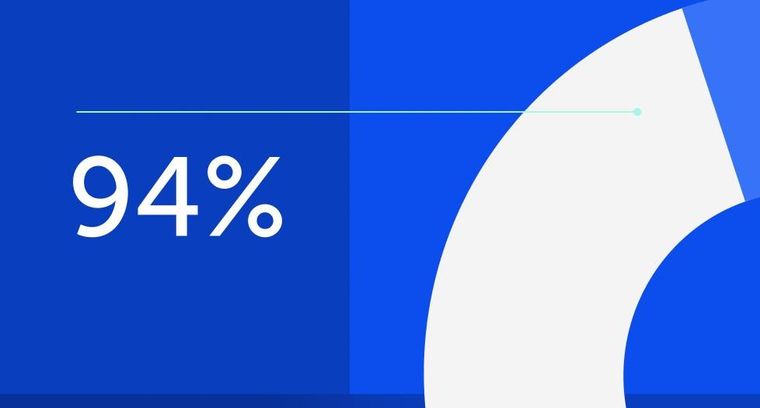
94% of researchers rate our articles as excellent or good
Learn more about the work of our research integrity team to safeguard the quality of each article we publish.
Find out more
REVIEW article
Front. Cell. Neurosci., 28 October 2014
Sec. Cellular Neurophysiology
Volume 8 - 2014 | https://doi.org/10.3389/fncel.2014.00350
This article is part of the Research TopicDynamics of cyclic nucleotide signaling in neuronsView all 10 articles
The second messenger cyclic AMP (cAMP) is a major intracellular mediator of many hormones and neurotransmitters and regulates a myriad of cell functions, including synaptic plasticity in neurons. Whereas cAMP can freely diffuse in the cytosol, a growing body of evidence suggests the formation of cAMP gradients and microdomains near the sites of cAMP production, where cAMP signals remain apparently confined. The mechanisms responsible for the formation of such microdomains are subject of intensive investigation. The development of optical methods based on fluorescence resonance energy transfer (FRET), which allow a direct observation of cAMP signaling with high temporal and spatial resolution, is playing a fundamental role in elucidating the nature of such microdomains. Here, we will review the optical methods used for monitoring cAMP and protein kinase A (PKA) signaling in living cells, providing some examples of their application in neurons, and will discuss the major hypotheses on the formation of cAMP/PKA microdomains.
The concept of second messenger made its first appearance nearly 60 years ago after the identification of cyclic AMP (cAMP) as the heat-stable and dialyzable molecule that mediated the intracellular actions of the hormones glucagon and epinephrine in the liver (Rall and Sutherland, 1958). Subsequently, cAMP has been shown to regulate a myriad of cellular processes such as gene expression, proliferation, apoptosis, exocytosis or migration as well as a wide range of physiological functions including immune responses, cardiac contractility and memory formation (Beavo and Brunton, 2002).
The events triggering the production of cAMP have long been believed to begin exclusively at the plasma membrane, where a variety of ligands are capable of binding to and activating several members of the large family of G protein-coupled receptors (GPCRs; Pierce et al., 2002; Lefkowitz, 2013). Subsequently, active GPCRs induce the exchange of GTP for GDP on the α-subunit of the Gs protein, which in turn activates adenylyl cyclases (AC). These enzymes, which exist in different isoforms, convert ATP to cAMP, which ultimately stimulates different effector proteins, namely cyclic-nucleotide-gated (CNG) channels, guanine-nucleotide exchanging proteins activated by cAMP (Epac) and protein kinase A (PKA). A group of phosphodiesterases (PDEs), which degrade cAMP to AMP, are finally responsible for bringing the concentration of cAMP back to the basal level, so that the cell can respond to a new stimulus (Beavo and Brunton, 2002).
For many years after the discovery of cAMP, its intracellular concentration has been thought to increase uniformly after stimulation of a receptor located on the plasma membrane. This view was based on at least two important observations: first, cAMP is a small soluble molecule with a high diffusion coefficient in solution; second, dissimilarly from Ca2+ ions, which are buffered by binding proteins in the cytosol, cAMP remains largely free in the cytoplasm. However, this view has been challenged by an increasing number of experimental observations, which provide convincing evidence that cAMP may remain confined in microdomains near the sites of its synthesis, thus leading to the selective activation of a subset of effectors located in their proximity.
First indirect evidence for such compartmentation in cAMP/PKA signaling was provided by Corbin et al. (1977) working on heart lysates. Few years later, it was reported that prostaglandin E1 (PGE1) and the β-adrenergic agonist isoproterenol caused similar elevations of cAMP in heart tissue, but only isoproterenol was able to activate glycogen phosphorylase (Brunton et al., 1979, 1981; Hayes et al., 1979, 1980). Interestingly, it was subsequently found that PGE1 activated PKA only in a soluble fraction, whereas isoproterenol could also activate PKA in a particulate fraction, which was apparently required for its effects on glycogen phosphorylase (Buxton and Brunton, 1983). A number of analogous observations of hormones inducing dissimilar effects in the same tissue have been reported by several groups (Steinberg and Brunton, 2001; Zaccolo, 2011; Lefkimmiatis and Zaccolo, 2014). Moreover, Jurevicius and Fischmeister performed an elegant experiment in which they used a set-up that allowed perfusing the two halves of a frog cardiomyocyte with two different solutions and at the same time record L-type calcium currents induced by β-adrenergic stimulation. Based on their results, they concluded that β-adrenergic receptors (β-AR) induced local cAMP elevations, which caused the preferential activation of nearby Ca2+ channels (Jurevicius and Fischmeister, 1996).
However, it was only later, thanks to the introduction of optical methods for monitoring cAMP/PKA signaling in living cells, that a direct observation and investigation of cAMP microdomains became possible. This review will focus on the currently available tools for monitoring cAMP levels and PKA activation in living cells, providing some illustrative examples of their application in neurons. Moreover, we will discuss the major hypotheses on the mechanisms responsible for the formation of such microdomains, which have emerged from these studies.
The currently available optical methods for monitoring cAMP signaling in living cells are largely based on fluorescence resonance energy transfer (FRET; Förster, 1948). FRET is a physical phenomenon that occurs between two fluorophores located in close proximity: if the distance between two fluorophores is less than approximately 10 nm and there is a certain overlap between their fluorescence spectra, the energy in the excited state of the first fluorophore (donor) can be transferred without emission of a photon to the second fluorophore (acceptor), which will in turn emit a photon of a lower energy. The resulting red-shift of the emitted light, or, alternatively, the shortening of the time the donor resides in the excited state can be used to monitor FRET. Such measurements can be performed in living cells with the spatial and temporal resolution of fluorescence microscopy. This approach has been exploited to follow all the major steps of GPCR signaling, including the generation of cAMP and the activation of PKA (Adams et al., 1991; Zaccolo et al., 2000; Zhang et al., 2001; DiPilato et al., 2004; Nikolaev et al., 2004; Ponsioen et al., 2004)—for a comprehensive review on sensors for monitoring other steps in GPCR signaling see Lohse et al. (2008) and Lohse et al. (2012). Here we will focus on the sensors used for monitoring cAMP concentrations and PKA activation.
The first FRET sensor used to monitor cAMP was based on PKA. This sensor consisted of two PKA catalytic (C) subunits labeled with fluorescein as FRET donor and two PKA regulatory (R) subunits labeled with rhodamine as FRET acceptor—hence the name FICRhR (fluorescein-labeled catalytic subunit and rhodamine-labeled regulatory subunit, pronounced “flicker”). In the presence of low cAMP concentrations, the R and C subunits are associated and FRET can occur between the two nearby fluorophores. When cAMP concentrations increase, cAMP binds to the R subunits causing dissociation of the C subunits and a concomitant loss of FRET. This sensor was used to monitor cAMP signaling in different neuronal preparations, such as invertebrate neurons (Bacskai et al., 1993; Hempel et al., 1996), Xenopus embryonic spinal neurons (Gorbunova and Spitzer, 2002) or murine thalamic neurons (Goaillard and Vincent, 2002), as well as in oocytes (Webb et al., 2002; Takeda et al., 2006) and frog ventricular myocytes (Goaillard et al., 2001). A drawback of this sensor was that it had to be injected into the cytoplasm, which was a challenging task, particularly for cells of small size. Moreover, it has been suggested that the injection pipette might work as a “cAMP sink”, artificially reducing the cAMP concentration inside the cell (Vincent and Brusciano, 2001).
These problems were later overcome by Manuela Zaccolo and Tullio Pozzan with the introduction of a genetically-encoded PKA-based sensor (Zaccolo et al., 2000). In this sensor, the PKA R and C subunits were fused with two color variants of GFP—BFP and GFP, later replaced by CFP and YFP, respectively (Zaccolo and Pozzan, 2002). This sensor could now be introduced into cells by co-transfection of two plasmids, each coding for one of the two fluorescently labeled PKA subunit. Although this represented a major advancement, some limitations and possible drawbacks remained. Most importantly, this sensor retained PKA catalytic activity, which can alter intracellular signaling and various cellular functions. Moreover, both labeled subunits had to be overexpressed and present in comparable amounts in order to associate into a functional sensor, which complicated the use of this sensor and increased the chances that it might interfere with the functions of the cell (Rich and Karpen, 2002; Haugh, 2012; Saucerman et al., 2013; Rich et al., 2014).
In an attempt to circumvent the remaining limitations of PKA-based sensors, the group of Martin Lohse in Würzburg developed a new type of cAMP sensor that was single-chain and catalytically inactive. The first version of this sensor was based on a cAMP-binding domain derived from Epac1, a GTP exchange factor for Rap1 activated by cAMP, which was fused on either side to CFP and YFP (Nikolaev et al., 2004)—binding of cAMP to this sensor causes a decrease of FRET between CFP and YFP. In parallel, two other groups generated similar types of sensors that were based on full-length or partially truncated version of Epac proteins (DiPilato et al., 2004; Ponsioen et al., 2004; Dunn et al., 2006). A whole range of single-chain cAMP sensors with improved characteristics have been developed by utilizing cAMP binding domains derived from other proteins, deleting functional domains of Epac proteins, introducing point mutations in the cAMP binding domains and/or using improved donor:acceptor pairs (Nikolaev et al., 2005, 2006; Violin et al., 2006, 2008; Norris et al., 2009; Klarenbeek et al., 2011; Polito et al., 2013).
In addition, endogenous PKA signaling can be monitored by FRET sensors known as AKARs, standing for A-kinase activity reporters (Zhang et al., 2001). This family of biosensors has been generated by sandwiching a PKA substrate sequence and a phosphoamino acid binding domain between a FRET donor (e.g., CFP) and a FRET acceptor (e.g., YFP or Venus). Once phosphorylated by PKA, the PKA substrate contained in the sensor interacts with the phosphoamino acid binding domain, leading to an increase in FRET between the two fluorophores. Like for cAMP sensors, several generations of AKAR sensors with progressively improved characteristics have been produced by replacing the initial fluorophores with brighter and more photostable ones (Zhang et al., 2005; Allen and Zhang, 2006; Depry et al., 2011; Erard et al., 2013; Chen et al., 2014) or introducing a long flexible linker between the PKA substrate and the phosphoamino acid binding domain (Komatsu et al., 2011). It should be noted that, since the phosphorylation of AKAR sensors and the accompanying FRET changes are rapidly reversed by the action of endogenous phosphatases, these sensors measure the equilibrium between phosphorylation and dephosphorylation and not just PKA activity.
Although FRET-based methods for monitoring cAMP/PKA signaling offer several advantages compared to other approaches, there are still some limitations and potential pitfalls that deserve careful consideration—for a detailed review see Rich and Karpen (2002), Haugh (2012) and Rich et al. (2014). One is the already mentioned catalytic activity of some sensors. In addition, all sensors have the potential of interacting with other proteins present in the cell. This risk should be particularly low with the use of small sensors that contain no specific interacting domains. Finally, all sensors have the potential of buffering intracellular cAMP. Performing measurements at different levels of expression of the sensor (Castro et al., 2013) appears to be a good strategy for evaluating the presence of such an unwanted effect.
One of the first applications of the new method was to study cAMP signaling in the giant sensory neurons of the see snail Aplysia californica, a model organism that had been extensively used by the group of Eric Kandel to demonstrate the importance of cAMP in synaptic plasticity (Kandel, 2009; Kandel et al., 2014). In this model, serotonin released by interneurons activates a Gs-coupled receptor located on pre-synaptic termini, where it induces both short-term and long-term facilitation. Since the latter requires phosphorylation of CREB as well as mRNA and protein synthesis, it was postulated that the cAMP signals produced in the periphery must reach the soma in order to induce long-term changes in synaptic transmission. Using Aplysia sensory neurons injected with FlCRhR, Bacskai et al. (1993) found that bath stimulation with saturating serotonin concentrations induced PKA activation in the distal processes but only a minimal PKA activation near the nucleus. Interestingly, such cAMP gradient between the periphery and the central compartment was observed also with forskolin stimulation and was not prevented by treatment with IBMX, a PDE inhibitor. At the same time, injected cAMP was found to be freely diffusible, with a diffusion coefficient of 780 µm2/s. Based on these observations, the authors hypothesized that the observed gradient between the periphery and the soma was due to the higher surface-to-volume ratio of the fine processes compared to the cell body. However, it has been suggested that buffering by FICRhR might artificially favor the formation of cAMP gradients, an effect that could be bypassed if the concentration of injected cAMP should exceed the buffering capacity of the sensor (Rich et al., 2001). This factor may complicate the interpretation of the results of Bacskai et al. (Rich et al., 2001).
Subsequent experiments performed in neurons of the lobster stomatogastric ganglion injected with the same PKA sensor revealed that electric stimulation of afferent fibers, presumably through release of endogenous modulators, is capable of inducing local increases of cAMP in fine neurite branches (Hempel et al., 1996). Interestingly, after repeated electric stimulation for 2–3 min, a unidirectional propagation of cAMP signals from the fine neurites towards the soma was observed. The authors explained this as the intracellular diffusion of cAMP from its peripheral site of production towards the central compartment (Hempel et al., 1996). However, measurements of the time required for the axonal propagation of the cAMP signal gave values that are consistently lower than it would be expected for the free diffusion of cAMP and about 6–8 times lower than those experimentally observed after local cAMP microinjection (Bacskai et al., 1993). Moreover, the results obtained with lobster stomatogastric ganglion neurons were inconsistent with the simple hypothesis that differences in the surface-to-volume ratio might be the major cause for the formation of cAMP gradients between fine peripheral structures and the soma.
Subsequently, optical measurements of cAMP levels and PKA activation in neurons have been performed in various mammalian preparations (Nikolaev et al., 2004; Gervasi et al., 2007; Dunn and Feller, 2008; Neves et al., 2008; Mironov et al., 2009; Castro et al., 2010, 2013; Shelly et al., 2010; Hu et al., 2011; Nicol et al., 2011). Some of these studies provided further evidence for the existence of cAMP/PKA microdomains. For instance, Nikolaev et al. (2004) followed the formation of transient cAMP gradients in hippocampal neurons transfected with the Epac1-camps sensor and locally stimulated with the β-adrenergic agonist isoproterenol. They measured a very rapid diffusion of cAMP inside neurons (diffusion coefficient ~500 µm2/s), which was ~20-fold slower if measured with a PKA-based sensor (Nikolaev et al., 2004). These data confirmed the high diffusibility of cAMP in neurons.
In an elegant study, Neves et al. (2008) performed a series of spatial simulations of the propagation of cAMP signals in hippocampal CA1 neurons, which they subsequently validated in rat hippocampal slices transfected with the Epac1-camps sensor. This study confirmed the possible generation of cAMP gradients between fine processes and the cell body as a consequence of different surface-to-volume ratios, despite a homogenous distribution of receptors and AC on the entire plasma membrane (Neves et al., 2008). In agreement with these findings, a study by Castro et al. (2010) in pyramidal cortical neurons imaged by two-photon microscopy revealed higher cAMP and PKA responses to isoproterenol stimulation in small distal dendrites compared to the bulk cytosol.
Another emerging model to study the spatiotemporal dynamics of cAMP/PKA signaling in neurons is represented by Drosophila melanogaster, in which several genetic studies have revealed the importance of cAMP/PKA signaling for memory formation and synaptic plasticity (Kandel, 2009). Interestingly, a number of studies have employed FRET sensors to directly monitor cAMP/PKA signaling in semi-intact larval and adult preparations as well as in intact flies (Shafer et al., 2008; Tomchik and Davis, 2009; Gervasi et al., 2010; Duvall and Taghert, 2012; Boto et al., 2014). For example, flies expressing the Epac1-camps sensor have been used to investigate cAMP signaling in the pacemaker neurons of the central nervous system that are involved in circadian rhythms (Shafer et al., 2008; Duvall and Taghert, 2012). These studies provide evidence that the response to the neuropeptide Pigment Dispersing Factor (PDF) in the pacemaker M cells requires the presence of AC3 and the A-kinase anchoring protein (AKAP) Nervy. Interestingly, signaling by other GPCRs in M cells or by the PDF receptor in other pacemaker cells was not affected by depletion of either AC3 or Nervy. These findings suggest a high degree of compartmentation of PDF signaling in M cells.
Another interesting line of research in Drosophila focuses on the involvement of cAMP/PKA signaling in olfactory memory, which is processed in specialized brain regions called Mushroom Bodies (MBs). In an interesting study, Tomchik and Davis (2009) used a FRET sensor to monitor cAMP signaling in two regions of the MBs called α-lobe and calix. They found that the responses to dopamine and octopamine differed substantially in the two regions, with the calyx being particularly sensitive to octopamine while dopamine inducing the largest response in the α-lobe. In a subsequent study, Gervasi et al. (2010) analyzed PKA signaling in the different regions of MBs using an AKAR sensor. They suggested that, whereas octopamine can induce a generalized PKA response in MBs, dopamine induces a localized PKA activation in the α-lobe. Interestingly, the selective localization of PKA signals in α-lobes in response to dopamine was lost in mutant flies that carried an inactive Dunce PDE (Gervasi et al., 2010). These studies are consistent with the hypothesis that cAMP/PKA signals might be spatially restricted in MB neurons.
Furthermore, targeting of cAMP or PKA sensors to different signaling complexes by fusion with one of their components, such as type-I and type-II PKA regulatory subunits (Di Benedetto et al., 2008), PDEs (Herget et al., 2008) and ACs (Wachten et al., 2010), has provided additional evidence for the existence of cAMP microdomains in different types of non-neuronal cells. The application of similar approaches to neurons might allow to further investigate the organization of cAMP microdomains in these cells. For instance, Herbst et al. (2011) used an AKAR sensor targeted to the plasma membrane via a K-ras domain to show the existence of a distinct sub-membrane compartment for cAMP signaling in PC12 cells.
This topic has been thoroughly discussed elsewhere (Willoughby and Cooper, 2007; Conti et al., 2014; Lefkimmiatis and Zaccolo, 2014; Saucerman et al., 2013; Rich et al., 2014). Here we will highlight some general aspects and provide a few selected examples.
Different mechanisms have been put forward to explain the compartmentation of cAMP/PKA signaling in spite of the high diffusibility of cAMP in solution. These include the formation of cAMP gradients due to reaction-diffusion mechanisms, a different subcellular localization of signaling molecules (receptors, ACs, PDEs, etc.), factors (e.g., fiscal barriers, buffering, higher viscosity) capable of hindering the diffusion of cAMP, and the association of signaling molecules into supramolecular complexes, often through the intervention of scaffolding proteins (Figure 1). Indeed, all these mechanisms could cause local variations in cAMP concentrations, ultimately leading to activation of PKA and downstream targets in a spatially restricted manner.
The formation of cAMP gradients from the plasma membrane to the cell interior has been predicted on the basis of the spatial segregation of ACs on the plasma membrane and PDEs in the cytosol long before the advent of optical methods (Fell, 1980; Kholodenko, 2006; Kholodenko and Kolch, 2008). In addition, differences in the surface-to-volume ratio among different parts of a cell, for instance between the fine neurites and the soma of a neuron, have been claimed for similar reasons to generate a gradient, with cAMP being higher in the finer structures (Kholodenko and Kolch, 2008; Neves et al., 2008). Such gradients have been directly visualized by FRET microscopy in cardiomyocytes (Zaccolo and Pozzan, 2002; Nikolaev et al., 2006, 2010) and in neurons (Bacskai et al., 1993; Neves et al., 2008; Castro et al., 2010; Gervasi et al., 2010).
Another mechanism that has been advocated for the formation of cAMP microdomains involves the non-uniform localization of the proteins implicated in cAMP/PKA signaling in different subcellular structures and/or microdomains. For instance, in cardiomyocytes β1-adrenergic receptors (β1-ARs) have been suggested to be localized in both caveolar and non-caveolar membrane fractions, whereas β2-ARs appear to be prevalently localized in caveolae and to exit caveolae upon activation (Rybin et al., 2000). Interestingly, pharmacological disruption of caveolae enhances and prolongs β2-AR-mediated cAMP accumulation (DiPilato and Zhang, 2009). Moreover, combining FRET and scanning ion conductance microscopy, it was shown that functional β2-ARs are localized selectively in transverse tubules of cardiomyocytes, whereas β1-ARs are distributed across the entire cell surface (Nikolaev et al., 2010). Such selective localization on the surface of cardiomyoctes might explain why stimulation of β1- and β2-ARs produce different effects although both receptors activate the cAMP signaling pathway. More recently, we and another group have independently found that GPCRs can not only activate ACs once located at the cell surface, but can continue stimulating cAMP production after internalization to an endosomal compartment that contains G-proteins and ACs (Calebiro et al., 2009, 2010; Ferrandon et al., 2009). Very recently, such a phenomenon has also been described for the β2-AR (Irannejad et al., 2013). Interestingly, the authors of this study were able to directly visualize active β2-ARs and Gαs-subunits on endosomal membranes by taking advantage of fluorescently labeled nanobodies that bind to these proteins only when they are in an active state (Irannejad et al., 2013). Similarly, there is increasing evidence for a functional role of GPCRs located on the nuclear membrane (for a review see Tadevosyan et al., 2012). These findings provide a new basis to explain compartmentation in GPCR signaling, and could allow reconciling the notion of spatially confined cAMP/PKA signaling with the known phosphorylation by PKA of targets located deep inside the cell or distant from the site of receptor activation, as might occur in neurons.
A hindered diffusion of cAMP has been advocated by Rich et al. (2000) to explain the results of whole-cell patch clamp experiments in which cAMP concentrations near the plasma membrane were measured using CNG channels. Since they found a low exchange of cAMP between the cytoplasm immediately below the plasma membrane and the bulk cytosol, they hypothesized the presence of a 3D barrier, possibly involving the endoplasmic reticulum, which would limit diffusion of cAMP between these two compartments.
Finally, a number of studies have revealed that different components of GPCR signaling cascades can assemble into functional molecular complexes, thus allowing the formation of signaling domains of the size of a few nanometers (for a detailed review see Lefkimmiatis and Zaccolo, 2014). An illustrative example of such concept is represented by the reported formation of a signaling complex involving the β2-AR, heterotrimeric G-proteins, AC, PKA and its target, the L-type Ca2+ channel CaV 1.2 (Davare et al., 2001). Such compartmentalization could explain electrophysiological data obtained in frog ventricular cardiomyocytes that support the preferential functional coupling of β-ARs to nearby L-type Ca2+ channels (Jurevicius and Fischmeister, 1996). An important role in this context is played by AKAPs. The over 50 AKAPs identified so far are capable of localizing PKA to multiple subcellular structures via interaction with a specific domain localized at the N-terminus of PKA regulatory subunits. Interestingly, AKAPs do not only interact with both PKA-I and II isoforms, but can bind several other proteins involved in GPCR signaling, such as receptors, ACs, PDEs and phosphatases (Esseltine and Scott, 2013). Thus, these scaffolding proteins appear to play a fundamental role in assembling focal cAMP/PKA signaling complexes. An elegant demonstration of this concept comes from a study by Di Benedetto et al. (2008) in cardiomyocytes. In this study, a FRET sensor for cAMP was fused to either RI- or RII-derived AKAP-binding domains, thus allowing its targeted localization to different compartments. Interestingly, it was found that isoproterenol stimulation causes a larger cAMP elevation at the level of the RII-tethered sensor. In contrast, the RI-tethered sensor was preferentially responding to PGE1. Moreover, the two PKA pools had a different sensitivity to different isoform-selective PDE inhibitors and were coupled to different downstream targets (Di Benedetto et al., 2008). This study provided a possible molecular explanation for the observations made by Buxton and Brunton (1983) nearly 25 years before.
Although all these studies have provided fundamental insights into the possible mechanisms of cAMP compartmentation, putting all the pieces of the puzzle together is not trivial. Moreover, some hypotheses on the formation of cAMP gradients are difficult to verify, due to the lack of adequate tools. Here, an important help may come from mathematical models and simulations capable of predicting the behavior of signaling cascades (Kholodenko, 2006; Kholodenko and Kolch, 2008) and, thus, of verifying, in a systematic way, the possible contribution of different mechanisms to cAMP compartmentation. Indeed, several groups have built a series of mathematical models of GPCR/cAMP signaling pathways and performed different types of simulations (e.g., single/multiple compartment vs. spatial, or deterministic vs. stochastic), a topic that has been recently reviewed by Saucerman et al. (2013).
Most of these simulations confirm the importance of having differentially localized AC and PDE activities for the generation of cAMP gradients. Some simulations have suggested that the presence of ACs on the plasma membrane and a higher PDE activity in the cytosol is sufficient to produce a cAMP gradient in the cell. For instance, Oliveira et al. (2010) performed a stochastic simulation of cAMP production and degradation in HEK293 cells, which they validated with previous cAMP FRET measurements. They concluded that the formation of cAMP gradients requires a pool of PDE4D anchored in the cytosol as well as PDE4D phosphorylation by PKA. In a subsequent paper, the same group simulated dopamine D1 signaling in a segment of a spiny projection neuron dendrite, and evaluated the effect of anchoring the receptor/AC and/or PKA in the spine heads vs. the dendrite. The results indicated that when receptors and ACs were located on the spine heads, cAMP was significantly higher there than in the dendrite. In addition, PKA activation was predicted to be more efficient when PKA was located together with AC in the same compartment (Oliveira et al., 2012). However, it has been pointed out that these simulations utilized rather high AC and PDE activities, which may not reflect the physiological ones (Saucerman et al., 2013; Conti et al., 2014). On the other hand, simulations by other groups suggest that the formation of a cAMP gradient requires the presence of additional factors capable of reducing the effective cAMP diffusion. Proposed factors include the presence of physical barriers, buffering of cAMP and local changes in the viscosity of the cytoplasm (Saucerman et al., 2013). For instance, Feinstein et al. (2012) applied a mathematical model to study the contribution of all the major mechanisms proposed for the formation of cAMP microdomains in pulmonary microvascular endothelia cells. They concluded that, with experimentally measured PDE and AC activities in these cells, the formation of a gradient could be observed only using a diffusion coefficient for cAMP of 3 µm2/s or less, i.e., at least 100-fold lower than experimentally measured in the bulk cytoplasm. However, it should be mentioned that, while mathematical simulations are a very powerful tool for interpreting experimental results and formulating new hypotheses, they also have a number of limitations. First, they are based on simplified models of cellular biochemistry. Second, they also have spatial and temporal limits. In particular, since the size of signaling complexes is in the range of tens to hundreds of nanometers, it would be important to be able to predict the behavior of signaling molecules at such short distances. Third, the results of simulations are largely dependent on the kinetics parameters and initial concentrations used. Unfortunately, for several of these parameters we possess only rough estimations, which are mostly based on in vitro measurements, and therefore do not necessarily reflect the values in intact cells. Further efforts to better estimate these parameters would help improving the reliability and predictive power of such simulations.
The development of optical methods that allow monitoring cAMP/PKA signaling in living cells played a fundamental role in revealing an unexpected level of organization in GPCR signaling cascades. On the one hand, receptors and downstream signaling molecules appear to be highly organized in micro- or nano-domains on biological membranes. Contrary to what originally thought, such high degree of compartmentation appears to also involve a rapidly diffusing molecule such as cAMP. On the other hand, the finding of receptor signaling on endosomes and possibly other intracellular compartments adds another level of organization, which could help explaining why receptors coupled to the same downstream signaling pathway can induce dissimilar biological effects. It is likely that new technological developments, as is often the case, will play a fundamental role in further elucidating the organization of signaling microdomains located on the cell surface and on other intracellular membranes. Indeed, optical methods with higher temporal and spatial resolution would permit to better dissect the nature of such microdomains. In this context, methods based on single molecule microscopy, which have been recently applied by us and other groups to GPCRs (Hern et al., 2010; Kasai et al., 2011; Calebiro et al., 2013), appear particularly indicated to analyze the composition of protein complexes on the surface of living cells and to capture dynamic events such as protein-protein interactions with high temporal and spatial resolution. Furthermore, new models such as Drosophila and new methods such as two-photon microscopy are hopefully going to permit a direct observation of these phenomena in vivo, thus allowing to address the role of cAMP compartmentation under highly physiological conditions. After all, almost six decades after the discovery of cAMP, there are still many good reasons to expect novel and exciting discoveries in this field.
The authors declare that the research was conducted in the absence of any commercial or financial relationships that could be construed as a potential conflict of interest.
This work was supported by the Deutsche Forschungsgemeinschaft (DFG grant CA 1014/1-1 to Davide Calebiro).
Adams, S. R., Harootunian, A. T., Buechler, Y. J., Taylor, S. S., and Tsien, R. Y. (1991). Fluorescence ratio imaging of cyclic AMP in single cells. Nature 349, 694–697. doi: 10.1038/349694a0
Pubmed Abstract | Pubmed Full Text | CrossRef Full Text | Google Scholar
Allen, M. D., and Zhang, J. (2006). Subcellular dynamics of protein kinase A activity visualized by FRET-based reporters. Biochem. Biophys. Res. Commun. 348, 716–721. doi: 10.1016/j.bbrc.2006.07.136
Pubmed Abstract | Pubmed Full Text | CrossRef Full Text | Google Scholar
Bacskai, B. J., Hochner, B., Mahaut-Smith, M., Adams, S. R., Kaang, B. K., Kandel, E. R., et al. (1993). Spatially resolved dynamics of cAMP and protein kinase A subunits in Aplysia sensory neurons. Science 260, 222–226. doi: 10.1126/science.7682336
Pubmed Abstract | Pubmed Full Text | CrossRef Full Text | Google Scholar
Beavo, J. A., and Brunton, L. L. (2002). Cyclic nucleotide research—still expanding after half a century. Nat. Rev. Mol. Cell Biol. 3, 710–718. doi: 10.1038/nrm911
Pubmed Abstract | Pubmed Full Text | CrossRef Full Text | Google Scholar
Boto, T., Louis, T., Jindachomthong, K., Jalink, K., and Tomchik, S. M. (2014). Dopaminergic modulation of cAMP drives nonlinear plasticity across the Drosophila mushroom body lobes. Curr. Biol. 24, 822–831. doi: 10.1016/j.cub.2014.03.021
Pubmed Abstract | Pubmed Full Text | CrossRef Full Text | Google Scholar
Brunton, L. L., Hayes, J. S., and Mayer, S. E. (1979). Hormonally specific phosphorylation of cardiac troponin I and activation of glycogen phosphorylase. Nature 280, 78–80. doi: 10.1038/280078a0
Pubmed Abstract | Pubmed Full Text | CrossRef Full Text | Google Scholar
Brunton, L. L., Hayes, J. S., and Mayer, S. E. (1981). Functional compartmentation of cyclic AMP and protein kinase in heart. Adv. Cyclic Nucleotide Res. 14, 391–397.
Buxton, I. L., and Brunton, L. L. (1983). Compartments of cyclic AMP and protein kinase in mammalian cardiomyocytes. J. Biol. Chem. 258, 10233–10239.
Calebiro, D., Nikolaev, V. O., Gagliani, M. C., de Filippis, T., Dees, C., Tacchetti, C., et al. (2009). Persistent cAMP-signals triggered by internalized G-protein-coupled receptors. PLoS Biol. 7:e1000172. doi: 10.1371/journal.pbio.1000172
Pubmed Abstract | Pubmed Full Text | CrossRef Full Text | Google Scholar
Calebiro, D., Nikolaev, V. O., Persani, L., and Lohse, M. J. (2010). Signaling by internalized G-protein-coupled receptors. Trends Pharmacol. Sci. 31, 221–228. doi: 10.1016/j.tips.2010.02.002
Pubmed Abstract | Pubmed Full Text | CrossRef Full Text | Google Scholar
Calebiro, D., Rieken, F., Wagner, J., Sungkaworn, T., Zabel, U., Borzi, A., et al. (2013). Single-molecule analysis of fluorescently labeled G-protein-coupled receptors reveals complexes with distinct dynamics and organization. Proc. Natl. Acad. Sci. U S A 110, 743–748. doi: 10.1073/pnas.1205798110
Pubmed Abstract | Pubmed Full Text | CrossRef Full Text | Google Scholar
Castro, L. R., Brito, M., Guiot, E., Polito, M., Korn, C. W., Hervé, D., et al. (2013). Striatal neurones have a specific ability to respond to phasic dopamine release. J. Physiol. 591, 3197–3214. doi: 10.1113/jphysiol.2013.252197
Pubmed Abstract | Pubmed Full Text | CrossRef Full Text | Google Scholar
Castro, L. R., Gervasi, N., Guiot, E., Cavellini, L., Nikolaev, V. O., Paupardin-Tritsch, D., et al. (2010). Type 4 phosphodiesterase plays different integrating roles in different cellular domains in pyramidal cortical neurons. J. Neurosci. 30, 6143–6151. doi: 10.1523/JNEUROSCI.5851-09.2010
Pubmed Abstract | Pubmed Full Text | CrossRef Full Text | Google Scholar
Chen, Y., Saulnier, J. L., Yellen, G., and Sabatini, B. L. (2014). A PKA activity sensor for quantitative analysis of endogenous GPCR signaling via 2-photon FRET-FLIM imaging. Front. Pharmacol. 5:56. doi: 10.3389/fphar.2014.00056
Pubmed Abstract | Pubmed Full Text | CrossRef Full Text | Google Scholar
Conti, M., Mika, D., and Richter, W. (2014). Cyclic AMP compartments and signaling specificity: role of cyclic nucleotide phosphodiesterases. J. Gen. Physiol. 143, 29–38. doi: 10.1085/jgp.201311083
Pubmed Abstract | Pubmed Full Text | CrossRef Full Text | Google Scholar
Corbin, J. D., Sugden, P. H., Lincoln, T. M., and Keely, S. L. (1977). Compartmentalization of adenosine 3′,5′-monophosphate and adenosine 3′,5′-monophosphate-dependent protein kinase in heart tissue. J. Biol. Chem. 252, 3854–3861.
Davare, M. A., Avdonin, V., Hall, D. D., Peden, E. M., Burette, A., Weinberg, R. J., et al. (2001). A β2 adrenergic receptor signaling complex assembled with the Ca2+ channel Cav1.2. Science 293, 98–101. doi: 10.1126/science.293.5527.98
Pubmed Abstract | Pubmed Full Text | CrossRef Full Text | Google Scholar
Depry, C., Allen, M. D., and Zhang, J. (2011). Visualization of PKA activity in plasma membrane microdomains. Mol. Biosyst. 7, 52–58. doi: 10.1039/c0mb00079e
Pubmed Abstract | Pubmed Full Text | CrossRef Full Text | Google Scholar
Di Benedetto, G., Zoccarato, A., Lissandron, V., Terrin, A., Li, X., Houslay, M. D., et al. (2008). Protein kinase A type I and type II define distinct intracellular signaling compartments. Circ. Res. 103, 836–844. doi: 10.1161/CIRCRESAHA.108.174813
Pubmed Abstract | Pubmed Full Text | CrossRef Full Text | Google Scholar
DiPilato, L. M., Cheng, X., and Zhang, J. (2004). Fluorescent indicators of cAMP and Epac activation reveal differential dynamics of cAMP signaling within discrete subcellular compartments. Proc. Natl. Acad. Sci. U S A 101, 16513–16518. doi: 10.1073/pnas.0405973101
Pubmed Abstract | Pubmed Full Text | CrossRef Full Text | Google Scholar
DiPilato, L. M., and Zhang, J. (2009). The role of membrane microdomains in shaping β2-adrenergic receptor-mediated cAMP dynamics. Mol. Biosyst. 5, 832–837. doi: 10.1039/b823243a
Pubmed Abstract | Pubmed Full Text | CrossRef Full Text | Google Scholar
Dunn, T. A., and Feller, M. B. (2008). Imaging second messenger dynamics in developing neural circuits. Dev. Neurobiol. 68, 835–844. doi: 10.1002/dneu.20619
Pubmed Abstract | Pubmed Full Text | CrossRef Full Text | Google Scholar
Dunn, T. A., Wang, C. T., Colicos, M. A., Zaccolo, M., Dipilato, L. M., Zhang, J., et al. (2006). Imaging of cAMP levels and protein kinase A activity reveals that retinal waves drive oscillations in second-messenger cascades. J. Neurosci. 26, 12807–12815. doi: 10.1523/jneurosci.3238-06.2006
Pubmed Abstract | Pubmed Full Text | CrossRef Full Text | Google Scholar
Duvall, L. B., and Taghert, P. H. (2012). The circadian neuropeptide PDF signals preferentially through a specific adenylate cyclase isoform AC3 in M pacemakers of Drosophila. PLoS Biol. 10:e1001337. doi: 10.1371/journal.pbio.1001337
Pubmed Abstract | Pubmed Full Text | CrossRef Full Text | Google Scholar
Erard, M., Fredj, A., Pasquier, H., Beltolngar, D. B., Bousmah, Y., Derrien, V., et al. (2013). Minimum set of mutations needed to optimize cyan fluorescent proteins for live cell imaging. Mol. Biosyst. 9, 258–267. doi: 10.1039/c2mb25303h
Pubmed Abstract | Pubmed Full Text | CrossRef Full Text | Google Scholar
Esseltine, J. L., and Scott, J. D. (2013). AKAP signaling complexes: pointing towards the next generation of therapeutic targets? Trends Pharmacol. Sci. 34, 648–655. doi: 10.1016/j.tips.2013.10.005
Pubmed Abstract | Pubmed Full Text | CrossRef Full Text | Google Scholar
Feinstein, W. P., Zhu, B., Leavesley, S. J., Sayner, S. L., and Rich, T. C. (2012). Assessment of cellular mechanisms contributing to cAMP compartmentalization in pulmonary microvascular endothelial cells. Am. J. Physiol. Cell Physiol. 302, C839–C852. doi: 10.1152/ajpcell.00361.2011
Pubmed Abstract | Pubmed Full Text | CrossRef Full Text | Google Scholar
Fell, D. A. (1980). Theoretical analyses of the functioning of the high- and low-Km cyclic nucleotide phosphodiesterases in the regulation of the concentration of adenosine 3′,5′-cyclic monophosphate in animal cells. J. Theor. Biol. 84, 361–385. doi: 10.1016/s0022-5193(80)80011-7
Pubmed Abstract | Pubmed Full Text | CrossRef Full Text | Google Scholar
Ferrandon, S., Feinstein, T. N., Castro, M., Wang, B., Bouley, R., Potts, J. T., et al. (2009). Sustained cyclic AMP production by parathyroid hormone receptor endocytosis. Nat. Chem. Biol. 5, 734–742. doi: 10.1038/nchembio.206
Pubmed Abstract | Pubmed Full Text | CrossRef Full Text | Google Scholar
Förster, T. (1948). Zwischenmolekulare Energiewanderung und Fluoreszenz. Ann. Phys. 437, 55–75. doi: 10.1002/andp.19484370105
Gervasi, N., Hepp, R., Tricoire, L., Zhang, J., Lambolez, B., Paupardin-Tritsch, D., et al. (2007). Dynamics of protein kinase A signaling at the membrane, in the cytosol and in the nucleus of neurons in mouse brain slices. J. Neurosci. 27, 2744–2750. doi: 10.1523/jneurosci.5352-06.2007
Pubmed Abstract | Pubmed Full Text | CrossRef Full Text | Google Scholar
Gervasi, N., Tchénio, P., and Preat, T. (2010). PKA dynamics in a Drosophila learning center: coincidence detection by rutabaga adenylyl cyclase and spatial regulation by dunce phosphodiesterase. Neuron 65, 516–529. doi: 10.1016/j.neuron.2010.01.014
Pubmed Abstract | Pubmed Full Text | CrossRef Full Text | Google Scholar
Goaillard, J. M., and Vincent, P. (2002). Serotonin suppresses the slow afterhyperpolarization in rat intralaminar and midline thalamic neurones by activating 5-HT7 receptors. J. Physiol. 541, 453–465. doi: 10.1113/jphysiol.2001.013896
Pubmed Abstract | Pubmed Full Text | CrossRef Full Text | Google Scholar
Goaillard, J. M., Vincent, P. V., and Fischmeister, R. (2001). Simultaneous measurements of intracellular cAMP and L-type Ca2+ current in single frog ventricular myocytes. J. Physiol. 530, 79–91. doi: 10.1111/j.1469-7793.2001.0079m.x
Pubmed Abstract | Pubmed Full Text | CrossRef Full Text | Google Scholar
Gorbunova, Y. V., and Spitzer, N. C. (2002). Dynamic interactions of cyclic AMP transients and spontaneous Ca2+ spikes. Nature 418, 93–96. doi: 10.1038/nature00835
Pubmed Abstract | Pubmed Full Text | CrossRef Full Text | Google Scholar
Haugh, J. M. (2012). Live-cell fluorescence microscopy with molecular biosensors: what are we really measuring? Biophys. J. 102, 2003–2011. doi: 10.1016/j.bpj.2012.03.055
Pubmed Abstract | Pubmed Full Text | CrossRef Full Text | Google Scholar
Hayes, J. S., Brunton, L. L., Brown, J. H., Reese, J. B., and Mayer, S. E. (1979). Hormonally specific expression of cardiac protein kinase activity. Proc. Natl. Acad. Sci. U S A 76, 1570–1574. doi: 10.1073/pnas.76.4.1570
Pubmed Abstract | Pubmed Full Text | CrossRef Full Text | Google Scholar
Hayes, J. S., Brunton, L. L., and Mayer, S. E. (1980). Selective activation of particulate cAMP-dependent protein kinase by isoproterenol and prostaglandin E1. J. Biol. Chem. 255, 5113–5119.
Hempel, C. M., Vincent, P., Adams, S. R., Tsien, R. Y., and Selverston, A. I. (1996). Spatio-temporal dynamics of cyclic AMP signals in an intact neural circuit. Nature 384, 166–169. doi: 10.1038/384166a0
Pubmed Abstract | Pubmed Full Text | CrossRef Full Text | Google Scholar
Herbst, K. J., Allen, M. D., and Zhang, J. (2011). Spatiotemporally regulated protein kinase A activity is a critical regulator of growth factor-stimulated extracellular signal-regulated kinase signaling in PC12 cells. Mol. Cell Biol. 31, 4063–4075. doi: 10.1128/MCB.05459-11
Pubmed Abstract | Pubmed Full Text | CrossRef Full Text | Google Scholar
Herget, S., Lohse, M. J., and Nikolaev, V. O. (2008). Real-time monitoring of phosphodiesterase inhibition in intact cells. Cell. Signal. 20, 1423–1431. doi: 10.1016/j.cellsig.2008.03.011
Pubmed Abstract | Pubmed Full Text | CrossRef Full Text | Google Scholar
Hern, J. A., Baig, A. H., Mashanov, G. I., Birdsall, B., Corrie, J. E., Lazareno, S., et al. (2010). Formation and dissociation of M1 muscarinic receptor dimers seen by total internal reflection fluorescence imaging of single molecules. Proc. Natl. Acad. Sci. U S A 107, 2693–2698. doi: 10.1073/pnas.0907915107
Pubmed Abstract | Pubmed Full Text | CrossRef Full Text | Google Scholar
Hu, E., Demmou, L., Cauli, B., Gallopin, T., Geoffroy, H., Harris-Warrick, R. M., et al. (2011). VIP, CRF and PACAP act at distinct receptors to elicit different cAMP/PKA dynamics in the neocortex. Cereb. Cortex 21, 708–718. doi: 10.1093/cercor/bhq143
Pubmed Abstract | Pubmed Full Text | CrossRef Full Text | Google Scholar
Irannejad, R., Tomshine, J. C., Tomshine, J. R., Chevalier, M., Mahoney, J. P., Steyaert, J., et al. (2013). Conformational biosensors reveal GPCR signalling from endosomes. Nature 495, 534–538. doi: 10.1038/nature12000
Pubmed Abstract | Pubmed Full Text | CrossRef Full Text | Google Scholar
Jurevicius, J., and Fischmeister, R. (1996). cAMP compartmentation is responsible for a local activation of cardiac Ca2+ channels by β-adrenergic agonists. Proc. Natl. Acad. Sci. U S A 93, 295–299. doi: 10.1073/pnas.93.1.295
Pubmed Abstract | Pubmed Full Text | CrossRef Full Text | Google Scholar
Kandel, E. R. (2009). The biology of memory: a forty-year perspective. J. Neurosci. 29, 12748–12756. doi: 10.1523/JNEUROSCI.3958-09.2009
Pubmed Abstract | Pubmed Full Text | CrossRef Full Text | Google Scholar
Kandel, E. R., Dudai, Y., and Mayford, M. R. (2014). The molecular and systems biology of memory. Cell 157, 163–186. doi: 10.1016/j.cell.2014.03.001
Pubmed Abstract | Pubmed Full Text | CrossRef Full Text | Google Scholar
Kasai, R. S., Suzuki, K. G., Prossnitz, E. R., Koyama-Honda, I., Nakada, C., Fujiwara, T. K., et al. (2011). Full characterization of GPCR monomer-dimer dynamic equilibrium by single molecule imaging. J. Cell Biol. 192, 463–480. doi: 10.1083/jcb.201009128
Pubmed Abstract | Pubmed Full Text | CrossRef Full Text | Google Scholar
Kholodenko, B. N. (2006). Cell-signalling dynamics in time and space. Nat. Rev. Mol. Cell Biol. 7, 165–176. doi: 10.1038/nrm1838
Pubmed Abstract | Pubmed Full Text | CrossRef Full Text | Google Scholar
Kholodenko, B. N., and Kolch, W. (2008). Giving space to cell signaling. Cell 133, 566–567. doi: 10.1016/j.cell.2008.04.033
Pubmed Abstract | Pubmed Full Text | CrossRef Full Text | Google Scholar
Klarenbeek, J. B., Goedhart, J., Hink, M. A., Gadella, T. W., and Jalink, K. (2011). A mTurquoise-based cAMP sensor for both FLIM and ratiometric read-out has improved dynamic range. PLoS One 6:e19170. doi: 10.1371/journal.pone.0019170
Pubmed Abstract | Pubmed Full Text | CrossRef Full Text | Google Scholar
Komatsu, N., Aoki, K., Yamada, M., Yukinaga, H., Fujita, Y., Kamioka, Y., et al. (2011). Development of an optimized backbone of FRET biosensors for kinases and GTPases. Mol. Biol. Cell 22, 4647–4656. doi: 10.1091/mbc.E11-01-0072
Pubmed Abstract | Pubmed Full Text | CrossRef Full Text | Google Scholar
Lefkimmiatis, K., and Zaccolo, M. (2014). cAMP signaling in subcellular compartments. Pharmacol. Ther. 143, 295–304. doi: 10.1016/j.pharmthera.2014.03.008
Pubmed Abstract | Pubmed Full Text | CrossRef Full Text | Google Scholar
Lefkowitz, R. J. (2013). A brief history of G-protein coupled receptors (Nobel Lecture). Angew. Chem. Int. Ed. Engl. 52, 6366–6378. doi: 10.1002/anie.201301924
Pubmed Abstract | Pubmed Full Text | CrossRef Full Text | Google Scholar
Lohse, M. J., Nikolaev, V. O., Hein, P., Hoffmann, C., Vilardaga, J. P., and Bünemann, M. (2008). Optical techniques to analyze real-time activation and signaling of G-protein-coupled receptors. Trends Pharmacol. Sci. 29, 159–165. doi: 10.1016/j.tips.2007.12.002
Pubmed Abstract | Pubmed Full Text | CrossRef Full Text | Google Scholar
Lohse, M. J., Nuber, S., and Hoffmann, C. (2012). Fluorescence/bioluminescence resonance energy transfer techniques to study G-protein-coupled receptor activation and signaling. Pharmacol. Rev. 64, 299–336. doi: 10.1124/pr.110.004309
Pubmed Abstract | Pubmed Full Text | CrossRef Full Text | Google Scholar
Mironov, S. L., Skorova, E., Taschenberger, G., Hartelt, N., Nikolaev, V. O., Lohse, M. J., et al. (2009). Imaging cytoplasmic cAMP in mouse brainstem neurons. BMC Neurosci. 10:29. doi: 10.1186/1471-2202-10-29
Pubmed Abstract | Pubmed Full Text | CrossRef Full Text | Google Scholar
Neves, S. R., Tsokas, P., Sarkar, A., Grace, E. A., Rangamani, P., Taubenfeld, S. M., et al. (2008). Cell shape and negative links in regulatory motifs together control spatial information flow in signaling networks. Cell 133, 666–680. doi: 10.1016/j.cell.2008.04.025
Pubmed Abstract | Pubmed Full Text | CrossRef Full Text | Google Scholar
Nicol, X., Hong, K. P., and Spitzer, N. C. (2011). Spatial and temporal second messenger codes for growth cone turning. Proc. Natl. Acad. Sci. U S A 108, 13776–13781. doi: 10.1073/pnas.1100247108
Pubmed Abstract | Pubmed Full Text | CrossRef Full Text | Google Scholar
Nikolaev, V. O., Bünemann, M., Hein, L., Hannawacker, A., and Lohse, M. J. (2004). Novel single chain cAMP sensors for receptor-induced signal propagation. J. Biol. Chem. 279, 37215–37218. doi: 10.1074/jbc.c400302200
Pubmed Abstract | Pubmed Full Text | CrossRef Full Text | Google Scholar
Nikolaev, V. O., Bünemann, M., Schmitteckert, E., Lohse, M. J., and Engelhardt, S. (2006). Cyclic AMP imaging in adult cardiac myocytes reveals far-reaching β1-adrenergic but locally confined β2-adrenergic receptor-mediated signaling. Circ. Res. 99, 1084–1091. doi: 10.1161/01.res.0000250046.69918.d5
Pubmed Abstract | Pubmed Full Text | CrossRef Full Text | Google Scholar
Nikolaev, V. O., Gambaryan, S., Engelhardt, S., Walter, U., and Lohse, M. J. (2005). Real-time monitoring of the PDE2 activity of live cells: hormone-stimulated cAMP hydrolysis is faster than hormone-stimulated cAMP synthesis. J. Biol. Chem. 280, 1716–1719. doi: 10.1074/jbc.c400505200
Pubmed Abstract | Pubmed Full Text | CrossRef Full Text | Google Scholar
Nikolaev, V. O., Moshkov, A., Lyon, A. R., Miragoli, M., Novak, P., Paur, H., et al. (2010). β2-adrenergic receptor redistribution in heart failure changes cAMP compartmentation. Science 327, 1653–1657. doi: 10.1126/science.1185988
Pubmed Abstract | Pubmed Full Text | CrossRef Full Text | Google Scholar
Norris, R. P., Ratzan, W. J., Freudzon, M., Mehlmann, L. M., Krall, J., Movsesian, M. A., et al. (2009). Cyclic GMP from the surrounding somatic cells regulates cyclic AMP and meiosis in the mouse oocyte. Development 136, 1869–1878. doi: 10.1242/dev.035238
Pubmed Abstract | Pubmed Full Text | CrossRef Full Text | Google Scholar
Oliveira, R. F., Kim, M., and Blackwell, K. T. (2012). Subcellular location of PKA controls striatal plasticity: stochastic simulations in spiny dendrites. PLoS Comput. Biol. 8:e1002383. doi: 10.1371/journal.pcbi.1002383
Pubmed Abstract | Pubmed Full Text | CrossRef Full Text | Google Scholar
Oliveira, R. F., Terrin, A., Di Benedetto, G., Cannon, R. C., Koh, W., Kim, M., et al. (2010). The role of type 4 phosphodiesterases in generating microdomains of cAMP: large scale stochastic simulations. PLoS One 5:e11725. doi: 10.1371/journal.pone.0011725
Pubmed Abstract | Pubmed Full Text | CrossRef Full Text | Google Scholar
Pierce, K. L., Premont, R. T., and Lefkowitz, R. J. (2002). Seven-transmembrane receptors. Nat. Rev. Mol. Cell Biol. 3, 639–650. doi: 10.1038/nrm908
Pubmed Abstract | Pubmed Full Text | CrossRef Full Text | Google Scholar
Polito, M., Klarenbeek, J., Jalink, K., Paupardin-Tritsch, D., Vincent, P., and Castro, L. R. (2013). The NO/cGMP pathway inhibits transient cAMP signals through the activation of PDE2 in striatal neurons. Front. Cell. Neurosci. 7:211. doi: 10.3389/fncel.2013.00211
Pubmed Abstract | Pubmed Full Text | CrossRef Full Text | Google Scholar
Ponsioen, B., Zhao, J., Riedl, J., Zwartkruis, F., van der Krogt, G., Zaccolo, M., et al. (2004). Detecting cAMP-induced Epac activation by fluorescence resonance energy transfer: Epac as a novel cAMP indicator. EMBO Rep. 5, 1176–1180. doi: 10.1038/sj.embor.7400290
Pubmed Abstract | Pubmed Full Text | CrossRef Full Text | Google Scholar
Rall, T. W., and Sutherland, E. W. (1958). Formation of a cyclic adenine ribonucleotide by tissue particles. J. Biol. Chem. 232, 1065–1076.
Rich, T. C., Fagan, K. A., Nakata, H., Schaack, J., Cooper, D. M., and Karpen, J. W. (2000). Cyclic nucleotide-gated channels colocalize with adenylyl cyclase in regions of restricted cAMP diffusion. J. Gen. Physiol. 116, 147–161. doi: 10.1085/jgp.116.2.147
Pubmed Abstract | Pubmed Full Text | CrossRef Full Text | Google Scholar
Rich, T. C., and Karpen, J. W. (2002). Review article: cyclic AMP sensors in living cells: what signals can they actually measure? Ann. Biomed. Eng. 30, 1088–1099. doi: 10.1114/1.1511242
Pubmed Abstract | Pubmed Full Text | CrossRef Full Text | Google Scholar
Rich, T. C., Tse, T. E., Rohan, J. G., Schaack, J., and Karpen, J. W. (2001). In vivo assessment of local phosphodiesterase activity using tailored cyclic nucleotide-gated channels as cAMP sensors. J. Gen. Physiol. 118, 63–78. doi: 10.1085/jgp.118.1.63
Pubmed Abstract | Pubmed Full Text | CrossRef Full Text | Google Scholar
Rich, T. C., Webb, K. J., and Leavesley, S. J. (2014). Can we decipher the information content contained within cyclic nucleotide signals? J. Gen. Physiol. 143, 17–27. doi: 10.1085/jgp.201311095
Pubmed Abstract | Pubmed Full Text | CrossRef Full Text | Google Scholar
Rybin, V. O., Xu, X., Lisanti, M. P., and Steinberg, S. F. (2000). Differential targeting of β-adrenergic receptor subtypes and adenylyl cyclase to cardiomyocyte caveolae. A mechanism to functionally regulate the cAMP signaling pathway. J. Biol. Chem. 275, 41447–41457. doi: 10.1074/jbc.m006951200
Pubmed Abstract | Pubmed Full Text | CrossRef Full Text | Google Scholar
Saucerman, J. J., Greenwald, E. C., and Polanowska-Grabowska, R. (2013). Perspectives on: cyclic nucleotide microdomains and signaling specificity: mechanisms of cyclic amp compartmentation revealed by computational models. J. Gen. Physiol. 143, 39–48. doi: 10.1085/jgp.201311044
Shafer, O. T., Kim, D. J., Dunbar-Yaffe, R., Nikolaev, V. O., Lohse, M. J., and Taghert, P. H. (2008). Widespread receptivity to neuropeptide PDF throughout the neuronal circadian clock network of Drosophila revealed by real-time cyclic AMP imaging. Neuron 58, 223–237. doi: 10.1016/j.neuron.2008.02.018
Pubmed Abstract | Pubmed Full Text | CrossRef Full Text | Google Scholar
Shelly, M., Lim, B. K., Cancedda, L., Heilshorn, S. C., Gao, H., and Poo, M. M. (2010). Local and long-range reciprocal regulation of cAMP and cGMP in axon/dendrite formation. Science 327, 547–552. doi: 10.1126/science.1179735
Pubmed Abstract | Pubmed Full Text | CrossRef Full Text | Google Scholar
Steinberg, S. F., and Brunton, L. L. (2001). Compartmentation of G protein-coupled signaling pathways in cardiac myocytes. Annu. Rev. Pharmacol. Toxicol. 41, 751–773. doi: 10.1146/annurev.pharmtox.41.1.751
Pubmed Abstract | Pubmed Full Text | CrossRef Full Text | Google Scholar
Tadevosyan, A., Vaniotis, G., Allen, B. G., Hebert, T. E., and Nattel, S. (2012). G protein-coupled receptor signalling in the cardiac nuclear membrane: evidence and possible roles in physiological and pathophysiological function. J. Physiol. 590, 1313–1330. doi: 10.1113/jphysiol.2011.222794
Pubmed Abstract | Pubmed Full Text | CrossRef Full Text | Google Scholar
Takeda, N., Kyozuka, K., and Deguchi, R. (2006). Increase in intracellular cAMP is a prerequisite signal for initiation of physiological oocyte meiotic maturation in the hydrozoan Cytaeis uchidae. Dev. Biol. 298, 248–258. doi: 10.1016/j.ydbio.2006.06.034
Pubmed Abstract | Pubmed Full Text | CrossRef Full Text | Google Scholar
Tomchik, S. M., and Davis, R. L. (2009). Dynamics of learning-related cAMP signaling and stimulus integration in the Drosophila olfactory pathway. Neuron 64, 510–521. doi: 10.1016/j.neuron.2009.09.029
Pubmed Abstract | Pubmed Full Text | CrossRef Full Text | Google Scholar
Vincent, P., and Brusciano, D. (2001). Cyclic AMP imaging in neurones in brain slice preparations. J. Neurosci. Methods 108, 189–198. doi: 10.1016/s0165-0270(01)00393-4
Pubmed Abstract | Pubmed Full Text | CrossRef Full Text | Google Scholar
Violin, J. D., Dewire, S. M., Barnes, W. G., and Lefkowitz, R. J. (2006). G protein-coupled receptor kinase and β-arrestin-mediated desensitization of the angiotensin II type 1A receptor elucidated by diacylglycerol dynamics. J. Biol. Chem. 281, 36411–36419. doi: 10.1074/jbc.m607956200
Pubmed Abstract | Pubmed Full Text | CrossRef Full Text | Google Scholar
Violin, J. D., Dipilato, L. M., Yildirim, N., Elston, T. C., Zhang, J., and Lefkowitz, R. J. (2008). β2-adrenergic receptor signaling and desensitization elucidated by quantitative modeling of real time cAMP dynamics. J. Biol. Chem. 283, 2949–2961. doi: 10.1074/jbc.m707009200
Pubmed Abstract | Pubmed Full Text | CrossRef Full Text | Google Scholar
Wachten, S., Masada, N., Ayling, L. J., Ciruela, A., Nikolaev, V. O., Lohse, M. J., et al. (2010). Distinct pools of cAMP centre on different isoforms of adenylyl cyclase in pituitary-derived GH3B6 cells. J. Cell Sci. 123, 95–106. doi: 10.1242/jcs.058594
Pubmed Abstract | Pubmed Full Text | CrossRef Full Text | Google Scholar
Webb, R. J., Marshall, F., Swann, K., and Carroll, J. (2002). Follicle-stimulating hormone induces a gap junction-dependent dynamic change in [cAMP] and protein kinase A in mammalian oocytes. Dev. Biol. 246, 441–454. doi: 10.1006/dbio.2002.0630
Pubmed Abstract | Pubmed Full Text | CrossRef Full Text | Google Scholar
Willoughby, D., and Cooper, D. M. (2007). Organization and Ca2+ regulation of adenylyl cyclases in cAMP microdomains. Physiol. Rev. 87, 965–1010. doi: 10.1152/physrev.00049.2006
Pubmed Abstract | Pubmed Full Text | CrossRef Full Text | Google Scholar
Zaccolo, M. (2011). Spatial control of cAMP signalling in health and disease. Curr. Opin. Pharmacol. 11, 649–655. doi: 10.1016/j.coph.2011.09.014
Pubmed Abstract | Pubmed Full Text | CrossRef Full Text | Google Scholar
Zaccolo, M., De Giorgi, F., Cho, C. Y., Feng, L., Knapp, T., Negulescu, P. A., et al. (2000). A genetically encoded, fluorescent indicator for cyclic AMP in living cells. Nat. Cell Biol. 2, 25–29. doi: 10.1038/71345
Pubmed Abstract | Pubmed Full Text | CrossRef Full Text | Google Scholar
Zaccolo, M., and Pozzan, T. (2002). Discrete microdomains with high concentration of cAMP in stimulated rat neonatal cardiac myocytes. Science 295, 1711–1715. doi: 10.1126/science.1069982
Pubmed Abstract | Pubmed Full Text | CrossRef Full Text | Google Scholar
Zhang, J., Hupfeld, C. J., Taylor, S. S., Olefsky, J. M., and Tsien, R. Y. (2005). Insulin disrupts β-adrenergic signalling to protein kinase A in adipocytes. Nature 437, 569–573. doi: 10.1038/nature04140
Pubmed Abstract | Pubmed Full Text | CrossRef Full Text | Google Scholar
Zhang, J., Ma, Y., Taylor, S. S., and Tsien, R. Y. (2001). Genetically encoded reporters of protein kinase A activity reveal impact of substrate tethering. Proc. Natl. Acad. Sci. U S A 98, 14997–15002. doi: 10.1073/pnas.211566798
Pubmed Abstract | Pubmed Full Text | CrossRef Full Text | Google Scholar
Keywords: G protein-coupled receptor, cyclic AMP, signaling microdomain, fluorescence resonance energy transfer, neurons
Citation: Calebiro D and Maiellaro I (2014) cAMP signaling microdomains and their observation by optical methods. Front. Cell. Neurosci. 8:350. doi: 10.3389/fncel.2014.00350
Received: 28 July 2014; Accepted: 07 October 2014;
Published online: 28 October 2014.
Edited by:
Pierre Vincent, Centre National de la Recherche Scientifique, FranceReviewed by:
Thomas Rich, University of South Alabama College of Medicine, USACopyright © 2014 Calebiro and Maiellaro. This is an open-access article distributed under the terms of the Creative Commons Attribution License (CC BY). The use, distribution and reproduction in other forums is permitted, provided the original author(s) or licensor are credited and that the original publication in this journal is cited, in accordance with accepted academic practice. No use, distribution or reproduction is permitted which does not comply with these terms.
*Correspondence: Davide Calebiro, Institute of Pharmacology and Toxicology, University of Würzburg, Versbacherstr. 9, 97078 Würzburg, Germany e-mail:ZGF2aWRlLmNhbGViaXJvQHRveGkudW5pLXd1ZXJ6YnVyZy5kZQ==
Disclaimer: All claims expressed in this article are solely those of the authors and do not necessarily represent those of their affiliated organizations, or those of the publisher, the editors and the reviewers. Any product that may be evaluated in this article or claim that may be made by its manufacturer is not guaranteed or endorsed by the publisher.
Research integrity at Frontiers
Learn more about the work of our research integrity team to safeguard the quality of each article we publish.