- 1Department of Advanced Neuroimaging, Integrative Brain Imaging Center, National Center of Neurology and Psychiatry, Tokyo, Japan
- 2Computational, Cognitive and Clinical Neuroimaging Laboratory, Division of Restorative Neurosciences, Imperial College London, London, UK
- 3Research Fellow of the Japan Society for the Promotion of Science, Tokyo Japan
There has been an explosion of research using transcranial direct current stimulation (tDCS) for investigating and modulating human cognitive and motor function in healthy populations. It has also been used in many studies seeking to improve deficits in disease populations. With the slew of studies reporting “promising results” for everything from motor recovery after stroke to boosting memory function, one could be easily seduced by the idea of tDCS being the next panacea for all neurological ills. However, huge variability exists in the reported effects of tDCS, with great variability in the effect sizes and even contradictory results reported. In this review, we consider the interindividual factors that may contribute to this variability. In particular, we discuss the importance of baseline neuronal state and features, anatomy, age and the inherent variability in the injured brain. We additionally consider how interindividual variability affects the results of motor-evoked potential (MEP) testing with transcranial magnetic stimulation (TMS), which, in turn, can lead to apparent variability in response to tDCS in motor studies.
Introduction
Non-invasive brain stimulation (NIBS) techniques, in particular transcranial direct current stimulation (tDCS), have become increasingly popular methods for temporary modulation of behavior. However, there is increasing recognition of the high variability in the reported effects of tDCS, even when using the same stimulation parameters, making the effect of tDCS on behavior and cognition anything but predictable (Jacobson et al., 2012; Horvath et al., 2014a, 2015; López-Alonso et al., 2014; Wiethoff et al., 2014; Strube et al., 2015a). The observed effect of tDCS is dependent on the electrical dose administered, the biological response to that dose, and the way that response is assessed. Electrical dose is defined by “what is externally applied (and therefore fully controlled) rather than by any physiologic or behavioral response to stimulation” (Peterchev et al., 2012). There is lack of standardization in the electrical dose administered across studies, particularly in the cognitive field but even in the motor field where recommended protocols exist (Nitsche and Paulus, 2000, 2001). This significantly affects reproducibility of study results. Recent papers have also highlighted several other issues with tDCS research, which can confound outcomes, such as blinding techniques, task (outcome assessment) selection and absence of proper control conditions (Jacobson et al., 2012; Horvath et al., 2014a; de Aguiar et al., 2015). However, the factors affecting biological response to electrical current, and causing interindividual variability in response to tDCS, are highly important yet under-appreciated. Understanding the biological response to tDCS is both crucial for developing tDCS for wider use and for providing insights into neurophysiological function.
Early animal studies have demonstrated that anodal stimulation increases neuronal excitability whilst cathodal stimulation decreases neuronal excitability (e.g., Purpura and McMurtry, 1965), and later Nitsche and colleagues confirmed this effect in the human primary motor cortex (M1) (Nitsche and Paulus, 2000; Stagg and Nitsche, 2011). The majority of subsequent tDCS protocols have been based on this premise – anodal stimulation causing neuronal, and therefore behavioral, facilitation, with cathodal stimulation causing the opposite. However, a recent meta-analysis of tDCS studies found that the probability of achieving the classical “anodal-facilitatory/cathodal-inhibitory” effect on motor outcomes was only 0.67, and for cognitive outcomes only 0.16 (Jacobson et al., 2012). It is becoming increasingly clear that this classical view of a dichotomous tDCS effect is far too simplistic.
The pitfalls of an excessively simplistic view have been seen with other brain stimulation techniques, such as transcranial magnetic stimulation (TMS). For example, we now recognize that repetitive or patterned TMS protocols aimed at inducing neuroplasticity does not always have the “classical effect” in all participants (Müller-Dahlhaus et al., 2008; Ridding and Ziemann, 2010; Hamada et al., 2013; Hinder et al., 2014; López-Alonso et al., 2014). Studies specifically setting out to address the underlying physiological reasons for this variation have found numerous factors, including age, genetic polymorphisms, and variability in inhibitory circuitry (Cheeran et al., 2008; Ridding and Ziemann, 2010; Hamada et al., 2013).
The variability in reported results is therefore likely to arise from variability in stimulation protocols, interindividual variability and the interaction of the two. Understanding the contribution of protocol variability to the observed results in the tDCS literature is also crucial for deciphering the impact of interindividual variability to tDCS response. This topic is worthy of extensive review and investigation in itself and is outside the scope of this review. In this review, we focus on interindividual biological differences that can confound tDCS studies, that is, factors which would cause variability in behavioral responses even under identical electrical doses. In particular, we discuss neurophysiological state and features, anatomy, genetics, age, the injured brain and also the variability introduced by TMS assessment techniques. We will primarily consider the evidence from healthy subjects in both motor and cognitive tDCS studies, and also discuss the special case of the injured brain. We make suggestions for how studies can address interindividual variability and how future research can investigate this important issue.
Literature Search Strategy
There are extremely few published studies specifically addressing interindividual variability in tDCS so a specific literature search was not possible. Instead, we searched PubMed with “transcranial direct current stimulation” as a MeSH® term and also as a string, as well as performing searches with the following strings: “tDCS anatomy,” “tDCS genetics,” “tDCS task,” “tDCS age,” “tDCS GABA,” “tDCS dopamine,” “tDCS injury,” “tDCS stroke.” Relevant papers were identified by all authors, and reference lists were examined for any other relevant studies.
Anatomy
Interindividual differences in cranial and brain anatomy can influence the impact of tDCS by creating variability in the actual current received by the brain, even when the same electrical dose is administered. The distribution and extent of current density is the most common measure for assessing this current received by the brain, and computer simulations based on anatomical data have provided useful insights into how the factors which modulate current density. A comprehensive discussion of the development of such simulations is outside the scope of this paper and is reviewed in detail elsewhere (Bikson et al., 2012, 2013; Wagner et al., 2014).
Simulation studies modeling factors derived from individual neuroimaging have demonstrated that gross anatomical features and microarchitectural features influence current distribution (Bikson et al., 2012; Datta et al., 2012a). These factors include: skull thickness (Opitz et al., 2015), cerebrospinal fluid (CSF) thickness (Opitz et al., 2015), subcutaneous fat (Truong et al., 2013), gyral pattern (Datta et al., 2012a; Halko et al., 2012; Opitz et al., 2015), local tissue heterogeneities (Shahid et al., 2012; Russell et al., 2013), and orientation of neurons (Arlotti et al., 2012). Moreover, a recent study modeled the current density induced by an electrode over the left dorsolateral prefrontal cortex (dlPFC), and found that the improvement in a working memory task correlated with the simulated current density, suggesting that the work from simulations has real functional relevance (Kim et al., 2014a).
Cortical anatomy shows high variability in gyrus and sulcus patterns between individuals (Ono et al., 1990), and is likely to produce high interindividual variability in tDCS response (Rademacher et al., 1993). This is because the orientation of neurons is a particularly important determinant of the polarizing effect of direct current. Radial current flow appears to be most effective at causing somatic polarization, whereas tangential current flow appears to be most effective at causing terminal polarization (Bikson et al., 2004; Rahman et al., 2013).
Individual differences in anatomical fiber connectivity between brain regions likely influence tDCS effects. In fact, current distribution of tDCS is influenced by integrity of white matter indexed by fractional anisotropy (FA) of diffusion-weighted MRI (Metwally et al., 2012; Shahid et al., 2012; Suh et al., 2012; Russell et al., 2013; Shahid and Ahfock, 2014a). Rosso et al., used cathodal tDCS on the right hemispheric Broca homolog to increase picture naming speed after stroke, and showed that the extent of improvement correlated with tract size and functional connectivity between the right supplementary motor area and right inferior frontal gyrus (Rosso et al., 2014). Given that widespread cortical networks underlie many cognitive functions, this effect is unsurprising, particularly as tDCS has been shown to affect functional connectivity (Park et al., 2013a). However, the influence of structural and functional connectivity on the effect of tDCS has not been extensively studied.
These studies have also found that anatomical factors do not always have the expected influence. For example, it might be assumed that current density is inversely related to skull thickness; however, a recent study demonstrates that the bigger proportion of highly conducting spongy bone in thicker skull areas results in a more complex relationship between skull thickness and current density (Opitz et al., 2015).
The computer models based on anatomical information will be most helpful if they can guide experimental design. Not all modeled factors included in a simulation significantly alter protocol decisions. For example, although thickness of subcutaneous fat alters the spread of tDCS current, its effect is trivial compared to other anatomical variables (Truong et al., 2013). Similarly, including a detailed FA map in a model of tDCS current from an electrode over the primary motor cortex (M1) alters the modeling output but not in a way that would necessitate changes in stimulation parameters (Shahid and Ahfock, 2014a). Future applications of stimulations may be to help individualize electrode placement in patients with large anatomical variations, such as stroke patients with lesions.
Functional Organization of Local Circuits
The functional organization of local inhibitory and excitatory circuits within the cortex appears to contribute to variability of response to tDCS. TMS protocols have traditionally been used to assess the function of these local circuits. TMS over the M1 produce a motor-evoked potential (MEP), which has been widely used as a measure of corticospinal excitability. An MEP is composed of several components. The earliest MEP component, called a D-wave, originates “directly” from axonal activity of pyramidal tract neurons (PTNs). The subsequent MEP components are called “indirect” waves (I-waves), which originate from mono-synaptic (early I-waves) and poly-synaptic (later I-waves) activity of interneurons projecting onto PTNs within the M1 (Di Lazzaro et al., 1998, 2012; Di Lazzaro and Rothwell, 2014) (Figure 1). Therefore, the measurement of I-waves gives detailed information about the conditions of local circuits within the M1. Crucially, the measurements of I-waves may refute the commonly held notion that cathodal tDCS is simply the opposite of anodal tDCS. An epidural recording study found that M1 anodal tDCS facilitates both the earliest I-wave (the I1-wave) and later I-waves whereas cathodal tDCS suppresses later I-waves only (Lang et al., 2011). Despite its potential significance, however, further studies are necessary to establish the relationships between I-wave components and response to tDCS since evidence is available only from a single study consisting of a small sample size (n = 8).
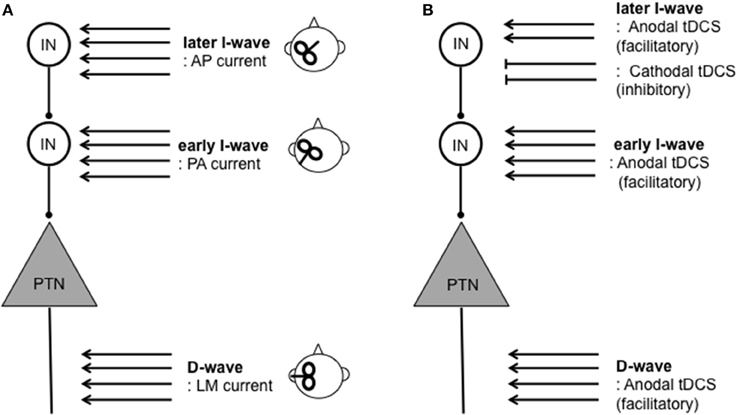
Figure 1. Schematic illustration of assessment and modulation of direct (D-) and indirect (I-) waves resulting from corticospinal activity. D-waves originate from activity of pyramidal tract neurons (PTNs). I-waves originate from activity of mono- (early I-wave) and poly- (later I-wave) synaptic activity of interneurons (INs) projecting onto PTNs. (A) TMS inducing lateral-medial (LM) currents results in D-waves. TMS inducing the posterior-anterior (PA) current and anterior-posterior (AP) current primarily results in early I-wave and later I-waves, respectively. (B) Modulation of D- and I-waves by tDCS protocols. Anodal tDCS can facilitate both D- and later I-waves whereas cathodal tDCS suppresses later I-waves only. For further detail on I-waves physiology and relationships between tDCS on D- and I-waves (see Di Lazzaro et al., 2004; Lang et al., 2011; Di Lazzaro et al., 2012; Di Lazzaro and Rothwell, 2014).
New evidence suggests that interindividual differences in I-wave components may signify differences in local circuit architecture, and predict response to brain stimulation. I-wave components such as early and later I-waves can be distinguished by changes in MEP latency caused by changes in orientation of the TMS coil (Day et al., 1989; Rothwell, 1997; Sakai et al., 1997). That is, the latency of MEPs evoked by an antero-posteriorly (AP)-directed coil differs from that evoked by a latero-medially (LM)-directed coil (AP-LM latency difference) (Day et al., 1989; Sakai et al., 1997; Di Lazzaro et al., 2012). The AP-LM latency difference is considered to reflect later I-wave and D-wave activity, which is taken as a measure of organization of local circuits. Taking advantage of this technique, Wiethoff et al. (2014) demonstrated that AP-LM latency difference correlated with the effects of anodal tDCS, but not those of cathodal tDCS.
Although individual differences in functional organization of local circuits may be an important factor underlying individual differences in response to tDCS, there are few relevant studies so far. Further research is necessary to test their utility for predicting response to tDCS.
Baseline Level of Motor and Cognitive Function
A participant's initial level of function can have a significant impact on both the motor and cognitive effects of tDCS. Cathodal tDCS to the M1 ipsilateral to the tasked limb has been used to improve motor coordination in people who vary in motor coordination of the upper limb (McCambridge et al., 2011; Uehara et al., 2015). In these studies, participants with poor baseline motor coordination show clear improvement after tDCS, whereas those with superior motor coordination prior to tDCS do not improve as much. Additionally, when opposite-polarity tDCS was applied to the bilateral M1 in both expert musicians and non-musicians, the non-musicians improved fine motor control of the hands whereas the expert musicians experienced paradoxical deterioration of performance (Furuya et al., 2014).
The effect of baseline function on tDCS response has also been observed in cognitive studies. Performance on a visual short-term memory task (VSTM) was improved by anodal tDCS to the right posterior parietal cortex (PPC) only in participants who had initially poor performance. It did not alter performance for those participants with initially high performance (Tseng et al., 2012). Furthermore, the improvement in VSTM performance after tDCS was accompanied by increased amplitude of event-related potentials (ERPs), implying improvement of attention deployment, from concurrent EEG recordings. However, those who did not improve already had large amplitude ERPs even before tDCS. Though by no means comprehensive, these studies imply the existence of ceiling effects on neuronal modulation with tDCS, which, in turn, may explain the variable effects of tDCS on behavior.
Task-related Neurophysiology
Interindividual differences in the neurophysiological response to tasks performed during tDCS can produce variability in the assessed effect of tDCS (Antal et al., 2007). Similarly, although only specifically addressed in a handful of studies, individual differences in the recruitment of brain regions during task performance can contribute to variability in response to tDCS. The extent to which a cognitive function is lateralized can be measured by task activation during functional magnetic resonance imaging (fMRI), and this factor appears to influence response to bi-hemispheric tDCS to the posterior parietal cortices (PPC) (Kasahara et al., 2013). Related to this, handedness also modulates the time-course and amplitude of the MEP response to M1 tDCS, though not the direction of response (Schade et al., 2012).
Differences in task strategy depending on baseline ability and resulting differences in recruitment of brain regions may also produce variability of response to tDCS. Targeting a similar area (right PPC) using a similar task, but with variable degrees of difficulty, Jones and colleagues demonstrated that tDCS improved task performance, but only on difficult tasks, and only in people with initially high task performance (Jones and Berryhill, 2012). Additionally, in this group of people, both anodal and cathodal tDCS produced improvements. However, tDCS had no significant effect on the performance in those with initially low task performance. The authors explain these findings as an interaction of baseline function with task difficulty, possibly because of different task strategies and recruitment of brain regions depending on baseline ability. Other studies have also found a similar interaction of tDCS function with task difficulty (Sandrini et al., 2012; Wu et al., 2014).
Psychological Status
As well as baseline task performance, other physiological and psychological factors influence tDCS effects. A study on maths performance found that tDCS improved performance, and decreased serum cortisol, in high “maths anxiety” participants but had the opposite effect in those with low “maths anxiety” (Sarkar et al., 2014). Abstinent methamphetamine users reported decreased cravings following 10 min of right DLPFC anodal tDCS alone, but increased cravings following if the tDCS was accompanied by the presentation of methamphetamine-related cues (Shahbabaie et al., 2014).
Neurochemistry
Response variances depending on psychological status may reflect different levels of neurotransmitters and receptor sensitivity. The ability to study neurotransmitter levels with magnetic resonance spectroscopy (MRS) has led to studies of the relationship between the level of γ-aminobutyric acid (GABA) and the effect of tDCS. Anodal tDCS reduces local GABA levels (Stagg et al., 2009) and the extent of this reduction correlates to the degree of motor learning and also to fMRI signal change during tDCS-induced learning (Stagg et al., 2011; Kim et al., 2014b). It should also be noted that neurochemical factors, especially GABA, have a close relationship with the functionality of local cortical circuits, as discussed above. Furthermore, the influence of baseline GABA levels on tDCS outcome may be linked to functional connectivity, as baseline GABA is indirectly correlated to resting functional connectivity (Stagg et al., 2014).
It has thus been mooted that there exists an “optimal” level of GABA for brain performance, similar to the idea of frontal cortex “optimal dopamine” levels. In the case of dopamine, it has been hypothesized that there is a normal range of dopamine in the prefrontal cortex, which can be represented by an “inverted-U”-shaped relationship between dopamine transmission and working memory performance or motor cortex plasticity (Monte-silva et al., 2010; Cools and D'Esposito, 2012). An animal study demonstrates that cathodal tDCS increases striatal dopamine levels (Tanaka et al., 2013). Additionally, the facilitatory effect of anodal tDCS on the M1 is dependent on the baseline activity of D1 receptors (Fresnoza et al., 2014). Thus, depending on a participant's baseline GABA or dopamine, either anodal or cathodal tDCS could shift their levels to the optimum, and may account for why some studies have reported unexpected effects (Wiethoff et al., 2014) of tDCS at a group level (Krause et al., 2013).
There have been several studies assessing how neuroactive drugs affect the neuroplastic effect of tDCS and other non-invasive stimulation techniques (for detailed review, see Nitsche et al., 2012). These studies not only help to elucidate the mechanisms of tDCS work but, in combination with studies on neurotransmitter levels and tDCS effect, can provide interesting insights into how interindividual variability in neurotransmitter levels impacts on the action of tDCS. For example, studying the contribution of local GABA, dopamine, and other neurotransmitters from the viewpoint of their “optimal” levels may prove a fruitful line of investigation in tDCS studies.
Baseline Neurophysiological State including Circadian Rhythms
Physiological states can impact response to tDCS even within the same individual. For instance, the difference in physiological states between “eyes open” and “eyes closed” influences the extent to which electroencephalographic (EEG) α oscillation is increased by occipital transcranial alternative current stimulation (tACS) (Neuling et al., 2013). More intriguingly, an improvement in declarative memory with tDCS was only seen when tDCS was applied during sleep, thought to be an important brain state for consolidating declarative memories (Marshall et al., 2004). Serum cortisol, which shows circadian variations across the day, is thought to have an impact on brain function and neuroplasticity (Nader et al., 2010). It has recently been demonstrated that cortisol levels influence TMS-induced neuroplasticity; higher cortisol levels predict greater response to a neuroplasticity protocol based on repetitive TMS (Clow et al., 2014). In fact, TMS studies typically have paid particular attention to testing subjects at similar times of day, and this may be a consideration that is also important for tDCS-mediated plasticity.
Genetics
A study found that non-schizophrenic first-degree relatives of schizophrenia patients had altered MEP response to cathodal tDCS of M1, as compared with non-related healthy participants (Hasan et al., 2013). Given the high heritability of schizophrenia, these findings support the idea that genetic variability may be an important reason underlying interindividual variability in response to tDCS.
The impact of genotype on neuroplasticity was initially demonstrated for TMS neuroplasticity protocols. The PAS25 neuroplastic TMS protocol, which may act on similar neural circuits to tDCS, showed high heritability in a twin study (0.68 heritability) (Missitzi et al., 2011). A study comparing carriers of the Val66Met polymorphism of the brain-derived neurotrophic factor (BDNF) with carriers of the Val66Val polymorphism found that only those with the Val66Val polymorphism displayed the expected neural response to a range of TMS neuroplasticity protocols (Cheeran et al., 2008). The BDNF polymorphism also appears to influence response to tDCS. Healthy participants with the Met66Val or Met66Met BDNF polymorphism showed a later facilitation of MEP amplitude after anodal tDCS of M1 (Teo et al., 2014). In a cognitive domain, however, a large study of combined tDCS and sertraline (a selective serotonin reuptake inhibitor) in depression found that BDNF polymorphism did not predict response to treatment, but that serotonin-transporter-linked polymorphism (5HT-TLPR) did (Brunoni et al., 2013). The BDNF polymorphism can also interact with other factors to produce unpredictable effects on neuroplasticity, for example, the finding that cathodal tDCS reduced short-interval intracortical inhibition (SICI) in Val66Met schizophrenia patients but increased SICI in Val66Met healthy controls (Strube et al., 2015b). In brief, SICI is thought to be mediated by the GABAA receptors of the M1 interneurons that modulate M1 excitability (Kujirai et al., 1993; Ziemann et al., 1996; Hanajima et al., 1998; for review see Rothwell et al., 2009; Ziemann et al., 2015). These studies imply that the relative impact of genetic polymorphisms on tDCS-induced effects may be task or disease specific. It is also possible that differences in genotype alter tDCS effect through impact on anatomical and neurophysiological states in individuals. For example, Met BDNF polymorphism carriers (Val66Met or Met66Met) showed differences in regional brain volumes and task-specific synchrony on EEG, which predicted performance on an error-processing task (Soltesz et al., 2014).
The Met/Val158Met/Val polymorphism of the catechol-O-methyltransferase (COMT) gene has also been explored. When tDCS was applied to the left dlPFC in a go-nogo task, anodal tDCS was unexpectedly inhibitory in Met58Met carriers, whilst cathodal tDCS was inhibitory in Val58Val carriers only (Plewnia et al., 2012; Nieratschker et al., 2014). The authors of these studies linked this to the aforementioned “inverted-U” theory of cognition of frontal dopamine levels. This theory suggests that optimal cognitive function depends on an optimal level of frontal dopamine and both too high and too low a level results in poorer cognitive function (Aguilera et al., 2008; Cools and D'Esposito, 2012). Therefore, the reason anodal tDCS inhibits in Met58Met carriers is because it is further increasing the frontal dopamine level in individuals who already have a high level, and vice versa for cathodal tDCS in Val58Val carriers.
Development and Aging
The effect of age on response to tDCS is probably non-trivial in both older and younger populations. Fujiyama et al. (2014) assessed age-related modulation of M1 excitability following anodal tDCS over the M1 in young (mean age 22.7 years) and elderly adults (mean age 68.3 years). They found that the facilitatory effect of anodal tDCS was initially greater in young adults, but that the facilitatory effect lasted much longer in elderly adults. Heise et al. (2014) demonstrated that anodal tDCS led to differential effects on SICI between young and elderly adults. Specifically, elderly adults showed increased SICI after anodal M1 tDCS, whereas young adults showed the “classical” finding of decreased SICI. These findings indicate that age-related variability of tDCS effects may occur through age-related functional changes in local circuits.
Although in the literature on applications of tDCS in infants and children are limited as compared with those to adults, tDCS is gradually being employed as a therapeutic tool for children with cerebral palsy, language disorder and dystonia (Andrade et al., 2014; Grecco et al., 2014; Young et al., 2014). Moliadze et al. (2014) reported that both anodal and cathodal tDCS at an intensity of 1 mA increased M1 excitability in healthy children (11–16 years old). However, at a 0.5-mA intensity, cathodal tDCS decreased M1 excitability whilst anodal tDCS had no effect, suggesting an interaction between tDCS intensity and developmental stage.
Several reasons may underlie the influence of age on tDCS. The process of normal development and aging leads to substantial changes in the structure, connectivity, and function of the brain at both microscopic and macroscopic levels. Accumulating evidence indicates that aging leads to modulations of synaptic connectivity, myelination, gene expression, and neurotransmission (Anderson and Rutledge, 1996; Zimerman and Hummel, 2010; for review see Burke and Barnes, 2006). Macroscopically, age-related changes are typically seen as atrophy of the brain, resulting in dilatation of sulci and ventricles, which would directly influence the current flow induced by tDCS as discussed above. In particular, aging leads to an increased distance between the skull and brain, and an increased proportion of CSF (Beauchamp et al., 2011; Kessler et al., 2013; Lockhart and Decarli, 2014). This is problematic because CSF has high conductivity compared to brain substance and may cause shunting of the current. Increases in extra-axial CSF space may therefore decrease current intensity at the cortical surface. Additionally, simulation of current distribution in children suggests that changes in the skull thickness and head circumference through childhood development affects current density of tDCS (Kessler et al., 2013). Specifically, the thinner skull appears to result in higher peak electrical fields in children than adults with the same tDCS intensity.
Given these potential sources of age-related variability in tDCS effects, studies should either seek to match ages between experimental groups or to account for the age effect during analysis.
Brain Lesions after Stroke and Injury
Using tDCS to improve outcomes, particularly motor and speech deficits after stroke, has proven a popular area of exploration (Holland and Crinion, 2012; Stagg and Johansen-Berg, 2013). However, there has been great variability in reported benefit (Hummel and Cohen, 2006; Tanaka et al., 2011a,b; Grefkes and Fink, 2012; Fusco et al., 2014; Lüdemann-Podubecká et al., 2014; O'Shea et al., 2014; de Aguiar et al., 2015). As well as great variability in protocols used in stroke studies, such as montage positioning and stimulation intensity, it is evident from stroke literature that interindividual heterogeneity is also a huge issue for using tDCS after injury. Indeed, appropriate understanding of participant characteristics is likely to be of even greater importance than in healthy populations studies, because of additional variability in injury type, injury extent and initial recovery.
Several stroke studies have attempted to elucidate the factors predicting behavioral improvement after tDCS. Patients with larger deficits and less surviving brain, assessed by lesion size (Bolognini et al., 2014), white matter tract integrity (Bradnam et al., 2012; Lindenberg et al., 2012), or level of impairment (Bradnam et al., 2012; Marquez et al., 2013; O'Shea et al., 2014), appear to experience less benefit with tDCS. In some cases, it has been reported that tDCS can even worsen the function of those with high levels of impairment (Bradnam et al., 2012). Specifically, using inhibitory cathodal tDCS on the contralesional hemisphere in severely impaired patients can produce a worsening effect. A possible explanation is that, in severely impaired patients, the contralesional hemisphere activity is having a compensatory effect that improves function rather than impairing recovering of the lesioned hemisphere (O'Shea et al., 2014). Other factors, such as a longer time post-injury (Marquez et al., 2013; O'Shea et al., 2014), greater preservation of key white matter tracts and increased baseline functional connectivity (Rosso et al., 2014), also appears to confer better response to tDCS. These studies suggest that having enough surviving brain, which is the neural substrate for tDCS-related improvement, is a crucial factor for achieving a good response to tDCS.
Electrode location is an especially important consideration with lesions. If large lesion size predicts poor response to tDCS, then this would imply that tDCS should have greatest benefit when targeting surviving brain. Indeed, a small study of chronic post-stroke aphasic patients demonstrated that the greatest behavioral benefit was seen in those patients who had peri-lesional areas closest to the tDCS electrode (Baker et al., 2010). Another study using inhibitory cathodal tDCS of the Broca's area homolog only led to improvements if patients had a Broca's lesion (Rosso et al., 2014).
Simulations of current distribution can assist in post-injury tDCS studies because the model can factor in the effect of a lesion, and researchers can adjust the montage accordingly (Datta et al., 2012b). A single-subject case study of tDCS with combined visual rehabilitation training after stroke demonstrated that the modeled electrical field correlated with areas of increased task-related fMRI activation, as well as improved behavioral outcomes (Halko et al., 2012). Post-injury MRI studies have also identified various markers of deficit and recovery, such as task-related fMRI activation with recovery after stroke (Grefkes and Fink, 2012; Stagg et al., 2012; Liew et al., 2014), and default mode network disruption after traumatic brain injury (Sharp et al., 2011). Therefore, combined neuroimaging-tDCS studies can help identify targets for tDCS after injury.
A unique consideration for injury studies is the interaction of tDCS and rehabilitative training. In healthy control studies, tDCS has been variously reported to boost or inhibit learning and performance of motor and cognitive tasks (Reis et al., 2009; Meinzer et al., 2014; Orban de Xivry and Shadmehr, 2014; Bortoletto et al., 2015). There has been similar variability in the reports of combined rehabilitative training and tDCS in the injured population. Several studies have reported greater behavioral improvement with combined tDCS and training, compared with training alone, in motor function (Middleton et al., 2014; Kasashima-shindo et al., 2015), motor function associated electrophysiology measures (Kim and Ko, 2013), aphasia (Wu et al., 2015), neglect (single case report) (Brem et al., 2014), and attention (Park et al., 2013b). However, some of the positive studies were conducted on extremely small numbers (n < 10). There here have also been many studies which have not found a benefit of tDCS over training alone, whether for motor (Geroin et al., 2011; Fusco et al., 2014; Viana et al., 2014) or cognitive effects (Leśniak et al., 2014), after stroke and traumatic brain injury. Furthermore, because tDCS is thought to act on the networks and brain regions concurrently active, the type of activity is likely to also be extremely important in determining final effect. Grossly similar tasks can differ in subtle but meaningful ways that lead to apparent variability in response to tDCS (McCambridge et al., 2011; Bardi et al., 2013; Miyaguchi et al., 2013; Horvath et al., 2014b, 2015). Other important considerations when combining tDCS with rehabilitative training include the timing of tDCS in relation to training, and how soon after injury to start training and tDCS.
Apparent Variability due to Variability in Outcome Assessment
Variability between the outcome measures used in different studies can create apparent differences in response to tDCS. This is certainly a problem in cognitive studies, where a wide variety of cognitive tasks are used. This issue is reviewed in detail elsewhere (Jacobson et al., 2012; Horvath et al., 2014b; de Aguiar et al., 2015). Motor studies have almost exclusively depended on change in MEP amplitude, evoked by TMS, as an outcome measure and the MEP amplitude is generally regarded as a reliable measurement of M1 excitability (Hallett, 2000, 2007). Indeed, changes in MEP amplitude are the only consistent outcome reported by motor tDCS studies (Horvath et al., 2014a).
However, researchers using tDCS should recognize that MEPs do actually exhibit high inter- and intra-individual variability. Technical factors include coil position or orientation, number of trials, the intertrial interval (ITI) and stimulus intensity. Physiological and psychological factors include attention, background muscle activity, and muscle fatigue. All these are potential confounds in assessing MEPs and can introduce inter and intra-individual variability that is independent of the effect of tDCS (Kiers et al., 1993; Darling et al., 2006).
For example, longer ITIs result in greater MEP amplitudes. Vaseghi et al. (2014) investigated effects of short- (4 s) and long- (10 s) ITIs on MEP sizes, and showed that sizes of the MEP amplitudes were around 1.3 times greater in the long ITI condition than the short ITI condition. Intraclass correlation (ICC) reflecting intra-trial reliability was 0.80–0.91 for the short ITI and 0.79–0.96 for the long ITI condition. Inter-session reliability was also tested by two MEP measurements with at least 48-h inter-session intervals. ICC for the inter-session reliability was 0.87 and 0.80 for the short and long ITI conditions, respectively. Likewise, Julkunen et al. (2012) showed that MEP amplitudes were greater in long ITI (5–10 s) than short ITI (1–5 s). Moreover, high intra-individual variability of MEPs was seen in the first 10 trials irrespective of the ITI. These findings indicate that, although the difference in ITI affects MEP amplitudes, intra-trial and inter-session reliability is relatively high irrespective of ITI. Nevertheless, we recommend using the same ITI between sessions and subjects for MEP measurement, and including a sufficient number of trials (>20 trials). Given that unstable MEPs are frequently seen in the first 10 trials (Julkunen et al., 2012), exclusion of these trials from averaged data is highly recommended to reduce variability.
Differences in intensity of the TMS test stimulus used to elicit an MEP may also lead to apparent variability of the MEP response to tDCS. Researchers in the field mostly use one of the two intensity criteria for MEP measurement: an intensity required to produce an MEP amplitude of 1 mV, or an intensity corresponding to 1.2 times (120%) of the resting motor threshold (RMT) that often produces MEPs <1 mV. The former method is frequently employed for assessing tDCS-induced changes in MEPs. Wiethoff et al. (2014) demonstrated that the size of the pre-tDCS MEP, a function of the intensity of the TMS test stimulus, affected response of the MEP to tDCS. M1 tDCS showed the classical anodal-excitatory/cathodal-inhibitory effect when using a TMS intensity that produced baseline MEP amplitudes of 1 mV. However, when a TMS intensity that produced small baseline MEPs (around 0.5 mV amplitude) was used, both anodal and cathodal tDCS increased MEP size. Furthermore, most studies only use a single intensity to evoke MEPs, and this convention may not always be adequate for testing the effect of a tDCS intervention. It may be better to employ a more refined measurement of corticospinal tract excitability that overcomes the limitation of the single-point sampling of stimulus intensity, for example by recording stimulus-response (S-R) curves of MEPs, called an MEP recruitment curve. The S-R curve is created by plotting MEP amplitudes against test stimulus intensity ranging from 80 to 160% of RMT in steps of 20%. A sigmoid-shaped S-R curve is obtained from healthy subjects, and is thought to reflect net corticospinal tract excitability as a function of stimulus intensity (Pitcher et al., 2003; Werhahn et al., 2007). An S-R curve allows researchers to assess MEP amplitudes in response to stimulation ranging over minimal and maximal intensities (Groppa et al., 2012; Temesi et al., 2014), which may serve a better measurement of the corticospinal tract excitability overall for detecting tDCS effects than a single-point MEP measurement. Variability in outcome measurements arising from a TMS protocol is a relatively controllable factor by a researcher to reduce inter- and individual variability in response to the tDCS.
Neural oscillations in the cortex beneath a coil may introduce variability in the response to TMS. Romei et al. (2008a,b) demonstrated that neural responses to TMS were dependent on fluctuations of EEG α activity. Indeed, Bergmann et al. (2012) developed an EEG-triggered TMS technique based on the spontaneous neocortical slow oscillations below 1 Hz from the C3 EEG electrode (left sensorimotor area). TMS stimuli were delivered selectively to the hand representation of M1 during either the up-states (i.e., peak of oscillations) or the down-states of the slow oscillation (i.e., trough of oscillations). They found that MEP amplitudes were significantly greater during the up-states than during the down-states, and the latency of MEP onset was significantly shorter during the up-states than during the down-states. That is, fluctuations of slow oscillatory brain activity appears to introduce variability in MEP amplitude, which may introduce variance when using MEPs as an outcome measure in tDCS motor studies, at both intra- and interindividual levels. Using temporal neuronavigation systems to deliver TMS based on online EEG signals may enable more controlled studies in the future.
Discussion
This review has provided an overview of the main interindividual biological factors currently known to produce variability in response to tDCS (Table 1). There are many proposed clinical and practical applications of tDCS, one of which is to use it as a biomarker for the neuroplastic effects of drugs acting on the central nervous system, as a screen for drug efficacy (Nitsche et al., 2012). However, the interpretation of such studies should take into account that variability of results reflects not only variability in response to the drug, but also in response to tDCS and an interaction of drug and tDCS response. Therefore, better understanding of how interindividual factors affect response to tDCS is an absolute prerequisite for successful application outside the lab.

Table 1. Summary of studies which have reported on the impact of interindividual features on tDCS effect.
Few studies have specifically sought to investigate the impact of physiological factors on inter- and intra-individual variability in tDCS response and the studies summarized in our review (Table 1) report the effect of interindividual factors in many different ways. For example, some studies report correlation coefficients between a biological factor and tDCS effect whilst other studies group participants based on a biological factor and report an ANOVA result of the interaction between participant groups and tDCS effects. Therefore, it is difficult to draw conclusions on the relative impact of such factors and grade them in order of importance. However, factors resulting in differences in the direction of tDCS-induced change are likely to introduce more variability at group level analyses than factors that only affect the size of tDCS-induced change (Table 1). It is these factors which most urgently need investigating, in order to better quantify their effects and thus to better decide the extent to which experimental designs need to account for them. For now, the best practice may be to homogenize participant recruitment in the factors that are easiest to control, such as age, handedness, baseline ability and, for injury studies, time after injury.
Unlike TMS, tDCS does not cause immediate depolarization of stimulated neurons. Rather, it is thought that tDCS modulates excitability of stimulated areas by interacting selectively with simultaneously active neuronal populations (Nitsche et al., 2008; Stagg and Nitsche, 2011). Therefore, the state of neuron populations during stimulation is likely to be one of the most important factors influencing the final behavioral effect. It is crucial to give proper consideration to these factors because they can influence the direction, as well as the extent, of behavioral modulation. This is likely to be especially important for studies of higher-level cognitive functions. Unfortunately, the pre- and post-stimulation states of participants is often not controlled, or even reported, in previous studies. Detailed reporting of participant characteristics, strict monitoring and control of participant neurophysiological states and behaviors are important first steps to teasing apart the complexity of interaction between baseline state, task features, and stimulation.
The impact of interindividual variability is also dependent on experimental design. In crossover designs, the assessment of tDCS effects requires outcome assessment at least two time-points, which introduces a within-subject factor of time. This makes the data acquired vulnerable to confounding by learning or order effects. For this reason, and for reasons of practicality, many studies recruit different cohorts for different intervention types. However, cross-section studies are particularly vulnerable to the effects of interindividual variability because, in these studies, a tDCS intervention (anodal, cathodal, and sham) becomes a between-subject factor. The variance of a between-subject factor (i.e., the variance between subject means) is largely down to interindividual variability (Lee et al., 2002). This may mask the effects of tDCS intervention and make the factor of interest (i.e., time-intervention interactions) harder to detect. However, using crossover designs, which minimize the effect of interindividual variability, requires the researchers to seek ways to either reduce learning and order effects (e.g., training on the task, randomization of stimulation order) or to incorporate learning into the study design.
Although this study has primarily discussed interindividual variability, many methodological factors can produce substantial variability across studies. These range from the seemingly trivial to absolute crucial, for example, how electrodes are attached, electrode size, amount of fluid in a sponge, montage (where, single/ opposition), and return electrode placement. There is little standardization in many of these parameters, especially in the cognitive field, but they can greatly influence current dose (Peterchev et al., 2012). Therefore, two studies aiming to produce the same effect may produce different results because their protocols vary. This lack of reproducibility of studies due to inconsistent methodology in the field may be mistakenly attributed to interindividual biological differences. The aim should be to standardize methodology where practicable and to promote the publication of methodology studies specifically exploring the impact of different technical parameters (such as montage, intensity, and duration) and protocols (such as timing of assessment). Seeking to understand and reduce the noise introduced by methodological variability is as important as investigating interindividual factors.
Several approaches can produce more useful studies. Encouraging the publication of negative studies (providing they are well-designed) would help reduce a publication bias that prevents an objective assessment of tDCS effect. Developing “biomarkers” for tDCS activity, such as MRS imaging of neurotransmitters, EEG and fMRI measures of local network changes, will enable more informative interpretation of results. For example, a negative behavioral result can be explained in physiological terms. Introducing the use of control tasks or control stimulation sites would increase the validity of results. Optimizing technical factors, such as using simulations based on individual metrics to maximize current density, could make the current density at the cortex sufficiently high such that its effect can overcome the interindividual variability. Finally, where practicable, sufficiently large samples should be recruited to avoid the risk of underpowered studies and to enable sub-group analyses that can elucidate the participant characteristics that are best associated with response.
Conclusion
We are all cognizant of how inter and intraindividual factors can alter the pharmacokinetics and pharmacodynamics of a drug, and thus its overall effect. It stands to reason that inter and intraindividual variability will have a similar impact on tDCS, which is an intervention that also interacts with individual physiology. It is often stated that tDCS has huge potential for clinical applications. We must now take more care in the designing, performing, analyzing, and reporting of studies, if we are to realize this potential and to not let it become consigned as a curious blip in the annals of scientific endeavor.
Conflict of Interest Statement
The authors declare that the research was conducted in the absence of any commercial or financial relationships that could be construed as a potential conflict of interest.
Acknowledgments
LL is supported by a Wellcome Trust Clinical Training Research Fellowship (UK). KU is supported by a postdoctoral fellow from Japan Society for the Promotion of Science (26·119). TH receives grants from Strategic Research Program for Brain Sciences (13021041) and Brain Mapping by Integrated Neurotechnologies for Disease Studies (14029038) from MEXT, JSPS KAKENHI Grant Number 26120008, Health and Labor Sciences Research Grants, and Intramural Research Grant for Neurological and Psychiatric Disorders of National Center of Neurology and Psychiatry, Japan.
References
Aguilera, M., Barrantes-Vidal, N., Arias, B., Moya, J., Villa, H., Ibanez, M. I., et al. (2008). Putative role of the COMT gene polymorphism (Val158Met) on verbal working memory functioning in a healthy population. Am. J. Med. Genet. B Neuropsychiatr. Genet. 147B, 898–902. doi: 10.1002/ajmg.b.30705
Anderson, B., and Rutledge, V. (1996). Age and hemisphere effects on dendritic structure. Brain 119, 1983–1990. doi: 10.1093/brain/119.6.1983
Andrade, A. C., Magnavita, G. M., Allegro, J. V., Neto, C. E., Lucena, R. C., and Fregni, F. (2014). Feasibility of transcranial direct current stimulation use in children aged 5 to 12 years. J. Child Neurol. 29, 1360–1365. doi: 10.1177/0883073813503710
Antal, A., Terney, D., Poreisez, C., and Paulus, W. (2007). Towards unravelling task-related modulations of neuroplastic changes induced in the human motor cortex. Eur. J. Neurosci. 26, 2687–2691. doi: 10.1111/j.1460-9568.2007.05896.x
Arlotti, M., Rahman, A., Minhas, P., and Bikson, M. (2012). Axon terminal polarization induced by weak uniform DC electric fields: a modeling study. Conf. Proc. IEEE Eng. Med. Biol. Soc. 2012, 4575–4578. doi: 10.1109/EMBC.2012.6346985
Baker, J. M., Rorden, C., and Fridriksson, J. (2010). Using transcranial direct-current stimulation to treat stroke patients with aphasia. Stroke 41, 1229–1236. doi: 10.1161/STROKEAHA.109.576785
Bardi, L., Kanai, R., Mapelli, D., and Walsh, V. (2013). Direct current stimulation (tDCS) reveals parietal asymmetry in local/global and salience-based selection. Cortex 49, 850–860. doi: 10.1016/j.cortex.2012.04.016
Beauchamp, M. S., Beurlot, M. R., Fava, E., Nath, A. R., Parikh, N. A., Saad, Z. S., et al. (2011). The developmental trajectory of brain-scalp distance from birth through childhood: implications for functional neuroimaging. PLoS ONE 6:e24981. doi: 10.1371/journal.pone.0024981
Bergmann, T. O., Molle, M., Schmidt, M. A., Lindner, C., Marshall, L., Born, J., et al. (2012). EEG-guided transcranial magnetic stimulation reveals rapid shifts in motor cortical excitability during the human sleep slow oscillation. J. Neurosci. 32, 243–253. doi: 10.1523/JNEUROSCI.4792-11.2012
Berryhill, M. E., and Jones, K. T. (2012). tDCS selectively improves working memory in older adults with more education. Neurosci. Letts. 521, 148–151. doi: 10.1016/j.neulet.2012.05.074
Bikson, M., Inoue, M., Akiyama, H., Deans, J. K., Fox, J. E., Miyakawa, H., et al. (2004). Effects of uniform extracellular DC electric fields on excitability in rat hippocampal slices in vitro. J. Physiol. 557, 175–190. doi: 10.1113/jphysiol.2003.055772
Bikson, M., Rahman, A., and Datta, A. (2012). Computational models of transcranial direct current stimulation. Clin. EEG Neurosci. 43, 176–183. doi: 10.1177/1550059412445138
Bikson, M., Rahman, A., Datta, A., Fregni, F., and Merabet, L. (2013). High-resolution modeling assisted design of customised and individualised transcranial direct current stimulation protocols. Neuromodulation 15, 306–315. doi: 10.1111/j.1525-1403.2012.00481.x
Bolognini, N., Convento, S., Banco, E., Mattioli, F., Tesio, L., and Vallar, G. (2014). Improving ideomotor limb apraxia by electrical stimulation of the left posterior parietal cortex. Brain 138, 428–439. doi: 10.1093/brain/awu343
Bortoletto, M., Pellicciari, M. C., Rodella, C., and Miniussi, C. (2015). The interaction with task-induced activity is more important than polarization: a tDCS study. Brain Stimul. 8, 269–276. doi: 10.1016/j.brs.2014.11.006
Bradnam, L. V., Stinear, C. M., Barber, P. A., and Byblow, W. D. (2012). Contralesional hemisphere control of the proximal paretic upper limb following stroke. Cereb. Cortex 22, 2662–2671. doi: 10.1093/cercor/bhr344
Brem, A. K., Unterburger, E., Speight, I., and Jancke, L. (2014). Treatment of visuospatial neglect with biparietal tDCS and cognitive training: a single-case study. Front. Syst. Neurosci. 8:180. doi: 10.3389/fnsys.2014.00180
Brunoni, A. R., Kemp, A. H., Shiozawa, P., Cordeiro, Q., Valiengo, L. C., Goulart, A. C., et al. (2013). Impact of 5-HTTLPR and BDNF polymorphisms on response to sertraline versus transcranial direct current stimulation: implications for the serotonergic system. Eur. Neuropsychopharmacol. 23, 1530–1540. doi: 10.1016/j.euroneuro.2013.03.009
Burke, S. N., and Barnes, C. A. (2006). Neural plasticity in the ageing brain. Nat. Rev. Neurosci. 7, 30–40. doi: 10.1038/nrn1809
Cheeran, B., Talelli, P., Mori, F., Suppa, A., Edwards, M., Houlden, H., et al. (2008). A common polymorphism in the brain-derived neurotrophic factor gene (BDNF) modulates human cortical plasticity and the response to rTMS. J. Physiol. 586, 5717–5725. doi: 10.1113/jphysiol.2008.159905
Clow, A., Law, R., Evans, P., Vallence, A. M., Hodyl, N. A., Goldsworthy, M. R., et al. (2014). Day differences in the cortisol awakening response predict day differences in synaptic plasticity in the brain. Stress 17, 219–223. doi: 10.3109/10253890.2014.905533
Cools, R., and D'Esposito, M. (2012). Inverted U-shaped dopamine actions on human working memory and cognitive control. Biol. Psychiatry 69, 113–125. doi: 10.1016/j.biopsych.2011.03.028
Darling, W. G., Wolf, S. L., and Butler, A. J. (2006). Variability of motor potentials evoked by transcranial magnetic stimulation depends on muscle activation. Exp. Brain Res. 174, 376–385. doi: 10.1007/s00221-006-0468-9
Datta, A., Baker, J. M., Bikson, M., and Fridriksson, J. (2012b). Individualized model predicts brain current flow during transcranial direct-current stimulation treatment in response stroke patient. Brain Stimul. 4, 169–174. doi: 10.1016/j.brs.2010.11.001
Datta, A., Truong, D., Minhas, P., Parra, L. C., and Bikson, M. (2012a). Interindividual variation during transcranial direct current stimulation and normalization of dose using MRI-derived computational models. Front. Psychiatry 3:91. doi: 10.3389/fpsyt.2012.00091
Day, B. L., Dressler, D., Maertens de Noordhout, A., Marsden, C. D., Nakashima, K., Rothwell, J. C., et al. (1989). Electric and magnetic stimulation of human motor cortex: surface EMG and single motor unit responses. J. Physiol. 412, 449–473. doi: 10.1113/jphysiol.1989.sp017626
de Aguiar, V., Paolazzi, C. L., and Miceli, G. (2015). tDCS in post-stroke aphasia: the role of stimulation parameters, behavioral treatment and patient characteristics. Cortex 63, 296–316. doi: 10.1016/j.cortex.2014.08.015
Di Lazzaro, V., Oliviero, A., Pilato, F., Saturno, E., Dileone, M., Mazzone, P., et al. (2004). The Physiological basis of transcranial motor cortex stimulation in conscious humans. Clin. Neurophysiol. 115, 255–266. doi: 10.1016/j.clinph.2003.10.009
Di Lazzaro, V., Oliviero, A., Profice, P., Saturno, E., Pilato, F., Insola, A., et al. (1998). Comparison of descending volleys evoked by transcranial magnetic and electric stimulation in conscious humans. Electroencephalogr. Clin. Neurophysiol. 109, 397–401. doi: 10.1016/S0924-980X(98)00038-1
Di Lazzaro, V., Profice, P., Ranieri, F., Capone, F., Dileone, M., Oliviero, A., et al. (2012). I-wave origin and modulation. Brain Stimul. 5, 512–525. doi: 10.1016/j.brs.2011.07.008
Di Lazzaro, V., and Rothwell, J. C. (2014). Corticospinal activity evoked and modulated by non-invasive stimulation of the intact human motor cortex. J. Physiol. 19, 4115–4128. doi: 10.1113/jphysiol.2014.274316
Fresnoza, S., Paulus, W., Nitsche, M. A., and Kuo, M. F. (2014). Nonlinear dose-dependent impact of d1 receptor activation on motor cortex plasticity in humans. J. Neurosci. 34, 2744–2753. doi: 10.1523/JNEUROSCI.3655-13.2014
Fujiyama, H., Hyde, J., Hinder, M. R., Kim, S. J., McCormack, G. H., Vickers, J. C., et al. (2014). Delayed plastic responses to anodal tDCS in older adults. Front. Aging Neurosci. 6:115. doi: 10.3389/fnagi.2014.00115
Furuya, S., Klaus, M., Nitsche, M. A., Paulus, W., and Altenmuller, E. (2014). Ceiling effects prevent further improvement of transcranial stimulation in skilled musicians. J. Neurosci. 34, 13834–13839. doi: 10.1523/JNEUROSCI.1170-14.2014
Fusco, A., Assenza, F., Iosa, M., Izzo, S., Altavilla, R., Paolucci, S., et al. (2014). The ineffective role of cathodal tDCS in enhancing the functional motor outcomes in early phase of stroke rehabilitation: an experimental trial. Biomed. Res. Int. 2014:547290. doi: 10.1155/2014/547290
Geroin, C., Picelli, A., Munari, D., Waldner, A., Tomelleri, C., and Smania, N. (2011). Combined transcranial direct current stimulation and robot-assisted gait training in patients with chronic stroke: a preliminary comparison. Clin. Rehabil. 25, 537–548. doi: 10.1177/0269215510389497
Grecco, L. A. C., de Almeida Carvalho Duarte, N., Mendonça, M. E., Cimolin, V., Galli, M., Fregni, F., et al. (2014). Transcranial direct current stimulation during treadmill training in children with cerebral palsy: a randomized controlled double-blind clinical trial. Res. Dev. Disabil. 35, 2840–2848. doi: 10.1016/j.ridd.2014.07.030
Grefkes, C., and Fink, G. R. (2012). Disruption of motor network connectivity post-stroke and its noninvasive neuromodulation. Curr. Opin. Neurol. 25, 670–675. doi: 10.1097/WCO.0b013e3283598473
Groppa, S., Werner-Petroll, N., Münchau, A., Deuschl, G., Ruschworth, M. F. S., and Siebner, H. R. (2012). A novel dual-site transcranial magnetic stimulation paradigm to probe fast facilitatory inputs from ipsilateral dorsal premotor cortex to primary motor cortex. Neuroimage 62, 500–509. doi: 10.1016/j.neuroimage.2012.05.023
Halko, D. A., Datta, A., Plow, E. B., Scaturro, J., Bikson, M., and Merabet, L. B. (2012). Neuroplastic changes following rehabilitative training correlate with regional electrical field induced with tDCS. Neuroimage 57, 885–891. doi: 10.1016/j.neuroimage.2011.05.026
Hallett, M. (2000). Transcranial magnetic stimulation and the human brain. Nature 406, 147–150. doi: 10.1038/35018000
Hallett, M. (2007). Transcranial magnetic stimulation: a primer. Neuron 55, 187–199. doi: 10.1016/j.neuron.2007.06.026
Hamada, M., Murase, N., Hasan, A., Baiaratnam, M., and Rothwell, J. C. (2013). The role of interneuron networks in driving human motor cortical plasticity. Cereb. Cortex 23, 1593–1605. doi: 10.1093/cercor/bhs147
Hanajima, R., Ugawa, Y., Terao, Y., Sasaki, K., Furubayashi, T., Machii, K., et al. (1998). Paired−pulse magnetic stimulation of the human motor cortex: differences among I waves. J. Physiol. 509, 607–618. doi: 10.1111/j.1469-7793.1998.607bn.x
Hasan, A., Misewitsch, K., Nitsche, M. A., Gruber, O., Padberg, F., Falkai, P., et al. (2013). Impaired motor cortex responses in non-psychotic first-degree relatives of schizophrenia patients: a cathodal tDCS pilot study. Brain Stimul. 6, 821–829. doi: 10.1016/j.brs.2013.03.001
Heise, K. F., Niehoff, M., Feldheim, J. F., Liuzzi, G., Gerloff, C., and Hummel, F. C. (2014). Differential behavioral and physiological effects of anodal transcranial direct current stimulation in healthy adults of younger and older age. Front. Aging Neurosci. 6:146. doi: 10.3389/fnagi.2014.00146
Hinder, M. R., Goss, E. L., Fujiyama, H., Canty, A. J., Garry, M. I., Rodger, J., et al. (2014). Inter- and Intra-individual variability following intermittent theta burst stimulation: implications for rehabilitation and recovery. Brain Stimul. 7, 365–371. doi: 10.1016/j.brs.2014.01.004
Holland, R., and Crinion, J. (2012). Can tDCS enhance treatment of aphasia after stroke? Aphasiology 26, 1169–1191. doi: 10.1080/02687038.2011.616925
Horvath, J. C., Carter, O., and Forte, J. D. (2014b). Transcranial direct current stimulation: five important issues we aren't discussing (but probably should be). Front. Syst. Neurosci. 8:2. doi: 10.3389/fnsys.2014.00002
Horvath, J. C., Forte, J. D., and Carter, O. (2014a). Evidence that transcranial direct current stimulation (tDCS) generates little-to-no reliable neurophysiologic effect beyond MEP amplitude modulation in healthy human subjects: a systematic review. Neuropsychologia, 66, 213–236. doi: 10.1016/j.neuropsychologia.2014.11.021
Horvath, J. C., Forte, J. D., and Carter, O. (2015). Quantitative review finds no evidence of cognitive effects in healthy populations from single-session transcranial direct current stimulation (tDCS). Brain Stimul. doi: 10.1016/j.brs.2015.01.400. [Epub ahead of print].
Hummel, F. C., and Cohen, L. G. (2006). Non-invasive brain stimulation: a new strategy to improve neurorehabilitation after stroke? Lancet Neurol. 5, 708–712. doi: 10.1016/S1474-4422(06)70525-7
Jacobson, L., Koslowsky, M., and Lavidor, M. (2012). tDCS polarity effects in motor and cognitive domains: a meta-analytical review. Exp. Brain Res. 216, 1–10. doi: 10.1007/s00221-011-2891-9
Jones, K. T., and Berryhill, M. E. (2012). Parietal contributions to visual working memory depend on task difficulty. Front. Psychiatry 3:81. doi: 10.3389/fpsyt.2012.00081
Julkunen, P., Säisänen, L., Hukkanen, T., Danner, N., and Könönen, M. (2012). Does second-scale intertrial interval affect motor evoked potentials induced by single-pulse transcranial magnetic stimulation? Brain Stimul. 5, 526–532. doi: 10.1016/j.brs.2011.07.006
Kasahara, K., Tanaka, S., Hanakawa, T., Senoo, A., and Honda, M. (2013). Lateralization of activity in the parietal cortex predicts the effectiveness of bilateral transcranial direct current stimulation on performance of a mental calculation task. Neurosci. Lett. 545, 86–90. doi: 10.1016/j.neulet.2013.04.022
Kasashima-shindo, Y., Fujiwara, T., Ushiba, J., Matsushika, Y., Kamatani, D., Oto, M., et al. (2015). Brain-computer interface training combined with transcranial direct current stimulation in patients with chronic severe hemiparesis: proof of concept study. J. Rehabil. Med. 47, 318–324. doi: 10.2340/16501977-1925
Kessler, S. K., Minhas, P., Woods, A. J., Rosen, A., Gorman, C., and Bikson, M. (2013). Dosage considerations for transcranial direct current stimulation in children: a computational modeling study. PLoS ONE 8:e76112. doi: 10.1371/journal.pone.0076112
Kiers, L., Cros, D., Chiappa, K. H., and Fang, J. (1993). Variability of motor potentials evoked by transcranial magnetic stimulation. Electroencephalogr. Clin. Neurophysiol. 89, 415–423. doi: 10.1016/0168-5597(93)90115-6
Kim, G. W., and Ko, M. H. (2013). Facilitation of corticospinal tract excitability by transcranial direct current stimulation combined with voluntary grip exercise. Neurosci. Lett. 548, 181–184. doi: 10.1016/j.neulet.2013.05.037
Kim, J. H., Kim, D. W., Chan, W. H., Kim, Y. H., Kim, K., and Im, C. H. (2014a). Inconsistent outcomes of transcranial direct current stimulation may originate from anatomical differences among individuals: electric field simulation using individual MRI data. Neurosci. Lett. 564, 6–10. doi: 10.1016/j.neulet.2014.01.054
Kim, S., Stephenson, M., Morris, P. G., and Jackson, S. R. (2014b). tDCS-induced alterations in GABA concentration within primary motor cortex predict motor learning and motor memory: A 7T magnetic resonance spectroscopy study. Neuroimage 99, 237–243. doi: 10.1016/j.neuroimage.2014.05.070
Krause, B., Márquez-ruiz, J., and Kadosh, R. C. (2013). The effect of transcranial direct current stimulation: a role for cortical excitation/inhibition balance? Front. Hum. Neurosci. 7:602. doi: 10.3389/fnhum.2013.00602
Kujirai, T., Caramia, M., Rothwell, J., Day, B., Thompson, P., Ferbert, A., et al. (1993). Corticocortical inhibition in human motor cortex. J. Physiol. 471, 501–519. doi: 10.1113/jphysiol.1993.sp019912
Lang, N., Nitsche, M. A., Dileone, M., Mazzone, P., De Andrés-Arés, J., Diaz-Jara, L., et al. (2011). Transcranial direct current stimulation effects on I-wave activity in humans. J. Neurophysiol. 105, 2802–2810. doi: 10.1152/jn.00617.2010
Lee, Y., Shao, J., Chow, S.-C., and Wang, H. (2002). Tests for inter-subject and total variabilities under crossover designs. J. Biopharm. Stat. 12, 503–534. doi: 10.1081/BIP-120016233
Leśniak, M., Polanowska, K., Seniow, J., and Czlonkowska, A. (2014). Effects of repeated anodal tDCS coupled with cognitive training for patients with severe traumatic brain injury: a pilot randomized controlled trial. J. Head Trauma Rehabil. 29, E20–E29. doi: 10.1097/HTR.0b013e318292a4c2
Liew, S. L., Santernecchi, E., Buch, E. R., and Cohen, L. G. (2014). Non-invasive brain stimulation in neurorehabilitation: local and distant effects for motor recovery. Front. Hum. Neurosci. 8:378. doi: 10.3389/fnhum.2014.00378
Lindenberg, R., Zhu, L. L., Rüber, T., and Schlaug, G. (2012). Predicting functional motor potential in chronic stroke patients using diffusion tensor imaging. Hum. Brain Mapp. 33, 1040–1051. doi: 10.1002/hbm.21266
Lockhart, S. N., and Decarli, C. (2014). Structural imaging measures of brain aging. Neuropsychol. Rev. 271–289. doi: 10.1007/s11065-014-9268-3
López-Alonso, V., Cheeran, B., Río-Rodríguez, D., and Fernández-Del-Olmo, M. (2014). Interindividual variability in response to non-invasive brain stimulation paradigms. Brain Stimul. 7, 372–380. doi: 10.1016/j.brs.2014.02.004
Lüdemann-Podubecká, J., Bösl, K., Rothhardt, S., Verheyden, G., and Nowak, D. A. (2014). Transcranial direct current stimulation for motor recovery of upper limb function after stroke. Neurosci. Biobehav. Rev. 47, 245–259. doi: 10.1016/j.neubiorev.2014.07.022
Marquez, J., van Vliet, P., McElduff, P., Lagopoulos, J., and Parsons, M. (2013). Transcranial direct current stimulation (tDCS): does it have merit in stroke rehabilitation? A systematic review. Int. J. Stroke 10, 306–316. doi: 10.1111/ijs.12169
Marshall, L., Möll, M., Hallschmid, M., and Born, J. (2004). Transcranial direct current stimulation during sleep improves declarative memory. J. Neurosci. 24, 9985–9992. doi: 10.1523/JNEUROSCI.2725-04.2004
McCambridge, A. B., Bradnam, L. V., Stinear, C., and Byblow, W. D. (2011). Cathodal transcranial direct current stimulation of the primary motor cortex improves selective muscle activation in the ipsilateral arm. J. Neurophysiol. 105, 2937–2942. doi: 10.1152/jn.00171.2011
Meinzer, M., Jähnigen, S., Copland, D. A., Darkow, R., Grittner, U., Avirame, K., et al. (2014). Transcranial direct current stimulation over multiple days improves learning and maintenance of a novel vocabulary. Cortex 50, 137–147. doi: 10.1016/j.cortex.2013.07.013
Metwally, M. K., Cho, Y. S., Park, H. J., and Kim, T. S. (2012). Investigation of the electric field components of tDCS via anisotropically conductive gyri-specific Finite Element Head Models. Conf. Proc. IEEE Eng. Med. Biol. Soc. 2012, 5514–5517. doi: 10.1109/EMBC.2012.6347243
Middleton, A., Fritz, S. L., Liuzzo, D. M., Newman-Norlund, R., Herter, T. M., et al. (2014). Using clinical and robotic assessment tools to examine the feasibility of pairing tDCS with upper extremity physical therapy in patients with stroke and TBI: a consideration-of-concept pilot study. Neurorehabilitation 35, 741–754. doi: 10.3233/NRE-141178
Missitzi, J., Gentner, R., Geladas, N., Politis, P., Karandreas, N., Classen, J., et al. (2011). Plasticity in human motor cortex is in part genetically determined. J. Physiol. 589, 297–306. doi: 10.1113/jphysiol.2010.200600
Miyaguchi, S., Onishi, H., Kojima, S., Sugawara, K., Tsubaki, A., Kirimoto, H., et al. (2013). Corticomotor excitability induced by anodal transcranial direct current stimulation with and without non-exhaustive movement. Brain Res. 1529, 83–91. doi: 10.1016/j.brainres.2013.07.026
Moliadze, V., Schmanke, T., Andreas, S., Lyzhko, E., Freitag, C. M., and Siniatchkin, M. (2014). Stimulation intensities of transcranial direct current stimulation have to be adjusted in children and adolescents. Clin. Neurophysiol. doi: 10.1016/j.clinph.2014.10.142. [Epub ahead of print].
Monte-silva, K., Liebetanz, D., Grundey, J., Paulus, W., and Nitsche, M. A. (2010). Dosage-dependent non-linear effect of L-dopa on human motor cortex plasticity. J. Physiol. 588, 3415–3424. doi: 10.1113/jphysiol.2010.190181
Müller-Dahlhaus, J. F. M., Orekhov, Y., Liu, Y., and Ziemann, U. (2008). Interindividual variability and age-dependency of motor cortical plasticity induced by paired associative stimulation. Exp. Brain Res. 187, 467–475. doi: 10.1007/s00221-008-1319-7
Nader, N., Chrousos, G. P., and Kino, T. (2010). Interactions of the Circadian CLOCK system and the HPA axis. Trends Endocrinol. Metab. 21, 277–286. doi: 10.1016/j.tem.2009.12.011
Neuling, T., Rach, S., and Herrmann, C. S. (2013). Orchestrating neuronal networks: sustained after-effects of transcranial alternating current stimulation depend upon brain states. Front. Hum. Neurosci. 7:161. doi: 10.3389/fnhum.2013.00161
Nieratschker, V., Kiefer, C., Giel, K., Krüger, R., and Plewnia, C. (2014). The COMT val/met polymorphism modulates effects of tDCS on response inhibition. Brain Stimul. 8, 283–288. doi: 10.1016/j.brs.2014.11.009
Nitsche, M. A., Cohen, L. G., Wasserman, E. M., Priori, A., Lang, N., Antal, A., et al. (2008). Transcranial direct current stimulation: state of the art. Brain Stimul. 1, 206–223. doi: 10.1016/j.brs.2008.06.004
Nitsche, M. A., Muller-Dahlhaus, F., Paulus, W., and Ziemann, U. (2012). The pharmacology of neuroplasticity induced by non-invasive brain stimulation: building models for the clinical use of CNS active drugs. J. Physiol. 290, 4641–4662. doi: 10.1113/jphysiol.2012.232975
Nitsche, M. A., and Paulus, W. (2000). Excitability changes induced in the human motor cortex by weak transcranial direct current stimulation. J. Physiol. 527, 633–639. doi: 10.1111/j.1469-7793.2000.t01-1-00633.x
Nitsche, M. A., and Paulus, W. (2001). Sustained excitability elevations induced by transcranial DC motor cortex stimulation in humans. Neurology 57, 1899–1901. doi: 10.1212/WNL.57.10.1899
O'Shea, J., Boudrias, M. H., Stagg, C. J., Bachtair, V., Kischka, U., Blicher, J. U., et al. (2014). Predicting behavioural response to TDCS in chronic motor stroke. Neuroimage 85, 924–933. doi: 10.1016/j.neuroimage.2013.05.096
Ono, M., Kubik, S., and Abernathy, C. D. (1990). Atlas of the Cerebral Sulci, 1st Edn. Stuttgart; New York, NY: George Thieme Verlag.
Opitz, A., Paulus, W., Will, S., Antunes, A., and Thielscher, A. (2015). Determinants of the electrcial field during transcranial direct current stimulation. Neuroimage 109, 140–150. doi: 10.1016/j.neuroimage.2015.01.033
Orban de Xivry, J. J., and Shadmehr, R. (2014). Electrifying the motor engram: effects of tDCS on motor learning and control. Exp. Brain Res. 232, 3379–3395. doi: 10.1007/s00221-014-4087-6
Park, C. H., Chang, W. H., Park, J. Y., Shin, Y. I., Kim, S. T., and Kim, Y. H. (2013a). Transcranial direct current stimulation increases resting state interhemispheric connectivity. Neurosci. Lett. 539, 7–10. doi: 10.1016/j.neulet.2013.01.047
Park, S. H., Koh, E. J., Choi, H. Y., and Ko, M. H. (2013b). A double-blind, sham-controlled, pilot study to assess the effects of the concomitant use of transcranial direct current stimulation with the computer assisted cognitive rehabilitation to the prefrontal cortex on cognitive functions in patients with stroke. J. Korean Neurosurg. Soc. 54, 484–488. doi: 10.3340/jkns.2013.54.6.484
Peterchev, A. V., Wagner, T. A., Miranda, P. C., Nitsche, M. A., Paulus, W., Lisanby, S. H., et al. (2012). Fundamentals of transcranial electrical and magnetic stimulation dose: definition, selection and reporting practices. Brain Stimul 5, 435–453. doi: 10.1016/j.brs.2011.10.001
Pitcher, J. B., Ogston, K. M., and Miles, T. S. (2003). Age and sex differences in human motor cortex input-output characteristics. J. Physiol. 546, 605–613. doi: 10.1113/jphysiol.2002.029454
Plewnia, C., Zwissler, B., Längst, I., Maurer, B., Giel, K., and Krüger, R. (2012). Effects of transcranial direct current stimulation (tDCS) on executive functions: influence of COMT Val/Met polymorphism. Cortex 9, 3–9. doi: 10.1016/j.cortex.2012.11.002
Purpura, D. P., and McMurtry, J. G. (1965). Intracellular activities and evoked potential changes during polarization of motor cortex. J. Neurophysiol. 28, 166–185
Rademacher, J., Caviness, V. S. Jr. Steinmetz, H., and Galaburda, A. M. (1993). Topographical variation of the human primary cortices: implications for neuroimaging, brain mapping, and neurobiology. Cereb. Cortex 3, 313–329. doi: 10.1093/cercor/3.4.313
Rahman, A., Reato, D., Arlotti, M., Gasca, F., Datta, A., Parra, L. C., et al. (2013). Cellular effects of acute direct current stimulation: somatic and synaptic terminal effects. J. Physiol. 591, 2563–2578. doi: 10.1113/jphysiol.2012.247171
Reis, J., Schambra, H. M., Cohen, L. G., Buch, E. R., Fritsch, B., and Zarahn, E. (2009). Noninvasive cortical stimulation enhances motor skill acquisition over multiple days through an effect on consolidation. Proc. Natl. Acad. Sci. U.S.A. 106, 1590–1595. doi: 10.1073/pnas.0805413106
Ridding, M. C., and Ziemann, U. (2010). Determinants of the induction of cortical plasticity by non-invasive brain stimulation in healthy subjects. J. Physiol. 588, 2291–2304. doi: 10.1113/jphysiol.2010.190314
Romei, V., Brodbeck, V., Michel, C., Amedi, A., Pascual-Leone, A., and Thut, G. (2008a). Spontaneous fluctuations in posterior α-band EEG activity reflect variability in excitability of human visual areas. Cereb. Cortex 18, 2010–2018. doi: 10.1093/cercor/bhm229
Romei, V., Rihs, T., Brodbeck, V., and Thut, G. (2008b). Resting electroencephalogram alpha-power over posterior sites indexes baseline visual cortex excitability. Neuroreport 19, 203–208. doi: 10.1097/WNR.0b013e3282f454c4
Rosso, C., Valabregu, R., Arbizu, C., Ferrieux, S., Vargas, P., and Humbert, F. (2014). Connectivity between right inferior frontal gyrus and supplementary motor area predicts after-effects of right frontal cathodal tDCS on picture naming speed. Brain Stimul. 7, 122–129. doi: 10.1016/j.brs.2013.08.007
Rothwell, J. C. (1997). Techniques and mechanisms of action of transcranial stimulation of the human motor cortex. J. Neurosci. Methods 74, 113–122. doi: 10.1016/S0165-0270(97)02242-5
Rothwell, J. C., Day, B. L., Thompson, P. D., and Kujirai, T. (2009). Short latency intracortical inhibition: one of the most popular tools in human motor neurophysiology. J. Physiol. 587, 11–12. doi: 10.1113/jphysiol.2008.162461
Russell, M. J., Goodman, T., Pierson, R., Shepherd, S., Wang, Q., Groshong, B., et al. (2013). Individual differences in transcranial electrical stimulation current density. J. Biomed. Res. 27, 495–508. doi: 10.7555/JBR.27.20130074
Sakai, K., Ugawa, Y., Terao, Y., Hanajima, R., Furubayashi, T., and Kanazawa, I. (1997). Preferential activation of different I waves by transcranial magnetic stimulation with a figure-of-eight-shaped coil. Exp. Brain Res. 113, 24–32. doi: 10.1007/BF02454139
Sandrini, M., Fertonani, A., Cohen, L. G., and Miniussi, C. (2012). Double dissociation of working memory load effects induced by bilateral parietal modulation. Neuropsychologia 50, 396–402. doi: 10.1016/j.neuropsychologia.2011.12.011
Sarkar, A., Dowker, A., and Kadosh, R. C. (2014). Cognitive enhancement or cognitive cost: trait-specific outcomes of brain stimulation in the case of mathematics anxiety. J. Neurosci. 34, 16605–16610. doi: 10.1523/JNEUROSCI.3129-14.2014
Schade, S., Moliadze, V., Paulus, W., and Antal, A. (2012). Modulating neuronal excitability in the motor cortex with tDCS shows moderate hemispheric asymmetry due to subject's handedness: a pilot study. Restor. Neurol. Neurosci. 30, 1–8. doi: 10.3233/RNN-2012-110175
Shahbabaie, A., Golesorkhi, M., Zamanian, B., Ebrahimpoor, M., Keshvari, F., Nejati, V., et al. (2014). State dependent effect of transcranial direct current stimulation (tDCS) on methamphetamine craving. Int. J. Neuropsychopharm. 17, 1591–1598. doi: 10.1017/S1461145714000686
Shahid, S., Wen, P., and Ahfock, T. (2014a). Assessment of electric field distribution in anisotropic cortical and subcortical regions under the influence of tDCS. Bioelectromagnetics 35, 41–57. doi: 10.1002/bem.21814
Shahid, S. S., Bikson, M., Salman, H., Wen, P., and Ahfock, T. (2014b) The value and cost of complexity in predictive modelling: role of tissue anisotropy conductivity and fibre tracts in neuromodulation. J. Neural. Eng. 11:036002. doi: 10.1088/1741-2560/11/3/036002
Shahid, S., Wen, P., and Ahfock, T. (2012). Numerical investigation of white matter anisotropic conductivity in defining current distribution under tDCS. Comput. Methods Prog. Biomed. 109, 48–64. doi: 10.1016/j.cmpb.2012.09.001
Sharp, D. J., Beckmann, C. F., Greenwood, R., Kinnunen, K. M., Bonnelle, V., De Boissezon, X., et al. (2011). Default mode network functional and structural connectivity after traumatic brain injury. Brain 134, 2233–2247. doi: 10.1093/brain/awr175
Soltesz, F., Suckling, J., Lawrence, P., Tait, R., Ooi, C., Bentley, G., et al. (2014). Identification of BDNF sensitive electrophysiological markers of synaptic activity and their structural correlates in healthy subjects using a genetic approach utilizing the functional BDNF Val66Met polymorphism. PLoS ONE 9:e955558. doi: 10.1371/journal.pone.0095558
Stagg, C. J., Bachtair, V., Amadi, U., Gudberg, C. A., Ilie, A. S., Sampaio-Baptista, C., et al. (2014). Local GABA concentration is related to network-level resting functional connectivity. Elife 3:e01465. doi: 10.7554/eLife.01465
Stagg, C. J., Bachtair, V., O'Shea, J., Allman, C., Bosnell, R. A., Kischka, U., et al. (2012). Cortical changes underlying stimulation-induced behavioural gains in chronic stroke. Brain 135, 276–284. doi: 10.1093/brain/awr313
Stagg, C. J., Bachtiar, V., and Johansen-Berg, H. (2011). The role of GABA in human motor learning. Curr. Biol. 21, 480–484. doi: 10.1016/j.cub.2011.01.069
Stagg, C. J., Best, J. G., Stephensen, M. C., O'Shea, J., Wylezinska, M., Kincses, Z. T., et al. (2009). Polarity-sensitive modulation of cortical neurotransmitters by transcranial stimulation. J. Neurosci. 29, 5202–5206. doi: 10.1523/JNEUROSCI.4432-08.2009
Stagg, C. J., and Johansen-Berg, H. (2013). Studying the effects of transcranial direct-current stimulation in stroke recovery using magnetic resonance imaging. Front. Hum. Neurosci. 7:857. doi: 10.3389/fnhum.2013.00857
Stagg, C. J., and Nitsche, M. A. (2011). Physiological basis of transcranial direct current stimulation. Neuroscientist 17, 37–53. doi: 10.1177/1073858410386614
Strube, W., Bunse, T., Malchow, B., and Hasan, A. (2015b). Efficacy and interindividual variability in motor-cortex plasticity following anodal tDCS and paired-associated stimulation. Neural Plast. 2015:530423. doi: 10.1155/2015/530423
Strube, W., Nitsche, M. A., Wobrock, T., Bunse, T., Rein, B., Herrmann, M., et al. (2015a). BDNF-Val66Met-polymorphism impact on cortical plasticity in schizophrenia patients: a proof of concept study. Int. J. Neuropsychopharmacol. 18, 1–11. doi: 10.1093/ijnp/pyu040
Suh, H. S., Lee, W. H., and Kim, T. (2012). Influence of anisotropic conductivity in the skull and white matter on transcranial direct current stimulation via an anatomically realistic finite element head model. Phys. Med. Biol. 57, 6961–6980. doi: 10.1088/0031-9155/57/21/6961
Tanaka, S., Sandrini, M., and Cohen, L. G. (2011b). Modulation of motor learning and memory formation by non-invasive cortical stimulation of the primary motor cortex. Neuropsychol. Rehabil. 21, 650–675. doi: 10.1080/09602011.2011.605589
Tanaka, S., Takeda, K., Otaka, Y., Kita, K., Osu, R., Honda, M., et al. (2011a). Single session of transcranial direct-current stimulation transiently increases knee extensor force in patients with hemiparetic stroke. Neurorehabil. Neural Repair 25, 565–569. doi: 10.1177/1545968311402091
Tanaka, T., Takano, Y., Tanaka, S., Hironaka, N., Kobayashi, K., Hanakawa, T., et al. (2013). Transcranial direct-current stimulation increases extracellular dopamine levels in the rat striatum. Front. Syst. Neurosci. 7:6. doi: 10.3389/fnsys.2013.00006
Temesi, J., Gruet, M., Rupp, T., Verges, S., and Millet, G. Y. (2014). Resting and active motor thresholds versus stimulus-response curves to determine transcranial magnetic stimulation intensity in quadriceps femoris. J. Neuroeng. Rehabil. 11:40. doi: 10.1186/1743-0003-11-40
Teo, J. T. H., Bentley, G., Lawrence, P., Soltesz, F., Miller, S., Willé, D., et al. (2014). Late cortical plasticity in motor and auditory cortex: role of met-allele in BDNF Val66Met polymorphism. Int. J. Psychopharmacol. 17, 705–713. doi: 10.1017/S1461145713001636
Truong, D. Q., Magerowski, G., Blackburn, G. L., Biksom, M., and Alonso-Alonso, M. (2013). Clinical computational modeling of transcranial direct current stimulation (tDCS) in obesity: Impact of head fat and dose guidelines Neuroimage 2, 759–766. doi: 10.1016/j.nicl.2013.05.011
Tseng, P., Hsu, T. Y., Chang, C. F., Tzeng, O. J. L., Hung, D. L., Muggleton, N. G., et al. (2012). Unleashing potential: transcranial direct current stimulation over the right posterior parietal cortex improves change detection in low-performing individuals. J. Neurosci. 32, 10554–10561. doi: 10.1523/JNEUROSCI.0362-12.2012
Uehara, K., Coxon, J. P., and Byblow, W. D. (2015). Transcranial direct current stimulation improves ipsilateral selective muscle activation in a frequency dependent manner. PLoS ONE 10:e0122434. doi: 10.1371/journal.pone.0122434
Vaseghi, B., Zoghi, M., and Jaberzadeh, S. (2014). Inter-pulse interval affects the size of single-pulse TMS-induced motor evoked potentials: a reliability study. Basic Clin. Neurosci. 6, 44–51.
Viana, R. T., Laurentino, G. E. C., Souza, R. J. P., Fonseca, J. B., Silva Filho, E. M., Dias, S. N., et al. (2014). Effects of the addition of transcranial direct current stimulation to virtual reality therapy after stroke: a pilot randomized controlled trial. NeuroRehabilitation 34, 437–446. doi: 10.3233/NRE-141065
Wagner, S., Rampersad, S. M., Aydin, Ü., Vorwerk, J., Oostendorp, T. F., Neuling, T., et al. (2014). Investigation of tDCS volume conduction effects in a highly realistic head model. J. Neural Eng. 11, 1–14. doi: 10.1088/1741-2560/11/1/016002
Werhahn, K. J., Behrang-Nia, M., Bott, M. C., and Klimpe, S. (2007). Does the recruitment of excitation and inhibition in the motor cortex differ? J. Clin. Neurophysiol. 24, 419–423. doi: 10.1097/WNP.0b013e3181379a69
Wiethoff, S., Hamada, M., and Rothwell, J. C. (2014). Variability in response to transcranial direct current stimulation of the motor cortex. Brain Stimul. 7, 468–475. doi: 10.1016/j.brs.2014.02.003
Wu, D., Wang, J., and Yuan, Y. (2015). Effects of transcranial direct current stimulation on naming and cortical excitability in stroke patients with aphasia. Neurosci. Lett. 589, 115–120. doi: 10.1016/j.neulet.2015.01.045
Wu, Y., Tseng, P., Chang, C., Pai, M., Hsu, K., Lin, C., et al. (2014). Modulating the interference effect on spatial working memory by applying transcranial direct current stimulation over the right dorsolateral prefrontal cortex. Brain Cogn. 91, 87–94. doi: 10.1016/j.bandc.2014.09.002
Young, S. J., Bertucco, M., and Sanger, T. D. (2014). Cathodal transcranial direct current stimulation in children with dystonia: a sham-controlled study. J. Child Neurol. 29, 232–239. doi: 10.1177/0883073813492385
Ziemann, U., Lönnecker, S., Steinhoff, B. J., and Paulus, W. (1996). Effects of antiepileptic drugs on motor cortex excitability in humans: a transcranial magnetic stimulation study. Ann. Neurol. 40, 367–378. doi: 10.1002/ana.410400306
Ziemann, U., Reis, J., Schwenkreis, P., Rosanova, M., Strafella, A., Badawy, R., et al. (2015). TMS and drugs revised 2014. Clin. Neurophysiol. doi: 10.1016/j.clinph.2014.08.028. [Epub ahead of print].
Keywords: transcranial direct current stimulation, interindividual variability, transcranial magnetic stimulation, cognition, motor-evoked potential
Citation: Li LM, Uehara K and Hanakawa T (2015) The contribution of interindividual factors to variability of response in transcranial direct current stimulation studies. Front. Cell. Neurosci. 9:181. doi: 10.3389/fncel.2015.00181
Received: 29 March 2015; Accepted: 25 April 2015;
Published: 12 May 2015.
Edited by:
Michael A. Nitsche, Georg-August-University, GermanyReviewed by:
Marom Bikson, The City College of New York of The City University of New York, USAAlkomiet Hasan, Ludwig-Maximilians-University Munich, Germany
Copyright © 2015 Li, Uehara and Hanakawa. This is an open-access article distributed under the terms of the Creative Commons Attribution License (CC BY). The use, distribution or reproduction in other forums is permitted, provided the original author(s) or licensor are credited and that the original publication in this journal is cited, in accordance with accepted academic practice. No use, distribution or reproduction is permitted which does not comply with these terms.
*Correspondence: Takashi Hanakawa, Department of Advanced Neuroimaging, Integrative Brain Imaging Center, National Center of Neurology and Psychiatry, 4-1-1 Ogawahigashi, Kodaira, Tokyo 187-8551, Japan,aGFuYWthd2FAbmNucC5nby5qcA==
†These authors have contributed equally to this work and are co-first authors.