- 1Department of Brain and Behavioral Sciences, University of Pavia, Pavia, Italy
- 2Museo Storico Della Fisica e Centro Studi e Ricerche Enrico Fermi, Rome, Italy
- 3Institute of Pharmacology and Toxicology, University of Zurich, Zurich, Switzerland
- 4Brain Connectivity Center, C. Mondino National Neurological Institute, Pavia, Italy
- 5Department of Computer Architecture and Technology, University of Granada, Granada, Spain
The way long-term potentiation (LTP) and depression (LTD) are integrated within the different synapses of brain neuronal circuits is poorly understood. In order to progress beyond the identification of specific molecular mechanisms, a system in which multiple forms of plasticity can be correlated with large-scale neural processing is required. In this paper we take as an example the cerebellar network, in which extensive investigations have revealed LTP and LTD at several excitatory and inhibitory synapses. Cerebellar LTP and LTD occur in all three main cerebellar subcircuits (granular layer, molecular layer, deep cerebellar nuclei) and correspondingly regulate the function of their three main neurons: granule cells (GrCs), Purkinje cells (PCs) and deep cerebellar nuclear (DCN) cells. All these neurons, in addition to be excited, are reached by feed-forward and feed-back inhibitory connections, in which LTP and LTD may either operate synergistically or homeostatically in order to control information flow through the circuit. Although the investigation of individual synaptic plasticities in vitro is essential to prove their existence and mechanisms, it is insufficient to generate a coherent view of their impact on network functioning in vivo. Recent computational models and cell-specific genetic mutations in mice are shedding light on how plasticity at multiple excitatory and inhibitory synapses might regulate neuronal activities in the cerebellar circuit and contribute to learning and memory and behavioral control.
Introduction
Various persistent modifications in neuronal and synaptic functioning provide the biological basis of learning and memory in neuronal circuits and, among these, long-term synaptic plasticity (Bliss and Collingridge, 1993) and intrinsic neuronal excitability (Linden, 1999; Hansel et al., 2001; Xu and Kang, 2005) are thought to play a primary role. Long-term synaptic plasticity appears in various forms of potentiation (LTP) and depression (LTD). Although different forms and mechanisms of LTP and LTD have been revealed, often along with forms of intrinsic excitability changes occurring in the same neurons, plasticity in inhibitory subcircuits is still poorly understood. Moreover, the way inhibitory and excitatory mechanisms cooperate in determining brain circuit computations remains unclear. What is most critical is to understand how excitatory and inhibitory plasticity impinging on the same neuron regulate its function, and how excitatory and inhibitory plasticity contribute to microcircuit computation as a whole. This lack of knowledge is somewhat surprising if one considers that long-term synaptic plasticity is largely believed to play a key role in regulating neuronal and microcircuit operations.
In the cerebellum, long-term synaptic plasticity was initially predicted on theoretical grounds to occur only in the form of LTD or LTP (Marr, 1969; Albus, 1971) at the parallel fiber—Purkinje cell (PF-PC) synapse, but now synaptic plasticity is known to be distributed in the granular layer, molecular layer and deep cerebellar nuclear (DCN; Hansel et al., 2001; Gao et al., 2012) involving both excitatory and inhibitory synaptic transmission as well as neuronal intrinsic excitability. These different forms of plasticity eventually impinge on three main neurons, namely GrCs, Purkinje cells (PCs), and DCN cells, which act therefore as nodes integrating excitatory and inhibitory plasticity (Figure 1). Thus, the cerebellum is an ideal system in which the interplay of excitatory and inhibitory plasticity can be investigated. Following their discovery, the possible role of cerebellar plasticities has been hypothesized:
1. Synaptic plasticity in the granular layer may serve to improve spatio-temporal recoding of mossy fiber (MF) inputs into new GrC spike patterns [expansion recoding (D’Angelo and De Zeeuw, 2009)]. Plasticity in the inhibitory Golgi cell (GoC) loop has still to be fully investigated but, based on modeling predictions, it may provide a powerful regulatory mechanism for transmission of appropriate spike trains to PCs.
2. Synaptic plasticity in the molecular layer may serve to store correlated granular layer spike patterns under the teaching signal generated by climbing fibers (CFs) although this latter point is controversial (D’Angelo et al., 2011). This plasticity is in fact composed of multiple mechanisms: different forms of PF-PC LTD and LTP occur together with plasticity in the molecular layer inhibitory interneuron (MLI) network involving GABAergic synapses. For example, PF-PC LTD may occur together with PF-MLI LTP and MLI-PC LTP globally raising PC responses, while PF-PC LTP may occur together with PF-MLI LTD and MLI-PC LTD globally reducing PC responses (Gao et al., 2012).
3. Synaptic plasticity in the DCN may serve to store MF spike patterns (Bagnall and du Lac, 2006; Pugh and Raman, 2006) depending on control signals generated through the cerebellar cortical loop (Figure 1). The inhibitory PCs synapses, which regulate DCN activity (Hansel et al., 2001; Boyden et al., 2004; Gao et al., 2012), develop their own LTP and LTD (Morishita and Sastry, 1996; Aizenman et al., 1998; Ouardouz and Sastry, 2000). Recent works (Medina and Mauk, 1999, 2000; Masuda and Amari, 2008) have suggested the importance for MF-DCN and PC-DCN plasticity in controlling cerebellar learning in eye-blink conditioning and vestibulo-ocular reflex (VOR).
4. Long-term changes in intrinsic excitability in GrCs, PCs and DCN cells could further contribute to change the global activity level in these neurons contributing to homeostasis and plasticity (e.g., see Schweighofer et al., 2001).
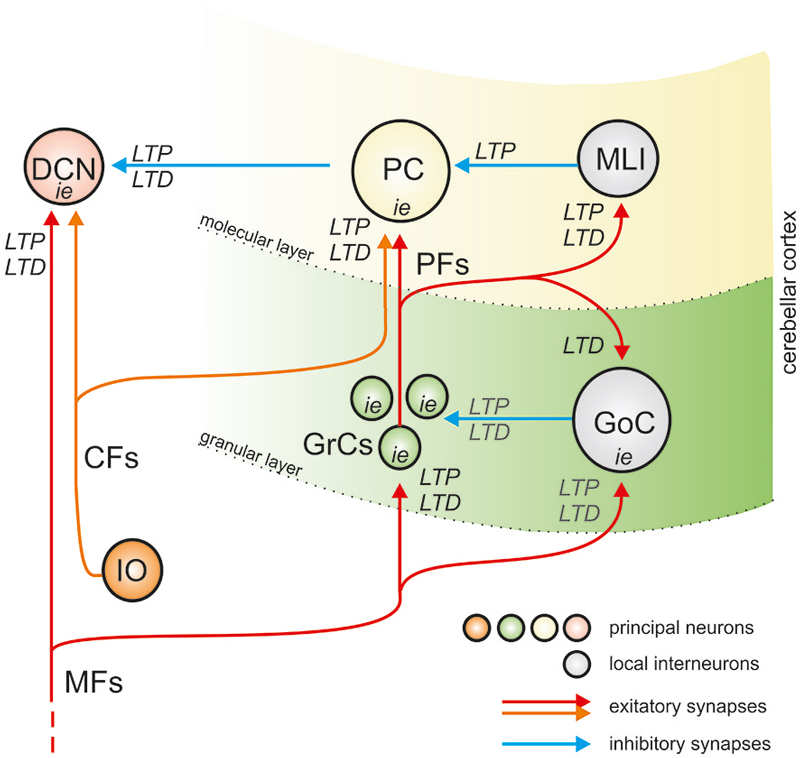
Figure 1. The organization of plasticity in the cerebellar circuit. The drawing shows that the cerebellum is made of three main sub-circuits, comprising the granular layer, molecular layer and deep cerebellar nuclear (DCN). The granular layer and molecular layer form the cerebellar cortex. Both the cerebellar cortex and DCN are activated by MFs, and the cerebellar cortex output inhibits the DCN. Therefore, the cerebellar cortex forms a large inhibitory loop for DCN. Inside cerebellar cortex, in turn, MFs activate GrCs which emit PFs activating Purkinje cells (PCs) and local interneurons inhibit the principal neurons (GoCs inhibit GrCs, MLIs inhibit PCs). PCs and DCN cells are also activated by Climbing fibers (CFs). Therefore, a similar feed-forward inhibitory scheme is implemented in all the three cerebellar subcircuits. LTP, LTD and plasticity of intrinsic excitability (i.e.,) have been either observed or predicted in all subcircuits. The forms of plasticity determined experimentally are reported in black, those predicted by computational modeling are reported in gray. Excitatory and inhibitory synapses are represented using red and blue arrows.
In this review we evaluate the integrated impact of plasticity at inhibitory and excitatory synapses along with long-term changes in intrinsic excitability in the cerebellar circuit and highlight their implications for cerebellar computation.
Long-Term Synaptic Plasticity and Learning in the Cerebellar Circuit
The cerebellum is classically associated with motor control, and learning is thought to subserve the role of calibrating synaptic weights for appropriate response gain regulation and timing. The cerebellum is thought to act through cerebro-cerebellar loops involving the motor cortices (Eccles et al., 1972; Ito, 1972). The critical role in executing precise movements becomes evident when studying patients with cerebellar malfunctioning and diseases, who manifest a sensori-motor syndrome called ataxia. Nevertheless, in the last decade a growing body of evidence supported the cerebellar involvement in non-motor, cognitive and emotional functions (Schmahmann, 2010; Schraa-Tam et al., 2012; Voogd, 2012). It is likely that the cerebro-cerebellar loops involved in motor control represent also a model of how the cerebellum takes part in higher functions through reciprocal connections with non-motor brain areas (D’Angelo and Casali, 2012).
The cerebellum controls movements on the millisecond time scale. The motor commands descending from upstream brain areas are relayed to the cerebellum through the pontine nuclei. Once elaborated in the cerebellar circuits, these signals are sent back to the motor cortex through the thalamus to trigger motor acts with appropriate timing (Timmann et al., 1999). The ability to elaborate temporal information on the millisecond time scale led to consider the cerebellum as a timing machine (Eccles et al., 1967; Eccles, 1973; Ivry, 1997). As a site of procedural memory, the cerebellum has been predicted to operate as a learning machine (Marr, 1969; Ito, 2006). It receives the motor commands from cerebral cortex and, through internal memory of movement inverse dynamics, it is able to elaborate a prediction of sensory consequences of motor acts. The sensory prediction is then compared to the sensory feedback to produce a sensory discrepancy signal (Blakemore et al., 2001; Ivry et al., 2002; Ivry and Spencer, 2004). This triad—namely learning, timing and prediction—emerges as a crucial determinant in adaptive behavior under cerebellar control (De Zeeuw et al., 2011; D’Angelo and Casali, 2012).
Afferent signals are conveyed to the cerebellum through two excitatory pathways composed of the MFs and the CFs. Both these fiber systems send collaterals to the DCN before entering the cerebellar cortex. The MFs contact GrC dendrites in the granular layer of the cerebellar cortex. The GrCs axons generate the PFs, that ascend to the molecular layer and relay the signals onto PCs dendritic arbors. Moreover, PCs directly receive the CFs input and in turn inhibit the DCN. Inhibition in the cerebellar cortex is provided by GoCs interneurons in the granular layer, and stellate (SCs) and basket cells (BCs) in the molecular layer. The DCN cells are inhibited by PCs axons and activated by MF and CF collaterals (Fredette and Mugnaini, 1991; Teune et al., 1995, 2000; Medina et al., 2002). While the PCs represent the only output of the cerebellar cortex, the DCN neurons integrate the PC inhibitory input with the excitatory inputs carried by CFs and MFs collaterals and provide the sole output of the cerebellum.
The regular architecture of the cerebellum has inspired several theories, aiming at understanding how the cerebellum processes incoming information and performs timing and learning functions. According to the motor-learning theory (Marr, 1969; Albus, 1971), the property of learning motor skills relies on the cerebellar cortex ability to store stimulus-response associations, by linking inputs with the appropriate motor output. The theory implied that only PF-PC synapses may be modified by experience and that the CF acting as a teacher signal calibrates the PC responsiveness and thus leads the encoding of stimulus-responses associations. The motor-learning theory in the Marr’s version implies that, when MFs carry inappropriate information, the PF-PC synapse should be silenced by the olivary input (the opposite would occur according to Albus’ version). The hypothetical plasticity of PF synapses postulated by the Motor Learning Theory was observed in vivo as a persistent attenuation of PF-PC transmission (PF-PC long term depression, LTD) produced when PF and CF inputs are stimulated together at low frequency (Ito, 1972, 1989). Miles and Lisberger proposed an alternative model (valid at least for the VOR), in which motor learning is achieved through synaptic plasticity at a different site. The instructive signal conveyed by the PC to the vestibular nuclei triggers a change in synaptic efficacy in the connection between MF collaterals and vestibular nuclei (Miles and Lisberger, 1981).
Experimental data provided support for and against each of the two hypotheses, indicating that the explanation of cerebellar motor learning is likely to involve a more complex picture than plasticity at a single synapse. The cellular basis of cerebellar motor learning is generally assumed to be mediated by long-term modifications in the strength of synaptic transmission (for review see Martin et al., 2000). However, the information storage may also involve activity dependent changes in neuronal intrinsic excitability (Armano et al., 2000; Hansel et al., 2001; Zhang and Linden, 2003; Frick and Johnston, 2005; Mozzachiodi and Byrne, 2010).
Different forms of synaptic and non-synaptic plasticity have been described in excitatory and inhibitory neurons of the granular layer, the molecular layer and the DCN (Hansel et al., 2001; Boyden et al., 2004; Gao et al., 2012). Thus, it is likely that cerebellar learning emerges as an integrated process involving various synaptic sites that elaborate, over different time courses, different components of learning (Medina and Mauk, 2000; Medina et al., 2000; van Alphen and De Zeeuw, 2002; Jörntell and Ekerot, 2003; Yang and Lisberger, 2013). However, how remodeling of synaptic weights generates the complex properties of cerebellar learning remains to be understood.
Mathematical models (Mauk and Donegan, 1997; Medina and Mauk, 1999, 2000; Medina et al., 2000, 2001; Ohyama et al., 2003b; Mauk and Buonomano, 2004; Lepora et al., 2010) incorporating more and more details on synaptic connectivity and plasticity at different network sites, may help determining the impact of the different sites of plasticity on cerebellar learning (Shadmehr et al., 2010). These computational approaches have generated several hypotheses, many of which require validation through experimental assessment. Specific tests can be performed either by using mutant mice with alterations in specific plasticity mechanisms (Gao et al., 2012) or by embedding a cerebellar model with multiple learning rules into the control loop of a robotic simulator (Garrido et al., 2013a; Casellato et al., 2014, 2015).
Excitatory and Inhibitory Plasticity in the Granular Layer
In vitro, LTP and LTD at the MF-GrC synapse are associated with changes of GrCs intrinsic excitability (D’Angelo et al., 1999; Armano et al., 2000; Sola et al., 2004; Gall et al., 2005; Nieus et al., 2006; D’Errico et al., 2009). In vivo, LTP and LTD can be induced in the granular layer by facial tactile stimulation and intra-cerebellar electrical stimulation (Roggeri et al., 2008). In mathematical models using reconvolution algorithms of granular layer local field potentials, the synaptic and non-synaptic changes reported in vitro turned out to be necessary and sufficient to explain those observed in vivo (Diwakar et al., 2011). Information on potential changes in the inhibitory circuit are poor at the moment, but they may be synergistic or antagonistic with respect to those at the MF-GrC relay and regulate information transfer through the granular layer (Arleo et al., 2010; Garrido et al., 2013b).
Plasticity at the MF-GrC Synapse
MF-GrC LTP induction is driven by the coactivation of NMDA receptors (NMDARs) and metabotropic glutamate receptors (mGluRs) (Rossi et al., 1996; D’Angelo et al., 1999; Maffei et al., 2002). The NMDARs are the main source of Ca2+ influx that drives synaptic plasticity induction at the MF-GrC relay, while mGluRs represent an amplifying mechanism acting through the IP3 pathway (Finch et al., 1991; Irving et al., 1992a). Voltage-Dependent Calcium Channels (VDCCs) activation, following membrane depolarization and repetitive spike discharges, may also favor MF-GrC LTP (Armano et al., 2000). The intracellular Ca2+ signals may be remarkably protracted and amplified by Ca2+-induced Ca2+ release (CICR) mechanisms (Irving et al., 1992a,b; Simpson et al., 1996).
Knowing the mechanisms underlying LTP/LTD balance is fundamental to understand how the information is processed and retransmitted by the granular layer. Several intrinsic and extrinsic factors could regulate bidirectional plasticity (for review see D’Angelo, 2014).
First, the patterns of MFs stimulation determine LTP-LTD balance. According to the BCM learning rule (Bienenstock et al., 1982), long-term synaptic plasticity is correlated with the duration of stimulus trains through postsynaptic Ca2+ regulation (Gall et al., 2005). Long and repeated MF bursts induce a relatively large Ca2+ influx that drives LTP. Instead, LTD is induced by short isolated burst stimulation that causes relatively small Ca2+ changes (Gall et al., 2005). Similarly, bidirectional plasticity is influenced by MF stimulation frequencies (D’Errico et al., 2009).
Secondly, nitric oxide (NO) may orchestrate the LTP/LTD balance. High frequency MF stimulation generates a significant NMDAR-dependent and NOS-dependent release of NO in the granular layer (Maffei et al., 2003). As a retrograde messenger, NO regulates the presynaptic release probability, thus driving LTP expression (Maffei et al., 2002). NO release inhibition shifts the balance toward LTD, suggesting that NO is critical for determining plasticity orientation (Maffei et al., 2003).
Thirdly, gating by neuromodulators has been proposed to control LTP and LTD induction at the MF-GrC relay (Schweighofer et al., 2001, 2004). Indeed, it has recently been shown that the cholinergic system enhances MF-GrC LTP through α7-nAChRs activation by shifting the Ca2+-plasticity relationship. In this way, in the presence of nicotine a short MF burst that normally induces MF-GrC LTD, is able to induce LTP, both in acute brain slices and in vivo (Prestori et al., 2013). The cholinergic facilitation of LTP induction could be critical for controlling adaptive behaviors like VOR (Schweighofer et al., 2001, 2004; Prestori et al., 2013).
Finally, the combination of synaptic response (excitatory post-synaptic potential, EPSP) and spikes has itself a role in determining plasticity, giving rise to the so-called spike-timing-dependent-plasticity (STDP; Song et al., 2000). Preliminary evidence suggests that STDP could indeed exist at the MF-GrC relay, although the underlying mechanisms remain to be clarified (Sgritta et al., 2014).
Plasticity in the GoC Inhibitory Circuit
Plasticity in the GoC inhibitory circuit may regulate information transfer at the MF-GrC synapse. Following protracted high frequency activation of the MF bundle (typically a theta burst stimulation, TBS) the long-term synaptic plasticity in the granular layer shows a specific spatial organization (Mapelli and D’Angelo, 2007). In particular, LTP and LTD are organized in center-surround structures: more active centers that tend to generate LTP, and less active surrounds that preferentially generate LTD. The sign of plasticity depends on the excitatory/inhibitory balance and therefore it is sensitive to the inhibitory circuit activity. Therefore, GoCs activity may modulate the center-surround organization of signal transmission and bidirectional plasticity at the MF-GrC relay (Mapelli and D’Angelo, 2007; Mapelli et al., 2010, 2014). Although a form of long-term plasticity in the inhibitory GoC-GrC connection has not been described, a recent model suggests that it could represent a potent regulatory mechanism for MF-GrC plasticity (Garrido et al., 2013b). A form of LTD at PF-GoC synapse, following high-frequency burst stimulation of PFs (Robberechts et al., 2010) and long term enhancement of spontaneous GoC firing rate after hyperpolarization (Hull et al., 2013) have been reported (with the first potentially being synergistic and the second homeostatic with respect to the MF-GrC pathway). Also forms of adaptation and long-lasting regulation at the GoC-GrC synapse have been described (Rossi et al., 2006; Mapelli et al., 2009; Brandalise et al., 2012), operating a disinhibition of GrCs in case of high GoCs activation rates, in that being presumably synergistic in our case. However, plasticity at this level remains to be fully investigated. A control of GoC inhibitory activity could also come from GoC-GoC inhibitory synapses (Hull and Regehr, 2012) and gap-junctions (Vervaeke et al., 2010), although the potential impact of these mechanisms on plasticity in the inhibitory circuit is unclear.
Plasticity of GrC Intrinsic Excitability
High frequency activation of the MF-GrC relay (either through a TBS or a high frequency/protracted stimulation) has been shown to induce long-term modifications of GrC intrinsic excitability, along with the LTP of synaptic efficacy. In particular, high frequency stimulation (HFS) is able to determine a long-lasting increase in neuronal responsiveness, increasing the GrC input resistance and reducing the spike threshold. This form of plasticity had been described for the first time in the hippocampus by Bliss and Lomo in 1973 (Bliss and Lomo, 1973). At the MF-GrC synapse, protracted high-frequency stimulations, weaker than the TBS, determine the increase of the intrinsic excitability, leaving unaltered the postsynaptic response amplitude. As for the synaptic LTP, the plasticity of GrC intrinsic excitability is dependent on NMDARs activation (Armano et al., 2000). Indeed, a previous work assessed the role of the NMDA current in enhancing synaptic depolarization and GrC output firing, in particular in response to HFSs (D’Angelo and Rossi, 1998). The mechanism involved in the NMDAR-dependence probably relies on the calcium ions influx through these receptors (and the consequent activation of calcium-dependent intracellular pathways), rather than on the depolarization consequent to NMDARs opening (Armano et al., 2000). The spike threshold reduction is probably related to a modification of the persistent sodium current and potassium currents (Nieus et al., 2006). The potentiation of GrC responsiveness, and the consequent increase in the number of emitted spikes, reasonably facilitates the development of synaptic LTP. Plasticity of GrC responsiveness could have an additional compensatory role, by restoring granular layer level of excitability in case of weak synaptic excitation (Frégnac, 1998; Armano et al., 2000). Both these mechanisms (synaptic long-term plasticity and plasticity of intrinsic excitability) cooperate in determining the granular layer processing of MF input. Indeed, GrC electrotonic compactness (Silver et al., 1992; D’Angelo et al., 1993) presumably determines that a change in intrinsic excitability would affect neuronal responsiveness as a whole, including synaptic efficacy. Synaptic inhibition mediated by GoCs reasonably affects GrC excitability, through tonic and phasic mechanisms (Armano et al., 2000; D’Angelo et al., 2005). Indeed, GABAergic inhibition modulates GrC excitability in different ways ((Brickley et al., 1996; Rossi et al., 2006); for review (Mapelli et al., 2014)).
Excitatory and Inhibitory Plasticity in the Molecular Layer
The Marr-Albus-Ito hypothesis of cerebellar motor learning implies that the PF input to PCs is the only site of learning in the cerebellar network. However, multiple sites of synaptic plasticity in the molecular layer have been described (Hansel et al., 2001; Boyden et al., 2004; Coesmans et al., 2004; Ito, 2006). The picture emerging from the latest evidences shows that several forms of synaptic plasticity, not only the classical PF-PCs LTD, appear to be involved in cerebellar learning. Here we summarize the principal features of molecular layer plasticity (for a detailed review see Gao et al., 2012; D’Angelo, 2014).
Plasticity at the PF-PC Synapse
Different forms of LTP and LTD, either entirely postsynaptically or presynaptically expressed, have been observed at the PF-PC relay. Thus, four different forms of plasticity may be described: a postsynaptic LTD, a postsynaptic LTP, a presynaptic LTP and a presynaptic LTD.
Postsynaptic PF-PC LTD is induced by paired PF and CF stimulations and involves complex signal transduction pathways. The activation of AMPARs and mGluRs following PF stimulations induces, through different mechanisms, a postsynaptic Ca2+ transient that, over a certain threshold, may activate protein kinase C (PKC; Hartell, 2002). Active PKC phosphorylates the AMPARs at the PC terminals and drives their desensitization and internalization, resulting in LTD of PF-PC relays (Wang and Linden, 2000; Xia et al., 2000). CF stimulations contribute to generate large widespread Ca2+ transients, through AMPARs, NMDARs, and VGCCs activation (Konnerth et al., 1992; Piochon et al., 2010). In particular, postsynaptic CF-PC NMDARs are necessary for LTD (but not for LTP) at the PF-PC synapse, when PF activation is paired with CF activation (Piochon et al., 2010). CaMKIV (Boyden et al., 2006) and α/βCaMKII (Hansel et al., 2006; van Woerden et al., 2009) are necessary both for PF-PC LTD and for motor learning. Nevertheless, this form of LTD does not strictly require CF activity and may be induced through PF stimulation alone (Ohtsuki et al., 2009). Other mechanisms could amplify local Ca2+ signals and allow LTD induction, as somatic depolarization (Linden et al., 1991) or strong PFs activation (Hartell, 1996; Eilers et al., 1997). Indeed, the simultaneous activation of several PFs stimulated at 1 Hz, at relatively high stimulus intensity, may generate postsynaptic Ca2+ transients that remain confined to spines (Midtgaard et al., 1993; Denk et al., 1995), reaching the levels for the LTD induction (Hartell, 1996). However, when the CFs are stimulated a lower stimulus strength is sufficient for LTD induction (Han et al., 2007). Therefore, although it is clear that CF activity facilitates PF-PC LTD, CF involvement is not strictly required (Ohtsuki et al., 2009). Additionally, intense PF stimulations (as brief burst of 2–5 pulses at 10–50 Hz for 1–2 min) may induce heterosynaptic LTD (Marcaggi and Attwell, 2007), in which the LTD may spread to PF synapses tens of microns away from the original site, through second messengers such as NO (Reynolds and Hartell, 2000; Wang et al., 2000) and arachidonic acid (Reynolds and Hartell, 2001). The NO pathway is necessary for the heterosynaptic LTD induction (Crepel and Jaillard, 1990; Shibuki and Okada, 1991; Daniel et al., 1993) and provides another important mechanism involved in plasticity induction at the PF-PC synapses. Indeed, the NO produced by PFs (Southam et al., 1992; Kimura et al., 1998) or by MLIs (Carter and Regehr, 2000) activates a NO-dependent form of guanylate cyclase (GC) in PCs, thus activating the cGMP/PKG pathway, whose effect is to prevent the dephosphorilation of AMPARs by blocking the PP2/PP1/PP2B cascade and therefore unblocking PKC (Lev-Ram et al., 1995, 1997; Linden et al., 1995; Gao et al., 2012).
Postsynaptic PF-PC LTP is induced by single pulses PF stimulation at 1 Hz for 5 min, driving GluR2 AMPARs subunit insertion in the spine membrane, through a mechanism dependent on the activation of the PKA, PKC and CAMKII pathways (Lev-Ram et al., 2002; Coesmans et al., 2004; Belmeguenai and Hansel, 2005; Kakegawa and Yuzaki, 2005; van Woerden et al., 2009). The use of selective mutant mice can help investigating the mechanisms underlying these forms of plasticity and their role in vivo. In particular, the L7-PP2B mice, in which the PP2B was deleted only in cerebellar PCs, allowed to determine that this molecule is necessary for PF-PC LTP and for correct VOR and eye-blink conditioning (Schonewille et al., 2010). Postsynaptic LTP and LTD are mutually reversible, modulating the AMPARs desensitization and membrane expression (Lev-Ram et al., 2003; Coesmans et al., 2004). The sign of plasticity is determined by several factors, depending on the different induction mechanisms, the NO pathway and the postsynaptic Ca2+ transients. In general, stimulation patterns that generate a relatively low Ca2+ influx drive LTP while relatively high Ca2+ transients are associated with LTD (Coesmans et al., 2004). This is an opposite scenario of that predicted by the “BCM rule”, in which lower and higher Ca2+ transients are associated with the induction of LTD and LTP respectively (Bienenstock et al., 1982). This property of PF-PC plasticity may have profound impact on cerebellar information processing.
Presynaptic PF-PC LTP may be induced by low-frequency (2–8 Hz) PF stimulations (Sakurai, 1987; Crepel and Jaillard, 1990; Hirano, 1991; Shibuki and Okada, 1992), determining an increase of presynaptic Ca2+ influx that activates the adenyl cyclase (AC1) pathway. The consequent activation of PKA determines the phophorilation of the vesicle-release related proteins thus increasing neurotransmitter release (Salin et al., 1996; Kimura et al., 1998; Storm et al., 1998; Powell et al., 2004). In addition, NO released by neighboring synapses may regulate the probability of glutamate release and LTP induction in non-activated PF terminals (Hartell, 2002; Qiu and Knöpfel, 2007; Le Guen and De Zeeuw, 2010). On the contrary, the endocannabinoids released after a high frequency bursts, suppress in PF terminals the AC1 pathway, activating the cannabinoid 1 (CB1) receptors, thereby preventing the induction of presynaptic LTP (van Beugen et al., 2006). A cannabinoid-mediated affect at this level has been described as consequent of the activation of the cholinergic system, mediated by muscarinic receptors (Rinaldo and Hansel, 2013).
Presynaptic PF-PC LTD also requires the activation of CB1 receptors. This LTD may emerge after a low-frequency stimulation when presynaptic LTP is pharmacologically prevented, providing a mechanism of bidirectional plasticity at the presynaptic site (Qiu and Knöpfel, 2007).
In conclusion, the cholinergic system and endocannabinoid receptors are able to deeply modulate synaptic plasticity at the PF-PC connection, at various levels. Notably, these two system proved able to interact (Rinaldo and Hansel, 2013). As it is true also for other cerebellar regions, cholinergic activation is able to modulate synaptic activity and plasticity, also mediating the release of other neurotransmitters, therefore influencing local neuronal activity (Turner et al., 2011).
Plasticity at the CF-PC Synapse
The CFs activity may play an important role in regulation of LTP/LTD balance at the PF-PC synapses. The CF stimulation facilitates postsynaptic PF-PC LTD induction by enhancing dendritic Ca2+ signals and by releasing the neuropeptide CRF. Moreover, CF activity triggers the release of endocannabinoids from PC dendrites and suppresses the presynaptic PF-PC LTP (Ohtsuki et al., 2009). The high probability of neurotransmitter release at the CF terminals (Dittman and Regehr, 1998) as well as the all-or-none character of CF signaling (Eccles et al., 1966) has induced to consider the CF-PC synapses as “unmodified” synapses. However, low-frequency (5 Hz, 30 s) CF stimulation may induce LTD of PC responses (Hansel and Linden, 2000; Carta et al., 2006). The CF-LTD is postsynaptically induced and expressed (Shen et al., 2002) and it is associated with an alteration in the complex spike waveform (Hansel and Linden, 2000), a reduction in the complex spike afterhyperpolarization (Schmolesky et al., 2005), and a depression of CF-evoked dendritic Ca2+ transient (Weber et al., 2003). The CF-LTD has a significant effect on the probability of induction of postsynaptic LTD and LTP at PF-PC synapses (Coesmans et al., 2004). The reduction in complex spike-associated Ca2+ transients following the CF-LTD is sufficiently strong to reverse the polarity of postsynaptic plasticity at the PF-PC relay. Indeed, when CF-LTD is induced first, subsequent application of PF-PC LTD induction protocol results in LTP (Coesmans et al., 2004). A form of CF-PC LTP has been described during development in mice (around 4–11 postnatal days) (Bosman et al., 2008; Ohtsuki and Hirano, 2008). This LTP requires large CF inputs and is dependent on postsynaptic Ca2+ increase although being independent on NMDARs activation (Bosman et al., 2008). Since CF innervations on PC shows a 1:1 ratio in adult animals (while more CF impinge on the same PC during development), the CF-PC LTP observed in newborn mice could help strengthen one CF connection, while determining the pruning of the others (Bosman et al., 2008; Ohtsuki and Hirano, 2008).
Plasticity in the MLI Inhibitory Circuit
PF-MLI synapses and MLI-PC synapses are both sites of plasticity. Different forms of long-term plasticity have been described in PF-MLI relays: a postsynaptic LTD, a postsynaptic LTP and a presynaptic LTP.
Postsynaptic PF-MLI LTD can be induced by sustained PFs stimulations (repeated sequences of 4 × 25 stimuli at 30 Hz) and requires the activation of Ca2+-permeable AMPARs, mGlur1Rs and CB1Rs. The postsynaptic Ca2+ influx that drives LTD induction is confined at activated synapses (Soler-Llavina and Sabatini, 2006). Moreover, this synapse-specific plasticity drives the membrane expression of GluR2-containg Ca2+-impermeable AMPARs, thus providing a self-limiting mechanism (Liu and Cull-Candy, 2000; Sun and June Liu, 2007). PFs stimulation paired with SCs depolarization, which could follow CFs activations (Szapiro and Barbour, 2007), can induce a postsynaptic PF-MLI LTP (Rancillac and Crépel, 2004). This LTP depends on NO and/or cAMP (Rancillac and Crépel, 2004). In vivo PF-MLI LTP may be induced by simultaneous activation of PFs and CFs inputs, resulting in long-lasting increases in receptive fields of MLIs (Jörntell and Ekerot, 2002).
Presynaptic LTP at PF-MLI synapses has been described after PF stimulations (at 8 Hz for 30 s (Bender et al., 2009)) and provides a positive feedback mechanism. Indeed, GABA released from MLI diffuses in the extracellular space and activates GABAARs at nearby PF terminals. GABAARs activation leads to an increase in PF release probability and an increase of the excitability of the axon and soma/initial segment, potentiating synaptic transmission onto MLI (Pugh and Jahr, 2011).
CF activation can induce a long-lasting potentiation of PCs spontaneous and evoked inhibitory post-synaptic currents (IPSCs), a phenomenon that is called rebound potentiation (Kano et al., 1992). This form of MLI-PCs LTP requires the increase of intracellular Ca2+ concentration and is caused by the upregulation of GABAAR activity on PCs (Kano et al., 1996; Hashimoto et al., 2001; Kawaguchi and Hirano, 2007).
There are therefore several mechanisms that could come into play to make synaptic plasticity in the MLI inhibitory circuit either synergistic or antagonistic with respect to plastic changes occurring at the PF-PC synapse.
Plasticity of PC Intrinsic Excitability
PC excitability may be enhanced by somatic current injection or the PFs stimulation protocols that induce PF-LTP (Belmeguenai et al., 2010). The PCs intrinsic plasticity shares with LTP the activation of protein phosphatises 1, 2A and 2B for the induction (Belmeguenai et al., 2010). It also requires PKA and casein kinase 2 (CK2) activity and it is mediated by the downregulation of different K+ channel-mediated conductances, such as A-type K+ channels and probably Ca2+-activated K+ currents (Schreurs et al., 1998). PC intrinsic plasticity, resulting in enhanced spine Ca2+ signaling, lowers the probability of subsequent LTP induction. Thus, intrinsic PC plasticity follows LTP of active PF synapses and reduces the probability of subsequent LTP at weaker, non-potentiated synapses.
Excitatory and Inhibitory Plasticity in DCN
The DCN (as well as the vestibular nuclei, VN) are strategically located within the cerebellar circuitry, in a position ideal to integrate the information coming from brain stem, inferior olive (IO) and spinal cord with the PCs output coming from the cerebellar cortical loop, and provide the sole output of the cerebellum. Experimental investigations using pharmacological tools and focal lesions have revealed that the DCN play an important role in associative learning, such as in eyelid conditioning or VOR adaptation (Lavond et al., 1985; Steinmetz et al., 1992). Evidence that these forms of cerebellar motor learning induce plasticity in DCN and VN (Lisberger, 1994; du Lac et al., 1995; Kleim et al., 2002; Ohyama et al., 2006) suggested that memory storage was not limited to the cerebellar cortex. Long-term modifications in synaptic strength have been described in the inhibitory synapses between PCs and DCN neurons and in the excitatory synapses between MFs and DCN. In addition, persistent changes of the intrinsic excitability have been observed in DCN neurons. Recently, it has been suggested that PC-DCN and MF-DCN synapses are plastic on a slow time scale and store persistent memory. Conversely, plasticity in cerebellar cortex could operate on a shorter time scale, storing transient memory that could then be transferred downstream and consolidated through DCN plasticity in slow phases of learning (Medina and Mauk, 1999; Medina et al., 2000; Masuda and Amari, 2008).
Plasticity at the MF-DCN Synapse (Excitatory)
DCN neurons show a robust post-inhibitory rebound spike burst after stimulation of inhibitory PCs synapses (Gardette et al., 1985a,b; Aizenman and Linden, 1999). This rebound hallmark induced by PC activity drives the plastic changes of the MF-DCN glutamatergic synapse. A MFs high-frequency burst that precedes a DCN post-inhibitory rebound depolarization induces a synapse-specific MF-DCN LTP (Pugh and Raman, 2006). This LTP induction protocol mimics the predicted time course of excitation and inhibition during delay eyelid conditioning. The MFs convey the conditioned stimulus, while the unconditioned stimulus is carried by the CFs. The DCN neurons receive excitation directly from the MFs collaterals, followed by the indirect inhibition via the GrC-PC-DCN circuit (Mauk et al., 1986; Hesslow et al., 1999). The PCs respond to the unconditioned stimulus with a complex spike, followed by a brief pause that allows the post-inhibitory firing in the nuclei. During cerebellar learning of associative tasks, the acquisition of the conditioned response depends on the correct timing between the MF-mediated excitation and the PC-mediated inhibition that drives excitatory post-synaptic currents (EPSCs) potentiation (Ohyama et al., 2003a). The PCs fire as a response to MF activation, but when the conditioned and unconditioned stimuli are paired and repeated, PCs firing slows down during the final phase of the conditioned stimulus. This would generate a disinhibition in DCN neurons, allowing the generation of the excitatory response that elicits a blink (Jirenhed et al., 2007). LTP cannot be induced when the timing of synaptic excitation and hyperpolarization is modified. With longer intervals between excitation and inhibition, or with a reverse sequence, EPSCs tend to depress (Pugh and Raman, 2008).
MF-DCN LTP depends on both NMDAR and low-voltage-activated Ca2+ channels, activated respectively by synaptic excitation and inhibition (Pugh and Raman, 2006). DCN are spontaneously active neurons and express NR2D subunit-containing NMDARs, generating channels weakly blocked by Mg2+ (Akazawa et al., 1994). Therefore, unlike other brain regions, the MF-DCN LTP is not consequent to the coincidence detection of signals that generates a suprathreshold increase in the intracellular Ca2+ level. MF-DCN plasticity rather depends on the timing of two different signals that act independently to activate distinct intracellular signaling pathways. This mechanism may be adequate to encode temporal information that is required for non-Hebbian and adaptive plasticity during associative learning tasks (Medina and Mauk, 1999; Pugh and Raman, 2009). The excitation drives the Ca2+ influx in individual synapses, with NMDARs providing the priming signal. The inhibition generates a global signal that triggers LTP induction only in the primed synapses (Pugh and Raman, 2008). Multiple signaling cascades may be activated by priming and trigger signals. The Ca2+ influx through NMDARs activates the calcium-dependent phosphatase calcineurin, while the Ca2+ influx through the high-voltage-activated Ca2+ channels activates the calmodulin-dependent protein kinase II (CaMKKII). At the same time, the potentiation of the primed synapse is triggered only if the suppressive effect of L-type Ca2+ current is reduced by hyperpolarization (Person and Raman, 2010). This provides evidence that synaptic inhibition plays an active role in the induction of MF-DCN LTP.
Moreover, a form of MF-DCN LTD has been reported, which can be induced by MFs high-frequency burst stimulation, either alone or paired with postsynaptic depolarization. Again, a postsynaptic Ca2+ transient is needed to the induction of this plasticity, that it is blocked by Ca2+ chelators (Zhang and Linden, 2006). MF-DCN LTD is NMDAR independent and requires the activation of the group I metabotropic glutamate receptor 1 (mGluR1) and protein translation (Zhang and Linden, 2006).
Plasticity at the PC-DCN Synapse (Inhibitory)
LTP of IPSCs in DCN neurons can be induced after HFS at 100 Hz (Ouardouz and Sastry, 2000) of PC axons, while stimulation at lower frequencies (as 10 Hz), induces LTD (Morishita and Sastry, 1996). The PC-DCN tetanus-induced long-term plasticity does not require GABARs activation (Morishita and Sastry, 1996; Ouardouz and Sastry, 2000). LTP and LTD appear to depend on NMDAR activation and on intracellular Ca2+ increase, as they are both blocked by NMDAR antagonist APV and/or the calcium chelator BAPTA. Moreover, depolarizing pulses that activate VGCCs in DCN neurons induce LTP when given at 2 Hz or LTD when given at 0.1 Hz (Morishita and Sastry, 1996; Aizenman et al., 1998; Ouardouz and Sastry, 2000). Therefore, a large Ca2+ influx through NMDAR or L-Type Ca2+ channels drives LTP of IPSCs, while LTD is induced by moderate Ca2+ increases. The plasticity induced by depolarization pulses is weaker than that induced by tetanus (HFS). The Ca2+ increase that follows the depolarizing pulses driving a smaller LTP, remains mainly located in the DCN soma and proximal dendrites (Muri and Knöpfel, 1994; Aizenman et al., 1998). In contrast, HFS may act also on CFs and MFs collaterals, whose excitatory synapses are distributed on DCN soma as well as on proximal and distal dendrites (Ikeda and Matsushita, 1973, 1974). The consequent NMDARs activation leads to a larger increase of intracellular calcium, both in the soma and in the dendritic tree, inducing a stronger IPSCs LTP.
PCs activity drives the plasticity of the inhibitory synapses in DCN neurons, but the sign of the bidirectional plasticity strikingly depends on excitatory synapses activation level. Therefore, the activation of MF or CF collaterals influences the induction of LTP (Ouardouz and Sastry, 2000) or LTD (Morishita and Sastry, 1996), by regulating the Ca2+ influx through the NMDARs.
Plasticity of DCN Intrinsic Excitability
High-frequency MF bursts induce potentiation of intrinsic excitability in DCN neurons (Aizenman and Linden, 2000; Zhang et al., 2004). After the EPSP bursts, the input resistance and the number of action potentials evoked by a depolarization pulse increase, while the spike threshold decreases. Also the rebound depolarization that follows a hyperpolarization step is increased. All these mechanisms enhance DCN neurons excitability. Similar to the GrC intrinsic excitability increase following MF-GrC LTP (Armano et al., 2000), the changes in DCN intrinsic excitability depend on NMDARs activation and require an increase in intracellular Ca2+ concentration. MF stimulation may also drive LTP in DCN (Pugh and Raman, 2006), suggesting that potentiation of intrinsic excitability coexists with potentiation of synaptic efficacy, again similar to MF-GrCs LTP. The increase in neuronal excitability amplifies synaptic potentiation, enhancing the ability of DCN neurons to respond to MF inputs (Zheng and Raman, 2010). Also PC inhibitory post-synaptic potential (IPSP) bursts can induce a persistent and Ca2+-dependent increase of DCN intrinsic excitability (Zhang et al., 2004). The changes in DCN neurons excitability caused by PC firing increase followed by a brief pause, might play an important role in motor learning tasks (Zhang et al., 2004).
Coordination of Multiple Forms of Excitatory and Inhibitory Plasticity
As described above, the three main cerebellar subcircuits (granular layer, molecular layer and DCN) are all sites of complex forms of plasticity, some occurring at excitatory and some at inhibitory synapses (D’Angelo, 2014). However, despite numerous hypotheses have been formulated, the main question remains: how does plasticity at excitatory and inhibitory synapses interact in controlling cerebellar circuit functioning? There are four general considerations that need to be taken into account in order to answer the questions.
First, the granular and molecular layer subcircuits share a similar inhibitory architecture, with a feed-forward inhibitory loop passing through the local inhibitory interneurons and controlling retransmission through the primary neuron (in addition, the granular layer also has a feed-back inhibitory loop). Moreover, the whole cerebellar cortex acts as a third large feed-forward inhibitory loop controlling retransmission through the DCN (Figure 1).
Secondly, synaptic plasticity is present at both excitatory and inhibitory synapses, distributed at several connections at the granular layer, molecular layer and DCN. These different forms of synaptic plasticity are expected to develop in a coordinated manner following signal inputs to the cerebellum.
Thirdly, in each subcircuit, inhibitory interneurons fine-tune the principal neuron output and the critical issue is whether inhibitory plasticity tends to compensate and rebalance changes (homeostatic effect) or rather reinforces and amplifies the effects of excitatory plasticity occurring in the main neuronal pathway (synergistic effect).
Finally, plasticity is probably dynamically transferred through the cerebellar circuit synapses into deep structures and possibly also outside the cerebellum, e.g., in the cerebral cortex and brainstem (Koch et al., 2008). Cerebellar plasticity seems therefore unavoidably bound to local circuit dynamics (D’Angelo and De Zeeuw, 2009) and to the extended recurrent networks formed by the cerebellum with extracerebellar areas.
Insight from Experimental Recordings
In order to provide a key to interpret the role of the various plastic mechanisms reported in the cerebellar circuit (see above), it would be helpful to develop a plausible hypothesis of excitatory and inhibitory plasticity interaction using a prototypical demonstration. It is already known that a sensory stimulus like the TSS (theta-sensory stimulus) delivered to the rat whisker pad is able to induce LTD of granular layer response to MF input in vivo (Roggeri et al., 2008). Since this effect is expected on the basis of MF-GrC plasticity rules in vitro (Armano et al., 2000; Sola et al., 2004; D’Errico et al., 2009), it can be hypothesized that synaptic plasticity at the inhibitory GoC connections does not counterbalance MF-GrC LTD. Therefore, plasticity in the Golgi cell loop may be synergistic with that developed at the MF-GrC relay and may effectively increase GrC inhibition. As a consequence, PFs would convey a decreased level of excitation to the molecular layer. Given the inverse “BCM rule” at the PF-PC connection, a weak PFs activation pattern could lead to PF-PC LTP, as suggested by preliminary data (Ramakrishnan and D’Angelo, 2012). Similarly to the granular layer, the inhibitory feed-forward loop in the molecular layer (PF-MLI-PC) could act synergistically with the PF-PC synapse through the induction of PF-MLI LTD, further boosting PC responses. Little is known about the CFs activation following the TSS, although it is likely that it would considerably affect PF-PC behavior. The consequent increase in PC responsiveness could lead to LTP at the PC-DCN connection, increasing PC inhibition of DCN cells. This, in turn, would favor the onset of post-inhibitory rebound depolarization colliding with MFs high-frequency burst activity conveyed by MFs. Since MF activity precedes DCN post-inhibitory rebound depolarization, MF-DCN LTP would be favored (Pugh and Raman, 2006). This example shows a concatenation of events providing a plausible hypothesis of how excitatory and inhibitory plasticity could act synergistically to modify MF input processing and integration through the cerebellar cortex and DCN. This picture, although deliberately oversimplified, provides a working hypothesis on the events that might develop in cerebellar cortex following patterned inputs on the afferent MF pathway. Clearly, introducing CF inputs and their potential instructive role on PF-PC plasticity is another primary factor that should be considered to reshape the landscape of plasticity and signal transmission through the cerebellar network.
Insight from Cerebellar Network Models
Cerebellar modeling has traditionally focused on the classical Marr-Albus’ hypothesis of cerebellar learning (Marr, 1969; Albus, 1971), accounting for plasticity only at the PF-PC connection. According to the Marr-Albus’ hypothesis, the cerebellum operates like a perceptron (Albus, 1971). The PF-PC synapses adapt their weights depending on CF activity (assumed to carry error-related signals) and GrC activity (assumed to perform expansion recoding of sensory inputs reaching the cerebellum through the MFs). Although the Marr-Albus’ hypothesis does not account for the numerous forms of cerebellar plasticity and totally ignored any potential role for inhibitory plasticity, it has inspired most cerebellar models elaborated so far. Surprisingly, in these models based on the Marr-Albus’ hypothesis, the cerebellum succeeded in solving different kinds of tasks, including eyelid conditioning (Medina and Mauk, 2000), VOR adaptation (Masuda and Amari, 2008) and object manipulation (Luque et al., 2011) or even multiple tasks together demonstrating generalization (Casellato et al., 2014). The fact is that learning in these tasks was oversimplified and far from biological realism, so that these models provided a proof of principle that the cerebellum requires plasticity to perform sensory-motor control rather than explaining how its internal plasticity mechanisms operate.
Plasticity in the granular layer has long been neglected in computational models. The GrCs have been supposed to sparsify the MFs incoming signals based on a combinatorial principle (Yamazaki and Tanaka, 2007), exploiting their huge number and connectivity. However, some models have proposed that MF-GrCs and GoC-GrC plasticity may improve granular layer sparse coding of MF inputs (Coenen et al., 2001; Schweighofer et al., 2001; Philipona and Coenen, 2004; Rössert et al., 2014). According to these models, Hebbian learning in the MF-GrC synapses, operating in conjunction with anti-Hebbian learning in the GoC-GrC synapses and homeostatic intrinsic plasticity in both GrCs and GoCs, maximizes the information transfer between the MFs and the GrCs, generating a sparse representation of the MF input. Recent models suggested how cerebellar granular-layer coding could take advantage of spike-timing and distributed plasticity (Garrido et al., 2013b; Rössert et al., 2014). Variations in multiple weights distributed among different connections succeeded to regulate the number of GrC spikes and their positioning with millisecond precision in response to MF bursts. The weight at MF-GrC synapses (main transmission pathway) effectively controlled the first-spike delay, as previously shown experimentally (Arleo et al., 2010). Modeling of weight changes at the inhibitory GoC-GrC together with the excitatory MF-GrC connections revealed the key role of inhibition in shaping the timing and precision of GrC firing (Nieus et al., 2014). The weight at MF-GoC synapses (feed-forward inhibitory loop) and PF-GoC synapses (feed-back inhibitory loop) regulated the duration of the excitatory time-window during which the first spike could be emitted. Moreover, the weights at the GoC-GrC synapses (common inhibitory loop) and GoC-GoC (inhibitory interneuron network) weights controlled the intensity and duration of GrC inhibition and the number of emitted spikes. Therefore, plasticity in the inhibitory circuit of the granular layer could effectively shape the spatio-temporal time-windows of PF discharge.
Distributed plasticity, depending on different combinations of weights at excitatory and inhibitory synapses, proved able to change information flow through the main MF-GrC excitatory pathway favoring different aspects of network processing in turn (Figure 2): (i) increasing transmission (when inhibition on GrCs is depressed); (ii) filtering (in case of inhibition increase and simultaneous LTD at the MF-GrC relay); (iii) maximize time precision (when LTP prevails at all connections in the subcircuit); and (iv) maximize bursting (when inhibition is depressed while the MF-GrC relay potentiates). This model is of particular relevance, since it defines the different functional states that could be achieved by the granular layer circuit in different phases of the learning process. While increasing transmission may be useful to enable the learning process, maximizing timing, filtering or bursting could be the end-point of a specific circuit learning process (Garrido et al., 2013b).
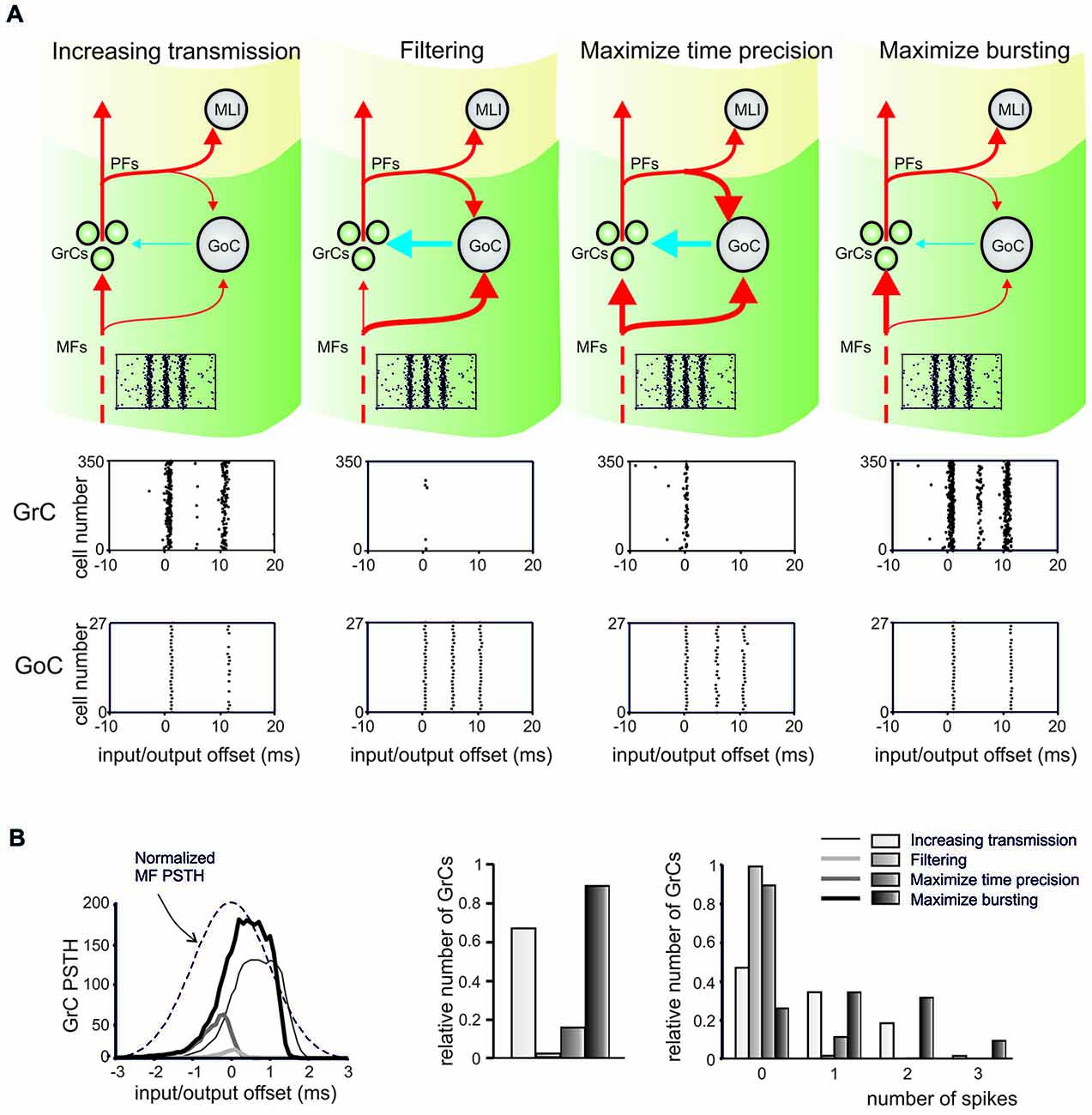
Figure 2. Integrated regulation of microcircuit functions by synaptic plasticity at excitatory and inhibitory synapses. This figure shows the effect of results of integrated regulation of microcircuit functions by synaptic plasticity at excitatory and inhibitory synapses in a computational model of the cerebellar granular layer. (A) The line thickness in the circuit schemes illustrates the relative synaptic weights for the four different conditions (same colors and circuit elements as in Figure 1) and the raster plots indicate the Mossy fiber (MF) input. Systematic changes in synaptic weights could generate four different effects: (1) increase transmission; (2) signal filtering; (3) maximize time precision; and (4) maximize bursting. The GrC and GoC firing in response to the MF input burst are shown in the raster plots for each condition. (B) The peri-stimulus time histograms (PSTH) show the relative number of GrCs generating spikes in response to the input. The PSTHs change in the four different conditions. The nature of changes is illustrated in the histograms, showing the relative number of GrCs responding to the input pattern with 0, 1, 2, or 3 spikes. Modified from Garrido et al. (2013b).
Other models have long hypothesized the role of plasticity in DCN afferent synapses (Medina et al., 2001; Masuda and Amari, 2008; Garrido et al., 2013a; Clopath et al., 2014). These models generally agree that MF-DCN plasticity consolidates the information that has previously been acquired due to the molecular layer plasticity. The separation of learning in two stages (fast learning and consolidation) has been shown to enhance the learning capabilities in eyeblink conditioning (Medina et al., 2001; Monaco et al., 2014), VOR (Masuda and Amari, 2008; Clopath et al., 2014) and complex manipulation tasks (Garrido et al., 2013a). However, this last model has proposed that distributed plasticity in the DCN (including both the MF-DCN and PC-DCN plasticity) could also store gain information, keeping the PCs operating in their optimal firing range and avoiding their saturation. According to this model, while the PF-PC synapses stored information related with the correlation between sensorial state representations (along the sparse GrC activity) and the associated error in the task under development (represented in the CF activity), the DCN afferents could store information about the gain of the task, enhancing the generalization capabilities of the cerebellum. The IO-DCN connection was not considered until a recent computational model proposed that it could act as an internal feed-back loop (Luque et al., 2013), accelerating the convergence of learning without conflicting with the generalization capabilities previously suggested to the MF-DCN and PC-DCN synapses. Moreover, the hypothesized existence of short-term plasticity in that connection could effectively enable/disable this feedback loop based on the error evolution.
These computational models are therefore providing new hypotheses on how inhibitory and excitatory plasticity could integrate to generate the cerebellar output. What is most interesting is that, in closed-loop robotic simulations, the multiple forms of long-term synaptic plasticity can effectively enable adaptive motor control with properties—prediction, timing and learning (Ivry, 1997; Ivry et al., 2002; Shadmehr et al., 2010)—and temporal dynamics similar to those observed in humans (Casellato et al., 2014, 2015; Luque et al., 2014). The main inhibitory plasticity in these neurorobots was located in the PC-DCN synapse (Figure 3). In these robotic tests, the PF-PC synapse could rapidly learn the contextual information needed to compensate for movement errors. With a slower kinetics, the PC-DCN and MF-DCN synapses were able to store this information in a stable form leaving the PF-PC synapse capable of readapting rapidly. This gave the system a remarkable flexibility preventing PF-PC synaptic weight saturation and allowing its reuse in different tasks, for example when the same muscle district was engaged in different motor tasks and when signal rescaling was needed. Therefore, distributed plasticity seems essential to endow the neuronal circuit with biologically effective properties. Moreover, plasticity at the inhibitory PC-DCN synapse appears to be critical to effectively tune transmission along the large side-loop formed by the cerebellar cortex onto DCN. In future works, more realistic representations of neuronal circuits and learning rules will have to be included into closed-loop robotic systems in order to improve our understanding on how integrated plasticity at inhibitory and excitatory synapses controls functioning of the cerebellar circuit.
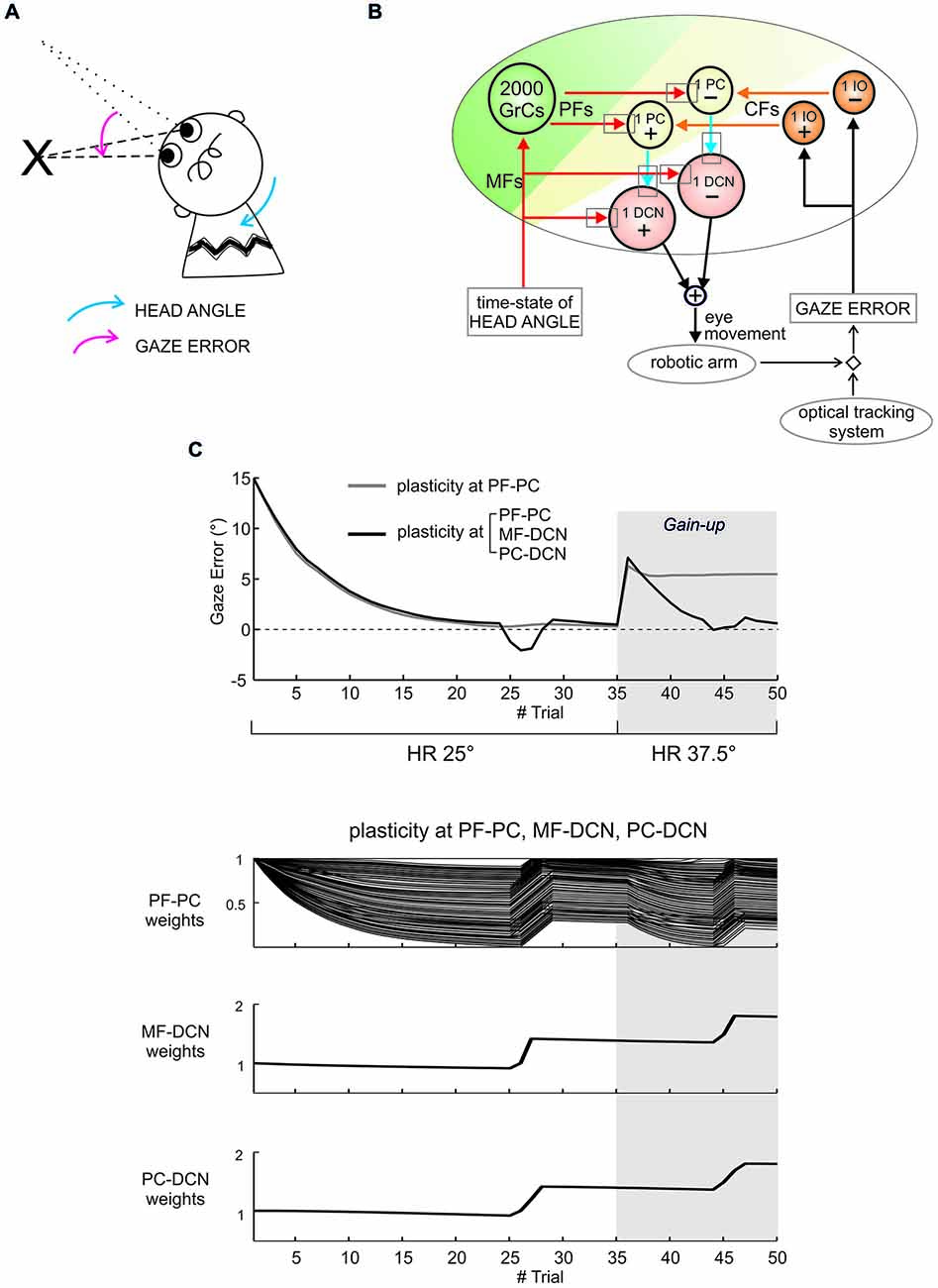
Figure 3. Distributed cerebellar plasticity in real-robot sensorimotor vestibulo-ocular reflex (VOR) task. (A) Human-like VOR task: the arrows show the head angle rotation (blue arrow) and the angle of gaze error (pink arrow). (B) Cerebellar model with VOR-specific input and output signals. The plasticity sites are indicated by the gray rectangles (all plasticities are bidirectional with LTP and LTD rules defined and calibrated according to experimental observations, see (Casellato et al., 2014, 2015; Luque et al., 2014)). Red and orange arrows indicate excitatory connections from mossy fibers (MFs) and CFs, respectively. Blue arrows indicate inhibitory connections. The head vestibular stimulus represents the system time-state, decoded by the granular layer. The gaze error is fed into the CF pathway, and the DCN neurons modulate compensatory eye movements. (C) Gain-up VOR test: after 35 trials, the head rotation (HR) was increased 1.5 times (from 25° to 37.5°), and imposed for other 15 trials. The curves report the gaze error within each of the total 50 trials, implementing plasticity at a single site (PF-PC connection, in gray) or at multiple sites (PF-PC, MF-DCN and PC-DCN connections, in black). With one or three plasticities, the robot compensated equally well for HR. However, while plasticity at the PF-PC connection alone proved unable to change the gain and to correct for the increased HR, combined plasticities at PF-PC, MF-DCN and PC-DCN were able to rescale the response and adapt to the new HR angle. The three bottom plots show synaptic weights at the end of each trial for the three synapses involved, referring to the case of plasticity at the three connections. Indeed, the transfer from cortical to nuclear sites made the PF-PC synapses ready for further plasticity, making them able to react to perturbations suddenly presented to the system. Modified from Casellato et al. (2015).
Conclusions
This revisitation of cerebellar network plasticity shows that LTP and LTD at inhibitory synapses, and more in general in the inhibitory loops, are needed to fine tune the activity at crucial neuronal nodes located along the main circuit transmission pathway. The combination of synaptic weight change at excitatory and inhibitory synapses can effectively shape the network activity states. These states could change dynamically in order to enable different phases of the learning process and transfer of plasticity inside and outside the local circuit. Experimental and modeling evidence suggests that, in certain conditions, plasticity at inhibitory and excitatory synapses could have synergistic effects. Therefore, introduction of inhibitory plasticity allows to draw a new picture of cerebellar circuit functioning beyond the original intuition that learning has just to occur through plasticity at the PF-PC synapse. There is now the need for several critical demonstrations to fully understand the integrated role of inhibitory and excitatory plasticity in the cerebellar circuit. First, learning rules at inhibitory and excitatory synapses and their interdependence need to be determined experimentally. This investigation has to take into account the modulatory states and input patterns relevant to control plasticity and may make use of mutant mice with alteration in specific synaptic mechanisms. Secondly, the effective occurrence of LTP and LTD in vivo in response to specific stimuli or learning protocols needs to be clarified. Thirdly, network models incorporating realistic learning rules need to be extended in order to simulate plasticity dynamics in the circuit. Finally, closed-loop robotic simulations are needed to determine the effective engagement of network learning mechanisms during complex tasks. In this framework, the cerebellar network is likely to provide a very effective workbench.
Conflict of Interest Statement
The authors declare that the research was conducted in the absence of any commercial or financial relationships that could be construed as a potential conflict of interest.
Acknowledgments
This work was supported by European Union grants to ED [CEREBNET FP7-ITN238686, REALNET FP7-ICT270434, Human Brain Project (HBP-604102)] and by Centro Fermi grant [13(14)] to LM.
References
Aizenman, C. D., and Linden, D. J. (1999). Regulation of the rebound depolarization and spontaneous firing patterns of deep nuclear neurons in slices of rat cerebellum. J. Neurophysiol. 82, 1697–1709.
Aizenman, C. D., and Linden, D. J. (2000). Rapid, synaptically driven increases in the intrinsic excitability of cerebellar deep nuclear neurons. Nat. Neurosci. 3, 109–111. doi: 10.1038/72049
PubMed Abstract | Full Text | CrossRef Full Text | Google Scholar
Aizenman, C. D., Manis, P. B., and Linden, D. J. (1998). Polarity of long-term synaptic gain change is related to postsynaptic spike firing at a cerebellar inhibitory synapse. Neuron 21, 827–835. doi: 10.1016/s0896-6273(00)80598-x
PubMed Abstract | Full Text | CrossRef Full Text | Google Scholar
Akazawa, C., Shigemoto, R., Bessho, Y., Nakanishi, S., and Mizuno, N. (1994). Differential expression of five N-methyl-D-aspartate receptor subunit mRNAs in the cerebellum of developing and adult rats. J. Comp. Neurol. 347, 150–160. doi: 10.1002/cne.903470112
PubMed Abstract | Full Text | CrossRef Full Text | Google Scholar
Albus, J. S. (1971). A theory of cerebellar function. Math. Biosci. 10, 25–61. doi: 10.1016/0025-5564(71)90051-4
Arleo, A., Nieus, T., Bezzi, M., D’Errico, A., D’Angelo, E., and Coenen, O. J. M. D. (2010). How synaptic release probability shapes neuronal transmission: information-theoretic analysis in a cerebellar granule cell. Neural Comput. 22, 2031–2058. doi: 10.1162/NECO_a_00006-Arleo
PubMed Abstract | Full Text | CrossRef Full Text | Google Scholar
Armano, S., Rossi, P., Taglietti, V., and D’Angelo, E. (2000). Long-term potentiation of intrinsic excitability at the mossy fiber-granule cell synapse of rat cerebellum. J. Neurosci. 20, 5208–5216.
Bagnall, M. W., and du Lac, S. (2006). A new locus for synaptic plasticity in cerebellar circuits. Neuron 51, 5–57. doi: 10.1016/j.neuron.2006.06.014
PubMed Abstract | Full Text | CrossRef Full Text | Google Scholar
Belmeguenai, A., and Hansel, C. (2005). A role for protein phosphatases 1, 2A and 2B in cerebellar long-term potentiation. J. Neurosci. 25, 10768–10772. doi: 10.1523/jneurosci.2876-05.2005
PubMed Abstract | Full Text | CrossRef Full Text | Google Scholar
Belmeguenai, A., Hosy, E., Bengtsson, F., Pedroarena, C. M., Piochon, C., Teuling, E., et al. (2010). Intrinsic plasticity complements long-term potentiation in parallel fiber input gain control in cerebellar Purkinje cells. J. Neurosci. 30, 13630–13643. doi: 10.1523/jneurosci.3226-10.2010
PubMed Abstract | Full Text | CrossRef Full Text | Google Scholar
Bender, V. A., Pugh, J. R., and Jahr, C. E. (2009). Presynaptically expressed long-term potentiation increases multivesicular release at parallel fiber synapses. J. Neurosci. 29, 10974–10978. doi: 10.1523/jneurosci.2123-09.2009
PubMed Abstract | Full Text | CrossRef Full Text | Google Scholar
Bienenstock, E. L., Cooper, L. N., and Munro, P. W. (1982). Theory for the development of neuron selectivity: orientation specificity and binocular interaction in visual cortex. J. Neurosci. 2, 32–48.
Blakemore, S. J., Frith, C. D., and Wolpert, D. M. (2001). The cerebellum is involved in predicting the sensory consequences of action. Neuroreport 12, 1879–1884. doi: 10.1097/00001756-200107030-00023
PubMed Abstract | Full Text | CrossRef Full Text | Google Scholar
Bliss, T. V., and Collingridge, G. L. (1993). A synaptic model of memory: long-term potentiation in the hippocampus. Nature 361, 31–39. doi: 10.1038/361031a0
PubMed Abstract | Full Text | CrossRef Full Text | Google Scholar
Bliss, T. V., and Lomo, T. (1973). Long-lasting potentiation of synaptic transmission in the dentate area of the anaesthetized rabbit following stimulation of the perforant path. J. Physiol. 232, 331–356. doi: 10.1113/jphysiol.1973.sp010273
PubMed Abstract | Full Text | CrossRef Full Text | Google Scholar
Bosman, L. W., Takechi, H., Hartmann, J., Eilers, J., and Konnerth, A. (2008). Homosynaptic long-term synaptic potentiation of the “winner” climbing fiber synapse in developing Purkinje cells. J. Neurosci. 28, 798–807. doi: 10.1523/jneurosci.4074-07.2008
PubMed Abstract | Full Text | CrossRef Full Text | Google Scholar
Boyden, E. S., Katoh, A., Pyle, J. L., Chatila, T. A., Tsien, R. W., and Raymond, J. L. (2006). Selective engagement of plasticity mechanisms for motor memory storage. Neuron 51, 823–834. doi: 10.1016/j.neuron.2006.08.026
PubMed Abstract | Full Text | CrossRef Full Text | Google Scholar
Boyden, E. S., Katoh, A., and Raymond, J. L. (2004). Cerebellum-dependent learning: the role of multiple plasticity mechanisms. Annu. Rev. Neurosci. 27, 581–609. doi: 10.1146/annurev.neuro.27.070203.144238
PubMed Abstract | Full Text | CrossRef Full Text | Google Scholar
Brandalise, F., Mapelli, L., Gerber, U., and Rossi, P. (2012). Golgi cell-mediated activation of postsynaptic GABA(B) receptors induces disinhibition of the Golgi cell-granule cell synapse in rat cerebellum. PLoS One 7:e43417. doi: 10.1371/journal.pone.0043417
PubMed Abstract | Full Text | CrossRef Full Text | Google Scholar
Brickley, S. G., Cull-Candy, S. G., and Farrant, M. (1996). Development of a tonic form of synaptic inhibition in rat cerebellar granule cells resulting from persistent activation of GABAA receptors. J. Physiol. 497(Pt. 3), 753–759. doi: 10.1113/jphysiol.1996.sp021806
PubMed Abstract | Full Text | CrossRef Full Text | Google Scholar
Carta, M., Mameli, M., and Valenzuela, C. F. (2006). Alcohol potently modulates climbing fiber–>Purkinje neuron synapses: role of metabotropic glutamate receptors. J. Neurosci. 26, 1906–1912. doi: 10.1523/jneurosci.4430-05.2006
PubMed Abstract | Full Text | CrossRef Full Text | Google Scholar
Carter, A. G., and Regehr, W. G. (2000). Prolonged synaptic currents and glutamate spillover at the parallel fiber to stellate cell synapse. J. Neurosci. 20, 4423–4434.
Casellato, C., Antonietti, A., Garrido, J. A., Carrillo, R. R., Luque, N. R., Ros, E., et al. (2014). Adaptive robotic control driven by a versatile spiking cerebellar network. PLoS One 9:e112265. doi: 10.1371/journal.pone.0112265
PubMed Abstract | Full Text | CrossRef Full Text | Google Scholar
Casellato, C., Antonietti, A., Garrido, J. A., Ferrigno, G., D’Angelo, E., and Pedrocchi, A. (2015). Distributed cerebellar plasticity implements generalized multiple-scale memory components in real-robot sensorimotor tasks. Front. Comput. Neurosci. 9:24. doi: 10.3389/fncom.2015.00024
PubMed Abstract | Full Text | CrossRef Full Text | Google Scholar
Clopath, C., Badura, A., De Zeeuw, C. I., and Brunel, N. (2014). A cerebellar learning model of vestibulo-ocular reflex adaptation in wild-type and mutant mice. J. Neurosci. 34, 7203–7215. doi: 10.1523/jneurosci.2791-13.2014
PubMed Abstract | Full Text | CrossRef Full Text | Google Scholar
Coenen, O. J. M. D., Arnold, M. P., Sejnowski, T. J., and Jabri, M. A. (2001). Parallel fiber coding in the cerebellum for life-long learning. Auton. Robots 11, 291–297. doi: 10.1023/A:1012403510221
Coesmans, M., Weber, J. T., De Zeeuw, C. I., and Hansel, C. (2004). Bidirectional parallel fiber plasticity in the cerebellum under climbing fiber control. Neuron 44, 691–700. doi: 10.1016/j.neuron.2004.10.031
PubMed Abstract | Full Text | CrossRef Full Text | Google Scholar
Crepel, F., and Jaillard, D. (1990). Protein kinases, nitric oxide and long-term depression of synapses in the cerebellum. Neuroreport 1, 133–136. doi: 10.1097/00001756-199010000-00013
PubMed Abstract | Full Text | CrossRef Full Text | Google Scholar
D’Angelo, E. (2014). The organization of plasticity in the cerebellar cortex: from synapses to control. Prog. Brain Res. 210, 31–58. doi: 10.1016/b978-0-444-63356-9.00002-9
PubMed Abstract | Full Text | CrossRef Full Text | Google Scholar
D’Angelo, E., and Casali, S. (2012). Seeking a unified framework for cerebellar function and dysfunction: from circuit operations to cognition. Front. Neural Circuits 6:116. doi: 10.3389/fncir.2012.00116
PubMed Abstract | Full Text | CrossRef Full Text | Google Scholar
D’Angelo, E., and De Zeeuw, C. I. (2009). Timing and plasticity in the cerebellum: focus on the granular layer. Trends Neurosci. 32, 30–40. doi: 10.1016/j.tins.2008.09.007
PubMed Abstract | Full Text | CrossRef Full Text | Google Scholar
D’Angelo, E., Mazzarello, P., Prestori, F., Mapelli, J., Solinas, S., Lombardo, P., et al. (2011). The cerebellar network: from structure to function and dynamics. Brain Res. Rev. 66, 5–15. doi: 10.1016/j.brainresrev.2010.10.002
PubMed Abstract | Full Text | CrossRef Full Text | Google Scholar
D’Angelo, E., and Rossi, P. (1998). Integrated regulation of signal coding and plasticity by NMDA receptors at a central synapse. Neural Plast. 6, 8–16. doi: 10.1155/np.1998.8
PubMed Abstract | Full Text | CrossRef Full Text | Google Scholar
D’Angelo, E., Rossi, P., Armano, S., and Taglietti, V. (1999). Evidence for NMDA and mGlu receptor-dependent long-term potentiation of mossy fiber-granule cell transmission in rat cerebellum. J. Neurophysiol. 81, 277–287.
D’Angelo, E., Rossi, P., Gall, D., Prestori, F., Nieus, T., Maffei, A., et al. (2005). Long-term potentiation of synaptic transmission at the mossy fiber-granule cell relay of cerebellum. Prog. Brain Res. 148, 69–80. doi: 10.1016/s0079-6123(04)48007-8
PubMed Abstract | Full Text | CrossRef Full Text | Google Scholar
D’Angelo, E., Rossi, P., and Taglietti, V. (1993). Different proportions of N-methyl-D-aspartate and non-N-methyl-D-aspartate receptor currents at the mossy fibre-granule cell synapse of developing rat cerebellum. Neuroscience 53, 121–130. doi: 10.1016/0306-4522(93)90290-v
PubMed Abstract | Full Text | CrossRef Full Text | Google Scholar
Daniel, H., Hemart, N., Jaillard, D., and Crepel, F. (1993). Long-term depression requires nitric oxide and guanosine 3′:5′ cyclic monophosphate production in rat cerebellar Purkinje cells. Eur. J. Neurosci. 5, 1079–1082. doi: 10.1111/j.1460-9568.1993.tb00961.x
PubMed Abstract | Full Text | CrossRef Full Text | Google Scholar
Denk, W., Sugimori, M., and Llinás, R. (1995). Two types of calcium response limited to single spines in cerebellar Purkinje cells. Proc. Natl. Acad. Sci. U S A 92, 8279–8282. doi: 10.1073/pnas.92.18.8279
PubMed Abstract | Full Text | CrossRef Full Text | Google Scholar
D’Errico, A., Prestori, F., and D’Angelo, E. (2009). Differential induction of bidirectional long-term changes in neurotransmitter release by frequency-coded patterns at the cerebellar input. J. Physiol. 587, 5843–5857. doi: 10.1113/jphysiol.2009.177162
PubMed Abstract | Full Text | CrossRef Full Text | Google Scholar
De Zeeuw, C. I., Hoebeek, F. E., Bosman, L. W., Schonewille, M., Witter, L., and Koekkoek, S. K. (2011). Spatiotemporal firing patterns in the cerebellum. Nat. Rev. Neurosci. 12, 327–344. doi: 10.1038/nrn3011
PubMed Abstract | Full Text | CrossRef Full Text | Google Scholar
Dittman, J. S., and Regehr, W. G. (1998). Calcium dependence and recovery kinetics of presynaptic depression at the climbing fiber to Purkinje cell synapse. J. Neurosci. 18, 6147–6162.
Diwakar, S., Lombardo, P., Solinas, S., Naldi, G., and D’Angelo, E. (2011). Local field potential modeling predicts dense activation in cerebellar granule cells clusters under LTP and LTD control. PLoS One 6:e21928. doi: 10.1371/journal.pone.0021928
PubMed Abstract | Full Text | CrossRef Full Text | Google Scholar
du Lac, S., Raymond, J. L., Sejnowski, T. J., and Lisberger, S. G. (1995). Learning and memory in the vestibulo-ocular reflex. Annu. Rev. Neurosci. 18, 409–441. doi: 10.1146/annurev.neuro.18.1.409
PubMed Abstract | Full Text | CrossRef Full Text | Google Scholar
Eccles, J. C. (1973). The cerebellum as a computer: patterns in space and time. J. Physiol. 229, 1–32. doi: 10.1113/jphysiol.1973.sp010123
PubMed Abstract | Full Text | CrossRef Full Text | Google Scholar
Eccles, J. C., Ito, M., and Szentagothai, J. (1967). The Cerebellum as a Neuronal Machine. Berlin, Heidelberg, New York: Springer-Verlag, 337–347.
Eccles, J. C., Llinás, R., and Sasaki, K. (1966). The excitatory synaptic action of climbing fibres on the Purkinje cells of the cerebellum. J. Physiol. 182, 268–296. doi: 10.1113/jphysiol.1966.sp007824
PubMed Abstract | Full Text | CrossRef Full Text | Google Scholar
Eccles, J. C., Sabah, N. H., Schmidt, R. F., and Táboríková, H. (1972). Mode of operation of the cerebellum in the dynamic loop control of movement. Brain Res. 40, 73–80. doi: 10.1016/0006-8993(72)90109-6
PubMed Abstract | Full Text | CrossRef Full Text | Google Scholar
Eilers, J., Takechi, H., Finch, E. A., Augustine, G. J., and Konnerth, A. (1997). Local dendritic Ca2+ signaling induces cerebellar long-term depression. Learn. Mem. 4, 159–168. doi: 10.1101/lm.4.1.159
PubMed Abstract | Full Text | CrossRef Full Text | Google Scholar
Finch, E. A., Turner, T. J., and Goldin, S. M. (1991). Calcium as a coagonist of inositol 1,4,5-trisphosphate-induced calcium release. Science 252, 443–446. doi: 10.1126/science.2017683
PubMed Abstract | Full Text | CrossRef Full Text | Google Scholar
Fredette, B. J., and Mugnaini, E. (1991). The GABAergic cerebello-olivary projection in the rat. Anat. Embryol. (Berl.) 184, 225–243. doi: 10.1007/bf01673258
PubMed Abstract | Full Text | CrossRef Full Text | Google Scholar
Frick, A., and Johnston, D. (2005). Plasticity of dendritic excitability. J. Neurobiol. 64, 100–115. doi: 10.1002/neu.20148
PubMed Abstract | Full Text | CrossRef Full Text | Google Scholar
Gall, D., Prestori, F., Sola, E., D’Errico, A., Roussel, C., Forti, L., et al. (2005). Intracellular calcium regulation by burst discharge determines bidirectional long-term synaptic plasticity at the cerebellum input stage. J. Neurosci. 25, 4813–4822. doi: 10.1523/jneurosci.0410-05.2005
PubMed Abstract | Full Text | CrossRef Full Text | Google Scholar
Gao, Z., van Beugen, B. J., and De Zeeuw, C. I. (2012). Distributed synergistic plasticity and cerebellar learning. Nat. Rev. Neurosci. 13, 619–635. doi: 10.1038/nrn3312
PubMed Abstract | Full Text | CrossRef Full Text | Google Scholar
Gardette, R., Debono, M., Dupont, J. L., and Crepel, F. (1985a). Electrophysiological studies on the postnatal development of intracerebellar nuclei neurons in rat cerebellar slices maintained in vitro. I. Postsynaptic potentials. Brain Res. 351, 47–55. doi: 10.1016/0165-3806(85)90230-5
PubMed Abstract | Full Text | CrossRef Full Text | Google Scholar
Gardette, R., Debono, M., Dupont, J. L., and Crepel, F. (1985b). Electrophysiological studies on the postnatal development of intracerebellar nuclei neurons in rat cerebellar slices maintained in vitro. II. Membrane conductances. Brain Res. 352, 97–106. doi: 10.1016/0165-3806(85)90091-4
PubMed Abstract | Full Text | CrossRef Full Text | Google Scholar
Garrido, J. A., Luque, N. R., D’Angelo, E., and Ros, E. (2013a). Distributed cerebellar plasticity implements adaptable gain control in a manipulation task: a closed-loop robotic simulation. Front. Neural Circuits 7:159. doi: 10.3389/fncir.2013.00159
PubMed Abstract | Full Text | CrossRef Full Text | Google Scholar
Garrido, J. A., Ros, E., and D’Angelo, E. (2013b). Spike timing regulation on the millisecond scale by distributed synaptic plasticity at the cerebellum input stage: a simulation study. Front. Comput. Neurosci. 7:64. doi: 10.3389/fncom.2013.00064
PubMed Abstract | Full Text | CrossRef Full Text | Google Scholar
Han, V. Z., Zhang, Y., Bell, C. C., and Hansel, C. (2007). Synaptic plasticity and calcium signaling in Purkinje cells of the central cerebellar lobes of mormyrid fish. J. Neurosci. 27, 13499–13512. doi: 10.1523/jneurosci.2613-07.2007
PubMed Abstract | Full Text | CrossRef Full Text | Google Scholar
Hansel, C., de Jeu, M., Belmeguenai, A., Houtman, S. H., Buitendijk, G. H., Andreev, D., et al. (2006). αCaMKII is essential for cerebellar LTD and motor learning. Neuron 51, 835–843. doi: 10.1016/j.neuron.2006.08.013
PubMed Abstract | Full Text | CrossRef Full Text | Google Scholar
Hansel, C., and Linden, D. J. (2000). Long-term depression of the cerebellar climbing fiber–Purkinje neuron synapse. Neuron 26, 473–482. doi: 10.1016/s0896-6273(00)81179-4
PubMed Abstract | Full Text | CrossRef Full Text | Google Scholar
Hansel, C., Linden, D. J., and D’Angelo, E. (2001). Beyond parallel fiber LTD: the diversity of synaptic and non-synaptic plasticity in the cerebellum. Nat. Neurosci. 4, 467–475.
Hartell, N. A. (1996). Strong activation of parallel fibers produces localized calcium transients and a form of LTD that spreads to distant synapses. Neuron 16, 601–610. doi: 10.1016/s0896-6273(00)80079-3
PubMed Abstract | Full Text | CrossRef Full Text | Google Scholar
Hartell, N. A. (2002). Parallel fiber plasticity. Cerebellum 1, 3–18. doi: 10.1080/147342202753203041
PubMed Abstract | Full Text | CrossRef Full Text | Google Scholar
Hashimoto, K., Ichikawa, R., Takechi, H., Inoue, Y., Aiba, A., Sakimura, K., et al. (2001). Roles of glutamate receptor delta 2 subunit (GluRdelta 2) and metabotropic glutamate receptor subtype 1 (mGluR1) in climbing fiber synapse elimination during postnatal cerebellar development. J. Neurosci. 21, 9701–9712.
Hesslow, G., Svensson, P., and Ivarsson, M. (1999). Learned movements elicited by direct stimulation of cerebellar mossy fiber afferents. Neuron 24, 179–185. doi: 10.1016/s0896-6273(00)80831-4
PubMed Abstract | Full Text | CrossRef Full Text | Google Scholar
Hirano, T. (1991). Differential pre- and postsynaptic mechanisms for synaptic potentiation and depression between a granule cell and a Purkinje cell in rat cerebellar culture. Synapse 7, 321–333. doi: 10.1002/syn.890070408
PubMed Abstract | Full Text | CrossRef Full Text | Google Scholar
Hull, C. A., Chu, Y., Thanawala, M., and Regehr, W. G. (2013). Hyperpolarization induces a long-term increase in the spontaneous firing rate of cerebellar Golgi cells. J. Neurosci. 33, 5895–5902. doi: 10.1523/jneurosci.4052-12.2013
PubMed Abstract | Full Text | CrossRef Full Text | Google Scholar
Hull, C., and Regehr, W. G. (2012). Identification of an inhibitory circuit that regulates cerebellar Golgi cell activity. Neuron 73, 149–158. doi: 10.1016/j.neuron.2011.10.030
PubMed Abstract | Full Text | CrossRef Full Text | Google Scholar
Ikeda, M., and Matsushita, M. (1973). Electron microscopic observations on the spinal projections to the cerebellar nuclei in the cat and rabbit. Experientia 29, 1280–1282. doi: 10.1007/bf01935117
PubMed Abstract | Full Text | CrossRef Full Text | Google Scholar
Ikeda, M., and Matsushita, M. (1974). Electron microscopic observations on the olivary projections to the cerebellar nuclei in the cat. Experientia 30, 536–538. doi: 10.1007/bf01926338
PubMed Abstract | Full Text | CrossRef Full Text | Google Scholar
Irving, A. J., Collingridge, G. L., and Schofield, J. G. (1992a). Interactions between Ca2+ mobilizing mechanisms in cultured rat cerebellar granule cells. J. Physiol. 456, 667–680. doi: 10.1113/jphysiol.1992.sp019360
PubMed Abstract | Full Text | CrossRef Full Text | Google Scholar
Irving, A. J., Collingridge, G. L., and Schofield, J. G. (1992b). L-glutamate and acetylcholine mobilise Ca2+ from the same intracellular pool in cerebellar granule cells using transduction mechanisms with different Ca2+ sensitivities. Cell Calcium 13, 293–301. doi: 10.1016/0143-4160(92)90064-y
PubMed Abstract | Full Text | CrossRef Full Text | Google Scholar
Ito, M. (1972). Neural design of the cerebellar motor control system. Brain Res. 40, 81–84. doi: 10.1016/0006-8993(72)90110-2
PubMed Abstract | Full Text | CrossRef Full Text | Google Scholar
Ito, M. (1989). Long-term depression. Annu. Rev. Neurosci. 12, 85–102. doi: 10.1146/annurev.ne.12.030189.000505
PubMed Abstract | Full Text | CrossRef Full Text | Google Scholar
Ito, M. (2006). Cerebellar circuitry as a neuronal machine. Prog. Neurobiol. 78, 272–303. doi: 10.1016/j.pneurobio.2006.02.006
PubMed Abstract | Full Text | CrossRef Full Text | Google Scholar
Ivry, R. (1997). Cerebellar timing systems. Int. Rev. Neurobiol. 41, 555–573. doi: 10.1016/s0074-7742(08)60370-0
PubMed Abstract | Full Text | CrossRef Full Text | Google Scholar
Ivry, R. B., and Spencer, R. M. (2004). Evaluating the role of the cerebellum in temporal processing: beware of the null hypothesis. Brain 127:E13; author reply E14. doi: 10.1093/brain/awh226
PubMed Abstract | Full Text | CrossRef Full Text | Google Scholar
Ivry, R. B., Spencer, R. M., Zelaznik, H. N., and Diedrichsen, J. (2002). The cerebellum and event timing. Ann. N Y Acad. Sci. 978, 302–317. doi: 10.1111/j.1749-6632.2002.tb07576.x
PubMed Abstract | Full Text | CrossRef Full Text | Google Scholar
Jirenhed, D. A., Bengtsson, F., and Hesslow, G. (2007). Acquisition, extinction and reacquisition of a cerebellar cortical memory trace. J. Neurosci. 27, 2493–2502. doi: 10.1523/jneurosci.4202-06.2007
PubMed Abstract | Full Text | CrossRef Full Text | Google Scholar
Jörntell, H., and Ekerot, C. F. (2002). Reciprocal bidirectional plasticity of parallel fiber receptive fields in cerebellar Purkinje cells and their afferent interneurons. Neuron 34, 797–806. doi: 10.1016/s0896-6273(02)00713-4
PubMed Abstract | Full Text | CrossRef Full Text | Google Scholar
Jörntell, H., and Ekerot, C. F. (2003). Receptive field plasticity profoundly alters the cutaneous parallel fiber synaptic input to cerebellar interneurons in vivo. J. Neurosci. 23, 9620–9631.
Kakegawa, W., and Yuzaki, M. (2005). A mechanism underlying AMPA receptor trafficking during cerebellar long-term potentiation. Proc. Natl. Acad. Sci. U S A 102, 17846–17851. doi: 10.1073/pnas.0508910102
PubMed Abstract | Full Text | CrossRef Full Text | Google Scholar
Kano, M., Fukunaga, K., and Konnerth, A. (1996). Ca(2+)-induced rebound potentiation of gamma-aminobutyric acid-mediated currents requires activation of Ca2+/calmodulin-dependent kinase II. Proc. Natl. Acad. Sci. U S A 93, 13351–13356. doi: 10.1073/pnas.93.23.13351
PubMed Abstract | Full Text | CrossRef Full Text | Google Scholar
Kano, M., Rexhausen, U., Dreessen, J., and Konnerth, A. (1992). Synaptic excitation produces a long-lasting rebound potentiation of inhibitory synaptic signals in cerebellar Purkinje cells. Nature 356, 601–604. doi: 10.1038/356601a0
PubMed Abstract | Full Text | CrossRef Full Text | Google Scholar
Kawaguchi, S. Y., and Hirano, T. (2007). Sustained structural change of GABA(A) receptor-associated protein underlies long-term potentiation at inhibitory synapses on a cerebellar Purkinje neuron. J. Neurosci. 27, 6788–6799. doi: 10.1523/jneurosci.1981-07.2007
PubMed Abstract | Full Text | CrossRef Full Text | Google Scholar
Kimura, S., Uchiyama, S., Takahashi, H. E., and Shibuki, K. (1998). cAMP-dependent long-term potentiation of nitric oxide release from cerebellar parallel fibers in rats. J. Neurosci. 18, 8551–8558.
Kleim, J. A., Freeman, J. H., Bruneau, R., Nolan, B. C., Cooper, N. R., Zook, A., et al. (2002). Synapse formation is associated with memory storage in the cerebellum. Proc. Natl. Acad. Sci. U S A 99, 13228–13231. doi: 10.1073/pnas.202483399
PubMed Abstract | Full Text | CrossRef Full Text | Google Scholar
Koch, G., Mori, F., Marconi, B., Codecà, C., Pecchioli, C., Salerno, S., et al. (2008). Changes in intracortical circuits of the human motor cortex following theta burst stimulation of the lateral cerebellum. Clin. Neurophysiol. 119, 2559–2569. doi: 10.1016/s1388-2457(08)60115-7
PubMed Abstract | Full Text | CrossRef Full Text | Google Scholar
Konnerth, A., Dreessen, J., and Augustine, G. J. (1992). Brief dendritic calcium signals initiate long-lasting synaptic depression in cerebellar Purkinje cells. Proc. Natl. Acad. Sci. U S A 89, 7051–7055. doi: 10.1073/pnas.89.15.7051
PubMed Abstract | Full Text | CrossRef Full Text | Google Scholar
Lavond, D. G., Hembree, T. L., and Thompson, R. F. (1985). Effect of kainic acid lesions of the cerebellar interpositus nucleus on eyelid conditioning in the rabbit. Brain Res. 326, 179–182. doi: 10.1016/0006-8993(85)91400-3
PubMed Abstract | Full Text | CrossRef Full Text | Google Scholar
Le Guen, M. C., and De Zeeuw, C. I. (2010). Presynaptic plasticity at cerebellar parallel fiber terminals. Funct. Neurol. 25, 141–151.
Lepora, N. F., Porrill, J., Yeo, C. H., and Dean, P. (2010). Sensory prediction or motor control? Application of marr-albus type models of cerebellar function to classical conditioning. Front. Comput. Neurosci. 4:140. doi: 10.3389/fncom.2010.00140
PubMed Abstract | Full Text | CrossRef Full Text | Google Scholar
Lev-Ram, V., Jiang, T., Wood, J., Lawrence, D. S., and Tsien, R. Y. (1997). Synergies and coincidence requirements between NO, cGMP and Ca2+ in the induction of cerebellar long-term depression. Neuron 18, 1025–1038. doi: 10.1016/s0896-6273(00)80340-2
PubMed Abstract | Full Text | CrossRef Full Text | Google Scholar
Lev-Ram, V., Makings, L. R., Keitz, P. F., Kao, J. P., and Tsien, R. Y. (1995). Long-term depression in cerebellar Purkinje neurons results from coincidence of nitric oxide and depolarization-induced Ca2+ transients. Neuron 15, 407–415. doi: 10.1016/0896-6273(95)90044-6
PubMed Abstract | Full Text | CrossRef Full Text | Google Scholar
Lev-Ram, V., Mehta, S. B., Kleinfeld, D., and Tsien, R. Y. (2003). Reversing cerebellar long-term depression. Proc. Natl. Acad. Sci. U S A 100, 15989–15993. doi: 10.1073/pnas.2636935100
PubMed Abstract | Full Text | CrossRef Full Text | Google Scholar
Lev-Ram, V., Wong, S. T., Storm, D. R., and Tsien, R. Y. (2002). A new form of cerebellar long-term potentiation is postsynaptic and depends on nitric oxide but not cAMP. Proc. Natl. Acad. Sci. U S A 99, 8389–8393. doi: 10.1073/pnas.122206399
PubMed Abstract | Full Text | CrossRef Full Text | Google Scholar
Linden, D. J. (1999). The return of the spike: postsynaptic action potentials and the induction of LTP and LTD. Neuron 22, 661–666. doi: 10.1016/S0896-6273(00)80726-6
PubMed Abstract | Full Text | CrossRef Full Text | Google Scholar
Linden, D. J., Dawson, T. M., and Dawson, V. L. (1995). An evaluation of the nitric oxide/cGMP/cGMP-dependent protein kinase cascade in the induction of cerebellar long-term depression in culture. J. Neurosci. 15, 5098–5105.
Linden, D. J., Dickinson, M. H., Smeyne, M., and Connor, J. A. (1991). A long-term depression of AMPA currents in cultured cerebellar Purkinje neurons. Neuron 7, 81–89. doi: 10.1016/0896-6273(91)90076-c
PubMed Abstract | Full Text | CrossRef Full Text | Google Scholar
Lisberger, S. G. (1994). Neural basis for motor learning in the vestibuloocular reflex of primates. III. Computational and behavioral analysis of the sites of learning. J. Neurophysiol. 72, 974–998.
Liu, S. Q., and Cull-Candy, S. G. (2000). Synaptic activity at calcium-permeable AMPA receptors induces a switch in receptor subtype. Nature 405, 454–458. doi: 10.1038/35013064
PubMed Abstract | Full Text | CrossRef Full Text | Google Scholar
Luque, N. R., Garrido, J. A., Carrillo, R. R., Coenen, O. J.-M. D., and Ros, E. (2011). Cerebellarlike corrective model inference engine for manipulation tasks. IEEE Trans. Syst. Man Cybern. B Cybern. 41, 1299–1312. doi: 10.1109/tsmcb.2011.2138693
Luque, N. R., Garrido, J. A., Carrillo, R. R., D’Angelo, E., and Ros, E. (2014). Fast convergence of learning requires plasticity between inferior olive and deep cerebellar nuclei in a manipulation task: a closed-loop robotic simulation. Front. Comput. Neurosci. 8:97. doi: 10.3389/fncom.2014.00097
PubMed Abstract | Full Text | CrossRef Full Text | Google Scholar
Luque, N. R., Garrido, J. A., Carrillo, R. R., and Ros, E. (2013). Connection control implications in a distributed plasticity cerebellar model. BMC Neurosci. 14:P329.
Maffei, A., Prestori, F., Rossi, P., Taglietti, V., and D’Angelo, E. (2002). Presynaptic current changes at the mossy fiber-granule cell synapse of cerebellum during LTP. J. Neurophysiol. 88, 627–638.
Maffei, A., Prestori, F., Shibuki, K., Rossi, P., Taglietti, V., and D’Angelo, E. (2003). NO enhances presynaptic currents during cerebellar mossy fiber-granule cell LTP. J. Neurophysiol. 90, 2478–2483. doi: 10.1152/jn.00399.2003
PubMed Abstract | Full Text | CrossRef Full Text | Google Scholar
Mapelli, J., and D’Angelo, E. (2007). The spatial organization of long-term synaptic plasticity at the input stage of cerebellum. J. Neurosci. 27, 1285–1296. doi: 10.1523/jneurosci.4873-06.2007
PubMed Abstract | Full Text | CrossRef Full Text | Google Scholar
Mapelli, J., Gandolfi, D., and D’Angelo, E. (2010). Combinatorial responses controlled by synaptic inhibition in the cerebellum granular layer. J. Neurophysiol. 103, 250–261. doi: 10.1152/jn.00642.2009
PubMed Abstract | Full Text | CrossRef Full Text | Google Scholar
Mapelli, L., Rossi, P., Nieus, T., and D’Angelo, E. (2009). Tonic activation of GABAB receptors reduces release probability at inhibitory connections in the cerebellar glomerulus. J. Neurophysiol. 101, 3089–3099. doi: 10.1152/jn.91190.2008
PubMed Abstract | Full Text | CrossRef Full Text | Google Scholar
Mapelli, L., Solinas, S., and D’Angelo, E. (2014). Integration and regulation of glomerular inhibition in the cerebellar granular layer circuit. Front. Cell. Neurosci. 8:55. doi: 10.3389/fncel.2014.00342
PubMed Abstract | Full Text | CrossRef Full Text | Google Scholar
Marcaggi, P., and Attwell, D. (2007). Short- and long-term depression of rat cerebellar parallel fibre synaptic transmission mediated by synaptic crosstalk. J. Physiol. 578, 545–550. doi: 10.1113/jphysiol.2006.115014
PubMed Abstract | Full Text | CrossRef Full Text | Google Scholar
Marr, D. (1969). A theory of cerebellar cortex. J. Physiol. 202, 437–470. doi: 10.1113/jphysiol.1969.sp008820
Martin, S. J., Grimwood, P. D., and Morris, R. G. (2000). Synaptic plasticity and memory: an evaluation of the hypothesis. Annu. Rev. Neurosci. 23, 649–711. doi: 10.1146/annurev.neuro.23.1.649
PubMed Abstract | Full Text | CrossRef Full Text | Google Scholar
Masuda, N., and Amari, S. (2008). A computational study of synaptic mechanisms of partial memory transfer in cerebellar vestibulo-ocular-reflex learning. J. Comput. Neurosci. 24, 137–156. doi: 10.1007/s10827-007-0045-7
PubMed Abstract | Full Text | CrossRef Full Text | Google Scholar
Mauk, M. D., and Buonomano, D. V. (2004). The neural basis of temporal processing. Annu. Rev. Neurosci. 27, 307–340. doi: 10.1146/annurev.neuro.27.070203.144247
PubMed Abstract | Full Text | CrossRef Full Text | Google Scholar
Mauk, M. D., and Donegan, N. H. (1997). A model of Pavlovian eyelid conditioning based on the synaptic organization of the cerebellum. Learn. Mem. 4, 130–158. doi: 10.1101/lm.4.1.130
PubMed Abstract | Full Text | CrossRef Full Text | Google Scholar
Mauk, M. D., Steinmetz, J. E., and Thompson, R. F. (1986). Classical conditioning using stimulation of the inferior olive as the unconditioned stimulus. Proc. Natl. Acad. Sci. U S A 83, 5349–5353. doi: 10.1073/pnas.83.14.5349
PubMed Abstract | Full Text | CrossRef Full Text | Google Scholar
Medina, J. F., Garcia, K. S., and Mauk, M. D. (2001). A mechanism for savings in the cerebellum. J. Neurosci. 21, 4081–4089.
Medina, J. F., Garcia, K. S., Nores, W. L., Taylor, N. M., and Mauk, M. D. (2000). Timing mechanisms in the cerebellum: testing predictions of a large-scale computer simulation. J. Neurosci. 20, 5516–5525.
Medina, J. F., and Mauk, M. D. (1999). Simulations of cerebellar motor learning: computational analysis of plasticity at the mossy fiber to deep nucleus synapse. J. Neurosci. 19, 7140–7151.
Medina, J. F., and Mauk, M. D. (2000). Computer simulation of cerebellar information processing. Nat. Neurosci. 3(Suppl.), 1205–1211. doi: 10.1038/81486
PubMed Abstract | Full Text | CrossRef Full Text | Google Scholar
Medina, J. F., Nores, W. L., and Mauk, M. D. (2002). Inhibition of climbing fibres is a signal for the extinction of conditioned eyelid responses. Nature 416, 330–333. doi: 10.1038/416330a
PubMed Abstract | Full Text | CrossRef Full Text | Google Scholar
Midtgaard, J., Lasser-Ross, N., and Ross, W. N. (1993). Spatial distribution of Ca2+ influx in turtle Purkinje cell dendrites in vitro: role of a transient outward current. J. Neurophysiol. 70, 2455–2469.
Miles, F. A., and Lisberger, S. G. (1981). The “error” signals subserving adaptive gain control in the primate vestibulo-ocular reflex. Ann. N Y Acad. Sci. 374, 513–525. doi: 10.1111/j.1749-6632.1981.tb30896.x
PubMed Abstract | Full Text | CrossRef Full Text | Google Scholar
Monaco, J., Casellato, C., Koch, G., and D’Angelo, E. (2014). Cerebellar theta burst stimulation dissociates memory components in eyeblink classical conditioning. Eur. J. Neurosci. 40, 3363–3370. doi: 10.1111/ejn.12700
PubMed Abstract | Full Text | CrossRef Full Text | Google Scholar
Morishita, W., and Sastry, B. R. (1996). Postsynaptic mechanisms underlying long-term depression of GABAergic transmission in neurons of the deep cerebellar nuclei. J. Neurophysiol. 76, 59–68.
Mozzachiodi, R., and Byrne, J. H. (2010). More than synaptic plasticity: role of nonsynaptic plasticity in learning and memory. Trends Neurosci. 33, 17–26. doi: 10.1016/j.tins.2009.10.001
PubMed Abstract | Full Text | CrossRef Full Text | Google Scholar
Muri, R., and Knöpfel, T. (1994). Activity induced elevations of intracellular calcium concentration in neurons of the deep cerebellar nuclei. J. Neurophysiol. 71, 420–428.
Nieus, T. R., Mapelli, L., and D’Angelo, E. (2014). Regulation of output spike patterns by phasic inhibition in cerebellar granule cells. Front. Cell. Neurosci. 8:246. doi: 10.3389/fncel.2014.00246
PubMed Abstract | Full Text | CrossRef Full Text | Google Scholar
Nieus, T., Sola, E., Mapelli, J., Saftenku, E., Rossi, P., and D’Angelo, E. (2006). LTP regulates burst initiation and frequency at mossy fiber-granule cell synapses of rat cerebellum: experimental observations and theoretical predictions. J. Neurophysiol. 95, 686–699. doi: 10.1152/jn.00696.2005
PubMed Abstract | Full Text | CrossRef Full Text | Google Scholar
Ohtsuki, G., and Hirano, T. (2008). Bidirectional plasticity at developing climbing fiber-Purkinje neuron synapses. Eur. J. Neurosci. 28, 2393–2400. doi: 10.1111/j.1460-9568.2008.06539.x
PubMed Abstract | Full Text | CrossRef Full Text | Google Scholar
Ohtsuki, G., Piochon, C., and Hansel, C. (2009). Climbing fiber signaling and cerebellar gain control. Front. Cell. Neurosci. 3:4. doi: 10.3389/neuro.03.004.2009
PubMed Abstract | Full Text | CrossRef Full Text | Google Scholar
Ohyama, T., Nores, W. L., and Mauk, M. D. (2003a). Stimulus generalization of conditioned eyelid responses produced without cerebellar cortex: implications for plasticity in the cerebellar nuclei. Learn. Mem. 10, 346–354. doi: 10.1101/lm.67103
PubMed Abstract | Full Text | CrossRef Full Text | Google Scholar
Ohyama, T., Nores, W. L., Medina, J. F., Riusech, F. A., and Mauk, M. D. (2006). Learning-induced plasticity in deep cerebellar nucleus. J. Neurosci. 26, 12656–12663. doi: 10.3410/f.1053945.505900
PubMed Abstract | Full Text | CrossRef Full Text | Google Scholar
Ohyama, T., Nores, W. L., Murphy, M., and Mauk, M. D. (2003b). What the cerebellum computes. Trends Neurosci. 26, 222–227. doi: 10.1016/s0166-2236(03)00054-7
PubMed Abstract | Full Text | CrossRef Full Text | Google Scholar
Ouardouz, M., and Sastry, B. R. (2000). Mechanisms underlying LTP of inhibitory synaptic transmission in the deep cerebellar nuclei. J. Neurophysiol. 84, 1414–1421.
Person, A. L., and Raman, I. M. (2010). Deactivation of L-type Ca current by inhibition controls LTP at excitatory synapses in the cerebellar nuclei. Neuron 66, 550–559. doi: 10.1016/j.neuron.2010.04.024
PubMed Abstract | Full Text | CrossRef Full Text | Google Scholar
Philipona, D., and Coenen, O. J.-M. D. (2004). Model of granular layer encoding in the cerebellum. Neurocomputing 58, 575–580. doi: 10.1016/j.neucom.2004.01.097
Piochon, C., Levenes, C., Ohtsuki, G., and Hansel, C. (2010). Purkinje cell NMDA receptors assume a key role in synaptic gain control in the mature cerebellum. J. Neurosci. 30, 15330–15335. doi: 10.1523/jneurosci.4344-10.2010
PubMed Abstract | Full Text | CrossRef Full Text | Google Scholar
Powell, C. M., Schoch, S., Monteggia, L., Barrot, M., Matos, M. F., Feldmann, N., et al. (2004). The presynaptic active zone protein RIM1alpha is critical for normal learning and memory. Neuron 42, 143–153. doi: 10.1016/s0896-6273(04)00146-1
PubMed Abstract | Full Text | CrossRef Full Text | Google Scholar
Prestori, F., Bonardi, C., Mapelli, L., Lombardo, P., Goselink, R., De Stefano, M. E., et al. (2013). Gating of long-term potentiation by nicotinic acetylcholine receptors at the cerebellum input stage. PLoS One 8:e64828. doi: 10.1371/journal.pone.0064828
PubMed Abstract | Full Text | CrossRef Full Text | Google Scholar
Pugh, J. R., and Jahr, C. E. (2011). Axonal GABAA receptors increase cerebellar granule cell excitability and synaptic activity. J. Neurosci. 31, 565–574. doi: 10.1523/jneurosci.4506-10.2011
PubMed Abstract | Full Text | CrossRef Full Text | Google Scholar
Pugh, J. R., and Raman, I. M. (2006). Potentiation of mossy fiber EPSCs in the cerebellar nuclei by NMDA receptor activation followed by postinhibitory rebound current. Neuron 51, 113–123. doi: 10.1016/j.neuron.2006.05.021
PubMed Abstract | Full Text | CrossRef Full Text | Google Scholar
Pugh, J. R., and Raman, I. M. (2008). Mechanisms of potentiation of mossy fiber EPSCs in the cerebellar nuclei by coincident synaptic excitation and inhibition. J. Neurosci. 28, 10549–10560. doi: 10.1523/jneurosci.2061-08.2008
PubMed Abstract | Full Text | CrossRef Full Text | Google Scholar
Pugh, J. R., and Raman, I. M. (2009). Nothing can be coincidence: synaptic inhibition and plasticity in the cerebellar nuclei. Trends Neurosci. 32, 170–177. doi: 10.1016/j.tins.2008.12.001
PubMed Abstract | Full Text | CrossRef Full Text | Google Scholar
Qiu, D. L., and Knöpfel, T. (2007). An NMDA receptor/nitric oxide cascade in presynaptic parallel fiber-Purkinje neuron long-term potentiation. J. Neurosci. 27, 3408–3415. doi: 10.1523/jneurosci.4831-06.2007
PubMed Abstract | Full Text | CrossRef Full Text | Google Scholar
Ramakrishnan, K. B., and D’Angelo, E. (2012). Theta-Sensory input induced long-term potentiation (Ltp) In Purkinje cell layer of rat cerebellum. FENS Abstr. 6, 153.11.
Rancillac, A., and Crépel, F. (2004). Synapses between parallel fibres and stellate cells express long-term changes in synaptic efficacy in rat cerebellum. J. Physiol. 554, 707–720. doi: 10.1113/jphysiol.2003.055871
PubMed Abstract | Full Text | CrossRef Full Text | Google Scholar
Reynolds, T., and Hartell, N. A. (2000). An evaluation of the synapse specificity of long-term depression induced in rat cerebellar slices. J. Physiol. 527(Pt. 3), 563–577. doi: 10.1111/j.1469-7793.2000.00563.x
PubMed Abstract | Full Text | CrossRef Full Text | Google Scholar
Reynolds, T., and Hartell, N. A. (2001). Roles for nitric oxide and arachidonic acid in the induction of heterosynaptic cerebellar LTD. Neuroreport 12, 133–136. doi: 10.1097/00001756-200101220-00034
PubMed Abstract | Full Text | CrossRef Full Text | Google Scholar
Rinaldo, L., and Hansel, C. (2013). Muscarinic acetylcholine receptor activation blocks long-term potentiation at cerebellar parallel fiber-Purkinje cell synapses via cannabinoid signaling. Proc. Natl. Acad. Sci. U S A 110, 11181–11186. doi: 10.1073/pnas.1221803110
PubMed Abstract | Full Text | CrossRef Full Text | Google Scholar
Robberechts, Q., Wijnants, M., Giugliano, M., and De Schutter, E. (2010). Long-term depression at parallel fiber to Golgi cell synapses. J. Neurophysiol. 104, 3413–3423. doi: 10.1152/jn.00030.2010
PubMed Abstract | Full Text | CrossRef Full Text | Google Scholar
Roggeri, L., Rivieccio, B., Rossi, P., and D’Angelo, E. (2008). Tactile stimulation evokes long-term synaptic plasticity in the granular layer of cerebellum. J. Neurosci. 28, 6354–6359. doi: 10.1523/jneurosci.5709-07.2008
PubMed Abstract | Full Text | CrossRef Full Text | Google Scholar
Rössert, C., Solinas, S., D’Angelo, E., Dean, P., and Porrill, J. (2014). Model cerebellar granule cells can faithfully transmit modulated firing rate signals. Front. Cell. Neurosci. 8:304. doi: 10.3389/fncel.2014.00304
PubMed Abstract | Full Text | CrossRef Full Text | Google Scholar
Rossi, P., D’Angelo, E., and Taglietti, V. (1996). Differential long-lasting potentiation of the NMDA and non-NMDA synaptic currents induced by metabotropic and NMDA receptor coactivation in cerebellar granule cells. Eur. J. Neurosci. 8, 1182–1189. doi: 10.1111/j.1460-9568.1996.tb01286.x
PubMed Abstract | Full Text | CrossRef Full Text | Google Scholar
Rossi, P., Mapelli, L., Roggeri, L., Gall, D., de Kerchove d’Exaerde, A., Schiffmann, S. N., et al. (2006). Inhibition of constitutive inward rectifier currents in cerebellar granule cells by pharmacological and synaptic activation of GABA receptors. Eur. J. Neurosci. 24, 419–432. doi: 10.1111/j.1460-9568.2006.04914.x
PubMed Abstract | Full Text | CrossRef Full Text | Google Scholar
Sakurai, M. (1987). Synaptic modification of parallel fibre-Purkinje cell transmission in in vitro guinea-pig cerebellar slices. J. Physiol. 394, 463–480. doi: 10.1113/jphysiol.1987.sp016881
PubMed Abstract | Full Text | CrossRef Full Text | Google Scholar
Salin, P. A., Malenka, R. C., and Nicoll, R. A. (1996). Cyclic AMP mediates a presynaptic form of LTP at cerebellar parallel fiber synapses. Neuron 16, 797–803. doi: 10.1016/s0896-6273(00)80099-9
PubMed Abstract | Full Text | CrossRef Full Text | Google Scholar
Schmahmann, J. D. (2010). The role of the cerebellum in cognition and emotion: personal reflections since 1982 on the dysmetria of thought hypothesis and its historical evolution from theory to therapy. Neuropsychol. Rev. 20, 236–260. doi: 10.1007/s11065-010-9142-x
PubMed Abstract | Full Text | CrossRef Full Text | Google Scholar
Schmolesky, M. T., De Zeeuw, C. I., and Hansel, C. (2005). Climbing fiber synaptic plasticity and modifications in Purkinje cell excitability. Prog. Brain Res. 148, 81–94. doi: 10.1016/s0079-6123(04)48008-x
PubMed Abstract | Full Text | CrossRef Full Text | Google Scholar
Schonewille, M., Belmeguenai, A., Koekkoek, S. K., Houtman, S. H., Boele, H. J., van Beugen, B. J., et al. (2010). Purkinje cell-specific knockout of the protein phosphatase PP2B impairs potentiation and cerebellar motor learning. Neuron 67, 618–628. doi: 10.3410/f.5461957.5425055
PubMed Abstract | Full Text | CrossRef Full Text | Google Scholar
Schraa-Tam, C. K., Rietdijk, W. J., Verbeke, W. J., Dietvorst, R. C., van den Berg, W. E., Bagozzi, R. P., et al. (2012). fMRI activities in the emotional cerebellum: a preference for negative stimuli and goal-directed behavior. Cerebellum 11, 233–245. doi: 10.1007/s12311-011-0301-2
PubMed Abstract | Full Text | CrossRef Full Text | Google Scholar
Schreurs, B. G., Gusev, P. A., Tomsic, D., Alkon, D. L., and Shi, T. (1998). Intracellular correlates of acquisition and long-term memory of classical conditioning in Purkinje cell dendrites in slices of rabbit cerebellar lobule HVI. J. Neurosci. 18, 5498–5507.
Schweighofer, N., Doya, K., and Kuroda, S. (2004). Cerebellar aminergic neuromodulation: towards a functional understanding. Brain Res. Brain Res. Rev. 44, 103–116. doi: 10.1016/j.brainresrev.2003.10.004
PubMed Abstract | Full Text | CrossRef Full Text | Google Scholar
Schweighofer, N., Doya, K., and Lay, F. (2001). Unsupervised learning of granule cell sparse codes enhances cerebellar adaptive control. Neuroscience 103, 35–50. doi: 10.1016/s0306-4522(00)00548-0
PubMed Abstract | Full Text | CrossRef Full Text | Google Scholar
Sgritta, M., Prestori, F., and D’Angelo, E. (2014). Spike-timing dependent plasticity at the cerebellar mossy fiber–granule cell synapse. FENS Abstr. 7, 1396.57.
Shadmehr, R., Smith, M. A., and Krakauer, J. W. (2010). Error correction, sensory prediction and adaptation in motor control. Annu. Rev. Neurosci. 33, 89–108. doi: 10.1146/annurev-neuro-060909-153135
PubMed Abstract | Full Text | CrossRef Full Text | Google Scholar
Shen, Y., Hansel, C., and Linden, D. J. (2002). Glutamate release during LTD at cerebellar climbing fiber-Purkinje cell synapses. Nat. Neurosci. 5, 725–726. doi: 10.1038/nn895
PubMed Abstract | Full Text | CrossRef Full Text | Google Scholar
Shibuki, K., and Okada, D. (1991). Endogenous nitric oxide release required for long-term synaptic depression in the cerebellum. Nature 349, 326–328. doi: 10.1038/349326a0
PubMed Abstract | Full Text | CrossRef Full Text | Google Scholar
Shibuki, K., and Okada, D. (1992). Cerebellar long-term potentiation under suppressed postsynaptic Ca2+ activity. Neuroreport 3, 231–234. doi: 10.1097/00001756-199203000-00003
PubMed Abstract | Full Text | CrossRef Full Text | Google Scholar
Silver, R. A., Traynelis, S. F., and Cull-Candy, S. G. (1992). Rapid-time-course miniature and evoked excitatory currents at cerebellar synapses in situ. Nature 355, 163–166. doi: 10.1038/355163a0
PubMed Abstract | Full Text | CrossRef Full Text | Google Scholar
Simpson, P. B., Nahorski, S. R., and Challiss, R. A. (1996). Agonist-evoked Ca2+ mobilization from stores expressing inositol 1,4,5-trisphosphate receptors and ryanodine receptors in cerebellar granule neurones. J. Neurochem. 67, 364–373. doi: 10.1046/j.1471-4159.1996.67010364.x
PubMed Abstract | Full Text | CrossRef Full Text | Google Scholar
Sola, E., Prestori, F., Rossi, P., Taglietti, V., and D’Angelo, E. (2004). Increased neurotransmitter release during long-term potentiation at mossy fibre-granule cell synapses in rat cerebellum. J. Physiol. 557, 843–861. doi: 10.1113/jphysiol.2003.060285
PubMed Abstract | Full Text | CrossRef Full Text | Google Scholar
Soler-Llavina, G. J., and Sabatini, B. L. (2006). Synapse-specific plasticity and compartmentalized signaling in cerebellar stellate cells. Nat. Neurosci. 9, 798–806. doi: 10.1038/nn1698
PubMed Abstract | Full Text | CrossRef Full Text | Google Scholar
Song, S., Miller, K. D., and Abbott, L. F. (2000). Competitive Hebbian learning through spike-timing-dependent synaptic plasticity. Nat. Neurosci. 3, 919–926. doi: 10.1038/78829
PubMed Abstract | Full Text | CrossRef Full Text | Google Scholar
Southam, E., Morris, R., and Garthwaite, J. (1992). Sources and targets of nitric oxide in rat cerebellum. Neurosci. Lett. 137, 241–244. doi: 10.1016/0304-3940(92)90413-2
PubMed Abstract | Full Text | CrossRef Full Text | Google Scholar
Steinmetz, J. E., Lavond, D. G., Ivkovich, D., Logan, C. G., and Thompson, R. F. (1992). Disruption of classical eyelid conditioning after cerebellar lesions: damage to a memory trace system or a simple performance deficit? J. Neurosci. 12, 4403–4426.
Storm, D. R., Hansel, C., Hacker, B., Parent, A., and Linden, D. J. (1998). Impaired cerebellar long-term potentiation in type I adenylyl cyclase mutant mice. Neuron 20, 1199–1210. doi: 10.1016/s0896-6273(00)80500-0
PubMed Abstract | Full Text | CrossRef Full Text | Google Scholar
Sun, L., and June Liu, S. (2007). Activation of extrasynaptic NMDA receptors induces a PKC-dependent switch in AMPA receptor subtypes in mouse cerebellar stellate cells. J. Physiol. 583, 537–553. doi: 10.1113/jphysiol.2007.136788
PubMed Abstract | Full Text | CrossRef Full Text | Google Scholar
Szapiro, G., and Barbour, B. (2007). Multiple climbing fibers signal to molecular layer interneurons exclusively via glutamate spillover. Nat. Neurosci. 10, 735–742. doi: 10.1038/nn1907
PubMed Abstract | Full Text | CrossRef Full Text | Google Scholar
Teune, T. M., van der Burg, J., and Ruigrok, T. J. (1995). Cerebellar projections to the red nucleus and inferior olive originate from separate populations of neurons in the rat: a non-fluorescent double labeling study. Brain Res. 673, 313–369. doi: 10.1016/0006-8993(94)01431-g
PubMed Abstract | Full Text | CrossRef Full Text | Google Scholar
Teune, T. M., van der Burg, J., van der Moer, J., Voogd, J., and Ruigrok, T. J. (2000). Topography of cerebellar nuclear projections to the brain stem in the rat. Prog. Brain Res. 124, 141–172. doi: 10.1016/s0079-6123(00)24014-4
PubMed Abstract | Full Text | CrossRef Full Text | Google Scholar
Timmann, D., Watts, S., and Hore, J. (1999). Failure of cerebellar patients to time finger opening precisely causes ball high-low inaccuracy in overarm throws. J. Neurophysiol. 82, 103–114.
Turner, J. R., Ortinski, P. I., Sherrard, R. M., and Kellar, K. J. (2011). Cerebellar nicotinic cholinergic receptors are intrinsic to the cerebellum: implications for diverse functional roles. Cerebellum 10, 748–757. doi: 10.1007/s12311-011-0285-y
PubMed Abstract | Full Text | CrossRef Full Text | Google Scholar
van Alphen, A. M., and De Zeeuw, C. I. (2002). Cerebellar LTD facilitates but is not essential for long-term adaptation of the vestibulo-ocular reflex. Eur. J. Neurosci. 16, 486–490. doi: 10.1046/j.1460-9568.2002.02094.x
PubMed Abstract | Full Text | CrossRef Full Text | Google Scholar
van Beugen, B. J., Nagaraja, R. Y., and Hansel, C. (2006). Climbing fiber-evoked endocannabinoid signaling heterosynaptically suppresses presynaptic cerebellar long-term potentiation. J. Neurosci. 26, 8289–8294. doi: 10.1523/jneurosci.0805-06.2006
PubMed Abstract | Full Text | CrossRef Full Text | Google Scholar
van Woerden, G. M., Hoebeek, F. E., Gao, Z., Nagaraja, R. Y., Hoogenraad, C. C., Kushner, S. A., et al. (2009). βCaMKII controls the direction of plasticity at parallel fiber-Purkinje cell synapses. Nat. Neurosci. 12, 823–825. doi: 10.1038/nn.2329
PubMed Abstract | Full Text | CrossRef Full Text | Google Scholar
Vervaeke, K., Lorincz, A., Gleeson, P., Farinella, M., Nusser, Z., and Silver, R. A. (2010). Rapid desynchronization of an electrically coupled interneuron network with sparse excitatory synaptic input. Neuron 67, 435–451. doi: 10.1016/j.neuron.2010.06.028
PubMed Abstract | Full Text | CrossRef Full Text | Google Scholar
Voogd, J. (2012). A note on the definition and the development of cerebellar Purkinje cell zones. Cerebellum 11, 422–425. doi: 10.1007/s12311-012-0367-5
PubMed Abstract | Full Text | CrossRef Full Text | Google Scholar
Wang, S. S., Denk, W., and Häusser, M. (2000). Coincidence detection in single dendritic spines mediated by calcium release. Nat. Neurosci. 3, 1266–1273. doi: 10.1038/81792
PubMed Abstract | Full Text | CrossRef Full Text | Google Scholar
Wang, Y. T., and Linden, D. J. (2000). Expression of cerebellar long-term depression requires postsynaptic clathrin-mediated endocytosis. Neuron 25, 635–647. doi: 10.1016/s0896-6273(00)81066-1
PubMed Abstract | Full Text | CrossRef Full Text | Google Scholar
Weber, J. T., De Zeeuw, C. I., Linden, D. J., and Hansel, C. (2003). Long-term depression of climbing fiber-evoked calcium transients in Purkinje cell dendrites. Proc. Natl. Acad. Sci. U S A 100, 2878–2883. doi: 10.1073/pnas.0536420100
PubMed Abstract | Full Text | CrossRef Full Text | Google Scholar
Xia, J., Chung, H. J., Wihler, C., Huganir, R. L., and Linden, D. J. (2000). Cerebellar long-term depression requires PKC-regulated interactions between GluR2/3 and PDZ domain-containing proteins. Neuron 28, 499–510. doi: 10.1016/s0896-6273(00)00128-8
PubMed Abstract | Full Text | CrossRef Full Text | Google Scholar
Xu, J., and Kang, J. (2005). The mechanisms and functions of activity-dependent long-term potentiation of intrinsic excitability. Rev Neurosci 16, 311–323. doi: 10.1515/revneuro.2005.16.4.311
PubMed Abstract | Full Text | CrossRef Full Text | Google Scholar
Yamazaki, T., and Tanaka, S. (2007). The cerebellum as a liquid state machine. Neural Networks 20, 290–297. doi: 10.1016/j.neunet.2007.04.004
PubMed Abstract | Full Text | CrossRef Full Text | Google Scholar
Yang, Y., and Lisberger, S. G. (2013). Interaction of plasticity and circuit organization during the acquisition of cerebellum-dependent motor learning. Elife 2:e01574. doi: 10.7554/elife.01574
PubMed Abstract | Full Text | CrossRef Full Text | Google Scholar
Zhang, W., and Linden, D. J. (2003). The other side of the engram: experience-driven changes in neuronal intrinsic excitability. Nat. Rev. Neurosci. 4, 885–900. doi: 10.1038/nrn1248
PubMed Abstract | Full Text | CrossRef Full Text | Google Scholar
Zhang, W., and Linden, D. J. (2006). Long-term depression at the mossy fiber-deep cerebellar nucleus synapse. J. Neurosci. 26, 6935–6944. doi: 10.1523/jneurosci.0784-06.2006
PubMed Abstract | Full Text | CrossRef Full Text | Google Scholar
Zhang, W., Shin, J. H., and Linden, D. J. (2004). Persistent changes in the intrinsic excitability of rat deep cerebellar nuclear neurones induced by EPSP or IPSP bursts. J. Physiol. 561, 703–719. doi: 10.1113/jphysiol.2004.071696
PubMed Abstract | Full Text | CrossRef Full Text | Google Scholar
Zheng, N., and Raman, I. M. (2010). Synaptic inhibition, excitation and plasticity in neurons of the cerebellar nuclei. Cerebellum 9, 56–66. doi: 10.1007/s12311-009-0140-6
PubMed Abstract | Full Text | CrossRef Full Text | Google Scholar
Keywords: cerebellum, inhibitory synapse, excitatory synapse, LTP, LTD
Citation: Mapelli L, Pagani M, Garrido JA and D‘Angelo E (2015) Integrated plasticity at inhibitory and excitatory synapses in the cerebellar circuit. Front. Cell. Neurosci. 9:169. doi: 10.3389/fncel.2015.00169
Received: 16 February 2015; Accepted: 16 April 2015;
Published online: 05 May 2015.
Edited by:
Andrea Barberis, Fondazione Istituto Italiano di Tecnologia, ItalyReviewed by:
Stéphane Dieudonné, Institut de Biologie de l’École Normale Supérieure, FranceLaurens Bosman, Erasmus MC, Netherlands
Copyright © 2015 Mapelli, Pagani, Garrido and D‘Angelo. This is an open-access article distributed under the terms of the Creative Commons Attribution License (CC BY). The use, distribution and reproduction in other forums is permitted, provided the original author(s) or licensor are credited and that the original publication in this journal is cited, in accordance with accepted academic practice. No use, distribution or reproduction is permitted which does not comply with these terms.
*Correspondence: Egidio D’Angelo, Department of Brain and Behavioral Sciences, University of Pavia, Via Forlanini 6, I-27100, Pavia, Italy,ZGFuZ2Vsb0B1bmlwdi5pdA==
† These authors have contributed equally to this work.