- 1Institut National de la Santé et de la Recherche Médicale (INSERM U930) “Imagerie et Cerveau”, CHRU de Tours, Université François-Rabelais, Tours, France
- 2Laboratoire de Biochimie et de Biologie Moléculaire, Hôpital Bretonneau, CHRU de Tours, Tours, France
- 3Institut National de la Santé et de la Recherche Médicale (INSERM U1069) “Nutrition, Growth and Cancer”, Université François-Rabelais de Tours, Tours, France
- 4Centre SLA, Service de Neurologie, CHRU de Tours, Tours, France
- 5The Neuroscience Institute Montpellier, Institut National de la Santé et de la Recherche Médicale (INSERM UMR1051), Saint Eloi Hospital, Montpellier, France
Glutamate-induced excitotoxicity is a major contributor to motor neuron degeneration in the pathogenesis of amyotrophic lateral sclerosis (ALS). The spinal cord × Neuroblastoma hybrid cell line (NSC-34) is often used as a bona fide cellular model to investigate the physiopathological mechanisms of ALS. However, the physiological response of NSC-34 to glutamate remains insufficiently described. In this study, we evaluated the relevance of differentiated NSC-34 (NSC-34D) as an in vitro model for glutamate excitotoxicity studies. NSC-34D showed morphological and physiological properties of motor neuron-like cells and expressed glutamate receptor subunits GluA1–4, GluN1 and GluN2A/D. Despite these diverse characteristics, no specific effect of glutamate was observed on cultured NSC-34D survival and morphology, in contrast to what has been described in primary culture of motor neurons (MN). Moreover, a small non sustained increase in the concentration of intracellular calcium was observed in NSC-34D after exposure to glutamate compared to primary MN. Our findings, together with the inability to obtain cultures containing only differentiated cells, suggest that the motor neuron-like NSC-34 cell line is not a suitable in vitro model to study glutamate-induced excitotoxicity. We suggest that the use of primary cultures of MN is more suitable than NSC-34 cell line to explore the pathogenesis of glutamate-mediated excitotoxicity at the cellular level in ALS and other motor neuron diseases.
Introduction
Amyotrophic lateral sclerosis (ALS) is one of the most common neurodegenerative diseases in adults, caused by the selective death of motor neurons (MN). Studies of the physiology of ALS support the involvement of genetic factors and micro-environmental factors with mechanisms such as glutamate excitotoxicity and oxidative stress, but these mechanisms await elucidation. Glutamate excitotoxicity is a major contributor to dysfunction and death of MN in the pathogenesis of ALS (Heath and Shaw, 2002; Van Den Bosch et al., 2006; Spalloni et al., 2013; Blasco et al., 2014). Among the many drugs targeting this pathogenic mechanism tested in clinical trials (Blasco et al., 2014), one treatment (riluzole) is used in common practice to slow the progression of the disease by blocking glutamatergic neurotransmission in the central nervous system. However, the mechanisms of toxicity caused by glutamate and their links with other physiopathological pathways remain poorly understood.
Overstimulation of glutamate receptors facilitates the entry and consequently the excess of calcium (Ca2+) in cell compartments, leading to a cascade of destructive events by calcium-dependent enzymatic pathways and mitochondrial dysfunction with the generation of free radicals (Van Den Bosch et al., 2000; Blasco et al., 2014). Glutamate receptors are divided into two families: the ligand-gated cation channels (ionotropic; Lodge, 2009) and G protein-coupled receptors (metabotropic; Niswender and Conn, 2010). Ionotropic receptors are further divided into three categories according to their non-natural preferred agonists, i.e., AMPA (α-amino-3-hydroxy-5-methyl-4-isoxazole propionic acid), NMDA (N-methyl-D-aspartate) and kainate (AK; Lodge, 2009). AMPA and NMDA receptors are largely responsible for calcium flux across neuronal cell membranes (Hollmann et al., 1991; Van Den Bosch et al., 2000).
Primary cultures of MN (Krugman et al., 1999) and cultures of different cell lines are used to study the molecular mechanisms of neurotoxicity induced by glutamate in MN. The most common cell line used in ALS research is the spinal cord neuron × neuroblastoma hybrid cell line (NSC-34), which was originally described as having several morphological and physiological properties of MN (Eggett et al., 2000; Rembach et al., 2004; Benkler et al., 2013; Maier et al., 2013; Valbuena et al., 2015). We required validation of this model to study the metabolic effects of glutamate excitotoxicity in a neurological disease. NSC-34 is an hybrid cell line produced by the fusion of MN from the spinal cords of mouse embryos with mouse neuroblastoma cells N18TG2 (Cashman et al., 1992). These cells exhibit properties of MN when subjected to protocols of differentiation and maturation. Although NSC-34 cells share many features with MN such as long processes and S-laminin with leucine-arginine-glutamate (LRE) adhesion motif (a specific basal lamina glycoprotein concentrated at the neuromuscular synapse; Hunter et al., 1991), the formation of contacts with myotubes in culture (Cashman et al., 1992) and increased survival in the presence of neurotrophic factors (NTFs; Turner et al., 2004), their functional properties when stimulated by glutamate have rarely been studied (Durham et al., 1993; Eggett et al., 2000).
The selective motor neuronal cell death in ALS is highly dependent on intracellular Ca2+ and is insensitive to inhibitors of voltage-operated Ca2+ and Na+ channels (MacDermott et al., 1986; Van Den Bosch et al., 2000, 2006; Heath and Shaw, 2002). Thus, the permeability of glutamate receptors to Ca2+ seems crucial to validate the NSC-34 model as appropriate for the exploration of glutamate excitotoxicity. In order to evaluate whether NSC34 represents a relevant model for studies on glutamate-induced neurotoxicity, we differentiated the NSC-34 cells by serum depletion in the presence or absence of all-trans retinoic acid (RA; Clagett-Dame et al., 2006; Maden, 2007), and analyzed the expression and functionality of glutamate receptors. We compared for the first time the usefulness of NSC-34 cells (using various differentiation protocols) and primary motor neuron cultures (used as the reference) as models for glutamate induced-excitotoxicity in the same study.
Materials and Methods
Materials
Dulbecco’s modified Eagle’s medium (DMEM, Gibco), DMEM-Ham’s F12 and Alpha-modified Eagle’s medium (α-MEM), modified Eagle’s medium non-essential amino acid (MEM-NEAA), penicillin/streptomycin (P/S), Trizol, Superscript II reverse transcriptase kit and Fura-2 acetoxymethyl ester were all obtained from Invitrogen (Life Technologies, Saint Aubin, France), L-glutamic acid and all-trans RA from Sigma Aldrich (Saint-Quentin Fallavier, France) and fetal calf serum (FCS) from Eurobio (Courtaboeuf, France).
Culture of NSC-34
The NSC-34 was obtained from Cedarlane Laboratories (via Tebu-Bio, Le Perray en Yvelines, France). Cells were cultured as described previously (Madji Hounoum et al., 2015). Cultures were used 5–15 passages. Each type of experiment was performed on the same passage. No sub-culture passage was performed for differentiated NSC-34.
For differentiation, NSC-34 cells were grown to confluence and the proliferation medium (DMEM plus 10% FCS) was exchanged for fresh differentiation medium every 3 days. Cells were allowed to differentiate for up to 4 weeks. Three differentiation media were investigated: (1) 1:1 DMEM/Ham’s F12 plus 1% FCS, 1% P/S and 1% MEM-NEAA, the most commonly used medium for NSC-34 differentiation (Kruman et al., 1999; Eggett et al., 2000; Rembach et al., 2004; Benkler et al., 2013); (2) α-MEM [the medium used for another neuron-like cell line (P19; MacPherson et al., 1997)] plus 1% FCS, 1% P/S and 1% MEM-NEAA; and (3) DMEM (the classic medium for NSC-34 culture) plus 1% FCS and 1% P/S.
Two conditions were investigated for all differentiation media, i.e., with or without RA [1 μM, as used previously (Johann et al., 2011; Maier et al., 2013)]. NSC-34 cells, maintained on proliferation medium, served as the undifferentiated control group. The average length of neurites in the differentiation media was quantified using Sholl’s method for quantification of dendritic branching in hippocampal neurons (Sholl, 1956). Briefly, concentric circles at 25 μm intervals between adjacent circles were drawn on Powerpoint, at the same magnification of cell pictures. The center of the circle centered on the soma of the cell, the lengths of the processes were measured from the soma by multiplying the number of intersections (neurite-circle) every 25 μm. Cells with neurites longer than 50 μm were considered as differentiated. Neurite length was analyzed by imaging a minimum of 10 cells per experiment, four experiments for each condition.
Primary Motor Neuron Cultures
Studies were conducted using primary cultures of motor neuron from the spinal cords of C57BL/6 mice at embryonic day 12.5 (Centre d’Elevage Roger Janvier, France). Cultures were grown as described previously (Camu et al., 2014; Dangoumau et al., 2015). MN were plated on poly-ornithine/laminin-treated wells in the presence of NTFs (0.1 ng/mL GDNF, 1 ng/mL BDNF, and 10 ng/mL CNTF in supplemented neurobasal medium (Invitrogen, Carlsbad, CA, USA)). Supplemented neurobasal medium contained 2% horse serum, L-glutamate (25 mM), β-mercaptoethanol (25 mM), L-glutamine (0.5 mM), and 2% B-27 supplement (Invitrogen, Life Technologies, Saint Aubin, France). The use of appropriate culture medium combined with multiple purification steps using density gradient (BSA cushion and Optiprep density centrifugation) and magnetic cell sorting with an indirect microbeads technique promoted the enrichment of MN as well as the elimination of astrocytes and microglia cells from the culture (Arce et al., 1999).
Immunocytochemistry
To assess morphological characterization of primary motor neuron culture derived from embryonic spinal cord, expression of βIII-tubulin and p75 neurotrophic receptor were analyzed (Rembach et al., 2004). Cells were fixed in 4% paraformaldehyde and then incubated in a blocking and permeabilizing solution (10% donkey serum and 2% Triton X-100) for 1 h. The cells were stained with rabbit anti-βIII-tubulin (1:200, Covance, Princeton, NJ, USA) and mouse anti-nerve growth factor receptor (p75NTR 1:60, Chemicon MAB357) for 1 h. After being washed with phosphate buffered saline (PBS), the cells were incubated with secondary antibodies for 1 h. The secondary antibodies used were donkey anti-rabbit Alexa-488 (1:300, Life Technologies, Saint Aubin, France) and donkey anti-mouse Alexa-594 (1:300, Life Technologies, Saint Aubin, France). Nuclear DNA was observed by 4,6-diamino-2-phenylindole (DAPI) contained in ProLong® (Life Technologies, Saint Aubin, France). Cells were photographed using a confocal microscope (Olympus FV500).
Cell Viability Assay
To assess the effect of glutamate-induced excitotoxicity in NSC-34D, cells were exposed to glutamate at different concentrations (100 μM, 500 μM, 1 mM and 10 mM) for 48 h (Eggett et al., 2000; Rembach et al., 2004; Maier et al., 2013) before determination of cell viability by trypan blue assay. A control condition with no excitotoxic agent (PBS) was included for comparison. Four replicates per condition were obtained. The cells were harvested with trypsin and re-suspended in culture medium before addition of trypan blue (0.4% w/v; Molecular Probes-Invitrogen, Carlsbad, CA, USA). Living and dead cells were counted accurately with a Countess Automated Cell Counter (Invitrogen, Carlsbad, CA, USA). Motor neuron survival was assessed by direct counting after exposing cells to 100 μM glutamate for 48 h.
RNA Extraction, Reverse Transcription PCR (RT-PCR) and Real-Time Polymerase Chain Reaction (RT-qPCR)
Following a wash in PBS, RNA samples were extracted from NSC-34 cells and primary MN using Trizol and treated with DNase I (Proteigene®). Reverse transcription was performed on 2.5 μg of treated RNA using the superscript II reverse transcriptase kit according to the manufacturer’s instructions. cDNA samples were analyzed using primers for subunits of AMPA receptors (GluA1–4), NMDA receptors (GluN1, GluN2A-D, GluN3A), and standard markers for MN such as choline acetyltransferase (ChAT), p75 (Cashman et al., 1992; Matusica et al., 2008; Maier et al., 2013; see Table A1 in Supplementary Material). Actin was used as internal standard. cDNA samples were amplified using GoTaq® Flexi DNA polymerase (Promega) in 25 μL reaction mixture containing 125 ng cDNA following the manufacturer’s protocol. Amplification consisted of 34 cycles at 95°C for 1 min, 59°C for 1 min and 72°C for 1.5 min. Aliquots of 10 μL of these products underwent electrophoresis on 1.5% agarose gel before capture with a ChemiDoc XRS camera and quantification by Quantity One Software (BioRad). Mouse brain extract was used as positive control for amplifications.
The gene expression of glutamate receptor subunits, ChAT and p75NTR, was measured by semi quantitative real-time PCR (RT-qPCR) using the Brilliant III Ultra-Fast SYBR® QPCR MM kit (AgilentTM) and the same primers as for conventional RT-PCR (see Table A1 in Supplementary Material). The reaction was performed in a LightCycler 480 (Roche Diagnostic, Meylan, France) at Tm 60–62°C. The efficiency of amplification was calculated on cDNA at concentrations ranging from 3.75 to 60 ng/mL. To ensure the absence of genomic DNA contamination, a control sample of non-reverse-transcribed RNA was run for each set of RNA extractions. The expression’s stability of reference genes was determined following MIQE guidelines (Bustin et al., 2009). We showed that β-actin and GAPDH genes were stably expressed (data not shown). Relative quantification was obtained by calculating the ratio between the values obtained for each gene of interest and the reference genes (β-actin, GAPDH). Melting curves were routinely performed to determine the specificity of the qPCR reaction. The 2−ΔΔCt method was used for analysis.
Measurement of Calcium Influx
Cells were seeded on coverslips at a density of 3 × 104 cells/mL and allowed to grow for 2 days. They were loaded with a fluorescent probe (1 μM Fura-2 AM) in culture medium for 45 min at 37°C. Cells were then washed with medium and allowed to de-esterify for at least 15 min at room temperature. The dish was then placed on the stage of a Nikon Eclipse TE2000-S inverted fluorescence microscope (Nikon, France) equipped with a 75 W Xenon Arc Lamp (Ushio, Japan), an Optoscan Monochromator (Cairn Optoscan, Kent, UK), and an ORCA-03G (CCD) camera (Hamamatsu, Japan). The coverslips were placed in a perfusion chamber containing physiological solution with 2 mM calcium (see Table A2 in Supplementary Material).
Excitation light was chopped by the monochromator at the two excitation wavelength maxima of fura-2 (340/380 nm). The excitation protocol used was a 500 ms excitation at each wavelength every 4 s. Fluorescence emission at 510 was detected by the CCD digital camera. The software (Imaging workbench 6) excited cells and allowed acquisition of images. The acute effects of glutamate at 100 μM and 1 mM (for primary MN and NSC-34D, respectively) on calcium entry were measured in physiological solution for 200 s. For primary motor neuron cells, we first determined the optimal time window allowing the experiment by measuring calcium entry every 2 days on independent replicates (cells that had not been exposed to fura-2 experiment before).
Statistical Analysis
Data was expressed as the mean ± SEM values. Statistical significance was determined using the Mann-Withney test or one-way Anova. For multiple comparisons, Tukey’s tests were used as post hoc tests or Kruskal-wallis test for appropriate analyses. For all analyses, p values < 0.05 were considered significant. The type of statistical test is specified in each figure legend.
Results
Efficient Differentiation of NSC-34 Cells into Motor Neuron-Like Cells
When NSC-34 cells were cultured in the differentiation media (NSC-34D), we distinguished two morphologically distinct populations: cells with short neurites, and cells with phenotypic characterization of MN with long processes (Figure 1A). However, this differentiation was preceded by a massive loss of cells (>50%) as described by Eggett et al. (2000), with surviving cells sprouting extensive neuron-like projections.
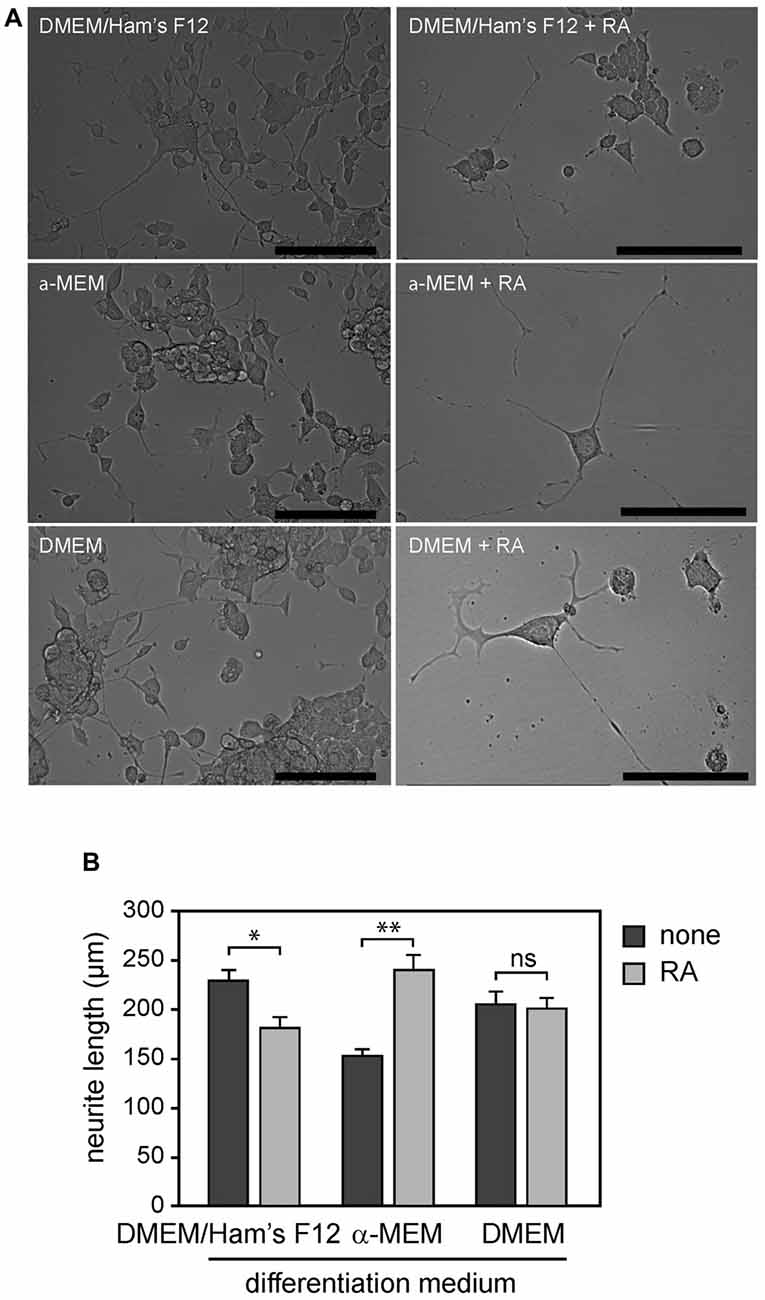
Figure 1. (A) NSC-34 cells grown for 4 weeks in differentiation media with or without addition of all-trans retinoic acid (RA): DMEM/Ham’s F12, α-MEM and DMEM. (B) Morphological differentiation of NSC-34 cells determined by measurement of neurite length. Statistically significant difference from differentiated cells without addition of RA was defined using Mann-Whitney test, according to the different media: DMEM/Ham’s F12, α-MEM and DMEM. Values are means ± SEM (10–11 cells per experiment and four experiments for each condition), *p < 0.001, **p < 0.0001, ns = not significant, Mann-Whitney test + RA vs. none.
We next investigated whether the potent morphogen RA could enhance differentiation of NSC-34 into motor neuron-like cells. We found that addition of RA (1 μM) in differentiation medium resulted in a significant decrease (60–70%) in proliferation at 4 days. Surviving cells showed a very low rate of proliferation compared to conditions without RA, as previously shown (Johann et al., 2011). However, we observed that a significant proportion of surviving cells had a motor neuron-like phenotype (~80%) in the presence of RA as determined by morphological criteria (Figure 1A).
We then assessed whether differentiation conditions could potentiate the effects of RA on process outgrowth of NSC-34 cells. We determined the average neurite length of NSC-34 cells when they are maintained for 4 weeks in three differentiation media (α-MEM, DMEM/Ham’s F12 and DMEM) in the presence or absence of RA. We found that the average neurite length has increased by 55% when they are cultured in α-MEM media with RA compared to α-MEM media without RA (240.00 ± 15.89 and 154.16 ± 7.01, respectively, p < 0.0001). While it has decreased by 20% when cells were cultured in DMEM/Ham’s F12 media with RA compared to media without RA (181.82 ± 11.29 and 229.69 ± 12.09, respectively, p < 0.001; Figure 1B). We did not find any significant change when NSC-34 cells were cultured in DMEM with or without RA (200.45 ± 11.95 and 206.25 ± 13.13, respectively, p = 0.46). Therefore, the effects of RA on NSC-34D cell morphology were highly dependent on the differentiation medium (Figure 1B).
Differentiated NSC-34 Cells Expressed Glutamate Receptor Subunits
We first set up conditions for optimal and specific amplification of glutamate receptor subunits GluA1–4, GluN1, GluN2A-D and GluN3A using total RNA obtained from mouse brain extract (see Figure A1 in Supplementary Material). We next studied the expression profiles of all glutamate receptors promoted by NSC-34 differentiation (up for 4 weeks) and in primary MN. We found that the differentiation of NSC-34 in the conditions that we had previously described induced a significant increase in mRNA expression of glutamate receptor subunits GluN1, GluN2A and GluN2D compared to undifferentiated cells (Figures 2B–I). The example of GluN2A protein confirms this statement: the result obtained by western blot analysis showed that the GluN2A protein level in NSC-34D was higher than in undifferentiated NSC-34 (see Figure A2 in Supplementary Material). Levels of GluA4 subunits mRNA have increased in DMEM without RA compared to the other conditions without RA (Figures 2C,E,G). At more discrete levels, we also observed increases of GluA2 and GluA3 mRNA in DMEM/Ham’s F12 and α-MEM but not in DMEM. We noted the expression of all glutamate subunits in primary MN, as observed in mouse brain extracts (Figure 2A and Figure A1, see Supplementary Material).
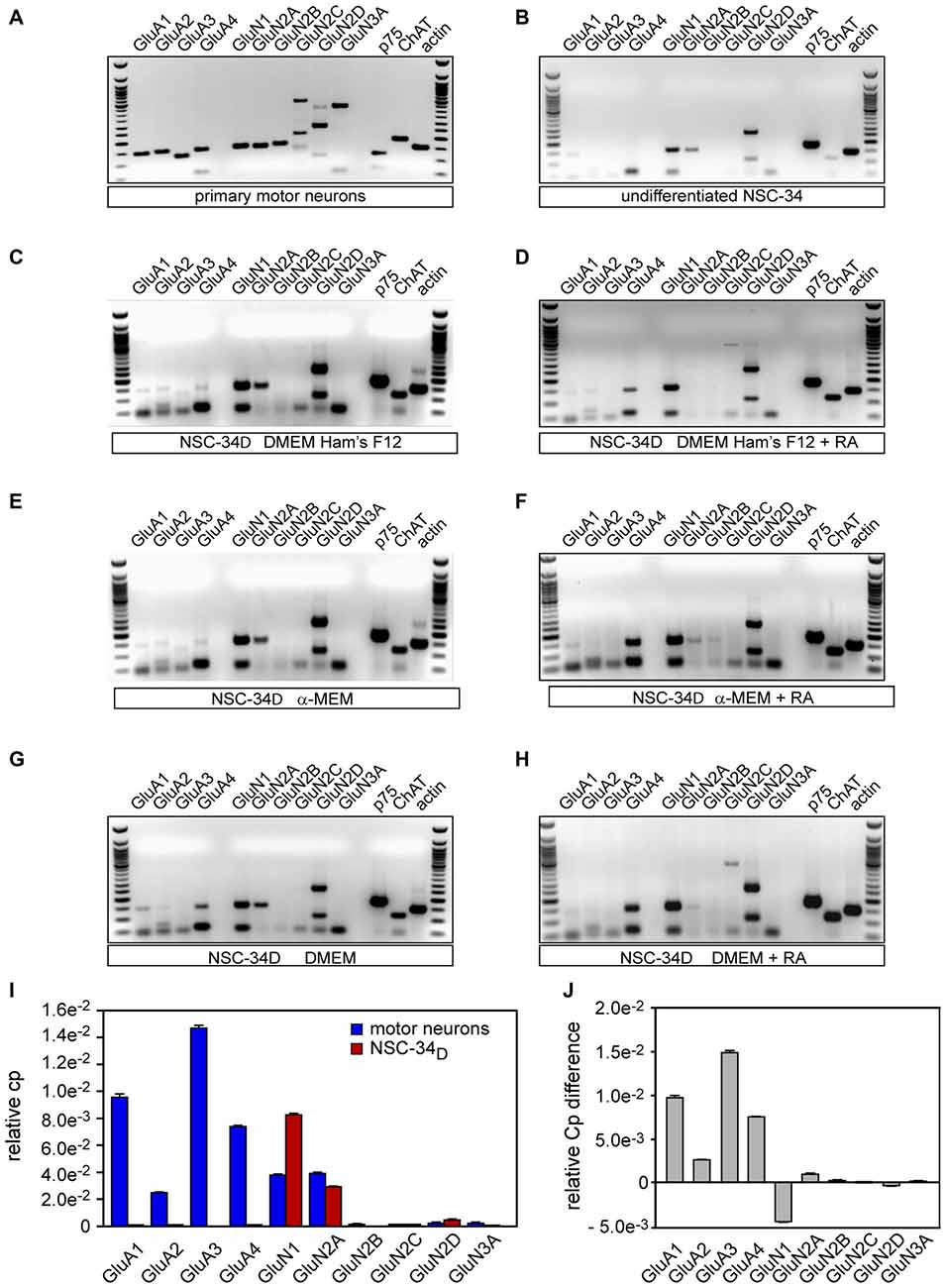
Figure 2. Characterization of the expression of glutamatergic receptors in motor neurons (MNs) and NSC-34D cells. Conventional RT-PCR on RNA isolated from NSC-34 cells maintained in the proliferation medium (undifferentiated) (A), from MNs (B), and from NSC-34 cells grown in three differentiation media with or without all trans-retinoic-acid (AR) for 4 weeks: (C,D) DMEM/Ham’s F12, (E,F) MEM and (G,H) DMEM. (I) Semi-quantitative RT-qPCR analysis of gene expression of glutamate receptor subunits in MN and NSC-34 differentiated in DMEM/Ham’s F12 media without RA (mean ± SEM, n = 3). (J) Semi quantification of the differences in RT-qPCR between MN and NSC-34D in DMEM/Ham’s F12 media without RA (mean ± SEM, n = 3).
We found that the presence of RA promoted the expression of GluA4 mRNA in every differentiation medium (Figures 2C–H). However, mRNA expression of the other subunits of glutamate receptors was not modified by the presence of RA in the culture medium. The differentiation condition that promoted most of the glutamate receptors and that generated less heterogeneity bias was thus DMEM-Ham’s F12 without RA. Indeed, this differentiation condition led to expression of all AMPR subunits (GluA1–4) and half of the NMDAR subunits (GluN1, GluN2A, and GluN2D), as summarized in Table A3 (see Supplementary Material). However, expression of AMPR subunits was lower compared to primary MN (Figures 2A,C,I,J). DMEM-Ham’s F12 without RA medium is used for further explorations. Our results also showed that NSC-34D cells expressed many motor neuron properties such as ChAT and p75NTR (Figures 2C–H). RA-differentiated NSC-34 did not express more receptor subunits than NSC-34D without RA (Figures 2C–H and Table A3 in Supplementary Material). We next semi quantified expression of glutamate receptor subunits in NSC-34D differentiated in DMEM-Ham’s F12 without RA condition compared to MN.
Expression of Receptor Subunits
RT-qPCR analysis revealed high levels of expression of GluN1, GluN2A and GluN2D mRNA (CP values were 8.35 × 10−3 ± 4.96 × 10−5, 3.02 × 10−3 ± 6.30 × 10−8 and 4.95 × 10−4 ± 1.83 × 10−6, respectively) in NSC-34 differentiated in DMEM/Ham’s F12 without RA, while expression of GluA1, GluA2, GluA4, GluN2C and GluN3A mRNA (9.85 × 10−7 ± 4.02 × 10−9, 4.45 × 10−7 ± 5.78 × 10−9, 7.45 × 10−6 ± 5.77 × 10−9, 3.22 × 10−6 ± 1.80 × 10−7 and 9.74 × 10 −6 ± 2.01 × 10−8, respectively) was low in NSC-34D (Figures 2I,J), i.e., as for the results of conventional RT-PCR (Figure 2C). GluA3, and GluN2B mRNA levels were almost zero in NSC-34D (Figures 2I,J). It is of note that only 20% of the cells did not express motor neuron-like morphology. The results showed that primary MN expressed all glutamate receptor subunits at different levels, unlike to NSC-34D. Consistently with RT-PCR data, the transcript profile of NSC-34D glutamate receptor subunits is different from that of MN.
Differentiated NSC-34 Cells did not Show Susceptibility to Glutamate-Induced Death
We investigated whether glutamate could trigger death of differentiated NSC-34 cells. NSC-34D cells were exposed to increasing concentrations of glutamate ranging from 0.1 to 10 mM. We found 102.04 ± 3.63%, 104.08 ± 8.03%, 94.90 ± 1.44%, 86.73 ± 4.17% and 64.28 ± 2.89% of relative cell viability when cells exposed to 0.1 mM, 0.5 mM, 1 mM, 5 mM and 10 mM glutamate, respectively (Figure 3A). We also evaluated excitotoxicity death of MN at 8 DIV (Days In Vitro) exposed to 0.1 mM glutamate and we found 53.99 ± 3.04% of relative cell viability (Figure 3B). We observed that 48 h exposure to 0–5 mM glutamate did not elicit any toxic response to NSC-34D, and a non-specific effect of about 30% on cells survival was found when glutamate was added at 100-fold higher concentrations (10 mM; Figure 3A) than that necessary to induce 50% death of primary MN (0.1 mM; Figure 3B). The results show that NSC-34D cells do not show the same susceptibility to glutamate-induced excitotoxicity compared to MN.
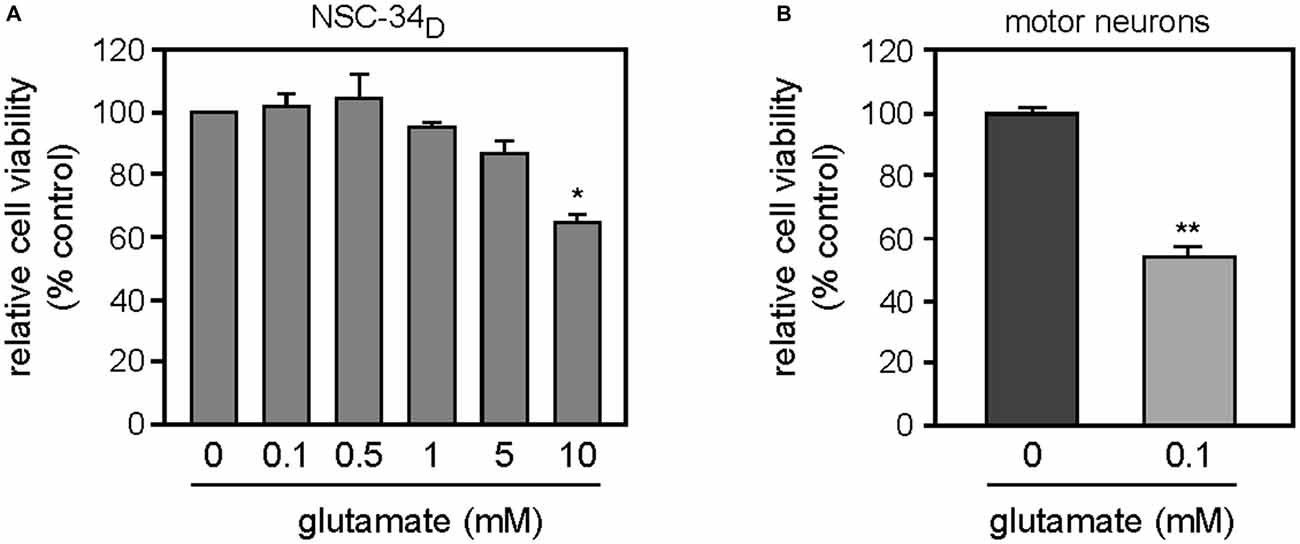
Figure 3. (A) Effects of glutamate on NSC-34D cell cultured in DMEM/Ham’s F12 without RA. Cells were exposed to increasing concentrations of glutamate (ranging from 0.1 to 10 mM) for 48 h before counting. (B) Effects of glutamate on survival of primary MN, cells were exposed at 8 DIV to 0.1 mM glutamate for 48 h before counting. Data were analyzed by Mann-Whitney test or Kruskal-Wallis (multiple comparisons) to detect statistical significance. Statistically significant difference from untreated cells *p < 0.05, **p < 0.01. Data are mean ± SEM (n = 3).
Glutamate did not Elicit Sustained Calcium Entry into NSC-34D
Glutamate-induced excitotoxicity resulted in elevated intracellular calcium levels via ionotropic glutamate receptors and activation of cell death signaling pathways. To address the effects of glutamate on Ca2+ transients in NSC-34D, we used ratiometric Ca2+ measurement with the imaging dye Fura-2AM. To establish sensitive and accurate measurement of intracellular Ca2+, we used motor neuron-enriched cultures. When MN were purified from E12.5 embryos as described above, a culture containing about 70% MN was identified using the p75NTR generic marker (Figure A3-A see Supplementary Material). The phase-bright soma with long motor neuron processes made them clearly distinguishable from other cells (Figure A3 see Supplementary Material). Their maturity was tested every 2 days by measuring [Ca2+] influx to determine the optimal time window allowing the experiment. We found that younger MN did not efficiently load Fura-2AM before 5 DIV (data not shown). We observed that MN showed robust fluorescence signal from 5 DIV and glutamate induced a robust Ca2+ influx in MN at 12 DIV (Figure A3-B, see Supplementary Material). The maximum fura-2 fluorescence intensity relative to baseline was significantly higher (p < 0.005) in MN at 12 DIV (0.0293 ± 0.0026) and 13 DIV (0.0320 ± 0.0004) compared to those at 5 DIV (0.0019 ± 0.0002). There was no significant difference between MN at 12 DIV and 13 DIV. Subsequent experiments were therefore performed using 13-day-old cultures (Figure A3-B, see Supplementary Material). At 13 DIV, the MN are fully mature with electrical activity (Jackson et al., 1982; Nicola et al., 1992; Chang and Martin, 2011).
We then investigated the functionality of glutamate receptors expressed in NSC-34D growing in DMEM/Ham’s F12 medium without RA for 4 weeks through Ca2+ permeability. The baseline recordings (first 0.5 min in all trace graphs) demonstrate stable cytosolic calcium levels in both MN and NSC-34D cells before treatment with glutamate. The addition of glutamate immediately increased the fluorescence intensity of the calcium-sensitive dye Fura-2, indicating increased cytosolic Ca2+ flux in MN (Figure 4A). Whereas MN presented a sustained calcium entry following acute stimulation by glutamate (0.1 mM), glutamate stimulation in NSC34D resulted in a small transient calcium entry (Figure 4B). However, in the presence of glutamate, Ca2+ entry into MN was significantly higher than in NSC-34D cells, and the signal was as consistent as that obtained by fura-2 fluorescence intensity relative to baseline (0.032 ± 0.0004 vs. 0.009 ± 0.0012; *p < 0.001; Figure 4C). Taken together, these results showed that an increased extracellular glutamate concentration (1 mM) did not elicit the same calcium response in NSC-34D cells (Figure 4C) as in MN.
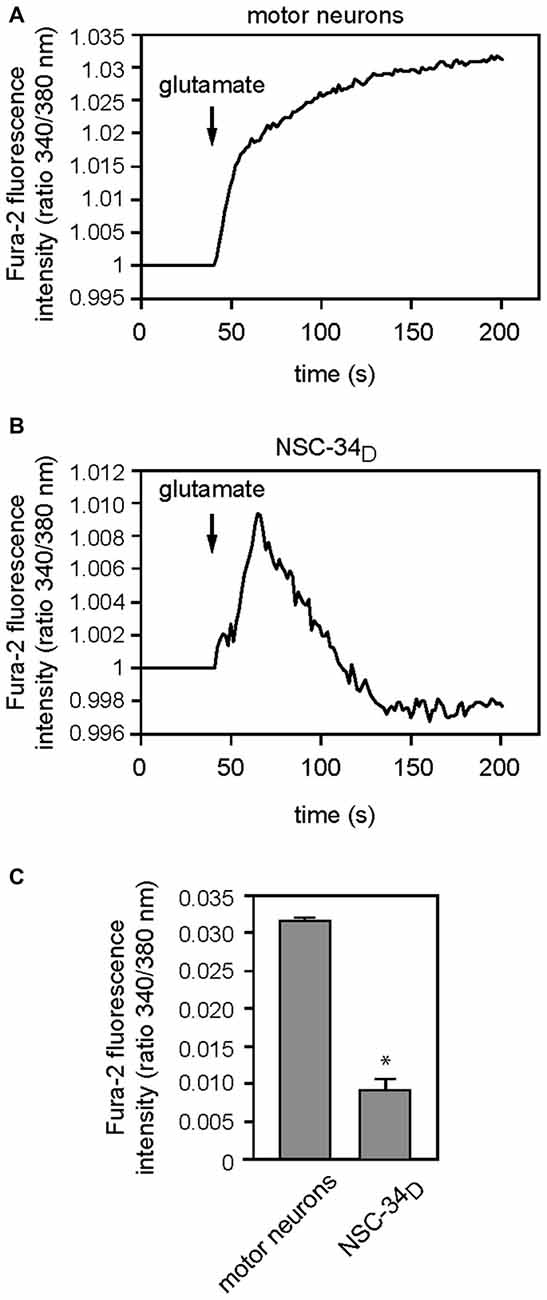
Figure 4. Acute effects of 100 μM and 1 mM glutamate (for primary MN and NSC-34D, respectively) for 200 s on calcium entry. Representative fluorescence measurement of Ca2+ entry in MN (A) and NSC-34D (B) after acute application of glutamate. Glutamate was added at the time indicated by the arrow. (A) Mean traces of cytosolic Ca2+ flux of MN and (B) NSC-34D in response to glutamate. (C) Histograms showing maximum fluorescence intensity following glutamate application in MN and NSC-34D. Mean data ± SEM from three coverslips per cell type with at least five cells per coverslip analyzed. *p < 0.001 (Mann-Whitney test MN vs. NSC-34D).
Discussion
Although glutamate-mediated excitotoxicity is known to be involved in ALS pathogenesis (Beal, 1992; Mattson et al., 1992), the precise mechanisms leading to electrical, metabolic or signaling dysfunction in the motor neuron diseases remain to be determined. Excitotoxicity mechanisms have been described following in vitro studies using either primary motor neuron cultures (Kruman et al., 1999; Van Den Bosch et al., 2000; Dolga et al., 2011; Joshi et al., 2011) or cell lines (Rembach et al., 2004; Benkler et al., 2013). The advantage of primary cultures is the development of similar in situ counterpart morphology and physiology over time. The neurotoxicity of a variety of toxins in situ has also been reported (Durham et al., 1993). However, due to the low yields of motor neuron cultures, the NSC-34 cell line has been widely used and tacitly considered to be the most stable motor neuron cell line to model ALS pathophysiology (Veyrat-Durebex et al., 2014). Indeed, many studies have shown that the differentiation process can induce NSC-34 cells to express certain motor neuron properties and have shown some toxic effects following exposure to glutamate (Eggett et al., 2000; Benkler et al., 2013; Maier et al., 2013). The differentiation and the maturation of NSC-34 cells are generally characterized by extension of neurites and expression of certain motor neuron-specific proteins. In this study, we investigated the reliability of these cells as an in vitro model to study glutamate-mediated neurotoxicity, using an approach from receptor expression to the Ca2+ influx.
Differentiation of NSC-34 Cells into Motor Neuron-Like Cells
Differentiation of cell lines frequently requires modification of the culture medium through serum depletion and/or and use of chemical reagents or metabolites such as RA to obtain more neuron-like properties, including neurite outgrowth and morphological changes (MacPherson et al., 1997; Eggett et al., 2000; Maier et al., 2013). As reported in the literature, we found that the differentiation process induced NSC-34 cells to express phenotypic MN with long processes (Figure 1A and Figure A3-A, see Supplementary Material). We found that the RA effects on NSC-34D cell morphology were highly dependent on the differentiation medium (Figure 1B). Importantly, we did not observe any effect on undifferentiated NSC-34 cells as previously demonstrated (Cashman et al., 1992; Maier et al., 2013). We also evaluated the RA effects on the expression of genes encoding certain specific motor neuron proteins and glutamate receptor subunits, and we found that RA-differentiated NSC-34 did not express more receptor subunits than NSC-34D without RA (Figures 2C–H and Table A3, see Supplementary Material), thus suggesting that using RA is not required in NSC-34 differentiation processes in the context of excitotoxicity studies. This conclusion is supported by the results obtained by Cheung et al. (2009) who showed that RA differentiation conferred higher tolerance of SH-SY5Y cells to neurotoxin (6-hydroxydopamine) compared to undifferentiated SH-SY5Y cells, and they suggested that RA-differentiated SH-SY5Y was not appropriate for neurotoxicity or neuroprotection studies.
The Receptor Subunits Expressed by NSC-34D
NMDARs and AMPARs have critical roles in excitatory synaptic transmission, plasticity and excitotoxicity in the CNS. We found that NSC-34D expressed more receptor subunits in DMEM/Ham’s F12 medium without RA than in other media (Figures 2C–H and Table A3, see Supplementary Material). NSC-34D expressed all AMPAR (GluA1–4) and some NMDAR (GluN1, GluN2A/B) subunits, as reported in the literature (Eggett et al., 2000; Rembach et al., 2004). We therefore chose DMEM/Ham’s F12 medium without RA for further experiments. Then we compared expression of glutamate receptor subunits on primary MN and NSC-34D using RT-qPCR (Figures 2I,J). The results showed that primary MN expressed all glutamate receptor subunits at different levels, in contrast to NSC-34D which expressed some of them at times and in very small quantities. This clearly showed that the transcript profile of NSC-34D glutamate receptor subunits was different from that of MN and very consistent with RT-PCR and RT-qPCR data, indicating that NSC-34D could not be used as a model of primary MN for glutamate-mediated excitotoxicity.
No Glutamate-Induced Toxicity in NSC-34D
Glutamate concentrations in the extracellular fluid are normally around 0.8–2.9 μM (Lerma et al., 1986). A rise in the extracellular glutamate concentration to 2–5 μM is considered sufficient to cause degeneration of neurons through excessive stimulation of glutamate receptors (Rosenberg et al., 1992). When investigating glutamate-induced excitotoxicity at the cellular level, the glutamate concentrations used to obtain effects varied according to the in vitro models investigated and exposure times. For primary cultures, the range of glutamate concentrations used to act on ionotropic glutamate receptors (Van Den Bosch et al., 2000) was between 10 μM–1 mM, and cultures were exposed for less than 24 h (Mattson et al., 1995; Kruman et al., 1999; Sen et al., 2008). As in our study (Figure 3B), it had previously been demonstrated that exposure of primary embryonic MN to glutamate (0.1 mM) for 24 or 48 h led to approximately 50% cell death (Metzger et al., 1998; Urushitani et al., 1998), whereas for cell lines, this glutamate concentration range was increased to 10 mM with longer exposure times (24–72 h; Eggett et al., 2000; Rembach et al., 2004; Benkler et al., 2013; Maier et al., 2013). In our study, we investigated the toxicity of glutamate on NSC-34D and motor neuron survival. The results showed approximately 50% cell death at 100 μM glutamate for primary MN (Figure 3B), as described in the literature (Metzger et al., 1998; Urushitani et al., 1998). For NSC-34D cells, we found a significant loss of viable cells at a concentration of only 10 mM glutamate (Figure 3A). This result is consistent with previous excitotoxicity studies on NSC-34D where treatment with glutamate (up to 1 mM) failed to induce vacuolation of NSC-34D, in contrast to the effects on primary MN (Durham et al., 1993).
Among the different neuroprotective mechanisms in neurons, neurotrophin factors may play a key role. Literature reported that NTFs and glutamate interact to regulate developmental and adult neuroplasticity (Mattson et al., 1995; Mattson, 2008). Consequently, neurotrophin factors attenuated elevation of intracellular calcium concentrations induced by glutamate (Mattson et al., 1995), thus promoting the survival of neurons (McKay et al., 1996; Giménez y Ribotta et al., 1997; Vincent et al., 2004; Mattson, 2008) via the MAPK and PI-3K/Akt pathways (Vincent et al., 2004).
To validate the glutamate-sensitive motor neuron NSC-34 cell line, many studies have used modified growth conditions through serum depletion to induce differentiated NSC-34 cells and to investigate the cytotoxicity of glutamate or other excitotoxins (AMPA, 5-fluorowillardiine, H2O2, TNF-α, etc.) in the presence or absence of receptor antagonists by using viability tests (Rembach et al., 2004; Hemendinger et al., 2012; Benkler et al., 2013; Maier et al., 2013). In some cases, they evaluated the expression of certain genes which encode cholinergic phenotype-related proteins (ChAT, acetylcholine esterase and vesicular acetylcholine transferase; Maier et al., 2013). It should be emphasized that most studies that used cell line models to investigate glutamate neurotoxicity focused predominantly on its toxic action and not on the functional properties of glutamatergic receptors such as Ca2+ permeability (Rembach et al., 2004; Hemendinger et al., 2012; Benkler et al., 2013; Maier et al., 2013).
No Sustained Calcium Entry in NSC-34D
The stoichiometry of the receptor complexes in mammalian cells seems to be largely controlled by the level of the individual subunits expression that determine their functional properties including Ca2+ permeability via glutamate receptors (Hollmann et al., 1991; Monyer et al., 1992; Das et al., 1998; Dingledine et al., 1999; Sobolevsky et al., 2009). According to the literature evidence, the expression of GluA1–4, GluN1 and GluN2A or GluN2B subunits induces functional receptors. Surprisingly, in our study, despite the expression of GluA, GluN1 and GluN2A/D subunits in NSC-34D (Figure 2I), we did not observe a significant sustained calcium entry as demonstrated in MN (Figure 4C). In this study, we globally evaluated the intracellular calcium concentrations (including the interplay between extracellular and intracellular origin) provoked by glutamate, through glutamate receptors by using the Fura-2-AM. However, we cannot exclude the possibility that part of the increase of cytosolic calcium could come from internal stores. Using SH-SY5Y cells line and measurement of calcium release from different compartments, Jaiswal et al. (2009) provided evidences of the existence of two separate intracellular Ca2+ stores (endoplasmic reticulum (ER) and mitochondrial intracellular pools) which may participate in the generation of intracellular Ca2+ signals. Calcium permeability via glutamate receptors has also been shown to initiate a self-perpetuating process of intracellular Ca2+ dysregulation with consecutive ER Ca2+ release and mitochondrial Ca2+ overload (Jahn et al., 2006; Grosskreutz et al., 2007, 2010). The ER and mitochondria form a highly dynamic interconnected network that is involved in the generation of Ca2+ signals (Tadic et al., 2014). A recent article reported a Ca2+ influx in NSC-34D cells compared to a “negative control” undifferentiated NSC-34 (Liu et al., 2015), but authors did not compare calcium influx of these NSC-34D to that of MN (as “positive control”) which are the parent motor neuron for NSC-34, as performed here in our study. We postulate that this shift of Ca2+ entry in NSC-34D could be due to the fact that some glutamate receptor subunits were not expressed or very weakly expressed, in contrast to MN which showed a sustained calcium entry following glutamate application.
Limitations in the Use of the NSC-34 Cell Line
As mentioned above, Eggett et al. (2000) evaluated the use of NSC-34 cells as a glutamate-sensitive motor neuron model. Using immunocytochemistry, they demonstrated the presence of glutamate receptor proteins GluN1, GluN2A/B, GluA1–4, GluK2/3 and GluK5. Exposure to glutamate (1 mM) for 24 h induced significant cell death (~30%), and changes in calcium cytosolic levels were observed. As in many other studies (Eggett et al., 2000; Rembach et al., 2004; Benkler et al., 2013; Maier et al., 2013; Liu et al., 2015) authors suggested that, because of their motor neuron origin, the NSC-34 cell line could be used to investigate excitotoxicity mechanisms. However, as reported by Durham et al. (1993), in our study we found limitations in the use of the NSC-34 cell line for neurotoxicity testing. Durham et al. (1993) have evaluated the value of these cells by following exposure of cultures to chemicals known to be neurotoxic for MN. Authors showed that NSC-34 responded to agents that affect voltage-gated ion channels, cytoskeleton organization and axonal transport. However, no electrophysiological evidence was shown, and exposure to glutamate (1 mM) had no effect on cell morphology or potential production. They concluded that the NSC-34 cell line was not a good model to investigate agents that affect synaptic transmission (Durham et al., 1993). Hemendinger et al. (2012) have demonstrated that riluzole in the neurorescue paradigm was unable to reduce cell death induced by neurotoxins that increased intracellular calcium levels independently of ER stress in NSC-34D cells, but they did not provide information on the molecular basis. This finding may potentially be explained by the fact that NSC-34 is not a suitable in vitro model to investigate excitotoxicity. All these results together suggest that NSC-34 cells may not be appropriate as an in vitro model to study glutamatergic toxicity in motor neuron degeneration. Motor neuronal properties evaluated on differentiated NSC-34 cells and literature findings were summarized in the Appendix (Table A4, see Supplementary Material).
Importantly, the phenotypes of NSC-34 may vary, according to the repeated culture passage or to the origin of the cell line [provided by Dr Neil Cashman (Durham et al., 1993; Maier et al., 2013; Liu et al., 2015) or purchased (Hemendinger et al., 2012)]. So, results may not be reproducible over time, from one laboratory to another, and even within the same laboratory. We cannot exclude the possibility that this contradictory findings may be explained by the differences between basal features of NSC-34 cells, rarely detailed in the studies (Eggett et al., 2000; Rembach et al., 2004; Benkler et al., 2013). There may be various differentiation processes with various media and chemicals known to induce various NSC-34-expressed motor neuron properties, and the culture conditions were not systematically explored to seek optimal toxicity to glutamate. However, in agreement with the literature, in our study we did not find any condition which promoted expression of all glutamatergic properties. The aim of this work was not to focus on optimization of differentiation processes which should involve several parameters (medium composition, differentiation time, time of changing medium, cell density, cell passage, etc.) but rather to evaluate the versatility of the NSC-34 cell line to model motor neuron susceptibility to glutamate-induced excitotoxicity. Reliable validation is a crucial step before using a cell line as an in vitro model in glutamate-induced excitotoxicity studies. Such validation requires the evaluation of the biochemical and electrophysiological processes of MN that are targets of glutamate such as glutamate receptors and Ca2+ influx with a “positive control”.
We could not exclude the possibility that the maturity was insufficient to induce calcium influx in NSC-34D cells under the present experimental conditions. Even if organotypic spinal cord cultures remain the models that closely reflect in situ reality, we suggest that the use of primary motor neuron culture is more suitable than NCS-34 cell line to explore the pathogenesis of glutamate-mediated excitotoxicity at the cellular level, in ALS and other motor neuron diseases. However, different authors have shown that NSC-34 cells remain a suitable model of vulnerability to neurotoxins (Maier et al., 2013) and to investigate the effects of cerebrolysin (Keilhoff et al., 2014). NSC-34 cells line is a viable in vitro cell model for screening therapeutic candidates against nerve agents (Kanjilal et al., 2014), investigating the expression of transcription factors (Prell et al., 2012) and could thus be a relevant model for some specific experiments.
Author Contributions
BMH, RF, FP and MG conducted all experiments, analyzed the data and BMH wrote the manuscript. HB, SM, PV, CR and CRA designed the project, analyzed the data and wrote the manuscript. All authors listed, have made substantial, direct and intellectual contribution to the work, and approved it for publication.
Funding
This work was supported by the “Institut National de la Santé et de la Recherche” INSERM, the University François-Rabelais de Tours, and ARSLA (“Association pour la Recherche sur la Sclérose Latérale Amyotrophique et autres maladies du motoneurone”). BMH was supported by “La Région Centre” with a PhD graduate grant.
Conflict of Interest Statement
The authors declare that the research was conducted in the absence of any commercial or financial relationships that could be construed as a potential conflict of interest.
Acknowledgments
We thank the staff of the PPF ASB platform of the Université François-Rabelais de Tours, France, and Catherine Cherpi-Antar for technical assistance.
Abbreviations
α-MEM, Alpha-modified Eagle’s medium; ALS, amyotrophic lateral sclerosis; AMPA, α-amino-3-hydroxy-5-methyl-4-isoxazole propionic acid; Ca2+, Calcium ion; DAPI, 4,6-diamino-2-phenylindole; DMEM, Dulbecco’s modified Eagle’s medium; FCS, fetal calf serum; KA, kainite; MEM-NEAA, modified Eagle’s medium non-essential amino acids; NSC, Neuroblastoma × Spinal Cord; NSC-34D, differentiated NSC-34; MN, motor neurons; NTFs, neurotrophic factors; PBS, phosphate buffered saline; P/S, penicillin/streptomycin; RA, all-trans retinoic acid.
Supplementary Material
The Supplementary Material for this article can be found online at: http://journal.frontiersin.org/article/10.3389/fncel.2016.00118/abstract
References
Arce, V., Garces, A., de Bovis, B., Filippi, P., Henderson, C., Pettmann, B., et al. (1999). Cardiotrophin-1 requires LIFRβ to promote survival of mouse motoneurons purified by a novel technique. J. Neurosci Res 55, 119–126. doi: 10.1002/(sici)1097-4547(19990101)55:1<119::aid-jnr13>3.0.co;2-6
Beal, M. F. (1992). Does impairment of energy metabolism result in excitotoxic neuronal death in neurodegenerative illnesses? Ann. Neurol. 31, 119–130. doi: 10.1002/ana.410310202
Benkler, C., Ben-Zur, T., Barhum, Y., and Offen, D. (2013). Altered astrocytic response to activation in SOD1G93A mice and its implications on amyotrophic lateral sclerosis pathogenesis. Glia 61, 312–326. doi: 10.1002/glia.22428
Blasco, H., Mavel, S., Corcia, P., and Gordon, P. H. (2014). The glutamate hypothesis in ALS: pathophysiology and drug development. Curr. Med. Chem. 21, 3551–3575. doi: 10.2174/0929867321666140916120118
Bustin, S. A., Benes, V., Garson, J. A., Hellemans, J., Huggett, J., Kubista, M., et al. (2009). The MIQE guidelines: minimum information for publication of quantitative real-time PCR experiments. Clin. Chem. 55, 611–622. doi: 10.1373/clinchem.2008.112797
Camu, W., Tremblier, B., Plassot, C., Alphandery, S., Salsac, C., Pageot, N., et al. (2014). Vitamin D confers protection to motoneurons and is a prognostic factor of amyotrophic lateral sclerosis. Neurobiol. Aging 35, 1198–1205. doi: 10.1016/j.neurobiolaging.2013.11.005
Cashman, N. R., Durham, H. D., Blusztajn, J. K., Oda, K., Tabira, T., Shaw, I. T., et al. (1992). Neuroblastoma × spinal cord (NSC) hybrid cell lines resemble developing motor neurons. Dev. Dyn. 194, 209–221.
Chang, Q., and Martin, L. J. (2011). Glycine receptor channels in spinal motoneurons are abnormal in a transgenic mouse model of amyotrophic lateral sclerosis. J. Neurosci. 31, 2815–2827. doi: 10.1523/jneurosci.2475-10.2011
Cheung, Y.-T., Lau, W. K.-W., Yu, M.-S., Lai, C. S.-W., Yeung, S.-C., So, K.-F., et al. (2009). Effects of all-trans-retinoic acid on human SH-SY5Y neuroblastoma as in vitro model in neurotoxicity research. Neurotoxicology 30, 127–135. doi: 10.1016/j.neuro.2008.11.001
Clagett-Dame, M., McNeill, E. M., and Muley, P. D. (2006). Role of all-trans retinoic acid in neurite outgrowth and axonal elongation. J. Neurobiol. 66, 739–756. doi: 10.1002/neu.20241
Dangoumau, A., Deschamps, R., Veyrat-Durebex, C., Pettmann, B., Corcia, P., Andres, C. R., et al. (2015). A novel p.E121G SOD1 mutation in slowly progressive form of amyotrophic lateral sclerosis induces cytoplasmic aggregates in cultured motor neurons and reduces cell viability. Amyotroph. Lateral Scler. Frontotemporal Degener. 16, 131–134. doi: 10.3109/21678421.2014.965179
Das, S., Sasaki, Y. F., Rothe, T., Premkumar, L. S., Takasu, M., Crandall, J. E., et al. (1998). Increased NMDA current and spine density in mice lacking the NMDA receptor subunit NR3A. Nature 393, 377–381. doi: 10.1016/s0168-0102(98)82331-6
Dingledine, R., Borges, K., Bowie, D., and Traynelis, S. F. (1999). The glutamate receptor ion channels. Pharmacol Rev 51, 7–62.
Dolga, A. M., Terpolilli, N., Kepura, F., Nijholt, I. M., Knaus, H. G., D’Orsi, B., et al. (2011). KCa2 channels activation prevents Cai2+ deregulation and reduces neuronal death following glutamate toxicity and cerebral ischemia. Cell Death Dis. 2:e147. doi: 10.1038/cddis.2011.30
Durham, H. D., Dahrouge, S., and Cashman, N. R. (1993). Evaluation of the spinal cord neuron × neuroblastoma hybrid cell line NSC-34 as a model for neurotoxicity testing. Neurotoxicology 14, 387–395.
Eggett, C. J., Crosier, S., Manning, P., Cookson, M. R., Menzies, F. M., McNeil, C. J., et al. (2000). Development and characterisation of a glutamate-sensitive motor neurone cell line. J. Neurochem. 74, 1895–1902. doi: 10.1046/j.1471-4159.2000.0741895.x
Giménez y Ribotta, M., Revah, F., Pradier, L., Loquet, I., Mallet, J., and Privat, A. (1997). Prevention of motoneuron death by adenovirus-mediated neurotrophic factors. J. Neurosci. Res. 48, 281–285. doi: 10.1002/(sici)1097-4547(19970501)48:3<281::aid-jnr11>3.0.co;2-4
Grosskreutz, J., Haastert, K., Dewil, M., Van Damme, P., Callewaert, G., Robberecht, W., et al. (2007). Role of mitochondria in kainate-induced fast Ca2+ transients in cultured spinal motor neurons. Cell Calcium 42, 59–69. doi: 10.1016/j.ceca.2006.11.010
Grosskreutz, J., Van Den Bosch, L., and Keller, B. U. (2010). Calcium dysregulation in amyotrophic lateral sclerosis. Cell Calcium 47, 165–174. doi: 10.1016/j.ceca.2009.12.002
Heath, P. R., and Shaw, P. J. (2002). Update on the glutamatergic neurotransmitter system and the role of excitotoxicity in amyotrophic lateral sclerosis. Muscle Nerve 26, 438–458. doi: 10.1002/mus.10186
Hemendinger, R. A., Armstrong Iii, E. J., Radio, N., and Brooks, B. R. (2012). Neurotoxic injury pathways in differentiated mouse motor neuron-neuroblastoma hybrid (NSC-34D) cells in vitro—Limited effect of riluzole on thapsigargin, but not staurosporine, hydrogen peroxide and homocysteine neurotoxicity. Toxicol. Appl. Pharm. 258, 208–215. doi: 10.1016/j.taap.2011.10.022
Hollmann, M., Hartley, M., and Heinemann, S. (1991). Ca2+ permeability of KA-AMPA–gated glutamate receptor channels depends on subunit composition. Science 252, 851–853. doi: 10.1126/science.1709304
Hunter, D. D., Cashman, N., Morris-Valero, R., Bulock, J. W., Adams, S. P., and Sanes, J. R. (1991). An LRE (leucine-arginine-glutamate)-dependent mechanism for adhesion of neurons to S-laminin. J. Neurosci. 11, 3960–3971.
Jackson, M. B., Lecar, H., Brenneman, D. E., Fitzgerald, S., and Nelson, P. G. (1982). Electrical development in spinal cord cell culture. J. Neurosci. 2, 1052–1061.
Jahn, K., Grosskreutz, J., Haastert, K., Ziegler, E., Schlesinger, F., Grothe, C., et al. (2006). Temporospatial coupling of networked synaptic activation of AMPA-type glutamate receptor channels and calcium transients in cultured motoneurons. Neuroscience 142, 1019–1029. doi: 10.1016/j.neuroscience.2006.07.034
Jaiswal, M. K., Zech, W.-D., Goos, M., Leutbecher, C., Ferri, A., Zippelius, A., et al. (2009). Impairment of mitochondrial calcium handling in a mtSOD1 cell culture model of motoneuron disease. BMC Neurosci. 10:64. doi: 10.1186/1471-2202-10-64
Johann, S., Dahm, M., Kipp, M., Zahn, U., and Beyer, C. (2011). Regulation of choline acetyltransferase expression by 17 β-oestradiol in NSC-34 cells and in the spinal cord. J. Neuroendocrinol. 23, 839–848. doi: 10.1111/j.1365-2826.2011.02192.x
Joshi, D. C., Singh, M., Krishnamurthy, K., Joshi, P. G., and Joshi, N. B. (2011). AMPA induced Ca2+ influx in motor neurons occurs through voltage gated Ca2+ channel and Ca2+ permeable AMPA receptor. Neurochem. Int. 59, 913–921. doi: 10.1016/j.neuint.2011.06.023
Kanjilal, B., Keyser, B. M., Andres, D. K., Nealley, E., Benton, B., Melber, A. A., et al. (2014). Differentiated NSC-34 cells as an in vitro cell model for VX. Toxicol. Mech. Methods 24, 488–494. doi: 10.3109/15376516.2014.943442
Keilhoff, G., Lucas, B., Pinkernelle, J., Steiner, M., and Fansa, H. (2014). Effects of cerebrolysin on motor-neuron-like NSC-34 cells. Exp. Cell Res. 327, 234–255. doi: 10.1016/j.yexcr.2014.06.020
Kruman, I. I., Pedersen, W. A., Springer, J. E., and Mattson, M. P. (1999). ALS-Linked Cu/Zn-SOD mutation increases vulnerability of motor neurons to excitotoxicity by a mechanism involving increased oxidative stress and perturbed calcium homeostasis. Exp. Neurol. 160, 28–39. doi: 10.1006/exnr.1999.7190
Lerma, J., Herranz, A. S., Herreras, O., Abraira, V., and Martin del Rio, R. (1986). In vivo determination of extracellular concentration of amino acids in the rat hippocampus. A method based on brain dialysis and computerized analysis. Brain Res. 384, 145–155. doi: 10.1016/0006-8993(86)91230-8
Liu, X., Xu, S., Wang, P., and Wang, W. (2015). Transient mitochondrial permeability transition mediates excitotoxicity in glutamate-sensitive NSC34 motor neuron-like cells. Exp. Neurol. 271, 122–130. doi: 10.1016/j.expneurol.2015.05.010
Lodge, D. (2009). The history of the pharmacology and cloning of ionotropic glutamate receptors and the development of idiosyncratic nomenclature. Neuropharmacology 56, 6–21. doi: 10.1016/j.neuropharm.2008.08.006
MacDermott, A. B., Mayer, M. L., Westbrook, G. L., Smith, S. J., and Barker, J. L. (1986). NMDA-receptor activation increases cytoplasmic calcium concentration in cultured spinal cord neurones. Nature 321, 519–522. doi: 10.1038/321519a0
MacPherson, P. A., Jones, S., Pawson, P. A., Marshall, K. C., and McBurney, M. W. (1997). P19 cells differentiate into glutamatergic and glutamate-responsive neurons in vitro. Neuroscience 80, 487–499. doi: 10.1016/s0306-4522(97)00102-4
Maden, M. (2007). Retinoic acid in the development, regeneration and maintenance of the nervous system. Nat. Rev. Neurosci. 8, 755–765. doi: 10.1038/nrn2212
Madji Hounoum, B., Blasco, H., Nadal-Desbarats, L., Diémé, B., Montigny, F., Andres, C., et al. (2015). Analytical methodology for metabolomics study of adherent mammalian cells using NMR, GC-MS and LC-HRMS. Anal. Bioanal. Chem. 407, 8861–8872. doi: 10.1007/s00216-015-9047-x
Maier, O., Böhm, J., Dahm, M., Brück, S., Beyer, C., and Johann, S. (2013). Differentiated NSC-34 motoneuron-like cells as experimental model for cholinergic neurodegeneration. Neurochem. Int. 62, 1029–1038. doi: 10.1016/j.neuint.2013.03.008
Mattson, M., Cheng, B., Davis, D., Bryant, K., Lieberburg, I., and Rydel, R. (1992). beta-Amyloid peptides destabilize calcium homeostasis and render human cortical neurons vulnerable to excitotoxicity. J. Neurosci. 12, 376–389.
Mattson, M. P. (2008). Glutamate and neurotrophic factors in neuronal plasticity and disease. Ann. N. Y. Acad. Sci. 1144, 97–112. doi: 10.1196/annals.1418.005
Mattson, M. P., Lovell, M. A., Furukawa, K., and Markesbery, W. R. (1995). Neurotrophic factors attenuate glutamate-induced accumulation of peroxides, elevation of intracellular Ca2+ concentration and neurotoxicity and increase antioxidant enzyme activities in hippocampal neurons. J. Neurochem. 65, 1740–1751. doi: 10.1046/j.1471-4159.1995.65041740.x
Matusica, D., Fenech, M. P., Rogers, M.-L., and Rush, R. A. (2008). Characterization and use of the NSC-34 cell line for study of neurotrophin receptor trafficking. J. Neurosci. Res. 86, 553–565. doi: 10.1002/jnr.21507
McKay, S. E., Garner, A., Caldero, J., Tucker, R. P., Large, T., and Oppenheim, R. W. (1996). The expression of trkB and p75 and the role of BDNF in the developing neuromuscular system of the chick embryo. Development 122, 715–724. doi: 10.1007/bf02532343
Metzger, F., Wiese, S., and Sendtner, M. (1998). Effect of glutamate on dendritic growth in embryonic rat motoneurons. J. Neurosci. 18, 1735–1742.
Monyer, H., Sprengel, R., Schoepfer, R., Herb, A., Higuchi, M., Lomeli, H., et al. (1992). Heteromeric NMDA receptors: molecular and functional distinction of subtypes. Science 256, 1217–1221. doi: 10.1126/science.256.5060.1217
Nicola, M. A., Becker, C. M., and Triller, A. (1992). Development of glycine receptor alpha subunit in cultivated rat spinal neurons: an immunocytochemical study. Neurosci. Lett. 138, 173–178. doi: 10.1016/0304-3940(92)90499-w
Niswender, C. M., and Conn, P. J. (2010). Metabotropic glutamate receptors: physiology, pharmacology, and disease. Annl. Rev. Pharmacol. 50, 295–322. doi: 10.1146/annurev.pharmtox.011008.145533
Prell, T., Lautenschlager, J., Witte, O. W., Carri, M. T., and Grosskreutz, J. (2012). The unfolded protein response in models of human mutant G93A amyotrophic lateral sclerosis. Eur. J. Neurosci. 35, 652–660. doi: 10.1111/j.1460-9568.2012.08008.x
Rembach, A., Turner, B. J., Bruce, S., Cheah, I. K., Scott, R. L., Lopes, E. C., et al. (2004). Antisense peptide nucleic acid targeting GluR3 delays disease onset and progression in the SOD1 G93A mouse model of familial ALS. J. Neurosci. Res. 77, 573–582. doi: 10.1002/jnr.20191
Rosenberg, P. A., Amin, S., and Leitner, M. (1992). Glutamate uptake disguises neurotoxic potency of glutamate agonists in cerebral cortex in dissociated cell culture. J. Neurosci. 12, 56–61.
Sen, I., Joshi, D. C., Joshi, P. G., and Joshi, N. B. (2008). NMDA and non-NMDA receptor-mediated differential Ca2+ load and greater vulnerability of motor neurons in spinal cord cultures. Neurochem. Int. 52, 247–255. doi: 10.1016/j.neuint.2007.06.028
Sholl, D. A. (1956). The measurable parameters of the cerebral cortex and their significance in its organization. Prog. Neurobiol. 2, 324–333. doi: 10.1016/s0079-6123(08)62112-3
Sobolevsky, A. I., Rosconi, M. P., and Gouaux, E. (2009). X-ray structure, symmetry and mechanism of an AMPA-subtype glutamate receptor. Nature 462, 745–756. doi: 10.1038/nature08624
Spalloni, A., Nutini, M., and Longone, P. (2013). Role of the N-methyl-d-aspartate receptors complex in amyotrophic lateral sclerosis. Biochim. Biophys. Acta 1832, 312–322. doi: 10.1016/j.bbadis.2012.11.013
Tadic, V., Prell, T., Lautenschlaeger, J., and Grosskreutz, J. (2014). The ER mitochondria calcium cycle and ER stress response as therapeutic targets in amyotrophic lateral sclerosis. Front. Cell Neurosci. 8:147. doi: 10.3389/fncel.2014.00147
Turner, B. J., Murray, S. S., Piccenna, L. G., Lopes, E. C., Kilpatrick, T. J., and Cheema, S. S. (2004). Effect of p75 neurotrophin receptor antagonist on disease progression in transgenic amyotrophic lateral sclerosis mice. J. Neurosci. Res. 78, 193–199. doi: 10.1002/jnr.20256
Urushitani, M., Shimohama, S., Kihara, T., Sawada, H., Akaike, A., Ibi, M., et al. (1998). Mechanism of selective motor neuronal death after exposure of spinal cord to glutamate: involvement of glutamate-induced nitric oxide in motor neuron toxicity and nonmotor neuron protection. Ann. Neurol. 44, 796–807. doi: 10.1002/ana.410440514
Valbuena, G. N., Rizzardini, M., Cimini, S., Siskos, A. P., Bendotti, C., Cantoni, L., et al. (2015). Metabolomic analysis reveals increased aerobic glycolysis and amino acid deficit in a cellular model of amyotrophic lateral sclerosis. Mol. Neurobiol. 53, 2222–2240. doi: 10.1007/s12035-015-9165-7
Van Den Bosch, L., Van Damme, P., Bogaert, E., and Robberecht, W. (2006). The role of excitotoxicity in the pathogenesis of amyotrophic lateral sclerosis. Biochim. Biophys. Acta 1762, 1068–1082. doi: 10.1016/j.bbadis.2006.05.002
Van Den Bosch, L., Vandenberghe, W., Klaassen, H., Van Houtte, E., and Robberecht, W. (2000). Ca2+-permeable AMPA receptors and selective vulnerability of motor neurons. J. Neurol. Sci. 180, 29–34. doi: 10.1016/s0022-510x(00)00414-7
Veyrat-Durebex, C., Corcia, P., Dangoumau, A., Laumonnier, F., Piver, E., Gordon, P. H., et al. (2014). Advances in cellular models to explore the pathophysiology of amyotrophic lateral sclerosis. Mol. Neurobiol. 49, 966–983. doi: 10.1007/s12035-013-8573-9
Keywords: ALS, Ca2+ influx, differentiation, NSC34, glutamate receptors, NMDA
Citation: Madji Hounoum B, Vourc’h P, Felix R, Corcia P, Patin F, Guéguinou M, Potier-Cartereau M, Vandier C, Raoul C, Andres CR, Mavel S and Blasco H (2016) NSC-34 Motor Neuron-Like Cells Are Unsuitable as Experimental Model for Glutamate-Mediated Excitotoxicity. Front. Cell. Neurosci. 10:118. doi: 10.3389/fncel.2016.00118
Received: 16 February 2016; Accepted: 25 April 2016;
Published: 09 May 2016.
Edited by:
Manoj Kumar Jaiswal, Columbia University Medical Center, USAReviewed by:
Joe Tauskela, National Research Council Canada, CanadaTatsuro Mutoh, Fujita Health University School of Medicine, Japan
Andrea Nistri, Scuola Internazionale Superiore di Studi Avanzati (SISSA), Italy
Copyright © 2016 Madji Hounoum, Vourc’h, Felix, Corcia, Patin, Guéguinou, Potier-Cartereau, Vandier, Raoul, Andres, Mavel and Blasco. This is an open-access article distributed under the terms of the Creative Commons Attribution License (CC BY). The use, distribution and reproduction in other forums is permitted, provided the original author(s) or licensor are credited and that the original publication in this journal is cited, in accordance with accepted academic practice. No use, distribution or reproduction is permitted which does not comply with these terms.
*Correspondence: Hélène Blasco, aGVsZW5lLmJsYXNjb0B1bml2LXRvdXJzLmZy