- The School of Pharmacy, University of London, London, UK
The mature neocortex contains many different classes of GABAergic inhibitory interneurons, distributed, with some degree of selectivity, through six layers, and through many different regions. Some of the events in the early lives of these neurones that may determine their ultimate destination, their maturation and their selective innervation of targets appropriate for each subtype, are discussed. Both time and place of birth influence the class of interneuron that an early post-mitotic interneuronal precursor will become, driven by the selective expression of different combinations of transcription factors in different regions of their birth places in the ganglionic eminence and ventricular zone. The long distance migration of these precursors along tangential routes in marginal, subventricular, and intermediate zones and their final radial movement, into the developing cortex, is regulated by chemical cues, both attractant and repellent. Once they arrive at their final destination, they must integrate into the developing circuitry. As they mature within the cortex, their axons grow and branch in highly specific patterns that may be partially determined by the genetic blueprint for each interneuronal class and partly by the environment in which they find themselves. Finally, as each interneuron class begins to form synapses with only certain postsynaptic targets, cell–cell recognition, most probably via protein–protein interactions across the synaptic cleft, facilitate the formation of appropriate synapses.
What Can Mature Neuronal Structure Suggest about Development?
Anyone who has spent hours at the microscope, reconstructing cortical axons at high power, will be aware of the immense complexity of these axonal arbors and their huge diversity. A number of anatomical properties become apparent very rapidly. Firstly that pyramidal cell axons (with the exception of the drum stick like branches of layer 6 cortico-thalamic pyramidal axons in layer 4, Zhang and Deschênes, 1997), run almost straight through the neuropil, deviating significantly only as they encounter large obstacles, like blood vessels. They may have a gross structure that is peculiar to just one particular class of pyramidal cell, innervating only certain layers for example, but whatever the pattern of their branches, they run more or less straight through the cortex. They do not therefore give the impression of axons in search of a target. Pyramidal dendrites, on the other hand, are far from straight. Not only are they studded with spines of different lengths and shapes, which project in all possible directions from the shaft, but the dendritic shafts themselves take tortuous routes through the neuropil. The impression gained is that each class of pyramidal cell may have a preordained shape, in terms of the number, length, and diameter of its dendrites, whether or not it has well developed oblique dendrites, or an apical tuft, but that within these constraints, the dendritic spines and even the shafts modify this basic plan to seek appropriate excitatory axonal contacts.
In striking contrast, the axons of many classes of interneurons are often extremely convoluted, as though they were seeking targets, rather than waiting to be found. Moreover, each class of inhibitory interneuron has its own branching pattern, some having branches that exit almost at right angles, some branching at acute-, and others branching at oblique-angles. The following section explores the possibility that these differences result in part from the order in which different types of neurones arrive in the neocortex; spiny excitatory cells typically arriving before the GABAergic inhibitory interneurons that are assigned, at their birth, to the same layer (Miller, 1986a,b; Rymar and Sadikot, 2007).
Origins and Fates of Cortical Neurones
Origins and Fates of Spiny Excitatory Neurones
The spiny glutamatergic cells of the cortex (pyramidal and spiny stellate cells) are born in the ventricular zone. After their last cell division in the ventral zone, pyramidal neurones migrate to the cortical plate (the future cortex) along a common radial glial fascicle (Rakic, 1972). The radial unit model (Rakic, 1988) proposes that the position of a neuron’s precursor in the ventral zone determines its final horizontal coordinates, while its birth date determines its radial position, i.e., the layer, or sublayer, it is destined to occupy. This is the anatomical basis for the columnar structure of cortex first proposed by Mountcastle et al. (1957). Later born spiny cells that are destined for more superficial layers, must pass through the layers of older neurones, as they migrate radially to their final positions. The cortex develops “inside out,” laying down the pyramidal cells of the deepest layers first. The secreted signal, Reelin, its receptors and their downstream signaling pathways are thought to control/promote first this radial migration, then its termination (Huang, 2009a; Rakic, 2009; Vitalis and Rossier, 2011, for reviews). Recent studies using in utero intraventricular injection of EGFP-expressing retroviruses, confirm this hypothesis, by demonstrating that sister neurones take up radially aligned positions in the cortex, across layers. Moreover, sister cells have a stronger propensity to form chemical synapses with each other, than with neighboring cells of differing lineage (Yu et al., 2009; Costa and Hedin-Pereira, 2010). That these sister cells end up in different layers and will therefore develop different morphological and physiological characteristics and make synaptic connections with different cortical and sub-cortical targets, demonstrates that the impact of lineage, while crucial, is modified by birth date and environment.
Origins and Fates of Inhibitory Interneurons
The extent to which interneuronal properties are modified after their arrival in the cortex has been less studied to date. The vast majority of cortical interneurons are not born in the ventricular zone, but in the medial and caudal ganglionic eminence in the ventral forebrain and, in primates, in the subventricular zone (see also Inta et al., 2008).
Influence of Birth Place
Most of the well characterized types of inhibitory interneurons are born within the ventral telencephalone (subpallium), a region comprising distinct morphological zones referred to as lateral, medial, caudal, and septal ganglionic eminence (LGE, MGE, CGE, and SGE, respectively, Figure 1; Wonders and Anderson, 2006; Batista-Brito and Fishell, 2009; Vitalis and Rossier, 2011). In addition, interneurons can originate from the endopeduncular and preoptic area (Gelman et al., 2009), and from the cortical subventricular zone (Inta et al., 2008).
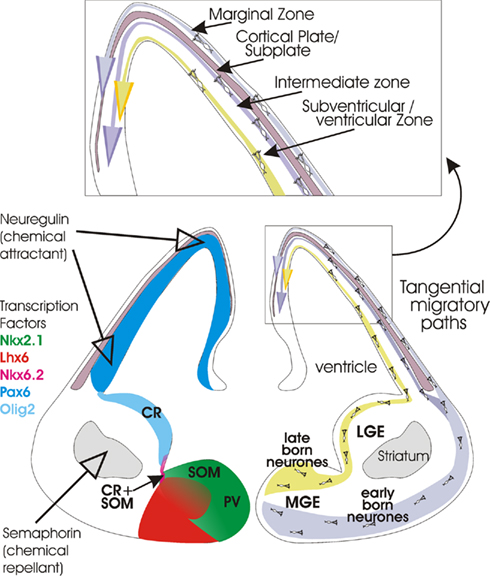
Figure 1. Cartoon (lower right) summarizing the origins of cortical interneurons and the tangential migratory paths they follow to the developing neocortex (cortical plate and subplate at this stage of development). At first, early born interneurons follow a more ventral route before migrating along the marginal zone overlying the developing cortex. Once the cortical plate has developed, cells in this pathway also migrate along the intermediate zone. Later born neurones follow a more dorsal route and then migrate along the subventricular zone. See insert (top) for layers. On the left, expression patterns of some of the transcription factors that appear to play a role in differentiation and migration are indicated (see color key). The expression of two important extracellular signals, Semaphorin (3A and 3F) and Neuregulin-1 are also indicated. The birth places of somatostatin-containing (SOM), parvalbumin- containing (PV), calretinin- (CR), and calretinin- plus somatostatin-containing (CR + SOM) interneurons are also indicated. Coronal section through the brain of an embryonic mouse (approximately E14). Figure modified from Hernández-Miranda et al. (2010), Heng et al. (2007), Huang (2009b).
The identity of newborn interneurons is regulated by the overlapping expression of specific transcription factors which is coordinated both spatially and temporally. The initial commitment to the GABAergic lineage is determined by the activity of Dlx1/2 transcription factors. The expression of these factors is however under control of a proneural gene Mash1 (Casarosa et al., 1999; Stuhmer et al., 2002). These genes are expressed widely throughout the subpallium, and they play a crucial role in development of cortical and olfactory bulb interneurons (Anderson et al., 1997a; Bulfone et al., 1998), and the striatum (Anderson et al., 1997b).
Parvalbumin-positive interneurons originate from the ventral area of the MGE (Wonders and Anderson, 2006) and their development relies on the activity of the Lhx6 transcription factor which is itself controlled by the Nkx2.1 transcription factor in this region (Figure 1; Liodis et al., 2007; Du et al., 2008). Although under the control of the same transcription factors, the dorsal area of the MGE is somehow specialized to give rise to a different class of interneurons, those that will express somatostatin/calretinin. Cell proliferation in both regions is under the regulation of β-catenin-dependent Wnt signaling pathway (Gulacsi and Anderson, 2008) and under the influence of sonic hedgehog (Shh) signaling which shows a gradual decrease in expression from dorsal to ventral MGE (Wonders et al., 2008; Xu et al., 2010).
In contrast, the cortical interneurons that express calretinin in combination with vasoactive intestinal polypeptide (VIP), VIP alone, or VIP, and cholecystokinine (CCK; Yozu et al., 2004; Butt et al., 2005; Fogarty et al., 2007) are generated in the CGE areas where the Lhx6 transcription factor is not expressed (Figure 1; Flames and Marin, 2005). Their lineage is postulated to be regulated by Nkx6.2 and CoupTF1/2, transcription factors that are widely, but not selectively expressed in this region (Sousa et al., 2009). It is currently unknown whether the LGE gives rise to any specific class of cortical interneurons (Wichterle et al., 1999). This region is, however, the main source of GABAergic striatal projection neurones (Anderson et al., 1997b), which originate from the ventral area, and of olfactory bulb interneurons (Waclaw et al., 2006), which originate from the dorsal area of LGE. Interneuronal progenitors in the endopeduncular and preoptic area give rise to NPY-containing interneurons including neurogliaform cells, under the guidance of the transcription factor Nkx5.1 (Figure 1; Gelman et al., 2009). In addition, a small population of calretinin-positive interneurons, destined for deeper cortical layers, originate from the cortical subventricular zone at postnatal stages (Inta et al., 2008). The functional and spatial overlap in the expression levels of specific transcription factors and gradients of these factors across the ganglionic eminence, are likely to play crucial roles in determining the lineage of different types of cortical interneurons.
Influence of Birth Date
In addition to the place of birth, the temporal order of interneuronal specification is critical. It is now well established that different classes of interneurons are born at different times within the ganglionic eminence. According to the current model of neurogenic divisions in the MGE, neural progenitor division in the ventricular zone is mostly asymmetric, giving rise either to radial glia cells or to intermediate progenitor cells and neurones. The progression through the cell cycle in this zone is known to be regulated by the cyclin D1/cdk4/6 enzyme complex which is generally expressed in proliferating progenitors but also in post-mitotic neurones (Glickstein et al., 2007a).
In contrast, the intermediate progenitor cells located within the subventricular zone of the MGE are under the guidance of the cyclin D2/cdk4/6 complex and divide symmetrically to give rise to two neurones or two glia cells, which at this stage acquire the ability to migrate to the cerebral cortex or striatum (Ross, 2011). Following the temporal order of events, the first classes of interneurons born in this region between E9.5 and E15 are parvalbumin-positive and somatostatin-positive interneurons, followed by calretinin-positive interneurons which are born significantly later (between E12.4 and E15.5; Butt et al., 2007). Among these three interneuronal types however, only the future parvalbumin-positive interneurons in this region appear to be under selective regulation by the cyclin D2 complex. The deletion of cyclin D2 gene leads not only to a prominent reduction in the abundance of parvalbumin-positive interneurons in the more mature cortex, but also to a microcephalic phenotype with general impairments in cortical development (Glickstein et al., 2007b). This distinct phenotype appears to be a consequence of a decrease in the proliferation potential of cells within the subventricular zone of the MGE, leading to an increase in the number of cells exiting the cell cycle prematurely. This phenotype also suggests that the cell fate of future parvalbumin-positive interneurons is already specified at the stage of their proliferating intermediate precursors. Thus, regulation of the cell cycle of progenitors in the MGE is critical for determining the final numbers of specific interneuronal types in the cortex and may influence their final fate.
Does GABA Signaling Influence the Fate of Interneurons?
A role for GABAergic signaling in the control of proliferation of interneuronal precursors within the regions of ganglionic eminence has not been demonstrated. However, evidence from other brain regions indicates that GABA plays a potent role in determining the proliferating potential of neuronal progenitors in general. GABAergic signaling mediated by GABAA receptors was shown to inhibit proliferation of neural crest stem cells early in development (Andang et al., 2008). Proliferation of neocortical progenitors in the ventricular and subventricular zones of the developing cortex is down-regulated by GABA and glutamate, both of which can lead to depolarization of the plasma membrane and an increase in intracellular calcium. The number of progenitor cells able to synthesize DNA was reported to be reduced as a consequence of this increase in calcium (LoTurco et al., 1995). Subsequently, similar effects of GABA and glutamate on cell proliferation were reported for the subventricular zone progenitors, while the opposite was observed in the ventricular zone (Haydar et al., 2000). The effects of GABA and glutamate were mimicked by specific agonists for GABAA and NMDA/AMPA receptors, respectively, and blocked by specific receptor antagonists in both studies. Furthermore, neuronal progenitors isolated from the ventricular and subventricular zones were shown to express GABAA receptors with different functional properties; the former showing a higher affinity for GABA, slow desensitization, small currents and no evidence of any synaptic activity. In contrast to this, the later post-mitotic neurones in the subventricular zone showed spontaneous synaptic activity mediated by GABAA receptors, which was dependent on action potentials and arising from the local interneurons (Owens et al., 1999).
The extracellular concentrations of GABA in the ganglionic eminence regions may be as high as 0.5 ± 0.1 μM (Cuzon et al., 2006). Early interneuronal precursors and progenitors appear to be the main cellular source of this GABA, since GABA (Bellion and Metin, 2005) and GABA-synthesizing enzymes are demonstrable by immunolocalisation in the ganglionic eminence regions. At the same time, these cells may be regulated by GABA via an autocrine feed-back mechanism. By analogy with immature pyramidal neurones (Demarque et al., 2002), interneuronal precursors may be able to release GABA tonically in a calcium- and soluble NSF-attachment protein receptor (SNARE)-independent fashion, possibly from their growth cones, since the anatomically defined presynaptic elements are not yet established at this early stage (Bourgeois and Rakic, 1993; Balslev et al., 1996). It is of interest however to note that these cells express some proteins known to regulate the neurotransmitter release machinery such as NXPH1 (Batista-Brito and Fishell, 2009), a peptide that binds to α-neurexins (see below).
Interneuronal precursors express functional GABAA receptors, predominantly containing the α4/β1/γ1 or γ2 subunits, GABA transporters VIAAT and GAT1, as well as chloride transporters NKCC1 and KCC2 (Laurie et al., 1992; Ma and Barker, 1995; Batista-Brito and Fishell, 2009). GABAergic markers GAD65/67 and GABAB receptors are also expressed at the earliest stages of neuronal lineage progression, and the autocrine activity of GABA appears to regulate the neurite outgrowth in cells cultured from these regions (Maric et al., 2001). Although GABA transporters are expressed they are suggested to be non-functional at this stage (Demarque et al., 2002), as the excitatory GABAergic currents recorded are slow tonic currents, unaffected by the presence of GABA transporter inhibitors.
Thus, interneuronal precursors within the proliferating zones of the ganglionic eminence not only synthesize and probably release GABA, but also express all the components of the molecular machinery necessary to respond to the secreted GABA. By analogy with the well established regulation of both embryonic (LoTurco et al., 1995; Haydar et al., 2000) and adult neuronal progenitors (Ge et al., 2007), a modulatory role for GABAergic signaling at the early stages of interneuron proliferation can be proposed. Additional direct evidence is, however, required.
Migration of Interneurons
Following their birth and early specialization in the ganglionic eminence, post-mitotic cortical interneuronal precursors embark on a long journey to their final destinations within the cortex. With a remarkable precision, they first follow tangential routes along the cortical marginal, subventricular, or intermediate zones (Figure 1). They then migrate into the growing cortical plate in a radial direction (Huang, 2009b). Their progression along these defined routes is regulated by a number of chemical cues which, in a highly coordinated fashion, either attract or repel the migrating interneurons. One such cue is semaphorine (Sema 3A and 3F), a factor expressed in the lateral ganglionic eminence which inhibits the entry of the migrating interneurons into this region (Marín et al., 2001). Another example of factors influencing the directionality of migrating interneurons are Slit proteins and their Robo (Roundabout) receptors expressed throughout the ventricular and subventricular zone of the ganglionic eminence. Although the exact function of these proteins is unclear, knock out mice studies have revealed miss-localization of calbindin-positive interneurons in the striatum and the embryonic cortex, and altered morphology of these cells (Andrews et al., 2008).
Ephrin EphA5/EphA4 receptors are expressed in the ventricular zone and are chemo-repellent for MGE-derived neurones (Zimmer et al., 2008). The other powerful cues include attractant neuregulin-1 (Yau et al., 2003; Flames et al., 2004), hepatocyte growth factor/scatter factor (Powell et al., 2001), and chemokine stromal-derived factor 1 (SDF-1; also known as CXCL12) which was shown to influence tangential migration in the subventricular/intermediate zone and integration of interneurons into their appropriate cortical layers (Stumm et al., 2003; Tiveron et al., 2006; Li et al., 2008; Lopez-Bendito et al., 2008). The migration is strongly enhanced by neurotrophins (BDNF and NT4; Polleux et al., 2002), glia-derived neurotrophic factor (GDNF; Pozas and Ibanez, 2005), and glutamate acting on AMPA receptors (Poluch et al., 2003). BDNF was also found to regulate the distribution of Cajal–Retzius cells in the medial zone and migration of interneurons within the cortex (Alcantara et al., 2006).
Cortical entry of tangentially migrating interneuronal precursors arriving from the medial ganglionic eminence is enhanced by GABA via GABAA receptors. As these cells progress toward the cortex, they also show increased sensitivity to GABA (Cuzon et al., 2006), in parallel with increased levels of expression of α1, α2, α5, γ2, and γ3 subunits of GABAA receptors (Cuzon Carlson and Yeh, 2011). Enhanced motility of interneurons is dependent on GABAA receptor-mediated depolarisation and downstream activation of L-type calcium channels (Bortone and Polleux, 2009). However, soon after interneurons enter the cortex their spontaneous intracellular calcium oscillations and their migration terminate. This was shown to be caused by an increase in the expression of KCC2 transporter which reduces the intracellular chloride concentration and terminates depolarizing activity of GABAA receptors (Bortone and Polleux, 2009). Possibly guided by intrinsic genetic cues or extrinsic environmental cues such as GABA itself (Ganguly et al., 2001; Kriegstein and Owens, 2001; Ludwig et al., 2003; Titz et al., 2003; Toyoda et al., 2003; Rivera et al., 2004; Leitch et al., 2005; Bortone and Polleux, 2009) or BDNF (Rivera et al., 2002, 2004), this change in the functional outcome of GABAA receptors expressed by migrating interneurons was suggested to play a key role in their correct positioning within the cortical layers. Once at the right place, further development of specific characteristics of some interneuronal classes, for example, those that express NPY, may also be modified by activation of GABAA receptors, as well as by BDNF released from their target neurones (Marty et al., 1996).
A Delay in the Marginal Zone
Thus, interneurons migrate tangentially from the regions in which they are born, through the marginal zone and along the subventricular and intermediate zones, reaching their final positions by radial migration within the cortex (Ang et al., 2003; Marín and Rubenstein, 2001, for review). There is, however, a delay en route. Interneurons are held in the marginal zone for a few days before entering the cortical plate (Tanaka et al., 2006). Interactions between the chemokine SDF-1 (CXCL12) and receptors expressed by the interneurons (CXCR4) delay interneuron entry into the cortex (Stumm et al., 2003; Tiveron et al., 2006; Li et al., 2008; Lopez-Bendito et al., 2008). It is proposed that changes in responsiveness to CXCL12 are responsible for controlling the timing of interneuron invasion of the cortical plate and that delayed entry is essential for the proper integration of interneurons into the cortical circuitry (Huang, 2009b, for review).
Since both pyramidal cells and at least some of the interneurons that are destined for the same layer are born at the same time (Miller, 1986a,b; Rymar and Sadikot, 2007), the delay in the marginal zone would ensure that interneurons enter each layer after the projection neurones have populated it (Pla et al., 2006). From their birthdays it would appear that parvalbumin, somatostatin, and somatostatin/calretinin-containing interneurons from the dorsal and ventral medial ganglionic eminence respectively (Butt et al., 2005; Wonders and Anderson, 2006; Wonders et al., 2008 for review) might be the first interneurons to enter the cortex, following an inside-out pattern similar to that of the pyramidal cells. Later, the VIP, calretinin/VIP, calbindin, VIP/Chat (Choline acetyltransferase) containing interneurons and NPY (neuropeptide Y) containing neurogliaform cells from the caudal ganglionic eminence (Yozu et al., 2004; Vucurovic et al., 2010; see also Batista-Brito et al., 2008; Gelman et al., 2009; Vitalis and Rossier, 2011, for review) would enter, but may not follow the conventional inside out pattern of destination. Since other interneurons may be the preferred targets of some of these later born VIP and calretinin-containing interneurons (by analogy with the CA1 region of the hippocampus, Freund and Buzsáki, 1996; Somogyi and Klausberger, 2005 for reviews), it seems reasonable to propose that interneurons like to “make an entrance,” entering their final layer destination only after their preferred targets have arrived.
Interneurons do not therefore have, as it were, a blank cortical canvas upon which to organize themselves. The complexity of their axonal arbors most probably reflects their need to provide many opportunities for the formation of synaptic contacts with appropriate targets. An important recent study (Wierenga et al., 2008, discussed in more detail below) confirms predictions that might be made from detailed examination of mature axonal and dendritic structures (see above), that inhibitory synapses do not exploit exploratory postsynaptic filopodia as many cortical excitatory synapses do, but must rely upon close physical associations between presynaptic axons and postsynaptic dendritic shafts. Whether and where such close associations occur and therefore where inhibitory synapses have opportunities to form, will be dependent upon the interneuronal axon arborisation within the context of the local target distribution (see also Stepanyants et al., 2004).
Inputs to Interneuronal Dendrites
Before leaving the question of target versus input selection, it is of interest to note that interneuronal dendrites do not typically exhibit the convoluted trajectories seen in pyramidal cells, nor are mature interneurons more than very sparsely spiny. With the exception of neurogliaform cells, each class, in each layer, appears to develop a stereotypical dendritic branching pattern, with relatively straight trajectories and few, if any spines. If (as suggested above) the excitatory axons are not seeking targets, but positioning themselves to receive the “correct” postsynaptic contacts and since the interneuronal dendrites are not convoluted or spiny, there is perhaps an anatomical explanation for the less finely tuned response properties of interneurons in primary sensory regions. Naturally, whether a synapse forms and even more importantly during development, whether it is maintained and strengthened, depends on additional factors, such as coincident activity. However, the opportunities available to interneuronal dendrites to “select” inputs, may be more limited by their own structure than those available to pyramidal dendrites.
Where Do Inhibitory Synapses Form?
In postnatal hippocampal cultures, the formation of synapses can be observed over hours. Excitatory, glutamatergic connections that formed between exploratory dendritic filopodia and axons often became stable and exhibited structural and immunocytochemical properties of mature synapses after a few hours. This was not, however, the case for GABAergic synapses. Contacts did form between dendritic filopodia and inhibitory axons, but these contacts were always short-lived. Only those that formed between an axon and a dendritic shaft, where these elements were in close proximity, i.e., at cross-over points, developed into stable, mature synapses. Within 1 h, the new presynaptic bouton had accumulated VGAT, though accumulation of postsynaptic scaffold proteins such as gephyrin did not occur within this time frame (Wierenga et al., 2008).
That there is a strong recognition signal between the pre and postsynaptic components of a potential future synapse and that excitatory and inhibitory cortical synapses employ different mechanisms and signals, is demonstrated by a calcium imaging study in early postnatal hippocampal cultures. Calcium activity was much higher in dendrites whose filopodia had made successful contacts with excitatory axons. It was independent of glutamate receptor activation suggesting that other, probably protein–protein interactions across the future synaptic cleft mediate this response. Moreover, this activity occurred within the first minute of the contact forming and was higher in those contacts that subsequently became stable. Activity in dendrites whose filopodia had failed to make contacts was much lower and contact with GABAergic axons produced no calcium signal (Lohmann and Bonhoeffer, 2008). Exploratory filopodia therefore recognize appropriate excitatory axons and respond to contact with them with a strong calcium signal, which is proposed to contribute to their stabilization.
A large body of work, some of which is summarized below, has studied GABAergic synapse formation in neuronal cultures and co-cultures. These studies have provided extremely important insight, but differences between in vivo and in vitro should not be overlooked. One interesting point is the weeks it can take for innervation to mature during development in vivo, compared with the minutes to hours needed to form a functional (if not fully mature) synapse in culture (Ahmari et al., 2000; Bresler et al., 2004; see also Kirov et al., 1999). In addition to the many factors that promote synaptogenesis, there must be some very strong controls exerted to ensure that uncontrolled aberrant synaptogenesis does not occur, either in the temporal, or in the spatial domain (see L1CAM, PSA–NCAM below).
Cell Adhesion Molecules
The first critical step in the formation of a mature inhibitory synapse in cortical structures appears, therefore, to be close proximity of pre and postsynaptic elements and does not involve postsynaptic filopodia. It has yet to be determined whether these opportunities occur near randomly and are successful only at “appropriate” cross-over points, i.e., when the class of interneuron coincides with the appropriate postsynaptic compartment, or whether each class of axonal arborisation forms these cross-over points preferentially with appropriate potential targets. Further, if the latter is true, is it the fine structure of the axonal arbor predetermined genetically, or is it the environment in which it finds itself, that dominates, or it is a combination of the two?
The L1 Family of Immunoglobulin Cell Adhesion Molecules
Cell adhesion molecules can play an important role in determining the direction of inhibitory axon outgrowth (Panicker et al., 2003, for review). This has been demonstrated in the developing cerebellum. Basket cell axons first make contact with Purkinje cell somata. Whether they then extend processes along the axon initial segment to form pinceau synapses there, or make aberrant synapses elsewhere, depends on a subcellular gradient of neurofascin186 (NF186). This is a member of the L1 family of immunoglobulin cell adhesion molecules (L1CAM), which is recruited to the initial segment by ankyrin G, a membrane adaptor protein that is restricted to the axon initial segment (Ango et al., 2004). More recently, interactions between L1CAM and ankyrin have also been shown to be important for the elaboration and branching of GABAergic basket cell axons around postsynaptic somata in the prefrontal and cingulate cortex (Guan and Maness, 2010).
The L1 family of immunoglobulin cell adhesion molecules phosphorylation (on Tyr-1229) which is very high at P0, decreases postnatally, to become nearly undetectable in the adult (at P60). This decrease in phosphorylation is correlated with increased L1CAM-ankyrin binding and coincides with periods of synaptogenesis and synapse remodeling (Guan and Maness, 2010). Another cell adhesion molecule, CHL1 (Close Homolog of L1) which is localized to cerebellar Bergmann glial cells and to stellate neurones while their axons are developing, plays a critical role in the targeting of stellate axons to Purkinje cell dendrites. Without CHL1, the axons show atypical branching and orientation, synaptogenesis is reduced and synapses are not maintained (Ango et al., 2008). A role for CHL1 in organizing the chaperoning of the presynaptic SNARE complex, through interactions between the intracellular domain of CHL1 and Hsc70, has also been demonstrated (Andreyeva et al., 2010).
Neuronal Cell Adhesion Molecules
Neuronal cell adhesion molecules (NCAMs) have also been shown to influence neurite outgrowth, axon branching, and GABAergic synaptogenesis. Cell densities were not altered in a mouse schizophrenia model in which the shedding of soluble extracellular domains (ectodomains) of NCAM (NCAM-EC) is enhanced. There were, however, abnormalities in cortical GABAergic interneurons and reduced puncta immuno-positive for the presynaptic GABAergic markers, GAD65, GAD67 and GAT1 (GABA transporter 1), suggesting that fewer GABAergic synapses had formed (Pillai-Nair et al., 2005). In a study that focused on cortical basket cells, over-expression of NCAM-EC (which would block interactions with membrane-bound NCAM) disrupted neurite arborisation at the time when maximum growth is expected. This resulted in a reduction in the numbers of perisomatic synapses in vivo, while in cortical neurone cultures, soluble NCAM-EC acted as a dominant inhibitor of NCAM-dependent neurite branching and outgrowth (Brennaman and Maness, 2008). In support of the suggestion that NCAM promotes neurite development and synaptogenesis, while its soluble ectodomain inhibits these processes, inhibition of the shedding of NCAM-EC in cortical neurone cultures promoted neurite outgrowth and branching (Hinkle et al., 2006).
Polysialic acid (PSA) masks NCAM function; a control that appears critical for normal brain development (Weinhold et al., 2005). Disruption of this control results in a severe phenotype, including progressive hydrocephalus, postnatal growth retardation, and precocious death, despite the presence of NCAM. In addition, wiring defects included accumulation of cells in the anterior subventricular zone and rostral migratory stream. PSA concentrations fall just after eye opening in the rodent, a decline that does not occur if the eyes remain closed. This change in PSA-masking of NCAM is therefore proposed to be activity-dependent and to promote perisomatic inhibitory synaptogenesis and the onset of ocular dominance plasticity. It is therefore proposed that a major function of PSA is to down regulate NCAM function at specific stages of development (Di Cristo et al., 2007).
GABA
Widespread remodeling of cortical circuitry during certain critical periods, such as that which follows eye opening in carnivores and rodents, is essential for the establishment of mature function. Activity in the circuit, driven by sensory input, is an essential contributor to this developmental plasticity. For example, although the targeting of specific postsynaptic subcellular compartments by different classes of interneurons occurs in the absence of sensory input (Di Cristo et al., 2004), visual deprivation retards the maturation of perisomatic inhibitory innervation of pyramidal cells (Chattopadhyaya et al., 2004) and the normal threefold increase in GABAergic input, during the critical period, is prevented (Morales et al., 2002). Moreover, this is a two way process. Evidence is growing that the functional maturation of inhibitory synapses triggers activity-dependent changes during critical periods and that development of perisomatic inhibition by parvalbumin-containing basket cells acting through α1-GABAA receptors drives these changes (Hensch, 2005, for review).
Knock out of one isoform of glutamic acid decarboxylase (GAD65) prevented the loss of visual responsiveness that is the normal consequence of eye closure during the critical period. This function was restored by infusion of the positive GABAA receptor modulator Diazepam into the visual cortex (Hensch et al., 1998). GABA itself, or its interactions with GABAA receptors also appear to influence axonal branching and synapse formation. In adolescent visual cortex, conditional knock down of GAD67 (but not of GAD65) in parvalbumin-containing interneurons, resulted in axonal branching defects in these neurones and a reduction in inhibitory innervation of pyramidal somata. Maintenance of those synapses that did form was not, however affected. Over-expression of GAD67 in these cells accelerated the maturation of somatic innervation. Since the knock down affected only PV-cells and since a less severe modification (deletion of only one GAD1 allele), also resulted in reduced somatic innervation, it appears that “spill-over” of GABA from neighboring synapses is insufficient to promote axonal branching and synaptogenesis. It does, however appear, that GABA itself is an essential component of the signal, since somatic innervation could be rescued by blocking GABA-uptake or by adding Diazepam. Moreover, activation of GABAB receptors also appears to contribute, since addition of baclofen rescued somatic innervation, at least in part, although terminal branching remained reduced (Chattopadhyaya et al., 2007).
Importance of GABAA Receptors in Synapse Formation and Stabilization
There is a general consensus that cortical synaptic GABAA receptors contain two α-subunits, two β-subunits, and one γ-subunit. While the β-subunits are required for transport of the pentomeric receptors to the cell surface and may confer dendritic versus somatic targeting of GABAA receptors and α-subunits may determine their localisation at specific synaptic subtypes (Thomson and Jovanovic, 2010, for review), the γ-subunit is thought to be essential for synaptic versus extrasynaptic localisation. When the expression of γ-subunits is suppressed, the clustering of GABAA receptors becomes disrupted and the GABAergic innervation of neurones lacking γ2-receptors is profoundly reduced (Schweizer et al., 2003; Li et al., 2005). That it is the clustering of GABAA receptors at potential synaptic sites that is important for establishing GABAergic innervation, was also indicated by experiments in which neurones were transfected with dominant negative GODZ [Golgi-specific DHHC (Asp-His-His-Cys) zinc finger protein], a member of the DHHC palmitoyl acyltransferase family. Without the palmitoylation of the γ2 subunit by GODZ, trafficking of GABAA receptors to the cell surface is disrupted and GABAergic innervation of transfected cells is reduced (Fang et al., 2006).
Clusters of GABAA receptors appear, therefore, to promote the stabilization and maturation of presynaptic GABAergic terminals. The converse is also true. Extrasynaptic GABAA receptors exhibit much greater lateral mobility in the plasma membrane than synaptic receptors. The presence of a presynaptic terminal reduces lateral mobility, enhancing the stabilization of GABAA receptor clusters at synapses. Although it is unclear whether gephyrin controls the formation or the stability of receptor clusters, or in some way regulates the numbers expressed on the cell surface, an important role for gephyrin in facilitating the accumulation of GABAA receptors at inhibitory synapses was indicated by the reduction in receptor clusters and greater mobility of those that existed when gephyrin was knocked down (Jacob et al., 2005).
Interactions between Neuroligin (NL) and Neurexin (Nx)
Clearly, interactions between the presynaptic axon and postsynaptic cell are required for GABAergic synapses to form, mature and survive. There has recently been intense interest in the interactions between presynaptic neurexins (Nx) and postsynaptic neuroligins (NL); interactions that are thought to be fundamental to the formation of synapses. Of the four neuroligins, NL1, NL3, and NL4 are found primarily at excitatory and NL2 at inhibitory synapses (Graf et al., 2004; Chih et al., 2005). In immature neurones, NL2 aggregates with GABAA receptors that do not (yet) face a GABAergic nerve terminal, suggesting a role for NL2 in determining the future locations of GABAergic synapses (Varoqueaux et al., 2004; see also Ziv and Garner, 2004; Gerrow et al., 2006).
In sufficiently reduced systems, NL2 appears to play a near essential role in the formation of functional GABAergic synapse-like contacts. In neurone-HEK293 cell co-cultures, the transfected HEK293 cells that express NL2 and GABAA receptors acquired functional GABAergic axonal contacts, while those expressing only GABAA receptors, or NL1 and GABAARs, did not (Dong et al., 2007). Indeed, NL2 alone, expressed in non-neuronal cells, or attached to beads (Graf et al., 2004), appears able to promote the formation of presynaptic boutons. NL2 does not, however, appear to be an absolute requirement for GABAergic synapse-formation in vivo (Varoqueaux et al., 2006; Chubykin et al., 2007). In triple NL-knock out mice (NL1, NL2, NL3), the number of synapses appeared normal, but mismatches between pre and postsynaptic proteins developed. For example, VGlut was found co-localized with gephyrin and VIAAT with PSD95. Moreover, a range of excitatory, and inhibitory synaptic vesicle markers were reduced in these triple knock outs, e.g., the soluble SNARE regulators complexin 2 and (SNAP, as well as KCC2 were reduced. However, the presynaptic active zone proteins Munc13-1 and RIM1/2, SNAP-25, and calbindin appeared unchanged, as did postsynaptic gephyrin, β-dystroglycan and α1, and β2/3 GABAAR subunits (Varoqueaux et al., 2006).
Neurorexins appear to recruit a number of important proteins to the active zone, including presynaptic Ca2+-channels (O’Connor et al., 1993). Indeed, in the non-viable triple α-neurexin knock out, N and P/Q Ca2+-channels do not cluster at active zones and action potential-triggered release fails (Missler et al., 2003; Zhang et al., 2005). The stabilization of Nxs, particularly Nx-1β, at the active zone is also regulated by its interactions with postsynaptic proteins (like NLs) and the rate at which these proteins turnover is reduced by neuronal activity and by presynaptic GABAB receptors (Fu and Huang, 2010). NLs interact with and possibly localize and stabilize Nxs, these cross-cleft interactions may therefore promote and modify a range of synaptic properties. Again, a role for specifying the location and types of synapse and more subtle aspects of their function, rather than an absolute requirement for their presence or absence, is suggested for the NL–Nx interactions.
Alternative Splicing and Synapse Specificity
Presynaptic Neurexins (1α, 2α, 3α, 1β, 2β, 3β) exhibit extensive alternative splicing (>2000 potential variants, Missler and Sudhof, 1998; Tabuchi and Sudhof, 2002) particularly within their laminin neurexin sex (LNS) hormone binding protein domains. Six LNS domains in α-neurexin and one in β-neurexin exhibit Ca2+-dependent binding to extracellular domains of NLs, dystroglycan, and neurexophilin and provide a high affinity α-latrotoxin binding site (Craig and Kang, 2007, for review). By altering Ca2+-binding affinity, splice insertions at these sites alter their interactions with other proteins (Sheckler et al., 2006).
Alternative splicing of NLs is less extensive, but powerfully influences their interactions with other proteins. Commensurate with its localisation at GABAergic synapses, NL2 is not normally alternatively spliced at splice site B. Splice insertion at this site restricts the activity of neuroligins to glutamatergic synapses and negates binding to the β-neurexin isoform that contains an insertion in site 4 [Nx-1β4(+)]. The selectivity imposed by the B insertion requires the N-glycosylation site in B (Boucard et al., 2005). Neuroligin lacking the B-site insert also interacts with Nx-1α, with or without a neurexin-site 4 (S4) insertion (Chih et al., 2005). When expressed in COS cells, Nx-1β4(+) and Nx-1α4(−) induced the inclusion of gephyrin and NL2, but not of PSD95 in postsynaptic densities in co-cultured neurones. Recombinant Nx-1β4(+)-Fc, designed to block extracellular interactions with NL, selectively reduced the density of VGAT-positive terminals with no effect on the density of vGlut1-positive puncta (Chih et al., 2006). Thus the S4 insert in Nx-1β permits binding to NL2, which typically does not include the B-site insert, and promotes clustering of postsynaptic GABAergic proteins. The S4 insert in Nx-1β also decreases the ability of Nx-1β to cluster NL1, NL3, and NL4 and postsynaptic glutamatergic scaffold proteins (Graf et al., 2006). A developmental and possibly activity-driven decrease in the inclusion of the S4 insert correlates with glutamatergic versus GABAergic synaptogenesis (Kang et al., 2008).
Finally, the impact of deleting NL2 in the adult appears to vary with the type of synapse involved. In adult CA1, gephyrin- and GABAA receptor-positive puncta were significantly reduced in stratum pyramidale (primarily basket cell synapses on pyramidal somata), but not in stratum radiatum (innervation from a range of dendrite-preferring interneuronal subtypes). This was despite the continuing presence of VIAAT immuno-labeling, suggesting that inhibitory innervation of stratum pyramidale was maintained, but with reduced postsynaptic specialization (Poulopoulos et al., 2009).
Final remarks
From the early proliferating precursors within the ganglionic eminence to morphologically and functionally diverse, and synaptically connected interneurons within the cortex, interneurons undergo a process of remarkable transformation in time and space guided by the activity of transcription factors, but shaped by continuous signaling to and from their surrounding environment mediated by specific protein–protein interactions at the cell surface. Although the main outline of this journey is increasingly clear, a number of key steps toward the final complex phenotype of interneurons remain uncharacterized. One of the least known, yet perhaps the most critical of these, is the outgrowth and the coordination of developing axonal and dendritic arbors of interneurons once they have reached their final destinations in the cortex. This step remains challenging to tackle experimentally and will require systematic in vivo structural and functional analysis. The step that follows this, during which interneurons approach their synaptic partners with a remarkable selectivity and form morphologically and functionally highly specialized synapses is equally challenging. A number of cell adhesion proteins discussed here have been implicated in this step. However, the molecular key to the synaptic specificity remains to be uncovered.
Conflict of Interest Statement
The authors declare that the research was conducted in the absence of any commercial or financial relationships that could be construed as a potential conflict of interest.
Acknowledgments
The work from our own laboratories that is discussed here and much of the insight we have gained is the result of studies that were funded by the MRC, the BBSRC and the Wellcome Trust.
References
Ahmari, S. E., Buchanan, J., and Smith, S. J. (2000). Assembly of presynaptic active zones from cytoplasmic transport packets. Nat. Neurosci. 3, 445–451.
Alcantara, S., Pozas, E., Ibanez, C. F., and Soriano, E. (2006). BDNF-modulated spatial organization of Cajal-Retzius and GABAergic neurons in the marginal zone plays a role in the development of cortical organization. Cereb. Cortex 16, 487–499.
Andang, M., Hjerling-Leffler, J., Moliner, A., Lundgren, T. K., Castelo-Branco, G., Nanou, E., Pozas, E., Bryja, V., Halliez, S., Nishimaru, H., Wilbertz, J., Arenas, E., Koltzenburg, M., Charnay, P., El, M. A., Ibanez, C. F., and Ernfors, P. (2008). Histone H2AX-dependent GABA(A) receptor regulation of stem cell proliferation. Nature 451, 460–464.
Anderson, S. A., Eisenstat, D. D., Shi, L., and Rubenstein, J. L. (1997a). Interneuron migration from basal forebrain to neocortex: dependence on Dlx genes. Science 278, 474–476.
Anderson, S. A., Qiu, M., Bulfone, A., Eisenstat, D. D., Meneses, J., Pedersen, R., and Rubenstein, J. L. (1997b). Mutations of the homeobox genes Dlx-1 and Dlx-2 disrupt the striatal subventricular zone and differentiation of late born striatal neurons. Neuron 19, 27–37.
Andrews, W., Barber, M., Hernadez-Miranda, L. R., Xian, J., Rakic, S., Sundaresan, V., Rabbitts, T. H., Pannell, R., Rabbitts, P., Thompson, H., Erskine, L., Murakami, F., and Parnavelas, J. G. (2008). The role of Slit-Robo signaling in the generation, migration and morphological differentiation of cortical interneurons. Dev. Biol. 313, 648–658.
Andreyeva, A., Leshchyns’ka, I., Knepper, M., Betzel, C., Redecke, L., Sytnyk, V., and Schachner, M. (2010). CHL1 is a selective organizer of the presynaptic machinery chaperoning the SNARE complex. PLoS ONE 5, e12018. doi: 10.1371/journal.pone.0012018
Ang, E. S. B. C. Jr., Haydar, T. F., Gluncic, V., and Rakic, P. (2003). Four-dimensional migratory coordinates of GABAergic interneurons in the developing mouse cortex. J. Neurosci. 23, 5805–5815.
Ango, F., di Cristo, G., Higashiyama, H., Bennett, V., Wu, P., and Huang, Z. J. (2004). Ankyrin-based subcellular gradient of neurofascin, an immunoglobulin family protein, directs GABAergic innervation at purkinje axon initial segment. Cell 119, 257–272.
Ango, F., Wu, C., Van der Want, J. J., Wu, P., Schachner, M., and Huang, Z. J. (2008). Bergmann glia and the recognition molecule CHL1 organize GABAergic axons and direct innervation of Purkinje cell dendrites. PLoS Biol. 6, e103. doi: 10.1371/journal.pbio.0060103
Balslev, Y., Saunders, N. R., and Mollgard, K. (1996). Synaptogenesis in the neocortical anlage and early developing neocortex of rat embryos. Acta Anat. (Basel) 156, 2–10.
Batista-Brito, R., and Fishell, G. (2009). The developmental integration of cortical interneurons into a functional network. Curr. Top. Dev. Biol. 87, 81–118.
Batista-Brito, R., Machold, R., Klein, C., and Fishell, G. (2008). Gene expression in cortical interneuron precursors is prescient of their mature function. Cereb. Cortex 18, 2306–2317.
Bellion, A., and Metin, C. (2005). Early regionalisation of the neocortex and the medial ganglionic eminence. Brain Res. Bull. 66, 402–409.
Bortone, D., and Polleux, F. (2009). KCC2 expression promotes the termination of cortical interneuron migration in a voltage-sensitive calcium-dependent manner. Neuron 62, 53–71.
Boucard, A. A., Chubykin, A. A., Comoletti, D., Taylor, P., and Südhof, T. C. (2005). A splice code for trans-synaptic cell adhesion mediated by binding of neuroligin 1 to alpha- and beta-neurexins. Neuron 48, 229–236.
Bourgeois, J. P., and Rakic, P. (1993). Changes of synaptic density in the primary visual cortex of the macaque monkey from fetal to adult stage. J. Neurosci. 13, 2801–2820.
Brennaman, L. H., and Maness, P. F. (2008). Developmental regulation of GABAergic interneuron branching and synaptic development in the prefrontal cortex by soluble neural cell adhesion molecule. Mol. Cell. Neurosci. 37, 781–793.
Bresler, T. Shapira M., Boeckers, T., Dresbach, T., Futter, M., Garner, C. C., Rosenblum, K., Gundelfinger, E. D., and Ziv, N. E. (2004). Postsynaptic density assembly is fundamentally different from presynaptic active zone assembly. J. Neurosci. 24, 1507–1520.
Bulfone, A., Wang, F., Hevner, R., Anderson, S., Cutforth, T., Chen, S., Meneses, J., Pedersen, R., Axel, R., and Rubenstein, J. L. (1998). An olfactory sensory map develops in the absence of normal projection neurons or GABAergic interneurons. Neuron 21, 1273–1282.
Butt, S. J., Cobos, I., Golden, J., Kessaris, N., Pachnis, V., and Anderson, S. A. (2007). Transcriptional regulation of cortical interneuron development. J. Neurosci. 27, 11847–11850.
Butt, S. J., Fuccillo, M., Nery, S., Noctor, S., Kriegstein, A., Corbin, J. G., and Fishell, G. (2005). The temporal and spatial origins of cortical interneurons predict their physiological subtype. Neuron 48, 591–604.
Casarosa, S., Fode, C., and Guillemot, F. (1999). Mash1 regulates neurogenesis in the ventral telencephalon. Development 126, 525–534.
Chattopadhyaya, B., Di Cristo, G., Higashiyama, H., Knott, G. W., Kuhlman, S. J., Welker, E., and Huang, Z. J. (2004). Experience and activity-dependent maturation of perisomatic GABAergic innervation in primary visual cortex during a postnatal critical period. J. Neurosci. 24, 9598–9611.
Chattopadhyaya, B., Di Cristo, G., Wu, C. Z., Knott, G., Kuhlman, S., Fu, Y., Palmiter, R. D., and Huang, Z. J. (2007). GAD67-mediated GABA synthesis and signaling regulate inhibitory synaptic innervation in the visual cortex. Neuron 54, 889–903.
Chih, B., Engelman, H., and Scheiffele, P. (2005). Control of excitatory and inhibitory synapse formation by neuroligins. Science 307, 1324–1328.
Chih, B., Gollan, L., and Scheiffele, P. (2006). Alternative splicing controls selective trans-synaptic interactions of the neuroligin–neurexin complex. Neuron 51, 171–178.
Chubykin, A. A., Atasoy, D., Etherton, M. R., Brose, N., Kavalali, E. T., Gibson, J. R., and Sudhof, T. C. (2007). Activity-dependent validation of excitatory versus inhibitory synapses by neuroligin-1 versus neuroligin-2. Neuron 54, 919–931.
Costa, M. R., and Hedin-Pereira, C. (2010). Does cell lineage in the developing cerebral cortex contribute to its columnar organization? Front. Neuroanat. 4:26. doi: 10.3389/fnana.2010.00026
Craig, A. M., and Kang, Y. (2007). Neurexin-neuroligin signalling in synapse development. Curr. Opin. Neurobiol. 17, 43–52.
Cuzon, V. C., Yeh, P. W., Cheng, Q., and Yeh, H. H. (2006). Ambient GABA promotes cortical entry of tangentially migrating cells derived from the medial ganglionic eminence. Cereb. Cortex 16, 1377–1388.
Cuzon Carlson, V. C., and Yeh, H. H. (2011). GABAA Receptor subunit profiles of tangentially migrating neurons derived from the medial ganglionic eminence. Cereb. Cortex. e 247. doi:10.1093/cercor/bhq247. [Epub ahead of print].
Demarque, M., Represa, A., Becq, H., Khalilov, I., Ben Ari, Y., and Aniksztejn, L. (2002). Paracrine intercellular communication by a Ca2+- and SNARE-independent release of GABA and glutamate prior to synapse formation. Neuron 36, 1051–1061.
Di Cristo, G., Chattopadhyaya, B., Kuhlman, S. J., Fu, Y., Belanger, M. C., Wu, C. Z., Rutishauser, U., Maffei, L., and Huang, Z. J. (2007). Activity-dependent PSA expression regulates inhibitory maturation and onset of critical period plasticity. Nat. Neurosci. 10, 1569–1577.
Di Cristo, G., Wu, C., Chattopadhyaya, B., Ango, F., Knott, G., Welker, E., Svoboda, K., and Huang, Z. J. (2004). Subcellular domain-restricted GABAergic innervation in primary visual cortex in the absence of sensory and thalamic inputs. Nat. Neurosci. 7, 1184–1186.
Dong, N., Qi, J., and Chen, G. (2007). Molecular reconstitution of functional GABAergic synapses with expression of neuroligin-2 and GABAA receptors. Mol. Cell. Neurosci. 35, 14–23.
Du, T., Xu, Q., Ocbina, P. J., and Anderson, S. A. (2008). NKX2.1 specifies cortical interneuron fate by activating Lhx6. Development 135, 1559–1567.
Fang, C., Deng, L., Keller, C. A., Fukata, M., Fukata, Y., Chen, G., and Luscher, B. (2006). GODZ-mediated palmitoylation of GABAA receptors is required for normal assembly and function of GABAergic inhibitory synapses. J. Neurosci. 26, 12758–12768.
Flames, N., Long, J. E., Garratt, A. N., Fischer, T. M., Gassmann, M., Birchmeier, C., Lai, C., Rubenstein, J. L., and Marin, O. (2004). Short- and long-range attraction of cortical GABAergic interneurons by neuregulin-1. Neuron 44, 251–261.
Flames, N., and Marin, O. (2005). Developmental mechanisms underlying the generation of cortical interneuron diversity. Neuron 46, 377–381.
Fogarty, M., Grist, M., Gelman, D., Marin, O., Pachnis, V., and Kessaris, N. (2007). Spatial genetic patterning of the embryonic neuroepithelium generates GABAergic interneuron diversity in the adult cortex. J. Neurosci. 27, 10935–10946.
Fu, Y., and Huang, Z. J. (2010). Differential dynamics and activity-dependent regulation of alpha- and beta-neurexins at developing GABAergic synapses. Proc. Natl. Acad. Sci. U.S.A. 107, 22699–22704.
Ganguly, K., Schinder, A. F., Wong, S. T., and Poo, M. M. (2001). GABA itself promotes the developmental switch of neuronal GABAergic responses from excitation to inhibition. Cell 105, 521–532.
Ge, S., Pradhan, D. A., Ming, G. L., and Song, H. (2007). GABA sets the tempo for activity-dependent adult neurogenesis. Trends Neurosci. 30, 1–8.
Gelman, D. M., Martini, F. J., Nóbrega-Pereira, S., Pierani, A., Kessaris, N., and Marín, O. (2009). The embryonic preoptic area is a novel source of cortical GABAergic interneurons. J. Neurosci. 29, 9380–9389.
Gerrow, K., Romorini, S., Nabi, S. M., Colicos, M. A., Sala, C., and El-Husseini, A. (2006). A preformed complex of postsynaptic proteins is involved in excitatory synapse development. Neuron 49, 547–562.
Glickstein, S. B., Alexander, S., and Ross, M. E. (2007a). Differences in cyclin D2 and D1 protein expression distinguish forebrain progenitor subsets. Cereb. Cortex 17, 632–642.
Glickstein, S. B., Moore, H., Slowinska, B., Racchumi, J., Suh, M., Chuhma, N., and Ross, M. E. (2007b). Selective cortical interneuron and GABA deficits in cyclin D2-null mice. Development 134, 4083–4093.
Graf, E. R., Kang, Y., Hauner, A. M., and Craig, A. M. (2006). Structure function and splice site analysis of the synaptogenic activity of the neurexin-1 beta LNS domain. J. Neurosci. 26, 4256–4265.
Graf, E. R., Zhang, X., Jin, S. X., Linhoff, M. W., and Craig, A. M. (2004). Neurexins induce differentiation of GABA and glutamate postsynaptic specializations via neuroligins. Cell 119, 1013–1026.
Guan, H., and Maness, P. F. (2010). Perisomatic GABAergic innervation in prefrontal cortex is regulated by ankyrin interaction with the L1 cell adhesion molecule. Cereb. Cortex 20, 2684–2693.
Gulacsi, A. A., and Anderson, S. A. (2008). Beta-catenin-mediated Wnt signaling regulates neurogenesis in the ventral telencephalon. Nat. Neurosci. 11, 1383–1391.
Haydar, T. F., Wang, F., Schwartz, M. L., and Rakic, P. (2000). Differential modulation of proliferation in the neocortical ventricular and subventricular zones. J. Neurosci. 20, 5764–5774.
Heng, J. I., Moonen, G., and Nguyen, L. (2007). Neurotransmitters regulate cell migration in the telencephalon. Eur. J. Neurosci. 26, 537–546.
Hensch, T. K. (2005). Critical period plasticity in local cortical circuits. Nat. Rev. Neurosci. 6, 877–888.
Hensch, T. K., Fagiolini, M., Mataga, N., Stryker, M. P., Baekkeskov, S., and Kash, S. F. (1998). Local GABA circuit control of experience-dependent plasticity in developing visual cortex. Science 282, 1504–1508.
Hernández-Miranda, L. R., Parnavelas, J. G., and Chiara, F. (2010) Molecules and mechanisms involved in the generation and migration of cortical interneurons. ASN Neuro. 2, e00031.
Hinkle, C. L., Diestel, S., Lieberman, J., and Maness, P. F. (2006). Metalloprotease-induced ectodomain shedding of neural cell adhesion molecule (NCAM). J. Neurobiol. 66, 1378–1395.
Huang, Z. J. (2009a). Molecular regulation of neuronal migration during neocortical development. Mol. Cell. Neurosci. 42, 11–22.
Huang, Z. J. (2009b). Activity-dependent development of inhibitory synapses and innervation pattern: role of GABA signalling and beyond. J. Physiol. 587, 1881–1888.
Inta, D., Alfonso, J., von Engelhardt, J., Kreuzberg, M. M., Meyer, H., van Hooft, J. A., and Monyer, H. (2008). Neurogenesis and widespread forebrain migration of distinct GABAergic neurons from the postnatal subventricular zone. Proc. Natl. Acad. Sci. U.S.A. 105, 20994–20999.
Jacob, T. C., Bogdanov, Y. D., Magnus, C., Saliba, R. S., Kittler, J. T., Haydon, P. G., and Moss, S. J. (2005). Gephyrin regulates the cell surface dynamics of synaptic GABAA receptors. J. Neurosci. 25, 10469–10478.
Kang, Y., Zhang, X., Dobie, F., Wu, H., and Craig, A. M. (2008). Induction of GABAergic postsynaptic differentiation by α-Neurexins. J. Biol. Chem. 283, 2323–2334.
Kirov, S. A., Sorra, K. E., and Harris, K. M. (1999). Slices have more synapses than perfusion-fixed hippocampus from both young and mature rats. J. Neurosci. 19, 2876–2886.
Kriegstein, A. R., and Owens, D. F. (2001). GABA may act as a self-limiting trophic factor at developing synapses. Sci. STKE 2001, pe1.
Laurie, D. J., Wisden, W., and Seeburg, P. H. (1992). The distribution of thirteen GABAA receptor subunit mRNAs in the rat brain. III. Embryonic and postnatal development. J. Neurosci. 12, 4151–4172.
Leitch, E., Coaker, J., Young, C., Mehta, V., and Sernagor, E. (2005). GABA type-A activity controls its own developmental polarity switch in the maturing retina. J. Neurosci. 25, 4801–4805.
Li, G., Adesnik, H., Li, J., Long, J., Nicoll, R. A., Rubenstein, J. L., and Pleasure, S. J. (2008). Regional distribution of cortical interneurons and development of inhibitory tone are regulated by Cxcl12/Cxcr4 signaling. J. Neurosci. 28, 1085–1098.
Li, R. W., Yu, W., Christie, S., Miralles, C. P., Bai, J., Loturco, J. J., and De Blas, A. L. (2005). Disruption of postsynaptic GABA receptor clusters leads to decreased GABAergic innervation of pyramidal neurons. J. Neurochem. 95, 756–770.
Liodis, P., Denaxa, M., Grigoriou, M., Akufo-Addo, C., Yanagawa, Y., and Pachnis, V. (2007). Lhx6 activity is required for the normal migration and specification of cortical interneuron subtypes. J. Neurosci. 27, 3078–3089.
Lohmann, C., and Bonhoeffer, T. (2008). A role for local calcium signaling in rapid synaptic partner selection by dendritic filopodia. Neuron 59, 253–260.
Lopez-Bendito, G., Sanchez-Alcaniz, J. A., Pla, R., Borrell, V., Pico, E., Valdeolmillos, M., and Marin, O. (2008). Chemokine signaling controls intracortical migration and final distribution of GABAergic interneurons. J. Neurosci. 28, 1613–1624.
LoTurco, J. J., Owens, D. F., Heath, M. J., Davis, M. B., and Kriegstein, A. R. (1995). GABA and glutamate depolarize cortical progenitor cells and inhibit DNA synthesis. Neuron 15,1287–1298.
Ludwig, A., Li, H., Saarma, M., Kaila, K., and Rivera, C. (2003). Developmental up-regulation of KCC2 in the absence of GABAergic and glutamatergic transmission. Eur. J. Neurosci. 18, 3199–3206.
Ma, W., and Barker, J. L. (1995). Complementary expressions of transcripts encoding GAD67 and GABAA receptor alpha 4, beta 1, and gamma 1 subunits in the proliferative zone of the embryonic rat central nervous system. J. Neurosci. 15, 2547–2560.
Maric, D., Liu, Q. Y., Maric, I., Chaudry, S., Chang, Y. H., Smith, S. V., Sieghart, W., Fritschy, J. M., and Barker, J. L. (2001). GABA expression dominates neuronal lineage progression in the embryonic rat neocortex and facilitates neurite outgrowth via GABA(A) autoreceptor/Cl− channels. J. Neurosci. 21, 2343–2360.
Marín, O., and Rubenstein, J. L. (2001). A long, remarkable journey: tangential migration in the telencephalon. Nat. Rev. Neurosci. 2, 780–790.
Marín, O., Yaron, A., Bagri, A., Tessier-Lavigne, M., and Rubenstein, J. L. (2001). Sorting of striatal and cortical interneurons regulated by semaphorin-neuropilin interactions. Science 293, 872–875.
Marty, S., Berninger, B., Carroll, P., and Thoenen, H. (1996). GABAergic stimulation regulates the phenotype of hippocampal interneurons through the regulation of brain-derived neurotrophic factor. Neuron 16, 565–570.
Miller, M. W. (1986a). Maturation of rat visual cortex. III. Postnatal morphogenesis and synaptogenesis of local circuit neurons. Brain Res. 390, 271–285.
Miller, M. W. (1986b). The migration and neurochemical differentiation of gamma-aminobutyric acid (GABA)-immunoreactive neurons in rat visual cortex as demonstrated by a combined immu nocyto-chemical-autoradio-graphic technique. Brain Res. 393, 41–46.
Missler, M., and Sudhof, T. C. (1998). Neurexins: three genes and 1001 products. Trends Genet. 14, 20–26.
Missler, M., Zhang, W., Rohlmann, A., Kattenstroth, G., Hammer, R. E., Gottmann, K., and Sudhof, T. C. (2003). a-neurexins couple Ca2+ channels to synaptic vesicle exocytosis. Nature 424, 939–948.
Morales, B., Choi, S. Y., and Kirkwood, A. (2002). Dark rearing alters the development of GABAergic transmission in visual cortex. J. Neurosci. 22, 8084–8090.
Mountcastle, V. B., Davies, P. W., and Berman, A. L. (1957). Response properties of neurons of cats somatic sensory cortex to peripheral stimuli. J. Neurophysiol. 20, 374–407.
O’Connor, V., Shamotienko, O., Grishin, E., and Betz, H. (1993). On the structure of the synaptosecretosome: evidence for a neurexin/synaptotagmin/syntaxin/Ca2+ channel complex FEBS Lett. 326, 255–260.
Owens, D. F., Liu, X., and Kriegstein, A. R. (1999). Changing properties of GABA(A) receptor-mediated signaling during early neocortical development. J. Neurophysiol. 82, 570–583.
Panicker, A. K., Bulusi, M., Theleu, K., and Maness, P. F. (2003). Cellular signalling mechanisms of neural cell adhesion molecules. Front. Biosci. 8, 900–911.
Pillai-Nair, N., Panicker, A. K., Rodriguiz, R. M., Gilmore, K. L., Demyanenko, G. P., Huang, J. Z., Wetsel, W. C., and Maness, P. F. (2005). Neural cell adhesion molecule-secreting transgenic mice display abnormalities in GABAergic interneurons and alterations in behaviour. J. Neurosci. 25, 4659–4671.
Pla, R., Borrell, V., Flames, N., and Marin, O. (2006). Layer acquisition by cortical GABAergic interneurons is independent of Reelin signalling. J. Neurosci. 26, 6924–6934.
Polleux, F., Whitford, K. L., Dijkhuizen, P. A., Vitalis, T., and Ghosh, A. (2002). Control of cortical interneuron migration by neurotrophins and PI3-kinase signaling. Development 129, 3147–3160.
Poluch, S., Rossel, M., and Konig, N. (2003). AMPA-evoked ion influx is strongest in tangential neurons of the rat neocortical intermediate zone close to the front of the migratory stream. Dev. Dyn. 227, 416–421.
Poulopoulos, A., Aramuni, G., Meyer, G., Soykan, T., Hoon, M., Papadopoulos, T., Zhang, M., Paarmann, I., Fuchs, C., Harvey, K., Jedlicka, P., Schwarzacher, S. W., Betz, H., Harvey, R. J., Brose, N., Zhang, W., and Varoqueaux, F. (2009). Neuroligin 2 drives postsynaptic assembly at perisomatic inhibitory synapses through gephyrin and collybistin. Neuron 63, 628–642.
Powell, E. M., Mars, W. M., and Levitt, P. (2001). Heptocyte growth factor/scatter factor is a motogen for interneurons migrating from the ventral to dorsal telencephalon. Neuron 30, 79–89.
Pozas, E., and Ibanez, C. F. (2005). GDNF and GFRalpha1 promote differentiation and tangential migration of cortical GABAergic neurons. Neuron 45, 701–713.
Rakic, P. (1972). Mode of cell migration to the superficial layers of fetal monkey neocortex. J. Comp. Neurol. 145, 61–83.
Rakic, P. (2009). Evolution of the neocortex: a perspective from developmental biology. Nat. Rev. Neurosci. 10, 724–735.
Rivera, C., Li, H., Thomas-Crusells, J., Lahtinen, H., Viitanen, T., Nanobashvili, A., Kokaia, Z., Airaksinen, M. S., Voipio, J., Kaila, K., and Saarma, M. (2002). BDNF-induced TrkB activation down-regulates the K+-Cl− cotransporter KCC2 and impairs neuronal Cl− extrusion. J. Cell Biol. 159, 747–752.
Rivera, C., Voipio, J., Thomas-Crusells, J., Li, H., Emri, Z., Sipila, S., Payne, J. A., Minichiello, L., Saarma, M., and Kaila, K. (2004). Mechanism of activity-dependent downregulation of the neuron-specific K-Cl cotransporter KCC2. J. Neurosci. 24, 4683–4691.
Rymar, V. V., and Sadikot, A. F. (2007). Laminar fate of cortical GABAergic interneurons is dependent on both birthdate and phenotype. J. Comp. Neurol. 501, 369–380.
Schweizer, C., Balsiger, S., Bluethmann, H., Mansuy, I. M., Fritschy, J. M., Mohler, H., and Lüscher, B. (2003). The gamma 2 subunit of GABA(A) receptors is required for maintenance of receptors at mature synapses. Mol. Cell. Neurosci. 24, 442–450.
Sheckler, L. R., Henry, L., Sugita, S., Sudhof, T. C., and Rudenko, G. (2006). Crystal structure of the second LNS/LG domain from neurexin1a: Ca2+ binding and the effects of alternative splicing. J. Biol. Chem. 281, 22896–22905.
Somogyi, P., and Klausberger, T. (2005). Defined types of cortical interneurone structure space and spike timing in the hippocampus. J. Physiol. (Lond.) 562, 9–26.
Sousa, V. H., Miyoshi, G., Hjerling-Leffler, J., Karayannis, T., and Fishell, G. (2009). Characterization of Nkx6-2-derived neocortical interneuron lineages. Cereb. Cortex 19(Suppl. 1), i1–i10.
Stepanyants, A., Tamàs, G., and Chklovskii, D. B. (2004). Class-specific features of neuronal wiring. Neuron 43, 251–259.
Stuhmer, T., Anderson, S. A., Ekker, M., and Rubenstein, J. L. (2002). Ectopic expression of the Dlx genes induces glutamic acid decarboxylase and Dlx expression. Development 129, 245–252.
Stumm, R. K., Zhou, C., Ara, T., Lazarini, F., Dubois-Dalcq, M., Nagasawa, T., Hollt, V., and Schulz, S. (2003). CXCR4 regulates interneuron migration in the developing neocortex. J. Neurosci. 23, 5123–5130.
Tabuchi, K., and Sudhof, T. C. (2002). Structure and evolu-tion of neurexin genes: insight into the mechanism of alternative splicing. Genomics 79, 849–859.
Tanaka, D. H., Maekawa, K., Yanagawa, Y., Obata, K., and Murakami, F. (2006). Multidirectional and multizonal tangential migration of GABAergic interneurons in the developing cerebral cortex. Development 133, 2167–2176.
Thomson, A. M., and Jovanovic, J. N. (2010). Mechanisms underlying synapse-specific clustering of GABAA receptors. Eur. J. Neurosci. 31, 2193–2203.
Titz, S., Hans, M., Kelsch, W., Lewen, A., Swandulla, D., and Misgeld, U. (2003). Hyperpolarizing inhibition develops without trophic support by GABA in cultured rat midbrain neurons. J. Physiol. 550, 719–730.
Tiveron, M. C., Rossel, M., Moepps, B., Zhang, Y. L., Seidenfaden, R., Favor, J., Konig, N., and Cremer, H. (2006). Molecular interaction between projection neuron precursors and invading interneurons via stromal-derived factor 1 (CXCL12)/CXCR4 signaling in the cortical subventricular zone/intermediate zone. J. Neurosci. 26,13273–13278.
Toyoda, H., Ohno, K., Yamada, J., Ikeda, M., Okabe, A., Sato, K., Hashimoto, K., and Fukuda, A. (2003). Induction of NMDA and GABAA receptor-mediated Ca2+ oscillations with KCC2 mRNA downregulation in injured facial motoneurons. J. Neurophysiol. 89,1353–1362.
Varoqueaux, F., Aramuni, G., Rawson, R. L., Mohrmann, R., Missler, M., Gottmann, K., Zhang, W., Südhof, T. C., and Brose, N. (2006). Neuroligins determine synapse maturation and function. Neuron 51, 741–754.
Varoqueaux, F., Jamain, S., and Brose, N. (2004). Neuroligin 2 is exclusively localized to inhibitory synapses. Eur. J. Cell Biol. 83, 449–456.
Vitalis, T., and Rossier, J. (2011). New insights into cortical interneurons development and classification: contribution of developmental studies. Dev. Neurobiol. 71, 34–44.
Vucurovic, K., Gallopin, T., Ferezou, I., Rancillac, A., Chameau, P., van Hooft, J. A., Geoffroy, H., Monyer, H., Rossier, J., and Vitalis, T. (2010). Serotonin 3A receptor subtype as an early and protracted marker of cortical interneuron subpopulations. Cereb. Cortex 20, 2333–2347.
Waclaw, R. R., Allen, Z. J., Bell, S. M., Erdelyi, F., Szabo, G., Potter, S. S., and Campbell, K. (2006). The zinc finger transcription factor Sp8 regulates the generation and diversity of olfactory bulb interneurons. Neuron 49, 503–516.
Weinhold, B., Seidenfaden, R., Rockle, I., Muhlenhoff, M., Schertzinger, F., Conzelmann, S., Marth, J. D., Gerardy-Schahn, R., and Hildebrandt, H. (2005). Genetic ablation of polysialic acid causes severe neurodevelopmental defects rescued by deletion of the neural cell adhesion molecule. J. Biol. Chem. 280, 42971–42977.
Wichterle, H., Garcia-Verdugo, J. M., Herrera, D. G., and Alvarez-Buylla, A. (1999). Young neurons from medial ganglionic eminence disperse in adult and embryonic brain. Nat. Neurosci. 2, 461–466.
Wierenga, C. J., Becker, N., and Bonhoeffer, T. (2008). GABAergic synapses are formed without the involvement of protrusions. Nat. Neurosci. 11, 1044–1052.
Wonders, C. P., and Anderson, S. A. (2006). The origin and specification of cortical interneurons. Nat. Rev. Neurosci. 7, 687–696.
Wonders, C. P., Taylor, L., Welagen, J., Mbata, I. C., Xiang, J. Z., and Anderson, S. A. (2008). A spatial bias for the origins of interneuron subgroups within the medial ganglionic eminence. Dev. Biol. 314, 127–136.
Xu, Q., Guo, L., Moore, H., Waclaw, R. R., Campbell, K., and Anderson, S. A. (2010). Sonic hedgehog signaling confers ventral telencephalic progenitors with distinct cortical interneuron fates. Neuron 65, 328–340.
Yau, H. J., Wang, H. F., Lai, C., and Liu, F. C. (2003). Neural development of the neuregulin receptor ErbB4 in the cerebral cortex and the hippocampus: preferential expression by interneurons tangentially migrating from the ganglionic eminences. Cereb. Cortex 13, 252–264.
Yozu, M., Tabata, H., and Nakajima, K. (2004). Birth-date dependent alignment of GABAergic neurons occurs in a different pattern from that of non-GABAergic neurons in the developing mouse visual cortex. Neurosci. Res. 49, 395–403.
Yu, Y. C., Bultje, R. S., Wang, X., and Shi, S. H. (2009). Specific synapses develop preferentially among sister excitatory neurons in the neocortex. Nature 458, 501–504.
Zhang, W., Rohlmann, A., Sargsyan, V., Aramuni, G., Hammer, R. E., Südhof, T. C., and Missler, M. (2005). Extracellular domains of alpha-neurexins participate in regulating synaptic transmission by selectively affecting N- and P/Q-type Ca2+ channels. J. Neurosci. 25, 4330–4342.
Zhang, Z. W., and Deschênes, M. (1997). Intracortical axonal projections of lamina VI cells of the primary somatosensory cortex in the rat: a single-cell labeling study. J. Neurosci. 17, 6365–6379.
Zimmer, G., Garcez, P., Rudolph, J., Niehage, R., Weth, F., Lent, R., and Bolz, J. (2008). Ephrin-A5 acts as a repulsive cue for migrating cortical interneurons. Eur. J. Neurosci. 28, 62–73.
Keywords: synapse, GABA, inhibition, development, interneuron, cortex, synaptogenesis, ganglionic eminence
Citation: Jovanovic JN and Thomson AM (2011) Development of cortical GABAergic innervation. Front. Cell. Neurosci. 5:14. doi: 10.3389/fncel.2011.00014
Received: 27 May 2011;
Paper pending published: 13 June 2011;
Accepted: 28 June 2011;
Published online: 14 July 2011.
Edited by:
Enrico Cherubini, International School for Advanced Studies, ItalyReviewed by:
Graziella DiCristo, University of Montreal, CanadaLaura Cancedda, Istituto Italiano di Tecnologia, Italy
Copyright: © 2011 Jovanovic and Thomson. This is an open-access article subject to a non-exclusive license between the authors and Frontiers Media SA, which permits use, distribution and reproduction in other forums, provided the original authors and source are credited and other Frontiers conditions are complied with.
*Correspondence: Alex M. Thomson, Department of Pharmacology, The School of Pharmacy, 29-39 Brunswick Square, London WC1N 1AX, UK. e-mail: alex.thomson@pharmacy.ac.uk