- 1Life and Health Sciences Research Institute (ICVS), School of Health Sciences, University of Minho, Braga, Portugal
- 2ICVS/3B’s, PT Government Associate Laboratory, Braga/Guimarães, Portugal
Neural stem cells (NSCs) and mesenchymal stem cells (MSCs) share few characteristics apart from self-renewal and multipotency. In fact, the neurogenic and osteogenic stem cell niches derive from two distinct embryonary structures; while the later originates from the mesoderm, as all the connective tissues do, the first derives from the ectoderm. Therefore, it is highly unlikely that stem cells isolated from one niche could form terminally differentiated cells from the other. Additionally, these two niches are associated to tissues/systems (e.g., bone and central nervous system) that have markedly different needs and display diverse functions within the human body. Nevertheless they do share common features. For instance, the differentiation of both NSCs and MSCs is intimately associated with the bone morphogenetic protein family. Moreover, both NSCs and MSCs secrete a panel of common growth factors, such as nerve growth factor (NGF), glial derived neurotrophic factor (GDNF), and brain derived neurotrophic factor (BDNF), among others. But it is not the features they share but the interaction between them that seem most important, and worth exploring; namely, it has already been shown that there are mutually beneficially effects when these cell types are co-cultured in vitro. In fact the use of MSCs, and their secretome, become a strong candidate to be used as a therapeutic tool for CNS applications, namely by triggering the endogenous proliferation and differentiation of neural progenitors, among other mechanisms. Quite interestingly it was recently revealed that MSCs could be found in the human brain, in the vicinity of capillaries. In the present review we highlight how MSCs and NSCs in the neurogenic niches interact. Furthermore, we propose directions on this field and explore the future therapeutic possibilities that may arise from the combination/interaction of MSCs and NSCs.
Introduction
Injury and disease within the central nervous system (CNS) frequently induce chronic and acute insults leading to irreversible processes of neuronal cell death. Understanding how neurogenesis can be modulated, either through drugs or interaction with other cell types, and neural progenitors recruited to the site of injury, is of the utmost importance for the development of novel strategies that may impact the current state of the art. In recent years it has become evident that a population with a non-neural phenotype known for their role in the osteogenic niche, mesenchymal stem cells (MSCs), is able to regulate important phenomena within the CNS, including neural progenitor cells proliferation and differentiation. This quite unexpected and surprising function of MSCs brought closer the neurogenic and osteogenic niches, and prompted a new field of research that aims at understanding their interaction, and how both may impact on CNS regenerative medicine as we know it. Having this in mind the objective of the present paper is to review the most relevant advances in this field. It will first give an overview of neurogenic niches and how neurogenesis is regulated within them, then give an introduction to the osteogenic niches and MSCs, and end with a review on the most important works on the interactions between MSCs, neurogenic niches and disease models within the CNS.
Neurogenesis in the Adult Brain
Neuroanatomists have long believed Cajal’s assumptions on the immutability of the CNS. This dogma has been challenged due to growing evidence that endow the brain with considerable regenerative potential and neuroplastic capacity, essential to promote brain homeostasis (Lemaire et al., 2012). It is now well established that adult neurogenesis occurs throughout life in specific brain regions where neurons are constantly generated (Doetsch et al., 1999; Gage, 2002).
Globally, this neuroadaptative phenomenon occurs by the re-organization of the neuromorphological and electrophysiological properties of post-mitotic cells and the generation of new neuronal or glial cells that will incorporate the pre-existing networks, a process therefore called neuro- or gliogenesis, respectively (Guan et al., 2009). This complex process involves several steps beyond cell division; these include the commitment of the new cell to a neuronal phenotype, the migration and morphophysiological maturation of the neuroblasts, and the establishment of appropriate synaptic contacts that culminate with a full integration on the pre-existent network. These spatially defined brain regions where neurogenesis occurs display a permissive microenvironment for maintenance, proliferation and differentiation of Neural stem cells (NSCs). Admittedly, at least two defined neurogenic brain regions are broadly recognized in the adult mammalian brain (Figure 1): the subependymal zone (SEZ) of the lateral ventricles, and the subgranular zone (SGZ) of the hippocampal dentate gyrus (DG; Zhao et al., 2008). In both regions, astroglial cells act as the source of adult progenitor cells (Seri et al., 2001).
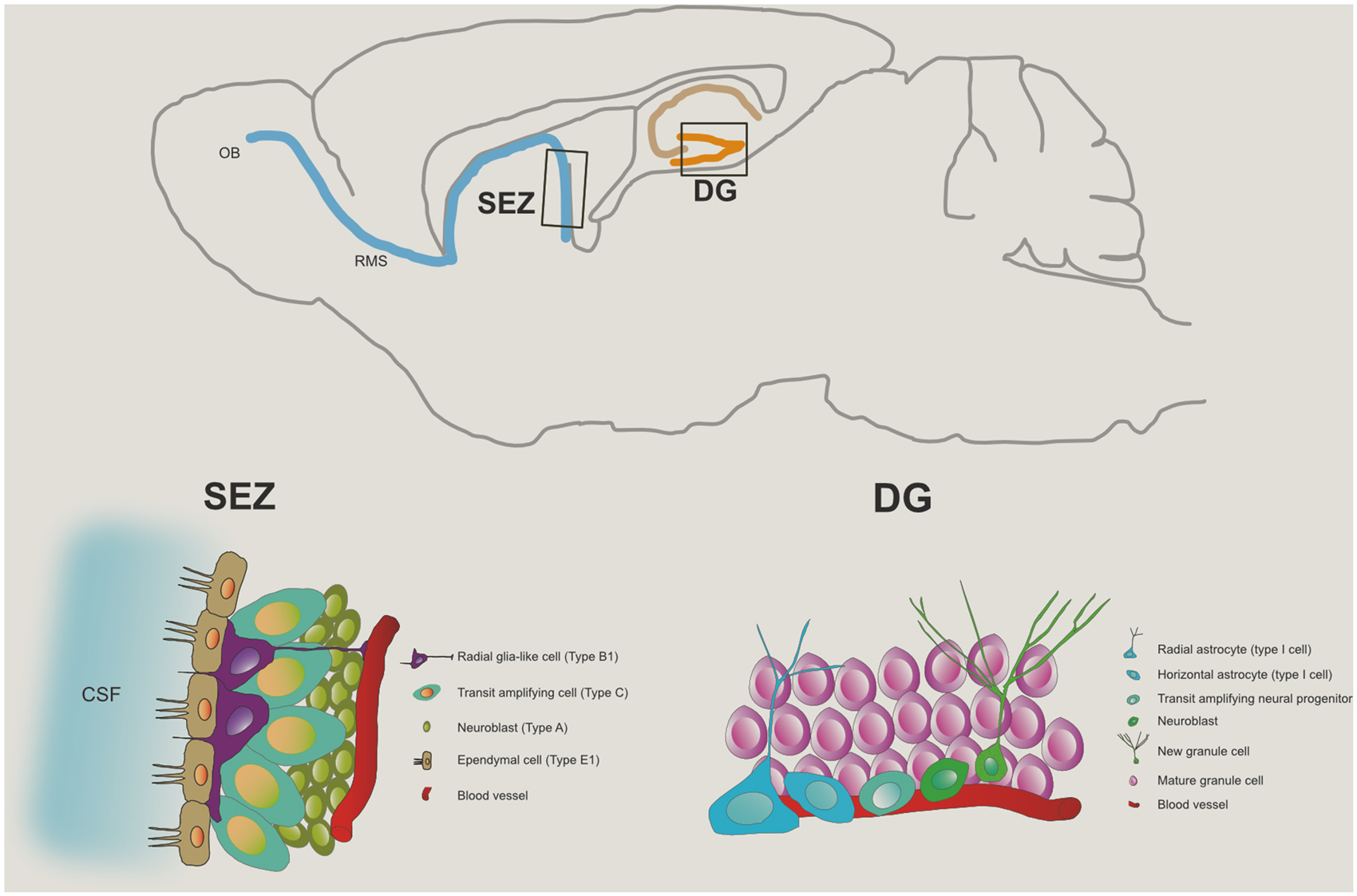
Figure 1. Neurogenic niches in the adult brain. The top panel represents, in a saggital section of the rodent brain, the two major niches of neural progenitor cells in the adult brain: one, in the sub granular zone of the dentate gyrus (DG) of the hippocampus, and the other, the subependymal zone (SEZ), from where progenitor cells committed to the neuronal lineage migrate via the rostral migratory stream (RMS) towards the olfactory bulb (OB). The bottom left panel illustrates the typical cytoarchitecture of the SEZ niche while the cell population in the DG niche is presented in the bottom right panel.
In the adult hippocampal neurogenic region, the progenitor cells reside in the SGZ, with defined gradients (Silva et al., 2006). Newly-born cells generated in the SGZ, become committed to a neuronal lineage and migrate into the granular cell layer (GCL), where they mature to become glutamatergic granule neurons (Seri et al., 2004; Zhao et al., 2008; Brill et al., 2009). The neuroblasts born in the SEZ migrate anteriorly along the rostral migratory stream (RMS), becoming mostly mature GABAergic granule neurons and periglomerular interneurons in the olfactory bulb (OB; Chumley et al., 2007; Zhao et al., 2008). Besides these two well-accepted adult neurogenic regions, although disputable, some reports have shown evidences for the generation of new neurons on other brain regions of the adult brain, including the amygdala (Bernier et al., 2002; Fowler et al., 2005; Gonçalves et al., 2008), the hypothalamus (Fowler et al., 2002; Kokoeva et al., 2005), the cortex (Gould et al., 1999; Kodama et al., 2004), the striatum (Dayer et al., 2005; Bédard et al., 2006) and the substantia nigra (SN; Zhao et al., 2003; Yoshimi et al., 2005). Importantly, it appears that neurogenesis in these regions occurs at very low levels or under non-physiological conditions (von Bohlen und Halbach, 2011).
Importantly, the neurogenesis process in the adult brain constitutes a new dimension of plasticity, with great impact on neuronal remodeling and repair, being now considered by the biomedical field as a promising therapeutical target in several neuropathological contexts. For instance, abnormal alterations in the hippocampal neurogenesis process have been implicated in an assortment of neuropsychiatric disorders (Sapolsky, 2000; Eisch et al., 2008; Kobayashi, 2009). Indeed, impairments in neuroplasticity are increasingly considered central to the ethiopathogenesis of depression (Bessa et al., 2009; Mateus-Pinheiro et al., 2013a,b). Studies have also shown the contribution of new neurons to a subset of hippocampal functions, influencing mood control, learning and memory (Hanson et al., 2011; Eisch and Petrik, 2012; Konefal et al., 2013). In fact, a clear connection between adult neurogenesis and learning/memory was demonstrated, as diminished neurogenesis decreases learning/memory, while enhanced neurogenesis improves it (Eisch and Petrik, 2012; Nakashiba et al., 2012). These examples prompt for the relevance of modulating the neurogenic niches as a potential therapeutic strategy to treat the symptoms of neurodegenerative disorders such as Parkinson’s disease (PD), which we will later develop in the context of MSCs derived therapies.
We will next refer to the structural and functional organization specificities of the adult SGZ and SEZ neurogenic niches.
Adult Hippocampal Neurogenesis
As referred above, the adult brain is capable of generating new cells that can incorporate into its established complex circuitry (Trujillo et al., 2009). This process of adult neurogenesis highly recapitulates the embryonic neurogenic process, with the important difference that new neurons are generated in an already mature microenvironment and have to integrate in pre-existing neural circuits. Adult hippocampal neurogenesis consists of several highly regulated sequential phases (Kempermann et al., 2004; Ming and Song, 2005) characterized by morphological distinct cells: (i) proliferation of neural progenitor cells residing in a narrow layer of about three nuclei wide, the SGZ; (ii) generation of amplifying progenitors; (iii) cell migration; (iv) differentiation; and (v) maturation at the final destination with axon and dendrites formation and establishment of new synapses (Kempermann et al., 2004; Steiner et al., 2006; Balu and Lucki, 2009).
The adult SGZ contains heterogeneous progenitor cells, which can be distinguished and identified by a particular set of molecules expressed by each progenitor population. The first type of progenitors are the quiescent neural progenitors (QNPs), described to be multipotent stem cells (Seri et al., 2001, 2004) and also known as NSCs or type-1 progenitor cells (Type-1 cells). These cells have morphological and antigenic glial properties, expressing markers such as the intermediate filament protein nestin, brain lipid binding-protein (BLPB), the glutamate aspartate transporter (GLAST; Steiner et al., 2006) and glial fibrillary acidic protein (GFAP), among others; it can be further distinguishable into two subtypes, based on their spatial orientation in the SGZ: radial astrocytes (rA) and horizontal astrocytes (hA). Radial astrocytes are characterized by having a single radial process, being also slowly dividing cells, whereas hA present a short horizontal process and divide faster (Lugert et al., 2010; Hodge et al., 2012). These cells divide asymmetrically giving rise to transient amplifying neural progenitors (tANPs, also designated as type-2 progenitor cells or TAPs). It is important to notice that this phase of the neurogenic process comprises a decisive point in the determination of neural progenitors cell-fate (neuronal or non-neuronal lineage commitment; Steiner et al., 2006). This latter progenitor cells, TAPs, are already committed to a neuronal lineage, being mitotically active (Encinas et al., 2006) and dividing symmetrically to give rise to neuroblasts (also known as type-3 cells). Neuroblasts are intermediate progenitors in the generation of new glutamatergic granule neurons, corresponding to a stage of transition from a slowly proliferating neuroblast, which is exiting the cell cycle, to a postmitotic immature neuron, that will migrate into the GCL of the DG. These neuroblasts express markers of the neuronal lineage, such as the polysialylated-neural cell adhesion molecule (PSA-NCAM), calcium-binding protein calretinin and doublecortin (DCX), that are crucial for further maturation and migration of these cells (Pleasure et al., 2000; Ehninger and Kempermann, 2003; Balu and Lucki, 2009). When reaching the GCL, newborn cells will fully maturate, elongating their axons towards the CA3 region (von Bohlen und Halbach, 2011) and establishing new functional connections (Balu and Lucki, 2009), thus becoming mature granule neurons, which express neuronal nuclei protein (NeuN). The cell markers described above are not all exclusive to the SGZ; as will be described next, some are also characteristic of cells from the SEZ niche (Table 1). Moreover, similarly to the SEZ, only some of these markers allow cell-specific phenotypic characterization, as indicated in Table 1. Approximately 2–3 weeks after exiting the cell cycle, they express calbindin, a marker of mature granule cells (Kempermann et al., 2004). Newly formed neurons enter a period of enhanced synaptic plasticity in which their electrophysiological properties resemble those of neurons in the early postnatal period in juvenile animals (Ge et al., 2007). This phase lasts around 4–6 weeks after the original cell division, resulting in a total of approximately 7–8 weeks required for newborn cells to become functionally indistinguishable from the older granule cell population (Carlén et al., 2002; Abrous et al., 2005; Zhao et al., 2008; Snyder et al., 2009; Hanson et al., 2011). Newborn neurons display very different characteristics than mature ones, such as enhanced excitability, reduced threshold to induction of long-term potentiation (LTP) and an excitatory response to GABAergic input, since this neurotransmitter induces depolarization instead of hyperpolarization that is seen in adult neurons, which is related to a specific pattern of expression of some ionic co-transporters. This clearly indicates that adult-born neurons possess specific properties associated with plasticity (Schmidt-Hieber et al., 2004; Saxe et al., 2006; Ge et al., 2007; Hanson et al., 2011).
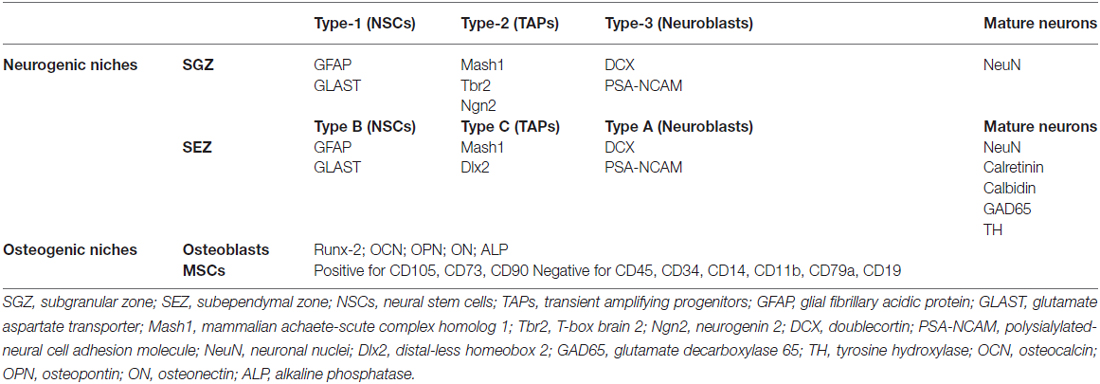
Table 1. Summary of markers that specifically allow phenotypic characterization of major cell types found in both neurogenic and osteogenic niches.
Noticeably, neurogenesis is a fine tuned process, in which not all cells expressing immature neuronal markers develop into fully mature neurons (Kempermann et al., 2003) and most newly-born neurons are eliminated by apoptosis (Biebl et al., 2000). The mechanisms that regulate this clearance of neurons are still to be fully understood, however, very recently a report showed that DCX-neuronal progenitors present phagocytic activity in the hippocampal and SEZ neurogenic niches and have great impact in the neurogenic process (Lu et al., 2011).
The harmonization of the several processes and cellular activities that occurs during the generation of new neurons in the adult mammalian brain is thus paramount. Several studies propose a complex transcriptional and epigenetic orchestration of the adult hippocampal neurogenic process, with both intrinsic and extrinsic factors being ultimately responsible for the modulation of this phenomenon. Therefore, the niche, where adult neurogenesis occurs is also crucial for the modulation and fine-tuning of this process.
Subependymal Zone Neurogenesis
The SEZ, also referred in the literature as adult subventricular zone (SVZ), is the site of the adult brain where neurogenesis is most intense. In rodents, the SEZ is seldom described as a thin layer of cells located below the ependymal layer that lines the lateral walls of the lateral ventricles, but it also extends to the dorsal and medial ventricular walls (Alvarez-Buylla et al., 2008). As in the SGZ niche, the cell populations in the SEZ are heterogeneous, containing several cell types that are identifiable by cell-specific markers. In general terms it might be described as being composed of slow-dividing type B cells (the NSCs) that originate fast-dividing type C cells, that in turn give rise to neuroblasts (type A cells). Nevertheless, given the complexity of these cell populations they, and respective phenotypic markers, will next be described with further detail (see also Table 1).
Type B cells are astrocytic cells and express the intermediate filament GFAP. In the SEZ two types of GFAP positive cells were distinguished according to ultrastructural differences: type B2 astrocytes, or niche astrocytes, display a highly branched morphology and are frequently found in the interface of the SEZ and the striatum (Doetsch et al., 1997); type B1 astrocytes are radial-glia like that organize in pinwheel structures with the apical ending, the primary cilium, turned towards the brain ventricles—and hence in bathed in the cerebrospinal fluid—and is surrounded by ependymal cells (Mirzadeh et al., 2008). The type B1 cells are recognized as the NSCs of the SEZ. Type C cells, or TAPs, originate from the NSCs. These rapidly dividing cells are organized in clusters of immature precursors that express distal-less homeobox 2 (Dlx2), achaete-scute complex homolog 1 (Ascl1or Mash1) and epidermal growth factor receptor (EFGR; Ciccolini et al., 2005; Ming and Song, 2011). A short pulse (24 h) of the timidine analog BrdU mainly labels TAPs indicating that these cells are the largest pool of proliferating cells in the SEZ. Type A cells, or neuroblasts, are born from type C cells and constitute the neuronal precursors cells. Most type A cells express PSA-NCAM and DCX, which are associated to their migratory properties (Ming and Song, 2011). Under physiological conditions neuroblasts migrate tangentially from the SEZ, via the RMS to the OBs where they become fully mature neurons. Neuroblasts divide actively in the SEZ but also in the RMS. Once in the OBs, neuroblasts migrate radially, give rise to mature neurons and are integrated in distinct layers of the OB. They form new granular cells (deep, superficial and calretin positive) and periglomerular cells (calretin positive, calbidin positive and tyrosine hydroxylase positive; Lledo et al., 2008; Kriegstein and Alvarez-Buylla, 2009). Most of these new neurons are granule cells integrated in the granule cell layer and are GABAergic, but a small group of glutamatergic neurons was also identified (Brill et al., 2009).
Also of relevance in the SEZ are the ependymal cells (type E cells) that, as indicated above, form a monolayer that outlines the ventricular wall. These cells constitute a physical barrier that diminishes the direct and free exchange of molecules between the CSF and brain parenchyma (Falcão et al., 2012a). Two distinct ependymal cells have been described: the most common type E1 ependymal cells that are multiciliated, and the E2 ependymal cells that display two long cilia and represent solely 5% of the type E cells (Mirzadeh et al., 2008). Under physiological conditions these cells proliferate rarely (Coskun et al., 2008) or do not proliferate at all (Mirzadeh et al., 2008).
Tanycytes (Doetsch et al., 1997; Chojnacki et al., 2009), microglia (in response to injury; Ekdahl et al., 2009) and endothelial cells of the blood vessels (Tavazoie et al., 2008) are also relevant cellular components of the SEZ niche. These later cell types contribute to the specific microenvironment that constitute the SEZ NSCs niche; for instance, endothelial cells secrete several factors (pigment epithelium-derived factor, PEDF; NT3, among others) that induce proliferation and migration of NSCs (Ramírez-Castillejo et al., 2006; Delgado et al., 2014). Hence their interaction with proliferating cells should be taken into account when considering the modulation of the SEZ NSCs namely if one targets, for neuroregenerative purposes, the application of exogenous cells and/or protein/molecular factors, as will be further discussed in later sections.
In addition to the SEZ cellular heterogeneity, there is a further level of complexity in the form of topographical heterogeneity. A simple observation on the topography of the SEZ discloses major anatomical differences (Falcão et al., 2012b). It is now evident that even in the above described cell populations lays a remarkable heterogeneity either due to inherited intrinsic or epigenetic factors (Alvarez-Buylla et al., 2008) and/or an additional diversity in the surrounding microenvironment cues. Several studies showed that the NSCs pool is highly heterogeneous both in the origin and in cellular fate (Merkle et al., 2007; Alvarez-Buylla et al., 2008). For instance, while the common fate of SEZ born cells is the OB where they become interneurons, it was shown that it also generates a small pool of glutamatergic neurons steming from NSCs that reside in the adult dorsal wall of the lateral (Brill et al., 2009). Moreover, neuroblasts born either in ventral, dorsal, anterior or posterior regions are distinct, produce different neuronal types and are integrated in different layers of the OB (Alvarez-Buylla et al., 2008). As an example, neuroblasts from dorsal regions mostly originate superficial granule cells; while ventral derived neuroblasts give rise mostly to deep granule cells (Merkle et al., 2007). Also of notice, SEZ NSCs also originate oligodendrocyte precursors that migrate to the striatum and the corpus callosum and differentiate into oligodendrocytes (Nait-Oumesmar et al., 1999; Picard-Riera et al., 2002). The reason for why different regionally placed NSCs give rise to distinct progeny might reside in the distribution pattern of specific transcription factors, adding another layer of complexity in the regulation of cell proliferation in the SEZ, and thus in cell fate. All of these cell intrinsic and extrinsic aspects must be taken into account when considering putative therapeutic approaches for CNS regeneration.
Transcriptional Regulation of Adult Neurogenesis
Adult neurogenesis gives rise to both glutamatergic and GABAergic neurons. In the hippocampus changes in the rates of generation of glutamatergic neurons might contribute to several pathologies. In this context, the discovery of new factors important for the generation of glutamatergic neurons is needed. Interestingly, adult glutamatergic neurogenesis recapitulates the sequential expression of transcription factors found in the developing cerebral cortex (Pax6→Neurogenin2→Tbr2→Tbr1), demonstrating that this transcription network is maintained postnatally (Brill et al., 2009). For example, Pax6, a crucial determinant for the specification of glutamatergic neurons during development, is essential for adult neurogenesis (Hack et al., 2005) and is sufficient to instruct postnatal neocortical astrocytes towards neurogenesis in vitro (Heins et al., 2002). It was also shown during development, that one of the downstream targets of Pax6, the transcription factor AP2γ, is important for the specification of glutamatergic neocortical neurons and their progenitors (Pinto et al., 2009), and also for the differentiation of glutamatergic neurons in the adult neurogenic regions. Furthermore, AP2γ regulates Tbr2, which was shown to be important for glutamatergic neurogenesis during development (Pinto et al., 2009).
As described above, generation of specific cell types (neuronal or glial type) in the adult SEZ is topographically heterogeneous and this might be bound to transcriptional regulation. In fact, the expression of distinct transcription factors in both overlapping and non-overlapping regions of the SEZ is described. Similarly to the SGZ, some of these transcription factors were correlated with the SEZ embryonic origin (Waclaw et al., 2006; Young et al., 2007). In fact, a topographical pattern of transcription factors expression in the SEZ is associated with NSCs embryonic origin and adult neuronal fate. Generally, NSCs in the lateral ventricular wall ubiquitously express Dlx1, 2, 5 and Mash1, while Emx1 expression is exclusive to the dorsal wall of the ventricle (Young et al., 2007). Furthermore, the transcription factors Nkx2.1 and Pax6 outline the ventral and dorsal regions of the lateral wall, respectively (Alvarez-Buylla et al., 2008; Weinandy et al., 2011). Thus, in the SEZ, an additional challenge is to understand how to modulate different combinations of transcription factors so as to result in production of specific neuronal types.
A targeted induction of neurogenesis, by stimulating endogenous neural progenitors in the adult brain, could represent an important cellular therapy to treat neurodegenerative disorders. A major challenge in our days is to improve survival and induce differentiation of newborn neurons after acute lesions. For instance, it was already shown that Pax6 can induce neurogenesis from non-neurogenic astrocytes in vivo, when overexpressed after stab-wound lesion (Buffo et al., 2005). These experiments provide proof of principle that neurons can be newly generated from endogenous sources of the adult mammalian brain. However, these induced neurons are very few in number and fail to mature. Therefore, new cues are needed to efficiently instruct neurogenesis and repair after neuronal insult.
The Microenvironment of the Neurogenic Niches
The interplay between extrinsic and intrinsic factors determines the NSCs niche homeostasis. Intrinsic factors are a set of signals produced by the progenitors that together with exterior microenvironment cues (extrinsic factors) instruct distinct neurogenic phases and ultimately the cellular fate. Many of the mechanisms regulating NSCs proliferation and neurogenesis during embryonic development, appear to be conserved in adulthood, and both intrinsic and extrinsic factors important for embryonic neurogenesis are also involved in the regulation of neurogenesis in the adult brain (Ming and Song, 2011). However, there are relevant differences between them, especially regarding the properties of the cellular and molecular niche. Whereas during development, the cellular environment is highly specialized to support proliferation, in the adult neurogenic niches the environmental context is concomitantly able to maintain a population of fully mature neurons (Zhao et al., 2008; Jessberger et al., 2009), thus providing a different set of both intrinsic and extrinsic signals.
Extrinsic signals, for instance, for the SEZ regulation include several trophic and growth factors, neurotransmitters, morphogens, hormones and cytokines (Falcão et al., 2012a). These extracellular signaling molecules are of diverse origins, namely from ependymal cells, endothelial cells, neural progenitor cells and neurons. The neurotransmitters are examples of key extrinsic factors of neuronal origin. For instance, the neurotransmitter GABA produced by niche neuroblasts is reported to inhibit NSCs proliferation but serotonine stimulates NSCs proliferation (Banasr et al., 2004, and conflicting results were presented for the effects of dopamine (DA) in the SEZ niche (Berg et al., 2013).
This important role of the microenvironment in the neurogenic niches for the regulation of NSCs has been shown by many in vivo and in vitro studies. For example, SEZ derived neuroblasts can change their fate and differentiate into oligodendrocytes upon a change in the microenvironment induced by demyelination of the corpus callosum (Picard-Riera et al., 2002; Jablonska et al., 2010). Additionally, glial progenitor cells may change to a neuronal fate when transplanted into a neurogenic region (Shihabuddin et al., 2000), while mouse SEZ neural progenitors committed to the neuronal lineage, changed to glial differentiation upon transplantation into regions outside the neurogenic niche (Seidenfaden et al., 2006).
The microenvironment of the neurogenic niches is thus essential for fate determination and cell differentiation, as well as for self-renewal, proliferation, migration and maturation of NSCs. This microenvironment is comprised of local cell types, cell signals, extracellular matrix and microvasculature. Indeed, the SEZ and SGZ niches are highly vascularized by a network of specialized capillaries (Goldberg and Hirschi, 2009) and NSCs closely interact with the microvasculature (Palmer et al., 2000; Mirzadeh et al., 2008; Shen et al., 2008; Tavazoie et al., 2008). This microvasculature has been shown to be essential in maintaining the function of the neurogenic niches, namely by regulating the proliferation and quiescence of NSCs (Palmer et al., 2000; Shen et al., 2004, 2008; Tavazoie et al., 2008; Culver et al., 2013), as well as NSCs self-renewal and neurogenesis through soluble factors secreted by the endothelial cells (Shen et al., 2004; Ramírez-Castillejo et al., 2006; Gómez-Gaviro et al., 2012). Noteworthy is the recent report of the existence of MSCs in the brain microvasculature (Paul et al., 2012), which paves way for the usage of MSCs secretome to modulate the neurogenic niches cells. One further example of NSCs microenvironment modulators are microglia cells, the brain resident macrophages, have also a crucial role in the regulation and maintenance of neurogenesis in the SGZ neurogenic niche (Sierra et al., 2010) given that they impact on the proliferation of neural stem/progenitor cells (Gebara et al., 2013); also they are particularly relevant in modulating the SEZ in response to brain injury (Thored et al., 2009).
In this way, signaling from and into the niche is suggested to be responsible for key processes in the regulation of homeostasis of adult neurogenesis including the balance between quiescence vs. proliferation, the mode of cell division, and the prevention of stem cell depletion.
The existence of NSCs in the adult neurogenic niches prompted research for their usage in adult brain regeneration. Nevertheless, their intrinsic and extrinsinc properties, which we have summarized above, pose also major challenges to mount adequate therapeutic approaches. MSCs, and specifically the interaction of their properties with NSCs, might be ideal candidates for this purpose. We will next describe the major characteristics of MSCs and how they might promote brain regeneration.
The Osteogenic Niche
The osteogenic niche is a highly vascularized and dynamic environment in which four cell types play an important role on the maintenance and renewal of bone tissue: MSCs, osteoblasts, osteocytes and osteoclasts.
Osteoblasts (Table 1) arise from osteoprogenitor and MSCs (further details on MSCs biology are discussed in “MSCs and CNS Therapies” Section) present in the bone marrow and periosteum. They are known to be involved in the synthesis and regulation of extracellular matrix elaboration (ECM) and mineralization (Sommerfeldt and Rubin, 2001; Salgado et al., 2004). Furthermore, it is also known that basic cellular functions and responsiveness to metabolic and mechanical stimuli demand are maintained through extensive cell-matrix and cell-cell contacts via a variety of transmembranous proteins and specific receptors (Sommerfeldt and Rubin, 2001). Osteocytes represent osteoblasts that became incorporated in the newly elaborated extracellular matrix, being enclosed in spaces called lacunae. They maintain direct contact with neighboring osteocytes, osteoblasts and bone lining cells through cellular processes that are created before and during matrix synthesis (Sommerfeldt and Rubin, 2001; Knothe et al., 2004). In mature bone these cell processes are contained in channels called the canaliculi. The communication and interaction between neighboring osteocytes is achieved through the establishment of gap junctions (Sommerfeldt and Rubin, 2001; Knothe et al., 2004). This is an absolute need for osteocytes because is the only way by which they can assure the access to oxygen and nutrients. They are known to be involved in the calcification of osteoid matrix, blood-calcium homeostasis and to be the mechanosensor cells of bone (Sikavitsas et al., 2001; Knothe et al., 2004). Finally, osteoclasts, are multinucleated polarized cells involved in the bone remodeling process, that belong to the monocyte/macrophage lineage. Their main function is to resorb mineralised bone. For this purpose they are enriched in intracellular structures such as pleomorphic mithocondria, vacuoles, and lysossomes, as well as alterations, namely at the structural level, in its cell membrane (Vaananen, 1996).
Mesenchymal Stem Cells
Mesenchymal Stem Cells, The Secretome and Neurogenic Niches
The first reports on the possible existence of a population with a Mesenchymal progenitor character are attributed to Friedenstein et al. (1974b). Indeed, Friedenstein et al. identified and defined these cells as plastic-adherent fibroblast colony-forming units with clonogenic capacity (Friedenstein et al., 1974a). Later, these cells were also named as marrow “stromal cells”, on the basis of their possible use as a feeder layer for hematopoietic stem cells (Eaves et al., 1991; Glavaski-Joksimovic and Bohn, 2013). Additionally other reports also referred to them as MSCs because of their clonogenicity capacity and ability to undergo multilineage differentiation (Caplan, 1991; Bluguermann et al., 2013). Currently MSCs have been defined, according with the International Society for Cellular Therapy (ISCT) criteria, as multipotent cells (with the ability of at least differentiating towards the osteogenic, chondrogenic and adipogenic lineages), capable of self-renewal, able to adhere to tissue culture plastic and to display the presence of surface markers (CD105, CD73, CD90), as well as the lack of hematopoietic cell surface markers (CD45, CD34, CD14 or CD11b, CD79a or CD19 and Human Leukocyte Antigen DR; Table 1; Dominici et al., 2006). So far, MSCs have been isolated from bone marrow (BMSCs), adipose tissue (ASCs), dental pulp, placenta, amniotic fluid, umbilical cord blood, umbilical cord Wharton’s jelly (bulk—WJ-MSCs; perivascular region—human umbilical cord perivascular cells, HUCPVCs), liver, lung and spleen, and brain (for an extensive review see Teixeira et al., 2013). As potential therapeutic agents, MSCs display a number of key characteristics that are believed to be advantageous when compared to other cell populations. For instance they can be isolated with minimal invasive procedures, easily cultured and expanded in vitro for several passages, can be used for allogenous transplantation in virtue of their hypoimmunogenicity, decreased tumorigenic potential and, as adult cells, are not hindered by ethical concerns (Salgado et al., 2006; Kishk and Abokrysha, 2011; Seo and Cho, 2012; Teixeira et al., 2013). These MSCs features have made them attractive tools for CNS neurodegenerative diseases.
Initially it was considered that the true therapeutic potential of these cells relied on their multilineage differentiation. Indeed most of the literature of the 90 s and early 21st century was focused on the differentiation of these cells towards mesodermal lineages, such as the osteogenic, mainly within 3D matrices known as scaffolds, to induce regeneration in the affected areas. Around the same time it was also suggested that MSCs even had a greater differentiation potential than was originally predicted, as several reports indicated that these cells could be differentiated beyond the mesodermal lineages (Dominici et al., 2006). In 2005, Gnecchi et al. (2005) put forward a new concept that lately would change the paradigm of how MSCs could be used in regenerative medicine, by showing that their therapeutic potential was mostly related to the growth factors that they secreted to the extracellular milieu, rather than to their differentiation potential.
Indeed, in recent years it is becoming increasingly accepted that the regenerative effects promoted by MSCs are mainly associated with their secretome. As discussed by Teixeira et al. (Teixeira et al., 2013) the secretome of MSCs is composed by a proteic soluble fraction, constituted by growth factors and cytokines, and a vesicular fraction composed by microvesicles and exosomes, which are involved in the transference of proteins and genetic material (e.g., miRNA) to other cells. The protective actions promoted by MSCs secreted molecules are closely related with therapeutic plasticity in the CNS. Indeed several authors have reported the presence of a plethora of growth factors with a known influence on neuronal survival, differentiation, neurite outgrowth and immunomodulation of microglial cells; these factors are BDNF, glial derived neurotrophic factor (GDNF), nerve growth factor (NGF), hepatocyte growth factor (HGF), vascular endothelial growth factor (VEGF), VEGF-receptor 3 (VEGF-R3), angiopoietin 1, insulin-like growth factor 1 (IGF-1), insulin-like growth factor 2 (IGF-2), epidermal growth facto (EGF), basic fibroblast growth factor (bFGF), FGF 20, granulocyte colony-stimulating factor (G-CSF), platelet-derived growth factor AA (PDGF-AA), chemokine ligand 16 (CXCL 16), neutrophil-activating-protein-2 (NAP 2) and neurotrophin-3 (NT-3) growth factors, as well as interleukin-6 (IL-6), interleukin-10 (IL-10), transforming growth factor beta 1 (TGF β1), stem cell factor (SCF), stromal cell-derived factor 1 (SDF-1) and monocyte chemotactic protein 1 (MCP-1) cytokines (Rehman et al., 2004; Caplan and Dennis, 2006; Chen et al., 2008b; Bonfield et al., 2010; Meyerrose et al., 2010; Nakano et al., 2010; Ribeiro et al., 2012). Other proteins such as 14-3-3, ubiquitin carboxyl-terminal esterase L1 (UCHL1), hsp70 and peroxiredoxin-6 have also been related to the neuroregulatory character of the secretome of MSCs (Fraga et al., 2013).
The action of MSCs and their secretome in neurogenic niches (Figure 2) such as the SGZ has been previously described. For instance, Munoz et al. (2006) transplanted BMSCs into the DG of immunodeficient mice. Results revealed that the transplanted MSCs markedly increased the proliferation of endogenous NSCs that expressed the stem cell marker Sox2, as well as their differentiation, a fact that was attributed to a local increase on the expression of growth factors such as VEGF, ciliary neurotrophic factor (CNTF), neurotrophin-4/5 and NGF. More recently it was also shown that the injection of the secretome of MSCs itself, was also able to modulate both neuronal survival and differentiation within the adult rat hippocampus. Teixeira et al. (2015) show that the injection of the secretome of HUCPVCs (a MSC population that resides in the perivascular region of the umbilical cord) was able to induce an increased number of DCX+ cells. This observation was then related with a higher expression of FGF-2 and NGF in the injected area.
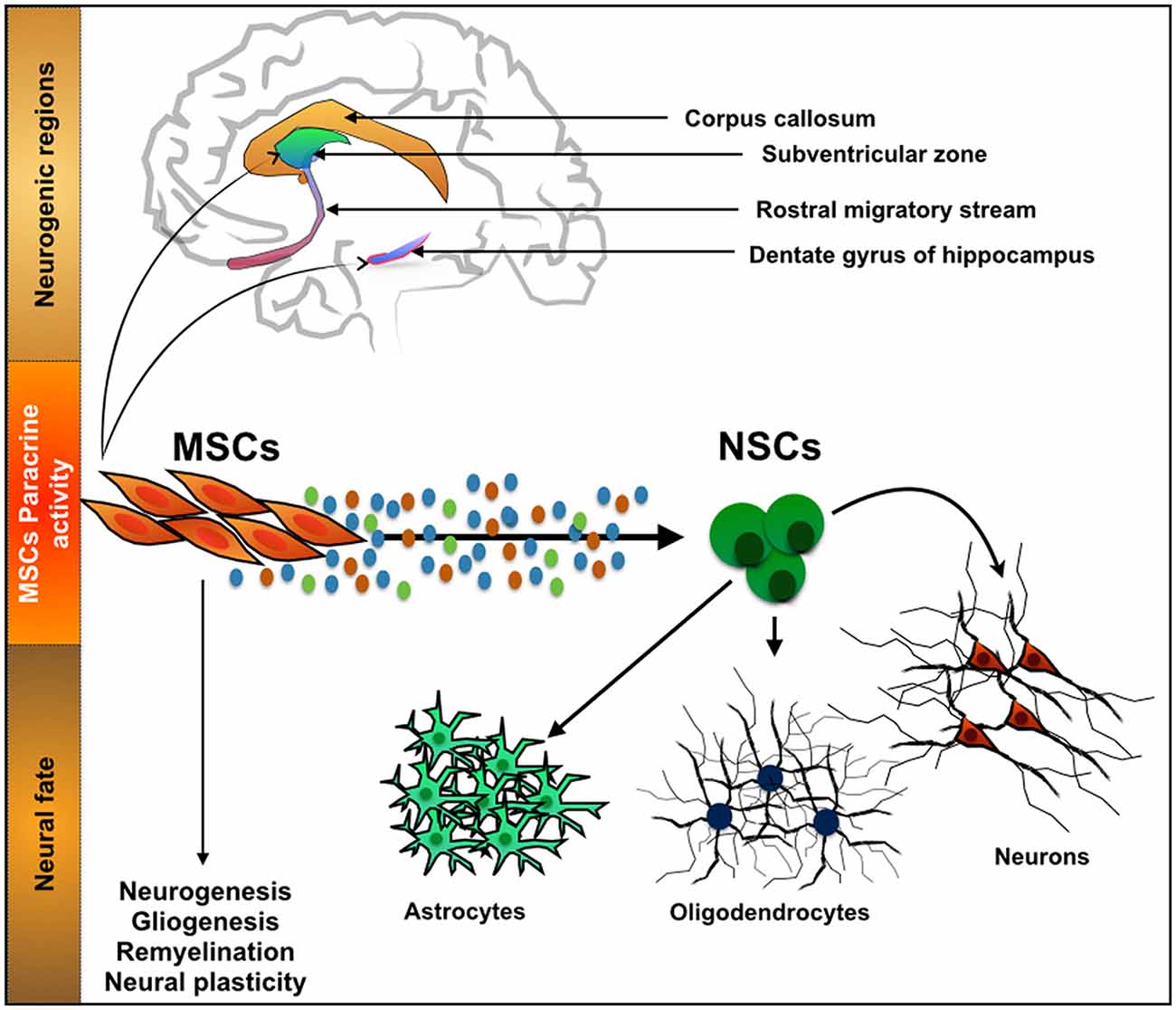
Figure 2. Interaction between mesenchymal stem cells (MSCs) and neurogenic niches. MSCs, a cell population with a known function in the osteogenic niche, is able to modulate the action of Neural stem cells (NSCs) by means of their secretome. Through the secretion of neuroregulatory molecules, either soluble or in the form of vesicles, MSCs are able to influence processes such as neurogenesis, gliogenesis, remyelination and neural plasticity. With it important developments have been recently witnessed in CNS regenerative medicine strategies.
As a consequence of this, the multiple faces of MSCs and their secretome have prompted a number of different experimental therapeutic strategies in CNS regenerative medicine. Such strategies rely on a strong interplay between neuroregulatory molecules secreted by MSCs and the different niches with the CNS.
In disorders such as multiple sclerosis (MS), available data, both from animal models and human patient related studies, indicates that the immunomodulatory properties of the secretome of MSCs regulate the immune/oligodendrogenic niches. For instance Wang et al. (2010) revealed that MSCs derived from human embryonic stem cells (hES-MSCs) significantly reduce clinical symptoms and prevent neuronal demyelination in a mouse experimental autoimmune encephalitis (EAE) mouse model of MS, by reducing the frequency of CD4+ and CD8+ cells infiltration in the CNS. A similar trend was described by Li et al. (2014) and Llufriu et al. (2014) in studies with human patients, in which the administration of MSCs from different sources, alone or combined with pharmacotherapies, positively impacted the condition of the patients, by modulating MS related inflammatory events.
On other hand, in disorders such as PD, Ischemic Stroke (IS) and Glioblastoma Multiforme (GBM) it is believed that the action of MSCs goes beyond the neuro-immunomodulation, and in fact, some of the reported benefits may be closely related with their direct interaction with the neurogenic niches. Due to the nature and objectives of this review, this topic will be further explored in the following section.
MSCs and CNS Therapies
Parkinson’s Disease
Among CNS disorders, PD is the most common motor-related disorder in middle or late-life affecting millions (Pereira and Aziz, 2006) worldwide. It is a slowly progressive neurodegenerative disease that is primarily characterized by the loss of dopaminergic (DAergic) neurons in several dopaminergic networks, most intensively in the ventral tier of the substantia nigra pars compacta (SNpc) within the mesostriatal/nigrostriatal pathway (Koller, 2003; Pereira and Aziz, 2006; Cummins and Barker, 2012; Teixeira et al., 2013). The depletion of SN neurons leads to the loss of DAergic innervations and consequently to striatal DA deficiency, which is responsible for the major sensory-motor symptoms of PD (Dauer and Przedborski, 2003).
A considerable body of evidence has revealed the potential of MSCs to promote protection and/or recovery of DAergic neurons against neurotoxin-induced nigrostriatal degeneration. Indeed, several studies have demonstrated that BMSCs secretome protect and/or regenerate DAergic neurons in in vitro and in vivo models of PD, through the secretion of growth factors and cytokines (summarized in Table 2; Weiss et al., 2006; Shintani et al., 2007; McCoy et al., 2008; Kim et al., 2009; Sadan et al., 2009; Blandini et al., 2010; Cova et al., 2010; Wang et al., 2010; Danielyan et al., 2011; Park et al., 2012). For instance, Shintani and coworkers demonstrated that BMSCs conditioned media (CM) was able to promote survival of tyrosine hydroxylase (TH)-positive DAergic neurons in rat primary cultures of ventral mesencephalic cells (Shintani et al., 2007). Moreover, intrastriatal transplantation of fetal mesencephalic cells treated with human BMSCs CM, during steps of donor preparation and implantation, induced survival of DAergic grafted cells and promoted functional recovery in a 6-OHDA rat model of PD (Shintani et al., 2007). The observed protection of DAergic neurons was attributed to BMSCs secretion of BDNF, GDNF and bFGF. Similarly, Sadan et al. showed that human BMSCs (hBMSCs) cultured in the presence of growth factors, not only significantly increased the viability of the SH-SY5Y neuroblastoma cell line exposed to 6-OHDA, but also that BMSCs transplanted into the striatum of a 6-OHDA rat model of PD, migrated to the lesion site, and increased the numbers of TH-positive cells and DA levels (Sadan et al., 2009). These neuroprotective and neuro-regenerative effects were accompanied by an improvement in animals’ motor behavior and were correlated with BMSCs secretion of BDNF and GDNF. This expression pattern is in accordance with data published by Blandini and co-workers using the same animal model (Blandini et al., 2010). On the other hand, Wang and colleagues associated rat-derived BMSCs expression of stromal cell-derived factor 1 (SDF)-1α with the DAergic neurons protection against 6-OHDA neurotoxin both in vitro and in vivo, through anti-apoptotic based mechanisms (Wang et al., 2010). Moreover, Cova et al. demonstrated that BMSCs transplanted in the striatum of a 6-OHDA rodent model of PD were able to survive and interact with the lesion site surroundings, thus enhancing the survival of DAergic terminals and neurogenesis in the SVZ in a sustained manner (Cova et al., 2010). Finally, the secretion of BDNF in vivo by BMSCs, was correlated with the activation of endogenous stem cells (Cova et al., 2010).
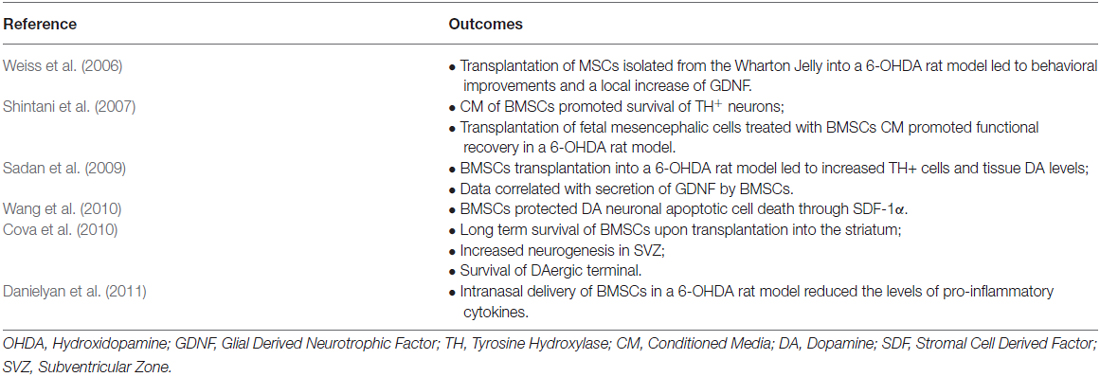
Table 2. Summary of the studies focused on the impact of MSCs on multiple aspects of PD regenerative medicine.
In addition to the capability of BMSCs to induce survival of DAergic neurons, its effects have also been related with their immunomodulatory properties. In this context, intranasally delivered rat BMSCs into 6-OHDA hemi-parkinsonian rats migrated toward the SN and the striatum and reduced the overall expression of pro-inflammatory cytokines, such as IL-1β, IL-2; IL-12; tumor necrosis factor alpha (TNF-α) and interferon γ (INF γ). Moreover, their presence also revert the loss of nigral DAergic neurons and striatal fibers (Danielyan et al., 2011).
From the above-referred studies, it is clear that there is increasing evidence indicating that the neuroprotective and neuroregenerative effects of MSCs observed in PD are attributed to the secretion of soluble growth factors and cytokines. The secretion of these factors by MSCs not only protects DAergic neurons from further degeneration and enhances endogenous restorative processes (e.g., neurogenesis), but also acts as inflammation and immune response modulators. Moreover, recent reports have shown that besides soluble growth factors and cytokines, MSCs also secrete microvesicles and exosomes containing mRNAs and/or miRNAs (microRNAs), which are believed to mediate cell-to-cell communication and act as reparative agents (Baglio et al., 2012). Indeed exosomes secreted by BMSCs in vitro not only mediate communication with neurons and astrocytes, but also regulate neurite outgrowth by transfer of miRNA (miR-133b) to neural cells (Xin et al., 2012).
Ischemic Stroke (IS)
Cerebrovascular diseases, such as stroke, result from blood vessel occlusion or damage, leading to focal tissue loss and death of endothelial cells and multiple neural populations (Lindvall and Björklund, 2004; Lindvall and Kokaia, 2010).
It has been proposed that the transplantation of MSCs (summarized in Table 3) can represent a feasible therapeutic option for IS (Locatelli et al., 2009). Indeed, studies have shown that after intravenous administration of BM-MSCs, these have the capacity to migrate to the lesion site promoting tissue regeneration and behavioral improvement (Komatsu et al., 2010). Moreover, these cells were able to promote neurogenesis, increase the survival of neuroblasts and to reduce the volume of lesion after IS (Keimpema et al., 2009; Zheng et al., 2010). According to Wakabayashi and colleagues the secretion of molecules such as IGF-1, VEGF, EGF, BNDF and bFGF mediate some of the observed effects, namely the reduction of lesion size and the modulation of the inflammatory environment for host cells (Wakabayashi et al., 2010). Leu et al. (2010) also proposed that like BM-MSCs, adipose stroma/stem cells (ASCs) therapy also enhances angiogenic and neurogenic processes. Although the exact mechanism of these cells remains still unclear, other studies have suggested that homing properties, cytokines (SDF-1α, IL-1, IL-8) effects, and paracrine mediators (HGF, BDNF, IGF-1, VEGF) could pinpoint ASCs effects, contributing to tissue regeneration and functional behavior (Tang et al., 2005; Banas et al., 2008; Chen et al., 2008a). On the other hand Koh et al. (2008) also demonstrated that MSCs exhibited a migratory tropism to the lesion site, which might foster the creation of new networks between the host neural and transplanted stem cells (Koh et al., 2008). Additionally exosomes secreted by MSCs were also shown to mediate important actions in these environments. Xin et al. (2013b) suggested that the observed improvements were due to the presence of miRNA-133b in the exosomal fraction of MSCs that were transplanted into a middle cerebral artery occlusion (MCAo) rat model. Similarly, the same authors also demonstrated that after systemic administration of MSCs-derived exosomes, there was an increase in neurovascular plasticity, which led to an enhancement of the functional recovery of an animal model of stroke (Xin et al., 2013a,b).
Glioblastoma Multiforme (GBM)
Malignant gliomas are particularly dramatic cancers of the CNS, ranking first among all human tumor types for tumor-related average years of life lost (Burnet et al., 2005). GBM is the most common and most malignant subtype (Ohgaki and Kleihues, 2007), typically treated with surgery, radiotherapy and temozolomide (TMZ)-based chemotherapy (Stupp et al., 2005). Despite this multimodal approach, virtually all GBMs eventually recur and are fatal. GBMs present critical hallmark features that largely contribute to treatment failure, including their high invasive capacity, the presence of the bood-brain barrier, and remarkable genetic and epigenetic heterogeneity. Additionally, GBMs present a small population of cells with neural stem cell-like properties (Singh et al., 2003), called glioma stem cells (GSC), which display remarkable features in the context of glioma pathophysiology, including self-renewal capacity (generating both GSCs and non-GSCs cancer cells necessary for tumor maintenance), multipotency (differentiating into diverse cell population lineages), and prominent tumorigenic potential in vivo. In resemblance with NSCs that are located in specific highly-vascularized neurogenic niches of the adult brain, GSCs also accumulate and depend on the prominent vasculature of these regions to control their stemness and differentiation processes (Folkins et al., 2007; Calabrese et al., 2007; Gilbertson and Rich, 2007; Hadjipanayis and van Meir, 2009). GSCs have been shown to be more resistant to radiation and conventional chemotherapeutic drugs, and are believed to be responsible for tumor relapse observed almost universally in GBM patients (Singh et al., 2003; Bao et al., 2006; Calabrese et al., 2007; Chalmers, 2007). Since the clinical prognosis of GBM patients has not improved significantly in the last years, it is urgent to develop novel unconventional therapeutic strategies.
Like in other cancer types, a relatively new and promising therapeutic approach to tackle malignant gliomas is based on the use of (normal) stem cells. The most unique and critical feature of stem cells that renders them as attractive tools for cancer therapy is their intrinsic capacity to migrate towards pathologic tissues, including malignant tumors. Indeed, this selective cancer-tropism has been shown for various stem cell types, including embryonic, hematopoietic, mesenchymal, neural, endothelial, and experimentally-induced stem cells (e.g., inducible pluripotent stem cells, iPSCs; Stuckey and Shah, 2014). Whether this innate tropism of normal stem cells is associated with cancer promotion or suppression functions is still controversial and a matter of debate, particularly in the case of MSCs, as reported by contradicting findings in many studies (Klopp et al., 2011). Nonetheless, it is widely consensual that the rational engineering of stem cells to express or deliver anticancer therapeutic agents, while taking advantage of their innate tumor tropism and immunosuppressive properties, may be a promising strategy to target cancer.
Aboody et al. (2000) first showed that NSCs are able to migrate towards the major tumor site and track along with invading glioma cells that form small satellite tumor masses (Aboody et al., 2000). Importantly, this tumor-tropism by NSCs was also later observed towards brain metastasis derived from breast cancer (Joo et al., 2009) and melanoma (Aboody et al., 2006), highlighting the potential application of NSCs as therapeutic vehicles for primary and metastatic brain tumors. In this context, and because stem cells are relatively easy to be genetically modified, many studies have explored them as cargo delivery vehicles for therapeutic agents, including cytokines, pro-drug converting enzymes, oncolytic viruses, nanoparticles, and antibodies, as summarized below.
Cytokines. Many recent studies have explored NSCs as efficient delivery systems of soluble tumor necrosis factor-related apoptosis-inducing ligand (sTRAIL), a cytokine that promotes apoptosis by binding to death receptors commonly present in the cellular membrane of tumor cells. These engineered NSCs can track tumor cells and deliver sTRAIL to glioma cells in vivo, resulting in significant anti-tumor effects. Combinations of sTRAIL-secreting NSCs with anticancer drugs, including bortezomib (a proteasome inhibitor), PI-103 (a dual PI3K/mTOR inhibitor), and lanatoside C (a cardiac glycoside), resulted in synergistic therapeutic effects, emphasizing the potential clinical value of sensitizing glioma cells to TRAIL-induced NSC-mediated cell death (Hingtgen et al., 2010; Bagci-Onder et al., 2011; Balyasnikova et al., 2011; Teng et al., 2014). Importantly, studies with MSCs engineered to deliver sTRAIL showed equally promising results, as these cells efficiently tracked and successfully induced a caspase-dependent cell death in glioma cells, resulting in increased survival of glioma mice models (Shah et al., 2004; Menon et al., 2009; Sasportas et al., 2009; Choi et al., 2011).
NSCs have also been genetically modified to express and secrete IL-12, a cytokine that does not act directly in tumor cells, but is involved in the enhancement of T-cell-mediated antitumor immune responses. Using intracranial glioma mice models, Ehtesham et al. showed that IL-12-secreting NSCs injected directly in the tumor significantly prolong the survival of mice (Ehtesham et al., 2002). Similarly, MSCs genetically engineered to express a modified IL-12 also prolonged the survival of glioma mice models when injected intratumorally (Ryu et al., 2011). Similar approaches were used to engineer NSCs, MSCs, and bone marrow-derived stem cells to produce pro-inflammatory cytokines, including IL-4, IL-7, IL-23, and IFN-β, which were shown to increase the infiltration of anti-tumor T-cells and natural killer (NK)-cells in glioma murine models (Benedetti et al., 2000; Nakamizo et al., 2005; Yuan et al., 2006; Gunnarsson et al., 2010). These studies provide important proof-of-concept on the potential of modulating immune mediators with different types of stem cells in order to achieve increased therapeutic responses.
Enzymes/pro-drugs. Another novel approach involves the modification of stem cells to express enzymes that convert inactive pro-drugs into toxic compounds, in order to increase tumor tissue selectivity. One of the most popular pro-drug/enzyme therapeutic systems is the herpes simplex virus type 1 thymidine kinase (HSV-tk) in combination with the pro-drug ganciclovir (GCV), based on the HSV-tk-mediated phosphorylation of inert GCV into a cytotoxic product that kills HSV-tk-positive cells and neighboring cells (via the so-called bystander effect). Taking advantage of the tumor-tropism of stem cells, many recent studies have explored the incorporation of HSV-tk into NSCs, MSCs, and bone marrow-derived progenitor cells as therapeutic strategies for glioma, showing promising results (Li et al., 2005; Uhl et al., 2005; Miletic et al., 2007; Uchibori et al., 2009; Matuskova et al., 2010). Other enzyme/pro-drug systems that have been explored as anti-cancer therapeutic tools for stem cells include the cytosine deaminase (CD), which converts inactive 5-fluorocytosine (5-FC) into the cytotoxic 5-fluorouracil (5-FU), and the rabbit carboxylesterase enzyme (rCE), which converts the pro-drug CTP-11 (irinotecan) into the anticancer topoisomerase I inhibitor SN-38 (7-ethyl-10-hydroxycamptothecin). These approaches have been tested with promising therapeutic results in stem cells of different origin (NSCs and MSCs) and distinct glioma models (including rat and mice models), either alone or in combination with other anticancer drugs (Aboody et al., 2000, 2006; Lim et al., 2011; Yin et al., 2011; Choi et al., 2012; Fei et al., 2012; Kim et al., 2012; Kosaka et al., 2012; Ryu et al., 2012; Zhao et al., 2012), emphasizing the potential of these enzyme/pro-drug systems as stem cell-mediated anti-tumor therapies.
Oncolytic viruses. The use of oncolytic viruses as therapeutic agents has been extensively studied for cancer, taking advantage of their capacity to infect, replicate within, and ultimately kill cancer cells. Despite many promising pre-clinical studies, including in gliomas (Wollmann et al., 2012), the clinical application of oncolytic viruses presents critical obstacles, including sub-optimal distribution throughout the major tumor cores and particularly to invading cancer cells, low infection rates, and host anti-viral immune responses (Yamamoto and Curiel, 2010). Critically, these shortcomings can be largely surpassed by the incorporation of oncolytic viruses within tumor-trophic stem cells. Indeed, recent work has been performed in NSCs, MSCs and ASCs that were used as oncolytic viral carriers to treat in vivo models of glioma, showing that these cells retain tumor-tropism, permit continued viral replication for several days, and cause glioma cell death in vivo more efficiently than viral delivery alone (Herrlinger et al., 2000; Sonabend et al., 2008; Tyler et al., 2009; Yong et al., 2009; Josiah et al., 2010; Ahmed et al., 2011; Thaci et al., 2012).
Nanoparticles and antibodies. In the last 4 years, some studies also started to explore MSCs as delivery vehicles of drug-loaded nanoparticles and antibodies to target glioma. This strategy aims to improve the capacity of these agents to cross the blood-brain barrier, while minimizing toxic side effects caused by intravenous administrations. The results obtained to date indicate that these cells can successfully deliver nanoparticles (e.g., lipid nanocapsules loaded with ferrociphenol and membrane-anchored silica nanorattle–doxorubicin) and antibodies (e.g., cell surface-bound single-chain anti-EGFRvIII) to glioma cells in vivo, resulting in increased anti-tumor responses (Balyasnikova et al., 2010; Roger et al., 2010, 2012; Li et al., 2011).
In conclusion, a wide variety of stem cells hold great promise as novel therapeutic tools for the treatment of therapy-insensitive malignant brain gliomas. Some hallmarks of these cells that are critical for this purpose include their high tumor-trophic migration and tracking capacity, peculiar immunosuppressive properties, and easy genetic manipulation for cargo delivery. Nonetheless, inherently to its innovative nature and similarly to other experimental glioma therapies attempted in the past, several issues will certainly need to be addressed in order to translate these promising pre-clinical findings into clinically-relevant therapies for patients. Some of the obstacles that may be envisaged include the proper selection of the best stem cell type/origin, choice of the most appropriate cargo for each tumor type or personalized to specific patients, optimization of administration routes and dosing, evaluation of the long-term cell fate of engrafted stem cells (which may conceptually also form tumors or differentiate aberrantly in the target tissue/organ), and development of real-time imaging systems for therapeutic stem cells in vivo. The recent literature on this topic is very promising, but a concerted and integrated effort in this field will still be crucial to definitely pave the way to better treat patients, most likely integrating the rational use of particular stem cell-based approaches to act synergistically in concert with surgery, radiation and chemotherapy.
Conclusion
It is now evident that cells derived from the osteogenic and neurogenic niches present important interactions that may impact the future development of CNS related therapies. As discussed in the present review there is robust evidence showing that MSCs and their secretome are able to modulate the action of neurogenic niches and neural progenitors. Their usage was shown to promote the functional recovery of animal models of PD and stroke, as well as the application of novel paradigms for glioblastoma therapies. Nevertheless, it is still a largely unexplored field, with many questions yet to be addressed. For instance, are the traditional growth factors the main mediators of the actions promoted by the MSCs secretome; or, instead, do MSCs-derived unknown neuroregulatory molecules modulate such actions? Can we modulate the tropism that these cells display towards gliobastomas? So far, most of the studies focused on the action of MSCs towards the neurogenic niches, namely NSCs. However, few address if and how the neurogenic niches, and within them NSCs, modulate the action of MSCs. In fact a bidirectional communication between both cell types is most likely to occur. The answer to this and other questions will be important to further define this field in the future, and its impact in future CNS regenerative strategies.
Conflict of Interest Statement
The authors declare that the research was conducted in the absence of any commercial or financial relationships that could be construed as a potential conflict of interest.
Acknowledgments
Portuguese Foundation for Science and Technology (FCT; IF Development Grant to AJS; IF Starting Grant to BMC); Bial Foundation (Grant 217/12 to JCS); co-funded by Programa Operacional Regional do Norte (ON.2 – O Novo Norte), ao abrigo do Quadro de Referência Estratégico Nacional (QREN), através do Fundo Europeu de Desenvolvimento Regional (FEDER).
References
Aboody, K. S., Brown, A., Rainov, N. G., Bower, K. A., Liu, S., Yang, W., et al. (2000). Neural stem cells display extensive tropism for pathology in adult brain: evidence from intracranial gliomas. Proc. Natl. Acad. Sci. U S A 97, 12846–12851. doi: 10.1073/pnas.97.23.12846
Aboody, K. S., Najbauer, J., Schmidt, N. O., Yang, W., Wu, J. K., Zhuge, Y., et al. (2006). Targeting of melanoma brain metastases using engineered neural stem/progenitor cells. Neuro Oncol. 8, 119–126. doi: 10.1215/15228517-2005-012
Abrous, D. N., Koehl, M., and Le Moal, M. (2005). Adult neurogenesis: from precursors to network and physiology. Physiol. Rev. 85, 523–569. doi: 10.1152/physrev.00055.2003
Ahmed, A. U., Thaci, B., Alexiades, N. G., Han, Y., Qian, S., Liu, F., et al. (2011). Neural stem cell-based cell carriers enhance therapeutic efficacy of an oncolytic adenovirus in an orthotopic mouse model of human glioblastoma. Mol. Ther. 19, 1714–1726. doi: 10.1038/mt.2011.100
Alvarez-Buylla, A., Kohwi, M., Nguyen, T. M., and Merkle, F. T. (2008). The heterogeneity of adult neural stem cells and the emerging complexity of their niche. Cold Spring Harb. Symp. Quant. Biol. 73, 357–365. doi: 10.1101/sqb.2008.73.019
Bagci-Onder, T., Wakimoto, H., Anderegg, M., Cameron, C., and Shah, K. (2011). A dual PI3K/mTOR inhibitor, PI-103, cooperates with stem cell-delivered TRAIL in experimental glioma models. Cancer Res. 71, 154–163. doi: 10.1158/0008-5472.CAN-10-1601
Baglio, S. R., Pegtel, D. M., and Baldini, N. (2012). Mesenchymal stem cell secreted vesicles provide novel opportunities in (stem) cell-free therapy. Front. Physiol. 3:359. doi: 10.3389/fphys.2012.00359
Balu, D. T., and Lucki, I. (2009). Adult hippocampal neurogenesis: regulation, functional implications and contribution to disease pathology. Neurosci. Biobehav. Rev. 33, 232–252. doi: 10.1016/j.neubiorev.2008.08.007
Balyasnikova, I. V., Ferguson, S. D., Han, Y., Liu, F., and Lesniak, M. S. (2011). Therapeutic effect of neural stem cells expressing TRAIL and bortezomib in mice with glioma xenografts. Cancer Lett. 310, 148–159. doi: 10.1016/j.canlet.2011.06.029
Balyasnikova, I. V., Ferguson, S. D., Sengupta, S., Han, Y., and Lesniak, M. S. (2010). Mesenchymal stem cells modified with a single-chain antibody against EGFRvIII successfully inhibit the growth of human xenograft malignant glioma. PLoS One 5:e9750. doi: 10.1371/journal.pone.0009750
Banas, A., Teratani, T., Yamamoto, Y., Tokuhara, M., Takeshita, F., Osaki, M., et al. (2008). IFATS collection: in vivo therapeutic potential of human adipose tissue mesenchymal stem cells after transplantation into mice with liver injury. Stem Cells 26, 2705–2712. doi: 10.1634/stemcells.2008-0034
Banasr, M., Hery, M., Printemps, R., and Daszuta, A. (2004). Serotonin-induced increases in adult cell proliferation and neurogenesis are mediated through different and common 5-HT receptor subtypes in the dentate gyrus and the subventricular zone. Neuropsychopharmacology 29, 450–460. doi: 10.1038/sj.npp.1300320
Bao, S., Wu, Q., Sathornsumetee, S., Hao, Y., Li, Z., Hjelmeland, A. B., et al. (2006). Stem cell-like glioma cells promote tumor angiogenesis through vascular endothelial growth factor. Cancer Res. 66, 7843–7848. doi: 10.1158/0008-5472.can-06-1010
Bédard, A., Gravel, C., and Parent, A. (2006). Chemical characterization of newly generated neurons in the striatum of adult primates. Exp. Brain Res. 170, 501–512. doi: 10.1007/s00221-005-0233-5
Benedetti, S., Pirola, B., Pollo, B., Magrassi, L., Bruzzone, M. G., Rigamonti, D., et al. (2000). Gene therapy of experimental brain tumors using neural progenitor cells. Nat. Med. 6, 447–450. doi: 10.1038/74710
Berg, D. A., Belnoue, L., Song, H., and Simon, A. (2013). Neurotransmitter-mediated control of neurogenesis in the adult vertebrate brain. Development 140, 2548–2561. doi: 10.1242/dev.088005
Bernier, P. J., Bedard, A., Vinet, J., Levesque, M., and Parent, A. (2002). Newly generated neurons in the amygdala and adjoining cortex of adult primates. Proc. Natl. Acad. Sci. U S A 99, 11464–11469. doi: 10.1073/pnas.172403999
Bessa, J. M., Ferreira, D., Melo, I., Marques, F., Cerqueira, J. J., Palha, J. A., et al. (2009). The mood-improving actions of antidepressants do not depend on neurogenesis but are associated with neuronal remodeling. Mol. Psychiatry 14, 764–773, 739. doi: 10.1038/mp.2008.119
Biebl, M., Cooper, C. M., Winkler, J., and Kuhn, H. G. (2000). Analysis of neurogenesis and programmed cell death reveals a self-renewing capacity in the adult rat brain. Neurosci. Lett. 291, 17–20. doi: 10.1016/s0304-3940(00)01368-9
Blandini, F., Cova, L., Armentero, M. T., Zennaro, E., Levandis, G., Bossolasco, P., et al. (2010). Transplantation of undifferentiated human mesenchymal stem cells protects against 6-hydroxydopamine neurotoxicity in the rat. Cell Transplant. 19, 203–217. doi: 10.3727/096368909x479839
Bluguermann, C., Wu, L., Petrigliano, F., Mcallister, D., Miriuka, S., and Evseenko, D. A. (2013). Novel aspects of parenchymal-mesenchymal interactions: from cell types to molecules and beyond. Cell Biochem. Funct. 31, 271–280. doi: 10.1002/cbf.2950
Bonfield, T. L., Nolan Koloze, M. T., Lennon, D. P., and Caplan, A. I. (2010). Defining human mesenchymal stem cell efficacy in vivo. J. Inflamm. (Lond) 7:51. doi: 10.1186/1476-9255-7-51
Brill, M. S., Ninkovic, J., Winpenny, E., Hodge, R. D., Ozen, I., Yang, R., et al. (2009). Adult generation of glutamatergic olfactory bulb interneurons. Nat. Neurosci. 12, 1524–1533. doi: 10.1038/nn.2416
Buffo, A., Vosko, M. R., Erturk, D., Hamann, G. F., Jucker, M., Rowitch, D., et al. (2005). Expression pattern of the transcription factor Olig2 in response to brain injuries: implications for neuronal repair. Proc. Natl. Acad. Sci. U S A 102, 18183–18188. doi: 10.1073/pnas.0506535102
Burnet, N. G., Jefferies, S. J., Benson, R. J., Hunt, D. P., and Treasure, F. P. (2005). Years of life lost (YLL) from cancer is an important measure of population burden—and should be considered when allocating research funds. Br. J. Cancer 92, 241–245. doi: 10.1038/sj.bjc.6602321
Calabrese, C., Poppleton, H., Kocak, M., Hogg, T. L., Fuller, C., Hamner, B., et al. (2007). A perivascular niche for brain tumor stem cells. Cancer Cell 11, 69–82. doi: 10.1016/j.ccr.2006.11.020
Caplan, A. I. (1991). Mesenchymal stem cells. J. Orthop. Res. 9, 641–650. doi: 10.1002/jor.1100090504
Caplan, A. I., and Dennis, J. E. (2006). Mesenchymal stem cells as trophic mediators. J. Cell. Biochem. 98, 1076–1084. doi: 10.1002/jcb.20886
Carlén, M., Cassidy, R. M., Brismar, H., Smith, G. A., Enquist, L. W., and Frisen, J. (2002). Functional integration of adult-born neurons. Curr. Biol. 12, 606–608. doi: 10.1016/s0960-9822(02)00771-6
Chalmers, A. J. (2007). Radioresistant glioma stem cells—therapeutic obstacle or promising target? DNA Repair (Amst) 6, 1391–1394. doi: 10.1016/j.dnarep.2007.03.019
Chen, J. R., Cheng, G. Y., Sheu, C. C., Tseng, G. F., Wang, T. J., and Huang, Y. S. (2008a). Transplanted bone marrow stromal cells migrate, differentiate and improve motor function in rats with experimentally induced cerebral stroke. J. Anat. 213, 249–258. doi: 10.1111/j.1469-7580.2008.00948.x
Chen, L., Tredget, E. E., Wu, P. Y., and Wu, Y. (2008b). Paracrine factors of mesenchymal stem cells recruit macrophages and endothelial lineage cells and enhance wound healing. PLoS One 3:e1886. doi: 10.1371/journal.pone.0001886
Choi, S. A., Hwang, S. K., Wang, K. C., Cho, B. K., Phi, J. H., Lee, J. Y., et al. (2011). Therapeutic efficacy and safety of TRAIL- producing human adipose tissue-derived mesenchymal stem cells against experimental brainstem glioma. Neuro Oncol. 13, 61–69. doi: 10.1093/neuonc/noq147
Choi, S. A., Lee, J. Y., Wang, K. C., Phi, J. H., Song, S. H., Song, J., et al. (2012). Human adipose tissue-derived mesenchymal stem cells: characteristics and therapeutic potential as cellular vehicles for prodrug gene therapy against brainstem gliomas. Eur. J. Cancer 48, 129–137. doi: 10.1016/j.ejca.2011.04.033
Chojnacki, A. K., Mak, G. K., and Weiss, S. (2009). Identity crisis for adult periventricular neural stem cells: subventricular zone astrocytes, ependymal cells or both? Nat. Rev. Neurosci. 10, 153–163. doi: 10.1038/nrn2616
Chumley, M. J., Catchpole, T., Silvany, R. E., Kernie, S. G., and Henkemeyer, M. (2007). EphB receptors regulate stem/progenitor cell proliferation, migration and polarity during hippocampal neurogenesis. J. Neurosci. 27, 13481–13490. doi: 10.1523/jneurosci.4158-07.2007
Ciccolini, F., Mandl, C., Hölzl-Wenig, G., Kehlenbach, A., and Hellwig, A. (2005). Prospective isolation of late development multipotent precursors whose migration is promoted by EGFR. Dev. Biol. 284, 112–125. doi: 10.1016/j.ydbio.2005.05.007
Coskun, V., Wu, H., Blanchi, B., Tsao, S., Kim, K., Zhao, J., et al. (2008). CD133+ neural stem cells in the ependyma of mammalian postnatal forebrain. Proc. Natl. Acad. Sci. U S A 105, 1026–1031. doi: 10.1073/pnas.0710000105
Cova, L., Armentero, M. T., Zennaro, E., Calzarossa, C., Bossolasco, P., Busca, G., et al. (2010). Multiple neurogenic and neurorescue effects of human mesenchymal stem cell after transplantation in an experimental model of Parkinson’s disease. Brain Res. 1311, 12–27. doi: 10.1016/j.brainres.2009.11.041
Culver, J. C., Vadakkan, T. J., and Dickinson, M. E. (2013). A specialized microvascular domain in the mouse neural stem cell niche. PLoS One 8:e53546. doi: 10.1371/journal.pone.0053546
Cummins, G., and Barker, R. A. (2012). What is the most promising treatment for Parkinson’s disease: genes, cells, growth factors or none of the above? Regen. Med. 7, 617–621. doi: 10.2217/rme.12.47
Danielyan, L., Schäfer, R., von Ameln-Mayerhofer, A., Bernhard, F., Verleysdonk, S., Buadze, M., et al. (2011). Therapeutic efficacy of intranasally delivered mesenchymal stem cells in a rat model of Parkinson disease. Rejuvenation Res. 14, 3–16. doi: 10.1089/rej.2010.1130
Dauer, W., and Przedborski, S. (2003). Parkinson’s disease: mechanisms and models. Neuron 39, 889–909. doi: 10.1016/S0896-6273(03)00568-3
Dayer, A. G., Cleaver, K. M., Abouantoun, T., and Cameron, H. A. (2005). New GABAergic interneurons in the adult neocortex and striatum are generated from different precursors. J. Cell Biol. 168, 415–427. doi: 10.1083/jcb.200407053
Delgado, A. C., Ferrón, S. R., Vicente, D., Porlan, E., Perez-Villalba, A., Trujillo, C. M., et al. (2014). Endothelial NT-3 delivered by Vasculature and CSF promotes Quiescence of Subependymal neural stem cells through Nitric Oxide induction. Neuron 83, 572–585. doi: 10.1016/j.neuron.2014.06.015
Doetsch, F., Caillé, I., Lim, D. A., García-Verdugo, J. M., and Alvarez-Buylla, A. (1999). Subventricular zone astrocytes are neural stem cells in the adult mammalian brain. Cell 97, 703–716. doi: 10.1016/s0092-8674(00)80783-7
Doetsch, F., García-Verdugo, J. M., and Alvarez-Buylla, A. (1997). Cellular composition and three-dimensional organization of the subventricular germinal zone in the adult mammalian brain. J. Neurosci. 17, 5046–5061.
Dominici, M., Le Blanc, K., Mueller, I., Slaper-Cortenbach, I., Marini, F., Krause, D., et al. (2006). Minimal criteria for defining multipotent mesenchymal stromal cells. The international society for cellular therapy position statement. Cytotherapy 8, 315–317. doi: 10.1080/14653240600855905
Eaves, C. J., Cashman, J. D., Sutherland, H. J., Otsuka, T., Humphries, R. K., Hogge, D. E., et al. (1991). Molecular analysis of primitive hematopoietic cell proliferation control mechanisms. Ann. N Y Acad. Sci. 628, 298–306. doi: 10.1111/j.1749-6632.1991.tb17260.x
Ehninger, D., and Kempermann, G. (2003). Regional effects of wheel running and environmental enrichment on cell genesis and microglia proliferation in the adult murine neocortex. Cereb. Cortex 13, 845–851. doi: 10.1093/cercor/13.8.845
Ehtesham, M., Kabos, P., Kabosova, A., Neuman, T., Black, K. L., and Yu, J. S. (2002). The use of interleukin 12-secreting neural stem cells for the treatment of intracranial glioma. Cancer Res. 62, 5657–5663.
Eisch, A. J., Cameron, H. A., Encinas, J. M., Meltzer, L. A., Ming, G. L., and Overstreet-Wadiche, L. S. (2008). Adult neurogenesis, mental health and mental illness: hope or hype? J. Neurosci. 28, 11785–11791. doi: 10.1523/JNEUROSCI.3798-08.2008
Eisch, A. J., and Petrik, D. (2012). Depression and hippocampal neurogenesis: a road to remission? Science 338, 72–75. doi: 10.1126/science.1222941
Ekdahl, C. T., Kokaia, Z., and Lindvall, O. (2009). Brain inflammation and adult neurogenesis: the dual role of microglia. Neuroscience 158, 1021–1029. doi: 10.1016/j.neuroscience.2008.06.052
Encinas, J. M., Vaahtokari, A., and Enikolopov, G. (2006). Fluoxetine targets early progenitor cells in the adult brain. Proc. Natl. Acad. Sci. U S A 103, 8233–8238. doi: 10.1073/pnas.0601992103
Falcão, A. M., Marques, F., Novais, A., Sousa, N., Palha, J. A., and Sousa, J. C. (2012a). The path from the choroid plexus to the subventricular zone: go with the flow! Front. Cell. Neurosci. 6:34. doi: 10.3389/fncel.2012.00034
Falcão, A. M., Palha, J. A., Ferreira, A. C., Marques, F., Sousa, N., and Sousa, J. C. (2012b). Topographical analysis of the subependymal zone neurogenic niche. PLoS One 7:e38647. doi: 10.1371/journal.pone.0038647
Fei, S., Qi, X., Kedong, S., Guangchun, J., Jian, L., and Wei, Q. (2012). The antitumor effect of mesenchymal stem cells transduced with a lentiviral vector expressing cytosine deaminase in a rat glioma model. J. Cancer Res. Clin. Oncol. 138, 347–357. doi: 10.1007/s00432-011-1104-z
Folkins, C., Man, S., Xu, P., Shaked, Y., Hicklin, D. J., and Kerbel, R. S. (2007). Anticancer therapies combining antiangiogenic and tumor cell cytotoxic effects reduce the tumor stem-like cell fraction in glioma xenograft tumors. Cancer Res. 67, 3560–3564. doi: 10.1158/0008-5472.can-06-4238
Fowler, C. D., Johnson, F., and Wang, Z. (2005). Estrogen regulation of cell proliferation and distribution of estrogen receptor-alpha in the brains of adult female prairie and meadow voles. J. Comp. Neurol. 489, 166–179. doi: 10.1002/cne.20638
Fowler, C. D., Liu, Y., Ouimet, C., and Wang, Z. (2002). The effects of social environment on adult neurogenesis in the female prairie vole. J. Neurobiol. 51, 115–128. doi: 10.1002/neu.10042
Fraga, J. S., Silva, N. A., Lourenço, A. S., Gonçalves, V., Neves, N. M., Reis, R. L., et al. (2013). Unveiling the effects of the secretome of mesenchymal progenitors from the umbilical cord in different neuronal cell populations. Biochimie 95, 2297–2303. doi: 10.1016/j.biochi.2013.06.028
Friedenstein, A. J., Chailakhyan, R. K., Latsinik, N. V., Panasyuk, A. F., and Keiliss-Borok, I. V. (1974a). Stromal cells responsible for transferring the microenvironment of the hemopoietic tissues. Cloning in vitro and retransplantation in vivo. Transplantation 17, 331–340. doi: 10.1097/00007890-197404000-00001
Friedenstein, A. J., Deriglasova, U. F., Kulagina, N. N., Panasuk, A. F., Rudakowa, S. F., Luriá, E. A., et al. (1974b). Precursors for fibroblasts in different populations of hematopoietic cells as detected by the in vitro colony assay method. Exp. Hematol. 2, 83–92.
Ge, S., Yang, C. H., Hsu, K. S., Ming, G. L., and Song, H. (2007). A critical period for enhanced synaptic plasticity in newly generated neurons of the adult brain. Neuron 54, 559–566. doi: 10.1016/j.neuron.2007.05.002
Gebara, E., Sultan, S., Kocher-Braissant, J., and Toni, N. (2013). Adult hippocampal neurogenesis inversely correlates with microglia in conditions of voluntary running and aging. Front. Neurosci. 7:145. doi: 10.3389/fnins.2013.00145
Gilbertson, R. J., and Rich, J. N. (2007). Making a tumour’s bed: glioblastoma stem cells and the vascular niche. Nat. Rev. Cancer 7, 733–736. doi: 10.1038/nrc2246
Glavaski-Joksimovic, A., and Bohn, M. C. (2013). Mesenchymal stem cells and neuroregeneration in Parkinson’s disease. Exp. Neurol. 247, 25–38. doi: 10.1016/j.expneurol.2013.03.016
Gnecchi, M., He, H., Liang, O. D., Melo, L. G., Morello, F., Mu, H., et al. (2005). Paracrine action accounts for marked protection of ischemic heart by Akt-modified mesenchymal stem cells. Nat. Med. 11, 367–368. doi: 10.1038/nm0405-367
Goldberg, J. S., and Hirschi, K. K. (2009). Diverse roles of the vasculature within the neural stem cell niche. Regen. Med. 4, 879–897. doi: 10.2217/rme.09.61
Gómez-Gaviro, M. V., Scott, C. E., Sesay, A. K., Matheu, A., Booth, S., Galichet, C., et al. (2012). Betacellulin promotes cell proliferation in the neural stem cell niche and stimulates neurogenesis. Proc. Natl. Acad. Sci. U S A 109, 1317–1322. doi: 10.1073/pnas.1016199109
Gonçalves, L., Silva, R., Pinto-Ribeiro, F., Pêgo, J. M., Bessa, J. M., Pertovaara, A., et al. (2008). Neuropathic pain is associated with depressive behaviour and induces neuroplasticity in the amygdala of the rat. Exp. Neurol. 213, 48–56. doi: 10.1016/j.expneurol.2008.04.043
Gould, E., Reeves, A. J., Graziano, M. S., and Gross, C. G. (1999). Neurogenesis in the neocortex of adult primates. Science 286, 548–552. doi: 10.1126/science.286.5439.548
Guan, J. S., Haggarty, S. J., Giacometti, E., Dannenberg, J. H., Joseph, N., Gao, J., et al. (2009). HDAC2 negatively regulates memory formation and synaptic plasticity. Nature 459, 55–60. doi: 10.1038/nature07925
Gunnarsson, S., Bexell, D., Svensson, A., Siesjö, P., Darabi, A., and Bengzon, J. (2010). Intratumoral IL-7 delivery by mesenchymal stromal cells potentiates IFN gamma-transduced tumor cell immunotherapy of experimental glioma. J. Neuroimmunol. 218, 140–144. doi: 10.1016/j.jneuroim.2009.10.017
Hack, M. A., Saghatelyan, A., de Chevigny, A., Pfeifer, A., Ashery-Padan, R., Lledo, P. M., et al. (2005). Neuronal fate determinants of adult olfactory bulb neurogenesis. Nat. Neurosci. 8, 865–872. doi: 10.1038/nn1479
Hadjipanayis, C. G., and van Meir, E. G. (2009). Tumor initiating cells in malignant gliomas: biology and implications for therapy. J. Mol. Med. (Berl) 87, 363–374. doi: 10.1007/s00109-009-0440-9
Hanson, N. D., Owens, M. J., and Nemeroff, C. B. (2011). Depression, antidepressants and neurogenesis: a critical reappraisal. Neuropsychopharmacology 36, 2589–2602. doi: 10.1038/npp.2011.220
Heins, N., Malatesta, P., Cecconi, F., Nakafuku, M., Tucker, K. L., Hack, M. A., et al. (2002). Glial cells generate neurons: the role of the transcription factor Pax6. Nat. Neurosci. 5, 308–315. doi: 10.1038/nn828
Herrlinger, U., Woiciechowski, C., Sena-Esteves, M., Aboody, K. S., Jacobs, A. H., Rainov, N. G., et al. (2000). Neural precursor cells for delivery of replication-conditional HSV-1 vectors to intracerebral gliomas. Mol. Ther. 1, 347–357. doi: 10.1006/mthe.2000.0046
Hingtgen, S. D., Kasmieh, R., van de Water, J., Weissleder, R., and Shah, K. (2010). A novel molecule integrating therapeutic and diagnostic activities reveals multiple aspects of stem cell-based therapy. Stem Cells 28, 832–841. doi: 10.1002/stem.313
Hodge, R. D., Kahoud, R. J., and Hevner, R. F. (2012). Transcriptional control of glutamatergic differentiation during adult neurogenesis. Cell. Mol. Life Sci. 69, 2125–2134. doi: 10.1007/s00018-011-0916-y
Jablonska, B., Aguirre, A., Raymond, M., Szabo, G., Kitabatake, Y., Sailor, K. A., et al. (2010). Chordin-induced lineage plasticity of adult SVZ neuroblasts after demyelination. Nat. Neurosci. 13, 541–550. doi: 10.1038/nn.2536
Jessberger, S., Gage, F. H., Eisch, A. J., and Lagace, D. C. (2009). Making a neuron: Cdk5 in embryonic and adult neurogenesis. Trends Neurosci. 32, 575–582. doi: 10.1016/j.tins.2009.07.002
Joo, K. M., Park, I. H., Shin, J. Y., Jin, J., Kang, B. G., Kim, M. H., et al. (2009). Human neural stem cells can target and deliver therapeutic genes to breast cancer brain metastases. Mol. Ther. 17, 570–575. doi: 10.1038/mt.2008.290
Josiah, D. T., Zhu, D., Dreher, F., Olson, J., McFadden, G., and Caldas, H. (2010). Adipose-derived stem cells as therapeutic delivery vehicles of an oncolytic virus for glioblastoma. Mol. Ther. 18, 377–385. doi: 10.1038/mt.2009.265
Keimpema, E., Fokkens, M. R., Nagy, Z., Agoston, V., Luiten, P. G., Nyakas, C., et al. (2009). Early transient presence of implanted bone marrow stem cells reduces lesion size after cerebral ischaemia in adult rats. Neuropathol. Appl. Neurobiol. 35, 89–102. doi: 10.1111/j.1365-2990.2008.00961.x
Kempermann, G., Gast, D., Kronenberg, G., Yamaguchi, M., and Gage, F. H. (2003). Early determination and long-term persistence of adult-generated new neurons in the hippocampus of mice. Development 130, 391–399. doi: 10.1242/dev.00203
Kempermann, G., Jessberger, S., Steiner, B., and Kronenberg, G. (2004). Milestones of neuronal development in the adult hippocampus. Trends Neurosci. 27, 447–452. doi: 10.1016/j.tins.2004.05.013
Kim, J. H., Kim, J. Y., Kim, S. U., and Cho, K. G. (2012). Therapeutic effect of genetically modified human neural stem cells encoding cytosine deaminase on experimental glioma. Biochem. Biophys. Res. Commun. 417, 534–540. doi: 10.1016/j.bbrc.2011.11.155
Kim, Y. J., Park, H. J., Lee, G., Bang, O. Y., Ahn, Y. H., Joe, E., et al. (2009). Neuroprotective effects of human mesenchymal stem cells on dopaminergic neurons through anti-inflammatory action. Glia 57, 13–23. doi: 10.1002/glia.20731
Kishk, N., and Abokrysha, N. (2011). “Stem cell in neurological disorders,” in Stem Cells in Clinic and Research, ed. A. Gholamrezanezhad (In Tech),
Klopp, A. H., Gupta, A., Spaeth, E., Andreeff, M., and Marini, F. (2011). Concise review: dissecting a discrepancy in the literature: do mesenchymal stem cells support or suppress tumor growth? Stem Cells 29, 11–19. doi: 10.1002/stem.559
Knothe, T. M. L., Adamson, J. R., Tami, A. E., and Bauer, T. W. (2004). The osteocyte. Int. J. Biochem. Cell Biol. 36, 1–8. doi: 10.1016/S1357-2725(03)00241-3
Kobayashi, K. (2009). Targeting the hippocampal mossy fiber synapse for the treatment of psychiatric disorders. Mol. Neurobiol. 39, 24–36. doi: 10.1007/s12035-008-8049-5
Kodama, M., Fujioka, T., and Duman, R. S. (2004). Chronic olanzapine or fluoxetine administration increases cell proliferation in hippocampus and prefrontal cortex of adult rat. Biol. Psychiatry 56, 570–580. doi: 10.1016/j.biopsych.2004.07.008
Koh, S. H., Kim, K. S., Choi, M. R., Jung, K. H., Park, K. S., Chai, Y. G., et al. (2008). Implantation of human umbilical cord-derived mesenchymal stem cells as a neuroprotective therapy for ischemic stroke in rats. Brain Res. 1229, 233–248. doi: 10.1016/j.brainres.2008.06.087
Kokoeva, M. V., Yin, H., and Flier, J. S. (2005). Neurogenesis in the hypothalamus of adult mice: potential role in energy balance. Science 310, 679–683. doi: 10.1126/science.1115360
Komatsu, K., Honmou, O., Suzuki, J., Houkin, K., Hamada, H., and Kocsis, J. D. (2010). Therapeutic time window of mesenchymal stem cells derived from bone marrow after cerebral ischemia. Brain Res. 1334, 84–92. doi: 10.1016/j.brainres.2010.04.006
Konefal, S., Elliot, M., and Crespi, B. (2013). The adaptive significance of adult neurogenesis: an integrative approach. Front. Neuroanat. 7:21. doi: 10.3389/fnana.2013.00021
Kosaka, H., Ichikawa, T., Kurozumi, K., Kambara, H., Inoue, S., Maruo, T., et al. (2012). Therapeutic effect of suicide gene-transferred mesenchymal stem cells in a rat model of glioma. Cancer Gene Ther. 19, 572–578. doi: 10.1038/cgt.2012.35
Kriegstein, A., and Alvarez-Buylla, A. (2009). The glial nature of embryonic and adult neural stem cells. Annu. Rev. Neurosci. 32, 149–184. doi: 10.1146/annurev.neuro.051508.135600
Lemaire, V., Tronel, S., Montaron, M. F., Fabre, A., Dugast, E., and Abrous, D. N. (2012). Long-lasting plasticity of hippocampal adult-born neurons. J. Neurosci. 32, 3101–3108. doi: 10.1523/jneurosci.4731-11.2012
Leu, S., Lin, Y. C., Yuen, C. M., Yen, C. H., Kao, Y. H., Sun, C. K., et al. (2010). Adipose-derived mesenchymal stem cells markedly attenuate brain infarct size and improve neurological function in rats. J. Transl. Med. 8:63. doi: 10.1186/1479-5876-8-63
Li, L., Guan, Y., Liu, H., Hao, N., Liu, T., Meng, X., et al. (2011). Silica nanorattle-doxorubicin-anchored mesenchymal stem cells for tumor-tropic therapy. ACS Nano 5, 7462–7470. doi: 10.1021/nn202399w
Li, S., Tokuyama, T., Yamamoto, J., Koide, M., Yokota, N., and Namba, H. (2005). Bystander effect-mediated gene therapy of gliomas using genetically engineered neural stem cells. Cancer Gene Ther. 12, 600–607. doi: 10.1038/sj.cgt.7700826
Li, J. F., Zhang, D. J., Geng, T., Chen, L., Huang, H., Yin, H. L., et al. (2014). The potential of human umbilical cord-derived mesenchymal stem cells as a novel cellular therapy for multiple sclerosis. Cell Transplant. 23, S113–S122. doi: 10.3727/096368914x685005
Lim, S. H., Choi, S. A., Lee, J. Y., Wang, K. C., Phi, J. H., Lee, D. H., et al. (2011). Therapeutic targeting of subdural medulloblastomas using human neural stem cells expressing carboxylesterase. Cancer Gene Ther. 18, 817–824. doi: 10.1038/cgt.2011.52
Lindvall, O., and Björklund, A. (2004). Cell replacement therapy: helping the brain to repair itself. NeuroRx 1, 379–381. doi: 10.1602/neurorx.1.4.379
Lindvall, O., and Kokaia, Z. (2010). Stem cells in human neurodegenerative disorders–time for clinical translation? J. Clin. Invest. 120, 29–40. doi: 10.1172/jci40543
Lledo, P.-M., Merkle, F. T., and Álvarez-Buylla, A. (2008). Origin and function of olfactory bulb interneuron diversity. Trends Neurosci. 31, 392–400. doi: 10.1016/j.tins.2008.05.006
Llufriu, S., Sepúlveda, M., Blanco, Y., Marín, P., Moreno, B., Berenguer, J., et al. (2014). Randomized placebo-controlled phase II trial of autologous mesenchymal stem cells in multiple sclerosis. PLoS One 9:e113936. doi: 10.1371/journal.pone.0113936
Locatelli, F., Bersano, A., Ballabio, E., Lanfranconi, S., Papadimitriou, D., Strazzer, S., et al. (2009). Stem cell therapy in stroke. Cell. Mol. Life Sci. 66, 757–772. doi: 10.1007/s00018-008-8346-1
Lu, Z., Elliott, M. R., Chen, Y., Walsh, J. T., Klibanov, A. L., Ravichandran, K. S., et al. (2011). Phagocytic activity of neuronal progenitors regulates adult neurogenesis. Nat. Cell Biol. 13, 1076–1083. doi: 10.1038/ncb2299
Lugert, S., Basak, O., Knuckles, P., Haussler, U., Fabel, K., Gotz, M., et al. (2010). Quiescent and active hippocampal neural stem cells with distinct morphologies respond selectively to physiological and pathological stimuli and aging. Cell Stem Cell 6, 445–456. doi: 10.1016/j.stem.2010.03.017
Mateus-Pinheiro, A., Patrício, P., Bessa, J. M., Sousa, N., and Pinto, L. (2013a). Cell genesis and dendritic plasticity: a neuroplastic pas de deux in the onset and remission from depression. Mol. Psychiatry 18, 748–750. doi: 10.1038/mp.2013.56
Mateus-Pinheiro, A., Pinto, L., Bessa, J. M., Morais, M., Alves, N. D., Monteiro, S., et al. (2013b). Sustained remission from depressive-like behavior depends on hippocampal neurogenesis. Transl. Psychiatry 3:e210. doi: 10.1038/tp.2012.141
Matuskova, M., Hlubinova, K., Pastorakova, A., Hunakova, L., Altanerova, V., Altaner, C., et al. (2010). HSV-tk expressing mesenchymal stem cells exert bystander effect on human glioblastoma cells. Cancer Lett. 290, 58–67. doi: 10.1016/j.canlet.2009.08.028
McCoy, M. K., Martinez, T. N., Ruhn, K. A., Wrage, P. C., Keefer, E. W., Botterman, B. R., et al. (2008). Autologous transplants of Adipose-Derived Adult Stromal (ADAS) cells afford dopaminergic neuroprotection in a model of Parkinson’s disease. Exp. Neurol. 210, 14–29. doi: 10.1016/j.expneurol.2007.10.011
Menon, L. G., Kelly, K., Yang, H. W., Kim, S. K., Black, P. M., and Carroll, R. S. (2009). Human bone marrow-derived mesenchymal stromal cells expressing S-TRAIL as a cellular delivery vehicle for human glioma therapy. Stem Cells 27, 2320–2330. doi: 10.1002/stem.136
Merkle, F. T., Mirzadeh, Z., and Álvarez-Buylla, A. (2007). Mosaic organization of neural stem cells in the adult brain. Science 317, 381–384. doi: 10.1126/science.1144914
Meyerrose, T., Olson, S., Pontow, S., Kalomoiris, S., Jung, Y., Annett, G., et al. (2010). Mesenchymal stem cells for the sustained in vivo delivery of bioactive factors. Adv. Drug Deliv. Rev. 62, 1167–1174. doi: 10.1016/j.addr.2010.09.013
Miletic, H., Fischer, Y., Litwak, S., Giroglou, T., Waerzeggers, Y., Winkeler, A., et al. (2007). Bystander killing of malignant glioma by bone marrow-derived tumor- infiltrating progenitor cells expressing a suicide gene. Mol. Ther. 15, 1373–1381. doi: 10.1038/sj.mt.6300155
Ming, G. L., and Song, H. (2005). Adult neurogenesis in the mammalian central nervous system. Annu. Rev. Neurosci. 28, 223–250. doi: 10.1146/annurev.neuro.28.051804.101459
Ming, G. L., and Song, H. (2011). Adult neurogenesis in the mammalian brain: significant answers and significant questions. Neuron 70, 687–702. doi: 10.1016/j.neuron.2011.05.001
Mirzadeh, Z., Merkle, F. T., Soriano-Navarro, M., Garcia-Verdugo, J. M., and Álvarez-Buylla, A. (2008). Neural stem cells confer unique pinwheel architecture to the ventricular surface in neurogenic regions of the adult brain. Cell Stem Cell 3, 265–278. doi: 10.1016/j.stem.2008.07.004
Munoz, J. R., Stoutenger, B. R., Robinson, A. P., Spees, J. L., and Prockop, D. J. (2006). Human stem/progenitor cells from bone marrow promote neurogenesis of endogenous neural stem cells in the hippocampus of mice. Proc. Natl. Acad. Sci. U S A 102, 18171–18176. doi: 10.1073/pnas.0508945102
Nait-Oumesmar, B., Decker, L., Lachapelle, F., Avellana-Adalid, V., Bachelin, C., and Baron-Van Evercooren, A. (1999). Progenitor cells of the adult mouse subventricular zone proliferate, migrate and differentiate into oligodendrocytes after demyelination. Eur. J. Neurosci. 11, 4357–4366. doi: 10.1046/j.1460-9568.1999.00873.x
Nakamizo, A., Marini, F., Amano, T., Khan, A., Studeny, M., Gumin, J., et al. (2005). Human bone marrow-derived mesenchymal stem cells in the treatment of gliomas. Cancer Res. 65, 3307–3318. doi: 10.1158/0008-5472.CAN-04-1874
Nakano, N., Nakai, Y., Seo, T. B., Yamada, Y., Ohno, T., Yamanaka, A., et al. (2010). Characterization of conditioned medium of cultured bone marrow stromal cells. Neurosci. Lett. 483, 57–61. doi: 10.1016/j.neulet.2010.07.062
Nakashiba, T., Cushman, J. D., Pelkey, K. A., Renaudineau, S., Buhl, D. L., McHugh, T. J., et al. (2012). Young dentate granule cells mediate pattern separation, whereas old granule cells facilitate pattern completion. Cell 149, 188–201. doi: 10.1016/j.cell.2012.01.046
Ohgaki, H., and Kleihues, P. (2007). Genetic pathways to primary and secondary glioblastoma. Am. J. Pathol. 170, 1445–1453. doi: 10.2353/ajpath.2007.070011
Palmer, T. D., Willhoite, A. R., and Gage, F. H. (2000). Vascular niche for adult hippocampal neurogenesis. J. Comp. Neurol. 425, 479–494. doi: 10.1002/1096-9861(20001002)425:4<479::aid-cne2>3.0.co;2-3
Park, H. J., Shin, J. Y., Lee, B. R., Kim, H. O., and Lee, P. H. (2012). Mesenchymal stem cells augment neurogenesis in the subventricular zone and enhance differentiation of neural precursor cells into dopaminergic neurons in the substantia nigra of a parkinsonian model. Cell Transplant. 21, 1629–1640. doi: 10.3727/096368912x640556
Paul, G., Özen, I., Christophersen, N. S., Reinbothe, T., Bengzon, J., Visse, E., et al. (2012). The adult human brain harbors multipotent perivascular mesenchymal stem cells. PLoS One 7:e35577. doi: 10.1371/journal.pone.0035577
Pereira, E. A., and Aziz, T. Z. (2006). Surgical insights into Parkinson’s disease. J. R. Soc. Med. 99, 238–244. doi: 10.1258/jrsm.99.5.238
Picard-Riera, N., Decker, L., Delarasse, C., Goude, K., Nait-Oumesmar, B., Liblau, R., et al. (2002). Experimental autoimmune encephalomyelitis mobilizes neural progenitors from the subventricular zone to undergo oligodendrogenesis in adult mice. Proc. Natl. Acad. Sci. U S A 99, 13211–13216. doi: 10.1073/pnas.192314199
Pinto, L., Drechsel, D., Schmid, M. T., Ninkovic, J., Irmler, M., Brill, M. S., et al. (2009). AP2gamma regulates basal progenitor fate in a region- and layer-specific manner in the developing cortex. Nat. Neurosci. 12, 1229–1237. doi: 10.1038/nn.2399
Pleasure, S. J., Collins, A. E., and Lowenstein, D. H. (2000). Unique expression patterns of cell fate molecules delineate sequential stages of dentate gyrus development. J. Neurosci. 20, 6095–6105.
Ramírez-Castillejo, C., Sánchez-Sánchez, F., Andreu-Agulló, C., Ferrón, S. R., Aroca-Aguilar, J. D., Sánchez, P., et al. (2006). Pigment epithelium-derived factor is a niche signal for neural stem cell renewal. Nat. Neurosci. 9, 331–339. doi: 10.1038/nn1657
Rehman, J., Traktuev, D., Li, J., Merfeld-Clauss, S., Temm-Grove, C. J., Bovenkerk, J. E., et al. (2004). Secretion of angiogenic and antiapoptotic factors by human adipose stromal cells. Circulation 109, 1292–1298. doi: 10.1161/01.cir.0000121425.42966.f1
Ribeiro, C. A., Fraga, J. S., Grãos, M., Neves, N. M., Reis, R. L., Gimble, J. M., et al. (2012). The secretome of stem cells isolated from the adipose tissue and Wharton jelly acts differently on central nervous system derived cell populations. Stem Cell Res. Ther. 3:18. doi: 10.1186/scrt109
Roger, M., Clavreul, A., Huynh, N. T., Passirani, C., Schiller, P., Vessières, A., et al. (2012). Ferrociphenol lipid nanocapsule delivery by mesenchymal stromal cells in brain tumor therapy. Int. J. Pharm. 423, 63–68. doi: 10.1016/j.ijpharm.2011.04.058
Roger, M., Clavreul, A., Venier-Julienne, M. C., Passirani, C., Sindji, L., Schiller, P., et al. (2010). Mesenchymal stem cells as cellular vehicles for delivery of nanoparticles to brain tumors. Biomaterials 31, 8393–8401. doi: 10.1016/j.biomaterials.2010.07.048
Ryu, C. H., Park, K. Y., Kim, S. M., Jeong, C. H., Woo, J. S., Hou, Y., et al. (2012). Valproic acid enhances anti-tumor effect of mesenchymal stem cell mediated HSV-TK gene therapy in intracranial glioma. Biochem. Biophys. Res. Commun. 421, 585–590. doi: 10.1016/j.bbrc.2012.04.050
Ryu, C. H., Park, S. H., Park, S. A., Kim, S. M., Lim, J. Y., Jeong, C. H., et al. (2011). Gene therapy of intracranial glioma using interleukin 12-secreting human umbilical cord blood-derived mesenchymal stem cells. Hum. Gene Ther. 22, 733–743. doi: 10.1089/hum.2010.187
Sadan, O., Bahat-Stromza, M., Barhum, Y., Levy, Y. S., Pisnevsky, A., Peretz, H., et al. (2009). Protective effects of neurotrophic factor-secreting cells in a 6-OHDA rat model of Parkinson disease. Stem Cells Dev. 18, 1179–1190. doi: 10.1089/scd.2008.0411
Salgado, A. J., Coutinho, O. P., and Reis, R. L. (2004). Bone tissue engineering: state of the art and future trends. Macromol. Biosci. 4, 743–765. doi: 10.1002/mabi.200400026
Salgado, A. J., Oliveira, J. T., Pedro, A. J., and Reis, R. L. (2006). Adult stem cells in bone and cartilage tissue engineering. Curr. Stem Cell Res. Ther. 1, 345–364. doi: 10.2174/157488806778226803
Sapolsky, R. M. (2000). Glucocorticoids and hippocampal atrophy in neuropsychiatric disorders. Arch. Gen. Psychiatry 57, 925–935. doi: 10.1001/archpsyc.57.10.925
Sasportas, L. S., Kasmieh, R., Wakimoto, H., Hingtgen, S., van de Water, J. A., Mohapatra, G., et al. (2009). Assessment of therapeutic efficacy and fate of engineered human mesenchymal stem cells for cancer therapy. Proc. Natl. Acad. Sci. U S A 106, 4822–4827. doi: 10.1073/pnas.0806647106
Saxe, M. D., Battaglia, F., Wang, J. W., Malleret, G., David, D. J., Monckton, J. E., et al. (2006). Ablation of hippocampal neurogenesis impairs contextual fear conditioning and synaptic plasticity in the dentate gyrus. Proc. Natl. Acad. Sci. U S A 103, 17501–17506. doi: 10.1073/pnas.0607207103
Schmidt-Hieber, C., Jonas, P., and Bischofberger, J. (2004). Enhanced synaptic plasticity in newly generated granule cells of the adult hippocampus. Nature 429, 184–187. doi: 10.1038/nature02553
Seidenfaden, R., Desoeuvre, A., Bosio, A., Virard, I., and Cremer, H. (2006). Glial conversion of SVZ-derived committed neuronal precursors after ectopic grafting into the adult brain. Mol. Cell. Neurosci. 32, 187–198. doi: 10.1016/j.mcn.2006.04.003
Seo, J. H., and Cho, S. R. (2012). Neurorestoration induced by mesenchymal stem cells: potential therapeutic mechanisms for clinical trials. Yonsei Med. J. 53, 1059–1067. doi: 10.3349/ymj.2012.53.6.1059
Seri, B., Garcia-Verdugo, J. M., Collado-Morente, L., McEwen, B. S., and Alvarez-Buylla, A. (2004). Cell types, lineage and architecture of the germinal zone in the adult dentate gyrus. J. Comp. Neurol. 478, 359–378. doi: 10.1002/cne.20288
Seri, B., García-Verdugo, J. M., McEwen, B. S., and Alvarez-Buylla, A. (2001). Astrocytes give rise to new neurons in the adult mammalian hippocampus. J. Neurosci. 21, 7153–7160.
Shah, K., Tung, C. H., Yang, K., Weissleder, R., and Breakefield, X. O. (2004). Inducible release of TRAIL fusion proteins from a proapoptotic form for tumor therapy. Cancer Res. 64, 3236–3242. doi: 10.1158/0008-5472.can-03-3516
Shen, Q., Goderie, S. K., Jin, L., Karanth, N., Sun, Y., Abramova, N., et al. (2004). Endothelial cells stimulate self-renewal and expand neurogenesis of neural stem cells. Science 304, 1338–1340. doi: 10.1126/science.1095505
Shen, Y., Mishra, R., Mani, S., and Meiri, K. F. (2008). Both cell-autonomous and cell non-autonomous functions of GAP-43 are required for normal patterning of the cerebellum in vivo. Cerebellum 7, 451–466. doi: 10.1007/s12311-008-0049-5
Shihabuddin, L. S., Horner, P. J., Ray, J., and Gage, F. H. (2000). Adult spinal cord stem cells generate neurons after transplantation in the adult dentate gyrus. J. Neurosci. 20, 8727–8735.
Shintani, A., Nakao, N., Kakishita, K., and Itakura, T. (2007). Protection of dopamine neurons by bone marrow stromal cells. Brain Res. 1186, 48–55. doi: 10.1016/j.brainres.2007.09.086
Sierra, A., Encinas, J. M., Deudero, J. J., Chancey, J. H., Enikolopov, G., Overstreet-Wadiche, L. S., et al. (2010). Microglia shape adult hippocampal neurogenesis through apoptosis-coupled phagocytosis. Cell Stem Cell 7, 483–495. doi: 10.1016/j.stem.2010.08.014
Sikavitsas, V. I., Temenoff, J. S., and Mikos, A. G. (2001). Biomaterials and bone mechanotransduction. Biomaterials 22, 2581–2593. doi: 10.1016/s0142-9612(01)00002-3
Silva, R., Lu, J., Wu, Y., Martins, L., Almeida, O. F., and Sousa, N. (2006). Mapping cellular gains and losses in the postnatal dentate gyrus: implications for psychiatric disorders. Exp. Neurol. 200, 321–331. doi: 10.1016/j.expneurol.2006.02.119
Singh, S. K., Clarke, I. D., Terasaki, M., Bonn, V. E., Hawkins, C., Squire, J., et al. (2003). Identification of a cancer stem cell in human brain tumors. Cancer Res. 63, 5821–5828.
Snyder, J. S., Choe, J. S., Clifford, M. A., Jeurling, S. I., Hurley, P., Brown, A., et al. (2009). Adult-born hippocampal neurons are more numerous, faster maturing and more involved in behavior in rats than in mice. J. Neurosci. 29, 14484–14495. doi: 10.1523/JNEUROSCI.1768-09.2009
Sommerfeldt, D. W., and Rubin, C. T. (2001). Biology of bone and how it orchestrates the form and function of the skeleton. Eur. Spine J. 10, S86–S95. doi: 10.1007/s005860100283
Sonabend, A. M., Ulasov, I. V., Tyler, M. A., Rivera, A. A., Mathis, J. M., and Lesniak, M. S. (2008). Mesenchymal stem cells effectively deliver an oncolytic adenovirus to intracranial glioma. Stem Cells 26, 831–841. doi: 10.1634/stemcells.2007-0758
Steiner, B., Klempin, F., Wang, L., Kott, M., Kettenmann, H., and Kempermann, G. (2006). Type-2 cells as link between glial and neuronal lineage in adult hippocampal neurogenesis. Glia 54, 805–814. doi: 10.1002/glia.20407
Stuckey, D. W., and Shah, K. (2014). Stem cell-based therapies for cancer treatment: separating hope from hype. Nat. Rev. Cancer 14, 683–691. doi: 10.1038/nrc3798
Stupp, R., Mason, W. P., van den Bent, M. J., Weller, M., Fisher, B., Taphoorn, M. J., et al. (2005). Radiotherapy plus concomitant and adjuvant temozolomide for glioblastoma. N. Engl. J. Med. 352, 987–996. doi: 10.1056/NEJMoa043330
Tang, Y. L., Zhao, Q., Qin, X., Shen, L., Cheng, L., Ge, J., et al. (2005). Paracrine action enhances the effects of autologous mesenchymal stem cell transplantation on vascular regeneration in rat model of myocardial infarction. Ann. Thorac. Surg. 80, 229–236. doi: 10.1016/j.athoracsur.2005.02.072
Tavazoie, M., Van der Veken, L., Silva-Vargas, V., Louissaint, M., Colonna, L., Zaidi, B., et al. (2008). A specialized vascular niche for adult neural stem cells. Cell Stem Cell 3, 279–288. doi: 10.1016/j.stem.2008.07.025
Teixeira, F. G., Carvalho, M. M., Neves-Carvalho, A., Panchalingam, K. M., Behie, L. A., Pinto, L., et al. (2015). Secretome of Mesenchymal progenitors from the Umbilical cord acts as modulator of neural/glial proliferation and differentiation. Stem Cell Rev. 11, 288–897. doi: 10.1007/s12015-014-9576-2
Teixeira, F. G., Carvalho, M. M., Sousa, N., and Salgado, A. J. (2013). Mesenchymal stem cells secretome: a new paradigm for central nervous system regeneration? Cell Mol. Life Sci. 70, 3871–3882. doi: 10.1007/s00018-013-1290-8
Teng, J., Hejazi, S., Badr, C. E., and Tannous, B. A. (2014). Systemic anticancer neural stem cells in combination with a cardiac glycoside for glioblastoma therapy. Stem Cells 32, 2021–2032. doi: 10.1002/stem.1727
Thaci, B., Ahmed, A. U., Ulasov, I. V., Tobias, A. L., Han, Y., Aboody, K. S., et al. (2012). Pharmacokinetic study of neural stem cell-based cell carrier for oncolytic virotherapy: targeted delivery of the therapeutic payload in an orthotopic brain tumor model. Cancer Gene Ther. 19, 431–442. doi: 10.1038/cgt.2012.21
Thored, P., Heldmann, U., Gomes-Leal, W., Gisler, R., Darsalia, V., Taneera, J., et al. (2009). Long-term accumulation of microglia with proneurogenic phenotype concomitant with persistent neurogenesis in adult subventricular zone after stroke. Glia 57, 835–849. doi: 10.1002/glia.20810
Trujillo, C. A., Schwindt, T. T., Martins, A. H., Alves, J. M., Mello, L. E., and Ulrich, H. (2009). Novel perspectives of neural stem cell differentiation: from neurotransmitters to therapeutics. Cytometry A 75, 38–53. doi: 10.1002/cyto.a.20666
Tyler, M. A., Ulasov, I. V., Sonabend, A. M., Nandi, S., Han, Y., Marler, S., et al. (2009). Neural stem cells target intracranial glioma to deliver an oncolytic adenovirus in vivo. Gene Ther. 16, 262–278. doi: 10.1038/gt.2008.165
Uchibori, R., Okada, T., Ito, T., Urabe, M., Mizukami, H., Kume, A., et al. (2009). Retroviral vector-producing mesenchymal stem cells for targeted suicide cancer gene therapy. J. Gene Med. 11, 373–381. doi: 10.1002/jgm.1313
Uhl, M., Weiler, M., Wick, W., Jacobs, A. H., Weller, M., and Herrlinger, U. (2005). Migratory neural stem cells for improved thymidine kinase-based gene therapy of malignant gliomas. Biochem. Biophys. Res. Commun. 328, 125–129. doi: 10.1016/j.bbrc.2004.12.164
von Bohlen und Halbach, O. (2011). Immunohistological markers for proliferative events, gliogenesis and neurogenesis within the adult hippocampus. Cell Tissue Res. 345, 1–19. doi: 10.1007/s00441-011-1196-4
Waclaw, R. R., Allen, Z. J. II, Bell, S. M., Erdèlyi, F., Szabó, G., Potter, S. S., et al. (2006). The zinc finger transcription factor Sp8 regulates the generation and diversity of olfactory bulb interneurons. Neuron 49, 503–516. doi: 10.1016/j.neuron.2006.01.018
Wakabayashi, K., Nagai, A., Sheikh, A. M., Shiota, Y., Narantuya, D., Watanabe, T., et al. (2010). Transplantation of human mesenchymal stem cells promotes functional improvement and increased expression of neurotrophic factors in a rat focal cerebral ischemia model. J. Neurosci. Res. 88, 1017–1025. doi: 10.1002/jnr.22279
Wang, F., Yasuhara, T., Shingo, T., Kameda, M., Tajiri, N., Yuan, W. J., et al. (2010). Intravenous administration of mesenchymal stem cells exerts therapeutic effects on parkinsonian model of rats: focusing on neuroprotective effects of stromal cell-derived factor-1alpha. BMC Neurosci. 11:52. doi: 10.1186/1471-2202-11-52
Weinandy, F., Ninkovic, J., and Götz, M. (2011). Restrictions in time and space–new insights into generation of specific neuronal subtypes in the adult mammalian brain. Eur. J. Neurosci. 33, 1045–1054. doi: 10.1111/j.1460-9568.2011.07602.x
Weiss, M. L., Medicetty, S., Bledsoe, A. R., Rachakatla, R. S., Choi, M., Merchav, S., et al. (2006). Human umbilical cord matrix stem cells: preliminary characterization and effect of transplantation in a rodent model of Parkinson’s disease. Stem Cells 24, 781–792. doi: 10.1634/stemcells.2005-0330
Wollmann, G., Ozduman, K., and van den Pol, A. N. (2012). Oncolytic virus therapy for glioblastoma multiforme: concepts and candidates. Cancer J. 18, 69–81. doi: 10.1097/PPO.0b013e31824671c9
Xin, H., Li, Y., Buller, B., Katakowski, M., Zhang, Y., Wang, X., et al. (2012). Exosome-mediated transfer of miR-133b from multipotent mesenchymal stromal cells to neural cells contributes to neurite outgrowth. Stem Cells 30, 1556–1564. doi: 10.1002/stem.1129
Xin, H., Li, Y., Cui, Y., Yang, J. J., Zhang, Z. G., and Chopp, M. (2013a). Systemic administration of exosomes released from mesenchymal stromal cells promote functional recovery and neurovascular plasticity after stroke in rats. J. Cereb. Blood Flow Metab. 33, 1711–1715. doi: 10.1038/jcbfm.2013.152
Xin, H., Li, Y., Liu, Z., Wang, X., Shang, X., Cui, Y., et al. (2013b). MiR-133b promotes neural plasticity and functional recovery after treatment of stroke with multipotent mesenchymal stromal cells in rats via transfer of exosome-enriched extracellular particles. Stem Cells 31, 2737–2746. doi: 10.1002/stem.1409
Yamamoto, M., and Curiel, D. T. (2010). Current issues and future directions of oncolytic adenoviruses. Mol. Ther. 18, 243–250. doi: 10.1038/mt.2009.266
Yin, J., Kim, J. K., Moon, J. H., Beck, S., Piao, D., Jin, X., et al. (2011). hMSC-mediated concurrent delivery of endostatin and carboxylesterase to mouse xenografts suppresses glioma initiation and recurrence. Mol. Ther. 19, 1161–1169. doi: 10.1038/mt.2011.28
Yong, R. L., Shinojima, N., Fueyo, J., Gumin, J., Vecil, G. G., Marini, F. C., et al. (2009). Human bone marrow-derived mesenchymal stem cells for intravascular delivery of oncolytic adenovirus Delta24-RGD to human gliomas. Cancer Res. 69, 8932–8940. doi: 10.1158/0008-5472.CAN-08-3873
Yoshimi, K., Ren, Y. R., Seki, T., Yamada, M., Ooizumi, H., Onodera, M., et al. (2005). Possibility for neurogenesis in substantia nigra of parkinsonian brain. Ann. Neurol. 58, 31–40. doi: 10.1002/ana.20506
Young, K. M., Fogarty, M., Kessaris, N., and Richardson, W. D. (2007). Subventricular zone stem cells are heterogeneous with respect to their embryonic origins and neurogenic fates in the adult olfactory bulb. J. Neurosci. 27, 8286–8296. doi: 10.1523/jneurosci.0476-07.2007
Yuan, X., Hu, J., Belladonna, M. L., Black, K. L., and Yu, J. S. (2006). Interleukin-23-expressing bone marrow-derived neural stem-like cells exhibit antitumor activity against intracranial glioma. Cancer Res. 66, 2630–2638. doi: 10.1158/0008-5472.can-05-1682
Zhao, C., Deng, W., and Gage, F. H. (2008). Mechanisms and functional implications of adult neurogenesis. Cell 132, 645–660. doi: 10.1016/j.cell.2008.01.033
Zhao, D., Najbauer, J., Annala, A. J., Garcia, E., Metz, M. Z., Gutova, M., et al. (2012). Human neural stem cell tropism to metastatic breast cancer. Stem Cells 30, 314–325. doi: 10.1002/stem.784
Zhao, M., Momma, S., Delfani, K., Carlen, M., Cassidy, R. M., Johansson, C. B., et al. (2003). Evidence for neurogenesis in the adult mammalian substantia nigra. Proc. Natl. Acad. Sci. U S A 100, 7925–7930. doi: 10.1073/pnas.1131955100
Keywords: mesenchymal stem cells, neural stem cells, niche, neurogenesis, secretome, regenerative medicine, interactions
Citation: Salgado AJ, Sousa JC, Costa BM, Pires AO, Mateus-Pinheiro A, Teixeira FG, Pinto L and Sousa N (2015) Mesenchymal stem cells secretome as a modulator of the neurogenic niche: basic insights and therapeutic opportunities. Front. Cell. Neurosci. 9:249. doi: 10.3389/fncel.2015.00249
Received: 19 March 2015; Accepted: 18 June 2015;
Published: 13 July 2015.
Edited by:
Wanda Lattanzi, Università Cattolica del Sacro Cuore, ItalyReviewed by:
Alexander K. Murashov, East Carolina University, USARobert Weissert, University of Regensburg, Germany
Copyright © 2015 Salgado, Sousa, Costa, Pires, Mateus-Pinheiro, Teixeira, Pinto and Sousa. This is an open-access article distributed under the terms of the Creative Commons Attribution License (CC BY). The use, distribution and reproduction in other forums is permitted, provided the original author(s) or licensor are credited and that the original publication in this journal is cited, in accordance with accepted academic practice. No use, distribution or reproduction is permitted which does not comply with these terms.
*Correspondence: Nuno Sousa, Life and Health Sciences Research Institute (ICVS), School of Health Sciences, Campus de Gualtar, University of Minho, 4710-057 Braga, Portugal, njcsousa@ecsaude.uminho.pt