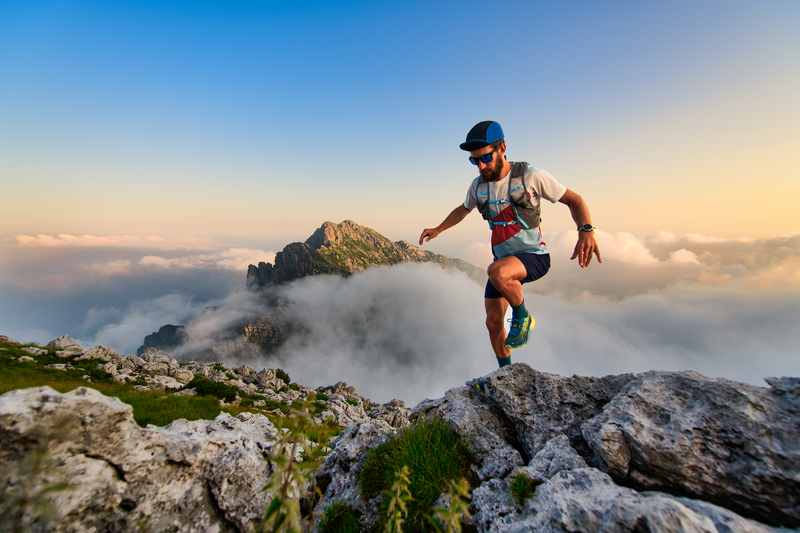
95% of researchers rate our articles as excellent or good
Learn more about the work of our research integrity team to safeguard the quality of each article we publish.
Find out more
ORIGINAL RESEARCH article
Front. Cell. Neurosci. , 19 July 2017
Sec. Cellular Neuropathology
Volume 11 - 2017 | https://doi.org/10.3389/fncel.2017.00213
Transected spinal cord injury (SCT) is a devastating clinical disease that strongly affects a patient’s daily life and remains a great challenge for clinicians. Stem-cell therapy has been proposed as a potential therapeutic modality for SCT. To investigate the effects of hematopoietic stem cells (HSCs) on the recovery of structure and function in SCT rats and to explore the mechanisms associated with recovery, 57 adult Sprague-Dawley rats were randomly divided into sham (n = 15), SCT (n = 24), and HSC transplantation groups (n = 15). HSCs (passage 3) labeled by Hoechst 33342, were transplanted intraspinally into the rostral, scar and caudal sites of the transected lesion at 14 days post-operation. Both in vitro and in vivo, HSCs exhibited a capacity for cell proliferation and differentiation. Following HSC transplantation, the animals’ Basso, Beattie, and Bresnahan (BBB). locomotion scale scores increased significantly between weeks 4 and 24 post-SCT, which corresponded to an increased number of 5-hydroxytryptamine (5-HT) fibers and oligodendrocytes. The amount of astrogliosis indicated by immunohistochemical staining, was markedly decreased. Moreover, the decreased expression of neurotrophin- 3 (NT-3) and mitogen-activated protein kinase kinase-1 (MEK-1) after SCT was effectively restored by HSC transplantation. The data from the current study indicate that intraspinally administered HSCs in the chronic phase of SCT results in an improvement in neurological function. Further, the results indicate that intraspinally administered HSCs benefit the underlying mechanisms involved in the enhancement of 5-HT-positive fibers and oligogenesis, the suppression of excessive astrogliosis and the upregulation of NT3-regulated MEK-1 activation in the spinal cord. These crucial findings reveal not only the mechanism of cell therapy, but may also contribute to a novel therapeutic target for the treatment of spinal cord injury (SCI).
Spinal cord injury (SCI) is a devastating clinical disorder that usually results severe damage to sensory, motor and autonomic functions distal to the level of the trauma (Hirano et al., 2012; Kamei et al., 2012; Vawda and Fehlings, 2013; Dasari et al., 2014). Current treatments consist of decompressing and stabilizing the injury, preventing secondary complications and rehabilitating function (Bavaria et al., 2007; Tederko et al., 2009), but the efficacy of these treatments is limited. As a result, most SCI patients suffer from substantial neurological dysfunction and lifelong disability (Coutts and Keirstead, 2008; Bracken, 2012). Therefore, it is important to study the underlying mechanisms of and find new therapies for SCI.
Stem cell transplantation, an effective method of biotherapy, has been used to improve nervous system disorders (Mothe and Tator, 2012; Vawda and Fehlings, 2013). Hematopoietic stem cells (HSCs; Aggarwal et al., 2012), a type of multipotent stem cell that can give rise to all types of blood cells and lymphoid lineages, have been used to treat various hematological disorders such as severe combined immunodeficiency, congenital neutropenia (Tyndall et al., 1999; Burt et al., 2008), and malignancies (Mendez et al., 2005). Recently, HSC transplantation (Huang et al., 2010), has also been used as a valuable therapeutic intervention for stroke, traumatic brain injury and multiple sclerosis (MS; Burt et al., 1995), and to study the underlying mechanisms involved in transdifferentiation, neuroprotection through trophic support and cell fusion (Haas et al., 2005), as well as the replacement of lost or damaged cell populations (Nishio et al., 2006; Schwarting et al., 2008; Xu and Onifer, 2009; Mothe and Tator, 2012; Boulland et al., 2013; Vawda and Fehlings, 2013; Nicaise et al., 2015; Singh, 2015). In addition, several previous studies have indicated that transplantation of HSCs from bone marrow or human umbilical cord blood (HUCB) could effectively promote the repair of the spinal cord in animals 1 week post-injury (Koshizuka et al., 2004; Cabanes et al., 2007; Dasari et al., 2008; Deda et al., 2008). Moreover, HSCs have been used in the treatment of SCI patients in clinic, and have shown beneficial effects in reducing deterioration after SCI (Deda et al., 2008; Bryukhovetskiy and Bryukhovetskiy, 2015; Thakkar et al., 2016). Bryukhovetskiy and Bryukhovetskiy (2015) demonstrated the safety and effectiveness of HSCs transplantation in 202 cases of SCI, showing that the administration of HSC can effectively improve the quality of life for SCI patients. However, the concrete mechanisms underlying the effect of HSC transplantation for both morphological remodeling and molecular alternation remain to be understood. Previously, it has been shown that vascular endothelial growth factor (VEGF), and stromal cell-derived factor-1 (SDF1), and its receptor, Cxc chemokine receptor 4 (CXCR4) were involved in the effects caused by HSC transplantation (Deda et al., 2008). However, crucial evidence linking HSCs to improvement in neural behavior in terms of both morphology and at the molecular level is too limited.
Neurotrophic factors (NTFs) play a vital role in nerve regeneration, neovascularization and growth and differentiation of neurons and non-neuronal cells (Lambert et al., 2004; Feng et al., 2005). Persistent delivery of NTFs is crucial for creating a microenvironment for cell survival and nerve regeneration in SCI (Li et al., 2016). Accumulating evidence has demonstrated a crucial role for NTFs in the treatment of neurological diseases after cell transplantation (Isele et al., 2007; Müller et al., 2009; Abbaszadeh et al., 2015), but the role of Neurotrophin-3 (NT-3), an important member of the NTF family, in SCI following with HSC transplantation needs to be explored.
As previous reports have revealed that NT-3 can promote proprioceptive axon regeneration in the injured spinal cord (Li et al., 2016; Liu et al., 2016; Keefe et al., 2017) and that the mitogen-activated protein kinase kinase/extracellular signal-regulated kinase/cAMP-response element binding protein (MEK/ERK/CREB) signaling pathway is crucial for neuroprotection (Lebesgue et al., 2009), the current study was designed to evaluate the role of HSC transplantation in the transected spinal cord, and to explore associated mechanisms involving NT-3-MEK signaling so as to provide translational evidence for the usage of HSCs in future clinical trials. The results revealed that HSC transplantation can improve the neurological function of transected spinal cord injury (SCT) rats by enhancing 5-hydroxytryptamine (5-HT) positive fibers and oligogenesis, suppressing excessive astrogliosis, and upregulating NT3-regulated MEK-1 activation in the spinal cord. These findings reveal the mechanism of cell therapy and contribute to a novel therapeutic target for the treatment of SCI.
Fifty-seven adult male Sprague-Dawley (SD) rats, weighing 220 ± 20 g, were provided by the Center of Experimental Animals, Kunming Medical University. Animals were provided access to pellet chow and water ad libitum and were housed in individual cages in a temperature (21–25°C) and humidity (45%–50%) controlled room with a 12-h light/dark cycle. In addition, following SCT, rats were placed in warm condition to keep body temperatures and the bladders were manually massaged three times a day to enhance their function.This study was carried out in accordance with the recommendations of guidelines for laboratory animal care and safety from the United States National Institutes of Health. The protocol was approved by the guidelines of the Institutional Medical Experimental Animal Care Committee of Sichuan University, West China Hospital, China.
The rats were randomly divided into three groups. The sham group (n = 15) received no transection or transplantation; the SCT group (n = 15) had SCT performed and treated with Dulbecco’s Modified Eagle Medium: nutrient mixture F-12 (DMEM/F12; Hyclone, Logan, UT, USA); the transplantation group (n = 24) were subjected to SCT and then injected with an HSC suspension. The assignment of cases is shown in Table 1.
The SCT procedure was established as previously described (Liu et al., 2014). Briefly, rats were anesthetized intraperitoneally with 2% sodium pentobarbital sodium at a dose of 30 mg/kg. A laminectomy of T9-11 was subsequently performed, and the dura was opened with a surgical blade to expose a length of spinal cord about approximately 1.5 cm long. Complete transection of the T10 spinal cord was performed and the intervening tissue was removed. The completeness of the transection was assured by lifting the cut ends with small forceps. Sham animals underwent the same surgical procedures except for the T10 transection. After transecting the spinal cord, the surgery incision was sutured. The animals’ hind limbs became motionless after their surgery, remaining in a condition of paraplegia (Gruner, 1992). Following the procedure, 5% cefotaxime sodium salt (diluted 10× with normal saline) was injected intraperitoneally (0.5 μl per rat) once per day until the post-operative day 7. The bladders were manually massaged three times a day until recovery of micturition reflex.
Three SD rats were sacrificed routinely after being anesthetized as described above, and the femurs were removed. Bone marrow was obtained from femoral bones as previously reported (Sasaki et al., 2001; Koda et al., 2005). Briefly, the epiphyses of the femurs were removed, and the femurs were dissected down the midline. The marrow was then extruded using a syringe filled with DMEM/F12 containing 10% fetal bovine serum (FBS, Gibco, Carlsbad, CA, USA) to obtain the amount of marrow from the head of the femur. Afterward, the bone marrow was beaten into a single cell suspension with 5 ml DMEM/F12 containing 10% FBS and 10,000 U/L penicillin and 10 mg/L streptomycin. After centrifuging (1000 rpm, 5 min) and re-suspending, cells were plated in a 75 cm2 culture flask at a density of 1 × 106 cells/ml and incubated for 12 h (37°C, 95% humidity, 5% CO2). The suspended cells were then collected in a flask and transferred to another culture flask for further incubating. Culture media was changed twice per week. When the cells grew to a density of between 4 and 5 × 106 cells/cm2, they were passaged. Only suspended cells were collected for 2–3 weeks following cultivation due to a lack of additional adherent cells any more. Thus, following the third passage, we were left with pure suspended HSCs. After culturing for 3 days, the HSCs were identified by CD34 enzyme histo-cytochemical staining.
HSCs were labeled by Hoechst 33342 2 h prior to transplantation. Briefly, a fluorescent dye was added after replacing the media, then the cells were washed with phosphate-buffered saline (PBS; no Ca2+ or Mg2+) after being incubated at 37°C with 5% CO2 for 2 h. After incubation, DMEM/F12 was added and the cells were counted and concentrated at a final concentration of 2.0 × 104/μl.
Each rat was anesthetized and placed in a stereotaxic frame. Six sites around the rostral, scar and caudal area of the transected spinal cord were injected using the following coordinates: two sites 5 mm rostral and two sites 5 mm caudal to the injured site, two sites located in the injured site. At a rate of 600 nl/min, a total of 6 μl of the cell suspension was injected into the spinal cord through a glass micropipette positioned at 60 degrees, with 1 μl of the cell suspension (2.0 × 104/μl) injected at each site. After injection, the glass pipette remained in its position for 5 min before being slowly retracted. The control group underwent the same procedure using DMEM/F12. Then, the rat was removed from the device, and the incision was sutured. For suppressing the immunoreaction, cyclosporin-A (10 mg/kg per day) was intraperitoneally used from the third day before transplantation, and kept till the animals were sacrificed.
From week 1 to 24 post-SCT, Basso, Beattie and Bresnahan. (BBB) locomotor rating scale values were recorded in an open enclosure (99 cm in diameter, 23 cm deep) with scores graded from 0 points (absence of any hind limb movement) to 21 points (normal mobility Basso et al., 1995). In order to avoid motionlessness upon introduction to a new environment, subjects were acclimated to the observation fields for 3 days prior to surgery for 5 min per day. During testing, each subject was placed in the open field and observed for 4 min (He et al., 2016). The final score was the average of three individual researchers, who were blinded to the experimental treatment.
Twenty-four weeks after SCT, rats were anesthetized with 3.6% chloral hydrate (1 ml/100 g, intraperitoneal injection) and transcardially perfused with heparinized physiological saline followed by 4% paraformaldehyde in 0.1 M ice-cold phosphate buffer, pH 7.4. Immediately after perfusion, a length of spinal cord extending from 10 mm rostral to 10 mm caudal to the injured site was collected. Samples for quantitative real-time polymerase chain reaction (qRT-PCR) were harvested and stored in 1.5 ml RNase-free Eppendorf tubes at −80°C. Spinal cords for immunohistochemical staining were post-fixed for 5 h at 4°C. The tissues were stored in 30% sucrose in 0.1 M phosphate buffer, pH 7.4, for 72 h at 4°C. Then the rostral, scar and caudal segments of transected spinal cord from the different groups were embedded in the same paraffin block and sectioned at 5 μm thickness. After routinely deparaffinized and rehydrated, immunohistochemistry was performed on the slices of spinal cord tissue. Some slices were placed under a fluorescent microscope for direct observation of the state of the transplanted cells.
To observe the differentiation of HSCs into neurons and astrocytes in vitro and in vivo, immunofluorescence staining of Tuj1 and aldehyde dehydrogenase 1 family, member L1 (ALDH1L1) was performed, respectively. In brief, the primary antibodies directed against Tuj1 (1:100, Rabbit, ABclonal, College Park, MD, USA) and ALDH1L1 (1:100, Rabbit, ABclonal Biotech), as well as the secondary antibody Dylight 594 (1:100, goat anti-rabbit, Abbkine) were applied progressively as previously described (Liu et al., 2014). A negative control was performed by using PBS in place of the primary antibodies. Slides were then viewed and photographed using a fluorescence microscope (Leica, Germany).
Immunohistochemistry was used to determine the purity of cultured HSCs (CD34 immunohistochemical staining) and observe the alternations in the oligodendrocyte precursor cells after HSC transplantation into the spinal cord. Oligodendrocytes, neurons and astrocytes were identified using APC, NeuN and GFAP antibodies, respectively. In addition, 5-HT fibers were also identified. Briefly, slices were washed in 0.01 mol/L PBS repeated three times for 5 min each, then incubated at 37.5°C in 3% hydrogen peroxide for 30 min in the dark to block the action of endogenous peroxidases. This was followed by a 5-min immersion in PBS, repeated three times, then immersion in 5% goat serum at 37.5°C for 30 min. Afterwards, sections were incubated overnight at 4°C in the primary antibody solutions shown in Table 2. A negative control was prepared by using PBS in place of the primary antibodies. Next, sections were washed three times with 0.01 mol/L PBS Tween-20 (PBST-20) and incubated with PV-9000 reagent 1 for 30 min at 37.5°C, followed by PV-9000 reagent 2 for 30 min at 37.5°C. Subsequently, sections were in incubated in the chromogenic agent 3,3′-Diaminobenzidine (DAB) for 3–7 min in the dark. The sections were rinsed with water, then counter-stained with hematoxylin. Following dehydration, sealing took place using a transparent and neutral gum, and then positive staining was visualized under an inverted phase contrast microscope imaging system. The cell numbers and sizes were analyzed using Image-Pro Plus 6.0 software (Media Cybernetics, Silver Spring, MD, USA).
Twenty-four weeks post-SCT, sections of spinal cord (10-mm in length, containing the injury and the graft) from the HSC, SCT and sham groups were collected and homogenized to determine the level of MEK-1 and NT3 mRNA. Total RNA was isolated with Trizol reagent (Takara Bio Inc., Otsu, Japan) and was reverse transcribed into cDNA. Subsequently, qRT-PCR of cDNA was performed using the following primers (TaKaRa Company (Japan)): NT3 (forward) 5′-GTCCATCTTGTTTTAT GTGAT-3′, (reverse) 5′-GTGCTCTGGAATTTTCCTT-3′; MEK-1 (forward) 5′-GCAATCCGGAACCAGATCAT-3′, (reverse) 5′-CAGGAATTCTTCCAGCTTTCT-3′. β-actin was used as the internal control. PCR conditions were as follows: initial denaturation at 95°C for 2 min, denaturation at 95°C for 15 s and amplification at 53°C for 20 s, followed by extension at 60°C for 30 s for a total of 40 cycles. The threshold cycle (Ct) of each sample was recorded, and data were analyzed by normalization to β-actin values using the 2−ΔΔCt method (Livak and Schmittgen, 2001; Liu et al., 2014).
All statistical analyses were performed with SPSS19.0 software (IBM Corporation, NY, USA). Data were analyzed by Student’s t test between two groups. For multiple group comparison, one-way ANOVA with Tukey’s post hoc multiple comparisons was applied. P < 0.05 was considered statistically significant. Data are expressed as mean ± standard deviation (SD).
At 3 days post-culture, HSCs were round in shape with bright edges and exhibited a floating growth status (Figure 1A). They increased greatly in number 7 days later (Figure 1B). CD34+, a specific marker for HSCs, was used to identify the cultured HSCs at 3 days post-culture. The results showed that CD34+ positive staining was expressed in these cells, and that the positive rate was >90% at 3 days post-culture (Figure 1C), which further confirmed the purity of the HSCs. As cultured HSCs would be transplanted into the host, Hoechst 33342 was also used to label the cultured cells, which showed a blue color with nucleus staining (Figure 1D).
Figure 1. Characterization and identification of hematopoietic stem cells (HSCs) cultured in vitro. (A) Under a phase contrast microscopy, HSCs exhibited a floating growth status at day 3, and the number increased greatly at day 7 (B). (C) Enzyme histo-cytochemical staining at 3 days showed most of the cultured HSCs expressed CD34 demonstrating the purity of the cultured HSC. (D) HSCs were labeled by Hoechst 33342 with nucleus staining, showing a blue color. Bar = 50 μm.
In order to detect the differentiation of HSCs into neurons and astrocytes, an immunofluorecence staining of Tuj1 (Figures 2A–C) and ALDH1L1 (Figures 2D–F), respectively was performed in the cultured HSCs. The results showed that HSCs have the ability to differentiate into neurons and astrocytes. In these experiments, PBS was used as negative control instead of the primary antibody, and resulted in unspecific staining except for a blue nucleus (Figures 2G–I).
Figure 2. Cultured HSCs differentiate towards neurons and glia cells in vitro. Cultured HSCs differentiated toward neurons (A–C) and astrocytes (D–F), which were identified by immunofluorecent staining of Tuj1 and aldehyde dehydrogenase 1 family, member L1 (ALDH1L1) antibodies, respectively, while the negative control showed no positive staining (G–I). Bar = 25 μm. White arrows represented the positive cells.
There were no Hoechst-positive cells found in the SCT control group. Comparatively, HSCs with blue staining in the nucleus could be seen in HSC transplanted rats at the first month (Figure 3A), third month (Figure 3B) and sixth month (Figure 3C) post-operation. Implanted HSCs also migrated towards the caudal and rostral ends of the cord, and the number of surviving cells was approximately 90% of the total number of transplanted cells at sixth months post-SCT (Figure 3D).
Figure 3. Transplanted HSCs survived and migrated in the host spinal cord. There are no positive HSCs with blue staining marked by Hoechst 33342 in the spinal cord without HSC transplantation. While, HSCs with blue staining in nucleus could be seen at the first month (A), third month (B) and sixth month post transected spinal cord injury (SCT) (C), indicating the survival and migration of the transplanted HSCs in the host spinal cord. (D) Bar chart for quantitative analysis of the survived HSCs in vivo using Image-Pro Plus 6.0 software. Data are presented as the mean ± SD (n = 3, one-way ANOVA). Bar = 50 μm. m, month.
Implanted HSCs with blue staining labeled by Hoechst 33342 could be found in the host spinal cord, which confirmed the survival of HSCs (Figures 4A,D,G). Simultaneously, some HSCs exhibited positive Tuj1 staining (Figures 4B,C) or ALDH1L1 staining (Figures 4E,F). Quantitative analysis showed that 3.1% of the HSCs were positive for Tuj1 and 18.3% were positive for ALDH1L1. These data demonstrate that the transplanted HSCs can develop into astrocytes in vivo, with fewer neuronal differentiations. The negative control showed no positive staining (Figures 4G–I).
Figure 4. Transplanted HSCs could differentiate into neurons and glia like cells in vivo. (A) Positive HSCs with blue staining in nucleus marked by Hoechst 33342 in the spinal cord could be seen after HSC transplantation. (B) A few of HSCs could differentiate into neurons with Tuj1 positive staining (red). (C) The merged picture of Tuj1 and Hoechst 33342, the positive ratio of Tuj1/ Hoechst 33342 is about 3.1%. (D) Positive HSCs with Hoechst 33342 blue staining. (E) A few of HSCs could differentiate into astrocytes with ALDH1L1 positive staining (red). (F) The merged picture of ALDH1L1 and Hoechst 33342, which showed the positive ratio is about 18.3%. (G) Hoechst 33342 blue staining in the negative control (no primary antibody). (H) There is no positive red staining in the negative control (no primary antibody). (I) The merged picture. Bar = 50 μm. White arrows represented the positive cells. The positive ratio of Tuj1 (Tuj1/ Hoechst 33342) and ALDH1L1 (ALDH1L1/Hoechst 33342) was quantified using Image-Pro Plus 6.0 software (n = 7).
BBB scores from week 1 to 24 showed that SCT resulted in a complete locomotor function deficit, and that locomotor function could be partially restored over time. Comparatively, BBB scores in the HSC group were higher than in the SCT group from week 4 to 24 post-SCT. The behavioral evaluation from the BBB scores demonstrated that HSC transplantation dramatically improves the neurological function of SCT rats (P < 0.05, Figure 5).
Figure 5. HSCs transplantation improved the neural behavior in rats subjected to SCT. BBB score of the sham group was 21. There was a significant decrease of BBB score at week 1–24 after operation in SCT and HSC group (n = 15/group). HSC transplantation group displayed a significantly improved scores compared with SCT group beginning at week 2–22 after transplantation. Three independent experiments were performed and the data are presented as the mean ± SD (n = 15). Student’s t test was used to analyze the data. *P < 0.05 vs. SCT. Arrow indicates the HSC transplantation time.
The spinal cord appeared normal in shape, with a plum shape and normal volume, at both the rostral or caudal sides in the sham group at sixth months post-SCT (Figure 6A), while both the rostral and caudal spinal cord in the SCT group exhibited a narrow shape as compared with the sham group. A decreased volume at the center of the injury was most apparent (Figures 6B,C). In contrast, HSC transplantation markedly restored the volume of the spinal cord, and promoted the morphological recovery of the injured spinal cord (Figures 6B,D).
Figure 6. HSC transplantation restored the volume of spared spinal cord. After SCT, compared with the sham one (A), both rostral and caudal spinal cord exhibited a narrow shape, structure was damaged. Moreover, the volume in injured center decreased apparently with the narrowest shape (B) upper, (C). Comparatively, HSC transplantation resulted in a significant increase on the volume of spared spinal cord (B) down, (D). Bar = 200 μm.
Following SCT, the number and size of neurons in layer VIII and IX of the ventral horn became much fewer and smaller than in the sham and HSC group (Figures 7A–D). However, quantitative analysis showed that the cellular number and size in the HSC group was not significantly greater than in the SCT group (P > 0.05; Figures 7G,H), which indicated that administration of HSCs did not increase the number and size of neurons in the ventral horn (Figures 7E,F).
Figure 7. The effect of HSC transplantation on the neural survival. Compared with the sham group (A,B,G,H), the number and size of neurons in ventral horn in the SCT group (C,D,G,H) became markedly less. However, quantitative analysis showed no differences on the number and size of neurons between SCT and HSC groups, P > 0.05 (E–H). Data are presented as the mean ± SD (n = 7). One-way ANOVA was used to analyze the data. *P < 0.05 vs. sham. Bar = 100 μm in (A,C,E); 50 μm in (B,D,F).
To investigate whether astrocytes were changed after HSC transplantation, double staining of Hoechst 33342 and GFAP was performed in the injured center, and in the rostral and caudal regions 6 months post-SCT. SCT induced a marked increase in the number of GFAP+ cells in the injured center, and rostral and caudal ends of the spinal cord (Figures 8A–D,I–L), compared with the sham group. This finding was confirmed in a previous observation (data not shown). Following HSC transplantation, the number of Hoechst 33342/GFAP-labeled cells effectively decreased in the observed areas (injured center, and rostral and caudal ends of the spinal cord), when compared with the SCT group (P < 0.05; Figures 8E–L).
Figure 8. HSC transplantation decreased the number of astrocytes. SCT greatly increased the number of GFAP+ cells in the rostral, scar and caudal sites of the transected cord (A–D). While HSCs transplantation effectively impressed the number of astrocytes in these segments (E–H). Histogram showed that the number of astrocytes in HSC transplanted group was lower than that of SCT one (I–L). (A,E,I) Scar center; (B,F,J) Posterior Funiculus of rostral scar; (C,G,K) gray matter of rostral scar; (D,H,I) Dorsal horn of caudal scar. Data are presented as the mean ± SD (n = 7). Student’s t test was used to analyze the data. Data in the sham group were not shown. *P < 0.05 vs. SCT. Bar = 50 μm.
Double staining of Hoechst 33342 and APC showed a distinct decrease in the number of olidgoendrocytes in SCT rats compared with the sham group from a previous observation (sham data not shown, Figures 9A–D,I–L). On the contrary, in the HSC group, the number of oligodendrocytes was increased conspicuously when compared with the SCT group (P < 0.05; Figures 9E–L). The increase was observed in the scar area as well as neighboring spinal tissue, including the rostral and caudal cords (Figures 9E–L).
Figure 9. HSC transplantation promoted oligogenesis. Compared with the sham group, the number of APC positive cells was largely decreased in the injured center, rostral and caudal spinal cord near the injured site post SCT (A–D). Comparatively, the number of oligodendrocytes was effectively increased at 24 weeks post-SCT (E–H). Quantitative histogram showed that the number of oligodendrocytes in HSC group was higher than that of the SCT one (I–L). (A,E,I) Scar center; (B,F,J) Posterior Funiculus of rostral scar; (C,G,K) gray matter of rostral scar; (D,H,I) Dorsal horn of caudal scar. Data are presented as the mean ± SD (n = 7). Student’s t test was used to analyze the data. Data in the sham group were not shown. *P < 0.05 vs. SCT. Bar = 50 μm.
5-HT fibers exhibited a weak regeneration capacity post-SCT compared with the sham group from a previous observation (data not shown). Moreover, 24 weeks post-SCT, the number of 5-HT-positive fibers had increased compared with the SCT group. Descending 5-HT fibers, known for transducing motor signals from the brain to the end effectors, regenerated approximately 2 cm into the scar area (Figure 10).
Figure 10. HSC transplantation enhanced the regeneration of 5-HT fibers. After SCT, 5-HT fiber exhibited weak regeneration capacity (A), while HSC transplantation largely increased the number of 5-HT positive fibers (B), the length is near 2 cm (C). Data are presented as the mean ± SD (n = 7). Student’s t test was used to analyze the data. *P < 0.05 vs. SCT. Bar = 50 μm.
Twenty-four weeks post-SCT, qRT-PCR showed that the mRNA expression of NT3 and MEK-1 in the SCT group was significantly decreased, as compared with the sham group (p < 0.05). The levels of NT-3 and MEK-1 mRNA in the HSC group were markedly increased (p < 0.05), but were not significantly different from the sham group (p > 0.05), when compared with the SCT group. Therefore, increased expression of NT-3 and MEK-1 may be associated with the protection of HSC transplantation (Figures 11A,B).
Figure 11. Detection of neurotrophin-3 (NT-3) and mitogenactivated protein kinase kinase-1 (MEK-1) expression after HSC transplantation. The expressional changes of MEK-1 and NT-3 mRNA among the sham, SCT and HSC group at 24 weeks post-SCT were respectively shown in (A,B). *p < 0.05 compared with SCT group. Data are presented as the means ± SEM (n = 8). One-way ANOVA was used to analyze the data.
In the current study, we found that HSC transplantation promotes functional improvement after SCT in rats, and enhances nerve regeneration and oligogenesis while suppressing excessive astrogliosis. Importantly, NT3-regulated MEK-1 activation may be responsible for the success of HSC grafts in SCI rats.
In this study, we found that HSCs transplanted into SCT rats could survive and migrate around the injured site and ameliorate behavior deficits after SCT. We also found that the decrease in the spinal cord volume of HSC-treated rats was smaller than in SCT rats. It has been shown that the compromised transected tissue and the subsequent cavity formation are characteristics of progressive tissue necrosis following initial primary cell destruction in SCT (Park et al., 2012). Therefore, reduction of the structural injury or cavity volume indicates that the transplanted HSCs have a neuroprotective effect after SCT. Moreover, the results of the present study demonstrate the therapeutic effect of HSCs in chronic SCT rats, which differed from findings of previous studies (Deda et al., 2008; Sasaki et al., 2009). The morphological mechanisms for improving the functional deficits involved in promoting nerve regeneration and oligogenesis, together with astrogliosis inhibition, were shown.
In the previous studies involving animals, reactive astrocytosis was shown to promote the regeneration of severed axons, and that this may have occurred through glial scar-associated extracellular matrix proteins (GrandPré et al., 2000; Bradbury et al., 2002; Kamei et al., 2010, 2012). While the issue of whether reactive astrocytosis is beneficial or detrimental remains controversial, it may play a critical and necessary role in the early stages of destructive CNS processes, but may be harmful in latter stages by contributing to an inhibition of axonal regeneration. Previous studies have also shown that in the repair process of central nervous system damage, an excessive increase in astrocytes promotes the secretion of inhibitory molecules and the formation of fibrotic scarring in the injured spinal cord, which then prevents axonal or nerve regeneration (Frisén et al., 1995; Yu et al., 2000; Shearer and Fawcett, 2001; Fields and Stevens-Graham, 2002). In the current study, we found that the BBB score was improved, astrogliosis was inhibited and 5-HT fibers were increased at 24 weeks post-SCT. These data suggest that the moderate decrease in the number of astrocytes following HSC transplantation may provide an opportunity for nerve regeneration. Our data indirectly show that reactive astrocytosis may be harmful in the latter stages of destructive CNS processes. As our observations lasted 6 months and a decreased number of astrocytes were found, our findings support the hypothesis that HSCs grafts have a protective role in chronic SCI, which is different from previous observations (Deda et al., 2008; Sasaki et al., 2009; Kamei et al., 2010).
Enhanced oligogenesis was also observed after HSC transplantation. It has been suggested that oligogenesis by and survival of endogenous oligodendrocyte progenitor cells (OPCs) can contribute to self-repair after myelin loss (Deda et al., 2008; Park et al., 2012). This finding indicates that oligodendrocytes are important myelin-formation cells responsible for the formation of myelin surrounding axons in the central nervous system. Previous studies have reported that transplanted peripheral blood stem cells mobilized by granulocyte colony-stimulating factor promote hindlimb functional recovery following SCI in mice by suppressing oligodendrocyte apoptosis (Takahashi et al., 2016). Similarly, in the current study, we found that HSC transplantation effectively increased the number of oligodendrocytes and 5-HT fibers in vivo. Therefore, the results revealed that HSC transplantation is very important for promotion of functional improvement after SCT by increasing the number of oligodendrocytes and 5-HT fibers. It has been shown that 5-HT fibers promote surviving nerve axons to extend their lateral branches towards damaged axons, and that oligodendrocytes can repair demyelinated CNS neurons and improve the function of spinal cord nerves (Sasaki et al., 2001; Bjugstad et al., 2005; Garbuzova-Davis et al., 2006; Leu et al., 2010), which further supports their role in the functional recovery of the transected spinal cord.
Although HSCs have been proposed as a potential source of neural cells for repairing brain lesions (Sigurjonsson et al., 2005), we found no significant changes in the number or size of neurons in the transected spinal cords treated with HSC transplantation in our research. The degeneration and atrophy of injured neurons post-SCT were difficult to evaluate (Dobkin and Havton, 2004). Moreover, HSC engraftment exhibited the potential to restore injured spinal cord and promote functional recovery, similar to marrow stromal cells in contusion conditions (Koda et al., 2005). The underlying mechanism was involved in promoting the oligogenesis and nerve regeneration from 5-HT fibers, as well as inhibiting excessive astrogliosis.
Although the roles of HSC transplantation have been preliminarily determined, the source of HSCs is still an obstacle for their wide application. Thus, the molecular mechanism of HSC transplantation must be explored in order to find alternative treatments. Previous studies have reported that VEGF, SDF1, CXCR4, PI3-K/Akt and MAPK signaling may be involved in protection following HSC transplantation (Isele et al., 2007; Deda et al., 2008). Based on the neuroprotective role of NT-3 and the MEK/ERK/CREB signaling pathway (Isele et al., 2007; Lebesgue et al., 2009; Li et al., 2016; Liu et al., 2016; Keefe et al., 2017), we speculate that HSC transplantation may promote functional improvement after SCT by increasing the expression of NT3 and MEK-1 signaling. Our findings show that SCT induced a significant decline in NT3 and MEK-1 mRNA levels, while HSC transplantation was able to restore this decrease. This indicates that upregulation of NT3 and MEK-1 signaling may be a potential molecular mechanism underlying protection following HSC transplantation. Therefore, activation of NT-3 or MEK-1 signaling may present a new alternative strategy for the treatment of SCI in future clinical practice.
Our study revealed that HSC transplantation promotes functional improvement in SCT rats and found possible mechanisms that are involved in morphological remodeling and the activation of NT-3 and MEK-1 signaling. These findings may contribute to the understanding of the mechanism of SCI and provide a novel treatment strategy for neural repair following SCI.
L-LX, FL, Q-JX and T-HW designed the experiments. L-LX, FL, S-KD, JL, Q-QD and YZ performed the experiments. L-LX, PZ and Q-JX analyzed the data. L-LX, FL and T-HW wrote the manuscript. All authors read and approved the final manuscript.
The authors declare that the research was conducted in the absence of any commercial or financial relationships that could be construed as a potential conflict of interest.
This study was supported by the Grant of National Science foundation (No.81471268, No.81601074) and the Program Innovative Research Team in Science and Technology in Yunnan province and IRTSTYN.
Abbaszadeh, H. A., Tiraihi, T., Noori-Zadeh, A., Delshad, A. R., Sadeghizade, M., and Taheri, T. (2015). Human ciliary neurotrophic factor-overexpressing stable bone marrow stromal cells in the treatment of a rat model of traumatic spinal cord injury. Cytotherapy 17, 912–921. doi: 10.1016/j.jcyt.2015.03.689
Aggarwal, R., Lu, J., Pompili, V. J., and Das, H. (2012). Hematopoietic stem cells: transcriptional regulation, ex vivo expansion and clinical application. Curr. Mol. Med. 12, 34–49. doi: 10.2174/156652412798376125
Basso, D. M., Beattie, M. S., and Bresnahan, J. C. (1995). A sensitive and reliable locomotor rating scale for open field testing in rats. J. Neurotrauma 12, 1–21. doi: 10.1089/neu.1995.12.1
Bavaria, J. E., Appoo, J. J., Makaroun, M. S., Verer, J., Yu, Z. F., and Mitchell, R. S. (2007). Endovascular stent grafting versus open surgical repair of descending thoracic aortic aneurysms in low-risk patients: a multicenter comparative trial. J. Thorac. Cardiovasc. Surg. 133, 369–377. doi: 10.1016/j.jtcvs.2006.07.040
Bjugstad, K. B., Redmond, D. E. Jr., Teng, Y. D., Elsworth, J. D., Roth, R. H., Blanchard, B. C., et al. (2005). Neural stem cells implanted into MPTP-treated monkeys increase the size of endogenous tyrosine hydroxylase positive cellsfound in the striatum: a return to control measures. Cell Transplant. 14, 183–192. doi: 10.3727/000000005783983098
Boulland, J. L., Lambert, F. M., Züchner, M., Strom, S., and Glover, J. C. (2013). A neonatal mouse spinal cord injury model for assessing post-injury adaptive plasticity and human stem cell integration. PLoS One. 8:e71701. doi: 10.1371/journal.pone.0071701
Bracken, M. B. (2012). Steroids for acute spinal cord injury. Cochrane Database Syst. Rev. 1:CD001046. doi: 10.1002/14651858.cd001046.pub2
Bradbury, E. J., Moon, L. D., Popat, R. J., King, V. R., Bennett, G. S., Patel, P. N., et al. (2002). Chondroitinase ABC promotes functional recovery after spinal cord injury. Nature 416, 636–640. doi: 10.1038/416636a
Bryukhovetskiy, A. S., and Bryukhovetskiy, I. S. (2015). Effectiveness of repeated transplantations of hematopoietic stem cells in spinal cord injury. World J. Transplant. 5, 110–128. doi: 10.5500/wjt.v5.i3.110
Burt, R. K., Burns, W., and Hess, A. (1995). Bone marrow transplantation for multiple sclerosis. Bone Marrow Transplant. 16, 1–6.
Burt, R. K., Loh, Y., Pearce, W., Beohar, N., Barr, W. G., Craig, R., et al. (2008). Clinical applications of blood-derived and marrow-derived stem cells for nonmalignant diseases. JAMA 299, 925–926. doi: 10.1001/jama.299.8.925
Cabanes, C., Bonilla, S., Tabares, L., and Martínez, S. (2007). Neuroprotective effect of adult hematopoietic stem cells in a mouse model of motoneuron degeneration. Neurobiol. Dis. 26, 408–418. doi: 10.1016/j.nbd.2007.01.008
Coutts, M., and Keirstead, H. S. (2008). Stem cells for the treatment of spinal cord injury. Exp. Neurol. 209, 368–377. doi: 10.1016/j.expneurol.2007.09.002
Dasari, V. R., Spomar, D. G., Li, L., Gujrati, M., Rao, J. S., and Dinh, D. H. (2008). Umbilical cord blood stem cell mediated downregulation of fas improves functional recovery of rats after spinal cord injury. Neurochem. Res. 33, 134–149. doi: 10.1007/s11064-007-9426-6
Dasari, V. R., Veeravalli, K. K., and Dinh, D. H. (2014). Mesenchymal stem cells in the treatment of spinal cord injuries: a review. World J. Stem Cells 6, 120–133. doi: 10.4252/wjsc.v6.i2.120
Deda, H., Inci, M. C., Kürekci, A. E., Kayihan, K., Ozgün, E., Ustünsoy, G. E., et al. (2008). Treatment of chronic spinal cord injured patients with autologousbone marrow-derived hematopoietic stem cell transplantation: 1-year follow-up. Cytotherapy 10, 565–574. doi: 10.1080/14653240802241797
Dobkin, B. H., and Havton, L. A. (2004). Basic advances and new avenues in therapy of spinal cord injury. Annu. Rev. Med. 55, 255–282. doi: 10.1146/annurev.med.55.091902.104338
Feng, S. Q., Kong, X. H., Guo, S. F., Wang, P., Li, L., Zhong, J. H., et al. (2005). Treatment of spinal cord injury with co-grafts of genetically modified Schwann cells and fetal spinal cord cell suspension in the rat. Neurotox. Res. 7, 169–177. doi: 10.1007/bf03033785
Fields, R. D., and Stevens-Graham, B. (2002). New insights into neuron-glia communication. Science 298, 556–562. doi: 10.1126/science.298.5593.556
Frisén, J., Hægerstrand, A., Risling, M., Fried, K., Johansson, C. B., Hammarberg, H., et al. (1995). Spinal axons in central nervous system scar tissue are closely related to laming in immunoreactive astrocytes. Neuroscience 65, 293–304. doi: 10.1016/0306-4522(94)00467-j
Garbuzova-Davis, S., Willing, A. E., Saporta, S., Bickford, P. C., Gemma, C., Chen, N., et al. (2006). Novel cell therapy approaches for brain repair. Prog. Brain Res. 157, 207–222. doi: 10.1016/s0079-6123(06)57014-1
GrandPré, T., Nakamura, F., Vartanian, T., and Strittmatter, S. M. (2000). Identification of the Nogo inhibitor of axon regeneration as a Reticulon protein. Nature 403, 439–444. doi: 10.1038/35000226
Gruner, J. A. (1992). A monitored contusion model of spinal cord injury in the rat. J. Neurotrauma. 9, 123–126; discussion 126–128. doi: 10.1089/neu.1992.9.123
Haas, S., Weidner, N., and Winkler, J. (2005). Adult stem cell therapy in stroke. Curr. Opin. Neurol. 18, 59–64. doi: 10.1097/00019052-200502000-00012
He, Q. Q., Xiong, L. L., Liu, F., He, X., Feng, GY., Shang, F. F., et al. (2016). MicroRNA-127 targeting of mitoNEET inhibits neurite outgrowth, induces cell apoptosis and contributes to physiological dysfunction after spinal cord transection. Sci. Rep. 6:35205. doi: 10.1038/srep35205
Hirano, K., Wagner, K., Mark, P., Pittermann, E., Gäbel, R., Furlani, D., et al. (2012). Erythropoietin attenuates the sequels of ischaemic spinal cord injurywithenhanced recruitment of CD34+ cells in mice. J. Cell. Mol. Med. 16, 1792–1802. doi: 10.1111/j.1582-4934.2011.01489.x
Huang, H. Y., Chen, L., and Sanberg, P. (2010). Cell therapy from bench to bedside translation in CNS neurorestoratology era. Cell Med. 1, 15–46. doi: 10.3727/215517910x516673
Isele, N. B., Lee, H. S., Landshamer, S., Straube, A., Padovan, C. S., Plesnila, N., et al. (2007). Bone marrow stromal cells mediate protection through stimulation of PI3-K/Akt and MAPK signaling in neurons. Neurochem. Int. 50, 243–250. doi: 10.1016/j.neuint.2006.08.007
Kamei, N., Kwon, S. M., Alev, C., Ishikawa, M., Yokoyama, A., Nakanishi, K., et al. (2010). Lnk deletion reinforces the function of bone marrow progenitors in promoting neovascularization and astrogliosis following spinal cord injury. Stem Cells 28, 365–375. doi: 10.1002/stem.243
Kamei, N., Kwon, S. M., Kawamoto, A., Ii, M., Ishikawa, M., Ochi, M., et al. (2012). Contribution of bone marrow-derived endothelial progenitor cells toneovascularization and astrogliosis following spinal cord injury. J. Neurosci. Res. 90, 2281–2292. doi: 10.1002/jnr.23113
Keefe, K. M., Sheikh, I. S., and Smith, G. M. (2017). Targeting neurotrophins to specific populations of neurons: NGF, BDNF, and NT-3 and their relevance for treatment of spinal cord injury. Int. J. Mol. Sci. 18:E548. doi: 10.3390/ijms18030548
Koda, M., Okada, S., Nakayama, T., Koshizuka, S., Kamada, T., Nishio, Y., et al. (2005). Hematopoietic stem cell and marrow stromal cell for spinal cord injury in mice. Neuroreport 16, 1763–1767. doi: 10.1097/01.wnr.0000183329.05994.d7
Koshizuka, S., Okada, S., Okawa, A., Koda, M., Murasawa, M., Hashimoto, M., et al. (2004). Transplanted hematopoietic stem cells from bone marrow differentiate into neural lineage cells and promote functional recovery after spinal cord injury in mice. J. Neuropathol. Exp. Neurol. 263, 64–67. doi: 10.1093/jnen/63.1.64
Lambert, W. S., Clark, A. F., and Wordinger, R. J. (2004). Neurotrophin and Trkexpression by cells of the human lamina cribrosa following oxygen-glucose deprivation. BMC Neurosci. 5:51. doi: 10.1186/1471-2202-5-51
Lebesgue, D., Chevaleyre, V., Zukin, R. S., and Etgen, A. M. (2009). Estradiol rescues neurons from global ischemia-induced cell death: multiple cellular pathways of neuroprotection. Steroids 74, 555–561. doi: 10.1016/j.steroids.2009.01.003
Leu, S., Lin, Y. C., Yuen, C. M., Yen, C. H., Kao, Y. H., Sun, C. K., et al. (2010). Adipose-derived mesenchymal stem cells markedly attenuate brain infarct size and improve neurological function in rats. J. Transl. Med. 8:63. doi: 10.1186/1479-5876-8-63
Li, G., Che, M. T., Zhang, K., Qin, L. N., Zhang, Y. T., Chen, R. Q., et al. (2016). Graft of the NT-3 persistent delivery gelatin sponge scaffold promotes axonregeneration, attenuates inflammation, and indu. Biomaterials 83, 233–248. doi: 10.1016/j.biomaterials.2015.11.059
Liu, Y., Kelamangalath, L., Kim, H., Han, S. B., Tang, X., Zhai, J., et al. (2016). NT-3 promotes proprioceptive axon regeneration when combined with activation of the mTor intrinsic growth pathway but not with reduction of myelin extrinsic inhibitors. Exp. Neurol. 283, 73–84. doi: 10.1016/j.expneurol.2016.05.021
Liu, R., Zhao, W., Zhao, Q., Liu, S. J., Liu, J., He, M., et al. (2014). Endoplasmic reticulum protein 29 protects cortical neurons from apoptosis and promoting corticospinal tract regeneration to improve neural behavior via caspaseand Erk signal in rats with spinal cord transection. Mol. Neurobiol. 50, 1035–1048. doi: 10.1007/s12035-014-8681-1
Livak, K. J., and Schmittgen, T. D. (2001). Analysis of relative gene expression data using real-time quantitative PCR and the 2−ΔΔCT method. Methods 25, 402–408. doi: 10.1006/meth.2001.1262
Mendez, I., Sanchez-Pernaute, R., Cooper, O., Viñuela, A., Ferrari, D., Björklund, L., et al. (2005). Cell type analysis of functional fetal dopamine cellsuspension transplants in the striatum and substantianigra of patients with Parkinson’s disease. Brain 128, 1498–1510. doi: 10.1093/brain/awh510
Mothe, A. J., and Tator, C. H. (2012). Advances in stem cell therapy for spinal cord injury. J. Clin. Invest. 122, 3824–3834. doi: 10.1172/JCI64124
Müller, A., Hauk, T. G., Leibinger, M., Marienfeld, R., and Fischer, D. (2009). Exogenous CNTF stimulates axon regeneration of retinal ganglion cells part-ially via endogenous CNTF. Mol. Cell. Neurosci. 41, 233–246. doi: 10.1016/j.mcn.2009.03.002
Nicaise, C., Mitrecic, D., Falnikar, A., and Lepore, A. C. (2015). Transplantation of stem cell-derived astrocytes for the treatment of amyotrophic lateral scl-erosis and spinal cord injury. World J. Stem Cells 7, 380–398. doi: 10.4252/wjsc.v7.i2.380
Nishio, Y., Koda, M., Kamada, T., Someya, Y., Yoshinaga, K., Okada, S., et al. (2006). The use of hemopoietic stem cells derived from human umbilical cord blood to promote restoration of spinal cord tissue and recovery of hindlimb function in adult rats. J. Neurosurg. Spine 5, 424–433. doi: 10.3171/spi.2006.5.5.424
Park, S. I., Lim, J. Y., Jeong, C. H., Kim, S. M., Jun, J. A., Jeun, S. S., et al. (2012). Human umbilical cord blood-derived mesenchymal stem cell therapy promotes functional recovery of contused rat spinal cord through enhancement of endogenous cell proliferation and oligogenesis. J. Biomed. Biotechnol. 2012:362473. doi: 10.1155/2012/362473
Sasaki, M., Honmou, O., Akiyama, Y., Uede, T., Hashi, K., and Kocsis, J. D. (2001). Transplantation of an acutely isolated bone marrow fraction repairs demyelinated adult rat spinal cord axons. Glia 35, 26–34. doi: 10.1002/glia.1067
Sasaki, H., Ishikawa, M., Tanaka, N., Nakanishi, K., Kamei, N., Asahara, T., et al. (2009). Administration of human peripheral blood-derived CD133+ cells accelerates functional recovery in a rat spinal cord injury model. Spine 34, 249–254. doi: 10.1097/BRS.0b013e3181913cde
Schwarting, S., Litwak, S., Hao, W., Bähr, M., Weise, J., and Neumann, H. (2008). Hematopoietic stem cells reduce post ischemic inflammation and ameliorate ischemic brain injury. Stroke 39, 2867–2875. doi: 10.1161/STROKEAHA.108.513978
Shearer, M. C., and Fawcett, J. W. (2001). The astrocyte/meningeal cell interfaceabarrier to successful nerve regeneration. Cell Tissue Res. 305, 267–273. doi: 10.1007/s004410100384
Sigurjonsson, O. E., Perreault, M. C., Egeland, T., and Glover, J. C. (2005). Adulthuman hematopoietic stem cells produce neurons efficiently in the regenerating chicken embryo spinal cord. Proc. Natl. Acad. Sci. U S A 102, 5227–5232. doi: 10.1073/pnas.0501029102
Singh, S. (2015). Stem cell therapy in spinal trauma: does it have scientific validity? Indian J. Orthop. 49:485. doi: 10.4103/0019-5413.159683
Takahashi, H., Koda, M., Hashimoto, M., Furuya, T., Sakuma, T., Kato, K., et al. (2016). Transplanted peripheral blood stem cells mobilized by granulo-cyte colony-stimulating factor promoted hindlimb functional recoveryafterspinal cord injury in mice. Cell Transplant. 25, 283–292. doi: 10.3727/096368915x688146
Tederko, P., Krasuski, M., Kiwerski, J., Nyka, I., and Białoszewski, D. (2009). Strategies for neuroprotection following spinal cord injury. Ortop. Traumatol. Rehabil. 11, 103–110.
Thakkar, U. G., Vanikar, A. V., Trivedi, H. L., Shah, V. R., Dave, S. D., Dixit, S. B., et al. (2016). Infusion of autologous adipose tissue derived neuronal differentiated mesenchymal stem cells and hematopoietic stem cells in post-traumaticparaplegia offers aviable therapeutic approach. Adv. Biomed. Res. 5:51. doi: 10.4103/2277-9175.178792
Tyndall, A., Fassas, A., Passweg, J., Ruiz de Elvira, C., Attal, M., Brooks, P., et al. (1999). Autologous hematopoietic stem cell transplants for autoimmune disease-feasibility and transplant-related mortality. Bone Marrow Transplant. 24, 729–734. doi: 10.1038/sj.bmt.1701987
Vawda, R., and Fehlings, M. G. (2013). Mesenchymal cells in the treatment of spinal cord injury: current & future perspectives. Curr. Stem Cell Res. Ther. 8, 25–38. doi: 10.2174/1574888x11308010005
Xu, X. M., and Onifer, S. M. (2009). Transplantation-mediated strategies to promote axonal regeneration following spinal cord injury. Respir. Physiol. Neurobiol. 169, 171–182. doi: 10.1016/j.resp.2009.07.016
Keywords: hematopoietic stem cells, spinal cord transection, cell transplantation, neurological behavior, neurotrophin 3, MEK-1
Citation: Xiong L-L, Liu F, Deng S-K, Liu J, Dan Q-Q, Zhang P, Zou Y, Xia Q-J and Wang T-H (2017) Transplantation of Hematopoietic Stem Cells Promotes Functional Improvement Associated with NT-3-MEK-1 Activation in Spinal Cord-Transected Rats. Front. Cell. Neurosci. 11:213. doi: 10.3389/fncel.2017.00213
Received: 12 March 2017; Accepted: 04 July 2017;
Published: 19 July 2017.
Edited by:
Kempuraj Duraisamy, University of Missouri, United StatesReviewed by:
Guang Li, Stanford University, United StatesCopyright © 2017 Xiong, Liu, Deng, Liu, Dan, Zhang, Zou, Xia and Wang. This is an open-access article distributed under the terms of the Creative Commons Attribution License (CC BY). The use, distribution or reproduction in other forums is permitted, provided the original author(s) or licensor are credited and that the original publication in this journal is cited, in accordance with accepted academic practice. No use, distribution or reproduction is permitted which does not comply with these terms.
*Correspondence: Qing-Jie Xia, eGlhcWoyMDA1QDEyNi5jb20=
Ting-Hua Wang, dGluZ2h1YV9uZXVyb25AMjYzLm5ldA==
† These authors have contributed equally to this work.
Disclaimer: All claims expressed in this article are solely those of the authors and do not necessarily represent those of their affiliated organizations, or those of the publisher, the editors and the reviewers. Any product that may be evaluated in this article or claim that may be made by its manufacturer is not guaranteed or endorsed by the publisher.
Research integrity at Frontiers
Learn more about the work of our research integrity team to safeguard the quality of each article we publish.