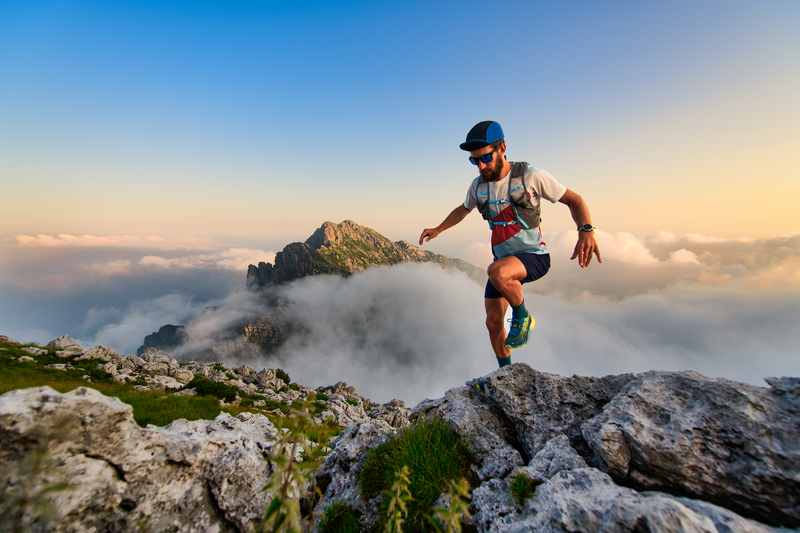
94% of researchers rate our articles as excellent or good
Learn more about the work of our research integrity team to safeguard the quality of each article we publish.
Find out more
REVIEW article
Front. Cell. Neurosci. , 30 January 2012
Sec. Cellular Neurophysiology
Volume 6 - 2012 | https://doi.org/10.3389/fncel.2012.00003
This article is part of the Research Topic GABA signaling in health and disease View all 18 articles
Mature macroglia and almost all neural progenitor types express γ-aminobutyric (GABA) A receptors (GABAARs), whose activation by ambient or synaptic GABA, leads to influx or efflux of chloride (Cl−) depending on its electro-chemical gradient (ECl). Since the flux of Cl− is indissolubly associated to that of osmotically obliged water, GABAARs regulate water movements by modulating ion gradients. In addition, since water movements also occur through specialized water channels and transporters, GABAAR signaling could affect the movement of water by regulating the function of the channels and transporters involved, thereby affecting not only the direction of the water fluxes but also their dynamics. We will here review recent observations indicating that in neural cells GABAAR-mediated osmotic regulation affects the cellular volume thereby activating multiple intracellular signaling mechanisms important for cell proliferation, maturation, and survival. In addition, we will discuss evidence that the osmotic regulation exerted by GABA may contribute to brain water homeostasis in physiological and in pathological conditions causing brain edema, in which the GABAergic transmission is often altered.
In the mammalian central nervous system (CNS) three classes of GABARs have been identified, two of which, GABAARs and GABACRs, are ligand activated pentameric anion channels. Besides the differences in subunit composition, biophysical characteristics, and pharmacological properties (Chebib and Johnston, 1999), the two classes of ionotropic receptors also exhibit a different pattern of expression. Whereas GABAARs are present throughout the CNS, including non-neuronal cells and precursors, GABAcRs are mainly found in neurons in a few brain structures (Frazao et al., 2007). As yet GABAcRs have not been detected in mature glial cells or in oligodendrocyte progenitors (OPCs; Williamson et al., 1998). Although the transcripts of ρ subunits are expressed in some populations of neural progenitors (Fukui et al., 2008; Cesetti et al., 2010), the information concerning the functional role of GABACRs in these cells is still scarce. Therefore, we will here focus only on GABAARs. Moreover, since the presence GABAARs in microglia cells in vivo is still controversial (Velez-Fort et al., 2011), we will not include this cell population in our discussion.
With a few exceptions, in mature neurons activation of GABAARs leads to Cl− influx and hyperpolarization, whereas in immature neuronal cells it generally causes a depolarizing efflux of Cl−. This in turn triggers a voltage-dependent influx of Ca2+, which is essential for the morphological and electrical maturation of young neurons (Ben-Ari et al., 1989).
The consequence of GABAAR activation in non-neuronal cells is far less predictable than in neurons. Moreover its functional significance is still tentative. In non-neuronal cells, Cl− fluxes via GABAARs occur in both directions according to the cellular electro-chemical Cl− gradient (ECl), thereby contributing to the regulation of osmotic tension. Therefore, activation of GABAARs in these cells may directly affect the cell volume and indirectly control neuronal excitability by regulating the extracellular space and the concentration of Cl−. Whereas in neurons changes in cell size and osmotic tension are often associated to cell death and apoptosis (Pasantes-Morales and Tuz, 2006), in non-neuronal cells such changes may activate several intracellular signaling mechanisms important for cell survival, proliferation, and maturation.
We will here review evidence indicating that in the adult brain GABAAR activation regulates osmotic tension as, despite its potential importance both at the cellular and systemic level, this function of GABAARs has been so far less investigated than its role in neurotransmission. After introducing the basic concepts of tissue and cell volume regulation in the brain (Figure 1), we will then describe the molecular machinery involved in water movements and the anionic fluxes activated by GABAAR with a special focus on non-neural cells, i.e. macroglia and different precursor types (Figure 2). In the second part of the review we will discuss the role of GABA in the context of cell volume regulation and water exchange in the brain, its physiological significance and potential clinical relevance.
Figure 1. Basic properties of water and anions fluxes. (A) Water can diffuse according to the osmotic pressure through the membrane lipid bilayer or via the dedicated channels AQP. Additionally it can be transported against its gradient by cotransporters, such as those for GABA and glutamate that use the driving force of Na+ to move the neurotransmitters and eventually water against their gradient. (B,C) The activity of different transporters and exchangers determines the steady-state gradient for Cl− and consequently regulates the . Cl− transport via cation–chloride cotransporters is fueled by the Na+ and K+ gradients generated by the Na–K ATPase. Two of the major players in neural cells are NKCC1 and KCC2, by which Cl− is transported inside and outside the cells respectively. When
(see Box 1), is more positive that the EM, opening of GABAAR would mediate an efflux of Cl− (for convention called inward current) that depolarizes the cells (B). Conversely when
is more negative that the EM, GABAergic currents (outward) bring the membrane potential close to these values and are hyperpolarizing (C). In this case to determine the EGABA is important to consider also the flux of
since GABAARs are permeable to this anion. Due to its higher level inside the cells, opening of GABAAR would drive a depolarizing efflux of
that, counteracting the influx of Cl−, contributes to deviate EGABA to value more positive than that of
(For Rev, see Andrew et al., 2007; Blaesse et al., 2009; Risher et al., 2009).
Figure 2. GABA-mediated osmotic regulation in non-neuronal cells. Schematic representation summarizing the current knowledge concerning the expression of the neurotransmitter GABA, the synthesizing enzyme GAD, the membrane transporter GAT, which can work in both directions, and the ionic GABAAR in the indicated non-neuronal cell types of the mammalian CNS. Depicted is also the direction of water flux and of the Cl− gradient, as estimated in resting physiological conditions. The scheme also illustrates the expression of the aquaporin (AQP) water channels and of the Na+/K+/Cl− (NKCC) and K+/Cl− (KCC) transporters in those cell types where their expression has been directly investigated. As in neurons, GABA promotes swelling in neural stem cells (NSC) but not in NG2+ oligodendrocyte precursors (OPCs) and in mature macroglia, where GABAAR activation induces Cl− exit and water efflux. Note that here the information concerning neural stem cells (NSCs) is gathered from the analysis of neural precursors isolated from the postnatal SVZ on the basis of Prominin expression. We did not include in this diagram the information concerning GFAP expressing cells in neurogenic regions of the adult brain because they mainly consist of niche astrocytes. Moreover, the properties illustrated in the diagram were not directly investigated in these populations. However, as discussed in the text, the available information indicates that these GFAP populations resemble mature astrocytes with respect to the direction of the GABA evoked Cl− currents.
Normal brain function is inextricably coupled to water homeostasis, which is the result of central osmoreception, osmolarity compensation, and cell volume regulation. More than 75% of the adult mammalian brain weight is represented by water subdivided in four distinct compartments: the blood of the cerebral vasculature, the cerebrospinal fluid (CSF) in the ventricular system and subarachnoid space, the extracellular fluid (ECF) in the brain parenchyma, and the intracellular fluid (ICF). Three main barriers maintain a distinct fluidic composition among these compartments: the blood–brain barrier (BBB), the blood–CSF barrier (BCSFB) formed by the surface of the arachnoidea and choroid plexus epithelial cells, and the plasma membranes of the neural cells. Although the bulk of the ECF is generated from the metabolism of neural cells, around 30% is secreted from the endothelial cells of the brain capillary. The composition of the ECF depends on the interaction between the BBB, the BCSFB, and the activity of transporters on the membrane of neural cells, primarily astrocytes. The bulk of the CSF is largely the result of its secretion by the choroids plexus epithelium and its re-adsorption into the blood plasma at the dural sinuses in the subarachnoid space. In addition, according to recent evidence there is a flow of fluid from the ECF to the CSF. Although its composition displays regional variation, compared to the plasma, the CSF is generally slightly hypertonic containing moderately higher Na+ and and lower K+ and Cl− concentration. The K+ concentration, which is critical for the regulation of the neuronal resting potential, is even lower in the ECF but it is increased in the ICF, which also contains lower Ca2+ and Na+ concentrations than the ECF. The volume and ionic composition of the ICF depend on cellular metabolic activity and active transport of ions, and therefore, the ICF compartment is particularly sensitive to traumatic or ischemic injury. In general, the maintenance of these compartments depends on the existence of ionic gradients and water transport that are tightly regulated. For a comprehensive discussion of this complex topic we refer the reader to recently published excellent reviews (Strange, 1993; Kahle et al., 2009; Oreskovic and Klarica, 2010; Redzic, 2011).
Water homeostasis in the brain is necessary to prevent changes in the brain volume that could critically affect intracerebral pressure. As in other tissues, also in the brain changes in the extracellular or intracellular content of osmolytes are coupled to movements of osmotically obliged water. In normal conditions a redistribution of water between the intra and extracellular space occurs, which modifies the volume of the neural cells but not of the total brain. These changes in cell volume are referred to as isosmotic or anisosmotic depending on whether they originate from a change in the intracellular solute content or of the extracellular osmotic pressure, respectively. For example, neural activity determines isosmotic volume changes as a consequence of the ionic fluxes across the cell membrane occurring during neuronal firing. Cells counteract a decrease or an increase in volume by activating accordingly the processes of regulatory volume increase (RVI) and decrease (RVD). These processes of volume regulation involve increase or decrease of intracellular ionic and organic osmolytes achieved by modifying the expression and activity of ion channels and transporters and by metabolic changes. Different pathologies lead to isosmotic cytotoxic swelling. For example, energetic failure and dissipation of Na+ gradients during hypoxia/ischemia, increase in the extracellular K+ concentration such as during ischemia, epilepsies, and cortical spreading depression or ammonium accumulation occurring during hepatic encephalopathy, they all lead to cytotoxic swelling. Swelling in isosmotic conditions alters neuronal activity since changes in the extracellular/intracellular ionic equilibrium, which determine the resting membrane potential and the driving force for the different ions (see Box 1), directly impact the discharge pattern of the neurons. Neurons are very sensitive to isosmotic swelling which is usually detrimental for these cells, since they cannot recover their original volume. Astrocytes instead should be better geared than neurons to counteract cytotoxic swelling, as they can undergo RVD. However, in intact tissue under physiologically relevant osmotic conditions, swelled astrocytes recover their volume only when the osmotic challenge is removed and control condition are re-established (Risher et al., 2009).
Box 1. Basic elements of electrophysiology
The direction of the current flow is conventionally defined as the movements of positive charges: an inward currents (depolarizing) means that cations enter the cells or/and anions exit. Respectively an outward current (hyperpolarizing) is determined by the efflux of cations or influx of anions. By convention, an inward current is displayed in voltage clamp as a downward deflection, while an outward current (positive charge moving out of the cell) is shown as an upward deflection.
The concentration gradient (ΔC = [X]o/[X]i) depends on the concentration of the ion outside and inside of the cells and it determines the reversal potential (EX) for a defined ion (X) according to the Nernst Equation:
At the reversal potential (EX) the electrical force (membrane potential) counteracts the chemical force (concentration gradient ΔC) so that the ion influx is equal to the efflux. Changes in the extracellular and/or intracellular volume would impact ΔC and thus the EX.
The resting membrane potential (EM) in general depend on the driving force of Cl−, Na+, and K+ and their relative permeability (PX) according to the Goldmann-Hodgkin-Katz equation:
In resting condition, having K+ the higher permeability, the EM is closer to the EK that is about −80 mV.
The current (i) through a single ionic channel is dependent on the channel conductance (g) and the “driving force” of the permeant ion. The driving force is determined according to the EX and the resting membrane potential (EM). The single channel current is calculated as follow:
The whole-cell current (I) through specific ion channels is proportional to the single channel current (i) and the number of opened channels at the membrane (n) according to the equation:
In whole-cell patch-clamp measurements, due to dialysis of the cytoplasm, the intracellular ionic composition is altered therefore the measure of EM in current-clamp does not correspond to the real value. The real EM can be better estimated by measuring the amplitude of the current of a single K+ channel versus the voltage in cell-attached configuration (Soltesz and Mody, 1994). Another approach takes advantage of voltage-sensitive dyes: changes in fluorescence visualized with live microscopic imaging or fluorescent activated cell sorting (FACS) correspond to changes in the membrane potential. Membrane potential can be calibrated by permeabilizing the cells with gramicidin and applying different Na+ concentrations (Maric et al., 2000). Recently also voltage-sensitive genetically encoded sensors have been developed (Mutoh et al., 2011).
It can be experimentally determined in whole-cell perforated patch-clamp recording using gramicidin, which is not permeable to Cl− and therefore does not alter the intracellular Cl− concentration. Using a ramp or step protocol in the presence of a GABAergic agonist the current versus voltage relationship is measured: the EGABA corresponds to the voltage at which the currents is zero (Ge et al., 2006). Another possibilty is to block Na+, Ca2+, and K+ currents and, in perforated current-clamp recording, measure the maximum depolarization produced by the application of a saturating concentration of a GABAergic agonist (Owens et al., 1996). EGABA can also be measured on the basis of the reversal potential of single GABA and NMDA receptor channels (Tyzio et al., 2006).
It can be established with Cl− sensitive intracellular microelectrodes (Kettenmann et al., 1987) and radioactive studies (Kimelberg, 1981). Additionally it can be estimated with Cl− sensitive dye, such as MEQ, (Bevensee et al., 1997) or genetically encoded probes, such as Clomeleon (Kuner and Augustine, 2000): both allow the analysis, with cellular resolution, of real-time changes in [Cl−]i by microscopic imaging.
Since in cells undergoing osmotic changes the intensity of light scattering varies inversely with the cell volume, changes in forward scattering measured by FACS analysis, can be related to changes in cell volume of a whole-cell population (McGann et al., 1988). To measure changes in the volume of single cells, optical measurement of calcein fluorescence quenching can be employed (Solenov et al., 2004). Also in slices intrinsic optic signals are a read out of volume changes: when cells swell light scattering decreases and the tissue shows increased light transmittance (MacVicar and Hochman, 1991; Andrew and MacVicar, 1994; Holthoff and Witte, 1996). However, with this technique it is not possible to distinguish which cell type changes its volume. On the contrary with the two-photon microscopic technique the time course of swelling in slice and in living brain can be monitored at cellular and subcellular levels (Andrew et al., 2007; Risher et al., 2009). Additionally with electrophysiology, the amplitude of evoked field potential is an indirect way to measure tissue swelling: since the extracellular resistance is inversely proportional to the osmolarity, a reduction of the latter induces an increase in the evoked field potential (Andrew et al., 2007).
In vivo changes in human brain volume can be revealed by monitoring intracranial pressure, by computed tomography and by MRI. These techniques provide a measure of the total water content at a given anatomical location.
The blood plasma osmolality is strictly regulated and the solute content of the ECF and CSF is kept constant by the balanced influx/efflux across the plasma membrane and production/removal of osmotically active substances. Thus, under normal physiological conditions, neural cells are relatively protected against drastic anisosmotic volume changes. However, many pathological processes can cause drastic changes in the blood plasma osmolarity that in turn can affect brain volume. In particular, hypoosmolar states can lead to brain swelling whereas hyperosmotic changes cause brain dehydration. For example, hyponatremia associated with clinical conditions such as heart failure, nephritic syndromes, and hepatic cirrhosis may cause hyposmotic swelling of neural cells. Hyponatremia, even if drastic, rarely results in neuronal death, since neurons use compensatory mechanisms to retain their volume such as unconventional release of neurotransmitters (Tuz et al., 2004). On the contrary, hyposmolarity causes astrocytes swelling due to water fluxes across the membrane. Water can also diffuse to neighbor astrocytes via gap-junctions. Swelling of astrocytes may represent a protective mechanism for neurons since they clear from the extracellular space not only water but also the neurotransmitters in excess. This function of the astroglia is crucial to synaptic transmission since it counteracts the effect that hyposmolarity may have on the extracellular concentration of neurotransmitters and the size of the extracellular space.
Cell membranes are highly permeable to water and cannot resist hydrostatic pressure. Therefore, water movements occurring by diffusion across the cell membrane and through the aquaporin (AQP) water channels, are largely driven by the transmembrane difference in chemical potential. However, water can also be actively transported in the brain, and it is widely accepted that some cotransporters and uniporters contribute to this flux exchange (Agre, 2004).
AQPs are a family of tetrameric water channels assembled at the cell membrane or, as in the case of AQP6, inside the cell. Thirteen homologs of AQPs (AQP0–AQP12) have been identified so far in mammals (Verkman, 2005). AQPs display a variable tissue distribution, depending on their distinct physiological functions. They mediate movements of water and small solutes, such as glycerol, across membranes according to osmotic gradients and differences in hydrostatic pressures (Verkman, 2005). AQPs have recently been subdivided into three functional groups based on permeability characteristics (Verkman, 2000): the water selective aquaporins, including AQP0, AQP1, AQP2, AQP4, AQP5, AQP6; the aquaglyceroporins, including AQP3, AQP7, AQP8, permeable to water, glycerol, and urea; the neutral solute channels, including AQP9, allowing the passage of water, glycerol, urea, purines, pyrimidines, and monocarboxylates. AQP10, like AQP9, is permeable to water and neutral solutes, but not to urea and glycerol (Hatakeyama et al., 2001).
Three AQPs have been functionally involved in the regulation of water movements in the CNS: AQP1, AQP4, and AQP9. Among these, AQP4 is the most abundant. It is strongly expressed at the borders between the brain parenchyma and major fluid compartments, including the foot processes of astrocytes, the glia limitans, and the ependyma lining the lateral ventricle. AQP4 is also expressed in the astrocytes of the two major neurogenic regions in the postnatal CNS: the subventricular zone (SVZ; Rash et al., 1998) and the hippocampal dentate gyrus (DG; Venero et al., 2001). In the brain, AQP4 normally displays a polarized cellular distribution, being expressed in astroglial foot processes adjacent to the endothelial cells (Nielsen et al., 1997). In general, AQPs are not expressed in neurons and it is still unclear whether these cells possess a dedicated molecular machinery mediating water movements (Andrew et al., 2007).
The levels of AQP expression are not constant but functionally regulated. For example they are increased in brain regions where the BBB is disrupted following brain injury, ischemia, or tumor (Vizuete et al., 1999; Taniguchi et al., 2000; Saadoun et al., 2002). The amount of AQPs expressed at the cell surface is regulated both at the levels of RNA transcription (Wen et al., 1999) and of channel assembly. Multiple phosphorylation sites and different kinases have been involved in this complex regulation (Zelenina et al., 2002; Carmosino et al., 2007) and the precise regulatory mechanisms of AQP expression in different brain cell types remain unclear.
AQP4 has been involved in brain water homeostasis. Deficiency of AQP4 in mouse markedly reduces brain swelling in cytotoxic brain edema and tissue swelling mediated by physiological neuronal activity (Papadopoulos and Verkman, 2005), while it worsens the outcome in vasogenic brain edema (Zador et al., 2007), indicating that AQP4 facilitates the redistribution and absorption of excessive brain fluid. Several evidences point at functional and physical interaction between AQPs and ion channels in the regulation of water homeostasis. For example, in astrocytes AQP4 interacts with the inward rectifier K+-channel (Kir 4.1; Nagelhus et al., 2004). Tetraethylammonium, a blocker of voltage-dependent K+-channel, also inhibits water permeability of AQP1 (Brooks et al., 2000). Lack (Binder et al., 2006) or mislocation (Amiry-Moghaddam et al., 2003) of AQP4 causes impaired K+ clearance following neuronal stimulation, suggesting that K+ clearance is mediated by the AQP4–Kir4.1 complex. In astrocytes, the complex between AQP4 and the transient receptor potential vanilloid 4 (TRPV4) is essential to induce [Ca2+]i increase and promote RVD upon hypotonic challenge (Benfenati and Ferroni, 2010).
Besides the systemic regulation of water exchange, AQP4 contributes to multiple steps of adult neurogenesis (i.e., proliferation, migration, and differentiation). Adult neural stem cells express AQP4 (Cavazzin et al., 2006) and its level of expression in neural precursors changes during brain development (Wen et al., 1999). Genetic ablation of AQP4 impairs proliferation, migration, and neuronal differentiation of adult neural stem cells (Kong et al., 2008). Using microarray and quantitative mRNA analysis in neural precursors prospectively isolated from the neonatal SVZ, we have also recently confirmed that neural stem cells, and particularly activated neural stem cells, express AQP4 at higher levels in comparison to later stages of differentiation (Li and Ciccolini, unpublished observations). Our observations also indicate a similar expression pattern for AQP4 and GABAAR in the neonatal SVZ. We showed that activation of GABAARs induces Cl− entry and osmotic swelling of neural stem cells (Cesetti et al., 2010), opening up to the possibility that GABAARs and water channels interact to mediate cell volume changes in the neonatal SVZ niche.
Interestingly, GABAARs and AQPs are involved in the regulation of similar processes. For example, they both enhance migration in response to a chemotactic stimulus in vitro in various neural cell types (Behar et al., 1998; Saadoun et al., 2005). They also modulate Ca2+ homeostasis and promote neuronal differentiation in neural precursors. Lack of AQP4 in adult neural stem cells significantly decreases their ability to generate neurons, alters spontaneous Ca2+ oscillations, and suppresses depolarization-induced Ca2+ influx (Kong et al., 2008). Similarly GABAAR signaling increases [Ca2+]i and promotes neuronal differentiation of progenitors in the adult hippocampus (Tozuka et al., 2005). Moreover, we found that GABAAR activation modulates spontaneous Ca2+ oscillations in culture of neural stem cells isolated from the neonatal SVZ (Figure 2; Cesetti et al., 2010). The analysis of mice lacking the intracellular membrane protein dystrophin provides a further hint of a possible connection between GABAAR and AQPs. Dystrophin in the brain is important for clustering and stabilizing GABAAR in neurons (Brunig et al., 2002). It is also responsible for anchoring AQP4 at the membrane of perivascular astrocytes (Nicchia et al., 2008). The expression of AQP4 in ependymal cells and in astrocytic endfeet of the lateral ventricle is reduced in a dystrophic mice model (mdx; Frigeri et al., 2001) and hippocampal neurogenesis is altered in these mice (Deng et al., 2009), suggesting that dystrophin may be important for stem cell function.
However, despite being coexpressed in non-neuronal cells and regulating similar mechanisms, a direct functional interaction between AQPs and GABAAR has not been yet demonstrated.
It has been recently recognized that some cotransporters and uniporters also transport water (MacAulay et al., 2001). Extensive data show that water molecules move in association with the transport of ions and substrates.
Cotransporters are a group of membrane-spanning transport proteins which can couple ion and substrate transport. For example, it is well known that Na+ is employed as the principal cotransported ion for its large inwardly directed electro-chemical gradient. In this process, Na+ can force the uptake of a substrate against its chemical gradient. The ratio between the various fluxes is a fixed property of the transporter protein and the energy for the water transport can be derived from the transport of the non-aqueous substrates. Thus, cotransport may carry a fixed number of water molecules together with each transported solute against the osmotic gradients.
Various cotransporters are able to transport water against the osmotic gradient, such as for example the K+/Cl− cotransporter (KCC) in the choroid plexus (Zeuthen, 1994), the Na+/K+/2Cl− (NKCC; Hamann et al., 2005), the glial Na+-coupled glutamate (EAAT1; MacAulay et al., 2001), and the Na+/GABA (GAT-1) cotransporters (MacAulay et al., 2002). Similar phenomena are also associated to glucose uniporters (GLUT1 and GLUT2; Zeuthen and Zeuthen, 2007). For some ionic cotransporters, water transport is closely coupled to the transport of the other substrates. Other cotransporters, such as EAAT1 and GAT-1, not only cotransport water but also have water channel properties. Therefore, the total water transported is the sum of the cotransported and the osmotic components. In GAT-1 expressing oocytes, water can move passively through GAT-1 under external osmotic challenge. However, upon addition of GABA the influx of water increases and it is strictly coupled to the transport of GABA through GAT-1, independent of the external osmotic gradient (MacAulay et al., 2002). Thus, ambient GABA in the brain could also affect osmotic gradients by enhancing water transport via GATs activation.
Despite the difficulties in detecting GABAergic currents in astrocytes due to their electrical coupling, it has been proved that glial cells express GABAARs in a functionally significant amount.
The astrocytic GABAARs have many pharmacological similarities to the receptors on neuronal cells, such as barbiturate- and benzodiazepine-mediated potentiation. Differently from neurons, the inverse benzodiazepine agonist DMCM enhances the GABAergic currents of some subpopulations of astrocytes, suggesting that the subunit composition of GABAARs among different populations of astrocytes is heterogeneous (Bormann and Kettenmann, 1988). Analyses in vitro and in vivo have shown that in the SVZ niche glial fibrillary acidic protein (GFAP)/nestin immunopositive cells, pre-neuroblasts, and especially neuroblasts also express functional GABAARs (Stewart et al., 2002; Liu et al., 2005; Cesetti et al., 2010). GABAARs are also expressed in mature oligodendrocytes (Von Blankenfeld et al., 1991) and the mRNAs for GABAAR α2–5, γ2–3 and to a lesser extent γ1 subunits have been found in NG2+ OPCs. Different sources of GABA activate GABAARs in non-neuronal cells. Some progenitor cells, such as the OPCs in the gray and cerebellar white matter, receive direct GABAergic synaptic input, which regulates their proliferation and differentiation (Lin and Bergles, 2004). Newly born granule cells in the adult DG of the hippocampus also display during their maturation first tonic and then phasic GABAergic currents. These currents are evoked by GABA released from mature interneurons, which modulates morphological development and connectivity of new born granule cells (Ge et al., 2006; Markwardt et al., 2009). Despite these examples of phasic currents, in non-neuronal cells GABAAR activation is mostly tonic, mediated by GABA derived from non-synaptic release or synaptic spillover. Neurons may release GABAAR modulators such as taurine and GABA upon osmotic stress, via a mechanism similar to Ca2+ mediated exocytosis that can be blocked by tetanus toxin (Tuz et al., 2004). Additionally, alternative mechanisms, such as reverse operation of the transporters have been reported (Koch and Magnusson, 2009). Glial cells are also a source of ambient GABA, although it is unclear whether this reflects uptake/release dynamics or synthesis and whether GABA acts as an autocrine factor on glial cells. The fact that glial cells can release GABA in culture has been long known. Already 40 years ago low activity of the glutamic acid decarboxylase (GAD), the rate-limiting enzyme in the synthesis of GABA, and modest production of GABA (Wu et al., 1979) were observed in astrocytes and GABA concentration in culture was estimated to be 3.5 mM (Bardakdjian et al., 1979). The finding that astrocytes in situ are immunopositive for GABA (Blomqvist and Broman, 1988) and for GAD (Martinez-Rodriguez et al., 1993) has been recently confirmed also in human astrocytes (Benagiano et al., 2000). Recent evidence suggests that GABA released by astrocytes can modulate the neuronal network by inducing either tonic or transient currents in neurons (Angulo et al., 2008). It was originally proposed that GABA may be released by cultured cerebellar astrocytes via the GABA transporter working in the reverse mode (Gallo et al., 1991). This mechanism has been later confirmed in Bergman glial cells in acute slices (Barakat and Bordey, 2002). Outwardly directed GABA currents occur when the cells are filled with GABA 10 mM and Na+ 12.5 mM. However, it is still unclear whether in physiological conditions the intracellular concentration of GABA is enough to activate this mechanism (Barakat and Bordey, 2002). Astrocytes of the olfactory bulb can release not only glutamate but also GABA that leads to a long lasting synchronous inhibition of both mitral and granule cells (Kozlov et al., 2006). As the frequency of these inhibitory slow outward currents is sensitive to extracellular osmolarity, the mechanism proposed involved a release from volume activated anion channels. Such a mechanism has been confirmed only recently in the cerebellum Bergmann glial cells that have been shown to release GABA via the Bestrophin 1 anion channel, providing a tonic inhibition for cerebellar granule cells (Lee et al., 2010). Bestrophins are enigmatic anion channels, permeable to , large anions, and even to glutamate; they are activated by increases in the intracellular Ca2+ and cell swelling, but they are active also at resting Ca2+ levels and normal cell volume.
In the neonatal SVZ both neuroblasts and neural precursors are immunopositive for GABA, GAD65, and GAD67. However, mRNA levels are much higher in neuroblasts than in neural precursors (Cesetti et al., 2010). On the contrary, GABA has not been detected in SVZ astrocytes. It is believed that in this region GABA is manly synthesized and released by neuroblasts via an unknown non-synaptic mechanism which is SNARE-independent but mediated by depolarization, acting as “volume neurotransmitter” (Liu et al., 2005). Despite the presence of GAD65/67 in this region, GABA in neonatal tissue is mainly produced via monoacetylation of putrescine (Sequerra et al., 2007). Similarly, O2-A progenitor cells of the optic nerve synthesize GABA from putrescine (Barres et al., 1990).
The concept that astrocytes regulate K+ homeostasis by clearing it from the extracellular space (K+ siphoning) has been proposed a long time ago along with the idea that astrocytes have no resting Cl− conductance (Ballanyi et al., 1987; Walz and Wuttke, 1999). Glial cells in resting condition are indeed permeable to K+ therefore their resting potential (EM) is more negative than −75 mV. However, it is quite intuitive that in order to maintain the EM and the osmotic pressure constant, K+ fluxes must be followed by Cl− and water fluxes. During neuronal activity, astrocytes accumulate K+, which causes an influx of Cl− and osmotically obliged water, thereby increasing their cell volume. Astrocytes counteract this volume increase by releasing Cl− and other anions. Thus, K+ and Cl− homeostasis are crucially linked. In astrocytes the regulation of Cl− fluxes is quite complex involving a large number of exchangers, transporters as well as ion channels (Walz, 2002). Although reactive, neoplastic, and deformed astrocytes can express a significant resting Cl− conductance (Walz and Wuttke, 1999), astrocytes have generally a low permeability to Cl− and are able to accumulate it. When 30 years ago functional GABAARs were firstly identified in astrocytes in situ, the depolarizing effect of GABA was thought to be indirect, due to the increase in [K+]o upon its release from adjacent neurons (Hosli et al., 1978, 1981). Later evidence proved that GABAARs in glial cells in situ mediate Cl− outward currents (MacVicar et al., 1989; Steinhauser et al., 1992; Pastor et al., 1995), in agreement with previous data in vitro (Kettenmann et al., 1987). Confirming that astrocytes have a ECl much more depolarized than their EM, the analysis of GABAAR-mediated currents (see Box 1) showed that the ECl of astrocytes in cultures is around −40 mV that corresponds to a [Cl−]i of 29 mM (Bekar and Walz, 2002). Using more direct experimental approaches, such as intracellular microelectrodes (Kettenmann et al., 1987), radioactive studies (Kimelberg, 1981), and Cl−-sensitive dyes (Bevensee et al., 1997), both in cell culture and in vivo, the intracellular Cl− concentration has been calculated between 30 and 40 mM. In contrast to neurons, where GABAergic currents shift from depolarizing to hyperpolarizing during the second week after birth, the direction and the density of GABAergic currents in astrocytes do not change during development (Bekar et al., 1999). The absence of phasic GABAARs activation in astrocytes suggests that they would mainly sense ambient GABA rather than transient high concentrations from synaptic release.
Analyses in vitro have implicated two major transport systems in the accumulation of Cl− in astrocytes: NKCC1 (Jayakumar and Norenberg, 2010) and the Cl−/ exchanger (Kimelberg, 1981). NKCC1 plays a major role in setting Cl− gradients in resting condition as well as during astrocytes swelling which occurs in pathological conditions such as brain edema, ischemia, and trauma (Chen et al., 2005). As the activity of NKCC1 is strongly stimulated by cell swelling (Mongin et al., 1994), a positive feedforward mechanism further contributes to cell volume increase. The K+/Cl− (KCC) cotransporter is also responsible for the maintenance and regulation of the cell volume of cultured astrocytes, but it is mainly involved in RVD (Ringel and Plesnila, 2008). The expression of these carriers in astrocytes in vivo is still controversial: whereas previous works failed to detect them (Plotkin et al., 1997; Clayton et al., 1998), one recent study reported the expression of NKCC1 and KCC1 mRNAs also in glial and ependymal cells (Kanaka et al., 2001), which is consistent with functional evidence (Tas et al., 1987). Indeed, application of GABA after pharmacological blockade of NKCC1 no longer depolarizes the membrane of astrocytes, since the outwardly directed Cl− gradient is dissipated (Kimelberg and Frangakis, 1985).
The role of GABAARs in astrocytes is still an open question. Interestingly, GABA signaling regulates the differentiation of immature astrocytes (Matsutani and Yamamoto, 1997; Mong et al., 2002). In the brain there is a positive correlation between the numbers of GABAergic axonal terminals and the expression of GFAP, suggesting that GABA released by neurons may promote GFAP expression and stellation of astrocytes. This hypothesis has been strengthened by the observation that increasing GABAergic signaling in vivo induces a striking modification of the structural organization of GFAP+ astrocytes, increasing the number of branches. The mechanism underlying these modifications is not known but it could be direct and mediated by the efflux of Cl− (Runquist and Alonso, 2003). We also showed that a systemic administration of diazepam increased the number of GFAP expressing cells in the SVZ of the lateral ventricle, where GFAP expression identifies both niche astrocytes and stem cells (Cesetti et al., 2010).
Similar to astrocytes, oligodendrocytes actively accumulate Cl− via NKCC1 and their [Cl−]i, measured with microelectrodes, is 2–3 mM higher than the one expected for a passive distribution (Hoppe and Kettenmann, 1989). Cumulative evidence indicates that a higher resting permeability accounts for the lower [Cl−]i observed in oligodendrocytes as compared to astrocytes. A resting Cl− conductance was detected in most cultured oligodendrocytes and the ECl (−61 mV) is only slightly more positive than the EM (−64 mV), in line with the observation that GABA depolarizes oligodendrocytes by 4 mV (Kettenmann et al., 1984). GABAergic currents were recorded in oligodendrocytes in brain slices in the corpus callosum (Berger et al., 1992) and in the hippocampus (Von Blankenfeld et al., 1991). However, GABAAR-mediated currents in oligodendrocytes are smaller than in astrocytes, probably depending on both a reduced expression of GABAARs and a smaller outwardly directed driving force for Cl−. It was first shown that oligodendrocytes in explant cultures of the spinal cord can be directly depolarized by the activation of GABAARs (Kettenmann et al., 1984). Later on it was shown that the mechanism underlying the depolarization is an efflux of Cl− via GABAARs (Wang et al., 2003). However, only a subpopulation of the oligodendrocytes investigated was GABA-sensitive, indicating heterogeneity in the cell population in culture or in its differentiation stage. Indeed, NG2+ OPCs have larger GABAergic currents compared to their differentiated counterpart: the density of GABAARs decreases by a factor 100 when OPCs in culture maturate along the oligodendrocytic lineage, indicating a developmental down-regulation of GABAAR expression (Von Blankenfeld et al., 1991). A difference in Cl− driving force could also contribute to the larger GABAergic currents in OPCs. In line with this, in OPCs of the adult neocortex [Cl−]i was estimated to be 45 mM and the ECl to be −30 mV (Tanaka et al., 2009). Furthermore, a robust expression of NKCC1 was reported in satellite NG2+ glial cells (Price et al., 2006). These developmental changes in the GABAergic signaling suggest that GABA may exert different physiological roles during oligodendrocytes lineage progression. Unlike astrocytes, GABAARs in OPCs are transiently activated by synaptically released GABA, since it was shown that in the hippocampus OPCs form real functional synapses with neuronal axons thereby sensing the activity of the dense inhibitory network surrounding them. Despite the depolarizing ECl, GABA regulates OPCs membrane excitability with a shunting effect on AMPA currents due to an increase in membrane conductance and alteration of [Cl]i (Lin and Bergles, 2004). In cultured oligodendrocytes muscimol promoted cell survival upon growth factor withdrawal by reducing [Cl−]i, which in turn induced cell shrinkage and a subsequent Ca2+ signal. NKCC1 activity was required for both effects, confirming the importance of NKCC1 for maintaining [Cl−]i above the electro-chemical gradient (Wang et al., 2003). Thus, in OPCs the signaling pathways downstream GABAAR involve alternative mechanisms such as shunting inhibition, modulation of transporter activity and control of ion homeostasis and cell volume.
In progenitors of the embryonic (E16) ventricular zone [Cl−]i has been estimated to be 37 mM, based on the measurement of an EGABA of −30 mV (see Box 1). The EGABA becomes progressively more negative during development reaching about −60 mV at P16. This gradient is dissipated upon pharmacological blockade of NKCC1 (Owens et al., 1996) or by the overexpression of exogenous KCC2 (Cancedda et al., 2007). The development of neuronal progenitors is ubiquitously characterized by changes in Cl− transporters expression. This was also observed in the neurogenic niche of the adult DG. The measurement of the EGABA in DG precursors at different stages of lineage progression revealed that at early stages of neuronal differentiation the EGABA is more positive than the EM. With the progressive maturation of granule neurons, EGABA gradually decreases to finally switch to values more negative than the EM. The estimated change in [Cl−]i during this process, i.e., from about 30–10 mM, is likely due to the fact that the pattern of cotransporter expression changes from high NKCC1/low KCC2 to low NKCC1/high KCC2 between the beginning and the end of this maturation period. Indeed, down-regulation of NKCC1 expression resulted in a shift of EGABA from depolarizing to hyperpolarizing at all time-points analyzed (Ge et al., 2006). However, even if depolarizing, in differentiating granule neurons GABAergic currents have a shunting effect (Overstreet Wadiche et al., 2005). Within the DG, GABA released by interneurons evokes currents not only in newborn neurons but also in progenitors, albeit there is still controversy over the developmental stage at which the switch from tonic to phasic currents occurs (Overstreet Wadiche et al., 2005; Tozuka et al., 2005; Wang et al., 2005; Ge et al., 2006).
In the postnatal SVZ, GABA elicits Cl− currents in neuroblasts and pre-neuroblasts and in two cell populations defined as stem cells on the basis of the expression of either Prominin (Cesetti et al., 2010) or GFAP (Wang et al., 2003; Liu et al., 2005). The largest currents were observed in neuroblasts (Wang et al., 2003; Cesetti et al., 2010). In these cells EGABA, estimated via gramicidin perforated whole-cell recording (see Box 1), is about −40 mV, which is more positive than their EM and corresponds to a [Cl−]i of 26.7 mM (Wang et al., 2003). Considering their very small size, the current density is also high (in vitro estimated about 190 pA/pF) and consistent with the very strong GABAAR immunoreactivity displayed by these cells (Cesetti et al., 2010). These huge GABAergic currents depolarize very efficiently neuroblasts (Wang et al., 2003), which have already a depolarized EM (−55 mV) and a very high RM (1–3 GΩ). Indeed, spontaneous depolarizations of 15–20 mV were previously observed in neuroblasts (Liu et al., 2005). It is very likely that GABA regulates the osmotic pressure in neuroblasts since GABAARs are present at high density and are tonically activated. Thus, a persistent Cl− efflux may affect the osmolarity of the intracellular milieu from such small cells.
In contrast to neuroblasts, the Cl− gradient of SVZ stem cells is still unclear. Compared to neuroblasts, neonatal Prominin+ precursors have a much smaller (30 times) density of GABAergic current (5 pA/pF), which likely reflects the differences in levels of receptor expression between the two populations (Cesetti et al., 2010). However, larger GABAergic currents were measured from precursor cells contacting each other in vitro, possibly due to electrical coupling. A similar phenomenon was previously observed in precursors of the embryonic ventricular zone, where GABA elicited small (around 5 pA) currents on isolated cells and almost 100 times larger currents in coupled cells (Owens et al., 1996). Although the exact [Cl−]i and the absolute value of EM are not known, using a voltage-sensitive dye (see Box 1) we showed that muscimol hyperpolarizes precursors, suggesting that GABAAR activation leads to Cl− influx in this cell population (Cesetti et al., 2010). Instead, in GFAP+ cells of the adult SVZ GABAAR activation elicited Ca2+ increases (Young et al., 2010). This indirectly suggests that, as in mature astrocytes, also in GFAP+ cells of the SVZ GABA has a depolarizing effect.
Osmotic swelling may affect brain physiology by different mechanisms. For example, it may change the ionic gradients, which are key for setting the resting membrane potential and the driving force of the ionic currents. It may modulate the extracellular concentration of neurotransmitters, either inducing their release or regulating the size of the extracellular space. Osmotic swelling can also represent a signaling mechanism, by activating different intracellular pathways. While the first two scenarios are more relevant to the regulation of systemic processes, for example water balance and synaptic transmission, the last one can transduce signals regulating cell growth and proliferation as it can modulate the activity of membrane and intracellular signaling molecules.
Below, we will suggest ways in which GABAAR activation can modulate these different processes and discuss a few studies that have indicated a physiological role for GABAAR-mediated osmotic regulation.
A few studies have indicated that GABAAR activation may affect brain water homeostasis. For example the anti-epileptic drug vigabatrin, which raises extracellular GABA levels by inhibiting the GABA degrading enzyme GABA transaminase, can cause swelling and loss of myelin (Sidhu et al., 1997), suggesting that excessive activation of GABAARs in the myelinating processes of oligodendrocytes may damage them. In one patient it was also found that cerebral edema induced by valproate overdose can be aggravated by diazepam (Rupasinghe and Jasinarachchi, 2011). Since conditions such as energy deprivation and brain trauma lead to a temporary increase in the concentration of extracellular GABA (Hagberg et al., 1985; Shimada et al., 1993), GABAAR-mediated osmotic regulation may also play a significant role in the regulation of water balance in pathological conditions. For neurons an excess of GABA may have contrasting consequences. On one hand, it will protect neurons by decreasing the membrane depolarization caused by glutamate. On the other hand, it will induce entry of Cl− ions through GABAARs, leading to water influx and cell swelling (Chen et al., 1999; Allen et al., 2004). Indeed, diazepam elicits opposite responses depending on the concentration and time of application (Ricci et al., 2007). Benzodiazepines can also ameliorate the effect of ammonia-induced swelling in vitro (Bender and Norenberg, 1998). Additionally, in an in vitro model of ischemic insult, the inhibitory effect of taurine on water gain required active GABAAR, indicating a potential involvement of GABAAR on osmotic regulation (Ricci et al., 2009). However these studies have neither investigated the direct target of the treatment nor elucidated the mechanisms underlying its effect and they cannot be used as evidence that GABAAR signaling plays a role in osmotic regulation. Also in astrocytes, the activation of GABAARs generates physiologically important changes in ion distribution. For example, GABAAR function in astrocytes may be important for the maintenance of the extracellular Cl− concentration in the vicinity of neuronal GABAergic synapses, to prevent the sink in [Cl−]o during high inhibitory activity. In addition, GABA modulates voltage-dependent K+ channels within astrocytes themselves, by depressing A-type outward K+-currents (Bekar and Walz, 2002). Such modulation of K+ conductance may have important consequences for the progression of spreading depression through the brain and for astrocytic swelling in pathological conditions. In contrast to neurons, activation of GABAARs in conditions of cytotoxic swelling may be beneficial to astrocytes since their is depolarizing. Therefore, the activation of GABAAR will decrease the intracellular osmolarity of these cells, thereby preventing cellular edema.
However, so far activation of GABAAR in astrocytes has not been directly associated to osmotic regulation. Moreover, although there is good evidence that following ischemic or traumatic brain injury the levels of ambient GABA increase, the rapid release of GABA is followed in various cases by a decrease in its synthesis (Green et al., 1992). Thus it remains still unclear whether GABAergic function is effectively increased in these conditions (Green et al., 2000). Therefore, the hypothesis that GABAAR contributes to the regulation of osmotic tension in the CNS needs experimental confirmation.
A functional connection between swelling and neural activity was indirectly suggested by the observation that the intake of water in healthy subjects leads to an increased synchronization of the spontaneous magnetoencephalogram during hyperventilation with an increase in the spectral power in all frequency bands (Muller et al., 2002). Moreover, hyposmolarity induces hyperexcitability and increases evoked epileptiform activity (Rosen and Andrew, 1990; Saly and Andrew, 1993). Neuron swelling occurs also in physiological conditions. For example, changes in tissue volume in the brain related to neuronal activity and cell swelling may occur in vivo in the human visual cortex (Darquie et al., 2001). In hippocampal slices, high frequency stimulation of the Schaffer collateral fibers increases the transmittance of the somatic and dendritic CA1 regions, in concomitance with the evoked postsynaptic field potential (fEPSP), indicating a relationship between tissue swelling and neuronal activity. GABA negatively modulates the generation of fEPSP and at the same time promotes swelling. Moreover, lowering the [Cl−]o to create an outwardly directed driving force for Cl− produces the opposite effect on transmittance, leading to the interpretation that neuronal swelling is mediated mainly by Cl− synaptic influx via GABAAR (Takagi et al., 2002). However, so far the change in neuronal volume has not been directly analyzed at the cellular level and it is still unknown to which extent GABA modulates cell volume during normal network activity. A functional consequence of the osmotic pressure generated by GABA may include the modulation of neuronal plasticity and the generation of an alternative way of signal propagation in a network of neurons and glia.
Prolonged activation of GABAARs, such as during high frequency activity of inter-neuronal network, induces in postnatal cerebellar interneurons an increase in [Ca2+]i, which can be observed up to 3 weeks after birth (Chavas et al., 2004). Since the membrane potential measured in these cells in such conditions is more negative than the activation threshold of VDCCs, it has been suggested that the increase in [Ca2+]i, is triggered by a Ca2+ influx independent from VDCCs and/or by a release from intracellular stores in response to the rising osmotic tension within the neuronal dendrites. According to this hypothesis, it was suggested that prolonged opening of GABAAR causes a persistent efflux, which in turn exerts a continuous depolarizing force favoring a large Cl− influx. As Cl− carriers correct variations in the [Cl−]i on a slow time scale, this large Cl− influxes cannot be immediately compensated and they lead to water entry and swelling. Consistently with this hypothesis, the application of a GABAAR agonist increases the dimension of the dendrites (Chavas et al., 2004). However, the exact link between osmotic tension and Ca2+ elevations remains so far unknown.
Despite the intense scrutiny, many aspects of the regulation of GABA on neurogenesis are still unclear. This concerns especially the downstream mechanisms involved, the role of this regulation in vivo and the differences associated to age and regional factors. The concept that GABA regulates neural progenitor behavior mainly via GABAAR-mediated depolarization and consequent activation of VDCCs has become widely accepted. However, considering the wide range of cell types within the stem cell niches and the relative differences in their GABAergic currents and in the source of GABA, it is likely that several downstream transduction mechanisms are involved. Moreover, often the conclusion that VDCCs are activated by GABAARs-mediated depolarization has been indirectly drawn from the observation that VDCC antagonists impact the physiological effect mediated by GABA. Although some studies have provided evidence that GABAARs activation depolarizes neural precursors in the embryonic and adult neurogenic niche (Owens et al., 1996; Liu et al., 2005; Young et al., 2010), it is often unclear whether this effect can be obtained at physiological concentrations of GABA, whether functional VDCCs are present and are activated by the GABA-induced depolarization. This analysis is further complicated by the technical challenge posed by the simultaneous measurement of membrane potential and Ca2+ signals without interfering with Cl− homeostasis, and by the identification of the different cell types within the adult neurogenic niche. By combining antigenic and functional characterization we have previously detected VDCCs in neuroblasts, but not in more immature precursor cells of the neonatal SVZ (Cesetti et al., 2009). Consistently, GABA evoked a rapid Ca2+ increase in neuroblasts but not in precursor cells (Cesetti et al., 2010). However, spontaneous Ca2+ signals occur in vitro both in embryonic (Ciccolini et al., 2003; Gakhar-Koppole et al., 2008) and in neonatal neural progenitors (Cesetti et al., 2009) and they can be modulated by GABA (Figure 3). GABAergic modulation of spontaneous Ca2+ signals was also observed in situ in GFAP expressing cells of the SVZ; these spontaneous Ca2+ signals depended on IP3 mediated Ca2+ release from the stores. In about half of these cells the GABAAR antagonist bicuculline reduced the frequency of the Ca2+ transients while in the other half it produced an increase (Young et al., 2010). Likewise, GABA evoked Ca2+ signals mediated by VDCCs only in half of the GFAP+ cells. Since all GFAP+ cells respond to GABA (Liu et al., 2005), these results underscore the heterogeneity of the GFAP+ cell population that, beside a small fraction of stem cells, mostly consists of niche astrocytes. They also suggest the involvement of different downstream signaling cascades in the GABAergic response.
Figure 3. GABAAR signaling promotes regular Ca2+ transients on proliferating neural precursors. Analysis of spontaneous Ca2+ transients in a population enriched in neural stem cells, purified by fluorescence activated cell sorting from the postnatal SVZ on the basis of the expression of high levels of EGFR (EGFRhigh cells; Ciccolini et al., 2005). After isolation the cells were plated on coverslips and maintained in medium containing EGF and FGF-2 for 2 days (Cesetti et al., 2009). Upon loading with the Ca2+ indicator fluo-3, in control conditions the EGFRhigh cells displayed a very low incidence of regular Ca2+ oscillation (regular oscillation defined as at least three spikes with similar amplitude and interspike interval within 15 min recording) (A,B). However the addition of GABA (100 μM) to the medium before (15 min) and during the imaging led to a dramatic increase in the incidence of the cells displaying regular Ca2+ oscillation (A,B), an effect that was blocked by the GABAAR bicuculline (50 μM) (A). In (B) three representative traces of Ca2+ changes are shown, respectively in control condition and upon GABA treatment, after background subtraction, and normalization to baseline values.
Indeed we have shown that in stem cells of the neonatal SVZ, identified according Prominin and GFAP expression, activation of GABAARs produces a Cl− influx which promotes osmotic swelling and insertion of epidermal growth factor receptor (EGFR) in the cell membrane. In the presence of EGF, this event leads to a change in the expression of the phosphatase and tensin homolog deleted on chromosome 10 (PTEN) tumor suppressor and Cyclin D1 and promotes cell cycle entry (Cesetti et al., 2010). Since osmotic swelling can spread through gap junction coupling, potentially this mechanism could synchronize the cell cycle of several progenitor cells (Cesetti et al., 2009). However, GABA evokes very small Cl− currents in these progenitor cells, raising the question of whether GABAAR activation alone is enough to cause swelling and stem cell proliferation in vivo. Supporting this possibility we found that a single intraperitoneal injection of diazepam promotes massive stem cell proliferation and increased EGFR expression (Cesetti et al., 2010). It is possible that the amplitude of GABAergic currents measured in vitro does not reflect the degree of activation in vivo. This could be due to the modulation of GABAAR by endogenous ligands such as neurosteroids. Indeed, using gene array analysis we have recently found that precursors express the E18-kDa translocator protein (Tspo) and the 11-kDa diazepam binding inhibitor, a polypeptide that is a well-characterized ligand for Tspo (Obernier and Ciccolini, unpublished observation). Activation of Tspo results in an increased production of the neurosteroid precursor pregnenolone that in turn potentiates GABAergic currents (Mellon and Griffin, 2002).
In embryonic and peripheral neural crest stem cells, activation of GABAAR also induced swelling associated to hyperpolarization, but it had an opposite effect on cell proliferation, as it led to the generation of phosphorylated histone γH2AX, which in turn inhibited cell cycle progression (Andang et al., 2008). It has been proposed that GABA prevents proliferation in the adult SVZ by a similar mechanism (Fernando et al., 2011). However, we found that activation of GABAAR in the neonatal SVZ did not lead to the generation of γH2AX (Cesetti et al., 2010), suggesting that the responses of neonatal and adult SVZ precursors to GABA may differ. Thus, in more primitive neural precursors GABAAR-mediated cell swelling plays an important role in proliferation control.
So far only a few studies have clearly indicated a role for GABAAR activation in the osmotic regulation of brain.
One possible reason of such limited number of observations is the difficulty to discriminate in vivo between the effect of GABA as neurotransmitter and as osmotic modulator due to the interdependency between neural activity and osmotic regulation in the brain. Another limiting factor may be the technical difficulties associated to the measurement of cell volume changes in situ or in vivo. The regulation of water and ion balance in the brain is crucial for normal functions and for recovery from pathological swelling. A constant redistribution of water occurs across the membrane of the different neural cell types, accompanying fluxes of ions and release and uptake of neurotransmitters. However the measurement of water diffusion/transport with cellular resolution is still technically challenging. Magnetic resonance imaging (MRI) permits the quantification of global or local increase in water content at specific anatomical location but without cell specificity. Only recently, with the development of two-photon laser scanning microscopy and of transgenic mice with intrinsic fluorescent neurons or glia, it has been possible to monitor real-time changes in cell volume in brain slice and in vivo with cellular and even subcellular resolution. However the molecular mechanism involved in cell volume changes and the downstream activated signaling have been mainly investigated in isolated cell systems which do not reflect the complexity of the situation in vivo. Although the picture is still fragmented and incomplete, new concepts start to emerge. GABA regulates not only inter-neuronal communication but also the communication between neurons and non-neuronal cells. GABAergic signaling between astrocytes and neurons can be bidirectional, with astrocytes sensing extrasynaptic GABA and releasing GABA and taurine upon osmotic challenge, providing a feedback mechanism of volume regulation. Oligodendrocytes instead receive a dedicated synaptic GABAergic input. Adult neural precursors in the DG and SVZ of the adult brain sense synaptic or ambient GABA, respectively.
Thus, although many questions are still open, recent evidence indicates that GABAAR-mediated osmotic regulation may have consequences at the cellular and at the systemic level. Therefore, GABAergic osmotic regulation should be taken into account during the treatment of pathologies requiring the administration of GABAAR modulators and for the development of therapies for diseases causing water unbalance in the brain.
The authors declare that the research was conducted in the absence of any commercial or financial relationships that could be construed as a potential conflict of interest.
We would like to thank Prof. A. Draguhn for critical reading of the manuscript.
Allen, N. J., Rossi, D. J., and Attwell, D. (2004). Sequential release of GABA by exocytosis and reversed uptake leads to neuronal swelling in simulated ischemia of hippocampal slices. J. Neurosci. 24, 3837–3849.
Amiry-Moghaddam, M., Williamson, A., Palomba, M., Eid, T., de Lanerolle, N. C., Nagelhus, E. A., Adams, M. E., Froehner, S. C., Agre, P., and Ottersen, O. P. (2003). Delayed K+ clearance associated with aquaporin-4 mislocalization: phenotypic defects in brains of alpha-syntrophin-null mice. Proc. Natl. Acad. Sci. U.S.A. 100, 13615–13620.
Andang, M., Hjerling-Leffler, J., Moliner, A., Lundgren, T. K., Castelo-Branco, G., Nanou, E., Pozas, E., Bryja, V., Halliez, S., Nishimaru, H., Wilbertz, J., Arenas, E., Koltzenburg, M., Charnay, P., El Manira, A., Ibanez, C. F., and Ernfors, P. (2008). Histone H2AX-dependent GABA(A) receptor regulation of stem cell proliferation. Nature 451, 460–464.
Andrew, R. D., Labron, M. W., Boehnke, S. E., Carnduff, L., and Kirov, S. A. (2007). Physiological evidence that pyramidal neurons lack functional water channels. Cereb. Cortex 17, 787–802.
Andrew, R. D., and MacVicar, B. A. (1994). Imaging cell volume changes and neuronal excitation in the hippocampal slice. Neuroscience 62, 371–383.
Angulo, M. C., Le Meur, K., Kozlov, A. S., Charpak, S., and Audinat, E. (2008). GABA, a forgotten gliotransmitter. Prog. Neurobiol. 86, 297–303.
Ballanyi, K., Grafe, P., and ten Bruggencate, G. (1987). Ion activities and potassium uptake mechanisms of glial cells in guinea-pig olfactory cortex slices. J. Physiol. (Lond.) 382, 159–174.
Barakat, L., and Bordey, A. (2002). GAT-1 and reversible GABA transport in Bergmann glia in slices. J. Neurophysiol. 88, 1407–1419.
Bardakdjian, J., Tardy, M., Pimoule, C., and Gonnard, P. (1979). GABA metabolism in cultured glial cells. Neurochem. Res. 4, 517–527.
Barres, B. A., Koroshetz, W. J., Swartz, K. J., Chun, L. L., and Corey, D. P. (1990). Ion channel expression by white matter glia: the O-2A glial progenitor cell. Neuron 4, 507–524.
Behar, T. N., Schaffner, A. E., Scott, C. A., O’Connell, C., and Barker, J. L. (1998). Differential response of cortical plate and ventricular zone cells to GABA as a migration stimulus. J. Neurosci. 18, 6378–6387.
Bekar, L. K., Jabs, R., and Walz, W. (1999). GABAA receptor agonists modulate K+ currents in adult hippocampal glial cells in situ. Glia 26, 129–138.
Bekar, L. K., and Walz, W. (2002). Intracellular chloride modulates A-type potassium currents in astrocytes. Glia 39, 207–216.
Benagiano, V., Flace, P., Virgintino, D., Rizzi, A., Roncali, L., and Ambrosi, G. (2000). Immunolocalization of glutamic acid decarboxylase in postmortem human cerebellar cortex. A light microscopy study. Histochem. Cell Biol. 114, 191–195.
Ben-Ari, Y., Cherubini, E., Corradetti, R., and Gaiarsa, J. L. (1989). Giant synaptic potentials in immature rat CA3 hippocampal neurones. J. Physiol. (Lond.) 416, 303–325.
Bender, A. S., and Norenberg, M. D. (1998). Effect of benzodiazepines and neurosteroids on ammonia-induced swelling in cultured astrocytes. J. Neurosci. Res. 54, 673–680.
Benfenati, V., and Ferroni, S. (2010). Water transport between CNS compartments: functional and molecular interactions between aquaporins and ion channels. Neuroscience 168, 926–940.
Berger, T., Walz, W., Schnitzer, J., and Kettenmann, H. (1992). GABA- and glutamate-activated currents in glial cells of the mouse corpus callosum slice. J. Neurosci. Res. 31, 21–27.
Bevensee, M. O., Apkon, M., and Boron, W. F. (1997). Intracellular pH regulation in cultured astrocytes from rat hippocampus. II. Electrogenic Na/HCO3 cotransport. J. Gen. Physiol. 110, 467–483.
Binder, D. K., Yao, X., Zador, Z., Sick, T. J., Verkman, A. S., and Manley, G. T. (2006). Increased seizure duration and slowed potassium kinetics in mice lacking aquaporin-4 water channels. Glia 53, 631–636.
Blaesse, P., Airaksinen, M. S., Rivera, C., and Kaila, K. (2009). Cation-chloride cotransporters and neuronal function. Neuron 61, 820–838.
Blomqvist, A., and Broman, J. (1988). Light and electron microscopic immunohistochemical demonstration of GABA-immunoreactive astrocytes in the brain stem of the rat. J. Neurocytol. 17, 629–637.
Bormann, J., and Kettenmann, H. (1988). Patch-clamp study of gamma-aminobutyric acid receptor Cl- channels in cultured astrocytes. Proc. Natl. Acad. Sci. U.S.A. 85, 9336–9340.
Brooks, H. L., Regan, J. W., and Yool, A. J. (2000). Inhibition of aquaporin-1 water permeability by tetraethylammonium: involvement of the loop E pore region. Mol. Pharmacol. 57, 1021–1026.
Brunig, I., Suter, A., Knuesel, I., Luscher, B., and Fritschy, J. M. (2002). GABAergic terminals are required for postsynaptic clustering of dystrophin but not of GABA(A) receptors and gephyrin. J. Neurosci. 22, 4805–4813.
Cancedda, L., Fiumelli, H., Chen, K., and Poo, M. M. (2007). Excitatory GABA action is essential for morphological maturation of cortical neurons in vivo. J. Neurosci. 27, 5224–5235.
Carmosino, M., Procino, G., Tamma, G., Mannucci, R., Svelto, M., and Valenti, G. (2007). Trafficking and phosphorylation dynamics of AQP4 in histamine-treated human gastric cells. Biol. Cell 99, 25–36.
Cavazzin, C., Ferrari, D., Facchetti, F., Russignan, A., Vescovi, A. L., La Porta, C. A., and Gritti, A. (2006). Unique expression and localization of aquaporin-4 and aquaporin-9 in murine and human neural stem cells and in their glial progeny. Glia 53, 167–181.
Cesetti, T., Fila, T., Obernier, K., Bengtson, C. P., Li, Y., Mandl, C., Holzl-Wenig, G., and Ciccolini, F. (2010). GABA(A) receptor signalling induces osmotic swelling and cell cycle activation of neonatal prominin(+) precursors. Stem Cells 29, 307–319.
Cesetti, T., Obernier, K., Bengtson, C. P., Fila, T., Mandl, C., Holzl-Wenig, G., Worner, K., Eckstein, V., and Ciccolini, F. (2009). Analysis of stem cell lineage progression in the neonatal subventricular zone identifies EGFR+/NG2− cells as transit-amplifying precursors. Stem Cells 27, 1443–1454.
Chavas, J., Forero, M. E., Collin, T., Llano, I., and Marty, A. (2004). Osmotic tension as a possible link between GABA(A) receptor activation and intracellular calcium elevation. Neuron 44, 701–713.
Chebib, M., and Johnston, G. A. (1999). The ‘ABC’ of GABA receptors: a brief review. Clin. Exp. Pharmacol. Physiol. 26, 937–940.
Chen, H., Luo, J., Kintner, D. B., Shull, G. E., and Sun, D. (2005). Na(+)-dependent chloride transporter (NKCC1)-null mice exhibit less gray and white matter damage after focal cerebral ischemia. J. Cereb. Blood Flow Metab. 25, 54–66.
Chen, Q., Moulder, K., Tenkova, T., Hardy, K., Olney, J. W., and Romano, C. (1999). Excitotoxic cell death dependent on inhibitory receptor activation. Exp. Neurol. 160, 215–225.
Ciccolini, F., Collins, T. J., Sudhoelter, J., Lipp, P., Berridge, M. J., and Bootman, M. D. (2003). Local and global spontaneous calcium events regulate neurite outgrowth and onset of GABAergic phenotype during neural precursor differentiation. J. Neurosci. 23, 103–111.
Ciccolini, F., Mandl, C., Holzl-Wenig, G., Kehlenbach, A., and Hellwig, A. (2005). Prospective isolation of late development multipotent precursors whose migration is promoted by EGFR. Dev. Biol. 284, 112–125.
Clayton, G. H., Owens, G. C., Wolff, J. S., and Smith, R. L. (1998). Ontogeny of cation-Cl- cotransporter expression in rat neocortex. Brain Res. Dev. Brain Res. 109, 281–292.
Darquie, A., Poline, J. B., Poupon, C., Saint-Jalmes, H., and Le Bihan, D. (2001). Transient decrease in water diffusion observed in human occipital cortex during visual stimulation. Proc. Natl. Acad. Sci. U.S.A. 98, 9391–9395.
Deng, B., Glanzman, D., and Tidball, J. G. (2009). Nitric oxide generated by muscle corrects defects in hippocampal neurogenesis and neural differentiation caused by muscular dystrophy. J. Physiol. (Lond.) 587(Pt 8), 1769–1778.
Fernando, R. N., Eleuteri, B., Abdelhady, S., Nussenzweig, A., Andang, M., and Ernfors, P. (2011). Cell cycle restriction by histone H2AX limits proliferation of adult neural stem cells. Proc. Natl. Acad. Sci. U.S.A. 108, 5837–5842.
Frazao, R., Nogueira, M. I., and Wassle, H. (2007). Colocalization of synaptic GABA(C)-receptors with GABA (A)-receptors and glycine-receptors in the rodent central nervous system. Cell Tissue Res. 330, 1–15.
Frigeri, A., Nicchia, G. P., Nico, B., Quondamatteo, F., Herken, R., Roncali, L., and Svelto, M. (2001). Aquaporin-4 deficiency in skeletal muscle and brain of dystrophic mdx mice. FASEB J. 15, 90–98.
Fukui, M., Nakamichi, N., Yoneyama, M., Ozawa, S., Fujimori, S., Takahata, Y., Nakamura, N., Taniura, H., and Yoneda, Y. (2008). Modulation of cellular proliferation and differentiation through GABA(B) receptors expressed by undifferentiated neural progenitor cells isolated from fetal mouse brain. J. Cell. Physiol. 216, 507–519.
Gakhar-Koppole, N., Bengtson, C. P., Parlato, R., Horsch, K., Eckstein, V., and Ciccolini, F. (2008). Depolarization promotes GAD 65-mediated GABA synthesis by a post-translational mechanism in neural stem cell-derived neurons. Eur. J. Neurosci. 27, 269–283.
Gallo, V., Patrizio, M., and Levi, G. (1991). GABA release triggered by the activation of neuron-like non-NMDA receptors in cultured type 2 astrocytes is carrier-mediated. Glia 4, 245–255.
Ge, S., Goh, E. L., Sailor, K. A., Kitabatake, Y., Ming, G. L., and Song, H. (2006). GABA regulates synaptic integration of newly generated neurons in the adult brain. Nature 439, 589–593.
Green, A. R., Cross, A. J., Snape, M. F., and De Souza, R. J. (1992). The immediate consequences of middle cerebral artery occlusion on GABA synthesis in mouse cortex and cerebellum. Neurosci. Lett. 138, 141–144.
Green, A. R., Hainsworth, A. H., and Jackson, D. M. (2000). GABA potentiation: a logical pharmacological approach for the treatment of acute ischaemic stroke. Neuropharmacology 39, 1483–1494.
Hagberg, H., Lehmann, A., Sandberg, M., Nystrom, B., Jacobson, I., and Hamberger, A. (1985). Ischemia-induced shift of inhibitory and excitatory amino acids from intra- to extracellular compartments. J. Cereb. Blood Flow Metab. 5, 413–419.
Hamann, S., Herrera-Perez, J. J., Bundgaard, M., Alvarez-Leefmans, F. J., and Zeuthen, T. (2005). Water permeability of Na+-K+-2Cl− cotransporters in mammalian epithelial cells. J. Physiol. (Lond.) 568(Pt 1), 123–135.
Hatakeyama, S., Yoshida, Y., Tani, T., Koyama, Y., Nihei, K., Ohshiro, K., Kamiie, J. I., Yaoita, E., Suda, T., Hatakeyama, K., and Yamamoto, T. (2001). Cloning of a new aquaporin (AQP10) abundantly expressed in duodenum and jejunum. Biochem. Biophys. Res. Commun. 287, 814–819.
Holthoff, K., and Witte, O. W. (1996). Intrinsic optical signals in rat neocortical slices measured with near-infrared dark-field microscopy reveal changes in extracellular space. J. Neurosci. 16, 2740–2749.
Hoppe, D., and Kettenmann, H. (1989). Carrier-mediated Cl- transport in cultured mouse oligodendrocytes. J. Neurosci. Res. 23, 467–475.
Hosli, L., Andres, P. F., and Hosli, E. (1978). Neuron-glia interactions: indirect effect of GABA on cultured glial cells. Exp. Brain Res. 33, 425–434.
Hosli, L., Hosli, E., Andres, P. F., and Landolt, H. (1981). Evidence that the depolarization of glial cells by inhibitory amino acids is caused by an efflux of K+ from neurones. Exp. Brain Res. 42, 43–48.
Jayakumar, A. R., and Norenberg, M. D. (2010). The Na-K-Cl co-transporter in astrocyte swelling. Metab. Brain Dis. 25, 31–38.
Kahle, K. T., Simard, J. M., Staley, K. J., Nahed, B. V., Jones, P. S., and Sun, D. (2009). Molecular mechanisms of ischemic cerebral edema: role of electroneutral ion transport. Physiology (Bethesda) 24, 257–265.
Kanaka, C., Ohno, K., Okabe, A., Kuriyama, K., Itoh, T., Fukuda, A., and Sato, K. (2001). The differential expression patterns of messenger RNAs encoding K-Cl cotransporters (KCC1,2) and Na-K-2Cl cotransporter (NKCC1) in the rat nervous system. Neuroscience 104, 933–946.
Kettenmann, H., Backus, K. H., and Schachner, M. (1987). Gamma-aminobutyric acid opens Cl-channels in cultured astrocytes. Brain Res. 404, 1–9.
Kettenmann, H., Gilbert, P., and Schachner, M. (1984). Depolarization of cultured oligodendrocytes by glutamate and GABA. Neurosci. Lett. 47, 271–276.
Kimelberg, H. K. (1981). Active accumulation and exchange transport of chloride in astroglial cells in culture. Biochim. Biophys. Acta 646, 179–184.
Kimelberg, H. K., and Frangakis, M. V. (1985). Furosemide- and bumetanide-sensitive ion transport and volume control in primary astrocyte cultures from rat brain. Brain Res. 361, 125–134.
Koch, U., and Magnusson, A. K. (2009). Unconventional GABA release: mechanisms and function. Curr. Opin. Neurobiol. 19, 305–310.
Kong, H., Fan, Y., Xie, J., Ding, J., Sha, L., Shi, X., Sun, X., and Hu, G. (2008). AQP4 knockout impairs proliferation, migration and neuronal differentiation of adult neural stem cells. J. Cell. Sci. 121(Pt 24), 4029–4036.
Kozlov, A. S., Angulo, M. C., Audinat, E., and Charpak, S. (2006). Target cell-specific modulation of neuronal activity by astrocytes. Proc. Natl. Acad. Sci. U.S.A. 103, 10058–10063.
Kuner, T., and Augustine, G. J. (2000). A genetically encoded ratiometric indicator for chloride: capturing chloride transients in cultured hippocampal neurons. Neuron 27, 447–459.
Lee, S., Yoon, B. E., Berglund, K., Oh, S. J., Park, H., Shin, H. S., Augustine, G. J., and Lee, C. J. (2010). Channel-mediated tonic GABA release from glia. Science 330, 790–796.
Lin, S. C., and Bergles, D. E. (2004). Synaptic signaling between GABAergic interneurons and oligodendrocyte precursor cells in the hippocampus. Nat. Neurosci. 7, 24–32.
Liu, X., Wang, Q., Haydar, T. F., and Bordey, A. (2005). Nonsynaptic GABA signaling in postnatal subventricular zone controls proliferation of GFAP-expressing progenitors. Nat. Neurosci. 8, 1179–1187.
MacAulay, N., Gether, U., Klaerke, D. A., and Zeuthen, T. (2001). Water transport by the human Na+-coupled glutamate cotransporter expressed in Xenopus oocytes. J. Physiol. (Lond.) 530(Pt 3), 367–378.
MacAulay, N., Zeuthen, T., and Gether, U. (2002). Conformational basis for the Li(+)-induced leak current in the rat gamma-aminobutyric acid (GABA) transporter-1. J. Physiol. (Lond.) 544(Pt 2), 447–458.
MacVicar, B. A., and Hochman, D. (1991). Imaging of synaptically evoked intrinsic optical signals in hippocampal slices. J. Neurosci. 11, 1458–1469.
MacVicar, B. A., Tse, F. W., Crichton, S. A., and Kettenmann, H. (1989). GABA-activated Cl- channels in astrocytes of hippocampal slices. J. Neurosci. 9, 3577–3583.
Maric, D., Maric, I., and Barker, J. L. (2000). Dual video microscopic imaging of membrane potential and cytosolic calcium of immunoidentified embryonic rat cortical cells. Methods 21, 335–347.
Markwardt, S. J., Wadiche, J. I., and Overstreet-Wadiche, L. S. (2009). Input-specific GABAergic signaling to newborn neurons in adult dentate gyrus. J. Neurosci. 29, 15063–15072.
Martinez-Rodriguez, R., Tonda, A., Gragera, R. R., Paz-Doel, R., Garcia-Cordovilla, R., Fernandez-Fernandez, E., Fernandez, A. M., Gonzalez-Romero, F., and Lopez-Bravo, A. (1993). Synaptic and non-synaptic immunolocalization of GABA and glutamate acid decarboxylase (GAD) in cerebellar cortex of rat. Cell. Mol. Biol. (Noisy-le-grand) 39, 115–123.
Matsutani, S., and Yamamoto, N. (1997). Neuronal regulation of astrocyte morphology in vitro is mediated by GABAergic signaling. Glia 20, 1–9.
McGann, L. E., Walterson, M. L., and Hogg, L. M. (1988). Light scattering and cell volumes in osmotically stressed and frozen-thawed cells. Cytometry 9, 33–38.
Mellon, S. H., and Griffin, L. D. (2002). Neurosteroids: biochemistry and clinical significance. Trends Endocrinol. Metab. 13, 35–43.
Mong, J. A., Nunez, J. L., and McCarthy, M. M. (2002). GABA mediates steroid-induced astrocyte differentiation in the neonatal rat hypothalamus. J. Neuroendocrinol. 14, 45–55.
Mongin, A. A., Aksentsev, S. L., Orlov, S. N., Slepko, N. G., Kozlova, M. V., Maximov, G. V., and Konev, S. V. (1994). Swelling-induced K+ influx in cultured primary astrocytes. Brain Res. 655, 110–114.
Muller, V., Birbaumer, N., Preissl, H., Braun, C., and Lang, F. (2002). Effects of water on cortical excitability in humans. Eur. J. Neurosci. 15, 528–538.
Mutoh, H., Perron, A., Akemann, W., Iwamoto, Y., and Knopfel, T. (2011). Optogenetic monitoring of membrane potentials. Exp. Physiol. 96, 13–18.
Nagelhus, E. A., Mathiisen, T. M., and Ottersen, O. P. (2004). Aquaporin-4 in the central nervous system: cellular and subcellular distribution and coexpression with KIR4.1. Neuroscience 129, 905–913.
Nicchia, G. P., Rossi, A., Nudel, U., Svelto, M., and Frigeri, A. (2008). Dystrophin-dependent and -independent AQP4 pools are expressed in the mouse brain. Glia 56, 869–876.
Nielsen, S., King, L. S., Christensen, B. M., and Agre, P. (1997). Aquaporins in complex tissues. II. Subcellular distribution in respiratory and glandular tissues of rat. Am. J. Physiol. 273(5 Pt 1), C1549–C1561.
Oreskovic, D., and Klarica, M. (2010). The formation of cerebrospinal fluid: nearly a hundred years of interpretations and misinterpretations. Brain Res. Rev. 64, 241–262.
Overstreet Wadiche, L., Bromberg, D. A., Bensen, A. L., and Westbrook, G. L. (2005). GABAergic signaling to newborn neurons in dentate gyrus. J. Neurophysiol. 94, 4528–4532.
Owens, D. F., Boyce, L. H., Davis, M. B., and Kriegstein, A. R. (1996). Excitatory GABA responses in embryonic and neonatal cortical slices demonstrated by gramicidin perforated-patch recordings and calcium imaging. J. Neurosci. 16, 6414–6423.
Papadopoulos, M. C., and Verkman, A. S. (2005). Aquaporin-4 gene disruption in mice reduces brain swelling and mortality in pneumococcal meningitis. J. Biol. Chem. 280, 13906–13912.
Pasantes-Morales, H., and Tuz, K. (2006). Volume changes in neurons: hyperexcitability and neuronal death. Contrib. Nephrol. 152, 221–240.
Pastor, A., Chvatal, A., Sykova, E., and Kettenmann, H. (1995). Glycine- and GABA-activated currents in identified glial cells of the developing rat spinal cord slice. Eur. J. Neurosci. 7, 1188–1198.
Plotkin, M. D., Kaplan, M. R., Peterson, L. N., Gullans, S. R., Hebert, S. C., and Delpire, E. (1997). Expression of the Na(+)-K(+)-2Cl- cotransporter BSC2 in the nervous system. Am. J. Physiol. 272(1 Pt 1), C173–C183.
Price, T. J., Hargreaves, K. M., and Cervero, F. (2006). Protein expression and mRNA cellular distribution of the NKCC1 cotransporter in the dorsal root and trigeminal ganglia of the rat. Brain Res. 1112, 146–158.
Rash, J. E., Yasumura, T., Hudson, C. S., Agre, P., and Nielsen, S. (1998). Direct immunogold labeling of aquaporin-4 in square arrays of astrocyte and ependymocyte plasma membranes in rat brain and spinal cord. Proc. Natl. Acad. Sci. U.S.A. 95, 11981–11986.
Redzic, Z. (2011). Molecular biology of the blood-brain and the blood-cerebrospinal fluid barriers: similarities and differences. Fluids Barriers CNS 8, 3.
Ricci, L., Valoti, M., Sgaragli, G., and Frosini, M. (2007). Neuroprotection afforded by diazepam against oxygen/glucose deprivation-induced injury in rat cortical brain slices. Eur. J. Pharmacol. 561, 80–84.
Ricci, L., Valoti, M., Sgaragli, G., and Frosini, M. (2009). Protection by taurine of rat brain cortical slices against oxygen glucose deprivation- and reoxygenation-induced damage. Eur. J. Pharmacol. 621, 26–32.
Ringel, F., and Plesnila, N. (2008). Expression and functional role of potassium-chloride cotransporters (KCC) in astrocytes and C6 glioma cells. Neurosci. Lett. 442, 219–223.
Risher, W. C., Andrew, R. D., and Kirov, S. A. (2009). Real-time passive volume responses of astrocytes to acute osmotic and ischemic stress in cortical slices and in vivo revealed by two-photon microscopy. Glia 57, 207–221.
Rosen, A. S., and Andrew, R. D. (1990). Osmotic effects upon excitability in rat neocortical slices. Neuroscience 38, 579–590.
Runquist, M., and Alonso, G. (2003). Gabaergic signaling mediates the morphological organization of astrocytes in the adult rat forebrain. Glia 41, 137–151.
Rupasinghe, J., and Jasinarachchi, M. (2011). Progressive encephalopathy with cerebral oedema and infarctions associated with valproate and diazepam overdose. J. Clin. Neurosci. 18, 710–711.
Saadoun, S., Papadopoulos, M. C., Davies, D. C., Bell, B. A., and Krishna, S. (2002). Increased aquaporin 1 water channel expression in human brain tumours. Br. J. Cancer 87, 621–623.
Saadoun, S., Papadopoulos, M. C., Watanabe, H., Yan, D., Manley, G. T., and Verkman, A. S. (2005). Involvement of aquaporin-4 in astroglial cell migration and glial scar formation. J. Cell. Sci. 118(Pt 24), 5691–5698.
Saly, V., and Andrew, R. D. (1993). CA3 neuron excitation and epileptiform discharge are sensitive to osmolality. J. Neurophysiol. 69, 2200–2208.
Sequerra, E. B., Gardino, P., Hedin-Pereira, C., and de Mello, F. G. (2007). Putrescine as an important source of GABA in the postnatal rat subventricular zone. Neuroscience 146, 489–493.
Shimada, N., Graf, R., Rosner, G., and Heiss, W. D. (1993). Ischemia-induced accumulation of extracellular amino acids in cerebral cortex, white matter, and cerebrospinal fluid. J. Neurochem. 60, 66–71.
Sidhu, R. S., Del Bigio, M. R., Tuor, U. I., and Seshia, S. S. (1997). Low-dose vigabatrin (gamma-vinyl GABA)-induced damage in the immature rat brain. Exp. Neurol. 144, 400–405.
Solenov, E., Watanabe, H., Manley, G. T., and Verkman, A. S. (2004). Sevenfold-reduced osmotic water permeability in primary astrocyte cultures from AQP-4-deficient mice, measured by a fluorescence quenching method. Am. J. Physiol. Cell Physiol. 286, C426–C432.
Soltesz, I., and Mody, I. (1994). Patch-clamp recordings reveal powerful GABAergic inhibition in dentate hilar neurons. J. Neurosci. 14, 2365–2376.
Steinhauser, C., Berger, T., Frotscher, M., and Kettenmann, H. (1992). Heterogeneity in the Membrane current pattern of identified glial cells in the hippocampal slice. Eur. J. Neurosci. 4, 472–484.
Stewart, R. R., Hoge, G. J., Zigova, T., and Luskin, M. B. (2002). Neural progenitor cells of the neonatal rat anterior subventricular zone express functional GABA(A) receptors. J. Neurobiol. 50, 305–322.
Strange, K. (1993). Maintenance of cell volume in the central nervous system. Pediatr. Nephrol. 7, 689–697.
Takagi, S., Obata, K., and Tsubokawa, H. (2002). GABAergic input contributes to activity-dependent change in cell volume in the hippocampal CA1 region. Neurosci. Res. 44, 315–324.
Tanaka, Y., Tozuka, Y., Takata, T., Shimazu, N., Matsumura, N., Ohta, A., and Hisatsune, T. (2009). Excitatory GABAergic activation of cortical dividing glial cells. Cereb. Cortex 19, 2181–2195.
Taniguchi, M., Yamashita, T., Kumura, E., Tamatani, M., Kobayashi, A., Yokawa, T., Maruno, M., Kato, A., Ohnishi, T., Kohmura, E., Tohyama, M., and Yoshimine, T. (2000). Induction of aquaporin-4 water channel mRNA after focal cerebral ischemia in rat. Brain Res. Mol. Brain Res. 78, 131–137.
Tas, P. W., Massa, P. T., Kress, H. G., and Koschel, K. (1987). Characterization of an Na+/K+/Cl- co-transport in primary cultures of rat astrocytes. Biochim. Biophys. Acta 903, 411–416.
Tozuka, Y., Fukuda, S., Namba, T., Seki, T., and Hisatsune, T. (2005). GABAergic excitation promotes neuronal differentiation in adult hippocampal progenitor cells. Neuron 47, 803–815.
Tuz, K., Pena-Segura, C., Franco, R., and Pasantes-Morales, H. (2004). Depolarization, exocytosis and amino acid release evoked by hyposmolarity from cortical synaptosomes. Eur. J. Neurosci. 19, 916–924.
Tyzio, R., Cossart, R., Khalilov, I., Minlebaev, M., Hubner, C. A., Represa, A., Ben-Ari, Y., and Khazipov, R. (2006). Maternal oxytocin triggers a transient inhibitory switch in GABA signaling in the fetal brain during delivery. Science 314, 1788–1792.
Velez-Fort, M., Audinat, E., and Angulo, M. C. (2011). Central role of GABA in neuron-glia interactions. Neuroscientist. doi: 10.1177/1073858411403317. [Epub ahead of print]
Venero, J. L., Vizuete, M. L., Machado, A., and Cano, J. (2001). Aquaporins in the central nervous system. Prog. Neurobiol. 63, 321–336.
Verkman, A. S. (2000). Physiological importance of aquaporins: lessons from knockout mice. Curr. Opin. Nephrol. Hypertens. 9, 517–522.
Verkman, A. S. (2005). More than just water channels: unexpected cellular roles of aquaporins. J. Cell. Sci. 118(Pt 15), 3225–3232.
Vizuete, M. L., Venero, J. L., Vargas, C., Ilundain, A. A., Echevarria, M., Machado, A., and Cano, J. (1999). Differential upregulation of aquaporin-4 mRNA expression in reactive astrocytes after brain injury: potential role in brain edema. Neurobiol. Dis. 6, 245–258.
Von Blankenfeld, G., Trotter, J., and Kettenmann, H. (1991). Expression and developmental regulation of a GABAA receptor in cultured murine cells of the oligodendrocyte lineage. Eur. J. Neurosci. 3, 310–316.
Walz, W., and Wuttke, W. A. (1999). Independent mechanisms of potassium clearance by astrocytes in gliotic tissue. J. Neurosci. Res. 56, 595–603.
Wang, H., Yan, Y., Kintner, D. B., Lytle, C., and Sun, D. (2003). GABA-mediated trophic effect on oligodendrocytes requires Na-K-2Cl cotransport activity. J. Neurophysiol. 90, 1257–1265.
Wang, L. P., Kempermann, G., and Kettenmann, H. (2005). A subpopulation of precursor cells in the mouse dentate gyrus receives synaptic GABAergic input. Mol. Cell. Neurosci. 29, 181–189.
Wen, H., Nagelhus, E. A., Amiry-Moghaddam, M., Agre, P., Ottersen, O. P., and Nielsen, S. (1999). Ontogeny of water transport in rat brain: postnatal expression of the aquaporin-4 water channel. Eur. J. Neurosci. 11, 935–945.
Williamson, A. V., Mellor, J. R., Grant, A. L., and Randall, A. D. (1998). Properties of GABA(A) receptors in cultured rat oligodendrocyte progenitor cells. Neuropharmacology 37, 859–873.
Wu, P. H., Durden, D. A., and Hertz, L. (1979). Net production of gamma-aminobutyric acid in astrocytes in primary cultures determined by a sensitive mass spectrometric method. J. Neurochem. 32, 379–390.
Young, S. Z., Platel, J. C., Nielsen, J. V., Jensen, N. A., and Bordey, A. (2010). GABA(A) Increases calcium in subventricular zone astrocyte-like cells through L- and T-type voltage-gated calcium channels. Front. Cell. Neurosci. 4:8. doi:10.3389/fncel.2010.00008
Zador, Z., Bloch, O., Yao, X., and Manley, G. T. (2007). Aquaporins: role in cerebral edema and brain water balance. Prog. Brain Res. 161, 185–194.
Zelenina, M., Zelenin, S., Bondar, A. A., Brismar, H., and Aperia, A. (2002). Water permeability of aquaporin-4 is decreased by protein kinase C and dopamine. Am. J. Physiol. Renal Physiol. 283, F309–F318.
Zeuthen, T. (1994). Cotransport of K+, Cl- and H2O by membrane proteins from choroid plexus epithelium of Necturus maculosus. J. Physiol. (Lond.) 478(Pt 2), 203–219.
Keywords: GABA, GABAAR, osmotic swelling, chloride, cell volume
Citation: Cesetti T, Ciccolini F and Li Y (2012) GABA not only a neurotransmitter: osmotic regulation by GABAAR signaling. Front. Cell. Neurosci. 6:3. doi: 10.3389/fncel.2012.00003
Received: 30 September 2011;
Accepted: 10 January 2012;
Published online: 30 January 2012.
Edited by:
Yehezkel Ben-Ari, Institut National de la Santé et de la Recherche Médicale, FranceReviewed by:
Valentin Nägerl, Université de Bordeaux, FranceCopyright: © 2012 Cesetti, Ciccolini and Li. This is an open-access article distributed under the terms of the Creative Commons Attribution Non Commercial License, which permits non-commercial use, distribution, and reproduction in other forums, provided the original authors and source are credited.
*Correspondence: Francesca Ciccolini, Department of Neurobiology, Interdisciplinary Center for Neurosciences, University of Heidelberg, INF 364 Heidelberg, Germany. e-mail:Y2ljY29saW5pQG5iaW8udW5pLWhlaWRlbGJlcmcuZGU=
Disclaimer: All claims expressed in this article are solely those of the authors and do not necessarily represent those of their affiliated organizations, or those of the publisher, the editors and the reviewers. Any product that may be evaluated in this article or claim that may be made by its manufacturer is not guaranteed or endorsed by the publisher.
Research integrity at Frontiers
Learn more about the work of our research integrity team to safeguard the quality of each article we publish.