- Center for Substance Abuse Research, Lewis Katz School of Medicine at Temple University, Philadelphia, PA, USA
Exposure to stress increases vulnerability to drug abuse, as well as relapse liability in addicted individuals. Chronic drug use alters stress response in a manner that increases drug seeking behaviors and relapse. Drug exposure and withdrawal have been shown to alter stress responses, and corticosteroid mediators of stress have been shown to impact addiction-related brain function and drug-seeking behavior. Despite the documented interplay between stress and substance abuse, the mechanisms by which stress exposure and drug seeking interact remain largely unknown. Recent studies indicate that microRNAs (miRNA) play a significant role in stress modulation as well as addiction-related processes including neurogenesis, synapse development, plasticity, drug acquisition, withdrawal and relapse. MiRNAs are short non-coding RNAs that function as bidirectional epigenetic modulators of gene expression through imperfect sequence targeted degradation and/or translational repression of mRNAs. They serve as dynamic regulators of CNS physiology and pathophysiology, and facilitate rapid and long-lasting changes to complex systems and behaviors. MiRNAs function in glucocorticoid signaling and the mesolimbic dopamine reward system, as well as mood disorders related to drug withdrawal. The literature suggests miRNAs play a pivotal role in the interaction between exposures to stress, addiction-related processes, and negative affective states resulting from extended drug withdrawal. This manuscript reviews recent evidence for the role of miRNAs in the modulation of stress and cocaine responses, and discusses potential mediation of the interaction of these systems by miRNAs. Uncovering the mechanism behind the association of stress and drug taking has the potential to impact the treatment of drug abuse and prevention of relapse. Further comprehension of these complex interactions may provide promising new targets for the treatment of drug addiction.
Neurocircuits and Pathways Common to Stress and Cocaine Abuse
Considerable evidence supports the overlap between the stress and reward systems of the brain and that alterations in stress systems may contribute to increased liability to abuse drugs including cocaine (de Jong and de Kloet, 2004). Both repeated stress and psychostimulant dependence are associated with alterations in the mesocorticolimbic dopamine system, the medial prefrontal cortex glutamatergic corticolimbic circuit, and corticotropin-releasing factor (CRF) signaling in the ventral tegmental area (VTA; Piazza and Le Moal, 1997; Everitt and Wolf, 2002).
The transition from drug-taking behavior to addiction is characterized by a shift from positive drug reinforcement involving dopamine signaling to negative reinforcement involving the stress systems of the brain where drug-taking now removes the dysphoria, anxiety and negative emotional state experienced during abstinence/withdrawal (Koob and Le Moal, 2001). The extended amygdala, comprised of the bed nucleus of the stria terminalis (BNST), central nucleus of the amygdala and the nucleus accumbens shell, serves as a common circuit between drug reward and the negative emotional state experienced during abstinence/withdrawal (Alheid et al., 1998; Koob and Le Moal, 2001). The extended amygdala receives afferent connections form limbic brain structures including the basolateral amygdala and hypothalamus, and sends efferent projections to the medial ventral pallidum and the lateral hypothalamus (Heimer et al., 1991). The extended amygdala also has interconnections with the VTA and ventral striatum. The BNST contains a large number of dopamine and norepinephrine terminals, CRF terminals and cell bodies, neuropeptide Y terminals, and receives afferent connections from the prefrontal cortex (Allen et al., 1984; Phelix and Paull, 1990; Pacak et al., 1995; Kozicz, 2001; Koob, 2003).
The extended amygdala functions in both the positive reinforcing effects of drugs of abuse and the negative reinforcing effects of drug abstinence and withdrawal. Drugs of abuse including cocaine increase extracellular levels of dopamine in the nucleus accumbens shell (Pontieri et al., 1995). Further, withdrawal from cocaine increases extracellular concentrations of CRF in the extended amygdala (Koob et al., 1994). Rats receiving repeated injections of corticosterone acquire cocaine self-administration at lower cocaine doses relative to rats receiving vehicle (Deroche et al., 1997), and blockade of glucocorticoid and CRF receptors suppresses cocaine self-administration in rats (Piazza and Le Moal, 1996; Goeders, 1997). Taken together, these findings suggest considerable overlap between and cross-regulation of the stress and reward systems of the brain. Interaction between the stress and reward systems can contribute to responding to drugs of abuse and abstinence/withdrawal following exposure to drugs of abuse. Long-lasting changes in the stress and reward systems of the brain are known to play a crucial role in the transition from recreational drug taking to compulsive drug abuse. Understanding of the role of miRNAs in the maintenance of homeostasis within and between these brain systems will significantly improve our understanding of the etiology of compulsive drug use and further provide new genetic targets for the treatment of substance abuse.
Micrornas Modulate Cocaine Reward and Withdrawal
Several recent studies demonstrate that miRNAs play a direct and crucial role in the modulation of cocaine intake in rodent models (Table 1). MiRNAs exert their regulatory translational repression and degradation of mRNA through the RNA-induced silencing complex (RISC). A core component of the RISC complex is the Argonaute (Ago) miRNA binding proteins, particularly Ago2 which mediates miRNA-dependent degradation and translational repression of target mRNAs (Hammond et al., 2001; Liu et al., 2004; Song et al., 2004) and functions in the generation of selective miRNAs from their precursors (Diederichs and Haber, 2007; O’Carroll et al., 2007). Mice deficient in Ago2 expression in dopamine D2 (Drd2) expressing neurons in the striatum self-administer significantly fewer infusions of cocaine with a downward shift in the cocaine dose-response curve relative to control animals expressing normal levels of Ago2 (Schaefer et al., 2010). Further, in contrast to wild-type control mice, Ago2-deficient mice show no preference for cocaine paired environments in conditioned place preference experiments (Schaefer et al., 2010). Deficient expression of Ago2 in the striatum results in decreased expression of ~25% of the examined miRNA species, providing strong evidence for the modulation of cocaine self-administration and reward by miRNAs through the action of Ago2 in the RISC complex. Thus, Ago2 plays a critical role in cocaine reward and motivation to self-administer cocaine. Further, these studies demonstrate that miRNAs function to modulate complex behavioral responses through region specific post-transcriptional regulation of gene expression.
Exposure to cocaine significantly alters miRNA expression in many regions of the brain (Figures 1, 2). MiR-134 and miR-135a are upregulated in the hippocampus following exposure to cocaine (Chen et al., 2013). MiR-181a (Chandrasekar and Dreyer, 2009), miR-212 (Hollander et al., 2010), and miR-375 (Jonkman and Kenny, 2013) are upregulated in the striatum following cocaine exposure. MiR-9 and miR-124 are upregulated, whereas miR-183 is downregulated in striatal post-synaptic densities (Eipper-Mains et al., 2011). Further, altered expression of these miRNAs has been demonstrated to have profound effects on cocaine reward and intake (Table 1). For instance over-expression of miR-181a in the nucleus accumbens increases cocaine-induced conditioned place preference, whereas miR-181a knockdown has the opposite effect (Chandrasekar and Dreyer, 2011). Over-expression of miR-124 in the nucleus accumbens attenuates cocaine conditioned place preference (Chandrasekar and Dreyer, 2011). MiR-135a is upregulated 2.5-fold and miR-134 is upregulated greater than 7-fold following extinction of cocaine conditioned place preference (Chen et al., 2013). MiR-134 functions in memory formation and synaptic plasticity through sirtuin 1 (SIRT1), and regulates expression of cAMP response element binding protein (CREB) and brain-derived neurotrophic factor (BDNF) (Gao et al., 2010). Chronic cocaine induces SIRT1 expression in the nucleus accumbens (Ferguson et al., 2013). Over-expression of SIRT1 in the accumbens increases the rewarding effects of cocaine, and knockdown of SIRT1 has the inverse effect on cocaine reward (Ferguson et al., 2013). Further, a recent study by Quinn et al. (2015) identified several miRNAs predicted to function in synaptic plasticity which show differential expression in the dorsolateral and dorsomedial striatum of rats with high versus low propensity to self-administer cocaine. MiRs 101b, and 431 are significantly overexpressed, whereas miR-212 is significantly underexpressed in the dorsomedial striatum of the high cocaine responders. In the dorsolateral striatum, miRs 101b, 132, 181a, 431, and 708 are significantly overexpressed in vulnerable animals relative to those that show resilience to cocaine seeking (Quinn et al., 2015). These miRNAs may modulate responding to drugs of abuse through regulation of LTD, LTP, and specifically activity-regulated cytoskeleton-associated protein (Arc), a master regulator of synaptic plasticity.
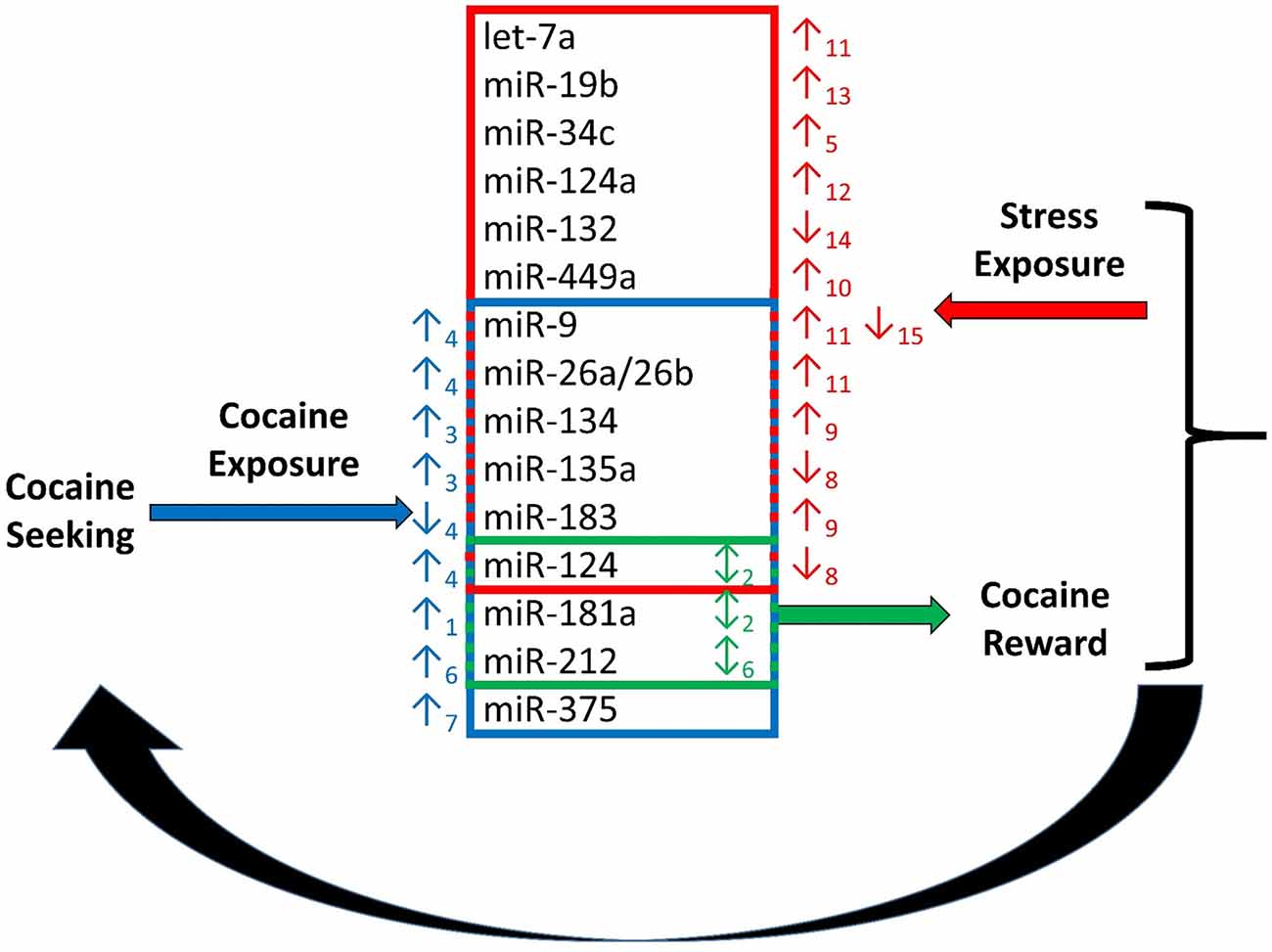
Figure 1. Regulation of cocaine reward and stress by microRNAs (miRNAs). Chronic cocaine exposure has been shown to alter expression of a number of miRNAs in various regions of the brain resulting in altered expression of down-stream molecular targets (Chandrasekar and Dreyer, 2009)1, (Chen et al., 2013)3, (Eipper-Mains et al., 2011)4, (Hollander et al., 2010)6, (Jonkman and Kenny, 2013)7. This in turn effects responding in the stress and reward systems of the brain. Exposure to stress regulates expression of miRNAs, and exogenous alteration of expression of several miRNAs regulated by cocaine and stress exposure alters the rewarding effects of cocaine. Alteration of miRNA expression in the stress and reward systems together modulate cocaine intake in a feed-back regulatory loop (Chandrasekar and Dreyer, 2011)2, (Haramati et al., 2011)5, (Hollander et al., 2010)6, (Mannironi et al., 2013)8, (Meerson et al., 2010)9, (Nemoto et al., 2012)10, (Rinaldi et al., 2010)11, (Shimizu et al., 2015)12, (Volk et al., 2014)13, (Yi et al., 2014)14, (Zhang et al., 2015)15.
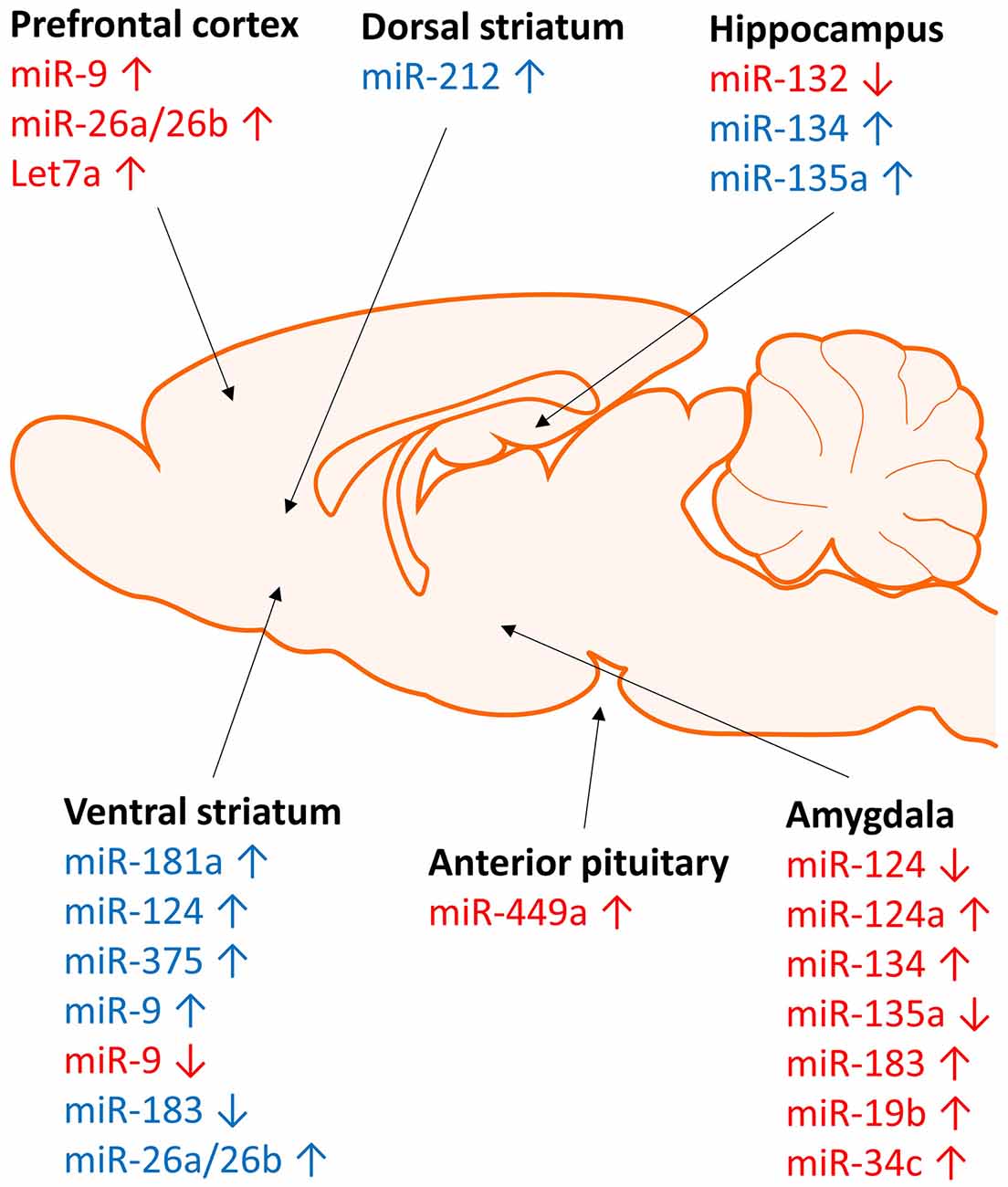
Figure 2. Brain region specific regulation of micrRNA expression by stress and cocaine. Regulation of microRNA expression by stress (red) and cocaine (blue) occurs in a region specific manner. Stress and cocaine exposure alters microRNA expression in brain regions involved in stress (amygdala), reward (striatum), and learning and memory (hippocampus and frontal cortex).
Evidence shows that miR-212 plays a particularly important role in the modulation of cocaine intake. MiR-212 is upregulated 1.75-fold in the dorsal striatum of rats provided extended daily access to cocaine self-administration compared to restricted access and yoked rats (Hollander et al., 2010). Rats overexpressing miR-212 in the dorsal striatum have lower cocaine self-administration rates and a downward shift in cocaine self-administration dose response under extended access conditions relative to control animals, indicating a decreased motivation to self-administer cocaine. Inhibition of miR-212 increases cocaine intake and increases non-reinforced responding suggesting that miR-212 modulates cocaine intake and compulsive-like responding (Hollander et al., 2010). These actions are mediated by the regulation of CREB:TORC signaling by miR-212 in response to cocaine exposure (Hollander et al., 2010). The regulation of CREB signaling by cocaine is an established mechanism contributing to the modulation of the rewarding effects of cocaine (Carlezon et al., 1998; McClung and Nestler, 2003).
The kappa opioid system also plays a significant role in the modulation of cocaine reward. Dynorphin, an endogenous kappa opioid peptide, as well as exogenous kappa opioid agonists decrease dopaminergic tone in the striatum resulting in dysphoria and negative affective state (Tejeda et al., 2012). Chronic exposure to cocaine upregulates kappa opioid receptors and decrease dopaminergic activity (Unterwald et al., 1994; Maisonneuve et al., 1995). Further, stress induced activation of kappa opioid receptors through release of dynorphin potentiates the rewarding effects of cocaine as measured by conditioned place preference (McLaughlin et al., 2003). Thus far, there is little data in the literature on the regulation of kappa opioid receptors or prodynorphin by miRNAs. However, it is known that CREB regulates prodynorphin expression in rodents. Overexpression of CREB increases the expression of dynorphin whereas expression of mutant CREB has the opposite effect (Carlezon et al., 1998). Several of the miRNAs discussed herein regulate CREB, and likely function to regulate kappa opioid receptors and dynorphin through modulation of CREB activity.
In addition to CREB signaling, miRNAs also regulate BDNF which is known to function in the rewarding and reinforcing actions of cocaine. The effects of BDNF on cocaine reward and seeking behavior are brain region specific. BDNF infusion into the nucleus accumbens and VTA enhance the rewarding effects of cocaine (Lu et al., 2004; Graham et al., 2007). In contrast, BDNF infusion into the prefrontal cortex following cocaine self-administration attenuates cocaine reinstatement via modulation of ERK, CREB and glutamate signaling (Berglind et al., 2007, 2009; Whitfield et al., 2011). MiR-212 indirectly regulates BDNF levels in the striatum of mice undergoing extended access cocaine self-administration through interaction with MeCP2 (Im et al., 2010). MeCP2 expression is positively correlated with BDNF expression, and miR-212 expression is negatively correlated to BDNF expression (Im et al., 2010). Increased expression of CREB in the nucleus accumbens shell significantly increases cocaine self-administration and motivation to self-administer cocaine (Larson et al., 2011) and this may be related to BDNF regulation. Animals overexpressing CREB have increased expression of BDNF and a short-term upward and long-term leftward shift in the cocaine dose-response curve for IV self-administration. Further, increased CREB expression after withdrawal reinforced cocaine-stimulated reinstatement (Larson et al., 2011). Expression of BDNF is increased in midbrain and amygdala during withdrawal from cocaine self-administration, and is hypothesized to contribute to heightened motivation to self-administer cocaine (Grimm et al., 2003; Lu et al., 2004).
Increased BDNF activity in brain regions involved in drug reward potentiates the reinforcing effects of cocaine. BDNF infusions into the nucleus accumbens increases sensitivity to the psychomotor stimulant effects of cocaine (Horger et al., 1999) and increases cocaine self-administration behavior in rodents (Graham et al., 2007). Further, knockdown of BDNF in the accumbens decreases cocaine self-administration (Graham et al., 2009). Knockdown of MeCP2 results in increased expression of miR-212, whereas increased miR-212 expression decreased the expression of MeCP2 (Im et al., 2010). Striatal MeCP2 knockdown decreases cocaine self-administration and shifts the cocaine self-administration dose-response curve down in animals given extended access. Knockdown of miR-212 expression in the striatum of MeCP2 deficient mice restores cocaine self-administration and shifts the cocaine dose response curve back up to control levels (Im et al., 2010). This suggests miR-212 represses expression of MeCP2 and is itself repressed by MeCP2 thereby regulating cocaine effects on striatal BDNF expression. This negative homeostatic balance in turn regulates the rewarding properties of cocaine and may contribute to the escalation to compulsive drug seeking. Taken together, these data provide strong evidence that miRNAs serve as critical short-term and long-term epigenetic modulators of cocaine exposure and reward through regulation of canonical drug reward signaling cascades. Cocaine exposure alters miRNA expression in several brain regions, and modulation of regional expression of these miRNA species alters responding to cocaine in several behavioral paradigms of drug reward.
Interactions of Cocaine-Seeking and Stress
In addition to the reward system, it is known that the stress systems of the brain play a vital role in drug seeking behavior. It is hypothesized that dysregulation of both the reward and stress pathways of the brain lead to the transition from recreational to compulsive drug seeking, and long-term dysregulation of these systems leads to vulnerability to relapse. Dopaminergic projections from the VTA to the medial prefrontal cortex have been implicated in the stress-induced relapse of cocaine-seeking in rodent reinstatement models (Vranjkovic et al., 2014). Dopamine D1 receptor activation in the VTA increases the activity of glutamatergic pathways leading to the nucleus accumbens, thereby increasing cocaine seeking (McFarland et al., 2004). Foot-shock stress increases CRF release in the VTA which in turn increases glutamate release activating mesocorticolimbic dopamine neurons and inducing cocaine reinstatement in rats previously exposed to cocaine self-administration (Wang et al., 2005). The VTA functions in the modulation of reward as part of the dopamine reward pathway and also receives inputs from brain regions involved in the modulation of stress response, including the extended amygdala (Phillipson, 1979). Of particular interest is the BNST which functions in the integration of stress and the reward system. Inhibition of the central nucleus of the amygdala, ventral BNST, and nucleus accumbens shell via co-infusion of baclofen and muscimol prevents reinstatement of cocaine seeking by foot shock stress (McFarland et al., 2004). Further, the BNST is critical in swim-stress induced reinstatement of cocaine seeking through CREB signaling (Briand et al., 2010). These data provide strong evidence that the extended amygdala is a critical region for the integration of stress and reward signaling in the brain and plays an important role in stress-induced drug-seeking behaviors. Recent evidence shows that miRNA expression is significantly altered in regions of the extended amygdala in response to acute and chronic stress. Many of these miRNAs are also modulated by cocaine exposure in reward regions thus suggesting that miRNAs regulate both reward and stress signaling in the brain. These complex interconnected regulatory cascades likely contribute to the long-term dysregulation of the reward and stress systems hypothesized to drive compulsive drug seeking.
Micrornas Modulate Stress Response and Negative Affective States
MiRNAs play a role in the short-term and long-term modulation of stress response and contribute to the etiology of anxiety and depression-like behaviors. Several miRNAs have been identified that function in the modulation of stress responses including miRNAs also shown to function in cocaine reward acting through similar signaling pathways to affect stress response as well as drug reward (Table 1). Mir-134 and miR-183 expression is increased in the central nucleus of the amygdala in response to acute immobilization stress, and miR-183 modulates expression of SC35, a protein which regulates stress-induced alternative splicing of acetylcholinesterase in vitro (Meerson et al., 2010). Mannironi et al. (2013) demonstrated that miR-135a and miR-124 are significantly down-regulated in mouse amygdala following 2 h restraint and directly regulate expression of the mineralocorticoid receptor, a regulator of early stress response. In addition, miR-124 is upregulated by cholinergic agonists, and plays a critical role in the cholinergic anti-inflammatory pathway (Sun et al., 2013). Further, miR-375, which is upregulated by repeated cocaine exposure, inhibits proopiomelanocortin (POMC) expression by targeting MAP3K8 and mediating CRF signaling (Zhang et al., 2013). Acute stress has also been shown to alter expression of let-7a, miR-9 and miR-26a/b in the frontal cortex (Rinaldi et al., 2010), and miR-124a (Shimizu et al., 2015) in mouse corpus callosum. Expression of these same miRNAs is also altered in the striatum by cocaine exposure (Eipper-Mains et al., 2011).
The literature shows that these miRNAs, which are modulated by both drug and stress exposure, in turn modulate drug seeking and stress response through transcriptional regulation of downstream targets in interacting canonical pathways (Table 1). For instance, chronic unpredictable stress decreases expression of miR-9 in the nucleus accumbens leading to increased expression of the dopamine D2 receptor (Zhang et al., 2015). Restraint stress significantly increases expression of miR-449a, increases expression of POMC mRNA, and decreases expression of CRF-R1 mRNA and protein in the anterior pituitary of rats (Nemoto et al., 2012). Further, over-expression of miR-449a results in suppression of CRF-R1 mRNA and protein, and down-regulation of miR-449a attenuates suppression of CRF-R1 by dexamethasone in cultured anterior pituitary cells (Nemoto et al., 2012). This suggests that miR-449a contributes to the stress-induced down-regulation of CRF-R1 by glucocorticoids in the anterior pituitary. MiR-132 is upregulated by BDNF (Numakawa et al., 2011) and down-regulated by glucocorticoids (Kawashima et al., 2010) in cultured cells. Yi et al. (2014) have shown that miR-132 is down-regulated in the hippocampus of mice exposed to chronic unpredictable mild stress. Further, evidence suggests that miR-132 expression is regulated by the ERK signaling pathway (Remenyi et al., 2010). MiR-132 also modulates Toll-like receptor (TLR) signaling pathways via regulation of acetylcholinesterase to increase acetylcholine-mediated negative regulation of TLR-signaling pathways (Shaked et al., 2009).
MiR-34c is upregulated in the amygdala of mice exposed to acute restraint stress and repeated social defeat stress, and over-expression of miR-34c in the amygdala protects against the anxiogenic effects of acute restraint stress possibly through regulation of CRF-R1 (Haramati et al., 2011). MiR-19b selectively associates with Ago2, is significantly upregulated in the amygdala of mice exposed to chronic social defeat stress, and regulates expression of adrenergic receptor β1 in vitro (Volk et al., 2014). MiR-124a is upregulated in the hippocampus of adult rats exposed to social defeat stress (Bahi et al., 2014). MiR-124a is a direct regulator of BDNF expression, and as expected, BDNF is down-regulated in hippocampus of rats after social defeat stress, and over-expression of miR-124a in rat hippocampus exacerbates social defeat stress-induced depression-like behaviors as measured by novelty suppressed feeding, sucrose preference and force swim tests (Bahi et al., 2014). Recently, it was demonstrated that both activation of VTA- accumbens neurons and subthreshold social defeat stress stimulation are required to induce upregulation of BDNF in a CRF receptor dependent manner in the nucleus accumbens (Walsh et al., 2014). Further, accumbens BDNF promotes increases in cocaine self-administration and relapse (Graham et al., 2007). Dwivedi et al. (2015) have shown that chronic administration of corticosterone differentially regulates expression of 26 miRNAs in the prefrontal cortex, several of which (miRs 19b, 124, 181a, 135a) regulate responding to cocaine exposure. The majority of these miRNAs show binding sites for glucocorticoid receptor elements, suggesting a common regulatory pathway for miRNA regulation of corticosterone able to cross-talk with reward and synaptic plasticity pathways. MiR-181 suppresses TNF-induced cytokine production through regulation of p300/cyclic AMP response element binding protein-associated factor, demonstrating an integral role of miRNAs in inflammatory and immune responses (Zhao et al., 2012). These data suggest miRNAs shown to modulate cocaine-seeking behaviors, also contribute to the regulation of stress response, anxiety-like behaviors and anhedonia. Further this strongly suggests a role for miRNAs in the modulation of the observed interactions between stress exposure/response and drug reward.
Summary
MicroRNAs (miRNAs) play significant roles in the modulation of both the stress and reward systems of the brain. The literature has long documented that both the reward and stress systems function in responding to the acute effects of drugs of abuse, as well as withdrawal/abstinence from chronic drug exposure. Chronic exposure to cocaine results in dysregulation of brain reward circuitry and recruitment of the brain stress systems leading to long-term cocaine dependence. Long lasting changes in both reward and stress systems lead to increased vulnerability to relapse during periods of abstinence. Several miRNAs have been identified which are co-regulated by chronic cocaine exposure and various models of rodent stress responding. These include, but are not limited to miR-134, 135a, 375, and the miR-212/132 family. Further, these miRNAs function in signaling pathways long known to regulate reward and stress response such as dopamine and CRF signaling, and glutamate transmission. It is well-established that CREB, CRF, and BDNF, among other molecules, play key roles in both stress and drug seeking/abuse. These molecules are therefore widely theorized to mediate the interaction between stress and drug seeking/abuse. Further, many of the miRNAs shown to regulate these molecules also function in immune and inflammatory responses, mechanisms known to play vital roles in the etiology of addiction. For instance, miR-132 and miR-212, shown to play a critical role in cocaine self-administration, also play important roles in TLR2 ligand-mediated TNF-α secretion (Nahid et al., 2013). The kappa opioid system is also known to modulate both stress response and the rewarding properties of drugs of abuse. However, to date, few studies have examined the regulation of kappa opioid signaling by miRNAs. These interactions are currently not very well understood, however, deciphering these complex signaling pathways will be vital to furthering our understanding of the addicted brain.
This review posits that miRNAs serve as master regulators of both the stress and reward systems through coordinated regulation of a large number key molecules and facilitate cross-talk between stress, reward, synaptic plasticity, and immune/inflammatory responses. Through integration of these signaling pathways, miRNAs serve as master regulators of downstream behavioral and cellular responses to drugs of abuse. In turn, miRNA expression is itself regulated by external stimuli including stress and drug exposure. MicroRNAs are highly conserved between species, however, species specific differences do exist. Within species, there are tissue and cell type differences in expression regulated by post-transcriptional modification of more ubiquitously expressed pre-miRNAs. This is likely necessary due to the ability of a single miRNA to regulate numerous target genes. While the complexity of miRNA regulation of gene expression poses a daunting challenge, it is an area of study that holds immense promise for future advancement of drug abuse research and the biomedical sciences as a whole. We argue that miRNAs co-regulated by stress and chronic cocaine represent prime targets for study in order to further elucidate the etiology of the transition from casual drug use to drug dependence. Further, this population of miRNAs represents promising targets for identification of novel treatments for drug abuse.
Author Contributions
MBD and EMU wrote the manuscript.
Conflict of Interest Statement
The authors declare that the research was conducted in the absence of any commercial or financial relationships that could be construed as a potential conflict of interest.
Acknowledgments
We would like to acknowledge the following NIDA funded grants T32DA007237 and R01 DA018326.
Abbreviations
Ago, argonaute; Arc; activity-regulated cytoskeleton-associated protein; BDNF, brain derived neurotrophic factor; BNST, bed nucleus of the stria terminalis; CREB, cAMP response element-binding protein; CRF, corticotrophin releasing factor; Drd2, D2 dopamine receptors; ERK, Extracellular signal-regulated kinase; MAP3K8, mitogen-activated protein kinase kinase kinase 8; MeCP2, methyl CpG binding protein 2; POMC, proopiomelanocortin; RISC, RNA-induced silencing complex; SIRT, NAD-dependent deacetylase sirtuin; TLR, Toll-like receptor; TNF, tumor necrosis factor; TORC, target of rapamycin complex; VTA, ventral tegmental area.
References
Abdelmohsen, K., Hutchison, E. R., Lee, E. K., Kuwano, Y., Kim, M. M., Masuda, K., et al. (2010). miR-375 inhibits differentiation of neurites by lowering HuD levels. Mol. Cell Biol. 30, 4197–4210. doi: 10.1128/MCB.00316-10
Alheid, G. F., Beltramino, C. A., De Olmos, J. S., Forbes, M. S., Swanson, D. J., and Heimer, L. (1998). The neuronal organization of the supracapsular part of the stria terminalis in the rat: the dorsal component of the extended amygdala. Neuroscience 84, 967–996. doi: 10.1016/s0306-4522(97)00560-5
Allen, Y. S., Roberts, G. W., Bloom, S. R., Crow, T. J., and Polak, J. M. (1984). Neuropeptide Y in the stria terminalis: evidence for an amygdalofugal projection. Brain Res. 321, 357–362. doi: 10.1016/0006-8993(84)90193-8
Bahi, A., Chandrasekar, V., and Dreyer, J. L. (2014). Selective lentiviral-mediated suppression of microRNA124a in the hippocampus evokes antidepressants-like effects in rats. Psychoneuroendocrinology 46, 78–87. doi: 10.1016/j.psyneuen.2014.04.009
Berglind, W. J., See, R. E., Fuchs, R. A., Ghee, S. M., Whitfield, T. W., Jr., Miller, S. W., et al. (2007). A BDNF infusion into the medial prefrontal cortex suppresses cocaine seeking in rats. Eur. J. Neurosci. 26, 757–766. doi: 10.1111/j.1460-9568.2007.05692.x
Berglind, W. J., Whitfield, T. W., Jr., LaLumiere, R. T., Kalivas, P. W., and McGinty, J. F. (2009). A single intra-PFC infusion of BDNF prevents cocaine-induced alterations in extracellular glutamatewithin the nucleus accumbens. J. Neurosci. 29, 3715–3719. doi: 10.1523/JNEUROSCI.5457-08.2009
Briand, L. A., Vassoler, F. M., Pierce, R. C., Valentino, R. J., and Blendy, J. A. (2010). Ventral tegmental afferents in stress-induced reinstatement: the role of cAMP response element-binding protein. J. Neurosci. 30, 16149–16159. doi: 10.1523/JNEUROSCI.2827-10.2010
Caputo, V., Sinibaldi, L., Fiorentino, A., Parisi, C., Catalanotto, C., Pasini, A., et al. (2011). Brain derived neurotrophic factor (BDNF) expression is regulated by microRNAs miR-26a and miR-26b allele-specific binding. PLoS One 6:e28656. doi: 10.1371/journal.pone.0028656
Carlezon, W. A. Jr., Thome, J., Olson, V. G., Lane-Ladd, S. B., Brodkin, E. S., Hiroi, N., et al. (1998). Regulation of cocaine reward by CREB. Science 282, 2272–2275. doi: 10.1126/science.282.5397.2272
Chandrasekar, V., and Dreyer, J. L. (2009). microRNAs miR-124, let-7d and miR-181a regulate cocaine-induced plasticity. Mol. Cell. Neurosci. 42, 350–362. doi: 10.1016/j.mcn.2009.08.009
Chandrasekar, V., and Dreyer, J. L. (2011). Regulation of MiR-124, Let-7d and MiR-181a in the accumbens affects the expression, extinction and reinstatement of cocaine-induced conditioned place preference. Neuropsychopharmacology 36, 1149–1164. doi: 10.1038/npp.2010.250
Chen, C. L., Liu, H., and Guan, X. (2013). Changes in microRNA expression profile in hippocampus during the acquisition and extinction of cocaine-induced conditioned place preference in rats. J. Biomed. Sci. 20:96. doi: 10.1186/1423-0127-20-96
Dajas-Bailador, F., Bonev, B., Garcez, P., Stanley, P., Guillemot, F., and Papalopulu, N. (2012). microRNA-9 regulates axon extension and branching by targeting Map1b in mouse cortical neurons. Nat. Neurosci. doi: 10.1038/nn.3082 [Epub ahead of print].
de Jong, I. E., and de Kloet, E. R. (2004). Glucocorticoids and vulnerability to psychostimulant drugs: toward substrate and mechanism. Ann. N. Y. Acad. Sci. 1018, 192–198. doi: 10.1196/annals.1296.022
Delaloy, C., Liu, L., Lee, J. A., Su, H., Shen, F., Yang, G. Y., et al. (2010). MicroRNA-9 coordinates proliferation and migration of human embryonic stem cell-derived neural progenitors. Cell Stem Cell 6, 323–335. doi: 10.1016/j.stem.2010.02.015
Deroche, V., Marinelli, M., Le Moal, M., and Piazza, P. V. (1997). Glucocorticoids and behavioral effects of psychostimulants. II: cocaine intravenous self-administration and reinstatement depend on glucocorticoid levels. J. Pharmacol. Exp. Ther. 281, 1401–1407.
Diederichs, S., and Haber, D. A. (2007). Dual role for argonautes in microRNA processing and posttranscriptional regulation of microRNA expression. Cell 131, 1097–1108. doi: 10.1016/j.cell.2007.10.032
Dwivedi, Y., Roy, B., Lugli, G., Rizavi, H., Zhang, H., and Smalheiser, N. R. (2015). Chronic corticosterone-mediated dysregulation of microRNA network in prefrontal cortex of rats: relevance to depression pathophysiology. Transl. Psychiatry 15:e682. doi: 10.1038/tp.2015.175
Eipper-Mains, J. E., Kiraly, D. D., Palakodeti, D., Mains, R. E., Eipper, B. A., and Graveley, B. R. (2011). microRNA-Seq reveals cocaine-regulated expression of striatal microRNAs. RNA 17, 1529–1543. doi: 10.1261/rna.2775511
Everitt, B. J., and Wolf, M. E. (2002). Psychomotor stimulant addiction: a neural systems perspective. J. Neurosci. 22, 3312–3320.
Ferguson, D., Koo, J. W., Feng, J., Heller, E., Rabkin, J., Heshmati, M., et al. (2013). Essential role of SIRT1 signaling in the nucleus accumbens in cocaine and morphine action. J. Neurosci. 33, 16088–16098. doi: 10.1523/JNEUROSCI.1284-13.2013
Gao, J., Wang, W. Y., Mao, Y. W., Gräff, J., Guan, J. S., Pan, L., et al. (2010). A novel pathway regulates memory and plasticity via SIRT1 and miR-134. Nature 466, 1105–1109. doi: 10.1038/nature09271
Goeders, N. E. (1997). A neuroendocrine role in cocaine reinforcement. Psychoneuroendocrinology 22, 237–259. doi: 10.1016/s0306-4530(97)00027-9
Graham, D. L., Edwards, S., Bachtell, R. K., Dileone, R. J., Rios, M., and Self, D. W. (2007). Dynamic BDNF activity in nucleus accumbens with cocaine use increases self-administration and relapse. Nat. Neurosci. 10, 1029–1037. doi: 10.1038/nn1929
Graham, D. L., Krishnan, V., Larson, E. B., Graham, A., Edwards, S., Bachtell, R. K., et al. (2009). Tropomyosin-related kinase B in the mesolimbic dopamine system: region-specific effects on cocaine reward. Biol. Psychiatry 65, 696–701. doi: 10.1016/j.biopsych.2008.09.032
Grimm, J. W., Lu, L., Hayashi, T., Hope, B. T., Su, T. P., and Shaham, Y. (2003). Time-dependent increases in brain-derived neurotrophic factor protein levels within the mesolimbic dopamine system after withdrawal from cocaine: implications for incubation of cocaine craving. J. Neurosci. 23, 742–747.
Hammond, S. M., Boettcher, S., Caudy, A. A., Kobayashi, R., and Hannon, G. J. (2001). Argonaute2, a link between genetic and biochemical analyses of RNAi. Science 293, 1146–1150. doi: 10.1126/science.1064023
Haramati, S., Navon, I., Issler, O., Ezra-Nevo, G., Gil, S., Zwang, R., et al. (2011). MicroRNA as repressors of stress-induced anxiety: the case of amygdalar miR-34. J. Neurosci. 31, 14191–14203. doi: 10.1523/JNEUROSCI.1673-11.2011
Heimer, L., Zahm, D. S., Churchill, L., Kalivas, P. W., and Wohltmann, C. (1991). Specificity in the projection patterns of accumbal core and shell in the rat. Neuroscience 41, 89–125. doi: 10.1016/0306-4522(91)90202-y
Helwak, A., Kudla, G., Dudnakova, T., and Tollervey, D. (2013). Mapping the human miRNA interactome by CLASH reveals frequent noncanonical binding. Cell 153, 654–665. doi: 10.1016/j.cell.2013.03.043
Hollander, J. A., Im, H. I., Amelio, A. L., Kocerha, J., Bali, P., Lu, Q., et al. (2010). Striatal microRNA controls cocaine intake through CREB signalling. Nature 466, 197–202. doi: 10.1038/nature09202
Horger, B. A., Iyasere, C. A., Berhow, M. T., Messer, C. J., Nestler, E. J., and Taylor, J. R. (1999). Enhancement of locomotor activity and conditioned reward to cocaine by brain-derived neurotrophic factor. J. Neurosci. 19, 4110–4122.
Im, H. I., Hollander, J. A., Bali, P., and Kenny, P. J. (2010). MeCP2 controls BDNF expression and cocaine intake through homeostatic interactions with microRNA-212. Nat. Neurosci. 13, 1120–1127. doi: 10.1038/nn.2615
Issler, O., Haramati, S., Paul, E. D., Maeno, H., Navon, I., Zwang, R., et al. (2014). MicroRNA 135 is essential for chronic stress resiliency, antidepressant efficacy and intact serotonergic activity. Neuron 83, 344–360. doi: 10.1016/j.neuron.2014.05.042
Jonkman, S., and Kenny, P. J. (2013). Molecular, cellular and structural mechanisms of cocaine addiction: a key role for microRNAs. Neuropsychopharmacology 38, 198–211. doi: 10.1038/npp.2012.120
Kawashima, H., Numakawa, T., Kumamaru, E., Adachi, N., Mizuno, H., Ninomiya, M., et al. (2010). Glucocorticoid attenuates brain-derived neurotrophic factor-dependent upregulation of glutamate receptors via the suppression of microRNA-132 expression. Neuroscience 165, 1301–1311. doi: 10.1016/j.neuroscience.2009.11.057
Koob, G. F. (2003). Neuroadaptive mechanisms of addiction: studies on the extended amygdala. Eur. Neuropsychopharmacol. 13, 442–452. doi: 10.1016/j.euroneuro.2003.08.005
Koob, G. F., Caine, B., Markou, A., Pulvirenti, L., and Weiss, F. (1994). Role for the mesocortical dopamine system in the motivating effects of cocaine. NIDA Res. Monogr. 145, 1–18. doi: 10.1037/e495842006-002
Koob, G. F., and Le Moal, M. (2001). Drug addiction, dysregulation of reward and allostasis. Neuropsychopharmacology 24, 97–129. doi: 10.1016/s0893-133x(00)00195-0
Kozicz, T. (2001). Axon terminals containing tyrosine hydroxylase- and dopamine-beta-hydroxylase immunoreactivity form synapses with galanin immunoreactive neurons in the lateral division of the bed nucleus of the stria terminalis in the rat. Brain Res. 914, 23–33. doi: 10.1016/s0006-8993(01)02770-6
Kye, M. J., Niederst, E. D., Wertz, M. H., Gonçalves, I. O. C., Akten, B., Dover, K. Z., et al. (2014). SMN regulates axonal local translation via miR-183/mTOR pathway. Hum. Mol. Genet. 23, 6318–6331. doi: 10.1093/hmg/ddu350
Larson, E. B., Graham, D. L., Arzaga, R. R., Buzin, N., Webb, J., Green, T. A., et al. (2011). Overexpression of CREB in the nucleus accumbens shell increases cocaine reinforcement in self-administering rats. J. Neurosci. 31, 16447–16457. doi: 10.1523/JNEUROSCI.3070-11.2011
Liu, J., Carmell, M. A., Rivas, F. V., Marsden, C. G., Thomson, J. M., Song, J. J., et al. (2004). Argonaute2 is the catalytic engine of mammalian RNAi. Science 305, 1437–1441. doi: 10.1126/science.1102513
Lu, L., Dempsey, J., Liu, S. Y., Bossert, J. M., and Shaham, Y. (2004). A single infusion of brain-derived neurotrophic factor into the ventral tegmental area induces long-lasting potentiation of cocaine seeking after withdrawal. J. Neurosci. 24, 1604–1611. doi: 10.1523/JNEUROSCI.5124-03.2004
Maisonneuve, I. M., Ho, A., and Kreek, M. J. (1995). Chronic administration of a cocaine “binge” alters basal extracellular levels in male rats: an in vivo microdialysis study. J. Pharmacol. Exp. Ther. 272, 652–657.
Mannironi, C., Camon, J., De Vito, F., Biundo, A., De Stefano, M. E., Persiconi, I., et al. (2013). Acute stress alters amygdala microRNA miR-135a and miR-124 expression: inferences for corticosteroid dependent stress response. PLoS One 8:e73385. doi: 10.1371/journal.pone.0073385
McClung, C. A., and Nestler, E. J. (2003). Regulation of gene expression and cocaine reward by CREB and DeltaFosB. Nat. Neurosci. 6, 1208–1215. doi: 10.1038/nn1143
McFarland, K., Davidge, S. B., Lapish, C. C., and Kalivas, P. W. (2004). Limbic and motor circuitry underlying footshock-induced reinstatement of cocaine-seeking behavior. J. Neurosci. 24, 1551–1560. doi: 10.1523/JNEUROSCI.4177-03.2004
McLaughlin, J. P., Marton-Popovici, M., and Chavkin, C. (2003). Kappa opioid receptor antagonism and prodynorphin gene disruption block stress-induced behavioral responses. J. Neurosci. 23, 5674–5683.
Meerson, A., Cacheaux, L., Goosens, K. A., Sapolsky, R. M., Soreq, H., and Kaufer, D. (2010). Changes in brain MicroRNAs contribute to cholinergic stress reactions. J. Mol. Neurosci. 40, 47–55. doi: 10.1007/s12031-009-9252-1
Nahid, M. A., Yao, B., Dominguez-Gutierrez, P. R., Satoh, M., and Chan, E. K. L. (2013). Regulation of TLR2-mediated tolerance and cross-tolerance through IRAK4 modulation by miR-132/-212. J. Immunol. 190, 1250–1263. doi: 10.4049/jimmunol.1103060
Nemoto, T., Mano, A., and Shibasaki, T. (2012). Increased expression of miR-325–3p by urocortin 2 and its involvement in stress-induced suppression of LH secretion in rat pituitary. Am. J. Physiol. Endocrinol. Metab. 302, E781–E787. doi: 10.1152/ajpendo.00616.2011
Numakawa, T., Yamamoto, N., Chiba, S., Richards, M., Ooshima, Y., Kishi, S., et al. (2011). Growth factors stimulate expression of neuronal and glial miR-132. Neurosci. Lett. 505, 242–247. doi: 10.1016/j.neulet.2011.10.025
O’Carroll, D., Mecklenbrauker, I., Das, P. P., Santana, A., Koenig, U., Enright, A. J., et al. (2007). A Slicer-independent role for Argonaute 2 in hematopoiesis and the microRNA pathway. Genes. Dev. 21, 1999–2004. doi: 10.1101/gad.1565607
Olive, V., Bennett, M. J., Walker, J. C., Ma, C., Jiang, I., Cordon-Cardo, C., et al. (2009). miR-19 is a key oncogenic component of mir-17–92. Genes. Dev. 23, 2839–2849. doi: 10.1101/gad.1861409
Pacak, K., McCarty, R., Palkovits, M., Kopin, I. J., and Goldstein, D. S. (1995). Effects of immobilization on in vivo release of norepinephrine in the bed nucleus of the stria terminalis in conscious rats. Brain Res. 688, 242–246. doi: 10.1016/0006-8993(95)00566-9
Phelix, C. F., and Paull, W. K. (1990). Demonstration of distinct corticotropin releasing factor-containing neuron populations in the bed nucleus of the stria terminalis: a light and electron microscopic immunocytochemical study in the rat. Histochemistry 94, 345–364. doi: 10.1007/bf00266441
Phillipson, O. T. (1979). Afferent projections to the ventral tegmental area of Tsai and interfascicular nucleus: a horseradish peroxidase study in the rat. J. Comp. Neurol. 187, 117–143. doi: 10.1002/cne.901870108
Piazza, P. V., and Le Moal, M. L. (1996). Pathophysiological basis of vulnerability to drug abuse: role of an interaction between stress, glucocorticoids and dopaminergic neurons. Annu. Rev. Pharmacol. Toxicol. 36, 359–378. doi: 10.1146/annurev.pharmtox.36.1.359
Piazza, P. V., and Le Moal, M. (1997). Glucocorticoids as a biological substrate of reward: physiological and pathophysiological implications. Brain Res. Brain Res. Rev. 25, 359–372. doi: 10.1016/s0165-0173(97)00025-8
Pontieri, F. E., Mainero, C., La Riccia, M., Passarelli, F., and Orzi, F. (1995). Functional correlates of repeated administration of cocaine and apomorphine in the rat. Eur. J. Pharmacol. 284, 205–209. doi: 10.1016/0014-2999(95)00441-m
Quinn, R. K., Brown, A. L., Goldie, B. J., Levi, E. M., Dickson, P. W., Smith, D. W., et al. (2015). Distinct miRNA expression in dorsal striatal subregions is associated with 581 risk for addiction in rats. Transl. Psychiatry 5:e503. doi: 10.1038/tp.2014.144
Remenyi, J., Hunter, C. J., Cole, C., Ando, H., Impey, S., Monk, C. E., et al. (2010). Regulation of the miR-212/132 locus by MSK1 and CREB in response to neurotrophins. Biochem. J. 428, 281–291. doi: 10.1042/BJ20100024
Rinaldi, A., Vincenti, S., De Vito, F., Bozzoni, I., Oliverio, A., Presutti, C., et al. (2010). Stress induces region specific alterations in microRNAs expression in mice. Behav. Brain Res. 208, 265–269. doi: 10.1016/j.bbr.2009.11.012
Rivetti di Val Cervo, P., Lena, A. M., Nicoloso, M., Rossi, S., Mancini, M., Zhou, H., et al. (2012). p63-microRNA feedback in keratinocyte senescence. Proc. Natl. Acad. Sci. U S A 109, 1133–1138. doi: 10.1073/pnas.1112257109
Saba, R., Störchel, P. H., Aksoy-Aksel, A., Kepura, F., Lippi, G., Plant, T. D., et al. (2012). Dopamine-regulated microRNA MiR-181a controls GluA2 surface expression in hippocampal neurons. Mol. Cell Biol. 32, 619–632. doi: 10.1128/MCB.05896-11
Schaefer, A., Im, H. I., Venø, M. T., Fowler, C. D., Min, A., Intrator, A., et al. (2010). Argonaute 2 in dopamine 2 receptor-expressing neurons regulates cocaine addiction. J. Exp. Med. 207, 1843–1851. doi: 10.1084/jem.20100451
Shaked, I., Meerson, A., Wolf, Y., Avni, R., Greenberg, D., Gilboa-Geffen, A., et al. (2009). MicroRNA-132 potentiates cholinergic anti-inflammatory signaling by targeting acetylcholinesterase. Immunity 31, 965–973. doi: 10.1016/j.immuni.2009.09.019
Shimizu, S., Tanaka, T., Tohyama, M., and Miyata, S. (2015). Yokukansan normalizes glucocorticoid receptor protein expression in oligodendrocytes of the corpus callosum by regulating microRNA-124a expression after stress exposure. Brain Res. Bull. 114, 49–55. doi: 10.1016/j.brainresbull.2015.03.007
Sõber, S., Laan, M., and Annilo, T. (2010). MicroRNAs miR-124 and miR-135a are potential regulators of the mineralocorticoid receptor gene (NR3C2) expression. Biochem. Biophys. Res. Commun. 391, 727–732. doi: 10.1016/j.bbrc.2009.11.128
Song, J. J., Smith, S. K., Hannon, G. J., and Joshua-Tor, L. (2004). Crystal structure of Argonaute and its implications for RISC slicer activity. Science 305, 1434–1437. doi: 10.1126/science.1102514
Sun, Y., Li, Q., Gui, H., Xu, D.-P., Yang, Y.-L., Su, D.-F., et al. (2013). MicroRNA-124 mediates the cholinergic anti-inflammatory action through inhibiting the production of pro-inflammatory cytokines. Cell Res. 23, 1270–1283. doi: 10.1038/cr.2013.116
Strum, J. C., Johnson, J. H., Ward, J., Xie, H., Feild, J., Hester, A., et al. (2009). MicroRNA 132 regulates nutritional stress-induced chemokine production through repression of SirT1. Mol. Endocrinol. 23, 1876–1884. doi: 10.1210/me.2009-0117
Tejeda, H. A., Shippenberg, T. S., and Henriksson, R. (2012). The dynorphin/κ-opioid receptor system and its role in psychiatric disorders. Cell. Mol. Life Sci. 69, 857–896. doi: 10.1007/s00018-011-0844-x
Unterwald, E. M., Rubenfeld, J. M., and Kreek, M. J. (1994). Repeated cocaine administration upregulates kappa and mu, but not delta, opioid receptors. Neuroreport 5, 1613–1616. doi: 10.1097/00001756-199408150-00018
Volk, N., Paul, E. D., Haramati, S., Eitan, C., Fields, B. K., Zwang, R., et al. (2014). MicroRNA-19b associates with Ago2 in the amygdala following chronic stress and regulates the adrenergic receptor beta 1. J. Neurosci. 34, 15070–15082. doi: 10.1523/JNEUROSCI.0855-14.2014
Vranjkovic, O., Gasser, P. J., Gerndt, C. H., Baker, D. A., and Mantsch, J. R. (2014). Stress-induced cocaine seeking requires a β-2 adrenergic receptor-regulated pathway from the ventral bed nucleus of the stria terminalis that regulates CRF actions in the ventral tegmental area. J. Neurosci. 34, 12504–12514. doi: 10.1523/JNEUROSCI.0680-14.2014
Walsh, J. J., Friedman, A. K., Sun, H., Heller, E. A., Ku, S. M., Juarez, B., et al. (2014). Stress and CRF gate neural activation of BDNF in the mesolimbic reward pathway. Nat. Neurosci. 17, 27–29. doi: 10.1038/nn.3591
Wang, B., Shaham, Y., Zitzman, D., Azari, S., Wise, R. A., and You, Z. B. (2005). Cocaine experience establishes control of midbrain glutamate and dopamine by corticotropin-releasing factor: a role in stress-induced relapse to drug seeking. J. Neurosci. 25, 5389–5396. doi: 10.1523/JNEUROSCI.0955-05.2005
Whitfield, T. W., Jr., Shi, X., Sun, W. L., and McGinty, J. F. (2011). The suppressive effect of an intra-prefrontal cortical infusion of BDNF on cocaine-seeking is Trk receptor and extracellular signal-regulated protein kinase mitogen-activated protein kinase dependent. J. Neurosci. 31, 834–842. doi: 10.1523/JNEUROSCI.4986-10.2011
Wu, J., and Xie, X. (2006). Comparative sequence analysis reveals an intricate network among REST, CREB and miRNA in mediating neuronal gene expression. Genome Biol. 7:R85. doi: 10.1186/gb-2006-7-9-r85
Yi, L. T., Li, J., Liu, B. B., Luo, L., Liu, Q., and Geng, D. (2014). BDNF-ERK-CREB signalling mediates the role of miR-132 in the regulation of the effects of oleanolic acid in male mice. J. Psychiatry Neurosci. 39, 348–359. doi: 10.1503/jpn.130169
Zhang, N., Lin, J. K., Chen, J., Liu, X. F., Liu, J. L., Luo, H. S., et al. (2013). MicroRNA 375 mediates the signaling pathway of corticotropin-releasing factor (CRF) regulating pro-opiomelanocortin (POMC) expression by targeting mitogen-activated protein kinase 8. J. Biol. Chem. 288, 10361–10373. doi: 10.1074/jbc.M112.425504
Zhang, Y., Wang, Y., Wang, L., Bai, M., Zhang, X., and Zhu, X. (2015). Dopamine receptor D2 and associated microRNAs are involved in stress susceptibility and resistance to escitalopram treatment. Int. J. Neuropsychopharmacol. 18:pyv025. doi: 10.1093/ijnp/pyv025
Keywords: microRNA, stress, cocaine, extended amygdala, corticotropin-releasing factor, brain-derived neurotrophic factor
Citation: Doura MB and Unterwald EM (2016) MicroRNAs Modulate Interactions between Stress and Risk for Cocaine Addiction. Front. Cell. Neurosci. 10:125. doi: 10.3389/fncel.2016.00125
Received: 11 March 2016; Accepted: 29 April 2016;
Published: 26 May 2016.
Edited by:
Hansen Wang, University of Toronto, CanadaReviewed by:
Hermona Soreq, The Hebrew University of Jerusalem, IsraelBronwyn Maree Kivell, Victoria University of Wellington, New Zealand
Christopher V. Dayas, University of Newcastle, Australia
Copyright © 2016 Doura and Unterwald. This is an open-access article distributed under the terms of the Creative Commons Attribution License (CC BY). The use, distribution and reproduction in other forums is permitted, provided the original author(s) or licensor are credited and that the original publication in this journal is cited, in accordance with accepted academic practice. No use, distribution or reproduction is permitted which does not comply with these terms.
*Correspondence: Menahem B. Doura, dHVlODcyOTJAdGVtcGxlLmVkdQ==