- 1 Department of Pharmacology and Physiology, Georgetown University School of Medicine, Washington, DC, USA
- 2 Interdisciplinary Program in Neuroscience, Georgetown University School of Medicine, Washington, DC, USA
The striatum is a key structure for movement control, but the mechanisms that dictate the output of distinct subpopulations of medium spiny projection neurons (MSNs), striatonigral projecting and dopamine D1 receptor- (D1+) or striatopallidal projecting and dopamine D2 receptor- (D2+) expressing neurons, remains poorly understood. GABA-mediated tonic inhibition largely controls neuronal excitability and action potential firing rates, and we previously suggested with pharmacological analysis that the GABAA receptor β3 subunit plays a large role in the basal tonic current seen in D2+ MSNs from young mice (Ade et al., 2008; Janssen et al., 2009). In this study, we demonstrated the essential role of the β3 GABAA receptor subunit in mediating MSN tonic currents using conditional β3 subunit knock-out (β3f/fDrd2) mice. Cre-lox genetics were used to generate mice where Cre recombinase was expressed under the D2 receptor (Drd2) promoter. We show that while the wild-type MSN tonic current pattern demonstrates a high degree of variability, tonic current patterns from β3f/fDrd2 mice are narrow, suggesting that the β3 subunit is essential to striatal MSN GABA-mediated tonic current. Our data also suggest that a distinct population of synaptic receptors upregulate due to β3 subunit removal. Further, deletion of this subunit significantly decreases the D2+ MSN excitability. These results offer insight for target mechanisms in Parkinson’s disease, where symptoms arise due to the imbalance in striatal D1+ and D2+ MSN excitability and output.
Introduction
Medium spiny neurons (MSNs) are GABAergic projection neurons of the striatum, and project either to the substantia nigra pars reticulata though a direct pathway or the globus pallidus through an indirect pathway (Gerfen et al., 1990). MSNs of the direct pathway express the dopamine D1 receptors (D1+); their activity is thought to facilitate movement. Dopamine D2 receptors (D2+) are expressed on indirect pathway MSNs that inhibit movement initiation.
Proper motor control relies on a complex balance of D1+ and D2+ MSN excitation. Further, MSN excitability and synchrony is thought to be altered in dopamine-depleted animal models of Parkinson’s disease (Costa et al., 2006; Fino et al., 2007; Azdad et al., 2009; Burkhardt et al., 2009; Jáidar et al., 2010). Although overly simplified, it is thought that D2+ MSNs experience increased excitability in the disease state (Day et al., 2006, 2008; Mallet et al., 2006; Shen et al., 2007; Tian et al., 2010), while D1+ MSNs show little changes (Day et al., 2006; Tian et al., 2010). Indeed, optogenetic activation of striatal D2+ MSNs in vivo mimicked Parkinson’s disease with decreased movement initiation and increased freezing behavior (Kravitz et al., 2010). Thus, the intrinsic mechanisms that control MSN output are of crucial interest in understanding the underlying factors that mediate altered neuronal excitability.
GABAergic inhibition is mediated by a combination of fast, phasic inhibition and a slower, tonic inhibition. Synaptic release of neurotransmitter mediates phasic inhibition through low-affinity synaptic GABAA receptors and is effective in generating neuronal rhythmic activity and synchronicity (Cobb et al., 1995). Tonic inhibition, due to GABA spillover, is mediated through high-affinity receptors located in the extrasynaptic space, and increases a cell’s input conductance, affecting neuronal excitability (Brickley et al., 1996). Therefore, the subunits that mediate striatal MSN tonic inhibition are of crucial interest in understanding the underlying factors that control MSN output and altered neuronal excitability. Our previous study used pharmacology to identify the GABAA receptor β3 subunit as an important regulator of both striatal D1+ and D2+ MSN tonic current (Janssen et al., 2009).
In general, β3 subunit knock-out (KO) mice show an increase in seizure activity and serve as an animal model for Angelman’s syndrome (DeLorey et al., 1998). Studies from global β3 subunit KO mice have illustrated the subunit’s importance in synaptic transmission and oscillatory behavior or synchronicity (Huntsman et al., 1999; Nusser et al., 2001; Hentschke et al., 2009). Cortical cultures from β3 subunit KO animals further showed that this subunit is crucial for synaptic inhibition (Ramadan et al., 2003). To our knowledge, no study has investigated the role of the β3 subunit in striatal inhibition using a genetic approach. Therefore, we investigated the role of β3 GABAA receptor subunits on striatal MSN phasic and tonic currents by selectively excising the subunit from D2-expressing neurons (Drd2-Cre; Gong et al., 2007; i.e., striatal D2+ MSNs) using the Cre-lox system. This conditional KO approach allowed us to more accurately determine the importance of this subunit without more global compensatory mechanisms.
We found that the β3 GABAA receptor subunit is fundamental in both phasic and tonic inhibition as miniature inhibitory post-synaptic current (mIPSC) decay kinetics and tonic current were significantly altered in conditional KO animals. In addition, deletion of this subunit significantly decreased the excitability of MSNs, suggesting the GABAA receptor β3 subunit may be an important pharmacological target in the treatment of striatal disorders.
Materials and Methods
Animals
Bacterial artificial chromosome (BAC) mice that identify the indirect striatal output pathway (BAC-D2-EGFP; Gong et al., 2003) were crossed with BAC mice that identify the direct striatal output pathway (Drd1a-tdTomato; Shuen et al., 2008) so progeny (BAC-D2-EGFP; Drd1a-tdTomato) contained fluorescent markers for simultaneous visualization of both indirect and direct pathways.
Conditional β3 subunit knock-out (KO) mice were produced by crossing floxed β3 mice (β3f/f; Jackson Labs #008310; Ferguson et al., 2007) with transgenic mice that expressed Cre recombinase under the Drd2 promoter (GENSAT, ER44; Gong et al., 2007) to yield β3f/+Drd2 mice. These mice were then crossed with β3f/f mice to generate β3f/f, β3f/+Drd2, and β3f/fDrd2 mice. To determine the integrity of the Drd2-Cre expression, Drd2-Cre mice were crossed with a Cre reporter mouse expressing tdTomato (ROSA-tdTomato, Jackson Labs #007914; Madisen et al., 2010). Parvalbumin-expressing interneurons (zolpidem control experiments) were identified by a cross between Parv-Cre (Jackson Labs #008069; Murray et al., 2011) and ROSA-tdTomato reporter mice. All mice were genotyped through standard PCR procedures. The following primers were used to genotype tail DNA: Cre – F5′-GGATGAGGTTCGCAAGAACC-3′, R5′-CCATGAGTGAACGAACCTGG-3′ with a PCR product at 400 bp; Floxedβ3 – F5′-ATTCGCCTGAGACCCGACT-3′, R5′-GTTCATCCCCACGCAGAC-3′ with PCR products of ~250 bp for wild-type β3 subunit allele and ~300 bp for the floxedβ3 allele.
Immunohistochemistry
Progeny of mouse matings between Drd2-Cre and ROSA-tdTomato reporter mice were used to assess the fidelity of the Drd2-Cre expression. Whole mouse brains (~18 days) were fixed and coronally sliced at 100 μm using a Lancer Vibratome (Series 1000, Sherwood Medical, St. Louis, MO, USA). Free-floating tissue sections were blocked with 4% normal donkey serum in PBS for 1 h at room temperature and washed for 30 min in PBS/0.1% Triton-X100 (Tx). Rabbit α-DARPP-32 (19A3, Cell Signaling) 1° antibody (1:200) was diluted in PBS/Tx/1% BSA and slices were incubated at room temperature for approximately 18 h. Slices were washed for 30 min in PBS/Tx. Goat α-rabbit 2° antibody conjugated to FITC (Invitrogen, Carlsbad, CA, USA) was diluted 1:500 in PBS/Tx/BSA and exposed to tissue for 1.5 h at room temperature. The 2° antibody was washed with PBS/Tx, and sections were placed on microscope slides with VECTASHIELD H-1000 mounting media (Vector Labs, Burlingame, CA, USA) and sealed with glass coverslips. For imaging, a Nikon Eclipse E600 microscope (Nikon Instruments, Melville, NY, USA) was used to excite endogenous tdTomato and FITC fluorophores. Colocalization of immunostaining was performed by manually tracing regions of interest corresponding to cell bodies of tdTomato+ neurons and/or FITC+ neurons using MetaMorph software (Molecular Devices).
Western Blots
Striata and cortex were dissected from 7 β3f/+ and β3f/f (Cre−) and 6 β3f/fDrd2 (Cre+) adult mice (>30 days) and flash-frozen on dry ice. Tissue homogenates were prepared in TEE buffer containing (in mM): Tris-HCl (10), pH 7.4, EDTA (1) and (1), centrifuged (30,000×g) and crude membrane pellets were resuspended. Each sample was diluted down to a protein concentration of 1 μg/μL in TEE buffer. Equivalent amounts of protein (10 μg) were run on a sodium dodecyl sulfate-polyacrylamide gel (10% polyacrylamide) and transferred to the polyscreen polyvinylidene fluoride (New England Nuclear, Boston, MA, USA) membranes in transfer buffer containing (in mM): Tris-HCl (25), glycine (192), 20% methanol. Blots were blocked in PBST buffer containing (in mM): NaPO4 (10), pH 7.4, NaCl (140), and 0.1% v/v Tween (20) containing 1% bovine serum albumin (Sigma) and incubated with α−β3 antibody (1:1000; NB300-119; Novus Biologicals, Littleton, CO, USA) overnight at 4°C. Blots were then washed with PBST buffer and incubated with α-rabbit horseradish peroxidase antibody (1:5000; Amersham, Piscataway, NJ, USA) for 30 min at room temperature. After several washes, individual bands were visualized on Hyperfilm (Amersham, Piscataway, NJ, USA) with enhanced chemiluminescence SuperSignal West Pico Enhancer Solution (Pierce, Rockford, IL, USA). Membranes were stripped of all antibodies for 1 h in elution buffer and incubated in α−β-actin antibody (Sigma) overnight at 4°C. Films were scanned and quantified using ImageJ software (http://rsb.info.nih.gov/ij/).
Slice Preparation
Young male and female mice (15–23 days) were sacrificed by decapitation in agreement with the guidelines of the AMVA Panel on Euthanasia and the Georgetown University ACUC. Adult animals (>30 days) were used in some experiments, where noted. The whole brain was removed and placed in an ice-cold slicing solution containing (in mM): NaCl (85), KCl (2.5), CaCl2 (1), MgCl2 (4), NaH2PO4 (1), NaHCO3 (25), glucose (25), sucrose (75; all from Sigma). Corticostriatal coronal slices (250 μm) were prepared using a Vibratome 3000 Plus Sectioning System (Vibratome, St Louis, MO, USA) in slicing solution. They were incubated in artificial cerebrospinal fluid (aCSF) containing (in mM): NaCl (124), KCl (4.5), Na2HPO4 (1.2), NaHCO3 (26), CaCl2 (2.0), MgCl2 (1), and dextrose (10.0) at 305 mOsm at 32°C for 30 min. Slices recovered for an additional 30 min in aCSF, at room temperature, 22–24°C. All solutions were maintained at pH 7.4 by continuous bubbling with 95% O2, 5% CO2.
Slices were visualized under an upright microscope (E600FN, Nikon) equipped with Nomarski optics and an electrically insulated 60× water immersion objective with a long working distance (2 mm) and high numerical aperture (1.0). Recording pipettes were pulled on a vertical pipette puller from borosilicate glass capillaries (Wiretrol II; Drummond) and filled with cesium chloride (CsCl)- or potassium gluconate (Kgluc)-based internal solutions. The CsCl-based internal solution contained (in mM): CsCl (145), HEPES (10), ATP–Mg (5), GTP–Na (0.2), EGTA (10), adjusted to pH 7.2 with CsOH. This high chloride internal solution enhanced the detection of GABAergic events, placing their reversal potential near 0 mV. In Kgluc-based internal solutions, CsCl was replaced with equimolar (145 mM) K gluconate and pH was adjusted with KOH. Kgluc internal solutions did not alter the chloride concentration and the reversal potential for GABAergic events was around −60 mV.
Whole-Cell Recordings
In BAC-D2-EGFP; Drd1a-tdTomato mice, MSNs were classified as being either striatopallidal dopamine D2 receptor positive (D2+) or striatonigral dopamine D1 receptor positive (D1+) based on their expression of EGFP and tdTomato, respectively. In β3 subunit transgenic mice, MSNs were not tagged with fluorescent proteins (to blind the study), so the sample population likely contains both D1+ and D2+ MSNs.
All recordings were performed at room temperature, 22–24°C. Voltage-clamp recordings were achieved using the whole-cell configuration of the patch-clamp technique at a holding voltage of −60 mV using the Axopatch 200B amplifier (Molecular Device Co., Sunnyvale, CA, USA). Access resistance was monitored during the recordings and experiments with >20% change were discarded. When Kgluc internal solutions were used, the baseline membrane potential for current-clamp recordings was set at −70 mV before each series of current step injection protocols. Rheobase current was defined as the first current step, within a series of 20 pA steps, that elicited an action potential. Recordings were not corrected for liquid junction potential.
Stock solutions of bicuculline methobromide (BMR), GABA (both from Sigma), and tetrodotoxin (TTX; Alomone Labs) were prepared in water. Etomidate, zolpidem, flumazenil, and diazepam (Sigma) were dissolved in dimethylsulfoxide (<0.01% final concentration). All stock solutions were diluted to the desired concentration in aCSF and applied locally through a Y tube (Murase et al., 1989) modified for optimal solution exchange in brain slices (Hevers and Lüddens, 2002).
Currents were filtered at 2 kHz with a low-pass Bessel filter and digitized at 5–10 kHz using a personal computer equipped with Digidata 1322A data acquisition board and pCLAMP10 software (both from Molecular Devices). Off-line data analysis, curve fitting, and figure preparation were performed with Clampfit10 software (Molecular Devices). Spontaneous and miniature inhibitory post-synaptic currents (sIPSCs and mIPSCs) were identified using a semi-automated threshold-based mini detection software (Mini Analysis, Synaptosoft Inc., Fort Lee, NJ, USA) and were visually confirmed. IPSC averages were based on more than 50 non-overlapping events, and decay kinetics were determined with averaged IPSC traces using double exponential curve fittings and reported as weighted time constants (Tw):

where τx is the decay time constant for a particular component of the curve and Ax is the peak amplitude of the corresponding component. All detected events were used for event frequency analysis, but superimposing events were eliminated for the amplitude and decay kinetic analysis.
Tonic current was primarily measured with an all-points histogram that measured the mean holding current 10 s before and during BMR application (Janssen et al., 2009). Tonic current is represented as the change in baseline amplitude. When indicated, tonic current was also measured by changes in RMS noise before and during BMR application (Glykys and Mody, 2007). These analyses were based on an all-points histogram that was fitted to the Gaussian function (Fleming et al., 2007):

where σ represents the RMS noise during the 10 s period before and during drug application and μ represents the mean holding current. Tonic noise was measured as a difference in RMS noise between two conditions.
Statistics
Box and whisker plots were generated for more accurate representation of critical data. The whiskers include the minimum and maximum values, while the box outlines the 25th and 75th percentile of data points. The median value is represented by a bar inside the box. Scatterplots of individual data points are also included in these plots to further show data spread.
Cumulative probability plots were constructed by averaging the single cell probability distribution, as calculated in Mini Analysis, for amplitudes and frequencies across all cells. Dotted lines denote the SEM for the data set.
Statistical significance was determined using the paired two-tailed Student’s t-test to compare pre-drug conditions with recordings made under drug conditions of the same cell population. Unpaired two-tailed Student’s t-test was used for comparisons across cell groups as well as for comparisons of striata and cortex from β3f/+ and β3f/f (Cre−) and β3f/fDrd2 (Cre+) mice for β3 subunit and actin protein in Western blots. Significance criteria was set at p < 0.05, and all values in text and figures are expressed as mean ± SEM. In text and figures, *p < 0.05, **p < 0.005, and ***p < 0.0005, unless noted otherwise.
Results
Generation of the Conditional β3 GABAA Receptor Subunit Knock-Out in D2+ MSNs
To study the role of the β3 GABAA receptor subunit in striatal MSN tonic current, the subunit was rendered non-functional by deleting exon 3 (Ferguson et al., 2007). We deleted this sequence by crossing floxed β3 subunit (β3f/f) mice with Drd2-Cre mice, where Cre recombinase is selectively expressed in dopamine receptor 2 (D2+) neurons. β3f/fDrd2 mice had the β3 subunit selectively deleted from all Drd2-expressing neurons, while β3f/+Drd2 and β3f/f mice had the incomplete combination of alleles and served as controls.
To verify the integrity of Drd2-Cre expression, we crossed this animal with a ROSA-tdTomato reporter mouse; any cell that expresses Cre recombinase will also express tdTomato. Counterstaining to DARPP-32 (a general marker for MSNs) showed that all tdTomato+ cells were also DARPP-32+ (Figure 1A). Further, approximately half of the DARPP-32+ cells were also positive for tdTomato, consistent with previous reports that show similar numbers of striatal D2+ and D1+ MSNs (Shuen et al., 2008). The adjacent cortex showed minimal tdTomato and DARPP-32 expression (data not shown).
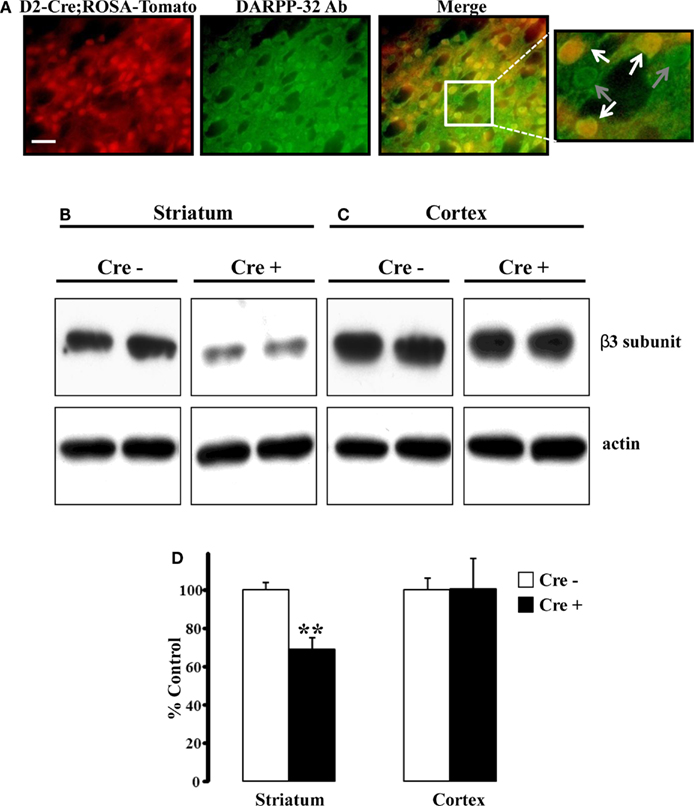
Figure 1. Striatal β3 subunit protein reduced conditional KO mice. (A) Fluorescent images demonstrating colocalization between endogenous tdTomato fluorescence and DARPP-32 expression. Approximately half of the DARPP-32+ cells expressed endogenous tdTomato, indicative of Cre expression. Scale bar is 25 μm. (B) Representative western blot analysis of the GABAA receptor β3 subunit from individual 30-day-old mice revealed reduced amounts of β3 subunit protein in striatum (B) from Cre positive (β3f/fDrd2) animals compared to Cre negative (β3f/+ and β3f/f) control animals. The amount of β3 protein in cortex (C) did not differ between genotypes. Blots were reprobed for β-actin. (D) Summary graph of western blot analysis demonstrating a significant reduction in β3 protein in striatum, but not cortex. Data are expressed as percent change in band intensity relative to Cre negative controls following normalization to actin.
To examine the degree to which the β3 subunit was reduced in the striatum of β3f/fDrd2 mice, western blot analysis of cortex and striatum were performed using an antibody specific for the GABAA receptor β3 subunit (Figures 1B–D). As shown in Figure 1B, β3 subunit protein was significantly reduced in striatum of Cre positive mice compared to Cre negative controls (31% reduction). This reduction was expected as striatal D2+ MSNs constitute approximately half of the striatal MSN population (Shuen et al., 2008; personal observations). Since the cortex does not contain Drd2-expressing neurons, it serves as an ideal control. Indeed, β3 subunit protein levels were not reduced in cortical tissue from Cre positive mice (Figure 1C).
To investigate the consequences of β3 GABAA receptor subunit ablation in Drd2-expressing neurons, we first examined whether this deletion was affecting litter size. The floxed β3 GABAA receptor subunit mice were originally developed (Ferguson et al., 2007) to avoid neonatal lethality associated with global knock-out (KO) of the β3 subunit (Homanics et al., 1997). To determine whether our conditional KO animals experienced enhanced lethality, the number of β3f/+Drd2 mice surviving to p7 was compared to the number of β3f/fDrd2mice from several litters. The ratio of β3f/+Drd2 to β3f/fDrd2 mice was similar to the 1:1 ratio (n = 10) predicted by Mendelian genetics with our breeding scheme, suggesting that β3f/fDrd2 mice do not experience premature death. All mice lacked any abnormal phenotypes like cleft palate or hyperactivity, which were observed in global β3 subunit KOs (Homanics et al., 1997), but not pan-neuronal KOs (Ferguson et al., 2007). Under general observation, β3f/fDrd2 animals did not display gross behavioral abnormalities compared to control littermates. Further tests need to be conducted to determine more subtle behavioral abnormalities.
Conditional β3 Subunit KO Mice Lack Typical MSN Tonic Current Expression Pattern
Because our previous work suggested that striatal MSN GABAergic tonic current may be mediated (at least in part) through the β3 GABAA receptor subunit (Janssen et al., 2009), it was important to determine MSN tonic current expression patterns in these conditional β3 subunit KO mice. Although dopamine receptor expression was not identified, many cells were sampled, and therefore the sample population likely contains both D1+ and D2+ MSNs. Because cell identity was not known, box and whisker plots are presented to demonstrate the tonic current expression spread and pattern. Averages were used to determine significance in these cells.
Striatal MSN GABAergic tonic inhibition was measured and compared between wild-type BAC-D2-EGFP; Drd1a-tdTomato mice, β3f/f, β3f/+Drd2, and β3f/fDrd2 mice to verify that any differences observed in tonic current were due to the lack of the β3 subunit. It is possible that the floxed β3 allele alters β3 subunit function, also affecting tonic current expression in neurons. Therefore, MSN GABA-mediated tonic currents were measured from mice that lacked Cre recombinase expression, but were homozygous for the floxed β3 allele (β3f/f). Suggesting inclusion of unaltered D1+ and D2+ MSNs in the sample population, β3f/f mice showed quite varied tonic current amplitudes (0–34 pA; Figure 2B), similar to the varied tonic current amplitudes in BAC-D2-EGFP; Drd1a-tdTomato wild-type mice (Figure 2B, right). These functional data support western blot analysis which suggested that β3 subunits are not affected by the inclusion of loxP sites (Figure 1B; Ferguson et al., 2007). Further, the presence of the wide box representing the 25th and 75th percentile, shown in Figure 2B, shows a high degree of variability in tonic current amplitudes (0–25 pA, n = 14) from striatal MSNs in β3f/+Drd2 mice. On the other hand, β3f/fDrd2 mice had tonic current amplitudes that were confined to a much more narrow range (0–10 pA, n = 29). The average GABA-mediated tonic current amplitude was significantly smaller in conditional β3 subunit KO mice compared to both types of control mice (β3f/fDrd2: 3.1 ± 0.5 pA, n = 29; β3f/f: 11.3 ± 2.7 pA, n = 13, p < 0.0005; β3f/+Drd2: 11.0 ± 2.3 pA, n = 14, p < 0.0005). In addition, RMS noise was significantly lower in β3f/fDrd2 mice (0.8 ± 0.1 pA, n = 24) compared to β3f/+Drd2 (1.4 ± 0.2 pA, n = 14; p < 0.05) and β3f/f littermates (1.9 ± 0.5 pA, n = 11, p < 0.005). Because results from these two control mice did not differ, the data were pooled for Figures 3–5 (although labeled in text and figures as β3f/f for clarity). Since all MSNs in the sample population showed little to no tonic current, these results suggest D2+ MSN tonic current expression is largely dependent upon β3 subunit expression.
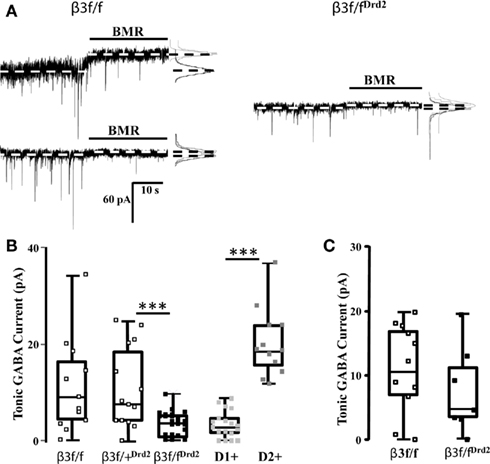
Figure 2. Conditional β3 subunit KO mice show reduced GABA-mediated tonic current in MSNs. (A) Individual representative current traces displaying MSN BMR-sensitive (25 μM) tonic current in control β3f/f mice (left) and β3f/fDrd2 mice (right). The dichotomous pattern of tonic current was not present in β3f/fDrd2 mice. (B) Summary box and whisker plots displaying the tonic current patterns in β3f/f (open square, left; n = 13), β3f/+Drd2 (open square, middle; n = 14), β3f/fDrd2 (black square, middle; n = 29), and wild-type BAC-D2-EGFP;Drd1a-tdTomato D1+ (light gray, right n = 15) and D2+ (dark gray, right n = 10) MSNs from at least three mice in each group. (C) Summary box and whisker plots displaying the tonic current patterns in adult β3f/f (open square, left; n = 12), β3f/fDrd2 (black square, right; n = 7) from at least three mice in each group.
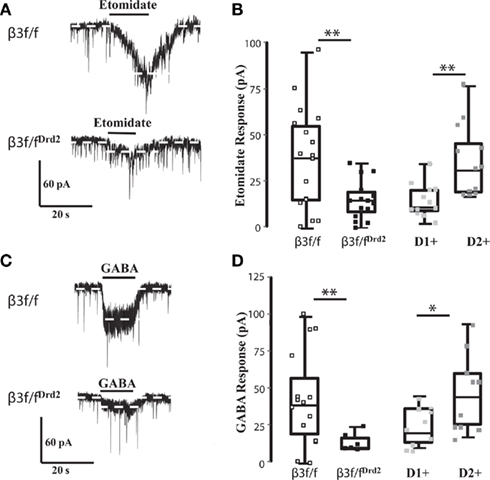
Figure 3. β3 GABAA receptor subunit affects GABA sensitivity. (A) Representative traces of etomidate (3 μM) responses in TTX (0.5 μM) from β3f/f (top) and β3f/fDrd2 (bottom) mice. (B) Summary box and whisker plots showing effects of etomidate in TTX from 3 β3f/f (open square; n = 17), 5 β3f/fDrd2 (black; n = 15), and 6 BAC-Drd2-EGFP; Drd1a-tdTomato mice with D1+ (light gray; n = 12) and D2+ (dark gray; n = 12) neurons. (C) Representative traces of GABA (1 μM) responses in TTX (0.5 μM) from β3f/f (top) and β3f/fDrd2 (bottom) mice. (D) Summary box and whisker plots showing effects of GABA in TTX from 5 β3f/f (open square; n = 15), 3 β3f/fDrd2 (black; n = 6), and 6 wild-type BAC-D2-EGFP; Drd1a-tdTomato mice from D1+ (light gray; n = 10) and D2+ (dark gray; n = 11) neurons.
Our immunohistochemistry and western blot data suggest that the β3 subunit was deleted from a select group of striatal MSNs, but does not address the subtype. Because the MSN GABAergic tonic current pattern shifts through development such that adult D1+ MSNs express the majority of the GABAergic tonic conductance (Janssen et al., 2009; Santhakumar et al., 2010), we recorded tonic current from adult β3f/fDrd2 and β3f/f mice (>30 days). Indicative of a population of D1+ and D2+ MSNs, BMR-sensitive tonic current from β3f/f MSNs was highly variable (0–18 pA), with an average of 10.9 ± 2.0 pA (n = 12; Figure 2C). Tonic current expression from adult β3f/fDrd2 MSNs showed a similar, variable pattern (0–19 pA), with an average of 7.6 ± 2.8 pA (n = 7; p = 0.3). Both data sets were significantly different from tonic current averages from young β3f/fDrd2 mice (adult β3f/f p < 0.0005; β3f/fDrd2p < 0.05). These data further suggest that the β3 subunit was only deleted from D2+ MSNs since adult striatal tonic current from KO mice resembled that of the controls.
Conditional Deletion of β3 Subunit Alters MSN Etomidate and GABA Response
To functionally verify that the β3 subunit was successfully excised from striatal D2+ MSNs, the effects of etomidate in TTX were tested on β3f/f (and β3f/+Drd2 with pooled data) and β3f/fDrd2 mice. This general anesthetic is a selective pharmacological agent at β2/3-containing GABAA receptors (Hill-Venning et al., 1997). In striatal MSNs, however, etomidate identifies β3 subunit-containing GABAA receptors (Janssen et al., 2009) due to their lack of β2 subunit expression (Flores-Hernandez et al., 2000).
Responses to etomidate (3 μM) in TTX averaged 37.7 ± 6.8 pA (n = 17) in β3f/f mice, with a wide range of amplitudes (1–95 pA), suggesting that this population contains both relatively etomidate-insensitive D1+ MSNs and etomidate-sensitive D2+ MSNs, as seen in wild-type BAC-D2-EGFP; Drd1a-tdTomato mice (Figure 3B). The range of etomidate responses was more narrow from β3f/fDrd2 mice (0–35 pA), and the average was significantly smaller (14.9 ± 2.7 pA, n = 15, p < 0.005; Figure 3B), and was similar to etomidate currents recorded from wild-type D1+ MSNs. These data functionally confirm that the β3 subunit was deleted in etomidate-sensitive D2+ MSNs.
Post-synaptic GABA responses were also assessed in β3f/fDrd2 mice (in the presence of TTX) to determine if the β3 subunit plays a role in the differential D1+ and D2+ MSN GABA sensitivity (Ade et al., 2008) seen in wild-type mice. The average response to GABA (1 μM) in control β3f/f mice was 44.2 ± 8.5 pA (n = 15) with a range of 1–101 pA. The wide range of GABA responses were similar in current amplitude to identified D2+ and D1+ MSNs from BAC-D2-EGFP; Drd1a-tdTomato mice (Figure 3D). In contrast, β3f/fDrd2 mice expressed an average inward GABA current of just 14.0 ± 2.7 pA (n = 6, p < 0.05), with a more narrow range of amplitudes (9–24 pA; Figure 3D). These data suggest that the absence β3 subunit may affect GABAA receptor number and agonist sensitivity at D2+ MSN receptors, as previously shown in HEK293 cells (Janssen et al., 2009).
Deletion of the β3 Subunit Affects Synaptic Receptor Composition
Although D1+ and D2+ MSNs do not differ in their mIPSC profile (Ade et al., 2008), mIPSC characteristics from unidentified MSN subtypes were compared between β3f/fDrd2 and control β3f/f mice to evaluate changes in the composition of synaptically located GABAA receptors. As shown in Figure 4A and quantified in Figures 4B,C and Table 1, mIPSC frequency and amplitude did not change with β3 subunit deletion. However, mIPSC decay kinetics from β3f/fDrd2 mice were significantly faster than those from β3f/f mice (Figure 4D).
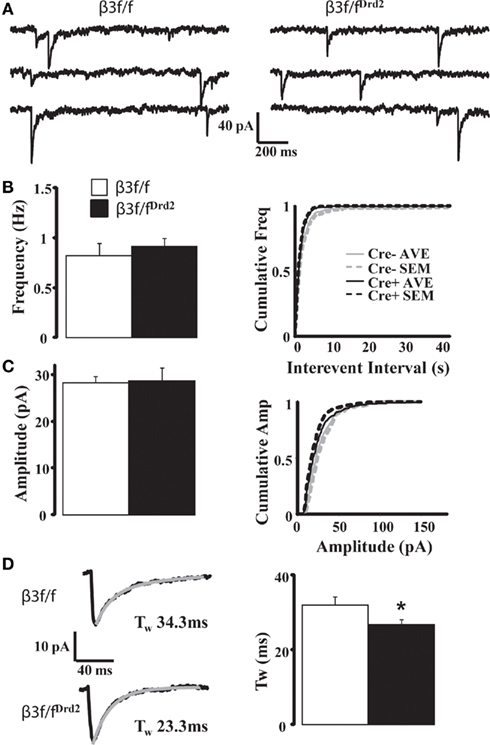
Figure 4. Synaptic decay is altered in MSNs from conditional KO mice. (A) Representative mIPSC traces from β3f/f and β3f/fDrd2 mice. (B,C) Summary mIPSC frequency and amplitude graphs (left) and averaged cumulative distribution plots for all cells (right) in β3f/f and β3f/fDrd2 mice. (D) Representative individual mIPSCs (left) with double exponential decay fittings in gray from β3f/f and β3f/fDrd2 mice. Summary of decay fittings, right. Data derive from 16 to 17 cells from at least three mice in each group.
Since decreased numbers of synaptic receptors are reflected by decreased mIPSC amplitude, the data presented here suggest homeostatic changes of GABAA receptors at MSN inhibitory synapses upon β3 subunit deletion. Further, the faster decay of mIPSCs in β3f/fDrd2 animals suggests that this subunit deletion uncovers a distinct pool of synaptic receptors and/or causes a compensatory upregulation of synaptic GABAA receptors with faster kinetic properties.
Because α subunits largely determine synaptic receptor decay properties (Picton and Fisher, 2007), we used the imidazopyridine zolpidem (1 μM), effective at GABAA receptor α1–3 subunits, to determine GABAA receptor subunit differences between β3f/f and β3f/fDrd2 mice (Figure 5A). Cells that displayed decay prolongation more than 110% control were defined as “zolpidem sensitive.” Zolpidem prolonged the average mIPSC decay kinetics in 15 of 24 (62%) neurons from β3f/f mice (134 ± 5% control; Figure 5B). The remaining nine neurons did not respond (97.9 ± 3% control). mIPSC potentiation in zolpidem sensitive cells was suppressed with co-application of the benzodiazepine antagonist flumazenil (10 μM), bringing mIPSC decay kinetics to their original states (zolpidem: 142.3 ± 8% control; flumazenil + zolpidem: 107.6 ± 14% control, n = 6). Flumazenil application alone failed to prolong the kinetics of zolpidem sensitive and insensitive mIPSCs (85 ± 5%, n = 14 and 68.9 ± 2%, n = 2), suggesting a minimal contribution from α4 subunits (Wafford et al., 1996). Diazepam (5 μM) prolonged the kinetics in all cells (sensitive: 138.4 ± 11%, n = 12; insensitive: 141.5 ± 11%, n = 3), supporting previous findings (Ade et al., 2008) suggesting the presence of α5-containing synaptic receptors in MSNs.
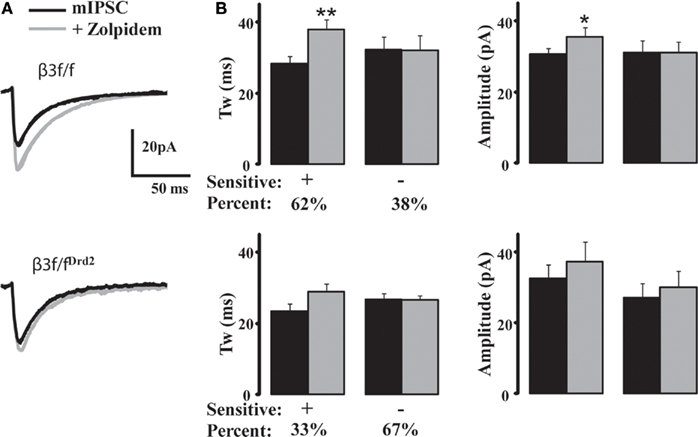
Figure 5. Zolpidem fails to modulate majority of mIPSCs in MSNs from conditional KO mice. (A) Averaged representative mIPSC traces from a β3f/f (top) and a β3f/fDrd2 (bottom) neuron with zolpidem (1 μM). (B) Summary graphs showing zolpidem sensitivity on β3f/f (top) and β3f/fDrd2 (bottom) MSN kinetics (left) and amplitude (right). Cells were determined to be responders if their mIPSC decay increased at least 110% of control. The majority of neurons from control β3f/f mice showed a significant mIPSC prolongation (n = 15/24), while the majority of neurons from β3f/fDrd2 mice did not (n = 6/9). Cells derive from at least two mice in each group.
Zolpidem affected fewer neurons from β3f/fDrd2 mice (three of nine, 33%); the increased decay kinetics in these cells did not reach significance (124.1 ± 1% control; Figure 5B). Most cells (six of nine, 67%) from β3f/fDrd2 mice showed no response to zolpidem application (100.6 ± 5% control). Therefore, zolpidem failed to affect the majority of MSNs from β3f/fDrd2 mice, suggesting the upregulation of a zolpidem-insensitive α subunit.
To test the sensitivity of zolpidem on a cell-type that is known to express an abundance of synaptic α1-containing GABAA receptors, we recorded from parvalbumin- (PV+) expressing striatal interneurons (Riedel et al., 1998). The PV+ interneuron mIPSC decay was faster (15.9 ± 1.5 ms, n = 4) than MSNs, and was prolonged 221.7 ± 50% control with zolpidem (1 μM) application.
β3 Subunit Responsible for Differences in Excitability between D1+ and D2+ MSNs
Our previous work suggested that GABA-mediated tonic current affects striatal MSN excitability (Ade et al., 2008; Janssen et al., 2009), and therefore it is possible that by modulating GABAergic tonic current, β3 subunit expression also contributes to differences in D1+ and D2+ MSN excitability (Ade et al., 2008; Cepeda et al., 2008; Gertler et al., 2008; Janssen et al., 2009). Thus, we compared MSN excitability in identified D1+ and D2+ MSNs in BAC-D2-EGFP; Drd1a-tdTomato mice to MSNs from β3f/fDrd2 mice. For these experiments, a Kgluc-based internal solution was used with the current-clamp technique. Cells were held at a pre-determined membrane potential (−70 mV), and subjected to 1 s, 20 pA steps of hyperpolarizing and depolarizing current injections.
As previously shown (Ade et al., 2008; Janssen et al., 2009), D2+ MSNs were significantly more excitable than D1+ MSNs (Figure 6). A substantial MSN sample size from β3f/fDrd2 mice showed that these cells were significantly less excitable than wild-type MSNs (Figure 6). The input–output curves and rheobase measurements from this mutant mouse were similar to wild-type D1+ MSNs and support the finding that the β3 GABAA receptor subunit regulates and contributes to the increased excitability of striatal D2+ MSNs.
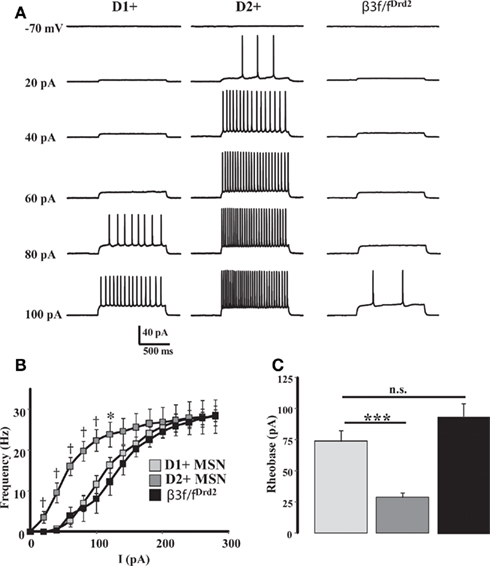
Figure 6. β3 subunit determines cell excitability. (A) Representative current-clamp recordings from a D1+ MSN (left), D2+ MSN (middle), β3f/fDrd2 MSN (right), illustrating the responses to a series of depolarizing current injections (20 pA steps) from a set potential of −70 mV. (B) Summary of action potential firing frequency in response to increasing depolarizing current injections in D1+ (n = 10), D2+ (n = 9), and β3f/fDrd2 (n = 10) MSNs. * Denotes p < 0.05 and † denotes p < 0.005 for clarity. (C) Summary graph showing the averaged rheobase current in D1+, D2+, and β3f/fDrd2 MSNs. Data derive from the same cells in (B), from at least three mice in each group.
Discussion
Pharmacological evidence suggested that β3 GABAA receptor subunits contribute to differences in GABA-mediated tonic currents between D2+ and D1+ MSNs (Janssen et al., 2009). The present study sought to complement and confirm those results with a genetic approach: the β3 subunit was conditionally removed from Drd2-expressing neurons using the Cre-lox system (β3f/fDrd2).
Western blot data and etomidate-mediated currents from β3f/fDrd2 mice verified that the β3 subunit was reduced in a subpopulation, presumably D2+, of MSNs. The most significant, yet anticipated, finding with β3f/fDrd2 mice was the dramatic reduction in tonic current amplitude and spread, a consequence of GABAA receptor β3 subunits deletion in D2+ MSNs.
β3f/fDrd2 mice also showed substantially less GABA-mediated inward currents in response to exogenous GABA application than β3f/f littermates. Therefore, it is likely that high-affinity β3 subunit-containing GABAA receptors contribute to the greater GABA-sensitivity of D2+ MSNs (Ade et al., 2008). This reduced GABA sensitivity may be due to a reduction of highly sensitive α5β3-containing extrasynaptic receptors (Homanics et al., 1997; Ade et al., 2008).
Synaptic current properties in β3f/fDrd2 MSNs suggest that β3 subunit deletion affects α subunit composition; receptors with faster decay kinetics were uncovered or upregulated. Previous studies of IPSCs in distinct neuronal populations from β3 subunit KO mice (Huntsman et al., 1999; Ramadan et al., 2003; Hentschke et al., 2009) showed stronger changes in IPSCs than observed here. Faster decay and increased zolpidem sensitivity of mIPSCs in cortical neurons of primary cultures from β3 subunit KO mice suggested that the β3 subunit preferentially assembles with α2/3 subunits (Ramadan et al., 2003) with slower decay properties, as has been shown through immunoprecipitation (Benke et al., 1994). It has been hypothesized that removal of the β3 subunit leaves α1β2 subunit-containing synaptic receptors with faster decay and greater zolpidem sensitivity (Ramadan et al., 2003). All available data, however, suggest β3 subunit-containing GABAA receptors are characterized by slow synaptic decay (Figure 4; Huntsman et al., 1999; Ramadan et al., 2003; Hentschke et al., 2009).
We previously showed that MSN synaptic receptors do not differ between D1+ and D2+ MSNs, are comprised of α2 and α5 subunits, and are slightly sensitive to zolpidem (Ade et al., 2008). As zolpidem had no effect on the majority of rapidly decaying mIPSCs from β3f/fDrd2 mice, we suggest that deletion of the β3 subunit uncovered or upregulated synaptic receptors that contain the α4 or α5 subunit, characterized by faster decay kinetics than those with α2 or α1 subunits (Picton and Fisher, 2007). It is plausible that removal of the β3 subunit uncovers or upregulates α4/5β1-containing synaptic receptors since the β2 subunit is not expressed in MSNs (Flores-Hernandez et al., 2000).
Our data also support a homeostatic upregulation of GABAA receptors upon β3 subunit deletion since mIPSC amplitude remained unchanged. A select population of sIPSCs in the hippocampus of β3 subunit KO mice also showed unaltered amplitude with faster decay although receptor upregulation was not specifically investigated (Hentschke et al., 2009). Yet, it appears that β3 subunit deletion does not result in receptor upregulation in many brain regions (Homanics et al., 1997; Huntsman et al., 1999; Nusser et al., 2001; Wong et al., 2001; Ramadan et al., 2003; Ferguson et al., 2007). Receptor upregulation may occur in the striatum following β3 subunit deletion because receptor composition is highly dependent upon the abundance of both β1 and β3 subunits.
MSN excitability was significantly lower in MSNs from β3f/fDrd2 mice, suggesting that D2+ MSN output was decreased to levels that are normally characteristic of D1+ MSNs. This data indicates that disruption of β3 subunit expression, and therefore MSN GABAA-mediated tonic current, significantly affects MSN output, as previously reported and discussed (Ade et al., 2008; Janssen et al., 2009).
This study verifies that D2+ MSN tonic current is mediated through the β3 GABAA receptor subunit, but offers no direct indication that the subunit plays an essential role in D1+ MSN current since the subunit was selectively deleted from D2+ MSNs. However, our previous study suggested that the β3 subunit was also essential in modulating D1+ MSN tonic current in both young and adult animals. Because tonic current from both cell-types could be pharmacologically modulated with similar conditions, the β3 subunit may mediate tonic current in both D1+ and D2+ MSNs, albeit in different situations and developmental stages.
This study primarily focuses on GABA-mediated tonic current in young mice, yet it is important to note the developmental changes in GABAA receptor composition (Laurie et al., 1992) and their potential impact on striatal MSN tonic current. The developmental regulation of tonic current is clearly demonstrated by the shifts observed in MSN tonic current patterns (Janssen et al., 2009; Santhakumar et al., 2010). The α5 subunit mediates tonic current in young D2+ MSNs (Ade et al., 2008), but pharmacological manipulation of this subunit did not affect D1+ or D2+ MSN tonic current in adult mice (Santhakumar et al., 2010). On the other hand, the δ subunit has only been found to mediate striatal tonic current in adult animals (Janssen et al., 2009; Santhakumar et al., 2010). mRNA expression studies have shown that young striatal tissue primarily expresses α2, α5, β3, and γX GABAA receptor subunits, while adult tissue abundantly expresses α2, α4, β3, and δ subunits (Laurie et al., 1992). Importantly, striatal β subunit expression remains relatively intact throughout striatal development, although it is more developmentally regulated in other brain regions (Laurie et al., 1992). Based on the available data, MSN tonic current in the young animal is predominately mediated through α5β3γ2 receptors, while tonic current in older MSNs is likely mediated through αXβ3δ receptors.
Many studies have noted the crucial importance of the GABAA receptor β3 subunit; this subunit has been linked to many autism spectrum disorders (Buxbaum et al., 2002) and childhood absence epilepsy (Feucht et al., 1999; Urak et al., 2006). Although the mechanism that links the β3 subunit with these developmental disorders is unknown, the data presented here suggest that the GABAA receptor β3 subunit largely regulates both phasic and tonic striatal GABAergic inhibitory currents and neuronal output, a plausible target mechanism for some aspects of these disorders. Our data also provide significant insight into the etiology and treatment of movement disorders like Parkinson’s disease that manifest due to the imbalance of output from D2+ and D1+ MSNs. Pharmacological manipulation aimed at the β3 subunit may restore proper MSN output and alleviate movement disturbances.
Conflict of Interest Statement
The authors declare that the research was conducted in the absence of any commercial or financial relationships that could be construed as a potential conflict of interest.
Acknowledgments
We thank Dr. David Lovinger at the National Institute on Alcoholism and Alcohol Abuse for providing the BAC-D2-EGFP mice, Dr. Nicole Calakos from Duke University for the Drd1a-tdTomato mice, and Dr. Baoji Xu at Georgetown University for providing the Drd2-Cre mice. The authors also appreciate the technical assistance of Patrick Forcelli and Patrick Hussman from Georgetown University. Supported by: NIH grant MH64797.
References
Ade, K. K., Janssen, M. J., Ortinski, P. I., and Vicini, S. (2008). Differential tonic GABA conductances in striatal medium spiny neurons. J. Neurosci. 28, 1185–1197.
Azdad, K., Chàvez, M., Don Bischop, P., Wetzelaer, P., Marescau, B., De Deyn, P. P., Gall, D., and Schiffmann, S. N. (2009). Homeostatic plasticity of striatal neurons intrinsic excitability following dopamine depletion. PLoS ONE 4, 6908.
Benke, D., Fritschy, J.-M., Trzeciak, A., Bannwarth, W., and Möhler, H. (1994). Distribution, prevalence and drug binding profile of γ-aminobutyric acid type A receptor subtypes differing in the β-subunit variant. J. Biol. Chem. 269, 27100–27107.
Brickley, S., Cull-Candy, S., and Farrant, M. (1996). Development of a tonic form of synaptic inhibition in rat cerebellar granule cells resulting from persistent activation of GABAA receptors. J. Physiol. (Lond.) 497, 753–759.
Burkhardt, J. M., Jin, X., and Costa, R. M. (2009). Dissociable effects of dopamine on neuronal firing rate and synchrony in the dorsal striatum. Front. Integr. Neurosci. 3:28.
Buxbaum, J. D., Silverman, J. M., Smith, C. J., Greenberg, D. A., Kilifarski, M., Reichert, J., Cook, E. H. Jr., Fang, Y., Song, C. Y., and Vitale, R. (2002). Association between a GABRB3 polymorphism and autism. Mol. Psychiatry 7, 311–316.
Cepeda, C., André, V. M., Yamazaki, I., Wu, N., Kleiman-Weiner, M., and Levine, M. S. (2008). Differential electrophysiological properties of dopamine D1 and D2 receptor-containing striatal medium-sized spiny neurons. Eur. J. Neurosci. 27, 671–682.
Cobb, S. R., Buhl, E. H., Halasy, K., Paulsen, O., and Somogyi, P. (1995). Synchronization of neuronal activity in hippocampus by individual GABAergic interneurons. Nature 378, 75–78.
Costa, R. M., Lin, S. C., Sotnikova, T. D., Cyr, M., Gainetdinov, R. R., Caron, M. G., and Nicolelis, M. A. (2006). Rapid alterations in corticostriatal ensemble coordination during acute dopamine-dependent motor dysfunction. Neuron 52, 359–369.
Day, M., Wang, Z., Ding, J., An, X., Ingham, C. A., Shering, A. F., Wokosin, D., Ilijic, E., Sun, Z., Sampson, A. R., Mugnaini, E., Deutch, A. Y., Sesack, S. R., Arbuthnott, G. W., and Surmeier, D. J. (2006). Selective elimination of glutamatergic synapses on striatopallidal neurons in Parkinson disease models. Nat. Neurosci. 9, 251–259.
Day, M., Wokosin, D., Plotkin, J. L., Tian, X., and Surmeier, D. J. (2008). Differential excitability and modulation of striatal medium spiny neuron dendrites. J. Neurosci. 28, 11603–11614.
DeLorey, T. M., Handforth, A., Anagnostaras, S. G., Homanics, G. E., Minassian, B. A., Asatourian, A., Fanselow, M. S., Delgado-Escueta, A., Ellison, G. D., and Olsen, R. W. (1998). Mice lacking the beta3 subunit of the GABAA receptor have the epilepsy phenotype and many of the behavioral characteristics of Angelman syndrome. J. Neurosci. 18, 8505–8514.
Ferguson, C., Hardy, S. L., Werner, D. F., Hileman, S. M., Delorey, T. M., and Homanics, G. E. (2007). New insight into the role of the beta3 subunit of the GABAA-R in development, behavior, body weight regulation, and anesthesia revealed by conditional gene knockout. BMC Neurosci. 8, 85.
Feucht, M., Fuchs, K., Pichlbauer, E., Hornik, K., Scharfetter, J., Goessler, R., Fureder, T., Cvetkovic, N., Sieghart, W., Kasper, S., and Aschauer, H. (1999). Possible association between childhood absence epilepsy and the gene encoding GABRB3. Biol. Psychiatry 46, 997–1002.
Fino, E., Glowinski, J., and Venance, L. (2007). Effects of acute dopamine depletion on the electrophysiological properties of striatal neurons. Neurosci. Res. 58, 305–316.
Fleming, R. L., Wilson, W. A., and Swartzwelder, H. S. (2007). Magnitude and ethanol sensitivity of tonic GABAA receptor-mediated inhibition in dentate gyrus changes from adolescence to adulthood. J. Neurophysiol. 97, 3806–3811.
Flores-Hernandez, J., Hernandez, S., Snyder, G. L., Yan, Z., Fienberg, A. A., Moss, S. J., Greengard, P., and Surmeier, D. J. (2000). D(1) dopamine receptor activation reduces GABA(A) receptor currents in neostriatal neurons through a PKA/DARPP-32/PP1 signaling cascade. J. Neurophysiol. 83, 2996–3004.
Gerfen, C. R., Engber, T. M., Mahan, L. C., Susel, Z., Chase, T. N., Monsma, F. J. Jr., and Sibley, D. R. (1990). D1 and D2 dopamine receptor-regulated gene expression of striatonigral and striatopallidal neurons. Science 250, 1429–1432.
Gertler, T. S., Chan, C. S., and Surmeier, D. J. (2008). Dichotomous anatomical properties of adult striatal medium spiny neurons. J. Neurosci. 28, 10814–10824.
Glykys, J., and Mody, I. (2007). Activation of GABAA receptors: views from outside the synaptic cleft. Neuron 56, 763–770.
Gong, S., Doughty, M., Harbaugh, C. R., Cummins, A., Hatten, M. E., Heintz, N., and Gerfen, C. R. (2007). Targeting Cre recombinase to specific neuron populations with bacterial artificial chromosome constructs. J. Neurosci. 2, 9817–9823.
Gong, S., Zheng, C., Doughty, M. L., Losos, K., Didkovsky, N., Schambra, U. B., Nowak, N. J., Joyner, A., Leblanc, G., Hatten, M. E., and Heintz, N. (2003). A gene expression atlas of the central nervous system based on bacterial artificial chromosomes. Nature 425, 917–925.
Hentschke, H., Benkwitz, C., Banks, M. I., Perkins, M. G., Homanics, G. E., and Pearce, R. A. (2009). Altered GABAA,slow inhibition and network oscillations in mice lacking the GABAA receptor beta3 subunit. J. Neurophysiol. 102, 3643–3655.
Hevers, W., and Lüddens, H. (2002). Pharmacological heterogeneity of gamma-aminobutyric acid receptors during development suggests distinct classes of rat cerebellar granule cells in situ. Neuropharmacology 42, 34–47.
Hill-Venning, C., Belelli, D., Peters, J. A., and Lambert, J. J. (1997). Subunit-dependent interaction of the general anaesthetic etomidate with the gamma-aminobutyric acid type A receptor. Br. J. Pharmacol. 120, 749–756.
Homanics, G. E., DeLorey, T. M., Firestone, L. L., Quinlan, J. J., Handforth, A., Harrison, N. L., Krasowski, M. D., Rick, C. E., Korpi, E. R., Mäkelä, R., Brilliant, M. H., Hagiwara, N., Ferguson, C., Snyder, K., and Olsen, R. W. (1997). Mice devoid of gamma-aminobutyrate type A receptor beta3 subunit have epilepsy, cleft palate, and hypersensitive behavior. Proc. Natl. Acad. Sci. U.S.A. 94, 4143–4148.
Huntsman, M. M., Porcello, D. M., Homanics, G. E., DeLorey, T. M., and Huguenard, J. R. (1999). Reciprocal inhibitory connections and network synchrony in the mammalian thalamus. Science 283, 541–543.
Jáidar, O., Carrillo-Reid, L., Hernández, A., Drucker-Colín, R., Bargas, J., and Hernández-Cruz, A. (2010). Dynamics of the Parkinsonian striatal microcircuit: entrainment into a dominant network state. J. Neurosci. 30, 11326–11336.
Janssen, M. J., Ade, K. K., Fu, Z., and Vicini, S. (2009). Dopamine modulation of GABA tonic conductance in striatal output neurons. J. Neurosci. 29, 5116–5126.
Kravitz, A. V., Freeze, B. S., Parker, P. R., Kay, K., Thwin, M. T., Deisseroth, K., and Kreitzer, A. C. (2010). Regulation of parkinsonian motor behaviours by optogenetic control of basal ganglia circuitry. 29, 622–626.
Laurie, D. J., Wisden, W., and Seeburg, P. H. (1992). The distribution of thirteen GABAA receptor subunit mRNAs in the rat brain. III. Embryonic and postnatal development. J. Neurosci. 12, 4151–4172.
Madisen, L., Zwingman, T. A., Sunkin, S. M., Oh, S. W., Zariwala, H. A., Gu, H., Ng, L. L., Palmiter, R. D., Hawrylycz, M. J., Jones, A. R., Lein, E. S., and Zeng, H. (2010). A robust and high-throughput Cre reporting and characterization system for the whole mouse brain. Nat. Neurosci. 13, 133–140.
Mallet, N., Ballion, B., Le Moine, C., and Gonon, F. (2006). Cortical inputs and GABA interneurons imbalance projection neurons in the striatum of parkinsonian rats. J. Neurosci. 26, 3875–3884.
Murase, K., Ryu, P. D., and Randic, M. (1989). Excitatory and inhibitory amino acids and peptide-induced responses in acutely isolated rat spinal dorsal horn neurons. Neurosci. Lett. 103, 56–63.
Murray, A. J., Sauer, J. F., Riedel, G., McClure, C., Ansel, L., Cheyne, L., Bartos, M., Wisden, W., and Wulff, P. (2011). Parvalbumin-positive CA1 interneurons are required for spatial working but not for reference memory. Nat. Neurosci. 14, 297–299.
Nusser, Z., Kay, L. M., Laurent, G., Homanics, G. E., and Mody, I. (2001). Disruption of GABA(A) receptors on GABAergic interneurons leads to increased oscillatory power in the olfactory bulb network. J. Neurophysiol. 86, 2823–2833.
Picton, A. J., and Fisher, J. L. (2007). Effect of the alpha subunit subtype on the macroscopic kinetic properties of recombinant GABA(A) receptors. Brain Res. 1165, 40–49.
Ramadan, E., Fu, Z., Losi, G., Homanics, G. E., Neale, J. H., and Vicini, S. (2003). GABA(A) receptor beta3 subunit deletion decreases alpha2/3 subunits and IPSC duration. J. Neurophysiol. 89, 128–134.
Riedel, A., Härtig, W., Fritschy, J. M., Brückner, G., Seifert, U., and Brauer, K. (1998). Comparison of the rat dorsal and ventral striatopallidal system. A study using the GABA(A)-receptor alpha1-subunit and parvalbumin immunolabeling. Exp. Brain Res. 121, 215–221.
Santhakumar, V., Jones, R. T., and Mody, I. (2010). Developmental regulation and neuroprotective effects of striatal tonic GABA(A) currents. Neuroscience 167, 644–655.
Shen, W., Tian, X., Day, M., Ulrich, S., Tkatch, T., Nathanson, N. M., and Surmeier, D. J. (2007). Cholinergic modulation of Kir2 channels selectively elevates dendritic excitability in striatopallidal neurons. Nat. Neurosci. 10, 1458–1466.
Shuen, J. A., Chen, M., Gloss, B., and Calakos, N. (2008). Drd1a-tdTomato BAC transgenic mice for simultaneous visualization of medium spiny neurons in the direct and indirect pathways of the basal ganglia. J. Neurosci. 28, 2681–2685.
Tian, X., Kai, L., Hockberger, P. E., Wokosin, D. L., and Surmeier, D. J. (2010). MEF-2 regulates activity-dependent spine loss in striatopallidal medium spiny neurons. Mol. Cell. Neurosci. 44, 94–108.
Urak, L., Feucht, M., Fathi, N., Hornik, K., and Fuchs, K. (2006). A GABRB3 promoter haplotype associated with childhood absence epilepsy impairs transcriptional activity. Hum. Mol. Genet. 15, 2533–2541.
Wafford, K. A., Thompson, S. A., Thomas, D., Sikela, J., Wilcox, A. S., and Whiting, P. J. (1996). Functional characterization of human gamma-aminobutyric acidA receptors containing the alpha 4 subunit. Mol. Pharmacol. 50, 670–678.
Keywords: GABA, tonic inhibition, striatum, patch-clamp, Cre-lox genetics
Citation: Janssen MJ, Yasuda RP and Vicini S (2011) GABAA receptor β3 subunit expression regulates tonic current in developing striatopallidal medium spiny neurons. Front. Cell. Neurosci. 5:15. doi: 10.3389/fncel.2011.00015
Received: 16 March 2011; Paper pending published: 11 April 2011;
Accepted: 09 July 2011; Published online: 28 July 2011.
Edited by:
Enrico Cherubini, International School for Advanced Studies, ItalyReviewed by:
John Huguenard, Stanford University School of Medicine, USAIstvan Mody, University of California Los Angeles, USA
Copyright: © 2011 Janssen, Yasuda and Vicini. This is an open-access article subject to a non-exclusive license between the authors and Frontiers Media SA, which permits use, distribution and reproduction in other forums, provided the original authors and source are credited and other Frontiers conditions are complied with.
*Correspondence: Megan J. Janssen, Section on Molecular Neurobiology, NICHD, Building 35, Room 2C-1001, 35 Lincoln Drive, National Institutes of Health, Bethesda, MD 20892, USA. e-mail: megan.janssen-schroeder@nih.gov