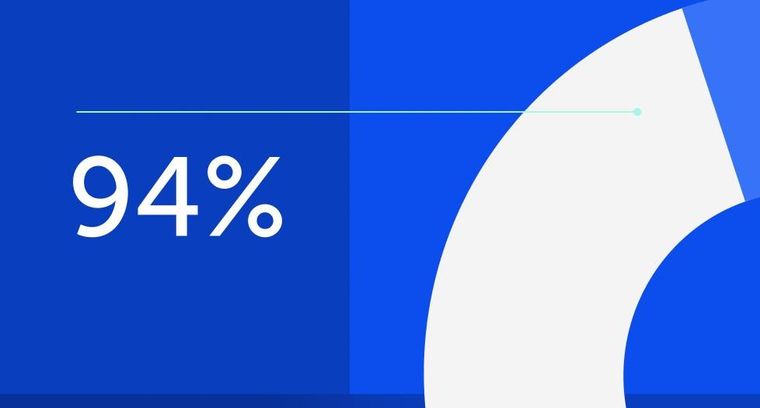
94% of researchers rate our articles as excellent or good
Learn more about the work of our research integrity team to safeguard the quality of each article we publish.
Find out more
ORIGINAL RESEARCH article
Front. Behav. Neurosci., 30 October 2024
Sec. Individual and Social Behaviors
Volume 18 - 2024 | https://doi.org/10.3389/fnbeh.2024.1476501
Introduction: Exposure to blue-enriched light from electronic devices is an emergent disruptor of human sleep, especially at particular times of day. Further dissection of this phenomenon necessitates modeling in a tractable model organism.
Methods: Thus, we investigated the effects of light color on sleep in Drosophila melanogaster. We measured sleep in red-eyed Canton-S (CS) and white-eyed w1118 flies in baseline 12:12 light/dark conditions and experimental conditions with light-color (blue, red, or green) exposure for all 12 h of daylight or 3 h in the morning or evening.
Results: Blue light reduced daytime and nighttime sleep in CS but not in w1118, potentially indicating a role for the compound eye in blue light’s effects on fruit fly sleep. Red light, especially in the evening, reduced sleep during exposure in both strains. Green light had minimal effects on sleep in CS flies, but evening exposure reduced sleep in w1118 flies, mimicking red light’s effects.
Discussion: In conclusion, light’s effects on sleep in D. melanogaster are dependent on wavelength and time-of-day. Future studies will aim to dissect these mechanisms genetically.
The study of life reached places as remote as Antarctica and the ocean floor long before researchers scientifically examined a phenomenon so universal it encompasses about one-third of our lives: sleep. As a physio-behavioral state, sleep is conserved across phyla from cnidarians to complex vertebrates (Kanaya et al., 2020; Nath et al., 2017), and overwhelming evidence supports nightly sleep’s importance for human health. Sleep disruption is a symptom of myriad neuro-psychiatric disorders (Breen et al., 2014; Jagannath et al., 2013; Ju et al., 2014; Wisor et al., 2005), and impaired sleep may play a role in the development of several common fatal diseases (Altman et al., 2012; Engeda et al., 2013; Medic et al., 2017). Hence, widespread sources of sleep disruption are serious public health concerns. With the omnipresence of modern technology, a new disruptor of sleep health has emerged: blue light-emitting visual display units, or screens.
The light emitted from screens on smartphones, TVs, and computers is enriched in blue wavelengths, which delay sleep onset and reduce both total and slow-wave sleep (Chang et al., 2015; Chellappa et al., 2013; Hale and Guan, 2015). Blue light’s sleep effects are time-of-day dependent, as evening exposure disrupts sleep that night and impairs wakefulness the following morning (Chang et al., 2015). The discovery of blue light’s deleterious impact on sleep has led to studies on how other light colors might affect sleep. Namely, exposure to red light may positively impact sleep health. For example, red light increases melatonin secretion and improves next-day wakefulness, as well as self-reported sleep quality scores (Kennedy et al., 2023; Zhao et al., 2012). In response to these discoveries, technology companies have developed software that mitigates negative sleep effects of blue light by enabling users to shift the spectral profile of their screens to longer wavelengths, but the efficacy of these technologies at improving sleep health remains unclear (Jin et al., 2021; Nagare et al., 2019). Clearly, light color and sleep have a complex relationship. However, we still lack a basic understanding of this important interaction, underscoring the need for a systematic investigation.
Studying sleep in human participants has immediate relevance to understanding broad phenomena related to human physiology, behavior, and disease; however, human subjects are unideal for dissecting the cellular and molecular pathways underlying sleep due to species-wide genetic and environmental variation. The fruit fly Drosophila melanogaster overcomes those barriers as an effective small model organism for studying environmental, genetic, and circuit-level control of complex behavior. Fly genetics are well-documented (Adams et al., 2000; Myers et al., 2000), and D. melanogaster has a fast generation time, allowing investigators to simultaneously monitor the sleep of large, inbred populations over a short timespan (Dissel, 2020). Unlike in humans, sleep in D. melanogaster has a bimodal pattern, with a daytime peak known as the “siesta” in addition to their nighttime sleep (see Figure 2B, e.g.). Nevertheless, sleep in flies shares core physiological and behavioral similarities with human sleep, such as: physical immobility, postural changes, lack of responsiveness, preferred location, dynamic stages, characteristic electrical patterns in the brain, and importantly, regulation by both circadian rhythms and a homeostatic response (Hendricks et al., 2000; Huber et al., 2004; Nitz et al., 2002; Shang et al., 2008; Shaw et al., 2000; Tainton-Heap et al., 2020; van Alphen et al., 2021; van Alphen et al., 2013).
Given these similarities, D. melanogaster has been employed as a model to study sleep behavior for decades (Hendricks et al., 2000; Shaw et al., 2002). However, to our knowledge, only one report has directly assayed the relationship between light color and sleep behavior in flies (Krittika and Yadav, 2022). Surprisingly, the authors found that blue light increased daytime sleep while red light moderately reduced daytime sleep, relative to white light. However, each individual fly was only exposed to one color of light throughout their adult lives, making it impossible to examine how animals adjust sleep behavior in response to environmental changes. Additionally, the study by Krittika and Yadav (2022) only utilized a full 12 h of light color exposure, providing little insight into how light color signaling differs across the day. While the effects of light color on fruit fly sleep remain relatively unknown, blue light shortens the overall lifespan of flies (Krittika and Yadav, 2022; Nash et al., 2019) and causes oxidative stress which leads to retinal degeneration, similar to humans (Chen et al., 2017; Nash et al., 2019; Shibuya et al., 2018). Coinciding with these negative health consequences, flies unsurprisingly possess an innate aversion to daytime blue light as well as a positive attraction to red light (Lazopulo et al., 2019). These phase-dependent color preferences are at least partially circadian clock-driven, and both the blue avoidance and red attraction behaviors peak midday when sunlight would typically be brightest (Lazopulo et al., 2019). Green light—which falls between blue and red light on the visual spectrum—may be beneficial to flies, as it extends their lifespan relative to blue or red light (Shen et al., 2021). Additionally, flies exhibit a clock- and compound eye-controlled preference for green light during the morning and evening hours (Lazopulo et al., 2019). Taken together, prior findings demonstrate that light color affects the physiology and behavior of D. melanogaster (likely in a time-dependent manner), but there remains a gap in the literature regarding how these three light colors—blue, red, and green light—specifically impact sleep/wake behavior in fruit flies across the day.
Therefore, we systematically tested how blue-, red-, and green-light exposure at different times of day affect sleep in D. melanogaster. In our study, we measured the sleep of two common D. melanogaster laboratory strains (Canton-S and w1118) in a baseline 12:12 white light/dark cycle before exposing them to one of the three light colors (blue, red, or green) during either all 12 h of the daytime, the first 3 h of daylight (“morning”), or the last 3 h of daylight (“evening”) for 6 experimental days. Here we provide a comprehensive, phenomenological portrait of how a core environmental stimulus—the color of light—regulates complex behavior in a tractable model organism.
Two commonly used laboratory strains of Drosophila melanogaster were studied: the wild-type Canton-S (CS), as well as homozygous white mutants (w1118). w1118 animals were backcrossed 6 times onto a CS background to ensure genotypes had equivalent genetic backgrounds except for the white mutation. Homozygous female w1118 were identified phenotypically by their white eye color, and male w1118 with the mutation (carrying only one white allele) were identified similarly. All flies were raised at 25°C with a 12:12 white light/dark (LD) photoperiod on cornmeal/yeast food as previously described (Juneau et al., 2019). Young adult flies were ≥ 3 d post-eclosion at the start of the experiment, as adult sleep behavior stabilizes by day 3 (Shaw et al., 2000). Within a given study, all flies had eclosed within 4 days of one another. Female flies were mated with males for at least 2 days prior to experimentation to ensure that no virgin females, which have unique sleep patterns (Dove et al., 2017; Isaac et al., 2010), were included in the female sleep data.
After allowing them to age and mate, flies were anesthetized with CO2 and carefully loaded into polycarbonate tubes (5 mm diameter × 65 mm length) with food (5% sucrose w/v, 2% agar w/v). Tubes were then placed into Drosophila Activity Monitor (DAM2) boards (Trikinetics, Inc., Waltham, MA, USA). Infrared beams within the DAM2 boards record each time a fly crosses the center of tube. All activity monitors, along with an environmental monitor, were loaded into the same incubator. The incubator maintained an internal environment of ∼25.0°C and ∼50–75% humidity during the sleep studies. For each experiment, monitors were loaded into the incubator the day before experimentation began. The activity monitors recorded each fly’s activity for two 12 h:12 h white light/dark (WD) baseline days, followed by 6 experimental days of light color treatment, and then 2 WD recovery days.
On the 6 experimental days of each experiment, flies were illuminated with either blue light (peak λ = 466 nm), green light (peak λ = 521 nm), or red light (peak λ = 629 nm) during their subjective daytime (Figure 1A). Zeitgeber time (ZT) 0 was set to be the onset of light each day. Light color exposure was studied during all 12 h of the daytime (ZT0–ZT12), the first 3 h of the day (ZT0–ZT3), and the last 3 h before the dark period (ZT9–ZT12). For the 3 h-exposure experiments, flies were illuminated with white light (3 peaks at 468, 521, and 629 nm) during the other 9 h of the light period. All spectral measurements were taken using a Red Tide USB650 spectrometer (Ocean Insight, Inc., Orlando, FL, USA). White, blue, green, and red light over all 10 d of each experiment were emitted from the same source: a homemade RGB-emitting light box located directly below the activity monitors (Figure 1B). The RGB light box contained three LEDs per bulb (one red, one green, and one blue), as well as a white diffuser sheet placed over the LEDs. The homemade light box’s color changes were controlled with an Arduino microcontroller (Arduino LLC, Boston, MA, USA), which in turn, received time-of-day signals via electrical pulses from the DAM System (Figure 1B). A one-second electrical pulse was sent to the Arduino every 12 h to maintain the 12:12 LD photoperiod in all experiments. Additionally, the 3-h exposure experiments shown in Figures 3, 5, 7 also had a one-second electrical pulse every 24 h to shift between white light and colored light during the day for 3 h. These electrical signals initiated transitions between steps in the lighting paradigms established in Arduino scripts (“sketches”) designed in our lab that also dictated the color and intensity of the light to be delivered. Intensities ranged from ∼250–300 lx for blue and green light, whereas the red LED had a lower intensity (∼150 lx). By adjusting the electrical output strength in the Arduino sketch, we lowered the LED emission intensity during the white-light condition until the overall light intensity in the incubator was ∼230 lx, approximating levels seen with the individual light colors.
Figure 1. Experimental design and methodology. (A) Emission spectra of four light colors (white, blue, red, and green) used during the experiment. (B) Experimental setup illustrating locations of hardware controlling the light emission, as well as the Drosophila Activity Monitors (DAMs) in and around our sleep testing incubator. The desktop computer sends electrical signals to a circuit that then connects to our Arduino microcontroller (atop the incubator), which is wired to the light grid (inside the incubator). The light grid—which contains red, green, and blue LEDs, is located below the DAMs, which track individual flies’ sleep/wake behavior. The DAMs are wired out of the incubator to provide data to the desktop computer.
Sleep and circadian data were extracted from the activity dataset with the Sleep and Circadian Analysis MATLAB Program (SCAMP), versions 2–4 (Donelson et al., 2012; Vecsey et al., 2024). Sleep was defined as ≥ 5 min of inactivity (no beam crosses), as is widely done in the field (Hendricks et al., 2000). Data were only used from flies that survived the entirety of the experiment.
Each 24-h day’s sleep/activity data were divided into 12-h, 3-h, and 30-min bins to enable both broad day/night sleep analysis (12-h bins) and finer-tuned dissection of sleep patterns. Next, total minutes of sleep were calculated for each bin on baseline and experimental days. Each group’s binned data across days were subjected to Kolmogorov–Smirnov and Shapiro–Wilk normality tests. For normally distributed data, we carried out a within-group, repeated-measures ANOVA to analyze how sleep changed across days. For non-Gaussian data, a nonparametric equivalent of repeated-measures ANOVA (Friedman’s test) was performed. If a significant effect was identified via ANOVA or Friedman’s test, we performed post-hoc Tukey or Dunn tests, respectively, to ascertain within a particular group which experimental/recovery days differed significantly from baseline sleep. A p-value < 0.05 was considered significant for all statistical tests. Significant results for post-hoc tests are indicated with asterisks (*) in panel D of Figures 2–10, while ANOVA and Friedman’s test results are reported in Supplementary Tables 1, 2 respectively. Statistical analysis was carried out using GraphPad Prism 10 (Dotmatics, Boston, MA, USA).
Figure 2. Blue-light exposure for 12 h reduces daytime and nighttime sleep in male and female Canton-S (CS) D. melanogaster, but not white (w1118) mutants. (A) Timeline of entire experiment, showing 2 d of baseline 12:12 white/dark (WD), 6 d experimental 12:12 blue/dark (BD), and 2 d recovery WD. (B) Sleep patterns for male and female CS (red) and w1118 (gray) flies. Sleep (min) during each 30-min bin of the day was averaged across flies for each group and plotted to show overall sleep profiles over the second baseline and first four experimental days. (C) Differences in sleep between experimental days and baseline of male and female CS and w1118. Mean sleep duration (min) in each 30-min bin of the baseline day was subtracted from the mean sleep duration in each respective bin on the first four experimental days. (D) Total sleep duration (min) during day (ZT0–12) and night (ZT12–24) for all groups. All experimental and recovery days of the experiment are shown, along with the second baseline day. Sexes and genotypes were analyzed separately with a repeated-measures ANOVA or nonparametric alternative, followed by post-hoc tests. Asterisks indicates significant difference in day/night sleep (per post-hoc test) within group between experimental/recovery and baseline day (*P < 0.05, **P < 0.01, ***P < 0.001, ****P < 0.0001). Ns were 30, 31, 29, 29 for CS female, w1118 female, CS male, w1118 male, respectively.
Figure 3. Morning blue light for 3 h marginally impacts sleep in CS or w1118 flies. (A) Timeline of entire experiment, showing 2 d of baseline 12:12 WD, 6 d experimental 3:9:12 blue/white/dark (BWD), 2 d recovery WD. (B) Sleep patterns for male and female CS (red) and w1118 (gray) flies. Sleep (min) during each 30-min bin of the day was averaged for across flies for each group and plotted to show the overall sleep profiles over the second baseline and first four experimental days. (C) Differences in sleep between experimental days and baseline of male and female CS and w1118. Mean sleep duration (min) in each 30-min bin of the baseline day was subtracted from the mean sleep duration in each respective bin on the first four experimental days. (D) Total sleep duration (min) during blue light exposure (ZT0–3) and immediately after (ZT3–6). Sexes and genotypes were analyzed separately with a repeated-measures ANOVA or nonparametric alternative, followed by post-hoc tests. Asterisks indicates significant difference in sleep during 3-h bin (per post-hoc test) within group between experimental/recovery and baseline day (*P < 0.05, **P < 0.01). Ns were 27, 24, 29, 29 for CS female, w1118 female, CS male, w1118 male, respectively.
Figure 4. Evening blue light for 3 h acutely reduces sleep in CS flies and increases nighttime sleep both CS and w1118. (A) Timeline of entire experiment, showing 2 d of baseline 12:12 WD, 6 d experimental 9:3:12 white/blue/dark (WBD), 2 d recovery WD. (B) Sleep patterns for male and female CS (red) and w1118 (gray) flies. Sleep (min) during each 30-min bin of the day was averaged across flies for each group and plotted to show overall sleep profiles over the second baseline and first four experimental days. (C) Differences in sleep between experimental days and baseline of male and female CS and w1118. Mean sleep duration (min) in each 30-min bin of the baseline day was subtracted from the mean sleep duration in each respective bin on the first four experimental days. (D) Total sleep duration (min) during the 3-h blue light exposure (ZT9–12) and the 3 h immediately after (ZT12–15). Sexes and genotypes were analyzed separately with a repeated-measures ANOVA or nonparametric alternative, followed by post-hoc tests. Asterisks indicates significant difference in sleep during 3-h bin (per post-hoc test) within group between experimental/recovery and baseline day (*P < 0.05, **P < 0.01, ***P < 0.001, ****P < 0.0001). Ns were 31, 25, 29, 29 for CS female, w1118 female, CS male, w1118 male, respectively.
Figure 5. Green-light exposure for 12 h does not alter sleep in CS flies but does in w1118. (A) Timeline of entire experiment, showing 2 d of baseline 12:12 white/dark (WD), 6 d experimental 12:12 green/dark (GD), 2 d recovery WD. (B) Sleep patterns for male and female CS (red) and w1118 (gray) flies. Sleep (min) during each 30-min bin of the day was averaged across flies for each group and plotted to show overall sleep profiles over the second baseline and first four experimental days. (C) Differences in sleep between experimental days and baseline of male and female CS and w1118. Mean sleep duration (min) in each 30-min bin of the baseline day was subtracted from the mean sleep duration in each respective bin on the first four experimental days. (D) Total sleep duration (min) during day (ZT0–12) and night (ZT12–24) for all groups. All experimental and recovery days of the experiment are shown, along with the second baseline day. Sexes and genotypes were analyzed separately with a repeated-measures ANOVA or nonparametric alternative, followed by post-hoc tests. Asterisks indicates significant difference in day/night sleep (per post-hoc test) within group between experimental/recovery and baseline day (*P < 0.05, **P < 0.01, ***P < 0.001, ****P < 0.0001). Ns were 29, 26, 27, 29 for CS female, w1118 female, CS male, w1118 male, respectively.
Figure 6. Morning green light for 3 h has little effect on sleep in CS and w1118 flies. (A) Timeline of entire experiment, showing 2 d of baseline 12:12 WD, 6 d experimental 3:9:12 green/white/dark (GWD), 2 d recovery WD. (B) Sleep patterns for male and female CS (red) and w1118 (gray) flies. Sleep (min) during each 30-min bin of the day was averaged for all groups and plotted to show the flies’ overall sleep profiles over the second baseline and first four experimental days. (C) Difference in sleep between experimental days and baseline of male and female CS and w1118. Mean sleep duration (min) in each 30-min bin of the baseline day was subtracted from the mean sleep duration in each respective bin on the first four experimental days. (D) Total sleep duration (min) during green-light exposure (ZT0–3) and the 3 h preceding exposure after (ZT21–24). Sexes and genotypes were analyzed separately with a repeated-measures ANOVA or nonparametric alternative, followed by post-hoc tests. Asterisks indicates significant difference in sleep during 3-h bin (per post-hoc test) within group between experimental/recovery and baseline day (**P < 0.01, ***P < 0.001, ****P < 0.0001). Ns were 29, 27, 21, 29 for CS female, w1118 female, CS male, w1118 male, respectively.
Figure 7. Evening green light for 3 h acutely reduces sleep in male and female w1118 but not in CS flies. (A) Timeline of entire experiment, showing 2 d of baseline 12:12 WD, 6 d experimental 9:3:12 white/green/dark (WGD). (B) Sleep patterns for male and female CS (red) and w1118 (gray) flies. Sleep (min) during each 30-min bin of the day was averaged across flies for each group and plotted to show overall sleep profiles over the second baseline and first four experimental days. (C) Differences in sleep between experimental days and baseline of male and female CS and w1118. Mean sleep duration (min) in each 30-min bin of the baseline day was subtracted from the mean sleep duration in each respective bin on the first four experimental days. (D) Total sleep duration (min) during green-light exposure (ZT9–12) and immediately after (ZT12–15). Sexes and genotypes were analyzed separately with a repeated-measures ANOVA or nonparametric alternative, followed by post-hoc tests. Asterisks indicates significant difference in sleep during 3-h bin (per post-hoc test) within group between experimental/recovery and baseline day (*P < 0.05, **P < 0.01, ***P < 0.001, ****P < 0.0001). Ns were 31, 31, 21, 32 for CS female, w1118 female, CS male, w1118 male, respectively.
Figure 8. Red-light exposure for 12 h acutely reduces daytime sleep and remodels overall sleep patterns in CS and w1118. (A) Timeline of entire experiment, showing 2 d of baseline 12:12 white/dark (WD), 6 d experimental 12:12 red/dark (RD), 2 d recovery WD. (B) Sleep patterns for male and female CS (red) and w1118 (gray) flies. Sleep (min) during each 30-min bin of the day was averaged across flies for each group and plotted to show overall sleep profiles over the second baseline and first four experimental days. (C) Differences in sleep between experimental days and baseline of male and female CS and w1118. Mean sleep duration (min) in each 30-min bin of the baseline day was subtracted from the mean sleep duration in each respective bin on the first four experimental days. (D) Total sleep duration (min) during day (ZT0–12) and night (ZT12–24) for all groups. All experimental and recovery days of the experiment are shown, along with the second baseline day. Sexes and genotypes were analyzed separately with a repeated-measures ANOVA or nonparametric alternative, followed by post-hoc tests. Asterisks indicates significant difference in day/night sleep (per post-hoc test) within group between experimental/recovery and baseline day (*P < 0.05, **P < 0.01, ***P < 0.001, ****P < 0.0001). Ns were 29, 29, 32, 27 for CS female, w1118 female, CS male, w1118 male, respectively.
Figure 9. Morning red light extends the siesta and delays nighttime sleep in CS and w1118. (A) Timeline of entire experiment, showing 2 d of baseline 12:12 WD, 6 d experimental 3:9:12 red/white/dark (RWD), 2 d recovery WD. (B) Sleep patterns for male and female CS (red) and w1118 (gray) flies. Sleep (min) during each 30-min bin of the day was averaged across flies for each group and plotted to show overall sleep profiles over the second baseline and first four experimental days. (C) Differences in sleep between experimental days and baseline of male and female CS and w1118. Mean sleep duration (min) in each 30-min bin of the baseline day was subtracted from the mean sleep duration in each respective bin on the first four experimental days. (D) Total sleep duration (min) during red-light exposure (ZT0–3) and during the last 3 h of daytime (ZT9–12). Sexes and genotypes were analyzed separately with a repeated-measures ANOVA or nonparametric alternative, followed by post-hoc tests. Asterisks indicates significant difference in sleep during 3-h bin (per post-hoc test) within group between experimental/recovery and baseline day (*P < 0.05, **P < 0.01, ***P < 0.001, ****P < 0.0001). Ns were 29, 27, 32, 31 for CS female, w1118 female, CS male, w1118 male, respectively.
Figure 10. Evening red light for 3 h acutely reduces sleep in both CS and w1118 flies, but stronger effects are observed in CS. (A) Timeline of entire experiment, showing 2 d of baseline 12:12 WD, 6 d experimental 9:3:12 white/red/dark (WRD), 2 d recovery WD. (B) Sleep patterns for male and female CS (red) and w1118 (gray) flies. Sleep (min) during each 30-min bin of the day was averaged across flies for each group and plotted to show overall sleep profiles over the second baseline and first four experimental days. (C) Differences in sleep between experimental days and baseline of male and female CS and w1118. Mean sleep duration (min) in each 30-min bin of the baseline day was subtracted from the mean sleep duration in each respective bin on the first four experimental days. (D) Total sleep duration (min) during red-light exposure (ZT9–12) and immediately after (ZT12–15). Sexes and genotypes were analyzed separately with a repeated-measures ANOVA or nonparametric alternative, followed by post-hoc tests. Asterisks indicates significant difference in sleep during 3-h bin (per post-hoc test) within group between experimental/recovery and baseline day (*P < 0.05, **P < 0.01, ***P < 0.001, ****P < 0.0001). Ns were 32, 31, 30, 28 for CS female, w1118 female, CS male, w1118 male, respectively.
Because experiments were performed with either 12-h (Figures 2, 5, 8) or 3-h light-color exposures (Figures 3, 4, 6, 7, 9, 10), data were analyzed and displayed accordingly (see panel D for each figure). For instance, data in 12-h bins (day and night) are shown and discussed for 12-h light exposures (e.g., Figure 2), while data in 3-h bins (ZT0–3, ZT3–6, etc.) are shown for 3-h exposure experiments to demonstrate acute effects during (and immediately after) the light color exposure (see panel D for Figures 3, 4, 6, 7, 9, 10).
To investigate the effects of blue-light exposure on D. melanogaster sleep, we measured the baseline sleep of CS and w1118 flies in 12:12 white/dark (WD) for 2 days before transitioning to 12:12 blue/dark (BD) for 6 days and then back to WD for 2 days of recovery (Figure 2A). As sleep is sexually dimorphic in flies, the two sexes were analyzed separately. For this experiment and all others, daytime sleep effects for each sex and genotype will be discussed prior to nighttime sleep effects.
Female CS flies tended to sleep less during the daytime under blue light exposure compared to the baseline day (Figures 2B–D). In contrast, no acute reductions in sleep during the blue-light exposure were observed in w1118 females (Figures 2B–D). Like females, male CS flies, but not w1118, exhibited substantial daytime sleep effects due to 12 h of blue light (Figures 2B–D). Notably, the sleep-reducing effect of 12-h blue-light exposure on CS females and males was primarily observed during the morning hours (Figures 2B, C).
We had expected that blue light exposure would delay sleep onset. However, CS and w1118 flies surprisingly showed significant increases in nighttime sleep between baseline and the first day of blue/dark treatment (BD1) (Figures 2B–D). Substantial nighttime sleep loss began to emerge on BD2, as both male and female CS flies reduced their nighttime sleep by > 25% (Figures 2B–D). Over subsequent days, nighttime sleep gradually returned back to baseline levels. In contrast, w1118 flies of both sexes did not show any reduction in nighttime sleep on BD2, and instead generally slept more at night following blue-light exposure than during the baseline day (Figure 2D). Taken together, these findings indicate a potent daytime- and nighttime-sleep-inhibiting effect of blue light that is present in CS flies but not w1118 flies.
We next sought to examine how 3 h of blue-light exposure during the flies’ subjective morning or evening alters their sleep (Figures 3, 4). These 3-h experiments allowed us to test for time-specific effects of blue light on sleep, potentially to better model human screen use when waking up vs. before bed.
Unlike the 12-h exposure, blue light restricted to 3 h in the morning did not globally alter CS sleep patterns in an overt manner (Figure 3B). Accordingly, limited effects were observed on total sleep within 3-h bins in females nor males, including the 3-h light exposure (ZT0–3) and the following 3-h bin (ZT3–6) (Figure 3D), suggesting that blue light’s sleep effects on flies may depend on what time of day the exposure occurs.
After testing the effects of morning blue light, we sought to examine how evening exposure to blue light affected D. melanogaster sleep patterns (Figure 4). On the first day of evening blue light exposure, both male and female CS flies had reduced sleep during the 3 h of blue-light exposure, compared to baseline sleep across that same 3-h period (ZT9–12), although this only reached significance in males (Figures 4B–D). This sleep reduction was not observed in either male or female CS over subsequent blue-light exposures, suggesting that flies acclimated to the transition to blue light. In fact, males began to sleep more during the blue light exposure over successive days. In contrast to CS flies, sleep in w1118 males and females was increased from baseline across blue-light treatment days, including from the first exposure (Figures 4B–D).
We expected that evening exposure to blue light—close to the onset of nighttime sleep—would decrease CS nighttime sleep as was observed in response to 12 h of blue light exposure. However, our results indicated the opposite. Blue light induced an increase in nighttime sleep for about 3 h following the onset of darkness in both CS and w1118 females (Figures 4B, C). In male flies, this effect was stronger in w1118 than CS (Figure 4C), but this greater magnitude appeared to stem from w1118 males having a longer sleep latency (or later sleep onset) at night than CS males (Figure 4B). Overall, evening exposure to blue light caused an acute sleep reduction during the exposure that was specific to CS flies and subsided over successive days, and caused increased sleep in the first portion of the dark period in both genotypes that persisted throughout the experimental days.
We next investigated the effects of green light on D. melanogaster sleep using a 12:12 green/dark (GD) schedule (Figure 5A). Green light elicited little change in daytime sleep in male and female flies in both CS and w1118 strains (Figures 5B–D). As with daytime sleep, no changes in nighttime sleep in CS males or females were observed due to the 12-h green-light exposure (Figures 5B–D). However, w1118 males and females had an increase in sleep early in the night due to an earlier sleep onset (Figures 5B–D). Overall, switching from white-light to green-light had less of an effect on sleep than switching to blue-light exposure.
To study the effects of morning green-light exposure on D. melanogaster sleep, we used the same experimental design as our morning blue-light experiment but with a 3:9:12 green/white/dark (GWD) photoperiod during the experimental phase (Figure 6A). This morning exposure to green light produced little change in daytime or nighttime sleep of male and female CS and w1118 flies (Figures 6B–D). These results were very similar to what we observed in response to morning exposure to blue light (Figure 3).
We next performed an evening green-light exposure experiment, as we did with blue light. During the experimental period, flies were housed on a photoperiod of 9:3:12 WGD; however, no recovery data in WD were able to be obtained (Figure 7A). Because 12 h of green light had little effect on daytime sleep in CS females (Figure 5), we anticipated similar results with the evening exposure. Indeed, no significant effects of the evening green-light exposure were observed in CS males or females (Figures 7B–D). However, we found that w1118 males and females were acutely sensitive to evening green light, with sharp reductions in sleep occurring during the 3-h exposure from ZT9–12 (Figures 7B–D).
Nighttime sleep was not substantially altered in either CS or w1118 flies. This was notably different from the response to 12 h of green-light exposure, which had resulted in an increase in sleep in w1118 flies in the early nighttime.
We next investigated the effects of red light on D. melanogaster sleep using a 12:12 red/dark (RD) schedule (Figure 8A). In CS and w1118 female flies under red-light conditions, the siesta peaked earlier in the day as compared with baseline white-light conditions (Figure 8B). In addition to this change in pattern, total daytime sleep was significantly reduced in both CS and w1118 females across most experimental days (Figure 8D). After returning to a WD photoperiod in the recovery phase of the experiment, CS flies sharply increased their daytime sleep, far surpassing their baseline levels (Figure 8D)—a possible rebound effect.
Male CS flies also had changes in daytime sleep under red-light conditions that were even more obvious than in female flies. Their siesta was strongly phase-advanced, resulting in the loss of their morning arousal period and an earlier start to their evening arousal period (Figure 8B). Interestingly, male w1118 flies exhibited an opposite effect on morning sleep as compared to CS flies, having stronger morning arousal as the experiment progressed (Figures 8B, C). The end result of these observed potent sleep effects was that both CS and w1118 male flies had significantly reduced daytime sleep on all 6 red-light days (Figure 8D). Male and female w1118 flies also began to lose their period of wakefulness at dusk across days of red-light exposure (Figure 8B).
Nighttime sleep effects of red light were less conclusive and consistent than those observed in the daytime. In females, the two genotypes showed opposing effects. For instance, w1118 flies progressively increased their total nighttime sleep, reaching peak levels on RD5 (Figure 8D). This increased total nighttime sleep was driven mainly by increases early in the night (Figures 8B, C). On the contrary, CS females showed a delay in their nighttime sleep onset during the RD days (Figure 8B). With the return the WD, CS females recovered to their baseline nighttime sleep amount (Figure 8D).
The most notable change to nighttime sleep in CS males was that sleep time gradually increased across red-light exposure days. This seemed to be driven by a phase advance in which flies experienced a mild morning arousal at approximately ZT21, followed by an increase in sleep that continued through “lights-on” at ZT0 (Figure 8B). w1118 males showed no significant changes relative to baseline in their total nighttime sleep during the red-light days (Figure 8D); however, their sleep patterns changed dramatically (Figure 8B). w1118 males gradually began to show a more gradual sleep onset, achieving peak nighttime sleep levels much later during the RD days than during the WD baseline (Figures 8B, C). Overall, these data demonstrate that red light has a genotype- and sex-independent, inhibitory effect on daytime sleep but genotype-specific nighttime sleep effects. The timing of sleep/activity is also affected by red-light exposure, with red light causing a phase advance in daytime sleep in all groups, but especially males, and delays in sleep onset in male w1118 flies.
We next tested the effects of morning red-light exposure using a 3:9:12 red/white/dark (RWD) photoperiod during the six experimental days (Figure 9A). The results demonstrated that morning red-light exposure elicited only minor effects during the exposure, but, in females in particular, caused an immediate increase in sleep as soon as the light condition was switched from red-light to white-light—this is most noticeable on RWD days 2–4 (Figure 9B). In RWD conditions, both CS females and males also experienced an extension of the siesta into the afternoon/evening, and then a delay in sleep onset after lights-out (Figures 9B, C), which was quite different from the response to 12 h of red-light exposure (Figures 8B, C). This phase delay resulted in an increase in sleep toward the end of the light period and a decrease in sleep in the beginning of the dark period (Figure 9C). This overall pattern was also observed in both female and male w1118 flies. Taken together, these results show that a 3 h morning red-light pulse has little acute effects on sleep but may remodel both CS and w1118 sleep patterns by delaying their sleep/activity timing later in the day.
During our 12-h experiment with red light, flies showed substantial reductions in daytime sleep late during the siesta (Figure 8). Thus, we expected that exposing flies to evening red light using a 9:3:12 white/red/dark photoperiod (WRD) (Figure 10A) would elicit strong sleep reductions during the evening red-light exposure. Our results matched this expectation, as both female and male CS flies exhibited potent sleep reductions during the WRD portion of the experiment, especially at the onset of red light at ZT9 (Figures 10B–D). CS females and males also had increased sleep in the morning, an effect that increased over successive WRD days (Figure 10C). While w1118 flies followed qualitatively similar patterns to those observed in CS flies, they were not as completely aroused by the onset of the evening red-light period (Figures 10B–D).
In terms of the effects of evening red-light exposure on nighttime sleep patterns, both w1118 and CS females and males had increased sleep soon after the onset of darkness (Figure 10C). Although w1118 showed a weaker sleep reduction during the red-light exposure, this genotype exhibited a robust nighttime sleep increase immediately after red-light offset (Figure 10C). Indeed, all groups showed significant increases in sleep in the ZT12–15 period during WRD days (Figure 10D). Despite similar effects of evening red light on sleep early in the night, the two genotypes diverged during the latter portion of the night. As the experiment progressed, CS females began to have reduced nighttime sleep (ZT18–24), while w1118 females did not deviate from their baseline late-night sleep pattern (Figures 10B, C). In the experiment involving 12 h of red light exposure, CS males had appeared to phase-advance their morning anticipation activity bout (Figure 8B), and a similar effect was noted with the 3-h evening exposure (Figure 10B). w1118 males, on the other hand, exhibited little change to their sleep late at night after the evening red-light exposure (Figure 10C).
It is notable, especially in CS females, that evening red-light exposure tended to increase sleep at both the beginning of the day and beginning of the night, while decreasing sleep toward the end of the day and the end of the night (Figure 10C). In contrast, morning red-light exposure had the opposite effect (Figure 9C). These changes seem to stem from a phase advance due to evening red-light exposure and a phase-delay due to morning red-light exposure. Additionally, the responses of w1118 flies to red light were generally more similar to CS flies’ than their responses to other colors, although there were still some differences between the two genotypes.
Blue light appears to act as an alerting stimulus in humans (Chang et al., 2015), and the fruit fly clock is particularly sensitive to blue light, with cryptochrome (CRY) as its primary photoreceptor (Emery et al., 2000). Therefore, we expected that transitioning from white/dark to blue/dark lighting patterns would have an acute wake-promoting effect during exposure. Our results support this hypothesis, as blue light disrupts sleep and has an acute arousal effect in wild-type flies of both sexes (Figures 2–4). This acute arousal could act as an adaptive response, signaling the animal to initiate locomotion and avoid the high-frequency irradiation. This wavelength-specific behavioral plasticity aligns with findings from Lazopulo et al. (2019), who demonstrated that flies have a daytime-specific aversion to blue light. Exposure to blue light generally has negative effects on the health and lifespan of flies, including neurodegeneration, likely through oxidative stress (Arthaut et al., 2017; Hall et al., 2018; Krittika and Yadav, 2022; Nash et al., 2019). Thus, adaptive behavioral responses to avoid the adverse effects of short-wavelength light could provide a fitness advantage.
Daytime behavioral aversion to blue light in flies is mediated by Rhodopsin 7 (Rh7) and CRY, the two internal blue-light photoreceptors in the Drosophila brain—both of which can entrain the circadian clock and are mediate some behavioral responses to UV light, another form of short-wavelength light (Baik et al., 2019). Blue light-induced CRY activation in the l-LNvs, master clock cells in the fly brain, stimulates release of the wake-promoting neuropeptide pigment-dispersing factor (PDF), which is essential for light-based arousal (Parisky et al., 2008; Shang et al., 2008). CRY and Rh7 are attractive molecules as mediators for the sleep response to blue light. However, blue-light input pathways in the compound eye also exist, as Rh4, Rh5, and Rh1 also respond to blue wavelengths (Sharkey et al., 2020). Future studies should aim to determine which specific photoreceptors are involved in blue light’s regulation of sleep.
The acute arousing effect of blue light we observed differs from findings by Krittika and Yadav (2022), who noted that flies exposed long-term to a 12:12 BD cycle slept more during the day than those in a traditional WD condition. Our 12:12 BD condition led to acute reductions in sleep (relative to baseline) among CS flies. These conflicting results may stem from distinctions in experimental design—our study used flies as their own controls by assessing baseline sleep in WD before switching to BD (“within-subjects design”), as opposed to comparing between two sets of test groups. On a related note, Krittika and Yadav (2022) experimental design included a more chronic BD photoperiod (10 d) that began immediately post-eclosion, and data from all 10 d were averaged together, so the elevated daytime sleep they observed in BD could have been a progressive compensatory response against chronic light-induced cellular stress, as sleep has an intimate relationship with the restoration of oxidative stress (Haynes et al., 2024; Hill et al., 2018; Vaccaro et al., 2020). Unlike prior studies (Krittika and Yadav, 2022; Shen et al., 2021), we did not note any differences in mortality across light-color experiments, with most rates < 10%. This lack of effect may stem from our shorter experimental timeline of only 6 d of light-color treatment, which was much shorter than the exposure period in the other studies mentioned.
In addition to a daytime sleep reduction during the exposure, we also hypothesized that blue light would impact nighttime sleep (after “lights off”). Our results also support this hypothesis, as we noted that 12 h of blue light profoundly reduces nighttime sleep on the second and third days of exposure in CS flies (Figure 2). Interestingly, this decrease in sleep was only observed with 12 h of treatment, as neither 3-h morning nor evening exposures was sufficient to elicit the same effect (Figures 3, 4). Surprisingly, evening exposure to blue light increased nighttime sleep in CS flies (Figure 4), suggesting that a 3-h evening exposure to blue light is insufficient to model the sleep effects of VDU exposure on humans, and instead, 12 h of blue light is needed to elicit robust nighttime sleep reductions in wild-type flies.
We have consistently found that w1118 flies show no daytime sleep inhibition from blue light (Figures 2–4). Our w1118 flies possess the w1118 allele, a null mutation in white, the first gene identified in Drosophila (Morgan, 1910). White encodes an ATP binding cassette (ABC) transporter that dimerizes with either Scarlet or Brown to traffic metabolic precursors throughout the animal, with highest expression in the compound eye (Ferreiro et al., 2017). The White-Scarlet dimer transports tryptophan to the eye and through the brain, while White-Brown carries guanine (Ferreiro et al., 2017). In the compound eye, guanine and tryptophan serve as precursors to drosopterin and ommochrome—the two pigments which give wild-type flies their brick-red appearance (Tomlinson, 2012). The primary function of these screening pigments is to improve visual acuity by maintaining photons within each ommatidium, so they do not activate photoreceptors in other nearby cells (Tomlinson, 2012). They may also protect photoreceptors from damage associated with excessive light exposure, as white mutants exhibit signs of retinal degeneration at just 5 days old (Ferreiro et al., 2017). White-transported guanine and tryptophan are essential precursors in the synthesis of biogenic amines, such as serotonin, dopamine, octopamine, and histamine; consequently, white mutants exhibit especially low levels of histamine in the brain (Borycz et al., 2008). Intriguingly, histamine is a primary neurotransmitter used by the Drosophila visual system, which could underlie w1118 flies’ lack of an acute reaction to blue light. In other words, w1118 flies may sense the blue light in the compound eye but then be unable to transmit this information to the central brain due to histamine deficiency. Ultimately, the lack of a daytime sleep-inhibiting effect of blue light in w1118 flies suggests that the compound eye and downstream pathways may play a role in mediating the sleep effects of blue light. This would fit with a recent report demonstrating that neither just the compound eye nor CRY is sufficient for blue light-induced l-LNv activity (Au et al., 2023). Both pathways (and Rh7) are also important for acute arousal from sleep with a blue light pulse, with an especially outsized role for Rh7 at higher light intensities (Au et al., 2023). However, it has also been shown that other neurotransmitter imbalances caused by mutations to the white gene, can be behaviorally relevant (Alekseyenko and Kravitz, 2014; Kain et al., 2012; Sitaraman et al., 2008). Thus, those changes could affect sleep independently of effects on function of the compound eyes.
As with daytime effects of blue light, w1118 flies did not exhibit the same nighttime sleep reduction that was observed in CS flies (Figure 2), possibly implicating the compound eye in this nighttime sleep suppression as well. As the sleep loss in CS flies was spread across the night (Figures 2B, C), it appeared that it was not a defect in nighttime sleep onset, but rather in sleep maintenance. Interestingly, visual experience regulates sleep need in flies, as complex visual stimuli increase nighttime sleep consolidation (Kirszenblat et al., 2019), but any role for light color in regulating sleep need has yet to be elucidated. Nighttime sleep maintenance in Drosophila is regulated through a myriad of signals, including NPF release and GABAergic inhibition of the PDFergic l-LNvs (Gmeiner et al., 2013; He et al., 2013). l-LNvs are activated by CRY phototransduction but also by compound eye inputs (Au et al., 2023; Schlichting et al., 2016), which we expect to be involved in this given absent effect in w1118. Like with daytime sleep, the lack of effect in w1118 flies could result from disrupted phototransduction due to the absence of screening pigment or neurotransmitter monoamines downstream from photoreception.
To validate the compound eye’s role in mediating these blue light’s effects on both daytime and nighttime sleep, future studies could examine how a 12-h blue light exposure influences sleep in glass or norpA mutants. Glass encodes a transcription factor critical for cell-fate decisions underlying photoreceptor identity in the developing retina and HB eyelet, rendering glass mutants completely reliant on internal sensors like CRY to entrain to environmental light (Bernardo-Garcia et al., 2016; Helfrich-Forster et al., 2001). On the other hand, norpA encodes a phospholipase-Cβ involved in rhodopsin signal transduction in compound eye photoreceptors, leading norpA mutants to also have dysfunctional vision and altered photoentrainment (Bloomquist et al., 1988; Breda et al., 2020).
Perhaps an even stronger effect than what was observed with blue light was the daytime sleep suppression induced by red light (Figures 8–11). With 12 h of red light, we observed a consistent daytime sleep reduction not only across sexes, but also in both CS and w1118 flies (Figure 8). Moreover, CS and w1118 flies returned to WD sleep levels during the recovery period, ruling out the possibility that sleep changes earlier in the experiment were merely developmental (Figures 8, 11). Lower daytime sleep in the presence of red light aligns with findings from Krittika and Yadav (2022), but our effects reached a statistically significant level. Along with reduced evening (ZT9–12) sleep, an overall phase advance in sleep patterns was noted due to both 12 h and evening red-light exposures (Figures 8, 10). Despite this phase advance, CS flies did maintain rhythmicity, corroborating prior evidence that wild-type Drosophila can entrain to red light (Hanai et al., 2008; Zordan et al., 2001). Ethologically, the evening wake-promoting effect of red light may represent a behavioral adaptation of Drosophila to natural daily changes in sunlight’s spectral composition. While present across the whole day, long wavelengths like red light are especially enriched at sunset (Hut et al., 2000; Moon et al., 2016). Thus, the crepuscular Drosophila may have evolved to coordinate its evening activity with red-enriched light at sunset, such that greater ambient red-light composition acts as a time-of-day cue to the animal to awaken from its midday rest and pursue food, mates, etc.
Figure 11. Diagram summarizing the effects of light color on sleep in CS and w1118 flies. Effects of exposure to blue, green, and red light on red-eyed CS flies are shown at the top, whereas effects on white-eyed w1118 flies are shown at the bottom. Schematic generated using BioRender.com.
We also noted that the evening 3-h exposure to red light potently inhibited sleep during the exposure in both CS and w1118 flies (Figure 10). The arousal response to a low-intensity pulse of red light has been shown to be dependent on the compound eye (Au et al., 2023). In our study, a similar acute sleep suppression was noted in w1118 flies with an evening exposure to green light (Figure 7). Indeed, w1118 males also showed reduced daytime sleep in the presence of 12 h of green light, with peak sleep loss occurring in the evening (Figure 5). Taken together, these findings suggest that the same downstream sleep effects elicited by evening exposure to red light in CS flies may be elicited by green light in w1118. Sharkey et al. (2020) demonstrated that the presence of screening pigment shifts the spectral sensitivity of particular rhodopsins in the compound eye, which may account for the similar effects of green light on w1118 and red light on CS. Specifically, Rh6 shows peak sensitivity to 600-nm (red) light in red-eyed flies but to 510 nm (green) in white-eyed flies. Importantly, Rh6 along with Rh1 mediate circadian entrainment to red light (Hanai et al., 2008). Upcoming studies will directly test our hypothesis that Rh6 is essential for the acute sleep effects of evening red or green light in CS and w1118 animals, respectively. The evening-specific nature of this effect could imply the existence of a sleep-regulating pathway in which Rh6-dependent phototransduction (via either the compound eye or the Rh6+ HB eyelet) sits upstream of E cells, the clock neuron subtype in the fly brain responsible for controlling the circadian activity rhythms during the evening (Brown et al., 2024).
It is worth noting that experiments presented in Figures 2–10 represent one replicate of each experiment; however, our main results closely resemble several additional renditions of the same experiments from our lab, using either the same light delivery system or completely distinct lighting units—underscoring the reliability and reproducibility of our light color-specific findings. For example, nighttime sleep reductions after 12 h blue light reliably occur on days 2–3 of exposure regardless of light delivery system. Moreover, slight differences in light intensity between the white light and light color conditions could account for some effects, but unpublished results from our lab show that altering light intensity alone has negligible impact on sleep. Another potential limitation to our study design is the difficulty to ascertain if effects across days are due to the light color exposure or simply aging-associated changes. Considering that sleep behavior largely stabilizes at 3 d post-eclosion (Shaw et al., 2000), and all flies tested were ≥ 3 d old, the latter appears unlikely. Further, the transience and specificity of our sleep effects also disputes this interpretation. For example, if blue light-associated nighttime sleep reductions observed in Figure 2 were strictly due to age, one would expect the effect to persist and progress as the experiment continued. Rather, this effect largely disappears after ∼2 d. Lastly, the nature of our experimental design makes it difficult to assess if light color-specific sleep phenomena are directly caused by the light color being emitted or rather by the absence of others. For example, are daytime sleep reductions in RD conditions due to the red light itself or the absence of blue-green input? Studies that just remove one of these light color inputs (e.g., only green-red input without blue) could help answer this question. Nevertheless, our results unequivocally indicate that light color is an important environmental modulator of fruit fly sleep behavior.
Taken together, our results illustrate that fruit fly sleep, much like human sleep, is subject to light color-specific regulation (Figure 11), and demonstrate time-of-day dependency for the effects of specific light colors on sleep. This work further establishes D. melanogaster as an effective model to study how environmental cues are received by the brain and integrated to facilitate complex behavior. Moreover, our within-subject experimental design provides the first direct evidence of light color-dependent sleep/wake behavioral plasticity in flies. Researchers commonly use red or blue light to optogenetically activate specific neuronal populations in Drosophila. However, our findings underscore that experimentation combining optogenetics with behavioral analysis must be interpreted knowing that the optic stimulus may elicit behavioral changes, independent of the transgenic light-gated ion channel. Additionally, white-eyed w1118 flies are often employed as a “wild-type” strain in sleep studies, but our results indicate a substantially different behavioral response to blue light as compared with red-eyed CS flies. This finding should caution the use of white mutants as wild-type animals when investigating the interplay between environmental cues and sleep behavior. These findings in w1118 flies additionally provide mechanistic insight into how light color affects sleep, pointing to transmission of light color information from the compound eye to central brain sleep circuits. Future experiments should seek to elucidate the logic behind how the fly brain encodes light color information in these sleep circuits and how that code is translated into behavior. Ultimately, further work employing Drosophila to study mechanisms surrounding light color’s sleep effects will undoubtedly provide novel insights into how the environment modulates neural circuits to effect behavioral change and may lead to improved technologies to combat the negative impacts of blue light-enriched screen use.
The raw data supporting the conclusions of this article will be made available by the authors, without undue reservation.
The manuscript presents research on animals that do not require ethical approval for their study.
SB: Writing – review and editing, Writing – original draft, Software, Methodology, Investigation, Formal analysis, Conceptualization. AP: Writing – original draft, Investigation, Formal analysis. DS: Writing – review and editing, Methodology, Investigation, Formal analysis, Conceptualization. DAS: Writing – review and editing, Methodology, Investigation, Formal analysis, Conceptualization. HH: Writing – review and editing, Software, Methodology. DP: Writing – review and editing, Investigation, Formal analysis. CV: Writing – review and editing, Writing – original draft, Supervision, Software, Resources, Methodology, Funding acquisition, Conceptualization.
The authors declare that financial support was received for the research, authorship, and/or publication of this article. Funding was provided by the National Institutes of Health (NINDS) in the form of AREA Grants 1R15NS101692-01A1 and 2R15NS101692-02A1 (to CV), the Skidmore College Summer Faculty/Student Collaborative Research program (to SB and DAS), and to the Skidmore College S3M program (to AP).
We thank John Tagariello, Maya Wyse, and Matthew Grega for their help with maintaining fly stocks, making fly food, and carrying out many other necessary tasks around the lab. Figures 1, 11 were generated with the assistance of BioRender.com.
The authors declare that the research was conducted in the absence of any commercial or financial relationships that could be construed as a potential conflict of interest.
All claims expressed in this article are solely those of the authors and do not necessarily represent those of their affiliated organizations, or those of the publisher, the editors and the reviewers. Any product that may be evaluated in this article, or claim that may be made by its manufacturer, is not guaranteed or endorsed by the publisher.
The Supplementary Material for this article can be found online at: https://www.frontiersin.org/articles/10.3389/fnbeh.2024.1476501/full#supplementary-material
Adams, M. D., Celniker, S. E., Holt, R. A., Evans, C. A., Gocayne, J. D., Amanatides, P. G., et al. (2000). The genome sequence of Drosophila melanogaster [Research Support, Non-U.S. Gov’t Research Support, U.S. Gov’t, P.H.S.]. Science 287, 2185–2195.
Alekseyenko, O. V., and Kravitz, E. A. (2014). Serotonin and the search for the anatomical substrate of aggression. Fly (Austin) 8, 200–205. doi: 10.1080/19336934.2015.1045171
Altman, N. G., Izci-Balserak, B., Schopfer, E., Jackson, N., Rattanaumpawan, P., Gehrman, P. R., et al. (2012). Sleep duration versus sleep insufficiency as predictors of cardiometabolic health outcomes. Sleep Med. 13, 1261–1270. doi: 10.1016/j.sleep.2012.08.005
Arthaut, L. D., Jourdan, N., Mteyrek, A., Procopio, M., El-Esawi, M., d’Harlingue, A., et al. (2017). Blue-light induced accumulation of reactive oxygen species is a consequence of the Drosophila cryptochrome photocycle. PLoS One 12:e0171836. doi: 10.1371/journal.pone.0171836
Au, D. D., Liu, J. C., Park, S. J., Nguyen, T. H., Dimalanta, M., Foden, A. J., et al. (2023). Drosophila photoreceptor systems converge in arousal neurons and confer light responsive robustness. Front. Neurosci. 17:1160353. doi: 10.3389/fnins.2023.1160353
Baik, L. S., Recinos, Y., Chevez, J. A., Au, D. D., and Holmes, T. C. (2019). Multiple phototransduction inputs integrate to mediate UV light-evoked avoidance/attraction behavior in Drosophila. J. Biol. Rhythms 34, 391–400. doi: 10.1177/0748730419847339
Bernardo-Garcia, F. J., Fritsch, C., and Sprecher, S. G. (2016). The transcription factor Glass links eye field specification with photoreceptor differentiation in Drosophila. Development 143, 1413–1423. doi: 10.1242/dev.128801
Bloomquist, B. T., Shortridge, R. D., Schneuwly, S., Perdew, M., Montell, C., Steller, H., et al. (1988). Isolation of a putative phospholipase C gene of Drosophila, norpA, and its role in phototransduction. Cell 54, 723–733. doi: 10.1016/s0092-8674(88)80017-5
Borycz, J., Borycz, J. A., Kubów, A., Lloyd, V., and Meinertzhagen, I. A. (2008). Drosophila ABC transporter mutants white, brown and scarlet have altered contents and distribution of biogenic amines in the brain. J. Exp. Biol. 211, 3454–3466. doi: 10.1242/jeb.021162
Breda, C., Rosato, E., and Kyriacou, C. P. (2020). Norpa signalling and the seasonal circadian locomotor phenotype in Drosophila. Biology (Basel) 9:130. doi: 10.3390/biology9060130
Breen, D. P., Vuono, R., Nawarathna, U., Fisher, K., Shneerson, J. M., Reddy, A. B., et al. (2014). Sleep and circadian rhythm regulation in early Parkinson disease. JAMA Neurol. 71, 589–595. doi: 10.1001/jamaneurol.2014.65
Brown, M. P., Verma, S., Palmer, I., Guerrero Zuniga, A., Mehta, A., Rosensweig, C., et al. (2024). A subclass of evening cells promotes the switch from arousal to sleep at dusk. Curr. Biol. 34:2186–2199.e2183. doi: 10.1016/j.cub.2024.04.039
Chang, A. M., Aeschbach, D., Duffy, J. F., and Czeisler, C. A. (2015). Evening use of light-emitting eReaders negatively affects sleep, circadian timing, and next-morning alertness. Proc. Natl. Acad. Sci. U.S.A. 112, 1232–1237. doi: 10.1073/pnas.1418490112
Chellappa, S. L., Steiner, R., Oelhafen, P., Lang, D., Götz, T., Krebs, J., et al. (2013). Acute exposure to evening blue-enriched light impacts on human sleep. J. Sleep Res. 22, 573–580. doi: 10.1111/jsr.12050
Chen, X., Hall, H., Simpson, J. P., Leon-Salas, W. D., Ready, D. F., and Weake, V. M. (2017). Cytochrome b5 protects photoreceptors from light stress-induced lipid peroxidation and retinal degeneration. NPJ Aging Mech. Dis. 3:18. doi: 10.1038/s41514-017-0019-6
Dissel, S. (2020). Drosophila as a model to study the relationship between sleep, plasticity, and memory. Front. Physiol. 11:533. doi: 10.3389/fphys.2020.00533
Donelson, N., Kim, E. Z., Slawson, J. B., Vecsey, C. G., Huber, R., and Griffith, L. C. (2012). High-resolution positional tracking for long-term analysis of Drosophila sleep and locomotion using the “tracker” program [Research Support, N.I.H., Extramural]. PLoS One 7:e37250. doi: 10.1371/journal.pone.0037250
Dove, A. E., Cook, B. L., Irgebay, Z., and Vecsey, C. G. (2017). Mechanisms of sleep plasticity due to sexual experience in Drosophila melanogaster. Physiol. Behav. 180, 146–158. doi: 10.1016/j.physbeh.2017.08.020
Emery, P., Stanewsky, R., Hall, J. C., and Rosbash, M. (2000). A unique circadian-rhythm photoreceptor. Nature 404, 456–457. doi: 10.1038/35006558
Engeda, J., Mezuk, B., Ratliff, S., and Ning, Y. (2013). Association between duration and quality of sleep and the risk of pre-diabetes: Evidence from NHANES. Diabet. Med. 30, 676–680. doi: 10.1111/dme.12165
Ferreiro, M. J., Pérez, C., Marchesano, M., Ruiz, S., Caputi, A., Aguilera, P., et al. (2017). Drosophila melanogaster white mutant w1118 undergo retinal degeneration. Front. Neurosci. 11:732. doi: 10.3389/fnins.2017.00732
Gmeiner, F., Kolodziejczyk, A., Yoshii, T., Rieger, D., Nassel, D. R., and Helfrich-Forster, C. (2013). GABA(B) receptors play an essential role in maintaining sleep during the second half of the night in Drosophila melanogaster. J. Exp. Biol. 216, 3837–3843. doi: 10.1242/jeb.085563
Hale, L., and Guan, S. (2015). Screen time and sleep among school-aged children and adolescents: A systematic literature review. Sleep Med. Rev. 21, 50–58. doi: 10.1016/j.smrv.2014.07.007
Hall, H., Ma, J., Shekhar, S., Leon-Salas, W. D., and Weake, V. M. (2018). Blue light induces a neuroprotective gene expression program in Drosophila photoreceptors. BMC Neurosci. 19:43. doi: 10.1186/s12868-018-0443-y
Hanai, S., Hamasaka, Y., and Ishida, N. (2008). Circadian entrainment to red light in Drosophila: Requirement of Rhodopsin 1 and Rhodopsin 6. Neuroreport 19, 1441–1444. doi: 10.1097/WNR.0b013e32830e4961
Haynes, P. R., Pyfrom, E. S., Li, Y., Stein, C., Cuddapah, V. A., Jacobs, J. A., et al. (2024). A neuron-glia lipid metabolic cycle couples daily sleep to mitochondrial homeostasis. Nat. Neurosci. 27, 666–678. doi: 10.1038/s41593-023-01568-1
He, C., Yang, Y., Zhang, M., Price, J. L., and Zhao, Z. (2013). Regulation of sleep by neuropeptide Y-like system in Drosophila melanogaster. PLoS One 8:e74237. doi: 10.1371/journal.pone.0074237
Helfrich-Forster, C., Winter, C., Hofbauer, A., Hall, J. C., and Stanewsky, R. (2001). The circadian clock of fruit flies is blind after elimination of all known photoreceptors [Research Support, Non-U.S. Gov’t Research Support, U.S. Gov’t, P.H.S.]. Neuron 30, 249–261.
Hendricks, J. C., Finn, S. M., Panckeri, K. A., Chavkin, J., Williams, J. A., Sehgal, A., et al. (2000). Rest in Drosophila is a sleep-like state. Neuron 25, 129–138.
Hill, V. M., O’Connor, R. M., Sissoko, G. B., Irobunda, I. S., Leong, S., Canman, J. C., et al. (2018). A bidirectional relationship between sleep and oxidative stress in Drosophila. PLoS Biol. 16:e2005206. doi: 10.1371/journal.pbio.2005206
Huber, R., Hill, S. L., Holladay, C., Biesiadecki, M., Tononi, G., and Cirelli, C. (2004). Sleep homeostasis in Drosophila melanogaster. Sleep 27, 628–639.
Hut, R. A., Scheper, A., and Daan, S. (2000). Can the circadian system of a diurnal and a nocturnal rodent entrain to ultraviolet light? J. Comp. Physiol. A 186, 707–715. doi: 10.1007/s003590000124
Isaac, R. E., Li, C., Leedale, A. E., and Shirras, A. D. (2010). Drosophila male sex peptide inhibits siesta sleep and promotes locomotor activity in the post-mated female. Proc. Biol. Sci. 277, 65–70. doi: 10.1098/rspb.2009.1236
Jagannath, A., Peirson, S. N., and Foster, R. G. (2013). Sleep and circadian rhythm disruption in neuropsychiatric illness. Curr. Opin. Neurobiol. 23, 888–894. doi: 10.1016/j.conb.2013.03.008
Jin, M., Li, X., Yan, F., Chen, W., Jiang, L., and Zhang, X. (2021). The effects of low-color-temperature dual-primary-color light-emitting diodes on three kinds of retinal cells. J. Photochem. Photobiol. B 214, 112099. doi: 10.1016/j.jphotobiol.2020.112099
Ju, Y. E., Lucey, B. P., and Holtzman, D. M. (2014). Sleep and Alzheimer disease pathology–a bidirectional relationship. Nat. Rev. Neurol. 10, 115–119. doi: 10.1038/nrneurol.2013.269
Juneau, Z. C., Stonemetz, J. M., Toma, R. F., Possidente, D. R., Heins, R. C., and Vecsey, C. G. (2019). Optogenetic activation of short neuropeptide F (sNPF) neurons induces sleep in Drosophila melanogaster. Physiol. Behav. 206, 143–156. doi: 10.1016/j.physbeh.2019.03.027
Kain, J. S., Stokes, C., and de Bivort, B. L. (2012). Phototactic personality in fruit flies and its suppression by serotonin and white. Proc. Natl. Acad. Sci. U.S.A. 109, 19834–19839. doi: 10.1073/pnas.1211988109
Kanaya, H. J., Park, S., Kim, J. H., Kusumi, J., Krenenou, S., Sawatari, E., et al. (2020). A sleep-like state in Hydra unravels conserved sleep mechanisms during the evolutionary development of the central nervous system. Sci. Adv. 6:abb9415. doi: 10.1126/sciadv.abb9415
Kennedy, K. E. R., Wills, C. C. A., Holt, C., and Grandner, M. A. (2023). A randomized, sham-controlled trial of a novel near-infrared phototherapy device on sleep and daytime function. J. Clin. Sleep Med. 19:1669–1675. doi: 10.5664/jcsm.10648
Kirszenblat, L., Yaun, R., and van Swinderen, B. (2019). Visual experience drives sleep need in Drosophila. Sleep 42:zsz102. doi: 10.1093/sleep/zsz102
Krittika, S., and Yadav, P. (2022). Alterations in lifespan and sleep: Wake duration under selective monochromes of visible light in Drosophila melanogaster. Biol. Open 11:73. doi: 10.1242/bio.059273
Lazopulo, S., Lazopulo, A., Baker, J. D., and Syed, S. (2019). Daytime colour preference in Drosophila depends on the circadian clock and TRP channels. Nature 574, 108–111. doi: 10.1038/s41586-019-1571-y
Medic, G., Wille, M., and Hemels, M. E. (2017). Short- and long-term health consequences of sleep disruption. Nat. Sci. Sleep 9, 151–161. doi: 10.2147/NSS.S134864
Moon, S., Kwon, S., and Lim, J. (2016). Implementation of smartphone-based color temperature and wavelength control LED lighting system. Cluster Comput. 19, 949–966.
Morgan, T. H. (1910). Sex limited inheritance in Drosophila. Science 32, 120–122. doi: 10.1126/science.32.812.120
Myers, E. W., Sutton, G. G., Delcher, A. L., Dew, I. M., Fasulo, D. P., Flanigan, M. J., et al. (2000). A whole-genome assembly of Drosophila. Science 287, 2196–2204.
Nagare, R., Plitnick, B., and Figueiro, M. G. (2019). Does the iPad Night Shift mode reduce melatonin suppression? Light Res. Technol. 51, 373–383. doi: 10.1177/1477153517748189
Nash, T. R., Chow, E. S., Law, A. D., Fu, S. D., Fuszara, E., Bilska, A., et al. (2019). Daily blue-light exposure shortens lifespan and causes brain neurodegeneration in. NPJ Aging Mech. Dis. 5:8. doi: 10.1038/s41514-019-0038-6
Nath, R. D., Bedbrook, C. N., Abrams, M. J., Basinger, T., Bois, J. S., Prober, D. A., et al. (2017). The jellyfish cassiopea exhibits a sleep-like state. Curr. Biol. 27:2984–2990.e2983. doi: 10.1016/j.cub.2017.08.014
Nitz, D. A., van Swinderen, B., Tononi, G., and Greenspan, R. J. (2002). Electrophysiological correlates of rest and activity in Drosophila melanogaster. Curr. Biol. 12, 1934–1940.
Parisky, K. M., Agosto, J., Pulver, S. R., Shang, Y., Kuklin, E., Hodge, J. J., et al. (2008). PDF cells are a GABA-responsive wake-promoting component of the Drosophila sleep circuit [Research Support, N.I.H., Extramural Research Support, U.S. Gov’t, Non-P.H.S.]. Neuron 60, 672–682. doi: 10.1016/j.neuron.2008.10.042
Schlichting, M., Menegazzi, P., Lelito, K. R., Yao, Z., Buhl, E., Dalla Benetta, E., et al. (2016). A neural network underlying circadian entrainment and photoperiodic adjustment of sleep and activity in Drosophila. J. Neurosci. 36, 9084–9096. doi: 10.1523/JNEUROSCI.0992-16.2016
Shang, Y., Griffith, L. C., and Rosbash, M. (2008). Light-arousal and circadian photoreception circuits intersect at the large PDF cells of the Drosophila brain. Proc. Natl. Acad. Sci. U.S.A. 105, 19587–19594.
Sharkey, C. R., Blanco, J., Leibowitz, M. M., Pinto-Benito, D., and Wardill, T. J. (2020). The spectral sensitivity of Drosophila photoreceptors. Sci. Rep. 10:18242. doi: 10.1038/s41598-020-74742-1
Shaw, P. J., Cirelli, C., Greenspan, R. J., and Tononi, G. (2000). Correlates of sleep and waking in Drosophila melanogaster. Science 287, 1834–1837.
Shaw, P. J., Tononi, G., Greenspan, R. J., and Robinson, D. F. (2002). Stress response genes protect against lethal effects of sleep deprivation in Drosophila. Nature 417, 287–291. doi: 10.1038/417287a
Shen, J., Yang, P., Luo, X., Li, H., Xu, Y., Shan, J., et al. (2021). Green light extends Drosophila longevity. Exp. Gerontol. 147:111268. doi: 10.1016/j.exger.2021.111268
Shibuya, K., Onodera, S., and Hori, M. (2018). Toxic wavelength of blue light changes as insects grow. PLoS One 13:e0199266. doi: 10.1371/journal.pone.0199266
Sitaraman, D., Zars, M., Laferriere, H., Chen, Y. C., Sable-Smith, A., Kitamoto, T., et al. (2008). Serotonin is necessary for place memory in Drosophila. Proc. Nat.l Acad. Sci. U.S.A. 105, 5579–5584. doi: 10.1073/pnas.0710168105
Tainton-Heap, L. A. L., Kirszenblat, L. C., Notaras, E. T., Grabowska, M. J., Jeans, R., Feng, K., et al. (2020). A Paradoxical Kind of Sleep in Drosophila melanogaster. Curr. Biol. 31:578–590.e6. doi: 10.1016/j.cub.2020.10.081
Tomlinson, A. (2012). The origin of the Drosophila subretinal pigment layer. J. Comp. Neurol. 520, 2676–2682. doi: 10.1002/cne.23063
Vaccaro, A., Kaplan Dor, Y., Nambara, K., Pollina, E. A., Lin, C., Greenberg, M. E., et al. (2020). Sleep loss can cause death through accumulation of reactive oxygen species in the gut. Cell 181:1307–1328.e1315. doi: 10.1016/j.cell.2020.04.049
van Alphen, B., Semenza, E. R., Yap, M., van Swinderen, B., and Allada, R. (2021). A deep sleep stage in Drosophila with a functional role in waste clearance. Sci. Adv. 7:abc2999. doi: 10.1126/sciadv.abc2999
van Alphen, B., Yap, M. H., Kirszenblat, L., Kottler, B., and van Swinderen, B. (2013). A dynamic deep sleep stage in Drosophila. J. Neurosci. 33, 6917–6927. doi: 10.1523/jneurosci.0061-13.2013
Vecsey, C. G., Koochagian, C., Porter, M. T., Roman, G., and Sitaraman, D. (2024). Analysis of sleep and circadian rhythms from Drosophila activity-monitoring data using SCAMP. Cold Spring Harb. Protoc. doi: 10.1101/pdb.prot108182 [Epub ahead of print].
Wisor, J. P., Edgar, D. M., Yesavage, J., Ryan, H. S., McCormick, C. M., Lapustea, N., et al. (2005). Sleep and circadian abnormalities in a transgenic mouse model of Alzheimer’s disease: A role for cholinergic transmission. Neuroscience 131, 375–385. doi: 10.1016/j.neuroscience.2004.11.018
Zhao, J., Tian, Y., Nie, J., Xu, J., and Liu, D. (2012). Red light and the sleep quality and endurance performance of Chinese female basketball players. J. Athl. Train 47, 673–678. doi: 10.4085/1062-6050-47.6.08
Keywords: sleep, Drosophila melanogaster, light color, red, blue, green
Citation: Bond SM, Peralta AJ, Sirtalan D, Skeele DA, Huang H, Possidente DR and Vecsey CG (2024) Differential regulation of sleep by blue, green, and red light in Drosophila melanogaster. Front. Behav. Neurosci. 18:1476501. doi: 10.3389/fnbeh.2024.1476501
Received: 05 August 2024; Accepted: 18 October 2024;
Published: 30 October 2024.
Edited by:
Shogo Sato, Texas A and M University, United StatesReviewed by:
Sheyum Syed, University of Miami, United StatesCopyright © 2024 Bond, Peralta, Sirtalan, Skeele, Huang, Possidente and Vecsey. This is an open-access article distributed under the terms of the Creative Commons Attribution License (CC BY). The use, distribution or reproduction in other forums is permitted, provided the original author(s) and the copyright owner(s) are credited and that the original publication in this journal is cited, in accordance with accepted academic practice. No use, distribution or reproduction is permitted which does not comply with these terms.
*Correspondence: Christopher G. Vecsey, Y3ZlY3NleUBza2lkbW9yZS5lZHU=
Disclaimer: All claims expressed in this article are solely those of the authors and do not necessarily represent those of their affiliated organizations, or those of the publisher, the editors and the reviewers. Any product that may be evaluated in this article or claim that may be made by its manufacturer is not guaranteed or endorsed by the publisher.
Research integrity at Frontiers
Learn more about the work of our research integrity team to safeguard the quality of each article we publish.