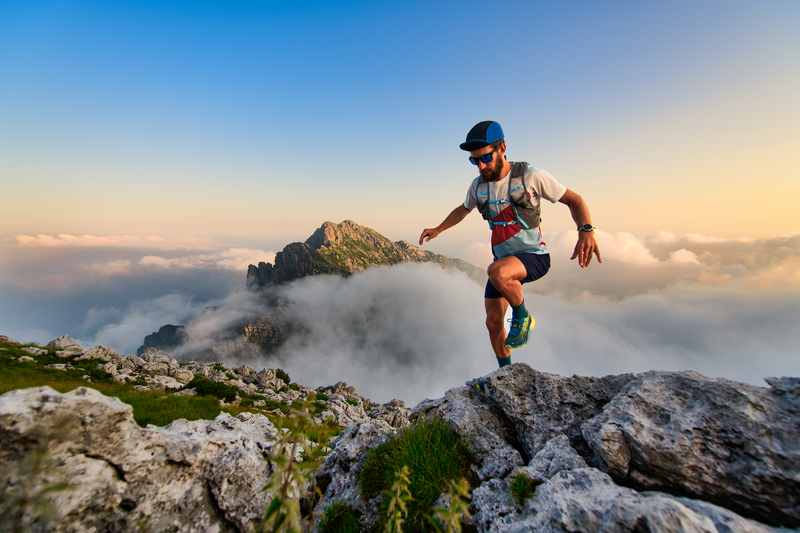
95% of researchers rate our articles as excellent or good
Learn more about the work of our research integrity team to safeguard the quality of each article we publish.
Find out more
REVIEW article
Front. Cell. Neurosci. , 13 September 2013
Sec. Cellular Neurophysiology
Volume 7 - 2013 | https://doi.org/10.3389/fncel.2013.00134
This article is part of the Research Topic Neuronal mechanisms of epileptogenesis View all 18 articles
A commentary has been posted on this article:
Erratum: K+ channelepsy: progress in the neurobiology of potassium channels and epilepsy
K+ channels are important determinants of seizure susceptibility. These membrane proteins, encoded by more than 70 genes, make the largest group of ion channels that fine-tune the electrical activity of neuronal and non-neuronal cells in the brain. Their ubiquity and extremely high genetic and functional diversity, unmatched by any other ion channel type, place K+ channels as primary targets of genetic variations or perturbations in K+-dependent homeostasis, even in the absence of a primary channel defect. It is therefore not surprising that numerous inherited or acquired K+ channels dysfunctions have been associated with several neurologic syndromes, including epilepsy, which often generate confusion in the classification of the associated diseases. Therefore, we propose to name the K+ channels defects underlying distinct epilepsies as “K+ channelepsies,” and introduce a new nomenclature (e.g., Kx.y-channelepsy), following the widely used K+ channel classification, which could be also adopted to easily identify other channelopathies involving Na+ (e.g., Navx.y-phenotype), Ca2+ (e.g., Cavx.y-phenotype), and Cl− channels. Furthermore, we discuss novel genetic defects in K+ channels and associated proteins that underlie distinct epileptic phenotypes in humans, and analyze critically the recent progress in the neurobiology of this disease that has also been provided by investigations on valuable animal models of epilepsy. The abundant and varied lines of evidence discussed here strongly foster assessments for variations in genes encoding for K+ channels and associated proteins in patients with idiopathic epilepsy, provide new avenues for future investigations, and highlight these proteins as critical pharmacological targets.
Epilepsy is a brain disorder due to abnormal firing of neuronal networks in the brain that often causes convulsions, muscle spasms, and loss of consciousness. Seizures sometimes cause brain damage, particularly if they are severe. More than 2 million people in the United States—about 1 percent—have experienced an unprovoked seizure or been diagnosed with epilepsy. For about 80 percent of those diagnosed with epilepsy, seizures can be controlled pharmacologically, or treated surgically. However, the rest of people with epilepsy—intractable epilepsy—will continue to experience seizures even with the best available treatment. Although the causes of epilepsy are numerous, the fundamental disorder is secondary to abnormal synchronous discharges of a network of neurons, either due to neuronal membrane instability, or an imbalance between excitatory and inhibitory influences. Neurons store and convey information in the form of electrical impulses generated by ion channels. Neuronal excitability can be controlled by both the intrinsic activity of K+ channels and the receptors-mediated modulation of their activity (Pessia et al., 1994; Imbrici et al., 2000; Pessia, 2004; D'Adamo et al., 2013). Their opening and resulting outward K+ flux dampen neuronal excitability and therefore they are viewed as inhibitory channels. However, contrary to this general notion, increased K+ channel activity may also result in enhanced cell excitability. Hence, K+ channels are critical for neuronal excitability, as they control the resting membrane potentials and enable rapid repolarization of the action potentials. Moreover, they are essential effectors of neurotransmitter-mediated signaling, regulator of Ca2+ homeostasis and cell survival. To fulfill these pivotal functions efficiently, K+ channels are found in virtually every cell of the human body, are distinguished by being the largest and most diverse class of ion channels, and are encoded by more than 70 genes (http://www.genenames.org/genefamily/kcn.php). An additional reason for their large diversity resides in the fact that they form macromolecular complexes, involving several proteins. The need for such a large number of K+ channels remains unclear.
In the past few years, several types of epilepsies have been associated to dysfunction of K+ channels, resulting from mutations in their encoding genes (Table 1), which appear prime elements potentially underlying idiopathic epilepsy. Indeed, the extremely high molecular and functional diversity of K+ channels, unmatched by any other types of channels, places them (by statistical probability alone) as primary targets of genetic variations. Besides the intrinsic K+ channels' gene defects associated with epilepsy, it is increasingly clear that also disruption/modification in K+ channel properties, even in the absence of a primary channel defect, may underlie increased susceptibility to seizures. The association between K+ channel dysfunctions and epileptic phenotypes is also confirmed by a multitude of animal models of epilepsy, that is, animals carrying K+ channel mutations or genetic manipulations and displaying spontaneous seizures or increased susceptibility to stimulus-induced seizure (Table 2). The extremely high diversity of K+ channels and the numerous variations identified in their genes often generate confusion in the classification of the associated diseases. Therefore, we propose to name the K+ channels defects underlying distinct epilepsies as “K+ channelepsies,” and offer a new classification according to a widely used K+ channel nomenclature (e.g., KVx.y). Moreover, here we discuss the different aspects of K+ channels dysfunctions underlying distinct epileptic phenotypes and describe the recent progress in the neurobiology of seizure susceptibility in animal models of epilepsy. Comprehensive knowledge of the neurobiological processes altered by K+ channel defects is a pivotal step to identify original therapeutic solutions for this devastating disease. Full understanding of how mutations in K+ channels give rise to distinct human and animal epileptic phenotypes requires a basic knowledge of their molecular features, expression pattern, and physiological roles. Thus, brief overviews on these topics for each K+ channel subfamily have been included.
Voltage-gated K+ channels (Kv) are generally closed at the resting membrane potential of nerve cells (ca.–70 mV) and open following membrane depolarization. At a single channel level, membrane depolarizations elicit channel opening and closing (a process named gating) visible as upwards and downwards deflections of current trace (Pessia, 2004). The first Kv channel was cloned from the Shaker mutant of Drosophila melanogaster in 1987 (Tempel et al., 1987). The human ortholog of Shaker K+ channel is encoded by the gene KCNA1(Kv1.1). Since the first cloning, several other genes encoding for Kv channels have been identified from many different species. Based on sequence relatedness, Kv channels have been classified in subfamilies by using the abbreviation Kvy.x (Chandy and Gutman, 1993). According to this standardized nomenclature Shaker-related channels have been classified in the subfamily Kv1.x and each member numbered Kv1.1 through Kv1.8. The same criteria have been used to classify channels related to the Drosophila subfamilies Shab (Kv2.1 and Kv2.2), Shaw (Kv3.1 to Kv3.4), and Shal (Kv4.1 to Kv4.3). These channels may exist as homomers, whenever four identical α-subunits are assembled. However, different types of α-subunits may heteropolymerize to form channels with functional and pharmacological properties that are different from the parental homomeric channels (Isacoff et al., 1990; Ruppersberg et al., 1990).
The predicted 496 amino acids of the Kv1.1 α subunit form six transmembrane segments (TM) with the N- and C-termini residing inside the cell. TM5, TM6, and the H5 loop linking them contribute to the ion-conducting pore, and the GYG residues, that reside within the loop, control the K+ selectivity of the channel. The TM4 segment of each Kv1.1 α subunit is made of regularly spaced positively charged arginines and lysines and embodies the main voltage-sensor region that opens the channel by undergoing a conformational rearrangement upon membrane depolarization (Pessia, 2004). The full crystal structure, provided for a Kv channel, confirmed that this channel is composed of four homologous pore-forming α subunits (Jiang et al., 2003a,b). The description of the membrane-delimited Kv channel structure, T1 domain and β subunits allowed elucidation of many biophysical mechanisms controlling channel function. The Kv1 family members exhibit diverse expression patterns in the central and peripheral nervous system and are found tightly clustered within distinct neuronal compartments (Trimmer and Rhodes, 2004). Kv channels regulate the duration of action potentials, modulate the release of neurotransmitters, control the excitability, electrical properties, and firing pattern of central and peripheral neurons (Pessia, 2004). Moreover, the activity of Kv channels can be dynamically modulated by several events, including neurotransmitter- stimulated biochemical cascades (Imbrici et al., 2000; D'Adamo et al., 2013). Knowledge of their precise targeting and neurophysiological functions has important implications for defining the roles played by each Kv channel type in the pathophysiology of epilepsy.
Episodic ataxia type 1 (EA1) [OMIM 160120] is a Shaker-like K+ channels disease characterized by constant myokymia and dramatic episodes of spastic contractions of the skeletal muscles of the head, arms, and legs with loss of both motor coordination and balance (D'Adamo et al., 2012). EA1 was clearly described during the mid '70s by van Dyke and colleagues who first reported electroencephalographic (EEG) recordings characterized by runs of paroxysmal slow waves and generalized motor seizures in the proband's mother (van Dyke et al., 1975). The subsequent genetic analysis revealed that the individuals displaying epilepsy carried the F184C mutation in their KCNA1 gene that profoundly altered the channel's properties (Browne et al., 1994; Adelman et al., 1995). Confirmations of an increased susceptibility to seizures in EA1 came from several subsequent studies (Table 1). Isolated photosensitive generalized tonic–clonic seizure (Imbrici et al., 2008) and abnormal EEGs have been observed in individuals with EA1 (Zuberi et al., 1999). EEGs may be characterized by intermittent and generalized slow activity, frequently intermingled with spikes. Zuberi et al. (1999) described a 3-year-old boy who presented with an ictal EEG with rhythmical slow-wave activity over the right hemisphere, becoming spike-and-wave complexes that then spread to the left hemisphere. Neuronal circuit dysfunctions within the hippocampus have been postulated to play a role in seizures and cognitive dysfunctions associated with EA1. Indeed, the hippocampus is a major brain region of the limbic system which plays an important role in the consolidation of information and in spatial memory, and it is often the focus of epileptic seizures. In rodent hippocampus, Kv1.1, Kv1.2, and Kv1.4 are found in Schaffer collateral axons and are highly expressed in axons and terminals of the medial perforant path in the middle third of the molecular layer of the dentate gyrus. In particular, Kv1.1, Kv1.4, and Kvβ1.1 subunits are expressed in mossy fiber boutons (swellings of mossy fiber axons) that form en passant synapses with pyramidal neurons in CA3. The macromolecular channel complex, composed of these subunits, regulates the activity-dependent spike broadening of hippocampal mossy fiber boutons and, consequently, the amount of neurotransmitter released during high-frequency stimuli (Geiger and Jonas, 2000). Mutations associated with EA1 profoundly alter the function of heteromeric channels composed of Kv1.1, Kv1.2, Kv1.4, and Kvβ1.1 subunits (D'Adamo et al., 1999; Imbrici et al., 2006) that likely contributes to seizures susceptibility and cognitive symptoms (Imbrici et al., 2006) in EA1. Notably, epileptiform brain activity has also been associated with intracranial administration of Zn2+ salts, and changes in Zn2+ modulation of GABA receptors have been implicated in the etiology of epilepsy. Zn2+ is released from mossy fiber terminals in the hippocampus, and from the basket cell terminals of the cerebellum (Assaf and Chung, 1984) where Kv1 channel activity likely is subjected to Zn2+ modulation. Indeed, homomeric and heteromeric channels containing Kv1 subunits are inhibited by extracellular Zn2+, and a distinct EA1 mutation increases several folds the Zn2+ sensitivity of these channels (Cusimano et al., 2004; Imbrici et al., 2007). Whether Zn2+ plays a role in triggering epilepsy-like symptoms in EA1 remains an intriguing hypothesis. A murine model that recapitulates the EA1 phenotype (mKv1.1V408A/+) has been generated by inserting in the mouse Kcna1(mKv1.1) a very conservative valine to alanine substitution (V408A) previously identified in patients (Herson et al., 2003; Brunetti et al., 2012, Table 2). Although data concerning the mKv1.1V408A/+ are not yet available, the role of Kv1.1 channels in the neurobiology of epilepsy has been investigated by using Kv1.1 knockout mice (mKv1.1−/−). The hippocampus of these animals displays morphological characteristics typical of epilepsy, with neural loss, astrocytosis, and mossy fiber sprouting (Rho et al., 1999). Moreover, mKv1.1−/− also exhibits frequent spontaneous seizures throughout adult life, although the intrinsic passive properties of CA3 pyramidal cells are normal (Table 2). Antidromic action potentials were recruited at lower thresholds in mKv1.1−/− slices, and mossy fiber stimulation triggered synaptically-mediated long-latency epileptiform burst discharges. These data indicate that loss of Kv1.1 results in increased excitability in the CA3 recurrent axon collateral system, perhaps contributing to the limbic and tonic–clonic components of the observed epileptic phenotype of EA1 (Smart et al., 1998). Recently, in vitro extracellular recordings were performed by using a multielectrode array to characterize spontaneous sharp waves and high frequency oscillations in mKv1.1−/− hippocampi. This study showed that the mossy fibers and medial perforant path axons of mKv1.1−/− were hyperexcitable and produced greater pre- and post-synaptic responses with reduced paired-pulse ratios. Microdissection of mossy fibers and perforant path in mKv1.1−/− hippocampal slices ameliorated the abnormal oscillatory pattern and improved spike timing. In contrast, blockade of Kv1.1 channels with dendrotoxin-K reproduced these effects in WT slices. These findings suggest that loss of Kv1.1 enhances synaptic neurotransmitter release in the CA3 region, which reduces spike timing precision of individual neurons, leading to disorganization of network oscillatory activity and promoting the emergence of fast ripples (Simeone et al., 2013).
N-ethyl-N-nitrosourea (ENU) mutagenesis has been widely used to generate animal models of human diseases. An ENU-mutagenized rat strain has been recently generated and named “autosomal dominant myokymia and seizures” (ADMS) rats (Ishida et al., 2012). Genetic analysis of these animals resulted in the identification of the missense mutation S309T in the voltage-sensor domain of Kv1.1 channels (rKv1.1S309T/+). This heterozygous mutation resulted in 80% smaller current amplitudes with dominant-negative effects (Table 2). From 16 weeks of age severe periodic seizures were observed, and by 30 weeks of age, 84% of rKv1.1S309T/+ had died. Cooling induced severe neuromyotonia, ataxia and aberrant spike-and-wave discharges (2–3 Hz) associated with clonus behaviors. Spontaneous convulsive seizures from 10 to 16 weeks of age were aggravated by stress (cage changing or animal handling). Cortical and hippocampal EEG recordings identified aberrant large spike activity associated with falling-down behavior, low-voltage fast wave discharges detected during the tonic stage, and spike-and-wave discharges (2 Hz) detected during the clonic convulsive stage (Ishida et al., 2012). The behavioral phenotypes and abnormal discharge patterns in rKv1.1S309T/+ are similar to other rodent models of temporal lobe epilepsy (TLE). Carbamazepine (CBZ) administration ameliorated seizures. The videos related to this animal model of EA1 are available online at doi:10.1016/j.brainres.2011.11.023.
The megencephaly mice, mceph/mceph, are characterized by increased brain volume, hypertrophic brain cells and slight hippocampal astrocytosis. They display a complex behavioral phenotype including running seizures, complex partial seizures and postanaesthetic tonic-clonic seizures (Donahue et al., 1996, Table 2). Remarkably, an 11-basepair deletion in the Kcna1 gene of mceph/mceph mice has been identified (Petersson et al., 2003). The mutation leads to a frame shift and a premature stop codon that was predicted to truncate the protein at amino acid 230 (out of 495). Therefore, mceph/mceph mice express Kv1.1 subunits lacking the last five TMs and the C-terminal domain: The absence of Kv1.1 channels with intact C-terminal domains was confirmed by Western blotting analysis of whole protein extracts from brain. Electrophysiological investigations from mceph/mceph brain slices revealed hippocampal mceph/mceph brain slices revealed hippocampal hyperexcitability consistent with limbic status epilepticus (SE) C-teminal domain of Kv1.1 channels (R417stop) has also been found in a EA1 proband displaying episodes of ataxia precipitated by exercise, stress, startle or high temperature occurring after a hot bath or when using a hairdryer but, absence of epilepsy (Eunson et al., 2000).
Kv1.2 knockout mice display increased seizure susceptibility (Brew et al., 2007, Table 2). It should be recalled that Kv1.1 and Kv1.2 are closely coupled K+ channel subunits, as they form heteromeric channels in several brain regions. Indeed, biochemical and electrophysiological studies have shown that Kv1.1/Kv1.2 channels control neuronal excitability, action potentials propagation and synaptic transmission. Notably, EA1 mutations alter the function of heteromeric channels composed of Kv1.1 and Kv1.2 subunits (D'Adamo et al., 1999). In conclusion, these investigations with animal models of Kv1.1 channelepsy highlighted the crucial brain regions that are likely the site of origin of abnormal discharges in EA1 and the relevant mechanisms underlying their susceptibility to seizures.
Kv1.1 mRNA is target for enzymatic deamination by adenosine deaminase acting on RNA (ADAR2). Knockout mice for the ADAR2 gene are prone to epileptic seizures and die within a few weeks after birth (Higuchi et al., 2000, Table 2). Kv1.1 editing by ADAR2 results in channels with an I400V exchange in the S6 segment (Kv1.1I400V). In vitro, an increase in increase in Kv1.1I400V editing increases K+ outward current upon membrane depolarization and accelerates recovery from inactivation at negative membrane potentials (Bhalla et al., 2004). Interestingly, increased levels of Kv1.1I400V editing were found in chronic epileptic rats. It has also been reported a reduced ability of 4-AP to trigger seizure-like effects in brain slices dissected from a kainic acid rat model of chronic epilepsy (Zahn et al., 2008; Streit et al., 2011). A similar phenomenon was observed in human brain slices of patients with pharmacoresistant TLE (Gabriel et al., 2004). The postulated ictiogenic mechanism of 4-AP action is its ability to block Kv1 and Kv3 channels, which results in increased transmitter release. Kv1.1I400V editing results in 4-AP–insensitive homomeric Kv1.1 channels, and alters the pharmacology of heteromeric channels (Decher et al., 2010; Streit et al., 2011). These findings suggest that mRNA editing of Kv1.1 in chronic epilepsy may to the reorganization of the entorhinal cortex and have anticonvulsive effects. The editing of Kv1.1 mRNA could therefore represent a compensatory mechanism in brain areas where epileptic seizures originate. TLE, namely spontaneous seizures involving the hippocampal formation, is the most prevalent refractory epilepsy. TLE can be sub-classified into mesial, lateral and neocortical. Mesial TLE (MTLE) is defined by clinic-anatomical observations and characterized by seizures arising from the hippocampus or parahippocampal structures, and frequent pharmacotherapy resistance. A recent screening study identified a significant negative correlation between epilepsy duration in patients with MTLE plus hippocampal sclerosis and the I/V editing site of Kv1.1 channels. This may be the result of the epileptic process itself, together with the medication history of the patient, and may thus reflect compensatory mechanisms to either of these factors (Krestel et al., 2013).
Kv4 channels underlie the main dendritic A-type Kv currents in hippocampal neurons and play a critical role in regulating the extent to which back-propagating action potentials invade the dendritic tree. They also impact the propagation of synaptic potentials from the dendritic arbor to the soma (Jerng et al., 2004). The relevance of Kv4.2 to epilepsy comes from the identification of a mutation in a patient with TLE, which results in the expression of a truncated Kv4.2 subunit (Singh et al., 2006, Table 1), and the observation that pharmacological blockade of Kv4 channels is epileptogenic (Avoli, 2001). These studies support the concept that Kv4.2 deficiency may contribute to aberrant network excitability and regulate seizure threshold. Intriguingly, it has been reported that Kv4.2 knockout mice do not exhibit an overt seizure phenotype (Hu et al., 2006), perhaps due to posttranslational up-regulation of other Kv currents (Nerbonne et al., 2008). A more recent study, however, suggests that loss of Kv4.2 channels is associated with enhanced susceptibility to seizures after kainate injection (Barnwell et al., 2009, Table 2).
Systemic administration of the muscarinic agonist pilocarpine to rats recapitulates the features of human limbic seizures and SE. The expression of a number of different K+ channels, including Kv (Monaghan et al., 2008) and KCa (BK) channels (Pacheco Otalora et al., 2008) is altered in response to pilocarpine-induced SE. The amplitude of A-type currents in the dendrites of hippocampal CA1 pyramidal neurons is reduced in response to pilocarpine-induced seizures (Bernard et al., 2004). In addition to Kv4.2, Kv4.3 and KChIP2 current decrease, pilocarpine-treated rats exhibited staining changes for these proteins in the molecular layer of the dentate gyrus from being uniformly distributed across the molecular layer to become concentrated in just the outer two-thirds (Monaghan et al., 2008). As a consequence, an increased dendritic excitability was found in CA1 pyramidal cell dendrites in response to the same pilocarpine model of TLE (Bernard et al., 2004). In situ hybridization studies revealed that generalized seizures induced by pentylenetetrazol (Tsaur et al., 1992) or kainic acid (Francis et al., 1997) also altered the regional hippocampal gene expression of rat Kv4.2. A decrease of A-current density in this proximal region of the granule cell dendrite—a location that receives massive aberrant excitatory mossy fiber input following induction of SE and as spontaneous seizures develop—would lower the firing threshold and thus contribute to the development of spontaneous recurrent seizures. On the other hand, seizures in vivo and glutamate in vitro induce a rapid surface recruitment of Kv4.2 channels in neurons. Thus, seizure would induce dampening of phasic firing, generated by glutamatergic synaptic transmission, by enhancing the surface expression of Kv4.2 channels. Interestingly, mutant LGI1 blocks this homeostatic neuronal response (Smith et al., 2012). Thus, it seems that convulsants may affect Kv4 currents in different ways and compensatory pathways may be recruited to dampen hyper-excitability.
One of the most pronounced anatomical effects of TLE, exhibited in the pilocarpine animal model, is mossy fiber sprouting. The expansion of Kv1.4 staining in stratum lucidum of CA3 in pilocarpine-SE animals suggests that expansion of the mossy fiber terminal field, due to SE-induced sprouting, is accompanied by parallel increases in targeting of Kv1.4 to the newly sprouted mossy fiber axons and terminals. Changes in Kv1.4 immunoreactivity were observed also in the molecular layer of the dentate gyrus. In conclusion, variations in A-type Kv1.4-containing channels in mossy fiber presynaptic terminals, and Kv4.2-containing channels in dentate granule cell and CA1 dendrites, represent important mechanisms intervening in the acquisition of the permanent epileptic phenotype in this animal model of human TLE and attractive therapeutic targets. Moreover, these studies highlighted the essential nature of Kv4.2 and the specific contributions of its auxiliary KChIP subunits (see below) in regulating the seizure susceptibility associated with epileptogenesis.
Five members of the KCNQ gene family have been identified (KCNQ1-5) which form homomeric or heteromeric K+ channels. In many brain regions, heteromeric KCNQ2(Kv7.2)/KCNQ3(Kv7.3) channels seem the major determinant of “M” currents that are inhibited by several neurotransmitters, including acetylcholine (ACh) through the muscarinic receptors (Devaux et al., 2004; Pan et al., 2006). Functionally, these slow-gating K+ channels that are open at subthreshold voltages, contribute to setting the resting membrane potential of neurons, prevent repetitive firing, and control spike-frequency adaptation. Indeed, blockade of M-currents is associated with depolarization of the resting membrane potential, and generation of trains of action potentials (Brown and Adams, 1980; Delmas and Brown, 2005). Mutations in KCNQ2 or KCNQ3 cause a form of juvenile epilepsy called benign familial neonatal convulsions (Biervert et al., 1998; Charlier et al., 1998; Schroeder et al., 1998; Singh et al., 1998, Table 1). The clinical features of KCNQ2-related benign familial neonatal epilepsy (KCNQ2-BFNE) are characterized by tonic or apneic episodes, focal clonic activity, or autonomic changes that start between the second and eighth day of life and spontaneously disappear between the first and the sixth to 12th month. The KCNQ2-related epileptic encephalopathy (KCNQ2-NEE) is characterized by multiple daily seizures that begin the first week of life, are mostly tonic, with motor and autonomic features, and cease after 9 months to 4 years. Most affected individuals are intellectually impaired (Bellini et al., 1993-2013). Just a 25% decrease in M-current amplitude is sufficient to drive human neurons to epileptogenic levels, and causes neonatal epilepsy (Schroeder et al., 1998). The clinical severity of the disease may be related to the extent of mutation-induced functional K+ channel impairment (Miceli et al., 2013). Drugs that enhance neuronal M-currents, such as retigabine, represent a valuable therapeutic treatment for certain hyperexcitatory diseases, including epilepsy (Porter et al., 2012). Flupirtine, a structural analogue of retigabine, which also activates channels formed by neuronal Kv7 subunits, has been shown to be effective in animal models of neonatal convulsion (Raol et al., 2009). Kv7.1 (KCNQ1) channels are mainly expressed in the heart where they contribute to termination of the action potential. Mutations in KCNQ1(Kv7.1) are responsible for one form of long QT syndrome (LQT1) (Wang et al., 1996). However, Kv7.1 channels are also expressed in the brain. Intriguingly, LQT1 patients exhibit an increased risk of epilepsy, suggesting a relationship between these two diseases (see below). Notably, M-channels are also highly sensitive to intracellular Ca2+ variations, being inhibited by Ca2+ with an IC50 of ~100 nM in sympathetic neurons (Selyanko and Brown, 1996; Gamper and Shapiro, 2003). Recently, it has been proposed that abnormal [Ca2+]i transients induced by Kv1.1 channel dysfunction may result in repetitive discharges in myelinated nerves (Brunetti et al., 2012). This mechanism may underlie not only the neuromyotonic/myokymic discharges typically observed in EA1 individuals, but also their susceptibility to seizures.
The neuronal serum- and glucocorticoid-regulated kinase 1 (SGK1.1) appears to be a physiological M-current regulator, as it enhances Kv7.2/3 current levels. Transgenic mice expressing a constitutively active form of SGK1.1 are resistant to kainic acid-induced seizures which occur mainly in the temporal lobe. These findings indicate that SGK1.1 activity can regulate neuronal excitability through M-current modulation and protects against seizures (Miranda et al., 2013).
A number of subunits have been cloned and classified in the K+ channel subfamilies Kv6, Kv8, and Kv9. Although they possess the hallmarks of Kv subunits they do not appear to form functional homomeric K+ channels when expressed alone and, therefore, have been named “silent” subunits. However, these proteins do co-assemble with other Kv subunits (e.g., Kv2), and confer distinct biophysical properties to heteromeric channels Kv2/Kv6, Kv2/Kv8, or Kv2/Kv9. Thus, these gene products are also known as “silent modifiers” of Kv channels. In particular, Kv8.2 (KCNV2) co-assembles with Kv2.1 as a heterotetramer, significantly reducing the surface expression of the resulting channels and influencing their biophysical properties. Within the hippocampus, Kv2.1 and Kv8.2 co-localize in pyramidal neurons and in the principal excitatory neurons of the pyramidal cell layers and the dentate gyrus. Moreover, both are expressed in the cortex, with high levels of transcript in layers 2/3 and 5 (Allen brain atlas; http://www.brain-map.org). These regions are critically involved in seizure generation and propagation. It has been shown that Kv2.1 channels contribute significantly to the delayed-rectifier K+ currents in hippocampal neurons (Murakoshi and Trimmer, 1999). Importantly, knockdown of Kv2.1 in hippocampal slices resulted in increased CA1 pyramidal neuron excitability under conditions of high-frequency stimulation (Du et al., 2000). Thus, the Kv2.1 current reduction, mediated by the inclusion of Kv8.2 subunits in heterotetramers, could regulate the membrane repolarization and excitability of hippocampal neurons, contributing to seizure susceptibility. Indeed, mutations in two related silent subunits have been associated with neurological disorders (Table 1). In particular Kv8.2 (KCNV2) with epilepsy (Jorge et al., 2011) and Kv9.1 (KCNS1) with chronic pain (Costigan et al., 2010). Recently, an individual affected by febrile and afebrile partial seizures has been reported to carry a genetic variation in KCNV2, inherited from his unaffected mother. This resulted in the substitution of a highly conserved lysine for an arginine (R7K) in the cytoplasmic amino terminus of Kv8.2 subunits. An additional patient who inherited a methionine to arginine mutation (M285R) in Kv8.2 subunits from his unaffected mother was likewise identified with an epileptic encephalopathy and with severe refractory epilepsy (Jorge et al., 2011). The pathogenic relevance of these variants was assessed by electrophysiological recordings from cells co-expressing the human Kv2.1 with Kv8.2 wild-type or its mutated subunit (R7K or M285R). This assay showed that both variants suppressed Kv2.1-mediated current more than the wild-type; in addition, the M285R impaired the voltage-dependence of the channel. These results suggest that both variants enhance seizure susceptibility of affected patients by reducing neuronal delayed-rectifier K+ channel function in brain regions critically involved in seizure generation and propagation (Jorge et al., 2011).
The severity of seizures in mouse models of epilepsy is highly dependent on their genetic background. In transgenic mouse models of sodium channel-dependent epilepsy, the phenotype is more severe in SJL/J compared with the C57BL/6J strain (Bergren et al., 2005). Interestingly, it has been shown that the hippocampal Kv8.2 (Kcnv2) transcript is ~3-fold greater in SJL/J compared with the C57BL/6J strain (Jorge et al., 2011). The enhanced availability of Kv8.2 subunits for co-assembling with Kv2.1 would remarkably reduce the surface expression of the resulting channels in hippocampal neurons of SJL/J mice contributing to their greater susceptibility to seizures.
In conclusion, these studies implicate Kv8.2 (Kcnv2) as an epilepsy gene in rodents and humans. Moreover, although silent subunits have been mostly ignored since they were first cloned a decade or more ago, their potential clinical relevance is now becoming fully evident and therefore represents an attractive new avenue of investigation in neurologic channelopathies research.
The human ether-a-go-go-related gene (HERG) encodes for voltage-gated K+ channels. HERG channels exhibit functional properties remarkably different from other K+ channels (Tristani-Firouzi and Sanguinetti, 2003). They are widely expressed in the brain where they contribute to setting the frequency and the discharge stability of neurons, and to adapting their intrinsic properties to signal processing (Pessia et al., 2008). They also modulate the excitability of dopaminergic and GABAergic neurons (Nedergaard, 2004; Canavier et al., 2007). HERG channels are expressed in the heart where they control the repolarization of ventricular action potentials. Loss-of-function mutations in KCNH2 (HERG) cause type 2 long QT syndrome (LQT2), a condition in which the induced delayed repolarization of the heart following a heartbeat increases the risk of episodes of sudden death due to ventricular fibrillation. However, LQT syndrome is closely associated with seizure and frequently it is misdiagnosed as epilepsy (Table 1). Sudden unexpected death in epilepsy is the most frequent epilepsy-related cause of death for which an underlying arrhythmogenic predisposition has been suggested. Several clinical reports have recently described seizures and arrhythmic events in LQT2 triggered by visual or acoustic stimuli (Keller et al., 2009; Omichi et al., 2010; Tu et al., 2011; Zamorano-León et al., 2012). Considering that HERG channels control several neuronal electrical features, including discharge dynamics (Pessia et al., 2008), these clinical findings raise the possibility that alteration in KCNH2-encoded K+ channels may confer susceptibility for epilepsy and cardiac LQT2 arrhythmia.
K+ channels expressed by distinct cell types may be formed by unique hetero-oligomeric complexes comprising auxiliary subunits. Several types of these subunits have been identified including beta-subunits (Kvβ), minK (minimal K+ channel peptide), MiRP (minK-related peptide), KChAP (K+ channel-associated protein), KChIP (K+ channel-interacting protein) and neuronal calcium sensor (NCS). Each type of auxiliary subunit modulates the activity of the associated K+ channel in distinct ways. Defects in these subunits may alter the function of the channel and result in increased seizure susceptibility.
Auxiliary subunits such as Kvβ1.1 and Kvβ1.2 confer fast N-type or A-type inactivation to non-inactivating Kv1 channels by means of a “ball-and-chain” mechanism of pore occlusion whereby the tethered (chain) positively charged inactivation particle (ball) in the amino-terminus of the Kvβ1 subunit binds to the intracellular entrance of the pore discontinuing the outflow of K+ ions. The fast inactivation of delayed-rectifier K+ channels is a physiologically relevant process, as it controls the firing properties of neurons and their response to input stimuli (Pessia, 2004). Surprisingly, Kvβ1.1 knockout mice do not display an overt epileptic phenotype. On the other hand, Kvβ2 knockout and Kvβ1/Kvβ2 double-knockout mice are characterized by an increased neuronal excitability, occasional seizures, cold swim-induced tremors and a reduced life span (McCormack et al., 2002; Connor et al., 2005, Table 2). Clinical investigations have found an association between the severity of seizures, including infantile spasms and the loss of the Kvβ2 gene. Moreover, the hemizygosity of this gene in epileptic patients suggests that haploinsufficiency for KCNAB2 is a significant risk factor for epilepsy (Heilstedt et al., 2001). Notably, Kvβ2 subunits do not confer fast inactivation properties to Kv1 channels, since they lack the inactivation particles. Thus, a chaperone-like function has been proposed for these subunits. Although these findings are consistent with a role for accessory subunits in regulating central nervous system excitability, further functional assays are necessary to determine thoroughly how loss or haploinsufficiency of the Kvβ2 gene affects distinct network excitability and causes Kvβ2 channelepsy.
The leucine-rich glioma-inactivated-1 (LGI1) is a secreted neuronal protein, complexed with Kv channels, and highly expressed in neocortex and hippocampus. LGI1 mutations—e.g., the point mutation E383A that prevents the neuronal secretion of LGI1—have been found in patients with autosomal dominant lateral temporal lobe epilepsy (ADLTE), a syndrome characterized by partial seizures with acoustic or other sensory hallucinations (Kalachikov et al., 2002; Morante-Redolat et al., 2002; Fukata et al., 2010, Table 1). Moreover, loss of Lgi1 in mice causes lethal epilepsy (Fukata et al., 2010, Table 2). In the hippocampus, both Kv1.1 and Lgi1 appear co-assembled with Kv1.4 and Kvβ1 in axonal terminals. In A-type channels composed of these subunits, Lgi1 prevents N-type inactivation mediated by the Kvβ1 subunit. In contrast, defective LGI1 molecules identified in ADLTE patients fail to exert this effect, which results in channels with rapid inactivation kinetics. These data suggest that these changes in inactivation gating of presynaptic A-type channels may promote epileptic activity (Schulte et al., 2006).
Specific auto-antibodies underlie an emerging class of seizures named “autoimmune epilepsy” that involves K+ channels. Indeed, several of these auto-antibodies do not bind directly with Kv1.1, Kv1.2, or Kv1.6 channels, as previously believed, but rather to associated proteins such as LGI1, contactin-associated protein 2 (CASPR2), contactin-2, or others to be identified (Irani et al., 2010; Lai et al., 2010; Lancaster et al., 2011).
Cytosolic Kv channel-interacting proteins KChIP1–KChIP4 (An et al., 2000), which belong to the NCS family of calcium binding EF-hand proteins, co-assemble with the N-terminus of Kv4 subunits (Zhou et al., 2004) to form a native complex that encodes major components of neuronal somatodendritic A-type K+ current (IA). KChIP2 expression is high in the hippocampus, particularly within the apical dendrites of pyramidal cells. KChIP2 gene deletion in mice (Kchip2−/−) affected the IA in hippocampus, namely reduced current density, decreased channel availability, and slowed recovery from inactivation. This results in chronic hyper-excitability in hippocampal pyramidal neurons and Kchip2−/− mice exhibited increased susceptibility to seizures induced by kindling (Table 2). However, a compensatory up-regulation of inhibitory synaptic activity (up-regulation of GABA currents) was also observed (Wang et al., 2013). These findings indicated that KChIP2 is essential for homeostasis in hippocampal neurons and mutations in these K+ channel auxiliary subunits may be loci for epilepsy (Wang et al., 2013). Interestingly, Kchip2−/− mice are highly susceptible to cardiac arrhythmias (Kuo et al., 2001). Thus, this evidence suggests that loss-of-function mutations in KChIP2 could confer an increased susceptibility to both seizures and cardiac arrhythmias, increasing the risk to sudden unexpected death in epileptic patients.
In conclusion, these studies demonstrated that the auxiliary subunits play important roles in the pathogenesis of epilepsy by affecting K+ channel function and network excitability in distinct ways (see also KCa1.1 channelepsy).
The calcium-activated K+ (KCa) channels are highly conserved across species, and widely expressed in the human brain. The phylogenetic tree of the KCa channels shows that they are made of two genetically well-distinct groups (Wei et al., 2005), the large conductance (BK; KCa1.1), and the small/intermediate-conductance (SK/IK; KCa2.1, KCa2.2, KCa2.3, KCa3.1) KCa channels. With regard to gating mechanism, the Ca2+ sensitivity of SK/IK channels is provided by tightly bound calmodulin (Xia et al., 1998; Fanger et al., 1999), in contrast to the direct binding of Ca2+ at specific internal sites on the channel protein of KCa1.1 channels (Lee and Cui, 2010). Moreover, unlike the SK/IK channels, KCa1.1 channels are also activated by voltage.
In brain neurons KCa channels are widely distributed in the axons plasma membrane and at the presynaptic terminals (Knaus et al., 1996; Blank et al., 2004), and often located close to voltage-gated Ca2+ channels (Cav; Marrion and Tavalin, 1998). The Ca2+ influx that follows neuronal excitation activates KCa channels whose outward K+ flux contributes to terminate the action potential and establish the afterhyperpolarization (AHP) that closes Cav channels. This negative feedback control has been generally assumed to make KCa channels critical players in opposing repetitive firing and hyperexcitability typical of epileptic disorders. To date only mutations in the KCa1.1 channel have been clearly associated to epilepsy.
The KCa1.1 channel, originally cloned from the Drosophila slowpoke locus, thus the name slo (Atkinson et al., 1991), is coded by one single gene, the KCNMA1 (Wei et al., 2005). The variety observed in biophysical and pharmacologic properties of the channel derives from the extensive alternative splicing of its mRNA (Tseng-Crank et al., 1994), and the type of accessory β subunit (KCaβ) that associates with the channel (Jiang et al., 1999). The KCa1.1 channel is composed of four identical pore-forming α subunits, each displaying a transmembrane portion very much like the Kv channel, with the voltage sensing domain made by segments S1–S4, and the permeating pore domain by the S5, P, and S6 segments. Unlike Kv channels, the KCa1.1 channel has an additional transmembrane domain (S0), thought to subserve for (KCaβ) subunit interaction and modulation of the channel (Morrow et al., 2006). The channel has also a large C-terminal cytosolic domain that confers Ca2+ sensitivity to the channel. Notably, the amino-acid sequence of the C-terminal domain contains no conventional Ca2+ binding motif such as EF hands or C2 domains. The two putative high affinity Ca2+ binding sites of the channel are formed, respectively, by two closely located (five amino acids apart) aspartate residues (Xia et al., 2002) on the RCK1 (regulator of K+ conductance) domain, and by a series of aspartate residues in a region known as the Ca2+ bowl, located in the RCK2 domain (Wei et al., 1994; Schreiber and Salkoff, 1997).
KCa1.1 channels expression predominates in axons and pre-synaptic terminals of excitatory neurons located in epileptic relevant structures, such as cortex and hippocampus (Knaus et al., 1996; Hu et al., 2001; Misonou et al., 2006; Martire et al., 2010). In brain neurons, the Ca2+ that activates KCa1.1 channels enters primarily through Cav channels (Berkefeld et al., 2006), with which KCa1.1 channels strictly co-localize in order to be activated during an action potential by the Ca2+ microdomains that form around the Ca2+ source (Müller et al., 2007). The KCa1.1 channel activity is thus limited by the duration of the action potential-evoked Ca2+ transients, and consequently restricted to the action potential repolarization phase and the fast portion of the after-hyperpolarization (fAHP; Sah and Faber, 2002), and generally assumed to reduce neuronal excitability. Recent findings point, however, to a role of KCa1.1 channels in promoting high frequency firing, an effect likely attributed to fast spike repolarization, fAHP generation and the consequent reduction in the activation of other slower Kv channels and of the inactivation of Na+ channels (Storm, 1987a,b; Gu et al., 2007). Whereas they appear to be virtually uninfluential to synaptic release modulation under physiologic conditions (Hu et al., 2001; Raffaelli et al., 2004; Shruti et al., 2008; Martire et al., 2010).
Given the role of KCa1.1 channels in promoting high neuronal firing frequency and their predominant expression in excitatory neurons of cortex and hippocampus, it is no surprising that several lines of evidence from animal models point to a pro-epileptic role for KCa1.1 channels. For example, spontaneous cortical bursting in mice with high susceptibility to convulsions was completely inhibited by the KCa1.1 channel blocker iberiotoxin (IbTX) (Jin et al., 2000). A similar inhibitory effect of KCa1.1 channel antagonists was reported on chemoconvulsant-induced seizures in vitro and in vivo (Jin et al., 2000; Shruti et al., 2008; Sheehan et al., 2009). Finally, KCaβ4 knockout mice displayed temporal cortex seizures and a gain-of-function of KCa1.1 channels in dentate gyrus slices, resulting in higher firing rate (Brenner et al., 2005, Table 2).
Evidence for an association of human epilepsy with the KCa1.1 channel has also been found. A missense mutation (D434G) in the KCNMA1 gene coding for the α subunit has been found in family patients suffering from generalized epilepsy and paroxysmal diskinesia (Du et al., 2005, Table 1). Expression studies indicated that the D434G mutant channel displayed markedly greater macroscopic currents and single channel open probability, resulting from a 5-fold increase in Ca2+ sensitivity (Du et al., 2005). In accordance, the mutation appeared to be located in the RCK1 domain, close to the putative Ca2+ binding site and the segments subserving the allosteric coupling between Ca2+ binding site and the activation gate (Yang et al., 2010). Subsequent studies showed that the effects of the D434G mutation on the KCa1.1 channel properties were similar or even more pronounced in the presence of the KCaβ1, KCaβ2, or KCaβ4 subunit, highly expressed in the brain (Díez-Sampedro et al., 2006; Lee and Cui, 2009). Notably, a polymorphism in the KCaβ4 subunit has been associated to human MTLE in an Irish cohort, but this has not been confirmed in other populations (Cavalleri et al., 2007; Manna et al., 2013). By contrast, the α subunit coexpressed with KCaβ3b (a splicing variant of KCaβ3) was modified by the mutation with a slowing of the activation, a reduction in the voltage-dependence, but no change in Ca2+-dependence, suggesting a loss-of-function of the KCa1.1 current (Lee and Cui, 2009). Interestingly, a KCaβ3 single-nucleotide mutation, causing a loss-of-function of KCa1.1 current containing the KCaβ3b subunit, displays a small but significant association with idiopathic generalized epilepsy (Hu et al., 2003; Lorenz et al., 2007, Table 1). Taken together, these data suggest that both a loss-of-function of KCaβ3b-containing KCa1.1 channels and a gain-of-function of KCaβ1, KCaβ2, or KCaβ4-containing KCa1.1 channels would favor the epileptic phenotype, but more information on the location and function of KCaβ3b subunits is needed to clarify this point.
A modulation of KCa1.1 channel expression in epilepsy models has also been found. A gain-of-function of KCa1.1 currents associated with increased spontaneous and evoked firing rates occurs in mouse neocortical pyramidal neurons 24 h after chemoconvulsant-induced generalized tonic-clonic seizures (Shruti et al., 2008). Conversely, in a model of pilocarpine-induced MTLE KCa1.1 channel α subunit was down-regulated at the protein and mRNA level in hyppocampal mossy fibers originating from the dentate gyrus (Ermolinsky et al., 2008; Miceli et al., 2013). Notably, KCa1.1 channel proteins remaining after seizure induction were mostly changed to the STREX splicing isoform, displaying an increased Ca2+-sensitivity with respect to the ZERO splice variant normally present (Ermolinsky et al., 2008). The functional consequence of the observed changes is thus not clear.
Members of the inwardly-rectifying family of K+ channels (Kir) are found in virtually every cell type where they are major regulators of K+ fluxes across membranes (Hibino et al., 2010). The principal role of most Kir channels is the maintenance of the resting membrane potential and thereby the control of cell excitability, while others subserve the transport and recycling of K+ across membranes. Like other K+ channels, Kir subunits assemble as tetramers, and their ability to heteromultimerise adds functional diversity to a limited number of gene products. Kir subunits possess two transmembrane domains and approximately 15 distinct Kir clones have been identified so far, forming seven major subfamilies: Kir1–Kir7 (Bond et al., 1994; Hibino et al., 2010). Important physiological roles have been established for nearly all of these subfamilies. Generally, a Kir channel acts as a diode whereby the inward current through these channels is greater at potentials more negative than the EK, as compared to more positive values, where the outward flow is inhibited and the membrane potential free to change. The rectifying nature of Kir channels is due to a voltage-dependent block of the intracellular side of the pore by cytoplasmic polyamines and Mg2+ ions (Matsuda et al., 1987; Lopatin et al., 1994; Lu and MacKinnon, 1994; Stanfield et al., 1994). Several studies highlighted the role of inwardly-rectifying K+ channels' dysfunction in neuropsychiatric disorders and epilepsy although the relevant mechanisms in some instances await clarification.
Kir2.1 channels are highly expressed in brain, particularly in hippocampus, caudate, putamen, nucleus accumbens, and to lower levels in habenula and amygdala (Karschin et al., 1996), where they contribute to control neuronal excitability. In particular, the amplitude of Kir2.1 currents is small in young dentate granule neurons (DGCs), and increases ~3-fold in mature DGCs to optimize their excitability. Thus, Kir2.1 channels play an important role in DGCs firing properties during development (Mongiat et al., 2009). Moreover, Kir2.1 channels in combination with Kir4.1 control the astrocyte-mediated K+ buffering (Bordey and Sontheimer, 1998; Jabs et al., 2008; Chever et al., 2010). It has been proposed that up-regulation of Kir2.1 in DGCs would counterbalance the hyper-excitability observed in TLE, thus functioning as an anti-convulsant (Young et al., 2009). Individuals harboring loss-of-function mutations in KCNJ2 (e.g., Andersen-Tawil syndrome; OMIM 170390) may present with mood disorders and seizures (Haruna et al., 2007; Chan et al., 2010, Table 1), suggesting a possible role for Kir2.1 channels in the pathogenesis of neuropsychiatric disorders and epilepsy (Haruna et al., 2007; Chan et al., 2010).
Several neurotransmitters, including dopamine, opioid, somatostatin, acetylcholine, serotonin, adenosine, and GABA exert their actions by modulating the activity of G protein-coupled Kir channels (GIRK) belonging to the subfamily 3 (Kir3). Four subunits have been cloned: GIRK1-GIRK4, also known as Kir3.1–Kir3.4, that may heteropolimerize. Generally, receptors activation of intracellular heterotrimeric G proteins αβγ leads to stimulation of heteromeric Kir3 channels activity, resulting in an outward flux of K+ ions that causes membrane hyperpolarization and inhibition of cell excitability (Krapivinsky et al., 1995; Slesinger et al., 1995; Tucker et al., 1996a). The crystal structure of this channel type has been resolved, recently (Whorton and Mackinnon, 2013). Gain-of-function of Kir3 channels can considerably reduce neuronal activity, whereas loss-of-function can lead to excessive neuronal excitability and epilepsy. Indeed, ablation of the gene encoding for Kir3.2 channels (GIRK2) results in spontaneous convulsions and increased susceptibility for generalized seizures in rodents (Signorini et al., 1997, Table 2). The principal phenotype of weaver mice (wv/wv) is an ataxic gait, due to severe hypoplasia of the cerebellum, learning deficits and epileptic seizures. Weaver mice carry a deleterious mutation in the pore of Kir3.2 channels (G156S; Patil et al., 1995, Table 2). This mutation alters the K+ selectivity of the channel, induces calcium overload in cells, and reduces channel availability (Slesinger et al., 1996; Tucker et al., 1996b) with a mechanism different from heteromeric subunit degradation (Tucker et al., 1996c). It is likely that these molecular defects, induced by the G156S mutation, would lead to neurodegeneration and seizures susceptibility that characterize the phenotype of weaver mice.
Kir3 channel inhibition, induced by intrathecal administration of tertiapin, is pro-convulsant (Mazarati et al., 2006). Moreover, several drugs used in clinics—desimipramine, fluoxetine, haloperidol, thioridazine, pimozide and clozapine—inhibit Kir3 channel activity, and cause seizures as side effect. Conversely, electroconvulsive shock leads to increased expression of Kir3 channels (Pei et al., 1999), which may provide compensatory mechanisms against excessive electrical activity leading to neuroprotection. In support of this hypothesis, stimulation of galanin type 2 receptors that activate GIRK channels prevents kindled epileptogenesis in rats (Mazarati et al., 2006). In conclusion, these studies point out that distinct changes in Kir3 channel activity or availability throughout the brain may result in pro-convulsant or anti-convulsant effects.
Kir4.1 subunits (KCNJ10; BIR10; Bond et al., 1994; Lagrutta et al., 1996) may form homomeric channels or may polymerize with Kir5.1 (KCNJ16) to form heterotetramers (Pessia et al., 1996) highly sensitive to pH (Tucker et al., 2000; Pessia et al., 2001; Casamassima et al., 2003; D'Adamo et al., 2011). Kir4.1 channels are expressed primarily in oligodendrocytes and astrocytes surrounding synapses and blood vessels, mainly in the cortex, thalamus, hippocampus, and brainstem (Takumi et al., 1995; Higashi et al., 2001). Kir4.1 channel activity shows a profound developmental regulation, which correlates with both cell differentiation and the developmental regulation of extracellular K+ dynamics (Connors et al., 1982; MacFarlane and Sontheimer, 2000; Neusch et al., 2001). Kir4.1 controls primarily the resting membrane potential of astrocytes, and maintains the extracellular ionic and osmotic environment by promoting K+ transport from regions of high [K+]o, which results from synaptic excitation, to those of low [K+]o. This polarized transport of K+ in astrocytes, referred to as “spatial buffering of K+” is essential for normal neuronal activity, excitability, and synaptic functions. Among the genes associated with different forms of epilepsy Kir4.1 is receiving increasing interest. Genetic studies have indicated a linkage between missense variations in Kir4.1 and seizure susceptibility (Buono et al., 2004; Connors et al., 2004, Table 1). The DBA/2 mouse strain exhibits a greater susceptibility to induced seizures compared to the C57BL/6 strain. Previous QTL mapping identified the seizure susceptibility locus (Szs1) on the distal region of mouse chromosome 1 and further fine mapping studies suggested that a missense variation (T262S) in Kcnj10 was the likely candidate for this linkage (Ferraro et al., 2004, Table 2). In a second linkage study, a variation in the human KCNJ10 gene (R271C) was associated with seizure resistance in groups of patients with either focal or generalized epilepsy (Buono et al., 2004). However, a functional study demonstrated that these variations (T262S and R271C) do not produce any observable change in channel function or in predicted channel structure (Shang et al., 2005). It is therefore unlikely that the seizure susceptibility phenotypes associated with these missense variations are caused by changes in the intrinsic functional properties of Kir4.1. However, this study was unable to comprehensively disprove this association, and alterations in Kir4.1 channel activity remain an attractive mechanistic hypothesis. Future investigations willing to prove the association between these variants and seizure susceptibility phenotypes should include examination of how these variants could produce subtle changes in their interaction with cell-specific trafficking or regulatory proteins, or other possible pathways.
Recordings from surgical specimens of patients with intractable epilepsies have demonstrated a reduction of Kir conductance in astrocytes (Bordey and Sontheimer, 1998) and potassium clearance (Jauch et al., 2002). Moreover, loss-of-function recessive mutations of KCNJ10 (Kir4.1) have been recently associated with a disease, named EAST syndrome or SeSAME syndrome, consisting of seizures, ataxia, sensorineural deafness, mental retardation, and renal salt-losing tubulopathy (Bockenhauer et al., 2009; Scholl et al., 2009, Table 1). A number of additional elements substantiate the hypothesis that variants in KCNJ10 might contribute to brain dysfunction and seizures susceptibility. Conditional knockout mice lacking Kir4.1 exhibit stress-induced seizures, severe ataxia, spongiform vacuolation, axonal swellings, and degeneration, in addition to hearing loss and premature lethality (Neusch et al., 2001; Djukic et al., 2007, Table 2). In Kir4.1 knockout glial cells, no variations in membrane potential were observed during increases in [K+]o induced by nerve stimulations (Chever et al., 2010). Hence, it has been proposed that the loss-of-function of glial K+ conductance would favor extracellular K+ accumulation, contributing to neuronal hyperexcitability and epilepsy (Orkand et al., 1966; Chever et al., 2010).
Epilepsy and autism spectrum disorders (ASD) are strongly associated. The prevalence of seizures is highly represented in ASD (5–46%) (Bryson et al., 1988; Hughes and Melyn, 2005), compared with the general population (0.5–1%). The prevalence of autism in the epilepsy population is ~32%, which is about 50 times higher than in the general population (Clarke et al., 2005). An “autism–epilepsy phenotype” has been identified (Tuchman et al., 2009). Recently we reported a mutational screening of KCNJ10 in 52 children with cryptogenic epilepsy that resulted in the identification of two heterozygous KCNJ10 mutations in two identical twins (R18Q) and in a 14-year-old child (V84M; Sicca et al., 2011, Table 1). Clinically, the two 8-year-old identical twins showed impaired social interaction, sleep difficulties, hypotonia and both exhibited epileptic spasms within the same 24 h period. Other symptoms, typical of ASD, included clumsiness, absence of speech, severe disorder of social interaction, stereotypies, repetitive behaviors, symptoms of anxiety, depression, obsessive compulsive disorder and intellectual disability (IQ: 58). The 14-year-old child showed normal psychomotor development until 12 months of age, when ASD symptoms such as poor social gaze, no response to name, absence of language development, and withdrawal behaviors became evident. At the age of 6, he experienced complex partial seizures. EEG recordings showed synchronous and asynchronous paroxysmal abnormalities over frontal regions in both hemispheres tending to spread. The functional consequences of these heterozygous mutations were gain-of-function of either Kir4.1 or Kir4.1/Kir5.1 channels (Sicca et al., 2011). To date, we have identified several new probands displaying an autism–epilepsy phenotype who carry mutations in KCNJ10 that cause gain-of-function effects, assessed by using astrocytoma cell lines. Collectively, our findings point to a new class of genes that should be examined in autism-epilepsy patients, and disclose novel molecular mechanisms that may increase the susceptibility to this distinct neuropsychiatric phenotype by altering the K+ homeostasis in the brain (D'Adamo et al., 2011; Sicca et al., 2011). Indeed, Kir4.1 is the main glial inward conductance, astrocytes make up 90% of all human brain cells, and each astrocyte controls the activity of many thousands of synapses (about 140,000 in the hippocampus; Benarroch, 2009). Co-occurrence of epilepsy and ASD in patients harboring KCNJ10 gain-of-function mutations suggests that dysfunction in the astrocytic-dependent K+ buffering may be a common mechanism contributing to seizures as well as the core behavioral features of ASD. It has been shown that an isolated episode of local neuronal hyperactivity triggers a large and synchronous Ca2+ elevation in closely associated astrocytes. Activated astrocytes signal back to neurons favoring their recruitment into a coherent activity that underlines the hypersynchronous ictal discharge (Gómez-Gonzalo et al., 2010). It is possible that an increased and faster influx of K+ into astrocytes through high functioning Kir4.1-containing channels may lead, during intense neuronal activity, to larger membrane depolarization and higher intracellular Ca2+ elevations in these cells. Ca2+ elevations in astrocytes are associated with the release of gliotransmitters, such as glutamate and D-serine, which trigger discharges in neurons, promote local neuronal synchrony and epileptic activity (Parpura et al., 1994; Bezzi et al., 1998; Pasti et al., 2001; Angulo et al., 2004; Fellin et al., 2004; Mothet et al., 2005; Tian et al., 2005). Speculatively, a recurrent neuron-astrocyte-neuron excitatory loop may develop at a restricted brain site, as a consequence of gain-of-function of Kir4.1 channels, and contribute to initiation of seizures. These mutations may alter the noradrenergic (NA) system of the brain as well, since Kir4.1/Kir5.1 channels control the excitability of locus coeruleus (LC) neurons (D'Adamo et al., 2011b). Indeed, a developmental dysregulation of this LC-NA network (Samuels and Szabadi, 2008) has been suggested to underlie epilepsy. From a therapeutic perspective, these studies indicate that, alike neurons, astrocytes may represent a crucial target for the pharmacological control of abnormal electrical discharge and synaptic function.
The adenosine triphosphate (ATP)-sensitive K+ (KATP) channels are octamers composed of four pore-forming subunits, consisting of Kir6.1 or Kir6.2, with four regulatory sulfonylurea receptors such as SUR1, SUR2A, or SUR2B, which are members of the ATP-binding-cassette transporter family (Aguilar-Bryan et al., 1995; Inagaki et al., 1995; Nichols et al., 1996). The secretion of insulin from pancreatic β-cells is mediated by the closure of these channels caused by increased levels of cytoplasmic ATP. Neuronal KATP channels are predominantly composed of Kir6.2/SUR1, although Kir6.1/SUR2B and Kir6.2/SUR2B are also found. These channels link the metabolic state of neurons to their excitability by sensing changes in intracellular phosphate potential (i.e., ATP/ADP ratio). DEND syndrome (OMIM 606176) is an inherited disease characterized by developmental delay, epilepsy and neonatal diabetes mellitus. Twenty percent of these patients have associated neurologic defects, the most severe of which are generalized epilepsy, marked delay of motor and social development, including late development of speech, and learning difficulties. Numerous gain-of-function mutations have been identified in the genes encoding Kir6.2 (KCNJ11) or the associated regulatory SUR1 subunit (ABCC8) of patients affected by DEND syndrome (Table 1). To date, all mutations in KCNJ11 that have been characterized functionally, produce marked decrease in the ability of ATP to inhibit the KATP channel when expressed in heterologous systems or enhance the activatory effects of Mg2+-nucleotides. This reduction in ATP sensitivity translates in more fully openings of the channel at physiologically relevant concentrations of ATP, increased KATP current, hyperpolarization of the β-cell plasma membrane, and consequent suppression of Ca2+ influx and insulin secretion (Hattersley and Ashcroft, 2005). Clinical severity of the disorder correlates with the magnitude of shift in the ATP affinity. Sulfonylureas, which block opened channels and restore glucose homeostasis, ameliorate some of the neurological symptoms of DEND syndrome. How KATP channel over-activity in the central nervous system results in epilepsy is unclear. Whereas insights have been provided on the mechanisms linking loss of KATP channel function to increased seizure susceptibility. Notably, generalized seizures can be evoked by metabolic stresses such as hypoxia and hypoglycemia. Kir6.2 knockout mice exhibited high-voltage sharp-wave bursts in the EEG recordings, myoclonic jerks followed by severe tonic-clonic convulsion and death upon exposure to hypoxia (Table 2). However, the wild-type mice remained sedated during this challenge and revived normally (Yamada et al., 2001). Substantia nigra pars reticulata (SNr) and its efferents act as a central gating system in the propagation of seizure activity (Iadarola and Gale, 1982). Remarkably, wild-type neurons in brain slices from the substantia nigra pars reticulata (SNr) were hyperpolarized by hypoxia, whereas the membrane potential of Kir6.2 knockout neurons were depolarized by the perfusion with hypoxic solutions. The SNr and its efferents act as a central gating system in the propagation of seizure activity (Iadarola and Gale, 1982). Therefore, hyperpolarization of SNr neurons upon opening of KATP channels has been proposed to protect against seizure propagation during metabolic stress, although other brain regions could be involved in this process (Yamada et al., 2001).
Solving the puzzle of epilepsy is an extremely difficult task. Here we have put several new pieces in the puzzle by describing novel genetic defects in K+ channels and related proteins that underlie distinct human epileptic phenotypes. We have also analyzed critically the new insights in the neurobiology of this disease that have been provided by investigations on valuable animal models of epilepsy. It is becoming increasingly clear that mutations in K+ channel genes or perturbations in K+ channel function, even in the absence of a primary channel defect, underlie an increased susceptibility to epilepsy. Despite the abundance of genes encoding for K+ channels and associated subunits, and their established crucial functional roles, these genes have been greatly overlooked in the search for the causes underlying idiopathic epilepsy. The extremely high diversity of K+ channels and the numerous mutations identified in their genes often generate confusion in the classification of the associated diseases. Therefore, we proposed to name the K+ channels defects underlying distinct epilepsies as “K+ channelepsies” and suggested a new classification according to a widely used K+ channel nomenclature (Chandy and Gutman, 1993; Kubo et al., 2005; Wei et al., 2005). This original classification could be also adopted to easily unify, identify and describe multiple organ dysfunction related to a single ion channel gene defect (e.g., Kx.y-phenotype; Navx.y-phenotype; Cavx.y-phenotype) (Catterall et al., 2005a,b). K+ channels represent crucial targets for novel pharmacological control of abnormal electrical discharges and synaptic function in the brain. Much greater efforts should thus be made to find new K+ channel modulators and gene therapies to ameliorate the symptoms of this devastating disease. On the other hand, the effects of newly developed drugs on the activity of most K+ channel types should be tested in order to predict their pro-convulsant side effects. Research on K+ channelepsies is clearly providing important knowledge on the signaling pathways and circuits involved in epilepsy. The understanding of these “experiments” of Nature will also help us to uncover, in a much broader sense, the physiological workings of the human body.
The authors declare that the research was conducted in the absence of any commercial or financial relationships that could be construed as a potential conflict of interest.
This work was supported by Telethon (GGP11188), Ministero della Salute (GR-2009-1580433); MIUR-PRIN 20108WT59Y_004; COMPAGNIA di San Paolo (Turin) “Programma Neuroscienze,” and Fondazione Cassa di Risparmio di Perugia.
Adelman, J. P., Bond, C. T., Pessia, M., and Maylie, J. (1995). Episodic ataxia results from voltage-dependent potassium channels with altered functions. Neuron 15, 1449–1454. doi: 10.1016/0896-6273(95)90022-5
Aguilar-Bryan, L., Nichols, C. G., Wechsler, S. W., Clement, J. P., Boyd, A. E., González, G., et al. (1995). Cloning of the beta cell high-affinity sulfonylurea receptor: a regulator of insulin secretion. Science 268, 423–426. doi: 10.1126/science.7716547
An, W. F., Bowlby, M. R., Betty, M., Cao, J., Ling, H. P., Mendoza, G., et al. (2000). Modulation of A-type potassium channels by a family of calcium sensors. Nature 403, 553–556. doi: 10.1038/35000592
Angulo, M. C., Kozlov, A. S., Charpak, S., and Audinat, E. (2004). Glutamate released from glial cells synchronizes neuronal activity in the hippocampus. J. Neurosci. 24, 6920–6927. doi: 10.1523/JNEUROSCI.0473-04.2004
Assaf, S. Y., and Chung, S. H. (1984). Release of endogenous Zn2+ from brain tissue during activity. Nature 308, 734–736. doi: 10.1038/308734a0
Atkinson, N. S., Robertson, G. A., and Ganetzky, B. (1991). A component of calcium-activated potassium channels encoded by the Drosophila slo locus. Science 253, 551–555. doi: 10.1126/science.1857984
Avoli, M. (2001). Do interictal discharges promote or control seizures? Experimental evidence from an in vitro model of epileptiform discharge. Epilepsia 42, 2–4. doi: 10.1046/j.1528-1157.2001.042suppl.3002.x
Barnwell, L. F., Lugo, J. N., Lee, W. L., Willis, S. E., Gertz, S. J., Hrachovy, R. A., et al. (2009). Kv4.2 knockout mice demonstrate increased susceptibility to convulsant stimulation. Epilepsia 50, 1741–1751. doi: 10.1111/j.1528-1167.2009.02086.x
Behrens, R., Nolting, A., Reimann, F., Schwarz, M., Waldschütz, R., and Pongs, O. (2000). hKCNMB3 and hKCNMB4, cloning and characterization of two members of the large-conductance calcium-activated potassium channel beta subunit family. FEBS Lett. 474, 99–106.
Bellini, G., Miceli, F., and Soldovieri, M. V. (1993-2013). “Benign familial neonatal seizures 2,” in GeneReviews™ [Internet], eds R. A. Pagon, M. P. Adam and T. D. Bird (Seattle, WA). Available online at: http://www.ncbi.nlm.nih.gov/books/NBK1116/
Benarroch, E. E. (2009). Astrocyte-neuron interactions: implications for epilepsy. Neurology 73, 1323–1327. doi: 10.1212/WNL.0b013e3181bd432d
Bergren, S. K., Chen, S., Galecki, A., and Kearney, J. A. (2005). Genetic modifiers affecting severity of epilepsy caused by mutation of sodium channel Scn2a. Mamm. Genome 16, 683–690. doi: 10.1007/s00335-005-0049-4
Berkefeld, H., Sailer, C. A., Bildl, W., Rohde, V., Thumfart, J. O., Eble, S., et al. (2006). BKCa-Cav channel complexes mediate rapid and localized Ca2+-activated K+ signaling. Science 314, 615–620. doi: 10.1126/science.1132915
Bernard, C., Anderson, A., Becker, A., Poolos, N. P., Beck, H., and Johnston, D. (2004). Acquired dendritic channelopathy in temporal lobe epilepsy. Science 305, 532–535. doi: 10.1126/science.1097065
Bezzi, P., Carmignoto, G., Pasti, L., Vesce, S., Rossi, D., Rizzini, B. L., et al. (1998). Prostaglandins stimulate calcium-dependent glutamate release in astrocytes. Nature 391, 281–285. doi: 10.1038/34651
Bhalla, T., Rosenthal, J. J., Holmgren, M., and Reenan, R. (2004). Control of human potassium channel inactivation by editing of a small mRNA hairpin. Nat. Struct. Mol. Biol. 11, 950–956. doi: 10.1038/nsmb825
Biervert, C., Schroeder, B. C., Kubisch, C., Berkovic, S. F., Propping, P., Jentsch, T. J., et al. (1998). A potassium channel mutation in neonatal human epilepsy. Science 279, 403–406. doi: 10.1126/science.279.5349.403
Blank, T., Nijholt, I., Kye, M. J., and Spiess, J. (2004). Small conductance Ca2+-activated K+ channels as targets of CNS drug development. Curr. Drug Targets CNS Neurol. Disord. 3, 161–167. doi: 10.2174/1568007043337472
Bockenhauer, D., Feather, S., Stanescu, H. C., Bandulik, S., Zdebik, A. A., Reichold, M., et al. (2009). Epilepsy, ataxia, sensorineural deafness, tubulopathy, and KCNJ10 mutations. N. Engl. J. Med. 360, 1960–1970. doi: 10.1056/NEJMoa0810276
Bond, C. T., Pessia, M., Xia, X. M., Lagrutta, A., Kavanaugh, M. P., and Adelman, J. P. (1994). Cloning and expression of a family of inward rectifier potassium channels. Receptors Channels 2, 183–191.
Bordey, A., and Sontheimer, H. (1998). Properties of human glial cells associated with epileptic seizure foci. Epilepsy Res. 32, 286–303. doi: 10.1016/S0920-1211(98)00059-X
Brenner, R., Chen, Q. H., Vilaythong, A., Toney, G. M., Noebels, J. L., and Aldrich, R. W. (2005). BK channel beta4 subunit reduces dentate gyrus excitability and protects against temporal lobe seizures. Nat. Neurosci. 8, 1752–1759. doi: 10.1038/nn1573
Brew, H. M., Gittelman, J. X., Silverstein, R. S., Hanks, T. D., Demas, V. P., Robinson, L. C., et al. (2007). Seizures and reduced life span in mice lacking the potassium channel subunit Kv1.2, but hypoexcitability and enlarged Kv1 currents in auditory neurons. J. Neurophysiol. 98, 1501–1525. doi: 10.1152/jn.00640.2006
Brown, D. A., and Adams, P. R. (1980). Muscarinic suppression of a novel voltage-sensitive K+ current in a vertebrate neurone. Nature 283, 673–676. doi: 10.1038/283673a0
Browne, D. L., Gancher, S. T., Nutt, J. G., Brunt, E. R., Smith, E. A., Kramer, P., et al. (1994). Episodic ataxia/myokymia syndrome is associated with point mutations in the human potassium channel gene KCNA1. Nat. Genet. 8, 136–140. doi: 10.1038/ng1094-136
Brunetti, O., Imbrici, P., Botti, F. M., Pettorossi, V. E., D'Adamo, M. C., Valentino, M., et al. (2012). Kv11 knock-in ataxic mice exhibit spontaneous myokymic activity exacerbated by fatigue ischemia and low temperature. Neurobiol. Dis. 47, 310–321. doi: 10.1016/j.nbd.2012.05.002
Bryson, S. E., Clark, B. S., and Smith, I. M. (1988). First report of a Canadian epidemiological study of autistic syndromes. J. Child Psychol. Psychiatry 29, 433–445. doi: 10.1111/j.1469-7610.1988.tb00735.x
Buono, R. J., Lohoff, F. W., Sander, T., Sperling, M. R., O'Connor, M. J., Dlugos, D. J., et al. (2004). Association between variation in the human KCNJ10 potassium ion channel gene and seizure susceptibility. Epilepsy Res. 58, 175–183. doi: 10.1016/j.eplepsyres.2004.02.003
Canavier, C. C., Oprisan, S. A., Callaway, J. C., Ji, H., and Shepard, P. D. (2007). Computational model predicts a role for ERG current in repolarizing plateau potentials in dopamine neurons: implications for modulation of neuronal activity. J. Neurophysiol. 9, 3006–3022. doi: 10.1152/jn.00422.2007
Casamassima, M., D'Adamo, M. C., Pessia, M., and Tucker, S. J. (2003). Identification of a heteromeric interaction which influences the rectification gating and pH-sensitivity of Kir41/Kir51 potassium channels. J. Biol. Chem. 278, 43533–43540. doi: 10.1074/jbc.M306596200
Catterall, W. A., Goldin, A. L., and Waxman, S. G. (2005). International Union of Pharmacology. XLVII. Nomenclature and structure-function relationships of voltage-gated sodium channels. Pharmacol. Rev. 57, 397–409. doi: 10.1124/pr.57.4.4
Catterall, W. A., Perez-Reyes, E., Snutch, T. P., and Striessnig, J. (2005). International Union of Pharmacology. XLVIII. Nomenclature and structure-function relationships of voltage-gated calcium channels. Pharmacol. Rev. 57, 411–425. doi: 10.1124/pr.57.4.5
Cavalleri, G. L., Weale, M. E., Shianna, K. V., Singh, R., Lynch, J. M., Grinton, B., et al. (2007). Multicentre search for genetic susceptibility loci in sporadic epilepsy syndrome and seizure types: a case-control study. Lancet Neurol. 6, 970–980. doi: 10.1016/S1474-4422(07)70247-8
Chan, H. F., Chen, M. L., Su, J. J., Ko, L. C., Lin, C. H., and Wu, R. M. (2010). A novel neuropsychiatric phenotype of KCNJ2 mutation in one Taiwanese family with Andersen-Tawil syndrome. J. Hum. Genet. 55, 186–188. doi: 10.1038/jhg.2010.2
Chandy, K. G., and Gutman, G. A. (1993). Nomenclature for mammalian potassium channel genes. Trends Pharmacol. Sci. 14, 434. doi: 10.1016/0165-6147(93)90181-I
Charlier, C., Singh, N. A., Ryan, S. G., Lewis, T. B., Reus, B. E., Leach, R. J., et al. (1998). A pore mutation in a novel KQT-like potassium channel gene in an idiopathic epilepsy family. Nat. Genet. 18, 53–55. doi: 10.1038/ng0198-53
Chever, O., Djukic, B., McCarthy, K. D., and Amzica, F. (2010). Implication of Kir4.1 channel in excess potassium clearance: an in vivo study on anesthetized glial-conditional Kir4.1 knock-out mice. J. Neurosci. 30, 15769–15777. doi: 10.1523/JNEUROSCI.2078-10.2010
Clarke, D. F., Roberts, W., Daraksan, M., Dupuis, A., McCabe, J., Wood, H., et al. (2005). The prevalence of autistic spectrum disorder in children surveyed in a tertiary care epilepsy clinic. Epilepsia 46, 1970–1977. doi: 10.1111/j.1528-1167.2005.00343.x
Connor, J. X., McCormack, K., Pletsch, A., Gaeta, S., Ganetzky, B., Chiu, S. Y., et al. (2005). Genetic modifiers of the Kv beta2-null phenotype in mice. Genes Brain Behav. 4, 77–88. doi: 10.1111/j.1601-183X.2004.00094.x
Connors, B. W., Ransom, B. R., Kunis, D. M., and Gutnick, M. J. (1982). Activity-dependent K+ accumulation in the developing rat optic nerve. Science 216, 1341–1343. doi: 10.1126/science.7079771
Connors, N. C., Adams, M. E., Froehner, S. C., and Kofuji, P. (2004). The Potassium channel Kir41 associates with the dystrophin–glycoprotein complex via α-syntrophin in glia. J. Biol. Chem. 279, 28387–28392. doi: 10.1074/jbc.M402604200
Costigan, M., Belfer, I., Griffin, R. S., Dai, F., Barrett, L. B., Coppola, G., et al. (2010). Multiple chronic pain states are associated with a common amino acid-changing allele in KCNS1. Brain 13, 2519–2527. doi: 10.1093/brain/awq195
Cusimano, A., D'Adamo, M. C., and Pessia, M. (2004). An episodic ataxia type-1 mutation in the S1 segment sensitises the hKv11 potassium channel to extracellular Zn2+. FEBS Lett. 576, 237–244. doi: 10.1016/j.febslet.2004.09.018
D'Adamo, M. C., Hanna, M. G., Di Giovanni, G., and Pessia, M. (2012). “Episodic ataxia type 1,” in GeneReviews™ [Internet] Internet Second Edition NCBI Bookshelf, eds R. A. Pagon, M. P. Adam, and T. D. Bird (Seattle, WA). Available online at: http://wwwncbinlmnihgov/books/NBK25442
D'Adamo, M. C., Imbrici, P., Sponcichetti, F., and Pessia, M. (1999). Mutations in the KCNA1 gene associated with episodic ataxia type-1 syndrome impair heteromeric voltage-gated K+ channel function. FASEB J. 13, 1335–1345.
D'Adamo, M. C., Liu, Z., Adelman, J. P., Maylie, J., and Pessia, M. (1998). Episodic ataxia type-1 mutations in the hKv1.1 cytoplasmic pore region alter the gating properties of the channel. EMBO J. 17, 1200–1207. doi: 10.1093/emboj/17.5.1200
D'Adamo, M. C., Moro, F., Imbrici, P., Martino, D., Roscini, M., Santorelli, F. M., et al. (2011). The emerging role of the inwardly rectifying K+ channels in autism spectrum disorders and epilepsy. Malta Med. J. 23, 10–14. Available online at: http://www.um.edu.mt/umms/mmj/PDF/327.pdf
D'Adamo, M. C., Servettini, I., Guglielmi, L., Di Matteo, V., Di Maio, R., Di Giovanni, G., et al. (2013). 5-HT2 receptors-mediated modulation of voltage-gated K+ channels and neurophysiopathological correlates. Exp. Brain Res. doi: 10.1007/s00221-013-3555-8. [Epub ahead of print].
D'Adamo, M. C., Shang, L., Imbrici, P., Brown, S. D. M., Pessia, M., and Tucker, S. (2011). Genetic inactivation of Kcnj16 identifies Kir 5.1 as an important determinant of neuronal PCO2/pH sensitivity. J. Biol. Chem. 286, 192–198. doi: 10.1074/jbc.M110.189290
Decher, N., Streit, A. K., Rapedius, M., Netter, M. F., Marzian, S., Ehling, P., et al. (2010). RNA editing modulates the binding of drugs and highly unsaturated fatty acids to the open pore of Kv potassium channels. EMBO J. 29, 2101–2113. doi: 10.1038/emboj.2010.88
Delmas, P., and Brown, D. A. (2005). Pathways modulating neural KCNQ/M (Kv7) potassium channels. Nat. Rev. Neurosci. 6, 850–862. doi: 10.1038/nrn1785
Devaux, J. J., Kleopa, K. A., Cooper, E. C., and Scherer, S. S. (2004). KCNQ2 is a nodal K+ channel. J. Neurosci. 24, 1236–1244. doi: 10.1523/JNEUROSCI.4512-03.2004
Díez-Sampedro, A., Silverman, W. R., Bautista, J. F., and Richerson, G. B. (2006). Mechanism of increased open probability by a mutation of the BK channel. J. Neurophysiol. 96, 1507–1516. doi: 10.1152/jn.00461.2006
Djukic, B., Casper, K. B., Philpot, B. D., Chin, L. S., and McCarthy, K. D. (2007). Conditional knock-out of Kir41 leads to glial membrane depolarization inhibition of potassium and glutamate uptake and enhanced short-term synaptic potentiation. J. Neurosci. 27, 11354–11365. doi: 10.1523/JNEUROSCI.0723-07.2007
Donahue, L. R., Cook, S. A., Johnson, K. R., Bronson, R. T., and Davisson, M. T. (1996). Megencephaly: a new mouse mutation on chromosome 6 that causes hypertrophy of the brain. Mamm. Genome 7, 871–876. doi: 10.1007/s003359900259
Du, J., Haak, L. L., Phillips-Tansey, E., Russell, J. T., and McBain, C. J. (2000). Frequency-dependent regulation of rat hippocampal somato-dendritic excitability by the K+ channel subunit Kv21. J. Physiol. 522, 19–31. doi: 10.1111/j.1469-7793.2000.t01-2-00019.xm
Du, W., Bautista, J. F., Yang, H., Diez-Sampedro, A., You, S. A., Wang, L., et al. (2005). Calcium-sensitive potassium channelopathy in human epilepsy and paroxysmal movement disorder. Nat. Genet. 37, 733–738. doi: 10.1038/ng1585
Ermolinsky, B., Arshadmansab, M. F., Pacheco Otalora, L. F., Zarei, M. M., and Garrido-Sanabria, E. R. (2008). Deficit of Kcnma1 mRNA expression in the dentate gyrus of epileptic rats. Neuroreport 19, 1291–1294. doi: 10.1097/WNR.0b013e3283094bb6
Eunson, L. H., Rea, R., Zuberi, S. M., Youroukos, S., Panayiotopoulos, C. P., Liguori, R., et al. (2000). Clinical genetic and expression studies of mutations in the potassium channel gene KCNA1 reveal new phenotypic variability. Ann. Neurol. 48, 647–656.
Fanger, C. M., Ghanshani, S., Logsdon, N. J., Rauer, H., Kalman, K., Zhou, J., et al. (1999). Calmodulin mediates calcium-dependent activationof the intermediate conductance KCa channel IKCa1. J. Biol. Chem. 274, 5746–5754. doi: 10.1074/jbc.274.9.5746
Fellin, T., Pascual, O., Gobbo, S., Pozzan, T., Haydon, P. G., and Carmignoto, G. (2004). Neuronal synchrony mediated by astrocytic glutamate through activation of extrasynaptic NMDA receptors. Neuron 43, 729–743. doi: 10.1016/j.neuron.2004.08.011
Ferraro, T. N., Golden, G. T., Smith, G. G., Martin, J. F., Lohoff, F. W., Gieringer, T. A., et al. (2004). Fine mapping of a seizure susceptibility locus on mouse Chromosome 1: nomination of Kcnj10 as a causative gene. Mamm. Genome 15, 239–251. doi: 10.1007/s00335-003-2270-3
Francis, J., Jugloff, D. G., Mingo, N. S., Wallace, M. C., Jones, O. T., Burnham, W. M., et al. (1997). Kainic acid-induced generalized seizures alter the regional hippocampal expression of the rat Kv42 potassium channel gene. Neurosci. Lett. 232, 91–94. doi: 10.1016/S0304-3940(97)00593-4
Fukata, Y., Lovero, K. L., Iwanaga, T., Watanabe, A., Yokoi, N., Tabuchi, K., et al. (2010). Disruption of LGI1-linked synaptic complex causes abnormal synaptic transmission and epilepsy. Proc. Natl. Acad. Sci. U.S.A. 107, 3799–3804. doi: 10.1073/pnas.0914537107
Gabriel, S., Njunting, M., Pomper, J. K., Merschhemke, M., Sanabria, E. R., Eilers, A., et al. (2004). Stimulus and potassium-induced epileptiform activity in the human dentate gyrus from patients with and without hippocampal sclerosis. J. Neurosci. 24, 10416–10430. doi: 10.1523/JNEUROSCI.2074-04.2004
Gamper, N., and Shapiro, M. S. (2003). Calmodulin mediates Ca2+-dependent modulation of M-type K+ channels. J. Gen. Physiol. 122, 17–31. doi: 10.1085/jgp.200208783
Geiger, J. R., and Jonas, P. (2000). Dynamic control of presynaptic Ca2+ inflow by fast-inactivating K+ channels in hippocampal mossy fiber boutons. Neuron 28, 927–939. doi: 10.1016/S0896-6273(00)00164-1
Gómez-Gonzalo, M., Losi, G., Chiavegato, A., Zonta, M., Cammarota, M., Brondi, M., et al. (2010). An excitatory loop with astrocytes contributes to drive neurons to seizure threshold. PLoS Biol. 8:e1000352. doi: 10.1371/journal.pbio.1000352
Gu, N., Vervaeke, K., and Storm, J. F. (2007). BK potassium channels facilitate high-frequency firing and cause early spike frequency adaptation in rat CA1 hippocampal pyramidal cells. J. Physiol. 580, 859–882. doi: 10.1113/jphysiol.2006.126367
Guan, D., Lee, J. C., Tkatch, T., Surmeier, D. J., Armstrong, W. E., and Foehring, R. C. (2006). Expression and biophysical properties of Kv1 channels in supragranular neocortical pyramidal neurones. J. Physiol. 571, 371–389.
Haruna, Y., Kobori, A., Makiyama, T., Yoshida, H., Akao, M., Doi, T., et al. (2007). Genotype-phenotype correlations of KCNJ2 mutations in Japanese patients with Andersen-Tawil syndrome. Hum. Mutat. 28, 208. doi: 10.1002/humu.9483
Hattersley, A. T., and Ashcroft, F. M. (2005). Activating mutations in Kir6.2 and neonatal diabetes: new clinical syndromes new scientific insights and new therapy. Diabetes 54, 2503–2513. doi: 10.2337/diabetes.54.9.2503
Heilstedt, H. A., Burgess, D. L., Anderson, A. E., Chedrawi, A., Tharp, B., Lee, O., et al. (2001). Loss of the potassium channel betasubunit gene KCNAB2 is associated with epilepsy in patients with 1p36 deletion syndrome. Epilepsia 42, 1103–1111. doi: 10.1046/j.1528-1157.2001.08801.x
Herson, P. S., Virk, M., Rustay, N. R., Bond, C. T., Crabbe, J. C., Adelman, J. P., et al. (2003). A mouse model of episodic ataxia type-1. Nat. Neurosci. 6, 378–383. doi: 10.1038/nn1025
Hibino, H., Inanobe, A., Furutani, K., Murakami, S., Findlay, I., and Kurachi, Y. (2010). Inwardly rectifying potassium channels: their structure function and physiological roles. Physiol. Rev. 90, 291–366. doi: 10.1152/physrev.00021.2009
Higashi, K., Fujita, A., Inanobe, A., Tanemoto, M., Doi, K., Kubo, T., et al. (2001). An inwardly rectifying K+ channel Kir4.1 expressed in astrocytes surrounds synapses and blood vessels in brain. Am. J. Physiol. Cell Physiol. 281, C922–C931.
Higuchi, M., Maas, S., Single, F. N., Hartner, J., Rozov, A., Burnashev, N., et al. (2000). Point mutation in an AMPA receptor gene rescues lethality in mice deficient in the RNA-editing enzyme ADAR2. Nature 406, 78–81. doi: 10.1038/35017558
Hu, H., Shao, L. R., Chavoshy, S., Gu, N., Trieb, M., Behrens, R., et al. (2001). Presynaptic Ca2+-activated K+ channels in glutamatergic hippocampal terminals and their role in spike repolarization and regulation of transmitter release. J. Neurosci. 21, 9585–9597.
Hu, H. J., Carrasquillo, Y., Karim, F., Jung, W. E., Nerbonne, J. M., Schwarz, T. L., et al. (2006). The Kv4.2 potassium channel subunit is required for pain plasticity. Neuron 50, 89–100. doi: 10.1016/j.neuron.2006.03.010
Hu, S., Labuda, M. Z., Pandolfo, M., Goss, G. G., McDermid, H. E., and Ali, D. W. (2003). Variants of the KCNMB3 regulatory subunit of maxi BK channels affect channel inactivation. Physiol. Genomics 15, 191–198.
Hughes, J. R., and Melyn, M. (2005). EEG and seizures in autistic children and adolescents: further findings with therapeutic implications. Clin. EEG Neurosci. 36, 15–20. doi: 10.1177/155005940503600105
Iadarola, M. J., and Gale, K. (1982). Substantia nigra: site of anticonvulsant activity mediated by gamma-aminobutyric acid. Science 218, 1237–1240. doi: 10.1126/science.7146907
Imbrici, P., D'Adamo, M. C., Cusimano, A., and Pessia, M. (2007). Episodic ataxia type 1 mutation F184C alters Zn2+-induced modulation of the human K+ channel Kv1.4-Kv1.1/Kvβ1.1. Am. J. Physiol. Cell Physiol. 292, C778–C787. doi: 10.1152/ajpcell.00259.2006
Imbrici, P., D'Adamo, M. C., Kullmann, D. M., and Pessia, M. (2006). Episodic ataxia type 1 mutations in the KCNA1 Gene impair the fast inactivation properties of the human K+ Channels Kv1.4-1.1/Kvβ1.1 and Kv1.4-1.1/Kvβ1.2. Eur. J. Neurosci. 24, 3073–3083. doi: 10.1111/j.1460-9568.2006.05186.x
Imbrici, P., Gualandi, F., D'Adamo, M. C., Masieri, M. T., Cudia, P., De Grandis, D., et al. (2008). A novel KCNA1 mutation identified in an italian family affected by episodic ataxia type 1. Neuroscience 157, 577–587. doi: 10.1016/j.neuroscience.2008.09.022
Imbrici, P., Tucker, S. J., D'Adamo, M. C., and Pessia, M. (2000). Role of RPTPα and tyrosine phosphorylation in the serotonergic inhibition of voltage-dependent potassium channels. Pflügers Archiv.Eur. J. Physiol. 441, 257–262. doi: 10.1007/s004240000406
Inagaki, N., Gonoi, T., Clement, J. P., Namba, N., Inazawa, J., Gonzalez, G., et al. (1995). Reconstitution of IKATP: an inward rectifier subunit plus the sulfonylurea receptor. Science 270, 1166–1170. doi: 10.1126/science.270.5239.1166
Inyushin, M., Kucheryavykh, L. Y., Kucheryavykh, Y. V., Nichols, C. G., Buono, R. J., Ferraro, T. N., et al. (2010). Potassium channel activity and glutamate uptake are impaired in astrocytes of seizure-susceptible DBA/2 mice. Epilepsia 51, 1707–1713. doi: 10.1111/j.1528-1167.2010.02592.x
Irani, S. R., Alexander, S., Waters, P., Kleopa, K. A., Pettingill, P., Zuliani, L., et al. (2010). Antibodies to Kv1 potassium channel-complex proteins leucine-rich glioma inactivated 1 protein and contactin-associated protein-2 in limbic encephalitis Morvan's syndrome and acquired neuromyotonia. Brain 133, 2734–2748. doi: 10.1093/brain/awq213
Isacoff, E. Y., Jan, Y. N., and Jan, L. Y. (1990). Evidence for the formation of heteromultimeric potassium channels in Xenopus oocytes. Nature 345, 530–534. doi: 10.1038/345530a0
Ishida, S., Sakamoto, Y., Nishio, T., Baulac, S., Kuwamura, M., Ohno, Y., et al. (2012). Kcna1-mutant rats dominantly display myokymia neuromyotonia and spontaneous epileptic seizures. Brain Res. 1435, 154–166. doi: 10.1016/j.brainres.2011.11.023
Jabs, R., Seifert, G., and Steinhäuser, C. (2008). Astrocytic function and its alteration in the epileptic brain. Epilepsia 49, 3–12. doi: 10.1111/j.1528-1167.2008.01488.x
Jauch, R., Windmuller, O., Lehmann, T. N., Heinemann, U., and Gabriel, S. (2002). Effects of barium furosemide ouabaine and 44′-diisothiocyanatostilbene-22′-disulfonic acid (DIDS) on ionophoretically-induced changes in extracellular potassium concentration in hippocampal slices from rats and from patients with epilepsy. Brain Res. 925, 18–27. doi: 10.1016/S0006-8993(01)03254-1
Jerng, H. H., Pfaffinger, P. J., and Covarrubias, M. (2004). Molecular physiology and modulation of somatodendritic A-type potassium channels. Mol. Cell. Neurosci. 27, 343–369. doi: 10.1016/j.mcn.2004.06.011
Jiang, Y., Lee, A., Chen, J., Ruta, V., Cadene, M., Chait, B. T., et al. (2003a). X-ray structure of a voltage-dependent K+ channel. Nature 423, 33–41. doi: 10.1038/nature01580
Jiang, Y., Ruta, V., Chen, J., Lee, A., and MacKinnon, R. (2003b). The principle of gating charge movement in a voltage-dependent K+ channel. Nature 423, 42–48. doi: 10.1038/nature01581
Jiang, Z., Wallner, M., Meera, P., and Toro, L. (1999). Human and rodent MaxiK channel beta-subunit genes: cloning and characterization. Genomics 55, 57–67. doi: 10.1006/geno.1998.5627
Jin, W., Sugaya, A., Tsuda, T., Ohguchi, H., and Sugaya, E. (2000). Relationship between large conductance calcium-activated potassium channel and bursting activity. Brain Res. 860, 21–28. doi: 10.1016/S0006-8993(00)01943-0
Jorge, B. S., Campbell, C. M., Miller, A. R., Rutter, E. D., Gurnett, C. A., Vanoye, C. G., et al. (2011). Voltage gated potassium channel KCNV2 (Kv8.2) contributes to epilepsy susceptibility. Proc. Natl. Acad. Sci. U.S.A. 108, 5443–5448. doi: 10.1073/pnas.1017539108
Kalachikov, S., Evgrafov, O., Ross, B., Winawer, M., Barker-Cummings, C., Martinelli Boneschi, F., et al. (2002). Mutations in LGI1 cause autosomal-dominant partial epilepsy with auditory features. Nat. Genet. 30, 335–341. doi: 10.1038/ng832
Karschin, C., Dissmann, E., Stühmer, W., and Karschin, A. (1996). IRK(1-3) and GIRK(1-4) inwardly rectifying K+ channel mRNAs are differentially expressed in the adult rat brain. J. Neurosci. 16, 3559–3570.
Karschin, C., Ecke, C., Ashcroft, F. M., and Karschin, A. (1997). Overlapping distribution of K(ATP) channel-forming Kir6.2 subunit and the sulfonylurea receptor SUR1 in rodent brain. FEBS Lett. 401, 59–64. doi: 10.1016/S0014-5793(96)01438-X
Keller, D. I., Grenier, J., Christé, G., Dubouloz, F., Osswald, S., Brink, M., et al. (2009). Characterization of novel KCNH2 mutations in type 2 long QT syndrome manifesting as seizures. Can. J. Cardiol. 25, 455–462. doi: 10.1016/S0828-282X(09)70117-5
Knaus, H. G., Schwarzer, C., Koch, R. O., Eberhart, A., Kaczorowski, G. J., Glossmann, H., et al. (1996). Distribution of high-conductance Ca2+-activated K+ channels in rat brain: targeting to axons and nerve terminals. J. Neurosci. 16, 955–963.
Krapivinsky, G., Gordon, E. A., Wickman, K., Velimirovic, B., Krapivinsky, L., and Clapham, D. E. (1995). The G-protein-gated atrial K+ channel IKACh is a heteromultimer of two inwardly rectifying K+-channel proteins. Nature 374, 135–141. doi: 10.1038/374135a0
Krestel, H., Raffel, S., von Lehe, M., Jagella, C., Moskau-Hartmann, S., Becker, A., et al. (2013). Differences between RNA and DNA due to RNA editing in temporal lobe epilepsy. Neurobiol. Dis. 56, 66–73. doi: 10.1016/j.nbd.2013.04.006
Kubo, Y., Adelman, J. P., Clapham, D. E., Jan, L. Y., Karschin, A., Kurachi, Y., et al. (2005). International Union of Pharmacology. LIV. Nomenclature and molecular relationships of inwardly rectifying potassium channels. Pharmacol. Rev. 57, 509–526. doi: 10.1124/pr.57.4.11
Kuo, H. C., Cheng, C. F., Clark, R. B., Lin, J. J., Lin, J. L., Hoshijima, M., et al. (2001). A defect in the Kv channel-interacting protein 2 (KChIP2) gene leads to a complete loss of I(to) and confers susceptibility to ventricular tachycardia. Cell 107, 801–813. doi: 10.1016/S0092-8674(01)00588-8
Lagrutta, A. A., Bond, C. T., Xia, X. M., Pessia, M., Tucker, S. J., and Adelman, J. P. (1996). inward rectifier potassium channels: cloning expression and structure-function studies. Jpn. Heart J. 37, 651–660. doi: 10.1536/ihj.37.651
Lai, M., Huijbers, M. G., Lancaster, E., Graus, F., Bataller, L., Balice-Gordon, R., et al. (2010). Investigation of LGI1 as the antigen in limbic encephalitis previously attributed to potassium channels: a case series. Lancet Neurol. 9, 776–785. doi: 10.1016/S1474-4422(10)70137-X
Lancaster, E., Martinez-Hernandez, E., and Dalmau, J. (2011). Encephalitis and antibodies to synaptic and neuronal cell surface proteins. Neurology 77, 179–189. doi: 10.1212/WNL.0b013e318224afde
Lee, U. S., and Cui, J. (2009). β subunit-specific modulations of BK channel function by a mutation associated with epilepsy and dyskinesia. J. Physiol. 587, 1481–1498. doi: 10.1113/jphysiol.2009.169243
Lee, U. S., and Cui, J. (2010). BK channel activation: structural and functional insights. Trends Neurosci. 33, 415–423. doi: 10.1016/j.tins.2010.06.004
Lopatin, A. N., Makhina, E. N., and Nichols, C. G. (1994). Potassium channel block by cytoplasmic polyamines as the mechanism of intrinsic rectification. Nature 372, 366–369. doi: 10.1038/372366a0
Lorenz, S., Heils, A., Kasper, J. M., and Sander, T. (2007). Allelic association of a truncation mutation of the KCNMB3 gene with idiopathic generalized epilepsy. Am. J. Med. Genet. B Neuropsychiatr. Genet. 144B, 10–13. doi: 10.1002/ajmg.b.30369
Lu, Z., and MacKinnon, R. (1994). Electrostatic tuning of Mg2+ affinity in an inward-rectifier K+ channel. Nature 371, 243–246. doi: 10.1038/371243a0
MacFarlane, S. N., and Sontheimer, H. (2000). Changes in ion channel expression accompany cell cycle progression of spinal cord astrocytes. Glia 30, 39–48.
Manna, I., Labate, A., Mumoli, L., Ferlazzo, E., Aguglia, U., Quattrone, A., et al. (2013). Failure to confirm association of a polymorphism in KCNMB4 gene with mesial temporal lobe epilepsy. Epilepsy Res. doi: 10.1016/j.eplepsyres.2013.03.014. [Epub ahead of print].
Marrion, N. V., and Tavalin, S. J. (1998). Selective activation of Ca2+-activated K+ channels by co-localized Ca2+ channels in hippocampal neurons. Nature 395, 900–905. doi: 10.1038/27674
Martire, M., Barrese, V., D'Amico, M., Iannotti, F. A., Pizzarelli, R., Samengo, I., et al. (2010). Pre-synaptic BK channels selectively control glutamate versus GABA release from cortical and hippocampal nerve terminals. J. Neurochem. 115, 411–422. doi: 10.1111/j.1471-4159.2010.06938.x
Matsuda, H., Saigusa, A., and Irisawa, H. (1987). Ohmic conductance through the inwardly rectifying K channel and blocking by internal Mg2+. Nature 325, 156–159. doi: 10.1038/325156a0
Mazarati, A., Lundström, L., Sollenberg, U., Shin, D., Langel, U., and Sankar, R. (2006). Regulation of kindling epileptogenesis by hippocampal galanin type 1 and type 2 receptors: the effects of subtype-selective agonists and the role of G-protein-mediated signaling. J. Pharmacol. Exp. Ther. 318, 700–718. doi: 10.1124/jpet.106.104703
McCormack, K., Connor, J. X., Zhou, L., Ho, L. L., Ganetzky, B., Chiu, S. Y., et al. (2002). Genetic analysis of the mammalian K+ channel beta subunit Kvbeta 2 (Kcnab2). J. Biol. Chem. 277, 13219–13228. doi: 10.1074/jbc.M111465200
Miceli, F., Soldovieri, M. V., Ambrosino, P., Barrese, V., Migliore, M., Cilio, M. R., et al. (2013). Genotype-phenotype correlations in neonatal epilepsies caused by mutations in the voltage sensor of Kv7.2 potassium channel subunits. Proc. Natl. Acad. Sci. U.S.A. 110, 4386–4391. doi: 10.1073/pnas.1216867110
Miranda, P., Cadaveira-Mosquera, A., González-Montelongo, R., Villarroel, A., González-Hernández, T., Lamas, J. A., et al. (2013). The neuronal serum- and glucocorticoid-regulated kinase 1.1 reduces neuronal excitability and protects against seizures through upregulation of the M-current. J. Neurosci. 33, 2684–2696. doi: 10.1523/JNEUROSCI.3442-12.2013
Misonou, H., Menegola, M., Buchwalder, L., Park, E. W., Meredith, A., Rhodes, K. J., et al. (2006). Immunolocalization of the Ca2+-activated K+ channel Slo1 in axons and nerve terminals of mammalian brain and cultured neurons. J. Comp. Neurol. 496, 289–302. doi: 10.1002/cne.20931
Monaghan, M. M., Menegola, M., Vacher, H., Rhodes, K. J., and Trimmer, J. S. (2008). Altered expression and localization of hippocampal A-type potassium channel subunits in the pilocarpine-induced model of temporal lobe epilepsy. Neuroscience 156, 550–562. doi: 10.1016/j.neuroscience.2008.07.057
Mongiat, L. A., Espòsito, M. S., Lombardi, G., and Schinder, A. F. (2009). Reliable activation of immature neurons in the adult hippocampus. PLoS ONE 4:e5320. doi: 10.1371/journal.pone.0005320
Morante-Redolat, J. M., Gorostidi-Pagola, A., Piquer-Sirerol, S., Saenz, A., Poza, J. J., Galan, J., et al. (2002). Mutations in the LGI1/Epitempin gene on 10q24 cause autosomal dominant lateral temporal epilepsy. Hum. Mol. Genet. 11, 1119–1128. doi: 10.1093/hmg/11.9.1119
Morrow, J. P., Zakharov, S. I., Liu, G., Yang, L., Sok, A. J., and Marx, S. O. (2006). Defining the BK channel domains required for beta1-subunit modulation. Proc. Natl. Acad. Sci. U.S.A. 103, 5096–5101. doi: 10.1073/pnas.0600907103
Mothet, J. P., Pollegioni, L., Ouanounou, G., Martineau, M., Fossier, P., and Baux, G. (2005). Glutamate receptor activation triggers a calcium-dependent and SNARE protein-dependent release of the gliotransmitter D-serine. Proc. Natl. Acad. Sci. U.S.A. 102, 5606–5611. doi: 10.1073/pnas.0408483102
Müller, A., Kukley, M., Uebachs, M., Beck, H., and Dietrich, D. (2007). Nanodomains of single Ca2+ channels contribute to action potential repolarization in cortical neurons. J. Neurosci. 27, 483–495. doi: 10.1523/JNEUROSCI.3816-06.2007
Murakoshi, H., and Trimmer, J. S. (1999). Identification of the Kv2.1 K+ channel as a major component of the delayed rectifier K+ current in rat hippocampal neurons. J. Neurosci. 19, 1728–1735.
Nedergaard, S. A. (2004). Ca2+-independent slow afterhyperpolarization in substantia nigra compacta neurons. Neuroscience 125, 841–852. doi: 10.1016/j.neuroscience.2004.02.030
Nerbonne, J. M., Gerber, B. R., Norris, A., and Burkhalter, A. (2008). Electrical remodelling maintains firing properties in cortical pyramidal neurons lacking KCND2-encoded A-type K+ currents. J. Physiol. 586, 1565–1579. doi: 10.1113/jphysiol.2007.146597
Neusch, C., Rozengurt, N., Jacobs, R. E., Lester, H. A., and Kofuji, P. (2001). Kir4.1 potassium channel subunit is crucial for oligodendrocyte development and in vivo myelination. J. Neurosci. 21, 5429–5438.
Nichols, C. G., Shyng, S. L., Nestorowicz, A., Glaser, B., Clement, J. P., Gonzalez, G., et al. (1996). Adenosine diphosphate as an intracellular regulator of insulin secretion. Science 272, 1785–1787. doi: 10.1126/science.272.5269.1785
Omichi, C., Momose, Y., and Kitahara, S. (2010). Congenital long QT syndrome presenting with a history of epilepsy: misdiagnosis or relationship between channelopathies of the heart and brain? Epilepsia 51, 289–292. doi: 10.1111/j.1528-1167.2009.02267.x
Orkand, R. K., Nicholls, J. G., and Kuffler, S. W. (1966). Effect of nerve impulses on the membrane potential of glial cells in the central nervous system of amphibia. J. Neurophysiol. 29, 788–806.
Pacheco Otalora, L. F., Hernandez, E. F., Arshadmansab, M. F., Francisco, S., Willis, M., Ermolinsky, B., et al. (2008). Down-regulation of BK channel expression in the pilocarpine model of temporal lobe epilepsy. Brain Res. 1200, 116–131. doi: 10.1016/j.brainres.2008.01.017
Pan, Z., Kao, T., Horvath, Z., Lemos, J., Sul, J. Y., Cranstoun, S. D., et al. (2006). A common ankyrin-G-based mechanism retains KCNQ and Nav channels at electrically active domains of the axon. J. Neurosci. 26, 2599–2613. doi: 10.1523/JNEUROSCI.4314-05.2006
Parpura, V., Basarsky, T. A., Liu, F., Jeftinija, K., Jeftinija, S., and Haydon, P. G. (1994). Glutamate-mediated astrocyte-neuron signaling. Nature 369, 744–747. doi: 10.1038/369744a0
Pasti, L., Zonta, M., Pozzan, T., Vicini, S., and Carmignoto, G. (2001). Cytosolic calcium oscillations in astrocytes may regulate exocytotic release of glutamate. J. Neurosci. 21, 477–484.
Patil, N., Cox, D. R., Bhat, D., Faham, M., Myers, R. M., and Peterson, A. S. (1995). A potassium channel mutation in weaver mice implicates membrane excitability in granule cell differentiation. Nat. Genet. 11, 126–129. doi: 10.1038/ng1095-126
Pei, Q., Lewis, L., Grahame-Smith, D. G., and Zetterstrom, T. S. C. (1999). Alteration in expression of G-protein-activated inward rectifier K+-channel subunits GIRK 1 and GIRK 2 in the rat brain following electroconvulsive shock. Neuroscience 90, 621–627. doi: 10.1016/S0306-4522(98)00453-9
Perkowski, J. J., and Murphy, G. G. (2011). Deletion of the mouse homolog of KCNAB2, a gene linked to monosomy 1p36, results in associative memory impairments and amygdala hyperexcitability. J. Neurosci. 31, 46–54.
Pessia, M. (2004). “Ion channels and electrical activity,” in Molecular Biology of the Neuron, 2nd Edn., eds W. R. Davies and B. J. Morris (Oxford, UK: Oxford University Press), 103–137. ISBN 0-19-850998-7. doi: 10.1093/acprof:oso/9780198509981.003.0005
Pessia, M., Imbrici, P., D'Adamo, M. C., Salvatore, L., and Tucker, S. J. (2001). Differential pH-sensitivity of Kir4.1 and Kir4.2 and modulation by heteropolymerisation with Kir5.1. J. Physiol. 532, 359–367. doi: 10.1111/j.1469-7793.2001.0359f.x
Pessia, M., Jiang, Z. G., North, R. A., and Johnson, S. W. (1994). Actions of 5-hydroxytryptamine on ventral tegmental area neurons of the rat in vitro. Brain Res. 654, 324–330. doi: 10.1016/0006-8993(94)90495-2
Pessia, M., Servettini, I., Panichi, R., Guasti, L., Grassi, S., Arcangeli, A., et al. (2008). ERG voltage-gated K+ channels regulate excitability and discharge dynamics of the medial vestibular nucleus neurons. J. Physiol. 586, 4877–4890. doi: 10.1113/jphysiol.2008.155762
Pessia, M., Tucker, S. J., Lee, K., Bond, C. T., and Adelman, J. P. (1996). Subunit positional effects revealed by novel heteromeric inwardly rectifying K+ channels. EMBO J. 15, 2980–2987.
Petersson, S., Persson, A. S., Johansen, J. E., Ingvar, M., Nilsson, J., Klement, G., et al. (2003). Truncation of the Shaker-like voltage-gated potassium channel Kv1.1 causes megencephaly. Eur. J. Neurosci. 18, 3231–3240. doi: 10.1111/j.1460-9568.2003.03044.x
Porter, R. J., Burdette, D. E., Gil-Nagel, A., Hall, S. T., White, R., Shaikh, S., et al. (2012). Retigabine as adjunctive therapy in adults with partial-onset seizures: integrated analysis of three pivotal controlled trials. Epilepsy Res. 101, 103–112. doi: 10.1016/j.eplepsyres.2012.03.010
Raffaelli, G., Saviane, C., Mohajerani, M. H., Pedarzani, P., and Cherubini, E. (2004). BK potassium channels control transmitter release at CA3-CA3 synapses in the rat hippocampus. J. Physiol. 557, 147–157. doi: 10.1113/jphysiol.2004.062661
Raol, Y. H., Lapides, D. A., Keating, J. G., Brooks-Kayal, A. R., and Cooper, E. C. (2009). A KCNQ channel opener for experimental neonatal seizures and status epilepticus. Ann. Neurol. 65, 326–336. doi: 10.1002/ana.21593
Rho, J. M., Szot, P., Tempel, B. L., and Schwartzkroin, P. A. (1999). Developmental seizure susceptibility of Kv1.1 potassium channel knockout mice. Dev. Neurosci. 21, 320–327. doi: 10.1159/000017381
Ruppersberg, J. P., Schröter, K. H., Sakmann, B., Stocker, M., Sewing, S., and Pongs, O. (1990). Heteromultimeric channels formed by rat brain potassium-channel proteins. Nature 345, 535–537. doi: 10.1038/345535a0
Sah, P., and Faber, E. S. (2002). Channels underlying neuronal calcium-activated potassium currents Prog. Neurobiol. 66, 345–353. doi: 10.1016/S0301-0082(02)00004-7
Samuels, E. R., and Szabadi, E. (2008). Functional neuroanatomy of the noradrenergic locus coeruleus: its roles in the regulation of arousal and autonomic function part I: principles of functional organization. Curr. Neuropharmacol. 6, 235–253. doi: 10.2174/157015908785777229
Scholl, U. I., Choi, M., Liu, T., Ramaekers, V. T., Häusler, M. G., Grimmer, J., et al. (2009). Seizures sensorineural deafness ataxia mental retardation and electrolyte imbalance (SeSAME syndrome) caused by mutations in KCNJ10. Proc. Natl. Acad. Sci. U.S.A. 106, 5842–5847. doi: 10.1073/pnas.0901749106
Schreiber, M., and Salkoff, L. (1997). A novel calcium-sensing domain in the BK channel. Biophys. J. 73, 1355–1363. doi: 10.1016/S0006-3495(97)78168-2
Schroeder, B. C., Kubisch, C., Stein, V., and Jentsch, T. J. (1998). Moderate loss of function of cyclic-AMP-modulated KCNQ2/KCNQ3 K+ channels causes epilepsy. Nature 396, 687–690. doi: 10.1038/25367
Schulte, U., Thumfart, J. O., Klöcker, N., Sailer, C. A., Bildl, W., Biniossek, M., et al. (2006). The epilepsy-linked Lgi1 protein assembles into presynaptic Kv1 channels and inhibits inactivation by Kvbeta1. Neuron 49, 697–706. doi: 10.1016/j.neuron.2006.01.033
Selyanko, A. A., and Brown, D. A. (1996). Intracellular calcium directly inhibits potassium M channels in excised membrane patches from rat sympathetic neurons. Neuron 16, 151–162. doi: 10.1016/S0896-6273(00)80032-X
Shang, L., Lucchese, C. J., Haider, S., and Tucker, S. J. (2005). Functional characterisation of missense variations in the Kir4.1 potassium channel (KCNJ10) associated with seizure susceptibility. Brain Res. Mol. Brain Res. 139, 178–183. doi: 10.1016/j.molbrainres.2005.05.003
Sheehan, J. J., Benedetti, B. L., and Barth, A. L. (2009). Anticonvulsant effects of the BK-channel antagonist paxilline. Epilepsia 50, 711–720. doi: 10.1111/j.1528-1167.2008.01888.x
Shruti, S., Clem, R. L., and Barth, A. L. (2008). A seizure-induced gain-of-function in BK channels is associated with elevated firing activity in neocortical pyramidal neurons. Neurobiol. Dis. 30, 323–330. doi: 10.1016/j.nbd.2008.02.002
Sicca, F., Imbrici, P., D'Adamo, M. C., Moro, F., Bonatti, F., Brovedani, P., et al. (2011). Autism with seizures and intellectual disability: possible causative role of gain-of-function of the inwardly-rectifying K+ Channel Kir4.1. Neurobiol. Dis. 43, 239–247. doi: 10.1016/j.nbd.2011.03.016
Signorini, S., Liao, Y. J., Duncan, S. A., Jan, L. Y., and Stoffel, M. (1997). Normal cerebellar development but susceptibility to seizures in mice lacking G protein coupled inwardly rectifying K+ channel GIRK2 Proc. Natl. Acad. Sci. U.S.A. 94, 923–927. doi: 10.1073/pnas.94.3.923
Simeone, T. A., Simeone, K. A., Samson, K. K., Kim do, Y., and Rho, J. M. (2013). Loss of the Kv1.1 potassium channel promotes pathologic sharp waves and high frequency oscillations in in vitro hippocampal slices. Neurobiol. Dis. 54, 68–81. doi: 10.1016/j.nbd.2013.02.009
Singh, B., Ogiwara, I., Kaneda, M., Tokonami, N., Mazaki, E., Baba, K., et al. (2006). Kv4.2 truncation mutation in a patient with temporal lobe epilepsy. Neurobiol. Dis. 24, 245–253. doi: 10.1016/j.nbd.2006.07.001
Singh, N. A., Charlier, C., Stauffer, D., DuPont, B. R., Leach, R. J., Melis, R., et al. (1998). A novel potassium channel gene KCNQ2 is mutated in an inherited epilepsy of newborns. Nat. Genet. 18, 25–29. doi: 10.1038/ng0198-25
Slesinger, P. A., Patil, N., Liao, Y. J., Jan, Y. N., Jan, L. Y., and Cox, D. R. (1996). Functional effects of the mouse weaver mutation on G protein-gated inwardly rectifying K+ channels. Neuron 16, 321–331. doi: 10.1016/S0896-6273(00)80050-1
Slesinger, P. A., Reuveny, E., Jan, Y. N., and Jan, L. Y. (1995). Identification of structural elements involved in G protein gating of the GIRK1 potassium channel. Neuron 15, 1145–1156. doi: 10.1016/0896-6273(95)90102-7
Smart, S. L., Lopantsev, V., Zhang, C. L., Robbins, C. A., Wang, H., Chiu, S. Y., et al. (1998). Deletion of the Kv1.1 potassium channel causes epilepsy in mice. Neuron 20, 809–819. doi: 10.1016/S0896-6273(00)81018-1
Smith, S. E., Xu, L., Kasten, M. R., and Anderson, M. P. (2012). Mutant LGI1 inhibits seizure-induced trafficking of Kv4.2 potassium channels. J. Neurochem. 120, 611–621. doi: 10.1111/j.1471-4159.2011.07605.x
Stanfield, P. R., Davies, N. W., Shelton, P. A., Khan, I. A., Brammar, W. J., Standen, N. B., et al. (1994). The intrinsic gating of inward rectifier K+ channels expressed from the murine IRK1 gene depends on voltage K+ and Mg2+. J. Physiol. 475, 1–7.
Storm, J. F. (1987a). Action potential repolarization and a fast after-hyperpolarization in rat hippocampal pyramidal cells. J. Physiol. 385, 733–759.
Storm, J. F. (1987b). Intracellular injection of a Ca2+ chelator inhibits spike repolarization in hippocampal neurons. Brain Res. 435, 387–392. doi: 10.1016/0006-8993(87)91631-3
Streit, A. K., Derst, C., Wegner, S., Heinemann, U., Zahn, R. K., and Decher, N. (2011). RNA editing of Kv1.1 channels may account for reduced ictogenic potential of 4-aminopyridine in chronic epileptic rats Epilepsia 52, 645–648. doi: 10.1111/j.1528-1167.2011.02986.x
Takumi, T., Ishii, T., Horio, Y., Morishige, K., Takahashi, N., Yamada, M., et al. (1995). A novel ATP-dependent inward rectifier potassium channel expressed predominantly in glial cells. J. Biol. Chem. 270, 16339–16346. doi: 10.1074/jbc.270.27.16339
Tempel, B. L., Papazian, D. M., Schwarz, T. L., Jan, Y. N., and Jan, L. Y. (1987). Sequence of a probable potassium channel component encoded at Shaker locus of Drosophila. Science 237, 770–775. doi: 10.1126/science.2441471
Tian, G. F., Azmi, H., Takano, T., Xu, Q., Peng, W., Lin, J., et al. (2005). An astrocytic basis of epilepsy. Nat. Med. 11, 973–981.
Trimmer, J. S., and Rhodes, K. J. (2004). Localization of voltage-gated ion channels in mammalian brain. Annu. Rev. Physiol. 66, 477–519. doi: 10.1146/annurev.physiol.66.032102.113328
Tristani-Firouzi, M., and Sanguinetti, M. C. (2003). Structural determinants and biophysical properties of HERG and KCNQ1 channel gating. J. Mol. Cell. Cardiol. 35, 27–35. doi: 10.1016/S0022-2828(02)00286-9
Tsaur, M. L., Sheng, M., Lowenstein, D. H., Jan, Y. N., and Jan, L. Y. (1992). Differential expression of K+ channel mRNAs in the rat brain and down-regulation in the hippocampus following seizures. Neuron 8, 1055–1067. doi: 10.1016/0896-6273(92)90127-Y
Tseng-Crank, J., Foster, C. D., Krause, J. D., Mertz, R., Godinot, N., DiChiara, T. J., et al. (1994). Cloning expression and distribution of functionally distinct Ca2+-activated K+ channel isoforms from human brain. Neuron 13, 1315–1330. doi: 10.1016/0896-6273(94)90418-9
Tu, E., Bagnall, R. D., Duflou, J., and Semsarian, C. (2011). Post-mortem review and genetic analysis of sudden unexpected death in epilepsy (SUDEP) cases. Brain Pathol. 21, 201–208. doi: 10.1111/j.1750-3639.2010.00438.x
Tuchman, R., Moshé, S. L., and Rapin, I. (2009). Convulsing toward the pathophysiology of autism. Brain Dev. 31, 95–103. doi: 10.1016/j.braindev.2008.09.009
Tucker, J. S., Imbrici, P., Salvatore, L., D'Adamo, M. C., and Pessia, M. (2000). pH-dependence of the inwardly rectifying potassium channel Kir5.1 and localisation in renal tubular epithelia. J. Biol. Chem. 275, 16404–16407. doi: 10.1074/jbc.C000127200
Tucker, S. J., Pessia, M., and Adelman, J. P. (1996a). Muscarine-gated K+ channel: subunit stoichiometry and structural domains essential for G protein stimulation. Am. J. Physiol. 271, H379–H385.
Tucker, S. J., Pessia, M., Moorhouse, A. J., Gribble, F., Ashcroft, F. M., Maylie, J., et al. (1996b). Heteromeric channel formation and Ca2+-free media reduce the toxic effect of the weaver Kir 3.2 Allele. FEBS Lett. 390, 253–257. doi: 10.1016/0014-5793(96)00635-7
Tucker, S. J., Bond, C. T., Herson, P., Pessia, M., and Adelman, J. P. (1996c). Inhibitory interactions between two inward rectifier K+ channel subunits mediated by transmembrane domains. J. Biol. Chem. 271, 5866–5870. doi: 10.1074/jbc.271.10.5866
van Dyke, D. H., Griggs, R. C., Murphy, M. J., and Goldstein, M. N. (1975). Hereditary myokymia and periodic ataxia. J. Neurol. Sci. 25, 109–118. doi: 10.1016/0022-510X(75)90191-4
Wang, H. G., He, X. P., Li, Q., Madison, R. D., Moore, S. D., McNamara, J. O., et al. (2013). The auxiliary subunit KChIP2 is an essential regulator of homeostatic excitability. J. Biol. Chem. 288, 13258–13268. doi: 10.1074/jbc.M112.434548
Wang, Q., Curran, M. E., Splawski, I., Burn, T. C., Millholland, J. M., VanRaay, T. J., et al. (1996). Positional cloning of a novel potassium channel gene: KVLQT1 mutations cause cardiac arrhythmias. Nat. Genet. 12, 17–23. doi: 10.1038/ng0196-17
Wei, A. D., Gutman, G. A., Aldrich, R., Chandy, K. G., Grissmer, S., and Wulff, H. (2005). International Union of Pharmacology LII Nomenclature and molecular relationships of calcium-activated potassium channels. Pharmacol. Rev. 57, 463–472. doi: 10.1124/pr.57.4.9
Wei, A., Solaro, C., Lingle, C., and Salkoff, L. (1994). Calcium sensitivity of BK-type KCa channels determined by a separable domain. Neuron 13, 671–681. doi: 10.1016/0896-6273(94)90034-5
Whorton, M. R., and Mackinnon, R. (2013). X-ray structure of the mammalian GIRK2-β γ G-protein complex. Nature 498, 190–197. doi: 10.1038/nature12241
Xia, X. M., Fakler, B., Rivard, A., Wayman, G., Johnson-Pais, T., Keen, J. E., et al. (1998). Mechanism of calcium gating in small-conductance calcium-activated potassium channels. Nature 395, 503–507. doi: 10.1038/26758
Xia, X. M., Zeng, X., and Lingle, C. J. (2002). Multiple regulatory sites in large-conductance calcium-activated potassium channels. Nature 418, 880–884. doi: 10.1038/nature00956
Yamada, K., Ji, J. J., Yuan, H., Miki, T., Sato, S., Horimoto, N., et al. (2001). Protective role of ATP-sensitive potassium channels in hypoxia-induced generalized seizure. Science 292, 1543–1546. doi: 10.1126/science.1059829
Yang, J., Krishnamoorthy, G., Saxena, A., Zhang, G., Shi, J., Yang, H., et al. (2010). An epilepsy/dyskinesia-associated mutation enhances BK channel activation by potentiating Ca2+ sensing. Neuron 66, 871–883. doi: 10.1016/j.neuron.2010.05.009
Young, C. C., Stegen, M., Bernard, R., Müller, M., Bischofberger, J., Veh, R. W., et al. (2009). Upregulation of inward rectifier K+ (Kir2) channels in dentate gyrus granule cells in temporal lobe epilepsy. J. Physiol. 587, 4213–4233. doi: 10.1113/jphysiol.2009.170746
Zahn, R. K., Tolner, E. A., Derst, C., Gruber, C., Veh, R. W., and Heinemann, U. (2008). Reduced ictogenic potential of 4-aminopyridine in the perirhinal and entorhinal cortex of kainate-treated chronic epileptic rats. Neurobiol. Dis. 29, 186–200. doi: 10.1016/j.nbd.2007.08.013
Zamorano-León, J. J., Yañez, R., Jaime, G., Rodriguez-Sierra, P., Calatrava-Ledrado, L., Alvarez-Granada, R. R., et al. (2012). KCNH2 gene mutation: a potential link between epilepsy and long QT-2 syndrome. J. Neurogenet. 26, 382–386. doi: 10.3109/01677063.2012.674993
Zhou, W., Qian, Y., Kunjilwar, K., Pfaffinger, P. J., and Choe, S. (2004). Structural insights into the functional interaction of KChIP1 with Shal-type K+channels. Neuron 41, 573–586. doi: 10.1016/S0896-6273(04)00045-5
Keywords: Potassium channels: [Kv1, Kv2, Kv3, Kv4, Kv8, Kv11(HERG), KCa1.1, Kvβ1, Kvβ2, KChIP LGI1, Kir1-Kir7 (GIRK, KATP)], temporal lobe epilepsy, autism–epilepsy, channelopathies
Citation: D'Adamo MC, Catacuzzeno L, Di Giovanni G, Franciolini F and Pessia M (2013) K+ channelepsy: progress in the neurobiology of potassium channels and epilepsy. Front. Cell. Neurosci. 7:134. doi: 10.3389/fncel.2013.00134
Received: 12 June 2013; Accepted: 06 August 2013;
Published online: 13 September 2013.
Edited by:
Roberto Di Maio, University of Pittsburgh, USAReviewed by:
Enrico Cherubini, International School for Advanced Studies, ItalyCopyright © 2013 D'Adamo, Catacuzzeno, Di Giovanni, Franciolini and Pessia. This is an open-access article distributed under the terms of the Creative Commons Attribution License (CC BY). The use, distribution or reproduction in other forums is permitted, provided the original author(s) or licensor are credited and that the original publication in this journal is cited, in accordance with accepted academic practice. No use, distribution or reproduction is permitted which does not comply with these terms.
*Correspondence: Maria Cristina D'Adamo and Mauro Pessia, Faculty of Medicine, Section of Human Physiology, Department of Internal Medicine, University of Perugia, P.le Gambuli, Edificio D - Piano 1, 06132 San Sisto, Perugia, Italy e-mail:bWFyaWEuZGFkYW1vQHVuaXBnLml0;bWF1cm8ucGVzc2lhQHVuaXBnLml0
Disclaimer: All claims expressed in this article are solely those of the authors and do not necessarily represent those of their affiliated organizations, or those of the publisher, the editors and the reviewers. Any product that may be evaluated in this article or claim that may be made by its manufacturer is not guaranteed or endorsed by the publisher.
Research integrity at Frontiers
Learn more about the work of our research integrity team to safeguard the quality of each article we publish.