reorganization. The top panel shows the somatotopic areas corresponding to the forepaw somatosensory (FLs1) and motor (cFLm1) representations. Under the pre-stroke condition, a cortical response in FLs1 emerges after forelimb stimulation (blue concentric dashed lines), which subsequently is transmitted to cFLm1 (green concentric dashed lines) indicating the dependency of both cortical maps on a functional circuit (yellow arrows). After stroke, the transplantation of mSCs encapsulated in silk fibroin hydrogels decreased the area of the affected cortical territory (white gaps represent the damaged loss tissue), partially preserving the evoked activity of both cortical representations in response to forelimb stimulation (illustrated as a reduction in the number of concentric blue and green lines). Several weeks after treatment, coinciding with the phase of significant sensorimotor recovery, a functional disconnection was produced between both cortical maps (red line). In this stage, the cFLm1 area represented the sensory information after forelimb stimulation (transition from green to blue lines in cFLm1 area), whereas a gradual loss of responsiveness was observed in the FLs1 map. In contrast, the stroke animals injected with mSCs alone or PBS showed a significant loss of cortical territory and complete abolition of activity in both cortical maps that was translated into profound and permanent sensorimotor behavioral deficits and recovery was not observed in the time period examined (left panel of the figure).
Discussion
For most patients, effective therapies for repairing the damaged brain are not currently available. Conceptually, behavioral improvement might be related to (i) cortical reorganization of peri-infarcted regions in the affected (Brown et al., 2009) and non-affected (Dijkhuizen et al., 2001) hemispheres; (ii) the reestablishment/restitution of circuitry in the damaged tissue (Taguchi et al., 2004; Ramos-Cabrer et al., 2010; Wang et al., 2016); and (iii) compensatory postural strategies that are particularly relevant in patients with large cerebral infarcts involving the motor cortex (Farr and Whishaw, 2002; Knieling et al., 2009; Moon et al., 2009). Regarding the first mechanism, in most cases, researchers have not been able to define whether cortical reorganization is maladaptive (Kim et al., 2015), otherwise unrelated (Xerri et al., 1998) or participates (Castro-Alamancos and Borrel, 1995; Nudo et al., 1996b; Dijkhuizen et al., 2001; Shanina et al., 2006; Andres et al., 2011) in the temporal pattern of functional recovery, making the establishment of appropriate intervention programs to drive efficient cortical plasticity difficult.
In our study, we did not detect significant signs of recovery in the stroke animals that had been acutely injected with PBS or mSCs alone; these animals showed permanent sensorimotor deficits (examined with the grid walking test) and complete lack of evoked activity in the somatosensory and motor cortices. The lack of evoked responses is mostly compatible with extensive damage to the cortical territory, which might justify the poor functional outcomes characteristic of large infarctions (Whishaw, 2000; Moon et al., 2009). In contrast, progressive and significant recovery was exclusively observed in mice implanted with mSCs encapsulated in SF hydrogels. Two sequential phenomena might tentatively explain the positive effect of this therapy on this group of animals. First, the reduced cortical damage detected after treatment probably ascribed to the well-known neuroprotective properties of mSCs (Steinbeck and Studer, 2015; Trounson and McDonald, 2015), properties that were visibly potentiated when this cell population was implanted together the biomaterial. The lower injury on the cortex was translated into a partial preservation of evoked responses in FLs1 and cFLm1 areas and reduced sensorimotor deficits from the first weeks after treatment. Second, and probably more relevant for recovery, the lower amount of cortical damage in the mSCs-SF animals might constitute a prerequisite to promote delayed cortical plasticity in the tissue surrounding the lesion, leading to behavioral improvements. Current evidence favors the existence of reorganization of the peri-infarct areas in infarctions covering small regions of cortical territory (Gonzalez and Kolb, 2003; Dancause et al., 2006; Murphy and Corbett, 2009). This finding is consistent with the lack of emerging sensory activity in cFLm1 or any evidence of plasticity examined under our experimental conditions in the animals injected with PBS or mSCs alone, which showed large damage in the sensorimotor cortex and poor recovery, as described above. Although the reorganization of cortical maps in the peri-lesional tissues in most studies with human and animals have been examined with intracortical stimulation or transcranial magnetic stimulation (humans), remodeling events were inferred in our study from the recordings of evoked cortical activity after forelimb stimulation, recapitulating the peri-lesional reorganization previously described in animals that exhibited spontaneously recovery or recovered after physical rehabilitation (Nudo et al., 1996b; Dancause, 2006; Brown et al., 2009).
Different engineering approaches are being used to increase stem cell survival and potentiate the neuro-secretome ability of different stem cells. Genetic engineering, pre-conditioning and cell encapsulation into biomaterial scaffolds constitute technological opportunities in the field (Bernstock et al., 2017). Particularly interesting is the ability of several biomaterials to enhance the survival, retention and integration of therapeutic cells in the brain tissue (González-Nieto et al., 2018). This is the case for hyaluronic acid (Ballios et al., 2015; Moshayedi et al., 2016), PLGA (Bible et al., 2009), matrigel (Jin et al., 2010), collagen (Yu et al., 2010), hyaluronan-methylcellulose (Ballios et al., 2015) or thermoreversible polymers (Osanai et al., 2010) among others. This nervous tissue-biomaterial-cells triad has been preferably exercised with hydrogel-based biomaterials, which show unique mechanical properties in the range of soft tissues like the nervous tissue and therefore might mimic with certain similarity the in vivo microenvironment. Because the list of biomaterials for brain repair is rapidly expanding there is an important need to identify the more suitable materials in terms of efficacy and compatibility. In our study, we tested for first time the efficacy of combining mSCs with SF to repair the stroke-damaged brain. Among the spectrum of possible biomaterials, SF is a flexible and adaptable natural polymer that has been used for years in many clinical applications including surgical sutures, mechanical support for cruciate ligament or reconstructive and aesthetic surgery. Its null immunogenic response, non-toxic properties and inertness respect other biomaterials makes it a strong biomaterial candidate for tissue regeneration. In addition this biomaterial shows good compatibility and tolerability with central nervous system (Fernandez-Garcia et al., 2016). Alternative engineering materials under development have strong potential but also are subjected to certain limitations. For example, hyaluronic acid (HA), a very common biomaterial used in experimental stroke (Ballios et al., 2015; Nih et al., 2018) might exerts different effects depending of the size of the polymer. The fragments generated after degradation might trigger immuno-reactivity and inflammatory events (Petrey and de la Motte, 2014). Furthermore, the accumulation of HA has been associated with aging and demyelinating disorders such as multiple sclerosis (Back et al., 2005) and HA might be detrimental for remyelination of neural circuits. Biomaterials like chitosan or PLGA have been associated with inflammation and allergic reactions; matrigel, a mix of extracellular matrix proteins from mouse sarcoma is not suitable for human subjects. Collagen shows important safety problems in relation to the possible contamination with viruses and prions and degrades rapidly.
In comparison with the implantation of mSCs alone a greater content of EGFP-mSCs was observed in the brain after transplantation with SF hydrogels. Despite their known immunosuppressive and anti-inflammatory properties it has been extensively reported that the engraftment of mSCs is quite limited in the host brain (Munoz et al., 2005; Coyne et al., 2006, 2007; Ohtaki et al., 2008; Moloney et al., 2010). A strong reduction of mSCs survival was found in hippocampus and striatum as soon as 1 week after implantation (Munoz et al., 2005; Ohtaki et al., 2008; Moloney et al., 2010). Rapid rejection of mSCs has been attributed to inflammatory responses mostly mediated by microglia and macrophages although these mechanisms are not completely elucidate. In our study, the EGFP transgene might tentatively contribute to this detrimental effect since a cytotoxic T lymphocyte response against the EGFP antigen cells has been detected after transplantation of EGFP-lymphoma cells in non-EGFP immunocompetent syngeneic mice (Stripecke et al., 1999) and treatment with cyclosporine A enhances the survival of EGFP-mSCs implanted in the striatum and the subarachnoideal space (Cobacho et al., 2009; Moloney et al., 2010). However, GFP/EGFP reporters has been commonly used to track axonal projections and neuronal connectivity for example after cerebral implantation of GFP expressing embryonic stem cells into non-EGFP mouse brains (Gaillard et al., 2007; Michelsen et al., 2015) and in the absence of cyclosporine A no differences in survival were observed between non-EGFP- and EGFP-mSCs implanted into the brain striatum (Moloney et al., 2010). In any case, the biomaterial used in the present study might plausibly constitute a structural scaffold to partially protect mSCs from a possible inflammatory response avoiding their rapid rejection by the host brain.
Because a main objective of our study was to establish the mechanistic aspects of recovery at functional level (connectivity between somatotopic maps), a limitation of our study relates to the analysis of the cellular/molecular mechanisms behind the neuroprotective ability of mSCs encapsulated into SF. The neuroprotection exerted by mSCs-SF might be inferred from the reduction of the damage area after the occlusion of the MCA and from the partial preservation of evoked cortical activity from the first week post-implantation. The therapeutic action of mSCs embedded into SF might be due to a synergic effect involving a higher long-term survival of mSCs in the host tissue and modifications of their secretome function. Recent in vitro results from our group (in preparation) indicates that this biomaterial reduces the secretion of BDNF, SDF1-Alpha and VEGF from mSCs, while substantially augments (up to 10 times more) the secretion of TGF-Beta1, a known anti-inflammatory and angiogenic factor that is strongly induced after brain injury polarizing microglia to an anti-inflammatory phenotype (Rustenhoven et al., 2016) increasing functional recovery after acute brain injury (Taylor et al., 2017). In relation to the angiogenic potential of TGF-Beta1, brain plasticity and post-stroke neurological recovery have been related with increasing angiogenesis (Chen et al., 2003). In this context it is also interesting the strong ability of SF to support endothelial cell survival and growth as well as the formation of microvascular networks in vitro and in vivo (Unger et al., 2007; Stoppato et al., 2013).
By contrast, a controversial aspect of our study relies in the lack of any post-stroke therapeutic effect ascribed to the mSCs implanted without the biomaterial in relation to the injection of PBS. Numerous studies have reported favorable outcomes after transplantation of mSCs (Vu et al., 2014). However, the effects of mSCs are largely variable depending of administration route, temporal window of administration, cell dosage and tissue source, animal model and type of stroke. In alternative studies no functional recovery has been observed after treatment with mSCs (Qu et al., 2009; Scheibe et al., 2012; Quittet et al., 2015). Discrepancies between positive and negative results are not yet clarified, although application route, cell dosage and final read outs employed to examine functional outcomes might contribute. Across the literature the highest therapeutic effects of mSCs were observed when this cell population was implanted in the early hours after stroke, typically 24 h, in our case 72 h. Another possible variable might be related with the area of brain implantation, striatum in our study in comparison with other brain regions (stroke cavity, peri-lesional cortex in cortical stroke models). We believe that a main factor is the mouse strain used in this study, CD-1. In contrast to the more popular mouse strain (C57Bl6) used in the majority of studies, in our work we choose this strain (CD-1) because in our hands the infarction size and the functional deficits (examined by behavioral testing and electrophysiology) were more consistent and reproducible than in C57Bl6 (Barios et al., 2016). Infarction volumes in CD-1 are generally higher because of poor collateral vasculature and higher susceptibility to hypoxia. Thus, our election was to test the efficacy of this experimental treatment in a very restricted condition, which could have repercussion in the ability of mSCs to exert a significant response. The cortical tissue loss tended to be smaller after implantation of mSCs alone although this effect was not statistically significant (Supplementary Figure S5). Under this condition we found a complete abolition of cortical evoked response that contrasted with the partial preservation (from the first week post-treatment) of cortical function in mice treated with mSCs and SF together.
In humans and in a majority of stroke models, the reorganization of cortical maps has mainly been observed between motor representations surrounding the damage area (Dancause, 2006). In stroke rodent models affecting the somatosensory forepaw area, a delayed emerging sensory activity (several weeks after stroke) was observed in peri-lesional areas corresponding to the motor cortex, coinciding with the temporal pattern of spontaneous sensorimotor recovery (Brown et al., 2009; Harrison et al., 2013). In our case, the fundamental mechanisms by which cFLm1 regulates direct sensory processing and whether the canonic function of the remaining (non-affected) motor cortical map is still preserved or also extended to other nearby motor areas under this condition are not known. A probable first requirement for this vicarious process is associated with the reciprocal functions between cortical maps that share similar elements of neuronal network architecture, regardless of whether they are involved in direct sensory or motor processing. Considering the functional organization of the barrel cortex as an example, the somatosensory barrel representation might physiologically contribute to motor control of whisker retraction in rodents (Matyas et al., 2010). Thus, the promiscuity of cFLm1 for sensorial and motor processing may not be surprising if somatosensory and motor areas are functionally interchangeable (Brown et al., 2009; Harrison et al., 2013). However, some exceptions to this “rule” have been reported, since infarctions restricted to the motor cortex do not produce substantial reorganization of sensory representations (Harrison et al., 2013). Several possibilities have been proposed to explain the emerging sensory activity in peri-lesional cFLm1 areas, such as the unmasking of somatosensory circuitry (Jacobs and Donoghue, 1991). Alterations in GABAergic inhibitory neurotransmission have been proposed to be primarily responsible for the somatotopic reorganization to define cortical territories (Foeller et al., 2005; Hensch, 2005). Inhibition of GABAergic signaling restricted to the forelimb motor cortex causes fast reorganization (in the range of minutes) of cortical maps in adult rats, which showed evoked forelimb movement when the vibrissa representation was electrically stimulated (Jacobs and Donoghue, 1991). Moreover, the inhibition of GABAergic signaling favors the rearrangement of neural networks in the peri-infarct region supporting functional recovery. For example, when a α5-GABAA antagonist was chronically applied beginning on day 3 after stroke, functional recovery was observed as early as 7 days after ischemia (Clarkson et al., 2010), a time period observed in other studies of therapeutic post-stroke GABAergic signaling inhibition (Alia et al., 2016). Early inhibition of GABAergic signaling appears to accelerate functional recovery compared with the findings from alternative studies showing spontaneous recovery (Brown et al., 2009) or a neural stem cells transplantation-induced (Andres et al., 2011) delay in functional recovery, which similar to our study, were characterized by a significant sensorimotor improvement weeks after stroke. Different structural changes (synaptogenesis, dendritic length and branching, axonal sprouting, dendritic spine turnover) might also explain the delayed emergence of sensory activity in cFLm1 (Stroemer et al., 1993, 1995; Carmichael et al., 2001; Gonzalez and Kolb, 2003; Brown et al., 2007, 2008, 2009; Li et al., 2010, 2015; Andres et al., 2011; Overman et al., 2012). In addition, mSCs might be involved in the paracrine release of diverse factors that stimulate axonal and dendritic plasticity as well as synaptogenesis (Li et al., 2006; Liu et al., 2008, 2010; van Velthoven et al., 2012). The structural rearrangement in the peri-lesional cortex might be favored by structural modifications in pre-existing neuronal/glia networks, as well as the functional integration of newborn neurons that facilitate functional plasticity (Shiromoto et al., 2017) due to the secretory activity of mSCs (Munoz et al., 2005).
In a recent study, delayed post-stroke recovery occurred after the combined transplantation of neural stem cells and progenitors (nSCs) and the 3K3A-APC protease to enhance the survival and proliferation of the cerebrally transplanted cells (Wang et al., 2016). Behavioral improvements resulted from the functional integration of nSCs-derived neurons in the territory affected by the infarction in parallel with cortical hyperplasia (Wang et al., 2016). In another example, a delay in the functional restoration of the damaged representation was also observed after treatment with a C17.2 stem cell line, but no evidence of brain reorganization was detected in peri-lesional areas (Ramos-Cabrer et al., 2010). In any case, if endogenous neuronal integration supported the delayed recovery of our treated animals, it would functionally occur through modifications in the perilesional cortex, but not in the damaged forepaw sensory area (FLS1), since a gradual, unexplained loss of activity, was observed in the FLS1 map over time after stroke, reaching values of residual activity that did not correlate with sensorimotor abilities during the significant recovery phase.
We are still far from obtaining a comprehensive picture of the molecular and cellular substrates underlying cortical reorganization in the peri-lesional cortex and how these mechanisms are cleverly orchestrated to restore damaged functions in response to post-stroke signaling. In this study, we have revealed at functional level how the infarcted brain might recover from injury after the combined transplantation of stem cells and SF hydrogels. We report for the first time to our knowledge, that cortical maps can be reshaped after an experimental paradigm based on the transplantation of stem cells of mesenchymal origin, whose neuroprotective function was potentiated by this innocuous polymer, confirming the suitability of this biomaterial in this specific application. Our findings contribute to the rational search for therapies designed to enhance cortical plasticity and recovery after cerebral damage, expanding the list of revolutionary biomaterials that serve as a support for the engraftment and function of stem cells with neuro-therapeutic abilities. Finally, our results may pave the way for studies aiming to understand how specific types of peri-lesional plasticity contribute to functional recovery.
Author Contributions
GG and DG-N conceived the idea and supervised the whole project. LF-G performed the majority of the experiments with the help of JP-R, MR, RM-M, FP, and DG-N. LF-G and DG-N analyzed the data. DG-N interpreted the data and wrote the paper.
Funding
This study was funded by the Ministerio de Economia y Competitividad Grants MAT2016-79832-R (to GG and DG-N) and MAT2016-79832-R/MAT2015-66666-C3-3-R (to FP) and funds from the community of Madrid (grant Neurocentro-B2017/BMD-3760).
Conflict of Interest Statement
The authors declare that the research was conducted in the absence of any commercial or financial relationships that could be construed as a potential conflict of interest.
Acknowledgments
We would like to thank Soledad Martinez for the excellent technical assistance. We gratefully acknowledge the technical assistance of Juan A. Barios and the support with the Micromed system. We also express our gratitude to Professor Masaru Okabe (Osaka University, Japan) and Professor Jose Carlos Segovia (CIEMAT, Madrid, Spain) who donate us the C57BL/6-Tg(CAG-EGFP)C14-Y01-FM131Osb mice. Cocoons were kindly provided by Professor Jose Luis Cenis (IMIDA, Murcia, Spain).
Supplementary Material
The Supplementary Material for this article can be found online at: https://www.frontiersin.org/articles/10.3389/fncel.2018.00296/full#supplementary-material
References
Albers, G. W., Goldstein, L. B., Hess, D. C., Wechsler, L. R., Furie, K. L., Gorelick, P. B., et al. (2011). Stroke Treatment Academic Industry Roundtable (STAIR) recommendations for maximizing the use of intravenous thrombolytics and expanding treatment options with intra-arterial and neuroprotective therapies. Stroke 42, 2645–2650. doi: 10.1161/STROKEAHA.111.618850
Alia, C., Spalletti, C., Lai, S., Panarese, A., Micera, S., and Caleo, M. (2016). Reducing GABAA-mediated inhibition improves forelimb motor function after focal cortical stroke in mice. Sci. Rep. 6:37823. doi: 10.1038/srep37823
Andres, R. H., Horie, N., Slikker, W., Keren-Gill, H., Zhan, K., Sun, G., et al. (2011). Human neural stem cells enhance structural plasticity and axonal transport in the ischaemic brain. Brain 134, 1777–1789. doi: 10.1093/brain/awr094
Back, S. A., Tuohy, T. M., Chen, H., Wallingford, N., Craig, A., Struve, J., et al. (2005). Hyaluronan accumulates in demyelinated lesions and inhibits oligodendrocyte progenitor maturation. Nat. Med. 11, 966–972. doi: 10.1038/nm1279
Ballios, B. G., Cooke, M. J., Donaldson, L., Coles, B. L., Morshead, C. M., van der Kooy, D., et al. (2015). A hyaluronan-based injectable hydrogel improves the survival and integration of stem cell progeny following transplantation. Stem Cell Reports 4, 1031–1045. doi: 10.1016/j.stemcr.2015.04.008
Barios, J. A., Pisarchyk, L., Fernandez-Garcia, L., Barrio, L. C., Ramos, M., Martinez-Murillo, R., et al. (2016). Long-term dynamics of somatosensory activity in a stroke model of distal middle cerebral artery oclussion. J. Cereb. Blood Flow Metab. 36, 606–620. doi: 10.1177/0271678X15606139
Benjamin, E. J., Blaha, M. J., Chiuve, S. E., Cushman, M., Das, S. R., Deo, R., et al. (2017). Heart disease and stroke statistics-2017 update: a report from the American heart association. Circulation 135, e146–e603. doi: 10.1161/CIR.0000000000000485
Bernstock, J. D., Peruzzotti-Jametti, L., Ye, D., Gessler, F. A., Maric, D., Vicario, N., et al. (2017). Neural stem cell transplantation in ischemic stroke: a role for preconditioning and cellular engineering. J. Cereb. Blood Flow Metab. 37, 2314–2319. doi: 10.1177/0271678X17700432
Bible, E., Chau, D. Y., Alexander, M. R., Price, J., Shakesheff, K. M., and Modo, M. (2009). The support of neural stem cells transplanted into stroke-induced brain cavities by PLGA particles. Biomaterials 30, 2985–2994. doi: 10.1016/j.biomaterials.2009.02.012
Bliss, T. M., Andres, R. H., and Steinberg, G. K. (2010). Optimizing the success of cell transplantation therapy for stroke. Neurobiol. Dis. 37, 275–283. doi: 10.1016/j.nbd.2009.10.003
Borlongan, C. V. (2016). Preliminary reports of stereotaxic stem cell transplants in chronic stroke patients. Mol. Ther. 24, 1710–1711. doi: 10.1038/mt.2016.186
Brown, C. E., Aminoltejari, K., Erb, H., Winship, I. R., and Murphy, T. H. (2009). In vivo voltage-sensitive dye imaging in adult mice reveals that somatosensory maps lost to stroke are replaced over weeks by new structural and functional circuits with prolonged modes of activation within both the peri-infarct zone and distant sites. J. Neurosci. 29, 1719–1734. doi: 10.1523/JNEUROSCI.4249-08.2009
Brown, C. E., Li, P., Boyd, J. D., Delaney, K. R., and Murphy, T. H. (2007). Extensive turnover of dendritic spines and vascular remodeling in cortical tissues recovering from stroke. J. Neurosci. 27, 4101–4109. doi: 10.1523/JNEUROSCI.4295-06.2007
Brown, C. E., Wong, C., and Murphy, T. H. (2008). Rapid morphologic plasticity of peri-infarct dendritic spines after focal ischemic stroke. Stroke 39, 1286–1291. doi: 10.1161/STROKEAHA.107.498238
Calford, M. B., and Tweedale, R. (1988). Immediate and chronic changes in responses of somatosensory cortex in adult flying-fox after digit amputation. Nature 332, 446–448. doi: 10.1038/332446a0
Carmichael, S. T., Wei, L., Rovainen, C. M., and Woolsey, T. A. (2001). New patterns of intracortical projections after focal cortical stroke. Neurobiol. Dis. 8, 910–922. doi: 10.1006/nbdi.2001.0425
Castro-Alamancos, M. A., and Borrel, J. (1995). Functional recovery of forelimb response capacity after forelimb primary motor cortex damage in the rat is due to the reorganization of adjacent areas of cortex. Neuroscience 68, 793–805. doi: 10.1016/0306-4522(95)00178-L
Chen, D. C., Lin, S. Z., Fan, J. R., Lin, C. H., Lee, W., Lin, C. C., et al. (2014). Intracerebral implantation of autologous peripheral blood stem cells in stroke patients: a randomized phase II study. Cell Transplant. 23, 1599–1612. doi: 10.3727/096368914X678562
Chen, J., Li, Y., Wang, L., Lu, M., Zhang, X., and Chopp, M. (2001a). Therapeutic benefit of intracerebral transplantation of bone marrow stromal cells after cerebral ischemia in rats. J. Neurol. Sci. 189, 49–57. doi: 10.1016/S0022-510X(01)00557-3
Chen, J., Li, Y., Wang, L., Zhang, Z., Lu, D., Lu, M., et al. (2001b). Therapeutic benefit of intravenous administration of bone marrow stromal cells after cerebral ischemia in rats. Stroke 32, 1005–1011. doi: 10.1161/01.STR.32.4.1005
Chen, J., Zhang, Z. G., Li, Y., Wang, Y., Wang, L., Jiang, H., et al. (2003). Statins induce angiogenesis, neurogenesis, and synaptogenesis after stroke. Ann. Neurol. 53, 743–751. doi: 10.1002/ana.10555
Clarkson, A. N., Huang, B. S., Macisaac, S. E., Mody, I., and Carmichael, S. T. (2010). Reducing excessive GABA-mediated tonic inhibition promotes functional recovery after stroke. Nature 468, 305–309. doi: 10.1038/nature09511
Cobacho, N., Serano, A. B., Casarejos, M. J., Mena, M. A., and Paino, C. L. (2009). Use of transduced adipose tissue stromal cells as biologic minipumps to deliver levodopa for the treatment of neuropathic pain: possibilities and limitations. Cell Transplant. 18, 1341–1358. doi: 10.3727/096368909X12483162197367
Coyne, T. M., Marcus, A. J., Reynolds, K., Black, I. B., and Woodbury, D. (2007). Disparate host response and donor survival after the transplantation of mesenchymal or neuroectodermal cells to the intact rodent brain. Transplantation 84, 1507–1516. doi: 10.1097/01.tp.0000288185.09601.4d00007890-200712150-00018
Coyne, T. M., Marcus, A. J., Woodbury, D., and Black, I. B. (2006). Marrow stromal cells transplanted to the adult brain are rejected by an inflammatory response and transfer donor labels to host neurons and glia. Stem 24, 2483–2492. doi: 10.1634/stemcells.2006-0174
Dancause, N. (2006). Vicarious function of remote cortex following stroke: recent evidence from human and animal studies. Neuroscientist 12, 489–499. doi: 10.1177/1073858406292782
Dancause, N., Barbay, S., Frost, S. B., Zoubina, E. V., Plautz, E. J., Mahnken, J. D., et al. (2006). Effects of small ischemic lesions in the primary motor cortex on neurophysiological organization in ventral premotor cortex. J. Neurophysiol. 96, 3506–3511. doi: 10.1152/jn.00792.2006
Dijkhuizen, R. M., Ren, J., Mandeville, J. B., Wu, O., Ozdag, F. M., Moskowitz, M. A., et al. (2001). Functional magnetic resonance imaging of reorganization in rat brain after stroke. Proc. Natl. Acad. Sci. U.S.A. 98, 12766–12771. doi: 10.1073/pnas.231235598
Du, G., Liu, Y., Dang, M., Zhu, G., Su, R., Fan, Y., et al. (2014). Comparison of administration routes for adipose-derived stem cells in the treatment of middle cerebral artery occlusion in rats. Acta Histochem. 116, 1075–1084. doi: 10.1016/j.acthis.2014.05.002
Du, S., Guan, J., Mao, G., Liu, Y., Ma, S., Bao, X., et al. (2014). Intra-arterial delivery of human bone marrow mesenchymal stem cells is a safe and effective way to treat cerebral ischemia in rats. Cell Transplant. 23(Suppl. 1), S73–S82. doi: 10.3727/096368914X685023
Elbert, T., Pantev, C., Wienbruch, C., Rockstroh, B., and Taub, E. (1995). Increased cortical representation of the fingers of the left hand in string players. Science 270, 305–307. doi: 10.1126/science.270.5234.305
Elbert, T., Sterr, A., Flor, H., Rockstroh, B., Knecht, S., Pantev, C., et al. (1997). Input-increase and input-decrease types of cortical reorganization after upper extremity amputation in humans. Exp. Brain Res. 117, 161–164. doi: 10.1007/s002210050210
Farkas, T., Kis, Z., Toldi, J., and Wolff, J. R. (1999). Activation of the primary motor cortex by somatosensory stimulation in adult rats is mediated mainly by associational connections from the somatosensory cortex. Neuroscience 90, 353–361. doi: 10.1016/S0306-4522(98)00451-5
Farr, T. D., and Whishaw, I. Q. (2002). Quantitative and qualitative impairments in skilled reaching in the mouse (Mus musculus) after a focal motor cortex stroke. Stroke 33, 1869–1875. doi: 10.1161/01.STR.0000020714.48349.4E
Ferezou, I., Haiss, F., Gentet, L. J., Aronoff, R., Weber, B., and Petersen, C. C. (2007). Spatiotemporal dynamics of cortical sensorimotor integration in behaving mice. Neuron 56, 907–923. doi: 10.1016/j.neuron.2007.10.007
Fernandez-Garcia, L., Mari-Buye, N., Barios, J. A., Madurga, R., Elices, M., Perez-Rigueiro, J., et al. (2016). Safety and tolerability of silk fibroin hydrogels implanted into the mouse brain. Acta Biomater. 45, 262–275. doi: 10.1016/j.actbio.2016.09.003
Foeller, E., Celikel, T., and Feldman, D. E. (2005). Inhibitory sharpening of receptive fields contributes to whisker map plasticity in rat somatosensory cortex. J. Neurophysiol. 94, 4387–4400. doi: 10.1152/jn.00553.2005
Gaillard, A., Prestoz, L., Dumartin, B., Cantereau, A., Morel, F., Roger, M., et al. (2007). Reestablishment of damaged adult motor pathways by grafted embryonic cortical neurons. Nat. Neurosci. 10, 1294–1299. doi: 10.1038/nn1970
George, P. M., and Steinberg, G. K. (2015). Novel stroke therapeutics: unraveling stroke pathophysiology and its impact on clinical treatments. Neuron 87, 297–309. doi: 10.1016/j.neuron.2015.05.041
Gervois, P., Wolfs, E., Ratajczak, J., Dillen, Y., Vangansewinkel, T., Hilkens, P., et al. (2016). Stem cell-based therapies for ischemic stroke: preclinical results and the potential of imaging-assisted evaluation of donor cell fate and mechanisms of brain regeneration. Med. Res. Rev. 36, 1080–1126. doi: 10.1002/med.21400
Gonzalez, C. L., and Kolb, B. (2003). A comparison of different models of stroke on behaviour and brain morphology. Eur. J. Neurosci. 18, 1950–1962. doi: 10.1046/j.1460-9568.2003.02928.x
González-Nieto, D., Fernández-García, L., Pérez-Rigueiro, J., Guinea, G., and Panetsos, F. (2018). Hydrogels-assisted cell engraftment for repairing the stroke-damaged brain: chimera or reality. Polymers 10:184. doi: 10.3390/polym10020184
Graziano, A., and Jones, E. G. (2009). Early withdrawal of axons from higher centers in response to peripheral somatosensory denervation. J. Neurosci. 29, 3738–3748. doi: 10.1523/JNEUROSCI.5388-08.2009
Guan, J., Zhu, Z., Zhao, R. C., Xiao, Z., Wu, C., Han, Q., et al. (2013). Transplantation of human mesenchymal stem cells loaded on collagen scaffolds for the treatment of traumatic brain injury in rats. Biomaterials 34, 5937–5946. doi: 10.1016/j.biomaterials.2013.04.047
Harrison, T. C., Silasi, G., Boyd, J. D., and Murphy, T. H. (2013). Displacement of sensory maps and disorganization of motor cortex after targeted stroke in mice. Stroke 44, 2300–2306. doi: 10.1161/STROKEAHA.113.001272
Hensch, T. K. (2005). Critical period plasticity in local cortical circuits. Nat. Rev. Neurosci. 6, 877–888. doi: 10.1038/nrn1787
Hermann, D. M., Peruzzotti-Jametti, L., Schlechter, J., Bernstock, J. D., Doeppner, T. R., and Pluchino, S. (2014). Neural precursor cells in the ischemic brain - integration, cellular crosstalk, and consequences for stroke recovery. Front. Cell. Neurosci. 8:291. doi: 10.3389/fncel.2014.00291
Hess, D. C., Wechsler, L. R., Clark, W. M., Savitz, S. I., Ford, G. A., Chiu, D., et al. (2017). Safety and efficacy of multipotent adult progenitor cells in acute ischaemic stroke (MASTERS): a randomised, double-blind, placebo-controlled, phase 2 trial. Lancet Neurol. 16, 360–368. doi: 10.1016/S1474-4422(17)30046-7
Hira, R., Ohkubo, F., Ozawa, K., Isomura, Y., Kitamura, K., Kano, M., et al. (2013a). Spatiotemporal dynamics of functional clusters of neurons in the mouse motor cortex during a voluntary movement. J. Neurosci. 33, 1377–1390. doi: 10.1523/JNEUROSCI.2550-12.2013
Hira, R., Ohkubo, F., Tanaka, Y. R., Masamizu, Y., Augustine, G. J., Kasai, H., et al. (2013b). In vivo optogenetic tracing of functional corticocortical connections between motor forelimb areas. Front. Neural Circuits 7:55. doi: 10.3389/fncir.2013.00055
Hira, R., Terada, S., Kondo, M., and Matsuzaki, M. (2015). Distinct functional modules for discrete and rhythmic forelimb movements in the mouse motor cortex. J. Neurosci. 35, 13311–13322. doi: 10.1523/JNEUROSCI.2731-15.2015
Jacobs, K. M., and Donoghue, J. P. (1991). Reshaping the cortical motor map by unmasking latent intracortical connections. Science 251, 944–947. doi: 10.1126/science.2000496
Jaillard, A., Martin, C. D., Garambois, K., Lebas, J. F., and Hommel, M. (2005). Vicarious function within the human primary motor cortex? A longitudinal fMRI stroke study. Brain 128, 1122–1138. doi: 10.1093/brain/awh456
Jendelova, P., Kubinova, S., Sandvig, I., Erceg, S., Sandvig, A., and Sykova, E. (2016). Current developments in cell- and biomaterial-based approaches for stroke repair. Expert Opin. Biol. Ther. 16, 43–56. doi: 10.1517/14712598.2016.1094457
Jenkins, W. M., Merzenich, M. M., Ochs, M. T., Allard, T., and Guic-Robles, E. (1990). Functional reorganization of primary somatosensory cortex in adult owl monkeys after behaviorally controlled tactile stimulation. J. Neurophysiol. 63, 82–104. doi: 10.1152/jn.1990.63.1.82
Jin, K., Mao, X., Xie, L., Greenberg, R. B., Peng, B., Moore, A., et al. (2010). Delayed transplantation of human neural precursor cells improves outcome from focal cerebral ischemia in aged rats. Aging Cell 9, 1076–1083. doi: 10.1111/j.1474-9726.2010.00638.x
Jones, E. G. (1993). GABAergic neurons and their role in cortical plasticity in primates. Cereb. Cortex 3, 361–372. doi: 10.1093/cercor/3.5.361-a
Kelly, S., Bliss, T. M., Shah, A. K., Sun, G. H., Ma, M., Foo, W. C., et al. (2004). Transplanted human fetal neural stem cells survive, migrate, and differentiate in ischemic rat cerebral cortex. Proc. Natl. Acad. Sci. U.S.A. 101, 11839–11844. doi: 10.1073/pnas.0404474101
Kim, S. Y., Allred, R. P., Adkins, D. L., Tennant, K. A., Donlan, N. A., Kleim, J. A., et al. (2015). Experience with the “good” limb induces aberrant synaptic plasticity in the perilesion cortex after stroke. J. Neurosci. 35, 8604–8610. doi: 10.1523/JNEUROSCI.0829-15.2015
Knieling, M., Metz, G. A., Antonow-Schlorke, I., and Witte, O. W. (2009). Enriched environment promotes efficiency of compensatory movements after cerebral ischemia in rats. Neuroscience 163, 759–769. doi: 10.1016/j.neuroscience.2009.07.004
Li, S., Nie, E. H., Yin, Y., Benowitz, L. I., Tung, S., Vinters, H. V., et al. (2015). GDF10 is a signal for axonal sprouting and functional recovery after stroke. Nat. Neurosci. 18, 1737–1745. doi: 10.1038/nn.4146
Li, S., Overman, J. J., Katsman, D., Kozlov, S. V., Donnelly, C. J., Twiss, J. L., et al. (2010). An age-related sprouting transcriptome provides molecular control of axonal sprouting after stroke. Nat. Neurosci. 13, 1496–1504. doi: 10.1038/nn.2674
Li, Y., McIntosh, K., Chen, J., Zhang, C., Gao, Q., Borneman, J., et al. (2006). Allogeneic bone marrow stromal cells promote glial-axonal remodeling without immunologic sensitization after stroke in rats. Exp. Neurol. 198, 313–325. doi: 10.1016/j.expneurol.2005.11.029
Lim, D. H., LeDue, J. M., Mohajerani, M. H., and Murphy, T. H. (2014). Optogenetic mapping after stroke reveals network-wide scaling of functional connections and heterogeneous recovery of the peri-infarct. J. Neurosci. 34, 16455–16466. doi: 10.1523/JNEUROSCI.3384-14.2014
Liu, Z., Li, Y., Zhang, X., Savant-Bhonsale, S., and Chopp, M. (2008). Contralesional axonal remodeling of the corticospinal system in adult rats after stroke and bone marrow stromal cell treatment. Stroke 39, 2571–2577. doi: 10.1161/STROKEAHA.107.511659
Liu, Z., Li, Y., Zhang, Z. G., Cui, X., Cui, Y., Lu, M., et al. (2010). Bone marrow stromal cells enhance inter- and intracortical axonal connections after ischemic stroke in adult rats. J. Cereb. Blood Flow Metab. 30, 1288–1295. doi: 10.1038/jcbfm.2010.8
Matyas, F., Sreenivasan, V., Marbach, F., Wacongne, C., Barsy, B., Mateo, C., et al. (2010). Motor control by sensory cortex. Science 330, 1240–1243. doi: 10.1126/science.1195797
Merzenich, M. M., Nelson, R. J., Stryker, M. P., Cynader, M. S., Schoppmann, A., and Zook, J. M. (1984). Somatosensory cortical map changes following digit amputation in adult monkeys. J. Comp. Neurol. 224, 591–605. doi: 10.1002/cne.902240408
Michelsen, K. A., Acosta-Verdugo, S., Benoit-Marand, M., Espuny-Camacho, I., Gaspard, N., Saha, B., et al. (2015). Area-specific reestablishment of damaged circuits in the adult cerebral cortex by cortical neurons derived from mouse embryonic stem cells. Neuron 85, 982–997. doi: 10.1016/j.neuron.2015.02.001S0896-6273(15)00090-2
Moloney, T. C., Dockery, P., Windebank, A. J., Barry, F. P., Howard, L., and Dowd, E. (2010). Survival and immunogenicity of mesenchymal stem cells from the green fluorescent protein transgenic rat in the adult rat brain. Neurorehabil. Neural Repair 24, 645–656. doi: 10.1177/15459683093577451545968309357745
Moon, S. K., Alaverdashvili, M., Cross, A. R., and Whishaw, I. Q. (2009). Both compensation and recovery of skilled reaching following small photothrombotic stroke to motor cortex in the rat. Exp. Neurol. 218, 145–153. doi: 10.1016/j.expneurol.2009.04.021
Mora-Lee, S., Sirerol-Piquer, M. S., Gutierrez-Perez, M., Gomez-Pinedo, U., Roobrouck, V. D., Lopez, T., et al. (2012). Therapeutic effects of hMAPC and hMSC transplantation after stroke in mice. PLoS One 7:e43683. doi: 10.1371/journal.pone.0043683
Moshayedi, P., Nih, L. R., Llorente, I. L., Berg, A. R., Cinkornpumin, J., Lowry, W. E., et al. (2016). Systematic optimization of an engineered hydrogel allows for selective control of human neural stem cell survival and differentiation after transplantation in the stroke brain. Biomaterials 105, 145–155. doi: 10.1016/j.biomaterials.2016.07.028
Munoz, J. R., Stoutenger, B. R., Robinson, A. P., Spees, J. L., and Prockop, D. J. (2005). Human stem/progenitor cells from bone marrow promote neurogenesis of endogenous neural stem cells in the hippocampus of mice. Proc. Natl. Acad. Sci. U.S.A. 102, 18171–18176. doi: 10.1073/pnas.0508945102
Murphy, T. H., and Corbett, D. (2009). Plasticity during stroke recovery: from synapse to behaviour. Nat. Rev. Neurosci. 10, 861–872. doi: 10.1038/nrn2735
Nih, L. R., Carmichael, S. T., and Segura, T. (2016). Hydrogels for brain repair after stroke: an emerging treatment option. Curr. Opin. Biotechnol. 40, 155–163. doi: 10.1016/j.copbio.2016.04.021
Nih, L. R., Gojgini, S., Carmichael, S. T., and Segura, T. (2018). Dual-function injectable angiogenic biomaterial for the repair of brain tissue following stroke. Nat. Mater. 17, 642–651. doi: 10.1038/s41563-018-0083-8
Nishibe, M., Urban, E. T. III, Barbay, S., and Nudo, R. J. (2015). Rehabilitative training promotes rapid motor recovery but delayed motor map reorganization in a rat cortical ischemic infarct model. Neurorehabil. Neural Repair 29, 472–482. doi: 10.1177/1545968314543499
Nudo, R. J., and Milliken, G. W. (1996). Reorganization of movement representations in primary motor cortex following focal ischemic infarcts in adult squirrel monkeys. J. Neurophysiol. 75, 2144–2149. doi: 10.1152/jn.1996.75.5.2144
Nudo, R. J., Milliken, G. W., Jenkins, W. M., and Merzenich, M. M. (1996a). Use-dependent alterations of movement representations in primary motor cortex of adult squirrel monkeys. J. Neurosci. 16, 785–807.
Nudo, R. J., Wise, B. M., SiFuentes, F., and Milliken, G. W. (1996b). Neural substrates for the effects of rehabilitative training on motor recovery after ischemic infarct. Science 272, 1791–1794.
Ohtaki, H., Ylostalo, J. H., Foraker, J. E., Robinson, A. P., Reger, R. L., Shioda, S., et al. (2008). Stem/progenitor cells from bone marrow decrease neuronal death in global ischemia by modulation of inflammatory/immune responses. Proc. Natl. Acad. Sci. U.S.A. 105, 14638–14643. doi: 10.1073/pnas.08036701050803670105
Osanai, T., Kuroda, S., Yasuda, H., Chiba, Y., Maruichi, K., Hokari, M., et al. (2010). Noninvasive transplantation of bone marrow stromal cells for ischemic stroke: preliminary study with a thermoreversible gelation polymer hydrogel. Neurosurgery 66, 1140–1147; discussion 1147. doi: 10.1227/01.NEU.0000369610.76181.CF
Overman, J. J., Clarkson, A. N., Wanner, I. B., Overman, W. T., Eckstein, I., Maguire, J. L., et al. (2012). A role for ephrin-A5 in axonal sprouting, recovery, and activity-dependent plasticity after stroke. Proc. Natl. Acad. Sci. U.S.A. 109, E2230–E2239. doi: 10.1073/pnas.1204386109
Panetsos, F., Nunez, A., and Avendano, C. (1995). Local anaesthesia induces immediate receptive field changes in nucleus gracilis and cortex. Neuroreport 7, 150–152. doi: 10.1097/00001756-199512290-00036
Paxinos, G., and Franklin, K. B. J. (eds) (2004). The Mouse Brain in Stereotaxic Coordinates. New York, NY: Elsevier Science.
Petrey, A. C., and de la Motte, C. A. (2014). Hyaluronan, a crucial regulator of inflammation. Front. Immunol. 5:101. doi: 10.3389/fimmu.2014.00101
Prasad, K., Sharma, A., Garg, A., Mohanty, S., Bhatnagar, S., Johri, S., et al. (2014). Intravenous autologous bone marrow mononuclear stem cell therapy for ischemic stroke: a multicentric, randomized trial. Stroke 45, 3618–3624. doi: 10.1161/STROKEAHA.114.007028
Qu, C., Xiong, Y., Mahmood, A., Kaplan, D. L., Goussev, A., Ning, R., et al. (2009). Treatment of traumatic brain injury in mice with bone marrow stromal cell-impregnated collagen scaffolds. J. Neurosurg. 111, 658–665. doi: 10.3171/2009.4.JNS081681
Quittet, M. S., Touzani, O., Sindji, L., Cayon, J., Fillesoye, F., Toutain, J., et al. (2015). Effects of mesenchymal stem cell therapy, in association with pharmacologically active microcarriers releasing VEGF, in an ischaemic stroke model in the rat. Acta Biomater. 15, 77–88. doi: 10.1016/j.actbio.2014.12.017
Ramos-Cabrer, P., Justicia, C., Wiedermann, D., and Hoehn, M. (2010). Stem cell mediation of functional recovery after stroke in the rat. PLoS One 5:e12779. doi: 10.1371/journal.pone.0012779
Rustenhoven, J., Aalderink, M., Scotter, E. L., Oldfield, R. L., Bergin, P. S., Mee, E. W., et al. (2016). TGF-beta1 regulates human brain pericyte inflammatory processes involved in neurovasculature function. J. Neuroinflammation 13, 37. doi: 10.1186/s12974-016-0503-0
Saver, J. L., Goyal, M., Bonafe, A., Diener, H. C., Levy, E. I., Pereira, V. M., et al. (2015). Stent-retriever thrombectomy after intravenous t-PA vs. t-PA alone in stroke. N. Engl. J. Med. 372, 2285–2295. doi: 10.1056/NEJMoa1415061
Schaechter, J. D., Moore, C. I., Connell, B. D., Rosen, B. R., and Dijkhuizen, R. M. (2006). Structural and functional plasticity in the somatosensory cortex of chronic stroke patients. Brain 129, 2722–2733. doi: 10.1093/brain/awl214
Scheibe, F., Ladhoff, J., Huck, J., Grohmann, M., Blazej, K., Oersal, A., et al. (2012). Immune effects of mesenchymal stromal cells in experimental stroke. J. Cereb. Blood Flow Metab. 32, 1578–1588. doi: 10.1038/jcbfm.2012.55
Shanina, E. V., Schallert, T., Witte, O. W., and Redecker, C. (2006). Behavioral recovery from unilateral photothrombotic infarcts of the forelimb sensorimotor cortex in rats: role of the contralateral cortex. Neuroscience 139, 1495–1506. doi: 10.1016/j.neuroscience.2006.01.016
Shiromoto, T., Okabe, N., Lu, F., Maruyama-Nakamura, E., Himi, N., Narita, K., et al. (2017). The role of endogenous neurogenesis in functional recovery and motor map reorganization induced by rehabilitative therapy after stroke in rats. J. Stroke Cerebrovasc. Dis. 26, 260–272. doi: 10.1016/j.jstrokecerebrovasdis.2016.09.016
Simoes, E. L., Bramati, I., Rodrigues, E., Franzoi, A., Moll, J., Lent, R., et al. (2012). Functional expansion of sensorimotor representation and structural reorganization of callosal connections in lower limb amputees. J. Neurosci. 32, 3211–3220. doi: 10.1523/JNEUROSCI.4592-11.2012
Steinbeck, J. A., and Studer, L. (2015). Moving stem cells to the clinic: potential and limitations for brain repair. Neuron 86, 187–206. doi: 10.1016/j.neuron.2015.03.002
Steinberg, G. K., Kondziolka, D., Wechsler, L. R., Lunsford, L. D., Coburn, M. L., Billigen, J. B., et al. (2016). Clinical outcomes of transplanted modified bone marrow-derived mesenchymal stem cells in stroke: a phase 1/2a study. Stroke 47, 1817–1824. doi: 10.1161/STROKEAHA.116.012995
Stoppato, M., Stevens, H. Y., Carletti, E., Migliaresi, C., Motta, A., and Guldberg, R. E. (2013). Effects of silk fibroin fiber incorporation on mechanical properties, endothelial cell colonization and vascularization of PDLLA scaffolds. Biomaterials 34, 4573–4581. doi: 10.1016/j.biomaterials.2013.02.009
Stripecke, R., Carmen Villacres, M., Skelton, D., Satake, N., Halene, S., and Kohn, D. (1999). Immune response to green fluorescent protein: implications for gene therapy. Gene Ther. 6, 1305–1312. doi: 10.1038/sj.gt.3300951
Stroemer, R. P., Kent, T. A., and Hulsebosch, C. E. (1993). Acute increase in expression of growth associated protein GAP-43 following cortical ischemia in rat. Neurosci. Lett. 162, 51–54. doi: 10.1016/0304-3940(93)90557-2
Stroemer, R. P., Kent, T. A., and Hulsebosch, C. E. (1995). Neocortical neural sprouting, synaptogenesis, and behavioral recovery after neocortical infarction in rats. Stroke 26, 2135–2144. doi: 10.1161/01.STR.26.11.2135
Taguchi, A., Soma, T., Tanaka, H., Kanda, T., Nishimura, H., Yoshikawa, H., et al. (2004). Administration of CD34+ cells after stroke enhances neurogenesis via angiogenesis in a mouse model. J. Clin. Invest. 114, 330–338. doi: 10.1172/JCI20622
Taylor, R. A., Chang, C. F., Goods, B. A., Hammond, M. D., Mac Grory, B., Ai, Y., et al. (2017). TGF-beta1 modulates microglial phenotype and promotes recovery after intracerebral hemorrhage. J. Ciln. Invest. 127, 280–292. doi: 10.1172/JCI88647
Tennant, K. A., Adkins, D. L., Donlan, N. A., Asay, A. L., Thomas, N., Kleim, J. A., et al. (2011). The organization of the forelimb representation of the C57BL/6 mouse motor cortex as defined by intracortical microstimulation and cytoarchitecture. Cereb. Cortex 21, 865–876. doi: 10.1093/cercor/bhq159
Tennant, K. A., Kerr, A. L., Adkins, D. L., Donlan, N., Thomas, N., Kleim, J. A., et al. (2015). Age-dependent reorganization of peri-infarct “premotor” cortex with task-specific rehabilitative training in mice. Neurorehabil. Neural Repair 29, 193–202. doi: 10.1177/1545968314541329
Traversa, R., Cicinelli, P., Bassi, A., Rossini, P. M., and Bernardi, G. (1997). Mapping of motor cortical reorganization after stroke. A brain stimulation study with focal magnetic pulses. Stroke 28, 110–117. doi: 10.1161/01.STR.28.1.110
Trounson, A., and McDonald, C. (2015). Stem cell therapies in clinical trials: progress and challenges. Cell Stem Cell 17, 11–22. doi: 10.1016/j.stem.2015.06.007
Unger, R. E., Sartoris, A., Peters, K., Motta, A., Migliaresi, C., Kunkel, M., et al. (2007). Tissue-like self-assembly in cocultures of endothelial cells and osteoblasts and the formation of microcapillary-like structures on three-dimensional porous biomaterials. Biomaterials 28, 3965–3976. doi: 10.1016/j.biomaterials.2007.05.032
van Velthoven, C. T., van de Looij, Y., Kavelaars, A., Zijlstra, J., van Bel, F., Huppi, P. S., et al. (2012). Mesenchymal stem cells restore cortical rewiring after neonatal ischemia in mice. Ann. Neurol. 71, 785–796. doi: 10.1002/ana.23543
Vanni, M. P., and Murphy, T. H. (2014). Mesoscale transcranial spontaneous activity mapping in GCaMP3 transgenic mice reveals extensive reciprocal connections between areas of somatomotor cortex. J. Neurosci. 34, 15931–15946. doi: 10.1523/JNEUROSCI.1818-14.2014
Vu, Q., Xie, K., Eckert, M., Zhao, W., and Cramer, S. C. (2014). Meta-analysis of preclinical studies of mesenchymal stromal cells for ischemic stroke. Neurology 82, 1277–1286. doi: 10.1212/WNL.0000000000000278
Wang, Y., Zhao, Z., Rege, S. V., Wang, M., Si, G., Zhou, Y., et al. (2016). 3K3A-activated protein C stimulates postischemic neuronal repair by human neural stem cells in mice. Nat. Med. 22, 1050–1055. doi: 10.1038/nm.4154
Whishaw, I. Q. (2000). Loss of the innate cortical engram for action patterns used in skilled reaching and the development of behavioral compensation following motor cortex lesions in the rat. Neuropharmacology 39, 788–805. doi: 10.1016/S0028-3908(99)00259-2
Xerri, C., Merzenich, M. M., Peterson, B. E., and Jenkins, W. (1998). Plasticity of primary somatosensory cortex paralleling sensorimotor skill recovery from stroke in adult monkeys. J. Neurophysiol. 79, 2119–2148. doi: 10.1152/jn.1998.79.4.2119
Yang, B., Strong, R., Sharma, S., Brenneman, M., Mallikarjunarao, K., Xi, X., et al. (2011). Therapeutic time window and dose response of autologous bone marrow mononuclear cells for ischemic stroke. J. Neurosci. Res. 89, 833–839. doi: 10.1002/jnr.22614
Keywords: brain remapping and plasticity, sensorimotor recovery, stroke, mesenchymal stem cells, silk fibroin hydrogels, somatosensory and motor cortex
Citation: Fernández-García L, Pérez-Rigueiro J, Martinez-Murillo R, Panetsos F, Ramos M, Guinea GV and González-Nieto D (2018) Cortical Reshaping and Functional Recovery Induced by Silk Fibroin Hydrogels-Encapsulated Stem Cells Implanted in Stroke Animals. Front. Cell. Neurosci. 12:296. doi: 10.3389/fncel.2018.00296
Received: 05 May 2018; Accepted: 16 August 2018;
Published: 06 September 2018.
Edited by:
Dirk M. Hermann, Universität Duisburg-Essen, GermanyReviewed by:
Afsaneh Gaillard, University of Poitiers, FranceJoshua D. Bernstock, National Institutes of Health, United States and University of Cambridge, United Kingdom
Copyright © 2018 Fernández-García, Pérez-Rigueiro, Martinez-Murillo, Panetsos, Ramos, Guinea and González-Nieto. This is an open-access article distributed under the terms of the Creative Commons Attribution License (CC BY). The use, distribution or reproduction in other forums is permitted, provided the original author(s) and the copyright owner(s) are credited and that the original publication in this journal is cited, in accordance with accepted academic practice. No use, distribution or reproduction is permitted which does not comply with these terms.
*Correspondence: Daniel González-Nieto, ZGFuaWVsLmdvbnphbGV6QGN0Yi51cG0uZXM=