- 1Swiss Center for Design and Health, Nidau, Switzerland
- 2Department of Ophthalmology, Jules-Gonin Eye Hospital, University of Lausanne, Lausanne, Switzerland
- 3Swiss Center for Game Design Studies, Institute of Design Research, Academy of the Arts, Bern University of Applied Science, Bern, Switzerland
Digital immersive technologies have become increasingly prominent in clinical research and practice, including medical communication and technical education, serious games for health, psychotherapy, and interfaces for neurorehabilitation. The worldwide enthusiasm for digital health and digital therapeutics has prompted the development and testing of numerous applications and interaction methods. Nevertheless, the lack of consistency in the approaches and the peculiarity of the constructed environments contribute to an increasing disparity between the eagerness for new immersive designs and the long-term clinical adoption of these technologies. Several challenges emerge in aligning the different priorities of virtual environment designers and clinicians. This article seeks to examine the utilization and mechanics of medical immersive interfaces based on extended reality and highlight specific design challenges. The transfer of skills from virtual to clinical environments is often confounded by perceptual and attractiveness factors. We argue that a multidisciplinary approach to development and testing, along with a comprehensive acknowledgement of the shared mechanisms that underlie immersive training, are essential for the sustainable integration of extended reality into clinical settings. The present review discusses the application of a multilevel sensory framework to extended reality design, with the aim of developing brain-centered immersive interfaces tailored for therapeutic and educational purposes. Such a framework must include broader design questions, such as the integration of digital technologies into psychosocial care models, clinical validation, and related ethical concerns. We propose that efforts to bridge the virtual gap should include mixed methodologies and neurodesign approaches, integrating user behavioral and physiological feedback into iterative design phases.
1 Introduction
Digital immersive technologies have attracted considerable interest in the landscape of biomedical research, due to their versatile range of applications, spanning from patient data visualization, digital Games for Health (GfH) to neurorehabilitation. The use of digital virtual interfaces and augmented reality-assisted technologies have especially gained traction within clinical settings. These technologies are utilized not only to train medical professionals but also for research, education, and patient-centered purposes, including occupational therapy, cognitive, visual, and motor rehabilitation and diagnosis (Yeung et al., 2021). A distinction can be drawn among applications that serve preventive, therapeutic, diagnostic, informative, or educational purposes (Sawyer, 2008). Nevertheless, the primary focus of technology developers tends to revolve around primary therapeutic outcomes and overall usability, but often neglect fundamental plasticity mechanisms, end-user inputs and social context (Mummah et al., 2016; Birckhead et al., 2019).
Biomedical engineers often overlook the intricate user-interface interactions and their long-term interplay with visual and cognitive functions, learning and rehabilitation mechanisms. The chosen technology, intermediate objectives, immersive universes, and communication modalities are more often design choices than evidence-based decisions (Birckhead et al., 2019). There is a limited number of studies addressing the discursive, social construction of immersive interfaces as a novel medium within the field of the social system of medicine. Despite the innovative advancement of various immersive interfaces for diagnosis, education, or rehabilitation purposes, studies are often not designed to effectively compare digital approaches with non-digital methods. Numerous trials investigating the clinical efficiency of immersive interfaces lack sham group, and more comprehensive randomized trials are still in an early assessment phase (Gerber et al., 2017, 2019a,b; Saraiva et al., 2018; Yeung et al., 2021; Jiménez-Rodríguez et al., 2023). Furthermore, the existing comparison studies most fail to demonstrate an advantage of digital over non-digital approaches. Pilot studies including conventional therapy or a placebo control group have demonstrated limited and individually variable advantages of digital methods, with the notable exception of chronic pain alleviation (Maddox et al., 2022) and surgical training (Grantcharov et al., 2004; Lohre et al., 2020; Sadek et al., 2023). Finally, there is a lack of publicly accessible insights into the production processes of such applications, which would make their development traceable and provide insight on detailed design evaluation.
Currently, the success of a given immersive biomedical approach cannot be differentiated from the success of the interaction design choices that have been made (Garrett et al., 2018). Designing for immersive interaction requires consideration of human sensory perception, cognition, and sensorimotor systems and social context. This knowledge has to be carefully integrated into the design process. This applies all the more if the interface is to be used in a clinical setting, as design choices have the potential to impact healthcare processes and patient outcomes negatively (Garzón et al., 2019; Chang et al., 2022). It is today’s challenge to determine which immersive approach and design choices are advisable for which goal and learning context, and to create a general framework of understanding to facilitate further Digital Therapeutics (DTx) development, and design (or neurodesign) brain-centered interfaces (Ahram et al., 2016; Auernhammer et al., 2023).
Despite yet mixed outcomes, the growing number of projects developing and analyzing immersive gaming approaches in the clinics shows the professionalization and institutionalization of the field. The emergence of general interest networks, such as the German “Netzwerk Serious Games und Gamification for Health” signals that the field reaches a stage where tools are moving beyond prototyping. A shared understanding of effective practices is beginning to form, with increased collaboration and dissemination of results. Our objectives with the current review are to contribute to this ongoing process; to further stimulate cross-disciplinary interest and dialogue between the different disciplines involved in that field; and to include social, ethics, and neuroscience perspectives in the emerging voices formalizing future solutions. We propose to specially put in perspective the concepts and recent advancements in immersive technologies, perceptual neuroscience, game design and neurodesign domains.
To achieve this, we will first explore the key components and usages of immersive interfaces (2). We will differentiate and define involvement, immersion, and presence as three distinct aspects of the user experience (2.1, Figure 1). Next, we will provide an overview of the technologies in use, including Virtual, Augmented and Mixed realities (2.2, Figure 2). Given the broad range of clinical applications, we will examine how these technologies are currently used (2.3, Figure 3) and identify the primary mechanisms that support this use in practice and favor learning from the perspective of various disciplines (2.3). We will then address the design of actual applications and their challenges (3, Figure 4), focusing on sensory design issues (3.1), cognition and game design (3.2), methods for assessing user progress and behavior (3.3), social (3.4) and regulatory challenges (3.5).
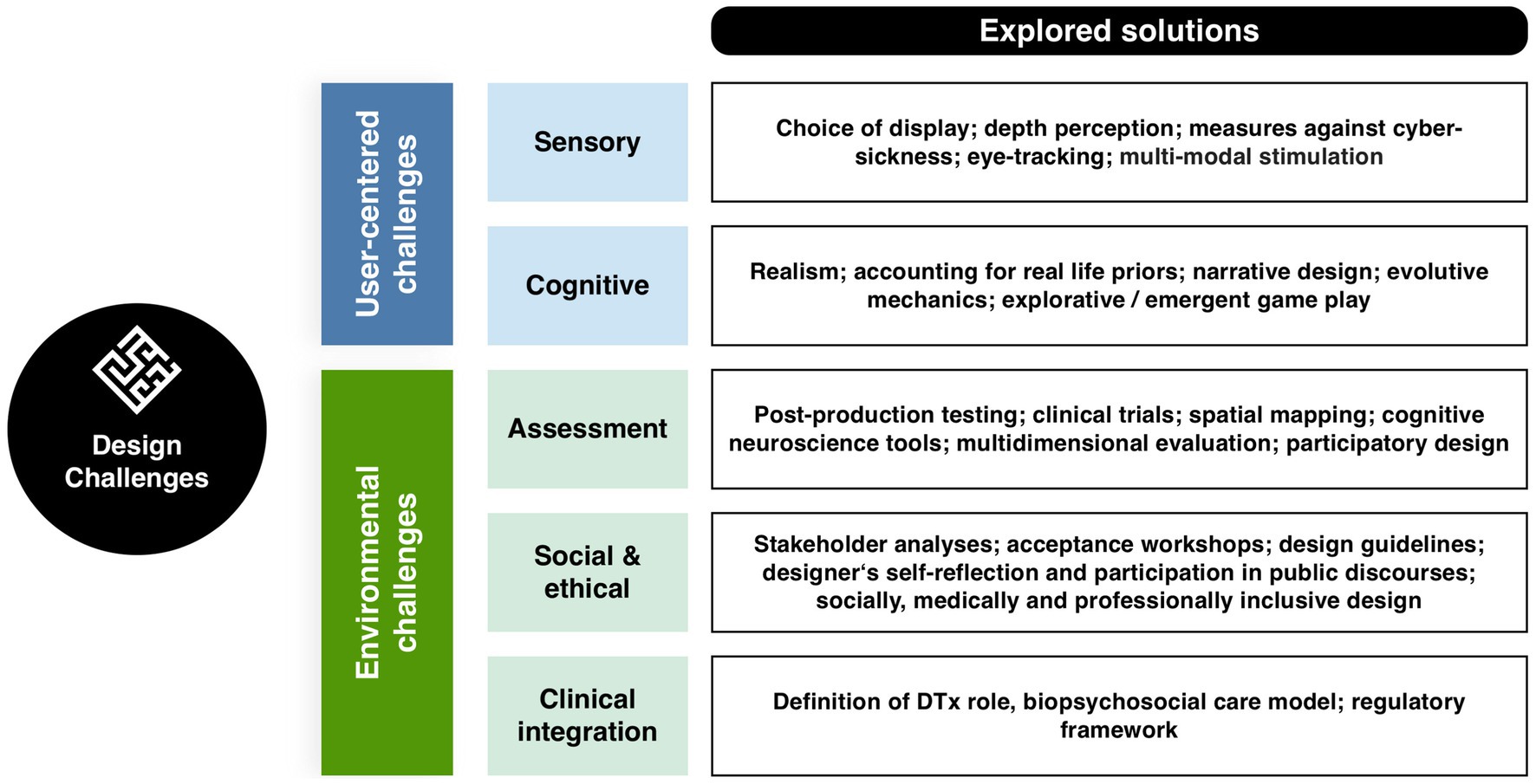
Figure 4. Overview of the design challenges associated with the development of XR interfaces development and their integration into healthcare.
2 Immersive interfaces for clinical applications: development and definitions
2.1 The immersive experience
Immersive technologies such as Virtual Reality (VR), Augmented Reality (AR), GfH, and other interactive virtual mediums, have been progressively integrating into the realm of healthcare. These technologies, initially created for entertainment purposes, have started to permeate medical practices, and unlocked novel ways to engage the patient, the clinician, and the medical team. The common ground of these technologies is to create a positive user experience (UX) by integrating digital elements into the user’s physical world, thereby enhancing the perceptual and cognitive aspects of user interactions. Yildirim et al. state that three interconnected factors must be ensured for a positive UX: presence, immersion and involvement (Yildirim et al., 2018; see Figure 1).
Presence or the sense of “being there” are much-explored cognitive psychology and consciousness research concepts, often used as subjective metrics to evaluate participants experience in Virtual Environments (VEs) (Riva et al., 2003; Pillai et al., 2013; Grassini and Laumann, 2020; Cypress and Caboral-Stevens, 2022). Presence refers to “a mental state in which an individual feels like they are in an environment other than the one they are physically occupying” (Yildirim et al., 2018). Such mental state is not specifically bound to a type of immersive technology but rather “formed through an interplay of raw (multi-)sensory data and various cognitive processes […] in which attentional factors play a crucial role” (Riva et al., 2003). Sense of presence (SoP) thus describes the minimal emotional and experiential access to another reality—that of the VE for instance. This makes the philosopher de Vignemont describe it as a buffer zone “between the self and the external world […], a place in which objects and events have a unique immediate significance for the subject because they may soon be in contact with [them],” triggering an “illusion of non-mediation” (Lombard and Ditton, 2006; De Vignemont, 2021). Presence therefore relates to the perception of one’s peripersonal space and the likelihood of interaction with the media elements (Riva et al., 2003; De Vignemont, 2021). Precisely because it lies at the interface between perceptual, cognitive and emotional experience, presence is a key component to empower users and reinforce learning, notably in clinical settings. The extent to which users experience SoP in a VE depends on both their involvement and immersion in the environment (Witmer and Singer, 1998).
Involvement can be described as “a mental state in which attentional resources are allocated to the processing of perceptual stimuli rendered in the VE” (Yildirim et al., 2018).,This relates to a much more diffuse aspect of immersive GfH: they are entertaining. Games are designed to generate elevated levels of motivation and engagement in the players, which means that the motivation to use them may be significantly greater in comparison to conventional medical practices (Watters et al., 2006). Yet, GfH and immersive technologies are susceptible to the criticism of being “sugar-coated broccoli,” meaning that the UX differentiates from what GfH promise to offer, namely a gaming experience that can compete with commercial games (Baranowski et al., 2016). In that way, the user involvement depends on the immersion and presence brought by both the interaction design and the narrative of the GfH. From a UX perspective, the product of involvement and immersion has been conceptualized as flow, i.e., the absorption in an activity, during which irrelevant thoughts and perceptions are being screened out (Jennett et al., 2008). Flow is associated with the following components: “clear goals; high degree of concentration; a loss of the feeling of self-consciousness (sense of serenity); distorted sense of time; direct and immediate feedback; balance between ability level and challenge; sense of personal control; intrinsically rewarding.”
Finally, immersion is a “a psychological construct concerned with the extent to which individuals feel encapsulated by the VE and perceive themselves as an integrated component of the environment” (Yildirim et al., 2018). The two major components of immersion are, on the one hand, interactivity, i.e., the user’s possibility to meaningfully influence the media content and alter it within the framework and possibility spaces granted by the media (Bodi and Thon, 2020); and on the other hand, procedural narratives (Bogost, 2010). Such narratives unfold primarily in the form of systemic processes: users must do action x to get result y. Ultimately, interactivity and narrative allow for immersion from a story building perspective and make GfH suitable for use in a medical context. Translating this definition into a visual communication and digital implementation perspective, immersion becomes the sense that digital virtual objects belong to the real world (Stevens, 2021). This implies the viewer to integrate together perceptions from the digital and physical worlds, and to interact with digital or physical elements equivalently. This equivalence is a key factor to the generalization of learning when using serious games. The crucial aspect in this context is the perception of immersion as a bottom-up phenomenon. Donghee Shin highlights that “[t]he meaning of immersion depends on the users’ idiosyncrasies, and the influence of immersion substantially depends on the users’ contexts such as their pre-existing conditions and personal traits. […] Immersion is a form of awareness in the eye of the beholder, and its degree reflects the intensity of the users’ cognitive, emotional, and sensory connections to both the content and form of the product” (Shin, 2019).
The various forms of existing extended reality technologies reflect not only the available technological advances, but also users’ presumed needs in term of involvement, immersion and presence. Focus is either made on physical immersion (“from every angle”), sensory immersion (“from all the senses”), involvement (“that do not allow distracting information”) or presence (“that does not provide diverging information”).
2.2 Current immersive technologies
There are several types of immersive technologies that differ in their degree of immersion and the way they blend virtual and physical elements (Milgram and Kishino, 1994; see Figure 2). Extended reality (XR) is an overarching term that refers to hybridization of virtual and physical elements into a more complex immersive perceptual space. XR comprises virtual reality (VR), augmented reality (AR), and mixed reality (MR). All XR modalities are explored for health and clinical applications, though VR has been the format preferred for GfH development in the past decade.
VR immerses users in computer-generated or digitally-presented environments. Presentation is typically achieved through head-mounted displays (HMDs) or computer-assisted virtual environments (CAVEs). VR typically isolates users from their physical surroundings. This isolation primarily concerns visual and auditory systems, but efforts are made to create congruent haptics (Jerald, 2015; Stevens, 2021). This multisensory isolation perspective hallmarks VR for multiple clinical applications, particularly in sensory training, but it has been investigated for its potential to serve as a positive distraction during medical procedures (Dumoulin et al., 2019; Faruki et al., 2022) or to create calming environments (Gerber et al., 2017, 2019b).
AR overlays virtual content onto the real-world environment, either directly projecting cues onto the physical world, via mobile digital interface, or wearable devices. The added information can be visual cues enhancers, text, or local distortions, audio stimuli and audio-description of visual cues. AR can be implemented on personal mobile devices or through projection mapping: visual information can be projected to 360° onto multiple physical displays, such as circular screens or projection floors, eventually paired with spatial audio rendering. Projection mapping limits multisensory immersion but promotes interpersonal interaction and communication. For this reason, it has been used to explore medical communication (Aliwi et al., 2023; Song et al., 2023), medical architectural design (Afzali et al., 2023) and to facilitate social communication between children with auditory impairment, cognitive impairment, or autism spectrum disorders (ASD) and their relatives (Richard et al., 2007; Liu et al., 2017; Sahin et al., 2018; Tenesaca et al., 2019). In clinical contexts, AR can also be implemented on optical see-through mode (OST). The user perceives unaltered real-world environment through a see-through device, typically goggles, and the supplementary digital content is directly projected onto the retina through the lens. OST is key to visuomotor training and ophthalmology applications as it allows the user to use their normal head and eyes movements to scan the visual scene, what facilitates the processing of visual inputs and the process of learning to use the interface (Hafed et al., 2016; Thorn et al., 2022).
MR combines elements of both VR and AR, mapping virtual and real-world environments together, and foremost allowing user to interact with both worlds. MR differs by its dynamic multidimensional technology and flexibility. MR relies on real time scanning of the user’s visual space with a 3D laser technology, usually integrated on the image acquiring device: a virtual mesh of the real world is built on which virtual objects to be manipulated and interacted with. The visual environment is continuously adjusted and aligned with the user’s visual field, its motion speed, and the mesh adapts to create depth and proportion perception through occlusion, shadows, aerial or linear perspective. The latter two features are key for establishing plausibility (Jerald, 2015). Some MR models also incorporate real world mapping, such as GPS coordinates or known floor plans (Jerald, 2015; Syahputra et al., 2020; Verma et al., 2020), substituting visual scan to positional and kinematic information.
2.3 Users and applications
In clinical health, immersive technologies have primarily three categories of users: medical professionals, patients or potential patients, and healthcare planners. Interfaces for medical staff training are designed for multidirectional thinking, enabling the retrieval of diverse health data and parameters, processing of knowledge, generation of decisions in the form of a coordinated care plan or medical actions, and provision of feedback (see Figure 3). Most interfaces are designed to provide information related to diagnosis (such as rehabilitation interfaces), to train medical communication skills (especially in nursing and psychotherapy fields), or to train technical sensorimotor skills (through anatomical simulation) (Hussey, 2021; Tene et al., 2024). Conversely, most interfaces geared for patients are designed for goal-oriented thinking; and focus on transformational outcomes, i.e., lasting changes in the user behavior, knowledge, priors, cognitive, emotional or sensorimotor skills (see Figure 3). XR interfaces have been developed for prevention and awareness, relaxation, and DTx—especially in neurorehabilitation and psychiatry fields. Finally, interfaces used by health planners are intended to provide insights, observational feedback, and design guidelines. This field of application is still emerging to date, but research efforts are made to develop XR tools for evidence-based healthcare environment design (Bianco et al., 2016; Aoyama and Aflatoony, 2020; Hwang and Shim, 2021; Afzali et al., 2023).
A substantial number of immersive interfaces developed over the past 15 years are geared towards health professional education. Immersive interfaces have been developed to train various core aspects of clinical care: anatomy knowledge, anamnesis, diagnosis, interpersonal communication during care, ethical decision making, medical acts, and surgical procedures (Grantcharov et al., 2004; Bhoopathi and Sheoran, 2006; Garzón et al., 2019; Lohre et al., 2020; Chang et al., 2022; Sadek et al., 2023). XR environments are acknowledged valid methods for clinical education and training, but evidence suggests that they should rather be envisioned as an auxiliary consolidating medium for related learning (Kyaw et al., 2019; Kim and Ahn, 2021; Sadek et al., 2023). Kyaw and Tudor Cal state in their meta-analysis that “only low-quality evidence show(s) that digital education is as effective as traditional learning in medical students’ communication skills training. Blended digital education appears to be at least as effective as, and potentially more effective than, traditional learning for developing communication skills and knowledge” (Kyaw et al., 2019). For anatomy learning and specific technical and surgical skills acquisition, XR immersive learning generates a more nuanced picture. Students perform equally when taught with immersive or traditional methods, but acquire skills significantly faster with virtual immersive environments, (Jordan et al., 2001; Eichenberg, 2012; Lohre et al., 2020). For rare or high-risk neurological or ophthalmic surgical procedures, XR environments are often the only way trainees can have hands-on realistic training (Butt et al., 2018; Muñoz et al., 2022). Finally, XR training can be exerted without ethical considerations of the patients’ consent to be an in-vivo or post-mortem exercise subject. This suggest that the main interest of medical educational immersive interfaces do not reside in clinical efficacy, but in resources. They might offer a fast, reliable, mistake-allowing training to students and reduce the pressure on training time, availabilities, and ethics. Importantly, VE learning alone is not the preferred learning tool for medical students, but still needs observation and mentorship as complimentary didactical approaches (Engum et al., 2003; Williams, 2019; Vizcaya-Moreno and Pérez-Cañaveras, 2020). Last, the above-mentioned studies all focus on knowledge and skill retrieval from a user perspective. Patient-related outcomes, as well as adverse effects, and cost-effectiveness of immersive digital education are still case-specific open questions.
Immersive technologies and GfH intended for (potential) patients focus on the transformational potential of the user’s experience, and on the real-word transfer and persistence of the acquired skills. All GfH can be described as transformational, but the intricacy between entertainment and learning is more pronounced when game mechanics is part of therapeutic process (Culyba, 2018). XR technologies for patients most often fall into two main categories: DTx, and preventive applications. DTx are evidence-based digital products aiming at preventing, managing, or treating treat health conditions (Digital Therapeutics Alliance, 2023), and lie within the broader category of digital medicine (Wang et al., 2023). In this article, the term DTx is used to denote prescription-only interventions that have been developed with clinical evidence and are intended for the treatment of specific medical conditions, particularly major chronic diseases.
Preventive applications are developed for either general or risk-specific audiences, sometimes in collaboration with entertainment studios. They aim at transforming the user cognitive priors and habits on a health topic, through medical information delivery or training. Examples of preventive GfH include the niche application Playforward - developed to reduce HIV exposure in at-risk teen populations (Fiellin et al., 2016), and the more popular WiiFit exergames (Tripette et al., 2017). In such GfH, participatory methods are made possible by two key factors: a healthy target group—limiting the need of medical supervision and multidisciplinary safety evaluation, while allowing a large dataset acquisition-; and overly broad expected outcomes—allowing exploratory methods to evaluate potential clinical benefits. In the hospital settings, preventive applications aim at patient relaxation and distraction, helping patients to manage pain and anxiety during medical procedures. Research efforts have notably focused on stress reduction in the emergency department and intensive care unit (Gerber et al., 2017, 2019a; Dumoulin et al., 2019; Hill et al., 2022; Vlake et al., 2022). Preventive applications only represent 4.4% of the clinical trials in digital medicine (Wang et al., 2023). Commercial interfaces are often evaluated post hoc by independent research groups (Li et al., 2011; Bonnechère et al., 2016; Tripette et al., 2017).
XR-based DTx focuses on chronic neurological and psychiatric diseases, that require continuous interaction. They indirectly interface with patient nervous system through behavioral intervention, to favor plastic changes in sensorimotor and cognitive loops, and allow modification or acquisition of new behavioral, sensory, and motor skills. Their mechanisms do not essentially differ from that of educative immersive interfaces, however, their regulatory framework do. DTx represents the majority (65%) of clinical trials conducted in the digital medicine sector worldwide and are usually classified as class II medical devices (Wang et al., 2023; Watson et al., 2023).
Neurorehabilitation is a major application field of immersive DTx: specific interfaces have notably been developed for motor (Vinolo Gil et al., 2021; Chen et al., 2022; Gorman and Gustafsson, 2022; Yang Z-Q et al., 2022), cognitive (Richard et al., 2007; Laver et al., 2017; Miskowiak et al., 2022), speech (Da Silva et al., 2015; Xu et al., 2020; Bu et al., 2022) and visual rehabilitation (Li et al., 2011; Ghali et al., 2012; Boon et al., 2017; Saraiva et al., 2018; Jiménez-Rodríguez et al., 2023). A major advantage of XR training is to provide tailored and realistic sensory feedback on motor actions, which is essential for sensorimotor training. Continuous feedback can be visually delivered through avatar posture and gestures; on–off targeting accuracy feedback can be provided visually or haptically through controllers; and proprioceptive feedback can eventually be provided through flooring force plates and postural platform. The early development of preventive balance exergames for general audience paved the way for DTx applications. But these commercial exergames are often used with little adaptation for clinical monitoring and disabled population (Chao et al., 2015; Bonnechère et al., 2016). Further research is needed to understand how various feedback modality and types of visual feedback and point of views contribute to sensorimotor reeducation, and which plastic mechanisms can be exploited for tailored XR rehabilitation interfaces. A notable advantage of XR therapy is the opportunity to pair it with robot-assistance for more reliable quantitative measures of patient performance and online tasks adjustment during sessions (Vidrios-Serrano et al., 2015). XR technology is also exploited for sensory substitution training in the visually impaired (Dragos Bogdan Moldoveanu et al., 2017).
The second major clinical application for immersive DTx is psychotherapy. XR technology is a first-class tool to design behavioral interventions, particularly for exposure therapy, exposure with response prevention, and cognitive-behavioral therapy (Grochowska et al., 2019), notably in contexts of Post-Traumatic Stress Disorders (PTSD) (Rothbaum and Schwartz, 2002; Kothgassner et al., 2019; Eshuis et al., 2021), eating disorders’ body disturbance (Ferrer-García and Gutiérrez-Maldonado, 2012; Riva et al., 2021; Behrens et al., 2022), phobias and social anxiety (Schoneveld et al., 2018; Boeldt et al., 2019; Wechsler et al., 2019). It can help the therapist understand the fictive situation, and assess patient behavior, addressing significant shortcomings of existing therapies (Boeldt et al., 2019). AR behavioral interventions have also been specifically developed for collaborative therapies in children and adults with ASD. Contrary to VR exposure therapy, this application merges real-life situations and virtual information, with the objective of assisting and training social cognition skills in ASD users (Da Silva et al., 2015; Liu et al., 2017; Sahin et al., 2018). Last, VR GfH has been developed for avatar therapy in schizophrenic patients (Mayor, 2017; Ward et al., 2020; Verma et al., 2020).
To date, the majority of immersive interfaces for neurorehabilitation and psychotherapy uses VR, with the notable exception of sensory substitution and sensory pain applications, whose intrinsic mechanisms rely on a combination of virtual and real-life stimulations.
2.4 Exploited mechanisms
Immersive applications and GfH are extremely tailored to their user group and their intended use. To implement this focus effectively, a series of game and UX mechanisms should be implemented in a targeted manner.
2.4.1 Embodiment and presence
Presence and SoP are a core mechanism that mediates positive immersive UX, XR-trained skills acquisition, transfer, and retention. SoP refers to spatial and plausibility illusions, also referred to as illusion of non-mediation in XR (Slater, 2009; Slater et al., 2010a). This misperception has been foreseen as multimodal perceptual illusion of self’s body and peripersonal space (Riva et al., 2003; De Vignemont, 2021). Embodiment and SoP are intricated in XR, although only recently conceptualized together (De Vignemont, 2011; Forster et al., 2022). Embodiment describes the process of integrating external bodily entities (as an avatar) into self-body representation – in a similar way external entities can be integrated into peripersonal space representation. In VR in particular, SoP mostly depends on embodiment users have to embody the virtual body to feel present enough in the VE. This mechanism is directly exploited in digital therapy to alleviate phantom limb pain (Hunter, 2003; Foell et al., 2014; Romano et al., 2016; Osumi et al., 2017), and avatar therapy for schizophrenic and psychotic patients (Carter et al., 2017; Mayor, 2017; Ward et al., 2020). Interfacing with VE and avatar can teach latter patients to question the boundaries between external and internal percepts, or alternatively provides them with a virtual object to which they attribute the hallucinated percepts (Ward et al., 2020).
Embodiment may be limited by either bottom-up (Slater et al., 2010b), or top-down factors, such as virtual body characteristics and point of view (Ehrsson et al., 2004; Tsakiris, 2010; Romano et al., 2016), and its plausible connection to the user body. Third-person point of view, despite facilitating visual design, hampers SoP (Jerald, 2015). However, preferred avatar modalities for given applications are still unclear. The term “avatar” describes a user’s representative in the VE, as an immersion medium, “persona” refers to the product of such immersion and embodiment, i.e., the self-perception of the user in the virtual or extended environment. A recent study showed that an unrealistic but highly controllable first person-perspective avatar allowed higher embodiment than realistic but less flexible avatar (Fribourg et al., 2020). Third-person point of view personas can also be especially important for psychotherapy applications, allowing a trade-off between high emotional engagement and limited SoP (Ward et al., 2020). Personas are also a valuable tool for co-design process and empathy generation (Ventura et al., 2020).
The major contributing factors to embodiment and SoP are sensory realism and crossmodal perception (Lopez et al., 2008; De Vignemont, 2011; Blanke, 2012). Most VR and AR systems are designed to provide a virtual stimulus to the dominant visual sensory modality, unless they specifically target visually impaired audience, or are oriented towards specific haptic uses (Ghali et al., 2012; Borja et al., 2018). However, the integration of multisensory inputs, and the congruence of various cues are key to self-perception, embodiment, and SoP (Lopez et al., 2008; Blanke, 2012; Cao et al., 2019). A major challenge of XR is to integrate and provide multiple sensory modalities together. One specific challenge is integrating haptics with immersive visuals: few haptic devices today are wireless, what hampers the free movement of the user in the XR environment. A proposed solution is to integrate passive haptic feedback and control into augmented floors: such passive haptics have a positive impact on SoP and allow multiuser interaction (Law et al., 2008; Goncalves et al., 2020), but has yet not been implemented for clinical applications.
Social factors also influence embodiment and SoP. Openness to virtual immersion, medical education and occupation, confidence in medical preparation, and previous exposure to the simulated situation influence SoP during medical XR simulation (Paquay et al., 2022). SoP is also influenced by social factors, such as gender (Grassini et al., 2020). Higher SoP was reported in women during medical XR training (Grassini et al., 2020), and possibly relate to their better learning performance (Yang et al., 2016). Conversely, the lower SoP in man students may be associated to increased confidence in educational preparation and medical proficiency (Blanch et al., 2008; Flyckt et al., 2017; Vajapey et al., 2020).
2.4.2 Procedural learning
Procedural learning pertains to the acquisition of complex and adaptive motor or cognitive skills, i.e., skills that involve constant decision and action rules update. Procedural learning is required for sensorimotor, cognitive, emotional and social competencies acquisition during clinical education, physical or cognitive therapies. According to the adaptive control of thought model (Anderson, 1982, 2000), training pattern plays a significant role in automatizing the learnt procedural skills (Miyake et al., 2000; Shahar and Meiran, 2015). Training through simulation has shown satisfying efficiency for medical procedural training (Nestel et al., 2011), and is routinely used in medical education for developing technical motor skills, as well as for learning analysis pathways and communication methods learning.
XR technology offers several advantages for simulation-based procedural learning. First, XR offers the possibility to overlay the simulated situation with explicit information. It enables to combine exploratory learning with on request explicit information display. Indeed, the two main methods to acquire procedural skills are instruction-based training and exploration-based training. Exploration-based training, also referred to as active learning, facilitates the induction of abstract representations and procedural knowledge by employing analogical reasoning (Kamouri et al., 1986). Exploration-based learning is centered on the active engagement of the user, and both gamification and immersion technologies are privileged tools to achieve so. Second, procedural learning requires constant shift between environmental action cues in a goal-directed manner. Working memory, information update, and cognitive inhibition are key to initiate procedural learning (Miyake et al., 2000; Shahar and Meiran, 2015). Real-life environment holds a host of action cues; but in VEs, the dimensionality of available cues is intentionally reduced and carefully selected by design. This reduced dimensionality might facilitate the information sorting process and reduce the cognitive load during the cognitive phase, which can explain the fastening of procedural learning in VR-based surgery training or communication skills training.
Meta-reviews point out that the 3D design of a XR interface is a major predictor of procedural learning performance (Garzón et al., 2019; Chang et al., 2022). Importantly, procedural learning abilities can be altered in populations using XR interfaces for cognitive therapies training, such as neurodegenerative patients (Soliveri et al., 1992; Muslimovic et al., 2007; Clark and Lum, 2017), but also users with ASDs, though diverging evidence (Mostofsky et al., 2000; Clark and Lum, 2017; Boucher and Anns, 2018).
2.4.3 Perceptual learning
Perceptual learning refers to a lasting alteration in perception resulting from experience. It can be categorized as a form of implicit or procedural learning involving sensory systems. Perceptual learning differs from sensitization and habituation, as it leads to permanent improvements in perception and perceptual thresholds. Importantly, it can occur independently of both attention to the stimulus and conscious perception (Watanabe et al., 2001). Perceptual learning underlies active sensory rehabilitation trainings, such as low vision or sensory substitution trainings, but also education trainings related to discrimination tasks—such as medical images interpretation (Seitz, 2017; Serrada et al., 2019). In a clinical context, the process may entail distinguishing between simple and complex classes of stimuli, such as the histology of various tissues for surgical training, or distinct types of auditory information during low-vision training (Fahle, 2009). In adults, perceptual learning depends on prolonged and repeated exposure, strength of exposure, and various additional factors, including attention, reinforcement, and interactions of multiple sensory systems (Seitz, 2017). These factors are actively investigated into perceptual learning research field, but receive less attention when designing applied XR and GfH interfaces. Consequently, there is currently no strong evidence regarding the effectiveness of XR-based training on perceptual thresholds in sensory-impaired patients (Polat, 2009; Serrada et al., 2019).
Due to the visually dominant nature of most XR outputs, visual learning is the most prone to occur with XR training. Perceptual learning can lead to very fast improvements of discrimination performance in visual tasks. Yet, perceptual improvement is often highly specific for the trained task, stimulus orientation and position in the visual field (Fahle, 2004, 2009), what limits the generalization of the perceptual improvements to other tasks. The positive influence of top-down factors such as feedback and attentional control (Ahissar and Hochstein, 1993; Herzog and Fahle, 1997, 1998; Fahle, 2004; Seitz, 2017) open opportunities for better perceptual training design.
2.4.4 Positive reinforcement learning
Positive reinforcement is a common feedback-driven mechanism for multiple forms of procedural, associative and perceptual learning, including purely implicit sensorimotor learning (Law and Gold, 2009; Wächter et al., 2009). Reinforcement learning is an adaptive process in which the user’s previous experiences are used to predict the outcome of possible action and make a choice accordingly. Action choices can be made at various levels: among alternative single motor outcomes, among different complex actions or objects selection, or among different interaction strategies. Reward-based learning is an essential element of gamification in GfH and immersive interfaces. Rewarding outcomes can be explicitly included in the game mechanics; visual, auditory, or haptic feedback of task completion or accuracy can also be considered as a rewarding element. In educational and DTx, the rewarding elements can also arise from patient-therapist verbal interactions, and from other users’ interaction in collaborative training. Reward type, timing, and predictability matter for serious games impact. Reward-based game mechanics based on badges and trophies was found to have greater positive influence on learning than points scoring and meaningful educational messages delivery (Whittaker et al., 2021). Granting rewards after an unpredictable number of correct trials and adjusting rewards to the user individual occurrence preference were associated with higher enjoyment, improved learning performance, and longer durations of gameplay (Nagle et al., 2014). Comprehensive and generalizable studies on the use of serious games and DTx rewards-based reinforcement are still required.
However, educational technologies based solely on positive reinforcement learning raise several concerns, not unlike those raised by Skinner’s teaching machines (Skinner, 1961). Skinner’s radical behaviorism was a tempting education research program until the beginnings of the cognitive science revolution in the 60s. It postulated that behaviors could be fully understood as overt actions and motor outcomes from physical environmental stimuli (Abrahamsen and Bechtel, 2012). This reductive approach is rooted in experimental approaches of operant conditioning and has long been criticized as oversimplifying human cognition (Cranmore, 2022). Behaviorism survived in several psychological approaches and biomedical fields, such as behavior therapy (notably for autistic and schizophrenic patients, at the origin of today’s XR applications) (Lovaas and Newson, 1976; Stahl and Leitenberg, 1976; Lovaas and Smith, 1988). While a reductionist approach might be relevant to model learning from a fundamental perspective, it is questionable to apply such framework for educational technology. XR applications are still based on experimental approaches, and clinically validated with assessable behavioral or motor outcomes. These plays a crucial role in understanding behavior and training success, particularly in tasks like surgical gesture training or physical rehabilitation. Yet, higher cognitive and emotional processes cannot be neglected in the numerous applications involving therapeutic relationship building.
2.4.5 Symbolic enactment
In transformational XR interfaces with behavioral, psychological, empathy, social communication frameworks, the gaming and immersive aspects can be seen as forms of symbolic enactment. Symbolic enactment is a powerful tool for personal transformation within digital games (Rusch and Phelps, 2020), akin to its role in experimental psychotherapy that incorporates drama, role play, and user active and spontaneous performance (Moreno, 1987; Rusch and Phelps, 2020). Symbolic actions and metaphors in games, performance, and simulations are thought to transcend abstract representation (Thompson et al., 2009; Rusch, 2017). D.C Rusch postulates that gaming metaphors and iconic symbols convey intangible aspects of human experience, constituting a shortcut to experience complex abstract concepts enactment (Rusch, 2017). In immersive interfaces, the experimental metaphor and SoP converge, resulting in a more potent symbolic and enactive experience. Metaphor design holds particular significance in XR interfaces developed for clinical psychotherapy, particularly in the context of exposure therapies for PSTD, eating disorders (Boeldt et al., 2019; Eshuis et al., 2021; Herz, 2021; Behrens et al., 2022), or social immersive training (Liu et al., 2017; Sahin et al., 2018). However, the benefits of metaphorical exposure and virtual immersive exposure—yet perceived as experienced are difficult to distinguish in XR.
In this first part, we have shown how immersive technologies as VR, AR and MR are increasingly used in healthcare, particularly for clinical education, rehabilitation, and therapeutic interventions, and how they can directly or indirectly mediate the medical practices and exchanges between patients and health professionals. These technologies enhance UX by combining virtual and physical artifacts and actions. VR is the most commonly applied technology in serious GfH and for behavioral or cognitive therapy. AR and MR overlays digital elements onto the real world, and are offer additional possibilities for interactive and sensorimotor trainings. These technologies relying on three key factors for users to emotionally and experientially access a VE: presence, involvement, and immersion. Their efficacy for clinical training takes advantage of several learning mechanisms, that could be further addressed by designers. A core element is SoP, influenced by embodiment, where users integrate avatars into their self-representation. Procedural learning in XR benefits from reduced sensory overload and targeted cues, and supports development of complex skill in medical education. Positive reinforcement mechanisms are central to gamification in XR. Lastly, symbolic enactment is particularly key in psychotherapy applications. The interaction between users and the VE are multidimensional: from early sensory processing to complex sensorimotor, emotional and behavioral responses, until transformation of user representations. In the following section, we will analyze these interactions of increasing complexity, the theoretical framework and technologies that make it possible to design them. Design challenges and discussed solutions are summarized in Figure 4.
3 Human-computer interaction design
3.1 Sensory design challenges
The effectiveness of immersive interfaces in training, rehabilitation, or education hinges on their ability to interact with human senses, cognition, and to generate motor or cognitive actions. To exploit the full potential of recent technological innovation in XR, a deeper understanding of user interaction mechanisms is imperative for maximizing therapeutic outcomes. This observation led to several efforts to integrate cognitive psychology education into developers and computer scientists training (Jerald, 2015; Hodent, 2017). Most XR developers focus on spatial visual and auditory stimulation as fundamental human factors (LaViola et al., 2017). Indeed, space perception is a fundamental prerequisite for immersion and interactivity.
3.1.1 Visual simulation
Visual displays are by far the predominant display devices utilized in XR technologies. Various projection techniques include HMD, tabletop, single screen display, surround screen, multiscreen, or arbitrary surface display. Single-screen displays—conventional monitors, smartphone, or tablet display—are commonly used in clinical XR applications, including GfH, diagnosis and information applications. Surround screens allow users to rely on their peripheral vision, and to move freely within the VE. Such advantages can be essential for biomechanical tracking, visual behavior tracking, and communication studies. Nevertheless, the encoding of depth encoding and 3D objects manipulations in such environments is often inaccurate.
HMDs can either display virtual objects on a head-worn screen or project images directly on the user’s retina (Kollin and Tidwell, 1995). In such virtual retinal display systems, patterned monochromatic light beams are projected through a goggle-mounted OST system. In DTx, virtual retinal displays are predominantly envisioned for ophthalmic applications, in combination with retinal prosthesis or vision restoration interventions (Palanker et al., 2005; Bloch and Da Cruz, 2019; Muqit et al., 2020; Chenais et al., 2021). HMDs can achieve a finer and more naturalistic stereopsis control: stereopsis is directly achieved by the simultaneous projection of one image per eye. However, the tradeoff between constant focal depths and different virtual depths can cause accommodation and vergence conflicts, leading to eye strain and discomfort. Consequently, HMD is not the preferred display source for clinical ophthalmologic applications: diagnosis and visual training commonly rely on non-digital or screen-based digital displays; though multiple VR initiatives are emerging to facilitate diagnosis (Moon et al., 2021; Rajavi et al., 2021; Vicat, 2021; Ma et al., 2022). Comparative advantages of screen-based MR and AR have not been investigated to our knowledge.
In HMDs, integrated eye tracking allows to adjust projected images or virtual elements to a fixed retinal location. The virtual retinal display can increase SoP, reduce cyber-sickness, accommodation, and vergence issues (Jerald, 2015). Integrated adjusting lenses and micro-deformable optics have also been proposed to dynamically adjust the focal plane, and limit vergence and accommodation issues (Love et al., 2009; Zhan et al., 2020; Zhou et al., 2021). These technologies allow users to use their natural accommodative response for depth perception and are promising tools to further develop ophthalmic training applications. However, users suffering from amblyopia or other conditions affecting depth perception cannot perceive 3D effects (Knopf et al., 2017), and compensatory design or evolutive design throughout the visual training protocol must be considered. Neck muscular fatigue and discomfort resulting from prolonged HMD use must also be considered: it is not suitable for physically vulnerable patients, claustrophobic or dement patients, nor for prolonged surgery training.
3.1.2 Auditory cues and audio-visual integration
In the untrained healthy brain, converging information from auditory, visual, and sensory cortices are integrated together to form meaningful multimodal percepts (Marks, 1978; Blattner and Glinert, 1996; Graziano, 2001; Zmigrod and Hommel, 2013). Yet, the different sensory modalities are not equal in VE information integration. Vision dominates auditory and haptic sensory modalities in numerous experimental settings (Colavita, 1974; Blattner and Glinert, 1996; Spence et al., 2012; Bruns, 2019), including virtual reality multisensory display (Gonzalez-Franco et al., 2017). However, visual dominance disappears in a visuo-audio-haptics sensory combinations, and auditory stimulation can be critical to achieve a balanced multimodal information processing and limit the user dependency on visual display (Hecht and Reiner, 2009).
Furthermore, sensory dominance is modified by specific sensorimotor skills acquisition and perceptual training (Colavita, 1974; Powers et al., 2009); and it is altered in specific user groups: patients with neurodegenerative diseases (Murray et al., 2018), motor or sensory disabilities, children with ASD (Hermelin and O’Connor, 1964; Feldman et al., 2018; Ostrolenk et al., 2019). This makes audio-visual integration testing in VE an interesting tool for diagnosis, and a necessary design question. Spatial and timing congruency of stimuli are necessary to audio-visual multimodal integration (Teder-Sälejärvi et al., 2005; Bruns, 2019). In VR, multimodal stimulation, either combining auditory and visual stimulation, or auditory, tactile, and visual stimulation, can decrease the cognitive load of users (Marucci et al., 2021). Audio-visual integration has been identified as promoting embodiment in VEs, and surround auditory stimulation was found to be the preferred display modality to elicit presence neural correlates (Langiulli et al., 2023).
The technological challenge for open VEs and multiple-users VEs is to provide surround ambient sound that dynamically matches with the user spatial location and can serve as a multidimensional information cue (Raghuvanshi and Lin, 2007; Kapralos et al., 2008; Verron et al., 2010; Mehra et al., 2015; Yang J. et al., 2022; Liang et al., 2023).
3.1.3 Olfactive cues
Inclusion of olfactory stimulation has been for long envisioned to develop fully immersive multisensory experience (LaViola et al., 2017). Olfactory stimulation has been identified as a potential contributor to the efficient recall of memories in PSTD exposure therapy (Herz, 2021). The delivery of olfactory cues for medical diagnosis training in humans and animals has been investigated, but the approach success has been limited (Krueger, 1996). The digitalization and controlled delivery of olfactory cues also present new opportunities for sensory substitution training. Nevertheless, the primary issues associated with olfactory cues include their chemical synthesis, user’s fundamental attribution and attention bias to other sensory modalities, and perceptual cross-cultural differences (Spence et al., 2017). In addition, the neural mechanisms behind odor coding, such as odor valence and intensity perception, are not clearly elucidated, what makes it difficult to exploit for controlled clinical and DTx applications (Mainland et al., 2014; Sagar et al., 2023).
3.1.4 Vestibular system
The vestibular system provides multidimensional positional and self-motion information, thanks to inner ear mechanoreceptors responding to vertical, linear, and angular acceleration of the head. Vestibular information is critical in self-representation and embodiment mechanisms (Lopez et al., 2008). The vestibular system interplays with visual system, notably through the vestibular-ocular reflex, allowing to adjust eyes movements in response to motion to keep visual focus. In XR, the incongruency between vestibular cues and visual cues, for instance self-motion, plays a major role in cybersickness. Conversely, providing minimal amount of vestibular feedback through user motion or minimal ambulation can help reduce it and improve SoP during XR experience (Kruijff et al., 2015, 2016).
Furthermore, vestibular feedback and ocular torsion tracking in XR are interesting tools for clinical diagnosis and treatment of visual-vestibular dysfunctions and neurodegenerative diseases. Measurement of ocular torsion and skewing responses in response to vestibular cues changes are possible clinical examination tools to detect aberrant processing of visual information (Wibble and Pansell, 2019), diagnose vestibular dysfunctions and monitor optometric or balance rehabilitative therapies (Cohen, 2013). Vestibular dysfunctions are also associated with multiple neurodegenerative diseases and have a major impact on patients’ mortality and morbidity (Cronin et al., 2017; Kouris et al., 2018). Vestibular rehabilitation has shown positive impact in Parkinson disease patients’ motor control (Rossi-Izquierdo et al., 2009; Basta et al., 2011). XR interfaces with dissociable avatar, direct full body visual feedback and paired stabilometry could be a potent tool for such motor and balance therapies. Visual feedback strategies indeed have significant impact on balance therapy efficiency (Walker et al., 2016; Noh et al., 2019).
3.1.5 Haptics and proprioception
Elementary haptic interactions are frequently included in XR interfaces for feedback—to underlie a cue or an action, or for controlling—through specific fingers gestures. Hand-based techniques are the most common approach to implementing grasping, rotation and manipulating metaphors. Visuo-tactile integration often occurs under highly dynamic conditions requiring constant sensorimotor update, such as dexterity tasks. However, manual control and haptic feedback in most current XR interfaces do not mimic human haptics, but require a new task-specific learning, what can impact the transfer of trained skills to real-life. In surgery simulators and manual motor control recovery interfaces, realistic haptic control and feedback are core to the learning process and the skills transfer. Yet, haptic feedback in XR interfaces mostly covers vibratile input through hand controllers, whose complexity merely addresses that of real-life mechanoreceptors, that integrates temperature, pain, and pressure patterns sensing. Most 3D interaction systems do not support the ability to track the individual fingers (Jerald, 2015). This unnaturalistic use of haptic feedback, including in dexterity training applications, is questioned by multiple groups striving to integrate texture and temperature perception into XR haptic feedback (Kato et al., 2019; Junput et al., 2020; Keef et al., 2020), and allow precise finger and naturalistic grip and push movements through manual controllers (Dorfmuller-Ulhaas and Schmalstieg, 2001; Voigt-Antons et al., 2020).
Incongruent visuo-haptic information dilemmas are often solved in favor of visual information (Farnè et al., 2000; Tsakiris and Haggard, 2005). This might explain the relatively low troubles created by over-simplistic haptic feedback when associated with rich visual content. This mechanism is also exploited in clinical XR interfaces addressing phantom limb syndrome in amputee patients (Hunter, 2003; Foell et al., 2014). However, the visual dominance is less prominent during active haptics and self-generated motor tasks (Tsakiris et al., 2006; Rognini et al., 2013; Boban et al., 2022), what highlights the interference with proprioceptive signals, and the need for realistic feedback solutions for sensorimotor trainings. The implications of visuotactile integration in body ownership and out-of-body experience also suggest potential for further avatar therapy, awareness, and immersion research (Pavani et al., 2000; Rognini et al., 2013).
Proprioception through tendons, muscle spindle and joint mechanoreceptors, is frequently regarded as a distinct secondary haptic system. It provides crucial information for self-body perception, such as muscle tension and joints angles, what informs users on their body angle, and whether their movements are self or passively induced. This information is central to body ownership (Tsakiris et al., 2006; Lopez et al., 2008; Ehrsson, 2012; Butler et al., 2017). Incongruent proprioceptive cues in VR are contributing to cybersickness, lower SoP, and can potentially have a negative impact on motor control rehabilitation processes (Pritchard et al., 2016; Gallagher and Ferrè, 2018; Schlienger et al., 2023). XR interfaces allowing users ambulation and full body kinematics seem a more suitable alternative (Slater et al., 1995), but comparative research still misses. VR interfaces for specific upper limb proprioceptive rehabilitation have been parallelly developed for post-stroke and movement disorders patients (Wong et al., 2012; Abbruzzese et al., 2014), but proprioceptive feedback is not systematically implemented in motor rehabilitation processes. Yet, the occurrence of visuo-proprioceptive integration during joint visual and proprioceptive stimulation and its positive effect on motor learning provides new perspectives for rehabilitation (Wong et al., 2012; Schlienger et al., 2023).
Haptic feedback is also developed as an accessibility feature, for instance to allow deaf audience to feel ambient sounds through vibratile floors or vest (Shibasaki et al., 2016; Hashizume et al., 2018), or to provide information to visually impaired VR users through augmented interactive white cane or braille display (Ghali et al., 2012; Kim, 2020). Such features could ultimately be integrated into occupational therapy practices, but also in XR interfaces for diverse applications and general patient users.
3.1.6 Sensorimotor interactions
Active interaction thinking is critical in XR design. Immersive design does not only comprise 3D visualization of virtual objects but also techniques to interact, manipulate, and gain knowledge from these objects. To interact with the virtual objects in XR, the user is requested to perform real-world actions or symbolic “magical” actions. For non-motor-skill-specific tasks, such as cognitive games or data exploration, sticking to a close real-world-action for every interactive task might lose user’s engagement and slow down its progression and focus. A common trade-off design is to allow magical license for contextual or navigating interactions and focus on realism for perceptual and motor tasks (LaViola et al., 2017). Traditional sensorimotor interaction techniques from 2D interfaces rely on a set of manual simple interactions (pinch, drag, rotate). 3D interactions can also be performed through handles joysticks, what allows simple but limited interaction. Verbal or gaze interaction control have been marginally investigated in 2D (SpecialEffect Studio, 2023). Their extension into 3D has an enormous potential for disabled user accessibility, but also to prioritize the sensory modalities to be trained or investigated. In large MR environments, telerobotic is also explored for 3D interaction, notably to provide personal assistance to medical students during care simulations (Sampsel et al., 2014; Molloy et al., 2016; Gobron, 2020).
Interaction is often limited to rotating the viewpoint, zooming, targeting, or releasing text information, what leads to lack of usability, low quality experience, and limited engagement and training efficacy (LaViola et al., 2017). The best solution implemented to date originates in the development of realistic shooting games: by combining 3D display and 2D interaction designers force users to successfully train 3D cognition, situational probability and anticipation skills, transferable to real-life situations (Green and Bavelier, 2003; Feng et al., 2007; Basak et al., 2008; Zelinski and Reyes, 2009). These benefits were also found to be partially transferable to overall executive function improvement in older adults (Basak et al., 2008), and are exploited as a GfH in geriatric psychology (Stern et al., 2011). In psychiatric applications, the passive exposition to virtual stimuli is thought to be a more central therapeutic component than the interaction itself (Grochowska et al., 2019). However, for pedagogic or rehabilitation applications, this lack of complex and realistic interaction can be a major struggle. Sensory feedback is most lacking. Efforts towards realistic and operable sensory feedback has been made by neurosurgery simulators developers, who notably introduced various force feedback depending on the mechanical properties of the biological tissues and the force exerted by the users (Alaraj et al., 2011, 2013). Though, the majority of currently used interfaces for surgery training only provide visual and rough vibratile haptic feedback (Mao et al., 2021).
3.2 Cognition and game design
Working and long-term memories of situation, decisions, actions, and their consequences are essential for interacting and training, either for complex declarative tasks (such as informative educative interfaces), or procedural tasks. Initial immersive exposure and training allow users to acquire situational awareness, i.e., to internalize a cognitive model of themselves within the trained environment. Situational awareness includes time and space awareness, understanding of the spatial relationships, of the other players of the interaction, and possible action outcomes evaluation. Situational immersion is often the only way to acquire awareness of precise sensorimotor actions, such as surgical gestures or fine coordinated motor control: only the physical experience and sensorimotor feedback can build the cognitive and proprioceptive representations necessary for such learning. Conventionally, these skills are typically acquired through experimentation. The closed sensory information-processing feedback loop is crucial, as concomitant motor actions and positive sensory and proprioceptive feedback serve to strengthen action loops at both the associative and local circuit scale (Schouenborg, 2004; Makino et al., 2016). By adding multiple components to this action-perception loop, such as verbal cognition (e.g., explicit rules, knowledge, mention of the goal), the learning process can be declarative, conscious and proprioceptive at the same time, and the user leverage multiple cognitive strategies (McDougle and Taylor, 2019).
From a game design perspective, the initial situational awareness can be promoted by realism, storytelling, and use of user’s real-life priors. XR environments are characterized by a set of rules that define virtual objects’ physics, actions goals and narrative; providing the user with a framework to analyze, anticipate and act with the virtual objects. Rules are the gateway to both XR interaction and serious objectives, as they “set up potential actions, actions that are meaningful inside the game, but meaningless outside” (Juul, 2005).
Game mechanics include abstract mechanics—the physics and probabilistic rules governing the gameplay; and representational mechanics,—the elements that are directly tied to the game context, storytelling, and progression systems. Representational mechanics include features such as visual level of detail, time space, and point of view, which can exert a significant influence on embodiment (Juul, 2005; Romano et al., 2016). In XR-based clinical applications, whether explicitly gamified or not, abstract and narrative representational mechanics are deeply intertwined. One on hand the narrative coherence and the adhesion to the immersive environment is key to skills acquisition. However, the generalization of these skills in real-life settings depends on interaction realism. Abstract game mechanics can be implicit or explicit—through goals declaration, or tutorials; common or game-specific; magical or relying on real-world rules—e.g., gravity, probability of adverse events, emotional faces, trust relationship building. In recreational video or immersive games, users adapt and perform faster when they can apply their real-life priors to the game actions (Johnson and Wiles, 2003; Bodi and Thon, 2020). This prior transfer is considered as a core element of flow (Johnson and Wiles, 2003; Jennett et al., 2008). In GfH for medical education and skills training, medical prior knowledge impacts performance, and vice versa, gaming experience updates students’ priors (Lee et al., 2019; Hofmann et al., 2020). In GfH, real-life physics and social behaviors are crucial for, respectively, motor and communication skills transfer. Nonetheless, XR application focusing on sensorimotor skills acquisition can accommodate fictive magical environments, declarative goals or even magical interaction with secondary objects or controllers. On the opposite, behavioral interventions or psychotherapy applications can tolerate some space, time, or haptic feedback detour, but they should encompass a realistic narrative sequence, realistic characters interaction, body movements and facial expression cues, making realistic visual display inseparable from realistic storytelling.
Today, most GfH and XR-based interfaces used for clinical applications are system-driven games of progression, focused on abstract game mechanics. Compelling storylines and narrative gaming universes, though rarely implemented in clinical applications, have the potential to enhance immersion, long-term engagement, and enactment of the user, that has a meaningful impact on the story (Juul, 2005; Bodi and Thon, 2020). This can be especially important in vulnerable populations. In pediatrics and disabled populations, demotivation and disengagement often lead to discontinuation of therapy, depression, and worsens patient outcome (Zihl, 2010; Zahi et al., 2016; Finn et al., 2018; Levac, 2023). This is especially true in therapeutic domains that require repetitive drills, such as motor, speech, and visual rehabilitation therapies. Narrative design may also be of particular interest for psychotherapeutic and communication applications. On the other hand, games of emergence offer higher cognitive complexity and decision branching trees that are essential to numerous clinical applications, such as clinical education, exploratory learning for motor or sensory rehabilitation, and behavioral interventions. Specifically, exploratory games offer interesting models for puzzle-solving design in the perspective of diagnosing cognitive functions, as well as for preventive and rehabilitative training (LaViola et al., 2017; Chicchi Giglioli et al., 2018). Games of emergence also offer unpredictable challenges and rewards. Nevertheless, it poses significant challenges in terms of reproducibility, standardization, and monitoring when considered for educational and therapeutic applications. The impact of challenge rarity and variability on sensorimotor plasticity in the context of serious games remains unknown. The design mechanics of serious games is a relatively new field that necessitates careful consideration and cross-evaluation with clinical outcomes, particularly in the case of DTx.
Last, evolutive game mechanics can benefit clinical GfH and DTx. Level design strategies are pertinent to create engaging experiences for users, but also provide a framework to monitor their outcomes and assist in clinical decisions. In application fields such as occupational therapy or visual reeducation, therapists play a pivotal role in analyzing clinical progress and level progression. Paired evolutive mechanics and professional monitoring offer opening for better standardized, equitable, and evidence-based level design. It has the capability to incorporate numerous quantitative variables derived from user behavior and history, while reducing therapist implicit biases (Hall et al., 2015; FitzGerald and Hurst, 2017; Backhus et al., 2019; Garb, 2021).
3.3 Assessment
The assessment of efficiency and usability of clinical interfaces typically occurs concurrently during a post-production testing phase or clinical trials for DTx. Irrespective of the particular uses, the evaluation of clinical efficiency is assessed through broad functional outcomes, success rate, or memory tasks, while the usability is assessed through questionnaires and eventually medical sociology methodology (Culyba, 2018; Wang et al., 2023). Immersive interfaces could benefit from detailed assessment and analysis of users sensory, behavioral, and cognitive responses during interactions, proper to neurodesign studies (Auernhammer et al., 2023). In tailored applications development, intermediate psychomotor, physiological, and electrophysiological correlates would though be critical evaluation variables (Liu et al., 2017; Chicchi Giglioli et al., 2018; Kim, 2020; Miskowiak et al., 2022; Jiménez-Rodríguez et al., 2023).
In current neurodesign toolkit, eye tracking stands out as the most widely utilized and accessible method for evaluating user behavior and human factors. Usage includes safety studies (Manhartsberger and Zellhofer, 2005; Han et al., 2020), health design validation (Champlin et al., 2014; Erol Barkana and Açık, 2014), and to a lower extend visual communication (King et al., 2019; Kredel et al., 2023) and medical education (Ashraf et al., 2018; Lévêque et al., 2018) research. Visual behavior tracking can provide critical information about attention, spatial orientation strategies, pattern recognition strategies, and visual functions (King et al., 2019; Titchener et al., 2019). However, most design, communication, and usability concentrate on the locations of gaze fixation (Manhartsberger and Zellhofer, 2005). Downscaling gaze patterns analysis is an interesting research opportunity for the advancement of visuo-cognitive analysis in clinical applications.
Sensory assessment and adjustment allow to further link the virtual and real-world environments, closing the loop between real-life perception and virtual action through the provision of real-life sensory consequences. In contemporary MR interfaces, eye tracking and spatial mapping allow to align the virtual actions with real-world perceptual rules, at least on the visual sensory modality. The next generation of interfaces is focused on expanding this mapping to include other sensory modalities. Hand-tracking has been the subject of particular investigation (Dorfmuller-Ulhaas and Schmalstieg, 2001; Xiao et al., 2018; Voigt-Antons et al., 2020). K. Dorfmuller-Ulhaas pioneered the research on detailed optical kinematic hand tracking, which enabled the grasping of objects with natural finger closure movements (Dorfmuller-Ulhaas and Schmalstieg, 2001). The potential to circumvent controllers and naturally interact with objects provides great opportunities for complex surgical training and motor control rehabilitation (Buń et al., 2022). However, the current reported usability does not exceed that of traditional controls (Voigt-Antons et al., 2020).
In DTx, sensory and cognitive responses are the bottom line of the clinical evaluation. Their assessment and design are particularly challenging, as the nervous system interfaced can be impaired at multiple levels—e.g., sensory or motor nerve degeneration, cognitive impairment, attention deficits, circuits that have undergone plastic adaptation to sensory deprivation. It is essential to comprehend the ways in which particular users interact with and utilize virtual content, as well as to identify the specific adverse effects and safety concerns that may arise from immersion, HMDs, visual simulation, and wearable technology used for evaluation in these users. Risks encompass worsening of condition, hallucinations, epilepsy outbreaks, loss of balance, falls and physical injury (Sutcliffe, 2003; Garrett et al., 2018; Tychsen and Thio, 2020). The inclusion of at-risk patients poses an ethical challenge for DTx evaluation: interfaces are either clinically evaluated as a class III device on risky population (Wang et al., 2023), what renders co-design, multiple versions, and iterative design almost impossible, either evaluated based on general usability outcomes. A first framework has been developed by the Spatial Perception and Cognitive Experience (SPACE) Lab design research group. To design visual space for epileptic residents, they conducted preliminary exploratory study was conducted to gather evidence on the perception of various spatial features in their end-user epileptic population, based on their visual and interaction behavior. They used in-situ eye tracking during VR sessions, and psychological evaluations in complement to participatory design discussion (Kwon et al., 2023). The singularity of this methodology – that is referred to as Participatory Neurodesign framework, and that of its design outcomes, underscores the current lack of evidence and joined studies interfacing perceptual sciences, design research, and patient populations. Simultaneously, it presents a novel applied neurodesign framework for evidence-based design intended to neurodiverse and patient populations.
3.4 Social and ethical design challenges
With the increasing use of XR and gaming in the healthcare sector, there is also an increasing analytical observation of these applications by medical and technology ethicists. Schmitt-Rüth and Simon develop a socio-ethical model to evaluate GfH design process (Schmitt-Rüth and Simon, 2020). Four fields are to be included in such evaluations:
- Safety: the basic need for user safety describes the integrity of their health, physical and psychological well-being. Interface usage should be free from harm, respect privacy and confidentiality.
- Equity and Participation: this evaluation domain captures concepts such as solidarity, fairness, equality and inequality, discrimination, stigma, rights, inclusion and exclusion, accessibility, affordability, ownership, universal access, employment.
- Sustainability: aspects such as efficiency, effectiveness, social sustainability, economic sustainability, environment, profitability, and cost are the focus of this category. The technology impact of the user’s living environment is of particular importance.
- Self-determination: this field evaluates dependence, controllability, and ease of assigns of the developed technology, but also confidentiality, privacy, and data protection.
A sustainable development in the sense of these four fields is achieved by comprehensive stakeholder analyses and “acceptance workshops,” in which the mentioned problem fields are analyzed and considered case-specifically, possibly with end-users’ participation. Arora and Razavian postulate that the overlay of virtual and real norms, and the conflict “between the interests of individuals subjected to gamification and those who provide or design gamification elements” are the two primary reasons for the prima facie ethical issues related to gamification (Arora and Razavian, 2021). They therefore propose a model that attributes “[r]esponsibilities for proper design” to designers: designers should facilitate “proper use ensuring proper embedding of the apps within the larger social context.” Designers thus need to share responsibilities with stakeholders such as the public, patients, physicians, biomedical engineers, and health insurers, in order to enhance the outcomes of applications and effectively educate users. Active participation in democratic social discourse and self-reflection by designers regarding the social and economic implications of their technologies are also crucial.
In addition to ethical considerations, the specific nature of XR as an audiovisual, haptic, and interactive experience also raises some very narrow questions. First, virtual embodiment can lead to induce emotional, cognitive, and behavioral changes, intended and unintended, and could ultimately lead users to develop addiction symptoms (Slater et al., 2010b, 2020; Neyret et al., 2020). Owing to the high persuasive power of artificial environments, not all effects are foreseeable (e.g., in the form of unintentional retraumatization); this possibility must also be factored into the ethical and responsibility considerations. Second, long-term vision safety concerns were raised regarding XR therapeutic use, especially in children; however no long-term effect on visual functions were observed (Ha et al., 2016; Turnbull and Phillips, 2017; Tychsen and Foeller, 2020; Iskander et al., 2021). Last, the sensory aspects of interaction design are subject to sociocultural biases: sensory dominance varies with specific population cognition (Feldman et al., 2018; Murray et al., 2018). And the social hierarchy of senses subjective relevance is not universal (Sharma, 2023). Multisensory design and evaluation should therefore consider the cultural aspects of the user population. At present, the clinical testing of XR-based DTx does not include multinational clinical trials, and very few trials were held in Asia to date (Wang et al., 2023). Extending the tested population of clinical users would enable the identification and mitigation of cultural biases in UX design (Slater et al., 2020; Oliva et al., 2022).
The ethical consideration of accessibility is a significant issue in contemporary XR interfaces. XR interfaces include specific accessibility features when they address specifically impaired populations in a rehabilitation perspective—but limited to the specific sensory modality addressed. The vast majority of educational, exploratory, and informative technologies lack general accessibility features (Wang et al., 2023). Including and balancing accessibility in XR is a major challenge, mostly originating from hardware settings, controllers design and sensory feedback design. Quite interestingly, these challenges technically overlap with the need for multisensorial modalities integration. Indeed, a major principle of inclusive design is to provide redundant sources of control and information, so that diverse sensory modalities can be used in alternation or in custom combinations (Dudley et al., 2023). The generalization of inclusive design effort has tremendous benefit potential to both abled and disabled user population: allowing social, professional, and medical inclusion to the former, and enhancing immersive experience and clinical outcomes in the latter. This design aspect could therefore be a relevant indicator that XR technologies are achieving maturity and are ready to take root in educational and clinical settings.
One challenge researchers face is accessing enough users with specific disabilities. One potential solution is to collaborate with organizations representing disabled users and to leverage the participation of a diverse audience for web-based platforms. Such user-centric approach is increasingly adopted in healthcare design, predominantly in the development of digital health tools intended to assist clinical staff documentation (Mummah et al., 2016; Marwaha et al., 2022). However, the validation of DTx and diagnosis technologies is based on clinical trials outcomes and evidence cross-validation: this methodology typically excludes iterative processes and the involvement of multiple stakeholders, or limits their involvement to the preliminary conception phases.
3.5 Clinical status challenge
The development of home-based digital rehabilitation interfaces raises some concerns. XR interfaces can be seen as potential solutions for addressing the shortage of clinical staff and medical isolation (Khan et al., 2023). Importantly, current immersive DTx are not intended to substitute clinical therapists. XR interfaces can only offer a facilitated environment for patient-therapist interaction, and for exercises repetitions. In physical and sensory rehabilitation, synchronous validation and monitoring of exercises by a reeducation specialist are crucial, as poorly executed exercises are a major threat to therapy efficacy. Finally, human care relations are central to patient engagement, commitment, and long-term therapeutical outcomes (Beach et al., 2006). The biopsychosocial (BPS) care model postulates that multiple levels participate to disease, healing and rehabilitation, and that these multiple levels—physiological to social—must be addressed together in the clinical practice (Engel, 1977; Adler, 2009). BPS model strongly influenced medical education and nursing practices over the past 50 years: XR simulation-based nursing education can be seen as a direct heritage of this framework. However, the integration of digital health in BPS and relationship-centered care is still to be defined.
DTx products have been approved and commercialized for evidence-based therapeutic interventions in the US since 1999 and in Europe since the early 2010s (US Department of Health and Human Services, 1999; Hong et al., 2021; Wang et al., 2023). However, their implementation into current clinical practices is limited by insufficient efficacy evidence, and lack of appropriate control groups and comparative studies. The absence of regulatory post-approval studies contributes to this information gap. A recent systematic review underlined the notorious absence of multinational clinical trials; the sparsity of clinical trials held in Asia; and the challenges to design blind conditions (Wang et al., 2023). The legal framework and practical availability of DTx greatly vary significantly from one country to another, posing risks of exacerbating care inequalities (European Federation of Pharmaceutical Industries and Associations, 2023). Furthermore, the utilization of DTx interfaces requires prior basic digital education, openness, and some level of cognitive ability, what is a barrier for elderly, isolated, or cognitively impaired patient populations.
4 Discussion
XR interfaces have numerous technical potentials to train, assist and develop clinical practice. Its sensory dimensionality reduction promotes attentional focus and targeted learning; it offers explicit and exploratory learning overlay; it can offer multisensory control for accessibility, tracking and closed-loop feedback; it beneficiates from technological and theoretical progresses from entertainment, that facilitates immersion, embodiment, and provide insights for successful gamification. However, the benefits of most of these aspects for clinical outcomes are still unverified. Available studies so far demonstrated that specific XR technologies could teach sensorimotor skills, social skills, and declarative knowledge; that they were well accepted by clinicians, health stakeholders and patients; and that recourse to VR could enhance user motivation and engagement. But in numerous sensorimotor trainings or DTx applications, there is no evidence that XR technologies outperform traditional training methods. Multiple XR applications accommodate the traditional role-play, information navigation or physical therapy methods to the digital world and explore their outcomes, rather than taking advantage of new digital learning mechanisms. This exploratory method is complexified by the time and resources needed for both design and evaluation steps. As a result, XR design choices are more often retrospectively evaluated as a whole, rather than subjects to evidence-based iterative processes. The current limitations of XR interfaces for clinical applications likely originate in this retrospective and generalist assessment of efficiency and usability. An approach involving evidence-based design of the multiple interaction mechanics is crucial to align interface functionality with therapeutic or learning goals. Co-design and participative framework can help designers focus on and assess application-specific parameters. There is also room in the current framework for a better integration of the fundamental mechanisms of nervous system plasticity and learning, at the core of clinical training and rehabilitation processes.
A central problem to a comprehensive implementation is the multiplicity and collide of disciplines involved in XR technologies development and medical integration. Only a few studies deal with the social construction of digital games as a new medium in the field of the social system of medicine. The new category “DTx” was introduced for the FDA approval of the game EndeavorRx as a “medical device” for behavioral intervention (Hooker and Karnes, 2022). This discursive shift classified games no longer as entertainment, but as instruments, and only in this way could they be strategically positioned for economic, medical, and governmental stakeholders (Hooker and Karnes, 2022). This positioning requires a second level of multidisciplinary collaboration. In the realm of game production, medical researchers should “be engaged before serious games for health are developed in order to place serious games for health in the best position to have a measurable impact on health outcomes” (Kato, 2013). On the other hand, it is the developers who bring the aesthetic and technological competence to GfH construction, and “are very user-centric and tended to focus almost equally on the problem and the solution spaces when approaching game design” (Cheng et al., 2016). Moreover, many of current XR projects are “driven by game designers and developers, for whom creating a new game is their area of expertise, their comfort zone. When immersed into a healthcare setting, other factors come into play, such as testing, validation, patient-centered outcomes, and evidence-based practice; but how well equipped are the gaming and healthcare professionals to recognize the underlying nature of each other’s field?” (Gendle, 2012). Transdisciplinary knowledge transfer on scientific methodologies is key to uncovering the potential of currently developed XR technologies.
The testing and validation of game mechanisms and technology are the cornerstones of this intertwining. There are few windows in the traditional game development pipeline that allow detailed clinical evaluation and behavioral feedback. White box stage and pre-production playtest may allow designers to validate level design, representational mechanics, and identify specific features needs. Yet, for medical applications, both the clinical approach and its concrete implementation influence its efficacy. An early white-box validation of game mechanics could guarantee the relevance of the VE and framework for the clinical approach and learning mechanisms; while a late evaluation of polished displays and interactions can address realism, immersion, and technical accuracy. However, for DTx, playtesting and redesign iterations are limited by time and resources and design features are often subjectively driven by designers. Iterative clinical evaluation is usually not possible, because robust intervention studies require blinding to prevent bias. This includes avoiding the introduction of bias by engaging users in favor of the intervention asking them active feedback (Birckhead et al., 2019; Chidambaram and Josephson, 2019). In DTx, the clinical efficacy can only be evaluated with a beta version, which is already tremendously late in the development process. It also raises experimental evaluation problems: which game features are participating in the clinical outcome, and need to be evaluated as clinical variables? No study to our knowledge has dissected game mechanics into clinical variables. Another major problem of testing clinical outcomes during playtesting is that user transformation—sensorimotor skills acquisition, perceptual learning, and plastic changes in the nervous system—requires extensive amount of time and repetition. In GfH testing, the solution adopted is to focus on user engagement, and discard clinical evolution. Transformational success in GfH is often measured through game progression, suggesting that completion of the game leads to transformation (Culyba, 2018). However, completion of the tasks itself tells nothing of the retention, generalization of the acquired knowledge and skills.
Solutions are needed to conciliate the requirements, expertise, and procedures from both worlds. Assessment plans with mixed methodologies—including sensory, behavioral, and cognitive neuroscience tools, together with ethical considerations and participatory evaluation windows should be considered. Participatory frameworks offer a base to incorporate behavioral and physiological evaluation in both the co-design and post-design phases. In particular, Participatory Neurodesign (PND) framework represents an initial effort to facilitate the convergence of disciplines (Kwon et al., 2023). PND framework originates in applied research from built environment and wayfinding studies (Edelstein and Macagno, 2012; Rohra et al., 2021; Othman et al., 2023; Dornelese, 2024). In these fields, designers have regular recourse to user involvement and participatory methods, but new challenges arise when wayfinding or environment are designed for healthcare practices and users with specific medical and/or cognitive needs. The healthcare design field is in the search for more evidence-based methodologies, notably for domains peripheral to care, such as built environment, management of care and therapy, occupational therapy, digital health, and among it XR applications. This research accelerated the development of neurodesign as a discipline bridging cognitive sciences with UX. Design of XR interfaces able to exploit complex learning mechanisms require this multidisciplinary consideration. Indeed, while XR systems inherently simplify sensory experiences due to their reductionist nature, this does not confine them to a purely behaviorist perspective in their transformative action on the user. We have discussed earlier how our understanding of interactions and learning mechanisms is bounded to disciplines and scales, and the risks of reductionist or metaphorical perspectives alones. PND framework is one way to combine reductionist validation—intrinsic to sensorimotor training and necessary to optimize interactions, cognitive and experiential validation—intrinsic to XR media and necessary to immersive learning, and user involvement—necessary for integration of the media in the healthcare or health education practices. It opens up the design process, ensuring that the technologies developed reflect both the clinical and experiential needs of the users. This is especially valuable in healthcare, where new digital technologies often face resistance from both patients and professionals due to their integration challenges and the complexity of demonstrating immediate, critical improvements. Participatory design is key to address these acceptation and integration challenges. In GfH development, participatory design improved the effectiveness of complex games, notably when users where involved in game dynamics, levels, and game challenge design (DeSmet et al., 2016). Integrating a data-driven neuroscience component into participatory design further connects this discussion with formal clinical metrics. This is essential to balance the usability and the effectiveness of the technology; whose ultimate objective is a positive patient clinical outcome—either direct or indirect. Open metrics integration also empowers users in discussions with designers, and later facilitates conversations between patients and health professionals. This aligns with the view that health data serve as a medium for care dialogue, and reinforces the place of the patient at the center of their own care journey.
Author contributions
NC: Conceptualization, Project administration, Writing – original draft, Writing – review & editing. AG: Writing – original draft, Writing – review & editing, Conceptualization.
Funding
The author(s) declare that financial support was received for the research, authorship, and/or publication of this article. This work has been funded by the Swiss State Secretariat for Education Research and Innovation, Bern Canton, and the Swiss National Science Foundation (“Confederatio Ludens. Swiss History of Games, Play and Game Design 1968-2000”, Project-ID: 501100001711-209248).
Conflict of interest
The authors declare that the research was conducted in the absence of any commercial or financial relationships that could be construed as a potential conflict of interest.
Publisher’s note
All claims expressed in this article are solely those of the authors and do not necessarily represent those of their affiliated organizations, or those of the publisher, the editors and the reviewers. Any product that may be evaluated in this article, or claim that may be made by its manufacturer, is not guaranteed or endorsed by the publisher.
References
Abbruzzese, G., Trompetto, C., Mori, L., and Pelosin, E. (2014). Proprioceptive rehabilitation of upper limb dysfunction in movement disorders: a clinical perspective. Front. Hum. Neurosci. 8:961. doi: 10.3389/fnhum.2014.00961
Abrahamsen, A., and Bechtel, W. (2012). History and core themes. In: The Cambridge handbook of cognitive science. Eds. K. Frankish and W. Ramsey. 9–28.
Adler, R. H. (2009). Engel’s biopsychosocial model is still relevant today. J. Psychosom. Res. 67, 607–611. doi: 10.1016/j.jpsychores.2009.08.008
Afzali, M., Chenais, N., Eckert, J., Inauen, R., and Wollschlegel, D. (2023). Spital Bülach AG. Neubau Ambulatorium: OP-Bereich.
Ahissar, M., and Hochstein, S. (1993). Attentional control of early perceptual learning. Proc. Natl. Acad. Sci. 90, 5718–5722. doi: 10.1073/pnas.90.12.5718
Ahram, T., Falcão, C., Barros, R. Q., Soares, M. M., and Karwowski, W. (2016). “Neurodesign: applications of neuroscience in design and human–system interactions” in Ergonomics in design (Boca Raton, FL, USA: CRC Press).
Alaraj, A., Charbel, F. T., Birk, D., Tobin, M., Luciano, C., Banerjee, P. P., et al. (2013). Role of cranial and spinal virtual and augmented reality simulation using immersive touch modules in neurosurgical training. Neurosurgery 72, A115–A123. doi: 10.1227/NEU.0b013e3182753093
Alaraj, A., Lemole, M., Finkle, J., Yudkowsky, R., Wallace, A., Luciano, C., et al. (2011). Virtual reality training in neurosurgery: review of current status and future applications. Surg. Neurol. Int. 2:52. doi: 10.4103/2152-7806.80117
Aliwi, I., Schot, V., Carrabba, M., Duong, P., Shievano, S., Caputo, M., et al. (2023). The role of immersive virtual reality and augmented reality in medical communication: a scoping review. J. Patient. Exp. 10:23743735231171562. doi: 10.1177/23743735231171562
Anderson, J. R. (1982). Acquisition of cognitive skill. Psychol. Rev. 89, 369–406. doi: 10.1037/0033-295X.89.4.369
Anderson, J. R. (2000). Learning and memory: An integrated approach. 2nd ed. (Hoboken, NJ, US: John Wiley & Sons Inc.) p487.
Aoyama, H., and Aflatoony, L. (2020). “HomeModAR: a home intervention augmented reality tool for occupational therapists” in Extended abstracts of the 2020 CHI conference on human factors in computing systems (Honolulu, HI: ACM), 1–7.
Arora, C., and Razavian, M. (2021). Ethics of gamification in health and fitness-tracking. Int. J. Environ. Res. Public Health 18:11052. doi: 10.3390/ijerph182111052
Ashraf, H., Sodergren, M. H., Merali, N., Mylonas, G., Singh, H., and Darzi, A. (2018). Eye-tracking technology in medical education: a systematic review. Med. Teach. 40, 62–69. doi: 10.1080/0142159X.2017.1391373
Auernhammer, J., Bruno, J., Booras, A., McIntyre, C., Hasegan, D., and Saggar, M. (2023). “NeuroDesign: greater than the sum of its parts” in Design thinking research. eds. C. Meinel and L. Leifer (Cham: Springer Nature Switzerland), 197–211.
Backhus, L. M., Lui, N. S., Cooke, D. T., Bush, E. L., Enumah, Z., and Higgins, R. (2019). Unconscious bias. Thorac. Surg. Clin. 29, 259–267. doi: 10.1016/j.thorsurg.2019.03.004
Baranowski, T., Blumberg, F., Buday, R., DeSmet, A., Fiellin, L. E., Green, C. S., et al. (2016). Games for health for children—current status and needed research, Institute of Digital Media and Child Development Working Group on games for health. Games Health J. 5, 1–12. doi: 10.1089/g4h.2015.0026
Basak, C., Boot, W. R., Voss, M. W., and Kramer, A. F. (2008). Can training in a real-time strategy video game attenuate cognitive decline in older adults? Psychol. Aging 23, 765–777. doi: 10.1037/a0013494
Basta, D., Rossi-Izquierdo, M., Soto-Varela, A., Greters, M. E., Bittar, R. S., Steinhagen-Thiessen, E., et al. (2011). Efficacy of a Vibrotactile neurofeedback training in stance and gait conditions for the treatment of balance deficits: a double-blind, placebo-controlled multicenter study. Otol. Neurotol. 32, 1492–1499. doi: 10.1097/MAO.0b013e31823827ec
Beach, M. C., and Inui, T.The Relationship-Centered Care Research Network (2006). Relationship-centered care. A constructive reframing. J. Gen. Intern. Med. 21, S3–S8. doi: 10.1111/j.1525-1497.2006.00302.x
Behrens, S. C., Streuber, S., Keizer, A., and Giel, K. E. (2022). How immersive virtual reality can become a key tool to advance research and psychotherapy of eating and weight disorders. Front. Psych. 13:1011620. doi: 10.3389/fpsyt.2022.1011620
Bhoopathi, P. S., and Sheoran, R. (2006). Educational games for mental health professionals. Cochrane Database Syst. Rev. 2006:CD001471. doi: 10.1002/14651858.CD001471.pub2
Bianco, M. L., Pedell, S., and Renda, G. (2016) Augmented reality and home modifications: a tool to empower older adults in fall prevention. In: Proceedings of the 28th Australian Conference on Computer-Human Interaction - OzCHI ‘16, pp. 499–507. Launceston: ACM Press.
Birckhead, B., Khalil, C., Liu, X., Conovitz, S., Rizzo, A., Danovitch, I., et al. (2019). Recommendations for methodology of virtual reality clinical trials in health care by an international working group: iterative study. JMIR Ment. Health 6:e11973. doi: 10.2196/11973
Blanch, D. C., Hall, J. A., Roter, D. L., and Frankel, R. M. (2008). Medical student gender and issues of confidence. Patient Educ. Couns. 72, 374–381. doi: 10.1016/j.pec.2008.05.021
Blanke, O. (2012). Multisensory brain mechanisms of bodily self-consciousness. Nat. Rev. Neurosci. 13, 556–571. doi: 10.1038/nrn3292
Blattner, M. M., and Glinert, E. P. (1996). Multimodal integration. IEEE Multimed. 3, 14–24. doi: 10.1109/93.556457
Bloch, E., and Da Cruz, L. (2019) The argus II retinal prosthesis system. In IntechOpen. doi: 10.5772/intechopen.84947
Boban, L., Pittet, D., Herbelin, B., and Boulic, R. (2022). Changing finger movement perception: influence of active haptics on visual dominance. Front. Virtual Real. 3:860872. doi: 10.3389/frvir.2022.860872
Bodi, B., and Thon, J.-N. (2020). Playing stories? Narrative-dramatic agency in disco Elysium (2019) and Astroneer (2019). Front. Narrat. Stud. 6, 157–190. doi: 10.1515/fns-2020-0012
Boeldt, D., McMahon, E., McFaul, M., and Greenleaf, W. (2019). Using virtual reality exposure therapy to enhance treatment of anxiety disorders: identifying areas of clinical adoption and potential obstacles. Front. Psych. 10:773. doi: 10.3389/fpsyt.2019.00773
Bogost, I. (2010). Persuasive games: the expressive power of videogames. paperback Edn. Cambridge: MIT Press.
Bonnechère, B., Jansen, B., Omelina, L., and Van Sint, J. S. (2016). The use of commercial video games in rehabilitation: a systematic review. Int. J. Rehabil. Res. 39, 277–290. doi: 10.1097/MRR.0000000000000190
Boon, M. Y., Asper, L., Jiao, N., and Ryan, M. (2017). “Vision training; comparing a novel virtual reality game of snakes with a conventional clinical therapy” in 2017 IEEE Life Sciences Conference (LSC) (Sydney, Australia: IEEE), 43–46.
Borja, E. F., Lara, D. A., Quevedo, W. X., and Andaluz, V. H. (2018) Haptic stimulation glove for fine motor rehabilitation in virtual reality environments. In: Augmented reality, virtual reality, and computer graphics, L. T. PaolisDe and P. Bourdot, eds, pp. 211–229 Lecture Notes in Computer Science. Cham: Springer International Publishing.
Boucher, J., and Anns, S. (2018). Memory, learning and language in autism spectrum disorder. Autism Dev. Lang. Impair. 3:239694151774207. doi: 10.1177/2396941517742078
Bruns, P. (2019). The ventriloquist illusion as a tool to study multisensory processing: an update. Front. Integr. Neurosci. 13:51. doi: 10.3389/fnint.2019.00051
Bu, X., Ng, P. H., Tong, Y., Chen, P. Q., Fan, R., Tang, Q., et al. (2022). A Mobile-based virtual reality speech rehabilitation app for patients with aphasia after stroke: development and pilot usability study. JMIR Serious Games 10:e30196. doi: 10.2196/30196
Buń, P., Husár, J., and Kaščak, J. (2022). “Hand tracking in extended reality educational applications” in Advances in manufacturing III. eds. J. Trojanowska, A. Kujawińska, J. Machado, and I. Pavlenko (Cham: Springer International Publishing), 317–325 Lecture Notes in Mechanical Engineering.
Butler, A. A., Héroux, M. E., and Gandevia, S. C. (2017). Body ownership and a new proprioceptive role for muscle spindles. Acta Physiol. 220, 19–27. doi: 10.1111/apha.12792
Butt, A. L., Kardong-Edgren, S., and Ellertson, A. (2018). Using game-based virtual reality with haptics for skill acquisition. Clin. Simul. Nurs. 16, 25–32. doi: 10.1016/j.ecns.2017.09.010
Cao, Y., Summerfield, C., Park, H., Giordano, B. L., and Kayser, C. (2019). Causal inference in the multisensory brain. Neuron 102, 1076–1087.e8. doi: 10.1016/j.neuron.2019.03.043
Carter, O., Bennett, D., Nash, T., Arnold, S., Brown, L., Cai, R. Y., et al. (2017). Sensory integration deficits support a dimensional view of psychosis and are not limited to schizophrenia. Transl. Psychiatry 7, –e1118. doi: 10.1038/tp.2017.69
Champlin, S., Lazard, A., Mackert, M., and Pasch, K. E. (2014). Perceptions of design quality: an eye tracking study of attention and appeal in health advertisements. J. Commun. Healthc. 7, 285–294. doi: 10.1179/1753807614Y.0000000065
Chang, H.-Y., Binali, T., Liang, J.-C., Chiou, G.-L., Cheng, K.-H., Lee, S. W.-Y., et al. (2022). Ten years of augmented reality in education: a meta-analysis of (quasi-) experimental studies to investigate the impact. Comput. Educ. 191:104641. doi: 10.1016/j.compedu.2022.104641
Chao, Y.-Y., Scherer, Y. K., and Montgomery, C. A. (2015). Effects of using Nintendo Wii™ Exergames in older adults: a review of the literature. J. Aging Health 27, 379–402. doi: 10.1177/0898264314551171
Chen, J., Or, C. K., and Chen, T. (2022). Effectiveness of using virtual reality–supported exercise therapy for upper extremity motor rehabilitation in patients with stroke: systematic review and Meta-analysis of randomized controlled trials. J. Med. Internet Res. 24:e24111. doi: 10.2196/24111
Chenais, N. A. L., Leccardi, M. J. I. A., and Ghezzi, D. (2021). Photovoltaic retinal prosthesis restores high-resolution responses to single-pixel stimulation in blind retinas. Commun. Mater. 2, 1–16. doi: 10.1038/s43246-021-00133-2
Cheng, J., Putnam, C., and Guo, J. (2016). ““Always a tall order” values and practices of professional game designers of serious games for health” in Proceedings of the 2016 Annual Symposium on Computer-Human Interaction in Play, (CHI PLAY ‘16). New York, NY, USA: Association for Computing Machinery. 217–228.
Chicchi Giglioli, I. A., De Juan, R. C., Parra, E., and Alcañiz Raya, M. (2018). EXPANSE: a novel narrative serious game for the behavioral assessment of cognitive abilities Kotozaki Y, ed. PLoS One 13:e0206925. doi: 10.1371/journal.pone.0206925
Chidambaram, A. G., and Josephson, M. (2019). Clinical research study designs: the essentials. Pediatr. Investig. 3, 245–252. doi: 10.1002/ped4.12166
Clark, G. M., and Lum, J. A. G. (2017). Procedural learning in Parkinson’s disease, specific language impairment, dyslexia, schizophrenia, developmental coordination disorder, and autism spectrum disorders: a second-order meta-analysis. Brain Cogn. 117, 41–48. doi: 10.1016/j.bandc.2017.07.004
Cohen, A. H. (2013). Vision rehabilitation for visual-vestibular dysfunction: the role of the neuro-optometrist. Neurorehabilitation 32, 483–492. doi: 10.3233/NRE-130871
Colavita, F. B. (1974). Human sensory dominance. Percept. Psychophys. 16, 409–412. doi: 10.3758/BF03203962
Cranmore, J. L. (2022). “B. F. Skinner: lasting influences in education and behaviorism” in The Palgrave handbook of educational thinkers. ed. B. A. Geier (Cham: Springer International Publishing), 1–16.
Cronin, T., Arshad, Q., and Seemungal, B. M. (2017). Vestibular deficits in neurodegenerative disorders: balance, dizziness, and spatial disorientation. Front. Neurol. 8:538. doi: 10.3389/fneur.2017.00538
Culyba, S. (2018) The transformational framework: a process tool for the development of transformational games. Pittsburgh, PA, USA: Carnegie Mellon University, ETC press.
Cypress, B. S., and Caboral-Stevens, M. (2022). “Sense of presence” in immersive virtual reality environment: an evolutionary concept analysis. Dimens. Crit. Care Nurs. 41, 235–245. doi: 10.1097/DCC.0000000000000538
Da Silva, C. A., Fernandes, A. R., and Grohmann, A. P. (2015). “STAR: speech therapy with augmented reality for children with autism spectrum disorders” in Enterprise information systems. eds. J. Cordeiro, S. Hammoudi, L. Maciaszek, O. Camp, and J. Filipe (Cham: Springer International Publishing), 379–396 Lecture Notes in Business Information Processing.
De Vignemont, F. (2011). Embodiment, ownership and disownership. Conscious. Cogn. 20, 82–93. doi: 10.1016/j.concog.2010.09.004
De Vignemont, F. (2021). A minimal sense of Here-ness. J. Philos. 118, 169–187. doi: 10.5840/jphil2021118413
DeSmet, A., Thompson, D., Baranowski, T., Palmeira, A., Verloigne, M., and De Bourdeaudhuij, I. (2016). Is participatory design associated with the effectiveness of serious digital games for healthy lifestyle promotion? A meta-analysis. J. Med. Internet Res. 18:e94. doi: 10.2196/jmir.4444
Digital Therapeutics Alliance (2023) DTx: digital therapeutics alliance. Available at: dtxalliance.org (Accessed November 15, 2023).
Dorfmuller-Ulhaas, K., and Schmalstieg, D. (2001). “Finger tracking for interaction in augmented environments” in Proceedings IEEE and ACM International Symposium on Augmented Reality (New York, NY: IEEE Comput. Soc), 55–64.
Dornelese, A. A. (2024). “Vulnerability and neuroarchitecture, approaches to care in healthcare environments: a narrative review” in In-presence/The body and the space, Eds. M. Bovati, A. Moro, and D. Villa (Alghero, IT: Publica, Dipartimento di Architettura, Urbanistica e Design Università degli Studi di Sassari) 615–618.
Dragos Bogdan Moldoveanu, A., Stanica, I., Dascalu, M.-I., Nicoleta Bodea, C., Flamaropol, D., Moldoveanu, F., et al. (2017). “Virtual environments for training visually impaired for a sensory substitution device” in 2017 Zooming Innovation in Consumer Electronics International Conference (ZINC) (Novi Sad, Serbia: IEEE), 26–29.
Dudley, J., Yin, L., Garaj, V., and Kristensson, P. O. (2023). Inclusive immersion: a review of efforts to improve accessibility in virtual reality, augmented reality and the metaverse. Virtual Real. 27, 2989–3020. doi: 10.1007/s10055-023-00850-8
Dumoulin, S., Bouchard, S., Ellis, J., Lavoie, K. L., Vézina, M.-P., Charbonneau, P., et al. (2019). A randomized controlled trial on the use of virtual reality for needle-related procedures in children and adolescents in the emergency department. Games Health J. 8, 285–293. doi: 10.1089/g4h.2018.0111
Edelstein, E. A., and Macagno, E. (2012). “Form follows function: bridging neuroscience and architecture” in Sustainable environmental design in architecture. eds. S. T. Rassia and P. M. Pardalos (New York, NY: Springer New York), 27–41 Springer Optimization and Its Applications.
Ehrsson, H. H. (2012). “The concept of body ownership and its relation to multisensory integration” in New handbook of multisensory process. Ed. B. E. Stein (Cambridge, MA, USA: The MIT press).
Ehrsson, H. H., Spence, C., and Passingham, R. E. (2004). That’s my hand! Activity in premotor cortex reflects feeling of ownership of a limb. Science 305, 875–877. doi: 10.1126/science.1097011
Eichenberg, C. (2012). Virtual reality in psychological, medical and pedagogical applications : IntechOpen. Norderstedt, GE: BoD - Books on Demand GmbH.
Engel, G. L. (1977). The need for a new medical model: a challenge for biomedicine. Science 196, 129–136. doi: 10.1126/science.847460
Engum, S. A., Jeffries, P., and Fisher, L. (2003). Intravenous catheter training system: computer-based education versus traditional learning methods. Am. J. Surg. 186, 67–74. doi: 10.1016/S0002-9610(03)00109-0
Erol Barkana, D., and Açık, A. (2014). Improvement of design of a surgical interface using an eye tracking device. Theor. Biol. Med. Model. 11, 1–18. doi: 10.1186/1742-4682-11-S1-S4
Eshuis, L. V., Van Gelderen, M. J., Van Zuiden, M., Nijdam, M. J., Vermetten, E., Olff, M., et al. (2021). Efficacy of immersive PTSD treatments: a systematic review of virtual and augmented reality exposure therapy and a meta-analysis of virtual reality exposure therapy. J. Psychiatr. Res. 143, 516–527. doi: 10.1016/j.jpsychires.2020.11.030
European Federation of Pharmaceutical Industries and Associations (2023). Improving access to digital therapeutics in Europe. Brussels: EFPIA Available at: www.efpia.eu/media/677347/improving-access-to-digital-therapeutics-in-europe.pdf (Accessed November 27, 2024).
Fahle, M. (2009). “Perceptual learning and sensory plasticity” in Encyclopedia of neuroscience. Eds. L. R. Squire, F. E. Bloom, N. C. Spitzer, F. Gage, and T. Albright (Amsterdam, NL: Elsevier), 523–533.
Farnè, A., Pavani, F., Meneghello, F., and Làdavas, E. (2000). Left tactile extinction following visual stimulation of a rubber hand. Brain 123, 2350–2360. doi: 10.1093/brain/123.11.2350
Faruki, A. A., Nguyen, T. B., Gasangwa, D.-V., Levy, N., Proeschel, S., Yu, J., et al. (2022). Virtual reality immersion compared to monitored anesthesia care for hand surgery: a randomized controlled trial. PLoS One 17:e0272030. doi: 10.1371/journal.pone.0272030
Feldman, J. I., Dunham, K., Cassidy, M., Wallace, M. T., Liu, Y., and Woynaroski, T. G. (2018). Audiovisual multisensory integration in individuals with autism spectrum disorder: a systematic review and meta-analysis. Neurosci. Biobehav. Rev. 95, 220–234. doi: 10.1016/j.neubiorev.2018.09.020
Feng, J., Spence, I., and Pratt, J. (2007). Playing an action video game reduces gender differences in spatial cognition. Psychol. Sci. 18, 850–855. doi: 10.1111/j.1467-9280.2007.01990.x
Ferrer-García, M., and Gutiérrez-Maldonado, J. (2012). The use of virtual reality in the study, assessment, and treatment of body image in eating disorders and nonclinical samples: a review of the literature. Body Image 9, 1–11. doi: 10.1016/j.bodyim.2011.10.001
Fiellin, L. E., Kyriakides, T. C., Hieftje, K. D., Pendergrass, T. M., Duncan, L. R., Dziura, J. D., et al. (2016). The design and implementation of a randomized controlled trial of a risk reduction and human immunodeficiency virus prevention videogame intervention in minority adolescents: PlayForward: Elm City stories. Clin. Trials 13, 400–408. doi: 10.1177/1740774516637871
Finn, A. P., Grewal, D. S., and Vajzovic, L. (2018). Argus II retinal prosthesis system: a review of patient selection criteria, surgical considerations, and post-operative outcomes. Clin. Ophthalmol. Auckl. NZ 12, 1089–1097. doi: 10.2147/OPTH.S137525
FitzGerald, C., and Hurst, S. (2017). Implicit bias in healthcare professionals: a systematic review. BMC Med. Ethics 18:19. doi: 10.1186/s12910-017-0179-8
Flyckt, R. L., White, E. E., Goodman, L. R., Mohr, C., Dutta, S., and Zanotti, K. M. (2017). The use of laparoscopy simulation to explore gender differences in resident surgical confidence. Obstet. Gynecol. Int. 2017, 1–7. doi: 10.1155/2017/1945801
Foell, J., Bekrater-Bodmann, R., Diers, M., and Flor, H. (2014). Mirror therapy for phantom limb pain: brain changes and the role of body representation. Eur. J. Pain 18, 729–739. doi: 10.1002/j.1532-2149.2013.00433.x
Forster, P.-P., Karimpur, H., and Fiehler, K. (2022). Why we should rethink our approach to embodiment and presence. Front. Virtual. Real. 3:838369. doi: 10.3389/frvir.2022.838369
Fribourg, R., Argelaguet, F., Lecuyer, A., and Hoyet, L. (2020). Avatar and sense of embodiment: studying the relative preference between appearance, control and point of view. IEEE Trans. Vis. Comput. Graph. 26, 2062–2072. doi: 10.1109/TVCG.2020.2973077
Gallagher, M., and Ferrè, E. R. (2018). Cybersickness: a multisensory integration perspective. Multisens. Res. 31, 645–674. doi: 10.1163/22134808-20181293
Garb, H. N. (2021). Race bias and gender bias in the diagnosis of psychological disorders. Clin. Psychol. Rev. 90:102087. doi: 10.1016/j.cpr.2021.102087
Garrett, B., Taverner, T., Gromala, D., Tao, G., Cordingley, E., and Sun, C. (2018). Virtual reality clinical research: promises and challenges. JMIR Serious Games 6:e10839. doi: 10.2196/10839
Garzón, J., Pavón, J., and Baldiris, S. (2019). Systematic review and meta-analysis of augmented reality in educational settings. Virtual. Real. 23, 447–459. doi: 10.1007/s10055-019-00379-9
Gendle, E. (2012). 2nd annual games for health Europe conference: let’s start playing! Int. J. Ther. Rehabil. 19, 670–671. doi: 10.12968/ijtr.2012.19.12.670
Gerber, S. M., Jeitziner, M.-M., Knobel, S. E. J., Mosimann, U. P., Müri, R. M., Jakob, S. M., et al. (2019a). Perception and performance on a virtual reality cognitive stimulation for use in the intensive care unit: a non-randomized trial in critically ill patients. Front. Med. 6:287. doi: 10.3389/fmed.2019.00287
Gerber, S. M., Jeitziner, M.-M., Sänger, S. D., Knobel, S. E. J., Marchal-Crespo, L., Müri, R. M., et al. (2019b). Comparing the relaxing effects of different virtual reality environments in the intensive care unit: observational study. JMIR Perioper. Med. 2:e15579. doi: 10.2196/15579
Gerber, S. M., Jeitziner, M.-M., Wyss, P., Chesham, A., Urwyler, P., Müri, R. M., et al. (2017). Visuo-acoustic stimulation that helps you to relax: a virtual reality setup for patients in the intensive care unit. Sci. Rep. 7:13228. doi: 10.1038/s41598-017-13153-1
Ghali, N. I., Soluiman, O., El-Bendary, N., Nassef, T. M., Ahmed, S. A., Elbarawy, Y. M., et al. (2012). “Virtual reality Technology for Blind and Visual Impaired People: reviews and recent advances” in Advances in robotics and virtual reality. eds. T. Gulrez and A. E. Hassanien (Berlin, Heidelberg: Springer Berlin Heidelberg), 363–385 Intelligent Systems Reference Library.
Gobron, S. (2020) Proceedings by Stéphane Gobron of the 5th gamification and serious game symposium, organized by the HE-arc, HES-SO with the NIFFF. Neuchâtel, CH: Éditions HE-Arc, HES-SO.
Goncalves, G., Melo, M., Vasconcelos-Raposo, J., and Bessa, M. (2020). Impact of different sensory stimuli on presence in credible virtual environments. IEEE Trans. Vis. Comput. Graph. 26, 3231–3240. doi: 10.1109/TVCG.2019.2926978
Gonzalez-Franco, M., Maselli, A., Florencio, D., Smolyanskiy, N., and Zhang, Z. (2017). Concurrent talking in immersive virtual reality: on the dominance of visual speech cues. Sci. Rep. 7:3817. doi: 10.1038/s41598-017-04201-x
Gorman, C., and Gustafsson, L. (2022). The use of augmented reality for rehabilitation after stroke: a narrative review. Disabil. Rehabil. Assist. Technol. 17, 409–417. doi: 10.1080/17483107.2020.1791264
Grantcharov, T. P., Kristiansen, V. B., Bendix, J., Bardram, L., Rosenberg, J., and Funch-Jensen, P. (2004). Randomized clinical trial of virtual reality simulation for laparoscopic skills training. Br. J. Surg. 91, 146–150. doi: 10.1002/bjs.4407
Grassini, S., and Laumann, K. (2020). Questionnaire measures and physiological correlates of presence: a systematic review. Front. Psychol. 11:349. doi: 10.3389/fpsyg.2020.00349
Grassini, S., Laumann, K., and Rasmussen Skogstad, M. (2020). The use of virtual reality alone does not promote training performance (but sense of presence does). Front. Psychol. 11:1743. doi: 10.3389/fpsyg.2020.01743
Graziano, M. S. (2001). A system of multimodal areas in the primate brain. Neuron 29, 4–6. doi: 10.1016/S0896-6273(01)00174-X
Green, C. S., and Bavelier, D. (2003). Action video game modifies visual selective attention. Nature 423, 534–537. doi: 10.1038/nature01647
Grochowska, A., Wichniak, A., and Jarema, M. (2019). Virtual reality – a valuable tool to advance treatment of mental disorders. Arch. Psychiatry Psychother. 21, 65–73. doi: 10.12740/APP/101654
Ha, S.-G., Na, K.-H., Kweon, I.-J., Suh, Y.-W., and Kim, S.-H. (2016). Effects of head-mounted display on the oculomotor system and refractive error in normal adolescents. J. Pediatr. Ophthalmol. Strabismus 53, 238–245. doi: 10.3928/01913913-20160511-01
Hafed, Z. M., Stingl, K., Bartz-Schmidt, K.-U., Gekeler, F., and Zrenner, E. (2016). Oculomotor behavior of blind patients seeing with a subretinal visual implant. Vis. Res. 118, 119–131. doi: 10.1016/j.visres.2015.04.006
Hall, W. J., Chapman, M. V., Lee, K. M., Merino, Y. M., Thomas, T. W., Payne, B. K., et al. (2015). Implicit racial/ethnic Bias among health care professionals and its influence on health care outcomes: a systematic review. Am. J. Public Health 105, e60–e76. doi: 10.2105/AJPH.2015.302903
Han, Y., Yin, Z., Zhang, J., Jin, R., and Yang, T. (2020). Eye-tracking experimental study investigating the influence factors of construction safety hazard recognition. J. Constr. Eng. Manag. 146:04020091. doi: 10.1061/(ASCE)CO.1943-7862.0001884
Hashizume, S., Sakamoto, S., Suzuki, K., and Ochiai, Y. (2018) Livejacket: wearable music experience device with multiple speakers. In: Distributed, Ambient and Pervasive Interactions: Understanding Humans: 6th International Conference, DAPI 2018, Held as Part of HCI International 2018, Las Vegas, NV, July 15–20, 2018, 359–371 Springer.
Hecht, D., and Reiner, M. (2009). Sensory dominance in combinations of audio, visual and haptic stimuli. Exp. Brain Res. 193, 307–314. doi: 10.1007/s00221-008-1626-z
Hermelin, B., and O’Connor, N. (1964). Effects of sensory input and sensory dominance on severely disturbed autistic children and on subnormal controls. Br. J. Psychol. 55, 201–206. doi: 10.1111/j.2044-8295.1964.tb02719.x
Herz, S. (2021). Olfactory virtual reality: a new frontier in the treatment and prevention of posttraumatic stress disorder. Brain Sci. 11:1070. doi: 10.3390/brainsci11081070 R
Herzog, M. H., and Fahle, M. (1997). The role of feedback in learning a Vernier discrimination task. Vis. Res. 37, 2133–2141. doi: 10.1016/S0042-6989(97)00043-6
Herzog, M. H., and Fahle, M. (1998). Modeling perceptual learning: difficulties and how they can be overcome. Biol. Cybern. 78, 107–117. doi: 10.1007/s004220050418
Hill, J. E., Twamley, J., Breed, H., Kenyon, R., Casey, R., Zhang, J., et al. (2022). Scoping review of the use of virtual reality in intensive care units. Nurs. Crit. Care 27, 756–771. doi: 10.1111/nicc.12732
Hodent, C. (2017). The Gamer’s brain: How neuroscience and UX can impact video game design. Boca Raton, FL, USA: CRC Press.
Hofmann, M., Pietraß, M., Eichenberg, C., Hofmann, J., Karagkasidis, A., Küsel, C., et al. (2020). “Games for Health: Herausforderungen einer sachgerechten Entwicklung von Lernspielen am Beispiel von SanTrain” in Medical humanities. eds. A. Görgen and S. H. Simond. 1st ed (Bielefeld, Germany: transcript Verlag), 387–416.
Hong, J. S., Wasden, C., and Han, D. H. (2021). Introduction of digital therapeutics. Comput. Methods Prog. Biomed. 209:106319. doi: 10.1016/j.cmpb.2021.106319
Hooker, T. B., and Karnes, M. S. (2022). More than serious: medicine, games, and care. Comput. Compos. 65:102727. doi: 10.1016/j.compcom.2022.102727
Hunter, J. P. (2003). The effect of tactile and visual sensory inputs on phantom limb awareness. Brain 126, 579–589. doi: 10.1093/brain/awg054
Hussey, P. (2021). “Connecting health immersion of digital into eHealth” in Introduction to nursing informatics. eds. P. Hussey and M. A. Kennedy (Cham: Springer International Publishing), 15–53 Health informatics.
Hwang, N.-K., and Shim, S.-H. (2021). Use of virtual reality technology to support the home modification process: a scoping review. Int. J. Environ. Res. Public Health 18:11096. doi: 10.3390/ijerph182111096
Iskander, M., Ogunsola, T., Ramachandran, R., McGowan, R., and Al-Aswad, L. A. (2021). Virtual reality and augmented reality in ophthalmology: a contemporary prospective. Asia Pac. J. Ophthalmol. 10, 244–252. doi: 10.1097/APO.0000000000000409
Jennett, C., Cox, A. L., Cairns, P., Dhoparee, S., Epps, A., Tijs, T., et al. (2008). Measuring and defining the experience of immersion in games. Int. J. Hum. Comput. Stud. 66, 641–661. doi: 10.1016/j.ijhcs.2008.04.004
Jerald, J. (2015). The VR book: human-centered design for virtual reality. New York, USA: Association for Computing Machinery (ACM).
Jiménez-Rodríguez, C., Yélamos-Capel, L., Salvestrini, P., Pérez-Fernández, C., Sánchez-Santed, F., and Nieto-Escámez, F. (2023). Rehabilitation of visual functions in adult amblyopic patients with a virtual reality videogame: a case series. Virtual. Real. 27, 385–396. doi: 10.1007/s10055-021-00605-3
Johnson, D., and Wiles, J. (2003). Effective affective user interface design in games. Ergonomics 46, 1332–1345. doi: 10.1080/00140130310001610865
Jordan, J. A., Gallagher, A. G., McGuigan, J., and McClure, N. (2001). Virtual reality training leads to faster adaptation to the novel psychomotor restrictions encountered by laparoscopic surgeons. Surg. Endosc. 15, 1080–1084. doi: 10.1007/s004640000374
Junput, B., Farkhatdinov, I., and Jamone, L. (2020). “Touch it, rub it, feel it! Haptic rendering of physical textures with a low cost wearable system” in Towards autonomous robotic systems. eds. A. Mohammad, X. Dong, and M. Russo (Cham: Springer International Publishing), 274–286 Lecture Notes in Computer Science.
Juul, J. (2005) Half-real. Video games between real rules and fictional worlds. Cambridge, MA, USA: The MIT press.
Kamouri, A. L., Kamouri, J., and Smith, K. H. (1986). Training by exploration: facilitating the transfer of procedural knowledge through analogical reasoning. Int. J. Man. Mach. Stud. 24, 171–192. doi: 10.1016/S0020-7373(86)80047-5
Kapralos, B., Jenkin, M. R., and Milios, E. (2008). Virtual audio systems. Presence Teleoperat. Virtual. Environ. 17, 527–549. doi: 10.1162/pres.17.6.527
Kato, P. M. (2013). “The role of the researcher in making serious games for health” in Serious games for healthcare: applications and implications (New York, USA: GI Global Scientific Publishing), 213–231.
Kato, F., Inoue, Y., and Tachi, S. (2019). “Haptic display glove capable of force/vibration/temperature” in 2019 IEEE International Symposium on Measurement and Control in Robotics (ISMCR) (Houston, TX: IEEE), D2-2-1–D2-2–5.
Keef, C. V., Kayser, L. V., Tronboll, S., Carpenter, C. W., Root, N. B., Finn, M., et al. (2020). Virtual texture generated using elastomeric conductive block copolymer in a wireless multimodal haptic glove. Adv. Intell. Syst. 2:2000018. doi: 10.1002/aisy.202000018
Khan, N., Gilliar, W., Bamrah, J. S., and Dave, S. (2023). Post-COVID-19: can digital solutions lead to a more equitable global healthcare workforce? BJPsych Int. 20, 18–23. doi: 10.1192/bji.2022.12
Kim, J. (2020). VIVR: presence of immersive interaction for visual impairment virtual reality. IEEE Access 8, 196151–196159. doi: 10.1109/ACCESS.2020.3034363
Kim, Y.-J., and Ahn, S.-Y. (2021). Factors influencing nursing students’ immersive virtual reality media technology-based learning. Sensors 21:8088. doi: 10.3390/s21238088
King, A. J., Bol, N., Cummins, R. G., and John, K. K. (2019). Improving visual behavior research in communication science: an overview, review, and reporting recommendations for using eye-tracking methods. Commun. Methods Meas. 13, 149–177. doi: 10.1080/19312458.2018.1558194
Knopf, N. A., Boon, M.-Y., Suaning, G. J., Zapf, M. P. H., and Grigg, J. (2017). “Initial mobility behaviors of people with visual impairment in a virtual environment using a mixed methods design” in 2017 IEEE Life Sciences Conference (LSC) (Sydney, Australia: IEEE), 153–156.
Kollin, J. S., and Tidwell, M. R. (1995). “Optical engineering challenges of the virtual retinal display,” Proc. SPIE 2537, Novel Optical Systems Design and Optimization, in. ed. J. M. Sasian (San Diego, CA), 48–60.
Kothgassner, O. D., Goreis, A., Kafka, J. X., Van Eickels, R. L., Plener, P. L., and Felnhofer, A. (2019). Virtual reality exposure therapy for posttraumatic stress disorder (PTSD): a meta-analysis. Eur. J. Psychotraumatol. 10:1654782. doi: 10.1080/20008198.2019.1654782
Kouris, I., Sarafidis, M., Androutsou, T., and Koutsouris, D. (2018). “HOLOBALANCE: an augmented reality virtual trainer solution forbalance training and fall prevention” in 2018 40th Annual International Conference of the IEEE Engineering in Medicine and Biology Society (EMBC) (Honolulu, HI: IEEE), 4233–4236.
Kredel, R., Hernandez, J., Hossner, E.-J., and Zahno, S. (2023). Eye-tracking technology and the dynamics of natural gaze behavior in sports: an update 2016–2022. Front. Psychol. 14:1130051. doi: 10.3389/fpsyg.2023.1130051
Krueger, M. (1996). Addition of olfactory stimuli to virtual reality simulations for medical training applications. Frederick: U.S. Army Medical Research and Materiel Command Fort Detrick.
Kruijff, E., Marquardt, A., Trepkowski, C., Lindeman, R. W., Hinkenjann, A., Maiero, J., et al. (2016). “On your feet! Enhancing vection in leaning-based interfaces through multisensory stimuli” in Proceedings of the 2016 Symposium on Spatial User Interaction, New York, NY, USA: Association for Computing Machinery (ACM) 149–158.
Kruijff, E., Riecke, B., Trekowski, C., and Kitson, A. (2015). “Upper body leaning can affect forward self-motion perception in virtual environments” in Proceedings of the 3rd ACM Symposium on Spatial User Interaction, New York, NY, USA: Association for Computing Machinery (ACM) 103–112.
Kwon, J., Linihan, S., Iedema, A., Schmidt, A., Luo, C., and Marrufo, K. (2023). How interior design responds to neurodiversity: implementing wearable technologies in neurodesign processes. Front. Built Environ. 9:1211519. doi: 10.3389/fbuil.2023.1211519
Kyaw, B. M., Posadzki, P., Paddock, S., Car, J., Campbell, J., and Tudor Car, L. (2019). Effectiveness of digital education on communication skills among medical students: systematic review and Meta-analysis by the digital health education collaboration. J. Med. Internet Res. 21:e12967. doi: 10.2196/12967
Langiulli, N., Calbi, M., Sbravatti, V., Umiltà, M. A., and Gallese, V. (2023). The effect of surround sound on embodiment and sense of presence in cinematic experience: a behavioral and HD-EEG study. Front. Neurosci. 17:1222472. doi: 10.3389/fnins.2023.1222472
Laver, K. E., Lange, B., George, S., Deutsch, J. E., Saposnik, G., and Crotty, M. (2017). Virtual reality for stroke rehabilitation. Cochrane Database Syst. Rev. 11:CD008349. doi: 10.1002/14651858.CD008349.pub4
LaViola, J. J., Kruijff, E., McMahan, R. P., Bowman, D., and Poupyrev, I. P. (2017). 3D user interfaces: theory and practice. Boston, MA, USA: Addison-Wesley, Pearson Education, Inc.
Law, C.-T., and Gold, J. I. (2009). Reinforcement learning can account for associative and perceptual learning on a visual-decision task. Nat. Neurosci. 12, 655–663. doi: 10.1038/nn.2304
Law, A. W., Peck, B. V., Visell, Y., Kry, P. G., and Cooperstock, J. R. (2008). “A multi-modal floor-space for experiencing material deformation underfoot in virtual reality” in 2008 IEEE International Workshop on Haptic Audio Visual Environments and Games (Ottawa, ON: IEEE), 126–131.
Lee, J. Y., Donkers, J., Jarodzka, H., and Van Merriënboer, J. J. G. (2019). How prior knowledge affects problem-solving performance in a medical simulation game: using game-logs and eye-tracking. Comput. Hum. Behav. 99, 268–277. doi: 10.1016/j.chb.2019.05.035
Levac, D. E. (2023). Individual and contextual factors influencing children’s effort in pediatric rehabilitation interventions. Dev. Med. Child. Neurol. 66, 23–31. doi: 10.1111/dmcn.15609
Lévêque, L., Bosmans, H., Cockmartin, L., and Liu, H. (2018). State of the art: eye-tracking studies in medical imaging. Ieee Access 6, 37023–37034. doi: 10.1109/ACCESS.2018.2851451
Li, R. W., Ngo, C., Nguyen, J., and Levi, D. M. (2011). Video-game play induces plasticity in the visual system of adults with amblyopia. PLoS Biol. 9:e1001135. doi: 10.1371/journal.pbio.1001135
Liang, B. S., Liang, A. S., Roman, I., Weiss, T., Duinkharjav, B., Bello, J. P., et al. (2023). Reconstructing room scales with a single sound for augmented reality displays. J. Inf. Disp. 24, 1–12. doi: 10.1080/15980316.2022.2145377
Liu, R., Salisbury, J. P., Vahabzadeh, A., and Sahin, N. T. (2017). Feasibility of an autism-focused augmented reality Smartglasses system for social communication and behavioral coaching. Front. Pediatr. 5:145. doi: 10.3389/fped.2017.00145
Lohre, R., Bois, A. J., Athwal, G. S., and Goel, D. P.on behalf of the Canadian Shoulder and Elbow Society (CSES) (2020). Improved complex skill acquisition by immersive virtual reality training: a randomized controlled trial. J. Bone Jt Surg. 102:e26. doi: 10.2106/JBJS.19.00982
Lombard, M., and Ditton, T. (2006). At the heart of it all: the concept of presence. J. Comput.-Mediat. Commun. 3:JCMC321. doi: 10.1111/j.1083-6101.1997.tb00072.x
Lopez, C., Halje, P., and Blanke, O. (2008). Body ownership and embodiment: vestibular and multisensory mechanisms. Neurophysiol. Clin. Neurophysiol. 38, 149–161. doi: 10.1016/j.neucli.2007.12.006
Lovaas, O. I., and Newsom, C. D. (1976). “Behavior modification with psychotic children” in International Handbook of Behavior Modification and Therapy. Eds. A. S. Bellack, M. Hersen, and A. E. Kazdin (Berlin, GE: Springer) 303–360.
Lovaas, O. I., and Smith, T. (1988). “Intensive behavioral treatment for young autistic children” in Advances in clinical child psychology. eds. B. B. Lahey and A. E. Kazdin (Boston, MA: Springer US), 285–324.
Love, G. D., Hoffman, D. M., Hands, P. J. W., Gao, J., Kirby, A. K., and Banks, M. S. (2009). High-speed switchable lens enables the development of a volumetric stereoscopic display. Opt. Express 17, 15716–15725. doi: 10.1364/OE.17.015716
Ma, M. K. I., Saha, C., Poon, S. H. L., Yiu, R. S. W., Shih, K. C., and Chan, Y. K. (2022). Virtual reality and augmented reality— emerging screening and diagnostic techniques in ophthalmology: a systematic review. Surv. Ophthalmol. 67, 1516–1530. doi: 10.1016/j.survophthal.2022.02.001
Maddox, T., Garcia, H., French, K., Maddox, R., Garcia, L., Krishnamurthy, P., et al. (2022). In-home virtual reality program for chronic low back pain: durability of a randomized, placebo-controlled clinical trial to 18 months post-treatment. Reg. Anesth. Pain Med. 49, 373–375. doi: 10.1136/rapm-2022-104093
Mainland, J. D., Lundström, J. N., Reisert, J., and Lowe, G. (2014). From molecule to mind: an integrative perspective on odor intensity. Trends Neurosci. 37, 443–454. doi: 10.1016/j.tins.2014.05.005
Makino, H., Hwang, E. J., Hedrick, N. G., and Komiyama, T. (2016). Circuit mechanisms of sensorimotor learning. Neuron 92, 705–721. doi: 10.1016/j.neuron.2016.10.029
Manhartsberger, M., and Zellhofer, N. (2005) Eye tracking in usability research: what users really see. In Usability symposium, (Wien, AT: Oesterreichische Computer Gesellschaft OCG Publication) 141–152.
Mao, R. Q., Lan, L., Kay, J., Lohre, R., Ayeni, O. R., Goel, D. P., et al. (2021). Immersive virtual reality for surgical training: a systematic review. J. Surg. Res. 268, 40–58. doi: 10.1016/j.jss.2021.06.045
Marks, L. E. (1978). “Multimodal perception” in Perceptual coding (Amsterdam, NL: Elsevier), 321–339.
Marucci, M., Di Flumeri, G., Borghini, G., Sciaraffa, N., Scandola, M., Pavone, E. F., et al. (2021). The impact of multisensory integration and perceptual load in virtual reality settings on performance, workload and presence. Sci. Rep. 11:4831. doi: 10.1038/s41598-021-84196-8
Marwaha, J. S., Landman, A. B., Brat, G. A., Dunn, T., and Gordon, W. J. (2022). Deploying digital health tools within large, complex health systems: key considerations for adoption and implementation. NPJ. Digit. Med. 5:13. doi: 10.1038/s41746-022-00557-1
Mayor, S. (2017). Avatar therapy reduces auditory hallucinations in schizophrenia, trial finds. Br. Med. J. 359:J5458. doi: 10.1136/bmj.j5458
McDougle, S. D., and Taylor, J. A. (2019). Dissociable cognitive strategies for sensorimotor learning. Nat. Commun. 10:40. doi: 10.1038/s41467-018-07941-0
Mehra, R., Rungta, A., Golas, A., Lin, M., and Manocha, D. (2015). WAVE: interactive Wave-based sound propagation for virtual environments. IEEE Trans. Vis. Comput. Graph. 21, 434–442. doi: 10.1109/TVCG.2015.2391858
Milgram, P., and Kishino, F. (1994). A taxonomy of mixed reality visual displays. IEICE Trans. Inf. Syst. 7, 1321–1329.
Miskowiak, K. W., Jespersen, A. E., Kessing, L. V., Aggestrup, A. S., Glenthøj, L. B., Nordentoft, M., et al. (2022). Cognition assessment in virtual reality: validity and feasibility of a novel virtual reality test for real-life cognitive functions in mood disorders and psychosis spectrum disorders. J. Psychiatr. Res. 145, 182–189. doi: 10.1016/j.jpsychires.2021.12.002
Miyake, A., Friedman, N. P., Emerson, M. J., Witzki, A. H., Howerter, A., and Wager, T. D. (2000). The Unity and Diversity of executive functions and their contributions to complex “frontal lobe” tasks: a latent variable analysis. Cognit. Psychol. 41, 49–100. doi: 10.1006/cogp.1999.0734
Molloy, M., Shaw, R. J., Vaughn, J., and Hueckel, R. (2016). “An innovative use of telepresence robots for educating healthcare professional” in Nursing informatics 2016 (Amsterdam, NL: IOS Press), 989–990.
Moon, H. S., Yoon, H. J., Park, S. W., Kim, C. Y., Jeong, M. S., Lim, S. M., et al. (2021). Usefulness of virtual reality-based training to diagnose strabismus. Sci. Rep. 11:5891. doi: 10.1038/s41598-021-85265-8
Moreno, J. L. (1987). The essential Moreno: writings on psychodrama, group method, and spontaneity. New York, NY, USA: Springer Publishing Company.
Mostofsky, S. H., Goldberg, M. C., Landa, R. J., and Denckla, M. B. (2000). Evidence for a deficit in procedural learning in children and adolescents with autism: implications for cerebellar contribution. J. Int. Neuropsychol. Soc. 6, 752–759. doi: 10.1017/S1355617700677020
Mummah, S. A., Robinson, T. N., King, A. C., Gardner, C. D., and Sutton, S. (2016). IDEAS (integrate, design, assess, and share): a framework and toolkit of strategies for the development of more effective digital interventions to change health behavior. J. Med. Internet Res. 18:e317. doi: 10.2196/jmir.5927
Muñoz, E. G., Fabregat, R., Bacca-Acosta, J., Duque-Méndez, N., and Avila-Garzon, C. (2022). Augmented reality, virtual reality, and game technologies in ophthalmology training. Information 13:222. doi: 10.3390/info13050222
Muqit, M. M., Hubschman, J. P., Picaud, S., McCreery, D. B., Van Meurs, J. C., Hornig, R., et al. (2020). PRIMA subretinal wireless photovoltaic microchip implantation in non-human primate and feline models. PLoS One 15:e0230713. doi: 10.1371/journal.pone.0230713
Murray, M. M., Eardley, A. F., Edginton, T., Oyekan, R., Smyth, E., and Matusz, P. J. (2018). Sensory dominance and multisensory integration as screening tools in aging. Sci. Rep. 8:8901. doi: 10.1038/s41598-018-27288-2
Muslimovic, D., Post, B., Speelman, J. D., and Schmand, B. (2007). Motor procedural learning in Parkinson’s disease. Brain 130, 2887–2897. doi: 10.1093/brain/awm211
Nagle, A., Wolf, P., Riener, R., and Novak, D. (2014). The use of player-centered positive reinforcement to schedule in-game rewards increases enjoyment and performance in a serious game. Int. J. Serious Games 1, 35–47. doi: 10.17083/ijsg.v1i4.47
Nestel, D., Groom, J., Eikeland-Husebø, S., and O’Donnell, J. M. (2011). Simulation for learning and teaching procedural skills: the state of the science. Simul. Healthc. 6, S10–S13. doi: 10.1097/SIH.0b013e318227ce96
Neyret, S., Bellido Rivas, A. I., Navarro, X., and Slater, M. (2020). Which body would you like to have? The impact of embodied perspective on body perception and body evaluation in immersive virtual reality. Front. Robot. AI 7:31. doi: 10.3389/frobt.2020.00031
Noh, H.-J., Lee, S.-H., and Bang, D.-H. (2019). Three-dimensional balance training using visual feedback on balance and walking ability in subacute stroke patients: a single-blinded randomized controlled pilot trial. J. Stroke Cerebrovasc. Dis. 28, 994–1000. doi: 10.1016/j.jstrokecerebrovasdis.2018.12.016
Oliva, A., Grassi, S., Vetrugno, G., Rossi, R., Della Morte, G., Pinchi, V., et al. (2022). Management of Medico-Legal Risks in digital health era: a scoping review. Front. Med. 8:821756. doi: 10.3389/fmed.2021.821756
Ostrolenk, A., Bao, V. A., Mottron, L., Collignon, O., and Bertone, A. (2019). Reduced multisensory facilitation in adolescents and adults on the autism spectrum. Sci. Rep. 9:11965. doi: 10.1038/s41598-019-48413-9
Osumi, M., Ichinose, A., Sumitani, M., Wake, N., Sano, Y., Yozu, A., et al. (2017). Restoring movement representation and alleviating phantom limb pain through short-term neurorehabilitation with a virtual reality system. Eur. J. Pain 21, 140–147. doi: 10.1002/ejp.910
Othman, F. Z., Taib, M. N., Shaari, M. F., and Zaiki, Y. (2023) Potential application of brainwaves to optimise evacuation wayfinding performance during fires. In: 2023 19th IEEE International Colloquium on Signal Processing & its Applications (CSPA), pp. 190–194. Kedah: IEEE. Available at: https://ieeexplore.ieee.org/document/10087615/ (Accessed October 24, 2024).
Palanker, D., Vankov, A., Huie, P., and Baccus, S. (2005). Design of a high-resolution optoelectronic retinal prosthesis. J. Neural Eng. 2, S105–S120. doi: 10.1088/1741-2560/2/1/012
Paquay, M., Goffoy, J., Chevalier, S., Servotte, J.-C., and Ghuysen, A. (2022). Relationships between internal factors, social factors and the sense of presence in virtual reality-based simulations. Clin. Simul. Nurs. 62, 1–11. doi: 10.1016/j.ecns.2021.09.006
Pavani, F., Spence, C., and Driver, J. (2000). Visual capture of touch: out-of-the-body experiences with rubber gloves. Psychol. Sci. 11, 353–359. doi: 10.1111/1467-9280.00270
Pillai, J. S., Schmidt, C., and Richir, S. (2013). Achieving presence through evoked reality. Front. Psychol. 4:86. doi: 10.3389/fpsyg.2013.00086
Polat, U. (2009). Making perceptual learning practical to improve visual functions. Vis. Res. 49, 2566–2573. doi: 10.1016/j.visres.2009.06.005
Powers, A. R., Hillock, A. R., and Wallace, M. T. (2009). Perceptual training narrows the temporal window of multisensory binding. J. Neurosci. 29, 12265–12274. doi: 10.1523/JNEUROSCI.3501-09.2009
Pritchard, S. C., Zopf, R., Polito, V., Kaplan, D. M., and Williams, M. A. (2016). Non-hierarchical influence of visual form, touch, and position cues on embodiment, agency, and presence in virtual reality. Front. Psychol. 7:1649. doi: 10.3389/fpsyg.2016.01649
Raghuvanshi, N., and Lin, M. C. (2007). Physically based sound synthesis for large-scale virtual environments. IEEE Comput. Graph. Appl. 27, 14–18. doi: 10.1109/MCG.2007.16
Rajavi, Z., Soltani, A., Vakili, A., Sabbaghi, H., Behradfar, N., Kheiri, B., et al. (2021). Virtual reality game playing in amblyopia therapy: a randomized clinical trial. J. Pediatr. Ophthalmol. Strabismus 58, 154–160. doi: 10.3928/01913913-20210108-02
Richard, E., Billaudeau, V., Richard, P., and Gaudin, G. (2007). “Augmented reality for rehabilitation of cognitive disabled children: a preliminary study” in 2007 virtual rehabilitation (Venice, Italy: IEEE), 102–108.
Riva, G., Davide, F., and Ijsselsteijn, W. (2003). “Being there: the experience of presence in mediated environments” in Being there: concepts, effects and measurement of user presence in synthetic environments. eds. G. Riva, F. Davide, and W. A. IJsselsteijn (Ios Press: Amsterdam).
Riva, G., Malighetti, C., and Serino, S. (2021). Virtual reality in the treatment of eating disorders. Clin. Psychol. Psychother. 28, 477–488. doi: 10.1002/cpp.2622
Rognini, G., Sengül, A., Aspell, J. E., Salomon, R., Bleuler, H., and Blanke, O. (2013). Visuo-tactile integration and body ownership during self-generated action. Eur. J. Neurosci. 37, 1120–1129. doi: 10.1111/ejn.12128
Rohra, H., Mann, J., Rommerskirch-Manietta, M., Roes, M., and Kuliga, S. (2021). Wayfinding and Urban Design from the perspective of people living with dementia – a call for participatory research. J. Urban Des. Ment. Health 7:4.
Romano, D., Llobera, J., and Blanke, O. (2016). Size and viewpoint of an embodied virtual body affect the processing of painful stimuli. J. Pain 17, 350–358. doi: 10.1016/j.jpain.2015.11.005
Rossi-Izquierdo, M., Soto-Varela, A., Santos-Pérez, S., Sesar-Ignacio, A., and Labella-Caballero, T. (2009). Vestibular rehabilitation with computerised dynamic posturography in patients with Parkinson’s disease: improving balance impairment. Disabil. Rehabil. 31, 1907–1916. doi: 10.1080/09638280902846384
Rothbaum, B. O., and Schwartz, A. C. (2002). Exposure therapy for posttraumatic stress disorder. Am. J. Psychother. 56, 59–75. doi: 10.1176/appi.psychotherapy.2002.56.1.59
Rusch, D. C. (2017). Making deep games: designing Games with Meaning and Purpose. New York, NY, USA: Routledge.
Rusch, D. C., and Phelps, A. M. (2020). Existential transformational game design: harnessing the “Psychomagic” of symbolic enactment. Front. Psychol. 11:571522. doi: 10.3389/fpsyg.2020.571522
Sadek, O., Baldwin, F., Gray, R., Khayyat, N., and Fotis, T. (2023). Impact of virtual and augmented reality on quality of medical education during the COVID-19 pandemic: a systematic review. J. Grad. Med. Educ. 15, 328–338. doi: 10.4300/JGME-D-22-00594.1
Sagar, V., Shanahan, L. K., Zelano, C. M., Gottfried, J. A., and Kahnt, T. (2023). High-precision mapping reveals the structure of odor coding in the human brain. Nat. Neurosci. 26, 1595–1602. doi: 10.1038/s41593-023-01414-4
Sahin, N. T., Abdus-Sabur, R., Keshav, N. U., Liu, R., Salisbury, J. P., and Vahabzadeh, A. (2018) Augmented reality intervention for social communication in autism in a school classroom: rated by teachers and parents as effective and usable in a controlled, longitudinal pilot study, PsyArXiv.
Sampsel, D., Vermeersch, P., and Doarn, C. R. (2014). Utility and effectiveness of a remote telepresence robotic system in nursing education in a simulated care environment. Telemed. E-Health 20, 1015–1020. doi: 10.1089/tmj.2014.0038
Saraiva, A., Barros, M., Nogueira, A., Fonseca Ferreira, N., and Valente, A. (2018). Virtual interactive environment for low-cost treatment of mechanical strabismus and amblyopia. Information 9:175. doi: 10.3390/info9070175
Sawyer, B. (2008). From cells to cell processors: the integration of health and video games. IEEE Comput. Graph. Appl. 28, 83–85. doi: 10.1109/MCG.2008.114
Schlienger, R., De Giovanni, C., Guerraz, M., and Kavounoudias, A. (2023). When proprioceptive feedback enhances visual perception of self-body movement: rehabilitation perspectives. Front. Hum. Neurosci. 17:1144033. doi: 10.3389/fnhum.2023.1144033
Schmitt-Rüth, S., and Simon, M. (2020). Sozio-ethische Betrachtungen in Technikentwicklungsprojekten – Zeitverschwendung oder Erfolgsfaktor für Nutzerakzeptanz? HMD Prax Wirtsch 57, 541–557. doi: 10.1365/s40702-020-00607-w
Schoneveld, E. A., Lichtwarck-Aschoff, A., and Granic, I. (2018). Preventing childhood anxiety disorders: is an applied game as effective as a cognitive behavioral therapy-based program? Prev. Sci. 19, 220–232. doi: 10.1007/s11121-017-0843-8
Schouenborg, J. (2004). Learning in sensorimotor circuits. Curr. Opin. Neurobiol. 14, 693–697. doi: 10.1016/j.conb.2004.10.009
Serrada, I., Hordacre, B., and Hillier, S. L. (2019). Does sensory retraining improve sensation and sensorimotor function following stroke: a systematic review and Meta-analysis. Front. Neurosci. 13:402. doi: 10.3389/fnins.2019.00402
Shahar, N., and Meiran, N. (2015). Learning to control actions: transfer effects following a procedural cognitive control computerized training. PLoS One 10:e0119992. doi: 10.1371/journal.pone.0119992
Sharma, C. (2023). Language of smell: tracing some cross-cultural insights from past and present. Front. Food. Sci. Technol. 3:1091355. doi: 10.3389/frfst.2023.1091355
Shibasaki, M., Kamiyama, Y., and Minamizawa, K. (2016). “Designing a haptic feedback system for hearing-impaired to experience tap dance” in Adjunct Proceedings of the 29th Annual ACM Symposium on User Interface Software and Technology, (New York, NY, USA: Association for Computing Machinery (ACM)) 97–99.
Shin, D. (2019). How does immersion work in augmented reality games? A user-centric view of immersion and engagement. Inf. Commun. Soc. 22, 1212–1229. doi: 10.1080/1369118X.2017.1411519
Skinner, B. F. (1961). Teaching Machines. Sci. Am. 205, 90–102. doi: 10.1038/scientificamerican1161-90
Slater, M. (2009). Place illusion and plausibility can lead to realistic behaviour in immersive virtual environments. Philos. Trans. R. Soc. B Biol. Sci. 364, 3549–3557. doi: 10.1098/rstb.2009.0138
Slater, M., Gonzalez-Liencres, C., Haggard, P., Vinkers, C., Gregory-Clarke, R., Jelley, S., et al. (2020). The ethics of realism in virtual and augmented reality. Front. Virtual. Real. 1:1. doi: 10.3389/frvir.2020.00001
Slater, M., Spanlang, B., and Corominas, D. (2010a). Simulating virtual environments within virtual environments as the basis for a psychophysics of presence. ACM Trans. Graph. 29, 1–9. doi: 10.1145/1778765.1778829
Slater, M., Spanlang, B., Sanchez-Vives, M. V., and Blanke, O. (2010b). First person experience of body transfer in virtual reality. PLoS One 5:e10564. doi: 10.1371/journal.pone.0010564
Slater, M., Usoh, M., and Steed, A. (1995). Taking steps: the influence of a walking technique on presence in virtual reality. ACM Trans. Comput. Hum. Interact. 2, 201–219. doi: 10.1145/210079.210084
Soliveri, P., Brown, R. G., Jahanshahi, M., and Marsden, C. D. (1992). Procedural memory and neurological disease. Eur. J. Cogn. Psychol. 4, 161–193. doi: 10.1080/09541449208406181
Song, T., Yu, K., Eck, U., and Navab, N. (2023). Augmented reality collaborative medical displays (ARC-MeDs) for multi-user surgical planning and intra-operative communication. Comput. Methods Biomech. Biomed. Eng. Imaging Vis. 11, 1042–1049. doi: 10.1080/21681163.2022.2150892
SpecialEffect Studio (2023) Eye Gaze Games. Eye Gaze Games Available at: www.specialeffect.org.uk (Accessed November 22, 2023).
Spence, C., Obrist, M., Velasco, C., and Ranasinghe, N. (2017). Digitizing the chemical senses: possibilities and pitfalls. Int. J. Hum.-Comput. Stud. 107, 62–74. doi: 10.1016/j.ijhcs.2017.06.003
Spence, C., Parise, C., and Chen, Y.-C. (2012). “The Colavita visual dominance effect” in The neural bases of multisensory processes (Boca Raton, FL, USA: CRC Press/Taylor & Francis).
Stahl, J. R., and Leitenberg, H. (1976). “Behavioral treatment of the chronic mental hospital patient” in International Handbook of Behavior Modification and Therapy. Eds. A. S. Bellack, M. Hersen, A. E. Kazdin (Berlin, GE: Springer) 211–241.
Stern, Y., Blumen, H. M., Rich, L. W., Richards, A., Herzberg, G., and Gopher, D. (2011). Space fortress game training and executive control in older adults: a pilot intervention. Aging Neuropsychol. Cogn. 18, 653–677. doi: 10.1080/13825585.2011.613450
Stevens, R. C. (2021). Designing immersive 3D experiences: a designer’s guide to creating realistic 3D experiences for extended reality. Indianapolis, IN, USA: New Riders.
Sutcliffe, A. (2003). Multimedia and virtual reality: designing multisensory user interfaces. London, UK: Psychology Press.
Syahputra, M. F., Aulia, M. R., and Arisandy, D. (2020). Augmented reality technologies for interior design planning using a simultaneous localization and mapping method. IOP Conf Ser Mater. Sci. Eng. 851:012067. doi: 10.1088/1757-899X/851/1/012067
Teder-Sälejärvi, W. A., Russo, F. D., McDonald, J. J., and Hillyard, S. A. (2005). Effects of spatial congruity on audio-visual multimodal integration. J. Cogn. Neurosci. 17, 1396–1409. doi: 10.1162/0898929054985383
Tene, T., Vique López, D. F., Valverde Aguirre, P. E., Orna Puente, L. M., and Vacacela Gomez, C. (2024). Virtual reality and augmented reality in medical education: an umbrella review. Front. Digit. Health 6:1365345. doi: 10.3389/fdgth.2024.1365345
Tenesaca, A., Oh, J. Y., Lee, C., Hu, W., and Bai, Z. (2019). “Augmenting communication between hearing parents and deaf children” in 2019 IEEE International Symposium on Mixed and Augmented Reality Adjunct (ISMAR-adjunct) (Beijing: IEEE), 431–434.
Thompson, J. J., Ritenbaugh, C., and Nichter, M. (2009). Reconsidering the placebo response from a broad anthropological perspective. Cult. Med. Psychiatry 33, 112–152. doi: 10.1007/s11013-008-9122-2
Thorn, J. T., Chenais, N. A. L., Hinrichs, S., Chatelain, M., and Ghezzi, D. (2022). Virtual reality validation of naturalistic modulation strategies to counteract fading in retinal stimulation. J. Neural Eng. 19:026016. doi: 10.1088/1741-2552/ac5a5c
Titchener, S. A., Ayton, L. N., Abbott, C. J., Fallon, J. B., Shivdasani, M. N., Caruso, E., et al. (2019). Head and gaze behavior in retinitis Pigmentosa. Investig. Opthalmol. Vis. Sci. 60, 2263–2273. doi: 10.1167/iovs.18-26121
Tripette, J., Murakami, H., Ryan, K. R., Ohta, Y., and Miyachi, M. (2017). The contribution of Nintendo Wii fit series in the field of health: a systematic review and meta-analysis. PeerJ 5:e3600. doi: 10.7717/peerj.3600
Tsakiris, M. (2010). My body in the brain: a neurocognitive model of body-ownership. Neuropsychologia 48, 703–712. doi: 10.1016/j.neuropsychologia.2009.09.034
Tsakiris, M., and Haggard, P. (2005). The rubber hand illusion revisited: Visuotactile integration and self-attribution. J. Exp. Psychol. Hum. Percept. Perform. 31, 80–91. doi: 10.1037/0096-1523.31.1.80
Tsakiris, M., Prabhu, G., and Haggard, P. (2006). Having a body versus moving your body: how agency structures body-ownership. Conscious. Cogn. 15, 423–432. doi: 10.1016/j.concog.2005.09.004
Turnbull, P. R. K., and Phillips, J. R. (2017). Ocular effects of virtual reality headset wear in young adults. Sci. Rep. 7:16172. doi: 10.1038/s41598-017-16320-6
Tychsen, L., and Foeller, P. (2020). Effects of immersive virtual reality headset viewing on Young children: Visuomotor function, postural stability, and motion sickness. Am. J. Ophthalmol. 209, 151–159. doi: 10.1016/j.ajo.2019.07.020
Tychsen, L., and Thio, L. L. (2020). Concern of photosensitive seizures evoked by 3D video displays or virtual reality headsets in children: current perspective. Eye Brain 12, 45–48. doi: 10.2147/EB.S233195
US Department of Health and Human Services (1999). “Off-the-shelf-software use in medical devices” in Guidance for Industry FDA Review Compliance, 1–26.
Vajapey, S. P., Weber, K. L., and Samora, J. B. (2020). Confidence gap between men and women in medicine: a systematic review. Curr. Orthop. Pract. 31, 494–502. doi: 10.1097/BCO.0000000000000906
Ventura, S., Badenes-Ribera, L., Herrero, R., Cebolla, A., Galiana, L., and Baños, R. (2020). Virtual reality as a medium to elicit empathy: a meta-analysis. Cyberpsychol. Behav. Soc. Netw. 23, 667–676. doi: 10.1089/cyber.2019.0681
Verma, P., Agrawal, K., and Sarasvathi, V. (2020). “Indoor navigation using augmented reality” in Proceedings of the 2020 4th International Conference on Virtual and Augmented Reality Simulations (Sydney, NSW: ACM), 58–63.
Verron, C., Aramaki, M., Kronland-Martinet, R., and Pallone, G. (2010). A 3-D immersive synthesizer for environmental sounds. IEEE Trans. Audio Speech Lang. Process. 18, 1550–1561. doi: 10.1109/TASL.2009.2037402
Vicat, S. (2021). Cas Clinique Eyesoft: utilisation du casque EMAA d’Eyesoft dans ma pratique orthoptique: la réalité virtuelle au service de l’orthoptie. Rev. Francoph. Orthopt. 14, 181–183. doi: 10.1016/j.rfo.2021.09.011
Vidrios-Serrano, C., Bonilla, I., Vigueras-Gomez, F., and Mendoza, M. (2015). “Development of a haptic interface for motor rehabilitation therapy using augmented reality” in 2015 37th Annual International Conference of the IEEE Engineering in Medicine and Biology Society (EMBC) (Milan: IEEE), 1156–1159.
Vinolo Gil, M. J., Gonzalez-Medina, G., Lucena-Anton, D., Perez-Cabezas, V., Ruiz-Molinero, M. D. C., and Martín-Valero, R. (2021). Augmented reality in physical therapy: systematic review and meta-analysis. JMIR Serious Games 9:e30985. doi: 10.2196/30985
Vizcaya-Moreno, M. F., and Pérez-Cañaveras, R. M. (2020). Social media used and teaching methods preferred by generation Z students in the nursing clinical learning environment: a cross-sectional research study. Int. J. Environ. Res. Public Health 17:8267. doi: 10.3390/ijerph17218267
Vlake, J. H., Van Bommel, J., Wils, E.-J., Bienvenu, J., Hellemons, M. E., Korevaar, T. I., et al. (2022). Intensive care unit–specific virtual reality for critically ill patients with COVID-19: multicenter randomized controlled trial. J. Med. Internet Res. 24:e32368. doi: 10.2196/32368
Voigt-Antons, J.-N., Kojic, T., Ali, D., and Moller, S. (2020). “Influence of hand tracking as a way of interaction in virtual reality on user experience” in 2020 Twelfth International Conference on Quality of Multimedia Experience (QoMEX) (Athlone: IEEE), 1–4.
Wächter, T., Lungu, O. V., Liu, T., Willingham, D. T., and Ashe, J. (2009). Differential effect of reward and punishment on procedural learning. J. Neurosci. 29, 436–443. doi: 10.1523/JNEUROSCI.4132-08.2009
Walker, E. R., Hyngstrom, A. S., and Schmit, B. D. (2016). Influence of visual feedback on dynamic balance control in chronic stroke survivors. J. Biomech. 49, 698–703. doi: 10.1016/j.jbiomech.2016.01.028
Wang, C., Lee, C., and Shin, H. (2023). Digital therapeutics from bench to bedside. Npj. Digit. Med. 6:38. doi: 10.1038/s41746-023-00777-z
Ward, T., Rus-Calafell, M., Ramadhan, Z., Soumelidou, O., Fornells-Ambrojo, M., Garety, P., et al. (2020). AVATAR therapy for distressing voices: a comprehensive account of therapeutic targets. Schizophr. Bull. 46, 1038–1044. doi: 10.1093/schbul/sbaa061
Watanabe, T., Náñez, J. E., and Sasaki, Y. (2001). Perceptual learning without perception. Nature 413, 844–848. doi: 10.1038/35101601
Watson, A., Chapman, R., Shafai, G., and Maricich, Y. A. (2023). FDA regulations and prescription digital therapeutics: evolving with the technologies they regulate. Front. Digit. Health 5:1086219. doi: 10.3389/fdgth.2023.1086219
Watters, C., Oore, S., Shepherd, M., Abouzied, A., Cox, A., Kellar, M., et al. (2006). “Extending the use of games in health care” in Proceedings of the 39th Annual Hawaii International Conference on System Sciences (HICSS’06) (Kauia, HI: IEEE), 88b.
Wechsler, T. F., Kümpers, F., and Mühlberger, A. (2019). Inferiority or even superiority of virtual reality exposure therapy in phobias?—a systematic review and quantitative Meta-analysis on randomized controlled trials specifically comparing the efficacy of virtual reality exposure to Gold standard in vivo exposure in agoraphobia, specific phobia, and social phobia. Front. Psychol. 10:1758. doi: 10.3389/fpsyg.2019.01758
Whittaker, L., Russell-Bennett, R., and Mulcahy, R. (2021). Reward-based or meaningful gaming? A field study on game mechanics and serious games for sustainability. Psychol. Mark. 38, 981–1000. doi: 10.1002/mar.21476
Wibble, T., and Pansell, T. (2019). Vestibular eye movements are heavily impacted by visual motion and are sensitive to changes in visual intensities. Investig. Opthalmol. Vis. Sci. 60, 1021–1027. doi: 10.1167/iovs.19-26706
Williams, C. A. (2019). Nurse educators meet your new students: generation Z. Nurse Educ. 44, 59–60. doi: 10.1097/NNE.0000000000000637
Witmer, B. G., and Singer, M. J. (1998). Measuring presence in virtual environments: a presence questionnaire. Presence Teleoperat. Virt. Environ. 7, 225–240. doi: 10.1162/105474698565686
Wong, J. D., Kistemaker, D. A., Chin, A., and Gribble, P. L. (2012). Can proprioceptive training improve motor learning? J. Neurophysiol. 108, 3313–3321. doi: 10.1152/jn.00122.2012
Xiao, R., Schwarz, J., Throm, N., Wilson, A. D., and Benko, H. (2018). MRTouch: adding touch input to head-mounted mixed reality. IEEE Trans. Vis. Comput. Graph. 24, 1653–1660. doi: 10.1109/TVCG.2018.2794222
Xu, D., Ma, Z., Jian, Z., Shi, L., Wang, L., and Gao, J. (2020). “Speech rehabilitation system for hearing impaired children based on virtual reality technology” in 2020 International Conference on Virtual Reality and Visualization (ICVRV) (Recife: IEEE), 209–212.
Yang, J., Barde, A., and Billinghurst, M. (2022). Audio augmented reality: a systematic review of technologies, applications, and future research directions. J. Audio Eng. Soc. 70, 788–809. doi: 10.17743/jaes.2022.0048
Yang, Z.-Q., Du, D., Wei, X.-Y., and Tong, R. K.-Y. (2022). Augmented reality for stroke rehabilitation during COVID-19. J. Neuroeng. Rehabil. 19:136. doi: 10.1186/s12984-022-01100-9
Yang, J. C., Quadir, B., Chen, N.-S., and Miao, Q. (2016). Effects of online presence on learning performance in a blog-based online course. Internet High. Educ. 30, 11–20. doi: 10.1016/j.iheduc.2016.04.002
Yeung, A. W. K., Tosevska, A., Klager, E., Eibensteiner, F., Laxar, D., Stoyanov, J., et al. (2021). Virtual and augmented reality applications in medicine: analysis of the scientific literature. J. Med. Internet Res. 23:e25499. doi: 10.2196/25499
Yildirim, C., Carroll, M., Hufnal, D., Johnson, T., and Pericles, S. (2018). “Video game user experience: to VR, or not to VR?” in 2018 IEEE Games, Entertainment, Media Conference (GEM) (Galway: IEEE), 1–9.
Zahi, S., Mahir, L., Azanmasso, H., Lmidmani, F., and El Fatimi, A. (2016). Anxiety and depression after stroke: report of 64 cases. Ann. Phys. Rehabil. Med. 59:e76. doi: 10.1016/j.rehab.2016.07.178
Zelinski, E. M., and Reyes, R. (2009). Cognitive benefits of computer games for older adults. Geron 8, 220–235. doi: 10.4017/gt.2009.08.04.004.00
Zhan, T., Xiong, J., Zou, J., and Wu, S.-T. (2020). Multifocal displays: review and prospect. PhotoniX 1:10. doi: 10.1186/s43074-020-00010-0
Zhou, Y., Zhang, J., and Fang, F. (2021). Vergence-accommodation conflict in optical see-through display: review and prospect. Results Opt. 5:100160. doi: 10.1016/j.rio.2021.100160
Zihl, J. (2010). Rehabilitation of visual disorders after brain injury. 2nd Edn. London, UK: Psychology Press.
Keywords: immersive technologies, neurodesign, serious games, extended reality, virtual reality, augmented reality, digital education, digital therapeutics
Citation: Chenais N and Görgen A (2024) Immersive interfaces for clinical applications: current status and future perspective. Front. Neurorobot. 18:1362444. doi: 10.3389/fnbot.2024.1362444
Edited by:
Ker-Jiun Wang, University of Pittsburgh, United StatesReviewed by:
Andrea Demofonti, Campus Bio-Medico University, ItalySteve Gallagher, Dunedin School of Medicine, New Zealand
Copyright © 2024 Chenais and Görgen. This is an open-access article distributed under the terms of the Creative Commons Attribution License (CC BY). The use, distribution or reproduction in other forums is permitted, provided the original author(s) and the copyright owner(s) are credited and that the original publication in this journal is cited, in accordance with accepted academic practice. No use, distribution or reproduction is permitted which does not comply with these terms.
*Correspondence: Naïg Chenais, bmFpZy5jaGVuYWlzQHVuaWwuY2g=